- Infectious Diseases Service, Department of Medicine, Lausanne University Hospital and University of Lausanne, Epalinges, Switzerland
Sepsis is a clinical syndrome defined as a dysregulated host response to infection resulting in life-threatening organ dysfunction. Sepsis is a major public health concern associated with one in five deaths worldwide. Sepsis is characterized by unbalanced inflammation and profound and sustained immunosuppression, increasing patient susceptibility to secondary infections and mortality. microRNAs (miRNAs) play a central role in the control of many biological processes, and deregulation of their expression has been linked to the development of oncological, cardiovascular, neurodegenerative and metabolic diseases. In this review, we discuss the role of miRNAs in sepsis pathophysiology. Overall, miRNAs are seen as promising biomarkers, and it has been proposed to develop miRNA-based therapies for sepsis. Yet, the picture is not so straightforward because of the versatile and dynamic features of miRNAs. Clearly, more research is needed to clarify the expression and role of miRNAs in sepsis, and to promote the use of miRNAs for sepsis management.
1 Introduction
1.1 Innate immune sensing
Innate immune cells sense signals of microbial origin (microbial-associated molecular patterns or MAMPs, also known as pathogen-associated molecular patterns or PAMPs) or endogenous components released by injured or stressed cells (damage or danger-associated molecular patterns or DAMPs) through pattern-recognition receptors (PRRs). Lipopolysaccharide (LPS), peptidoglycan, flagellin, β-glucan, lipoproteins, glycoproteins, double-stranded and single-stranded RNA, and unmethylated CpG motif containing DNA from bacteria, mycoplasma, mycobacteria, fungi, parasites and viruses are MAMPs/PAMPs. The best described DAMPs are high mobility group box-1 (HMGB1), fibrinogen, fibronectin, nucleic acids, histones, heat shock proteins (HSPs), uric acid, ATP, cytochrome c, S100 molecules and serum amyloid A. The main families of PRRs comprise Toll-like receptors (TLRs), NOD-like receptors (NLRs), c-type lectin receptors, RIG-I-like receptors, cytosolic DNA sensors and scavenger receptors (1–4). The triggering of PRRs by MAMPs/DAMPs activates intracellular signal transduction pathways such as the nuclear factor-κB (NF-κB), interferon (IFN) response factor (IRF), mitogen-activated protein kinase (MAPK) and phosphoinositide 3-kinase/Akt/mammalian target of rapamycin (PI3K/Akt/mTOR) pathways regulating the expression of cytokines, acute phase proteins, and adhesion, co-stimulatory and major histocompatibility complex molecules as well as metabolism. A fine control of these pathways is essential to restore homeostasis following injury.
1.2 Sepsis
Sepsis-3 alliance redefined sepsis as “a life-threatening organ dysfunction caused by a dysregulated host response to infection” (5). Sepsis remains one of the leading causes of mortality worldwide. Recent estimations indicate that sepsis affects around 50 million people and is responsible of at least 11 million deaths annually worldwide (6). These numbers increased during the COVID-19 pandemic. Indeed, most patients dying from COVID-19 present respiratory failure (mostly acute respiratory distress syndrome, ARDS) and multi-organ failure, which are manifestations of sepsis (5). Despite progresses in basic, clinical and translational research, the pathophysiology of sepsis remains not fully understood. Sepsis-specific targeting strategies tested in clinical trials failed to show benefit for patients (7–19).
Sepsis is characterized by an exacerbation of antimicrobial defense mechanisms responsible for collateral tissue injury, organ dysfunctions and early mortality involved in around 10% of all fatal cases (Figure 1). The hyper-inflammatory response is associated with a concurrent shift towards inflammation resolution and tissue repair involved in immuno-paralysis or immunosuppression. The suppressive phase is related to the depletion of dendritic cells (DCs), T cells and B cells through apoptosis, a reduced expression of proinflammatory cytokines, costimulatory and antigen-presenting molecules, and an increased expression of anti-inflammatory cytokines and inhibitory checkpoint molecules. Immunosuppression can persist for months to years (Figure 1). A subset of patients with prolonged stay in intensive care units (ICUs) suffer from persistent inflammation, immunosuppression and catabolism syndrome (PICS) (20). Dysregulated immune responses favor the development of secondary infections, viral reactivation and long-term immune disabilities accounting for late morbidity and mortality (7–13, 20). Delayed mortality associated with viral reactivation and nosocomial infections represent 20-40% and long-term mortality 50-70% of total fatal sepsis cases. Twenty percent of sepsis survivors develop secondary infections within 30 days, and nearly half of sepsis survivors are re-hospitalized within a year.
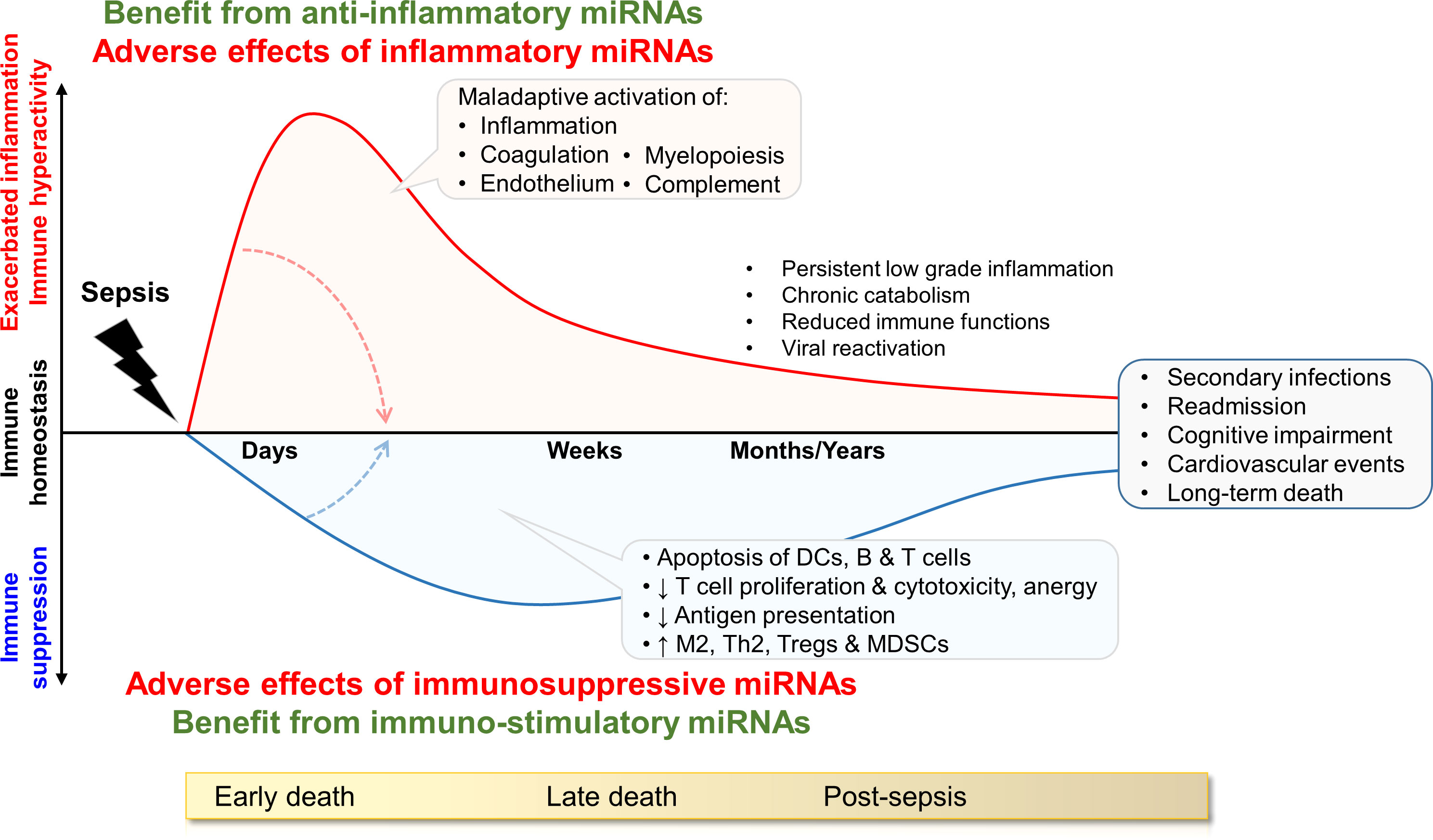
Figure 1 Model of immune status during sepsis and potential impact of miRNAs. The drawing shows the dysregulation of immune homeostasis over time, and lists pathophysiological consequences. The inflammatory and immunosuppressive responses are represented concurrently. Early deaths are mainly attributed to organ failure due to overwhelming inflammation. Late deaths are associated to immunosuppression causing increased susceptibility to (nosocomial) infections, viral reactivation and cardiovascular diseases. The influence of miRNAs may fluctuate over time. During the hyper-inflammatory phase of early sepsis, anti-inflammatory miRNAs can provide benefit to the host by dampening excessive immune reactions. In the immunosuppressive late phase of sepsis, inflammatory/immuno-stimulatory miRNAs can be beneficial by sustaining immune activity and protecting from nosocomial infections and reinfections. DCs: dendritic cells, MDSCs: myeloid derived suppressor cells; Th2: T helper 2, Tregs: regulatory T cells. M2 are pro-resolving/anti-inflammatory M2 macrophages.
The identification of biomarkers and targets is one the most burning areas of research in the sepsis field. A biomarker is “any substance, structure, or process that can be measured in the body or its products and influence or predict the incidence of outcome or disease” (21). The identification of diagnostic, prognostic and theragnostic biomarkers to distinguish sepsis, identify patients who may benefit from host-targeted therapies, predict responsiveness and monitor the effectiveness of treatment holds great promise for improving patient management (10, 12, 22–29). In the last years, microRNAs (miRNAs) have been suggested to be potential biomarkers and targets for sepsis.
In this review we aim to shed light on the role of miRNAs involved in the pathogenesis of severe infections and sepsis. We will start by briefly summarizing the biogenesis, modes of action, circulation and delivery of miRNAs, which are described comprehensively elsewhere (30–35).
2 miRNAs
2.1 Identification
Non-coding RNAs (ncRNAs) comprise a growing list of RNA species, including miRNAs, small interfering RNAs, long non-coding RNAs (lncRNA), Piwi-interacting RNAs, small nuclear RNAs, small nucleolar RNAs, extracellular RNAs and small Cajal body-specific RNAs. ncRNAs regulate numerous biological and pathological processes such as cancer and autoimmune, cardiovascular and metabolic diseases.
In 1993, Lee et al. and Wightman et al. described a small RNA of 22 nucleotides, lin-4, with antisense complementarity to the heterochronic gene lin-14 in Caenorhabditis elegans (36, 37). In 2000, the description of let-7, a small RNA conserved in diverse species and with silencing abilities, highlighted the critical role of this category of RNA molecules (38–40). The following year, the term microRNA was coined by Tuschl et al. (41). Along with other groups, they paved the way for the discovery of numerous miRNAs. About 38’600 miRNAs have been identified in 271 species (http://www.mirbase.org). Around 2’600 human mature miRNAs are encoded in the human genome, with half annotated in miRBase V22 (42). The expression atlas of miRNAs generated by the Functional Annotation of the Mammalian Genome (FANTOM5) consortium revealed that the five most expressed miRNAs represent around 50% of the miRNA pool in a given human cell type (43). About half of miRNAs are cell type-enriched, a quarter are broadly expressed, and a quarter are expressed at small levels regardless the cell type.
2.2 Biogenesis
miRNAs can be encoded in non-coding (intergenic miRNAs) and intronic regions of genes. miRNAs are generated through canonical and non-canonical pathways (32, 44, 45) (Figure 2). In the canonical pathway, a long primary transcript (pri-miRNA) of hundreds to thousands nucleotides is generated by RNA polymerase II (Pol-II) or Pol-III and cleaved through the action of the RNA-binding protein DiGeorge syndrome critical region gene 8 (DGCR8) and the nuclear RNase III enzyme Drosha into a precursor-miRNA (pre-miRNA) of approximately 70 nucleotides (46–49). Intronic pri-miRNAs are generated from host RNA transcripts (pre-mRNAs) by RNA splicing and excised into pre-miRNAs by spliceosomal components. Their expression relies on transcription factors and Pol-II (50). pre-miRNAs are exported into the cytoplasm in an exportin-5/RanGTP-dependent manner. Pre-miRNAs are converted into active miRNAs of approximatively 22 nucleotides by a complex composed of the cytoplasmic RNase III Dicer and cofactors including transactivation response (TAR) RNA binding protein (TRBP) and the protein kinase RNA activator (PACT) (49, 51, 52). Of note, miRNAs (-5p and -3p) can be generated from the 5’ and 3’ arms of a pre-miRNA precursor, and co-expression of miRNA-5p and -3p species have been repeatedly reported. Non-canonical miRNA biogenesis pathways use different combinations of proteins, and are grouped into DGCR8/Drosha-independent and Dicer-independent pathways (Figure 2). Small hairpin RNA (shRNA) are cleaved by the DGCR8/Drosha complex and exported into the cytoplasm as in the canonical pathway, while pre-miRNA can be exported into the cytoplasm through exportin-1. A more detailed description of miRNA biogenesis pathways is beyond the scope of this review, but available in excellent reviews (32–35).
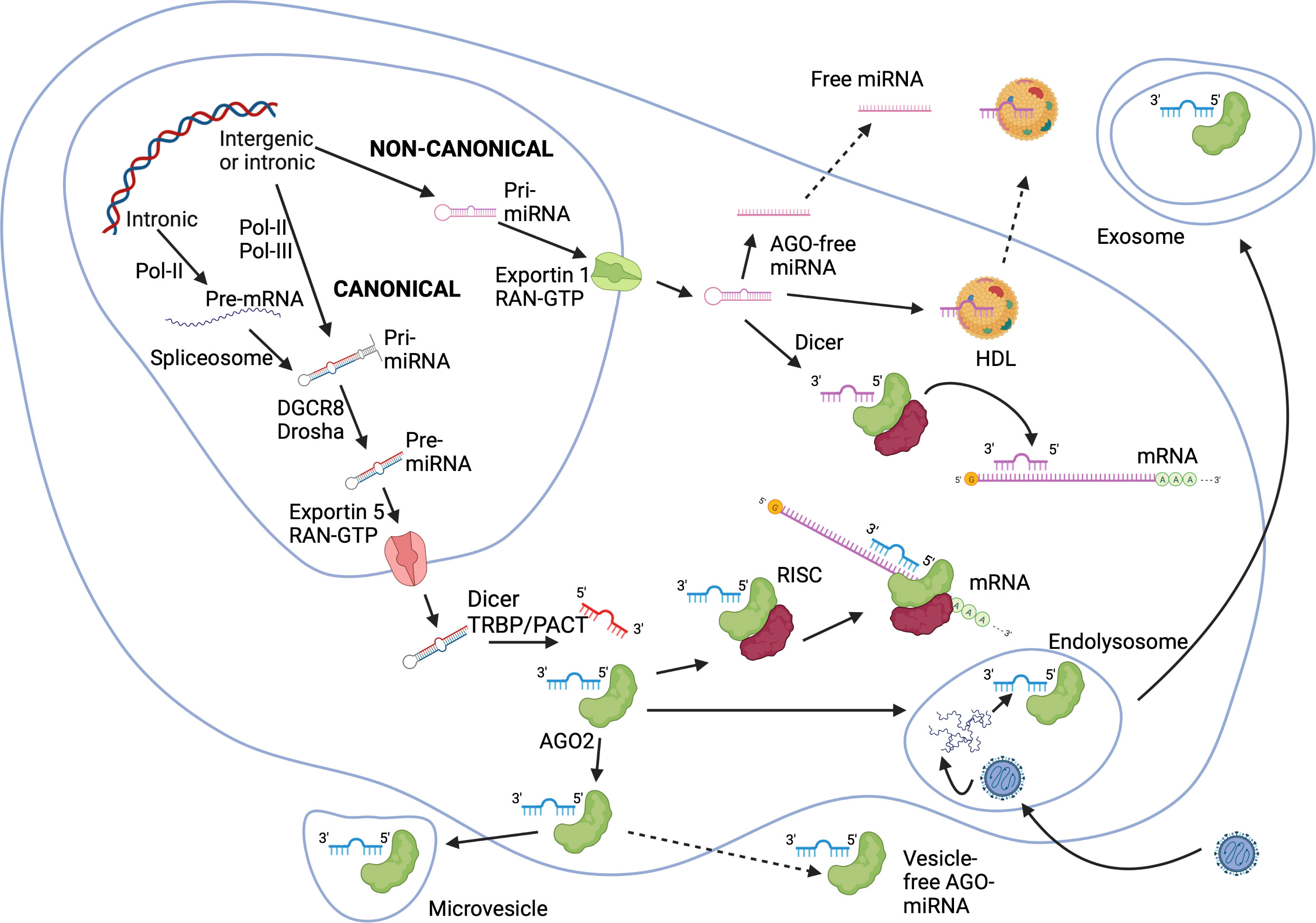
Figure 2 miRNA biogenesis via canonical and non-canonical pathways. In the canonical pathway, pri-miRNAs are turned into pre-miRNAs by the action of DGCR8 and Drosha within the nucleus. Intronic miRNAs can originate from host mRNA transcripts and processed into pre-miRNA by the spliceosome. Pre-miRNAs are exported into the cytoplasm through an exportin-5/RanGTP-dependent way, and are processed into mature miRNAs by Dicer with eventually RNA binding protein cofactors TRBP or PACT. In non-canonical pathways, shRNAs are cleaved by the DGCR8/Drosha complex and exported into the cytoplasm by exportin-1 before Dicer processing. Mature miRNAs bind to AGO proteins forming RISCs, which in turn silence or cleave mRNAs. Alternatively, miRNA-AGO complexes are exported out of the cell via vesicles (exosomes or microvesicles) or as vesicle-free complexes. miRNAs binding to HDLs are actively secreted. AGO-free miRNAs can be exported out of the cell as well. AGO, Argonaute; DGCR8, DiGeorge syndrome critical region gene 8; HDL, high density lipoproteins; miRNA, microRNA; mRNA, messenger RNA; PACT, protein kinase RNA activator; pre-miRNA, precursor-miRNA; Ran, Ras-related nuclear protein; RISC, RNA induced silencing complex; shRNA, small hairpin RNA; TRBP, transactivation response RNA binding protein. The Figure was created on BioRender.com.
2.3 Modes of action
miRNAs interact with the 3′-untranslated region (3’-UTR) of mRNAs to induce mRNA degradation and translational repression. Additionally, miRNAs can interact with gene promoter, 5′-untranslated region (5’-UTR) and coding sequence, and can activate transcription in a phenomenon known as RNA activation (53). Finally, miRNA can interact with proteins to modify their activity.
Crosslinking and immunoprecipitation analyses revealed that most miRNA binding events have little functional consequences (54). miRNAs do not possess catalytic functions, but form effector ribonucleoprotein complexes known as RNA induced silencing complexes (RISCs) (55). Mature miRNA molecules bind with proteins of the Argonaute (AGO) family in an ATP-dependent manner. Four AGO proteins (AGO1-4) playing a key role in the formation of RISCs are expressed in humans (56). RISCs bind to target mRNA molecules based on complementarity of miRNA (Figure 2). The result can be translational inhibition by interfering with the eukaryotic initiation factor 4F (eIF4F) followed by the decay of the target mRNA. Moreover, AGO2 initiates mRNA deadenylation by poly(A)-deadenylases, uncapping and 5′−3′ degradation by an exoribonuclease (32, 55, 57). While full complementarity with the target mRNA triggers AGO2 and mRNA degradation, partial complementarity results in transient binding to RISC. It induces the unloading of miRNA from AGO2 (57, 58). AGO-free miRNA molecules and endogenous miRNA-mRNA duplexes have been studied during the past years (59, 60). Mature miRNAs may adopt secondary structures like hairpin and homoduplex that may increase their half-life, affinity and specificity for targets (61).
Free miRNAs interact with proteins, but the prevalence and outcome of such interactions are poorly described. For instance, miR-130b-3p binds to extracellular cold-inducible RNA binding protein (eCIRP) (62). miR-130b-3p and eCIRP are increased in the blood of septic mice and sepsis patient. eCIRP acts as a DAMP sensed through TLR4, promoting the release of inflammatory mediators. Upon binding to eCIRP, miR-130b-3p inhibits eCIRP/TLR4 interaction and cytokine release by immune cells. Injection of a miR-130b-3p mimic reduces cecal ligation and puncture (CLP)-induced inflammation and acute lung injury (ALI) in mice (62). Moreover, miR-130b-3p has been shown to inhibit M1 macrophage polarization (63). A single miRNA can thus interfere with immune responses through multiple ways.
2.4 Circulation and delivery
miRNA secretion and release are intrinsic to cell response to hypoxia, starvation, heat, triggering of PRRs and cytokine/growth factor receptors, and other environmental factors (32, 59, 64, 65). miRNAs are present in biological fluids like blood, plasma, serum, urine, tears, saliva, semen, cerebrospinal fluid, bronchial and peritoneal fluids, and breast milk (32, 59, 64). An important feature of extracellular miRNAs is their stability and resistance to RNaseA-mediated degradation (64, 66). miRNAs in fluids exert paracrine or endocrine effects as signal transducers of intracellular communication (32, 65).
miRNAs are released passively accompanying apoptotic bodies or cell debris (from one to few μm) or secreted actively (59, 65). Secretion occurs through microvesicles of 100 to 1000 nm usually containing a RISC or a miRNA-AGO complex, and through exosomes (59). MAMPs or DAMPs trigger the release of exosomes containing miRNAs as well as DAMPs such as HMGB1, HSPs and histones, and cytokines, interleukins (ILs), chemokines and IFNγ. Early endosomes gradually turn to multivesicular bodies that integrate miRNAs and a RISC or similar complexes through mechanisms regulated by ceramide synthesis and neutral sphingomyelinase 2 (65, 67). Multivesicular bodies merge with lysosomes inducing the degradation of trapped material, or fuse with the cell membrane expelling exosomes containing miRNAs (59, 68). Exosomes can carry oncogenic miRNAs promoting tumor invasiveness (69), or anti-oncogenic and anti-angiogenic miRNAs inhibiting the growth of malignant cells (70). Similarly, exosomes can carry miRNAs that enhance or decrease cellular responses to MAMPs as reported for miR-155 and miR-146a in LPS-stimulated DCs (71). High-density lipoproteins (HDL) act as alternative carriers of miRNAs in the blood (Figure 2). This is an active and energy dependent procedure to differentiate from the passive release of miRNAs upon cell death (65).
