- 1Department of Microbiology, Immunology and Parasitology, Faculty of Medical Sciences, Rio de Janeiro State University, Rio de Janeiro, Brazil
- 2Sorbonne Université, Centre de Recherche Saint-Antoine, Inserm, Institut Pasteur, Mucoviscidose et Bronchopathies Chroniques, Département Santé Globale, Paris, France
The opportunistic pathogen Pseudomonas aeruginosa is one of the most common agents of respiratory infections and has been associated with high morbidity and mortality rates. The ability of P. aeruginosa to cause severe respiratory infections results from the coordinated action of a variety of virulence factors that promote bacterial persistence in the lungs. Several of these P. aeruginosa virulence mechanisms are mediated by bacterial lipids, mainly lipopolysaccharide, rhamnolipid, and outer membrane vesicles. Other mechanisms arise from the activity of P. aeruginosa enzymes, particularly ExoU, phospholipase C, and lipoxygenase A, which modulate host lipid signaling pathways. Moreover, host phospholipases, such as cPLA2α and sPLA2, are also activated during the infectious process and play important roles in P. aeruginosa pathogenesis. These mechanisms affect key points of the P. aeruginosa-host interaction, such as: i) biofilm formation that contributes to bacterial colonization and survival, ii) invasion of tissue barriers that allows bacterial dissemination, iii) modulation of inflammatory responses, and iv) escape from host defenses. In this mini-review, we present the lipid-based mechanism that interferes with the establishment of P. aeruginosa in the lungs and discuss how bacterial and host lipids can impact the outcome of P. aeruginosa respiratory infections.
Introduction
Pseudomonas aeruginosa is a major etiological agent of both acute and chronic respiratory infections in immunocompromised and critically ill individuals. Several features explain the success of P. aeruginosa as an opportunistic pathogen, including the wide distribution of these bacteria in the environment (1, 2), the high frequency of multidrug-resistant strains (3–7), and the ability to produce an extensive and adaptable set of virulence factors, which are expressed depending on environmental conditions (8, 9).
In hospitalized patients, P. aeruginosa is usually associated with acute infections, representing one of the most common causes of hospital-acquired pneumonia (HAP) and the most isolated pathogen in ventilator-associated pneumonia (VAP) (10–12). Additionally, P. aeruginosa can persist in the lungs of individuals suffering from chronic respiratory diseases, such as cystic fibrosis (CF) or chronic obstructive pulmonary disease (COPD). In fact, P. aeruginosa is the most frequently detected and longest-lasting microorganism found in CF lungs, representing the main cause of morbidity and mortality for these patients (13–17).
The capacity to cause acute and chronic infections relies on the multifactorial nature of P. aeruginosa pathogenicity, which is supported by a wide range of proteins, carbohydrates, and lipids that allow colonization of abiotic surfaces and host cells, invasion of tissue barriers, killing of other bacterial species, and escape from the immune system. To highlight the role of lipids in the pathogenesis of respiratory infections caused by P. aeruginosa, this mini-review will focus on virulence mechanisms that use bacterial lipids or interfere with host lipids to favor the establishment and persistence of P. aeruginosa in the airways.
Bacterial Lipids Acting as Virulence Factors
Lipopolysaccharide (LPS)
pt?>LPS is composed of three domains: lipid A, the core oligosaccharide, and the O-antigen polysaccharide. P. aeruginosa lipid A consists of an acylated glucosamine disaccharide phosphorylated at the 1 and 4’ positions which can undergo several modifications, such as phosphorylation, hydroxylation, and addition of a palmitate acyl chain or aminoarabinose (18–22).
Lipid A is highly variable among P. aeruginosa isolates and also differs under planktonic and biofilm growth conditions (22, 23). Lipid A modifications are under the control of the two-component regulatory systems PhoP-PhoQ and PmrA-PmrB, which sense changes in environmental conditions and activate the expression of lipid A-modifying enzymes (20, 24, 25). In addition, PagL, which encodes a lipid A 3-O-deacylase, is particularly susceptible to mutations and is one of the hot spot loci detected in CF isolates (23). Mutations in PagL can lead to increased acylation of lipid A over time, with the penta-acylated lipid A seen in bacteria that initially colonize CF lungs being replaced by hexa- or, in the late stages of CF disease, hepta-acylated forms (26, 27).
During infection, lipid A modifications may confer greater resistance to cationic antimicrobial peptides or activate the inflammatory response (20, 25, 28, 29). It is interesting to note that the P. aeruginosa penta-acylated LPS binds TLR2 and is predominantly found in isolates from non-CF and early CF disease (30), whereas the hexa- and hepta-acylated forms that prevail in well-established P. aeruginosa infections, with higher acylation pattern been associated with higher CF disease severity in late stages, efficiently bind and activate the human TLR4-MD2-CD14 complex, inducing a more robust inflammatory response (31–34). Since CF individuals acquire P. aeruginosa infection from environment early in their lives, the inability to respond strongly to the penta-acylated LPS of environmental strains may facilitate the initial colonization of CF lungs by P. aeruginosa.
In mice lungs, TLR4 activation by P. aeruginosa LPS was able to induce NF-kB activation, secretion of proinflammatory cytokines and chemokines, and neutrophil recruitment, through a mechanism involving GM-CSF and the transcription factor PU.1 (35). It remains to be elucidated whether chronic exposure to P. aeruginosa lipid A contributes to CF morbidity by stimulating neutrophils to release mediators that promote lung damage or whether it induces LPS-hyporesponsiveness to reduce the inflammatory injury.
Rhamnolipids
P. aeruginosa rhamnolipids are biosurfactants that consist of a dimer of fatty acids (3-(3-hydroxyalkanoyloxy) alkanoic acids - HAA), mainly composed of 10 carbon chains, linked to one or two molecules of L-rhamnose. The biosynthesis of rhamnolipids is under the control of various transcriptional and post-transcriptional regulators, with a critical role of the Rhl quorum sensing (QS) system that directly induces the transcription of the rhlAB operon and rhlC, which encode enzymes involved in HAA production and L-rhamnose transfer (36–38).
Rhamnolipids were first detected in sputum from CF patients chronically infected with P. aeruginosa (39), although a later study showed higher levels of rhamnolipids in P. aeruginosa isolates from intermittently colonized individuals than in isolates from chronically infected CF individuals (40). Curiously, when isolates from either chronic or acute infections were compared, a positive association between rhamnolipid production and acute infection was found (41).
In the airways, rhamnolipids favor the invasion of the epithelial barrier by P. aeruginosa and reduce bacterial clearance through innate immunity. On the respiratory epithelial surface, rhamnolipids slow down ciliary beat frequency and impair mucociliary transport, thus reducing the bacterial clearance (42, 43). Rhamnolipids initially interact with the apical membrane of epithelial cells and then progressively reach the basolateral membrane, displacing ezrin and disrupting the tight junctions, thus opening a paracellular route to invading bacteria (44). In the lungs, rhamnolipids inhibit phagocytosis by macrophages (45) and induce necrosis of neutrophils (46, 47), which play a key role in the defense against P. aeruginosa.
Several other effects related to rhamnolipid production may affect the respiratory infections caused by P. aeruginosa, since rhamnolipids can modulate swarming motility, participate in biofilm architecture by promoting the maintenance of channels that diffuse nutrients and oxygen, and mediate biofilm disruption by promoting the seeding dispersal of motile bacteria (48–51). Furthermore, rhamnolipids increase the bioactivity of the Pseudomonas quinolone signal (PQS) (52), a QS signaling molecule that controls several virulence factors (53), and can be detected in the lungs of CF patients infected with P. aeruginosa (54, 55). Importantly, rhamnolipids inhibit the growth of microorganisms that colonize CF lungs along with P. aeruginosa, such as Staphylococcus aureus and Aspergillus fumigatus, conferring them a competitive advantage in this environment (56–58).
Outer Membrane Vesicles (OMVs)
Outer membrane vesicles (OMVs) are spherical nanoparticles with a lipid bilayer produced by blebbing of the bacterial outer membrane, containing a variety of lipids, sugars, DNA, RNA, and proteins. Depending on their content, which differs among P. aeruginosa strains (59, 60), OMVs can be involved in diverse biological processes, such as horizontal gene transfer (61–63), protection against phages (64), cell-cell communication (65), biofilm architecture (66, 67), antibiotic resistance (68, 69), escape from the immune system (70), and delivery of virulence factors into host cells (71).
The lipid membrane protects the vesicle content from extracellular degradative enzymes, enabling long-distance transport, and upon contact with host cells, fuses with cholesterol-rich host membrane microdomains known as lipid rafts, delivering their contents into the cell cytoplasm (72). The aminopeptidase PaAP, which is associated with the surface of OMVs from CF strains (59, 73), participates in the interaction with lung epithelial cells, optimizing the delivery of OMV content (73).
In P. aeruginosa respiratory infections, OMVs can release important virulence factors, such as the cystic CFTR inhibitory factor (Cif) (72). Cif decreases the apical membrane expression of CFTR and chloride secretion, altering mucociliary clearance (74), and inhibits TAP1, reducing MHC class I antigen presentation in the airways (75). OMVs are also associated with macrophage apoptosis (76) and can induce inflammation since they stimulate CXCL8 secretion by lung epithelial cells (59), as well as secretion of TNF-α, IL-6, MIP-2, CXCL1, CXCL-8, CCL2, IL-1β, and IFN-γ, and activation of the inflammasome in macrophages (77–79). Moreover, Park et al., 2013 showed in vivo that OMVs can cause dose-dependent pulmonary inflammation, with greater cellular recruitment and higher chemokine and cytokine secretion in mice lungs than in live bacteria (78). In contrast, release of sRNA by P. aeruginosa OMVs is associated with reduced LPS- and OMV-induced CXCL8 secretion by human airway epithelial cells along with decreased OMV-induced KC secretion in the bronchoalveolar fluid and reduced neutrophil recruitment in mouse lungs (80).
