- 1Farm Animal Genetic Resources Exploration and Innovation Key Laboratory of Sichuan Province, Sichuan Agricultural University, Chengdu, China
- 2State Key Laboratory of Southwestern Chinese Medicine Resources, Ministry of Education, Chengdu University of Traditional Chinese Medicine, Chengdu, China
- 3Department of Basic Veterinary Medicine, Sichuan Agricultural University, Chengdu, China
Quercetin, a naturally non-toxic flavonoid within the safe dose range with antioxidant, anti-apoptotic and anti-inflammatory properties, plays an important role in the treatment of aging-related diseases. Sirtuin 1 (SIRT1), a member of NAD+-dependent deacetylase enzyme family, is extensively explored as a potential therapeutic target for attenuating aging-induced disorders. SIRT1 possess beneficial effects against aging-related diseases such as Alzheimer’s disease (AD), Parkinson’s disease (PD), Huntington’s disease (HD), Depression, Osteoporosis, Myocardial ischemia (M/I) and reperfusion (MI/R), Atherosclerosis (AS), and Diabetes. Previous studies have reported that aging increases tissue susceptibility, whereas, SIRT1 regulates cellular senescence and multiple aging-related cellular processes, including SIRT1/Keap1/Nrf2/HO-1 and SIRTI/PI3K/Akt/GSK-3β mediated oxidative stress, SIRT1/NF-κB and SIRT1/NLRP3 regulated inflammatory response, SIRT1/PGC1α/eIF2α/ATF4/CHOP and SIRT1/PKD1/CREB controlled phosphorylation, SIRT1-PINK1-Parkin mediated mitochondrial damage, SIRT1/FoxO mediated autophagy, and SIRT1/FoxG1/CREB/BDNF/Trkβ-catenin mediated neuroprotective effects. In this review, we summarized the role of SIRT1 in the improvement of the attenuation effect of quercetin on aging-related diseases and the relationship between relevant signaling pathways regulated by SIRT1. Moreover, the functional regulation of quercetin in aging-related markers such as oxidative stress, inflammatory response, mitochondrial function, autophagy and apoptosis through SIRT1 was discussed. Finally, the prospects of an extracellular vesicles (EVs) as quercetin loading and delivery, and SIRT1-mediated EVs as signal carriers for treating aging-related diseases, as well as discussed the ferroptosis alleviation effects of quercetin to protect against aging-related disease via activating SIRT1. Generally, SIRT1 may serve as a promising therapeutic target in the treatment of aging-related diseases via inhibiting oxidative stress, reducing inflammatory responses, and restoring mitochondrial dysfunction.
Highlights:
1. Quercetin, a naturally non-toxic flavonoid within the safe dose range with antioxidant, anti-apoptotic, and anti-inflammatory properties, plays an important role in the treatment of aging-related diseases.
2. Quercetin exerts neuroprotective effects against chronic aging-related diseases via targeting SIRT1 to regulate cellular senescence and multiple aging-related cellular processes such as SIRT1/Keap1/Nrf2/HO-1 and PI3K/Akt/GSK-3β mediated oxidative stress, SIRT1/NF-κB mediated inflammatory response, SIRT1/PGC1α/eIF2α/ATF4/CHOP mediated mitochondrial damage, and SIRT1/FoxO mediated autophagy.
3. Studies on the preventive and therapeutic effects and clinical application of natural SIRT1 activator or synthetic SIRT1 activator on aging-related diseases could provide a strong foundation and basis for investigating further potential target drugs to attenuate aging-related diseases.
1 Introduction
Aging in both animals and humans is positively correlated with a decline in the physiological functions, thereby increasing the occurrence of aging-related diseases; such as neurodegenerative diseases (NDS) (1) including Alzheimer’s disease (AD) (2), Parkinson’s disease (PD) (3), Huntington’s disease (HD) (4), and Depression (5); as well as other related diseases such as Osteoporosis (6), Myocardial ischemia (M/I) and reperfusion (MI/R) (7), Atherosclerosis (AS) (8), and Diabetes (9). A study reported that aging-related NDS caused significant morbidity and economic burden of approximately $25 billion annually in the USA (10). Therefore, several researchers engaged in studies to explore the molecular mechanism of various aging related diseases.
Chronological aging cause subtle changes in the neuronal structure and function of specific neuronal circuits, resulting in significant decrease in dopamine receptors in the striatum and reactive microglia and astrocytes, and the overactivation of proinflammatory microglia which triggers chronic inflammation (11). The production and accumulation of free radicals causes oxidative stress which increases the production of proinflammatory cytokines, leading to neuronal cell death and glial cell activation (12, 13). However, Sirtuin 1 (SIRT1) regulates the expression of proinflammatory cytokines such as tumor necrosis factor (TNF), interleukin-1 β (IL-1β), and interferon γ (IFN-γ) during microglial activation (14), as well as alleviates degeneration of dopaminergic neurons (15).
Sirtuin (SIRT), a member of the NAD+-dependent deacetylase enzyme family, regulates various cellular targets and functions. SIRT1 is the most widely studied Sirtuin in mammals and is considered as a potential therapeutic target for aging-associated disorders (16–18). SIRT1 activation was reported to attenuate other age-associated disorders such as NDS (19–21). Several aging-related markers such as neuroinflammation, oxidative stress, activation of glial cell, inhibition of adaptive neuroplasticity, dysregulation of neuronal Ca2+ homeostasis, mitochondrial dysfunction, and cellular senescence elevate the risk of aging-related diseases (22). Studies have indicated that SIRT1 silencing intensify the formation of aging-related inclusions containing alpha-synuclein, increase the number of age-dependent degeneration of dopaminergic neurons, which intensify the progression of related diseases (23). Moreover, excessive production of free radicals causes cell damage, leading to oxidative and nitrosative stress, which disrupts the immune function and hence, induces series of aging-related diseases. SIRT1 modulates the production and accumulation of reactive oxygen species (ROS) in vitro and in vivo, thereby attenuating oxidative stress associated with neurodegeneration (15, 24).
ROS accumulation cause mitochondrial dysfunction and cell death due to excessive inflammation (25), this allows the development of aging-related diseases (26). However, SIRT1 promotes mitochondrial function and regulates mitochondrial homeostasis. Studies have indicated that overexpression of SIRT1 can effectively inhibit cell death, promote cell survival, and prolong the lifespan of cells (27). However, available strategies such as chemical drugs to treat diseases are very expensive, less clinically effective, and have obvious toxic effects. Therefore, researchers are currently studying to develop potent, cheap, safe, and more effective natural alternatives or novel targets to attenuate aging-induced diseases.
Quercetin (2-(3,4-dihydroxy phenyl)-3,5,7-trihydroxy-4H-1-benzopyran-4-one) (Figure 1) is one of the major naturally nontoxic flavonoids which is widely found in fruits (grapes, peaches) and vegetables (onions, garlic). Quercetin is a lipophilic compound that could be absorbed by simple diffusion across the intestinal membrane, however, it is ingested primarily as a glycoside, which is converted to a glycoside ligand in the intestine, and then release by absorption into the intestinal epithelium through the action of β-glycosidase, and both the intestinal and oral bacteria are involved in this enzymatic hydrolysis. Studies have shown that poor oral bioavailability of single doses (28–30). The poor aqueous solubility of quercetin (~1 mg/mL in water, ~5.5 mg/mL in simulated gastric fluid, and ~28.9 mg/mL in simulated intestinal fluid) and its instability in the physiological media have limited its application in pharmacology (31, 32), great efforts have been made in its drug delivery systems to address the problem of limited application (33, 34).
Quercetin plays many beneficial roles such as antioxidant (35), anti-inflammatory (36), anti-apoptotic (37) and other biological activities (38) in neuroprotection (39). Reports have indicated that quercetin contain anti-aging properties (31, 40–43). However, the mechanisms through which quercetin exert these functions are complex and the signaling pathways are intertwined. Therefore, our aim in this review was to clarify how quercetin targets SIRT1 to prevent aging-related diseases, elucidate the relationships between the relevant signaling pathways, provide the possible targets and the theoretical basis for quercetin to serve as an effective drug for aging-related diseases.
2 Effects of quercetin on neurodegenerative diseases
2.1 Alzheimer’s disease (AD)
Alzheimer’s disease (AD) is a neurodegenerative disorder, characterized by learning and memory dysfunction at an early stage and eventually evolves into a cognitive disorder. Due to the progression of the disease and limited treatment options, AD has become one of the greatest threats to the modern population, accounting for approximately 45 million patients worldwide. Studies have predicted that by the year 2050, approximately 150 million people may suffer from AD (2).
The mechanisms of AD are related to the deposition of β-amyloid (Aβ) peptides and intracellular neurofibrillary tangles consisting of hyperphosphorylated tau protein, which are important characteristics of AD and can lead to serial neuronal loss and brain atrophy (44). SIRT1 regulates the expression of Aβ (45) and tau (46), which are associated with AD. A study using a three-transgenic AD (3XTG-AD) mice model, reported that quercetin (100 mg/kg) significantly improved the biomarkers of neurodegeneration and cognitive and emotional deficits (47).
2.1.1 Regulation of oxidative stress
In addition to its direct toxic effects on neurons, Aβ increases the sensitivity of neurons to harmful factors such as free radicals and oxidative stress, which play important role during AD. Quercetin supplementation reduce microglia aggregation around amyloid plaques in AD mice (48) through various excess protein pathways (49). Activation of SIRT1 was reported to counteract oxidative stress induced by Aβ aggregation (50). In addition, Yu et al. reported that quercetin regulates SIRT1/Nrf2/HO-1 pathway thereby exerting neuroprotective effects against AD (51). In HT22 hippocampal neurons, quercetin was reported to show a neuroprotective effect by promoting phosphoinositide 3-kinase (PI3K)/Akt which could downregulate glycogen synthase kinase 3beta (GSK-3β) activity (52), thereby reducing oxidative stress-mediated neuronal death.
2.1.2 Regulation of inflammatory response
Aβ promotes the activation of microglia and the release of inflammatory cytokines. Chronic inflammation is associated with age-related Alzheimer’s disease. Studies have shown that quercetin exerts an anti-neuroinflammatory effects on LPS-activated BV-2 microglial cells (53), by attenuating the production of inflammatory mediators, including nitric oxide (NO) and TNF, as well as reduce the level of inducible NO synthase (iNOS) (54). Quercetin reduces ROS production by promoting the activity of SIRT1 and enhancing the anti-inflammatory activity of NF-κB acetylation in the neural and glial cells, thereby reducing neuronal cell death (55).
2.1.3 Regulation of mitochondrial function
Mitochondria are organelles responsible for ATP production, calcium regulation, and regulation of redox homeostasis. Mitochondrial dysfunction is regarded as the main causative factor of the pathogenesis aging-related AD (56), as a result could inflict damage to neurons, microglia, and astrocytes (57). Microglia phagocytose Aβ and the surrounding Aβ plaques promote the synaptic and neuronal loss in the chronic AD process in the mitochondrial dysfunction and hence, increase LAMP1 immunoreactivity, thereby preventing the diffusion of soluble amyloid into the surrounding thin-walled brain (58). In addition, quercetin was found to improve the cognitive function in the APPswe/PS1De9 transgenic mice model during chronic AD, by reducing mitochondrial dysfunction via the activation of AMP-activated protein kinase (AMPK) (59). Moreover, SIRT1 regulates mitochondrial biogenesis and function by directly controlling the activity of proliferator-activated receptor gamma coactivator 1alpha (PGC-1α) through phosphorylation and deacetylation (60), whereas quercetin cause a reduction in the phosphorylation of the eukaryotic initiation factor 2 alpha (eIF2α), as well as activate the expression of transcription factor 4 (ATF4) through the GADD34 induction in the brain, leading to memory improvement in aged mice and delayed memory deterioration at the early stage of APP23 AD model mice (61).
2.1.4 Regulation of autophagy function
Autophagy is the main mechanism underlying the onset and progression of AD (62). FoxO proteins, key substrates of SIRT1, are prominent and necessary factors in the formation of memory and cognitive function (63). Studies in a drosophila AD model have shown that quercetin significantly alleviate chronic AD by restoring the expression of cell cycle protein including cell cycle proteins B located in the FoxO signaling pathway perturbed by Aβ accumulation (64).
In summary, quercetin exerts neuroprotective effects against chronic AD by targeting SIRT1 to regulate cellular senescence and aging-related multiple cellular processes, including SIRT1/Keap1/Nrf2/HO-1 and PI3K/Akt/GSK-3β mediated oxidative stress, SIRT1/NF-κB mediated inflammatory response, SIRT1/PGC1α/eIF2α/ATF4/CHOP mediated mitochondrial damage, and SIRT1/FoxO mediated autophagy (Figure 2 and Table 1).
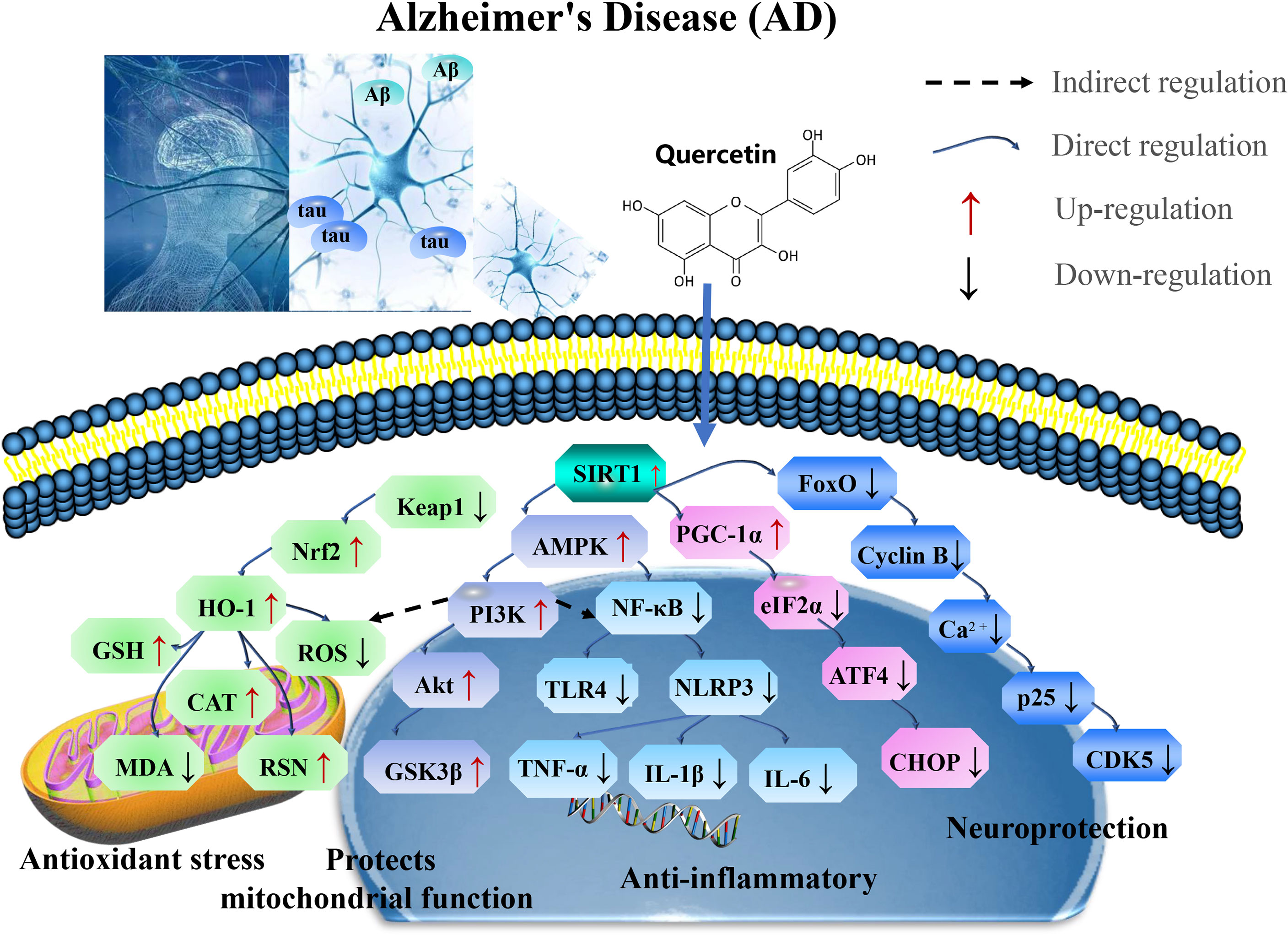
Figure 2 Mechanisms of quercetin on Alzheimer’s disease (AD). Quercetin exert neuroprotective effects against chronic AD by targeting SIRT1 to regulate cellular senescence and aging-related multiple cellular processes, including SIRT1/Keap1/Nrf2/HO-1 and PI3K/Akt/GSK-3β mediated oxidative stress, SIRT1/NF-κB mediated inflammatory response, SIRT1/PGC1α/eIF2α/ATF4/CHOP mediated mitochondrial damage, and SIRT1/FoxO mediated autophagy. CAT, catalase; GSH-Px, glutathione peroxidase; PI3K, phosphoinositide 3-kinase; GSK-3β, glycogen synthase kinase 3beta; iNOS, inducible nitric oxide synthase; TNF, tumor necrosis factor; TLR, toll-like receptors; PGC-1α, proliferator-activated receptor gamma coactivator 1alpha; AMPK, AMP-activated protein kinase; eIF2α, eukaryotic initiation factor 2 alpha; and ATF4, activating transcription factor 4.
2.2 Parkinson’s disease (PD)
Parkinson’s disease (PD) is a common progressive neurodegenerative disease after AD, which affects approximately 1-2% of people at the age ≥ 65 (65). PD is characterized by dopaminergic (DAergic) neuronal deficits and glial dysfunction in the substantia nigra (SN), caused by neuroglial dysfunction and neuroinflammation (66). Studies have shown that α-synuclein (α-SYN) regulates pathological events that cascade the response in PD (67), whereas SIRT1 proteins modulates PD (68).
2.2.1 Regulation of oxidative stress
Oxidative stress is a major inducers of PD pathogenesis. This is because ROS activates α-syn aggregation cascade, together with Lewy bodies, promotes neurodegeneration (69). In a 6-hydroxydopamine (6-OHDA) PD rat model, it was observed that quercetin alleviated the unilateral medial forebrain tract (annigra lesion) or the striatum (part lesion) through the antioxidant, anti-inflammatory, and protective neurotransmitter mechanisms (70–72). The efficacy of quercetin was tested in MitoPark transgenic chronic PD mice model, to ameliorate striatal dopamine depletion and 5-TH neuronal cell loss (73). Other studies have shown that different doses of quercetin reduce oxidative damage by regulating SIRT1/HO-1/Nrf2 pathway, thereby increasing neuronal density (74). Furthermore, Nrf2 modulates ARE/PINK1 expression (75), to restore mitochondrial homeostasis in chronic PD (76).
2.2.2 Regulation of inflammatory response
PD activates microglia to promote inflammatory processes by releasing inflammatory cytokines and chemokines (77). Moreover, degeneration of dopaminergic neurons is induced by the synergy of ROS, neuroinflammation, and loss of other trophic factors (78). In the microglial (N9)-neuronal (PC12) co-culture systems, quercetin downregulates the expression of proinflammatory cytokines in the N9 microglia, and reduce apoptosis in the post-neuronal cells (79). Studies have reported that quercetin alleviates manganese-induced neuroinflammation by inhibiting apoptosis and oxidative stress through the SIRT1/iNOS/NF-κB and HO-1/Nrf2 pathways (80, 81). NLRP3 inflammatory vesicle is one the most characterized inflammatory vesicles, which can be activated by highly diverse stimuli. NLRP3 is activated by procaspase-1, which in turn induces maturation and secretion of inflammatory cytokines, as well as plays an important role in PD (82). Studies have shown that quercetin impedes microglial activation by inhibiting the interaction between the NLRP3 inflammasome and mitochondrial autophagy to reduce neurotoxicity (39). In aged mice, quercetin was reported to attenuate neuroinflammation by modulating the SIRT1/NLRP3 pathway (83).
