- 1Transplant Surgery Research Laboratory and Division of Transplant Surgery, Department of Surgery, Brigham and Women’s Hospital, Harvard Medical School, Boston, MA, United States
- 2Regenerative Medicine and Experimental Surgery, Department of General, Visceral and Transplant Surgery, Hannover Medical School, Hannover, Germany
- 3Institute of Medical Immunology, Charite Universitaetsmedizin Berlin, Berlin, Germany
- 4Department of Urology, Osaka Medical and Pharmaceutical University, Takatsuki City, Japan
Myeloid-derived suppressor cells (MDSC) are defined as a group of myeloid cells with potent immunoregulatory functions that have been shown to be involved in a variety of immune-related diseases including infections, autoimmune disorders, and cancer. In organ transplantation, MDSC promote tolerance by modifying adaptive immune responses. With aging, however, substantial changes occur that affect immune functions and impact alloimmunity. Since the vast majority of transplant patients are elderly, age-specific modifications of MDSC are of relevance. Furthermore, understanding age-associated changes in MDSC may lead to improved therapeutic strategies. Here, we provide a comprehensive update on the effects of aging on MDSC and discuss potential consequences on alloimmunity.
Introduction
Myeloid-derived suppressor cells (MDSC) are a heterogenous group of immature myeloid cells that exhibit immunosuppressive functions affecting various immune cells (1). First observed in patients with cancer, MDSC are generated under chronic pathological conditions including persistent inflammation or malignancies where continuous stimulation and inhibition of standard myelopoiesis pathways result in the formation of undifferentiated cells. Reflecting this heterogeneity, MDSC in mice are phenotyped as CD11b+Gr-1+ with two major subsets: granulocytic MDSC (G-MDSC) (CD11b+Gr-1+Ly6G+Ly6Clow) and monocytic MDSC (M-MDSC) (CD11b+Gr-1+Ly6G-Ly6Chi), based on their expression of Ly6C and Ly6G (1–4). In humans, the classification is more complex with M-MDSC phenotyped as CD11b+CD33+CD14+CD66b+HLA-DRlow and G-MDSC as CD11b+CD33+CD14-CD15+HLA-DRlow cells (5). The functional capacities of these cells have gained increasing attention in the recent past. Most importantly, MDSC have shown a strong capacity to suppress CD4+ and CD8+ T-cell functions while promoting the activation and expansion of regulatory T cells (Tregs) (5–9). The mechanisms of immune modulation have also been shown to vary depending on the cell subset. Generally, G-MDSC suppress the immune function via reactive oxygen species (ROS) whereas the effects of M-MDSC are primarily mediated through the up-regulation of inducible nitric oxide synthase (iNOS), arginase, and immunosuppressive cytokines (10). However, immune modulation is not limited on the effects on T cells but also impacts innate immune cells including neutrophils, monocytes, macrophages, and dendritic cells (DCs) (11), many of which play a critical role in alloimmunity (12–16).
Clinical Impact
In organ transplantation, MDSC may promote allograft acceptance and have therefore been of clinical interest in and beyond organ transplantation.
In various clinical settings including kidney, lung, and intestinal transplantation, significantly elevated levels of MDSC have been reported suppressing T-cell proliferation and pro-inflammatory cytokine levels when cultured in vitro (17–19). In addition, clinical studies investigating the role of MDSC in acute T-cell-mediated rejection (ATCMR) have shown an improved allograft function in parallel with increasing peripheral MDSC counts. In renal transplant biopsies, tissue injury has been attenuated with high peripheral MDSC counts, corroborating the immunosuppressive potential of MDSC in alloimmunity. Interestingly, MDSC collected from patients had the capacity to expand Tregs while inhibiting IL-17 production in vitro (20). Moreover, MDSC expansion correlated linearly with an increase in Tregs in vivo and Treg induction in vitro (21). Notably, transplant recipients with infections or chronic lung allograft dysfunction (CLAD) have shown lower G-MDSC levels when compared to patients with stable transplant function (18). Cytomegalovirus (CMV) infection, a major risk factor for developing CLAD, has been linked to an impaired MDSC differentiation (22, 23). In addition, hepatic stellate cells have been shown to induce MDSC both in vivo and in vitro, indicating the potential to promote liver allograft acceptance (24–27). Of additional interest, numbers of MDSC increased in intestinal transplant patients with acute cellular rejection (ACR) and positively correlated with serum IL-6 levels, a cytokine that has previously been shown to induce MDSC expansion (1). Furthermore, corresponding in vitro experiments have shown that IL-6 and methylprednisolone promoted the differentiation of bone marrow cells into MDSC. An accumulation of MDSC in the intestinal mucosa was also observed, and MDSC were able to suppress the T-cell-mediated destruction of donor intestinal epithelial organoids. Thus, MDSC may play an important role in suppressing the pathogenic T-cell response in the context of intestinal transplants and control ACR (19).
Impact of Immunosuppressive Drugs on MDSC
In addition to the potential effects of MDSC on allograft survival, interactions with commonly used immunosuppressive drugs have been reported (Table 1). Clinically, circulating numbers of MDSC increased rapidly after applying calcineurin inhibitors, rapamycin, or corticosteroids (34, 35). Long-term, granulocytic subsets (G-MDSC) fluctuated in numbers whereas monocytic subsets (M-MDSC) remained relatively stable (38). Interestingly, M-MDSC from tacrolimus-treated but not from rapamycin-treated kidney transplant recipients were able to suppress the proliferation of CD4+ T cells, indicating that rapamycin may curtail the immunosuppressive abilities of M-MDSC (30). The correlation of MDSC activity with CNI levels may be explained by mechanistic findings that show an increased expression of indoleamine 2,3-dioxygenase (IDO), an enzyme regulating the immunosuppressive activity of MDSC, thereby inducing the suppressive functions of recipient MDSC (28). Conversely, rapamycin has been shown to downregulate IDO expression and may therefore also attenuate iNOS expression in rapamycin-exposed M-MDSC (30, 39).
In other experiments, however, rapamycin has been shown to increase MDSC levels, enhancing cardiac and hepatic allograft survival in mouse models potentially facilitated through the induction of inducible iNOS (31, 32). Intriguingly, the adoptive transfer of MDSC from rapamycin-treated recipients prolonged allograft survival in third-party recipient mice (31). Most recently, delayed allograft rejection following MDSC induction by rapamycin has been shown in obese transplant recipients, emphasizing on the effectiveness of rapamycin in transplant recipients with preexisting conditions (33). As obesity has been linked to cellular senescence, those findings are of particular interest for the assessment of the role that MDSC may play in aging (40–42). Taken together, these findings may indicate that mTOR inhibition may have dual effects—both immunogenic as well as tolerogenic—on MDSC (30). Steroids have also been shown to impact MDSC numbers and functions (35). Steroid treatment correlates with increasing numbers of MDSC and Tregs in cardiac transplant models. Moreover, levels of Tregs were diminished when anti-Gr-1 antibodies targeting MDSC were administered in this study, emphasizing on the potential of MDSC affecting Tregs (36).
In addition, supplementing a granulocyte-macrophage colony-stimulating factor (GM-CSF) system with dexamethasone promoted MDSC with an enhanced immunosuppressive function specifically in vitro, and, when transferred into cardiac allograft recipients, prolonged allograft survival and Treg expansion. Mechanistically, iNOS and glucocorticoid receptor (GR) signaling pathways have been shown to be essential for mediating these processes. iNOS signaling was required for MDSC to regulate T-cell responses, whereas GR signaling was essential for mediating the recruitment of MDSC into allografts (37). The effects on MDSC were not only limited to rapamycin and corticosteroids as cyclosporine A also significantly increased the numbers and immunosuppressive functions of MDSC, ameliorating alloimmune responses (29).
