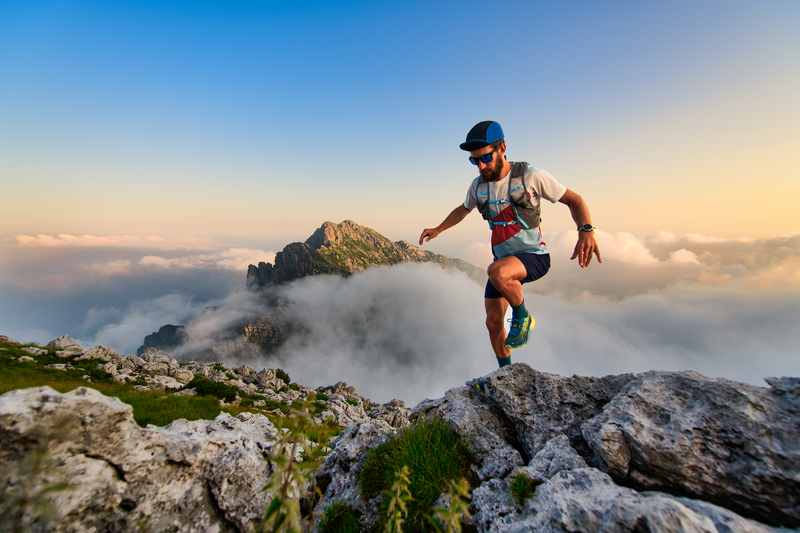
94% of researchers rate our articles as excellent or good
Learn more about the work of our research integrity team to safeguard the quality of each article we publish.
Find out more
REVIEW article
Front. Immunol. , 28 July 2022
Sec. Inflammation
Volume 13 - 2022 | https://doi.org/10.3389/fimmu.2022.922377
This article is part of the Research Topic Pharmacological Approaches Targeting Neutrophilic Inflammation: Volume II View all 11 articles
Neutrophils are the most abundant leukocytes in human peripheral blood. They form the first line of defense against invading foreign pathogens and might play a crucial role in malaria. According to World Health Organization (WHO), malaria is a globally significant disease caused by protozoan parasites from the Plasmodium genus, and it’s responsible for 627,000 deaths in 2020. Neutrophils participate in the defense response against the malaria parasite via phagocytosis and reactive oxygen species (ROS) production. Neutrophils might also be involved in the pathogenesis of malaria by the release of toxic granules and the release of neutrophil extracellular traps (NETs). Intriguingly, malaria parasites inhibit the anti-microbial function of neutrophils, thus making malaria patients more susceptible to secondary opportunistic Salmonella infections. In this review, we will provide a summary of the role of neutrophils during malaria infection, some contradicting mouse model neutrophil data and neutrophil-related mechanisms involved in malaria patients’ susceptibility to bacterial infection.
According to the WHO, malaria is a globally significant disease caused by protozoan parasites from the Plasmodium genus. There are five species of the protozoan genus Plasmodium known to infect humans: P. falciparum, P. vivax, P. malariae, P. ovale, and P. knowlesi, of which P. falciparum is responsible for most cases of severe malaria and death (1). The parasites caused over 241 million clinical cases and 627,000 deaths in 2020; this represents about 14 million more cases in 2020 compared to 2019 and 69 000 more deaths. Sub-Saharan Africa continues to carry the heaviest malaria burden, accounting for about 95% of all malaria cases and 96% of all deaths in 2020 (2). There are three clinical presentations of malaria identified: severe or complicated, mild or uncomplicated (3) and asymptomatic (4). The host immune response to malaria infection varies depending on factors. Factors like the genetic make-up of parasite proteins, co-infections, host genetics, host ethnic background, and geographical locations (5, 6). The response starts with physical barriers, progresses to an innate immune response, and leads to more adaptive responses.
The malaria parasites have a complex life cycle requiring a human and mosquito host (7). During the blood meal of the female Anopheles mosquito, sporozoites are transmitted into the human hosts (8). The pre-erythrocytic developmental stage is initiated when the released sporozoites migrate to the host liver. In the liver, the released sporozoites infect the hepatocytes (liver cells) in a process known as the liver stage. Within the hepatocytes the parasites grow and replicate as hepatic schizonts over a period of 10–12 days after which they are released as merozoites. The blood stage of the parasite starts when the merozoites rapidly invades the red blood cells (RBCs) in the bloodstream. During the blood stage, the merozoites replicates to produce more daughter parasites which are then released from the host cell upon parasite egress and subsequently re-invade RBCs to start a new asexual replication cycle (9). A small proportion of the malaria parasites will eventually differentiate into gametocytes to begin the sexual cycle which are subsequently taken up by mosquitos during the next blood meal (10).
Neutrophils are the most abundant white blood cell accounting for up to 70% of all blood leukocytes (11). They are also known as polymorphonuclear cells (PMNs) and are terminally differentiated leukocytes. Neutrophils are professional phagocytes, which use receptor mediated phagocytosis to internalize pathogens into phagolysosomes (12). Cytoplasmic granules include cathepsins, elastases, and myeloperoxidases that fuse with the phagolysosome to digest phagocytosed pathogens, in a process known as degranulation (12). Release of reactive oxygen species (ROS), produced via an NADPH oxidase-dependent process is a crucial bactericidal mechanism. Neutrophils can also kill extracellular pathogens by degranulation, secretion of ROS, or the release of neutrophil extracellular traps (NETs). NETs is the release of decondensed chromatin laced with granular proteins and histones to prevent the spread of pathogens (13).
Neutrophils are crucial for the body’s innate immune response (14, 15) and are involved in various disease processes, including pathogen infection (16), pulmonary diseases (17), cardiovascular diseases (18), inflammatory disorders (19) and cancer (20). They are challenging to study because they are short-lived effector cells of the innate immune system. Upon sensing infection, neutrophils are the first cells to migrate to the infection site (21). At the affected tissues, neutrophils use multiple antimicrobial functions such as engulfing foreign matter for internal digestion, reactive oxygen species (ROS) production and releasing NETs. The immune system plays a vital role in controlling the parasite’s growth (22). Clinical data has shown that the number of circulating neutrophils is high in patients with acute uncomplicated malaria (23), in contrast to circulating lymphocytes, which decrease during P. falciparum infections. During Plasmodium infection, parasites components and cytokines are produced and might activate circulating neutrophils. Activated neutrophils are equipped with several weapons to mount an immune defense against the parasite. While on the other hand, these neutrophil weapons might also be involved in the pathogenesis of severe malaria, though the underlying mechanism is still unclear.
