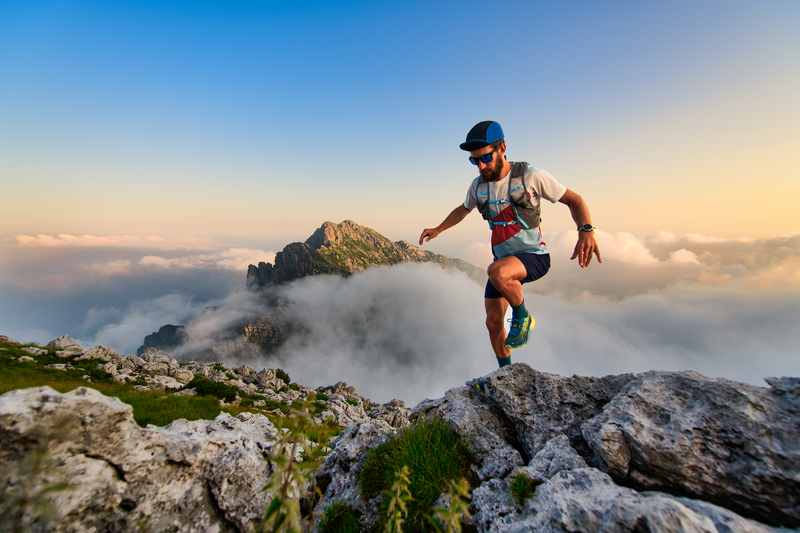
95% of researchers rate our articles as excellent or good
Learn more about the work of our research integrity team to safeguard the quality of each article we publish.
Find out more
ORIGINAL RESEARCH article
Front. Immunol. , 15 September 2022
Sec. Parasite Immunology
Volume 13 - 2022 | https://doi.org/10.3389/fimmu.2022.926446
Mucosal-associated invariant T (MAIT) cells are restricted by MR1 and are known to protect against bacterial and viral infections. Our understanding of the role of MAIT cells in parasitic infections, such as visceral leishmaniasis (VL) caused by protozoan parasites of Leishmania donovani, is limited. This study showed that in response to L. infantum, human peripheral blood MAIT cells from children with leishmaniasis produced TNF and IFN-γ in an MR1-dependent manner. The overall frequency of MAIT cells was inversely correlated with alanine aminotransferase levels, a specific marker of liver damage strongly associated with severe hepatic involvement in VL. In addition, there was a positive correlation between total protein levels and the frequency of IL-17A+ CD8+ MAIT cells, whereby reduced total protein levels are a marker of liver and kidney damage. Furthermore, the frequencies of IFN-γ+ and IL-10+ MAIT cells were inversely correlated with hemoglobin levels, a marker of severe anemia. In asymptomatic individuals and VL patients after treatment, MAIT cells also produced IL-17A, a cytokine signature associated with resistance to visceral leishmaniasis, suggesting that MAIT cells play important role in protecting against VL. In summary, these results broaden our understanding of MAIT-cell immunity to include protection against parasitic infections, with implications for MAIT-cell-based therapeutics and vaccines. At last, this study paves the way for the investigation of putative MAIT cell antigens that could exist in the context of Leishmania infection.
Mucosal-associated invariant T (MAIT) cells are a subset of unconventional T-cells that expresses an invariant TCR α-chain, composed of the variable region 1-2 and joining region 33 (TRAV1-2-TRAJ33 in humans and TRAV1-TRAJ33 in mice), while TRAJ12 and TRAJ20 are also commonly incorporated in humans. Human MAIT cells can be identified by their expression of the α-chain along with a high expression of CD161 (1–8). MAIT cells are present in most tissues (9), are abundant in the human peripheral blood, where they represent on average 3% of T-cells (10) and are the most dominant T-cell subset in the human liver, where they comprise 20-50% of all T-cells (2, 11). MAIT cells are stimulated by a modified intermediate of bacterial/fungal vitamin B2 biosynthesis presented by the MHC-I-like MHC-I related protein 1 (MR1) (12–15). Upon activation, MAIT cells rapidly secrete T helper (Th)1 and Th17 cytokines (10, 16, 17) and cytotoxic granules (18) and provide first-line surveillance against lung bacteria (19–24). Despite advances in our understanding of MAIT cell biology, little is known about the role of these cells in protozoan infectious diseases.
Leishmania is a protozoan parasite that infects vertebrates, including humans, and causes leishmaniasis. Two strains of Leishmania, L. infantum and L. donovani, can cause the most severe form of leishmaniasis, i.e., visceral leishmaniasis (VL), which affects tropical areas such as Africa, India, and Brazil (25, 26). Although 80-90% of L. infantum/donovani-infected individuals remain asymptomatic, a severe form of the disease can occur, in which patients may develop severe anemia and leukopenia, persistent fever, hepatosplenomegaly, hemorrhagic manifestations and, ultimately, sepsis (27, 28). This aggravated state is characterized by a failure to generate an immediate cellular immune response against the pathogen, leading to the accumulation of malfunctioning T-cells and an exaggerated inflammatory cytokine response (29, 30). Overall, the role of conventional T-cells in visceral leishmaniasis has been described as either dysfunctional or deleterious to disease outcome, and restoration of T-cell responses is important for human recovery and resistance to reinfection in mice (31–35).
Here, we describe MR1-dependent activation of MAIT cells in the context of Leishmania infection and shed light on the putative role of MAIT cells in possibly limiting pathology in visceral leishmaniasis. To our knowledge, this is the first report of MR1-restricted responses induced by leishmania parasites.
Peripheral blood samples from 2 to 10 years old children with visceral leishmaniasis (Leish), comprised of asymptomatic (AS) and active (VL) cases as well as non-infected endemic healthy controls (NI), were evaluated. The frequencies of total MAIT cells and CD8- and CD8+ MAIT cell subsets were assessed (Figures 1A, B). In the absence of tetramers, MAIT cells were identified based on surrogate markers (CD161highTRAV1-2+) (Figure 1A). A significant decrease in total MAIT cells in the peripheral blood of Leish patients - AS and VL subgroups - was observed compared to NI. In the VL subgroup, there was a decrease in the fraction of MAIT cells compared to the AS and NI subgroups (Figures 1B, C). Notably, the frequency of the CD8+ MAIT cell subset was reduced in VL compared to NI (Figure 1B). To determine whether age impacted the percentage of circulating MAIT cells and subsets in VL children, comparative linear regression analyses were conducted for each MAIT cell subset frequency according to age group. This analysis revealed that older children presented the most significant decrease in MAIT cell frequencies when comparing VL with AS and NI (Figure 1D).
Figure 1 Frequency and profile of MAIT cell subsets in samples from children with visceral leishmaniasis. Multicolor flow cytometric analyses of TRAV1-2+CD161high T-cells in peripheral whole blood samples of children with visceral leishmaniasis (VL = 14), asymptomatic carriers (AS = 18), and endemic non-infected controls (NI = 15). (A) Representative flow cytometric pseudocolor plots of NI, AS, and VL samples showing the gating strategy to identify TRAV1-2+CD161high T-cells (referred to here as MAIT cells) and contour plots of CD8+ versus CD8- TRAV1-2+CD161high T-cells. (B) Scatter plot distribution of individual values with the median lines of the frequencies (%) of total CD3+ cells – MAIT cells (TRAV1-2+CD161high), and CD8α-, and CD8α+ MAIT-cell subsets in NI, Leish (AS+VL), AS, and VL samples. Significant differences amongst groups (p < 0.05) based on the Mann-Whitney test for comparing two groups are indicated by connecting lines and asterisks (*p ≤ 0.05). (C) Pie charts displaying the mean proportions of MAIT cells (black), non-MAIT (TRAV1-2- CD161low/-) CD8- T-cells (light grey), non-MAIT CD8+ T-cells (dark grey), and other CD161+ T-cells (mid-grey) in peripheral blood amongst VL, AS, and NI samples. (D) Linear regression analyses for MAIT cell frequency according to the age group for VL (continuous line), AS (dashed line), and NI (dotted line), assessing the impact of age on the percentage of circulating TRAV1-2+ CD161high T-cell subsets.
