- 1Department of Biochemistry and Chemistry, La Trobe Institute for Molecular Science, La Trobe University, Melbourne, VIC, Australia
- 2Department of Molecular Microbiology, Amsterdam Institute of Molecular and Life Sciences (AIMMS), Vrije Universiteit, Amsterdam, Netherlands
Autotransporters are the core component of a molecular nano-machine that delivers cargo proteins across the outer membrane of Gram-negative bacteria. Part of the type V secretion system, this large family of proteins play a central role in controlling bacterial interactions with their environment by promoting adhesion to surfaces, biofilm formation, host colonization and invasion as well as cytotoxicity and immunomodulation. As such, autotransporters are key facilitators of fitness and pathogenesis and enable co-operation or competition with other bacteria. Recent years have witnessed a dramatic increase in the number of autotransporter sequences reported and a steady rise in functional studies, which further link these proteins to multiple virulence phenotypes. In this review we provide an overview of our current knowledge on classical autotransporter proteins, the archetype of this protein superfamily. We also carry out a phylogenetic analysis of their functional domains and present a new classification system for this exquisitely diverse group of bacterial proteins. The sixteen phylogenetic divisions identified establish sensible relationships between well characterized autotransporters and inform structural and functional predictions of uncharacterized proteins, which may guide future research aimed at addressing multiple unanswered aspects in this group of therapeutically important bacterial factors.
1 Introduction
Many processes essential for bacterial survival require proteins located extracellularly or at the bacterial surface (1, 2). To facilitate their transport across the cell envelope, bacteria have evolved a diverse range of secretion systems. This includes the secretion of virulence factors that promote bacterial pathogenesis via functions such as invasion, adherence, dissemination, and immune evasion (3, 4). Accordingly, these secretion systems are fundamental for bacterial pathogenesis. The most ubiquitous are the Sec and Tat systems, which transport a large variety of proteins across the phospholipid biolayer of the inner membrane (IM) (5). In Gram-negative bacteria, the outer membrane (OM), with phospholipid and lipopolysaccharide leaflets, presents a second barrier to secretion. To overcome the multilayered cell envelope, Gram-negative bacteria possess additional secretion machineries including the chaperone usher system and those classified as type 1 to type 9 secretion systems (T1SS to T9SS) (1, 6). In addition to these established secretion systems, other secretory systems are likely present in Gram-negative bacteria and this list is expected to grow to include further members (7, 8). These systems may directly secrete proteins outside the cell (T1SS and T7SS), traverse multiple membranes and deliver them into the cytoplasm of recipient cells (T3SS, T4SS, T6SS), or transport them across the OM in two steps assisted by the Sec or Tat IM transportation systems (T2SS, T5SS, T8SS, T9SS) (9). Because the periplasm lacks ATP, most of these machineries are large complexes including IM components to access cytoplasmic ATP (10). By comparison, the T5SS does not require ATP and is remarkably simple, typically involving a single dedicated protein (2, 11, 12). This review focuses on the T5SS, alternatively called the autotransporter system reflecting its uniquely simple and energy-efficient transport mechanism.
1.1 The T5SS: Autotransporters (ATs)
The type 5 secretion system (T5SS) is the largest group of secreted proteins in Gram-negative bacteria (13–15). While it encompasses functionally diverse proteins, their journey from cytoplasm to OM is similar (Figure 1A) (16, 17). T5SS proteins are termed autotransporters (ATs) because each contains both, secretion machinery (translocator) and functional cargo (passenger) (17). In the cytoplasm, ATs carry an N-terminal signal peptide (SP) for Sec-mediated transport across the IM where the SP is cleaved (23, 24). Periplasmic chaperones keep ATs unfolded until reaching the OM (25–28). The translocator forms a pore in the OM to facilitate the transport of the passenger to the cell surface (29). The passengers are frequently comprised of repetitive secondary structure elements, the sequential folding of which on the bacterial surface may provide a driving force for AT translocation (30–33). The first model of an autotransport mechanism was proposed in 1987 (29) and this has remained an active area of research with several recent reviews on the topic (19, 34, 35). While these basic transport steps are largely consistent with the initial model, later studies revealed the process is not entirely autonomous. Most notably, the barrel assembly machinery (BAM) complex, which catalyzes folding of many OM proteins, is required for insertion of the translocator into the OM and may also facilitate passenger translocation directly (25, 36–39). Significant advances have also been made in our understanding of passenger functions, and these are reviewed in the current work.
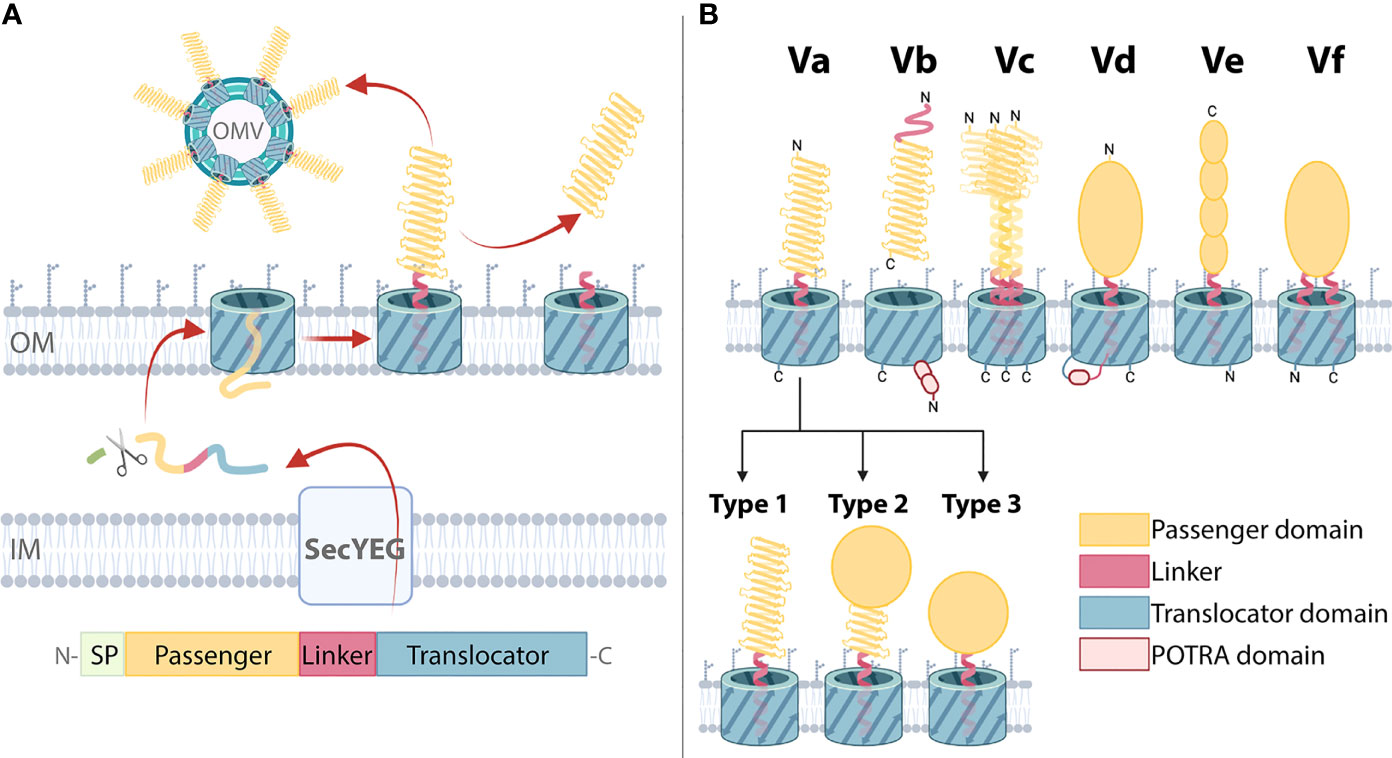
Figure 1 Biogenesis and domain architecture of the type 5 secretion system (T5SS). (A) AT secretion mechanism modelled on classical ATs with the following domain organization: The N-terminal signal peptide (SP) is followed by the passenger, linker, and translocator. The SP targets the ATs for inner membrane (IM) secretion via the SecYEG translocon which is subsequently cleaved by a periplasmic peptidase. The translocator inserts into the outer membrane (OM), forming a β-barrel with the α-helical linker spanning its pore. The passenger is translocated to the OM surface where it folds into its tertiary structure. In some ATs, the passenger is cleaved and secreted into the external milieu. Release can also occur through outer membrane vesicles (OMVs). (B) T5SS subtypes Va-Vf. Three basic domains (the passenger, linker, and translocator) are present in all T5SS subtypes with variations in topology, domain order, and oligomeric states producing six different subtypes (16–18). These AT classes include: the classical ATs (Va), where the translocator that forms a 12-stranded β-barrel in the outer membrane, and a mostly β-helical passenger, are part of one polypeptide; the two-partner secretion systems (Vb), which are unique because the β-helical passenger is encoded by a separate gene from the translocator, which forms a 16-stranded β-barrel that harbors two polypeptide-transport-associated (POTRA) domains that facilitate the interaction of the passenger and translocators; trimeric ATs (Vc), which require three polypeptides to constitute a full 12-stranded β-barrel translocator to secrete the passengers which includes a coiled-coil stalk and β-helical head regions; patatin-like ATs (Vd), with similar domain architecture to Va but where the translocator is a 16-stranded β-barrel that contains a POTRA domain; inverse ATs (Ve), which comprise an inverted domain organization with an N-terminal signal sequence followed by the translocator, then the linker and a C-terminal passenger; and Hop-family ATs (Vf) possessing an interrupted β-barrel translocator where the passenger is inserted in the loop joining the 1st and second β-strands, and therefore resembling a prolonged loop protruding from the 8-stranded β-barrel. Outer membrane (OM) is indicated. Within classical Va ATs, passengers can adopt various structural configurations: Type 1 passenger structures consist of a β-helix, which may be decorated with functional loops and are connected to the translocator via the α-helical linker; in Type 2 structures a catalytic domain is present at the β-helix N-terminus; Type 3 structures lack a β-helix, instead a catalytic domain is directly connected to the translocator via the linker. This visual representation of T5SS subtype domain organization is consistent with other reviews (16, 17, 19–22).
While all T5SS members contain both a passenger and translocator, there are variations in their domain arrangement dividing them into subtypes Va to Vf (Figure 1B). The Va ATs include, from the N- to C-terminus, a signal peptide, passenger and translocator. The Vc ATs, that include YadA from Yersinia ssp. are similar except that their passenger and translocator form trimers, with three ATs forming a single passenger-translocator in the bacterial outer membrane (40, 41). By comparison the Ve ATs represented by intimin from enteropathogenic and enterohaemorrhagic Escherichia coli are similar to that of the Va subtype except that their passenger and translocator are switched in position (42). In contrast, the passenger and translocator of Vb ATs such as Bordetella pertussis FHA, are expressed as separate proteins. Their translocators include two periplasmic polypeptide-transport-associated (POTRA) domains (20, 43). Similarly, the Vd ATs such as PlpD from Pseudomonas aeruginosa and FplA from Fusobacterium nucleatum also include a POTRA domain, but only a single POTRA domain exists between the passenger and translocator which are expressed as a single protein (44, 45). Lastly, the type Vf ATs represented by BapA from Helicobacter pylori are the most distant subtype, whereby its inclusion into the T5SS is still unclear (18). The likely passenger of the Vf ATs derives from a loop that is part of its putative β-barrel translocator. The Va ATs are the focus of this study, where for clarity, the term ‘AT’ will hereafter refer to this group.
1.2 Type Va ATs
ATs are highly diverse outer membrane proteins that are distributed widely throughout Gram-negative bacteria, including the phylum Fusobacteria, the order Chlamydiales and all classes of Proteobacteria (14). However, each AT exhibits a similar domain organization consisting of an N-terminal SP followed by a passenger, linker, and C-terminal translocator (Figure 1A) (29, 46, 47).
1.2.1 Translocator: Conserved Sequence, Structure, and Function
Translocators exhibit sequence conservation corresponding to the Pfam entry PF03797 (48) and form β-barrel structures that span the OM and facilitate passenger translocation (14, 47, 49–53). The first translocator crystal structure, NalP from Neisseria meningitidis, revealed a monomeric, 12-stranded β-barrel forming a 10 Å by 12.5 Å pore (47). Homologous structures have since been determined for distantly related ATs AIDA-I, Hbp/Tsh, EspP, EstA, NalP, and BrkA (50–54). Along with the observation that chaperones are required for proper secretion, the narrow pore size suggests passengers are unfolded during translocation (19, 27, 36, 47). However, folded passengers may be secreted through a larger pore formed by the translocator together with the BamA insertase (19, 25, 55). Despite this, there are limitations on the complexity of folded regions tolerated (31, 56, 57).
1.2.2 The Linker Domain, Cleavage, and Release
The linker connects the passenger and translocator, where after transport of the passenger to the bacterial surface, the linker forms an α-helix spanning the translocator pore (54). In many cases, the passenger is cleaved from the translocator either within the linker or at a nearby site. Cleavage is catalyzed by separate proteases or by the AT itself via its own protease subdomain contained within the passenger, or through an autoproteolytic mechanism within the β-barrel (58–64). Many ATs remain at the bacterial surface, either covalently attached to the translocator or through non-covalent interactions after cleavage (65–68). These ATs influence the surface properties of bacteria such as AIDA-I promoting bacterial aggregation through self-adhesion (65). Other ATs are released into the external milieu to act on targets away from the bacterial surface, for example the passenger of IgA1 protease is proteolytically released and moves away to cleave host immunoglobulins (29). ATs can also be released via outer membrane vesicles (OMVs) that pinch off from the OM, for example Vag8 released in OMVs activates and depletes host immune factors away from the bacterial surface (68, 69).
1.2.3 Passenger: Common Structural Themes
Passengers execute the specific function of each AT, and thus show more sequence variation compared to the translocators (49). Despite their sequence and functional diversity, passenger structures are strikingly similar. Most are predicted to include β-solenoid content, with over 90% of published passenger structures comprising a right-handed three-stranded β-helix (70–81). Although the β-helix structure predominates, variations include β-helices with curved or extended sections and the addition of subdomains and loops that protrude out from the β-helix (70–78, 80, 81). The passenger β-helix facilitates multifunctionality as it may directly function as a binding domain specialized to interact with specific host or bacterial factors (70, 71) and can act as a scaffold for catalytic subdomains (72–75, 77, 81). Notably, some ATs lack β-helical structure entirely, for instance, EstA from P. aeruginosa is the only published passenger structure comprised of a globular catalytic domain attached directly to the linker (54). Taken together, published AT passenger structures can be divided into three broad types: Type 1, β-helix only; Type 2, globular enzymatic domain supported by a β-helix stalk; Type 3, enzymatic domain without a β-helix (Figure 1B). However, given the small proportion of AT structures available the full extent of structural variation within this family remains to be fully uncovered.
1.3 Functional Properties of AT Proteins
ATs are multifunctional proteins that contribute to supporting bacterial survival and growth in different environments. Of significance is that many of these functions are virulence traits that enhance bacterial pathogenic potential (14, 82–87). AT passengers exhibit highly varied sequences, consistent with the variety of functions they perform (88). Some examples of the roles executed by ATs include host adhesion, auto-aggregation, biofilm formation, hemagglutination, invasion, intracellular motility, toxicity, and immune evasion, along with enzymatic functions such as protease, lipase, and sialidase activities (16). In many cases, these ATs are expressed by bacterial pathogens where these activities promote disease.
Based on functional properties, some classical AT proteins are classified into four broad groups. These are the serine protease ATs of Enterobacteriaceae (SPATEs) (87), subtilisin-like ATs (17), self-associating ATs (SAATs) (89), and GDSL-lipases (90).
SPATEs are a family of secreted AT toxins that cleave a variety of host substrates including fodrin, hemoglobin, mucin and Factor V, among others (91). SPATEs are probably the best-studied group of ATs where several reviews have covered current knowledge about SPATE functions (87, 91–94). The passenger of these ATs incorporates a β-helical scaffold with an N-terminal chymotrypsin-like subdomain corresponding to the S6 serine protease family in the MEROPS database (49, 95). Detailed phylogenetic analysis performed on SPATEs have divided these proteins into Class-1 cytotoxins that degrade intracellular substrates and Class-2 immunomodulators that degrade extracellular substrates (87).
Another group of AT proteases are the subtilisin-like ATs, which may be anchored to the bacterial surface or released into the extracellular environment (96–98). These ATs are predicted to contain a β-helical stalk with an N-terminal subtilisin-like subdomain corresponding to the S8A serine protease family in the MEROPS database (17, 95). Overall, subtilisin-like AT functions are poorly understood, but have been associated with surface maturation of other virulence factors to promote virulence functions like cytotoxicity, aggregation, and hemagglutination (17).
Self-associating ATs (SAATs) are a prominent functional subgroup in the AT superfamily (89). These diverse OM-anchored adhesins are predicted to share β-helix architecture in their passenger, as shown for two canonical SAATs, Ag43 and TibA (71, 80). Although ATs in this group can have different functions, all promote bacterial aggregation and biofilm formation through self-association between passengers on neighboring bacteria (71, 89).
Another class of ATs with catalytic activity are the GDSL-lipase ATs. These ATs lack the archetypal β-helix scaffold found in the majority of ATs (54, 90) and are primarily membrane anchored where they hydrolyze ester bonds in host or bacterial lipids (90). Although their natural substrates are unknown, it is assumed they hydrolyze membrane lipids, where they have been shown to affect host cell lysis, lipid and phosphate metabolism, adhesion, and motility (90).
While the identification and definition of these functional groups has provided an important framework for understanding AT proteins, many ATs have been characterized that do not belong to these established functional group.
2 Phylogenetic Classification of AT Proteins
Over the past decades, different groups have devoted considerable effort to the phylogenetic characterization of AT proteins. Henderson, et al. (17) published a landmark phylogenetic analysis of ATs with described phenotypes. This analysis used the sequences of the more conserved AT translocator resulting in the division into 11 subgroups. This enabled comparison and description of the functions within each phylogenetic group and has provided a guiding principle for AT research for the last 18 years. Since this time Celik, et al. (14) using a bioinformatics strategy, presented a large-scale phylogenetic analysis with hundreds of predicted AT passenger sequences, which highlighted the anticipated diversity and widespread distribution of these proteins. Additionally, other phylogenetic analyses have been reported focused on specific AT subgroups (21, 87, 88, 99). With the advent of genome sequencing techniques, the past years have seen a substantial increase in the number of AT sequences reported in public databases along with a steady rise in AT functional characterization, to the point where there is now sufficient data for functional phylogenetic classification studies.
2.1 Sequence Alignment of Characterized ATs
In this work we sought to carry out a comprehensive analysis of functionally characterized ATs. Given the passenger of ATs is the region primarily responsible for facilitating the associated bacterial phenotype through its interactions with the host and/or environment, our analysis concentrated on AT passengers alone to gain insights into the functional relationships between ATs.
