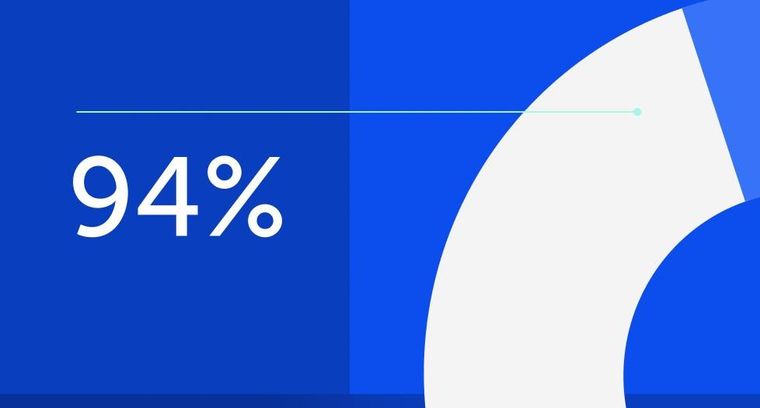
94% of researchers rate our articles as excellent or good
Learn more about the work of our research integrity team to safeguard the quality of each article we publish.
Find out more
REVIEW article
Front. Immunol., 30 June 2022
Sec. Microbial Immunology
Volume 13 - 2022 | https://doi.org/10.3389/fimmu.2022.899992
This article is part of the Research TopicFrom microbial immunology to microbial therapeutics via targeted delivery technologyView all 8 articles
Pathogenic bacterial infections are the second highest cause of death worldwide and bring severe challenges to public healthcare. Antibiotic resistance makes it urgent to explore new antibacterial therapy. As an essential metal element in both humans and bacteria, zinc ions have various physiological and biochemical functions. They can stabilize the folded conformation of metalloproteins and participate in critical biochemical reactions, including DNA replication, transcription, translation, and signal transduction. Therefore, zinc deficiency would impair bacterial activity and inhibit the growth of bacteria. Interestingly, excess zinc ions also could cause oxidative stress to damage DNA, proteins, and lipids by inhibiting the function of respiratory enzymes to promote the formation of free radicals. Such dual characteristics endow zinc ions with unparalleled advantages in the direction of antibacterial therapy. Based on the fascinating features of zinc ions, nanomaterial-based zinc ion interference therapy emerges relying on the outstanding benefits of nanomaterials. Zinc ion interference therapy is divided into two classes: zinc overloading and zinc deprivation. In this review, we summarized the recent innovative zinc ion interference strategy for the treatment of bacterial infections and focused on analyzing the antibacterial mechanism of zinc overloading and zinc deprivation. Finally, we discuss the current limitations of zinc ion interference antibacterial therapy and put forward problems of clinical translation for zinc ion interference antibacterial therapy.
Bacterial infections, especially multidrug-resistant (MDR) bacteria, are considered a major threat to global healthcare due to the emergence and rapid spread of antibiotic resistance (1–3). According to the WHO in 2019, antimicrobial resistance (AMR) had been declared a top 10 global health threat. It is predicted that around 10 million annual deaths are expected by 2050 if the situation is not improved (4). Infection by these bacteria could cause many types of serious diseases such as pneumonia and sepsis. The bacteria could invade the human with the help of the motility of bacterial flagella and trigger a severe cytokine-mediated immune response (5–7). This severe immune response could damage the host’s normal organs, causing organ dysfunction (8). Moreover, some important virulence factors released by bacteria could also cause a series of toxicities (9, 10). With the aggravation of local bacterial infection, systemic sepsis is developed, which is defined as the host inflammatory response to severe, life-threatening infection (11–13). During sepsis, the release of pathogen-associated molecular patterns (PAMPs) and damage-associated molecular patterns (DAMPs) perpetuates systemic inflammation, a collapse of cardiovascular function, multiple organ dysfunction, and septic shock. Despite advanced supportive care, the mortality rates in severe sepsis and septic shock reach up to 40% (14, 15). Currently, the clinical treatment options for MDR bacteria are extremely limited, and it is difficult to develop novel antibiotics. Therefore, it is urgently needed to treat the infection caused by this bacterium.
Many metal ions (16, 17) have been reported to possess a broad spectrum of antibacterial activity. Silver and copper metals have been used as antibacterial agents for thousands of years. Due to the unique physical and chemical properties of nanomaterials, metal-based nanoparticles (NPs), such as silver NPs, and copper NPs, have emerged as promising options in the antibacterial field (16, 18, 19). Many studies have confirmed that the antibacterial effect of these metals silver and copper is mainly due to the ions produced (20). The antibacterial mechanisms of silver and copper ions are involved in reactive oxygen species (ROS) production, membrane damage, and destruction of ATP synthesis (21). In this framework, silver- and copper-based nano-antibacterial therapy has been widely studied by researchers and used in cosmetics, medical devices coating, and other fields (18). However, antibacterial therapies based on heavy metal NPs also have some limitations. I) Silver and copper NPs could induce cell membrane perforation, ROS damage, and liver dysfunction (18). II) These heavy metal ions would accumulate in mammalian cells, causing serious toxicity that impairs the physiology of the normal cell. III) Recently, some studies (22) have shown that bacteria are becoming resistant to silver ions, which means that the antibacterial potency of silver ions/silver NPs is dropping. For TiO2 NPs, it generally acts as a photocatalytic agent (23, 24) to enhance the antibacterial efficacy. The penetration depth of UV-light or near-IR (NIR) still severely limits the application of the TiO2-based nanosystems. Therefore, a new strategy needs to be developed to fight against bacteria.
Competition of transition metal ions between host and pathogen is a key battleground for infectious diseases (25). The result of the competition could determine whether the infection is successfully established. As one of the most important essential metals in the human body, Zn plays a key role in many signal transduction processes of organisms and acts as a key structural cofactor in the biological functions of many proteins (26–29). Firstly, Zn(II) is a cofactor of many bacterial proteins; for example, New Delhi metallo-β-lactamase 1 (NDM-1) (30), a β-lactamase with double Zn(II) as its active center, activates nucleophilic water molecule to destroy the β-lactam ring of antibiotics (31). Then, Zn(II) is related to bacterial transcriptional regulation and the maintenance of gene stability by different paths. It could also stabilize the structure of chromatin and affect the replication of DNA (32). Zinc-sensitive modulators are used to alter gene expression in response to changes in intracellular zinc (33). Zn(II) is also responsible for the virulence of bacteria by the zinc uptake regulator. For example, ZnuABC (a zinc uptake system) plays a critical role in facilitating bacterial adhesion to epithelial cells (25, 34). Therefore, bacterial intracellular zinc imbalance would result in the dysfunction of a range of functional proteins (35), organism metabolic disorders (36), and transcription imbalance (33). For example, small molecule inhibitors such as disodium ethylenediaminetetraacetate (EDTA) (37) and aspergillomarasmine A (AMA) (38) could chelate zinc ions in bacteria, resulting in the inactivation of NDM-1. Interestingly, excess Zn(II) naturally possesses superior antibacterial activity. Bacteria intracellular accumulation of Zn(II) would interact with the thiol group of bacterial respiratory enzymes and result in the inhibition of their function. This inactivation of respiratory enzymes causes a mismatch during the electron transfer process in the bacterial respiratory chain, resulting in the production of intracellular ROS (39, 40). The intracellular ROS would irreversibly induce bacterial DNA damage and induce bacterial membranes lipid peroxidation, leading to bacterial death (39). Moreover, compared to heavy ions/NPs, zinc is safer for normal cells. Zinc is a mineral element essential to humans (41) and exhibits strong antibacterial activity even when administered in small doses. Zinc-based nanomaterials are easy to decompose, so they could be better metabolized from the body. Those degradable nanomaterials radically reduce the inherent toxicity of nanomaterials. Moreover, zinc ions are versatile. They could not only exert an excellent antibacterial function but also act as nutrients or stimulus signals for damaged tissues. In wound and osteomyelitis mouse models, Zn(II) accelerates wound healing and improves osteogenic differentiation of bone marrow mesenchymal stem cells (42). Therefore, with the ever-developing MDR bacteria and the slow pace of development of efficient antibiotics, Zn(II) interference therapy might offer an alternative option to combat bacterial infection. Based on the dual characteristics of zinc ion, zinc ion interference therapy could be divided into two strategies: zinc overloading and zinc deprivation. For the first, the Zn(II) overloading strategy could increase locally zinc ion concentration and achieve excellent antibacterial effects (Figure 1A). The Zn(II) deprivation strategy is used to break the zinc homeostasis by chelating the bacterial microenvironment or intracellular Zn(II) to kill bacterial species (Figure 1B).
Figure 1 Schematic diagram of the antibacterial mechanism of zinc ion interference therapy. (A) Zinc ion overloading strategy. Zinc-loaded nanodelivery systems release large amounts of zinc ions at the site of bacterial infection. The excess zinc ions produce the following antibacterial effects: I) interaction with the thiol group of bacterial respiratory enzymes resulting in the production of intracellular ROS, thus damaging bacterial DNA: II) interference with bacterial transcriptional regulation; III) inactivation of functional proteins leading to metabolic dysfunction of bacteria. (B) Zinc ion deprivation strategy. Zinc chelating agents deprive intracellular zinc ions, resulting in the imbalance of zinc ion homeostasis. The lack of zinc ions could lead to the inactivation of Zn2+-dependent enzymes, affecting the structure of chromatin and inhibiting the replication of DNA, thus causing bacterial death.
In the past decades, nanoscience and nanotechnology have flourished with outstanding progress (43, 44). Various antibacterial nanomaterials, including liposomes (45, 46), carbon-based nanomaterials (47, 48), noble metal NPs (49, 50), semiconductor NPs (51), and polymeric nanomaterials (52), are being developed as alternative therapeutic options to combat against bacterial infections. These nanomaterials not only could exert a good antibacterial activity by themselves (53) but also are loaded with antibiotics (54) or antimicrobial peptides (55). After further modification (56, 57), they could achieve targeted drug delivery to exert a synergistic antibacterial effect. In the post-antibiotic era, nanomaterial-based antimicrobial therapy is a promising antibacterial strategy.
Recently, owing to zinc-based antibacterial materials with a large surface/volume ratio (58, 59), pH-responsive release nanostructure (60), and good biocompatibility, nanomaterial-based zinc ion interference therapy against bacterial infection has attracted much attention and is widely used in various fields (61–63). It could be designed as targeting, environmentally responsive antibacterial delivery systems. It could also serve as a platform for integrating multiple modes of antimicrobial action against pathogens. Here, we review the recent advances in antibacterial research of zinc ion interference therapy and focus on its main physical and chemical properties and various mechanisms of antibacterial action. Finally, we discuss the challenges and prospects of utilizing zinc ion interference therapy in practical antibacterial applications.
More and more studies have shown that the bactericidal mechanism of Zn(II) involves the inactivation of many functional proteins and the production of ROS (36). The excess Zn(II) will bind the active-site thiols to inhibit the activity of functional proteins (35, 39). Moreover, the excess Zn(II) induces the production of large amounts of ROS in bacterial cells via interacting with the thiol group of bacterial respiratory enzymes (39). The ROS mediated by Zn(II) could damage cellular macromolecules and contribute to protein oxidation, lipid peroxidation, and DNA damage (64). Considering the excellent antibacterial property of Zn(II), the Zn(II) overloading strategy by delivering local excess of Zn(II) to fight against bacterial infections has attracted increasing attention from researchers, and numerous Zn(II)-based nanodelivery systems were developed to combat bacterial infections (65, 66). However, certain challenges still limit their application and therapeutic efficacy. Therefore, in the following, we will discuss the limitations of the Zn(II) overloading strategy and how to overcome the challenges.
