- 1Center for Hematology and Regenerative Medicine, Karolinska Institutet, Stockholm, Sweden
- 2Department of Laboratory Medicine, Karolinska Institutet, Stockholm, Sweden
- 3Department of Microbiology Tumor and Cell Biology, Karolinska Institutet, Stockholm, Sweden
- 4Department of Medicine, Huddinge, Karolinska Institutet, Stockholm, Sweden
- 5Department of Medicine, Solna, Karolinska Institutet, Stockholm, Sweden
- 6Department of Cell and Molecular Biology, National Bioinformatics Infrastructure Sweden, Science for Life Laboratory, Uppsala University, Uppsala, Sweden
- 7Hematology Center, Karolinska University Hospital, Stockholm, Sweden
The development of B cells relies on an intricate network of transcription factors critical for developmental progression and lineage commitment. In the B cell developmental trajectory, a temporal switch from predominant Foxo3 to Foxo1 expression occurs at the CLP stage. Utilizing VAV-iCre mediated conditional deletion, we found that the loss of FOXO3 impaired B cell development from LMPP down to B cell precursors, while the loss of FOXO1 impaired B cell commitment and resulted in a complete developmental block at the CD25 negative proB cell stage. Strikingly, the combined loss of FOXO1 and FOXO3 resulted in the failure to restrict the myeloid potential of CLPs and the complete loss of the B cell lineage. This is underpinned by the failure to enforce the early B-lineage gene regulatory circuitry upon a predominantly pre-established open chromatin landscape. Altogether, this demonstrates that FOXO3 and FOXO1 cooperatively govern early lineage restriction and initiation of B-lineage commitment in CLPs.
Introduction
The development of highly specialized cell types from hematopoietic stem cells depends on the expression of lineage specific transcription factors that together orchestrate the establishment of specific transcriptional programs and lineage commitment. Lymphoid development is initiated with the generation of lymphoid-primed multipotent progenitors (LMPP) (1, 2) that subsequently give rise to common lymphoid progenitor (CLP) (3). The heterogeneous CLP compartment can be divided into LY6D- CLPs (ALP) that maintain the potential to generate all lymphoid lineages and LY6D+ B-lineage specified CLPs (BLP) (4, 5). Within the BLP compartment the potential to generate NK- and T-lineage cells is restricted and B- lineage commitment occurs (6, 7). The committed BLPs differentiate into CD19 expressing B cell progenitors that undergo V(D)J rearrangement of first the Ig heavy (Igh) chain gene in proB cells and subsequently Ig light (Igl) chain genes in CD25 expressing preB cells (8–10). The formation of a functional B cell receptor (BCR) results in the generation of immature B cells that further mature into follicular and marginal zone B cells.
The initiation of lymphopoiesis and early B cell development is critically dependent on the stage-specific expression of an interdependent network of transcription factors (11, 12). At the CLP stage, early B cell factor 1 (EBF1) is needed for the establishment of the early B cell transcriptional program (13, 14) and subsequently for the activation of PAX5 (15). With EBF1 and PAX5 active, a mutually enforcing positive feedback loop is established (12, 15, 16) that locks down B cell identity (17–19) and promotes the development of mature B cells (20, 21).
The Forkhead box O (FOXO) family of transcription factors are major effectors of the PI3K-AKT signaling pathway and out of the four family members, Foxo1, Foxo3, and Foxo4 are expressed in the hematopoietic system. Foxo1 is central to the B cell lineage (22, 23). In a developmental context, the loss of FOXO1 was initially reported to have a mild phenotype in early B cell development (24) with no significant reductions in proB and preB cell numbers despite the role of FOXO1 in regulating the Rag genes critical for V(D)J recombination (24, 25). In addition to demonstrating that the loss of FOXO1 impairs the initiation of B cell specification in CLPs, our later study using earlier conditional deletion found a more severe reduction in B cell numbers and indicated that B cell development was blocked at the proB cell stage (26). However, the existence of B cells in both the BM and spleen suggests that FOXO1 is not an absolute requirement for B cell commitment nor for developmental progression past the proB cell stage (26). In addition to FOXO1, the loss of FOXO3 has been shown to impact early B cell development by causing reduced preB cell numbers (27) while a potential role in earlier lymphoid progenitors remains to be explored.
Here we utilized VAV-iCre mediated conditional deletion of FOXO1 and FOXO3 throughout the hematopoietic system to study their roles in B cell development. We show that the loss of FOXO3 impacts B lymphopoiesis at a much earlier stage than previously reported with FOXO3 being required for the generation of LMPPs, CLPs, and B cell precursors while being dispensable for the mature B cell subsets. In addition, we show without ambiguity that the loss of FOXO1 causes a developmental block at the proB cell stage mirroring that of RAG deficient mice. Strikingly, we further show that the combined loss of FOXO1 and FOXO3 resulted in a failure to restrict the myeloid potential of CLPs and to initiate the early B cell program. This resulted in the complete loss of the B cell lineage and demonstrates that cooperatively FOXO1 and FOXO3 are indispensable for the initiation of B cell development.
Materials and Methods
Mice
To generate mice lacking FOXO1 and/or FOXO3 throughout the hematopoietic system, Vav-iCre (28) was utilized in combination with conditional (floxed) Foxo1 (29) and Foxo3 (30) alleles. In addition, RAG2 knockout (ko) (31) and wildtype (WT) C57BL/6 mice were used. Genetically modified strains were maintained on a C57BL/6 background. Both male and female mice were included in the study and analyzed at an age of 8-14 weeks. All animal experiments were approved by the local animal ethical committee.
Cell Preparation, Flow Cytometry and Cell Sorting
BM, spleen and thymus were dissected, crushed in PBS with 2% FCS and passed through a 70µm filter. Cells were counted using a Sysmex hematology analyzer (Sysmex). Cells were incubated with Fc-blocking antibodies (CD16/32, clone: 93, BD) prior to staining with fluorescently labeled antibodies. For antibody panels see Table S1. Dead cell discrimination was performed using propidium iodide (PI), 7AAD or live/dead fixable Aqua dead cell stain (Thermo Fisher Scientific). For FACS sorting of progenitors from BM, mature cells were depleted using purified antibodies against TER119, CD19, CD3, GR1 and MAC1 in combination with sheep anti-rat IgG Dynabeads (Thermo Fisher Scientific) prior to the specific antibody staining. For FACS sorting of B cell progenitors, total BM cells were either depleted as described above (omitting CD19) or enriched using anti-B220 beads (Miltenyi) prior to the specific antibody staining. Flow cytometry and cell sorting were performed using mainly the LSRFortessa and FACSARIAIIu/III platforms (BD Biosciences). Analysis of FACS data was done using FlowJo (BD Biosciences) and statistical analysis performed in R.
Transplantation
Transplantation was performed by tail-vein injection of lethally irradiated 10-16 weeks old CD45.1 (B6.SJL) mice. The full irradiation dose (1000cGy) was given as two split doses. Each transplanted mouse was given 5x106 BM cells from control or FOXOdko mice (CD45.2, donor cells) and 0.2x106 BM cells from B6.SJL mice (CD45.1, support cells). Analysis of reconstitution in peripheral blood (PB) and spleen was performed 12 weeks post-transplantation using FACS. For antibody panels see Table S1.
Cell Cycle and Apoptosis Analysis
For cell cycle analysis, fully surface stained cells (stained as described above, for staining panel see Table S1) were fixed with 1% formaldehyde (Thermo Fisher Scientific), permeabilized with 0.05% Triton X-100 (Sigma) and subsequently stained with Ki67 AlexaFluor488 (BD Biosciences) and DAPI (Thermo Fisher Scientific). For analysis of apoptosis, cells were surface stained (for antibody panel see Table S1) and subsequently stained with Annexin V (Biolegend).
ELISA
Serum was collected by tail bleeding and kept at -80°C until use. To perform the ELISA assays, MaxiSorp ELISA plates (Thermo Fisher Scientific) were coated with 10-20µg/ml of anti-mouse immunoglobulin (Ig) (H+L) (Southern Biotech) to capture total Ig in the serum. Serum Ig was detected using a secondary HRP-conjugated anti-mouse IgM (Southern Biotech) or IgG (Southern Biotech) antibody. Phosphatase substrate (Merck) was added to generate a signal subsequently measured by an ELISA plate reader.
Immunohistochemistry
Cryostat sections (10 μm) were fixed in ice-cold acetone and then stained in phosphate-buffer saline with 5% fetal calf serum (FCS) and 0.3% Tween. The following reagents were used: B220 (RA3-6B2), TCRβ (H57-597, BioLegend); CD169 (MOMA-1); MARCO (EPR22944, AbCam), biotinylated peanut agglutinin (Vector Laboratories); goat anti-rat-AlexaFluor488, Strepavidin-AlexaFluor555 (Thermo Fisher Scientific). Images were collected with a Leica DM IRBE confocal laser scanning microscope (Leica Microsystems) equipped with 1 argon and 2 HeNe lasers, using an HC PL APO lens at 10x/0.40 CS and 90% glycerol (MP Biomedicals) and processed with Adobe Photoshop CS5 (Adobe).
RNAseq
3-10k cells were FACS sorted into buffer RLT with β-mercaptoethanol and total RNA prepared using RNAeasy Micro (Qiagen) with on column DNase I treatment. Stranded RNAseq libraries were prepared using the TotalScript RNAseq kit (Epicenter). Libraries were sequenced paired-end (2x50 cycles) on the Illumina platform (Illumina). Reads were mapped using STAR (v 2.5.2b) (32), strand-specific reads in exons quantified using HOMER (33) and significant changes identified using EdgeR (34). Only genes with ≥30 reads in at least two samples were considered in the analysis. Data was log transformed and quantile normalized for display. PCA and visualization were performed using R (v3.3.3). GO term analysis was done using Metascape (35) with standard settings.
ATACseq
3-10k cells were FACS sorted into cold 200μl PBS with 2% FCS and ATACseq libraries immediately prepared as previously described (36). Libraries were sequenced paired-end (2x50 cycles) on the Illumina platform (Illumina). To obtain differential ATACseq peaks, data was first trimmed and mapped to mm10 using bowtie2 (v2.3.3.1) (37). Elimination of PCR duplicates, identification of peaks, annotation of peaks, quantification of reads in peaks and motif enrichment analysis was done using HOMER. Identification of significant changes to chromatin accessibility was done using EdgeR considering peaks with ≥30 reads in at least two samples. For visualization of data in heatmaps and box plots, data was log transformed and quantile normalized. Tracks were prepared for visualization in the UCSC genome browser (38) by calculating the median IP efficiency-normalized signal of biological replicas. Cut-profiles were made using the HOMER function annotatePeaks.pl with parameters -fragLength 9 -hist 1 inputting regions with differential accessibility (as identified by EdgeR) and containing transcription factor binding sites (TFBS) of any expressed TF (FPKM ≥1 in ≥2 samples) from the analyzed TF family. The cut-profiles obtained for each sample were subsequently normalized to the IP efficiency and the average across biological replicates plotted.
