- 1Molecular Cardiology Laboratory, IRCCS-Policlinico San Donato, San Donato Milanese, Milan, Italy
- 2Immunology and General Pathology Laboratory, Department of Biotechnology and Life Sciences, University of Insubria, Varese, Italy
- 3Medical Oncology Clinic, Oncology Institute of Southern Switzerland, Bellinzona, Switzerland
- 4Center for Thoracic Surgery, Department of Medicine and Surgery, University of Insubria, Varese, Italy
- 5Department of Basic Medical Sciences, Neurosciences and Sensory Organs, University of Bari Aldo Moro Medical School, Bari, Italy
Despite some significant therapeutic breakthroughs leading to immunotherapy, a high percentage of patients with non-small cell lung cancer (NSCLC) do not respond to treatment on relapse, thus experiencing poor prognosis and survival. The unsatisfying results could be related to the features of the tumor immune microenvironment and the dynamic interactions between a tumor and immune infiltrate. Host–tumor interactions strongly influence the course of disease and response to therapies. Thus, targeting host-associated factors by restoring their physiologic functions altered by the presence of a tumor represents a new therapeutic approach to control tumor development and progression. In NSCLC, the immunogenic tumor balance is shifted negatively toward immunosuppression due to the release of inhibitory factors as well as the presence of immunosuppressive cells. Among these cells, there are myeloid-derived suppressor cells, regulatory T cells that can generate a tumor-permissive milieu by reprogramming the cells of the hosts such as tumor-associated macrophages, tumor-associated neutrophils, natural killer cells, dendritic cells, and mast cells that acquire tumor-supporting phenotypes and functions. This review highlights the current knowledge of the involvement of host-related factors, including innate and adaptive immunity in orchestrating the tumor cell fate and the primary resistance mechanisms to immunotherapy in NSCLC. Finally, we discuss combinational therapeutic strategies targeting different aspects of the tumor immune microenvironment (TIME) to prime the host response. Further research dissecting the characteristics and dynamic interactions within the interface host–tumor is necessary to improve a patient fitness immune response and provide answers regarding the immunotherapy efficacy, with the aim to develop more successful treatments for NSCLC.
Introduction
Lung cancer has become a leading cause of cancer death worldwide. The global incidence sees polarized differences according to the economic development of different countries. There is a decrease in the incidence among men in high-income countries due to public health measures and a gradual and progressive increase in both genders in low-income countries where public health initiatives for smoking cessation have lagged and access to healthcare is scarce (1–3). During the first decade of the present century, the outcomes of at least a subset of patients have seen substantial improvements, thanks to a general understanding of disease biology, the application of predictive biomarkers, and refinements in specific treatments (4).
Non-small cell lung cancer (NSCLC) represents approximately 85% of all lung cancer cases. It includes three major histologic classifications: adenocarcinoma (ADC), representing the most common subtype of lung cancer, followed by squamous-cell carcinoma (SCC) and large-cell carcinoma (LCC) (5).
Treatment is highly dependent on many parameters of the patient, particularly their general functional status, comorbidities, the tumor stage, and the molecular features of the disease. The primary treatment for stages I and II and in selected cases for stage III A disease is curative surgery, chemotherapy, radiation therapy (RT), or a combined modality approach. Postoperative adjuvant cisplatin-based chemotherapy is recommended in patients with completely resected stage II–IIIA disease and selected patients with stage IB disease (6). However, this therapy is associated with only a 16% decrease in the risk of disease recurrence or death; for 5 years, it is associated with a 5% decrease in the risk of death (7, 8).
Systemic therapy is pursued in the cases of patients with stage IV disease and in presence of metastases or in the presence of relapse after initial management.
Over a median follow-up of approximately five years, the percentage of patients who have disease recurrence or who die after surgery remains high (ranging from 45% among patients with stage IB disease to 76% among those with stage III disease), regardless of the use of postoperative chemotherapy (8). The opportunity for improving survival is pronounced in early-stage disease and is driving studies integrating targeted therapies and immune checkpoint inhibitors (ICIs). As a result, after the revolutionary data on the metastatic setting of epidermal growth factor receptor (EGFR) inhibitors (9–13), we, nowadays, have the impressive results of the Adaura trial, which led to an important improvement of disease-free survival (DFS) in a subset of patients with EGFR-mutated early-stage lung cancer when osimertinib was added as an adjuvant treatment to the main treatment for the duration of 3 years (14). Unfortunately, this subgroup is limited to only patients with EGFR-targetable mutations.
From the past decade to the present, with additional activating genomic alterations such as those affecting anaplastic lymphoma kinase (ALK), ROS1 proto-oncogene receptor tyrosine kinase, class 1 B-Raf proto-oncogene (BRAF) mutations (V600), mesenchymal-epithelial transition factor (MET), and neurotrophic receptor tyrosine kinase (NTRK) ALK, ROS1, B-Raf V600, MET, and NTRK alterations and the availability of an increasing number of specific tyrosine-kinase inhibitors (TKIs) of various generations, the proportion of patients with an improved prognosis has further increased (15–17).
The second pilar of the modern treatment of metastatic NSCLC is taken by immunotherapy (PD-1 and PD-L1 monoclonal antibodies), which is nowadays in the frontline of treatment in oncogenic driver–negative NSCLC and has produced response and survival rates that were unreachable a few years ago (18, 19).
Patients whose tumors express PD-L1 in at least 50% of the cells are more likely to attain a response and survive longer if treated with these compounds.
After breakthrough immune checkpoint inhibitor data in an advance setting, we now have the first results of immunotherapy in an adjuvant setting. The study IMpower 010 (20) addressed some of the unmet needs for adjuvant treatment oncodriver-negative tumors, adding immunotherapy in the plethora of new approvals in the early setting of NSCLC for patients expressing PD-L1 >1% on tumor cells (20).
In the meantime, immunotherapy continues to demonstrate a significant overall survival (OS) benefit in advanced NSCLC. In particular, pembrolizumab or atezolizumab monotherapies are superior to first-line chemotherapy in tumors with a higher expression of the PD-L1 molecule (21, 22). Interestingly, different chemo-immunotherapy combinations have been shown to be superior to chemotherapy, regardless of PD-L1 expression (23, 24).
Even though immunotherapy can produce great and long-lasting results, not all NSCLC patients seem to benefit from this approach (25). Many attempts have been made to identify predictive biomarkers to select responding patients who would benefit from ICIs. Tumor mutational burden (TMB) is a critical predictive factor for response to immunotherapy, but the available results need further confirmation in prospective randomized trials (26). A critical factor underlying the poor response to immunotherapies is the heterogeneity in the immune cell response to NSCLC and the existence of multiple mechanisms mediating tumor immune suppression (27). Indeed, a limited knowledge of the characteristics of the TME, to a great extent, hinders the development of new targets for immunotherapy. Here, we review the biological functions of immune cells within the tumor immune microenvironment (TIME) and their roles in cancer immunotherapy and discuss the perspectives of the basic and translational studies for improving the effectiveness of the clinical use.
Overview of NSCLC Tumor Immune Microenvironment
Taking advantage of new technologies (e.g., single-cell RNA sequencing), multiple ongoing studies are now identifying new subtypes of tumor-associated immune cells to predict the clinical efficacy of different immunotherapy approaches. The study of immune tumor cell contexture in cancer patients to target the multiple immune-suppressive factors might ameliorate response rates and contribute to develop the era of personalized immune-based therapies. The immune cell populations present in the TIME possess both tumor-killing potentials and may alternatively promote or suppress immune cell activity. Tolerogenic immune cell populations such as regulatory T cells (Tregs) and myeloid-derived suppressor cells (MDSCs) create an immune-suppressive milieu that, in turn, favors the polarization toward a protumor phenotype of other immune cells such as neutrophils, dendritic cells (DCs), and natural killer (NK) cells (Figure 1 and Table 1). Understanding these immunologic states and the mechanisms underpinning them may provide the key to restore an effective anti-tumor immune response and improve the survival rate of NSCLC patients.
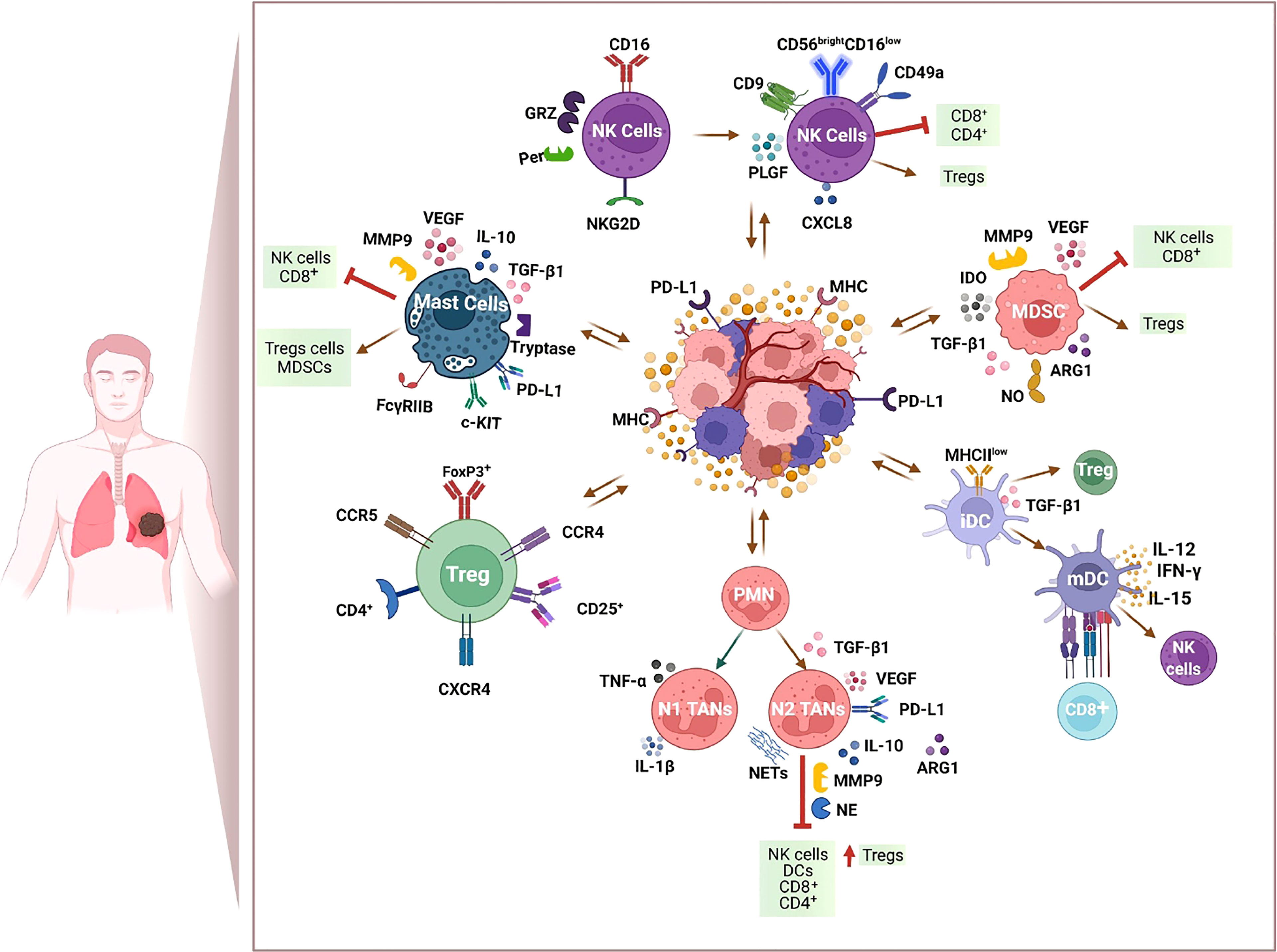
Figure 1 Immunosuppressive milieu within the tumor immune microenvironment (TIME) of NSCLC. Immunotherapy resistance is marked by an immunosuppressive TIME and includes tumor-derived factors, infiltration of T regulatory cells (Tregs), myeloid-derived suppressor cells (MDSCs), and mast cells that, in turn, favor the polarization toward a protumor phenotype of other immune cells such as neutrophils, dendritic cells (DCs), and natural killer (NK) cells. Figure created with http://biorender.com.
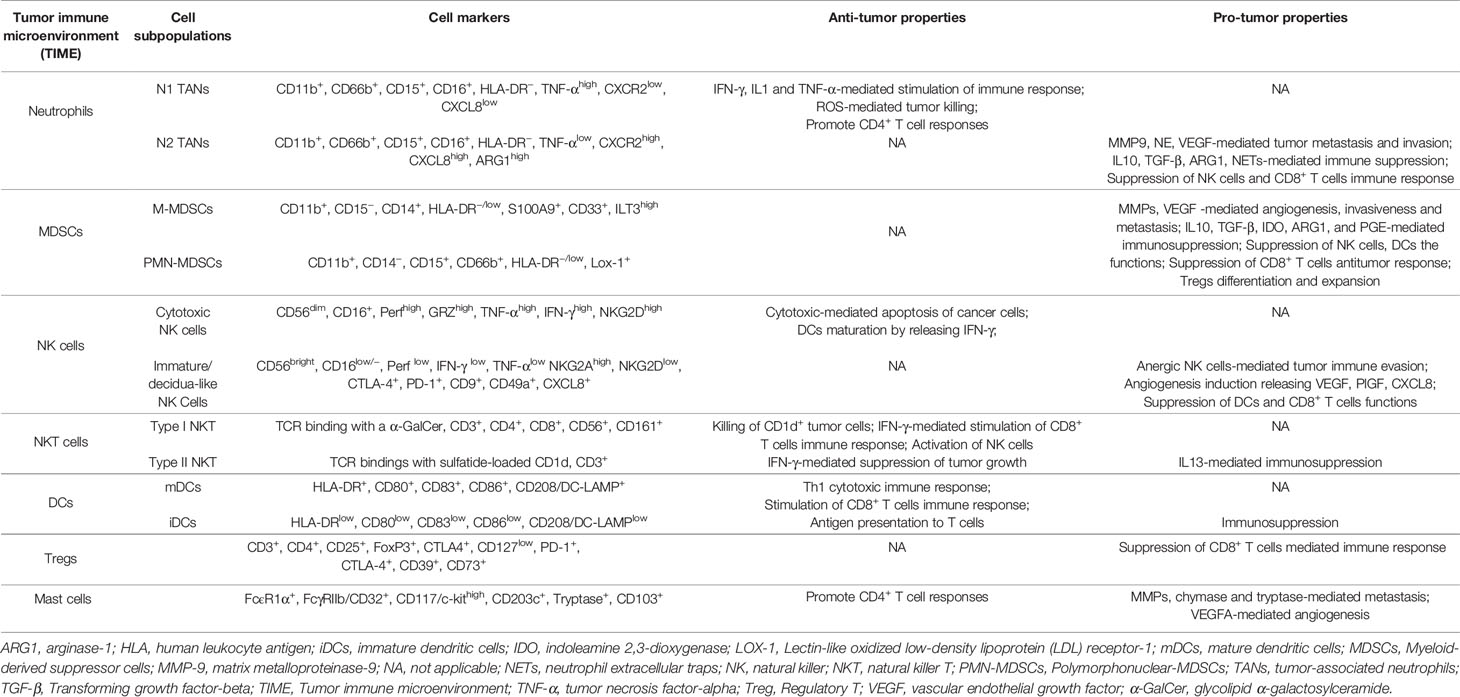
Table 1 NSCLC immune landscape: anti- and pro-tumorigenic phenotypes and activities of immune cell populations within the tumor microenvironment.
