- Shanghai Key Laboratory of Maternal-Fetal Medicine, Department of Biobank, Clinical and Translational Research Center, Shanghai First Maternity and Infant Hospital, School of Medicine, Tongji University, Shanghai, China
Macrophages are versatile immune cells associated with various diseases, and their phenotypes and functions change on the basis of the surrounding environments. Reprogramming of metabolism is required for the proper polarization of macrophages. This review will focus on basic metabolic pathways, the effects of key enzymes and specific products, relationships between cellular metabolism and macrophage polarization in different diseases and the potential prospect of therapy targeted key metabolic enzymes. In particular, the types and characteristics of macrophages at the maternal-fetal interface and their effects on a successful conception will be discussed.
Overview of Macrophages
Origination of Macrophages
It is believed that macrophages belong to the mononuclear phagocytic system, mainly derived from bone marrow progenitor cells. When macrophages are within the bloodstream, they are called mononuclear cells. When inflammation or injury occurs, monocytes are recruited to tissues and induced into macrophages based on the condition of the local environment (1, 2). As a matter of fact, most adult tissue-resident macrophages are seeded during embryonic development, have self-renewal capacity and are maintained without depending on monocytes (2–4). The most majority of tissue-resident macrophages are derived from erythro-myeloid progenitors that develop in vitellus capsule embryos. However, the existence of tissue-specific macrophage progenitors that can promote the maintenance of adult macrophages has not been clarified (4). When the quantity of monocytes in the blood is reduced, those in the tissues are largely unaffected. However, monocytes and their progenitors can supplement classical tissue mononuclear phagocytes as needed (2). Embryonic macrophages are involved in tissue remodeling while monocyte-derived macrophages mainly play a role in host immune response (2). The activation status and the function of tissue macrophages under different stimuli are largely dependent on the local tissue microenvironment (3, 5). The importance of the local microenvironment has been identified through studies based on transcriptomics, epigenetics and open chromatin regions (3).
Currently, comparative studies of gene expression have been conducted in macrophages from the most commonly used cell sources: murine bone marrow, human peripheral monocytes, and human leukemic monocytic cell line THP-1, as well as those derived from induced pluripotent stem cells (iPSCs) which were generated from differentiated cells by upregulating pluripotency factors (Oct3/4, Sox2, c-Myc, and Klf4) (6). MCP1, IL6, TNF, IL10, CXCL12, IL1β and IL6 are expressed in human blood-derived macrophages; meanwhile, IL1β, TNFα, iNOS, IL12β, Arg1, VEGFA and IL10 are expressed in murine bone marrow-derived M1 and M2 macrophages (7). IL1 and CD36 are expressed in THP-1 cells in vitro (7), which can be treated as a model of human macrophages when studing relatively simple biological processes, but cannot be used in more comprehensive immunopharmacology and drug screening programs (8). Human peripheral blood-derived macrophages and human induced pluripotent stem cell (iPSC)-derived macrophages showed similar gene expression patterns, reminding that iPSC-derived monocytes can be used as a credible cell source of human macrophages for in vitro studies (7, 9). Further studies are needed to determine how the heterogeneity of macrophages is reflected, whether the functions and activities of tissue-specific macrophages are different from those of peripheral migration-induced cells, and the concordance between in vitro and in vivo experiments.
Classification of Macrophages
Diversity and plasticity are hallmarks of macrophages. In response to IFN-γ/lipopolysaccharide(LPS) (10) or IL-4/IL-13, macrophages undergo M1 or M2 activation (11), which represent two extremes of a continuum of functional states. M1 macrophages have proinflammatory abilities and are able to initiate and maintain inflammatory reactions (12, 13), induce Th1 response activation (14), activate endothelial cells, amplify antigen presenting capacity (12, 14, 15), secrete proinflammatory cytokines and recruit other immune cells into inflammatory tissue. Nevertheless, M2 macrophages release anti-inflammatory mediators (16), support angiogenesis, induce adaptive Th2 immunity, refurnish and repair of scavenge debris and damaged tissue, take part in tumor progression, allergic reactions, and response to helminths (15). Some molecules are relative to both M1 and M2 macrophage polarization, such as NF‐κB (17), IRF, AP1, PPAR‐γ, AMPK and SIRPα (18). Some pathways are mainly involved in one polarization process of macrophages, JAK/STAT1 (12), JAK/STAT5 (19), extracellular signal-regulated kinase (ERK) (19), and Notch-RBP-J signaling pathway (18) take part in M1 polarization. JAK/STAT6 (12) and IL-4-JNK-c-Myc pathway (20) participate in M2 polarization. The common transcription factors and the differences in M1 and M2 macrophages are described in Figure 1.
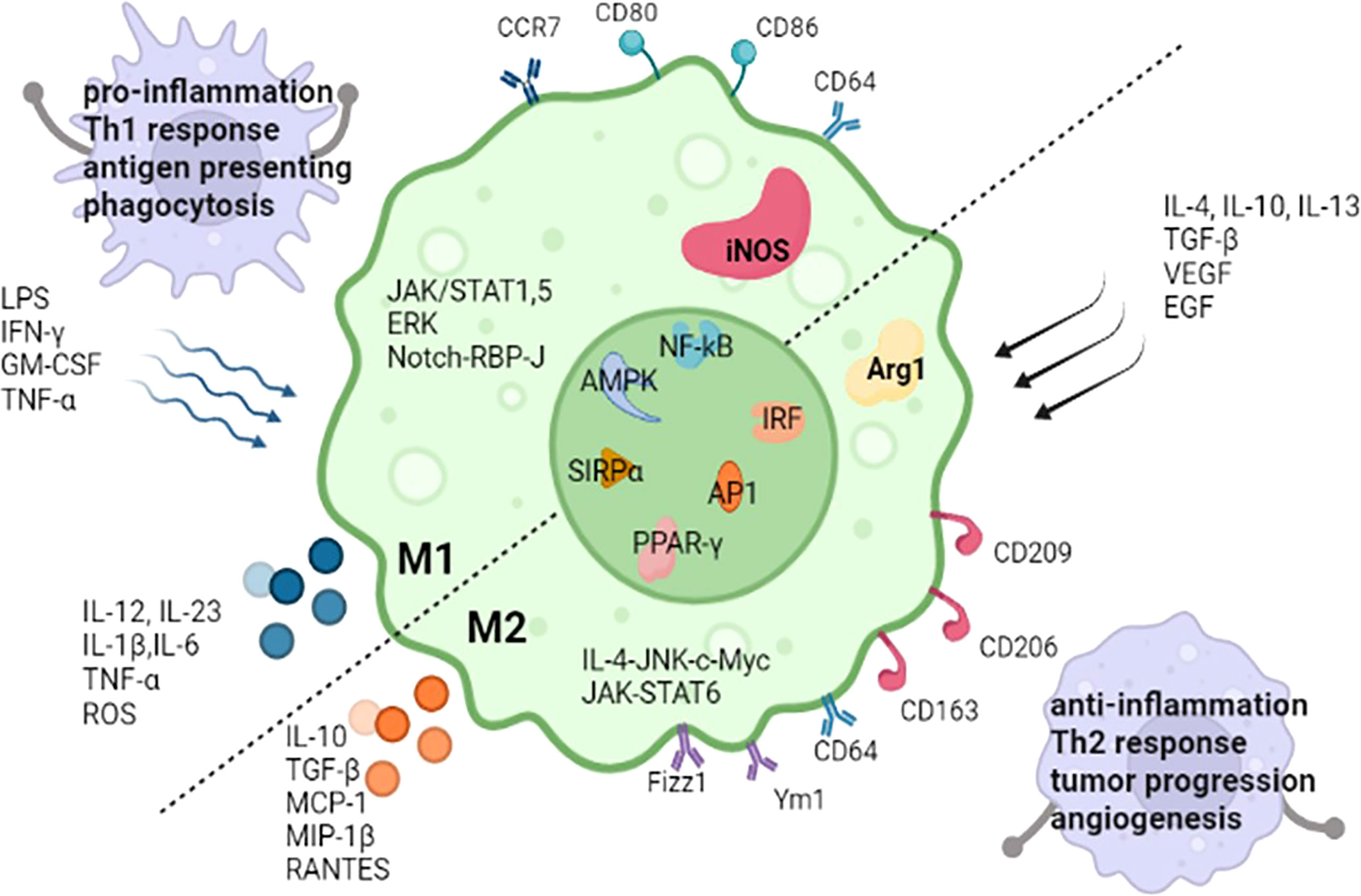
