- 1Department of Neurology, The College of Medicine, The Ohio State University, Columbus, OH, United States
- 2Pelotonia Institute for Immuno-Oncology, The James Comprehensive Cancer Center, The Ohio State University, Columbus, OH, United States
- 3Department of Microbial Infection and Immunity, The College of Medicine, The Ohio State University, Columbus, OH, United States
The concept of cancer immunotherapy has gained immense momentum over the recent years. The advancements in checkpoint blockade have led to a notable progress in treating a plethora of cancer types. However, these approaches also appear to have stalled due to factors such as individuals’ genetic make-up, resistant tumor sub-types and immune related adverse events (irAE). While the major focus of immunotherapies has largely been alleviating the cell-intrinsic defects of CD8+ T cells in the tumor microenvironment (TME), amending the relationship between tumor specific CD4+ T cells and CD8+ T cells has started driving attention as well. A major roadblock to improve the cross-talk between CD4+ T cells and CD8+ T cells is the immune suppressive action of tumor infiltrating T regulatory (Treg) cells. Despite their indispensable in protecting tissues against autoimmune threats, Tregs have also been under scrutiny for helping tumors thrive. This review addresses how Tregs establish themselves at the TME and suppress anti-tumor immunity. Particularly, we delve into factors that promote Treg migration into tumor tissue and discuss the unique cellular and humoral composition of TME that aids survival, differentiation and function of intratumoral Tregs. Furthermore, we summarize the potential suppression mechanisms used by intratumoral Tregs and discuss ways to target those to ultimately guide new immunotherapies.
Introduction
Tregs constitute a small yet significant subset of CD4+ T cells with a distinct immune suppressive role (1). Their primary function is enforcing peripheral tolerance and is largely dictated by the activity of transcription factor Foxp3. Treg ontogeny involves thymic Tregs (tTregs), that develop in the thymus from precursors of CD4+ helper T (Th) cells and peripheral Tregs (pTregs), that differentiate from mature CD4+ Th cells in the periphery. A third Treg type is known as induced Treg (iTregs) that can be produced ex-vivo from mature CD4+ Th cells by providing T cell receptor (TCR) stimulus and TGF-β (2). A dual expression of Foxp3 and IL-2 receptor α chain (CD25) accompanied by a low IL-7 receptor (CD127) expression is widely accepted as the hallmark of Treg ontogeny in mammals (3). Suppression mechanisms of Tregs can be grouped into active and counteractive modes, where active mode represents the production of immune suppressive cytokines by Tregs such as IL-10, TGF-β, IL-35, adenosine and counteractive mode includes removal of crucial elements for the activation and survival of effector T cells such as peptide-MHC class II, CD80-CD86, and IL-2 (4–12) (Figure 1). While it is largely unknown which mechanism(s) predominate in vivo, it is widely accepted that stimulation of Treg TCR precedes the suppressive activity.
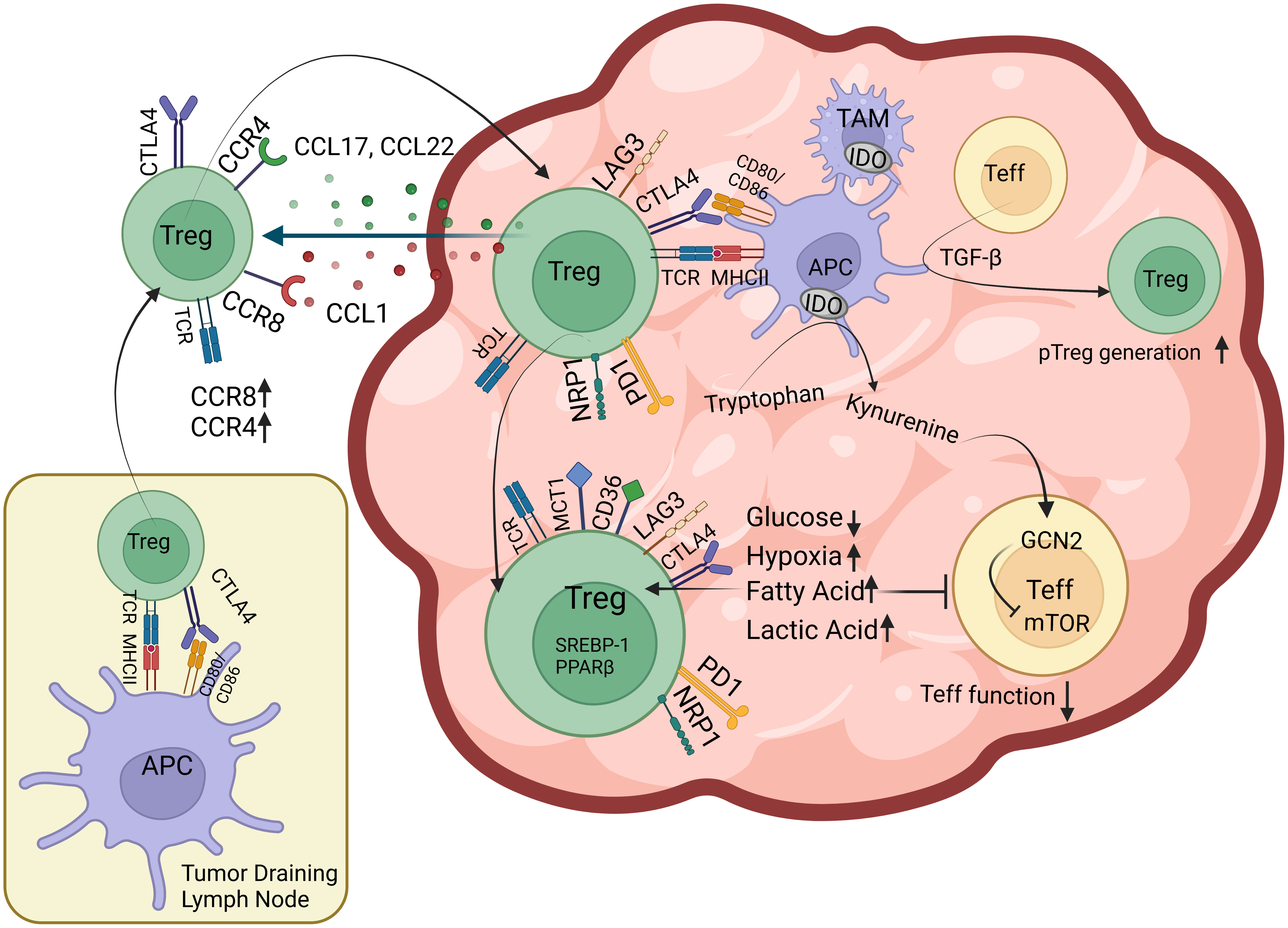
Figure 1 Factors that facilitate Treg infiltration of the TME Tregs that are presented tumor antigens by professional APCs in the tumor draining lymph node upregulate chemokine receptors including CCR4 and CCR8 and migrate towards CCL17, CCL22, CCL1 gradient created by TME. Once in tumor tissue, Tregs have subsequent encounters with intratumoral APCs where they upregulate surface expression of coinhibitory receptors including PD-1 and LAG3. Intratumoral Tregs also express CTLA-4, Nrp-1, lactic acid transporter MCT-1 and free fatty acid (FFA) scavenging receptor CD36 on their surface as well as nuclear factors such as PPAR family of transcription factors and SREBP-1 responsible for FFA oxidation. TGF-β from tumor cells, stroma and immune cells induce intratumoral pTreg generation. Tumor and immune cell IDO activity and reduced glucose concentration of TME inhibits mTOR in effector T cells (Teff) and inhibit their function.
The major role played by Tregs is to prevent autoimmunity as evidenced by the fatal multiorgan autoimmunity in patients with IPEX (Immune dysregulation, Polyendocrinopathy, Enteropathy, X-linked) syndrome and Scurfy mice that harbor deleterious Foxp3 mutations (13, 14). Despite critical importance, Treg activity is not desired in certain niches, such as sites of chronic infection and the tumor microenvironment (TME). It has been shown that the changes associated with tumor growth, such as altered nutrient composition and oxygen availability, cytokines and chemokines released by tumor cells, stroma and immune cells also favor Treg infiltration and effector T cell exhaustion. These also is a correlation with disease progression and resistance to immune checkpoint inhibitors (ICI), hence Treg infiltration of the TME has started to be appreciated as a biomarker and predictive factor for tumor progression and therapy response (15, 16). Moreover, this highlights the need for more specific approaches to reduce Treg to effector T cell ratio in the TME. To help achieve this goal, this review focuses on the factors that recruit Tregs to the tumor site, promote their differentiation and activity in the TME as well as biomarkers that distinguish tumor-specific Tregs from tumor-specific CD4+ effector T cells and self-specific Tregs that protect against autoimmunity.
How do Tregs populate the tumor microenvironment?
Antigen-T cell receptor interactions
TCR and antigen-MHC interactions constitute the essence of T cell development, function, and tolerance. T cell precursors start to recognize antigens in thymic cortex as soon as they successfully assemble a pre-TCR complex on their membrane. Pre-TCRs that are capable of binding self pMHC, get through positive selection, continue rearranging TCRα loci in both alleles, then enter thymic medulla and encounter another set of self pMHCs. This encounter subjects fully rearranged TCRαβ chains to a negative selection, which then determines the T cell fate. Although it is still debated, traditionally it has been assumed that the affinity of a single interaction between individual TCR molecule and self pMHC determines the fate of T cells precursors. In this affinity-based model for negative selection, the strongest signal leads to elimination of the T cell clone altogether, weak signals lead to generation of conventional (effector) T cells while the signals in between tend to produce Tregs (1, 17–19). However, more recent studies support an avidity-based model where the fate of CD4+ T cell precursor is based on the timing and density of the interactions between TCR and self-antigens as the major determinant (20–22). This allows for T cells that have fewer reactions with antigens to become conventional T cells, T cells that have the highest number of interactions with self-antigens to be deleted, and the T cells that have moderate amounts of interaction with self-antigen to become Tregs (20). A common feature for both models, is that they demonstrate the TCR-pMHCII interactions as the key determining factor for T cell fate. However, it’s also possible that costimulatory pathways, integrins, and cytokines further tune the TCR signals and ultimately contribute to the lineage decision for Tregs vs. effector T cells (23–25).
Antigens presented at the TME have been heavily investigated due to their potential use as cancer vaccines (26). These antigens can be divided into two large groups according to their expression in normal tissues. Tumor associated antigens (TAAs) can be expressed in normal tissues hence, the high affinity T cell clones specific for TAAs are mostly deleted from the repertoire during thymic development. Therefore, their use for immunotherapies is limited due to the lack of good effectors that would use them and the possibility of off-target toxicity against normal tissues. Because TAA-specific Tregs that are present in the repertoire unlike effector T cells, it is possible TAA presentation can selectively recruit them. The second group of antigens is tumor specific antigens (TSA), also known as neoantigens. These occur due to mutations in tumor genome, thus perceived as foreign by immune cells. They can induce significant anti-tumor T cell response as TCR repertoire can display a full spectrum of affinities for foreign antigens. However, their role in Treg recruitment and conversion is largely unknown (27–29).
TCR-pMHCII interactions are also critical for mature Tregs to home and function in the periphery. Because mature Treg repertoire is equipped to detect self pMHCII, a pathological expression of tissue antigens on MHCII could possibly engage and expand particular Treg clones in the secondary lymphoid organs (30, 31). Depending on the inflammatory context that tissue-specific Tregs see their antigen, they can unleash a myriad of suppressive mechanisms such as the removal of self pMHCII and CD80-86 from APC surface, IL-2 from potential autoimmune clonal escapees and an active production of anti-inflammatory mediators (4–12, 32). Tumor site gradually diverges from adjacent healthy tissue due to increasing mutational burden, emerging tumor stroma, and new blood vessels. Hence, antigenic profile of tumor deviates from normal tissue where those antigens can be presented on MHCI and MHCII by both tumor itself and the APCs within tumor and draining lymph node (33). This potentially helps tumor-reactive bona fide tTregs to migrate into tumor site. An alternative way tumor site can be enriched in Tregs is the differentiation of tumor-reactive effector CD4+ T cells into pTregs. This path is mostly attributed to the role of TGF-β in inducing Foxp3 expression in effector CD4+ T cells in addition to its prominent role in transforming the architectural and immune scapes of TME (34–39)
Tregs display a limited TCR repertoire within the TME, suggestive of a clonal enrichment for Tregs that recognize TAAs and TSAs (40, 41). Due to a lack of reliable markers that distinguish the origin of Tregs, it is largely unclear to what extent pTregs vs tTregs make up these clones (42). Based on limited overlap between the TCR repertoires of tumor infiltrating effector CD4+ T cells and Tregs, some have argued that pTregs do not play a prominent role in the TME (15, 43, 44). However, a single snapshot of the TIL repertoire may be misleading due to a number of factors including differences in the metabolic adaptation and survival of Tregs and effector T cells in TME. It is possible that TME imposes a bottle-neck for the incoming tumor-specific CD4+ T cells due to hostile metabolic environment where the ones that differentiate into pTregs survive and others face their demise. This may gradually build a tumor-specific Treg repertoire composed of metabolically adapted tTregs and pTregs, while CD4+ effector T cell repertoire may reflect a constantly replenished pool from periphery. Hence a single repertoire analysis can potentially capture relatively older Treg clones together with freshly migrating CD4+ effector T cells reacting to a changing antigen scape. Moreover, as tumor progresses, clonal representation of effector T cells may be actively skewed by Tregs via antigen-specific suppression mechanisms. We have previously shown that antigen-specific Tregs can steal their cognate pMHCII from DCs, resulting in an antigen-biased suppression in vivo (45). If this is a prominent suppression mechanism in the TME, it may be another reason why some effector CD4+ and CD8+ T cell clones vanish from TIL repertoire while others remain (46, 47).
