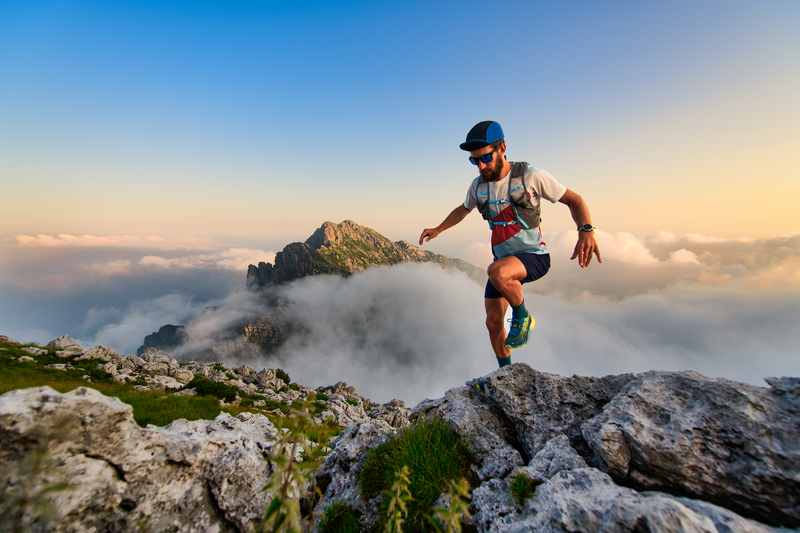
95% of researchers rate our articles as excellent or good
Learn more about the work of our research integrity team to safeguard the quality of each article we publish.
Find out more
REVIEW article
Front. Immunol. , 29 June 2022
Sec. Nutritional Immunology
Volume 13 - 2022 | https://doi.org/10.3389/fimmu.2022.911951
This article is part of the Research Topic Effects of Alcohol Use on Immunity and Immune Responses View all 12 articles
Drinking alcohol, even in moderation, can affect the immune system. Studies have shown disproportionate effects of alcohol on circulating and tissue-resident myeloid cells (granulocytes, monocytes, macrophages, dendritic cells). These cells orchestrate the body’s first line of defense against microbial challenges as well as maintain tissue homeostasis and repair. Alcohol’s effects on these cells are dependent on exposure pattern, with acute drinking dampening but chronic drinking enhancing production of inflammatory mediators. Although chronic drinking is associated with heightened systemic inflammation, studies on tissue resident macrophage populations in several organs including the spleen, liver, brain, and lung have also shown compromised functional and metabolic capacities of these cells. Many of these effects are thought to be mediated by oxidative stress caused by alcohol and its metabolites which can directly impact the cellular epigenetic landscapes. In addition, since myeloid cells are relatively short-lived in circulation and are under constant repopulation from the bone marrow compartment, alcohol’s effects on bone marrow progenitors and hematopoiesis are important for understanding the impact of alcohol systemically on these myeloid populations. Alcohol-induced disruption of progenitor, circulating, and tissue resident myeloid populations contribute to the increased susceptibility of patients with alcohol use disorders to viral and bacterial infections. In this review, we provide an overview of the impact of chronic alcohol consumption on the function of monocytes and macrophages in host defense, tissue repair and inflammation. We then summarize our current understanding of the mechanisms underlying alcohol-induced disruption and examine changes in transcriptome and epigenome of monocytes and mcrophages. Overall, chronic alcohol consumption leads to hyper-inflammation concomitant with decreased microbial and wound healing responses by monocytes/macrophages due to a rewiring of the epigentic and transcriptional landscape. However, in advanced alcoholic liver disease, myeloid cells become immunosuppressed as a response to the surrounding hyper-inflammatory milieu. Therefore, the effect of chronic alcohol on the inflammatory response depends on disease state and the immune cell population.
Per the National Institute on Alcohol Abuse and Alcoholism (NIAAA), alcohol use disorder (AUD) is “a medical condition characterized by an impaired ability to stop or control alcohol use despite adverse social, occupational, or health consequences” ranging from mild to severe. Lasting changes in the brain caused by alcohol misuse perpetuate AUD and make individuals vulnerable to relapse (1). AUD exerts a heavy burden on people’s health and the economy. According to the 2020 National Survey on Drug Use and Health (NSDUH), alcohol consumption is widespread in the USA with 138.5 million people 12 and older reporting drinking alcohol in 2020 and 50% of them drinking alcohol in the month preceding the survey. While a majority of individuals are considered moderate drinkers defined as consuming 2 alcoholic drinks/day or less for men and 1 drink/day for women, 6.4% of people reported engaging in heavy alcohol use in the month preceding the survey. Heavy alcohol use is defined as binge drinking on 5 or more days in the past 30 days with binge drinking defined as consuming ≥5 or more drinks in one sitting for men and ≥4 drinks in one sitting for women. Furthermore, it is estimated that 10.2% of American age 12 and older and 2.8% of adolescents ages 12–17 met the diagnosis of AUD in 2020 (2).
In the United States (U.S.), alcohol is the third-leading preventable cause of death, with an estimated annual death toll of 95,000 people (3). Furthermore, the economic cost of alcohol misuse was estimated to be $249.0 billion in 2010 (4). Adverse outcomes associated with heavy drinking are not limited to the U.S. In 2016, 5.3% of all global deaths, were attributable to alcohol drinking and alcohol abuse was the seventh-leading risk factor for premature death and disability worldwide. Of alcohol-related deaths in 2016, 21.3% were due to digestive diseases (liver cirrhosis, pancreatitis), 19% to cardiovascular diseases, and 12.9% to infectious diseases (including tuberculosis, pneumonia, and HIV/AIDS) (3). These data suggest dysregulated anti-microbial and inflammatory responses with chronic alcohol consumption.
During infection or inflammation, macrophages and monocytes are recruited rapidly to the affected tissues where they play a crucial role in the antimicrobial immune response via phagocytosis and secretion of immune mediators (cytokines, chemokines, and growth factors). Furthermore, monocytes and macrophages coordinate the recruitment of additional immune cell populations including lymphocytes (5, 6). Monocytes and macrophages play a critical role in tissue repair and wound healing by participating in the initial inflammatory response, clearance of injured tissue and invading pathogens, and the resolution phase (7).
Myeloid cell embryonic development occurs in three distinct waves in humans and in mice, involving first the yolk sac then the fetal liver, and finally the fetal bone marrow (5, 8). Yolk-sac derived macrophages seed multiple tissues where they persist for the life of the organism (9). In the adult bone marrow, monocytes develop from hematopoietic progenitor stem cells (HPSC) via progressively restricted lineage-committed progenitors (10, 11). Monopoeisis is well characterized in adult humans and mice, where two independent developmental pathways exist from common myeloid progenitors (CMP): via granulocyte-monocyte progenitors (GMP) to monocyte progenitors (MP) or via monocyte-DC progenitors (MDP) to common monocyte progenitors (cMoP). Mature monocytes develop from the MP and cMoP progenitor populations into the three well-defined subsets of classical, intermediate, and non-classical monocytes (8) based on their expression of CD14 and CD16. The three subsets have distinct yet sometimes overlapping functions. Classical monocytes (CD14++ CD16-) are involved in phagocytosis, innate sensing/immune responses, and migration. Intermediate monocytes (CD14++ CD16+) main functions are antigen presentation, cytokine secretion (TNF-α, IL-1β, and IL-6, following TLR stimulation), differentiation, and regulation of apoptosis. Non-classical monocytes (CD14dim CD16++) are involved in anti-viral responses, complement and Fcγ-mediated phagocytosis, and adhesion (6).
While monocytes are relatively short-lived blood circulating phagocytes, macrophages are longer-lived cells that reside in tissues, including the brain, lung, liver, and intestine, where they perform a central role in antimicrobial responses as well as in tissue homeostasis and repair. Microglia are the tissue-resident macrophages in the brain where they comprise approximately 5-15% of cells (12). Microglia originate from yolk-sac progenitor cells and are maintained in adulthood by self-replenishment without the involvement of progenitor cells from the bone marrow (13, 14). Microglia have two main functions: immune defense of the central nervous system (CNS) via phagocytosis and mediator production as well as CNS maintenance via their control of neuronal proliferation (15). Lung macrophages are the most abundant immune cell population in the healthy lung and are composed of alveolar macrophages in the lumen and interstitial macrophages in the tissue (16, 17). Alveolar macrophages arise from fetal liver-derived monocytes and populate alveoli after birth. They are maintained by self-renewal without the contribution of bone marrow (16, 17). Alveolar macrophages participate in lung host defense and in gas exchange (16, 17). Kupffer cells are the liver tissue-resident macrophages and also are self-renewing populations originating from fetal-liver precursors (18). Other tissue-resident macrophage populations, including in the intestine (where they reside primarily in the lamina propria) (19) and the dermis (20), develop via fetal liver monocyte intermediates and are replenished in adulthood by monocyte-derived macrophages.
While chronic moderate alcohol consumption and acute alcohol exposure affect multiple cell populations and their functions, this review will focus on the impact of chronic heavy alcohol use on circulating monocytes and macrophages that reside within the brain, liver, intestine, and lung since a rich body of literature indicates that heavy alcohol consumption disproportionally impacts their phenotype and function (21, 22).
Findings from clinical studies are confounded by the presence of organ damage, smoking, use of recreational or illicit drugs, nutritional deficiencies, and by self-reported alcohol intake. To address these limitations, different experimental models have been developed to study the effects of alcohol on cell function in more defined settings. The main model systems are described below and listed in Table 1.
To model ethanol consumption, primary and immortalized cells/cell lines are exposed in vitro to ethanol at different concentrations and for various durations (23, 24). Due to their ease of manipulation, several macrophage cell lines have been used to study the in vitro effect of alcohol exposure on cellular processes. Some notable examples include cell lines to model lung alveolar macrophages [mouse AM cell line MH-S cells (25), rat alveolar macrophage cell line NR8383 cells (26), and mouse macrophage cell line RAW264.7 (27)]; Kupffer cells [HepG2 human liver hepatocellular carcinoma (28), Huh7 human hepatoma cells (29), rat Kupffer cell line 1 (RKC1) (30), human macrophage cell lines MonoMac6 (31), and mouse J774.1 cell line (32)]; microglia [mouse BV2 cell line (33)], rat cell line Microglia-SV40 (34)). In addition, THP-1 human cells differentiated into macrophages have been extensively used (35). Primary cells, including alveolar macrophages, hepatocytes and peripheral blood mononuclear cells (PBMCs) obtained from control and alcohol-exposed animals and humans have also been used (24).
However, transformed cell lines respond differently to immunological challenges compared to primary cells (36) and in vitro exposure to ethanol does not accurately recapitulate the complexity of in vivo alcohol consumption such as the generation of ethanol metabolites (37). To address some of these challenges, organoid models have been developed, offering new opportunities to explore the impact of chronic ethanol exposure using three-dimensional (3D) in vitro structures that better replicate the cellular complexity as well as the morphological and functional features of in vivo tissues compared to cells grown in a monolayer (38). Organoid models for organs heavily impacted by alcohol consumption (including liver, brain, intestine, colon, lung) are now available (39–45). A recent liver organoid model replicates alcoholic liver disease (ALD)-associated pathophysiologic changes upon ethanol treatment, including oxidative stress response, steatosis, inflammatory mediators release, and fibrosis in hepatocytes (39). Brain organoids (40) were recently used to assess the effect of alcohol binge drinking on neurons and astrocytes (46). Alcohol-mediated neurotoxicity was unveiled at the structural, functional, and transcriptional levels, revealing that alcohol-induced dose-dependent apoptosis was more severe in neurons than in astrocytes (46). Gut organoids have also been developed including a colon organoid based on a normal colon epithelium (41, 42). Jejunum and colon organoids showed that alcohol’s effects mainly targeted colon cells leading to gut leakiness (43) in line with findings showing that ALD patients with the worse intestinal dysbiosis exhibit the greatest gut leakiness (47). While alcohol’s effect on epithelial and endothelial cells was assessed in these various organoids, the role of macrophages in alcohol-mediated disease was not investigated even though they are a prominent cell subset in the colon, brain, and liver (18).
Mice and rats are the animal models most commonly used to study the impact of chronic alcohol consumption on several organ systems. Depending on the model, ethanol is administered via different modalities (liquid diet, ethanol in water, gavage, or injection) in combination with dietary, chemical, or genetic manipulations (48, 49). Two prominent mouse models are the Lieber–DeCarli liquid diet (LD) and the Meadows-Cook diet (MC) of ad libitum ethanol feeding (50, 51). The LD model, designed to enhance the alcoholic liver injury phenotype in mice (50, 52), is based on an isocaloric liquid diet with alcohol concentration usually increased from 0% to 3.395% w/v for a period of 25 days to 8 weeks, with a 4-week duration most commonly used. In contrast, in the MC diet where alcohol is delivered in the water, exposure to alcohol consists of a 2-week ramping up phase from 0% to 20% ethanol followed by a maintenance phase of 4–16 weeks with a 12-week duration most commonly used (51). Some cellular immunological changes are model-dependent and limited common shared alcohol-induced transcriptional abnormalities are observed between LD and MC models (53). In addition, these mouse models of chronic alcohol consumption are characterized by minimal liver inflammation (52). Thus, both MC and LD mouse models are models of early alcoholic liver injury (steatosis) and not models of more advanced liver diseases such as fibrosis, cirrhosis, or alcoholic hepatitis. Moreover, the aversion to self-administration of ethanol in some mouse strains (BALB/c resistance vs C57BL/6) as well rodents’ higher alcohol catabolism rate compared to humans, make the analysis of organ damage challenging in a self-administration setting (54, 55).
