- Division of Gastroenterology and Hepatology, Key Laboratory of Gastroenterology and Hepatology, Ministry of Health, Inflammatory Bowel Disease Research Center, Renji Hospital, School of Medicine, Shanghai Institute of Digestive Disease, Shanghai Jiao Tong University, Shanghai, China
The human intestine contains a complex network of innate and adaptive immune cells that provide protective immunity. The dysfunction of this network may cause various chronic diseases. A large number of T cells in the human intestine have been identified as tissue-resident memory T cells (TRM). TRM are present in the peripheral tissues, and they do not recirculate through the blood. It is known that TRM provide rapid immune responses at the frontline of pathogen invasion. Recent evidence also suggests that these cells play a role in tumor surveillance and the pathogenesis of autoimmune diseases. In this review, we discuss the general features of intestinal TRM together with their role in intestinal infection, colorectal cancer (CRC), and inflammatory bowel disease (IBD).
Introduction
The intestinal mucosa is predominantly exposed to environmental antigens, such as food, innocuous microbes, and enteric pathogens. A complex network of innate and adaptive immune cells in the intestine mediates the protective immune responses against harmful pathogens and immune unresponsiveness to benign antigens. Among these immune cells, memory T cells provide effective and efficient immune responses to antigens previously encountered (1).
Memory T cells were primarily divided into two subsets: central memory T cells (TCM) and effector memory T cells (TEM) (2). TCM express high levels of secondary lymphoid organ homing receptors CCR7 and CD62L and recirculate between the blood and secondary lymphoid organs (3). On the other hand, TEM express integrins and chemokine receptors and circulate through the non-lymphoid tissues (4). With advances in technology, another lineage of memory T cells called tissue resident-memory T cells (TRM) (5) has been identified. Unlike TCM and TEM, TRM are permanently retained in the peripheral tissues and do not recirculate via the bloodstream. Apart from the location and migration patterns, they mediate local immune response to reencountered pathogens (5). In fact, most memory T cells in the intestinal mucosa possess a resident nature with distinct phenotypes and transcriptional profiles (6–8), implicating the significance of TRM in intestinal immunity.
Several studies focused on the intestinal immune system have explored the potential roles of TRM in pathogen defense (9), cancer immunosurveillance (10), and immunopathies (11). Understanding how TRM function in intestinal immunity is vital for developing novel therapies and vaccines targeting TRM. Thus, this review aims to summarize the features of intestinal TRM and their role in intestinal health and disease.
TRM In the Intestine
Intestinal TRM are phenotypically different from TEM. CD69 and CD103 are the two key cell surface markers identified for TRM (12, 13). Lectin CD69, a marker for early T cell activation, is reexpressed in the intestinal TRM, and it can be used to distinguish TRM from their circulating counterparts (7). CD69 can promote T cell retention by downregulating the expression of the sphingosine-1-phosphate receptor 1 (S1PR1) protein and interacting with the transmembrane domains of the S1PR1 to suppress its function, subsequently preventing the T cells from sensing the sphingosine-1-phosphate (S1P) gradient and exiting from the gut (14–17). However, according to a recent report, the elevated CD69 expression may only serve as a passive marker rather than an essential functional regulator in driving TRM cell formation in the gut (18). Another marker for TRM, CD103 (αE integrin), is expressed by most CD8+ cells and few CD4+ cells in the intestine (7, 12, 13, 19, 20). The αEβ7 integrin binds to E-cadherin expressed on the intestinal epithelial cells, promoting TRM retention in the epithelium (21–23). However, the absence of CD103 does not rule out long-term maintenance of T cells in the intestine. Evidence showed that a small population of CD8+CD69+CD103- T cells displayed persistence in the human intestine transplantation model for more than one year (12, 24). The immune repertoire of CD8+CD69+CD103- T cells showed low clonal overlap with the CD8+CD103+ subset and was rather similar to T cells from peripheral blood in terms of phenotype and function (12, 24). Therefore, it can be speculated that the CD8+CD103- TRM subset represents an intermediate state between recently recruited T cells and CD8+CD103+ TRM. Interestingly, CD8+CD103- TRM express high levels of β2-integrin, but whether it plays a role in CD8+CD103- TRM differentiation remains unclear (24). Furthermore, a fraction of CD8+CD103- TRM express KLRG1, another ligation of E-cadherin (12, 24, 25). The comparison of CD103+ TRM and CD103- TRM is summarized in Table 1. For CD4+ TRM and CD8+ TRM, the phenotypical and functional differences between their CD103+ and CD103- subsets are similar (24). In addition to CD69 and CD103, other molecules have been described as potential phenotypical markers of intestinal TRM, including CD49a (integrin α1 chain) (8, 12, 13), CD101 (8) and CD161 (13, 24, 28).
TRM have a distinct pattern of transcription factor expression that coordinates TRM development and survival. Hobit and Blimp-1 have been identified as two key transcription factors expressed in mice TRM (29). Hobit and Blimp-1 work in synergy to repress genes required for tissue egress by binding to the S1pr1, Tcf7, and Ccr7 loci (29). Additionally, Blimp-1 alone downregulates the expression of KLF2 and subsequently S1PR1 (29). Runx3 plays a primary role in CD8+ TRM differentiation by enhancing the expression of core residency signature and repressing the expression of signature genes of TCM (30, 31). Downregulation of T-box transcription factor Eomes and T-bet is also necessary for early-stage TRM differentiation (32, 33). Recent studies have found that effector-like and memory-like CD8+ TRM subsets can be distinguished by differential expression of two transcriptional factors Blimp-1 and Id3. It was shown that Blimp-1hiId3loCD8+ TRM displayed an effector gene signature and dominated the early phase of infections, whereas Blimp-1loId3hiCD8+ TRM exhibited enhanced memory potential and accumulated at the late stage of infections (34).
The differentiation and maintenance of intestinal TRM are highly regulated by the local microenvironment of the gut (26, 35). Upon antigen encounter, naïve CD8 T cells become activated and differentiate into short-lived effector cells (SLEC) and memory precursor cells (MPEC) (36). Early adoptive transfer experiments in mice identified the MPEC with low KLRG1 expression as the precursors of TRM (21). However, a fate-mapping study on KLRG1 reporter mice revealed that more than half of the TRM population in the small intestine of mice originate from the effector T cell population previously expressing KLRG1 (37). In addition, CD103- TRM within the intraepithelial (IE) preferentially developed from T cells that transiently expressed KLRG1 rather than those that never express KLRG1 (37). The expression of KLRG1 may be downregulated by the cytokine transforming growth factor β (TGF-β) and T cell receptor (TCR) triggering in the intestine (38). TGF-β plays a vital role in the development of the intestinal TRM (39). TGF-β selectively accelerates the apoptosis of SLEC in the intestine, thus contributing to the rapid MPEC phenotype formation (26). TGF-β also downregulates KLF2 expression and subsequently downregulates the expression of S1PR1 expression (40). Additionally, TGF-β upregulates the expression of CD103 directly through Smad3 and indirectly through counteracting suppression of CD103 expression mediated by Eomes, T-bet, and TCF1 (26, 32, 41). IL-12 and TNF-β produced by inflammatory monocytes can suppress the TGF-β-induced CD103 expression, leading to the formation of CD103- TRM cells in the lamina propria (LP) with different transcriptional profiles (27). When exposed to extracellular ATP, the purinergic receptor P2RX7 can promote TGF-β receptor expression in CD8+ TRM precursor, suggesting the importance of P2RX7 in TRM generation (42). Recently, Peng et al. found that local ICOS signaling also promotes the generation of intestinal TRM by activating the PI3K pathway and downregulating KLF2 expression (43). While the maintenance of TCM and TEM depends on the presence of IL-15, IL-15 is not necessary for TRM retention (44).
