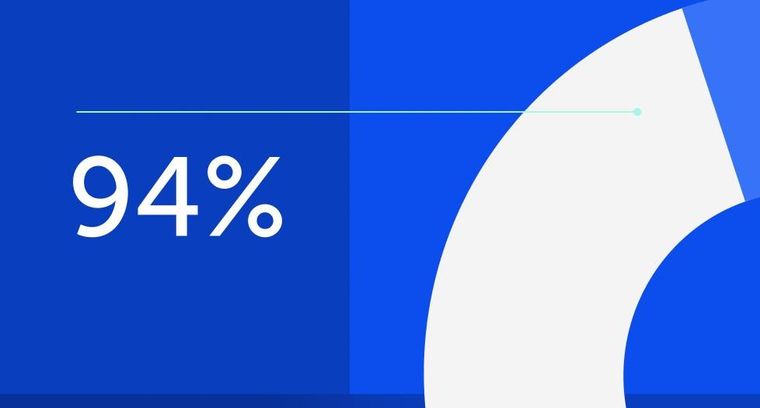
94% of researchers rate our articles as excellent or good
Learn more about the work of our research integrity team to safeguard the quality of each article we publish.
Find out more
REVIEW article
Front. Immunol., 06 July 2022
Sec. Viral Immunology
Volume 13 - 2022 | https://doi.org/10.3389/fimmu.2022.889196
The dynamics of host-virus interactions, and impairment of the host’s immune surveillance by dengue virus (DENV) serotypes largely remain ambiguous. Several experimental and preclinical studies have demonstrated how the virus brings about severe disease by activating immune cells and other key elements of the inflammatory cascade. Plasmablasts are activated during primary and secondary infections, and play a determinative role in severe dengue. The cross-reactivity of DENV immune responses with other flaviviruses can have implications both for cross-protection and severity of disease. The consequences of a cross-reactivity between DENV and anti-SARS-CoV-2 responses are highly relevant in endemic areas. Here, we review the latest progress in the understanding of dengue immunopathogenesis and provide suggestions to the development of target strategies against dengue.
Dengue is an infectious disease transmitted between humans by Aedes mosquitoes, especially across the tropical and subtropical latitudes afflicting ~400 million people annually, of which 100 million manifests clinically (1). Estimates by the World Health Organization (WHO) suggest that the global consequence of dengue is exponentially increasing and almost half of the global population is at risk for contracting the infection (2). Dengue is caused by at least four different dengue virus (DENV) serotypes, DENV1, DENV2, DENV3, and DENV4. In recent years, most endemic countries, e.g., Asia-Pacific and Latin American nations, are reporting almost all the four different DENV serotypes (3), which altogether cause ~20000 deaths annually (4). The surge in endemicity is attributed to rapid urbanization, increasing population density and a rise in vector-breeding sites (5). Aedes aegypti (A. aegypti) represents the major vector that transmits dengue in urban areas, whereas the density of A. albopictus, the secondary vector, is dramatically expanding globally (6, 7). Given the context of global warming, the environment appears to be appropriate for the breeding of Aedes mosquitos, that in turn, would drive the dissemination of the dengue disease further (8).
DENV is a member of the Flavivirus genus of the Flaviviridae family. DENV has a spherical shape with icosahedral symmetry. It is a single-stranded positive sense RNA virus with a genome size of ~11 kb (9). It has a single long open reading frame (ORF) that encodes for three structural and seven non-structural (NS) proteins. The structural proteins are capsid (C), pre-membrane/membrane (prM/M), and envelope glycoproteins (E), and the NS proteins are NS1, NS2A, NS2B, NS3, NS4A, NS4B, and NS5. The NS proteins are not present in the virion, but contribute to viral replication and immune evasion within an infected cell (10–12). Of all the NS proteins, only NS1 is displayed on the infected cell surfaces, and is eventually secreted into the systemic circulation making it an appropriate diagnostic marker. While detection of DENV genomic RNA (by RT-PCR) and NS1 are the mainstay of laboratory diagnosis, detection of NS1 has an edge over the detection of DENV genomic RNA. Albeit being highly sensitive, the RT-PCR viral detection rate has a finer window period where detection rate appears to drop dramatically by day 4 onwards following the onset of clinical symptoms (13, 14). Conversely, NS1 can be detected in the serum for a wider time range, viz., from the first day of symptom onset, with the concentration average of 2 µg/ml (can reach as high as 50 µg/ml in the same cases) (15) that remains detectable between 9 and 18 days (16, 17). Furthermore, the level of NS1 appears to correlate with disease severity, rendering it an ideal biomarker, both for diagnosis as well as prognosis in dengue (12, 18, 19).
Dengue infection results in clinical manifestations ranging from a predominantly asymptomatic or a symptomatic, mild undifferentiated febrile illness to severe life-threatening dengue hemorrhagic fever (DHF) and dengue shock syndrome (DSS) that can be fatal (6). The hallmarks of severe dengue are coagulopathy and leaky vasculature that eventually can lead to life-threatening hemodynamic shock and organ failure (20, 21). Evidence suggests that young age, female biological sex, high body-mass index, virus strain, and genetic variants of the human MHC class I–related sequence B, and phospholipase C epsilon 1 genes could serve as risk factors for development of severe dengue (6).
The human and economic burden caused by dengue fever remains enormous as specific antiviral drugs, or effective vector-control mechanisms is lacking. Although no specific treatment is available, prompt hospital admission, triage, and fluid restoration are critical to prevent death (22). In 2016, (CYD-TDV) DengVaxia®, a tetravalent vaccine was licensed to prevent severe secondary dengue in seropositive individuals. However, the vaccine was not recommended for seronegative individuals as the levels of vaccine-induced antibodies reportedly decreased over time (23). The current review will focus on some of the hitherto poorly understood disease immunopathogenesis mechanisms as well as potential interventions against DENV infection.
The onset of severe dengue often occurs during the defervescence stage after peak viremia suggesting that the host immune responses are implicated in viral clearance (6, 24), inferring that life-threatening dengue involves a complex interplay between virus and the host (25). Natural infection with one of the serotypes confers long-lasting immunity to subsequent infection with the same serotype. However, subsequent infection with heterotypic serotypes often results in severe immunopathological manifestations, triggered early during the course of disease (26). This, at least in part, could be attributed to a phenomenon known as original antigenic sin that engenders ineffective T and B cell responses and potentially harmful manifestations, particularly during secondary infection. The complex interplay between these factors may eventually lead to both antibody-dependent enhancement (ADE), antibody-dependent cellular cytotoxicity (ADCC), cytokine storm (hypercytokinemia), aberrant activation of the complement system (CS), as well as endothelial dysfunction, culminating in severe clinical dengue (27, 28).
Although both T and B cell responses play a paramount role in combating DENV infection (29), they could be pathological during secondary infection due to original antigenic sin. Because the four DENV serotypes share ~80% homology in amino acid sequences, cross-reactivity is common (30). Hence, during a heterotypic infection, the preexisting memory T and B cells rapidly become activated to proliferate to enter into the effector phase (26). As protective adaptive immunity is more efficient against homotypic than heterotypic reinfection (31), seeing that cross-reactive responses may have suboptimal avidity and affinity towards the epitopes of the secondary-infecting virus (27). These cross-reactive T cells often exhibit lower cytotoxicity yet secreting higher abundance of several pro-inflammatory cytokines (32), rendering viral control ineffective as well as exaggerated release of pro-inflammatory cytokines leading to cytokine storm and endothelial dysfunction (26, 33) (Figure 1).
Figure 1 Original antigenic sin and antibody-dependent enhancement in DENV infection. (A) When primary infection occurs with e,g. DENV1, resulting in activation of adaptive immune responses (both T and B cells) DENV1-specific T cells are selected, activated, and clonally expanded to combat infection. Upon termination of primary infection, memory DENV1–specific T cells and B cells are formed and are retained with higher frequency compared to other naïve cells. (B) A secondary infection with the same serotype of DENV (e.g. DENV1) for the second time (homotypic infection), the virus will evoke a memory response that entails in the effective containment of DENV1 by highly specific T and B cell responses. (C) A secondary challenge with a different serotype of DENV (e.g. DENV2) (heterotypic infection), there is a chance that the cross-reactive memory T and B cells get preferentially activated, proliferated over the DENV2-specific T and B cells. The cross-reactive DENV1–specific adaptive immune responses outcompete naïve T cells that would be more specific for DENV2, resulting in an expanded memory T cell pool that is of low specificity for DENV2 and poor viral clearance. Antibody-dependent enhanced replication also has the potential to occur during a secondary, heterologous infection.
Akin to T cells, the titer of DENV-specific antibodies produced from prior infection increases substantially during secondary dengue, and they are predominantly non-neutralizing. Binding of these cross-reactive non-neutralizing antibodies with DENV virions could set in motion both extrinsic and intrinsic forms of ADE. Extrinsic ADE occurs when non-neutralizing antibodies forming a virus-antibody complex are recognized and engulfed by other uninfected cells, e.g., monocytes, macrophages, dendritic cells (DCs) and mast cells, via their gamma Fc receptors (FcγR), particularly FcγRI (CD64) and FcγRII (CD32), resulting in an increase in the frequency of DENV-infected cells, and subsequent upsurge in viral production (28, 34). Intrinsic ADE on the other hand, was first observed in Ross River virus (RRV) where incubation of RRV anti-RRV IgG had resulted in ADE-mediated persistent productive infection of macrophages for extended time periods. Further investigations showed that the entry of virus via Fcγ-antibody complexes will bypass TLR3 and TLR7 signaling leading to a Th2-biased immune responses and increased viral production (35, 36). Later the same phenomena was also observed in DENV (37, 38), where viral entry via FcγR often produces <10-fold virions than through host receptors (e.g mannose receptor; CD205), CD14-associated protein, heat shock protein (HSP79/HSP90), DC-SIGN (CD209)) (39, 40). While intrinsic ADE increases the quantity of new infectious virions produced by an infected cell, extrinsic ADE increases the mass of cells infected by DENV, both contributing to enhanced viral replication and disease severity (41).
Apart from enhanced viral replication, the FcγR-bearing immune cells also become hyperactivated, leading to augmented production of cytokines and vasoactive mediators entailing in cytokine storm (hypercytokinemia) and endothelial dysfunction (25, 42, 43). The presence of reactive, non-neutralizing IgG is the greatest risk factor for development of DSS. These antibody isotypes have enhanced affinity for the FcγRIIIA (CD16) due to a-fucosylated FC glycans, which results in rapid reduction of platelets leading to significant thrombocytopenia (44, 45). Recently, B cells has been shown to be directly susceptible to DENV infection. The infected B cells can support low viral replication via CD300a (46). Interestingly, direct DENV infection of B cell induces their proliferation in vivo and formation of plasmablasts in vitro (46). Though ADE is not observed in B cells, the production of massive amounts of non-neutralizing antibodies during secondary infection likely has an association with disease severity (46).
The complement system composed of ~50 plasma proteins is pivotal to first-line immune surveillance and are found in inactive forms in the circulation. The proteins can be activated via three pathways, i.e. the classical, (binding of C1q with antigen-antibody complex), lectin (binding by mannose binding lectins (MBLs) and the alternate (via spontaneous activation of C3 protein) pathways. Once activated, the complement proteolytic cascade will initiate the expression of chemokines, recruitment of monocytes and granulocytes via anaphylatoxins C3a and C5a, opsonization, release of pro-inflammatory cytokines, and direct killing of infected cells by deposition of membrane attack complex (MAC).
