- Department of Neurology, Medical Faculty, Heinrich-Heine University, Düsseldorf, Germany
Posttranslational modifications (PTMs) allow to control molecular and cellular functions in response to specific signals and changes in the microenvironment of cells. They regulate structure, localization, stability, and function of proteins in a spatial and temporal manner. Among them, specific thiol modifications of cysteine (Cys) residues facilitate rapid signal transduction. In fact, Cys is unique because it contains the highly reactive thiol group that can undergo different reversible and irreversible modifications. Upon inflammation and changes in the cellular microenvironment, many extracellular soluble and membrane proteins undergo thiol modifications, particularly dithiol–disulfide exchange, S-glutathionylation, and S-nitrosylation. Among others, these thiol switches are essential for inflammatory signaling, regulation of gene expression, cytokine release, immunoglobulin function and isoform variation, and antigen presentation. Interestingly, also the redox state of bacterial and viral proteins depends on host cell-mediated redox reactions that are critical for invasion and infection. Here, we highlight mechanistic thiol switches in inflammatory pathways and infections including cholera, diphtheria, hepatitis, human immunodeficiency virus (HIV), influenza, and coronavirus disease 2019 (COVID-19).
Introduction
Following transcription and translation, synthesized proteins can undergo posttranslational modifications (PTMs). These constitute regulatory mechanisms to control molecular and cellular functions in response to signals and changes in the microenvironment. Astonishingly, 300–400 different modifications have been described, including catalytic cleavage, phosphorylation, glucosylation, ubiquitinylation, methylation, and oxidation (1, 2). The spatiotemporal and the interconnectivity analysis of different PTMs is demanding, even though biochemical methods based, e.g., on specific antibodies, large-scale experimental tools such as mass spectrometry (3–5), and computational methods including the analysis of putative modifications are available (1, 2). Particularly, the analysis of PTMs in the extracellular space is challenging. However, it is clear that PTMs, e.g., i) of the extracellular matrix (ECM) play an essential role in signal transduction (6) and ii) are involved in extracellular vesicle (EV) biogenesis, cargo sorting, and vesicular uptake (7, 8).
Posttranslational Thiol Modifications
The highly reactive thiol group of cysteines (Cys) is special because it can undergo many different reversible and irreversible modifications that control protein activity, interaction, and/or distribution (9). Single Cys modifications include S-glutathionylation [addition of glutathione (GSH)] and S-nitrosylation [incorporation of nitric oxide (NO)]. Moreover, two Cys residues can be oxidized and form a disulfide bond that has additionally been linked to protein structure and conformation. As such, they depend on the presence of enzymatically produced second messengers like hydrogen peroxide (H2O2) and NO and the catalysis by Thioredoxin (Trx) family proteins such as Trx, Glutaredoxins (Grx), Peroxiredoxins (Prx), and Protein disulfide isomerases (PDIs) (10). All these modifications are important not only for intracellular but also for extracellular signal transduction (i.e., oxidative eustress), also for regulating membrane, soluble, and vesicular proteins (11, 12).
Thiol Modification of Extracellular Proteins in Inflammatory Signaling
Thiol modifications are essential in the innate and adaptive immune response, controlling various pathways and functions, by distinct catalytical mechanisms in different cellular and extracellular compartments and specialized cell types (13). Here, we focus on extracellular dithiol–disulfide exchange, S-glutathionylation, and S-nitrosylation of key proteins in inflammation and infection, as well as the redox proteins involved in the regulation of these PTMs (Table 1).
Dithiol–Disulfide Exchange
Disulfide formation occurs between two thiols of one (intramolecular) or two (intermolecular) proteins. Conformational disulfides are important for the structure and function of specific proteins and their regulation. Several intracellular thiol switches were identified that involve the regulated formation or reduction of disulfide bonds, e.g., Collapsin Response Mediator Protein 2 (Cys504-Cys504) (40, 41), Glyceraldehyde 3-phosphate dehydrogenase (GAPDH) (42), Heat shock protein (HSP) 33 (Cys232–Cys234) (43, 44), H2O2-inducible gene activator (Cys199-Cys208) (45, 46), and yes-associated protein 1 (Cys303-Cys598) (47). Interestingly, the extracellular space was considered to be rather oxidizing and disulfides inert, not engaging in redox regulation. However, it is an exciting time when this view is challenged. Redox regulation of proteins not only occurs extracellularly but also is in fact an important part of various signaling cascades. Even though the function of many proteins was shown to be sensitive toward oxidation by H2O2, not many extracellular thiol switches have been identified and thoroughly characterized so far. Known examples were described in recent review articles (12, 48). A well-characterized soluble protein is High-mobility group box 1 (HMGB1). In its disulfide form (Cys23-Cys45), HMGB1 binds to Toll-like receptors (TLRs) and the receptor for advanced glycation end products (RAGE), inducing the signal cascade that leads to Nuclear factor kappa B (NFκB) activation and cytokine expression and release (49, 50). The interaction of HMGB1 with TLRs also depends on the redox state of the third Cys residue (51). Dithiol–disulfide exchange in membrane proteins such as A disintegrin and metalloprotease 17 (ADAM17) (Cys600-Cys635; Cys630-Cys641) (52, 53), cluster of differentiation 4 (CD4) (intermolecular, Cys130-Cys159) (20), beta-1 defensin (15), various integrins (36, 54–56), tumor necrosis factor receptor superfamily member 8 (CD30) (57), and transient receptor potential canonical channels (58, 59) affects ligand binding, protein function, and cell signaling. Beta-1 defensin increases its antimicrobial activity when reduced. Inhibition and knockout of Trx decreased the rate of Beta-1 defensin degradation, directly affecting its antimicrobial function. CD4, CD30, and α7β1-integrin are also substrates for extracellular Trx (36, 60). ADAM17 is regulated by PDIs (52).
Conformational disulfides are particularly important in the adaptive immunity, as they are essential for the structure and function of antibodies and antigen presentation. PDIs have been associated with increased production of immunoglobulin heavy chains (61), including most of the IgG isoforms. In vitro, PDIs are able to replicate disulfide bonds in antibody formation as it occurs in vivo, and this is even more upon oxidizing conditions (62). PDIs are involved in the modification of immunoglobulins, including IgG (63) and IgM (64). The IgM pentameric structure is the first antibody produced by B-cells before undergoing class switching. PDIs regulate the biogenesis of this multimeric immunoglobulin by catalyzing the formation of disulfide bonds, structures that are later modified before secretion (64). IgG is vital for immunological memory. Several isoforms have been described with differences in their constant regions (63). Disulfide bonds vary across the chains in different IgG isoforms, changing the conformation and subsequently antibody stability and antigen affinity (33). Remarkably, all IgG isoforms can also present non-classical disulfide conformations, such as trisulfides (65). One of the IgG isoforms most studied for its non-classical conformation is IgG4. A change in amino acid in the hinge region makes it different from IgG1 and more prone to form intrachain bonds, rather than interchain bonds. This change in IgG4 has been hypothesized to be key in its role as a monovalent antibody (66) and has been associated with protective effects after prolonged immunizations (67). Interestingly, its production does not seem to be affected by PDI expression (68). Oxidation of immunoglobulins is a problem that has been studied particularly in vitro, especially concerning protein storage for medical treatments or experimental purposes. Environmental oxidizing conditions lead to changes in amino acid charges and specificity of the antibody for its antigen (69). Researchers have been actively working on solving this problem in the production and distribution of immunoglobulins by using Trx1 (70), Trx-like proteins (71), and a combination of GSH and Trx1 (72). Not many in vivo experiments have shown the impact of redox reactions on antibody function. However, it has been shown that the redox environment could affect the functionality of antibodies and potentially disease diagnosis (73). In vivo, modifications to the heavy-chain disulfide bonds of, e.g., IgG1 subclass proteins alter the binding site of the C1 complement protein (74). This interaction is key for the complement classical pathway activation. We can hypothesize that these changes are constantly occurring in vivo, but how this is affecting and modulating the immune response remains to be elucidated. Oxidation of the immunoglobulins might not be the only factor affecting the adaptive immune response. Peptides presented to T-cell receptors via major histocompatibility complex (MHC) class II can be modified if they contain Cys residues (75, 76). Changes in antigen recognition can change when these residues form disulfide structures with peptides in the vicinity (75), or are S-glutathionylated, which modulates the immune response against viruses and T cell-mediated tumor cell recognition (76). Future research is needed to determine if these changes are active mechanisms for the immune escape of infected or neoplastic cells.