The mechanisms of uptake of miRNAs by recipient cells is not fully deciphered (65). The uptake of microvesicles and exosomes occurs by endocytosis, phagocytosis or fusion with the plasma membrane. Endocytosis of microvesicles requires a docking step mediated by specific or non-specific molecules (72, 73). Because of their small size, microvesicles are also taken-up by micropinocytosis, which does not require a docking step (74). Exosomes and smaller extracellular vesicles are engulfed by phagocytosis mediated by TLRs and complement receptors. Exosomes released during sepsis impact on organs including lungs, kidneys, liver, heart and brain (75).
3 miRNAs in sepsis
Sepsis shows features of early immune hyper-activation and late immunosuppression. Accordingly, we may suggest that miRNAs having anti-inflammatory activities may be beneficial during early sepsis but detrimental during late sepsis. On the contrary, miRNAs having proinflammatory activities may be detrimental during early sepsis but beneficial during late sepsis (Figure 1).
Given that infection and stress modulate the expression of miRNAs, it is not surprising that miRNAs have been the focus of much interest. The stability, simple structure and expression of miRNAs in blood and other biological fluids represent an opportunity to stem new sepsis biomarkers (76). We will focus on promising miRNAs in sepsis. We will summarize observations about the modulation and the role of miRNAs in vitro and in vivo in models of sepsis (Table 1), and miRNAs as potential biomarkers in human sepsis (Table 2).
3.1 miRNAs and sepsis pathophysiology
3.1.1 miRNAs and innate immune cells
First, it should be recalled that miRNAs are not acting only as brakes, but also as promoters of inflammatory and innate immune responses. Cues to how miRNAs weight the inflammatory response have been obtained in studies using Dicer 1-deficient mouse macrophages depleted of miRNAs. Contrary to expectations, Dicer 1-deficient macrophages produce reduced levels of tumor necrosis factor (TNF), IL-6 and IL-12 in response to TLR1/2, TLR4, and TLR9 stimulation (84). It has been proposed that miRNAs expressed constitutively repress innate immune genes to preserve homeostasis, while stimulus-induced miRNAs fine-tune inflammatory responses and return to homeostasis (266).
miRNAs modulate immune signals by targeting positive or negative players of immune signaling pathways. This process is highly dynamic for several reasons. First, miRNAs are differentially expressed in innate and non-innate immune cell types. Second, miRNA expression is upregulated or downregulated in response to MAMPs/DAMPs/cytokines, and subjected to circadian rhythm (267). For example, miR-146a and miR-155 are upregulated while miR-27a and miR-532-5p are downregulated in macrophages exposed to LPS. Third, miRNAs regulate their own expression. The proinflammatory miR-375 inhibits the expression of the anti-inflammatory miR-21 by targeting the Janus kinase (JAK) 2-signal transducer and activator of transcription protein (STAT) 3 signaling pathway (185). Fourth, one miRNA targets many mRNAs, and one mRNA is regulated by various miRNAs. Consequently, miRNAs have additive or antagonistic effects on their targets. Fifth, one miRNA either inhibits or activates immune signaling, participating to feedback loop mechanisms controlling gene expression. Sixth, miRNAs circulate in fluids and act at a distance (268–272).
miRNAs target transcription factors, signaling proteins and growth factors to influence hematopoiesis and modulate the development of innate and adaptive immune cells. miRNAs regulate the functions of mature innate immune cells, including migration, phagocytosis, efferocytosis, production of cytokines, tolerance, tissue remodeling and promotion of tumor development (273–278). Macrophages display a continuum of functional states, ranging from proinflammatory M1 macrophages to pro-resolving/anti-inflammatory M2 macrophages. miR-155 is up-regulated in M1 macrophages. The knockout of mir155 and miR-155 antagomir reduces the expression of Inos, Il1b, Il6, Il12, and Tnf in M1 macrophages. In fact, around half of the 650 genes that make up the M1 signature rely on miR-155 (279). miR-130b-3p inhibits IRF1 expression, M1 macrophage polarization and the production of C‐C motif chemokine ligand 5 (CCL5), C‐X‐C motif chemokine ligand 10 (CXCL10), inducible nitric oxide (NO) synthase (iNOS) and TNF (63). miR-223 regulates peroxisome proliferator-activated receptor-γ mediated M2 macrophage activation (280). Overall, many miRNAs have been associated with the polarization/activity of M1 macrophages (miR-9, miR-26a-2, miR-125a-3p, miR-125b, miR-127, miR-155-5p, miR-181a, miR-204-5p, miR-451) and M2 macrophages (miR-27a, miR-29b-1, miR-34a, miR-124, miR-125a-5p, miR-132, miR-143-3p, miR-145-5p miR-146a-3p, miR-193b, miR-222, miR-223, let-7c) (278, 281–283). miRNAs influence the differentiation, expansion and biological activities of myeloid-derived suppressor cells (MDSCs) that are associated with sepsis morbidity and mortality (17, 28, 284, 285). Thus, miRNAs shape both inflammation-associated antimicrobial defenses, anti-inflammatory and pro-resolving immune reactions and immunosuppression. The two facets can be driven by a single miRNA entity. miR-466l expression in polymorphonuclear neutrophils (PMNs) induces inflammation and precedes miR-466l expression in macrophages acquiring pre-resolving functions (286).
3.1.2 miRNAs and endothelium and coagulation activation in sepsis
DAMPs and MAMPs released during sepsis activate the complement and coagulation systems. Disseminated intravascular coagulation (DIC) affects around 35% of sepsis patients. Beside thrombosis, DIC is associated with bleeding due to the consumption of clotting factors, anticoagulant proteins, and platelets (9, 11). Thrombocytopenia develops in about 50% of sepsis patients. Signaling through PRRs and cytokine receptors triggers endothelial cells, increasing the expression of adhesion molecules, vascular permeability, transcellular migration, microcirculation lesions, tissue ischemia and organ failure (287, 288). Many endogenous and microvesicles-derived miRNAs regulate endothelial cell functions acting on apoptosis, proliferation, migration and inflammation (289–292). For example, miR-155 is increased in pulmonary endothelial cells of sepsis mice, targets the tight junction protein Claudin-1 and induces capillary leakage during infection (159). In a model of ALI, endothelial cell-derived exosomal miRNA-125b-5p downregulates topoisomerase II α resulting in reduced lung injury and inflammatory cell infiltration in the pulmonary mesenchyme (112). Decreased exosomal miR-125b (and miR-30a-5p) is associated with mortality in sepsis patients (197).
Platelets play a role beyond thrombosis and hemostasis, regulating innate immune cells including PMNs, monocytes and macrophages (287, 293, 294). Platelets are an important sources of miRNAs that are released through microvesicles or exosomes and are taken up by endothelial cells and macrophages (113, 295). Reduced miR-26b in platelets is associated with increased P-selectin expression, and with severity and mortality in sepsis patients (92). In fact, miR-26b reduces platelet adhesion and aggregation in mice (296). An increased miR-320a/miR-127 ratio in platelets could help detecting sepsis (236). Platelet microvesicles containing miR-126-3p are taken up by macrophages, strongly affecting the transcriptome and decreasing the expression of cytokines/chemokines/growth factors in the cells (113). Additionaly, miR-126-3p is associated with platelet activation (297). miR15b-5p and miR-378a-3p in platelet-derived exosomes obtained from sepsis patients induce the formation of neutrophil extracellular traps (NETs) involved in organ injury (80). On the contrary, platelet microparticles containing miR-223 reduce intercellular adhesion molecule 1 (ICAM-1) expression and binding to peripheral blood mononuclear cells by endothelial cells, providing a possible protective role against excessive sepsis-induced vascular inflammation (183).
3.1.3 miRNAs and host response to endotoxin (LPS)
Many studies analyzed miRNAs selected based on prior knowledge or miRNA screenings. While very instructive on a case-by-case basis, reductionist explorations tackle a small part of the role of miRNAs. A good illustration comes from reports on endotoxin, which is used as a model system to study host response to Gram-negative bacteria (Figure 3). The sensing of extracellular LPS by innate immune cells involves LPS binding protein (LBP), CD14, MD-2 and TLR4 (298, 299). TLR4, anchored at the cell membrane, recruits the adaptor molecule myeloid differentiation primary response 88 protein (MyD88). MyD88 activates a cascade of phosphorylation initiated at the level of IL-1 receptor (IL-1R)-associated kinase-1 (IRAK1) and TNF receptor-associated factor 6 (TRAF6), filling the NF-κB, IRFs and MAPK signaling pathways. These pathways control the transcription of immune response genes. Note that TLR4 shuttling to late endosome induces an alternative signaling through the adaptor molecule TIR domain-containing adaptor inducing IFNβ (TRIF). TRIF initiates IRF3 and late NF-κB activation, involved in the production of type I IFNs and IFN-inducible genes. For reasons of simplicity, this pathway is not described on Figure 3.
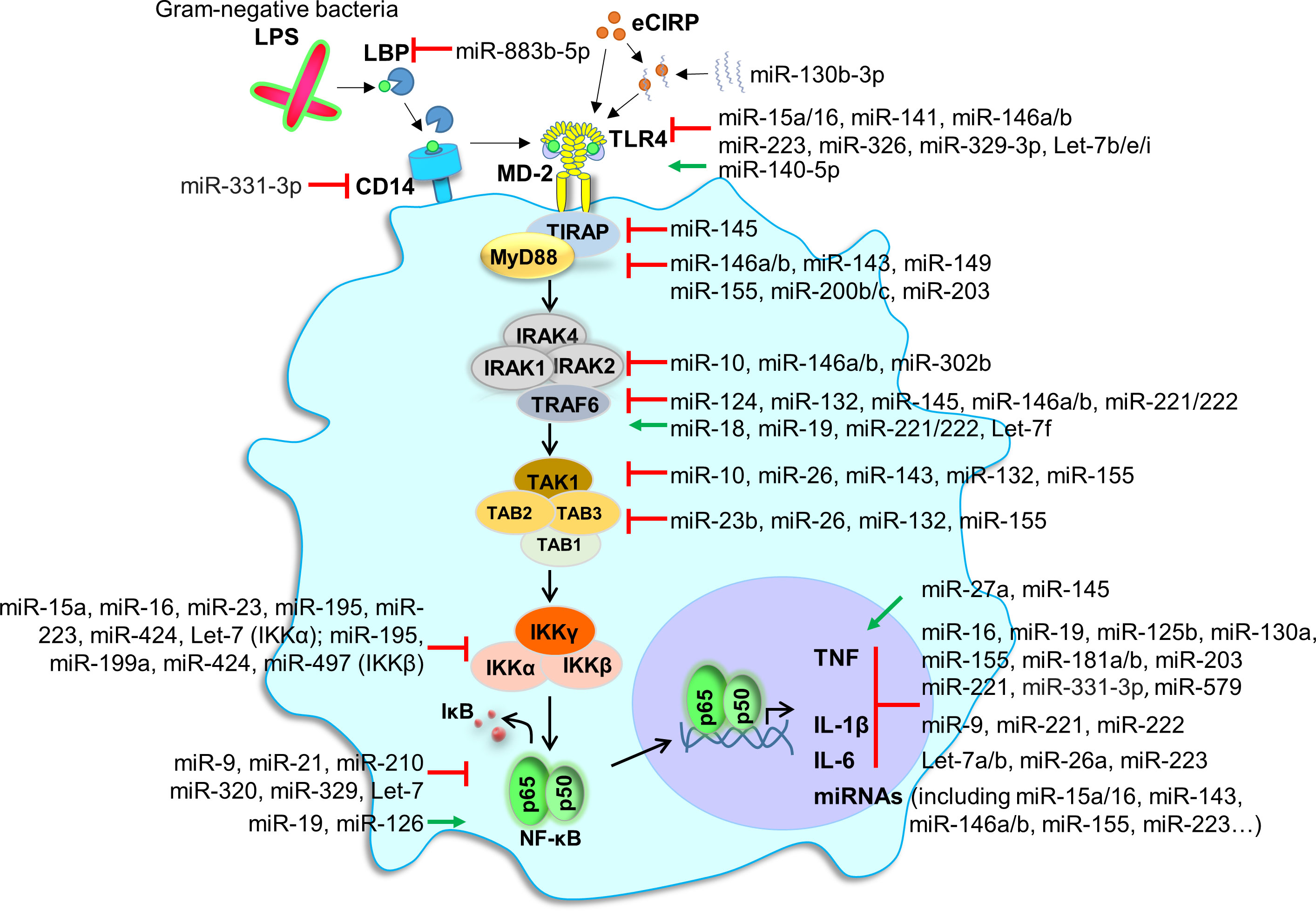
Figure 3 miRNAs and endotoxin sensing and signaling. The figure shows the recognition and intracellular signaling events following the sensing by monocytic cells of LPS from Gram-negative bacteria. LPS aggregates are dissociated by the LPS-binding protein (LBP). LPS/LBP complexes are transferred to CD14, a glycosylphosphatidylinositol-anchored molecule expressed on the membrane of monocytic cells. CD14 transfers LPS to TLR4 together with MD-2. This induces the recruitment of TIR domain-containing adaptor protein (TIRAP) and myeloid differentiation primary response gene (MyD88). MyD88 is involved in early nuclear factor-κB (NF-κB) activation and pro-inflammatory gene expression. NF-κB signaling is involved in the expression of many miRNAs. For reasons of simplicity, we did not depict the TIR domain-containing adaptor inducing IFNβ (TRIF)-dependent, MyD88 independent, pathway involved in IRF signaling and late NF-κB activation. Red lines depict inhibition, while green lines depict activation (by miRNAs). eCIRP, extracellular cold-inducible RNA binding protein; IκB, inhibitory kappa B; IKK, IκB kinase; IRAK, IL-1 receptor-associated kinase-1; TAB, transforming growth factor-β (TGF-β) activated kinase 1; TRAF, TNF receptor-associated factor; TAK1, TGF-β activated kinase-1.
A few dozen of miRNAs, among which miR-15a, miR-16, miR-17-5p, miR-21, miR-25, miR-31, miR-98, miR-124-5p, miR-125b, miR-140-5p, miR-141, miR-146a, miR-149-5p, miR-155 miR-181c, miR-203-5p, miR-221, miR-326, miR-378, miR-448 and miR-466I, interfere at different levels with LPS sensing and LPS-induced signaling pathways (Figure 3). Note that miR-15a/16, miR-17-5p, miR-25, miR-125b, miR-141, miR-326 and miR-448 inhibit TLR4 expression, while miR-140-5p increases TLR4 expression. In addition, dozens of miRNAs among which miR-9-5p, miR-19a-5p, miR-21, miR-29, miR-93, miR-98, miR-125, miR-221, miR-222, miR-223 and let-7a-5p target the expression of downstream proinflammatory and anti-inflammatory cytokines. Finally, the inflammatory response itself regulates the expression of proinflammatory and anti-inflammatory miRNAs (273–277, 300–302). These observations provide insight into the complexity of miRNAs interactions during host antimicrobial responses, and highlight the challenge of taking a comprehensive and integrated view of the impact of miRNAs on immune responses.
3.1.4 miRNAs and endotoxin tolerance
Exposure of isolated innate immune cells or whole body to low amounts of LPS induces a transient period of refractory response to subsequent exposure to LPS, generally attested by inhibition of cytokine production. This phenomenon is known as endotoxin tolerance. Expression studies suggest that miR-146a and miR-146b are involved in endotoxin tolerance in THP-1 human monocytic cells (303–305). miR-146a disrupts both transcription and translation of TNF gene in tolerant THP-1 cells (305). miR-146b is induced by the anti-inflammatory cytokines IL-10 and transforming growth factor (TGF)-β, but repressed by IFNγ which reverses endotoxin tolerance (304). Tolerance extends beyond LPS and TLR4 signaling. Bacterial lipoproteins recognized through TLR2 increase miR-146a expression and render THP-1 cells hypo-responsive to subsequent stimulation by Salmonella typhimurium. This is associated with a strong reduction of IRAK-1, phosphorylated inhibitory kappa B α (IκBα), and TNF production in tolerant THP-1 cells (306). Epigenetic mechanisms are involved in the establishment of tolerance. miR-146a and miR-155 are co-regulated in naïve and tolerant RAW 264.7 mouse macrophages. LPS stimulation induces histone 3 lysine 4 trimethylation (H3K4me3, a mark of transcriptionally active genes) and NF-κB p65 binding to miR-146a and miR-155 gene loci. The induction of tolerance is associated with a shift towards repressive H3K9me3 mark and the recruitment of CCAAT/enhancer-binding protein (C/EBP) β and p50 inhibitory component of the NF-κB complex to miR-146a and miR-155 genes (307).
3.2 Examples of miRNAs studied as modulators of innate immune responses and biomarkers of sepsis
Table 1 summarizes observations about miRNAs obtained in cells exposed to MAMPs/DAMPs, and in animals and humans with sepsis. Table 2 summarizes observations about miRNAs as potential biomarkers of human sepsis. We will not describe all studies because it would be tedious if not impossible. We will focus on miR-15a, miR-16, miR-122, miR-143, miR-146a/b, miR-150, miR-155 and miR-223 taken as examples of important and versatile miRNAs, and because these miRNAs are discussed in several publications in the sepsis field. This selection is arbitrary, but we will nevertheless see that even a limited sample of miRNAs provides insight into the complexity by which miRNAs on impact sepsis. Observations reported in precedent chapters will not be repeated.
3.2.1 miR-15a/16
miR-15a and miR-16 are members of the miR-15 family comprising miR-15a, miR-15b, miR-16-1, miR-16-2, miR-195, and miR-497. miR-15a/16-1 cluster resides on human chromosome 13. miR-15a and miR-16 share the same seed sequence suggesting that they mediate similar biological functions.
3.2.1.1. Anti-inflammatory activity
miR-15a and miR-16 are commonly viewed as anti-inflammatory miRNAs. Bacterial infection and LPS increase miR-15a/16 in mouse bone-marrow derived macrophages, and in mouse lungs. miR-15a/16 target TLR4 and IRAK-1 in RAW 264.7 mouse macrophages exposed to LPS (78). In agreement, miR-15a/16 deficiency increases the expression of TLR4 through PU.1 (a transcription factor essential for TLR4 expression (308), and the phagocytosis and killing of E. coli by macrophages (77). Accordingly, miR-15a/16 knockout mice are resistant to CLP, E. coli and LPS-induced lethal sepsis (77). As an example of the connection between ncRNAs, the lncRNA SNHG16 downregulates the expression of miR-15a/16 and counter-regulates the inhibitory effects of miR-15a/16 on the expression of TLR4 in RAW 264.7 macrophages (209).
3.2.1.2 Inflammatory activity
LPS increases miR-16 expression in human monocytic cells and biliary epithelial cells through the MAPK pathway. In a counter-regulatory manner, miR-16 suppresses silencing mediator for retinoid and thyroid hormone receptor, and increases NF-κB transcriptional activity and expression of IL-1α, IL-6 and IL-8 in LPS-stimulated cells (81). Similarly, miR−15a−5p is increased in RAW 264.7 macrophages exposed to LPS, targets TNF-induced protein 3−interacting protein 2, activates the NF-κB pathway and increases cytokine production (79). A miR-15a-5p inhibitor reduces IL-1β, IL-6 and TNF and inflammatory response in mice challenged with LPS (79).
3.2.1.3 Biomarker value
miR-15a and miR-16 are increased in patients with systemic inflammatory response syndrome (SIRS) and sepsis patients when compared to healthy controls (n = 66, 32, 24). miR-15a levels are higher in SIRS than in sepsis patients (206). The screening of 13 miRNAs (miR-15a, miR-16, miR-21, miR-27a, miR-34a, miR-126, miR-150, miR-155 miR-181b, miR-223, miR-125b, miR-146a, miR-486) in 62 adult sepsis patients and 32 healthy controls shows that miR-15a, miR-16, miR-21, miR-125b, miR-126, miR-146a, miR-155, miR-181b, miR-223 are increased in sepsis patients. miR-15a is lower in patients with shock than in patients without shock (309). In a prospective observational study (117 survivors and 97 non-survivors with sepsis), a miRNome analysis shows that miR-15a (together with miR122, miR-193b* and miR483-5p) is increased in sepsis non-survivors, while miR-16 (and miR-223) is decreased (195).
Among seven miRNAs (miR-15a, miR-15b, miR-16, miR-206, miR-223, miR-378 and miR-451) measured in 46 neonatal sepsis patients, only miR-15a and miR-16 are increased, while miR-378 and miR-451 are decreased. Receiver operating characteristic (ROC) curve analyses suggest that miR-15a and miR-16 serum levels are good predictors of neonatal sepsis with area under the curves (AUCs) of 0.85 and 0.87 (78). miR-15a and miR-16 are increased in the serum of neonates with sepsis when compared to neonates with respiratory infection or pneumonia without sepsis (n = 62 and 32) (309). Finally, two recent studies report increased miR-15b and miR-16a in small cohorts of sepsis neonates (25 sepsis and 25 controls) (211, 212).
Overall, miR-15a/16 drive anti-inflammatory or inflammatory action, and are commonly increased in sepsis patients. A link with disease severity seems more uncertain.
3.2.2 miR-122
miR-122 was identified 20 years ago as a liver specific miRNA in mice (310). miR-122 is encoded on chromosome 18 in humans, and has no close paralog. miR-122 has been especially studied in the context of host response to liver-tropic viruses.
3.2.2.1 Anti-inflammatory activity
miR-122 is decreased in the liver of patients with hepatocellular carcinoma (HCC). The upregulation of miR-122 in HepG2 human hepatocellular carcinoma cell lines inhibits TLR4 expression. Moreover, miR-122 decreases the proliferation and the production of TNF and IL-6 by HepG2 and Huh7 hepatocellular carcinoma cell lines (107).
