- Department of Critical Care Medicine, The Third Xiangya Hospital, Central South University, Changsha, China
Sepsis, a systemic inflammatory response disease, is the most severe complication of infection and a deadly disease. High mobility group proteins (HMGs) are non-histone nuclear proteins binding nucleosomes and regulate chromosome architecture and gene transcription, which act as a potent pro-inflammatory cytokine involved in the delayed endotoxin lethality and systemic inflammatory response. HMGs increase in serum and tissues during infection, especially in sepsis. A growing number of studies have demonstrated HMGs are not only cytokines which can mediate inflammation, but also potential therapeutic targets in sepsis. To reduce sepsis-related mortality, a better understanding of HMGs is essential. In this review, we described the structure and function of HMGs, summarized the definition, epidemiology and pathophysiology of sepsis, and discussed the HMGs-related mechanisms in sepsis from the perspectives of non-coding RNAs (microRNA, long non-coding RNA, circular RNA), programmed cell death (apoptosis, necroptosis and pyroptosis), drugs and other pathophysiological aspects to provide new targets and ideas for the diagnosis and treatment of sepsis.
1 Introduction
Sepsis is a syndrome caused by maladjusted body response to infection with no effective treatment (1). Globally, it’s estimated that there are 31.5 million sepsis patients and potentially 5.3 million mortalities every year, hospital mortality rates for general and severe sepsis in high-income countries were 17% and 26% respectively (2). It is estimated that the annual medical cost of 230,000 patients with sepsis treated in the ICU is about 4.6 billion US dollars, and the medical and social burden is heavy (3). The pathogenesis of sepsis is very complex, involving infection, inflammation, immune, blood coagulation, dysfunction of vascular endothelium cells (ECs) and tissue damage (4). The early stage of sepsis is characterized by the excessive systemic inflammatory immune response, and the late stage of sepsis is characterized by continuous immunosuppression (5). These processes may lead to cell dysfunction and eventually organ failure (6).
High mobility group proteins (HMGs) are isolated from mammalian nuclei firstly and named according to their electrophoretic mobility (7). HMGs are the most abundant nuclear proteins except histones, and are widely expressed in tissues and organs (8). HMGs can bind distorted DNA uniquely and play a key role in transcription, recombination and DNA repair (9). HMGs are implicated in the pathogenesis of multiple diseases, including traumatic shock, infection, cancer, diabetes and autoimmune diseases (10–12). According to the structural and biological characteristics of different proteins, the HMGs are divided into three groups: HMGA, HMGB, and HMGN proteins (13). Each group of HMGs is characterized by a distinguished functional sequence motif: HMGA with “AT-hook”, HMGB with “HMG-box” and HMGN with “nucleosome binding domain (NBD)” (14) (Figure 1). Through these functional motifs, HMGs bind to specific structures in DNA or chromatin. HMGs are important inflammatory mediators in the fatal systemic reaction of sepsis, affect genomic functions not only by directly binding to chromatin but also by interacting with regulatory factors that affect gene expression.
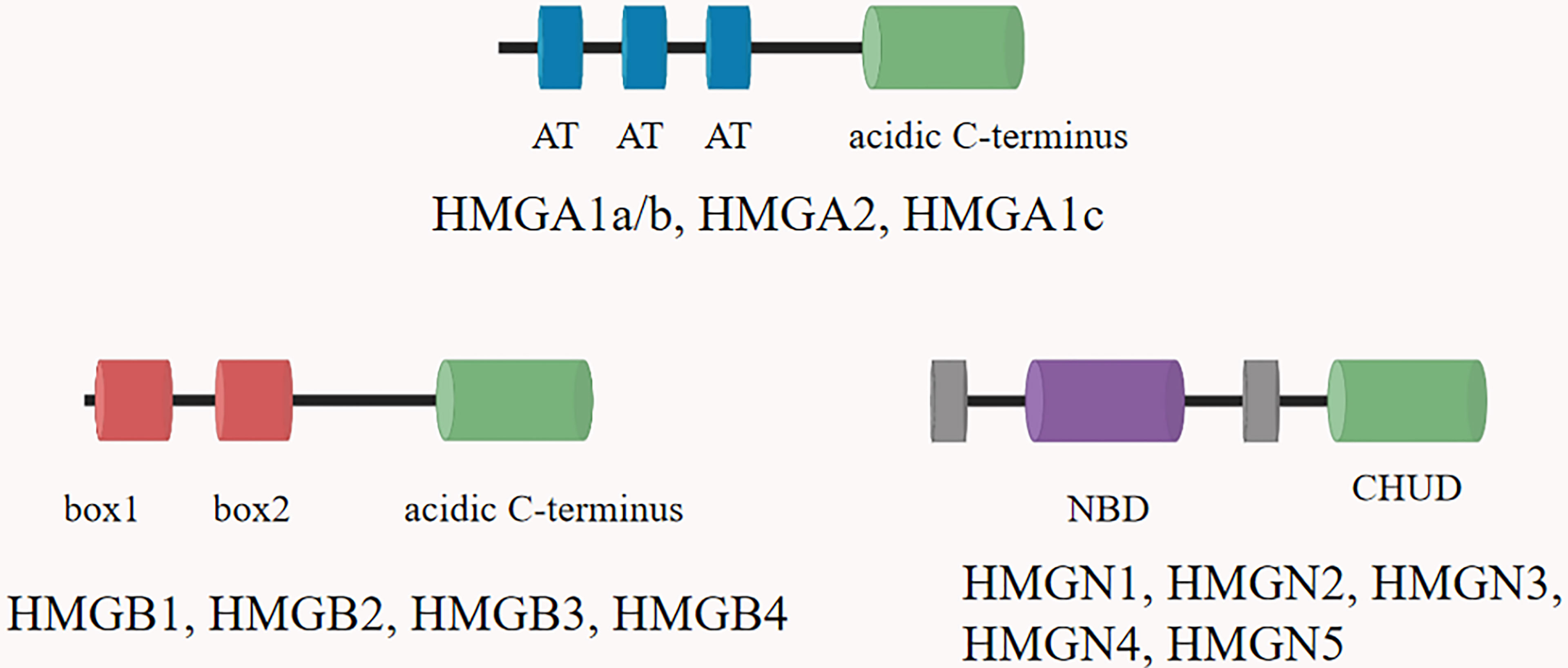
Figure 1 The structural features of the HMGs. Family members are listed above each diagram. All HMGAs contain three AT hooks and an acidic C-terminal part, except HMGA1c, which contains only two hooks. Each of the three members of the HMGB family contains two HMG boxes and an extended acidic C-terminus. The HMGN proteins are characterized by a positively charged nucleosomal binding domain (NBD) and a negatively charged C-terminal region named chromatin unfolding domain (CHUD).
Although the pathophysiology of sepsis is now much better understood, the search for pharmacotherapies for modulating the septic response has been unsuccessful, and the incidence and mortality of sepsis have not significantly decreased over the past two decades (15). More than 80 phase II and III clinical randomized controlled trials of drug therapy on sepsis have emerged around the world. In these trials, only a few drugs showed positive effect on the increase of survival rate in patients with sepsis. However, Chinese herbs spark interest in their use as a potential therapeutic agent. A summary of the linkage between HMGs and drug therapy in sepsis is necessary.
In this review, we summarized the roles of HMGs in sepsis. Understanding the role of HMGs in the pathogenesis of sepsis may help to propose potential effective treatment strategies, shorten the course of sepsis and improve the prognosis and survival rate of sepsis patients.
2 High Mobility Group Proteins
2.1 HMGA
The typical DNA-binding domain of HMGA is a palindrome amino motif, which preferentially binds to small grooves of short fragments of A/T-rich B-form DNA by recognizing structures rather than nucleotide sequences (16). There are two HMGA protein subgroups in mammals, HMGA1 and HMGA2. The HMGA1 subgroup consists of three proteins (HMGA1a, HMGA1b, and HMGA1c) (7).
2.1.1 HMGA1
HMGA1 gene is located on human chromosome 6p21 (17). The HMGA protein family includes HMGA1a (107 amino acids, 11.7 kDa), HMGA1b (96 amino acids, 10.6 kDa) and HMGA1c (179 amino acids, 19.7 kDa) (18). HMGA1 plays an important role in chromatin change, chromatin remodeling, regulating gene transcription and DNA replication. HMGA1 regulates gene expression and transcription, improves the ability of cell proliferation and invasion and makes gene overexpressed, which will lead to the deterioration of cell proliferation and promote the formation of tumors. Moreover, HMGA1 plays an important role in the pathogenesis and treatment of sepsis (19–21).
2.1.2 HMGA2
Human HMGA2 gene is composed of 5 exons and 4 introns, located in 12q13-15 (22). Most of its expression is located in the nucleus and plays a crucial role in regulating gene expression (22). It contains three independent DNA binding regions and an acidic hydroxyl terminal (22). Its amino acid composition is usually characterized by rich proline, basic amino acids and acidic amino acids, showing a high phosphorylation state (23). There are two phosphorylation sites on both sides of “AT-hook”. In S phase and G2/M phase, Ser can be phosphorylated by cyclin or cyclin-dependent kinase, which further affects its affinity with DNA or selectively regulates nuclear input (23). HMGA2 connects its AT-hook’ with specific DNA sequence to change the spatial conformation of its chromatin, further stretch, bend, loop or unzip its structure, and then regulate the transcription process of target gene (23). Its C-terminal can bind to H1 histone, affecting the conformation of nucleosome and participating in the formation of active chromatin. Therefore, HMGA2 is also known as structural transcription factor (24).
2.2 HMGB
HMGB is the most abundant protein in HMGs with molecular weight less than 30 kDa, and binds DNA specifically with DNA structure. In mammals, HMGB is highly conserved and contains four members, namely HMGB1, HMGB2, HMGB3 and HMGB4 (25). Although the protein encoded by HMGB has about 80% amino acid sequence homology, mice with knockout of HMGB1, HMGB2 or HMGB3 genes showed a recognizable phenotype (25). Each HMGB gene contains two DNA binding domains, named HMG box-A and HMG box-B. HMGB1-3 contain an acidic C-terminal, while HMGB4 lacks this acidic C-terminal (26). HMGB4 is a mammalian specific protein, which contains two HMG box domains, but lacks an acidic tail. Its nucleotide sequence is not conserved compared with other HMGB genes (27). HMG box in each HMGB can bind to DNA without any sequence specificity, to induce changes in DNA structure. HMG protein is widely expressed in all nucleated cells. The expression levels of HMGB1 and HMGB2 are the highest in immune cells among all kinds of cells. The expression of HMGB3 is relatively high in placenta, while the expression of HMGB4 is testicular specific (27).
2.2.1 HMGB1
HMGB1 is the most studied member of HMGs. HMGB1 is encoded by the HMGB1 gene (13q12) in human beings (28). HMGB1 is the most expressed of HMGs family members and the most abundant component in all mammalian nuclei. It could be used as a construction factor to promote DNA folding and protein assembly on specific DNA targets (28). HMGB1 is the fastest migrating protein in the nucleus. It takes only 1-2s to cross from organelle to cell fluid (29). Because of its high mobility, HMGB1 could be found in cellular solutes such as mitochondria and lysosomes. HMGB1 protein has many biological functions in mammalian cells. Nuclear HMGB1 is involved in many DNA dependent biological activities, such as gene replication, repair, recombination, transcription and genome stability. HMGB1 plays an important role in cell growth, proliferation and death (30). When cells die or are about to die, HMGB1 is released into the extracellular space. Extracellular HMGB1 could act as damage-associated molecular patterns (DAMPs) to remind the immune system by recruiting inflammation, smooth muscle cells, vascular cells and stem cells (31). In addition, extracellular HMGB1 could act as an immune adjuvant to induce the activation or inhibition of cells (32–34). The activity of extracellular HMGB1 is regulated not only by the receptor, but also by its redox state and structure. In addition to the nuclear and extracellular roles of HMGB1, cytoplasmic HMGB1 could bind many proteins involved in autophagy, cancer evolution and unconventional secretory pathways (35).
HMGB1 involved in diseases and signaling pathways (Figure 2). HMGB1 can be passively released during various types of cell death, such as pyroptosis, autophagy, ferroptosis, necrosis, necroptosis, apoptosis, lysosome-dependent cell death (LCD) and NETosis. HMGB1 binding with toll-like receptors2 (TLR2), TLR4 and TLR5 receptors activates Myd88, which finally responsible for the activation of inflammatory transcription factor NF-κB by phosphorylation and ubiquitin mediated degradation of IκB (36). HMGB1 and mtDNA activate TLR9 signaling during hypoxia to induce tumor growth (37). HMGB1 binds to receptor for advanced glycation end-products (RAGE) receptors to activate Ras-oncogenes, leading to MAP kinase-mediated activation of NF-κB in inflammatory pathways (38). Moreover, activated NF-κB promotes the expression of different downstream pro-inflammatory cytokines such as IL-1β, IL-6, IL-18 and TNF-α, which is responsible for diseases progress (39). HMGB1 released by hepatocytes binds to LPS and is internalized into lysosomes of macrophages and endothelial cells through RAGE. Subsequently, HMGB1 permeates the phospholipid bilayer in the acidic environment of lysosomes. This causes LPS to leak into the cytoplasm and activate caspase-11. Loss of hepatocyte HMGB1, inhibition of hepatocyte HMGB1 release, neutralization of extracellular HMGB1 or RAGE deficiency can prevent caspase-11-dependent pyroptosis and death in endotoxemia and bacterial sepsis (40).