ChIPseq
ChIPseq was performed as previously described (39) using H3K27Ac antibodies (Diagenode Cat #C15410196, lot A1723-0041D/2) and the ThruPLEX DNA-seq (Rubicon Genomics) library preparation kit. Reads were mapped using bowtie2. Elimination of PCR duplicates and generation of tracks was performed using HOMER. Visualization was performed using the UCSC genome browser.
In Vitro Evaluation of Myeloid Potential and Colony RT-PCRs
To evaluate myeloid potential of progenitors, 150 cells were FACS sorted into 3ml of medium and single cells manually plated (20µl/well) in Terasaki plates. Medium constituted IMDM (with GlutaMax, Thermo Fisher Scientific) supplemented with 100U/ml penicillin-streptomycin (Hyclone), 100µM β−mercaptoethanol, 20% Fetal Calf Serum (Merck), 25ng/ml murine KL, 25ng/ml murine GM-CSF, 25ng/ml human TPO, 25ng/ml human G-CSF, 25ng/ml human FL and 10ng/ml murine IL3 with or without 25ng/ml murine M-CSF (all from PeproTech). No apparent difference was observed between cultures with or without M-CSF and data was combined. Size of CLP- and LMPP-derived colonies was scored as low (colonies containing 2-99 cells), intermediate (≥100 cells to cells covering <10% of the well) or high (cells covered ≥10%-100% of the well) proliferating under a microscope after 5 and 10 days of culture, respectively. Cloning frequency was calculated considering the Poisson distribution (which predicts that 63% of wells should contain 1 cell following manual plating). The composition of the generated cells was evaluated on May-Grünwald/Giemsa (Merck) stained cytospin slides prepared from pooled colonies. To perform colony RT-PCRs, individual colonies were transferred to a 96-well PCR plate with PBS. After spinning down (1000g 10min), the supernatant was aspirated (leaving approximately 5μl), 5μl 2x lysis buffer (0.8% NP40, 0.125mM dNTPs, 5mM DTT and 0.005U RNaseOUT) added. Plates were frozen at -80°C till further use. Colony RT-PCRs was performed as previously described but using DreamTaq DNA Polymerase (Thermo Fisher Scientific) (1, 5, 6, 40).
In Vitro Evaluation of T Cell Potential on OP9-DL1
Single cells were FACS sorted onto pre-seeded OP9-DL1 stromal cells in 96-well plates and evaluated as previously described (2). In brief, OP9-DL1 co-cultures were performed in OptiMEM (with GlutaMax, Gibco) supplemented with 100U/ml penicillin-streptomycin (Hyclone), 100μM β-mercaptoethanol, 10% Fetal Calf Serum (Merck), 5ng/ml murine SCF and 5ng/ml human FL (PeproTech). Additional FL (5ng/ml) was added weekly. The clonal readout was evaluated by flow cytometry after 3 weeks of culture (for antibody panel see Table S1). Colonies with CD90.2/Thy1.2+CD25+ cells were considered to contain T cell (2, 6, 41).
Results
To analyze the expression of the FOXO-family genes, we performed RNA sequencing on wild-type (WT) cells representing the B cell developmental pathway ranging from hematopoietic stem cells to mature peripheral B cells. This revealed a clear temporal switch where Foxo3 is highly expressed in stem- and multipotent progenitor cells but downregulated in CLPs where Foxo1 is upregulated instead and subsequently becomes the predominantly expressed Foxo gene in the B cell lineage (Figure 1A). With both CLPs and B cells co-expressing significant levels of Foxo3 and Foxo1, this prompted us to investigate more closely their role in B cell development. To this end, we utilized Vav-iCre (28) to achieve conditional loss of FOXO1 (FOXO1ko) and FOXO3 (FOXO3ko) alone or in combination (FOXOdko) throughout the hematopoietic system. This model provided effective deletion of the loxP flanked (floxed) DNA binding domains of both Foxo1 and Foxo3 (Figures S1A–C). Mice lacking the Vav-iCre transgene (mainly Foxo1flox/floxFoxo3flox/flox mice) were utilized as normal littermate controls (CTRL).
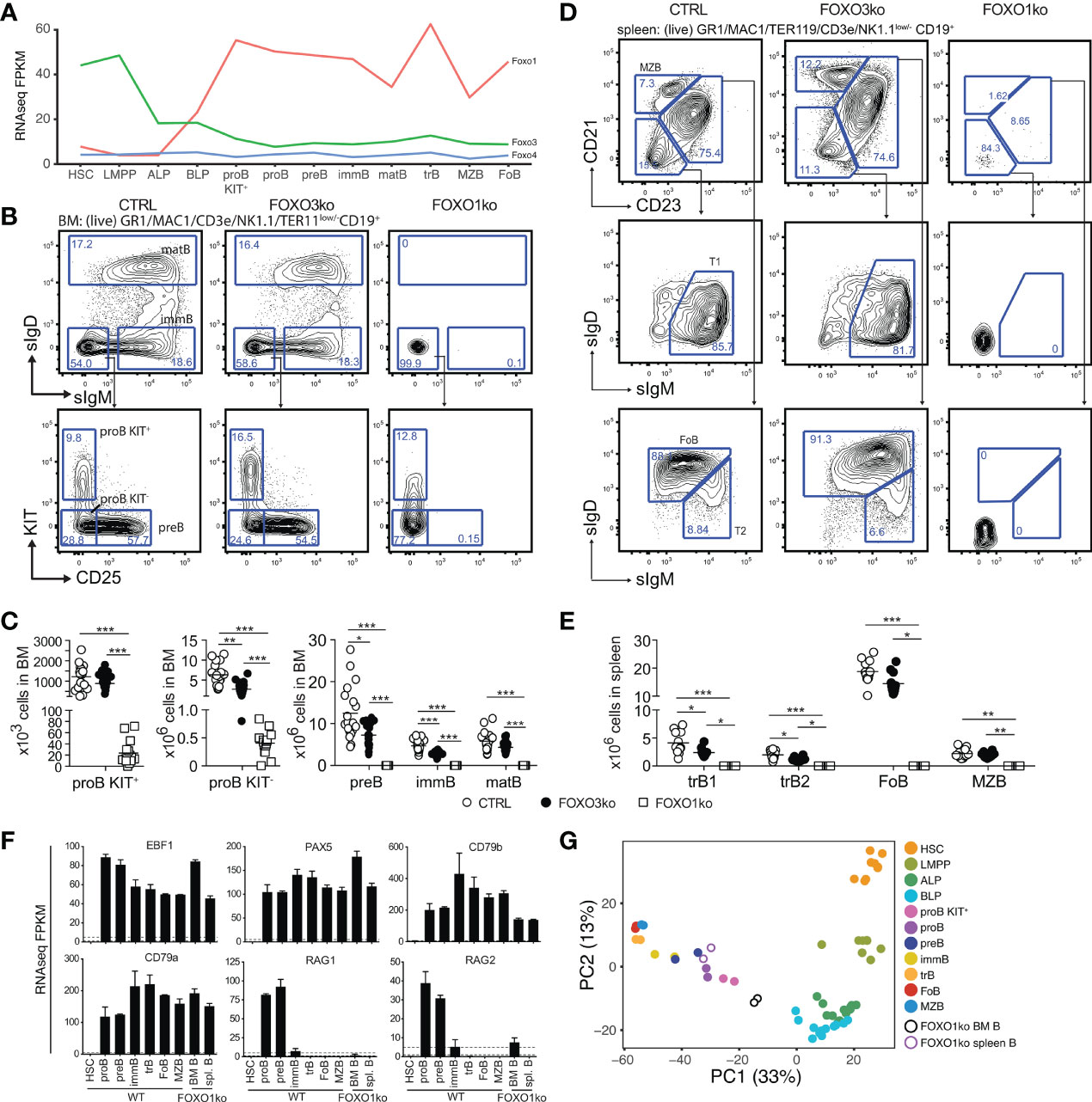
Figure 1 Loss of FOXO1 or FOXO3 impairs B cell development. (A) Gene expression (FPKM) of Foxo1, Foxo3, and Foxo4. HSC, hematopoietic stem cell; LMPP, lymphoid primed multipotent progenitor; ALP, LY6D- common lymphoid progenitor; BLP, LY6D+ common lymphoid progenitors; BLP, LY6D+ common lymphoid progenitors; immB, immature B; matB, mature B in BM; trB, transitional B; FoB, follicular B; MZB, marginal zone B. (B) Gating strategy for identification of BM B lineage cells. (C) Total number of B lineage cells in BM. In panels C and E: each dot represents data from an individual mouse; p-values were calculated using the Kruskal Wallis test with Dunn’s test of multiple comparisons; *, ** and *** indicating p-values <0.05, <0.01, and <0.001 respectively. (D) Gating strategy for identification of spleen B lineage cells. (E) Total number of B lineage cells in spleen. (F) Expression of indicated genes. (G) Principal component analysis of RNAseq data.
B Cell Development Is Blocked at the proB Cell Stage in the Absence of FOXO1
In line with our earlier observations (26), we found that the FOXO1ko mice displayed diminished proB cell numbers (Figures 1B, C), a complete lack of B cell progenitors expressing CD25 (Figures 1B, C), and a preBCR/BCR either on the cell surface (Figures 1B, C) or intracellularly (Figure S2A). Considering the apparent lack of cells passing beyond the proB cell stage in the BM, we next confirmed that the FOXO1ko mice had a splenic B cell population (Figures 1D, E) (26). To investigate if the remaining B cells represented cells that had matured despite the lack of FOXO1, we carefully analyzed the remaining B cells. We found no expression of the conditionally deleted Foxo1 exon (Figure S1C), suggesting that this was not caused by escape from VAV-iCre mediated deletion. The remaining FOXO1ko splenic B cells did not generate apparent CD21/CD23-expressing populations (Figures 1D, E) and, like the FOXO1ko BM B cells, lacked both surface (Figures 1D, E) and intracellular (Figure S2B) BCR expression. Further supporting the notion that the remaining splenic FOXO1ko B cells were not functional mature B cells, we found that the splenic architecture was not maintained (Figure S2C) and no discernable serum levels of IgM or IgG (Figure S2D). This indicated that the splenic B cells in the FOXO1ko represent a B cell progenitor population.
To confirm the cellular identity of the remaining B cells, we performed RNAseq on the FOXO1ko CD19+ cells from both BM and spleen. As expected, the FOXO1ko B cells maintained expression of the identity defining transcription factors Pax5 and Ebf1 (17, 19) as well as their target genes Cd79a and Cd79b (42–44) (Figure 1F). Consistent with the immature cell-surface phenotype of the remaining B cells, principal component analysis (PCA) positioned the BM and spleen FOXO1ko B cells adjacent to KIT+ proB and total (KIT unfractionated) proB respectively (Figure 1G). Further, in line with a developmental block in cells lacking pre-BCR expression and the known role of FOXO1 in regulating the recombinase activating genes (Rag1 and Rag2) (25), we found that the expression of both Rag genes was dramatically reduced with Rag1 expression essentially being abolished (Figure 1F).