MDSC in Alloimmunity
Organ tpransplantation initiates a complex immunological cascade composed of cellular and humoral components, ultimately leading, if not successfully treated or modified, to an irreversible rejection. In addition to the adaptive immune system, several components of the innate immune response including dendritic cells (DCs), natural killer (NK) cells, and macrophages play critical roles in this process (43, 44). Accumulating evidence suggests that MDSC may also play an important role in allorecognition. Experimental models including kidney, heart, and skin transplantation have shown elevated numbers of MDSC that can suppress T-cell proliferation while inducing apoptosis, linked to graft prolongation (39, 45–47). It has been demonstrated that MDSC are recruited from the bone marrow, migrating into the graft early after transplantation (47). Interestingly, graft survival was prolonged in old mice and those exhibiting high serum levels of TGF-β, an immunosuppressive cytokine that has been shown to increase with age (39, 46).. When entinostat, a histone deacetylase inhibitor, was administered to block MDSC function, graft survival was abbreviated comparable to survival times observed in young mice (48). In a pre-sensitized transplant model, massively elevated levels of MDSC were found in the peripheral blood of recipient mice. Notably, particularly the CD11b+Gr-1(-low) subtype has been shown to prevent allograft injury after prolonged ischemia (49). In vitro experiments have shown that these effects are dependent on iNOS, an enzyme that is upregulated in graft-infiltrating MDSC (16, 45). Additional supporting evidence comes from findings showing that the suppressive ability of MDSC is mediated by nitric oxide (NO), secreted subsequent to receiving signals from activated T cells including IFN-γ and contact-dependent stimuli (16). Additional experiments in iNOS knockout mice demonstrated that the inhibition of activated T cells in lymphoid organs depended on NO (50). In support of clinical findings, MDSC play also a critical role in initiating the beneficial effects of Tregs with CCL5 secreted by graft infiltrating MDSC promoting the accumulation of Tregs in tolerant kidney allografts (20, 21, 51). Conversely, boosting Tregs increased the numbers of CD11b+Gr-1(-low) MDSC in recipient peripheral blood, spleen, and the graft itself (49).
Adoptive transfer experiments demonstrated that the expansion of MDSC is initiated by the inhibitory receptor immunoglobulin-like transcript (ILT)-2 and its ligands leading to prolonged allograft survival (52). Other cytokines including IL-2C, IL-33, and TNF-α have also been shown to induce MDSC resulting in a suppression of T-cell activation (53–56). Additional studies confirmed these findings; however, no alterations of antigen-specific CD8+ T-cell proliferation and cytotoxicity were found (57, 58). Based on previous findings, the combinatorial application of induced MDSC and Tregs exhibited superior immunosuppressive capabilities with prolonged graft survival compared to the treatment with individual cell populations (53). Notably, other regulatory myeloid cell types including tolerogenic dendritic cells and regulatory/suppressor macrophages have also been shown to prolong skin allograft survival by distinct mechanisms of action (59).
The Role of MDSC in Ischemia–Reperfusion Injury
Ischemia–reperfusion injury (IRI) is an inevitable component of solid organ transplantation. In general, pro-inflammatory events are initiated upon blood flow cessation and resumption causing a myriad of immune cells to migrate into the graft. As an immediate response, innate immune cells including neutrophils and macrophages infiltrate the organ, secreting pro-inflammatory cytokines while initiating phagocytosis and complement activation (60–62). Adaptive immune cells such as T and B cells contribute to the deleterious events by producing pro-inflammatory cytokines, lysosomal enzymes, and ROS (63–65). Recent data indicate that MDSC may also play an important role in IRI. In murine kidney transplant models, MDSC have been found to aggravate IRI. These findings may be explained by the differentiation of M-MDSC into harmful macrophages and dendritic cells (66). However, the immunosuppressive effects that MDSC exert on adaptive CD4+ and CD8+ T cells were not sufficient to prevent an impairment of renal function. Supporting evidence comes from findings that pharmacological depletion of MDSC in kidney IRI models using anti-Gr-1 antibodies entailed beneficial effects. Interestingly, human C-reactive protein (CRP), which has previously been found to exacerbate renal IRI, has been shown to specifically promote the kidney infiltration of G-MDSC, a subtype displaying augmented immunosuppressive activities. Consistently, blocking CRP reduced the numbers of G-MDSC and attenuated albuminuria, suggesting a regulating role of CRP in MDSC activation (67). In contrast, increased renal infiltration of MDSC upon granulocyte colony-stimulating factor (G-CSF) administration improved renal function after IRI and attenuated acute tissue injury as well as chronic renal fibrosis (68, 69). In addition, further mechanistic studies have shown that renal fibrosis can be alleviated by MDSC through CCL5-CCR-5 axis regulation and TGF-β1/Smad/Snail signaling pathway inhibition (70).
Changes of MDSC in Aging
Aging is characterized by an increased accumulation of proinflammatory cytokines together with modifications of the composition and the effects of various immune cell types of both the adaptive and innate immune systems (71, 72). In general, adaptive immune function appears modified in aging, manifesting clinically with a compromised response to vaccines and a limited capacity to combat infections (73). At a cellular level, thymic involution leads to a decreased production of naïve T cells in the elderly with a restricted T-cell receptor repertoire. In addition, functional impairments of naïve CD4 T cells that include a compromised proliferation upon stimulation by antigen-presenting cells in addition to a limited cytokine production have been observed. Consistently, effector functions have been inferior when compared to cells derived from young progenitors (74). In parallel, B-cell production also declines with age and an accumulation of phenotypically distinct, age-associated B cells has been reported (75–77). These findings are in line with functional limitations, leading to an impaired capacity to mount sufficient antibody responses (78). Furthermore, functional changes in natural killer T cells (NKT cells) have been observed in elderly individuals (79). In addition to changes in the adaptive immune response, alterations of innate immune cells have also been found. Macrophages from old mice have shown a reduced production of pro-inflammatory cytokines following stimulation by lipopolysaccharides (LPS) (80–83). These findings may be attributed to various age-related changes including a reduced Toll-like receptor (TLR) expression, decreased nuclear factor kappa B (NF-κB) and mitogen-activated protein kinase (MAPK) activation, and increased levels of the signaling protein A20, which blocks TRAF6 signaling, thus interfering with the TLR4 pathway (82, 84–88). In addition, an impaired cytokine production by DCs and monocytes upon stimulation has been reported (89, 90). MDSC are considered progenitors of innate immune cells and increase in numbers with aging (Figure 1) (91, 92). Elevated numbers of MDSC have been shown to accumulate in the blood, bone marrow, and secondary lymphoid organs in aging and may, in fact, through the initiation of a defective PI3K-Akt signaling pathway contribute themselves to immune senescence (93, 94). Further experimental studies suggest that the expansion of MDSC with aging relies, at least in part, on NF-κB activation (95). In addition, a shift toward myelopoiesis in bone marrow hematopoietic stem cells (HSC) occurs with aging and may further promote the increase of MDSC in elderly individuals (96). Moreover, MDSC could increase myelopoiesis themselves by secreting TGF-β, a cytokine which promotes the differentiation of hematopoietic stem cells into myeloid cells (97). Consistently, elevated numbers of MDSC have also been shown in older humans with elevated levels of proinflammatory cytokines including TNF-α, IL-6, and IL-1β necessary for MDSC differentiation. Interestingly, elderly individuals with a history of cancer showed significantly higher levels of MDSC in the peripheral blood, pointing toward a role of MDSC in cancer development (6–8, 98). Additional evidence comes from clinical studies that also observed elevated numbers of MDSC with a predominance of the granulocytic subtype in old individuals (99). At a functional level, older MDSC isolated from spleen and bone marrow have shown an augmented ability to inhibit T-cell functions when compared with MDSC from young donors. Consistently, removal of MDSC from aged splenocyte cultures restored T-cell proliferation in vitro and was associated with reduced NO levels (93). Additional supporting evidence comes from studies showing a correlation between increased MDSC levels in old mice and limited T-cell tumor cytotoxicity. In addition, MDSC also delayed the response to tumor cells when adoptively transferred to young mice. Mechanistically, age-associated induction of arginase 1 in MDSC may play an important role in suppressing T-cell functions (100). Thus, age-related changes in MDSC and their subsequent impact on other immune cells may influence allograft tolerance in various ways. In addition, age-associated limited Th1 alloimmunity may enhance the effects by older MDSC (101). Moreover, suppressive capacities of Tregs have been found to be well-preserved experimentally, thus promoting graft acceptance (101). At a molecular level, an augmented expression of senescent cell markers p16 and p21 has been found in aging MDSC. However, other senescence-associated phenotypes including the accumulation of γH2AX and 53BP1 foci, reduced lamin B1 expression, and induced IL-6 expression have not been detected. Moreover, senolytics (ABT-263) were unable to eliminate these cells (102).