3.2.2.2 Inflammatory activity
miR-122 targets suppressor of cytokine signaling protein (SOCS) 1 and SOCS3, inducing IFNα/β expression and decreasing hepatitis B virus (HBV) replication (103, 104). miR-122 targets the receptor tyrosine kinases (RTKs) insulin like growth factor 1 receptor (IGF1R), fibroblast growth factor receptor and myeloid-epithelial-reproductive tyrosine kinase. Then, miR-122 decreases STAT3 phosphorylation and increases IRF1 signaling and the expression of IFNs in response to hepatitis C virus (HCV) and the synthetic analog of doubled stranded RNA poly(I:C) (105). miR-122 targets heme oxygenase-1 and decreases HBV expression in hepatoma cells (106). Related to sepsis, miR-122-5p is increased in the heart of rats and in H9c2 rat cardiomyocytes challenged with LPS. Inhibition of miR-122-5p reduces myocardial injury through inhibition of inflammation, oxidative stress and apoptosis in endotoxemic rats (108).
3.2.2.3 Biomarker value
At least four studies have reported decreased miR-122 levels in patients with sepsis when compared to healthy controls (201, 207, 225, 227). miR-122 levels are lower in ARDS than in non-ARDS patients and show a negative correlation with 28-days mortality (227). In contrast with these observations, miR-122 is increased in sepsis patients and is an independent risk factor for 30-day mortality (195, 226). Moreover, the levels of miR-122 (but not miR-15a, miR-16, miR-193b*, miR-223 and miR-483-5p) are higher in patients with coagulation abnormalities than in patients with normal coagulation tested at days 1, 3, 7 and 10 of ICU admission (208). Finally, other studies do not point to miR-122 differential expression in sepsis patients and healthy controls (198, 228). Hence, the biomarker value of miR-122 remains questionable.
3.2.3 miR-143
miR-143 is encoded in a bicistronic locus with miR-145, but has no homology with miR-145. miR-143 is considered as an anti-inflammatory miRNA. Few studies looked at the mechanisms of action of miR-143 in the context of innate immune response and sepsis.
3.2.3.1 Anti-inflammatory activity
The quantification of 455 miRNAs in blood leukocytes from heathy volunteers infused 4 hours with endotoxin identified miR-143 as the only upregulated miRNA. High levels of miR-143 are linked to decreased expression of B-cell CLL/lymphoma 2, a regulator of apoptosis an innate immune signaling, and the silencing of inflammation-related target genes (128). Mycobacterial cell wall glycolipid (Ac2PIM) and muramyl dipeptide (MDP) are recognized by TLR2 and NOD2. In mouse macrophages, Ac2PIM induces miR-143. In turn, miR-143 targets the NOD2 signaling adaptors TGF-β activated kinase-1 (TAK1) and receptor-interacting protein kinase 2. miR-143 suppresses PI3K/PKCδ/MAPK/β-catenin-mediated expression of cyclooxygenase-2 (COX-2), SOCS3 and matrix metalloproteinase (MMP)-9 induced by MDP (129). Thus, miR-143 negatively regulates the NOD2 pathway, which may have consequences on the development of vaccines and Gram-positive bacteria sepsis.
miR-143 is the most significantly downregulated miRNA in nasal mucosal tissues from patients with allergic rhinitis (311). miR-143 dampens inflammatory responses in upper airways (130). Bronchial epithelium cells exposed to angiotensin II (AngII) and LPS increase miR-143 which targets angiotensin converting enzyme 2 (ACE2). A miR-143-3p inhibitor increases ACE2 and decreases inflammatory cytokines and apoptosis in cells exposed to AngII and LPS (132). ACE2 protects mice from ALI induced by sepsis (312), so miR-143 may be used to decrease lung inflammation involved in ARDS. In a mouse model of mycoplasma pneumonia, a miR-143-3p mimic reduces IL-2 and TNF, increases IL-10 and reduces alveolar epithelial cell apoptosis. A miR-143 mimic decreases TLR4, MyD88 and phosphorylated NF-κB p50 in lungs. miR-143 might be used to inhibit the TLR4/MyD88/NF-κB signaling pathway and normalize pulmonary inflammation during pneumonia (133).
Mesenchymal stem/stromal cells (MSCs) therapy improves sepsis outcome. Treating human umbilical cord MSCs with poly(I:C) decreases miR-143 and increases the anti-inflammatory power of MSCs on macrophages. miR-143 targets TAK1 involved in TLR3 signaling and COX-2. The infusion of poly(I:C)-activated MSCs improves survival of CLP mice, while the co-delivery of miR-143 reduces the survival benefit provided by MSCs (134). Targeting miR-143 might have therapeutic potential in dampening inflammatory responses in sepsis. No study reported inflammatory activity of miR-143.
3.2.3.2 Biomarker value
Microarray and RT-qPCR analyses have been used to explore miRNAs in T cells and whole blood in 34 healthy controls and 31 sepsis patients. Thirty five miRNAs are differentially regulated in sepsis patients. miR-143 (and miR-15a, miR-16, miR-93, miR-223 and miR-424) is increased in sepsis patients. miR-143 levels correlate with T cell immuno-paralysis. The discriminatory power of miR-143 in T cells performs well, with an AUC of 0.95. miR-143 correlates positively with sequential organ failure assessment (SOFA; a clinical score based on the assessment of 6 variables representing an organ system: respiration, coagulation, liver, cardiovascular, central nervous system, renal) score (204). Another study reports higher blood levels of miR-143 in patients with sepsis than in patients with SIRS, and in SIRS patients than in healthy controls (n = 103/95/40). miR-143 levels correlate with disease severity, evaluated by SOFA and Acute Physiology And Chronic Health Evaluation (APACHE) II (a clinical score that estimates ICU mortality based on laboratory values, age and previous health conditions) scores (240).
In a prospective observational study, miR-143 is similarly expressed in sepsis survivors and non-survivors (n = 117/97) (195). In a cohort of 218 critically ill patients, among which 135 sepsis patients, miR-143 levels are similar to those measured in healthy controls (n = 76). In ICU patients, miR-143 levels do not correlate with inflammatory markers, but correlate with indicators of organ failure (239).
Contrary to the above, miR-143 serum levels are higher in sepsis survivors than in sepsis non-survivors. The performance of miR-143 is rather modest (AUC = 0.628), yet it is higher than that of C-reactive protein (CRP), leukocyte count, creatinine and international normalization ratio value (239). In a subsequent report, the same team analyzed the prognostic scoring of combinations of miR-143 (and miR-122, miR-133a, miR-150, miR-155, miR-192, miR-223) in 204 ICU patients of whom 127 with sepsis (228). A “3 miRNAs” score based on higher miR-133a or lower miR-143 and miR-223 levels predicts patient survival in ICU. A “2 miRNAs” score (higher miR-133a and lower miR-150 levels) predicts patient long-term prognosis. The predictive power of the scores is increased by adding age into the calculation.
Overall, miR-143 is consensually anti-inflammatory. It is almost invariably increased in sepsis patients. Its usage as a biomarker remains unsure. miR-143 might be valuable incorporated in combined scores, but this should be confirmed in independent studies.
3.2.4 miR-146a/b
miR-146a and miR-146b are encoded on human chromosomes 5 and 10, respectively. They have nearly identical sequences and might share targets (313).
3.2.4.1 Anti-inflammatory activity
The group of David Baltimore reported in 2006 the negative impact of miR-146a/b on signaling in innate immune cells (314). miR-146a/b is an immediate early-response NF-κB-dependent gene induced by microbial components and proinflammatory mediators. IRAK1 and TRAF6 are targets of miR-146a/b (314). Macrophages from miR-146a knockout mice are hyper-responsive to LPS, and miR-146a restrains inflammation, myeloid cell proliferation, and oncogenic transformation in vivo (315). miR-146a inhibits NF-κB signaling and expression of cytokines, ICAM-1 and E-selectin, and trafficking induced by MAMPs in monocytes, macrophages, DCs, endothelial cells and keratinocytes (137, 138, 159, 316, 317). miR-146a inhibits the expression of STAT1, IFNγ and TNF, and the cytotoxicity of natural killer cells (318). A miR-146a agomir (a synthetic chemically modified double-strand miRNA) inhibits macrophage inflammatory response and protects mice from LPS-mediated organ damage (141). The delivery of a miR-146a-expressing plasmid decreases inflammatory cytokines and organ injury, and increases survival of mice subjected to CLP (138).
3.2.4.2 Inflammatory activity
Exogenous single stranded miR-146a-5p induces inflammatory responses through activation of TLR7 and proteasome, and downregulation of IRAK-1. miR-146a knockout mice show reduced inflammation and organ injury, improved cardiac function, and increased survival to acute sepsis induced by CLP (144). miR-146a-5p-mediated activation of TLR7 induces TNF, pulmonary inflammation, endothelial barrier disruption and ARDS in sepsis mice (145).
3.2.4.3 Biomarker value
miR-146a is increased in the blood of healthy subjects infused with endotoxin (251). Among 7 miRNAs measured in the serum of healthy controls, SIRS patients, and sepsis patients (n = 20/30/50), miR-146a and miR-223 are lower in sepsis patients (AUC = 0.804 and 0.858) (242). Similarly, reduced miR-146a levels discriminate sepsis from SIRS patients (AUC = 0.813) (243). In a pediatric study (n = 60/55 healthy and sepsis patients), miR-146a is decreased in blood and negatively correlated with the levels of C-reactive protein, procalcitonin (PCT), IL-6 and TNF. miR-146a levels correlate with sepsis severity and mortality, showing lower levels of miR-146a in non‐surviving than in surviving patients (244). However, another study does not report differential expression of miR-146a in newborns with or without early-onset sepsis (n = 25/group) (237).
In contrast, miR-146a is increased in two studies analyzing adult patients (241, 245). In the first study (19 healthy controls, 102 sepsis, 44 severe sepsis), the AUCs of miR-146a and miR-155 for predicting 30-day mortality in ALI patients are 0.733 and 0.782 (241). In the second study (180 healthy controls, 180 sepsis patients), miR-146a and miR-146b expression levels are predictors of sepsis risk (AUC = 0.774 and 0.897) (245). miR-146a and miR-146b positively correlate with APACHE II score, SOFA score, creatinine, CRP, IL-1β, IL-6, IL-17 and TNF. miR-146a and miR-146b are higher in survivors than in 28-day non-survivors. miR-146b has a better predictive value than miR-146a (AUC = 0.703 vs 0.599).
Overall, miR-146a is traditionally considered as anti-inflammatory, but 2 recent studies seem to contradict the uniform view. In the same manner, it remains unclear how miR-146a/b are modulated in human sepsis.
3.2.5 miR-150
miR-150 is encoded on human chromosome 19. miR-150 plays a role in hematopoiesis (319). miR-150 affects apoptosis, maturation and differentiation of lymphocytes and NK cells, and autoimmune diseases (320, 321). miR-150 is one of the four miRNAs (with miR-146b, miR-342, and let-7g) down-regulated in healthy subjects infused with LPS (128).
3.2.5.1 Anti-inflammatory activity
miR-150 targets notch receptor 1, STAT1 and NF-κB to inhibit LPS-induced apoptosis and IL-1β, IL-6 and TNF, ICAM-1, VCAM-1 and E-selectin in RAW 264.7 macrophages, THP-1 monocytic cells and endothelial cells (150, 154, 155). miR-150-5p is decreased in the heart of rats challenged with LPS. miR-150 decreases Akt2, cleaved caspase-3, Bax and apoptosis in rat heart and H9C2 cardiomyocytes (152). In a similar way, miR-150 binding to MALAT1 lncRNA inhibits the NF-κB pathway, cytokine production, ER stress and apoptosis in LPS-stimulated human umbilical endothelial cells, H9c2 cardiomyocytes, IL-1β-stimulated chondrocytes, and pulmonary arterial endothelial cells from CLP mice (150, 151, 153). miR-150-5p interacts with X-inactive specific transcript lncRNA to regulate the c-Fos axis, thioredoxin-interacting protein-mediated pyroptosis and sepsis-induced myocardial injury (157). In sepsis mice with acute kidney injury (AKI), miR-150 targets MEKK3, inhibits LPS-induced c-Jun N-terminal kinase (JNK) pathway, apoptosis and inflammation (156). miR-150-/- mice show increased mortality from LPS and CLP. Rescuing miR-150 in lung endothelial cells decreases EGR2-dependent Ang2 expression, restores adherent junction reannealing and endothelial barrier function, and reduces mortality (149). miR-150 inhibits ARG1 and the expansion and immunosuppressive function of MDSCs (146) that expand during severe infections and have been associated with nosocomial infections, morbidity, and mortality in critically ill patients (17, 28, 322). miR-150-3p may have similar expression pattern and activity as miR-150-5p. miR-150-3p is one of the most downregulated exosomal miRNAs (with 146a-5p, 150-3p, 151a-3p) in heat stroke, associated with inflammatory response and coagulation cascade (323).
3.2.5.2 Inflammatory activity
There is no formal demonstration of a proinflammatory activity of miR-150. Though, miR-150 is increased (and not decreased) in the serum of mice ongoing CLP-induced sepsis and in rats challenged with LPS (147, 148).
3.2.5.3 Biomarker value
Many studies have reported decreased miR-150 expression in sepsis conditions. An initial miRNome study identifies 17 differentially expressed miRNAs in sepsis patients and healthy subjects (n = 17/32). miR-150 is decreased in patients. miR-150 positively correlates with diseases severity evaluated by SOFA score, and inversely correlates with cytokine levels (203). The authors propose that miR-150 could be used as a biomarker of early sepsis.
miR-150 is decreased in healthy subjects infused with endotoxin (251), and in patients with urosepsis (250), sepsis with AKI (156), and other sepsis conditions (146, 150, 204, 249). Several studies have reported negative correlations between miR-150-5p and IL-1β and TNF serum levels, renal dysfunction and T cells immunoparalysis (150, 156, 204). Accordingly, sepsis patients with fatal outcomes have reduced miR-150 levels (150, 248, 251, 252). A combination of miR-150 and SOFA score improves prognosis prediction (252). However, while patients with sepsis show lower levels of miR-150 than patients with SIRS and non-sepsis trauma patients (146, 249), no significant difference is observed between critically ill patients with and without sepsis (248). It is proposed that miR-150 may be a useful biomarker or target in the diagnosis, prognosis and treatment of sepsis. This suggestion should be tempered since miR-150 is not differentially expressed in adult sepsis patients tested for 13 miRNAs (309) and, more annoying, in unbiased studies looking at miRNome (195, 196, 198, 202).
Several reasons explain why miRNAs biomarkers are not confirmed in miRNome studies. In any case, it shows that we could increase robustness of the methodology (including cohort constitution) to accurately demonstrate miRNA differential expression in sepsis. On the other side, all studies so far reported anti-inflammatory mode of action of miR-150.
3.2.6 miR-155
miR-155 is encoded on human chromosome 21. Its expression is increased by MAMPs, bacteria, viruses and parasites (267, 324–333). Captivatingly, the induction of miR-155 in macrophages is controlled by the molecular clock controller Bmal1, which in turn is repressed by miR-155. Thus, miR-155 is a regulatory component of the circadian rhythm, and of the circadian control of inflammation (267).
3.2.6.1 Anti-inflammatory activity
miR-155 targets TGF-β activated kinase 1 binding protein 2 (TAB2) and negatively regulates the TLR/IL-1 signaling cascade in human DCs exposed to microbial stimuli (271). miR-155 inhibits caspase 1 and IL-1β by increasing autophagy through inhibition of TAB2. miR-155 agomir reduces lung pathology in mice with CLP (254). miR-155 inhibits IRF8-mediated antiviral response in Japanese encephalitis virus infected microglial cells (333). In Francisella tularensis-infected human macrophages, miR-155 downregulates MyD88 (327). The delivery of miR-155 inhibitor to mice challenged with LPS increases SOCS1, and reduces JAK and STAT3, cytokines, and kidney injury (325). In mice with CLP, a miR-155 mimic decreases JNK and β-arrestin 2 expression, reduces infiltration of macrophages and PMNs in the myocardium, and attenuates late sepsis-induced cardiac dysfunction (162). miR-155-deficient mice infected with H1N1 influenza virus and challenged 5 days later with Staphylococcus aureus show a robust induction of IL-17 and IL-23 and reduced bacterial burden in lungs. In a similar way, a miR-155 antagomir (i.e. anti-miRNAs, in the form of oligonucleotides silencing endogenous miRNAs) enhances lung bacterial clearance in mice (326). This could be relevant since post influenza bacterial pneumonia is an important cause of morbidity and mortality.
The infection of astrocytes with Escherichia coli induces miR-146a and miR-155 expression. In a feedback loop mechanism, miR-146a and miR-155 inhibit TLR- and epithelial growth factor receptor (EGFR)-mediated NF-κB signaling pathway and inflammation. miR-146a and miR-155 antagomirs increase brain inflammation in mice infected with E. coli. Thus, miR-155 acts coordinately with miR-146a to safeguard the central nervous system from neuroinflammatory damages (334).
3.2.6.2 Inflammatory activity
Pioneer studies published in late 2000’s linked miR-155 with inflammation and innate immunity. miR-155 has been identified as a target induced by inflammatory mediators in macrophages (324). Subsequently, miR-155 is shown to repress SOCS1 and Src homology 2 domain containing inositol polyphosphate 5-phosphatase 1 to increase LPS-induced cytokine production by mouse macrophages (269, 270).
miR-155 transgenic mice produce more TNF in response to LPS and are more sensitive to endotoxemia (335). miR-155 deficient mice have a reduced capacity to clear Streptococcus pneumoniae colonization from the nasopharynx, which is associated with impaired recruitment of macrophages and induction of protective T helper (Th) 17 immune responses (328). PMNs from miR-155-deficient septic mice express less NETs. miR-155 deficiency is associated with reduced accumulation of PMNs, NETs, edema and lung damage in mice with CLP (158). miR-155 is increased in endothelial cells from endotoxemic mice, and in the serum and bronchoalveolar lavage fluid (BALF) from septic patients with ARDS. miR-155 promotes vascular permeability and capillary leakage (159). miR-155 deficiency reduces endothelial activation and leukocyte adhesion and infiltration into the myocardium, myocardial edema and dysfunction, vasoplegia, and mortality in mice with endotoxemia or CLP. miR-155 targets CD47 and angiotensin type 1 receptor to promote nitric oxide (NO)-mediated vasorelaxation and vasoplegia (161). Injection of a miR-155 inhibitor reduces inflammation and intestinal barrier dysfunction in mice with CLP (160).
3.2.6.3 Biomarker value
The measure of 13 miRNAs in the plasma of 32 healthy controls and 62 patients with sepsis shows that 11 miRNAs including miR-155 are increased in patients. miR-155 levels are not associated with severity or outcome (309). A miRNome identifies 11 differentially expressed miRNAs in sepsis patients compared to healthy controls (n = 60/30), but only miR-155 is confirmed by PCR. miR-155 is elevated in patients, and positively correlates with SOFA score. miR-155 shows a good prediction value of 28-day survival (AUC = 0.763). Interestingly. miR-155 levels are proportional to the percentage of CD39+ regulatory T cells (201). Another study reports that miR-155 is increased in septic patients and is a valuable predictor of mortality (241). In a study analyzing 10 healthy controls and 10 sepsis patients with ARDS, miR-155 levels are elevated in BALF samples from sepsis patients (254). In a cohort of 156 sepsis patients of whom 41 with ALI and 32 with ARDS, miR-155 levels are higher in patients with ALI or ARDS, positively correlate with IL-1β and TNF, and negatively correlate with PaO2/FiO2 ratio. miR-155 AUC for diagnosing sepsis with ALI/ARDS is 0.87 (253). A study comparing 218 critically ill patients (135 with sepsis) with 76 healthy controls shows that, in critically ill patients ≤ 65 years, high miR-155 levels are associated with increased survival. This is not the case in patients older than 65 years (336). Finally, miR-155 is similarly expressed in peripheral blood from newborns with or without sepsis (237).
To summarize, there are strong arguments in favor of anti-inflammatory and proinflammatory activities of miR-155. miR-155 is usually increased in adults with sepsis, and associated with worse outcome. This is not observed in elderly and newborns, suggesting that miRNA-based biomarkers should be interpreted according to patient’s age.
3.2.7 miR-223
miR-233 is encoded on chromosome X in mammals, and is highly conserved among species. miR-223 regulates hematopoiesis and triggers granulopoiesis and macrophage differentiation (337–340). miR-223 targets NLRP3, IGF1R, HSP90, C/EBPα, C/EBPβ, E2F1, forkhead box protein O1, NF-κB p65, nuclear factor I A, PBX/knotted 1 homeobox 1, STAT3 and STAT5, which accounts for a broad range of biological effects (338).
3.2.7.1 Anti-inflammatory activity
miR-223 is predominantly expressed in myeloid cells and drives anti-inflammatory functions. miR-223 is involved in macrophage polarization and activation, and negatively regulates neutrophil functions. miR-223 inhibits NF-κB p65 phosphorylation and IL-1β, IL-6, TNF and IL-12p40 expression in U-937 human monocytic cells stimulated with LPS and IFNγ (341). NLRP3 is a sensor of the classical inflammasome involved in gasdermin-D processing, pyroptosis and secretion of IL-1β and IL-18 (342). miR-223 suppresses NLRP3 expression and IL-1β production in mouse macrophages and PMNs (343). Stimulation of macrophages with LPS, CpG DNA or poly(I:C) decreases miR-233 expression, which results in increased STAT3, NF-κB and MAPK signaling and production of IL-1β, IL-6 and TNF (344, 345). In the same line, PMN-derived miR-223 inhibits NLRP3 and IL-1β expression, and reduces pathogenesis in mice with DAMPs-induced ALI (346). miRNA-223 is upregulated in blood and lung parenchyma during experimental and human tuberculosis (347), and in monocytes from patients with tuberculosis (341). In a mouse model, miR-223 restricts the expression of CCL3, CXCL2 and IL-6 and the recruitment of PMNs into the lungs. miR-223 knockdown sensitizes mice to Mycobacterium tuberculosis lung infection through exacerbated PMN-dependent lethal inflammation (347). miR-223 promotes MMP-1 and MMP-9 activity in macrophages. M. tuberculosis infection increases the expression of miR-223, MMP-1 and MMP-9 in lungs. In doing so, it favors bacteria dissemination. On the contrary, miR-223 impedes BMAL1, which influences the expression of the circadian clock genes CLOCK, PER1 and PER2. Thus, Mycobacterium tuberculosis interferes with circadian rhythm via a miR-223/BMAL1 axis to subvert host defenses (348). Mechanical ventilation and Staphylococcus aureus-induced ALI is increased in miR-223 deficient mice. Pulmonary delivery of miR-223 using nanoparticles inhibits ALI. Interestingly, the transfer of miR-223 from PMNs to alveolar epithelial cells may be involved in attenuating lung inflammation (349).