Migration and homing of Tregs to tumor site
Tregs that are primed by dendritic cells in secondary lymphoid organs upregulate an array of chemokine receptors and adhesion molecules can then migrate into tissue (48, 49). CCR4 and CCR8 expression on Tregs have long been implicated in Treg recruitment to TME (39, 50–53). CCR4 responds to CCL17 and CCL22, mostly released from several immunologically active “hot” tumors, while CCR8 responds to CCL1 gradient (51, 54, 55). It has been shown that inhibition of CCR4 signaling diminishes Treg infiltration of the TME while Treg numbers are maintained in the periphery (54). Furthermore, depleting CCR4+ Tregs seems to reduce the tumor burden both as a standalone therapy and in combination with checkpoint blockade in certain tumor settings (54, 56–60). Despite the potential of targeting CCR4 for immunotherapies, exact role of CCR4 in Treg development, function and tumor-Treg crosstalk remains elusive (61). To properly address these points, future studies using constitutive and inducible conditional knockout animal models, where CCR4 deficiency is restricted to Tregs, are required. Similar to CCR4, CCR8 has also been noted for its specificity to Treg that have infiltrated the TME (39, 62). While antibody mediated depletion of CCR8+ Tregs via antibody dependent cell cytotoxicity (ADCC) can ameliorate the tumor burden, blocking CCR8 activity using antibodies that are devoid of ADCC capabilities doesn’t seem to create the same effect (52). Furthermore, comparison of CCR8 knockout and wild type mice revealed that Tregs infiltrate the TME similarly, suggesting a trivial role for CCR8 in Treg recruitment and function (63). Similar to CCR4, the contribution of CCR8 to Treg recruitment needs to be further elucidated in mouse models that specifically lacks CCR8 in Tregs. Hypoxic environment of TME has also been implicated in recruitment of Tregs along with myeloid derived stromal cells (MDSCs) and tumor-associated macrophages (TAMs) (64–66). Hypoxia driven release of CCL28 from tumors has been shown to recruit CCR10 expressing Tregs that contribute to angiogenesis by VEGF production (67–69). Tregs also require cell adhesion molecules including ICAM-1, L-selectin, P-selectin, integrin αE (CD103) and VLA-4 to home to secondary lymphoid organs and inflammation site (70). While majority of these factors also play role in effector T cells homing, Nrp-1-Semaphorin-4a axis has recently been recognized for its unique role in enriching a stable, suppressive intratumoral Treg population in mice and human (71–74). Although Nrp-1 is also known as a co-receptor for vascular endothelial growth factor (VEGF) that regulates endothelial cell adhesion and angiogenesis, to what extent it plays role in Treg homing to TME remains to be elucidated (75).
Metabolism
Changing nutrient, metabolite and oxygen composition of the tumor affect presence and function of anti- and pro-tumor immune cells (76–78). Upon activation, naïve T cells switch from oxidative phosphorylation (OXPHOS) to aerobic glycolysis to meet the needs of activated T cells (79). This metabolic adaptation is similar to the Warburg effect seen in cancer cells, where tumor prefers quick yet less efficient energy supply from glycolysis over OXPHOS regardless of oxygen tension (80). However, not only this depletes glucose in TME and puts effector T cells in a hyporesponsive state, but also hinders T cell wellbeing via increasing lactate (80, 81). While effector T cells suffer in this hostile landscape, Tregs thrive largely due to their adaptations for utilizing fatty acids and lactate (82–87) (Figure 1). T cell activation in the presence of CD28 stimulation upregulates machinery for glycolysis via PI3K/Akt/mTOR (the mammalian target of rapamycin) pathway. mTOR activity increases glucose and amino acid transporters on cell membrane, stimulates the hypoxia-inducible factor-α (HIF1-α), c-Myc, altogether maximizing nutrient uptake, glycolysis and glutaminolysis (88–90). Foxp3 suppresses c-Myc expression, thus limits glucose uptake and glycolysis of Tregs while promoting OXPHOS (91, 92). Hypoxia-driven HIF1-α activation has been shown to increase Treg fragility by binding Foxp3 and targeting it for proteosomal degradation (73, 93, 94). However, there is conflicting evidence on the overall effects of hypoxia on Tregs as, it also promotes TGF-β expression and signaling, hence can facilitate pTreg generation (95–97). In highly glycolytic tumors, glucose is broken down into lactate that can be taken up by Tregs via MCT1 lactic acid transporter. Tregs have been shown to metabolize lactic acid into phosphoenolpyruvate (PEP) in lactic acid rich, glucose poor environments. This has been shown to increase intracellular calcium and NFAT translocation in Tregs, but not in CD8+ T cells, and preserved suppressive abilities of Tregs in vitro (87). Mice with specific deletion of MCT1 lactic acid transporter in Treg compartment, displayed increased effector CD8+ and CD4+ T cell activity in the TME that was further enhanced upon PD-1 blockade (87). Tumor growth is also associated with increasing free fatty acid (FFA) content of TME (98–100). It has been shown that both tumor-associated myeloid cells and Tregs take up FFAs via scavenger receptor CD36 (101). CD36-mediated FFA signals through PPAR-β and promotes mitochondrial fitness and intratumoral Treg survival. Treg-specific deletion of CD36 selectively impairs intratumoral Tregs and reduces tumor burden without overt autoimmunity (102). Furthermore, comprehensive transcriptome analyses of Tregs from tumor and peripheral tissues of mouse melanoma, breast cancer and head and neck squamous cell carcinoma revealed that lipid metabolism is among the top enriched pathways among intratumoral Tregs (86, 103). This analysis also identified sterol-regulatory-element-binding proteins (SREBPs) as crucial transcription factors that control lipid metabolism and homeostasis of Tregs in the TME. Tregs deficient in SREBP1 cleavage activating protein (SCAP) were found less able to maintain a suppressive phenotype within the TME due to impaired SREBP activity (86, 104). Phenotype of Treg-specific SREBP1 deletion is similar to that of CD36, where mice don’t develop systemic inflammatory disorder, and have improved anti-tumor responses. It is worthwhile noting that SREBPs also play role in metabolism of healthy tissues, therefore broad targeting of SREBPs may pose serious risks for off-target toxicity (104). Lastly, Indoleamine 2,3-dioxygenase (IDO) is an enzyme expressed by APCs, epithelial cells, vascular endothelium and tumor cells that contributes to tolerogenic milieu in TME. It metabolizes the essential amino acid tryptophan into kynurenine, thus induces the stress-response kinase GCN2 that suppresses mTOR activity. Reduced mTOR activity can cause Foxp3 induction in effector T cells (105). Kynurenine has also been found to activate transcription of the aryl hydrocarbon receptor (AHR) that further promotes Treg differentiation. IDO is another attractive target for monotherapy and combination therapies with ICI (105–107).
Targeting the suppression mechanisms used by Tregs in the TME
While reducing Treg infiltration of the TME is a desirable goal for cancer immunotherapy, targeting suppression mechanisms of Tregs may also provide good outcomes. This may especially be helpful in treating established tumors that have already been enriched with Tregs. Tregs have been shown to require an antigen encounter in the tumor-draining lymph node and subsequently within the tumor to optimally exert their suppressive functions (31). Activated Tregs can suppress APCs and effector T cells using both antigen-specific and bystander mechanisms. While their bystander effects are mostly mediated through suppressive cytokines, antigen-specific suppression relies on a contact-dependent removal of antigenic peptides (4). The use of these mechanisms by Tregs is dictated, for the most part, by antigen density and the inflammatory context it is presented in. Compared to a pathogen activated immune response, anti-tumoral immune response is surrounded by an immunosuppressive milieu that inhibits antigen presentation and overall lymphocyte function (108). In such a microenvironment, some Treg clones seem to proliferate and can possibly reach substantial numbers at later stages, outcompeting the effector T cells. Although reduced clonal diversity of Tregs may point out to a Treg response against particular tumor-derived antigens, it is hard to say that they operate in an antigen-specific manner (15, 40, 109). Instead, there may be unique combinations of antigen-specific and bystander mechanisms that Tregs tailor for the type and stage of the tumor based on the microenvironmental cues they receive. Although we are still a long way from deciphering the multiverse of suppression mechanisms, some useful clues from mouse models have started to point us in the right direction for finding Treg mechanisms that may be worth targeting.
Contribution of Tregs to the regulatory milieu of TME
Tregs have been reported to release a plethora of cytokines that inhibit immune responses. Among those, IL-10, IL-35 and TGF-β have been intensely investigated for their contribution to Treg mediated suppression. While systemic effects of Treg specific deletion of these cytokines are controversial, their production by Tregs seem to be critical in particular niches such as epithelial barriers and TME (110–116).
IL-10 is a pleitropic cytokine that has immunoregulatory and immune activating effects. Treg-derived IL-10 has been shown to suppress antigen presentation and effector T cell function in vitro, and promote tolerance at the intestinal lamina propria in vivo. Although it is mostly produced by tumor associated myeloid cells and Tregs, overall effects IL-10 on tumor immunity remain elusive (117–119). It has been reported that tumor associated M2-type macrophages produce IL-10 to weaken the anti-tumor immunity in various cancer types (120, 121). Treg-derived IL-10 was shown to facilitate CD4+ and CD8+ T cell exhaustion by activating Prdm1 locus and upregulating BLIMP-1 mediated expression of PD-1, LAG3, TIM3, TIGIT, 2B4 (113, 122). On the other hand, studies that introduced PEGylated IL-10 for therapy elicited improved CD8+ TIL function and metabolic profile in mouse models and patients with solid tumors (123–127). Moreover, tumor targeted delivery of IL10-cetuximab fusion protein reduced apoptosis of intratumoral CD8+ T cells, boosted IFN-γ production and decreased tumor burden (124). A multicenter phase 1 clinical trial for PEGylated IL-10 monotherapy and combination therapy with anti-PD-1 also demonstrated expansion of antigen experienced CD8+ T cells in advanced tumor patients in both groups, further supporting an activating role for IL-10 in tumor immunity (128). However, the overall effect of Treg-derived IL-10 in TME and the strategies to target it require further elucidation.
IL-35 is a suppressive cytokine that belongs to IL-12 family. It is a heterodimer composed of Epstein-Barr-virus-induced gene (Ebi-3) and IL-12α chain (p35) and its expression has been detected in a variety of tumors (129, 130). A Treg specific Ebi3 deletion was shown to ameliorate anti-tumor responses similar to the effects observed in anti-IL35 treatment of wild type mice suggesting that depletion of IL-35 expressing Tregs and/or blocking IL-35 activity can be developed as potential immunotherapies (131, 132). IL-35 has been shown to act together with IL-10 in TME to induce BLIMP-1 mediated upregulation of inhibitory receptors on tumor-reactive CD4+ and CD8+ T cells (122). Furthermore, IL-35 release by Tregs has been shown to confer a regulatory phenotype to Foxp3- effector T cells in an IL-10 dependent manner (131). These cells, called iTr35, represent a stable suppressive population and can perform infectious tolerance in TME and transplant settings (133). More recently, IL-35 expression has been attributed a role in tumor cell extravasation and metastatic processes (134–136). Whether IL-35 producing Tregs are involved in metastasis remains elusive.
TGF-β is another cytokine that has important roles in immunoregulation and malignant transformation (137–139). High plasma TGF-β concentration and tumor TGF-β expression are used as indicators of poor prognosis in late stages of cancer and may help predict immunotherapy resistance in cancer patients (140–142). TGF-β blockade was shown to have a synergistic effect with checkpoint inhibitors in animal models, therefore, multiple clinical trials have investigated therapeutic effects of TGF-β inhibition as a monotherapy and in combination with ICIs (140). While TGF-β is secreted mostly by tumor and tumor associated fibroblasts, it can also be released by activated Tregs in a paracrine fashion. Upon activation, Tregs express the surface receptor glycoprotein-A repetitions predominant (GARP), which tethers inactive latent TGF-β complex to the Treg membrane. Upon cellular interactions, αVβ8 integrins can activate TGF-β in-cis and trans (143, 144). This provides a local, niche-restricted delivery of biologically active TGF-β by Tregs. Among T cells, GARP expression is limited to activated Tregs and in mice with Treg-specific GARP deletion, mice develop spontaneous colitis resembling the phenotype seen in Treg-specific TGF-β deletion. Furthermore, deletion of GARP in Tregs confer protection from colorectal cancer, suggesting that GARP may be an ideal drug target for inhibiting TGF-β mediated immunosuppressive effects of Tregs in colorectal tumors (145–149). GARP is also expressed by tumor cells, providing a reservoir of TGF-β, thus GARP inhibition may have broad tumor-directed effects beyond limiting Treg suppression (146, 150–152).