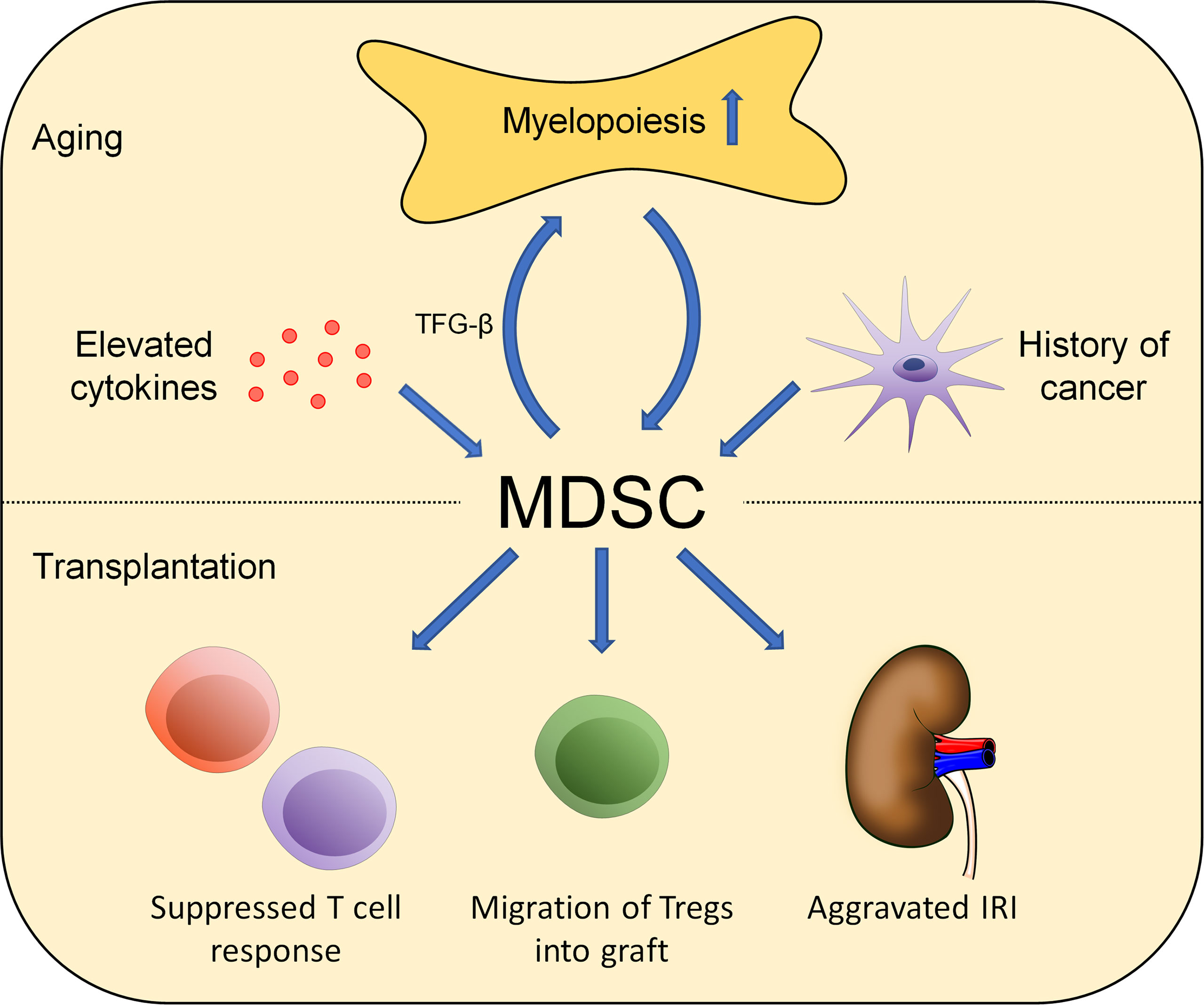
Figure 1 Aging affects the role of MDSC in alloimmunity. Changes in MDSC numbers and functionality occur during aging. Environmental factors including elevated cytokine levels, increased myelopoiesis, and malignancies impact MDSC numbers. Those effects impact alloimmunity in multiple ways including T-cell suppression, Treg activation and migration, and aggravating IRI.
Overall, senescence of MDSC remains ill-defined currently. Age-related changes in MDSC favoring a generally immunosuppressive environment may have beneficial effects in transplantation, ameliorating alloimmunity in older recipients.
Therapeutic Strategies
Over the recent past, therapeutic strategies utilizing MDSC have been proposed for various immune-related diseases (103). In cancer, MDSC contribute to tumor progression, metastasis development, and resistance to immunotherapeutic drugs by establishing an immunosuppressive microenvironment (104, 105). Thus, various therapeutic strategies including the depletion of MDSC, blocking MDSC migration, and attenuating their immunosuppressive capacities have been tested (106–109). Autoimmune diseases such as multiple sclerosis, myasthenia gravis, and rheumatoid arthritis have also been shown to be associated with changes in MDSC, and corresponding therapeutic strategies have been proposed (110–113). In transplantation, adoptive transfer of MDSC has yielded promising results in various experimental models (56, 114, 115). Promoting the accumulation of MDSC through nanoimmunotherapy targeting myeloid cell precursors demonstrated graft tolerance in most recipient animals. Intriguingly, the effect was already present after short-term administration of the nanobiologics (116). Further supporting evidence comes from other experimental models in which adoptively transferred MDSC were found to expand after transplantation, migrating into the graft and prolonging allograft survival (117). Moreover, MDSC facilitated the recruitment of Tregs into cardiac allografts by inducing programmed death ligand-1 (PD-L1) (118). It needs to be stressed, however, that not all studies confirmed the effects of MDSC on graft prolongation (45, 119). MDSC have thus far not been tested clinically in transplant patients. Notably, MDSC may lose their immunosuppressive functions in an already immune-activated environment as MDSC transferred into patients with acute graft-versus-host-disease lost their suppressive capacity and their potential to improve transplant outcomes. Mechanistically, an inflammasome-induced differentiation of MDSC into mature cells may play a role (120). In addition to bone marrow-derived MDSC, induced pluripotent stem cells (iPSC) have been evaluated for their immunosuppressive potential. Interestingly, fibroblast-derived iPSC cultured in medium containing GM-CSF, M-CSF, IL-4, and LPS have been able to suppress allogeneic T- as well as B-cell response while reducing alloantibody production in vivo (111, 121).
Taken together, MDSC cell therapy may, at least in theory, have immunosuppressive potential in organ transplantation; however, confirmatory clinical studies are missing (58).
Conclusion
MDSC have a profound impact on immune responses. While the immunosuppressive capacities of MDSC may have potential in clinical transplantation, effects need to be confirmed. Side effects including an increased risk for malignancies need to be carefully assessed (122). Aging impacts both MDSC numbers and functionality with potential consequences on their capacity to modulate immune responses. Understanding those aspects in greater detail may contribute to novel therapeutic strategies for improving allograft survival in an age-specific fashion (45, 56, 58, 123, 124). Moreover, monitoring the frequencies of MDSC as biomarkers in organ transplant recipients may provide additional valuable diagnostic information.