S-Glutathionylation
S-glutathionylation is the formation of a disulfide bond between the thiol group of GSH and a protein (77), catalyzed by Grxs (78). Intracellular proteins that can be glutathionylated include actin (Cys374) (79), Fatty Acid Binding Protein 5 (80), Major vault protein (81), NFκB (82, 83), sarco/endoplasmic reticulum Ca2+-ATPase (Cys674) (84), Signal transducer and activator of transcription 1 (STAT1) (Cys324 and Cys492) (39, 85), STAT3 (Cys328 and Cys542) (85), and members of the Trx family (17, 86, 87). Glutathionylation is crucial for signaling pathways and cellular processes like proliferation, differentiation, apoptosis, cytokine production (76), and metabolic changes in response to inflammatory signals (88). Also, the release of glutathionylated proteins upon inflammation and infection has been described (17). The functional characterization of extracellular proteins that undergo glutathionylation, however, is considerably rare. Examples include several soluble proteins and receptors that function in signal transduction and inflammation such as Intercellular Adhesion Molecule 1 (89), α4β1 integrin (90), HMGB1 (29), interleukin 1 β (IL-1β) (37), and Paraoxonase-1 (91). Glutathionylation of IL-1β (Cys188) does not directly control the bioactivity under physiopathological conditions, but it protects IL-1β from H2O2-induced irreversible deactivation, which is regulated by Grx1 (37). Trx1 (Cys73) is also glutathionylated, which prevents dimerization or limited proteolysis. The latter gives rise to the truncated form Trx80. This form is released into the extracellular space and has cytokine-like activity (86, 92). Prx1 and Prx2 are glutathionylated (17, 87) and are known to act as danger signals by binding to TLR4 (93, 94) (Figure 1). Other ligands of TLR2 and 4 like HSPs 60 and 70 (Cys574 and Cys603) (95) can be glutathionylated in T-lymphocytes in response to inflammatory stimuli and H2O2 (96–98). Extracellular HMGB1 also binds to TLRs and RAGE, triggering the production of pro-inflammatory cytokines (Figure 1). Downstream signal transduction also involves glutathionylated mediators, such as IKKβ, which reduces nuclear translocation of ReIA (p65), inhibiting DNA binding. ReIA (p65) and the p50 subunit of NFκB also undergo glutathionylation, resulting in decreased ability to bind DNA (83) (Figure 1). Of note, the pro-inflammatory response triggered by IL-17a is also associated with glutathionylation of ReIA (p65) and IKKα (99).
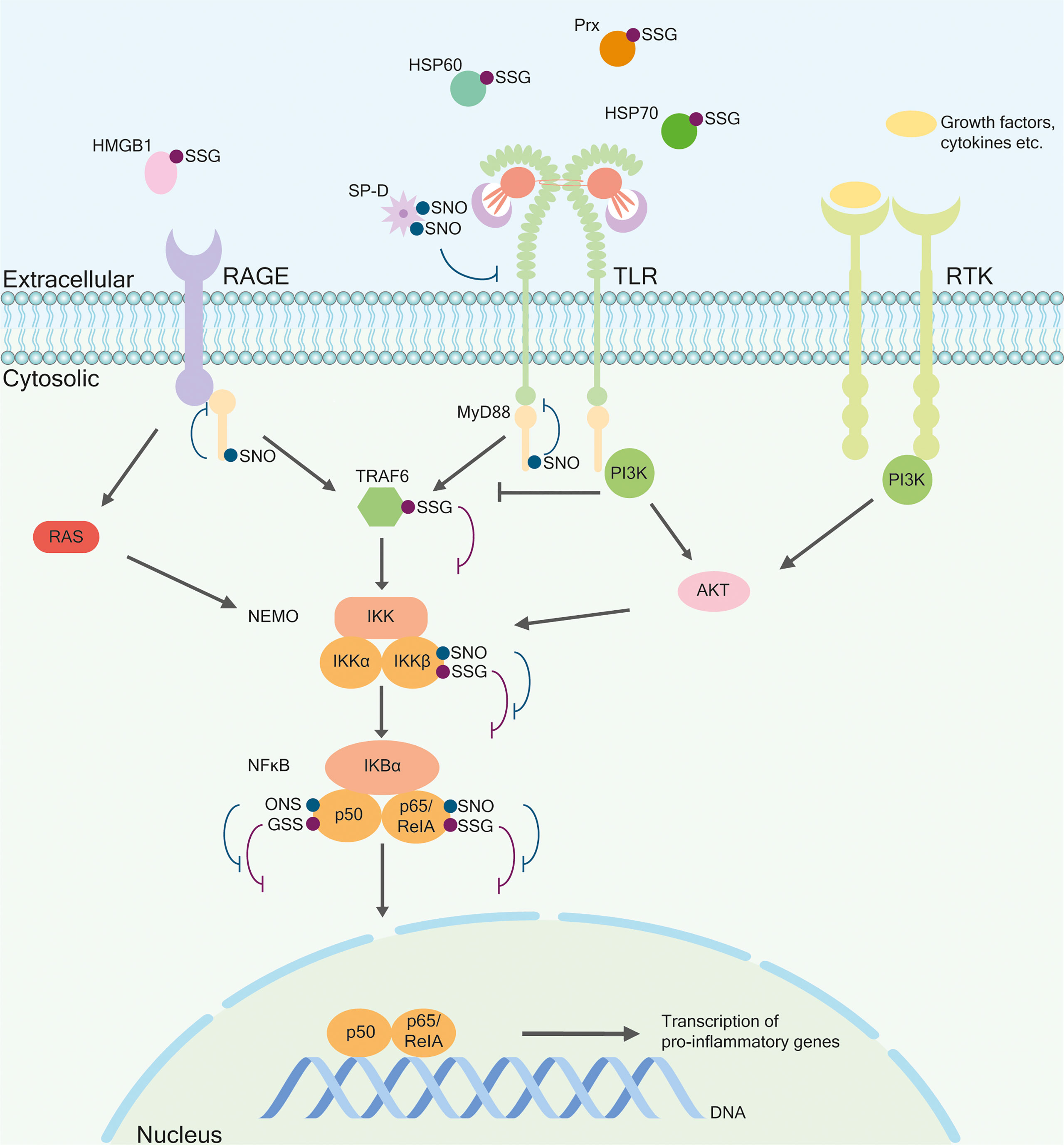
Figure 1 Selected thiol modifications in the regulation of the inflammatory response. Posttranslational modifications such as S-glutathionylation and S-nitrosylation occur on intracellular and extracellular proteins. Many ligands for membrane-bound receptors such as receptors for advanced glycation end products (RAGE), Toll-like receptor (TLR), and receptor tyrosine kinase (RTK) undergo redox regulation. Nitrosylated, oligomeric Surfactant protein D (SP-D) binds and inhibits TLR. Heat shock proteins (HSPs) 60 and 70, High-mobility group box 1 (HMGB1), and Peroxiredoxin (Prx) are glutathionylated. They bind to RAGE or TLR, inducing similar downstream-signaling components Tumor necrosis factor receptor–associated factor (TRAF), Nuclear Factor-kappa-B essential modulator (NEMO), Nuclear Factor-kappa-B (NFκB) and eventually a pro-inflammatory response. The RAGE receptor can also activate the NEMO complex via RAS. Also, the TLR pathway can be activated through the Phosphoinositide 3-kinases (PI3K) pathway. PI3K binds to the receptor, which inhibits TRAF6 and activates the serine/threonine-protein kinases (Akt) pathway by activating NEMO. Different components of this pathway can be glutathionylated or nitrosylated. Nitrosylated myeloid differentiation primary response 88 (MyD88) leads to the detaching of the receptor whereby the signal is inhibited. Nitrosylated or glutathionylated IKKβ, p50, and ReIA/p65 lead to their inactivation, eventually inhibiting gene expression.
S-Nitrosylation
S-nitrosylation is the covalent binding of NO to a thiol group and regulates >3,000 proteins (100), affecting protein structure, function, and the interconnectivity with other PTMs like phosphorylation, acetylation, and disulfide formation (101–104). Identified intracellular S-nitrosylated proteins include Cofilin-1 (Cys80, Cys139) (105, 106), GAPDH (Cys150, Cys247) (107–109), HSP60 (Cys237) (31, 105), HSP90 (Cys521) (110, 111), MyD88 (Cys113, Cys216) (112, 113), NFκB (114, 115), Nitric oxide synthase (NOS) (116, 117), and calcium- and zinc-binding proteins S100A8 (Cys42) (117) and S100A9 (108). Interestingly, Trx1 and PDIs can be nitrosylated and act as transnitrosylases (32, 118). Furthermore, Trx1 catalyzes the denitrosylation of substrate proteins like HSP60 and cofilin-1 (105). S-nitrosylation is involved in various physiological and pathophysiological processes, including apoptosis, DNA damage repair, inflammation, mitochondrial energy metabolism, proliferation, and regulation of transcription (119, 120). Extracellular proteins and receptors include Aquaporin-1 (AQP-1) (121), CD40 (122), Epidermal Growth Factor Receptor (123), insulin-like growth factor type 1 receptor (124), and Surfactant Protein D (SP-D) (Cys15, Cys20) (125–127). S-nitrosylation of SP-D leads to oligomerization of the protein (125) and TLR4 binding and inhibition (126, 127) (Figure 1). Additional nitrosylation of SP-D (Cys15/20) separates the oligomeric form into trimers, exposing the N-terminal domain and leading to chemotaxis of macrophages (125). S-nitrosylation of MyD88 within the TLR pathway disrupts its binding to upstream Toll/IL-1R adaptor protein (TIRAP) but not to downstream Interleukin-1 receptor-associated kinase 1 (IRAK-1). This could influence the delayed development of the acute immune response (112). The subunits p65 and p50 of NFκB can become nitrosylated, which has a similar inhibitory effect on DNA binding as glutathionylation. Nitrosylation of both subunits leads to inactivation of the complex (114, 115) (Figure 1). In addition, IKKβ is nitrosylated at Cys179, and this PTM is reversed upon Tumor necrosis factor α activation (128). Among membrane proteins, channels can be nitrosylated such as AQP-1 at Cys189, located within the functional pore, allowing potential negative feedback regulation by inhibiting the function of AQP-1 (reviewed in 121). Nitrosylation of CD40 prohibits binding and activation by CD40L. This modification occurs in the extracellular domain in resting macrophages and monocytes. Denitrosylation occurs after activation by CD40L, resulting in the activation of the NFκB pathway (122).