Figure 1 The common transcription factors and the differences of stimuli, markers, secreted cytokines, the main regulate pathways and functions between M1 and M2 macrophages. iNOS, inducible nitric oxide synthase; Arg1, Arginase 1; LPS, lipopolysaccharides; GM-CSF, granulocyte-macrophage colony stimulating factor; IFN-γ, interferon-gamma; IL, interleukin; TNF-α, tumor necrosis factor-α; TGF‐β, transforming growth factor‐β; ROS, reactive oxygen species; VEGF, vascular endothelial growth factor; EGF, epidermal growth factor; Ym1, chitinase 3-like 3; Fizz1, resistin-like-α; MIP-1β, macrophages inflammatory protein; MCP-1, monocyte chemo-attractant protein-1; RANTES, regulated on activation, normal T cell expressed and secreted; ERK, extracellular signal-regulated kinase; RBP-J, recombination signal-binding protein Jk; IRF, interferon‐regulatory factors; NF‐κB, nuclear factor‐κB; AP1, activator protein1; AMPK, adenosine monophosphate kinase; PPAR‐γ, peroxisome proliferator‐activated receptor‐γ; SIRPα, signal regulatory protein α. RBP-J, recombination signal-binding protein Jk.
Recent studies have found that the M2 macrophages are further sub-categorized into M2a, M2b, M2c, and M2d subtypes (21). M2a macrophages can be induced by IL-4 or IL-13, expressing high levels of arginase 1 (Arg1), FIZZ1 and Ym1 (22), playing a role in anti-inflammatory activity and tissue remodeling. M2b macrophages are activated by IL‐1 receptor ligand, immune complexes (ICs) and Toll-like receptor (TLR) agonists and produce both anti-inflammatory and proinflammatory cytokines, including IL-6, IL-10, CCL1, CD86, TNF-α and SpHK1 (23), playing a role in immunoregulation. The M2c subset of macrophages is induced by IL-10, TGF-β or glucocorticoids (24), secreting high levels of IL-10, TGF-β, CCL16, CCL18, MMP7, MMP8, and TIMP1, playing crucial roles in the phagocytosis of apoptotic cells (2) and wound healing (25). M2d macrophages, also called tumor-associated macrophages (TAMs), can be induced by IL-6 or combined exposure to adenosine 2A receptor (A2AR) agonists and TLR agonists. M2d macrophages secrete high levels of TGF-β, VEGF and IL-10, and low levels of IL-1β, IL-12 and TNF-α, taking part in tumor growth, angiogenesis and metastasis (26). It has been demonstrated that several stimuli in the tumor microenvironment, such as hypoxia, may contribute to the tumoral heterogeneity of TAMs. The integrated use of new technologies, such as single-cell RNA-seq, spatial transcriptomics, mass cytometry, and systems biology approaches, is promising to strongly reveal the tumoral heterogeneity of TAMs, potentially redefining TAMs with new valuable biomarkers (27). Excessive activity of either polarized phenotype is responsible for tissue damage, inflammatory disease, fibrosis, or tumor growth. Fortunately, macrophages sustain plasticity after activation and can change phenotypes according to the microenvironment (15).
Functional macrophage polarization has been reported in vivo, under physiological conditions (embryogenesis and pregnancy) and pathological conditions (chronic inflammation, infection and cancer). In some conditions, such as infection or pregnancy, macrophage polarizations express mixed or unique phenotypes. in vitro Because of the complexity of tissue macrophages, the in vitro-based M1/M2 dichotomous classification does not fully capture the classification of them. Some researchers have hypothesized that an M3 switch phenotype exists under the background of M1 and M2 phenotypes based on studies in lung diseases (28). M3 switch phenotype reprograms toward the anti-inflammatory M2 phenotype with proinflammatory stimuli, contrarily, M3 switches to proinflammatory M1 phenotype with anti-inflammatory stimuli. Therefore, understanding the role of coexisting phenotypes of macrophages and the mechanisms driving their dynamic modulation to adjust to the microenvironmental changes will be a key challenge in the coming years. The effort might provide a great prospective for designing macrophage-centered diagnostic and therapeutic strategies.
Tissue Specific Macrophages: Maternal-Fetal Interface Macrophages
The maternal-fetal interface consist of various types of immune cells, such as decidual macrophages (dMϕs), natural killer (dNK) cells, T cells, dendritic cells, B cells and NKT cells. dMϕs account for a proportion of ~20% of decidual immune cells, and is the second most common immune cells during early human pregnancy (29). dMϕs are present in all stages of pregnancy and cannot be simply distinguished according to the M1 or M2 types. dMϕs constantly switch in the spectrum of continuous changes between the M1 and M2 types throughout the pregnancy. It has been found that dMϕs around the blastocyst are inclined toward M1 polarization before implantation. While trophoblast cells begin to invade the myometrium, dMϕ are inclined toward M1/M2 mixed-type polarization. M2 phenotype predominates during the late first trimester and maintenances of pregnancy during the second trimester. Then during the third trimester and parturition, M2 phenotype begins to decline and M1 phenotype again increases. Successful pregnancy requires that the dMϕs activation states remain regulated throughout pregnancy. Imbalanced M1/M2 dynamics are associated with complications, such as fetal growth restriction, preeclampsia and preterm delivery.
Different from dMϕs, which are primarily recruited from the maternal peripheral circulation, there is another homogeneous population of macrophages of fetal origin at the maternal-fetal interface, which are called Hofbauer cells (HBCs) (30). HBCs exist in the placental villi starting from Day 11 in mice and Day 18 in humans, persisting until parturition and representing a highly pleiomorphic cell population (31). HBCs phenotypically and functionally resemble M2 macrophages and are thought to have broad roles in immune regulation, placental morphogenesis, stromal water content and ion transport across the maternal–fetal barrier (32). In vitroAlterations of HBCs have been associated with several pregnancy disorders, such as Villitis and preterm delivery (33).