TRM and Protection Against Pathogen
Numerous studies have revealed that the intestinal CD4+ and CD8+ TRM can be generated by intravenous or oral infections and provide enhanced regional immunity (19, 20, 26). In mice, CD4+ and CD8+ TRM have been shown to provide strong protection against oral infection with Listeria monocytogenes (19, 26). In humans, attenuated oral typhoid vaccine can elicit activated CD4+ and CD8+ TRM response (45, 46). Here, we review the two possible mechanisms by which the intestinal TRM protect against pathogens rapidly and efficiently upon reinfection (Figure 1).
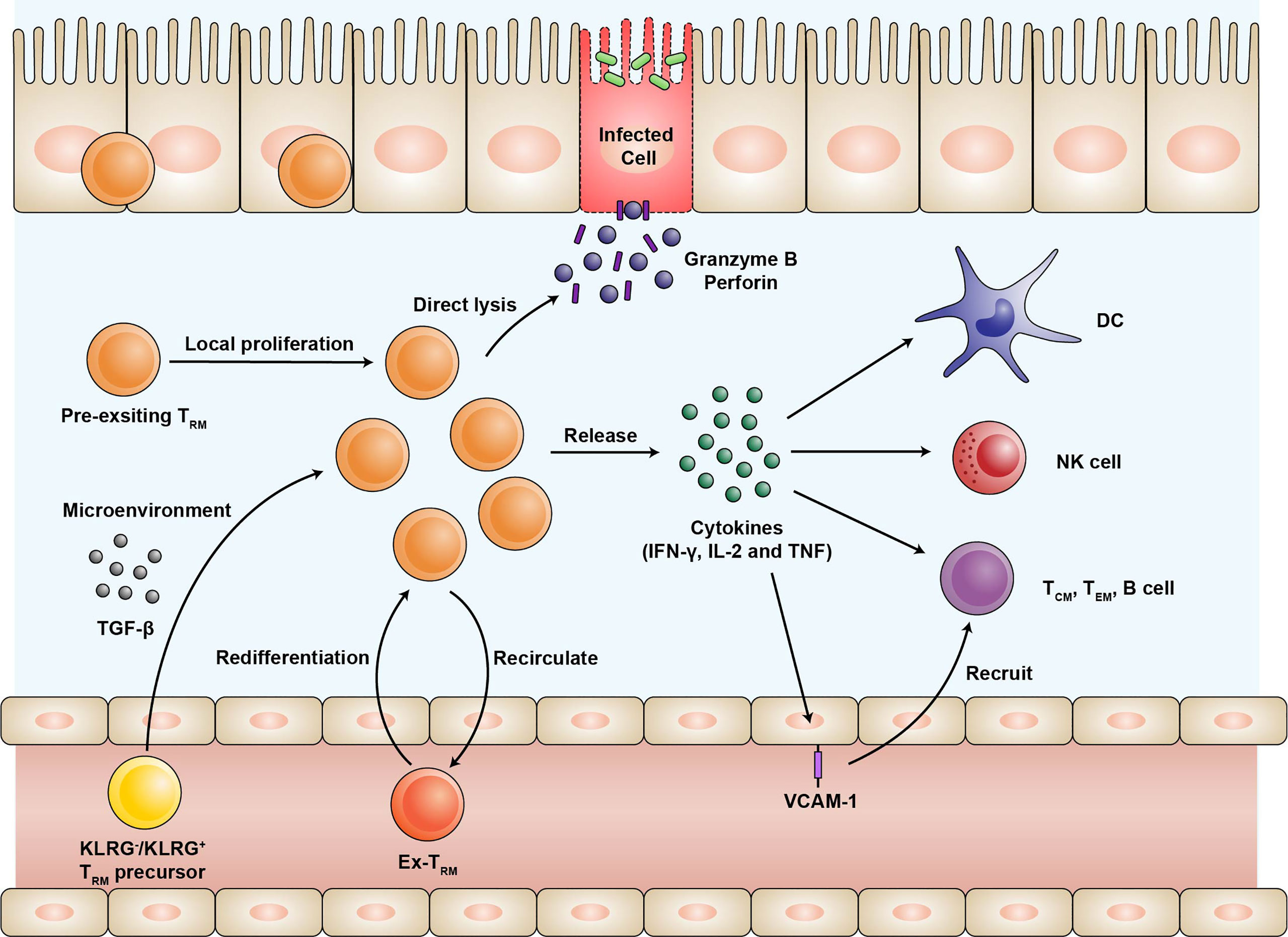
Figure 1 The expansion of the TRM population and their protective function during infection. Effector T cells expressing KLRG or not are recruited into intestinal mucosa and differentiate into TRM. The differentiation of TRM depends on the microenvironment of the intestine, especially TGF-β. Upon reinfection, pre-existing TRM undergo local proliferation and dominate efficient recall responses. A fraction of TRM may rejoin the circulation with the preference of migrating back and the potential of re-differentiating into TRM. TRM release proinflammatory cytokines such as IFN-γ, IL-2, and TNF-α, thus activating natural killer (NK) cells and dendritic cells (DC), as well as recruiting other immune cells through upregulation of the vascular cell adhesion molecule-1 (VCAM-1) on the endothelial cells. In addition, TRM can directly lyse the infected cells by producing high levels of granzyme B and perforin. TRM, tissue-resident memory T cells; KLRG, killer cell lectin-like receptor G1; TGF, transforming growth factor; IFN, interferon; IL, Interleukin; TNF, tumor necrosis factor; NK, natural killer; DC, dendritic cells; VCAM, vascular cell adhesion molecule.