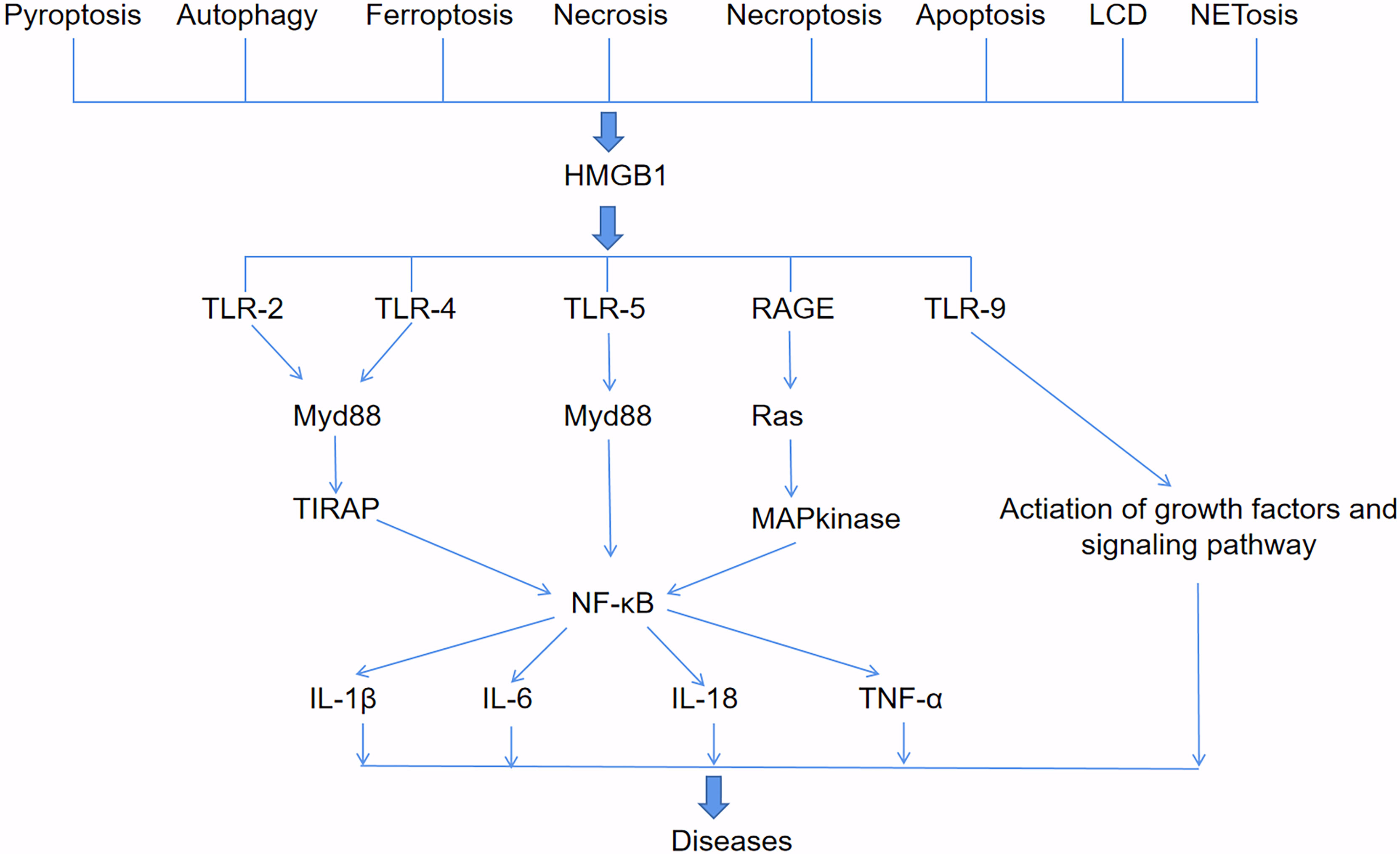
Figure 2 HMGB1-involved signaling pathways. HMGB1 can be passively released during various types of cell death, such as pyroptosis, autophagy, ferroptosis, necrosis, necroptosis, apoptosis, lysosome-dependent cell death (LCD) and NETosis. HMGB1 binds to TLR2, TLR4, TLR5, RAGE and TLR9 receptors to activate downstream pathways that produce pro-inflammatory cytokines such as IL-1β, IL-6, IL-18, and TNF-α, and lead to diseases.
2.2.2 HMGB2
The expression of HMGB2 is mainly limited to adult mouse lymphoid organs and testis (41). HMGB2 exists in all cultured cells and is abundant in thymus. Male HMGB2-/- mice showed defects in spermatogenesis, which was caused by the degeneration of Sertoli cells and germ cells in seminiferous tubules and sperm immobility (42). The proinflammatory activity of extracellular HMGB2 was significantly lower than that of HMGB1 (43). In experimental and clinical acute lung injury (ALI), the content of extracellular HMGB2 increases, which means that HMGB2 has a potential role in tissue injury (43). Küchler R et al. identified competent antibacterial activity of HMGB2 against Escherichia coli, and demonstrated that the two DNA-binding domains (HMG boxes A and B) are crucial for the antibiotic function (44). Thought measure titers of anti-HMGB1/HMGB2 antibodies and anti-Saccharomyces cerevisiae antibodies (ASCA) in the sera of 213 patients with ulcerative colitis (UC) and 93 with Crohn’s disease (CD), using enzyme-linked immunosorbent assays. The presence of serum anti-HMGB2 antibody contributes to inflammatory bowel disease, which indicates that extracellular HMGB2 plays a role in regulating autoimmunity (45).
2.2.3 HMGB3
HMGB3 was first discovered by Marco Bianchi and his colleagues in 1998, it could be used as an expression sequence tag (EST) for preferential expression in embryonic tissues (46). HMGB3 protein was highly expressed in embryos (46), most lymphocytes and bone marrow progenitor cells. HMGB3 is related to B cell differentiation and hematopoietic stem cell self-renewal and proliferation (47, 48).
2.2.4 HMGB4
HMGB4 was a mammalian specific protein discovered by Irwin Davidson in 2009, it could be used as a new member of mammalian HMGB family (27). The molecular weight of HMGB4 protein is about 21 kDa. It contains a pair of DNA domains of HMG box and lacks acidic tail. Its nucleotide sequence is more conservative than that of other HMGB family members (27). Compare with HMGB1, HMGB4 is usually a transcription inhibitor encoded by intron free genes. Similar to other HMGBs, HMGB4 has a potential role in tumorigenesis. Overexpression of HMGB4 could inhibit the proliferation of breast cancer cells (49). HMGB4 has a potential role in regulating the anticancer activity of cisplatin (50). Up to now, there is no report of HMGB4 in sepsis.
2.3 HMGN
The HMGN group includes five members: HMGN1, HMGN2, HMGN3, HMGN4 and HMGN5 (51). The molecular structure of this group includes three obvious functional domains, including bilateral nuclear localization signal (NLS), nucleosome binding domain (NBD) and regulation domain (RD). HMGN gene is mainly expressed in vertebrates and located on different chromosomes. The HMGN1 gene in human is located on 21q22, HMGN2 on 1p36.1, HMGN3 on 6q14, HMGN4 on 6p21 and HMGN5 on Xp13. HMGN protein could interact with nucleosome particles to participate in DNA replication, transcription, repair and recombination (52). Most studies on HNGNs focus on tumors (53–55).
2.3.1 HMGN1
HMGN1 was first extracted from porcine thymus by Graham in 1977 (56). Then Johns isolated HMGN1 from chicken erythrocyte nuclei (57). Human HMGN1 protein is composed of 100 amino acids with a size of about 10.6 kDa (57). Its coding gene is located on human chromosome 21p22 and mouse chromosome 16 (57). In addition to binding to DNA and affecting its replication and transcription, HMGN1 also plays a role in DNA damage repair, organ differentiation and development, disease occurrence (57).
2.3.2 HMGN2
HMGN2 is the most conservative member of HMGN group. Its molecular weight is about 9.2kDa and consists of 90 amino acid residues. HMGN2 coding gene is located on human chromosome 1p36 and mouse chromosome 4. It was first extracted from chicken red cell nucleus. HMGN2 has many functions, such as changing chromosome activity, participating in organ differentiation and development, regulating DNA damage repair (58).
2.3.3 HMGN3
The structure of HMGN3 is highly conserved and contains two splice variants, HMGN3a and HMGN3b. HMGN3a has classical HMGN regions (HLS, NBD and RD), while HMGN3b lacks regions (30). HMGN3 coding gene is located on human chromosome 6p14 (30). Fan Y et al. identified HMGN3 as a marker gene for sepsis through bioinformatics analysis, but no clinical verification was carried out (59).
2.3.4 HMGN4
HMGN4 was the least studied component in HMGN group. Birger Y et al. first found a transcript in the gene bank during data search, and then confirmed it as HMGN4 (60). Its coding gene is an intron free gene which located on human chromosome 6p21. HMGN4 exists only in primates and consists of 90 amino acids with a size of about 9.5 kDa (61).
2.3.5 HMGN5
Shirakawa H et al. found HMGN5 by searching GenBank database in 2000 (62). King and Francomano in 2001 discovered the structure of HMGN5 protein-coding gene (63). HMGN5 gene is located on human chromosome Xp13. The protein is composed of 282 amino acids with a size of about 31.5 kDa (30). During embryonic development, the expression level of HMGN5 protein in different cells or tissues is very different (64).
3 HMGs in Sepsis
The definition of Sepsis 3.0 was used since 2016, which includes patients with life-threatening organ dysfunction caused by an uncontrolled host response to infection, and sequential organ failure assessment (SOFA) score ≥ 2 (65). The fundamental pathogenesis of sepsis is not fully clear, including imbalance between pro-inflammation and anti-inflammation, coagulation disorders, immunosuppression, multiple organ dysfunction, mitochondrial disorder and gene polymorphism (Figure 3). HMGs participate in the occurrence and development of sepsis through most of the above mechanisms. After infection, the host immune system-mediated pro-inflammatory response will respond within a few hours. Previous studies have suggested that with the development of pro-inflammatory response, the body initiated compensatory anti-inflammatory response syndrome (CARS) (66). In fact, the process is more complex, and the pro-inflammatory reaction and anti-inflammatory reaction are more likely to occur simultaneously in the early stage (67, 68).
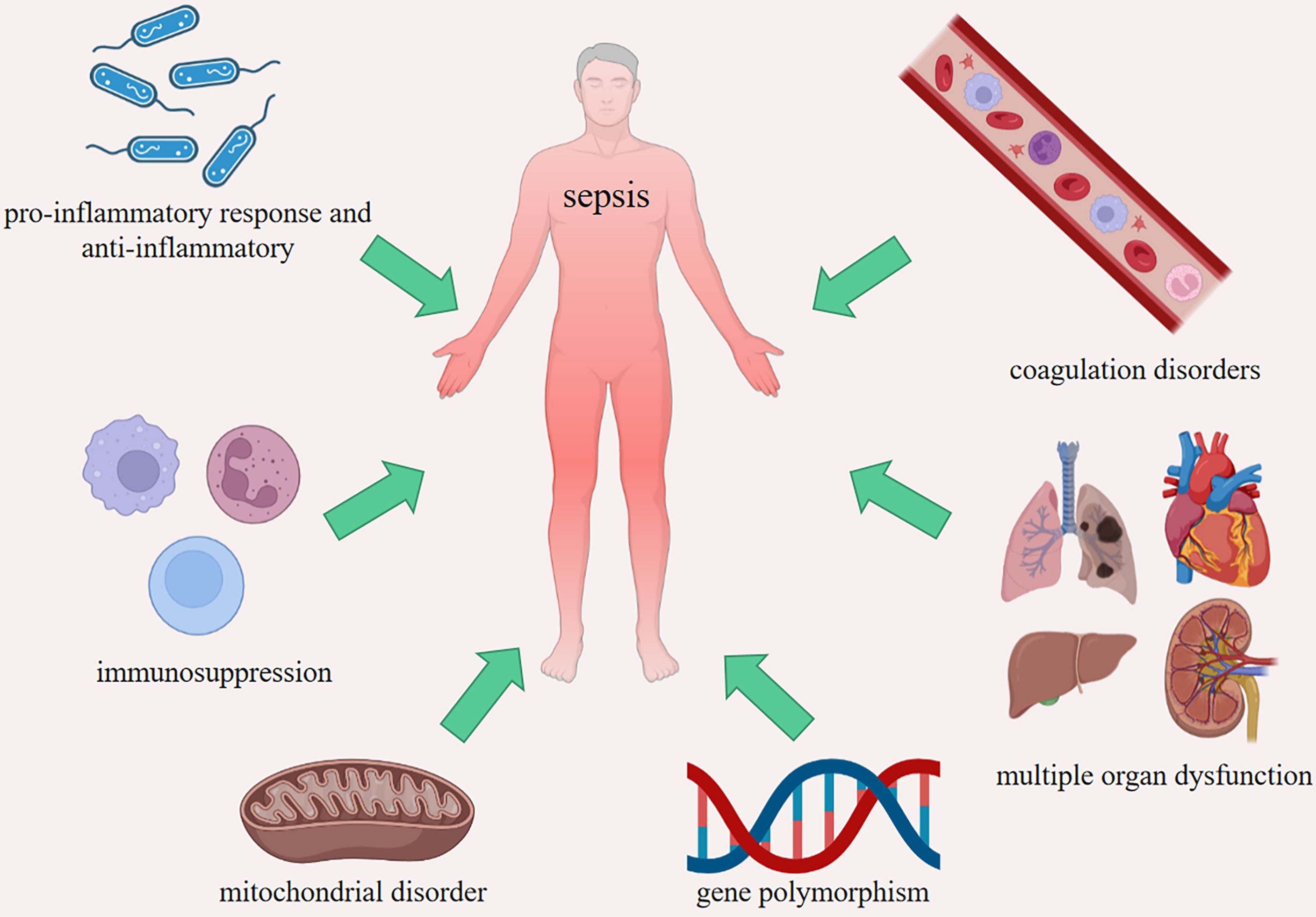
Figure 3 The fundamental pathogenesis of sepsis. Imbalance between pro-inflammatory response and anti-inflammatory, coagulation disorders, immunosuppression, multiple organ dysfunction, mitochondrial disorder and gene polymorphism.