To establish if the loss of FOXO1 was permissive for BCR negative B cell progenitors to appear in the spleen, we next analyzed RAG2 knockout (RAG2ko) mice (31). Mirroring the FOXO1ko phenotype, the RAG2 deficient mice displayed a developmental block at the proB cell stage in the BM while still maintaining a population of BCR negative B cells in the spleen (Figures S2E–G). Hence, the loss of FOXO1 is not a prerequisite for BCR negative B cell progenitors to reach the spleen.
We conclude that early loss of FOXO1, as previously suggested (26), results in a complete developmental block at the proB cell stage. Further, we conclude that the splenic B cells in the FOXO1ko are not a sign of developmental progression but rather that FOXO1ko mice, like RAG deficient mice, harbor proB cells in the spleen.
Loss of FOXO3 Impairs the Generation of LMPPs, CLPs, and B Cell Progenitors
To investigate the functional impact of the loss of FOXO3, we characterized the composition of lymphoid progenitors and B-lineage cells in BM and spleen. Looking at the B cell progenitors in the BM of FOXO3ko mice, we found that the numbers of KIT+ proB cells was not reduced but a significant reduction in KIT- proB cells, preB, and immature B cells was found (Figures 1B, C). In contrast to the B cell progenitors, mature B cell numbers in the BM were not affected (Figures 1B, C). In line with the observations from the BM, transitional B cells were reduced while follicular and marginal zone B cells were present in normal numbers in the spleen of FOXO3ko mice (Figures 1D, E). Hence, the loss of FOXO3 impairs the generation of immature and transitional B cells from KIT+ proB cells while being dispensable for the maintenance of the mature B cell pool.
With Foxo3 being highly expressed in LMPPs and CLPs (Figure 1A), we next analyzed the progenitor compartment to investigate if FOXO3 also impacted the development of early lymphoid progenitors. Analyzing the BM of FOXO3ko mice, we found that the loss of FOXO3 caused a decrease in LMPP, ALP, and BLP numbers (Figures 2A–C).
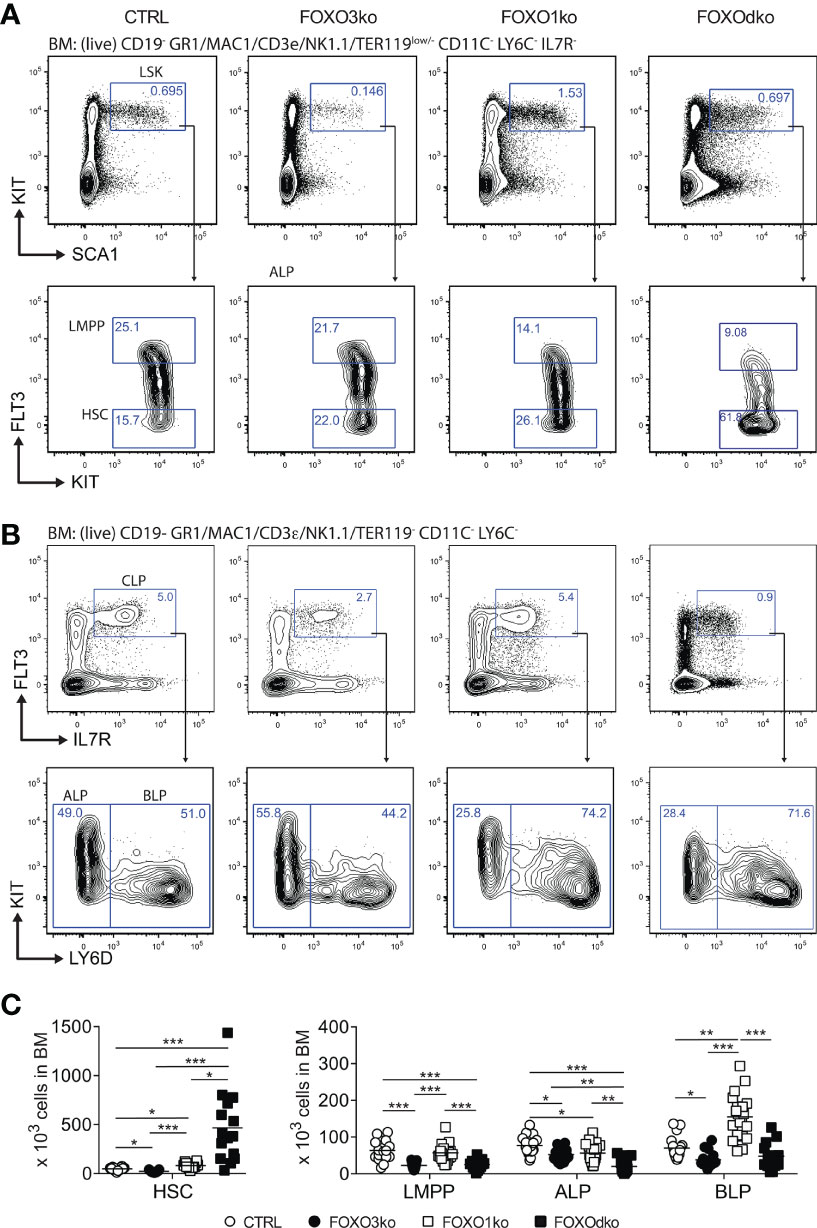
Figure 2 Development of lymphoid progenitors is perturbed by the loss of FOXO1 and FOXO3. (A, B) Gating strategy for identification of stem and lymphoid progenitor cells. For complete gating see Fig. S3A. (C) Total number of progenitor cells in BM. In panel (C) each dot represents data from an individual mouse; p-values were calculated using the Kruskal Wallis test with Dunn’s test of multiple comparisons; *, ** and *** indicating p-values <0.05, <0.01, and <0.001 respectively.
Hence, in keeping with the Foxo3 expression pattern (Figure 1A), loss of FOXO3 impacts the generation of the lymphoid progenitor branch from LMPPs to transitional B cells. Altogether, this shows that FOXO3 has a previously undescribed role in the generation of lymphoid progenitors and that the role in B cell progenitors is not restricted only to the preB cell stage (27).
Loss of FOXO1 and FOXO3 Results in Developmental Block at the BLP Stage and a Complete Loss of the B Cell Lineage
Given the co-expression of Foxo1 and Foxo3 at the CLP stage (Figure 1A), we hypothesized that functional redundancy could mask a critical role of the FOXO-family in early B cell development. The FOXOdko mice displayed increased numbers of HSCs (LSK FLT3-) directly reflected in increased number of LSK cells (Figure S3B), and a similar decrease in LMPPs as observed in the FOXO3ko mice (Figures 2B, C). While FOXO1 has been implicated in the regulation of Il7r gene (24, 45, 46), we found that IL7R expression readily allowed for identifying CLPs regardless of the loss of FOXO1 and FOXO3 (Figure 2B). The combined loss of FOXO1 and FOXO3 (FOXOdko) exacerbated the loss of ALPs observed in the single FOXO3ko while BLP numbers were seemingly normal (Figures 2B, C). This indicates that the expansion of the BLP compartment observed after the loss of FOXO1 (26) (Figures 2B, C) still occurs in the FOXOdko mice. In line with this as well as the close link between the FOXO family and the regulation of proliferation, the FOXOdko mice displayed markedly increased proliferation in the CLP compartment (Figure S3C). We found no increase in the frequency of apoptotic CLPs (Figure S3D).
With both FOXO1 and FOXO3 impacting early B cell progenitors, we next analyzed BM and spleen for B cells also in the FOXOdko mice. Strikingly, we found that the FOXOdko mice displayed a complete lack of the B cells in BM and spleen (Figures 3A–D). This was recapitulated upon the adoptive transfer of FOXOdko progenitor cells into normal hosts (Figures S3E, F). Further in line with the loss of the B cell lineage, we were unable to detect serum Ig in FOXOdko animals (Figure S2D). Hence, the combined loss of FOXO1 and FOXO3 results in a complete block in B cell differentiation at the BLP stage and a loss of the B cell lineage.
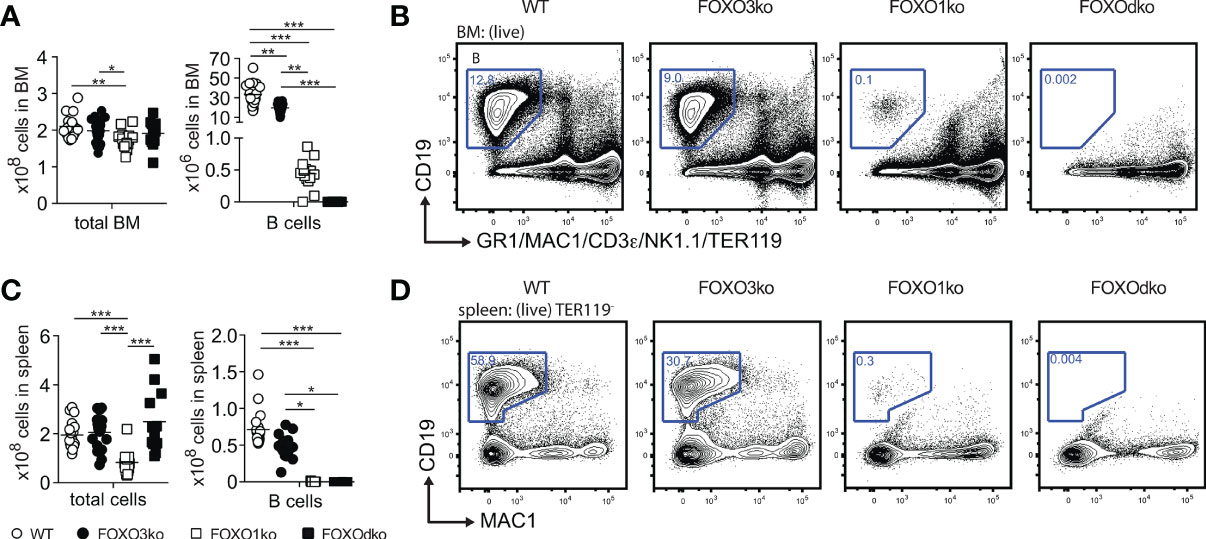
Figure 3 The B lineage developmental is critically dependent on FOXO1 and FOXO3. (A) Total number of cells and B cells in BM. In panel (A, C): each dot represents data from an individual mouse; p-values were calculated using the Kruskal Wallis test with Dunn’s test of multiple comparisons; *, ** and *** indicating p-values <0.05, <0.01, and <0.001 respectively. (B) Gating strategy for identification of B cells in BM. (C) Total number of cells and B cells in spleen. (D) Gating strategy for identification of B cells in spleen.