First, TRM upregulate the production of proinflammatory cytokines, serving as an alarm for both innate and adaptive immune responses. Previous experiments have demonstrated the robust cytokine polyfunctionality of certain pathogen-specific CD4+ and CD8+ TRM that produce high levels of interferon-γ (IFN-γ), IL-2, and tumor necrosis factor-α (TNF-α) (20, 47). The functions of TRM primarily rely on the cytokines they secrete. Upon cognate peptide rechallenge, the released IFN-γ induces the expression of various antiviral and antibacterial genes in the surrounding cells to limit the initial pathogen invasion (48, 49). Furthermore, TRM-induced IFN-γ is required for the rapid recruitment of the circulating B and memory T cells via vascular cell adhesion molecule-1 (VCAM-1) upregulation on the endothelial cells (50). IL-2 stimulates granzyme B expression in the natural killer cells and the bystander memory T cells. The proinflammatory cytokine TNF-α facilitates the maturation of dendritic cells by upregulating CCR7 and other co-stimulatory molecules (49). Comparing subsets of intestinal TRM, LP CD8+CD103+ TRM are more polyfunctional in cytokine production than LP CD8+CD103- TRM and IE CD8+CD103+ TRM (12, 25). For LP CD4+ TRM, both CD103+ and CD103- subsets are very potent producers of cytokines IFN-γ, IL-2, and TNF-α (13).
Second, some TRM exhibit direct effector function. A fraction of intestinal TRM can directly lyse the infected cells by expressing high levels of cytotoxic granules such as granzyme B at an early stage (23, 51). This effector-like phenotype of the intestinal TRM mainly depends on the cytokine milieu of the intestinal microenvironment rather than the persistent antigen stimulation (23). Interestingly, it was found that the expression of cytotoxic granules is quite similar between CD4+ and CD8+ TRM (19). CD8+CD103- TRM, especially those still expressing KLRG1, exhibit high cytotoxic and proliferative potentials (12, 25). As mentioned above, they may represent TRM populations recently recruited and maintained in the intestinal mucosa. Previous studies using two-photon laser scanning microscopy have shown restricted motility of the intestinal TRM (52). They may stay at the sites of previous infections to surveil potential recurring pathogens. The actual contribution of the effector functions in immunologic protection mediated by the intestinal TRM needs further evaluation.
As shown in the mouse infection model, intestinal TRM undergo proliferation in situ in response to antigen rechallenging (Figure 1) (19, 53). Two recent studies have demonstrated that reactivated TCM maintain the potential to form CD103- TRM and few CD103+ TRM, but the efficiency of reactivated TCM is extremely lower than naïve T cells (53, 54). Under steady-state conditions, the intestinal TRM populations are maintained mainly by longevity rather than local proliferation (12).
Recent evidence suggested that intestinal TRM are not terminally differentiated as previously thought (Figure 1) (54). After pathogen rechallenge, intestinal TRM are able to egress from the intestine and differentiate into circulating memory T cells. And some “ex-TRM” can migrate to the draining lymph nodes and give rise to the local TRM (54–56). Such a process may be initiated by the antigen-driven downregulation of Hobit expression (56). In addition, “ex-TRM” that re-joined the circulating pool are advantaged to migrate back and re-differentiated into intestinal TRM (54). A TRM differentiation program is epigenetically maintained for those cells despite their developmental plasticity (54, 55). The differentiation potential of TRM enables them to shape systemic T cell responses after reinfection (56).
In general, the intestinal TRM can exert protective functions locally by expressing proinflammatory cytokine and cytolytic granules. Based on these findings, developing vaccines that induce TRM to combat recurrent infections may be a promising strategy to enhance immunological protection.
TRM and Colorectal Cancer
CD8+ T cells contribute to the immunosurveillance against cancer, and their protective effects are highly correlated to their ability to enter and survive in the immunosuppressive microenvironment of the tumor compartments (10). Therefore, TRM present within the tumor may be critical for controlling tumor growth. Recent reports have recognized the immunosurveillance function and prognostic significance of TRM in CRC.
Over a decade ago, large numbers of CD103+CD8+ tumor-infiltrating lymphocytes (TIL) were observed in the microsatellite instability (MSI) sporadic CRC (57). Further investigations suggested that these TRM-like TIL may be divided into two subsets based on the expression of CD39 (58). CD39, an ectonucleotidase that hydrolyzes ATP and ADP, marks CD8 T cells for chronic antigen stimulation. The expression level of CD39 in the TIL varies significantly in the CRC patients and is remarkably high in patients with high MSI (59). CD39+CD103+ TIL are enriched for tumor antigen-specific T cells which may contribute to the control of tumor growth. In comparison, CD39-CD103+ TIL recognize cancer-unrelated epitopes and are named “bystander” T cells (59, 60). When activated in vitro, the CD8+CD39+CD103+ TIL are highly capable of killing the tumor cells in an MHC-dependent manner (59). One possible explanation is that the interaction between the CD103 on the TIL and E-cadherin on the tumor cells induces the polarization and exocytosis of cytotoxic granules at the immune synapse. As a result, the effector function of the tumor-resident memory T cells is enhanced (61). Consistent with that, a co-culture system of intestinal tumor organoids and T cells showed the significance of CD103/E-cadherin signals for antitumor immune response (62). However, whether intestinal TRM are able to suppress tumors in vivo by target cell killing has not been validated. In addition to direct cell killing, intestinal TRM may suppress tumor cell growth by secreting cytokine TNF-α and IFN-γ (63, 64). Current studies also confirmed that infiltration of CD103+CD8+ TIL is an independent predictor of survival for patients with CRC (65).
CD39+CD103+ TIL promote the gene transcription of typical markers related to exhaustion and immunomodulation (PD-1, CTLA-4, and TIM-3) (59), which implies a possible immune escape mechanism of tumor cells. Such TRM-like TIL are an appealing target of immune checkpoint inhibitor (ICI) therapy due to their expression of immunomodulatory markers and proliferative potential (58, 59, 66). In fact, CD103 expression highly correlates with clinical response to anti-PD-L1 immunotherapy in patients with lung and bladder cancer (67). A recent study also identified IFN-γ-producing CD8+ TRM as a potential therapeutic target of ICI–colitis (68). It was found that most activated T cells in ICI-colitis co-express CD69 and CD103 (68). Moreover, the proportion of activated CD8+ TRM in intestinal mucosa is highly correlated with clinical and endoscopic scores of ICI-colitis (68).
Taken together, TRM contribute to the tumor immunosurveillance in the intestinal mucosa and act as a prominent target of ICI therapy and ICI-colitis. A more comprehensive understanding of the identification, regulation, and function of TRM-like TIL is required for improving current cancer treatment.
TRM and Inflammatory Bowel Disease
IBD, including Crohn’s disease (CD) and ulcerative colitis (UC), is a chronic inflammatory disease of the intestinal tract characterized by local relapsing flares (69). The pathogenesis of IBD involves abnormal immune responses against intestinal microbiome (70). Some properties of TRM suggest that they may be the key player in the onset and relapse of IBD. TRM possess the capacity to secrete pro-inflammatory cytokine and recruit other leukocytes. Also, TRM in the intestine mucosa are confined to certain regions with limited ability to migrate, which may explain the localized pattern of flares seen in IBD (52).