HBCs may be DC-SIGN+/CD163+, which may be connected with the relation between the high expression of IL-10 in the chorionic villi and the maintenance of immune regulation (34). CD28 expression has also been found in Hofbauer cells, which may adjust the immune function of leukocytes positively or negatively, and may have a very important influence on physiological or pathological processes (35). HBCs expressd higher levels of SPP1, PLIN2, HMOX1, CD36, and LYVE1, whereas dMϕs showed higher expression of HLA genes (HLA-DRA, HLA-DPA1, HLA-DRB1, HLA-DPB1, HLA-DQA1, and HLA-DMA) and invariant chain CD74, indicating strong antigen-presenting capacity of dMϕs. What’s more, dMϕ sspecifically expressed MS4A4A, STAB1, SEPP1, and MS4A7 (36). In a recent study, CD74 was regarded as a critical marker in Hofbauer cells. CD45+CD68+CD74- cells were determined as HBC-like-1 cells and CD45+CD68+CD74+ cells were determined as HBC-like-2 cells (37). The expression of these markers is regulated by environmental signals, such as cytokines and hormones,. Meanwhile, it is suggested that differential epigenetic regulatory patterns might be critical for the functional and characteristic differences between dMϕ and HBCs (38). Epigenetic patterns will give clues for further understanding of the immunological characteristics of dMϕ and HBCs during human pregnancy.
Metabolic Signature of Macrophage Polarization in Physiological and Pathological Situations
In the 1920s, Warburg reported the “Warburg effect” in which cancer cells “ferment” glucose to produce lactic acid in aerobic environment, whereas noncancer cells rely primarily on oxidative phosphorylation in the presence of adequate oxygen levels (39). Since then, studies have confirmed lipopolysaccharide which is the cell wall components of gram-negative bacteria can stimulate aerobic glycolysis and produce the Warburg effect in macrophages (40). In 2011, Mathis and Shoelson introduced the term immunometabolism, which was defined as the interaction between inflammation and metabolic diseases (40). Recently, the term immunometabolism has been given new meanings that incorporate the following components: contributions of metabolic pathways to the development, maturity, destiny, and behavior of immune cells; the changes in intracellular metabolic pathways that alter the functions of immune cells; and the metabolic reprogramming of immune cells (39). Immunometabolism opens new perspectives for modulating immune responses. Thus, targeting metabolic machineries is a potential treatment for immune-related diseases (17). This review mainly describes the metabolic characteristics of activated macrophages, the roles of specific metabolites, and the crucial steps in metabolic processes. In addition, metabolic features in decidual macrophages were summarized. The main metabolic specialties of M1 and M2 macrophages are exhibited in Table 1. The main metabolic pathways and crucial metabolites in macrophages are shown in Figure 2.
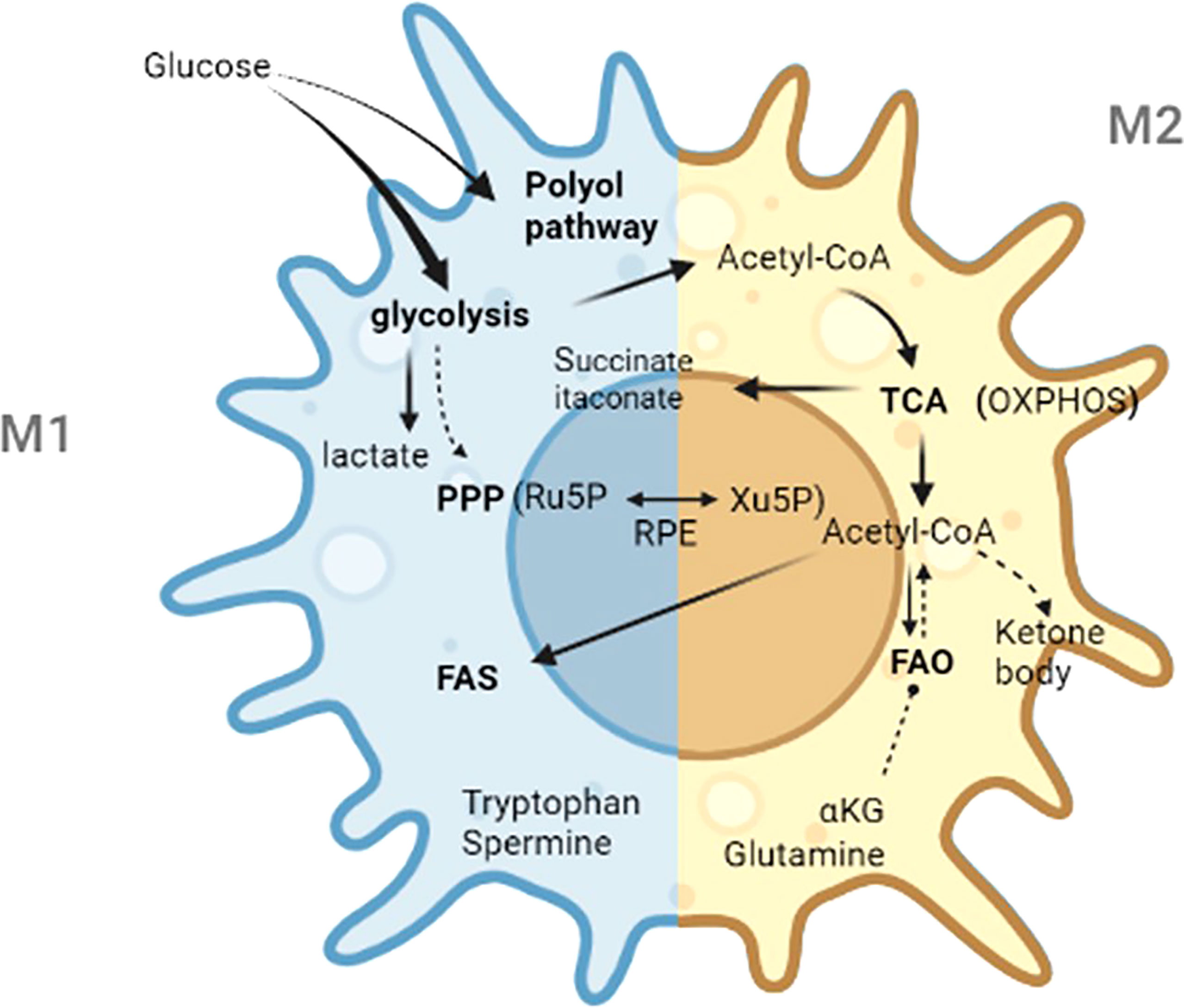
Figure 2 The main metabolic pathways and crucial metabolites in macrophages. Ru5P, d-ribulose-5-phosphate; Xu5P, d-xylulose-5-phosphate; RPE, ribulose 5-phosphate 3-epimerase; PPP, pentose phosphate pathway; TCA, tricarboxylic acid; FAS, fatty acid synthesis; FAO, fatty acid oxidation; Acetyl-CoA, acetyl-Coenzyme A; OXPHOS, oxidative phosphorylation; α-KG, α-ketoglutarate.