Thiol Switches in Bacterial and Viral Infections
PTMs of thiols, i.e., mainly regulatory disulfides, mediate viral entry into host cells. Specific thiol switches occur in major viral infections including hepatitis, human immunodeficiency virus (HIV), and influenza. Recent studies also imply the presence of a relevant thiol switch that facilitates the entry of SARS-CoV-2. Even though there is more evidence on viral infections, critical thiol modifications also occur in bacterial infections.
Bacterial Infections
The Gram-positive Corynebacterium diphtheriae is the cause of diphtheria that affects the respiratory tract and skin. Diphtheria toxin (DT) is released as a virulence factor and enters cells via receptor-mediated endocytosis. It contains an intermolecular disulfide bridge (Cys186, Cys201) and depends on host cell-mediated reduction (24), which occurs on the cell surface (25), and is catalyzed by PDIs (25). In vitro, Trx1 reduces DT at pH 5 and GSH or Cys at neutral pH (26). Following reduction, the N-terminal part containing the ADP-ribosyltransferase activity enters the cytoplasm—a process that among others depends on Trx reductase—and catalyzes the ADP-ribosylation of elongation factor (EF) 2 and thereby inhibits protein synthesis, which eventually induces apoptosis (24). A comparable mechanism was found for the Gram-negative Pseudomonas aeruginosa. Released Exotoxin A contains a disulfide (Cys265, Cys287) that is cleaved by PDIs and eventually leads to inhibition of protein synthesis. Interestingly, the reduction does not occur on the cell surface, but intracellularly (129, 130). For the Gram-negative Vibrio cholera, a disulfide bond (Cys-187, Cys-199) in cholera toxin was identified that is also a substrate for intracellular PDIs (131–133).
Besides activating bacterial toxins, some bacterial strains were shown to rely on disulfide reductases on the cell surface for invasion and infection. The Gram-negative Anaplasma phagocytophilum, the cause of anaplasmosis, expresses the adhesin Asp14 required for host invasion. Asp14 binds and brings PDI proximal to the bacterial surface. The substrate disulfide bonds on the bacterial surface have not been identified; however, a reduction is needed. Interestingly, extracellularly, membrane-associated Trx1 mediates bacterial entry (134). Similarly, the Gram-negative Ehrlichia chaffeensia, the cause of human monocytic ehrlichiosis, expresses an adhesin EplA that binds PDIs and mediates bacterial entry into host cells. Inhibition of Trx1 prohibits infection (135).
Viral Infections
The enveloped HIV is the cause of acquired immunodeficiency syndrome (AIDS). The viral envelope glycoprotein 120 (gp120) is rich in disulfide bonds and is essential for viral attachment to the host lymphocyte cell surface. Following binding to receptor CD4 and coreceptor CXCR4, conformational changes, i.e., the reduction of two intramolecular disulfides, allow fusion, viral entry, and infection. The reduction is catalyzed by PDIs (27, 28), Grx1 (136), and Trx1 (137). Interestingly, PDI is mainly involved in infection of T-lymphocytes and Trx1 in infection of macrophages. Trx1 is particularly elevated in chronic phases of HIV infection. The authors concluded that Trx1 may enable sustained viremia, when T-lymphocytes are declining (138). Another mechanistic thiol switch was identified for the enveloped hepatitis C virus that causes hepatitis C. The envelope glycoproteins E1 and E2 contain several conserved Cys residues. Cellular attachment is enabled by free thiol groups in E1 and E2 and not by disulfide formation (139). Mutagenesis screenings of E1 (140) and E2 (141) revealed the contributions of Cys residues on structure and function. All 18 Cys residues of E2 are needed for viral entry, even though the contribution to structure and function is different (141). Thiol switches in other viral strains have been identified and analyzed (142), including the Hepatitis A Virus-2B (HAV-2B) peptide (142), vaccinia virus proteins (143), and adenoviral capsid protein VI (144). PDIs play an important role in viral infection via diverse mechanisms. PDIs were shown to be involved in viral entry of dengue virus by interaction with nonstructural protein 1 (145) and regulating integrin activity (146). PDIs bind capsid spike proteins of human astroviruses, the cause of gastroenteritis, thereby inhibiting viral entry, more precisely uncoating of the viral genome (147). PDIs regulate the redox state of hemagglutinin and neuraminidase and thereby virus attachment and influenza infection (148, 149). Also, in coronavirus disease 2019 (COVID-19), the spike glycoprotein of severe acute respiratory syndrome coronavirus type 2 (SARS-CoV-2) that binds to its receptor angiotensin-converting enzyme 2 contains two disulfide bonds (Cys379-Cys432 and Cys391-Cys525). To our knowledge, an interaction with PDI or Trx1 has so far not been determined; however, reducing agents showed antiviral properties, inhibiting viral entry (150).
Conclusions
● The impact of extracellular thiol switches has long been underestimated.
● Extracellular thiol modifications of key proteins and receptors mediate i) signal transduction; ii) bacterial toxicity, adhesion, and invasion; and iii) viral invasion and infection.
● Extracellular thiol modifications regulate the adaptive immune response by modulating immunoglobulin antigen affinity and T-cell receptor epitope recognition. Whether this is an active mechanism of immune tampering or immune escape or even explains individual responses to pathogens remains to be elucidated.
● Members of the Trx family are involved in the modulation of thiol PTMs.
● Thiol modifications are novel targets for diagnosis and/or prognosis of inflammatory and infectious diseases and constitute potential therapeutic targets.
Author Contributions
Conceptualization: KAB and E-MH. Writing, review, and editing: KAB, PLP, E-MH. All authors contributed to the article and approved the submitted version.
Funding
This study was supported by the German Research Council (DFG: SPP1710) to E-MH (HA 8334/2-2). We acknowledge support by the Heinrich Heine University Düsseldorf.
Conflict of Interest
The authors declare that the research was conducted in the absence of any commercial or financial relationships that could be construed as a potential conflict of interest.
Publisher’s Note
All claims expressed in this article are solely those of the authors and do not necessarily represent those of their affiliated organizations, or those of the publisher, the editors and the reviewers. Any product that may be evaluated in this article, or claim that may be made by its manufacturer, is not guaranteed or endorsed by the publisher.