Recent evidence suggests that CD4+ TRM may serve as a driver for IBD (71). Zundler et al. reported that knockout of Hobit and Blimp-1, the two core transcriptional factors of TRM, prevented the development of colitis in several experimental mouse models (71). Furthermore, in the T cell transfer colitis model, the mouse with Hobit-Blimp-1 double knockout CD4+ T cells showed impaired secretion of pro-inflammatory cytokines (IFN-γ, IL-13, and IL-17A) and recruitment of granulocytes and macrophages (71). In humans, the same authors observed that CD4+CD69+ T cells in the LP of IBD patients produce markedly more pro-inflammatory cytokines (IFN-γ, IL-13, IL-17A, and TNF) when compared to CD4+CD69- T cells. High CD4+CD69+CD103+ TRM proportion in LP was prospectively associated with earlier relapse in both UC and CD patients (71). Consistently, a study by Lamb et al. showed that the high expression of CD103 in CD4+ T cells is associated with elevated pro-inflammatory cytokine production and lowered regulatory markers expression in UC patients (72). Furthermore, CD4+CD103+ TRM are enriched for Th17/Th1 lineage cells, which co-express IL-17A and IFN-γ (72). Bishu et al. investigated colon samples of CD patients and found that CD4+ TRM in CD patients expresses higher levels of IFN-γ and IL-17A relative to controls (73). Particularly, CD4+ TRM are identified as the major mucosal TNF-α-producing T cell subsets in the CD patients (73). Despite the pathogenic role of CD4+ TRM, it remains controversial whether CD4+ CD103+ TRM are increased in the gut of IBD patients (71, 74).
The heterogeneity and functional profile of CD8+ TRM in IBD were also investigated. Bottois et al. observed two distinct subsets of CD8+ TRM in the ileum of CD patients, defined by the mutually exclusive expression of CD103 and KLRG1 (25). CD8+ CD103+ TRM were decreased in inflamed mucosa from IBD patients when compared to non-inflamed mucosa and controls, while CD8+KLRG1+ TRM showed a significant increase (25). CD8+CD103+ TRM in CD patients expressed higher levels of IL22, IL26, and CCL20. These three Th17-related cytokines are involved in tissue homeostasis and innate immune response (25). Similarly, single-cell RNA-sequencing of CD8+ T cells from the colon of UC patients showed that the expression of IL26 was enriched in the CD8+CD103+ population (75). In addition, CD8+CD103+ TRM express high levels of CD39 and CD73, two key functional markers of regulatory T cells (76). CD39 and CD73 can hydrolyze extracellular ATP into adenosine, which is a potent immunoregulator (77). Roosenboom et al. observed that CD8+CD103+ TRM were significantly decreased in patients with active IBD compared to patients with endoscopic remission and healthy controls (74). These findings suggest that CD8+CD103+ TRM may contribute to tissue homeostasis and immunoregulation. By contrast, CD8+KLRG+ TRM have higher proliferative and cytotoxic potential (12, 25).
The pathogenic role of TRM makes them a promising target for IBD treatment. For instance, etrolizumab is a humanized monoclonal antibody that targets the β7 subunits of αEβ7 and α4β7 integrins. In a completed phase III trial in UC, etrolizumab met its primary endpoint in two induction studies but not in any maintenance studies (78–81). Phase III trials assessing etrolizumab in CD are still ongoing with positive results in an exploratory induction cohort (82). The clinical efficacy of Etrolizumab may be partially explained by impairing the retention of CD103+ TRM. In fact, a phase II trial in UC showed that the response to etrolizumab is associated with the number of CD103+ cell in the colon samples (83). However, CD103 is also expressed on a subset of intestinal dendritic cells (84), so it remains to be investigated whether TRM are the real target of etrolizumab. Another pharmacological that might target TRM is S1PR modulators. Recently, the S1PR modulator ozanimod has been approved for UC (85). By triggering the internalization and degradation of the S1P receptors, S1PR modulators inhibit lymphocyte egress from lymphoid organs and may impair local generation of intestinal TRM (86).
Collectively, a growing body of evidence implicates that TRM participate in the pathogenesis of IBD. Therefore, they might be the potential target of some currently developed drugs, especially etrolizumab and S1PR modulators. It is not surprising that TRM have also been identified as key mediators in other intestinal immunopathologies, such as celiac disease (87) and acute graft-versus-host disease (88). Further exploration is needed to better understand the role TRM play in different intestinal immunopathologies and to develop therapies that specifically target pathogenic TRM.
Conclusions
A large number of CD8+ and CD4+ TRM are permanently located in the human intestinal LP and IE (12, 13). After developing within the gut microenvironment, CD8+ and CD4+ TRM differ from their circulating counterpart both at the phenotypical level and transcriptional level (29). In recent years, studies in mouse models and human biopsies have demonstrated a crucial role for intestine TRM in the protection against enteric pathogens, immunosurveillance of intestinal malignancy, and probably inducing intestinal chronic inflammation (26, 59, 71). Advancing our knowledge of the properties and functions of intestinal TRM may provide insights into developing efficacious vaccines and therapies against intestinal diseases.
Author Contributions
YL and YZ collected the papers and data, analyzed the conclusions, and drafted the manuscript; JS presented the idea of this paper, supported the funding, analyzed the conclusions, drafted and revised the manuscript. All authors contributed to the article and approved the submitted version.
Funding
Supported by grants from Shanghai Science and Technology Innovation Initiative (21SQBS02302), and Cultivated Funding for Clinical Research Innovation, Renji Hospital, School of Medicine, Shanghai Jiaotong University (RJPY-LX-004).
Conflict of Interest
The authors declare that the research was conducted in the absence of any commercial or financial relationships that could be construed as a potential conflict of interest.
Publisher’s Note
All claims expressed in this article are solely those of the authors and do not necessarily represent those of their affiliated organizations, or those of the publisher, the editors and the reviewers. Any product that may be evaluated in this article, or claim that may be made by its manufacturer, is not guaranteed or endorsed by the publisher.