Neutrophils
Neutrophils are considered the first line of innate immune defense and are recognized as a critical targetable cellular feature of NSCLC TIME (28). Several preclinical and clinical studies have linked neutrophil trafficking and degranulation with various stages of tumor progression and the attenuation of treatment efficacy (29, 30). A high neutrophil/lymphocyte ratio (NLR) is now considered as a useful predictor associated with a negative clinical outcome, as well as with poor responsiveness to PD-1-/PD-L1 inhibitors (30). In accordance, Gentles et al. discovered that the neutrophil transcript signature was the strongest predictor of mortality and major infiltrating immune cells in adenocarcinoma NSCLC patients (31). In attempts to provide a clear description of the immune cell types present in NSCLC, Kargl et al. implicated neutrophils as the most abundant and dominant immune-suppressive factors associated with the depletion of CD4+ and CD8+ T lymphocytes within TIME (32). Consistent with previous findings, another study demonstrated a positive correlation between an increased tumor burden, high levels of neutrophil-related cytokines, and a dampened T-cell response associated with reduced CD3+CD8+ T-cell infiltration (33). However, the recruitment of neutrophils to the tumor microenvironment might depend on NSCLC subtypes and the smoking status, and larger studies are needed to define their role and association with survival (34).
Neutrophils in cancer consist of multiple heterogeneous cell populations and retain plasticity. A high expression of lectin-type oxidized LDL receptor 1 (LOX-1) distinguishes PMN-MDSCs from neutrophils (Table 1) (35). LOX-1+ PMN-MDSC numbers increased with anti-PD-1 therapy in non-responders, suggesting immunosuppressive functions in patients with NSCLC (36). A poor NSCLC prognosis and recurrence after surgery have been associated with increased circulating CD15+ LOX-1+ PMN-MDSCs, thus displaying potential as a diagnostic marker for NSCLC. In patients with advanced stages of lung cancer, there have been reports of the accumulation of low-density neutrophils (LDNs), CD66b+ PMNs, a subset of circulating neutrophils based on their sedimentation properties (37). Following tumor tissue infiltration and under specific tumor microenvironment cues, TANs can acquire a tumor- suppressive (N1) phenotype or become tumor-promoting/tolerogenic (N2) (Figure 1 and Table 1). At the early stages of tumor development, N1 TANs predominate. A cytotoxic action of TANs and tumor regression was reported in a recent work employing a patient-derived xenograft (PDX) mouse model of early-stage NSCLC that received anti-PD-1 ICI, as a monotherapy or with cisplatin (38).
However, tumor growth, together with a shifted balance between IFN-β and TGF-β, can favor N2 neutrophils and/or PMN-MDSC accumulation. The release of tumor recruitment–soluble factors, such as CXCL8, CXCL1, CXCL5, CXCL7, IL-6, and IL-1β, enhances immunosuppressive neutrophil chemotaxis through CXCR2 sensing, found to be highly expressed in NSCLC patients (39, 40). In a murine lung cancer model, CXCR1/2 neutrophil receptor inhibition granted access to CD8+ T cells to the malignant tumor. Notably, the IFN-γ signature was restored, thus overcoming neutrophil-mediated immunosuppression and an associated mitigation of the effectiveness of PD-1-targeted immunotherapy (41). N2 TANS enhances the immunosuppressive milieu by expressing high levels of PD-L1, arginase-1 (ARG1), reactive oxygen species (ROS), nitric oxide (NO), IL-10, and TGF-β1, shaping the tumor landscape and impairing T-cell-mediated cytotoxicity (42) The expression of both CXCL8 and Arg-1 by neutrophils is correlated with ICI therapy failure and poor prognosis in NSCLC (43, 44). N2 TANs also increase angiogenesis by releasing pro-angiogenic factors such as vascular endothelial growth factor (VEGF), enhance extracellular matrix (ECM) remodeling, and foster a pre-metastatic niche formation by directly acting as the primary source of proteolytic enzymes (45, 46). High levels of an MMP9:tissue inhibitor of metalloproteinase-3 (TIMP-3) ratio have been found significantly elevated in NSCLC biopsies. Furthermore, neutrophil elastase (NE) and myeloperoxidase (MPO) high degranulation induce the formation of neutrophil extracellular traps (NETs), directly implicated in metastasis (47, 48) (Figure 1). Accordingly, NETs are involved in a vascular endothelium injury mediated by an inflammatory response (48), as well as the wrapping and shielding of tumor cells from cytotoxicity mediated by CD8+ T cells and NK cells (47, 49). The Inhibition of NETosis sensitizes tumors to PD-1 plus CTLA-4 inhibition (47).
Several therapeutic strategies to suppress N2 tumor-promoting phenotypes or reactivate their cytotoxic features toward cancer cells are in preclinical and clinical phases of evaluation (28). Main neutrophil- targeting approaches neutralize tumor-derived chemokines, promoting their influx within the tumor microenvironment and conversion to an MDSC-like phenotype/N2 TANS. Two recent studies suggested that targeting MDSCs via the antagonism of GM-CSF and fatty acid transport protein 2 (FATP2) by using lipofermata decreased ROS and PGE2-levels and their immunosuppressive functions in tumor-bearing mice (50, 51). Importantly, FATP2 enhanced anti-PD-L1 tumor immunotherapy and inhibited tumor progression (50, 51). Metformin also targets FATP2, disabling the suppressive capacity of granulocytic myeloid-derived suppressor cells eliciting Th1 and cytotoxic T lymphocytes (CTLs) responses (52). Retrospective studies suggest the synergistic actions of metformin and conventional chemotherapy, improving the survival and outcomes of patients with NSCLC (53, 54). Targeting key immunosuppressive factors in TIME such as TGF-β1 and chemokine receptors CXCR1/2 through pharmacological antagonists represent some of the strategies to block the immunosuppressive milieu, leading to tumor growth and the nonsuccess of immunotherapies.
Myeloid-Derived Suppressor Cells
Myeloid-derived suppressor cells (MDSCs) represent a group of heterogeneous cells derived from immature myeloid progenitors with strong immunosuppressive features and functions. According to their phenotypic and morphological features, MDSCs have been classified into two major subsets: monocytic MDSCs (M-MDSCs) expressing CD14 and granulocytic or polymorphonuclear (PMN-MDSCs) expressing CD15 and CD66b; both types express CD33 in addition to CD11b with the absence of HLA-DR (Table 1). Even though PMN-MDSCs and neutrophils share similar phenotypic cell surface markers in humans, they have a distinct unique transcriptomic/phenotypic profile and functions that reflect the different roles within the tumor setting: PMN-MDSC but not neutrophils display immunosuppressive activities (55–57). A specific expression of LOX-1, fewer granules, and a reduced expression of CD16 can distinguish PMN-MDSCs phenotypically from neutrophils (Table 1) (35, 57, 58). In addition, neutrophils are high-density cells, whereas PMN-MDSCs are enriched in a low-density mononuclear cell fraction (55, 59). On the other hand, M-MDSCs can be distinguished from monocytes by detecting MHC class II, expressed only on monocytes (HLA-DR+) (60).
M-MDSCs and PMN-MDSCs share similar features; both enable immune response suppression but use different immunosuppressive mechanisms. For instance, PMN-MDSCs express high levels of ROS and low levels of NO, whereas M-MDSCs are the opposite. M-MDSCs preferentially exert their immunosuppressive functions by releasing IL-10, TGF-β, iNOS, and Arg-1 (56, 61).
Several studies have reported an accumulation of M-MDSCs in NSCLC patients (62–64). An increased pool of CD11b+CD14⁻CD15+CD33+ MDSCs and decreased CD8+ T cytotoxic lymphocytes have been reported in the peripheral blood of NSCLC patients (65). Another study reported a correlation between a subset of MDSC CD14+S100A9+, T-cell suppression mediated by arginase, iNOS, IL-13/IL-4Rα axis, and poor response to chemotherapy (66). Goeje et al. described increased levels of MDSCs expressing immunoglobulin-like transcript 3 (ILT3), identified as CD11b+CD14− CD33+CD15+ HLA-DR−ILT3high, associated with the immunosuppressive function of ILT3 on DCs and with reduced survival (67).
MDSCs are recruited to the tumor site via chemokines such as CCL2 and CXCL8 (68, 69). Of notice, CXCL8 is linked to the recruitment and activation of MDSCs and neutrophils. Indeed, the serum levels of CXCL8 may predict the responses to immunotherapies (68, 70, 71). TGF-β signaling has also been reported to promote the recruitment of MDSCs into tumors (72) and directly induce the generation of CD39+CD73+ myeloid cells in NSCLC patients via the activation of mTOR-HIF-1 signaling (73, 74) (Figure 1). CD39+CD73+ MDSCs are a distinct immunosuppressive subset, and their frequency in NSCLC patients may be sufficient to predict the chemotherapeutic response (74).
MDSCs are the largest producer of indoleamine 2,3-dioxygenase (IDO), directly acting on the immunosuppressive pathway of anti-tumor CD8+ T lymphocytes and the increase of Treg cell activity in the lung tumor microenvironment (75) (Figure 1). In a preclinical model of lung cancer, it was demonstrated that MDSC-associated IDO modulates the in vivo and ex vivo differentiation of B regulatory cells (Bregs), an IL-10 producing subset of B cells, found to be reduced in tumor-bearing IDO deficient mice (IDO-/-) (76). The anti-immune functions of MDSCs involve different mechanisms such as the production of NO, ROS, and the elimination of arginine required for T lymphocyte functions. In a KrasG12D GEMM of a lung adenocarcinoma model, the suppression of MDSC arginase activity by an ARG1 inhibitor restored T-cell function by increasing arginine (77). MDSCs could also enhance angiogenesis and metastasis through the production of MMP9 and VEGF (Figure 1). A tight association of PMN-MDSC number with a patient response to the ICI anti-PD-1 has been reported (36). An enhanced APC activity and increased frequency of CD8+ T or NK intracytoplasmic expression of IFN-γ, perforin, and granzyme were found following MDSC depletion (36). Accordingly, another study demonstrated an increased number and function of NK- and T-cell effectors in the tumor and enhanced therapeutic vaccination responses after the depletion of MDSCs (78). Therefore, the inhibition of MDSC functions represents the key therapeutic solution to restore anti-tumor T lymphocyte effector responses and successful immunotherapy. Until today, only a few preparations endorsed by the U.S. Food and Drug Administration (FDA) have been described to have prominent effects on the recruitment and function of MDSCs (e.g., ATRA, vitamin D, gemcitabine, and bevacizumab). Considering the promising results of targeting MDSCs in murine models of lung cancer, various clinical trials are now ongoing in NSCLC patients (NCT02922764; NCT03846310; NCT03801304; NCT04262388). Breakthroughs in this research area should promote the rational design of new strategies to target MDSCs to improve clinical responses to current immunotherapies.
Natural Killer Cells
NK cells represent innate effector lymphocytes with abilities to counteract or limit both tumor cells and virus-infected cells (79). In humans, the cell surface expression of the CD56 marker is the main phenotype marker for NK cells in association with a negative lineage-defining signature (CD3−, CD14−, CD19−, and TCR−), whereas in mice, it is the NK1.1 marker. NK cells do not need specific antigen stimulation but are activated toward neoplastic or stressed cells through the fine balance between multiple invariant activating and inhibitory receptors. The major inhibitory receptors are represented by the killer cell immunoglobulin–like receptor (KIR) family, which represents 17 distinct genes endowed with a high polymorphism, and the CD94/NKG2A heterodimer. Recognizing MHC Class I (MHC-I) molecules on a target cell, inhibitory receptors block NK cell activation (80).
When MHC-I are lost, or their expression is diminished, and this is the case of most tumor developments, NK cells become more susceptible to activation through the involvement of multiple activating receptors such as NKp30, NKp46, NKp44, CD16, NKG2D, DNAX accessory molecule1 (DNAM1), 2B4, and NKp80. Human peripheral blood NK cells can be classified into two subsets in relation to the expression of CD56 and CD16 markers: CD56dimCD16+ NK cells (comprising 90%–95% of total blood NK cells), characterized by their cytotoxic activity exerted by perforin and granzyme release and mediating antibody-dependent cellular cytotoxicity (ADCC) and CD56brightCD16– NK cells (5%–10% of total circulating NK cells), endowed with the capacity of proinflammatory cytokine production, such as IFN-γ and TNF-α and regulatory cytokines like IL-10 (81).
In NSCLC, it has been reported that intratumor NK cells profoundly modify their phenotype and functions, with the expansion of a CD56brightCD16– NK cell subset, impairment of cytotoxicity, inhibition of IFN-γ release, and acquisition of pro-angiogenic features (Table 1) (82, 83). This tumor-dependent NK cell subset has similarities with a different NK cell subset termed decidual NK (dNK) cells that was identified within decidua. This dNK cell subset was identified as CD56superbrightCD16– NK cell, and it was shown to be an important regulatory cell in the maternal–fetal interface because of its ability to release not only several pro-angiogenic cytokines and growth factors such as VEGF, PlGF, and CXCL8 but also IFN-γ, which are essential to driving the spiral artery formation during the embryo development (84).
Also, in the context of other types of solid cancers, NK cells accumulating within the tumor microenvironment had immature features and a CD56brightCD16low/−Perflow phenotype (Table 1).
Several soluble factors derived from tumor cells or neighboring innate immune or stromal cells can inhibit and alter NK-cell functions such as TGF-β, PGE2, IDO, adenosine, and IL-10 (85).
We were the first to characterize the decidual-like CD56brightCD16− of NSCLC patients with the ability to release pro-angiogenic factors: VEGF, PlGF, and CXCL8 (Figure 1) (Table 1). The NK-cell subset had the in vitro ability to trigger human umbilical vein endothelial cell (HUVEC) migration and the formation of capillary-like structures (86, 87). These peculiar functions are not restricted to the intratumoral NK cells but are also present in their peripheral blood counterpart, suggesting that vascular network induction occurs at a systemic level, too. Moreover, these pro-angiogenic features were detected at a higher intensity in NK cells from patients with squamous cell carcinomas than those with adenocarcinomas. Interestingly, the expansion of pro-angiogenic and decidual-like NK cells was also detected in malignant pleural effusions, colorectal cancer, and prostate cancer patients (88–90).
Recently, Russick et al. analyzed the gene expression profile of intratumoral NK cells and found that in comparison to non-tumorous NK cells, immune cells had a significant decrease of sphingosine-1-phosphate receptor 1 (S1PR1) and CX3CR1 with a concomitant increase of CXCR5 and CXCR6. Intriguingly, they also showed that intratumoral NK cells express inhibitory molecules: CTLA-4 and killer cell lectin like receptor (KLRC1), together with a high expression of CD69 and NKp44, conferring inhibitory capabilities in the context of TIME (91). Indeed, the co-culturing of purified NSCLC NK cells with tumor cells and CD11c+ peripheral blood autologous DC in the presence of LPS resulted in the impairment of DC maturation expressed as a percentage of MHC class II and CD86 on DCs. Interestingly, this phenomenon was partially counteracted by the addition of CTLA-4-blocking antibodies. However, the precise mechanism is still not clear, and beyond CTLA-4 expression, other mechanisms could be involved, such as yet-unidentified secreted molecules from NK-cell-derived tumor cells. However, in a tumor mouse model, another possible mechanism of NK-cell-dependent DC inhibition has been identified via PD-L1 with PD-1 expressed on DCs (92).
