- 1Department of Hematology, Sir Run Run Shaw Hospital, Zhejiang University School of Medicine, Hangzhou, China
- 2State Key Laboratory for Diagnosis and Treatment of Infectious Diseases, National Clinical Research Center for Infectious Diseases, National Medical Center for Infectious Diseases, Collaborative Innovation Center for Diagnosis and Treatment of Infectious Diseases, The First Affiliated Hospital, Zhejiang University School of Medicine, Hangzhou, China
Adoptive cell therapy (ACT) with engineered T cells has emerged as a promising strategy for the treatment of malignant tumors. Among them, there is great interest in engineered γδ T cells for ACT. With both adaptive and innate immune characteristics, γδ T cells can be activated by γδ TCRs to recognize antigens in a MHC-independent manner, or by NK receptors to recognize stress-induced molecules. The dual recognition system enables γδ T cells with unique activation and cytotoxicity profiles, which should be considered for the design of engineered γδ T cells. However, the current designs of engineered γδ T cells mostly follow the strategies that used in αβ T cells, but not making good use of the specific characteristics of γδ T cells. Therefore, it is no surprising that current engineered γδ T cells in preclinical or clinical trials have limited efficacy. In this review, we summarized the patterns of antigen recognition of γδ T cells and the features of signaling pathways for the functions of γδ T cells. This review will additionally discuss current progress in engineered γδ T cells and provide insights in the design of engineered γδ T cells based on their specific characteristics.
1 Introduction
Immunotherapy has become one of important pillars of cancer treatment, as it can trigger and augment the power of patients’ immunity to attack malignant cells. Among immunotherapy strategies, adoptive cell therapy (ACT) with engineered T cells, such as chimeric antigen receptor (CAR)-T and T cell receptor (TCR)-T cells, has gained considerable attention (1, 2). A good example is that CAR-T therapy has advanced the furthest in clinical development and three CAR-T products (Kymriah, Yescarta, and Tecartus) have gained commercial approval in the United States.
Over the past decades, a variety of researches of γδ T cells have added to the established understanding in highlighting conspicuous roles of γδ T cells in cancers. Although some researches point the potential tumorigenic effector functions of γδ T cells (3, 4), increasing translational researches have shown great interest in the therapeutic use of certain subsets of γδ T cells, especially engineered γδ T cells. In fact, the momentum of engineered γδ T cell therapy may have been generated, as U.S. Food and Drug Administration (FDA) has cleared investigational new drug application for ADI-001 that comprises CD22-allogenic γδ CAR-T cell therapy in 2020.
Activation of γδ T cells is in a TCR-dependent process as similar as that of αβ T cells, yet in independence of major histocompatibility complex (MHC). In addition to γδ TCR signals, γδ T cells mediate multiple responses via receptor-ligand interaction of innate signals, similar to NK cells. They bear a variety of NK cell receptors (NKRs) such as NKG2D and NK cytotoxicity receptors (NCRs) including NKp30, NKp44, and NKp46 (5). These receptors may fine-tune the γδ T cell activation threshold, enhance γδ T cells to recognize tumor target, prompt γδ T cells to mediate an immediate immune reaction against tumor target, and release cytotoxic granules such as perforin and granzyme B. In cancer, the down-regulation of MHC-I may prompt ‘missing-self recognition’, which unlock the binding between MHC-I and inhibitory receptors on γδ T cells, making γδ T cells unhindered to attack tumor cells in a NK-like manner (6). Dual recognition and stimulation system endows γδ T cells distinct anti-tumor effect. However, current design of engineered γδ T cells is a me-too engineered αβ T cells, such as using the same single-chain fragment variable (scFv) and co-stimulation molecules which are proved to help kill tumor cells effectively in αβ T cells but not completely confirmed in γδ T cells. This kind of design may take some advantages of γδ T cells such as GVHD absence, however, this raises the question that how to make the best use of dual recognition and stimulation system of γδ T cells to endow engineered γδ T maximum anti-tumor effect.
In this review, we provided a comprehensive and deep summary of the unique patterns of γδ T recognition and signaling pathways. Based on these underlying mechanisms, this review further discussed valuable insights in the design of engineered γδ T cells. It is promising that an intelligent design that considers the specific characteristics of γδ T cells will be beneficial for the utility of engineered γδ T cells.
2 γδ TCR Antigen Recognition
Like αβ T cells and B cells, γδ T cells generate their specific T cell receptors (TCRs) via recombination activating gene (RAG)-mediated V(D)J recombination, which contributes to the high diversity up to 1017 theoretically possible combinations of TCR repertoires (7). Since γδ T cells have been discovered in 1980s, what antigens γδ TCRs can recognize remains an outstanding question in this field. Nowadays, it has been known that γδ TCRs antigen recognition pattern is unrestricted by MHC. The ligands that they can recognize include self-antigens, such as MHC-like molecules, B7-like molecules, and foreign-antigens, such as haptens, virus protein, phycobiliproteins (8–12). Recent researches have shown that γδ TCRs most likely take part in complicated mechanisms that involves multiple ligands on the tumor cells, as well as the sensation of spatial and conformational changes through the γδ TCRs and potentially associated molecules.
The comprehensive description of antigen recognition by γδ TCRs has already been summarized in published articles (13, 14). Here, we only briefly review tumor-related antigens recognized by γδ TCRs, which are summarized in Figure 1.
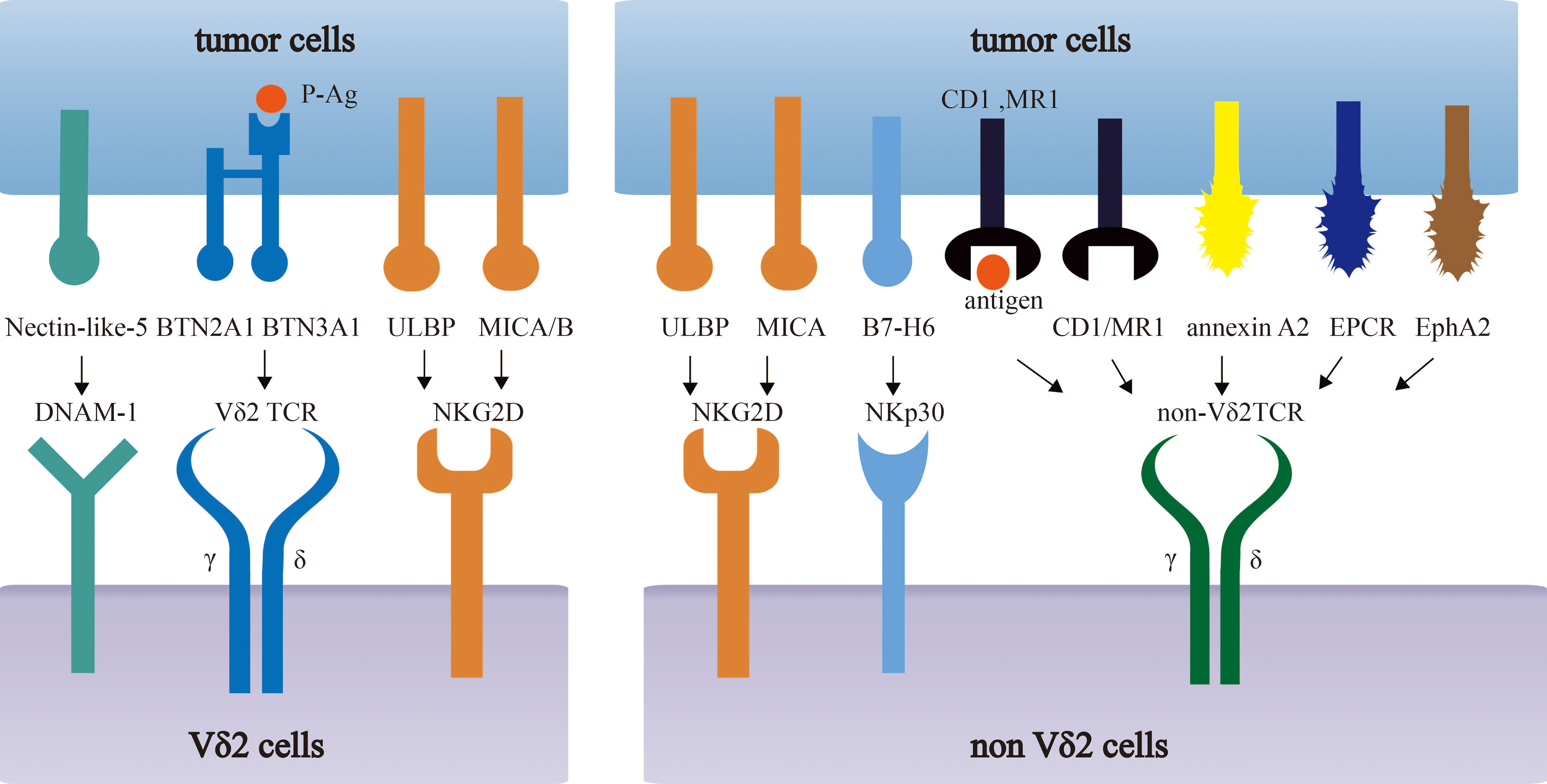
Figure 1 Recognition of tumor-associated antigens (TAAs) by different subsets of γδ T cells. Vδ2 T cells recognize phosphoantigens (P-Ag) modified Butyrophilin 2A1 (BTN2A1)-Butyrophilin 3A1 (BTN3A1) complex. Non-Vδ2 TCRs recognize CD1 family members and MR1 in either antigen dependent or independent manner. Besides, NK receptors (NKG2D, NKp30, DNAM-1) in γδ T cells recognize MICA/B, ULBP and B7-H6, Nectin-like-5 respectively.
Vγ9Vδ2 T cells recognize phosphoantigens (P-Ag) modified Butyrophilin 2A1 (BTN2A1)-Butyrophilin 3A1 (BTN3A1) complex in an MHC-independent, but TCR-dependent manner. In tumor, the dysregulation of mevalonate pathway accounts for the accumulation of phosphorylated mevalonate metabolites, such as Isopentenyl pyrophosphate (IPP) that was identified as a kind of P-Ag, and thus activate the Vγ9Vδ2 T cells (15–17). On the other hand, zoledronate (ZOL) can inhibit IPP-metabolizing enzyme, farnesyl-diphosphate-synthase (FPPS), and increase IPP level, which contributes to an enhanced IPP-induced γδ T cell activation (16, 18). ZOL has been widely used in cancer therapies such as renal cell carcinoma (RCC) and prostate cancer (19, 20). Many clinical trials have found it significantly inhibit the cancer progression and even completely cure cancers.
However, the defined molecular mechanisms of Vγ9Vδ2 T cell activation by phosphoantigens still remains to be discovered. The binding of P-Ags and the intracellular B30.2 domain of BTN3A1 leads to the conformational changes of the BTN3A1 extracellular domain, which can take part in the activation of Vγ9Vδ2+ TCRs (9, 21, 22). BTN2A1, a BTN molecule that is associated with BTN3A1 in extracellular and intracellular domains, directly binds to Vγ9 domain of the TCRs, potentiating Vγ9Vδ2-mediated P-Ag sensing. In addition, another Vγ9Vδ2 TCR direct interaction, mediated by BTN3A1 or an unknown ligand, is also essential in the response of Vγ9Vδ2 T cell to P-Ag (23, 24).
In addition to BTN3A molecules, the recognition of MHC or MHC-like molecules by γδ T cells has been intensively studied. γδ T cells can recognize molecules such as HLA-A24, HLA-B27 and HLA-A2 and may specifically recognize certain MHC molecules in tumor cells (13). For example, the engineered αβ T cells, which expressed Vγ5Vδ1+ TCRs, could be activated by HLA-A*24:02+ tumor cells and significantly decreased the tumor burden and enhanced survival rate of HLA-A*24:02+ tumor-bearing mice (25). On the other hand, some MHC-like molecules, such as MHC-related protein 1 (MR1), CD1, has a preference to specifically bind to Vδ1 TCRs in most cases (8, 26–29). Interestingly, loading different lipids may have different influences on the binding affinity of CD1d/CD1c and γδ TCRs, suggesting the loaded lipids on CD1 molecule contribute to γδ TCR antigen recognition (8, 28). MR1, another MHC-like molecule can also be recognized by γδ TCRs. γδ T cells co-cultured with MR1-transduced cells, MR1-restrict γδ TCRs transduced Jurkat-76 cell lines, can be activated with up-regulating CD69 and ERK1/2 phosphorylation (29). Although CD1/MR1-restricted NKT or αβ T cells were reported to induce specific tumor killing ability in many kinds of tumor cells (30–32), there is no evidence that γδ T cells can also lead to antitumor activity by recognizing CD1 or MR1 molecules. The role of CD1 or MR1-restricted γδ T cells in cancer immune surveillance still needs to be further studied.
Recently, more novel tumor-associated molecules that can be recognized by γδ TCRs have been revealed, including annexin A2 (33), EPCR (an MHC-like molecule) (34, 35), and ephrin type-A receptor 2 (EphA2) (36, 37). The expression of EphA2 is up-regulated in cervical cancer and colon cancer cells, which is mediated by the metabolic changes (AMP-activated protein kinase (AMPK)–dependent metabolic reprogramming) in tumor cells. γδ T cells play increasing tumor-killing ability by recognizing EphA2. This ability can be reduced by blocking EphA2 in endometrial carcinoma cells or knockout of EPHA2 gene in renal and colon tumor cells, which indicates the interaction of EphA2 and γδ T cells play an important role in enhancing the susceptibility of γδ T cytotoxic reactivity (36, 37).