2.2.3 Regulation of mitochondrial function
Excessive ROS production and accumulation cause mitochondrial dysfunction by α-SYN, which subsequently results in neurodegeneration (80, 84, 85). However, quercetin is significantly induced in MN9D dopaminergic neuronal cells, two major cell survival kinases, and the activation of PD protein kinase D1 (PKD1) and Akt (86). The cAMP response element-binding protein (CREB) and a transcriptional activator of brain-derived neurotrophic factor (BDNF), are involved in microglial activation. Interestingly, quercetin (10 μM) was found to upregulate BDNF gene expression in the dopaminergic neuronal cells through phosphorylating CREB by PKD1 or through stimulating mitochondrial biology in the dopaminergic neuronal cells via regulating SIRT1/PGC-1α transcriptional activity through CREB generation. Moreover, mitochondria act as a key regulator for NLRP3 inflammatory vesicles, whereas mitochondrial dysfunction leads to NLRP3 assembly and activation. Mitochondrial dysfunction in the astrocytes amplifies the activity of the NLRP3 inflammasome, as well as promotes IL-1β production.
2.2.4 Regulation of autophagy function
α-SYN regulates autophagosome synthesis, leading to defective autophagy. Quercetin acts as an autophagy enhancer in aged PD rat models and modulate the microenvironment leading to neuronal death (87). PTEN is responsible for inducing putative kinase 1 (PINK1) that contributes to the development of astrocyte and proliferation, as well as was identify as an essential protein for the removal of the damaged mitochondria via autophagy (88). PINK1-parkin-mediated dysfunction of the mitochondrial autophagy in the astrocytes can impair the integrity of the mitochondria (89), which leads neuronal dysfunction and degeneration (90). Reports have shown that quercetin activates SIRT1, promotes autophagic PINK1 activation, and reduces cytochrome c release, as well as enable cystatinase activation to maintain mitochondrial integrity, thereby preventing apoptosis (91). Quercetin regulates the protein expression of Bcl2/Bax and the release of cytochrome c, and nuclear translocation of apoptosis-inducing factor (AIF) (92). Quercetin also promotes autophagy and modulates the microenvironment which leads to neuronal death (87).
Therefore, quercetin is a potential therapeutic strategy for alleviating PD by targeting SIRT1. Developing therapies have shown that SIRT1/Nrf2/HO-1 mediated oxidative stress, SIRT1/NF-κB/NLRP3 pathway ameliorates neuroinflammation SIRT1-mediated PKD1/CREB phosphorylation and BDNF gene expression, and regulates mitochondrial disorders in the dopaminergic neurons and SIRT1-PINK1-Parkin mediated mitochondrial autophagy in the astrocytes to maintain mitochondrial function (Figure 3 and Table 2).
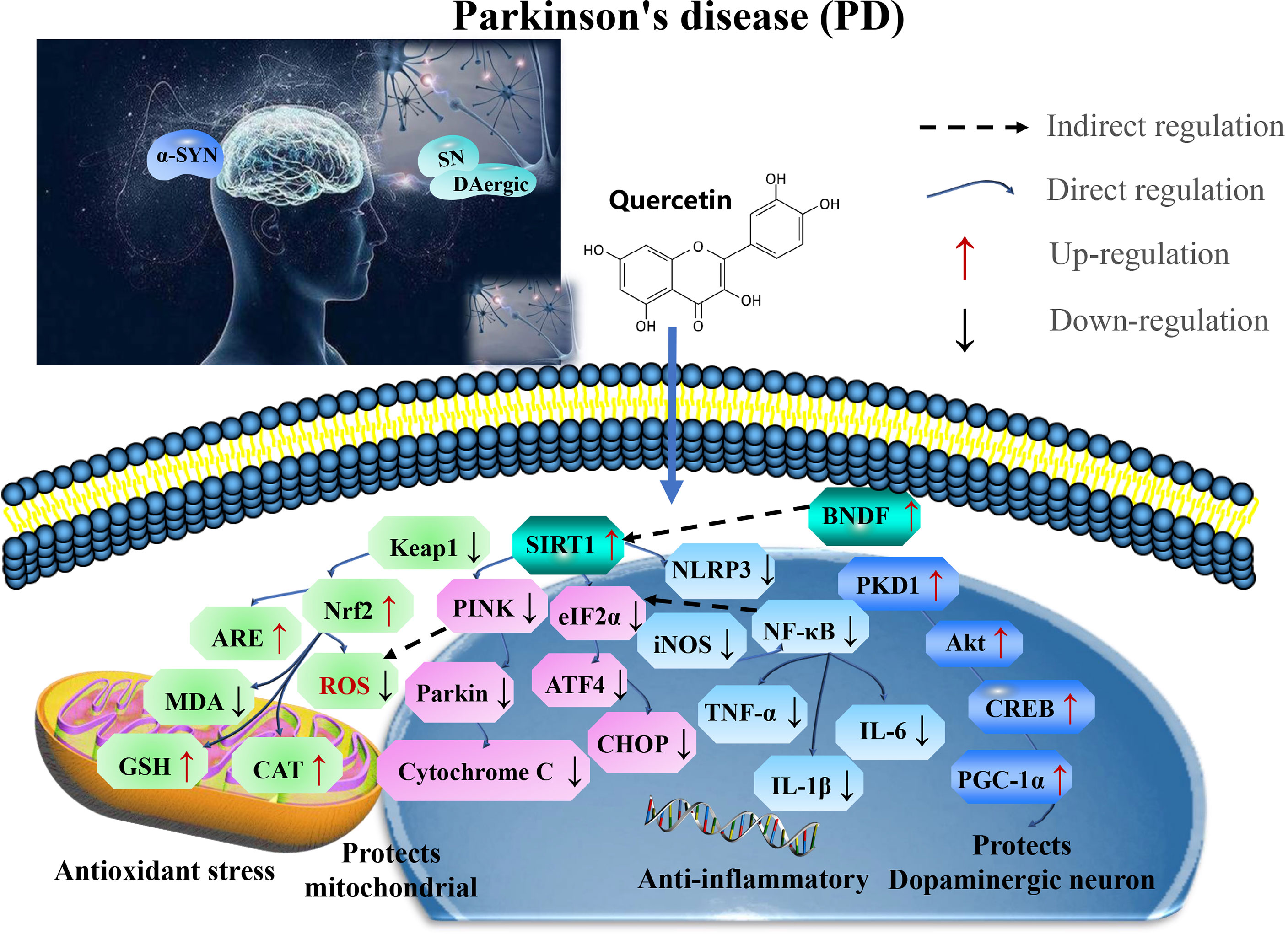
Figure 3 Mechanisms of quercetin on attenuating Parkinson’s disease (PD). Quercetin is a potential therapeutic strategy for PD by targeting SIRT1. Developing therapies have shown that SIRT1/Nrf2/HO-1 mediated oxidative stress, SIRT1/NF-κB/NLRP3 pathway ameliorates neuroinflammation SIRT1-mediated PKD1/CREB phosphorylation and BDNF gene expression, regulates mitochondrial disorders in dopaminergic neurons and SIRT1-PINK1-Parkin mediated mitochondrial autophagy in the astrocytes to maintain mitochondrial function. ROS, reactive oxygen species; 5-HT, 5-hydroxytryptamine; CAT, catalase; GSH-Px, glutathione peroxidase; TNF, tumor necrosis factor; NLRP3, NOD-like receptor protein 3; IL-1β, interleukin-1 β; CREB, cAMP response element binding protein; MDA, malondialdehyde; BDNF, brain-derived neurotrophic factor; and GFAP, glial fibrillary acidic protein.
2.3 Effects of quercetin on Huntington’s disease (HD)
Huntington’s disease (HD) is a neurodegenerative disorder characterized by a progressive loss of dopaminergic neurons in the substantia nigra, as well as progressive motor dysfunction, chorea, dystonia, mood disturbances, memory, and weight loss. Symptoms of HD in humans usually appear between the ages 30-50 years and increase in severity with chronological age (93). Current disease palliative therapies for HD focus on reducing the levels of mutant huntingtin (mHTT) in brain cells (94). The overexpression of SIRT1 was reported to increase the survival in R6/2 HD mice and improve neuropathology and reduced mHTT aggregation in R6/2 models (95).
2.3.1 Regulation of mitochondrial function
In 3-nitropropionic acid (3-NP)-induced HD rat models, quercetin maintain mitochondrial function by attenuating oxidative stress and neurobehavioral disorder (96). In addition, dysfunction of mitochondrial metabolism of mHTT play major role in the pathogenesis of HD which is primarily associated with impair respiratory chain function, thereby increasing ROS production and subsequently results in cell death. Furthermore, the ubiquitin-proteasome system (UPS) or autophagy reduce the formation of toxic mHTT aggregates (97). A study showed that inducement of mHTT could decrease the proteasomal activity of UPS which could be reverse by the quercetin (20 µM), thereby attenuating mitochondrial membrane potential damage (98).
2.3.2 Regulation of inflammatory response
It was established that quercetin could alleviate HD in rat model by regulating inflammatory changes (IL-1β, IL-6, and TNF) associated with growth factors released from astrocytes by the reduction of microglial proliferation, as well as increasing astrocyte numbers (99). In addition, quercetin reduce anxiety and depression in patients with 3-NP-induced chronic HD (100). This indicate that quercetin plays neuroprotective role in HD by targeting SIRT1 to relieve mHTT aggregation in patients, and restore mitochondrial function and reduce inflammation (Figure 4 and Table 3).
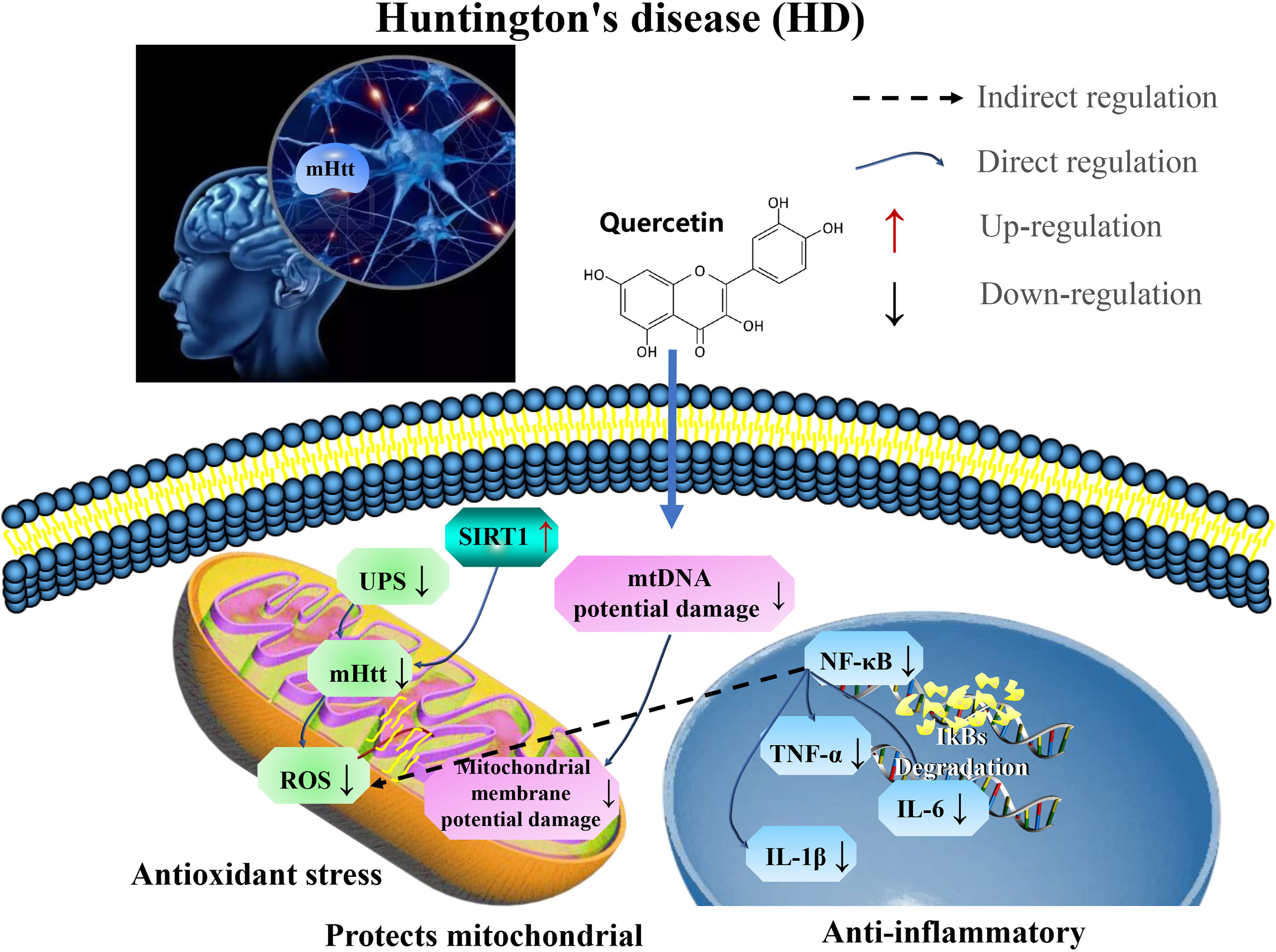
Figure 4 Mechanisms of quercetin in attenuating Huntington’s disease (HD). Quercetin plays neuroprotective role in HD by targeting SIRT1 to relieve aggregation of mHTT in patients, restore mitochondrial function, and reduce inflammation. UPS, ubiquitin-proteasome system; mHtt, Huntington’s protein; ROS, reactive oxygen species; TNF-α, tumor necrosis factor-α; and IL-1β, interleukin-1 β.
2.4 Effects of quercetin on depression
Major depressive disorder may be associated with volumetric indications of accelerated brain aging (101). Disorders of depression and anxiety are common mental ailments that imposes a significant global health challenge. However, current conventional antidepressants have limited efficacy, significant side effects and expensive. The structural and functional integrity of the hippocampus is critical for cognitive functions, as well as producing antidepressant effects in response to adverse factors (environmental changes, stress) (102, 103). Moreover, reports have indicated that SIRT1 inhibitors reverse sleep deprivation (SD)-induced depressive and anxiety-like behaviors and hippocampal neuroinflammation (104). The role and possible mechanisms of SIRT1 have revealed novel therapeutic strategies for clinical treatment of depression (105, 106). SIRT1 is required for normal neuronal excitability and regulates depression-related behaviors in a sex-specific manner (107). Activation of the estrogen receptor alpha (ERα)/SIRT1/NF-κB pathway was involved in LPS-induced depression in aged female mice (108).
Fork head box transcription factor G1 (FOXG1) play neuroprotective roles by regulating adult hippocampal neurogenesis (AHN). Quercetin promotes AHN via FoxG1/CREB/BDNF signaling pathway to improve chronic unpredictable mild stress (CUMS)-induced depression-like behaviors (109). In addition, it has been shown that microglial inhibitory pathways exert neuroprotective effects, and astrocyte activation, whereas quercetin significantly reduce the frequency of spontaneous excitatory postsynaptic currents (sEPSCs) and spontaneous inhibitory postsynaptic currents (sIPSCs) to antidepressants (110). Astrocytes secrete neurotrophic factors such as GDNF and BDNF, of which BDNF combined with exercise training attenuated 1,2-dimethylhydrazine-induced depression in rats with colorectal cancer by modulating the BDNF/tyrosine receptor kinase A (TrKβ)/β-catenin axis in the prefrontal cortex significantly reduced tumorigenesis and improve depression-like behavior (111), as well as attenuates LPS-induced depression-like behavior in rats by modulating the BDNF-related Copine 6 and TREM1/2 imbalance in the hippocampus and PFC (112), whereas reports showed that quercetin exert antidepressant and cardioprotective effects (113).
Quercetin reduce doxorubicin-induced anxiety by enhancing immune function and reducing oxidative stress in the brain (114). Alteration in the metabolism of monoamine oxidase (MAO) is associated with aging (115), which catalyzes monoamine-containing neurotransmitters such as serotonin 5-hydroxytryptamine (5-HT) (116). Quercetin possess antidepressant-like effects through its antioxidant, anti-inflammatory activities, and also reduce excitotoxicity, as well as increase 5-HT levels (117). Quercetin significantly reduced MAOs activity and increase the activity of antioxidant enzymes (Cu-Zn, SOD, CAT, and GSH-Px) in depressed rats (118). Some studies in olfactory bulbectomy (OB), the surgical removal of the olfactory bulbs, cause specific set of behavioral changes in social behavior, cognitive function, and activity; whereas quercetin shows an antidepressant-like and antioxidant effects in bulbectomized mice model via the glutamatergic and oxidonitrergic pathways (119), and mediate neuroinflammatory-apoptotic cascade response (120).
Furthermore, quercetin acts as an antidepressant by targeting SIRT1 to reverse depressive and anxiety-like behaviors and hippocampal neuroinflammation. The FoxG1/CREB/BDNF/Trkβ-catenin axis clarify the underlying mechanisms (Figure 5 and Table 4).
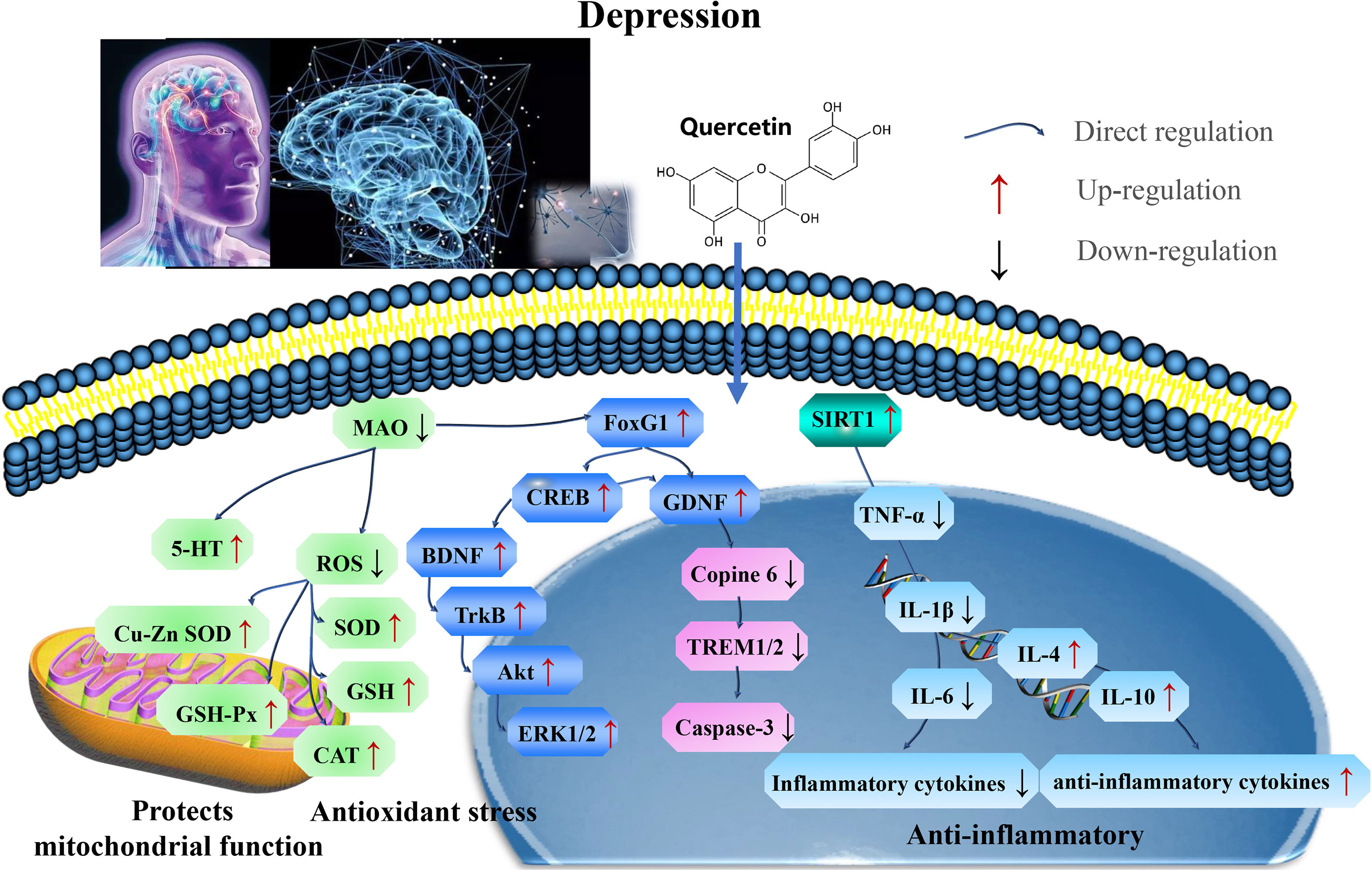
Figure 5 Mechanisms of quercetin on Depression. Quercetin act as an antidepressant by targeting SIRT1 to reverse depressive and anxiety-like behaviors and hippocampal neuroinflammation. The FoxG1/CREB/BDNF/Trkβ-catenin axis clarifies these mechanisms. FOXG1, Forkhead box transcription factor G1; CREB, cAMP response element binding protein; TrKβ, tyrosine receptor kinase A. SOD, superoxide dismutase; CAT, catalase; GSH-Px, glutathione peroxidase; TNF, tumor necrosis factor; IL-1β, interleukin-1 β; MDA, malondialdehyde; and BDNF, brain-derived neurotrophic factor.