CLPs Lacking FOXO Fail to Initiate Expression of the Early B Cell Program
To investigate the gene regulatory consequences of the loss of the FOXO proteins, we performed RNAseq on FACS sorted progenitor cells from the FOXO knock-out animals, WTs, and CTRLs. Using PCA, we found that the overall expression profiles of the progenitor populations from the knock-out animals corresponded well to that of their normal counterparts (Figure 4A). Looking only at the progenitors, the populations similarly organized in developmental order (PC1) (Figure 4B). However, the second component (PC2) clearly separated the FOXOdko cells from the other genotypes, suggesting that the combined loss of FOXO1 and FOXO3 is needed to cause a significant impact on the overall transcriptional program across the progenitor hierarchy. With B cell development being blocked at the CLP stage in the FOXOdko, we utilized EdgeR to identify significant expression changes occurring in ALP and BLP. This identified 319 genes differentially expressed in FOXOdko CLPs (Figures 4C, D and Table S2). A substantial number of these genes overlapped with genes significantly up-regulated in the normal ALP to BLP transition (Figures 4C and S4A). Overall, the loss of FOXO3 gave milder gene expression changes than the loss of FOXO1 and these changes were exacerbated by the combined loss of both FOXO proteins (Figure 4E). This suggests that the FOXO-proteins cooperatively regulate these genes. The usage of gene ontology analysis on the different gene clusters (Figure 4D), revealed that these were associated with leukocyte biology (cluster I); B cell activation and primary immunodeficiency (cluster II-III); and response to oxidative stress (cluster IV) (47) (Figure S4B). Looking at specific genes, we found that the loss of the Foxo genes impaired the expression of genes critical for the B cell lineage including the surrogate light chain genes (Igll1, VpreB1, and Vpreb3), signaling molecules (Cd79a, Blk, and Blnk), and transcription factors (Pou2af1, Irf4, and Ets1) (Figures 4D, F). Further, in agreement with both disruption of the positive Ebf1-Foxo1 feedback loop (26) and the loss of the B cell lineage, Ebf1 and Foxo1 expression was dramatically reduced while Pax5 expression was lost (Figures 4D, F). In conclusion, the combined loss of FOXO1 and FOXO3 results in a failure to initiate the B cell program in CLPs.
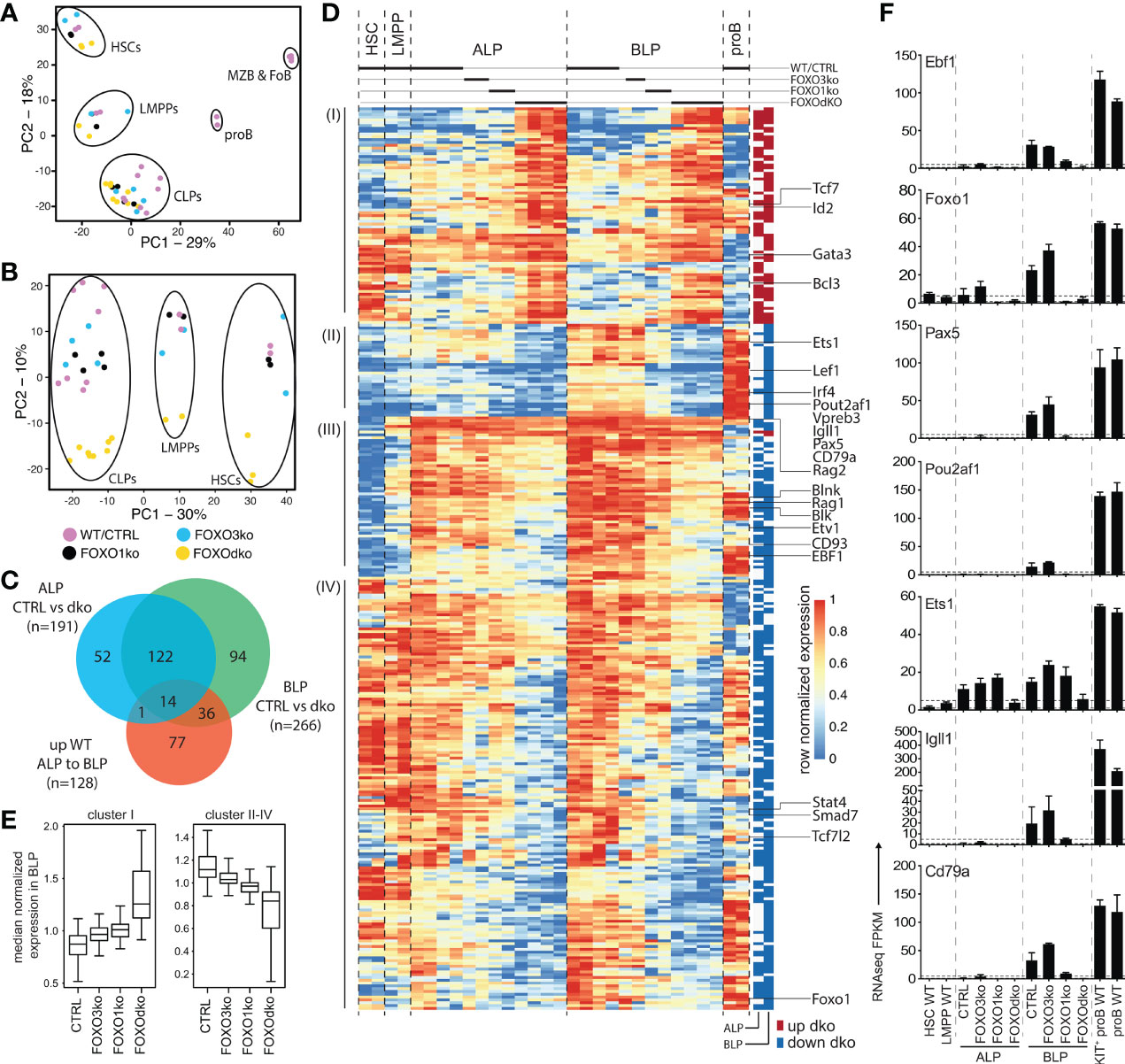
Figure 4 FOXOdko CLPs maintain cellular identity but fails to up-regulate B lineage related genes. (A, B) Principal component analysis of RNAseq data. (C) Venn diagram displaying the overlap between genes differentially expressed (Bonferroni corrected p-value <0.05 and 2-fold difference comparing FOXOdko to CTRL and ≥30 reads in at least two samples) in the FOXOdko at the indicated CLP stage or in the ALP to BLP developmental transition. (D) Hierarchical clustering of expression for genes displaying differential expression in FOXOdko CLPs. The stage where a significant change occurs is indicated to the right. (E) Median normalized expression of genes within clusters I and cluster II-IV from panel (D). (F) Expression of indicated genes.
FOXO Is Required for the Establishment of the B-Lineage Gene Regulatory Landscape
To understand the gene regulatory mechanisms that cause the transcriptional changes observed in the FOXOdko CLPs, we analyzed chromatin accessibility using ATACseq (36). This identified approximately 40 000 open chromatin regions (ATACseq peaks) per investigated population, with the majority of the peaks being localized distal to known promoters (Figure S4C). Subjecting the ATACseq data to PCA, we found that the progenitor subsets organized together and in a developmental trajectory regardless of the loss of FOXO activity (Figure 5A, left). Similar to the PCA of RNAseq data, the effect of the combined loss of FOXO1 and FOXO3 was observable when looking specifically at the progenitors (PC2) (Figure 5A, right). Next, we used EdgeR to identify regions with highly significant changes in chromatin accessibility in the FOXOdko CLPs. This identified 297 and 1068 differentially accessible regions (DARs) at the ALP and BLP developmental stages, respectively (Figure 5B). The majority of the DARs were localized outside of promoter regions (Figure 5C).
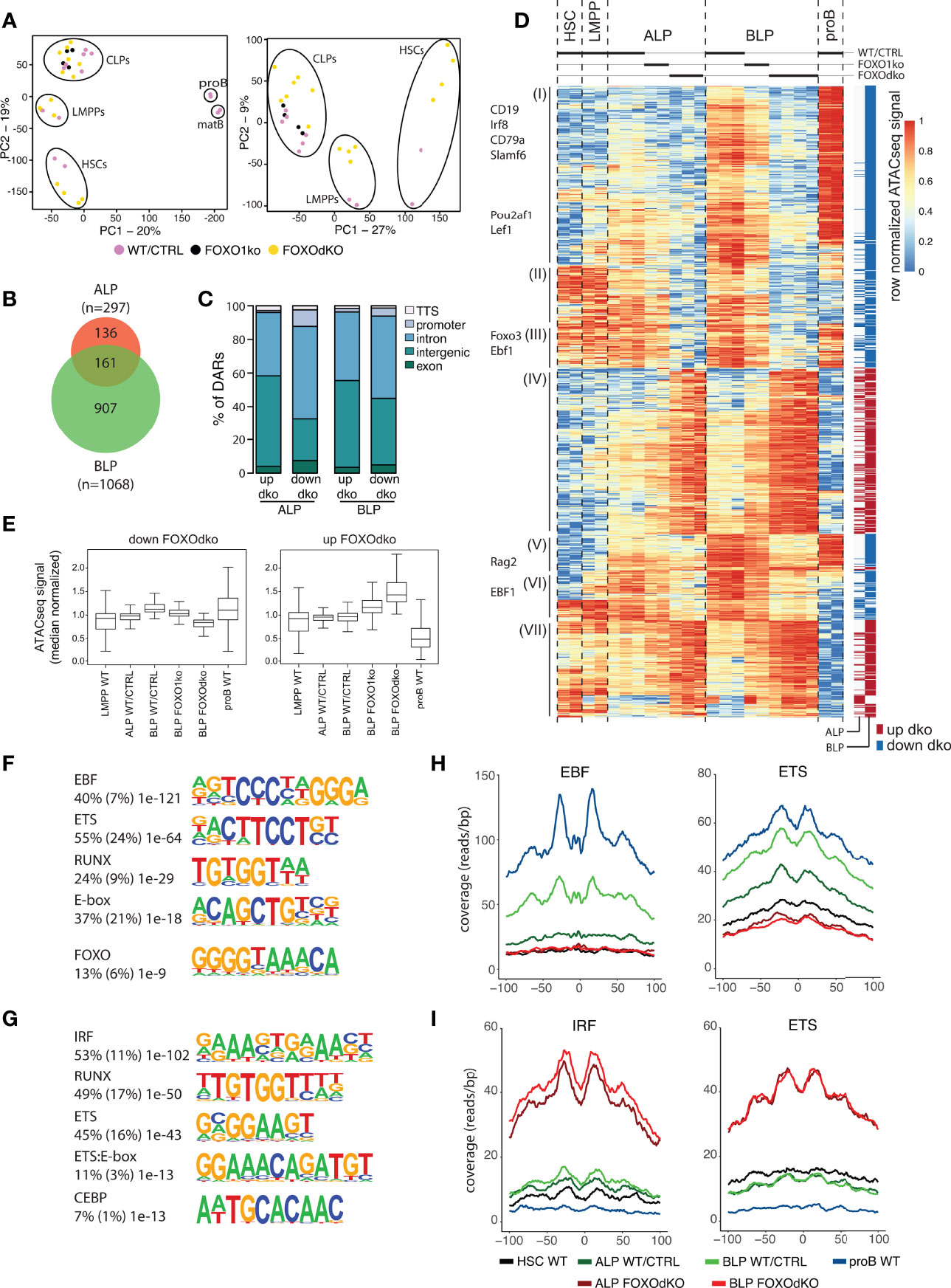
Figure 5 Chromatin accessibility is altered by the lack of FOXO. (A) Principal component analysis of ATACseq data. (B) Venn diagram displaying the overlap between differentially accessible regions (DARs) (adjusted p-value <0.01 and 2-fold change comparing FOXOdko to WT/CTRL and ≥30 reads in at least two samples) in the FOXOdko at the indicated CLP stage. (C) Bar graph illustrating distribution of DARs amongst indicated genomic locations. (D) Hierarchical clustering of ATACseq signal in DARs. The stage where a significant change occurs is indicated to the right. DAR proximal genes are indicated to the left. (E) Median normalized ATACseq signal in DARs with decreased (left) and increased (right). (F, G) De novo motif enrichment in DARs with decreased (F) and increased (G) ATACseq signal in FOXOdko BLP. Percentage of sequences containing the motif, percentage of background sequences containing the motif (in parenthesis) and p-value of the enrichment are displayed to the left of the enriched motifs. (H, I) Transposase integration-based cut-profiles of DARs with: (H) decreased ATACseq signal containing EBF and ETS binding sites; or (I) increased ATACseq signals containing IRF and ETS binding sites.