Functionally characterized ATs were identified from the literature, particularly focusing on previous reviews (16, 17, 19, 94) and by searching published databases (PubMed and Web of Science) using the keywords “autotransporter” and “T5SS”. After eliminating those lacking experimental characterization, 112 ATs were identified from 32 species across 24 genera of Gram-negative bacteria. Proteobacteria accounted for 97 ATs including classes α-proteobacteria (8 ATs), β-proteobacteria (16 ATs), ϵ-proteobacteria (7 ATs), and γ-proteobacteria (66 ATs, including 31 from E. coli). Twelve ATs from Chlamydiae and 3 ATs from Fusobacteria are also represented. Full-length amino acid sequences were retrieved from the National Centre of Biotechnology (NCBI) for prediction of the SP, α-helical linker, and translocators using SignalP 4.1 (100), PSIPRED (101), and InterPro (102), respectively. Table S1 details the accession numbers for all 112 ATs analyzed. Passenger sequences were identified and recorded as the region flanked by the SP and α-helical linker. PSIPRED secondary structure predictions were also used to predict the secondary structure of the passengers. Clustal Omega (103) was used to generate a multiple sequence alignment of the passengers, which demonstrated high diversity within the AT family. Consistent with previous reports (14), we found that passenger lengths were highly varied, ranging from 193 to 3,374 aa with an average of 945 aa (Supplementary Figure S1). This diversity of sequence lengths between ATs may have skewed some of the phylogenetic relationships, particularly for very short and very long sequences. A heatmap of pairwise identities (Supplementary Figure S2) from the alignment identified 15 high-identity groups, with low identities between the groups, indicating that each group is highly unique.
2.2 Functional Phylogenetic Classification of ATs
To obtain a phylogenetic classification that reflects AT function, following sequence alignment of the 112 curated passengers, an unrooted consensus tree was generated using PhyML (104) with 100 bootstrap iterations and visualized using the interactive tree of life (iTOL) (105). The consensus PhyML tree found the 112 AT passengers formed 16 homologous groupings (Figure 2) with 15 of these corresponding to the high-identity groups seen in the multiple sequence alignment pairwise identity heatmap (Supplementary Figure S2). The rationale for grouping ATs together took into consideration strong phylogenetic relationships on the tree (cladding together, short branch lengths, and strong bootstrapping support values) as well as similar reported functions and structural features. More distant similarities between nearby groups that share functional themes are considered together as larger clusters. The 16 phylogenetic groups are organized into broad AT functional themes, and importantly show that previously established functional groups form distinct clades: SPATEs (Group 1-2), SAATs (Group 4), GDSL-lipases (Group 6), and subtilisin-like ATs (Group 15). Furthermore, several of these individual clades form part of larger functionally related clusters (Clusters A-C).
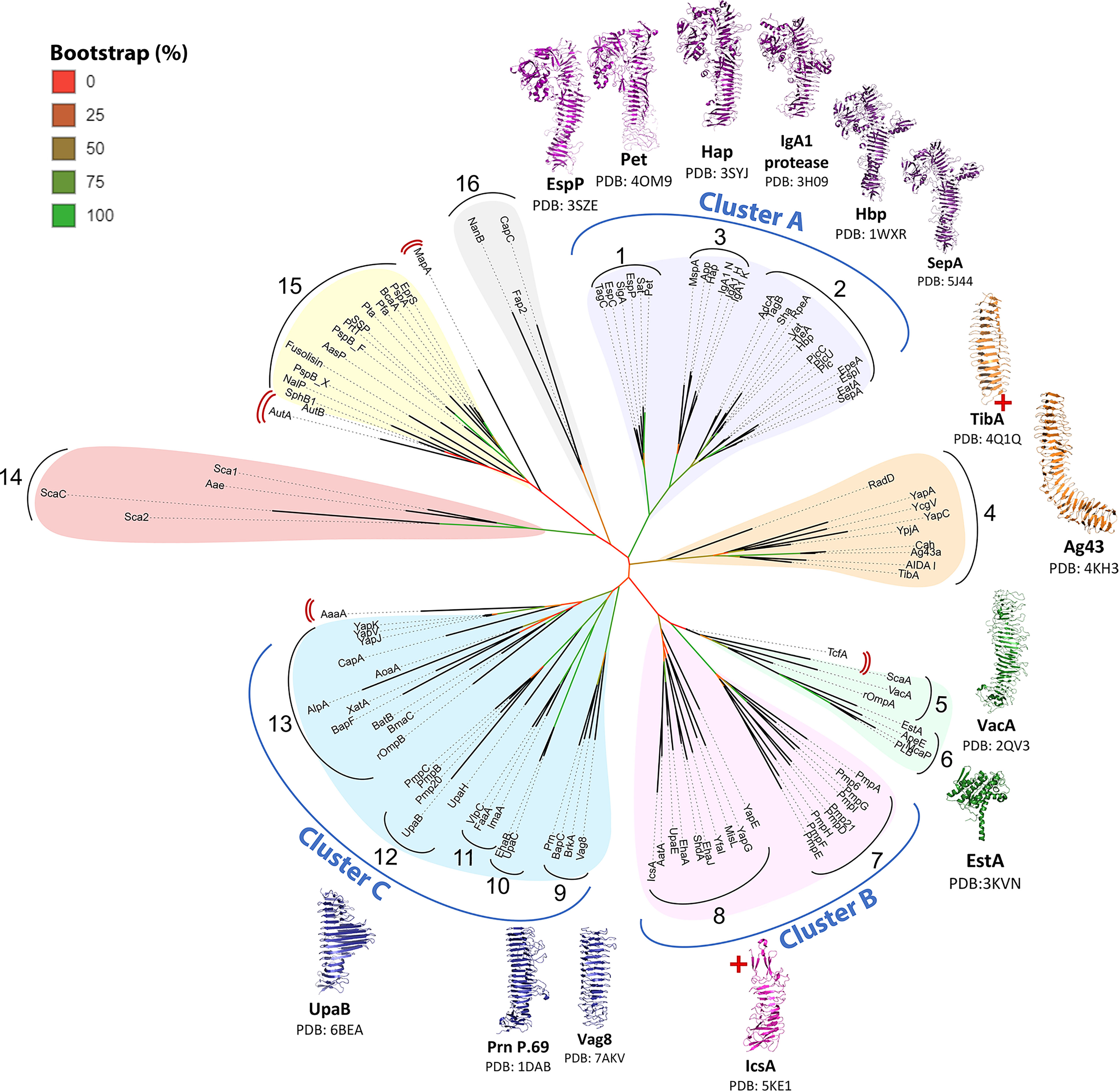
Figure 2 Phylogenetic tree of AT passengers. Unrooted maximum-likelihood phylogenetic tree using Clustal Omega MSA and PhyML with 100 bootstrap iterations and visualized using the interactive tree of life (iTOL). Branch color (red to green) indicates branch support values of 0–90%. Phylogenetic groups are numbered 1─16 with major functional categories indicated by colored shading. 14 published passenger structures are mapped onto the consensus tree, highlighting gaps in structural knowledge. AT structures (54, 70–77, 79–81, 106, 107) were visualized with PyMOL Molecular Graphics System (Schrödinger, LLC) (108). Red cross (+) indicates incomplete passenger structure. Red double brackets indicate ungrouped ATs.
Successful identification of these established groups validates the ability of this phylogenetics strategy to distinguish AT groups that share functional and structural similarities. This in turn supports the interpretation of novel groups identified here as functionally related AT classes. The groupings are discussed below, with overall functional themes assigned to each group. Table S1 provides a comprehensive list of the ATs and their experimentally defined functions.
2.2.1 Cluster A (Groups 1–3): Chymotrypsin-Like Serine Proteases
Cluster A contains Groups 1–3 totaling 26 ATs belonging to the chymotrypsin-like serine protease family (95). This includes Class-1 SPATEs (Group 1) and Class-2 SPATEs (Group 2) as defined by Ruiz–Perez and Nataro (87). These are now brought together with SPATE-like ATs (SLATs) from outside of the Enterobacteriaceae (Group 3). This is the first time to our knowledge that the close relationship between the SPATEs and SLATs has been shown. This relationship can be interpreted with confidence considering the high branch support values connecting Groups 1–3 (88–95%) and the conservation of well-defined structures among all Cluster A proteases. These are probably the best characterized ATs including six passenger structures (Pet, EspP, IgA1, Hap, SepA, and Hbp) exhibiting similar Type 2 architecture (Figure 1B) with a β-helix supporting an N-terminal serine protease subdomain (d1) (72–75, 77, 81). Extended loops arising from the β-helical stalk give rise to further smaller subdomains d2–d4 where d2 resembles a chitin-binding domain, d3 forms an α-helix, and d4 forms a β-hairpin (Figure 3B) (87). Recent work revealed that subdomain d3 mediates host cell internalization of Pet from Group 1 by binding cytokeratin-8 to initiate receptor-mediated endocytosis, an essential step in Pet-mediated virulence (109). Currently, no functions have been associated with d2 and d4 subdomains. The finding that the β-helix extended loop that forms d3 is involved in cell binding interactions is consistent with research on the AT adhesins, where their β-helices directly participate in binding interactions (70, 71, 106).
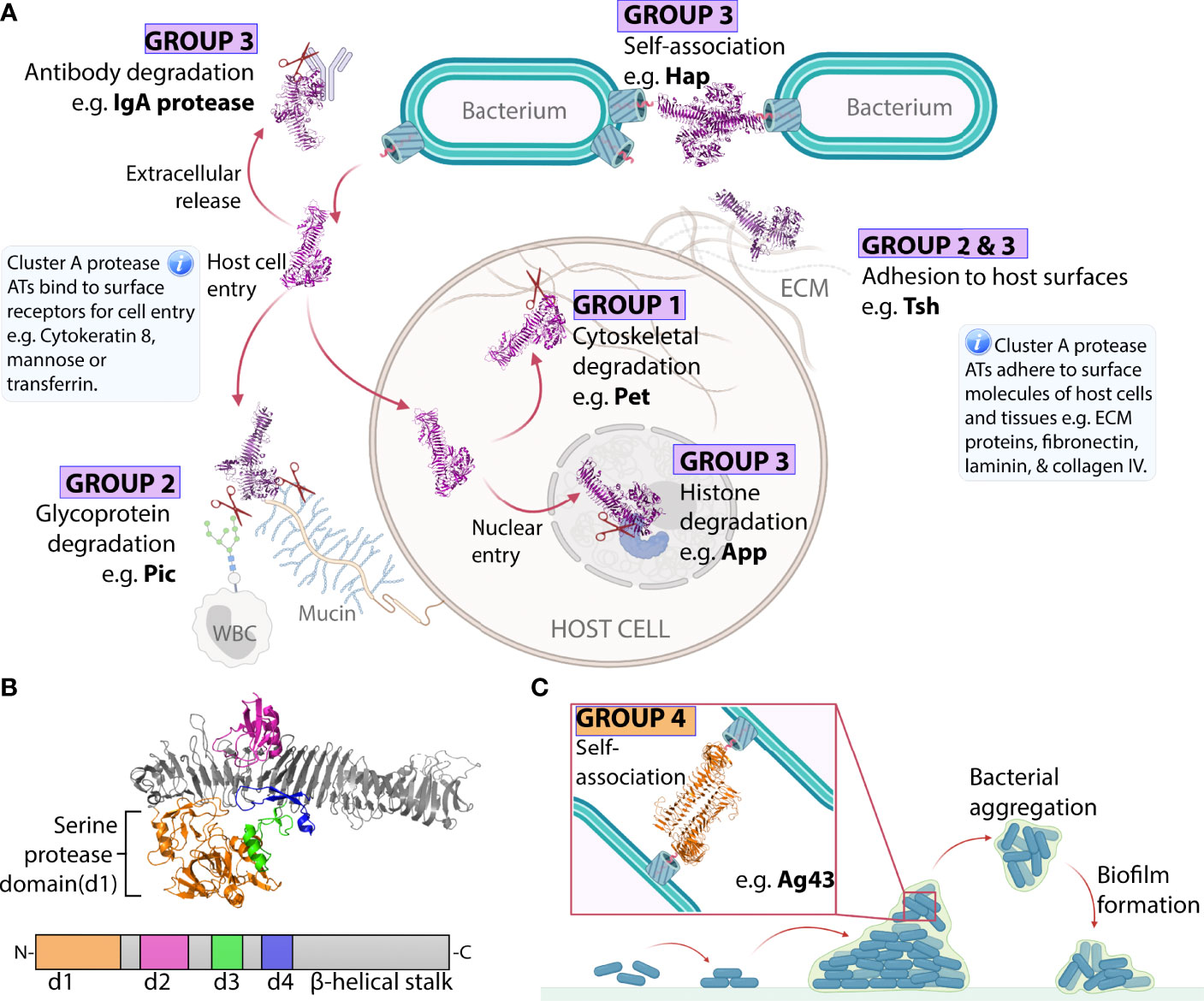
Figure 3 Virulence functions of ATs from Groups 1-4. (A) Cluster A chymotrypsin-like protease AT mechanisms. Cluster A protease ATs (Groups 1–3) are released into the extracellular space and move away from the bacterial surface to degrade host proteins. Group 1 proteases then enter host cells and degrade intracellular cytoskeletal components, triggering cytotoxicity. Group 2 proteases remain in the extracellular space where they degrade large host glycoproteins. Group 3 proteases degrade extracellular immunoglobulins or enter host nuclei to degrade nuclear proteins, triggering cell death. Some Cluster A proteases can execute additional functions if they remain at the bacterial surface where they contribute to adhesion to host and bacterial molecules. This includes some members of Group 2 and Group 3, which can promote bacteria-bacteria or bacteria-host adhesion interactions. (B) Subdomain organization of a representative Cluster A protease AT. Structure of the Hbp (Group 2) passenger showing the structural elements that are conserved across Cluster A proteases including the β-helical stalk (grey) which acts as a scaffold supporting the globular d1 protease subdomain (orange), the d2 subdomain which resembles a chitin-binding domain (pink), the α-helical loop of the d3 subdomain (green), and the β-hairpin loop of the d4 subdomain (blue). These subdomains are highly conserved, except d2, which is absent from Group 1 proteases. (C) Group 4 Self-associating ATs (SAATs) adhesion mechanism. The SAAT Ag43 on adjacent bacterial surfaces self-associate in a molecular Velcro-like manner. This bacteria-bacteria contact contributes to aggregation and biofilm formation. The structures of Hbp (PDB: 1WXR) (75) and Ag43 (PDB: 4KH3) (71) were visualized with PyMOL Molecular Graphics System (Schrödinger, LLC) (108).
While their clustering together reflects structural conservation, the division of Cluster A proteases into Groups 1–3 reflects their differences.
Group 1 contains six ATs (SigA, EspP, EspC, Pet, Sat, TagC) and encompasses the Class-1 SPATEs described by Ruiz–Perez and Nataro (87). These ATs enter host cells and degrade a vast range of large intracellular host proteins, including cytoskeletal components, which causes cytotoxicity and tissue damage at the site of infection (Figure 3A) (110–115). Most originate from diarrheagenic pathogens of the Enterobacteriaceae family where cytotoxicity contributes to cell exfoliation that is characteristic of diarrheal disease. This includes SigA from Shigella flexneri (112) alongside EspP, EspC, and Pet from enterohemorrhagic E. coli (EHEC), enteropathogenic E. coli (EPEC), and enteroaggregative E. coli (EAEC) strains, respectively (115–117). Meanwhile, Sat and TagC are expressed by E. coli strains associated with urinary tract infections (Sat is also expressed in other pathogens such as enteroaggregative E. coli (EAEC) and Shigella flexneri) (114, 118).
Group 2 contains 14 ATs (TagB, AdcA, RpeA, Sha, Vat, Hbp/Tsh, TleA, PicC, Pic, PicU, EspI, EpeA, SepA, EatA) and encompasses the Class-2 SPATEs described by Ruiz–Perez and Nataro (87). These ATs primarily cleave extracellular targets including mucin and immune glycoproteins (Figure 3A) (91, 119–123). Most originate from enteric pathogens responsible for intestinal infections where mucin degradation increases penetration into the protective mucous layer covering intestinal tissue. This includes PicC and AdcA from Citrobacter rodentium (119, 124), SepA from Shigella flexneri (125), alongside ATs from E. coli strains including EpeA from EHEC (122), TleA and EatA from enterotoxigenic E. coli (ETEC) (120, 126), EspI from Shiga toxin-producing E. coli (STEC) (127), Pic from Shigella flexneri and EAEC (128), and RpeA from rabbit-specific EPEC (REPEC) (129). Meanwhile, ATs such as Sha, TagB, PicC, Hbp, and Vat derive from extraintestinal pathogenic E. coli strains (114, 124, 130, 131), that cause urinary tract infections and wound formation (132). Hbp (haemoglobin protease), first found in a human E. coli pathogen (EB1) isolated from a peritoneal would infection, shares 99.8% identity with Tsh (temperature-sensitive hemagglutinin), which originates from the avian pathogenic E. coli which causes severe respiratory disease in avian populations (75, 130).
Group 3 contains five ATs and encompasses the SPATE-like ATs (SLATs) (MspA, Hap, App, IgA1 proteases). SLATs have properties found in both Class-1 and Class-2 SPATEs (Figure 3A). These ATs are expressed by pathogens that infect mucosal epithelia and may become invasive to cause severe disease. For example, App and MspA derive from Neisseria meningitidis, while IgA protease and Hap derive from Haemophilus influenzae (133–135). These are respiratory pathogens that can disseminate to cause meningitis (136–138). IgA protease is also expressed by Neisseria gonorrhoeae, a urogenital pathogen that can spread to cause septic arthritis and endocarditis (139, 140). SLAT functions are well-suited to such pathogens including immune evasion and adhesion to host and bacterial surfaces, which promotes mucosal colonization, as well as tissue damage, which is often required for dissemination.
Specifically, Hap has been shown to adhere to host surfaces and increase aggregation, while App and MspA bind to and enter host cells, degrade histone proteins in the nucleus, and trigger cell death which likely causes tissue damage (81, 141–145). Meanwhile, the IgA1 proteases degrade IgA, which is the most abundant immunoglobulin and an important line of defense at mucosal surfaces (141, 146, 147).
2.2.2 Group 4: Biofilm Forming AT Adhesins
Perhaps the most striking feature of AT adhesins is their sequence diversity despite overall conservation of Type 1 β-helical passenger architecture (Figure 1B) in all published structures (Figure 2) (70, 71, 76, 79, 80, 106). This diversity underlies their dispersal into 11 phylogenetic groups. Of these, the best studied adhesins are the SAATs encompassed by Group 4. SAATs Ag43, Cah, TibA, and AIDA-I are expressed by E. coli where they self-associate with other SAATs on adjacent bacterial surfaces to promote aggregation and biofilm formation (Figure 3C) (65, 89, 148–150). These prototypical SAATs are close together within Group 4, which reflects their functional and structural similarities (71, 80, 150–153). Group 4 includes four additional ATs YapC, YpjA, YcgV, YapA, and RadD, all of which are associated with biofilm formation except YapA for which no biofilm studies have been published (154–158). These proteins may be novel members of the SAAT class given their proximity to prototypical SAATs and functional role in biofilm formation. However, the mechanism used to promote biofilm formation remains unknown and structural studies have not been published for YpjA, YcgV, YapA, or RadD. Using PSIPRED (101) we predict a β-helix structure along the full length of the passenger for each of these proteins, which is consistent with the Type 1 AT structure observed in SAATs.