3 Effects of quercetin on osteoporosis
Osteoporosis is also a major aging-related disease (121). The rate of reduction of osteogenic differentiation and bone formation is the major cause of aging-related osteoporosis (122). Bone can act indirectly on the brainstem, midbrain and hippocampus, thereby affect the synthesis of multiple neurotransmitters, resulting in neurodegenerative diseases (123), suggesting that improving the conditions affecting neurodegenerative process may be a novel target for the treatment of bone injury. Osteoporosis is characterized by a reduction in the bone mass and bone mineral loss, and deterioration of bone microarchitecture, with a greater impact on postmenopausal women. In the United States, approximately 53 million people are at risk of bone mineral loss, however, this number will gradually increase resulting in an economic loss of an annual cost of $25.3 billion (124).
Quercetin is a potential drug for the clinical treatment of osteoporosis and has a significant effect on the structure and conformation of bone morphogenetic protein-2 (BMP-2) via upregulation of bone mineralization which promotes differentiation of bone marrow mesenchymal stem cells (BMSCs), as well as osteoblast-specific genes such as osterix (OSX), dwarf-related transcription factor 2 (Runx2), alkaline phosphatase (ALP), osteocalcin (OCN), and serum c-terminal type I collagen cross-linked telopeptides at the mRNA and protein expression levels (40, 125). Quercetin promotes osteogenic differentiation and inhibits lipogenic differentiation of mouse bone marrow mesenchymal stem cells (mBMSCs), enhancing AMPK protein phosphorylation and upregulating SIRT1 expression to exert antioxidant effects (126). Thereby promoting bone marrow mesenchymal stem cell proliferation and osteogenic differentiation. Rodent oophorectomy is extensively studied for to determine a reduction in bone mass and an increase in bone turnover in cancellous bone, which is similar to osteoporosis in postmenopausal women (127).
The activation of nicotine and muscarinic receptors (nAchR) in an osteoclast inhibits the receptor activator of nuclear factor-kappa B ligand (RANKL)-dependent osteoclast development, suggesting that it is closely related to sympathetic nerve activity (128). Quercetin has been shown to inhibit RANKL-mediated osteoclastogenesis, osteoblast apoptosis, and inflammatory response, nuclear factor κB (NF-κB) and activator protein 1 (AP-1) are transcription factors that regulates osteoclast differentiation, and signaling pathways associated with β-catenin degradation (129). Quercetin is inhibits osteoclast differentiation in vitro (130). The abundance of phosphorylated p38 MAPK and phosphorylated extracellular signal-regulated kinase (ERK) could be reverse after quercetin treatment, and trigger significant restoration of the Wnt/β-catenin pathway by enhancing the expression of Wnt3, β-catenin, Bax and cytochrome c expression and decrease the expression of Bcl-2, Bcl-xL and caspase-3 (131), regulating autophagy and apoptosis to prevent osteoporosis (132).
In summary, quercetin acts as a therapeutic measure for treating aging-related osteoporosis by targeting SIRT1, via antioxidants pathways, thereby inhibits osteoblast apoptosis, autophagy, and inflammatory responses (Figure 6 and Table 5).
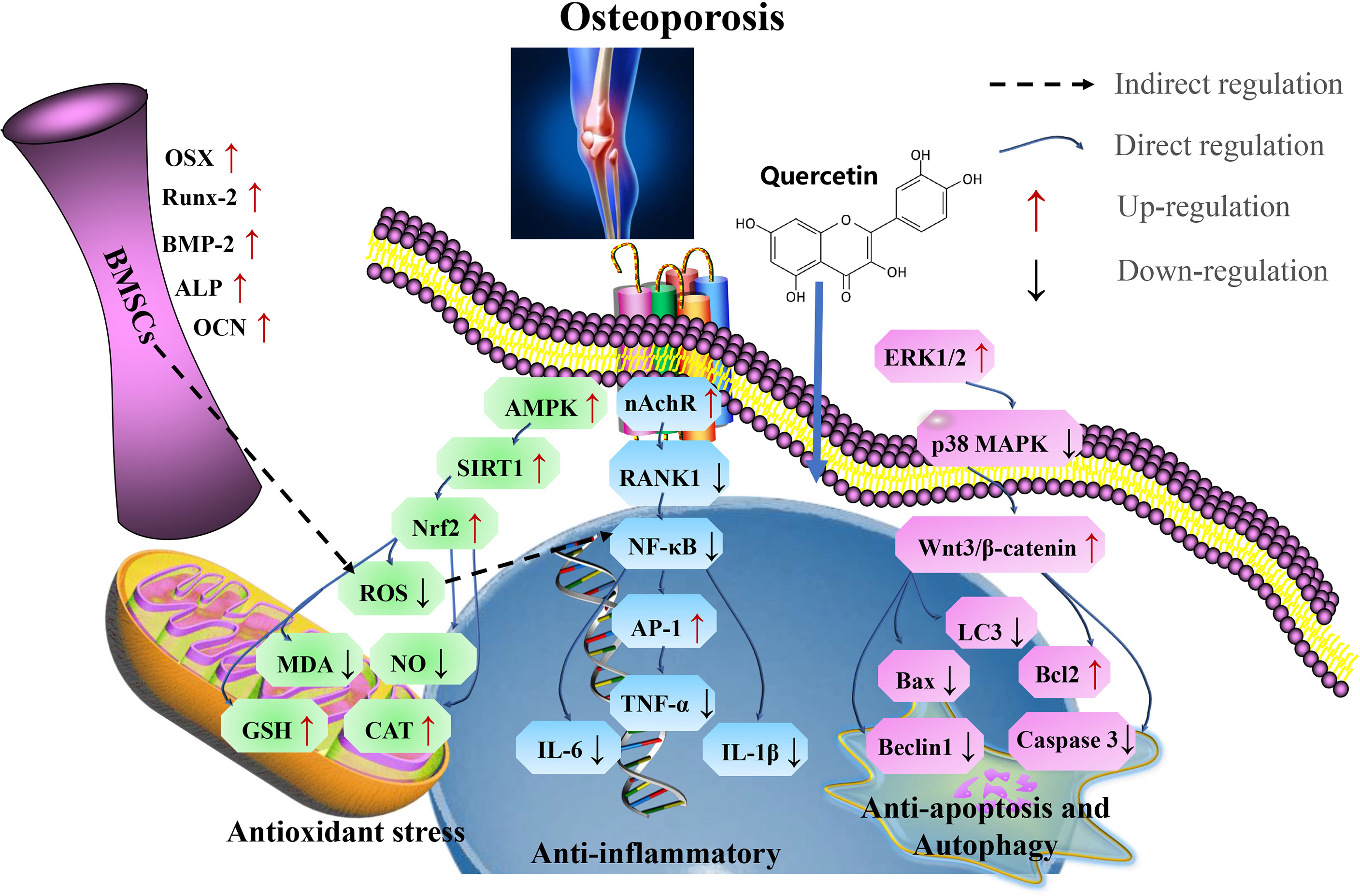
Figure 6 Mechanism of quercetin on osteoporosis. Quercetin as a therapeutic strategy for the treatment of aging-related osteoporosis by targeting SIRT1, via antioxidant pathways, thereby inhibits osteoblast apoptosis, autophagy, and inflammatory responses. Runx2, related transcription factor 2; OSX, Osterix; OCN, osteocalcin; Cx43, connexin 43; RANKL, receptor activator of nuclear factor-kappa B ligand; TNF, tumor necrosis factor; IL-6, interleukin-6; IFN-γ, interferon γ; SOD, superoxide dismutase; CAT, catalase; GSH, glutathione; ALP, alkaline phosphatase; LC3, microtubule-associated protein light chain 3; OPG, osteoprotegerin; CTX-1, C-terminal telopeptide of type I collagen; P1NP, N-terminal propeptide of type I procollagen; TRAP, Tartrate-resistant acid phosphatase; Runx2, related transcription factor 2.
4 Effect of quercetin on aging-related cardiovascular disease
4.1 Myocardial ischemia (M/I) and reperfusion (MI/R)
Myocardial ischemia is an aging-related cardiovascular disease and causes sudden death worldwide (136). Myocardial ischemia (M/I) infarction is caused by an inadequate supply of oxygen to the heart, leading to apoptosis (137). However, cardiomyocytes damage is caused by calcium overload, large amounts of free radical production, and infiltration of inflammatory cells cause reperfusion. Mitochondrial dysfunction is considered an important marker of neuronal death during cerebral MI/R (7).
The cardioprotective effect of quercetin is associated with attenuating oxidative stress induced by aging (138). In a mice model, quercetin was reported to attenuate cardiac damage induced by a high-fat diet (HFD) by restoring myocardial microcirculation, reducing infarct size and improving left ventricular function, myofibrillar and mitochondrial structure (139). Quercetin also improve the tricarboxylic acid cycle and respiratory chain-related enzyme activity in rats with myocardial infarction, as well as decrease the expression of biomarkers of myocardial induced oxidative stress in rats with myocardial infarction (140). In addition, quercetin protects AC16 cells from HG-induced oxidative stress by elevating p-SIRT1, endothelial NOS, and decreasing iNOS (141) through the PI3K/Akt/Nrf2 signaling pathway (142).
Pretreatment with quercetin significantly inhibit inflammatory cascade responses through the downregulation of HMGB1-TLR4-NF-κB signaling pathway (143). Quercetin protects against myocardial apoptosis in vivo via phosphorylation of JNK and p38, increasing Bcl-2 expression and directly or indirectly inhibiting the activation of Bax and caspase 3 (144, 145), as well as ameliorates MI/R-induced apoptosis through SIRT1/PGC-1α signaling (146).
Evidence shows that sympathetic excitatory reflexes exacerbate myocardial injury (147). In a neuropathic pain model a central mechanism through the inhibition of mitochondrial permeability transition pore (mPTP) opening (148) or involving the activation of paraventricular thalamic (PVA) neurons (149) induce cardioprotective effects.
Therefore, quercetin could be used as a potential therapeutic drug for reducing MI/R injury via SIRT1/PI3K/Akt/Nrf2 mediated oxidative stress, SIRT1/PGC1α and HMGB1/TLR4/NF-κB mediated inflammatory response, and SIRT1/p38 MAPK mediated apoptosis pathways (Figure 7 and Table 6).
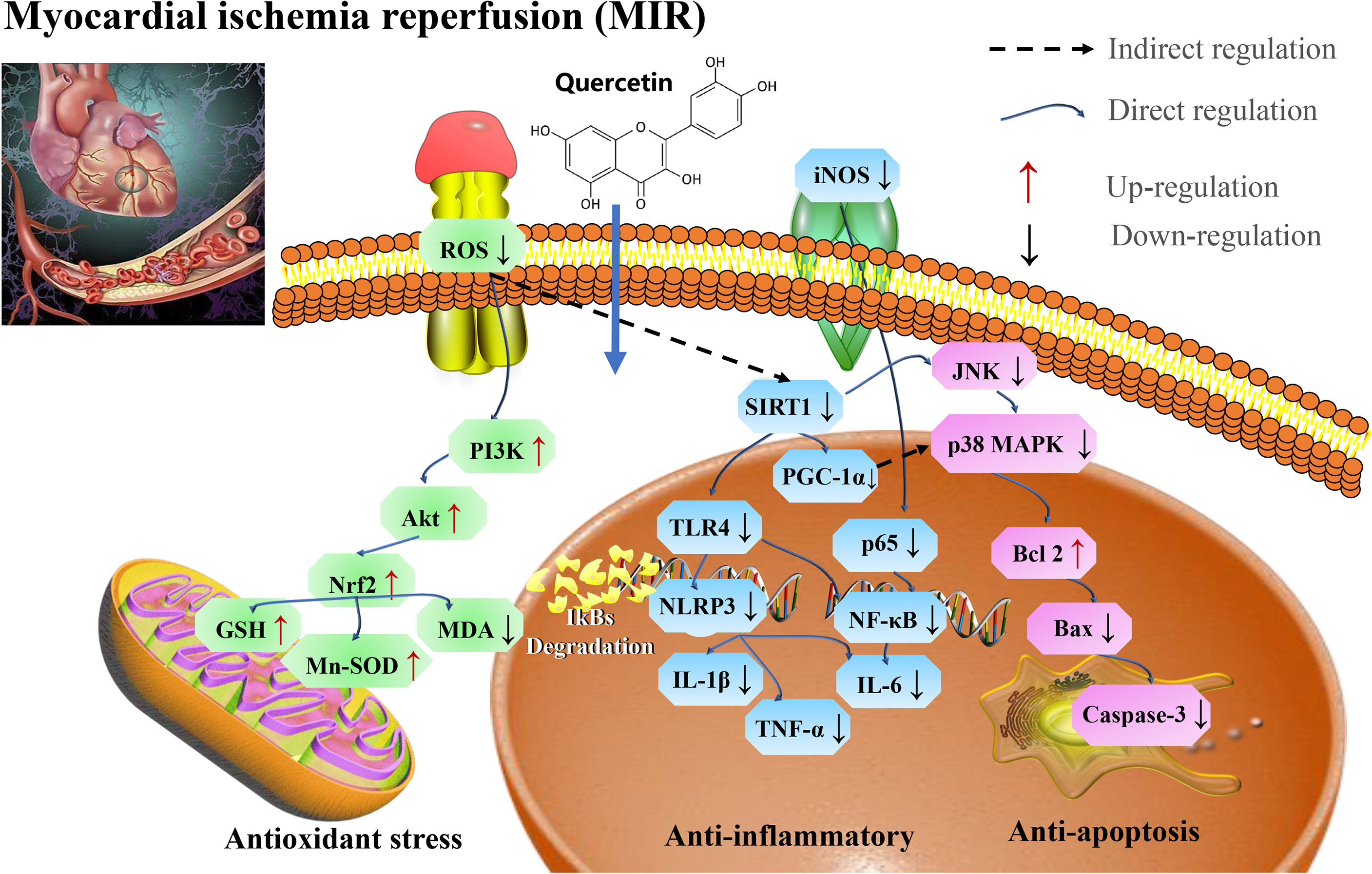
Figure 7 Mechanisms of quercetin on Myocardial ischemia (M/I) and reperfusion (MI/R). Quercetin is potential therapeutic drug that play roles in reducing MI/R injury via SIRT1/PI3K/Akt/Nrf2 mediated oxidative stress, SIRT1/PGC1α and HMGB1/TLR4/NF-κB mediated inflammatory response, and SIRT1/p38 MAPK mediated apoptosis pathways. MDA, malondialdehyde; SOD, superoxide dismutase; TNF-α, tumor necrosis factor-α; IL-1β, interleukin-1 β; iNOS, inducible nitric oxide synthase; GSH-Px, glutathione peroxidase; TLR4, toll-like receptor 4; and PGC-1α, proliferator-activated receptor gamma coactivator, 1alpha.
4.2 Atherosclerosis (AS)
Atherosclerosis promotes mortality and morbidity in aging-related cardiovascular diseased models (8, 153). Endothelial dysfunction is an important process involved in atherosclerosis (154). A solute exchange between the blood and nerve tissues have a direct contact and are intact by the blood-nerve barrier (BNB), consisting of the endothelium surrounding peripheral nerve substructures (155, 156). Oxidized low-density lipoprotein (ox-LDL)-induced oxidative damage to endothelial cells cause atherosclerosis. Thus, oxLDL-induces the formation of RAW264.7 macrophage-derived foam cells that exacerbate cellular lipid accumulation, and elevate the levels of ROS that lead to oxidation of LDL particles to produce ox-LDL, whereas quercetin inhibits cholesterol accumulation-induced apoptosis of macrophages, thereby reducing atherosclerosis (157, 158). In addition, quercetin enhances cellular antioxidant function through the Nrf2 pathway (154). Further studies have showed that quercetin inhibits ox-LDL induced oxidative damage in AS via activating SIRT1 and modulating the AMPK/NADPH/AKT signaling pathway (159), as well as inhibits inflammatory/oxidative stress responses in AS via AMPK/SIRT1/NF-κB pathway (160), and attenuated AS by increasing the density of SIRT1 (161). Another reports in mice have indicated that quercetin attenuates high fat diet-induced atherosclerosis in apolipoprotein E knockout mice by alleviating systemic oxidative stress and also inhibit aortic P47phox by blocking the activation of NADPH oxidase-P47phox membrane translocation (162).
During aging, chronic atherosclerosis promotes ROS production and accumulation which eventually cause mitochondria damage, due to mtDNA damage. OxLDL molecules are immunogenic and are associated with innate immunity to pattern recognition receptors, including scavenger receptors and TLR, and NF-κB, whereas quercetin significantly inhibit NLRP3 inflammatory vesicle activation in the ox-LDL-containing macrophages, thereby attenuating cell lipoatrophy and IL-1β secretion (163). Ex vivo studies have reported that quercetin (25 μM) reduce the HUVEC expression of VCAM-1, where ICAM-1 was significantly enhanced, and then attenuate oxLDL induced endothelial leukocyte adhesion, and effectively modulate the TLR-NF-κB signaling pathway by attenuating the inflammatory process in atherosclerosis (164). Quercetin inhibits the formation of atherosclerotic plaque, and the main mechanism of action is related to the PI3K/AKT-related regulation of caspase-3 and NF-κB activation (165). Moreover, quercetin decrease the mRNA expression of TNF-α and TLR4 in atherosclerotic rats, and the mechanism of action is related to the TLR4-mediated MAPK pathway by reducing the levels of expression of pro-inflammatory cytokines (IL-1β, TNF-α, and IL-10) (166). Dysregulation of TLRs has been reported to increase inflammation and metabolic syndrome which cause the development and progression of atherosclerosis (167).