Moreover, a high intratumor density of NK cells is correlated with an improved clinical outcome only in patients with a low infiltration of CD8+ T cells, while in patients with elevated CD8+ T lymphocyte counts, NK cells conferred a negative impact (91). At later stages, lung tumoral NK cells showed significantly attenuated cytotoxicity, the reduction of levels of granzyme B, perforin, CD107a, IFN-γ, TNF-α, cytotoxic receptor CD27, activating receptor NKG2D, and a higher expression of the inhibitory receptor NKG2A (93).
Natural Killer T Cells
Natural killer T cells (NKT) cells are a subset of heterogeneous innate-like T lymphocytes CD1d-restricted, recognizing lipid antigens and co-expressing both the T-cell receptor and NK-cell markers, such as CD56, CD16, and NKp46 in humans and NKp46 and NK1.1 in mice. NKT cells can be subdivided into two major subsets: type I and type II NKT cells according to TCR rearrangements and glycolipid reactivity (94, 95).
Type I or invariant NKT (iNKT) cells are cytotoxic cells that express an invariant TCRα chain rearrangement, whereas TCRβ chains present a restricted repertoire. These cells include several subsets called NKT1, NKT2, and NKT17, with similarities to Th1, Th2, and Th17 T-cell subsets, respectively. Type II NKT cells, conversely, display a more diverse repertoire of Vα rearrangements (96, 97). Whereas it is well documented that iNKT cells participate in the anti-tumor response (98), type II NKT cells, on the contrary, enhance tumor growth and metastasis, thus indicating a pro-tumor activity.
The predominant anti-tumor feature of iNKT cells mainly resides in their capacity to release large amounts of Th1 cytokines, such as IFN-γ, in addition to their ability to kill CD1d-positive tumor cells (99, 100) (Table 1).
Several studies have shown a relationship between the number and activity of iNKT cells and clinical outcomes, making these cells an interesting therapeutic tool against cancer development and metastasis (99, 101).
However, in NSCLCs, it has been shown that iNKT cells were diminished both in blood and in bronchial lavage samples from patients (102). Moreover, the lung CD1d expression is lowered in NSCLC patients and weak CD1d mRNA expression is significantly associated with poor prognosis. Together, this could indicate a role played by these cells in immunity against NSCLC (102). In vitro studies using DNA methyltransferase and histone deacetylase inhibitors on two CD1d-negative NSCLC cell lines: A549 and SK-MES-1, showed the induction of CD1d expression and cytotoxicity directed toward them by iNKT cells, making epigenetic manipulation an interesting immunotherapeutic approach against NSCLC.
A study protocol was mentioned in an ongoing exploring phase I/II clinic trial on 30 patients with EGFR mutation–positive stage III/IV NSCLC that will evaluate the efficacy and safety of using allogeneic CD3+CD8+ iNKT cells in combination with EGFR-TKIs such as gefitinib (103).
Dendritic Cells
Dendritic cells (DCs) are antigen-presenting cells (APCs) and consist of three major subsets: myeloid conventional DC1s (cDC1s), myeloid conventional DC2s (cDC2s), and plasmacytoid DCs (pDCs) (104). Several lines of evidence point out that all DC subsets have the capacity to trigger anti-tumor T- cell responses and that DC1s need cooperativity with the other DC subsets (105). Interestingly, it has been shown that DC1s regulate the response to ICIs in mouse models and correlated with better OS in patients with cancer; however, DC1s can be expanded in tumors that resist checkpoint treatment, suggesting that these cells may be altered in their functions (106). Maier et al., using single-cell RNA sequencing in human and mouse NSCLC specimens identified a type of DCs nominated “mature DCs abundant in immunoregulatory factors” (mregDCs), which possessed both immunoregulatory genes (Cd200, Cd274, and Pdcd1lg2) and maturation genes (Cd40, Ccr7, and Il12b). The mregDC function was detected in both DC1 and DC2 subsets upon interaction with tumor antigens and can exert a dual role, both regulatory and immunogenic. It has been shown that the two key steps crucial for regulatory effects driven by mregDCs were the upregulation of PD-L1 and of IL-12, the first was under the control of the receptor tyrosine kinase AXL while the second under the control of IL-4 signaling (106).
Moreover, immature DCs (imDCs), which are present sometimes in high numbers in the tumor microenvironment, can coordinate an immunosuppressive microenvironment together with other innate cells, such as Tregs, MDSCs, and TAMs (Figure 1 and Table 1) (107, 108). Several lung patients could present tertiary lymphoid structures in the stroma of NSCLC, representing a well-organized compartment with lymphocytes and a rise in the density of DC-LAMP+ mature DCs, suggesting that these structures might participate in antitumoral immunity. Indeed, several studies showed that these structures were associated with a favorable clinical outcome, together with a Th1 cytotoxic immune response and effective infiltrating CD8+ T cells (109, 110).
Interestingly, Inoshima et al. reported an immunohistochemical study in which they analyzed 132 lung cancer specimens showing that a high expression of VEGF and microvessel density is associated with low intratumoral DC infiltration and worse prognosis, whereas low VEGF and high DC are correlated with a better prognosis (111). VEGF that could be produced not only by tumor cells but also by TAMs and NK cells, in addition to having a role in tumor vascular formation, also has a role as an inhibitory molecule for several classes of immune cells, including DCs. Therefore, the subtle regulatory mechanisms involved in the TIME between NK and DC interactions, not yet fully elucidated, as seen above via CTLA-4 or PD-1 on NK cells, could underlie the divergent functions of DCs and, in some cases, therefore lead to negative outcomes for the immune response, that is, the expansion of TAMs and Tregs, with a protumoral effect (91, 92).
Mast Cells
Mast cells are bone marrow–derived immune cells with multiple protective functions against invading microorganisms and harmful agents. These long-lived immune cells exert their regulatory functions in immunity and inflammation by producing key inflammatory mediators, such as tryptase, VEGF, IL-10, TGF-β1, and MMP9, and the relevant data of their anti-tumor or pro-tumor features have been reported (Figure 1 and Table 1). Interestingly, Fontanini et al. investigated the relationship between tumor angiogenesis and survival in 407 NSCLC patients (112). In this study, a worse prognosis was significantly correlated with the increase of the tumor blood vessel network. However, in 2007, a meta-analysis did not confirm an independent prognostic role of vascular density in patients with non-metastatic-treated NSCLC patients (113). The expression of VEGF-A, VEGF-C, and VEGFR-1 was associated with a worse outcome in patients with NSCLC (114). A significant prognostic value of the overexpression of FGF-2 has been reported in patients with operable NSCLC (115). Mast cells are correlated with angiogenesis and a poor outcome in lung adenocarcinoma (116, 117). Angiogenesis assessed by microvessel counts is related with a poor outcome in stage I NSCLC (112, 118–120). Other authors have shown no significant correlations with respect to survival in patients with NSCLC for microvessel density or mast cell infiltration. (121–127). Niczyporuk et al. did not show any correlation between the mast cell count, microvascular count, and survival rate in NSCLC (128). There is no correlation between intratumoral mast cells and angiogenesis in NSCLC (129) and between mast cells and survival in NSCLC (125).
Mast cells present in tumor cell islets are correlated with a marked survival advantage in NSCLC (130). Indeed, whereas mast cell numbers are similar in the tumor stroma of patients with surgically resected NSCLC with no difference to their survival status, there is a substantial survival advantage when mast cells are localized within the clusters of tumor epithelial cells or tumor cell islets (130, 131).
Furthermore, Tomita et al. (132) and Welsh et al. (130) determined a strict correlation between the number of mast cells and a good prognosis in NSCLC. Mast cells have a pro-tumorigenic effect on lung tumor cell lines and an anti-tumorigenic effect in vivo (133). Conversely, Stoyanov et al. (133) have reported a significant effect of mast cells and histamine in enhancing NSCLC cell proliferation in vitro, whereas in the Lewis lung mouse carcinoma model, they have found that mast cells are crucial negative regulators of cancer development.
Tregs
Regulatory T lymphocytes (Tregs) are involved in the homeostasis of the immune system, inhibiting autoimmune disorders. Moreover, these cells collaborate with other cells and factors in establishing immunosuppressive TIME (Figure 1) (134–136).
The transcription factor forkhead box P3 (FoxP3) is crucial for peripheral naïve T cells to become competent Treg cells (Table 1). In lung cancer, Foxp3+ Tregs, which suppress auto-reactive T cells to maintain immunological self-tolerance and inhibit autoimmunity, are associated with advanced tumor growth and poor prognosis (137–139). In patients with NSCLC, augmented numbers of blood and intratumoral Tregs are correlated with worse prognosis and a higher risk or recurrence (140).
Several investigations reported significantly higher percentages of CD4+CD25+FoxP3+ Tregs in patients with advanced metastatic NSCLC compared to healthy donors (141–144), whereas the high percentage of CD152+CD4+CD25highFoxP3+ Tregs is correlated with a more advanced stage of disease (141, 145). Moreover, two studies reported a prognostic value of blood CD4+FoxP3+ Tregs in stage I–III NSCLC patients (146, 147).
In NSCLC patients, CD4+CD25+ Treg subtype functions were associated with their FoxP3, CTLA-4, and IL-7Rα expression, and their blood levels were correlated with the clinical outcome of the patients. Conversely, no difference was found in the percentage of CD4+CD25+FoxP3+ Treg between the entire NSCLC patients and healthy donors (148). Interestingly Tao et al. (139) demonstrated that in NSCLC, there was no significant relationship between the Treg number and the tumor Foxp3 status. However, increased numbers of Tregs were associated with worse overall and relapse-free survival, whereas there was no correlation between the tumor FoxP3 status and survival. In the meantime, when FoxP3+ cells were detected within the tumor, the Treg expansion was correlated with the attenuation of worse prognosis. Conversely, the patients in which there was no tumor FoxP3 expression and elevated Treg count had significantly worse overall and relapse-free survival. Collectively, these findings suggest that tumor FoxP3 expression has a better prognostic potential in NSCLC and that, in combination with intratumoral Tregs, the absence of the tumor FoxP3 is correlated with high-risk patients.
The Lung TIME as a Target for Therapy
The microenvironment of lung cancers is heterogeneous and plays an important role in determining the outcome. The lungs present a unique milieu in which tumors progress in synergy with the TIME, as evidenced by the regions of aberrant angiogenesis, inflammation, and hypoxia. The altered vasculature seen in lung cancers contributes to hypoxia and makes it difficult to efficiently deliver agents through the bloodstream. Hypoxia is associated with an increased risk of metastases as well as resistance to radiation therapy and perhaps chemotherapy. Neutrophils dominate the tumor microenvironment of NSCLC, suppressing T cells and promoting immunosuppression. The multifaceted microenvironment of lung tumors represents many potential targets for the development of novel anticancer agents. As with other cancers, in NSCLC, chronic inflammation represents a major risk factor for the development and progression of cancer.
Tumor-infiltrating CD8+ T lymphocytes were associated with improved anti-tumor immunity, as well as with better prognosis in the advanced stage of NSCLC patients (149). Other cell types, such as TAMs and TANs and their subtypes, have their own prognostic effects in NSCLC (150). Furthermore, Tuminello et al. demonstrated the positive role of CD8+ T cytotoxic cells, CD20+ B cells, and NK cells with survival in patients with early resectable NSCLC (151).
The assessment of tumor inflammation is also of interest, but again, various approaches are being pursued, including a histological assessment of immune cell infiltrates and the mRNA-based expression signatures of immune-related genes. Increased numbers of antitumor CD8+ and CD4+ T cells have been associated with responding tumors and improved survival, whereas elevated frequencies of Tregs render tumors refractory to immune effector cells (152). The altered vasculature in NSCLC contributes to hypoxia and makes it difficult to efficiently deliver agents through the bloodstream. We have a variety of clinically applicable agents that can modulate the TIME in a way that might improve the response to cytotoxic therapy.
Molecular-targeted therapy represents a fundamental aspect in the treatment of advanced NSCLC. In the past few years, the identification of new molecular subtypes, the search for tumor driver gene mutations, and the development of molecular targeted drugs, such as agents that are able to suppress tumor angiogenesis and regulate tumor immune response, have been the main directions of NSCLC research, clinical diagnosis, and treatment.
In metastatic NSCLC, cytotoxic chemotherapy has been replaced with targeted therapy or immunotherapy. The gene mutation status of EGFR in the tumor tissues of NSCLC is closely related to the efficacy of the TKIs. Getifinib was the first EGFR-TKI tested in patients with advanced NSCLC. The discovery of EGFR mutations provided the biological explanation for the clinical predictors of response to EGFR-TKIs (153). Virtually, all EGFR mutation patients developed acquired resistance to therapy. EMT is implicated in mediating resistance to EGFR inhibitors, chemotherapy, and other targeted drugs in lung cancer (154). In NSCLC, invasive tumor growth is associated with a desmoplastic stroma reaction and the upregulation of EMT markers at the invasive front (155). The inflammatory component of the tumor microenvironment stimulates EMT in lung cancer by contributing to hypoxia, angiogenesis, and the different regulations of miRNAs (156).
Second-generation EGFR TKIs, including afatinib, dacomitinib, and neratinib, have been developed with the intent to delay or overcome acquired resistance (157). Afatinib and dacomitinib resulted in more efficacy than gefitinib in terms of progression-free survival (PFS) and the response rate, whereas gefitinib is associated with fewer side effects (157).
Immunotherapy with anti-PD-1/PD-L1 antibodies has modified the treatment of locally advanced and metastatic NSCLC. The approval of the anti-PD-1 agent pembrolizumab as a standard-of-care first-line treatment in selected patients has made PD-L1 immunohistochemistry a mandatory test in all patients with advanced NSCLC. Immunotherapy alone (pembrolizumab) or in combination with chemotherapy (pembrolizumab or atezolizumab) is the standard of care for first-line therapy in stage IV NSCLC.
In the mouse models of lung cancer, the anti-PD-L1 approach is associated with a rise in exhausted CD8+ T lymphocytes (158). At the same time, enhanced numbers of PD-1+CD8+ T lymphocytes were correlated with reduced survival in stage II and III patients (149). The increased expression of CD38 on T cells after PD-1/PD-L1 ICI favors to acquired resistance by inhibiting CD8+ T lymphocyte proliferation and inducing an exhausted phenotype (159). Koh et al. (160) analyzed the correlation between Foxp3+ T cells with clinical outcomes before and after anti-PD-1 immunotherapy in patients with advanced NSCLC and found that a higher frequency of blood Tregs 1 week after immunotherapy was associated with prolonged PFS and OS when compared with patients with a low frequency of Tregs. In the meantime, a high expression of TGF-β was correlated with high levels of Tregs and with a favorable clinical outcome.