References
1. Khoury GA, Baliban RC, Floudas CA. Proteome-Wide Post-Translational Modification Statistics: Frequency Analysis and Curation of the Swiss-Prot Database. Sci Rep (2011) 1:1–5. doi: 10.1038/srep00090
2. Ramazi S, Zahiri J. Post-Translational Modifications in Proteins: Resources, Tools and Prediction Methods. Database (2021) 2021(7):1–20. doi: 10.1093/database/baab012
3. Larsen MR, Trelle MB, Thingholm TE, Jensen ON. Analysis of Posttranslational Modifications of Proteins by Tandem Mass Spectrometry. Biotechniques (2006) 40(6):790–8. doi: 10.2144/000112201
4. Yakubu RR, Nieves E, Weiss LM. The Methods Employed in Mass Spectrometric Analysis of Posttranslational Modifications (PTMs) and Protein-Protein Interactions (PPIs). Adv Exp Med Biol (2019) 1140(5):169–98. doi: 10.1007/978-3-030-15950-4_10
5. Leutert M, Entwisle SW, Villén J. Decoding Post-Translational Modification Crosstalk With Proteomics. Mol Cell Proteomics (2021) 20:0–11. doi: 10.1016/j.mcpro.2021.100129
6. Leeming DJ, Bay-Jensen AC, Vassiliadis E, Larsen MR, Henriksen K, Karsdal MA. Post-Translational Modifications of the Extracellular Matrix are Key Events in Cancer Progression: Opportunities for Biochemical Marker Development. Biomarkers (2011) 16(3):193–205. doi: 10.3109/1354750X.2011.557440
7. Szabó-Taylor K, Ryan B, Osteikoetxea X, Szabó TG, Sódar B, Holub M, et al. Oxidative and Other Posttranslational Modifications in Extracellular Vesicle Biology. Semin Cell Dev Biol (2015) 40:8–16. doi: 10.1016/j.semcdb.2015.02.012
8. Atukorala I, Mathivanan S. The Role of Post-Translational Modifications in Targeting Protein Cargo to Extracellular Vesicles. Subcellular Biochem (2021) 97:45–60. doi: 10.1007/978-3-030-67171-6_3
9. Ckless K. Redox Proteomics: From Bench to Bedside. Adv Exp Med Biol (2014) 806:301–17. doi: 10.1007/978-3-319-06068-2_13
10. Hanschmann E-M, Godoy JR, Berndt C, Hudemann C, Lillig CH. Thioredoxins, Glutaredoxins, and Peroxiredoxins—Molecular Mechanisms and Health Significance: From Cofactors to Antioxidants to Redox Signaling. Antioxid Redox Signal (2013) 19(13):1539–605. doi: 10.1089/ars.2012.4599
11. Sies H. Hydrogen Peroxide as a Central Redox Signaling Molecule in Physiological Oxidative Stress: Oxidative Eustress. Redox Biol (2017) 11:613–9. doi: 10.1016/j.redox.2016.12.035
12. Lorenzen I, Eble JA, Hanschmann EM. Thiol Switches in Membrane Proteins - Extracellular Redox Regulation in Cell Biology. Biol Chem (2021) 402(3):253–69. doi: 10.1515/hsz-2020-0266
13. Lorenzen I, Mullen L, Bekeschus S, Hanschmann E-M. Redox Regulation of Inflammatory Processes Is Enzymatically Controlled. Oxid Med Cell Longev (2017) 2017:8459402. doi: 10.1155/2017/8459402
14. Lechowicz U, Rudzinski S, Jezela-Stanek A, Janciauskiene S, Chorostowska-Wynimko J. Post-Translational Modifications of Circulating Alpha-1-Antitrypsin Protein. Int J Mol Sci (2020) 21(23):9187. doi: 10.3390/ijms21239187
15. Jaeger SU, Schroeder BO, Meyer-Hoffert U, Courth L, Fehr SN, Gersemann M, et al. Cell-Mediated Reduction of Human β-Defensin 1: A Major Role for Mucosal Thioredoxin. Mucosal Immunol (2013) 6(6):1179–90. doi: 10.1038/mi.2013.17
16. Tang YQ, Selsted ME. Characterization of the Disulfide Motif in BNBD-12, an Antimicrobial Beta-Defensin Peptide From Bovine Neutrophils. J Biol Chem (1993) 268(9):6649–53. doi: 10.1016/S0021-9258(18)53299-3
17. Checconi P, Salzano S, Bowler L, Mullen L, Mengozzi M, Hanschmann EM, et al. Redox Proteomics of the Inflammatory Secretome Identifies a Common Set of Redoxins and Other Glutathionylated Proteins Released in Inflammation, Influenza Virus Infection and Oxidative Stress. PLoS One (2015) 10(5):1–21. doi: 10.1371/journal.pone.0127086
18. Vidak E, Javoršek U, Vizovišek M, Turk B. Cysteine Cathepsins and Their Extracellular Roles: Shaping the Microenvironment. Cells (2019) 8(3):264. doi: 10.3390/cells8030264
19. Pillay CS, Dennison C. Cathepsin B Stability, But Not Activity, is Affected in Cysteine:Cysteine Redox Buffers. Biol Chem (2002) 383(7–8):1199–204. doi: 10.1515/BC.2002.132
20. Bourgeois R, Mercier J, Paquette-Brooks I, Cohen ÉA. Association Between Disruption of CD4 Receptor Dimerization and Increased Human Immunodeficiency Virus Type 1 Entry. Retrovirology (2006) 3:1–8. doi: 10.1186/1742-4690-3-31
21. Cerutti N, Killick M, Jugnarain V, Papathanasopoulos M, Capovilla A. Disulfide Reduction in CD4 Domain 1 or 2 is Essential for Interaction With HIV Glycoprotein 120 (Gp120), Which Impairs Thioredoxin-Driven CD4 Dimerization. J Biol Chem (2014) 289(15):10455–65. doi: 10.1074/jbc.M113.539353
22. Kim SF, Huri DA, Snyder SH. Medicine: Inducible Nitric Oxide Synthase Binds, S-Nitrosylates, and Activates Cyclooxygenase-2. Science (80- ) (2005) 310(5756):1966–70. doi: 10.1126/science.1119407.
23. Shin MJ, Kim DW, Choi YJ, Cha HJ, Lee SH, Lee S, et al. PEP-1-GLRX1 Protein Exhibits Anti-Inflammatory Effects by Inhibiting the Activation of MAPK and NF-κb Pathways in Raw 264. 7 Cells BMB Rep (2020) 53(2):106–11. doi: 10.5483/BMBRep.2020.53.2.180
24. Murphy JR. Mechanism of Diphtheria Toxin Catalytic Domain Delivery to the Eukaryotic Cell Cytosol and the Cellular Factors That Directly Participate in the Process. Toxins (2011) 3(3):294–308. doi: 10.3390/toxins3030294
25. Ryser HJP, Mandel R, Ghani F. Cell Surface Sulfhydryls are Required for the Cytotoxicity of Diphtheria Toxin But Not of Ricin in Chinese Hamster Ovary Cells. J Biol Chem (1991) 266(28):18439–42. doi: 10.1016/S0021-9258(18)55080-8
26. Moskaug JO, Sandvig K, Olsnes S. Cell-Mediated Reduction of the Interfragment Disulfide in Nicked Diphtheria Toxin. A New System to Study Toxin Entry at Low Ph. J Biol Chem (1987) 262(21):10339–45. doi: 10.1016/S0021-9258(18)61118-4
27. Barbouche R, Miquelis R, Jones IM, Fenouillet E. Protein-Disulfide Isomerase-Mediated Reduction of Two Disulfide Bonds of HIV Envelope Glycoprotein 120 Occurs Post-CXCR4 Binding and is Required for Fusion. J Biol Chem (2003) 278(5):3131–6. doi: 10.1074/jbc.M205467200
28. Fenouillet E, Barbouche R, Courageot J, Miquelis R. The Catalytic Activity of Protein Disulfide Isomerase is Involved in Human Immunodeficiency Virus Envelope-Mediated Membrane Fusion After CD4 Cell Binding. J Infect Dis (2001) 183(5):744–52. doi: 10.1086/318823
29. Hoppe G, Talcott KE, Bhattacharya SK, Crabb JW, Sears JE. Molecular Basis for the Redox Control of Nuclear Transport of the Structural Chromatin Protein Hmgb1. Exp Cell Res (2006) 312(18):3526–38. doi: 10.1016/j.yexcr.2006.07.020
30. Kwak MS, Kim HS, Lkhamsuren K, Kim YH, Han MG, Shin JM, et al. Peroxiredoxin-Mediated Disulfide Bond Formation is Required for Nucleocytoplasmic Translocation and Secretion of HMGB1 in Response to Inflammatory Stimuli. Redox Biol (2019) 24:101203. doi: 10.1016/j.redox.2019.101203
31. Suliman HB, Babiker A, Withers CM, Sweeney TE, Carraway MS, Tatro LG, et al. Nitric Oxide Synthase-2 Regulates Mitochondrial Hsp60 Chaperone Function During Bacterial Peritonitis in Mice. Free Radic Biol Med (2010) 48(5):736–46. doi: 10.1016/j.freeradbiomed.2009.12.019
32. Wu C, Liu T, Chen W, Oka SI, Fu C, Jain MR, et al. Redox Regulatory Mechanism of Transnitrosylation by Thioredoxin. Mol Cell Proteomics (2010) 9(10):2262–75. doi: 10.1074/mcp.M110.000034
33. Kawade R, Akiba H, Entzminger K, Maruyama T, Okumura CJ, Tsumoto K. Roles of the Disulfide Bond Between the Variable and the Constant Domains of Rabbit Immunoglobulin Kappa Chains in Thermal Stability and Affinity. Protein Eng Des Sel (2018) 31(7–8):243–7. doi: 10.1093/protein/gzy008
34. Magnusson CGM, Björnstedt M, Holmgren A. Human IgG is Substrate for the Thioredoxin System: Differential Cleavage Pattern of Interchain Disulfide Bridges in IgG Subclasses. Mol Immunol (1997) 34(10):709–17. doi: 10.1016/S0161-5890(97)00092-8
35. You Y, Chen J, Zhu F, Xu Q, Han L, Gao X, et al. Glutaredoxin 1 Up-Regulates Deglutathionylation of 4 Integrin and Thereby Restricts Neutrophil Mobilization From Bone Marrow. J Biol Chem (2019) 294(8):2616–27. doi: 10.1074/jbc.RA118.006096
36. Bergerhausen L, Grosche J, Meißner J, Hecker C, Caliandro MF, Westerhausen C, et al. Extracellular Redox Regulation of α7β Integrin-Mediated Cell Migration is Signaled via a Dominant Thiol-Switch. Antioxidants (2020) 9(3):1–23. doi: 10.3390/antiox9030227