Therefore, quercetin play an important role in the treatment of atherosclerosis by preventing endothelial cell damage via SIRT1/AMPK/Nrf2 mediated oxidative stress, SIRT1/PI3K/Akt/NF-κB and SIRT1/TLRs/MAPK mediated inflammatory response (Figure 8 and Table 7).
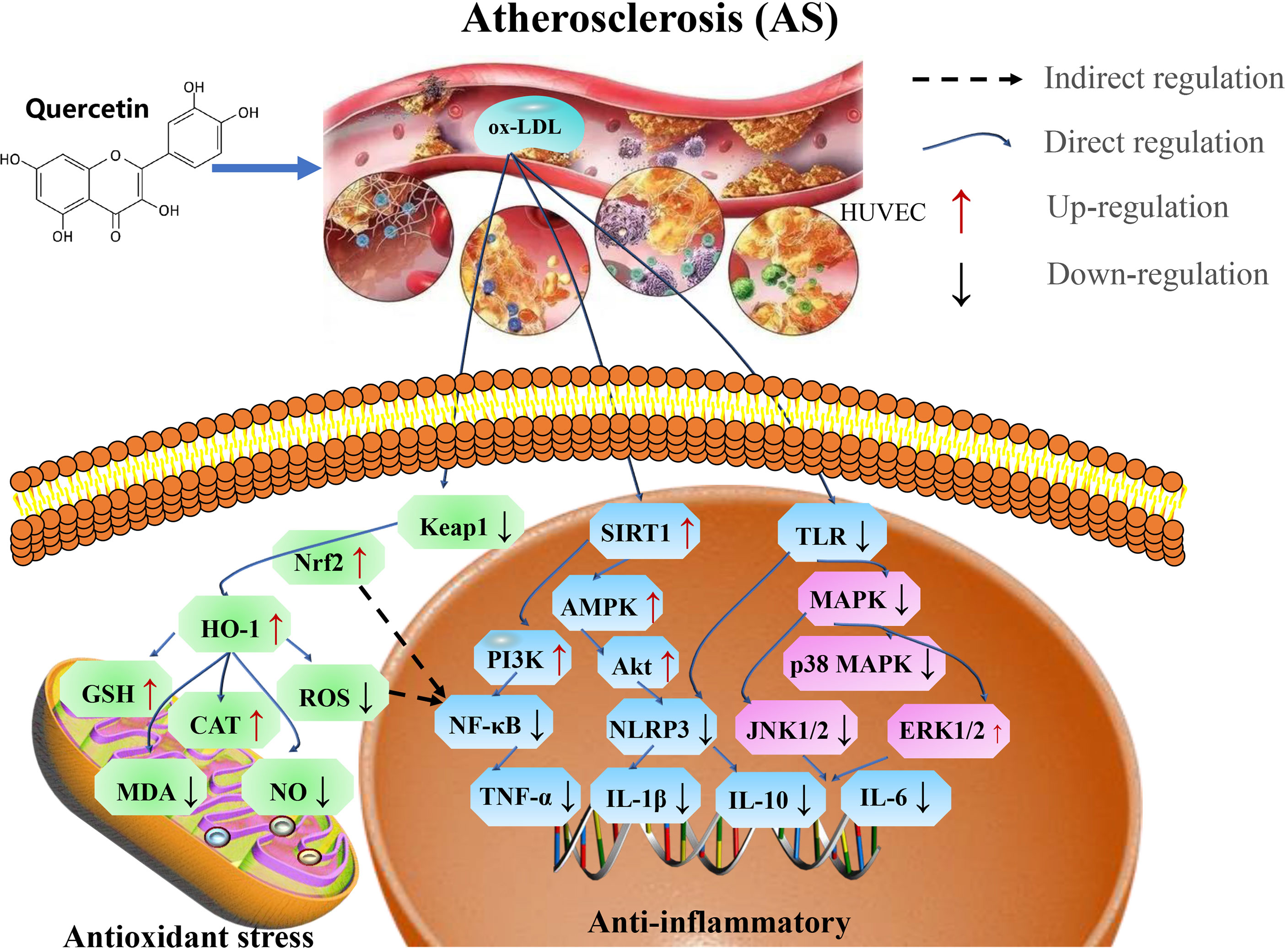
Figure 8 Mechanisms of quercetin on Atherosclerosis. Quercetin is an important target in the treatment of atherosclerosis by preventing endothelial cell damage via SIRT1/AMPK/Nrf2 mediated oxidative stress, SIRT1/PI3K/Akt/NF-κB and SIRT1/TLRs/MAPK mediated inflammatory response. TNF-α, tumor necrosis factor-α; AMPK, AMP-activated protein kinase; GSH-Px, glutathione peroxidase; MDA, malondialdehyde; NLRP3, NOD-like receptor protein 3; ox-LDL, oxidized low-density lipoprotein; and TLR4, toll-like receptor 4.
5 Effects of quercetin on diabetes
Diabetes is a metabolic disorder that cause death in human populace worldwide (mostly in elderly people) (169). The International Diabetes Federation reported that people suffering from diabetes equally exhibit neuropathy and neuropathic pain (170). The most common manifestation of diabetic neuropathy is distal symmetric polyneuropathy (DSPN), which affects approximately 30% of diabetic patients with the most relevant clinical manifestations, whereas the incidence of DSPN is approximately 2% per year (171). However, the pathogenesis is unclear, and clinical and epidemiological studies have indicated that oxidative stress and inflammatory processes are important pathological mechanisms in diabetic neuropathy, which is associated with distal symmetric sensorimotor polyneuropathy.
ROS is associated with the development of neuropathy in an experimental diabetes. The findings in streptozotocin (STZ)-injected diabetic rats suggest that oxidative stress cause neurotransmission defects (172–174). Quercetin has been shown to have a beneficial effect on nicotinamide/streptozotocin-induced antidiabetic and antioxidant capacity in Wistar diabetic rats (175, 176), reducing dorsal root ganglion (DRG) neurons damage by oxidative stress (177). Overproduction and accumulation of ROS and reactive carbonyl compounds induces endoplasmic reticulum stress (ERs). Quercetin was found to induce lysosomal defects which triggered ERs and ROS generation, thereby contributing to glioma cell death (29, 178). Quercetin can bypass the GLUT4 translocation insulin regulatory system through the AMPK signaling pathway and its downstream target p38 MAPK, thereby contributing to the correction of insulin resistance (179).An increase AMPK, insulin receptor substrate 1 (IRS-1) and AS160 phosphorylation increase GSK3β under insulin-stimulated conditions (180). Quercetin induces insulin secretion by direct activation of L-type calcium channels in pancreatic beta cells, thus, quercetin interacts with L-type Ca2+ channels at a location different from that of Bay K 8644 to increase Ca2+ influx, which stimulates insulin secretion (181) and thus, reduce the insulin levels (182). Quercetin improves endothelial function by inhibiting endoplasmic reticulum stress-mediated disruptions leading to the degeneration of islet initiation of the UPR response, and calcium homeostasis, thereby reducing oxidative stress in diabetic rats (183).
SOD is the main antioxidant parameter which prevents neuronal damage (184). Quercetin enhance the expression of antioxidant indices, thereby protects the mitochondrial function by increasing intracellular nicotinamide-adenine dinucleotide (NAD+) (185) and may be involved in regulating NF-κB and SIRT1 levels (186, 187). Further mechanisms may be related to the upregulation of SIRT1 activity and protein levels, and its effects on the Akt signaling pathway (188). In diabetic neuropathy, axonal and sensory neuron degeneration pathways are activated, leading to distal axonal lesions. The NAD+-dependent deacetylase SIRT1 prevents the activation of these pathways and promotes axonal regeneration (189).
Recent studies have demonstrated that ferroptosis, a newly identified form of regulated cell death characterized by iron-dependent dependence on the overproduction of ROS leading to irreparable lipid peroxidation, is involved in β-cell death leading to reduced insulin secretion. The protective effect of quercetin on mice pancreas occurs partly through the inhibition of hypertrophy (190).
In general, the application of quercetin at the appropriate dose could serve as a potential therapeutic agent in treating diabetes, by attenuating oxidative stress and ferroptosis (Figure 9 and Table 8).
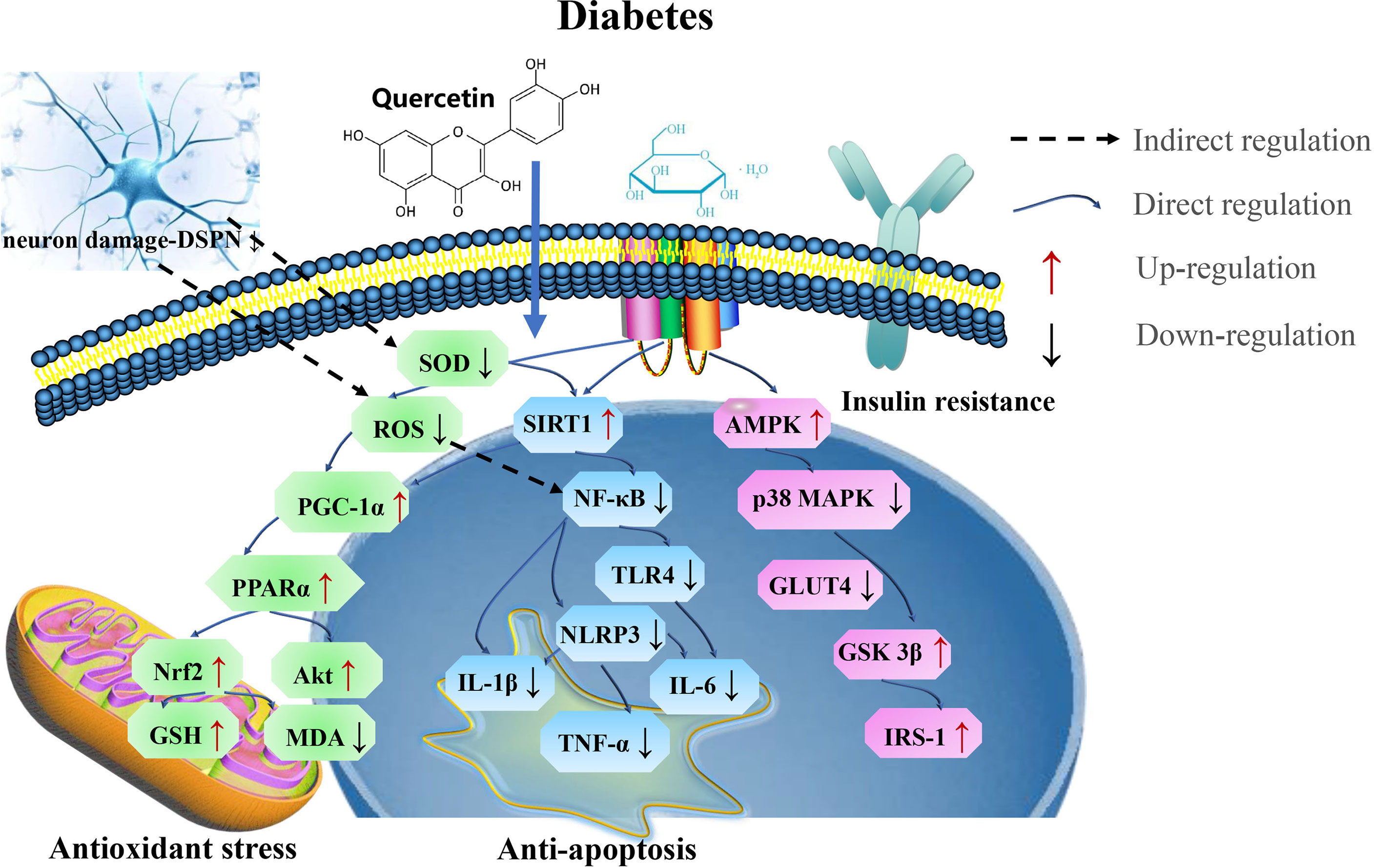
Figure 9 The mechanism of quercetin on diabetes. Quercetin play potential therapeutic roles in treating diabetes by targeting SIRT1 via PGC-1α/PPARα/Nrf2 mediated oxidative stress and SIRT1/NF-κB/NLRP3 mediated ferroptosis. SOD, superoxide dismutase; MDA, malondialdehyde; IL-6, interleukin-6; GST, glutathione S-transferases; PGC-1α, proliferator-activated receptor gamma coactivator; IRS-1, insulin receptor substrate-1; GSK3β, glycogen synthase kinase 3 beta.
6 Discussion
Aging is an irreversible physiological process, and the incidence of neurological diseases increases with age (192). Neurodegenerative diseases have been recognized as a manifestation of chronological aging (193). Therefore, it is important to identify suitable anti-aging drugs and explore their therapeutic mechanisms. Oxidative stress, neuroinflammation, apoptosis, autophagy, and mitochondrial dysfunction are significant causative factors related to aging-related diseases (194–197). Recently, numerous studies have reported the protective effects of SIRT1 in aging-related diseases, such as protective effect against neurodegeneration, regulation of oxidative stress, inflammatory response, mitochondrial biogenesis, cell death, and autophagy (17, 18, 198–200). Quercetin is an anti-aging flavonoid, because it possesses antioxidant, anti-apoptotic, anti-inflammatory properties, as well as can actively participate in the improvement of mitochondrial dysfunctions, thereby could be used as a novel therapeutic measure in treating aging-related diseases (29, 59, 96, 196, 201, 202). Therefore, recently researchers focus on investigating the medicinal effects of quercetin and its mechanism in attenuating aging-related diseases.
Several studies have shown that quercetin protects against neurodegenerative diseases by enhancing the mechanism of SIRT1 deacetylase (203, 204). However, low bioavailability and solubility of quercetin limits its clinical application (205). In addition, most of the current studies only focused on quercetin glycosides, however, the functions of the gut micobiota helps to breakdown quercetin into serious biological metabolites such as phloroglucinol, 3,4-dihydroxyphenylacetic acid, 4-hydroxyphenylacetic acid, and 3,4-dihydroxybenzaldehyde (206). Therefore, it is important to examine the potential therapeutic effects of these intestinal metabolites obtained from quercetin.
6.1 Quercetin regulates oxidative stress via SIRT1
Oxidative stress, mitochondrial dysfunction, inflammatory, and cell autophagy and apoptosis are pathophysiological factors responsible for initiating aging-related diseases (207). There is a correlation between oxidative stress and aging (208). Thus, with chronological aging, ROS production and accumulation from multiple sources increases, whereas the levels of antioxidant enzymes and repair systems (proteasomal degradation) decline. Reports have shown that quercetin improve the concentration antioxidant enzymes and anti-inflammatory cytokines, as well as improve mitochondrial function by targeting SIRT1 activity via the SIRT1/AMPK/NF-κB, SIRT1/Keap1/Nrf2/HO-1, and SIRT1/PI3K/Akt pathways (159, 203).
In a rat model, it was indicated that quercetin inhibited ER stress to attenuate oxidative stress-induced apoptosis and prevent the progression of osteoarthritis by activating the SIRT1/AMPK pathway in rat chondrocytes (209), and inhibited oxidative stress responses in diabetic high fat diet-induced atherosclerosis in the carotid artery of rats by modulating AMPK/SIRT1/NF-κB pathway (160). Quercetin inhibited oxidative stress to ameliorate diabetic encephalopathy via SIRT1/ER stress pathway in db/db mice (210), furthermore, it inhibited oxidative stress damage to regulate mitophagy and ER stress via SIRT1/TMBIM6 (transmembrane BAX inhibitor-1 motif-containing 6) in cardiovascular diseases (211), as well as attenuated collagen-induced oxidative stress in mice arthritis by mediating SIRT1 activation (212). Moreover, Quercetin was found to reduce oxLDL-induced oxidative damage by upregulating AMPK and SIRT1 activity (159); and reduce oxidative damage in the liver and kidney tissues, and NF-kB levels by increasing SIRT1 in diabetes model (187).
6.2 Quercetin regulates inflammatory response via SIRT1
The attenuation of the inflammatory response is a potential anti-aging strategy. Senescent cell expression is the most pronounced in post-mitotic cells, whereas mitochondria and lysosomes suffer the most significant aging-related alterations in all organelles. Studies have confirmed that SIRT1 activation can inhibit NF-κB, TLRs, and NLRP3 pathways to reduce inflammation. Activation of these SIRT1-dependent signaling pathways by quercetin result in the modulation of the levels and functions of the inflammatory cytokines (213). Quercetin modulates AMPK/SIRT1/NF-κB pathway to inhibit inflammatory responses in diabetic high fat diet-induced atherosclerosis in the carotid artery of rats (160). In addition, quercetin alleviates inflammatory responses by activating SIRT1 and inhibit NF-κB pathway (159), furthermore, it contributes to anti-inflammatory effect by modulating SIRT1 activation via AMPK/SIRT1/Nfr2/TNFα pathway (203), as well as counteracts cholesterol-induced activation of the NF-κB pathway in the pancreas and normalized the expression of pro-inflammatory cytokines by increasing SIRTI expression (214), and reduce inflammation response in obesity mice through AMPKα1/SIRT1 pathway (215).
6.3 Quercetin regulates mitochondrial function via SIRT1
Mitochondrial dysfunction contributes significantly to aging-related diseases (216–218). However, treatment with quercetin could reduce ROS generation and mitigate mitochondrial dysfunction, thereby maintaining the mitochondrial homeostasis and normal function. Quercetin suppressed oxLDL-induced mitochondrial dysfunction and ROS formation by activating SIRT1 and modulating the AMPK/NADPH/AKT pathway (159), and attenuate mitochondrial dysfunction via activating AMPK/SIRT1 pathway in osteoarthritis rats (219). Moreover, quercetin mitigate cerebral ischemia reperfusion injury and reduce ROS generation in the mitochondria via SIRT1/Nrf2/HO-1 pathway (220).
Quercetin, as SIRT1 agonists, promotes mitochondrial biogenesis thereby attenuates mitochondrial diseases (221), prevented cholesterol-induced mitochondrial bioenergetic dysfunction by upregulating the expression of SIRT1 in the Min6 cells (214), and promotes mitochondrial biogenesis in the brain by activating the transcription of SIRT1 (222, 223). Quercetin as SIRT1/PGC1-α activator was reported to improved cardiac function in aged Mdx/Utrn+/- mice by increasing the protein contents and decrease inflammatory markers NF-κB in the mitochondria (224).
6.4 Quercetin regulates autophagy and apoptosis via SIRT1
During the occurrence of aging-related diseases, cell become vulnerable to the accumulation of abnormal proteins and damage to the phagocytic lysosomal system, which eventually cause cell death (225). Quercetin-induced autophagy contributes to apoptosis via SIRT1/AMPK pathway in lung cancer cells (226), moreover, quercetin inhibits apoptosis and attenuates intervertebral disc degeneration by promoting SIRT1-dependent autophagy (227), as well as improve MI/R-induced cardiomyocyte apoptosis via SIRT1/PGC-1α pathway (146), and regulate autophagy and mitochondrial ROS homeostasis via Nrf2/PGC-1α/SIRT1 pathway in sodium iodate-induced retinal damage (228). A study identify that quercetin could rescue cardiomyocyte hypoxia by regulating SIRT1/TMBIM6-related mitophagy (211), and reduce renal tubular epithelial cell senescence by activating SIRT1/PINK1/Parkin-mediated mitophagy (229). In addition, other studies have showed that quercetin exerts protective effects against cholesterol-induced apoptosis by improving the expression of SIRTI and inhibits cytochrome c release in Min6 cells (214).
7 Conclusions and future perspectives
The pharmacological efficacy of quercetin has shown that it possesses promising therapeutic potentials. In this review, we highlighted the role of quercetin in targeting SIRT1 with the aim of preventing aging-related diseases via oxidative stress and inflammation alleviation, as well as the restoration of mitochondrial dysfunction. Therefore, SIRT1 may serve as a potential therapeutic target for the treatment of aging-related diseases.