3 The Characteristics of γδ TCR and the Related Co-Stimulation Signals in γδ T Cells
3.1 γδ TCR Signal
Since γδ T cells eliminate tumor cells via recognizing a variety of tumor-associated antigens, γδ TCR signals play a key role in regulating γδ T cell activation. Like conventional αβTCR, γδ TCR is a complex of a clonotypic heterodimer TCRδ/TCRγ, two CD3 dimers (CD3δϵ or/and CD3γϵ), and a ζζ dimer (38). The CD3ϵ-deficient patients had complete deficiencies in peripheral T cells, suggesting that the ϵ subunit plays a pivotal role in the αβ T cell development (39). However, some CD3 molecules may play different roles in the functions of γδ T cells. For example, CD3δ-/- mice have normal numbers of γδ T cells (40, 41). In addition, mouse γδ TCRs, which are naturally CD3δ-deficient, can induces calcium mobilization and ERK activation (42). On the contrary, if CD3δ is deficient in human or mice, the development of αβ T cells are failed (40, 41), and did not induce signaling events by the engagement of CD3δ-deficient αβ TCRs (43). Another important CD3 molecule, CD3γ, only blocks, but not significantly impairs the development of γδ T cells in human, as CD3δ gene may rescue the γδ T cell development (44). Current researches reported the function of TCR/CD3 complex components in signaling transmitting in αβ T cells, which was applied in engineered αβ T cells and engineered γδ T cells. For example, CD3ζ chain was determined to transmit signals in the absence of CD3 γ, δ, and ϵ in αβ T cells (45), which was widely used to deliver a major activation signal in both αβ T cell and CAR-T cells. In addition, in absence of CD3ζ chain, the CD3 γϵ/δϵ, or CD3ϵ alone were also able to independently activate αβ T cells (46, 47). However, the specific signaling function of TCR/CD3 complex components have not been precisely reported in γδ T cells, which needs to be explored in the future. As a whole, signals transmitted by TCR in αβ T cells and γδ T cells are not always the same. A clinical test of 60 samples from hospitalized and healthy individuals demonstrated that human γδ T cells constitutively expressed higher density of TCR/CD3 complex (2.12 ± 0.33 fold) than that in αβ T cells (48). Furthermore, by analyzing the ability to induce calcium mobilization, ERK activation, and cellular proliferation in mouse γδ T cells, it revealed a superior effect on γδ T cells in the aspect of signal transduction than that in αβ T cells with the same stimulation using immobilized anti-CD3 monoclonal antibody (mAb), which revealed that γδ T cells have a better signal-transducing complex than αβ T cells (42). Interestingly, in steady state, compared with αβ T cells, mouse γδ T cells have higher phosphorylation levels of ERK1/2 and stronger proliferation ability. Therefore, it suggested that γδ T cells possess a more “primed for action” status at baseline even in the absence of any external stimulation (49).
TCR conformation also influences the signal. CD3 conformational change (CD3 CC), which takes advantage of the increased accessibility of a proline-rich sequence (PRS) in the CD3ϵ cytoplasmic tail, was required for T cell activation (50, 51). In αβ T cells, cholesterol bound to the transmembrane region of TCRβ keeps the TCR in a resting and inactive conformation that cannot be phosphorylated by active kinases. Only αβ TCRs that spontaneously detached from cholesterol could switch to the active conformation (termed primed TCRs) and then be phosphorylated (52). Moreover, αβ TCR signaling could be inhibited by cholesterol sulfate, suggesting an important role of cholesterol in the conformation of αβ TCR (53). But γδ TCRs does not bind to cholesterol, accounting for a higher percentage of γδ TCRs in the active conformation compared to αβ TCRs (52). In addition, the CD3 CC in Vγ9Vδ2 T cells induced by anti-CD3ϵ mAb stimulation, which dramatically enhanced target cell lysis of the pancreatic tumor cell line Panc89 (54). The better reactivity of γδ T cells provides a better application of engineered γδ T cell therapy.
3.2 Co-Stimulation Molecules
Apart from TCR-dependent stimulation, the co-stimulation signals are also important and widely applied into the 2nd generation of CAR-T therapy. To date, almost all engineered γδ T cells follow the co-stimulation design in αβ T. However, whether these co-stimulation signals are applicable to engineered γδ T cells requires further investigation. The comparison of co-stimulatory molecules and their induced effector functions between αβ T cells and γδ T cells is summarized in Figure 2.
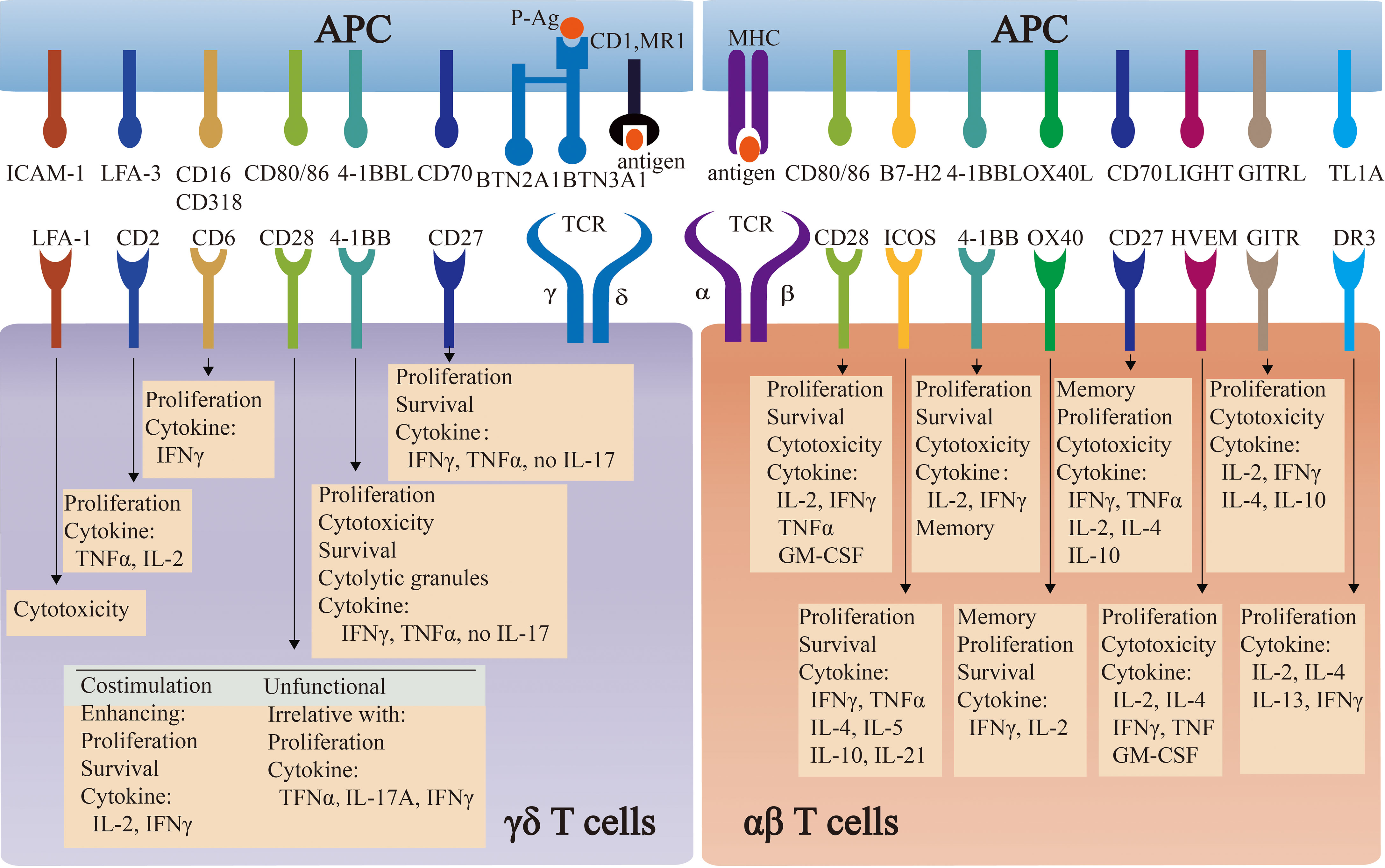
Figure 2 The comparison of co-stimulatory molecules and their induced effector functions between αβ T cells and γδ T cells. Co-stimulatory molecules induce the effector functions of αβ T cells and γδ T cells, by engaging respective ligands and counter-receptors on APCs. The specific functions of some molecules are commonly well-understood in αβ T cells, whereas they are controversial in γδ T cells.
3.2.1 CD28
Almost all engineered γδ T cell, especially CAR-γδ T cell, utilize CD28 as a co-stimulation molecule. CD28 is an important co-stimulation molecule that express on most CD4+ and half of CD8+ αβ T cells. It has been widely accepted that CD28 mediates costimulatory signal to amplify signaling generated by TCRs ligation, promoting proliferation, survival and cytokine production of αβ T cells (55). Thus, CD28 has been widely applied in CAR-αβ T cells to help exert better effect. However, co-stimulatory function of CD28 in γδ T cells is still under debate. Some studies indicated that CD28 functioned as a costimulatory molecule in γδ T cells. CD28+ γδ T cells have the better activation, proliferation, survival and production of IL-2 when they were stimulated with anti-CD28 mAb (56–58). It also showed that almost no γδ T cells, especially CD69+ γδ T cells, could expand in CD28-deficient malaria mouse model. Along this line, CD28-/- γδ T cells failed to produce cytokines, such as IFNγ and IL-17. In human, the blockage of CD28 ligand led to the impairment in γδ T cell proliferation and survival (56). However, since CD28 signal is extremely important for αβ T cells, including CD4+ T help cells, neither the CD28-/- mice infection model nor the CD28 ligand blockade experiment can exclude the possibility that blocking CD28 signal reduced the function of CD4+ T help cells, thereby affecting γδ T cells indirectly. On the contrary, some studies disagreed with the co-stimulatory function of CD28 in γδ T cells. Some researchers found that CD28 was not expressed in resting mouse splenic, intestinal intraepithelial, and vaginal γδ T cells, revealing the dispensable role of CD28 in mouse (57, 59, 60). In addition, the proliferation of γδ T was unchanged when they were stimulated with anti-CD3 mAb with or without anti-CD28 mAb (42). Consistently, CD28+/+ and CD28-/- mice were revealed to have equivalent increases in the percentage and quantity of the γδ T cells and IL-17A+/IFNγ+ γδ T cells in a listeria model of Infection (61). In human, although 40-60% freshly isolated human γδ T cells expressed CD28, this subset was diminished to 10% during in vitro culture, and even disappeared in long-term culture (62, 63). Moreover, human Vγ9Vδ2 T cells produced TNFα via direct TCR-induced p38 kinase and MEK/ERK activation pathway, but irrelative with CD28 (63). As is discussed above, since the co-stimulatory function of CD28 remains controversial in different stimulating conditions or infection models, it is still unclear whether γδ T cell function requires transient or continuous CD28 signals. Comprehensive studies to investigate the role of CD28 signals in γδ T cells are required, which will benefit for the better design of engineered γδ T cells.
3.2.2 4-1BB
4-1BB, also known as CD137, is an inducible T cell costimulatory molecule. It can be detected after stimulation and reaches the peak of expression at 48h in human αβ T cells, and functions as a conventional co-stimulatory molecule (64). 4-1BB has been widely applied in engineered αβ T cells, but not in γδ T cells currently. Many researches pointed that 4-1BB preferred to help expand memory CTLs, up-regulated NKG2D expression and rendered enhanced cytolysis. More importantly, the 4-1BB provided a stronger cytotoxicity than CD28 in some experiments (65). However, no research has specifically evaluated the advantages and disadvantages of 4-1BB in engineered γδ T cells, which raises the question of whether 4-1BB can exert as an efficient co-stimulator in engineered γδ T cells. Existing researches have revealed the co-stimulation function of 4-1BB in γδ T cell in different disease models. For example, 20% 4-1BB+ Vγ9Vδ2 T cells were observed in influenza virus infection model, and most importantly, such cell subset showed an enhanced ex vivo effector function such as more intensive granule release, more cytokine production (e.g. IFNγ), and superior cytotoxic activity towards virus-infected cells comparing to the 4-1BB- counterparts. Furthermore, the co-stimulation effect of 4-1BB was determined to induce better proliferation and enhance the survival of Vγ9Vδ2 T cells (66). On the other hand, in influenza virus infection mouse model, the transfer of 4-1BB+ γδ T cells was beneficial to maintain the body weight, enhance the survival rate, and reduce virus titers. With the co-stimulated of 4-1BB, only γδ T cells, but not other subsets of PBMCs, had improved therapeutic outcome in this disease model (66). In addition to influenza infection model, Listeria Monocytogenes infected mouse model indicated that compared to γδ T cells without 4-1BB stimulation, γδ T cells with 4-1BB stimulation showed the decreased bacterial load in vivo and enhanced survival. To be more specific, anti-4-1BB treatment in adoptive γδ T cell treatment, rather than adoptive αβ T cell treatment, significantly increased the cytokine production such as IFNγ and TNFα, and the augmented number of γδ T cells (67).