Metal-organic framework (MOF) materials are a kind of coordination polymers developed rapidly in the past two decades. They are crystalline porous materials with periodic network structures formed by the self-assembly of transition metal ions and organic ligands (67, 68). They have high porosity, low density, large specific surface area, regular pore size, and diversified topological structure. They are a new type of porous material and have been widely used in catalysis (69, 70), energy storage (71), and biomedicine (72). Among the MOFs, the zinc-dimethylimidazole MOF-8 (ZIF-8) is made up of Zn(II) and dimethylimidazole and is one of the most widely studied MOFs. Given the simple synthesis method (72), high porosity (73), and pH-triggered structural collapse (60, 74), ZIF-8 has been widely used as a pH-responsive nanodelivery system for antibacterial applications, as shown in Table 1. Meanwhile, ZIF-8 could absorb a great deal of Zn(II) in the cavities and be an ideal candidate for Zn(II)-overloading therapy. Wu et al. (78) reported a biodegradable BSA@ZIF-8 for simultaneously ablating tumors and inhibiting infection. As a Zn(II) reservoir, BSA@ZIF-8 could release plenty of Zn(II) for antibacterial therapy. The excess Zn(II) could disrupt the metabolic activity of bacteria. Moreover, a Mn-doped ZIF-8 (79) was prepared to simultaneously achieve a favorable antibacterial effect and regulation of inflammatory response (Figure 2). Therefore, all of the above articles demonstrate the excellent antibacterial efficiency attributed to Zn(II) overloading therapy.
Figure 2 Schematic illustration of Mn-ZIF-8 nanocomposite for antibacterial effects on infected wound model by eliminating bacteria (79). Copyright 2021, WILEY.
Although pH-triggered structural collapse is considered a manner of controlled drug release, uncontrolled drug and Zn(II) leaks also plague researchers. Therefore, how to weaken the potential toxicity of the prematurely released Zn(II) and drugs to mammalian cells or normal organs is in the spotlight. To improve drug release behaviors and prevent the drug and Zn(II) leakage in the physiological environment, various biocompatible macromolecules have been modified on the surface of ZIF-8. Tu et al. (90) defined a unique Zn(II) releasing behavior to avoid the cytotoxicity of the prematurely released Zn(II) by constructing core-shell ZIF-8@PDA NPs. polydopamine (PDA) (91, 92), as a good biocompatible biopolymer, has a strong coordination effect on metal ions to limit the leakage of Zn(II). With the help of the bioadhesion of PDA coating to the bacterial surface, Zn(II) burst releasing was performed in a pH-dependent manner. Such a controlled Zn(II) releasing manner presented a promising prospect for antibacterial applications, but there is a distinct limitation, which is the lack of animal experimental support. A series of animal experiments need to be conducted to prove that this unique release behavior is feasible in vivo. Moreover, the targeting ability of ZIF-8 is still too weak to be applied. To achieve the precise target of pathogenic intracellular bacteria, hyaluronic acid (HA) as an anionic chain glycosaminoglycan (93) was reported to enhance targeting ability (94). Therefore, Zhang et al. (76) reported a pH-responsive MOF/antibiotic synergistic three-in-one nanocarrier tetracycline (Tet)@ZIF-8@HA (TZH). The ZIF-8 was functionalized by using the coordination between the carboxyl group of tetracycline to obtain TZH for the treatment of intracellular infections. In this work, the TZH is endocytosed by cells with the help of the HA-mediated pathway. Under acidic conditions, TZH releases Zn(II) and antibiotics, which synergistically inhibit intracellular bacterial infections.
Gastritis caused by Helicobacter pylori is a very serious problem that might lead to gastric cancer (95, 96). Due to the gastric acid microenvironment and the unique physiological state of the gastric inflammation site, Zhang et al. (75) developed a pH-responsive Zn(II) MOF hydrogen-generation system (Pd(H)@ZIF-8@AP). The Pd(H)@ZIF-8@AP was further coated by negatively charged ascorbyl palmitate (AP) hydrogel for targeting the H. pylori infection site (Figure 3A). The coating of Pd(H)@ZIF-8@AP endows inflammation-targeting ability by electrostatic adsorption with positively charged matrix metalloproteinase (MMP; IV collagenase) (97) (Figure 3B). Moreover, the AP ensures the release of Pd(H)@ZIF-8 in situ and prevents the leakage of Zn(II). The HA and AP coating endow the ZIF-8 with good biocompatibility and enhance targeting ability in vivo. They have great potential to be developed as effective, safe, and precise coatings for antibacterial applications.
Figure 3 Helicobacter pylori treatment. (A, B) Schematic diagram showing the process of H pylori and restoring impaired gastric mucosa (75). Copyright 2021, WILEY-VCH.
In addition to pH-responsive ZIF-8, it is also designed as a light-triggered controllable nanoplatform. NIR as a trigger could collapse the nanostructure of ZIF-8 by photothermal effect and release Zn(II) to effectively kill bacteria. The light-triggered Zn(II) release manner has many advantages including high spatial resolution, fast effect, and controllability. This mode of release behavior has better specificity and is used for site-specific drug delivery. Yang et al. (83) designed a light-triggered ZIF-8-based chemical photothermal composite ICG@ZIF-8/PDA/Ag. The composite was constructed by encapsulating the photothermal agent (PTT) ICG inside and by decorating the surface with a PDA shell and Ag. Then, it could generate Ag ions in situ for a fast, long-term, and efficient antibacterial system. Local high temperature and metal ion release induced by NIR light cause damage to the bacteria. The antibacterial therapeutic effect was enhanced by the photothermal effect and the damage mediated by the release of excess Ag(I) and Zn(II). Wang et al. (98) proposed a combination therapy of Zn-doped MoS2 nanosheets and ZIF-8 (ZnDMZ) for photocatalytic sterilization. Under 660-nm light radiation, the ZnDMZ presented antibacterial efficacy of 99.9% against Staphylococcus aureus due to the synergy of photocatalytic effect, photothermal effect, and the released Zn(II). The pH-responsive and light-triggered ZIF-8 has been proved to improve drug release curves both in vitro and in vivo to varying degrees. To further improve Zn(II) release and enhance the therapeutic effects of Zn(II), dual stimulus-responsive nanodelivery systems (99) have been aggressively explored. The pH-responsive (75) and light-triggered ZIF-8 could accomplish enhanced drug release in spatial control and/or temporal control manner. It could respond to two physical stimuli to trigger drug release at the exact spot. It also provides many advantages including unprecedented control over drug delivery, sequential drug release manner, and excellent antibacterial potency. Song et al. (87) reported a pH-responsive and light-triggered MOF for antibacterial therapy, designated as o-NBA@ZIF-8 (Figure 4). The o-NBA@ZIF-8 is made up of “light-triggered” o-NBA (a pH-jump reagent), ZIF-8, and antibacterial agent rifampicin (RFP). The o-NBA could serve as a blocking cap to control the opening/closing of pore and a light-responsive functional component that produces acid in situ under the UV-light (365 nm) treatment. The acid produced by light stimulation leads to the degradation of ZIF-8 and the controlled release of antibiotics and Zn(II).
Figure 4 (A) Transmission electron microscopy (TEM) characterization. (B) X-ray diffraction (XRD). (C) Fourier transform IR (FT-IR). (D) (87). Copyright 2018, Wiley. Elemental mapping of RFP&o-NBA@ZIF-8.
The sequentially responsive ZIF-8 showed a synergistic antibacterial effect against methicillin-resistant S. aureus (MRSA) attributed to the release of large amounts of Zn(II) and antibiotics. This cascade reaction triggers the release of Zn(II), which is innovative and provides a new perspective on the transformation of ZIF-8 nanosystems. Moreover, Su et al. (88) designed a NIR/pH dual stimulus-responsive nanoplatform Van@ZIF-8@PDA based on an imidazolate zeolite framework for synergistic photothermal/pharmacological antimicrobial therapy. In the article, Van@ZIF-8@PDA was modified with PDA to achieve high NIR photothermal conversion performance. The PDA coating improves its biocompatibility, dispersibility, and NP stability. The Van@ZIF-8@PDA released the encapsulated drugs through NIR triggering and pH-responsive manner, which could significantly reduce the off-target toxicity of drugs. The platform exhibited superior antibacterial ability and significantly reduced the survival rates of both Mu50 and Escherichia coli through the combined effect of local photothermal damage, antibiotic membrane disruption, and Zn(II) overloading. As a result, the dual stimulus-responsive ZIF-8 nanoplatform provides a paradigm for optimizing the Zn(II) release curves via NIR triggering and pH-responsive mechanisms. Moreover, it is of high significance for enhancing the therapeutic efficacy and reducing side effects. This dual stimulus-responsive ZIF-8 nanoplatform as potential biomedicine with great biocidal property suggests great potential for treating drug-resistant bacterial infection.
As a widely used porous nanostructure, ZIF-8 has a homogeneous size and controllable pores (73, 74). Therefore, a variety of coatings could be modified to improve antibacterial efficiency. Different coatings endow ZIF-8 with different properties to overcome the challenges. With the development of polymer material science, the continuous innovation of these polymer coatings provides the possibility for the further development of ZIF-8. They could improve Zn(II) release curves, prevent the leak of Zn(II), and achieve the controlled release of Zn(II). However, the lack of active targeting remains an obstacle to its effective and precise drug delivery. For example, the collapse of ZIF-8 in the non-bacterial inflammatory sites would lead to the release of Zn(II) and drugs and severe off-target toxicity. In consequence, specifically targeting pathogens is necessary for improving therapeutic effectiveness. Next, we should focus on developing ZIF-8-based nanosystems with specific bacteria-targeting capabilities. Some materials that specifically target bacteria could be incorporated into ZIF-8 nanoplatform such as maltohexaose (100) and aptamer (101).
Bulk ZnO is recognized as a safe material (39) by the Food and Drug Administration (FDA) and could be biodegraded in the body (102). Nano-zinc oxide was explored by many researchers, as a form of zinc-based nanomaterials, and showed remarkable antibacterial activity in a variety of bacterial species (58, 103–105). Therefore, it is widely used for medical instruments (106), oral health products, the food industry, and other antibacterial applications (Table 2).
The antibacterial mechanism of ZnO NPs involves direct contact with the cell wall, destroying bacterial cell integrity (107), the release of antibacterial abundant Zn(II), and ROS formation (108). Previous studies (58, 103) reported that ZnO NPs directly interact with bacterial membrane structure leading to the injury and rupture of the membrane (111). The increased bacterial membrane permeability mediated by ZnO NPs could facilitate the bacterial internal accumulation of Zn(II) and amplify the antibacterial effect of Zn(II) overloading therapy. Wang et al. (112) prepared ZnO/graphene oxide composites by a simple one-pot reaction using ZnO and graphene oxide (GO) and showed excellent antibacterial properties. The synergistic effect of GO and ZnO NPs resulted in unprecedented antibacterial activity of the composites. GO contributed to the dispersion of ZnO NPs, slowed down the dissolution of ZnO, and brought E. coli into close contact with ZnO NPs and Zn(II). The close contact enhanced the permeability of the bacterial membrane and the local Zn(II) concentration, thus leading to bacterial death. ZnO has demonstrated superior antibacterial properties by combination action of disrupting their membrane structure and leading to local Zn(II) burst.
