- 1Department of Molecular Immunology, Ruhr-University Bochum, Bochum, Germany
- 2Department of Molecular Immunology and Cell Biology, Life and Medical Sciences Institute, University of Bonn, Bonn, Germany
- 3Department of Neurology, Ruhr-University Bochum, St. Josef-Hospital, Bochum, Germany
γδ T cells are unconventional T cells, distinguished from αβ T cells in a number of functional properties. Being small in number compared to αβ T cells, γδ T cells have surprised us with their pleiotropic roles in various diseases. γδ T cells are ambiguous in nature as they can produce a number of cytokines depending on the (micro) environmental cues and engage different immune response mechanisms, mainly due to their epigenetic plasticity. Depending on the disease condition, γδ T cells contribute to beneficial or detrimental response. In this review, we thus discuss the dichotomous nature of γδ T cells in cancer, neuroimmunology and infectious diseases. We shed light on the importance of equal consideration for systems immunology and personalized approaches, as exemplified by changes in metabolic requirements. While providing the status of immunotherapy, we will assess the metabolic (and other) considerations for better outcome of γδ T cell-based treatments.
Introduction
T cells and B cells have emerged as primary lymphocytes lineages throughout 500 million years of evolutionary conservation, mainly generating antigen receptor diversity through somatic recombination (1, 2). The broad range of diversity is achieved by the recombination events occurring on human chromosome 7 for TCR γ and β chain genes, and on human chromosome 14 for TCR α and δ chain genes. TCR γ and δ genes in mice are located on chromosomes 13 and 14, respectively (3, 4). The variable regions of TCR chains comprising of variable (V), diversity (D), and joining (J) elements give rise to the broad range of diversity which enables recognition of foreign molecular patterns (3). Conventionally, TCR α and β chains are rearranged and expressed on the surface to become αβ T cells (~95% of CD3+ T cells in human peripheral blood), while TCR γ and δ chain-expressing cells become γδ T cells (~5% of CD3+ T cells in human peripheral blood). TCR γ and δ chain genes are further classified into subfamilies, consequently, the multiple combinations of these TCR family genes generate many functional γδ T-cell subsets such as Vγ9+Vδ2, Vγ9-Vδ2, Vδ1 and Vδ3 which can be paired with various Vγ chains. Depending on the ontogeny of the subset, the phenotypic distribution and ligand recognition change dramatically (5). The Vδ1 subset is abundant in the intestine and gut, but it is a minor population in the peripheral blood. This is in contrast to the Vδ2 subset which is a major population in circulation and a minor subset in the mucosa. The features and functions of both αβ and γδ T cells differ remarkably well depending on the thymic and extrathymic origin, as extrathymic T cells are more functionally “innate” immune cells (6, 7). Mouse γδ T-cell subsets develop through successive but coordinated waves and reside in most of the peripheral tissues. These murine γδ T cells, classified based on TCR γ chains, are mainly found in two functional states depending on interferon-γ (IFN-γ) or interleukin-17 (IL-17) production (8).
γδ T cells possess a unique potential of functional plasticity. Within the tumor milieu, γδ T cells produce cytokines (e.g. IFN-γ or IL-17), which are associated with the prognosis of different kinds of cancers. This dual roles of human γδ T cells in cancer has been recently reviewed (9). Since γδ T cells possess a dichotomous nature in cancer, autoimmunity and infections, this review will focus on mechanisms of γδ T cells in those areas; however, being aware about the fact there are important advances in other fields such as modes of antigen recognition (5, 10), fetal ontology (11, 12), and involvement in hepatic or gastro-intestinal diseases (13–16), which is out of the scope of this review. Apart from the basic understanding of γδ T-cell subsets and their function in health and diseases, the use of γδ T cells for immunotherapeutic applications is of great interest. Additionally, other recent technological advances such as single-cell omics, 3D organoid models or humanized mice will facilitate the progress in harnessing the therapeutic potential of human γδ T cells. In this review, we discuss the pivotal features of γδ T cells and their potential for therapeutic approaches.
Dichotomy of γδ T Cells
γδ T cells in Cancers – a Double-Edged Sword
Due to their potential of immunoserveillance and anti-tumor response, γδ T cells are found to be involved in several types of cancer including hematological malignancies (17), glioblastoma (18), gastric (19), colorectal (20) and breast cancer (21). Dysregulated mevalonate metabolism in cancer cells often leads to the accumulation of phosphoantigens (pAg) such as Isopentenyl Pyrophosphate (IPP), which potentiates Vγ9Vδ2 T-cell cytotoxicity (22). IPP can be released to the extracellular space where it is recognized by Vδ2 T cells via ATP-binding cassette transporter A1 (ABCA1) and apoliprotein A-I (apoA-1) (23). Recent studies have shown that phosphoantigens are bound by butyrophilins (BTN), specifically BTN3A1 and BTN2A1, which then interact with the TCR of Vδ2 T cells. Formation of such a signaling complex results in Vδ2 T-cell activation and in the anti-tumor activity (24, 25). Though the molecular details of butyrophilins- γδ TCR signaling complex is largely unknown, a landmark study showed that BTN3A1 (an isoform of CD277) and its intracellular B30.2 domain are absolutely essential for inside-out signaling to activate Vδ2 T cells (26), which is further modulated by Rho-GTPase (27). Dissecting this molecular complexity further, it was revealed that Vγ9Vδ2 TCR is required initially for T-cell activation and formation of immune synapse (IS) with CD277 (recruiting BTN3A1 and BTN2A1, independent of pAg), upon which latter provides mandatory coactivation signal and stabilizes IS in a pAg-dependent manner (28).
In addition to the butyrophilins (as mentioned above) and B7 superfamily-like proteins, major histocompatibility class (MHC) - like antigens and immunoglobulin (Ig) -like antigens have also been identified as antigens for γδ T-cell subsets [extensively reviewed in (5)]. For example, Vδ1 T cells recognize self-derived or foreign lipids bound by the CD1d molecule on the surface of target cells (29, 30). Other interesting examples of MHC-like antigens are MHC-related protein 1 (MR1), ephrin type-A receptor 2 (EphA2) and endothelial protein C receptor (EPCR) (31–33). MR1 is a Vitamin B precursor and known antigen for mucosal associated invariant T cells, but recently shown to be recognized by Vδ1 T cells from healthy individuals and in some diseases (31). EphA2 and EPCR are well known stress-ligands. EPCR serves as a ligand for human Vγ4Vδ5 subset-specific recognition of endothelial cells infected by cytomegalovirus and epithelial tumors (33). Not only EPCR, annexin A2 (an Ig-like antigen) is also recognized by Vγ8Vδ3 subset during cellular stress surveillance (34). Interestingly, non-physiological molecules like red algal protein phycoerythrin have also been reported as antigens for human and murine IL-17-producing γδ T cells (35). Furthermore, the functional plasticity of γδ T cells includes a response mediated by CD16 and thus participates in the antibody-dependent cellular cytotoxicity (ADCC). It has been shown to enhance Vδ2 T cell function towards lymphoma cells with the use of anti-CD20 (36, 37). Vγ9Vδ2 T-cell cytotoxicity can also be mediated by the production of cytokines (e.g. IFN-γ and TNF-α), cytotoxic (e.g. granzymes) and apoptotic molecules (e.g. TRAIL), and/or via NKG2D receptor-ligand axis (22).
γδ T cells are highly pleiotropic in function as they possess both anti-tumor and pro-tumor activities in the tumor microenvironment (TME). γδ T cells are the early producers of IFN-γ during tumorigenesis (38), while IL-2 and IL-15 are the potent inducers of cytotoxic potential (39, 40), which provide an important cancer immunomodulating factor to promote other cytotoxic T lymphocyte responses. Tumor-infiltrating γδ T cells preferentially produce IFN-γ and are positively associated with better patient outcome in case of colon cancer (20). Also, intracellular IFN-γ expression only after phorbol ester and ionomycin (PMA/Iono) stimulation was remarkable in γδ T cells from TME of ovarian cancer (41). IFN-γ producing γδ T cells exert their anti-tumor functions by upregulating MHC class I molecules and CD54, thus further enhancing CD8 T-cell-mediated killing (42). Conversely, γδ T cells producing IL-17 have been suggested to negatively impact the progression of colon (43), gallbladder (44), and breast cancer (45), either by suppressing immune cell functions, promoting immune cell pro-tumor activity, or by inducing angiogenesis. Hypoxia, which is commonly found in solid tumors, was attributed to reduce cytotoxic activity of γδ T cells in oral cancer patients (46) and enhance IL-17 production. Furthermore, γδ T cells provide pro-tumor inflammatory conditions and thus favor tumor progression, participating in most of the hallmarks of cancer (47, 48).
γδ T Cells in Autoimmune Disease of the Central Nervous System
Classically, γδ T cells are known to possess the properties of innate immune cells such as rapid expression of IFN-γ or IL-17 in response to cytokine supplementation without TCR engagement. TME drives IL-17 production in γδ T cells and hence provide evidence for the γδ T-cell function as a consequence of (micro) environmental signals. The capacity to produce IL-17 is attributed to epigenetic regulation (49). IL-17 producing γδ T cells are implicated in autoimmunity and inflammatory conditions. Results from experimental autoimmune encephalomyelitis (EAE), a mouse model of multiple sclerosis (MS), provide evidence that γδ T cells serve as important source of cytokines IL-17 and IL-23 and consequently amplify IL-17 production by Th17 cells (50). Vice versa, IL-17A is also important for the recruitment of IL-1β secreting myeloid cells that prime pathogenic γδT17 and Th17 cells in EAE (51), suggesting a regulatory loop. One effect of IL-17 producing γδ T cells is to interfere with regulatory T cells (Treg) development by preventing the conversion of conventional T cells into Foxp3+ Treg cells as elicited using IL23R reporter mice (52). This observation was additionally supported by enhanced antigen-specific T cell responses by γδ T cells. In EAE, Vγ4+IL-17 producing γδ T cells differentiate in the draining lymph nodes, mediated by IL23R and through activation of Il17 locus, but not via IL-1R1 (53).