Most Group 4 ATs derive from pathogenic E. coli including diarrheagenic strains. This includes YpjA from EHEC (155), TibA from ETEC (159), and AIDA-I from EPEC (160). Meanwhile, Ag43 is one of the most prevalent AT adhesins across many E. coli subtypes (21) and YcgV was first identified in the E. coli K-12 laboratory strain (156). Conversely, YapC and YapA are expressed by Yersinia pestis, the causative agent of pneumonic, septicemic, and bubonic plague (154, 157). Finally, RadD is the only member of Group 4 originating outside the Proteobacteria phylum, being expressed by Fusobacterium nucleatum, which contributes to periodontal disease (158). Notably, the SAAT mechanism has only been characterized for ATs from E. coli (71, 161, 162). Future studies should determine if YapC, YapA, and RadD use an Ag43-like dimerization mechanism to expand our understanding of ATs adhesins in important pathogens other than E. coli (70, 76, 106).
Ag43 is possibly one of the best studied AT in Group 4 and the AT family more broadly. A high-resolution structure of the Ag43a passenger from uropathogenic E. coli revealed an L-shaped β-helix forming head-to-tail homodimers through ‘Velcro-like’ non-covalent interactions along the β-helix (71). Ag43 homologues from other E. coli pathogens are now known to follow a similar mechanism of interaction to that of Ag43a (161, 162). It is expected that similar modes of action exist for the other ATs in this group such as TibA and AIDA-1 (89). Apart from self-interactions, some of the ATs in this group can also promote binding to host surfaces (152, 153, 159). How the self-interaction binding is coordinated with binding to host surfaces is unknown. Nevertheless, the Ag43a self-interaction mechanism was one of the first clear indications that the β-helix can directly participate in AT function, and since this time AT β-helices from other groups have been shown to participate in diverse binding interactions (70, 106).
2.2.3 Group 5 VacA and Homologs
The best characterized protein in Group 5 is VacA, owing to its important role as a pore-forming toxin during Helicobacter pylori gastric infection (163–165). The VacA mechanism of action has been reviewed extensively elsewhere (166). Briefly, after being released from the OM, VacA enters host cells to form oligomeric pores in intracellular host membranes, thereby causing vacuolating cytotoxicity (166). A crystal structure of a VacA fragment (residues 388–844), revealed a β-helical passenger structure (78). This was validated by a cryo-EM structure of full-length VacA, which showed that the remainder of the passenger continued into a right-handed β-helix. Importantly, cryo-EM showed that the VacA membrane pore is formed by homo-hexameric rings through interactions between the N-terminal region of each β-helix, with this region also responsible for making contact with the host membrane (107, 167) (Figure 4). Other Group 5 ATs include, ScaA from Orientia tsutsugamushi, which causes scrub typhus, and rOmpA from Rickettsia rickettsii, which causes rocky mountain spotted fever (169, 170). Although less is known about these proteins, both mediate adhesion to host epithelial cells (169–171) and PSIPRED (101) predictions indicate β-helix structure along both passengers, suggesting structural similarity to the β-helical VacA.
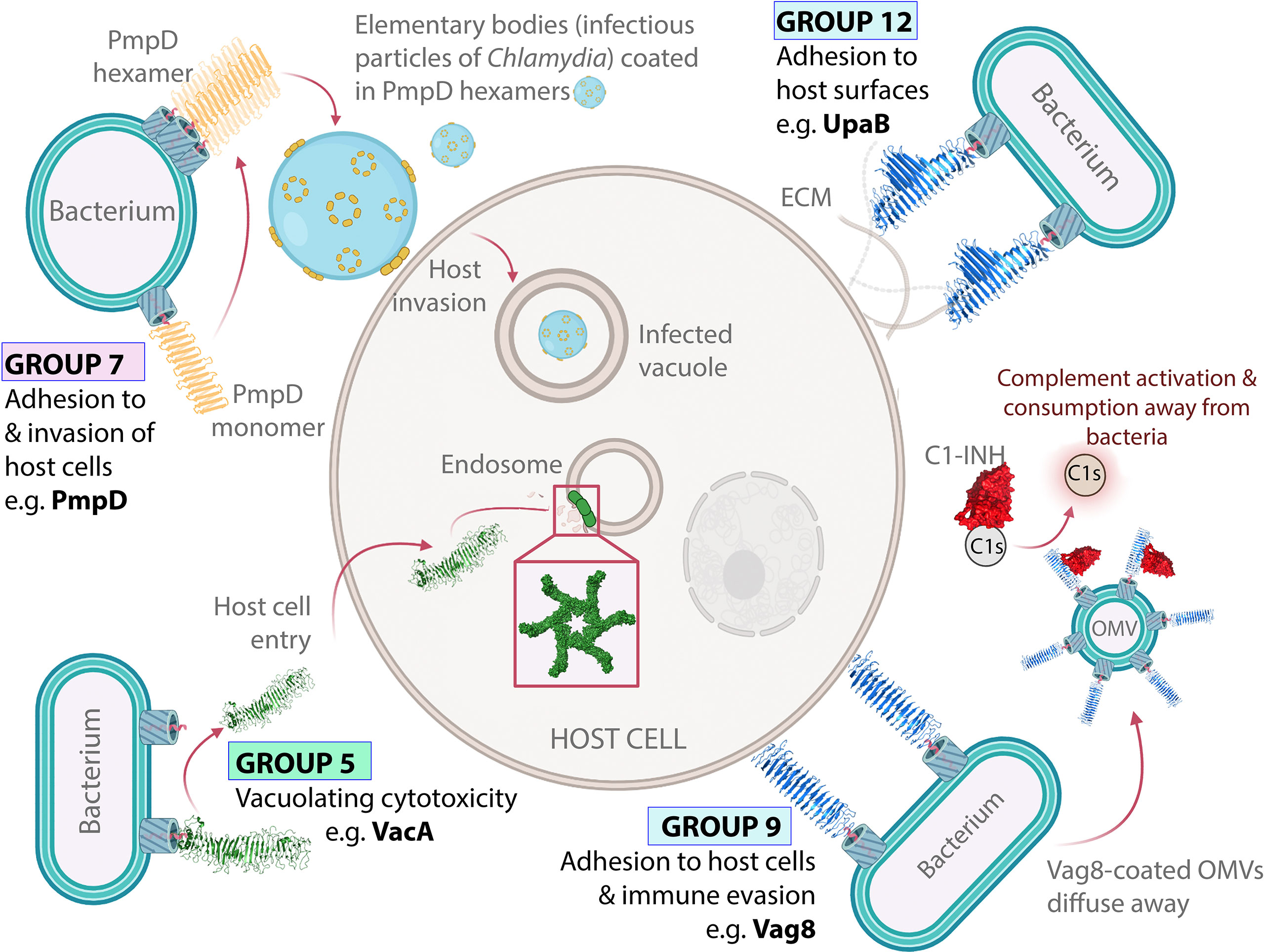
Figure 4 Virulence functions of ATs from Groups 5–12. VacA forms oligomeric pores in intracellular host membranes. VacA (Group 5) forms oligomeric pores in host intracellular membranes including endosomes through horizontal interactions in the lipid bilayer. PmpD is an oligomeric host adhesin. PmpD (Group 7) forms oligomeric rings within the bacterial OM and facilitates host cell invasion. Oligomeric ring structures based on electron microscopy images published by Swanson, et al. (168). Vag8 displays dual immunomodulation and adhesion activities. Vag8 (Group 9) binds to and inhibits the host immune regulator C1-inhibitor (C1-INH), which perturbs the host immune response. Vag8 also promotes adhesion to host cells through an unknown binding interaction. UpaB allows uropathogenic E. coli to bind directly to the urogenital epithelia. UpaB (Group 12) binds to ECM proteins on the surface of epithelial cells, which allows bacteria to bind directly to host surfaces within the urogenital tract, thus promoting disease (70). The structures of VacA (PDB: 6NYF) (107), Vag8 (PDB: 7AKV) (106), and UpaB (PDB: 7AKV) (70) were visualized with PyMOL Molecular Graphics System (Schrödinger, LLC) (108).
2.2.4 Group 6 GDSL-Lipases
Group 6 encompasses the GDSL-lipases EstA, ApeE, PLB, and McaP, all of which exhibit esterase activity catalyzing the hydrolysis of generic lipid substrates (172–175). Although their biological substrates remain unknown, Group 6 ATs may have a broad role in damaging the phospholipids of host cell membranes (90). Given their small size (<300 aa) and that they largely remain tethered to the outer membrane, the activities of these lipases are likely restricted to the immediate bacterial surface (172–176). The lipolytic activity of EstA has been associated with lipid biosynthesis, bacterial motility, and biofilm regulation (172). Meanwhile, McaP in addition to lipolytic activity also promotes bacterial adhesion to host cells (175, 176). The EstA crystal structure revealed the first non-β-helical AT passenger, whereby the protein is predominantly α-helical due to the GDSL-lipase domain which is directly connected to the α-helical AT linker domain (54). Among published structures, EstA is the only example of Type 3 passenger architecture comprising a catalytic domain without a β-helical stalk (Figure 1B). InterPro (102) predicted the lipase domain occupies the entire length of the passenger for ApeE, PLB, and McaP while PSIPRED (101) did not predict β-solenoid structure in this region, suggesting a non-β-helix structure similar to that of EstA. Although this is the only structural evidence of classical ATs lacking a β-helix, this is not uncommon in the wider T5SS. However, outside of the Va group, α-helical ATs tend to form much larger overall structures (17). All Group 6 ATs derive from γ-proteobacteria including EstA from Pseudomonas aeruginosa, an opportunistic pathogen associated with nosocomial infections (172), ApeE from Salmonella enterica Typhimurium, which causes the diarrheal disease salmonellosis (173), PLB from Moraxella bovis, which causes infectious bovine conjunctivitis (174), and McaP from Moraxella catarrhalis, which causes otitis media and upper respiratory tract infections (175, 176).
Notably, the clades for Groups 5 and 6 are close together, linked with strong branch supports in the phylogenetic tree (Figure 2) and can share up to 20% local amino acid identity. However, they are not known to share structural or functional similarities. The proximity of these distinct groups is therefore striking, and their sequence similarities are not confined to local regions or motifs, but rather spread throughout the sequences, possibly inferring a distant evolutionary relationship (data not shown).
Not shown within the tree but included within this group is the GDSL-lipase BatA from Burkholderia (177). BatA with only up to 28% sequence identity to members of this group, positions at its margins. Notably, BatA also shares significant sequence identity to the Group 13 adhesins.
2.2.5 Cluster B (Groups 7–8): Adhesins
Cluster B encompasses Groups 7 and 8 containing ATs that function as adhesins. Host binding is common to all Cluster B ATs while many Group 8 ATs also contribute to bacterial aggregation and/or biofilm formation (155, 156, 178–195). Furthermore, PSIPRED (101) predicted β-helix structure for all Cluster B passengers, which is consistent with the β-helical structure observed in the partial structure of IcsA (79).
Group 7 contains nine ATs designated ‘polymorphic membrane proteins’ (Pmps) including Pmp6 and Pmp21 from Chlamydia pneumoniae along with PmpA, PmpD, PmpE, PmpF, PmpG, PmpH, and PmpI from Chlamydia trachomatis. These are typically OM-anchored ATs that promote host cell adhesion and invasion, consistent with the intracellular lifestyle of the Chlamydia spp. from which they are derived (178, 179, 181, 196). Beyond this broad function, most Pmps are poorly characterized with no published structures. However, PmpD and Pmp21 have been observed to form higher-order oligomers (168, 197, 198). For PmpD, these oligomers appear as flower-like rings in the bacterial OM (168) (Figure 4). Notably, VacA, which is placed nearby in Group 5, is also known to form flower-like oligomers within lipid bilayers (199). This oligomerization may be important in the Pmp binding mechanism, however, the functional significance of PmpD and Pmp21 oligomerization has not been well established. Pmp21 is the only Group 7 AT where the binding partner required for host cell entry is known as it has been shown to promote invasion of host cells by binding to epidermal growth factor receptor (EGFR) (180).
Group 8 consists of ten proteins, YapE, MisL, YapG, Yfal, ShdA, EhaJ, UpaE, EhaA, IcsA and AatA, most of which derive from Enterobacteriaceae that cause diarrheal disease. This includes EhaA and EhaJ from diarrheagenic E. coli (155, 194), ShdA and MisL from Salmonella enterica Typhimurium (184, 193), and IcsA from Shigella flexneri (200). Others including AatA, YfaL, and UpaE derive from extraintestinal E. coli (156, 183, 195). Group 8 ATs that are found outside the Enterobacteriaceae family, include YapE from Yersinia pestis and YapG from Yersinia pseudotuberculosis, the latter causing Far East scarlet-like fever (157, 188).
Group 8 proteins are outer membrane anchored and primarily act as adhesins, with many having dual binding abilities to both host and bacterial targets. Specifically, most, including YapE, MisL, ShdA, EhaJ, UpaE, EhaA, IcsA and AatA mediate host adhesion (155, 183, 184, 186–189, 191–195, 201). For ShdA, MisL, EhaJ, and UpaE, this involves binding to extracellular matrix (ECM) proteins (184, 186, 187, 193–195). Whether ECM binding is a common host binding mechanism across Group 8 remains unknown as binding partners on host epithelial surfaces have not been published for YapE, EhaA, IcsA, and AatA. However, a host intracellular target of IcsA is known, Neural Wiskott–Aldrich syndrome protein (N-WASP), which contributes to the regulation of actin polymerisation as part of the cell cytoskeleton (202). IcsA activates N-WASP to promote intracellular actin-based spread of S. flexneri through the colonic epithelial layer. Regarding bacterial aggregation and/or biofilm formation, all but ShdA are associated with this phenotype (155, 156, 182, 188, 190, 192, 194, 195). However, the mechanism by which these ATs promote bacterial aggregation/biofilm formation has not been determined. IcsA promotes both biofilm formation and forms homodimers, which has raised the possibility of self-association similar to that of Ag43a (190, 203). However, a link between IcsA dimerisation and biofilm formation has not been established and dimerisation has not been demonstrated for other group members. Furthermore, the only passenger structure for Group 8 is a small IcsA fragment (residues 419–758) in the monomeric form, providing no insight into self-association (79).
2.2.6 Cluster C (Groups 9–13): Adhesins
Cluster C (Groups 9–13) contain a separate cluster of adhesin ATs that are primarily anchored to the outer membrane where their predominant function is adhesion to host cells and/or surfaces. Currently, Groups 10, 11, and 13 lack published structures.
Group 9 contains four ATs (Vag8, BrkA, Prn, and BapC), all of which derive from Bordetella spp. and exhibit high conservation in sequence, structure, and function. The reported crystal structure of Prn (76) and the cryo-EM structure of Vag8 (106) both reveal Type 1 AT β-helices. Meanwhile, PSIPRED (101) predicts β-helical passengers for BrkA and BapC, which is also consistent with Type 1 AT β-helices.
Group 9 ATs exhibit dual host adhesion and immune evasion activities (69, 204–206). For Prn, host binding involves its RGD integrin-binding motif (205). BrkA, BapC, and Vag8 also contain RGD motifs, suggesting a possible common host binding mechanism (206–208). To date, the host factors recognized by Group 9 ATs to promote cell adhesion are unknown. Furthermore, while evasion of the innate immune response is also common among Group 9 ATs, each is unique in its approach. Prn affords protection from the inflammatory response and neutrophil-mediated clearance (209, 210). Meanwhile, BapC, Vag8, and BrkA promote serum resistance by reducing complement-mediated killing (68, 208, 211, 212). The Vag8 immune evasion mechanism is the best understood. Vag8 enhances serum resistance by inhibiting the serpin C1-inhibitor (C1-INH) (106, 212), which regulates the complement system (68, 212). Structural studies have shown that Vag8 binds C1-INH using extended loops lining one face of its β-helix (106), thus providing further evidence that β-helix structures can directly participate in AT functions.
Although Group 9 ATs are present at the outer membrane, growing evidence suggests Bordetella may deploy ATs (i.e., Prn, BrkA, and Vag8) in OMVs, disseminating AT function away from the bacterial surface (68, 213, 214). This finding has been crucial for understanding Vag8 function. Hovingh, et al. (68) proposed that OMVs coated with Vag8 block C1-INH and enable unregulated complement activation away from the bacterial surface, thus protecting bacteria by depleting complement factors before they can be deposited on the bacterial surface (Figure 4).
Group 10 contains two ATs derived from pathogenic E. coli, UpaC and EhaB, both of which promote biofilm formation (215, 216). In addition, EhaB also mediates host adhesion by binding to ECM proteins (155). Group 11 contains three ATs (FaaA, VlpC, ImaA) that increase murine gastric colonization by H. pylori (217). Their placement in Cluster C suggests their contribution to colonization may involve host adhesion, aggregation, or biofilm formation. Unfortunately, to date, little is known about the mechanism of action of Group 10 and 11 ATs.
Group 12 comprises five ATs that promote host adhesion, UpaB, UpaH, PmpB, PmpC and Pmp20 (178, 179, 215, 218, 219). For UpaB and UpaH, both of which derive from uropathogenic E. coli, this involves binding to host ECM proteins (215, 218, 219). Meanwhile, the less-defined members PmpB, PmpC, and Pmp20 promote adhesion and entry of Chlamydia into host cells (178, 179). However, ECM binding or biofilm formation studies have not been conducted for the Pmps. The best-studied member of Group 12 is UpaB, which promotes bladder colonization through direct adhesion to urogenital epithelia (215). The crystal structure of the UpaB passenger is consistent with a Type 1 AT β-helix (70). However, its structure reveals unique features, in particular long loops and β-strand extensions projecting out from the β-helix, which form a long hydrophilic groove (70). UpaB was found to bind polysaccharides at this site, and in silico modelling and the resemblance of this groove to the active site of glycosaminoglycan (GAG) lyases, suggests that UpaB binds GAGs lining the human uroepithelium using this binding groove (70). In addition, on the opposite side of UpaB’s β-helix is a second binding site which was shown to bind human fibronectin. Altogether, this demonstrates that residues within the UpaB β-helix contribute to two host binding sites that promote urinary tract colonization. UpaB is therefore an excellent example of an AT β-helix exhibiting multiple direct contributions to the virulence phenotype.
Group 13 contains 11 ATs (CapA, YapJ, YapK, YapV, rOmpB, BatB, BmaC, XatA, BapF, AoaA, AlpA), most of which are anchored to the bacterial surface and function as adhesins. Notably, this is the largest adhesin group in the present study and the most diverse in sequence identity (ranging from 0–81%), passenger length (ranging from 280–3333 aa), and taxonomically with ATs deriving from ten Genera: Yersinia, Campylobacter, Pseudomonas, Brucella, Bordetella, Rickettsia, Helicobacter, Azorhizobium, Burkholderia, and Xylella (83, 177, 220–227). This covers a wide range of bacteria, from H. pylori, among the most widespread and oldest human pathogens and a major cause of stomach cancer worldwide (227), to Xylella fastidiosa, a genus of plant pathogens that is rapidly spreading across the globe and destroying important agricultural crops with huge economic impacts (225). This diversity is reflected by the bootstrapping values with Group 13 showing the lowest within-group bootstrapping among the Cluster C adhesins (Figure 2).
Consistent with the rest of Cluster C, PSIPRED (101) predictions indicate β-helical passenger structure for the majority of Group 13 ATs. However, notable exceptions include AlpA which has been predicted to be α-helical. Another unusual feature only shared by AlpA and CapA in this group includes the lipidation at the N-terminus of their mature passengers (220, 227). Lipidation is thought to allow the passengers to remain associated with the bacterial surface (98), a characteristic which would be favorable for an adhesin.