3.2.3 CD27
CD27 is also a stimulatory molecule in αβ T cells, which interacts with CD70 and induces the activation, proliferation, and survival of αβ T cells (68). It has been applied in CAR-αβ T which can promote the proliferation, anti-tumor effect, and survival both in vitro and in vivo (69). However, it has not been applied in engineered γδ T cells. CD27 has been found to be widely expressed in γδ T cells. It is expressed in 70-90% of γδ T cells in mouse spleen and lymph nodes (70), 81% of activated Vγ9+ T cells, and even some Vδ1+ T cells in peripheral blood in human (71). Many researches have showed its co-stimulation function as it can promote proliferation, survival and cytokine production in γδ T cells (56, 71). Interestingly, CD27 was used to distinguish mouse γδ T cells with different cytokine production. In this case, CD27- γδ T cells produce IL-17, whereas CD27+ γδ T cells produce IFNγ. In addition, 90% IFNγ and 70% TNFα-producing cells were CD27+ γδ T cells in naïve and malaria-infected mice (70). Apart from these in vitro researches, γδ T cells failed to expand in CD27 deficient mice when infected with MuHV-4, compared to that in WT mice. Moreover, the deficiency of CD27 related to the anergy of IFNγ production (72). In human, comparing to CD27- γδ T cells, CD27+ Vγ9Vδ2 T cells showed higher level of proliferation and up-regulation of BCL2A1 gene after being cultured with HDMAPP. Vγ9Vδ2 T cells had a stronger ability of proliferation and IFNγ, LT-α secretion under sCD70 stimulation, and CD70 blockade prevented efficient expansion of Vγ9Vδ2 T cells and reduced production of TNFα and LT-α (71). As summarized, CD27 may be a potential co-simulation molecule that can be applied in engineered γδ T cells.
3.2.4 Potential Co-Stimulation Molecules
Recent researches have revealed some other potential co-stimulation molecules. For example, CD6 (ligands to CD166 and CD318), a costimulatory receptor, is expressed on virtually all T cells, especially activated human γδ T cells (73–75). After stimulated by CD166, human Vδ2+ T cells showed increased proliferative capability and IFNγ production. In addition, both CD6 and CD166 were observed to locate at center of synapses in activation process (75). In αβ T cells, a CAR with CD6 showed increased release of IFNγ and enhanced anti-tumor effect when compared with the CAR without CD6 (76). However, CD6 has not been applied in engineered γδ T cell therapy. In addition to CD6, CD2 and LFA1, as adhesion molecules, also have costimulatory function in activated αβ T cells (77, 78). Ligation of CD2 and its ligand was applied in first-generation CD19-specific CAR to drives IL-2 production (79).There are several researches about its costimulatory function in γδ T cells. The stimulation by anti-CD2 mAb promotes IL-2 secretion and/or proliferation of γδ T cells (80). Correspondingly, the blockage of CD2 or LFA1 inhibited the effector function, especially reduced TNFα production, of Vδ2− T cells (34). However, LFA1 and CD2 signals affected the function of Vγ9Vδ2 T cells differently. CD2 blockade strongly inhibited proliferation of γδ T cells and release of TNFα/IL-2, but had no effect on the lytic activity of γδ T cells, whereas LFA-1 blockade had no effect on cell proliferation and cytokine production, but could effectively inhibited target cell lysis (81). Consequently, CD2/LFA1 co-stimulation may differently influence the effector function of engineered γδ T cells.
4 The Characteristics of NK Cell Receptor Signals in γδ T Cells
The expression of a variety of NK cell receptors, including NKRs and NCRs, is an important feature of γδ T cells, which endows γδ T cells innate immune characteristics like NK cells. Also, the two kinds of lymphocytes share similar characteristics in the perspective of immune responses. Compared to αβ T cells, involvement in innate immune reaction is beneficial for γδ T cells to recognize a more extended spectrum of antigens on tumor cells, reduce the risk of tumor immune escape by losing single tumor-associated antigen, and provide available chances for novel immunotherapies for cancers that lack tumor specific antigens.
4.1 Natural Killer Group 2D (NKG2D)
As one of the most important receptors, NKG2D is a C-type, lectic-like, type II transmembrane glycoprotein, which is expressed on NK cells, γδ T cells and some narrowed subsets of αβ T cells (82, 83). In human peripheral blood, almost all γδ T cells expressed NKG2D, but compared with NK cells, the expression level of NKG2D is about 10-times lower (82). In addition, the intestinal intraepithelial γδ T cells originally express relatively low level of NKG2D. Interestingly, the expression of NKG2D can be upregulated in response to IL-15 stimulation or 4-1BB signals (84).
4.1.1 NKG2D Recognition
Like NK cells, NKG2D on γδ T cells can also recognize ligands including MHC class I-like molecules [e.g. MHC I chain-related molecules A and B (MICA/B) and UL16-binding protein (ULBP1-6)] in human, and retinoic acid early transcripts (Rae1) α-ϵ, murine UL16-binding protein-like transcript 1 MULT1, H60a, H60b, and H60c in mouse. These ligands can be induced in infected and oncogenic transformed cells (85, 86). Therefore, NKG2D is frequently involved in the tumor cell recognition, induces cytokine release, and triggers degranulation. NKG2D is reported to trigger cytotoxicity of γδ T cells against tumor cells and bacterial or virus-infected cells in a NK-like and TCR-independent manner (87–91). Stimulated by anti-NKG2D mAb or NKG2D ligand protein (NKG2DL), human Vγ9Vδ2 T cells and mouse dendritic epidermal T cells (DETCs) released cytotoxic granules and cytokines such as TNFα. The blockade of NKG2D completely abolished such cytotoxicity to tumor cells induced by Vγ9Vδ2 T cells (83, 87, 91).
4.1.2 NKG2D Signal
The pivotal role of NKG2D in γδ T cells has attracted researchers to explore the underlying signaling molecules and specific signaling pathways. The NKG2D signals are much similar between γδ T cells and NK cells. In NK cells, NKG2D is associated with adapter molecules DAP10 to transmit signals in PI3K or Vav/SOS signaling pathway to trigger cytotoxicity, but without IFNγ production. Alternatively, NKG2D connects to DAP12 to recruit Syk and ZAP70 to downstream signaling events to trigger cytotoxicity, along with the secretion of IFNγ in mice (92–96). In γδ T cells, NKG2D has been observed to act in a PI3K-dependent signaling pathway that responds to target cells in a TCR-independent manner (88, 91, 97). DAP10, rather than DAP12, was reported to strongly express in resting and activated human Vγ9Vδ2 T cells (82, 96), while DAP10/DAP12 constitutively expressed in mouse DETCs (83). Human Vγ9Vδ2 T cells stimulated by ULBP proteins could produce IFNγ, TNFα, and released cytolytic granules usually accompanying PKB (a PI3K kinase substrate) phosphorylation (88, 97). The knockdown of either DAP10 or NKG2D in Vγ9Vδ2 T cells showed the similar impaired anti-bacterial effect, when cocultured with infected macrophages. This indicated that DAP10 is involved in NKG2D signaling during bacterial infection (88). In mouse DETCs, NKG2D could trigger a PI3K-dependent signaling pathway by DAP10 to increase phosphorylation of Akt, trigger degranulation and induce cytotoxicity, which could be completely inhibited by PI3K inhibitor. In addition, in the absence of both NKG2D-S-DAP12 (a shorter protein isoform that is produced by alternative splicing of killer cell lectin-like receptor K1(Klrk1)) and TCR signals, only NKG2D/DAP10 signals through the PI3K/Grb2/Vav1 pathway is sufficient to trigger cytotoxicity of DETCs against target cells (91). Of note, although γδ T cells could produce IFNγ and were cytotoxic under the stimulation of anti-NKG2D mAb (83), these cells failed to produce IFNγ, TNFα, IL-13 and induced Syk/ZAP70 activation when stimulated by recombinant NKG2DL protein. It indicated that NKG2DLs may not be able to engage enough activation of NKG2D/DAP12 signaling, which might be weaker than NKG2D/DAP10 on DETCs in the aspects of triggering Syk/ZAP70 signaling (91).
4.1.3 Signal Difference Between NKG2D and TCR in γδ T Cells
Apart from the signaling mechanism, another interesting question is the different activation level through NKG2D and TCR in activating γδ T cells. Some experiments compared effector function in cytokine production, degranulation, killing ability induced by γδ T cells with specific TCR stimulation to that with NKG2D stimulation. For example, comparison of cytokines production in different stimulation groups, Vγ9Vδ2 T cells induced the similar level of TNFα when stimulated by anti-NKG2D mAb or recombinant MICA-Fc or Daudi cells plus IPP, although which is weaker than that of stimulation with anti-CD3ϵ mAb (87). Furthermore, by stimulating NKG2D or TCRs pathways with antibodies or cell lines respectively, Vγ9Vδ2 T cells showed the similar level of degranulation, IFNγ production and cytotoxicity (87). In the real situation, tumor cells can express ligands that could bind both NKG2D and γδ TCRs. Therefore, researchers conducted inhibition experiment to compare the contribution of NKG2D and TCR to the cytotoxicity of γδ T cells. The inhibitory effect of TCR signal blocking on Vγ9Vδ2 T cell cytotoxicity was much stronger than that of NKG2D signal blocking (87, 97). However, TCR signal or NKG2D signal blockade had similar inhibitory effects on cytotoxicity of DETCs, indicating that the signaling pathways in human and mouse γδ T cells are different (91). Interestingly, some ligands that can be recognized by both γδ TCRs and NKG2D, such as ULBP4, can be killed by Vγ9Vδ2 T cells in both TCRs-based and NKG2D-based activation pathways. Blocking one of these pathways could only induce minor inhibitory effect on degranulation of Vγ9Vδ2 T cells and cytotoxicity to EL4-ULBP4+ cells, but almost completely inhibited IFNγ production by Vγ9Vδ2 T cells. However, blocking both of these pathways could significantly reduce the cytotoxicity of Vγ9Vδ2 T cells (97). In addition, NKG2D signal in γδ T cells could enhance TCR-dependent signals, which increased cytokine production and cytotoxicity of γδ T cells, and extended survival of γδ T cells (98–101). For calcium response, compared with αβ T cells and iNKT cells, which showed a strong and rapid TCR-induced Ca2+ response, Vγ9Vδ2 T cells showed a delayed and sustained Ca2+ response. However, when NKG2D signal was simultaneously activated, Ca2+ responses in γδ T cells induced by TCR signal could be accelerated. Besides, NKG2D signal alone could not induce significant Ca2+ activation signals, indicating NKG2D signal could enhance TCR signal in γδ T cells. Furthermore, PKCθ were found to play an important role in the NKG2D mediated costimulatory function. Vγ9Vδ2 T cells have significantly improved cytolytic ability to tumor cells with NKG2D signal, which could be blocked by PKCθ inhibitor. It is worth noting that PKCθ inhibitor could inhibit the acceleration of Ca2+ response induced by NKG2D, indicating that NKG2D signal could shape Ca2+ response and potentiate antitumor CTL activity of Vγ9Vδ2 T cells in a PKCθ-dependent manner (99). Taken together, regulation of NKG2D on DAP10 and/or DAP12 signals alone or together with TCR signals should be carefully designed for the application of engineered γδ T cell therapies, especially for the characteristic of cytotoxicity, proliferation, exhaustion, memory and cytokines production of engineered γδ T cells.
4.2 Natural Cytotoxicity Receptors (NCRs)
Like NKRs, NCRs are mostly detected on Vδ1 population, and can activate γδ T cells by recognizing ligands on tumor cells (102–104). They exert potent anti-tumor activities in a TCR-independent way (103–105), and sometimes enhance effector function of γδ T cells (106). Similar to NKG2D, NCRs ligated with adaptor proteins, such as CD3ζ, FcRγ and DAP12, to transmit intracellular activating signaling in NK cells. Similarly, NKp44 is detected to couple with DAP12 in stimulated human γδ T cells (106). But the specific function of these adapters associating with NCRs in γδ T cells remains unclear and needs further exploration.
The comparison of NKG2D and NCR signaling pathways between γδ T cells and NK cells is summarized in Figure 3.