References
1. Ahmed R, Gray D. Immunological Memory and Protective Immunity: Understanding Their Relation. Science (1996) 272(5258):54–60. doi: 10.1126/science.272.5258.54
2. Sallusto F, Lenig D, Forster R, Lipp M, Lanzavecchia A. Two Subsets of Memory T Lymphocytes With Distinct Homing Potentials and Effector Functions. Nature (1999) 401(6754):708–12. doi: 10.1038/44385
3. von Andrian UH, Mackay CR. T-Cell Function and Migration. Two Sides of the Same Coin. N Engl J Med (2000) 343(14):1020–34. doi: 10.1056/NEJM200010053431407
4. Butcher EC, Picker LJ. Lymphocyte Homing and Homeostasis. Science (1996) 272(5258):60–6. doi: 10.1126/science.272.5258.60
5. Mueller SN, Mackay LK. Tissue-Resident Memory T Cells: Local Specialists in Immune Defence. Nat Rev Immunol (2016) 16(2):79–89. doi: 10.1038/nri.2015.3
6. Masopust D, Choo D, Vezys V, Wherry EJ, Duraiswamy J, Akondy R, et al. Dynamic T Cell Migration Program Provides Resident Memory Within Intestinal Epithelium. J Exp Med (2010) 207(3):553–64. doi: 10.1084/jem.20090858
7. Sathaliyawala T, Kubota M, Yudanin N, Turner D, Camp P, Thome JJ, et al. Distribution and Compartmentalization of Human Circulating and Tissue-Resident Memory T Cell Subsets. Immunity (2013) 38(1):187–97. doi: 10.1016/j.immuni.2012.09.020
8. Kumar BV, Ma W, Miron M, Granot T, Guyer RS, Carpenter DJ, et al. Human Tissue-Resident Memory T Cells Are Defined by Core Transcriptional and Functional Signatures in Lymphoid and Mucosal Sites. Cell Rep (2017) 20(12):2921–34. doi: 10.1016/j.celrep.2017.08.078
9. Muruganandah V, Sathkumara HD, Navarro S, Kupz A. A Systematic Review: The Role of Resident Memory T Cells in Infectious Diseases and Their Relevance for Vaccine Development. Front Immunol (2018) 9:1574. doi: 10.3389/fimmu.2018.01574
10. Park SL, Gebhardt T, Mackay LK. Tissue-Resident Memory T Cells in Cancer Immunosurveillance. Trends Immunol (2019) 40(8):735–47. doi: 10.1016/j.it.2019.06.002
11. Wu HJ, Liao W, Li QW, Long H, Yin H, Zhao M, et al. Pathogenic Role of Tissue-Resident Memory T Cells in Autoimmune Diseases. Autoimmun Rev (2018) 17(9):906–11. doi: 10.1016/j.autrev.2018.03.014
12. Bartolome-Casado R, Landsverk OJB, Chauhan SK, Richter L, Phung D, Greiff V, et al. Resident Memory CD8 T Cells Persist for Years in Human Small Intestine. J Exp Med (2019) 216(10):2412–26. doi: 10.1084/jem.20190414
13. Bartolomé-Casado R, Landsverk OJB, Chauhan SK, Sætre F, Hagen KT, Yaqub S, et al. CD4(+) T Cells Persist for Years in the Human Small Intestine and Display a T(H)1 Cytokine Profile. Mucosal Immunol (2020) 14(2):402–10. doi: 10.1038/s41385-020-0315-5
14. Shiow LR, Rosen DB, Brdickova N, Xu Y, An J, Lanier LL, et al. CD69 Acts Downstream of Interferon-Alpha/Beta to Inhibit S1P1 and Lymphocyte Egress From Lymphoid Organs. Nature (2006) 440(7083):540–4. doi: 10.1038/nature04606
15. Bankovich AJ, Shiow LR, Cyster JG. CD69 Suppresses Sphingosine 1-Phosophate Receptor-1 (S1P(1)) Function Through Interaction With Membrane Helix 4. J Biol Chem (2010) 285(29):22328–37. doi: 10.1074/jbc.M110.123299
16. Mackay LK, Braun A, Macleod BL, Collins N, Tebartz C, Bedoui S, et al. Cutting Edge: CD69 Interference With Sphingosine-1-Phosphate Receptor Function Regulates Peripheral T Cell Retention. J Immunol (2015) 194(5):2059–63. doi: 10.4049/jimmunol.1402256
17. Skon CN, Lee JY, Anderson KG, Masopust D, Hogquist KA, Jameson SC. Transcriptional Downregulation of S1pr1 is Required for the Establishment of Resident Memory CD8(+) T Cells. Nat Immunol (2013) 14(12):1285. doi: 10.1038/ni.2745
18. Walsh DA, Borges da Silva H, Beura LK, Peng C, Hamilton SE, Masopust D, et al. The Functional Requirement for CD69 in Establishment of Resident Memory CD8(+) T Cells Varies With Tissue Location. J Immunol (2019) 203(4):946–55. doi: 10.4049/jimmunol.1900052
19. Beura LK, Fares-Frederickson NJ, Steinert EM, Scott MC, Thompson EA, Fraser KA, et al. CD4(+) Resident Memory T Cells Dominate Immunosurveillance and Orchestrate Local Recall Responses. J Immunol (2019) 216(5):1214–29. doi: 10.1084/jem.20181365
20. Romagnoli PA, Fu HH, Qiu Z, Khairallah C, Pham QM, Puddington L, et al. Differentiation of Distinct Long-Lived Memory CD4 T Cells in Intestinal Tissues After Oral Listeria Monocytogenes Infection. Mucosal Immunol (2017) 10(2):520–30. doi: 10.1038/mi.2016.66
21. Mackay LK, Rahimpour A, Ma JZ, Collins N, Stock AT, Hafon ML, et al. The Developmental Pathway for CD103(+)CD8+ Tissue-Resident Memory T Cells of Skin. Nat Immunol (2013) 14(12):1294–301. doi: 10.1038/ni.2744
22. Cepek KL, Shaw SK, Parker CM, Russell GJ, Morrow JS, Rimm DL, et al. Adhesion Between Epithelial Cells and T Lymphocytes Mediated by E-Cadherin and the Alpha E Beta 7 Integrin. Nature (1994) 372(6502):190–3. doi: 10.1038/372190a0
23. Casey KA, Fraser KA, Schenkel JM, Moran A, Abt MC, Beura LK, et al. Antigen-Independent Differentiation and Maintenance of Effector-Like Resident Memory T Cells in Tissues. J Immunol (2012) 188(10):4866–75. doi: 10.4049/jimmunol.1200402
24. FitzPatrick MEB, Provine NM, Garner LC, Powell K, Amini A, Irwin SL, et al. Human Intestinal Tissue-Resident Memory T Cells Comprise Transcriptionally and Functionally Distinct Subsets. Cell Rep (2021) 34(3):108661. doi: 10.1016/j.celrep.2020.108661
25. Bottois H, Ngollo M, Hammoudi N, Courau T, Bonnereau J, Chardiny V, et al. KLRG1 and CD103 Expressions Define Distinct Intestinal Tissue-Resident Memory CD8 T Cell Subsets Modulated in Crohn's Disease. Front Immunol (2020) 11:896. doi: 10.3389/fimmu.2020.00896