Those alterations hold also true for MS. Using single-cell RNA-seq and spatial transcriptomics Th17/Tfh cells have been identified as cellular marker of MS disease progression (54). Though EAE is skewed towards IL-17 producing γδ T cells, studies in human have shown a more remarkable association of MS with IFN-γ producing γδ T cells. Vδ1 T cells were shown to produce a high amount of IFN-γ in newly diagnosed, untreated MS patients, which was decreased by treatment with natalizumab (55). Contrarily, single or dual expression of IFN-γ and IL-17 by Vδ2 T cells is lower in MS patients compared to healthy controls (56). There is also evidence of direct cytotoxicity towards oligodendrocytes by γδ T cells (57). γδ T cells could therefore serve as marker of disease activity. Circulating CCR5+ γδ T cells are decreased during MS relapse in line with higher frequency of IFN-γ+ γδ T cells, assuming a Th1 profile (58). Hence, beside other nonconventional immune cells, single-cell resolution identified specific γδ T cell subsets as contributor of MS disease activity with potential as therapeutic target.
γδ T Cells in Infection
IL-17 production by Th17 cells is usually associated with protection against bacterial and fungal infection through their effector function (59). In 2009, the pivotal role of CCR6+ γδ T cells characterized by IL-17 production, innate receptor expression and recruitment of neutrophils was identified for the first time as first line response to mycobacteria and Candida albicans (60). Unlike αβ T cells, IL-17 producing γδ T cells are not associated with the engagement of TCR (50, 60, 61). These observations are highly intriguing, since transcriptionally distinct αβ-γδ co-expressing T cells have been discovered, which produce IL-17 upon stimulation by IL-1β and IL-23 and play a pathogenic role in the CNS autoimmunity in EAE. The characterization of TCR revealed that these hybrid αβ-γδ T cells are mainly Vγ4+ and TCRβ+ and importantly, provide protection against Staphylococcus aureus infection (62). This is consistent with findings showing that Vγ6+Vδ4+ T cells are clonally expanded in skin-draining lymph nodes after S. aureus infection in mice. RNA-seq analysis of TRG and TRD sequences revealed the clonal expansion of TRGV5, TRGV6, and TRDV4 (63). In contrast to murine γδ T cells, human γδ T cells play a diverse role in infection immunity. Human Vδ2 T cells have been known to respond strongly to phosphoantigens such as (E)-4-hydroxy-3-methyl-but-2-enyl pyrophosphate (HMBPP) which is a metabolite produced by microbes via the 2-c-methyl-D-erythritol 4-phosphate (MEP) pathway (64). Vδ2 cells are protective in Plasmodium falciparum infection (65). Recently, it was shown that γδ T cells kill infected red blood cells by phagocytosis and opsonization via CD16, in addition to the BTN3A1-TCR mediated degranulation process (66). This host defense mechanism adds a new aspect to the γδ T-cell function and engagement during immune response. Whether the phagocytotic machinery of γδ T cells is also involved during a response to other pathogens remains to be determined.
For a long time, the role of γδ T cells in mycobacterial infection has been studied in human and animal models. Mycobacterium tuberculosis (Mtb) was one of the first bacteria described to induce γδ T cell immune responses (67) by recognizing Mtb antigens. Bacillus Calmette-Guérin (BCG) vaccine, the only vaccine protecting against tuberculosis, has been broadly administered worldwide and it has been shown to generate a protective Vδ2 T cell memory response against Mtb infection (68). BCG has been also suggested to provide heterologous protection against infections that are not related to Mtb (69–72). In fact, in the early 90s, it was shown that in vitro pre-expanded γδ T cells with M. tuberculosis were able to proliferate in response to re-challenge with unrelated pathogens such as Listeria monocytogenes, group A streptococci or S. aureus (73). These results indicate that human γδ T cell responses are not pathogen-specific therefore raising the question, whether BCG-induced γδ T cells contribute to their cross-protective effect and whether they can develop innate immune cell memory referred to as “trained immunity”. Trained immunity was first described in monocytes and macrophages (which have a shorter half-life) (74, 75). Therefore, those might be less suitable vaccination targets to provide long-term protection. The characterization of trained immunity in long-lived γδ T cells could potentially open new avenues in designing effective vaccines with cross-protective effects. Whether immune memory responses of γδ T cells contribute to the protection induced by other vaccines still needs to be explored.
Altogether, it is crucial to broaden the mechanistic knowledge about the role of γδ T cells in infections using systems immunology approaches such as single-cell multi-omics to provide better therapeutic interventions. This is especially vital for the development of new generation vaccines, which would not only trigger αβ T cell memory responses but also harness the therapeutic potential of γδ T cells.
γδ T Cells in Systems Immunology – a Holistic Approach
The systems approach is defined by the use of a broad strategy for understanding the outcome of a complex set of components (76). Multi-omics methods and systems immunology measures help to investigate changes in the proteome, phenome, transcriptome, epigenome, metabolome, microbiome as well as cell-to-cell communication, all of which shape immune cell responses. A decade ago, these measurements were performed on a bulk cell population. Nowadays, it is possible to use these methods at a single-cell resolution. Such datasets are made publicly available by international consortia such as ImmGen (https://www.immgen.org) for mouse immune cells and the cell atlas for humans (https://www.humancellatlas.org). Additionally, due to increasing efforts to combine interdisciplinary approaches such as computational biology together with data science and machine learning (77), a comprehensive study of immune cells and their responses is feasible in defining disease severity/progression, therapeutic response, or even vaccine effects. Indeed, a new field of “systems vaccinology” has emerged with the aim to comprehensively analyze the immune response to vaccination and understand potential new mechanisms of protection (78). This will allow immunologists to consider the individual human variation, possibly identifying the reasons driving differential immune responses. This holds specifically true for γδ T cell responses, which are largely shaped by environmental factors and not genetic control (79). A milestone study for chimeric antigen receptor (CAR) T cells by Melenhorst et al. (2022) shows an impeccable use of single-cell multi-omics and systems immunology methods (80). In this longitudinal study, authors analyzed CD19 chimeric antigen receptor (CAR) T cells in two chronic lymphocytic leukemia patients over 10 years after successful CAR T cell transfer. In the earlier time-points, CD4-CD8-Helioshi CAR T cells in one of these patients were found using cytometry-by-flight (CyTOF) method. Further characterization using 5’cellular indexing of transcriptomes and epitopes by sequencing (CITE-seq) with TCR-seq found that these cells were γδ CAR T cells with specific TRDV1 and TRGV4 gene expression. However, long-term surviving CAR T cells were predominated by the CD4+ T cell population with cytotoxic properties especially at later time points. Yet, the origins and contribution of these cells to the remission remains to be determined. Despite a limited number of patients analyzed, this study is the first to show the potential of systems biology and single cell omics to understand the efficacy of CAR T-cell immunotherapy.
Another good example emphasizing the importance of the systems immunology approach is to evaluate the prognostic significance of immune cells in various cancers using CIBERSORT (a machine learning-based algorithm), where γδ T cells have emerged as the most favorable leukocyte with global prognostic association across 25 human cancers (81). Optimizing this computational identification approach further, tumor-infiltrating Vγ9Vδ2 T cells were variably associated with disease outcome due to considerable high inter-individual variation in its abundance (82). A combination of flow cytometry and sequencing results with the help of single sample gene set enrichment analysis (ssGSEA) method has inferred abundance of 24 immune cell types in cancer including γδ T cells. This algorithm called “Immune Cell Abundance Identifier (ImmuneCellAI)” could accurately predict response to anti-PD1 immunotherapy (83). To a limited extent, we have previously used a comprehensive approach to assess the disease progression and therapeutic response in patients with γδ T cells malignancies (84, 85). Though the transcriptome and epigenome of γδ T cells are already available, the focus is now shifted to single-cell studies (Table 1) as it allows to create a compendium of cell types as exemplified in mice (104) and humans (105, 106).
An emerging area in system immunology and single-cell methodology is to decipher the metabolic changes in γδ T cells during the development and differentiation process. Mechanistic target of rapamycin complex 1 (mTORC1) regulates a distinct metabolic requirement for thymic development of αβ and γδ T cells. Interestingly, mTORC1 signaling further coordinates developmental signals with TCR and NOTCH pathways (107). Diving into the details of metabolic requirements at a single-cell level, Single Cell ENergetIc metabolism (SCENITH) has been recently developed and used to assess γδ T cell energy metabolism (108, 109). Consequently, this study found that the metabolic requirements of IL-17+ γδ T cells are imprinted during early thymic development and are maintained in the periphery and tumor of obese mice (109). A distinct metabolic usage by IFN-γ+ versus IL-17+ γδ T cells shows a need for glycolysis versus oxidative metabolism, respectively. Interestingly, glucose supplementation elevated the anti-tumor function of IFN-γ+ γδ T cells (109). Similarly, altered tumor metabolism also needs to be studied as it is sensed by γδ T cells (32), which may ultimately implicates γδ T cell responses in TME causing hypoxia (46, 110) or tumor resistance (111). Moreover, recent reports highlight a crucial role of γδ T cells in thermogenesis and sympathetic innervation (112, 113). Furthermore, the ketogenic diet has been shown to expand protective γδ T cells during an infection with influenza virus in the lungs (114) and which restrain inflammation in adipose tissues (97). However, prolonged intake of the ketogenic diet causes obesity and significantly reduces the adipose tissue-resident γδ T cells (97). Thus, targeting metabolic changes together with transcriptional changes will help to understand the γδ T-cell differentiation process and its implications in diseases.