37. Zhang X, Liu P, Zhang C, Chiewchengchol D, Zhao F, Yu H, et al. Positive Regulation of Interleukin-1β Bioactivity by Physiological ROS-Mediated Cysteine S-Glutathionylation. Cell Rep (2017) 20(1):224–35. doi: 10.1016/j.celrep.2017.05.070
38. Guo L, Chen S, Liu Q, Ren H, Li Y, Pan J, et al. Glutaredoxin 1 Regulates Macrophage Polarization Through Mediating Glutathionylation of STAT1. Thorac Cancer (2020) 11(10):2966–74. doi: 10.1111/1759-7714.13647
39. Butturini E, Boriero D, Carcereri de Prati A, Mariotto S. STAT1 Drives M1 Microglia Activation and Neuroinflammation Under Hypoxia. Arch Biochem Biophys (2019) 669:22–30. doi: 10.1016/j.abb.2019.05.011
40. Bräutigam L, Schütte LD, Godoy JR, Prozorovski T, Gellert M, Hauptmann G, et al. Vertebrate-Specific Glutaredoxin is Essential for Brain Development. Proc Natl Acad Sci U S A (2011) 108(51):20532–7. doi: 10.1073/pnas.1110085108
41. Gellert M, Venz S, Mitlöhner J, Cott C, Hanschmann EM, Lillig CH. Identification of a Dithiol-Disulfide Switch in Collapsin Response Mediator Protein 2 (CRMP2) That is Toggled in a Model of Neuronal Differentiation. J Biol Chem (2013) 288(49):35117–25. doi: 10.1074/jbc.M113.521443
42. Cumming RC, Schubert D. Amyloid-β Induces Disulfide Bonding and Aggregation of GAPDH in Alzheimer’s Disease. FASEB J (2005) 19(14):2060–2. doi: 10.1096/fj.05-4195fje
43. Leichert LI, Gehrke F, Gudiseva HV, Blackwell T, Ilbert M, Walker AK, et al. Quantifying Changes in the Thiol Redox Proteome Upon Oxidative Stress In Vivo. Proc Natl Acad Sci U S A (2008) 105(24):8197–202. doi: 10.1073/pnas.0707723105
44. Barbirz S, Jakob U, Glocker MO. Mass Spectrometry Unravels Disulfide Bond Formation as the Mechanism That Activates a Molecular Chaperone. J Biol Chem (2000) 275(25):18759–66. doi: 10.1074/jbc.M001089200
45. Zheng M, Åslund F, Storz G. Activation of the OxyR Transcription Factor by Reversible Disulfide Bond Formation. Science (80- ) (1998) 279(5357):1718–21. doi: 10.1126/science.279.5357.1718
46. Lee C, Lee SM, Mukhopadhyay P, Kim SJ, Lee SC, Ahn WS, et al. Redox Regulation of OxyR Requires Specific Disulfide Bond Formation Involving a Rapid Kinetic Reaction Path. Nat Struct Mol Biol (2004) 11(12):1179–85. doi: 10.1038/nsmb856
47. Brandes N, Schmitt S, Jakob U. Thiol-Based Redox Switches in Eukaryotic Proteins. Antioxidants Redox Signal (2009) 11(5):997–1014. doi: 10.1089/ars.2008.2285
48. Tsermpini EE, Zhang Y, Niola P, Chillotti C, Ardau R, Bocchetta A, et al. Pharmacogenetics of Lithium Effects on Glomerular Function in Bipolar Disorder Patients Under Chronic Lithium Treatment: A Pilot Study. Neurosci Lett (2017) 638:1–4. doi: 10.1016/j.neulet.2016.12.001
49. Kokkola R, Andersson Å, Mullins G, Östberg T, Treutiger CJ, Arnold B, et al. RAGE is the Major Receptor for the Proinflammatory Activity of HMGB1 in Rodent Macrophages. Scand J Immunol (2005) 61(1):1–9. doi: 10.1111/j.0300-9475.2005.01534.x
50. Park JS, Svetkauskaite D, He Q, Kim JY, Strassheim D, Ishizaka A, et al. Involvement of Toll-Like Receptors 2 and 4 in Cellular Activation by High Mobility Group Box 1 Protein. J Biol Chem (2004) 279(9):7370–7. doi: 10.1074/jbc.M306793200
51. Yang H, Lundbäck P, Ottosson L, Erlandsson-Harris H, Venereau E, Bianchi ME, et al. Redox Modifications of Cysteine Residues Regulate the Cytokine Activity of HMGB1. Mol Med (2021) 27(1):58. doi: 10.1186/s10020-021-00307-1
52. Düsterhöft S, Jung S, Hung CW, Tholey A, Sönnichsen FD, Grötzinger J, et al. Membrane-Proximal Domain of a Disintegrin and Metalloprotease-17 Represents the Putative Molecular Switch of its Shedding Activity Operated by Protein-Disulfide Isomerase. J Am Chem Soc (2013) 135(15):5776–81. doi: 10.1021/ja400340u
53. Krossa S, Scheidig AJ, Grötzinger J, Lorenzen I. Redundancy of Protein Disulfide Isomerases in the Catalysis of the Inactivating Disulfide Switch in A Disintegrin and Metalloprotease. Sci Rep (2018) 8(1):2–10. doi: 10.1038/s41598-018-19429-4
54. Zhang K, Pan YD, Qi JP, Yue J, Zhang MB, Xu CQ, et al. Disruption of Disulfide Restriction at Integrin Knees Induces Activation and Ligand-Independent Signaling of α4β7. J Cell Sci (2013) 126(21):5030–41. doi: 10.1242/jcs.134528
55. Passam F, Chiu J, Ju L, Pijning A, Jahan Z, Mor-Cohen R, et al. Mechano-Redox Control of Integrin De-Adhesion. Elife (2018) 7:1–22. doi: 10.7554/eLife.34843
56. Laragione T, Bonetto V, Casoni F, Massignan T, Bianchi G, Gianazza E, et al. Redox Regulation of Surface Protein Thiols: Identification of Integrin α-4 as a Molecular Target by Using Redox Proteomics. Proc Natl Acad Sci U S A (2003) 100(25):14737–41. doi: 10.1073/pnas.2434516100
57. Schwertassek U, Balmer Y, Gutscher M, Weingarten L, Preuss M, Engelhard J, et al. Selective Redox Regulation of Cytokine Receptor Signaling by Extracellular Thioredoxin-1. EMBO J (2007) 26(13):3086–97. doi: 10.1038/sj.emboj.7601746
58. Yoshida T, Inoue R, Morii T, Takahashi N, Yamamoto S, Hara Y, et al. Nitric Oxide Activates TRP Channels by Cysteine S-Nitrosylation. Nat Chem Biol (2006) 2(11):596–607. doi: 10.1038/nchembio821
59. Xu SZ, Sukumar P, Zeng F, Li J, Jairaman A, English A, et al. TRPC Channel Activation by Extracellular Thioredoxin. Nature (2008) 451(7174):69–72. doi: 10.1038/nature06414
60. Moolla N, Killick M, Papathanasopoulos M, Capovilla A. Thioredoxin (Trx1) Regulates CD4 Membrane Domain Localization and is Required for Efficient CD4-Dependent HIV-1 Entry. Biochim Biophys Acta - Gen Subj (2016) 1860(9):1854–63. doi: 10.1016/j.bbagen.2016.05.030
61. Borth N, Mattanovich D, Kunert R, Katinger H. Effect of Increased Expression of Protein Disulfide Isomerase and Heavy Chain Binding Protein on Antibody Secretion in a Recombinant CHO Cell Line. Biotechnol Prog (2005) 21(1):106–11. doi: 10.1021/bp0498241
62. Lilie H, McLaughlin S, Freedman R, Buchnert J. Influence of Protein Disulfide Isomerase (PDI) on Antibody Folding In Vitro. J Biol Chem (1994) 269(19):14290–6. doi: 10.1016/S0021-9258(17)36787-X
63. Vidarsson G, Dekkers G, Rispens T. IgG Subclasses and Allotypes: From Structure to Effector Functions. Front Immunol (2014) 5:1–17. doi: 10.3389/fimmu.2014.00520
64. Giannone C, Chelazzi MR, Orsi A, Anelli T, Nguyen T, Buchner J, et al. Biogenesis of Secretory Immunoglobulin M Requires Intermediate non-Native Disulfide Bonds and Engagement of the Protein Disulfide Isomerase Erp44. EMBO J (2022) 41(3):e108518. doi: 10.15252/embj.2021108518
65. Liu H, May K. Disulfide Bond Structures of IgG Molecules: Structural Variations, Chemical Modifications and Possible Impacts to Stability and Biological Function. MAbs (2012) 4(1):17–23. doi: 10.4161/mabs.4.1.18347
66. Aalberse ROBC, Schuurman J. IgG4 Breaking the Rules. Immunology (2002) 105(1):9–19. doi: 10.1046/j.0019-2805.2001.01341.x
67. Trampert DC, Hubers LM, van de Graaf SFJ, Beuers U. On the Role of IgG4 in Inflammatory Conditions: Lessons for IgG4-Related Disease. Biochim Biophys Acta - Mol Basis Dis (2018) 1864(4):1401–9. doi: 10.1016/j.bbadis.2017.07.038
68. Hayes NVL, Smales CM, Klappa P. Protein Disulfide Isomerase Does Not Control Recombinant IgG4 Productivity in Mammalian Cell Lines. Biotechnol Bioeng (2010) 105(4):770–9. doi: 10.1002/bit.22587
69. Hmiel LK, Brorson KA, Boyne MT. Post-Translational Structural Modifications of Immunoglobulin G and Their Effect on Biological Activity. Analytical Bioanalytical Chem (2015) 407(1):79–94. doi: 10.1007/s00216-014-8108-x
70. Koterba KL, Borgschulte T, Laird MW. Thioredoxin 1 is Responsible for Antibody Disulfide Reduction in CHO Cell Culture. J Biotechnol (2012) 157(1):261–7. doi: 10.1016/j.jbiotec.2011.11.009
71. Kao YH, Hewitt DP, Trexler-Schmidt M, Laird MW. Mechanism of Antibody Reduction in Cell Culture Production Processes. Biotechnol Bioeng (2010) 107(4):622–32. doi: 10.1002/bit.22848
72. Handlogten MW, Zhu M, Ahuja S. Glutathione and Thioredoxin Systems Contribute to Recombinant Monoclonal Antibody Interchain Disulfide Bond Reduction During Bioprocessing. Biotechnol Bioeng (2017) 114(7):1469–77. doi: 10.1002/bit.26278
73. McIntyre JA, Wagenknecht DR, Faulk WP. Autoantibodies Unmasked by Redox Reactions. J Autoimmun (2005) 24(4):311–7. doi: 10.1016/j.jaut.2005.03.005
74. Isenman DE, Dorrington KJ, Painter RH. The Structure and Function of Immunoglobulin Domains. II. The Importance of Interchain Disulfide Bonds and the Possible Role of Molecular Flexibility in the Interaction Between Immunoglobulin G and Complement. J Immunol (1975) 114(6):1726–9.