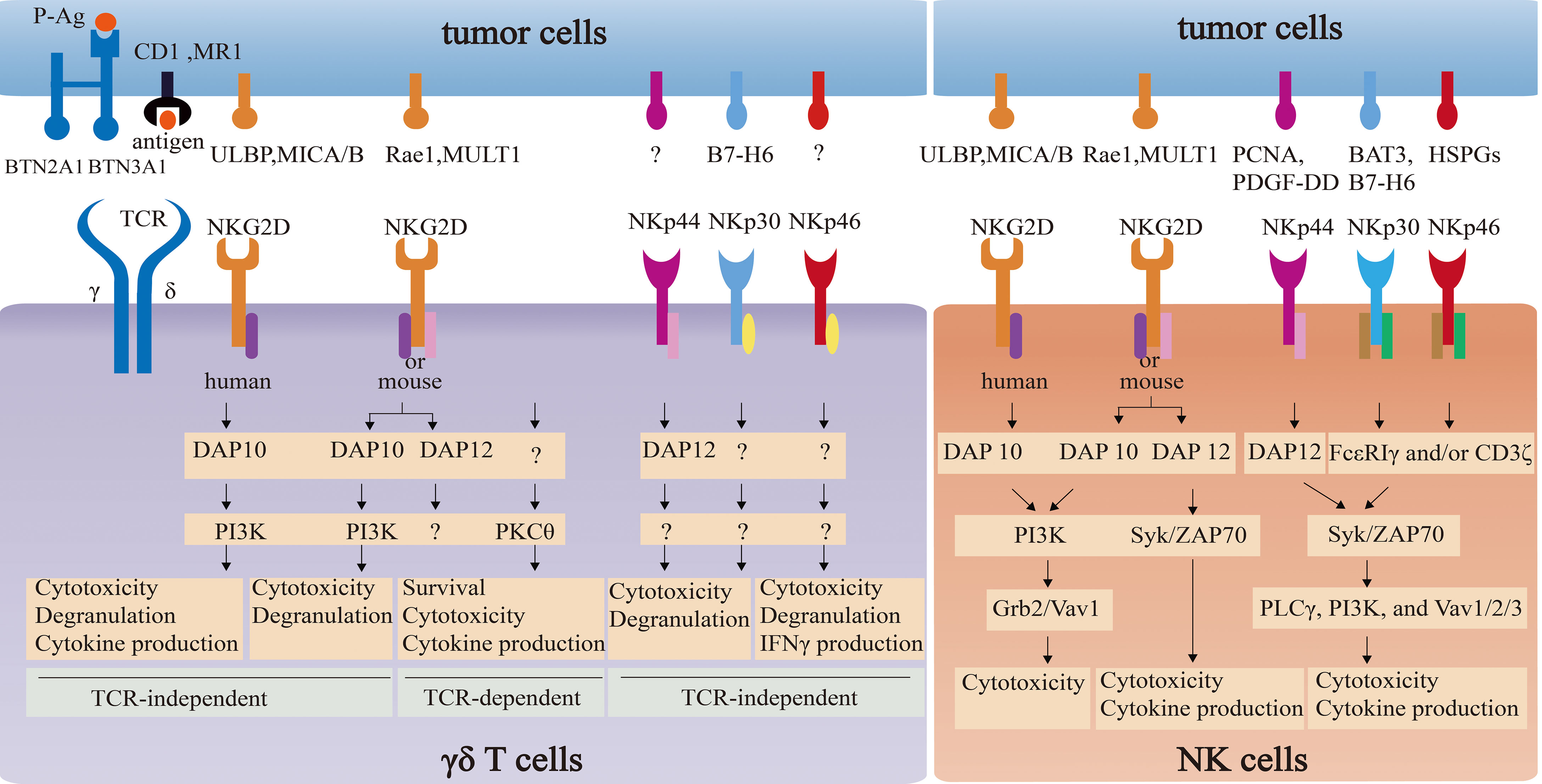
Figure 3 The comparison of NKG2D and NCR signaling pathways between γδ T cells and NK cells. NKG2D in NK cells associates with adapter DAP10 (PI3K and Grb2-Vav1 signaling) or DAP12 (Syk/ZAP70 signaling) to directly induce cytotoxicity and/or cytokine release. NKG2D in γδ T cells not only directly triggers cytotoxicity via PI3K-dependent pathway by coupling with DAP10, but also enhances the effector function in a TCR-independent way via PKCθ. However, the impact of NKG2D-DAP12 complex on the function on γδ T cells remain elusive. NCRs in NK cells and γδ T cells can induce cytotoxicity activity. However, signaling pathways of NCRs-ligands in NK cells are well-understood, which in γδ T cells remain unclear.
5 Other Receptors Delivering Signals in γδ T Cells
γδ T cells also express cytokine receptors, like IL-2Rβγ, IL-18R, IL-7Rα, IL23R (107–111), which can deliver activated signals by binding to interleukins. Stimulation of these cytokine receptors can not only enhance the effect function of γδ T cells, but also directly trigger the activation of γδ T cells even in the absence of TCR signal. For example, after initial stimulation by P-Ag or γδ TCR antibody, IL-15, IL-12, IL-2, IL-18, IL-33 and IL-7 could additionally enhance the proliferation, cytokine production, cytotoxic effect of γδ T cells (112–118). Furthermore, some cytokines alone or combination, such as IL-15, IL-2, IL-12, IL-18, IL-7, IL-1 and IL-23, were found to induce proliferation, cytokine production and killing ability in the absence of TCR signal (107, 110, 111, 115). Besides, γδ T-induced effector molecules were impacted by cytokines. IL-2, IL-12, IL-18, IL-15 and IL-21 were found to promote IFN-γ-production of γδ T cell (113, 115, 119, 120), whereas IL-17-production of γδ T cell was driven by IL-1, IL-23 and IL-7 (111, 118, 121). Interestingly, IL-18 could replace IL-1β and cooperate with IL-23 to induce IL-17 production in γδ T cells (108). In addition, toll-like receptors (TLRs) were also reported to deliver activated signals in γδ T cells. The simultaneous stimulation of TLRs (e.g. TLR1/2/6, 3, and 5) and TCR significantly enhanced the activation and effect function of γδ T cells. Furthermore, γδ T cells can also directly respond to TLR2 ligands to act effect function in a TCR-independent manner (122).
6 Application of γδ T Cells in Engineered T Cell Therapies
Although γδ T cells have limited ability to expand and proliferate in vivo, which may affect the antitumor efficacy of γδ T cells, γδ T cells remain good candidates for engineered therapies with many advantages. Firstly, since engineered γδ T cells can exploit the endogenous receptors (TCRs and innate immune receptors) and engineered receptors, the current CAR-γδ T therapies induce a significantly stronger potential to kill targeted cells and cytokine production, which contributes to more significant reductions of tumor burden and suppression of tumor growth compared with γδ T cells (123–126). These endogenous receptors enable γδ T cells not only to recognize a myriad of tumor-specific or associated ligands as described above, but also to prevent tumor escape caused by antigen loss or downregulation (127). In this scenario, the downregulation of MHC-I in tumors helps tumor cells to escape surveillance of αβ T cells, but it does not inhibit non-MHC-restrict γδ T cell activation and even enhances the consecutive γδ T cell activation (128). Indeed, compared with CAR-αβ T cells, CAR-γδ T cells targeting CD19 or melanoma cell surface chondroitin sulfate proteoglycan (MSCP) showed a significantly higher cytotoxicity against tumor associated antigen (TAA) negative target cells, or β2-microglobuline-deficient Daudi cells that lacks the expression of MHC-I (125, 129). Secondly, activated human Vδ2+ T cells can present the characteristics of professional antigen-presenting cells like dendritic cells, which can take up, process, and present soluble antigens to αβ T cells. HLA-A0201+V2δ+GD2-CAR-γδ T cells can present the epitopes of melanoma antigen recognized by T cells 1 (MART-1) to αβ T cells to promote expansion and cytotoxicity (130). Thirdly, since allogeneic αβ T cell therapies have side-effects of host-versus-graft activities (HVGA) and graft-versus-host disease (GVHD), current engineered αβ T cell products are individualized and have many limitations, such as high cost, time consuming, and unstable quality or quantity of T cells (131). However, engineered γδ T cells with MHC-unrestricted recognition pattern can avoid of GVHD, which makes it possible for engineered γδ T cells to become universal cell products to circumvent many disadvantages of above-mentioned individualized CAR-T cell products. Many clinical cases and trials were trying to assess the safety of allogeneic γδ-TCR T cell therapies to confirm the absence of GVHD by γδ T cells. For example, after receiving allogeneic γδ T cell immunotherapy, a patient with cholangiocarcinoma had improved peripheral immune function, reduced tumor activity, and prolonged life span, and more importantly, without side-effects (132), indicating the safety of γδ T cells and its potential to be universal. Besides, 3 clinical trials (NCT04107142, NCT04735471, NCT04911478) were conducted to evaluate the safety and tolerability of allogeneic CAR-γδ T cells targeting NKG2D ligand (NKG2DL) and CD20. However, HVGA and the persistence remained to be the challenge for engineered γδ T cell products. Taken together, engineered γδ T cells take advantage of recognizing antigens by endogenous receptors as well as engineered receptors, processing and presenting antigens to activate αβ T cells and avoiding GVHD. To sum, γδ T cells can be a more efficient and wider-applied antitumor candidate to produce engineered products.
6.1 CAR Transfer to γδ T Cells
CAR-αβ T therapy has shown unprecedented success in hematologic malignancies, but poor efficacy in solid tumors. Many studies found higher infiltration of γδ T cells in solid tumors than that of αβ T cells, and the frequency of infiltrated γδ T cells in solid tumors positively correlated with prognosis (133–135), indicating a promising application of CAR-γδ T cells in solid tumors. Thus, CAR-γδ T cells have been designed to target many solid tumor antigens, such as disialoganglioside 2 (GD2) on neuroblastoma and Ewing sarcoma (136), melanoma chondroitin sulfate proteoglycan (MCSP) on melanoma lesions (137), original or glycosylated Mucin 1 (MUC1) on breast cancer, head and neck squamous cell carcinoma (138, 139). Current ongoing clinical trials involving engineered γδ T products are summarized in Table 1.
However, current CAR-γδ T cells fails to show better efficacy of tumor immunotherapy than CAR-αβ T cells. One of reasons is the design of intracellular signaling domain of CAR-γδ T cells is less optimized. The intracellular signaling domains applied in CAR-γδ T cells are almost as same as what used in CAR-αβ T cells. Indeed, CAR-γδ T cells are reported to have a significant effector function against tumor cells. But it is controversial in different studies comparing CAR-γδ T cells with CAR-αβ T cells, particularly in solid and hematologic tumors. Meir Rozenbaum et al. pointed the superiority of CAR-αβ T cells in leukemia in vivo. To be more specific, treatment with CAR-γδ T or CAR-αβ T cells led to a respective 5% and 0.1% tumor cell residue in the bone marrow of mice, demonstrating the higher load of leukemia cells in recipients of CAR-γδ T cells compared to the CAR-αβ T treated mice (125). This phenomenon suggested that CAR-γδ T cells with suboptimal design have lower efficiency to eliminate tumor cells than that by CAR-αβ T cells. Furthermore, recent study reported the persistence of CAR-Vγ9Vδ2 T cells was worse than that of CAR-αβ T cells. While CAR-αβ T cells still effectively eliminate all the tumor cells in the fourth round of tumor stimulation, CAR-Vγ9Vδ2 T cells almost lost their cytotoxicity. Fortunately, the cytotoxicity of γδ T cells can be restored by the addition of IL-2 (126). Although compared with CAR-αβ T cells, CAR-Vδ1 and Vδ2 T cells secreted higher levels of granzyme B and cytokines, and exhibited similar or stronger cytotoxicity against some kind of solid tumors in vitro (126), the specific effector function against solid tumor in vivo should be comprehensively investigated in the future. Therefore, it is extremely important to investigate the optimal use of activation signals for CAR-γδ T cells. Recent studies have made some modifications to simultaneously take advantage of the natural endogenous signal properties of γδ T cells. For example, DAP10 was used in engineered γδ T cells and engaged in the antitumor response. Except for the signal induced by TCRs, GD2-DAP10 CAR transferred γδ T cells used the solitary endodomain derived from the NKG2D adaptor DAP10 to mimic NKG2D co-stimulation, which induced significant cytokine production and equivalent killing as CD28-CD3ζ-CAR-γδ T cells against GD2+ Neuroblastoma and Ewing Sarcoma (140). Interestingly, this example also promoted the utilize of “AND gate” system in engineered γδ T cells to minimize on-target off-tumor toxicity. It was only activated in presence of antigen through γδ TCR and GD2, whereas only GD2 could activate CD28-CD3ζ-CAR-γδ T cells (140).
6.2 αβ TCR Transfer to γδ T Cells
Engineered γδ T cells not only included CAR-γδ T cells, but also TCR-γδ T cells. For example, αβ TCRs were reported to be transferred to γδ T cells, making αβ TCR-γδ T cells sensitive to tumor cells with antigen-negative or tumor escape variants with MHC-downregulating. γδ T cells which expressed an HLA-A*0101 restricted αβ TCR targeting the adenovirus hexon protein of HAdV-species C, released more IFNγ and TNFα than CD8+ αβ T cells with the same αβ TCR, and had comparable cytotoxicity against adenovirus-infected dendritic cells (141). Interestingly, while most γδ T cells lack the expression of the co-receptors CD4 or CD8, some researches transferred the co-receptors along with αβ TCRs to γδ T cells and found the enhanced specific functional activity. Comparing to HA-2-TCR-γδ T cells without the additional transfer of CD8, co-transferring of CD8 and HA-2-TCR to γδ T cells significantly increased IFN-γ and IL-4 production and exerted more efficient cytotoxicity against the HA-2-expressing CML and AML cells (142). In addition, transferring αβ TCRs that recognized the same antigen as endogenous γδ TCRs could improve TCR-γδ T cell antigen recognition and cytotoxicity efficiency. For example, transferring αβ TCRs derived from invariant natural killer T (iNKT) cells, which recognized glycolipid antigens presented by CD1d, the TCR-γδ T cells were found to respond to CD1d via both endogenous γδ TCRs and transferred αβ TCRs, and had increasing antitumor effect against the CD1d positive leukemia cell line K562 (143). Of note, the transfer of αβ TCRs to γδ T cells did not show any mispairing of endogenous and transgenic TCRs (144), which significantly avoided autoimmunity (145, 146). Along this line, in order to obtain better anti-tumor efficacy, CAR-γδ T cells or TCR-γδ T cells can be designed so that endogenous γδ TCR and engineered CAR/TCR can recognize the same antigen, such as CAR-γδ T cell targeting NKG2DL or BTN3A, TCR-γδ T cell targeting HLA-A24, HLA-B27 and HLA-A2, all of which can be investigated in the future.