γδ T Cell-Based Immunotherapy: Missing Links and Unexplored Avenues
Due to their unique characteristics distinct from conventional αβ T cells, γδ T cells are an attractive cellular target for allogeneic transfer as exemplified by the recent phase I clinical trial in 132 late-stage cancer patients (115) and ongoing clinical trial on patients with solid tumors (https://clinicaltrials.gov/ct2/show/NCT04765462). The concept of γδ T cell-based immunotherapy has been under development for more than a decade. Earlier, the immunotherapy with γδ T cells for cancer was mainly based on two approaches: in vivo activation of γδ T cells using aminobisphonates (e.g. zoledronate) and adoptive transfer of in vitro expanded γδ T cells (116). The approach of in vivo activation of γδ T cells has extended its toolbox. Because of the basic research on γδ T-cell activation (26), the molecular mediators of activation (e.g. butyrophilins) can be targeted to improve immunotherapy outcome. In an ongoing clinical trial, monoclonal anti-BTN3A1 antibodies (ICT01) are administered alone or in combination with the checkpoint-inhibitor pembrolizumab for hematological cancers (https://www.clinicaltrials.gov/ct2/show/NCT04243499). Besides checkpoint inhibitors (117), other immunomodulatory agents that could be used in combination with γδ T cell-based immunotherapy include epigenetic drugs (118, 119), toll-like receptor ligands (120), or even bispecific antibodies targeting Vγ9 chains of Vγ9Vδ2 T cells (121). Since most of these modulators have been proposed or shown clinical relevance in vitro, many are being tested in a clinical trial. Likewise, the approach of using adoptive transfer of in vitro expanded γδ T cells is now suggested to be supplemented with biomaterials such as cytokines [e.g. TGF-β (122)] or nutrients [e.g. Vitamin C (123)]. Furthermore, engineered cytokines have emerged as an attractive tool to improve T cell immunotherapy by modulating cell expansion, persistence, tumor homing and adaptation to TME (124). As engineered IL-2 and IL-15 are already in clinical trials, their use in γδ T cell-based therapies might also be beneficial. The use of naturally occurring nutrient supplementation such as Vitamin C could potentially be beneficial too. However, the metabolic requirement and effect of these nutrients need to be considered given the inter-individual variation in γδ T cell frequency and their subset distribution due to age, gender, and race (125). These needs can be complemented for a more personalized approach to immunotherapy to provide benefit to patients.
γδ T cell-based immunotherapy has been progressing over the last decades along with the development of T cell-based therapies (Figure 1). The novel first-in-class approach is being exploited to apply genetically modified γδ T cells in human (138). Previously, the Vδ1 subset of γδ T cells were designed and applied as a new cellular product called “DOT cells” for adoptive immunotherapy of leukemia patients (17, 139). Further advancing the approach of genetic modifications, CAR γδ T cell therapy is under investigation (114, 140, 141). The patient-derived xenograft model showed anti-leukemic activity and IL-15-mediated long-term persistence of CD123-CAR-DOTs in acute myeloid leukemia (142). Following the United States Food and Drug Administration (US FDA) approval for ADI-01 in the year 2020, an allogenic CAR γδ T cell therapy targeting CD20 protein was manufactured at clinical scale. As observed in a preclinical study with B-cell malignancies, CAR γδ T cell therapy generated an innate and adaptive anti-tumor immune response but no xenogeneic graft-versus-host disease (143). This CD20 CAR γδ T cell therapy is now in a phase I clinical trial (https://www.clinicaltrials.gov/ct2/show/NCT04735471). The interim analysis of the ADI-01 phase I clinical trial showed that 50% patients achieved complete response (CR) while 75% patients achieved objective response rate (ORR) without any ADI-01-related serious adverse events using CD20-CAR γδ T cell therapy (press release dated on December 6, 2021; https://www.adicetbio.com). Furthermore, the ADI-01 therapy has been just granted the Fast Track Designation by the US FDA raising hopes for even faster implementation of γδ T cell-based therapies into daily clinical practice (press release dated on April 19, 2022; https://www.adicetbio.com). CAR T cell therapy is being broadened towards T cells engineered with γδTCR (TEG) (138, 144). With approval by US FDA, genetically modified γδ T cells might benefit a broad spectrum of cancer patients. In the future, targeting γδ T cells with tailored immunotherapies might also be a potential new avenue for the treatment of other diseases such as MS and infections.
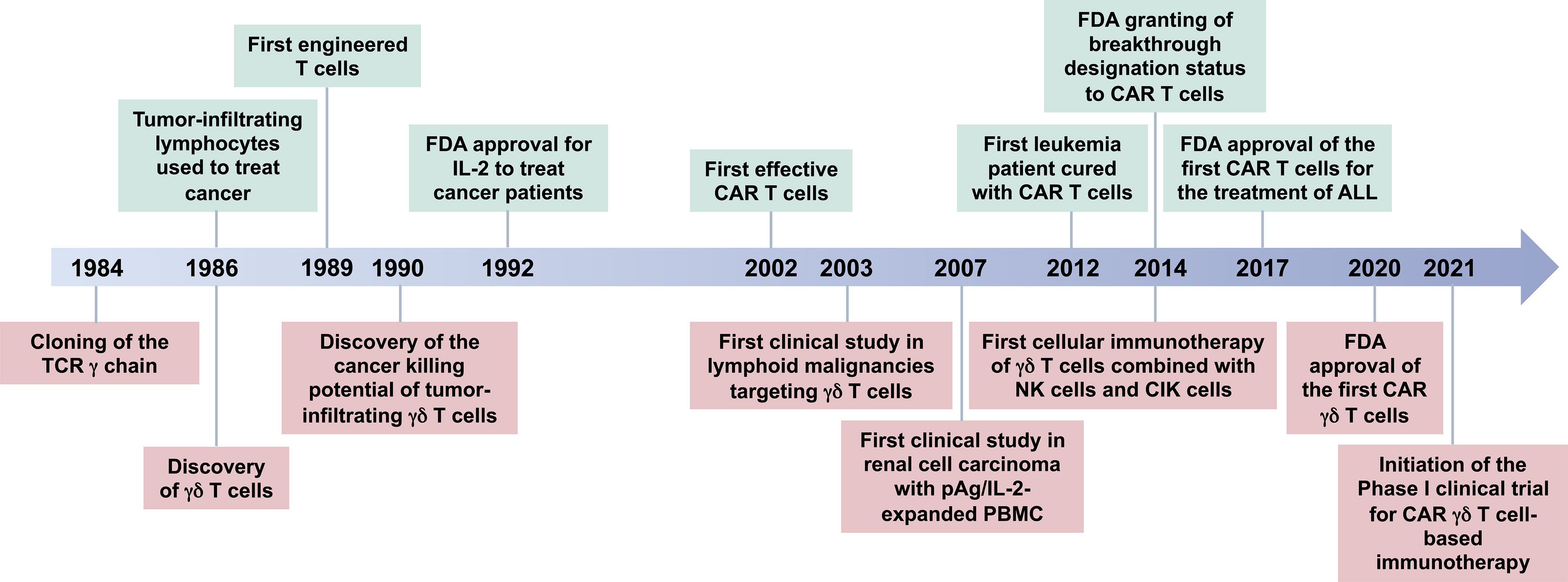
Figure 1 Timeline for γδ T-cell-based immunotherapy. A brief history of the breakthrough findings that led to the development of γδ T cell-based immunotherapies. γδ T cells were discovered in 1986 (126, 127), after accidental cloning of the gamma chain of the T cell receptor (TCR) in 1984 (128). At the same time, Rosenberg’s group started to treat cancer patients with their own tumor-infiltrating lymphocytes leading to the first patient to be cured from cancer using this method (129, 130). Fast forward from 1989 to year 2017 (131–137), the FDA approved the first CAR T cells for the treatment of B-cell lymphomas, Kymriah® and Yescarta® developed by Novartis Pharmaceuticals Corp. (https://www.hcp.novartis.com/home/) and Kite Pharma, Inc. (https://www.kitepharma.com/), respectively. The first big success came in 2020 for CAR therapy with γδ T cells, when the FDA cleared an investigational new drug (IND) application and orphan drug designation for GDX012 (an allogenic Vδ1 T-cell-based therapy) developed by Lymphact and later GammaDelta Therapeutics (https://gammadeltatx.com/). Also, at the same time, the Adicet Bio (https://www.adicetbio.com/) received the FDA approval for an IND application ADI-01, an allogenic CAR γδ T cell therapy targeting CD20 protein in non-Hodgkin lymphomas. In 2021, the first Phase I Clinical Trials of γδ T-cell-based immunotherapies were initiated. TCR, T-cell receptor; FDA, The United States Food and Drug Administration; IL-2, interleukin-2; CAR, chimeric antigen receptor; pAg, phosphoantigen; PBMC, peripheral blood mononuclear cells; ALL, acute lymphoblastic leukemia; NK cells, natural killer cells; CIK cells, cytokine-induced killer cells.