Author Contributions
All authors listed have made a substantial, direct, and intellectual contribution to the work, and approved it for publication.
Funding
This work was supported by the Biomedical Education Program BMEP (to AS and MJR), NIH grants R01AG064165 and U01AI132898 (to SGT) and P30AG031679 (to HZ).
Conflict of Interest
The authors declare that the research was conducted in the absence of any commercial or financial relationships that could be construed as a potential conflict of interest.
Publisher’s Note
All claims expressed in this article are solely those of the authors and do not necessarily represent those of their affiliated organizations, or those of the publisher, the editors and the reviewers. Any product that may be evaluated in this article, or claim that may be made by its manufacturer, is not guaranteed or endorsed by the publisher.
References
1. Gabrilovich DI, Nagaraj S. Myeloid-Derived Suppressor Cells as Regulators of the Immune System. Nat Rev Immunol (2009) 9(3):162–74. doi: 10.1038/nri2506
2. Marvel D, Gabrilovich DI. Myeloid-Derived Suppressor Cells in the Tumor Microenvironment: Expect the Unexpected. J Clin Invest (2015) 125(9):3356–64. doi: 10.1172/JCI80005
3. Sonda N, Chioda M, Zilio S, Simonato F, Bronte V. Transcription Factors in Myeloid-Derived Suppressor Cell Recruitment and Function. Curr Opin Immunol (2011) 23(2):279–85. doi: 10.1016/j.coi.2010.12.006
4. Condamine T, Gabrilovich DI. Molecular Mechanisms Regulating Myeloid-Derived Suppressor Cell Differentiation and Function. Trends Immunol (2011) 32(1):19–25. doi: 10.1016/j.it.2010.10.002
5. Shao L, Pan S, Zhang QP, Jamal M, Rushworth GM, Xiong J, et al. Emerging Role of Myeloid-Derived Suppressor Cells in the Biology of Transplantation Tolerance. Transplantation (2020) 104(3):467–75. doi: 10.1097/TP.0000000000002996
6. Young MR, Newby M, Wepsic HT. Hematopoiesis and Suppressor Bone Marrow Cells in Mice Bearing Large Metastatic Lewis Lung Carcinoma Tumors. Cancer Res (1987) 47(1):100–5.
7. Buessow SC, Paul RD, Lopez DM. Influence of Mammary Tumor Progression on Phenotype and Function of Spleen and in Situ Lymphocytes in Mice. J Natl Cancer Inst (1984) 73(1):249–55. doi: 10.1093/jnci/73.1.249
8. Seung LP, Rowley DA, Dubey P, Schreiber H. Synergy Between T-Cell Immunity and Inhibition of Paracrine Stimulation Causes Tumor Rejection. Proc Natl Acad Sci U.S.A. (1995) 92(14):6254–8. doi: 10.1073/pnas.92.14.6254
9. Nagaraj S, Gabrilovich DI. Regulation of Suppressive Function of Myeloid-Derived Suppressor Cells by CD4+ T Cells. Semin Cancer Biol (2012) 22(4):282–8. doi: 10.1016/j.semcancer.2012.01.010
10. Youn JI, Gabrilovich DI. The Biology of Myeloid-Derived Suppressor Cells: The Blessing and the Curse of Morphological and Functional Heterogeneity. Eur J Immunol (2010) 40(11):2969–75. doi: 10.1002/eji.201040895
11. Sinha P, Clements VK, Bunt SK, Albelda SM, Ostrand-Rosenberg S. Cross-Talk Between Myeloid-Derived Suppressor Cells and Macrophages Subverts Tumor Immunity Toward a Type 2 Response. J Immunol (2007) 179(2):977–83. doi: 10.4049/jimmunol.179.2.977
12. Scalea JR, Tomita Y, Lindholm CR, Burlingham W. Transplantation Tolerance Induction: Cell Therapies and Their Mechanisms. Front Immunol (2016) 7:87. doi: 10.3389/fimmu.2016.00087
13. Boros P, Ochando JC, Chen SH, Bromberg JS. Myeloid-Derived Suppressor Cells: Natural Regulators for Transplant Tolerance. Hum Immunol (2010) 71(11):1061–6. doi: 10.1016/j.humimm.2010.08.001
14. Dilek N, van Rompaey N, Le Moine A, Vanhove B. Myeloid-Derived Suppressor Cells in Transplantation. Curr Opin Organ Transplant (2010) 15(6):765–8. doi: 10.1097/MOT.0b013e3283401742
15. Lees JR, Azimzadeh AM, Bromberg JS. Myeloid Derived Suppressor Cells in Transplantation. Curr Opin Immunol (2011) 23(5):692–7. doi: 10.1016/j.coi.2011.07.004
16. Ochando J, Conde P, Utrero-Rico A, Paz-Artal E. Tolerogenic Role of Myeloid Suppressor Cells in Organ Transplantation. Front Immunol (2019) 10:374. doi: 10.3389/fimmu.2019.00374
17. Hock BD, Mackenzie KA, Cross NB, Taylor KG, Currie MJ, Robinson BA, et al. Renal Transplant Recipients Have Elevated Frequencies of Circulating Myeloid-Derived Suppressor Cells. Nephrol Dial Transplant (2012) 27(1):402–10. doi: 10.1093/ndt/gfr264
18. Heigl T, Singh A, Saez-Gimenez B, Kaes J, Van Herck A, Sacreas A, et al. Myeloid-Derived Suppressor Cells in Lung Transplantation. Front Immunol (2019) 10:900. doi: 10.3389/fimmu.2019.00900
19. Okano S, Abu-Elmagd K, Kish DD, Keslar K, Baldwin WM 3rd, Fairchild RL, et al. Myeloid-Derived Suppressor Cells Increase and Inhibit Donor-Reactive T Cell Responses to Graft Intestinal Epithelium in Intestinal Transplant Patients. Am J Transplant (2018) 18(10):2544–58. doi: 10.1111/ajt.14718
20. Meng F, Chen S, Guo X, Chen Z, Huang X, Lai Y, et al. Clinical Significance of Myeloid-Derived Suppressor Cells in Human Renal Transplantation With Acute T Cell-Mediated Rejection. Inflammation (2014) 37(5):1799–805. doi: 10.1007/s10753-014-9910-5
21. Luan Y, Mosheir E, Menon MC, Wilson D, Woytovich C, Ochando J, et al. Monocytic Myeloid-Derived Suppressor Cells Accumulate in Renal Transplant Patients and Mediate CD4(+) Foxp3(+) Treg Expansion. Am J Transplant (2013) 13(12):3123–31. doi: 10.1111/ajt.12461
22. Dangi A, Zhang L, Zhang X, Luo X. Murine CMV Induces Type 1 IFN That Impairs Differentiation of MDSCs Critical for Transplantation Tolerance. Blood Adv (2018) 2(6):669–80. doi: 10.1182/bloodadvances.2017012187
23. Paraskeva M, Bailey M, Levvey BJ, Griffiths AP, Kotsimbos TC, Williams TP, et al. Cytomegalovirus Replication Within the Lung Allograft is Associated With Bronchiolitis Obliterans Syndrome. Am J Transplant (2011) 11(10):2190–6. doi: 10.1111/j.1600-6143.2011.03663.x
24. Kamada N, Brons G, Davies HS. Fully Allogeneic Liver Grafting in Rats Induces a State of Systemic Nonreactivity to Donor Transplantation Antigens. Transplantation (1980) 29(5):429–31. doi: 10.1097/00007890-198005000-00021