The researches regarding HMGs in sepsis involve many aspects, including cellular pathways, molecular mechanisms and potential drug screening (69–71). Most studies regarding HMGs in sepsis were of the interaction between HMGs and non-coding RNAs, programmed cell death and drugs, so we summarized the roles of HMGs in sepsis mainly from the aspects of non-coding RNAs (microRNA, long non-coding RNA, circular RNA), programmed cell death (apoptosis, necroptosis and pyroptosis), drugs and mechanism of sepsis so as to provide some new targets and ideas for the diagnosis and treatment of sepsis.
3.1 HMGs and Non-Coding RNAs in Sepsis
Non-coding RNA refers to RNA that does not encode protein, including microRNA (miRNA), long non-coding RNA (lncRNA) and circular RNA (circRNA) (72). Non-coding RNAs interact with proteins, DNA and RNA, participate in a variety of cellular activities, including gene activation, RNA splicing and protein translation (73). Structural features and function of miRNA, lncRNA and circRNA are shown in Table S1. Studies on HMGs and sepsis involving non-coding RNAs are shown in Table 1.
3.1.1 miRNA and HMGs in Sepsis
miRNA is a kind of small single-stranded RNA molecule encoded by endogenous genes, with a length of about 22 nucleotides. It is not translated into protein, but participates in the process of protein translation (74). miRNA has three main characteristics: time and space specificity, high selectivity and conservation of structure and sequence, which makes it an ideal biomarker for medical clinical diagnosis (75). Compare with other biomarkers, miRNA remains stable and highly conservative in circulation (75). The existence of miRNA in body fluids resulted from multiple mechanisms: the release of miRNA from cell fragments after apoptosis or necroptosis, the active shedding of microcapsules (including exosomes), and the secretion of protein complex (76–78).
In sepsis patients, Zhu C et al. found that inhibiting the expression of miR-25 up-regulate the expression of HMGB1 and promote the secretion of inflammatory cytokines such as TNF-α and IL-6, resulting in sepsis (71). In sepsis mice model induced by cecum ligation and puncture (CLP), miR-103a-3p had a protection role in sepsis-induced liver injury by inhibiting the expression of HMGB1 (79). The rescue of miR-181-5p expression by lentivirus expression vector protects against sepsis-induced renal and hepatic dysfunction. miR-181-5p might function as an HMGB1 antagonist for alleviating sepsis-induced systemic inflammation (80). In sepsis rat model induced by CLP, Sun J et al. detected the expression profiles of SNHG16, miR-128-3p and HMGB3 by quantitative reverse transcription PCR and western blot. The levels of proinflammatory factors and proteins were detected by ELISA and western blot. The results showed that sepsis-mediated ALI was regulated by miR-128-3p/HMGB3 (81). miR-17-5p aggravated mtDNA oxidative damage, mitochondrial dysfunction and cardiomyocyte apoptosis during sepsis by activating HMGB1 (82). miR-181c-5p-HMGB1 signaling pathway could regulate sepsis-induced microglia activation and hippocampal neuron apoptosis (83). In another study, septic mice were injected with miR-193-3p agomir, miR-193-3p antagomir or siRNA-STAT3, and then the expressions of miR-193-3p, STAT3 and HMGB1 in myocardium of septic mice were detected. The researchers confirmed that miR193-3p reduced septic myocardial injury by reducing the expression of HMGB1 (84). In the cellular inflammation model, miR-103a-3p alleviated LPS-induced sepsis and multiple organ dysfunction syndrome (MODS) in vitro by reducing the expression of HMGB1 (85). LncRNA GAS5 could bind to miR-449b and improve the expression of HMGB1, thereby activating NF-κB signal, Gao H et al. found that miR-449b/HMGB1 axis could promote sepsis-induced myocardial inhibition (86). In sepsis mice model induced by CLP, the expression levels of miR-129-5p and HMGB1 were measured by quantitative real-time polymerase chain reaction and western blot, and the correlation between miR-129-5p and HMGB1 was verified by luciferase assay and RNA immunoprecipitation. The result indicated that miR-129-5p protected against sepsis-induced ALI by decreasing HMGB1 expression (87). miR-98 protected sepsis mice from cardiac dysfunction, liver and lung injury by negatively mediating HMGA2 (88). miR-205 influenced renal injury in sepsis rats through inhibiting HMGB1 (89). miR129-5p attenuated LPS-induced podocyte apoptosis, inflammation, and acute kidney injury (AKI) through HMGB1, and plays a protective role in sepsis models in vivo and in vitro (90). Through estimating the effects of paclitaxel, lncRNA-MALAT1, miR-370-3p and HMGB1 on the proliferation and apoptosis of HK-2 cells injured by LPS, Xu L et al. found that knockdown of miR-370-3p inhibited the expression of HMGB1 (91). Overexpression of miR-381-3p could inhibit the mRNA expression of HMGB1, repress H9c2 cells apoptosis and inflammatory response, and stimulate and promote the vitality of H9c2 cells. The result indicated that miR-381-3p restored sepsis-induced inflammatory response and myocardial dysfunction through HMGB1 (92). Xu HP et al. discovered that circRNA TLK1 enhanced HMGB1 expression by sponging miR-106a-5p in the HK-2 cells, and miR-106a-5p and HMGB1 were involved in circTLK1-mediated injury of LPS-treated cells, miR-106a-5p/HMGB1 axis could regulate inflammation, oxidative stress and promote sepsis-related AKI (93). Overexpression of miR-212-3p inhibited the cytoplasmic translocation of HMGB1 in LPS-induced RAW264.7 cells, which indicated that miR-212-3p directly targeted HMGB1 to suppress inflammatory response in LPS-treated macrophage RAW264.7 cells (94). HMGB1 expression negatively correlated with miR−205−5b expression in LPS-induced sepsis. By contrast, HMGB1 expression in LPS-stimulated RAW264.7 cells was increased following transfection with miR−205−5b inhibitor, which indicated that miR-205−5b inhibited HMGB1 expression in LPS-induced sepsis (95). Altogether, these findings indicate that HMGs play important roles in sepsis by regulating miRNAs.
3.1.2 LncRNA and HMGs in Sepsis
LncRNA is a kind of RNA transcribed by RNA polymerase II, with a length of more than 200 nucleotides and no clear protein-coding effect (96). The current gene coding database shows that there are about 17904 lncRNAs and 14739 pseudogenes in the human genome. According to the relative position between lncRNA and coding gene, it could be divided into sense lncRNA, antisense lncRNA, bidirectional lncRNA, intergenic lncRNA and intron lncRNA.
In human studies, as reported, lncRNA IGF2-AS binds HMGA1 to regulate nucleotide metabolism, promoting pyroptosis of endothelial progenitor cells (EPCs) in sepsis patients (21). In LPS-induced ALI mice models, lncRNA nuclear paraspeckle assembly transcript 1 (NEAT1) may induce alveolar epithelial cell injury and inflammation through HMGB1, thereby aggravating the progression of ALI and acute respiratory distress syndrome (ARDS) (97). Down-regulation of lncRNA PVT1 by miRNA-29a/HMGB1 axis attenuates the polarization of macrophage M1 and alleviates sepsis-induced myocardial injury (98). LncRNA GAS5 might through HMGB1 and subsequent NF-κB promote sepsis-induced myocardial inhibition (86). LncRNA HOTAIR promotes HK-2 cell apoptosis in sepsis-induced renal injury through HMGB1 (99). In vitro, paclitaxel could prevent LPS-induced AKI by regulating lncRNA MALAT1/miR-370-3p/HMGB1 axis, suggesting that paclitaxel may be used as a therapeutic drug to reduce sepsis-related AKI (91). These findings also suggest that HMG plays an important role in sepsis by regulating lncRNA. Up to now, there is no report about HMGN linked with lncRNA in sepsis.
3.1.3 CircRNA and HMGs in Sepsis
CircRNA is a kind of non-coding RNA with single-stranded closed-loop structure (100). It was once considered as an intermediate or wrong by-product in the process of RNA splicing (101). Recent studies have confirmed that circRNA is a non-random product with extensive existence, rich expression, stability and conservation (102). CircRNA has become a research hotspot in recent years. circRNAs are closed long non-coding RNAs, in which the 5’ and 3’ termini are covalently linked by back-splicing of exons from a single pre-mRNA. There are three kinds of circRNA: exonic circRNA (ecircRNA), exonic-intronic circRNA (EIciRNA) and circular intronic RNA (ciRNA) (103). CircRNAs have great potential as a new diagnostic biomarker and target for treatment of various diseases, including type 2 diabetes, cancer and sepsis. In sepsis rat model induced by CLP, circRNA circTLK1 regulates inflammation and oxidative stress through HMGB1, resulting in sepsis-related AKI (93). Li M et al. found CircRNA hsa_circ_0008305 (circPTK2) regulates sepsis-induced microglia activation and hippocampal neuron apoptosis through HMGB1 (83). In vitro and in vivo, circR-TLK1 aggravated mtDNA oxidative damage, mitochondrial dysfunction and cardiomyocyte apoptosis during sepsis by activating HMGB1 (82). There are relatively few researches about CircRNA and HMGs in sepsis. This area needs to be explored further. Up to now, there is no report about HMGA or HMGN linked with circRNA in sepsis.
3.2 HMGs and Programmed Cell Death in Sepsis
Sepsis can cause programmed cell death, including apoptosis, necroptosis and pyroptosis. Moreover, cell death is essential for understanding the pathophysiological basis of sepsis. HMGs are considered as the leading triggers of programmed cell death (PCD). Many studies have provided sufficient evidence linking HMGs’ role in cell death and sepsis (40, 104–106). The study on HMGs and PCD in sepsis facilitates the development of effective prevention and treatment for sepsis. The characteristics of apoptosis, necroptosis and pyroptosis are shown in Table S2.
3.2.1 Apoptosis and HMGs in Sepsis
Apoptosis is a physiological and active death process of cells under certain physiological or pathological conditions through the activation, expression and regulation of a series of genes. It plays an important role in the life process and the maintenance of body homeostasis. During the process of cell death, DNA fragments and other cell wastes arise after chromosome or chromatin pyknosis and rupture which produces apoptotic body. Apoptotic body is quickly swallowed by phagocytes, so as to avoid the diffusion of cell contents to the surrounding and subsequent inflammation (107). Therefore, there is no extravasation of cell contents and inflammatory reaction in apoptosis. To date, research indicated that there are four main apoptotic pathways: the extrinsic or death receptor pathway, the intrinsic or mitochondrial pathway, intrinsic endoplasmic reticulum pathway and perforin/granzyme pathway (108).
As a dynamic regulator of gene transcription and chromatin remodeling, HMGA1 played an important role in the pathological process of sepsis-induced cardiomyopathy. In sepsis-induced cardiomyopathy models induced by LPS, the overexpression of HMGA1 exacerbates the inflammation and apoptosis of cardiomyocytes, while the silencing of HMGA1 alleviates the inflammation, but aggregates the apoptosis of cardiomyocytes (109). In order to study the potential causal relationship between apoptosis and organ injury, Qin et al. used the broad-spectrum caspase inhibitor Z-VAD-FMK in septic mice. They found that Z-VAD-FMK treated septic mice had decreased levels of HMGB1 which inhibited macrophage apoptosis in the spleen and thymus (69). CircTLK1 aggravates mtDNA oxidative damage, mitochondrial dysfunction and cardiomyocyte apoptosis by activating HMGB1 during sepsis (82). miR-181b directly targets HMGB1, and down-regulating HMGB1 in sepsis could reduce inflammatory factors and myocardial injury and inhibit cardiomyocyte apoptosis (110). miR-22-3p is significantly down-regulated in a rat model of sepsis-induced AKI and LPS-induced sepsis model in HK-2 cells. Overexpression of miR-22-3p remarkably suppresses the inflammatory response and apoptosis throught down-regulating HMGB1, p-p65, TLR4 and pro-inflammatory factors such as IL-1β, IL-6, TNF-α and NO (111). LncRNA Hox transcriptional antisense RNA (HOTAIR) is up-regulated in sepsis-induced renal injury and promotes HK-2 cell apoptosis through HMGB1 (99). Overexpression of miR-381-3p could repress the expression of HMGB1, inhibit apoptosis and inflammatory response, and promote the viability of septic cells (92). Down-regulation of SNHG16 could reduce apoptosis and inflammation in sepsis-induced ALI model by regulating HMGB3 (81). Mitochondrial dysfunction, which is responsible for energy metabolism, intrinsic apoptotic pathway, oxidative stress, and systemic inflammatory responses, is closely related with severe sepsis-induced death (112). In vitro and in vivo, the researches of HMGNs in the field of apoptosis mainly focus on the tumorigenesis of human cancer and the development of multidrug resistance (113, 114). There is still a lack of sepsis-related research regarding HMGNs.
3.2.2 Necroptosis and HMGs in Sepsis
Necroptosis is programmed death, and is mainly caused by cytokines (TNF-α, IFN-α, IFN-γ), toll-like receptors (TLR3, TLR4, TLR9) and nucleic acid (DNA, RNA) sensors. Receptor interacting serine/threonine protein kinase 1 (RIPK1), receptor inter-acting serine/threonine protein kinase 3 (RIPK3) and mixed lineage kinase domain like pseudokinase (MLKL) are involved, and MLKL is the key molecule. The current research found that necroptosis has been linked to infectious diseases, neurological diseases, inflammatory diseases and cancer (115–118).
In human studies, Yoo et al. demonstrated that plasma HMGB1 was positively correlated with RIPK3 and MLKL, suggesting that HMGB1 is related to necroptosis (119). In vitro and in vivo, red blood cell (RBC) transfusion enhances the susceptibility to pulmonary inflammation and induces pulmonary endothelial cell necroptosis by releasing HMGB1 (120). The studies of sepsis and HMGs in necroptosis mainly focus on HMGB1, while the studies of other HMGs in necroptosis are still few at present.