Conclusion
Though neglected for a long time, γδ T cells have emerged as a key immune cell type, especially in cancer biology and are already investigated in clinical trials. Its pleiotropic role is further being investigated in other disorders including immune diseases such as MS, infections or transplantation. Use of “big data” and integrative multi-omics approaches enable us to more specifically unravel molecular mechanisms. This is complemented by the implementation of new methods such as 3D organoids combined with state-of-the-art technologies such as spatial transcriptomics. However, γδ T cells still require a clinical testing model for development of immunotherapy. Altogether, targeting γδ T cells will allow us to more precisely address a broad range of conditions, eventually allowing a γδ T cell targeted personalized immunotherapy.
Author Contributions
JB and KP conceptualized and wrote the first draft of manuscript. JB, KP, and SF contributed to the discussion and made final corrections. All authors contributed to the article and approved the submitted version.
Funding
This work was supported by the Ruhr University Bochum, Germany to JB. KP has received funding from the European Union’s Horizon 2020 research and innovation programme under the Marie Skłodowska-Curie grant agreement No. 798582 and from the Deutsche Forschungsgemeinschaft (DFG, German Research Foundation under Germany's Excellence Strategy - EXC2151 - 390873048.
Conflict of Interest
The authors declare that the research was conducted in the absence of any commercial or financial relationships that could be construed as a potential conflict of interest.
Publisher’s Note
All claims expressed in this article are solely those of the authors and do not necessarily represent those of their affiliated organizations, or those of the publisher, the editors and the reviewers. Any product that may be evaluated in this article, or claim that may be made by its manufacturer, is not guaranteed or endorsed by the publisher.
Acknowledgments
We thank Prof. Ingo Schmitz for reading and support to the manuscript. We apologize to all the colleagues whose studies cannot be cited due to space constraint. We acknowledge support by the Open Access Publication Funds of the Ruhr-Universität Bochum.
References
1. Hirano M, Guo P, McCurley N, Schorpp M, Das S, Boehm T, et al. Evolutionary Implications of a Third Lymphocyte Lineage in Lampreys. Nature (2013) 501(7467):435–8. doi: 10.1038/nature12467
2. Willcox BE, Willcox CR. γδ TCR Ligands: The Quest to Solve a 500-Million-Year-Old Mystery. Nat Immunol (2019) 20(2):121–8. doi: 10.1038/s41590-018-0304-y
3. Glusman G, Rowen L, Lee I, Boysen C, Roach JC, Smit AFA, et al. Comparative Genomics of the Human and Mouse T Cell Receptor Loci. Immunity (2001) 15(3):337–49. doi: 10.1016/S1074-7613(01)00200-X
4. Baum T-P, Hierle V, Pasqual N, Bellahcene F, Chaume D, Lefranc M-P, et al. IMGT/GeneInfo: T Cell Receptor Gamma TRG and Delta TRD Genes in Database Give Access to All TR Potential V(D)J Recombinations. BMC Bioinf (2006) 7(1):224. doi: 10.1186/1471-2105-7-224
5. Deseke M, Prinz I. Ligand Recognition by the γδ TCR and Discrimination Between Homeostasis and Stress Conditions. Cell Mol Immunol (2020) 17(9):914–24. doi: 10.1038/s41423-020-0503-y
6. Blais M-È, Brochu S, Giroux M, Bélanger M-P, Dulude G, Sékaly R-P, et al. Why T Cells of Thymic Versus Extrathymic Origin Are Functionally Different. J Immunol (2008) 180(4):2299–312. doi: 10.4049/jimmunol.180.4.2299
7. Melandri D, Zlatareva I, Chaleil RAG, Dart RJ, Chancellor A, Nussbaumer O, et al. The γδtcr Combines Innate Immunity With Adaptive Immunity by Utilizing Spatially Distinct Regions for Agonist Selection and Antigen Responsiveness. Nat Immunol (2018) 19(12):1352–65. doi: 10.1038/s41590-018-0253-5
8. Ribot JC, Lopes N, Silva-Santos B. γδ T Cells in Tissue Physiology and Surveillance. Nat Rev Immunol (2021) 21(4):221–32. doi: 10.1038/s41577-020-00452-4
9. Li Y, Li G, Zhang J, Wu X, Chen X. The Dual Roles of Human γδ T Cells: Anti-Tumor or Tumor-Promoting. Front Immunol (2021) 11. doi: 10.3389/fimmu.2020.619954
10. Herrmann T, Karunakaran MM. Butyrophilins: γδ T Cell Receptor Ligands, Immunomodulators and More. Front Immunol (2022) 13. doi: 10.3389/fimmu.2022.876493
11. Clark BL, Thomas PG. A Cell for the Ages: Human γδ T Cells Across the Lifespan. Int J Mol Sci (2020) 21(23):8903. doi: 10.3390/ijms21238903
12. Xu W, Lau ZW, Fulop T, Larbi A. The Aging of γδ T Cells. Cells (2020) 9(5):1181. doi: 10.3390/cells9051181
13. Wang X, Tian Z. γδ T Cells in Liver Diseases. Front Med (2018) 12(3):262–8. doi: 10.1007/s11684-017-0584-x
14. Rajoriya N, Fergusson JR, Leithead JA, Klenerman P. Gamma Delta T-Lymphocytes in Hepatitis C and Chronic Liver Disease. Front Immunol (2014) 5. doi: 10.3389/fimmu.2014.00400
15. Catalan-Serra I, Sandvik AK, Bruland T, Andreu-Ballester JC. Gammadelta T Cells in Crohn’s Disease: A New Player in the Disease Pathogenesis? J Crohn's Colitis (2017) 11(9):1135–45. doi: 10.1093/ecco-jcc/jjx039
16. Suzuki T, Hayman L, Kilbey A, Edwards J, Coffelt SB. Gut Gammadelta T Cells as Guardians, Disruptors, and Instigators of Cancer. Immunol Rev (2020) 298(1):198–217. doi: 10.1111/imr.12916
17. Di Lorenzo B, Simões AE, Caiado F, Tieppo P, Correia DV, Carvalho T, et al. Broad Cytotoxic Targeting of Acute Myeloid Leukemia by Polyclonal Delta One T Cells. Cancer Immunol Res (2019) 7(4):552–8. doi: 10.1158/2326-6066.CIR-18-0647
18. Chauvin C, Joalland N, Perroteau J, Jarry U, Lafrance L, Willem C, et al. NKG2D Controls Natural Reactivity of Vγ9vδ2 T Lymphocytes Against Mesenchymal Glioblastoma Cells. Clin Cancer Res (2019) 25(23):7218–28. doi: 10.1158/1078-0432.CCR-19-0375
19. Wang J, Lin C, Li H, Li R, Wu Y, Liu H, et al. Tumor-Infiltrating γδt Cells Predict Prognosis and Adjuvant Chemotherapeutic Benefit in Patients With Gastric Cancer. Oncoimmunology (2017) 6(11):e1353858. doi: 10.1080/2162402X.2017.1353858
20. Meraviglia S, Lo Presti E, Tosolini M, La Mendola C, Orlando V, Todaro M, et al. Distinctive Features of Tumor-Infiltrating γδ T Lymphocytes in Human Colorectal Cancer. Oncoimmunology (2017) 6(10):e1347742. doi: 10.1080/2162402X.2017.1347742
21. Boissière-Michot F, Chabab G, Mollevi C, Guiu S, Lopez-Crapez E, Ramos J, et al. Clinicopathological Correlates of γδ T Cell Infiltration in Triple-Negative Breast Cancer. Cancers (2021) 13(4):765. doi: 10.3390/cancers13040765
22. Todaro M, D'Asaro M, Caccamo N, Iovino F, Francipane MG, Meraviglia S, et al. Efficient Killing of Human Colon Cancer Stem Cells by γδ T Lymphocytes. J Immunol (2009) 182(11):7287–96. doi: 10.4049/jimmunol.0804288
23. Castella B, Kopecka J, Sciancalepore P, Mandili G, Foglietta M, Mitro N, et al. The ATP-Binding Cassette Transporter A1 Regulates Phosphoantigen Release and Vγ9vδ2 T Cell Activation by Dendritic Cells. Nat Commun (2017) 8(1):15663. doi: 10.1038/ncomms15663
24. Sandstrom A, Peigne CM, Leger A, Crooks JE, Konczak F, Gesnel MC, et al. The Intracellular B30.2 Domain of Butyrophilin 3A1 Binds Phosphoantigens to Mediate Activation of Human Vgamma9Vdelta2 T Cells. Immunity (2014) 40(4):490–500. doi: 10.1016/j.immuni.2014.03.003