25. Qian S, Demetris AJ, Murase N, Rao AS, Fung JJ, Starzl TE. Murine Liver Allograft Transplantation: Tolerance and Donor Cell Chimerism. Hepatology (1994) 19(4):916–24. doi: 10.1002/hep.1840190418
26. Chou HS, Hsieh CC, Yang HR, Wang L, Arakawa Y, Brown K, et al. Hepatic Stellate Cells Regulate Immune Response by Way of Induction of Myeloid Suppressor Cells in Mice. Hepatology (2011) 53(3):1007–19. doi: 10.1002/hep.24162
27. Yu MC, Chen CH, Liang X, Wang L, Gandhi CR, Fung JJ, et al. Inhibition of T-Cell Responses by Hepatic Stellate Cells via B7-H1-Mediated T-Cell Apoptosis in Mice. Hepatology (2004) 40(6):1312–21. doi: 10.1002/hep.20488
28. Wang X, Bi Y, Xue L, Liao J, Chen X, Lu Y, et al. The Calcineurin-NFAT Axis Controls Allograft Immunity in Myeloid-Derived Suppressor Cells Through Reprogramming T Cell Differentiation. Mol Cell Biol (2015) 35(3):598–609. doi: 10.1128/MCB.01251-14
29. Han C, Wu T, Na N, Zhao Y, Li W, Zhao Y. The Effect of Immunosuppressive Drug Cyclosporine A on Myeloid-Derived Suppressor Cells in Transplanted Mice. Inflammation Res (2016) 65(9):679–88. doi: 10.1007/s00011-016-0949-7
30. Iglesias-Escudero M, Sansegundo-Arribas D, Riquelme P, Merino-Fernández D, Guiral-Foz S, Pérez C, et al. Myeloid-Derived Suppressor Cells in Kidney Transplant Recipients and the Effect of Maintenance Immunotherapy. Front Immunol (2020) 11:643. doi: 10.3389/fimmu.2020.00643
31. Nakamura T, Nakao T, Yoshimura N, Ashihara E. Rapamycin Prolongs Cardiac Allograft Survival in a Mouse Model by Inducing Myeloid-Derived Suppressor Cells. Am J Transplant (2015) 15(9):2364–77. doi: 10.1111/ajt.13276
32. Hamdani S, Thiolat A, Naserian S, Grondin C, Moutereau S, Hulin A, et al. Delayed and Short Course of Rapamycin Prevents Organ Rejection After Allogeneic Liver Transplantation in Rats. World J Gastroenterol (2017) 23(38):6962–72. doi: 10.3748/wjg.v23.i38.6962
33. Deissler A, Della Penna A, van Geffen C, Gonzalez-Menendez I, Quintanilla-Martinez L, Gunther A, et al. Rapamycin Delays Allograft Rejection in Obese Graft Recipients Through Induction of Myeloid-Derived Suppressor Cells. Immunol Lett (2021) 236:1–11. doi: 10.1016/j.imlet.2021.05.003
34. Liao J, Wang X, Bi Y, Shen B, Shao K, Yang H, et al. Dexamethasone Potentiates Myeloid-Derived Suppressor Cell Function in Prolonging Allograft Survival Through Nitric Oxide. J Leukoc Biol (2014) 96(5):675–84. doi: 10.1189/jlb.2HI1113-611RR
35. Yang F, Li Y, Zhang Q, Tan L, Peng L, Zhao Y. The Effect of Immunosuppressive Drugs on MDSCs in Transplantation. J Immunol Res (2018) 2018:5414808. doi: 10.1155/2018/5414808
36. Nakao T, Nakamura T, Masuda K, Matsuyama T, Ushigome H, Ashihara E, et al. Dexamethasone Prolongs Cardiac Allograft Survival in a Murine Model Through Myeloid-Derived Suppressor Cells. Transplant Proc (2018) 50(1):299–304. doi: 10.1016/j.transproceed.2017.11.014
37. Zhao Y, Shen XF, Cao K, Ding J, Kang X, Guan WX, et al. Dexamethasone-Induced Myeloid-Derived Suppressor Cells Prolong Allo Cardiac Graft Survival Through iNOS- and Glucocorticoid Receptor-Dependent Mechanism. Front Immunol (2018) 9:282. doi: 10.3389/fimmu.2018.00282
38. Hock BD, McKenzie JL, Cross NB, Currie MJ. Dynamic Changes in Myeloid Derived Suppressor Cell Subsets Following Renal Transplant: A Prospective Study. Transpl Immunol (2015) 32(3):164–71. doi: 10.1016/j.trim.2015.05.001
39. Wu T, Zhao Y, Wang H, Li Y, Shao L, Wang R, et al. mTOR Masters Monocytic Myeloid-Derived Suppressor Cells in Mice With Allografts or Tumors. Sci Rep (2016) 6:20250. doi: 10.1038/srep20250
40. Liu Z, Wu KKL, Jiang X, Xu A, Cheng KKY. The Role of Adipose Tissue Senescence in Obesity- and Ageing-Related Metabolic Disorders. Clin Sci (Lond) (2020) 134(2):315–30. doi: 10.1042/CS20190966
41. Conley SM, Hickson LJ, Kellogg TA, McKenzie T, Heimbach JK, Taner T, et al. Human Obesity Induces Dysfunction and Early Senescence in Adipose Tissue-Derived Mesenchymal Stromal/Stem Cells. Front Cell Dev Biol (2020) 8:197. doi: 10.3389/fcell.2020.00197
42. Hale M, Itani F, Buchta CM, Wald G, Bing M, Norian LA. Obesity Triggers Enhanced MDSC Accumulation in Murine Renal Tumors via Elevated Local Production of CCL2. PloS One (2015) 10(3):e0118784. doi: 10.1371/journal.pone.0118784
43. Liu W, Xiao X, Demirci G, Madsen J, Li XC. Innate NK Cells and Macrophages Recognize and Reject Allogeneic Nonself In Vivo via Different Mechanisms. J Immunol (2012) 188(6):2703–11. doi: 10.4049/jimmunol.1102997
44. Morelli AE, Thomson AW. Dendritic Cells: Regulators of Alloimmunity and Opportunities for Tolerance Induction. Immunol Rev (2003) 196:125–46. doi: 10.1046/j.1600-065X.2003.00079.x
45. Dugast AS, Haudebourg T, Coulon F, Heslan M, Haspot F, Poirier N, et al. Myeloid-Derived Suppressor Cells Accumulate in Kidney Allograft Tolerance and Specifically Suppress Effector T Cell Expansion. J Immunol (2008) 180(12):7898–906. doi: 10.4049/jimmunol.180.12.7898
46. Scalea JR, Lee YS, Davila E, Bromberg JS. Myeloid-Derived Suppressor Cells and Their Potential Application in Transplantation. Transplantation (2018) 102(3):359–67. doi: 10.1097/TP.0000000000002022
47. Garcia MR, Ledgerwood L, Yang Y, Xu J, Lal G, Burrell B, et al. Monocytic Suppressive Cells Mediate Cardiovascular Transplantation Tolerance in Mice. J Clin Invest (2010) 120(7):2486–96. doi: 10.1172/JCI41628
48. Lee WC, Wang YC, Hsu HY, Hsu PY, Cheng CH, Lee CF, et al. Immunological Discrepancy in Aged Mice Facilitates Skin Allograft Survival. Aging (Albany NY) (2021) 13(12):16219–28. doi: 10.18632/aging.203152
49. Gong W, Ge F, Liu D, Wu Y, Liu F, Kim BS, et al. Role of Myeloid-Derived Suppressor Cells in Mouse Pre-Sensitized Cardiac Transplant Model. Clin Immunol (2014) 153(1):8–16. doi: 10.1016/j.clim.2014.03.013