A potential mechanism whereby Tregs can alter TME is the removal of extracellular ATP by its surface ectonucleotidases, CD39 and CD73. Rising extracellular ATP largely reflects tissue injury and hypoxia and is perceived by the immune system as a danger signal (153). While low and moderate levels of extracellular ATP provide activating signals to T cells via purinergic P2XR receptors, high levels can be toxic leading to mitochondrial dysfunction and cell death. However, this is not a Treg-specific mechanism. In fact, a plethora of innate and adaptive immune cells, including myeloid cells, DCs, B cells, effector T cells and Tregs, can metabolize ATP via CD39 and CD73 (154). While CD39 degrades ATP into AMP, CD73 metabolizes AMP into immunoregulatory adenosine (155, 156). Adenosine binds to adenosine receptor 2a (A2a) on CD8+ T cells and NK cells and inhibit their anti-tumor activity (157, 158). Overall, targeting CD39, CD73 and/or A2a receptor one by one or in combination are attractive strategies to enhance anti-tumor immune responses.
Costimulatory and coinhibitory pathways used by Tregs
Tregs express a plethora of costimulatory and coinhibitory molecules, mainly members of the CD28-B7 family and the tumor necrosis factor receptor superfamily of receptors (159, 160). CTLA-4 is one of the most well-known coinhibitory receptors of CD28-B7 family that is constitutively expressed on Tregs while its expression on the effector CD4+ and CD8+ T cells is induced following activation (161). CTLA-4 regulates effector T-cell responses in cell intrinsic and extrinsic manners, cell-extrinsic functions primarily being operated by Tregs (162). CTLA-4 has been shown to quench the signals downstream of TCR and CD28 at the effector immune synapse, compete with costimulatory receptor CD28 for binding CD80-CD86, downregulate CD80-CD86 expression and remove CD80-CD86 from APCs via trogocytosis and transendocytosis (Figure 2) (7, 11, 162–167). However, whether Tregs use CTLA-4 as a major player in maintaining peripheral tolerance or suppressing anti-tumoral immunity is still debated. While a Treg specific deletion of CTLA-4 from birth causes severe autoimmunity and death, an induced deletion during adulthood displays no overt autoimmunity with a slightly enhanced resistance to experimental autoimmune encephalomyelitis (111). This discrepancy possibly points out the importance of CTLA-4 in Treg development instead of function, and also suggests a role for CTLA-4 in tuning the CD28-driven homeostatic Treg proliferation (111, 168). Furthermore, suppressive ability of CTLA-4 deleted Tregs were found unchanged, suggesting that CTLA-4 is not used as a standalone mechanism by Tregs (45, 111). However, the overall effect of Treg CTLA-4, if any, seems to be diminishing the duration and quality of the contacts between APCs and effector T cells within the TME (163). While the role played by CTLA-4 in the TME is still obscure, the depletion of Tregs via anti-CTLA-4 antibodies seems to be an efficient strategy to boost the anti-tumoral immune responses. Yet, due to the well-established role of CTLA-4 in maintaining peripheral tolerance, its broad inhibition poses a high risk for serious irAE (169). This highlights the need for developing more specific strategies and/or targeted drug delivery systems for anti-tumoral immunotherapeutics.
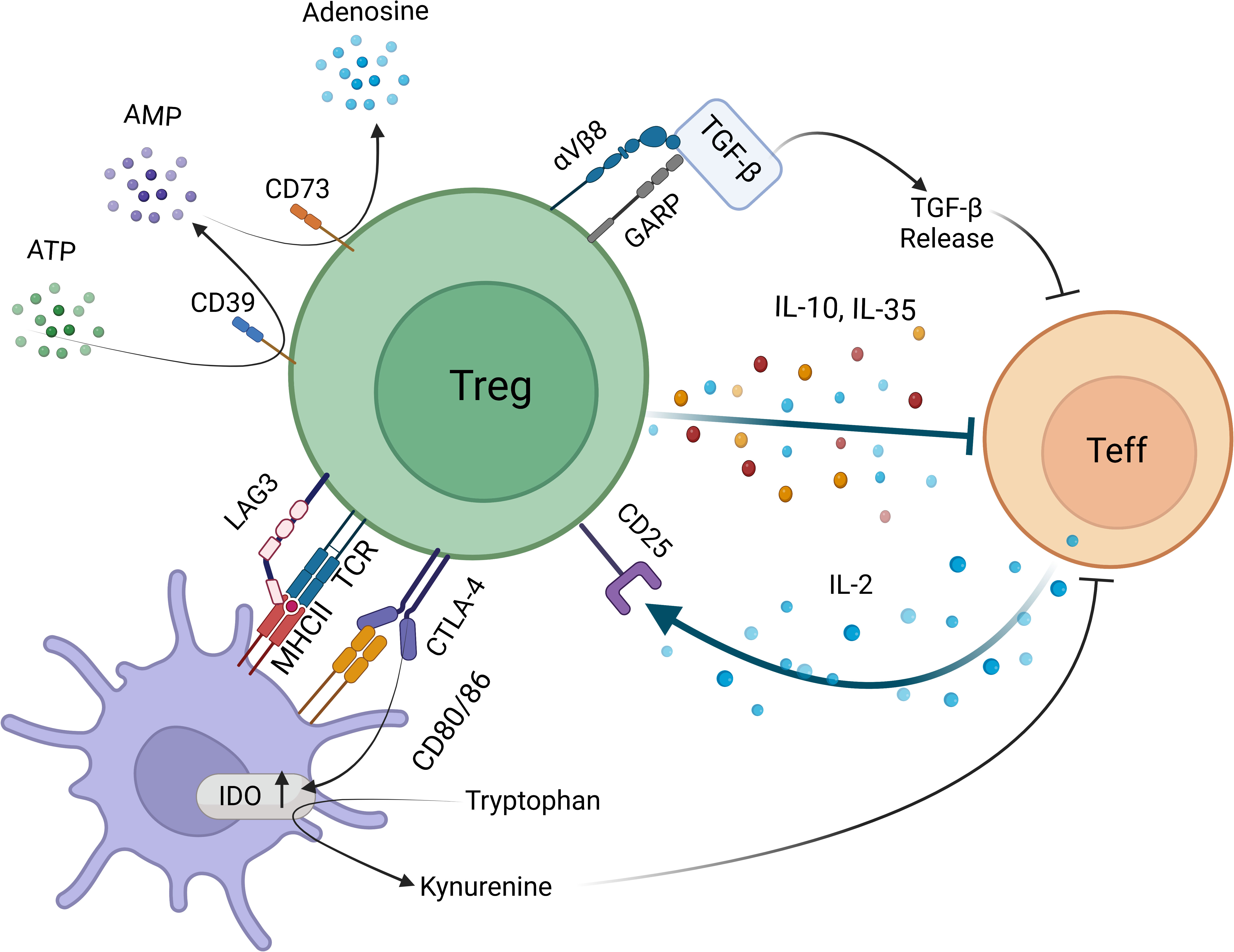
Figure 2 Targets for limiting intratumoral Treg activity based on in vivo mechanisms of suppression Treg-APC encounters activate antigen-specific and bystander suppression mechanisms. Tregs interfere with effector T cells function directly via producing inhibitory cytokines IL-10 and IL-35, delivering bioactive TGF-β via GARP-αVβ8 integrin axis, degrading proinflammatory ATP into AMP by surface 5’ ectonucleotidase CD39 and cleaving AMP into tolerogenic adenosine by 3’ ectonucleotidase CD73. Tregs can also deplete IL-2 by high affinity IL-2Rα (CD25), thus lead to effector T cell apoptosis. Indirect suppressive mechanisms include removal of antigen-MHCII and CD80-CD86 via TCR and CTLA-4 mediated transendocytosis and trogocytosis events. Tregs can also activate IDO activity via CTLA-4 reverse signaling into APC.
Similar to CTLA-4, PD-1 is also tasked to quench the activation signals transmitted by TCR and CD28 and plays a central role in the immunosuppressive nature of the TME (170). High PD-1 expression is one of the hallmarks of exhausted phenotype in T cells, hence the blockade of PD-1-PD-L1 axis has been adapted as another ICI modality (171, 172). Inhibiting PD-1 activity using antibodies against PD-1 or PD-L1 has been shown to rejuvenate exhausted effector T cells and reduce tumor burden (169, 171). While this therapy has already made its ways to clinics, our understanding of its action is largely limited to CD8+ T cells. However, a number of recent studies attempted to lay out role of PD-1 in Treg biology and maintenance in the TME (170). For instance, mice with Treg specific deletion of PD-1 displayed an activated phenotype and their increased suppressive abilities ameliorated autoimmune pathology (173). In the TME, high PD-1 expression on Tregs seems to be associated with reduced suppressive ability, therefore, blocking PD-1 pathway may revive those Tregs helping them more effectively compete with the effector T cells (174). Recent findings demonstrated that PD-1 blockade is less effective in tumors that are enriched with PD-1high Tregs, supporting the risks associated with activating Tregs in the TME (171, 175, 176). This may also explain why ICI is more effective when it is combined with Treg-depleting strategies. The synergistic effect of anti-CTLA-4 (Ipilimumab) and anti-PD-1 (nivolumab) may also point out to the benefit of removing Tregs to make ICI more effective (177). However, clinicians should carefully evaluate the options and timing for monotherapy vs combination therapies case by case to keep irAE at bay (177, 178). In the context of new combination therapies, dual PD-1 and LAG3 inhibition has gained attention to reverse T cell exhaustion (179, 180). Similar to PD-1, LAG3 expression also correlates with CD8+ T cell dysfunction that is characterized by reduced capacity for proliferation and cytokine production (181–183). As a corollary, LAG3 blockade reinvigorates CD8+ T cell function however, its role in CD4+ effector T cells is rather complex. Extracellular portion of LAG3 is cleaved off by metalloprotease enzymes ADAM10 and 17 upon activation and released as soluble monomeric LAG3 (sLAG3). LAG3 shedding was found critical for CD4+ T cell function in an elegant mouse model where CD4+ T cells were introduced a non-cleavable LAG3 mutant (184). This may be due to an incessant inhibitory signal through mutant LAG3 which would otherwise stop because of shedding. Alternatively, sLAG3 shed by CD4+ T cells has a local stimulating effect on CD4+ T cells or DCs in an autocrine or paracrine fashion. LAG3 harbors further complexities in Treg compartment. A Treg-specific deletion of LAG3 from birth was found to reduce autoimmune diabetes incidence and severity in NOD mice and diminish immune infiltration of pancreas (185). This was possibly due to exhausting effect of LAG3 expression on Tregs, similar to that of PD-1 due to chronic stimulation within tissue (175, 176). On the other hand, in experimental autoimmune encephalomyelitis and adoptive transfer colitis models, adoptive transfer of LAG3 deficient Tregs failed to protect against disease despite normal trafficking and stable foxp3 expression suggesting that LAG3 can be required for Treg mediated suppression in certain autoimmune settings while it can be detrimental in others (186, 187). However, we still don’t know which category tumor-specific Tregs fall in the spectrum of LAG3 mediated effects.
Another costimulatory molecule that plays role in fine-tuning Treg activity is GITR (188). GITR is a member of the TNFR superfamily of coreceptors that is constitutively expressed at high levels on Tregs (189). Naïve effector T cells also harbor a low surface expression that is upregulated upon activation (190). GITR seems to play a complex, context-dependent role in the immune system and so far, it has been shown to promote effector T cell activity in a cell-intrinsic manner, induce Treg expansion and inhibit Treg suppressive function (160, 188, 191, 192). In the context of tumors, stimulation of GITR pathway has been shown to destabilize Tregs, equipping them with cytotoxic abilities (192). While the effect of GITR monotherapy appears to be limited and varies with the tumor type, adding GITR agonists to PD-1 blockade has been shown to potentiate its anti-tumor effect (160, 192). Therefore, GITR agonism presents a promising new strategy for cancer immunotherapy hence, there are multiple ongoing clinical trials investigating the effects of GITR agonism and its combination with ICI in different cancer types (193, 194).
Strategies harnessing TCR and CD25 for eliminating tumor-specific Tregs
CD25 is the high affinity IL-2 receptor expressed constitutively at a high level by Tregs. It also gets expressed on effector T cells, B cells, NK cells upon activation (195–197). While a constitutive high expression seems to make it a reliable marker for murine and human Tregs, CD25 is not enough on its own and needs to be combined with other indicators such as Foxp3, Helios and/or lack or low expression of CD127 to determine Treg lineage (198). Likewise, failure of CD25 in distinguishing Tregs and activated effector T cells at sites with an ongoing immune response, such as the TME, makes it an unreliable target for immunotherapy. Indeed, CD25-targeting agents can hamper the anti-tumor immunity by depleting activated effectors or restricting their access to IL-2 and this may explain, at least partially, why anti-CD25 antibodies that have been used to deplete Tregs from the TME have not yielded optimal results (196, 197, 199, 200). Another possible reason could be the selection of an anti-CD25 antibody that suboptimally engages the Fc receptors in the TME, leading to a reduced ADCC (196). These setbacks have led to an effort to develop antibodies with better characteristics such as an improved intratumoral ADCC capability and an incessant IL-2 signaling in the effector T cells. These strategies, while not completely specific for Tregs, have been shown to be effective in boosting anti-tumor T cell response and reducing tumor burden in mice (197). Furthermore, their combination with PD-1 inhibitors has demonstrated significant synergistic effect, somewhat similar to the combination approaches using CTLA-4 (196). Yet, these new approaches must be carefully tested in clinical trials as the evidence for their ability to discriminate peripheral vs. intratumoral Tregs in human body are still lacking. This is a key safety point that should not be overlooked as the attempts to elicit better Treg depletion strategies may lead to intractable irAE.