3.2.3 Pyroptosis and HMGs in Sepsis
Pyroptosis is inflammatory PCD, which is induced by microbial infection and endogenous injury-related signals (121). Pyroptosis relies on aspartate specific cysteine proteases (Caspase-1, Caspase-4, Caspase-5, Caspase-11) to cleave Gasdermin D (GSDMD) and oligomerize its active N-terminal fragment (GSDMD-NT) in the plasma membrane, then form membrane pores and release proinflammatory factor (IL-1β, IL-18) was the main feature (122). Pyroptosis is one of the natural immune defense mechanisms of the host against pathogen infection (123). According to the different initial activation modes of pyroptosis, which can be divided into three types: the caspase-1-mediated canonical pathway, the caspase-4/5/11-mediated noncanonical pathway, and the pathway of transforming caspase-3-dependent apoptosis into pyroptosis activated by tumor-related chemotherapeutic drugs (124).
LncRNA IGF2-AS regulated nucleotide metabolism by mediating HMGA1 to promote pyroptosis of EPCs in sepsis patients (21). In sepsis mice model induced by CLP, HMGB1 might promote the pyroptosis of liver macrophages and mediate the acute liver injury of sepsis (125). In endotoxemia and bacterial sepsis, HMGB1 released by hepatocytes is necessary for caspase-11 dependent pyroptosis and mortality. In terms of mechanism, HMGB1 released by hepatocytes binds to LPS and is internalized into lysosomes of macrophages and endothelial cells through RAGE. Subsequently, HMGB1 permeates the phospholipid bilayer in a lysosomal acidic environment. This causes LPS to leak into the cytoplasm and activates caspase-11. Loss of hepatocyte HMGB1, inhibition of hepatocyte HMGB1 release, neutralization of extracellular HMGB1 or RAGE deficiency could prevent caspase-11-dependent pyroptosis and death in endotoxemia and bacterial sepsis. These results suggest that the interaction between HMGB1 and LPS mediates the pyroptosis of caspase-11 dependent fatal sepsis (40). There is no report regarding HMGs in pyroptosis except HMGA1 and HMGB1 at present.
3.3 HMGs and Drugs in Sepsis
The treatment of sepsis requires different drugs, including anti-inflammatory drugs, anticoagulants, immunomodulatory drugs and herbal medicines. Drugs are the cornerstone of sepsis treatment, often involving a variety of pathways, among which the pathway involving HMGs has gradually become a research hotspot. The deep understanding of the HMGs and sepsis in drug-related research could facilitate the development of effective treatments and preventive methods for sepsis. Studies on HMGs and sepsis involving drugs are shown in Table 2.
3.3.1 Anti-Inflammation Drugs and HMGs in Sepsis
In recent years, through the continuous research on the pathogenesis of sepsis, researchers have gradually realized that sepsis is a pathophysiological abnormality of the body and its own tissue damage caused by infection, rather than the result of the direct action of bacteria or toxins. The uncontrolled excessive inflammatory response and the accompanying cytokine storm in the early stage of sepsis are the main factors that promote the development of the disease and lead to multiple organ dysfunction and death of patients (126). Therefore, timely and appropriate antagonistic excessive release of inflammatory mediators has become one of the mainstream ideas of drug discovery for the treatment of sepsis. As an important late inflammatory factor, HMGB1 is involved in the pathogenesis of sepsis. Compare with the early inflammatory factors, HMGB1 appears later and lasts longer, and its function is not limited to the simple pro-inflammatory response, but also closely relates to the cellular immune dysfunction of the body, suggesting that HMGB1 intervention may contribute to sepsis (127).
In sepsis mice model induced by CLP, Indoprofen is a non-steroidal anti-inflammatory drug (NSAID), Bi X et al. demonstrated that indoprofen inhibited inflammatory responses and protected mice from lethal organ damages by down-regulating HMGB1 (128). Glucan phosphate (GP) is a carbohydrate ligand that modulates innate immunity and proinflammatory signaling in sepsis (129). GP could inhibit myocardial HMGB1 translocation in severe sepsis and result in attenuation of cardiac dysfunction and improve outcome (130). In vivo, GP could attenuate sepsis-induced cardiac dysfunction by inhibiting the release of HMGB-1 (131). Hydrogen gas (H2), which has anti-oxidative, anti-inflammatory, and anti-apoptotic effects (132). Xie K et al. found that the beneficial effects of H2 treatment on sepsis and sepsis-associated organ damage were associated with the decreased levels of oxidative product, increased activities of antioxidant enzymes, and reduced levels of HMGB1 in serum and tissue (133). Similarly, Yu Y et al. also demonstrated that 2% H2 inhalation might be a promising therapeutic strategy for intestinal injuries caused by severe sepsis through the regulation of HO-1 and HMGB1 release (134).
3.3.2 Herbal Medicines and HMGs in Sepsis
Drugs from herbal medicines have great immunomodulatory potential by inhibiting pro-inflammatory and anti-inflammatory cytokines, showing few unnecessary secondary reactions. At the same time, in inflammatory diseases, herbs tend to regulate the level of oxidative stress and inflammation (135). Natural compounds have attracted much attention for the treatment of a variety of clinical complications. Considering that medicinal plants have less side effects and are easy to obtain, comprehensive research on the treatment of sepsis by medicinal plants should be considered.
There are many herbal medicines that have been used as agents in sepsis, including Xuebijing (136, 137) and Ulinastatin (138). Xuebijing is a Chinese patent medicine, which had the advantages of detoxification and toning, elimination of bacteria and viruses, supplementation of vital energy, and improved blood circulation (136, 137). Ulinastatin is an important intrinsic broad-spectrum protease inhibitor, and is generally believed to manage a series of proinflammatory mediators and cytokines (138). Reduning (RDN), a popular traditional Chinese medicine, formulated by three herbs, has been widely used to treat upper respiratory infectious diseases in China (70). Wang Z et al. found that RDN and its effective constituent luteoloside protect against sepsis partly via inhibition of HMGB1/TLR4/NF-κB/MAPKs signaling pathways (70). Glycyrrhizin (GL), a natural triterpene glycoside derived from licorice, which protected rats from sepsis by blocking HMGB1 signaling (139). Sesamin is a lignin component isolated from sesame oil and is commonly considered a healthy food with anti-hypertensive, anti-inflammatory, anti-viral and anti-oxidative properties, Li ZL et al. demonstrated that sesamin attenuates intestinal injury in sepsis through the HMGB1/TLR4/IL-33 signaling pathway (140). Quercetin exerts protective effects in a sepsis rat model by quenching ROS, activating of the enzymic antioxidant system, and reducing HMGB1 expression (141). Other herbal medicines, including angelica sinensis (142), maslinic acid (143), baicalein (144), aloin (145) and zingerone (146), play a protective role in sepsis through different mechanisms. The mechanisms of these herbal medicines-related HMGs in sepsis are shown in Table 2.
3.3.3 Other Drugs and HMGs in Sepsis
The pathophysiology of sepsis involves infection, coagulation disorder and immunosuppression. Antibiotics, anticoagulant drugs and immunomodulators are effective in the treatment of sepsis. With the deepening of research, drugs for the targeted inhibition of HMGB1 in sepsis have been issued, including painkillers, anti-diabetic drugs and anesthetics (147–149).
Biapenem is an antibiotic that reduces HMGB1 release and septic mortality (150). Cilostazol is anti-platelet agent that inhibits HMGB1’s release in LPS-activated RAW 264.7 cells and increases the survival of septic mice (151). Hagiwara S et al. showed that intravenous immunoglobulin improves pulmonary pathology and overall survival in sepsis by inhibiting the inflammatory response, in particular HMGB1 (152). Ketamine, an intravenous anesthetic agent, has been shown to possess anti-inflammatory effects (153). It attenuates sepsis-induced ALI through HMGB1-RAGE pathways (154), and reduces LPS-induced HMGB1 through the activation of the Nrf2/HO-1 pathway and NF-κB suppression (148). Metformin is one of the most widely prescribed drugs for the treatment of type 2 diabetes (155). It improves the survival in mice model of lethal endotoxaemia by inhibiting HMGB1 release (149). Remifentanil (a painkiller) (147), magnesium sulfate (an anti-convulsive drug) (156), chloroquine (an anti-tumor drug) (157) and paclitaxel (an anti-tumor drug) (91) are involved in HMGs and sepsis-related researches. The mechanisms of drugs described above used in sepsis are shown in Table 2.
Sepsis is an extremely complex clinical syndrome, systemic inflammatory response and immune dysfunction are very prominent in the course of clinical manifestation. They often coexist in sepsis pathological process at the same time. The precision of sepsis inflammation and immune regulation make the choose of appropriate drugs great difficult. Prior to entering clinical trials, many sepsis drugs have been shown to improve survival in one or more sepsis animal models. However, when most of these compounds are tested in patients, the improvement in survival is not significant and there is no statistical significance (158, 159). In sepsis, the use of HMGs related drugs also may have the above problems, which need to be solved by researchers.
3.4 Other Studies Regarding HMGs in Sepsis
Sepsis is a SIRS caused by infection, which may lead to multiple organ dysfunction. The pathophysiology of sepsis involves inflammatory response and immune response. HMGs act as a potent pro-inflammatory cytokine involved in the delayed endotoxin lethality and systemic inflammatory response (40). HMGs increase in serum and tissues during infection, especially in sepsis. A growing number of studies have demonstrated that HMGs are potential therapeutic targets in the experimental model of sepsis (40). The mechanisms of sepsis are related to HMGs and have aroused extensive interest of researchers.
3.4.1 HMGs in Imbalance Between Pro-Inflammation and Anti-Inflammation
Overexpression of HMGA1 aggravates cardiomyocytes inflammation in sepsis-induced cardiomyopathy (109). HMGB1 is a late proinflammatory cytokine, the circulating HMGB1 level can reflect the disease severity and is potent in augmenting systemic inflammation (160). HMGB1 is an important factor that might link gut bacterial translocation and systemic inflammation in severe acute pancreatitis (161). Comparisons of the necrotic cell debris from HMGB1-deficient and wild-type cells demonstrated that HMGB1-deficient cells had a profoundly reduced capacity to induce cytokines (162). Toddalolactone protectes LPS-induced sepsis and attenuates LPS-induced inflammatory response by modulating HMGB1-NF-κB translocation (163). HMGB1 antibody alters inflammation in murine sepsis model and reduces sepsis mortality (164). ATF3 is a negative regulator of TLR4 signaling, up-regulation of ATF3 contributes to the reduced release of inflammatory molecules (especially HMGB1), which increases the survival rate of mice after LPS challenge (165).
3.4.2 HMGs in Coagulation Disorders
The release and activation of F3 (the main initiator of coagulation) from myeloid or epithelial cells is facilitated by activating inflammasomes and consequent gasdermin D (GSDMD)-mediated pyroptosis, coupled to signal through HMGB1, stimulator of interferon response CGAMP interactor 1 (STING1), or sequestosome 1 (SQSTM1) (166). Extracellular HMGB1 markedly increases the procoagulant activity of tissue factor by promoting the externalization of phosphatidylserine to the outer cell surface. Type 1 interferons (IFNs), a widely expressed family of cytokines that orchestrate innate antiviral and antibacterial immunity, mediates bacterial infection-induced DIC by amplifying the release of HMGB1 into the bloodstream. Inhibition of the expression of type 1 IFNs and disruption of their receptor IFN-α/βR or downstream effector (e.g., HMGB1) uniformly decrease gram-negative bacteria-induced DIC (167).
3.4.3 HMGs in Immunosuppression
HMGB1 can actively affect the immune functions of many types of cells including T lymphocytes, regulatory T cells (Tregs), dendritic cells (DCs), macrophages, and natural killer cells (NK cells). Various cellular responses can be mediated by HMGB1 which binds to cell-surface receptors [e.g., the receptor for advanced glycation end products (RAGE), cTLR2 and TLR4]. Anti-HMGB1 treatment, such as anti-HMGB1 polyclonal or monoclonal antibodies, inhibitors (e.g., ethyl pyruvate) and antagonists (e.g., A box), can protect against sepsis lethality (168). HMGB1 activates the innate immune system and promotes inflammation in conditions such as sepsis (169). HMGB1 regulates intracellular cascades influencing immune cell functions, including chemotaxis and immune modulation (170). HMGB1 secretion is critical for the immunity system because dendritic cells, when reaching the lymph nodes, secrete HMGB1, sustaining the proliferation of antigen-specific T-cells, to prevent their activation-dependent apoptosis, and to promote their polarization toward a T-helper 1 phenotype (171). Targeted expression of a dominant negative HMGA1 transgene is able to improve outcomes in models of endotoxin exposure and microbial sepsis, in part by modulating the immune response (19). Human macrophage and dendritic cell-specific silencing of HMGB1 ameliorates sepsis in a humanized mice model (172). Anti-brain HMGB1 could improve dendritic cell dysfunction in sepsis mice (173).
3.4.4 HMGs in Multiple Organ Dysfunction
In cellular inflammation models, decreasing HMGB1 alleviates LPS-induced sepsis and MODS in vitro (85). The inhibition of cerebral HMGB1 significantly alleviates multiple organ damage under septic exposure, including alleviating the damage to the heart, liver, lungs, and kidneys, which increases the survival rate of septic rats (174). HMGB1 level is an independent risk factor for sepsis and MODS in patient with severe blunt chest trauma (175). HMGB1 is involved in bone marrow mesenchymal stem cells (BMSCs) treatment for MODS, through regulation of the TLR2, TLR4-mediated NF-κB signal pathway (176).