Recent studies on aging-related diseases have indicated that ferroptosis contributes in the pathogenesis of Alzheimer’s disease (230–232), Parkinson’s disease (233, 234), Huntington’s disease (235), Depression (236–238), Osteoarthritis (239), Myocardial ischemia and reperfusion (MI/R) (240–242), Atherosclerosis (243, 244), and Diabetes (245, 246). Notably, quercetin shows iron-chelating activity and effectively decrease iron deposition in the hearts, kidneys and liver of iron-dextran-overloaded mice (247), and protects bone marrow-derived mesenchymal stem cells from erastin-induced ferroptosis through antioxidant pathway (248). Therefore, quercetin was identified as a ferroptosis inhibitor to alleviate acute kidney injury (249). Moreover, activation of SIRT1 inhibits excess iron-induced ferroptosis of foam cells, thereby providing a novel therapeutic target for atherosclerosis (250), and fisetin attenuated doxorubicin-induced cardiomyopathy by inhibiting ferroptosis via the activation of SIRT1/Nrf2 signaling pathway (251). Thus, acting as an intracellular iron chelators, as well as stimulating cellular degradation systems provide novel mechanisms for aging research and may be potential diagnostic biomarkers for aging-related disease in the future (252). The future challenge is to establish models of ferroptosis on disease-specific basis so that the underlying mechanisms of action can be investigated in detail.
Recently, various studies have suggested that extracellular vesicles (EVs) have multiple advantages over currently available drug delivery vehicles, opening new frontiers for modern drug delivery (253–255). Therefore, EVs can be considered for quercetin loading and delivery for treating aging-related disease. In addition, EVs can successfully communicate between cells and organs in the aging microenvironment and during the occurrence of aging-related diseases (256). In particular, neuron-derived EVs can breach the blood-brain barrier (BBB) and become important carriers of signals between cell types in the central nervous system (CNS) (257).
Recent studies have shown that EVs can reduce glutamate expression in the neurons to improve cognitive function and reduce Aβ plaques in AD model mice by activating the SIRT1 pathway (258). The loss of SIRT1 support could accelerated EV production, which carry pathological α-SYN and preferentially target microglia to induce microglial inflammation (259). The release of circulating EVs with oxidative contents alters redox and mitochondrial homeostasis in the brains of rats, suggesting that SIRT1-mediated EVs obtained from different donors may also be promising materials and tools for anti-aging disease therapy.
In this comprehensive review, we have found clues from separate studies and found that quercetin could regulate oxidative stress, inflammatory response, mitochondrial dysfunction, autophagy and apoptosis by activating SIRT1 in aging-related disease. However, there are limited in vivo clinical studies on this subject matter, due to limitations such as low bioavailability and solubility of quercetin and disease complexity, therefore, several clinical in vivo studies should be carried out to explore the pharmacological effects and the pharmacokinetics of metabolites to establish and identify useful clinical metabolites released from quercetin catabolism by the gut microbiota. Moreover, whether EVs could be considered for quercetin loading and delivery for treating aging-related disease, and whether quercetin could alleviate ferroptosis to protect against aging-related disease by activating SIRT1 requires further studies.
Author contributions
Conceptualization, ZC and XZ (2nd author); Literature review, YW, GS, YT, XD, and DL; Writing – original draft, ZC and XZ (2nd author); Writing – review & editing, ZC, FA, and XZ (2nd author). All authors have read and agreed to the published version of the manuscript.
Funding
This study was financially funded by Sichuan Science and Technology Program (2020JDRC0104), the Key Research & Development Plan of the Department of Science and Technology of Tibet Autonomous Region (XZ202101ZY0002N), the Local Projects Guided by the Central Government from Razi County, Tibet Autonomous Region, and the Projects Funded by the Central Government to Guide Local Scientific and Technological Development from Guizhou province (QIANKEZHONGYINDI[2021]4003).
Conflict of interest
The authors declare that the research was conducted in the absence of any commercial or financial relationships that could be construed as a potential conflict of interest.
Publisher’s note
All claims expressed in this article are solely those of the authors and do not necessarily represent those of their affiliated organizations, or those of the publisher, the editors and the reviewers. Any product that may be evaluated in this article, or claim that may be made by its manufacturer, is not guaranteed or endorsed by the publisher.
References
1. Zhang W, Qu J, Liu GH, Belmonte JCI. The ageing epigenome and its rejuvenation. Nat Rev Mol Cell Biol (2020) 21(3):137–50. doi: 10.1038/s41580-019-0204-5
2. Kalaria RN, Maestre GE, Arizaga R, Friedland RP, Galasko D, Hall K, et al. Alzheimer's disease and vascular dementia in developing countries: prevalence, management, and risk factors. Lancet Neurol (2008) 7(9):812–26. doi: 10.1016/s1474-4422(08)70169-8
3. Zhang M, Bian Z. The emerging role of circular rnas in alzheimer's disease and parkinson's disease. Front Aging Neurosci (2021) 13:691512. doi: 10.3389/fnagi.2021.691512
4. Šonský I, Vodička P, Vodičková Kepková K, Hansíková H. Mitophagy in huntington's disease. Neurochem Int (2021) 149:105147. doi: 10.1016/j.neuint.2021.105147
5. Terao T. Neglected but not negligible aspects of antidepressants and their availability in bipolar depression. Brain Behav (2021) 11(8):e2308. doi: 10.1002/brb3.2308
6. Kalinkovich A, Livshits G. Biased and allosteric modulation of bone cell-expressing g protein-coupled receptors as a novel approach to osteoporosis therapy. Pharmacol Res (2021) 171:105794. doi: 10.1016/j.phrs.2021.105794
7. Chouchani ET, Pell VR, Gaude E, Aksentijević D, Sundier SY, Robb EL, et al. Ischaemic accumulation of succinate controls reperfusion injury through mitochondrial ros. Nature (2014) 515(7527):431–5. doi: 10.1038/nature13909
8. Gueguen N, Lenaers G, Reynier P, Weissig V, Edeas M. Mitochondrial dysfunction in mitochondrial medicine: current limitations, pitfalls, and tomorrow. Methods Mol Biol (Clifton NJ) (2021) 2276:1–29. doi: 10.1007/978-1-0716-1266-8_1
9. Cuadros DF, Li J, Musuka G, Awad SF. Spatial epidemiology of diabetes: methods and insights. World J Diabetes (2021) 12(7):1042–56. doi: 10.4239/wjd.v12.i7.1042
10. Hou Y, Dan X, Babbar M, Wei Y, Hasselbalch SG, Croteau DL, et al. Ageing as a risk factor for neurodegenerative disease. Nat Rev Neurol (2019) 15(10):565–81. doi: 10.1038/s41582-019-0244-7
11. López-Otín C, Blasco MA, Partridge L, Serrano M, Kroemer G. The hallmarks of aging. Cell (2013) 153(6):1194–217. doi: 10.1016/j.cell.2013.05.039
12. Emmerzaal TL, Nijkamp G, Veldic M, Rahman S, Andreazza AC, Morava E, et al. Effect of neuropsychiatric medications on mitochondrial function: for better or for worse. Neurosci Biobehav R (2021) 127:555–71. doi: 10.1016/j.neubiorev.2021.05.001
13. Ferrucci L, Fabbri E. Inflammageing: chronic inflammation in ageing, cardiovascular disease, and frailty. Nat Rev Cardiol (2018) 15(9):505–22. doi: 10.1038/s41569-018-0064-2
14. Mulica P, Grünewald A, Pereira SL. Astrocyte-neuron metabolic crosstalk in neurodegeneration: a mitochondrial perspective. Front Endocrinol (2021) 12:668517. doi: 10.3389/fendo.2021.668517
15. Singh V, Ubaid S. Role of silent information regulator 1 (sirt1) in regulating oxidative stress and inflammation. Inflammation (2020) 43(5):1589–98. doi: 10.1007/s10753-020-01242-9
16. Seto E, Yoshida M. Erasers of histone acetylation: the histone deacetylase enzymes. CSH Perspect Biol (2014) 6(4):a018713. doi: 10.1101/cshperspect.a018713
17. Osborne B, Bentley NL, Montgomery MK, Turner N. The role of mitochondrial sirtuins in health and disease. Free Radical Bio Med (2016) 100:164–74. doi: 10.1016/j.freeradbiomed.2016.04.197
18. Shahgaldi S, Kahmini FR. A comprehensive review of sirtuins: with a major focus on redox homeostasis and metabolism. Life Sci (2021) 282:119803. doi: 10.1016/j.lfs.2021.119803
19. Fang Y, Wang X, Yang D, Lu Y, Wei G, Yu W, et al. Relieving cellular energy stress in aging, neurodegenerative, and metabolic diseases, sirt1 as a therapeutic and promising node. Front Aging Neurosci (2021) 13:738686. doi: 10.3389/fnagi.2021.738686
20. Bai L, Liu R, Wang R, Xin Y, Wu Z, Ba Y, et al. Attenuation of pb-induced aβ generation and autophagic dysfunction via activation of sirt1: neuroprotective properties of resveratrol. Ecotox Environ Safe (2021) 222:112511. doi: 10.1016/j.ecoenv.2021.112511
21. Pratiwi R, Nantasenamat C, Ruankham W, Suwanjang W, Prachayasittikul V, Prachayasittikul S, et al. Mechanisms and neuroprotective activities of stigmasterol against oxidative stress-induced neuronal cell death via sirtuin family. Front Nutr (2021) 8:648995. doi: 10.3389/fnut.2021.648995
22. Zia A, Pourbagher-Shahri AM, Farkhondeh T, Samarghandian S. Molecular and cellular pathways contributing to brain aging. Behav Brain Funct (2021) 17(1):6. doi: 10.1186/s12993-021-00179-9
23. Tripodi F, Lombardi L, Guzzetti L, Panzeri D, Milanesi R, Leri M, et al. Protective effect of vigna unguiculata extract against aging and neurodegeneration. Aging (2020) 12(19):19785–808. doi: 10.18632/aging.104069
24. Finkel T, Holbrook NJ. Oxidants, oxidative stress and the biology of ageing. Nature (2000) 408(6809):239–47. doi: 10.1038/35041687
25. Teng WL, Huang PH, Wang HC, Tseng CH, Yen FL. Pterostilbene attenuates particulate matter-induced oxidative stress, inflammation and aging in keratinocytes. Antioxidants (Basel Switzerland) (2021) 10(10):1552. doi: 10.3390/antiox10101552
26. Fontana L, Ghezzi L, Cross AH, Piccio L. Effects of dietary restriction on neuroinflammation in neurodegenerative diseases. J Exp Med (2021) 218(2):e20190086. doi: 10.1084/jem.20190086
27. Kitada M, Ogura Y, Monno I, Koya D. Sirtuins and type 2 diabetes: role in inflammation, oxidative stress, and mitochondrial function. Front Endocrinol (2019) 10:187. doi: 10.3389/fendo.2019.00187
28. Zhao X, Wang J, Deng Y, Liao L, Zhou M, Peng C, et al. Quercetin as a protective agent for liver diseases: a comprehensive descriptive review of the molecular mechanism. Phytother Res (2021) 35(9):4727–47. doi: 10.1002/ptr.7104
29. Grewal AK, Singh TG, Sharma D, Sharma V, Singh M, Rahman MH, et al. Mechanistic insights and perspectives involved in neuroprotective action of quercetin. BioMed Pharmacother (2021) 140:111729. doi: 10.1016/j.biopha.2021.111729
30. Luca SV, Macovei I, Bujor A, Miron A, Skalicka-Woźniak K, Aprotosoaie AC, et al. Bioactivity of dietary polyphenols: the role of metabolites. Crit Rev Food Sci Nutr (2020) 60(4):626–59. doi: 10.1080/10408398.2018.1546669
31. Dabeek WM, Marra MV. Dietary quercetin and kaempferol: bioavailability and potential cardiovascular-related bioactivity in humans. Nutrients (2019) 11:2288. doi: 10.3390/nu11102288
32. Fasolo D, Schwingel L, Holzschuh M, Bassani V, Teixeira H. Validation of an isocratic lc method for determination of quercetin and methylquercetin in topical nanoemulsions. J Pharm BioMed Anal (2007) 44(5):1174–7. doi: 10.1016/j.jpba.2007.04.026
33. Rodríguez-Félix F, Del-Toro-Sánchez CL, Javier Cinco-Moroyoqui F, Juárez J, Ruiz-Cruz S, López-Ahumada GA, et al. Preparation and characterization of quercetin-loaded zein nanoparticles by electrospraying and study of in vitro bioavailability. J Food Sci (2019) 84(10):2883–97. doi: 10.1111/1750-3841.14803
34. Lee YJ, Park Y. Green synthetic nanoarchitectonics of gold and silver nanoparticles prepared using quercetin and their cytotoxicity and catalytic applications. J Nanosci Nanotechnol (2020) 20(5):2781–90. doi: 10.1166/jnn.2020.17453
35. Xu D, Hu MJ, Wang YQ, Cui YL. Antioxidant activities of quercetin and its complexes for medicinal application. Molecules (Basel Switzerland) (2019) 24:1123. doi: 10.3390/molecules24061123
36. Tang SM, Deng XT, Zhou J, Li QP, Ge XX, Miao L. Pharmacological basis and new insights of quercetin action in respect to its anti-cancer effects. BioMed Pharmacother (2020) 121:109604. doi: 10.1016/j.biopha.2019.109604
37. Hu Y, Gui Z, Zhou Y, Xia L, Lin K, Xu Y. Quercetin alleviates rat osteoarthritis by inhibiting inflammation and apoptosis of chondrocytes, modulating synovial macrophages polarization to m2 macrophages. Free Radical Bio Med (2019) 145:146–60. doi: 10.1016/j.freeradbiomed.2019.09.024
38. Andres S, Pevny S, Ziegenhagen R, Bakhiya N, Schäfer B, Hirsch-Ernst KI, et al. Safety aspects of the use of quercetin as a dietary supplement. Mol Nutr Food Res (2018) 62(1):1700447. doi: 10.1002/mnfr.201700447
39. Han X, Xu T, Fang Q, Zhang H, Yue L, Hu G, et al. Quercetin hinders microglial activation to alleviate neurotoxicity via the interplay between Nlrp3 inflammasome and mitophagy. Redox Biol (2021) 44:102010. doi: 10.1016/j.redox.2021.102010
40. Huang YY, Wang ZH, Deng LH, Wang H, Zheng Q. Oral administration of quercetin or its derivatives inhibit bone loss in animal model of osteoporosis. Oxid Med Cell Longev (2020) 2020:6080597. doi: 10.1155/2020/6080597
41. Sato S, Mukai Y. Modulation of chronic inflammation by quercetin: the beneficial effects on obesity. J Inflammation Res (2020) 13:421–31. doi: 10.2147/jir.S228361
42. Boccellino M, D'Angelo S. Anti-obesity effects of polyphenol intake: current status and future possibilities. Int J Mol Sci (2020) 21(16):5642. doi: 10.3390/ijms21165642
43. Zhao X, Gong L, Wang C, Liu M, Hu N, Dai X, et al. Quercetin mitigates ethanol-induced hepatic steatosis in zebrafish via P2x7r-mediated Pi3k/ Keap1/Nrf2 signaling pathway. J Ethnopharmacol (2021) 268:113569. doi: 10.1016/j.jep.2020.113569
44. Shen XY, Luo T, Li S, Ting OY, He F, Xu J, et al. Quercetin inhibits okadaic acid-induced tau protein hyperphosphorylation through the Ca2+−Calpain−P25−Cdk5 pathway in Ht22 cells. Int J Mol Med (2018) 41(2):1138–46. doi: 10.3892/ijmm.2017.3281
45. Yin Z, Geng X, Zhang Z, Wang Y, Gao X. Rhein relieves oxidative stress in an aβ(1-42) oligomer-burdened neuron model by activating the sirt1/Pgc-1α-regulated mitochondrial biogenesis. Front Pharmacol (2021) 12:746711. doi: 10.3389/fphar.2021.746711
46. Yin X, Zhou Z, Qiu Y, Fan X, Zhao C, Bao J, et al. Sirt1 regulates tau expression and tau synaptic pathology. J Alzheimer's Dis (2021) 84(2):895–904. doi: 10.3233/jad-215118
47. Paula PC, Angelica Maria SG, Luis CH, Gloria Patricia CG. Preventive effect of quercetin in a triple transgenic alzheimer's disease mice model. Molecules (Basel Switzerland) (2019) 24(12):2287. doi: 10.3390/molecules24122287
48. Keren-Shaul H, Spinrad A, Weiner A, Matcovitch-Natan O, Dvir-Szternfeld R, Ulland TK, et al. A unique microglia type associated with restricting development of alzheimer's disease. Cell (2017) 169(7):1276–90.e17. doi: 10.1016/j.cell.2017.05.018
49. Burns JC, Cotleur B, Walther DM, Bajrami B, Rubino SJ, Wei R, et al. Differential accumulation of storage bodies with aging defines discrete subsets of microglia in the healthy brain. eLife (2020) 9:e57495. doi: 10.7554/eLife.57495
50. Ye F, Wu A. The protective mechanism of sirt1 in the regulation of mitochondrial biogenesis and mitochondrial autophagy in alzheimer's disease. J Alzheimer's Dis (2021) 82(1):149–57. doi: 10.3233/jad-210132
51. Yu X, Li Y, Mu X. Effect of quercetin on Pc12 alzheimer's disease cell model induced by aβ (25-35) and its mechanism based on Sirtuin1/Nrf2/Ho-1 pathway. BioMed Res Int (2020) 2020:8210578. doi: 10.1155/2020/8210578
52. Zaplatic E, Bule M, Shah SZA, Uddin MS, Niaz K. Molecular mechanisms underlying protective role of quercetin in attenuating alzheimer's disease. Life Sci (2019) 224:109–19. doi: 10.1016/j.lfs.2019.03.055
53. Mrvová N, Škandík M, Kuniaková M, Račková L. Modulation of bv-2 microglia functions by novel quercetin pivaloyl ester. Neurochem Int (2015) 90:246–54. doi: 10.1016/j.neuint.2015.09.005
54. Delage CI, Šimončičová E, Tremblay M. Microglial heterogeneity in aging and alzheimer's disease: Is sex relevant? J Pharmacol Sci (2021) 146(3):169–81. doi: 10.1016/j.jphs.2021.03.006
55. Lu Y, Liu Q, Yu Q. Quercetin enrich diet during the early-middle not middle-late stage of alzheimer's disease ameliorates cognitive dysfunction. Am J Transl Res (2018) 10(4):1237–46.