One poorly understood characteristic of the TME that future therapies can focus is the oligoclonal, potentially tumor-specific, expansion of the intratumoral Tregs. As was shown for secondary lymphoid organs in vivo, it is possible that the TME harbors a competition between the effector T and Treg clones for the tumor neoantigens (45). If so, a slow take over by the neoantigen-specific Tregs may gradually transform the clonal landscape of the TME for the intratumoral neoantigen-specific T cells via mechanisms such as antigen-specific suppression by pMHCII depletion and/or conversion into tumor-specific pTregs due to suboptimal antigen stimulus and elevated TGF-β as well as bystander mechanisms of suppression (40, 109). Unfortunately, it has so far been difficult to capture these possibilities due to a lack of tools such as reliable markers to distinguish tTreg and pTregs and time-sensitive sampling and analysis for the clonal representation and antigen-specificity of Tregs and effector T cells. Until we have a better understanding for how bystander and antigen-specific suppression mechanisms operate in the TME, we can utilize TCR sequence and antigen specificity of intratumoral Treg clones as a proxy to understand the type of response that needs to be potentiated (Figure 3). This would facilitate new antigen-targeted cellular biotherapies such as TCR-transgenic T cell, CAR-T cell and antigen-loaded immunogenic DCs and would ultimately revive or deliver right set of effectors that can outcompete intratumoral Tregs.
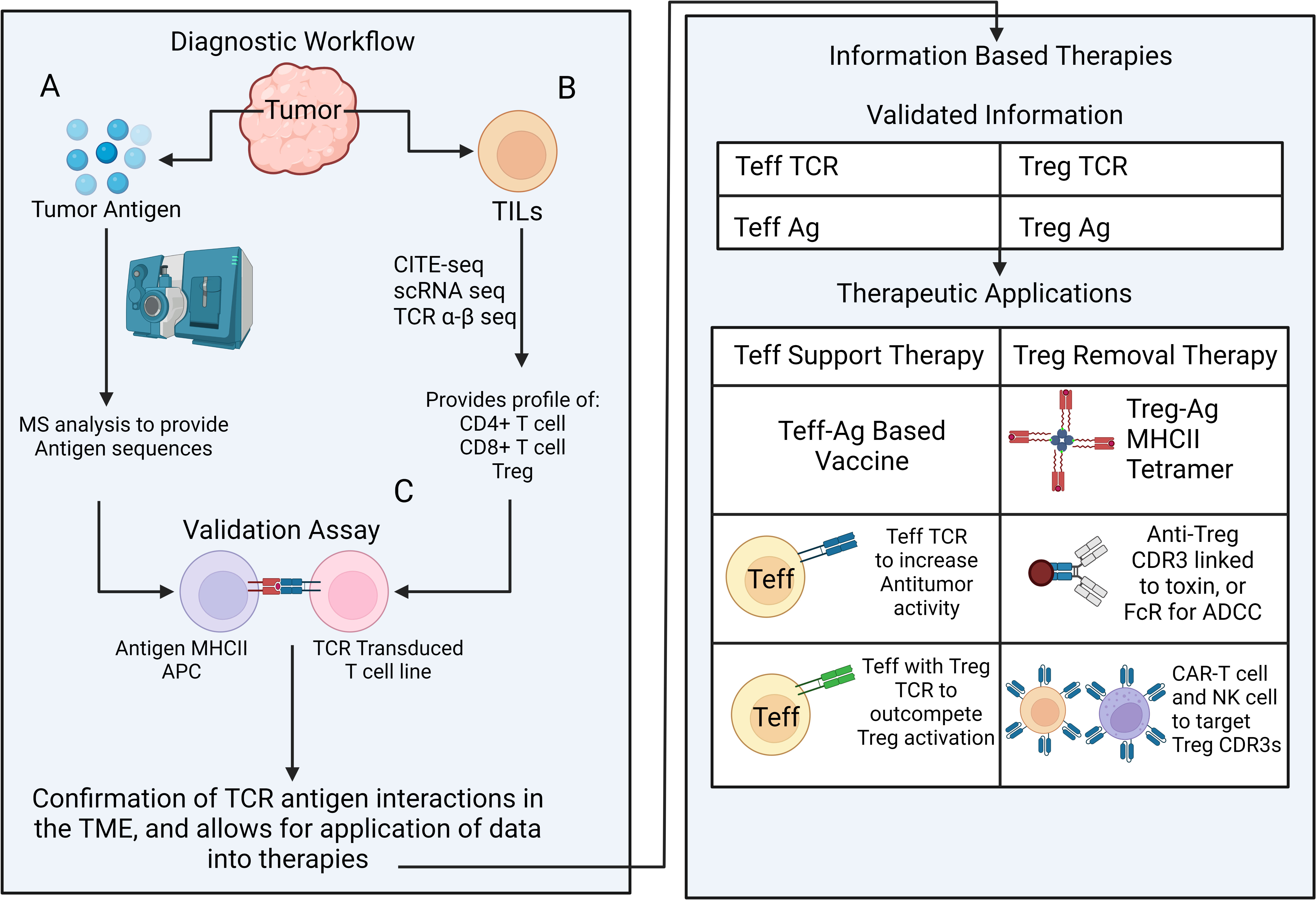
Figure 3 A multipronged approach for outcompeting vs. eliminating tumor-specific Tregs Diagnostic workflow for revealing antigen-specific elements in tumor tissue includes a multi-pronged approach; (A) Mass spectrometry-based detection of candidate antigens that potentially drive effector or regulatory T cell expansion, (B) Single cell RNA and TCR αβ VDJ sequencing for identifying and phenotyping the most frequently represented CD4+, CD8+ and Treg clones. (C) Next step aims at selecting biologically relevant antigen-TCR combinations in vitro. Validated antigen and TCR information can be used to develop strategies to selectively boost effector T cells and/or eliminate Tregs. Strategies for effector T cell support (Teff support therapy) include antigen vaccines, adoptive transfer of TCR transgenic effector T cells that contain select effector T or Treg TCRs. Strategies for Treg inactivation/removal consist of Treg antigen (ag) -MHCII tetramer to cease Treg-APC contacts, antibodies targeting select Treg TCR-CDR3 for removal (conjugated to a toxin or via FcR mediated ADCC) and CAR-T or CAR-NK cells targeting Treg TCR-CDR3 for Treg removal.
Conclusion
The fine balance between intratumoral Tregs and effector T cells is closely linked to disease prognosis. Tregs have a myriad of adaptations that facilitate their survival and function in TME. They can metabolize FFAs and lactic acid, hence survive in low glucose, low oxygen environments. Tolerogenic milieu enriched in TGF-β, adenosine and kynurenine can also induce Foxp3 expression in effector CD4+ T cells, further contributing to intratumoral Treg pool. Several contact-dependent and paracrine mechanisms have been proposed for Treg mediated suppression. However, strategies targeting Tregs have so far been hampered by our limited success in delineating how they suppress in-situ. This has been compounded by a lack of reliable markers that discern tumor-specific Tregs from tumor-specific effector CD4+ T cells and self-reactive Tregs, taking us back to the drawing board due to unpredictable risks of harming peripheral tolerance. Although antigen-targeted precision therapies have a long way from clinics, they appear to be a safer route to limit immune related adverse events. By unraveling immune interactions taking place in TME thoroughly, we would be able to lend a hand to the right effectors in their tug of war with intratumoral Tregs.
Author contributions
BA conceived the manuscript. BRM and BA drafted the manuscript. BA performed the critical revision and finalization of the manuscript. All authors contributed to the article and approved the submitted version.
Funding
This work was supported by the National Institutes of Health grant number DP2AI154451.
Conflict of interest
The authors declare that the research was conducted in the absence of any commercial or financial relationships that could be construed as a potential conflict of interest.
The handling editor declared a shared affiliation with the authors at the time of the review.
Publisher’s note
All claims expressed in this article are solely those of the authors and do not necessarily represent those of their affiliated organizations, or those of the publisher, the editors and the reviewers. Any product that may be evaluated in this article, or claim that may be made by its manufacturer, is not guaranteed or endorsed by the publisher.
References
1. Josefowicz SZ, Lu L-F, Rudensky AY. Regulatory T cells: Mechanisms of differentiation and function. Annu Rev Immunol (2012) 30(1):531–64. doi: 10.1146/annurev.immunol.25.022106.141623
2. Shevach EM, Thornton AM. tTregs, pTregs, and iTregs: Similarities and differences. Immunol Rev (2014) 259(1):88–102. doi: 10.1111/imr.12160
3. Liu W, Putnam AL, Xu-yu Z, Szot GL, Lee MR, Zhu S, et al. CD127 expression inversely correlates with FoxP3 and suppressive function of human CD4+ T reg cells. J Experiment Med (2006) 203(7):1701–11. doi: 10.1084/jem.20060772
4. Akkaya B, Shevach EM. Regulatory T cells: Master thieves of the immune system. Cell Immunol (2020) 355:104160. doi: 10.1016/j.cellimm.2020.104160
5. Shevach EM. Mechanisms of Foxp3+ T regulatory cell-mediated suppression. Immunity (2009) 30(5):636–45. doi: 10.1016/j.immuni.2009.04.010
6. Pandiyan P, Zheng L, Ishihara S, Reed J, Lenardo MJ. CD4+CD25+Foxp3+ regulatory T cells induce cytokine deprivation–mediated apoptosis of effector CD4+ T cells. Nat Immunol (2007) 8(12):1353–62. doi: 10.1038/ni1536
7. Wing K, Onishi Y, Prieto-Martin P, Yamaguchi T, Miyara M, Fehervari Z, et al. CTLA-4 control over Foxp3+ regulatory T cell function. Science (2008) 322(5899):271–5. doi: 10.1126/science.1160062
8. Collison LW, Workman CJ, Kuo TT, Boyd K, Wang Y, Vignali KM, et al. The inhibitory cytokine IL-35 contributes to regulatory T-cell function. Nature (2007) 450(7169):566–9. doi: 10.1038/nature06306
9. Andersson J, Tran DQ, Pesu M, Davidson TS, Ramsey H, O’Shea JJ, et al. CD4+FoxP3+ regulatory T cells confer infectious tolerance in a TGF-β–dependent manner. J Exp Med (2008) 205(9):1975–81. doi: 10.1084/jem.20080308
10. Borsellino G, Kleinewietfeld M, Di Mitri D, Sternjak A, Diamantini A, Giometto R, et al. Expression of ectonucleotidase CD39 by Foxp3+ treg cells: hydrolysis of extracellular ATP and immune suppression. Blood (2007) 110(4):1225–32. doi: 10.1182/blood-2006-12-064527
11. Qureshi Omar S, Zheng Y, Nakamura K, Attridge K, Manzotti C, Schmidt Emily M, et al. Trans-endocytosis of CD80 and CD86: A molecular basis for the cell-extrinsic function of CTLA-4. Science (2011) 332(6029):600–3. doi: 10.1126/science.1202947
12. Cao X, Cai SF, Fehniger TA, Song J, Collins LI, Piwnica-Worms DR, et al. Granzyme b and perforin are important for regulatory T cell-mediated suppression of tumor clearance. Immunity (2007) 27(4):635–46. doi: 10.1016/j.immuni.2007.08.014
13. Brunkow ME, Jeffery EW, Hjerrild KA, Paeper B, Clark LB, Yasayko S-A, et al. Disruption of a new forkhead/winged-helix protein, scurfin, results in the fatal lymphoproliferative disorder of the scurfy mouse. Nature Genetics (2001) 27(1):68–73. doi: 10.1038/83784
14. Bennett CL, Christie J, Ramsdell F, Brunkow ME, Ferguson PJ, Whitesell L, et al. The immune dysregulation, polyendocrinopathy, enteropathy, X-linked syndrome (IPEX) is caused by mutations of FOXP3. Nature Genetics (2001 27(1):20–1. doi: 10.1038/83713
15. Plitas G, Konopacki C, Wu K, Bos PD, Morrow M, Putintseva Ekaterina V, et al. Regulatory T cells exhibit distinct features in human breast cancer. Immunity (2016) 45(5):1122–34. doi: 10.1016/j.immuni.2016.10.032
16. Verma A, Mathur R, Farooque A, Kaul VA-O, Gupta SA-OX, Dwarakanath BA-O. T-regulatory cells in tumor progression and therapy. Cancer Management Research 11:1179–322. doi: 10.2147/CMAR.S228887
17. Wong J, Obst R, Correia-Neves M, Losyev G, Mathis D, Benoist C. Adaptation of TCR repertoires to self-peptides in regulatory and nonregulatory CD4+ T cells. J Immunol (2007) 178(11):7032. doi: 10.4049/jimmunol.178.11.7032
18. Le Borgne M, Ladi E, Dzhagalov I, Herzmark P, Liao YF, Chakraborty AK, et al. The impact of negative selection on thymocyte migration in the medulla. Nat Immunol (2009) 10(8):823–30. doi: 10.1038/ni.1761
19. Kishimoto H, Sprent J. Negative selection in the thymus includes semimature T cells. J Exp Med (1997) 185(2):263–72. doi: 10.1084/jem.185.2.263
20. Klein L, Robey EA, Hsieh C-S. Central CD4+ T cell tolerance: deletion versus regulatory T cell differentiation. Nat Rev Immunol (2019) 19(1):7–18. doi: 10.1038/s41577-018-0083-6
21. Relland LM, Mishra MK, Haribhai D, Edwards B, Ziegelbauer J, Williams CB. Affinity-based selection of regulatory T cells occurs independent of agonist-mediated induction of Foxp3 expression. J Immunol (2009) 182(3):1341. doi: 10.4049/jimmunol.182.3.1341
22. Simons DM, Picca CC, Oh S, Perng OA, Aitken M, Erikson J, et al. How specificity for self-peptides shapes the development and function of regulatory T cells. J Leukocyte Biol (2010) 88(6):1099–107. doi: 10.1189/jlb.0310183
23. Hinterberger M, Wirnsberger G, Klein L. B7/CD28 in central tolerance: Costimulation promotes maturation of regulatory T cell precursors and prevents their clonal deletion. Front Immunol (2011) 2. doi: 10.3389/fimmu.2011.00030
24. Savage PA, Klawon DEJ, Miller CH. Regulatory T cell development. Annu Rev Immunol (2020) 38(1):421–53. doi: 10.1146/annurev-immunol-100219-020937
25. Konkel JE, Jin W, Abbatiello B, Grainger JR, Chen W. Thymocyte apoptosis drives the intrathymic generation of regulatory T cells. Proc Natl Acad Sci (2014) 111(4):E465–E73. doi: 10.1073/pnas.1320319111
26. Finn OJ. Human tumor antigens yesterday, today, and tomorrow. Cancer Immunol Res (2017) 5(5):347–54. doi: 10.1158/2326-6066.CIR-17-0112
27. Jiang T, Shi T, Zhang H, Hu J, Song Y, Wei J, et al. Tumor neoantigens: from basic research to clinical applications. J Hematol Oncol (2019) 12(1):93. doi: 10.1186/s13045-019-0787-5
28. Leko V, Rosenberg SA. Identifying and targeting human tumor antigens for T cell-based immunotherapy of solid tumors. Cancer Cell (2020) 38(4):454–72. doi: 10.1016/j.ccell.2020.07.013
29. Haen SP, Löffler MW, Rammensee H-G, Brossart P. Towards new horizons: characterization, classification and implications of the tumour antigenic repertoire. Nat Rev Clin Oncol (2020) 17(10):595–610. doi: 10.1038/s41571-020-0387-x
