- 1Department of Medical Oncology, Cancer Center Amsterdam, Amsterdam Infection and Immunity Institute, Amsterdam University Medical Center (UMC), Vrije Universiteit Amsterdam, Amsterdam, Netherlands
- 2LAVA Therapeutics, Utrecht, Netherlands
- 3LAVA Therapeutics, Philadelphia, PA, United States
- 4Department of Immunology, Leiden University Medical Center, Leiden, Netherlands
γδ T-cells directly recognize and kill transformed cells independently of HLA-antigen presentation, which makes them a highly promising effector cell compartment for cancer immunotherapy. Novel γδ T-cell-based immunotherapies, primarily focusing on the two major γδ T-cell subtypes that infiltrate tumors (i.e. Vδ1 and Vδ2), are being developed. The Vδ1 T-cell subset is enriched in tissues and contains both effector T-cells as well as regulatory T-cells with tumor-promoting potential. Vδ2 T-cells, in contrast, are enriched in circulation and consist of a large, relatively homogeneous, pro-inflammatory effector T-cell subset. Healthy individuals typically harbor in the order of 50-500 million Vγ9Vδ2 T-cells in the peripheral blood alone (1-10% of the total CD3+ T-cell population), which can rapidly expand upon stimulation. The Vγ9Vδ2 T-cell receptor senses intracellular phosphorylated metabolites, which accumulate in cancer cells as a result of mevalonate pathway dysregulation or upon pharmaceutical intervention. Early clinical studies investigating the therapeutic potential of Vγ9Vδ2 T-cells were based on either ex vivo expansion and adoptive transfer or their systemic activation with aminobisphosphonates or synthetic phosphoantigens, either alone or combined with low dose IL-2. Immune-related adverse events (irAE) were generally \mild, but the clinical efficacy of these approaches provided overall limited benefit. In recent years, critical advances have renewed the excitement for the potential of Vγ9Vδ2 T-cells in cancer immunotherapy. Here, we review γδ T-cell-based therapeutic strategies and discuss the prospects of those currently evaluated in clinical studies in cancer patients as well as future therapies that might arise from current promising pre-clinical results.
Introduction
In humans, γδ T-cells represent 1 to 10% of total CD3+ T-cells (1, 2), and express a combination of either of 7 different Vγ TCR chains (Vγ2, 3, 4, 5, 8, 9, and 11), paired with either of 4 Vδ (Vδ1, 2, 3, and 5) chains (2–4). γδ T-cells are considered to bridge the innate and adaptive immune systems (3). Activated γδ T-cells display strong cytotoxic activity through the release of granzyme B and perforin, by membrane bound TRAIL and Fas (CD95) ligands or production of IFNγ or TNFα to amplify the immune response (12), thereby counteracting tumor development. Using γδ T-cell-deficient mice in a cutaneous carcinogenesis model, γδ T-cells were first shown to prevent malignancy formation (5). High γδ T-cell frequency in tumor infiltrates from cancer patients correlates with better clinical outcome in different malignancies (6–10) and γδ T-cells were identified as the prognostically most favorable immune cell subset in tumor infiltrates from 18,000 tumors across 39 malignancies (11). A more recent study confirmed the relative abundance of Vγ9Vδ2 T-cells in TILs and their association with improved patient outcome (12). These results highlight the relevance of γδ T-cells in tumor control and their potential for cancer therapy. γδ T-cells express several receptors shared with natural killer (NK) cells that participate in enhanced tumor cell recognition of which FcγRIIIa (CD16a), DNAM-1, and NKG2D are a few examples (13) (Figure 1A).
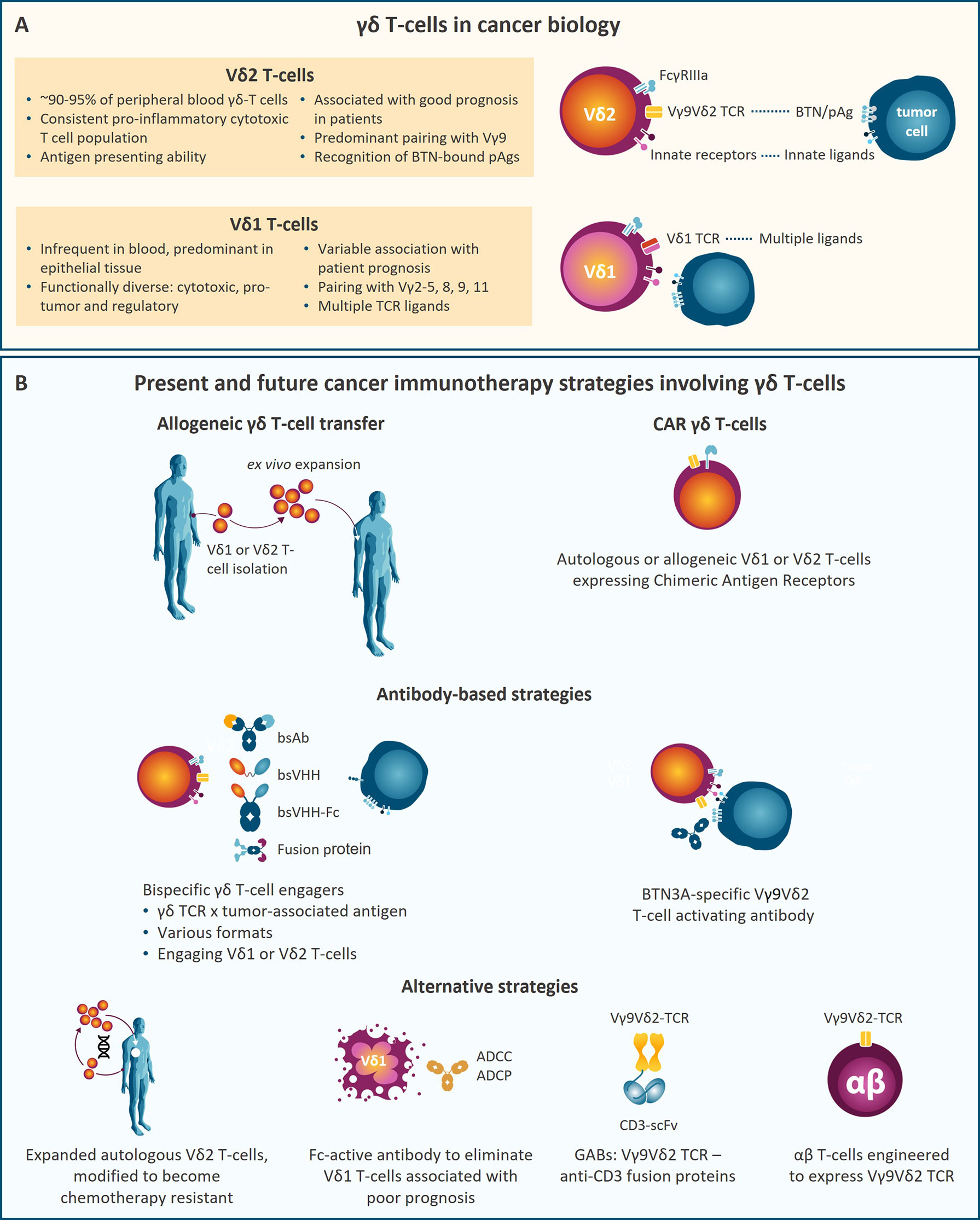
Figure 1 (A) Key characteristics of the two main γδ T-cell subsets, Vδ2 and Vδ1 T-cells, in cancer biology. (B) Schematic representation of therapeutic strategies involving γδ T-cells that are currently being developed. ADCC, Antibody-dependent cellular cytotoxicity; ADCP, antibody-dependent cellular phagocytosis; bsAb, bispecific antibody; bsVHH, bispecific variable domain of heavy-chain only antibody; BTN, Butyrophilin; CAR, chimeric antigen receptor; pAg, phosphoantigen; scFv, single-chain variable fragment.