56. Wu Y, Chen M, Jiang J. Mitochondrial dysfunction in neurodegenerative diseases and drug targets via apoptotic signaling. Mitochondrion (2019) 49:35–45. doi: 10.1016/j.mito.2019.07.003
57. Baik SH, Kang S, Lee W, Choi H, Chung S, Kim JI, et al. A breakdown in metabolic reprogramming causes microglia dysfunction in alzheimer's disease. Cell Metab (2019) 30(3):493–507.e6. doi: 10.1016/j.cmet.2019.06.005
58. Spangenberg E, Severson PL, Hohsfield LA, Crapser J, Zhang J, Burton EA, et al. Sustained microglial depletion with Csf1r inhibitor impairs parenchymal plaque development in an alzheimer's disease model. Nat Commun (2019) 10(1):3758. doi: 10.1038/s41467-019-11674-z
59. Wang DM, Li SQ, Wu WL, Zhu XY, Wang Y, Yuan HY. Effects of long-term treatment with quercetin on cognition and mitochondrial function in a mouse model of alzheimer's disease. Neurochem Res (2014) 39(8):1533–43. doi: 10.1007/s11064-014-1343-x
60. Shi L, Zhang J, Wang Y, Hao Q, Chen H, Cheng X. Sirt1 regulates oxidative stress in oxygen-glucose deprived hippocampal neurons. Front Pediatr (2020) 8:455. doi: 10.3389/fped.2020.00455
61. Hayakawa M, Itoh M, Ohta K, Li S, Ueda M, Wang MX, et al. Quercetin reduces Eif2α phosphorylation by Gadd34 induction. Neurobiol Aging (2015) 36(9):2509–18. doi: 10.1016/j.neurobiolaging.2015.05.006
62. Chen H, Chen F, Zhang M, Chen Y, Cui L, Liang C. A review of apoe genotype-dependent autophagic flux regulation in alzheimer's disease. J Alzheimer's Dis (2021) 84(2):535–55. doi: 10.3233/jad-210602
63. Goswami S, Kareem O, Goyal RK, Mumtaz SM, Tonk RK, Gupta R, et al. Role of forkhead transcription factors of the O class (Foxo) in development and progression of alzheimer's disease. CNS Neurol Disord-DR (2020) 19(9):709–21. doi: 10.2174/1871527319666201001105553
64. Kong Y, Li K, Fu T, Wan C, Zhang D, Song H, et al. Quercetin ameliorates aβ toxicity in drosophila ad model by modulating cell cycle-related protein expression. Oncotarget (2016) 7(42):67716–31. doi: 10.18632/oncotarget.11963
65. Morales V, McConnell J, Pérez-Garnes M, Almendro N, Sanz R, García-Muñoz RA. L-dopa release from mesoporous silica nanoparticles engineered through the concept of drug-Structure-Directing agents for parkinson's disease. J Mater Chem B (2021) 9(20):4178–89. doi: 10.1039/d1tb00481f
66. Tamtaji OR, Hadinezhad T, Fallah M, Shahmirzadi AR, Taghizadeh M, Behnam M, et al. The therapeutic potential of quercetin in parkinson's disease: Insights into its molecular and cellular regulation. Curr Drug Targets (2020) 21(5):509–18. doi: 10.2174/1389450120666191112155654
67. Zoey FL, Palanivel M, Padmanabhan P, Gulyás B. Parkinson's disease: A nanotheranostic approach targeting alpha-synuclein aggregation. Front Cell Dev Biol (2021) 9:707441. doi: 10.3389/fcell.2021.707441
68. Koo JH, Cho JY. Treadmill exercise attenuates A-synuclein levels by promoting mitochondrial function and autophagy possibly via Sirt1 in the chronic Mptp/P-induced mouse model of parkinson's disease. Neurotox Res (2017) 32(3):473–86. doi: 10.1007/s12640-017-9770-5
69. Picca A, Guerra F, Calvani R, Romano R, Coelho-Júnior HJ, Bucci C, et al. Mitochondrial dysfunction, protein misfolding and neuroinflammation in parkinson's disease: Roads to biomarker discovery. Biomolecules (2021) 11(10):1508. doi: 10.3390/biom11101508
70. Kääriäinen TM, Piltonen M, Ossola B, Kekki H, Lehtonen S, Nenonen T, et al. Lack of robust protective effect of quercetin in two types of 6-Hydroxydopamine-Induced parkinsonian models in rats and dopaminergic cell cultures. Brain Res (2008) 1203:149–59. doi: 10.1016/j.brainres.2008.01.089
71. Magalingam KB, Radhakrishnan A, Haleagrahara N. Protective effects of quercetin glycosides, rutin, and isoquercetrin against 6-hydroxydopamine (6-Ohda)-Induced neurotoxicity in rat pheochromocytoma (Pc-12) cells. Int J Immunopath Ph (2016) 29(1):30–9. doi: 10.1177/0394632015613039
72. Singh S, Kumar P. Piperine in combination with quercetin halt 6-ohda induced neurodegeneration in experimental rats: Biochemical and neurochemical evidences. Neurosci Res (2018) 133:38–47. doi: 10.1016/j.neures.2017.10.006
73. Karuppagounder SS, Madathil SK, Pandey M, Haobam R, Rajamma U, Mohanakumar KP. Quercetin up-regulates mitochondrial complex-I activity to protect against programmed cell death in rotenone model of parkinson's disease in rats. Neuroscience (2013) 236:136–48. doi: 10.1016/j.neuroscience.2013.01.032
74. Sriraksa N, Wattanathorn J, Muchimapura S, Tiamkao S, Brown K, Chaisiwamongkol K. Cognitive-enhancing effect of quercetin in a rat model of parkinson's disease induced by 6-hydroxydopamine. Evid-Based Compl Alt (2012) 2012:823206. doi: 10.1155/2012/823206
75. Gureev AP, Popov VN. Nrf2/Are pathway as a therapeutic target for the treatment of Parkinson diseases. Neurochem Res (2019) 44(10):2273–9. doi: 10.1007/s11064-018-02711-2
76. Liu Y, Yan J, Sun C, Li G, Li S, Zhang L, et al. Ameliorating mitochondrial dysfunction restores carbon ion-induced cognitive deficits via Co-activation of Nrf2 and Pink1 signaling pathway. Redox Biol (2018) 17:143–57. doi: 10.1016/j.redox.2018.04.012
77. Guzman-Martinez L, Maccioni RB, Andrade V, Navarrete LP, Pastor MG, Ramos-Escobar N. Neuroinflammation as a common feature of neurodegenerative disorders. Front Pharmacol (2019) 10:1008. doi: 10.3389/fphar.2019.01008
78. Picca A, Calvani R, Coelho-Junior HJ, Landi F, Bernabei R, Marzetti E. Mitochondrial dysfunction, oxidative stress, and neuroinflammation: Intertwined roads to neurodegeneration. Antioxidants (Basel Switzerland) (2020) 9(8):647. doi: 10.3390/antiox9080647
79. Bournival J, Plouffe M, Renaud J, Provencher C, Martinoli MG. Quercetin and sesamin protect dopaminergic cells from mpp+-induced neuroinflammation in a microglial (N9)-neuronal (Pc12) coculture system. Oxid Med Cell Longev (2012) 2012:921941. doi: 10.1155/2012/921941
80. Bahar E, Kim JY, Yoon H. Quercetin attenuates manganese-induced neuroinflammation by alleviating oxidative stress through regulation of apoptosis, Inos/Nf-Kb and ho-1/Nrf2 pathways. Int J Mol Sci (2017) 18(9):1989. doi: 10.3390/ijms18091989
81. Sharma S, Raj K, Singh S. Neuroprotective effect of quercetin in combination with piperine against rotenone- and iron supplement-induced parkinson's disease in experimental rats. Neurotox Res (2020) 37(1):198–209. doi: 10.1007/s12640-019-00120-z
82. Zahid A, Li B, Kombe AJK, Jin T, Tao J. Pharmacological inhibitors of the Nlrp3 inflammasome. Front Immunol (2019) 10:2538. doi: 10.3389/fimmu.2019.02538
83. Li H, Chen FJ, Yang WL, Qiao HZ, Zhang SJ. Quercetin improves cognitive disorder in aging mice by inhibiting Nlrp3 inflammasome activation. Food Funct (2021) 12(2):717–25. doi: 10.1039/d0fo01900c
84. Gupta R, Shukla RK, Chandravanshi LP, Srivastava P, Dhuriya YK, Shanker J, et al. Protective role of quercetin in cadmium-induced cholinergic dysfunctions in rat brain by modulating mitochondrial integrity and map kinase signaling. Mol Neurobiol (2017) 54(6):4560–83. doi: 10.1007/s12035-016-9950-y
85. Grünewald A, Kumar KR, Sue CM. New insights into the complex role of mitochondria in parkinson's disease. Prog Neurobiol (2019) 177:73–93. doi: 10.1016/j.pneurobio.2018.09.003
86. Ay M, Luo J, Langley M, Jin H, Anantharam V, Kanthasamy A, et al. Molecular mechanisms underlying protective effects of quercetin against mitochondrial dysfunction and progressive dopaminergic neurodegeneration in cell culture and mitopark transgenic mouse models of parkinson's disease. J Neurochem (2017) 141(5):766–82. doi: 10.1111/jnc.14033
87. El-Horany HE, El-Latif RN, ElBatsh MM, Emam MN. Ameliorative effect of quercetin on neurochemical and behavioral deficits in rotenone rat model of parkinson's disease: Modulating autophagy (Quercetin on experimental parkinson's disease). J Biochem Mol Toxic (2016) 30(7):360–9. doi: 10.1002/jbt.21821
88. Choi I, Kim J, Jeong HK, Kim B, Jou I, Park SM, et al. Pink1 deficiency attenuates astrocyte proliferation through mitochondrial dysfunction, reduced akt and increased P38 mapk activation, and downregulation of egfr. Glia (2013) 61(5):800–12. doi: 10.1002/glia.22475
89. Lazarou M, Sliter DA, Kane LA, Sarraf SA, Wang C, Burman JL, et al. The ubiquitin kinase Pink1 recruits autophagy receptors to induce mitophagy. Nature (2015) 524(7565):309–14. doi: 10.1038/nature14893
90. Thomas KJ, McCoy MK, Blackinton J, Beilina A, van der Brug M, Sandebring A, et al. Dj-1 acts in parallel to the Pink1/Parkin pathway to control mitochondrial function and autophagy. Hum Mol Genet (2011) 20(1):40–50. doi: 10.1093/hmg/ddq430
91. Wang C, Liu K, Cao J, Wang L, Zhao Q, Li Z, et al. Pink1-mediated mitophagy maintains pluripotency through optineurin. Cell Prolif (2021) 54(5):e13034. doi: 10.1111/cpr.13034
92. Bournival J, Quessy P, Martinoli MG. Protective effects of resveratrol and quercetin against mpp+ -induced oxidative stress act by modulating markers of apoptotic death in dopaminergic neurons. Cell Mol Neurobiol (2009) 29(8):1169–80. doi: 10.1007/s10571-009-9411-5
93. Choudhary S, Kumar P, Malik J. Plants and phytochemicals for huntington's disease. Pharmacogn Rev (2013) 7(14):81–91. doi: 10.4103/0973-7847.120505
94. Bailus BJ, Scheeler SM, Simons J, Sanchez MA, Tshilenge KT, Creus-Muncunill J, et al. Modulating Fkbp5/Fkbp51 and autophagy lowers htt (Huntingtin) levels. Autophagy (2021) 17(12):4119–40. doi: 10.1080/15548627.2021.1904489
95. Jiang M, Wang J, Fu J, Du L, Jeong H, West T, et al. Neuroprotective role of Sirt1 in mammalian models of huntington's disease through activation of multiple Sirt1 targets. Nat Med (2011) 18(1):153–8. doi: 10.1038/nm.2558
96. Sandhir R, Mehrotra A. Quercetin supplementation is effective in improving mitochondrial dysfunctions induced by 3-nitropropionic acid: Implications in huntington's disease. Biochim Biophys Acta (2013) 1832(3):421–30. doi: 10.1016/j.bbadis.2012.11.018
97. Wild EJ, Tabrizi SJ. Therapies targeting DNA and rna in huntington's disease. Lancet Neurol (2017) 16(10):837–47. doi: 10.1016/s1474-4422(17)30280-6
98. Chakraborty J, Rajamma U, Jana N, Mohanakumar KP. Quercetin improves the activity of the ubiquitin-proteasomal system in 150q mutated huntingtin-expressing cells but exerts detrimental effects on neuronal survivability. J Neurosci Res (2015) 93(10):1581–91. doi: 10.1002/jnr.23618
99. Chakraborty J, Singh R, Dutta D, Naskar A, Rajamma U, Mohanakumar KP. Quercetin improves behavioral deficiencies, restores astrocytes and microglia, and reduces serotonin metabolism in 3-nitropropionic acid-induced rat model of huntington's disease. CNS Neurosci Ther (2014) 20(1):10–9. doi: 10.1111/cns.12189
100. Jain D, Gangshettiwar A. Combination of lycopene, quercetin and poloxamer 188 alleviates anxiety and depression in 3-nitropropionic acid-induced huntington's disease in rats. J Intercult Ethnopharmacol (2014) 3(4):186–91. doi: 10.5455/jice.20140903012921
101. Ballester PL, Suh JS, Nogovitsyn N, Hassel S, Strother SC, Arnott SR, et al. Accelerated brain aging in major depressive disorder and antidepressant treatment response: A can-bind report. NeuroImage Clin (2021) 32:102864. doi: 10.1016/j.nicl.2021.102864
102. Masse C, Chopard G, Bennabi D, Haffen E, Vandel P. [Cognitive functions in late life depression]. Geriatr Psychol Neuropsychiatr Vieil (2021) 19(2):202–10. doi: 10.1684/pnv.2021.0939
103. Anacker C, Hen R. Adult hippocampal neurogenesis and cognitive flexibility - linking memory and mood. Nat Rev Neurosci (2017) 18(6):335–46. doi: 10.1038/nrn.2017.45
104. Kang X, Jiang L, Lan F, Tang YY, Zhang P, Zou W, et al. Hydrogen sulfide antagonizes sleep deprivation-induced depression- and anxiety-like behaviors by inhibiting neuroinflammation in a hippocampal Sirt1-dependent manner. Brain Res Bull (2021) 177:194–202. doi: 10.1016/j.brainresbull.2021.10.002
105. Lu G, Li J, Zhang H, Zhao X, Yan LJ, Yang X. Role and possible mechanisms of Sirt1 in depression. Oxid Med Cell Llongev (2018) 2018:8596903. doi: 10.1155/2018/8596903
106. Guo W, Xiao X, Tian YT, Yang JJ. [the role and mechanism of Sirt1 gene in depression]. Sheng li xue bao: [Acta physiol Sinica] (2021) 73(5):828–34. doi: 10.13294/j.aps.2021.0028
107. Lei Y, Wang J, Wang D, Li C, Liu B, Fang X, et al. Sirt1 in forebrain excitatory neurons produces sexually dimorphic effects on depression-related behaviors and modulates neuronal excitability and synaptic transmission in the medial prefrontal cortex. Mol Psychiatr (2020) 25(5):1094–111. doi: 10.1038/s41380-019-0352-1
108. Jiang X, Chen Z, Yu X, Chen J, Sun C, Jing C, et al. Lipopolysaccharide-induced depression is associated with estrogen receptor-A/Sirt1/Nf-Kb signaling pathway in old female mice. Neurochem Int (2021) 148:105097. doi: 10.1016/j.neuint.2021.105097
109. Ma ZX, Zhang RY, Rui WJ, Wang ZQ, Feng X. Quercetin alleviates chronic unpredictable mild stress-induced depressive-like behaviors by promoting adult hippocampal neurogenesis via Foxg1/Creb/ bdnf signaling pathway. Behav Brain Res (2021) 406:113245. doi: 10.1016/j.bbr.2021.113245
110. Zhang J, Ning L, Wang J. Dietary quercetin attenuates depressive-like behaviors by inhibiting astrocyte reactivation in response to stress. Biochem Bioph Res Co (2020) 533(4):1338–46. doi: 10.1016/j.bbrc.2020.10.016
111. Sadighparvar S, Darband SG, Yousefi B, Kaviani M, Ghaderi-Pakdel F, Mihanfar A, et al. Combination of quercetin and exercise training attenuates depression in rats with 1,2-Dimethylhydrazine-Induced colorectal cancer: Possible involvement of inflammation and bdnf signalling. Exp Physiol (2020) 105(9):1598–609. doi: 10.1113/ep088605
112. Fang K, Li HR, Chen XX, Gao XR, Huang LL, Du AQ, et al. Quercetin alleviates lps-induced depression-like behavior in rats via regulating bdnf-related imbalance of copine 6 and Trem1/2 in the hippocampus and pfc. Front Pharmacol (2019) 10:1544. doi: 10.3389/fphar.2019.01544
113. Wang G, Li Y, Lei C, Lei X, Zhu X, Yang L, et al. Quercetin exerts antidepressant and cardioprotective effects in estrogen receptor A-deficient female mice via bdnf-Akt/Erk1/2 signaling. J Steroid Biochem (2021) 206:105795. doi: 10.1016/j.jsbmb.2020.105795
114. Merzoug S, Toumi ML, Tahraoui A. Quercetin mitigates adriamycin-induced anxiety- and depression-like behaviors, immune dysfunction, and brain oxidative stress in rats. Naunyn-Schmiedeberg's Arch Pharmacol (2014) 387(10):921–33. doi: 10.1007/s00210-014-1008-y
115. Rahman MS, Uddin MS, Rahman MA, Samsuzzaman M, Behl T, Hafeez A, et al. Exploring the role of monoamine oxidase activity in aging and alzheimer's disease. Curr Pharm Design (2021) 27(38):4017–29. doi: 10.2174/1381612827666210612051713
116. Ramesh M, Muthuraman A. Quantitative structure-activity relationship (Qsar) studies for the inhibition of maos. Comb Chem High Throughput Screen (2020) 23(9):887–97. doi: 10.2174/1386207323666200324173231
117. Khan K, Najmi AK, Akhtar M. A natural phenolic compound quercetin showed the usefulness by targeting inflammatory, oxidative stress markers and augment 5-ht levels in one of the animal models of depression in mice. Drug Res (2019) 69(7):392–400. doi: 10.1055/a-0748-5518
118. Guan T, Cao C, Hou Y, Li Y, Wei X, Li S, et al. Effects of quercetin on the alterations of serum elements in chronic unpredictable mild stress-induced depressed rats. Biometals (2021) 34(3):589–602. doi: 10.1007/s10534-021-00298-w
119. Holzmann I, da Silva LM, Corrêa da Silva JA, Steimbach VM, de Souza MM. Antidepressant-like effect of quercetin in bulbectomized mice and involvement of the antioxidant defenses, and the glutamatergic and oxidonitrergic pathways. Pharmacol Biochem Be (2015) 136:55–63. doi: 10.1016/j.pbb.2015.07.003
120. Rinwa P, Kumar A. Quercetin suppress microglial neuroinflammatory response and induce antidepressent-like effect in olfactory bulbectomized rats. Neuroscience (2013) 255:86–98. doi: 10.1016/j.neuroscience.2013.09.044
121. Morris JA, Kemp JP, Youlten SE, Laurent L, Logan JG, Chai RC, et al. An atlas of genetic influences on osteoporosis in humans and mice. Nat Genet (2019) 51(2):258–66. doi: 10.1038/s41588-018-0302-x
122. Yin C, Tian Y, Hu L, Yu Y, Wu Z, Zhang Y, et al. Macf1 alleviates aging-related osteoporosis via Hes1. J Cell Mol Med (2021) 25(13):6242–57. doi: 10.1111/jcmm.16579
123. Gerosa L, Lombardi G. Bone-to-Brain: A round trip in the adaptation to mechanical stimuli. Front Physiol (2021) 12:623893. doi: 10.3389/fphys.2021.623893
124. Pandey MK, Gupta SC, Karelia D, Gilhooley PJ, Shakibaei M, Aggarwal BB. Dietary nutraceuticals as backbone for bone health. Biotechnol Adv (2018) 36(6):1633–48. doi: 10.1016/j.biotechadv.2018.03.014
125. Zhang Q, Chang B, Zheng G, Du S, Li X. Quercetin stimulates osteogenic differentiation of bone marrow stromal cells through mirna-206/Connexin 43 pathway. Am J Transl Res (2020) 12(5):2062–70.