30. Hsieh C-S, Liang Y, Tyznik AJ, Self SG, Liggitt D, Rudensky AY. Recognition of the peripheral self by naturally arising CD25+ CD4+ T cell receptors. Immunity (2004) 21(2):267–77. doi: 10.1016/j.immuni.2004.07.009
31. Bauer CA, Kim EY, Marangoni F, Carrizosa E, Claudio NM, Mempel TR. Dynamic treg interactions with intratumoral APCs promote local CTL dysfunction. J Clin Invest (2014) 124(6):2425–40. doi: 10.1172/JCI66375
32. Fu H, Kishore M, Gittens B, Wang G, Coe D, Komarowska I, et al. Self-recognition of the endothelium enables regulatory T-cell trafficking and defines the kinetics of immune regulation. Nat Commun (2014) 5(1):3436. doi: 10.1038/ncomms4436
33. Accolla RS, Ramia E, Tedeschi A, Forlani G. CIITA-driven MHC class II expressing tumor cells as antigen presenting cell performers: Toward the construction of an optimal anti-tumor vaccine. Front Immunol (2019) 10. doi: 10.3389/fimmu.2019.01806
34. Chen W, Jin W, Hardegen N, Lei K-J, Li L, Marinos N, et al. Conversion of peripheral CD4+CD25- naive T cells to CD4+CD25+ regulatory T cells by TGF-beta induction of transcription factor Foxp3. Journal of Experimental Medicine (2003) 198(12):1875–86. doi: 10.1084/jem.20030152
35. Fantini MC, Becker C, Monteleone G, Pallone F, Galle PR, Neurath MF. Cutting edge: TGF-beta induces a regulatory phenotype in CD4+CD25- T cells through Foxp3 induction and down-regulation of Smad7. The Journal of Immunology (2004) 172(9):5149. doi: 10.4049/jimmunol.172.9.5149
36. Takimoto T, Wakabayashi Y, Sekiya T, Inoue N, Morita R, Ichiyama K, et al. Smad2 and Smad3 are redundantly essential for the TGF-beta-mediated regulation of regulatory T plasticity and Th1 development. J Immunol (2010) 185(2):842. doi: 10.4049/jimmunol.0904100
37. Tone Y, Furuuchi K, Kojima Y, Tykocinski ML, Greene MI, Tone M. Smad3 and NFAT cooperate to induce Foxp3 expression through its enhancer. Nature Immunology (2008) 9(2):194–202. doi: 10.1038/ni1549
38. Ahmadzadeh M, Felipe-Silva A, Heemskerk B, Powell DJ Jr, Wunderlich JR, Merino MJ, et al. FOXP3 expression accurately defines the population of intratumoral regulatory T cells that selectively accumulate in metastatic melanoma lesions. Blood (2008) 112(13):4953–60. doi: 10.1182/blood-2008-06-163048
39. De Simone M, Arrigoni A, Rossetti G, Gruarin P, Ranzani V, Politano C, et al. Transcriptional landscape of human tissue lymphocytes unveils uniqueness of tumor-infiltrating T regulatory cells. Immunity (2016) 45(5):1135–47. doi: 10.1016/j.immuni.2016.10.021
40. Ahmadzadeh M, Pasetto A, Jia L, Deniger Drew C, Stevanović S, Robbins Paul F, et al. Tumor-infiltrating human CD4+ regulatory T cells display a distinct TCR repertoire and exhibit tumor and neoantigen reactivity. Sci Immunol (2019) 4(31):eaao4310. doi: 10.1126/sciimmunol.aao4310
41. Nishikawa H, Sakaguchi S. Regulatory T cells in tumor immunity. International Journal of Cancer (2010) 127(4):759–67. doi: 10.1002/ijc.25429
42. Thornton AM, Shevach EM. Helios: Still behind the clouds. Immunology. (2019) 158(3):161–70. doi: 10.1111/imm.13115
43. Malchow S, Leventhal DS, Nishi S, Fischer BI, Shen L, Paner GP, et al. Aire-dependent thymic development of tumor-associated regulatory T cells. Science (2013) 339(6124):1219–24. doi: 10.1126/science.1233913
44. Sainz-Perez A, Lim A, Lemercier B, Leclerc C. The T-cell receptor repertoire of tumor-infiltrating regulatory T lymphocytes is skewed toward public sequences. Cancer Research (2012) 72(14):3557–69. doi: 10.1158/0008-5472.CAN-12-0277
45. Akkaya B, Oya Y, Akkaya M, Al Souz J, Holstein AH, Kamenyeva O, et al. Regulatory T cells mediate specific suppression by depleting peptide–MHC class II from dendritic cells. Nat Immunol (2019) 20(2):218–31. doi: 10.1038/s41590-018-0280-2
46. Noyes D, Bag A, Oseni S, Semidey-Hurtado J, Cen L, Sarnaik AA, et al. Tumor-associated tregs obstruct antitumor immunity by promoting T cell dysfunction and restricting clonal diversity in tumor-infiltrating CD8+ T cells. J ImmunoTher Cancer (2022) 10(5):e004605. doi: 10.1136/jitc-2022-004605
47. Woolaver RA, Wang X, Krinsky AL, Waschke BC, Chen SMY, Popolizio V, et al. Differences in TCR repertoire and T cell activation underlie the divergent outcomes of antitumor immune responses in tumor-eradicating versus tumor-progressing hosts. J ImmunoTher Cancer. (2021) 9(1):e001615. doi: 10.1136/jitc-2020-001615
48. Campbell DJ. Control of regulatory T cell migration, function, and homeostasis. J Immunol (2015) 195(6):2507. doi: 10.4049/jimmunol.1500801
49. Sather BD, Treuting P, Perdue N, Miazgowicz M, Fontenot JD, Rudensky AY, et al. Altering the distribution of Foxp3+ regulatory T cells results in tissue-specific inflammatory disease. J Exp Med (2007) 204(6):1335–47. doi: 10.1084/jem.20070081
50. Iellem A, Mariani M, Lang R, Recalde H, Panina-Bordignon P, Sinigaglia F, et al. Unique chemotactic response profile and specific expression of chemokine receptors Ccr4 and Ccr8 by Cd4+Cd25+ regulatory T cells. J Exp Med (2001) 194(6):847–54. doi: 10.1084/jem.194.6.847
51. Barsheshet Y, Wildbaum G, Levy E, Vitenshtein A, Akinseye C, Griggs J, et al. CCR8+FOXp3+ treg cells as master drivers of immune regulation. Proc Natl Acad Sci (2017) 114(23):6086–91. doi: 10.1073/pnas.1621280114
52. Van Damme H, Dombrecht B, Kiss M, Roose H, Allen E, Van Overmeire E, et al. Therapeutic depletion of CCR8+ tumor-infiltrating regulatory T cells elicits antitumor immunity and synergizes with anti-PD-1 therapy. J ImmunoTher Cancer (2021) 9(2):e001749. doi: 10.1136/jitc-2020-001749
53. Curiel TJ, Coukos G, Zou L, Alvarez X, Cheng P, Mottram P, et al. Specific recruitment of regulatory T cells in ovarian carcinoma fosters immune privilege and predicts reduced survival. Nat Med (2004) 10(9):942–9. doi: 10.1038/nm1093
54. Marshall LA, Marubayashi S, Jorapur A, Jacobson S, Zibinsky M, Robles O, et al. Tumors establish resistance to immunotherapy by regulating t(reg) recruitment via CCR4. J immunother Cancer (2020) 8(2):e000764. doi: 10.1136/jitc-2020-000764
55. Hoelzinger DB, Smith SE, Mirza N, Dominguez AL, Manrique SZ, Lustgarten J. Blockade of CCL1 inhibits T regulatory cell suppressive function enhancing tumor immunity without affecting T effector responses. J Immunol (2010) 184(12):6833. doi: 10.4049/jimmunol.0904084
56. Jackson JJ, Ketcham JM, Younai A, Abraham B, Biannic B, Beck HP, et al. Discovery of a potent and selective CCR4 antagonist that inhibits treg trafficking into the tumor microenvironment. J Medicinal Chem (2019) 62(13):6190–213. doi: 10.1021/acs.jmedchem.9b00506
57. Sarkar T, Dhar S, Chakraborty D, Pati S, Bose S, Panda AK, et al. FOXP3/HAT1 axis controls treg infiltration in the tumor microenvironment by inducing CCR4 expression in breast cancer. Front Immunol (2022) 13. doi: 10.3389/fimmu.2022.740588
58. Gobert M, Treilleux I, Bendriss-Vermare N, Bachelot T, Goddard-Leon S, Arfi V, et al. Regulatory T cells recruited through CCL22/CCR4 are selectively activated in lymphoid infiltrates surrounding primary breast tumors and lead to an adverse clinical outcome. Cancer Res (2009) 69(5):2000–9. doi: 10.1158/0008-5472.CAN-08-2360
59. Ishida T, Ishii T, Inagaki A, Yano H, Komatsu H, Iida S, et al. Specific recruitment of CC chemokine receptor 4–positive regulatory T cells in Hodgkin lymphoma fosters immune privilege. Cancer Res (2006) 66(11):5716–22. doi: 10.1158/0008-5472.CAN-06-0261
60. Sugiyama D, Nishikawa H, Maeda Y, Nishioka M, Tanemura A, Katayama I, et al. Anti-CCR4 mAb selectively depletes effector-type FoxP3+CD4+ regulatory T cells, evoking antitumor immune responses in humans. Proc Natl Acad Sci United States America. (2013) 110(44):17945–50. doi: 10.1073/pnas.1316796110
61. Kohli K, Pillarisetty VG, Kim TS. Key chemokines direct migration of immune cells in solid tumors. Cancer Gene Ther (2022) 29(1):10–21. doi: 10.1038/s41417-021-00303-x
62. Magnuson Angela M, Kiner E, Ergun A, Park Jun S, Asinovski N, Ortiz-Lopez A, et al. Identification and validation of a tumor-infiltrating treg transcriptional signature conserved across species and tumor types. Proc Natl Acad Sci (2018) 115(45):E10672–E81. doi: 10.1073/pnas.1810580115
63. Whiteside SK, Grant FM, Gyori DS, Conti AG, Imianowski CJ, Kuo P, et al. CCR8 marks highly suppressive treg cells within tumours but is dispensable for their accumulation and suppressive function. Immunology. (2021) 163(4):512–20. doi: 10.1111/imm.13337
64. Sarkar T, Dhar S, Sa G. Tumor-infiltrating T-regulatory cells adapt to altered metabolism to promote tumor-immune escape. Curr Res Immunol (2021) 2:132–41. doi: 10.1016/j.crimmu.2021.08.002
65. Korbecki J, Kojder K, Kapczuk P, Kupnicka P, Gawrońska-Szklarz B, Gutowska I, et al. The effect of hypoxia on the expression of CXC chemokines and CXC chemokine receptors-a review of literature. International Journal of Molecular Sciences (2021) 22(2):843. doi: 10.3390/ijms22020843
66. Abou Khouzam R, Brodaczewska K, Filipiak A, Zeinelabdin NA, Buart S, Szczylik C, et al. Tumor hypoxia regulates immune Escape/Invasion: Influence on angiogenesis and potential impact of hypoxic biomarkers on cancer therapies. Front Immunol (2021) 11. doi: 10.3389/fimmu.2020.613114
67. Facciabene A, Peng X, Hagemann IS, Balint K, Barchetti A, Wang L-P, et al. Tumour hypoxia promotes tolerance and angiogenesis via CCL28 and treg cells. Nature (2011) 475(7355):226–30. doi: 10.1038/nature10169
68. Ren L, Yu Y, Wang L, Zhu Z, Lu R, Yao Z. Hypoxia-induced CCL28 promotes recruitment of regulatory T cells and tumor growth in liver cancer. Oncotarget (2016) 7(46). doi: 10.18632/oncotarget.12409
69. Suthen S, Lim CJ, Nguyen PHD, Dutertre C-A, Lai HLH, Wasser M, et al. Hypoxia-driven immunosuppression by treg and type-2 conventional dendritic cells in HCC. Hepatology (2022). doi: 10.1002/hep.32419
70. Harjunpää H, Llort Asens M, Guenther C, Fagerholm SC. Cell adhesion molecules and their roles and regulation in the immune and tumor microenvironment. Frontiers in Immunol (2019) 10. doi: 10.3389/fimmu.2019.01078
71. Delgoffe GM, Woo S-R, Turnis ME, Gravano DM, Guy C, Overacre AE, et al. Stability and function of regulatory T cells is maintained by a neuropilin-1–semaphorin-4a axis. Nature. (2013) 501(7466):252–6. doi: 10.1038/nature12428
72. Chuckran CA, Cillo AR, Moskovitz J, Overacre-Delgoffe A, Somasundaram AS, Shan F, et al. Prevalence of intratumoral regulatory T cells expressing neuropilin-1 is associated with poorer outcomes in patients with cance. Science Translational Medicine (2021) 13(623):eabf8495. doi: 10.1126/scitranslmed.abf8495
73. Overacre-Delgoffe AE, Chikina M, Dadey RE, Yano H, Brunazzi EA, Shayan G, et al. Interferon-γ drives treg fragility to promote anti-tumor immunity. Cell. (2017) 169(6):1130–41.e11. doi: 10.1016/j.cell.2017.05.005
74. Chuckran CA, Liu C, Bruno TC, Workman CJ, Vignali DAA. Neuropilin-1: A checkpoint target with unique implications for cancer immunology and immunotherapy. J ImmunoTher Cancer (2020) 8(2):e000967.