The complete repertoire of antigens recognized by γδ-TCRs and the specificity of each γδ T subset is still not fully understood. Vγ9Vδ2 T-cells represent the predominant γδ T-cell subset (95%) in peripheral blood (14). Vγ9Vδ2 T-cells participate in the defense against malignant cells by sensing small phosphorylated metabolites (phosphoantigen (pAg) molecules) produced in cholesterol synthesis [isopentenyl pyrophosphate (IPP)] or by pathogens [e.g. (E)-4-hydroxy-3-methyl-but-2-enyl-pyrophosphate (HMBPP)] (5, 15–19). Unlike conventional αβ T-cells, ligand recognition by Vγ9Vδ2 and most γδ T-cells does not involve antigen presentation by human leukocyte antigen (HLA) molecules (15, 20). Ligand recognition by Vγ9Vδ2 T-cells requires butyrophilin (BTN) 3A1 (21) and BTN2A1 (22–24). Intracellular pAg levels are increased under stress conditions like infection or malignant transformation or by aminobisphosphonates (ABP) (16, 17, 25–27). Vγ9Vδ2 T-cells sense increased intracellular pAg levels causing their activation and target cell killing. Recent studies show that pAg-bound BTN3A1 associates with BTN2A1 which directly interacts with non-variable regions of the Vγ9 chain on γδ T-cells. Besides Vγ9Vδ2 T-cell recognition of pAgs, some subsets of Vδ1 and Vδ3 T-cells detect pathogenic and self-lipids presented by CD1d through their TCR (28, 29). Vδ1 T-cells are less abundant in circulation than Vγ9Vδ2 T-cells, but they are enriched in epithelia (30) and among tumor infiltrating lymphocytes (TILs). While cultured Vδ1 T-cells may have higher cytotoxic capacity than Vγ9Vδ2 T-cells, Vδ1 T-cells can be pro-tumoral in certain malignancies (6, 31, 32) (Figure 1A).
In this review we discuss γδ T-cell-based therapeutic strategies with a focus on recent developments of bispecific γδ T-cell engagers (bsTCEs) and chimeric antigen receptor (CAR) γδ T-cells, and point towards approaches that may develop into therapies in the near future (Figure 1B).
Past Clinical Studies With Vγ9Vδ2 T-Cells
In the year 2000, ABP drugs, already approved to treat patients with excessive bone resorption, were shown to cause systemic Vγ9Vδ2 T-cell stimulation and to increase their antitumor activity in a preclinical study (26). Following this observation, studies explored ABP treatment as a systemic γδ T-cell stimulant or as an ex vivo tool to expand them for subsequent adoptive cell transfer (ACT) for cancer immunotherapy.
The ABPs pamidronate (PAM) and zoledronate (ZOL), and synthetic pAg analogues, mainly bromohydrin pyrophosphate (BrHPP) and 2-methyl-3-butenyl-1-pyrophosphate (2M3B1PP), have been used alone or in combination with IL-2 to activate Vγ9Vδ2 T-cells (33, 34). ABP treatment has been evaluated in cancer patients (e.g. with multiple myeloma (MM), non-Hodgkin lymphoma (NHL), acute myeloid leukemia (AML), prostate cancer, renal cell carcinoma, colorectal cancer, breast cancer, melanoma or neuroblastoma) (33, 35–39). Additionally, ex vivo expansion of autologous γδ T-cells with ABPs or synthetic pAg followed by ACT has been tested in a wide range of malignancies (e.g. in MM, renal cell carcinoma, non-small cell lung cancer, gastric cancer, hepatocellular carcinoma, melanoma, ovarian cancer, colon cancer and pancreatic cancer) (40–51). While these approaches were well tolerated, clinical responses typically were found to be infrequent and not long-lasting, though sporadic meaningful responses were achieved (52–54). The overall moderate clinical antitumor effect of systemic γδ T-cell activation with ABP or synthetic pAg and of autologous γδ T transfer, negatively impacted further development of these Vγ9Vδ2 T-cell-directed cancer immunotherapeutic approaches.
Present and Future Studies Involving γδ T-Cells
γδ T-Cell-Based Cellular Strategies
Allogeneic γδ T-Cell Transfer
As mentioned above, most γδ T-cells recognize target cells independently of HLA antigen presentation, suggesting that allogeneic donor derived γδ T-cells can be relatively safe for ACT due to low risk of graft-versus-host disease (GvHD). Taking advantage of this, current strategies exploring the use of ex vivo expanded γδ T-cell infusion have shifted towards allogeneic origin (Table 1). Increased frequency of γδ T-cells in leukemia patients that underwent αβ-depleted allogeneic stem cell transplantation from partially HLA-mismatched donors, was associated with a higher 5-year and overall survival (OS) (55, 56). A single infusion of allogeneic Vγ9Vδ2 T-cells, expanded ex vivo with ZOL plus IL-2, is being administered in a clinical trial (NCT03533816) to maximize antitumor response and reduce GvHD, after allogeneic hematopoietic cell transplant (alloHCT) and cyclophosphamide for hematologic malignancies. Moreover, allogeneic Vγ9Vδ2 T-cell infusion after lymphodepletion is being tested independently of alloHCT for hematologic malignancies and solid tumors. Some of these studies have already been completed with no major adverse effects reported, highlighting the safety of Vγ9Vδ2 T-cell transfer (57, 58). Importantly, patients receiving Vγ9Vδ2 T-cell infusion had increased OS compared to control patients and repeated Vγ9Vδ2 T-cell infusions resulted in higher OS when compared to single infusion. Future approaches are based on allogeneic γδ T-cells derived from healthy donors, either unmodified or CAR-transfected (see below) (Table 2).
Application of non-Vγ9Vδ2 T-cell subsets, like Vδ1 T-cells, is of interest but lagged behind because of lack of proper expansion protocols. In 2016, Almeida et al. described a 3 week culture protocol based on stimulation of γδ T-cells from healthy donors or CLL patients with a combination of cytokines and anti-CD3 monoclonal antibody (mAb) clone OKT-3, resulting in 2000-fold expansion and 60-80% enrichment of Vδ1 T-cells (59). Expanded cells expressed the NK receptors NKp30 and NKp40, displayed cytotoxic activity, produced IFNγ, TNFα and no IL-17. Application of this protocol led to the development of different “delta one T” (DOT) cell products. Gamma Delta Therapeutics initiated a first-in-human phase I clinical trial in AML patients after lymphodepletion with fludarabine and cyclophosphamide (NCT05001451) (Table 1). This study will analyse safety and maximum tolerated dose of GDX012 and its effect on minimal residual disease, progression free survival (PFS) and OS.
Chimeric Antigen Receptor γδ T-Cells
Another therapeutic approach to harness the potent anti-tumor effects of γδ T-cells consists of adoptive transfer of γδ CAR-T-cells (60). CARs are chimeric antigen-recognition receptors, consisting of an ectodomain, which binds a tumor specific cell surface receptor, and endodomains, consisting of CD3ζ as the signaling domain with co-stimulatory domains to provide robust activation (e.g. CD28, 4-1BB, or ICOS) (61). In recent years, CAR-T-cell therapy has been extensively investigated in preclinical and clinical studies, primarily focused on conventional αβ T-cells (62–64). These autologous CAR-T-cells have triggered encouraging remission rates in patients refractory to standard treatments against, in particular, B-lymphoid malignancies. This resulted in FDA approvals of CAR-T-cell therapies for the treatment of B-cell NHL, ALL, and MM (65–69). The remarkable success of CAR-T-cell therapy revolutionized the field of adoptive cell therapy for treating hematologic malignancies and resulted in numerous ongoing clinical trials. However, CAR-T-cell therapy can be complicated by severe, potentially life-threatening, toxicities such as cytokine release syndrome (CRS), immune effector cell-associated neurotoxicity syndrome (ICANS) and other ‘on-target off-tumor’ toxicities (70). Moreover, in contrast to the results seen in hematologic malignancies, only limited antitumor effects have been obtained in patients with solid tumors.