Overall, the reported functions for Group 13 ATs broadly resemble those of other Cluster C adhesins. Specifically, BapF and XatA promote bacterial aggregation and/or biofilm formation (225, 226). Meanwhile, YapJ, YapK, YapV, CapA, BmaC, rOmpA, and AlpA promote host adhesion, including ECM binding for the Yaps and BmaC (220–222, 224, 226–228). Additionally, BatB binds immunoglobulins to perturb the human immune response (223), while AoaA promotes the symbiotic relationship between legume root nodules and rhizobia by dampening plant defenses (83). While these immunomodulatory activities are somewhat reminiscent of the dual action adhesins and immunomodulators of Group 10, the adhesive properties of BatB and AoaA have not been reported.
Collectively, although Group 13 ATs display related functional properties, these proteins are very diverse and their phylogenetic relationships with well characterized ATs are uncertain, which warrants further studies on this AT grouping.
2.2.7 Group 14: α-Helical Adhesins
Our phylogenetic analysis identified a separate clade containing four surface-bound ATs that contribute to host adhesion including Aae from Acintobacillus actinomycetemcomitans (96) alongside Sca1, Sca2, and ScaC from Rickettsiaceae (229–231). Other functions associated with Group 14 include biofilm formation for Aae and intracellular invasion and motility for Sca2 (232, 233). Mechanistically, ATs in this group are poorly characterized and no structures are currently available in the PDB. Interestingly, PSIPRED (101) analysis predicts α-helical passenger structures for all Group 14 ATs, distinguishing this group as a type Va AT subfamily composed only of α-helical adhesins.
2.2.8 Group 15: Subtilisin-Like Serine Proteases
Group 15 contains 13 subtilisin-like protease ATs with remarkably diverse taxonomic backgrounds primarily deriving from β- and γ-proteobacteria. This includes PspB_F, Pfa, BcaA, EprS, and PspA from Pseudomonas spp. (234–238), SSP and PrtT from Serratia marcescens (239, 240), NalP from N. meningitidis (59), SphB1 from B. pertussis (241), AasP from Actinobacillus pleuropneumoniae (242), PspB_X from X. fastidiosa (243), along with Pta from P. mirabilis (97). These subtilisin-like ATs are also present in bacteria outside the Proteobacteria phylum as evidenced by the presence of Fusolisin from Fusobacterium nucleatum (61). In stark contrast to the Cluster A proteases, the subtilisin-like proteases of Group 15 are among the least understood ATs. Based on secondary structure and conserved domains predicted with PSIPRED (101) and InterPro (102), these ATs are thought to comprise of an ~400 aa N-terminal protease domain followed by an ~200 aa β-helix structure, thus following a Type 2 AT organization similar to the Cluster A proteases. Subtilisin-like ATs are known to have dual roles in bacteria, both at the bacterial surface and when released into the host environment. At the bacterial surface, protease activities of Pfa1, EprS, SphB1, AasP, and NalP are used to process other extra-cytoplasmic proteins including virulence factors (59, 235, 241, 242, 244–246). For example, NalP is responsible for proteolytic maturation of Cluster A protease ATs App, MspA, and IgA1 protease (59, 246). Meanwhile, SphB1 indirectly modifies host adhesion by modifying filamentous hemagglutinin adhesion molecules (241, 245). The capacity of NalP and SphB1 to process these virulence factors, is thought to rely on their abilities to remain temporarily associated with the bacterial surface via their lipidation at their N-terminus similar to members of Group 13 (98, 245). After their release from the bacterial surface, subtilisin-like protease activity appears responsible altering host processes. For example, Pta and Pfa promote host cell cytotoxicity (97, 235) and Fusolisin, EprS, PspB_F, Pfa, and NalP contribute to immunomodulation (234, 235, 237, 247, 248). This likely results from degradation of host proteins as Fusolisin degrades IgA whereas NalP cleaves C3 of the complement system (247, 248). Meanwhile, NalP can also enter a range of host cell types where it alters cellular metabolism (249). Notably, cytotoxicity, host cell internalization, and immunomodulation are also features of the Cluster A chymotrypsin-like proteases.
2.2.9 Group 16: Adhesins and a Sialidase
Group 16 contains three bacterial surface associated ATs including CapC from Campylobacter jejuni and Fap2 from Fusobacterium nucleatum, which promote host adhesion and mediate bacterial aggregation (250, 251). This group also includes NanB from Pasteurella multocida, the only AT with defined sialidase activity, thought to benefit in nutrient acquisition (252). PSIPRED (101) analysis predicted β-helix passenger structure for all members, however, this group is poorly characterized in terms of both structure and function. Accordingly, future research may further define the functional classification of the Group 16 ATs. Importantly, unlike all other phylogenetic groups reviewed here, Group 16 did not form a high-identity cluster on the multiple sequence alignment heatmap (Supplementary Figure S2). This suggests that Group 16 may be an outgroup of proteins lacking strong homologs in the current pool of functionally investigated ATs.
2.2.10 Ungrouped ATs
Our phylogenetic analysis also uncovered several ATs without strong relationships to any clade, as evidenced by low sequence identity across the AT pool in the sequence alignment heatmap (Supplementary Figure S2) and low bootstrap values within the phylogenetic tree (Figure 2). For example, the passenger of TcfA, an adhesin from B. pertussis, does not share significant identity with any other passenger included in this study. PSIPRED (101) analysis predicted a predominantly unstructured passenger for TcfA, which is consistent with its unusually high proline content (17%). TcfA has been shown to promote B. pertussis adhesion to the respiratory tract (69).
The adhesins AutA and AutB share homology with one another but showed no similarity to other AT adhesin groups in either the sequence alignment heatmap (Supplementary Figure S2) or the phylogenetic tree (Figure 2). These proteins are positioned within the subtilisin-like protease clade (Group 15) but with extremely low branch support values (13%). As such, AutA and AutB remain ungrouped. Functionally, AutA and AutB promote aggregation and biofilm formation in N. meningitidis (84, 253, 254). PSIPRED (101) analysis of both AutA and AutB predicts substantial β-helical passenger structure. This is typical of AT adhesins, however their distinction from other adhesins at the sequence level suggests unique structural and functional features.
In addition to the ungrouped adhesins, we found three enzyme classes on the tree with a single AT representative that did not therefore form a large functional group. This includes two enzymes that remain ungrouped: AaaA, a surface-bound arginine-specific aminopeptidase (255), and MapA, an acid phosphatase (256). These enzymes encompass two of the five enzyme classes observed in the phylogenetic analysis with the others being proteases, esterases, and the lone sialidase, NanB (252). NanB is part of Group 16, a probable outgroup of mostly unrelated proteins. Catalytic domain and secondary structure predictions using InterPro (102) and PSIPRED (101), respectively, indicate MapA may adopt a Type 2 AT architecture encompassing an N-terminal catalytic domain with a β-helix C-terminus, while AaaA appears to take on Type 3 AT architecture wherein the catalytic domain spans the full length of the passenger (Supplementary Figure S3).
Future structure-function studies on additional proteins in the Type Va AT family may shed some light as to whether these to date unrelated ATs proteins form part of other functional phylogenetic groups yet to be identified.
3 Discussion
The T5SS, which involves self-mediated transport of autotransporter (AT) proteins outside the cell, is the simplest system for extracellular secretion in Gram-negative bacteria (13–15). Transport relies on a modular architecture wherein each AT contains a signal peptide, translocator module and a functional passenger. Passenger functions vary widely, conferring functional diversity to this large family of bacterial secreted proteins. Comparatively, translocators are highly conserved where each promotes translocation of a passenger that may possess various structural elements and catalytic domains. This combination of variation and uniformity underlies the robustness of this secretion system: by leveraging both the passenger’s functional flexibility and the translocator’s simple and energetically economical secretion capacity, ATs have evolved into highly specialized molecular tools that promote many aspects of bacterial fitness and pathogenesis.
Steadily increasing numbers of publicly available ATs sequences and publications describing their functional properties prompted us to re-evaluate the classification of this protein family, focusing on their diverse passengers. In this study we show that 112 functionally characterized ATs can be divided into 16 phylogenetic groups. By using the passenger sequences alone, the divisions best reflect common passenger functions, many of which contribute to bacterial virulence. Overall, we found AT enzymes form three main divisions: chymotrypsin-like proteases (Cluster A), subtilisin-like proteases (Group 15), and GDSL-lipase esterases (Group 6). In addition to different enymatic actions, these AT enzymes also exhibited diverse structural compositions. Protease ATs adopt Type 2 passenger structures (Figure 1B) wherein an N-terminal protease subdomain responsible for cleaving target proteins sits atop a β-helix for which the functional role is less clear (94). Meanwhile, GDSL-lipases represent Type 3 structure (Figure 1B) which includes an esterase domain responsible for hydrolyzing target lipids without any β-helical content (54). Beyond these three main divisions, we observed a further three enzyme classes with a single representative in the pool of characterized ATs, including the aminopeptidase AaaA (ungrouped), the acid phosphatase MapA (ungrouped), and the sialidase NanB (Group 16). Future phylogenetic studies may reveal additional groups that capture these enzyme functions. Most of the remaining ATs are adhesins distributed into 11 groups reflecting a wide range of specialized functions. Based on limited published structural studies, AT adhesins typically exhibit Type 1 structure (Figure 1B) with long β-helical passengers (70, 71, 76, 79, 80, 106). Where adhesion mechanisms have been studied at the molecular level, the long β-helix forms an extended binding interface with specific host or bacterial targets, achieving high affinity through the additive effect of many non-covalent interactions (70, 71, 106). In some cases, the β-helix forms a groove along the binding interface to further facilitate specific binding (70, 106). Furthermore, ATs may bind multiple targets using different faces of the β-helix (70). Through these interactions adhesins promote adherence to host surfaces, biofilm formation, or bacterial aggregation. Biofilm formation is most strongly associated with the Group 4 SAATs but is also observed in some Group 8 and Group 10 ATs. Meanwhile, most Cluster B adhesins (Groups 7–8) promote adhesion to host surfaces yet some, including the Group 7 Pmps and IcsA from Group 8, also self-associate to form homo-oligomers. Furthermore, Cluster C adhesins (Groups 9–13) that are not known to oligomerize, include an array of ATs that promote adhesion to host surfaces and less frequently bacterial surfaces. A handful of poorly characterized adhesins are also present in Groups 5 and 16. Meanwhile, Group 14 is predicted to encompass adhesins with α-helical passengers, which has not been described previously for Type Va ATs and requires experimental verification. Importantly, Group 1 and 2 (SPATEs), Group 4 (SAATs), Group 15 (subtilisin-like proteases) and Group 6 (GDSL-lipases) represent previously established classes, which authenticated the phylogeny along with the 11 new groups.
4 Conclusion and Future Perspectives
Our work through providing a better understanding into the relationships of AT structure and function has revealed insights into the mechanisms and diversity of ATs, that, importantly, sheds light on the lesser-known ATs. We anticipate that this will aid in the characterization of further ATs and has also identified groups of ATs that require further research attention. This is particularly true of the six functional groups that entirely lack published structures and detailed mechanisms of action (Groups 7, 11, 13, 14, 15, and 16). Following the trend observed for other groups, we would expect these six groups to reveal new types of AT structures and modes of action. Although our pool of 112 sequences only represents a fraction of the >1500 ATs that have already been identified (14), our use of only ATs with some functional characterization performed should increase the reliability of our findings. This in itself also highlights the overall lack of knowledge regarding ATs, with most still uncharacterized especially outside of E. coli. Unfortunately, this may have also created some bias in our study and also contributed to the findings such as the lack of characterized homologs for functional outliers such as NanB (sialidase), MapA (acid phosphatase) and AaaA (aminopeptidase), which are likely representatives of separate functional groups. Apart from an increased awareness surrounding ATs, our work has also shed further light on bacterial pathogenesis and could be used to develop new technologies including antimicrobials and vaccines. Currently, the classical AT Prn is used in pertussis vaccines including Boostrix®, Infantrix®, and Adacel® (257–259), and the trimeric AT NadA is included in the meningococcal vaccine Bexsero® (260). ATs have also been identified as useful targets for anti-virulence antimicrobials (261). However, efforts to target ATs have been perhaps hampered by the scarcity of molecular-level knowledge. This can be observed in the biotechnological applications of ATs, which primarily exploit the relatively well-defined translocation mechanism for secretion or surface display of recombinant proteins such as β-lactamase (262) and DNA polymerase (263) amongst others (264–266). Further, the ATs have been used to engineer live bacteria that secrete a peptide therapeutic (267). The detailed protein structure for Hbp also allowed engineering of the passenger for multivalent antigen display on OMV-based vaccines (268–270). Overall, this work has provided an updated perspective of AT classification, that informs on AT functional relationships, which could benefit antimicrobial and vaccine research, but above all hopefully inspire further research into this area of widespread and abundant bacterial proteins.
Author Contributions
BH and JP contributed to conception of the study. KC, JP, and BH contributed to the design of the study. KC compiled the database of protein sequences and functions and performed the bioinformatics and phylogenetic analyses. KC wrote the first draft of the manuscript. JP, BH, and LH wrote sections of the manuscript. All authors contributed to manuscript revision, read, and approved the submitted version.
Funding
This work was supported by the Australian Research Council (ARC) project grants (DP180102987, DP210100673), a National Health and Medical Research Council (NHMRC) Project Grant (GNT1143638) and an La Trobe Strategic Innovation Fund project.
Conflict of Interest
The authors declare that the research was conducted in the absence of any commercial or financial relationships that could be construed as a potential conflict of interest.
Publisher’s Note
All claims expressed in this article are solely those of the authors and do not necessarily represent those of their affiliated organizations, or those of the publisher, the editors and the reviewers. Any product that may be evaluated in this article, or claim that may be made by its manufacturer, is not guaranteed or endorsed by the publisher.
Acknowledgments
We acknowledge the support of the Australian Research Council, the National Health and Medical Research Council, The Amsterdam Institute of Molecular and Life Sciences (Vrije Universiteit), and the La Trobe Institute for Molecular Science (La Trobe University).