Virulence Factors Targeting Host Lipids
ExoU
ExoU, a phospholipase A2 (PLA2)-like enzyme that is injected into host cytosol by the type III secretion system machinery (81), is of special interest for acute respiratory infections caused by P. aeruginosa, since potent ExoU-mediated virulence is particularly associated with bloodstream invasion and increased morbidity and mortality in hospitalized patients, especially those suffering from HAP (70, 82–86).
ExoU and its chaperone SpcU are encoded in the PAPI-2 pathogenicity island (87–89), and are detected in about 20-40% of isolates of acute nosocomial infections, such as pneumonia and bacteremia (83–86, 90–92). A recent study performed with 243 isolates from P. aeruginosa bloodstream infection, including 50 with an exoU-positive genotype, showed that patients infected with exoU-positive strains had a higher proportion of respiratory infections, greater severity of illness, septic shock, and increased mortality compared with those infected with exoU-negative strains (85).
After injection into host cytosol, the ExoU C-terminal domain promotes localization of ExoU to the host cell membrane (93) through binding to the lipid phosphatidylinositol 4,5-bisphosphate (PI(4,5)P2) followed by conformational change and oligomerization of ExoU (94–96). Furthermore, both ubiquitin and PI(4,5)P2 binding is necessary for full ExoU PLA2 activity and cytotoxicity (96–98). Hence, although the N-terminal domain interacts with SpcU and has enzymatic activity, the C-terminal domain, which promotes ExoU-membrane lipid interaction, is also essential for ExoU-mediated virulence (81, 87, 99–102).
Animal models of acute pneumonia showed that, after infection, ExoU is rapidly expressed in mice lungs and that its levels increase over time (103). In these models, ExoU promotes a bacterial burden in the lungs, enhances dissemination of P. aeruginosa from the bloodstream to other organs, and reduces survival of infected mice (102–106).
PLA2 catalyzes the hydrolysis of the sn-2 position of membrane glycerophospholipids to release arachidonic acid (AA) and lysophospholipids, both potent lipid mediators. In the lungs, the ExoU PLA2 activity on membrane phospholipids generates free AA (107, 108) that is used to produce PGE2 (109, 110), whereas lysophospholipids (102, 110) produce PAF, which binds to PAFR in airway epithelial cells and activates NF-kB, stimulating a potent proinflammatory response characterized by secretion of CXCL8, as well its murine homologue KC, and a marked influx of neutrophils (109, 111, 112). However, ExoU kills neutrophils, as well as other phagocytic cells, causing a state of local immunosuppression that favors the persistence of ExoU+ and ExoU- bacterial strains (113–115).
Although ExoU injection causes reactive oxygen species (ROS) imbalance (116) and is cytotoxic for airway epithelial cells (102, 117), the remaining non-infected cells activate several transcriptional regulators, such as AP1 and NF-kB, modulating the host response (111, 112, 118, 119). Furthermore, the cytotoxic activity of ExoU also promotes endothelial cell damage, which is associated with ROS generation, membrane lipid peroxidation, and caspase-1 activation (107, 120). The ability to break down cellular barriers to bacterial dissemination, such as epithelium and endothelium, helps explain why ExoU is a predictor of invasive infections and has been associated with severe pneumonia followed by bacteremia and sepsis.
Phospholipase C
P. aeruginosa synthesizes three types of phospholipases C (PLCs), the hemolytic PlcH, the non-hemolytic PlcN, and PlcB. All three PLCs hydrolyze phosphatidylcholine, the main component of cell membranes and lung surfactant, as well as other phospholipids found in eukaryotic membranes: PlcH also hydrolyzes sphingomyelin, PlcN targets phosphatidylserine, and PlcB, phosphatidylethanolamine (121, 122). To reach the extracellular medium, all three P. aeruginosa PLCs are secreted by the type II secretion system. However, to be transported across the inner membrane, PlcH and PlcN use the Tat system (123) whereas PlcB uses the Sec pathway (122). Furthermore, PlcH can be delivered into airway epithelial cells by OMVs (72).
In contrast to PlcN and PlcB, the role of PlcH in P. aeruginosa respiratory infections has been studied. Both intratracheal instillation of purified PlcH from P. aeruginosa and infection with a PlcH-producing strain, but not with its PlcH-defective isogenic mutant, were able to alter the respiratory mechanics during infection, with decreased pulmonary surfactant activity and impaired lung function (124).
Moreover, hydrolysis of phosphatidylcholine and sphingomyelin by PlcH yields diacylglycerol and ceramide, which are involved in signal transduction cascades that result in cellular processes such as cell death and inflammation (125, 126). Actually, intranasal administration of P. aeruginosa PlcH increases secretion of the proinflammatory cytokines and chemokines IL-6, IL-1β, TNF-α, MIP-1α, and MIP-2, as well as cellular infiltration, in mice lungs (127).
Despite the activation of the proinflammatory response, PlcH seems to favor P. aeruginosa persistence in the lungs (128). PlcH can increase the colonization of biotic and abiotic surfaces, since it contributes to P. aeruginosa attachment to CF bronchial epithelial cells and promotes biofilm formation on plastic when bacteria are grown in lung surfactant (129). In addition, PlcH is cytotoxic to macrophages (130) and suppresses the respiratory burst activity of human neutrophils (131), thus promoting bacterial survival in the lungs. Although PlcH is also cytotoxic to endothelial cells and inhibits angiogenesis (132), its role in bloodstream invasion remains to be determined.
The role of PlcN and PlcB in the pathogenesis of P. aeruginosa respiratory infections is unclear, although some properties can contribute to successful infection. Both PlcN and PlcB participate in the formation of P. aeruginosa biofilms (133), whereas PlcB is also associated with twitching motility (122).
LoxA
Lipoxygenases play an important role in eukaryotic organisms since they metabolize polyunsaturated fatty acids (PUFAs), allowing the subsequent production of lipid mediators with strong immunomodulatory effects. Although lipoxygenases are rare in prokaryotes, Vance and colleagues reported in 2004 that P. aeruginosa secretes lipoxygenase A (LoxA), a functional homolog of the eukaryotic 15-lipoxygenase (134).
Lipoxygenase activity was detected in 34% of isolates from lungs of non-CF patients and in 18,3% of isolates from lungs of CF individuals, suggesting that LoxA may be secreted during P. aeruginosa respiratory infections (135). In vitro studies showed that, after interaction with host cell membranes and peroxidation of phospholipids, P. aeruginosa LoxA promotes biofilm growth on the surface of airway epithelial cells, helps bacterial invasion, and triggers arachidonoyl phosphatidylethanolamine-dependent ferroptosis (136–139). Furthermore, in a murine model of acute pneumonia, LoxA increased the production of the 15-LOX-dependent metabolites 13-hydroxy-octadecadienoic acid (13-HODE), 15- hydroxyeicosatetraenoic acid (15-HETE), and 17-hydroxydocosahexaenoic acid (17-HDoHE), which were then used to produce lipoxin A4 (LXA4), a bioactive lipid mediator with anti-inflammatory properties. Additionally, LoxA inhibited the secretion of the chemokines MIP-1α/CCL-3, MIP-1β/CCL-4, MIP-2/CXCL-2, CXCL-1, and KC in BALF, reduced the recruitment of inflammatory leukocytes, and promoted the persistence of P. aeruginosa in the lungs (135).
Host PLA2 Enzymes and Their Role in P. aeruginosa Infection
In addition to the PLA2 activity of P. aeruginosa ExoU, host cells also exhibit PLA2 enzymes that can mediate P. aeruginosa-induced toxicity (Figure 1). Among these enzymes, the host cytosolic PLA2α (cPLA2α), which hydrolyzes host membrane phospholipids releasing lysophospholipids and AA, plays a key role in P. aeruginosa-induced mouse mortality, mainly through cPLA2α-derived AA metabolites (141). In addition, it is likely that the accumulation of highly cytotoxic lysophospholipids, such as lysophosphatidylcholine, may participate in the deleterious effects of P. aeruginosa. This may indicate that cPLA2α represents a potentially interesting therapeutic target for the treatment of lung injury induced by P. aeruginosa infection and that a cPLA2α inhibitor can be used as a new strategy against inflammation.