Glycometabolism in Macrophages
Glycolysis
Glycolysis includes aerobic and anaerobic glycolysis. Pyruvate is the end product of glycolysis (62). Glycolysis not only provides rapid production of ATP, but also produces metabolic intermediates, including material used for the biosynthesis of nucleotides and proteins in macrophages (63). When LPS-inducible glycolysis is attenuated in macrophages, macrophages switch to the M2 phenotype and is accompanied by a decrease in the inflammatory response (41). However, elevated glucose levels could enhance the expression of inflammatory factors, such as IL-6, IL-1β and TNF-α, in monocytes (42). TAMs are a heterogeneous cell population dominated by M2-type macrophages, corresponding to the increased mitochondrial respiration and reduced glycolysis (64). It has been shown that chloroquine, a proven antimalarial drug, reprogrammed the metabolism of TAMs from oxidative phosphorylation to glycolysis and switched TAMs to the tumor-killing M1 phenotype (65). What’s more, glycolysis contributes to the proinflammation of macrophages in adipose tissue of obesities (66). M1 macrophages use glycolysis to rapidly kill other cells (43), while M2 macrophages obtain energy from mitochondrial oxidative phosphorylation (OXPHOS) and fatty acid oxidation (FAO) (4, 49, 67). Glycolysis is pivotal to M1-type macrophages, but its effects on M2-type macrophages remain unclear. While glycolytic activity is effectively suppressed, there is no effective suppression of the expression of M2 differentiation markers, intracellular ATP levels, oxidative phosphorylation and STAT6 phosphorylation (68). A recent study found that HIF1α-dependent glycolysis is associated with M2 macrophage differentiation, indicating that glycolysis is also essential to the M2 macrophages polarization (44). Mitochondrial elimination, also known as mitochondrial autophagy is the process of eliminating damaged mitochondria (69). It has been found that mitochondrial elimination is essential for the glycolytic switch during macrophage polarization toward the M1 phenotype (70).
There are three irreversible reactions catalyzed by hexokinase, 6-phosphofructokinase 1 (PFK1), and pyruvate kinase (PK) in the glycolytic pathway. These enzymes are three regulatory points of the glycolytic pathway. Recent studies have mainly focused on the isozymes of these enzymes. For example, researchers found that glucose uptake and the expression of proinflammatory cytokines were attenuated by inhibiting hexokinase 2 (HK2) expression in macrophages (71). Likewise, targeting inhibition the expression of glycolysis limiting enzyme PFK-m by miR-21 was associated with the suppression of macrophage glycolysis (72) Pyruvate kinase M (PKM) is glycolytic enzyme, containing two isoforms (PKM1 and PKM2), and also take part in the metabolic changes in activated macrophages. PKM1 converts phosphoenolpyruvate to pyruvate, which is the final step of glycolysis. Pkm2 was identified as a partner of proinflammatory Hdac7 and Pkm2-Hdac7 complex acts as an immunometabolism signaling hub in macrophages. Disrupting the Pkm2-Hdac7 complex attenuates LPS-inducible glycolysis and the accompanying inflammatory responses (41). From above findings, we can see that the regulation of the isozymes is crucial to the process of glucose metabolism and is a promising field for future research.
Lactate has been considered a waste product of glycolysis for decades. Studies in the recent years have shown otherwise. Lactate is an active signaling metabolite working through transporter- and receptor-mediated signaling, which directly regulate functional polarization (73). It has been demonstrated that cancer cell-deprived lactate is a Gpr132 activator which stimulates the macrophage M2 phenotype in a Gpr132-dependent manner (74). In addition, tumor-derived lactate is sufficient to increse the expression of M2-labeled MGL1, FizZ1 and MGL2 in BMDMs (75). The results in the study conducted by Ohashi et al. showed that more macrophages aggregate at the tumor site when the intratumoral concentration of lactate is lower; however, a higher concentration of lactate inhibits monocyte migration in vitro (76). It is possible to say that the effects of lactic acid on immunity depend on the concentration of lactate. Hyperchloremia and lactic acidosis are two common metabolic acidosis, both of which are relative to distinct patterns of immune response. HCl is intrinsically proinflammatory through increased LPS-induced NF-κB DNA binding and NO release. Conversly, lactic acid significantly decreases LPS-induced IL-6, NO and IL-10 expression in a dose-dependent manner and inhibits LPS-induced NF-κB DNA binding (77). It is suggested that lactic acidos has an anti-inflammatory effect. From the functional comparison of these two situations, lactic acid possibly acts as a regulator unrelated to acidity. In addition, blocking the lactic acid signaling pathway can directly improve macrophage functions, even if the concentration of lactic acid in the tumor is not reduced (78). The pathway regulating lactic acid production can also influence the polarization of macrophages. Lactic acid clearance can be used for synergistic immunotherapy of tumors (79). Overall, we can predict that lactic acid is associated with M2-like macrophage polarization as a signaling mediator or through the regulatory pathway of its production. Lactate and the related signaling pathways reveal potential new therapeutic targets for disease amelioration.
Pentose Phosphate Pathway
PPP branches from glycolysis and contributes to inflammatory responses in macrophages (49, 57). The PPP contributes to amplifying the specific effector functions of LPS-activated macrophages, mainly providing two types of materials: pentose phosphates, which can participate in nucleotide and amino acid metabolism, and nicotinamide-adenine dinucleotide phosphate (NADPH), which is a critical factor for NADPH oxidase-dependent ROS (47). Nucleotide metabolism can be involved in the production of active substances, which are closely related to the function of macrophages. ROS plays a crucial role in the activation and maintenance of M1 macrophages, because ROS has strong oxidative characteristics and can damage intracellular constituents, such as nucleic acids, proteins and lipids. Mitochondrial ROS-induced lysosomal dysfunction contributes to M1 macrophage polarization (80) under diabetic conditions (81) and abamectin-induced cytotoxic disposition (82). Additionally, bacterial killing in macrophages is damaged when mitochondrial ROS (mROS) levels are attenuated (83). Researchers found that intracellular ROS production was significantly attenuated when the PPP was restricted, while M2 phenotype macrophage polarization was sensitized (48). Surprisingly, ROS also takes part in the anti-inflammatory reactions of macrophage phenotypes (84). In addition, glucose 6-phosphate dehydrogenase (G6PD) is highly active in macrophages and is the key enzyme in the PPP. The generation of ROS can be promoted by G6PD in macrophages under stressful conditions (80). In another trial, researchers found that proinflammatory responses were suppressed after the inhibition of G6PD in macrophages (85). It is reported that overexpression of G6PD enhanced the activation of the p38-MAPK and NF-κB signaling pathways and promoted the production of proinflammatory cytokines and ROS in a macrophage cell line (86, 87). Thus, the modulation of G6PD activity appears to provide a novel method for researchers to regulate macrophage activity and develop alternative therapies.
Tricarboxylic Acid Cycle
The TCA cycle, also named the citric acid or the Krebs cycle, uses a series of chemical reactions to generate energy in aerobic organisms (88). M2 macrophages can obtain enough ATP from OXPHOS through the TCA cycle. By contrast, M1 depends on ROS and intermediate metabolites (56). Mitochondrial ROS promotes macrophages swift to an inflammatory type through damaging the autophagy-lysosome system (81). OXPHOS deficiency in mitochondria is a feature of M1 macrophages. There are two breakpoints in M1 macrophages (49, 50): one breakpoint causes the accumulation of citric acid at the level of isocitrate dehydrogenase (IDH), which produces itaconate, and the other breakpoint causes the accumulation of succinate at the level of succinate dehydrogenase (SDH), which in turn induces HIF-1α mediated upregulation of glycolysis and IL-1β production (4, 51). In addition, SDH drives ROS generation (54, 55).