Supplementary Material
The Supplementary Material for this article can be found online at: https://www.frontiersin.org/articles/10.3389/fimmu.2022.921272/full#supplementary-material
References
1. Green ER, Mecsas J. Bacterial Secretion Systems: An Overview. Microbiol Spectr (2016) 4(1):10.1128/microbiolspec.VMBF-0012-2015. doi: 10.1128/microbiolspec.VMBF-0012-2015
2. Costa TR, Felisberto-Rodrigues C, Meir A, Prevost MS, Redzej A, Trokter M, et al. Secretion Systems in Gram-Negative Bacteria: Structural and Mechanistic Insights. Nat Rev Microbiol (2015) 13(6):343–59. doi: 10.1038/nrmicro3456
3. Subashchandrabose S, Mobley HLT. Virulence and Fitness Determinants of Uropathogenic. Escherichia coli. Microbiol Spectr (2015) 3(4). doi: 10.1128/microbiolspec.UTI-0015-2012
4. Beceiro A, Tomas M, Bou G. Antimicrobial Resistance and Virulence: A Successful or Deleterious Association in the Bacterial World? Clin Microbiol Rev (2013) 26(2):185–230. doi: 10.1128/CMR.00059-12
5. Natale P, Bruser T, Driessen AJ. Sec- and Tat-Mediated Protein Secretion Across the Bacterial Cytoplasmic Membrane—Distinct Translocases and Mechanisms. Biochim Biophys Acta (2008) 1778(9):1735–56. doi: 10.1016/j.bbamem.2007.07.015
6. Lasica AM, Ksiazek M, Madej M, Potempa J. The Type IX Secretion System (T9SS): Highlights and Recent Insights Into its Structure and Function. Front Cell Infect Microbiol (2017) 7:215. doi: 10.3389/fcimb.2017.00215
7. Palmer T, Finney AJ, Saha CK, Atkinson GC. Sargent F. A holin/peptidoglycan hydrolase-dependent Protein secretion system. Mol Microbiol (2021) 115(3):345–55. doi: 10.1111/mmi.14599
8. Grossman AS, Mauer TJ, Forest KT. Goodrich-Blair H. A Widespread Bacterial Secretion System Diverse Substrates. mBio (2021) 12(4):e0195621. doi: 10.1128/mBio.01956-21
9. Rapisarda C, Tassinari M, Gubellini F, Fronzes R. Using Cryo-EM to Investigate Bacterial Secretion Systems. Annu Rev Microbiol (2018) 72:231–54. doi: 10.1146/annurev-micro-090817-062702
10. Thanassi DG, Stathopoulos C, Karkal A, Li H. Protein Secretion in the Absence of ATP: The Autotransporter, Two-Partner Secretion and Chaperone/Usher Pathways of Gram-Negative Bacteria. Mol Membr Biol (2005) 22(1-2):63–72. doi: 10.1080/09687860500063290
11. Christie PJ. The Rich Tapestry of Bacterial Protein Translocation Systems. Protein J (2019) 38(4):389–408. doi: 10.1007/s10930-019-09862-3
12. Leo JC, Grin I, Linke D. Type V Secretion: Mechanism(s) of Autotransport Through the Bacterial Outer Membrane. Philos Trans R Soc Lond B Biol Sci (2012) 367(1592):1088–101. doi: 10.1098/rstb.2011.0208
13. Pallen MJ, Chaudhuri RR, Henderson IR. Genomic Analysis of Secretion Systems. Curr Opin Microbiol (2003) 6(5):519–27. doi: 10.1016/j.mib.2003.09.005
14. Celik N, Webb CT, Leyton DL, Holt KE, Heinz E, Gorrell R, et al. A Bioinformatic Strategy for the Detection, Classification and Analysis of Bacterial Autotransporters. PloS One (2012) 7(8):e43245. doi: 10.1371/journal.pone.0043245
15. Abby SS, Cury J, Guglielmini J, Neron B, Touchon M, Rocha EP. Identification of Protein Secretion Systems in Bacterial Genomes. Sci Rep (2016) 6:23080. doi: 10.1038/srep23080
16. Meuskens I, Saragliadis A, Leo JC, Linke D. Type V Secretion Systems: An Overview of Passenger Domain Functions. Front Microbiol (2019) 10:1163. doi: 10.3389/fmicb.2019.01163
17. Henderson IR, Navarro-Garcia F, Desvaux M, Fernandez RC, Ala’Aldeen D. Type V Protein Secretion Pathway: The Autotransporter Story. Microbiol Mol Biol Rev (2004) 68(4):692–744. doi: 10.1128/MMBR.68.4.692-744.2004
18. Coppens F, Castaldo G, Debraekeleer A, Subedi S, Moonens K, Lo A, et al. Hop-Family Helicobacter Outer Membrane Adhesins Form a Novel Class of Type 5-Like Secretion Proteins With an Interrupted Beta-Barrel Domain. Mol Microbiol (2018) 110(1):33–46. doi: 10.1111/mmi.14075
19. Bernstein HD. Type V Secretion in Gram-Negative Bacteria. EcoSal Plus (2019) 8(2). doi: 10.1128/ecosalplus.ESP-0031-2018
20. Guerin J, Bigot S, Schneider R, Buchanan SK, Jacob-Dubuisson F. Two-Partner Secretion: Combining Efficiency and Simplicity in the Secretion of Large Proteins for Bacteria-Host and Bacteria-Bacteria Interactions. Front Cell Infect Microbiol (2017) 7:148. doi: 10.3389/fcimb.2017.00148
21. Vo JL, Martinez Ortiz GC, Subedi P, Keerthikumar S, Mathivanan S, Paxman JJ, et al. Autotransporter Adhesins in Escherichia Coli Pathogenesis. Proteomics (2017) 17(23-24):1600431. doi: 10.1002/pmic.201600431
22. Wells TJ, Henderson IR. Type 1 and 5 Secretion Systems and Associated Toxin. Escherichia Coli: Pathotypes and Principles of Pathogenesis, 2nd Edition. (2013), 499–532. doi: 10.1016/B978-0-12-397048-0.00016-4
23. Sijbrandi R, Urbanus ML, ten Hagen-Jongman CM, Bernstein HD, Oudega B, Otto BR, et al. Signal Recognition Particle (SRP)-Mediated Targeting and Sec-Dependent Translocation of an Extracellular Escherichia Coli Protein. J Biol Chem (2003) 278(7):4654–9. doi: 10.1074/jbc.M211630200
24. Leyton DL, Rossiter AE, Henderson IR. From Self Sufficiency to Dependence: Mechanisms and Factors Important for Autotransporter Biogenesis. Nat Rev Microbiol (2012) 10(3):213–25. doi: 10.1038/nrmicro2733
25. Ieva R, Bernstein HD. Interaction of an Autotransporter Passenger Domain With BamA During its Translocation Across the Bacterial Outer Membrane. Proc Natl Acad Sci U.S.A. (2009) 106(45):19120–5. doi: 10.1073/pnas.0907912106
26. Ieva R, Tian P, Peterson JH, Bernstein HD. Sequential and Spatially Restricted Interactions of Assembly Factors With an Autotransporter β Domain. Proc Natl Acad Sci U.S.A. (2011) 108(31):E383–91. doi: 10.1073/pnas.1103827108
27. Ruiz-Perez F, Henderson IR, Leyton DL, Rossiter AE, Zhang Y, Nataro JP. Roles of Periplasmic Chaperone Proteins in the Biogenesis of Serine Protease Autotransporters of Enterobacteriaceae. J Bacteriol (2009) 191(21):6571–83. doi: 10.1128/JB.00754-09
28. Ruiz-Perez F, Henderson IR, Nataro JP. Interaction of FkpA, A Peptidyl-Prolyl Cis/Trans Isomerase With EspP Autotransporter Protein. Gut Microbes (2010) 1(5):339–44. doi: 10.4161/gmic.1.5.13436
29. Pohlner J, Halter R, Beyreuther K, Meyer TF. Gene Structure and Extracellular Secretion of Neisseria Gonorrhoeae IgA Protease. Nature (1987) 325(6103):458–62. doi: 10.1038/325458a0
30. Junker M, Schuster CC, McDonnell AV, Sorg KA, Finn MC, Berger B, et al. Pertactin Beta-Helix Folding Mechanism Suggests Common Themes for the Secretion and Folding of Autotransporter Proteins. Proc Natl Acad Sci U.S.A. (2006) 103(13):4918–23. doi: 10.1073/pnas.0507923103
31. Junker M, Besingi RN, Clark PL. Vectorial Transport and Folding of an Autotransporter Virulence Protein During Outer Membrane Secretion. Mol Microbiol (2009) 71(5):1323–32. doi: 10.1111/j.1365-2958.2009.06607.x
32. Sikdar R, Bernstein HD. Sequential Translocation of Polypeptides Across the Bacterial Outer Membrane Through the Trimeric Autotransporter Pathway. mBio (2019) 10(5):e01973–19. doi: 10.1128/mBio.01973-19
33. Leo JC, Oberhettinger P, Yoshimoto S, Udatha DB, Morth JP, Schutz M, et al. Secretion of the Intimin Passenger Domain Is Driven by Protein Folding. J Biol Chem (2016) 291(38):20096–112. doi: 10.1074/jbc.M116.731497
34. van Ulsen P, Zinner KM, Jong WSP, Luirink J. On Display: Autotransporter Secretion and Application. FEMS Microbiol Lett (2018) 365(18). doi: 10.1093/femsle/fny165
35. Albenne C, Ieva R. Job Contenders: Roles of the β-Barrel Assembly Machinery and the Translocation and Assembly Module in Autotransporter Secretion. Mol Microbiol (2017) 106(4):505–17. doi: 10.1111/mmi.13832
36. Pavlova O, Peterson JH, Ieva R, Bernstein HD. Mechanistic Link Between β Barrel Assembly and the Initiation of Autotransporter Secretion. Proc Natl Acad Sci U.S.A. (2013) 110(10):E938–47. doi: 10.1073/pnas.1219076110
37. Sauri A, Soprova Z, Wickstrom D, de Gier JW, van der Schors RC, Smit AB, et al. The Bam (Omp85) Complex is Involved in Secretion of the Autotransporter Haemoglobin Protease. Microbiol (Reading) (2009) 155(Pt 12):3982–91. doi: 10.1099/mic.0.034991-0
38. Roman-Hernandez G, Peterson JH, Bernstein HD. Reconstitution of Bacterial Autotransporter Assembly Using Purified Components. Elife (2014) 3:e04234. doi: 10.7554/eLife.04234
39. Soprova Z, Sauri A, van Ulsen P, Tame JR, den Blaauwen T, Jong WS, et al. A Conserved Aromatic Residue in the Autochaperone Domain of the Autotransporter Hbp is Critical for Initiation of Outer Membrane Translocation. J Biol Chem (2010) 285(49):38224–33. doi: 10.1074/jbc.M110.180505
40. Linke D, Riess T, Autenrieth IB, Lupas A, Kempf VA. Trimeric Autotransporter Adhesins: Variable Structure, Common Function. Trends Microbiol (2006) 14(6):264–70. doi: 10.1016/j.tim.2006.04.005
41. Kiessling AR, Malik A, Goldman A. Recent Advances in the Understanding of Trimeric Autotransporter Adhesins. Med Microbiol Immunol (2020) 209(3):233–42. doi: 10.1007/s00430-019-00652-3
42. Leo JC, Oberhettinger P, Schutz M, Linke D. The Inverse Autotransporter Family: Intimin, Invasin and Related Proteins. Int J Med Microbiol (2015) 305(2):276–82. doi: 10.1016/j.ijmm.2014.12.011
43. Jacob-Dubuisson F, Locht C, Antoine R. Two-Partner Secretion in Gram-Negative Bacteria: A Thrifty, Specific Pathway for Large Virulence Proteins. Mol Microbiol (2001) 40(2):306–13. doi: 10.1046/j.1365-2958.2001.02278.x
44. Casasanta MA, Yoo CC, Smith HB, Duncan AJ, Cochrane K, Varano AC, et al. A Chemical and Biological Toolbox for Type Vd Secretion: Characterization of the Phospholipase A1 Autotransporter FplA From Fusobacterium Nucleatum. J Biol Chem (2017) 292(49):20240–54. doi: 10.1074/jbc.M117.819144
45. Salacha R, Kovacic F, Brochier-Armanet C, Wilhelm S, Tommassen J, Filloux A, et al. The Pseudomonas Aeruginosa Patatin-Like Protein PlpD is the Archetype of a Novel Type V Secretion System. Environ Microbiol (2010) 12(6):1498–512. doi: 10.1111/j.1462-2920.2010.02174.x
46. Jose J, Jahnig F, Meyer TF. Common Structural Features of IgA1 Protease-Like Outer Membrane Protein Autotransporters. Mol Microbiol (1995) 18(2):378–80. doi: 10.1111/j.1365-2958.1995.mmi_18020378.x
47. Oomen CJ, Van Ulsen P, Van Gelder P, Feijen M, Tommassen J, Gros P. Structure of the Translocator Domain of a Bacterial Autotransporter. EMBO J (2004) 23(6):1257–66. doi: 10.1038/sj.emboj.7600148
48. Mistry J, Chuguransky S, Williams L, Qureshi M, Salazar GA, Sonnhammer ELL, et al. Pfam: The Protein Families Database in 2021. Nucleic Acids Res (2021) 49(D1):D412–D9. doi: 10.1093/nar/gkaa913
49. Henderson IR, Navarro-Garcia F, Nataro JP. The Great Escape: Structure and Function of the Autotransporter Proteins. Trends Microbiol (1998) 6(9):370–8. doi: 10.1016/S0966-842X(98)01318-3
50. Gawarzewski I, DiMaio F, Winterer E, Tschapek B, Smits SHJ, Jose J, et al. Crystal Structure of the Transport Unit of the Autotransporter Adhesin Involved in Diffuse Adherence From. Escherichia coli. J Struct Biol (2014) 187(1):20–9. doi: 10.1016/j.jsb.2014.05.003
51. Tajima N, Kawai F, Park SY, Tame JR. A Novel Intein-Like Autoproteolytic Mechanism in Autotransporter Proteins. J Mol Biol (2010) 402(4):645–56. doi: 10.1016/j.jmb.2010.06.068
52. Barnard TJ, Dautin N, Lukacik P, Bernstein HD, Buchanan SK. Autotransporter Structure Reveals Intra-Barrel Cleavage Followed by Conformational Changes. Nat Struct Mol Biol (2007) 14(12):1214–20. doi: 10.1038/nsmb1322
53. Zhai Y, Zhang K, Huo Y, Zhu Y, Zhou Q, Lu J, et al. Autotransporter Passenger Domain Secretion Requires a Hydrophobic Cavity at the Extracellular Entrance of the β-Domain Pore. Biochem J (2011) 435(3):577–87. doi: 10.1042/BJ20101548
54. van den Berg B. Crystal Structure of a Full-Length Autotransporter. J Mol Biol (2010) 396(3):627–33. doi: 10.1016/j.jmb.2009.12.061
55. Veiga E, de Lorenzo V, Fernandez LA. Structural Tolerance of Bacterial Autotransporters for Folded Passenger Protein Domains. Mol Microbiol (2004) 52(4):1069–80. doi: 10.1111/j.1365-2958.2004.04014.x
56. Jong WS, ten Hagen-Jongman CM, den Blaauwen T, Slotboom DJ, Tame JR, Wickstrom D, et al. Limited Tolerance Towards Folded Elements During Secretion of the Autotransporter Hbp. Mol Microbiol (2007) 63(5):1524–36. doi: 10.1111/j.1365-2958.2007.05605.x
57. Skillman KM, Barnard TJ, Peterson JH, Ghirlando R, Bernstein HD. Efficient Secretion of a Folded Protein Domain by a Monomeric Bacterial Autotransporter. Mol Microbiol (2005) 58(4):945–58. doi: 10.1111/j.1365-2958.2005.04885.x
58. van Ulsen P, Tommassen J. Protein Secretion and Secreted Proteins in Pathogenic. Neisseriaceae. FEMS Microbiol Rev (2006) 30(2):292–319. doi: 10.1111/j.1574-6976.2006.00013.x
59. van Ulsen P, van Alphen L, ten Hove J, Fransen F, van der Ley P, Tommassen JA. Neisserial Autotransporter NalP Modulating the Processing of Other Autotransporters. Mol Microbiol (2003) 50(3):1017–30. doi: 10.1046/j.1365-2958.2003.03773.x
60. Dautin N, Barnard TJ, Anderson DE, Bernstein HD. Cleavage of a Bacterial Autotransporter by an Evolutionarily Convergent Autocatalytic Mechanism. EMBO J (2007) 26(7):1942–52. doi: 10.1038/sj.emboj.7601638
61. Doron L, Coppenhagen-Glazer S, Ibrahim Y, Eini A, Naor R, Rosen G, et al. Identification and Characterization of Fusolisin, the Fusobacterium Nucleatum Autotransporter Serine Protease. PloS One (2014) 9(10):e111329. doi: 10.1371/journal.pone.0111329
62. Roussel-Jazede V, Arenas J, Langereis JD, Tommassen J, van Ulsen P. Variable Processing of the IgA Protease Autotransporter at the Cell Surface of Neisseria Meningitidis. Microbiology (2014) 160(Pt 11):2421–31. doi: 10.1099/mic.0.082511-0
63. Charbonneau ME, Janvore J, Mourez M. Autoprocessing of the Escherichia Coli AIDA-I Autotransporter: A New Mechanism Involving Acidic Residues in the Junction Region. J Biol Chem (2009) 284(25):17340–51. doi: 10.1074/jbc.M109.010108
64. Noriea NF, Clark TR, Mead D, Hackstadt T. Proteolytic Cleavage of the Immunodominant Outer Membrane Protein Rompa in Rickettsia Rickettsii. J Bacteriol (2017) 199(6):e00826–16. doi: 10.1128/JB.00826-16
65. Sherlock O, Schembri MA, Reisner A, Klemm P. Novel Roles for the AIDA Adhesin From Diarrheagenic Escherichia Coli: Cell Aggregation and Biofilm Formation. J Bacteriol (2004) 186(23):8058–65. doi: 10.1128/JB.186.23.8058-8065.2004
66. Hendrixson DR, St Geme JW 3rd. The Haemophilus Influenzae Hap Serine Protease Promotes Adherence and Microcolony Formation, Potentiated by a Soluble Host Protein. Mol Cell (1998) 2(6):841–50. doi: 10.1016/s1097-2765(00)80298-1
67. Caffrey P, Owen P. Purification and N-Terminal Sequence of the Alpha Subunit of Antigen 43, a Unique Protein Complex Associated With the Outer Membrane of. Escherichia coli. J Bacteriol (1989) 171(7):3634–40. doi: 10.1128/jb.171.7.3634-3640.1989
68. Hovingh ES, van den Broek B, Kuipers B, Pinelli E, Rooijakkers SHM, Jongerius I. Acquisition of C1 Inhibitor by Bordetella Pertussis Virulence Associated Gene 8 Results in C2 and C4 Consumption Away From the Bacterial Surface. PloS Pathog (2017) 13(7):e1006531. doi: 10.1371/journal.ppat.1006531
69. Gasperini G, Biagini M, Arato V, Gianfaldoni C, Vadi A, Norais N, et al. Outer Membrane Vesicles (OMV)-Based and Proteomics-Driven Antigen Selection Identifies Novel Factors Contributing to Bordetella Pertussis Adhesion to Epithelial Cells. Mol Cell Proteomics (2018) 17(2):205–15. doi: 10.1074/mcp.RA117.000045
70. Paxman JJ, Lo AW, Sullivan MJ, Panjikar S, Kuiper M, Whitten AE, et al. Unique Structural Features of a Bacterial Autotransporter Adhesin Suggest Mechanisms for Interaction With Host Macromolecules. Nat Commun (2019) 10(1):1967. doi: 10.1038/s41467-019-09814-6
71. Heras B, Totsika M, Peters KM, Paxman JJ, Gee CL, Jarrott RJ, et al. The Antigen 43 Structure Reveals a Molecular Velcro-Like Mechanism of Autotransporter-Mediated Bacterial Clumping. Proc Natl Acad Sci USA (2014) 111(1):457–62. doi: 10.1073/pnas.1311592111
72. Khan S, Mian HS, Sandercock LE, Chirgadze NY, Pai EF. Crystal Structure of the Passenger Domain of the Escherichia Coli Autotransporter EspP. J Mol Biol (2011) 413(5):985–1000. doi: 10.1016/j.jmb.2011.09.028
73. Domingo Meza-Aguilar J, Fromme P, Torres-Larios A, Mendoza-Hernandez G, Hernandez-Chinas U, Arreguin-Espinosa de Los Monteros RA, et al. X-Ray Crystal Structure of the Passenger Domain of Plasmid Encoded Toxin(Pet), an Autotransporter Enterotoxin From Enteroaggregative Escherichia Coli (EAEC). Biochem Biophys Res Commun (2014) 445(2):439–44. doi: 10.1016/j.bbrc.2014.02.016
74. Maldonado-Contreras A, Birtley JR, Boll E, Zhao Y, Mumy KL, Toscano J, et al. Shigella Depends on SepA to Destabilize the Intestinal Epithelial Integrity via Cofilin Activation. Gut Microbes (2017) 8(6):544–60. doi: 10.1080/19490976.2017.1339006
75. Otto BR, Sijbrandi R, Luirink J, Oudega B, Heddle JG, Mizutani K, et al. Crystal Structure of Hemoglobin Protease, a Heme Binding Autotransporter Protein From Pathogenic. Escherichia coli. J Biol Chem (2005) 280(17):17339–45. doi: 10.1074/jbc.M412885200
76. Emsley P, Charles IG, Fairweather NF, Isaacs NW. Structure of Bordetella Pertussis Virulence Factor P.69 Pertactin. Nature (1996) 381(6577):90–2. doi: 10.1038/381090a0
77. Johnson TA, Qiu J, Plaut AG, Holyoak T. Active-Site Gating Regulates Substrate Selectivity in a Chymotrypsin-Like Serine Protease the Structure of Haemophilus Influenzae Immunoglobulin A1 Protease. J Mol Biol (2009) 389(3):559–74. doi: 10.1016/j.jmb.2009.04.041
78. Gangwer KA, Mushrush DJ, Stauff DL, Spiller B, McClain MS, Cover TL, et al. Crystal Structure of the Helicobacter Pylori Vacuolating Toxin P55 Domain. Proc Natl Acad Sci U.S.A. (2007) 104(41):16293–8. doi: 10.1073/pnas.0707447104
79. Leupold S, Busing P, Mas PJ, Hart DJ, Scrima A. Structural Insights Into the Architecture of the Shigella Flexneri Virulence Factor IcsA/VirG and Motifs Involved in Polar Distribution and Secretion. J Struct Biol (2017) 198(1):19–27. doi: 10.1016/j.jsb.2017.03.003