Studies suggest that complement inhibits DENV infection through the classical pathway with C1q binding to E protein and the lectin pathways with MBL binding to virions (47), although DENV has evolved specific counterstrategies to avoid their activation. NS1 forms a multimeric structure that can be found in two forms, either in membrane-bound form (mNS1) or soluble form (sNS1). While mNS1 requires anti-NS1-specific antibodies to activate complement, sNS1 can activate it with or without anti-NS1-specific antibodies. The sNS1 is known to bind C1s and C4, which results in increased cleavage of C4 to C4a and C4b and sNS1 function as a “decoy” to limit the availability of complement to opsonize and lyse the DENV virions (47). Interestingly, sNS1 from DENV-infected insect cell line is able to bind to human MBL, which protect the virions from MBL-mediated neutralization, suggesting the role of NS1 in inhibiting complement activation at the site of the mosquito bite (48). Likewise, sNS1 present in human serum can enhance DENV acquisition by mosquitos (49). sNS1 can also inhibit MAC formation by interacting with vitronectin (VN), clusterin, and the terminal complement protein C9, thereby protecting the infected cells from complement-mediated lysis (47) (Figure 2).
Figure 2 Proposed mechanism of evasion of DENV and DENV-infected cells from classical and lectin pathway-mediated complement attack in the host. NS1 protein released by DENV-infected cells activates the classical pathway of complement activation resulting in the formation of a tri-partite C1S-NS1-C4 complex. C4 undergoes enzymatic cleavage forming C4a (anaphylatoxin and chemotaxin) and C4b present in the fluid phase becomes susceptible to spontaneous hydrolysis attributing to paucity of C4 in the circulation (33). NS1 recruits C4BP and binds with it leading to subsequent recruitment of C4b to engage with the NS1-C4BP complex to allow the ‘stepping-in’ of factor I. Factor I is a negative regulator of complement activation that cleaves C4b into C4c and C4d fragments to limit the classical as well as the lectin pathways (34). Competitive binding of NS1 to MBL prevents recognition of DENV by MBL that protects DENV from neutralization by MBL.
Following infection, B cells can be activated by DENV, via direct or indirect modes. Despite relatively poor permissibility, B cells are susceptible to DENV infection via a phosphatidylserine receptor, CD300a that serves as a portal for virus attachment and entry (46). Coculture of primary B cells with DENV gives rise of substantial amount of IgM release suggesting a heightened level of B cell activation (50). DENV activation of B cells is dependent on CD81 molecule that likely transmits signal via the ERK, p38 and JNK MAPK signalling pathways (50). An in vitro induction experiment with CD40L, IL-2 and IL-21 revealed that pre-infected B cells display enhanced rate of differentiation into plasmablasts and plasma cells (51). Nonetheless, B cells are not the ideally replicating host cells for DENV as low titers of infectious virions are still detectable in culture supernatants (46). Notably, NS3+ B cells could be found in the peripheral blood of acute DENV-infected patients, and other viral antigens can also be detected in the germinal centers of infected individuals (46, 52, 53). This collectively implies that B cells are not only susceptible to DENV infection, but their movement may promote viral dissemination in the body (46). On the other hand, B cells are also indirectly activated through a bystander reaction, by inflammatory mediators released by activated monocytes/macrophages that promote the differentiation of resting B cells into antibody-secreting plasmablasts (54, 55), secreting virus-specific neutralizing antibodies to eliminate the infection.
In the last decade, multiple clinical studies have reported on the expansion of DENV-specific plasmablasts following acute infection (56, 57). Although the phenomenon occurs in both primary and secondary infections, it is transient as the plasmablast population rapidly contracts to baseline level during convalescence (57). Interestingly, the magnitude of plasmablast response is reportedly unique for dengue fever as no such levels of plasmablasts can be seen in the patients with other febrile illnesses or individuals receiving influenza and yellow fever immunizations (56, 58). The total number of CD19+IgD-CD38highCD27high plasmablasts in DENV-infected patients increases strikingly over 1000-fold relative to the basal level in healthy individuals, reaching up to 1×106 plasmablasts per ml of blood within one week after the onset of symptoms (56). During acute DENV infection phase, the percentage of plasmablast population constitutes an average of 30% of the total lymphocyte, and around half of the total peripheral B cell populations (56).
Growing lines of evidence suggest a strong correlation between the number of plasmablasts and dengue severity. In patients with severe dengue, a significantly higher proportion of plasmablasts occupying ~87% of the total peripheral B cell pool has been described (59, 60). The plasmablast response peaks during severe illness and declines soon following recovery, coinciding with disease pathology (56, 61). The highly augmented plasmablast population in severe dengue implies a paramount role in dengue immunopathogenesis. However, further investigations are warranted to unveil role plasmablasts in the setting of DENV infection as differentiated from other viral infections.
B cell activation is driven primarily by antigen recognition through its surface B cell receptor (BCR) following infection or vaccination, which occurs either extrafollicular in a T-cell independent, or follicular in a T-cell dependent manner. Short-lived plasmablasts arise from extrafollicularly-activated B cells, whereas follicular B cells diverge into two distinct lineages, i.e., antibody secreting plasmablasts or memory B cells following T-dependent cell activation. Circulating plasmablasts constitute the major source of antibodies critical for virus clearance, whereas memory cells provide protection from secondary infection by differentiating robustly into antibody-secreting plasma cells. Plasmablast response in the context of DENV is characterized by concurrent T-dependent and T-independent B cell responses (62, 63) (Figure 3). High-throughput cDNA sequencing of the human Ig gene heavy chain variable region (VH) suggests a low percentage of plasmablasts displaying somatic hypermutated antibody genes even during secondary infection or severe dengue (62). The dominant population with hypomutated VH segments likely represents extrafollicular B cell-derived plasmablasts independent of T cells. Despite the lack of T cell help, the T-independent plasmablasts actively secreting antibodies particularly of IgG isotype with high neutralizing ability has been reported even after primary infection (57, 61). This suggests that germ-line encoded Ig genes give rise to poly-reactive antibodies that functions during acute dengue infection (62). By contrast, a high degree of somatic hypermutation in dengue plasmablasts has been reported, highlighting the role of T-dependent activity (64). A sub-optimal T cell response compared to B cell response occurs normally in dengue infection (65). Hospitalization is reportedly associated with lower frequencies of activated, terminally differentiated T cells and higher percentages of effector memory CD4+ T cells (61). Follicular helper T cells (Tfh) increase during acute DENV infection, peak at critical disease phase, and are higher during secondary compared to primary dengue (66, 67). Tfh cells express follicular homing chemokine receptor CXCR5, PD-1, ICOS and produce IL-21, which support the B cells in germinal centre (68).
Figure 3 T-dependent and T-independent plasmablast reactions elicited by dengue infection. Dengue virus infection activates B cells directly or indirectly, expanding the B cells into a vast number of circulating plasmablasts. Plasmablasts that are derived from extracellular B cells proliferate rapidly in an innate manner without the requirement of T cell help. The Immunoglobulin gene of this plasmablast population is hypomutated as it lacks germinal centre reaction and produces IgM, IgG and IgA. T-independent plasmablasts response vigorously during acute dengue infection regardless of primary or secondary infection. On the other hand, follicular B cells activated receive help from follicular helper T cells (TFH) and initiate the germinal centre reaction where clonal expansion, class switch and active somatic hypermutation (SHM) occurred. The plasmablast produced are long lived and predominant antibodies released are of IgG isotype. Surprisingly, these plasmablasts with evidence of T cell help secrete poor levels of antibodies compared to those without T cell help, but present with high oxidative phosphorylation, eukaryotic initiation factor (EIF2) pathway, and mitochondrial dysfunction. Memory B cells generated following dengue infection provides long term protection after recovery.
The percentage of Tfh also positively correlates with the frequency of plasmablasts and the titre of specific antibodies against DENV (66, 68). Surprisingly, the plasmablasts receiving T cell help following naïve B cell activation secrete low antibody levels, but have high oxidative phosphorylation, activation of eukaryotic initiation factor (EIF2) pathway, and mitochondrial dysfunction (63). A recent longitudinal analysis of DENV-infected patients has revealed distinct antibody responses and B cell activation during primary and secondary responses, suggesting the role of memory B cells in secondary dengue (61). Serotype-specific antibodies are retained in patients six-months post-infection and the serum antibody concentration correlates with the percentage of specific memory B cells (61, 69). Others have reported that ~8% of DENV-specific CD27+CD38- memory B cells undergo class-switching during acute and early convalescence (70). In fact, memory B cells secrete antibodies for a longer duration even after two decades post-infection (71). Despite this, plasmablasts and memory B cells in DENV infection are poorly linked to each other (72). Plasmablast expansion during acute dengue does not seem to be directly derived from memory B cell clones or contribute to memory B cell repertoire during convalescence. The preference of Ig heavy chain usage and complementarity-determining region (CDR3) length differs between plasmablasts and memory B cell clones (72). DENV-specific memory B cell clones predominantly express IgM, and likely requires a fresh round of germinal centre reaction during reinfection; whereas the plasmablasts that appear rapidly following infection predominantly express IgG (72).
The hallmark of severe dengue is the transient endothelial dysfunction leading to increased vascular permeability as well as the alteration in the coagulation cascade leading to bleeding, pleural effusion and hypovolemic shock (73). Although dengue pathogenesis is not completely understood, DENV NS1 is regarded as the “viral toxin” and the key mediator for severe disease. Firstly, NS1 activates the complement cascade leading to exaggerated release of vasoactive anaphylatoxins, which in turn cause aberrant activation of mast cells and histamine release leading to increased vascular permeability and endothelial dysfunction. This is evidenced by the presence of high levels of NS1, C3a, C5a and in the plasma of DHF patients before plasma leakage and in the pleural fluids of DSS patients, and their levels correlating with disease severity (47, 74). Secondly, the anti-NS1 antibodies (IgM and IgG) form complexes with both mNS1 and sNS1 leading to complement-dependent lysis of the host cell and ADCC, damaging the endothelial layer to necessitate vascular leakage (47). Furthermore, sNS1 also engages with TLR4 expressed on monocytes, macrophages and endothelial cells, leading to cytokine storm, further damaging the endothelium (75).
Bleeding tendency, plasma leakage and thrombocytopenia are the key clinical features of DHF/DSS. DENV infection induces polyclonal and highly cross-reactive antibodies (46, 50, 64). Studies have demonstrated that anti-NS1 antibodies are autoreactive, targeting various epitopes present on human including platelets and other proteins related to coagulation process (e.g. fibrinogen, plasminogen, thrombin) as well as extracellular matrix proteins of endothelial cells (76). During dengue infection, blood vessels may be injured due to autoreactive ADCC against mNS1. Activated platelets adhere to the injury site followed by changing shape, releasing granule contents, and eventually aggregating together through fibrin formation. It has been reported that the concentration of NS1 proportionally correlated with the disease severity (12, 15, 77) and could be as high as 50 μg/ml among DHF/DSS patients. During the defervescence, these NS1 is cleared from the circulation by antibody-mediated respond. Countless autoreactive antibodies are attacking self-component during the course of clearing NS1 (78–81) and the coagulation process is inhibited; further leading to bleeding, plasma leakage and shock. This is partly explain why plasma leakage is often happen during the defervescence phase. In a recent meta-analysis, the study showed that the activated partial thromboplastin time (APTT) and prothrombin time (PT) are significantly prolonged among patients with dengue infection where the APTT and PT were 42.91% (95% CI: 30.95, 54.87) and 16.48% (95% CI: 10.95, 22.01), respectively (82) compared to uninfected individuals, rendering them more prone to develop bleeding manifestations during dengue disease. NS1 has also been reported to form immune complexes with erythrocytes leading to complement-induced erythrocyte damage (76, 83).