75. Murga M, Fernandez-Capetillo O, Tosato G. Neuropilin-1 regulates attachment in human endothelial cells independently of vascular endothelial growth factor receptor-2. Blood (2005) 105(5):1992–9. doi: 10.1182/blood-2004-07-2598
76. Buck MD, Sowell RT, Kaech SM, Pearce EL. Metabolic instruction of immunity. Cell (2017) 169(4):570–86. doi: 10.1016/j.cell.2017.04.004
77. Chang C-H, Qiu J, O’Sullivan D, Buck Michael D, Noguchi T, Curtis Jonathan D, et al. Metabolic competition in the tumor microenvironment is a driver of cancer progression. Cell (2015) 162(6):1229–41. doi: 10.1016/j.cell.2015.08.016
78. Pearce Erika L, Poffenberger Maya C, Chang C-H, Jones Russell G. Fueling immunity: Insights into metabolism and lymphocyte function. Science (2013) 342(6155):1242454. doi: 10.1126/science.1242454
79. van der Windt GJ, Pearce EL. Metabolic switching and fuel choice during T-cell differentiation and memory development. Immunological Reviews (2012) 249(1):27–42. doi: 10.1111/j.1600-065X.2012.01150.x
80. MacIver NJ, Michalek RD, Rathmell JC. Metabolic regulation of T lymphocytes. Annu Rev Immunol (2013) 31(1):259–83. doi: 10.1146/annurev-immunol-032712-095956
81. Akkaya B, Roesler AS, Miozzo P, Theall BP, Al Souz J, Smelkinson MG, et al. Increased mitochondrial biogenesis and reactive oxygen species production accompany prolonged CD4(+) T cell activation. J Immunol (Baltimore Md: 1950) (2018) 201(11):3294–306. doi: 10.4049/jimmunol.1800753
82. Michalek RD, Gerriets VA, Jacobs SR, Macintyre AN, MacIver NJ, Mason EF, et al. Cutting edge: Distinct glycolytic and lipid oxidative metabolic programs are essential for effector and regulatory CD4+ T cell subsets. J Immunol (2011) 186(6):3299. doi: 10.4049/jimmunol.1003613
83. Almeida L, Lochner M, Berod L, Sparwasser T. Metabolic pathways in T cell activation and lineage differentiation. Semin Immunol (2016) 28(5):514–24. doi: 10.1016/j.smim.2016.10.009
84. Rao D, Verburg F, Renner K, Peeper DS, Lacroix R, Blank CU. Metabolic profiles of regulatory T cells in the tumour microenvironment. Cancer Immunol Immunother (2021) 70(9):2417–27. doi: 10.1007/s00262-021-02881-z
85. Kumagai S, Koyama S, Itahashi K, Tanegashima T, Y-t L, Togashi Y, et al. Lactic acid promotes PD-1 expression in regulatory T cells in highly glycolytic tumor microenvironments. Cancer Cell (2022) 40(2):201–18.e9. doi: 10.1016/j.ccell.2022.01.001
86. Lim SA, Wei J, Nguyen T-LM, Shi H, Su W, Palacios G, et al. Lipid signalling enforces functional specialization of treg cells in tumours. Nature. (2021) 591(7849):306–11. doi: 10.1038/s41586-021-03235-6
87. Watson MJ, Vignali PDA, Mullett SJ, Overacre-Delgoffe AE, Peralta RM, Grebinoski S, et al. Metabolic support of tumour-infiltrating regulatory T cells by lactic acid. Nature. (2021) 591(7851):645–51. doi: 10.1038/s41586-020-03045-2
88. Martini M, De Santis MC, Braccini L, Gulluni F, Hirsch E. PI3K/AKT signaling pathway and cancer: An updated review. Ann Med (2014) 46(6):372–83. doi: 10.3109/07853890.2014.912836
89. Sathe A, Nawroth R. Targeting the PI3K/AKT/mTOR pathway in bladder cancer. In: Urothelial carcinoma: Methods and protocols. New York, NY: Springer New York; (2018). p. 335–50.
90. Meric-Bernstam F, Gonzalez-Angulo AM. Targeting the mTOR signaling network for cancer therapy. Journal of Clinical Oncology (2009) 27(13):2278–87. doi: 10.1200/JCO.2008.20.0766
91. Waickman AT, Powell JD. mTOR, metabolism, and the regulation of T-cell differentiation and function. Immunological Reviews (2012) 249(1):43–58. doi: 10.1111/j.1600-065X.2012.01152.x
92. Angelin A, Gil-de-Gómez L, Dahiya S, Jiao J, Guo L, Levine MH, et al. Foxp3 reprograms T cell metabolism to function in low-glucose, high-lactate environments. Cell Metab (2017) 25(6):1282–93.e7. doi: 10.1016/j.cmet.2016.12.018
93. Dang Eric V, Barbi J, Yang H-Y, Jinasena D, Yu H, Zheng Y, et al. Control of TH17/Treg balance by hypoxia-inducible factor 1. Cell (2011) 146(5):772–84. doi: 10.1016/j.cell.2011.07.033
94. Hsiao H-W, Hsu T-S, Liu W-H, Hsieh W-C, Chou T-F, Wu Y-J, et al. Deltex1 antagonizes HIF-1α and sustains the stability of regulatory T cells in vivo. Nat Commun (2015) 6(1):6353. doi: 10.1038/ncomms7353
95. Clambey ET, McNamee EN, Westrich JA, Glover LE, Campbell EL, Jedlicka P, et al. Hypoxia-inducible factor-1 alpha–dependent induction of FoxP3 drives regulatory T-cell abundance and function during inflammatory hypoxia of the mucosa. Proc Natl Acad Sci (2012) 109(41):E2784–E93. doi: 10.1073/pnas.1202366109
96. Fong GH, Takeda K. Role and regulation of prolyl hydroxylase domain proteins. Cell Death Differentiation. (2008) 15(4):635–41. doi: 10.1038/cdd.2008.10
97. Deng B, Zhu J-M, Wang Y, Liu T-T, Ding Y-B, Xiao W-M, et al. Intratumor hypoxia promotes immune tolerance by inducing regulatory T cells via TGF-β1 in gastric cancer. PloS One (2013) 8(5):e63777. doi: 10.1371/journal.pone.0063777
98. Kumagai S, Togashi Y, Sakai C, Kawazoe A, Kawazu M, Ueno T, et al. An oncogenic alteration creates a microenvironment that promotes tumor progression by conferring a metabolic advantage to regulatory T cells. Immunity (2020) 53(1):187–203.e8. doi: 10.1016/j.immuni.2020.06.016
99. Pacella I, Procaccini C, Focaccetti C, Miacci S, Timperi E, Faicchia D, et al. Fatty acid metabolism complements glycolysis in the selective regulatory T cell expansion during tumor growth. Proc Natl Acad Sci (2018) 115(28):E6546–E55. doi: 10.1073/pnas.1720113115
100. Manzo T, Prentice BM, Anderson KG, Raman A, Schalck A, Codreanu GS, et al. Accumulation of long-chain fatty acids in the tumor microenvironment drives dysfunction in intrapancreatic CD8+ T cells. Journal of Experimental Medicine (2020) 217(8):e20191920.