Anti-Angiogenic Therapies
Angiogenesis has been strictly related with occurrence, proliferation, and metastasis (161). Targeting the angiogenesis process has been reported to be efficacious in diverse types of cancers, including NSCLC (22). Abnormal vasculature participates in the tumor escape. Anti-angiogenetic agents can normalize blood vessels and thereby reset the TIME from immunosuppressive into immunoreactive. Therefore, combining immunotherapy with anti-angiogenics seems to be a promising strategy for cancer treatments.
The mechanisms appear to be complex and quite a vicious circle where the abnormality of angiogenesis causes an increase in acidity, hypoxia, and interstitial pressure (161, 162), which, later on, are associated with modifications at the molecular and genetic level in blood vessel formation and proliferation, and thus exacerbating and feeding a hostile tumor microenvironment.
In clinical terms, we already have a few monoclonal antibodies approved by the FDA and EMA for the treatment of various cancer types (bevacizumab-binding to VEGF-A, ramucirumab-targeting VEGFR2). By inhibiting the interaction between the VEGF and VEGFR or targeting downstream signaling, these compounds could block tumor angiogenesis. Their efficacy has been proven as a combination therapy with other cytotoxic agents (carboplatin and paclitaxel plus bevacizumab (163), or docetaxel plus ramucirumab (164); meanwhile, as a monotherapy, it showed a limited therapeutic effect in cancer treatment (165).
Ideally, anti-angiogenesis reduces thevascular supply, and thereby impairs tumor cell replication by starving the tumor, but this phenomenon could also decrease the delivery of combination drugs.
Some recent attempts have been taken to solve this paradox. “Vessel normalization” stands at the basis of resetting the perfusion function and structure, enhancing the antitumor immune response by implementing immune cell infiltration (165–168). This procedure gives promises for anti-angiogenesis combined therapies.
Nonetheless, due to the cancer heterogeneity and the multiple aspects of the TIME, the global response rates to ICI therapy are still limited (169). One major factor decreasing the efficacy of ICIs seems to be the elevated recruited numbers of immunosuppressive cells and scarce infiltration of effector cells into the TIME (170).
Latest studies have indicated that pro-angiogenic factors in the tumor microenvironment favor and trigger the development of immunosuppressive cells, and in the meantime, neo vessels impair the infiltration of immune effector cell cancer (171–173).
The use of ICIs in combination with anti-angiogenic agents is hypothesized to be a promising strategy to enhance the global therapeutic efficacy.
There is a progressive and increased understanding on the possible effectivity of anti-angiogenic and IO combination. Nowadays, there are many preclinical and clinical trials suggesting that angiogenesis affects the TIME toward an immunosuppressive state by modifying the recruitment of immune cells (174–178). Later, clinical studies supported that the inhibition of the VEGF/VEGFR signaling can restore the anti-tumor T effector response (172). The use of bevacizumab (avastin) resulted in enhanced cytotoxic T lymphocyte functions in NSCSL as well as in CRC patients (179, 180).
It is well established that TIME is a complex, time-evolving ecosystem consisting not only of tumor cells but also of immune cell blood vessels, stroma cells, and different soluble factors, which turn off antitumor immune responses and favor ineffective immunotherapies (181).
Overstimulation by VEGF signaling in cancer leads to abnormal angiogenesis characterized by increased interstitial fluid pressure, hypoxia, and acidosis. This phenomenon leads to the suppression of the antitumor response through multiple distinct mechanisms (182, 183).
Hypoxia facilitates the infiltration of suppressive immune cells (Tregs, MDSCs, TAMs, and imDCs) by inducing the expression of chemokines (like CSF1, GM-CSF, IL-6, and IL-10) that recruit these immune cells (184); on the other hand, it also inhibits the infiltration of effector T cells through the activation of VEGF (185).
The stimulation and regulation of several key immune cells of TIME such as DCs, MDSCs, Tregs, and TAMs are under the control of VEGF signaling (186, 187). Immunosuppressive factors IL-10, IDO, and TGF-β released by these suppressive immune cells increase even more the immunosuppressive status of TIME (188).
Noteworthy, the inhibition of the VEGF signaling impairs the recruitment of suppressive cells into the tumor microenvironment and, at the same time, increases the infiltration of effector T cells (189). This fact implies that anti-VEGF/VEGFR therapy not only targets the blood vessel function but has the capacity to reactivate antitumor immune responses (173).
In addition to the above negative effects played by VEGF, another effect is related to their capacity to influence an enhanced expression of PD-1, Tim3, and CTLA-4 on activated CD8+ T lymphocytes (190). Moreover, VEGF inhibition could result in enhanced IFN-γ production and consequently the induction of PD-L1 expression on tumor cells. This phenomenon provides a strong promise for the anti-angiogenic and ICI drug combined treatment (172, 173).
Currently, we already have the clinical data of a phase III trial Impower 150 (191), which showed a clinical benefit of the combination of IO and bevacizumab plus chemotherapy in NSCLC; in the meantime, other clinical trials are ongoing to assess the safety and efficacy of this new combination therapy in NSCLC (NCT01454102 (CM 012), NCT03689855 (RamAtezo-1), NCT03836066 (TELMA), and others.
Immunotherapeutic Approaches
Current ICIs directed to CD28-CTLA4/B7 and PD-1/PD-L1 can unleash the power of T cells toward cancer cells by eliminating negative signals that block T-cell functions (192) (193, 194).
Several immune cells such as T cells, NK cells, B cells, and monocytes express PD-1 (195).
Monoclonal antibodies against PD-1, PD-L1, and CTLA-4 are the most used ICIs for NSCLC patients. A number of PD-1, PD-L1, and CTLA-4 inhibitors, including pembrolizumab (196), nivolumab (197), atezolizumab (198), durvalumab (199), avelumab (200), and ipilimumab (201), have been approved for the treatment of advanced NSCLC.
The anti-PD-1 agent pembrolizumab is approved for use as first- and second-line therapy in patients with advanced NSCLC whose tumors express PD-L1 in immunohistochemistry analysis. Nivolumab (anti-PD-1) and atezolizumab (anti-PD-L1) are both indicated for use as second-line therapies regardless of PD-L1 expression. Durvalumab (anti-PD-L1) is approved as a maintenance therapy in patients with unresectable stage III NSCLC whose disease has not progressed following concurrent platinum-based chemoradiotherapy.
Five randomized phase II–III trials testing three ICIs (nivolumab, pembrolizumab, and atezolizumab), all showed a clinically and statistically significant advantage over the same standard comparator docetaxel (21, 22, 197, 202, 203)
ICIs were tested in locoregional NSCLC. A phase III trial demonstrated that adjuvant durvalumab in stage III NSCLC non-progressing after concomitant chemo-radiotherapy improved not only PFS but also OS (204).
Pembrolizumab and nivolumab approval is strictly related with a positive PD-L1 expression.
Checkpoint inhibitors can be used as a combination therapy or as a monotherapy in first- and second-line treatments. The Pacific trial (205) brought immunotherapy in a locally advanced setting and later on, with the publication of IMpower 010 (20), immunotherapy will probably be a practice changing even in early-stage lung cancer.
Challenges and Future Directions
A prognostic role of many TIME biomarkers is not yet part of the current clinical practice, so further investigations that include larger patient cohorts will be necessary.
ICI alone or in combination with chemotherapy or in combination with other ICIs should be the first-line treatment of choice for patients with advanced NSCLC who do not have contraindications to immunotherapy and whose tumors do not harbor actionable driver mutations. Advances with immunotherapy have offered patients with lung cancer substantial improvements in survival and the quality of life. However, better predictive biomarkers are required to ameliorate the benefit of immunotherapy, and further investigations are needed to find out the mechanisms of resistance to ICIs and how to overcome it. Whereas the PD-1 and PD-L1 ICIs have received accelerated FDA approvals, the development of predictive and prognostic biomarkers for these agents have lagged far behind and remains a crucial area for future research.
The ability to increase the clinical benefit for higher numbers of NSCLC patients and preventing drug resistance will be essential prerequisites to achieve in the near future and related to the acquisition of more knowledge of the induced mechanisms underlying effective antitumor effector responses. The next step will be to better identify patients at the risk of primary or acquired resistance and use increasing amounts of translational research data to develop more effective combination therapies, making the promise of ICIs available to all patients with NSCLC. This is the only way to achieve further advances in cancer immunotherapy and succeed in making the promise of ICIs for all patients.
Author Contributions
Conceptualization: DB, DR and LM. Text drafting and editing: DB, EC, AI, DR, and LM. Critical revision: DB, EC, AI, and LM. Figure and table preparation: DB. Funds: DB and LM. All authors contributed to the article and approved the submitted version.
Funding
This study was supported by the Italian Ministry of Health-Grant Giovani Ricercatori 2019 (GR-019-12370076) to DB, and by Fondi di Ateneo per la Ricerca FAR2019 and FAR2020, University of Insubria to LM.
Conflict of Interest
The authors declare that the research was conducted in the absence of any commercial or financial relationships that could be construed as a potential conflict of interest.
Publisher’s Note
All claims expressed in this article are solely those of the authors and do not necessarily represent those of their affiliated organizations, or those of the publisher, the editors and the reviewers. Any product that may be evaluated in this article, or claim that may be made by its manufacturer, is not guaranteed or endorsed by the publisher.
Acknowledgments
The authors are grateful to Dr. Raffaella Bombelli and Dr. Marì De Leo for their helpful comments in the preparation of the manuscript.
References
1. Jemal A, Center MM, DeSantis C, Ward EM. Global Patterns of Cancer Incidence and Mortality Rates and Trends. Cancer Epidemiol Biomarkers Prev (2010) 19(8):1893–907. doi: 10.1158/1055-9965.EPI-10-0437
2. Siegel RL, Miller KD, Jemal A. Cancer Statistics, 2020. CA Cancer J Clin (2020) 70(1):7–30. doi: 10.3322/caac.21590
3. Giovino GA, Mirza SA, Samet JM, Gupta PC, Jarvis MJ, Bhala N, et al. Tobacco Use in 3 Billion Individuals From 16 Countries: An Analysis of Nationally Representative Cross-Sectional Household Surveys. Lancet (2012) 380(9842):668–79. doi: 10.1016/S0140-6736(12)61085-X
4. Howlader N, Forjaz G, Mooradian MJ, Meza R, Kong CY, Cronin KA, et al. The Effect of Advances in Lung-Cancer Treatment on Population Mortality. N Engl J Med (2020) 383(7):640–9. doi: 10.1056/NEJMoa1916623
5. Travis WD, Brambilla E, Nicholson AG, Yatabe Y, Austin JHM, Beasley MB, et al. The 2015 World Health Organization Classification of Lung Tumors: Impact of Genetic, Clinical and Radiologic Advances Since the 2004 Classification. J Thorac Oncol (2015) 10(9):1243–60. doi: 10.1097/JTO.0000000000000630
6. Ghysen K, Vansteenkiste J. Immunotherapy in Patients With Early Stage Resectable Nonsmall Cell Lung Cancer. Curr Opin Oncol (2019) 31(1):13–7. doi: 10.1097/CCO.0000000000000497
7. Kris MG, Gaspar LE, Chaft JE, Kennedy EB, Azzoli CG, Ellis PM, et al. Adjuvant Systemic Therapy and Adjuvant Radiation Therapy for Stage I to IIIA Completely Resected Non-Small-Cell Lung Cancers: American Society of Clinical Oncology/Cancer Care Ontario Clinical Practice Guideline Update. J Clin Oncol (2017) 35(25):2960–74. doi: 10.1200/JCO.2017.72.4401
8. Pignon JP, Tribodet H, Scagliotti GV, Douillard JY, Shepherd FA, Stephens RJ, et al. Lung Adjuvant Cisplatin Evaluation: A Pooled Analysis by the LACE Collaborative Group. J Clin Oncol (2008) 26(21):3552–9. doi: 10.1200/JCO.2007.13.9030