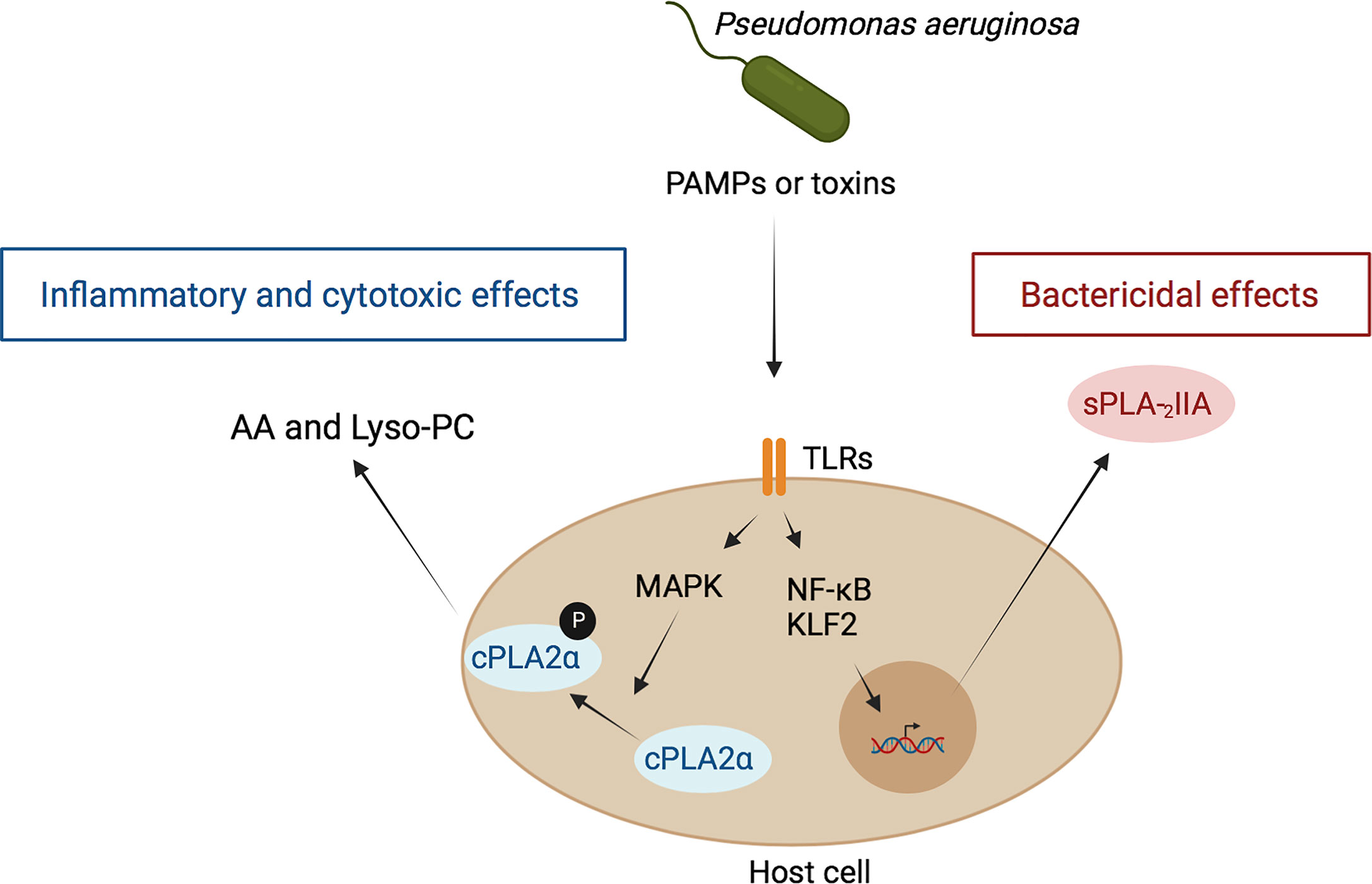
Figure 1 Role of host PLA2 in inflammation and antibacterial defense during P. aeruginosa infection. P. aeruginosa interacts with host cells through the binding of PAMPs of this bacterium, mainly LPS and flagellin to the host receptors TLR4 and TLR5, respectively, leading to NF-kB activation. In parallel, toxins, including ExoS, are also injected by this bacterium into host cells, leading to activation of the transcription factor KLF-2. Both processes result ultimately in the induction and secretion of sPLA2-IIA. Once in the extracellular media, this enzyme binds bacterial membranes and hydrolyzes their phospholipids leading to bacterial death (140). On the other hand, PAMPs stimulate cPLA2 translocation and activation via a MAPK-dependent mechanism. This leads to the hydrolysis of phospholipids of host cell membranes and subsequent release of free fatty acids, such as arachidonic acid (AA), and lysophospholipids, such as lysophosphatidyl-choline (Lyso-PC). AA is converted into pro-inflammatory eicosanoids and lyso-PC exert toxic effects on host cells (141, 142).
Conversely, the host also produces a family of secreted PLA2 (sPLA2) that play a key role in defense against invading bacteria. For example, sPLA2-IIA can kill Gram-positive bacteria at very low concentrations (below 10 ng/ml), due to the unique preference of sPLA2-IIA for anionic phospholipids, such as phosphatidylglycerol (140), the main phospholipid component of bacterial membranes. In contrast, much higher concentrations (> 10 µg/ml) of sPLA2-IIA are required for its action on host cell membranes mainly composed of phosphatidylcholine, a poor substrate for sPLA2-IIA. The ability of sPLA2-IIA to kill Gram-negative bacteria, including P. aeruginosa, depends on factors that disrupt bacterial outer membrane organization, such as the bactericidal/permeability-increasing protein (BPI), which predisposes bacterial membranes phospholipids to sPLA2-IIA attack. Additionally, sPLA2-IIA can directly kill clinical isolates of P. aeruginosa, which chronically colonizes the upper airways of CF patients, but this effect is not affected by the high salt concentrations observed in CF secretions. Studies have shown that sPLA2-IIA kills a laboratory strain of P. aeruginosa and that sPLA2-IIA transgenic mice are protected from mortality by both laboratory and clinical strains of P. aeruginosa isolated from CF patients. These findings suggest that sPLA2-IIA may play a role in host defense during episodes of pulmonary infection by P. aeruginosa in CF patients (140).
Discussion
P. aeruginosa uses multiple virulence factors to cause acute and chronic respiratory infections. As summarized in Figure 2, P. aeruginosa lipids are able to exert important effects during infection. Bacterial lipids can protect P. aeruginosa from antibiotics and phagocytosis, promote bacteria-bacteria communication, provide a competitive advantage, participate in biofilm development, and interfere with the host response. In addition, host and bacterial lipid-modifying enzymes induced during the infectious process may promote the direct lysis of membranes and manipulate eukaryotic signaling pathways, which may lead to modulation of the inflammatory response, invasion of host tissue barriers, escape from immune mechanisms, or bacterial clearance. Knowledge of lipid manipulation by P. aeruginosa that may facilitate its persistence is essential for understanding the mechanisms underlying its pathogenicity and may provide important insights to the control of P. aeruginosa infections.
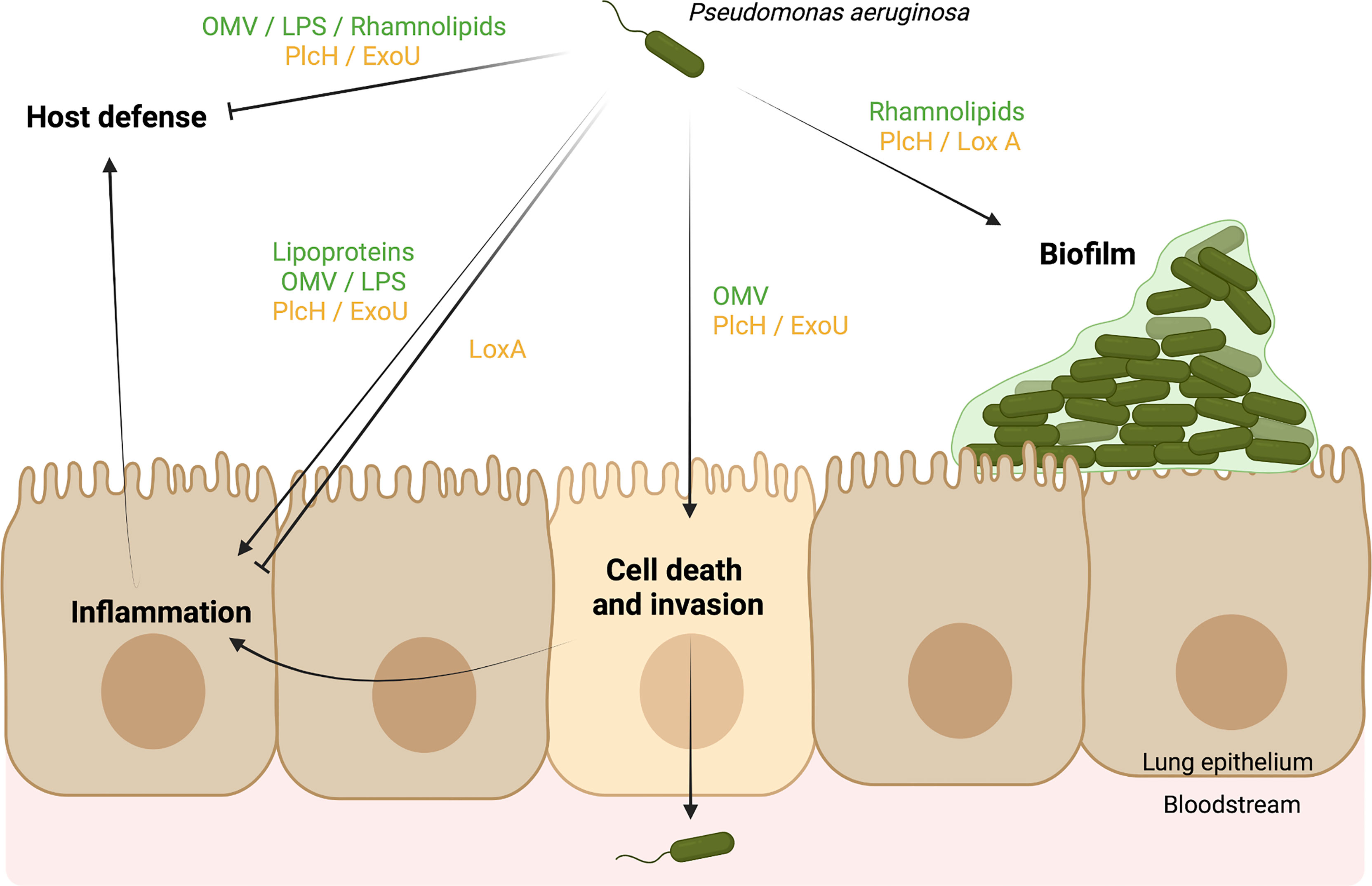
Figure 2 Scheme model of Pseudomonas aeruginosa virulence factors using a lipid-based mechanisms to cause respiratory infections. Bacterial lipids (green) and bacterial enzymes (yellow) targeting host lipids promote (→) or inhibit (˧) different biological functions during respiratory infections. They can (1) facilitate the biofilm formation of P. aeruginosa, (2) promote the death of host cells and the invasion of tissues, leading to the spread of P. aeruginosa in the bloodstream, (3) interfere with the inflammatory response, and (3) block the host defense (e.g. neutrophils, macrophages). As a result, P. aeruginosa can benefit from lipid mechanisms to persist in its host. Created in BioRender.com.