To address these limitations, models delivering ethanol via oral gavage or intragastric injection were established, including the Tsukamoto-French enteral feeding model that results in moderate liver inflammation and steatosis (55). To model more advanced ALD while using the oral delivery route and drinking patterns closer to those observed in humans, second-hit models were developed that combine ethanol exposure with a secondary stimulus including genetic knockout/overexpression, LPS stimulation, or high-fat diet (49). Furthermore, additional mouse models were established to study specific aspects of AUD such as binge drinking with the drinking in the dark model (56); a voluntary escalation in consumption with the chronic two-bottle choice drinking (57); and development of dependence with the chronic intermittent vapor model (58). In addition to mouse models, rat models of ethanol drinking have also been developed, including the alcohol-preferring (P) and the high-alcohol-drink (HAD) rat models which prefer an alcohol solution containing 10% ethanol over water (59).
Nonhuman primate (NHP) models are the gold standard to study human diseases due to their genetic proximity to humans; therefore different NHP models of ethanol delivery were developed to mimic human alcohol consumption. Models of oral ethanol self-administration include some with stress triggers including shock avoidance, food and water deprivation, or social stressors (60–62) as well as those with positive reinforcement triggers (63). In one of the models of voluntary ethanol self-administration using a positive reinforcement trigger, rhesus macaques are first induced to freely consume increasing daily doses of ethanol, ranging from 0.5 g/kg/day to 1.5 g/kg/day over a 3-month phase followed by “open access” to a 4% w/v ethanol solution for 22 h/day. This approach establishes consistent ethanol self-administration, and thus represents a true model of alcohol addiction in rhesus and cynomolgus macaques (64, 65) with animals being categorized as low drinkers (average ethanol intake less than 2 g/kg), binge drinkers (average intake of 2.4 g/kg), heavy drinkers (average intake of 2.8 g/kg) or very heavy drinkers (intake higher than 3 g/kg) (66). While this experimental model does not induce any overt liver damage over a 12-month period, signs of fatty liver disease were observed in cynomolgus macaques exposed to an open access period of 6 months followed by 12 months of abstinence that was followed by another open access period of up to 19 months (67). This rhesus macaque model was used to define the impact of chronic drinking on circulating and tissue-resident immune cells showing that the circulating innate immune cells bear the largest burden of chronic heavy drinking (21, 22, 68–73). However due to the inherent variation in alcohol consumed in the voluntary drinking models, a model of gastric infusion of alcohol was developed in order to deliver a consistent alcohol dose in treated rhesus macaques, where animals are infused with 30% ethanol in water (w/v) via an intragastric catheter either 4 days per week or 7 days per week during 3 months resulting in blood alcohol levels of 2.3 g/kg (74–76). This model has been used to study the impact of alcohol consumption on simian immunodeficiency virus (SIV) infection (74–76).
Chronic alcohol consumption weakens antimicrobial responses by impairing the immune system’s responses and by affecting the integrity of the mucosal barrier resulting in increased susceptibility to bacterial and viral infections (77–80). In particular, chronic alcohol consumption is a predisposing factor for severe respiratory infections, such as bacterial pneumonia, respiratory syncytial virus and SARS-CoV-2 infections (81–86). Several mechanisms contribute to increased disease severity, including alcohol-induced impairment of the innate immune response, chronic oxidative stress, and alcohol-induced organ damage due to excessive inflammation (82). In addition, chronic alcohol consumption affects lung physiology by desensitizing lung airway cilia and compromising the mucociliary escalator, and leading to impaired clearance of invading pathogens (82).
Community-acquired pneumonia is a leading cause of death in the USA and patients who suffer from AUD experience more severe pneumonia than non-AUD patients (77, 81, 83, 87). Streptococcus pneumoniae, usually a commensal bacterium in the upper respiratory tract, is the prevalent infectious agent of community-acquired pneumonia (81) but alcohol abuse is also associated with an increased incidence of pneumonia from gastric aspiration of Klebsiella pneumoniae (82, 83). Moreover, alcohol abuse is a risk factor for active tuberculosis (TB) with a three-fold risk increase of active TB associated with heavy drinking (80, 88) as well as reactivation of latent tuberculosis (80, 88, 89). AUD patients are also at a higher risk of viral infections, notably respiratory syncytial virus (RSV) (84) and SARS-CoV-2 (85, 86). Increased severity of COVID-19 is believed to be additionally mediated by increased prevalence of cardiovascular diseases and chronic respiratory diseases with alcohol abuse (85, 86). Finally, chronic alcohol consumption is an independent risk factor to develop acute respiratory distress syndrome (ARDS) (90). ARDS is characterized by endothelial and alveolar epithelial barrier dysfunction, severe inflammation, and pulmonary surfactant dysfunction leading to impaired gas exchange in the lung and insufficient oxygen levels in the blood and tissues resulting in organ failure (82).
Infectious diseases affecting the liver are also more prevalent in AUD patients as alcohol metabolism takes place in the liver (91). Specifically, the prevalence of hepatitis C virus (HCV) infection is up to 30-fold higher in AUD patients compared with the general population (92), with a 4.6% to 55.5% rate in AUD patients (93). Moreover, chronic alcohol has been reported to enhance the replication of hepatitis B virus and possibly HCV (93). The increased susceptibility is also mediated by an alcohol-induced increase in oxidative stress and impaired antiviral immune responses in the liver (91, 94). In addition to respiratory and liver diseases, alcohol abuse is also positively associated with HIV incidence and progression towards AIDS in part due to antiretroviral therapy failure (95). Moreover, alcohol increases the risk of multiple AIDS comorbidities including hepatic fibrosis, hepatic cirrhosis, neurocognitive impairment, and AIDS-related dementia (96).
Wound healing is a dynamic and tightly coordinated process defined by three interconnected phases: inflammation, proliferation, and remodeling. Studies have revealed the critical role played by myeloid cells at several steps of this process. Inflammation is the first phase where neutrophils and macrophages are recruited to the injury site to protect it from infection by phagocytosing invading pathogens and by producing reactive oxygen species (ROS) and pro-inflammatory cytokines and chemokines. These mediators are produced both by local tissue-resident macrophages and by circulating monocytes being recruited from bone marrow to differentiate into macrophages at the site of injury (7). After this initial pro-inflammatory response, macrophages switch to an anti-inflammatory phenotype and participate in wound resolution via their contribution to angiogenesis and formation of fibrous tissue (97). During the proliferative second phase, cell migration and proliferation are upregulated to repopulate the injured tissue with fibroblasts, endothelial and epithelial cells. Tissue reorganization takes place last during the remodeling phase so that the injured tissue can regain its functionality (98).
Alcohol abuse impairs wound healing by affecting each step of the repair process (97–99) resulting in AUD patients recovering poorly from surgeries and traumas. AUD patients with a burn injury require longer hospitalizations with more aggressive antibiotic treatments (100), exhibit higher rates of secondary bacterial infections, and are more likely to die compared to non-AUD burn patients (101, 102) even from smaller burn injuries (100). In case of other types of trauma, findings are conflicting with some studies showing that acute rather than chronic alcohol consumption accounts for septic complications after abdominal penetrating trauma while others found that chronic but not acute alcohol consumption influences the outcome from trauma (103). Alcohol consumption is associated with an increased risk for Staphylococcus aureus infection of the skin, further compromising the response to wound healing (82). Chronic alcohol exposure may impair wound healing by preventing macrophages from switching to a regulatory phenotype, a critical step to enable the subsequent resolution phase of the healing process (104). Indeed, alcohol exposure induces alterations in transcription and chromatin accessibility in alveolar macrophages indicative of decreased capability for tissue repair (105). In addition, alcohol-exposed macrophages have a reduced phagocytic capacity (26, 105) and this could further impair the wound healing process by reducing the clearance of invading pathogens (104).
The effect of chronic alcohol on the inflammatory response depends on the disease phase (early vs late) and the cell populations affected (tissue macrophages vs circulating monocytes). A pro-inflammatory cytokine profile (Figure 1) is observed in AUD patients, with elevated plasma levels of TNF-α, IL-1β, and IL-6 (106). Similarly, chronic alcohol results in a systemic pro-inflammatory response in ethanol-fed mice with increased circulating protein levels of TNF-α, IL-6 and MCP-1 (107). This heightened inflammatory state has been associated with multi-organ damage affecting the liver, intestine, lung, and brain (108–111). Prolonged in vitro exposure to ethanol upregulates TNF-α gene expression in human monocytes (112). Hyper-inflammation and heightened cell activation have also been observed in HIV patients suffering from AUD with increased plasma levels of soluble CD40 and TGF-β as well as upregulation of CD16, CD163 and TLR4 expression on monocytes (113). In addition, chronic alcohol consumption increases human monocyte sensitivity to LPS as indicated by elevated secretion of pro-inflammatory mediator TNF-α in part via downregulation of IRAK-M and enhanced activation of NF-κB and ERK kinases (23, 114).
Figure 1 Model capturing the impact of chronic alcohol consumption on the phenotype and function of monocytes and macrophages in absence of severe alcoholic liver disease. Figure created withBioRender.com.
Inflammatory responses are imbalanced in alcoholic hepatitis (AH) and acute-on-chronic liver failure (ACLF), two severe conditions with substantial mortality and morbidity observed in patients with significant AUD. While AH was initially associated with a proinflammatory phenotype due to higher circulating cytokines levels (115–117), recent studies revealed that AH leads to monocyte immuneparesis characterized by a downregulation of HLA-DR, TNF-α, Il-1β and IL-6; upregulation of regulatory IL-10, PPARγ and MERTK that were accompanied by a decrease in non-classical monocytes and increase in intermediate monocytes (118). Indeed, at the transcription level, monocytes from patients with severe AH display a phenotype characterized by immunosuppressive features including reduced expression of genes related to immune pathways (innate immune responses, cytokines, response to IFNs, antigenic presentation, phagocytosis) (118). Similarly, immuneparesis was also observed in ACLF monocytes with downregulation of HLA-DR and CD86; and upregulation of regulatory IL-10 and MERTK while classical monocyte populations decreased and intermediate monocytes increased (119, 120). The seemingly contradiction of a systemic pro-inflammatory milieu and immunosuppressed cells could be due to an environment with heightened release of soluble proinflammatory mediators by alcohol-injured tissues leading to expansion of mononuclear CD14+HLA-DR- myeloid-derived suppressor cells resulting in immunosuppression of monocytes in an attempt to reduce the inflammation (121–123). This immuneparesis extended to peritoneal and mesenteric lymph node macrophages and Kupffer cells in ACLF patients (119).
Furthermore, single-cell transcriptome analyses performed at rest revealed an unsuspected level of heterogeneity in classical monocyte subsets of AH patients with three distinct classical monocyte clusters (124). These three classical clusters differentially expressed markers including CD14, MHC class II, TLR8, and CD86 (124). Interestingly, this high level of heterogeneity in classical monocytes was also observed in alcohol-fed NHPs with the identification of seven classical monocyte clusters by single cell RNA sequencing analysis (68). Two of these clusters were exclusively composed of cells from chronic heavy drinking macaques: HIF1Ahi and SOD2hi. Marker genes of these clusters enriched to gene ontology terms related to myeloid cell differentiation, cytokine signaling, and response to alcohol. Trajectory analysis showed that chronic heavy drinking was associated with a specific lineage that culminated in the HIF1Ahi cluster, indicating that heavy drinking dysregulates monocyte differentiation states thereby affecting monocyte response to inflammation (68). In addition, chronic heavy drinking was associated with downregulated expression of MHC class II genes but upregulated expression of IFN-inducible genes and activation of NF-κB signaling pathway in classical monocytes (68).
In alcohol-fed animal models, chronic drinking also results in pro-inflammatory responses by Kupffer cells, microglia, splenic and lung alveolar macrophages (22, 82, 107, 125–129). Ethanol-fed mice upregulate the expression of Tlr1, 2, 4, 6-9 genes leading to an increase in Tnf gene expression (130). Furthermore, alcohol-fed mice deficient in TLR4, CD14, TNF-α or LPS-binding proteins (LBP) have reduced liver inflammation and do not develop liver injury (131–133). Anti-TNF-α antibody treatment prevented liver inflammation in alcohol-fed rats (134). Chronic heavy drinking in rhesus macaques is associated with higher gene expression of pattern recognition receptors (TLR4, FPR3, TLR2), chemokine receptors (CCR1, CCR5), differentiation and activation markers (CD163) in splenic macrophages (22). This effect is mediated by alcohol-induced enhanced chromatin accessibility of stress-responsive transcription factors that regulate genes involved in macrophage phagocytosis (GATA-2), activation (CEBPA), hypoxia (HIF-1α), polarization (GATA-3, NFAT5) and pro-inflammatory cytokine production (GATA-2, NRF-1, NFAT5) (22). In addition, chronic alcohol enhances chromatin accessibility of promoters that regulate genes involved in pro-inflammatory responses, including TLR4 and CCL2 genes (22). Chronic alcohol exposure also increases access to transcription factors that regulate expression of inflammatory genes in alveolar macrophages, including AP-1, IRF8, and NF-κB. In the lung, chronic alcohol also upregulates genes involved in granulocyte activation and degranulation (CTSG, SNAP25, MYO3A); and downregulates genes involved in anti-inflammation (CLEC1B) (105). At the single cell level, chronic alcohol increased the expression of genes involved in oxidative stress (LCN2), HIF-1α, and cytokine signaling (105).
Alcohol consumption also elicits functional and transcriptial changes in the microglia, with high expression of TLR2 gene in alcoholic human brains (135). Moreover, alcohol treatment of mice upregulates expression of Tlr3 in the cortex where microglia are found (136) and chronic intermittent ethanol vapor exposure leads to upregulation of type I interferon-stimulated genes in the prefrontal cortex of mice (137). Additionally, gene ontology analysis identified “toll-like receptor signaling pathway” and “activation of innate immune response” as the main processes in microglia affected by alcohol exposure (137, 138). Chronic alcohol induces transcription of pro-inflammatory IL-6, IL-1β, TNF-α, and MCP-1 in mouse brain tissue, cortex, and isolated microglial cells via NF-κB activation (136, 138). In addition, levels of anti-inflammatory IL-10 were decreased in a mouse model of chronic alcohol-induced neuroinflammation (139).