Monocytes are susceptible to DENV infection and produce several key proinflammatory cytokines contributing to endothelial dysfunction and vascular leakage. IL-1β, IL-18, IL-33 and high-mobility group B1 (HMGB1) represent the key inflammatory mediators produced during hypercytokinemia, via the inflammasome pathway and have been shown to correlate with dengue severity (20, 84, 85). Given that NS2A and NS2B can trigger the activation of NLR family pyrin domain containing 3 (NLRP3) (86), the main component of an NLRP3 inflammasome, the more the cells infected by DENV via ADE, the higher the release of IL-1β, IL-18 and HMGB1 (87). IL-1β and IL-18 are produced via the NLRP3 inflammasome-caspase-1 pathway. They bind to their cognate receptors (IL-1R1 and IL-18Rα/β) to promote Th1 cell activation, cytotoxic functions of CD8+ T cells and NK cells, release of cytokines, especially IFN-γ along with other mediators of inflammation namely IL-2, IL-12, TNF, and GM-CSF. IL-18 also induces the expression of chemokine and adhesion molecules on endothelial and smooth muscle cells (88). HMGB1 is, when released from cells, an ‘alarmin’ in peripheral circulation during pyroptosis, a pro-inflammatory cell death pathway induced by aberrant activation of inflammasome, and hence is a byproduct of inflammasome activation. In the circulation, HMGB1 binds to its cognate receptor, receptor of advanced glycation end-products (RAGE) resulting in the activation of an intracellular pathway that culminates in the formation of actin stress fibers, remodeling of cytoskeleton, contraction of endothelium and vascular leakage (89). Because RAGE is abundantly expressed along the lung endothelium, this likely could be the attribute behind why plasma leakage often manifests as pleural effusion in the lungs (89) (Figure 4).
Figure 4 Interplay between NS1, inflammasome activation, complement activation, autoreactive antibodies and cytokine storm in endothelial dysfunction. 1) DENV and NS1 can directly activate CS, the excessive conversion of C3, C4 and C5 to their active forms C3b, C4b and C5b will inevitably cause increased level of C3a, C4a and C5a (anaphylatoxin). Large amount of anaphylatoxin will cause aberrant activation of mast cells and release massive amount of histamine, along with other pro-inflammatory cytokines, leading to increase of the vascular permeability and vascular leakage. 2) anti-NS1-specific IgM and IgG have been found circulating in the blood stream forming immune complexes with the both forms of NS1 proteins. While mNS1 is expressed on DENV infected cells, the circulating sNS1 can subsequently bind to surface of infected or uninfected cells via heparan sulfate and chondroitin sulfate E (90). These antibodies will then bind to NS1 and leading complement-dependent lysis of cell and antibody-dependent cellular cytotoxicity, damaging the endothelial layer and leading to vascular leakage (47). 3) Secretion of IL-1β, IL-18 and HMGB1 results from NLRP3 inflammasome activation. 4) In the circulation, IL-1β and IL-18 binds to their cognate receptors (IL-1R1/acP and IL-18Rα/β) expressed on the surface of endothelial cells to activate intracellular signaling molecules, involving MyD88, IL-1 receptor-associated kinase 1/4 (IRAK1/4), and TNF receptor-associated factor (TRAF), which entails in NF-κB activation. NS1 can also activate the NF-κB signaling pathway via TLR4. The activation of NF-κB signaling pathway increases the secretion of pro-inflammatory cytokines and chemokines to mediate leukocyte adhesion and extravasation (diapedesis). Additionally, the binding of HMGB1 to the RAGE receptor leads to the downstream activation of p38 MAP kinase, resulting in phosphorylation of the actin-binding protein Hsp27 and caldesmon, which causes actin stress fibers to form, cytoskeletal remodeling, and endothelial contraction. All these reactions increase endothelial permeability by altering cell contractility and disruption of intercellular junctions. Given the ability of IL-18 to induce the production of various pro-inflammatory mediators including TLR4 and RAGE via activation of NFκ-B signaling (75, 91, 92), their higher expression will condition the endothelial cells to be more responsive to stimulation by NS1 and HMGB1, to further magnify inflammation and vascular permeability.
Since December 2019, coronavirus disease 2019 (COVID-19) caused by severe acute respiratory syndrome coronavirus 2 (SARS-CoV-2) has wreaked havoc globally and the pandemic continues to be a public health concern. Because certain features of clinical and laboratory presentation of dengue and COVID-19 seems to be overlapping, the correct diagnosis and treatment of co-infection is challenging, especially in tropical regions where they co-exist (93, 94). Table 1 shows the similarities between DENV and SARS-CoV-2. It is to be noted that both DENV and SARS-CoV-2 have similar incubation periods presenting with acute febrile phases. In such cases, respiratory symptoms such as cough and dyspnea should raise clinical suspicion towards COVID-19. While significant thrombocytopenia is a prominent feature observed in dengue, it is less common for COVID-19, reported in only about 5 to 12% of cases (94). A case report describes a patient with concurrent positivity for dengue serology and COVID-19 (by RT-PCR) raising concern on the possibility of co-infection with both viruses considering the dengue-endemic geography region of reports and call for rapid and sensitive diagnostic tests (105, 107). In a systematic review conducted between January and November 2020, it has been concluded that COVID-19 and dengue co-infection was associated with severe disease and fatal outcomes (108).
Organizing pneumonia, the prominent complication in COVID-19 patients results from lung injury caused by a surge in levels of proinflammatory cytokines. Various studies have demonstrated the correlation of hypercytokinemia with the breadth of lung injury, multiorgan failure, ICU admission and poor survival rates (especially with IL-6 levels) in COVID-19 patients (95–97). Cytokines such as IL-6, IL-2, IL-7 and many others have been demonstrated to be associated with the severity of COVID-19 (98). Similarly, elevation in the levels of proinflammatory cytokines leading to hypercytokinemia is observed in patients with DHF attributing to vascular leakage and disease pathogenesis (99–101). The role of hypercytokinemia in the dengue pathogenesis, similarities in certain clinical presentations and pathological changes in multiple organs are shared between DENV and SARS-CoV-2 (102). In both DENV and SARS-CoV-2 infections, elevated levels of proinflammatory cytokines and chemokines such as IL-6, IL-1β, IL-8, CXCL9, MCP-1 and IP-10 as well as IL-10 have been positively correlated with the severity of clinical illness (99, 103, 104, 106). A study that compared the responses in patients with varying severity of COVID-19 and acute dengue infection at different time-points showed significantly higher levels of IL-6, IL-10 and MIP-3α among those who developed COVID-19 pneumonia and DHF than those with mild manifestations (102). Interestingly, the finding points at the elevated levels of IL-10 in both COVID-19 as well as DENV infection during the early phases of illness as indicators of altered immune responses possibly lead to severe disease presentation and poor prognosis.
A recent study used a series of computational analyses to build host factor interaction networks and elucidated the biological process and molecular function categories for a better understanding of the underlying mechanisms of SARS-CoV-2 and DENV coinfection. Of the 460 shared host factors identified, CCL4 and AhR targets were significantly upregulated, and the study also demonstrated the role of the NOD-like receptor (NLR) and TLR signaling pathways during the interaction of the viruses with the host. The study reported that IL-1β, Hippo, p53, TNF-α and TLR were significantly upregulated. Furthermore, the tissue-specific enrichment analysis revealed an increase in the expression of ICAM-1 and CCL2 in the lungs (109). It has also been reported that SARS-CoV-2 and DENV coinfection could result in severe pulmonary impairment, hospitalization rates and grave clinical outcomes (110).
It also appears that the serological cross-reactivity between DENV and SARS-CoV-2 can have serious implications in the diagnosis point-of-view and can pose a diagnostic predicament in geographical locations endemic to dengue. A study from Singapore had reported a couple of cases with false-positive results for dengue based on rapid serological assays, which were later confirmed as COVID-19 positive (111). In a report from Thailand (where dengue infections are common), a COVID-19 patient presenting with petechial rash and low platelet count was initially misdiagnosed as dengue (112). A study from Indonesia had also reported false-positive results between dengue and COVID-19 despite the high specificity of the rapid diagnostic tests for COVID-19 (113). An interesting study from India wherein rapid dengue antibody detection tests were performed on archived serum samples pre-dating the COVID-19 pandemic and those positive for dengue were subjected to SARS-CoV-2 detection. Of the thirteen dengue positive sera tested, five produced false-positive results in SARS-CoV-2 lateral flow-based rapid tests. These results demonstrate the possibility of cross-reactivity and false-positive COVID-19 results in dengue-endemic countries like India. Interestingly, the study has also demonstrated that antibodies against DENV can cross-react with the receptor-binding domain (RBD) of SARS-CoV-2 spike protein based on computational analysis (114). A recent investigation from Brazil investigated 2351 COVID-19 patients to report that those with a history of dengue infection had a lower risk of mortality hinting the likely protection induced by DENV against severe COVID-19, which nevertheless remains a component warranting rather more evidence (115). It appears that although coinfection with SARS-CoV-2 and DENV combination can aggravate the clinical severity alongside diagnostic predicaments (in dengue-endemic areas), the interaction between the viruses remains a grey area of investigation. Further, the immune correlates of protection in DENV-SARS-CoV-2 coinfection needs thorough investigation, and must explore the possibilities of identification of improved therapeutic targets.
While our efforts to develop a safe and effective dengue vaccine are progressive albeit for many decades, we are yet to achieve significant success at these quarters. The live-attenuated, tetravalent DENV candidate vaccine developed by Sanofi Pasteur Inc., Swiftwater, PA, USA has shown varying levels of protective efficacy against all the four different DENV serotypes, viz., DENV1 (61.2%), DENV3 (81.3%), and DENV4 (89.9%), whereas no protection against DENV2 (116) has been reported. Owing to ADE (117), an increased risk of hospitalization and development of severe dengue among DengVaxia® recipients has been observed during the four Phase 3 clinical trials (viz., CYD17, CYD29, CYD32, and CYD33) conducted in the Philippines in 2019 (118). Perhaps vaccines are not the “silver bullets” against development of dengue disease (also with COVID-19). With the extensive understanding we have had on the immunopathogenesis of dengue disease, perhaps there are other potential interventions focusing on harnessing passive immunity, host-directed immunomodulation as well as alleviation of platelet loss, which likely could add to amelioration of dengue disease severity.
Recently, the U.S. Food and Drug Administration revised the emergency use authorization (EUA) for two neutralizing monoclonal antibodies, Bamlanivimab and Etesevimab for the emergency therapy against mild to moderate COVID-19 in pediatric patients, including neonates, who had a positive COVID-19 test and were also at an increased risk for development of severe COVID-19 (119). Similarly, monoclonal antibodies directed against all the four DENV serotypes could be developed and administrated in accordance with the infecting serotype in the corresponding group of patients. Such interventions where the corresponding drug is administered at optimum neutralizing levels, may effectively mitigate the risk of development of ADE in dengue patients.
Secondly, since DENV NS1 has been regarded as viral toxin implicated in the aberrant activation of the complement cascade, excessive release of anaphylatoxins C3a and C5a leading to eventual onset of increased vascular permeability and endothelial dysfunction, the neutralization of NS1 appears to be a promising strategy to reduce the severity of dengue illness and complications. Several lines of evidence show that the anti-NS1 antibodies can effectively block the NS1 from interacting with the surface of endothelial cells, thereby limiting the NS1-induced disruption of the intercellular junction complex (31, 120, 121).
More recently, by using a mouse model O’Donnell et al. (2020) demonstrated that the polyclonal avian IgY generated against a suspension of whole, killed DENV2 can confer protection against a lethal challenge of dengue infection without the development of ADE. This appears to be attributed to IgY’s ability to neutralize DENV notwithstanding the avian Fc does not bind with the mammalian Fc receptor (122). The above experiment provides us a viable clue for a promising therapeutic approach against DENV. Nonetheless, since anti-NS1 antibodies are known to be autoreactive and linked to coagulopathy and endothelial dysfunction (76), the use of anti-NS1 antobodies in treating dengue disease must be carefully selected to avoid antoreactivity. Further, with significant advances in antibody engineering, the Fc domain can be fine-tuned in such a way that it does not induce ADE or autoreactivity (123, 124).