75. Sachs A, Moore E, Kosaloglu-Yalcin Z, Peters B, Sidney J, Rosenberg SA, et al. Impact of Cysteine Residues on MHC Binding Predictions and Recognition by Tumor-Reactive T Cells. J Immunol (2020) 205(2):539–49. doi: 10.4049/jimmunol.1901173
76. Trujillo JA, Croft NP, Dudek NL, Channappanavar R, Theodossis A, Webb AI, et al. The Cellular Redox Environment Alters Antigen Presentation. J Biol Chem (2014) 289(40):27979–91. doi: 10.1074/jbc.M114.573402
77. Shelton MD, Kern TS, Mieyal JJ. Glutaredoxin Regulates Nuclear Factor κ-B and Intercellular Adhesion Molecule in Müller Cells: Model of Diabetic Retinopathy. J Biol Chem (2007) 282(17):12467–74. doi: 10.1074/jbc.M610863200
78. Lillig CH, Berndt C. Glutaredoxins in Thiol/Disulfide Exchange. Antioxid Redox Signal (2013) 18(13):1654–65. doi: 10.1089/ars.2012.5007
79. Sakai J, Li J, Subramanian KK, Mondal S, Bajrami B, Hattori H, et al. Reactive Oxygen Species-Induced Actin Glutathionylation Controls Actin Dynamics in Neutrophils. Immunity (2012) 37(6):1037–49. doi: 10.1016/j.immuni.2012.08.017
80. Guo Y, Liu Y, Zhao S, Xu W, Li Y, Zhao P, et al. Oxidative Stress-Induced FABP5 S-Glutathionylation Protects Against Acute Lung Injury by Suppressing Inflammation in Macrophages. Nat Commun (2021) 12(1):1–18. doi: 10.1038/s41467-021-27428-9
81. Das D, Wang Y-H, HSieh C-Y, Suzuki YJ. Major Vault Protein Regulates Cell Growth/Survival Signalling Through Oxidative Modifications Dividutta. Curr Drug Targets (2015) 16(7):700–10. doi: 10.1016/j.cellsig.2015.10.007.Major
82. Hoffman SM, Nolin JD, Jones JT, Lahue KG, Chapman DG, Aliyeva M, et al. Ablation of the Thiol Transferase Glutaredoxin-1 Augments Protein S-Glutathionylation and Modulates Type 2 Inflammatory Responses and IL-17 in a House Dust Mite Model of Allergic Airway Disease in Mice. Ann Am Thorac Soc (2016) 13(1):S97. doi: 10.1016/j.cellsig.2015.10.007.Major
83. Gorelenkova Miller O, Behring JB, Siedlak SL, Jiang S, Matsui R, Bachschmid MM, et al. Upregulation of Glutaredoxin-1 Activates Microglia and Promotes Neurodegeneration: Implications for Parkinson’s Disease. Antioxidants Redox Signal (2016) 25(18):967–82. doi: 10.1089/ars.2015.6598
84. Adachi T, Weisbrod RM, Pimentel DR, Ying J, Sharov VS, Schöneich C, et al. S-Glutathiolation by Peroxynitrite Activates SERCA During Arterial Relaxation by Nitric Oxide. Nat Med (2004) 10(11):1200–7. doi: 10.1038/nm1119
85. Butturini E, de Prati AC, Mariotto S. Redox Regulation of STAT1 and STAT3 Signaling. Int J Mol Sci (2020) 21(19):1–18. doi: 10.3390/ijms21197034
86. Casagrande S, Bonetto V, Fratelli M, Gianazza E, Eberini I, Massignan T, et al. Glutathionylation of Human Thioredoxin: A Possible Crosstalk Between the Glutathione and Thioredoxin Systems. Proc Natl Acad Sci U S A (2002) 99(15):9745–9. doi: 10.1073/pnas.152168599
87. Salzano S, Checconi P, Hanschmann EM, Lillig CH, Bowler LD, Chan P, et al. Linkage of Inflammation and Oxidative Stress via Release of Glutathionylated Peroxiredoxin-2, Which Acts as a Danger Signal. Proc Natl Acad Sci U S A (2014) 111(33):12157–62. doi: 10.1073/pnas.1401712111
88. Diotallevi M, Checconi P, Palamara AT, Celestino I, Coppo L, Holmgren A, et al. Glutathione Fine-Tunes the Innate Immune Response Toward Antiviral Pathways in a Macrophage Cell Line Independently of its Antioxidant Properties. Front Immunol (2017) 8. doi: 10.3389/fimmu.2017.01239
89. Mukherjee TK, Mishra AK, Mukhopadhyay S, Hoidal JR. High Concentration of Antioxidants N -Acetylcysteine and Mitoquinone-Q Induces Intercellular Adhesion Molecule 1 and Oxidative Stress by Increasing Intracellular Glutathione. J Immunol (2007) 178(3):1835–44. doi: 10.4049/jimmunol.178.3.1835
90. Liu SY, Tsai MY, Chuang KP, Huang YF, Shieh CC. Ligand Binding of Leukocyte Integrin Very Late Antigen-4 Involves Exposure of Sulfhydryl Groups and is Subject to Redox Modulation. Eur J Immunol (2008) 38(2):410–23. doi: 10.1002/eji.200737556
91. Rozenberg O, Aviram M. S-Glutathionylation Regulates HDL-Associated Paraoxonase 1 (PON1) Activity. Biochem Biophys Res Commun (2006) 351(2):492–8. doi: 10.1016/j.bbrc.2006.10.059
92. Pekkari K, Gurunath R, Arner ESJ, Holmgren A. Truncated Thioredoxin is a Mitogenic Cytokine for Resting Human Peripheral Blood Mononuclear Cells and is Present in Human Plasma. J Biol Chem (2000) 275(48):37474–80. doi: 10.1074/jbc.M001012200
93. Lu Y, Zhang XS, Zhang ZH, Zhou XM, Gao YY, Liu GJ, et al. Peroxiredoxin 2 Activates Microglia by Interacting With Toll-Like Receptor 4 After Subarachnoid Hemorrhage. J Neuroinflamm (2018) 15(1):1–10. doi: 10.1186/s12974-018-1118-4
94. Riddell JR, Bshara W, Moser MT, Spernyak JA, Foster BA, Gollnick SO. Peroxiredoxin 1 Controls Prostate Cancer Growth Through Toll-Like Receptor 4-Dependent Regulation of Tumor Vasculature. Cancer Res (2011) 71(5):1637–46. doi: 10.1158/0008-5472.CAN-10-3674
95. Yang J, Zhang H, Gong W, Liu Z, Wu H, Hu W, et al. S-Glutathionylation of Human Inducible Hsp70 Reveals a Regulatory Mechanism Involving the C-Terminal α-Helical Lid. J Biol Chem (2020) 295(24):37474–80. doi: 10.1074/jbc.RA119.012372
96. Fratelli M, Demol H, Puype M, Casagrande S, Eberini I, Salmona M, et al. Identification by Redox Proteomics of Glutathionylated Proteins in Oxidatively Stressed Human T Lymphocytes. Proc Natl Acad Sci U S A (2002) 99(6):3505–10. doi: 10.1073/pnas.052592699
97. Asea A, Rehli M, Kabingu E, Boch JA, Baré O, Auron PE, et al. Novel Signal Transduction Pathway Utilized by Extracellular HSP70. Role of Toll-Like Receptor (TLR) 2 and TLR4. J Biol Chem (2002) 277(17):15028–34. doi: 10.1074/jbc.M200497200
98. Vabulas RM, Ahmad-Nejad P, Da Costa C, Miethke T, Kirschning CJ, Häcker H, et al. Endocytosed HSP60s Use Toll-Like Receptor 2 (TLR2) and TLR4 to Activate the Toll/Interleukin-1 Receptor Signaling Pathway in Innate Immune Cells. J Biol Chem (2001) 276(33):31332–9. doi: 10.1074/jbc.M103217200
99. Nolin JD, Tully JE, Hoffman SM, Guala AS, van der Velden JL, Poynter ME, et al. The Glutaredoxin/S-Glutathionylation Axis Regulates Interleukin-17A-Induced Proinflammatory Responses in Lung Epithelial Cells in Association With S-Glutathionylation of Nuclear Factor κb Family Proteins. Free Radic Biol Med (2014) 73:143–53. doi: 10.1016/j.freeradbiomed.2014.04.028