Citrate is reported to support both proinflammatory and anti‐inflammatory macrophage functions, which are mainly shown by citrate’s mediated products. The accumulation of citric acid increases NADPH through ATP‐citrate lyase (ACLY)-mediated reactions, which directly act on NADPH‐dependent inducible nitric oxide synthase (iNOS) and increase the production of NO (89). NO was shown to be able to induce M1 marker expression on peritoneal macrophages under Con-A treatment (90). Remarkably, NO has the ability to inhibit the mitochondrial electron transport chain (ETC). Researchers have demonstrated that OXPHOS during M1 polarization can be repressed by NOS-derived NO in bone-marrow-derived macrophages (BMDMs) (91). What’s more, inhibiting NO production in mitochondrial contribute to macrophage M2 type convertion (67). In addition, NO modulates the productions of citrate and succinate, which are TCA cycle metabolites and inflammatory mediator, respectively (51). There is a complex relationship between metabolites. In further explorations, the network of metabolites should be taken into account.
Itaconate is also a vital metabolite rooted in redundant citrate and is synthesized through the intermediate cis-aconitate produced by cis-aconitate decarboxylase under regulation of immune responsive gene 1 (IRG1) (52, 53). In addition, some researchers found that IDH activity and itaconate synthesis were inhibited by endogenous type I IFN-driven IL-10 via in LPS-macrophages (92). At present, itaconate and itaconate derivatives, 4-octyl itaconate (4OI), dimethyl itaconate (DI), and 4-monoethyl itaconate (4EI), are mainly studied. Researchers found (93) that OI alleviated LPS-induced acute lung injury. Activation of nuclear factor erythroid 2-related factor 2 (NRF2) may contribute to the anti-inflammatory and antioxidant effects of OI. However, Sun, K. A., et al. (94) found that endogenous itaconate does not take part in inflammation induced by particulate matter (PM) or activation of NRF2 in macrophages either in vitro or in vivo. Conversely, OI attenuated PM-induced inflammation in macrophages. In another trial, DI could suppress the inflammatory responses of macrophages by triggering NRF2 (95). Meanwhile, DI increased the production of NRF2 and its downstream factors NQO-1 and HO-1 in sepsis (96). In addition, researchers found that DI is not metabolized into itaconate intracellularly (97). Recently, some researchers (98) confirmed that nonderivatized itaconate can efficiently concentrate in macrophages, and can be used for mechanistic studies through comparing unmodified itaconate and commonly used itaconate derivatives. By contrast, many of the ester derivatives were not detected with similar results as expected for nonderivatized itaconate. Beyond this, the results represented that itaconate should be regarded as an immunoregulatory metabolite rather than a simple immunosuppressive metabolite. Therefore, more research is required to identify the features and moderating effect of itaconate and its derivatives.
Succinate is synthesized from α-ketoglutarate (51). Extracellular succinate is sensed by succinate receptor 1 (SUNCR1) and works as a signaling metabolite. The activation of SUCNR1 in macrophages take part in anti-inflammatory responses (99) and can polarize macrophages into TAMs in the tumor microenvironment (100). Moreover, succinate stimulates osteoclastogenesis in osteoclastic lineage cells in vitro and in vivo (101). In addition, succinate stabilizes hypoxia-inducible factor-1α (HIF-1α) (102), a transcription factor, which plays a crucial role in mediating aerobic glycolysis and M1 differentiation (103). Moreover, researchers have found that TAMs enhance aerobic glycolysis by stabilizing HIF-1α protein. In diabetic nephropathy, TAB1/TAK1 can activate NF-κB in bone marrow mesenchymal stem cells to upregulate HIF-1α activity and enhance glycolytic metabolism (104). In another study, intraperitoneal exudate macrophages of HIF1α -/- mice showed significantly reduced glycolytic activity compared to those of wild-type mice; this reduced glycolytic activity was associated with a reduced proton production rate (PPR, a value used to indicate the cellular respiration rate) and glucose transporter 1 (GLUT1) expression. In addition, these peritoneal exudate macrophages (PEMs) from HIF1α -/- mice showed lower secretion of proinflammatory cytokines and iNOS expression than those from wild-type mice (44). In glucose metabolism of macrophages, GLUT1 is a key regulator. The expression of proinflammatory cytokines (IL-6, IL-1B and TNF-α) and glucose uptake are obviously increased when GLUT1 is overexpressed in macrophages, even in the absence of stimulus-specific activation (105). It has also been reported that HIF-1α affects glycolysis in macrophages in an AMPK-dependent manner (106). In addition, HIFs are key effector molecules regulating the metabolism and functional reprogramming through the PDPK1/Akt/mTOR pathway under neuroinflammatory conditions in M1/M2 microglia (107). HIF-1α is closely related to energetic metabolism and improves the activity of glycolytic enzymes such as 6-phosphate fructose-2-kinase/fructose-2, hexokinase-1 (HK1), lactate dehydrogenase A (LDHA) and 6-bisphosphatase 3 (PFKFB3) (108). Overall, HIF-1α participates in the metabolism of macrophages and regulates the switching of phenotypes; therefore HIF-1α is a potential intervening target to macrophages.
Lipid Metabolism in Macrophages
Fatty acid synthesis (FAS) takes part in the inflammatory reaction and signaling in macrophages (57). The proinflammatory response in macrophages can be inhibited through restraining fatty acid synthase (FASN), which is a key enzyme of FAS that catalyzes the production of long-chain fatty acids (58). Lipid droplets (LDs) is a feature of foamy macrophages that have been found in many diseases. In addition, polyunsaturated fatty acid (PUFA) metabolism in macrophages also influences pathological processes. In cardiovascular diseases, purified n-3 FA supplementation may be a potential strategy fortreatment and prevention (109). Therefore, understanding how immune cells handle and synthesize PUFAs is important. During macrophages activating, SREBPs and LXRs, the two master transcription factors of FAS, are upregulated. Upregulation in SREBPs enhances the maturation of pro-inflammatory precursors and causes macrophages to differentiate toward the pro-inflammatory M1 phenotype. In contrast, LXRα promotes cells towards an M2 phenotype (110).
FAO is essential in functional M2 macrophages by enhancing the secretion of IL-1β (59). Treatment with LPS attenuates the expression of inflammatory genes in macrophages when FAO is suppressed (111). FAO plays a crucial role in activation of NLRP3 inflammasome of M1 macrophages (112). It is implied that enhancing FAO in macrophages may be an underlying therapeutic method for patients with obesity, type 2 diabetes (113) or cancers (114). However, it remains unclear whether the increase in FAO correlates with M2 polarization (115). IL-4-induced polarization is not affected by the inhibition of FAO (116). The two subtypes of carnitine palmitoyltransferase (CPT) system, CPT1 and CPT2, are essential to the β-oxidation of long-chain aliphatic acid in mitochondria. Lacking CPT2, macrophages are unable to finish β-oxidation of fatty acids, but they can be polarized to M2 phenotype after stimulated by IL-4 in vitro and in vivo (117). Moreover, the CPT-1 inhibitor etomoxir in low concentrationcould suppress CPT-1 without changing M2 polarization.i However, high concentration of etomoxir can inhibit M2 polarization without affecting the activity of CPT-1 (56). Macrophage FAO likely plays a correlative rather than causative role in systemic metabolic dysfunction (118). Further exploration is needed to determine which is the dominant factor, the switchof cell phenotypes or the changes of metabolic transformation.