80. Lu Q, Yao Q, Xu Y, Li L, Li S, Liu Y, et al. An Iron-Containing Dodecameric Heptosyltransferase Family Modifies Bacterial Autotransporters in Pathogenesis. Cell Host Microbe (2014) 16(3):351–63. doi: 10.1016/j.chom.2014.08.008
81. Meng G, Spahich N, Kenjale R, Waksman G, St Geme JW 3rd. Crystal Structure of the Haemophilus Influenzae Hap Adhesin Reveals an Intercellular Oligomerization Mechanism for Bacterial Aggregation. EMBO J (2011) 30(18):3864–74. doi: 10.1038/emboj.2011.279
82. Shimoda Y, Nishigaya Y, Yamaya-Ito H, Inagaki N, Umehara Y, Hirakawa H, et al. The Rhizobial Autotransporter Determines the Symbiotic Nitrogen Fixation Activity of Lotus Japonicus in a Host-Specific Manner. Proc Natl Acad Sci U.S.A. (2020) 117(3):1806–15. doi: 10.1073/pnas.1913349117
83. Suzuki T, Aono T, Liu CT, Suzuki S, Iki T, Yokota K, et al. An Outer Membrane Autotransporter, AoaA, of Azorhizobium Caulinodans is Required for Sustaining High N2-Fixing Activity of Stem Nodules. FEMS Microbiol Lett (2008) 285(1):16–24. doi: 10.1111/j.1574-6968.2008.01215.x
84. Arenas J, Cano S, Nijland R, van Dongen V, Rutten L, van der Ende A, et al. The Meningococcal Autotransporter AutA Is Implicated in Autoaggregation and Biofilm Formation. Environ Microbiol (2015) 17(4):1321–37. doi: 10.1111/1462-2920.12581
85. Beatson SA, Ben Zakour NL, Totsika M, Forde BM, Watts RE, Mabbett AN, et al. Molecular Analysis of Asymptomatic Bacteriuria Escherichia Coli Strain VR50 Reveals Adaptation to the Urinary Tract by Gene Acquisition. Infect Immun (2015) 83(5):1749–64. doi: 10.1128/IAI.02810-14
86. Sandt CH, Hill CW. Four Different Genes Responsible for Nonimmune Immunoglobulin-Binding Activities Within a Single Strain of Escherichia Coli. Infect Immun (2000) 68(4):2205–14. doi: 10.1128/IAI.68.4.2205-2214.2000
87. Ruiz-Perez F, Nataro JP. Bacterial Serine Proteases Secreted by the Autotransporter Pathway: Classification, Specificity, and Role in Virulence. Cell Mol Life Sci (2014) 71(5):745–70. doi: 10.1007/s00018-013-1355-8
88. Wells TJ, Totsika M, Schembri MA. Autotransporters of Escherichia Coli: A Sequence-Based Characterization. Microbiol (Reading) (2010) 156(Pt 8):2459–69. doi: 10.1099/mic.0.039024-0
89. Klemm P, Vejborg RM, Sherlock O. Self-Associating Autotransporters, SAATs: Functional and Structural Similarities. Int J Med Microbiol (2006) 296(4-5):187–95. doi: 10.1016/j.ijmm.2005.10.002
90. Wilhelm S, Rosenau F, Kolmar H, Jaeger KE. Autotransporters With GDSL Passenger Domains: Molecular Physiology and Biotechnological Applications. Chembiochem (2011) 12(10):1476–85. doi: 10.1002/cbic.201100013
91. Dutta PR, Cappello R, Navarro-Garcia F, Nataro JP. Functional Comparison of Serine Protease Autotransporters of Enterobacteriaceae. Infect Immun (2002) 70(12):7105–13. doi: 10.1128/iai.70.12.7105-7113.2002
92. Pokharel P, Habouria H, Bessaiah H, Dozois CM. Serine Protease Autotransporters of the Enterobacteriaceae (SPATEs): Out and About and Chopping It Up. Microorganisms (2019) 7(12):594. doi: 10.3390/microorganisms7120594
93. Dautin N. Serine Protease Autotransporters of Enterobacteriaceae (SPATEs): Biogenesis and Function. Toxins (Basel) (2010) 2(6):1179–206. doi: 10.3390/toxins2061179
94. Yen YT, Kostakioti M, Henderson IR, Stathopoulos C. Common Themes and Variations in Serine Protease Autotransporters. Trends Microbiol (2008) 16(8):370–9. doi: 10.1016/j.tim.2008.05.003
95. Rawlings ND, Waller M, Barrett AJ, Bateman A. MEROPS: The Database of Proteolytic Enzymes, Their Substrates and Inhibitors. Nucleic Acids Res (2014) 42(Database issue):D503–9. doi: 10.1093/nar/gkt953
96. Rose JE, Meyer DH, Fives-Taylor PM. Aae, an Autotransporter Involved in Adhesion of Actinobacillus Actinomycetemcomitans to Epithelial Cells. Infect Immun (2003) 71(5):2384–93. doi: 10.1128/iai.71.5.2384-2393.2003
97. Alamuri P, Mobley HL. A Novel Autotransporter of Uropathogenic Proteus Mirabilis is Both a Cytotoxin and an Agglutinin. Mol Microbiol (2008) 68(4):997–1017. doi: 10.1111/j.1365-2958.2008.06199.x
98. Roussel-Jazede V, Grijpstra J, van Dam V, Tommassen J, van Ulsen P. Lipidation of the Autotransporter NalP of Neisseria Meningitidis is Required for its Function in the Release of Cell-Surface-Exposed Proteins. Microbiol (Reading) (2013) 159(Pt 2):286–95. doi: 10.1099/mic.0.063982-0
99. Zude I, Leimbach A, Dobrindt U. Prevalence of Autotransporters in Escherichia Coli: What is the Impact of Phylogeny and Pathotype? Int J Med Microbiol (2014) 304(3-4):243–56. doi: 10.1016/j.ijmm.2013.10.006
100. Petersen TN, Brunak S, von Heijne G, Nielsen H. SignalP 4.0: Discriminating Signal Peptides From Transmembrane Regions. Nat Methods (2011) 8(10):785–6. doi: 10.1038/nmeth.1701
101. Jones DT. Protein Secondary Structure Prediction Based on Position-Specific Scoring Matrices. J Mol Biol (1999) 292(2):195–202. doi: 10.1006/jmbi.1999.3091
102. Mitchell AL, Attwood TK, Babbitt PC, Blum M, Bork P, Bridge A, et al. InterPro in 2019: Improving Coverage, Classification and Access to Protein Sequence Annotations. Nucleic Acids Res (2019) 47(D1):D351–D60. doi: 10.1093/nar/gky1100
103. Sievers F, Wilm A, Dineen D, Gibson TJ, Karplus K, Li W, et al. Fast, Scalable Generation of High-Quality Protein Multiple Sequence Alignments Using Clustal Omega. Mol Syst Biol (2011) 7:539. doi: 10.1038/msb.2011.75
104. Guindon S, Dufayard JF, Lefort V, Anisimova M, Hordijk W, Gascuel O. New Algorithms and Methods to Estimate Maximum-Likelihood Phylogenies: Assessing the Performance of PhyML 3.0. Syst Biol (2010) 59(3):307–21. doi: 10.1093/sysbio/syq010
105. Letunic I, Bork P. Interactive Tree Of Life (iTOL) V4: Recent Updates and New Developments. Nucleic Acids Res (2019) 47(W1):W256–W9. doi: 10.1093/nar/gkz239
106. Dhillon A, Deme JC, Furlong E, Roem D, Jongerius I, Johnson S, et al. Molecular Basis for Bordetella Pertussis Interference With Complement, Coagulation, Fibrinolytic, and Contact Activation Systems: The Cryo-EM Structure of the Vag8-C1 Inhibitor Complex. mBio (2021) 12(2): e02823–20. doi: 10.1128/mBio.02823-20
107. Zhang K, Zhang H, Li S, Pintilie GD, Mou TC, Gao Y, et al. Cryo-EM Structures of Helicobacter Pylori Vacuolating Cytotoxin A Oligomeric Assemblies at Near-Atomic Resolution. Proc Natl Acad Sci U.S.A. (2019) 116(14):6800–5. doi: 10.1073/pnas.1821959116
108. Schrodinger LLC, DeLano W. PyMOL. Available at: http://www.pymol.org/pymol. (2020).
109. Chavez-Duenas L, Serapio-Palacios A, Nava-Acosta R, Navarro-Garcia F. Subdomain 2 of the Autotransporter Pet is the Ligand Site for Recognizing the Pet Receptor on the Epithelial Cell Surface. Infect Immun (2016) 84(7):2012–21. doi: 10.1128/IAI.01528-15
110. Cappello RE, Estrada-Gutierrez G, Irles C, Giono-Cerezo S, Bloch RJ, Nataro JP. Effects of the Plasmid-Encoded Toxin of Enteroaggregative Escherichia Coli on Focal Adhesion Complexes. FEMS Immunol Med Microbiol (2011) 61(3):301–14. doi: 10.1111/j.1574-695X.2010.00776.x
111. Navarro-Garcia F, Serapio-Palacios A, Vidal JE, Salazar MI, Tapia-Pastrana G. EspC Promotes Epithelial Cell Detachment by Enteropathogenic Escherichia Coli via Sequential Cleavages of a Cytoskeletal Protein and Then Focal Adhesion Proteins. Infect Immun (2014) 82(6):2255–65. doi: 10.1128/IAI.01386-13
112. Al-Hasani K, Navarro-Garcia F, Huerta J, Sakellaris H, Adler B. The Immunogenic SigA Enterotoxin of Shigella Flexneri 2a Binds to HEp-2 Cells and Induces Fodrin Redistribution in Intoxicated Epithelial Cells. PloS One (2009) 4(12):e8223. doi: 10.1371/journal.pone.0008223
113. Maroncle NM, Sivick KE, Brady R, Stokes FE, Mobley HL. Protease Activity, Secretion, Cell Entry, Cytotoxicity, and Cellular Targets of Secreted Autotransporter Toxin of Uropathogenic Escherichia Coli. Infect Immun (2006) 74(11):6124–34. doi: 10.1128/IAI.01086-06
114. Habouria H, Pokharel P, Maris S, Garenaux A, Bessaiah H, Houle S, et al. Three New Serine-Protease Autotransporters of Enterobacteriaceae (SPATEs) From Extra-Intestinal Pathogenic Escherichia Coli and Combined Role of SPATEs for Cytotoxicity and Colonization of the Mouse Kidney. Virulence (2019) 10(1):568–87. doi: 10.1080/21505594.2019.1624102
115. Djafari S, Ebel F, Deibel C, Kramer S, Hudel M, Chakraborty T. Characterization of an Exported Protease From Shiga Toxin-Producing Escherichia Coli. Mol Microbiol (1997) 25(4):771–84. doi: 10.1046/j.1365-2958.1997.5141874.x
116. Drago-Serrano ME, Parra SG, Manjarrez-Hernandez HA. EspC, an Autotransporter Protein Secreted by Enteropathogenic Escherichia Coli (EPEC), Displays Protease Activity on Human Hemoglobin. FEMS Microbiol Lett (2006) 265(1):35–40. doi: 10.1111/j.1574-6968.2006.00463.x
117. Navarro-Garcia F, Sears C, Eslava C, Cravioto A, Nataro JP. Cytoskeletal Effects Induced by Pet, the Serine Protease Enterotoxin of Enteroaggregative. Escherichia coli. Infect Immun (1999) 67(5):2184–92. doi: 10.1128/IAI.67.5.2184-2192.1999
118. Guyer DM, Radulovic S, Jones FE, Mobley HL. Sat, the Secreted Autotransporter Toxin of Uropathogenic Escherichia Coli, is a Vacuolating Cytotoxin for Bladder and Kidney Epithelial Cells. Infect Immun (2002) 70(8):4539–46. doi: 10.1128/iai.70.8.4539-4546.2002
119. Ayala-Lujan JL, Vijayakumar V, Gong M, Smith R, Santiago AE, Ruiz-Perez F. Broad Spectrum Activity of a Lectin-Like Bacterial Serine Protease Family on Human Leukocytes. PloS One (2014) 9(9):e107920. doi: 10.1371/journal.pone.0107920
120. Gutierrez D, Pardo M, Montero D, Onate A, Farfan MJ, Ruiz-Perez F, et al. TleA, a Tsh-Like Autotransporter Identified in a Human Enterotoxigenic Escherichia Coli Strain. Infect Immun (2015) 83(5):1893–903. doi: 10.1128/IAI.02976-14
121. Pokharel P, Díaz JM, Bessaiah H, Houle S, Guerrero-Barrera AL, Dozois CM. The Serine Protease Autotransporters TagB, TagC, and Sha From Extraintestinal Pathogenic Escherichia Coli Are Internalized by Human Bladder Epithelial Cells and Cause Actin Cytoskeletal Disruption. Int J Mol Sci (2020) 21(9):3047. doi: 10.3390/ijms21093047
122. Leyton DL, Sloan J, Hill RE, Doughty S, Hartland EL. Transfer Region of Po113 From Enterohemorrhagic Escherichia Coli: Similarity With R64 and Identification of a Novel Plasmid-Encoded Autotransporter, EpeA. Infect Immun (2003) 71(11):6307–19. doi: 10.1128/iai.71.11.6307-6319.2003
123. Kumar P, Luo Q, Vickers TJ, Sheikh A, Lewis WG, Fleckenstein JM. EatA, an Immunogenic Protective Antigen of Enterotoxigenic Escherichia Coli, Degrades Intestinal Mucin. Infect Immun (2014) 82(2):500–8. doi: 10.1128/IAI.01078-13
124. Bhullar K, Zarepour M, Yu H, Yang H, Croxen M, Stahl M, et al. The Serine Protease Autotransporter Pic Modulates Citrobacter Rodentium Pathogenesis and Its Innate Recognition by the Host. Infect Immun (2015) 83(7):2636–50. doi: 10.1128/IAI.00025-15
125. Benjelloun-Touimi Z, Sansonetti PJ, Parsot C. SepA, the Major Extracellular Protein of Shigella Flexneri: Autonomous Secretion and Involvement in Tissue Invasion. Mol Microbiol (1995) 17(1):123–35. doi: 10.1111/j.1365-2958.1995.mmi_17010123.x
126. Patel SK, Dotson J, Allen KP, Fleckenstein JM. Identification and Molecular Characterization of EatA, an Autotransporter Protein of Enterotoxigenic Escherichia Coli. Infect Immun (2004) 72(3):1786–94. doi: 10.1128/iai.72.3.1786-1794.2004
127. Schmidt H, Zhang WL, Hemmrich U, Jelacic S, Brunder W, Tarr PI, et al. Identification and Characterization of a Novel Genomic Island Integrated at selC in Locus of Enterocyte Effacement-Negative, Shiga Toxin-Producing Escherichia Coli. Infect Immun (2001) 69(11):6863–73. doi: 10.1128/IAI.69.11.6863-6873.2001
128. Henderson IR, Czeczulin J, Eslava C, Noriega F, Nataro JP. Characterization of Pic, a Secreted Protease of Shigella Flexneri and Enteroaggregative Escherichia Coli. Infect Immun (1999) 67(11):5587–96. doi: 10.1128/IAI.67.11.5587-5596.1999
129. Leyton DL, Adams LM, Kelly M, Sloan J, Tauschek M, Robins-Browne RM, et al. Contribution of a Novel Gene, Rpea, Encoding a Putative Autotransporter Adhesin to Intestinal Colonization by Rabbit-Specific Enteropathogenic Escherichia Coli. Infect Immun (2007) 75(9):4664–9. doi: 10.1128/IAI.00972-06
130. Otto BR, van Dooren SJ, Nuijens JH, Luirink J, Oudega B. Characterization of a Hemoglobin Protease Secreted by the Pathogenic Escherichia Coli Strain EB1. J Exp Med (1998) 188(6):1091–103. doi: 10.1084/jem.188.6.1091
131. Parreira VR, Gyles CL. A Novel Pathogenicity Island Integrated Adjacent to the thrW tRNA Gene of Avian Pathogenic Escherichia Coli Encodes a Vacuolating Autotransporter Toxin. Infect Immun (2003) 71(9):5087–96. doi: 10.1128/iai.71.9.5087-5096.2003
132. Gutiérrez-Lucas LR, Mendoza-Hernández G, González-Pedrajo B, Eslava-Campos C, Bustos-Martínez J, Sainz-Espuñes T. Identification of the Autotransporter Pet Toxin in Proteus Mirabilis Strain Isolated From Patients With Urinary Tract Infections. Adv Biol Chem (2012) 02(03):283–90. doi: 10.4236/abc.2012.23036
133. Dutta S, Oldfield N, Wooldridge K. Investigating the Nuclear Localisation and Proteolytic Activity of the Meningococcal App and MspA Autotransporters (2019). doi: 10.1099/acmi.ac2019.po0383
134. Beck SC, Meyer TF. IgA1 Protease From Neisseria Gonorrhoeae Inhibits TNFalpha-Mediated Apoptosis of Human Monocytic Cells. FEBS Lett (2000) 472(2-3):287–92. doi: 10.1016/s0014-5793(00)01478-2
135. Fink DL, Cope LD, Hansen EJ, Geme JW 3rd. The Hemophilus Influenzae Hap Autotransporter Is a Chymotrypsin Clan Serine Protease and Undergoes Autoproteolysis via an Intermolecular Mechanism. J Biol Chem (2001) 276(42):39492–500. doi: 10.1074/jbc.M106913200
136. Devakanthan B, Liyanapathirana V, Dissanayake N, Harasgama P, Punchihewa J. Identification of Bacterial Aetiology in Acute Meningitis. Ceylon Med J (2021) 66(2):65–72. doi: 10.4038/cmj.v66i2.9465
137. van Deuren M, Brandtzaeg P, van der Meer JW. Update on Meningococcal Disease With Emphasis on Pathogenesis and Clinical Management. Clin Microbiol Rev (2000) 13(1):144–66. doi: 10.1128/CMR.13.1.144
138. Ulanova M, Tsang RSW. Haemophilus Influenzae Serotype a as a Cause of Serious Invasive Infections. Lancet Infect Dis (2014) 14(1):70–82. doi: 10.1016/S1473-3099(13)70170-1
139. Humbert MV, Christodoulides M. Atypical, Yet Not Infrequent, Infections With Neisseria Species. Pathogens (2019) 9(1):10. doi: 10.3390/pathogens9010010
140. Mathew R, Chahin M, Isache C. Neisseria Gonorrhoeae: An Unexpected Cause of Polyarthritis and Meningitis. J Investig Med High Impact Case Rep (2021) 9:23247096211012194. doi: 10.1177/23247096211012194
141. Khairalla AS, Omer SA, Mahdavi J, Aslam A, Dufailu OA, Self T, et al. Nuclear Trafficking, Histone Cleavage and Induction of Apoptosis by the Meningococcal App and MspA Autotransporters. Cell Microbiol (2015) 17(7):1008–20. doi: 10.1111/cmi.12417
142. Serruto D, Adu-Bobie J, Scarselli M, Veggi D, Pizza M, Rappuoli R, et al. Neisseria Meningitidis App, a New Adhesin With Autocatalytic Serine Protease Activity. Mol Microbiol (2003) 48(2):323–34. doi: 10.1046/j.1365-2958.2003.03420.x
143. Turner DP, Marietou AG, Johnston L, Ho KK, Rogers AJ, Wooldridge KG, et al. Characterization of MspA, an Immunogenic Autotransporter Protein That Mediates Adhesion to Epithelial and Endothelial Cells in Neisseria Meningitidis. Infect Immun (2006) 74(5):2957–64. doi: 10.1128/IAI.74.5.2957-2964.2006
144. St Geme JW 3rd, de la Morena ML, Falkow S. A Haemophilus influenzae IgA protease-like Protein promotes intimate interaction Hum epithelial Cells. Mol Microbiol (1994) 14(2):217–33. doi: 10.1111/j.1365-2958.1994.tb01283.x
145. Fink DL, Buscher AZ, Green B, Fernsten P, St Geme JW 3rd. The Haemophilus Influenzae Hap Autotransporter Mediates Microcolony Formation and Adherence to Epithelial Cells and Extracellular Matrix via Binding Regions in the C-Terminal End of the Passenger Domain. Cell Microbiol (2003) 5(3):175–86. doi: 10.1046/j.1462-5822.2003.00266.x
146. Kilian M, Thomsen B, Petersen TE, Bleeg H. Molecular Biology of Haemophilus Influenzae IgA1 Proteases. Mol Immunol (1983) 20(9):1051–8. doi: 10.1016/0161-5890(83)90046-9
147. Plaut AG, Gilbert JV, Artenstein MS, Capra JD. Neisseria Gonorrhoeae and Neisseria Meningitidis: Extracellular Enzyme Cleaves Human Immunoglobulin a. Science (1975) 190(4219):1103–5. doi: 10.1126/science.810892
148. Danese PN, Pratt LA, Dove SL, Kolter R. The Outer Membrane Protein, Antigen 43, Mediates Cell-to-Cell Interactions Within Escherichia Coli Biofilms. Mol Microbiol (2000) 37(2):424–32. doi: 10.1046/j.1365-2958.2000.02008.x
149. Torres AG, Perna NT, Burland V, Ruknudin A, Blattner FR, Kaper JB. Characterization of Cah, a Calcium-Binding and Heat-Extractable Autotransporter Protein of Enterohaemorrhagic. Escherichia coli. Mol Microbiol (2002) 45(4):951–66. doi: 10.1046/j.1365-2958.2002.03094.x
150. Sherlock O, Vejborg RM, Klemm P. The TibA Adhesin/Invasin From Enterotoxigenic Escherichia Coli Is Self Recognizing and Induces Bacterial Aggregation and Biofilm Formation. Infect Immun (2005) 73(4):1954–63. doi: 10.1128/IAI.73.4.1954-1963.2005
151. Knudsen SK, Stensballe A, Franzmann M, Westergaard UB, Otzen DE. Effect of Glycosylation on the Extracellular Domain of the Ag43 Bacterial Autotransporter: Enhanced Stability and Reduced Cellular Aggregation. Biochem J (2008) 412(3):563–77. doi: 10.1042/BJ20071497
152. Sherlock O, Dobrindt U, Jensen JB, Munk Vejborg R, Klemm P. Glycosylation of the Self-Recognizing Escherichia Coli Ag43 Autotransporter Protein. J Bacteriol (2006) 188(5):1798–807. doi: 10.1128/JB.188.5.1798-1807.2006
153. Laarmann S, Schmidt MA. The Escherichia Coli AIDA Autotransporter Adhesin Recognizes an Integral Membrane Glycoprotein as Receptor. Microbiol (Reading) (2003) 149(Pt 7):1871–82. doi: 10.1099/mic.0.26264-0