It is now clear that exaggerated activation of the complement cascade could contribute to dengue severity and vascular leakage, and hence drug interventions that can regulate complement activation would likely be a valid target candidate. Eculizumab is an anti-C5 mAb that was initially approved to treat atypical hemolytic uremic syndrome (aHUS) (125, 126). Because the vasculopathy in dengue is mainly due to excessive release of C3a and C5a, the potential use of anti-C5 mAb may likely reduce vascular leakage and associated complications in severe dengue (127).
Having said that aberrant activation of inflammasome, especially NLRP3 inflammasomes could play an important role driving the onset of cytokine storm, inhibitors/blockers of the inflammasome would be an alternative promising approach against severe dengue-associated manifestations. MCC950 is a highly potent small molecule inhibitor of NLRP3, and binds directly to the NATCH (NAIP, CIITA, HET-E, and TP-1) domain of NLRP3 preventing it from undergoing conformational changes and assembly (128, 129). In vivo studies have shown that MCC950 reduced IL-1β production and attenuated the severity of experimental autoimmune encephalomyelitis (EAE) (130). Nonetheless, the applicability of treating clinical dengue with MCC950 has seldom been examined warranting in-depth investigations in the future.
IL-18 is an important (Th1 polarizing) cytokine produced during pyroptosis by the activation of NLRP3 inflammasome alongside IL-1β. However, the biological activity of IL-18 is regulated by a regulatory protein, named IL-18 binding protein (IL-18BP). IL-18BP is a natural-occurring soluble molecule that binds to IL-18 with a higher affinity, preventing IL-18 from engaging with the IL-18R expressed on cell surfaces, thereby regulating the bioavailability of IL-18 to other lymphocytes (131). In a previous study, we compared the plasma level of IL-18 and IL-18BP among dengue patients with and without warning signs as well as severe dengue. We also determined the bioavailability of IL-18, and found that the plasma levels of IL-18 was significantly higher in patients with severe dengue followed by those with dengue with warning signs as well as dengue without warning signs. However, the levels of IL-18BP were not significantly different. Having said that, the bioavailability of IL-18 is higher among patients with severe dengue as well as among those with warning signs as compared to dengue without warning signs (84). This suggests that the cytokine storm occurring in severe dengue disease may be due to inadequate regulation of IL-18 responses by IL-18BP.
In a more recent study, Li et al. (2020) used recombinant human IL-18 (rhIL-18BP) to decrease soluble IL-18 in irradiated experimental mouse. The administration of IL-18BP was found to inhibit the down-stream activities of IL-18, and the treated mouse showed low levels of IFN-γ, reactive oxygen species (ROS) as well as attenuated a stress responsive factor named growth differentiation factor-15 (GDF-15) (132). The study was encouraging as it showed that IL-18 played a paramount role in radiation-induced tissue damage and treatment with IL-18BP likely mitigates tissue injury/damage induced by radiation. Hence, the use IL-18BP appears to serve as a promising therapeutic strategy against severe dengue disease warranting investigations.
Thrombocytopenia and platelet dysfunction are commonly observed in DENV patients and dengue complications are usually preceded by a rapid drop in platelet count (133). Platelet, traditionally known for their key role in coagulation, but nowadays are well known to have important additional functions, including regulation of inflammation and host defense (134) and preservation of endothelial integrity (135), especially under inflammatory conditions (136). Circulating platelets of dengue patients are found to be over activated and exhausted (137), which likely contributes to platelet loss and coagulopathy. Other well-known mechanisms of platelet loss is the binding of von Willebrand factor (VWF) to platelets and loss of sialic acids from the platelet membrane (138). VWF is a multimeric protein that is present in plasma, platelets, and endothelial cells. Endothelial activation results in the release of VWF from Weibel-Palade bodies into the blood circulation with its primary function is to allow platelet adhesion and aggregation at sites of vessel injury (139). As dengue infection can cause endothelial activation, massive amount of VWF are released into circulation. Study showed that the VWF level was elevated in dengue infection and particularly heighten among DSS patients (139). Mechanistic studies revealed that the binding of VWF to platelets results in translocation of neuraminidase to the platelet membrane and subsequent cleavage the sialic acid off from the membrane (140). The good news is that this process could be inhibited by the neuraminidase inhibitor oseltamivir, or more commonly known as Tamiflu, the anti-influenza drug (141). The efficacy of oseltamivir in preventing thrombocytopenia in dengue disease should be investigated. The newly proposed approaches aforementioned above are summarized in Table 2.
The dengue pandemic was initially reported in Southeast Asia after World War II and has spread rapidly to almost all tropical countries. Dengue poses an enormous burden to public health systems worldwide as >40% of the global population is at risk of infection. Following infection, viruses undergo replication in the local tissues such as the skin, which leads to an activation of a cascade of events including the recruitment of skin resident cells, e.g., Langerhans cells, mast cells, and keratinocytes, and new cells, e.g., T cells and neutrophils, into the site of the infection. After infection of target cells, sensing of viral products results in the activation of innate immune responses, which establish the inflammatory and antiviral state intended to prevent the virus to replicate and spread. However, DENV utilizes several mechanisms to hijack these responses and escape from the normal immune recognition and processing, which results in its dissemination into the lymph nodes. There, DENV further replicates in monocytic cells, resulting in a primary viremia after its systemic disseminated through the circulatory bloodstream. This results in the subsequent infection of peripheral tissues, such as the liver, spleen, and kidney. Evidence also strongly supports the involvement of multiple cell death pathways following DENV infection leading to vascular dysfunction brought about by monocyte activation. Improved understanding of cell death pathways induced by DENV will help in the development of novel modalities of prevention of disease progression.
In endemic areas where multiple DENV serotypes circulate, distinct epidemiological studies found that an individual can become exposed to and can have sequential infections with distinct DENV serotypes, which poses a risk of developing severe manifestations such as DHF/DSS. This phenomenon has been attributed to the potential enhancement activity that the pre-existing antibody response elicited from a previous infection with one serotype may have on the infection with a different serotype. This process leads to an increased viral burden that triggers a series of immunological and cellular events, e.g., ADE, hypercytokinemia, skewed T cell responses, and complement pathways, which despite being intended to prevent the viral invasion and infection, can induce host tissue damage leading to pathology and disease. Hence, it is important to study the immunopathology of dengue fever, as we gain more insights into the pathogenic mechanisms of DENV infections, we can hope to improve our efforts towards providing better case management, reduce its overall morbidity and mortality, and assist in the development of safe and effective vaccines against the dreadful disease.
Maintenance of adequate hydration is key to dengue management. Patients also must be monitored for warning signs of severe dengue disease, and hence, prompt initiation of early management/treatment intervention is key to preventing dengue-associated complications such as prolonged shock and metabolic acidosis. Hence, the mainstay of successful management includes judicious and timely initiation of IV fluid replacement therapy with isotonic solutions and frequent monitoring of the hemodynamic status and vital signs during the critical phase. Patients should be administered with acetaminophen for pain as well as temperature management. Aspirin and non-steroidal, anti-inflammatory medications could aggravate the bleeding tendency in some patients and, in children, can be associated with the development of Reyes syndrome.
YKY, WFW, RV, IC, and EMS wrote the manuscript. YKY and EMS critically revised the article for important intellectual content, and approved publication of the article. VV, YZ, ML, and HYT provided critical inputs to the manuscript. All authors contributed to the article and approved the submitted version.
This work was supported by Xiamen University Malaysia Research Funding (XMUMRF), (XMUMRF/2018-C2/ILAB/0001) to YKY, (XMUMRF/2020-C5/ITCM/0003) to HYT and (XMURF/2018-C1/ENG/0005) to YZ. VV was supported in part by NIH R01AI148377 (to VV), Emory University CFAR grant P30 AI050409, NIH Office of Research Infrastructure Programs (ORIP) grants P51 OD011132 and U42 OD011023 (to ENPRC). The Swedish Research Council, The Swedish, Physicians against AIDS Research Foundation, The Swedish International Development Cooperation Agency; SIDA SARC, VINNMER for Vinnova, Linköping University Hospital Research Fund, CALF, and The Swedish Society of Medicine (AI52731) to ML, Funding support provided by the Department of Science and Technology-Science and Engineering Research Board, Government of India (CRG/2019/006096) (to EMS).
The content is solely the responsibility of the authors and does not necessarily represent the views of the official affiliations of the authors.
The authors declare that the research was conducted in the absence of any commercial or financial relationships that could be construed as a potential conflict of interest.
All claims expressed in this article are solely those of the authors and do not necessarily represent those of their affiliated organizations, or those of the publisher, the editors and the reviewers. Any product that may be evaluated in this article, or claim that may be made by its manufacturer, is not guaranteed or endorsed by the publisher.
We appreciate all those who have contributed substantially to the study of dengue immunopathogenesis from the Central University of Tamil Nadu (Jaisheela Vimali), and also from the Department of Microbiology, Government Theni Medical College and Hospitals (Amudhan Murugesan). We thank Rada Ellegård for the scientific editing, writing and support with the illustrations.
1. Messina JP, Brady OJ, Scott TW, Zou C, Pigott DM, Duda KA, et al. Global Spread of Dengue Virus Types: Mapping the 70 Year History. Trends Microbiol (2014) 22(3):138–46. doi: 10.1016/j.tim.2013.12.011
2. WHO. Dengue and Severe Dengue, Fact Sheets, World Health Organization (2020). Available at: https://www.who.int/news-room/fact-sheets/detail/Dengue-and-severe-Dengue.
3. Bhatt S, Gething PW, Brady OJ, Messina JP, Farlow AW, Moyes CL, et al. The Global Distribution and Burden of Dengue. Nature (2013) 496(7446):504–7. doi: 10.1038/nature12060
4. Bhatt S, Gething PW, Brady OJ, Messina JP, Farlow AW, Moyes CL, et al. The Global Burden of Dengue: An Analysis From the Global Burden of Disease Study 2013. Lancet Infect Dis (2016) 16(6):712–23. doi: 10.1016/S1473-3099(16)00026-8
5. Fukusumi M, Arashiro T, Arima Y, Matsui T, Shimada T, Kinoshita H, et al. Dengue Sentinel Traveler Surveillance: Monthly and Yearly Notification Trends Among Japanese Travelers, 2006-2014. PLoS Negl Trop Dis (2016) 10(8):e0004924. doi: 10.1371/journal.pntd.0004924
6. Simmons CP, Farrar JJ, Nguyen V, Wills B.. Dengue. N Engl J Med (2012) 366(15):1423–32. doi: 10.1056/NEJMra1110265
7. What Pay for Performance can Mean for Quality Managers. Healthcare Benchmarks Qual Improv (2004) 11(8):85–8.
8. Campbell LP, Luther C, Moo-Llanes D, Ramsey JM, Danis-Lozano R, Peterson AT., et al. Climate Change Influences on Global Distributions of Dengue and Chikungunya Virus Vectors. Philos Trans R Soc Lond B Biol Sci (2015) . 370(1665):20140135. doi: 10.1098/rstb.2014.0135
9. Guzman MG, Gubler DJ, Izquierdo A, Martinez E, Halstead SB. Dengue Infection. Nat Rev Dis Primers (2016) 2:16055. doi: 10.1038/nrdp.2016.55
10. Perera R, Kuhn RJ. Structural Proteomics of Dengue Virus. Curr Opin Microbiol (2008) 11(4):369–77. doi: 10.1016/j.mib.2008.06.004
11. Kuhn RJ, Zhang W, Rossmann MG, Pletnev SV, Corver J, Lenches E, et al. Structure of Dengue Virus: Implications for Flavivirus Organization, Maturation, and Fusion. Cell (2002) 108(5):717–25. doi: 10.1016/s0092-8674(02)00660-8
12. Libraty DH, Young PR, Pickering D, Endy TP, Kalayanarooj S, Green S, et al. High Circulating Levels of the Dengue Virus Nonstructural Protein NS1 Early in Dengue Illness Correlate With the Development of Dengue Hemorrhagic Fever. J Infect Dis (2002) 186(8):1165–8. doi: 10.1086/343813
13. Kong YY, Thay CH, Tin TC, Devi S.. Rapid Detection, Serotyping and Quantitation of Dengue Viruses by TaqMan Real-Time One-Step RT-PCR. J Virol Methods (2006) 138(1-2):123–30. doi: 10.1016/j.jviromet.2006.08.003
14. Yong YK, Thayan R, Chong HT, Tan CT, Sekaran SD.. Rapid Detection and Serotyping of Dengue Virus by Multiplex RT-PCR and Real-Time SYBR Green RT-PCR. Singapore Med J (2007) 48(7):662–8.