6.3 γδ TCRs Transfer to αβ T Cells
γδ TCRs transferred αβ T cells was also used to overcome the deficiency of cytotoxicity of particular types of HLA-restricted αβ T cells. This design has several advantages. Firstly, the γδ TCRs could target a broad range of solid and hematological tumors in MHC-independent manner. Secondly, compared with γδ T cells, the mechanism of effects and memory functions of CD4+ and CD8+ αβ T cells are better understood in vivo (7). Thirdly, this strategy can avoid the activity of inhibitory receptors like KIRs on γδ T cells. Indeed, αβ T cells expressing the Vγ9Vδ2 TCR clone G115 displayed a γδ T cell-like effector function, such as cytotoxicity against the Daudi cell line, cytokine release, enhanced cytotoxicity using amino-bisphosphonates, and the ability to induce dendritic cell maturation. Surprisingly, endogenous αβ TCRs were down-regulated after the transduction of γδ TCRs, leading to a lack of allo-reactive response (147). Besides, several types of tumor specific CDR3δ-grafted γδ TCRs were also used to modify αβ T cells and exhibited significant antitumor effects (148, 149). Moreover, a novel antibody-TCR (Ab-TCR) modified αβ T cells, combining Fab-based antigen recognition with γδ TCR signaling, showed a similar cytotoxicity and a less cytokine release comparing with CD28/CD3ζ CAR-T cells (150). Recently, TEG001, an engineered αβ T products expressing a defined γδ TCR, was proved to be safe and efficient against tumor models in vivo (151), and currently was applied in a first-in-human clinical study (NL6357).
7 Conclusion
Currently, increasing studies have confirmed the anti-tumor activities of γδ T cells in targeting various malignancies with their innate and adaptive immunities, which brings hopes to the engineered γδ T cells in cancer treatment. However, current engineered γδ T products almost copy the structure of engineered αβ T cells, owing to the ignore of the specific activating mechanism of γδ T cells. As discussed above, we detailed the activating and stimulating modes of γδ T cells via TCR signal, some important costimulatory signals, and innate signals from NK receptors, which were summarized in Figure 1. Furthermore, current engineered γδ T products and their characteristics are also depicted. Taken the basics of γδ T cells in previous sections together, this review will shed light on the optimal design of engineered γδ T cell to improve its efficacy. However, there are still numerous problems to be solved. More studies are supposed to be conducted to describe the specific activating mechanism of γδ T cells, which can be applied in engineered γδ T products.
Author Contributions
RD and YZ drafted the manuscript. XZ and HX take the primary responsibility for this paper as the corresponding authors. All authors contributed to the article and approved the submitted version.
Funding
This work was supported by the National Natural Science Foundation of China (No. 31870899 and 32070899 to XZ, No. 81870136 and 82170141 to HX), Natural Science Foundation of Zhejiang Province (No. LXZ22H080001), and the Independent Task of State Key Laboratory for Diagnosis and Treatment of Infectious Diseases (No.2022zz16).
Conflict of Interest
The authors declare that the research was conducted in the absence of any commercial or financial relationships that could be construed as a potential conflict of interest.
Publisher’s Note
All claims expressed in this article are solely those of the authors and do not necessarily represent those of their affiliated organizations, or those of the publisher, the editors and the reviewers. Any product that may be evaluated in this article, or claim that may be made by its manufacturer, is not guaranteed or endorsed by the publisher.
Abbreviations
ACT, Adoptive cell therapy; AMPK, AMP-activated protein kinase; BTN2A1, Butyrophilin 2A1; BTN3A1, Butyrophilin 3A1; CAR, chimeric antigen receptor; CD3 CC, CD3 conformational change; DETCs, dendritic epidermal T cells; EphA2, ephrin type-A receptor 2; FDA, Food and Drug Administration; FPPS, farnesyl-diphosphate-synthase; GD2, disialoganglioside 2; GVHD, graft-versus-host disease; HVGA, host-versus-graft activities; iNKT, invariant natural killer T; IPP, Isopentenyl pyrophosphate; Klrk1, killer cell lectin-like receptor K1; MART-1, melanoma antigen recognized by T cells 1; MHC, major histocompatibility complex; MICA/B, MHC I chain-related molecules A and B; MR1, MHC-related protein 1; MSCP, melanoma cell surface chondroitin sulfate proteoglycan; MUC1, Mucin 1; NCRs, NK cytotoxicity receptors; NKG2D, Natural killer group 2D; NKG2DL, NKG2D ligand; NKRs, NK cell receptors; P-Ag, phosphoantigens; RAG, recombination activating gene; PRS, proline-rich sequence; Rae1, retinoic acid early transcripts-1; RCC, renal cell carcinoma; scFv, single-chain fragment variable; TAA, tumor associated antigen; TCR, T cell receptors; ULBP, UL16-binding protein; ZOL, zoledronate.
References
1. Singh AK, McGuirk JP. CAR T Cells: Continuation in a Revolution of Immunotherapy. Lancet Oncol (2020) 21(3):e168–e78. doi: 10.1016/S1470-2045(19)30823-X
2. Jiang X, Xu J, Liu M, Xing H, Wang Z, Huang L, et al. Adoptive CD8(+) T Cell Therapy Against Cancer:Challenges and Opportunities. Cancer Lett (2019) 462:23–32. doi: 10.1016/j.canlet.2019.07.017
3. Silva-Santos B, Mensurado S, Coffelt SB. Gammadelta T Cells: Pleiotropic Immune Effectors With Therapeutic Potential in Cancer. Nat Rev Cancer (2019) 19(7):392–404. doi: 10.1038/s41568-019-0153-5
4. Liu Z, Eltoum IE, Guo B, Beck BH, Cloud GA, Lopez RD. Protective Immunosurveillance and Therapeutic Antitumor Activity of Gammadelta T Cells Demonstrated in a Mouse Model of Prostate Cancer. J Immunol (2008) 180(9):6044–53. doi: 10.4049/jimmunol.180.9.6044
5. Silva-Santos B, Strid J. Working in "NK Mode": Natural Killer Group 2 Member D and Natural Cytotoxicity Receptors in Stress-Surveillance by Gamma Delta T Cells. Front Immunol (2018) 9:851. doi: 10.3389/fimmu.2018.00851
6. Karre K, Ljunggren HG, Piontek G, Kiessling R. Selective Rejection of H-2-Deficient Lymphoma Variants Suggests Alternative Immune Defence Strategy. Nature (1986) 319(6055):675–8. doi: 10.1038/319675a0
7. Sebestyen Z, Prinz I, Dechanet-Merville J, Silva-Santos B, Kuball J. Translating Gammadelta (Gamma Delta) T Cells and Their Receptors Into Cancer Cell Therapies. Nat Rev Drug Discov (2020) 19(3):169–84. doi: 10.1038/s41573-019-0038-z
8. Uldrich AP, Le Nours J, Pellicci DG, Gherardin NA, McPherson KG, Lim RT, et al. CD1d-Lipid Antigen Recognition by the Gamma Delta TCR. Nat Immunol (2013) 14(11):1137–45. doi: 10.1038/ni.2713
9. Harly C, Guillaume Y, Nedellec S, Peigne CM, Monkkonen H, Monkkonen J, et al. Key Implication of CD277/butyrophilin-3 (BTN3A) in Cellular Stress Sensing by a Major Human Gamma Delta T-Cell Subset. Blood (2012) 120(11):2269–79. doi: 10.1182/blood-2012-05-430470
10. Zeng X, Meyer C, Huang J, Newell EW, Kidd BA, Wei YL, et al. Gamma Delta T Cells Recognize Haptens and Mount a Hapten-Specific Response. eLife (2014) 3:e03609. doi: 10.7554/eLife.03609
11. Doherty PC, Allan W, Eichelberger M, Carding SR. Heat-Shock Proteins and the Gamma Delta T Cell Response in Virus Infections: Implications for Autoimmunity. Springer Semin Immunopathol (1991) 13(1):11–24. doi: 10.1007/BF01225275
12. Zeng X, Wei YL, Huang J, Newell EW, Yu HX, Kidd BA, et al. Gamma Delta T Cells Recognize a Microbial Encoded B Cell Antigen to Initiate a Rapid Antigen-Specific Interleukin-17 Response. Immunity (2012) 37(3):524–34. doi: 10.1016/j.immuni.2012.06.011
13. Deseke M, Prinz I. Ligand Recognition by the Gamma Delta TCR and Discrimination Between Homeostasis and Stress Conditions. Cell Mol Immunol (2020) 17(9):914–24. doi: 10.1038/s41423-020-0503-y
14. Kabelitz D, Serrano R, Kouakanou L, Peters C, Kalyan S. Cancer Immunotherapy With Gamma Delta T Cells: Many Paths Ahead of Us. Cell Mol Immunol (2020) 17(9):925–39. doi: 10.1038/s41423-020-0504-x
15. Salim M, Knowles TJ, Baker AT, Davey MS, Jeeves M, Sridhar P, et al. BTN3A1 Discriminates Gamma Delta T Cell Phosphoantigens From Nonantigenic Small Molecules via a Conformational Sensor in Its B30.2 Domain. ACS Chem Biol (2017) 12(10):2631–43. doi: 10.1021/acschembio.7b00694
16. Gober HJ, Kistowska M, Angman L, Jeno P, Mori L, De Libero G. Human T Cell Receptor Gamma Delta Cells Recognize Endogenous Mevalonate Metabolites in Tumor Cells. J Exp Med (2003) 197(2):163–8. doi: 10.1084/jem.20021500
17. Freed-Pastor WA, Mizuno H, Zhao X, Langerod A, Moon SH, Rodriguez-Barrueco R, et al. Mutant P53 Disrupts Mammary Tissue Architecture via the Mevalonate Pathway. Cell (2012) 148(1-2):244–58. doi: 10.1016/j.cell.2011.12.017
18. Li JQ, Herold MJ, Kimmel B, Muller I, Rincon-Orozco B, Kunzmann V, et al. Reduced Expression of the Mevalonate Pathway Enzyme Farnesyl Pyrophosphate Synthase Unveils Recognition of Tumor Cells by V Gamma 9V Delta 2 T Cells. J Immunol (2009) 182(12):8118–24. doi: 10.4049/jimmunol.0900101
19. Kobayashi H, Tanaka Y, Yagi J, Minato N, Tanabe K. Phase I/II Study of Adoptive Transfer of Gamma Delta T Cells in Combination With Zoledronic Acid and IL-2 to Patients With Advanced Renal Cell Carcinoma. Cancer Immunol Immunother (2011) 60(8):1075–84. doi: 10.1007/s00262-011-1021-7
20. Dieli F, Vermijlen D, Fulfaro F, Caccamo N, Meraviglia S, Cicero G, et al. Targeting Human Gamma Delta T Cells With Zoledronate and Interleukin-2 for Immunotherapy of Hormone-Refractory Prostate Cancer. Cancer Res (2007) 67(15):7450–7. doi: 10.1158/0008-5472.CAN-07-0199
21. Sandstrom A, Peigne CM, Leger A, Crooks JE, Konczak F, Gesnel MC, et al. The Intracellular B30.2 Domain of Butyrophilin 3a1 Binds Phosphoantigens to Mediate Activation of Human V Gamma 9V Delta 2 T Cells. Immunity (2014) 40(4):490–500. doi: 10.1016/j.immuni.2014.03.003
22. Gu SY, Sachleben JR, Boughter CT, Nawrocka WI, Borowska MT, Tarrasch JT, et al. Phosphoantigen-Induced Conformational Change of Butyrophilin 3A1 (BTN3A1) and Its Implication on V Gamma 9V Delta 2 T Cell Activation. Proc Natl Acad Sci USA (2017) 114(35):E7311–E20. doi: 10.1073/pnas.1707547114
23. Karunakaran MM, Willcox CR, Salim M, Paletta D, Fichtner AS, Noll A, et al. Butyrophilin-2a1 Directly Binds Germline-Encoded Regions of the V Gamma 9V Delta 2 TCR and Is Essential for Phosphoantigen Sensing. Immunity (2020) 52(3):487–98.e6. doi: 10.1016/j.immuni.2020.02.014
24. Rigau M, Ostrouska S, Fulford TS, Johnson DN, Woods K, Ruan Z, et al. Butyrophilin 2A1 is Essential for Phosphoantigen Reactivity by Gamma Delta T Cells. Science (2020) 367(6478):eaay5516. doi: 10.1126/science.aay5516
25. Kierkels GJJ, Scheper W, Meringa AD, Johanna I, Beringer DX, Janssen A, et al. Identification of a Tumor-Specific Allo-HLA-Restricted Gamma Delta TCR. Blood Adv (2019) 3(19):2870–82. doi: 10.1182/bloodadvances.2019032409
26. Reijneveld JF, Ocampo TA, Shahine A, Gully BS, Vantourout P, Hayday AC, et al. Human Gamma Delta T Cells Recognize CD1b by Two Distinct Mechanisms. Proc Natl Acad Sci USA (2020) 117(37):22944–52. doi: 10.1073/pnas.2010545117
27. Spada FM, Grant EP, Peters PJ, Sugita M, Melian A, Leslie DS, et al. Self-Recognition of CD1 by Gamma/Delta T Cells: Implications for Innate Immunity. J Exp Med (2000) 191(6):937–48. doi: 10.1084/jem.191.6.937
28. Roy S, Ly D, Castro CD, Li NS, Hawk AJ, Altman JD, et al. Molecular Analysis of Lipid-Reactive V Delta 1 Gamma Delta T Cells Identified by CD1c Tetramers. J Immunol (2016) 196(4):1933–42. doi: 10.4049/jimmunol.1502202