50. Mazzoni A, Bronte V, Visintin A, Spitzer JH, Apolloni E, Serafini P, et al. Myeloid Suppressor Lines Inhibit T Cell Responses by an NO-Dependent Mechanism. J Immunol (2002) 168(2):689–95. doi: 10.4049/jimmunol.168.2.689
51. Dilek N, Vuillefroy de Silly R, Blancho G, Vanhove B. Myeloid-Derived Suppressor Cells: Mechanisms of Action and Recent Advances in Their Role in Transplant Tolerance. Front Immunol (2012) 3:208. doi: 10.3389/fimmu.2012.00208
52. Zhang W, Liang S, Wu J, Horuzsko A. Human Inhibitory Receptor Immunoglobulin-Like Transcript 2 Amplifies CD11b+Gr1+ Myeloid-Derived Suppressor Cells That Promote Long-Term Survival of Allografts. Transplantation (2008) 86(8):1125–34. doi: 10.1097/TP.0b013e318186fccd
53. Adeegbe D, Serafini P, Bronte V, Zoso A, Ricordi C, Inverardi L. In Vivo Induction of Myeloid Suppressor Cells and CD4(+)Foxp3(+) T Regulatory Cells Prolongs Skin Allograft Survival in Mice. Cell Transplant (2011) 20(6):941–54. doi: 10.3727/096368910X540621
54. Gajardo T, Morales RA, Campos-Mora M, Campos-Acuna J, Pino-Lagos K. Exogenous Interleukin-33 Targets Myeloid-Derived Suppressor Cells and Generates Periphery-Induced Foxp3(+) Regulatory T Cells in Skin-Transplanted Mice. Immunology (2015) 146(1):81–8. doi: 10.1111/imm.12483
55. Sido JM, Nagarkatti PS, Nagarkatti M. Delta(9)-Tetrahydrocannabinol Attenuates Allogeneic Host-Versus-Graft Response and Delays Skin Graft Rejection Through Activation of Cannabinoid Receptor 1 and Induction of Myeloid-Derived Suppressor Cells. J Leukoc Biol (2015) 98(3):435–47. doi: 10.1189/jlb.3A0115-030RR
56. Yang F, Li Y, Wu T, Na N, Zhao Y, Li W, et al. TNFalpha-Induced M-MDSCs Promote Transplant Immune Tolerance via Nitric Oxide. J Mol Med (Berl) (2016) 94(8):911–20. doi: 10.1007/s00109-016-1398-z
57. Drujont L, Carretero-Iglesia L, Bouchet-Delbos L, Beriou G, Merieau E, Hill M, et al. Evaluation of the Therapeutic Potential of Bone Marrow-Derived Myeloid Suppressor Cell (MDSC) Adoptive Transfer in Mouse Models of Autoimmunity and Allograft Rejection. PloS One (2014) 9(6):e100013. doi: 10.1371/journal.pone.0100013
58. Zhang W, Li J, Qi G, Tu G, Yang C, Xu M. Myeloid-Derived Suppressor Cells in Transplantation: The Dawn of Cell Therapy. J Transl Med (2018) 16(1):19. doi: 10.1186/s12967-018-1395-9
59. Carretero-Iglesia L, Bouchet-Delbos L, Louvet C, Drujont L, Segovia M, Merieau E, et al. Comparative Study of the Immunoregulatory Capacity of In Vitro Generated Tolerogenic Dendritic Cells, Suppressor Macrophages, and Myeloid-Derived Suppressor Cells. Transplantation (2016) 100(10):2079–89. doi: 10.1097/TP.0000000000001315
60. Salvadori M, Rosso G, Bertoni E. Update on Ischemia-Reperfusion Injury in Kidney Transplantation: Pathogenesis and Treatment. World J Transplant (2015) 5(2):52–67. doi: 10.5500/wjt.v5.i2.52
61. Nieuwenhuijs-Moeke GJ, Pischke SE, Berger SP, Sanders JSF, Pol RA, Struys M, et al. Ischemia and Reperfusion Injury in Kidney Transplantation: Relevant Mechanisms in Injury and Repair. J Clin Med (2020) 9(1). doi: 10.3390/jcm9010253
62. Slegtenhorst BR, Dor FJ, Rodriguez H, Voskuil FJ, Tullius SG. Ischemia/reperfusion Injury and its Consequences on Immunity and Inflammation. Curr Transplant Rep (2014) 1(3):147–54. doi: 10.1007/s40472-014-0017-6
63. Takada M, Nadeau KC, Shaw GD, Marquette KA, Tilney NL. The Cytokine-Adhesion Molecule Cascade in Ischemia/Reperfusion Injury of the Rat Kidney. Inhibition by a Soluble P-Selectin Ligand. J Clin Invest (1997) 99(11):2682–90. doi: 10.1172/JCI119457
64. Uehara M, Solhjou Z, Banouni N, Kasinath V, Xiaqun Y, Dai L, et al. Ischemia Augments Alloimmune Injury Through IL-6-Driven CD4(+) Alloreactivity. Sci Rep (2018) 8(1):2461. doi: 10.1038/s41598-018-20858-4
65. Solhjou Z, Uehara M, Bahmani B, Maarouf OH, Ichimura T, Brooks CR, et al. Novel Application of Localized Nanodelivery of Anti-Interleukin-6 Protects Organ Transplant From Ischemia-Reperfusion Injuries. Am J Transplant (2017) 17(9):2326–37. doi: 10.1111/ajt.14266
66. Ji J, Zhuang Y, Lin Z, Jiang Y, Wang W, Zhang X. Interferon-γ-Induced Myeloid-Derived Suppressor Cells Aggravate Kidney Ischemia-Reperfusion Injury by Regulating Innate Immune Cells. Nephron (2021) 146(1) 99–109. doi: 10.1159/000518876
67. Pegues MA, McWilliams IL, Szalai AJ. C-Reactive Protein Exacerbates Renal Ischemia-Reperfusion Injury: Are Myeloid-Derived Suppressor Cells to Blame? Am J Physiol Renal Physiol (2016) 311(1):F176–181. doi: 10.1152/ajprenal.00107.2016
68. Yan JJ, Ryu JH, Piao H, Hwang JH, Han D, Lee SK, et al. Granulocyte Colony-Stimulating Factor Attenuates Renal Ischemia-Reperfusion Injury by Inducing Myeloid-Derived Suppressor Cells. J Am Soc Nephrol (2020) 31(4):731–46. doi: 10.1681/ASN.2019060601
69. Li Y, Wu J, Shou Z, He Q, Zhang P, Han F, et al. Pretreatment With Granulocyte Colony-Stimulating Factor Attenuated Renal Ischaemia and Reperfusion Injury via Activation of PI3/Akt Signal Pathway. Nephrol (Carlton) (2008) 13(6):508–16. doi: 10.1111/j.1440-1797.2008.00928.x
70. Qiu Y, Cao Y, Tu G, Li J, Su Y, Fang F, et al. Myeloid-Derived Suppressor Cells Alleviate Renal Fibrosis Progression via Regulation of CCL5-CCR5 Axis. Front Immunol (2021) 12:698894. doi: 10.3389/fimmu.2021.698894
71. Frasca D, Blomberg BB. Inflammaging Decreases Adaptive and Innate Immune Responses in Mice and Humans. Biogerontology (2016) 17(1):7–19. doi: 10.1007/s10522-015-9578-8
72. Roesel MJ, Matsunaga T, Tullius SG. Opportunities and Challenges of Targeting an Aging Immune System. Transplantation (2021) 105(12):2515–6. doi: 10.1097/TP.0000000000003930
73. Mogilenko DA, Shchukina I, Artyomov MN. Immune Ageing at Single-Cell Resolution. Nat Rev Immunol (2021):1–15. doi: 10.1038/s41577-021-00646-4
74. Salam N, Rane S, Das R, Faulkner M, Gund R, Kandpal U, et al. T Cell Ageing: Effects of Age on Development, Survival & Function. Indian J Med Res (2013) 138(5):595–608.