Another huge advantage of ZnO is the synergistic effect of releasing large amounts of Zn(II) and producing ROS against bacterial infections (39). For ZnO, large numbers of valence band holes and/or conduction band electrons were thought to be available to trigger redox reactions and ROS generation in cellular environments (121, 122). With the production of ROS, Zn(II) is also released from ZnO NPs. Lucas-Gil et al. (108) prepared a ZnO cluster with a porous spherical structure by a soft chemical method. The ZnO clusters exhibited higher antibacterial and antifungal activities and lower cytotoxicity as compared to commercial ZnO NPs. Chai et al. (123) developed a hybrid NP-containing silica nanorattles (SNs) and ZnO, which could inhibit the growth of MRSA. SNs could gather ZnO NPs to enhance the production of free radicals and Zn(II) owing to the mesoporous nanomaterials, thus shortening the effective touching distance between free radicals and MRSA. The ZnO@SN NPs exhibit a higher inhibition efficiency than free ZnO NPs and SNs against MRSA in vitro and in vivo. Quantum dots (QDs) have been a hot topic since they have been discovered. Because of their water solubility, photostability, and easy functionalization, they have aroused great interest in various fields. Moussa et al. (124) reported that ZnO@APTMS/Cu QDs generate high amounts of H2O2 •OH and O2•− radicals. The production of Zn(II) and ROS gives the ZnO@APTMS/Cu QDs multimode antibacterial activity.
In addition to ZnO NPs, a variety of ZnO-based nanomaterials have been explored and developed for antimicrobial therapy. The sophisticated management of nanomaterials is essential for the efficiency of the antibacterial activities of ZnO. In recent studies, researchers designed various ZnO nanomaterials by morphological modulation including ZnO nanoflowers (109), nanowires (NWs) (114), nanorod (106, 116), nanopillar (110), and nanoneedles (115). Interestingly, Kim et al. (115), inspired by the sea urchin acicular structure, reported the super-hydrophilic and bactericidal surface of ZnO nanoneedles grown from micron-sized polylactic acid (PLA) beads and fibers (Figure 5). In this paper, the authors mainly focused on the physical behavior of sea urchin-like zinc oxide. There are a few contents for exploring the antibacterial mechanism of Zn(II). Future efforts will focus on biomedical applications. Bains et al. (117) prepared IL@ZnO nanosheets via a hydrothermal method and further functionalized them with an eco-friendly ionic liquid (IL). The IL@ZnO exhibits excellent antibacterial activity against E. coli and S. aureus. More importantly, the authors demonstrated that IL@ZnO material could serve as a potential antimicrobial coating surface for cotton fabric by fabricating IL@ZnO onto the surface of cotton fabric. Therefore, it might be utilized as a biomaterial for biomedical applications, especially in medical masks, to reduce the risk of the spread of infectious diseases. Despite showing a superior antibacterial performance, the lack of safety evaluation in vivo makes ZnO with different morphologies difficult in clinical practice. Researchers should focus on exploring the metabolic kinetics distribution of ZnO with different morphologies in vivo to lay a foundation for further application.
Figure 5 The formation process of artificial micro-urchin with ZnO nano-spicules (115). Copyright 2021, Wiley.
Although ZnO NPs and ZnO-based nanomaterials demonstrate excellent multi-mode antibacterial properties, ZnO NPs and ZnO-based nanomaterials as metal-based NPs inevitably produce cytotoxicity and serious adverse reactions. How to avoid the toxicity of ZnO-based nanomaterials is an important problem. Fang et al. (114) designed a photothermal antimicrobial platform that combines PDA-BP NSs/ZnO NWs on the titanium (Ti) matrix of the implant to eliminate biofilm-related infections. In this platform, PDA is modified on the implant to form a surface-adhesive nanocoating. With the synergistic effect of BP NSs and PDA, the PTT antimicrobial ability was significantly enhanced and made bacteria more sensitive to Zn(II) undergoing the 808-nm irradiation. The photothermal effect also amplified Zn(II)-overloading therapy against S. aureus and E. coli infection. The NIR synergistic antimicrobial strategy has good biosafety and can effectively control bacterial infection without damaging normal tissues. Li et al. (116) reported the hybrid ZnO/PDA/arginine-glycine-aspartic acid-cysteine (RGDC) nanorod (ZnO/PDA/RGDC) arrays on titanium (Ti) implants (Figure 6). The coating of titanium (Ti) implants was composed of ZnO/PDA/RGDC nanorods and had superior antibacterial properties and enhanced the osteoinductivity ascribed to the bio-functionalization of ZnO/PDA/RGDC nanorods. PDA, as a stabilization agent in the fabrication of diverse organic−inorganic materials with specific functionalities, has an excellent ability to manage the release of Zn(II) and balance antibacterial activity and cytotoxicity. The PDA coating endows the nanostructure with good biocompatibility, reducing the toxicity and enhancing the antibacterial performance of Zn(II).
Figure 6 (A) Schematic illustration of the fabrication process of the hybrid ZnO/PDA/RGDC NR arrays. Scanning electron microscopy (SEM) images of (B) Ti-ZnOs (scale bars = 100 nm), (C) Ti-ZnO, and (D)Ti-ZnO/PDA (116). Copyright © 2017 American Chemical Society. (E) SEM images of Ti-ZnO/PDA/RGDC; (E1) cross-sectional image of Ti-ZnO/PDA/ RGDC; (E2) Elemental mapping of Ti-ZnO/PDA/RGDC [scale bars = 100 nm (inset figures = 1 μm)].
ZnO NPs and ZnO-based nanomaterials exhibit strong antibacterial effects even when administered in small amounts. However, the antibacterial activity of zinc oxide is affected by many factors. Firstly, the antibacterial efficiency of ZnO NPs increased with the decreasing particle size (125). ZnO NPs exhibit enhanced antibacterial properties than bulk ZnO with micron-scale at the same concentration. The smaller-size ZnO NPs showed better antibacterial activity. The enhanced bactericidal efficacy of ZnO NPs attributes to a higher specific surface area. The smaller ZnO NPs could release more Zn(II) and ROS and result in a better antibacterial effect.
Various morphologies of ZnO also significantly impact the toxicity of ZnO to bacteria. The flower-shaped ZnO has revealed higher biocidal activity against S. aureus and E. coli than the spherical and rod-shaped ZnO (126). Such shape-dependent antibacterial activity behavior was explained that ZnO nanostructure with different morphologies has different active facets, which may lead to improved antibacterial activity (63). Therefore, these features that affect the antibacterial performance of ZnO nanostructure by the size and shape of ZnO provide us with ideas to design smaller, multi-active surface oxidizing nanomaterials.
Bulk ZnO particles are generally considered safe by the FDA and are widely used in food additives, cosmetics, and other fields (63, 125). However, toxicity occurs when the size of ZnO is reduced to the nanostructure. ZnO NPs are toxic to many mammalian cells, such as human bronchial epithelial cells, human kidney cells, and human alveolar adenocarcinoma cells (127–129). Therefore, the ZnO NPs are limited to ointment, medical dressing, and other forms to treat local skin infections. To further expand the antibacterial application of ZnO nanomaterials, the toxicological effect of ZnO nanomaterials should be elucidated. Moreover, some biomimetic or engineered biomimetic coatings like erythrocyte membrane (130, 131) and platelet (132) for ZnO nanomaterials could be applied to improve the biosafety of ZnO nanomaterials.
Nanogels could form a dense network through intermolecular cross-linking (65), which is an ideal delivery material for metal ions or small molecule drugs (133). However, the major limitation of nanogels or hydrogels is uncontrollable drug release. In order to achieve the controllable release of Zn(II), Malzahn et al. (66) reported enclosed nanogels based on diisocyanate chemistry reaction for the shell-forming. The enclosed nanogels could control the release pattern and reduce the Zn(II) release rate. In this paper, a dextran (DexMa)-polyacrylamide (PAAm) nanogel was prepared by a microemulsion method. Then, the multiple methacrylate portions of the dextran side chain would react with PAAm and thus act as a cross-linking agent to form the shell of the nanogel. This cross-linked shell limits water penetration and impedes the expansion of the nanogels, thus increasing the retention of Zn(II) and prolonging the release of Zn(II) for achieving sustained antibacterial action. The nanogels were modified to retain the Zn(II) over a longer period and be better suited for biomedical applications. This strategy of preparing nanogel with shell solves the problem of uncontrollable behavior of Zn(II) and achieves slow and long-term release of Zn(II). More important, it provides a promising treatment for repeated long-term infection of wounds, especially for diabetics. However, in this article, the nanogels only demonstrate the antibacterial effect of Zn(II), Zn(II) release behaviors, and kinetics at the theoretical level. Further research is needed on pharmacodynamics and animal models. Moreover, Dong et al. (120) have developed a nanofiber with the controllable release of Zn(II). In this paper, cellulose acetate was used to produce nanofibers by electrostatic spinning. Proto-porphyrin IX (PPIX) photosensitizer was fixed to the surface of the nanofibers by covalent grafting, and then the RC-TeTA-PPIX-Zn was obtained by conjugate chelating Zn(II) with porphyrin ring. Under xenon lamp irradiation, photosensitizer produces singlet oxygen (1O2) primary bactericide and releases Zn(II). The presence of the Zn(II) enhanced intersystem crossing, leading to higher singlet oxygen yield and improving the bactericidal efficiency. However, this light-responsive controlled release behavior of Zn(II) is severely limited to clinical translation owing to xenon lamp radiation. The researchers need to further optimize the nanosystems for practicality and safety.
For zinc ion overloading therapy, different zinc-based nanodelivery systems offer different advantages depending on their composition and structure. Compared with ZnO nanomaterial, ZIF-8 has many advantages. I) ZIF-8 has a homogeneous size and controllable pores. The controllable pores and high porosity allow ZIF-8 to act as an ideal nanocarrier for drug delivery. ZIF-8 could encapsulate a large number of antibiotics and zinc ions to play a synergistic antibacterial effect. II) ZIF-8 could respond to the collapse of nanomaterials caused by low pH. This pH-responsive feature of ZIF-8 allows the controlled release of Zn(II) at the infection site and reduces off-target toxicity. III) ZIF-8 is safer than ZnO nanomaterials. The ZnO could accumulate on the surface of the cell membrane and induce cell damage due to the positively charged Zn2+. IV) ZIF-8 has been extensively studied and developed. Recently, it was developed to deliver drugs, microRNA, and gas to enhance the antibacterial effect. ZIF-8, which is loaded with gas or microRNA, is highly effective against bacteria in combination with zinc ion overloading therapy. However, the antibacterial mechanism of ZnO nanomaterial is involved in Zn(II) release, membrane dysfunction, generation of ROS, etc. The combination of multiple antimicrobial modes makes the ZnO nanomaterial more lethal. Nanogels have been stranded out in recent years as innovative nanodelivery systems for overcoming the limitations of conventional nanodelivery (134). Compared with ZnO nanomaterial and ZIF-8, the advantages of nanogels include lower drug leakage, biocompatibility, and degradability (135). The nanogels formed by cross-linking of polysaccharides have been demonstrated to have greater safety for in vivo applications. Moreover, as an antibacterial Zn(II) carrier for Zn(II), it is rarely explored. This means that the antibacterial therapy of nanogels loaded with Zn(II) has broad prospects. The future goal of the usage and development of nanogels loaded with Zn(II) would be with robust biocompatible features and programmed responses. Moreover, inspired by nanosponge, nanogels formed by cross-linking molecules, peptides, and polymers with strong metal ion chelating abilities also show promising prospects for the application of the Zn(II) deprivation strategy.