26. Sheridan BS, Pham QM, Lee YT, Cauley LS, Puddington L, Lefrancois L. Oral Infection Drives a Distinct Population of Intestinal Resident Memory CD8(+) T Cells With Enhanced Protective Function. Immunity (2014) 40(5):747–57. doi: 10.1016/j.immuni.2014.03.007
27. Bergsbaken T, Bevan MJ, Fink PJ. Local Inflammatory Cues Regulate Differentiation and Persistence of CD8(+) Tissue-Resident Memory T Cells. Cell Rep (2017) 19(1):114–24. doi: 10.1016/j.celrep.2017.03.031
28. Fergusson JR, Hühn MH, Swadling L, Walker LJ, Kurioka A, Llibre A, et al. CD161(int)CD8+ T Cells: A Novel Population of Highly Functional, Memory CD8+ T Cells Enriched Within the Gut. Mucosal Immunol (2016) 9(2):401–13. doi: 10.1038/mi.2015.69
29. Mackay LK, Minnich M, Kragten NAM, Liao Y, Nota B, Seillet C, et al. Hobit and Blimp1 Instruct a Universal Transcriptional Program of Tissue Residency in Lymphocytes. Science (2016) 352(6284):459–63. doi: 10.1126/science.aad2035
30. Milner JJ, Toma C, Yu BF, Zhang K, Omilusik K, Phan AT, et al. Runx3 Programs CD8(+) T Cell Residency in non-Lymphoid Tissues and Tumours (Vol 552, Pg 253, 2017). Nature (2018) 552(7684):253–7. doi: 10.1038/nature24993
31. Grueter B, Petter M, Egawa T, Laule-Kilian K, Aldrian CJ, Wuerch A, et al. Runx3 Regulates Integrin Alpha E/CD103 and CD4 Expression During Development of CD4-/CD8+ T Cells. J Immunol (2005) 175(3):1694–705. doi: 10.4049/jimmunol.175.3.1694
32. Mackay LK, Wynne-Jones E, Freestone D, Pellicci DG, Mielke LA, Newman DM, et al. T-Box Transcription Factors Combine With the Cytokines TGF-β and IL-15 to Control Tissue-Resident Memory T Cell Fate. Immunity (2015) 43(6):1101–11. doi: 10.1016/j.immuni.2015.11.008
33. Parga-Vidal L, Behr FM, Kragten NAM, Nota B, Wesselink TH, Kavazović I, et al. Hobit Identifies Tissue-Resident Memory T Cell Precursors That are Regulated by Eomes. Sci Immunol (2021) 6(62). doi: 10.1126/sciimmunol.abg3533
34. Milner JJ, Toma C, He Z, Kurd NS, Nguyen QP, McDonald B, et al. Heterogenous Populations of Tissue-Resident CD8(+) T Cells Are Generated in Response to Infection and Malignancy. Immunity (2020) 52(5):808–24.e7. doi: 10.1016/j.immuni.2020.04.007
35. Masopust D, Vezys V, Wherry EJ, Barber DL, Ahmed R. Cutting Edge: Gut Microenvironment Promotes Differentiation of a Unique Memory CD8 T Cell Population. J Immunol (2006) 176(4):2079–83. doi: 10.4049/jimmunol.176.4.2079
36. Stemberger C, Huster KM, Koffler M, Anderl F, Schiemann M, Wagner H, et al. A Single Naive CD8+ T Cell Precursor can Develop Into Diverse Effector and Memory Subsets. Immunity (2007) 27(6):985–97. doi: 10.1016/j.immuni.2007.10.012
37. Herndler-Brandstetter D, Ishigame H, Shinnakasu R, Plajer V, Stecher C, Zhao J, et al. KLRG1(+) Effector CD8(+) T Cells Lose KLRG1, Differentiate Into All Memory T Cell Lineages, and Convey Enhanced Protective Immunity. Immunity (2018) 48(4): 716–29.e8. doi: 10.1016/j.immuni.2018.03.015
38. Schwartzkopff S, Woyciechowski S, Aichele U, Flecken T, Zhang N, Thimme R, et al. TGF-Beta Downregulates KLRG1 Expression in Mouse and Human CD8(+) T Cells. Eur J Immunol (2015) 45(8):2212–7. doi: 10.1002/eji.201545634
39. Qiu Z, Chu TH, Sheridan BS. TGF-Beta: Many Paths to CD103(+) CD8 T Cell Residency. Cells (2021) 10(5):989. doi: 10.3390/cells10050989
40. Bai A, Hu H, Yeung M, Chen J. Kruppel-Like Factor 2 Controls T Cell Trafficking by Activating L-Selectin (CD62L) and Sphingosine-1-Phosphate Receptor 1 Transcription. J Immunol (2007) 178(12):7632–9. doi: 10.4049/jimmunol.178.12.7632
41. Mokrani M, Klibi J, Bluteau D, Bismuth G, Mami-Chouaib F. Smad and NFAT Pathways Cooperate to Induce CD103 Expression in Human CD8 T Lymphocytes. J Immunol (2014) 192(5):2471–9. doi: 10.4049/jimmunol.1302192
42. Borges da Silva H, Peng C, Wang H, Wanhainen KM, Ma C, Lopez S, et al. Sensing of ATP via the Purinergic Receptor P2RX7 Promotes CD8(+) Trm Cell Generation by Enhancing Their Sensitivity to the Cytokine TGF-β. Immunity (2020) 53(1):158–71.e6. doi: 10.1016/j.immuni.2020.06.010
43. Peng C, Huggins MA, Wanhainen KM, Knutson TP, Lu H, Georgiev H, et al. Engagement of the Costimulatory Molecule ICOS in Tissues Promotes Establishment of CD8(+) Tissue-Resident Memory T Cells. Immunity (2022) 55(1):98–114.e5. doi: 10.1016/j.immuni.2021.11.017
44. Schenkel JM, Fraser KA, Casey KA, Beura LK, Pauken KE, Vezys V, et al. IL-15-Independent Maintenance of Tissue-Resident and Boosted Effector Memory CD8 T Cells. J Immunol (2016) 196(9):3920–6. doi: 10.4049/jimmunol.1502337
45. Booth JS, Goldberg E, Barnes RS, Greenwald BD, Sztein MB. Oral Typhoid Vaccine Ty21a Elicits Antigen-Specific Resident Memory CD4(+) T Cells in the Human Terminal Ileum Lamina Propria and Epithelial Compartments. J Transl Med (2020) 18(1):102. doi: 10.1186/s12967-020-02263-6
46. Booth JS, Patil SA, Goldberg E, Barnes RS, Greenwald BD, Sztein MB. Attenuated Oral Typhoid Vaccine Ty21a Elicits Lamina Propria and Intra-Epithelial Lymphocyte Tissue-Resident Effector Memory CD8 T Responses in the Human Terminal Ileum. Front Immunol (2019) 10:424. doi: 10.3389/fimmu.2019.00424
47. Kiniry BE, Li SB, Ganesh A, Hunt PW, Somsouk M, Skinner PJ, et al. Detection of HIV-1-Specific Gastrointestinal Tissue Resident CD8(+) T-Cells in Chronic Infection. Mucosal Immunol (2018) 11(3):909–20. doi: 10.1038/mi.2017.96