It was hypothesized that the efficacy of CAR-T-cells could be improved and its side effects mitigated by harnessing the innate properties of γδ T-cells as a backbone for CAR. CAR-modified γδ T-cells were first described by Rischer et al. (71), demonstrating specific in vitro tumor cell lysis using ZOL-expanded Vγ9Vδ2 T-cells with CD19- or GD2-directed CARs, followed by other studies confirming these findings using γδ T-cells containing CARs against a variety of targets (72–77). Interestingly, CAR-modified Vγ9Vδ2 T-cells maintained their ability to cross-present tumor antigens to αβ T-cells in vitro, which may prolong the anti-tumor efficacy (76). Furthermore, γδ T-cells bearing a CD19-CAR, unlike standard CD19-αβ CAR-T-cells, had reactivity against CD19-positive and negative tumor cells in vitro and in vivo, an effect that was enhanced by ZOL (78), suggesting that CD19-directed γδ CAR-T-cells may target leukemic cells also after antigen loss and retain pAg specificity via their TCR. More recently, Wallet et al. described the generation of induced pluripotent stem cell-derived γδ CAR-T-cells (γδ CAR-iT) (79). They demonstrated sustained in vitro tumor cell killing by γδ CAR-iT-cells in the presence of IL-15, with markedly less IFN-γ and other inflammatory cytokines being produced compared to conventional αβ CAR-T-cells, potentially resulting in lower risk of CRS. Moreover, a single dose of γδ CAR-iT-cells resulted in potent tumor growth inhibition in a xenograft mouse model (79). Table 2 summarizes the companies currently developing γδ CAR-T-cells.
Pre-clinical research on γδ CAR-T-cell based therapy initially focused on Vγ9Vδ2 T-cells, due to their dominant frequency in blood and their unique pAg response that allowed the specific expansion of this subset (80). Makkouk et al. recently showed the first example of genetically modified Vδ1 T-cells. They expanded PBMC-derived Vδ1 T-cells using an agonistic anti-Vδ1 antibody and genetically modified them to express a GPC-3 targeted CAR and to secrete IL-15 (81). In a HepG2 mouse model, these allogeneic Vδ1 CAR-T-cells primarily accumulated in the tumor and a single dose efficiently controlled tumor growth without evidence of xenogeneic GvHD. ADI-001 consists of CD20-targeting Vδ1 CAR-T-cells generated by a similar procedure by Adicet Bio (82) and is currently being used in a phase I clinical trial (NCT04735471). Recently reported interim data from this dose-escalation study showed complete responses in two and a partial response in one out of four evaluable patients already with low doses (30x106 cells) of ADI-001, indicating that relatively low amounts of γδ T-cells may suffice for activity (press release). To date, no dose-limiting toxicities, GvHD, or grade 3 or higher CRS has been reported. These encouraging first results underscore the potential of Vδ1 CAR-T-cell therapy in the clinic. A complete overview of the ongoing clinical trials evaluating CAR-modified γδ T-cells is listed in Table 1.
Antibody-Based Strategies
Imcheck develops ICT01, a Vγ9Vδ2 T-cell activating humanized IgG1 with a silent Fc that binds to all three BTN3A isoforms to trigger Vγ9Vδ2 T-cell activation and increased cytotoxicity against BTN3A+ tumor cell lines from diverse origin (21). However, this approach is not tumor specific as BTN3A is broadly expressed and could also be hampered by soluble BTN3A molecules potentially acting as decoy receptors (83). In immunodeficient NSG mice, treatment with ICT01 resulted in in vivo activation of adoptively transferred human Vγ9Vδ2 T-cells and delayed outgrowth of the AML cell line MOLM14 (84). The EVICTION trial is a Phase I/IIa clinical trial currently testing the effect of ICT01 in relapsed/refractory advanced-stage hematologic malignancies as a monotherapy and in a broad range of solid tumors as monotherapy or in combination with pembrolizumab (NCT04243499). Preliminary results show a good safety profile with activation of Vγ9Vδ2 T-cells and increased tumor infiltration in one melanoma patient. Stable disease has been achieved in 31% of patients treated with ICT01 as a monotherapy and in 62% in combination with pembrolizumab (84).
BsTCEs have emerged as a promising therapeutic approach for immune-oncology (85) and consist of a tumor antigen binding antibody linked to a T-cell engaging antibody fragment aiming to crosslink tumor cells and T-cells to elicit T-cell-mediated anti-tumor cytotoxicity (86, 87). Most efforts to generate bsTCEs have made use of CD3 as a T-cell engaging domain due to its role in T-cell activation. For CD3-based TCEs, proteins that are uniquely expressed or specifically overexpressed by tumor cells are the most attractive candidates for targeting, as this reduces on-target off-tumor toxicity. After approval of the CD19-CD3 bsTCE blinatumomab (88), multiple CD3-directed TCEs have been developed (89), but in many cases development has been complicated by the occurrence of adverse events such as on-target off-tumor toxicity, CRS or ICANS, highlighting the need for more tumor-selective targeting (90–92). Considering the clinical safety observed following systemic γδ T-cell activation and γδ T ACT, specific engagement of γδ T-cells using γδ bsTCEs might have an improved safety profile due to their tumor selectivity compared to CD3-bsTCEs. By avoiding detrimental co-activation of regulatory CD3+ T-cells observed with CD3 pan T-cell engagers (93) and their ability to bridge and engage components of both the innate and adaptive immune system, γδ bsTCEs could potentially result in increased antitumor activity.
Several γδ T-cell engaging formats are being developed and evaluated preclinically. Vγ9-TCR specific engagers directed against Her2 (94–96) and CD123 (97) were shown to cause killing of Her2 expressing cell lines and AML cell lines, respectively. The GADLEN platform (Shattuck Labs) consists of fusion proteins containing BTN heterodimers, to engage and activate Vγ9Vδ2 T-cells, bound to a tumor targeting scFv domain through an Fc linker (98). Vδ1 bsTCEs are also being developed by Adaptate Biotherapeutics. Heavy chain only antibodies occur naturally in camelids (99). Their antigen-binding fragments or variable heavy chain-only antibodies (VHH), are small, stable and with low inherent immunogenicity (100, 101). Lava Therapeutics` Gammabody™ platform combines Vδ2-specific and tumor-targeting VHHs as modules to generate bsTCE (102–105). In pre-clinical studies, Gammabody™ molecules targeting CD40, CD1d and EGFR efficiently engage Vγ9Vδ2 T-cells to kill tumor cells expressing these antigens (102–105). Two Gammabody™ molecules, are currently evaluated in clinical trials. LAVA-051, a Gammabody™ targeting CD1d is tested in a Phase I/IIa clinical trial (NCT04887259) in patients with therapy-refractory CLL, AML or MM. Preliminary data of the first 3 cohorts from this study showed a thus far good safety profile with no dose-limiting toxicities or CRS. In addition, LAVA-1207, a Gammabody™ targeting PSMA is tested in a phase I/IIa clinical trial (NCT05369000) in patients suffering from therapy-refractory metastatic castration-resistant prostate cancer. Table 2 summarizes companies developing antibody-based γδ T-cell therapies, and Table 1 contains clinical trials involving antibody-based γδ T-cell approaches.
Alternative γδ T-Cell-Related Strategies
A new γδ T-cell based approach being tested in clinical trials is DeltEx drug-resistant immunotherapy (DRI). IN8Bio`s first DeltEx DRI product, INB-200, consists of expanded autologous Vγ9Vδ2 T-cells genetically modified to express a methylguanine DNA methyltransferase (MGMT). MGMT confers them resistance to temozolomide (TMZ) allowing for simultaneous treatment with TMZ and immunotherapy (106). TMZ, which is the current standard of care for glioblastoma multiforme (GBM) together with radiotherapy after resection, might sensitize tumor cells to γδ T-cell recognition through upregulation of NKG2D ligands but it also causes lymphocytopenia that is avoided by MGMT expression (107). An ongoing clinical trial (NCT04165941) is testing intracranial administration of INB-200 to the tumor site after surgical resection, followed by TMZ treatment (Table 1). All 4 GBM patients enrolled in this study have been reported to exceed the expected PFS for TMZ alone treatment. This technology is based on expansion and modification of autologous γδ T-cells, however, other DeltEx DRI based on allogeneic γδ T-cells (INB-400) and γδ CAR-T-cells (INB-300) are being developed.
Interestingly, although Vδ1+ T-cells have cytotoxic capacity, Vδ1+ TIL associate with poor prognosis in certain malignancies, possibly through production of IL-17 (6, 32). LYT-210 is a mAb directed towards the Vδ1+ TCR with the aim of eliminating these pathogenic cells (Table 2). Gamma-delta TCR bispecific molecules (GABs) combine the extracellular domain of the Vγ9Vδ2 TCR fused with a CD3 binding domain, allowing conventional T-cells to recognize the presence of pAg on tumor cells (108). In the presence of GABs, αβ T-cells recognized and killed the squamous cell carcinoma cell line SCC9 in a pAg dependent manner and produced increased amounts of IFNγ when exposed to patient-derived AML blasts but not with healthy hematopoietic cells indicating preferential recognition of tumor cells.