Zn(II) deprivation strategy is used to break the bacterial intracellular Zn(II) homeostasis through the action of Zn(II) chelators. When the bacterial intracellular Zn(II) homeostasis is out of balance, it would seriously interfere with the normal physiological and metabolic processes of bacteria. Therefore, Zn(II) deprivation strategy has emerged as a promising approach to effectively kill bacteria or reverse antibiotic resistance. Zn(II) chelation agents, such as N,N,N′,N′-tetraacetate (EDTA) (136), AMA (29, 137), and other small molecules (138, 139), are powerful tools to chelate Zn(II) in the bacterial infection microenvironment and cause Zn(II) metabolism disorder to achieve dysregulation of related functional proteins and cause bacterial death. However, those Zn(II) chelation agents also face several challenges. Here, we will discuss the applications of the Zn(II) deprivation strategy.
Due to the lack of pathogen targeting, small-molecule Zn(II) chelation would result in indiscriminate chelation of Zn(II) in mammals, which impairs the biological functions of normal cells. Moreover, as a dense and strong barrier, the outer membrane of Gram-negative bacteria prevents many drugs from entering the bacteria (140, 141). How to break through the outer membrane barrier is also a very challenging problem. Wu et al. (142) first found that polyamidoamine (PAMAM) could efficiently break through the outer membrane barrier and reverse bacterial resistance through Zn(II) deprivation strategy. As a metal β-lactamase with Zn(II) as the active site (31, 143), NDM can hydrolyze almost all clinically available antibiotics and be a key factor in antibiotic resistance. There is an urgent need to find ways to overcome NDM-induced drug resistance. Therefore, they constructed a platelet membrane nanovesicle containing PAMAM and meropenem. The nanovesicle could target bacterial infection sites and adhere to the surface of the pathogen via the inflammatory targeting properties of platelets (Figure 7). Owing to the acid environment of bacterial infection, the amino groups of PAMAM are protonated and present a strong positive charge density, which mediates the formation of nanoscale pores in platelet membrane nanovesicle and bacterial outer membrane. In the bacteria periplasm, PAMAM inhibits NDM enzyme activity by competitively chelating Zn(II) of NDM active sites, thus preventing meropenem from hydrolysis. It provides a new angle for the treatment of NDM-producing bacterial infections. This nanovesicle solves two challenges: targeting and breaking through the outer membrane barrier. More important, this Zn(II) deprivation nanovesicle effectively inactivates NDM-1, reverses the antibiotic resistance, and revives meropenem. The nanovesicle offers hope for the treatment of patients with clinical infection NDM-1 and presents a great possibility for clinical translation.
Figure 7 Schematic illustration of adjuvant-like biomimetic nanovesicles could target the bacterial infection microenvironment and reverse carbapenem resistance for NDM-producing bacterial infections (142). Copyright 2021, Elsevier B.V.
Macromolecular substances like calprotectin (an antimicrobial protein released by neutrophils) (144) and antimicrobial peptides (145) also perform an excellent ability to chelate Zn(II). Ma et al. (145) developed an antimicrobial peptide named thanatin, which could break through the bacterial outer membrane barrier and competitively displace the Zn(II) on NDM-1. The results suggest that thanatin has the property to damage the bacterial outer membrane and restore the susceptibility of NDM-1-producing pathogens to β-lactam antibiotics by depriving the NDM-1 enzyme active site of Zn(II). The unique antibacterial mechanism of thanatin provides a strategy for combating infection by NDM-1 pathogens. Some studies (144, 146) found that calprotectin has broad-spectrum antimicrobial activity against many microorganisms including bacteria and fungi pathogens. The antibacterial mechanism of calprotectin (147) involves competition with many transition metal elements to limit the availability of essential metal nutrients such as Ca(II), Zn(II), and Cu(II). Moreover, although various hydrogels (148, 149) and microparticles (150) have been used to chelate Zn(II) in the microenvironment, they were rarely used in the field of antibacterial infection. Sonamuthu et al. (151) reported an MMP-9-responsive dipeptide-tempted natural protein hydrogel for infected diabetic wound therapy. The author explored a facile multifunctional silk protein hydrogel matrix encapsulating bioactive curcumin and L-carnosine dipeptide. The L-carnosine dipeptide inactivated the MMP-9 via its strong chelation action of Zn(II) from the MMP-9 active center. The curcumin biomolecules showed energetic antimicrobial efficacy, wound healing, and tissue regeneration ability. The L-car@cur/SF hydrogel matrix could be an effective diabetic wound dressing material to reinstate the functions of the chronic wound site.
Compared with the discovery of novel antibiotics, the development of the zinc ions deprivation strategy to reverse antibiotic resistance is considered to be the most cost-effective option against MDR bacteria. Although the zinc ion deprivation strategy shows promising prospects, certain challenges still limit its clinical translation and commercial application. Zn(II) chelators always indiscriminately deprive Zn(II) of bacterial and mammalian cells in vivo, resulting in multiple impaired biological functions and high off-target toxicity. Moreover, due to the influence of the complex bacterial infection microenvironment, the protonation characteristics of the PAMAM mentioned above are interfered with, thus significantly reducing its Zn(II) deprivation efficiency and specificity. How to effectively and accurately chelate Zn(II) in bacteria is the key to fighting drug-resistant bacteria in vivo and pushing Zn(II) chelators to clinical application.
In this paper, we review the applications of Zn(II) interference therapy in bacterial infection. Zn(II) is one of the most important transition elements in the metabolic activities of living organisms. Zn(II) homeostasis plays a decisive role in bacterial colonization, proliferation, and even the occurrence, development, and spread of antibiotic resistance. Therefore, Zn(II) deprivation could effectively inactivate the enzymes that perform important physiological functions and result in bacterial metabolic dysfunction or failure of the bacterial resistance mechanism. For Zn(II) overloading strategy, the excess Zn(II) could also cause different forms of damage to bacteria, such as oxidative stress, and protein dysfunction. Given the antibacterial mechanisms, Zn(II) interference therapy could avoid the antibiotic resistance mechanism or reverse the antibiotic resistance and provide a powerful weapon to kill MDR bacteria. The Zn(II) interference therapy with the help of bioactive nanomaterials is expected to amplify the effect of Zn(II) therapy and overcome antibiotic resistance. Zn(II) interference therapy provides an alternative means for the treatment of MDR bacterial infections.
For Zn(II) overloading strategy, as a zinc-centered MOF, ZIF-8 has been developed as an antibacterial delivery system due to its unique acid response mechanism and porous structure. Although it exhibits good antibacterial action, many organic ligands produced by dissociation of ZIF-8 show inherent toxicity and low biocompatibility, limiting their widespread application. Therefore, biosafe MOF should be explored. Some biosafe ligands and nucleobases such as amino acids should be developed as novel ligands of MOF. Moreover, various forms of ZnO and complexes have been also developed for antibacterial applications for Zn(II) overloading strategy. The antibacterial mechanism of ZnO involves the production of intracellular ROS, the release of Zn(II), and mechanical damage to bacterial membranes. The antibacterial activity of ZnO NPs is affected by many factors including particle size, concentration, morphology, surface modification, and other factors. However, the toxicity of ZnO NPs to mammalian cells should not be ignored, and more data are needed to determine long-term health risks. The long-term biosafety, biodistribution, and toxicity of various morphologies of ZnO nanomaterials remain to be further studied. To further expand the antibacterial application of ZnO nanomaterials, some biomimetic and engineered biomimetic coatings could be modified to ZnO nanomaterials. Moreover, hybrid ZnO nanomaterials have been proven to possess improved antimicrobial activity for synergistic effects. ZnO nanomaterials can be combined with enzymes or photosensitizers for reducing the dosage of ZnO.
For Zn(II) deprivation strategy, Zn(II) is an important transition element that both the human body and pathogens depend heavily on. How to deprive Zn(II) of bacteria more precisely is a very meaningful scientific problem. Fortunately, due to the demand for Zn(II) in the human body, there are measures to compete for Zn(II) in the human immune system. For example, calprotectin, as an antimicrobial protein released by neutrophils, could inhibit bacterial growth by limiting Zn(II) availability. It could be modified and designed into NPs that deliver antibiotics while chelating zinc ions. Moreover, improving the effectiveness of host-mediated Zn(II) restriction at the pathogen–host interface and reducing the acquisition of zinc by bacteria to limit the growth of bacteria are also promising solutions. In addition, some metal ions, such as Pb2+, Ni2+, and Bi3+, could act as “zinc traps” to replace zinc at active sites on functional proteins and embed themselves into the Zn(II)-utilization pathway and further damage the downstream Zn(II)-related metabolism state inside bacteria. In conclusion, Zn(II) interference therapy, based on nanodelivery systems, carves a new path for combating drug-resistant bacterial infections, benefiting from unique mechanisms, inherent targeting properties, and high therapeutic efficiency. With the development of advanced nanomaterials, nanomaterial-based zinc ion interference therapy will provide significant contributions to fighting MDR bacterial infection, and the clinical translation of Zn(II) interference therapy will be achieved in the near future.
YW: writing—original draft preparation. JW: writing—original draft preparation. SW: writing—original draft preparation. RZ: writing—original draft preparation. KZ: writing—review and editing. ZZ: writing—review and editing. JL: writing—review and editing. SQ: writing—review. JS: writing—review. All authors listed have made a substantial, direct, and intellectual contribution to the work and approved it for publication.
This work was supported by grants from the National Natural Science Foundation of China (Nos. 82172762, 31900991, 82073395, 82073787, U2004197, 22122409), Innovation Talent Support Program of Henan Province (No. 21HASTIT043), Postdoctoral Science Foundation of China (No. 2020TQ0288, 2021M690140), and Youth Talent Promotion Foundation of Henan Province (No. 2021HYTP047), Outstanding Youth Foundation of Henan Province (222300420020).
The authors declare that the research was conducted in the absence of any commercial or financial relationships that could be construed as a potential conflict of interest.
All claims expressed in this article are solely those of the authors and do not necessarily represent those of their affiliated organizations, or those of the publisher, the editors and the reviewers. Any product that may be evaluated in this article, or claim that may be made by its manufacturer, is not guaranteed or endorsed by the publisher.
We are indebted to members of the Key Laboratory of Targeting Therapy and Diagnosis for Critical Diseases for the discussion and investigation that contributed to this article. We apologize to colleagues whose works and publications could not be referenced owing to space constraints.