126. Wang N, Wang L, Yang J, Wang Z, Cheng L. Quercetin promotes osteogenic differentiation and antioxidant responses of mouse bone mesenchymal stem cells through activation of the Ampk/Sirt1 signaling pathway. Phytother Res (2021) 35(5):2639–50. doi: 10.1002/ptr.7010
127. Konar M, Sahoo H. Tyrosine mediated conformational change in bone morphogenetic protein - 2: Biophysical implications of protein - phytoestrogen interaction. Int J Biol Macromol (2020) 150:727–36. doi: 10.1016/j.ijbiomac.2020.02.113
128. Mandl P, Hayer S, Karonitsch T, Scholze P, Győri D, Sykoutri D, et al. Nicotinic acetylcholine receptors modulate osteoclastogenesis. Arthritis Res Ther (2016) 18:63. doi: 10.1186/s13075-016-0961-x
129. Wattel A, Kamel S, Prouillet C, Petit JP, Lorget F, Offord E, et al. Flavonoid quercetin decreases osteoclastic differentiation induced by rankl via a mechanism involving nf kappa b and ap-1. J Cell Biochem (2004) 92(2):285–95. doi: 10.1002/jcb.20071
130. Wong SK, Chin KY, Ima-Nirwana S. Quercetin as an agent for protecting the bone: A review of the current evidence. Int J Mol Sci (2020) 21(17):6448. doi: 10.3390/ijms21176448
131. Guo C, Yang RJ, Jang K, Zhou XL, Liu YZ. Protective effects of pretreatment with quercetin against lipopolysaccharide-induced apoptosis and the inhibition of osteoblast differentiation via the mapk and Wnt/B-catenin pathways in Mc3t3-E1 cells. Cell Physiol Biochem (2017) 43(4):1547–61. doi: 10.1159/000481978
132. Vakili S, Zal F, Mostafavi-Pour Z, Savardashtaki A, Koohpeyma F. Quercetin and vitamin e alleviate ovariectomy-induced osteoporosis by modulating autophagy and apoptosis in rat bone cells. J Cell Physiol (2021) 236(5):3495–509. doi: 10.1002/jcp.30087
133. Ruangsuriya J, Charumanee S, Jiranusornkul S, Sirisa-Ard P, Sirithunyalug B, Sirithunyalug J, et al. Depletion of B-sitosterol and enrichment of quercetin and rutin in cissus quadrangularis Linn fraction enhanced osteogenic but reduced osteoclastogenic marker expression. BMC Complement Med (2020) 20(1):105. doi: 10.1186/s12906-020-02892-w
134. Yuan Z, Min J, Zhao Y, Cheng Q, Wang K, Lin S, et al. Quercetin rescued tnf-Alpha-Induced impairments in bone marrow-derived mesenchymal stem cell osteogenesis and improved osteoporosis in rats. Am J Transl Res (2018) 10(12):4313–21.
135. Xing LZ, Ni HJ, Wang YL. Quercitrin attenuates osteoporosis in ovariectomized rats by regulating mitogen-activated protein kinase (Mapk) signaling pathways. BioMed Pharmacother (2017) 89:1136–41. doi: 10.1016/j.biopha.2017.02.073
136. Li T, Mu N, Yin Y, Yu L, Ma H. Targeting amp-activated protein kinase in aging-related cardiovascular diseases. Aging Dis (2020) 11(4):967–77. doi: 10.14336/ad.2019.0901
137. Krumholz HM, Normand SL, Wang Y. Trends in hospitalizations and outcomes for acute cardiovascular disease and stroke, 1999-2011. Circulation (2014) 130(12):966–75. doi: 10.1161/circulationaha.113.007787
138. Yu S, Kim SR, Jiang K, Ogrodnik M, Zhu XY, Ferguson CM, et al. Quercetin reverses cardiac systolic dysfunction in mice fed with a high-fat diet: Role of angiogenesis. Oxid Med Cell Longev (2021) 2021:8875729. doi: 10.1155/2021/8875729
139. Li B, Yang M, Liu JW, Yin GT. Protective mechanism of quercetin on acute myocardial infarction in rats. Genet Mol Res (2016) 15(1):15017117. doi: 10.4238/gmr.15017117
140. Chiş IC, Baltaru D, Dumitrovici A, Coseriu A, Radu BC, Moldovan R, et al. Protective effects of quercetin from Oxidative/Nitrosative stress under intermittent hypobaric hypoxia exposure in the rat's heart. Physiol Int (2018) 105(3):233–46. doi: 10.1556/2060.105.2018.3.23
141. Ma C, Jiang Y, Zhang X, Chen X, Liu Z, Tian X. Isoquercetin ameliorates myocardial infarction through anti-inflammation and anti-apoptosis factor and regulating Tlr4-Nf-Kb signal pathway. Mol Med Rep (2018) 17(5):6675–80. doi: 10.3892/mmr.2018.8709
142. Liu H, Zhang Z, Zhang L, Yao X, Zhong X, Cheng G, et al. Spiraeoside protects human cardiomyocytes against high glucose-induced injury, oxidative stress, and apoptosis by activation of Pi3k/Akt/Nrf2 pathway. J Biochem Mol Toxic (2020) 34(10):e22548. doi: 10.1002/jbt.22548
143. Dong LY, Chen F, Xu M, Yao LP, Zhang YJ, Zhuang Y. Quercetin attenuates myocardial ischemia-reperfusion injury via downregulation of the Hmgb1-Tlr4-Nf-Kb signaling pathway. Am J Transl Res (2018) 10(5):1273–83.
144. Li C, Wang T, Zhang C, Xuan J, Su C, Wang Y. Quercetin attenuates cardiomyocyte apoptosis via inhibition of jnk and P38 mitogen-activated protein kinase signaling pathways. Gene (2016) 577(2):275–80. doi: 10.1016/j.gene.2015.12.012
145. Liu H, Guo X, Chu Y, Lu S. Heart protective effects and mechanism of quercetin preconditioning on anti-myocardial ischemia reperfusion (Ir) injuries in rats. Gene (2014) 545(1):149–55. doi: 10.1016/j.gene.2014.04.043
146. Tang J, Lu L, Liu Y, Ma J, Yang L, Li L, et al. Quercetin improve Ischemia/Reperfusion-induced cardiomyocyte apoptosis in vitro and in vivo study via Sirt1/Pgc-1α signaling. J Cell Biochem (2019) 120(6):9747–57. doi: 10.1002/jcb.28255
147. Zou L, Gong Y, Liu S, Liang S. Natural compounds acting at P2 receptors alleviate peripheral neuropathy. Brain Res Bull (2019) 151:125–31. doi: 10.1016/j.brainresbull.2018.12.017
148. Kawai S, Yamada T, Matsuura T, Funao T, Nishikawa K. Neuropathic pain attenuates ischemia reperfusion injury through B2-adrenergic pathway. Life Sci (2017) 187:9–16. doi: 10.1016/j.lfs.2017.08.002
149. Cheng YF, Chang YT, Chen WH, Shih HC, Chen YH, Shyu BC, et al. Cardioprotection induced in a mouse model of neuropathic pain via anterior nucleus of paraventricular thalamus. Nat Commun (2017) 8(1):826. doi: 10.1038/s41467-017-00891-z
150. Albadrani GM, Binmowyna MN, Bin-Jumah MN, El-Akabawy G, Aldera H, Al-Farga AM. Quercetin protects against experimentally-induced myocardial infarction in rats by an antioxidant potential and concomitant activation of signal transducer and activator of transcription 3. J Physiol Pharmacol (2020) 71(6):875–90. doi: 10.26402/jpp.2020.6.11
151. Chan KR, Wang X, Saron W, Gan ES, Tan HC, Mok D, et al. Cross-reactive antibodies enhance live attenuated virus infection for increased immunogenicity. Nat Microbiol (2016) 1(12):16164. doi: 10.1038/nmicrobiol.2016.164
152. Liu X, Yu Z, Huang X, Gao Y, Wang X, Gu J, et al. Peroxisome proliferator-activated receptor Γ (Pparγ) mediates the protective effect of quercetin against myocardial ischemia-reperfusion injury via suppressing the nf-Kb pathway. Am J Transl Res (2016) 8(12):5169–86.
153. Ikehara S, Li M. Stem cell transplantation improves aging-related diseases. Front Cell Dev Biol (2014) 2:16. doi: 10.3389/fcell.2014.00016
154. Mirsafaei L, Reiner Ž, Shafabakhsh R, Asemi Z. Molecular and biological functions of quercetin as a natural solution for cardiovascular disease prevention and treatment. Plant Food Hum Nutr (Dordrecht Netherlands) (2020) 75(3):307–15. doi: 10.1007/s11130-020-00832-0
155. Lim TK, Shi XQ, Johnson JM, Rone MB, Antel JP, David S, et al. Peripheral nerve injury induces persistent vascular dysfunction and endoneurial hypoxia, contributing to the genesis of neuropathic pain. J Neurosci (2015) 35(8):3346–59. doi: 10.1523/jneurosci.4040-14.2015
156. Maiuolo J, Gliozzi M, Musolino V, Carresi C, Nucera S, Macrì R, et al. The role of endothelial dysfunction in peripheral blood nerve barrier: Molecular mechanisms and pathophysiological implications. Int J Mol Sci (2019) 20(12):3022. doi: 10.3390/ijms20123022
157. Cao H, Jia Q, Yan L, Chen C, Xing S, Shen D. Quercetin suppresses the progression of atherosclerosis by regulating Mst1-mediated autophagy in ox-Ldl-Induced Raw264.7 macrophage foam cells. Int J Mol Sci (2019) 20(23):6093. doi: 10.3390/ijms20236093
158. Garelnabi M, Mahini H, Wilson T. Quercetin intake with exercise modulates lipoprotein metabolism and reduces atherosclerosis plaque formation. J Int Soc Sport Nutr (2014) 11:22. doi: 10.1186/1550-2783-11-22
159. Hung CH, Chan SH, Chu PM, Tsai KL. Quercetin is a potent anti-atherosclerotic compound by activation of Sirt1 signaling under oxldl stimulation. Mol Nutr Food Res (2015) 59(10):1905–17. doi: 10.1002/mnfr.201500144
160. Zhang F, Feng J, Zhang J, Kang X, Qian D. Quercetin modulates Ampk/Sirt1/Nf-Kb signaling to inhibit Inflammatory/Oxidative stress responses in diabetic high fat diet-induced atherosclerosis in the rat carotid artery. Exp Ther Med (2020) 20(6):280. doi: 10.3892/etm.2020.9410
161. Jiang YH, Jiang LY, Wang YC, Ma DF, Li X. Quercetin attenuates atherosclerosis via modulating oxidized ldl-induced endothelial cellular senescence. Front Pharmacol (2020) 11:512. doi: 10.3389/fphar.2020.00512
162. Xiao L, Liu L, Guo X, Zhang S, Wang J, Zhou F, et al. Quercetin attenuates high fat diet-induced atherosclerosis in apolipoprotein e knockout mice: A critical role of nadph oxidase. Food Chem Toxicol (2017) 105:22–33. doi: 10.1016/j.fct.2017.03.048
163. Li H, Xiao L, He H, Zeng H, Liu J, Jiang C, et al. Quercetin attenuates atherosclerotic inflammation by inhibiting galectin-3-Nlrp3 signaling pathway. Mol Nutr Food Res (2021) 65(15):e2000746. doi: 10.1002/mnfr.202000746
164. Bhaskar S, Sudhakaran PR, Helen A. Quercetin attenuates atherosclerotic inflammation and adhesion molecule expression by modulating tlr-Nf-Kb signaling pathway. Cell Immunol (2016) 310:131–40. doi: 10.1016/j.cellimm.2016.08.011
165. Lu XL, Zhao CH, Yao XL, Zhang H. Quercetin attenuates high fructose feeding-induced atherosclerosis by suppressing inflammation and apoptosis via ros-regulated Pi3k/Akt signaling pathway. BioMed Pharmacother (2017) 85:658–71. doi: 10.1016/j.biopha.2016.11.077
166. Chekalina N, Burmak Y, Petrov Y, Borisova Z, Manusha Y, Kazakov Y, et al. Quercetin reduces the transcriptional activity of nf-kb in stable coronary artery disease. Indian Heart J (2018) 70(5):593–7. doi: 10.1016/j.ihj.2018.04.006
167. Bhaskar S, Helen A. Quercetin modulates toll-like receptor-mediated protein kinase signaling pathways in oxldl-challenged human pbmcs and regulates tlr-activated atherosclerotic inflammation in hypercholesterolemic rats. Mol Cell Biochem (2016) 423(1-2):53–65. doi: 10.1007/s11010-016-2824-9
168. Li H, Xiao L, He H, Zeng H, Liu J, Jiang C, et al. Quercetin attenuates atherosclerotic inflammation by inhibiting gal-3-Nlrp3 signaling pathway. Mol Nutr Food Res (2021) 65(15):e2000746. doi: 10.1002/mnfr.202000746
169. Zhang X, Li L. The significance of 8-oxogsn in aging-related diseases. Aging Dis (2020) 11(5):1329–38. doi: 10.14336/ad.2019.1021
170. Wild S, Roglic G, Green A, Sicree R, King H. Global prevalence of diabetes: Estimates for the year 2000 and projections for 2030. Diabetes Care (2004) 27(5):1047–53. doi: 10.2337/diacare.27.5.1047
171. Perkins B, Bril V. Electrophysiologic testing in diabetic neuropathy. Handb Clin Neurol (2014) 126:235–48. doi: 10.1016/b978-0-444-53480-4.00018-7
172. Cameron NE, Tuck Z, McCabe L, Cotter MA. Effect of the hydroxyl radical scavenger, dimethylthiourea, on peripheral nerve tissue perfusion, conduction velocity and nociception in experimental diabetes. Diabetologia (2001) 44(9):1161–9. doi: 10.1007/s001250100626
173. Yang DK, Kang HS. Anti-diabetic effect of cotreatment with quercetin and resveratrol in streptozotocin-induced diabetic rats. Biomol Ther (2018) 26(2):130–8. doi: 10.4062/biomolther.2017.254
174. Yao Z, Gu Y, Zhang Q, Liu L, Meng G, Wu H, et al. Estimated daily quercetin intake and association with the prevalence of type 2 diabetes mellitus in Chinese adults. Eur J Nutr (2019) 58(2):819–30. doi: 10.1007/s00394-018-1713-2
175. Ali AM, Gabbar MA, Abdel-Twab SM, Fahmy EM, Ebaid H, Alhazza IM, et al. Antidiabetic potency, antioxidant effects, and mode of actions of citrus reticulata fruit peel hydroethanolic extract, hesperidin, and quercetin in Nicotinamide/Streptozotocin-induced wistar diabetic rats. Oxid Med Cell Longev (2020) 2020:1730492. doi: 10.1155/2020/1730492
176. Ying L, Chaudhry MT, Xiao F, Mao Y, Wang M, Wang B, et al. The effects and mechanism of quercetin dietary supplementation in streptozotocin-induced hyperglycemic arbor acre broilers. Oxid Med Cell Longev (2020) 2020:9585047. doi: 10.1155/2020/9585047
177. Schmeichel AM, Schmelzer JD, Low PA. Oxidative injury and apoptosis of dorsal root ganglion neurons in chronic experimental diabetic neuropathy. Diabetes (2003) 52(1):165–71. doi: 10.2337/diabetes.52.1.165
178. Jang E, Kim IY, Kim H, Lee DM, Seo DY, Lee JA, et al. Quercetin and chloroquine synergistically kill glioma cells by inducing organelle stress and disrupting Ca(2+) homeostasis. Biochem Pharmacol (2020) 178:114098. doi: 10.1016/j.bcp.2020.114098
179. Dhanya R, Arya AD, Nisha P, Jayamurthy P. Quercetin, a lead compound against type 2 diabetes ameliorates glucose uptake via ampk pathway in skeletal muscle cell line. Front Pharmacol (2017) 8:336. doi: 10.3389/fphar.2017.00336
180. Eseberri I, Laurens C, Miranda J, Louche K, Lasa A, Moro C, et al. Effects of physiological doses of resveratrol and quercetin on glucose metabolism in primary myotubes. Int J Mol Sci (2021) 22(3):1384. doi: 10.3390/ijms22031384
181. Bardy G, Virsolvy A, Quignard JF, Ravier MA, Bertrand G, Dalle S, et al. Quercetin induces insulin secretion by direct activation of l-type calcium channels in pancreatic beta cells. Brit J Pharmacol (2013) 169(5):1102–13. doi: 10.1111/bph.12194
182. Roslan J, Giribabu N, Karim K, Salleh N. Quercetin ameliorates oxidative stress, inflammation and apoptosis in the heart of streptozotocin-Nicotinamide-Induced adult Male diabetic rats. BioMed Pharmacother (2017) 86:570–82. doi: 10.1016/j.biopha.2016.12.044
183. Suganya N, Dornadula S, Chatterjee S, Mohanram RK. Quercetin improves endothelial function in diabetic rats through inhibition of endoplasmic reticulum stress-mediated oxidative stress. Eur J Pharmacol (2018) 819:80–8. doi: 10.1016/j.ejphar.2017.11.034
184. Vincent AM, Russell JW, Sullivan KA, Backus C, Hayes JM, McLean LL, et al. Sod2 protects neurons from injury in cell culture and animal models of diabetic neuropathy. Exp Neurol (2007) 208(2):216–27. doi: 10.1016/j.expneurol.2007.07.017
185. Houghton MJ, Kerimi A, Tumova S, Boyle JP, Williamson G. Quercetin preserves redox status and stimulates mitochondrial function in metabolically-stressed Hepg2 cells. Free Radic Biol Med (2018) 129:296–309. doi: 10.1016/j.freeradbiomed.2018.09.037
186. Dokumacioglu E, Iskender H, Sen TM, Ince I, Dokumacioglu A, Kanbay Y, et al. The effects of hesperidin and quercetin on serum tumor necrosis factor-alpha and interleukin-6 levels in streptozotocin-induced diabetes model. Pharmacogn Mag (2018) 14(54):167–73. doi: 10.4103/pm.pm_41_17
187. Iskender H, Dokumacioglu E, Sen TM, Ince I, Kanbay Y, Saral S. The effect of hesperidin and quercetin on oxidative stress, nf-Kb and Sirt1 levels in a stz-induced experimental diabetes model. BioMed Pharmacother (2017) 90:500–8. doi: 10.1016/j.biopha.2017.03.102
188. Peng J, Li Q, Li K, Zhu L, Lin X, Lin X, et al. Quercetin improves glucose and lipid metabolism of diabetic rats: Involvement of akt signaling and Sirt1. J Diabetes Res (2017) 2017:3417306. doi: 10.1155/2017/3417306
189. Feldman EL, Nave KA, Jensen TS, Bennett DLH. New horizons in diabetic neuropathy: Mechanisms, bioenergetics, and pain. Neuron (2017) 93(6):1296–313. doi: 10.1016/j.neuron.2017.02.005
190. Li D, Jiang C, Mei G, Zhao Y, Chen L, Liu J, et al. Quercetin alleviates ferroptosis of pancreatic B cells in type 2 diabetes. Nutrients (2020) 12(10):2954. doi: 10.3390/nu12102954
191. Liu ZJ, Xu W, Han J, Liu QY, Gao LF, Wang XH, et al. Quercetin induces apoptosis and enhances gemcitabine therapeutic efficacy against gemcitabine-resistant cancer cells. Anti-cancer Drugs (2020) 31(7):684–92. doi: 10.1097/cad.0000000000000933
192. da Costa JP, Vitorino R, Silva GM, Vogel C, Duarte AC, Rocha-Santos T. A synopsis on aging-theories, mechanisms and future prospects. Ageing Res Rev (2016) 29:90–112. doi: 10.1016/j.arr.2016.06.005
193. Wyss-Coray T. Ageing, neurodegeneration and brain rejuvenation. Nature (2016) 539(7628):180–6. doi: 10.1038/nature20411