Two phase I dose-escalation clinical trials (NCT04688853; NTR6541) initiated by Gadeta are assessing the safety and tolerability of αβ T-cells engineered to express a defined Vγ9Vδ2 TCR (TEGs) in relapsed/refractory AML, MM, and high-risk myelodysplastic syndrome patients. These T-cells combine the tumor specificity of γδ T-cells with the tumor cell killing potential of αβ T-cells and show promising antitumor reactivity both in vitro and in vivo. Furthermore, chimeric PD-1 receptor (chPD1) γδ T-cells, turn PD-1 immune suppression into T-cell activation (109). The chPD1 γδ T-cells selectively killed PD-L1+ tumor cells in a xenograft murine model, without lysis of normal PD-L1+ cells or significant elevation of CRS-related cytokines. The authors reported that chPD1 γδ T-cell therapy will be assessed in a phase I/II clinical trial.
Conclusion
Past clinical trials have demonstrated that systemic activation of Vγ9Vδ2 T-cells or adoptive transfer of autologous Vγ9Vδ2 T-cells were well tolerated and could trigger antitumor immunity. These studies have been followed by a number of trials based on Vγ9Vδ2 and the first study with Vδ1 allogeneic T-cell transfer, which would allow for donor-derived therapies. Up to this date, these trials have not resulted in major adverse effects. Most strategies that are currently under evaluation profit from the safety of γδ T-cell activation and incorporate tumor-targeting mechanisms, e.g. CARs or bsTCEs, which might be key to obtain more robust and consistent clinical responses. Initial results from these targeted approaches, both cell and antibody-based, show great promise and confirm the safety of Vγ9Vδ2 and Vδ1 T-cell-based strategies. However, cell-based products present challenges that are not shared by antibody-based therapies, such as high cost, difficulty of production or need of specialized facilities, and preparatory lymphodepleting chemotherapy regimens. In the near future, the results obtained by the trials described in this review will determine whether the potential of γδ T-cells can be translated into clinical benefit.
Author Contributions
JS-E and MJ wrote the manuscript. HV co-wrote and reviewed the manuscript. LK, PP, EE, BW and TG reviewed the manuscript. All authors contributed to the article and approved the submitted version.
Funding
The authors declare that this study received funding from LAVA therapeutics. The funder had the following involvement with the study: providing research funding to Amsterdam UMC and in designing, writing and revising the text of the mini-review.
Conflict of Interest
JS-E, MJ and LK are funded by Lava therapeutics. HV, PP, EE, BW are employed by and hold stock of LAVA Therapeutics. TG holds stock of LAVA Therapeutics.
Publisher’s Note
All claims expressed in this article are solely those of the authors and do not necessarily represent those of their affiliated organizations, or those of the publisher, the editors and the reviewers. Any product that may be evaluated in this article, or claim that may be made by its manufacturer, is not guaranteed or endorsed by the publisher.
References
1. Kozbor D, Trinchieri G, Monos DS, Isobe M, Russo G, Haney JA, et al. Human TCR-Gamma+/Delta+, CD8+ T Lymphocytes Recognize Tetanus Toxoid in an MHC-Restricted Fashion. J Exp Med (1989) 169(5):1847–51. doi: 10.1084/jem.169.5.1847
2. Pistoia V, Tumino N, Vacca P, Veneziani I, Moretta A, Locatelli F, et al. Human γδ T-Cells: From Surface Receptors to the Therapy of High-Risk Leukemias. Front Immunol (2018) 9:984. doi: 10.3389/fimmu.2018.00984
3. Adams EJ, Gu S, Luoma AM. Human Gamma Delta T Cells: Evolution and Ligand Recognition. Cell Immunol (2015) 296(1):31–40. doi: 10.1016/j.cellimm.2015.04.008
4. Zhao Y, Niu C, Cui J. Gamma-Delta (Gammadelta) T Cells: Friend or Foe in Cancer Development? J Transl Med (2018) 16(1):3. doi: 10.1186/s12967-017-1378-2
5. Girardi M, Oppenheim DE, Steele CR, Lewis JM, Glusac E, Filler R, et al. Regulation of Cutaneous Malignancy by Gammadelta T Cells. Science (2001) 294(5542):605–9. doi: 10.1126/science.1063916
6. Meraviglia S, Lo Presti E, Tosolini M, La Mendola C, Orlando V, Todaro M, et al. Distinctive Features of Tumor-Infiltrating γδ T Lymphocytes in Human Colorectal Cancer. OncoImmunology (2017) 6(10):e1347742. doi: 10.1080/2162402X.2017.1347742
7. Wang J, Lin C, Li H, Li R, Wu Y, Liu H, et al. Tumor-Infiltrating γδt Cells Predict Prognosis and Adjuvant Chemotherapeutic Benefit in Patients With Gastric Cancer. OncoImmunology (2017) 6(11):e1353858. doi: 10.1080/2162402X.2017.1353858
8. Donia M, Ellebaek E, Andersen MH, Straten PT, Svane IM. Analysis of Vδ1 T Cells in Clinical Grade Melanoma-Infiltrating Lymphocytes. OncoImmunology (2012) 1(8):1297–304. doi: 10.4161/onci.21659
9. Lu H, Dai W, Guo J, Wang D, Wen S, Yang L, et al. High Abundance of Intratumoral γδ T Cells Favors a Better Prognosis in Head and Neck Squamous Cell Carcinoma: A Bioinformatic Analysis. Front Immunol (2020) 11. doi: 10.3389/fimmu.2020.573920
10. Zhao N, Dang H, Ma L, Martin SP, Forgues M, Ylaya K, et al. Intratumoral γδ T-Cell Infiltrates, Chemokine (C-C Motif ) Ligand 4/Chemokine (C-C Motif ) Ligand 5 Protein Expression and Survival in Patients With Hepatocellular Carcinoma. Hepatology (2020) 73(3):2021. doi: 10.1002/hep.31412
11. Gentles AJ, Newman AM, Liu CL, Bratman SV, Feng W, Kim D, et al. The Prognostic Landscape of Genes and Infiltrating Immune Cells Across Human Cancers. Nat Med (2015) 21(8):938–45. doi: 10.1038/nm.3909
12. Tosolini M, Pont F, Poupot M, Vergez F, Nicolau-Travers ML, Vermijlen D, et al. Assessment of Tumor-Infiltrating Tcrvγ9vδ2 γδ Lymphocyte Abundance by Deconvolution of Human Cancers Microarrays. OncoImmunology (2017) 6(3):e1284723. doi: 10.1080/2162402X.2017.1284723
13. Silva-Santos B, Serre K, Norell H. γδt Cells in Cancer. Nat Rev Immunol (2015) 15(11):683–91. doi: 10.1038/nri3904
14. Hinz T, Wesch D, Halary F, Marx S, Choudhary A, Arden B, et al. Identification of the Complete Expressed Human TCR V γ Repertoire by Flow Cytometry. Int Immunol (1997) 9(8):1065–72. doi: 10.1093/intimm/9.8.1065
15. Janis EM, Kaufmann SH, Schwartz RH, Pardoll DM. Activation of Gamma Delta T Cells in the Primary Immune Response to Mycobacterium Tuberculosis. Science (1989) 244(4905):713–6. doi: 10.1126/science.2524098