3.4.5 HMGs in Gene Polymorphism
Lee K et al. investigated the associations of a single nucleotide polymorphism (SNP; rs1045411) in HMGB1 with sepsis, and found that the variant A allele of rs1045411 appeared to be associated with a more severe inflammatory response than the GG genotype under specific conditions (177). The rs2249825 SNP and the haplotype TCG are significantly associated with LPS-induced HMGB1 production by peripheral blood leukocytes (178). There are also significant differences in sepsis morbidity rate and MODS scores among patients with different genotypes of the rs2249825. In addition, the patients with the wild-type haplotype TCG have lesser sepsis morbidity rate and MODS scores than those without the TCG haplotype (179).
4 Conclusion
Sepsis is an extremely complex clinical syndrome and remains a common and fatal problem worldwide. The discovery of HMGs as an effective cytokine mediator in sepsis has triggered a new field of investigation related to the treatment of sepsis. In the studies about HMGs and non-coding RNAs in sepsis, many miRNA/lncRNA and few circRNA participate in the mechanism of sepsis by targeting HMGs, especially HMGB1. In the studies about HMGs and programmed cell death in sepsis, HMGB1 and HMGA1 induce cell’s apoptosis and pyroptosis, and HMGB1 also induces necroptosis. Systemic inflammatory response and immune dysfunction often coexist in sepsis pathological process at the same time. The precision of sepsis inflammation and immune regulation make the choose of appropriate drugs great difficult. Although many compound drugs which related to sepsis have been shown to improve survival in one or more sepsis animal models, there is no positive effect on the survival improvement in patient. Chinese herbs spark interest in their use as a potential therapeutic agent. In short, HMGs, especially HMGA1 and HMGB1, are widely studied and demonstrated to be involved in the occurrence and development of sepsis. They can not only be used to evaluate the severity of sepsis, but also be potential therapeutic targets of sepsis. Although several lines of evidence have clarified the underlying mechanisms of HMGs in sepsis, the development of an effective treatment that includes HMGs has a long way to go. In order to provide more accurate targeted therapy for sepsis, more detailed studies on HMGs are needed.
Author Contributions
GL conceived the study, data analysis, and drafted the manuscript. ZH conceived the study, its design and critically revised the manuscript. All authors read and approved the final manuscript.
Funding
The work was supported by the Natural Science Foundation of Hunan Province (2021JJ31005).
Conflict of Interest
The authors declare that the research was conducted in the absence of any commercial or financial relationships that could be construed as a potential conflict of interest.
Publisher’s Note
All claims expressed in this article are solely those of the authors and do not necessarily represent those of their affiliated organizations, or those of the publisher, the editors and the reviewers. Any product that may be evaluated in this article, or claim that may be made by its manufacturer, is not guaranteed or endorsed by the publisher.
Supplementary Material
The Supplementary Material for this article can be found online at: https://www.frontiersin.org/articles/10.3389/fimmu.2022.911152/full#supplementary-material
References
1. Qiu P, Zhou J, Zhang J, Dong Y, Liu Y. Exosome: The Regulator of the Immune System in Sepsis. Front Pharmacol (2021) 12:671164. doi: 10.3389/fphar.2021.671164
2. Fleischmann C, Scherag A, Adhikari NK, Hartog CS, Tsaganos T, Schlattmann P, et al. Assessment of Global Incidence and Mortality of Hospital-Treated Sepsis. Curr Estimates Limitations Am J Respir Crit Care Med (2016) 193(3):259–72. doi: 10.1164/rccm.201504-0781OC
3. Xie J, Wang H, Kang Y, Zhou L, Liu Z, Qin B, et al. The Epidemiology of Sepsis in Chinese ICUs: A National Cross-Sectional Survey. Crit Care Med (2020) 48(3):e209–e18. doi: 10.1097/CCM.0000000000004155
4. Angus DC, van der Poll T. Severe Sepsis and Septic Shock. New Engl J Med (2013) 369(9):840–51. doi: 10.1056/NEJMra1208623
5. Steinhagen F, Schmidt SV, Schewe JC, Peukert K, Klinman DM, Bode C. Immunotherapy in Sepsis - Brake or Accelerate? Pharmacol Ther (2020) 208:107476. doi: 10.1016/j.pharmthera.2020.107476
6. Rodrigues PRS, Picco N, Morgan BP, Ghazal P. Sepsis Target Validation for Repurposing and Combining Complement and Immune Checkpoint Inhibition Therapeutics. Expert Opin Drug Discov (2021) 16(5):537–51. doi: 10.1080/17460441.2021.1851186
7. Papantonis A. HMGs as Rheostats of Chromosomal Structure and Cell Proliferation. Trends Genet TIG (2021) 37(11):986–94. doi: 10.1016/j.tig.2021.07.004
8. Bianchi ME, Agresti A. HMG Proteins: Dynamic Players in Gene Regulation and Differentiation. Curr Opin Genet Dev (2005) 15(5):496–506. doi: 10.1016/j.gde.2005.08.007
9. Amanzadeh E, Mohabatkar H, Biria D. Classification of DNA Minor and Major Grooves Binding Proteins According to the NLSs by Data Analysis Methods. Appl Biochem Biotechnol (2014) 174(1):437–51. doi: 10.1007/s12010-014-0926-y
10. Foti D, Chiefari E, Fedele M, Iuliano R, Brunetti L, Paonessa F, et al. Lack of the Architectural Factor HMGA1 Causes Insulin Resistance and Diabetes in Humans and Mice. Nat Med (2005) 11(7):765–73. doi: 10.1038/nm1254
11. Venereau E, De Leo F, Mezzapelle R, Careccia G, Musco G, Bianchi ME. HMGB1 as Biomarker and Drug Target. Pharmacol Res (2016) 111:534–44. doi: 10.1016/j.phrs.2016.06.031
12. Yu Y, Tang D, Kang R. Oxidative Stress-Mediated HMGB1 Biology. Front Physiol (2015) 6:93. doi: 10.3389/fphys.2015.00093
13. Ozturk N, Singh I, Mehta A, Braun T, Barreto G. HMGA Proteins as Modulators of Chromatin Structure During Transcriptional Activation. Front Cell Dev Biol (2014) 2:5. doi: 10.3389/fcell.2014.00005
14. Reeves R, Adair JE. Role of High Mobility Group (HMG) Chromatin Proteins in DNA Repair. DNA Repair (2005) 4(8):926–38. doi: 10.1016/j.dnarep.2005.04.010
15. Cecconi M, Evans L, Levy M, Rhodes A. Sepsis and Septic Shock. Lancet (2018) 392(10141):75–87. doi: 10.1016/S0140-6736(18)30696-2
16. Reeves R. Nuclear Functions of the HMG Proteins. Biochim Biophys Acta (2010) 1799(1-2):3–14. doi: 10.1016/j.bbagrm.2009.09.001
17. Lau KM, Chan QK, Pang JC, Ma FM, Li KK, Yeung WW, et al. Overexpression of HMGA1 Deregulates Tumor Growth via Cdc25a and Alters Migration/Invasion Through a Cdc25a-Independent Pathway in Medulloblastoma. Acta Neuropathol (2012) 123(4):553–71. doi: 10.1007/s00401-011-0934-8
18. Peluso S, Chiappetta G. High-Mobility Group A (HMGA) Proteins and Breast Cancer. Breast Care (Basel Switzerland) (2010) 5(2):81–5. doi: 10.1159/000297717
19. Baron RM, Kwon MY, Castano AP, Ghanta S, Riascos-Bernal DF, Lopez-Guzman S, et al. Frontline Science: Targeted Expression of a Dominant-Negative High Mobility Group A1 Transgene Improves Outcome in Sepsis. J Leuk Biol (2018) 104(4):677–89. doi: 10.1002/JLB.4HI0817-333RR
20. Kwon MY, Ghanta S, Ng J, Castano AP, Han J, Ith B, et al. Mesenchymal Stromal Cells Expressing a Dominant-Negative High Mobility Group A1 Transgene Exhibit Improved Function During Sepsis. J Leuk Biol (2021) 110(4):711–22. doi: 10.1002/JLB.4A0720-424R
21. Liang G, Zeng M, Gao M, Xing W, Jin X, Wang Q, et al. lncRNA IGF2-AS Regulates Nucleotide Metabolism by Mediating HMGA1 to Promote Pyroptosis of Endothelial Progenitor Cells in Sepsis Patients. Oxid Med Cell Longev (2022) 2022:9369035. doi: 10.1155/2022/9369035
22. Agaimy A, Ihrler S, Baněčková M, Costés Martineau V, Mantsopoulos K, Hartmann A, et al. HMGA2-WIF1 Rearrangements Characterize a Distinctive Subset of Salivary Pleomorphic Adenomas With Prominent Trabecular (Canalicular Adenoma-Like) Morphology. Am J Surg Pathol (2022) 46(2):190–9. doi: 10.1097/PAS.0000000000001783
23. Su L, Deng Z, Leng F. The Mammalian High Mobility Group Protein AT-Hook 2 (HMGA2): Biochemical and Biophysical Properties, and Its Association With Adipogenesis. Int J Mol Sci (2020) 21(10):3710. doi: 10.3390/ijms21103710
24. Reeves R, Beckerbauer L. HMGI/Y Proteins: Flexible Regulators of Transcription and Chromatin Structure. Biochim Biophys Acta (2001) 1519(1-2):13–29. doi: 10.1016/S0167-4781(01)00215-9
25. Wen B, Wei YT, Zhao K. The Role of High Mobility Group Protein B3 (HMGB3) in Tumor Proliferation and Drug Resistance. Mol Cell Biochem (2021) 476(4):1729–39. doi: 10.1007/s11010-020-04015-y
26. Stros M. HMGB Proteins: Interactions With DNA and Chromatin. Biochim Biophys Acta (2010) 1799(1-2):101–13. doi: 10.1016/j.bbagrm.2009.09.008
27. Catena R, Escoffier E, Caron C, Khochbin S, Martianov I, Davidson I. HMGB4, a Novel Member of the HMGB Family, Is Preferentially Expressed in the Mouse Testis and Localizes to the Basal Pole of Elongating Spermatids. Biol Reprod (2009) 80(2):358–66. doi: 10.1095/biolreprod.108.070243
28. Sohun M, Shen H. The Implication and Potential Applications of High-Mobility Group Box 1 Protein in Breast Cancer. Ann Trans Med (2016) 4(11):217. doi: 10.21037/atm.2016.05.36
29. Hamatani T, Daikoku T, Wang H, Matsumoto H, Carter MG, Ko MS, et al. Global Gene Expression Analysis Identifies Molecular Pathways Distinguishing Blastocyst Dormancy and Activation. Proc Natl Acad Sci USA (2004) 101(28):10326–31. doi: 10.1073/pnas.0402597101
30. Kang R, Chen R, Zhang Q, Hou W, Wu S, Cao L, et al. HMGB1 in Health and Disease. Mol Aspects Med (2014) 40:1–116. doi: 10.1016/j.mam.2014.05.001
31. Andersson U, Yang H, Harris H. High-Mobility Group Box 1 Protein (HMGB1) Operates as an Alarmin Outside as Well as Inside Cells. Semin Immunol (2018) 38:40–8. doi: 10.1016/j.smim.2018.02.011