LPS stimulation of alcohol-exposed monocytes and tissue macrophages further enhances the alcohol-induced hyper-inflammatory response. Upon LPS stimulation, monocytes of alcohol-fed NHPs upregulate genes associated with myeloid inflammatory pathways (TNFSF21, TLR2). These transcriptional pro-inflammatory changes were confirmed at the protein level with the increased production of pro-inflammatory mediators TNF-α, IL-6, IL-15, and CCL4 in LPS-stimulated monocytes (68). In splenic macrophages isolated from male macaques classified as chronic heavy drinkers, LPS stimulation induced greater production of TNF-α, MIP-1α, MIP-1β, IL-1β, IL-6, IL-8, and GM-CSF (22). From a transcriptional standpoint, LPS-responsive transcription factors NF-KB, p65, STAT1, SMAD1, and HIF-1 were upregulated along with genes associated with “innate immune response”, “TGF-β signaling” and “response to LPS” including CD80, NFKB1, JUN, IL6ST, IFNG, TNFRSF8 and TNFRSF10B and SOCS1 (22).
Alcohol-induced altered inflammatory state following LPS stimulation is not limited to circulating monocytes or macrophages from lymphoid tissues. Chronic heavy ethanol consumption for 12 months leads to a hyper-inflammatory response to LPS stimulation by NHP alveolar macrophages with increased production of IL-6, TNF-α, CXCL8, CXCL10, CCL2, and CCL4 (105). Similarly, chronic alcohol consumption increased protein levels of IFN-α and IFN-β in bronchoalveolar lavage (BAL) of mice. In addition, alveolar macrophages from alcohol-fed mice infected with RSV secrete higher levels of MCP-1 and TNF-α (84). In mice, chronic alcohol also leads to increased expression of pro-inflammatory mediators IL-6, TNF-α, CXCL-1 and MMP-9 by liver-resident Kupffer cells (107). Alcohol-induced liver inflammation in mice and rats is mediated by Kupffer cells, as suggested by upregulation of cell surface expression of CD14 and increased production of pro-inflammatory mediators TNFα, MCP-1, and reactive oxygen species (ROS) (140–145). Both alcohol-induced ROS and increased Kupffer-cell sensitization to endotoxin further exacerbate proinflammatory responses and are major drivers of ALD (91, 144). Intestinal macrophages from alcohol-exposed mice express higher levels of pro-inflammatory genes both at rest and following LPS-induced systemic inflammation, including Lcn2, Tnf, Il1b and Csf1 (146). Lcn2 gene encodes Lipocalin-2 which plays a pro-inflammatory role in metabolic diseases (147). Csf1 plays a role in the maintenance of intestinal macrophage populations (148), thus suggesting that alcohol-induced inflammation may increase the number of resident intestinal macrophages (147). In addition, LPS stimulation of alcohol-fed mice increases the expression of pro-inflammatory chemokine gene Cxcl1 (neutrophil recruitment) and cytokine signaling gene Il1a and its receptor Il1r in the ileum (146).
One of the potential drivers of the heightened inflammatory response following chronic alcohol exposure is microbial translocation and leakage of endotoxin in the portal circulation driven by impaired intestinal barrier function and increased gut permeability (149, 150). Additionally, alcohol abuse leads to bacterial overgrowth (151) and altered microbiome composition in the gut of alcoholic patients and alcohol-fed mice (152–154). Fecal transplants from alcohol-fed mice into germ-free mice induced intestinal inflammation, leaky gut, and liver injury (155), thus highlighting the role of dysbiosis in alcohol-induced inflammation. These effects are mediated by bacterial toxins including cytolysin as demonstrated by inhibition of alcohol-induced liver injury in humanized mice by bacteriophage treatment targeting cytolysin-producing E.faecalis (156). In addition, plasma endotoxin levels correlated with liver inflammation in alcohol-fed rats (157). While endotoxin leakage has been observed in some mouse models of chronic alcohol consumption (152, 158, 159), there is no consistent increase in endotoxin levels in plasma or in antibody-bound endotoxin levels in NHPs after 12 month of chronic ethanol consumption suggesting that a longer duration may be necessary to detect significant dysbiosis and translocation of microbial products in this model (68, 153).
Defects in anti-microbial functions of monocytes and macrophages (Figure 1) have been reported to play a critical role in the observed increased vulnerability to infectious diseases due to chronic drinking resulting in reduced phagocytic activity and pathogen-fighting function in Kupffer cells, microglia, alveolar and splenic macrophages (22, 107, 125–128). Under homeostatic conditions, chronic alcohol leads to a transcriptional downregulation of genes associated with immune and anti-viral responses (MHC class II, antigen processing and presentation, and IFNγ signaling pathways) in non-classical monocytes of alcohol-fed NHPs (68). In contrast to the hyper-inflammatory response to LPS generated by monocytes/macrophages from heavy drinking humans or animals, chronic alcohol exposure leads to a dampened production of immune mediators by macaque monocytes stimulated with E.coli bacteria, including reduced production of IL-1β, IL-5, IL-6, IL-15, CCL4 and CXCL11 (68). In addition, genes associated with adaptive immune activation (IL21R, CD40, MHC class II) are downregulated in LPS-stimulated monocytes from heavy drinking NHPs (68). Similarly, in splenic macrophages, LPS stimulation leads to downregulation of genes associated with “type I interferon signaling”, “defense response to virus” (22). Furthermore, monocytes from AH patients upregulate SOCS1 (160), a negative regulator of the JAK/STAT pathway (161), leading to impaired IFNγ signaling and impaired intracellular killing of phagocytosed bacteria in a subset of patients and is associated with poor survival outcomes (160). Chronic alcohol also led to reduced immune response to vaccination in NHPs and downregulation of several innate immune genes predicted to be highly expressed by myeloid cells including genes involved in antigen presentation (MHC class II genes), defense responses (type I IFN) as well as microbial sensors (CD14, TLR4, TLR5) (71).
In addition to impairing antimicrobial functions of monocytes and macrophages of lymphoid organs, chronic alcohol also alters the function of tissue-resident macrophage populations, notably alveolar macrophages, Kupffer cells, and microglia, resulting in increased disease severity (94). Patients with AUD are more susceptible to respiratory infections (81–86) and alcohol-fed mice infected with Mycobacterium tuberculosis have a higher lung bacterial load and a dampened IFN-γ production (162). In addition, chronic alcohol exposure impairs phagocytosis of bacteria by alveolar macrophages, such as E.coli by rat macrophages and S.aureus by NHP macrophages (26, 105). Chronic alcohol consumption also leads to blunted induction of interferon-stimulated genes (ISGs) by alveolar macrophages in response to RSV in NHPs suggesting disrupted anti-viral responses (105). Similarly, in a mouse model of chronic ethanol consumption, RSV infection resulted in increased viral loads in the lung and reduced IFNγ protein levels in bronchoalveolar lavage (84). Influenza infection of alcohol-fed mice leads to increased lung viral loads and disease severity (163). In addition, alcohol-fed mice infected with Mycobacterium avium have higher bacterial burden in the liver, spleen, and blood (164); and chronic alcohol has been shown to reduce E.coli phagocytosis by rat Kupffer cells and microglia (165, 166). Therefore, chronic alcohol dampens microbe phagocytosis by multiple tissue-resident macrophage populations, leading to defects in antimicrobial responses in rodents and NHPs exposed to chronic alcohol.
ROS are important mediators in cell signaling and apoptosis (167) and play a critical role in the pathogenesis of various diseases including respiratory infections. ROS are produced by NADPH oxidases (Noxes) (167, 168). Chronic drinking upregulates Nox expression resulting in elevated ROS production leading to heightened oxidative stress in Kupffer cells, microglia and brain cortex, alveolar and splenic macrophages (22, 107, 125–128, 136, 169). One proposed mechanism is via alcohol-mediated downregulation of PPARγ. This downregulation of PPARγ in alveolar macrophages following chronic alcohol exposure results in upregulation of Nox and an increase in oxidative stress response leading to heightened intracellular ROS levels (105). In addition, ROS increases the expression of transcription factors HIF-1α and HIF-2α which have important roles in the response to hypoxia in several tissues including mouse liver and rat brain cortex (170, 171). Furthermore, chronic alcohol elicits upregulation of genes enriched in the HIF-1α signaling pathway in alveolar and splenic macrophages as well as monocytes of ethanol-fed NHPs (22, 68, 105). Under homeostatic conditions, chronic alcohol also leads to increased differentiation of classical monocytes expressing high levels of HIF-1α (68). Alcohol’s effect on HIF-1α expression in the intestine is more complex with an increased HIF-1α expression in mice exposed to chronic alcohol for 28 days but a decreased expression when alcohol exposure is prolonged to 42 days (159). Therefore, overall chronic alcohol increases tissue hypoxia but its effect can be organ- and exposure-dependent.
The functional effects of alcohol on myeloid cells are dictated by changes at the epigenome level that affect gene transcription (172). Regulation of gene expression can be mediated by epigenetic changes resulting in DNA modification that does not alter the genome sequence. The main epigenetic modifications are provided by noncoding RNAs, DNA methylation, and modifications of histones. MicroRNA molecules (miRNA) are short (19-25 nt), highly conserved, single-stranded non-coding RNAs that modulate target proteins by regulating mRNA expression through decreased transcription or by post-transcriptionally induced mRNA decay (173). In addition to having an autocrine effect, miRNAs can have a paracrine effect via exosomal delivery (174). DNA methylation is an epigenetic mark that targets cytosine residues of cytosine-guanine dinucleotide repeats and is regulated by DNA methyltransferases (DNMT) and ten-eleven translocation (TET) enzymes. Hyper-methylation in promoter gene regions leads to transcriptional repression and down-regulation of protein production (175). Histone proteins play key structural and regulatory roles by enabling the formation of nucleosomes. Histone modifications (methylation, phosphorylation, acetylation, and deacetylation as well as ubiquitination) impact chromatin packing thereby modulating gene expression (176). Histone acetyltransferases (HAT) increase chromatin accessibility and promote transcription, whereas histone deacetylases (HDAC) decrease chromatin accessibility and inhibit transcription. In contrast, the result of histone methylation is more complex and depends on several factors including the degree of methylation, the histone and lysine residue targeted, the level of chromatin condensation as well as the function of the genome region targeted (i.e. promoter, enhancer). H3K4, H3K9, and H3K27 are some of the most prominent lysine residues targeted. Studies have reported that alcohol can interfere with the fundamental processes of epigenetic regulation in several cell types including monocytes and macrophages in a dose-, duration-, and time-dependent manner (176).
miRNAs play a critical role in regulating the function of macrophages and inflammatory pathways in alcoholic steatohepatitis (174). Indeed, several miRNAs modulate the alcohol-induced hyper-inflammatory response by inhibiting negative regulators of TLR signaling pathways (177) in multiple tissue-resident macrophages populations including Kupffer cells, microglia and alveolar macrophages. Alcohol exposure upregulates miR-155 and miR-132 in murine Kupffer cells (178–180) and microglia (181). miR-155 inhibits negative regulators of the TLR4 pathway, including IRAK-M, SHIP1, and PU.1, resulting in increased sensitization to LPS and increased production of pro-inflammatory TNF-α (179) in liver and TNF-α and MCP1 (CCL2) by microglia (181). In addition, miR-155 downregulates STAT3 and SOCS1 in murine Kupffer cells resulting in upregulation of pro-inflammatory cytokines TNF-α and IL-1β and downregulation of anti-inflammatory cytokine IL-10 (161, 179, 182). On the other hand, miR-132 regulates expression of TGF-β, IL-1β, and MCP-1 in Kupffer cells as well as TNF-α and MCP1 in the cerebellum (180, 181). Increased levels of TGF-β are key for the development of fibrogenesis in the liver, while increased levels of inflammatory mediators (IL-1β, TNF-α, MCP-1) contribute to increased hepatic inflammation and development of steatosis in alcohol-fed mice (183). Indeed, miR-132 is elevated in the liver of AUD patients with fibrosis/cirrhosis (180). Other alcohol-induced miRNAs contribute to dysregulation of monocyte/macrophage cytokine responses including miR-217 which also regulates TGF-β expression in Kupffer cells of alcohol-fed mice (184), as well as miR291b and miR-181b-3b both of which are negative regulators of the TLR signaling pathway (185, 186).
In addition to their effect on the production of cytokines and chemokines, alcohol-induced miRNAs affect other monocyte/macrophage cell functions including oxidative stress and phagocytosis. Chronic drinking downregulates miR-92a and upregulates miR-130a and miR-301a in mouse alveolar macrophages leading to increased oxidative stress and reduced bacterial phagocytosis (25, 187). Downregulation of miR-92a leads to upregulation of Nox4 (25) while upregulation of miR-130a and miR-301a reduces PPARγ expression leading to increased gene expression of Noxes 1, 2, and 4 (187). Nox upregulation results in increased oxidative stress response and increased production of TGF-β as well as decreased phagocytosis (25, 187, 188). ROS production by Kupffer cells is also upregulated by alcohol-induced miR-217 (184).
Alcohol-induced changes in miRNA expression can modulate macrophage polarization balance. LPS-stimulated Kupffer cells from alcohol-exposed rats show enrichment of miR-125a-5p (189), which inhibits TLR4-dependent signaling pathway and mediator production leading to anti-inflammatory polarization (189). Moreover, miR-27a, which regulates macrophage polarization towards a regulatory profile via IL-10, is downregulated in PBMCs of ethanol-fed NHPs (21) suggesting an alcohol-induced rewiring towards a pro-inflammatory profile.