3.2.7.2 Inflammatory activity
miR-223 increases in lungs of mice exposed to cigarette smoke and LPS and human in pulmonary cells and monocytes exposed to inflammatory cytokines. miR-223 targets histone deacetylase 2 (HDAC2), resulting in increased expression of fractalkine. miR-223 negatively correlates with HDAC2 expression in lungs from chronic obstructive pulmonary disease (COPD) patients (181). High miR-223 levels might contribute to stimulate the NF-κB pathway, and decrease corticosteroid response and disease severity in asthma and COPD (350).
3.2.7.3 Biomarker value
Studies evaluating miR-223 as a sepsis biomarker have generated contradictory results. When compared to healthy controls, miR-223 serum levels are either reduced (197, 237, 242), increased (182, 199, 204, 207) or not affected (259, 309) in patients. Observations using severity as a variable appear more consistent since miR-223 levels are lower in sepsis patients than in SIRS patients (242), and in patients with sepsis-induced cardiomyopathy than in healthy controls (238). Yet, miR-223 levels are either lower (182, 195) or higher (260) in sepsis non-survivors than in sepsis survivors (351). Finally, miRNome studies have not pointed to miR-223 as a differentially expressed miRNA in sepsis (195, 196, 198, 202).
Overall, miR-223 is considered anti-inflammatory, albeit it might drive inflammatory effects by targeting HDAC2 in specific conditions. Clinical studies yielded heterogeneous results when assessing the potential of miR-223 as a biomarker of sepsis. However, a meta-analysis of 22 records, including 2210 sepsis, 426 SIRS, and 1076 healthy controls suggested that miR-223 could be used as an indicator for sepsis (351). It should be stressed however that miR-223 values were available in a subset of 6/22 studies.
3.2.8 Other miRNAs
Finally, we will describe few studies analyzing miRNAs in an unsupervised manner or in the context of specific clinical questions. A miRNome analysis in critically ill patients with intra-abdominal sepsis or non-infective SIRS and healthy controls (n = 29/44/16) has detected 116 blood miRNAs increased in SIRS patients. miRNAs are more abundant in non-infectious SIRS than in sepsis patients. The top five differentially expressed miRNAs, miR-23a-5p, miR-26a-5p, miR-30a-5p, miR-30d-5p and miR-192-5p, discriminate severe sepsis from severe SIRS (AUC = 0.74-0.92). miRNA levels inversely correlate with IL-1, IL-6, IL-8, CRP and pancreatic stone protein (PSP), but not SOFA score. Hence, sepsis and non-infective SIRS are characterized by distinct changes in blood miRNAs, which may be used for diagnostic approaches in critically ill patients (196). However, except miR-23a, none of the short listed miRNAs are considered as sepsis biomarkers in previous studies (89, 249).
A recent study evaluated blood changes of miR-15a-5p, miR-155-5p, miR-192-5p, miR-423-5p in 46 sepsis patients treated with gentamicin, vancomycin (i.e. nephrotoxic antibiotics) or non-nephrotoxic antibiotics (n = 20/7/19). Small changes of miRNAs are observed in the different groups. miR-15a-5p at day 7 of gentamicin treatment provides good discrimination between AKI and non-AKI. miR-155-5p and miR-192-5p positively correlate with creatinine and neutrophil gelatinase-associated lipokalin in patients receiving vancomycin (210). These data suggest that miRNAs expression might be modulated by antimicrobials, and may serve as diagnostic markers in sepsis patients receiving nephrotoxic antibiotics.
The expression of miR-146-3p, miR-147b, miR-155 and miR-223 (associated with inflammation, see 3.2) was assessed in the plasma of patients with bacterial sepsis or dengue hemorrhagic fever and healthy controls (n = 130/69/82). miRNAs are increased in patients with sepsis when compared to patients with hemorrhagic fever or to healthy controls. miR-147b, alone or in combination with PCT, discriminates septic shock (AUC ≥ 0.8). Thus, miR-147b may be a biomarker to support clinical diagnosis of severe sepsis (247).
Necrotizing enterocolitis (NEC) is the most common and severe gastrointestinal pathology in preterm infants. A microarray-based screening has identified 230 upregulated miRNAs and 16 downregulated miRNAs in NEC when compared to sepsis and non-NEC/non-sepsis groups. Targeted analyses in a large cohort shows that miR-1290 can efficiently differentiate NEC from neonatal sepsis and neonatal inflammatory conditions such as bronchopulmonary dysplasia (200). Plasmatic miR-1290 expression may help differentiating NEC from neonatal sepsis.
4 Conclusions
Over the past decade, miRNAs have been the focus of intense research in the field of critical illness and sepsis. Our understanding of the modes of action and impact of miRNAs on host inflammatory and antimicrobial defenses has increased dramatically. However, this has not yet improved clinical management. Possibly, intervention strategies with miRNA mimics or miRNA antagomirs could rebalance the dysregulated host response during sepsis (Figure 1). Unfortunately, no miRNA-based clinical trials have been registered for sepsis so far.
The data summarized in Table 1 and Figure 3 illustrate the complexity and wide range of action of miRNAs in inflammatory and infectious conditions. Some miRNAs have been ascribed both anti-inflammatory and proinflammatory activities. Many reasons may account for diverse observations, including differences between in vitro, ex vivo and in vivo settings, sterile and infectious models, organs and cell types examined, and kinetics. In in vivo sepsis models, a mediator may be beneficial or harmful depending on disease condition. For example, inhibition of macrophage migration inhibitory factor (a pleiotropic cytokine and central regulator of innate immune responses (352, 353) increased susceptibility to infection but protected from lethal sepsis (354–357). Similarly, blocking TLR4 at the onset of infection induced mortality from otherwise non-lethal peritonitis, while therapeutic administration of anti-TLR4 antibodies protected mice from lethal Gram-negative bacterial sepsis (299).
Using miRNA as biomarker in sepsis holds more short-term potential than therapeutic opportunities. Many studies reported that miRNAs: 1) discriminate healthy donors from sepsis patients, 2) distinguish sepsis from non-infectious clinically-related diseases, 3) predict severity and/or the mortality, and 4) correlate with clinical parameters or cytokines. However, conflicting observations currently make translation to clinics challenging. So, how to use more efficiently miRNAs as biomarkers?
There is a crucial need for improvement and standardization of clinical studies in order to generate comprehensive views of miRNome during sepsis. We advocate for more stringent methodologies, in terms of both study design, clinical data collection, and miRNA investigation strategies. Importantly, small cohorts tends to exacerbate individual variations, whereas targeted techniques (e.g. RT-qPCR) fail to generate a global landscape of the miRNA fluctuations. Even if constraining, derivation and validation cohorts should be envisaged to corroborate and improve robustness of observations. A key objective would be to run unbiased miRNome analyses in large cohorts of well-defined critically-ill patients with or without sepsis.
Sepsis is a heterogeneous syndrome. Mediators detrimental during the overwhelming phase of sepsis might be beneficial during the later immunosuppressive phase, and miRNAs should not deviate from this principle (Figure 1). In fact, new types of clinical trials using a precision-medicine approach have been launched to adjust treatment (immunosuppressive or immuno-stimulant) given patients’ inflammatory status (see https://www.immunosep.eu/ as an example). We believe that studies should take into consideration the causative agent, the site of infection, medications and the inflammatory status to stratify patients. Bearing in mind disease progression, the timing of sampling should be recorded. Ideally, blood samples should be collected at hospital admission (ED, medical/surgical ICUs), and continued over time to have a longitudinal view of the expression miRNAs. For translational perspectives, it would be easier be detected miRNAs in serum or blood than in PBMCs or isolated vesicles.
Finally, miRNA expression levels are prone to be affected by individual parameters (age, sex, genetic, comorbidities…). Therefore, combination scores (including one or several miRNAs, demographic and/or clinical data) should also be considered. Along these lines, whole blood or single cell transcriptomic identified rather simple gene expression signatures to distinguish sterile inflammation from sepsis, sepsis from infection, viral infections from fungal and bacterial infections, peritonitis, and sepsis caused by community-acquired pneumonia (358–363). Ideally, polymorphisms affecting pri-, pre- and mature miRNA sequences or affecting the target gene sequence should be investigated as well (364).
Based on our current knowledge, clinical use of miRNA targeting in sepsis cannot be realistically envisaged. miRNAs might be used as biomarkers. However, further studies will be required to obtain robust results, in order to safely recommend the use of miRNAs as biomarkers of sepsis. This is a certainly an ambitious, but promising goal.
Author contributions
TR conceived the manuscript. TR and NA wrote the manuscript. NA, CG, CT, ITS and TR revised the manuscript. All authors contributed to the article and approved the submitted version.
Funding
TR is supported by the Swiss National Science Foundation (SNSF, grant number 310030_207418), by the Horizon 2020 Marie Skłodowska-Curie Action: Innovative Training Network (MSCA-ESA-ITN, grant number 676129) and Horizon 2020 ImmunoSep (grant number 847422), by the Fondation Carigest/Promex Stiftung für die Forschung (Geneva, Switzerland) and the Fondation de Recherche en Biochimie (Epalinges, Switzerland). NA received a scholarship from the Porphyrogenis Foundation (Lausanne, Switzerland). CT and IS received a scholarship from the Société Académique Vaudoise (Lausanne, Switzerland).
Acknowledgments
We apologize for those studies that were not mentioned in this review.
Conflict of interest
The authors declare that the research was conducted in the absence of any commercial or financial relationships that could be construed as a potential conflict of interest.
Publisher’s note
All claims expressed in this article are solely those of the authors and do not necessarily represent those of their affiliated organizations, or those of the publisher, the editors and the reviewers. Any product that may be evaluated in this article, or claim that may be made by its manufacturer, is not guaranteed or endorsed by the publisher.
Abbreviations
AKI, acute kidney injury; ALI, acute lung injury; APACHE, acute physiology and chronic health evaluation; ARDS, acute respiratory distress syndrome; CCL, C‐C motif chemokine ligand; CXCL, C‐X‐C motif chemokine ligand; DAMP, damage or danger-associated molecular pattern; DC, dendritic cell; HMGB1, high mobility group box-1; HSP, heat shock protein; ICU, intensive care unit; IFN, interferon; IL, interleukin; IRF, IFN response factor; IRAK, IL-1 receptor-associated kinase; lncRNA, long non-coding RNA; LPS, lipopolysaccharide; MAMP, microbial-associated molecular pattern; MAPK, mitogen-activated protein kinase; miRNA, microRNA; MyD88, myeloid differentiation primary response 88; NF-κB, nuclear factor-κB; PCT, procalcitonin; PRR, pattern-recognition receptor; SIRS, systemic inflammatory response syndrome; SOCS, suppressor of cytokine signaling; SOFA, sequential organ failure assessment; STAT, signal transducer and activator of transcription; TLR, Toll-like receptor; TNF, tumor necrosis factor.
References
1. Broz P, Monack DM. Newly described pattern recognition receptors team up against intracellular pathogens. Nat Rev Immunol (2013) 13:551–65. doi: 10.1038/nri3479
2. Savva A, Roger T. Targeting toll-like receptors: promising therapeutic strategies for the management of sepsis-associated pathology and infectious diseases. Front Immunol (2013) 4:387. doi: 10.3389/fimmu.2013.00387
3. Brubaker SW, Bonham KS, Zanoni I, Kagan JC. Innate immune pattern recognition: a cell biological perspective. Annu Rev Immunol (2015) 33:257–90. doi: 10.1146/annurev-immunol-032414-112240
4. Takeuchi O, Akira S. Pattern recognition receptors and inflammation. Cell (2010) 140:805–20. doi: 10.1016/j.cell.2010.01.022
5. Singer M, Deutschman CS, Seymour CW, Shankar-Hari M, Annane D, Bauer M, et al. The third international consensus definitions for sepsis and septic shock (sepsis-3). JAMA (2016) 315:801–10. doi: 10.1001/jama.2016.0287
6. Rudd KE, Johnson SC, Agesa KM, Shackelford KA, Tsoi D, Kievlan DR, et al. Global, regional, and national sepsis incidence and mortality, 1990-2017: analysis for the Global Burden of Disease Study. Lancet (2020) 395:200–11. doi: 10.1016/S0140-6736(19)32989-7
7. Prescott HC, Angus DC. Enhancing recovery from sepsis: a review. JAMA (2018) 319:62–75. doi: 10.1001/jama.2017.17687
8. Shankar-Hari M, Saha R, Wilson J, Prescott HC, Harrison D, Rowan K, et al. Rate and risk factors for rehospitalisation in sepsis survivors: systematic review and meta-analysis. Intensive Care Med (2020) 46:619–36. doi: 10.1007/s00134-019-05908-3
9. van der Poll T, Shankar-Hari M, Wiersinga WJ. The immunology of sepsis. Immunity (2021) 54:2450–64. doi: 10.1016/j.immuni.2021.10.012
10. Rubio I, Osuchowski MF, Shankar-Hari M, Skirecki T, Winkler MS, Lachmann G, et al. Current gaps in sepsis immunology: new opportunities for translational research. Lancet Infect Dis (2019) 19:e422–36. doi: 10.1016/S1473-3099(19)30567-5
11. Hotchkiss RS, Moldawer LL, Opal SM, Reinhart K, Turnbull IR, Vincent JL. Sepsis and septic shock. Nat Rev Dis Primers (2016) 2:16045. doi: 10.1038/nrdp.2016.45
12. Venet F, Monneret G. Advances in the understanding and treatment of sepsis-induced immunosuppression. Nat Rev Nephrol (2018) 14:121–37. doi: 10.1038/nrneph.2017.165
13. Torres LK, Pickkers P, van der Poll T. Sepsis-Induced Immunosuppression. Annu Rev Physiol (2022) 84:157–81. doi: 10.1146/annurev-physiol-061121-040214
14. Deutschman CS, Tracey KJ. Sepsis: current dogma and new perspectives. Immunity (2014) 40:463–75. doi: 10.1016/j.immuni.2014.04.001
15. Cohen J, Vincent JL, Adhikari NK, Machado FR, Angus DC, Calandra T, et al. Sepsis: a roadmap for future research. Lancet Infect Dis (2015) 15:581–614. doi: 10.1016/S1473-3099(15)70112-X
16. Ciarlo E, Savva A, Roger T. Epigenetics in sepsis: targeting histone deacetylases. Int J Antimicrob Agents (2013) 42 Suppl:S8–12. doi: 10.1016/j.ijantimicag.2013.04.004
17. Schrijver IT, Theroude C, Roger T. Myeloid-Derived Suppressor Cells in Sepsis. Front Immunol (2019) 10:327. doi: 10.3389/fimmu.2019.00327
18. Cecconi M, Evans L, Levy M, Rhodes A. Sepsis and septic shock. Lancet (2018) 392:75–87. doi: 10.1016/S0140-6736(18)30696-2
19. Schlapbach LJ, Truck J, Roger T. Editorial: the immunology of sepsis-understanding host susceptibility, pathogenesis of disease, and avenues for future treatment. Front Immunol (2020) 11:1263. doi: 10.3389/fimmu.2020.01263
20. MiRa JC, Gentile LF, Mathias BJ, Efron PA, Brakenridge SC, Mohr AM, et al. Sepsis pathophysiology, chronic critical illness, and persistent inflammation-immunosuppression and catabolism syndrome. Crit Care Med (2017) 45:253–62. doi: 10.1097/CCM.0000000000002074