32. Gougeon ML, Melki MT, Saïdi H. HMGB1, an Alarmin Promoting HIV Dissemination and Latency in Dendritic Cells. Cell Death Differ (2012) 19(1):96–106. doi: 10.1038/cdd.2011.134
33. Jia C, Zhang J, Chen H, Zhuge Y, Chen H, Qian F, et al. Endothelial Cell Pyroptosis Plays an Important Role in Kawasaki Disease via HMGB1/RAGE/cathespin B Signaling Pathway and NLRP3 Inflammasome Activation. Cell Death Dis (2019) 10(10):778. doi: 10.1038/s41419-019-2021-3
34. Tian Y, Cao Y, Chen R, Jing Y, Xia L, Zhang S, et al. HMGB1 A Box Protects Neurons by Potently Inhibiting Both Microglia and T Cell-Mediated Inflammation in a Mouse Parkinson's Disease Model. Clin Sci (London Engl 1979) (2020) 134(15):2075–90. doi: 10.1042/CS20200553
35. Chen R, Kang R, Tang D. The Mechanism of HMGB1 Secretion and Release. Exp Mol Med (2022) 54(2):91–102. doi: 10.1038/s12276-022-00736-w
36. Yamamoto Y, Gaynor RB. IkappaB Kinases: Key Regulators of the NF-kappaB Pathway. Trends Biochem Sci (2004) 29(2):72–9. doi: 10.1016/j.tibs.2003.12.003
37. Liu Y, Yan W, Tohme S, Chen M, Fu Y, Tian D, et al. Hypoxia Induced HMGB1 and Mitochondrial DNA Interactions Mediate Tumor Growth in Hepatocellular Carcinoma Through Toll-Like Receptor 9. J Hepatol (2015) 63(1):114–21. doi: 10.1016/j.jhep.2015.02.009
38. Sims GP, Rowe DC, Rietdijk ST, Herbst R, Coyle AJ. HMGB1 and RAGE in Inflammation and Cancer. Annu Rev Immunol (2010) 28:367–88. doi: 10.1146/annurev.immunol.021908.132603
39. Tripathi A, Shrinet K, Kumar A. HMGB1 Protein as a Novel Target for Cancer. Toxicol Rep (2019) 6:253–61. doi: 10.1016/j.toxrep.2019.03.002
40. Deng M, Tang Y, Li W, Wang X, Zhang R, Zhang X, et al. The Endotoxin Delivery Protein HMGB1 Mediates Caspase-11-Dependent Lethality in Sepsis. Immunity (2018) 49(4):740–53.e7. doi: 10.1016/j.immuni.2018.08.016
41. Abraham AB, Bronstein R, Chen EI, Koller A, Ronfani L, Maletic-Savatic M, et al. Members of the High Mobility Group B Protein Family are Dynamically Expressed in Embryonic Neural Stem Cells. Proteome Sci (2013) 11(1):18. doi: 10.1186/1477-5956-11-18
42. Taniguchi N, Kawakami Y, Maruyama I, Lotz M. HMGB Proteins and Arthritis. Hum Cell (2018) 31(1):1–9. doi: 10.1007/s13577-017-0182-x
43. Ueno H, Matsuda T, Hashimoto S, Amaya F, Kitamura Y, Tanaka M, et al. Contributions of High Mobility Group Box Protein in Experimental and Clinical Acute Lung Injury. Am J Respir Crit Care Med (2004) 170(12):1310–6. doi: 10.1164/rccm.200402-188OC
44. Küchler R, Schroeder BO, Jaeger SU, Stange EF, Wehkamp J. Antimicrobial Activity of High-Mobility-Group Box 2: A New Function to a Well-Known Protein. Antimicrobial Agents Chemother (2013) 57(10):4782–93. doi: 10.1128/AAC.00805-13
45. Takaishi H, Kanai T, Nakazawa A, Sugata F, Nikai A, Yoshizawa S, et al. Anti-High Mobility Group Box 1 and Box 2 Non-Histone Chromosomal Proteins (HMGB1/HMGB2) Antibodies and Anti-Saccharomyces Cerevisiae Antibodies (ASCA): Accuracy in Differentially Diagnosing UC and CD and Correlation With Inflammatory Bowel Disease Phenotype. J Gastroenterol (2012) 47(9):969–77. doi: 10.1007/s00535-012-0566-3
46. Vaccari T, Beltrame M, Ferrari S, Bianchi ME. Hmg4, a New Member of the Hmg1/2 Gene Family. Genomics (1998) 49(2):247–52. doi: 10.1006/geno.1998.5214
47. Nemeth MJ, Cline AP, Anderson SM, Garrett-Beal LJ, Bodine DM. Hmgb3 Deficiency Deregulates Proliferation and Differentiation of Common Lymphoid and Myeloid Progenitors. Blood (2005) 105(2):627–34. doi: 10.1182/blood-2004-07-2551
48. Nemeth MJ, Curtis DJ, Kirby MR, Garrett-Beal LJ, Seidel NE, Cline AP, et al. Hmgb3: An HMG-Box Family Member Expressed in Primitive Hematopoietic Cells That Inhibits Myeloid and B-Cell Differentiation. Blood (2003) 102(4):1298–306. doi: 10.1182/blood-2002-11-3541
49. Wang LL, Meng QH, Jiao Y, Xu JY, Ge CM, Zhou JY, et al. High-Mobility Group Boxes Mediate Cell Proliferation and Radiosensitivity via Retinoblastoma-Interaction-Dependent and -Independent Mechanisms. Cancer Biother Radiopharm (2012) 27(5):329–35. doi: 10.1089/cbr.2012.1199
50. Park S, Lippard SJ. Binding Interaction of HMGB4 With Cisplatin-Modified DNA. Biochemistry (2012) 51(34):6728–37. doi: 10.1021/bi300649v
51. Nanduri R, Furusawa T, Bustin M. Biological Functions of HMGN Chromosomal Proteins. Int J Mol Sci (2020) 21(2):449. doi: 10.3390/ijms21020449
52. Postnikov Y, Bustin M. Regulation of Chromatin Structure and Function by HMGN Proteins. Biochim Biophys Acta (2010) 1799(1-2):62–8. doi: 10.1016/j.bbagrm.2009.11.016
53. Chen J, Fan Y, Cui B, Li X, Yu Y, Du Y, et al. HMGN2: An Antitumor Effector Molecule of γδt Cells. J IImmunother (Hagerstown Md 1997) (2018) 41(3):118–24. doi: 10.1097/CJI.0000000000000211
54. Cui W, Liu Y, Tan Y, Peng X, Cui L, Cheng Z, et al. Prognostic Value of HMGN Family Expression in Acute Myeloid Leukemia. Future Oncol (London England) (2021) 17(5):541–8. doi: 10.2217/fon-2020-0555
55. Xu E, Ji Z, Jiang H, Lin T, Ma J, Zhou X. Hypoxia-Inducible Factor 1a Upregulates HMGN5 by Increasing the Expression of GATA1 and Plays a Role in Osteosarcoma Metastasis. BioMed Res Int (2019) 2019:5630124. doi: 10.1155/2019/5630124
56. Goodwin GH, Rabbani A, Nicolas PH, Johns EW. The Isolation of the High Mobility Group Non-Histone Chromosomal Protein HMG 14. FEBS Lett (1977) 80(2):413–6. doi: 10.1016/0014-5793(77)80488-2
57. Walker JM, Johns EW. The Isolation, Characterization and Partial Sequences of the Chicken Erythrocyte non-Histone Chromosomal Proteins HMG14 and HMG17. Comparison With the Homologous Calf Thymus Proteins. Biochem J (1980) 185(2):383–6. doi: 10.1042/bj1850383
58. Wu J, Kim S, Kwak MS, Jeong JB, Min HJ, Yoon HG, et al. High Mobility Group Nucleosomal Binding Domain 2 (HMGN2) SUMOylation by the SUMO E3 Ligase PIAS1 Decreases the Binding Affinity to Nucleosome Core Particles. J Biol Chem (2014) 289(29):20000–11. doi: 10.1074/jbc.M114.555425
59. Fan Y, Han Q, Li J, Ye G, Zhang X, Xu T, et al. Revealing Potential Diagnostic Gene Biomarkers of Septic Shock Based on Machine Learning Analysis. BMC Infect Dis (2022) 22(1):65. doi: 10.1186/s12879-022-07056-4
60. Birger Y, Ito Y, West KL, Landsman D, Bustin M. HMGN4, a Newly Discovered Nucleosome-Binding Protein Encoded by an Intronless Gene. DNA Cell Biol (2001) 20(5):257–64. doi: 10.1089/104454901750232454
61. Kugler JE, Deng T, Bustin M. The HMGN Family of Chromatin-Binding Proteins: Dynamic Modulators of Epigenetic Processes. Biochim Biophys Acta (2012) 1819(7):652–6. doi: 10.1016/j.bbagrm.2012.01.013
62. Shirakawa H, Landsman D, Postnikov YV, Bustin M. NBP-45, A Novel Nucleosomal Binding Protein With a Tissue-Specific and Developmentally Regulated Expression. J Biol Chem (2000) 275(9):6368–74. doi: 10.1074/jbc.275.9.6368
63. King LM, Francomano CA. Characterization of a Human Gene Encoding Nucleosomal Binding Protein NSBP1. Genomics (2001) 71(2):163–73. doi: 10.1006/geno.2000.6443
64. Li DD, Zhao SY, Yang ZQ, Duan CC, Guo CH, Zhang HL, et al. Hmgn5 Functions Downstream of Hoxa10 to Regulate Uterine Decidualization in Mice. Cell Cycle (Georgetown Tex) (2016) 15(20):2792–805. doi: 10.1080/15384101.2016.1220459
65. Rhodes A, Evans LE, Alhazzani W, Levy MM, Antonelli M, Ferrer R, et al. Surviving Sepsis Campaign: International Guidelines for Management of Sepsis and Septic Shock: 2016. Intensive Care Med (2017) 43(3):304–77. doi: 10.1007/s00134-017-4683-6
66. Yin J, Chen Y, Huang JL, Yan L, Kuang ZS, Xue MM, et al. Prognosis-Related Classification and Dynamic Monitoring of Immune Status in Patients With Sepsis: A Prospective Observational Study. World J Emergency Med (2021) 12(3):185–91. doi: 10.5847/wjem.j.1920-8642.2021.03.004
67. László I, Trásy D, Molnár Z, Fazakas J. Sepsis: From Pathophysiology to Individualized Patient Care. J Immunol Res (2015) 2015:510436. doi: 10.1155/2015/510436
68. Taeb AM, Hooper MH, Marik PE. Sepsis: Current Definition, Pathophysiology, Diagnosis, and Management. Nutr Clin Pract (2017) 32(3):296–308. doi: 10.1177/0884533617695243
69. Qin S, Wang H, Yuan R, Li H, Ochani M, Ochani K, et al. Role of HMGB1 in Apoptosis-Mediated Sepsis Lethality. J Exp Med (2006) 203(7):1637–42. doi: 10.1084/jem.20052203
70. Wang Z, Chen W, Li Y, Zhang S, Lou H, Lu X, et al. Reduning Injection and its Effective Constituent Luteoloside Protect Against Sepsis Partly via Inhibition of HMGB1/TLR4/NF-κb/MAPKs Signaling Pathways. J Ethnopharmacol (2021) 270:113783. doi: 10.1016/j.jep.2021.113783
71. Zhu C, Chen T, Liu B. Inhibitory Effects of miR-25 Targeting HMGB1 on Macrophage Secretion of Inflammatory Cytokines in Sepsis. Oncol Lett (2018) 16(4):5027–33. doi: 10.3892/ol.2018.9308
72. Fernandez-Diaz D, Rodriguez-Vidal C, Silva-Rodríguez P, Paniagua L, Blanco-Teijeiro MJ, Pardo M, et al. Applications of Non-Coding RNAs in Patients With Retinoblastoma. Front Genet (2022) 13:842509. doi: 10.3389/fgene.2022.842509
73. Maass PG, Luft FC, Bähring S. Long non-Coding RNA in Health and Disease. J Mol Med (Berlin Germany) (2014) 92(4):337–46. doi: 10.1007/s00109-014-1131-8
74. Kong H, Sun ML, Zhang XA, Wang XQ. Crosstalk Among circRNA/lncRNA, miRNA, and mRNA in Osteoarthritis. Front Cell Dev Biol (2021) 9:774370. doi: 10.3389/fcell.2021.774370
75. Condrat CE, Thompson DC, Barbu MG, Bugnar OL, Boboc A, Cretoiu D, et al. miRNAs as Biomarkers in Disease: Latest Findings Regarding Their Role in Diagnosis and Prognosis. Cells (2020) 9(2):276. doi: 10.3390/cells9020276
76. Barile L, Vassalli G. Exosomes: Therapy Delivery Tools and Biomarkers of Diseases. Pharmacol Ther (2017) 174:63–78. doi: 10.1016/j.pharmthera.2017.02.020
77. Cui Y, Lyu X, Ding L, Ke L, Yang D, Pirouz M, et al. Global miRNA Dosage Control of Embryonic Germ Layer Specification. Nature (2021) 593(7860):602–6. doi: 10.1038/s41586-021-03524-0