During malaria infection, the immune system is overwhelmed resulting in immune suppression thereby making malaria patients to be at risk of developing secondary infections. One well-documented risk factor for invasive bacterial infection is Plasmodium falciparum malaria (24, 25). In Sub-Saharan Africa countries, bacterial infection in children is highly associated with malaria infection (26–28). In this review, we assess the literature examining the role of neutrophils during malaria infection and the neutrophil related mechanism involved in malaria patients’ susceptibility to bacterial infection.
One of the ways by which neutrophil play a role in the clearance of malaria parasites is by phagocytosis. Neutrophils express immunoglobulin (Ig) binding receptors Fcγ receptors and complement receptor 1 (CR1) and complement receptor 3 (CR3) (29). Phagocytosis of the released sporozoites during malaria infection are facilitated by the FcγR-receptors and by the presence of antibodies against the circum-sporozoite protein, one of the main surface antigens on sporozoites (30). Phagocytosis of parasite infected red blood cells (iRBC) in vivo has been observed in children with malaria (31) and in bone marrow aspirates which show neutrophils with internalized merozoites and trophozoites (32). The interaction between neutrophils and iRBCs is mediated by PfEMP1 on the iRBC surface and ICAM-1 expressed on neutrophils (33).
Neutrophil phagocytosis of free merozoites could be in an antibody dependent manner or via a complement mediated opsonization manner (23, 34) and can be enhanced in the presence of immune sera or when cytokines such as interferon gamma and tumor necrosis factor was added (35, 36). Recently, it was demonstrated that at high antibody levels, neutrophils are more effective via the action of FcγRIIA and FcγRIIIB (37). This may suggest that neutrophils are responsible for the phagocytosis of parasites in immune patients. In addition, neutrophil uptake of serum opsonized merozoites has been demonstrated in vitro and ex vivo (38). In contrast to complement dependent merozoites phagocytosis, phagocytosis of iRBC in neutrophil is largely dependent on the presence of IgG (39). Neutrophils phagocytose gametes in vitro in conditions similar to those of the mosquito gut when immune sera is present especially IgG (40). However, ex vivo evidence of the specific role of neutrophil phagocytosis of intra erythrocytic gametes in human is still lacking.
Neutrophils can clear pathogens by producing ROS by converting oxygen to superoxide via nicotinamide adenine dinucleotide phosphate oxidase (NADPH) oxidase (NOX). This superoxide is converted into hydrogen peroxide (H2O2) and hydroxyl radicals (-OH), collectively known as ROS (41). Neutrophils may also be involved in the control of parasite growth through antibody-dependent respiratory burst (ADRB) (35). Neutrophils isolated from malaria patients have been shown to exhibit higher ADRB activity in vitro and promote parasite clearance by inhibiting parasite growth (42). ROS related parasite inhibition occurs during the parasite intra-erythrocytic development stage (43) rather than during the merozoite stage. This was further demonstrated by Dasari et al. that ROS production from stimulated neutrophils does not inhibit merozoite growth in vitro (34). Though the mechanism underlying the observed impaired ROS production in neutrophils during malaria is unclear but released hemozoins (23) and digestive vacuoles (DV) (34) from iRBC have been suggested to be responsible.
Neutrophil extracellular trap (NET) formation is an essential innate strategy for immobilizing and killing foreign pathogens by neutrophils. It occurs when activated neutrophils degranulate and release their antimicrobial factors into the extracellular environment. Several factors might induce NET formation during Plasmodium infections such as crystal uric acid is a potent inducer of NETosis (44) (Plasmodium cannot synthesize purines and imports hypoxanthine as a purine source (45). Upon erythrocyte rupture and release, xanthine dehydrogenase, which is normally present in the blood (46) will efficiently degrade it into uric acid and are released into circulation during malaria. Plasmodium-infected erythrocytes accumulate hypoxanthine, a precursor for uric acid), pro-inflammatory cytokines like TNF and IL8 increase during Plasmodium infections (47), H2O2 is secreted by immune cells stimulated by the malaria parasite and Plasmodium antigens induce NETosis in vitro (48). NETs may contribute to the host defense against sporozoites and merozoites (35). Studies have shown that in the peripheral blood of children with complicated and uncomplicated P. falciparum infections, NETs like structures are present (49, 50). The release of NETs might play a crucial role in controlling parasite dissemination, but on the other hand, it may also contribute to the development of severe complications. Few studies have reported the possible role of NETs in controlling parasite growth during malaria. For example, Kho and colleagues reported that NET formation was inversely associated with parasitemia levels in patients with asymptomatic malaria (51). Another study by Rodrigues et al. showed that Pulmozyme (active molecule: DNase 1) treatment to inhibit NETosis resulted in increased parasitemia levels in P. berghei infected mice and subsequently decreased survival rate (49). However, the group also reported that the same observation was not recorded when P. chabaudi-infected mice were treated with Pulmozyme. Interestingly, Plasmodium parasites express TatD-like DNases to cleave NETs. In vivo mouse data have shown that treatment of mice with recombinant TatD resulted in low parasitemia and ultimately increased survival rate (52). Knackstedt et al. demonstrated that NETs might be driving inflammatory pathogenesis in malaria (53). However, evidences that NETs released in response to malaria parasite and proof that NETs are present in tissues is still debatable. For example, Feintuch and colleagues reported that brain tissue sections from children with fatal cerebral malaria (CM) and associated retinopathy were stained with NET markers, neutrophil elastase, and citrullinated histones, with no evidence of NETs was observed (54). In contrast, a recent study by Knackstedt et al. examined and analyzed retinal tissue from fatal pediatric cases who had died of cerebral malaria. The authors showed the image of NETs by colocalizing citrullinated histone H3, elastase, and DAPI (53).