75. Dykstra B, de Haan G. Hematopoietic Stem Cell Aging and Self-Renewal. Cell Tissue Res (2008) 331(1):91–101. doi: 10.1007/s00441-007-0529-9
76. Hagen M, Derudder E. Inflammation and the Alteration of B-Cell Physiology in Aging. Gerontology (2020) 66(2):105–13. doi: 10.1159/000501963
77. Ma S, Wang C, Mao X, Hao Y. B Cell Dysfunction Associated With Aging and Autoimmune Diseases. Front Immunol (2019) 10:318. doi: 10.3389/fimmu.2019.00318
78. Frasca D, Blomberg BB. Aging Induces B Cell Defects and Decreased Antibody Responses to Influenza Infection and Vaccination. Immun Ageing (2020) 17(1):37. doi: 10.1186/s12979-020-00210-z
79. Seyda M, Elkhal A, Quante M, Falk CS, Tullius SG. T Cells Going Innate. Trends Immunol (2016) 37(8):546–56. doi: 10.1016/j.it.2016.06.004
80. Gomez CR, Goral J, Ramirez L, Kopf M, Kovacs EJ. Aberrant Acute-Phase Response in Aged Interleukin-6 Knockout Mice. Shock (2006) 25(6):581–5. doi: 10.1097/01.shk.000029553.39081.ec
81. Linton PJ, Dorshkind K. Age-Related Changes in Lymphocyte Development and Function. Nat Immunol (2004) 5(2):133–9. doi: 10.1038/ni1033
82. Murciano C, Yanez A, O'Connor JE, Gozalbo D, Gil ML. Influence of Aging on Murine Neutrophil and Macrophage Function Against Candida Albicans. FEMS Immunol Med Microbiol (2008) 53(2):214–21. doi: 10.1111/j.1574-695X.2008.00418.x
83. Ren Z, Gay R, Thomas A, Pae M, Wu D, Logsdon L, et al. Effect of Age on Susceptibility to Salmonella Typhimurium Infection in C57BL/6 Mice. J Med Microbiol (2009) 58(Pt 12):1559–67. doi: 10.1099/jmm.0.013250-0
84. Renshaw M, Rockwell J, Engleman C, Gewirtz A, Katz J, Sambhara S. Cutting Edge: Impaired Toll-Like Receptor Expression and Function in Aging. J Immunol (2002) 169(9):4697–701. doi: 10.4049/jimmunol.169.9.4697
85. Boehmer ED, Goral J, Faunce DE, Kovacs EJ. Age-Dependent Decrease in Toll-Like Receptor 4-Mediated Proinflammatory Cytokine Production and Mitogen-Activated Protein Kinase Expression. J Leukoc Biol (2004) 75(2):342–9. doi: 10.1189/jlb.0803389
86. van Duin D, Mohanty S, Thomas V, Ginter S, Montgomery RR, Fikrig E, et al. Age-Associated Defect in Human TLR-1/2 Function. J Immunol (2007) 178(2):970–5. doi: 10.4049/jimmunol.178.2.970
87. Hinojosa CA, Akula Suresh Babu R, Rahman MM, Fernandes G, Boyd AR, Orihuela CJ. Elevated A20 Contributes to Age-Dependent Macrophage Dysfunction in the Lungs. Exp Gerontol (2014) 54:58–66. doi: 10.1016/j.exger.2014.01.007
88. Shembade N, Ma A, Harhaj EW. Inhibition of NF-kappaB Signaling by A20 Through Disruption of Ubiquitin Enzyme Complexes. Science (2010) 327(5969):1135–9. doi: 10.1126/science.1182364
89. Mohanty S, Joshi SR, Ueda I, Wilson J, Blevins TP, Siconolfi B, et al. Prolonged Proinflammatory Cytokine Production in Monocytes Modulated by Interleukin 10 After Influenza Vaccination in Older Adults. J Infect Dis (2015) 211(7):1174–84. doi: 10.1093/infdis/jiu573
90. Panda A, Qian F, Mohanty S, van Duin D, Newman FK, Zhang L, et al. Age-Associated Decrease in TLR Function in Primary Human Dendritic Cells Predicts Influenza Vaccine Response. J Immunol (2010) 184(5):2518–27. doi: 10.4049/jimmunol.0901022
91. Salminen A, Kauppinen A, Kaarniranta K. AMPK Activation Inhibits the Functions of Myeloid-Derived Suppressor Cells (MDSC): Impact on Cancer and Aging. J Mol Med (Berl) (2019) 97(8):1049–64. doi: 10.1007/s00109-019-01795-9
92. Bueno V, Sant'Anna OA, Lord JM. Ageing and Myeloid-Derived Suppressor Cells: Possible Involvement in Immunosenescence and Age-Related Disease. Age (Dordr) (2014) 36(6):9729. doi: 10.1007/s11357-014-9729-x
93. Enioutina EY, Bareyan D, Daynes RA. A Role for Immature Myeloid Cells in Immune Senescence. J Immunol (2011) 186(2):697–707. doi: 10.4049/jimmunol.1002987
94. Jackaman C, Radley-Crabb HG, Soffe Z, Shavlakadze T, Grounds MD, Nelson DJ. Targeting Macrophages Rescues Age-Related Immune Deficiencies in C57BL/6J Geriatric Mice. Aging Cell (2013) 12(3):345–57. doi: 10.1111/acel.12062
95. Flores RR, Clauson CL, Cho J, Lee BC, McGowan SJ, Baker DJ, et al. Expansion of Myeloid-Derived Suppressor Cells With Aging in the Bone Marrow of Mice Through a NF-kappaB-Dependent Mechanism. Aging Cell (2017) 16(3):480–7. doi: 10.1111/acel.12571
96. Chinn IK, Blackburn CC, Manley NR, Sempowski GD. Changes in Primary Lymphoid Organs With Aging. Semin Immunol (2012) 24(5):309–20. doi: 10.1016/j.smim.2012.04.005
97. Challen GA, Boles NC, Chambers SM, Goodell MA. Distinct Hematopoietic Stem Cell Subtypes are Differentially Regulated by TGF-Beta1. Cell Stem Cell (2010) 6(3):265–78. doi: 10.1016/j.stem.2010.02.002
98. Verschoor CP, Johnstone J, Millar J, Dorrington MG, Habibagahi M, Lelic A, et al. Blood CD33(+)HLA-DR(-) Myeloid-Derived Suppressor Cells are Increased With Age and a History of Cancer. J Leukoc Biol (2013) 93(4):633–7. doi: 10.1189/jlb.0912461
99. Alves AS, Ishimura ME, Duarte YAO, Bueno V. Parameters of the Immune System and Vitamin D Levels in Old Individuals. Front Immunol (2018) 9:1122. doi: 10.3389/fimmu.2018.01122
100. Grizzle WE, Xu X, Zhang S, Stockard CR, Liu C, Yu S, et al. Age-Related Increase of Tumor Susceptibility Is Associated With Myeloid-Derived Suppressor Cell Mediated Suppression of T Cell Cytotoxicity in Recombinant Inbred BXD12 Mice. Mech Ageing Dev (2007) 128(11-12):672–80. doi: 10.1016/j.mad.2007.10.003
101. Denecke C, Bedi DS, Ge X, Kim IK, Jurisch A, Weiland A, et al. Prolonged Graft Survival in Older Recipient Mice Is Determined by Impaired Effector T-Cell But Intact Regulatory T-Cell Responses. PloS One (2010) 5(2):e9232. doi: 10.1371/journal.pone.0009232
102. Okuma A, Hanyu A, Watanabe S, Hara E. P16(Ink4a) and P21(Cip1/Waf1) Promote Tumour Growth by Enhancing Myeloid-Derived Suppressor Cells Chemotaxis. Nat Commun (2017) 8(1):2050. doi: 10.1038/s41467-017-02281-x
103. Law AMK, Valdes-Mora F, Gallego-Ortega D. Myeloid-Derived Suppressor Cells as a Therapeutic Target for Cancer. Cells (2020) 9(3):561. doi: 10.3390/cells9030561