1. Michael CA, Dominey-Howes D, Labbate M. The Antimicrobial Resistance Crisis: Causes, Consequences, and Management. Front Public Health (2014) 2:145. doi: 10.3389/fpubh.2014.00145
2. Nigam A, Gupta D, Sharma A. Treatment of Infectious Disease: Beyond Antibiotics. Microbiol Res (2014) 169(9-10):643–51. doi: 10.1016/j.micres.2014.02.009
3. Makabenta JMV, Nabawy A, Li CH, Schmidt-Malan S, Patel R, Rotello VM. Nanomaterial-Based Therapeutics for Antibiotic-Resistant Bacterial Infections. Nat Rev Microbiol (2020) 19(1):23–36. doi: 10.1038/s41579-020-0420-1
4. Willyard C. Drug-Resistant Bacteria Ranked. Nature (2017) 543(7643):15–5. doi: 10.1038/nature.2017.21550
5. Minamino T, Imada K. The Bacterial Flagellar Motor and its Structural Diversity. Trends Microbiol (2015) 23(5):267–74. doi: 10.1016/j.tim.2014.12.011
6. Lertsethtakarn P, Ottemann KM, Hendrixson DR. Motility and Chemotaxis in Campylobacter and Helicobacter. Annu Rev Microbiol (2011) 65:389–410. doi: 10.1146/annurev-micro-090110-102908
7. Takekawa N, Imada K, Homma M. Structure and Energy-Conversion Mechanism of the Bacterial Na(+)-Driven Flagellar Motor. Trends Microbiol (2020) 28(9):719–31. doi: 10.1016/j.tim.2020.03.010
8. Yao X, Smolka AJ. Gastric Parietal Cell Physiology and Helicobacter Pylori-Induced Disease. Gastroenterology (2019) 156(8):2158–73. doi: 10.1053/j.gastro.2019.02.036
9. Vogele M, Bhaskara RM, Mulvihill E, van Pee K, Yildiz O, Kuhlbrandt W, et al. Membrane Perforation by the Pore-Forming Toxin Pneumolysin. Proc Natl Acad Sci U S A (2019) 116(27):13352–7. doi: 10.1073/pnas.1904304116
10. Henry BD, Neill DR, Becker KA, Gore S, Bricio-Moreno L, Ziobro R, et al. Engineered Liposomes Sequester Bacterial Exotoxins and Protect From Severe Invasive Infections in Mice. Nat Biotechnol (2015) 33(1):81–8. doi: 10.1038/nbt.3037
11. van der Poll T, van de Veerdonk FL, Scicluna BP, Netea MG. The Immunopathology of Sepsis and Potential Therapeutic Targets. Nat Rev Immunol (2017) 17(7):407–20. doi: 10.1038/nri.2017.36
12. Torres LK, Pickkers P, van der Poll T. Sepsis-Induced Immunosuppression. Annu Rev Physiol (2022) 84:157–81. doi: 10.1146/annurev-physiol-061121-040214
13. Munford RS. Severe Sepsis and Septic Shock: The Role of Gram-Negative Bacteremia. Annu Rev Pathol (2006) 1:467–96. doi: 10.1146/annurev.pathol.1.110304.100200
14. Thamphiwatana S, Angsantikul P, Escajadillo T, Zhang Q, Olson J, Luk BT, et al. Macrophage-Like Nanoparticles Concurrently Absorbing Endotoxins and Proinflammatory Cytokines for Sepsis Management. Proc Natl Acad Sci U S A (2017) 114(43):11488–93. doi: 10.1073/pnas.1714267114
15. Shi C, Wang X, Wang L, Meng Q, Guo D, Chen L, et al. A Nanotrap Improves Survival in Severe Sepsis by Attenuating Hyperinflammation. Nat Commun (2020) 11(1):3384. doi: 10.1038/s41467-020-17153-0
16. Sambhy V, MacBride MM, Peterson BR, Sen A. Silver Bromide Nanoparticle/Polymer Composites: Dual Action Tunable Antimicrobial Materials. J Am Chem Soc (2006) 128(30):9798–808. doi: 10.1021/ja061442z
17. Ermini ML, Voliani V. Antimicrobial Nano-Agents: The Copper Age. ACS Nano (2021) 15(4):6008–29. doi: 10.1021/acsnano.0c10756
18. Chernousova S, Epple M. Silver as Antibacterial Agent: Ion, Nanoparticle, and Metal. Angew Chem Int Ed Engl (2013) 52(6):1636–53. doi: 10.1002/anie.201205923
19. Guo C, Cheng F, Liang G, Zhang S, Jia Q, He L, et al. Copper-Based Polymer-Metal–Organic Framework Embedded With Ag Nanoparticles: Long-Acting and Intelligent Antibacterial Activity and Accelerated Wound Healing. Chem Eng J (2022) 435:134915. doi: 10.1016/j.cej.2022.134915
20. Rizzello L, Pompa PP. Nanosilver-Based Antibacterial Drugs and Devices: Mechanisms, Methodological Drawbacks, and Guidelines. Chem Soc Rev (2014) 43(5):1501–18. doi: 10.1039/C3CS60218D
21. Rai M, Yadav A, Gade A. Silver Nanoparticles as a New Generation of Antimicrobials. Biotechnol Adv (2009) 27(1):76–83. doi: 10.1016/j.biotechadv.2008.09.002
22. Panacek A, Kvitek L, Smekalova M, Vecerova R, Kolar M, Roderova M, et al. Bacterial Resistance to Silver Nanoparticles and How to Overcome it. Nat Nanotechnol (2018) 13(1):65–71. doi: 10.1038/s41565-017-0013-y
23. Shi J, Li J, Wang Y, Zhang CY. TiO2-Based Nanosystem for Cancer Therapy and Antimicrobial Treatment: A Review. Chem Eng J (2022) 431:133714. doi: 10.1016/j.cej.2021.133714
24. Lagopati N, Evangelou K, Falaras P, Tsilibary EC, Vasileiou PVS, Havaki S, et al. Nanomedicine: Photo-Activated Nanostructured Titanium Dioxide, as a Promising Anticancer Agent. Pharmacol Ther (2021) 222:107795. doi: 10.1016/j.pharmthera.2020.107795
25. Corbett D, Wang J, Schuler S, Lopez-Castejon G, Glenn S, Brough D, et al. Two Zinc Uptake Systems Contribute to the Full Virulence of Listeria Monocytogenes During Growth In Vitro and In Vivo. Infect Immun (2012) 80(1):14–21. doi: 10.1128/IAI.05904-11
26. Yamasaki S, Sakata-Sogawa K, Hasegawa A, Suzuki T, Kabu K, Sato E, et al. Zinc is a Novel Intracellular Second Messenger. J Cell Biol (2007) 177(4):637–45. doi: 10.1083/jcb.200702081
27. Xia P, Lian S, Wu Y, Yan L, Quan G, Zhu G. Zinc is an Important Inter-Kingdom Signal Between the Host and Microbe. Vet Res (2021) 52(1):39. doi: 10.1186/s13567-021-00913-1
28. Rink L, Haase H. Zinc Homeostasis and Immunity. Trends Immunol (2007) 28(1):1–4. doi: 10.1016/j.it.2006.11.005
29. Maret W. Metallothionein Redox Biology in the Cytoprotective and Cytotoxic Functions of Zinc. Exp Gerontol (2008) 43(5):363–9. doi: 10.1016/j.exger.2007.11.005
30. Wang T, Xu K, Zhao L, Tong R, Xiong L, Shi J. Recent Research and Development of NDM-1 Inhibitors. Eur J Med Chem (2021) 223:113667. doi: 10.1016/j.ejmech.2021.113667
31. Feng H, Liu X, Wang S, Fleming J, Wang DC, Liu W. The Mechanism of NDM-1-Catalyzed Carbapenem Hydrolysis is Distinct From That of Penicillin or Cephalosporin Hydrolysis. Nat Commun (2017) 8(1):2242. doi: 10.1038/s41467-017-02339-w
32. Nejdl L, Ruttkay-Nedecky B, Kudr J, Krizkova S, Smerkova K, Dostalova S, et al. DNA Interaction With Zinc(II) Ions. Int J Biol Macromol (2014) 64:281–7. doi: 10.1016/j.ijbiomac.2013.12.013
33. Jung W, Sengupta K, Wendel BM, Helmann JD, Chen P. Biphasic Unbinding of a Metalloregulator From DNA for Transcription (De)Repression in Live Bacteria. Nucleic Acids Res (2020) 48(5):2199–208. doi: 10.1093/nar/gkaa056
34. Gabbianelli R, Scotti R, Ammendola S, Petrarca P, Nicolini L, Battistoni A. Role of Znuabc and Zint in Escherichia Coli O157: H7 Zinc Acquisition and Interaction with Epithelial Cells. BMC Microbiol (2011) 11(1):1–11. doi: 10.1186/1471-2180-11-36
35. Beard SJ, Hughes MN, Poole RK. Inhibition of the Cytochrome M-Terminated NADH Oxidase System in Escherichia Coli K-12 by Divalent Metal Cations. FEMS Microbiol Lett (1995) p:205–10. doi: 10.1111/j.1574-6968.1995.tb07778.x
36. Xu FF, Imlay JA. Silver(I), Mercury(II), Cadmium(II), and Zinc(II) Target Exposed Enzymic Iron-Sulfur Clusters When They Toxify Escherichia Coli. Appl Environ Microbiol (2012) 78(10):3614–21. doi: 10.1128/AEM.07368-11
37. Gao H, Ge Y, Jiang M-H, Chen C, Sun L-Y, Li J-Q, et al. Real-Time Monitoring and Inhibition of the Activity of Carbapenemase in Live Bacterial Cells: Application to Screening of β-Lactamase Inhibitors. New J Chem (2020) 44(46):20334–40. doi: 10.1039/D0NJ03475D
38. Guo Q, Wu D, Gao L, Bai Y, Liu Y, Guo N, et al. Identification of the AMA Synthase From the Aspergillomarasmine A Biosynthesis and Evaluation of Its Biocatalytic Potential. ACS Catal (2020) 10(11):6291–8. doi: 10.1021/acscatal.0c01187
39. Mishra PK, Mishra H, Ekielski A, Talegaonkar S, Vaidya B. Zinc Oxide Nanoparticles: A Promising Nanomaterial for Biomedical Applications. Drug Discovery Today (2017) 22(12):1825–34. doi: 10.1016/j.drudis.2017.08.006
40. Dwivedi S, Wahab R, Khan F, Mishra YK, Musarrat J, Al-Khedhairy AA. Reactive Oxygen Species Mediated Bacterial Biofilm Inhibition via Zinc Oxide Nanoparticles and Their Statistical Determination. PloS One (2014) 9(11):e111289. doi: 10.1371/journal.pone.0111289
41. Jarosz M, Olbert M, Wyszogrodzka G, Mlyniec K, Librowski T. Antioxidant and Anti-Inflammatory Effects of Zinc. Zinc-Dependent NF-kappaB Signaling. Inflammopharmacology (2017) 25(1):11–24.