Neutrophils may also contribute to the pathophysiology of malaria complications. Studies have associated high number of neutrophils with severe malaria cases (51, 53). The association between plasma levels of MPO, lysozyme and neutrophil lipocalin and malaria severity has been reported by several studies (35, 51, 53). In patients with severe malaria, neutrophil granule proteins such as neutrophil elastase and defensin have been observed to be increased compared to uncomplicated malaria patients (55). Another study also reported that during CM, neutrophil proteins in plasma are associated with CM and may contribute to CM pathology (endothelium damage via neutrophil elastase) (56) (Figure 1). Another indication for neutrophil activation is the release of matrix metalloproteinase (MMP)-8 and 9. For example, increased levels of plasma protein MMP-8 was reported in malaria patient, but no significant difference between uncomplicated and severe malaria. Furthermore, in Sub Saharan African children with CM, immunohistochemical staining revealed the presence of MMP-8 in the retina tissue accompanied with oedema, thus suggesting the role of MMP-8 in vascular endothelial barrier disruption (57). In addition, in vivo data further demonstrated that knocking out MMP-9 had no significant effect on CM development and survival in mice (58). Chemokines such as CXCLI and CXCL8 are known neutrophil chemoattractants, were at an increased level in the plasma of severe malaria patients (59). Furthermore, studies have associated a link between hemozoin laden neutrophils and disease severity has been reported in several malaria patients (60–62). In conclusion, these studies suggest a link between neutrophil activation and malaria severity.
Figure 1 Roles of neutrophils in defense and pathology of malaria. Neutrophils play crucial roles in the immune defense against malaria, through parasite clearance via neutrophil phenotypic functions such as phagocytosis, reactive oxygen species (ROS) production and NETs release. On the other hand, neutrophils might play a role in the development of malaria complications, and haemozoin-containing neutrophils are associated with malaria severity. In addition, the release of toxic granules, such as myeloperoxidase (MPO), neutrophil elastase (NE) and matrix metalloproteinase-8 (MMP-8), causes endothelial cell damage via apoptosis. Finally, the release of NETs may aggravate complications during malaria infection.
Animal models have been used to study the role of neutrophils in malaria complications including lung injury, CM and liver injury (17, 63, 64). Murine models have demonstrated the association of accumulated neutrophils in the lungs with lung injury. Murine models of CM have demonstrated that neutrophils express cytokines such as IL2, IL12, IL18, IFNγ, and TNF and chemoattractive-chemokines (65) suggesting a role for neutrophils in cytokine and chemokine secretion during CM. Nacer and colleagues reported that neutrophils in murine CM are detected in the vasculature (66) and their depletion prevented CM development (67). Using chimeric mice, Ioannidis and colleagues identified neutrophils as the main cellular sources of CXCL10, a CXCR3 binding chemokine which is essential for the attraction of pathogenic CD8+ T cells to the brain in murine CM (68). In addition, studies revealed that circulating levels of CXCL10 is the most accurate predictor of CM mortality (69) (70) and its neutralization with specific mAbs, significantly prevents brain intravascular inflammation and protects infected animals from CM by reducing peripheral parasitemia level (71–73).
Murine models have over the years played a valuable role in understanding the role of neutrophils in malaria (Table 1) however, there are contradicting data on the role of neutrophils using the mouse model, and as such, neutrophils’ role in malaria is still unclear. For example, Schumak et al. (74) suggested that the number of circulating neutrophils in the blood and brain increased in P. berghei ANKA infected mice, while in contrast, Pai et al. reported no increase in the number of circulating neutrophils in the brain (79) though the experimental approach to count neutrophils were different in the two studies. In Schumak study, brain tissue was fixed in buffered formalin and quantification of neutrophils in tissue sections was performed in 10 high power fields (HPF). While in Pai study, neutrophils were quantified in real time using 2-photon intravital microscopy. Another contradiction is the link between neutrophil depletion and survival rate in murine CM model. For example, some in vivo studies demonstrated that depletion of neutrophils using anti-CD11a resulted in an increased survival rate and slowed down the rate of CM development (67, 80) (Table 1). In these studies, depletion of neutrophils was done using anti-CD11A or anti-GR1 before parasite infection, which depleted neutrophils and other leukocytes. In contrast, when anti-GR1 was administered late during the infection, the authors still observed CM development in their model except for a study by Senaldi and colleague (76). In contrast, another study showed that when specific anti-Ly6G antibody was used for neutrophil depletion, no CM development was observed (74) (Table 1). Interestingly, neutrophil released CXCL10 is known to recruit pathogenic CD8+ T cells to the brain in murine CM (68). The indirect effect on CD8+ T cells in the murine CM by the anti-GR1 antibody might explain the contradicting data. Therefore, there is a need for more in depth studies to elucidate whether neutrophils count plays a role in the development of CM.
Salmonella co-infection is a common bacterial infection, and it remains a global health concern. One well-documented risk factor for Salmonella is Plasmodium falciparum malaria (25). It’s often a lethal complication of P. falciparum infection in Sub-Saharan Africa. In Gambia, the incidence of invasive NTS infection mirrors that of malaria, and about 43% of children with Salmonella bacteremia had concurrent P. falciparum infections (81). In Tanzania, invasive NTS in young children is highly associated with recent malaria infection (26). Interestingly evidence from co-infection models supports this idea (Table 2). For example, a study by Cunnington and colleagues demonstrated that prior infection of mice with non-lethal P. yoelii resulted in decreased survival of S. typhimurium in the mice. Though the authors suggested that the decrease in the survival rate of the mice was a result of impaired ROS production in neutrophils (85), the mechanism underlying the neutrophil-associated immune suppression remains unclear.