48. Ariotti S, Hogenbirk MA, Dijkgraaf FE, Visser LL, Hoekstra ME, Song JY, et al. T Cell Memory. Skin-Resident Memory CD8⁺ T Cells Trigger a State of Tissue-Wide Pathogen Alert. Science (2014) 346(6205):101–5. doi: 10.1126/science.1254803
49. Schenkel JM, Fraser KA, Vezys V, Masopust D. Sensing and Alarm Function of Resident Memory CD8⁺ T Cells. Nat Immunol (2013) 14(5):509–13. doi: 10.1038/ni.2568
50. Schenkel JM, Fraser KA, Beura LK, Pauken KE, Vezys V, Masopust D. T Cell Memory. Resident Memory CD8 T Cells Trigger Protective Innate and Adaptive Immune Responses. Science (2014) 346(6205):98–101. doi: 10.1126/science.1254536
51. Bergsbaken T, Bevan MJ. Proinflammatory Microenvironments Within the Intestine Regulate the Differentiation of Tissue-Resident CD8(+) T Cells Responding to Infection. Nat Immunol (2015) 16(4):406–14. doi: 10.1038/ni.3108
52. Thompson EA, Mitchell JS, Beura LK, Torres DJ, Mrass P, Pierson MJ, et al. Interstitial Migration of CD8 Alpha Beta T Cells in the Small Intestine Is Dynamic and Is Dictated by Environmental Cues. Cell Rep (2019) 26(11):2859–67. doi: 10.1016/j.celrep.2019.02.034
53. Behr FM, Beumer-Chuwonpad A, Kragten NAM, Wesselink TH, Stark R, van Gisbergen K. Circulating Memory CD8(+) T Cells are Limited in Forming CD103(+) Tissue-Resident Memory T Cells at Mucosal Sites After Reinfection. Eur J Immunol (2021) 51(1):151–66. doi: 10.1002/eji.202048737
54. Fonseca R, Beura LK, Quarnstrom CF, Ghoneim HE, Fan Y, Zebley CC, et al. Developmental Plasticity Allows Outside-in Immune Responses by Resident Memory T Cells. Nat Immunol (2020) 21(4):412–21. doi: 10.1038/s41590-020-0607-7
55. Beura LK, Wijeyesinghe S, Thompson EA, Macchietto MG, Rosato PC, Pierson MJ, et al. T Cells in Nonlymphoid Tissues Give Rise to Lymph-Node-Resident Memory T Cells. Immunity (2018) 48(2):327–38.e5. doi: 10.1016/j.immuni.2018.01.015
56. Behr FM, Parga-Vidal L, Kragten NAM, van Dam TJP, Wesselink TH, Sheridan BS, et al. Tissue-Resident Memory CD8(+) T Cells Shape Local and Systemic Secondary T Cell Responses. Nat Immunol (2020) 21(9):1070–81. doi: 10.1038/s41590-020-0723-4
57. Quinn E, Hawkins N, Yip YL, Suter C, Ward R. CD103+ Intraepithelial Lymphocytes–a Unique Population in Microsatellite Unstable Sporadic Colorectal Cancer. Eur J Cancer (2003) 39(4):469–75. doi: 10.1016/S0959-8049(02)00633-0
58. Simoni Y, Becht E, Fehlings M, Loh CY, Koo SL, Teng KWW, et al. Bystander CD8(+) T Cells are Abundant and Phenotypically Distinct in Human Tumour Infiltrates. Nature (2018) 557(7706):575–9. doi: 10.1038/s41586-018-0130-2
59. Duhen T, Duhen R, Montler R, Moses J, Moudgil T, de Miranda NF, et al. Co-Expression of CD39 and CD103 Identifies Tumor-Reactive CD8 T Cells in Human Solid Tumors. Nat Commun (2018) 9(1):2724. doi: 10.1038/s41467-018-05072-0
60. van den Bulk J, Verdegaal EME, Ruano D, Ijsselsteijn ME, Visser M, van der Breggen R, et al. Neoantigen-Specific Immunity in Low Mutation Burden Colorectal Cancers of the Consensus Molecular Subtype 4. Genome Med (2019) 11(1):87. doi: 10.1186/s13073-019-0697-8
61. Le Floc'h A, Jalil A, Vergnon I, Le Maux Chansac B, Lazar V, Bismuth G, et al. Alpha E Beta 7 Integrin Interaction With E-Cadherin Promotes Antitumor CTL Activity by Triggering Lytic Granule Polarization and Exocytosis. J Exp Med (2007) 204(3):559–70. doi: 10.1084/jem.20061524
62. Morikawa R, Nemoto Y, Yonemoto Y, Tanaka S, Takei Y, Oshima S, et al. Intraepithelial Lymphocytes Suppress Intestinal Tumor Growth by Cell-To-Cell Contact via CD103/E-Cadherin Signal. Cell Mol Gastroenterol Hepatol (2021) 11(5):1483–503. doi: 10.1016/j.jcmgh.2021.01.014
63. Becht E, Giraldo NA, Dieu-Nosjean MC, Sautès-Fridman C, Fridman WH. Cancer Immune Contexture and Immunotherapy. Curr Opin Immunol (2016) 39:7–13. doi: 10.1016/j.coi.2015.11.009
64. Park SL, Buzzai A, Rautela J, Hor JL, Hochheiser K, Effern M, et al. Tissue-Resident Memory CD8(+) T Cells Promote Melanoma-Immune Equilibrium in Skin. Nature (2019) 565(7739):366–71. doi: 10.1038/s41586-018-0812-9
65. Hu X, Li YQ, Li QG, Ma YL, Peng JJ, Cai SJ. ITGAE Defines CD8+ Tumor-Infiltrating Lymphocytes Predicting a Better Prognostic Survival in Colorectal Cancer. EBioMedicine (2018) 35:178–88. doi: 10.1016/j.ebiom.2018.08.003
66. Nizard M, Roussel H, Diniz MO, Karaki S, Tran T, Voron T, et al. Induction of Resident Memory T Cells Enhances the Efficacy of Cancer Vaccine. Nat Commun (2017) 8:15221. doi: 10.1038/ncomms15221
67. Banchereau R, Chitre AS, Scherl A, Wu TD, Patil NS, de Almeida P, et al. Intratumoral CD103+ CD8+ T Cells Predict Response to PD-L1 Blockade. J Immunother Cancer (2021) 9(4):e002231. doi: 10.1136/jitc-2020-002231
68. Sasson SC, Slevin SM, Cheung VTF, Nassiri I, Olsson-Brown A, Fryer E, et al. Interferon-Gamma-Producing CD8(+) Tissue Resident Memory T Cells Are a Targetable Hallmark of Immune Checkpoint Inhibitor-Colitis. Gastroenterology (2021) 161(4):1229–44.e9. doi: 10.1053/j.gastro.2021.06.025
69. Kaser A, Zeissig S, Blumberg RS. Inflammatory Bowel Disease. Annu Rev Immunol (2010) 28:573–621. doi: 10.1146/annurev-immunol-030409-101225
70. Zhang YZ, Li YY. Inflammatory Bowel Disease: Pathogenesis. World J Gastroenterol (2014) 20(1):91–9. doi: 10.3748/wjg.v20.i1.91
71. Zundler S, Becker E, Spocinska M, Slawik M, Parga-Vidal L, Stark R, et al. Hobit- and Blimp-1-Driven CD4(+) Tissue-Resident Memory T Cells Control Chronic Intestinal Inflammation. Nat Immunol (2019) 20(3):288–300. doi: 10.1038/s41590-018-0298-5