154. Felek S, Lawrenz MB, Krukonis ES. The Yersinia Pestis Autotransporter YapC Mediates Host Cell Binding, Autoaggregation and Biofilm Formation. Microbiol (Reading) (2008) 154(Pt 6):1802–12. doi: 10.1099/mic.0.2007/010918-0
155. Wells TJ, Sherlock O, Rivas L, Mahajan A, Beatson SA, Torpdahl M, et al. EhaA is a Novel Autotransporter Protein of Enterohemorrhagic Escherichia Coli O157:H7 That Contributes to Adhesion and Biofilm Formation. Environ Microbiol (2008) 10(3):589–604. doi: 10.1111/j.1462-2920.2007.01479.x
156. Roux A, Beloin C, Ghigo JM. Combined Inactivation and Expression Strategy to Study Gene Function Under Physiological Conditions: Application to Identification of New Escherichia Coli Adhesins. J Bacteriol (2005) 187(3):1001–13. doi: 10.1128/JB.187.3.1001-1013.2005
157. Yen YT, Karkal A, Bhattacharya M, Fernandez RC, Stathopoulos C. Identification and Characterization of Autotransporter Proteins of Yersinia Pestis KIM. Mol Membr Biol (2007) 24(1):28–40. doi: 10.1080/09687860600927626
158. Kaplan CW, Lux R, Haake SK, Shi W. The Fusobacterium Nucleatum Outer Membrane Protein RadD Is an Arginine-Inhibitable Adhesin Required for Inter-Species Adherence and the Structured Architecture of Multispecies Biofilm. Mol Microbiol (2009) 71(1):35–47. doi: 10.1111/j.1365-2958.2008.06503.x
159. Elsinghorst EA, Weitz JA. Epithelial Cell Invasion and Adherence Directed by the Enterotoxigenic Escherichia Coli Tib Locus is Associated With a 104-Kilodalton Outer Membrane Protein. Infect Immun (1994) 62(8):3463–71. doi: 10.1128/iai.62.8.3463-3471.1994
160. Benz I, Schmidt MA. Cloning and Expression of an Adhesin (AIDA-I) Involved in Diffuse Adherence of Enteropathogenic. Escherichia coli. Infect Immun (1989) 57(5):1506–11. doi: 10.1128/iai.57.5.1506-1511.1989
161. Ageorges V, Schiavone M, Jubelin G, Caccia N, Ruiz P, Chafsey I, et al. Differential Homotypic and Heterotypic Interactions of Antigen 43 (Ag43) Variants in Autotransporter-Mediated Bacterial Autoaggregation. Sci Rep (2019) 9(1):11100. doi: 10.1038/s41598-019-47608-4
162. Vo JL, Ortiz GCM, Totsika M, Lo AW, Hancock SJ, Whitten AE, et al. Variation of Antigen 43 Self-Association Modulates Bacterial Compacting Within Aggregates and Biofilms. NPJ Biofilms Microbiomes (2022) 8(1):20. doi: 10.1038/s41522-022-00284-1
163. Cover TL, Blaser MJ. Purification and Characterization of the Vacuolating Toxin From Helicobacter Pylori. J Biol Chem (1992) 267(15):10570–5. doi: 10.1016/S0021-9258(19)50054-0
164. Papini E, de Bernard M, Milia E, Bugnoli M, Zerial M, Rappuoli R, et al. Cellular Vacuoles Induced by Helicobacter Pylori Originate From Late Endosomal Compartments. Proc Natl Acad Sci U.S.A. (1994) 91(21):9720–4. doi: 10.1073/pnas.91.21.9720
165. Kimura M, Goto S, Wada A, Yahiro K, Niidome T, Hatakeyama T, et al. Vacuolating Cytotoxin Purified From Helicobacter Pylori Causes Mitochondrial Damage in Human Gastric Cells. Microb Pathog (1999) 26(1):45–52. doi: 10.1006/mpat.1998.0241
166. Foegeding NJ, Caston RR, McClain MS, Ohi MD, Cover TL. An Overview of Helicobacter Pylori VacA Toxin Biology. Toxins (Basel) (2016) 8(6):173. doi: 10.3390/toxins8060173
167. Su M, Erwin AL, Campbell AM, Pyburn TM, Salay LE, Hanks JL, et al. Cryo-EM Analysis Reveals Structural Basis of Helicobacter Pylori VacA Toxin Oligomerization. J Mol Biol (2019) 431(10):1956–65. doi: 10.1016/j.jmb.2019.03.029
168. Swanson KA, Taylor LD, Frank SD, Sturdevant GL, Fischer ER, Carlson JH, et al. Chlamydia Trachomatis Polymorphic Membrane Protein D Is an Oligomeric Autotransporter With a Higher-Order Structure. Infect Immun (2009) 77(1):508–16. doi: 10.1128/IAI.01173-08
169. Ha NY, Sharma P, Kim G, Kim Y, Min CK, Choi MS, et al. Immunization With an Autotransporter Protein of Orientia Tsutsugamushi Provides Protective Immunity Against Scrub Typhus. PloS Negl Trop Dis (2015) 9(3):e0003585. doi: 10.1371/journal.pntd.0003585
170. Li H, Walker DH. Rompa Is a Critical Protein for the Adhesion of Rickettsia Rickettsii to Host Cells. Microb Pathog (1998) 24(5):289–98. doi: 10.1006/mpat.1997.0197
171. Hillman RD Jr., Baktash YM, Martinez JJ. OmpA-Mediated Rickettsial Adherence to and Invasion of Human Endothelial Cells is Dependent Upon Interaction With α2β1 Integrin. Cell Microbiol (2013) 15(5):727–41. doi: 10.1111/cmi.12068
172. Wilhelm S, Gdynia A, Tielen P, Rosenau F, Jaeger KE. The Autotransporter Esterase EstA of Pseudomonas Aeruginosa is Required for Rhamnolipid Production, Cell Motility, and Biofilm Formation. J Bacteriol (2007) 189(18):6695–703. doi: 10.1128/JB.00023-07
173. Carinato ME, Collin-Osdoby P, Yang X, Knox TM, Conlin CA, Miller CG. The apeE Gene of Salmonella Typhimurium Encodes an Outer Membrane Esterase Not Present in Escherichia Coli. . J Bacteriol (1998) 180(14):3517–21. doi: 10.1128/JB.180.14.3517-3521.1998
174. Farn JL, Strugnell RA, Hoyne PA, Michalski WP, Tennent JM. Molecular Characterization of a Secreted Enzyme With Phospholipase B Activity From Moraxella Bovis. J Bacteriol (2001) 183(22):6717–20. doi: 10.1128/JB.183.22.6717-6720.2001
175. Timpe JM, Holm MM, Vanlerberg SL, Basrur V, Lafontaine ER. Identification of a Moraxella Catarrhalis Outer Membrane Protein Exhibiting Both Adhesin and Lipolytic Activities. Infect Immun (2003) 71(8):4341–50. doi: 10.1128/iai.71.8.4341-4350.2003
176. Lipski SL, Akimana C, Timpe JM, Wooten RM, Lafontaine ER. The Moraxella Catarrhalis Autotransporter McaP Is a Conserved Surface Protein That Mediates Adherence to Human Epithelial Cells Through its N-Terminal Passenger Domain. Infect Immun (2007) 75(1):314–24. doi: 10.1128/IAI.01330-06
177. Lafontaine ER, Chen Z, Huertas-Diaz MC, Dyke JS, Jelesijevic TP, Michel F, et al. The Autotransporter Protein BatA Is a Protective Antigen Against Lethal Aerosol Infection With Burkholderia Mallei and Burkholderia Pseudomallei. Vaccine: X (2019) 1:100002. doi: 10.1016/j.jvacx.2018.100002
178. Molleken K, Schmidt E, Hegemann JH. Members of the Pmp Protein Family of Chlamydia Pneumoniae Mediate Adhesion to Human Cells via Short Repetitive Peptide Motifs. Mol Microbiol (2010) 78(4):1004–17. doi: 10.1111/j.1365-2958.2010.07386.x
179. Becker E, Hegemann JH. All Subtypes of the Pmp Adhesin Family are Implicated in Chlamydial Virulence and Show Species-Specific Function. Microbiologyopen (2014) 3(4):544–56. doi: 10.1002/mbo3.186
180. Molleken K, Becker E, Hegemann JH. The Chlamydia Pneumoniae Invasin Protein Pmp21 Recruits the EGF Receptor for Host Cell Entry. PloS Pathog (2013) 9(4):e1003325. doi: 10.1371/journal.ppat.1003325
181. Wehrl W, Brinkmann V, Jungblut PR, Meyer TF, Szczepek AJ. From the Inside Out–Processing of the Chlamydial Autotransporter PmpD and its Role in Bacterial Adhesion and Activation of Human Host Cells. Mol Microbiol (2004) 51(2):319–34. doi: 10.1046/j.1365-2958.2003.03838.x
182. Wang S, Xia Y, Dai J, Shi Z, Kou Y, Li H, et al. Novel Roles for Autotransporter Adhesin AatA of Avian Pathogenic Escherichia Coli: Colonization During Infection and Cell Aggregation. FEMS Immunol Med Microbiol (2011) 63(3):328–38. doi: 10.1111/j.1574-695X.2011.00862.x
183. Li G, Feng Y, Kariyawasam S, Tivendale KA, Wannemuehler Y, Zhou F, et al. AatA is a Novel Autotransporter and Virulence Factor of Avian Pathogenic. Escherichia coli. Infect Immun (2010) 78(3):898–906. doi: 10.1128/IAI.00513-09
184. Kingsley RA, Santos RL, Keestra AM, Adams LG, Baumler AJ. Salmonella Enterica Serotype Typhimurium ShdA Is an Outer Membrane Fibronectin-Binding Protein That Is Expressed in the Intestine. Mol Microbiol (2002) 43(4):895–905. doi: 10.1046/j.1365-2958.2002.02805.x
185. Kingsley RA, Humphries AD, Weening EH, De Zoete MR, Winter S, Papaconstantinopoulou A, et al. Molecular and Phenotypic Analysis of the CS54 Island of Salmonella Enterica Serotype Typhimurium: Identification of Intestinal Colonization and Persistence Determinants. Infect Immun (2003) 71(2):629–40. doi: 10.1128/iai.71.2.629-640.2003
186. Kingsley RA, Abi Ghanem D, Puebla-Osorio N, Keestra AM, Berghman L, Baumler AJ. Fibronectin Binding to the Salmonella Enterica Serotype Typhimurium ShdA Autotransporter Protein Is Inhibited by a Monoclonal Antibody Recognizing the A3 Repeat. J Bacteriol (2004) 186(15):4931–9. doi: 10.1128/JB.186.15.4931-4939.2004
187. Kingsley RA, Keestra AM, de Zoete MR, Baumler AJ. The ShdA Adhesin Binds to the Cationic Cradle of the Fibronectin 13fniii Repeat Module: Evidence for Molecular Mimicry of Heparin Binding. Mol Microbiol (2004) 52(2):345–55. doi: 10.1111/j.1365-2958.2004.03995.x
188. Lawrenz MB, Lenz JD, Miller VL. A Novel Autotransporter Adhesin is Required for Efficient Colonization During Bubonic Plague. Infect Immun (2009) 77(1):317–26. doi: 10.1128/IAI.01206-08
189. Lawrenz MB, Pennington J, Miller VL. Acquisition of Omptin Reveals Cryptic Virulence Function of Autotransporter YapE in Yersinia Pestis. Mol Microbiol (2013) 89(2):276–87. doi: 10.1111/mmi.12273
190. Koseoglu VK, Hall CP, Rodriguez-Lopez EM, Agaisse H. The Autotransporter IcsA Promotes Shigella Flexneri Biofilm Formation in the Presence of Bile Salts. Infect Immun (2019) 87(7): e00861–18. doi: 10.1128/IAI.00861-18
191. Brotcke Zumsteg A, Goosmann C, Brinkmann V, Morona R, Zychlinsky A. IcsA Is a Shigella Flexneri Adhesin Regulated by the Type III Secretion System and Required for Pathogenesis. Cell Host Microbe (2014) 15(4):435–45. doi: 10.1016/j.chom.2014.03.001
192. Wang S, Yang D, Wu X, Wang Y, Wang D, Tian M, et al. Autotransporter MisL of Salmonella Enterica Serotype Typhimurium Facilitates Bacterial Aggregation and Biofilm Formation. FEMS Microbiol Lett (2018) 365(17):fny142. doi: 10.1093/femsle/fny142
193. Dorsey CW, Laarakker MC, Humphries AD, Weening EH, Baumler AJ. Salmonella Enterica Serotype Typhimurium MisL is an Intestinal Colonization Factor That Binds Fibronectin. Mol Microbiol (2005) 57(1):196–211. doi: 10.1111/j.1365-2958.2005.04666.x
194. Easton DM, Totsika M, Allsopp LP, Phan MD, Idris A, Wurpel DJ, et al. Characterization of EhaJ, a New Autotransporter Protein From Enterohemorrhagic and Enteropathogenic. Escherichia coli. Front Microbiol (2011) 2:120. doi: 10.3389/fmicb.2011.00120
195. Battaglioli EJ, Goh KGK, Atruktsang TS, Schwartz K, Schembri MA, Welch RA. Identification and Characterization of a Phase-Variable Element That Regulates the Autotransporter UpaE in Uropathogenic. Escherichia coli. mBio (2018) 9(4):e01360–18. doi: 10.1128/mBio.01360-18
196. Crane DD, Carlson JH, Fischer ER, Bavoil P, Hsia RC, Tan C, et al. Chlamydia Trachomatis Polymorphic Membrane Protein D is a Species-Common Pan-Neutralizing Antigen. Proc Natl Acad Sci U.S.A. (2006) 103(6):1894–9. doi: 10.1073/pnas.0508983103
197. Paes W, Dowle A, Coldwell J, Leech A, Ganderton T, Brzozowski A. The Chlamydia Trachomatis PmpD Adhesin Forms Higher Order Structures Through Disulphide-Mediated Covalent Interactions. PloS One (2018) 13(6):e0198662. doi: 10.1371/journal.pone.0198662
198. Luczak SE, Smits SH, Decker C, Nagel-Steger L, Schmitt L, Hegemann JH. The Chlamydia Pneumoniae Adhesin Pmp21 Forms Oligomers With Adhesive Properties. J Biol Chem (2016) 291(43):22806–18. doi: 10.1074/jbc.M116.728915
199. Palframan SL, Kwok T, Gabriel K. Vacuolating Cytotoxin A (VacA), a Key Toxin for Helicobacter Pylori Pathogenesis. Front Cell Infect Microbiol (2012) 2:92. doi: 10.3389/fcimb.2012.00092
200. Goldberg MB, Theriot JA. Shigella Flexneri Surface Protein IcsA is Sufficient to Direct Actin-Based Motility. Proc Natl Acad Sci U.S.A. (1995) 92(14):6572–6. doi: 10.1073/pnas.92.14.6572
201. Dai J, Wang S, Guerlebeck D, Laturnus C, Guenther S, Shi Z, et al. Suppression Subtractive Hybridization Identifies an Autotransporter Adhesin Gene of E. Coli IMT5155 Specifically Associated With Avian Pathogenic Escherichia Coli (APEC). BMC Microbiol (2010) 10:236. doi: 10.1186/1471-2180-10-236
202. Egile C, Loisel TP, Laurent V, Li R, Pantaloni D, Sansonetti PJ, et al. Activation of the CDC42 Effector N-WASP by the Shigella Flexneri IcsA Protein Promotes Actin Nucleation by Arp2/3 Complex and Bacterial Actin-Based Motility. J Cell Biol (1999) 146(6):1319–32. doi: 10.1083/jcb.146.6.1319
203. May KL, Grabowicz M, Polyak SW, Morona R. Self-Association of the Shigella Flexneri IcsA Autotransporter Protein. Microbiol (Reading) (2012) 158(Pt 7):1874–83. doi: 10.1099/mic.0.056465-0
204. Bokhari H, Bilal I, Zafar S. BapC Autotransporter Protein of Bordetella Pertussis Is an Adhesion Factor. J Basic Microbiol (2012) 52(4):390–6. doi: 10.1002/jobm.201100188
205. Leininger E, Roberts M, Kenimer JG, Charles IG, Fairweather N, Novotny P, et al. Pertactin, an Arg-Gly-Asp-Containing Bordetella Pertussis Surface Protein That Promotes Adherence of Mammalian Cells. Proc Natl Acad Sci U.S.A. (1991) 88(2):345–9. doi: 10.1073/pnas.88.2.345
206. Fernandez RC, Weiss AA. Cloning and Sequencing of a Bordetella Pertussis Serum Resistance Locus. Infect Immun (1994) 62(11):4727–38. doi: 10.1128/iai.62.11.4727-4738.1994
207. Finn TM, Amsbaugh DF. Vag8, a Bordetella Pertussis Bvg-Regulated Protein. Infect Immun (1998) 66(8):3985–9. doi: 10.1128/IAI.66.8.3985-3989.1998
208. Noofeli M, Bokhari H, Blackburn P, Roberts M, Coote JG, Parton R. BapC Autotransporter Protein is a Virulence Determinant of Bordetella Pertussis. Microb Pathog (2011) 51(3):169–77. doi: 10.1016/j.micpath.2011.04.004
209. Inatsuka CS, Xu Q, Vujkovic-Cvijin I, Wong S, Stibitz S, Miller JF, et al. Pertactin is Required for Bordetella Species to Resist Neutrophil-Mediated Clearance. Infect Immun (2010) 78(7):2901–9. doi: 10.1128/IAI.00188-10
210. Hovingh ES, Mariman R, Solans L, Hijdra D, Hamstra HJ, Jongerius I, et al. Bordetella Pertussis Pertactin Knock-Out Strains Reveal Immunomodulatory Properties of This Virulence Factor. Emerg Microbes Infect (2018) 7(1):39. doi: 10.1038/s41426-018-0039-8
211. Barnes MG, Weiss AA. BrkA Protein of Bordetella Pertussis Inhibits the Classical Pathway of Complement After C1 Deposition. Infect Immun (2001) 69(5):3067–72. doi: 10.1128/IAI.69.5.3067-3072.2001
212. Hovingh ES, de Maat S, Cloherty APM, Johnson S, Pinelli E, Maas C, et al. Virulence Associated Gene 8 of Bordetella Pertussis Enhances Contact System Activity by Inhibiting the Regulatory Function of Complement Regulator C1 Inhibitor. Front Immunol (2018) 9:1172. doi: 10.3389/fimmu.2018.01172
213. Raeven RH, van der Maas L, Tilstra W, Uittenbogaard JP, Bindels TH, Kuipers B, et al. Immunoproteomic Profiling of Bordetella Pertussis Outer Membrane Vesicle Vaccine Reveals Broad and Balanced Humoral Immunogenicity. J Proteome Res (2015) 14(7):2929–42. doi: 10.1021/acs.jproteome.5b00258
214. Raeven RHM, van Vlies N, Salverda MLM, van der Maas L, Uittenbogaard JP, Bindels THE, et al. The Role of Virulence Proteins in Protection Conferred by Bordetella Pertussis Outer Membrane Vesicle Vaccines. Vaccines (Basel) (2020) 8(3):429. doi: 10.3390/vaccines8030429
215. Allsopp LP, Beloin C, Ulett GC, Valle J, Totsika M, Sherlock O, et al. Molecular Characterization of UpaB and UpaC, Two New Autotransporter Proteins of Uropathogenic Escherichia Coli CFT073. Infect Immun (2012) 80(1):321–32. doi: 10.1128/IAI.05322-11
216. Wells TJ, McNeilly TN, Totsika M, Mahajan A, Gally DL, Schembri MA. The Escherichia Coli O157:H7 EhaB Autotransporter Protein Binds to Laminin and Collagen I and Induces a Serum IgA Response in O157:H7 Challenged Cattle. Environ Microbiol (2009) 11(7):1803–14. doi: 10.1111/j.1462-2920.2009.01905.x
217. Radin JN, Gaddy JA, Gonzalez-Rivera C, Loh JT, Algood HM, Cover TL. Flagellar Localization of a Helicobacter Pylori Autotransporter Protein. mBio (2013) 4(2):e00613–12. doi: 10.1128/mBio.00613-12
218. Allsopp LP, Beloin C, Moriel DG, Totsika M, Ghigo JM, Schembri MA. Functional Heterogeneity of the UpaH Autotransporter Protein From Uropathogenic. Escherichia coli. J Bacteriol (2012) 194(21):5769–82. doi: 10.1128/JB.01264-12
219. Allsopp LP, Totsika M, Tree JJ, Ulett GC, Mabbett AN, Wells TJ, et al. UpaH is a Newly Identified Autotransporter Protein That Contributes to Biofilm Formation and Bladder Colonization by Uropathogenic Escherichia Coli CFT073. Infect Immun (2010) 78(4):1659–69. doi: 10.1128/IAI.01010-09
220. Ashgar SS, Oldfield NJ, Wooldridge KG, Jones MA, Irving GJ, Turner DP, et al. CapA, an Autotransporter Protein of Campylobacter Jejuni, Mediates Association With Human Epithelial Cells and Colonization of the Chicken Gut. J Bacteriol (2007) 189(5):1856–65. doi: 10.1128/JB.01427-06
221. Nair MK, De Masi L, Yue M, Galvan EM, Chen H, Wang F, et al. Adhesive Properties of YapV and Paralogous Autotransporter Proteins of. Yersinia pestis. Infect Immun (2015) 83(5):1809–19. doi: 10.1128/IAI.00094-15
222. Uchiyama T, Kawano H, Kusuhara Y. The Major Outer Membrane Protein Rompb of Spotted Fever Group Rickettsiae Functions in the Rickettsial Adherence to and Invasion of Vero Cells. Microbes Infect (2006) 8(3):801–9. doi: 10.1016/j.micinf.2005.10.003
223. Williams CL, Haines R, Cotter PA. Serendipitous Discovery of an Immunoglobulin-Binding Autotransporter in Bordetella Species. Infect Immun (2008) 76(7):2966–77. doi: 10.1128/IAI.00323-08
224. Posadas DM, Ruiz-Ranwez V, Bonomi HR, Martin FA, Zorreguieta A. BmaC, a Novel Autotransporter of Brucella Suis, is Involved in Bacterial Adhesion to Host Cells. Cell Microbiol (2012) 14(6):965–82. doi: 10.1111/j.1462-5822.2012.01771.x
225. Matsumoto A, Huston SL, Killiny N, Igo MM. XatA, an AT-1 Autotransporter Important for the Virulence of Xylella Fastidiosa Temecula1. Microbiologyopen (2012) 1(1):33–45. doi: 10.1002/mbo3.6
226. Feitosa-Junior OR, Stefanello E, Zaini PA, Nascimento R, Pierry PM, Dandekar AM. Proteomic and Metabolomic Analyses of Xylella fastidiosa OMV-Enriched Fractions Reveal Association with Virulence Factors and Signaling Molecules of the DSF Family. Phytopathology (2019). 109(8):1344–1353. doi: 10.1094/PHYTO-03-19-0083-R.