During malaria infection, the parasite continuously breaks down RBCs followed by eryptosis of many uninfected RBCs (87). The direct destruction of RBC leads to the release of hemoglobin, or its breakdown product heme, into the plasma. Free heme is prooxidant and highly cytotoxic, contributing to endothelial injury (88). Intracellular heme is then degraded into equimolar amounts of iron, carbon monoxide, and biliverdin through the action of heme oxygenase (HO). Significantly, plasma heme is raised during both acute (89) and subclinical (90) P. falciparum malaria infections in humans and during acute P. yoelii infection in mice (85) https://jlb.onlinelibrary.wiley.com/doi/full/10.1002/JLB.3RI1018-400R - jlb10293-bib-0063. Studies have shown that hemolysis might be responsible for inhibiting neutrophil functions during malaria. For example, Cunnington and colleagues using a malaria mouse model demonstrated that neutrophils from malaria-infected mice could phagocytose S. typhimurium; however, their ability to kill was impaired.
The authors further reported that the phagocytosed bacteria remained viable and replicated within the phagosome due to deficient ROS production (85). In another study, neutrophils from children with acute malaria were observed to exhibit impaired ROS production. The authors recorded that the dysfunctional ROS production persists for up to 8 weeks after drug treatment (89). The authors suggested that this might explain why children with acute malaria remain susceptible to secondary bacterial infection (27). Several studies have shown that during malaria infection, the migration of neutrophils into the infected tissues, including blood (85), intestine (82), and liver (84) is impaired. Neutrophils precursors of Plasmodium-infected mice have been shown to express heme oxygenase-1(HO-1) (85), which has been reported to reduce neutrophil migration into the inflamed lung (91), thus suggesting an association between HO-1 and neutrophil migration.
During the parasite life cycle in the RBC, the malaria parasite feeds on the hemoglobin and packages the waste product hemozoin in an organelle designated the digestive vacuole (DV) (92). Large numbers of DVs are released into blood circulation during severe malaria infection. Some reports have implicated the role of DVs in suppressing the host immune system via the inhibition of neutrophil functions. For example, a study by Dasari and colleagues demonstrated that phagocytosed DVs induce oxidative burst in human neutrophils. However, the capacity to generate a subsequent ROS response to kill phagocytose bacteria was impaired (34). Thus, the anti-microbicidal activity was compromised. They suggested that DV might explain the risk of developing bacterial sepsis in patients with severe malaria. However, much more work is needed to fully characterize the content of these DVs and their effect on neutrophil migration during malaria infection.
Neutrophils in malaria remain understudied and they play a double-edge sword role in malaria. During malaria infection, neutrophils may be involved in defense mechanisms against parasites via phagocytosis and ROS production. On the other hand, neutrophils might also be involved in the pathogenesis of severe malaria via NETs and toxic granule proteins release. Therefore, more research studies are required to understand the specific roles of neutrophils in malaria.
During malaria, small extracellular vesicles (EVs) are secreted by Plasmodium infected RBC (iRBCs). EVs contribute to the immune regulation by transferring cargoes including RNAs from the iRBCs to immune cells, resulting in immune suppression or immune activation depending on the cellular context. Currently, it’s not clear if these EVs are involved in the observed immune suppression via the modulation of neutrophil functions in malaria patients. It will be interesting to know if EVs can deliver their biological cargoes to neutrophils which in turn maybe responsible for the observed immune suppression.
There seems to be a lot of contradicting neutrophil data using both in vitro and in vivo malaria models. We suggest that a novel ex vivo microfluidic platform, modelling the in vivo malaria infection microenvironment, will allow studying the interaction of neutrophils and malaria at single cell resolution and in real time. Such platform should allow the investigation of neutrophil migration, NETs release and parasite killing during neutrophil-parasite interaction.
KB conceptualized the review. KB and OA drafted the review. KB prepared the figure and we read and adapted the manuscript and approved the final version.
This work is supported by the Swiss National funding (P500PB_203002) and a grant from the Jubilee Foundation of the Swiss Life Insurance and Pension Fund for Public Health and Medical Research to KB.
The authors declare that the research was conducted in the absence of any commercial or financial relationships that could be construed as a potential conflict of interest.
All claims expressed in this article are solely those of the authors and do not necessarily represent those of their affiliated organizations, or those of the publisher, the editors and the reviewers. Any product that may be evaluated in this article, or claim that may be made by its manufacturer, is not guaranteed or endorsed by the publisher.