42. Wu Y, Liao Q, Wu L, Luo Y, Zhang W, Guan M, et al. ZnL2-BPs Integrated Bone Scaffold Under Sequential Photothermal Mediation: A Win-Win Strategy Delivering Antibacterial Therapy and Fostering Osteogenesis Thereafter. ACS Nano (2021) 15(11):17854–69. doi: 10.1021/acsnano.1c06062
43. Ferrari M. Cancer Nanotechnology: Opportunities and Challenges. Nat Rev Cancer (2005) 5(3):161–71. doi: 10.1038/nrc1566
44. Gupta A, Mumtaz S, Li CH, Hussain I, Rotello VM. Combatting Antibiotic-Resistant Bacteria Using Nanomaterials. Chem Soc Rev (2019) 48(2):415–27. doi: 10.1039/C7CS00748E
45. Thamphiwatana S, Gao W, Obonyo M, Zhang L. In Vivo Treatment of Helicobacter Pylori Infection With Liposomal Linolenic Acid Reduces Colonization and Ameliorates Inflammation. Proc Natl Acad Sci U S A (2014) 111(49):17600–5. doi: 10.1073/pnas.1418230111
46. Huang CM, Chen CH, Pornpattananangkul D, Zhang L, Chan M, Hsieh MF, et al. Eradication of Drug Resistant Staphylococcus Aureus by Liposomal Oleic Acids. Biomaterials (2011) 32(1):214–21. doi: 10.1016/j.biomaterials.2010.08.076
47. Zheng H, Ma R, Gao M, Tian X, Li Y-Q, Zeng L, et al. Antibacterial Applications of Graphene Oxides: Structure-Activity Relationships, Molecular Initiating Events and Biosafety. Sci Bull (2018) 63(2):133–42. doi: 10.1016/j.scib.2017.12.012
48. Xin Q, Shah H, Nawaz A, Xie W, Akram MZ, Batool A, et al. Antibacterial Carbon-Based Nanomaterials. Adv Mater (2019) 31(45):e1804838. doi: 10.1002/adma.201804838
49. Hu D, Li H, Wang B, Ye Z, Lei W, Jia F, et al. Surface-Adaptive Gold Nanoparticles With Effective Adherence and Enhanced Photothermal Ablation of Methicillin-Resistant Staphylococcus Aureus Biofilm. ACS Nano (2017) 11(9):9330–9. doi: 10.1021/acsnano.7b04731
50. Wang C, Wang Y, Zhang L, Miron RJ, Liang J, Shi M, et al. Pretreated Macrophage-Membrane-Coated Gold Nanocages for Precise Drug Delivery for Treatment of Bacterial Infections. Adv Mater (2018) 30(46):e1804023. doi: 10.1002/adma.201804023
51. Sen Karaman D, Pamukcu A, Karakaplan MB, Kocaoglu O, Rosenholm JM. Recent Advances in the Use of Mesoporous Silica Nanoparticles for the Diagnosis of Bacterial Infections. Int J Nanomed (2021) 16:6575–91. doi: 10.2147/IJN.S273062
52. Song Y, Elsabahy M, Collins CA, Khan S, Li R, Hreha TN, et al. Morphologic Design of Silver-Bearing Sugar-Based Polymer Nanoparticles for Uroepithelial Cell Binding and Antimicrobial Delivery. Nano Lett (2021) 21(12):4990–8. doi: 10.1021/acs.nanolett.1c00776
53. Hui S, Liu Q, Huang Z, Yang J, Liu Y, Jiang S. Gold Nanoclusters-Decorated Zeolitic Imidazolate Frameworks With Reactive Oxygen Species Generation for Photoenhanced Antibacterial Study. Bioconjug Chem (2020) 31(10):2439–45. doi: 10.1021/acs.bioconjchem.0c00485
54. Wu S, Huang Y, Yan J, Li Y, Wang J, Yang YY, et al. Bacterial Outer Membrane-Coated Mesoporous Silica Nanoparticles for Targeted Delivery of Antibiotic Rifampicin Against Gram-Negative Bacterial Infection In Vivo. Advanced Funct Mater (2021) 31(35):2103442. doi: 10.1002/adfm.202103442
55. Gera S, Kankuri E, Kogermann K. Antimicrobial Peptides - Unleashing Their Therapeutic Potential Using Nanotechnology. Pharmacol Ther (2021) p:107990.
56. Zhang CY, Gao J, Wang Z. Bioresponsive Nanoparticles Targeted to Infectious Microenvironments for Sepsis Management. Adv Mater (2018) 30(43):e1803618. doi: 10.1002/adma.201803618
57. Dehaini D, Wei X, Fang RH, Masson S, Angsantikul P, Luk BT, et al. Erythrocyte-Platelet Hybrid Membrane Coating for Enhanced Nanoparticle Functionalization. Adv Mater (2017) 29(16):1606209. doi: 10.1002/adma.201606209
58. Brayner R, Ferrari-Iliou R, Brivois N, Djediat S, Benedetti MF, Fievet F. Toxicological Impact Studies Based on Escherichia Coli Bacteria in Ultrafine ZnO Nanoparticles Colloidal Medium. Nano Lett (2006) 6(4):866–70. doi: 10.1021/nl052326h
59. Seil JT, Webster TJ. Antimicrobial Applications of Nanotechnology: Methods and Literature. Int J Nanomed (2012) 7:2767–81.
60. Wu S, Zhang K, Liang Y, Wei Y, An J, Wang Y, et al. Nano-Enabled Tumor Systematic Energy Exhaustion via Zinc (II) Interference Mediated Glycolysis Inhibition and Specific GLUT1 Depletion. Adv Sci (Weinh) (2021) 9(7):e2103534.
61. Xu B, Wang H, Wang W, Gao L, Li S, Pan X, et al. A Single-Atom Nanozyme for Wound Disinfection Applications. Angew Chem Int Ed Engl (2019) 58(15):4911–6. doi: 10.1002/anie.201813994
62. Guo Z, Zeng J, Liu W, Chen Y, Jiang H, Weizmann Y, et al. Formation of Bio-Responsive Nanocomposites for Targeted Bacterial Bioimaging and Disinfection. Chem Eng J (2021) 426:130726. doi: 10.1016/j.cej.2021.130726
63. Sirelkhatim A, Mahmud S, Seeni A, Kaus NHM, Ann LC, Bakhori SKM, et al. Review on Zinc Oxide Nanoparticles: Antibacterial Activity and Toxicity Mechanism. Nanomicro Lett (2015) 7(3):219–42. doi: 10.1007/s40820-015-0040-x
64. Ezraty B, Gennaris A, Barras F, Collet JF. Oxidative Stress, Protein Damage and Repair in Bacteria. Nat Rev Microbiol (2017) 15(7):385–96. doi: 10.1038/nrmicro.2017.26
65. Qi X, Simsek S, Chen B, Rao J. Alginate-Based Double-Network Hydrogel Improves the Viability of Encapsulated Probiotics During Simulated Sequential Gastrointestinal Digestion: Effect of Biopolymer Type and Concentrations. Int J Biol Macromol (2020) 165(Pt B):1675–85. doi: 10.1016/j.ijbiomac.2020.10.028
66. Malzahn K, Jamieson WD, Droge M, Mailander V, Jenkins ATA, Weiss CK, et al. Advanced Dextran Based Nanogels for Fighting Staphylococcus Aureus Infections by Sustained Zinc Release. J Mater Chem B (2014) 2(15):2175–83. doi: 10.1039/C3TB21335H
67. Kaur N, Tiwari P, Kapoor KS, Saini AK, Sharma V, Mobin SM. Metal–organic Framework Based Antibiotic Release and Antimicrobial Response: An Overview. CrystEngComm (2020) 22(44):7513–27. doi: 10.1039/D0CE01215G
68. Horcajada P, Chalati T, Serre C, Gillet B, Sebrie C, Baati T, et al. Porous Metal-Organic-Framework Nanoscale Carriers as a Potential Platform for Drug Delivery and Imaging. Nat Mater (2010) 9(2):172–8. doi: 10.1038/nmat2608
69. He H, Li R, Yang Z, Chai L, Jin L, Alhassan SI, et al. Preparation of MOFs and MOFs Derived Materials and Their Catalytic Application in Air Pollution: A Review. Catal Today (2021) 375:10–29. doi: 10.1016/j.cattod.2020.02.033
70. Wang C, An B, Lin W. Metal–Organic Frameworks in Solid–Gas Phase Catalysis. ACS Catal (2018) 9(1):130–46.
71. Makhanya N, Oboirien B, Ren J, Musyoka N, Sciacovelli A. Recent Advances on Thermal Energy Storage Using Metal-Organic Frameworks (MOFs). J Energy Storage (2021) 34:102179. doi: 10.1016/j.est.2020.102179
72. Yao X, Zhu G, Zhu P, Ma J, Chen W, Liu Z, et al. Omniphobic ZIF-8@Hydrogel Membrane by Microfluidic-Emulsion-Templating Method for Wound Healing. Advanced Funct Mater (2020) 30(13):1909389. doi: 10.1002/adfm.201909389
73. Wang Q, Sun Y, Li S, Zhang P, Yao Q. Synthesis and Modification of ZIF-8 and its Application in Drug Delivery and Tumor Therapy. RSC Adv (2020) 10(62):37600–20. doi: 10.1039/D0RA07950B
74. Sun CY, Qin C, Wang XL, Yang GS, Shao KZ, Lan YQ, et al. Zeolitic Imidazolate Framework-8 as Efficient pH-Sensitive Drug Delivery Vehicle. Dalton Trans (2012) 41(23):6906–9. doi: 10.1039/c2dt30357d
75. Zhang W, Zhou Y, Fan Y, Cao R, Xu Y, Weng Z, et al. Metal-Organic-Framework-Based Hydrogen-Release Platform for Multieffective Helicobacter Pylori Targeting Therapy and Intestinal Flora Protective Capabilities. Adv Mater (2021) p:e2105738.