9. Rosell R, Carcereny E, Gervais R, Vergnenegre A, Massuti B, Felip E, et al. Erlotinib Versus Standard Chemotherapy as First-Line Treatment for European Patients With Advanced EGFR Mutation-Positive Non-Small-Cell Lung Cancer (EURTAC): A Multicentre, Open-Label, Randomised Phase 3 Trial. Lancet Oncol (2012) 13(3):239–46. doi: 10.1016/S1470-2045(11)70393-X
10. Mok TS, Wu YL, Thongprasert S, Yang CH, Chu DT, Saijo N, et al. Gefitinib or Carboplatin-Paclitaxel in Pulmonary Adenocarcinoma. N Engl J Med (2009) 361(10):947–57. doi: 10.1056/NEJMoa0810699
11. Sequist LV, Yang JC, Yamamoto N, O'Byrne K, Hirsh V, Mok T, et al. Phase III Study of Afatinib or Cisplatin Plus Pemetrexed in Patients With Metastatic Lung Adenocarcinoma With EGFR Mutations. J Clin Oncol (2013) 31(27):3327–34. doi: 10.1200/JCO.2012.44.2806
12. Lee CK, Davies L, Wu YL, Mitsudomi T, Inoue A, Rosell R, et al. Gefitinib or Erlotinib vs Chemotherapy for EGFR Mutation-Positive Lung Cancer: Individual Patient Data Meta-Analysis of Overall Survival. J Natl Cancer Inst (2017) 109(6). doi: 10.1093/jnci/djw279
13. Kanitkar AA, Schwartz AG, George J, Soubani AO. Causes of Death in Long-Term Survivors of Non-Small Cell Lung Cancer: A Regional Surveillance, Epidemiology, and End Results Study. Ann Thorac Med (2018) 13(2):76–81. doi: 10.4103/atm.ATM_243_17
14. Wu YL, Tsuboi M, He J, John T, Grohe C, Majem M, et al. Osimertinib in Resected EGFR-Mutated Non-Small-Cell Lung Cancer. N Engl J Med (2020) 383(18):1711–23. doi: 10.1056/NEJMoa2027071
15. Hida T, Nokihara H, Kondo M, Kim YH, Azuma K, Seto T, et al. Alectinib Versus Crizotinib in Patients With ALK-Positive Non-Small-Cell Lung Cancer (J-ALEX): An Open-Label, Randomised Phase 3 Trial. Lancet (2017) 390(10089):29–39. doi: 10.1016/S0140-6736(17)30565-2
16. Shaw AT, Felip E, Bauer TM, Besse B, Navarro A, Postel-Vinay S, et al. Lorlatinib in Non-Small-Cell Lung Cancer With ALK or ROS1 Rearrangement: An International, Multicentre, Open-Label, Single-Arm First-in-Man Phase 1 Trial. Lancet Oncol (2017) 18(12):1590–9. doi: 10.1016/S1470-2045(17)30680-0
17. Camidge DR, Kim HR, Ahn MJ, Yang JCH, Han JY, Hochmair MJ, et al. Brigatinib Versus Crizotinib in Advanced ALK Inhibitor-Naive ALK-Positive Non-Small Cell Lung Cancer: Second Interim Analysis of the Phase III ALTA-1l Trial. J Clin Oncol (2020) 38(31):3592–603. doi: 10.1200/JCO.20.00505
18. Califano R, Gomes F, Ackermann CJ, Rafee S, Tsakonas G, Ekman S. Immune Checkpoint Blockade for non-Small Cell Lung Cancer: What is the Role in the Special Populations? Eur J Cancer (2020) 125:1–11. doi: 10.1016/j.ejca.2019.11.010
19. Xia B, Herbst RS. Immune Checkpoint Therapy for non-Small-Cell Lung Cancer: An Update. Immunotherapy (2016) 8(3):279–98. doi: 10.2217/imt.15.123
20. Felip E, Altorki N, Zhou C, Csoszi T, Vynnychenko I, Goloborodko O, et al. Adjuvant Atezolizumab After Adjuvant Chemotherapy in Resected Stage IB-IIIA Non-Small-Cell Lung Cancer (IMpower010): A Randomised, Multicentre, Open-Label, Phase 3 Trial. Lancet (2021) 398(10308):1344–57. doi: 10.1016/S0140-6736(21)02098-5
21. Herbst RS, Baas P, Kim DW, Felip E, Perez-Gracia JL, Han JY, et al. Pembrolizumab Versus Docetaxel for Previously Treated, PD-L1-Positive, Advanced Non-Small-Cell Lung Cancer (KEYNOTE-010): A Randomised Controlled Trial. Lancet (2016) 387(10027):1540–50. doi: 10.1016/S0140-6736(15)01281-7
22. Reck M, Rodriguez-Abreu D, Robinson AG, Hui R, Csoszi T, Fulop A, et al. Pembrolizumab Versus Chemotherapy for PD-L1-Positive Non-Small-Cell Lung Cancer. N Engl J Med (2016) 375(19):1823–33. doi: 10.1056/NEJMoa1606774
23. Gandhi L, Rodríguez-Abreu D, Gadgeel S, Esteban E, Felip E, De Angelis F, et al. Pembrolizumab Plus Chemotherapy in Metastatic Non–Small-Cell Lung Cancer. New Engl J Med (2018) 378(22):2078–92. doi: 10.1056/NEJMoa1801005
24. Paz-Ares L, Luft A, Vicente D, Tafreshi A, Gumus M, Mazieres J, et al. Pembrolizumab Plus Chemotherapy for Squamous Non-Small-Cell Lung Cancer. N Engl J Med (2018) 379(21):2040–51. doi: 10.1056/NEJMoa1810865
25. Massett HA, Mishkin G, Moscow JA, Gravell A, Steketee M, Kruhm M, et al. Transforming the Early Drug Development Paradigm at the National Cancer Institute: The Formation of NCI's Experimental Therapeutics Clinical Trials Network (ETCTN). Clin Cancer Res (2019) 25(23):6925–31. doi: 10.1158/1078-0432.CCR-19-1754
26. Addeo A, Banna GL, Weiss GJ. Tumor Mutation Burden-From Hopes to Doubts. JAMA Oncol (2019) 5(7):934–5. doi: 10.1001/jamaoncol.2019.0626
27. Lei X, Lei Y, Li JK, Du WX, Li RG, Yang J, et al. Immune Cells Within the Tumor Microenvironment: Biological Functions and Roles in Cancer Immunotherapy. Cancer Lett (2020) 470:126–33. doi: 10.1016/j.canlet.2019.11.009
28. Anderson R, Blidner AG, Rapoport BL. Frontiers in Pharmacology: Review Manuscript Targeting of the Neutrophil as an Adjunctive Strategy in Non-Small Cell Lung Cancer. Front Pharmacol (2021) 12. doi: 10.3389/fphar.2021.676399
29. Huang Y, Shen A. The Prediction Potential of Neutrophil-to-Lymphocyte Ratio for the Therapeutic Outcomes of Programmed Death Receptor-1/Programmed Death Ligand 1 Inhibitors in Non-Small Cell Lung Cancer Patients: A Meta-Analysis. Med (Baltimore) (2020) 99(34):e21718. doi: 10.1097/MD.0000000000021718
30. Rapoport BL, Theron AJ, Vorobiof DA, Langenhoven L, Hall JM, Van Eeden RI, et al. Prognostic Significance of the Neutrophil/Lymphocyte Ratio in Patients Undergoing Treatment With Nivolumab for Recurrent non-Small-Cell Lung Cancer. Lung Cancer Manage (2020) 9(3):LMT37. doi: 10.2217/lmt-2020-0014
31. Gentles AJ, Newman AM, Liu CL, Bratman SV, Feng W, Kim D, et al. The Prognostic Landscape of Genes and Infiltrating Immune Cells Across Human Cancers. Nat Med (2015) 21(8):938–45. doi: 10.1038/nm.3909
32. Kargl J, Busch SE, Yang GH, Kim KH, Hanke ML, Metz HE, et al. Neutrophils Dominate the Immune Cell Composition in Non-Small Cell Lung Cancer. Nat Commun (2017) 8:14381. doi: 10.1038/ncomms14381
33. Mitchell KG, Diao L, Karpinets T, Negrao MV, Tran HT, Parra ER, et al. Neutrophil Expansion Defines an Immunoinhibitory Peripheral and Intratumoral Inflammatory Milieu in Resected Non-Small Cell Lung Cancer: A Descriptive Analysis of a Prospectively Immunoprofiled Cohort. J Immunother Cancer (2020) 8(1). doi: 10.1136/jitc-2019-000405
34. Tamminga M, Hiltermann TJN, Schuuring E, Timens W, Fehrmann RS, Groen HJ. Immune Microenvironment Composition in non-Small Cell Lung Cancer and Its Association With Survival. Clin Transl Immunol (2020) 9(6):e1142. doi: 10.1002/cti2.1142
35. Condamine T, Dominguez GA, Youn JI, Kossenkov AV, Mony S, Alicea-Torres K, et al. Lectin-Type Oxidized LDL Receptor-1 Distinguishes Population of Human Polymorphonuclear Myeloid-Derived Suppressor Cells in Cancer Patients. Sci Immunol (2016) 1(2). doi: 10.1126/sciimmunol.aaf8943
36. Kim HR, Park SM, Seo SU, Jung I, Yoon HI, Gabrilovich DI, et al. The Ratio of Peripheral Regulatory T Cells to Lox-1(+) Polymorphonuclear Myeloid-Derived Suppressor Cells Predicts the Early Response to Anti-PD-1 Therapy in Patients With Non-Small Cell Lung Cancer. Am J Respir Crit Care Med (2019) 199(2):243–6. doi: 10.1164/rccm.201808-1502LE
37. Shaul ME, Eyal O, Guglietta S, Aloni P, Zlotnik A, Forkosh E, et al. Circulating Neutrophil Subsets in Advanced Lung Cancer Patients Exhibit Unique Immune Signature and Relate to Prognosis. FASEB J (2020) 34(3):4204–18. doi: 10.1096/fj.201902467R
38. Martin-Ruiz A, Fiuza-Luces C, Martinez-Martinez E, Arias CF, Gutierrez L, Ramirez M, et al. Effects of Anti-PD-1 Immunotherapy on Tumor Regression: Insights From a Patient-Derived Xenograft Model. Sci Rep (2020) 10(1):7078. doi: 10.1038/s41598-020-63796-w
39. Saintigny P, Massarelli E, Lin S, Ahn YH, Chen Y, Goswami S, et al. CXCR2 Expression in Tumor Cells Is a Poor Prognostic Factor and Promotes Invasion and Metastasis in Lung Adenocarcinoma. Cancer Res (2013) 73(2):571–82. doi: 10.1158/0008-5472.CAN-12-0263
40. Yuan M, Zhu H, Xu J, Zheng Y, Cao X, Liu Q. Tumor-Derived CXCL1 Promotes Lung Cancer Growth via Recruitment of Tumor-Associated Neutrophils. J Immunol Res (2016) 2016:6530410. doi: 10.1155/2016/6530410
41. Kargl J, Zhu X, Zhang H, Yang GHY, Friesen TJ, Shipley M, et al. Neutrophil Content Predicts Lymphocyte Depletion and Anti-PD1 Treatment Failure in NSCLC. JCI Insight (2019) 4(24). doi: 10.1172/jci.insight.130850
42. Jaillon S, Ponzetta A, Di Mitri D, Santoni A, Bonecchi R, Mantovani A. Neutrophil Diversity and Plasticity in Tumour Progression and Therapy. Nat Rev Cancer (2020) 20(9):485–503. doi: 10.1038/s41568-020-0281-y
43. Schalper KA, Carleton M, Zhou M, Chen T, Feng Y, Huang SP, et al. Elevated Serum Interleukin-8 Is Associated With Enhanced Intratumor Neutrophils and Reduced Clinical Benefit of Immune-Checkpoint Inhibitors. Nat Med (2020) 26(5):688–92. doi: 10.1038/s41591-020-0856-x
44. Chen JJ, Yao PL, Yuan A, Hong TM, Shun CT, Kuo ML, et al. Up-Regulation of Tumor Interleukin-8 Expression by Infiltrating Macrophages: Its Correlation With Tumor Angiogenesis and Patient Survival in non-Small Cell Lung Cancer. Clin Cancer Res (2003) 9(2):729–37.
45. Vannitamby A, Seow HJ, Anderson G, Vlahos R, Thompson M, Steinfort D, et al. Tumour-Associated Neutrophils and Loss of Epithelial PTEN can Promote Corticosteroid-Insensitive MMP-9 Expression in the Chronically Inflamed Lung Microenvironment. Thorax (2017) 72(12):1140–3. doi: 10.1136/thoraxjnl-2016-209389
46. Moroy G, Alix AJ, Sapi J, Hornebeck W, Bourguet E. Neutrophil Elastase as a Target in Lung Cancer. Anticancer Agents Med Chem (2012) 12(6):565–79. doi: 10.2174/187152012800617696
47. Teijeira A, Garasa S, Gato M, Alfaro C, Migueliz I, Cirella A, et al. CXCR1 and CXCR2 Chemokine Receptor Agonists Produced by Tumors Induce Neutrophil Extracellular Traps That Interfere With Immune Cytotoxicity. Immunity (2020) 52(5):856–71.e8. doi: 10.1016/j.immuni.2020.03.001
48. Cools-Lartigue J, Spicer J, McDonald B, Gowing S, Chow S, Giannias B, et al. Neutrophil Extracellular Traps Sequester Circulating Tumor Cells and Promote Metastasis. J Clin Invest (2013) (8):3446–58. doi: 10.1158/1538-7445.AM2012-2972
49. Demers M, Wong SL, Martinod K, Gallant M, Cabral JE, Wang Y, et al. Priming of Neutrophils Toward NETosis Promotes Tumor Growth. Oncoimmunology (2016) 5(5):e1134073. doi: 10.1080/2162402X.2015.1134073
50. Adeshakin AO, Liu W, Adeshakin FO, Afolabi LO, Zhang M, Zhang G, et al. Regulation of ROS in Myeloid-Derived Suppressor Cells Through Targeting Fatty Acid Transport Protein 2 Enhanced Anti-PD-L1 Tumor Immunotherapy. Cell Immunol (2021) 362:104286. doi: 10.1016/j.cellimm.2021.104286
51. Veglia F, Tyurin VA, Blasi M, De Leo A, Kossenkov AV, Donthireddy L, et al. Fatty Acid Transport Protein 2 Reprograms Neutrophils in Cancer. Nature (2019) 569(7754):73–8. doi: 10.1038/s41586-019-1118-2
52. Xu P, Yin K, Tang X, Tian J, Zhang Y, Ma J, et al. Metformin Inhibits the Function of Granulocytic Myeloid-Derived Suppressor Cells in Tumor-Bearing Mice. BioMed Pharmacother (2019) 120:109458. doi: 10.1016/j.biopha.2019.109458
53. Luo X, Chen X, Wang L, Yang B, Cai S. Metformin Adjunct With Antineoplastic Agents for the Treatment of Lung Cancer: A Meta-Analysis of Randomized Controlled Trials and Observational Cohort Studies. Front Pharmacol (2021) 12. doi: 10.3389/fphar.2021.639016
54. Levy A, Doyen J. Metformin for non-Small Cell Lung Cancer Patients: Opportunities and Pitfalls. Crit Rev Oncol Hematol (2018) 125:41–7. doi: 10.1016/j.critrevonc.2018.03.001
55. Dumitru CA, Moses K, Trellakis S, Lang S, Brandau S. Neutrophils and Granulocytic Myeloid-Derived Suppressor Cells: Immunophenotyping, Cell Biology and Clinical Relevance in Human Oncology. Cancer Immunol Immunother (2012) 61(8):1155–67. doi: 10.1007/s00262-012-1294-5
56. Veglia F, Sanseviero E, Gabrilovich DI. Myeloid-Derived Suppressor Cells in the Era of Increasing Myeloid Cell Diversity. Nat Rev Immunol (2021) 21(8):485–98. doi: 10.1038/s41577-020-00490-y
57. Zhou J, Nefedova Y, Lei A, Gabrilovich D. Neutrophils and PMN-MDSC: Their Biological Role and Interaction With Stromal Cells. Semin Immunol (2018) 35:19–28. doi: 10.1016/j.smim.2017.12.004
58. Bronte V, Brandau S, Chen SH, Colombo MP, Frey AB, Greten TF, et al. Recommendations for Myeloid-Derived Suppressor Cell Nomenclature and Characterization Standards. Nat Commun (2016) 7:12150. doi: 10.1038/ncomms12150