194. Tönnies E, Trushina E. Oxidative stress, synaptic dysfunction, and alzheimer's disease. J Alzheimer's Dis (2017) 57(4):1105–21. doi: 10.3233/jad-161088
195. Singh S, Singh TG. Role of nuclear factor kappa b (Nf-Kb) signalling in neurodegenerative diseases: An mechanistic approach. Curr Neuropharmacol (2020) 18(10):918–35. doi: 10.2174/1570159x18666200207120949
196. Elumalai P, Lakshmi S. Role of quercetin benefits in neurodegeneration. Adv Neurobiol (2016) 12:229–45. doi: 10.1007/978-3-319-28383-8_12
197. Depino AM, Earl C, Kaczmarczyk E, Ferrari C, Besedovsky H, del Rey A, et al. Microglial activation with atypical proinflammatory cytokine expression in a rat model of parkinson's disease. Eur J Neurosci (2003) 18(10):2731–42. doi: 10.1111/j.1460-9568.2003.03014.x
198. Chen C, Zhou M, Ge Y, Wang X. Sirt1 and aging related signaling pathways. Mech Ageing Dev (2020) 187:111215. doi: 10.1016/j.mad.2020.111215
199. Gräff J, Kahn M, Samiei A, Gao J, Ota KT, Rei D, et al. A dietary regimen of caloric restriction or pharmacological activation of Sirt1 to delay the onset of neurodegeneration. J Neurosci (2013) 33(21):8951–60. doi: 10.1523/jneurosci.5657-12.2013
200. Wang R, Li JJ, Diao S, Kwak YD, Liu L, Zhi L, et al. Metabolic stress modulates alzheimer's B-secretase gene transcription via Sirt1-Pparγ-Pgc-1 in neurons. Cell Metab (2013) 17(5):685–94. doi: 10.1016/j.cmet.2013.03.016
201. Muraoka K, Shimizu K, Sun X, Tani T, Izumi R, Miwa K, et al. Flavonoids exert diverse inhibitory effects on the activation of nf-kappab. Transpl P (2002) 34(4):1335–40. doi: 10.1016/s0041-1345(02)02795-1
202. Chen JC, Ho FM, Pei-Dawn Lee C, Chen CP, Jeng KC, Hsu HB, et al. Inhibition of inos gene expression by quercetin is mediated by the inhibition of ikappab kinase, nuclear factor-kappa b and Stat1, and depends on heme oxygenase-1 induction in mouse bv-2 microglia. Eur J Pharmacol (2005) 521(1-3):9–20. doi: 10.1016/j.ejphar.2005.08.005
203. Iside C, Scafuro M, Nebbioso A, Altucci L. Sirt1 activation by natural phytochemicals: An overview. Front Pharmacol (2020) 11:1225. doi: 10.3389/fphar.2020.01225
204. de Boer VC, de Goffau MC, Arts IC, Hollman PC, Keijer J. Sirt1 stimulation by polyphenols is affected by their stability and metabolism. Mech Ageing Dev (2006) 127(7):618–27. doi: 10.1016/j.mad.2006.02.007
205. Zhang L, Kong D, Wang H, Jiao L, Zhao X, Song J, et al. Cocrystal of apixaban-quercetin: Improving solubility and bioavailability of drug combination of two poorly soluble drugs. Molecules (Basel Switzerland) (2021) 26(9):2677. doi: 10.3390/molecules26092677
206. Porras D, Nistal E, Martínez-Flórez S, Pisonero-Vaquero S, Olcoz JL, Jover R, et al. Protective effect of quercetin on high-fat diet-induced non-alcoholic fatty liver disease in mice is mediated by modulating intestinal microbiota imbalance and related gut-liver axis activation. Free Radical Bio Med (2017) 102:188–202. doi: 10.1016/j.freeradbiomed.2016.11.037
207. Luo F, Sandhu AF, Rungratanawanich W, Williams GE, Akbar M, Zhou S, et al. Melatonin and autophagy in aging-related neurodegenerative diseases. Int J Mol Sci (2020) 21(19):7174. doi: 10.3390/ijms21197174
208. Papaconstantinou J. The role of signaling pathways of inflammation and oxidative stress in development of senescence and aging phenotypes in cardiovascular disease. Cells (2019) 8(11):1383. doi: 10.3390/cells8111383
209. Feng K, Chen Z, Pengcheng L, Zhang S, Wang X. Quercetin attenuates oxidative stress-induced apoptosis via Sirt1/Ampk-mediated inhibition of er stress in rat chondrocytes and prevents the progression of osteoarthritis in a rat model. J Cell Physiol (2019) 234(10):18192–205. doi: 10.1002/jcp.28452
210. Hu T, Shi JJ, Fang J, Wang Q, Chen YB, Zhang SJ. Quercetin ameliorates diabetic encephalopathy through Sirt1/Er stress pathway in Db/Db mice. Aging (2020) 12(8):7015–29. doi: 10.18632/aging.103059
211. Chang X, Zhang T, Meng Q, ShiyuanWang, Yan P, Wang X, et al. Quercetin improves cardiomyocyte vulnerability to hypoxia by regulating Sirt1/Tmbim6-related mitophagy and endoplasmic reticulum stress. Oxid Med Cell Longev (2021) 2021:5529913. doi: 10.1155/2021/5529913
212. Shen P, Lin W, Ba X, Huang Y, Chen Z, Han L, et al. Quercetin-mediated Sirt1 activation attenuates collagen-induced mice arthritis. J Ethnopharmacol (2021) 279:114213. doi: 10.1016/j.jep.2021.114213
213. Leyton L, Hott M, Acuña F, Caroca J, Nuñez M, Martin C, et al. Nutraceutical activators of Ampk/Sirt1 axis inhibit viral production and protect neurons from neurodegenerative events triggered during hsv-1 infection. Virus Res (2015) 205:63–72. doi: 10.1016/j.virusres.2015.05.015
214. Carrasco-Pozo C, Tan KN, Reyes-Farias M, de la Jara N, Ngo ST, Garcia-Diaz DF, et al. The deleterious effect of cholesterol and protection by quercetin on mitochondrial bioenergetics of pancreatic B-cells, glycemic control and inflammation: In vitro and in vivo studies. Redox Biol (2016) 9:229–43. doi: 10.1016/j.redox.2016.08.007
215. Dong J, Zhang X, Zhang L, Bian HX, Xu N, Bao B, et al. Quercetin reduces obesity-associated atm infiltration and inflammation in mice: A mechanism including Ampkα1/Sirt1. J Lipid Res (2014) 55(3):363–74. doi: 10.1194/jlr.M038786
216. Liu C, Bai J, Dan Q, Yang X, Lin K, Fu Z, et al. Mitochondrial dysfunction contributes to aging-related atrial fibrillation. Oxid Med Cell Longev (2021) 2021:5530293. doi: 10.1155/2021/5530293
217. Gan Z, Fu T, Kelly DP, Vega RB. Skeletal muscle mitochondrial remodeling in exercise and diseases. Cell Res (2018) 28(10):969–80. doi: 10.1038/s41422-018-0078-7
218. Lin Y, Xu Z. Fibroblast senescence in idiopathic pulmonary fibrosis. Front Cell Dev Biol (2020) 8:593283. doi: 10.3389/fcell.2020.593283
219. Qiu L, Luo Y, Chen X. Quercetin attenuates mitochondrial dysfunction and biogenesis via upregulated Ampk/Sirt1 signaling pathway in oa rats. BioMed Pharmacother (2018) 103:1585–91. doi: 10.1016/j.biopha.2018.05.003
220. Yang R, Shen YJ, Chen M, Zhao JY, Chen SH, Zhang W, et al. Quercetin attenuates ischemia reperfusion injury by protecting the blood-brain barrier through Sirt1 in mcao rats. J Asian Nat Prod Res (2022) 24(3):278–89. doi: 10.1080/10286020.2021.1949302
221. Valero T. Mitochondrial biogenesis: Pharmacological approaches. Curr Pharm Design (2014) 20(35):5507–9. doi: 10.2174/138161282035140911142118
222. Casuso RA, Martínez-López EJ, Hita-Contreras F, Camiletti-Moiron D, Martínez-Romero R, Cañuelo A, et al. The combination of oral quercetin supplementation and exercise prevents brain mitochondrial biogenesis. Genes Nutr (2014) 9(5):420. doi: 10.1007/s12263-014-0420-8
223. Liu P, Zou D, Yi L, Chen M, Gao Y, Zhou R, et al. Quercetin ameliorates hypobaric hypoxia-induced memory impairment through mitochondrial and neuron function adaptation via the pgc-1α pathway. Restor Neurol Neuros (2015) 33(2):143–57. doi: 10.3233/rnn-140446
224. Ballmann C, Denney TS, Beyers RJ, Quindry T, Romero M, Amin R, et al. Lifelong quercetin enrichment and cardioprotection in Mdx/Utrn+/- mice. Am J Physiol Heart C (2017) 312(1):H128–h40. doi: 10.1152/ajpheart.00552.2016
225. Tower J. Programmed cell death in aging. Ageing Res Rev (2015) 23(Pt A):90–100. doi: 10.1016/j.arr.2015.04.002
226. Guo H, Ding H, Tang X, Liang M, Li S, Zhang J, et al. Quercetin induces pro-apoptotic autophagy via Sirt1/Ampk signaling pathway in human lung cancer cell lines A549 and H1299 in vitro. Thorac Cancer (2021) 12(9):1415–22. doi: 10.1111/1759-7714.13925
227. Wang D, He X, Wang D, Peng P, Xu X, Gao B, et al. Quercetin suppresses apoptosis and attenuates intervertebral disc degeneration via the Sirt1-autophagy pathway. Front Cell Dev Biol (2020) 8:613006. doi: 10.3389/fcell.2020.613006
228. Hsu MY, Hsiao YP, Lin YT, Chen C, Lee CM, Liao WC, et al. Quercetin alleviates the accumulation of superoxide in sodium iodate-induced retinal autophagy by regulating mitochondrial reactive oxygen species homeostasis through enhanced deacetyl-Sod2 via the Nrf2-Pgc-1α-Sirt1 pathway. Antioxidants (Basel Switzerland) (2021) 10(7):1125. doi: 10.3390/antiox10071125
229. Liu T, Yang Q, Zhang X, Qin R, Shan W, Zhang H, et al. Quercetin alleviates kidney fibrosis by reducing renal tubular epithelial cell senescence through the Sirt1/Pink1/Mitophagy axis. Life Sci (2020) 257:118116. doi: 10.1016/j.lfs.2020.118116
230. Ayton S, Wang Y, Diouf I, Schneider JA, Brockman J, Morris MC, et al. Brain iron is associated with accelerated cognitive decline in people with Alzheimer pathology. Mol Psychiatr (2020) 25(11):2932–41. doi: 10.1038/s41380-019-0375-7
231. Lane DJR, Metselaar B, Greenough M, Bush AI, Ayton SJ. Ferroptosis and Nrf2: An emerging battlefield in the neurodegeneration of alzheimer's disease. Essays Biochem (2021) 65(7):925–40. doi: 10.1042/ebc20210017
232. Chen K, Jiang X, Wu M, Cao X, Bao W, Zhu LQ. Ferroptosis, a potential therapeutic target in alzheimer's disease. Front Cell Dev Biol (2021) 9:704298. doi: 10.3389/fcell.2021.704298
233. Wang ZL, Yuan L, Li W, Li JY. Ferroptosis in parkinson's disease: Glia-neuron crosstalk. Trends Mol Med (2022) 28(4):258–69. doi: 10.1016/j.molmed.2022.02.003
234. Zuo Y, Xie J, Li X, Li Y, Thirupathi A, Zhang J, et al. Ferritinophagy-mediated ferroptosis involved in paraquat-induced neurotoxicity of dopaminergic neurons: Implication for neurotoxicity in pd. Oxid Med Cellula Longev (2021) 2021:9961628. doi: 10.1155/2021/9961628
235. Reichert CO, de Freitas FA, Sampaio-Silva J, Rokita-Rosa L, Barros PL, Levy D, et al. Ferroptosis mechanisms involved in neurodegenerative diseases. Int J Mol Sci (2020) 21(22):8765. doi: 10.3390/ijms21228765
236. Portugal-Nunes C, Castanho TC, Amorim L, Moreira PS, Mariz J, Marques F, et al. Iron status is associated with mood, cognition, and functional ability in older adults: A cross-sectional study. Nutrients (2020) 12(11):3594. doi: 10.3390/nu12113594
237. Cao H, Zuo C, Huang Y, Zhu L, Zhao J, Yang Y, et al. Hippocampal proteomic analysis reveals activation of necroptosis and ferroptosis in a mouse model of chronic unpredictable mild stress-induced depression. Behav Brain Res (2021) 407:113261. doi: 10.1016/j.bbr.2021.113261
238. Jiao H, Yang H, Yan Z, Chen J, Xu M, Jiang Y, et al. Traditional Chinese formula xiaoyaosan alleviates depressive-like behavior in cums mice by regulating Pebp1-Gpx4-Mediated ferroptosis in the hippocampus. Neuropsych Dis Treat (2021) 17:1001–19. doi: 10.2147/ndt.S302443
239. Yang J, Hu S, Bian Y, Yao J, Wang D, Liu X, et al. Targeting cell death: Pyroptosis, ferroptosis, apoptosis and necroptosis in osteoarthritis. Front Cell Dev Biol (2021) 9:789948. doi: 10.3389/fcell.2021.789948
240. Yan HF, Tuo QZ, Yin QZ, Lei P. The pathological role of ferroptosis in Ischemia/Reperfusion-related injury. Zool Res (2020) 41(3):220–30. doi: 10.24272/j.issn.2095-8137.2020.042
241. Chen Y, Fan H, Wang S, Tang G, Zhai C, Shen L. Ferroptosis: A novel therapeutic target for ischemia-reperfusion injury. Front Cell Dev Biol (2021) 9:688605. doi: 10.3389/fcell.2021.688605
242. Li JY, Liu SQ, Yao RQ, Tian YP, Yao YM. A novel insight into the fate of cardiomyocytes in ischemia-reperfusion injury: From iron metabolism to ferroptosis. Front Cell Dev Biol (2021) 9:799499. doi: 10.3389/fcell.2021.799499
243. Vinchi F, Porto G, Simmelbauer A, Altamura S, Passos ST, Garbowski M, et al. Atherosclerosis is aggravated by iron overload and ameliorated by dietary and pharmacological iron restriction. Eur Heart J (2020) 41(28):2681–95. doi: 10.1093/eurheartj/ehz112
244. Lin L, Zhang MX, Zhang L, Zhang D, Li C, Li YL. Autophagy, pyroptosis, and ferroptosis: New regulatory mechanisms for atherosclerosis. Front Cell Dev Biol (2021) 9:809955. doi: 10.3389/fcell.2021.809955
245. Sha W, Hu F, Xi Y, Chu Y, Bu S. Mechanism of ferroptosis and its role in type 2 diabetes mellitus. J Diabetes Res (2021) 2021:9999612. doi: 10.1155/2021/9999612
246. Wang X, Chen X, Zhou W, Men H, Bao T, Sun Y, et al. Ferroptosis is essential for diabetic cardiomyopathy and is prevented by sulforaphane via Ampk/Nrf2 pathways. Acta Pharm Sin B (2022) 12(2):708–22. doi: 10.1016/j.apsb.2021.10.005
247. Zhang Y, Gao Z, Liu J, Xu Z. Protective effects of baicalin and quercetin on an iron-overloaded mouse: Comparison of liver, kidney and heart tissues. Nat Prod Res (2011) 25(12):1150–60. doi: 10.1080/14786419.2010.495070
248. Li X, Zeng J, Liu Y, Liang M, Liu Q, Li Z, et al. Inhibitory effect and mechanism of action of quercetin and quercetin diels-alder anti-dimer on erastin-induced ferroptosis in bone marrow-derived mesenchymal stem cells. Antioxidants (Basel Switzerland) (2020) 9(3):205. doi: 10.3390/antiox9030205
249. Wang Y, Quan F, Cao Q, Lin Y, Yue C, Bi R, et al. Quercetin alleviates acute kidney injury by inhibiting ferroptosis. J Adv Res (2021) 28:231–43. doi: 10.1016/j.jare.2020.07.007
250. Su G, Yang W, Wang S, Geng C, Guan X. Sirt1-autophagy axis inhibits excess iron-induced ferroptosis of foam cells and subsequently increases il-1β and il-18. Biochem Bioph Res Co (2021) 561:33–9. doi: 10.1016/j.bbrc.2021.05.011
251. Li D, Liu X, Pi W, Zhang Y, Yu L, Xu C, et al. Fisetin attenuates doxorubicin-induced cardiomyopathy in vivo and in vitro by inhibiting ferroptosis through Sirt1/Nrf2 signaling pathway activation. Front Pharmacol (2021) 12:808480. doi: 10.3389/fphar.2021.808480
252. Terman A, Brunk UT. Oxidative stress, accumulation of biological 'Garbage', and aging. Antioxid Redox Sign (2006) 8(1-2):197–204. doi: 10.1089/ars.2006.8.197
253. Vader P, Mol EA, Pasterkamp G, Schiffelers RM. Extracellular vesicles for drug delivery. Adv Drug Deliver Rev (2016) 106(Pt A):148–56. doi: 10.1016/j.addr.2016.02.006
254. Elsharkasy OM, Nordin JZ, Hagey DW, de Jong OG, Schiffelers RM, Andaloussi SE, et al. Extracellular vesicles as drug delivery systems: Why and how? Adv Drug Deliver Rev (2020) 159:332–43. doi: 10.1016/j.addr.2020.04.004
255. Herrmann IK, Wood MJA, Fuhrmann G. Extracellular vesicles as a next-generation drug delivery platform. Nat Nnanotechnol (2021) 16(7):748–59. doi: 10.1038/s41565-021-00931-2
256. Yin Y, Chen H, Wang Y, Zhang L, Wang X. Roles of extracellular vesicles in the aging microenvironment and age-related diseases. J Extracell Vesicles (2021) 10(12):e12154. doi: 10.1002/jev2.12154
257. Morad G, Carman CV, Hagedorn EJ, Perlin JR, Zon LI, Mustafaoglu N, et al. Tumor-derived extracellular vesicles breach the intact blood-brain barrier via transcytosis. ACS nano (2019) 13(12):13853–65. doi: 10.1021/acsnano.9b04397
258. Dou Y, Xie J, Tan Y, Zhang M, Zhao Y, Liu X. Neurotransmitter-stimulated neuron-derived sevs have opposite effects on amyloid B-induced neuronal damage. J Nanobiotechnol (2021) 19(1):324. doi: 10.1186/s12951-021-01070-5
Keywords: quercetin, aging-related diseases, sirtuin 1, oxidative stress, mitochondrial dysfunction, inflammatory response
Citation: Cui Z, Zhao X, Amevor FK, Du X, Wang Y, Li D, Shu G, Tian Y and Zhao X (2022) Therapeutic application of quercetin in aging-related diseases: SIRT1 as a potential mechanism. Front. Immunol. 13:943321. doi: 10.3389/fimmu.2022.943321
Received: 13 May 2022; Accepted: 27 June 2022;
Published: 22 July 2022.
Edited by:
Rama Vaidya, Medical Research Centre of the Kasturba Health Society (MRC-KHS), IndiaReviewed by:
Sun Young Park, Pusan National University, South KoreaKangkang Guo, Northwest A&F University, China
Copyright © 2022 Cui, Zhao, Amevor, Du, Wang, Li, Shu, Tian and Zhao. This is an open-access article distributed under the terms of the Creative Commons Attribution License (CC BY). The use, distribution or reproduction in other forums is permitted, provided the original author(s) and the copyright owner(s) are credited and that the original publication in this journal is cited, in accordance with accepted academic practice. No use, distribution or reproduction is permitted which does not comply with these terms.
*Correspondence: Xiaoling Zhao, emhhb3hpYW9saW5nQHNpY2F1LmVkdS5jbg==
†These authors have contributed equally to this work