21. Safety WIPoC. (2001). Available at: http://www.inchem.org/documents/ehc/ehc/ehc222.htm (Accessed 02.03.2021).
22. Pierrakos C, Vincent JL. Sepsis biomarkers: a review. Crit Care (2010) 14:R15. doi: 10.1186/cc8872
23. Stanski NL, Wong HR. Prognostic and predictive enrichment in sepsis. Nat Rev Nephrol (2020) 16:20–31. doi: 10.1038/s41581-019-0199-3
24. Barichello T, Generoso JS, Singer M, Dal-Pizzol F. Biomarkers for sepsis: more than just fever and leukocytosis-a narrative review. Crit Care (2022) 26:14. doi: 10.1186/s13054-021-03862-5
25. Opal SM, Wittebole X. Biomarkers of infection and sepsis. Crit Care Clin (2020) 36:11–22. doi: 10.1016/j.ccc.2019.08.002
26. Peters van Ton AM, Kox M, Abdo WF, Pickkers P. Precision immunotherapy for sepsis. Front Immunol (2018) 9:1926. doi: 10.3389/fimmu.2018.01926
27. Pierrakos C, Velissaris D, Bisdorff M, Marshall JC, Vincent JL. Biomarkers of sepsis: time for a reappraisal. Crit Care (2020) 24:287. doi: 10.1186/s13054-020-02993-5
28. Schrijver IT, Karakike E, Theroude C, Baumgartner P, Harari A, Giamarellos-Bourboulis EJ, et al. High levels of monocytic myeloid-derived suppressor cells are associated with favorable outcome in patients with pneumonia and sepsis with multi-organ failure. Intensive Care Med Exp (2022) 10:5. doi: 10.1186/s40635-022-00431-0
29. Slim MA, van Mourik N, Dionne JC, Oczkowski SJW, Netea MG, Pickkers P, et al. Personalised immunotherapy in sepsis: a scoping review protocol. BMJ Open (2022) 12:e060411. doi: 10.1136/bmjopen-2021-060411
30. Carthew RW, Sontheimer EJ. Origins and Mechanisms of MiRNAs and siRNAs. Cell (2009) 136:642–55. doi: 10.1016/j.cell.2009.01.035
31. Bartel DP. MicroRNAs: target recognition and regulatory functions. Cell (2009) 136:215–33. doi: 10.1016/j.cell.2009.01.002
32. O'Brien J, Hayder H, Zayed Y, Peng C. Overview of MicroRNA Biogenesis, Mechanisms of Actions, and Circulation. Front Endocrinol (Lausanne) (2018) 9:402. doi: 10.3389/fendo.2018.00402
33. Ha M, Kim VN. Regulation of microRNA biogenesis. Nat Rev Mol Cell Biol (2014) 15:509–24. doi: 10.1038/nrm3838
34. Treiber T, Treiber N, Meister G. Regulation of microRNA biogenesis and its crosstalk with other cellular pathways. Nat Rev Mol Cell Biol (2019) 20:5–20. doi: 10.1038/s41580-018-0059-1
35. Krol J, Loedige I, Filipowicz W. The widespread regulation of microRNA biogenesis, function and decay. Nat Rev Genet (2010) 11:597–610. doi: 10.1038/nrg2843
36. Lee RC, Feinbaum RL, Ambros V. The C. elegans heterochronic gene lin-4 encodes small RNAs with antisense complementarity to lin-14. Cell (1993) 75:843–54. doi: 10.1016/0092-8674(93)90529-y
37. Wightman B, Ha I, Ruvkun G. Posttranscriptional regulation of the heterochronic gene lin-14 by lin-4 mediates temporal pattern formation in C. elegans. Cell (1993) 75:855–62. doi: 10.1016/0092-8674(93)90530-4
38. Reinhart BJ, Slack FJ, Basson M, Pasquinelli AE, Bettinger JC, Rougvie AE, et al. The 21-nucleotide let-7 RNA regulates developmental timing in Caenorhabditis elegans. Nature (2000) 403:901–6. doi: 10.1038/35002607
39. Pasquinelli AE, Reinhart BJ, Slack F, Martindale MQ, Kuroda MI, Maller B, et al. Conservation of the sequence and temporal expression of let-7 heterochronic regulatory RNA. Nature (2000) 408:86–9. doi: 10.1038/35040556
40. Hammond SM, Bernstein E, Beach D, Hannon GJ. An RNA-directed nuclease mediates post-transcriptional gene silencing in Drosophila cells. Nature (2000) 404:293–6. doi: 10.1038/35005107
41. Lagos-Quintana M, Rauhut R, Lendeckel W, Tuschl T. Identification of novel genes coding for small expressed RNAs. Science (2001) 294:853–8. doi: 10.1126/science.1064921
42. Alles J, Fehlmann T, Fischer U, Backes C, Galata V, Minet M, et al. An estimate of the total number of true human MiRNAs. Nucleic Acids Res (2019) 47:3353–64. doi: 10.1093/nar/gkz097
43. de Rie D, Abugessaisa I, Alam T, Arner E, Arner P, Ashoor H, et al. An integrated expression atlas of MiRNAs and their promoters in human and mouse. Nat Biotechnol (2017) 35:872–8. doi: 10.1038/nbt.3947
44. Lee Y, Jeon K, Lee JT, Kim S, Kim VN. MicroRNA maturation: stepwise processing and subcellular localization. EMBO J (2002) 21:4663–70. doi: 10.1093/emboj/cdf476
45. Li Z, Rana TM. Therapeutic targeting of microRNAs: current status and future challenges. Nat Rev Drug Discovery (2014) 13:622–38. doi: 10.1038/nrd4359
46. Lee Y, Kim M, Han J, Yeom KH, Lee S, Baek SH, et al. MicroRNA genes are transcribed by RNA polymerase II. EMBO J (2004) 23:4051–60. doi: 10.1038/sj.emboj.7600385
47. Borchert GM, Lanier W, Davidson BL. RNA polymerase III transcribes human microRNAs. Nat Struct Mol Biol (2006) 13:1097–101. doi: 10.1038/nsmb1167
48. Denli AM, Tops BB, Plasterk RH, Ketting RF, Hannon GJ. Processing of primary microRNAs by the Microprocessor complex. Nature (2004) 432:231–5. doi: 10.1038/nature03049
49. Han J, Lee Y, Yeom KH, Kim YK, Jin H, Kim VN. The Drosha-DGCR8 complex in primary microRNA processing. Genes Dev (2004) 18:3016–27. doi: 10.1101/gad.1262504
50. Lin SL, Chang D, Wu DY, Ying SY. A novel RNA splicing-mediated gene silencing mechanism potential for genome evolution. Biochem Biophys Res Commun (2003) 310:754–60. doi: 10.1016/j.bbrc.2003.09.070
51. Lee Y, Hur I, Park SY, Kim YK, Suh MR, Kim VN. The role of PACT in the RNA silencing pathway. EMBO J (2006) 25:522–32. doi: 10.1038/sj.emboj.7600942
52. Redfern AD, Colley SM, Beveridge DJ, Ikeda N, Epis MR, Li X, et al. RNA-induced silencing complex (RISC) Proteins PACT, TRBP, and Dicer are SRA binding nuclear receptor coregulators. Proc Natl Acad Sci U.S.A. (2013) 110:6536–41. doi: 10.1073/pnas.1301620110
53. Ramchandran R, Chaluvally-Raghavan P. MiRNA-mediated RNA activation in mammalian cells. Adv Exp Med Biol (2017) 983:81–9. doi: 10.1007/978-981-10-4310-9_6
54. Agarwal V, Bell GW, Nam JW, Bartel DP. Predicting effective microRNA target sites in mammalian mRNAs. Elife (2015) 4:1–38. doi: 10.7554/eLife.05005
55. Kawamata T, Tomari Y. Making RISC. Trends Biochem Sci (2010) 35:368–76. doi: 10.1016/j.tibs.2010.03.009
56. Yoda M, Kawamata T, Paroo Z, Ye X, Iwasaki S, Liu Q, et al. ATP-dependent human RISC assembly pathways. Nat Struct Mol Biol (2010) 17:17–23. doi: 10.1038/nsmb.1733
57. Jo MH, Shin S, Jung SR, Kim E, Song JJ, Hohng S. Human argonaute 2 has diverse reaction pathways on target RNAs. Mol Cell (2015) 59:117–24. doi: 10.1016/j.molcel.2015.04.027
58. De N, Young L, Lau PW, Meisner NC, Morrissey DV, MacRae IJ. Highly complementary target RNAs promote release of guide RNAs from human Argonaute2. Mol Cell (2013) 50:344–55. doi: 10.1016/j.molcel.2013.04.001
59. Makarova JA, Shkurnikov MU, Wicklein D, Lange T, Samatov TR, Turchinovich AA, et al. Intracellular and extracellular microRNA: An update on localization and biological role. Prog Histochem Cytochem (2016) 51:33–49. doi: 10.1016/j.proghi.2016.06.001
60. Janas MM, Wang B, Harris AS, Aguiar M, Shaffer JM, Subrahmanyam YV, et al. Alternative RISC assembly: binding and repression of microRNA-mRNA duplexes by human Ago proteins. RNA (2012) 18:2041–55. doi: 10.1261/rna.035675.112
61. Belter A, Gudanis D, Rolle K, Piwecka M, Gdaniec Z, Naskret-Barciszewska MZ, et al. Mature MiRNAs form secondary structure, which suggests their function beyond RISC. PloS One (2014) 9:e113848. doi: 10.1371/journal.pone.0113848
62. Gurien SD, Aziz M, Jin H, Wang H, He M, Al-Abed Y, et al. Extracellular microRNA 130b-3p inhibits eCIRP-induced inflammation. EMBO Rep (2020) 21:e48075. doi: 10.15252/embr.201948075
63. Guo Q, Zhu X, Wei R, Zhao L, Zhang Z, Yin X, et al. MiR-130b-3p regulates M1 macrophage polarization via targeting IRF1. J Cell Physiol (2021) 236:2008–22. doi: 10.1002/jcp.29987
64. Wang K, Zhang S, Weber J, Baxter D, Galas DJ. Export of microRNAs and microRNA-protective protein by mammalian cells. Nucleic Acids Res (2010) 38:7248–59. doi: 10.1093/nar/gkq601
65. Chen X, Liang H, Zhang J, Zen K, Zhang CY. Secreted microRNAs: a new form of intercellular communication. Trends Cell Biol (2012) 22:125–32. doi: 10.1016/j.tcb.2011.12.001
66. Chen X, Ba Y, Ma L, Cai X, Yin Y, Wang K, et al. Characterization of microRNAs in serum: a novel class of biomarkers for diagnosis of cancer and other diseases. Cell Res (2008) 18:997–1006. doi: 10.1038/cr.2008.282
67. Kosaka N, Iguchi H, Yoshioka Y, Takeshita F, Matsuki Y, Ochiya T. Secretory mechanisms and intercellular transfer of microRNAs in living cells. J Biol Chem (2010) 285:17442–52. doi: 10.1074/jbc.M110.107821
68. Pfrieger FW, Vitale N. Cholesterol and the journey of extracellular vesicles. J Lipid Res (2018) 59:2255–61. doi: 10.1194/jlr.R084210
69. Yang M, Chen J, Su F, Yu B, Su F, Lin L, et al. Microvesicles secreted by macrophages shuttle invasion-potentiating microRNAs into breast cancer cells. Mol Cancer (2011) 10:117. doi: 10.1186/1476-4598-10-117
70. Hannafon BN, Carpenter KJ, Berry WL, Janknecht R, Dooley WC, Ding WQ. Exosome-mediated microRNA signaling from breast cancer cells is altered by the anti-angiogenesis agent docosahexaenoic acid (DHA). Mol Cancer (2015) 14:133. doi: 10.1186/s12943-015-0400-7
71. Alexander M, Hu R, Runtsch MC, Kagele DA, Mosbruger TL, Tolmachova T, et al. Exosome-delivered microRNAs modulate the inflammatory response to endotoxin. Nat Commun (2015) 6:7321. doi: 10.1038/ncomms8321
72. Mathieu M, Martin-Jaular L, Lavieu G, Thery C. Specificities of secretion and uptake of exosomes and other extracellular vesicles for cell-to-cell communication. Nat Cell Biol (2019) 21:9–17. doi: 10.1038/s41556-018-0250-9
73. Gonda A, Kabagwira J, Senthil GN, Wall NR. Internalization of Exosomes through Receptor-Mediated Endocytosis. Mol Cancer Res (2019) 17:337–47. doi: 10.1158/1541-7786.MCR-18-0891
74. Jadli AS, Ballasy N, Edalat P, Patel VB. Inside(sight) of tiny communicator: exosome biogenesis, secretion, and uptake. Mol Cell Biochem (2020) 467:77–94. doi: 10.1007/s11010-020-03703-z
75. Murao A, Brenner M, Aziz M, Wang P. Exosomes in Sepsis. Front Immunol (2020) 11:2140. doi: 10.3389/fimmu.2020.02140
76. Benz F, Roy S, Trautwein C, Roderburg C, Luedde T. Circulating MicroRNAs as Biomarkers for Sepsis. Int J Mol Sci (2016) 17:1–17. doi: 10.3390/ijms17010078
77. Moon HG, Yang J, Zheng Y, Jin Y. MiR-15a/16 regulates macrophage phagocytosis after bacterial infection. J Immunol (2014) 193:4558–67. doi: 10.4049/jimmunol.1401372
78. Wang X, Wang X, Liu X, Wang X, Xu J, Hou S, et al. MiR-15a/16 are upreuglated in the serum of neonatal sepsis patients and inhibit the LPS-induced inflammatory pathway. Int J Clin Exp Med (2015) 8:5683–90.
79. Lou Y, Huang Z. microRNA-15a-5p participates in sepsis by regulating the inflammatory response of macrophages and targeting TNIP2. Exp Ther Med (2020) 19:3060–8. doi: 10.3892/etm.2020.8547
80. Jiao Y, Li W, Wang W, Tong X, Xia R, Fan J, et al. Platelet-derived exosomes promote neutrophil extracellular trap formation during septic shock. Crit Care (2020) 24:380. doi: 10.1186/s13054-020-03082-3
81. Zhou R, Li X, Hu G, Gong AY, Drescher KM, Chen XM. MiR-16 targets transcriptional corepressor SMRT and modulates NF-kappaB-regulated transactivation of interleukin-8 gene. PloS One (2012) 7:e30772. doi: 10.1371/journal.pone.0030772
82. Su Y, Song X, Teng J, Zhou X, Dong Z, Li P, et al. Mesenchymal stem cells-derived extracellular vesicles carrying microRNA-17 inhibits macrophage apoptosis in lipopolysaccharide-induced sepsis. Int Immunopharmacol (2021) 95:107408. doi: 10.1016/j.intimp.2021.107408
83. Jiang Y, Zhou H, Ma D, Chen ZK, Cai X. MicroRNA-19a and CD22 Comprise a Feedback Loop for B Cell Response in Sepsis. Med Sci Monit (2015) 21:1548–55. doi: 10.12659/MSM.894321
84. Gantier MP, Stunden HJ, McCoy CE, Behlke MA, Wang D, Kaparakis-Liaskos M, et al. A MiR-19 regulon that controls NF-kappaB signaling. Nucleic Acids Res (2012) 40:8048–58. doi: 10.1093/nar/gks521
85. Xue Z, Xi Q, Liu H, Guo X, Zhang J, Zhang Z, et al. MiR-21 promotes NLRP3 inflammasome activation to mediate pyroptosis and endotoxic shock. Cell Death Dis (2019) 10:461. doi: 10.1038/s41419-019-1713-z
86. McClure C, Brudecki L, Ferguson DA, Yao ZQ, Moorman JP, McCall CE, et al. MicroRNA 21 (MiR-21) and MiR-181b couple with NFI-A to generate myeloid-derived suppressor cells and promote immunosuppression in late sepsis. Infect Immun (2014) 82:3816–25. doi: 10.1128/IAI.01495-14
87. McClure C, McPeak MB, Youssef D, Yao ZQ, McCall CE, El Gazzar M. Stat3 and C/EBPbeta synergize to induce MiR-21 and MiR-181b expression during sepsis. Immunol Cell Biol (2017) 95:42–55. doi: 10.1038/icb.2016.63
88. Xie W, Chen L, Chen L, Kou Q. Silencing of long non-coding RNA MALAT1 suppresses inflammation in septic mice: role of microRNA-23a in the down-regulation of MCEMP1 expression. Inflammation Res (2020) 69:179–90. doi: 10.1007/s00011-019-01306-z
89. Si X, Cao D, Chen J, Nie Y, Jiang Z, Chen MY, et al. MiR23a downregulation modulates the inflammatory response by targeting ATG12mediated autophagy. Mol Med Rep (2018) 18:1524–30. doi: 10.3892/mmr.2018.9081
90. Jiang T, Sun L, Zhu J, Li N, Gu H, Zhang Y, et al. MicroRNA-23a-3p promotes macrophage M1 polarization and aggravates lipopolysaccharide-induced acute lung injury by regulating PLK1/STAT1/STAT3 signalling. Int J Exp Pathol (2022). doi: 10.1111/iep.12445
91. Cheng Q, Tang L, Wang Y. Regulatory role of MiRNA-26a in neonatal sepsis. Exp Ther Med (2018) 16:4836–42. doi: 10.3892/etm.2018.6779
92. Szilagyi B, Fejes Z, Poliska S, Pocsi M, Czimmerer Z, Patsalos A, et al. Reduced MiR-26b Expression in Megakaryocytes and Platelets Contributes to Elevated Level of Platelet Activation Status in Sepsis. Int J Mol Sci (2020) 21:1–22. doi: 10.3390/ijms21030866
93. Wang L, Zhong Q, Feng Y, Tang X, Wang Q, Zou Y, et al. Long noncoding RNA TUG1 is downregulated in sepsis and may sponge MiR-27a to downregulate tumor necrosis factor-alpha. J Int Med Res (2020) 48:300060520910638. doi: 10.1177/0300060520910638
94. Wang Z, Ruan Z, Mao Y, Dong W, Zhang Y, Yin N, et al. MiR-27a is up regulated and promotes inflammatory response in sepsis. Cell Immunol (2014) 290:190–5. doi: 10.1016/j.cellimm.2014.06.006
95. Sun J, Sun X, Chen J, Liao X, He Y, Wang J, et al. microRNA-27b shuttled by mesenchymal stem cell-derived exosomes prevents sepsis by targeting JMJD3 and downregulating NF-kappaB signaling pathway. Stem Cell Res Ther (2021) 12:14. doi: 10.1186/s13287-020-02068-w
96. Yuan FH, Chen YL, Zhao Y, Liu ZM, Nan CC, Zheng BL, et al. microRNA-30a inhibits the liver cell proliferation and promotes cell apoptosis through the JAK/STAT signaling pathway by targeting SOCS-1 in rats with sepsis. J Cell Physiol (2019) 234:17839–53. doi: 10.1002/jcp.28410
97. Ling L, Zhang SH, Zhi LD, Li H, Wen QK, Li G, et al. MicroRNA-30e promotes hepatocyte proliferation and inhibits apoptosis in cecal ligation and puncture-induced sepsis through the JAK/STAT signaling pathway by binding to FOSL2. BioMed Pharmacother (2018) 104:411–9. doi: 10.1016/j.biopha.2018.05.042
98. Chen S, Ding R, Hu Z, Yin X, Xiao F, Zhang W, et al. MicroRNA-34a Inhibition Alleviates Lung Injury in Cecal Ligation and Puncture Induced Septic Mice. Front Immunol (2020) 11:1829. doi: 10.3389/fimmu.2020.01829
99. Cheng DL, Fang HX, Liang Y, Zhao Y, Shi CS. MicroRNA-34a promotes iNOS secretion from pulmonary macrophages in septic suckling rats through activating STAT3 pathway. BioMed Pharmacother (2018) 105:1276–82. doi: 10.1016/j.biopha.2018.06.063
100. Liu F, Peng W, Chen J, Xu Z, Jiang R, Shao Q, et al. Exosomes derived from alveolar epithelial cells promote alveolar macrophage activation mediated by MiR-92a-3p in sepsis-induced acute lung injury. Front Cell Infect Microbiol (2021) 11:646546. doi: 10.3389/fcimb.2021.646546
101. Zhu J, Lin X, Yan C, Yang S, Zhu Z. MicroRNA-98 protects sepsis mice from cardiac dysfunction, liver and lung injury by negatively regulating HMGA2 through inhibiting NF-kappaB signaling pathway. Cell Cycle (2019) 18:1948–64. doi: 10.1080/15384101.2019.1635869
102. Li Y, Zhu H, Pan L, Zhang B, Che H. MicroRNA-103a-3p confers protection against lipopolysaccharide-induced sepsis and consequent multiple organ dysfunction syndrome by targeting HMGB1. Infect Genet Evol (2021) 89:104681. doi: 10.1016/j.meegid.2020.104681
103. Li A, Song W, Qian J, Li Y, He J, Zhang Q, et al. MiR-122 modulates type I interferon expression through blocking suppressor of cytokine signaling 1. Int J Biochem Cell Biol (2013) 45:858–65. doi: 10.1016/j.biocel.2013.01.008
104. Gao D, Zhai A, Qian J, Li A, Li Y, Song W, et al. Down-regulation of suppressor of cytokine signaling 3 by MiR-122 enhances interferon-mediated suppression of hepatitis B virus. Antiviral Res (2015) 118:20–8. doi: 10.1016/j.antiviral.2015.03.001
105. Xu H, Xu SJ, Xie SJ, Zhang Y, Yang JH, Zhang WQ, et al. MicroRNA-122 supports robust innate immunity in hepatocytes by targeting the RTKs/STAT3 signaling pathway. Elife (2019) 8:1–26. doi: 10.7554/eLife.41159
106. Qiu L, Fan H, Jin W, Zhao B, Wang Y, Ju Y, et al. MiR-122-induced down-regulation of HO-1 negatively affects MiR-122-mediated suppression of HBV. Biochem Biophys Res Commun (2010) 398:771–7. doi: 10.1016/j.bbrc.2010.07.021
107. Shi L, Zheng X, Fan Y, Yang X, Li A, Qian J. The contribution of MiR-122 to the innate immunity by regulating toll-like receptor 4 in hepatoma cells. BMC Gastroenterol (2019) 19:130. doi: 10.1186/s12876-019-1048-3
108. Song W, Zhang T, Yang N, Zhang T, Wen R, Liu C. Inhibition of micro RNA MiR-122-5p prevents lipopolysaccharide-induced myocardial injury by inhibiting oxidative stress, inflammation and apoptosis via targeting GIT1. Bioengineered (2021) 12:1902–15. doi: 10.1080/21655979.2021.1926201
109. Pan W, Wei N, Xu W, Wang G, Gong F, Li N. MicroRNA-124 alleviates the lung injury in mice with septic shock through inhibiting the activation of the MAPK signaling pathway by downregulating MAPK14. Int Immunopharmacol (2019) 76:105835. doi: 10.1016/j.intimp.2019.105835
110. Le Y, Chen T, Xun K, Ding T. Expression of the long intergenic non-coding rna (lincrna) of the ned25 gene modulates the microrna-125b, stat3, nitric oxide, and procalcitonin signaling pathways in patients with sepsis. Med Sci Monit (2018) 24:4555–66. doi: 10.12659/MSM.907496
111. Zhang F, Fan X, Bai Y, Lu J, Zheng M, Chen J, et al. MiR-125b regulates procalcitonin production in monocytes by targeting Stat3. Microbes Infect (2016) 18:102–8. doi: 10.1016/j.micinf.2015.09.027
112. Jiang L, Ni J, Shen G, Xia Z, Zhang L, Xia S, et al. Upregulation of endothelial cell-derived exosomal microRNA-125b-5p protects from sepsis-induced acute lung injury by inhibiting topoisomerase II alpha. Inflammation Res (2021) 70:205–16. doi: 10.1007/s00011-020-01415-0
113. Laffont B, Corduan A, Rousseau M, Duchez AC, Lee CH, Boilard E, et al. Platelet microparticles reprogram macrophage gene expression and function. Thromb Haemost (2016) 115:311–23. doi: 10.1160/TH15-05-0389
114. Li Y, Song J, Xie Z, Liu M, Sun K. Long noncoding RNA colorectal neoplasia differentially expressed alleviates sepsis-induced liver injury via regulating MiR-126-5p. IUBMB Life (2020) 72:440–51. doi: 10.1002/iub.2230
115. Wang L, Wang K, Tian Z. MiR-128-3p Inhibits NRP1 Expression and Promotes Inflammatory Response to Acute Kidney Injury in Sepsis. Inflammation (2020) 43:1772–9. doi: 10.1007/s10753-020-01251-8
116. Yang P, Han J, Li S, Luo S, Tu X, Ye Z. MiR-128-3p inhibits apoptosis and inflammation in LPS-induced sepsis by targeting TGFBR2. Open Med (Wars) (2021) 16:274–83. doi: 10.1515/med-2021-0222
117. Yao W, Xu L, Jia X, Li S, Wei L. MicroRNA129 plays a protective role in sepsisinduced acute lung injury through the suppression of pulmonary inflammation via the modulation of the TAK1/NFkappaB pathway. Int J Mol Med (2021) 48:1–13. doi: 10.3892/ijmm.2021.4972
118. Huang X, Hou X, Chuan L, Wei S, Wang J, Yang X, et al. MiR-129-5p alleviates LPS-induced acute kidney injury via targeting HMGB1/TLRs/NF-kappaB pathway. Int Immunopharmacol (2020) 89:107016. doi: 10.1016/j.intimp.2020.107016
119. Yang P, Xiong W, Chen X, Liu J, Ye Z. Overexpression of MiR-129-5p mitigates sepsis-induced acute lung injury by targeting high mobility group box 1. J Surg Res (2020) 256:23–30. doi: 10.1016/j.jss.2020.05.101
120. Cui YL, Wang B, Gao HM, Xing YH, Li J, Li HJ, et al. Interleukin-18 and MiR-130a in severe sepsis patients with thrombocytopenia. Patient Prefer Adherence (2016) 10:313–9. doi: 10.2147/PPA.S95588
121. Liu F, Li Y, Jiang R, Nie C, Zeng Z, Zhao N, et al. MiR-132 inhibits lipopolysaccharide-induced inflammation in alveolar macrophages by the cholinergic anti-inflammatory pathway. Exp Lung Res (2015) 41:261–9. doi: 10.3109/01902148.2015.1004206
122. Qin LY, Wang MX, Zhang H. MiR-133a alleviates renal injury caused by sepsis by targeting BNIP3L. Eur Rev Med Pharmacol Sci (2020) 24:2632–9. doi: 10.26355/eurrev_202003_20532
123. Chen L, Xie W, Wang L, Zhang X, Liu E, Kou Q. MiRNA-133a aggravates inflammatory responses in sepsis by targeting SIRT1. Int Immunopharmacol (2020) 88:106848. doi: 10.1016/j.intimp.2020.106848
124. Zheng G, Pan M, Jin W, Jin G, Huang Y. MicroRNA-135a is up-regulated and aggravates myocardial depression in sepsis via regulating p38 MAPK/NF-kappaB pathway. Int Immunopharmacol (2017) 45:6–12. doi: 10.1016/j.intimp.2017.01.029