59. Kotsakis A, Harasymczuk M, Schilling B, Georgoulias V, Argiris A, Whiteside TL. Myeloid-Derived Suppressor Cell Measurements in Fresh and Cryopreserved Blood Samples. J Immunol Methods (2012) 381(1-2):14–22. doi: 10.1016/j.jim.2012.04.004
60. Mandruzzato S, Brandau S, Britten CM, Bronte V, Damuzzo V, Gouttefangeas C, et al. Toward Harmonized Phenotyping of Human Myeloid-Derived Suppressor Cells by Flow Cytometry: Results From an Interim Study. Cancer Immunol Immunother (2016) 65(2):161–9. doi: 10.1007/s00262-015-1782-5
61. Gabrilovich DI. Myeloid-Derived Suppressor Cells. Cancer Immunol Res (2017) 5(1):3–8. doi: 10.1158/2326-6066.CIR-16-0297
62. Zahran AM, Hetta HF, Zahran ZAM, Rashad A, Rayan A, Mohamed DO, et al. Prognostic Role of Monocytic Myeloid-Derived Suppressor Cells in Advanced Non-Small-Cell Lung Cancer: Relation to Different Hematologic Indices. J Immunol Res (2021) 2021:3241150. doi: 10.1155/2021/3241150
63. Huang A, Zhang B, Wang B, Zhang F, Fan KX, Guo YJ. Increased CD14(+)HLA-DR (-/Low) Myeloid-Derived Suppressor Cells Correlate With Extrathoracic Metastasis and Poor Response to Chemotherapy in Non-Small Cell Lung Cancer Patients. Cancer Immunol Immunother (2013) 62(9):1439–51. doi: 10.1007/s00262-013-1450-6
64. Vetsika EK, Koinis F, Gioulbasani M, Aggouraki D, Koutoulaki A, Skalidaki E, et al. A Circulating Subpopulation of Monocytic Myeloid-Derived Suppressor Cells as an Independent Prognostic/Predictive Factor in Untreated non-Small Lung Cancer Patients. J Immunol Res (2014) 2014:659294. doi: 10.1155/2014/659294
65. Liu CY, Wang YM, Wang CL, Feng PH, Ko HW, Liu YH, et al. Population Alterations of L-Arginase- and Inducible Nitric Oxide Synthase-Expressed CD11b+/CD14(-)/CD15+/CD33+ Myeloid-Derived Suppressor Cells and CD8+ T Lymphocytes in Patients With Advanced-Stage Non-Small Cell Lung Cancer. J Cancer Res Clin Oncol (2010) 136(1):35–45. doi: 10.1007/s00432-009-0634-0
66. Feng PH, Lee KY, Chang YL, Chan YF, Kuo LW, Lin TY, et al. CD14(+)S100A9(+) Monocytic Myeloid-Derived Suppressor Cells and Their Clinical Relevance in Non-Small Cell Lung Cancer. Am J Respir Crit Care Med (2012) 186(10):1025–36. doi: 10.1164/rccm.201204-0636OC
67. de Goeje PL, Bezemer K, Heuvers ME, Dingemans AC, Groen HJ, Smit EF, et al. Immunoglobulin-Like Transcript 3 is Expressed by Myeloid-Derived Suppressor Cells and Correlates With Survival in Patients With non-Small Cell Lung Cancer. Oncoimmunology (2015) 4(7):e1014242. doi: 10.1080/2162402X.2015.1014242
68. Alfaro C, Teijeira A, Onate C, Perez G, Sanmamed MF, Andueza MP, et al. Tumor-Produced Interleukin-8 Attracts Human Myeloid-Derived Suppressor Cells and Elicits Extrusion of Neutrophil Extracellular Traps (NETs). Clin Cancer Res (2016) 22(15):3924–36. doi: 10.1158/1078-0432.CCR-15-2463
69. Lesokhin AM, Hohl TM, Kitano S, Cortez C, Hirschhorn-Cymerman D, Avogadri F, et al. Monocytic CCR2(+) Myeloid-Derived Suppressor Cells Promote Immune Escape by Limiting Activated CD8 T-Cell Infiltration Into the Tumor Microenvironment. Cancer Res (2012) 72(4):876–86. doi: 10.1158/0008-5472.CAN-11-1792
70. Sanmamed MF, Carranza-Rua O, Alfaro C, Onate C, Martin-Algarra S, Perez G, et al. Serum Interleukin-8 Reflects Tumor Burden and Treatment Response Across Malignancies of Multiple Tissue Origins. Clin Cancer Res (2014) 20(22):5697–707. doi: 10.1158/1078-0432.CCR-13-3203
71. Sanmamed MF, Perez-Gracia JL, Schalper KA, Fusco JP, Gonzalez A, Rodriguez-Ruiz ME, et al. Changes in Serum Interleukin-8 (IL-8) Levels Reflect and Predict Response to Anti-PD-1 Treatment in Melanoma and Non-Small-Cell Lung Cancer Patients. Ann Oncol (2017) 28(8):1988–95. doi: 10.1093/annonc/mdx190
72. Ryzhov S, Novitskiy SV, Goldstein AE, Biktasova A, Blackburn MR, Biaggioni I, et al. Adenosinergic Regulation of the Expansion and Immunosuppressive Activity of CD11b+Gr1+ Cells. J Immunol (2011) 187(11):6120–9. doi: 10.4049/jimmunol.1101225
73. Ryzhov SV, Pickup MW, Chytil A, Gorska AE, Zhang Q, Owens P, et al. Role of TGF-Beta Signaling in Generation of CD39+CD73+ Myeloid Cells in Tumors. J Immunol (2014) 193(6):3155–64. doi: 10.4049/jimmunol.1400578
74. Li J, Wang L, Chen X, Li L, Li Y, Ping Y, et al. CD39/CD73 Upregulation on Myeloid-Derived Suppressor Cells via TGF-beta-mTOR-HIF-1 Signaling in Patients With Non-Small Cell Lung Cancer. Oncoimmunology (2017) 6(6):e1320011. doi: 10.1080/2162402X.2017.1320011
75. Sawant A, Schafer CC, Jin TH, Zmijewski J, Tse HM, Roth J, et al. Enhancement of Antitumor Immunity in Lung Cancer by Targeting Myeloid-Derived Suppressor Cell Pathways. Cancer Res (2013) 73(22):6609–20. doi: 10.1158/0008-5472.CAN-13-0987
76. Tousif S, Wang Y, Jackson J, Hough KP, Strenkowski JG, Athar M, et al. Indoleamine 2, 3-Dioxygenase Promotes Aryl Hydrocarbon Receptor-Dependent Differentiation Of Regulatory B Cells in Lung Cancer. Front Immunol (2021) 12. doi: 10.3389/fimmu.2021.747780
77. Miret JJ, Kirschmeier P, Koyama S, Zhu M, Li YY, Naito Y, et al. Suppression of Myeloid Cell Arginase Activity Leads to Therapeutic Response in a NSCLC Mouse Model by Activating Anti-Tumor Immunity. J Immunother Cancer (2019) 7(1):32. doi: 10.1186/s40425-019-0504-5
78. Srivastava MK, Zhu L, Harris-White M, Kar UK, Huang M, Johnson MF, et al. Myeloid Suppressor Cell Depletion Augments Antitumor Activity in Lung Cancer. PLoS One (2012) 7(7):e40677. doi: 10.1371/journal.pone.0040677
79. Vivier E, Tomasello E, Baratin M, Walzer T, Ugolini S. Functions of Natural Killer Cells. Nat Immunol (2008) 9(5):503–10. doi: 10.1038/ni1582
80. Cozar B, Greppi M, Carpentier S, Narni-Mancinelli E, Chiossone L, Vivier E. Tumor-Infiltrating Natural Killer Cells. Cancer Discov (2021) 11(1):34–44. doi: 10.1158/2159-8290.CD-20-0655
81. Freud AG, Mundy-Bosse BL, Yu J, Caligiuri MA. The Broad Spectrum of Human Natural Killer Cell Diversity. Immunity (2017) 47(5):820–33. doi: 10.1016/j.immuni.2017.10.008
82. Carrega P, Morandi B, Costa R, Frumento G, Forte G, Altavilla G, et al. Natural Killer Cells Infiltrating Human Nonsmall-Cell Lung Cancer are Enriched in CD56 Bright CD16(-) Cells and Display an Impaired Capability to Kill Tumor Cells. Cancer (2008) 112(4):863–75. doi: 10.1002/cncr.23239
83. Platonova S, Cherfils-Vicini J, Damotte D, Crozet L, Vieillard V, Validire P, et al. Profound Coordinated Alterations of Intratumoral NK Cell Phenotype and Function in Lung Carcinoma. Cancer Res (2011) 71(16):5412–22. doi: 10.1158/0008-5472.CAN-10-4179
84. Hanna J, Goldman-Wohl D, Hamani Y, Avraham I, Greenfield C, Natanson-Yaron S, et al. Decidual NK Cells Regulate Key Developmental Processes at the Human Fetal-Maternal Interface. Nat Med (2006) 12(9):1065–74. doi: 10.1038/nm1452
85. Morvan MG, Lanier LL. NK Cells and Cancer: You can Teach Innate Cells New Tricks. Nat Rev Cancer (2016) 16(1):7–19. doi: 10.1038/nrc.2015.5
86. Bruno A, Focaccetti C, Pagani A, Imperatori AS, Spagnoletti M, Rotolo N, et al. The Proangiogenic Phenotype of Natural Killer Cells in Patients With non-Small Cell Lung Cancer. Neoplasia (2013) 15(2):133–42. doi: 10.1593/neo.121758
87. Bassani B, Baci D, Gallazzi M, Poggi A, Bruno A, Mortara L. Natural Killer Cells as Key Players of Tumor Progression and Angiogenesis: Old and Novel Tools to Divert Their Pro-Tumor Activities Into Potent Anti-Tumor Effects. Cancers (Basel) (2019) 11(4). doi: 10.3390/cancers11040461
88. Gallazzi M, Baci D, Mortara L, Bosi A, Buono G, Naselli A, et al. Prostate Cancer Peripheral Blood NK Cells Show Enhanced CD9, CD49a, CXCR4, CXCL8, MMP-9 Production and Secrete Monocyte-Recruiting and Polarizing Factors. Front Immunol (2020) 11. doi: 10.3389/fimmu.2020.586126
89. Bosi A, Zanellato S, Bassani B, Albini A, Musco A, Cattoni M, et al. Natural Killer Cells From Malignant Pleural Effusion Are Endowed With a Decidual-Like Proangiogenic Polarization. J Immunol Res (2018) 2018:2438598. doi: 10.1155/2018/2438598
90. Bruno A, Bassani B, D'Urso DG, Pitaku I, Cassinotti E, Pelosi G, et al. Angiogenin and the MMP9-TIMP2 Axis are Up-Regulated in Proangiogenic, Decidual NK-Like Cells From Patients With Colorectal Cancer. FASEB J (2018) 32(10):5365–77. doi: 10.1096/fj.201701103R
91. Russick J, Joubert PE, Gillard-Bocquet M, Torset C, Meylan M, Petitprez F, et al. Natural Killer Cells in the Human Lung Tumor Microenvironment Display Immune Inhibitory Functions. J Immunother Cancer (2020) 8(2). doi: 10.1136/jitc-2020-001054
92. Iraolagoitia XL, Spallanzani RG, Torres NI, Araya RE, Ziblat A, Domaica CI, et al. NK Cells Restrain Spontaneous Antitumor CD8+ T Cell Priming Through PD-1/PD-L1 Interactions With Dendritic Cells. J Immunol (2016) 197(3):953–61. doi: 10.4049/jimmunol.1502291
93. Hu Z, Xu X, Wei H. The Adverse Impact of Tumor Microenvironment on NK-Cell. Front Immunol (2021) 12. doi: 10.3389/fimmu.2021.633361
94. Taniguchi M, Harada M, Kojo S, Nakayama T, Wakao H. The Regulatory Role of Valpha14 NKT Cells in Innate and Acquired Immune Response. Annu Rev Immunol (2003) 21:483–513. doi: 10.1146/annurev.immunol.21.120601.141057
95. Godfrey DI, MacDonald HR, Kronenberg M, Smyth MJ, Van Kaer L. NKT Cells: What's in a Name? Nat Rev Immunol (2004) 4(3):231–7. doi: 10.1038/nri1309
96. Smyth MJ, Godfrey DI. NKT Cells and Tumor Immunity–a Double-Edged Sword. Nat Immunol (2000) 1(6):459–60. doi: 10.1038/82698
97. Berzofsky JA, Terabe M. NKT Cells in Tumor Immunity: Opposing Subsets Define a New Immunoregulatory Axis. J Immunol (2008) 180(6):3627–35. doi: 10.4049/jimmunol.180.6.3627
98. Exley MA, Dellabona P, Casorati G. Exploiting CD1-Restricted T Cells for Clinical Benefit. Mol Immunol (2021) 132:126–31. doi: 10.1016/j.molimm.2020.12.015
99. Terabe M, Berzofsky JA. Tissue-Specific Roles of NKT Cells in Tumor Immunity. Front Immunol (2018) 9. doi: 10.3389/fimmu.2018.01838
100. Vivier E, Ugolini S, Blaise D, Chabannon C, Brossay L. Targeting Natural Killer Cells and Natural Killer T Cells in Cancer. Nat Rev Immunol (2012) 12(4):239–52. doi: 10.1038/nri3174
101. Nelson A, Lukacs JD, Johnston B. The Current Landscape of NKT Cell Immunotherapy and the Hills Ahead. Cancers (Basel) (2021) 13(20). doi: 10.3390/cancers13205174
102. Dockry E, O'Leary S, Gleeson LE, Lyons J, Keane J, Gray SG, et al. Epigenetic Induction of CD1d Expression Primes Lung Cancer Cells for Killing by Invariant Natural Killer T Cells. Oncoimmunology (2018) 7(6):e1428156. doi: 10.1080/2162402X.2018.1428156
103. Yu W, Ye F, Yuan X, Ma Y, Mao C, Li X, et al. A Phase I/II Clinical Trial on the Efficacy and Safety of NKT Cells Combined With Gefitinib for Advanced EGFR-Mutated Non-Small-Cell Lung Cancer. BMC Cancer (2021) 21(1):877. doi: 10.1186/s12885-021-08590-1
104. Collin M, Bigley V. Human Dendritic Cell Subsets: An Update. Immunology (2018) 154(1):3–20. doi: 10.1111/imm.12888
105. Del Prete A, Sozio F, Barbazza I, Salvi V, Tiberio L, Laffranchi M, et al. Functional Role of Dendritic Cell Subsets in Cancer Progression and Clinical Implications. Int J Mol Sci (2020) 21(11). doi: 10.3390/ijms21113930
106. Maier B, Leader AM, Chen ST, Tung N, Chang C, LeBerichel J, et al. A Conserved Dendritic-Cell Regulatory Program Limits Antitumour Immunity. Nature (2020) 580(7802):257–62. doi: 10.1038/s41586-020-2134-y
107. Bell D, Chomarat P, Broyles D, Netto G, Harb GM, Lebecque S, et al. In Breast Carcinoma Tissue, Immature Dendritic Cells Reside Within the Tumor, Whereas Mature Dendritic Cells are Located in Peritumoral Areas. J Exp Med (1999) 190(10):1417–26. doi: 10.1084/jem.190.10.1417
108. Perrot I, Blanchard D, Freymond N, Isaac S, Guibert B, Pacheco Y, et al. Dendritic Cells Infiltrating Human Non-Small Cell Lung Cancer are Blocked at Immature Stage. J Immunol (2007) 178(5):2763–9. doi: 10.4049/jimmunol.178.5.2763
109. Dieu-Nosjean MC, Antoine M, Danel C, Heudes D, Wislez M, Poulot V, et al. Long-Term Survival for Patients With Non-Small-Cell Lung Cancer With Intratumoral Lymphoid Structures. J Clin Oncol (2008) 26(27):4410–7. doi: 10.1200/JCO.2007.15.0284
110. Goc J, Germain C, Vo-Bourgais TK, Lupo A, Klein C, Knockaert S, et al. Dendritic Cells in Tumor-Associated Tertiary Lymphoid Structures Signal a Th1 Cytotoxic Immune Contexture and License the Positive Prognostic Value of Infiltrating CD8+ T Cells. Cancer Res (2014) 74(3):705–15. doi: 10.1158/0008-5472.CAN-13-1342
111. Inoshima N, Nakanishi Y, Minami T, Izumi M, Takayama K, Yoshino I, et al. The Influence of Dendritic Cell Infiltration and Vascular Endothelial Growth Factor Expression on the Prognosis of Non-Small Cell Lung Cancer. Clin Cancer Res (2002) 8(11):3480–6.