1. Schofield L, Grau GE. Immunological processes in malaria pathogenesis. Nat Rev Immunol (2005) 5:722–35. doi: 10.1038/nri1686
3. Adebayo JO, Krettli AU. Potential antimalarials from Nigerian plants: A review. J Ethnopharmacol (2011) 133:289–302. doi: 10.1016/j.jep.2010.11.024
4. Laishram DD, Sutton PL, Nanda N, Sharma VL, Sobti RC, Carlton JM, et al. The complexities of malaria disease manifestations with a focus on asymptomatic malaria. Malar J (2012) 11:1475–2875. doi: 10.1186/1475-2875-11-29
5. Balaji S, Deshmukh R, Trivedi V. Severe malaria: Biology, clinical manifestation, pathogenesis and consequences. J Vector Borne Dis (2020) 57:1–13. doi: 10.4103/0972-9062.308793
6. Moxon CA, Gibbins MP, McGuinness D, Milner DA, Marti M. New insights into malaria pathogenesis. Annu Rev Pathol Mech Dis (2020). doi: 10.1146/annurev-pathmechdis-012419-032640
7. Babatunde KA, Yesodha Subramanian B, Ahouidi AD, Martinez Murillo P, Walch M, Mantel PY. Role of extracellular vesicles in cellular cross talk in malaria. Front Immunol (2020) 11. doi: 10.3389/fimmu.2020.00022
8. Aly ASI, Vaughan AM, Kappe SHI. Malaria parasite development in the mosquito and infection of the mammalian host. Annu Rev Microbiol (2009) 63:195–221. doi: 10.1146/annurev.micro.091208.073403
9. Cowman AF, Crabb BS. Invasion of red blood cells by malaria parasites. Cell (2006) 124:755–86. doi: 10.1016/j.cell.2006.02.006
10. Baker DA. Malaria gametocytogenesis. Mol Biochem Parasitol (2010) 172:56–65. doi: 10.1016/j.molbiopara.2010.03.019
11. Mooney JP, Galloway LJ, Riley EM. Malaria, anemia, and invasive bacterial disease: A neutrophil problem? J Leukoc Biol (2019) 105:644–55. doi: 10.1002/JLB.3RI1018-400R
12. Kruger P, Saffarzadeh M, Weber ANR, Rieber N, Radsak M, von Bernuth H, et al. Neutrophils: Between host defence, immune modulation, and tissue injury. PloS Pathog (2015) 11:e1004651. doi: 10.1371/journal.ppat.1004651
13. Sollberger G, Tilley DO, Zychlinsky A. Neutrophil extracellular traps: The biology of chromatin externalization. Dev Cell (2018) 44:542–53. doi: 10.1016/j.devcel.2018.01.019
14. Rosales C. Neutrophil: A cell with many roles in inflammation or several cell types? Front Physiol (2018) 9. doi: 10.3389/fphys.2018.00113
15. Babatunde KA, Ayuso JM, Kerr SC, Huttenlocher A, Beebe DJ. Microfluidic systems to study neutrophil forward and reverse migration. Front Immunol (2021) 12. doi: 10.3389/fimmu.2021.781535
16. Jenne CN, Wong CHY, Zemp FJ, McDonald B, Rahman MM, Forsyth PA, et al. Neutrophils recruited to sites of infection protect from virus challenge by releasing neutrophil extracellular traps. Cell Host Microbe (2013) 13:169–80. doi: 10.1016/j.chom.2013.01.005
17. Sercundes MK, Ortolan LS, Debone D, Soeiro-Pereira PV, Gomes E, Aitken EH, et al. Targeting neutrophils to prevent malaria-associated acute lung Injury/Acute respiratory distress syndrome in mice. PloS Pathog (2016) 13:e1006730. doi: 10.1371/journal.ppat.1006054
18. Silvestre-Roig C, Braster Q, Wichapong K, Lee EY, Teulon JM, Berrebeh N, et al. Externalized histone H4 orchestrates chronic inflammation by inducing lytic cell death. Nature (2019) 569:263–240. doi: 10.1038/s41586-019-1167-6
19. Csepregi JZ, Orosz A, Zajta E, Kása O, Németh T, Simon E, et al. Myeloid-specific deletion of mcl-1 yields severely neutropenic mice that survive and breed in homozygous form. J Immunol (2018) 201:3793–803. doi: 10.4049/jimmunol.1701803
20. Hedrick CC, Malanchi I. Neutrophils in cancer: heterogeneous and multifaceted. Nat Rev Immunol (2022) 22:173–87. doi: 10.1038/s41577-021-00571-6
21. De Oliveira S, Rosowski EE, Huttenlocher A. Neutrophil migration in infection and wound repair: Going forward in reverse. Nat Rev Immunol (2016) 16:378–91. doi: 10.1038/nri.2016.49
22. Deroost K, Pham TT, Opdenakker G, Van den Steen PE. The immunological balance between host and parasite in malaria. FEMS Microbiol Rev (2016) 40:208–57. doi: 10.1093/femsre/fuv046
23. Aitken EH, Alemu A, Rogerson SJ. Neutrophils and malaria. Front Immunol (2018) 9:3005. doi: 10.3389/fimmu.2018.03005
24. Takem EN, Roca A, Cunnington A. The association between malaria and nontyphoid salmonella bacteraemia in children in sub-Saharan Africa: A literature review. Malar J (2014) 13:475–2875. doi: 10.1186/1475-2875-13-400
25. Church J, Maitland K. Invasive bacterial co-infection in African children with plasmodium falciparum malaria: A systematic review. BMC Med (2014) 12:1741–7015. doi: 10.1186/1741-7015-12-31
26. Mtove G, Amos B, Von Seidlein L, Hendriksen I, Mwambuli A, Kimera J, et al. Invasive salmonellosis among children admitted to a rural Tanzanian hospital and a comparison with previous studies. PloS One (2010) 5:e9244. doi: 10.1371/journal.pone.0009244
27. Biggs HM, Lester R, Nadjm B, Mtove G, Todd JE, Kinabo GD, et al. Invasive salmonella infections in areas of high and low malaria transmission intensity in Tanzania. Clin Infect Dis (2014) 58:638–47. doi: 10.1093/cid/cit798
28. Bronzan RN, Taylor TE, Mwenechanya J, Tembo M, Kayira K, Bwanaisa L, et al. Bacteremia in Malawian children with severe malaria: Prevalence, etiology, HIV coinfection, and outcome. J Infect Dis (2007) 195:895–904. doi: 10.1086/511437
29. Dale DC, Boxer L, Conrad Liles W. The phagocytes: Neutrophils and monocytes. Blood (2008) 112:935–45. doi: 10.1182/blood-2007-12-077917
30. Feng G, Wines BD, Kurtovic L, Chan JA, Boeuf P, Mollard V, et al. Mechanisms and targets of fcgamma-receptor mediated immunity to malaria sporozoites. Nat Commun (2021) 12:1742. doi: 10.1038/s41467-021-21998-4
31. Sun T, Chakrabarti C. Schizonts, merozoites, and phagocytosis in falciparum malaria. Ann Clin Lab Sci (1985) 15:465–9.