16. Gruenbacher G, Nussbaumer O, Gander H, Steiner B, Leonhartsberger N, Thurnher M. Stress-Related and Homeostatic Cytokines Regulate Vγ9vδ2 T-Cell Surveillance of Mevalonate Metabolism. OncoImmunology (2014) 3(8):1–12. doi: 10.4161/21624011.2014.953410
17. Tanaka Y, Morita CT, Tanaka Y, Nieves E, Brenner MB, Bloom BR. Natural and Synthetic non-Peptide Antigens Recognized by Human Gamma Delta T Cells. Nature (1995) 375(6527):155–8. doi: 10.1038/375155a0
18. Eberl M, Hintz M, Reichenberg A, Kollas AK, Wiesner J, Jomaa H. Microbial Isoprenoid Biosynthesis and Human γδ T Cell Activation. FEBS Lett (2003) 544(1-3):4–10. doi: 10.1016/S0014-5793(03)00483-6
19. Hintz M, Reichenberg A, Altincicek B, Bahr U, Gschwind RM, Kollas A-K, et al. Identi¢cation of (E)-4-Hydroxy-3-Methyl-But-2-Enyl Pyrophosphate as a Major Activator for Human QN T Cells in Escherichia Coli. FEBS Lett (2001) 509(2):317–22. doi: 10.1016/S0014-5793(01)03191-X
20. La Gruta NL, Gras S, Daley SR, Thomas PG, Rossjohn J. Understanding the Drivers of MHC Restriction of T Cell Receptors. Nat Rev Immunol (2018) 18(7):467–78. doi: 10.1038/s41577-018-0007-5
21. Harly C, Guillaume Y, Nedellec S, Peigné CM, Mönkkönen H, Mönkkönen J, et al. Key Implication of CD277/butyrophilin-3 (BTN3A) in Cellular Stress Sensing by a Major Human γδ T-Cell Subset. Blood (2012) 120(11):2269–79. doi: 10.1182/blood-2012-05-430470
22. Cano CE, Pasero C, De Gassart A, Kerneur C, Gabriac M, Fullana M, et al. BTN2A1, an Immune Checkpoint Targeting Vγ9vδ2 T Cell Cytotoxicity Against Malignant Cells. Cell Rep (2021) 36(2):109359. doi: 10.1016/j.celrep.2021.109359
23. Karunakaran MM, Willcox CR, Salim M, Paletta D, Fichtner AS, Noll A, et al. Butyrophilin-2a1 Directly Binds Germline-Encoded Regions of the Vγ9vδ2 TCR and Is Essential for Phosphoantigen Sensing. Immunity (2020) 52(3):487–98.e6. doi: 10.1016/j.immuni.2020.02.014
24. Rigau M, Ostrouska S, Fulford TS, Johnson DN, Woods K, Ruan Z, et al. Butyrophilin 2A1 Is Essential for Phosphoantigen Reactivity by Gd T Cells. Science (2020) 367(6478):eaay5516. doi: 10.1126/science.aay5516
25. Gober HJ, Kistowska M, Angman L, Jenö P, Mori L, De Libero G. Human T Cell Receptor γδ Cells Recognize Endogenous Mevalonate Metabolites in Tumor Cells. J Exp Med (2003) 197(2):163–8. doi: 10.1084/jem.20021500
26. Kunzmann V, Bauer E, Feurle J, Weissinger F, Tony HP, Wilhelm M. Stimulation of Gammadelta T Cells by Aminobisphosphonates and Induction of Antiplasma Cell Activity in Multiple Myeloma. Blood (2000) 96(2):384–92. doi: 10.1182/blood.V96.2.384
27. Dhar MK, Koul A, Kaul S. Farnesyl Pyrophosphate Synthase: A Key Enzyme in Isoprenoid Biosynthetic Pathway and Potential Molecular Target for Drug Development. N Biotechnol (2013) 30(2):114–23. doi: 10.1016/j.nbt.2012.07.001
28. Luoma AM, Castro CD, Mayassi T, Bembinster LA, Bai L, Picard D, et al. Crystal Structure of Vδ1t Cell Receptor in Complex With CD1d-Sulfatide Shows MHC-Like Recognition of a Self-Lipid by Human γδ T Cells. Immunity (2013) 39(6):1032–42. doi: 10.1016/j.immuni.2013.11.001
29. Mangan BA, Dunne MR, O’Reilly VP, Dunne PJ, Exley MA, O’Shea D, et al. Cutting Edge: CD1d Restriction and Th1/Th2/Th17 Cytokine Secretion by Human Vδ3 T Cells. J Immunol (2013) 191(1):30–4. doi: 10.4049/jimmunol.1300121
30. Deusch K, Pfeffer K, Reich K, Gstettenbauer M, Daum S, Luling F, et al. Phenotypic and Functional Characterization of Human TCR Gamma Delta+ Intestinal Intraepithelial Lymphocytes. Curr Top Microbiol Immunol (1991) 173:279–83.
31. Wu D, Wu P, Wu X, Ye J, Wang Z, Zhao S, et al. Ex Vivo Expanded Human Circulating Vdelta1 gammadeltaT Cells Exhibit Favorable Therapeutic Potential for Colon Cancer. Oncoimmunology (2015) 4(3):e992749. doi: 10.4161/2162402X.2014.992749
32. Li Y, Li G, Zhang J, Wu X, Chen X. The Dual Roles of Human γδ T Cells: Anti-Tumor or Tumor-Promoting. Front Immunol (2021) 11:619954. doi: 10.3389/fimmu.2020.619954
33. Bennouna J, Levy V, Sicard H, Senellart H, Audrain M, Hiret S, et al. Phase I Study of Bromohydrin Pyrophosphate (BrHPP, IPH 1101), a Vγ9vδ2 T Lymphocyte Agonist in Patients With Solid Tumors. Cancer Immunol Immunother (2010) 59(10):1521–30. doi: 10.1007/s00262-010-0879-0
34. Tanaka Y, Kobayashi H, Terasaki T, Toma H, Aruga A, Uchiyama T, et al. Synthesis of Pyrophosphate-Containing Compounds That Stimulate Vgamma2Vdelta2 T Cells: Application to Cancer Immunotherapy. Med Chem (2007) 3(1):85–99. doi: 10.2174/157340607779317544
35. Wilhelm M, Kunzmann V, Eckstein S, Reimer P, Weissinger F, Ruediger T, et al. T Cells for Immune Therapy of Patients With Lymphoid Malignancies. Blood (2003) 102:200–6. doi: 10.1182/blood-2002-12-3665
36. Dieli F, Vermijlen D, Fulfaro F, Caccamo N, Meraviglia S, Cicero G, et al. Targeting Human γδ T Cells With Zoledronate and Interleukin-2 for Immunotherapy of Hormone-Refractory Prostate Cancer. Cancer Res (2007) 67(15):7450–7. doi: 10.1158/0008-5472.CAN-07-0199
37. Lang JM, Kaikobad MR, Wallace M, Staab MJ, Horvath DL, Wilding G, et al. Pilot Trial of Interleukin-2 and Zoledronic Acid to Augment γδ T Cells as Treatment for Patients With Refractory Renal Cell Carcinoma. Cancer Immunol Immunother (2011) 60(10):1447–60. doi: 10.1007/s00262-011-1049-8
38. Kunzmann V, Smetak M, Kimmel B, Weigang-Koehler K, Goebeler M, Birkmann J, et al. Tumor-Promoting Versus Tumor-Antagonizing Roles of Gd T Cells in Cancer Immunotherapy: Results From a Prospective Phase I/II Trial. J Immunother (2012) 35(2):205–13. doi: 10.1097/CJI.0b013e318245bb1e
39. Pressey JG, Adams J, Harkins L, Kelly D, You Z, Lamb LS. In Vivo Expansion and Activation of Gd T Cells as Immunotherapy for Refractory Neuroblastoma A Phase 1 Study. Med (United States) (2016) 95(39):e4909. doi: 10.1097/MD.0000000000004909
40. Abe Y, Muto M, Nieda M, Nakagawa Y, Nicol A, Kaneko T, et al. Clinical and Immunological Evaluation of Zoledronate-Activated Vγ9γδ T-Cell-Based Immunotherapy for Patients With Multiple Myeloma. Exp Hematol (2009) 37(8):956–68. doi: 10.1016/j.exphem.2009.04.008
41. Kobayashi H, Tanaka Y, Yagi J, Osaka Y, Nakazawa H, Uchiyama T, et al. Safety Profile and Anti-Tumor Effects of Adoptive Immunotherapy Using Gamma-Delta T Cells Against Advanced Renal Cell Carcinoma: A Pilot Study. Cancer Immunol Immunother (2007) 56(4):469–76. doi: 10.1007/s00262-006-0199-6