15. Alcon S, Talarmin A, Debruyne M, Falconar A, Deubel V, Flamand M., et al. Enzyme-Linked Immunosorbent Assay Specific to Dengue Virus Type 1 Nonstructural Protein NS1 Reveals Circulation of the Antigen in the Blood During the Acute Phase of Disease in Patients Experiencing Primary or Secondary Infections. J Clin Microbiol (2002) 40(2):376–81. doi: 10.1128/JCM.40.02.376-381.2002
16. Watanabe S, Tan KH, Rathore AP, Rozen-Gagnon K, Shuai W, Ruedl C, et al. The Magnitude of Dengue Virus NS1 Protein Secretion is Strain Dependent and Does Not Correlate With Severe Pathologies in the Mouse Infection Model. J Virol (2012) 86(10):5508–14. doi: 10.1128/JVI.07081-11
17. Anand AM, Sistla S, Dhodapkar R, Hamide A, Biswal N, Srinivasan B.. Evaluation of NS1 Antigen Detection for Early Diagnosis of Dengue in a Tertiary Hospital in Southern India. J Clin Diagn Res (2016) 10(4):DC01–4. doi: 10.7860/JCDR/2016/15758.7562
18. Avirutnan P, Punyadee N, Noisakran S, Komoltri C, Thiemmeca S, Auethavornanan K, et al. Vascular Leakage in Severe Dengue Virus Infections: A Potential Role for the Nonstructural Viral Protein NS1 and Complement. J Infect Dis (2006) 193(8):1078–88. doi: 10.1086/500949
19. Hang VT, Nguyet NM, Trung DT, Tricou V, Yoksan S, Dung NM, et al. Diagnostic Accuracy of NS1 ELISA and Lateral Flow Rapid Tests for Dengue Sensitivity, Specificity and Relationship to Viraemia and Antibody Responses. PLoS Negl Trop Dis (2009) 3(1):e360. doi: 10.1371/journal.pntd.0000360
20. Oliveira ERA, Povoa TF, Nuovo GJ, Allonso D, Salomao NG, Basilio-de-Oliveira CA, et al. Dengue Fatal Cases Present Virus-Specific HMGB1 Response in Peripheral Organs. Sci Rep (2017) 7(1):16011. doi: 10.1038/s41598-017-16197-5
21. WHO. WHO Organisation (2009) Dengue Guidelines for Diagnosis, Treatment, Prevention and Control: New Edition. Geneva: WHO Organisation (2009).
22. Screaton G, Mongkolsapaya J, Yacoub S, Roberts C.. New Insights Into the Immunopathology and Control of Dengue Virus Infection. Nat Rev Immunol (2015) 15(12):745–59. doi: 10.1038/nri3916
23. Halstead SB. Dengvaxia Sensitizes Seronegatives to Vaccine Enhanced Disease Regardless of Age. Vaccine (2017) 35(47):6355–8. doi: 10.1016/j.vaccine.2017.09.089
24. Halstead SB. Controversies in Dengue Pathogenesis. Paediatr Int Child Health (2012) 32 Suppl 1:5–9. doi: 10.1179/2046904712Z.00000000045
25. Srikiatkhachorn A, Mathew A, Rothman AL. Immune-Mediated Cytokine Storm and its Role in Severe Dengue. Semin Immunopathol (2017) 39(5):563–74. doi: 10.1007/s00281-017-0625-1
26. Zompi S, Harris E. Original Antigenic Sin in Dengue Revisited. Proc Natl Acad Sci U S A (2013) 110(22):8761–2. doi: 10.1073/pnas.1306333110
27. Rothman AL. Immunity to Dengue Virus: A Tale of Original Antigenic Sin and Tropical Cytokine Storms. Nat Rev Immunol (2011) 11(8):532–43. doi: 10.1038/nri3014
28. Narayan R, Tripathi S. Intrinsic ADE: The Dark Side of Antibody Dependent Enhancement During Dengue Infection. Front Cell Infect Microbiol (2020) 10:580096. doi: 10.3389/fcimb.2020.580096
29. Guzman MG, Alvarez M, Rodriguez-Roche R, Bernardo L, Montes T, Vazquez S, et al. Neutralizing Antibodies After Infection With Dengue 1 Virus. Emerg Infect Dis (2007) 13(2):282–6. doi: 10.3201/eid1302.060539
30. Gallichotte EN, Baric TJ, Nivarthi U, Delacruz MJ, Graham R, Widman DG, et al. Genetic Variation Between Dengue Virus Type 4 Strains Impacts Human Antibody Binding and Neutralization. Cell Rep (2018) 25(5):1214–24. doi: 10.1016/j.celrep.2018.10.006
31. Waggoner JJ, Balmaseda A, Gresh L, Sahoo MK, Montoya M, Wang C, et al. Homotypic Dengue Virus Reinfections in Nicaraguan Children. J Infect Dis (2016) 214(7):986–93. doi: 10.1093/infdis/jiw099
32. Dong T, Moran E, Vinh Chau N, Simmons C, Luhn K, Peng Y, et al. High Pro-Inflammatory Cytokine Secretion and Loss of High Avidity Cross-Reactive Cytotoxic T-Cells During the Course of Secondary Dengue Virus Infection. PLoS One (2007) 2(12):e1192. doi: 10.1371/journal.pone.0001192
33. Mongkolsapaya J, Dejnirattisai W, Xu XN, Vasanawathana S, Tangthawornchaikul N, Chairunsri A, et al. Original Antigenic Sin and Apoptosis in the Pathogenesis of Dengue Hemorrhagic Fever. Nat Med (2003) 9(7):921–7. doi: 10.1038/nm887
34. Littaua R, Kurane I, Ennis FA. Human IgG Fc Receptor II Mediates Antibody-Dependent Enhancement of Dengue Virus Infection. J Immunol (1990) 144(8):3183–6.
35. Lidbury BA, Mahalingam S. Specific Ablation of Antiviral Gene Expression in Macrophages by Antibody-Dependent Enhancement of Ross River Virus Infection. J Virol (2000) 74(18):8376–81. doi: 10.1128/jvi.74.18.8376-8381.2000
36. Suhrbier A, La Linn M. Suppression of Antiviral Responses by Antibody-Dependent Enhancement of Macrophage Infection. Trends Immunol (2003) 24(4):165–8. doi: 10.1016/s1471-4906(03)00065-6
37. Chareonsirisuthigul T, Kalayanarooj S, Ubol S. Dengue Virus (DENV) Antibody-Dependent Enhancement of Infection Upregulates the Production of Anti-Inflammatory Cytokines, But Suppresses Anti-DENV Free Radical and Pro-Inflammatory Cytokine Production, in THP-1 Cells. J Gen Virol (2007) 88(Pt 2):365–75. doi: 10.1099/vir.0.82537-0
38. Ubol S, Phuklia W, Kalayanarooj S, Modhiran N.. Mechanisms of Immune Evasion Induced by a Complex of Dengue Virus and Preexisting Enhancing Antibodies. J Infect Dis (2010) 201(6):923–35. doi: 10.1086/651018
39. Cruz-Oliveira C, Freire JM, Conceicao TM, Higa LM, Castanho MA, Da Poian AT., et al. Receptors and Routes of Dengue Virus Entry Into the Host Cells. FEMS Microbiol Rev (2015) 39(2):155–70. doi: 10.1093/femsre/fuu004
40. Halstead SB, Mahalingam S, Marovich MA, Ubol S, Mosser DM.. Intrinsic Antibody-Dependent Enhancement of Microbial Infection in Macrophages: Disease Regulation by Immune Complexes. Lancet Infect Dis (2010) 10(10):712–22. doi: 10.1016/S1473-3099(10)70166-3
41. Flipse J, Diosa-Toro MA, Hoornweg TE, van dePol DP, Urcuqui-Inchima S, Smit JM., et al. Antibody-Dependent Enhancement of Dengue Virus Infection in Primary Human Macrophages; Balancing Higher Fusion Against Antiviral Responses. Sci Rep (2016) 6:29201. doi: 10.1038/srep29201
42. Anderson R, Wang S, Osiowy C, Issekutz AC.. Activation of Endothelial Cells via Antibody-Enhanced Dengue Virus Infection of Peripheral Blood Monocytes. J Virol (1997) 71(6):4226–32. doi: 10.1128/JVI.71.6.4226-4232.1997
43. Kuczera D, Assolini JP, Tomiotto-Pellissier F, Pavanelli WR, Silveira GF.. Highlights for Dengue Immunopathogenesis: Antibody-Dependent Enhancement, Cytokine Storm, and Beyond. J Interferon Cytokine Res (2018) 38(2):69–80. doi: 10.1089/jir.2017.0037
44. Uno N, Ross TM. Dengue Virus and the Host Innate Immune Response. Emerg Microbes Infect (2018) 7(1):167. doi: 10.1038/s41426-018-0168-0
45. Wu YW, Mettling C, Wu SR, Yu CY, Perng GC, Lin YS, et al. Autophagy-Associated Dengue Vesicles Promote Viral Transmission Avoiding Antibody Neutralization. Sci Rep (2016) 6:32243. doi: 10.1038/srep32243
46. Upasani V, Vo HTM, Auerswald H, Laurent D, Heng S, Duong V, et al. Direct Infection of B Cells by Dengue Virus Modulates B Cell Responses in a Cambodian Pediatric Cohort. Front Immunol (2020) 11:594813. doi: 10.3389/fimmu.2020.594813
47. Conde JN, Silva EM, Barbosa AS, Mohana-Borges R. The Complement System in Flavivirus Infections. Front Microbiol (2017) 8:213. doi: 10.3389/fmicb.2017.00213
48. Thiemmeca S, Tamdet C, Punyadee N, Prommool T, Songjaeng A, Noisakran S, et al. Secreted NS1 Protects Dengue Virus From Mannose-Binding Lectin-Mediated Neutralization. J Immunol (2016) 197(10):4053–65. doi: 10.4049/jimmunol.1600323
49. Liu J, Liu Y, Nie K, Du S, Qiu J, Pang X, et al. Flavivirus NS1 Protein in Infected Host Sera Enhances Viral Acquisition by Mosquitoes. Nat Microbiol (2016) 1(9):16087. doi: 10.1038/nmicrobiol.2016.87
50. Correa AR, Berbel AC, Papa MP, Morais AT, Pecanha LM, Arruda LB., et al. Dengue Virus Directly Stimulates Polyclonal B Cell Activation. PLoS One (2015) 10(12):e0143391. doi: 10.1371/journal.pone.0143391
51. Upasani V, Vo HTM, Ung S, Heng S, Laurent D, Choeung R, et al. Impaired Antibody-Independent Immune Response of B Cells in Patients With Acute Dengue Infection. Front Immunol (2019) 10:2500. doi: 10.3389/fimmu.2019.02500