In addition to regulating gene expression within the cells in which they are generated, miRNAs can be transferred into other target cells via exosomes, thus regulating the function of other cell populations including monocytes and macrophages in a paracrine manner (174, 190, 191). Of note, increased miR-122 levels have been measured in circulating exosomes of chronic alcohol-fed mice (140) and binge-drinking humans (191). miR-122 is liver-specific and is associated with lipid metabolism, stress response, and hepatitis C virus replication (192). In vitro, horizontal transfer of miRNA-122 from ethanol-treated hepatocytes to monocytic cells via exosomes upregulates the production of pro-inflammatory cytokines TNF-α and IL-1β (191). In PBMCs isolated from heavy drinking NHPs, alcohol exposure had a differential effect on several extracellular vesicle (EV)-derived miRNAs that regulate genes with roles in myeloid cell activation and angiogenesis. Gene ontology analyses revealed enrichment of putative target genes to TGF-β receptor signaling, histone, and chromatin modification, response to ROS, and myeloid leukocyte activation. Over-expression of candidates miR-155, miR-154, miR-34c, miR-450a, and miR-204, which are upregulated in EV with alcohol drinking, led to a heightened inflammatory response in PBMCs after stimulation (190).
DNA methylation plays an important role in ethanol-mediated dysfunction. In Kupffer cells, alcohol treatment leads to abnormal DNA methylation patterns and upregulation of methyl-transferases DNMT1, DNMT3a, and DNMT3b (193) which in turn hyper-methylate anti-inflammatory mediators, PSTPIP2, SOCS1, and ZSWIM3 leading to their inhibition and inhibition of downstream anti-inflammatory targets and promotion of a pro-inflammatory profile (193). Specifically, downregulation of PSTPIP2 leads to activation of the STAT1 pathway via increased STAT1 phosphorylation. Downregulation of PSTPIP2 also leads to activation of the NF-κB signaling pathway by affecting phosphorylation of p65 and IκBα as well as the nuclear transfer of p65. Activation of these pathways results in increased transcription of pro-inflammatory mediators IL-1β, TNF-α, IL-6, IL-17, and CCL2 and increased protein production of IL-1β, TNF-α, and IL-6. Thus, PSTPIP2 plays a crucial role in macrophage-induced inflammatory responses by regulating the STAT1 and NF-κB signaling pathways (193). In the same chronic-plus-binge mouse model, alcohol exposure also reduces the expression of ZSWIM3 in Kupffer cells due to DNMT3b-induced hypermethylation of ZSWIM3 promoter. Downregulation of ZSWIM3 leads to activation of adaptor protein TRAF2 which plays a crucial role in the activation of the NF-κB signaling pathway resulting in increased transcription of IL-1β, TNF-α, IL-6 and MCP-1 as well as increased protein production of IL-1β, TNF-α and IL-6 (194) thus eliciting a pro-inflammatory phenotype.
Chronic alcohol exposure leads to histone modifications that contribute to enhanced pro-inflammatory responses. Sirtuins are NAD+ dependent deacetylases that play a key role in inflammation and oxidative stress. Ethanol metabolism results in a decrease of NAD+ in the liver leading to sirtuin dysregulation. Additionally, alcohol consumption leads to the upregulation of miR-217 and miR-132 in Kupffer cells, which in turn leads to decreased levels of sirtuin SIRT1 (184, 195). Decreased SIRT1 gene and protein expression results in the downregulation of AMPK, an anti-inflammatory regulator, and the upregulation of NF-κB and NFATC4 through H3K9 acetylation. Activation of the NF-κB and NFATC4 signaling pathways results in increased production of pro-inflammatory IL-1β, TNF-α, IL-6 and MCP-1 (184). In addition to sirtuins, other classes of deacetylases have been involved in alcohol-induced effects. Histone deacetylase 11 (HDAC11) is induced in Kupffer cells in a mouse model of ALD via upregulation of miR-155 by alcohol metabolite acetaldehyde that leads to activation of the NF-κB signaling pathway, resulting in reduced expression of anti-inflammatory IL-10 (179). The mechanism of action of HDAC11 in macrophages has been elucidated. HDAC11 binds to the proximal site of the IL-10 promoter and modulates the recruitment of PU.1, Sp1, and STAT3 at late stages of LPS activation (196).
Histone methylation is also affected by alcohol consumption. H3K27me3, mediating epigenetic silencing, is enriched at the TGF-β promoter but reduced at the TNF-α promoter location in Kupffer cells of ACLF patients. Enhancer of zeste homolog 2 (EZH2) catalyzes the methylation of H3K27 loci and increased EZH2 expression and enhanced H3K27me3 are observed in PBMCs of ACLF patients. In addition, in a murine model of liver failure induced by administration of D-galactosamine, upregulation of EZH2 and H3K27me3 in Kupffer cells correlates with upregulation of pro-inflammatory cytokine TNF-α, IL-1β, and IL-6 (197). Furthermore, in splenic macrophages of alcohol-fed NHPs, chronic alcohol affects increased tri-methylation of H3K4, an active promoter mark. In addition, changes in overlapping regions enriched for cis-regulatory inactive enhancer H3K4me1 and active enhancer H3K27Ac are associated with host defense processes including “Immune System Process” and “regulation of cytokine production” in alcohol-exposed splenic macrophages (22).
Changes in histone modifications can lead to alterations in chromatin accessibility, resulting in changes in gene expression. Several studies have reported modulation of chromatin accessibility in monocytes and macrophages from alcoholic hepatitis (AH) patients and NHP chronically consuming ethanol leading to enhanced pro-inflammatory gene expression and reduced expression of genes involved in metabolic processes (22, 105, 118). In alveolar macrophages of alcohol-fed NHPs, these dysregulated responses are mediated by increased chromatin accessibility in intergenic regions that regulate inflammatory genes and binding motifs for transcription factors AP-1, IRF8, and NF-κB p-65 and reduced chromatin accessibility of promoters of genes important for endothelium development and cell junction assembly (105). In splenic macrophages of alcohol-fed NHPs, chronic alcohol increases chromatin accessibility of promoters and intergenic enhancer regions that regulate the expression of genes participating in immune activation, cytokine signaling pathways, myeloid cell activation (CD40), and cellular stress responses. Specifically, chronic alcohol increases accessibility in regions regulated by transcription factors involved in oxidative stress (NRF-1, AHR), hypoxia (HIF-1α), and inflammatory responses (NF-κB). Chronic alcohol is also associated with increased chromatin accessibility of genes engaged in inflammatory and immune responses including TLR4, CCL2, C3AR1, and LAMP1 (22).
In addition to its effect on tissue macrophages, chronic heavy alcohol consumption also increases chromatin accessibility at promoter regions that regulate the expression of genes involved in cytokine production and myeloid activation in monocytes of ethanol-fed NHPs, including regions containing binding sites for transcription factors important for monocyte activation and differentiation such as FOS, JUNB and PU.1 (68). Furthermore, studies reported increased accessibility to binding sites for transcription factors CEBP and MAF as well as regions associated with immunoregulatory genes such as PPARG in monocytes isolated from patients with severe AH. In contrast to what has been described for monocytes of heavy drinkers in the absence of liver disease, binding sites for NF-κB, IRF, and STAT1 were less accessible in enhancer regions as was chromatin accessibility in regions associated with genes involved in innate immune response, antigen presentation and cytokine secretion in monocytes obtained from patients with AH (118).
Monocytes develop continuously in the bone marrow from CD34+ hematopoietic stem and progenitor cells via increasingly restricted lineage-committed progenitors (10, 11). Inflammation and infection can affect monocyte production and induce emergency monopoiesis in the bone marrow (10, 198). AUD patients suffer from blood cell disorders including lymphopenia, anemia, and thrombocytopenia (199–203). In addition, almost half of alcoholics have foamy macrophages due to the storage of alcohol-induced lipids in the cytoplasm (204). Hemostasis is also perturbed in ALD, due to alcohol’s effects on the platelets leading to an increased risk of disseminated intravascular coagulation (205). These defects suggest that alcohol exposure impacts bone marrow progenitor cell populations and hematopoiesis in humans. Alcohol-induced effects on the myeloid progenitor compartment are wide-ranging, impacting hematopoietic precursor cell activation and differentiation as well as genes involved in inflammatory and metabolic responses. Multiple bone marrow precursor cell populations are affected by chronic alcohol, including erythrocytes and granulocytes where vacuolization is induced. This damage appears within a week of heavy drinking but is reversible (205).
Mice exposed to acute alcohol and infected with E.coli have a reduced number of bone marrow myeloid progenitor cells and alcohol treatment prevents switching to granulocyte phenotype. Furthermore, alcohol exposure increases the bacterial burden and mortality of E.coli-infected mice (206). In a chronic plus binge mouse model, alcohol administration triggers the release of granulocytes from the bone marrow compartment, resulting in a reduction of the granulocyte reserve in the marrow concomitant with an elevation of granulocytes in the circulation. This phenomenon is enhanced further following bacteremia, where alcohol impairs activation of granulopoietic precursor proliferation and response to LPS stimulation (207). These findings were confirmed in NHPs, where chronic alcohol exposure reduces the number of primitive hematopoietic stem and progenitor cells in the bone marrow and impairs their ability to proliferate and differentiate towards erythroid and granulocyte-monocyte cells (208). In addition, alcohol exposure leads to remodeling of the bone marrow niche and these alterations persisted after 1-month abstinence thus indicating that the effects of alcohol are long-lived (208) and may involve epigenetic rewiring of progenitor cells.
In summary, chronic alcohol consumption leads to a hyper-inflammatory response with heightened cytokine, chemokine, and ROS production concomitantly with decreased microbial and wound healing responses in circulating monocytes and tissue-resident macrophages. This profile is due to alcohol’s interference with the fundamental processes of epigenetic regulation at the transcriptome level.
All authors listed have made a substantial, direct, and intellectual contribution to the work and approved it for publication. IM secured funding for this work.
This work was funded by NIH Grant number R01 AA028735.
The authors declare that the research was conducted in the absence of any commercial or financial relationships that could be construed as a potential conflict of interest.
All claims expressed in this article are solely those of the authors and do not necessarily represent those of their affiliated organizations, or those of the publisher, the editors and the reviewers. Any product that may be evaluated in this article, or claim that may be made by its manufacturer, is not guaranteed or endorsed by the publisher.
We thank Dr. Suhas Sureshchandra for critical reading of the manuscript and Madison Blanton for help with Figure 1.