Looking at the chromatin accessibility of the DARs specifically in a B-lineage developmental context, the DARs with increased accessibility in the FOXOdko CLPs were generally regions that would lose accessibility upon developmental progression to the proB cell stage (Figure 5D, cluster IV and VII; and 5E, right). In contrast, DARs with reduced accessibility in the FOXOdko CLPs generally displayed increased accessibility in proB cells and were localized in proximity with B-lineage associated genes including Cd19, Cd79a, Pou2af1, Rag, and Ebf1 (Figure 5D, cluster I, III and V; and Figure 5E, left). This suggests that the affected elements are a critical part of the early B cell gene regulatory circuitry activated at the CLP stage.
To identify transcription factors (TFs) responsible for the changes in chromatin accessibility in the FOXOdko CLPs, we performed motif enrichment analysis on the DARs. The DARs with reduced accessibility in the FOXOdko CLPs displayed enrichment of transcription factor binding sites (TFBS) of TF families central to the B-lineage gene regulatory program including EBF, ETS, RUNX, E-box (E-protein), and FOXO (Figure 5F). To further support the loss of binding of these TFs to the DARs with reduced accessibility, we analyzed the transposase integration in the areas surrounding the TFBS to produce cut-profiles. In line with the loss of FOXO and EBF1 binding to the DARs with reduced accessibility, we found that the cut-profiles of these TFs were reduced or completely lost (Figures 5H and S4D). Similarly, the ETS footprint was clearly diminished (Figure 5H) and several ETS-family genes (including Ets1, Elk3, and Etv1) were downregulated in the FOXOdko CLPs (Figures 4D and S4E). We found no significant differences in the mRNA expression of the Runx-family and E-protein genes, nor clear cut-profiles in the regions surrounding the TFBS of these TFs (Figures S4D, E). Together, this suggests that the failure to express the early B-lineage program in the FOXOdko is caused by a combination of the loss of FOXO activity and the failure to properly up-regulate EBF1 and ETS-family genes.
Conversely, we found that the DARs with increased accessibility were associated with IRF, RUNX, ETS, ETS:E-box, and CEBP binding sites (Figure 5G). No corresponding up-regulation of genes within the respective transcription factor families was observed (Figure S4E). However, the IRF and ETS binding sites were associated with clear cut-profiles consistent with the binding of these TFs to the DARs with increased accessibility (Figure 5I). The other TFBS did not display clear cut-profiles (Figure S4D). Hence, this indicates that the gain of chromatin accessibility in the FOXOdko CLPs is related to the altered binding of TFs commonly expressed in CLPs.
Open Chromatin Is Largely Established Independent of FOXO in CLPs
Both FOXO1 and EBF1 have been shown to be pioneer factors (48, 49) that can remodel condensed chromatin and make it accessible for other TFs. Given the co-dependence of Foxo1 and Ebf1 expression in CLPs (26) and the essential loss of both Foxo1 and Ebf1 expression in the FOXOdko (Figure 4F), we next investigated if this was associated with a failure to establish chromatin accessibility at these loci. Somewhat surprisingly, we found that the chromatin accessibility landscape surrounding both the Foxo1 and Ebf1 genes was essentially maintained in the FOXOdko CLPs (Figures 6A, B). While a few DARs with decreased chromatin accessibility were identified ( ± 1Mbp of the respective promoter regions), accessibility was not lost in these regions (Figures 6A, B). Hence, the activation of the Foxo1-Ebf1 co-regulatory loop occurs via FOXO and EBF1 binding to pre-established open chromatin.
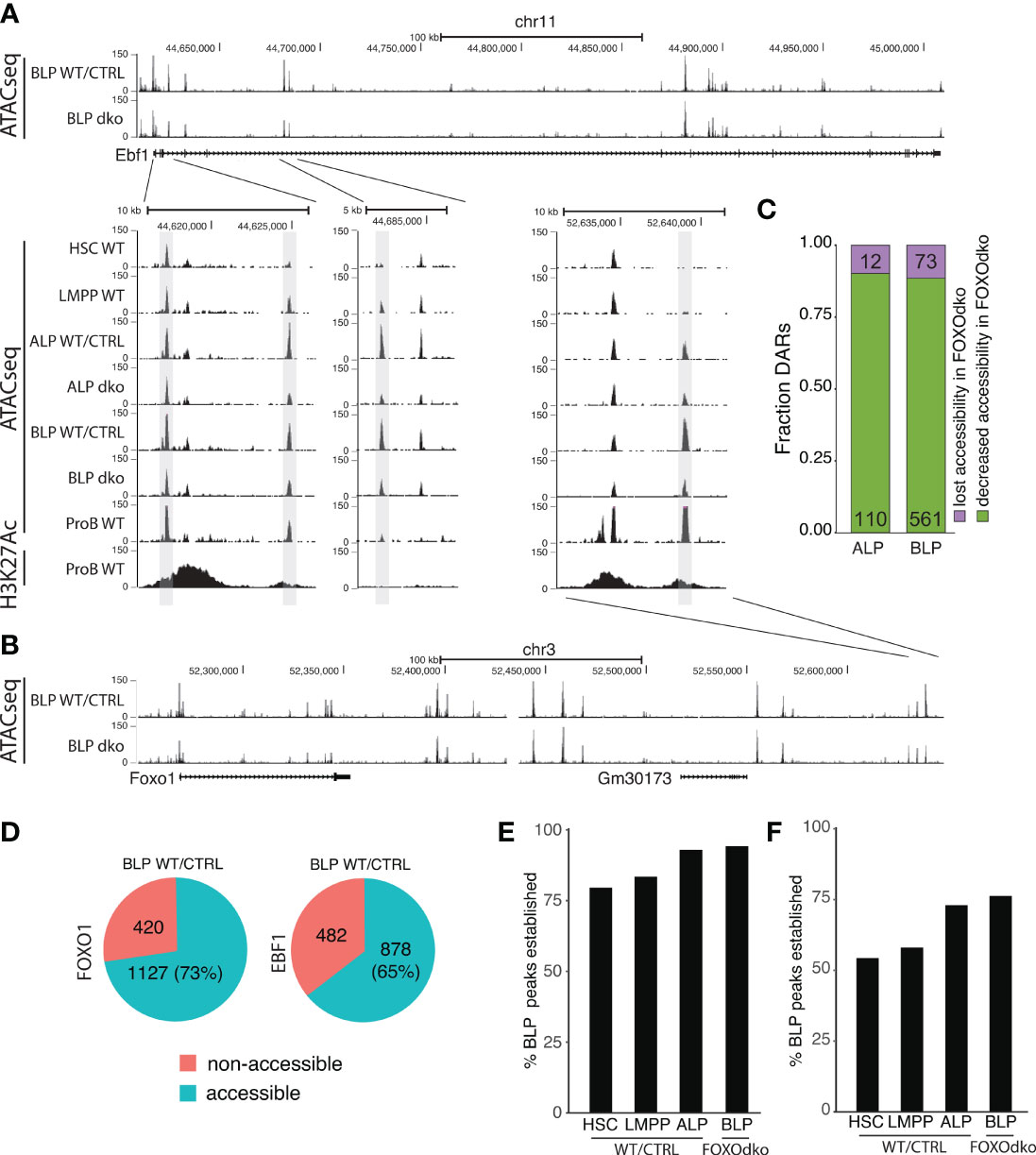
Figure 6 Loss of FOXO is not associated with a failure to establish open chromatin. (A, B) Tracks showing chromatin accessibility (ATACseq) and H3K27Ac (ChIPseq) for indicated cell- and genotypes at the (A) Ebf1 and (B) Foxo1 loci. (C) Fraction of DARs with reduced accessibility displaying either lost or decreased chromatin accessibility in the FOXOdko CLPs. (D) Venn diagram showing the overlap between FOXO1 and EBF1 binding in RAG1ko proB cells and open chromatin in WT/CTRL BLP. (E, F) Percentage of the open chromatin regions (consensus peaks) found in WT/CTRL BLP that overlap with (E) FOXO1 or (F) EBF1 binding and that constitute open chromatin (consensus peaks) at the indicated stages of development.
To address if a similar pattern was found on a broader level, we addressed if DARs with significant decreased chromatin accessibility (Figure 5D) were associated with a failure to establish open chromatin in the FOXOdko CLPs. To this end, we analyzed the extent to which DARs remained identified as open chromatin (peaks) in ATACseq from FOXOdko CLPs. We found that approximately 90% of the affected regions were identified as open chromatin (peaks) in FOXOdko CLPs (Figure 6C). Thus, the reductions in chromatin accessibility observed in the FOXOdko CLPs was generally associated with decreased but not lost chromatin accessibility. To look specifically at FOXO1 and EBF1 bound regions, we overlapped FOXO1 and EBF1 binding identified by ChIPseq in RAG1ko proB cells (50) with open chromatin (stage specific consensus peaks) in stem- and progenitor cells. We found that 73% of FOXO1 and 65% of EBF1 bound regions constituted open chromatin (peaks) at the BLP stage (Figure 6D). Out of the FOXO bound regions open in WT BLPs, we found that >75% of these regions were open chromatin already in HSCs and LMPPs while >90% of the regions were open chromatin in ALP and FOXOdko BLPs (Figure 6E). Similarly, analyzing the EBF1 bound regions, approximately 75% of the regions were accessible (identified as peaks) in the very low Ebf1 expressing ALP and FOXOdko BLP (Figure 6F). In addition, just over 50% of the regions constituted accessible chromatin already in LMPPs and HSCs prior to the expression of Ebf1 (Figure 6F).
Together, this suggests that the open chromatin at gene regulatory elements needed for activation of the early B cell transcriptional program at the CLP stage is, to a large extent, pre-established by FOXO and EBF1 independent mechanisms.
CLPs Devoid of FOXO Display Increased Ability to Generate Myeloid Cells
Given the altered expression of genes controlling B-lineage commitment and lineage restriction in the FOXOdko, we next analyze the potential of the FOXOdko CLPs to generate T- and myeloid-lineage cells. Using the OP9-DL1 system to evaluate the potential of single progenitor cells to generate T cells, we found that essentially all growing colonies contained T cell progeny (Figure 7A). This indicates that the ability to generate T cells is maintained in FOXOdko lymphoid progenitors. In agreement with this, analysis of the thymus revealed normal or slightly elevated numbers of γδ-, CD4, and CD8 T cells in the FOXOdko mice (Figures S5A, B).