125. Zhang X, Liu X, Chang R, Li Y. MiR-139-5p protects septic mice with acute lung injury by inhibiting Toll-like receptor 4/Myeloid differentiation factor 88/Nuclear factor-&mac_kgr;B signaling pathway. Clinics (Sao Paulo) (2021) 76:e2484. doi: 10.6061/clinics/2021/e2484
126. Lin X, Wang Y. MiR-141 is negatively correlated with TLR4 in neonatal sepsis and regulates LPS-induced inflammatory responses in monocytes. Braz J Med Biol Res (2021) 54:e10603. doi: 10.1590/1414-431X2020e10603
127. Zhen J, Chen W. MiR-142 inhibits cecal ligation and puncture (CLP)-induced inflammation via inhibiting PD-L1 expression in macrophages and improves survival in septic mice. BioMed Pharmacother (2018) 97:1479–85. doi: 10.1016/j.biopha.2017.11.058
128. Schmidt WM, Spiel AO, Jilma B, Wolzt M, Muller M. In vivo profile of the human leukocyte microRNA response to endotoxemia. Biochem Biophys Res Commun (2009) 380:437–41. doi: 10.1016/j.bbrc.2008.12.190
129. Prakhar P, Holla S, Ghorpade DS, Gilleron M, Puzo G, Udupa V, et al. Ac2PIM-responsive MiR-150 and MiR-143 target receptor-interacting protein kinase 2 and transforming growth factor beta-activated kinase 1 to suppress NOD2-induced immunomodulators. J Biol Chem (2015) 290:26576–86. doi: 10.1074/jbc.M115.662817
130. Teng Y, Zhang R, Liu C, Zhou L, Wang H, Zhuang W, et al. MiR-143 inhibits interleukin-13-induced inflammatory cytokine and mucus production in nasal epithelial cells from allergic rhinitis patients by targeting IL13Ralpha1. Biochem Biophys Res Commun (2015) 457:58–64. doi: 10.1016/j.bbrc.2014.12.058
131. Chen Y, Peng H, Zhou S, Zhuang Y. ADAR1 is targeted by MiR-143 to regulate IL-1beta-induced endothelial activation through the NFkappaB pathway. Int J Biochem Cell Biol (2017) 89:25–33. doi: 10.1016/j.biocel.2017.05.021
132. Wang S, Tan Y, Yang T, Liu C, Li R. Pulmonary AngII promotes LPS-induced lung inflammation by regulating microRNA-143. Cytotechnology (2021) 73:745–54. doi: 10.1007/s10616-021-00493-0
133. Wang Y, Li H, Shi Y, Wang S, Xu Y, Li H, et al. MiR-143-3p impacts on pulmonary inflammatory factors and cell apoptosis in mice with mycoplasmal pneumonia by regulating TLR4/MyD88/NF-kappaB pathway. Biosci Rep (2020) 40:1–10. doi: 10.1042/BSR20193419
134. Zhao X, Liu D, Gong W, Zhao G, Liu L, Yang L, et al. The toll-like receptor 3 ligand, poly(I:C), improves immunosuppressive function and therapeutic effect of mesenchymal stem cells on sepsis via inhibiting MiR-143. Stem Cells (2014) 32:521–33. doi: 10.1002/stem.1543
135. Cao X, Zhang C, Zhang X, Chen Y, Zhang H. MiR-145 negatively regulates TGFBR2 signaling responsible for sepsis-induced acute lung injury. BioMed Pharmacother (2019) 111:852–8. doi: 10.1016/j.biopha.2018.12.138
136. Ma F, Li Z, Cao J, Kong X, Gong G. A TGFBR2/SMAD2/DNMT1/MiR-145 negative regulatory loop is responsible for LPS-induced sepsis. BioMed Pharmacother (2019) 112:108626. doi: 10.1016/j.biopha.2019.108626
137. Gao N, Dong L. MicroRNA-146 regulates the inflammatory cytokines expression in vascular endothelial cells during sepsis. Pharmazie (2017) 72:700–4. doi: 10.1691/ph.2017.7600
138. Funahashi Y, Kato N, Masuda T, Nishio F, Kitai H, Ishimoto T, et al. MiR-146a targeted to splenic macrophages prevents sepsis-induced multiple organ injury. Lab Invest (2019) 99:1130–42. doi: 10.1038/s41374-019-0190-4
139. Pan Y, Wang J, Xue Y, Zhao J, Li D, Zhang S, et al. GSKJ4 Protects Mice Against Early Sepsis via Reducing Proinflammatory Factors and Up-Regulating MiR-146a. Front Immunol (2018) 9:2272. doi: 10.3389/fimmu.2018.02272
140. An R, Feng J, Xi C, Xu J, Sun L. MiR-146a Attenuates Sepsis-Induced Myocardial Dysfunction by Suppressing IRAK1 and TRAF6 via Targeting ErbB4 Expression. Oxid Med Cell Longev (2018) 2018:7163057. doi: 10.1155/2018/7163057
141. Bai X, Zhang J, Cao M, Han S, Liu Y, Wang K, et al. MicroRNA-146a protects against LPS-induced organ damage by inhibiting Notch1 in macrophage. Int Immunopharmacol (2018) 63:220–6. doi: 10.1016/j.intimp.2018.07.040
142. Mohnle P, Schutz SV, van der Heide V, Hubner M, Luchting B, Sedlbauer J, et al. MicroRNA-146a controls Th1-cell differentiation of human CD4+ T lymphocytes by targeting PRKCepsilon. Eur J Immunol (2015) 45:260–72. doi: 10.1002/eji.201444667
143. Pfeiffer D, Rossmanith E, Lang I, Falkenhagen D. MiR-146a, MiR-146b, and MiR-155 increase expression of IL-6 and IL-8 and support HSP10 in an In vitro sepsis model. PloS One (2017) 12:e0179850. doi: 10.1371/journal.pone.0179850
144. Wang S, Yang Y, Suen A, Zhu J, Williams B, Hu J, et al. Role of extracellular microRNA-146a-5p in host innate immunity and bacterial sepsis. iScience (2021) 24:103441. doi: 10.1016/j.isci.2021.103441
145. Huang H, Zhu J, Gu L, Hu J, Feng X, Huang W, et al. TLR7 Mediates Acute Respiratory Distress Syndrome in Sepsis by Sensing Extracellular MiR-146a. Am J Respir Cell Mol Biol (2022). doi: 10.1165/rcmb.2021-0551OC
146. Liu Q, Wang Y, Zheng Q, Dong X, Xie Z, Panayi A, et al. MicroRNA-150 inhibits myeloid-derived suppressor cells proliferation and function through negative regulation of ARG-1 in sepsis. Life Sci (2021) 278:119626. doi: 10.1016/j.lfs.2021.119626
147. Tacke F, Roderburg C, Benz F, Cardenas DV, Luedde M, Hippe HJ, et al. Levels of circulating MiR-133a are elevated in sepsis and predict mortality in critically ill patients. Crit Care Med (2014) 42:1096–104. doi: 10.1097/CCM.0000000000000131
148. Sari AN, Korkmaz B, Serin MS, Kacan M, Unsal D, Buharalioglu CK, et al. Effects of 5,14-HEDGE, a 20-HETE mimetic, on lipopolysaccharide-induced changes in MyD88/TAK1/IKKbeta/IkappaB-alpha/NF-kappaB pathway and circulating MiR-150, MiR-223, and MiR-297 levels in a rat model of septic shock. Inflammation Res (2014) 63:741–56. doi: 10.1007/s00011-014-0747-z
149. Rajput C, Tauseef M, Farazuddin M, Yazbeck P, Amin MR, Avin Br V, et al. MicroRNA-150 suppression of angiopoetin-2 generation and signaling is crucial for resolving vascular injury. Arterioscler Thromb Vasc Biol (2016) 36:380–8. doi: 10.1161/ATVBAHA.115.306997
150. Ma Y, Liu Y, Hou H, Yao Y, Meng H. MiR-150 predicts survival in patients with sepsis and inhibits LPS-induced inflammatory factors and apoptosis by targeting NF-kappaB1 in human umbilical vein endothelial cells. Biochem Biophys Res Commun (2018) 500:828–37. doi: 10.1016/j.bbrc.2018.04.168
151. Wei S, Liu Q. Long noncoding RNA MALAT1 modulates sepsis-induced cardiac inflammation through the MiR-150-5p/NF-kappaB axis. Int J Clin Exp Pathol (2019) 12:3311–9.
152. Zhu XG, Zhang TN, Wen R, Liu CF. Overexpression of MiR-150-5p Alleviates Apoptosis in Sepsis-Induced Myocardial Depression. BioMed Res Int (2020) 2020:3023186. doi: 10.1155/2020/3023186
153. Liu L, Yan LN, Sui Z. MicroRNA-150 affects endoplasmic reticulum stress via MALAT1-MiR-150 axis-mediated NF-kappaB pathway in LPS-challenged HUVECs and septic mice. Life Sci (2021) 265:118744. doi: 10.1016/j.lfs.2020.118744
154. Deng X, Lin Z, Zuo C, Fu Y. Upregulation of MiR-150-5p alleviates LPS-induced inflammatory response and apoptosis of RAW264. 7 macrophages by Targeting Notch1. Open Life Sci (2020) 15:544–52. doi: 10.1515/biol-2020-0058
155. Chen S, Zhu H, Sun J, Zhu L, Qin L, Wan J. Anti-inflammatory effects of MiR-150 are associated with the downregulation of STAT1 in macrophages following lipopolysaccharide treatment. Exp Ther Med (2021) 22:1049. doi: 10.3892/etm.2021.10483
156. Shi L, Zhang Y, Xia Y, Li C, Song Z, Zhu J. MiR-150-5p protects against septic acute kidney injury via repressing the MEKK3/JNK pathway. Cell Signal (2021) 86:110101. doi: 10.1016/j.cellsig.2021.110101
157. Wang X, Li XL, Qin LJ. The lncRNA XIST/MiR-150-5p/c-Fos axis regulates sepsis-induced myocardial injury via TXNIP-modulated pyroptosis. Lab Invest (2021) 101:1118–29. doi: 10.1038/s41374-021-00607-4
158. Hawez A, Taha D, Algaber A, Madhi R, Rahman M, Thorlacius H. MiR-155 regulates neutrophil extracellular trap formation and lung injury in abdominal sepsis. J Leukoc Biol (2022) 111:391–400. doi: 10.1002/JLB.3A1220-789RR
159. Etzrodt V, Idowu TO, Schenk H, Seeliger B, Prasse A, Thamm K, et al. Role of endothelial microRNA 155 on capillary leakage in systemic inflammation. Crit Care (2021) 25:76. doi: 10.1186/s13054-021-03500-0
160. Cao YY, Wang Z, Wang ZH, Jiang XG, Lu WH. Inhibition of MiR-155 alleviates sepsis-induced inflammation and intestinal barrier dysfunction by inactivating NF-kappaB signaling. Int Immunopharmacol (2021) 90:107218. doi: 10.1016/j.intimp.2020.107218
161. Vasques-Novoa F, Laundos TL, Cerqueira RJ, Quina-Rodrigues C, Soares-Dos-Reis R, Baganha F, et al. MicroRNA-155 amplifies nitric oxide/cgmp signaling and impairs vascular angiotensin ii reactivity in septic shock. Crit Care Med (2018) 46:e945–54. doi: 10.1097/CCM.0000000000003296
162. Zhou Y, Song Y, Shaikh Z, Li H, Zhang H, Caudle Y, et al. MicroRNA-155 attenuates late sepsis-induced cardiac dysfunction through JNK and beta-arrestin 2. Oncotarget (2017) 8:47317–29. doi: 10.18632/oncotarget.17636
163. Lv X, Zhang Y, Cui Y, Ren Y, Li R, Rong Q. Inhibition of microRNA155 relieves sepsisinduced liver injury through inactivating the JAK/STAT pathway. Mol Med Rep (2015) 12:6013–8. doi: 10.3892/mmr.2015.4188
164. Wang H, Bei Y, Huang P, Zhou Q, Shi J, Sun Q, et al. Inhibition of MiR-155 protects against lps-induced cardiac dysfunction and apoptosis in mice. Mol Ther Nucleic Acids (2016) 5:e374. doi: 10.1038/mtna.2016.80
165. Ma XF, Qin J, Guo XH. MiR-181-5p protects mice from sepsis via repressing HMGB1 in an experimental model. Eur Rev Med Pharmacol Sci (2020) 24:9712–20. doi: 10.26355/eurrev_202009_23063
166. Zhu J, Wang FL, Wang HB, Dong N, Zhu XM, Wu Y, et al. TNF-alpha mRNA is negatively regulated by microRNA-181a-5p in maturation of dendritic cells induced by high mobility group box-1 protein. Sci Rep (2017) 7:12239. doi: 10.1038/s41598-017-12492-3
167. Li W, Qiu X, Jiang H, Han Y, Wei D, Liu J. Downregulation of MiR-181a protects mice from LPS-induced acute lung injury by targeting Bcl-2. BioMed Pharmacother (2016) 84:1375–82. doi: 10.1016/j.biopha.2016.10.065
168. Ling L, Zhi L, Wang H, Deng Y, Gu C. MicroRNA-181b inhibits inflammatory response and reduces myocardial injury in sepsis by downregulating HMGB1. Inflammation (2021) 44:1263–73. doi: 10.1007/s10753-020-01411-w
169. Sun X, Icli B, Wara AK, Belkin N, He S, Kobzik L, et al. MicroRNA-181b regulates NF-kappaB-mediated vascular inflammation. J Clin Invest (2012) 122:1973–90. doi: 10.1172/JCI61495
170. Dan C, Jinjun B, Zi-Chun H, Lin M, Wei C, Xu Z, et al. Modulation of TNF-alpha mRNA stability by human antigen R and MiR181s in sepsis-induced immunoparalysis. EMBO Mol Med (2015) 7:140–57. doi: 10.15252/emmm.201404797
171. Li M, Li W, Ren FQ, Zhang ML. MiRNA-186 improves sepsis induced renal injury via PTEN/PI3K/AKT/P53 pathway. Open Med (Wars) (2020) 15:254–60. doi: 10.1515/med-2020-0036
172. Shen H, Xie K, Peng M, Wang X. MiR-186-5p Downregulates NAMPT and Functions as a Potential Therapeutic Target for Sepsis-Induced Coagulation Disorders. Comput Intell Neurosci (2022) 2022:1714041. doi: 10.1155/2022/1714041
173. Zhai Y, Ding N. MicroRNA-194 participates in endotoxemia induced myocardial injury via promoting apoptosis. Eur Rev Med Pharmacol Sci (2018) 22:2077–83. doi: 10.26355/eurrev_201804_14739
174. Zheng D, Yu Y, Li M, Wang G, Chen R, Fan GC, et al. Inhibition of MicroRNA 195 Prevents Apoptosis and Multiple-Organ Injury in Mouse Models of Sepsis. J Infect Dis (2016) 213:1661–70. doi: 10.1093/infdis/jiv760
175. Xia H, Zhao H, Yang W, Luo X, Wei J, Xia H. MiR-195-5p represses inflammation, apoptosis, oxidative stress, and endoplasmic reticulum stress in sepsis-induced myocardial injury by targeting activating transcription factor 6. Cell Biol Int (2022) 46:243–54. doi: 10.1002/cbin.11726
176. Du X, Tian D, Wei J, Yan C, Hu P, Wu X, et al. MiR-199a-5p exacerbated intestinal barrier dysfunction through inhibiting surfactant protein d and activating nf-kappab pathway in sepsis. Mediators Inflammation (2020) 2020:8275026. doi: 10.1155/2020/8275026
177. Liu Q, Du J, Yu X, Xu J, Huang F, Li X, et al. MiRNA-200c-3p is crucial in acute respiratory distress syndrome. Cell Discovery (2017) 3:17021. doi: 10.1038/celldisc.2017.21
178. Chen W, Ma X, Zhang P, Li Q, Liang X, Liu J. MiR-212-3p inhibits LPS-induced inflammatory response through targeting HMGB1 in murine macrophages. Exp Cell Res (2017) 350:318–26. doi: 10.1016/j.yexcr.2016.12.008
179. Sang Z, Zhang P, Wei Y, Dong S. MiR-214-3p attenuates sepsis-induced myocardial dysfunction in mice by inhibiting autophagy through PTEN/AKT/mTOR Pathway. BioMed Res Int (2020) 2020:1409038. doi: 10.1155/2020/1409038
180. Yang J, Do-Umehara HC, Zhang Q, Wang H, Hou C, Dong H, et al. MiR-221-5p-mediated downregulation of JNK2 aggravates acute lung injury. Front Immunol (2021) 12:700933. doi: 10.3389/fimmu.2021.700933
181. Leuenberger C, Schuoler C, Bye H, Mignan C, Rechsteiner T, Hillinger S, et al. MicroRNA-223 controls the expression of histone deacetylase 2: a novel axis in COPD. J Mol Med (Berl) (2016) 94:725–34. doi: 10.1007/s00109-016-1388-1
182. Liu D, Wang Z, Wang H, Ren F, Li Y, Zou S, et al. The protective role of MiR-223 in sepsis-induced mortality. Sci Rep (2020) 10:17691. doi: 10.1038/s41598-020-74965-2
183. Szilagyi B, Fejes Z, Rusznyak A, Fenyvesi F, Pocsi M, Halmi S, et al. Platelet microparticles enriched in mir-223 reduce icam-1-dependent vascular inflammation in septic conditions. Front Physiol (2021) 12:658524. doi: 10.3389/fphys.2021.658524
184. Wang Z, Yan J, Yang F, Wang D, Lu Y, Liu L. MicroRNA-326 prevents sepsis-induced acute lung injury via targeting TLR4. Free Radic Res (2020) 54:408–18. doi: 10.1080/10715762.2020.1781847
185. Sheng B, Zhao L, Zang X, Zhen J, Chen W. MiR-375 ameliorates sepsis by downregulating MiR-21 level via inhibiting JAK2-STAT3 signaling. BioMed Pharmacother (2017) 86:254–61. doi: 10.1016/j.biopha.2016.11.147
186. Liu Z, Tang C, He L, Yang D, Cai J, Zhu J, et al. The negative feedback loop of NF-kappaB/MiR-376b/NFKBIZ in septic acute kidney injury. JCI Insight (2020) 5:1–17. doi: 10.1172/jci.insight.142272
187. Ling Y, Li ZZ, Zhang JF, Zheng XW, Lei ZQ, Chen RY, et al. MicroRNA-494 inhibition alleviates acute lung injury through Nrf2 signaling pathway via NQO1 in sepsis-associated acute respiratory distress syndrome. Life Sci (2018) 210:1–8. doi: 10.1016/j.lfs.2018.08.037
188. Wang HF, Li Y, Wang YQ, Li HJ, Dou L. MicroRNA-494-3p alleviates inflammatory response in sepsis by targeting TLR6. Eur Rev Med Pharmacol Sci (2019) 23:2971–7. doi: 10.26355/eurrev_201904_17578
189. Wang C, Zhu Z. MiR-499a suppresses LPS-induced human vascular endothelial cell inflammatory response and apoptosis by regulating STAT1. Int J Clin Exp Pathol (2019) 12:4232–41.
190. Liu S, Zhao L, Zhang L, Qiao L, Gao S. Downregulation of MiR-574-5p inhibits HK-2 cell viability and predicts the onset of acute kidney injury in sepsis patients. Ren Fail (2021) 43:942–8. doi: 10.1080/0886022X.2021.1939051
191. Ling P, Tang R, Wang H, Deng X, Chen J. MiR-1184 regulates inflammatory responses and cell apoptosis by targeting TRADD in an LPS-induced cell model of sepsis. Exp Ther Med (2021) 21:630. doi: 10.3892/etm.2021.10062
192. Wang D, Han L. Downregulation of MiR-1184 serves as a diagnostic biomarker in neonatal sepsis and regulates LPS-induced inflammatory response by inhibiting IL-16 in monocytes. Exp Ther Med (2021) 21:350. doi: 10.3892/etm.2021.9781
193. Ma J, Xu LY, Sun QH, Wan XY, Bing L. Inhibition of MiR-1298-5p attenuates sepsis lung injury by targeting SOCS6. Mol Cell Biochem (2021) 476:3745–56. doi: 10.1007/s11010-021-04170-w
194. Zhou W, Wang J, Li Z, Li J, Sang M. MicroRNA-2055b inhibits HMGB1 expression in LPS-induced sepsis. Int J Mol Med (2016) 38:312–8. doi: 10.3892/ijmm.2016.2613
195. Wang H, Zhang P, Chen W, Feng D, Jia Y, Xie L. Serum microRNA signatures identified by Solexa sequencing predict sepsis patients' mortality: a prospective observational study. PloS One (2012) 7:e38885. doi: 10.1371/journal.pone.0038885
196. Caserta S, Kern F, Cohen J, Drage S, Newbury SF, Llewelyn MJ. Circulating plasma micrornas can differentiate human sepsis and systemic inflammatory response syndrome (SIRS). Sci Rep (2016) 6:28006. doi: 10.1038/srep28006
197. Reithmair M, Buschmann D, Marte M, Kirchner B, Hagl D, Kaufmann I, et al. Cellular and extracellular MiRNAs are blood-compartment-specific diagnostic targets in sepsis. J Cell Mol Med (2017) 21:2403–11. doi: 10.1111/jcmm.13162
198. Ge QM, Huang CM, Zhu XY, Bian F, Pan SM. Differentially expressed MiRNAs in sepsis-induced acute kidney injury target oxidative stress and mitochondrial dysfunction pathways. PloS One (2017) 12:e0173292. doi: 10.1371/journal.pone.0173292
199. Zhang W, Jia J, Liu Z, Si D, Ma L, Zhang G. Circulating microRNAs as biomarkers for Sepsis secondary to pneumonia diagnosed via Sepsis 3. 0. BMC Pulm Med (2019) 19:93. doi: 10.1186/s12890-019-0836-4
200. Ng PC, Chan KYY, Yuen TP, Sit T, Lam HS, Leung KT, et al. Plasma MiR-1290 is a novel and specific biomarker for early diagnosis of necrotizing enterocolitis-biomarker discovery with prospective cohort evaluation. J Pediatr (2019) 205:83–90 e10. doi: 10.1016/j.jpeds.2018.09.031
201. Liu J, Shi K, Chen M, Xu L, Hong J, Hu B, et al. Elevated MiR-155 expression induces immunosuppression via CD39(+) regulatory T-cells in sepsis patient. Int J Infect Dis (2015) 40:135–41. doi: 10.1016/j.ijid.2015.09.016
202. Zhu Z, Liang L, Zhang R, Wei Y, Su L, Tejera P, et al. Whole blood microRNA markers are associated with acute respiratory distress syndrome. Intensive Care Med Exp (2017) 5:38. doi: 10.1186/s40635-017-0155-0
203. Vasilescu C, Rossi S, Shimizu M, Tudor S, Veronese A, Ferracin M, et al. MicroRNA fingerprints identify MiR-150 as a plasma prognostic marker in patients with sepsis. PloS One (2009) 4:e7405. doi: 10.1371/journal.pone.0007405
204. Mohnle P, Hirschberger S, Hinske LC, Briegel J, Hubner M, Weis S, et al. MicroRNAs 143 and 150 in whole blood enable detection of T-cell immunoparalysis in sepsis. Mol Med (2018) 24:54. doi: 10.1186/s10020-018-0056-z
205. Zheng G, Qiu G, Ge M, Meng J, Zhang G, Wang J, et al. MiR-10a in Peripheral Blood Mononuclear Cells Is a Biomarker for Sepsis and Has Anti-Inflammatory Function. Mediators Inflammation (2020) 2020:4370983. doi: 10.1155/2020/4370983
206. Wang H, Zhang P, Chen W, Feng D, Jia Y, Xie LX. Evidence for serum MiR-15a and MiR-16 levels as biomarkers that distinguish sepsis from systemic inflammatory response syndrome in human subjects. Clin Chem Lab Med (2012) 50:1423–8. doi: 10.1515/cclm-2011-0826
207. Wang HJ, Zhang PJ, Chen WJ, Feng D, Jia YH, Xie LX. Four serum microRNAs identified as diagnostic biomarkers of sepsis. J Trauma Acute Care Surg (2012) 73:850–4. doi: 10.1097/TA.0b013e31825a7560
208. Wang HJ, Deng J, Wang JY, Zhang PJ, Xin Z, Xiao K, et al. Serum MiR-122 levels are related to coagulation disorders in sepsis patients. Clin Chem Lab Med (2014) 52:927–33. doi: 10.1515/cclm-2013-0899
209. Wang W, Lou C, Gao J, Zhang X, Du Y. LncRNA SNHG16 reverses the effects of MiR-15a/16 on LPS-induced inflammatory pathway. BioMed Pharmacother (2018) 106:1661–7. doi: 10.1016/j.biopha.2018.07.105
210. Petejova N, Martinek A, Zadrazil J, Klementa V, Pribylova L, Bris R, et al. Expression and 7-day time course of circulating microRNAs in septic patients treated with nephrotoxic antibiotic agents. BMC Nephrol (2022) 23:111. doi: 10.1186/s12882-022-02726-6
211. Fouda E, Elrazek Midan DA, Ellaban R, El-Kousy S, Arafat E. The diagnostic and prognostic role of MiRNA 15b and MiRNA 378a in neonatal sepsis. Biochem Biophys Rep (2021) 26:100988. doi: 10.1016/j.bbrep.2021.100988
212. El-Hefnawy SM, Mostafa RG, El Zayat RS, Elfeshawy EM, Abd El-Bari HM, El-Monem Ellaithy MA. Biochemical and molecular study on serum MiRNA-16a and MiRNA- 451 as neonatal sepsis biomarkers. Biochem Biophys Rep (2021) 25:100915. doi: 10.1016/j.bbrep.2021.100915
213. Xu H, Liu X, Ni H. Clinical significance of MiR-19b-3p in patients with sepsis and its regulatory role in the LPS-induced inflammatory response. Eur J Med Res (2020) 25:9. doi: 10.1186/s40001-020-00408-3
214. Na L, Ding H, Xing E, Zhang Y, Gao J, Liu B, et al. The predictive value of microRNA-21 for sepsis risk and its correlation with disease severity, systemic inflammation, and 28-day mortality in sepsis patients. J Clin Lab Anal (2020) 34:e23103. doi: 10.1002/jcla.23103
215. Sankar S, Maruthai K, Bobby Z, Adhisivam B. MicroRNA Expression in Neonates with Late-onset Sepsis - A Cross-sectional Comparative Study. Immunol Invest (2022) 1–13. doi: 10.1080/08820139.2021.2020282:1-13
216. Zhang H, Che L, Wang Y, Zhou H, Gong H, Man X, et al. Deregulated microRNA-22-3p in patients with sepsis-induced acute kidney injury serves as a new biomarker to predict disease occurrence and 28-day survival outcomes. Int Urol Nephrol (2021) 53:2107–16. doi: 10.1007/s11255-021-02784-z
217. Fatmi A, Rebiahi SA, Chabni N, Zerrouki H, Azzaoui H, Elhabiri Y, et al. MiRNA-23b as a biomarker of culture-positive neonatal sepsis. Mol Med (2020) 26:94. doi: 10.1186/s10020-020-00217-8
218. Yao L, Liu Z, Zhu J, Li B, Chai C, Tian Y. Clinical evaluation of circulating microRNA-25 level change in sepsis and its potential relationship with oxidative stress. Int J Clin Exp Pathol (2015) 8:7675–84.