32. Wickramasinghe SN, Phillips RE, Looareesuwan S, Warrell DA, Hughes M. The bone marrow in human cerebral malaria: parasite sequestration within sinusoids. Br J Haematol (1987) 66:295–306. doi: 10.1111/j.1365-2141.1987.00295.x
33. Zelter T, Strahilevitz J, Simantov K, Yajuk O, Adams Y, Jensen AR, et al. Neutrophils impose strong immune pressure against PfEMP1 variants implicated in cerebral malaria. EMBO Rep (2022) 23:e53641. doi: 10.15252/embr.202153641
34. Dasari P, Reiss K, Lingelbach K, Baumeister S, Lucius R, Udomsangpetch R, et al. Digestive vacuoles of plasmodium falciparum are selectively phagocytosed by and impair killing function of polymorphonuclear leukocytes. Blood (2011). 118:4946–56 doi: 10.1182/blood-2011-05-353920
35. Tannous S, Ghanem E. A bite to fight: front-line innate immune defenses against malaria parasites. Pathog Glob Health (2018) 112:1–12. doi: 10.1080/20477724.2018.1429847
36. Kumaratilake LM, Rathjen DA, Mack P, Widmer F, Prasertsiriroj V, Ferrante A. A synthetic tumor necrosis factor-α agonist peptide enhances human polymorphonuclear leukocyte-mediated killing of plasmodium falciparum in vitro and suppresses plasmodium chabaudi infection in mice. J Clin Invest (1995) 95:2315–23. doi: 10.1172/JCI117923
37. Garcia-Senosiain A, Kana IH, Singh S, Das MK, Dziegiel MH, Hertegonne S, et al. Neutrophils dominate in opsonic phagocytosis of p. falciparum blood-stage merozoites and protect against febrile malaria. Commun Biol (2021) 4:984. doi: 10.1038/s42003-021-02511-5
38. Kumaratilake LM, Ferrante A. Opsonization and phagocytosis of plasmodium falciparum merozoites measured by flow cytometry. Clin Diagn Lab Immunol (2000) 7:9–13. doi: 10.1128/cdli.7.1.9-13.2000
39. Celada A, Cruchaud A, Perrin LH. Phagocytosis of plasmodium falciparum-parasitized erythrocytes by human polymorphonuclear leukocytes. J Parasitol (1983) 69:49–53. doi: 10.2307/3281273
40. Healer J, Graszynski A, Riley E. Phagocytosis does not play a major role in naturally acquired transmission-blocking immunity to plasmodium falciparum malaria. Infect Immun (1999) 67:2334–9. doi: 10.1128/iai.67.5.2334-2339.1999
41. Dupré-Crochet S, Erard M, Nüβe O. ROS production in phagocytes: why, when, and where? J Leukoc Biol (2013) 94:657–70. doi: 10.1189/jlb.1012544
42. Llewellyn D, Miura K, Fay MP, Williams AR, Murungi LM, Shi J, et al. Standardization of the antibody-dependent respiratory burst assay with human neutrophils and plasmodium falciparum malaria. Sci Rep (2015) 5: 14081. doi: 10.1038/srep14081
43. Nnalue NA, Friedman MJ. Evidence for a neutrophil-mediated protective response in malaria. Parasite Immunol (1988) 10:47–58. doi: 10.1111/j.1365-3024.1988.tb00202.x
44. Hoppenbrouwers T, Autar ASA, Sultan AR, Abraham TE, Van Cappellen WA, Houtsmuller AB, et al. In vitro induction of NETosis: Comprehensive live imaging comparison and systematic review. PloS One (2017) 12:e0176472. doi: 10.1371/journal.pone.0176472
45. Asahi H, Kanazawa T, Kajihara Y, Takahashi K, Takahasht T. Hypoxanthine: A low molecular weight factor essential for growth of erythrocytic plasmodium falciparum in a serum-free medium. Parasitology (1996). doi: 10.1017/s0031182000066233
47. Dunst J, Kamena F, Matuschewski K. Cytokines and chemokines in cerebral malaria pathogenesis. Front Cell Infect Microbiol (2017) 7. doi: 10.3389/fcimb.2017.00324
48. Percário S, Moreira DR, Gomes BAQ, Ferreira MES, Gonçalves ACM, Laurindo PSOC, et al. Oxidative stress in malaria. Int J Mol Sci (2012) 13:16346–372. doi: 10.3390/ijms131216346
49. Rodrigues DAS, Prestes EB, Gama AMS, de Souza Silva L, Pinheiro AAS, Ribeiro JMC, et al. CXCR4 and MIF are required for neutrophil extracellular trap release triggered by plasmodium-infected erythrocytes. PloS Pathog (2020) 16:e1008230. doi: 10.1371/JOURNAL.PPAT.1008230
50. Baker VS, Imade GE, Molta NB, Tawde P, Pam SD, Obadofin MO, et al. Cytokine-associated neutrophil extracellular traps and antinuclear antibodies in plasmodium falciparum infected children under six years of age. Malar J (2008) 7: 41. doi: 10.1186/1475-2875-7-41
51. Kho S, Minigo G, Andries B, Leonardo L, Prayoga P, Poespoprodjo JR, et al. Circulating neutrophil extracellular traps and neutrophil activation are increased in proportion to disease severity in human malaria. J Infect Dis (2019) 219:1994–2004. doi: 10.1093/infdis/jiy661
52. Chang Z, Jiang N, Zhang Y, Lu H, Yin J, Wahlgren M, et al. The TatD-like DNase of plasmodium is a virulence factor and a potential malaria vaccine candidate. Nat Commun (2016) 7: 11537. doi: 10.1038/ncomms11537
53. Knackstedt SL, Georgiadou A, Apel F, Abu-Abed U, Moxon CA, Cunnington AJ, et al. Neutrophil extracellular traps drive inflammatory pathogenesis in malaria. Sci Immunol (2019) 4: 397. doi: 10.1126/SCIIMMUNOL.AAW0336
54. Feintuch CM, Saidi A, Seydel K, Chen G, Goldman-Yassen A, Mita-Mendoza NK, et al. Activated neutrophils are associated with pediatric cerebral malaria vasculopathy in Malawian children. MBio (2016) 7:e01300-–5. doi: 10.1128/mBio.01300-15
55. Lee HJ, Georgiadou A, Walther M, Nwakanma D, Stewart LB, Levin M, et al. Integrated pathogen load and dual transcriptome analysis of systemic host-pathogen interactions in severe malaria. Sci Transl Med (2018) 10:eaar3619. doi: 10.1126/scitranslmed.aar3619
56. Yang JJ, Kettritz R, Falk RJ, Jennette JC, Gaido ML. Apoptosis of endothelial cells induced by the neutrophil serine proteases proteinase 3 and elastase. Am J Pathol (1996) 149:1617–26.