25. Rigau M, Ostrouska S, Fulford TS, Johnson DN, Woods K, Ruan Z, et al. Butyrophilin 2A1 is Essential for Phosphoantigen Reactivity by γδ T Cells. Science (2020) 367:eaay5516. doi: 10.1126/science.aay5516
26. Harly C, Guillaume Y, Nedellec S, Peigne CM, Monkkonen H, Monkkonen J, et al. Key Implication of CD277/butyrophilin-3 (BTN3A) in Cellular Stress Sensing by a Major Human Gammadelta T-Cell Subset. Blood (2012) 120(11):2269–79. doi: 10.1182/blood-2012-05-430470
27. Sebestyen Z, Scheper W, Vyborova A, Gu S, Rychnavska Z, Schiffler M, et al. RhoB Mediates Phosphoantigen Recognition by Vgamma9Vdelta2 T Cell Receptor. Cell Rep (2016) 15(9):1973–85. doi: 10.1016/j.celrep.2016.04.081
28. Vyborova A, Beringer DX, Fasci D, Karaiskaki F, van Diest E, Kramer L, et al. γ9δ2t Cell Diversity and the Receptor Interface With Tumor Cells. J Clin Invest (2020) 130(9):4637–51. doi: 10.1172/JCI132489
29. Uldrich AP, Le Nours J, Pellicci DG, Gherardin NA, McPherson KG, Lim RT, et al. CD1d-Lipid Antigen Recognition by the Gammadelta TCR. Nat Immunol (2013) 14(11):1137–45. doi: 10.1038/ni.2713
30. Bai L, Picard D, Anderson B, Chaudhary V, Luoma A, Jabri B, et al. The Majority of CD1d-Sulfatide-Specific T Cells in Human Blood Use a Semiinvariant Vdelta1 TCR. Eur J Immunol (2012) 42(9):2505–10. doi: 10.1002/eji.201242531
31. Le Nours J, Gherardin Nicholas A, Ramarathinam H Sri, Awad W, Wiede F, Gully Benjamin S, et al. A Class of γδ T Cell Receptors Recognize the Underside of the Antigen-Presenting Molecule MR1. Science (2019) 366(6472):1522–7. doi: 10.1126/science.aav3900
32. Harly C, Joyce Stephen P, Domblides C, Bachelet T, Pitard V, Mannat C, et al. Human γδ T Cell Sensing of AMPK-Dependent Metabolic Tumor Reprogramming Through TCR Recognition of Epha2. Sci Immunol (2021) 6(61):eaba9010. doi: 10.1126/sciimmunol.aba9010
33. Willcox CR, Pitard V, Netzer S, Couzi L, Salim M, Silberzahn T, et al. Cytomegalovirus and Tumor Stress Surveillance by Binding of a Human γδ T Cell Antigen Receptor to Endothelial Protein C Receptor. Nat Immunol (2012) 13(9):872–9. doi: 10.1038/ni.2394
34. Marlin R, Pappalardo A, Kaminski H, Willcox Carrie R, Pitard V, Netzer S, et al. Sensing of Cell Stress by Human γδ TCR-Dependent Recognition of Annexin A2. Proc Natl Acad Sci (2017) 114(12):3163–8. doi: 10.1073/pnas.1621052114
35. Zeng X, Wei Y-L, Huang J, Newell Evan W, Yu H, Kidd Brian A, et al. γδ T Cells Recognize a Microbial Encoded B Cell Antigen to Initiate a Rapid Antigen-Specific Interleukin-17 Response. Immunity (2012) 37(3):524–34. doi: 10.1016/j.immuni.2012.06.011
36. Mounia Sabrina B, Bernard K, Geneviève F, Jean-François R. γδ T-Cell Killing of Primary Follicular Lymphoma Cells is Dramatically Potentiated by GA101, a Type II Glycoengineered Anti-CD20 Monoclonal Antibody. Haematologica (2011) 96(3):400–7. doi: 10.3324/haematol.2010.029520
37. Tokuyama H, Hagi T, Mattarollo SR, Morley J, Wang Q, So HF, et al. V Gamma 9 V Delta 2 T Cell Cytotoxicity Against Tumor Cells is Enhanced by Monoclonal Antibody Drugs–Rituximab and Trastuzumab. Int J Cancer (2008) 122(11):2526–34. doi: 10.1002/ijc.23365
38. Gao Y, Yang W, Pan M, Scully E, Girardi M, Augenlicht LH, et al. γδ T Cells Provide an Early Source of Interferon γ in Tumor Immunity. J Exp Med (2003) 198(3):433–42. doi: 10.1084/jem.20030584
39. Ribot JC, Ribeiro ST, Correia DV, Sousa AE, Silva-Santos B. Human γδ Thymocytes Are Functionally Immature and Differentiate Into Cytotoxic Type 1 Effector T Cells Upon IL-2/IL-15 Signaling. J Immunol (2014) 192(5):2237–43. doi: 10.4049/jimmunol.1303119
40. Silva-Santos B, Mensurado S, Coffelt SB. γδ T Cells: Pleiotropic Immune Effectors With Therapeutic Potential in Cancer. Nat Rev Cancer (2019) 19(7):392–404. doi: 10.1038/s41568-019-0153-5
41. Oberg HH, Janitschke L, Sulaj V, Weimer J, Gonnermann D, Hedemann N, et al. Bispecific Antibodies Enhance Tumor-Infiltrating T Cell Cytotoxicity Against Autologous HER-2-Expressing High-Grade Ovarian Tumors. J Leukocyte Biol (2020) 107(6):1081–95. doi: 10.1002/JLB.5MA1119-265R
42. Chen HC, Joalland N, Bridgeman JS, Alchami FS, Jarry U, Khan MWA, et al. Synergistic Targeting of Breast Cancer Stem-Like Cells by Human γδ T Cells and CD8(+) T Cells. Immunol Cell Biol (2017) 95(7):620–9. doi: 10.1038/icb.2017.21
43. Wu P, Wu D, Ni C, Ye J, Chen W, Hu G, et al. γδt17 Cells Promote the Accumulation and Expansion of Myeloid-Derived Suppressor Cells in Human Colorectal Cancer. Immunity (2014) 40(5):785–800. doi: 10.1016/j.immuni.2014.03.013
44. Patil RS, Shah SU, Shrikhande SV, Goel M, Dikshit RP, Chiplunkar SV. IL17 Producing γδt Cells Induce Angiogenesis and Are Associated With Poor Survival in Gallbladder Cancer Patients. Int J Cancer (2016) 139(4):869–81. doi: 10.1002/ijc.30134
45. Coffelt SB, Kersten K, Doornebal CW, Weiden J, Vrijland K, Hau C-S, et al. IL-17-Producing γδ T Cells and Neutrophils Conspire to Promote Breast Cancer Metastasis. Nature (2015) 522(7556):345–8. doi: 10.1038/nature14282
46. Sureshbabu SK, Chaukar D, Chiplunkar SV. Hypoxia Regulates the Differentiation and Anti-Tumor Effector Functions of γδt Cells in Oral Cancer. Clin Exp Immunol (2020) 201(1):40–57. doi: 10.1111/cei.13436
47. Hanahan D. Hallmarks of Cancer: New Dimensions. Cancer Discov (2022) 12(1):31–46. doi: 10.1158/2159-8290.CD-21-1059
48. Hanahan D, Weinberg Robert A. Hallmarks of Cancer: The Next Generation. Cell (2011) 144(5):646–74. doi: 10.1016/j.cell.2011.02.013
49. Schmolka N, Serre K, Grosso AR, Rei M, Pennington DJ, Gomes AQ, et al. Epigenetic and Transcriptional Signatures of Stable Versus Plastic Differentiation of Proinflammatory Gammadelta T Cell Subsets. Nat Immunol (2013) 14(10):1093–100. doi: 10.1038/ni.2702
50. Sutton CE, Lalor SJ, Sweeney CM, Brereton CF, Lavelle EC, Mills KHG. Interleukin-1 and IL-23 Induce Innate IL-17 Production From γδ T Cells, Amplifying Th17 Responses and Autoimmunity. Immunity (2009) 31(2):331–41. doi: 10.1016/j.immuni.2009.08.001
51. McGinley AM, Sutton CE, Edwards SC, Leane CM, DeCourcey J, Teijeiro A, et al. Interleukin-17a Serves a Priming Role in Autoimmunity by Recruiting IL-1beta-Producing Myeloid Cells That Promote Pathogenic T Cells. Immunity (2020) 52(2):342–56.e6. doi: 10.1016/j.immuni.2020.01.002
52. Petermann F, Rothhammer V, Claussen MC, Haas JD, Blanco LR, Heink S, et al. γδ T Cells Enhance Autoimmunity by Restraining Regulatory T Cell Responses via an Interleukin-23-Dependent Mechanism. Immunity (2010) 33(3):351–63. doi: 10.1016/j.immuni.2010.08.013
53. Papotto PH, Gonçalves-Sousa N, Schmolka N, Iseppon A, Mensurado S, Stockinger B, et al. IL-23 Drives Differentiation of Peripheral γδ17 T Cells From Adult Bone Marrow-Derived Precursors. EMBO Rep (2017) 18(11):1957–67. doi: 10.15252/embr.201744200
54. Kaufmann M, Evans H, Schaupp A-L, Engler JB, Kaur G, Willing A, et al. Identifying CNS-Colonizing T Cells as Potential Therapeutic Targets to Prevent Progression of Multiple Sclerosis. Med (2021) 2(3):296–312.e8. doi: 10.1016/j.medj.2021.01.006
55. Singh AK, Novakova L, Axelsson M, Malmeström C, Zetterberg H, Lycke J, et al. High Interferon-γ Uniquely in Vδ1 T Cells Correlates With Markers of Inflammation and Axonal Damage in Early Multiple Sclerosis. Front Immunol (2017) 8. doi: 10.3389/fimmu.2017.00260