Author Contributions
PC-T, AJ, LT, and AS contributed to the writing of the manuscript. All authors contributed to the article and approved the submitted version.
Conflict of Interest
The authors declare that the research was conducted in the absence of any commercial or financial relationships that could be construed as a potential conflict of interest.
Publisher’s Note
All claims expressed in this article are solely those of the authors and do not necessarily represent those of their affiliated organizations, or those of the publisher, the editors and the reviewers. Any product that may be evaluated in this article, or claim that may be made by its manufacturer, is not guaranteed or endorsed by the publisher.
Acknowledgments
We thank the Coordenação de Aperfeiçoamento de Pessoal de Nível Superior (CAPES) for the PhD fellowship to PC-T. We also thank the Fondation Air Liquide for supporting our studies on P. aeruginosa in CF.
References
1. Aditi, Shariff M, Chhabra SK, Rahman MU. Similar Virulence Properties of Infection and Colonization Associated Pseudomonas aeruginosa. J Med Microbiol (2017) 66:1489–98. doi: 10.1099/jmm.0.000569
2. Rutherford V, Yom K, Ozer EA, Pura O, Hughes A, Murphy KR, et al. Environmental Reservoirs for Exos+ and Exou+ Strains of Pseudomonas aeruginosa. Environ Microbiol Rep (2018) 10:485–92. doi: 10.1111/1758-2229.12653
3. Obritsch MD, Fish DN, MacLaren R, Jung R. National Surveillance of Antimicrobial Resistance in Pseudomonas aeruginosa Isolates Obtained From Intensive Care Unit Patients From 1993 to 2002. Antimicrob Agents Chemother (2004) 48:4606–10. doi: 10.1128/AAC.48.12.4606-4610.2004
4. Fazeli N, Momtaz H. Virulence Gene Profiles of Multidrug-Resistant Pseudomonas aeruginosa Isolated From Iranian Hospital Infections. Iran Red Crescent Med J (2014) 16:e15722. doi: 10.5812/ircmj.15722
5. Yaghi J, Fattouh N, Akkawi C, El Chamy L, Maroun RG, Khalil G. Unusually High Prevalence of Cosecretion of Ambler Class A and B Carbapenemases and Nonenzymatic Mechanisms in Multidrug-Resistant Clinical Isolates of Pseudomonas aeruginosa in Lebanon. Microb Drug Resist (2020) 26:150–9. doi: 10.1089/mdr.2019.0040
6. Pérez A, Gato E, Pérez-Llarena J, Fernández-Cuenca F, Gude MJ, Oviaño M, et al. High Incidence of MDR and XDR Pseudomonas aeruginosa Isolates Obtained From Patients With Ventilator-Associated Pneumonia in Greece, Italy and Spain as Part of the MagicBullet Clinical Trial. J Antimicrob Chemother (2019) 74:1244–52. doi: 10.1093/jac/dkz030
7. McAulay K, Schuetz AN, Fauntleroy K, Shen L, Merveille YM, Deroncelay A, et al. Multidrug-Resistant Pseudomonas aeruginosa in Healthcare Facilities in Port-Au-Prince, Haiti. J Glob Antimicrob Resist (2021) 25:60–5. doi: 10.1016/j.jgar.2021.02.016
8. Dötsch A, Schniederjans M, Khaledi A, Hornischer K, Schulz S, Bielecka A, et al. The Pseudomonas aeruginosa Transcriptional Landscape is Shaped by Environmental Heterogeneity and Genetic Variation. mBio (2015) 6:e00749–15. doi: 10.1128/mBio.00749-15
9. Wardell SJ, Gauthier J, Martin LW, Potvin M, Brockway B, Levesque RC, et al. Genome Evolution Drives Transcriptomic and Phenotypic Adaptation in Pseudomonas aeruginosa During 20 Years of Infection. Microb Genom (2021) 11:000681. doi: 10.1099/mgen.0.000681
10. Quartin AA, Scerpella EG, Puttagunta S, Kett DH. A Comparison of Microbiology and Demographics Among Patients With Healthcare-Associated, Hospital-Acquired, and Ventilator-Associated Pneumonia: A Retrospective Analysis of 1184 Patients From a Large, International Study. BMC Infect Dis (2013) 13:561. doi: 10.1186/1471-2334-13-561
11. Walter J, Haller S, Quinten C, Kärki T, Zacher B, Eckmanns T, et al. Healthcare-Associated Pneumonia in Acute Care Hospitals in European Union/European Economic Area Countries: An Analysis of Data From a Point Prevalence Survey, 2011 to 2012. Euro Surveill (2018) 23:1700843. doi: 10.2807/1560-7917.ES.2018.23.32.1700843
12. Litwin A, Rojek S, Gozdzik W, Duszynska W. Pseudomonas aeruginosa Device Associated – Healthcare Associated Infections and Its Multidrug Resistance at Intensive Care Unit of University Hospital: Polish, 8.5-Year, Prospective, Single-Centre Study. BMC Infect Dis (2021) 21:180. doi: 10.1186/s12879-021-05883-5
13. Cox MJ, Allgaier M, Taylor B, Baek MS, Huang YJ, Daly RA, et al. Airway Microbiota and Pathogen Abundance in Age-Stratified Cystic Fibrosis Patients. PLoS One (2010) 6:e11044. doi: 10.1371/journal.pone.0011044
14. Sibley CD, Grinwis ME, Field TR, Eshaghurshan CS, Faria MM, Dowd SE, et al. Culture Enriched Molecular Profiling of the Cystic Fibrosis Airway Microbiome. PLoS One (2011) 7:e22702. doi: 10.1371/journal.pone.0022702
15. Rodrigo-Troyano A, Suarez-Cuartin G, Peiró M, Barril S, Castillo D, Sanchez-Reus F, et al. Pseudomonas aeruginosa Resistance Patterns and Clinical Outcomes in Hospitalized Exacerbations of COPD. Respirol (2016) 21:1235–42. doi: 10.1111/resp.12825
16. Choi J, Oh JY, Lee YS, Hur GY, Lee SY, Shim JJ, et al. Pseudomonas aeruginosa Infection Increases the Readmission Rate of COPD Patients. Int J Chron Obstruct Pulmon Dis (2018) 13:3077–83. doi: 10.2147/COPD.S173759
17. Cuthbertson L, Walker AW, Oliver AE, Rogers GB, Rivett DW, Hampton TH, et al. Lung Function and Microbiota Diversity in Cystic Fibrosis. Microbiome (2020) 8:45. doi: 10.1186/s40168-020-00810-3
18. Ernst RK, Moskowitz SM, Emerson JC, Kraig GM, Adams KN, Harvey MD, et al. Unique Lipid A Modifications in Pseudomonas aeruginosa Isolated From the Airways of Patients With Cystic Fibrosis. J Infect Dis (2007) 196:1088–92. doi: 10.1086/521367
19. Thaipisuttikul I, Hittle LE, Chandra R, Zangari D, Dixon CL, Garrett TA, et al. A Divergent Pseudomonas aeruginosa Palmitoyltransferase Essential for Cystic Fibrosis-Specific Lipid a. Mol Microbiol (2014) 91:158–74. doi: 10.1111/mmi.12451
20. Ernst RK, Yi EC, Guo L, Lim KB, Burns JL, Hackett M, et al. Specific Lipopolysaccharide Found in Cystic Fibrosis Airway Pseudomonas aeruginosa. Science (1999) 286:1561–65. doi: 10.1126/science.286.5444.1561
21. Nowicky EM, O’Brien J, Brodbelt JS, Trent MS. Characterization of Pseudomonas aeruginosa LpxT Reveals Dual Positional Lipid A Kinase Activity and Co-Ordinated Control of Outer Membrane Modification. Mol Microbiol (2014) 94:728–41. doi: 10.1111/mmi.12796
22. Ciornei CD, Novikov A, Beloin C, Fitting C, Caroff M, Ghigo JM, et al. Biofilm-Forming Pseudomonas aeruginosa Bacteria Undergo Lipopolysaccharide Structural Modifications and Induce Enhanced Inflammatory Cytokine Response in Human Monocytes. Innate Immun (2010) 16:288–301. doi: 10.1177/1753425909341807
23. Fischer S, Klockgether J, Gonzalez Sorribes M, Dorda M, Wiehlmann L, Tümmler B. Sequence Diversity of the Pseudomonas aeruginosa Population in Loci That Undergo Microevolution in Cystic Fibrosis Airways. Access Microbiol (2021) 3:000286. doi: 10.1099/acmi.0.000286
24. McPhee JB, Lewenza S, Hancock REW. Cationic Antimicrobial Peptides Activate a Two-Component Regulatory System, PmrA-PmrB, That Regulates Resistance to Polymyxin B and Cationic Antimicrobial Peptides in Pseudomonas aeruginosa. Mol Microbiol (2003) 50:205–17. doi: 10.1046/j.1365-2958.2003.03673.x
25. Moskowitz SM, Ernst RK, Miller SI. PmrAB, a Two-Component Regulatory System of Pseudomonas aeruginosa That Modulates Resistance to Cationic Antimicrobial Peptides and Addition of Aminoarabinose to Lipid a. J Bacteriol (2004) 186:575–9. doi: 10.1128/JB.186.2.575-579.2004