29. Le Nours J, Gherardin NA, Ramarathinam SH, Awad W, Wiede F, Gully BS, et al. A Class of Gamma Delta T Cell Receptors Recognize the Underside of the Antigen-Presenting Molecule MR1. Science (2019) 366(6472):1522–7. doi: 10.1126/science.aav3900
30. Lepore M, de Lalla C, Gundimeda SR, Gsellinger H, Consonni M, Garavaglia C, et al. A Novel Self-Lipid Antigen Targets Human T Cells Against CD1c(+) Leukemias. J Exp Med (2014) 211(7):1363–77. doi: 10.1084/jem.20140410
31. Crowther MD, Dotlon G, Legut M, Caillaud ME, Lloyd A, Attaf M, et al. Genome-Wide CRISPR-Cas9 Screening Reveals Ubiquitous T Cell Cancer Targeting via the Monomorphic MHC Class I-Related Protein MR1. Nat Immunol (2020) 21(2):178–85. doi: 10.1038/s41590-019-0578-8
32. Gherardin NA, Loh L, Admojo L, Davenport AJ, Richardson K, Rogers A, et al. Enumeration, Functional Responses and Cytotoxic Capacity of MAIT Cells in Newly Diagnosed and Relapsed Multiple Myeloma. Sci Rep (2018) 8:4159. doi: 10.1038/s41598-018-22130-1
33. Marlin R, Pappalardo A, Kaminski H, Willcox CR, Pitard V, Netzer S, et al. Sensing of Cell Stress by Human Gamma Delta TCR-Dependent Recognition of Annexin A2. Proc Natl Acad Sci USA (2017) 114(12):3163–8. doi: 10.1073/pnas.1621052114
34. Willcox CR, Pitard V, Netzer S, Couzi L, Salim M, Silberzahn T, et al. Cytomegalovirus and Tumor Stress Surveillance by Binding of a Human Gamma Delta T Cell Antigen Receptor to Endothelial Protein C Receptor. Nat Immunol (2012) 13(9):872–9. doi: 10.1038/ni.2394
35. Scheffer GL, Flens MJ, Hageman S, Izquierdo MA, Shoemaker RH, Scheper RJ. Expression of the Vascular Endothelial Cell Protein C Receptor in Epithelial Tumour Cells. Eur J Cancer (2002) 38(11):1535–42. doi: 10.1016/S0959-8049(02)00108-9
36. Harly C, Joyce SP, Domblides C, Bachelet T, Pitard V, Mannat C, et al. Human Gamma Delta T Cell Sensing of AMPK-Dependent Metabolic Tumor Reprogramming Through TCR Recognition of Epha2. Sci Immunol (2021) 6(61):eaba9010. doi: 10.1126/sciimmunol.aba9010
37. Hudecek R, Kohlova B, Siskova I, Piskacek M, Knight A. Blocking of EphA2 on Endometrial Tumor Cells Reduces Susceptibility to V Delta 1 Gamma-Delta T-Cell-Mediated Killing. Front Immunol (2021) 12:12. doi: 10.3389/fimmu.2021.752646
38. Kuhns MS, Davis MM, Garcia KC. Deconstructing the Form and Function of the TCR/CD3 Complex. Immunity (2006) 24(2):133–9. doi: 10.1016/j.immuni.2006.01.006
39. Basile G, Geissmann F, Flori E, Uring-Lambert B, Soudais C, Cavazzana-Calvo M, et al. Severe Combined Immunodeficiency Caused by Deficiency in Either the δ or the ϵ Subunit of CD3. J Clin Invest (2004) 114(10):1512–7. doi: 10.1172/JCI200422588
40. Dave VP, Cao ZS, Browne C, Alarcon B, FernandezMiguel G, Lafaille J, et al. CD3 Delta Deficiency Arrests Development of the Alpha Beta But Not the Gamma Delta T Cell Lineage. EMBO J (1997) 16(6):1360–70. doi: 10.1093/emboj/16.6.1360
41. Dadi HK, Simon AJ, Roifman CM. Effect of CD3 Delta Deficiency on Maturation of Alpha/Beta and Gamma/Delta T-Cell Lineages in Severe Combined Immunodeficiency. N Engl J Med (2003) 349(19):1821–8. doi: 10.1056/NEJMoa031178
42. Hayes SM, Love PE. Distinct Structure and Signaling Potential of the Gamma Delta TCR Complex. Immunity (2002) 16(6):827–38. doi: 10.1016/S1074-7613(02)00320-5
43. Delgado P, Fernandez E, Dave V, Kappes D, Alarcon B. CD3 Delta Couples T-Cell Receptor Signalling to ERK Activation and Thymocyte Positive Selection. Nature (2000) 406(6794):426–30. doi: 10.1038/35019102
44. Siegers GM, Swamy M, Fernandez-Malave E, Minguet S, Rathmann S, Guardo AC, et al. Different Composition of the Human and the Mouse Gammadelta T Cell Receptor Explains Different Phenotypes of CD3gamma and CD3delta Immunodeficiencies. J Exp Med (2007) 204(11):2537–44. doi: 10.1084/jem.20070782
45. Irving BA, Weiss A. The Cytoplasmic Domain of the T Cell Receptor Zeta Chain is Sufficient to Couple to Receptor-Associated Signal Transduction Pathways. Cell (1991) 64(5):891–901. doi: 10.1016/0092-8674(91)90314-O
46. Pitcher LA, Mathis MA, Young JA, DeFord LM, Purtic B, Wulfing C, et al. The CD3 Gamma Epsilon/Delta Epsilon Signaling Module Provides Normal T Cell Functions in the Absence of the TCR Zeta Immunoreceptor Tyrosine-Based Activation Motifs. Eur J Immunol (2005) 35(12):3643–54. doi: 10.1002/eji.200535136
47. Letourneur F, Klausner RD. Activation of T Cells by a Tyrosine Kinase Activation Domain in the Cytoplasmic Tail of CD3 Epsilon. Science (1992) 255(5040):79–82. doi: 10.1126/science.1532456
48. Nicolas L, Monneret G, Debard AL, Blesius A, Gutowski MC, Salles G, et al. Human Gamma Delta T Cells Express a Higher TCR/CD3 Complex Density Than Alpha Beta T Cells. Clin Immunol (2001) 98(3):358–63. doi: 10.1006/clim.2000.4978
49. Cheng HY, Wu RP, Gebre AK, Hanna RN, Smith DJ, Parks JS, et al. Increased Cholesterol Content in Gammadelta (Gamma Delta) T Lymphocytes Differentially Regulates Their Activation. PloS One (2013) 8(5):e63746. doi: 10.1371/journal.pone.0063746
50. Gil D, Schamel WWA, Montoya M, Sanchez-Madrid F, Alarcon B. Recruitment of Nck by CD3 Epsilon Reveals a Ligand-Induced Conformational Change Essential for T Cell Receptor Signaling and Synapse Formation. Cell (2002) 109(7):901–12. doi: 10.1016/S0092-8674(02)00799-7
51. Minguet S, Swamy M, Alarcon B, Luescher IF, Schamel WWA. Full Activation of the T Cell Receptor Requires Both Clustering and Conformational Changes at CD3. Immunity (2007) 26(1):43–54. doi: 10.1016/j.immuni.2006.10.019
52. Swamy M, Beck-Garcia K, Beck-Garcia E, Hartl FA, Morath A, Yousefi OS, et al. A Cholesterol-Based Allostery Model of T Cell Receptor Phosphorylation. Immunity (2016) 44(5):1091–101. doi: 10.1016/j.immuni.2016.04.011
53. Wang F, Beck-Garcia K, Zorzin C, Schamel WW, Davis MM. Inhibition of T Cell Receptor Signaling by Cholesterol Sulfate, a Naturally Occurring Derivative of Membrane Cholesterol. Nat Immunol (2016) 17(7):844–50. doi: 10.1038/ni.3462
54. Dopfer EP, Hartl FA, Oberg HH, Siegers GM, Yousefi OS, Kock S, et al. The CD3 Conformational Change in the Gamma Delta T Cell Receptor Is Not Triggered by Antigens But Can Be Enforced to Enhance Tumor Killing. Cell Rep (2014) 7(5):1704–15. doi: 10.1016/j.celrep.2014.04.049
55. Acuto O, Michel F. CD28-Mediated Co-Stimulation: A Quantitative Support for TCR Signalling. Nat Rev Immunol (2003) 3(12):939–51. doi: 10.1038/nri1248
56. Ribot JC, deBarros A, Mancio-Silva L, Pamplona A, Silva-Santos B. B7-CD28 Costimulatory Signals Control the Survival and Proliferation of Murine and Human Gamma Delta T Cells via IL-2 Production. J Immunol (2012) 189(3):1202–8. doi: 10.4049/jimmunol.1200268
57. Sperling AI, Linsley PS, Barrett TA, Bluestone JA. CD28-Mediated Costimulation is Necessary for the Activation of T Cell Receptor-Gamma Delta+ T Lymphocytes. J Immunol (1993) 151(11):6043–50.
58. Testi R, Lanier LL. Functional Expression of CD28 on T Cell Antigen Receptor Gamma/Delta-Bearing T Lymphocytes. Eur J Immunol (1989) 19(1):185–8. doi: 10.1002/eji.1830190129
59. Ohteki T, Macdonald HR. Expression of the CD28 Costimulatory Molecule on Subsets of Murine Intestinal Intraepithelial Lymphocytes Correlates With Lineage and Responsiveness. Eur J Immunol (1993) 23(6):1251–5. doi: 10.1002/eji.1830230609
60. Rakasz E, Hagen M, Sandor M, Lynch RG. Gamma Delta T Cells of the Murine Vagina: T Cell Response In Vivo in the Absence of the Expression of CD2 and CD28 Molecules. Int Immunol (1997) 9(1):161–7. doi: 10.1093/intimm/9.1.161
61. Laird RM, Wolf BJ, Princiotta MF, Hayes SM. Gamma Delta T Cells Acquire Effector Fates in the Thymus and Differentiate Into Cytokine-Producing Effectors in a Listeria Model of Infection Independently of CD28 Costimulation. PloS One (2013) 8(5):e63178. doi: 10.1371/journal.pone.0063178
62. Wang Q, Sun Q, Chen QG, Li H, Liu D. Expression of CD27 and CD28 on Gamma Delta T Cells From the Peripheral Blood of Patients With Allergic Rhinitis. Exp Ther Med (2020) 20(6):224. doi: 10.3892/etm.2020.9354
63. Lafont V, Liautard J, Gross A, Liautard JP, Favero J. Tumor Necrosis Factor-Alpha Production is Differently Regulated in Gamma Delta and Alpha Beta Human T Lymphocytes. J Biol Chem (2000) 275(25):19282–7. doi: 10.1074/jbc.M910487199
64. Wen T, Bukczynski J, Watts TH. 4-1BB Ligand-Mediated Costimulation of Human T Cells Induces CD4 and CD8 T Cell Expansion, Cytokine Production, and the Development of Cytolytic Effector Function. J Immunol (2002) 168(10):4897–906. doi: 10.4049/jimmunol.168.10.4897
65. Zhang H, Snyder KM, Suhoski MM, Maus MV, Kapoor V, June CH, et al. 4-1BB is Superior to CD28 Costimulation for Generating CD8(+) Cytotoxic Lymphocytes for Adoptive Immunotherapy. J Immunol (2007) 179(7):4910–8. doi: 10.4049/jimmunol.179.7.4910
66. Pei YJ, Wen K, Xiang Z, Huang CY, Wang XW, Mu XF, et al. CD137 Costimulation Enhances the Antiviral Activity of V Gamma 9V Delta 2-T Cells Against Influenza Virus. Signal Transduct Target Ther (2020) 5(1):74. doi: 10.1038/s41392-020-0174-2
67. Lee SJ, Kim YH, Hwang SH, Kim YI, Han IS, Vinay DS, et al. 4-1BB Signal Stimulates the Activation, Expansion, and Effector Functions of Gamma Delta T Cells in Mice and Humans. Eur J Immunol (2013) 43(7):1839–48. doi: 10.1002/eji.201242842
68. Grant EJ, Nussing S, Sant S, Clemens EB, Kedzierska K. The Role of CD27 in Anti-Viral T-Cell Immunity. Curr Opin Virol (2017) 22:77–88. doi: 10.1016/j.coviro.2016.12.001
69. Song DG, Ye QR, Poussin M, Harms GM, Figini M, Powell DJ. CD27 Costimulation Augments the Survival and Antitumor Activity of Redirected Human T Cells In Vivo. Blood (2012) 119(3):696–706. doi: 10.1182/blood-2011-03-344275
70. Ribot JC, deBarros A, Pang DJ, Neves JF, Peperzak V, Roberts SJ, et al. CD27 is a Thymic Determinant of the Balance Between Interferon-Gamma-and Interleukin 17-Producing Gamma Delta T Cell Subsets. Nat Immunol (2009) 10(4):427–36. doi: 10.1038/ni.1717
71. deBarros A, Chaves-Ferreira M, d'Orey F, Ribot JC, Silva-Santos B. CD70-CD27 Interactions Provide Survival and Proliferative Signals That Regulate T Cell Receptor-Driven Activation of Human Gamma Delta Peripheral Blood Lymphocytes. Eur J Immunol (2011) 41(1):195–201. doi: 10.1002/eji.201040905
72. Ribot JC, Chaves-Ferreira M, d'Orey F, Wencker M, Goncalves-Sousa N, Decalf J, et al. Cutting Edge: Adaptive Versus Innate Receptor Signals Selectively Control the Pool Sizes of Murine IFN-Gamma- or IL-17-Producing Gamma Delta T Cells Upon Infection. J Immunol (2010) 185(11):6421–5. doi: 10.4049/jimmunol.1002283
73. Hassan NJ, Barclay AN, Brown MH. Optimal T Cell Activation Requires the Engagement of CD6 and CD166. Eur J Immunol (2004) 34(4):930–40. doi: 10.1002/eji.200424856
74. Ruth JH, Gurrea-Rubio M, Athukorala KS, Rasmussen SM, Weber DP, Randon PM, et al. CD6 is a Target for Cancer Immunotherapy. JCI Insight (2021) 6(5):e145662. doi: 10.1172/jci.insight.145662
75. Kato Y, Tanaka Y, Hayashi M, Okawa K, Minato N. Involvement of CD166 in the Activation of Human Gamma Delta T Cells by Tumor Cells Sensitized With Nonpeptide Antigens. J Immunol (2006) 177(2):877–84. doi: 10.4049/jimmunol.177.2.877
76. Breuning J, Philip B, Brown MH. Addition of the C-Terminus of CD6 to a Chimeric Antigen Receptor Enhances Cytotoxicity and Does Not Compromise Expression. Immunology (2019) 156(2):130–5. doi: 10.1111/imm.13009
77. Davignon D, Martz E, Reynolds T, Kurzinger K, Springer TA. Monoclonal Antibody to a Novel Lymphocyte Function-Associated Antigen (LFA-1): Mechanism of Blockade of T Lymphocyte-Mediated Killing and Effects on Other T and B Lymphocyte Functions. J Immunol (1981) 127(2):590–5.