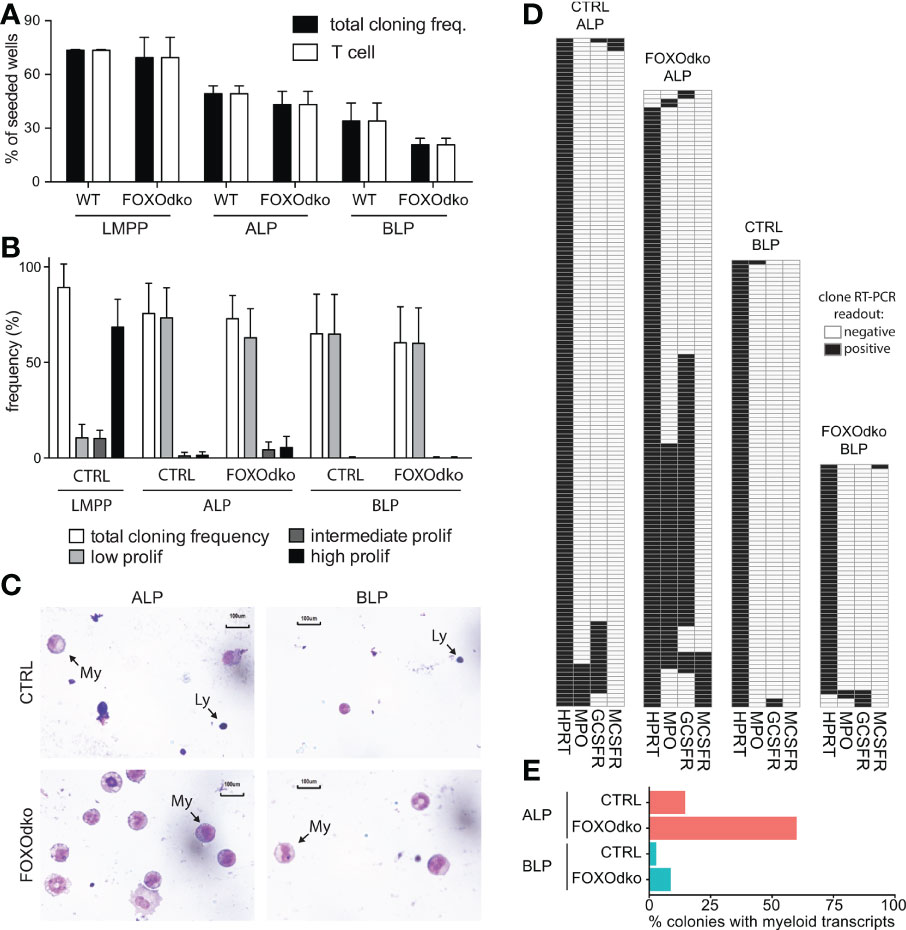
Figure 7 Loss of FOXO alleviates myeloid restriction in CLPs. (A) Frequency of single progenitors generating clones and T-cells (CD90.2/Thy1.2+CD25+) on OP9-DL1. (B) Frequency of single CLPs capable of generating colonies of the indicated size under myeloid growth conditions. Data is from seven independent experiments. (C) Morphology of cells derived from CLPs under myeloid conditions. Arrows indicate lymphoid (Ly) and myeloid (My) cells. (D) Heatmaps of gene expression in single-CLP derived colonies detected by multiplex RT-PCR. Data is from two independent experiments. (E) Percentage of colonies in panel D expressing myeloid transcripts (Mpo, Gcsfr and/or Mcsfr).
Subjecting CLPs to myeloid growth conditions, we found that the CLPs regardless of genotype gave rise to predominantly small (low proliferative) colonies with similar frequency (Figure 7B). To investigate the cellular composition of the colonies, we generated cytospins from pools of clones. As expected, CTRL CLPs mainly generated lymphocytes under myeloid conditions though limited numbers of myeloid cells could be observed, in particular in cytospins generated from ALP derived colonies (Figure 7C). Strikingly, we observed more abundant myeloid cells in cytospins generated from FOXOdko CLP colonies (Figure 7C). As we were unable to generate cytospins from individual colonies because of the low number of cells per colony, we instead performed RT-PCR on individual colonies to investigate the presence of myeloid restricted transcripts on the clonal level. Consistent with the increased generation of myeloid cells, we found that an increased frequency of colonies derived from FOXOdko ALP expressed myeloid transcripts (Figures 7D, E). Hence, the loss of FOXO activity results in the failure to restrict myeloid potential with FOXOdko ALPs, on a clonal level, displaying a higher propensity to generate myeloid cells. We did not find that this was associated with significant stage specific up-regulation of TFs including Spi1 (Pu.1) and the Cebp-family (Figure S5C) nor of myeloid growth factor receptors commonly associated with myeloid differentiation (Figure S5D). Interestingly, the expression of Gcsfr was down-regulated normally in the FOXOdko BLPs (Figure S5D). Together, this demonstrates that the loss of FOXO activity results in a failure to properly restrict the myeloid potential of ALP.
Discussion
The B cell developmental trajectory is critically dependent on TFs for developmental progression, establishing the B cell transcriptional program, and achieving B-lineage commitment. Analyzing the expression pattern of the FOXO TF family genes, we found that Foxo3 was the dominantly expressed FOXO gene at the HSC and LMPP stages. Subsequently, the simultaneous downregulation of Foxo3 and up-regulation of Foxo1 at the CLP stage left Foxo1 as the dominantly expressed Foxo-family gene in B-lineage cells. This temporal switch from Foxo3 to Foxo1 expression at the CLP stage together with the co-expression of Foxo3 and Foxo1, in particular at the BLP stage, prompted us to investigate how the loss of FOXO3 and/or FOXO1 from the HSC onwards impacts the B cell developmental pathway.
In line with Foxo3 being the dominantly expressed Foxo gene in early hematopoiesis, we found that the loss of FOXO3 significantly impaired the generation of LMPPs and CLPs adding FOXO3 to the list of TFs affecting early lymphopoiesis. While the loss of FOXO3 in the LMPPs had not previously been investigated, we also observed much broader effects on to the early B cell developmental pathway compared to prior studies (24, 27) with essentially all steps from proB to immature and transitional B cells being affected. Potentially, this can be explained by the use of KIT, CD25, IgM, and IgD allowing us to better resolve the subtle changes to early B cell development.
As expected from Foxo1 being upregulated at the CLP stage and in line with our previous report (26), we found that the loss of FOXO1 caused no discernable effect on LMPP generation while CLP numbers were perturbed. Our earlier study suggested that FOXO1ko mice displayed a more severe phenotype than initially reported (24) with a complete block at the proB cells stage (26). However, the identity of the residual B cells remained unverified on a molecular level and it remained unresolved if splenic B cells in the FOXO1ko represented mature cells that had developed in the absence of FOXO1. Here unambiguously show that the remaining FOXO1ko B cells represent proB cells and that the FOXO1ko mice, similar to RAG deficient mice, harbor proB also in the spleen. Hence, we show here FOXO1 is absolutely required for B cell development to progress past the CD25 negative proB cell stage and that likely this is directly caused by the failure of the residual proB cells to properly express the Rag genes (25). The difference between the Vav-iCre and Mb1-Cre models (24), likely is a reflection of the Mb1-Cre model (51) allowing B cell progenitors to proceed past early requirements for FOXO1 and EBF1 activity as the expression of Mb1 (Cd79a) depends on EBF1 (14, 43).
Interestingly, we found that the FOXO1ko proB cells maintained normal Ebf1 expression. This suggests that the Foxo1-Ebf1 positive feedback loop, critical for expression of Ebf1 at the CLP stage (26), is less important to maintain Ebf1 expression after B-lineage commitment and the development of proB cells. Potentially the establishment of the positive Ebf1-Pax5 feedforward loop (15, 16) supersedes Foxo1-Ebf1 regulation or simply decrease the need for FOXO activity to a level where FOXO3 can compensate for the loss of FOXO1 to maintain Ebf1 expression.
The BLP stage is the center of the temporal switch from Foxo3 to Foxo1 with the genes displaying similar expression on the mRNA level. This suggested that both could act to support B cell development and that compensation by FOXO3 allowed for developmental progression and B-lineage commitment to occur in the FOXO1ko mice. As hypothesized, the combined loss of FOXO1 and FOXO3 resulted in a complete block of B cell development at the BLP stage. The loss of the B cell lineage was associated with the failure to initiate the early B-lineage gene regulatory program on both the transcriptional and gene regulatory level. The more exacerbated changes observed in the FOXOdko CLPs, as compared to the loss of FOXO1 or FOXO3 alone, clearly show that FOXO1 and FOXO3 synergistically activate the B-lineage gene regulatory circuitry in CLPs. This effect is clearly observable on the reduced or lost expression of several TFs critical for the B-lineage in the FOXOdko CLPs, including Ebf1, Pax5, Pou2af1, and Ets1 (13, 17, 52, 53). Taken together, this suggests that the loss of the B cell lineage in the FOXOdko mice is caused by the failure to establish the coordinated expression of several transcription factors which ultimately results in a failure to express Pax5 and undergo B-lineage commitment.
Both FOXO1 and EBF1 have been implicated to be ‘pioneer factors’ (48, 49) that can open condensed chromatin and make it accessible for DNA binding proteins. While the pioneering function of EBF1 has been studied in the context of B cell development (48, 54–56), it remains to be investigated if the Foxo family has a similar function. With ATACseq, in essence, defining open chromatin through the enrichment of reads originating from accessible regions, we utilized this to characterize how the loss of FOXO and EBF1 in the FOXOdko CLPs affected the establishment of open chromatin. Somewhat surprisingly, we found that despite the close association between regions with highly significant reductions in chromatin accessibility in the FOXOdko CLPs and the binding sites of FOXO and EBF1, only a minor fraction of these regions did not remain open chromatin in the FOXOdko CLPs. In addition, the majority of both FOXO1 and EBF1 bound regions established as open chromatin at the BLP stage were already open chromatin in HSCs. While a role of the low expressed Foxo4 or residual Ebf1 expression for establishing open chromatin cannot be excluded, our data strongly suggests that both FOXO and EBF1 act mainly on pre-established open chromatin at the level of the CLP but that their binding causes further increased accessibility.
While the suppression of myeloid potential in committed B cells relies on PAX5, it remains unclear how the potential to give rise to myeloid cells is restricted in the LMPP to CLP developmental transition (10). Subjecting CLPs to myeloid growth conditions, we found that the ALP from the FOXOdko on the clonal level displayed substantially expanded ability to generate clones containing myeloid cells compared to ALP with normal FOXO function. While we did observe that gains in chromatin accessibility were associated with CEBP-family binding sites in the FOXOdko CLPs, we did not find significant changes to the expression of transcription factors (including Spi1 (Pu.1), Cebpa, and Cebpb) known to influence myeloid fate determination (57, 58) nor of myeloid growth factor receptors. This could potentially suggest that the regulatory changes that occur are simply too subtle for us to detect them or that the loss of FOXO activity alters the response of the CLPs to the myeloid growth conditions rather than directly influencing the expression of genes limiting the myeloid potential. While a role of EBF1 in restricting the myeloid potential in ALPs can’t be excluded, the already very low expression of Ebf1 in normal ALPs would argue against it playing a major role.