101. Su P, Wang Q, Bi E, Ma X, Liu L, Yang M, et al. Enhanced lipid accumulation and metabolism are required for the differentiation and activation of tumor-associated macrophages. Cancer Res (2020) 80(7):1438–50. doi: 10.1158/0008-5472.CAN-19-2994
102. Wang H, Franco F, Tsui Y-C, Xie X, Trefny MP, Zappasodi R, et al. CD36-mediated metabolic adaptation supports regulatory T cell survival and function in tumors. Nat Immunol (2020) 21(3):298–308. doi: 10.1038/s41590-019-0589-5
103. Liu C, Chikina M, Deshpande R, Menk AV, Wang T, Tabib T, et al. Treg cells promote the SREBP1-dependent metabolic fitness of tumor-promoting macrophages via repression of CD8+ T cell-derived interferon-γ. Immunity. (2019) 51(2):381–97.e6. doi: 10.1016/j.immuni.2019.06.017
104. Shimano H, Sato R. SREBP-regulated lipid metabolism: convergent physiology — divergent pathophysiology. Nat Rev Endocrinol (2017) 13(12):710–30. doi: 10.1038/nrendo.2017.91
105. Fatokun AA, Hunt NH, Ball HJ. Indoleamine 2,3-dioxygenase 2 (IDO2) and the kynurenine pathway: characteristics and potential roles in health and disease. Amino Acids (2013) 45(6):1319–29. doi: 10.1007/s00726-013-1602-1
106. Grohmann U, Orabona C, Fallarino F, Vacca C, Calcinaro F, Falorni A, et al. CTLA-4–ig regulates tryptophan catabolism in vivo. Nat Immunol (2002) 3(11):1097–101. doi: 10.1038/ni846
107. Tang K, Wu Y-H, Song Y, Yu B. Indoleamine 2,3-dioxygenase 1 (IDO1) inhibitors in clinical trials for cancer immunotherapy. J Hematol Oncol (2021) 14(1):68. doi: 10.1186/s13045-021-01080-8
108. Russo V, Protti MP. Tumor-derived factors affecting immune cells. Cytokine Growth Factor Rev (2017) 36:79–87. doi: 10.1016/j.cytogfr.2017.06.005
109. Xydia M, Rahbari R, Ruggiero E, Macaulay I, Tarabichi M, Lohmayer R, et al. Common clonal origin of conventional T cells and induced regulatory T cells in breast cancer patients. Nat Commun (2021) 12(1):1119–. doi: 10.1038/s41467-021-21297-y
110. Rubtsov YP, Rasmussen JP, Chi EY, Fontenot J, Castelli L, Ye X, et al. Regulatory T cell-derived interleukin-10 limits inflammation at environmental interfaces. Immunity (2008) 28(4):546–58. doi: 10.1016/j.immuni.2008.02.017
111. Paterson AM, Lovitch SB, Sage PT, Juneja VR, Lee Y, Trombley JD, et al. Deletion of CTLA-4 on regulatory T cells during adulthood leads to resistance to autoimmunity. J Exp Med (2015) 212(10):1603–21. doi: 10.1084/jem.20141030
112. David CJ, Massagué J. Contextual determinants of TGFβ action in development, immunity and cancer. Nat Rev Mol Cell Biol (2018) 19(7):419–35. doi: 10.1038/s41580-018-0007-0
113. Saraiva M, O’Garra A. The regulation of IL-10 production by immune cells. Nat Rev Immunol (2010) 10(3):170–81. doi: 10.1038/nri2711
114. Turner JA, Stephen-Victor E, Wang S, Rivas MN, Abdel-Gadir A, Harb H, et al. Regulatory T cell-derived TGF-β1 controls multiple checkpoints governing allergy and autoimmunity. Immunity (2020) 53(6):1202–14.e6. doi: 10.1016/j.immuni.2020.10.002
115. Gutcher I, Donkor Moses K, Ma Q, Rudensky Alexander Y, Flavell Richard A, Li Ming O. Autocrine transforming growth factor-β1 promotes in vivo Th17 cell differentiation. Immunity (2011) 34(3):396–408. doi: 10.1016/j.immuni.2011.03.005
116. Velegraki M, Salem M, Ansa-Addo EA, Wu BX, Li Z. Autocrine transforming growth factor β1 in regulatory T cell biology-gone but not missed. Immunity (2021) 54(3):395–6. doi: 10.1016/j.immuni.2021.02.007
117. Li C, Xu X, Wei S, Jiang P, Xue L, Wang J. Tumor-associated macrophages: potential therapeutic strategies and future prospects in cancer. J ImmunoTher Cancer. (2021) 9(1):e001341. doi: 10.1136/jitc-2020-001341
118. Rallis KS, Corrigan AE, Dadah H, George AM, Keshwara SM, Sideris M, et al. Cytokine-based cancer immunotherapy: Challenges and opportunities for IL-10. Anticancer Res (2021) 41(7):3247. doi: 10.21873/anticanres.15110
119. Zhang H, Li R, Cao Y, Gu Y, Lin C, Liu X, et al. Poor clinical outcomes and immunoevasive contexture in intratumoral IL-10-Producing macrophages enriched gastric cancer patients. Ann Surg (2022) 275(4):626–35. doi: 10.1097/SLA.0000000000004037
120. Qi L, Yu H, Zhang Y, Zhao D, Lv P, Zhong Y, et al. IL-10 secreted by M2 macrophage promoted tumorigenesis through interaction with JAK2 in glioma. Oncotarget (2016) 7(44). doi: 10.18632/oncotarget.12317
121. Chen L, Shi Y, Zhu X, Guo W, Zhang M, Che Y, et al. IL−10 secreted by cancer−associated macrophages regulates proliferation and invasion in gastric cancer cells via c−Met/STAT3 signaling. Oncol Rep (2019) 42(2):595–604. doi: 10.3892/or.2019.7206
122. Sawant DV, Yano H, Chikina M, Zhang Q, Liao M, Liu C, et al. Adaptive plasticity of IL-10+ and IL-35+ treg cells cooperatively promotes tumor T cell exhaustion. Nat Immunol (2019) 20(6):724–35. doi: 10.1038/s41590-019-0346-9
123. Guo Y, Xie Y-Q, Gao M, Zhao Y, Franco F, Wenes M, et al. Metabolic reprogramming of terminally exhausted CD8+ T cells by IL-10 enhances anti-tumor immunity. Nat Immunol (2021) 22(6):746–56. doi: 10.1038/s41590-021-00940-2
124. Qiao J, Liu Z, Dong C, Luan Y, Zhang A, Moore C, et al. Targeting tumors with IL-10 prevents dendritic cell-mediated CD8+ T cell apoptosis. Cancer Cell (2019) 35(6):901–15.e4. doi: 10.1016/j.ccell.2019.05.005
125. Emmerich J, Mumm JB, Chan IH, LaFace D, Truong H, McClanahan T, et al. IL-10 directly activates and expands tumor-resident CD8+ T cells without De novo infiltration from secondary lymphoid organs. Cancer Res (2012) 72(14):3570–81. doi: 10.1158/0008-5472.CAN-12-0721
126. Mumm John B, Emmerich J, Zhang X, Chan I, Wu L, Mauze S, et al. IL-10 elicits IFNγ-dependent tumor immune surveillance. Cancer Cell (2011) 20(6):781–96. doi: 10.1016/j.ccr.2011.11.003
127. Naing A, Papadopoulos KP, Autio KA, Ott PA, Patel MR, Wong DJ, et al. Safety, antitumor activity, and immune activation of pegylated recombinant human interleukin-10 (AM0010) in patients with advanced solid tumors. J Clin Oncol (2016) 34(29):3562–9. doi: 10.1200/JCO.2016.68.1106
128. Naing A, Wong DJ, Infante JR, Korn WM, Aljumaily R, Papadopoulos KP, et al. Pegilodecakin combined with pembrolizumab or nivolumab for patients with advanced solid tumours (IVY): A multicentre, multicohort, open-label, phase 1b trial. Lancet Oncol (2019) 20(11):1544–55. doi: 10.1016/S1470-2045(19)30514-5
129. Nicholl MB, Ledgewood CL, Chen X, Bai Q, Qin C, Cook KM, et al. IL-35 promotes pancreas cancer growth through enhancement of proliferation and inhibition of apoptosis: Evidence for a role as an autocrine growth factor. Cytokine (2014) 70(2):126–33. doi: 10.1016/j.cyto.2014.06.020
130. Tao Q, Pan Y, Wang Y, Wang H, Xiong S, Li Q, et al. Regulatory T cells-derived IL-35 promotes the growth of adult acute myeloid leukemia blasts. Int J Cancer (2015) 137(10):2384–93. doi: 10.1002/ijc.29563
131. Collison LW, Chaturvedi V, Henderson AL, Giacomin PR, Guy C, Bankoti J, et al. IL-35-mediated induction of a potent regulatory T cell population. Nat Immunol (2010) 11(12):1093–101. doi: 10.1038/ni.1952
132. Turnis ME, Sawant DV, Szymczak-Workman AL, Andrews LP, Delgoffe GM, Yano H, et al. Interleukin-35 limits anti-tumor immunity. Immunity (2016) 44(2):316–29. doi: 10.1016/j.immuni.2016.01.013
133. Sullivan JA, Tomita Y, Jankowska-Gan E, Lema DA, Arvedson MP, Nair A, et al. Treg-Cell-Derived IL-35-Coated extracellular vesicles promote infectious tolerance. Cell Rep (2020) 30(4):1039–51.e5. doi: 10.1016/j.celrep.2019.12.081
134. Lee C-C, Lin J-C, Hwang W-L, Kuo Y-J, Chen H-K, Tai S-K, et al. Macrophage-secreted interleukin-35 regulates cancer cell plasticity to facilitate metastatic colonization. Nat Commun (2018) 9(1):3763. doi: 10.1038/s41467-018-06268-0
135. Huang C, Li N, Li Z, Chang A, Chen Y, Zhao T, et al. Tumour-derived interleukin 35 promotes pancreatic ductal adenocarcinoma cell extravasation and metastasis by inducing ICAM1 expression. Nat Commun (2017) 8(1):14035. doi: 10.1038/ncomms14035
136. Sauer KA, Maxeiner JH, Karwot R, Scholtes P, Lehr HA, Birkenbach M, et al. Immunosurveillance of lung melanoma metastasis in EBI-3-Deficient mice mediated by CD8+ T cells. J Immunol (2008) 181(9):6148. doi: 10.4049/jimmunol.181.9.6148
137. Chen W, Wahl SM. TGF-β: the missing link in CD4+CD25+ regulatory T cell-mediated immunosuppression. Cytokine Growth Factor Rev (2003) 14(2):85–9. doi: 10.1016/S1359-6101(03)00003-0
138. Bayati F. The therapeutic potential of regulatory T cells: Challenges and opportunities. Front Immunol (2021) 11. doi: 10.3389/fimmu.2020.585819
139. Pickup M, Novitskiy S, Moses HL. The roles of TGFβ in the tumour microenvironment. Nat Rev Cancer. (2013) 13(11):788–99. doi: 10.1038/nrc3603
140. Ni Y, Soliman A, Joehlin-Price A, Rose PG, Vlad A, Edwards RP, et al. High TGF-β signature predicts immunotherapy resistance in gynecologic cancer patients treated with immune checkpoint inhibition. NPJ Precis Oncol (2021) 5(1):101–. doi: 10.1038/s41698-021-00242-8
141. Bai X, Yi M, Jiao Y, Chu Q, Wu K. Blocking TGF-β signaling to enhance the efficacy of immune checkpoint inhibitor. OncoTar Ther (2019) 12:9527–38. doi: 10.2147/OTT.S224013
142. Liu Z, Qi T, Li X, Yao Y, Othmane B, Chen J, et al. A novel TGF-β risk score predicts the clinical outcomes and tumour microenvironment phenotypes in bladder cancer. Front Immunol (2021) 12. doi: 10.3389/fimmu.2021.791924
143. Munger JS, Sheppard D. Cross talk among TGF-β signaling pathways, integrins, and the extracellular matrix. Cold Spring Harbor Perspect Biol (2011) 3(11):a005017–a. doi: 10.1101/cshperspect.a005017
144. Edwards JP, Thornton AM, Shevach EM. Release of active TGF-β1 from the latent TGF-β1/GARP complex on T regulatory cells is mediated by integrin β8. J Immunol (2014) 193(6):2843. doi: 10.4049/jimmunol.1401102
145. Metelli A, Wu BX, Riesenberg B, Guglietta S, Huck JD, Mills C, et al. Thrombin contributes to cancer immune evasion via proteolysis of platelet-bound GARP to activate LTGF-β. Sci Transl Med (2020) 12(525):eaay4860. doi: 10.1126/scitranslmed.aay4860
146. Bouchard A, Collin B, Garrido C, Bellaye P-S, Kohli E. GARP: A key target to evaluate tumor immunosuppressive microenvironment. Biology (2021) 10(9):836. doi: 10.3390/biology10090836
147. Salem M, Wallace C, Velegraki M, Li A, Ansa-Addo E, Metelli A, et al. GARP dampens cancer immunity by sustaining function and accumulation of regulatory T cells in the colon. Cancer Res (2019) 79(6):1178–90. doi: 10.1158/0008-5472.CAN-18-2623
148. Metelli A, Salem M, Wallace CH, Wu BX, Li A, Li X, et al. Immunoregulatory functions and the therapeutic implications of GARP-TGF-β in inflammation and cancer. J Hematol Oncol (2018) 11(1):24. doi: 10.1186/s13045-018-0570-z
149. Satoh K, Kobayashi Y, Fujimaki K, Hayashi S, Ishida S, Sugiyama D, et al. Novel anti-GARP antibody DS-1055a augments anti-tumor immunity by depleting highly suppressive GARP+ regulatory T cells. Int Immunol (2021) 33(8):435–46. doi: 10.1093/intimm/dxab027
150. Hahn SA, Neuhoff A, Landsberg J, Schupp J, Eberts D, Leukel P, et al. A key role of GARP in the immune suppressive tumor microenvironment. Oncotarget (2016) 7(28). doi: 10.18632/oncotarget.9598
151. Szepetowski P, Ollendorff V, Grosgeorge J, Courseaux A, Birnbaum D, Theillet C, et al. DNA Amplification at 11q13.5-q14 in human breast cancer. Oncogene (1993) 7:2513–7.