56. Maimaitijiang G, Shinoda K, Nakamura Y, Masaki K, Matsushita T, Isobe N, et al. Association of Decreased Percentage of Vδ2+Vγ9+ γδ T Cells With Disease Severity in Multiple Sclerosis. Front Immunol (2018) 9. doi: 10.3389/fimmu.2018.00748
57. Zeine R, Pon R, Ladiwala U, Antel JP, Filion LG, Freedman MS. Mechanism of γδ T Cell-Induced Human Oligodendrocyte Cytotoxicity: Relevance to Multiple Sclerosis. J Neuroimmunology (1998) 87(1):49–61. doi: 10.1016/S0165-5728(98)00047-2
58. Monteiro A, Cruto C, Rosado P, Martinho A, Rosado L, Fonseca M, et al. Characterization of Circulating Gamma-Delta T Cells in Relapsing vs Remission Multiple Sclerosis. J Neuroimmunol (2018) 318:65–71. doi: 10.1016/j.jneuroim.2018.02.009
59. Korn T, Bettelli E, Oukka M, Kuchroo VK. IL-17 and Th17 Cells. Annu Rev Immunol (2009) 27(1):485–517. doi: 10.1146/annurev.immunol.021908.132710
60. Martin B, Hirota K, Cua DJ, Stockinger B, Veldhoen M. Interleukin-17-Producing γδ T Cells Selectively Expand in Response to Pathogen Products and Environmental Signals. Immunity (2009) 31(2):321–30. doi: 10.1016/j.immuni.2009.06.020
61. Kapsenberg ML. γδ T Cell Receptors Without a Job. Immunity (2009) 31(2):181–3. doi: 10.1016/j.immuni.2009.08.004
62. Edwards SC, Sutton CE, Ladell K, Grant EJ, McLaren JE, Roche F, et al. A Population of Proinflammatory T Cells Coexpresses αβ and γδ T Cell Receptors in Mice and Humans. J Exp Med (2020) 217(5):e20190834. doi: 10.1084/jem.20190834
63. Marchitto MC, Dillen CA, Liu H, Miller RJ, Archer NK, Ortines RV, et al. Clonal Vγ6(+)Vδ4(+) T Cells Promote IL-17-Mediated Immunity Against Staphylococcus Aureus Skin Infection. Proc Natl Acad Sci USA (2019) 116(22):10917–26. doi: 10.1073/pnas.1818256116
64. Puan K-J, Jin C, Wang H, Sarikonda G, Raker AM, Lee HK, et al. Preferential Recognition of a Microbial Metabolite by Human Vγ2vδ2 T Cells. Int Immunol (2007) 19(5):657–73. doi: 10.1093/intimm/dxm031
65. Howard J, Zaidi I, Loizon S, Mercereau-Puijalon O, Déchanet-Merville J, Mamani-Matsuda M. Human Vγ9vδ2 T Lymphocytes in the Immune Response to P. Falciparum Infection. Front Immunol (2018) 9. doi: 10.3389/fimmu.2018.02760
66. Junqueira C, Polidoro RB, Castro G, Absalon S, Liang Z, Sen Santara S, et al. γδ T Cells Suppress Plasmodium Falciparum Blood-Stage Infection by Direct Killing and Phagocytosis. Nat Immunol (2021) 22(3):347–57. doi: 10.1038/s41590-020-00847-4
67. Ladel CH, Blum C, Dreher A, Reifenberg K, Kaufmann SH. Protective Role of Gamma/Delta T Cells and Alpha/Beta T Cells in Tuberculosis. Eur J Immunol (1995) 25(10):2877–81. doi: 10.1002/eji.1830251025
68. Hoft DF, Brown RM, Roodman ST. Bacille Calmette-Guerin Vaccination Enhances Human Gamma Delta T Cell Responsiveness to Mycobacteria Suggestive of a Memory-Like Phenotype. J Immunol (1998) 161(2):1045–54.
69. Garly ML, Martins CL, Balé C, Baldé MA, Hedegaard KL, Gustafson P, et al. BCG Scar and Positive Tuberculin Reaction Associated With Reduced Child Mortality in West Africa. A Non-Specific Beneficial Effect of BCG? Vaccine (2003) 21(21-22):2782–90. doi: 10.1016/S0264-410X(03)00181-6
70. Aaby P, Roth A, Ravn H, Napirna BM, Rodrigues A, Lisse IM, et al. Randomized Trial of BCG Vaccination at Birth to Low-Birth-Weight Children: Beneficial Nonspecific Effects in the Neonatal Period? J Infect Dis (2011) 204(2):245–52. doi: 10.1093/infdis/jir240
71. Benn CS, Netea MG, Selin LK, Aaby P. A Small Jab - A Big Effect: Nonspecific Immunomodulation by Vaccines. Trends Immunol (2013) 34(9):431–9. doi: 10.1016/j.it.2013.04.004
72. Higgins JP, Soares-Weiser K, López-López JA, Kakourou A, Chaplin K, Christensen H, et al. Association of BCG, DTP, and Measles Containing Vaccines With Childhood Mortality: Systematic Review. Bmj (2016) 355:i5170. doi: 10.1136/bmj.i5170
73. Munk ME, Gatrill AJ, Kaufmann SH. Target Cell Lysis and IL-2 Secretion by Gamma/Delta T Lymphocytes After Activation With Bacteria. J Immunol (1990) 145(8):2434–9.
74. Quintin J, Saeed S, Martens JHA, Giamarellos-Bourboulis EJ, Ifrim DC, Logie C, et al. Candida Albicans Infection Affords Protection Against Reinfection via Functional Reprogramming of Monocytes. Cell Host Microbe (2012) 12(2):223–32. doi: 10.1016/j.chom.2012.06.006
75. Saeed S, Quintin J, Kerstens HH, Rao NA, Aghajanirefah A, Matarese F, et al. Epigenetic Programming of Monocyte-to-Macrophage Differentiation and Trained Innate Immunity. Science (2014) 345(6204):1251086. doi: 10.1126/science.1251086
76. Davis MM, Tato CM, Furman D. Systems Immunology: Just Getting Started. Nat Immunol (2017) 18(7):725–32. doi: 10.1038/ni.3768
77. Villani A-C, Sarkizova S, Hacohen N. Systems Immunology: Learning the Rules of the Immune System. Annu Rev Immunol (2018) 36(1):813–42. doi: 10.1146/annurev-immunol-042617-053035
78. Pulendran B, Davis Mark M. The Science and Medicine of Human Immunology. Science (2020) 369(6511):eaay4014. doi: 10.1126/science.aay4014
79. Mangino M, Roederer M, Beddall MH, Nestle FO, Spector TD. Innate and Adaptive Immune Traits are Differentially Affected by Genetic and Environmental Factors. Nat Commun (2017) 8:13850. doi: 10.1038/ncomms13850
80. Melenhorst JJ, Chen GM, Wang M, Porter DL, Chen C, Collins MA, et al. Decade-Long Leukaemia Remissions With Persistence of CD4(+) CAR T Cells. Nature (2022) 602(7897):503–9. doi: 10.1038/s41586-021-04390-6
81. Gentles AJ, Newman AM, Liu CL, Bratman SV, Feng W, Kim D, et al. The Prognostic Landscape of Genes and Infiltrating Immune Cells Across Human Cancers. Nat Med (2015) 21(8):938–45. doi: 10.1038/nm.3909
82. Tosolini M, Pont F, Poupot M, Vergez F, Nicolau-Travers M-L, Vermijlen D, et al. Assessment of Tumor-Infiltrating Tcrvγ9vδ2 γδ Lymphocyte Abundance by Deconvolution of Human Cancers Microarrays. Oncoimmunology (2017) 6(3):e1284723. doi: 10.1080/2162402X.2017.1284723
83. Miao Y-R, Zhang Q, Lei Q, Luo M, Xie G-Y, Wang H, et al. ImmuCellAI: A Unique Method for Comprehensive T-Cell Subsets Abundance Prediction and Its Application in Cancer Immunotherapy. Advanced Sci (2020) 7(7):1902880. doi: 10.1002/advs.201902880
84. Bhat J, Bergmann AK, Waschina S, Nerl C, Kaleta C, Siebert R, et al. DNA Methylation Profile of a Hepatosplenic Gamma/Delta T-Cell Lymphoma Patient Associated With Response to Interferon-α Therapy. Cell Mol Immunol (2021) 18(5):1332–5. doi: 10.1038/s41423-020-0518-4
85. Mirji G, Bhat J, Kode J, Banavali S, Sengar M, Khadke P, et al. Risk Stratification of T-Cell Acute Lymphoblastic Leukemia Patients Based on Gene Expression, Mutations and Copy Number Variation. Leukemia Res (2016) 45:33–9. doi: 10.1016/j.leukres.2016.03.002
86. Watkin L, Anvari S, Hassan O, Schuster K, Davis C. Increased Costimulatory Molecule Expression in γδ-T Cells of Peanut Allergic Individuals. J Allergy Clin Immunol (2020) 145(2, Supplement):AB244. doi: 10.1016/j.jaci.2019.12.151
87. Boufea K, González-Huici V, Lindberg M, Symeonides S, Oikonomidou O, Batada NN. Single-Cell RNA Sequencing of Human Breast Tumour-Infiltrating Immune Cells Reveals a γδ T-Cell Subtype Associated With Good Clinical Outcome. Life Sci Alliance (2021) 4(1):e202000680. doi: 10.26508/lsa.202000680