52. Aye KS, Charngkaew K, Win N, Wai KZ, Moe K, Punyadee N, et al. Pathologic Highlights of Dengue Hemorrhagic Fever in 13 Autopsy Cases From Myanmar. Hum Pathol (2014) 45(6):1221–33. doi: 10.1016/j.humpath.2014.01.022
53. Yam-Puc JC, Garcia-Cordero J, Calderon-Amador J, Donis-Maturano L, Cedillo-Barron L, Flores-Romo L., et al. Germinal Center Reaction Following Cutaneous Dengue Virus Infection in Immune-Competent Mice. Front Immunol (2015) 6:188. doi: 10.3389/fimmu.2015.00188
54. Aguilar-Briseno JA, Upasani V, Ellen BMT, Moser J, Pauzuolis M, Ruiz-Silva M, et al. TLR2 on Blood Monocytes Senses Dengue Virus Infection and its Expression Correlates With Disease Pathogenesis. Nat Commun (2020) 11(1):3177. doi: 10.1038/s41467-020-16849-7
55. Kwissa M, Nakaya HI, Onlamoon N, Wrammert J, Villinger F, Perng GC, et al. Dengue Virus Infection Induces Expansion of a CD14(+)CD16(+) Monocyte Population That Stimulates Plasmablast Differentiation. Cell Host Microbe (2014) 16(1):115–27. doi: 10.1016/j.chom.2014.06.001
56. Wrammert J, Onlamoon N, Akondy RS, Perng GC, Polsrila K, Chandele A, et al. Rapid and Massive Virus-Specific Plasmablast Responses During Acute Dengue Virus Infection in Humans. J Virol (2012) 86(6):2911–8. doi: 10.1128/JVI.06075-11
57. Balakrishnan T, Bela-Ong DB, Toh YX, Flamand M, Devi S, Koh MB, et al. Dengue Virus Activates Polyreactive, Natural IgG B Cells After Primary and Secondary Infection. PLoS One (2011) 6(12):e29430. doi: 10.1371/journal.pone.0029430
58. Zompi S, Montoya M, Pohl MO, Balmaseda A, Harris E.. Dominant Cross-Reactive B Cell Response During Secondary Acute Dengue Virus Infection in Humans. PLoS Negl Trop Dis (2012) 6(3):e1568. doi: 10.1371/journal.pntd.0001568
59. Garcia-Bates TM, Cordeiro MT, Nascimento EJ, Smith AP, Soares deMelo KM, McBurney SP, et al. Association Between Magnitude of the Virus-Specific Plasmablast Response and Disease Severity in Dengue Patients. J Immunol (2013) 190(1):80–7. doi: 10.4049/jimmunol.1103350
60. Simmons CP, Popper S, Dolocek C, Chau TN, Griffiths M, Dung NT, et al. Patterns of Host Genome-Wide Gene Transcript Abundance in the Peripheral Blood of Patients With Acute Dengue Hemorrhagic Fever. J Infect Dis (2007) 195(8):1097–107. doi: 10.1086/512162
61. Rouers A, Chng MHY, Lee B, Rajapakse MP, Kaur K, Toh YX, et al. Immune Cell Phenotypes Associated With Disease Severity and Long-Term Neutralizing Antibody Titers After Natural Dengue Virus Infection. Cell Rep Med (2021) 2(5):100278. doi: 10.1016/j.xcrm.2021.100278
62. Godoy-Lozano EE, Tellez-Sosa J, Sanchez-Gonzalez G, Samano-Sanchez H, Aguilar-Salgado A, Salinas-Rodriguez A, et al. Lower IgG Somatic Hypermutation Rates During Acute Dengue Virus Infection is Compatible With a Germinal Center-Independent B Cell Response. Genome Med (2016) 8(1):23. doi: 10.1186/s13073-016-0276-1
63. Rouers A, Appanna R, Chevrier M, Lum J, Lau MC. CD27(hi)CD38(hi) Plasmablasts are Activated B Cells of Mixed Origin With Distinct Function. iScience (2021) 24(5):102482. doi: 10.1016/j.isci.2021.102482
64. Priyamvada L, Cho A, Onlamoon N, Zheng NY, Huang M, Kovalenkov Y, et al. B Cell Responses During Secondary Dengue Virus Infection Are Dominated by Highly Cross-Reactive, Memory-Derived Plasmablasts. J Virol (2016) 90(12):5574–85. doi: 10.1128/JVI.03203-15
65. Marcial-Juarez E, Garcia-Cordero J, Maqueda-Alfaro RA, Saucedo-Lopez RE, Sanchez-Torres LE, Cedillo- 17 Barron L, et al. Cutaneous Dengue Virus Inoculation Triggers Strong B Cell Reactions But Contrastingly Poor T Cell Responses. Virol Sin (2020) 35(5):575–87. doi: 10.1007/s12250-020-00213-6
66. Haltaufderhyde K, Srikiatkhachorn A, Green S, Macareo L, Park S, Kalayanarooj S, et al. Activation of Peripheral T Follicular Helper Cells During Acute Dengue Virus Infection. J Infect Dis (2018) 218(10):1675–85. doi: 10.1093/infdis/jiy360
67. Preeyaa SU, et al. Peripheral Follicular T Helper Cells and Mucosal-Associated Invariant T Cells Represent Activated Phenotypes During the Febrile Phase of Acute Dengue Virus Infection. Viral Immunol (2020) 33(9):610–5. doi: 10.1089/vim.2020.0149
68. Wijesinghe A, Gamage J, Goonewardena H, Gomes L, Jayathilaka D, Wijeratne DT, et al. Phenotype and Functionality of Follicular Helper T Cells in Patients With Acute Dengue Infection. J BioMed Sci (2020) 27(1):50. doi: 10.1186/s12929-020-00641-2
69. Nivarthi UK, Tu HA, Delacruz MJ, Swanstrom J, Patel B, Durbin AP, et al. Longitudinal Analysis of Acute and Convalescent B Cell Responses in a Human Primary Dengue Serotype 2 Infection Model. EBioMedicine (2019) 41:465–78. doi: 10.1016/j.ebiom.2019.02.060
70. Woda M, Friberg H, Currier JR, Srikiatkhachorn A, Macareo LR, Green S, et al. Dynamics of Dengue Virus (DENV)-Specific B Cells in the Response to DENV Serotype 1 Infections, Using Flow Cytometry With Labeled Virions. J Infect Dis (2016) 214(7):1001–9. doi: 10.1093/infdis/jiw308
71. Smith SA, Zhou Y, Olivarez NP, Broadwater AH, de Silva AM, Crowe JE Jr. Persistence of Circulating Memory B Cell Clones With Potential for Dengue Virus Disease Enhancement for Decades Following Infection. J Virol (2012) 86(5):2665–75. doi: 10.1128/JVI.06335-11
72. Appanna R, Kg S, Xu MH, Toh YX, Velumani S, Carbajo D, et al. Plasmablasts During Acute Dengue Infection Represent a Small Subset of a Broader Virus-Specific Memory B Cell Pool. EBioMedicine (2016) 12:178–88. doi: 10.1016/j.ebiom.2016.09.003
73. Green S, Rothman A. Immunopathological Mechanisms in Dengue and Dengue Hemorrhagic Fever. Curr Opin Infect Dis (2006) 19(5):429–36. doi: 10.1097/01.qco.0000244047.31135.fa
74. Nascimento EJ, Hottz ED, Garcia-Bates TM, Bozza F, Marques ET Jr, Barratt-Boyes SM., et al. Emerging Concepts in Dengue Pathogenesis: Interplay Between Plasmablasts, Platelets, and Complement in Triggering Vasculopathy. Crit Rev Immunol (2014) 34(3):227–40. doi: 10.1615/critrevimmunol.2014010212
75. Modhiran N, Watterson D, Muller DA, Panetta AK, Sester DP, Liu L, et al. Dengue Virus NS1 Protein Activates Cells via Toll-Like Receptor 4 and Disrupts Endothelial Cell Monolayer Integrity. Sci Transl Med (2015) 7(304):304ra142. doi: 10.1126/scitranslmed.aaa3863
76. Muller DA, Young PR. The Flavivirus NS1 Protein: Molecular and Structural Biology, Immunology, Role in Pathogenesis and Application as a Diagnostic Biomarker. Antiviral Res (2013) 98(2):192–208. doi: 10.1016/j.antiviral.2013.03.008
77. Young PR, Hilditch PA, Bletchly C, Halloran W.. An Antigen Capture Enzyme-Linked Immunosorbent Assay Reveals High Levels of the Dengue Virus Protein NS1 in the Sera of Infected Patients. J Clin Microbiol (2000) 38(3):1053–7. doi: 10.1128/JCM.38.3.1053-1057.2000
78. Yacoub S, Wills B. Predicting Outcome From Dengue. BMC Med (2014) 12:147. doi: 10.1186/s12916-014-0147-9
79. Robbiani DF, Ruzek D. A Dark Side to NS1 Antibodies? J Exp Med (2021) 218(9):e20211348. doi: 10.1084/jem.20211348
80. Jayathilaka D, Gomes L, Jeewandara C, Jayarathna GSB, Herath D, Perera PA, et al. Role of NS1 Antibodies in the Pathogenesis of Acute Secondary Dengue Infection. Nat Commun (2018) 9(1):5242. doi: 10.1038/s41467-018-07667-z
81. Lin CF, Lei HY, Liu CC, Liu HS, Yeh TM, Wang ST, et al. Generation of IgM Anti-Platelet Autoantibody in Dengue Patients. J Med Virol (2001) 63(2):143–9. doi: 10.1002/1096-9071(20000201)63:2<143::AID-JMV1009>3.0.CO;2-L
82. Adane T, Getawa S. Coagulation Abnormalities in Dengue Fever Infection: A Systematic Review and Meta-Analysis. PLoS Negl Trop Dis (2021) 15(8):e0009666. doi: 10.1371/journal.pntd.0009666
83. Patra G, Saha B, Mukhopadhyay S. High Titres of IgM-Bound Circulating Immune Complexes and Erythrocytic Oxidative Damage are Indicators of Dengue Severity. Clin Exp Immunol (2019) 198(2):251–60. doi: 10.1111/cei.13346
84. Yong YK, Tan HY, Jen SH, Shankar EM, Natkunam SK, Sathar J, et al. Aberrant Monocyte Responses Predict and Characterize Dengue Virus Infection in Individuals With Severe Disease. J Transl Med (2017) 15(1):121. doi: 10.1186/s12967-017-1226-4