Amino Acid Metabolism in Macrophages
Mills et al. first proposed the notion that arginine can be used to determine the functions of macrophages (119). The M1 macrophages are a product of the iNOS pathway, while the M2 macrophages come from the arginase pathway (60). L-Arginine takes part in initiating of intracellular signaling pathways in macrophages, such as triggering inflammatory responses and accelerating the sensitivity to bacterial endotoxin (120). Arginine deprivation attenuates osteoclastogenesis and also dampens generation of IL-4 induced multinucleated giant cells. Strikingly, in the absence of extracellular arginine, osteoclasts and IL-4-induced multinucleated giant cells display flexibility, since their formation can be restored by supplementation with select arginine precursors in vitro (121). The production of α-Ketoglutarate (αKG) is important for activation of M2 macrophages, including engagement of FAO and epigenetic reprogramming of M2 genes. The potential M2-promoting mechanism is demonstrated by the high αKG/succinate ratio,Whereas a low αKG/succinate ratio strengthens M1 polarization in macrophages (61). Nontargeted metabolomic analysis revealed that tryptophan metabolism is involved in M1 polarization (122). Ornithine decarboxylase (ODC), the rate-limiting enzyme in polyamine synthesis, leads to an increase in putrescine levels and imparis the transcription of M1 genes. In M1 macrophages, the translation of proinflammatory mediators can be regulated by spermidine and spermine (15). In applied research, there are no clear results that using amino acid auxotrophy to decrease the growth of cancerous lymphocyte, though attempting for decades (123). As for macrophages, there is also a long way to go. The detailed metabolic pathways in macrophages are shown in Figure 3.
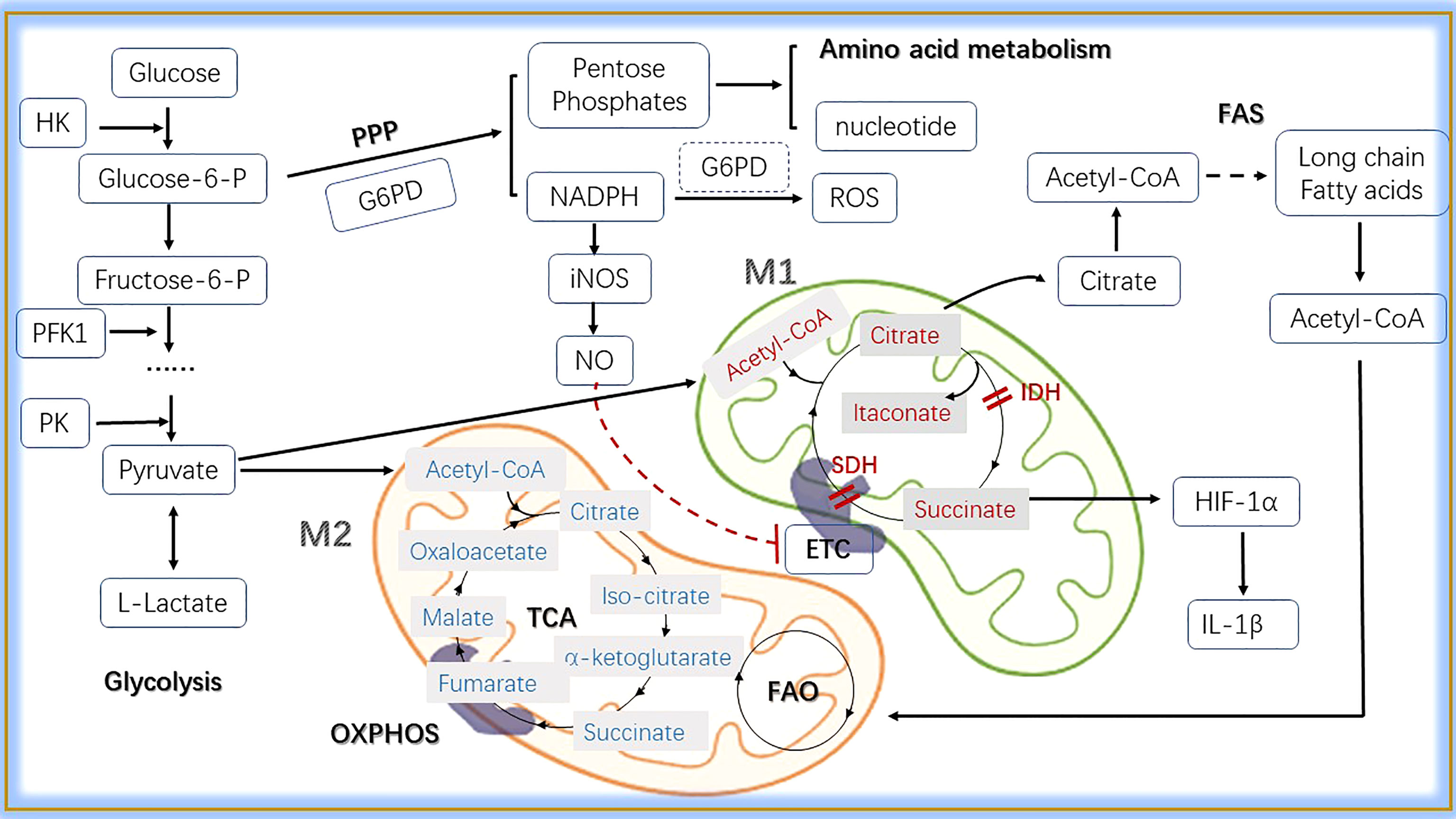
Figure 3 The main metabolic pathways in macrophages. In the cytosol, glucose is converted into L-lactate by glycolysis, in which HK, PFK1 and PK are key enzymes. In the progress of glycolysis, glucose-6-P can be shunted to PPP pathway sustaining pentose phosphates and NADPH production. Pentose phosphates are used for the synthesis of amnio acid and nucleotide, while NADPH contributes to the production of ROS and NO. Pyruvate is induced to lactate in hypoxic conditions, whereas decarboxylated into acetyl-CoA within the mitochondria in normoxic conditions. Here, acetyl-CoA enters into the TCA cycle, providing reducing agents to the ETC through OXPHOS to generate energy. Citrate, a metabolite in TCA, participates in fatty acid synthesis when exported to the cytoplasm. Acetyl-CoA produced from FAS can be transferred into mitochondria and take part in the FAO. In M1 macrophages, two breakpoints cause the production of itaconate and the accumulation of succinate that induces HIF-1α mediated upregulation of glycolysis and IL-1β production.What’s more, the production of NO inhibits ETC. M2 macrophages can obtain enough ATP from OXPHOS through the TCA cycle. As for energy gain, FAO is essential. HK, hexokinase; Glucose 6-P, glucose 6-phosphate; Fructose 6-P, fructose 6-phosphate; PFK1, 6-phosphofructokinase 1; PK, pyruvate kinase; PPP, pentose phosphate pathway; G6PD, glucose 6-phosphate dehydrogenase; iNOS, inducible nitric oxide synthase; NO, nitric oxide; ETC, electron transport chain; TCA, tricarboxylic acid; OXPHOS, oxidative phosphorylation; IDH, isocitrate dehydrogenase; NADPH, nicotinamide-adenine dinucleotide phosphate; SDH, succinate dehydrogenase; FAS, fatty acid synthesis; FAO, fatty acid oxidation; Acetyl-CoA, acetyl-Coenzyme A; IL-1β, interleukin-1β; HIF-1α, hypoxia inducible factor 1α.