88. Pizzolato G, Kaminski H, Tosolini M, Franchini D-M, Pont F, Martins F, et al. Single-Cell RNA Sequencing Unveils the Shared and the Distinct Cytotoxic Hallmarks of Human Tcrvδ1 and Tcrvδ2 γδ T Lymphocytes. Proc Natl Acad Sci (2019) 116(24):11906–15. doi: 10.1073/pnas.1818488116
89. Jaeger N, Gamini R, Cella M, Schettini JL, Bugatti M, Zhao S, et al. Single-Cell Analyses of Crohn’s Disease Tissues Reveal Intestinal Intraepithelial T Cells Heterogeneity and Altered Subset Distributions. Nat Commun (2021) 12(1):1921. doi: 10.1038/s41467-021-22164-6
90. Park J-E, Botting Rachel A, Domínguez Conde C, Popescu D-M, Lavaert M, Kunz Daniel J, et al. A Cell Atlas of Human Thymic Development Defines T Cell Repertoire Formation. Science (2020) 367(6480):eaay3224. doi: 10.1126/science.aay3224
91. Tan L, Fichtner Alina S, Bruni E, Odak I, Sandrock I, Bubke A, et al. A Fetal Wave of Human Type 3 Effector γδ Cells With Restricted TCR Diversity Persists Into Adulthood. Sci Immunol (2021) 6(58):eabf0125. doi: 10.1126/sciimmunol.abf0125
92. Reitermaier R, Krausgruber T, Fortelny N, Ayub T, Vieyra-Garcia PA, Kienzl P, et al. αβγδ T Cells Play a Vital Role in Fetal Human Skin Development and Immunity. J Exp Med (2021) 218(4):e20201189. doi: 10.1084/jem.20201189
93. Tan L, Sandrock I, Odak I, Aizenbud Y, Wilharm A, Barros-Martins J, et al. Single-Cell Transcriptomics Identifies the Adaptation of Scart1+ Vγ6+ T Cells to Skin Residency as Activated Effector Cells. Cell Rep (2019) 27(12):3657–71.e4. doi: 10.1016/j.celrep.2019.05.064
94. Sagar, Pokrovskii M, Herman JS, Naik S, Sock E, Zeis P, et al. Deciphering the Regulatory Landscape of Fetal and Adult γδ T-Cell Development at Single-Cell Resolution. EMBO J (2020) 39(13):e104159. doi: 10.15252/embj.2019104159
95. Lee M, Lee E, Han SK, Choi YH, D-i K, Choi H, et al. Single-Cell RNA Sequencing Identifies Shared Differentiation Paths of Mouse Thymic Innate T Cells. Nat Commun (2020) 11(1):4367. doi: 10.1038/s41467-020-18155-8
96. Alves de Lima K, Rustenhoven J, Da Mesquita S, Wall M, Salvador AF, Smirnov I, et al. Meningeal γδ T Cells Regulate Anxiety-Like Behavior via IL-17a Signaling in Neurons. Nat Immunol (2020) 21(11):1421–9. doi: 10.1038/s41590-020-0776-4
97. Goldberg EL, Shchukina I, Asher JL, Sidorov S, Artyomov MN, Dixit VD. Ketogenesis Activates Metabolically Protective γδ T Cells in Visceral Adipose Tissue. Nat Metab (2020) 2(1):50–61. doi: 10.1038/s42255-019-0160-6
98. Hu Y, Fang K, Wang Y, Lu N, Sun H, Zhang C. Single-Cell Analysis Reveals the Origins and Intrahepatic Development of Liver-Resident IFN-γ-Producing γδ T Cells. Cell Mol Immunol (2021) 18(4):954–68. doi: 10.1038/s41423-021-00656-1
99. Li Z, Yang Q, Tang X, Chen Y, Wang S, Qi X, et al. Single-Cell RNA-Seq and Chromatin Accessibility Profiling Decipher the Heterogeneity of Mouse γδ T Cells. Sci Bull (2022) 67(4):408–26. doi: 10.1016/j.scib.2021.11.013
100. Wang X, Chen Y, Li Z, Huang B, Xu L, Lai J, et al. Single-Cell RNA-Seq of T Cells in B-ALL Patients Reveals an Exhausted Subset With Remarkable Heterogeneity. Adv Sci (Weinh) (2021) 8(19):e2101447. doi: 10.1002/advs.202101447
101. Gherardin NA, Waldeck K, Caneborg A, Martelotto LG, Balachander S, Zethoven M, et al. γδ T Cells in Merkel Cell Carcinomas Have a Proinflammatory Profile Prognostic of Patient Survival. Cancer Immunol Res (2021) 9(6):612–23. doi: 10.1158/2326-6066.CIR-20-0817
102. Schafflick D, Xu CA, Hartlehnert M, Cole M, Schulte-Mecklenbeck A, Lautwein T, et al. Integrated Single Cell Analysis of Blood and Cerebrospinal Fluid Leukocytes in Multiple Sclerosis. Nat Commun (2020) 11(1):247. doi: 10.1038/s41467-019-14118-w
103. Cerapio J-P, Perrier M, Balança C-C, Gravelle P, Pont F, Devaud C, et al. Phased Differentiation of γδ T and T CD8 Tumor-Infiltrating Lymphocytes Revealed by Single-Cell Transcriptomics of Human Cancers. Oncoimmunology (2021) 10(1):1939518. doi: 10.1080/2162402X.2021.1939518
104. Schaum N, Karkanias J, Neff NF, May AP, Quake SR, Wyss-Coray T, et al. Single-Cell Transcriptomics of 20 Mouse Organs Creates a Tabula Muris. Nature (2018) 562(7727):367–72. doi: 10.1038/s41586-018-0590-4
105. Han X, Zhou Z, Fei L, Sun H, Wang R, Chen Y, et al. Construction of a Human Cell Landscape at Single-Cell Level. Nature (2020) 581(7808):303–9. doi: 10.1038/s41586-020-2157-4
106. Karlsson M, Zhang C, Méar L, Zhong W, Digre A, Katona B, et al. A Single–Cell Type Transcriptomics Map of Human Tissues. Sci Adv (2020) 7(31):eabh2169. doi: 10.1126/sciadv.abh2169
107. Yang K, Blanco DB, Chen X, Dash P, Neale G, Rosencrance C, et al. Metabolic Signaling Directs the Reciprocal Lineage Decisions of αβ and γδ T Cells. Sci Immunol (2018) 3(25):eaas9818. doi: 10.1126/sciimmunol.aas9818
108. Argüello RJ, Combes AJ, Char R, Gigan J-P, Baaziz AI, Bousiquot E, et al. SCENITH: A Flow Cytometry-Based Method to Functionally Profile Energy Metabolism With Single-Cell Resolution. Cell Metab (2020) 32(6):1063–75.e7. doi: 10.1016/j.cmet.2020.11.007
109. Lopes N, McIntyre C, Martin S, Raverdeau M, Sumaria N, Kohlgruber AC, et al. Distinct Metabolic Programs Established in the Thymus Control Effector Functions of γδ T Cell Subsets in Tumor Microenvironments. Nat Immunol (2021) 22(2):179–92. doi: 10.1038/s41590-020-00848-3
110. Li L, Cao B, Liang X, Lu S, Luo H, Wang Z, et al. Microenvironmental Oxygen Pressure Orchestrates an Anti- and Pro-Tumoral γδ T Cell Equilibrium via Tumor-Derived Exosomes. Oncogene (2019) 38(15):2830–43. doi: 10.1038/s41388-018-0627-z
111. Gonnermann D, Oberg HH, Kellner C, Peipp M, Sebens S, Kabelitz D, et al. Resistance of Cyclooxygenase-2 Expressing Pancreatic Ductal Adenocarcinoma Cells Against γδ T Cell Cytotoxicity. Oncoimmunology (2015) 4(3):e988460. doi: 10.4161/2162402X.2014.988460
112. Kohlgruber AC, Gal-Oz ST, LaMarche NM, Shimazaki M, Duquette D, Koay H-F, et al. γδ T Cells Producing Interleukin-17A Regulate Adipose Regulatory T Cell Homeostasis and Thermogenesis. Nat Immunol (2018) 19(5):464–74. doi: 10.1038/s41590-018-0094-2
113. Hu B, Jin C, Zeng X, Resch JM, Jedrychowski MP, Yang Z, et al. γδ T Cells and Adipocyte IL-17RC Control Fat Innervation and Thermogenesis. Nature (2020) 578(7796):610–4. doi: 10.1038/s41586-020-2028-z
114. Makkouk A, Yang X, Barca T, Lucas A, Turkoz M, Wong JTS, et al. Off-The-Shelf Vδ1 Gamma Delta T Cells Engineered With Glypican-3 (GPC-3)-Specific Chimeric Antigen Receptor (CAR) and Soluble IL-15 Display Robust Antitumor Efficacy Against Hepatocellular Carcinoma. J ImmunoTher Cancer (2021) 9(12):e003441. doi: 10.1136/jitc-2021-003441
115. Xu Y, Xiang Z, Alnaggar M, Kouakanou L, Li J, He J, et al. Allogeneic Vγ9vδ2 T-Cell Immunotherapy Exhibits Promising Clinical Safety and Prolongs the Survival of Patients With Late-Stage Lung or Liver Cancer. Cell Mol Immunol (2021) 18(2):427–39. doi: 10.1038/s41423-020-0515-7
116. Kabelitz D, Serrano R, Kouakanou L, Peters C, Kalyan S. Cancer Immunotherapy With γδ T Cells: Many Paths Ahead of Us. Cell Mol Immunol (2020) 17(9):925–39. doi: 10.1038/s41423-020-0504-x