To evaluate the impact of live L. infantum exposure on MAIT-cell function, including MR1 dependency, peripheral blood samples from NI and Leish (AS and VL) patients were stimulated with live L. infantum promastigotes in the presence or absence of anti-MR1 antibody, and the frequencies of proinflammatory (TNF, IFN-γ as well as IL-17A) and regulatory (IL-10) cytokine-producing MAIT cells were measured (Figure 2A). Overall, for all patient samples, similar percentages of MAIT cells produced TNF and IFN-γ upon in vitro L. infantum exposure, and cytokine production was abrogated by MR1 blockade (Figures 2A, B). MAIT cells also produced TNF and IFN-γ in an MR1-dependent manner upon in vitro L. infantum stimuli when NI, AS, and VL subgroups were evaluated individually (Figure 2C). In AS children, but not in VL or NI, MR1-independent IL-17A production was observed after L. infantum stimulation (Figure 2C). Therefore, NI, AS, and VL displayed a Type1 response, which was the major MR1-dependent response, while a Type17 MR1-independent response was observed exclusively in AS children (Figure 2C).
Figure 2 In vitro stimulation of MAIT cells from samples of children with asymptomatic and active visceral leishmaniasis. Plasma-depleted peripheral whole-blood samples of children with visceral leishmaniasis (VL = 14), asymptomatic carriers (AS = 18), and endemic non-infected controls (NI = 15), as in Figure 1, were short-term incubated with promastigotes with (Li+αMR1) or without (Li) MR1 blocking antibody and the expression of intracellular cytokine, as well as a surface activation marker, were evaluated. Significant differences amongst groups (p<0.05) based on the Mann-Whitney test for comparing two groups are indicated by connecting lines and asterisks (*p ≤ 0.05; **p ≤ 0.01). (A) Representative flow cytometry pseudocolor dot plots (large dots) showing gating of cytokine+ MAIT cells after in vitro culture – control (CC), Li and Li + αMR1. (B) Scattering distribution of individual values with the median lines showing the proportion of MAIT cells expressing TNF, IFN-γ, IL-17A, and IL-10 and CD69 expression (geometric mean fluorescence intensity-gMFI) as a marker of activation in all samples pooled together (NI, AS and VL) in CC, Li, and Li+αMR1 cultures. (C) Scattering distribution of individual values with the median lines showing the proportion of MAIT cells expressing TNF, IFN-γ, IL-17A and IL-10 in NI, AS, and VL samples for CC, Li and Li + αMR1 cultures. The number samples included in the Li + αMR1 condition for asymptomatic carriers and endemic non-infected controls groups was AS = 5 and NI = 7. (D) Scattering distribution of individual values with the median lines of CD69 expression (gMFI) ex vivo by MAIT cells, CD8α- and CD8α+ MAIT cells in NI, Leish, AS, and VL samples for control cultures (CC). (E) Representative flow cytometry histograms of CD69 expression in NI, AS, and VL samples, and box and whiskers plot with quartiles, median, and min to max values of the CD69 expression (gMFI) by MAIT cells in NI, AS, and VL samples after in vitro cultures CC, Li, and Li+αMR1.
MAIT cells, including the CD8- and CD8+ subsets, showed a decrease in CD69 expression levels in the VL group compared to the NI group, which was not observed in the AS group (Figures 2D, E). Following live L. infantum exposure, no differences in CD69 levels were observed in NI and AS groups, but an MR1-dependent upregulation of CD69 was found in the VL group (Figures 2D, E).
To understand whether the functional properties of MAIT cells in the VL group were associated with disease pathology, relevant biochemical and clinical parameters (aspartate transaminase-AST, alanine transaminase-ALT, alkaline phosphatase-AP, gamma-glutamyl transferase-GGT, total and direct bilirubin, total protein, albumin, creatinine, and hemoglobin-Hb) were evaluated (Table 1 and Figure 3A). Then, these biochemical and clinical parameters were correlated with the overall MAIT cell frequency and frequencies of various MAIT cell subsets, such as cytokine-producing MAIT cells. For this analysis, fold-changes in Leishmania-infected cultures according to the respective control culture were calculated and correlated with a range of biochemical parameters. The overall frequency of MAIT cells was negatively correlated with ALT, a classical laboratory marker of liver damage, strongly associated with severe hepatic involvement in VL (28, 36). In addition, there was a positive correlation between the total protein levels and the frequency of IL-17A+ CD8+ MAIT cells. The frequencies of IFN-γ+ and IL-10+ MAIT cells were inversely correlated with Hb levels, a marker of severe anemia (Figures 3B, C). Furthermore, VL patients presenting hepatosplenomegaly had severely reduced MAIT cell frequency and increased CD69 expression (Figure 3D). In Leishmania-infected cultures, VL patients had increased fold-change of TNF+ MAIT-cells compared to patients without those symptoms (Figure 3E). At the same time, no significant differences in the fold-change of IFN-γ+, IL-17A+ and IL-10+ MAIT cells were detected (Figure 3E). Overall, these findings reinforce the participation of MAIT cells in Leishmania-induced immunity during active disease, whereby MAIT cells appear to exert dual functions, i.e., protective and/or pathological inflammatory responses in VL patients.
Table 1 Demographic, clinical and laboratorial information of healthy endemic controls (NI) and Leishmaniasis patients classified as asymptomatic carriers (AS) and visceral leishmaniasis (VL).
Figure 3 Aspects of the MAIT cell profile are associated with biochemical and clinical findings related to the severity of visceral leishmaniasis. (A) Scattering distribution of individual values over box plots underscoring the median values, interquartile ranges along with min to max values of each biochemical liver biomarker (evaluated using standard clinical testing) in VL patients compared to reference values (light grey bars). Biochemical markers include aspartate transaminase-AST, alanine transaminase-ALT, alkaline phosphatase-AP, gamma-glutamyl transferase-GGT, total and direct bilirubin, total protein, albumin, creatinine, and hemoglobin-Hb. (B) Heatmap chart displaying the correlation between the phenotype/function of various MAIT cell subsets obtained by fold-changes of Leishmania-infected cultures and the respective control culture (Li/CC) and the biochemical liver biomarkers evaluated for each VL patient. Colors express the intensity of correlation (-1 < R < 1) as defined by the indicated color key. Black bordered squares highlight statistically significant correlations (p < 0.05). (C) Linear regression analysis of the biochemical liver biomarkers according to MAIT cell biomarkers. (D) Scattering distribution of individual values with the median lines of MAIT cell frequency amongst T-cells and CD69 expression (gMFI) in VL patients grouped based on the absence (-) and presence (+) of hepatosplenomegaly. (E) Fold-changes in cytokine expressing MAIT cells. Fold changes were calculated using results of Leishmania-infected cultures and the respective control culture (Li/CC) from VL patients grouped based on the absence (-) and presence (+) of hepatosplenomegaly. Significant differences amongst groups (p<0.05) based on Mann-Whitney test are indicated by connecting lines and asterisks (**p ≤ 0.01).
To determine the potential impact of MR1-dependent MAIT cell activation on non-MAIT T-cells, intracellular cytokine (TNF, IFN-γ, IL-17A, and IL-10) production by non-MAIT TRAV1-2- CD161low/- CD8- and CD8+ T-cells was evaluated upon stimulation with L. infantum in the absence or presence of MR1 blockade in all children (Figure 4A). Small proportions of CD8- and CD8+ non-MAIT T-cells in all children produced TNF and IFN-γ after L. infantum stimuli, which were not affected by MR1 blockade (Figure 4B). Pseudo-heatmap analysis (Supplementary Figure 1) indicated that while there was an increased number of subjects with higher proportions of proinflammatory cytokine-producing CD8- and CD8+ T-cells in AS after MR1 blocking, it correlated with fewer subjects with higher frequencies of TNF+ and IFN-γ+ MAIT-cells. Alternatively, these data may indicate that MAIT cells exert the role of the predominant proinflammatory T-cell subset upon Leishmania short-term stimuli in vitro.
Figure 4 L. infantum-induced production of proinflammatory cytokines by non-MAIT T-cells. (A) Representative flow cytometry pseudocolor plots showing gating of cytokine+ CD8α- and CD8α+ non- MAIT cells after in vitro culture – CC, Li, and Li+αMR1. (B) Scattering distribution of individual values with the median lines of frequencies (%) of intracellular cytokine-producing non-MAIT CD8- and CD8+ T-cells in all samples (NI, AS and VL), as part of the same experiment displayed in Figure 2B. Significant differences amongst groups (p<0.05) based on the Mann-Whitney test for comparing two groups are indicated by connecting lines and asterisks (*p ≤ 0.05).
Proinflammatory cytokines TNF, IFN-γ, IL-17A, IL-12, IL-15, and the regulatory cytokine IL-10 were quantified, and the results were expressed in pg/mL, as shown in Figure 5. The results demonstrate significant increase in IFN-γ, TNF, and IL-17A induced by L. infantum predominantly in NI and AS groups. In these groups, the increased IFN-γ and TNF production in vitro was abrogated by MR1 blockade, except for VL. For IL-17A, cytokine production was diminished in an MR1-dependent manner only in the AS group. No production of IL-10, IL-12, and IL-15 was observed after short-term culture with L. infantum. IL-10 and IL-15 were augmented consistently in VL compared to NI and AS, but with no alteration by L. infantum stimuli or MR1 blockade (Figure 5).
Figure 5 MR1-dependent abrogation of soluble cytokine secretion after short-term in vitro culture with L. infantum. Scattering distribution of individual values over bar charts with a median and interquartile range of TNF, IFN-γ, IL-17A, IL-10, IL-12, and IL-15 after in vitro culture – control (CC), Li, and Li + αMR1. Significant differences amongst groups (p < 0.05) based on the Mann-Whitney test for comparing two groups are indicated by connecting lines and asterisks (*p ≤ 0.05; **p ≤ 0.01).
To understand if leishmanicidal drug treatment could affect MAIT cell frequencies and function in VL children, we evaluated the patients after 1 to 2 months of treatment with Glucantime® or Amphotericin B (VL-AT) (Figures 6A, B). After in vitro stimulation with Leishmania, MAIT cells from VL-AT children produced exclusively IL-17A, suggesting that MAIT cells expressing IL-17A were selectively preserved after infection resolution (Figure 6B). Unlike IL-17A produced by MAIT cells in AS patients (Figure 2C), in VL patients upon drug treatment, IL-17A production was MR1-dependent. Additionally, MAIT cell frequencies were reduced after treatment, consistent with a tendency to decrease the absolute numbers of circulating MAIT cells (Figure 6C). In contrast, non-MAIT T-cells showed a rapid increase after treatment, mainly due to increased CD8α- non-MAIT T-cells (Figure 6C). In addition to the reduction of MAIT cells in VL-AT samples, there was a decrease in the frequency of IL-17A expressing MAIT cells ex vivo (Control culture) compared to samples before treatment (VL-BT). However, after in vitro stimulation with Leishmania, VL-AT samples presented an increased ability to produce IL-17A in response to the infection than VL-BT samples, in line with the hypothesis that IL-17A+ MAIT cells are preferentially selected after drug treatment (Figure 6D).
Figure 6 Impact of leishmanicidal therapy in MAIT cell activation in response to Leishmania. Multicolor flow cytometric analyses of TRAV1-2+CD161high T-cells in peripheral whole blood samples of children with visceral leishmaniasis before (VL-BT) and after (VL-AT) treatment with Glucantime® or Amphotericin B. (A) Representative flow cytometry pseudocolor dot plots showing gating of cytokine+ MAIT cells after in vitro culture in (A) it's Ctr in the panel, Li, and Li + αMR1. (B) Box and whisker plots with quartiles, median, and min to max values showing the proportion of MAIT cells expressing TNF, IFN-γ, IL-17A, and IL-10 in samples from VL-AT children in CC, Li, and Li + αMR1 cultures. (C) Scattering distribution of individual values with the median lines of the frequencies (%) for total CD3+ cells – MAIT cells (TRAV1-2+CD161high) and counts of MAIT cells and non-MAIT cells (TRAV1-2-CD161-), calculated based on lymphocyte differential counts from complete blood counts. (D) Scattering distribution of individual values with the median lines showing the proportion of MAIT cells expressing TNF, IFN-γ, IL-17A, and IL-10 in VL-BT and VL-AT in CC, Li and Li + αMR1 cultures. Significant differences amongst groups (p<0.05) based on the Mann-Whitney test for comparing two groups are indicated by connecting lines and asterisks (*p ≤ 0.05).
To assess whether the activation of MAIT cells by Leishmania is triggered in a time-, dose-, and MR1-restricted manner, whole blood samples from healthy adults were incubated with live Leishmania promastigotes and the expression of CD69 by MAIT cells (CD161highTRAV1-2+ cells) was monitored from 0 to 60 minutes (before 4h incubation with brefeldin) and at 100 to 0.1 multiplicity of infection (MOI). Data demonstrated that CD69 upregulation was time- and dose-dependent, reaching higher levels at 60 minutes using an MOI of 10 (Figure 7A), and CD69 upregulation and TNF production were dependent on MR1 (Figure 7B).
Figure 7 MAIT cell activation following in vitro Leishmania infection is dependent on MR1, infection time and dose. (A) Connecting line XY-graph showing the median with range (min and max) of surface-expressed CD69 (gMFI) on TRAV1-2+CD161high (MAIT) cells after in vitro stimulation with L. infantum, according to the time of infection and MOI before 4h incubation with brefeldin. MAIT cells were analyzed using plots similar to the ones shown in Figure 1. (B) Bar graphs representing the median with range (min and max) of surface CD69 expression (gMFI) and percentage of intracellular TNF-producing MAIT cells in plasma depleted whole-blood samples (healthy donors, n = 5) following in vitro L. infantum stimulation using the conditions highlighted with dashed rectangles in panel A, in the presence or absence of anti-MR1 blocking antibody (clone 26.5). The shaded grey represents the 95% confidence interval from control culture. (C) Flow cytometric dot plots of control (non-infected) and Leishmania-infected THP-1, THP-1.MR1 and THP-1.MR1.K0 cells. (D, E) Representative flow cytometric dot plots showing the gating strategy to identify MAIT cells amongst CD3+ T cells in peripheral blood mononuclear cells from healthy donors after in vitro stimulation with L. donovani based on TRAV1-2+CD161high (D) and 5-OP-RU-MR1 tetramer back-gated for TRAV1-2+ CD161high and CD161low expression before the selection of TNF-producing MAIT cells (E). (F) Bar graph displaying median with range (min and max) of the frequency of intracellular TNF-producing MAIT cells in conditions as indicated, using Leishmania donovani for infection of THP-1 cell lines, co-incubated with PBMCs samples (5 healthy donors). Significant differences (p<0.05), based on the Mann-Whitney test for comparing two groups, are highlighted by connecting lines and asterisks (*p ≤ 0.05; **p ≤ 0.01).
To confirm the MR1 dependency during activation of MAIT cells by live Leishmania promastigotes, L. donovani, a previously developed panel of THP-1 monocytic cell lines (20), was employed as antigen-presenting cells (APCs), which included wild-type, MR1 overexpressing (THP-1.MR1) and MR1 knock-out (THP-1.MR1KO) cells. THP-1 cells were co-cultured in the presence of live Leishmania promastigotes stained with an orange cell tracker to demonstrate similar levels of Leishmania infection across the THP-1 cell lines (Figure 7C). MAIT cells, identified by MR1-5-OP-RU tetramer, were also co-stained with surrogate MAIT cell markers (CD161high TRAV1-2+) (Figure 7D), suggesting that Leishmania-specific MR1-restricted MAIT cells are a subset of MR1-5-OP-RU specific T-cells (Figure 7E). After co-incubating Leishmania-infected THP-1 cells with peripheral blood mononuclear cells (PBMCs) from healthy adults, TNF production by MAIT cells (MR1-5-OP-RU tetramer+ cells) was dependent on MR1 with the highest frequency of TNF+ MAIT cells observed when co-incubated with Leishmania infected THP-1.MR1 cells as APCs (Figure 7F). Accordingly, MAIT cells responded to both L. infantum and L. donovani in an MR1-dependent manner.
MAIT cells have previously been shown to provide protective immunity against bacterial, fungal (16, 20, 22, 24, 37), and viral (38–40) infections. While their response to bacterial and fungal infections depends on the recognition of MR1 presentation of riboflavin pathway-derived antigens (14, 41), MAIT cell responses to viral agents are independent of MR1-Ag (39, 40). Here, we showed that MAIT cells also have a role in protecting from Leishmania parasites, thus broadening the spectrum of antimicrobial immune responses mediated by MAIT cells. Notably, the MAIT cell response against Leishmania was dose- and MR1-dependent. This response was characterized by TNF, IFN-γ, and IL-17A production, which was observed in intracellular staining and cytokines in culture supernatants. TNF, IFN-γ, and IL-17A have been strongly associated with protection during leishmaniasis (32, 33, 42–47). Given that Leishmania parasites do not code for riboflavin biosynthesis, this demonstrates the need for further study of new antigens in the context of Leishmania infection that might be similar between the two Leishmania species tested in this study (L. infantum and L. donovani). Cells responsive to parasite infection appeared to make up a fraction of MR1-5-OP-RU-restricted MAIT cells. The ability of the 5-OP-RU-specific MAIT TCR repertoire to cross-react with non-riboflavin pathway-derived antigens was previously observed for MAIT cells responding to small molecule drugs (48) and folate-based antigens (26). Whether there are also Leishmania responsive MR1-reactive T-cells outside the MR1-5-OP-RU-restricted repertoire remains to be investigated. Other MR1-reactive T-cells that do not respond to 5-OP-RU have previously been described (14, 15, 26). The variability observed for MAIT cell responses to Leishmania stimuli amongst individuals might imply differences in the repertoire of MAIT cells, potentially imposed by the microbiota or previous infections. The more pronounced response in AS compared to NI children to in vitro Leishmania infection suggests the pre-existence of larger numbers of Leishmania responsive clones.
Similar to previous studies on patients with acute or chronic diseases such as tuberculosis (6, 49, 50) and viral infections, including HIV, HCV, and Dengue virus (39, 40, 51), we also found a correlation between decreased frequencies of circulating MAIT cells and active disease in VL patients. Regarding the role of MAIT cells in active/chronic parasitic diseases, it was demonstrated that Plasmodium falciparum sporozoites may induce a reduction of circulating CD8-/+ MAIT cells (52). As speculated by previous studies, it is thus possible that also in active VL, MAIT cells migrate from the blood to the site of infection.
It is well established that active VL occurs following ineffective L. donovani or L. infantum control by infected cells, including macrophages, monocytes, and neutrophils (53–56). Active VL is characterized by a misbalance of TNF, IFN-γ, and regulatory IL-10, produced mainly by macrophages and regulatory T/B-cells (33, 42, 45, 57). In this study, the type-1 cytokine signature was strongly associated with MR1-dependent activation in the context of Leishmania infection in vitro. Although isotypic controls were not assessed for anti-MR1 blocking in the whole blood cultures of NI, AS, and VL due to children sampling restrictions, the results observed for the THP-1 cell lines corroborate the hypothesis of an MR1-dependent effect on MAIT cell response to Leishmania. While THP-1.MR1KO fails to induce cytokine production by MAIT cells, the over-expression of MR1 upregulates the TNF production by MAIT cells.
In addition, an exacerbated proinflammatory profile is associated with systemic inflammation in VL (29). Interestingly, MAIT cells from AS, but not NI or VL patients, also produced IL-17A in an MR1-dependent manner, which has been identified as beneficial in preventing the progression to VL (58).
In summary, distinct subsets of MAIT (Type1 and Type17) seem to have opposing roles during active L. infantum infection. MAIT cells did not produce intracellular or soluble IL-10 under live parasite stimulation. However, VL demonstrated consistently elevated levels of soluble IL-10 compared to NI and AS. IL-10 is the major suppressor of anti-Leishmania immune mechanisms in VL patients, promoting parasite persistence and susceptibility to severe disease (32, 33, 59–62). Other sources of IL-10, such as B-cells and macrophages, are possibly responsible for the total circulating IL-10 levels in VL children. In pre-exposed asymptomatic subjects, robust production of IFN-γ, TNF, and IL-17A indicates that in the absence of systemic inflammation, the two types of MAIT cell-mediated immune responses (Type1 and Type17) may synergize beneficially, counterbalanced by IL-10.
Regarding cytokines related to TCR-independent activation of MAIT cells, short-term culture with live parasite did not induce the production of IL-12 and IL-15, indicating that initial L. infantum activation of MAIT cells is strongly associated with a TCR-dependent activation pathway. These two cytokines may play a role in L. infantum-mediated MAIT cell activation in more extended incubation periods, which will be addressed in future studies.
In this study, no patient with active VL presented severe disease or mortality; yet our data suggested that MAIT cells contribute to this proinflammatory environment. In children with active VL, lower frequencies of circulating MAIT cells with increased levels of CD69 expression and higher proportions of TNF-producing cells were significantly associated with hepatosplenomegaly. The lower frequencies of MAIT cells also correlated with clinical markers of liver damage. It has been proposed that MAIT cells might contribute to acute liver injury (63). On the other hand, Jeffery et al. (64) proposed a protective role of MAIT cells in liver diseases associated with bacterial infection of different etiologies. Correlation analyses suggest that in active VL, the reduction of circulating MAIT cells can be related to an accumulation of this cell population in the liver and contribute to liver damage. More studies evaluating the profile of hepatic MAIT cells during human VL are needed to confirm this hypothesis. Moreover, the frequencies of circulating IFN-γ+ and IL-10+ MAIT cells were associated with a lower hemoglobin level, indicative of severe anemia. It has previously been shown that increased levels of circulating IL-10 in VL patients correlate with lower hemoglobin levels, which was directly associated with spleen and liver enlargement (29). However, we did not see a correlation between the frequency of IL-10-producing MAIT cells and liver/spleen enlargement. Thus, it is crucial to consider the subtle balance between immune response and clinical status in active VL.
Overall, our data expand the spectrum of the activity of MAIT cells by demonstrating its importance as the first line of defense against microbial and parasitic infections, specifically in asymptomatic visceral leishmaniasis. More studies are still needed to unveil the function of MAIT cells in the pivotal site of infection by parasites. These findings may shed light on the development of therapeutics and vaccines, an urgent necessity for controlling the spread of this fatal and highly neglected disease.
VL-infected symptomatic children (VL group) residing in the metropolitan region of Belo Horizonte, Minas Gerais, Brazil, were recruited at the João Paulo II Children’s Hospital. VL children presented symptoms of active VL, positive serology for VL diagnosis was determined by IT Leish® (Bio-Rad Laboratories, Hercules, CA, USA), and biochemical profiling was performed by conventional colorimetric methods at the hospital laboratory. Peripheral whole blood samples from 14 VL patients were collected before treatment. Asymptomatic children included in this study were positive in serological (rk39) or whole blood (buffy coat) real-time-PCR tests and lacked symptoms during clinical evaluation (within the last 3 years of follow-up before blood collection). Peripheral blood samples were collected from 18 asymptomatic children (AS group) and 15 non-infected (NI group) age and gender-matched healthy endemic controls recruited in the metropolitan region of Belo Horizonte. Demographic, clinical, and laboratory data are described in Table 1. All individuals included in this study agreed to participate and signed an informed written consent. This study followed the 466/2012 Brazilian resolution guidelines established by the National Commission on Research Ethics (CONEP) and was approved by the Ethics Committee at the René Rachou Institute (IRR/FIOCRUZ-MINAS protocol #782.042) and the João Paulo II Children’s Hospital (protocol #860.893).
Peripheral blood mononuclear cells (PBMCs) from buffy blood packs of 5 healthy donors in Australia were obtained from the Australian Red Blood Cross Service (authorized by Material Supply Agreement with The University of Melbourne), according to the University of Melbourne Ethics Committee approval (protocol #1239046.3). PBMCs were isolated using Ficoll-Paque, as previously described (14), stored in liquid nitrogen and thawed out in RPMI 10% of FCS one day before their use in activation assays.
Whole blood samples collected in EDTA-containing tubes were stained with anti-CD3-PerCP (clone: SP34-2), anti-TCRVα7.2/(TRAV1-2)-FITC (clone: 3C10), anti-CD161-BV605 (clone: DX12), anti-CD8-AF700 (clone: RPA-T8), and anti-CD69-APC-Cy7 (clone: FN50) for 20 minutes at room temperature (RT). After staining, the erythrocytes were lysed for 10 minutes using FACS lysing solution (BD Biosciences, San Jose, CA, USA). Then, cells were washed twice and resuspended in PBS. A minimum of 100,000 lymphocytes was acquired using a BD LSR Fortessa Flow Cytometer (BD Biosciences, San Jose, CA, USA) and the FACS DIVA software (BD Biosciences, San Jose, CA, USA). Data were analyzed using FlowJo software (version 9.3.2, TreeStar, San Diego, CA, USA).
L. infantum (MHOM/BR/1974/PP75) promastigotes were grown at 26°C in a biphasic medium consisting of rabbit blood agar (Novy-McNeal-Nicolle medium) and Liver Infusion Triptose medium (LIT) supplemented with 10% of heat-inactivated fetal calf serum (FCS). L. donovani (a human isolate from India) promastigotes were grown in RPMI 1640 medium (Sigma, MO, USA) supplemented with 10% of FCS at 27°C and used in the early stationary phase. Promastigotes were stained with CellTracker Orange CMRA (C34551; Thermo Fisher), as previously described (65).
Heparinized whole blood samples were centrifuged at 1,200 × g for 10 minutes at RT for plasma removal. Cells were resuspended in RPMI 1640 medium (Sigma, MO, USA) supplemented with 10% FCS at a final concentration of 1×107 cells/mL. Cell suspensions were incubated in the presence or absence of MR1 blocking (10µg/mL) antibody (clone: 26.5) prior to the addition of live promastigotes of Leishmania infantum at a 1:2 ratio (parasite:cells) to the cultures. After 60 minutes of incubation in the presence of parasites, 10μg/mL of Brefeldin A (Sigma, MO, USA) was added, and the samples were incubated for 4 hours at 37°C and 5% CO2. Cultures without MR1 blocking antibody and parasites were used as controls. Following incubation, the samples were treated with 20mmol/L of EDTA (Sigma, MO, USA) for 15 minutes at RT. Then, the cells were stained with anti-CD3-PerCP (clone: SP34-2), FITC/anti-TCRVα7.2/TRAV1-2 (clone: 3C10), BV605/anti-CD161 (clone: DX12), AF700/anti-CD8 (clone: RPA-T8), and APC-Cy7/anti-CD69 (clone: FN50) monoclonal antibodies. After 30 minutes of incubation at RT, erythrocytes were lysed for 10 minutes with FACS lysing solution (BD Biosciences, San Jose, CA, USA), and the samples were permeabilized for intracellular staining. For this, cells were incubated with PBS-P (PBS containing 0.5% BSA, 0.5% saponin and 0.1% sodium azide) for 10 minutes at RT. Following permeabilization, samples were stained with PE/anti-TNF (clone: mAb11), PE/anti-IFN-γ (clone: 4S.B3), PE/anti-IL-17A (clone: SCPL1362), and PE/anti-IL-10 (clone: JES3-9D7) monoclonal antibodies. Samples were washed with PBS-P and PBS-W (PBS containing 0.5% BSA and 0.1% sodium azide) and resuspended in PBS. A minimum of 100,000 lymphocytes were acquired using a BD LSR Fortessa Flow Cytometer and FACS DIVA software (BD Biosciences, San Jose, CA, USA). The data were analyzed by FlowJo (version 10.1, TreeStar Inc. Ashland, OR, USA).
After whole blood cell culture under different conditions (i.e., control, L. infantum-stimulated and cultures with MR1 blocking antibody), the levels of TNF, IFN-γ, IL-17A, IL-12, IL-15, and the regulatory cytokine IL-10 were quantified in the cell culture supernatants were measured by high-throughput microbeads array (Bio-Plex Pro™ Human Cytokine multiplex Assay, Bio-Rad Laboratories, Hercules, CA, USA), following the manufacturer’s instructions. The results were expressed in pg/mL according to standard curves for each immune mediator using a fifth parameter logistic fit analysis. Luminex analyses were run using the Bio-plex 200 System (Bio Rad Laboratories, CA, USA) at the Flow Cytometry core facility at FIOCRUZ-MINAS.
THP-1 cells (ATCC® TIB-202™) along with THP-1 cells over-expressing MR1 (THP-1.MR1) and THP-1 cells deficient in MR1 (THP-1.MR1K0) (20) were used as antigen-presenting cells (APC) for in vitro activation experiments. Cell lines were differentiated in 96-well plates (50,000/well) in RPMI 1640 medium (Sigma, MO, USA) supplemented with 10% FCS and phorbol myristate acetate (PMA - Sigma, MO, USA) at 50ng/mL for 2 days at 37°C and 5% CO2. Differentiated THP-1 cell lines were washed three times with RPMI 1640 medium (Sigma, MO, USA) supplemented with 10% FCS and infected with L. donovani using 10:1 multiplicities of infection (MOI) for 4 hours at 37°C and 5% CO2. After infection, cells were washed three times with RPMI 1640 medium (Sigma, MO, USA) with 10% FCS and incubated overnight at 37°C, 5% CO2. Cells were then washed three times with RPMI 1640 medium (Sigma, MO, USA) with 10% FCS and PBMCs were added to the cultures at a 10:1 ratio (PBMC : APC). Synthetic 5-OP-RU at a final concentration of 1nM was included as a positive control and prepared as previously described (73). Samples were incubated at 37°C and 5% CO2 for 1 hour prior to adding Brefeldin A (Sigma, MO, USA) (10μg/mL) and incubated for another 5 hours at 37°C and 5% CO2. The cells were washed with PBS and stained for 20 minutes at RT using Zombie Yellow Fixable Viability Kit (Biolegend), anti- PE-CF594/CD3 (clone UCHT1), BV785/anti-TRAV1-2 (clone 3C10), PEVio770/anti-CD161 (REA631) monoclonal antibodies, and human MR1–5-OP-RU tetramer, prepared in house as previously described (14, 74). Cells were then fixed with 1% Paraformaldehyde (PFA – Sigma, MO, USA) in PBS for 20 minutes at RT, washed twice with PBS and stained with APC/anti-TNF (clone: mAb11) monoclonal antibody in PBS supplemented with 0.3% Saponin overnight at 4°C. Cells were washed twice with PBS, and a minimum of 150,000 cells per sample were acquired using an BD LSR Fortessa Flow Cytometer and FACS DIVA software (BD Biosciences, San Jose, CA, USA). Data were analyzed with FlowJo (version 10.1, TreeStar Inc. Ashland, OR, USA).
Statistical analyses were performed using GraphPad Prism software (San Diego, USA, version 5.03). The non-parametric distribution of the data was confirmed by the Shapiro-Wilk test. The non-parametric Mann-Whitney test was used for comparative analyses between two groups. The Spearman test was employed for correlation analysis. Differences were considered statistically significant when the p-value was lower than 0.05. Categorical data analysis was performed to compare the expression of surface markers and the cytokine production by MAIT cell subsets, CD8- and CD8+ non-MAIT T-cells, using the global median of each biomarker as the cut-off to categorize low and high results. Heatmap analysis was employed to compile the frequency of subjects with high expression of markers (above the global median).
The raw data supporting the conclusions of this article will be made available by the authors, without undue reservation.
The studies involving human participants were reviewed and approved by Rene Rachou Institute - IRR/FIOCRUZ protocol #782.042. Written informed consent to participate in this study was provided by the participants’ legal guardian/next of kin.
VP-M and JC-d-R conceived the study and acquired funding. JC-d-R, ML, ÁLR, LB, VS, TP, ZC, and ES performed experiments in Brazil and Australia. MP-X, MSA, OM-F, AT-C, VP-M, and JC-d-R performed data analysis. ALC, MAM, FC, MB, and MC performed clinical sample acquisition and patient’s treatment and follow-up. MC, SE, MM, MT, and JM provided reagents, acquired additional funding and provided critical scientific advisory. VP, JC-d-R, and ML prepared figures and the first draft of manuscript. SE revised and modified the first drafts of manuscript. All authors revised and approved the final versions of the manuscript.
JC-d-R received financial support from the Conselho Nacional de Desenvolvimento Científico e Tecnológico –MCTI/CNPq/2014 - Grant# 458134/2014-7) and a fellowship from the Brazilian National Postdoctoral Fellowship Program (PNPD/CAPES). VP-M received financial support from the Fundação de Amparo à Pesquisa do Estado de Minas Gerais (FAPEMIG – Grant# APQ-01754-14 and PPM-00497-16). This study was financed in parts by the Coordenação de Aperfeiçoamento de Pessoal de–Nível Superior –Brasil (CAPES) - Finance Code 001. SE was supported by a fellowship from the Australian Research Council (ARC) (ARC DECRA DE170100407) and a fellowship and project grant from the Australian National Health and Medical Research Council (NHMRC fellowship APP1196881 and project grant GNT1157388). JC-d-R (Pq2), OM-F (Pq1B), AT-C (Pq1D), MC (Pq1D), and MSA (Pq2) thank CNPq for their Senior Research Productivity Fellowships. MT received financial support from the National Institutes of Health (R01-AI070258).
We thank Fernanda Vianni and Daniela Caldas Teixeira for medical assistance. We acknowledge the Melbourne Cytometry Platform (Doherty Institute node) for providing flow cytometry services.
JM, ZC, and SE are inventor of patents describing MR1 antigens and MR1 tetramers.
The remaining authors declare that the research was conducted in the absence of any commercial or financial relationships that could be construed as a potential conflict of interest.
All claims expressed in this article are solely those of the authors and do not necessarily represent those of their affiliated organizations, or those of the publisher, the editors and the reviewers. Any product that may be evaluated in this article, or claim that may be made by its manufacturer, is not guaranteed or endorsed by the publisher.
The Supplementary Material for this article can be found online at: https://www.frontiersin.org/articles/10.3389/fimmu.2022.926446/full#supplementary-material
1. Martin E, Treiner E, Duban L, Guerri L, Laude H, Toly C, et al. Stepwise development of MAIT cells in mouse and human. PloS Biol (2009) 7:525–36. doi: 10.1371/journal.pbio.1000054
2. Dusseaux M, Martin E, Serriari N, Péguillet I, Premel V, Louis D, et al. Human MAIT cells are xenobiotic-resistant, tissue-targeted, CD161hi IL-17–secreting T cells. Blood (2011) 117:1250–9. doi: 10.1182/blood-2010-08-303339
3. Porcelli S, Yockey CE, Brenner MB, Balk SP. Analysis of T cell antigen receptor (TCR) expression by human peripheral blood CD4-8- alpha/beta T cells demonstrates preferential use of several V beta genes and an invariant TCR alpha chain. J Exp Med (1993) 178:1–16. doi: 10.1084/jem.178.1.1
4. Tilloy F, Treiner E, Park S-H, Garcia C, Lemonnier F, de la Salle H, et al. An invariant T cell receptor α chain defines a novel TAP-independent major histocompatibility complex class ib–restricted α/β T cell subpopulation in mammals. J Exp Med (1999) 189:1907–21. doi: 10.1084/jem.189.12.1907
5. Reantragoon R, Corbett AJ, Sakala IG, Gherardin NA, Furness JB, Chen Z, et al. Antigen-loaded MR1 tetramers define T cell receptor heterogeneity in mucosal-associated invariant T cells. J Exp Med (2013) 210:2305–20. doi: 10.1084/jem.20130958
6. Gold MC, McLaren JE, Reistetter JA, Smyk-Pearson S, Ladell K, Swarbrick GM, et al. MR1-restricted MAIT cells display ligand discrimination and pathogen selectivity through distinct T cell receptor usage. J Exp Med (2014) 211:1601–10. doi: 10.1084/jem.20140507
7. Lepore M, Kalinichenko A, Colone A, Paleja B, Singhal A, Tschumi A, et al. Parallel T-cell cloning and deep sequencing of human MAIT cells reveal stable oligoclonal TCRβ repertoire. Nat Commun (2014) 5:3866. doi: 10.1038/ncomms4866
8. Eckle SB, Birkinshaw RW, Kostenko L, Corbett AJ, McWilliam HEG, Reantragoon R, et al. A molecular basis underpinning the T cell receptor heterogeneity of mucosal-associated invariant T cells. J Exp Med (2014) 211:1585–600. doi: 10.1084/jem.20140484
9. Kurioka A, Walker LJ, Klenerman P, Willberg CB. MAIT cells: new guardians of the liver. Clin Trans Immunol (2016) 5:e98. doi: 10.1038/cti.2016.51
10. Gherardin NA, Souter MN, Koay H, Mangas KM, Seemann T, Stinear TP, et al. Human blood MAIT cell subsets defined using MR1 tetramers. Immunol Cell Biol (2018) 96:507–25. doi: 10.1111/imcb.12021
11. Tang X, Jo J, Tan AT, Sandalova E, Chia A, Tan KC, et al. IL-7 licenses activation of human liver intrasinusoidal mucosal-associated invariant T cells. J Immunol (2013) 190:3142–52. doi: 10.4049/jimmunol.1203218
12. Treiner E, Duban L, Bahram S, Radosavljevic M, Wanner V, Tilloy F, et al. Selection of evolutionarily conserved mucosal-associated invariant T cells by MR1. Nature (2003) 422:164–9. doi: 10.1038/nature01433
13. Kjer-Nielsen L, Patel O, Corbett AJ, le Nours J, Meehan B, Liu L, et al. MR1 presents microbial vitamin b metabolites to MAIT cells. Nature (2012) 491:717–23. doi: 10.1038/nature11605
14. Corbett AJ, Eckle SBG, Birkinshaw RW, Liu L, Patel O, Mahony J, et al. T-Cell activation by transitory neo-antigens derived from distinct microbial pathways. Nature (2014) 509:361–5. doi: 10.1038/nature13160
15. Kjer-Nielsen L, Corbett AJ, Chen Z, Liu L, Mak JY, Godfrey DI, et al. An overview on the identification of MAIT cell antigens. Immunol Cell Biol (2018) 96:573–87. doi: 10.1111/imcb.12057
16. Le Bourhis L, Martin E, Péguillet I, Guihot A, Froux N, Coré M, et al. Antimicrobial activity of mucosal-associated invariant T cells. Nat Immunol (2010) 11:701–8. doi: 10.1038/ni.1890
17. Kurioka A, Jahun AS, Hannaway RF, Walker LJ, Fergusson JR, Sverremark-Ekström E, et al. Shared and distinct phenotypes and functions of human CD161++ Vα7.2+ T cell subsets. Front Immunol (2017) 8. doi: 10.3389/fimmu.2017.01031
18. Kurioka A, Ussher JE, Cosgrove C, Clough C, Fergusson JR, Smith K, et al. MAIT cells are licensed through granzyme exchange to kill bacterially sensitized targets. Mucosal Immunol (2015) 8:429–40. doi: 10.1038/mi.2014.81
19. Chen Z, Wang H, D’Souza C, Sun S, Kostenko L, Eckle SBG, et al. Mucosal-associated invariant T-cell activation and accumulation after in vivo infection depends on microbial riboflavin synthesis and co-stimulatory signals. Mucosal Immunol (2017) 10:58–68. doi: 10.1038/mi.2016.39
20. Wang H, D’Souza C, Lim XY, Kostenko L, Pediongco TJ, Eckle SBG, et al. MAIT cells protect against pulmonary legionella longbeachae infection. Nat Commun (2018) 9::3350.1-15. doi: 10.1038/s41467-018-05202-8
21. Meierovics A, Yankelevich W-JC, Cowley SC. MAIT cells are critical for optimal mucosal immune responses during in vivo pulmonary bacterial infection. Proc Natl Acad Sci (2013) 110:E3119–28. doi: 10.1073/pnas.1302799110
22. Meierovics AI, Cowley SC. MAIT cells promote inflammatory monocyte differentiation into dendritic cells during pulmonary intracellular infection. J Exp Med (2016) 213:2793–809. doi: 10.1084/jem.20160637
23. Georgel P, Radosavljevic M, Macquin C, Bahram S. The non-conventional MHC class I MR1 molecule controls infection by klebsiella pneumoniae in mice. Mol Immunol (2011) 48:769–75. doi: 10.1016/j.molimm.2010.12.002
24. Chua, Truscott SM, Eickhoff CS, Blazevic A, Hoft DF, Hansen TH, et al. Polyclonal mucosa-associated invariant T cells have unique innate functions in bacterial infection. Infect Immun (2012) 80:3256–67. doi: 10.1128/IAI.00279-12
25. Alvar J, Vélez ID, Bern C, Herrero M, Desjeux P, Cano J, et al. Leishmaniasis worldwide and global estimates of its incidence. PloS One (2012) 7:e35671. doi: 10.1371/journal.pone.0035671
26. Gherardin NA, Keller AN, Woolley RE, Le Nours J, Ritchie DS, Neeson PJ, et al. Diversity of T cells restricted by the MHC class I-related molecule MR1 facilitates differential antigen recognition. Immunity (2016) 44:32–45. doi: 10.1016/j.immuni.2015.12.005
27. Zacarias DA, Rolão N, de Pinho FA, Sene I, Silva JC, Pereira TC, et al. Causes and consequences of higher leishmania infantum burden in patients with kala-azar: a study of 625 patients. Trop Med Int Health (2017) 22:679–87. doi: 10.1111/tmi.12877
28. Costa CH, Werneck GL, Costa DL, Holanda TA, Aguiar GB, Carvalho AS, et al. Is severe visceral leishmaniasis a systemic inflammatory response syndrome? a case control study. Rev Soc Bras Med Trop (2010) 43:386–92. doi: 10.1590/S0037-86822010000400010
29. dos Santos PL, de Oliveira FA, Santos MLB, Cunha LCS, Lino MTB, de Oliveira MFS, et al. The severity of visceral leishmaniasis correlates with elevated levels of serum IL-6, IL-27 and sCD14. PloS Negl Trop Dis (2016) 10:e0004375. doi: 10.1371/journal.pntd.0004375
30. Sacks DL, S.L. L, Shrivastava SN, Blackwell J, Neva. FA. An analysis of T cell responsiveness in Indian kala-azar. J Immunol (1987) 138:908–13.
31. Potestio M, D’Agostino P, Romano GC, Milano S, Ferlazzo V, Aquino A, et al. CD4+ CCR5+ and CD4+ CCR3+ lymphocyte subset and monocyte apoptosis in patients with acute visceral leishmaniasis. Immunology (2004) 113:260–8. doi: 10.1111/j.1365-2567.2004.01948.x
32. Peruhype-Magalhaes V, Martins-Filho OA, Prata A, de Silva A. L, Rabello A, Teixeira-Carvalho A, et al. Immune response in human visceral leishmaniasis: Analysis of the correlation between innate immunity cytokine profile and disease outcome. Scandinavian J Immunol (2005) 62:487–95. doi: 10.1111/j.1365-3083.2005.01686.x
33. Peruhype-Magalhães V, Martins-Filho OA, Prata A, Silva LDA, Rabello A, Teixeira-Carvalho A, et al. Mixed inflammatory/regulatory cytokine profile marked by simultaneous raise of interferon-? and interleukin-10 and low frequency of tumour necrosis factor-a+ monocytes are hallmarks of active human visceral leishmaniasis due to leishmania chagasi infection. Clin Exp Immunol (2006) 146:124–32. doi: 10.1111/j.1365-2249.2006.03171.x
34. Bimal S, Singh SK, Sinha S, Pandey K, Sinha PK, Ranjan A, et al. Leishmania donovani: Role of CD2 on CD4+ T-cell function in visceral leishmaniasis. Exp Parasitol (2008) 118:238–46. doi: 10.1016/j.exppara.2007.08.009
35. Gautam S, Kumar R, Singh N, Singh AK, Rai M, Sacks D, et al. CD8 T cell exhaustion in human visceral leishmaniasis. J Infect Dis (2014) 209:290–9. doi: 10.1093/infdis/jit401
36. El Hag IA, Hashim FA, el Toum IA, Homeida M, el Kalifa M, el Hassan AM, et al. Liver morphology and function in visceral leishmaniasis (Kala-azar). J Clin Pathol (1994) 47:547–51. doi: 10.1136/jcp.47.6.547
37. Le Bourhis L, Dusseaux M, Bohineust A, Bessoles S, Martin E, Premel V, et al. MAIT cells detect and efficiently lyse bacterially-infected epithelial cells. PloS Pathog (2013) 9:e1003681. doi: 10.1371/journal.ppat.1003681
38. Leeansyah E, Ganesh A, Quigley MF, Sönnerborg A, Andersson J, Hunt PW, et al. Activation, exhaustion, and persistent decline of the antimicrobial MR1-restricted MAIT-cell population in chronic HIV-1 infection. Blood (2013) 121:1124–35. doi: 10.1182/blood-2012-07-445429
39. Loh L, Wang Z, Sant S, Koutsakos M, Jegaskanda S, Corbett AJ, et al. Human mucosal-associated invariant T cells contribute to antiviral influenza immunity via IL-18–dependent activation. Proc Natl Acad Sci (2016) 113:10133–8. doi: 10.1073/pnas.1610750113
40. Van Wilgenburg B, Scherwitzl I, Hutchinson EC, Leng T, Kurioka A, Kulicke C, et al. MAIT cells are activated during human viral infections. Nat Commun (2016) 7:11653. doi: 10.1038/ncomms11653
41. Eckle SB, Corbett AJ, Keller AN, Chen Z, Godfrey DI, Liu L, et al. Recognition of vitamin b precursors and byproducts by mucosal associated invariant T cells. J Biol Chem (2015) 290:30204–11. doi: 10.1074/jbc.R115.685990
42. Ansari NA, Ramesh V, Salotra P. Interferon (IFN)–γ, tumor necrosis factor–α, interleukin-6, and IFN-γ receptor 1 are the major immunological determinants associated with post–kala azar dermal leishmaniasis. J Infect Dis (2006) 194:958–65. doi: 10.1086/506624
43. Khoshdel A, Alborzi A, Rosouli M, Taheri E, Kiany S, Javadian MH, et al. Increased levels of IL-10, IL-12, and IFN- in patients with visceral leishmaniasis. Braz J Infect Dis (2009) 13:44–6. doi: 10.1590/S1413-86702009000100010
44. Pitta MGR, Romano A, Cabantous S, Henri S, Hammad A, Kouriba B, et al. IL-17 and IL-22 are associated with protection against human kala azar caused by leishmania donovani. J Clin Invest (2009) 119(8):2379–87. doi: 10.1172/JCI38813
45. Costa AS, Costa GC, Aquino DM, Mendonça VR, Barral A, Barral-Netto M, et al. Cytokines and visceral leishmaniasis: a comparison of plasma cytokine profiles between the clinical forms of visceral leishmaniasis. Memórias do Instituto Oswaldo Cruz (2012) 107:735–9. doi: 10.1590/S0074-02762012000600005
46. Nascimento MSL, Carregaro V, Lima-Júnior DS, Costa DL, Ryffel B, Duthie MS, et al. Interleukin 17A acts synergistically with interferon γ to promote protection against leishmania infantum infection. J Infect Dis (2015) 211:1015–26. doi: 10.1093/infdis/jiu531
47. Ramos PK, Carvalho KI, Rosa DS, Rodrigues AP, Lima LV, Campos MB, et al. Serum cytokine responses over the entire clinical-immunological spectrum of human leishmania (L.) infantum chagasi infection. BioMed Res Int (2016) 2016:1–8. doi: 10.1155/2016/6937980
48. Keller AN, Corbett AJ, Wubben JM, McCluskey J, Rossjohn J. MAIT cells and MR1-antigen recognition. Curr Opin Immunol (2017) 46:66–74. doi: 10.1016/j.coi.2017.04.002
49. Jiang J, Wang X, An H, Yang B, Cao Z, Liu Y, et al. MAIT cell function is modulated by PD-1 signaling in patients with active tuberculosis. Am J Respir Crit Care Med (2014) 190:140630082918004. doi: 10.1164/rccm.201401-0106OC
50. Kwon Y-S, Cho Y-N, Kim M-J, Jin H-M, Jung H-J, Kang J-H, et al. Mucosal-associated invariant T cells are numerically and functionally deficient in patients with mycobacterial infection and reflect disease activity. Tuberculosis (2015) 95:267–74. doi: 10.1016/j.tube.2015.03.004
51. Sattler A, Dang-Heine C, Reinke P, Babel N. IL-15 dependent induction of IL-18 secretion as a feedback mechanism controlling human MAIT-cell effector functions. Eur J Immunol (2015) 45:2286–98. doi: 10.1002/eji.201445313
52. Mpina M, Maurice NJ, Yajima M, Slichter CK, Miller HW, Dutta M, et al. Controlled human malaria infection leads to long-lasting changes in innate and innate-like lymphocyte populations. J Immunol (2017) 199:107–18. doi: 10.4049/jimmunol.1601989
53. Kima PE. The amastigote forms of leishmania are experts at exploiting host cell processes to establish infection and persist. Int J Parasitol (2007) 37:1087–96. doi: 10.1016/j.ijpara.2007.04.007
54. Gupta AK, Ghosh K, Palit S, Barua J, Das PK, Ukil A, et al. Leishmania donovani inhibits inflammasome-dependent macrophage activation by exploiting the negative regulatory proteins A20 and UCP2. FASEB J (2017) 31:5087–101. doi: 10.1096/fj.201700407R
55. Hurrell BP, Regli IB, Tacchini-Cottier. F. Different leishmania species drive distinct neutrophil functions. Trends Parasitol (2016) 32:392–401. doi: 10.1016/j.pt.2016.02.003
56. Gabriel C, McMaster WR, Girard D, Descoteaux. A. Leishmania donovani promastigotes evade the antimicrobial activity of neutrophil extracellular traps. J Immunol (2010) 185:4319–27. doi: 10.4049/jimmunol.1000893
57. Caldas A, Favali C, Aquino D, Vinhas V, van Weyenbergh J, Brodskyn C, et al. Balance of IL-10 and interferon-γ plasma levels in human visceral leishmaniasis: Implications in the pathogenesis. BMC Infect Dis (2005) 5:113. doi: 10.1186/1471-2334-5-113
58. Ghosh K, Sharma G, Saha A, Kar S, Das PK, Ukil A. Successful therapy of visceral leishmaniasis with curdlan involves T-helper 17 cytokines. J Infect Dis (2013) 207:1016–25. doi: 10.1093/infdis/jis771
59. Kumar R, Nylén S. Immunobiology of visceral leishmaniasis. Front Immunol (2012) 3. doi: 10.3389/fimmu.2012.00251
60. Nylén S, Sacks D. Interleukin-10 and the pathogenesis of human visceral leishmaniasis. Trends Immunol (2007) 28:378–84. doi: 10.1016/j.it.2007.07.004
61. Schaut RG, Lamb IM, Toepp AJ, Scott B, Mendes-Aguiar CO, Coutinho JF, et al. Regulatory IgD hi b cells suppress T cell function via IL-10 and PD-L1 during progressive visceral leishmaniasis. J Immunol (2016) 196:4100–9. doi: 10.4049/jimmunol.1502678
62. Bhattacharya P, Ghosh S, Ejazi SA, Rahaman M, Pandey K, Ravi Das VN, et al. Induction of IL-10 and TGFβ from CD4+CD25+FoxP3+ T cells correlates with parasite load in Indian kala-azar patients infected with leishmania donovani. PloS Negl Trop Dis (2016) 10:e0004422. doi: 10.1371/journal.pntd.0004422
63. Wigg AJ, Gunson BK, Mutimer DJ. Outcomes following liver transplantation for seronegative acute liver failure: experience during a 12-year period with more than 100 patients. Liver Transpl (2005) 11(1):27–34. doi: 10.1002/lt.20289
64. Jeffery HC, van Wilgenburg B, Kurioka A, Parekh K, Stirling K, Roberts S, et al. Biliary epithelium and liver b cells exposed to bacteria activate intrahepatic MAIT cells through MR1. J Hepatol (2016) 64:1118–27. doi: 10.1016/j.jhep.2015.12.017
Keywords: MAIT, Leishmania, anti-parasitic activity, MR1, IL-17+MAIT, IFN-γ+ MAIT, TNF+MAIT
Citation: Moreira MdL, Borges-Fernandes LO, Pascoal-Xavier MA, Ribeiro ÁL, Pereira VHS, Pediongco T, Araújo MSdS, Teixeira-Carvalho A, de Carvalho AL, Mourão MVA, Campos FA, Borges M, Carneiro M, Chen Z, Saunders E, McConville M, Tsuji M, McCluskey J, Martins-Filho OA, Eckle SBG, Coelho-dos-Reis JGA and Peruhype-Magalhães V (2022) The role of mucosal-associated invariant T cells in visceral leishmaniasis. Front. Immunol. 13:926446. doi: 10.3389/fimmu.2022.926446
Received: 22 April 2022; Accepted: 15 August 2022;
Published: 15 September 2022.
Edited by:
Brian S. Sheridan, Stony Brook University, United StatesReviewed by:
Kensuke Shibata, Yamaguchi University, JapanCopyright © 2022 Moreira, Borges-Fernandes, Pascoal-Xavier, Ribeiro, Pereira, Pediongco, Araújo, Teixeira-Carvalho, de Carvalho, Mourão, Campos, Borges, Carneiro, Chen, Saunders, McConville, Tsuji, McCluskey, Martins-Filho, Eckle, Coelho-dos-Reis and Peruhype-Magalhães. This is an open-access article distributed under the terms of the Creative Commons Attribution License (CC BY). The use, distribution or reproduction in other forums is permitted, provided the original author(s) and the copyright owner(s) are credited and that the original publication in this journal is cited, in accordance with accepted academic practice. No use, distribution or reproduction is permitted which does not comply with these terms.
*Correspondence: Vanessa Peruhype-Magalhães, dmFuZXNzYS5wYXNjb2FsQGZpb2NydXouYnI=; dmFuZXNzYXBlcnVoeXBlQGdtYWlsLmNvbQ==; Jordana Grazziela Alves Coelho-dos-Reis, anJlaXNAaWNiLnVmbWcuYnI=; cmVpc2pvcmRhbmFAZ21haWwuY29t
†These authors share first authorship
‡These authors share senior authorship
Disclaimer: All claims expressed in this article are solely those of the authors and do not necessarily represent those of their affiliated organizations, or those of the publisher, the editors and the reviewers. Any product that may be evaluated in this article or claim that may be made by its manufacturer is not guaranteed or endorsed by the publisher.
Research integrity at Frontiers
Learn more about the work of our research integrity team to safeguard the quality of each article we publish.