152. Carrillo-Gálvez AB, Quintero JE, Rodríguez R, Menéndez ST, Victoria González M, Blanco-Lorenzo V, et al. GARP promotes the proliferation and therapeutic resistance of bone sarcoma cancer cells through the activation of TGF-β. Cell Death & Disease (2020) 11(11):985. doi: 10.1038/s41419-020-03197-z
153. Di Virgilio F, Adinolfi E. Extracellular purines, purinergic receptors and tumor growth. Oncogene (2017) 36(3):293–303. doi: 10.1038/onc.2016.206
154. Gallerano D, Ciminati S, Grimaldi A, Piconese S, Cammarata I, Focaccetti C, et al. Genetically driven CD39 expression shapes human tumor-infiltrating CD8+ T-cell functions. Int J Cancer (2020) 147(9):2597–610. doi: 10.1002/ijc.33131
155. Schneider E, Winzer R, Rissiek A, Ricklefs I, Meyer-Schwesinger C, Ricklefs FL, et al. CD73-mediated adenosine production by CD8 T cell-derived extracellular vesicles constitutes an intrinsic mechanism of immune suppression. Nat Commun (2021) 12(1):5911. doi: 10.1038/s41467-021-26134-w
156. Timperi E, Barnaba V. CD39 regulation and functions in T cells. Int J Mol Sci (2021) 22(15). doi: 10.3390/ijms22158068
157. Ohta A, Gorelik E, Prasad SJ, Ronchese F, Lukashev D, Wong MKK, et al. A2A adenosine receptor protects tumors from antitumor T cells. Proc Natl Acad Sci (2006) 103(35):13132–7. doi: 10.1073/pnas.0605251103
158. Antonioli L, Fornai M, Pellegrini C, D’Antongiovanni V, Turiello R, Morello S, et al. Adenosine signaling in the tumor microenvironment. Tumor Microenvironment: Signaling Pathways – Part B Cham: Springer Int Publishing (2021) p:145–67. doi: 10.1007/978-3-030-47189-7_9
159. Hosseini A, Gharibi T, Marofi F, Babaloo Z, Baradaran B. CTLA-4: From mechanism to autoimmune therapy. Int Immunopharmacol (2020) 80:106221. doi: 10.1016/j.intimp.2020.106221
160. Zappasodi R, Sirard C, Li Y, Budhu S, Abu-Akeel M, Liu C, et al. Rational design of anti-GITR-based combination immunotherapy. Nat Med (2019) 25(5):759–66. doi: 10.1038/s41591-019-0420-8
161. Walker LSK. Treg and CTLA-4: Two intertwining pathways to immune tolerance. J Autoimmunity (2013) 45:49–57. doi: 10.1016/j.jaut.2013.06.006
162. Walker LSK, Sansom DM. Confusing signals: Recent progress in CTLA-4 biology. Trends Immunol (2015) 36(2):63–70. doi: 10.1016/j.it.2014.12.001
163. Marangoni F, Zhakyp A, Corsini M, Geels SN, Carrizosa E, Thelen M, et al. Expansion of tumor-associated treg cells upon disruption of a CTLA-4-dependent feedback loop. Cell (2021) 184(15):3998–4015.e19. doi: 10.1016/j.cell.2021.05.027
164. Brunner-Weinzierl MC, Rudd CE. CTLA-4 and PD-1 control of T-cell motility and migration: Implications for tumor immunotherapy. Front Immunol (2018) 9. doi: 10.3389/fimmu.2018.02737
165. Zappasodi R, Serganova I, Cohen IJ, Maeda M, Shindo M, Senbabaoglu Y, et al. CTLA-4 blockade drives loss of treg stability in glycolysis-low tumours. Nature (2021) 591(7851):652–8. doi: 10.1038/s41586-021-03326-4
166. Gu P, Fang Gao J, D’Souza CA, Kowalczyk A, Chou K-Y, Zhang L. Trogocytosis of CD80 and CD86 by induced regulatory T cells. Cell Mol Immunol (2012) 9(2):136–46. doi: 10.1038/cmi.2011.62
167. Tekguc M, Wing James B, Osaki M, Long J, Sakaguchi S. Treg-expressed CTLA-4 depletes CD80/CD86 by trogocytosis, releasing free PD-L1 on antigen-presenting cells. Proc Natl Acad Sci (2021) 118(30):e2023739118. doi: 10.1073/pnas.2023739118
168. Waterhouse P, Penninger Josef M, Timms E, Wakeham A, Shahinian A, Lee Kelvin P, et al. Lymphoproliferative disorders with early lethality in mice deficient in ctla-4. Science. (1995) 270(5238):985–8. doi: 10.1126/science.270.5238.985
169. Khattri R, Auger JA, Griffin MD, Sharpe AH, Bluestone JA. Lymphoproliferative disorder in CTLA-4 knockout mice is characterized by CD28-regulated activation of Th2 responses. J Immunol (1999) 162(10):5784.
170. Zou W, Wolchok Jedd D, Chen L. PD-L1 (B7-H1) and PD-1 pathway blockade for cancer therapy: Mechanisms, response biomarkers, and combinations. Sci Trans Med (2016) 8(328):328rv4–rv4. doi: 10.1126/scitranslmed.aad7118
171. Kumagai S, Togashi Y, Kamada T, Sugiyama E, Nishinakamura H, Takeuchi Y, et al. The PD-1 expression balance between effector and regulatory T cells predicts the clinical efficacy of PD-1 blockade therapies. Nat Immunol (2020) 21(11):1346–58. doi: 10.1038/s41590-020-0769-3
172. Patsoukis N, Wang Q, Strauss L, Boussiotis VA. Revisiting the PD-1 pathway. Sci Adv (2020) 6(38):eabd2712. doi: 10.1126/sciadv.abd2712
173. Tan CL, Kuchroo JR, Sage PT, Liang D, Francisco LM, Buck J, et al. PD-1 restraint of regulatory T cell suppressive activity is critical for immune tolerance. J Exp Med (2020) 218(1):e20182232. doi: 10.1084/jem.20182232
174. Lowther DE, Goods BA, Lucca LE, Lerner BA, Raddassi K, van Dijk D, et al. PD-1 marks dysfunctional regulatory T cells in malignant gliomas. JCI Insight (2019) 1(5):e85935. doi: 10.1172/jci.insight.85935
175. Kamada T, Togashi Y, Tay C, Ha D, Sasaki A, Nakamura Y, et al. PD-1+ regulatory T cells amplified by PD-1 blockade promote hyperprogression of cancer. Proc Natl Acad Sci (2019) 116(20):9999–10008. doi: 10.1073/pnas.1822001116
176. Aksoylar H-I, Boussiotis VA. PD-1+ treg cells: A foe in cancer immunotherapy? Nat Immunol (2020) 21(11):1311–2. doi: 10.1038/s41590-020-0801-7
177. Khalil DN, Smith EL, Brentjens RJ, Wolchok JD. The future of cancer treatment: immunomodulation, CARs and combination immunotherapy. Nat Rev Clin Oncol (2016) 13(5):273–90. doi: 10.1038/nrclinonc.2016.25
178. Johnson DB, Nebhan CA, Moslehi JJ, Balko JM. Immune-checkpoint inhibitors: long-term implications of toxicity. Nat Rev Clin Oncol (2022) 19(4):254–67. doi: 10.1038/s41571-022-00600-w
179. Woo S-R, Turnis ME, Goldberg MV, Bankoti J, Selby M, Nirschl CJ, et al. Immune inhibitory molecules LAG-3 and PD-1 synergistically regulate T-cell function to promote tumoral immune escape. Cancer Res (2012) 72(4):917–27. doi: 10.1158/0008-5472.CAN-11-1620
180. Robert C. LAG-3 and PD-1 blockade raises the bar for melanoma. Nat Cancer. (2021) 2(12):1251–3. doi: 10.1038/s43018-021-00276-8
181. Ruffo E, Wu RC, Bruno TC, Workman CJ, Vignali DAA. Lymphocyte-activation gene 3 (LAG3): The next immune checkpoint receptor. Seminars in Immunology (2019) 42:101305. doi: 10.1016/j.smim.2019.101305
182. Dong Y, Li X, Zhang L, Zhu Q, Chen C, Bao J, et al. CD4+ T cell exhaustion revealed by high PD-1 and LAG-3 expression and the loss of helper T cell function in chronic hepatitis b. BMC Immunol (2019) 20(1):27. doi: 10.1186/s12865-019-0309-9
183. Niu B, Zhou F, Su Y, Wang L, Xu Y, Yi Z, et al. Different expression characteristics of LAG3 and PD-1 in sepsis and their synergistic effect on T cell exhaustion: A new strategy for immune checkpoint blockade. Front Immunol (2019) 10. doi: 10.3389/fimmu.2019.01888
184. Andrews LP, Somasundaram A, Moskovitz JM, Szymczak-Workman AL, Liu C, Cillo AR, et al. Resistance to PD1 blockade in the absence of metalloprotease-mediated LAG3 shedding. Sci Immunol (2020) 5(49):eabc2728. doi: 10.1126/sciimmunol.abc2728
185. Zhang Q, Chikina M, Szymczak-Workman AL, Horne W, Kolls JK, Vignali KM, et al. LAG3 limits regulatory T cell proliferation and function in autoimmune diabetes. Science Immunology (2017) 2(9):eaah4569.
186. Js Do, Visperas A, Sanogo YO, Bechtel JJ, Dvorina N, Kim S, et al. An IL-27/Lag3 axis enhances Foxp3+ regulatory T cell–suppressive function and therapeutic efficacy. Mucosal Immunol (2016) 9(1):137–45. doi: 10.1038/mi.2015.45
187. Kim D, Le HT, Nguyen QT, Kim S, Lee J, Min B. Cutting edge: IL-27 attenuates autoimmune neuroinflammation via regulatory T Cell/Lag3–dependent but IL-10–independent mechanisms in vivo. J Immunol (2019) 202(6):1680. doi: 10.4049/jimmunol.1800898
188. Coe D, Begom S, Addey C, White M, Dyson J, Chai J-G. Depletion of regulatory T cells by anti-GITR mAb as a novel mechanism for cancer immunotherapy. Cancer Immunol Immunother (2010) 59(9):1367–77. doi: 10.1007/s00262-010-0866-5
189. Schaer DA, Murphy JT, Wolchok JD. Modulation of GITR for cancer immunotherapy. Curr Opin Immunol (2012) 24(2):217–24. doi: 10.1016/j.coi.2011.12.011
190. Kanamaru F, Youngnak P, Hashiguchi M, Nishioka T, Takahashi T, Sakaguchi S, et al. Costimulation via glucocorticoid-induced TNF receptor in both conventional and CD25+ regulatory CD4+ T cells. J Immunol (2004) 172(12):7306. doi: 10.4049/jimmunol.172.12.7306
191. Ephrem A, Epstein AL, Stephens GL, Thornton AM, Glass D, Shevach EM. Modulation of treg cells/T effector function by GITR signaling is context–dependent. Eur J Immunol (2013) 43(9):2421–9. doi: 10.1002/eji.201343451
192. Amoozgar Z, Kloepper J, Ren J, Tay RE, Kazer SW, Kiner E, et al. Targeting treg cells with GITR activation alleviates resistance to immunotherapy in murine glioblastomas. Nat Commun (2021) 12(1):2582. doi: 10.1038/s41467-021-22885-8
193. Li C, Jiang P, Wei S, Xu X, Wang J. Regulatory T cells in tumor microenvironment: New mechanisms, potential therapeutic strategies and future prospects. Mol Cancer (2020) 19(1):116. doi: 10.1158/1557-3125.HIPPO19-B11
194. Durham NM, Holoweckyj N, MacGill RS, McGlinchey K, Leow CC, Robbins SH. GITR ligand fusion protein agonist enhances the tumor antigen–specific CD8 T-cell response and leads to long-lasting memory. J ImmunoTher Cancer (2017) 5(1):47. doi: 10.1186/s40425-017-0247-0
195. Sakaguchi S, Sakaguchi N, Asano M, Itoh M, Toda M. Immunologic self-tolerance maintained by activated T cells expressing IL-2 receptor alpha-chains (CD25). breakdown of a single mechanism of self-tolerance causes various autoimmune diseases. J Immunol (1995) 155(3):1151.
196. Arce Vargas F, Furness AJS, Solomon I, Joshi K, Mekkaoui L, Lesko MH, et al. Fc-optimized anti-CD25 depletes tumor-infiltrating regulatory T cells and synergizes with PD-1 blockade to eradicate established tumors. Immunity (2017) 46(4):577–86. doi: 10.1016/j.immuni.2017.03.013
197. Solomon I, Amann M, Goubier A, Arce Vargas F, Zervas D, Qing C, et al. CD25-treg-depleting antibodies preserving IL-2 signaling on effector T cells enhance effector activation and antitumor immunity. Nat Cancer (2020) 1(12):1153–66. doi: 10.1038/s43018-020-00133-0
198. Josefowicz SZ, Rudensky A. Control of regulatory T cell lineage commitment and maintenance. Immunity (2009) 30(5):616–25. doi: 10.1016/j.immuni.2009.04.009
199. Amann M, Schnetzler G, Theresa K, Solomon I, Boetsch C, Marrer-Berger E, et al. Abstract 4553: The CD25 antibody RG6292 selectively depletes tregs while preserving IL-2 signaling and CTL activity for tumor control. Cancer Res (2020) 80(16_Supplement):4553. doi: 10.1158/1538-7445.AM2020-4553
Keywords: tumor, T cell exhaustion, immunotherapy, regulatory T cell, Treg, mechanism
Citation: McRitchie BR and Akkaya B (2022) Exhaust the exhausters: Targeting regulatory T cells in the tumor microenvironment. Front. Immunol. 13:940052. doi: 10.3389/fimmu.2022.940052
Received: 09 May 2022; Accepted: 14 September 2022;
Published: 30 September 2022.
Edited by:
Hazem E. Ghoneim, College of Medicine, The Ohio State University, United StatesReviewed by:
Khalil Hajiasgharzadeh, Tabriz University of Medical Sciences, IranFarhan Sachal Cyprian, Qatar University, Qatar
Copyright © 2022 McRitchie and Akkaya. This is an open-access article distributed under the terms of the Creative Commons Attribution License (CC BY). The use, distribution or reproduction in other forums is permitted, provided the original author(s) and the copyright owner(s) are credited and that the original publication in this journal is cited, in accordance with accepted academic practice. No use, distribution or reproduction is permitted which does not comply with these terms.
*Correspondence: Billur Akkaya, billur.akkaya@osumc.edu