78. Lee YS, Dutta A. MicroRNAs in Cancer. Annu Rev Pathol (2009) 4:199–227. doi: 10.1146/annurev.pathol.4.110807.092222
79. Chen L, Lu Q, Deng F, Peng S, Yuan J, Liu C, et al. miR-103a-3p Could Attenuate Sepsis-Induced Liver Injury by Targeting Hmgb1. Inflammation (2020) 43(6):2075–86. doi: 10.1007/s10753-020-01275-0
80. Ma XF, Qin J, Guo XH. MiR-181-5p Protects Mice From Sepsis via Repressing HMGB1 in an Experimental Model. Eur Rev Med Pharmacol Sci (2020) 24(18):9712–20. doi: 10.26355/eurrev_202009_23063
81. Sun J, Xin K, Leng C, Ge J. Down-Regulation of SNHG16 Alleviates the Acute Lung Injury in Sepsis Rats Through miR-128-3p/HMGB3 Axis. BMC Pulm Med (2021) 21(1):191. doi: 10.1186/s12890-021-01552-0
82. Qiu Y, Yu Y, Qin XM, Jiang T, Tan YF, Ouyang WX, et al. CircTLK1 Modulates Sepsis-Induced Cardiomyocyte Apoptosis via Enhancing PARP1/HMGB1 Axis-Mediated Mitochondrial DNA Damage by Sponging miR-17-5p. J Cell Mol Med (2021) 25(17):8244–60. doi: 10.1111/jcmm.16738
83. Li M, Hu J, Peng Y, Li J, Ren R. CircPTK2-miR-181c-5p-HMGB1: A New Regulatory Pathway for Microglia Activation and Hippocampal Neuronal Apoptosis Induced by Sepsis. Mol Med (Cambridge Mass) (2021) 27(1):45. doi: 10.1186/s10020-021-00305-3
84. Pan J, Alexan B, Dennis D, Bettina C, Christoph LIM, Tang Y. microRNA-193-3p Attenuates Myocardial Injury of Mice With Sepsis via STAT3/HMGB1 Axis. J Trans Med (2021) 19(1):386. doi: 10.1186/s12967-021-03022-x
85. Li Y, Zhu H, Pan L, Zhang B, Che H. microRNA-103a-3p Confers Protection Against Lipopolysaccharide-Induced Sepsis and Consequent Multiple Organ Dysfunction Syndrome by Targeting HMGB1. Infect Genet Evol (2021) 89:104681. doi: 10.1016/j.meegid.2020.104681
86. Gao H, Ma H, Gao M, Chen A, Zha S, Yan J. Long non-Coding RNA GAS5 Aggravates Myocardial Depression in Mice With Sepsis via the microRNA-449b/HMGB1 Axis and the NF-κb Signaling Pathway. Biosci Rep (2021) 41(4):BSR20201738. doi: 10.1042/BSR20201738
87. Yang P, Xiong W, Chen X, Liu J, Ye Z. Overexpression of miR-129-5p Mitigates Sepsis-Induced Acute Lung Injury by Targeting High Mobility Group Box 1. J Surg Res (2020) 256:23–30. doi: 10.1016/j.jss.2020.05.101
88. Zhu J, Lin X, Yan C, Yang S, Zhu Z. microRNA-98 Protects Sepsis Mice From Cardiac Dysfunction, Liver and Lung Injury by Negatively Regulating HMGA2 Through Inhibiting NF-κb Signaling Pathway. Cell Cycle (Georgetown Tex) (2019) 18(16):1948–64. doi: 10.1080/15384101.2019.1635869
89. Zhang Y, Xia F, Wu J, Yang AX, Zhang YY, Zhao H, et al. MiR-205 Influences Renal Injury in Sepsis Rats Through HMGB1-PTEN Signaling Pathway. Eur Rev Med Pharmacol Sci (2019) 23(24):10950–6. doi: 10.26355/eurrev_201912_19798
90. Huang X, Hou X, Chuan L, Wei S, Wang J, Yang X, et al. miR-129-5p Alleviates LPS-Induced Acute Kidney Injury via Targeting HMGB1/TLRs/NF-kappaB Pathway. Int Immunopharmacol (2020) 89(Pt A):107016. doi: 10.1016/j.intimp.2020.107016
91. Xu L, Hu G, Xing P, Zhou M, Wang D. Paclitaxel Alleviates the Sepsis-Induced Acute Kidney Injury via lnc-MALAT1/miR-370-3p/HMGB1 Axis. Life Sci (2020) 262:118505. doi: 10.1016/j.lfs.2020.118505
92. Liu J, Yang Y, Lu R, Liu Q, Hong S, Zhang Z, et al. MicroRNA-381-3p Signatures as a Diagnostic Marker in Patients With Sepsis and Modulates Sepsis-Steered Cardiac Damage and Inflammation by Binding HMGB1. Bioengineered (2021) 12(2):11936–46. doi: 10.1080/21655979.2021.2006967
93. Xu HP, Ma XY, Yang C. Circular RNA TLK1 Promotes Sepsis-Associated Acute Kidney Injury by Regulating Inflammation and Oxidative Stress Through miR-106a-5p/HMGB1 Axis. Front Mol Biosci (2021) 8:660269. doi: 10.3389/fmolb.2021.660269
94. Chen W, Ma X, Zhang P, Li Q, Liang X, Liu J. MiR-212-3p Inhibits LPS-Induced Inflammatory Response Through Targeting HMGB1 in Murine Macrophages. Exp Cell Res (2017) 350(2):318–26. doi: 10.1016/j.yexcr.2016.12.008
95. Zhou W, Wang J, Li Z, Li J, Sang M. MicroRNA-205−5b Inhibits HMGB1 Expression in LPS-Induced Sepsis. Int J Mol Med (2016) 38(1):312–8. doi: 10.3892/ijmm.2016.2613
96. Prensner JR, Chinnaiyan AM. The Emergence of lncRNAs in Cancer Biology. Cancer Discov (2011) 1(5):391–407. doi: 10.1158/2159-8290.CD-11-0209
97. Zhou H, Wang X, Zhang B. Depression of lncRNA NEAT1 Antagonizes LPS-Evoked Acute Injury and Inflammatory Response in Alveolar Epithelial Cells via HMGB1-RAGE Signaling. Mediators Inflam (2020) 2020:8019467. doi: 10.1155/2020/8019467
98. Luo YY, Yang ZQ, Lin XF, Zhao FL, Tu HT, Wang LJ, et al. Knockdown of lncRNA PVT1 Attenuated Macrophage M1 Polarization and Relieved Sepsis Induced Myocardial Injury via miR-29a/HMGB1 Axis. Cytokine (2021) 143:155509. doi: 10.1016/j.cyto.2021.155509
99. Shen J, Zhang J, Jiang X, Wang H, Pan G. LncRNA HOX Transcript Antisense RNA Accelerated Kidney Injury Induced by Urine-Derived Sepsis Through the miR-22/High Mobility Group Box 1 Pathway. Life Sci (2018) 210:185–91. doi: 10.1016/j.lfs.2018.08.041
100. Anastasiadou E, Jacob LS, Slack FJ. Non-Coding RNA Networks in Cancer. Nat Rev Cancer (2018) 18(1):5–18. doi: 10.1038/nrc.2017.99
101. Chan JJ, Tay Y. Noncoding RNA:RNA Regulatory Networks in Cancer. Int J Mol Sci (2018) 19(5):1310. doi: 10.3390/ijms19051310
102. Yu CY, Kuo HC. The Emerging Roles and Functions of Circular RNAs and Their Generation. J Biomed Sci (2019) 26(1):29. doi: 10.1186/s12929-019-0523-z
103. Wang M, Yu F, Wu W, Zhang Y, Chang W, Ponnusamy M, et al. Circular RNAs: A Novel Type of non-Coding RNA and Their Potential Implications in Antiviral Immunity. Int J Biol Sci (2017) 13(12):1497–506. doi: 10.7150/ijbs.22531
104. Cheng Z, Abrams ST, Toh J, Wang SS, Wang Z, Yu Q, et al. The Critical Roles and Mechanisms of Immune Cell Death in Sepsis. Front Immunol (2020) 11:1918. doi: 10.3389/fimmu.2020.01918
105. Denning NL, Aziz M, Gurien SD, Wang P. DAMPs and NETs in Sepsis. Front Immunol (2019) 10:2536. doi: 10.3389/fimmu.2019.02536
106. Zhou M, Aziz M, Wang P. Damage-Associated Molecular Patterns As Double-Edged Swords in Sepsis. Antioxid Redox Signaling (2021) 35(15):1308–23. doi: 10.1089/ars.2021.0008
107. Ramos GC, Fernandes D, Charão CT, Souza DG, Teixeira MM, Assreuy J. Apoptotic Mimicry: Phosphatidylserine Liposomes Reduce Inflammation Through Activation of Peroxisome Proliferator-Activated Receptors (PPARs) In Vivo. Br J Pharmacol (2007) 151(6):844–50. doi: 10.1038/sj.bjp.0707302
108. Obeng E. Apoptosis (Programmed Cell Death) and its Signals - A Review. Braz J Biol = Rev Brasleira Biol (2021) 81(4):1133–43. doi: 10.1590/1519-6984.228437
109. Cai ZL, Shen B, Yuan Y, Liu C, Xie QW, Hu TT, et al. The Effect of HMGA1 in LPS-Induced Myocardial Inflammation. Int J Biol Sci (2020) 16(11):1798–810. doi: 10.7150/ijbs.39947
110. Ling L, Zhi L, Wang H, Deng Y, Gu C. MicroRNA-181b Inhibits Inflammatory Response and Reduces Myocardial Injury in Sepsis by Downregulating Hmgb1. Inflammation (2021) 44(4):1263–73. doi: 10.1007/s10753-020-01411-w
111. Wang X, Wang Y, Kong M, Yang J. MiR-22-3p Suppresses Sepsis-Induced Acute Kidney Injury by Targeting PTEN. Biosci Rep (2020) 40(6):BSR20200527. doi: 10.1042/BSR20200527
112. Zhang L, Deng S, Zhao S, Ai Y, Zhang L, Pan P, et al. Intra-Peritoneal Administration of Mitochondrial DNA Provokes Acute Lung Injury and Systemic Inflammation via Toll-Like Receptor 9. Int J Mol Sci (2016) 17(9):1245. doi: 10.3390/ijms17091425
113. Fan B, Shi S, Shen X, Yang X, Liu N, Wu G, et al. Effect of HMGN2 on Proliferation and Apoptosis of MCF-7 Breast Cancer Cells. Oncol Lett (2019) 17(1):1160–6. doi: 10.3892/ol.2018.9668
114. Liu X, Ma W, Yan Y, Wu S. Silencing HMGN5 Suppresses Cell Growth and Promotes Chemosensitivity in Esophageal Squamous Cell Carcinoma. J Biochem Mol Toxicol (2017) 31(12). doi: 10.1002/jbt.21996
115. Huang WY, Lai YL, Liu KH, Lin S, Chen HY, Liang CH, et al. Tnfα-Mediated Necroptosis in Brain Endothelial Cells as a Potential Mechanism of Increased Seizure Susceptibility in Mice Following Systemic Inflammation. J Neuroinflam (2022) 19(1):29. doi: 10.1186/s12974-022-02406-0
116. Jiang W, Deng Z, Dai X, Zhao W. PANoptosis: A New Insight Into Oral Infectious Diseases. Front Immunol (2021) 12:789610. doi: 10.3389/fimmu.2021.789610
117. Shi H, Peng Q, Zhou X, He Y, Sun S. An Efficient Signature Based on Necroptosis-Related Genes for Prognosis of Patients With Pancreatic Cancer. Front Genet (2022) 13:848747. doi: 10.3389/fgene.2022.848747
118. Xu W, Huang Y. Regulation of Inflammatory Cell Death by Phosphorylation. Front Immunol (2022) 13:851169. doi: 10.3389/fimmu.2022.851169
119. Yoo H, Im Y, Ko RE, Lee JY, Park J, Jeon K. Association of Plasma Level of High-Mobility Group Box-1 With Necroptosis and Sepsis Outcomes. Sci Rep (2021) 11(1):9512. doi: 10.1038/s41598-021-88970-6
120. Qing DY, Conegliano D, Shashaty MG, Seo J, Reilly JP, Worthen GS, et al. Red Blood Cells Induce Necroptosis of Lung Endothelial Cells and Increase Susceptibility to Lung Inflammation. Am J Respir Crit Care Med (2014) 190(11):1243–54. doi: 10.1164/rccm.201406-1095OC
121. Man SM, Karki R, Kanneganti TD. Molecular Mechanisms and Functions of Pyroptosis, Inflammatory Caspases and Inflammasomes in Infectious Diseases. Immunol Rev (2017) 277(1):61–75. doi: 10.1111/imr.12534
122. Kovacs SB, Miao EA. Gasdermins: Effectors of Pyroptosis. Trends Cell Biol (2017) 27(9):673–84. doi: 10.1016/j.tcb.2017.05.005
123. Jorgensen I, Miao EA. Pyroptotic Cell Death Defends Against Intracellular Pathogens. Immunol Rev (2015) 265(1):130–42. doi: 10.1111/imr.12287
124. Li W, Sun J, Zhou X, Lu Y, Cui W, Miao L. Mini-Review: GSDME-Mediated Pyroptosis in Diabetic Nephropathy. Front Pharmacol (2021) 12:780790. doi: 10.3389/fphar.2021.780790
125. Huang Y, Zang K, Shang F, Guo S, Gao L, Zhang X. HMGB1 Mediates Acute Liver Injury in Sepsis Through Pyroptosis of Liver Macrophages. Int J Burns Trauma (2020) 10(3):60–7.