100. Seth D, Stamler JS. The SNO-Proteome: Causation and Classifications. Curr Opin Chem Biol (2011) 15:129–36. doi: 10.1016/j.cbpa.2010.10.012
101. Hess DT, Patterson SI, Smith DS, Skene JHP. Neuronal Growth Cone Collapse and Inhibition of Protein Fatty Acylation by Nitric Oxide. Nature (1993) 366(6455):562–5. doi: 10.1038/366562a0
102. Stamler JS, Simon DI, Osborne JA, Mullins ME, Jaraki O, Michel T, et al. S-Nitrosylation of Proteins With Nitric Oxide: Synthesis and Characterization of Biologically Active Compounds. Proc Natl Acad Sci U.S.A. (1992) 89(1):444–8. doi: 10.1073/pnas.89.1.444
103. Arnelle DR, Stamler JS. NO+, NO·, and NO– Donation by S-Nitrosothiols: Implications for Regulation of Physiological Functions by s-Nitrosylation and Acceleration of Disulfide Formation. Arch Biochem Biophysics (1995) 318:279–85. doi: 10.1006/abbi.1995.1231
104. Riccio A, Alvania RS, Lonze BE, Ramanan N, Kim T, Huang Y, et al. A Nitric Oxide Signaling Pathway Controls CREB-Mediated Gene Expression in Neurons. Mol Cell (2006) 21(2):283–94. doi: 10.1016/j.molcel.2005.12.006
105. Benhar M, Thompson JW, Moseley MA, Stamler JS. Identification of S-Nitrosylated Targets of Thioredoxin Using a Quantitative Proteomic Approach. Biochemistry (2010) 49(32):6963–9. doi: 10.1021/bi100619k
106. Zhang HH, Wang W, Feng L, Yang Y, Zheng J, Huang L, et al. S-Nitrosylation of Cofilin-1 Serves as a Novel Pathway for VEGF-Stimulated Endothelial Cell Migration. J Cell Physiol (2015) 230(2):406–17. doi: 10.1002/jcp.24724
107. Hara MR, Agrawal N, Kim SF, Cascio MB, Fujimuro M, Ozeki Y, et al. S-Nitrosylated GAPDH Initiates Apoptotic Cell Death by Nuclear Translocation Following Siah1 Binding. Nat Cell Biol (2005) 7(7):665–74. doi: 10.1038/ncb1268
108. Jia J, Arif A, Terenzi F, Willard B, Plow EF, Hazen SL, et al. Target-Selective Protein S-Nitrosylation by Sequence Motif Recognition. Cell (2014) 159(3):623–34. doi: 10.1016/j.cell.2014.09.032
109. Kornberg MD, Sen N, Hara MR, Juluri KR, Nguyen JVK, Snowman AM, et al. GAPDH Mediates Nitrosylation of Nuclear Proteins. Nat Cell Biol (2010) 12(11):1094–100. doi: 10.1038/ncb2114
110. Martínez-Ruiz A, Villanueva L, González de Orduña C, López-Ferrer D, Higueras MA, Tarín C, et al. S-Nitrosylation of Hsp90 Promotes the Inhibition of its ATPase and Endothelial Nitric Oxide Synthase Regulatory Activities. Proc Natl Acad Sci U S A (2005) 102(24):8525–30. doi: 10.1073/pnas.0407294102
111. Zhao S, Tang X, Miao Z, Chen Y, Cao J, Song T, et al. Hsp90 S-Nitrosylation at Cys521, as a Conformational Switch, Modulates Cycling of Hsp90-AHA1-CDC37 Chaperone Machine to Aggravate Atherosclerosis. Redox Biol (2022) 52:102290. doi: 10.1016/j.redox.2022.102290
112. Into T, Inomata M, Nakashima M, Shibata K, Häcker H, Matsushita K. Regulation of MyD88-Dependent Signaling Events by S Nitrosylation Retards Toll-Like Receptor Signal Transduction and Initiation of Acute-Phase Immune Responses. Mol Cell Biol (2008) 28(4):1338–47. doi: 10.1128/MCB.01412-07
113. Li BQ, Hu LL, Niu S, Cai YD, Chou KC. Predict and Analyze S-Nitrosylation Modification Sites With the mRMR and IFS Approaches. J Proteomics (2012) 75(5):1654–65. doi: 10.1016/j.jprot.2011.12.003
114. Marshall HE, Stamler JS. Inhibition of NF-Kappa B by S-Nitrosylation. Biochemistry (2001) 40(6):1688–93. doi: 10.1021/bi002239y
115. Kelleher ZT, Matsumoto A, Stamler JS, Marshall HE. NOS2 Regulation of NF-κb by S-Nitrosylation of P65. J Biol Chem (2007) 282(42):30667–72. doi: 10.1074/jbc.M705929200
116. Ravi K, Brennan LA, Levic S, Ross PA, Black SM. S-Nitrosylation of Endothelial Nitric Oxide Synthase is Associated With Monomerization and Decreased Enzyme Activity. Proc Natl Acad Sci U S A (2004) 101(8):2619–24. doi: 10.1073/pnas.0300464101
117. Lim SY, Raftery M, Cai H, Hsu K, Yan WX, Hseih H-L, et al. S-Nitrosylated S100A8: Novel Anti-Inflammatory Properties. J Immunol (2008) 181(8):5627–36. doi: 10.4049/jimmunol.181.8.5627
118. Bekendam RH, Iyu D, Passam F, Stopa JD, De Ceunynck K, Muse O, et al. Protein Disulfide Isomerase Regulation by Nitric Oxide Maintains Vascular Quiescence and Controls Thrombus Formation. J Thromb Haemost (2018) 16(11):2322–35. doi: 10.1111/jth.14291
119. Chao ML, Luo S, Zhang C, Zhou X, Zhou M, Wang J, et al. S-Nitrosylation-Mediated Coupling of G-Protein Alpha-2 With CXCR5 Induces Hippo/YAP-Dependent Diabetes-Accelerated Atherosclerosis. Nat Commun (2021) 12(1):1–17. doi: 10.1038/s41467-021-24736-y
120. Correa-Aragunde N, Graziano M, Chevalier C, Lamattina L. Nitric Oxide Modulates the Expression of Cell Cycle Regulatory Genes During Lateral Root Formation in Tomato. J Exp Bot (2006) 57(3):581–8. doi: 10.1093/jxb/erj045
121. Fernando V, Zheng X, Walia Y, Sharma V, Letson J, Furuta S. S-Nitrosylation: An Emerging Paradigm of Redox Signaling. Antioxidants (2019) 8(9):404. doi: 10.3390/antiox8090404
122. Godoy LC, Moretti AI, Jurado MC, Oxer D, Janiszewski M, Ckless K, et al. Loss of CD40 Endogenous S-Nitrosylation During Inflammatory Response in Endotoxemic Mice and Patients With Sepsis. Shock (2010) 33(6):626–33. doi: 10.1097/SHK.0b013e3181cb88e6
123. Switzer CH, Glynn SA, Cheng RY-S, Ridnour LA, Green JE, Ambs S, et al. S-Nitrosylation of EGFR and Src Activates an Oncogenic Signaling Network in Human Basal-Like Breast Cancer. Mol Cancer Res (2012) 10(9):1203–15. doi: 10.1158/1541-7786.MCR-12-0124
124. Okada K, Zhu B-T. S-Nitrosylation of the IGF-1 Receptor Disrupts the Cell Proliferative Action of IGF-1. Biochem Biophys Res Commun (2017) 491(4):870–5. doi: 10.1016/j.bbrc.2017.06.177
125. Guo CJ, Atochina-Vasserman EN, Abramova E, Foley JP, Zaman A, Crouch E, et al. S-Nitrosylation of Surfactant Protein-D Controls Inflammatory Function. PLoS Biol (2008) 6(11):e266. doi: 10.1371/journal.pbio.0060266
126. Ohya M, Nishitani C, Sano H, Yamada C, Mitsuzawa H, Shimizu T, et al. Human Pulmonary Surfactant Protein D Binds the Extracellular Domains of Toll-Like Receptors 2 and 4 Through the Carbohydrate Recognition Domain by a Mechanism Different From its Binding to Phosphatidylinositol and Lipopolysaccharide. Biochemistry (2006) 45(28):8657–64. doi: 10.1021/bi060176z