42. Bennouna J, Bompas E, Neidhardt EM, Rolland F, Philip I, Galéa C, et al. Phase-I Study of Innacell γδ™, an Autologous Cell-Therapy Product Highly Enriched in γ9δ2 T Lymphocytes, in Combination With IL-2, in Patients With Metastatic Renal Cell Carcinoma. Cancer Immunol Immunother (2008) 57(11):1599–609. doi: 10.1007/s00262-008-0491-8
43. Kobayashi H, Tanaka Y, Yagi J, Minato N, Tanabe K. Phase I/II Study of Adoptive Transfer of γδ T Cells in Combination With Zoledronic Acid and IL-2 to Patients With Advanced Renal Cell Carcinoma. Cancer Immunol Immunother (2011) 60(8):1075–84. doi: 10.1007/s00262-011-1021-7
44. Nakajima J, Murakawa T, Fukami T, Goto S, Kaneko T, Yoshida Y, et al. A Phase I Study of Adoptive Immunotherapy for Recurrent non-Small-Cell Lung Cancer Patients With Autologous γδ T Cells. Eur J Cardio-thoracic Surgery (2010) 37(5):1191–7. doi: 10.1016/j.ejcts.2009.11.051
45. Izumi T, Kondo M, Takahashi T, Fujieda N, Kondo A, Tamura N, et al. Ex Vivo Characterization of γδ T-Cell Repertoire in Patients After Adoptive Transfer of Vγ9vδ2 T Cells Expressing the Interleukin-2 Receptor β-Chain and the Common γ-Chain. Cytotherapy (2013) 15(4):481–91. doi: 10.1016/j.jcyt.2012.12.004
46. Kakimi K, Matsushita H, Masuzawa K, Karasaki T, Kobayashi Y, Nagaoka K, et al. Adoptive Transfer of Zoledronate-Expanded Autologous Vgamma9Vdelta2 T-Cells in Patients With Treatment-Refractory Non-Small-Cell Lung Cancer: A Multicenter, Open-Label, Single-Arm, Phase 2 Study. J Immunother Cancer (2s020) 8(2):e001185. doi: 10.1136/jitc-2020-001185
47. Sakamoto M, Nakajima J, Murakawa T, Fukami T, Yoshida Y, Murayama T, et al. Adoptive Immunotherapy for Advanced Non-Small Cell Lung Cancer Using Zoledronate-Expanded γδ T Cells: A Phase I Clinical Study. J Immunother (2011) 34(2):202–11. doi: 10.1097/CJI.0b013e318207ecfb
48. Wada I, Matsushita H, Noji S, Mori K, Yamashita H, Nomura S, et al. Intraperitoneal Injection of In Vitro Expanded Vγ9vδ2 T Cells Together With Zoledronate for the Treatment of Malignant Ascites Due to Gastric Cancer. Cancer Med (2014) 3(2):362–75. doi: 10.1002/cam4.196
49. Cui J, Wang N, Zhao H, Jin H, Wang G, Niu C, et al. Combination of Radiofrequency Ablation and Sequential Cellular Immunotherapy Improves Progression-Free Survival for Patients With Hepatocellular Carcinoma. Int J Cancer (2014) 134(2):342–51. doi: 10.1002/ijc.28372
50. Nicol AJ, Tokuyama H, Mattarollo SR, Hagi T, Suzuki K, Yokokawa K, et al. Clinical Evaluation of Autologous Gamma Delta T Cell-Based Immunotherapy for Metastatic Solid Tumours. Br J Cancer (2011) 105(6):778–86. doi: 10.1038/bjc.2011.293
51. Aoki T, Matsushita H, Hoshikawa M, Hasegawa K, Kokudo N, Kakimi K. Adjuvant Combination Therapy With Gemcitabine and Autologous γδ T-Cell Transfer in Patients With Curatively Resected Pancreatic Cancer. Cytotherapy (2017) 19(4):473–85. doi: 10.1016/j.jcyt.2017.01.002
52. Sebestyen Z, Prinz I, Déchanet-Merville J, Silva-Santos B, Kuball J. Translating Gammadelta (γδ) T Cells and Their Receptors Into Cancer Cell Therapies. Nat Rev Drug Discovery (2020) 19(3):169–84. doi: 10.1038/s41573-019-0038-z
53. Hoeres T, Smetak M, Pretscher D, Wilhelm M. Improving the Efficiency of Vγ9vδ2 T-Cell Immunotherapy in Cancer. Front Immunol (2018) 9:800. doi: 10.3389/fimmu.2018.00800
54. Wilhelm M, Smetak M, Schaefer-Eckart K, Kimmel B, Birkmann J, Einsele H, et al. Successful Adoptive Transfer and In Vivo Expansion of Haploidentical Gammadelta T Cells. J Transl Med (2014) 12:45. doi: 10.1186/1479-5876-12-45
55. Lamb LS Jr., Henslee-Downey PJ, Parrish RS, Godder K, Thompson J, Lee C, et al. Increased Frequency of TCR Gamma Delta + T Cells in Disease-Free Survivors Following T Cell-Depleted, Partially Mismatched, Related Donor Bone Marrow Transplantation for Leukemia. J Hematother (1996) 5(5):503–9. doi: 10.1089/scd.1.1996.5.503
56. Godder KT, Henslee-Downey PJ, Mehta J, Park BS, Chiang KY, Abhyankar S, et al. Long Term Disease-Free Survival in Acute Leukemia Patients Recovering With Increased γδ T Cells After Partially Mismatched Related Donor Bone Marrow Transplantation. Bone Marrow Transplantation (2007) 39(12):751–7. doi: 10.1038/sj.bmt.1705650
57. Lin M, Zhang X, Liang S, Luo H, Alnaggar M, Liu A, et al. Irreversible Electroporation Plus Allogenic Vγ9vδ2 T Cells Enhances Antitumor Effect for Locally Advanced Pancreatic Cancer Patients. Signal Transduction Targeted Ther (2020) 5(1):215. doi: 10.1038/s41392-020-00260-1
58. Xu Y, Xiang Z, Alnaggar M, Kouakanou L, Li J, He J, et al. Allogeneic Vγ9vδ2 T-Cell Immunotherapy Exhibits Promising Clinical Safety and Prolongs the Survival of Patients With Late-Stage Lung or Liver Cancer. Cell Mol Immunol (2021) 18(2):427–39. doi: 10.1038/s41423-020-0515-7
59. Almeida AR, Correia DV, Fernandes-Platzgummer A, da Silva CL, da Silva MG, Anjos DR, et al. Delta One T Cells for Immunotherapy of Chronic Lymphocytic Leukemia: Clinical-Grade Expansion/Differentiation and Preclinical Proof of Concept. Clin Cancer Res (2016) 22(23):5795–804. doi: 10.1158/1078-0432.CCR-16-0597
60. Mirzaei HR, Mirzaei H, Lee SY, Hadjati J, Till BG. Prospects for Chimeric Antigen Receptor (CAR) γδ T Cells: A Potential Game Changer for Adoptive T Cell Cancer Immunotherapy. Cancer Letters (2016) 380(2):413–23. doi: 10.1016/j.canlet.2016.07.001
61. Sadelain M, Riviere I, Riddell S. Therapeutic T Cell Engineering. Nature (2017) 545(7655):423–31. doi: 10.1038/nature22395
62. Lee DW, Kochenderfer JN, Stetler-Stevenson M, Cui YK, Delbrook C, Feldman SA, et al. T Cells Expressing CD19 Chimeric Antigen Receptors for Acute Lymphoblastic Leukaemia in Children and Young Adults: A Phase 1 Dose-escalation Trial. Lancet (2015) 385(9967):517–28. doi: 10.1016/S0140-6736(14)61403-3
63. Park JH, Riviere I, Gonen M, Wang X, Senechal B, Curran KJ, et al. Long-Term Follow-Up of CD19 CAR Therapy in Acute Lymphoblastic Leukemia. N Engl J Med (2018) 378(5):449–59. doi: 10.1056/NEJMoa1709919
64. Kochenderfer JN, Dudley ME, Kassim SH, Somerville RP, Carpenter RO, Stetler-Stevenson M, et al. Chemotherapy-Refractory Diffuse Large B-Cell Lymphoma and Indolent B-Cell Malignancies can be Effectively Treated With Autologous T Cells Expressing an Anti-CD19 Chimeric Antigen Receptor. J Clin Oncol (2015) 33(6):540–9. doi: 10.1200/JCO.2014.56.2025