In addition, the classic metabolic pathways, some bypasses, and the enzymes involved have also come into view in recent years. Glucose is converted to sorbitol by aldose reductase (AR) in Polyol pathway. When activating the Polyol pathway, the production of proinflammatory cytokines was increased in kidney cortex of diabetic mice (124). What’s more, upregulation of AR in human macrophages is proinflammatory in foam cells in atherosclerosis (125). When inhibiting the activity of AR, the phenotype was transferred to M2 (126). Ribulose 5-phosphate 3-epimerase (RPE) is an important enzyme for cellular response against oxidative stress and takes part in PPP, contributing to the reversible conversion of D-ribulose 5-phosphate to D-xylulose 5-phosphate (127). The gene coding RPE has exposed to be relative to poor outcome in cancer patients (128). Some metabolites also affect the function and polarization of macrophages. Lactate-derived lactylation of histone serves as an epigenetic modification and could directly stimulates M2 gene expression during M1 macrophage polarization (129). Ketone body, especially, β-hydroxybutyrate (BHB) promotes M2 macrophage polarization, contributing to the resolution of intestinal inflammation and providing ideas for the treatment of inflammatory bowel disease (130). Metabolites in shaping the phenotypes and functions of macrophages were summarized in Table 2.
Maternal-Fetal Interface Macrophages Remain Enigmatic
Houser (137, 138) found two distinct subsets of CD14+ dMϕs in the decidual tissues in early pregnancy: CD11cHI (CD11c high) and CD11cLO (CD11c low). CD11CHI dMϕs express genes related to lipid metabolism and inflammation, and may be the major APCs in the decidua. dMϕs (139) were further analyzed and three subtypes were identifiedin the first trimester of gestation: CCR2-CD11cLO (~80%), CCR2+CD11cHI (10–15%) and CCR2-CD11cHI (~5%). CCR2-CD11CHI macrophages were significantly different from the other two subsets in the heat map. CCR2-CD11cHI macrophages are specifically enriched in pathways related to cell metabolism and proliferation. The CCR2+CD11cHI subset wasparticularly involvedin the TNF signaling pathway and phagocytosome pathway. Few pathways were particularly enriched in the CCR2-CD11cLO subset. In addition, high expression levels incarbon metabolism genes and almost all genes related to heme metabolism, including NADPH,quinone oxidoreductase 1 (NQO1), ferritin heavy chain (FTH1) and HMOX1, were observed in CCR2-CD11cHI macrophages. HMOX1 is an enzyme that catalyzes the degradation of heme into carbon monoxide, ferrous iron, and biliverdin. Anti-inflammatory and antioxidant properties have been convincingly demonstrated in the above byproducts of heme metabolism (140). Thus, it is inferred that CCR2-CD11cHI macrophages may have an effect of anti-inflammation during the first trimester. Whetherand how the overall metabolic environment affects the function of macrophages are unanswered. Whether there is a special metabolic pattern in decidua macrophages remains to be explored. The association between disorders and metabolism in macrophages is shown in Table 3.
Therapeutic Perspective of Intervening in Metabolism via Macrophages
The different polarization types of macrophages have an obvious influence on the effects of therapies. At present, there are many ways to regulate the polarization of macrophages, and metabolic regulation is a new research field with great development. Moreover, the research on intervention in macrophages in metabolism as a therapeutic method mainly focuses on tumor-related fields. For example, interference with the phenotypes of TAMs, which are known to be immunosuppressive, is potential to be helpful combing with immunotherapy and/or chemotherapy (157). THP-1 M1 macrophages increased etoposide-induced cancer cell apoptosis, while M2 macrophages decreased apoptosis of cells (158). Recently, researchers found that itaconic acid is one attractive candidate for anti-tumor responses, since peritoneal tumors could be controlled by specifically targeting resident macrophage-associated itaconate levels (159). Although precise regulation is unclear, the targeting of resident macrophages is a potential perspective for future research. Moreover, it was found that administration of miR-223 over-expressed macrophages, with IL-4 preconditioning, attenuated sepsis severity in a LPS model, through the inhibition of the glycolysis pathway. These cells are thus proposed as a candidate for cell therapy during the pro-inflammatory phase of sepsis (160).
Metformin is one of the few drugs that clearly affect macrophage function by regulating its metabolism. Metformin can regulate glycometabolism, and is used as an antidiabetic agent. In metformin treated tumor tissue, M2-like macrophages decrease while M1-like macrophages increase. AMPK-NF-κB signaling, a pathway involved in regulating M1/M2 expression, can be activated by metformin and induce cytokines expression for macrophage polarization to an antitumor phenotype (161). In addition, macrophages could be transformed to foam cells through cholesterol transport and storage. Foam cells are present in all stages of atherosclerosis. Foam cell targeting anti-inflammatory therapies are known to indirectly regulate the actions of pro-atherogenic and anti-atherogenic cytokines (162). What’s more, in a murine model of pancreatic cancer, atorvastatin, a frequently used inhibitor for cholesterol synthesis and HMG-Coa reductase, facilitates TAMs to reprogram to M2-like phenotype and attenuates the chemotherapeutic efficacy of gemcitabine on pancreatic cancer (163).
Regulation of key enzymes in the metabolic process is also one of the main methods of treatment. In a recent trial of non-alcoholic fatty liver disease (NAFLD) (164), acetyl-coenzyme A carboxylase (ACC) inhibitor was used alone or cooperated with a diacylglycerol acyltransferase 2 (DGAT2) inhibitor in patients to observe the change of magnetic resonance imaging - proton density fat fraction in liver. ACC inhibitor monotherapy showed significant anti-liver steatosis, suggesting a potential clinical benefit for chronic treatment. Co-administration of these two trugs is possible to decrease some of the limitations of ACC inhibition alone. It can be seen from this study that the regulation in the metabolic process has the possibility of clinical application. But the influence of key enzymes on the functions of macrophages is still in the stage of scientific research. For example, lactate dehydrogenase (LDH) can catalyse the reversible conversion of pyruvate and lactate in glycolysis. When feeling tumor-derived miR-375, TAMs downregulated the expression of LDHB and increased aerobic glycolysis and lactagenesis (165). Then tumor-derived miR-375 was established as a novel regulator of macrophage metabolism in breast cancer. More research is needed in pregnancy-related diseases. Intervention in metabolism in macrophages is a very rational immunotherapeutic perspective, and it will bring good news for patients.
Author Contributions
J-XS and X-HX conceived of the manuscript. J-XS wrote the manuscript and prepared the figures. LJ and X-HX reviewed the manuscript. All authors listed have made a substantial, direct, and intellectual contribution to the work and approved it for publication.
Funding
This study was funded by National Natural Science Foundation of China (81730039, 82071653, 81971384, and 82171657).
Conflict of Interest
The authors declare that the research was conducted in the absence of any commercial or financial relationships that could be construed as a potential conflict of interest.