57. Dietmann A, Helbok R, Lackner P, Issifou S, Lell B, Matsiegui PB, et al. Matrix metalloproteinases and their tissue inhibitors (TIMPs) in plasmodium falciparum malaria: Serum levels of TIMP-1 are associated with disease severity. J Infect Dis (2008) 197:1614–20. doi: 10.1086/587943
58. Van Den Steen PE, Van Aelst I, Starckx S, Maskos K, Opdenakker G, Pagenstecher A. Matrix metalloproteinases, tissue inhibitors of MMPs and TACE in experimental cerebral malaria. Lab Investig (2006) 86:873–88. doi: 10.1038/labinvest.3700454
59. Amulic B, Moxon CA, Cunnington AJ. A more granular view of neutrophils in malaria. Trends Parasitol (2020) 36: 501–3. doi: 10.1016/j.pt.2020.03.003
60. Amodu OK, Adeyemo AA, Olumese PE, Gbadegesin RA. Intraleucocytic malaria pigment and clinical severity of malaria in children. Trans R Soc Trop Med Hyg (1998) 92:54–6. doi: 10.1016/S0035-9203(98)90952-X
61. Phu NH, Day N, Diep PT, Ferguson DJP, White NJ. Intraleucocytic malaria pigment and prognosis in severe malaria. Trans R Soc Trop Med Hyg (1995) 89:200–4. doi: 10.1016/0035-9203(95)90496-4
62. Lyke KE, Diallo DA, Dicko A, Kone A, Coulibaly D, Guindo A, et al. Association of intraleukocytic plasmodium falciparum malaria pigment with disease severity, clinical manifestations, and prognosis in severe malaria. Am J Trop Med Hyg (2003) 69:253–9. doi: 10.4269/ajtmh.2003.69.253
63. Lin JW, Sodenkamp J, Cunningham D, Deroost K, Tshitenge TC, McLaughlin S, et al. Signatures of malaria-associated pathology revealed by high-resolution whole-blood transcriptomics in a rodent model of malaria. Sci Rep (2017) 7:41722. doi: 10.1038/srep41722
64. Lovegrove FE, Gharib SA, Peña-Castillo L, Patel SN, Ruzinski JT, Hughes TR, et al. Parasite burden and CD36-mediated sequestration are determinants of acute lung injury in an experimental malaria model. PloS Pathog (2008) 4:e1000068. doi: 10.1371/journal.ppat.1000068
65. Chen L, Sendo F. Cytokine and chemokine mRNA expression in neutrophils from CBA/NSlc mice infected with plasmodium berghei ANKA that induces experimental cerebral malaria. Parasitol Int (2001) 50:139–43. doi: 10.1016/S1383-5769(01)00063-0
66. Nacer A, Movila A, Sohet F, Girgis NM, Gundra UM, Loke P, et al. Experimental cerebral malaria pathogenesis–hemodynamics at the blood brain barrier. PloS Pathog (2014) 10:e1004528. doi: 10.1371/journal.ppat.1004528
67. Chen L, Zhang ZH, Sendo F. Neutrophils play a critical role in the pathogenesis of experimental cerebral malaria. Clin Exp Immunol (2000) 120:125–33. doi: 10.1046/j.1365-2249.2000.01196.x
68. Ioannidis LJ, Nie CQ, Ly A, Ryg-Cornejo V, Chiu CY, Hansen DS. Monocyte- and neutrophil-derived CXCL10 impairs efficient control of blood-stage malaria infection and promotes severe disease. J Immunol (2016) 196:1227–38. doi: 10.4049/jimmunol.1501562
69. Jain V, Armah HB, Tongren JE, Ned RM, Wilson NO, Crawford S, et al. Plasma IP-10, apoptotic and angiogenic factors associated with fatal cerebral malaria in India. Malar J (2008) 7: 83. doi: 10.1186/1475-2875-7-83
70. Wilson NO, Jain V, Roberts CE, Lucchi N, Joel PK, Singh MP, et al. CXCL4 and CXCL10 predict risk of fatal cerebral malaria. Dis Markers (2011) 30:39–49. doi: 10.3233/DMA-2011-0763
71. Campanella GSV, Tager AM, El Khoury JK, Thomas SY, Abrazinski TA, Manice LA, et al. Chemokine receptor CXCR3 and its ligands CXCL9 and CXCL10 are required for the development of murine cerebral malaria. Proc Natl Acad Sci U.S.A. (2008) 105:4812–19. doi: 10.1073/pnas.0801544105
72. Wilson NO, Solomon W, Anderson L, Patrickson J, Pitts S, Bond V, et al. Pharmacologic inhibition of CXCL10 in combination with anti-malarial therapy eliminates mortality associated with murine model of cerebral malaria. PloS One (2013) 8:e60898. doi: 10.1371/journal.pone.0060898
73. Miu J, Mitchell AJ, Müller M, Carter SL, Manders PM, McQuillan JA, et al. Chemokine gene expression during fatal murine cerebral malaria and protection due to CXCR3 deficiency. J Immunol (2008) 180:1217–30. doi: 10.4049/jimmunol.180.2.1217
74. Schumak B, Klocke K, Kuepper JM, Biswas A, Djie-Maletz A, Limmer A, et al. Specific depletion of Ly6Chi inflammatory monocytes prevents immunopathology in experimental cerebral malaria. PloS One (2015) 10: e0124080. doi: 10.1371/journal.pone.0124080
75. Belnoue E, Kayibanda M, Vigario AM, Deschemin J-C, van Rooijen N, Viguier M, et al. On the pathogenic role of brain-sequestered αβ CD8 + T cells in experimental cerebral malaria. J Immunol (2002) 169:6369–75. doi: 10.4049/jimmunol.169.11.6369
76. Senaldi G, Vesin C, Chang R, Grau GE, Piguet PF. Role of polymorphonuclear neutrophil leukocytes and their integrin CD11a (LFA-1) in the pathogenesis of severe murine malaria. Infect Immun (1994) 62:1144–9. doi: 10.1128/iai.62.4.1144-1149.1994
77. Dey S, Bindu S, Goyal M, Pal C, Alam A, Iqbal MS, et al. Impact of intravascular hemolysis in malaria on liver dysfunction. J Biol Chem (2012) 287:26630–46. doi: 10.1074/jbc.m112.341255