26. Ernst RK, Adams KN, Moskowitz SM, Kraig GM, Kawasaki K, Stead CM, et al. The Pseudomonas aeruginosa Lipid A Deacylase: Selection for Expression and Loss Within the Cystic Fibrosis Airway. J Bacteriol (2006) 188:191–201. doi: 10.1128/JB.188.1.191-201.2006
27. Cigana C, Curcurù L, Leone MR, Ieranò T, Lorè NI, Bianconi I, et al. Pseudomonas aeruginosa Exploits Lipid A and Muropeptides Modification as a Strategy to Lower Innate Immunity During Cystic Fibrosis Lung Infection. PloS One (2009) 4:e8439. doi: 10.1371/journal.pone.0008439
28. Pamp SJ, Gjermansen M, Johansen HK, Tolker-Nielsen T. Tolerance to the Antimicrobial Peptide Colistin in Pseudomonas aeruginosa Biofilms Is Linked to Metabolically Active Cells, and Depends on the Pmr and mexAB-oprM Genes. Mol Microbiol (2008) 68:223–40. doi: 10.1111/j.1365-2958.2008.06152.x
29. Gellatly SL, Needham B, Madera L, Trent MS, Hancock RE. The Pseudomonas aeruginosa PhoP-PhoQ Two-Component Regulatory System Is Induced Upon Interaction With Epithelial Cells and Controls Cytotoxicity and Inflammation. Infect Immun (2012) 80:3122–31. doi: 10.1128/IAI.00382-12
30. Erridge C, Pridmore A, Eley A, Stewart J, Poxton IR. Lipopolysaccharides of Bacteroides Fragilis, Chlamydia Trachomatis and Pseudomonas aeruginosa Signal via Toll-Like Receptor 2. J Med Microbiol (2004) 53:735–40. doi: 10.1099/jmm.0.45598-0
31. Hajjar AM, Ernst RK, Tsai JH, Wilson CB, Miller SI. Human Toll-Like Receptor 4 Recognizes Host-Specific LPS Modifications. Nat Immunol (2002) 3:354–9. doi: 10.1038/ni777
32. Ernst RK, Hajjar AM, Tsai JH, Moskowitz SM, Wilson CB, Miller SI. Pseudomonas aeruginosa Lipid A Diversity and Its Recognition by Toll-Like Receptor 4. J Endotoxin Res (2003) 9:395–400. doi: 10.1177/09680519030090060201
33. SenGupta S, Hittle LE, Ernst RK, Uriarte SM, Mitchell TC. A Pseudomonas aeruginosa Hepta-Acylated Lipid A Variant Associated With Cystic Fibrosis Selectively Activates Human Neutrophils. J Leuk Biol (2016) 100:1047–59. doi: 10.1189/jlb.4VMA0316-101R
34. Hajjar AM, Ernst RK, Yi J, Yam CS, Miller SI. Expression Level of Human TLR4 Rather Than Sequence Is the Key Determinant of LPS Responsiveness. PLoS One (2017) 12:e0186308. doi: 10.1371/journal.pone.0186308
35. Berclaz PY, Carey B, Fillipi MD, Wernke-Dollries K, Geraci N, Cush S, et al. GM-CSF Regulates a PU.1-Dependent Transcriptional Program Determining the Pulmonary Response to LPS. Am J Resp Cell Mol Biol (2007) 36:114–21. doi: 10.1165/rcmb.2006-0174OC
36. Ochsner UA, Koch AK, Fiechter A, Reiser J. Isolation, Characterization, and Expression in Escherichia Coli of the Pseudomonas aeruginosa rhlAB Genes Encoding a Rhamnosyltransferase Involved in Rhamnolipid Biosurfactant Synthesis. J Bacteriol (1994) 176:2044–54. doi: 10.1128/jb.176.7.2044-2054.1994
37. Rahim R, Ochsner UA, Olvera C, Graninger M, Messner P, Lam JS, et al. Cloning and Functional Characterization of the Pseudomonas aeruginosa rhlC Gene That Encodes Rhamnosyltransferase 2, an Enzyme Responsible for Di-Rhamnolipid Biosynthesis. Mol Microbiol (2001) 40:708–18. doi: 10.1046/j.1365-2958.2001.02420.x
38. Soberón-Chávez G, González-Valdez A, Soto-Aceves MP, Cocotl-Yañez M. Rhamnolipids Produced by Pseudomonas: From Molecular Genetics to the Market. Microb Biotechnol (2021) 14:136–46. doi: 10.1111/1751-7915.13700
39. Kownatzki R, Tümmler B, Döring G. Rhamnolipid of Pseudomonas aeruginosa in Sputum of Cystic Fibrosis Patients. Lancet (1987) 1:1026–7. doi: 10.1016/S0140-6736(87)92286-0
40. Bjarnsholt T, Jensen PØ, Jakobsen TH, Phipps R, Nielsen AK, Rybtke MT, et al. Scandinavian Cystic Fibrosis Study Consortium. Quorum Sensing and Virulence of Pseudomonas aeruginosa During Lung Infection of Cystic Fibrosis Patients. PLoS One (2010) 5:e10115. doi: 10.1371/journal.pone.0010115
41. Laabei M, Jamieson WD, Lewis SE, Diggle SP, Jenkins ATA. A New Assay for Rhamnolipid Detection-Important Virulence Factors of Pseudomonas aeruginosa. Appl Microbiol Biotechnol (2014) 98:7199–209. doi: 10.1007/s00253-014-5904-3
42. Read RC, Roberts P, Munro N, Rutman A, Hastie A, Shryock T, et al. Effect of Pseudomonas aeruginosa Rhamnolipids on Mucociliary Transport and Ciliary Beating. J Appl Physiol (1992) 72:2271–7. doi: 10.1152/jappl.1992.72.6.2271
43. Kanthakumar K, Taylor GW, Cundell DR, Dowling RB, Johnson M, Cole PJ, et al. The Effect of Bacterial Toxins on Levels of Intracellular Adenosine Nucleotides and Human Ciliary Beat Frequency. Pulm Pharmacol Ther (1996) 9:223–30. doi: 10.1006/pulp.1996.0028
44. Zulianello L, Canard C, Koühler T, Caille D, Lacroix JS, Meda P. Rhamnolipids are Virulence Factors That Promote Early Infiltration of Primary Human Airway Epithelia by Pseudomonas aeruginosa. Infect Immun (2006) 74:3134–47. doi: 10.1128/IAI.01772-05
45. McClure CD, Schiller NL. Inhibition of Macrophage Phagocytosis by Pseudomonas aeruginosa Rhamnolipids In Vitro and In Vivo. Curr Microbiol (1996) 33:109–17. doi: 10.1007/s002849900084
46. Jensen PØ, Bjarnsholt T, Phipps R, Rasmussen TB, Calum H, Christoffersen L, et al. Rapid Necrotic Killing of Polymorphonuclear Leukocytes Is Caused by Quorum-Sensing-Controlled Production of Rhamnolipid by Pseudomonas aeruginosa. Microbiol (2007) 153:1329–38. doi: 10.1099/mic.0.2006/003863-0
47. Van Gennip M, Christense LD, Alhede M, Phipps R, Jensen PØ, Christophersen L, et al. Inactivation of the rhlA Gene in Pseudomonas aeruginosa Prevents Rhamnolipid Production, Disabling the Protection Against Polymorphonuclear Leukocytes. Apmis (2009) 117:537–46. doi: 10.1111/j.1600-0463.2009.02466.x
48. Davey ME, Caiazza NC, O'Toole GA. Rhamnolipid Surfactant Production Affects Biofilm Architecture in Pseudomonas aeruginosa PAO1. J Bacteriol (2003) 185:1027–36. doi: 10.1128/JB.185.3.1027-1036.2003
49. Caiazza NC, Shanks RM, O'Toole GA. Rhamnolipids Modulate Swarming Motility Patterns of Pseudomonas aeruginosa. J Bacteriol (2005) 187:7351–61. doi: 10.1128/JB.187.21.7351-7361.2005
50. Tremblay J, Richardson AP, Lépine F, Déziel E. Self-Produced Extracellular Stimuli Modulate the Pseudomonas aeruginosa Swarming Motility Behaviour. Environ Microbiol (2007) 9:2622–30. doi: 10.1111/j.1462-2920.2007.01396.x
51. Nickzad A, Déziel E. The Involvement of Rhamnolipids in Microbial Cell Adhesion and Biofilm Development - an Approach for Control? Lett Appl Microbiol (2014) 58:447–53. doi: 10.1111/lam.12211
52. Calfee MW, Shelton JG, McCubrey JA, Pesci EC. Solubility and Bioactivity of the Pseudomonas Quinolone Signal are Increased by a Pseudomonas aeruginosa-Produced Surfactant. Infect Immun (2005) 73:878–82. doi: 10.1128/IAI.73.2.878-882.2005
53. Lin J, Cheng J, Wang Y, Shen X. The Pseudomonas Quinolone Signal (PQS): Not Just for Quorum Sensing Anymore. Front Cell Infect Microbiol (2018) 8. doi: 10.3389/fcimb.2018.00230
54. Guina T, Purvine SO, Yi EC, Eng J, Goodlett DR, Aebersold R, et al. Quantitative Proteomic Analysis Indicates Increased Synthesis of a Quinolone by Pseudomonas aeruginosa Isolates From Cystic Fibrosis Airways. Proc Natl Acad Sci USA (2003) 100:2771–6. doi: 10.1073/pnas.0435846100
55. Barr HL, Halliday N, Cámara M, Barrett DA, Williams P, Forrester DL, et al. Pseudomonas aeruginosa Quorum Sensing Molecules Correlate With Clinical Status in Cystic Fibrosis. Eur Resp J (2015) 46:1046–54. doi: 10.1183/09031936.00225214