Interestingly, we found that the Gcsfr expression was sharply downregulated in the ALP to BLP transition in both normal and FOXOdko mice. Likely, in a similar manner to the PAX5 mediated down-regulation of Mcsfr in proB cells (17, 59), this acts to restrict the response to myeloid growth conditions of BLPs and at least in part could explain the maintained myeloid restriction in FOXOdko BLPs. As this occurs in the FOXOdko BLPs, which essentially lack FOXO activity as well as expression of Ebf1 and Pax5, this suggests that the gene regulatory circuitry mediating myeloid restriction at the CLP stage is independent of major transcription factors known to drive B-lineage commitment.
In sum, we show that both FOXO1 and FOXO3 are needed for the proper development of lymphoid progenitors and B-lineage cells. Strikingly, the combined loss of FOXO1 and FOXO3 in CLPs results in incomplete myeloid restriction, the failure to establish the early B cell gene regulatory program, and the complete loss of the B cell lineage. Future studies aimed at identifying additional factors responsible for establishing gene regulatory features and myeloid restriction at the level of the CLP will be paramount to understanding the early lymphoid specification and lineage restriction events.
Data Availability Statement
The ChIPseq, ATACseq and RNAseq datasets presented in this study are available from the European Nucleotide Archive (https://www.ebi.ac.uk/ena/) under accession numbers PRJEB41018 and PRJEB20316.
Ethics Statement
The animal studies were approved by the regional animal ethics committee in Stockholm.
Author Contributions
RM conceived the study. LP-P, SK, NF, and RM planned the study. SK, NF, MH, TB, and RM performed FACS phenotyping. SK, AK, XLW, and CG performed ATACseq and RNAseq. LP-P, SK, NF, TB, HQ, and RM performed in vitro colony assays. NF, ES, and SL performed transplantation experiments. SK and CD performed ELISA. LP-P, JH, and MK analyzed omics data. A-SJ, JW, NK, and PW assisted with animal studies. LW, SL, and RM supervised the study. LP-P and RM wrote the manuscript with input from the other authors. All authors contributed to the article and approved the submitted version.
Funding
This work was supported by funding from the Swedish Cancer Society (Cancerfonden), Swedish Research Council, King Gustav V Jubilee Fund (Radiumhemmet), the Swedish Foundation for Strategic Research, the Knut and Alice Wallenberg Foundation and generously, a donation by Björn and Lena Ulvaeus. Members of the Ulvaeus family were not involved in the study design, collection, analysis, interpretation of data, the writing of this article or the decision to submit it for publication. In addition, the Karolinska Institutet doctoral education program (KID) supported the doctoral studies of LP-P and JH.
Conflict of Interest
The authors declare that the research was conducted in the absence of any commercial or financial relationships that could be construed as a potential conflict of interest.
Publisher’s Note
All claims expressed in this article are solely those of the authors and do not necessarily represent those of their affiliated organizations, or those of the publisher, the editors and the reviewers. Any product that may be evaluated in this article, or claim that may be made by its manufacturer, is not guaranteed or endorsed by the publisher.
Acknowledgments
We would like to thank the staff at the animal facilities (Karolinska Institutet, KI) for animal care; the Centre for Cellular Analysis (CCA, Karolinska University Hospital) for assistance with cell sorting; the MedH Flow Cytometry core facility (KI) for access to equipment; the Swedish National Infrastructure for Computing (SNIC) at Uppmax for computational resources and data storage; National Bioinformatics Infrastructure Sweden (NBIS) for providing long-term informatics support and mentoring via the Swedish Bioinformatics Advisory program; the National Genomics Infrastructure for sequencing service; Professor Joakim Dillner (KI) with colleagues for access to the Illumina NextSeq 500 system; and Assistant professor Taras Kreslavskiy (KI) for critical reading of the manuscript.
Supplementary Material
The Supplementary Material for this article can be found online at: https://www.frontiersin.org/articles/10.3389/fimmu.2022.880668/full#supplementary-material
References
1. Adolfsson J, Mansson R, Buza-Vidas N, Hultquist A, Liuba K, Jensen CT, et al. Identification of Flt3+ Lympho-Myeloid Stem Cells Lacking Erythro-Megakaryocytic Potential. Cell (2005) 121:295–306. doi: 10.1016/j.cell.2005.02.013
2. Mansson R, Hultquist A, Luc S, Yang L, Anderson K, Kharazi S, et al. Molecular Evidence for Hierarchical Transcriptional Lineage Priming in Fetal and Adult Stem Cells and Multipotent Progenitors. Immunity (2007) 26:407–19. doi: 10.1016/j.immuni.2007.02.013
3. Kondo M, Weissman IL, Akashi K. Identification of Clonogenic Common Lymphoid Progenitors in Mouse Bone Marrow. Cell (1997) 91:661–72. doi: 10.1016/s0092-8674(00)80453-5
4. Inlay MA, Bhattacharya D, Sahoo D, Serwold T, Seita J, Karsunky H, et al. Ly6d Marks the Earliest Stage of B-Cell Specification and Identifies the Branchpoint Between B-Cell and T-Cell Development. Genes Dev (2009) 23:2376–81. doi: 10.1101/gad.1836009
5. Mansson R, Zandi S, Welinder E, Tsapogas P, Sakaguchi N, Bryder D, et al. Single-Cell Analysis of the Common Lymphoid Progenitor Compartment Reveals Functional and Molecular Heterogeneity. Blood (2010) 115:2601–9. doi: 10.1182/blood-2009-08-236398
6. Mansson R, Zandi S, Anderson K, Martensson I-L, Jacobsen SEW, Bryder D, et al. B-Lineage Commitment Prior to Surface Expression of B220 and CD19 on Hematopoietic Progenitor Cells. Blood (2008) 112:1048–55. doi: 10.1182/blood-2007-11-125385
7. Jensen CT, Åhsberg J, Sommarin MNE, Strid T, Somasundaram R, Okuyama K, et al. Dissection of Progenitor Compartments Resolves Developmental Trajectories in B-Lymphopoiesis. J Exp Med (2018) 215:1947–63. doi: 10.1084/jem.20171384
8. Rolink A, Grawunder U, Winkler TH, Karasuyama H, Melchers F. IL-2 Receptor Alpha Chain (CD25, TAC) Expression Defines a Crucial Stage in Pre-B Cell Development. Int Immunol (1994) 6:1257–64. doi: 10.1093/intimm/6.8.1257
9. Hardy RR, Kincade PW, Dorshkind K. The Protean Nature of Cells in the B Lymphocyte Lineage. Immunity (2007) 26:703–14. doi: 10.1016/j.immuni.2007.05.013
10. Rothenberg EV. Transcriptional Control of Early T and B Cell Developmental Choices. Annu Rev Immunol (2014) 32:283–321. doi: 10.1146/annurev-immunol-032712-100024
11. Mandel EM, Grosschedl R. Transcription Control of Early B Cell Differentiation. Curr Opin Immunol (2010) 22:161–7. doi: 10.1016/j.coi.2010.01.010
12. Sigvardsson M. Molecular Regulation of Differentiation in Early B-Lymphocyte Development. Int J Mol Sci (2018) 19(7):1928. doi: 10.3390/ijms19071928
13. Lin H, Grosschedl R. Failure of B-Cell Differentiation in Mice Lacking the Transcription Factor EBF. Nature (1995) 376:263–7. doi: 10.1038/376263a0
14. Zandi S, Mansson R, Tsapogas P, Zetterblad J, Bryder D, Sigvardsson M. EBF1 Is Essential for B-Lineage Priming and Establishment of a Transcription Factor Network in Common Lymphoid Progenitors. J Immunol (2008) 181:3364–72. doi: 10.4049/jimmunol.181.5.3364
15. Decker T, di Magliano MP, McManus S, Sun Q, Bonifer C, Tagoh H, et al. Stepwise Activation of Enhancer and Promoter Regions of the B Cell Commitment Gene Pax5 in Early Lymphopoiesis. Immunity (2009) 30:508–20. doi: 10.1016/j.immuni.2009.01.012
16. Roessler S, GyOry I, Imhof S, Spivakov M, Williams RR, Busslinger M, et al. Distinct Promoters Mediate the Regulation of Ebf1 Gene Expression by Interleukin-7 and Pax5. Mol Cell Biol (2007) 27:579–94. doi: 10.1128/MCB.01192-06
17. Nutt SL, Heavey B, Rolink AG, Busslinger M. Commitment to the B-Lymphoid Lineage Depends on the Transcription Factor Pax5. Nature (1999) 401:556–62. doi: 10.1038/44076
18. Cobaleda C, Jochum W, Busslinger M. Conversion of Mature B Cells Into T Cells by Dedifferentiation to Uncommitted Progenitors. Nature (2007) 449:473–7. doi: 10.1038/nature06159
19. Nechanitzky R, Akbas D, Scherer S, GyOry I, Hoyler T, Ramamoorthy S, et al. Transcription Factor EBF1 Is Essential for the Maintenance of B Cell Identity and Prevention of Alternative Fates in Committed Cells. Nat Immunol (2013) 14:867–75. doi: 10.1038/ni.2641
20. Gyory I, Boller S, Nechanitzky R, Mandel E, Pott S, Liu E, et al. Transcription Factor Ebf1 Regulates Differentiation Stage-Specific Signaling, Proliferation, and Survival of B Cells. Genes Dev (2012) 26:668–82. doi: 10.1101/gad.187328.112
21. Cobaleda C, Schebesta A, Delogu A, Busslinger M. Pax5: The Guardian of B Cell Identity and Function. Nat Immunol (2007) 8:463–70. doi: 10.1038/ni1454
22. Szydłowski M, Jabłońska E, Juszczyński P. FOXO1 Transcription Factor: A Critical Effector of the PI3K-AKT Axis in B-Cell Development. Int Rev Immunol (2014) 33:146–57. doi: 10.3109/08830185.2014.885022
23. Baracho GV, Miletic AV, Omori SA, Cato MH, Rickert RC. Emergence of the PI3-Kinase Pathway as a Central Modulator of Normal and Aberrant B Cell Differentiation. Curr Opin Immunol (2011) 23:178–83. doi: 10.1016/j.coi.2011.01.001