127. Yamazoe M, Nishitani C, Takahashi M, Katoh T, Ariki S, Shimizu T, et al. Pulmonary Surfactant Protein D Inhibits Lipopolysaccharide (LPS)-Induced Inflammatory Cell Responses by Altering LPS Binding to its Receptors. J Biol Chem (2008) 283(51):35878–88. doi: 10.1074/jbc.M807268200
128. Reynaert NL, Ckless K, Korn SH, Vos N, Guala AS, Wouters EFM, et al. Nitric Oxide Represses Inhibitory κb Kinase Through S-Nitrosylation. Proc Natl Acad Sci U S A (2004) 101(24):8945–50. doi: 10.1073/pnas.0400588101
129. Michalska M, Wolf P. Pseudomonas Exotoxin A: Optimized by Evolution for Effective Killing. Front Microbiol (2015) 6. doi: 10.3389/fmicb.2015.00963
130. McKee ML, FitzGerald DJ. Reduction of Furin-Nicked Pseudomonas Exotoxin A: An Unfolding Story. Biochemistry (1999) 38(50):16507–13. doi: 10.1021/bi991308+
131. Matsuda M, Barksdale L. System for the Investigation of the Bacteriophage-Directed Synthesis of Diphtherial Toxin. J Bacteriol (1967) 93(2):722–30. doi: 10.1128/jb.93.2.722-730.1967
132. Orlandi PA. Protein-Disulfide Isomerase-Mediated Reduction of the A Subunit of Cholera Toxin in a Human Intestinal Cell Line. J Biol Chem (1997) 272(7):4591–9. doi: 10.1016/S0021-9258(19)67333-3
133. Mekalanos JJ, Collier RJ, Romig WR. Enzymic Activity of Cholera Toxin. II. Relationships to Proteolytic Processing, Disulfide Bond Reduction, and Subunit Composition. J Biol Chem (1979) 254(13):5855–61. doi: 10.1016/S0021-9258(18)50491-9
134. Green RS, Naimi WA, Oliver LD, O’bier N, Cho J, Conrad DH, et al. Binding of Host Cell Surface Protein Disulfide Isomerase by Anaplasma Phagocytophilum Asp14 Enables Pathogen Infection. MBio (2020) 11(1):e03141–19 . doi: 10.1128/mBio.03141-19
135. Green RS, Izac JR, Naimi WA, O’Bier N, Breitschwerdt EB, Marconi RT, et al. Ehrlichia Chaffeensis EplA Interaction With Host Cell Protein Disulfide Isomerase Promotes Infection. Front Cell Infect Microbiol (2020) 10. doi: 10.3389/fcimb.2020.00500
136. Auwerx J, Isacsson O, Söderlund J, Balzarini J, Johansson M, Lundberg M. Human Glutaredoxin-1 Catalyzes the Reduction of HIV-1 Gp120 and CD4 Disulfides and its Inhibition Reduces HIV-1 Replication. Int J Biochem Cell Biol (2009) 41(6):1269–75. doi: 10.1016/j.biocel.2008.10.031
137. Reiser K, Franois KO, Schols D, Bergman T, Jörnvall H, Balzarini J, et al. Thioredoxin-1 and Protein Disulfide Isomerase Catalyze the Reduction of Similar Disulfides in HIV Gp120. Int J Biochem Cell Biol (2012) 44(3):556–62. doi: 10.1016/j.biocel.2011.12.015
138. Stantchev TS, Paciga M, Lankford CR, Schwartzkopff F, Broder CC, Clouse KA. Cell-Type Specific Requirements for Thiol/Disulfide Exchange During HIV-1 Entry and Infection. Retrovirology (2012) 9:97. doi: 10.1186/1742-4690-9-97
139. Fraser J, Boo I, Poumbourios P, Drummer HE. Hepatitis C Virus (HCV) Envelope Glycoproteins E1 and E2 Contain Reduced Cysteine Residues Essential for Virus Entry. J Biol Chem (2011) 286(37):31984–92. doi: 10.1074/jbc.M111.269605
140. Wahid A, Helle F, Descamps V, Duverlie G, Penin F, Dubuisson J. Disulfide Bonds in Hepatitis C Virus Glycoprotein E1 Control the Assembly and Entry Functions of E2 Glycoprotein. J Virol (2013) 87(3):1605–17. doi: 10.1128/JVI.02659-12
141. McCaffrey K, Boo I, Tewierek K, Edmunds ML, Poumbourios P, Drummer HE. Role of Conserved Cysteine Residues in Hepatitis C Virus Glycoprotein E2 Folding and Function. J Virol (2012) 86(7):3961–74. doi: 10.1128/JVI.05396-11
142. Sikdar S, Banerjee M, Vemparala S. Role of Disulphide Bonds in Membrane Partitioning of a Viral Peptide. J Membr Biol (2022) 255(2–3):129–142. doi: 10.1007/s00232-022-00218-0
143. Locker JK, Griffiths G. An Unconventional Role for Cytoplasmic Disulfide Bonds in Vaccinia Virus Proteins. J Cell Biol (1999) 144(2):267–79. doi: 10.1083/jcb.144.2.267
144. Moyer CL, Nemerow GR. Disulfide-Bond Formation by a Single Cysteine Mutation in Adenovirus Protein VI Impairs Capsid Release and Membrane Lysis. Virology (2012) 428(1):41–7. doi: 10.1016/j.virol.2012.03.024
145. Diwaker D, Mishra KP, Ganju L, Singh SB. Protein Disulfide Isomerase Mediates Dengue Virus Entry in Association With Lipid Rafts. Viral Immunol (2015) 28(3):153–60. doi: 10.1089/vim.2014.0095
146. Wan SW, Lin CF, Lu YT, Lei HY, Anderson R, Lin YS. Endothelial Cell Surface Expression of Protein Disulfide Isomerase Activates β1 and β3 Integrins and Facilitates Dengue Virus Infection. J Cell Biochem (2012) 113(5):1681–91. doi: 10.1002/jcb.24037
147. Aguilar-Hernández N, Meyer L, López S, Dubois RM, Arias CF. Protein Disulfide Isomerase A4 is Involved in Genome Uncoating During Human Astrovirus Cell Entry. Viruses (2021) 13(1):53. doi: 10.3390/v13010053
148. Chamberlain N, Korwin-Mihavics BR, Nakada EM, Bruno SR, Heppner DE, Chapman DG, et al. Lung Epithelial Protein Disulfide Isomerase A3 (PDIA3) Plays an Important Role in Influenzass Infection, Inflammation, and Airway Mechanics. Redox Biol (2019) 22:101129. doi: 10.1016/j.redox.2019.101129
149. Chamberlain N, Ruban M, Mark ZF, Bruno SR, Kumar A, Chandrasekaran R, et al. Protein Disulfide Isomerase A3 Regulates Influenza Neuraminidase Activity and Influenza Burden in the Lung. Int J Mol Sci (2022) 23(3):1078. doi: 10.3390/ijms23031078
Keywords: thiol switch, disulfide bond, redox signaling, extracellular, inflammation, infection, S-glutathionylation, S-nitrosylation
Citation: Brücksken KA, Loreto Palacio P and Hanschmann E-M (2022) Thiol Modifications in the Extracellular Space—Key Proteins in Inflammation and Viral Infection. Front. Immunol. 13:932525. doi: 10.3389/fimmu.2022.932525
Received: 04 May 2022; Accepted: 23 May 2022;
Published: 27 June 2022.
Edited by:
Gaby Palmer, Université de Genève, SwitzerlandReviewed by:
Rajib Sengupta, Amity University Kolkata, IndiaCopyright © 2022 Brücksken, Loreto Palacio and Hanschmann. This is an open-access article distributed under the terms of the Creative Commons Attribution License (CC BY). The use, distribution or reproduction in other forums is permitted, provided the original author(s) and the copyright owner(s) are credited and that the original publication in this journal is cited, in accordance with accepted academic practice. No use, distribution or reproduction is permitted which does not comply with these terms.
*Correspondence: Eva-Maria Hanschmann, RXZhLU1hcmlhLkhhbnNjaG1hbm5AbWVkLnVuaS1kdWVzc2VsZG9yZi5kZQ==