56. Radlinski L, Rowe SE, Kartchner LB, Maile R, Cairns BA, Vitko NP, et al. Pseudomonas aeruginosa Exoproducts Determine Antibiotic Efficacy Against Staphylococcus Aureus. PLoS Biol (2017) 15:e2003981. doi: 10.1371/journal.pbio.2003981
57. Gdaniec BG, Bonini F, Prodon F, Braschler T, Köhler T, van Delden C. Pseudomonas aeruginosa Rhamnolipid Micelles Deliver Toxic Metabolites and Antibiotics Into Staphylococcus Aureus. iScience (2021) 25:103669. doi: 10.1016/j.isci.2021.103669
58. Sass G, Nazik H, Chatterjee P, Shrestha P, Groleau MC, Déziel E, et al. Altered Pseudomonas Strategies to Inhibit Surface Aspergillus Colonies. Front Cell Infect Microbiol (2021) 11. doi: 10.3389/fcimb.2021.734296
59. Bauman SJ, Kuehn MJ. Purification of Outer Membrane Vesicles From Pseudomonas aeruginosa and Their Activation of an IL-8 Response. Microbes Infect (2006) 8:2400–8. doi: 10.1016/j.micinf.2006.05.001
60. Metruccio MME, Evans DJ, Gabriel MM, Kadurugamuwa JL, Fleiszig SMJ. Pseudomonas aeruginosa Outer Membrane Vesicles Triggered by Human Mucosal Fluid and Lysozyme Can Prime Host Tissue Surfaces for Bacterial Adhesion. Front Microbiol (2016) 7:871. doi: 10.3389/fmicb.2016.00871
61. Ciofu O, Beveridge TJ, Kadurugamuwa J, Walther-Rasmussen J, Hoiby N. Chromosomal Beta-Lactamase Is Packaged Into Membrane Vesicles and Secreted From Pseudomonas aeruginosa. J Antimicrob Chemother (2000) 45:9–13. doi: 10.1093/jac/45.1.9
62. Renelli M, Matias V, Lo RY, Beveridge TJ. DNA-Containing Membrane Vesicles of Pseudomonas aeruginosa PAO1 and Their Genetic Transformation Potential. Microbiol (2004) 150:2161–9. doi: 10.1099/mic.0.26841-0
63. Bitto NJ, Chapman R, Pidot S, Costin A, Lo C, Choi J, et al. Bacterial Membrane Vesicles Transport Their DNA Cargo Into Host Cells. Sci Rep (2017) 7:7072. doi: 10.1038/s41598-017-07288-4
64. Augustyniak D, Olszak T, Drulis-Kawa Z. Outer Membrane Vesicles (OMVs) of Pseudomonas aeruginosa Provide Passive Resistance But Not Sensitization to LPS-Specific Phages. Viruses (2022) 14:121. doi: 10.3390/v14010121
65. Zhao Z, Wang L, Miao J, Zhang Z, Ruan J, Xu L, et al. Regulation of the Formation and Structure of Biofilms by Quorum Sensing Signal Molecules Packaged in Outer Membrane Vesicles. Sci Total Environ (2022) 806:151403. doi: 10.1016/j.scitotenv.2021.151403
66. Esoda CN, Kuehn MJ. Pseudomonas aeruginosa Leucine Aminopeptidase Influences Early Biofilm Composition and Structure via Vesicle-Associated Antibiofilm Activity. mBio (2019) 10:e02548–19. doi: 10.1128/mBio.02548-19
67. Cooke AC, Florez C, Dunshee EB, Lieber AD, Terry ML, Light CJ, et al. Pseudomonas Quinolone Signal-Induced Outer Membrane Vesicles Enhance Biofilm Dispersion in Pseudomonas aeruginosa. mSphere (2020) 5:e01109–20. doi: 10.1128/mSphere.01109-20
68. López C, Ayala JA, Bonomo RA, González LJ, Vila AJ. Protein Determinants of Dissemination and Host Specificity of Metallo-β-Lactamases. Nat Commun (2019) 10:1–11. doi: 10.1038/s41467-019-11615-w
69. Martínez MMB, Bonomo RA, Vila AJ, Maffía PC, González LJ. On the Offensive: The Role of Outer Membrane Vesicles in the Successful Dissemination of New Delhi Metallo-β-Lactamase (NDM-1). mBio (2021) 12:e0183621. doi: 10.1128/mBio.01836-21
70. Wareham DW, Curtis MA. A Genotypic and Phenotypic Comparison of Type III Secretion Profiles of Pseudomonas aeruginosa Cystic Fibrosis and Bacteremia Isolates. Int J Med Microbiol (2007) 297:227–34. doi: 10.1016/j.ijmm.2007.02.004
71. Paulsson M, Che KF, Ahl J, Tham J, Sandblad L, Smith ME, et al. Bacterial Outer Membrane Vesicles Induce Vitronectin Release Into the Bronchoalveolar Space Conferring Protection From Complement-Mediated Killing. Front Microbiol (2018) 9:1559. doi: 10.3389/fmicb.2018.01559
72. Bomberger JM, MacEachran DP, Coutermarsh BA, Ye S, O'Toole GA, Stanton BA. Long-Distance Delivery of Bacterial Virulence Factors by Pseudomonas aeruginosa Outer Membrane Vesicles. PloS Pathog (2009) 5:e1000382. doi: 10.1371/journal.ppat.1000382
73. Bauman SJ, Kuehn MJ. Pseudomonas aeruginosa Vesicles Associate With and are Internalized by Human Lung Epithelial Cells. BMC Microbiol (2009) 9:26. doi: 10.1186/1471-2180-9-26
74. MacEachran DP, Ye S, Bomberger JM, Hogan DA, Swiatecka-Urban A, Stanton BA, et al. The Pseudomonas aeruginosa Secreted Protein PA2934 Decreases Apical Membrane Expression of the Cystic Fibrosis Transmembrane Conductance Regulator. Infect Immun (2007) 75:3902–12. doi: 10.1128/IAI.00338-07
75. Bomberger JM, Ely KH, Bangia N, Ye S, Green KA, Green WR, et al. Pseudomonas aeruginosa Cif Protein Enhances the Ubiquitination and Proteasomal Degradation of the Transporter Associated With Antigen Processing (TAP) and Reduces Major Histocompatibility Complex (MHC) Class I Antigen Presentation. J Biol Chem (2014) 289:152–62. doi: 10.1074/jbc.M113.459271
76. Deo P, Chow SH, Han ML, Speir M, Huang C, Schittenhelm RB, et al. Mitochondrial Dysfunction Caused by Outer Membrane Vesicles From Gram-Negative Bacteria Activates Intrinsic Apoptosis and Inflammation. Nat Microbiol (2020) 5:1418–27. doi: 10.1038/s41564-020-0773-2
77. Ellis TN, Leiman SA, Kuehn MJ. Naturally Produced Outer Membrane Vesicles From Pseudomonas aeruginosa Elicit a Potent Innate Immune Response via Combined Sensing of Both Lipopolysaccharide and Protein Components. Infect Immun (2010) 78:3822–31. doi: 10.1128/IAI.00433-10
78. Park KS, Lee J, Jang SC, Kim SR, Jang MH, Lötvall J, et al. Pulmonary Inflammation Induced by Bacteria-Free Outer Membrane Vesicles From Pseudomonas aeruginosa. Am J Respir Cell Mol Biol (2013) 49:637–45. doi: 10.1165/rcmb.2012-0370OC
79. Bitto NJ, Baker PJ, Dowling JK, Wray-McCann G, De Paoli A, Tran LS, et al. Membrane Vesicles From Pseudomonas aeruginosa Activate the Noncanonical Inflammasome Through Caspase-5 in Human Monocytes. Immunol Cell Biol (2018) 96:1120–30. doi: 10.1111/imcb.12190
80. Koeppen K, Hampton TH, Jarek M, Scharfe M, Gerber SA, Mielcarz DW, et al. A Novel Mechanism of Host-Pathogen Interaction Through sRNA in Bacterial Outer Membrane Vesicles. PLoS Pathog (2016) 12:e1005672. doi: 10.1371/journal.ppat.1005672
81. Sato H, Frank DW, Hillard CJ, Feix JB, Pankhaniya RR, Moriyama K, et al. The Mechanism of Action of the Pseudomonas aeruginosa-Encoded Type III Cytotoxin, ExoU. EMBO J (2003) 22:2959–69. doi: 10.1093/emboj/cdg290
82. El-Solh AA, Hattemer A, Hauser AR, Alhajhusain A, Vora H. Clinical Outcomes of Type III Pseudomonas aeruginosa Bacteremia. Crit Care Med (2012) 40:1157. doi: 10.1097/CCM.0b013e3182377906
83. Hauser AR, Cobb E, Bodí M, Mariscal D, Vallés J, Engel JN, et al. Type III Protein Secretion is Associated With Poor Clinical Outcomes in Patients With Ventilator-Associated Pneumonia Caused by Pseudomonas aeruginosa. Crit Care Med (2002) 30:521–8. doi: 10.1097/00003246-200203000-00005
84. Schulert GS, Feltman H, Rabin SD, Martin CG, Battle SE, Rello J, et al. Secretion of the Toxin ExoU Is a Marker for Highly Virulent Pseudomonas aeruginosa Isolates Obtained From Patients With Hospital-Acquired Pneumonia. J Infect Dis (2003) 188:1695–706. doi: 10.1086/379372
85. Recio R, Viedma E, González-Bodí S, Villa J, Orellana MÁ, Mancheño-Losa M, et al. Clinical and Bacterial Characteristics of Pseudomonas aeruginosa Affecting the Outcome of Patients With Bacteraemic Pneumonia. Int J Antimicrob Agents (2021) 58:106450. doi: 10.1016/j.ijantimicag.2021.106450