78. Theeß W, Sellau J, Steeg C, Klinke A, Baldus S, Cramer JP, et al. Myeloperoxidase attenuates pathogen clearance during plasmodium yoelii nonlethal infection. Infect Immun (2017) 85:e00475–16. doi: 10.1128/IAI.00475-16
79. Pai S, Qin J, Cavanagh L, Mitchell A, El-Assaad F, Jain R, et al. Real-time imaging reveals the dynamics of leukocyte behaviour during experimental cerebral malaria pathogenesis. PloS Pathog (2014) 10:e1004236. doi: 10.1371/journal.ppat.1004236
80. Porcherie A, Mathieu C, Peronet R, Schneider E, Claver J, Commere PH, et al. Critical role of the neutrophil-associated high-affinity receptor for IgE in the pathogenesis of experimental cerebral malaria. J Exp Med (2011) 208: 2225–236. doi: 10.1084/jem.20110845
81. Mabey DCW, Brown A, Greenwood BM. Plasmodium falciparum malaria and salmonella infections in gambian children. J Infect Dis (1987) 155:1319–21. doi: 10.1093/infdis/155.6.1319
82. Mooney JP, Butler BP, Lokken KL, Xavier MN, Chau JY, Schaltenberg N, et al. The mucosal inflammatory response to non-typhoidal salmonella in the intestine is blunted by IL-10 during concurrent malaria parasite infection. Mucosal Immunol (2014) 7:1302–11. doi: 10.1038/mi.2014.18
83. Mooney JP, Lokken KL, Byndloss MX, George MD, Velazquez EM, Faber F, et al. Inflammation-associated alterations to the intestinal microbiota reduce colonization resistance against non-typhoidal salmonella during concurrent malaria parasite infection. Sci Rep (2015) 5:14603. doi: 10.1038/srep14603
84. Lokken KL, Mooney JP, Butler BP, Xavier MN, Chau JY, Schaltenberg N, et al. Malaria parasite infection compromises control of concurrent systemic non-typhoidal salmonella infection via IL-10-Mediated alteration of myeloid cell function. PloS Pathog (2014) 10:e1004049. doi: 10.1371/journal.ppat.1004049
85. Cunnington AJ, de Souza JB, Walther M, Riley EM. Malaria impairs resistance to salmonella through heme- and heme oxygenase–dependent dysfunctional granulocyte mobilization. Nat Med (2011) 18:120–7. doi: 10.1038/nm.2601
86. Mooney JP, Lee SJ, Lokken KL, Nanton MR, Nuccio SP, McSorley SJ, et al. Transient loss of protection afforded by a live attenuated non-typhoidal salmonella vaccine in mice Co-infected with malaria. PloS Negl Trop Dis (2015) 9:e0004027. doi: 10.1371/journal.pntd.0004027
87. Price RN, Simpson JA, Nosten F, Luxemburger C, Hkirjaroen L, Kuile FT, et al. Factors contributing to anemia after uncomplicated falciparum malaria. Am J Trop Med Hyg (2001) 65:614–22. doi: 10.4269/ajtmh.2001.65.614
88. Jeney V, Balla J, Yachie A, Varga Z, Vercellotti GM, Eaton JW, et al. Pro-oxidant and cytotoxic effects of circulating heme. Blood (2002) 100:879–87. doi: 10.1182/blood.V100.3.879
89. Cunnington AJ, Njie M, Correa S, Takem EN, Riley EM, Walther M. Prolonged neutrophil dysfunction after plasmodium falciparum malaria is related to hemolysis and heme oxygenase-1 induction. J Immunol (2012) 189:5336–46. doi: 10.4049/jimmunol.1201028
90. Mooney JP, Barry A, Gonçalves BP, Tiono AB, Awandu SS, Grignard L, et al. Haemolysis and haem oxygenase-1 induction during persistent “asymptomatic” malaria infection in burkinabé children. Malar J (2018) 17: 253. doi: 10.1186/s12936-018-2402-6
91. Konrad FM, Knausberg U, Höne R, Ngamsri KC, Reutershan J. Tissue heme oxygenase-1 exerts anti-inflammatory effects on LPS-induced pulmonary inflammation. Mucosal Immunol (2016) 9:98–111. doi: 10.1038/mi.2015.39
Keywords: neutrophil, malaria, plasmodium, salmonella typhimurium, neutrophil extracellular traps (NETs)
Citation: Babatunde KA and Adenuga OF (2022) Neutrophils in malaria: A double-edged sword role. Front. Immunol. 13:922377. doi: 10.3389/fimmu.2022.922377
Received: 17 April 2022; Accepted: 01 July 2022;
Published: 28 July 2022.
Edited by:
Galina Sud’ina, Lomonosov Moscow State University, RussiaReviewed by:
Viviana Marin-Esteban, Université Paris-Saclay, FranceCopyright © 2022 Babatunde and Adenuga. This is an open-access article distributed under the terms of the Creative Commons Attribution License (CC BY). The use, distribution or reproduction in other forums is permitted, provided the original author(s) and the copyright owner(s) are credited and that the original publication in this journal is cited, in accordance with accepted academic practice. No use, distribution or reproduction is permitted which does not comply with these terms.
*Correspondence: Kehinde Adebayo Babatunde, a2VoaW5kZS5iYWJhdHVuZGVAdWNhbGdhcnkuY2E=
Disclaimer: All claims expressed in this article are solely those of the authors and do not necessarily represent those of their affiliated organizations, or those of the publisher, the editors and the reviewers. Any product that may be evaluated in this article or claim that may be made by its manufacturer is not guaranteed or endorsed by the publisher.
Research integrity at Frontiers
Learn more about the work of our research integrity team to safeguard the quality of each article we publish.