1. Available at: https://www.niaaa.nih.gov/publications/brochures-and-fact-sheets/understanding-alcohol-use-disorder.
2. Available at: https://www.samhsa.gov/data/report/2020-nsduh-annual-national-report.
3. Available at: https://www.niaaa.nih.gov/publications/brochures-and-fact-sheets/alcohol-facts-and-statistics.
4. Sacks JJ, Gonzales KR, Bouchery EE, Tomedi LE, Brewer RD. 2010 National and State Costs of Excessive Alcohol Consumption. Am J Prev Med (2015) 49(5):e73–e9. doi: 10.1016/j.amepre.2015.05.031
5. Mass E. Delineating the Origins, Developmental Programs and Homeostatic Functions of Tissue-Resident Macrophages. Int Immunol (2018) 30(11):493–501. doi: 10.1093/intimm/dxy044
6. Guilliams M, Mildner A, Yona S. Developmental and Functional Heterogeneity of Monocytes. Immunity (2018) 49(4):595–613. doi: 10.1016/j.immuni.2018.10.005
7. Snyder RJ, Lantis J, Kirsner RS, Shah V, Molyneaux M, Carter MJ. Macrophages: A Review of Their Role in Wound Healing and Their Therapeutic Use. Wound Repair Regen (2016) 24(4):613–29. doi: 10.1111/wrr.12444
8. Teh YC, Ding JL, Ng LG, Chong SZ. Capturing the Fantastic Voyage of Monocytes Through Time and Space. Front Immunol (2019) 10:834. doi: 10.3389/fimmu.2019.00834
9. Hoeffel G, Ginhoux F. Ontogeny of Tissue-Resident Macrophages. Front Immunol (2015) 6:486. doi: 10.3389/fimmu.2015.00486
10. Wolf AA, Yanez A, Barman PK, Goodridge HS. The Ontogeny of Monocyte Subsets. Front Immunol (2019) 10:1642. doi: 10.3389/fimmu.2019.01642
11. Kawamura S, Ohteki T. Monopoiesis in Humans and Mice. Int Immunol (2018) 30(11):503–9. doi: 10.1093/intimm/dxy063
12. Lawson LJ, Perry VH, Dri P, Gordon S. Heterogeneity in the Distribution and Morphology of Microglia in the Normal Adult Mouse Brain. Neuroscience (1990) 39(1):151–70. doi: 10.1016/0306-4522(90)90229-W
13. Zhang L, Cao Y, Zhang X, Gu X, Mao Y, Peng B. The Origin and Repopulation of Microglia. Dev Neurobiol (2022) 82(1):112–24. doi: 10.1002/dneu.22862
14. Huang Y, Xu Z, Xiong S, Sun F, Qin G, Hu G, et al. Repopulated Microglia are Solely Derived From the Proliferation of Residual Microglia After Acute Depletion. Nat Neurosci (2018) 21(4):530–40. doi: 10.1038/s41593-018-0090-8
15. Ginhoux F, Lim S, Hoeffel G, Low D, Huber T. Origin and Differentiation of Microglia. Front Cell Neurosci (2013) 7:45. doi: 10.3389/fncel.2013.00045
16. Liegeois M, Legrand C, Desmet CJ, Marichal T, Bureau F. The Interstitial Macrophage: A Long-Neglected Piece in the Puzzle of Lung Immunity. Cell Immunol (2018) 330:91–6. doi: 10.1016/j.cellimm.2018.02.001
17. Evren E, Ringqvist E, Willinger T. Origin and Ontogeny of Lung Macrophages: From Mice to Humans. Immunology (2020) 160(2):126–38. doi: 10.1111/imm.13154
18. Nobs SP, Kopf M. Tissue-Resident Macrophages: Guardians of Organ Homeostasis. Trends Immunol (2021) 42(6):495–507. doi: 10.1016/j.it.2021.04.007
19. Bain CC, Mowat AM. Macrophages in Intestinal Homeostasis and Inflammation. Immunol Rev (2014) 260(1):102–17. doi: 10.1111/imr.12192
20. Tamoutounour S, Guilliams M, Montanana Sanchis F, Liu H, Terhorst D, Malosse C, et al. Origins and Functional Specialization of Macrophages and of Conventional and Monocyte-Derived Dendritic Cells in Mouse Skin. Immunity (2013) 39(5):925–38. doi: 10.1016/j.immuni.2013.10.004
21. Sureshchandra S, Rais M, Stull C, Grant K, Messaoudi I. Transcriptome Profiling Reveals Disruption of Innate Immunity in Chronic Heavy Ethanol Consuming Female Rhesus Macaques. PloS One (2016) 11(7):e0159295. doi: 10.1371/journal.pone.0159295
22. Sureshchandra S, Stull C, Ligh BJK, Nguyen SB, Grant KA, Messaoudi I. Chronic Heavy Drinking Drives Distinct Transcriptional and Epigenetic Changes in Splenic Macrophages. EBioMedicine (2019) 43:594–606. doi: 10.1016/j.ebiom.2019.04.027
23. Mandrekar P, Bala S, Catalano D, Kodys K, Szabo G. The Opposite Effects of Acute and Chronic Alcohol on Lipopolysaccharide-Induced Inflammation Are Linked to IRAK-M in Human Monocytes. J Immunol (2009) 183(2):1320–7. doi: 10.4049/jimmunol.0803206
24. Liangpunsakul S, Toh E, Ross RA, Heathers LE, Chandler K, Oshodi A, et al. Quantity of Alcohol Drinking Positively Correlates With Serum Levels of Endotoxin and Markers of Monocyte Activation. Sci Rep (2017) 7(1):4462. doi: 10.1038/s41598-017-04669-7
25. Morris NL, Harris FL, Brown LAS, Yeligar SM. Alcohol Induces Mitochondrial Derangements in Alveolar Macrophages by Upregulating NADPH Oxidase 4. Alcohol (2021) 90:27–38. doi: 10.1016/j.alcohol.2020.11.004
26. Staitieh BS, Egea EE, Fan X, Amah A, Guidot DM. Chronic Alcohol Ingestion Impairs Rat Alveolar Macrophage Phagocytosis via Disruption of RAGE Signaling. Am J Med Sci (2018) 355(5):497–505. doi: 10.1016/j.amjms.2017.12.013
27. Shi L, Kishore R, McMullen MR, Nagy LE. Chronic Ethanol Increases Lipopolysaccharide-Stimulated Egr-1 Expression in RAW 264.7 Macrophages: Contribution to Enhanced Tumor Necrosis Factor Alpha Production. J Biol Chem (2002) 277(17):14777–85. doi: 10.1074/jbc.M108967200
28. Pochareddy S, Edenberg HJ. Chronic Alcohol Exposure Alters Gene Expression in HepG2 Cells. Alcohol Clin Exp Res (2012) 36(6):1021–33. doi: 10.1111/j.1530-0277.2011.01677.x
29. Plumlee CR, Lazaro CA, Fausto N, Polyak SJ. Effect of Ethanol on Innate Antiviral Pathways and HCV Replication in Human Liver Cells. Virol J (2005) 2:89. doi: 10.1186/1743-422X-2-89
30. Shen Z, Ajmo JM, Rogers CQ, Liang X, Le L, Murr MM, et al. Role of SIRT1 in Regulation of LPS- or Two Ethanol Metabolites-Induced TNF-Alpha Production in Cultured Macrophage Cell Lines. Am J Physiol Gastrointest Liver Physiol (2009) 296(5):G1047–53. doi: 10.1152/ajpgi.00016.2009
31. Zhang Z, Bagby GJ, Stoltz D, Oliver P, Schwarzenberger PO, Kolls JK. Prolonged Ethanol Treatment Enhances Lipopolysaccharide/Phorbol Myristate Acetate-Induced Tumor Necrosis Factor-Alpha Production in Human Monocytic Cells. Alcohol Clin Exp Res (2001) 25(3):444–9. doi: 10.1111/j.1530-0277.2001.tb02233.x
32. Jung F, Lippmann T, Brandt A, Jin CJ, Engstler AJ, Baumann A. Moderate Consumption of Fermented Alcoholic Beverages Diminishes Diet-Induced Non-Alcoholic Fatty Liver Disease Through Mechanisms Involving Hepatic Adiponectin Signaling in Mice. Eur J Nutr (2020) 59(2):787–99. doi: 10.1007/s00394-019-01945-2
33. Correa F, De Laurentiis A, Franchi AM. Ethanol Downregulates N-Acyl Phosphatidylethanolamine-Phospholipase D Expression in BV2 Microglial Cells via Epigenetic Mechanisms. Eur J Pharmacol (2016) 786:224–33. doi: 10.1016/j.ejphar.2016.06.004
34. Chiavari M, Ciotti GMP, Navarra P, Lisi L. Pro-Inflammatory Activation of A New Immortalized Human Microglia Cell Line. Brain Sci (2019) 9(5):111. doi: 10.3390/brainsci9050111
35. Nurmi K, Virkanen J, Rajamaki K, Niemi K, Kovanen PT, Eklund KK. Ethanol Inhibits Activation of NLRP3 and AIM2 Inflammasomes in Human Macrophages–a Novel Anti-Inflammatory Action of Alcohol. PloS One (2013) 8(11):e78537. doi: 10.1371/journal.pone.0078537
36. Andreu N, Phelan J, de Sessions PF, Cliff JM, Clark TG, Hibberd ML. Primary Macrophages and J774 Cells Respond Differently to Infection With Mycobacterium Tuberculosis. Sci Rep (2017) 7:42225. doi: 10.1038/srep42225
37. Pruett SB, Fan R, Zheng Q, Schwab C. Differences in IL-10 and IL-12 Production Patterns and Differences in the Effects of Acute Ethanol Treatment on Macrophages In Vivo and In Vitro. Alcohol (2005) 37(1):1–8. doi: 10.1016/j.alcohol.2005.09.004
38. Heydari Z, Moeinvaziri F, Agarwal T, Pooyan P, Shpichka A, Maiti TK, et al. Organoids: A Novel Modality in Disease Modeling. Biodes Manuf (2021) 4(4):689–716. doi: 10.1007/s42242-021-00150-7
39. Wang S, Wang X, Tan Z, Su Y, Liu J, Chang M, et al. Human ESC-Derived Expandable Hepatic Organoids Enable Therapeutic Liver Repopulation and Pathophysiological Modeling of Alcoholic Liver Injury. Cell Res (2019) 29(12):1009–26. doi: 10.1038/s41422-019-0242-8
40. Adams JW, Cugola FR, Muotri AR. Brain Organoids as Tools for Modeling Human Neurodevelopmental Disorders. Physiology (Bethesda) (2019) 34(5):365–75. doi: 10.1152/physiol.00005.2019
41. Devall M, Jennelle LT, Bryant J, Bien S, Peters U, Powell S, et al. Modeling the Effect of Prolonged Ethanol Exposure on Global Gene Expression and Chromatin Accessibility in Normal 3D Colon Organoids. PloS One (2020) 15(1):e0227116. doi: 10.1371/journal.pone.0227116
42. Devall M, Plummer SJ, Bryant J, Jennelle LT, Eaton S, Dampier CH, et al. Ethanol Exposure Drives Colon Location Specific Cell Composition Changes in a Normal Colon Crypt 3D Organoid Model. Sci Rep (2021) 11(1):432. doi: 10.1038/s41598-020-80240-1
43. Forsyth CB, Shaikh M, Bishehsari F, Swanson G, Voigt RM, Dodiya H, et al. Alcohol Feeding in Mice Promotes Colonic Hyperpermeability and Changes in Colonic Organoid Stem Cell Fate. Alcohol Clin Exp Res (2017) 41(12):2100–13. doi: 10.1111/acer.13519
44. Drakhlis L, Devadas SB, Zweigerdt R. Generation of Heart-Forming Organoids From Human Pluripotent Stem Cells. Nat Protoc (2021) 16(12):5652–72. doi: 10.1038/s41596-021-00629-8
45. Liberti DC, Morrisey EE. Organoid Models: Assessing Lung Cell Fate Decisions and Disease Responses. Trends Mol Med (2021) 27(12):1159–74. doi: 10.1016/j.molmed.2021.09.008
46. Arzua T, Yan Y, Jiang C, Logan S, Allison RL, Wells C, et al. Modeling Alcohol-Induced Neurotoxicity Using Human Induced Pluripotent Stem Cell-Derived Three-Dimensional Cerebral Organoids. Transl Psychiatry (2020) 10(1):347. doi: 10.1038/s41398-020-01029-4
47. Mutlu EA, Gillevet PM, Rangwala H, Sikaroodi M, Naqvi A, Engen PA, et al. Colonic Microbiome Is Altered in Alcoholism. Am J Physiol Gastrointest Liver Physiol (2012) 302(9):G966–78. doi: 10.1152/ajpgi.00380.2011
48. Bennett B, Downing C, Parker C, Johnson TE. Mouse Genetic Models in Alcohol Research. Trends Genet (2006) 22(7):367–74. doi: 10.1016/j.tig.2006.05.005
49. Ghosh Dastidar S, Warner JB, Warner DR, McClain CJ, Kirpich IA. Rodent Models of Alcoholic Liver Disease: Role of Binge Ethanol Administration. Biomolecules (2018) 8(1):3. doi: 10.3390/biom8010003
50. Lieber CS, DeCarli LM, Sorrell MF. Experimental Methods of Ethanol Administration. Hepatology (1989) 10(4):501–10. doi: 10.1002/hep.1840100417
51. Meadows GG, Wallendal M, Kosugi A, Wunderlich J, Singer DS. Ethanol Induces Marked Changes in Lymphocyte Populations and Natural Killer Cell Activity in Mice. Alcohol Clin Exp Res (1992) 16(3):474–9. doi: 10.1111/j.1530-0277.1992.tb01403.x
52. Nevzorova YA, Boyer-Diaz Z, Cubero FJ, Gracia-Sancho J. Animal Models for Liver Disease - A Practical Approach for Translational Research. J Hepatol (2020) 73(2):423–40. doi: 10.1016/j.jhep.2020.04.011
53. Vogle A, Qian T, Zhu S, Burnett E, Fey H, Zhu Z, et al. Restricted Immunological and Cellular Pathways Are Shared by Murine Models of Chronic Alcohol Consumption. Sci Rep (2020) 10(1):2451. doi: 10.1038/s41598-020-59188-9
54. Holmes RS, Duley JA, Algar EM, Mather PB, Rout UK. Biochemical and Genetic Studies on Enzymes of Alcohol Metabolism: The Mouse as a Model Organism for Human Studies. Alcohol Alcohol (1986) 21(1):41–56.