78. Kemenade E-v, Velde AAT, Boer AJD, Weening RS, Fischer A, Borst J, et al. Both LFA-1-Positive and -Deficient T Cell Clones Require the CD2/LFA-3 Interaction for Specific Cytolytic Activation. Eur J Immunol (1992) 22(6):1467–75. doi: 10.1002/eji.1830220620
79. Cheadle EJ, Rothwell DG, Bridgeman JS, Sheard VE, Hawkins RE, Gilham DE. Ligation of the CD2 Co-Stimulatory Receptor Enhances IL-2 Production From First-Generation Chimeric Antigen Receptor T Cells. Gene Ther (2012) 19(11):1114–20. doi: 10.1038/gt.2011.192
80. Wesselborg S, Janssen O, Pechhold K, Kabelitz D. Selective Activation of Gamma/Delta + T Cell Clones by Single Anti-CD2 Antibodies. J Exp Med (1991) 173(2):297–304. doi: 10.1084/jem.173.2.297
81. Wang P, Malkovsky M. Different Roles of the CD2 and LFA-1 T-Cell Co-Receptors for Regulating Cytotoxic, Proliferative, and Cytokine Responses of Human V Gamma 9/V Delta 2 T Cells. Mol Med (2000) 6(3):196–207. doi: 10.1007/BF03402114
82. Bauer S, Groh V, Wu J, Steinle A, Phillips JH, Lanier LL, et al. Activation of NK Cells and T Cells by NKG2D, a Receptor for Stress-Inducible MICA. Science (1999) 285(5428):727–9. doi: 10.1126/science.285.5428.727
83. Nitahara A, Shimura H, Ito A, Tomiyama K, Ito M, Kawai K. NKG2D Ligation Without T Cell Receptor Engagement Triggers Both Cytotoxicity and Cytokine Production in Dendritic Epidermal T Cells. J Invest Dermatol (2006) 126(5):1052–8. doi: 10.1038/sj.jid.5700112
84. Roberts AI, Lee L, Schwarz E, Groh V, Spies T, Ebert EC, et al. Cutting Edge: NKG2D Receptors Induced by IL-15 Costimulate CD28-Negative Effector CTL in the Tissue Microenvironment. J Immunol (2001) 167(10):5527–30. doi: 10.4049/jimmunol.167.10.5527
85. Stojanovic A, Correia MP, Cerwenka A. The NKG2D/NKG2DL Axis in the Crosstalk Between Lymphoid and Myeloid Cells in Health and Disease. Front Immunol (2018) 9:827. doi: 10.3389/fimmu.2018.00827
86. Lanier LL. NKG2D Receptor and Its Ligands in Host Defense. Cancer Immunol Res (2015) 3(6):575–82. doi: 10.1158/2326-6066.CIR-15-0098
87. Rincon-Orozco B, Kunzmann V, Wrobel P, Kabelitz D, Steinle A, Herrmann T. Activation of V Gamma 9V Delta 2 T Cells by NKG2D. J Immunol (2005) 175(4):2144–51. doi: 10.4049/jimmunol.175.4.2144
88. Bessoles S, Ni M, Garcia-Jimenez S, Sanchez F, Lafont V. Role of NKG2D and Its Ligands in the Anti-Infectious Activity of V Gamma 9V Delta 2 T Cells Against Intracellular Bacteria. Eur J Immunol (2011) 41(6):1619–28. doi: 10.1002/eji.201041230
89. Cimini E, Sacchi A, De Minicis S, Bordoni V, Casetti R, Grassi G, et al. V Delta 2 T-Cells Kill ZIKV-Infected Cells by NKG2D-Mediated Cytotoxicity. Microorganisms (2019) 7(9):350. doi: 10.3390/microorganisms7090350
90. Wrobel P, Shojaei H, Schittek B, Gieseler F, Wollenberg B, Kalthoff H, et al. Lysis of a Broad Range of Epithelial Tumour Cells by Human Gamma Delta T Cells: Involvement of NK62D Ligands and T-Cell Receptor- Versus NKG2D-Dependent Recognition. Scand J Immunol (2007) 66(2-3):320–8. doi: 10.1111/j.1365-3083.2007.01963.x
91. Ibusuki A, Kawai K, Yoshida S, Uchida Y, Nitahara-Takeuchi A, Kuroki K, et al. NKG2D Triggers Cytotoxicity in Murine Epidermal Gamma Delta T Cells via PI3K-Dependent, Syk/ZAP70-Independent Signaling Pathway. J Invest Dermatol (2014) 134(2):396–404. doi: 10.1038/jid.2013.353
92. Lanier LL. On Guard - Activating NK Cell Receptors. Nat Immunol (2001) 2(1):23–7. doi: 10.1038/83130
93. Billadeau DD, Upshaw JL, Schoon RA, Dick CJ, Leibson PJ. NKG2D-DAP10 Triggers Human NK Cell-Mediated Killing via a Syk-Independent Regulatory Pathway. Nat Immunol (2003) 4(6):557–64. doi: 10.1038/ni929
94. Zompi S, Hamerman JA, Ogasawara K, Schweighoffer E, Tybulewicz VLJ, Di Santo JP, et al. NKG2D Triggers Cytotoxicity in Mouse NK Cells Lacking DAP12 or Syk Family Kinases. Nat Immunol (2003) 4(6):565–72. doi: 10.1038/ni930
95. Diefenbach A, Tomasello E, Lucas M, Jamieson AM, Hsia JK, Vivier E, et al. Selective Associations With Signaling Proteins Determine Stimulatory Versus Costimulatory Activity of NKG2D. Nat Immunol (2002) 3(12):1142–9. doi: 10.1038/ni858
96. Gilfillan S, Ho EL, Cella M, Yokoyama WM, Colonna M. NKG2D Recruits Two Distinct Adapters to Trigger NK Cell Activation and Costimulation. Nat Immunol (2002) 3(12):1150–5. doi: 10.1038/ni857
97. Kong Y, Cao W, Xi XY, Ma C, Cui LX, He W. The NKG2D Ligand ULBP4 Binds to TCR Gamma 9/Delta 2 and Induces Cytotoxicity to Tumor Cells Through Both TCR Gamma Delta and NKG2D. Blood (2009) 114(2):310–7. doi: 10.1182/blood-2008-12-196287
98. Xiang Z, Liu YP, Zheng J, Liu M, Lv AZ, Gao YL, et al. Targeted Activation of Human V Gamma 9V Delta 2-T Cells Controls Epstein-Barr Virus-Induced B Cell Lymphoproliferative Disease. Cancer Cell (2014) 26(4):565–76. doi: 10.1016/j.ccr.2014.07.026
99. Nedellec S, Sabourin C, Bonneville M, Scotet E. NKG2D Costimulates Human V Gamma 9V Delta 2 T Cell Antitumor Cytotoxicity Through Protein Kinase C Theta-Dependent Modulation of Early TCR-Induced Calcium and Transduction Signals. J Immunol (2010) 185(1):55–63. doi: 10.4049/jimmunol.1000373
100. Girlanda S, Fortis C, Belloni D, Ferrero E, Ticozzi P, Sciorati C, et al. MICA Expressed by Multiple Myeloma and Monoclonal Gammopathy of Undetermined Significance Plasma Cells Costimulates Pamidronate-Activated Gamma Delta Lymphocytes. Cancer Res (2005) 65(16):7502–8. doi: 10.1158/0008-5472.CAN-05-0731
101. Das H, Groh V, Kuijl C, Sugita M, Morita CT, Spies T, et al. MICA Engagement by Human V Gamma 2V Delta 2 T Cells Enhances Their Antigen-Dependent Effector Function. Immunity (2001) 15(1):83–93. doi: 10.1016/S1074-7613(01)00168-6
102. Li YL, Wang QA, Mariuzza RA. Structure of the Human Activating Natural Cytotoxicity Receptor NKp30 Bound to Its Tumor Cell Ligand B7-H6. J Exp Med (2011) 208(4):703–14. doi: 10.1084/jem.20102548
103. Correia DV, Fogli M, Hudspeth K, da Silva MG, Mavilio D, Silva-Santos B. Differentiation of Human Peripheral Blood V Delta 1(+) T Cells Expressing the Natural Cytotoxicity Receptor NKp30 for Recognition of Lymphoid Leukemia Cells. Blood (2011) 118(4):992–1001. doi: 10.1182/blood-2011-02-339135
104. Mikulak J, Oriolo F, Bruni E, Roberto A, Colombo FS, Villa A, et al. NKp46-Expressing Human Gut-Resident Intraepithelial V Delta 1T Cell Subpopulation Exhibits High Antitumor Activity Against Colorectal Cancer. JCI Insight (2019) 4(24):e125884. doi: 10.1172/jci.insight.125884
105. Di Lorenzo B, Simoes AE, Caiado F, Tieppo P, Correia DV, Carvalho T, et al. Broad Cytotoxic Targeting of Acute Myeloid Leukemia by Polyclonal Delta One T Cells. Cancer Immunol Res (2019) 7(4):552–8. doi: 10.1158/2326-6066.CIR-18-0647
106. von Lilienfeld-Toal M, Nattermann J, Feldmann G, Sievers E, Frank S, Strehl J, et al. Activated Gamma Delta T Cells Express the Natural Cytotoxicity Receptor Natural Killer P44 and Show Cytotoxic Activity Against Myeloma Cells. Clin Exp Immunol (2006) 144(3):528–33. doi: 10.1111/j.1365-2249.2006.03078.x
107. Yamaguchi T, Suzuki Y, Katakura R, Ebina T, Yokoyama J, Fujimiya Y. Interleukin-15 Effectively Potentiates the In Vitro Tumor-Specific Activity and Proliferation of Peripheral Blood gammadeltaT Cells Isolated From Glioblastoma Patients. Cancer Immunol Immunother (1998) 47(2):97–103. doi: 10.1007/s002620050509
108. Lalor SJ, Dungan LS, Sutton CE, Basdeo SA, Fletcher JM, Mills KH. Caspase-1-Processed Cytokines IL-1beta and IL-18 Promote IL-17 Production by Gammadelta and CD4 T Cells That Mediate Autoimmunity. J Immunol (2011) 186(10):5738–48. doi: 10.4049/jimmunol.1003597
109. da Mota JB, Echevarria-Lima J, Kyle-Cezar F, Melo M, Bellio M, Scharfstein J, et al. IL-18R Signaling is Required for Gammadelta T Cell Response and Confers Resistance to Trypanosoma Cruzi Infection. J Leukoc Biol (2020) 108(4):1239–51. doi: 10.1002/JLB.4MA0420-568R
110. Hou L, Jie Z, Desai M, Liang Y, Soong L, Wang T, et al. Early IL-17 Production by Intrahepatic T Cells is Important for Adaptive Immune Responses in Viral Hepatitis. J Immunol (2013) 190(2):621–9. doi: 10.4049/jimmunol.1201970
111. Sutton CE, Lalor SJ, Sweeney CM, Brereton CF, Lavelle EC, Mills KH. Interleukin-1 and IL-23 Induce Innate IL-17 Production From Gammadelta T Cells, Amplifying Th17 Responses and Autoimmunity. Immunity (2009) 31(2):331–41. doi: 10.1016/j.immuni.2009.08.001
112. Zhang B, Li H, Liu W, Tian H, Li L, Gao C, et al. Adoptive Cell Therapy of Patient-Derived Renal Cell Carcinoma Xenograft Model With IL-15-Induced gammadeltaT Cells. Med Oncol (2021) 38(3):30. doi: 10.1007/s12032-021-01474-1
113. Garcia VE, Jullien D, Song M, Uyemura K, Shuai K, Morita CT, et al. IL-15 Enhances the Response of Human Gamma Delta T Cells to Nonpeptide [Correction of Nonpetide] Microbial Antigens. J Immunol (1998) 160(9):4322–9.