219. Zhang J, Wang CJ, Tang XM, Wei YK. Urinary MiR-26b as a potential biomarker for patients with sepsis-associated acute kidney injury: a Chinese population-based study. Eur Rev Med Pharmacol Sci (2018) 22:4604–10. doi: 10.26355/eurrev_201807_15518
220. Abdelaleem OO, Mohammed SR, El Sayed HS, Hussein SK, Ali DY, Abdelwahed MY, et al. Serum MiR-34a-5p and MiR-199a-3p as new biomarkers of neonatal sepsis. PloS One (2022) 17:e0262339. doi: 10.1371/journal.pone.0262339
221. Zhang C, Li X, Liu N, Feng Z, Zhang C. MicroRNA-96 is downregulated in sepsis neonates and attenuates lpsinduced inflammatory response by inhibiting il-16 in monocytes. Comb Chem High Throughput Screen (2022) 25:90–6. doi: 10.2174/1386207323666201211091312
222. Zhang J, Xu X, Wang M. Clinical significance of serum MiR-101-3p expression in patients with neonatal sepsis. Per Med (2021) 18:541–50. doi: 10.2217/pme-2020-0182
223. Yang M, Zhao L, Sun M. Diagnostic value of MiR-103 in patients with sepsis and noninfectious SIRS and its regulatory role in LPS-induced inflammatory response by targeting TLR4. Int J Genomics (2020) 2020:2198308. doi: 10.1155/2020/2198308
224. Wang Q, Feng Q, Zhang Y, Zhou S, Chen H. Decreased microRNA 103 and microRNA 107 predict increased risks of acute respiratory distress syndrome and 28-day mortality in sepsis patients. Med (Baltimore) (2020) 99:e20729. doi: 10.1097/MD.0000000000020729
225. Abou El-Khier NT, Zaki ME, Alkasaby NM. Study of MicroRNA-122 as a diagnostic biomarker of sepsis. Egypt J Immunol (2019) 26:105–16.
226. Rahmel T, Schafer ST, Frey UH, Adamzik M, Peters J. Increased circulating microRNA-122 is a biomarker for discrimination and risk stratification in patients defined by sepsis-3 criteria. PloS One (2018) 13:e0197637. doi: 10.1371/journal.pone.0197637
227. Wang H, Yu B, Deng J, Jin Y, Xie L. Serum MiR-122 correlates with short-term mortality in sepsis patients. Crit Care (2014) 18:704. doi: 10.1186/s13054-014-0704-9
228. Roderburg C, Benz F, Koch A, Loosen SH, Spehlmann M, Luedde M, et al. A combined score of circulating miRNAs allows outcome prediction in critically ill patients. J Clin Med (2019) 8:1–12. doi: 10.3390/jcm8101644
229. He F, Zhang C, Huang Q. Long noncoding RNA nuclear enriched abundant transcript 1/MiRNA-124 axis correlates with increased disease risk, elevated inflammation, deteriorative disease condition, and predicts decreased survival of sepsis. Med (Baltimore) (2019) 98:e16470. doi: 10.1097/MD.0000000000016470
230. Liu W, Geng F, Yu L. Long non-coding RNA MALAT1/microRNA 125a axis presents excellent value in discriminating sepsis patients and exhibits positive association with general disease severity, organ injury, inflammation level, and mortality in sepsis patients. J Clin Lab Anal (2020) 34:e23222. doi: 10.1002/jcla.23222
231. Zhao D, Li S, Cui J, Wang L, Ma X, Li Y. Plasma MiR-125a and MiR-125b in sepsis: Correlation with disease risk, inflammation, severity, and prognosis. J Clin Lab Anal (2020) 34:e23036. doi: 10.1002/jcla.23036
232. Zhu X. MiR-125b but not MiR-125a is upregulated and exhibits a trend to correlate with enhanced disease severity, inflammation, and increased mortality in sepsis patients. J Clin Lab Anal (2020) 34:e23094. doi: 10.1002/jcla.23094
233. Gui F, Peng H, Liu Y. Elevated circulating lnc-ANRIL/MiR-125a axis level predicts higher risk, more severe disease condition, and worse prognosis of sepsis. J Clin Lab Anal (2019) 33:e22917. doi: 10.1002/jcla.22917
234. Chen C, Zhang L, Huang H, Liu S, Liang Y, Xu L, et al. Serum MiR-126-3p level is down-regulated in sepsis patients. Int J Clin Exp Pathol (2018) 11:2605–12.
235. Lin R, Hu H, Li L, Chen G, Luo L, Rao P. The potential of microRNA-126 in predicting disease risk, mortality of sepsis, and its correlation with inflammation and sepsis severity. J Clin Lab Anal (2020) 34:e23408. doi: 10.1002/jcla.23408
236. Vieira Correa PCM, Carneiro DM, da Silva Valente LDS, Diogo FM, Lamarao LM, da Silva Maues JH, et al. Detection of Sepsis in Platelets Using MicroRNAs and Membrane Antigens. Genes (Basel) (2021) 12:1–11. doi: 10.3390/genes12121877
237. Dhas BB, Dirisala VR, Bhat BV. Expression Levels of Candidate Circulating microRNAs in Early-Onset Neonatal Sepsis Compared With Healthy Newborns. Genomics Insights (2018) 11:1178631018797079. doi: 10.1177/1178631018797079
238. Li Y, Lu B, Yu M, Zhai J, Yao Y, Chai Y. Diagnostic value and significance of serum MiR-132 combined with MiR-223 for sepsis-induced cardiomyopathy. Exp Ther Med (2021) 22:1396. doi: 10.3892/etm.2021.10832
239. Roderburg C, Koch A, Benz F, Vucur M, Spehlmann M, Loosen SH, et al. Serum levels of MiR-143 predict survival in critically Ill patients. Dis Markers (2019) 2019:4850472. doi: 10.1155/2019/4850472
240. Han Y, Dai QC, Shen HL, Zhang XW. Diagnostic value of elevated serum MiRNA-143 levels in sepsis. J Int Med Res (2016) 44:875–81. doi: 10.1177/0300060516645003
241. Han Y, Li Y, Jiang Y. The prognostic value of plasma MicroRNA-155 and MicroRNA-146a level in severe sepsis and sepsis-induced acute lung injury patients. Clin Lab (2016) 62:2355–60. doi: 10.7754/Clin.Lab.2016.160511
242. Wang JF, Yu ML, Yu G, Bian JJ, Deng XM, Wan XJ, et al. Serum MiR-146a and MiR-223 as potential new biomarkers for sepsis. Biochem Biophys Res Commun (2010) 394:184–8. doi: 10.1016/j.bbrc.2010.02.145
243. Wang L, Wang HC, Chen C, Zeng J, Wang Q, Zheng L, et al. Differential expression of plasma MiR-146a in sepsis patients compared with non-sepsis-SIRS patients. Exp Ther Med (2013) 5:1101–4. doi: 10.3892/etm.2013.937
244. Karam RA, Zidan HE, Karam NA, Abdel Rahman DM, El-Seifi OS. Diagnostic and prognostic significance of serum MiRNA-146-a expression in Egyptian children with sepsis in a pediatric intensive care unit. J Gene Med (2019) 21:e3128. doi: 10.1002/jgm.3128
245. Chen L, Yu L, Zhang R, Zhu L, Shen W. Correlation of microRNA-146a/b with disease risk, biochemical indices, inflammatory cytokines, overall disease severity, and prognosis of sepsis. Med (Baltimore) (2020) 99:e19754. doi: 10.1097/MD.0000000000019754
246. Chen W, Liu L, Yang J, Wang Y. MicroRNA-146b correlates with decreased acute respiratory distress syndrome risk, reduced disease severity, and lower 28-day mortality in sepsis patients. J Clin Lab Anal (2020) 34:e23510. doi: 10.1002/jcla.23510
247. Trung NT, Lien TT, Sang VV, Hoan NX, Manh ND, Thau NS, et al. Circulating MiR-147b as a diagnostic marker for patients with bacterial sepsis and septic shock. PloS One (2021) 16:e0261228. doi: 10.1371/journal.pone.0261228
248. Roderburg C, Luedde M, Vargas Cardenas D, Vucur M, Scholten D, Frey N, et al. Circulating microRNA-150 serum levels predict survival in patients with critical illness and sepsis. PloS One (2013) 8:e54612. doi: 10.1371/journal.pone.0054612
249. Ma Y, Vilanova D, Atalar K, Delfour O, Edgeworth J, Ostermann M, et al. Genome-wide sequencing of cellular microRNAs identifies a combinatorial expression signature diagnostic of sepsis. PloS One (2013) 8:e75918. doi: 10.1371/journal.pone.0075918
250. How CK, Hou SK, Shih HC, Huang MS, Chiou SH, Lee CH, et al. Expression profile of MicroRNAs in gram-negative bacterial sepsis. Shock (2015) 43:121–7. doi: 10.1097/SHK.0000000000000282
251. Braza-Boils A, Barwari T, Gutmann C, Thomas MR, Judge HM, Joshi A, et al. Circulating MicroRNA levels indicate platelet and leukocyte activation in endotoxemia despite platelet P2Y12 inhibition. Int J Mol Sci (2020) 21:1–13. doi: 10.3390/ijms21082897
252. Yang J, Liao Y, Dai Y, Hu L, Cai Y. Prediction of prognosis in sepsis patients by the SOFA score combined with MiR-150. Adv Clin Exp Med (2022) 31:9–15. doi: 10.17219/acem/142536
253. Wang ZF, Yang YM, Fan H. Diagnostic value of MiR-155 for acute lung injury/acute respiratory distress syndrome in patients with sepsis. J Int Med Res (2020) 48:300060520943070. doi: 10.1177/0300060520943070
254. Liu F, Nie C, Zhao N, Wang Y, Liu Y, Li Y, et al. MiR-155 Alleviates Septic Lung Injury by Inducing Autophagy Via Inhibition of Transforming Growth Factor-beta-Activated Binding Protein 2. Shock (2017) 48:61–8. doi: 10.1097/SHK.0000000000000839
255. Liu G, Liu W, Guo J. Clinical significance of MiR-181a in patients with neonatal sepsis and its regulatory role in the lipopolysaccharide-induced inflammatory response. Exp Ther Med (2020) 19:1977–83. doi: 10.3892/etm.2020.8408
256. Liang G, Wu Y, Guan Y, Dong Y, Jiang L, Mao G, et al. The correlations between the serum expression of MiR-206 and the severity and prognosis of sepsis. Ann Palliat Med (2020) 9:3222–34. doi: 10.21037/apm-20-1391
257. Li JM, Zhang H, Zuo YJ. MicroRNA-218 alleviates sepsis inflammation by negatively regulating VOPP1 via JAK/STAT pathway. Eur Rev Med Pharmacol Sci (2018) 22:5620–6. doi: 10.26355/eurrev_201809_15827
258. Wu X, Yang J, Yu L, Long D. Plasma MiRNA-223 correlates with risk, inflammatory markers as well as prognosis in sepsis patients. Med (Baltimore) (2018) 97:e11352. doi: 10.1097/MD.0000000000011352
259. Benz F, Tacke F, Luedde M, Trautwein C, Luedde T, Koch A, et al. Circulating microRNA-223 serum levels do not predict sepsis or survival in patients with critical illness. Dis Markers (2015) 2015:384208. doi: 10.1155/2015/384208
260. Li N, Wu S, Yu L. The associations of long non-coding RNA taurine upregulated gene 1 and microRNA-223 with general disease severity and mortality risk in sepsis patients. Med (Baltimore) (2020) 99:e23444. doi: 10.1097/MD.0000000000023444
261. Sun B, Luan C, Guo L, Zhang B, Liu Y. Low expression of microRNA-328 can predict sepsis and alleviate sepsis-induced cardiac dysfunction and inflammatory response. Braz J Med Biol Res (2020) 53:e9501. doi: 10.1590/1414-431X20209501
262. Zhang H, Li L, Xu L, Zheng Y. Clinical Significance of the Serum lncRNA NORAD Expression in Patients with Neonatal Sepsis and Its Association with MiR-410-3p. J Inflammation Res (2021) 14:4181–8. doi: 10.2147/JIR.S315985
263. Wang H, Cui W, Qiao L, Hu G. Overexpression of MiR-451a in sepsis and septic shock patients is involved in the regulation of sepsis-associated cardiac dysfunction and inflammation. Genet Mol Biol (2020) 43:e20200009. doi: 10.1590/1678-4685-GMB-2020-0009
264. Liu Z, Yang D, Gao J, Xiang X, Hu X, Li S, et al. Discovery and validation of MiR-452 as an effective biomarker for acute kidney injury in sepsis. Theranostics (2020) 10:11963–75. doi: 10.7150/thno.50093
265. Guo H, Tang L, Xu J, Lin C, Ling X, Lu C, et al. MicroRNA-495 serves as a diagnostic biomarker in patients with sepsis and regulates sepsis-induced inflammation and cardiac dysfunction. Eur J Med Res (2019) 24:37. doi: 10.1186/s40001-019-0396-3
266. Nejad C, Stunden HJ, Gantier MP. A guide to MiRNAs in inflammation and innate immune responses. FEBS J (2018) 285:3695–716. doi: 10.1111/febs.14482
267. Curtis AM, Fagundes CT, Yang G, Palsson-McDermott EM, Wochal P, McGettrick AF, et al. Circadian control of innate immunity in macrophages by MiR-155 targeting Bmal1. Proc Natl Acad Sci U.S.A. (2015) 112:7231–6. doi: 10.1073/pnas.1501327112
268. Cheng Y, Kuang W, Hao Y, Zhang D, Lei M, Du L, et al. Downregulation of MiR-27a* and MiR-532-5p and upregulation of MiR-146a and MiR-155 in LPS-induced RAW264. 7 macrophage Cells Inflammation (2012) 35:1308–13. doi: 10.1007/s10753-012-9443-8
269. Androulidaki A, Iliopoulos D, Arranz A, Doxaki C, Schworer S, Zacharioudaki V, et al. The kinase Akt1 controls macrophage response to lipopolysaccharide by regulating microRNAs. Immunity (2009) 31:220–31. doi: 10.1016/j.immuni.2009.06.024
270. O'Connell RM, Chaudhuri AA, Rao DS, Baltimore D. Inositol phosphatase SHIP1 is a primary target of MiR-155. Proc Natl Acad Sci U.S.A. (2009) 106:7113–8. doi: 10.1073/pnas.0902636106
271. Ceppi M, Pereira PM, Dunand-Sauthier I, Barras E, Reith W, Santos MA, et al. MicroRNA-155 modulates the interleukin-1 signaling pathway in activated human monocyte-derived dendritic cells. Proc Natl Acad Sci U.S.A. (2009) 106:2735–40. doi: 10.1073/pnas.0811073106
272. Tahamtan A, Teymoori-Rad M, Nakstad B, Salimi V. Anti-Inflammatory micrornas and their potential for inflammatory diseases treatment. Front Immunol (2018) 9:1377. doi: 10.3389/fimmu.2018.01377
273. Chen CZ, Schaffert S, Fragoso R, Loh C. Regulation of immune responses and tolerance: the microRNA perspective. Immunol Rev (2013) 253:112–28. doi: 10.1111/imr.12060
274. Mehta A, Baltimore D. MicroRNAs as regulatory elements in immune system logic. Nat Rev Immunol (2016) 16:279–94. doi: 10.1038/nri.2016.40
275. Kumar Kingsley SM, Vishnu Bhat B. Role of MicroRNAs in the development and function of innate immune cells. Int Rev Immunol (2017) 36:154–75. doi: 10.1080/08830185.2017.1284212
276. Zhou X, Li X, Wu M. MiRNAs reshape immunity and inflammatory responses in bacterial infection. Signal Transduct Target Ther (2018) 3:14. doi: 10.1038/s41392-018-0006-9
277. Wu CJ, Lu LF. MicroRNA in immune regulation. Curr Top Microbiol Immunol (2017) 410:249–67. doi: 10.1007/82_2017_65
278. Roy S. MiRNA in macrophage development and function. Antioxid Redox Signal (2016) 25:795–804. doi: 10.1089/ars.2016.6728
279. Jablonski KA, Gaudet AD, Amici SA, Popovich PG, Guerau-de-Arellano M. Control of the inflammatory macrophage transcriptional signature by MiR-155. PloS One (2016) 11:e0159724. doi: 10.1371/journal.pone.0159724
280. Ying W, Tseng A, Chang RC, Morin A, Brehm T, Triff K, et al. MicroRNA-223 is a crucial mediator of PPARgamma-regulated alternative macrophage activation. J Clin Invest (2015) 125:4149–59. doi: 10.1172/JCI81656
281. Graff JW, Dickson AM, Clay G, McCaffrey AP, Wilson ME. Identifying functional microRNAs in macrophages with polarized phenotypes. J Biol Chem (2012) 287:21816–25. doi: 10.1074/jbc.M111.327031
282. Zhang Y, Zhang M, Zhong M, Suo Q, Lv K. Expression profiles of MiRNAs in polarized macrophages. Int J Mol Med (2013) 31:797–802. doi: 10.3892/ijmm.2013.1260
283. Essandoh K, Li Y, Huo J, Fan GC. MiRNA-mediated macrophage polarization and its potential role in the regulation of inflammatory response. Shock (2016) 46:122–31. doi: 10.1097/SHK.0000000000000604
284. El Gazzar M. MicroRNAs as potential regulators of myeloid-derived suppressor cell expansion. Innate Immun (2014) 20:227–38. doi: 10.1177/1753425913489850
285. Veglia F, Sanseviero E, Gabrilovich DI. Myeloid-derived suppressor cells in the era of increasing myeloid cell diversity. Nat Rev Immunol (2021) 21:485–98. doi: 10.1038/s41577-020-00490-y
286. Li Y, Dalli J, Chiang N, Baron RM, Quintana C, Serhan CN. Plasticity of leukocytic exudates in resolving acute inflammation is regulated by MicroRNA and proresolving mediators. Immunity (2013) 39:885–98. doi: 10.1016/j.immuni.2013.10.011
287. Kerrigan SW, Devine T, Fitzpatrick G, Thachil J, Cox D. Early Host Interactions That Drive the Dysregulated Response in Sepsis. Front Immunol (2019) 10:1748. doi: 10.3389/fimmu.2019.01748
288. Dewitte A, Lepreux S, Villeneuve J, Rigothier C, Combe C, Ouattara A, et al. Blood platelets and sepsis pathophysiology: A new therapeutic prospect in critically [corrected] ill patients? Ann Intensive Care (2017) 7:115. doi: 10.1186/s13613-017-0337-7
289. Kuosmanen SM, Kansanen E, Sihvola V, Levonen AL. MicroRNA profiling reveals distinct profiles for tissue-derived and cultured endothelial cells. Sci Rep (2017) 7:10943. doi: 10.1038/s41598-017-11487-4
290. Krammer TL, Mayr M, Hackl M. MicroRNAs as promising biomarkers of platelet activity in antiplatelet therapy monitoring. Int J Mol Sci (2020) 21:1–23. doi: 10.3390/ijms21103477
291. Zhong L, Simard MJ, Huot J. Endothelial microRNAs regulating the NF-kappaB pathway and cell adhesion molecules during inflammation. FASEB J (2018) 32:4070–84. doi: 10.1096/fj.201701536R
292. Shu Z, Tan J, Miao Y, Zhang Q. The role of microvesicles containing microRNAs in vascular endothelial dysfunction. J Cell Mol Med (2019) 23:7933–45. doi: 10.1111/jcmm.14716
293. Mandel J, Casari M, Stepanyan M, Martyanov A, Deppermann C. Beyond hemostasis: platelet innate immune interactions and thromboinflammation. Int J Mol Sci (2022) 23:1–30. doi: 10.3390/ijms23073868
294. Graham SM, Liles WC. Platelets in sepsis: beyond hemostasis. Blood (2016) 127:2947–9. doi: 10.1182/blood-2016-03-706168
295. Laffont B, Corduan A, Ple H, Duchez AC, Cloutier N, Boilard E, et al. Activated platelets can deliver mRNA regulatory Ago2*microRNA complexes to endothelial cells via microparticles. Blood (2013) 122:253–61. doi: 10.1182/blood-2013-03-492801
296. Peters LJF, Baaten C, Maas SL, Lu C, Nagy M, Jooss NJ, et al. MicroRNA-26b attenuates platelet adhesion and aggregation in mice. Biomedicines (2022) 10:1–14. doi: 10.3390/biomedicines10050983