85. Wu MF, Chen ST, Yang AH, Lin WW, Lin YL, Chen NJ, et al. CLEC5A is Critical for Dengue Virus-Induced Inflammasome Activation in Human Macrophages. Blood (2013) 121(1):95–106. doi: 10.1182/blood-2012-05-430090
86. Shrivastava G, Visoso-Carvajal G, Garcia-Cordero J, Leon-Juarez M, Chavez-Munguia B, Lopez T, et al. Dengue Virus Serotype 2 and Its Non-Structural Proteins 2A and 2B Activate NLRP3 Inflammasome. Front Immunol (2020) 11:352. doi: 10.3389/fimmu.2020.00352
87. Pan P, Zhang Q, Liu W, Wang W, Yu Z, Lao Z, et al. Dengue Virus Infection Activates Interleukin-1beta to Induce Tissue Injury and Vascular Leakage. Front Microbiol (2019) 10:2637. doi: 10.3389/fmicb.2019.02637
88. Yasuda K, Nakanishi K, Tsutsui H. Interleukin-18 in Health and Disease. Int J Mol Sci (2019) . 20(3):649. doi: 10.3390/ijms20030649
89. Bai B, Yang Y, Wang Q, Li M, Tian C, Liu Y, et al. NLRP3 Inflammasome in Endothelial Dysfunction. Cell Death Dis (2020) 11(9):776. doi: 10.1038/s41419-020-02985-x
90. Avirutnan P, Zhang L, Punyadee N, Manuyakorn A, Puttikhunt C, Kasinrerk W, et al. Secreted NS1 of Dengue Virus Attaches to the Surface of Cells via Interactions With Heparan Sulfate and Chondroitin Sulfate E. PLoS Pathog (2007) 3(11):e183. doi: 10.1371/journal.ppat.0030183
91. Dias-Melicio LA, Fernandes RK, Rodrigues DR, Golim MA, Soares AM.. Interleukin-18 Increases TLR4 and Mannose Receptor Expression and Modulates Cytokine Production in Human Monocytes. Mediators Inflamm (2015) 2015:236839. doi: 10.1155/2015/236839
92. Tanaka N, Yonekura H, Yamagishi S, Fujimori H, Yamamoto Y, Yamamoto H., et al. The Receptor for Advanced Glycation End Products is Induced by the Glycation Products Themselves and Tumor Necrosis Factor-Alpha Through Nuclear Factor-Kappa B, and by 17beta-Estradiol Through Sp-1 in Human Vascular Endothelial Cells. J Biol Chem (2000) 275(33):25781–90. doi: 10.1074/jbc.M001235200
93. Navarro JC, Arrivillaga-Henriquez J, Salazar-Loor J, Rodriguez-Morales AJ.. COVID-19 and Dengue, Co-Epidemics in Ecuador and Other Countries in Latin America: Pushing Strained Health Care Systems Over the Edge. Travel Med Infect Dis (2020) 37:101656. doi: 10.1016/j.tmaid.2020.101656
94. Lam LTM, Chua YX, Tan DHY. Roles and Challenges of Primary Care Physicians Facing a Dual Outbreak of COVID-19 and Dengue in Singapore. Fam Pract (2020) 37(4):578–9. doi: 10.1093/fampra/cmaa047
95. Blanco-Melo D, Nilsson-Payant BE, Liu WC, Uhl S, Hoagland D, Moller R, et al. Imbalanced Host Response to SARS-CoV-2 Drives Development of COVID-19. Cell (2020) 181(5):1036–1045.e9. doi: 10.1016/j.cell.2020.04.026
96. Chen G, Wu D, Guo W, Cao Y, Huang D, Wang H, et al. Clinical and Immunological Features of Severe and Moderate Coronavirus Disease 2019. J Clin Invest (2020) 130(5):2620–9. doi: 10.1172/JCI137244
97. Ragab D, Salah Eldin H, Taeimah M, Khattab R, Salem R.. The COVID-19 Cytokine Storm; What We Know So Far. Front Immunol (2020) 11:1446. doi: 10.3389/fimmu.2020.01446
98. Lin SH, Zhao YS, Zhou DX, Zhou FC, Xu F.. Coronavirus Disease 2019 (COVID-19): Cytokine Storms, Hyper-Inflammatory Phenotypes, and Acute Respiratory Distress Syndrome. Genes Dis (2020) 7(4):520–7. doi: 10.1016/j.gendis.2020.06.009
99. Malavige GN, Huang LC, Salimi M, Gomes L, Jayaratne SD, Ogg GS., et al. Cellular and Cytokine Correlates of Severe Dengue Infection. PLoS One (2012) 7(11):e50387. doi: 10.1371/journal.pone.0050387
100. Nguyen TH, Nguyen TH, Vu TT, Farrar J, Hoang TL, Dong TH, et al. Corticosteroids for Dengue - Why Don't They Work? PLoS Negl Trop Dis (2013) 7(12):e2592. doi: 10.1371/journal.pntd.0002592
101. Tramontini Gomes de Sousa Cardozo F, Baimukanova G, Lanteri MC, Keating SM, Moraes Ferreira F, Heitman 56 J, et al. Serum From Dengue Virus-Infected Patients With and Without Plasma Leakage Differentially Affects Endothelial Cells Barrier Function In Vitro. PLoS One (2017) 12(6):e0178820. doi: 10.1371/journal.pone.0178820
102. Dayarathna S, Jeewandara C, Gomes L, Somathilaka G, Jayathilaka D, Vimalachandran V, et al. Similarities and Differences Between the 'Cytokine Storms' in Acute Dengue and COVID-19. Sci Rep (2020) 10(1):19839. doi: 10.1038/s41598-020-76836-2
103. Kamaladasa A, Gomes L, Jeewandara C, Shyamali NL, Ogg GS, Malavige GN., et al. Lipopolysaccharide Acts Synergistically With the Dengue Virus to Induce Monocyte Production of Platelet Activating Factor and Other Inflammatory Mediators. Antiviral Res (2016) 133:183–90. doi: 10.1016/j.antiviral.2016.07.016
104. Yang Y, Shen C, Li J, Yuan J, Wei J, Huang F, et al. Plasma IP-10 and MCP-3 Levels are Highly Associated With Disease Severity and Predict the Progression of COVID-19. J Allergy Clin Immunol (2020) 146(1):119–27.e4. doi: 10.1016/j.jaci.2020.04.027
105. Wu D, Lu J, Liu Q, Ma X, He W.. To Alert Coinfection of COVID-19 and Dengue Virus in Developing Countries in the Dengue-Endemic Area. Infect Control Hosp Epidemiol (2020) 41(12):1482. doi: 10.1017/ice.2020.187
106. Gong J, Dong H, Xia QS, Huang ZY, Wang DK, Zhao Y, et al. Correlation Analysis Between Disease Severity and Inflammation-Related Parameters in Patients With COVID-19: A Retrospective Study. BMC Infect Dis (2020) 20(1):963. doi: 10.1186/s12879-020-05681-5
107. Malibari AA, Al-Husayni F, Jabri A, Al-Amri A, Alharbi M.. A Patient With Dengue Fever and COVID-19: Coinfection or Not? Cureus (2020) 12(12):e11955. doi: 10.7759/cureus.11955
108. Tsheten T, Clements ACA, Gray DJ, Adhikary RK, Wangdi K.. Clinical Features and Outcomes of COVID-19 and Dengue Co-Infection: A Systematic Review. BMC Infect Dis (2021) 21(1):729. doi: 10.1186/s12879-021-06409-9
109. Zheng W, Wu H, Liu C, Yan Q, Wang T, Wu P, et al. Identification of COVID-19 and Dengue Host Factor Interaction Networks Based on Integrative Bioinformatics Analyses. Front Immunol (2021) 12:707287. doi: 10.3389/fimmu.2021.707287
110. Teotonio I, de Carvalho JL, Castro LC, Nitz N, Hagstrom L, Rios GG, et al. Clinical and Biochemical Parameters of COVID-19 Patients With Prior or Active Dengue Fever. Acta Trop (2021) 214:105782. doi: 10.1016/j.actatropica.2020.105782
111. Yan G, Lee CK, Lam LTM, Yan B, Chua YX, Lim AYN, et al. Covert COVID-19 and False-Positive Dengue Serology in Singapore. Lancet Infect Dis (2020) 20(5):536. doi: 10.1016/S1473-3099(20)30158-4
112. Joob B, Wiwanitkit V. COVID-19 can Present With a Rash and be Mistaken for Dengue. J Am Acad Dermatol (2020) 82(5):e177. doi: 10.1016/j.jaad.2020.03.036
113. Santoso MS, Masyeni S, Haryanto S, Yohan B, Hibberd ML, Sasmono RT., et al. Assessment of Dengue and COVID-19 Antibody Rapid Diagnostic Tests Cross-Reactivity in Indonesia. Virol J (2021) 18(1):54. doi: 10.1186/s12985-021-01522-2
114. Nath H, Mallick A, Roy S, Sukla S, Biswas S.. Computational Modelling Supports That Dengue Virus Envelope Antibodies can Bind to SARS-CoV-2 Receptor Binding Sites: Is Pre-Exposure to Dengue Virus Protective Against COVID-19 Severity? Comput Struct Biotechnol J (2021) 19:459–66. doi: 10.1016/j.csbj.2020.12.037
115. Silvestre OM, Costa LR, Lopes BVR, Barbosa MR, Botelho KKP, Albuquerque KLC, et al. Previous Dengue Infection and Mortality in COVID-19. Clin Infect Dis (2020). doi: 10.1093/cid/ciaa1895
116. Sabchareon A, Wallace D, Sirivichayakul C, Limkittikul K, Chanthavanich P, Suvannadabba S, et al. Protective Efficacy of the Recombinant, Live-Attenuated, CYD Tetravalent Dengue Vaccine in Thai Schoolchildren: A Randomised, Controlled Phase 2b Trial. Lancet (2012) 380(9853):1559–67. doi: 10.1016/S0140-6736(12)61428-7
117. Mahalingam S, Herring BL, Halstead SB. Call to Action for Dengue Vaccine Failure. Emerg Infect Dis (2013) 19(8):1335–7. doi: 10.3201/eid1908.121864
118. Wilder-Smith A, Flasche S, Smith PG. Vaccine-Attributable Severe Dengue in the Philippines. Lancet (2019) 394(10215):2151–2. doi: 10.1016/S0140-6736(19)32525-5
119. FDA Expands Authorization of Two Monoclonal Antibodies for Treatment and Post-Exposure Prevention of COVID-19 to Younger Pediatric Patients, Including Newborns (2021). Available at: https://www.fda.gov/news-events/press-announcements/fda-expands-authorization-two-monoclonal-antibodies-treatment-and-post-exposure-prevention-covid-19.