55. Mathews S, Xu M, Wang H, Bertola A, Gao B. Animals Models of Gastrointestinal and Liver Diseases. Animal Models of Alcohol-Induced Liver Disease: Pathophysiology, Translational Relevance, and Challenges. Am J Physiol Gastrointest Liver Physiol (2014) 306(10):G819–23. doi: 10.1152/ajpgi.00041.2014
56. Thiele TE, Navarro M. "Drinking in the Dark" (DID) Procedures: A Model of Binge-Like Ethanol Drinking in non-Dependent Mice. Alcohol (2014) 48(3):235–41. doi: 10.1016/j.alcohol.2013.08.005
57. Osterndorff-Kahanek E, Ponomarev I, Blednov YA, Harris RA. Gene Expression in Brain and Liver Produced by Three Different Regimens of Alcohol Consumption in Mice: Comparison With Immune Activation. PloS One (2013) 8(3):e59870. doi: 10.1371/journal.pone.0059870
58. Becker HC, Lopez MF. Increased Ethanol Drinking After Repeated Chronic Ethanol Exposure and Withdrawal Experience in C57BL/6 Mice. Alcohol Clin Exp Res (2004) 28(12):1829–38. doi: 10.1097/01.ALC.0000149977.95306.3A
59. McBride WJ, Rodd ZA, Bell RL, Lumeng L, Li TK. The Alcohol-Preferring (P) and High-Alcohol-Drinking (HAD) Rats–Animal Models of Alcoholism. Alcohol (2014) 48(3):209–15. doi: 10.1016/j.alcohol.2013.09.044
60. Clark R, Polish E. Avoidance Conditioning and Alcohol Consumption in Rhesus Monkeys. Science (1960) 132(3421):223–4. doi: 10.1126/science.132.3421.223
61. Mello NK, Mendelson JH. Evaluation of a Polydipsia Technique to Induce Alcohol Consumption in Monkeys. Physiol Behav (1971) 7(6):827–36. doi: 10.1016/0031-9384(71)90047-3
62. Higley JD, Hasert MF, Suomi SJ, Linnoila M. Nonhuman Primate Model of Alcohol Abuse: Effects of Early Experience, Personality, and Stress on Alcohol Consumption. Proc Natl Acad Sci USA (1991) 88(16):7261–5. doi: 10.1073/pnas.88.16.7261
63. Grant KA, Johanson CE. The Nature of the Scheduled Reinforcer and Adjunctive Drinking in Nondeprived Rhesus Monkeys. Pharmacol Biochem Behav (1988) 29(2):295–301. doi: 10.1016/0091-3057(88)90159-1
64. Grant KA, Johanson CE. Oral Ethanol Self-Administration in Free-Feeding Rhesus Monkeys. Alcohol Clin Exp Res (1988) 12(6):780–4. doi: 10.1111/j.1530-0277.1988.tb01345.x
65. Vivian JA, Green HL, Young JE, Majerksy LS, Thomas BW, Shively CA, et al. Induction and Maintenance of Ethanol Self-Administration in Cynomolgus Monkeys (Macaca Fascicularis): Long-Term Characterization of Sex and Individual Differences. Alcohol Clin Exp Res (2001) 25(8):1087–97. doi: 10.1111/j.1530-0277.2001.tb02321.x
66. Baker EJ, Farro J, Gonzales S, Helms C, Grant KA. Chronic Alcohol Self-Administration in Monkeys Shows Long-Term Quantity/Frequency Categorical Stability. Alcohol Clin Exp Res (2014) 38(11):2835–43. doi: 10.1111/acer.12547
67. Ivester P, Roberts LJ 2nd, Young T, Stafforini D, Vivian J, Lees C, et al. Ethanol Self-Administration and Alterations in the Livers of the Cynomolgus Monkey, Macaca Fascicularis. Alcohol Clin Exp Res (2007) 31(1):144–55. doi: 10.1111/j.1530-0277.2006.00276.x
68. Lewis SA, Sureshchandra S, Doratt B, Jimenez VA, Stull C, Grant KA, et al. Transcriptional, Epigenetic, and Functional Reprogramming of Monocytes From Non-Human Primates Following Chronic Alcohol Drinking. Front Immunol (2021) 12:724015. doi: 10.3389/fimmu.2021.724015
69. Barr T, Lewis SA, Sureshchandra S, Doratt B, Grant KA, Messaoudi I. Chronic Ethanol Consumption Alters Lamina Propria Leukocyte Response to Stimulation in a Region-Dependent Manner. FASEB J (2019) 33(6):7767–77. doi: 10.1096/fj.201802780R
70. Sureshchandra S, Raus A, Jankeel A, Ligh BJK, Walter NAR, Newman N, et al. Dose-Dependent Effects of Chronic Alcohol Drinking on Peripheral Immune Responses. Sci Rep (2019) 9(1):7847. doi: 10.1038/s41598-019-44302-3
71. Barr T, Girke T, Sureshchandra S, Nguyen C, Grant K, Messaoudi I. Alcohol Consumption Modulates Host Defense in Rhesus Macaques by Altering Gene Expression in Circulating Leukocytes. J Immunol (2016) 196(1):182–95. doi: 10.4049/jimmunol.1501527
72. Asquith M, Pasala S, Engelmann F, Haberthur K, Meyer C, Park B, et al. Chronic Ethanol Consumption Modulates Growth Factor Release, Mucosal Cytokine Production, and microRNA Expression in Nonhuman Primates. Alcohol Clin Exp Res (2014) 38(4):980–93. doi: 10.1111/acer.12325
73. Messaoudi I, Asquith M, Engelmann F, Park B, Brown M, Rau A, et al. Moderate Alcohol Consumption Enhances Vaccine-Induced Responses in Rhesus Macaques. Vaccine (2013) 32(1):54–61. doi: 10.1016/j.vaccine.2013.10.076
74. Bagby GJ, Stoltz DA, Zhang P, Kolls JK, Brown J, Bohm RP Jr., et al. The Effect of Chronic Binge Ethanol Consumption on the Primary Stage of SIV Infection in Rhesus Macaques. Alcohol Clin Exp Res (2003) 27(3):495–502. doi: 10.1097/01.ALC.0000057947.57330.BE
75. Winsauer PJ, Moerschbaecher JM, Brauner IN, Purcell JE, Lancaster JR Jr., Bagby GJ, et al. Alcohol Unmasks Simian Immunodeficiency Virus-Induced Cognitive Impairments in Rhesus Monkeys. Alcohol Clin Exp Res (2002) 26(12):1846–57. doi: 10.1111/j.1530-0277.2002.tb02492.x
76. Amedee AM, Nichols WA, Robichaux S, Bagby GJ, Nelson S. Chronic Alcohol Abuse and HIV Disease Progression: Studies With the Non-Human Primate Model. Curr HIV Res (2014) 12(4):243–53. doi: 10.2174/1570162X12666140721115717
77. Saitz R, Ghali WA, Moskowitz MA. The Impact of Alcohol-Related Diagnoses on Pneumonia Outcomes. Arch Intern Med (1997) 157(13):1446–52. doi: 10.1001/archinte.1997.00440340078008
78. Baum MK, Rafie C, Lai S, Sales S, Page JB, Campa A. Alcohol Use Accelerates HIV Disease Progression. AIDS Res Hum Retroviruses (2010) 26(5):511–8. doi: 10.1089/aid.2009.0211
79. Bhattacharya R, Shuhart MC. Hepatitis C and Alcohol: Interactions, Outcomes, and Implications. J Clin Gastroenterol (2003) 36(3):242–52. doi: 10.1097/00004836-200303000-00012
80. Lonnroth K, Williams BG, Stadlin S, Jaramillo E, Dye C. Alcohol Use as a Risk Factor for Tuberculosis - A Systematic Review. BMC Public Health (2008) 8:289. doi: 10.1186/1471-2458-8-289
81. Bhatty M, Pruett SB, Swiatlo E, Nanduri B. Alcohol Abuse and Streptococcus Pneumoniae Infections: Consideration of Virulence Factors and Impaired Immune Responses. Alcohol (2011) 45(6):523–39. doi: 10.1016/j.alcohol.2011.02.305
82. Simet SM, Sisson JH. Alcohol's Effects on Lung Health and Immunity. Alcohol Res (2015) 37(2):199–208.
83. Jong GM, Hsiue TR, Chen CR, Chang HY, Chen CW. Rapidly Fatal Outcome of Bacteremic Klebsiella Pneumoniae Pneumonia in Alcoholics. Chest (1995) 107(1):214–7. doi: 10.1378/chest.107.1.214
84. Jerrells TR, Pavlik JA, DeVasure J, Vidlak D, Costello A, Strachota JM, et al. Association of Chronic Alcohol Consumption and Increased Susceptibility to and Pathogenic Effects of Pulmonary Infection With Respiratory Syncytial Virus in Mice. Alcohol (2007) 41(5):357–69. doi: 10.1016/j.alcohol.2007.07.001
85. Vallecillo G, Perello R, Guerri R, Fonseca F, Torrens M. Clinical Impact of COVID-19 on People With Substance Use Disorders. J Public Health (Oxf) (2021) 43(1):9–12. doi: 10.1093/pubmed/fdaa181
86. Ojo AS, Balogun SA, Williams OT, Ojo OS. Pulmonary Fibrosis in COVID-19 Survivors: Predictive Factors and Risk Reduction Strategies. Pulm Med (2020) 2020:6175964. doi: 10.1155/2020/6175964
87. Gupta NM, Lindenauer PK, Yu PC, Imrey PB, Haessler S, Deshpande A, et al. Association Between Alcohol Use Disorders and Outcomes of Patients Hospitalized With Community-Acquired Pneumonia. JAMA Netw Open (2019) 2(6):e195172. doi: 10.1001/jamanetworkopen.2019.5172
88. Narasimhan P, Wood J, Macintyre CR, Mathai D. Risk Factors for Tuberculosis. Pulm Med (2013) 2013:828939. doi: 10.1155/2013/828939
89. Rehm J, Samokhvalov AV, Neuman MG, Room R, Parry C, Lonnroth K, et al. The Association Between Alcohol Use, Alcohol Use Disorders and Tuberculosis (TB). A Systematic Review. BMC Public Health (2009) 9:450. doi: 10.1186/1471-2458-9-450
90. Moss M, Bucher B, Moore FA, Moore EE, Parsons PE. The Role of Chronic Alcohol Abuse in the Development of Acute Respiratory Distress Syndrome in Adults. JAMA (1996) 275(1):50–4. doi: 10.1001/jama.1996.03530250054027
91. Hyun J, Han J, Lee C, Yoon M, Jung Y. Pathophysiological Aspects of Alcohol Metabolism in the Liver. Int J Mol Sci (2021) 22(11):5717. doi: 10.3390/ijms22115717
92. Singal AK, Anand BS. Mechanisms of Synergy Between Alcohol and Hepatitis C Virus. J Clin Gastroenterol (2007) 41(8):761–72. doi: 10.1097/MCG.0b013e3180381584
93. Xu HQ, Wang CG, Zhou Q, Gao YH. Effects of Alcohol Consumption on Viral Hepatitis B and C. World J Clin Cases (2021) 9(33):10052–63. doi: 10.12998/wjcc.v9.i33.10052
94. Chan C, Levitsky J. Infection and Alcoholic Liver Disease. Clin Liver Dis (2016) 20(3):595–606. doi: 10.1016/j.cld.2016.02.014
95. Azar MM, Springer SA, Meyer JP, Altice FL. A Systematic Review of the Impact of Alcohol Use Disorders on HIV Treatment Outcomes, Adherence to Antiretroviral Therapy and Health Care Utilization. Drug Alcohol Depend (2010) 112(3):178–93. doi: 10.1016/j.drugalcdep.2010.06.014
96. Yan J, Ouyang J, Isnard S, Zhou X, Harypursat V, Routy JP, et al. Alcohol Use and Abuse Conspires With HIV Infection to Aggravate Intestinal Dysbiosis and Increase Microbial Translocation in People Living With HIV: A Review. Front Immunol (2021) 12:741658. doi: 10.3389/fimmu.2021.741658
97. Radek KA, Ranzer MJ, DiPietro LA. Brewing Complications: The Effect of Acute Ethanol Exposure on Wound Healing. J Leukoc Biol (2009) 86(5):1125–34. doi: 10.1189/jlb.0209103
98. Jung MK, Callaci JJ, Lauing KL, Otis JS, Radek KA, Jones MK, et al. Alcohol Exposure and Mechanisms of Tissue Injury and Repair. Alcohol Clin Exp Res (2011) 35(3):392–9. doi: 10.1111/j.1530-0277.2010.01356.x
99. Benveniste K, Thut P. The Effect of Chronic Alcoholism on Wound Healing. Proc Soc Exp Biol Med (1981) 166(4):568–75. doi: 10.3181/00379727-166-41110
100. Jones JD, Barber B, Engrav L, Heimbach D. Alcohol Use and Burn Injury. J Burn Care Rehabil (1991) 12(2):148–52. doi: 10.1097/00004630-199103000-00012
101. Messingham KA, Faunce DE, Kovacs EJ. Alcohol, Injury, and Cellular Immunity. Alcohol (2002) 28(3):137–49. doi: 10.1016/S0741-8329(02)00278-1
102. Maier RV. Ethanol Abuse and the Trauma Patient. Surg Infect (Larchmt) (2001) 2(2):133–41; discussion 41-4. doi: 10.1089/109629601750469456
103. Jurkovich GJ, Rivara FP, Gurney JG, Fligner C, Ries R, Mueller BA, et al. The Effect of Acute Alcohol Intoxication and Chronic Alcohol Abuse on Outcome From Trauma. JAMA (1993) 270(1):51–6. doi: 10.1001/jama.1993.03510010057029
104. Guo S, Dipietro LA. Factors Affecting Wound Healing. J Dent Res (2010) 89(3):219–29. doi: 10.1177/0022034509359125
105. Lewis SA, Doratt B, Sureshchandra S, Jankeel A, Newman N, Shen W, et al. Ethanol Consumption Induces Non-Specific Inflammation and Functional Defects in Alveolar Macrophages. Am J Respir Cell Mol Biol (2022). doi: 10.1165/rcmb.2021-0346OC
107. Maraslioglu M, Oppermann E, Blattner C, Weber R, Henrich D, Jobin C, et al. Chronic Ethanol Feeding Modulates Inflammatory Mediators, Activation of Nuclear Factor-Kappab, and Responsiveness to Endotoxin in Murine Kupffer Cells and Circulating Leukocytes. Mediators Inflamm (2014) 2014:808695. doi: 10.1155/2014/808695
108. Bishehsari F, Magno E, Swanson G, Desai V, Voigt RM, Forsyth CB, et al. Alcohol and Gut-Derived Inflammation. Alcohol Res (2017) 38(2):163–71.