65. Wang M, Munoz J, Goy A, Locke FL, Jacobson CA, Hill BT, et al. KTE-X19 CAR T-Cell Therapy in Relapsed or Refractory Mantle-Cell Lymphoma. N Engl J Med (2020) 382(14):1331–42. doi: 10.1056/NEJMoa1914347
66. Munshi NC, Anderson LD Jr., Shah N, Madduri D, Berdeja J, Lonial S, et al. Idecabtagene Vicleucel in Relapsed and Refractory Multiple Myeloma. N Engl J Med (2021) 384(8):705–16. doi: 10.1056/NEJMoa2024850
67. Neelapu SS, Locke FL, Bartlett NL, Lekakis LJ, Miklos DB, Jacobson CA, et al. Axicabtagene Ciloleucel CAR T-Cell Therapy in Refractory Large B-Cell Lymphoma. N Engl J Med (2017) 377(26):2531–44. doi: 10.1056/NEJMoa1707447
68. Schuster SJ, Bishop MR, Tam CS, Waller EK, Borchmann P, McGuirk JP, et al. Tisagenlecleucel in Adult Relapsed or Refractory Diffuse Large B-Cell Lymphoma. N Engl J Med (2019) 380(1):45–56. doi: 10.1056/NEJMoa1804980
69. Abramson JS, Palomba ML, Gordon LI, Lunning MA, Wang M, Arnason J, et al. Lisocabtagene Maraleucel for Patients With Relapsed or Refractory Large B-Cell Lymphomas (TRANSCEND NHL 001): A Multicentre Seamless Design Study. Lancet (2020) 396(10254):839–52. doi: 10.1016/S0140-6736(20)31366-0
70. Sterner RC, Sterner RM. CAR-T-Cell Therapy: Current Limitations and Potential Strategies. Blood Cancer J (2021) 11(4):69. doi: 10.1038/s41408-021-00459-7
71. Rischer M, Pscherer S, Duwe S, Vormoor J, Jurgens H, Rossig C. Human Gammadelta T Cells as Mediators of Chimaeric-Receptor Redirected Anti-Tumour Immunity. Br J Haematol (2004) 126(4):583–92. doi: 10.1111/j.1365-2141.2004.05077.x
72. Harrer DC, Simon B, Fujii S-I, Shimizu K, Uslu U, Schuler G, et al. RNA-Transfection of γ/δ T Cells With a Chimeric Antigen Receptor or an α/β T-Cell Receptor: A Safer Alternative to Genetically Engineered α/β T Cells for the Immunotherapy of Melanoma. BMC Cancer (2017) 17(1):551. doi: 10.1186/s12885-017-3539-3
73. Ang WX, Ng YY, Xiao L, Chen C, Li Z, Chi Z, et al. Electroporation of NKG2D RNA CAR Improves Vgamma9Vdelta2 T Cell Responses Against Human Solid Tumor Xenografts. Mol Ther Oncolytics (2020) 17:421–30. doi: 10.1016/j.omto.2020.04.013
74. Xiao L, Chen C, Li Z, Zhu S, Tay JC, Zhang X, et al. Large-Scale Expansion of Vγ9vδ2 T Cells With Engineered K562 Feeder Cells in G-Rex Vessels and Their Use as Chimeric Antigen Receptor–Modified Effector Cells. Cytotherapy (2018) 20(3):420–35. doi: 10.1016/j.jcyt.2017.12.014
75. Deniger DC, Switzer K, Mi T, Maiti S, Hurton L, Singh H, et al. Bispecific T-Cells Expressing Polyclonal Repertoire of Endogenous Gammadelta T-Cell Receptors and Introduced CD19-Specific Chimeric Antigen Receptor. Mol Ther (2013) 21(3):638–47. doi: 10.1038/mt.2012.267
76. Capsomidis A, Benthall G, Van Acker HH, Fisher J, Kramer AM, Abeln Z, et al. Chimeric Antigen Receptor-Engineered Human Gamma Delta T Cells: Enhanced Cytotoxicity With Retention of Cross Presentation. Mol Ther (2018) 26(2):354–65. doi: 10.1016/j.ymthe.2017.12.001
77. Fleischer LC, Becker SA, Ryan RE, Fedanov A, Doering CB, Spencer HT. Non-Signaling Chimeric Antigen Receptors Enhance Antigen-Directed Killing by Gammadelta T Cells in Contrast to Alphabeta T Cells. Mol Ther Oncolytics (2020) 18:149–60. doi: 10.1016/j.omto.2020.06.003
78. Rozenbaum M, Meir A, Aharony Y, Itzhaki O, Schachter J, Bank I, et al. Gamma-Delta CAR-T-Cells Show CAR-Directed and Independent Activity Against Leukemia. Front Immunol (2020) 11:1347. doi: 10.3389/fimmu.2020.01347
79. Wallet M, Nishimura T, Del Casale C, Lebid A, Salantes B, Santostefano K, et al. Induced Pluripotent Stem Cell-Derived Gamma Delta CAR-T-Cells for Cancer Immunotherapy. Blood (2021) 138Supplement 1):2771. doi: 10.1182/blood-2021-149095
80. Siegers GM, Lamb LS Jr. Cytotoxic and Regulatory Properties of Circulating Vdelta1+ Gammadelta T Cells: A New Player on the Cell Therapy Field? Mol Ther (2014) 22(8):1416–22. doi: 10.1038/mt.2014.104
81. Makkouk A, Yang XC, Barca T, Lucas A, Turkoz M, Wong JTS, et al. Off-The-Shelf Vdelta1 Gamma Delta T Cells Engineered With Glypican-3 (GPC-3)-Specific Chimeric Antigen Receptor (CAR) and Soluble IL-15 Display Robust Antitumor Efficacy Against Hepatocellular Carcinoma. J Immunother Cancer (2021) 9(12):e003441. doi: 10.1136/jitc-2021-003441
82. Nishimoto KP, Barca T, Azameera A, Makkouk A, Romero JM, Bai L, et al. Allogeneic CD20-Targeted Gammadelta T Cells Exhibit Innate and Adaptive Antitumor Activities in Preclinical B-Cell Lymphoma Models. Clin Transl Immunol (2022) 11(2):e1373. doi: 10.1002/cti2.1373
83. Benyamine A, Loncle C, Foucher E, Blazquez JL, Castanier C, Chretien AS, et al. BTN3A Is a Prognosis Marker and a Promising Target for Vgamma9Vdelta2 T Cells Based-Immunotherapy in Pancreatic Ductal Adenocarcinoma (PDAC). Oncoimmunology (2017) 7(1):e1372080. doi: 10.1080/2162402X.2017.1372080
84. De Gassart A, Le KS, Brune P, Agaugue S, Sims J, Goubard A, et al. Development of ICT01, a First-in-Class, Anti-BTN3A Antibody for Activating Vgamma9Vdelta2 T Cell-Mediated Antitumor Immune Response. Sci Transl Med (2021) 13(616):eabj0835. doi: 10.1126/scitranslmed.abj0835
85. Wang S, Chen K, Lei Q, Ma P, Yuan AQ, Zhao Y, et al. The State of the Art of Bispecific Antibodies for Treating Human Malignancies. EMBO Mol Med (2021) 13(9):e14291. doi: 10.15252/emmm.202114291
86. Singh A, Dees S, Grewal IS. Overcoming the Challenges Associated With CD3+ T-Cell Redirection in Cancer. Br J Cancer (2021) 124(6):1037–48. doi: 10.1038/s41416-020-01225-5
87. Labrijn AF, Janmaat ML, Reichert JM, Parren P. Bispecific Antibodies: A Mechanistic Review of the Pipeline. Nat Rev Drug Discovery (2019) 18(8):585–608. doi: 10.1038/s41573-019-0028-1
88. Topp MS, Gokbuget N, Zugmaier G, Degenhard E, Goebeler ME, Klinger M, et al. Long-Term Follow-Up of Hematologic Relapse-Free Survival in a Phase 2 Study of Blinatumomab in Patients With MRD in B-Lineage ALL. Blood (2012) 120(26):5185–7. doi: 10.1182/blood-2012-07-441030
89. Einsele H, Borghaei H, Orlowski RZ, Subklewe M, Roboz GJ, Zugmaier G, et al. The BiTE (Bispecific T-Cell Engager) Platform: Development and Future Potential of a Targeted Immuno-Oncology Therapy Across Tumor Types. Cancer (2020) 126(14):3192–201. doi: 10.1002/cncr.32909