114. Domae E, Hirai Y, Ikeo T, Goda S, Shimizu Y. Cytokine-Mediated Activation of Human Ex Vivo-Expanded Vgamma9Vdelta2 T Cells. Oncotarget (2017) 8(28):45928–42. doi: 10.18632/oncotarget.17498
115. Schilbach K, Welker C, Krickeberg N, Kaisser C, Schleicher S, Hashimoto H. In the Absence of a TCR Signal IL-2/IL-12/18-Stimulated Gammadelta T Cells Demonstrate Potent Anti-Tumoral Function Through Direct Killing and Senescence Induction in Cancer Cells. Cancers (Basel) (2020) 12(1):130. doi: 10.3390/cancers12010130
116. Duault C, Betous D, Bezombes C, Roga S, Cayrol C, Girard JP, et al. IL-33-Expanded Human Vgamma9Vdelta2 T Cells Have Anti-Lymphoma Effect in a Mouse Tumor Model. Eur J Immunol (2017) 47(12):2137–41. doi: 10.1002/eji.201747093
117. Liang Y, Jie Z, Hou L, Yi P, Wang W, Kwota Z, et al. IL-33 Promotes Innate IFN-Gamma Production and Modulates Dendritic Cell Response in LCMV-Induced Hepatitis in Mice. Eur J Immunol (2015) 45(11):3052–63. doi: 10.1002/eji.201545696
118. Michel ML, Pang DJ, Haque SF, Potocnik AJ, Pennington DJ, Hayday AC. Interleukin 7 (IL-7) Selectively Promotes Mouse and Human IL-17-Producing Gammadelta Cells. Proc Natl Acad Sci USA (2012) 109(43):17549–54. doi: 10.1073/pnas.1204327109
119. Li W, Kubo S, Okuda A, Yamamoto H, Ueda H, Tanaka T, et al. Effect of IL-18 on Expansion of Gammadelta T Cells Stimulated by Zoledronate and IL-2. J Immunother (2010) 33(3):287–96. doi: 10.1097/CJI.0b013e3181c80ffa
120. Thedrez A, Harly C, Morice A, Salot S, Bonneville M, Scotet E. IL-21-Mediated Potentiation of Antitumor Cytolytic and Proinflammatory Responses of Human V Gamma 9V Delta 2 T Cells for Adoptive Immunotherapy. J Immunol (2009) 182(6):3423–31. doi: 10.4049/jimmunol.0803068
121. Lockhart E, Green AM, Flynn JL. IL-17 Production is Dominated by Gammadelta T Cells Rather Than CD4 T Cells During Mycobacterium Tuberculosis Infection. J Immunol (2006) 177(7):4662–9. doi: 10.4049/jimmunol.177.7.4662
122. Wesch D, Peters C, Oberg HH, Pietschmann K, Kabelitz D. Modulation of Gammadelta T Cell Responses by TLR Ligands. Cell Mol Life Sci (2011) 68(14):2357–70. doi: 10.1007/s00018-011-0699-1
123. Rischer M, Pscherer S, Duwe S, Vormoor J, Jurgens H, Rossig C. Human Gamma Delta T Cells as Mediators of Chimaeric-Receptor Redirected Anti-Tumour Immunity. Br J Haematol (2004) 126(4):583–92. doi: 10.1111/j.1365-2141.2004.05077.x
124. Deniger DC, Switzer K, Mi TJ, Maiti S, Hurton L, Singh H, et al. Bispecific T-Cells Expressing Polyclonal Repertoire of Endogenous Gamma Delta T-Cell Receptors and Introduced CD19-Specific Chimeric Antigen Receptor. Mol Ther (2013) 21(3):638–47. doi: 10.1038/mt.2012.267
125. Rozenbaum M, Meir A, Aharony Y, Itzhaki O, Schachter J, Bank I, et al. Gamma-Delta CAR-T Cells Show CAR-Directed and Independent Activity Against Leukemia. Front Immunol (2020) 11:1347. doi: 10.3389/fimmu.2020.01347
126. Zhai XC, You FT, Xiang SF, Jiang LC, Chen D, Li YF, et al. MUC1-Tn-Targeting Chimeric Antigen Receptor-Modified V Gamma 9V Delta 2 T Cells With Enhanced Antigen-Specific Anti-Tumor Activity. Am J Cancer Res (2021) 11(1):79–91.
127. Zhang C, Hu Y, Xiao WH, Tian ZG. Chimeric Antigen Receptor- and Natural Killer Cell Receptor-Engineered Innate Killer Cells in Cancer Immunotherapy. Cell Mol Immunol (2021) 18(9):2083–100. doi: 10.1038/s41423-021-00732-6
128. Halary F, Peyrat MA, Champagne E, LopezBotet M, Moretta A, Moretta L, et al. Control of Self-Reactive Cytotoxic T Lymphocytes Expressing Gamma Delta T Cell Receptors by Natural Killer Inhibitory Receptors. Eur J Immunol (1997) 27(11):2812–21. doi: 10.1002/eji.1830271111
129. Harrer DC, Simon B, Fujii S, Shimizu K, Uslu U, Schuler G, et al. RNA-Transfection of Gamma/Delta T Cells With a Chimeric Antigen Receptor or an Alpha/Beta T-Cell Receptor: A Safer Alternative to Genetically Engineered Alpha/Beta T Cells for the Immunotherapy of Melanoma. BMC Cancer (2017) 17:17. doi: 10.1186/s12885-017-3539-3
130. Capsomidis A, Benthall G, Van Acker HH, Fisher J, Kramer AM, Abeln Z, et al. Chimeric Antigen Receptor-Engineered Human Gamma Delta T Cells: Enhanced Cytotoxicity With Retention of Cross Presentation. Mol Ther (2018) 26(2):354–65. doi: 10.1016/j.ymthe.2017.12.001
131. Perez C, Gruber I, Arber C. Off-The-Shelf Allogeneic T Cell Therapies for Cancer: Opportunities and Challenges Using Naturally Occurring "Universal" Donor T Cells. Front Immunol (2020) 11:583716. doi: 10.3389/fimmu.2020.583716
132. Alnaggar M, Xu Y, Li JX, He JY, Chen JB, Li M, et al. Allogenic Vγ9vδ2 T Cell as New Potential Immunotherapy Drug for Solid Tumor: A Case Study for Cholangiocarcinoma. J Immunother Cancer (2019) 7(1):36. doi: 10.1186/s40425-019-0501-8
133. Chabab G, Boissiere-Michot F, Mollevi C, Ramos J, Lopez-Crapez E, Colombo PE, et al. Diversity of Tumor-Infiltrating, Gammadelta T-Cell Abundance in Solid Cancers. Cells (2020) 9(6):1537. doi: 10.3390/cells9061537
134. Gentles AJ, Newman AM, Liu CL, Bratman SV, Feng WG, Kim D, et al. The Prognostic Landscape of Genes and Infiltrating Immune Cells Across Human Cancers. Nat Med (2015) 21(8):938–45. doi: 10.1038/nm.3909
135. Tosolini M, Pont F, Poupot M, Vergez F, Nicolau-Travers ML, Vermijlen D, et al. Assessment of Tumor-Infiltrating TCRVgamma9Vdelta2 Gammadelta Lymphocyte Abundance by Deconvolution of Human Cancers Microarrays. Oncoimmunology (2017) 6(3):e1284723. doi: 10.1080/2162402X.2017.1284723
136. Kailayangiri S, Altvater B, Meltzer J, Pscherer S, Luecke A, Dierkes C, et al. The Ganglioside Antigen G(D2) is Surface-Expressed in Ewing Sarcoma and Allows for MHC-Independent Immune Targeting. Br J Cancer (2012) 106(6):1123–33. doi: 10.1038/bjc.2012.57
137. Campoli MR, Chang C-C, Kageshita T, Wang X, McCarthy JB, Ferrone S. Human High Molecular Weight-Melanoma-Associated Antigen (HMW-MAA): A Melanoma Cell Surface Chondroitin Sulfate Proteoglycan (MSCP) With Biological and Clinical Significance. Crit Rev Immunol (2004) 24(4):267–96. doi: 10.1615/CritRevImmunol.v24.i4.40
138. Rakha EA, Boyce RWG, Abd El-Rehim D, Kurien T, Green AR, Paish EC, et al. Expression of Mucins (MUC1, MUC2, MUC3, MUC4, MUC5AC and MUC6) and Their Prognostic Significance in Human Breast Cancer. Mod Pathol (2005) 18(10):1295–304. doi: 10.1038/modpathol.3800445
139. Mei Z, Zhang K, Lam AK, Huang J, Qiu F, Qiao B, et al. MUC1 as a Target for CAR-T Therapy in Head and Neck Squamous Cell Carinoma. Cancer Med (2020) 9(2):640–52. doi: 10.1002/cam4.2733
140. Fisher J, Abramowski P, Wisidagamage Don ND, Flutter B, Capsomidis A, Cheung GW, et al. Avoidance of On-Target Off-Tumor Activation Using a Co-Stimulation-Only Chimeric Antigen Receptor. Mol Ther (2017) 25(5):1234–47. doi: 10.1016/j.ymthe.2017.03.002
141. Dorrie J, Krug C, Hofmann C, Muller I, Wellner V, Knippertz I, et al. Human Adenovirus-Specific Gamma/Delta and CD8(+) T Cells Generated by T-Cell Receptor Transfection to Treat Adenovirus Infection After Allogeneic Stem Cell Transplantation. PloS One (2014) 9(10):e109944. doi: 10.1371/journal.pone.0109944
142. van der Veken LT, Hagedoorn RS, van Loenen MM, Willemze R, Falkenburg JH, Heemskerk MH. Alphabeta T-Cell Receptor Engineered Gammadelta T Cells Mediate Effective Antileukemic Reactivity. Cancer Res (2006) 66(6):3331–7. doi: 10.1158/0008-5472.CAN-05-4190
143. Shimizu K, Shinga J, Yamasaki S, Kawamura M, Dorrie J, Schaft N, et al. Transfer of mRNA Encoding Invariant NKT Cell Receptors Imparts Glycolipid Specific Responses to T Cells and gammadeltaT Cells. PloS One (2015) 10(6):e0131477. doi: 10.1371/journal.pone.0131477
144. van der Veken LT, Coccoris M, Swart E, Falkenburg JH, Schumacher TN, Heemskerk MH. Alpha Beta T Cell Receptor Transfer to Gamma Delta T Cells Generates Functional Effector Cells Without Mixed TCR Dimers In Vivo. J Immunol (2009) 182(1):164–70. doi: 10.4049/jimmunol.182.1.164
145. Zhang T, He XH, Tsang TC, Harris DT. Transgenic TCR Expression: Comparison of Single Chain With Full-Length Receptor Constructs for T-Cell Function. Cancer Gene Ther (2004) 11(7):487–96. doi: 10.1038/sj.cgt.7700703
146. Bendle GM, Linnemann C, Hooijkaas AI, Bies L, de Witte MA, Jorritsma A, et al. Lethal Graft-Versus-Host Disease in Mouse Models of T Cell Receptor Gene Therapy. Nat Med (2010) 16(5):565–70. doi: 10.1038/nm.2128
147. Marcu-Malina V, Heijhuurs S, van Buuren M, Hartkamp L, Strand S, Sebestyen Z, et al. Redirecting Alpha Beta T Cells Against Cancer Cells by Transfer of a Broadly Tumor-Reactive Gamma Delta T-Cell Receptor. Blood (2011) 118(1):50–9. doi: 10.1182/blood-2010-12-325993
148. Zhao H, Xi X, Cui L, He W. CDR3delta -Grafted Gamma9delta2t Cells Mediate Effective Antitumor Reactivity. Cell Mol Immunol (2012) 9(2):147–54. doi: 10.1038/cmi.2011.28
149. He K, You H, Li Y, Cui L, Zhang J, He W. TCRgamma4delta1-Engineered alphabetaT Cells Exhibit Effective Antitumor Activity. Mol Med (2016) 22:519–29. doi: 10.2119/molmed.2016.00023
150. Xu Y, Yang Z, Horan LH, Zhang P, Liu L, Zimdahl B, et al. A Novel Antibody-TCR (AbTCR) Platform Combines Fab-Based Antigen Recognition With Gamma/Delta-TCR Signaling to Facilitate T-Cell Cytotoxicity With Low Cytokine Release. Cell Discov (2018) 4:62. doi: 10.1038/s41421-018-0066-6
151. Johanna I, Straetemans T, Heijhuurs S, Aarts-Riemens T, Norell H, Bongiovanni L, et al. Evaluating In Vivo Efficacy - Toxicity Profile of TEG001 in Humanized Mice Xenografts Against Primary Human AML Disease and Healthy Hematopoietic Cells. J Immunother Cancer (2019) 7(1):69. doi: 10.1186/s40425-019-0558-4
Keywords: γδ T cells, engineering, stimulation, dual recognition, tumor
Citation: Dong R, Zhang Y, Xiao H and Zeng X (2022) Engineering γδ T Cells: Recognizing and Activating on Their Own Way. Front. Immunol. 13:889051. doi: 10.3389/fimmu.2022.889051
Received: 03 March 2022; Accepted: 11 April 2022;
Published: 06 May 2022.
Edited by:
Xiaoli Wu, Tianjin University, ChinaReviewed by:
Hui Chen, Chinese Academy of Medical Sciences and Peking Union Medical College, ChinaFeng Wang, Shanghai Jiao Tong University, China
Copyright © 2022 Dong, Zhang, Xiao and Zeng. This is an open-access article distributed under the terms of the Creative Commons Attribution License (CC BY). The use, distribution or reproduction in other forums is permitted, provided the original author(s) and the copyright owner(s) are credited and that the original publication in this journal is cited, in accordance with accepted academic practice. No use, distribution or reproduction is permitted which does not comply with these terms.
*Correspondence: Haowen Xiao, aGFvd2VueGlhb3hpYW9Aemp1LmVkdS5jbg==; Xun Zeng, eHVuemVuZ0B6anUuZWR1LmNu
†These authors have contributed equally to this work