76. Zhang X, Liu L, Huang L, Zhang W, Wang R, Yue T, et al. The Highly Efficient Elimination of Intracellular Bacteria via a Metal Organic Framework (MOF)-Based Three-in-One Delivery System. Nanoscale (2019) 11(19):9468–77. doi: 10.1039/C9NR01284B
77. Peng D, Liu G, He Y, Gao P, Gou S, Wu J, et al. Fabrication of a pH-Responsive Core-Shell Nanosystem With a Low-Temperature Photothermal Therapy Effect for Treating Bacterial Biofilm Infection. Biomater Sci (2021) 9(22):7483–91. doi: 10.1039/D1BM01329G
78. Wu Q, Li M, Tan L, Yu J, Chen Z, Su L, et al. A Tumor Treatment Strategy Based on Biodegradable BSA@ZIF-8 for Simultaneously Ablating Tumors and Inhibiting Infection. Nanoscale Horiz (2018) 3(6):606–15. doi: 10.1039/C8NH00113H
79. Wan Y, Fang J, Wang Y, Sun J, Sun Y, Sun X, et al. Antibacterial Zeolite Imidazole Frameworks With Manganese Doping for Immunomodulation to Accelerate Infected Wound Healing. Adv Healthc Mater (2021) 10(22):e2101515. doi: 10.1002/adhm.202101515
80. Wen T, Lin Z, Zhao Y, Zhou Y, Niu B, Shi C, et al. Bioresponsive Nanoarchitectonics-Integrated Microneedles for Amplified Chemo-Photodynamic Therapy Against Acne Vulgaris. ACS Appl Mater Interfaces (2021) 13(41):48433–48. doi: 10.1021/acsami.1c15673
81. Tao B, Zhao W, Lin C, Yuan Z, He Y, Lu L, et al. Surface Modification of Titanium Implants by ZIF-8@Levo/LBL Coating for Inhibition of Bacterial-Associated Infection and Enhancement of In Vivo Osseointegration. Chem Eng J (2020) 390:124621. doi: 10.1016/j.cej.2020.124621
82. Wang M, Nian L, Cheng Y, Yuan B, Cheng S, Cao C. Encapsulation of Colloidal Semiconductor Quantum Dots Into Metal-Organic Frameworks for Enhanced Antibacterial Activity Through Interfacial Electron Transfer. Chem Eng J (2021) 426:130832. doi: 10.1016/j.cej.2021.130832
83. Hui S, Liu Q, Han Y, Zhang L, Yang J, Jiang S, et al. ICG@ZIF-8/PDA/Ag Composites as Chemo-Photothermal Antibacterial Agents for Efficient Sterilization and Enhanced Wound Disinfection. J Mater Chem B (2021) 9(48):9961–70. doi: 10.1039/D1TB02107A
84. Li P, Li J, Feng X, Li J, Hao Y, Zhang J, et al. Metal-Organic Frameworks With Photocatalytic Bactericidal Activity for Integrated Air Cleaning. Nat Commun (2019) 10(1):2177. doi: 10.1038/s41467-019-10218-9
85. Yang Y, Deng Y, Huang J, Fan X, Cheng C, Nie C, et al. Size-Transformable Metal–Organic Framework–Derived Nanocarbons for Localized Chemo-Photothermal Bacterial Ablation and Wound Disinfection. Advanced Funct Mater (2019) 29(33):1900143. doi: 10.1002/adfm.201900143
86. Duan S, Zhao X, Su Z, Wang C, Lin Y. Layer-By-Layer Decorated Nanoscale ZIF-8 With High Curcumin Loading Effectively Inactivates Gram-Negative and Gram-Positive Bacteria. ACS Appl Bio Mater (2020) 3(6):3673–80. doi: 10.1021/acsabm.0c00300
87. Song Z, Wu Y, Cao Q, Wang H, Wang X, Han H. pH-Responsive, Light-Triggered on-Demand Antibiotic Release From Functional Metal-Organic Framework for Bacterial Infection Combination Therapy. Advanced Funct Mater (2018) 28(23):1800011. doi: 10.1002/adfm.201800011
88. Xiao Y, Xu M, Lv N, Cheng C, Huang P, Li J, et al. Dual Stimuli-Responsive Metal-Organic Framework-Based Nanosystem for Synergistic Photothermal/Pharmacological Antibacterial Therapy. Acta Biomater (2021) 122:291–305. doi: 10.1016/j.actbio.2020.12.045
89. Zhang S, Ye J, Liu X, Wang G, Qi Y, Wang T, et al. Dual Stimuli-Responsive Smart Fibrous Membranes for Efficient Photothermal/Photodynamic/Chemo-Therapy of Drug-Resistant Bacterial Infection. Chem Eng J (2022) 432:134351. doi: 10.1016/j.cej.2021.134351
90. Tu Y, Lei C, Deng F, Chen Y, Wang Y, Zhang Z. Core–shell ZIF-8@Polydopamine Nanoparticles Obtained by Mitigating the Polydopamine Coating Induced Self-Etching of MOFs: Prototypical Metal Ion Reservoirs for Sticking to and Killing Bacteria. New J Chem (2021) 45(19):8701–13. doi: 10.1039/D1NJ00461A
91. Ejima H, Richardson JJ, Liang K, Best JP, van Koeverden MP, Such GK, et al. One-Step Assembly of Coordination Complexes for Versatile Film and Particle Engineering. Science (2013) 341(6142):154–7. doi: 10.1126/science.1237265
92. Wang Z, Zou Y, Li Y, Cheng Y. Metal-Containing Polydopamine Nanomaterials: Catalysis, Energy, and Theranostics. Small (2020) 16(18):e1907042. doi: 10.1002/smll.201907042
93. Rostami S, Parsian H. Hyaluronic Acid: From Biochemical Characteristics to its Clinical Translation in Assessment of Liver Fibrosis. Hepat Mon (2013) 13(12):e13787. doi: 10.5812/hepatmon.13787
94. Xu X, Jha AK, Harrington DA, Farach-Carson MC, Jia X. Hyaluronic Acid-Based Hydrogels: From a Natural Polysaccharide to Complex Networks. Soft Matter (2012) 8(12):3280–94. doi: 10.1039/c2sm06463d
95. Ailloud F, Didelot X, Woltemate S, Pfaffinger G, Overmann J, Bader RC, et al. Within-Host Evolution of Helicobacter Pylori Shaped by Niche-Specific Adaptation, Intragastric Migrations and Selective Sweeps. Nat Commun (2019) 10(1):2273. doi: 10.1038/s41467-019-10050-1
96. Naumann M, Sokolova O, Tegtmeyer N, Backert S. Helicobacter Pylori: A Paradigm Pathogen for Subverting Host Cell Signal Transmission. Trends Microbiol (2017) 25(4):316–28. doi: 10.1016/j.tim.2016.12.004
97. Zhang S, Ermann J, Succi MD, Zhou A, Hamilton MJ, Cao B, et al. An Inflammation-Targeting Hydrogel for Local Drug Delivery in Inflammatory Bowel Disease. Sci Transl Med (2015) 7(300):300ra128. doi: 10.1126/scitranslmed.aaa5657
98. Wang C, Luo Y, Liu X, Cui Z, Zheng Y, Liang Y, et al. The Enhanced Photocatalytic Sterilization of MOF-Based Nanohybrid for Rapid and Portable Therapy of Bacteria-Infected Open Wounds. Bioact Mater (2022) 13:200–11. doi: 10.1016/j.bioactmat.2021.10.033
99. Cheng R, Meng F, Deng C, Klok HA, Zhong Z. Dual and Multi-Stimuli Responsive Polymeric Nanoparticles for Programmed Site-Specific Drug Delivery. Biomaterials (2013) 34(14):3647–57. doi: 10.1016/j.biomaterials.2013.01.084
100. Ning X, Lee S, Wang Z, Kim D, Stubblefield B, Gilbert E, et al. Maltodextrin-Based Imaging Probes Detect Bacteria In Vivo With High Sensitivity and Specificity. Nat Mater (2011) 10(8):602–7. doi: 10.1038/nmat3074
101. Al Mamun M, Wahab YA, Hossain MAM, Hashem A, Johan MR. Electrochemical Biosensors With Aptamer Recognition Layer for the Diagnosis of Pathogenic Bacteria: Barriers to Commercialization and Remediation. TrAC Trends Anal Chem (2021) 145:116458. doi: 10.1016/j.trac.2021.116458
102. Hanley C, Layne J, Punnoose A, Reddy KM, Coombs I, Coombs A, et al. Preferential Killing of Cancer Cells and Activated Human T Cells Using ZnO Nanoparticles. Nanotechnology (2008) 19(29):295103. doi: 10.1088/0957-4484/19/29/295103
103. Zhang L, Jiang Y, Ding Y, Povey M, York D. Investigation Into the Antibacterial Behaviour of Suspensions of ZnO Nanoparticles (ZnO Nanofluids). J Nanopart Res (2006) 9(3):479–89.
104. Magesh G, Bhoopathi G, Nithya N, Arun AP, Ranjith Kumar E. Structural, Morphological, Optical and Biological Properties of Pure ZnO and Agar/Zinc Oxide Nanocomposites. Int J Biol Macromol (2018) 117:959–66. doi: 10.1016/j.ijbiomac.2018.04.197
105. Kasraei S, Sami L, Hendi S, Alikhani MY, Rezaei-Soufi L, Khamverdi Z. Antibacterial Properties of Composite Resins Incorporating Silver and Zinc Oxide Nanoparticles on Streptococcus Mutans and Lactobacillus. Restor Dent Endod (2014) 39(2):109–14. doi: 10.5395/rde.2014.39.2.109
106. Ye J, Li B, Li M, Zheng Y, Wu S, Han Y. Formation of a ZnO Nanorods-Patterned Coating With Strong Bactericidal Capability and Quantitative Evaluation of the Contribution of Nanorods-Derived Puncture and ROS-Derived Killing. Bioact Mater (2022) 11:181–91. doi: 10.1016/j.bioactmat.2021.09.019
107. Jones N, Ray B, Ranjit KT, Manna AC. Antibacterial Activity of ZnO Nanoparticle Suspensions on a Broad Spectrum of Microorganisms. FEMS Microbiol Lett (2008) 279(1):71–6. doi: 10.1111/j.1574-6968.2007.01012.x
108. de Lucas-Gil E, Leret P, Monte-Serrano M, Reinosa JJ, Enríquez E, Del Campo A, et al. ZnO Nanoporous Spheres With Broad-Spectrum Antimicrobial Activity by Physicochemical Interactions. ACS Appl Nano Mater (2018) 1(7):3214–25. doi: 10.1021/acsanm.8b00402
109. Cai Q, Gao Y, Gao T, Lan S, Simalou O, Zhou X, et al. Insight Into Biological Effects of Zinc Oxide Nanoflowers on Bacteria: Why Morphology Matters. ACS Appl Mater Interfaces (2016) 8(16):10109–20. doi: 10.1021/acsami.5b11573
110. Yi G, Yuan Y, Li X, Zhang Y. ZnO Nanopillar Coated Surfaces With Substrate-Dependent Superbactericidal Property. Small (2018) 14(14):e1703159. doi: 10.1002/smll.201703159
111. Chen H, Zhang M, Li B, Chen D, Dong X, Wang Y, et al. Versatile Antimicrobial Peptide-Based ZnO Quantum Dots for In Vivo Bacteria Diagnosis and Treatment With High Specificity. Biomaterials (2015) 53:532–44. doi: 10.1016/j.biomaterials.2015.02.105
112. Wang YW, Cao A, Jiang Y, Zhang X, Liu JH, Liu Y, et al. Superior Antibacterial Activity of Zinc Oxide/Graphene Oxide Composites Originating From High Zinc Concentration Localized Around Bacteria. ACS Appl Mater Interfaces (2014) 6(4):2791–8. doi: 10.1021/am4053317
113. Gao D, Zhao P, Lyu B, Li Y, Hou Y, Ma J. Carbon Quantum Dots Decorated on ZnO Nanoparticles: An Efficient Visible-Light Responsive Antibacterial Agents. Appl Organometallic Chem (2020) 34(8):e5665. doi: 10.1002/aoc.5665
114. Fang J, Wan Y, Sun Y, Sun X, Qi M, Cheng S, et al. Near-Infrared-Activated Nanohybrid Coating With Black Phosphorus/Zinc Oxide for Efficient Biofilm Eradication Against Implant-Associated Infections. Chem Eng J (2022) 435:134935. doi: 10.1016/j.cej.2022.134935
115. Park BC, Byun SW, Ju Y, Lee DB, Shin JB, Yeon KM, et al. Zinc Oxide Nano-Spicules on Polylactic Acid for Super-Hydrophilic and Bactericidal Surfaces. Advanced Funct Mater (2021) 31(36):2100844. doi: 10.1002/adfm.202100844