110. Mukherjee S. Alcoholism and its Effects on the Central Nervous System. Curr Neurovasc Res (2013) 10(3):256–62. doi: 10.2174/15672026113109990004
111. Szabo G, Lippai D. Converging Actions of Alcohol on Liver and Brain Immune Signaling. Int Rev Neurobiol (2014) 118:359–80. doi: 10.1016/B978-0-12-801284-0.00011-7
112. Pang M, Bala S, Kodys K, Catalano D, Szabo G. Inhibition of TLR8- and TLR4-Induced Type I IFN Induction by Alcohol is Different From its Effects on Inflammatory Cytokine Production in Monocytes. BMC Immunol (2011) 12:55. doi: 10.1186/1471-2172-12-55
113. Underwood ML, Park B, Uebelhoer LS, Gu G, Kunkel LE, Korthuis PT, et al. Chronic Alcohol Exposure Among People Living With HIV Is Associated With Innate Immune Activation and Alterations in Monocyte Phenotype and Plasma Cytokine Profile. Front Immunol (2022) 13. doi: 10.3389/fimmu.2022.867937
114. Thakur V, McMullen MR, Pritchard MT, Nagy LE. Regulation of Macrophage Activation in Alcoholic Liver Disease. J Gastroenterol Hepatol (2007) 22 Suppl 1:S53–6. doi: 10.1111/j.1440-1746.2006.04650.x
115. Khoruts A, Stahnke L, McClain CJ, Logan G, Allen JI. Circulating Tumor Necrosis Factor, Interleukin-1 and Interleukin-6 Concentrations in Chronic Alcoholic Patients. Hepatology (1991) 13(2):267–76. doi: 10.1002/hep.1840130211
116. McClain CJ, Cohen DA. Increased Tumor Necrosis Factor Production by Monocytes in Alcoholic Hepatitis. Hepatology (1989) 9(3):349–51. doi: 10.1002/hep.1840090302
117. Bird GL, Sheron N, Goka AK, Alexander GJ, Williams RS. Increased Plasma Tumor Necrosis Factor in Severe Alcoholic Hepatitis. Ann Intern Med (1990) 112(12):917–20. doi: 10.7326/0003-4819-112-12-917
118. Weichselbaum L, Azouz A, Smolen KK, Das J, Splittgerber M, Lepida A, et al. Epigenetic Basis for Monocyte Dysfunction in Patients With Severe Alcoholic Hepatitis. J Hepatol (2020) 73(2):303–14. doi: 10.1016/j.jhep.2020.02.017
119. Bernsmeier C, Pop OT, Singanayagam A, Triantafyllou E, Patel VC, Weston CJ, et al. Patients With Acute-on-Chronic Liver Failure Have Increased Numbers of Regulatory Immune Cells Expressing the Receptor Tyrosine Kinase MERTK. Gastroenterology (2015) 148(3):603–15 e14. doi: 10.1053/j.gastro.2014.11.045
120. Korf H, du Plessis J, van Pelt J, De Groote S, Cassiman D, Verbeke L, et al. Inhibition of Glutamine Synthetase in Monocytes From Patients With Acute-on-Chronic Liver Failure Resuscitates Their Antibacterial and Inflammatory Capacity. Gut (2019) 68(10):1872–83. doi: 10.1136/gutjnl-2018-316888
121. Bernsmeier C, Triantafyllou E, Brenig R, Lebosse FJ, Singanayagam A, Patel VC, et al. CD14(+) CD15(-) HLA-DR(-) Myeloid-Derived Suppressor Cells Impair Antimicrobial Responses in Patients With Acute-on-Chronic Liver Failure. Gut (2018) 67(6):1155–67. doi: 10.1136/gutjnl-2017-314184
122. Casulleras M, Zhang IW, Lopez-Vicario C, Claria J. Leukocytes, Systemic Inflammation and Immunopathology in Acute-On-Chronic Liver Failure. Cells (2020) 9(12):2632. doi: 10.3390/cells9122632
123. Triantafyllou E, Woollard KJ, McPhail MJW, Antoniades CG, Possamai LA. The Role of Monocytes and Macrophages in Acute and Acute-On-Chronic Liver Failure. Front Immunol (2018) 9:2948. doi: 10.3389/fimmu.2018.02948
124. Kim A, Bellar A, McMullen MR, Li X, Nagy LE. Functionally Diverse Inflammatory Responses in Peripheral and Liver Monocytes in Alcohol-Associated Hepatitis. Hepatol Commun (2020) 4(10):1459–76. doi: 10.1002/hep4.1563
125. Coleman LG Jr., Crews FT. Innate Immune Signaling and Alcohol Use Disorders. Handb Exp Pharmacol (2018) 248:369–96. doi: 10.1007/164_2018_92
126. O'Halloran EB, Curtis BJ, Afshar M, Chen MM, Kovacs EJ, Burnham EL. Alveolar Macrophage Inflammatory Mediator Expression Is Elevated in the Setting of Alcohol Use Disorders. Alcohol (2016) 50:43–50. doi: 10.1016/j.alcohol.2015.11.003
127. He J, Crews FT. Increased MCP-1 and Microglia in Various Regions of the Human Alcoholic Brain. Exp Neurol (2008) 210(2):349–58. doi: 10.1016/j.expneurol.2007.11.017
128. Zhu X, Coleman RA, Alber C, Ballas ZK, Waldschmidt TJ, Ray NB, et al. Chronic Ethanol Ingestion by Mice Increases Expression of CD80 and CD86 by Activated Macrophages. Alcohol (2004) 32(2):91–100. doi: 10.1016/j.alcohol.2004.01.004
129. D'Souza NB, Nelson S, Summer WR, Deaciuc IV. Alcohol Modulates Alveolar Macrophage Tumor Necrosis Factor-Alpha, Superoxide Anion, and Nitric Oxide Secretion in the Rat. Alcohol Clin Exp Res (1996) 20(1):156–63. doi: 10.1111/j.1530-0277.1996.tb01059.x
130. Gustot T, Lemmers A, Moreno C, Nagy N, Quertinmont E, Nicaise C, et al. Differential Liver Sensitization to Toll-Like Receptor Pathways in Mice With Alcoholic Fatty Liver. Hepatology (2006) 43(5):989–1000. doi: 10.1002/hep.21138
131. Yin M, Bradford BU, Wheeler MD, Uesugi T, Froh M, Goyert SM, et al. Reduced Early Alcohol-Induced Liver Injury in CD14-Deficient Mice. J Immunol (2001) 166(7):4737–42. doi: 10.4049/jimmunol.166.7.4737
132. Kawaratani H, Tsujimoto T, Douhara A, Takaya H, Moriya K, Namisaki T, et al. The Effect of Inflammatory Cytokines in Alcoholic Liver Disease. Mediators Inflamm (2013) 2013:495156. doi: 10.1155/2013/495156
133. Wang H, Mehal W, Nagy LE, Rotman Y. Immunological Mechanisms and Therapeutic Targets of Fatty Liver Diseases. Cell Mol Immunol (2021) 18(1):73–91. doi: 10.1038/s41423-020-00579-3
134. Iimuro Y, Gallucci RM, Luster MI, Kono H, Thurman RG. Antibodies to Tumor Necrosis Factor Alfa Attenuate Hepatic Necrosis and Inflammation Caused by Chronic Exposure to Ethanol in the Rat. Hepatology (1997) 26(6):1530–7. doi: 10.1002/hep.510260621
135. Brenner E, Tiwari GR, Kapoor M, Liu Y, Brock A, Mayfield RD. Single Cell Transcriptome Profiling of the Human Alcohol-Dependent Brain. Hum Mol Genet (2020) 29(7):1144–53. doi: 10.1093/hmg/ddaa038
136. Qin L, Crews FT. Chronic Ethanol Increases Systemic TLR3 Agonist-Induced Neuroinflammation and Neurodegeneration. J Neuroinflammation (2012) 9:130. doi: 10.1186/1742-2094-9-130
137. McCarthy GM, Farris SP, Blednov YA, Harris RA, Mayfield RD. Microglial-Specific Transcriptome Changes Following Chronic Alcohol Consumption. Neuropharmacology (2018) 128:416–24. doi: 10.1016/j.neuropharm.2017.10.035
138. Lippai D, Bala S, Petrasek J, Csak T, Levin I, Kurt-Jones EA, et al. Alcohol-Induced IL-1beta in the Brain is Mediated by NLRP3/ASC Inflammasome Activation That Amplifies Neuroinflammation. J Leukoc Biol (2013) 94(1):171–82. doi: 10.1189/jlb.1212659
139. Qin L, He J, Hanes RN, Pluzarev O, Hong JS, Crews FT. Increased Systemic and Brain Cytokine Production and Neuroinflammation by Endotoxin Following Ethanol Treatment. J Neuroinflammation (2008) 5:10. doi: 10.1186/1742-2094-5-10
140. Bala S, Petrasek J, Mundkur S, Catalano D, Levin I, Ward J, et al. Circulating microRNAs in Exosomes Indicate Hepatocyte Injury and Inflammation in Alcoholic, Drug-Induced, and Inflammatory Liver Diseases. Hepatology (2012) 56(5):1946–57. doi: 10.1002/hep.25873
141. Mandrekar P, Ambade A, Lim A, Szabo G, Catalano D. An Essential Role for Monocyte Chemoattractant Protein-1 in Alcoholic Liver Injury: Regulation of Proinflammatory Cytokines and Hepatic Steatosis in Mice. Hepatology (2011) 54(6):2185–97. doi: 10.1002/hep.24599
142. Thakur V, Pritchard MT, McMullen MR, Wang Q, Nagy LE. Chronic Ethanol Feeding Increases Activation of NADPH Oxidase by Lipopolysaccharide in Rat Kupffer Cells: Role of Increased Reactive Oxygen in LPS-Stimulated ERK1/2 Activation and TNF-Alpha Production. J Leukoc Biol (2006) 79(6):1348–56. doi: 10.1189/jlb.1005613
143. Kono H, Wheeler MD, Rusyn I, Lin M, Seabra V, Rivera CA, et al. Gender Differences in Early Alcohol-Induced Liver Injury: Role of CD14, NF-Kappab, and TNF-Alpha. Am J Physiol Gastrointest Liver Physiol (2000) 278(4):G652–61. doi: 10.1152/ajpgi.2000.278.4.G652
144. Enomoto N, Ikejima K, Bradford BU, Rivera CA, Kono H, Goto M, et al. Role of Kupffer Cells and Gut-Derived Endotoxins in Alcoholic Liver Injury. J Gastroenterol Hepatol (2000) 15 Suppl:D20–5. doi: 10.1046/j.1440-1746.2000.02179.x
145. Nagy LE. Recent Insights Into the Role of the Innate Immune System in the Development of Alcoholic Liver Disease. Exp Biol Med (Maywood) (2003) 228(8):882–90. doi: 10.1177/153537020322800803
146. Hardesty JE, Warner JB, Song YL, Rouchka EC, McClain CJ, Warner DR, et al. Ileum Gene Expression in Response to Acute Systemic Inflammation in Mice Chronically Fed Ethanol: Beneficial Effects of Elevated Tissue N-3 PUFAs. Int J Mol Sci (2021) 22(4):1582. doi: 10.3390/ijms22041582
147. Moschen AR, Adolph TE, Gerner RR, Wieser V, Tilg H. Lipocalin-2: A Master Mediator of Intestinal and Metabolic Inflammation. Trends Endocrinol Metab (2017) 28(5):388–97. doi: 10.1016/j.tem.2017.01.003
148. Dai XM, Zong XH, Sylvestre V, Stanley ER. Incomplete Restoration of Colony-Stimulating Factor 1 (CSF-1) Function in CSF-1-Deficient Csf1op/Csf1op Mice by Transgenic Expression of Cell Surface CSF-1. Blood (2004) 103(3):1114–23. doi: 10.1182/blood-2003-08-2739
149. Tang Y, Banan A, Forsyth CB, Fields JZ, Lau CK, Zhang LJ, et al. Effect of Alcohol on miR-212 Expression in Intestinal Epithelial Cells and its Potential Role in Alcoholic Liver Disease. Alcohol Clin Exp Res (2008) 32(2):355–64. doi: 10.1111/j.1530-0277.2007.00584.x
150. Basuroy S, Sheth P, Mansbach CM, Rao RK. Acetaldehyde Disrupts Tight Junctions and Adherens Junctions in Human Colonic Mucosa: Protection by EGF and L-Glutamine. Am J Physiol Gastrointest Liver Physiol (2005) 289(2):G367–75. doi: 10.1152/ajpgi.00464.2004
151. Rao R. Endotoxemia and Gut Barrier Dysfunction in Alcoholic Liver Disease. Hepatology (2009) 50(2):638–44. doi: 10.1002/hep.23009
152. Bull-Otterson L, Feng W, Kirpich I, Wang Y, Qin X, Liu Y, et al. Metagenomic Analyses of Alcohol Induced Pathogenic Alterations in the Intestinal Microbiome and the Effect of Lactobacillus Rhamnosus GG Treatment. PloS One (2013) 8(1):e53028. doi: 10.1371/journal.pone.0053028
153. Barr T, Sureshchandra S, Ruegger P, Zhang J, Ma W, Borneman J, et al. Concurrent Gut Transcriptome and Microbiota Profiling Following Chronic Ethanol Consumption in Nonhuman Primates. Gut Microbes (2018) 9(4):338–56. doi: 10.1080/19490976.2018.1441663
154. Chen P, Starkel P, Turner JR, Ho SB, Schnabl B. Dysbiosis-Induced Intestinal Inflammation Activates Tumor Necrosis Factor Receptor I and Mediates Alcoholic Liver Disease in Mice. Hepatology (2015) 61(3):883–94. doi: 10.1002/hep.27489
155. Canesso MCC, Lacernada NL, Ferreira CM, Goncalves JL, Almeida D, Gamba C, et al. Comparing the Effects of Acute Alcohol Consumption in Germ-Free and Conventional Mice: The Role of the Gut Microbiota. BMC Microbiol (2014) 14:240. doi: 10.1186/s12866-014-0240-4
156. Duan Y, Llorente C, Lang S, Brandl K, Chu H, Jiang L, et al. Bacteriophage Targeting of Gut Bacterium Attenuates Alcoholic Liver Disease. Nature (2019) 575(7783):505–11. doi: 10.1038/s41586-019-1742-x
157. Nanji AA, Khettry U, Sadrzadeh SM, Yamanaka T. Severity of Liver Injury in Experimental Alcoholic Liver Disease. Correlation With Plasma Endotoxin, Prostaglandin E2, Leukotriene B4, and Thromboxane B2. Am J Pathol (1993) 142(2):367–73.
158. Yan AW, Fouts DE, Brandl J, Starkel P, Torralba M, Schott E, et al. Enteric Dysbiosis Associated With a Mouse Model of Alcoholic Liver Disease. Hepatology (2011) 53(1):96–105. doi: 10.1002/hep.24018
159. Shao T, Zhao C, Li F, Gu Z, Liu L, Zhang L, et al. Intestinal HIF-1alpha Deletion Exacerbates Alcoholic Liver Disease by Inducing Intestinal Dysbiosis and Barrier Dysfunction. J Hepatol (2018) 69(4):886–95. doi: 10.1016/j.jhep.2018.05.021
160. Vergis N, Khamri W, Beale K, Sadiq F, Aletrari MO, Moore C, et al. Defective Monocyte Oxidative Burst Predicts Infection in Alcoholic Hepatitis and Is Associated With Reduced Expression of NADPH Oxidase. Gut (2017) 66(3):519–29. doi: 10.1136/gutjnl-2015-310378
161. Norkina O, Dolganiuc A, Catalano D, Kodys K, Mandrekar P, Syed A, et al. Acute Alcohol Intake Induces SOCS1 and SOCS3 and Inhibits Cytokine-Induced STAT1 and STAT3 Signaling in Human Monocytes. Alcohol Clin Exp Res (2008) 32(9):1565–73. doi: 10.1111/j.1530-0277.2008.00726.x
162. Mason CM, Dobard E, Zhang P, Nelson S. Alcohol Exacerbates Murine Pulmonary Tuberculosis. Infect Immun (2004) 72(5):2556–63. doi: 10.1128/IAI.72.5.2556-2563.2004
163. Meyerholz DK, Edsen-Moore M, McGill J, Coleman RA, Cook RT, Legge KL. Chronic Alcohol Consumption Increases the Severity of Murine Influenza Virus Infections. J Immunol (2008) 181(1):641–8. doi: 10.4049/jimmunol.181.1.641
164. Bermudez LE, Young LS. Ethanol Augments Intracellular Survival of Mycobacterium Avium Complex and Impairs Macrophage Responses to Cytokines. J Infect Dis (1991) 163(6):1286–92. doi: 10.1093/infdis/163.6.1286
165. Bautista AP. Chronic Alcohol Intoxication Primes Kupffer Cells and Endothelial Cells for Enhanced CC-Chemokine Production and Concomitantly Suppresses Phagocytosis and Chemotaxis. Front Biosci (2002) 7:a117–25. doi: 10.2741/A746
166. Aroor AR, Baker RC. Ethanol Inhibition of Phagocytosis and Superoxide Anion Production by Microglia. Alcohol (1998) 15(4):277–80. doi: 10.1016/S0741-8329(97)00129-8
167. Forman HJ, Torres M. Reactive Oxygen Species and Cell Signaling: Respiratory Burst in Macrophage Signaling. Am J Respir Crit Care Med (2002) 166(12 Pt 2):S4–8. doi: 10.1164/rccm.2206007
168. Piotrowski WJ, Marczak J. Cellular Sources of Oxidants in the Lung. Int J Occup Med Environ Health (2000) 13(4):369–85.