24. Dengler HS, Baracho GV, Omori SA, Bruckner S, Arden KC, Castrillon DH, et al. Distinct Functions for the Transcription Factor Foxo1 at Various Stages of B Cell Differentiation. Nat Immunol (2008) 9:1388–98. doi: 10.1038/ni.1667
25. Amin RH, Schlissel MS. Foxo1 Directly Regulates the Transcription of Recombination-Activating Genes During B Cell Development. Nat Immunol (2008) 9:613–22. doi: 10.1038/ni.1612
26. Mansson R, Welinder E, Åhsberg J, Lin YC, Benner C, Glass CK, et al. Positive Intergenic Feedback Circuitry, Involving EBF1 and FOXO1, Orchestrates B-Cell Fate. PNAS (2012) 109:21028–33. doi: 10.1073/pnas.1211427109
27. Hinman RM, Nichols WA, Diaz TM, Gallardo TD, Castrillon DH, Satterthwaite AB. Foxo3-/- Mice Demonstrate Reduced Numbers of Pre-B and Recirculating B Cells But Normal Splenic B Cell Sub-Population Distribution. Int Immunol (2009) 21:831–42. doi: 10.1093/intimm/dxp049
28. de Boer J, Williams A, Skavdis G, Harker N, Coles M, Tolaini M, et al. Transgenic Mice With Hematopoietic and Lymphoid Specific Expression of Cre. Eur J Immunol (2003) 33:314–25. doi: 10.1002/immu.200310005
29. Paik J-H, Kollipara R, Chu G, Ji H, Xiao Y, Ding Z, et al. FoxOs Are Lineage-Restricted Redundant Tumor Suppressors and Regulate Endothelial Cell Homeostasis. Cell (2007) 128:309–23. doi: 10.1016/j.cell.2006.12.029
30. Castrillon DH, Miao L, Kollipara R, Horner JW, DePinho RA. Suppression of Ovarian Follicle Activation in Mice by the Transcription Factor Foxo3a. Science (2003) 301:215–8. doi: 10.1126/science.1086336
31. Shinkai Y, Rathbun G, Lam KP, Oltz EM, Stewart V, Mendelsohn M, et al. RAG-2-Deficient Mice Lack Mature Lymphocytes Owing to Inability to Initiate V(D)J Rearrangement. Cell (1992) 68:855–67. doi: 10.1016/0092-8674(92)90029-c
32. Dobin A, Davis CA, Schlesinger F, Drenkow J, Zaleski C, Jha S, et al. STAR: Ultrafast Universal RNA-Seq Aligner. Bioinformatics (2013) 29:15–21. doi: 10.1093/bioinformatics/bts635
33. Heinz S, Benner C, Spann N, Bertolino E, Lin YC, Laslo P, et al. Simple Combinations of Lineage-Determining Transcription Factors Prime Cis-Regulatory Elements Required for Macrophage and B Cell Identities. Mol Cell (2010) 38:576–89. doi: 10.1016/j.molcel.2010.05.004
34. Robinson MD, McCarthy DJ, Smyth GK. Edger: A Bioconductor Package for Differential Expression Analysis of Digital Gene Expression Data. Bioinformatics (2010) 26:139–40. doi: 10.1093/bioinformatics/btp616
35. Zhou Y, Zhou B, Pache L, Chang M, Khodabakhshi AH, Tanaseichuk O, et al. Metascape Provides a Biologist-Oriented Resource for the Analysis of Systems-Level Datasets. Nat Commun (2019) 10:1523. doi: 10.1038/s41467-019-09234-6
36. Buenrostro JD, Giresi PG, Zaba LC, Chang HY, Greenleaf WJ. Transposition of Native Chromatin for Fast and Sensitive Epigenomic Profiling of Open Chromatin, DNA-Binding Proteins and Nucleosome Position. Nat Meth (2013) 10:1213–8. doi: 10.1038/nmeth.2688
37. Langmead B, Salzberg SL. Fast Gapped-Read Alignment With Bowtie 2. Nat Publishing Group (2012) 9:357–9. doi: 10.1038/nmeth.1923
38. Kent WJ, Sugnet CW, Furey TS, Roskin KM, Pringle TH, Zahler AM, et al. The Human Genome Browser at UCSC. Genome Res (2002) 12:996–1006. doi: 10.1101/gr.229102
39. Bouderlique T, Peña Perez L, Kharazi S, Hils M, Li X, Krstic A, et al. The Concerted Action of E2-2 and HEB Is Critical for Early Lymphoid Specification. Front Immunol (2019) 10:455. doi: 10.3389/fimmu.2019.00455
40. Hu M, Krause D, Greaves M, Sharkis S, Dexter M, Heyworth C, et al. Multilineage Gene Expression Precedes Commitment in the Hemopoietic System. Genes Dev (1997) 11:774–85. doi: 10.1101/gad.11.6.774
41. Rumfelt LL, Zhou Y, Rowley BM, Shinton SA, Hardy RR. Lineage Specification and Plasticity in CD19- Early B Cell Precursors. J Exp Med (2006) 203:675–87. doi: 10.1084/jem.20052444
42. Sigvardsson M, Clark DR, Fitzsimmons D, Doyle M, Åkerblad P, Breslin T, et al. Early B-Cell Factor, E2A, and Pax-5 Cooperate To Activate the Early B Cell-Specific Mb-1 Promoter. Mol Cell Biol (2002) 22:8539–51. doi: 10.1128/MCB.22.24.8539-8551.2002
43. Maier H, Ostraat R, Gao H, Fields S, Shinton SA, Medina KL, et al. Early B Cell Factor Cooperates With Runx1 and Mediates Epigenetic Changes Associated With Mb-1 Transcription. Nat Immunol (2004) 5:1069–77. doi: 10.1038/ni1119
44. Akerblad P, Rosberg M, Leanderson T, Sigvardsson M. The B29 (Immunoglobulin Beta-Chain) Gene Is a Genetic Target for Early B-Cell Factor. Mol Cell Biol (1999) 19:392–401. doi: 10.1128/mcb.19.1.392
45. Kerdiles YM, Beisner DR, Tinoco R, Dejean AS, Castrillon DH, DePinho RA, et al. Foxo1 Links Homing and Survival of Naive T Cells by Regulating L-Selectin, CCR7 and Interleukin 7 Receptor. Nat Immunol (2009) 10:176–84. doi: 10.1038/ni.1689
46. Ouyang W, Beckett O, Flavell RA, Li MO. An Essential Role of the Forkhead-Box Transcription Factor Foxo1 in Control of T Cell Homeostasis and Tolerance. Immunity (2009) 30:358–71. doi: 10.1016/j.immuni.2009.02.003
47. Tothova Z, Kollipara R, Huntly BJ, Lee BH, Castrillon DH, Cullen DE, et al. FoxOs Are Critical Mediators of Hematopoietic Stem Cell Resistance to Physiologic Oxidative Stress. Cell (2007) 128:325–39. doi: 10.1016/j.cell.2007.01.003
48. Treiber T, Mandel EM, Pott S, GyOry I, Firner S, Liu ET, et al. Early B Cell Factor 1 Regulates B Cell Gene Networks by Activation, Repression, and Transcription- Independent Poising of Chromatin. Immunity (2010) 32:714–25. doi: 10.1016/j.immuni.2010.04.013
49. Hatta M, Cirillo LA. Chromatin Opening and Stable Perturbation of Core Histone:DNA Contacts by Foxo1*. J Biol Chem (2007) 282:35583–93. doi: 10.1074/jbc.M704735200
50. Lin YC, Jhunjhunwala S, Benner C, Heinz S, Welinder E, Mansson R, et al. A Global Network of Transcription Factors, Involving E2A, EBF1 and Foxo1, That Orchestrates B Cell Fate. Nat Immunol (2010) 11:635–43. doi: 10.1038/ni.1891
51. Hobeika E, Thiemann S, Storch B, Jumaa H, Nielsen PJ, Pelanda R, et al. Testing Gene Function Early in the B Cell Lineage in Mb1-Cre Mice. PNAS (2006) 103:13789–94. doi: 10.1073/pnas.0605944103
52. Schubart DB, Rolink A, Kosco-Vilbois MH, Botteri F, Matthias P. B-Cell-Specific Coactivator OBF-1/OCA-B/Bob1 Required for Immune Response and Germinal Centre Formation. Nature (1996) 383:538–42. doi: 10.1038/383538a0
53. Eyquem S, Chemin K, Fasseu M, Chopin M, Sigaux F, Cumano A, et al. The Development of Early and Mature B Cells Is Impaired in Mice Deficient for the Ets-1 Transcription Factor. Eur J Immunol (2004) 34:3187–96. doi: 10.1002/eji.200425352
54. Li R, Cauchy P, Ramamoorthy S, Boller S, Chavez L, Grosschedl R. Dynamic EBF1 Occupancy Directs Sequential Epigenetic and Transcriptional Events in B-Cell Programming. Genes Dev (2018) 32:96–111. doi: 10.1101/gad.309583.117
55. Wang Y, Zolotarev N, Yang C-Y, Rambold A, Mittler G, Grosschedl R. A Prion-Like Domain in Transcription Factor EBF1 Promotes Phase Separation and Enables B Cell Programming of Progenitor Chromatin. Immunity (2020) 53:1151–67. doi: 10.1016/j.immuni.2020.10.009
56. Strid T, Okuyama K, Tingvall-Gustafsson J, Kuruvilla J, Jensen CT, Lang S, et al. B Lymphocyte Specification Is Preceded by Extensive Epigenetic Priming in Multipotent Progenitors. J Immunol (2021) 206:2700–13. doi: 10.4049/jimmunol.2100048
57. DeKoter RP, Singh H. Regulation of B Lymphocyte and Macrophage Development by Graded Expression of PU.1. Science (2000) 288:1439–41. doi: 10.1126/science.288.5470.1439
58. Xie H, Ye M, Feng R, Graf T. Stepwise Reprogramming of B Cells Into Macrophages. Cell (2004) 117:663–76. doi: 10.1016/s0092-8674(04)00419-2
Keywords: B cell, FOXO (forkhead box protein O), lineage commitment/specification, myeloid restriction, gene regulation
Citation: Peña-Pérez L, Kharazi S, Frengen N, Krstic A, Bouderlique T, Hauenstein J, He M, Somuncular E, Li Wang X, Dahlberg C, Gustafsson C, Johansson A-S, Walfridsson J, Kadri N, Woll P, Kierczak M, Qian H, Westerberg L, Luc S and Månsson R (2022) FOXO Dictates Initiation of B Cell Development and Myeloid Restriction in Common Lymphoid Progenitors. Front. Immunol. 13:880668. doi: 10.3389/fimmu.2022.880668
Received: 21 February 2022; Accepted: 23 March 2022;
Published: 04 May 2022.
Edited by:
James R. Hagman, National Jewish Health, United StatesReviewed by:
Kay L. Medina, Mayo Clinic, United StatesTomokatsu Ikawa, Tokyo University of Science, Japan
Copyright © 2022 Peña-Pérez, Kharazi, Frengen, Krstic, Bouderlique, Hauenstein, He, Somuncular, Li Wang, Dahlberg, Gustafsson, Johansson, Walfridsson, Kadri, Woll, Kierczak, Qian, Westerberg, Luc and Månsson. This is an open-access article distributed under the terms of the Creative Commons Attribution License (CC BY). The use, distribution or reproduction in other forums is permitted, provided the original author(s) and the copyright owner(s) are credited and that the original publication in this journal is cited, in accordance with accepted academic practice. No use, distribution or reproduction is permitted which does not comply with these terms.
*Correspondence: Robert Månsson, cm9iZXJ0Lm1hbnNzb25Aa2kuc2U=; orcid.org/0000-0003-0738-0328
†These authors have contributed equally to this work