126. Cao C, Yu M, Chai Y. Pathological Alteration and Therapeutic Implications of Sepsis-Induced Immune Cell Apoptosis. Cell Death Dis (2019) 10(10):782. doi: 10.1038/s41419-019-2015-1
127. Yang K, Fan M, Wang X, Xu J, Wang Y, Tu F, et al. Lactate Promotes Macrophage HMGB1 Lactylation, Acetylation, and Exosomal Release in Polymicrobial Sepsis. Cell Death Differ (2022) 29(1):133–46. doi: 10.1038/s41418-021-00841-9
128. Bi X, Yan X, Jiang B, Liang J, Zhou J, Lu S, et al. Indoprofen Exerts a Potent Therapeutic Effect Against Sepsis by Alleviating High Mobility Group Box 1-Mediated Inflammatory Responses. Toxicol Appl Pharmacol (2021) 433:115778. doi: 10.1016/j.taap.2021.115778
129. Williams DL, Ha T, Li C, Kalbfleisch JH, Laffan JJ, Ferguson DA. Inhibiting Early Activation of Tissue Nuclear Factor-Kappa B and Nuclear Factor Interleukin 6 With (1–>3)-Beta-D-Glucan Increases Long-Term Survival in Polymicrobial Sepsis. Surgery (1999) 126(1):54–65. doi: 10.1067/msy.1999.99058
130. Ha T, Xia Y, Liu X, Lu C, Liu L, Kelley J, et al. Glucan Phosphate Attenuates Myocardial HMGB1 Translocation in Severe Sepsis Through Inhibiting NF-κb Activation. Am J Physiol Heart Circulatory Physiol (2011) 301(3):H848–55. doi: 10.1152/ajpheart.01007.2010
131. Wang H, Cui Z, Sun F, Ding H. Glucan Phosphate Inhibits HMGB-1 Release From Rat Myocardial H9C2 Cells in Sepsis via TLR4/NF-Кb Signal Pathway. Clin Invest Med Med Clin Experiment (2017) 40(2):E66–e72. doi: 10.25011/cim.v40i2.28197
132. Liang IC, Ko WC, Hsu YJ, Lin YR, Chang YH, Zong XH, et al. The Anti-Inflammatory Effect of Hydrogen Gas Inhalation and Its Influence on Laser-Induced Choroidal Neovascularization in a Mouse Model of Neovascular Age-Related Macular Degeneration. Int J Mol Sci (2021) 22(21):12049. doi: 10.3390/ijms222112049
133. Xie K, Yu Y, Pei Y, Hou L, Chen S, Xiong L, et al. Protective Effects of Hydrogen Gas on Murine Polymicrobial Sepsis via Reducing Oxidative Stress and HMGB1 Release. Shock (Augusta Ga) (2010) 34(1):90–7. doi: 10.1097/SHK.0b013e3181cdc4ae
134. Yu Y, Yang Y, Bian Y, Li Y, Liu L, Zhang H, et al. Hydrogen Gas Protects Against Intestinal Injury in Wild Type But Not NRF2 Knockout Mice With Severe Sepsis by Regulating HO-1 and HMGB1 Release. Shock (Augusta Ga) (2017) 48(3):364–70. doi: 10.1097/SHK.0000000000000856
135. Chen S, Chen H, Du Q, Shen J. Targeting Myeloperoxidase (MPO) Mediated Oxidative Stress and Inflammation for Reducing Brain Ischemia Injury: Potential Application of Natural Compounds. Front Physiol (2020) 11:433. doi: 10.3389/fphys.2020.00433
136. Chen S, Dai G, Hu J, Rong A, Lv J, Su L, et al. Discovery of Xuebijing Injection Exhibiting Protective Efficacy on Sepsis by Inhibiting the Expression of HMGB1 in Septic Rat Model Designed by Cecal Ligation and Puncture. Am J Ther (2016) 23(6):e1819–e25. doi: 10.1097/MJT.0000000000000296
137. Wang Q, Wu X, Tong X, Zhang Z, Xu B, Zhou W. Xuebijing Ameliorates Sepsis-Induced Lung Injury by Downregulating HMGB1 and RAGE Expressions in Mice. Evidence-Based Complement Altern Med eCAM (2015) 2015:860259. doi: 10.1155/2015/860259
138. Meng C, Qian Y, Zhang WH, Liu Y, Song XC, Liu H, et al. A Retrospective Study of Ulinastatin for the Treatment of Severe Sepsis. Medicine (2020) 99(49):e23361. doi: 10.1097/MD.0000000000023361
139. Zhao F, Fang Y, Deng S, Li X, Zhou Y, Gong Y, et al. Glycyrrhizin Protects Rats From Sepsis by Blocking HMGB1 Signaling. BioMed Res Int (2017) 2017:9719647. doi: 10.1155/2017/9719647
140. Li ZL, Gao M, Yang MS, Xiao XF, Liu JJ, Yang BC. Sesamin Attenuates Intestinal Injury in Sepsis via the HMGB1/TLR4/IL-33 Signalling Pathway. Pharm Biol (2020) 58(1):898–904. doi: 10.1080/13880209.2020.1787469
141. Cui W, Hu G, Peng J, Mu L, Liu J, Qiao L. Quercetin Exerted Protective Effects in a Rat Model of Sepsis via Inhibition of Reactive Oxygen Species (ROS) and Downregulation of High Mobility Group Box 1 (HMGB1) Protein Expression. Med Sci Monit (2019) 25:5795–800. doi: 10.12659/MSM.916044
142. Wang H, Li W, Li J, Rendon-Mitchell B, Ochani M, Ashok M, et al. The Aqueous Extract of a Popular Herbal Nutrient Supplement, Angelica Sinensis, Protects Mice Against Lethal Endotoxemia and Sepsis. J Nutr (2006) 136(2):360–5. doi: 10.1093/jn/136.2.360
143. Lee W, Lee H, Lee T, Park EK, Bae JS. Inhibitory Functions of Maslinic Acid, a Natural Triterpene, on HMGB1-Mediated Septic Responses. Phytomedicine (2020) 69:153200. doi: 10.1016/j.phymed.2020.153200
144. Chen HM, Liou SF, Hsu JH, Chen TJ, Cheng TL, Chiu CC, et al. Baicalein Inhibits HMGB1 Release and MMP-2/-9 Expression in Lipopolysaccharide-Induced Cardiac Hypertrophy. Am J Chin Med (2014) 42(4):785–97. doi: 10.1142/S0192415X14500505
145. Yang S, Lee W, Lee BS, Lee C, Park EK, Ku SK, et al. Aloin Reduces HMGB1-Mediated Septic Responses and Improves Survival in Septic Mice by Activation of the SIRT1 and PI3K/Nrf2/HO-1 Signaling Axis. Am J Chin Med (2019) 47(3):613–33. doi: 10.1142/S0192415X19500320
146. Lee W, Ku SK, Bae JS. Zingerone Reduces HMGB1-Mediated Septic Responses and Improves Survival in Septic Mice. Toxicol Appl Pharmacol (2017) 329:202–11. doi: 10.1016/j.taap.2017.06.006
147. Seo KH, Choi JW, Jung HS, Yoo H, Joo JD. The Effects of Remifentanil on Expression of High Mobility Group Box 1 in Septic Rats. J Korean Med Sci (2017) 32(3):542–51. doi: 10.3346/jkms.2017.32.3.542
148. Tan Y, Wang Q, She Y, Bi X, Zhao B. Ketamine Reduces LPS-Induced HMGB1 via Activation of the Nrf2/HO-1 Pathway and NF-κb Suppression. J Trauma Acute Care Surg (2015) 78(4):784–92. doi: 10.1097/TA.0000000000000588
149. Tsoyi K, Jang HJ, Nizamutdinova IT, Kim YM, Lee YS, Kim HJ, et al. Metformin Inhibits HMGB1 Release in LPS-Treated RAW 264.7 cells and increases survival rate of endotoxaemic mice. Br J Pharmacol (2011) 162(7):1498–508. doi: 10.1111/j.1476-5381.2010.01126.x
150. Kim J, Choo S, Sim H, Baek MC, Bae JS. Biapenem Reduces Sepsis Mortality via Barrier Protective Pathways Against HMGB1-Mediated Septic Responses. Pharmacol Rep PR (2021) 73(3):786–95. doi: 10.1007/s43440-020-00212-0
151. Chang KC. Cilostazol Inhibits HMGB1 Release in LPS-Activated RAW 264.7 Cells and Increases the Survival of Septic Mice. Thromb Res (2015) 136(2):456–64. doi: 10.1016/j.thromres.2015.06.017
152. Hagiwara S, Iwasaka H, Hasegawa A, Asai N, Noguchi T. High-Dose Intravenous Immunoglobulin G Improves Systemic Inflammation in a Rat Model of CLP-Induced Sepsis. Intensive Care Med (2008) 34(10):1812–9. doi: 10.1007/s00134-008-1161-1
153. Liu FL, Chen TL, Chen RM. Mechanisms of Ketamine-Induced Immunosuppression. Acta Anaesthesiol Taiwanica (2012) 50(4):172–7. doi: 10.1016/j.aat.2012.12.001
154. Li K, Yang J, Han X. Ketamine Attenuates Sepsis-Induced Acute Lung Injury via Regulation of HMGB1-RAGE Pathways. Int Immunopharmacol (2016) 34:114–28. doi: 10.1016/j.intimp.2016.01.021
155. Foretz M, Guigas B, Viollet B. Understanding the Glucoregulatory Mechanisms of Metformin in Type 2 Diabetes Mellitus. Nat Rev Endocrinol (2019) 15(10):569–89. doi: 10.1038/s41574-019-0242-2
156. Jiang J, Chen Q, Chen X, Li J, Li S, Yang B. Magnesium Sulfate Ameliorates Sepsis-Induced Diaphragm Dysfunction in Rats via Inhibiting HMGB1/TLR4/NF-κb Pathway. Neuroreport (2020) 31(12):902–8. doi: 10.1097/WNR.0000000000001478
157. Yang M, Cao L, Xie M, Yu Y, Kang R, Yang L, et al. Chloroquine Inhibits HMGB1 Inflammatory Signaling and Protects Mice From Lethal Sepsis. Biochem Pharmacol (2013) 86(3):410–8. doi: 10.1016/j.bcp.2013.05.013
158. Angus DC, Birmingham MC, Balk RA, Scannon PJ, Collins D, Kruse JA, et al. E5 Murine Monoclonal Antiendotoxin Antibody in Gram-Negative Sepsis: A Randomized Controlled Trial. E5 Study Investigators. Jama (2000) 283(13):1723–30. doi: 10.1001/jama.283.13.1723
159. Qiu P, Cui X, Sun J, Welsh J, Natanson C, Eichacker PQ. Antitumor Necrosis Factor Therapy is Associated With Improved Survival in Clinical Sepsis Trials: A Meta-Analysis. Crit Care Med (2013) 41(10):2419–29. doi: 10.1097/CCM.0b013e3182982add
160. Yang ZY, Ling Y, Yin T, Tao J, Xiong JX, Wu HS, et al. Delayed Ethyl Pyruvate Therapy Attenuates Experimental Severe Acute Pancreatitis via Reduced Serum High Mobility Group Box 1 Levels in Rats. World J Gastroenterol (2008) 14(28):4546–50. doi: 10.3748/wjg.14.4546
161. Yang R, Tenhunen J, Tonnessen TI. HMGB1 and Histones Play a Significant Role in Inducing Systemic Inflammation and Multiple Organ Dysfunctions in Severe Acute Pancreatitis. Int J Inflam (2017) 2017:1817564. doi: 10.1155/2017/1817564
162. Scaffidi P, Misteli T, Bianchi ME. Release of Chromatin Protein HMGB1 by Necrotic Cells Triggers Inflammation. Nature (2002) 418(6894):191–5. doi: 10.1038/nature00858
163. Ni J, Zhao Y, Su J, Liu Z, Fang S, Li L, et al. Toddalolactone Protects Lipopolysaccharide-Induced Sepsis and Attenuates Lipopolysaccharide-Induced Inflammatory Response by Modulating HMGB1-NF-κb Translocation. Front Pharmacol (2020) 11:109. doi: 10.3389/fphar.2020.00109
164. Stevens NE, Chapman MJ, Fraser CK, Kuchel TR, Hayball JD, Diener KR. Therapeutic Targeting of HMGB1 During Experimental Sepsis Modulates the Inflammatory Cytokine Profile to One Associated With Improved Clinical Outcomes. Sci Rep (2017) 7(1):5850. doi: 10.1038/s41598-017-06205-z
165. Lai PF, Cheng CF, Lin H, Tseng TL, Chen HH, Chen SH. ATF3 Protects Against LPS-Induced Inflammation in Mice via Inhibiting HMGB1 Expression. Evidence-Based Complement Altern Med eCAM (2013) 2013:716481. doi: 10.1155/2013/716481
166. Tang D, Wang H, Billiar TR, Kroemer G, Kang R. Emerging Mechanisms of Immunocoagulation in Sepsis and Septic Shock. Trends Immunol (2021) 42(6):508–22. doi: 10.1016/j.it.2021.04.001
167. Yang X, Cheng X, Tang Y, Qiu X, Wang Z, Fu G, et al. The Role of Type 1 Interferons in Coagulation Induced by Gram-Negative Bacteria. Blood (2020) 135(14):1087–100. doi: 10.1182/blood.2019002282
168. Huang LF, Yao YM, Sheng ZY. Novel Insights for High Mobility Group Box 1 Protein-Mediated Cellular Immune Response in Sepsis: A Systemic Review. World J Emergency Med (2012) 3(3):165–71. doi: 10.5847/wjem.j.issn.1920-8642.2012.03.001
169. Gauley J, Pisetsky DS. The Translocation of HMGB1 During Cell Activation and Cell Death. Autoimmunity (2009) 42(4):299–301. doi: 10.1080/08916930902831522
170. Martinotti S, Patrone M, Ranzato E. Emerging Roles for HMGB1 Protein in Immunity, Inflammation, and Cancer. ImmunoTargets Ther (2015) 4:101–9. doi: 10.2147/ITT.S58064
171. Bianchi ME, Manfredi AA. High-Mobility Group Box 1 (HMGB1) Protein at the Crossroads Between Innate and Adaptive Immunity. Immunol Rev (2007) 220:35–46. doi: 10.1111/j.1600-065X.2007.00574.x
172. Ye C, Choi JG, Abraham S, Wu H, Diaz D, Terreros D, et al. Human Macrophage and Dendritic Cell-Specific Silencing of High-Mobility Group Protein B1 Ameliorates Sepsis in a Humanized Mouse Model. Proc Natl Acad Sci U.S.A. (2012) 109(51):21052–7. doi: 10.1073/pnas.1216195109
173. Ren C, Yao RQ, Wang LX, Li JC, Chen KW, Wu Y, et al. Antagonism of Cerebral High Mobility Group Box 1 Ameliorates Dendritic Cell Dysfunction in Sepsis. Front Pharmacol (2021) 12:665579. doi: 10.3389/fphar.2021.665579
174. Ren C, Li XH, Wu Y, Dong N, Tong YL, Yao YM. Inhibition of Cerebral High-Mobility Group Box 1 Protein Attenuates Multiple Organ Damage and Improves T Cell-Mediated Immunity in Septic Rats. Mediators Inflam (2019) 2019:6197084. doi: 10.1155/2019/6197084
175. Wang XW, Karki A, Zhao XJ, Xiang XY, Lu ZQ. High Plasma Levels of High Mobility Group Box 1 Is Associated With the Risk of Sepsis in Severe Blunt Chest Trauma Patients: A Prospective Cohort Study. J Cardiothorac Surg (2014) 9:133. doi: 10.1186/s13019-014-0133-5
176. Xiu G, Sun J, Li X, Jin H, Zhu Y, Zhou X, et al. The Role of HMGB1 in BMSC Transplantation for Treating MODS in Rats. Cell Tissue Res (2018) 373(2):395–406. doi: 10.1007/s00441-018-2823-0
177. Lee K, Chang Y, Song K, Park YY, Huh JW, Hong SB, et al. Associations Between Single Nucleotide Polymorphisms of High Mobility Group Box 1 Protein and Clinical Outcomes in Korean Sepsis Patients. Yonsei Med J (2016) 57(1):111–7. doi: 10.3349/ymj.2016.57.1.111
178. Kornblit B, Munthe-Fog L, Petersen SL, Madsen HO, Vindeløv L, Garred P. The Genetic Variation of the Human HMGB1 Gene. Tissue Antigens (2007) 70(2):151–6. doi: 10.1111/j.1399-0039.2007.00854.x
Keywords: high mobility group, protein, sepsis, non-coding RNAs, programmed cell death, drugs
Citation: Liang G and He Z (2022) High Mobility Group Proteins in Sepsis. Front. Immunol. 13:911152. doi: 10.3389/fimmu.2022.911152
Received: 02 April 2022; Accepted: 05 May 2022;
Published: 02 June 2022.
Edited by:
Guo-Chang Fan, University of Cincinnati, United StatesReviewed by:
Lu Wang, Renmin Hospital of Wuhan University, ChinaPeter A. Ward, University of Michigan, United States
Copyright © 2022 Liang and He. This is an open-access article distributed under the terms of the Creative Commons Attribution License (CC BY). The use, distribution or reproduction in other forums is permitted, provided the original author(s) and the copyright owner(s) are credited and that the original publication in this journal is cited, in accordance with accepted academic practice. No use, distribution or reproduction is permitted which does not comply with these terms.
*Correspondence: Zhihui He, aHpoNzAzQGNzdS5lZHUuY24=