86. Sullivan E, Bensman J, Lou M, Agnello M, Shriner K, Wong-Beringer A. Risk of Developing Pneumonia Is Enhanced by the Combined Traits of Fluoroquinolone Resistance and Type III Secretion Virulence in Respiratory Isolates of Pseudomonas aeruginosa. Crit Care Med (2014) 42:48–56. doi: 10.1097/CCM.0b013e318298a86f
87. Finck-Barbançon V, Yahr TL, Frank DW. Identification and Characterization of SpcU, a Chaperone Required for Efficient Secretion of the ExoU Cytotoxin. J Bacteriol (1998) 180:6224–31. doi: 10.1128/JB.180.23.6224-6231.1998
88. He J, Baldini RL, Déziel E, Saucier M, Zhang Q, Liberati NT, et al. The Broad Host Range Pathogen Pseudomonas aeruginosa Strain PA14 Carries Two Pathogenicity Islands Harboring Plant and Animal Virulence Genes. Proc Natl Acad Sci U S A (2004) 101:2530–5. doi: 10.1073/pnas.0304622101
89. Halavaty AS, Borek D, Tyson GH, Veesenmeyer JL, Shuvalova L, Minasov G, et al. Structure of the Type III Secretion Effector Protein ExoU in Complex With its Chaperone SpcU. PLoS One (2012) 7:e49388. doi: 10.1371/journal.pone.0049388
90. Feltman H, Schulert G, Khan S, Jain M, Peterson L, Hauser AR. Prevalence of Type III Secretion Genes in Clinical and Environmental Isolates of Pseudomonas aeruginosa. Microbiol (Reading) (2001) 147:2659–69. doi: 10.1099/00221287-147-10-2659
91. Roy-Burman A, Savel RH, Racine S, Swanson BL, Revadigar NS, Fujimoto J, et al. Type III Protein Secretion is Associated With Death in Lower Respiratory and Systemic Pseudomonas aeruginosa Infections. J Infect Dis (2001) 183:1767–74. doi: 10.1086/320737
92. Al Dawodeyah HY, Obeidat N, Abu-Qatouseh LF, Shehabi AA. Antimicrobial Resistance and Putative Virulence Genes of Pseudomonas aeruginosa Isolates From Patients With Respiratory Tract Infection. Germs (2018) 8:31–40. doi: 10.18683/germs.2018.1130
93. Rabin SD, Veesenmeyer JL, Bieging KT, Hauser AR. A C-Terminal Domain Targets the Pseudomonas aeruginosa Cytotoxin ExoU to the Plasma Membrane of Host Cells. Infect Immun (2006) 74:2552–61. doi: 10.1128/IAI.74.5.2552-2561.2006
94. Zhang A, Veesenmeyer JL, Hauser AR. Phosphatidylinositol 4,5-Bisphosphate-Dependent Oligomerization of the Pseudomonas aeruginosa Cytotoxin ExoU. Infect Immun (2017) 86:e00402–17. doi: 10.1128/IAI.00402-17
95. Springer TI, Reid TE, Gies SL, Feix JB. Interactions of the Effector ExoU From Pseudomonas aeruginosa With Short-Chain Phosphatidylinositides Provide Insights Into ExoU Targeting to Host Membranes. J Biol Chem (2019) 294:19012–21. doi: 10.1074/jbc.RA119.010278
96. Tyson GH, Halavaty AS, Kim H, Geissler B, Agard M, Satchell KJ, et al. A Novel Phosphatidylinositol 4, 5-Bisphosphate Binding Domain Mediates Plasma Membrane Localization of ExoU and Other Patatin-Like Phospholipases. J Biol Chem (2015) 290:2919–37. doi: 10.1074/jbc.M114.611251
97. Anderson DM, Schmalzer KM, Sato H, Casey M, Terhune SS, Haas AL, et al. Ubiquitin and Ubiquitin-Modified Proteins Activate the Pseudomonas aeruginosa T3SS Cytotoxin, ExoU. Mol Microbiol (2011) 82:1454–67. doi: 10.1111/j.1365-2958.2011.07904.x
98. Sato H, Frank DW. Intoxication of Host Cells by the T3SS Phospholipase ExoU: PI (4, 5) P2-Associated, Cytoskeletal Collapse and Late Phase Membrane Blebbing. PLoS One (2014) 9:7. doi: 10.1371/journal.pone.0103127
99. Phillips RM, Six DA, Dennis EA, Ghosh P. In Vivo Phospholipase Activity of the Pseudomonas aeruginosa Cytotoxin ExoU and Protection of Mammalian Cells With Phospholipase A2 Inhibitors. J Biol Chem (2003) 42:41326–32. doi: 10.1074/jbc.M302472200
100. Rabin SD, Hauser AR. Functional Regions of the Pseudomonas aeruginosa Cytotoxin ExoU. Infect Immun (2005) 1:573–82. doi: 10.1128/IAI.73.1.573-582.2005
101. Veesenmeyer JL, Howell H, Halavaty AS, Ahrens S, Anderson WF, Hauser AR. Role of the Membrane Localization Domain of the Pseudomonas aeruginosa Effector Protein ExoU in Cytotoxicity. Infect Immun (2010) 78:3346–57. doi: 10.1128/IAI.00223-10
102. Pankhaniya RR, Tamura M, Allmond LR, Moriyama K, Ajayi T, Wiener-Kronish JP, et al. Pseudomonas aeruginosa Causes Acute Lung Injury via the Catalytic Activity of the Patatin-Like Phospholipase Domain of ExoU. Crit Care Med (2004) 32:2293–9. doi: 10.1097/01.CCM.0000145588.79063.07
103. Howell HA, Logan LK, Hauser AR. Type III Secretion of ExoU is Critical During Early Pseudomonas aeruginosa Pneumonia. mBio (2013) 4:e00032–13. doi: 10.1128/mBio.00032-13
104. Finck-Barbançon V, Goranson J, Zhu L, Sawa T, Wiener-Kronish JP, Fleiszig SM, et al. ExoU Expression by Pseudomonas aeruginosa Correlates With Acute Cytotoxicity and Epithelial Injury. Mol Microbiol (1997) 25:547–57. doi: 10.1046/j.1365-2958.1997.4891851.x
105. Allewelt M, Coleman FT, Grout M, Priebe GP, Píer GB. Acquisition of Expression of the Pseudomonas aeruginosa ExoU Cytotoxin Leads to Increased Bacterial Virulence in a Murine Model of Acute Pneumonia and Systemic Spread. Infect Immun (2000) 68:3998–4004. doi: 10.1128/IAI.68.7.3998-4004.2000
106. Shaver CM, Hauser AR. Relative Contributions of Pseudomonas aeruginosa ExoU, ExoS, and ExoT to Virulence in the Lung. Infect Immun (2004) 72:6969–77. doi: 10.1128/IAI.72.12.6969-6977.2004
107. Saliba AM, De Assis MC, Nishi R, Raymond B, Marques EA, Lopes UG, et al. Implications of Oxidative Stress in the Cytotoxicity of Pseudomonas aeruginosa ExoU. Microbes Infect (2006) 8:450–9. doi: 10.1016/j.micinf.2005.07.011
108. Plotkowski MC, Brandão BA, de Assis MC, Feliciano LFP, Raymond B, Freitas C, et al. Lipid Body Mobilization in the ExoU-Induced Release of Inflammatory Mediators by Airway Epithelial Cells. Microb Pathog (2008) 45:30–7. doi: 10.1016/j.micpath.2008.01.008
109. Saliba AM, Nascimento DO, Silva MCA, Assis MC, Gayer CRM, Raymond B, et al. Eicosanoid-Mediated Proinflammatory Activity of Pseudomonas aeruginosa ExoU. Cell Microbiol (2005) 7:1811–22. doi: 10.1111/j.1462-5822.2005.00635.x
110. Tamura M, Ajayi T, Allmond LR, Moriyama K, Wiener-Kronish JP, Sawa T. Lysophospholipase A Activity of Pseudomonas aeruginosa Type III Secretory Toxin ExoU. Biochem Biophys Res Commun (2004) 316:323–31. doi: 10.1016/j.bbrc.2004.02.050
111. de Lima CDM, Calegari-Silva TC, Pereira RMS, Santos SADOL, Lopes UG, Plotkowski MCM, et al. ExoU Activates NF-κb and Increases IL-8/KC Secretion During Pseudomonas aeruginosa Infection. PloS One (2012) 7:e41772. doi: 10.1073/pnas.0304622101
112. Mallet de Lima CD, da Conceição Costa J, de Oliveira Lima Santos SA, Carvalho S, de Carvalho L, Albano RM, et al. Central Role of PAFR Signalling in ExoU-Induced NF-κ B Activation. Cell Microbiol (2014) 16:1244–54. doi: 10.1111/cmi.12280
113. Diaz MH, Shaver CM, King JD, Musunuri S, Kazzaz JA, Hauser AR. Pseudomonas aeruginosa Induces Localized Immunosuppression During Pneumonia. Infect Immun (2008) 76:4414–21. doi: 10.1128/IAI.00012-08
114. Diaz MH, Hauser AR. Pseudomonas aeruginosa Cytotoxin ExoU is Injected Into Phagocytic Cells During Acute Pneumonia. Infect Immun (2010) 78:1447–56. doi: 10.1128/IAI.01134-09
115. Czechowska K, McKeithen-Mead S, Al Moussawi K, Kazmierczak BI. Cheating by Type 3 Secretion System-Negative Pseudomonas aeruginosa During Pulmonary Infection. Proc Natl Acad Sci USA (2014) 111:7801–6. doi: 10.1073/pnas.1400782111
116. da Cunha LG, Ferreira MF, de Moraes JA, Reis PA, Castro-Faria-Neto HC, Barja-Fidalgo C, et al. ExoU-Induced Redox Imbalance and Oxidative Stress in Airway Epithelial Cells During Pseudomonas aeruginosa Pneumosepsis. Med Microbiol Immunol (2015) 204:673–80. doi: 10.1007/s00430-015-0418-x