117. Bhat SA, Vedpathak DM, Chiplunkar SV. Checkpoint Blockade Rescues the Repressive Effect of Histone Deacetylases Inhibitors on Gammadelta T Cell Function. Front Immunol (2018) 9:1615. doi: 10.3389/fimmu.2018.01615
118. Bhat J, Kabelitz D. Gammadelta T Cells and Epigenetic Drugs: A Useful Merger in Cancer Immunotherapy? Oncoimmunology (2015) 4(6):e1006088. doi: 10.1080/2162402X.2015.1006088
119. Bhat J, Kouakanou L, Peters C, Yin Z, Kabelitz D. Immunotherapy With Human Gamma Delta T Cells—Synergistic Potential of Epigenetic Drugs? Front Immunol (2018) 9(512). doi: 10.3389/fimmu.2018.00512
120. Wang H, Chen H, Liu S, Zhang J, Lu H, Somasundaram R, et al. Costimulation of γδtcr and TLR7/8 Promotes Vδ2 T-Cell Antitumor Activity by Modulating mTOR Pathway and APC Function. J Immunother Cancer (2021) 9(12):e003339. doi: 10.1136/jitc-2021-003339
121. Ganesan R, Chennupati V, Ramachandran B, Hansen MR, Singh S, Grewal IS. Selective Recruitment of γδ T Cells by a Bispecific Antibody for the Treatment of Acute Myeloid Leukemia. Leukemia (2021) 35(8):2274–84. doi: 10.1038/s41375-021-01122-7
122. Peters C, Meyer A, Kouakanou L, Feder J, Schricker T, Lettau M, et al. TGF-β Enhances the Cytotoxic Activity of Vδ2 T Cells. Oncoimmunology (2019) 8(1):e1522471. doi: 10.1080/2162402X.2018.1522471
123. Kouakanou L, Peters C, Sun Q, Floess S, Bhat J, Huehn J, et al. Vitamin C Supports Conversion of Human γδ T Cells Into FOXP3-Expressing Regulatory Cells by Epigenetic Regulation. Sci Rep (2020) 10(1):6550. doi: 10.1038/s41598-020-63572-w
124. Yang Y, Lundqvist A. Immunomodulatory Effects of IL-2 and IL-15; Implications for Cancer Immunotherapy. Cancers (Basel) (2020) 12(12):3586. doi: 10.3390/cancers12123586
125. Cairo C, Armstrong CL, Cummings JS, Deetz CO, Tan M, Lu C, et al. Impact of Age, Gender, and Race on Circulating γδ T Cells. Hum Immunol (2010) 71(10):968–75. doi: 10.1016/j.humimm.2010.06.014
126. Brenner MB, McLean J, Dialynas DP, Strominger JL, Smith JA, Owen FL, et al. Identification of a Putative Second T-Cell Receptor. Nature (1986) 322(6075):145–9. doi: 10.1038/322145a0
127. Bank I, DePinho RA, Brenner MB, Cassimeris J, Alt FW, Chess L. A Functional T3 Molecule Associated With a Novel Heterodimer on the Surface of Immature Human Thymocytes. Nature (1986) 322(6075):179–81. doi: 10.1038/322179a0
128. Saito H, Kranz DM, Takagaki Y, Hayday AC, Eisen HN, Tonegawa S. A Third Rearranged and Expressed Gene in a Clone of Cytotoxic T Lymphocytes. Nature (1984) 312(5989):36–40. doi: 10.1038/312036a0
129. Rosenberg SA, Lotze MT, Muul LM, Leitman S, Chang AE, Ettinghausen SE, et al. Observations on the Systemic Administration of Autologous Lymphokine-Activated Killer Cells and Recombinant Interleukin-2 to Patients With Metastatic Cancer. N Engl J Med (1985) 313(23):1485–92. doi: 10.1056/NEJM198512053132327
130. Rosenberg SA, Spiess P, Lafreniere R. A New Approach to the Adoptive Immunotherapy of Cancer With Tumor-Infiltrating Lymphocytes. Science (1986) 233(4770):1318–21. doi: 10.1126/science.3489291
131. Gross G, Waks T, Eshhar Z. Expression of Immunoglobulin-T-Cell Receptor Chimeric Molecules as Functional Receptors With Antibody-Type Specificity. Proc Natl Acad Sci USA (1989) 86(24):10024–8. doi: 10.1073/pnas.86.24.10024
132. Maher J, Brentjens RJ, Gunset G, Riviere I, Sadelain M. Human T-Lymphocyte Cytotoxicity and Proliferation Directed by a Single Chimeric TCRzeta /CD28 Receptor. Nat Biotechnol (2002) 20(1):70–5. doi: 10.1038/nbt0102-70
133. Rosenbaum L. Tragedy, Perseverance, and Chance - The Story of CAR-T Therapy. N Engl J Med (2017) 377(14):1313–5. doi: 10.1056/NEJMp1711886
134. Zocchi MR, Ferrarini M, Rugarli C. Selective Lysis of the Autologous Tumor by Delta TCS1+ Gamma/Delta+ Tumor-Infiltrating Lymphocytes From Human Lung Carcinomas. Eur J Immunol (1990) 20(12):2685–9. doi: 10.1002/eji.1830201224
135. Wilhelm M, Kunzmann V, Eckstein S, Reimer P, Weissinger F, Ruediger T, et al. Gammadelta T Cells for Immune Therapy of Patients With Lymphoid Malignancies. Blood (2003) 102(1):200–6. doi: 10.1182/blood-2002-12-3665
136. Kobayashi H, Tanaka Y, Yagi J, Osaka Y, Nakazawa H, Uchiyama T, et al. Safety Profile and Anti-Tumor Effects of Adoptive Immunotherapy Using Gamma-Delta T Cells Against Advanced Renal Cell Carcinoma: A Pilot Study. Cancer Immunol Immunother CII (2007) 56(4):469–76. doi: 10.1007/s00262-006-0199-6
137. Cui J, Wang N, Zhao H, Jin H, Wang G, Niu C, et al. Combination of Radiofrequency Ablation and Sequential Cellular Immunotherapy Improves Progression-Free Survival for Patients With Hepatocellular Carcinoma. Int J Cancer (2014) 134(2):342–51. doi: 10.1002/ijc.28372
138. Sebestyen Z, Prinz I, Déchanet-Merville J, Silva-Santos B, Kuball J. Translating Gammadelta (γδ) T Cells and Their Receptors Into Cancer Cell Therapies. Nat Rev Drug Discov (2020) 19(3):169–84. doi: 10.1038/s41573-019-0038-z
139. Almeida AR, Correia DV, Fernandes-Platzgummer A, da Silva CL, da Silva MG, Anjos DR, et al. Delta One T Cells for Immunotherapy of Chronic Lymphocytic Leukemia: Clinical-Grade Expansion/Differentiation and Preclinical Proof of Concept. Clin Cancer Res (2016) 22(23):5795–804. doi: 10.1158/1078-0432.CCR-16-0597
140. Wawrzyniecka PA, Ibrahim L, Gritti G, Pule MA, Maciocia PM. Chimeric Antigen Receptor T Cells for Gamma–Delta T Cell Malignancies. Leukemia (2022) 36(2):577–9. doi: 10.1038/s41375-021-01385-0
141. Rozenbaum M, Meir A, Aharony Y, Itzhaki O, Schachter J, Bank I, et al. Gamma-Delta CAR-T Cells Show CAR-Directed and Independent Activity Against Leukemia. Front Immunol (2020) 11. doi: 10.3389/fimmu.2020.01347
142. Sánchez-Martínez D, Tirado N, Mensurado S, Martínez-Moreno A, Romecin P, Gutiérrez-Agüera F, et al. Generation and Proof-of-Concept for Allogeneic CD123 CAR-Delta One T (DOT) Cells in Acute Myeloid Leukemia. bioRxiv (2022) 2022:03.15.484289. doi: 10.1101/2022.03.15.484289
143. Nishimoto KP, Barca T, Azameera A, Makkouk A, Romero JM, Bai L, et al. Allogeneic CD20-Targeted Gammadelta T Cells Exhibit Innate and Adaptive Antitumor Activities in Preclinical B-Cell Lymphoma Models. Clin Transl Immunol (2022) 11(2):e1373. doi: 10.1002/cti2.1373
Keywords: gamma delta T cells, immunotherapy, cancers, infection, neuroimmunology, metabolism, multi-omics
Citation: Bhat J, Placek K and Faissner S (2022) Contemplating Dichotomous Nature of Gamma Delta T Cells for Immunotherapy. Front. Immunol. 13:894580. doi: 10.3389/fimmu.2022.894580
Received: 11 March 2022; Accepted: 22 April 2022;
Published: 20 May 2022.
Edited by:
Alice Cheung, Singapore General Hospital, SingaporeReviewed by:
Ilan Bank, Sheba Medical Center, IsraelCopyright © 2022 Bhat, Placek and Faissner. This is an open-access article distributed under the terms of the Creative Commons Attribution License (CC BY). The use, distribution or reproduction in other forums is permitted, provided the original author(s) and the copyright owner(s) are credited and that the original publication in this journal is cited, in accordance with accepted academic practice. No use, distribution or reproduction is permitted which does not comply with these terms.
*Correspondence: Jaydeep Bhat, amF5ZGVlcC5iaGF0QHJ1Yi5kZQ==
†These authors have contributed equally to this work and share first authorship