104. Burkholder B, Huang RY, Burgess R, Luo S, Jones VS, Zhang W, et al. Tumor-Induced Perturbations of Cytokines and Immune Cell Networks. Biochim Biophys Acta (2014) 1845(2):182–201. doi: 10.1016/j.bbcan.2014.01.004
105. Messmer MN, Netherby CS, Banik D, Abrams SI. Tumor-Induced Myeloid Dysfunction and its Implications for Cancer Immunotherapy. Cancer Immunol Immunother (2015) 64(1):1–13. doi: 10.1007/s00262-014-1639-3
106. Vincent J, Mignot G, Chalmin F, Ladoire S, Bruchard M, Chevriaux A, et al. 5-Fluorouracil Selectively Kills Tumor-Associated Myeloid-Derived Suppressor Cells Resulting in Enhanced T Cell-Dependent Antitumor Immunity. Cancer Res (2010) 70(8):3052–61. doi: 10.1158/0008-5472.CAN-09-3690
107. Elliott LA, Doherty GA, Sheahan K, Ryan EJ. Human Tumor-Infiltrating Myeloid Cells: Phenotypic and Functional Diversity. Front Immunol (2017) 8:86. doi: 10.3389/fimmu.2017.00086
108. Blattner C, Fleming V, Weber R, Himmelhan B, Altevogt P, Gebhardt C, et al. CCR5(+) Myeloid-Derived Suppressor Cells Are Enriched and Activated in Melanoma Lesions. Cancer Res (2018) 78(1):157–67. doi: 10.1158/0008-5472.CAN-17-0348
109. Veltman JD, Lambers ME, van Nimwegen M, Hendriks RW, Hoogsteden HC, Aerts JG, et al. COX-2 Inhibition Improves Immunotherapy and is Associated With Decreased Numbers of Myeloid-Derived Suppressor Cells in Mesothelioma. Celecoxib Influences MDSC Function. BMC Cancer (2010) 10:464. doi: 10.1186/1471-2407-10-464
110. Ioannou M, Alissafi T, Lazaridis I, Deraos G, Matsoukas J, Gravanis A, et al. Crucial Role of Granulocytic Myeloid-Derived Suppressor Cells in the Regulation of Central Nervous System Autoimmune Disease. J Immunol (2012) 188(3):1136–46. doi: 10.4049/jimmunol.1101816
111. Zhang J, Hodges A, Chen SH, Pan PY. Myeloid-Derived Suppressor Cells as Cellular Immunotherapy in Transplantation and Autoimmune Diseases. Cell Immunol (2021) 362:104300. doi: 10.1016/j.cellimm.2021.104300
112. Li Y, Tu Z, Qian S, Fung JJ, Markowitz SD, Kusner LL, et al. Myeloid-Derived Suppressor Cells as a Potential Therapy for Experimental Autoimmune Myasthenia Gravis. J Immunol (2014) 193(5):2127–34. doi: 10.4049/jimmunol.1400857
113. Kurko J, Vida A, Ocsko T, Tryniszewska B, Rauch TA, Glant TT, et al. Suppression of Proteoglycan-Induced Autoimmune Arthritis by Myeloid-Derived Suppressor Cells Generated In Vitro From Murine Bone Marrow. PloS One (2014) 9(11):e111815. doi: 10.1371/journal.pone.0111815
114. He Y, Wang B, Jia B, Guan J, Zeng H, Pan Z. Effects of Adoptive Transferring Different Sources of Myeloid-Derived Suppressor Cells in Mice Corneal Transplant Survival. Transplantation (2015) 99(10):2102–8. doi: 10.1097/TP.0000000000000749
115. He Y, Bei J, Zeng H, Pan Z. The Roles of Sepsis-Induced Myeloid Derived Suppressor Cells in Mice Corneal, Skin and Combined Transplantation. Transpl Immunol (2016) 34:8–13. doi: 10.1016/j.trim.2015.12.003
116. Braza MS, van Leent MMT, Lameijer M, Sanchez-Gaytan BL, Arts RJW, Perez-Medina C, et al. Inhibiting Inflammation With Myeloid Cell-Specific Nanobiologics Promotes Organ Transplant Acceptance. Immunity (2018) 49(5):819–828.e816. doi: 10.1016/j.immuni.2018.09.008
117. Lee YS, Zhang T, Saxena V, Li L, Piao W, Bromberg JS, et al. Myeloid-Derived Suppressor Cells Expand After Transplantation and Their Augmentation Increases Graft Survival. Am J Transplant (2020) 20(9):2343–55. doi: 10.1111/ajt.15879
118. Nakamura T, Nakao T, Ashihara E, Yoshimura N. Myeloid-Derived Suppressor Cells Recruit CD4(+)/Foxp3(+) Regulatory T Cells in a Murine Cardiac Allograft. Transplant Proc (2016) 48(4):1275–8. doi: 10.1016/j.transproceed.2015.10.060
119. Ezzelarab MB, Perez-Gutierrez A, Humar A, Wijkstrom M, Zahorchak AF, Lu-Casto L, et al. Preliminary Assessment of the Feasibility of Autologous Myeloid-Derived Suppressor Cell Infusion in non-Human Primate Kidney Transplantation. Transpl Immunol (2019) 56:101225. doi: 10.1016/j.trim.2019.101225
120. Koehn BH, Apostolova P, Haverkamp JM, Miller JS, McCullar V, Tolar J, et al. GVHD-Associated, Inflammasome-Mediated Loss of Function in Adoptively Transferred Myeloid-Derived Suppressor Cells. Blood (2015) 126(13):1621–8. doi: 10.1182/blood-2015-03-634691
121. Sasaki H, Wada H, Baghdadi M, Tsuji H, Otsuka R, Morita K, et al. New Immunosuppressive Cell Therapy to Prolong Survival of Induced Pluripotent Stem Cell-Derived Allografts. Transplantation (2015) 99(11):2301–10. doi: 10.1097/TP.0000000000000875
122. Utrero-Rico A, Laguna-Goya R, Cano-Romero F, Chivite-Lacaba M, Gonzalez-Cuadrado C, Rodríguez-Sánchez E, et al. Early Posttransplant Mobilization of Monocytic Myeloid-Derived Suppressor Cell Correlates With Increase in Soluble Immunosuppressive Factors and Predicts Cancer in Kidney Recipients. Transplantation (2020) 104(12):2599–608. doi: 10.1097/TP.0000000000003179
123. Blazar BR, MacDonald KPA, Hill GR. Immune Regulatory Cell Infusion for Graft-Versus-Host Disease Prevention and Therapy. Blood (2018) 131(24):2651–60. doi: 10.1182/blood-2017-11-785865
Keywords: MDSC, myeloid cells, organ transplantation, alloimmunity, aging, immunosenescence
Citation: Schroeter A, Roesel MJ, Matsunaga T, Xiao Y, Zhou H and Tullius SG (2022) Aging Affects the Role of Myeloid-Derived Suppressor Cells in Alloimmunity. Front. Immunol. 13:917972. doi: 10.3389/fimmu.2022.917972
Received: 11 April 2022; Accepted: 07 June 2022;
Published: 06 July 2022.
Edited by:
Stanislaw Stepkowski, University of Toledo, United StatesReviewed by:
Chenming Sun, Xian Jiaotong University, ChinaCopyright © 2022 Schroeter, Roesel, Matsunaga, Xiao, Zhou and Tullius. This is an open-access article distributed under the terms of the Creative Commons Attribution License (CC BY). The use, distribution or reproduction in other forums is permitted, provided the original author(s) and the copyright owner(s) are credited and that the original publication in this journal is cited, in accordance with accepted academic practice. No use, distribution or reproduction is permitted which does not comply with these terms.
*Correspondence: Stefan G. Tullius, c3R1bGxpdXNAYndoLmhhcnZhcmQuZWR1