117. Torres IM, Demirdjian S, Vargas J, Goodale BC, Berwin B. Acidosis Increases the Susceptibility of Respiratory Epithelial Cells to Pseudomonas aeruginosa-Induced Cytotoxicity. Am J Physiol Lung Cell Mol Physiol (2017) 313:126–37. doi: 10.1152/ajplung.00524.2016
118. McMorran B, Town L, Costelloe E, Palmer J, Engel J, Hume D, et al. Effector ExoU From the Type III Secretion System is an Important Modulator of Gene Expression in Lung Epithelial Cells in Response to Pseudomonas aeruginosa Infection. Infect Immun (2003) 71:6035–44. doi: 10.1128/IAI.71.10.6035-6044.2003
119. Cuzick A, Stirling FR, Lindsay SL, Evans TJ. The Type III Pseudomonal Exotoxin U Activates the C-Jun NH2-Terminal Kinase Pathway and Increases Human Epithelial Interleukin-8 Production. Infect Immun (2006) 74:4104–13. doi: 10.1128/IAI.02045-05
120. Hardy KS, Tuckey AN, Renema P, Patel M, Al-Mehdi AB, Spadafora D, et al. ExoU Induces Lung Endothelial Cell Damage and Activates Pro-Inflammatory Caspase-1 During Pseudomonas aeruginosa Infection. Toxins (2022) 14:152. doi: 10.3390/toxins14020152
121. Ostroff RM, Vasil AI, Vasil ML. Molecular Comparison of a Nonhemolytic and a Hemolytic Phospholipase C From Pseudomonas aeruginosa. J Bacteriol (1990) 172:5915–23. doi: 10.1128/jb.172.10.5915-5923.1990
122. Barker AP, Vasil AI, Filloux A, Ball G, Wilderman PJ, Vasil ML. A Novel Extracellular Phospholipase C of Pseudomonas aeruginosa is Required for Phospholipid Chemotaxis. Mol Microbiol (2004) 53:1089–98. doi: 10.1111/j.1365-2958.2004.04189.x
123. Voulhoux R, Ball G, Ize B, Vasil ML, Lazdunski A, Wu LF, et al. Involvement of the Twin-Arginine Translocation System in Protein Secretion via the Type II Pathway. EMBO J (2001) 20:6735–41. doi: 10.1093/emboj/20.23.6735
124. Wargo MJ, Gross MJ, Rajamani S, Allard JL, Lundblad LK, Allen GB, et al. Hemolytic Phospholipase C Inhibition Protects Lung Function During Pseudomonas aeruginosa Infection. Am J Respir Crit Care Med (2011) 184:345–54. doi: 10.1164/rccm.201103-0374OC
125. Toker A. The Biology and Biochemistry of Diacylglycerol Signalling. EMBO Rep (2005) 6:310–4. doi: 10.1038/sj.embor.7400378
126. Gomez-Muñoz A, Presa N, Gomez-Larrauri A, Rivera IG, Trueba M, Ordoñez M. Control of Inflammatory Responses by Ceramide, Sphingosine 1-Phosphate and Ceramide 1-Phosphate. Prog Lipid Res (2016) 61:51–62. doi: 10.1016/j.plipres.2015.09.002
127. Wieland CW, Siegmund B, Senaldi G, Vasil ML, Dinarello CA, Fantuzzi G. Pulmonary Inflammation Induced by Pseudomonas aeruginosa Lipopolysaccharide, Phospholipase C, and Exotoxin A: Role of Interferon Regulatory Factor 1. Infect Immun (2002) 70:1352–8. doi: 10.1128/IAI.70.3.1352-1358.2002
128. Wargo MJ. Choline Catabolism to Glycine Betaine Contributes to Pseudomonas aeruginosa Survival During Murine Lung Infection. PLoS One (2013) 8:e56850. doi: 10.1371/journal.pone.0056850
129. Jackson AA, Gross MJ, Daniels EF, Hampton TH, Hammond JH, Vallet-Gely I, et al. Anr and its Activation by PlcH Activity in Pseudomonas aeruginosa Host Colonization and Virulence. J Bacteriol (2013) 195:3093–104. doi: 10.1128/JB.02169-12
130. Kida Y, Shimizu T, Kuwano K. Cooperation Between LepA and PlcH Contributes to the In Vivo Virulence and Growth of Pseudomonas aeruginosa in Mice. Infect Immun (2011) 79:211–9. doi: 10.1128/IAI.01053-10
131. Terada LS, Johansen KA, Nowbar S, Vasil AI, Vasil ML. Pseudomonas aeruginosa Hemolytic Phospholipase C Suppresses Neutrophil Respiratory Burst Activity. Infect Immun (1999) 67:2371–6. doi: 10.1128/IAI.67.5.2371-2376.1999
132. Vasil ML, Stonehouse MJ, Vasil AI, Wadsworth SJ, Goldfine H, Bolcome IIIRE, et al. A Complex Extracellular Sphingomyelinase of Pseudomonas aeruginosa Inhibits Angiogenesis by Selective Cytotoxicity to Endothelial Cells. PloS Pathog (2009) 5:e1000420. doi: 10.1371/journal.ppat.1000420
133. Lewenza S, Charron-Mazenod L, Afroj S, van Tilburg Bernardes E. Hyperbiofilm Phenotype of Pseudomonas aeruginosa Defective for the PlcB and PlcN Secreted Phospholipases. Can J Microbiol (2017) 63:780–7. doi: 10.1139/cjm-2017-0244
134. Vance RE, Hong S, Gronert K, Serhan CN, Mekalanos JJ. The Opportunistic Pathogen Pseudomonas aeruginosa Carries a Secretable Arachidonate 15-Lipoxygenase. Proc Natl Acad Sci USA (2004) 101:2135–9. doi: 10.1073/pnas.0307308101
135. Morello E, Pérez-Berezo T, Boisseau C, Baranek T, Guillon A, Bréa D, et al. Pseudomonas aeruginosa Lipoxygenase LoxA Contributes to Lung Infection by Altering the Host Immune Lipid Signaling. Front Microbiol (2019) 10:1826. doi: 10.3389/fmicb.2019.01826
136. Garreta A, Val-Moraes SP, García-Fernández Q, Busquets M, Juan C, Oliver A, et al. Structure and Interaction With Phospholipids of a Prokaryotic Lipoxygenase From Pseudomonas aeruginosa. FASEB J (2013) 27:4811–21. doi: 10.1096/fj.13-235952
137. Deschamps JD, Ogunsola AF, Jameson JB, Yasgar A, Flitter BA, Freedman CJ, et al. Biochemical and Cellular Characterization and Inhibitor Discovery of Pseudomonas aeruginosa 15-Lipoxygenase. Biochemistry (2016) 55:3329–40. doi: 10.1021/acs.biochem.6b00338
138. Aldrovandi M, Banthiya S, Meckelmann S, Zhou Y, Heydeck D, O’Donnell VB, et al. Specific Oxygenation of Plasma Membrane Phospholipids by Pseudomonas aeruginosa Lipoxygenase Induces Structural and Functional Alterations in Mammalian Cells. Biochim Biophys Acta Mol Cell Biol Lipids (2018) 1863:152–64. doi: 10.1016/j.bbalip.2017.11.005
139. Dar HH, Tyurina YY, Mikulska-Ruminska K, Shrivastava I, Ting HC, Tyurin VA, et al. Pseudomonas aeruginosa Utilizes Host Polyunsaturated Phosphatidylethanolamines to Trigger Theft-Ferroptosis in Bronchial Epithelium. J Clin Invest (2018) 128:4639–5. doi: 10.1172/JCI99490
140. van Hensbergen VP, Wu Y, van Sorge NM, Touqui L. Type IIA Secreted Phospholipase A2 in Host Defense Against Bacterial Infections. Trends Immunol (2020) 41:313–26. doi: 10.1016/j.it.2020.02.003
141. Guillemot L, Medina M, Pernet E, Leduc D, Chignard M, Touqui L, et al. Cytosolic Phospholipase A2α Enhances Mouse Mortality Induced by Pseudomonas aeruginosa Pulmonary Infection via Interleukin 6. Biochimie (2014) 107:95–104. doi: 10.1016/j.biochi.2014.08.018
Keywords: lipid, respiratory infection, Pseudomonas aeruginosa, phospholipase, inflammation, virulence
Citation: Constantino-Teles P, Jouault A, Touqui L and Saliba AM (2022) Role of Host and Bacterial Lipids in Pseudomonas aeruginosa Respiratory Infections. Front. Immunol. 13:931027. doi: 10.3389/fimmu.2022.931027
Received: 29 April 2022; Accepted: 07 June 2022;
Published: 04 July 2022.
Edited by:
Gee W. Lau, University of Illinois at Urbana-Champaign, United StatesReviewed by:
Gloria Soberón-Chávez, National Autonomous University of Mexico, MexicoJon Audia, University of South Alabama, United States
Copyright © 2022 Constantino-Teles, Jouault, Touqui and Saliba. This is an open-access article distributed under the terms of the Creative Commons Attribution License (CC BY). The use, distribution or reproduction in other forums is permitted, provided the original author(s) and the copyright owner(s) are credited and that the original publication in this journal is cited, in accordance with accepted academic practice. No use, distribution or reproduction is permitted which does not comply with these terms.
*Correspondence: Alessandra Mattos Saliba, YWxlc3NhbmRyYS5zYWxpYmFAdWVyai5icg==