72. Lamb CA, Mansfield JC, Tew GW, Gibbons D, Long AK, Irving P, et al. Alphaebeta7 Integrin Identifies Subsets of Pro-Inflammatory Colonic CD4+ T Lymphocytes in Ulcerative Colitis. J Crohns Colitis (2017) 11(5):610–20. doi: 10.1093/ecco-jcc/jjw189
73. Bishu S, El Zaatari M, Hayashi A, Hou G, Bowers N, Kinnucan J, et al. CD4+ Tissue-Resident Memory T Cells Expand and Are a Major Source of Mucosal Tumour Necrosis Factor Alpha in Active Crohn's Disease. J Crohns Colitis (2019) 13(7):905–15. doi: 10.1093/ecco-jcc/jjz010
74. Roosenboom B, Wahab PJ, Smids C, Groenen MJM, van Koolwijk E, van Lochem EG, et al. Intestinal CD103+CD4+ and CD103+CD8+ T-Cell Subsets in the Gut of Inflammatory Bowel Disease Patients at Diagnosis and During Follow-Up. Inflammation Bowel Dis (2019) 25(9):1497–509. doi: 10.1093/ibd/izz049
75. Corridoni D, Antanaviciute A, Gupta T, Fawkner-Corbett D, Aulicino A, Jagielowicz M, et al. Single-Cell Atlas of Colonic CD8(+) T Cells in Ulcerative Colitis. Nat Med (2020) 26(9):1480–90. doi: 10.1038/s41591-020-1003-4
76. Noble A, Durant L, Hoyles L, McCartney AL, Man R, Segal J, et al. Deficient Resident Memory T Cell and CD8 T Cell Response to Commensals in Inflammatory Bowel Disease. J Crohns Colitis (2020) 14(4):525–37. doi: 10.1093/ecco-jcc/jjz175
77. Deaglio S, Dwyer KM, Gao W, Friedman D, Usheva A, Erat A, et al. Adenosine Generation Catalyzed by CD39 and CD73 Expressed on Regulatory T Cells Mediates Immune Suppression. J Exp Med (2007) 204(6):1257–65. doi: 10.1084/jem.20062512
78. Danese S, Colombel J, Lukas M, Gisbert J, D’Haens G, Hayee B. Etrolizumab Versus Infliximab for Treating Patients With Moderately to Severely Active Ulcerative Colitis: Results From the Phase 3 GARDENIA Study. United Eur Gastroenterol J (2020) 8:1258–75. doi: 10.1177/2050640620968709
79. Dotan I, Panés J, Duval A, Bouhnik Y, Radford-Smith G, Higgins P. Etrolizumab Compared With Adalimumab or Placebo as Induction Therapy for Ulcerative Colitis: Results From the Randomized, Phase 3 Hibiscus I & Ii Trials. United Eur Gastroenterol J (2020) 8:1258–75. doi: 10.1177/2050640620968709
80. Peyrin-Biroulet L, Hart A, Bossuyt P, Long M, Allez M, Juillerat P. Etrolizumab as Induction and Maintenance Therapy in Patients With Ulcerative Colitis Previously Exposed to Anti-Tumor Necrosis Factor Agent: The Randomized, Phase 3 HICKORY Trial. United Eur Gastroenterol J (2020) 8:1258–75. doi: 10.1177/2050640620968709
81. Vermeire S, Lakatos P, Ritter T, Hanauer S, Bressler B, Khanna R. Etrolizumab Versus Placebo in Tumor Necrosis Factor Antagonist Naive Patients With Ulcerative Colitis: Results From the Randomized Phase 3 Laurel Trial. United Eur Gastroenterol J (2020) 8:1258–75. doi: 10.1177/2050640620968709
82. William S, Julian P, Jennifer J, Azra H, Rhian J, Zaineb S, et al. Etrolizumab as Induction Therapy in Moderate to Severe Crohn's Disease: Results From BERGAMOT Cohort 1: P-011. Off J Am Coll Gastroenterol ACG (2018) 113:S3. doi: 10.14309/00000434-201802001-00011
83. Tew GW, Hackney JA, Gibbons D, Lamb CA, Luca D, Egen JG, et al. Association Between Response to Etrolizumab and Expression of Integrin αe and Granzyme A in Colon Biopsies of Patients With Ulcerative Colitis. Gastroenterology (2016) 150(2):477–87.e9. doi: 10.1053/j.gastro.2015.10.041
84. Bain CC, Montgomery J, Scott CL, Kel JM, Girard-Madoux MJH, Martens L, et al. Tgfβr Signalling Controls CD103(+)CD11b(+) Dendritic Cell Development in the Intestine. Nat Commun (2017) 8(1):620. doi: 10.1038/s41467-017-00658-6
85. Sandborn WJ, Feagan BG, D'Haens G, Wolf DC, Jovanovic I, Hanauer SB, et al. Ozanimod as Induction and Maintenance Therapy for Ulcerative Colitis. N Engl J Med (2021) 385(14):1280–91. doi: 10.1056/NEJMoa2033617
86. McGinley MP, Cohen JA. Sphingosine 1-Phosphate Receptor Modulators in Multiple Sclerosis and Other Conditions. Lancet (2021) 398(10306):1184–94. doi: 10.1016/S0140-6736(21)00244-0
87. Sollid LM. Gut Tissue-Resident Memory T Cells in Coeliac Disease. Scand J Immunol (2022) 95(1):e13120. doi: 10.1111/sji.13120
Keywords: autoimmune disease, colorectal carcinoma, human intestine, inflammatory bowel disease, memory T cells
Citation: Lyu Y, Zhou Y and Shen J (2022) An Overview of Tissue-Resident Memory T Cells in the Intestine: From Physiological Functions to Pathological Mechanisms. Front. Immunol. 13:912393. doi: 10.3389/fimmu.2022.912393
Received: 04 April 2022; Accepted: 02 May 2022;
Published: 31 May 2022.
Edited by:
Andreas Hutloff, University Hospital Schleswig-Holstein, GermanyReviewed by:
Juliana Barreto de Albuquerque, Massachusetts General Hospital and Harvard Medical School, United StatesKiyoshi Hirahara, Chiba University, Japan
Copyright © 2022 Lyu, Zhou and Shen. This is an open-access article distributed under the terms of the Creative Commons Attribution License (CC BY). The use, distribution or reproduction in other forums is permitted, provided the original author(s) and the copyright owner(s) are credited and that the original publication in this journal is cited, in accordance with accepted academic practice. No use, distribution or reproduction is permitted which does not comply with these terms.
*Correspondence: Jun Shen, c2hlbmp1bl9tZWQyMDAwQDE2My5jb20=
†These authors have contributed equally to this work and share first authorship