112. Fontanini G, Vignati S, Lucchi M, Mussi A, Calcinai A, Boldrini L, et al. Neoangiogenesis and P53 Protein in Lung Cancer: Their Prognostic Role and Their Relation With Vascular Endothelial Growth Factor (VEGF) Expression. Br J Cancer (1997) 75(9):1295–301. doi: 10.1038/bjc.1997.220
113. Trivella M, Pezzella F, Pastorino U, Harris AL, Altman DG. Prognosis In Lung Cancer Collaborative Study G. Microvessel Density as a Prognostic Factor in Non-Small-Cell Lung Carcinoma: A Meta-Analysis of Individual Patient Data. Lancet Oncol (2007) 8(6):488–99. doi: 10.1016/S1470-2045(07)70145-6
114. Zheng CL, Qiu C, Shen MX, Qu X, Zhang TH, Zhang JH, et al. Prognostic Impact of Elevation of Vascular Endothelial Growth Factor Family Expression in Patients With Non-Small Cell Lung Cancer: An Updated Meta-Analysis. Asian Pac J Cancer Prev (2015) 16(5):1881–95. doi: 10.7314/APJCP.2015.16.5.1881
115. Hu M, Hu Y, He J, Li B. Prognostic Value of Basic Fibroblast Growth Factor (bFGF) in Lung Cancer: A Systematic Review With Meta-Analysis. PLoS One (2016) 11(1):e0147374. doi: 10.1371/journal.pone.0147374
116. Imada A, Shijubo N, Kojima H, Abe S. Mast Cells Correlate With Angiogenesis and Poor Outcome in Stage I Lung Adenocarcinoma. Eur Respir J (2000) 15(6):1087–93. doi: 10.1034/j.1399-3003.2000.01517.x
117. Takanami I, Takeuchi K, Naruke M. Mast Cell Density Is Associated With Angiogenesis and Poor Prognosis in Pulmonary Adenocarcinoma. Cancer (2000) 88(12):2686–92. doi: 10.1002/1097-0142(20000615)88:12<2686::AID-CNCR6>3.0.CO;2-6
118. Duarte IG, Bufkin BL, Pennington MF, Gal AA, Cohen C, Kosinski AS, et al. Angiogenesis as a Predictor of Survival After Surgical Resection for Stage I non-Small-Cell Lung Cancer. J Thorac Cardiovasc Surg (1998) 115(3):652–8. doi: 10.1016/S0022-5223(98)70331-9
119. Shibusa T, Shijubo N, Abe S. Tumor Angiogenesis and Vascular Endothelial Growth Factor Expression in Stage I Lung Adenocarcinoma. Clin Cancer Res (1998) 4(6):1483–7.
120. Shijubo N, Uede T, Kon S, Maeda M, Segawa T, Imada A, et al. Vascular Endothelial Growth Factor and Osteopontin in Stage I Lung Adenocarcinoma. Am J Respir Crit Care Med (1999) 160(4):1269–73. doi: 10.1164/ajrccm.160.4.9807094
121. Brattstrom D, Wester K, Bergqvist M, Hesselius P, Malmstrom PU, Nordgren H, et al. HER-2, EGFR, COX-2 Expression Status Correlated to Microvessel Density and Survival in Resected non-Small Cell Lung Cancer. Acta Oncol (2004) 43(1):80–6. doi: 10.1080/02841860310017441
122. Chandrachud LM, Pendleton N, Chisholm DM, Horan MA, Schor AM. Relationship Between Vascularity, Age and Survival in Non-Small-Cell Lung Cancer. Br J Cancer (1997) 76(10):1367–75. doi: 10.1038/bjc.1997.562
123. Dazzi C, Cariello A, Maioli P, Solaini L, Scarpi E, Rosti G, et al. Prognostic and Predictive Value of Intratumoral Microvessels Density in Operable non-Small-Cell Lung Cancer. Lung Cancer (1999) 24(2):81–8. doi: 10.1016/S0169-5002(99)00036-7
124. Decaussin M, Sartelet H, Robert C, Moro D, Claraz C, Brambilla C, et al. Expression of Vascular Endothelial Growth Factor (VEGF) and its Two Receptors (VEGF-R1-Flt1 and VEGF-R2-Flk1/KDR) in non-Small Cell Lung Carcinomas (NSCLCs): Correlation With Angiogenesis and Survival. J Pathol (1999) 188(4):369–77. doi: 10.1002/(SICI)1096-9896(199908)188:4<369::AID-PATH381>3.0.CO;2-X
125. Dundar E, Oner U, Peker BC, Metintas M, Isiksoy S, Ak G. The Significance and Relationship Between Mast Cells and Tumour Angiogenesis in non-Small Cell Lung Carcinoma. J Int Med Res (2008) 36(1):88–95. doi: 10.1177/147323000803600112
126. Tanaka F, Otake Y, Yanagihara K, Kawano Y, Miyahara R, Li M, et al. Evaluation of Angiogenesis in Non-Small Cell Lung Cancer: Comparison Between Anti-CD34 Antibody and Anti-CD105 Antibody. Clin Cancer Res (2001) 7(11):3410–5.
127. Tsoli E, Zacharatos P, Dasiou-Plakida D, Peros J, Evangelou K, Zavras AI, et al. Growth Index Is Independent of Microvessel Density in non-Small-Cell Lung Carcinomas. Hum Pathol (2002) 33(8):812–8. doi: 10.1053/hupa.2002.125379
128. Niczyporuk M, Hermanowicz A, Matuszczak E, Dziadziuszko R, Knas M, Zalewska A, et al. A Lack of Correlation Between Mast Cells, Angiogenesis, and Outcome in Non-Small Cell Lung Cancer. Exp Lung Res (2012) 38(6):281–5. doi: 10.3109/01902148.2012.686559
129. Tataroglu C, Kargi A, Ozkal S, Esrefoglu N, Akkoclu A. Association of Macrophages, Mast Cells and Eosinophil Leukocytes With Angiogenesis and Tumor Stage in non-Small Cell Lung Carcinomas (NSCLC). Lung Cancer (2004) 43(1):47–54. doi: 10.1016/j.lungcan.2003.08.013
130. Welsh TJ, Green RH, Richardson D, Waller DA, O'Byrne KJ, Bradding P. Macrophage and Mast-Cell Invasion of Tumor Cell Islets Confers a Marked Survival Advantage in Non-Small-Cell Lung Cancer. J Clin Oncol (2005) 23(35):8959–67. doi: 10.1200/JCO.2005.01.4910
131. Shikotra A, Ohri CM, Green RH, Waller DA, Bradding P. Mast Cell Phenotype, TNFalpha Expression and Degranulation Status in Non-Small Cell Lung Cancer. Sci Rep (2016) 6:38352. doi: 10.1038/srep38352
132. Tomita M, Matsuzaki Y, Onitsuka T. Correlation Between Mast Cells and Survival Rates in Patients With Pulmonary Adenocarcinoma. Lung Cancer (1999) 26(2):103–8. doi: 10.1016/S0169-5002(99)00076-8
133. Stoyanov E, Uddin M, Mankuta D, Dubinett SM, Levi-Schaffer F. Mast Cells and Histamine Enhance the Proliferation of Non-Small Cell Lung Cancer Cells. Lung Cancer (2012) 75(1):38–44. doi: 10.1016/j.lungcan.2011.05.029
134. Liang J, Tian C, Zeng Y, Yang Q, Liu Y, Liu Y, et al. FOXA1(+) Regulatory T Cells: A Novel T Cell Subset That Suppresses Antitumor Immunity in Lung Cancer. Biochem Biophys Res Commun (2019) 514(1):308–15. doi: 10.1016/j.bbrc.2019.04.152
135. Sakaguchi S, Sakaguchi N, Asano M, Itoh M, Toda M. Immunologic Self-Tolerance Maintained by Activated T Cells Expressing IL-2 Receptor Alpha-Chains (CD25). Breakdown of a Single Mechanism of Self-Tolerance Causes Various Autoimmune Diseases. J Immunol (1995) 155(3):1151–64.
136. Beyer M, Schultze JL. Regulatory T Cells in Cancer. Blood (2006) 108(3):804–11. doi: 10.1182/blood-2006-02-002774
137. Petersen RP, Campa MJ, Sperlazza J, Conlon D, Joshi MB, Harpole DH Jr., et al. Tumor Infiltrating Foxp3+ Regulatory T-Cells Are Associated With Recurrence in Pathologic Stage I NSCLC Patients. Cancer (2006) 107(12):2866–72. doi: 10.1002/cncr.22282
138. Shimizu K, Nakata M, Hirami Y, Yukawa T, Maeda A, Tanemoto K. Tumor-Infiltrating Foxp3+ Regulatory T Cells Are Correlated With Cyclooxygenase-2 Expression and Are Associated With Recurrence in Resected Non-Small Cell Lung Cancer. J Thorac Oncol (2010) 5(5):585–90. doi: 10.1097/JTO.0b013e3181d60fd7
139. Tao H, Mimura Y, Aoe K, Kobayashi S, Yamamoto H, Matsuda E, et al. Prognostic Potential of FOXP3 Expression in Non-Small Cell Lung Cancer Cells Combined With Tumor-Infiltrating Regulatory T Cells. Lung Cancer (2012) 75(1):95–101. doi: 10.1016/j.lungcan.2011.06.002
140. Woo EY, Chu CS, Goletz TJ, Schlienger K, Yeh H, Coukos G, et al. Regulatory CD4(+)CD25(+) T Cells in Tumors From Patients With Early-Stage non-Small Cell Lung Cancer and Late-Stage Ovarian Cancer. Cancer Res (2001) 61(12):4766–72.
141. Chen C, Chen D, Zhang Y, Chen Z, Zhu W, Zhang B, et al. Changes of CD4+CD25+FOXP3+ and CD8+CD28- Regulatory T Cells in Non-Small Cell Lung Cancer Patients Undergoing Surgery. Int Immunopharmacol (2014) 18(2):255–61. doi: 10.1016/j.intimp.2013.12.004
142. Li L, Chao QG, Ping LZ, Xue C, Xia ZY, Qian D, et al. The Prevalence of FOXP3+ Regulatory T-Cells in Peripheral Blood of Patients With NSCLC. Cancer Biother Radiopharm (2009) 24(3):357–67. doi: 10.1089/cbr.2008.0612
143. Okita R, Saeki T, Takashima S, Yamaguchi Y, Toge T. CD4+CD25+ Regulatory T Cells in the Peripheral Blood of Patients With Breast Cancer and Non-Small Cell Lung Cancer. Oncol Rep (2005) 14(5):1269–73. doi: 10.3892/or.14.5.1269
144. Yannelli JR, Tucker JA, Hidalgo G, Perkins S, Kryscio R, Hirschowitz EA. Characteristics of PBMC Obtained From Leukapheresis Products and Tumor Biopsies of Patients With Non-Small Cell Lung Cancer. Oncol Rep (2009) 22(6):1459–71. doi: 10.3892/or_00000588
145. Erfani N, Mehrabadi SM, Ghayumi MA, Haghshenas MR, Mojtahedi Z, Ghaderi A, et al. Increase of Regulatory T Cells in Metastatic Stage and CTLA-4 Over Expression in Lymphocytes of Patients With Non-Small Cell Lung Cancer (NSCLC). Lung Cancer (2012) 77(2):306–11. doi: 10.1016/j.lungcan.2012.04.011
146. Hanagiri T, Shigematsu Y, Shinohara S, Takenaka M, Oka S, Chikaishi Y, et al. Clinical Significance of the Frequency of Regulatory T Cells in Regional Lymph Node Lymphocytes as a Prognostic Factor for Non-Small-Cell Lung Cancer. Lung Cancer (2013) 81(3):475–9. doi: 10.1016/j.lungcan.2013.07.001
147. Hasegawa T, Suzuki H, Yamaura T, Muto S, Okabe N, Osugi J, et al. Prognostic Value of Peripheral and Local Forkhead Box P3(+) Regulatory T Cells in Patients With Non-Small-Cell Lung Cancer. Mol Clin Oncol (2014) 2(5):685–94. doi: 10.3892/mco.2014.299
148. Kotsakis A, Koinis F, Katsarou A, Gioulbasani M, Aggouraki D, Kentepozidis N, et al. Prognostic Value of Circulating Regulatory T Cell Subsets in Untreated Non-Small Cell Lung Cancer Patients. Sci Rep (2016) 6:39247. doi: 10.1038/srep39247
149. Yang H, Shi J, Lin D, Li X, Zhao C, Wang Q, et al. Prognostic Value of PD-L1 Expression in Combination With CD8(+) TILs Density in Patients With Surgically Resected Non-Small Cell Lung Cancer. Cancer Med (2018) 7(1):32–45. doi: 10.1002/cam4.1243
150. Carus A, Ladekarl M, Hager H, Pilegaard H, Nielsen PS, Donskov F. Tumor-Associated Neutrophils and Macrophages in Non-Small Cell Lung Cancer: No Immediate Impact on Patient Outcome. Lung Cancer (2013) 81(1):130–7. doi: 10.1016/j.lungcan.2013.03.003
151. Tuminello S, Veluswamy R, Lieberman-Cribbin W, Gnjatic S, Petralia F, Wang P, et al. Prognostic Value of Immune Cells in the Tumor Microenvironment of Early-Stage Lung Cancer: A Meta-Analysis. Oncotarget (2019) 10(67):7142–55. doi: 10.18632/oncotarget.27392
152. Geng Y, Shao Y, He W, Hu W, Xu Y, Chen J, et al. Prognostic Role of Tumor-Infiltrating Lymphocytes in Lung Cancer: A Meta-Analysis. Cell Physiol Biochem (2015) 37(4):1560–71. doi: 10.1159/000438523
153. Lynch TJ, Bell DW, Sordella R, Gurubhagavatula S, Okimoto RA, Brannigan BW, et al. Activating Mutations in the Epidermal Growth Factor Receptor Underlying Responsiveness of Non-Small-Cell Lung Cancer to Gefitinib. N Engl J Med (2004) 350(21):2129–39. doi: 10.1056/NEJMoa040938
154. Garofalo M, Romano G, Di Leva G, Nuovo G, Jeon YJ, Ngankeu A, et al. EGFR and MET Receptor Tyrosine Kinase-Altered microRNA Expression Induces Tumorigenesis and Gefitinib Resistance in Lung Cancers. Nat Med (2011) 18(1):74–82. doi: 10.1038/nm.2577
155. Soltermann A. [Epithelial-Mesenchymal Transition in non-Small Cell Lung Cancer]. Pathologe (2012) 33 Suppl 2:311–7. doi: 10.1007/s00292-012-1635-3
156. Heinrich EL, Walser TC, Krysan K, Liclican EL, Grant JL, Rodriguez NL, et al. The Inflammatory Tumor Microenvironment, Epithelial Mesenchymal Transition and Lung Carcinogenesis. Cancer Microenviron (2012) 5(1):5–18. doi: 10.1007/s12307-011-0089-0
157. Yu HA, Riely GJ. Second-Generation Epidermal Growth Factor Receptor Tyrosine Kinase Inhibitors in Lung Cancers. J Natl Compr Canc Netw (2013) 11(2):161–9. doi: 10.6004/jnccn.2013.0024
158. Im SJ, Hashimoto M, Gerner MY, Lee J, Kissick HT, Burger MC, et al. Defining CD8+ T Cells That Provide the Proliferative Burst After PD-1 Therapy. Nature (2016) 537(7620):417–21. doi: 10.1038/nature19330
159. Chen L, Diao L, Yang Y, Yi X, Rodriguez BL, Li Y, et al. CD38-Mediated Immunosuppression as a Mechanism of Tumor Cell Escape From PD-1/PD-L1 Blockade. Cancer Discov (2018) 8(9):1156–75. doi: 10.1158/2159-8290.CD-17-1033