120. Olkowski S, Forshey BM, Morrison AC, Rocha C, Vilcarromero S, Halsey ES, et al. Reduced Risk of Disease During Postsecondary Dengue Virus Infections. J Infect Dis (2013) 208(6):1026–33. doi: 10.1093/infdis/jit273
121. Wan SW, Chen PW, Chen CY, Lai YC, Chu YT, Hung CY, et al. Therapeutic Effects of Monoclonal Antibody Against Dengue Virus NS1 in a STAT1 Knockout Mouse Model of Dengue Infection. J Immunol (2017) 199(8):2834–44. doi: 10.4049/jimmunol.1601523
122. O'Donnell KL, Espinosa DA, Puerta-Guardo H, Biering SB, Warnes CM, Schiltz J, et al. Avian Anti-NS1 IgY Antibodies Neutralize Dengue Virus Infection and Protect Against Lethal Dengue Virus Challenge. Antiviral Res (2020) 183:104923. doi: 10.1016/j.antiviral.2020.104923
123. Kang TH, Jung ST. Boosting Therapeutic Potency of Antibodies by Taming Fc Domain Functions. Exp Mol Med (2019) 51(11):1–9. doi: 10.1038/s12276-019-0345-9
124. Bournazos S, Gupta A, Ravetch JV. The Role of IgG Fc Receptors in Antibody-Dependent Enhancement. Nat Rev Immunol (2020) 20(10):633–43. doi: 10.1038/s41577-020-00410-0
125. Legendre CM, Licht C, Muus P, Greenbaum LA, Babu S, Bedrosian C, et al. Terminal Complement Inhibitor Eculizumab in Atypical Hemolytic-Uremic Syndrome. N Engl J Med (2013) 368(23):2169–81. doi: 10.1056/NEJMoa1208981
126. Cofiell R, Kukreja A, Bedard K, Yan Y, Mickle AP, Ogawa M, et al. Eculizumab Reduces Complement Activation, Inflammation, Endothelial Damage, Thrombosis, and Renal Injury Markers in aHUS. Blood (2015) 125(21):3253–62. doi: 10.1182/blood-2014-09-600411
127. Carr JM, Cabezas-Falcon S, Dubowsky JG, Hulme-Jones J, Gordon DL.. Dengue Virus and the Complement Alternative Pathway. FEBS Lett (2020) 594(16):2543–55. doi: 10.1002/1873-3468.13730
128. Tapia-Abellan A, Angosto-Bazarra D, Martinez-Banaclocha H, de Torre-Minguela C, Ceron-Carrasco JP, Perez-Sanchez H, et al. MCC950 Closes the Active Conformation of NLRP3 to an Inactive State. Nat Chem Biol (2019) 15(6):560–4. doi: 10.1038/s41589-019-0278-6
129. Coll RC, Hill JR, Day CJ, Zamoshnikova A, Boucher D, Massey NL, et al. MCC950 Directly Targets the NLRP3 ATP-Hydrolysis Motif for Inflammasome Inhibition. Nat Chem Biol (2019) 15(6):556–9. doi: 10.1038/s41589-019-0277-7
130. Coll RC, Robertson AA, Chae JJ, Higgins SC, Munoz-Planillo R, Inserra MC, et al. A Small-Molecule Inhibitor of the NLRP3 Inflammasome for the Treatment of Inflammatory Diseases. Nat Med (2015) 21(3):248–55. doi: 10.1038/nm.3806
131. Dinarello CA, Novick D, Kim S, Kaplanski G.. Interleukin-18 and IL-18 Binding Protein. Front Immunol (2013) 4:289 DOI|. doi: 10.3389/fimmu.2013.00289
132. Li X, Cui W, Hull L, Wang L, Yu T, Xiao M., et al. IL-18 Binding Protein (IL-18BP) as a Novel Radiation Countermeasure After Radiation Exposure in Mice. Sci Rep (2020) 10(1):18674. doi: 10.1038/s41598-020-75675-5
133. Potts JA, Rothman AL. Clinical and Laboratory Features That Distinguish Dengue From Other Febrile Illnesses in Endemic Populations. Trop Med Int Health (2008) 13(11):1328–40. doi: 10.1111/j.1365-3156.2008.02151.x
134. Vieira-de-Abreu A, Campbell RA, Weyrich AS, Zimmerman GA.. Platelets: Versatile Effector Cells in Hemostasis, Inflammation, and the Immune Continuum. Semin Immunopathol (2012) 34(1):5–30. doi: 10.1007/s00281-011-0286-4
135. Nachman RL, Rafii S. Platelets, Petechiae, and Preservation of the Vascular Wall. N Engl J Med (2008) 359(12):1261–70. doi: 10.1056/NEJMra0800887
136. Stokes KY, Granger DN. Platelets: A Critical Link Between Inflammation and Microvascular Dysfunction. J Physiol (2012) 590(5):1023–34. doi: 10.1113/jphysiol.2011.225417
137. Ojha A, Nandi D, Batra H, Singhal R, Annarapu GK, Bhattacharyya S, et al. Platelet Activation Determines the Severity of Thrombocytopenia in Dengue Infection. Sci Rep (2017) 7:41697. doi: 10.1038/srep41697
138. Quach ME, Chen W, Li R. Mechanisms of Platelet Clearance and Translation to Improve Platelet Storage. Blood (2018) 131(14):1512–21. doi: 10.1182/blood-2017-08-743229
139. Djamiatun K, van derVen AJ, de Groot PG, Faradz SM, Hapsari D, Dolmans WM, et al. Severe Dengue is Associated With Consumption of Von Willebrand Factor and Its Cleaving Enzyme ADAMTS-13. PLoS Negl Trop Dis (2012) 6(5):e1628. doi: 10.1371/journal.pntd.0001628
140. Ward S, O'Sullivan JM, O'Donnell JS. Von Willebrand Factor Sialylation-A Critical Regulator of Biological Function. J Thromb Haemost (2019) 17(7):1018–29. doi: 10.1111/jth.14471
141. Glanz VY, Myasoedova VA, Grechko AV, Orekhov AN.. Inhibition of Sialidase Activity as a Therapeutic Approach. Drug Des Devel Ther (2018) 12:3431–7. doi: 10.2147/DDDT.S176220
142. WHO. Vaccines and Immunization: Dengue (2018). Available at: https://www.who.int/news-room/questions-and-answers/item/dengue-vaccines.
143. Mullard A. NLRP3 Inhibitors Stoke Anti-Inflammatory Ambitions. Nat Rev Drug Discov (2019) 18(6):405–7. doi: 10.1038/d41573-019-00086-9
Alarmins: Also called as danger-associated molecular patterns, such as IL-33, Hsp70, HMGB1 and IL-1α, are released by injured or necrotic cells
Antibody-dependent cellular cytotoxicity: ADCC is an immune mechanism where an FcR-bearing effector cell recognizes and binds to Fc fragment of an antibody molecule already bound to an antigen displayed on a target cell (for instance, a host cell expressing pathogen-derived antigens on its surface), which entails in downstream signaling within the effector cell resulting in cytolytic granule release and consequent lysis of the target cell via the perforin-granzyme pathway.
Antibody-dependent enhancement: ADE represents a mechanism where a non-neutralizing antibody becomes bound to a viral particle (often during secondary infection) is recognized by the Fc gamma receptor IIa (FcγRIIa) expressed on a phagocytic cell leading to enhanced intracellular viral replication, or immune complex formation resulting in hyperinflammation and immunopathology. ADE occur when binding antibodies (non-neutralizing) or antibodies at sub-optimal levels bind to viral particles without necessitating viral clearance.
Atypical hemolytic uremic syndrome: A rare life-threatening disease resulting from dysregulated activation of the complement system culminating in the formation of thrombi in small blood vessels of visceral organs especially the kidneys progressing to end-stage renal disease (ESRD).
Cytokine: A wide range of proteins secreted by various host cells especially immune cells that facilitate intercellular signaling, and can exert localized or systemic biological effects.
Cytokine storm: A state of excessive systemic inflammation involving dramatically elevated levels of proinflammatory cytokines and inflammatory cells viz. macrophages, neutrophils, mast cells, eosinophils and basophils. Cytokine release syndrome can often result in the dysfunction of secondary organs, systemic multi-organ failure, and can be fatal.
Damage-associated molecular patterns (DAMPs): DAMPs are molecules released by injured and necrotized cells usually following an infection in the host, and are recognized by pattern recognition receptors (PRRs) expressed on host cells to activate innate immune responses.
Defervescence: A phase in dengue fever where the patient’s body temperature decreases rapidly.
Dengue hemorrhagic fever: DHF is currently defined by four WHO criteria viz., (1) Fever or recent history of fever lasting 2–7 days (2) Any hemorrhagic manifestation (3) Thrombocytopenia (platelet count of <100,000/mm3 (4) Evidence of increased vascular permeability. Common manifestations include a positive tourniquet test, skin hemorrhages (petechiae, hematomas), epistaxis, gingival bleeding, and occult hematuria. (https://www.cdc.gov/dengue/resources/denguedhf-information-for-health-care-practitioners_2009.pdf).
Dengue shock syndrome: DSS is defined as any case that meets the four criteria for DHF together with evidence of circulatory failure manifested by (1) rapid, weak pulse and narrow pulse pressure (≤20 mmHg [2.7 kPa]) or (2) hypotension for age, restlessness, and cold, clammy skin. Dengue can rapidly progress into DSS, which, if not diagnosed and treated promptly, can result in far-reaching consequences.
Experimental autoimmune encephalomyelitis: EAE refers to an autoimmune form of multiple sclerosis induced in animals resulting from aberrant activation of NLRP3 inflammasome as well as T cells against CNS myelin.
Fc receptor: Receptors expressed on certain cells of the host immune system, such as phagocytes, granulocytes, and lymphocytes (NK cells and B cells). These receptors permit the binding of the cells to the Fc regions of antibodies that have become bound to pathogenic surfaces or infected cells, rendering their timely elimination.
Hemodynamic shock: HS is an acute syndrome characterised by inadequate blood flow throughout the body leading to organ failure due to poor perfusion. Shock results from a fall in cardiac output (CO) (for e.g. cardiogenic shock, mechanical shock, hypovolaemic shock) or an ebb in total peripheral resistance (TPR) (distributive shock such as toxic/septic shock and anaphylactic shock. A fall in CO or a poor TPR results in low blood pressure culminating in decreased tissue perfusion and multi-organ failure.
Icosahedral symmetry: An icosahedron is a geometric shape with 20 sides, each composed of an equilateral triangle.
IgY: An evolutionary ancestor of mammalian IgG found in the circulation of aves, reptiles, and amphibians, and is considered to be the functional homolog of mammalian IgG.
Original antigenic sin: Alteration in adaptive immune response (Both T and B cells), when preexisting memory cells of the previous infection encountered a heterotypic antigen. Such immune response is often reduced in degranulation, but increase in pro-inflammatory cytokine production, rendering such immune response ineffective in controlling viral infection, but more prone to cytokine storm.
Pathogen-associated molecular patterns: PAMPs are small molecular motifs that are shared between classes of microorganisms, and are recognized by PRRs in host cells to activate innate immune responses.
Pattern recognition receptors: PRR refers to an array of germline-encoded molecules sensing molecular motifs (PAMPs) conserved among different classes of microbes, and also recognize endogenous molecules called alarmins (DAMPs), released from injured host cells.
Pyroptosis: It is a form of cell death that follows pro-inflammatory signaling especially in inflammatory cells such as macrophages triggered by microbial pathogens.
Tetravalent vaccine: A tetravalent CYD vaccine contains live-attenuated DENV serotypes expressing the structural genes (encoding the membrane and envelope proteins) of each of the four DENV serotypes together with the genetic backbone of an attenuated 17D yellow fever virus (YFV) strain.
Virion: A single infectious virus particle that interacts with the host cells.
Keywords: dengue (DENV), pathogenesis, plasmablasts, inflammasome, cytokine storm, endothelial dysfunction, antibody-dependent enhancement (ADE), COVID-19
Citation: Yong YK, Wong WF, Vignesh R, Chattopadhyay I, Velu V, Tan HY, Zhang Y, Larsson M and Shankar EM (2022) Dengue Infection - Recent Advances in Disease Pathogenesis in the Era of COVID-19. Front. Immunol. 13:889196. doi: 10.3389/fimmu.2022.889196
Received: 03 March 2022; Accepted: 30 May 2022;
Published: 06 July 2022.
Edited by:
Julie Olson, University of Minnesota Twin Cities, United StatesReviewed by:
Leticia Cedillo-Barron, Centro de Investigaciones y Estudios Avanzados, Instituto Politécnico Nacional de México (CINVESTAV), MexicoCopyright © 2022 Yong, Wong, Vignesh, Chattopadhyay, Velu, Tan, Zhang, Larsson and Shankar. This is an open-access article distributed under the terms of the Creative Commons Attribution License (CC BY). The use, distribution or reproduction in other forums is permitted, provided the original author(s) and the copyright owner(s) are credited and that the original publication in this journal is cited, in accordance with accepted academic practice. No use, distribution or reproduction is permitted which does not comply with these terms.
*Correspondence: Esaki M. Shankar, c2hhbmthcmVtQGN1dG4uYWMuaW4=; Yean Kong Yong, eW9uZy55ZWFua29uZ0BnbWFpbC5jb20=
†These authors have contributed equally to this work
Disclaimer: All claims expressed in this article are solely those of the authors and do not necessarily represent those of their affiliated organizations, or those of the publisher, the editors and the reviewers. Any product that may be evaluated in this article or claim that may be made by its manufacturer is not guaranteed or endorsed by the publisher.
Research integrity at Frontiers
Learn more about the work of our research integrity team to safeguard the quality of each article we publish.