297. Garcia A, Dunoyer-Geindre S, Zapilko V, Nolli S, Reny JL, Fontana P. Functional validation of microRNA-126-3p as a platelet reactivity regulator using human haematopoietic stem cells. Thromb Haemost (2019) 119:254–63. doi: 10.1055/s-0038-1676802
298. Heumann D, Roger T. Initial responses to endotoxins and Gram-negative bacteria. Clin Chim Acta (2002) 323:59–72. doi: 10.1016/s0009-8981(02)00180-8
299. Roger T, Froidevaux C, Le Roy D, Reymond MK, Chanson AL, Mauri D, et al. Protection from lethal gram-negative bacterial sepsis by targeting Toll-like receptor 4. Proc Natl Acad Sci U.S.A. (2009) 106:2348–52. doi: 10.1073/pnas.0808146106
300. Arenas-Padilla M, Mata-Haro V. Regulation of TLR signaling pathways by microRNAs: implications in inflammatory diseases. Cent Eur J Immunol (2018) 43:482–9. doi: 10.5114/ceji.2018.81351
301. O'Neill LA, Sheedy FJ, McCoy CE. MicroRNAs: the fine-tuners of Toll-like receptor signalling. Nat Rev Immunol (2011) 11:163–75. doi: 10.1038/nri2957
302. Ghafouri-Fard S, Khoshbakht T, Hussen BM, Taheri M, Arefian N. Regulatory role of non-coding RNAs on immune responses during sepsis. Front Immunol (2021) 12:798713. doi: 10.3389/fimmu.2021.798713
303. Nahid MA, Pauley KM, Satoh M, Chan EK. MiR-146a is critical for endotoxin-induced tolerance: Implication in innate immunity. J Biol Chem (2009) 284:34590–9. doi: 10.1074/jbc.M109.056317
304. Renzi TA, Rubino M, Gornati L, Garlanda C, Locati M, Curtale G. MiR-146b mediates endotoxin tolerance in human phagocytes. Mediators Inflammation (2015) 2015:145305. doi: 10.1155/2015/145305
305. El Gazzar M, Church A, Liu T, McCall CE. MicroRNA-146a regulates both transcription silencing and translation disruption of TNF-alpha during TLR4-induced gene reprogramming. J Leukoc Biol (2011) 90:509–19. doi: 10.1189/jlb.0211074
306. Quinn EM, Wang JH, O'Callaghan G, Redmond HP. MicroRNA-146a is upregulated by and negatively regulates TLR2 signaling. PloS One (2013) 8:e62232. doi: 10.1371/journal.pone.0062232
307. Doxaki C, Kampranis SC, Eliopoulos AG, Spilianakis C, Tsatsanis C. Coordinated regulation of MiR-155 and MiR-146a genes during induction of endotoxin tolerance in macrophages. J Immunol (2015) 195:5750–61. doi: 10.4049/jimmunol.1500615
308. Roger T, Miconnet I, Schiesser AL, Kai H, Miyake K, Calandra T. Critical role for Ets, AP-1 and GATA-like transcription factors in regulating mouse Toll-like receptor 4 (Tlr4) gene expression. Biochem J (2005) 387:355–65. doi: 10.1042/BJ20041243
309. Goodwin AJ, Guo C, Cook JA, Wolf B, Halushka PV, Fan H. Plasma levels of microRNA are altered with the development of shock in human sepsis: an observational study. Crit Care (2015) 19:440. doi: 10.1186/s13054-015-1162-8
310. Lagos-Quintana M, Rauhut R, Yalcin A, Meyer J, Lendeckel W, Tuschl T. Identification of tissue-specific microRNAs from mouse. Curr Biol (2002) 12:735–9. doi: 10.1016/s0960-9822(02)00809-6
311. Shaoqing Y, Ruxin Z, Guojun L, Zhiqiang Y, Hua H, Shudong Y, et al. Microarray analysis of differentially expressed microRNAs in allergic rhinitis. Am J Rhinol Allergy (2011) 25:e242–6. doi: 10.2500/ajra.2011.25.3682
312. Imai Y, Kuba K, Rao S, Huan Y, Guo F, Guan B, et al. Angiotensin-converting enzyme 2 protects from severe acute lung failure. Nature (2005) 436:112–6. doi: 10.1038/nature03712
313. Paterson MR, Kriegel AJ. MiR-146a/b: a family with shared seeds and different roots. Physiol Genomics (2017) 49:243–52. doi: 10.1152/physiolgenomics.00133.2016
314. Taganov KD, Boldin MP, Chang KJ, Baltimore D. NF-kappaB-dependent induction of microRNA MiR-146, an inhibitor targeted to signaling proteins of innate immune responses. Proc Natl Acad Sci U.S.A. (2006) 103:12481–6. doi: 10.1073/pnas.0605298103
315. Boldin MP, Taganov KD, Rao DS, Yang L, Zhao JL, Kalwani M, et al. MiR-146a is a significant brake on autoimmunity, myeloproliferation, and cancer in mice. J Exp Med (2011) 208:1189–201. doi: 10.1084/jem.20101823
316. Jurkin J, Schichl YM, Koeffel R, Bauer T, Richter S, Konradi S, et al. MiR-146a is differentially expressed by myeloid dendritic cell subsets and desensitizes cells to TLR2-dependent activation. J Immunol (2010) 184:4955–65. doi: 10.4049/jimmunol.0903021
317. Meisgen F, Xu Landen N, Wang A, Rethi B, Bouez C, Zuccolo M, et al. MiR-146a negatively regulates TLR2-induced inflammatory responses in keratinocytes. J Invest Dermatol (2014) 134:1931–40. doi: 10.1038/jid.2014.89
318. Xu D, Han Q, Hou Z, Zhang C, Zhang J. MiR-146a negatively regulates NK cell functions via STAT1 signaling. Cell Mol Immunol (2017) 14:712–20. doi: 10.1038/cmi.2015.113
319. He Y, Jiang X, Chen J. The role of MiR-150 in normal and malignant hematopoiesis. Oncogene (2014) 33:3887–93. doi: 10.1038/onc.2013.346
320. Cron MA, Maillard S, Truffault F, Gualeni AV, Gloghini A, Fadel E, et al. Causes and consequences of MiR-150-5p dysregulation in myasthenia gravis. Front Immunol (2019) 10:539. doi: 10.3389/fimmu.2019.00539
321. Hu Z, Cui Y, Qiao X, He X, Li F, Luo C, et al. Silencing MiR-150 ameliorates experimental autoimmune encephalomyelitis. Front Neurosci (2018) 12:465. doi: 10.3389/fnins.2018.00465
322. Schrijver IT, Theroude C, Antonakos N, Regina J, Le Roy D, Bart PA, et al. COVID-19 rapidly increases MDSCs and prolongs innate immune dysfunctions. Eur J Immunol (2022). doi: 10.1002/eji.202249827
323. Li Y, Wen Q, Chen H, Wu X, Liu B, Li H, et al. Exosomes derived from heat stroke cases carry MiRNAs associated with inflammation and coagulation cascade. Front Immunol (2021) 12:624753. doi: 10.3389/fimmu.2021.624753
324. O'Connell RM, Taganov KD, Boldin MP, Cheng G, Baltimore D. MicroRNA-155 is induced during the macrophage inflammatory response. Proc Natl Acad Sci U.S.A. (2007) 104:1604–9. doi: 10.1073/pnas.0610731104
325. Ren Y, Cui Y, Xiong X, Wang C, Zhang Y. Inhibition of microRNA-155 alleviates lipopolysaccharide-induced kidney injury in mice. Int J Clin Exp Pathol (2017) 10:9362–71.
326. Podsiad A, Standiford TJ, Ballinger MN, Eakin R, Park P, Kunkel SL, et al. MicroRNA-155 regulates host immune response to postviral bacterial pneumonia via IL-23/IL-17 pathway. Am J Physiol Lung Cell Mol Physiol (2016) 310:L465–75. doi: 10.1152/ajplung.00224.2015
327. Bandyopadhyay S, Long ME, Allen LA. Differential expression of microRNAs in Francisella tularensis-infected human macrophages: MiR-155-dependent downregulation of MyD88 inhibits the inflammatory response. PloS One (2014) 9:e109525. doi: 10.1371/journal.pone.0109525
328. Verschoor CP, Dorrington MG, Novakowski KE, Kaiser J, Radford K, Nair P, et al. MicroRNA-155 is required for clearance of Streptococcus pneumoniae from the nasopharynx. Infect Immun (2014) 82:4824–33. doi: 10.1128/IAI.02251-14
329. Bitar A, De R, Melgar S, Aung KM, Rahman A, Qadri F, et al. Induction of immunomodulatory MiR-146a and MiR-155 in small intestinal epithelium of Vibrio cholerae infected patients at acute stage of cholera. PloS One (2017) 12:e0173817. doi: 10.1371/journal.pone.0173817
330. Huck O, Al-Hashemi J, Poidevin L, Poch O, Davideau JL, Tenenbaum H, et al. Identification and Characterization of MicroRNA Differentially Expressed in Macrophages Exposed to Porphyromonas gingivalis Infection. Infect Immun (2017) 85:1–12. doi: 10.1128/IAI.00771-16
331. Rothchild AC, Sissons JR, Shafiani S, Plaisier C, Min D, Mai D, et al. MiR-155-regulated molecular network orchestrates cell fate in the innate and adaptive immune response to Mycobacterium tuberculosis. Proc Natl Acad Sci U.S.A. (2016) 113:E6172–81. doi: 10.1073/pnas.1608255113
332. Eren RO, Reverte M, Rossi M, Hartley MA, Castiglioni P, Prevel F, et al. Mammalian innate immune response to a leishmania-resident RNA virus increases macrophage survival to promote parasite persistence. Cell Host Microbe (2016) 20:318–28. doi: 10.1016/j.chom.2016.08.001
333. Pareek S, Roy S, Kumari B, Jain P, Banerjee A, Vrati S. MiR-155 induction in microglial cells suppresses Japanese encephalitis virus replication and negatively modulates innate immune responses. J Neuroinflamm (2014) 11:97. doi: 10.1186/1742-2094-11-97
334. Yang B, Yang R, Xu B, Fu J, Qu X, Li L, et al. MiR-155 and MiR-146a collectively regulate meningitic Escherichia coli infection-mediated neuroinflammatory responses. J Neuroinflamm (2021) 18:114. doi: 10.1186/s12974-021-02165-4
335. Tili E, Michaille JJ, Cimino A, Costinean S, Dumitru CD, Adair B, et al. Modulation of MiR-155 and MiR-125b levels following lipopolysaccharide/TNF-alpha stimulation and their possible roles in regulating the response to endotoxin shock. J Immunol (2007) 179:5082–9. doi: 10.4049/jimmunol.179.8.5082
336. Tacke F, Spehlmann ME, Vucur M, Benz F, Luedde M, Cardenas DV, et al. MiR-155 Predicts Long-Term Mortality in Critically Ill Patients Younger than 65 Years. Mediators Inflammation (2019) 2019:6714080. doi: 10.1155/2019/6714080
337. Yuan JY, Wang F, Yu J, Yang GH, Liu XL, Zhang JW. MicroRNA-223 reversibly regulates erythroid and megakaryocytic differentiation of K562 cells. J Cell Mol Med (2009) 13:4551–9. doi: 10.1111/j.1582-4934.2008.00585.x
338. Aziz F. The emerging role of MiR-223 as novel potential diagnostic and therapeutic target for inflammatory disorders. Cell Immunol (2016) 303:1–6. doi: 10.1016/j.cellimm.2016.04.003
339. Sun W, Shen W, Yang S, Hu F, Li H, Zhu TH. MiR-223 and MiR-142 attenuate hematopoietic cell proliferation, and MiR-223 positively regulates MiR-142 through LMO2 isoforms and CEBP-beta. Cell Res (2010) 20:1158–69. doi: 10.1038/cr.2010.134
340. Yuan X, Berg N, Lee JW, Le TT, Neudecker V, Jing N, et al. MicroRNA MiR-223 as regulator of innate immunity. J Leukoc Biol (2018) 104:515–24. doi: 10.1002/JLB.3MR0218-079R
341. Liu Y, Wang R, Jiang J, Yang B, Cao Z, Cheng X. MiR-223 is upregulated in monocytes from patients with tuberculosis and regulates function of monocyte-derived macrophages. Mol Immunol (2015) 67:475–81. doi: 10.1016/j.molimm.2015.08.006
342. Chen KW, Demarco B, Broz P. Beyond inflammasomes: emerging function of gasdermins during apoptosis and NETosis. EMBO J (2020) 39:e103397. doi: 10.15252/embj.2019103397
343. Bauernfeind F, Rieger A, Schildberg FA, Knolle PA, Schmid-Burgk JL, Hornung V. NLRP3 inflammasome activity is negatively controlled by MiR-223. J Immunol (2012) 189:4175–81. doi: 10.4049/jimmunol.1201516
344. Chen Q, Wang H, Liu Y, Song Y, Lai L, Han Q, et al. Inducible microRNA-223 down-regulation promotes TLR-triggered IL-6 and IL-1beta production in macrophages by targeting STAT3. PloS One (2012) 7:e42971. doi: 10.1371/journal.pone.0042971
345. Zhang N, Fu L, Bu Y, Yao Y, Wang Y. Downregulated expression of MiR-223 promotes Toll-like receptor-activated inflammatory responses in macrophages by targeting RhoB. Mol Immunol (2017) 91:42–8. doi: 10.1016/j.molimm.2017.08.026
346. Feng Z, Qi S, Zhang Y, Qi Z, Yan L, Zhou J, et al. Ly6G+ neutrophil-derived MiR-223 inhibits the NLRP3 inflammasome in mitochondrial DAMP-induced acute lung injury. Cell Death Dis (2017) 8:e3170. doi: 10.1038/cddis.2017.549
347. Dorhoi A, Iannaccone M, Farinacci M, Fae KC, Schreiber J, Moura-Alves P, et al. MicroRNA-223 controls susceptibility to tuberculosis by regulating lung neutrophil recruitment. J Clin Invest (2013) 123:4836–48. doi: 10.1172/JCI67604
348. Lou J, Wang Y, Zhang Z, Qiu W. Activation of MMPs in Macrophages by Mycobacterium tuberculosis via the MiR-223-BMAL1 Signaling Pathway. J Cell Biochem (2017) 118:4804–12. doi: 10.1002/jcb.26150
349. Neudecker V, Brodsky KS, Clambey ET, Schmidt EP, Packard TA, Davenport B, et al. Neutrophil transfer of MiR-223 to lung epithelial cells dampens acute lung injury in mice. Sci Transl Med (2017) 9:1–19. doi: 10.1126/scitranslmed.aah5360
350. Roffel MP, Bracke KR, Heijink IH, Maes T. MiR-223: A Key Regulator in the Innate Immune Response in Asthma and COPD. Front Med (Lausanne) (2020) 7:196. doi: 10.3389/fmed.2020.00196
351. Shen X, Zhang J, Huang Y, Tong J, Zhang L, Zhang Z, et al. Accuracy of circulating microRNAs in diagnosis of sepsis: a systematic review and meta-analysis. J Intensive Care (2020) 8:84. doi: 10.1186/s40560-020-00497-6
352. Calandra T, Roger T. Macrophage migration inhibitory factor: a regulator of innate immunity. Nat Rev Immunol (2003) 3:791–800. doi: 10.1038/nri1200
353. Froidevaux C, Roger T, Martin C, Glauser MP, Calandra T. Macrophage migration inhibitory factor and innate immune responses to bacterial infections. Crit Care Med (2001) 29:S13–5. doi: 10.1097/00003246-200107001-00006
354. Kerschbaumer RJ, Rieger M, Volkel D, Le Roy D, Roger T, Garbaraviciene J, et al. Neutralization of macrophage migration inhibitory factor (MIF) by fully human antibodies correlates with their specificity for the beta-sheet structure of MIF. J Biol Chem (2012) 287:7446–55. doi: 10.1074/jbc.M111.329664
355. Roger T, Delaloye J, Chanson AL, Giddey M, Le Roy D, Calandra T. Macrophage migration inhibitory factor deficiency is associated with impaired killing of gram-negative bacteria by macrophages and increased susceptibility to Klebsiella pneumoniae sepsis. J Infect Dis (2013) 207:331–9. doi: 10.1093/infdis/jis673
356. Roger T, Schneider A, Weier M, Sweep FC, Le Roy D, Bernhagen J, et al. High expression levels of macrophage migration inhibitory factor sustain the innate immune responses of neonates. Proc Natl Acad Sci U.S.A. (2016) 113:E997–1005. doi: 10.1073/pnas.1514018113
357. Savva A, Brouwer MC, Roger T, Valls Seron M, Le Roy D, Ferwerda B, et al. Functional polymorphisms of macrophage migration inhibitory factor as predictors of morbidity and mortality of pneumococcal meningitis. Proc Natl Acad Sci U.S.A. (2016) 113:3597–602. doi: 10.1073/pnas.1520727113
358. Sweeney TE, Shidham A, Wong HR, Khatri P. A comprehensive time-course-based multicohort analysis of sepsis and sterile inflammation reveals a robust diagnostic gene set. Sci Transl Med (2015). doi: 10.1126/scitranslmed.aaa5993
359. McHugh L, Seldon TA, Brandon RA, Kirk JT, Rapisarda A, Sutherland AJ, et al. A molecular host response assay to discriminate between sepsis and infection-negative systemic inflammation in critically ill patients: discovery and validation in independent cohorts. PloS Med (2015) 12:e1001916. doi: 10.1371/journal.pmed.1001916
360. Sweeney TE, Wong HR, Khatri P. Robust classification of bacterial and viral infections via integrated host gene expression diagnostics. Sci Transl Med (2016). doi: 10.1126/scitranslmed.aaf7165
361. Scicluna BP, Klein Klouwenberg PM, van Vught LA, Wiewel MA, Ong DS, Zwinderman AH, et al. A molecular biomarker to diagnose community-acquired pneumonia on intensive care unit admission. Am J Respir Crit Care Med (2015) 192:826–35. doi: 10.1164/rccm.201502-0355OC
362. Scicluna BP, van Vught LA, Zwinderman AH, Wiewel MA, Davenport EE, Burnham KL, et al. Classification of patients with sepsis according to blood genomic endotype: a prospective cohort study. Lancet Respir Med (2017) 5:816–26. doi: 10.1016/S2213-2600(17)30294-1
363. Reyes M, Filbin MR, Bhattacharyya RP, Billman K, Eisenhaure T, Hung DT, et al. An immune-cell signature of bacterial sepsis. Nat Med (2020) 26:333–40. doi: 10.1038/s41591-020-0752-4
Keywords: miRNA, sepsis, infection, innate immunity, biomarkers, critically ill
Citation: Antonakos N, Gilbert C, Théroude C, Schrijver IT and Roger T (2022) Modes of action and diagnostic value of miRNAs in sepsis. Front. Immunol. 13:951798. doi: 10.3389/fimmu.2022.951798
Received: 24 May 2022; Accepted: 08 July 2022;
Published: 05 August 2022.
Edited by:
Bertrand Kaeffer, Institut National de Recherche Pour L’agriculture, L’alimentation et L’environnement (INRAE), FranceReviewed by:
Ping Sun, School of Medicine, University of Pittsburgh, United StatesZsolt Fejes, University of Debrecen, Hungary
Copyright © 2022 Antonakos, Gilbert, Théroude, Schrijver and Roger. This is an open-access article distributed under the terms of the Creative Commons Attribution License (CC BY). The use, distribution or reproduction in other forums is permitted, provided the original author(s) and the copyright owner(s) are credited and that the original publication in this journal is cited, in accordance with accepted academic practice. No use, distribution or reproduction is permitted which does not comply with these terms.
*Correspondence: Thierry Roger, VGhpZXJyeS5Sb2dlckBjaHV2LmNo
†Present address: 4th Department of Internal Medicine, National and Kapodistrian University of Athens, Medical School, 124 62 Athens, Greece
‡These authors have contributed equally to this work