227. Odenbreit S, Till M, Hofreuter D, Faller G, Haas R. Genetic and Functional Characterization of the alpAB Gene Locus Essential for the Adhesion of Helicobacter Pylori to Human Gastric Tissue. Mol Microbiol (1999) 31(5):1537–48. doi: 10.1046/j.1365-2958.1999.01300.x
228. Chan YG, Cardwell MM, Hermanas TM, Uchiyama T, Martinez JJ. Rickettsial Outer-Membrane Protein B (Rompb) Mediates Bacterial Invasion Through Ku70 in an Actin, C-Cbl, Clathrin and Caveolin 2-Dependent Manner. Cell Microbiol (2009) 11(4):629–44. doi: 10.1111/j.1462-5822.2008.01279.x
229. Riley SP, Goh KC, Hermanas TM, Cardwell MM, Chan YG, Martinez JJ. The Rickettsia Conorii Autotransporter Protein Sca1 Promotes Adherence to Nonphagocytic Mammalian Cells. Infect Immun (2010) 78(5):1895–904. doi: 10.1128/IAI.01165-09
230. Cardwell MM, Martinez JJ. The Sca2 Autotransporter Protein From Rickettsia Conorii is Sufficient to Mediate Adherence to and Invasion of Cultured Mammalian Cells. Infect Immun (2009) 77(12):5272–80. doi: 10.1128/IAI.00201-09
231. Ha NY, Cho NH, Kim YS, Choi MS, Kim IS. An Autotransporter Protein From Orientia Tsutsugamushi Mediates Adherence to Nonphagocytic Host Cells. Infect Immun (2011) 79(4):1718–27. doi: 10.1128/IAI.01239-10
232. Nunes AC, Longo PL, Mayer MP. Influence of Aae Autotransporter Protein on Adhesion and Biofilm Formation by Aggregatibacter Actinomycetemcomitans. Braz Dent J (2016) 27(3):255–60. doi: 10.1590/0103-6440201600260
233. Haglund CM, Choe JE, Skau CT, Kovar DR, Welch MD. Rickettsia Sca2 is a Bacterial Formin-Like Mediator of Actin-Based Motility. Nat Cell Biol (2010) 12(11):1057–63. doi: 10.1038/ncb2109
234. Sun YY, Sun L. Pseudomonas Fluorescens: Iron-Responsive Proteins and Their Involvement in Host Infection. Vet Microbiol (2015) 176(3-4):309–20. doi: 10.1016/j.vetmic.2015.01.020
235. Hu YH, Liu CS, Hou JH, Sun L. Identification, Characterization, and Molecular Application of a Virulence-Associated Autotransporter From a Pathogenic Pseudomonas Fluorescens Strain. Appl Environ Microbiol (2009) 75(13):4333–40. doi: 10.1128/AEM.00159-09
236. Campos CG, Borst L, Cotter PA. Characterization of BcaA, a Putative Classical Autotransporter Protein in Burkholderia Pseudomallei. Infect Immun (2013) 81(4):1121–8. doi: 10.1128/IAI.01453-12
237. Kida Y, Taira J, Yamamoto T, Higashimoto Y, Kuwano K. EprS, an Autotransporter Protein of Pseudomonas Aeruginosa, Possessing Serine Protease Activity Induces Inflammatory Responses Through Protease-Activated Receptors. Cell Microbiol (2013) 15(7):1168–81. doi: 10.1111/cmi.12106
238. Liu L, Chi H, Sun L. Pseudomonas Fluorescens: Identification of Fur-Regulated Proteins and Evaluation of Their Contribution to Pathogenesis. Dis Aquat Organ (2015) 115(1):67–80. doi: 10.3354/dao02874
239. Ohnishi Y, Horinouchi S. Extracellular Production of a Serratia Marcescens Serine Protease in Escherichia Coli. Biosci Biotechnol Biochem (1996) 60(10):1551–8. doi: 10.1271/bbb.60.1551
240. Miyazaki H, Yanagida N, Horinouchi S, Beppu T. Characterization of the Precursor of Serratia Marcescens Serine Protease and COOH-Terminal Processing of the Precursor During its Excretion Through the Outer Membrane of Escherichia Coli. J Bacteriol (1989) 171(12):6566–72. doi: 10.1128/jb.171.12.6566-6572.1989
241. Coutte L, Antoine R, Drobecq H, Locht C, Jacob-Dubuisson F. Subtilisin-Like Autotransporter Serves as Maturation Protease in a Bacterial Secretion Pathway. EMBO J (2001) 20(18):5040–8. doi: 10.1093/emboj/20.18.5040
242. Ali T, Oldfield NJ, Wooldridge KG, Turner DP, Ala’Aldeen DA. Functional Characterization of AasP, a Maturation Protease Autotransporter Protein of Actinobacillus Pleuropneumoniae. Infect Immun (2008) 76(12):5608–14. doi: 10.1128/IAI.00085-08
243. Chen J, Civerolo E, Tubajika K, Livingston S, Higbee B. Hypervariations of a Protease-Encoding Gene, PD0218 (Pspb), in Xylella Fastidiosa Strains Causing Almond Leaf Scorch and Pierce’s Disease in California. Appl Environ Microbiol (2008) 74(12):3652–7. doi: 10.1128/AEM.02386-07
244. Kida Y, Taira J, Kuwano K. EprS, an Autotransporter Serine Protease, Plays an Important Role in Various Pathogenic Phenotypes of Pseudomonas Aeruginosa. Microbiol (Reading) (2016) 162(2):318–29. doi: 10.1099/mic.0.000228
245. Coutte L, Willery E, Antoine R, Drobecq H, Locht C, Jacob-Dubuisson F. Surface Anchoring of Bacterial Subtilisin Important for Maturation Function. Mol Microbiol (2003) 49(2):529–39. doi: 10.1046/j.1365-2958.2003.03573.x
246. van Ulsen P, Adler B, Fassler P, Gilbert M, van Schilfgaarde M, van der Ley P, et al. A Novel Phase-Variable Autotransporter Serine Protease, AusI, of Neisseria Meningitidis. Microbes Infect (2006) 8(8):2088–97. doi: 10.1016/j.micinf.2006.03.007
247. Bachrach G, Rosen G, Bellalou M, Naor R, Sela MN. Identification of a Fusobacterium Nucleatum 65 kDa Serine Protease. Oral Microbiol Immunol (2004) 19(3):155–9. doi: 10.1111/j.0902-0055.2004.00132.x
248. Del Tordello E, Vacca I, Ram S, Rappuoli R, Serruto D. Neisseria Meningitidis NalP Cleaves Human Complement C3, Facilitating Degradation of C3b and Survival in Human Serum. Proc Natl Acad Sci U.S.A. (2014) 111(1):427–32. doi: 10.1073/pnas.1321556111
249. Dufailu OA, Mahdavi J, Ala’Aldeen DAA, Wooldridge KG, Oldfield NJ. Uptake of Neisserial Autotransporter Lipoprotein (NalP) Promotes an Increase in Human Brain Microvascular Endothelial Cell Metabolic Activity. Microb Pathog (2018) 124:70–5. doi: 10.1016/j.micpath.2018.08.001
250. Mehat JW, Park SF, van Vliet AHM, La Ragione RM. CapC, a Novel Autotransporter and Virulence Factor of Campylobacter Jejuni. Appl Environ Microbiol (2018) 84(16):e01032–18. doi: 10.1128/AEM.01032-18
251. Coppenhagen-Glazer S, Sol A, Abed J, Naor R, Zhang X, Han YW, et al. Fap2 of Fusobacterium Nucleatum is a Galactose-Inhibitable Adhesin Involved in Coaggregation, Cell Adhesion, and Preterm Birth. Infect Immun (2015) 83(3):1104–13. doi: 10.1128/IAI.02838-14
252. Mizan S, Henk A, Stallings A, Maier M, Lee MD. Cloning and Characterization of Sialidases With 2-6’ and 2-3’ Sialyl Lactose Specificity From. Pasteurella multocida. J Bacteriol (2000) 182(24):6874–83. doi: 10.1128/jb.182.24.6874-6883.2000
253. Arenas J, Paganelli FL, Rodriguez-Castano P, Cano-Crespo S, van der Ende A, van Putten JP, et al. Expression of the Gene for Autotransporter AutB of Neisseria Meningitidis Affects Biofilm Formation and Epithelial Transmigration. Front Cell Infect Microbiol (2016) 6:162. doi: 10.3389/fcimb.2016.00162
254. Perez-Ortega J, Rodriguez A, Ribes E, Tommassen J, Arenas J. Interstrain Cooperation in Meningococcal Biofilms: Role of Autotransporters NalP and AutA. Front Microbiol (2017) 8:434. doi: 10.3389/fmicb.2017.00434
255. Luckett JC, Darch O, Watters C, Abuoun M, Wright V, Paredes-Osses E, et al. A Novel Virulence Strategy for Pseudomonas Aeruginosa Mediated by an Autotransporter With Arginine-Specific Aminopeptidase Activity. PloS Pathog (2012) 8(8):e1002854. doi: 10.1371/journal.ppat.1002854
256. Hoopman TC, Wang W, Brautigam CA, Sedillo JL, Reilly TJ, Hansen EJ. Moraxella Catarrhalis Synthesizes an Autotransporter That is an Acid Phosphatase. J Bacteriol (2008) 190(4):1459–72. doi: 10.1128/JB.01688-07
257. Chapman TM, Goa KL. Reduced-Antigen Combined Diphtheria-Tetanus-Acellular Pertussis Vaccine (Boostrix). Drugs (2003) 63(13):1407–13. doi: 10.2165/00003495-200363130-00005
258. Curran MP, Goa KL. DTPa-HBV-IPV/Hib Vaccine (Infanrix Hexa). Drugs (2003) 63(7):673–82. doi: 10.2165/00003495-200363070-00004
259. Pichichero ME, DeTora LM, Johnson DR. An Adolescent and Adult Formulation Combined Tetanus, Diphtheria and Five-Component Pertussis Vaccine. Expert Rev Vaccines (2006) 5(2):175–87. doi: 10.1586/14760584.5.2.175
260. Gorringe AR, Pajon R. Bexsero: A Multicomponent Vaccine for Prevention of Meningococcal Disease. Hum Vaccin Immunother (2012) 8(2):174–83. doi: 10.4161/hv.18500
261. Heras B, Scanlon MJ, Martin JL. Targeting Virulence Not Viability in the Search for Future Antibacterials. Br J Clin Pharmacol (2015) 79(2):208–15. doi: 10.1111/bcp.12356
262. Lattemann CT, Maurer J, Gerland E, Meyer TF. Autodisplay: Functional Display of Active Beta-Lactamase on the Surface of Escherichia Coli by the AIDA-I Autotransporter. J Bacteriol (2000) 182(13):3726–33. doi: 10.1128/jb.182.13.3726-3733.2000
263. Chung ME, Goroncy K, Kolesnikova A, Schonauer D, Schwaneberg U. Display of Functional Nucleic Acid Polymerase on Escherichia Coli Surface and its Application in Directed Polymerase Evolution. Biotechnol Bioeng (2020) 117(12):3699–711. doi: 10.1002/bit.27542
264. Ko HJ, Park E, Song J, Yang TH, Lee HJ, Kim KH, et al. Functional Cell Surface Display and Controlled Secretion of Diverse Agarolytic Enzymes by Escherichia Coli With a Novel Ligation-Independent Cloning Vector Based on the Autotransporter YfaL. Appl Environ Microbiol (2012) 78(9):3051–8. doi: 10.1128/AEM.07004-11
265. Dvorak P, Bayer EA, de Lorenzo V. Surface Display of Designer Protein Scaffolds on Genome-Reduced Strains of Pseudomonas Putida. ACS Synth Biol (2020) 9(10):2749–64. doi: 10.1021/acssynbio.0c00276
266. Stratker K, Haidar S, Dubiel M, Estevez-Braun A, Jose J. Autodisplay of Human PIP5K1alpha Lipid Kinase on Escherichia Coli and Inhibitor Testing. Enzyme Microb Technol (2021) 143:109717. doi: 10.1016/j.enzmictec.2020.109717
267. Mateos-Chavez AA, Munoz-Lopez P, Becerra-Baez EI, Flores-Martinez LF, Prada-Gracia D, Moreno-Vargas LM, et al. Live Attenuated Salmonella Enterica Expressing and Releasing Cell-Permeable Bax BH3 Peptide Through the MisL Autotransporter System Elicits Antitumor Activity in a Murine Xenograft Model of Human B Non-Hodgkin’s Lymphoma. Front Immunol (2019) 10:2562. doi: 10.3389/fimmu.2019.02562
268. Jong WS, Soprova Z, de Punder K, ten Hagen-Jongman CM, Wagner S, Wickstrom D, et al. A Structurally Informed Autotransporter Platform for Efficient Heterologous Protein Secretion and Display. Microb Cell Fact (2012) 11:85. doi: 10.1186/1475-2859-11-85
269. van den Berg van Saparoea HB, Houben D, Kuijl C, Luirink J, Jong WSP. Combining Protein Ligation Systems to Expand the Functionality of Semi-Synthetic Outer Membrane Vesicle Nanoparticles. Front Microbiol (2020) 11:890. doi: 10.3389/fmicb.2020.00890
Keywords: type V secretion system, virulence, bacterial pathogenesis, toxins, adhesins, secreted proteins
Citation: Clarke KR, Hor L, Pilapitiya A, Luirink J, Paxman JJ and Heras B (2022) Phylogenetic Classification and Functional Review of Autotransporters. Front. Immunol. 13:921272. doi: 10.3389/fimmu.2022.921272
Received: 15 April 2022; Accepted: 06 June 2022;
Published: 01 July 2022.
Edited by:
Mario M. D'Elios, University of Florence, ItalyReviewed by:
Arsenio M. Fialho, Universidade de Lisboa, PortugalJack Christopher Leo, Nottingham Trent University, United Kingdom
Copyright © 2022 Clarke, Hor, Pilapitiya, Luirink, Paxman and Heras. This is an open-access article distributed under the terms of the Creative Commons Attribution License (CC BY). The use, distribution or reproduction in other forums is permitted, provided the original author(s) and the copyright owner(s) are credited and that the original publication in this journal is cited, in accordance with accepted academic practice. No use, distribution or reproduction is permitted which does not comply with these terms.
*Correspondence: Begoña Heras, Yi5oZXJhc0BsYXRyb2JlLmVkdS5hdQ==; Jason J. Paxman, ai5wYXhtYW5AbGF0cm9iZS5lZHUuYXU=