160. Koh J, Hur JY, Lee KY, Kim MS, Heo JY, Ku BM, et al. Regulatory (FoxP3(+)) T Cells and TGF-Beta Predict the Response to Anti-PD-1 Immunotherapy in Patients With non-Small Cell Lung Cancer. Sci Rep (2020) 10(1):18994. doi: 10.1038/s41598-020-76130-1
161. Aggarwal C, Somaiah N, Simon G. Antiangiogenic Agents in the Management of non-Small Cell Lung Cancer: Where do We Stand Now and Where are We Headed? Cancer Biol Ther (2012) 13(5):247–63. doi: 10.4161/cbt.19594
162. Jain RK. Antiangiogenesis Strategies Revisited: From Starving Tumors to Alleviating Hypoxia. Cancer Cell (2014) 26(5):605–22. doi: 10.1016/j.ccell.2014.10.006
163. Sandler A, Gray R, Perry MC, Brahmer J, Schiller JH, Dowlati A, et al. Paclitaxel-Carboplatin Alone or With Bevacizumab for non-Small-Cell Lung Cancer. N Engl J Med (2006) 355(24):2542–50. doi: 10.1056/NEJMoa061884
164. Garon EB, Ciuleanu TE, Arrieta O, Prabhash K, Syrigos KN, Goksel T, et al. Ramucirumab Plus Docetaxel Versus Placebo Plus Docetaxel for Second-Line Treatment of Stage IV non-Small-Cell Lung Cancer After Disease Progression on Platinum-Based Therapy (REVEL): A Multicentre, Double-Blind, Randomised Phase 3 Trial. Lancet (2014) 384(9944):665–73. doi: 10.1016/S0140-6736(14)60845-X
165. Yi M, Jiao D, Qin S, Chu Q, Wu K, Li A. Synergistic Effect of Immune Checkpoint Blockade and Anti-Angiogenesis in Cancer Treatment. Mol Cancer (2019) 18(1):60. doi: 10.1186/s12943-019-0974-6
166. Winkler F, Kozin SV, Tong RT, Chae SS, Booth MF, Garkavtsev I, et al. Kinetics of Vascular Normalization by VEGFR2 Blockade Governs Brain Tumor Response to Radiation: Role of Oxygenation, Angiopoietin-1, and Matrix Metalloproteinases. Cancer Cell (2004) 6(6):553–63. doi: 10.1016/j.ccr.2004.10.011
167. Mazzone M, Dettori D, de Oliveira RL, Loges S, Schmidt T, Jonckx B, et al. Heterozygous Deficiency of PHD2 Restores Tumor Oxygenation and Inhibits Metastasis via Endothelial Normalization. Cell (2009) 136(5):839–51. doi: 10.1016/j.cell.2009.01.020
168. Stockmann C, Doedens A, Weidemann A, Zhang N, Takeda N, Greenberg JI, et al. Deletion of Vascular Endothelial Growth Factor in Myeloid Cells Accelerates Tumorigenesis. Nature (2008) 456(7223):814–8. doi: 10.1038/nature07445
169. Taube JM, Klein A, Brahmer JR, Xu H, Pan X, Kim JH, et al. Association of PD-1, PD-1 Ligands, and Other Features of the Tumor Immune Microenvironment With Response to Anti-PD-1 Therapy. Clin Cancer Res (2014) 20(19):5064–74. doi: 10.1158/1078-0432.CCR-13-3271
170. Guo H, Bai R, Cui J. [Advances in Combination Therapy of Immune Checkpoint Inhibitors for Lung Cancer]. Zhongguo Fei Ai Za Zhi (2020) 23(2):101–10. doi: 10.3779/j.issn.1009-3419.2020.02.05
171. Voron T, Marcheteau E, Pernot S, Colussi O, Tartour E, Taieb J, et al. Control of the Immune Response by Pro-Angiogenic Factors. Front Oncol (2014) 4. doi: 10.3389/fonc.2014.00070
172. Ren S, Xiong X, You H, Shen J, Zhou P. The Combination of Immune Checkpoint Blockade and Angiogenesis Inhibitors in the Treatment of Advanced Non-Small Cell Lung Cancer. Front Immunol (2021) 12. doi: 10.3389/fimmu.2021.689132
173. Mortara L, Benest AV, Bates DO, Noonan DM. Can the Co-Dependence of the Immune System and Angiogenesis Facilitate Pharmacological Targeting of Tumours? Curr Opin Pharmacol (2017) 35:66–74. doi: 10.1016/j.coph.2017.05.009
174. Alfaro C, Suarez N, Gonzalez A, Solano S, Erro L, Dubrot J, et al. Influence of Bevacizumab, Sunitinib and Sorafenib as Single Agents or in Combination on the Inhibitory Effects of VEGF on Human Dendritic Cell Differentiation From Monocytes. Br J Cancer (2009) 100(7):1111–9. doi: 10.1038/sj.bjc.6604965
175. Oyama T, Ran S, Ishida T, Nadaf S, Kerr L, Carbone DP, et al. Vascular Endothelial Growth Factor Affects Dendritic Cell Maturation Through the Inhibition of Nuclear Factor-Kappa B Activation in Hemopoietic Progenitor Cells. J Immunol (1998) 160(3):1224–32.
176. Gabrilovich DI, Ostrand-Rosenberg S, Bronte V. Coordinated Regulation of Myeloid Cells by Tumours. Nat Rev Immunol (2012) 12(4):253–68. doi: 10.1038/nri3175
177. Suzuki H, Onishi H, Wada J, Yamasaki A, Tanaka H, Nakano K, et al. VEGFR2 is Selectively Expressed by FOXP3high CD4+ Treg. Eur J Immunol (2010) 40(1):197–203. doi: 10.1002/eji.200939887
178. Wada J, Suzuki H, Fuchino R, Yamasaki A, Nagai S, Yanai K, et al. The Contribution of Vascular Endothelial Growth Factor to the Induction of Regulatory T-Cells in Malignant Effusions. Anticancer Res (2009) 29(3):881–8.
179. Manzoni M, Rovati B, Ronzoni M, Loupakis F, Mariucci S, Ricci V, et al. Immunological Effects of Bevacizumab-Based Treatment in Metastatic Colorectal Cancer. Oncology (2010) 79(3-4):187–96. doi: 10.1159/000320609
180. Martino EC, Misso G, Pastina P, Costantini S, Vanni F, Gandolfo C, et al. Immune-Modulating Effects of Bevacizumab in Metastatic non-Small-Cell Lung Cancer Patients. Cell Death Discovery (2016) 2:16025. doi: 10.1038/cddiscovery.2016.25
181. Cheng HS, Lee JXT, Wahli W, Tan NS. Exploiting Vulnerabilities of Cancer by Targeting Nuclear Receptors of Stromal Cells in Tumor Microenvironment. Mol Cancer (2019) 18(1):51. doi: 10.1186/s12943-019-0971-9
182. Huang Y, Kim BYS, Chan CK, Hahn SM, Weissman IL, Jiang W. Improving Immune-Vascular Crosstalk for Cancer Immunotherapy. Nat Rev Immunol (2018) 18(3):195–203. doi: 10.1038/nri.2017.145
183. Huang Y, Goel S, Duda DG, Fukumura D, Jain RK. Vascular Normalization as an Emerging Strategy to Enhance Cancer Immunotherapy. Cancer Res (2013) 73(10):2943–8. doi: 10.1158/0008-5472.CAN-12-4354
184. Movahedi K, Laoui D, Gysemans C, Baeten M, Stange G, Van den Bossche J, et al. Different Tumor Microenvironments Contain Functionally Distinct Subsets of Macrophages Derived From Ly6C(high) Monocytes. Cancer Res (2010) 70(14):5728–39. doi: 10.1158/0008-5472.CAN-09-4672
185. Motz GT, Santoro SP, Wang LP, Garrabrant T, Lastra RR, Hagemann IS, et al. Tumor Endothelium FasL Establishes a Selective Immune Barrier Promoting Tolerance in Tumors. Nat Med (2014) 20(6):607–15. doi: 10.1038/nm.3541
186. Terme M, Pernot S, Marcheteau E, Sandoval F, Benhamouda N, Colussi O, et al. VEGFA-VEGFR Pathway Blockade Inhibits Tumor-Induced Regulatory T-Cell Proliferation in Colorectal Cancer. Cancer Res (2013) 73(2):539–49. doi: 10.1158/0008-5472.CAN-12-2325
187. Huang Y, Chen X, Dikov MM, Novitskiy SV, Mosse CA, Yang L, et al. Distinct Roles of VEGFR-1 and VEGFR-2 in the Aberrant Hematopoiesis Associated With Elevated Levels of VEGF. Blood (2007) 110(2):624–31. doi: 10.1182/blood-2007-01-065714
188. Kudo M. Scientific Rationale for Combined Immunotherapy With PD-1/PD-L1 Antibodies and VEGF Inhibitors in Advanced Hepatocellular Carcinoma. Cancers (Basel) (2020) 12(5):1089. doi: 10.3390/cancers12051089
189. Huang Y, Yuan J, Righi E, Kamoun WS, Ancukiewicz M, Nezivar J, et al. Vascular Normalizing Doses of Antiangiogenic Treatment Reprogram the Immunosuppressive Tumor Microenvironment and Enhance Immunotherapy. Proc Natl Acad Sci USA (2012) 109(43):17561–6. doi: 10.1073/pnas.1215397109
190. Voron T, Colussi O, Marcheteau E, Pernot S, Nizard M, Pointet A-L, et al. VEGF-A Modulates Expression of Inhibitory Checkpoints on CD8+ T Cells in Tumors. J Exp Med (2015) 212(2):139–48. doi: 10.1084/jem.20140559
191. Socinski MA, Nishio M, Jotte RM, Cappuzzo F, Orlandi F, Stroyakovskiy D, et al. IMpower150 Final Overall Survival Analyses for Atezolizumab Plus Bevacizumab and Chemotherapy in First-Line Metastatic Nonsquamous NSCLC. J Thorac Oncol (2021) 16(11):1909–24. doi: 10.1016/j.jtho.2021.07.009
192. Francis DM, Manspeaker MP, Schudel A, Sestito LF, O'Melia MJ, Kissick HT, et al. Blockade of Immune Checkpoints in Lymph Nodes Through Locoregional Delivery Augments Cancer Immunotherapy. Sci Transl Med (2020) 12(563). doi: 10.1126/scitranslmed.aay3575
193. Corthay A. Does the Immune System Naturally Protect Against Cancer? Front Immunol (2014) 5. doi: 10.3389/fimmu.2014.00197
194. Callahan MK, Wolchok JD. At the Bedside: CTLA-4- and PD-1-Blocking Antibodies in Cancer Immunotherapy. J Leukoc Biol (2013) 94(1):41–53. doi: 10.1189/jlb.1212631
195. Dermani FK, Samadi P, Rahmani G, Kohlan AK, Najafi R. PD-1/PD-L1 Immune Checkpoint: Potential Target for Cancer Therapy. J Cell Physiol (2019) 234(2):1313–25. doi: 10.1002/jcp.27172
196. Herbst RS, Arkenau HT, Bendell J, Arrowsmith E, Wermke M, Soriano A, et al. Phase 1 Expansion Cohort of Ramucirumab Plus Pembrolizumab in Advanced Treatment-Naive NSCLC. J Thorac Oncol (2021) 16(2):289–98. doi: 10.1016/j.jtho.2020.10.004
197. Borghaei H, Paz-Ares L, Horn L, Spigel DR, Steins M, Ready NE, et al. Nivolumab Versus Docetaxel in Advanced Nonsquamous Non-Small-Cell Lung Cancer. N Engl J Med (2015) 373(17):1627–39. doi: 10.1056/NEJMoa1507643
198. Rittmeyer A, Barlesi F, Waterkamp D, Park K, Ciardiello F, von Pawel J, et al. Atezolizumab Versus Docetaxel in Patients With Previously Treated Non-Small-Cell Lung Cancer (OAK): A Phase 3, Open-Label, Multicentre Randomised Controlled Trial. Lancet (2017) 389(10066):255–65. doi: 10.1016/S0140-6736(16)32517-X
199. Shibata Y, Murakami S. Safety Evaluation of Durvalumab for the Treatment of non-Small-Cell Lung Cancer. Expert Opin Drug Saf (2020) 19(6):653–9. doi: 10.1080/14740338.2020.1764936
200. Barlesi F, Vansteenkiste J, Spigel D, Ishii H, Garassino M, de Marinis F, et al. Avelumab Versus Docetaxel in Patients With Platinum-Treated Advanced non-Small-Cell Lung Cancer (JAVELIN Lung 200): An Open-Label, Randomised, Phase 3 Study. Lancet Oncol (2018) 19(11):1468–79. doi: 10.1016/S1470-2045(18)30673-9
201. Pinto JA, Raez LE, Oliveres H, Rolfo CC. Current Knowledge of Ipilimumab and its Use in Treating non-Small Cell Lung Cancer. Expert Opin Biol Ther (2019) 19(6):509–15. doi: 10.1080/14712598.2019.1610380
202. Brahmer J, Reckamp KL, Baas P, Crinò L, Eberhardt WEE, Poddubskaya E, et al. Nivolumab Versus Docetaxel in Advanced Squamous-Cell Non–Small-Cell Lung Cancer. New Engl J Med (2015) 373(2):123–35. doi: 10.1056/NEJMoa1504627
203. Fehrenbacher L, Spira A, Ballinger M, Kowanetz M, Vansteenkiste J, Mazieres J, et al. Atezolizumab Versus Docetaxel for Patients With Previously Treated Non-Small-Cell Lung Cancer (POPLAR): A Multicentre, Open-Label, Phase 2 Randomised Controlled Trial. Lancet (2016) 387(10030):1837–46. doi: 10.1016/S0140-6736(16)00587-0
204. Antonia SJ, Villegas A, Daniel D, Vicente D, Murakami S, Hui R, et al. Overall Survival With Durvalumab After Chemoradiotherapy in Stage III NSCLC. New Engl J Med (2018) 379(24):2342–50. doi: 10.1056/NEJMoa1809697
Keywords: non-small cell lung cancer, tumor microenvironment, immunotherapy, immune checkpoint inhibitors, anti-angiogenic therapies
Citation: Baci D, Cekani E, Imperatori A, Ribatti D and Mortara L (2022) Host-Related Factors as Targetable Drivers of Immunotherapy Response in Non-Small Cell Lung Cancer Patients. Front. Immunol. 13:914890. doi: 10.3389/fimmu.2022.914890
Received: 07 April 2022; Accepted: 13 May 2022;
Published: 06 July 2022.
Edited by:
Keqiang Chen, National Cancer Institute at Frederick (NIH), United StatesReviewed by:
Yafeng He, National Heart, Lung, and Blood Institute (NIH), United StatesJi Ming Wang, National Cancer Institute at Frederick (NIH), United States
Copyright © 2022 Baci, Cekani, Imperatori, Ribatti and Mortara. This is an open-access article distributed under the terms of the Creative Commons Attribution License (CC BY). The use, distribution or reproduction in other forums is permitted, provided the original author(s) and the copyright owner(s) are credited and that the original publication in this journal is cited, in accordance with accepted academic practice. No use, distribution or reproduction is permitted which does not comply with these terms.
*Correspondence: Denisa Baci, ZGVuaXNhLmJhY2lAZ3J1cHBvc2FuZG9uYXRvLml0; Lorenzo Mortara, bG9yZW56by5tb3J0YXJhQHVuaW5zdWJyaWEuaXQ=