116. Li J, Tan L, Liu X, Cui Z, Yang X, Yeung KWK, et al. Balancing Bacteria-Osteoblast Competition Through Selective Physical Puncture and Biofunctionalization of ZnO/Polydopamine/Arginine-Glycine-Aspartic Acid-Cysteine Nanorods. ACS Nano (2017) 11(11):11250–63. doi: 10.1021/acsnano.7b05620
117. Bains D, Singh G, Singh N. Sustainable Synthesis of Ionic Liquid-Functionalized Zinc Oxide Nanosheets (IL@ZnO): Evaluation of Antibacterial Potential Activity for Biomedical Applications. ACS Appl Bio Mater (2022) 5(3):1239–51. doi: 10.1021/acsabm.1c01258
118. Sethi D, Sakthivel R. ZnO/TiO2 Composites for Photocatalytic Inactivation of Escherichia Coli. J Photochem Photobiol B (2017) 168:117–23. doi: 10.1016/j.jphotobiol.2017.02.005
119. Xiang Y, Mao C, Liu X, Cui Z, Jing D, Yang X, et al. Rapid and Superior Bacteria Killing of Carbon Quantum Dots/ZnO Decorated Injectable Folic Acid-Conjugated PDA Hydrogel Through Dual-Light Triggered ROS and Membrane Permeability. Small (2019) 15(22):e1900322. doi: 10.1002/smll.201900322
120. Dong J, Ghiladi RA, Wang Q, Cai Y, Wei Q. Protoporphyrin-IX Conjugated Cellulose Nanofibers That Exhibit High Antibacterial Photodynamic Inactivation Efficacy. Nanotechnology (2018) 29(26):265601. doi: 10.1088/1361-6528/aabb3c
121. Rasmussen JW, Martinez E, Louka P, Wingett DG. Zinc Oxide Nanoparticles for Selective Destruction of Tumor Cells and Potential for Drug Delivery Applications. Expert Opin Drug Delivery (2010) 7(9):1063–77. doi: 10.1517/17425247.2010.502560
122. Sharma H, Mishra PK, Talegaonkar S, Vaidya B. Metal Nanoparticles: A Theranostic Nanotool Against Cancer. Drug Discovery Today (2015) 20(9):1143–51. doi: 10.1016/j.drudis.2015.05.009
123. Chai Q, Wu Q, Liu T, Tan L, Fu C, Ren X, et al. Enhanced Antibacterial Activity of Silica Nanorattles With ZnO Combination Nanoparticles Against Methicillin-Resistant Staphylococcus Aureus. Sci Bull (2017) 62(17):1207–15. doi: 10.1016/j.scib.2017.08.016
124. Moussa H, Merlin C, Dezanet C, Balan L, Medjahdi G, Ben-Attia M, et al. Trace Amounts of Cu(2)(+) Ions Influence ROS Production and Cytotoxicity of ZnO Quantum Dots. J Hazard Mater (2016) 304:532–42. doi: 10.1016/j.jhazmat.2015.11.013
125. Padmavathy N, Vijayaraghavan R. Enhanced Bioactivity of ZnO Nanoparticles-an Antimicrobial Study. Sci Technol Adv Mater (2008) 9(3):035004. doi: 10.1088/1468-6996/9/3/035004
126. Talebian N, Amininezhad SM, Doudi M. Controllable Synthesis of ZnO Nanoparticles and Their Morphology-Dependent Antibacterial and Optical Properties. J Photochem Photobiol B (2013) 120:66–73. doi: 10.1016/j.jphotobiol.2013.01.004
127. Nair S, Sasidharan A, Divya Rani VV, Menon D, Nair S, Manzoor K, et al. Role of Size Scale of ZnO Nanoparticles and Microparticles on Toxicity Toward Bacteria and Osteoblast Cancer Cells. J Mater Sci Mater Med (2009) 20 Suppl 1:S235–41. doi: 10.1007/s10856-008-3548-5
128. Heng BC, Zhao X, Xiong S, Ng KW, Boey FY, Loo JS. Cytotoxicity of Zinc Oxide (ZnO) Nanoparticles is Influenced by Cell Density and Culture Format. Arch Toxicol (2011) 85(6):695–704. doi: 10.1007/s00204-010-0608-7
129. Pujalte I, Passagne I, Brouillaud B, Treguer M, Durand E, Ohayon-Courtes C, et al. Cytotoxicity and Oxidative Stress Induced by Different Metallic Nanoparticles on Human Kidney Cells. Part Fibre Toxicol (2011) 8:10. doi: 10.1186/1743-8977-8-10
130. Shao J, Abdelghani M, Shen G, Cao S, Williams DS, van Hest JCM. Erythrocyte Membrane Modified Janus Polymeric Motors for Thrombus Therapy. ACS Nano (2018) 12(5):4877–85. doi: 10.1021/acsnano.8b01772
131. Dai J, Chen Z, Wang S, Xia F, Lou X. Erythrocyte Membrane-Camouflaged Nanoparticles as Effective and Biocompatible Platform: Either Autologous or Allogeneic Erythrocyte-Derived. Mater Today Bio (2022). doi: 10.1016/j.mtbio.2022.100279
132. Huang R, Cai GQ, Li J, Li XS, Liu HT, Shang XL, et al. Platelet Membrane-Camouflaged Silver Metal-Organic Framework Drug System Against Infections Caused by Methicillin-Resistant Staphylococcus Aureus. J Nanobiotechnol (2021) 19(1):229. doi: 10.1186/s12951-021-00978-2
133. Fan Y, Lüchow M, Zhang Y, Lin J, Fortuin L, Mohanty S, et al. Nanogel Encapsulated Hydrogels As Advanced Wound Dressings for the Controlled Delivery of Antibiotics. Advanced Funct Mater (2020) 31(7):2006453. doi: 10.1002/adfm.202006453
134. Keskin D, Zu G, Forson AM, Tromp L, Sjollema J, van Rijn P. Nanogels: A Novel Approach in Antimicrobial Delivery Systems and Antimicrobial Coatings. Bioact Mater (2021) 6(10):3634–57. doi: 10.1016/j.bioactmat.2021.03.004
135. Pinelli F, Saadati M, Zare EN, Makvandi P, Masi M, Sacchetti A, et al. A Perspective on the Applications of Functionalized Nanogels: Promises and Challenges. Int Mater Rev (2022) p:1–25. doi: 10.1080/09506608.2022.2026864
136. Yoshizumi A, Ishii Y, Livermore DM, Woodford N, Kimura S, Saga T, et al. Efficacies of Calcium-EDTA in Combination With Imipenem in a Murine Model of Sepsis Caused by Escherichia Coli With NDM-1 Beta-Lactamase. J Infect Chemother (2013) 19(5):992–5. doi: 10.1007/s10156-012-0528-y
137. King AM, Reid-Yu SA, Wang W, King DT, De Pascale G, Strynadka NC, et al. Aspergillomarasmine A Overcomes Metallo-Beta-Lactamase Antibiotic Resistance. Nature (2014) 510(7506):503–6. doi: 10.1038/nature13445
138. Davies DT, Everett M. Designing Inhibitors of Beta-Lactamase Enzymes to Overcome Carbapenem Resistance in Gram-Negative Bacteria. Acc Chem Res (2021) 54(9):2055–64. doi: 10.1021/acs.accounts.0c00863
139. Chiou J, Wan S, Chan KF, So PK, He D, Chan EW, et al. Ebselen as a Potent Covalent Inhibitor of New Delhi Metallo-Beta-Lactamase (NDM-1). Chem Commun (Camb) (2015) 51(46):9543–6. doi: 10.1039/C5CC02594J
140. Rojas ER, Billings G, Odermatt PD, Auer GK, Zhu L, Miguel A, et al. The Outer Membrane is an Essential Load-Bearing Element in Gram-Negative Bacteria. Nature (2018) 559(7715):617–21. doi: 10.1038/s41586-018-0344-3
141. May KL, Grabowicz M. The Bacterial Outer Membrane is an Evolving Antibiotic Barrier. Proc Natl Acad Sci U S A (2018) 115(36):8852–4. doi: 10.1073/pnas.1812779115
142. Wu S, Yu T, Zhou R, Liang Y, Li Y, Yang J, et al. Adjuvant-Like Biomimetic Nanovesicles Combat New Delhi Metallo-β-Lactamases (NDMs) Producing Superbugs Infections. Nano Today (2021) 38:101185. doi: 10.1016/j.nantod.2021.101185
143. Gonzalez LJ, Bahr G, Nakashige TG, Nolan EM, Bonomo RA, Vila AJ. Membrane Anchoring Stabilizes and Favors Secretion of New Delhi Metallo-Beta-Lactamase. Nat Chem Biol (2016) 12(7):516–22. doi: 10.1038/nchembio.2083
144. Liu JZ, Jellbauer S, Poe AJ, Ton V, Pesciaroli M, Kehl-Fie TE, et al. Zinc Sequestration by the Neutrophil Protein Calprotectin Enhances Salmonella Growth in the Inflamed Gut. Cell Host Microbe (2012) 11(3):227–39. doi: 10.1016/j.chom.2012.01.017
145. Ma B, Fang C, Lu L, Wang M, Xue X, Zhou Y, et al. The Antimicrobial Peptide Thanatin Disrupts the Bacterial Outer Membrane and Inactivates the NDM-1 Metallo-Beta-Lactamase. Nat Commun (2019) 10(1):3517.
146. Kehl-Fie TE, Chitayat S, Hood MI, Damo S, Restrepo N, Garcia C, et al. Nutrient Metal Sequestration by Calprotectin Inhibits Bacterial Superoxide Defense, Enhancing Neutrophil Killing of Staphylococcus Aureus. Cell Host Microbe (2011) 10(2):158–64. doi: 10.1016/j.chom.2011.07.004
147. Zygiel EM, Nolan EM. Transition Metal Sequestration by the Host-Defense Protein Calprotectin. Annu Rev Biochem (2018) 87:621–43. doi: 10.1146/annurev-biochem-062917-012312
148. Wang L-Y, Wang M-J. Removal of Heavy Metal Ions by Poly(vinyl Alcohol) and Carboxymethyl Cellulose Composite Hydrogels Prepared by a Freeze–Thaw Method. ACS Sustain Chem Eng (2016) 4(5):2830–7. doi: 10.1021/acssuschemeng.6b00336
149. Panja S, Hanson S, Wang C. EDTA-Inspired Polydentate Hydrogels With Exceptionally High Heavy Metal Adsorption Capacity as Reusable Adsorbents for Wastewater Purification. ACS Appl Mater Interfaces (2020) 12(22):25276–85. doi: 10.1021/acsami.0c03689
150. Maia MT, Sena DN, Calais GB, Luna FMT, Beppu MM, Vieira RS. Effects of Histidine Modification of Chitosan Microparticles on Metal Ion Adsorption. Reactive Funct Polymers (2020) 154:104694. doi: 10.1016/j.reactfunctpolym.2020.104694
Keywords: antibacterial therapeutics, nanomaterials, zinc ion interference, targeted delivery technology, zinc homeostasis
Citation: Wei Y, Wang J, Wu S, Zhou R, Zhang K, Zhang Z, Liu J, Qin S and Shi J (2022) Nanomaterial-Based Zinc Ion Interference Therapy to Combat Bacterial Infections. Front. Immunol. 13:899992. doi: 10.3389/fimmu.2022.899992
Received: 19 March 2022; Accepted: 27 May 2022;
Published: 30 June 2022.
Edited by:
Luigina Romani, University of Perugia, ItalyReviewed by:
Chunhong Dong, Georgia State University, United StatesCopyright © 2022 Wei, Wang, Wu, Zhou, Zhang, Zhang, Liu, Qin and Shi. This is an open-access article distributed under the terms of the Creative Commons Attribution License (CC BY). The use, distribution or reproduction in other forums is permitted, provided the original author(s) and the copyright owner(s) are credited and that the original publication in this journal is cited, in accordance with accepted academic practice. No use, distribution or reproduction is permitted which does not comply with these terms.
*Correspondence: Junjie Liu, bGl1anVuamllQHp6dS5lZHUuY24=; Shangshang Qin, cWluc2hhbmdzaGFuZ0AxMjYuY29t; Jinjin Shi, c2hpamlueXh5QHp6dS5lZHUuY24=
Disclaimer: All claims expressed in this article are solely those of the authors and do not necessarily represent those of their affiliated organizations, or those of the publisher, the editors and the reviewers. Any product that may be evaluated in this article or claim that may be made by its manufacturer is not guaranteed or endorsed by the publisher.
Research integrity at Frontiers
Learn more about the work of our research integrity team to safeguard the quality of each article we publish.