90. Jain T, Litzow MR. Management of Toxicities Associated With Novel Immunotherapy Agents in Acute Lymphoblastic Leukemia. Ther Adv Hematol (2020) 11:2040620719899897. doi: 10.1177/2040620719899897
91. Strohl WR, Naso M. Bispecific T-Cell Redirection Versus Chimeric Antigen Receptor (CAR)-T Cells as Approaches to Kill Cancer Cells. Antibodies (Basel) (2019) 8(3):41. doi: 10.3390/antib8030041
92. Teachey DT, Rheingold SR, Maude SL, Zugmaier G, Barrett DM, Seif AE, et al. Cytokine Release Syndrome After Blinatumomab Treatment Related to Abnormal Macrophage Activation and Ameliorated With Cytokine-Directed Therapy. Blood (2013) 121(26):5154–7. doi: 10.1182/blood-2013-02-485623
93. Koristka S, Cartellieri M, Arndt C, Feldmann A, Seliger B, Ehninger G, et al. Tregs Activated by Bispecific Antibodies: Killers or Suppressors? OncoImmunology (2015) 4(3):1–3. doi: 10.4161/2162402X.2014.994441
94. Oberg HH, Janitschke L, Sulaj V, Weimer J, Gonnermann D, Hedemann N, et al. Bispecific Antibodies Enhance Tumor-Infiltrating T Cell Cytotoxicity Against Autologous HER-2-Expressing High-Grade Ovarian Tumors. J Leukoc Biol (2020) 107(6):1081–95. doi: 10.1002/JLB.5MA1119-265R
95. Oberg HH, Kellner C, Gonnermann D, Peipp M, Peters C, Sebens S, et al. Gammadelta T Cell Activation by Bispecific Antibodies. Cell Immunol (2015) 296(1):41–9. doi: 10.1016/j.cellimm.2015.04.009
96. Oberg HH, Peipp M, Kellner C, Sebens S, Krause S, Petrick D, et al. Novel Bispecific Antibodies Increase Gammadelta T-Cell Cytotoxicity Against Pancreatic Cancer Cells. Cancer Res (2014) 74(5):1349–60. doi: 10.1158/0008-5472.CAN-13-0675
97. Ganesan R, Chennupati V, Ramachandran B, Hansen MR, Singh S, Grewal IS. Selective Recruitment of Gammadelta T Cells by a Bispecific Antibody for the Treatment of Acute Myeloid Leukemia. Leukemia (2021) 35(8):2274–84. doi: 10.1038/s41375-021-01122-7
98. de Silva S, Lai A, Patel A, Evans KJ, Yoo K, Jin K, et al. Antigen-Specific Targeting of Tissue-Resident Gamma Delta T Cells With Recombinant Butyrophilin Heterodimeric Fusion Proteins. Am Assoc Cancer Res (2021) 81(13_Supplement):1736. doi: 10.1158/1538-7445.AM2021-1736
99. Arbabi-Ghahroudi M. Camelid Single-Domain Antibodies: Historical Perspective and Future Outlook. Front Immunol (2017) 8(NOV). doi: 10.3389/fimmu.2017.01589
100. Muyldermans S. Nanobodies: Natural Single-Domain Antibodies. Annu Rev Biochem (2013) 82:775–97. doi: 10.1146/annurev-biochem-063011-092449
101. Kunz P, Zinner K, Mucke N, Bartoschik T, Muyldermans S, Hoheisel JD. The Structural Basis of Nanobody Unfolding Reversibility and Thermoresistance. Sci Rep (2018) 8(1):e1375641. doi: 10.1038/s41598-018-26338-z
102. de Bruin RCG, Veluchamy JP, Lougheed SM, Schneiders FL, Lopez-Lastra S, Lameris R, et al. A Bispecific Nanobody Approach to Leverage the Potent and Widely Applicable Tumor Cytolytic Capacity of Vγ9vδ2-T Cells. OncoImmunology (2018) 7(1). doi: 10.1080/2162402X.2017.1375641
103. de Weerdt I, Lameris R, Scheffer GL, Vree J, de Boer R, Stam AG, et al. A Bispecific Antibody Antagonizes Prosurvival CD40 Signaling and Promotes Vγ9vδ2 T Cell-Mediated Antitumor Responses in Human B-Cell Malignancies. Cancer Immunol Res (2021) 9(1):50–61. doi: 10.1158/2326-6066.CIR-20-0138
104. de Weerdt I, Lameris R, Ruben JM, de Boer R, Kloosterman J, King LA, et al. A Bispecific Single-Domain Antibody Boosts Autologous Vgamma9Vdelta2-T Cell Responses Toward CD1d in Chronic Lymphocytic Leukemia. Clin Cancer Res (2021) 27(6):1744–55. doi: 10.1158/1078-0432.CCR-20-4576
105. Lameris R, de Bruin RC, van Bergen En Henegouwen PM, Verheul HM, Zweegman S, de Gruijl TD, et al. Generation and Characterization of CD1d-Specific Single-Domain Antibodies With Distinct Functional Features. Immunology (2016) 149(1):111–21. doi: 10.1111/imm.12635
106. Lamb LS, Pereboeva L, Youngblood S, Gillespie GY, Nabors LB, Markert JM, et al. A Combined Treatment Regimen of MGMT-Modified γδ T Cells and Temozolomide Chemotherapy Is Effective Against Primary High Grade Gliomas. Sci Rep (2021) 11(1):21133. doi: 10.1038/s41598-021-00536-8
107. Lamb LS, Bowersock J, Dasgupta A, Gillespie GY, Su Y, Johnson A, et al. Engineered Drug Resistant γδ T Cells Kill Glioblastoma Cell Lines During a Chemotherapy Challenge: A Strategy for Combining Chemo- and Immunotherapy. PloS One (2013) 8(1):e51805. doi: 10.1371/journal.pone.0051805
108. van Diest E, Hernandez Lopez P, Meringa AD, Vyborova A, Karaiskaki F, Heijhuurs S, et al. Gamma Delta TCR Anti-CD3 Bispecific Molecules (GABs) as Novel Immunotherapeutic Compounds. J Immunother Cancer (2021) 9(11):e003850. doi: 10.1136/jitc-2021-003850
109. Barber A, Wang X, Gopisetty A, Mirandola L, Chiriva-Internati M. Abstract LB148: Gamma Delta T Cells Engineered With a Chimeric PD-1 Receptor Effectively Control PD-L1 Positive Tumors In Vitro and In Vivo With Minimal Toxicities. Cancer Res (2021) 81Supplement):LB148–LB. doi: 10.1158/1538-7445.AM2021-LB148
Keywords: gamma delta T-cell, cancer, immunotherapy, phosphoantigens, aminobisphosphonates, adoptive cell transfer, bispecific t-cell engager, chimeric antigen receptor
Citation: Saura-Esteller J, de Jong M, King LA, Ensing E, Winograd B, de Gruijl TD, Parren PWHI and van der Vliet HJ (2022) Gamma Delta T-Cell Based Cancer Immunotherapy: Past-Present-Future. Front. Immunol. 13:915837. doi: 10.3389/fimmu.2022.915837
Received: 08 April 2022; Accepted: 05 May 2022;
Published: 16 June 2022.
Edited by:
Alice Cheung, Singapore General Hospital, SingaporeReviewed by:
Alessandro Poggi, San Martino Hospital (IRCCS), ItalyEmmanuel Scotet, Université de Nantes, France
Copyright © 2022 Saura-Esteller, de Jong, King, Ensing, Winograd, de Gruijl, Parren and van der Vliet. This is an open-access article distributed under the terms of the Creative Commons Attribution License (CC BY). The use, distribution or reproduction in other forums is permitted, provided the original author(s) and the copyright owner(s) are credited and that the original publication in this journal is cited, in accordance with accepted academic practice. No use, distribution or reproduction is permitted which does not comply with these terms.
*Correspondence: Hans J. van der Vliet, aC52YW5kZXJ2bGlldEBsYXZhdGhlcmFwZXV0aWNzLmNvbQ==; amoudmFuZGVydmxpZXRAYW1zdGVyZGFtdW1jLm5s