Publisher’s Note
All claims expressed in this article are solely those of the authors and do not necessarily represent those of their affiliated organizations, or those of the publisher, the editors and the reviewers. Any product that may be evaluated in this article, or claim that may be made by its manufacturer, is not guaranteed or endorsed by the publisher.
Glossary
References
1. Curi R, de Siqueira Mendes R, LA dCC, GD N, SC S, Newsholme P. A Past and Present Overview of Macrophage Metabolism and Functional Outcomes. Clin Sci (London England: 1979) (2017) 131(12):1329–42. doi: 10.1042/cs20170220
2. Shapouri-Moghaddam A, Mohammadian S, Vazini H, Taghadosi M, Esmaeili SA, Mardani F, et al. Macrophage Plasticity, Polarization, and Function in Health and Disease. J Cell Physiol (2018) 233(9):6425–40. doi: 10.1002/jcp.26429
3. Rajab N, Rutar M, Laslett AL, Wells CA. Designer Macrophages: Pitfalls and Opportunities for Modelling Macrophage Phenotypes From Pluripotent Stem Cells. Diff Res Biol Diversity (2018) 104:42–9. doi: 10.1016/j.diff.2018.10.001
4. Verdeguer F, Aouadi M. Macrophage Heterogeneity and Energy Metabolism. Exp Cell Res (2017) 360(1):35–40. doi: 10.1016/j.yexcr.2017.03.043
5. Yang HL, Wang CJ, Lai ZZ, Yang SL, Zheng ZM, Shi JW, et al. Decidual Stromal Cells Maintain Decidual Macrophage Homeostasis by Secreting IL-24 in Early Pregnancy. Am J Reprod Immunol (N Y NY: 1989) (2020) 84(2):e13261. doi: 10.1111/aji.13261
6. Deng W, Jacobson EC, Collier AJ, Plath K. The Transcription Factor Code in iPSC Reprogramming. Curr Opin Genet Dev (2021) 70:89–96. doi: 10.1016/j.gde.2021.06.003
7. Spiller KL, Wrona EA, Romero-Torres S, Pallotta I, Graney PL, Witherel CE, et al. Differential Gene Expression in Human, Murine, and Cell Line-Derived Macrophages Upon Polarization. Exp Cell Res (2016) 347(1):1–13. doi: 10.1016/j.yexcr.2015.10.017
8. Tedesco S, De Majo F, Kim J, Trenti A, Trevisi L, Fadini GP, et al. Convenience Versus Biological Significance: Are PMA-Differentiated THP-1 Cells a Reliable Substitute for Blood-Derived Macrophages When Studying In Vitro Polarization? Front Pharmacol (2018) 9:71. doi: 10.3389/fphar.2018.00071
9. Takata K, Kozaki T, Lee CZW, Thion MS, Otsuka M, Lim S, et al. Induced-Pluripotent-Stem-Cell-Derived Primitive Macrophages Provide a Platform for Modeling Tissue-Resident Macrophage Differentiation and Function. Immunity (2017) 47(1):183–98.e6. doi: 10.1016/j.immuni.2017.06.017
10. Li X, Lei Y, Wu M, Li N. Regulation of Macrophage Activation and Polarization by HCC-Derived Exosomal lncRNA Tuc339. Int J Mol Sci (2018) 19(10):2958. doi: 10.3390/ijms19102958
11. Wynn TA, Chawla A, Pollard JW. Macrophage Biology in Development, Homeostasis and Disease. Nature (2013) 496(7446):445–55. doi: 10.1038/nature12034
12. Arora S, Dev K, Agarwal B, Das P, Syed MA. Macrophages: Their Role, Activation and Polarization in Pulmonary Diseases. Immunobiology (2018) 223(4-5):383–96. doi: 10.1016/j.imbio.2017.11.001
13. Funes SC, Rios M, Escobar-Vera J, Kalergis AM. Implications of Macrophage Polarization in Autoimmunity. Immunology (2018) 154(2):186–95. doi: 10.1111/imm.12910
14. Atri C, Guerfali FZ, Laouini D. Role of Human Macrophage Polarization in Inflammation During Infectious Diseases. Int J Mol Sci (2018) 19(6):1801. doi: 10.3390/ijms19061801
15. Latour YL, Gobert AP, Wilson KT. The Role of Polyamines in the Regulation of Macrophage Polarization and Function. Amino Acids (2020) 52(2):151–60. doi: 10.1007/s00726-019-02719-0
16. Liu M, Yin L, Li W, Hu J, Wang H, Ye B, et al. C1q/TNF-Related Protein-9 Promotes Macrophage Polarization and Improves Cardiac Dysfunction After Myocardial Infarction. J Cell Physiol (2019) 234(10):18731–47. doi: 10.1002/jcp.28513
17. Liu PS, Ho PC. Determining Macrophage Polarization Upon Metabolic Perturbation. Methods Mol Biol (Clifton NJ) (2019) 1862:173–86. doi: 10.1007/978-1-4939-8769-6_13
18. Lin Y, Zhao JL, Zheng QJ, Jiang X, Tian J, Liang SQ, et al. Notch Signaling Modulates Macrophage Polarization and Phagocytosis Through Direct Suppression of Signal Regulatory Protein α Expression. Front Immunol (2018) 9:1744. doi: 10.3389/fimmu.2018.01744
19. Essandoh K, Li Y, Huo J, Fan GC. MiRNA-Mediated Macrophage Polarization and Its Potential Role in the Regulation of Inflammatory Response. Shock (2016) 46(2):122–31. doi: 10.1097/shk.0000000000000604
20. Hao J, Hu Y, Li Y, Zhou Q, Lv X. Involvement of JNK Signaling in IL4-Induced M2 Macrophage Polarization. Exp Cell Res (2017) 357(2):155–62. doi: 10.1016/j.yexcr.2017.05.010
21. Hsieh SW, Huang LC, Chang YP, Hung CH, Yang YH. M2b Macrophage Subset Decrement as an Indicator of Cognitive Function in Alzheimer’s Disease. Psychiatry Clin Neurosci (2020) 74(7):383–91. doi: 10.1111/pcn.13000
22. Kubota T, Inoue M, Kubota N, Takamoto I, Mineyama T, Iwayama K, et al. Downregulation of Macrophage Irs2 by Hyperinsulinemia Impairs IL-4-Indeuced M2a-Subtype Macrophage Activation in Obesity. Nat Commun (2018) 9(1):4863. doi: 10.1038/s41467-018-07358-9
23. Wang LX, Zhang SX, Wu HJ, Rong XL, Guo J. M2b Macrophage Polarization and Its Roles in Diseases. J Leukoc Biol (2019) 106(2):345–58. doi: 10.1002/jlb.3ru1018-378rr
24. Ferrante CJ, Leibovich SJ. Regulation of Macrophage Polarization and Wound Healing. Adv Wound Care (2012) 1(1):10–6. doi: 10.1089/wound.2011.0307
25. Lurier EB, Dalton D, Dampier W, Raman P, Nassiri S, Ferraro NM, et al. Transcriptome Analysis of IL-10-Stimulated (M2c) Macrophages by Next-Generation Sequencing. Immunobiology (2017) 222(7):847–56. doi: 10.1016/j.imbio.2017.02.006
26. Viola A, Munari F, Sánchez-Rodríguez R, Scolaro T, Castegna A. The Metabolic Signature of Macrophage Responses. