- 1Cell Death Investigation and Therapy (CDIT) Laboratory, Department of Human Structure and Repair, Ghent University, Ghent, Belgium
- 2Cancer Research Institute Ghent, Ghent, Belgium
- 3Cancer Translational Research Laboratory, Pole of Pharmacology and Therapeutics, Institut de Recherche Expérimentale et Clinique (IREC), UCLouvain, Brussels, Belgium
- 4Nano-BioTechnology Laboratory, Department of Biotechnology, Ghent University, Ghent, Belgium
- 5Institute of Biology and Biomedicine, National Research Lobachevsky State University of Nizhny Novgorod, Nizhny Novgorod, Russia
- 6Department of Pathophysiology, Sechenov First Moscow State Medical University (Sechenov University), Moscow, Russia
Immunogenic cell death (ICD) is a rapidly growing research area representing one of the emerging therapeutic strategies of cancer immunotherapy. ICD is an umbrella term covering several cell death modalities including apoptosis, necroptosis, ferroptosis and pyroptosis, and is the product of a balanced combination of adjuvanticity (damage-associated molecular patterns and chemokines/cytokines) and antigenicity (tumor associated antigens). Only a limited number of anti-cancer therapies are available to induce ICD in experimental cancer therapies and even much less is available for clinical use. To overcome this limitation, nanomaterials can be used to increase the immunogenicity of cancer cells killed by anti-cancer therapy, which in themselves are not necessarily immunogenic. In this review, we outline the current state of knowledge of ICD modalities and discuss achievements in using nanomaterials to increase the immunogenicity of dying cancer cells. The emerging trends in modulating the immunogenicity of dying cancer cells in experimental and translational cancer therapies and the challenges facing them are described. In conclusion, nanomaterials are expected to drive further progress in their use to increase efficacy of anti-cancer therapy based on ICD induction and in the future, it is necessary to validate these strategies in clinical settings, which will be a challenging research area.
Introduction
Cancer immunotherapy rearms the body’s immune system to fight cancer by mounting an effective anti-tumor immune response. Cancer immunotherapy has been recognized as the break-through technology of the year in 2013 (1). In 2018, the Nobel Prize in Physiology or Medicine was awarded to James Allison and Tasuku Honjo for their discovery of check-point inhibitors. Notably, one of the first attempts to treat cancer by activating the immune system goes back to 2600 BC, when the Egyptian pharaoh Imhotep, in order to induce infection and consequent inflammation, used poultice in combination with incision to treat cancer (2).
It is now well known that monotherapies and killing cancer cells simply by inducing apoptosis is not, by itself, particularly effective for treating cancer. Several anti-cancer approaches, including chemotherapy and radiotherapy need to be combined with immunotherapy to effectively induce activation of anti-tumor immunity leading to tumor eradication and generation of long-term immunity. Personalized immunotherapy is based on collecting tumor tissue by biopsy or during surgery and inducting cell death in vitro in the cells. After that, the cells are re-injected into the patient. This approach seems promising for raising the potency and tumor-specific immunity of cancer vaccines. The administration of cancer cell-based vaccines is particularly interesting because of the presence of all the cellular components, including tumor-associated antigens and ‘Damage-associated Molecular Patterns’ (DAMPs) released during cell death (3, 4) (Box 1). One of the emerging methods of immunotherapy involves the use of cancer cells undergoing immunogenic cell death (ICD) as a cell-based vaccine. ICD is an umbrella term covering several regulated cell death modalities. The immunogenic characteristics of ICD are mainly mediated by DAMPs and cytokines/chemokines (5–8). These molecules are intracellularly involved in fundamental biological processes and are normally not recognized by the immune system. But during ICD, these molecules are liberated into the extracellular environment, where they are recognized by corresponding pattern recognition receptors, leading to the activation of the innate and adaptive immune systems. The ICD concept has recently attracted special interest as a novel approach for personalized cancer immunotherapy.
Box 1. Definition of immunogenicity and antigenicity of ICD. Immunogenicity and antigenicity are two major determinants of ICD. Immunogenicity of dying cancer cells is mediated mainly by DAMPs and/or cytokines/chemokines that are emitted from the dying cancer cells, they function as adjuvants and are key elements in the induction of cellular and humoral anti-tumor immune responses. By contrast, antigenicity of ICD refers to relevant antigens (e.g., tumor-specific antigens, tumor-associated antigens or neoepitopes) which are revealed by the dying cancer cells, they account for specific recognition by antibodies generated as a result of the anti-tumor immune response. Both components need to be simultaneously present in dying cancer cells to efficiently support anti-tumor immunity.
Anti-cancer vaccines based on dead cells should have at least two key features: the cancer cells must be able to efficiently undergo cell death by one of the known modalities, and the cell death must be immunogenic. In other words, the dying cells must be able to induce significant immunological anti-tumor responses. However, it is important to emphasize that one of the hallmarks of cancer cells is resistance to (apoptotic) cell death manifested in the acquisition of properties allowing them to circumvent or limit the apoptotic death pathways (9). To overcome apoptotic cell death resistance, anti-cancer therapies have to be designed to induce other ICD modalities. Indeed, it has been shown that cancer cells undergoing necroptosis (10, 11), ferroptosis (12) and pyroptosis (13, 14) are all immunogenic, fulfilling the first criterion for anti-cancer vaccines based on dead cancer cells, at least in experimental mouse models. However, it is important to stress that only limited anti-cancer therapies in clinical use can induce immunogenic forms of cell death. Inducers of different regulated cell death modalities (necroptosis, pyroptosis and ferroptosis) are available mostly for experimental cancer therapy. Therefore, one possible approach to overcome this limitation is to use nanomaterials to increase the immunogenicity of cancer cells killed by conventional anti-cancer therapy, which in themselves are not necessarily immunogenic (6).
The use of a broad range of nanomaterials can help to address essential challenges and needs in controlling ICD and modulating the immunogenicity of cancer cell death modalities. Specifically, nanomaterials can be used to control the immunogenicity of cells (15), and they can also provide efficient, targeted delivery of immunomodulatory agents and their controlled release, which increases the efficiency of the treatment. Many nanomaterials, both organic-based and inorganic-based are available. In the context of cancer, nano-carriers were first applied to apoptosis. For over 20 years, drug delivery for apoptosis induction has stood out as a broad application area in which liposomes were among the first and most widely used carriers (Figure 1). Peculiarly, in the last ten years, almost all main classes of nanomaterials have been linked with immuno-oncology and immunogenicity and used to trigger apoptosis, including liposomes, micelles, dendrimers, organic nanoparticles (polymeric, hydrogel, lipid-based) and inorganic materials (metal, oxide, semiconductor, carbon) as well as special nanomaterials such as nanobubbles, cell penetrating peptides (CPP), antibodies, and exosomes (Figure 1). But since the discovery of other ICD modalities such as necroptosis, pyroptosis and ferroptosis, the use of nanomaterials to modulate the immunogenicity of cell death has been gaining pace.
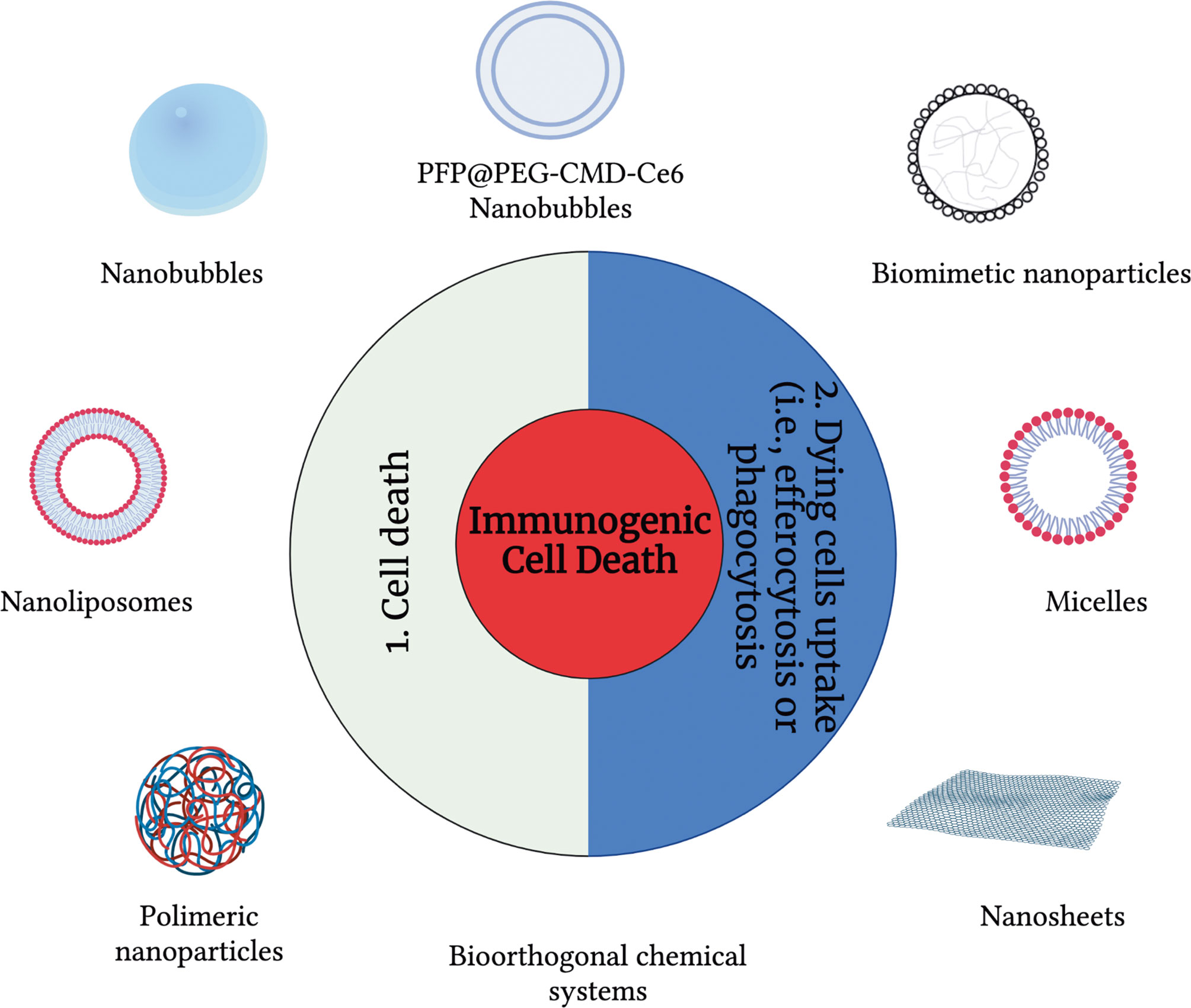
Figure 1 An overview of drug delivery carriers used to modulate immunogenic cell death modalities. To modulate the immunogenicity of cell death, drug delivery carriers can be used at three different levels: (1) cell death and (2) modulation of the dying cell’s uptake.
In this review, we first give a short overview on the recent advances and the current state of knowledge of ICD modalities, including apoptosis, necroptosis, ferroptosis and pyroptosis, as well as on the achievements in the application of nanoparticles, nanomaterials and nano-drug delivery carriers in this area. In particular, since the role of nanomaterials in increasing immunogenicity of apoptosis is widely described elsewhere (16, 17), we will main focus our attention on the non-canonical cell deaths. Next, we will discuss potential mechanisms by which nanoparticle-based technology can be used to increase the immunogenicity of dying cancer cells based on the interplay between the use of nanomaterials and interference with the clearance of dying cancer cells. Finally, we will outline the challenges and opportunities and highlight the emerging trends in the modulation of the immunogenicity of dying cancer cells in experimental and translational cancer therapies.
ICD Modalities at a Glance
Apoptosis is a physiological phenomenon that plays a crucial role in tissues development and cellular homeostasis. For instance, during organ development, tissue remodeling, or cellular replacement after injuries, the clearance of apoptotic cells by phagocytes (e.g., macrophages) is crucial and allows the removal of dead cells without the induction of an immune- or -inflammatory response (18, 19). Indeed, in these settings apoptotic cells are silently recognized and removed by professional and non-professional phagocytes, in a process called efferocytosis. Efferocytosis happens before dying cells lose membrane integrity and avoids the pouring of the cellular content in the extracellular milieu. For this reason, for long time apoptotic cell death has been considered strictly immunologically silent (20, 21). These notions were supported by studies published in the early nineties by the group of Peter Henson and others. These researchers showed that the loss of phospholipid asymmetry in the plasma membrane of apoptotic cells leads to phosphatidylserine (PS) exposure on the outer surface of the lipid bilayer early during apoptosis, and that PS is responsible for recognition of those cells by antigen-presenting cells and for the immune-suppressive characteristics of apoptotic cell death (21, 22). During the same time, the “danger theory” of immunity was postulated by Polly Matzinger, who claimed that the immune system can distinguish self from non-self and dangerous signals from innocuous ones (23). This theory suggested that immune responses can be triggered by “danger signals” or DAMPs, which are released by the damaged/dying cells. It became clear that programmed cell death can be silent or tolerogenic depending on several factors, such as the initiating stimuli or the tumor microenvironment status (24). For instance, the lack of T cell infiltration, or the presence of immunosuppressive subtypes of engulfing cells, such as M2 macrophages, and the consequent production of immunosuppressive cytokines dictate an overall tolerogenic effect (25).
As opposite, a new concept of ICD emerged, initially referred to as immunogenic apoptosis (5, 26–29), because at that time apoptosis was the only known regulated form of cell death. Indeed, in the early 2000s, the dogma indicating a strict silent or tolerogenic apoptosis started to fall apart. AB1 mesothelioma cells expressing hemagglutinin primed CD8+ T cells when going through gemcitabine-mediated apoptosis elicited an effective immune response (30). Anthracyclines, such as doxorubicin, but not mitomycin c (26), and oxaliplatin, but not cisplatin (31), were able to initiate the immune system. It has been then demonstrated that apoptotic ICD is triggered only when cell death is caused by a concerted induction of oxidative stress and endoplasmic reticulum stress, which in turn, promote the activation of the emission of DAMPs (5). DAMPs act as find-me and/or eating-me signals for antigen-presenting cells mainly by interacting with pattern recognition receptors (PRRs). Then, the antigen presentation, together with the concurrent release of cytokine/chemokines trigger the polarization of interferon-γ (IFNγ)-producing CD8+ T cells and the activation of the adaptive immune response (5, 32) (Box 1). Later it has become clear that many other non-apoptotic cell death modalities can also be regulated and experimentally triggered in cancer cells. In this section, we will give a bird’s eye view of the main non-apoptotic cell death modalities (necroptosis, pyroptosis and ferroptosis) with focus on recent advances supporting their immunogenicity (Figure 2). Interestingly, for all types of cell death, certain nanomaterials favor the onset of immune system activation and improves the efficiency of cell-death induced anti-tumor activity (33–45).
Necroptosis
Necroptosis is one of the best characterized cell death modalities. It is mediated by the kinase activities of receptor interacting protein kinase-1 (RIPK1) and RIPK3 and activation of the executioner protein mixed lineage kinase domain-like (MLKL), leading to rapid membrane permeabilisation and release of intracellular contents into the extracellular environment (46–50). Although it was initially shown that necroptotic cells do not induce pro-inflammatory responses (51, 52), several recent reports indicate that necroptotic cancer cells are immunogenic (Figure 2) and can induce effective anti-tumor immune responses (55, 61). It has been shown that necroptotic cells are engulfed by macropinocytosis which is characterized by formation of specious macropinosomes and co-ingestion of extracellular fluid (62, 63). When necroptotic cancer cells are used as a vaccine to protect mice against subsequent challenge with viable cancer cells, they release DAMPs such as HMGB1 and ATP and can induce activation and maturation of bone-marrow derived dendritic cells in vitro and in vivo (61). In that study, the authors showed that necroptotic cancer cells induce cross-priming of T cells in vivo. Also interesting is another study showing that necroptotic cancer cells can induce the release of pro-inflammatory cytokines and chemokines in addition to DAMPs (55). It has been demonstrated that transcriptional signaling downstream of RIPK1/RIPK3/NF-κB activation results in necroptotic cells producing pro-inflammatory cytokines/chemokines, which is a key determinant for the cross-priming of CD8+ T cells and critical for the immunogenicity of necroptotic cancer cells (55). A subsequent study clarified the mechanism by which effector immune cells interact with necroptotic cells (64). Intra-tumoral injection of necroptotic cells promotes BATF3+ cDC1- and CD8+ leukocyte-dependent anti-tumor immunity accompanied by increased tumor antigen loading by tumor-associated antigen-presenting cells. That study further confirmed an initial observation (55) that immune-mediated tumor control by necroptotic cells requires NF-κB activation within necroptotic cells but not MLKL-mediated and cell lysis-dependent DAMP release (64).
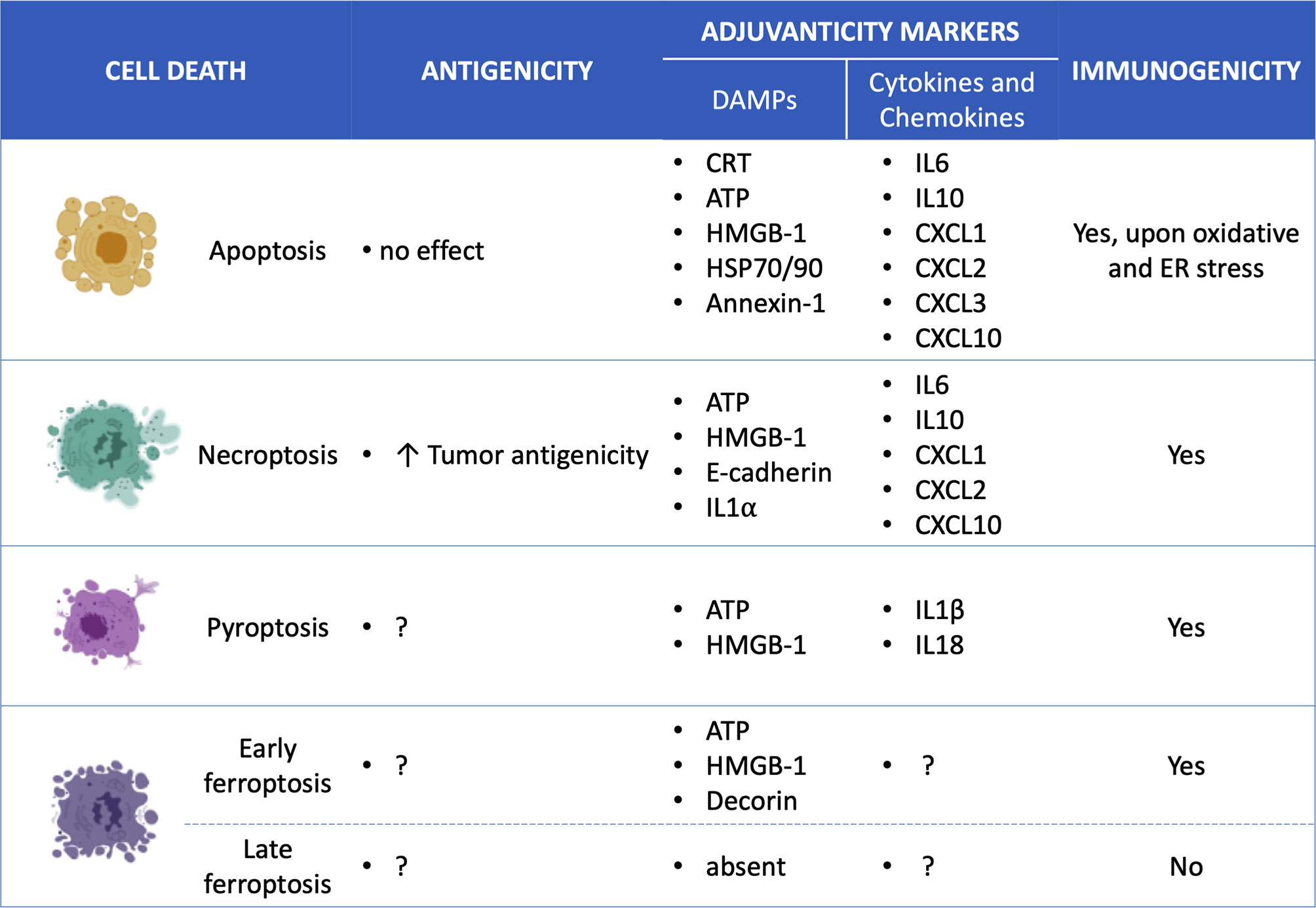
Figure 2 Overview of the specific ICD mediators for each cell death type. Apoptosis (7, 53, 54), Necroptosis (10, 53, 55, 56), Pyroptosis (14, 57–60); Ferroptosis (12) and; Damage-associated molecular patterns (DAMPs); Calreticulin (CRT); high mobility group box-1 (HMGB-1); interleukin (IL); chemokine (C-X-C motif) ligand (CXCL).
However, the role of NF-kB activation in necroptosis-induced activation of antitumor immune responses is still under discussion. It has been shown that the immunogenicity of necroptotic tumor cells is not correlated with the NF-κB activation status (56, 61). But what is more interesting in the mechanisms of necroptotic immunogenicity is the antigenic properties of necroptotic cancer cells. In fact, the ability of dying cancer cells to induce anti-cancer immunity requires both antigenicity and adjuvanticity (65, 66). Adjuvanticity is mediated by the release of DAMPs and pro-inflammatory cytokines/chemokines from the dying cancer cells, which stimulate corresponding pattern recognition receptors of the innate immune system (Figure 3). However, the presence of these adjuvant signals is not sufficient to elicit an effective immune response against cancer cells. The cancer cells should also have strong antigenic properties, including tumor associated antigens (TAA), which can trigger an adaptive immune response under specific conditions in the presence of adjuvants (i.e., DAMPs) (Box 1). A recent study highlighted this hidden aspect of the immunogenicity of necroptotic cancer cells (Figures 2, 3), i.e., their antigenicity, and underlined the tight correlation between the cell death type that cancer cells undergo and their antigenicity (56). That study showed in a murine prophylactic tumor vaccination model that when immune-dominant tumor antigen (AH1) was knocked out, necroptotic cancer cells were more immunogenic than apoptotic cells (56). That work suggests that the cell death modality may determine the strength of the immune response to alternative neoepitopes and that necroptosis is probably preferable to apoptosis in the treatment of cancer: necroptotic cancer cells can give rise to a broader antitumor immune response against tumor-intrinsic neoepitopes. Identification of the effects of different cell death modalities on the pattern of antigenic epitopes can become a highly valuable tool for future cancer immunotherapy.
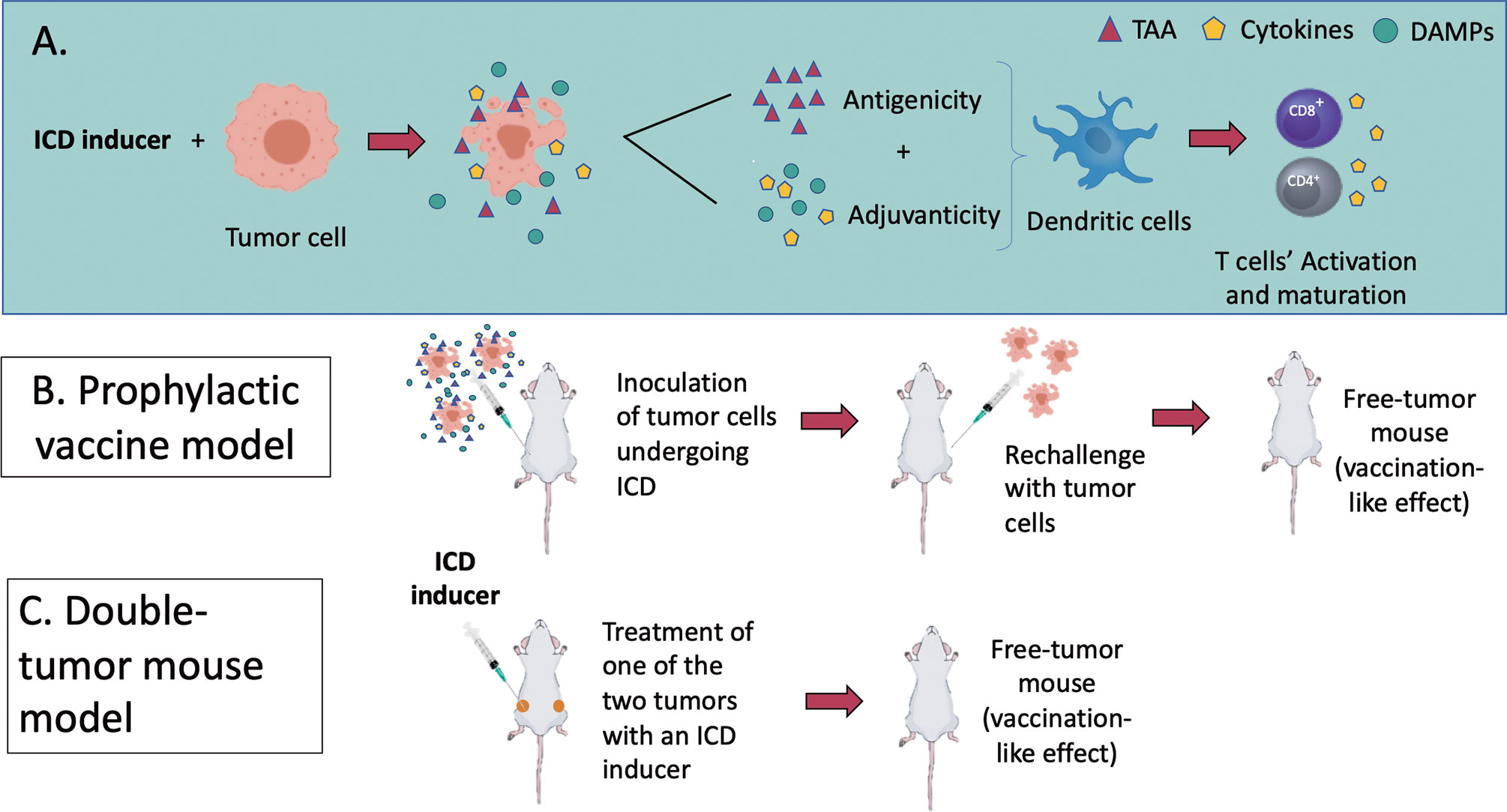
Figure 3 (A) An overview of the molecular mechanisms of ICD modalities and the in vivo models to assess it. ICD results as a balanced combination of adjuvanticity (induced by DAMPs and chemokines/cytokines) and antigenicity (tumour-associated antigens), which together promote the recruitment of antigen-presenting cells (APCs) and stimulate their ability to take up particulate material and cross-present dead cell-associated antigens to CD4+ and CD8+ cytotoxic T lymphocytes (Box 1). (B, C) represent the two recognized models to assess ICD in vivo. (B) In a tumour prophylactic vaccination model, cancer cells are first induced in vitro to undergo a particular form of ICD (apoptosis, necroptosis, pyroptosis or ferroptosis). They are then inoculated subcutaneously on one flank of the mice. Eight days later, the mice are challenged subcutaneously on the opposite flank with live cancer cells. Tumour growth at the challenge site is monitored. (C) In the bilateral tumour model, only the primary tumour is treated with the ICD inducers. The ability of mice to reject or limit the growth of primary and distant tumours is considered a sign of protective anticancer immunity.
Necroptosis can be also employed to modulate the immunogenicity of nanomaterials (Figure 1). For instance, necroptotic cell death contributes to the immunogenicity of near-infrared (NIR) responsive nanomaterials in combination with photoimmunotherapy (PTT). NIR-responsive nanomaterials have been shown to induce intratumor necroptosis that translates into the activation of both humoral and cell-mediated immune systems and photothermal tumor ablation. Black phosphorous nanosheets (BPs) excited with a specific laser is a vivid example of this. When injected into tumor-bearing mice, BPs localize in tumors, and their activation with laser promotes necroptosis, which triggers the immune response. On 4T1 breast cancer cells, BP-PTT modulates the most crucial markers of ICD, namely, exposure of calreticulin (CRT) and release of high mobility group box-1 (HMGB-1) and ATP. In addition, medium obtained from BP-PTT 4T1-treated cells, promoted macrophages to secrete tumor necrosis factor-α (TNF-α) and interleukin 6 (IL-6) which have positive immune stimulatory properties. In vivo studies confirmed that BP-PTT activates a comprehensive humoral and cellular immune response. 4T1 cells were injected in the left and right dorsal flank of Balb/c mice and one of them was irradiated with the NIR laser. The necroptosis occurring on one of the two tumor masses was sufficient to suppress tumor growth on both xenografts, which indicates systemic immune activation. Indeed, BP-PTT promoted the activation of both CD8+ and CD4+ T cells, together with downregulation of regulatory T cells, which are involved in negative immune regulation. Also, given the ease of structural manipulation of nanomaterials to further increase their immunogenicity, BP was conjugated with the adjuvant CpG to create a BPCP nanocomposite, significantly increasing BP immune stimulation (Table 1). Notably, no toxicity was observed, suggesting that BP nanomaterial is highly compatible as an antitumor and immunostimulant agent (67).
Nanobubbles (NBs) exposed to ultrasound represent another nanoparticle model for inducing immunogenic necroptosis. Acoustic cavitation generated by ultrasound in chlorine e 6 PEGylated carboxymethyl dextran (PEG-CMD-Ce6) nanobubbles triggers an intense RIPK3-independent necroptosis coupled with immunostimulant effects. In CT26 cells, the stimulated NBs promote HMGB1 release and DC maturation. In Balb/c mice, after systemic administration of NBs, they localize mainly in the tumor and limit tumor growth, especially when they are administered in combination with the immune check-point inhibitor PD-L1. Indeed, combining ultrasound-NBs-mediated necroptosis with PD-L1 considerably strengthened the immune response in terms of the number of CD8+ T lymphocytes entering into the tumor bed and the prevention of metastatic spread (68) (Table 1). What emerges from these studies is that the advantages of nanoparticles, such as tumor localization and the ability to conjugate them with different active molecules, is that they represent a safe and effective antitumor immunotherapy that induces necroptosis.
Pyroptosis
Pyroptosis is a proinflammatory form of regulated cell death triggered in response to pathogenic infections. It is caspase-dependent and mediated by pore-forming proteins named gasdermins (GSDMs) (13), but its role in cancer is still under investigation. Although it is a proinflammatory cell-death modality and could promote tumor progression, there is little evidence that induction of pyroptosis in cells undergoing carcinogenesis can suppress tumors (47, 72, 73). Nevertheless, pyroptosis is being explored as a new antitumor strategy to enhance antitumor immunity and overcome resistance to apoptosis (14, 47, 57, 74).
Morphologically, pyroptosis is characterized by DNA fragmentation and chromatin condensation accompanied by cellular swelling and bubble formation. The development of transmembrane pores caused by GSDM cleavage provokes the release of inflammatory cytokines and DAMPs and irreversible disruption of the osmotic equilibrium, which leads to cell membrane rupture (75, 76). Proinflammatory caspases such as caspases 1 and 11 and proapoptotic caspase 3 trigger different but intertwined pyroptotic pathways converging on the activation of GSDMs. The canonical pyroptotic pathway involves caspase 1 and is characterized by the formation of inflammasomes, while caspase 11 (the mouse orthologue of human caspases 4 and 5) and caspase 3 activate two non-canonical pathways directly targeting the effector protein (GSDMs), which are activated by cleavage into two fragments. The N-terminal fragment is involved in the formation of transmembrane pores linking the cytosol with the extracellular environment. The consequent potassium efflux and water influx alter the membrane potential and cellular homeostasis, causing cell rupture. Also, through the canonical pathway, caspase 1 activates the inflammatory cytokines pro-IL-1β and pro-IL-18, which are released through the pores and initiate the inflammatory response.
Given the pro-inflammatory nature of pyroptosis and the release of DAMPs and cytokines, this kind of cell death is highly immunogenic (Figure 2). Interestingly, the anticancer and immunostimulant activities are interconnected and interdependent. An intact and functioning immune system is required for pyroptotic tumor eradication, and the activation of GSDMs fosters the stimulation of both innate and adaptive tumor immunity (13, 14, 57). For instance, GSDME activation suppresses tumor growth by increasing the antitumor properties of tumor-infiltrating NK cells and CD4+ and CD8+ T lymphocytes (57). Only recently, it has been shown that the apoptosis effector caspase 3 can also promote the cleavage of GSDMs in cells with high levels of GSDMs (13, 47, 57). Thus, due to the connection between GSDMs and the apoptotic pathway, the activation of these proteins allows the conversion of non-inflammatory cell death (i.e. apoptosis) to inflammatory and immunogenic pyroptosis (13, 14, 57). The coexistence of apoptosis and pyroptosis could be considered as a new and noteworthy strategy to counteract apoptosis resistance, increase the chances of positive therapeutic outcomes, and, due to stimulation of the adaptive immune system, limit the incidence of metastasis and relapse. Of interest, the induction of mixed cell death types may provide a beneficial strategy to overcome the development of cancer resistance to a particular cell death type (29), increasing the chances for efficient cancer cell death killing.
However, GSDMs levels are often low in many tumors due to, for example, hypermethylation of mRNA (58, 69, 77). This notion together with the fact that pyroptosis can be used for antitumor therapy has prompted different research groups to exploit the efficiency and versatility of nanoparticles to directly increase GSDM levels in different tumor models to induce caspase-3 mediated pyroptosis (Figure 1). Zhao et al. (58) and Fan et al. (69) recently approached the problem by targeting the DFNA5 gene (which is responsible for hypermethylation of GSDME genes) with the methyltransferase inhibitor, decitabine (DAC) (69, 77). Fan et al. (69) loaded tumour-targeted nanoliposomes with the apoptotic inducer, cisplatin, and pre-treated tumours with DAC. The increased levels of GSDME enabled the nanoliposomes to trigger both apoptosis and pyroptosis, boosting cisplatin’s overall antitumor potential and remarkably stimulating the immune system. The nanoliposomes counteracted tumor growth and prevented metastasis in Balb/c mice bearing 4T1 tumors, while substantially increasing DC maturation in the lymph nodes. It also induced the transformation of native CD8+ T lymphocytes into central memory CD8+ cells in the spleen, showing that it increased the systemic immune response compared to the effect of cisplatin in the absence of DAC (69) (Table 1).
Using the same strategy, Zhao et al. (58) used a polymer-based nano-formulation to deliver both DAC and the proapoptotic indocyanine green to tumors. In this case, NIR sensitive biomimetic nanoparticles (BNP) were chosen to contain the two chemotherapeutic agents. Photoactivation of BNP promoted both apoptosis and pyroptosis (Figure 1). In vitro, BNP-photodynamic therapy induced the maturation of DCs and the secretion of IL-6 and TNF-α. In vivo, in a double xenograft model, photoactivating BNP prevented the growth of both irradiated and non-irradiated neoplastic lesions and induced a significantly larger amount of both CD4+ and CD8+ T cells in the spleen and in the non-irradiated tumor, together with active secretion of IFN-ɣ (58). It is notable that both nanomaterial constructs were well tolerated in the animal models, highlighting the high potential of this therapeutic strategy (58, 69).
Alternatively, instead of inducing the expression of GSDMs in cells, GSDMs can be transported into the tumor. Wang and colleagues created an elegant biorthogonal system to bring GSDMA3 into tumors and release it in a controlled way. They created an ortho-carbamoylmethylene silyl-phenolic ether system as a vehicle and attached to it GSDMA3 for delivery inside tumor cells. There, phenylalanine trifluoroborate (Phe-BF3) was used to release GSDMA3 from the system through a desilylation reaction. This system’s ability to induce pyroptosis was not astonishing (less than 15% of pyroptotic cells), but astonishing was that this small scale of cell death was sufficient to clear the entire 4T1 xenograft in Balb/c mice and to efficiently activate adaptive immunity. Notably, three administrations of nanoparticle-mediated delivery of GSDMA3 combined with Phe-BF3 were necessary to eradicate tumors, but just one round was effective when it was used together with PD-L1. In both situations, infiltration by NK cells and CD8+ and CD4+ T cells increased, while the levels of CD4+FOXP3+ T regulatory cells decreased. In parallel, the same treatment increased the M1 macrophage population whereas decreased the immunosuppressive M2 macrophage population (14). These results suggest that activation of GSDMs is an interesting strategy to increase the antitumor immunity-stimulating potential of silent cell death and that application of nanomaterials is an effective and safe method to achieve that (Figure 2).
Nonetheless, pyroptosis has not always proven to be advantageous for all the immunotherapy modalities. It has been shown that T cells with a CD19 chimeric antigen receptor against B cell malignancies trigger intense caspase 3/GSDME-dependent pyroptosis in targeted cells via granzyme B release. GSDME induces positive feedback resulting in the activation of the canonical pyroptotic pathway in macrophages, leading to a cytokine storm, which is a dangerous adverse reaction consisting of fever, hypotension, and respiratory insufficiency (78–80). In addition, pyroptosis is characterized by the active release of IL-1β, which is hyperinflammatory and could trigger detrimental effects in the long run, such as autoimmune diseases and cancer initiation and progression (81, 82). So far, the use of biomaterials seems to be useful for preventing pyroptosis resistance in cancer cells and increasing the overall immunotherapeutic potential of pyroptotic cancer cells. But as no chronic toxicity studies have been done, chronic adverse reactions cannot be excluded. Thus, future studies elucidating the mechanisms involved in the different pyroptosis pathways will enhance our understanding of the potential of pyroptosis as an anticancer strategy and of the use of pyroptosis to increase anticancer immunity, as well as delineating the toxicological profile.
Ferroptosis
Ferroptosis is another regulated form of cell death recently recognized for its effectiveness in cancer therapy (83). Ferroptosis execution is characterized by an iron-catalyzed excessive peroxidation of polyunsaturated fatty acids (PUFAs). Consecutive depletion of PUFAs from plasma membrane alters membrane fluidity and structure, thereby increasing membrane permeability and altering integrity (84, 85). By using atomic force microscopy we have also shown that ferroptosis is accompanied by a decrease in overall elasticity of the ferroptotic cells which distinguishes it from apoptosis (50). In addition, lipid hydroperoxides may decompose to reactive toxic aldehydes such as malondialdehydes (MDA) or 4-hydroxy-2-nonenals (4-HNE), which upon crosslinking may inactivate key membrane proteins and promote ferroptosis. Damages resulting from lipid peroxidation can also occur in other subcellular locations. Among them, mitochondria may undergo severe stress causing loss of function and cytological changes, such as shrinkage and reduction in mitochondrial cristae (86, 87).
Free PUFA or PUFA-containing phospholipids are oxidized through non-enzymatic oxidation by free radicals or enzymatic activity of lipoxygenases (LOX), cyclooxygenases (COX) or cytochrome P450 oxidoreductases (88–90). Moreover, polyunsaturated ether phospholipids act as substrates for lipid peroxidation, contributing to the ferroptosis induction (91). Among the various classes of phospholipids, specific oxidation of phosphatidylethanolamines is proposed to give rise to unique lipid death signals that drive the execution of ferroptosis (89). Still, regardless of the enzymatic or non-enzymatic modes of lipid peroxidation, the canonical ferroptotic pathway requires the inactivation of mechanisms protecting cells against peroxidation damage (50, 84, 85, 89, 92–94) and/or the presence of excess cellular PUFAs. The major detoxifying mechanism involves the activity of glutathione peroxidases (GPX). GPX4, the major GPX enzyme capable to detoxify hydroperoxides in lipids inserted into membranes, is inactivated upon depletion of intracellular glutathione (GSH) which may itself result from the blockade of the xc cystine/glutamate antiporter system. As for the excess PUFAs, it may result from an enhanced PUFA uptake or deficit in PUFA storage into lipid droplets that is thought to protect PUFA from peroxidation (94). We recently documented that cancer cells located in the acidic compartment of tumors exhibit a shift from glucose to lipid metabolism (95) and interestingly, that the consecutive stimulated uptake of FA represents a vulnerability for these cancer cells that undergo ferroptosis in response to an increase in dietary PUFAs (94). These data together with the higher oxidative stress usually observed in cancer cells contribute to make drugs inducing ferroptosis a valuable alternative to more conventional anticancer therapies leading to apoptosis or necroptosis.
Though many findings hint at the immunogenicity of ferroptosis, however, its immune-stimulating potential has not been fully elucidated. Nevertheless, there is definitely a strong connection between ferroptosis and immunomodulation. For instance, PD-L1-mediated immunotherapy on B16 cells enhanced CD8+ T cells, which in turn promoted lipid peroxidation and ferroptosis via INF-ɣ (96). But the inherent potential of ferroptosis as an anti-cancer immunotherapeutic agent is still under investigation. It is presumed that, like apoptosis or necroptosis, ferroptosis activates ICD by exploiting a pattern of events resembling those in other regulated cell death types, i.e. activation of DAMPs and release of cytokines (97, 98). Krysko’s research team was the first who was able to confirm this hypothesis (12). The authors demonstrated that murine fibrosarcoma MCA205 ferroptotic cells promote the phenotypic maturation of BMDCs and elicit a vaccination-like effect in vivo, proving the ability of ferroptosis to induce an adaptive immune response (Figure 2). ATP and HMGB-1 have proven to be the key DAMPs mediating the immunogenicity of ferroptosis (99, 100). The immunogenic nature of ferroptotic cancer cells has been recently confirmed also by other research groups. It has been identified that the inflammatory and immune response elicited by ferroptosis involves decorin as an essential DAMP that acts on the receptor advanced glycosylation end-product-specific receptor on macrophages to trigger the production of pro-inflammatory cytokines (98). Notably that using the same murine tumor prophylactic vaccination model as was used by Krysko’s team (12) but using another cancer cell line (i.e., pancreatic ductal adenocarcinoma KPC cells), the authors confirmed the immunogenic potential of ferroptotic cancer cells in vivo. Recently, by using another murine tumor model it has been shown that arachidonic acid and IFNɣ coordinately induce tumor cell ferroptosis via ACSL4 and ACSL4-mediated immunogenic tumor ferroptosis (101). Of interest, other evidence of ferroptosis immunogenicity is found in non-tumor models. For example, ferroptotic cells can attract neutrophils in a model of heart injury. They do so by activating the pathway of Toll-like receptor 4 (TLR4)-TIR domain containing adaptor inducing interferon beta (TRIF), corroborating the hypothesis implicating DAMPs in ferroptosis-mediated immunity (102). All these studies strongly confirm the hypothesis of the immunogenic potential of ferroptosis and point to the need to develop new therapeutic strategies for cancers based on induction of ferroptosis.
To facilitate ferroptosis and lessen therapy-related toxicity, several nanotherapeutic models have been designed and optimized. Different types of strategies have been developed using different nanocarriers (Figure 1). For instance, arginine-rich silicate-nanobubbles (AMSNs) have been built to inactivate GPX4 activity through GSH scavenger activity. The arginine delivers AMSN into the tumor while the manganese silicate moiety reacts with GSH, reducing its scavenging ability and inducing ferroptosis and tumor suppression in Huh7 xenografted Balb/c mice (70) (Table 1). Another strategy is to offer tumor cells an apparatus that favors the Fenton reaction by catalyzing the reaction or by intratumor delivery of reagents. The Fenton reaction takes place between intratumor hydrogen peroxide and ferric ions and leads to the formation of hydroxyl radicals, thereby causing lethal lipid peroxidation (103, 104). Numerous nanocatalysts that have been developed successfully triggered ferroptosis in cancer cells. The simplest is a nanocatalyst consisting of a single PEGylated iron atom (PLGA). In the acidic tumor microenvironment, the iron is released and can support the formation of lipid peroxides, which subsequently induce both ferroptosis and apoptosis (43). Besides, hydrogen peroxide can be inserted into a Fe3O4-PLGA nanocarrier and transported into the tumor. By using ultrasound, the system is disrupted, and the liberated reagents are set free to proceed with the Fenton reaction and lipid peroxidation (42). Another sophisticated nanocarrier involves the release of iron. Briefly, an amorphous calcium carbonate nanostructure is loaded with the proapoptotic agent, doxorubicin, chelated to Fe2+ (Fe2- doxorubicin core) and coated with a complex structure that guarantees the entrance of the intact core into the cells. There, Fe2+ triggers ferroptosis, which in turn is synergized by the oxidative stress induced by doxorubicin. In nude mice bearing 4T1 and A375 cells, this approach efficiently inhibited tumor growth and with a very safe profile (71) (Table 1). Besides, iron can be targeted into tumor cells by loading a magnetosome with Fe3O4 nanoclusters. On melanoma B16F10-xenografted mice, this system was able to accumulate in the tumor site, promote tumor suppression and the generation of an immunogenic tumor microenvironment. In particular, it ameliorated the CD4+ T/Treg cells, CD8+ T/Treg cells, and M1/M2 macrophages ratios (105).
Many other nano and micro drug delivery systems efficiently induce antitumor activity by inducing ferroptosis, but their immunogenic potential has not been thoroughly investigated. It will be interesting to understand if the specific target triggered to induce ferroptosis is crucial to ensure activation of the immune system and if some nanomaterials provide stronger activation of the immune system than others. Thus, the next step is to assess if and which nanoparticles induce immunogenic ferroptosis. If, unexpectedly, nano-induced ferroptosis turns out to be not immunogenic, other strategies could be tried to create the strong antitumor and immunogenic response (listed in the next section).
Interplay Between Nanomaterials and the Clearance of Dying Cancer Cells as a Strategy to Increase Their Immunogenicity
Recognition and efferocytosis of dying cancer cells (i.e., phagocytosis) determines the subsequent immunological effects (tolerogenic, neutral, or immunogenic) (18, 106). At the two extremes, tolerogenic phagocytosis leads to active immunosuppression, while immunogenic phagocytosis promotes immunostimulatory clearance of cancer cell corpses (107). The balance between pro- and anti-inflammatory stimuli released from both dying and immune cells in the tumor microenvironment (TME) dictate the fate of dying cells and the immunogenicity of the outcome. Also, the type of immune response mediators (immunostimulant or immunosuppressive) decides whether the TME will be immunogenic or not. For instance, neutrophils or M2 macrophages secreting the anti-inflammatory IL-10 and TGF-β promote tolerogenic phagocytosis, while inflammatory monocytes or DCs induce an immunogenic outcome (25). Phagocytosis passes through different phases: attraction, recognition, engulfment, and processing (106). Every step is critical to resolving the immunogenic outcome and can be modulated to increase the immunogenicity of dying cells (Figure 1).
One promising strategy to increase the immunogenicity of dying cells is blocking PS exposure during the early phases of cell death. For the best characterized regulated types of cell death, including apoptosis, necroptosis and pyroptosis, it has been ascertained that PS translocation to the outer side of the cell membrane acts as an early-stage “eat-me” signal (10, 108, 109). In each cell death type, the exposure mechanism is different, and its function can be slightly diverse. During PS exposure, necroptotic and pyroptotic cells release, respectively, proinflammatory DAMPs and cytokines such as IL-33 or IL-1β (108, 110, 111). PS-exposing necroptotic cells also cross-prime CD8+ T lymphocytes in a PS-dependent way. Thus, in these two cell death types, the PS exposure interval represents a window of opportunity to promote inflammation. Therefore, prolonging the time during which dying cells expose PS and blocking PS-mediated efferocytosis represents an interesting strategy to favor the development of an immunogenic TME (Figure 4). PS blocking also increases the immunogenicity of apoptotic cancer cells. During apoptosis, the removal of the PS “eat-me” signal impedes the phagocytosis of apoptotic cells and pushes early apoptotic cells through secondary necrosis, switching apoptosis to a proinflammatory ICD (112). The loss of the cell membrane due to secondary necrosis increases the possibility of DCs taking up tumor antigen epitopes. Consequently, blocking PS might be an appealing strategy to modulate the immunogenicity of dying cancer cells (112).
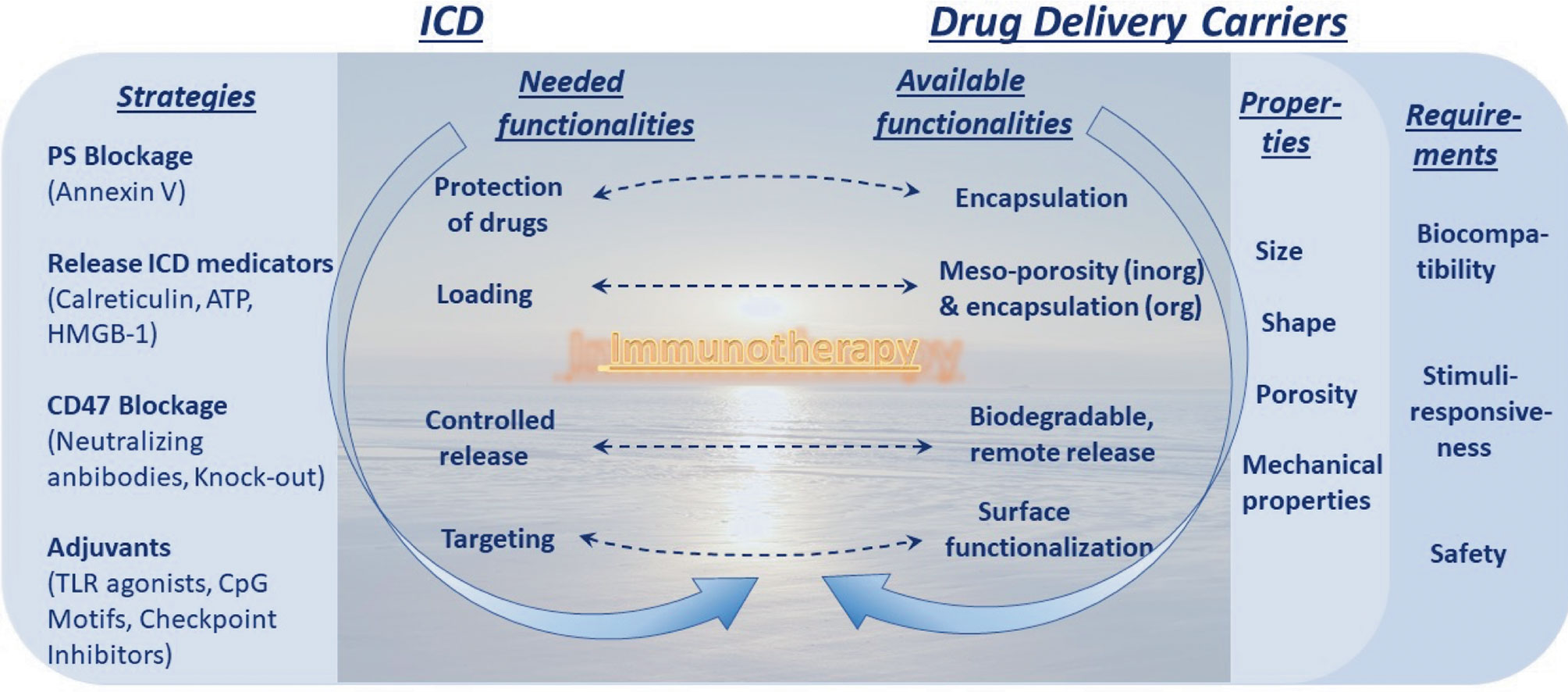
Figure 4 Dawn of immunotherapy spurred by immunogenic cell death (ICD) and enables by drug delivery carriers. Strategies to increase ICD and its requirements, the left column, are to be met by capabilities in the area of drug delivery carriers, the right column. This truly represents a dawn of a new era linked to immunotherapy (and this message is conveyed in the background).
The best-known PS ligand is Annexin-V (Ann-V), which can be used to block PS and hide dying cells from phagocytosis. In practice, Ann-V disrupts PS-dependent recognition of apoptotic dying cells by macrophages both in vivo and in vitro (51), but it does not affect the uptake by immature DCs and therefore induces CD8+ T cell-mediated immunogenic apoptosis (112, 113), confirming the pro-immunogenicity of this strategy. Indeed, it has been shown that shielding PS by Ann-V on the surface of apoptotic dying cancer cells is an attractive strategy for increasing the immunogenicity of dying cancer cells (113). However, as the exposure time of PS is short, it is difficult to catch the right moment to use Ann-V. Nano-formulations proved to overcome this inconvenience by co-delivery of an apoptosis-inducing agent and Ann-V, allowing on-demand release. In this way, optimal PS blocking was achieved on cue, maximizing the immunogenic potential. In a recent study, Ann-V was immobilized in A diselenide-bridged, hollow, mesoporous, organosilica nanocapsule to be released by biodegradation in the oxidative TME or reductive intracellular milieu. Apoptosis was induced in 4T1-bearing Balb/c mice by laser irradiation during Ann-V release. As above, macrophage phagocytic clearance was blocked and M2 macrophage levels decreased, while the phagocytosis of these apoptotic cells by DCs was not affected. Moreover, HMGB-1 release was increased, while myeloid-derived suppressor cells and immunosuppressor Treg cells decreased, with the consequence of favoring DC maturation and activation and boosting the INF-ɣ secreting-CD8+ T response. The overall effect was tumor regression in half of the mice. In other words, Ann-V shifted the apoptotic cell clearing program from tolerogenic to immunogenic, with a significant improvement in the antitumor antigen-specific cytotoxic T cell immune response (112), indicating that this strategy is very promising for increasing dying cell immunogenicity (Figure 4). Though this method has not been applied to cancer cells undergoing other modalities of cell death, these results portend a wide application of this strategy.
Since the balance between “eat-me” and “don’t eat me” signals defines whether dying cells will promote immunogenic or tolerogenic cell death, another strategy to improve the chances of stimulating the immune system is neutralization of negative mediators of efferocytosis. CD47 is a don’t eat-me signal and immune checkpoint inhibitor (21, 114, 115). The use of specific antibodies to disable CD47 significantly increased the recognition of dying cells by the immune system and its activation. Antigen presentation by DCs was improved, together with macrophage-mediated clearance of tumor cells (116–120). Furthermore, vaccination with tumor cells lacking CD47 or pre-coated with anti-CD47 antibodies triggered T cell-mediated antitumor responses (121). Furthermore, nanomaterials could be exploited for delivery of neutralizing antibodies as an intermediary in anti-CD47-related immune therapy. Besides that, several adjuvants can boost and drive immunity by promoting local inflammation and cytokine production, activating antigen-presenting cells, or increasing the magnitude and function of the T cell response (107). Among the many adjuvants, the ability of TLR agonists, CpG motifs and check-point inhibitors to boost the immunogenicity of dying cells has been also documented (Figure 4).
A different strategy to increase the immunogenicity of dying cells could emerge from deeper knowledge of the main ICD mediators, DAMPs. The molecular nature and the spatial-temporal frame in which these immunostimulant elements are presented to the immune system are indeed two critical factors in ICD induction (6, 25). For this reason, an ambition could be to engineer nanoparticles capable of delivering different compounds in a time-dependent way in order to deliver different “find-me” and “eat-me” factors in the same order that prompts ICD. It is well documented that the effectiveness of DAMPs requires release in a specific order, with CRT exposure being an early signal preceding even PS translocation, followed by ATP and HMGB-1 release (6, 12, 25). It is conceivable that the surface of nanoparticles could be loaded with ATP, and HMGB-1 could be conjugated with CRT to mimic, within the tumor, the appropriate spatial-temporal pattern of DAMPs release required to trigger ICD. In this way, nanoparticles would immediately expose CRT and then release ATP and HMGB-1 (Figure 4).
Taking all these notions together, it is evident that many strategies can be exploited to boost the immunogenicity of dying cells, and that they represent a concrete scope for improvement over the current immune anticancer therapy based on modulating the immunogenicity of dying cancer cells (Figure 4). By supporting cell death induction and increasing the overall immunogenic effect, nanoparticles make it possible to overcome every shortcoming of every type of cell death that usually limits its therapeutic use. The increased anticancer immune-efficiency of nanoparticle-mediated cell death is also due to the specificity of such vectors for tumor cells and the consequent limitation of toxicity. The lower toxicity could also be due to the possibility of lowering the doses of the anticancer agents because nanoparticles can boost the drugs’ potency. Although further pre-clinical and clinical studies will be needed to bring nano-mediated cancer vaccines from the bench to the bedside, the use of nanoparticles to boost the anticancer immunogenicity of dying cancer cells seems to raise the benefit/risk ratio of such therapies considerably, which indicates that this is a promising approach to pursue.
Conclusions and Final Remarks
Induction of ICD is a promising strategy for triggering effective anti-tumor immune responses. However, only a limited number of anti-cancer therapies are available to induce ICD in experimental cancer therapies and even much less is available for clinical use. Several different nanomaterials can be tailored to target specific immunological patterns in order to promote immunogenic apoptosis, necroptosis, ferroptosis or pyroptosis, or to synergize with other immunotherapies, such as checkpoint inhibition. Of note that drug delivery carriers can be used to increase the immunogenicity of cancer cells killed by anti-cancer therapy. They can be used at least at two different levels to increase immunogenicity of dying cancer cells (Figure 4). The first level is based on induction of a particular type of ICD which will allow to overcome often occurring in tumor cells resistance to a particular cell death type. The second level is based on modulation of the uptake (i.e., efferocytosis or phagocytosis) of dying cancer cells because recognition and phagocytosis of dying cancer cells determines the subsequent immunological effects to be either tolerogenic, neutral, or immunogenic and thus represent a critical decision point for mounting of an efficient anti-cancer immunity. These strategies allow to convert immuno-suppressive into immunostimulatory cancer cell death and such approach can be used for cell-based therapy in situ (therapeutic vaccination) to enhance anti-tumor immune responses.
Although pre-clinical studies on the use of nanomaterials to promote ICD have been conducted (122) and some are on-going, essential challenges exist in pre-clinical studies on nanomaterials and in their clinical translation (123, 124). One general yet essential requirement in clinical application is ensuring the safety of nanomaterials for patients, especially for any new class of materials. The seeming discrepancy between published research and approved drugs is explained by the substantial time it takes to complete safety trials and obtain clearance (125). Reflecting on the necessity of good regulation of nanomaterial safety, regulatory measures have been adopted by the FDA in the USA (126) and regulatory commissions in EU (127, 128). Indeed, the future perspectives are closely linked with on-going clinical trials, of which approximately 98% of about 379 trials are related to apoptosis. Taking into consideration that the other cell death modalities were discovered much later than apoptosis, this is not surprising. There are no clinical trials on ferroptosis while only a few clinical trials on necroptosis and pyroptosis. The safety of the newly introduced materials also remains a vital issue for performing pre-clinical and clinical trials on the application of ICD (129, 130). Further progress in this area is also linked with the possibility of determining which nanomaterials induce which cell death modalities, where new approaches are needed. Briefly, we can summarize the challenges for the application of nanomaterials to increase the immunogenicity of dying (dead) cancer cells for anti-cancer therapy as follow:
● It is important to be able to control the immunogenicity of nanomaterials. In this context, PLGA has been shown to be a suitable material (131), and efforts are being invested in extending the scope of new immunogenically relevant materials. Immunogenic peptides that can be presented by MHC Class I and II complexes for interaction with the T cell receptor (TCR) were recently reported (132).
● It is essential for nanomaterials to be biocompatible and, if necessary, biodegradable. Emphasizing these important attributes, a number of both synthetic and natural molecules can be used to modulate ICD (133). Another important aspect of the use of nanomaterials lies in combining the best properties of various constituents of nano-carriers (134). An interesting way to accomplish that could be by combining the organic and inorganic nanomaterials, as recently reported (135).
● More insight is needed into how the size and the surface properties of nanomaterials (charge, functionality) (136) influence their interaction with dying cancer cells. Although the size of nanoparticles affects their uptake by cells (137), limited data are available on the correlation between the size of nanoparticles administered at realistic doses (138) and clinically relevant toxicity patterns (139).
● Novel nanomaterials have been developed and applied for antigen presentation relevant for immunogenic cell death. For example, polyelectrolyte microparticles, which can be controlled by various external actions (140) and have advanced release capabilities (141), have been reported to be effective enhancer of antigen cross-presentation in porcine dendritic cells (142). Further, lipid-based formulations (143, 144), anisotropic (145), multicompartment (146), anisotropic multicompartment (147) carriers, soft and hard nanocarriers (148) are some of the candidates for incorporating advanced functionalities for immunology.
● Among different types of carriers, theragnostic drug delivery vehicles (149) are seen to be a particularly valuable type of carriers due to their dual function: diagnostic or sensoric (150) as well as therapeutic functions.
● Sufficient statistically significant data must be ensured in two adjacent areas. In biology and medicine, it is difficult to obtain sufficiently extensive statistical data from animal experiments and even more difficult from humans. Furthermore, if this approach is to be translated to the clinic, it is necessary to take into account the variability between patients, which incurs substantial time and financial costs. In addition, to assure broad application, medically relevant data need to be collected for sufficiently diverse populations. In nanotechnology, extensive efforts have been recently invested in addressing transparency and reproducibility, and a minimum information standard has been launched for reporting experimentation on bio−nano interactions in bio-nano experimental literature (MIRABEL) (151).
● To launch and implement clinical trials, it is vital to provide effective feedback from those trials to scientists developing novel materials for modulation of ICD. Moreover, the ethics of animal experimentation also have to be considered, particularly in view of the new regulations (152) that introduced new restrictions on the use of animals.
But despite all these challenges discussed, solid progress has already been achieved. This progress can be seen when examining examples of the application of photodynamic therapy based on novel photosensitizers (153, 154) and employing new materials and approaches to modulate the immunogenicity of dying cancer cells (Table 1). Various approaches and materials have been developed to target several cell death modalities including apoptosis, necroptosis, pyroptosis and ferroptosis. One example of such development is death-inducing gene therapy by using plasmid DNA (155), for which there is mounting preclinical evidence for its potential clinical application (156). Another development is repurposing nanoparticles for drug delivery to enhance ICD responses (157). Furthermore, nanoparticles with specific functions, for example, iron-catalysis, have been shown to induce ferroptosis (158).
The most significant hurdle to the implementation of nanotechnology and nanomedicine (159) in immunotherapy (i.e., in ICD) is defining the targets that result in the most effective immune response. Indeed, to improve the design of nanomaterials, deeper knowledge of the mechanisms of ICD is needed. Therefore, ICD basic research should go hand-in-hand with the development of nanoparticle-based therapeutics (Figure 4) which is largely driven by the availability of new nanomaterials. In the future new technologies are needed to be developed to distinguish different ICD modalities not only in vitro but also in in vivo context (50, 87, 160). In conclusion, interdisciplinarity, interconnectivity and interdependence of immuno-oncology on the one hand and nanomaterials and nanotechnology on the other hand are expected to drive further progress in the use of nanomaterial in anti-cancer therapy based on ICD induction.
Author Contributions
EC, OF, AS, and DK designed the manuscript and recommended a structure for the review. EC, AS, and DK wrote the initial draft and prepared the figures. All authors contributed to the article and approved the submitted version.
Funding
DK lab is supported by FWO-Flanders (G016221N). AS and DK acknowledge the support of FWO-Flanders (G043219N) and Ghent University BOF (Special Research Fund; IOP 01/O3618). AS acknowledges support of FWO-Flanders (I002620N) and BOF (Special Research Fund (BAS094-18, BOF14/IOP/003). This study is also supported by the Ministry of Science and Higher Education of the Russian Federation, agreement No. 075-15-2020-808. This project (40007488) has received funding from the FWO and F.R.S.-FNRS under the Excellence of Science (EOS) program to OF, AS, and DK.
Conflict of Interest
The authors declare that the research was conducted in the absence of any commercial or financial relationships that could be construed as a potential conflict of interest.
Publisher’s Note
All claims expressed in this article are solely those of the authors and do not necessarily represent those of their affiliated organizations, or those of the publisher, the editors and the reviewers. Any product that may be evaluated in this article, or claim that may be made by its manufacturer, is not guaranteed or endorsed by the publisher.
References
1. Couzin-Frankel J. Cancer Immunotherapy. Science. (2013) 342(6165):1432–3. doi: 10.1126/science.342.6165.1432
2. Kucerova P, Cervinkova M. Spontaneous Regression of Tumour and the Role of Microbial Infection – Possibilities for Cancer Treatment. Anticancer Drugs (2016) 27(4):269–77. doi: 10.1097/CAD.0000000000000337
3. Krysko O, Løve Aaes T, Bachert C, Vandenabeele P, Krysko DV. Many Faces of DAMPs in Cancer Therapy. Cell Death Dis (2013) 4:e631. doi: 10.1038/cddis.2013.156
4. Krysko DV, Agostinis P, Krysko O, Garg AD, Bachert C, Lambrecht BN, et al. Emerging Role of Damage-Associated Molecular Patterns Derived From Mitochondria in Inflammation. Trends Immunol (2011) 32(4):157–64. doi: 10.1016/j.it.2011.01.005
5. Krysko DV, Garg AD, Kaczmarek A, Krysko O, Agostinis P, Vandenabeele P. Immunogenic Cell Death and DAMPs in Cancer Therapy. Nat Rev Cancer (2012) 12(12):860–75. doi: 10.1038/nrc3380
6. Kepp O, Senovilla L, Vitale I, Vacchelli E, Adjemian S, Agostinis P, et al. Consensus Guidelines for the Detection of Immunogenic Cell Death. Oncoimmunology (2014) 3(9):e955691. doi: 10.4161/21624011.2014.955691
7. Galluzzi L, Vitale I, Warren S, Adjemian S, Agostinis P, Martinez AB, et al. Consensus Guidelines for the Definition, Detection and Interpretation of Immunogenic Cell Death. J Immunother Cancer (2020) 8(1):e000337. doi: 10.1136/jitc-2019-000337corr1
8. Krysko DV, Kaczmarek A, Krysko O, Heyndrickx L, Woznicki J, Bogaert P, et al. TLR-2 and TLR-9 are Sensors of Apoptosis in a Mouse Model of Doxorubicin-Induced Acute Inflammation. Cell Death Differ (2011) 18(8):1316–25. doi: 10.1038/cdd.2011.4
9. Hanahan D, Weinberg RA. Hallmarks of Cancer: The Next Generation. Cell. (2011) 144(5):646–74. doi: 10.1016/j.cell.2011.02.013
10. Krysko O, Aaes TL, Kagan VE, D’Herde K, Bachert C, Leybaert L, et al. Necroptotic Cell Death in Anti-Cancer Therapy. Immunol Rev (2017) 280(1):207–19. doi: 10.1111/imr.12583
11. Smith HG, Jamal K, Dayal JH, Tenev T, Kyula-Currie J, Guppy N, et al. RIPK1-Mediated Immunogenic Cell Death Promotes Anti-Tumour Immunity Against Soft-Tissue Sarcoma. EMBO Mol Med (2020) 12(6):e10979. doi: 10.15252/emmm.201910979
12. Efimova II, Catanzaro E, van der Meeren L, Turubanova VD, Hammad H, Mishchenko T, et al. Vaccination With Early Ferroptotic Cancer Cells Induces Efficient Anti-Tumour Immunity. J Immunother Cancer (2020) 8(2):e001369. doi: 10.1136/jitc-2020-001369
13. Shen C, Pandey A, Man SM. Gasdermins Deliver a Deadly Punch to Cancer. Cell Res (2020) 30(6):463–4. doi: 10.1038/s41422-020-0316-7
14. Wang Q, Wang Y, Ding J, Wang C, Zhou X, Gao W, et al. A Bioorthogonal System Reveals Antitumour Immune Function of Pyroptosis. Nature. (2020) 579(7799):421–6. doi: 10.1038/s41586-020-2079-1
15. Mishchenko T, Mitroshina E, Balalaeva I, Krysko O, Vedunova M, Krysko DV. An Emerging Role for Nanomaterials in Increasing Immunogenicity of Cancer Cell Death. Biochim Biophys Acta Rev Cancer (2019) 1871(1):99–108. doi: 10.1016/j.bbcan.2018.11.004
16. Fu L, Zhou X, He C. Polymeric Nanosystems for Immunogenic Cell Death-Based Cancer Immunotherapy. Macromol Biosci (2021) 21(7):e2100075. doi: 10.1002/mabi.202100075
17. Li Q, Liu Y, Huang Z, Guo Y, Li Q. Triggering Immune System With Nanomaterials for Cancer Immunotherapy. Front Bioeng Biotechnol (2022) 10:878524. doi: 10.3389/fbioe.2022.878524
18. Morioka S, Maueröder C, Ravichandran KS. Living on the Edge: Efferocytosis at the Interface of Homeostasis and Pathology. Immunity. (2019) 50(5):1149–62. doi: 10.1016/j.immuni.2019.04.018
19. Werfel TA, Cook RS. Efferocytosis in the Tumor Microenvironment. Semin Immunopathol (2018) 40(6):545–54. doi: 10.1007/s00281-018-0698-5
20. Zhang S, Weinberg S, DeBerge M, Gainullina A, Schipma M, Kinchen JM, et al. Efferocytosis Fuels Requirements of Fatty Acid Oxidation and the Electron Transport Chain to Polarize Macrophages for Tissue Repair. Cell Metab (2019) 29(2):443–456.e5. doi: 10.1016/j.cmet.2018.12.004
21. Krysko DV, D’Herde K, Vandenabeele P. Clearance of Apoptotic and Necrotic Cells and its Immunological Consequences. Apoptosis Int J Program Cell Death (2006) 11(10):1709–26. doi: 10.1007/s10495-006-9527-8
22. Fadok VA, Voelker DR, Campbell PA, Cohen JJ, Bratton DL, Henson PM. Exposure of Phosphatidylserine on the Surface of Apoptotic Lymphocytes Triggers Specific Recognition and Removal by Macrophages. J Immunol Baltim Md 1950. (1992) 148(7):2207–16.
23. Matzinger P. Tolerance, Danger, and the Extended Family. Annu Rev Immunol (1994) 12:991–1045. doi: 10.1146/annurev.iy.12.040194.005015
24. Green DR, Ferguson T, Zitvogel L, Kroemer G. Immunogenic and Tolerogenic Cell Death. Nat Rev Immunol (2009) 9(5):353–63. doi: 10.1038/nri2545
25. Garg AD, Romano E, Rufo N, Agostinis P. Immunogenic Versus Tolerogenic Phagocytosis During Anticancer Therapy: Mechanisms and Clinical Translation. Cell Death Differ (2016) 23(6):938–51. doi: 10.1038/cdd.2016.5
26. Casares N, Pequignot MO, Tesniere A, Ghiringhelli F, Roux S, Chaput N, et al. Caspase-Dependent Immunogenicity of Doxorubicin-Induced Tumor Cell Death. J Exp Med (2005) 202(12):1691–701. doi: 10.1084/jem.20050915
27. Apetoh L, Ghiringhelli F, Tesniere A, Obeid M, Ortiz C, Criollo A, et al. Toll-Like Receptor 4–Dependent Contribution of the Immune System to Anticancer Chemotherapy and Radiotherapy. Nat Med (2007) 13(9):1050–9. doi: 10.1038/nm1622
28. Garg AD, Krysko DV, Verfaillie T, Kaczmarek A, Ferreira GB, Marysael T, et al. A Novel Pathway Combining Calreticulin Exposure and ATP Secretion in Immunogenic Cancer Cell Death. EMBO J (2012) 31(5):1062–79. doi: 10.1038/emboj.2011.497
29. Turubanova VD, Balalaeva IV, Mishchenko TA, Catanzaro E, Alzeibak R, Peskova NN, et al. Immunogenic Cell Death Induced by a New Photodynamic Therapy Based on Photosens and Photodithazine. J Immunother Cancer (2019) 7(1):350. doi: 10.1186/s40425-019-0826-3
30. Nowak AK, Lake RA, Marzo AL, Scott B, Heath WR, Collins EJ, et al. Induction of Tumor Cell Apoptosis In Vivo Increases Tumor Antigen Cross-Presentation, Cross-Priming Rather Than Cross-Tolerizing Host Tumor-Specific CD8 T Cells. J Immunol Baltim Md 1950 (2003) 170(10):4905–13. doi: 10.4049/jimmunol.170.10.4905
31. Tesniere A, Schlemmer F, Boige V, Kepp O, Martins I, Ghiringhelli F, et al. Immunogenic Death of Colon Cancer Cells Treated With Oxaliplatin. Oncogene. (2010) 29(4):482–91. doi: 10.1038/onc.2009.356
32. Ghiringhelli F, Apetoh L, Tesniere A, Aymeric L, Ma Y, Ortiz C, et al. Activation of the NLRP3 Inflammasome in Dendritic Cells Induces IL-1beta-Dependent Adaptive Immunity Against Tumors. Nat Med (2009) 15(10):1170–8. doi: 10.1038/nm.2028
33. Kobayashi T. Cancer Hyperthermia Using Magnetic Nanoparticles. Biotechnol J (2011) 6(11):1342–7. doi: 10.1002/biot.201100045
34. Kobayashi T, Kakimi K, Nakayama E, Jimbow K. Antitumor Immunity by Magnetic Nanoparticle-Mediated Hyperthermia. Nanomed. (2014) 9(11):1715–26. doi: 10.2217/nnm.14.106
35. Yanase M, Shinkai M, Honda H, Wakabayashi T, Yoshida J, Kobayashi T. Antitumor Immunity Induction by Intracellular Hyperthermia Using Magnetite Cationic Liposomes. Jpn J Cancer Res (1998) 89(7):775–82. doi: 10.1111/j.1349-7006.1998.tb03283.x
36. Li L, Yang S, Song L, Zeng Y, He T, Wang N, et al. An Endogenous Vaccine Based on Fluorophores and Multivalent Immunoadjuvants Regulates Tumor Micro-Environment for Synergistic Photothermal and Immunotherapy. Theranostics. (2018) 8(3):860–73. doi: 10.7150/thno.19826
37. Chen Q, Xu L, Liang C, Wang C, Peng R, Liu Z. Photothermal Therapy With Immune-Adjuvant Nanoparticles Together With Checkpoint Blockade for Effective Cancer Immunotherapy. Nat Commun (2016) 21(7):13193. doi: 10.1038/ncomms13193
38. Kang T, Huang Y, Zhu Q, Cheng H, Pei Y, Feng J, et al. Necroptotic Cancer Cells-Mimicry Nanovaccine Boosts Anti-Tumor Immunity With Tailored Immune-Stimulatory Modality. Biomaterials. (2018) 164:80–97. doi: 10.1016/j.biomaterials.2018.02.033
39. Sharma B, McLeland CB, Potter TM, Stern ST, Adiseshaiah PP. Assessing NLRP3 Inflammasome Activation by Nanoparticles. Methods Mol Biol Clifton NJ (2018) 1682:135–47. doi: 10.1007/978-1-4939-7352-1_12
40. Wu D, Wang S, Yu G, Chen X. Cell Death Mediated by the Pyroptosis Pathway With the Aid of Nanotechnology: Prospects for Cancer Therapy. Angew Chem Int Ed (2021)133(15):8096–8112. doi: 10.1002/anie.202010281
41. Elion DL, Jacobson ME, Hicks DJ, Rahman B, Sanchez V, Gonzales-Ericsson PI, et al. Therapeutically Active RIG-I Agonist Induces Immunogenic Tumor Cell Killing in Breast Cancers. Cancer Res (2018) 78(21):6183–95. doi: 10.1158/0008-5472.CAN-18-0730
42. Li WP, Su CH, Chang YC, Lin YJ, Yeh CS. Ultrasound-Induced Reactive Oxygen Species Mediated Therapy and Imaging Using a Fenton Reaction Activable Polymersome. ACS Nano (2016) 10(2):2017–27. doi: 10.1021/acsnano.5b06175
43. Huo M, Wang L, Wang Y, Chen Y, Shi J. Nanocatalytic Tumor Therapy by Single-Atom Catalysts. ACS Nano (2019) 13(2):2643–53. doi: 10.1021/acsnano.9b00457
44. Liu T, Liu W, Zhang M, Yu W, Gao F, Li C, et al. Ferrous-Supply-Regeneration Nanoengineering for Cancer-Cell-Specific Ferroptosis in Combination With Imaging-Guided Photodynamic Therapy. ACS Nano (2018) 12(12):12181–92. doi: 10.1021/acsnano.8b05860
45. Zhou Z, Song J, Tian R, Yang Z, Yu G, Lin L, et al. Activatable Singlet Oxygen Generation From Lipid Hydroperoxide Nanoparticles for Cancer Therapy. Angew Chem Int Ed Engl (2017) 56(23):6492–6. doi: 10.1002/anie.201701181
46. Sprooten J, De Wijngaert P, Vanmeerbeerk I, Martin S, Vangheluwe P, Schlenner S, et al. Necroptosis in Immuno-Oncology and Cancer Immunotherapy. Cells. (2020) 9(8):1823. doi: 10.3390/cells9081823
47. Bedoui S, Herold MJ, Strasser A. Emerging Connectivity of Programmed Cell Death Pathways and its Physiological Implications. Nat Rev Mol Cell Biol (2020) 21(11):678–95. doi: 10.1038/s41580-020-0270-8
48. Kaczmarek A, Vandenabeele P, Krysko DV. Necroptosis: The Release of Damage-Associated Molecular Patterns and its Physiological Relevance. Immunity. (2013) 38(2):209–23. doi: 10.1016/j.immuni.2013.02.003
49. Verduijn J, van der Meeren L, Krysko DV, Skirtach AG. Deep Learning With Digital Holographic Microscopy Discriminates Apoptosis and Necroptosis. Cell Death Discovery (2021) 7(1):229. doi: 10.1038/s41420-021-00616-8
50. Van der Meeren L, Verduijn J, Krysko DV, Skirtach AG. AFM Analysis Enables Differentiation Between Apoptosis, Necroptosis, and Ferroptosis in Murine Cancer Cells. iScience. (2020) 23(12):101816. doi: 10.1016/j.isci.2020.101816
51. Brouckaert G, Kalai M, Krysko DV, Saelens X, Vercammen D, Ndlovu MN, et al. Phagocytosis of Necrotic Cells by Macrophages Is Phosphatidylserine Dependent and Does Not Induce Inflammatory Cytokine Production. Mol Biol Cell (2004) 15(3):1089–100. doi: 10.1091/mbc.e03-09-0668
52. Lohmann C, Muschaweckh A, Kirschnek S, Jennen L, Wagner H, Häcker G. Induction of Tumor Cell Apoptosis or Necrosis by Conditional Expression of Cell Death Proteins: Analysis of Cell Death Pathways and In Vitro Immune Stimulatory Potential. J Immunol Baltim Md 1950 (2009) 182(8):4538–46. doi: 10.4049/jimmunol.0803989
53. Yatim N, Cullen S, Albert ML. Dying Cells Actively Regulate Adaptive Immune Responses. Nat Rev Immunol (2017) 17(4):262–75. doi: 10.1038/nri.2017.9
54. Fucikova J, Moserova I, Truxova I, Hermanova I, Vancurova I, Partlova S, et al. High Hydrostatic Pressure Induces Immunogenic Cell Death in Human Tumor Cells. Int J Cancer (2014) 135(5):1165–77. doi: 10.1002/ijc.28766
55. Yatim N, Jusforgues-Saklani H, Orozco S, Schulz O, Barreira da Silva R, Reis e Sousa C, et al. RIPK1 and NF-κb Signaling in Dying Cells Determines Cross-Priming of CD8+ T Cells. Science. (2015) 350(6258):328–34. doi: 10.1126/science.aad0395
56. Aaes TL, Verschuere H, Kaczmarek A, Heyndrickx L, Wiernicki B, Delrue I, et al. Immunodominant AH1 Antigen-Deficient Necroptotic, But Not Apoptotic, Murine Cancer Cells Induce Antitumor Protection. J Immunol (2020) 204(4):775–87. doi: 10.4049/jimmunol.1900072
57. Zhang Z, Zhang Y, Xia S, Kong Q, Li S, Liu X, et al. Gasdermin E Suppresses Tumour Growth by Activating Anti-Tumour Immunity. Nature. (2020) 579(7799):415–20. doi: 10.1038/s41586-020-2071-9
58. Zhao P, Wang M, Chen M, Chen Z, Peng X, Zhou F, et al. Programming Cell Pyroptosis With Biomimetic Nanoparticles for Solid Tumor Immunotherapy. Biomaterials. (2020) 254:120142. doi: 10.1016/j.biomaterials.2020.120142
59. Frank D, Vince JE. Pyroptosis Versus Necroptosis: Similarities, Differences, and Crosstalk. Cell Death Differ (2019) 26(1):99–114. doi: 10.1038/s41418-018-0212-6
60. Tang R, Xu J, Zhang B, Liu J, Liang C, Hua J, et al. Ferroptosis, Necroptosis, and Pyroptosis in Anticancer Immunity. J Hematol OncolJ Hematol Oncol (2020) 13(1):110. doi: 10.1186/s13045-020-00946-7
61. Aaes TL, Kaczmarek A, Delvaeye T, De Craene B, De Koker S, Heyndrickx L, et al. Vaccination With Necroptotic Cancer Cells Induces Efficient Anti-Tumor Immunity. Cell Rep (2016) 15(2):274–87. doi: 10.1016/j.celrep.2016.03.037
62. Krysko DV, Brouckaert G, Kalai M, Vandenabeele P, D’Herde K. Mechanisms of Internalization of Apoptotic and Necrotic L929 Cells by a Macrophage Cell Line Studied by Electron Microscopy. J Morphol (2003) 258(3):336–45. doi: 10.1002/jmor.10161
63. Krysko DV, Denecker G, Festjens N, Gabriels S, Parthoens E, D’Herde K, et al. Macrophages Use Different Internalization Mechanisms to Clear Apoptotic and Necrotic Cells. Cell Death Differ (2006) 13(12):2011–22. doi: 10.1038/sj.cdd.4401900
64. Snyder AG, Hubbard NW, Messmer MN, Kofman SB, Hagan CE, Orozco SL, et al. Intratumoral Activation of the Necroptotic Pathway Components RIPK1 and RIPK3 Potentiates Antitumor Immunity. Sci Immunol (2019) 4(36):eaaw2004. doi: 10.1126/sciimmunol.aaw2004
65. Bloy N, Garcia P, Laumont CM, Pitt JM, Sistigu A, Stoll G, et al. Immunogenic Stress and Death of Cancer Cells: Contribution of Antigenicity vs Adjuvanticity to Immunosurveillance. Immunol Rev (2017) 280(1):165–74. doi: 10.1111/imr.12582
66. Galluzzi L, Buqué A, Kepp O, Zitvogel L, Kroemer G. Immunogenic Cell Death in Cancer and Infectious Disease. Nat Rev Immunol (2017) 17(2):97–111. doi: 10.1038/nri.2016.107
67. Zhao H, Chen H, Guo Z, Zhang W, Yu H, Zhuang Z, et al. In Situ Photothermal Activation of Necroptosis Potentiates Black Phosphorus-Mediated Cancer Photo-Immunotherapy. Chem Eng J (2020) 394:124314. doi: 10.1016/j.cej.2020.124314
68. Um W, Ko H, You DG, Lim S, Kwak G, Shim MK, et al. Necroptosis-Inducible Polymeric Nanobubbles for Enhanced Cancer Sonoimmunotherapy. Adv Mater (2020) 32(16):1907953. doi: 10.1002/adma.201907953
69. Fan JX, Deng RH, Wang H, Liu XH, Wang XN, Qin R, et al. Epigenetics-Based Tumor Cells Pyroptosis for Enhancing the Immunological Effect of Chemotherapeutic Nanocarriers. Nano Lett (2019) 19(11):8049–58. doi: 10.1021/acs.nanolett.9b03245
70. Wang S, Li F, Qiao R, Hu X, Liao H, Chen L, et al. Arginine-Rich Manganese Silicate Nanobubbles as a Ferroptosis-Inducing Agent for Tumor-Targeted Theranostics. ACS Nano (2018) 12(12):12380–92. doi: 10.1021/acsnano.8b06399
71. Xue CC, Li MH, Zhao Y, Zhou J, Hu Y, Cai KY, et al. Tumor Microenvironment-Activatable Fe-Doxorubicin Preloaded Amorphous CaCO3 Nanoformulation Triggers Ferroptosis in Target Tumor Cells. Sci Adv (2020) 6(18):eaax1346. doi: 10.1126/sciadv.aax1346
72. Xia X, Wang X, Cheng Z, Qin W, Lei L, Jiang J, et al. The Role of Pyroptosis in Cancer: Pro-Cancer or Pro-”Host”? Cell Death Dis (2019) 10(9):1–13. doi: 10.1038/s41419-019-1883-8
73. Broz P, Pelegrín P, Shao F. The Gasdermins, a Protein Family Executing Cell Death and Inflammation. Nat Rev Immunol (2020) 20(3):143–57. doi: 10.1038/s41577-019-0228-2
74. Minton K. Pyroptosis Heats Tumour Immunity. Nat Rev Drug Discovery (2020) 19(5):309–9. doi: 10.1038/d41573-020-00062-8
75. Galluzzi L, Vitale I, Aaronson SA, Abrams JM, Adam D, Agostinis P, et al. Molecular Mechanisms of Cell Death: Recommendations of the Nomenclature Committee on Cell Death 2018. Cell Death Differ (2018) 25(3):486–541. doi: 10.1038/s41418-018-0102-y
76. Yu J, Li S, Qi J, Chen Z, Wu Y, Guo J, et al. Cleavage of GSDME by Caspase-3 Determines Lobaplatin-Induced Pyroptosis in Colon Cancer Cells. Cell Death Dis (2019) 10(3):1–20. doi: 10.1038/s41419-019-1441-4
77. Rogers C, Fernandes-Alnemri T, Mayes L, Alnemri D, Cingolani G, Alnemri ES. Cleavage of DFNA5 by Caspase-3 During Apoptosis Mediates Progression to Secondary Necrotic/Pyroptotic Cell Death. Nat Commun (2017) 8:14128. doi: 10.1038/ncomms14128
78. Davila ML, Riviere I, Wang X, Bartido S, Park J, Curran K, et al. Efficacy and Toxicity Management of 19-28z CAR T Cell Therapy in B Cell Acute Lymphoblastic Leukemia. Sci Transl Med (2014) 6(224):224ra25. doi: 10.1126/scitranslmed.3008226
79. Liu Y, Fang Y, Chen X, Wang Z, Liang X, Zhang T, et al. Gasdermin E-Mediated Target Cell Pyroptosis by CAR T Cells Triggers Cytokine Release Syndrome. Sci Immunol (2020) 5(43):eaax7969. doi: 10.1126/sciimmunol.aax7969
80. Delvaeye T, De Smet MAJ, Verwaerde S, Decrock E, Czekaj A, Vandenbroucke RE, et al. Blocking Connexin43 Hemichannels Protects Mice Against Tumour Necrosis Factor-Induced Inflammatory Shock. Sci Rep (2019) 9(1):16623. doi: 10.1038/s41598-019-52900-4
81. Mantovani A, Dinarello CA, Molgora M, Garlanda C. Interleukin-1 and Related Cytokines in the Regulation of Inflammation and Immunity. Immunity. (2019) 50(4):778–95. doi: 10.1016/j.immuni.2019.03.012
82. Van Gorp H, Lamkanfi M. The Emerging Roles of Inflammasome-Dependent Cytokines in Cancer Development. EMBO Rep (2019) 20(6):e47575. doi: 10.15252/embr.201847575
83. Friedmann Angeli JP, Krysko DV, Conrad M. Ferroptosis at the Crossroads of Cancer-Acquired Drug Resistance and Immune Evasion. Nat Rev Cancer (2019) 19(7):405–14. doi: 10.1038/s41568-019-0149-1
84. Dixon SJ, Lemberg KM, Lamprecht MR, Skouta R, Zaitsev EM, Gleason CE, et al. Ferroptosis: An Iron-Dependent Form of Nonapoptotic Cell Death. Cell. (2012) 149(5):1060–72. doi: 10.1016/j.cell.2012.03.042
85. Stockwell BR, Angeli JPF, Bayir H, Bush AI, Conrad M, Dixon S, et al. Ferroptosis: A Regulated Cell Death Nexus Linking Metabolism, Redox Biology, and Disease. Cell. (2017) 171(2):273–85. doi: 10.1016/j.cell.2017.09.021
86. Neitemeier S, Jelinek A, Laino V, Hoffmann L, Eisenbach I, Eying R, et al. BID Links Ferroptosis to Mitochondrial Cell Death Pathways. Redox Biol (2017) 12:558–70. doi: 10.1016/j.redox.2017.03.007
87. Demuynck R, Efimova I, Lin A, Declercq H, Krysko DV. A 3D Cell Death Assay to Quantitatively Determine Ferroptosis in Spheroids. Cells. (2020) 9(3):703. doi: 10.3390/cells9030703
88. Doll S, Proneth B, Tyurina YY, Panzilius E, Kobayashi S, Ingold I, et al. ACSL4 Dictates Ferroptosis Sensitivity by Shaping Cellular Lipid Composition. Nat Chem Biol (2017) 13(1):91–8. doi: 10.1038/nchembio.2239
89. Kagan VE, Mao G, Qu F, Angeli JPF, Doll S, Croix CS, et al. Oxidized Arachidonic and Adrenic PEs Navigate Cells to Ferroptosis. Nat Chem Biol (2017) 13(1):81–90. doi: 10.1038/nchembio.2238
90. Yang WS, Kim KJ, Gaschler MM, Patel M, Shchepinov MS, Stockwell BR. Peroxidation of Polyunsaturated Fatty Acids by Lipoxygenases Drives Ferroptosis. Proc Natl Acad Sci U S A. (2016) 113(34):E4966–75. doi: 10.1073/pnas.1603244113
91. Zou Y, Henry WS, Ricq EL, Graham ET, Phadnis VV, Maretich P, et al. Plasticity of Ether Lipids Promotes Ferroptosis Susceptibility and Evasion. Nature. (2020) 585(7826):603–8. doi: 10.1038/s41586-020-2732-8
92. Gao M, Yi J, Zhu J, Minikes AM, Monian P, Thompson CB, et al. Role of Mitochondria in Ferroptosis. Mol Cell (2019) 73(2):354–363.e3. doi: 10.1016/j.molcel.2018.10.042
93. D’Herde K, Krysko DV. Ferroptosis: Oxidized PEs Trigger Death. Nat Chem Biol (2017) 13(1):4–5. doi: 10.1038/nchembio.2261
94. Dierge E, Debock E, Guilbaud C, Corbet C, Mignolet E, Mignard L, et al. Peroxidation of N-3 and N-6 Polyunsaturated Fatty Acids in the Acidic Tumor Environment Leads to Ferroptosis-Mediated Anticancer Effects. Cell Metab (2021) 33(8):1701–15. doi: 10.1016/j.cmet.2021.05.016
95. Corbet C, Bastien E, Santiago de Jesus JP, Dierge E, Martherus R, Vander Linden C, et al. Tgfβ2-Induced Formation of Lipid Droplets Supports Acidosis-Driven EMT and the Metastatic Spreading of Cancer Cells. Nat Commun (2020) 11(1):454. doi: 10.1038/s41467-019-14262-3
96. Wang W, Green M, Choi JE, Gijón M, Kennedy PD, Johnson JK, et al. CD8+ T Cells Regulate Tumour Ferroptosis During Cancer Immunotherapy. Nature. (2019) 569(7755):270–4. doi: 10.1038/s41586-019-1170-y
97. Demuynck R, Efimova I, Naessens F, Krysko DV. Immunogenic Ferroptosis and Where to Find it? J Immunother Cancer (2021) 9(12):e003430. doi: 10.1136/jitc-2021-003430
98. Liu J, Zhu S, Zeng L, Li J, Klionsky DJ, Kroemer G, et al. DCN Released From Ferroptotic Cells Ignites AGER-Dependent Immune Responses. Autophagy. (2021), 1–14. doi: 10.1080/15548627.2021.2008692
99. Wen Q, Liu J, Kang R, Zhou B, Tang D. The Release and Activity of HMGB1 in Ferroptosis. Biochem Biophys Res Commun (2019) 510(2):278–83. doi: 10.1016/j.bbrc.2019.01.090
100. Yu Y, Xie Y, Cao L, Yang L, Yang M, Lotze MT, et al. The Ferroptosis Inducer Erastin Enhances Sensitivity of Acute Myeloid Leukemia Cells to Chemotherapeutic Agents. Mol Cell Oncol (2015) 2(4):e1054549. doi: 10.1080/23723556.2015.1054549
101. Liao P, Wang W, Wang W, Kryczek I, Li X, Bian Y, et al. CD8+ T Cells and Fatty Acids Orchestrate Tumor Ferroptosis and Immunity via ACSL4. Cancer Cell (2022) 40(4):365–378.e6. doi: 10.1016/j.ccell.2022.02.003
102. Li W, Feng G, Gauthier JM, Lokshina I, Higashikubo R, Evans S, et al. Ferroptotic Cell Death and TLR4/Trif Signaling Initiate Neutrophil Recruitment After Heart Transplantation. J Clin Invest (2019) 129(6):2293–304. doi: 10.1172/JCI126428
103. Qian X, Zhang J, Gu Z, Chen Y. Nanocatalysts-Augmented Fenton Chemical Reaction for Nanocatalytic Tumor Therapy. Biomaterials. (2019) 211:1–13. doi: 10.1016/j.biomaterials.2019.04.023
104. Mishchenko TA, Balalaeva IV, Vedunova MV, Krysko DV. Ferroptosis and Photodynamic Therapy Synergism: Enhancing Anticancer Treatment. Trends Cancer (2021) 7(6):484–7. doi: 10.1016/j.trecan.2021.01.013
105. Zhang F, Li F, Lu GH, Nie W, Zhang L, Lv Y, et al. Engineering Magnetosomes for Ferroptosis/Immunomodulation Synergism in Cancer. ACS Nano (2019) 13(5):5662–73. doi: 10.1021/acsnano.9b00892
106. Krysko DV, Vandenabeele P. Clearance of Dead Cells: Mechanisms, Immune Responses and Implication in the Development of Diseases. Apoptosis Int J Program Cell Death (2010) 15(9):995–7. doi: 10.1007/s10495-010-0524-6
107. Hollingsworth RE, Jansen K. Turning the Corner on Therapeutic Cancer Vaccines. NPJ Vaccines (2019) 4(1):1–10. doi: 10.1038/s41541-019-0103-y
108. Shlomovitz I, Speir M, Gerlic M. Flipping the Dogma – Phosphatidylserine in non-Apoptotic Cell Death. Cell Commun Signal (2019) 17(1):139. doi: 10.1186/s12964-019-0437-0
109. Krysko O, De Ridder L, Cornelissen M. Phosphatidylserine Exposure During Early Primary Necrosis (Oncosis) in JB6 Cells as Evidenced by Immunogold Labeling Technique. Apoptosis Int J Program Cell Death (2004) 9(4):495–500. doi: 10.1023/B:APPT.0000031452.75162.75
110. Shlomovitz I, Erlich Z, Speir M, Zargarian S, Baram N, Engler M, et al. Necroptosis Directly Induces the Release of Full-Length Biologically Active IL-33 In Vitro and in an Inflammatory Disease Model. FEBS J (2019) 286(3):507–22. doi: 10.1111/febs.14738
111. Thornberry NA, Bull HG, Calaycay JR, Chapman KT, Howard AD, Kostura MJ, et al. A Novel Heterodimeric Cysteine Protease is Required for Interleukin-1βprocessing in Monocytes. Nature. (1992) 356(6372):768–74. doi: 10.1038/356768a0
112. Li L, Zou J, Dai Y, Fan W, Niu G, Yang Z, et al. Burst Release of Encapsulated Annexin A5 in Tumours Boosts Cytotoxic T-Cell Responses by Blocking the Phagocytosis of Apoptotic Cells. Nat BioMed Eng. (2020) 4(11):1102–16. doi: 10.1038/s41551-020-0599-5
113. Bondanza A, Zimmermann VS, Rovere-Querini P, Turnay J, Dumitriu IE, Stach CM, et al. Inhibition of Phosphatidylserine Recognition Heightens the Immunogenicity of Irradiated Lymphoma Cells In Vivo. J Exp Med (2004) 200(9):1157–65. doi: 10.1084/jem.20040327
114. Feng R, Zhao H, Xu J, Shen C. CD47: The Next Checkpoint Target for Cancer Immunotherapy. Crit Rev Oncol Hematol (2020) 152:103014. doi: 10.1016/j.critrevonc.2020.103014
115. Chao MP, Takimoto CH, Feng DD, McKenna K, Gip P, Liu J, et al. Therapeutic Targeting of the Macrophage Immune Checkpoint CD47 in Myeloid Malignancies. Front Oncol (2019) 9:1380. doi: 10.3389/fonc.2019.01380
116. Candas-Green D, Xie B, Huang J, Fan M, Wang A, Menaa C, et al. Dual Blockade of CD47 and HER2 Eliminates Radioresistant Breast Cancer Cells. Nat Commun (2020) 11(1):4591. doi: 10.1038/s41467-020-18245-7
117. Sikic BI, Lakhani N, Patnaik A, Shah SA, Chandana SR, Rasco D, et al. First-In-Human, First-in-Class Phase I Trial of the Anti-CD47 Antibody Hu5F9-G4 in Patients With Advanced Cancers. J Clin Oncol Off J Am Soc Clin Oncol (2019) 37(12):946–53. doi: 10.1200/JCO.18.02018
118. Veillette A, Chen J. Sirpα–CD47 Immune Checkpoint Blockade in Anticancer Therapy. Trends Immunol (2018) 39(3):173–84. doi: 10.1016/j.it.2017.12.005
119. Edris B, Weiskopf K, Volkmer AK, Volkmer JP, Willingham SB, Contreras-Trujillo H, et al. Antibody Therapy Targeting the CD47 Protein is Effective in a Model of Aggressive Metastatic Leiomyosarcoma. Proc Natl Acad Sci U S A. (2012) 109(17):6656–61. doi: 10.1073/pnas.1121629109
120. Chao MP, Alizadeh AA, Tang C, Myklebust JH, Varghese B, Gill S, et al. Anti-CD47 Antibody Synergizes With Rituximab to Promote Phagocytosis and Eradicate non-Hodgkin Lymphoma. Cell. (2010) 142(5):699–713. doi: 10.1016/j.cell.2010.07.044
121. Li Y, Zhang M, Wang X, Liu W, Wang H, Yang YG. Vaccination With CD47 Deficient Tumor Cells Elicits an Antitumor Immune Response in Mice. Nat Commun (2020) 11(1):581. doi: 10.1038/s41467-019-14102-4
122. Dobrovolskaia MA, Aggarwal P, Hall JB, McNeil SE. Preclinical Studies to Understand Nanoparticle Interaction With the Immune System and its Potential Effects on Nanoparticle Biodistribution. Mol Pharm (2008) 5(4):487–95. doi: 10.1021/mp800032f
123. Bobo D, Robinson KJ, Islam J, Thurecht KJ, Corrie SR. Nanoparticle-Based Medicines: A Review of FDA-Approved Materials and Clinical Trials to Date. Pharm Res (2016) 33(10):2373–87. doi: 10.1007/s11095-016-1958-5
124. Anselmo AC, Mitragotri S. Nanoparticles in the Clinic. Bioeng Transl Med (2016) 1(1):10–29. doi: 10.1002/btm2.10003
125. Venditto VJ, Szoka FC. Cancer Nanomedicines: So Many Papers and So Few Drugs! Adv Drug Delivery Rev (2013) 65(1):80–8. doi: 10.1016/j.addr.2012.09.038
126. American Food and Drug Administration. Nanotechnology Regulatory Science Research Plan (2013). Available at: https://www.fda.gov/scienceresearch/nanotechnology-programs-fda/2013-nanotechnology-regulatory-science-research-plan.
127. European Union. EU NanoSafety Cluster. Available at: https://www.nanosafetycluster.eu/.
128. Rauscher H, Rasmussen K, Sokull-Klüttgen B. Regulatory Aspects of Nanomaterials in the EU. Chem Ing Tech. (2017) 89(3):224–31. doi: 10.1002/cite.201600076
129. Andón FT, Fadeel B. Programmed Cell Death: Molecular Mechanisms and Implications for Safety Assessment of Nanomaterials. Acc Chem Res (2013) 46(3):733–42. doi: 10.1021/ar300020b
130. Lenders V, Koutsoumpou X, Sargsian A, Manshian BB. Biomedical Nanomaterials for Immunological Applications: Ongoing Research and Clinical Trials. Nanoscale Adv (2020) 2:5046–5089. doi: 10.1039/D0NA00478B
131. Silva AL, Soema PC, Slütter B, Ossendorp F, Jiskoot W. PLGA Particulate Delivery Systems for Subunit Vaccines: Linking Particle Properties to Immunogenicity. Hum Vaccines Immunother (2016) 12(4):1056–69. doi: 10.1080/21645515.2015.1117714
132. Snyder A, Chan TA. Immunogenic Peptide Discovery in Cancer Genomes. Curr Opin Genet Dev (2015) 30:7–16. doi: 10.1016/j.gde.2014.12.003
133. Diederich M. Natural Compound Inducers of Immunogenic Cell Death. Arch Pharm Res (2019) 42(7):629–45. doi: 10.1007/s12272-019-01150-z
134. Kolishetti N, Dhar S, Valencia PM, Lin LQ, Karnik R, Lippard SJ, et al. Engineering of Self-Assembled Nanoparticle Platform for Precisely Controlled Combination Drug Therapy. Proc Natl Acad Sci U S A. (2010) 107(42):17939–44. doi: 10.1073/pnas.1011368107
135. Saveleva MS, Eftekhari K, Abalymov A, Douglas TEL, Volodkin D, Parakhonskiy BV, et al. Hierarchy of Hybrid Materials-the Place of Inorganics-in-Organics in it, Their Composition and Applications. Front Chem (2019) 7:179. doi: 10.3389/fchem.2019.00179
136. Nel AE, Mädler L, Velegol D, Xia T, Hoek EMV, Somasundaran P, et al. Understanding Biophysicochemical Interactions at the Nano–Bio Interface. Nat Mater (2009) 8(7):543–57. doi: 10.1038/nmat2442
137. Chithrani BD, Ghazani AA, Chan WCW. Determining the Size and Shape Dependence of Gold Nanoparticle Uptake Into Mammalian Cells. Nano Lett (2006) 6(4):662–8. doi: 10.1021/nl052396o
138. Dobrovolskaia MA, McNeil SE. Understanding the Correlation Between In Vitro and In Vivo Immunotoxicity Tests for Nanomedicines. J Control Release Off J Control Release Soc (2013) 172(2):456–66. doi: 10.1016/j.jconrel.2013.05.025
139. Valsami-Jones E, Lynch I. Nanosafety. How Safe are Nanomaterials? Science. (2015) 350(6259):388–9. doi: 10.1126/science.aad0768
140. Delcea M, Möhwald H, Skirtach AG. Stimuli-Responsive LbL Capsules and Nanoshells for Drug Delivery. Adv Drug Delivery Rev (2011) 63(9):730–47. doi: 10.1016/j.addr.2011.03.010
141. Skirtach AG, Yashchenok AM, Möhwald H. Encapsulation, Release and Applications of LbL Polyelectrolyte Multilayer Capsules. Chem Commun (2011) 47(48):12736–46. doi: 10.1039/c1cc13453a
142. Devriendt B, Baert K, Dierendonck M, Favoreel H, De Koker S, Remon JP, et al. One-Step Spray-Dried Polyelectrolyte Microparticles Enhance the Antigen Cross-Presentation Capacity of Porcine Dendritic Cells. Eur J Pharm Biopharm (2013) 84(2):421–9. doi: 10.1016/j.ejpb.2012.11.016
143. Porter CJH, Trevaskis NL, Charman WN. Lipids and Lipid-Based Formulations: Optimizing the Oral Delivery of Lipophilic Drugs. Nat Rev Drug Discovery (2007) 6(3):231–48. doi: 10.1038/nrd2197
144. Filipczak N, Yalamarty SSK, Li X, Khan MM, Parveen F, Torchilin V. Lipid-Based Drug Delivery Systems in Regenerative Medicine. Materials. (2021) 14(18):5371. doi: 10.3390/ma14185371
145. Simone EA, Dziubla TD, Muzykantov VR. Polymeric Carriers: Role of Geometry in Drug Delivery. Expert Opin Drug Deliv. (2008) 5(12):1283–300. doi: 10.1517/17425240802567846
146. Delcea M, Yashchenok A, Videnova K, Kreft O, Möhwald H, Skirtach AG. Multicompartmental Micro- and Nanocapsules: Hierarchy and Applications in Biosciences. Macromol Biosci (2010) 10(5):465–74. doi: 10.1002/mabi.200900359
147. Delcea M, Madaboosi N, Yashchenok AM, Subedi P, Volodkin DV, Geest BGD, et al. Anisotropic Multicompartment Micro- and Nano-Capsules Produced via Embedding Into Biocompatible PLL/HA Films. Chem Commun (2011) 47(7):2098–100. doi: 10.1039/C0CC04820H
148. Huang Y, Cao L, Parakhonskiy BV, Skirtach AG. Hard, Soft, and Hard-And-Soft Drug Delivery Carriers Based on CaCO3 and Alginate Biomaterials: Synthesis, Properties, Pharmaceutical Applications. Pharmaceutics. (2022) 14(5):909. doi: 10.3390/pharmaceutics14050909
149. Xiong R, Soenen SJ, Braeckmans K, Skirtach AG. Towards Theranostic Multicompartment Microcapsules: in-Situ Diagnostics and Laser-Induced Treatment. Theranostics. (2013) 3(3):141–51. doi: 10.7150/thno.5846
150. Van der Meeren L, Li J, Parakhonskiy B, Krysko D, Skirtach A. Classification of Analytics, Sensorics, and Bioanalytics With Polyelectrolyte Multilayer Capsules. Anal Bioanal Chem (2020) 412:1–15. doi: 10.1007/s00216-020-02428-8
151. Leong HS, Butler KS, Brinker CJ, Azzawi M, Conlan S, Dufés C, et al. On the Issue of Transparency and Reproducibility in Nanomedicine. Nat Nanotechnol (2019) 14(7):629–35. doi: 10.1038/s41565-019-0496-9
152. European Medicines Agency. Ethical Use of Animals in Medicine Testing . Available at: https://www.ema.europa.eu/en/human-regulatory/research-development/ethical-use-animals-medicine-testing.
153. Turubanova VD, Mishchenko TA, Balalaeva IV, Efimova I, Peskova NN, Klapshina LG, et al. Novel Porphyrazine-Based Photodynamic Anti-Cancer Therapy Induces Immunogenic Cell Death. Sci Rep (2021) 11(1):7205. doi: 10.1038/s41598-021-86354-4
154. Alzeibak R, Mishchenko TA, Shilyagina NY, Balalaeva IV, Vedunova MV, Krysko DV. Targeting Immunogenic Cancer Cell Death by Photodynamic Therapy: Past, Present and Future. J Immunother Cancer (2021) 9(1):e001926. doi: 10.1136/jitc-2020-001926
155. Roacho−Perez JA, Gallardo−Blanco HL, Sanchez−Dominguez M, Garcia−Casillas PE, Chapa−Gonzalez C, Sanchez−Dominguez CN. Nanoparticles for Death−Induced Gene Therapy in Cancer (Review). Mol Med Rep (2018) 17(1):1413–20. doi: 10.3892/mmr.2017.8091
156. Gebremedhin S, Singh A, Koons S, Bernt W, Konopka K, Duzgunes N. Gene Delivery to Carcinoma Cells via Novel non-Viral Vectors: Nanoparticle Tracking Analysis and Suicide Gene Therapy. Eur J Pharm Sci Off J Eur Fed Pharm Sci (2014) 60:72–9. doi: 10.1016/j.ejps.2014.03.003
157. Lim S, Park J, Shim MK, Um W, Yoon HY, Ryu JH, et al. Recent Advances and Challenges of Repurposing Nanoparticle-Based Drug Delivery Systems to Enhance Cancer Immunotherapy. Theranostics. (2019) 9(25):7906–23. doi: 10.7150/thno.38425
158. Ruiz-de-Angulo A, Bilbao-Asensio M, Cronin J, Evans SJ, Clift MJD, Llop J, et al. Chemically Programmed Vaccines: Iron Catalysis in Nanoparticles Enhances Combination Immunotherapy and Immunotherapy-Promoted Tumor Ferroptosis. iScience. (2020) 23(9):101499. doi: 10.1016/j.isci.2020.101499
159. Pelaz B, Alexiou C, Alvarez-Puebla RA, Alves F, Andrews AM, Ashraf S, et al. Diverse Applications of Nanomedicine. ACS Nano (2017) 11(3):2313–81. doi: 10.1021/acsnano.6b06040
Keywords: ferroptosis apoptosis, necroptosis, pyroptosis, immunogenicity, immunogenic cell death, antitumor therapy, nanomaterials
Citation: Catanzaro E, Feron O, Skirtach AG and Krysko DV (2022) Immunogenic Cell Death and Role of Nanomaterials Serving as Therapeutic Vaccine for Personalized Cancer Immunotherapy. Front. Immunol. 13:925290. doi: 10.3389/fimmu.2022.925290
Received: 21 April 2022; Accepted: 02 June 2022;
Published: 30 June 2022.
Edited by:
Catherine Sautes-Fridman, U1138 Centre de Recherche des Cordeliers (CRC)(INSERM), FranceReviewed by:
Xianda Zhao, University of Minnesota, United StatesThierry Mp Gauthier, National Institutes of Health (NIH), United States
Copyright © 2022 Catanzaro, Feron, Skirtach and Krysko. This is an open-access article distributed under the terms of the Creative Commons Attribution License (CC BY). The use, distribution or reproduction in other forums is permitted, provided the original author(s) and the copyright owner(s) are credited and that the original publication in this journal is cited, in accordance with accepted academic practice. No use, distribution or reproduction is permitted which does not comply with these terms.
*Correspondence: Dmitri V. Krysko, RG1pdHJpLktyeXNrb0BVR2VudC5iZQ==; André G. Skirtach, QW5kcmUuU2tpcnRhY2hAVUdlbnQuYmU=
†These authors shared senior authorship