169. Liang Y, Harris FL, Brown LA. Alcohol Induced Mitochondrial Oxidative Stress and Alveolar Macrophage Dysfunction. BioMed Res Int (2014) 2014:371593. doi: 10.1155/2014/371593
170. Morris NL, Yeligar SM. Role of HIF-1alpha in Alcohol-Mediated Multiple Organ Dysfunction. Biomolecules (2018) 8(4):170. doi: 10.3390/biom8040170
171. Nath B, Levin I, Csak T, Petrasek J, Mueller C, Kodys K, et al. Hepatocyte-Specific Hypoxia-Inducible Factor-1alpha is a Determinant of Lipid Accumulation and Liver Injury in Alcohol-Induced Steatosis in Mice. Hepatology (2011) 53(5):1526–37. doi: 10.1002/hep.24256
172. Curtis BJ, Zahs A, Kovacs EJ. Epigenetic Targets for Reversing Immune Defects Caused by Alcohol Exposure. Alcohol Res (2013) 35(1):97–113.
173. Bushati N, Cohen SM. microRNA Functions. Annu Rev Cell Dev Biol (2007) 23:175–205. doi: 10.1146/annurev.cellbio.23.090506.123406
174. Gao B, Ahmad MF, Nagy LE, Tsukamoto H. Inflammatory Pathways in Alcoholic Steatohepatitis. J Hepatol (2019) 70(2):249–59. doi: 10.1016/j.jhep.2018.10.023
175. Jones PA. Functions of DNA Methylation: Islands, Start Sites, Gene Bodies and Beyond. Nat Rev Genet (2012) 13(7):484–92. doi: 10.1038/nrg3230
176. Bannister AJ, Kouzarides T. Regulation of Chromatin by Histone Modifications. Cell Res (2011) 21(3):381–95. doi: 10.1038/cr.2011.22
177. Lai L, Song Y, Liu Y, Chen Q, Han Q, Chen W, et al. MicroRNA-92a Negatively Regulates Toll-Like Receptor (TLR)-Triggered Inflammatory Response in Macrophages by Targeting MKK4 Kinase. J Biol Chem (2013) 288(11):7956–67. doi: 10.1074/jbc.M112.445429
178. Bala S, Marcos M, Kodys K, Csak T, Catalano D, Mandrekar P, et al. Up-Regulation of microRNA-155 in Macrophages Contributes to Increased Tumor Necrosis Factor {Alpha} (TNF{alpha}) Production via Increased mRNA Half-Life in Alcoholic Liver Disease. J Biol Chem (2011) 286(2):1436–44. doi: 10.1074/jbc.M110.145870
179. Bala S, Csak T, Kodys K, Catalano D, Ambade A, Furi I, et al. Alcohol-Induced miR-155 and HDAC11 Inhibit Negative Regulators of the TLR4 Pathway and Lead to Increased LPS Responsiveness of Kupffer Cells in Alcoholic Liver Disease. J Leukoc Biol (2017) 102(2):487–98. doi: 10.1189/jlb.3A0716-310R
180. Bala S, Szabo G. MicroRNA Signature in Alcoholic Liver Disease. Int J Hepatol (2012) 2012:498232. doi: 10.1155/2012/498232
181. Lippai D, Bala S, Csak T, Kurt-Jones EA, Szabo G. Chronic Alcohol-Induced microRNA-155 Contributes to Neuroinflammation in a TLR4-Dependent Manner in Mice. PloS One (2013) 8(8):e70945. doi: 10.1371/journal.pone.0070945
182. Bala S, Petrasek J, Csak T, Catalano D, Kodys K, Mundkur S, et al. MicroRNA-155 Regulates Inflammation in Alcoholic Liver Disease via Targeting SOCS1 and SHIP1. J Immunol (2012) 188(1):54.15.
183. Jager J, Aparicio-Vergara M, Aouadi M. Liver Innate Immune Cells and Insulin Resistance: The Multiple Facets of Kupffer Cells. J Intern Med (2016) 280(2):209–20. doi: 10.1111/joim.12483
184. Yin H, Liang X, Jogasuria A, Davidson NO, You M. miR-217 Regulates Ethanol-Induced Hepatic Inflammation by Disrupting Sirtuin 1-Lipin-1 Signaling. Am J Pathol (2015) 185(5):1286–96. doi: 10.1016/j.ajpath.2015.01.030
185. Saikia P, Roychowdhury S, Bellos D, Pollard KA, McMullen MR, McCullough RL, et al. Hyaluronic Acid 35 Normalizes TLR4 Signaling in Kupffer Cells From Ethanol-Fed Rats via Regulation of Microrna291b and its Target Tollip. Sci Rep (2017) 7(1):15671. doi: 10.1038/s41598-017-15760-4
186. Saikia P, Bellos D, McMullen MR, Pollard KA, de la Motte C, Nagy LE. MicroRNA 181b-3p and its Target Importin Alpha5 Regulate Toll-Like Receptor 4 Signaling in Kupffer Cells and Liver Injury in Mice in Response to Ethanol. Hepatology (2017) 66(2):602–15. doi: 10.1002/hep.29144
187. Yeligar SM, Mehta AJ, Harris FL, Brown LA, Hart CM. Peroxisome Proliferator-Activated Receptor Gamma Regulates Chronic Alcohol-Induced Alveolar Macrophage Dysfunction. Am J Respir Cell Mol Biol (2016) 55(1):35–46. doi: 10.1165/rcmb.2015-0077OC
188. Yeligar SM, Harris FL, Hart CM, Brown LA. Ethanol Induces Oxidative Stress in Alveolar Macrophages via Upregulation of NADPH Oxidases. J Immunol (2012) 188(8):3648–57. doi: 10.4049/jimmunol.1101278
189. Kim A, Saikia P, Nagy LE. miRNAs Involved in M1/M2 Hyperpolarization Are Clustered and Coordinately Expressed in Alcoholic Hepatitis. Front Immunol (2019) 10:1295. doi: 10.3389/fimmu.2019.01295
190. Lewis SA, Doratt B, Sureshchandra S, Pan T, Gonzales SW, Shen W, et al. Profiling of Extracellular Vesicle-Bound miRNA to Identify Candidate Biomarkers of Chronic Alcohol Drinking in Nonhuman Primates. Alcohol Clin Exp Res (2022) 46(2):221–31. doi: 10.1111/acer.14760
191. Momen-Heravi F, Saha B, Kodys K, Catalano D, Satishchandran A, Szabo G. Increased Number of Circulating Exosomes and Their microRNA Cargos are Potential Novel Biomarkers in Alcoholic Hepatitis. J Transl Med (2015) 13:261. doi: 10.1186/s12967-015-0623-9
192. Hsu SH, Wang B, Kota J, Yu J, Costinean S, Kutay H, et al. Essential Metabolic, Anti-Inflammatory, and Anti-Tumorigenic Functions of miR-122 in Liver. J Clin Invest (2012) 122(8):2871–83. doi: 10.1172/JCI63539
193. Xu JJ, Zhu L, Li HD, Du XS, Li JJ, Yin NN, et al. DNMT3a-Mediated Methylation of PSTPIP2 Enhances Inflammation in Alcohol-Induced Liver Injury via Regulating STAT1 and NF-kappaB Pathway. Pharmacol Res (2022) 177:106125. doi: 10.1016/j.phrs.2022.106125
194. Li HD, Chen X, Xu JJ, Du XS, Yang Y, Li JJ, et al. DNMT3b-Mediated Methylation of ZSWIM3 Enhances Inflammation in Alcohol-Induced Liver Injury via Regulating TRAF2-Mediated NF-kappaB Pathway. Clin Sci (Lond) (2020) 134(14):1935–56. doi: 10.1042/CS20200031
195. Momen-Heravi F, Catalano D, Talis A, Szabo G, Bala S. Protective Effect of LNA-anti-miR-132 Therapy on Liver Fibrosis in Mice. Mol Ther Nucleic Acids (2021) 25:155–67. doi: 10.1016/j.omtn.2021.05.007
196. Villagra A, Cheng F, Wang HW, Suarez I, Glozak M, Maurin M, et al. The Histone Deacetylase HDAC11 Regulates the Expression of Interleukin 10 and Immune Tolerance. Nat Immunol (2009) 10(1):92–100. doi: 10.1038/ni.1673
197. Zhou T, Sun Y, Li M, Ding Y, Yin R, Li Z, et al. Enhancer of Zeste Homolog 2-Catalysed H3K27 Trimethylation Plays a Key Role in Acute-on-Chronic Liver Failure via TNF-Mediated Pathway. Cell Death Dis (2018) 9(6):590. doi: 10.1038/s41419-018-0670-2
198. Takizawa H, Boettcher S, Manz MG. Demand-Adapted Regulation of Early Hematopoiesis in Infection and Inflammation. Blood (2012) 119(13):2991–3002. doi: 10.1182/blood-2011-12-380113
199. Shi X, DeLucia AL, Bao J, Zhang P. Alcohol Abuse and Disorder of Granulopoiesis. Pharmacol Ther (2019) 198:206–19. doi: 10.1016/j.pharmthera.2019.03.001
200. Panasiuk A, Kemona A. Bone Marrow Failure and Hematological Abnormalities in Alcoholic Liver Cirrhosis. Rocz Akad Med Bialymst (2001) 46:100–5.
201. Latvala J, Parkkila S, Niemela O. Excess Alcohol Consumption is Common in Patients With Cytopenia: Studies in Blood and Bone Marrow Cells. Alcohol Clin Exp Res (2004) 28(4):619–24. doi: 10.1097/01.ALC.0000122766.54544.3B
202. Ballard HS. The Hematological Complications of Alcoholism. Alcohol Health Res World (1997) 21(1):42–52.
203. Smith C, Gasparetto M, Jordan C, Pollyea DA, Vasiliou V. The Effects of Alcohol and Aldehyde Dehydrogenases on Disorders of Hematopoiesis. Adv Exp Med Biol (2015) 815:349–59. doi: 10.1007/978-3-319-09614-8_20
204. Budde R, Hellerich U. Alcoholic Dyshaematopoiesis: Morphological Features of Alcohol-Induced Bone Marrow Damage in Biopsy Sections Compared With Aspiration Smears. Acta Haematol (1995) 94(2):74–7. doi: 10.1159/000203977
205. Heermans EH. Booze and Blood: The Effects of Acute and Chronic Alcohol Abuse on the Hematopoietic System. Clin Lab Sci (1998) 11(4):229–32.
206. Zhang P, Welsh DA, Siggins RW 2nd, Bagby GJ, Raasch CE, Happel KI, et al. Acute Alcohol Intoxication Inhibits the Lineage- C-Kit+ Sca-1+ Cell Response to Escherichia Coli Bacteremia. J Immunol (2009) 182(3):1568–76. doi: 10.4049/jimmunol.182.3.1568
207. Shi X, Lin YP, Gao B, Zhang P. Impairment of Hematopoietic Precursor Cell Activation During the Granulopoietic Response to Bacteremia in Mice With Chronic-Plus-Binge Alcohol Administration. Infect Immun (2017) 85(11):e00369-17. doi: 10.1128/IAI.00369-17
Keywords: monocytes/macrophages, epigenetics, transcriptome (RNA-seq), inflammation, alcohol
Citation: Malherbe DC and Messaoudi I (2022) Transcriptional and Epigenetic Regulation of Monocyte and Macrophage Dysfunction by Chronic Alcohol Consumption. Front. Immunol. 13:911951. doi: 10.3389/fimmu.2022.911951
Received: 03 April 2022; Accepted: 27 May 2022;
Published: 29 June 2022.
Edited by:
Pinyi Lu, National Cancer Institute at Frederick (NIH), United StatesReviewed by:
Jingbo Pang, University of Illinois at Chicago, United StatesCopyright © 2022 Malherbe and Messaoudi. This is an open-access article distributed under the terms of the Creative Commons Attribution License (CC BY). The use, distribution or reproduction in other forums is permitted, provided the original author(s) and the copyright owner(s) are credited and that the original publication in this journal is cited, in accordance with accepted academic practice. No use, distribution or reproduction is permitted which does not comply with these terms.
*Correspondence: Ilhem Messaoudi, SWxoZW0uTWVzc2FvdWRpQHVreS5lZHU=
Disclaimer: All claims expressed in this article are solely those of the authors and do not necessarily represent those of their affiliated organizations, or those of the publisher, the editors and the reviewers. Any product that may be evaluated in this article or claim that may be made by its manufacturer is not guaranteed or endorsed by the publisher.
Research integrity at Frontiers
Learn more about the work of our research integrity team to safeguard the quality of each article we publish.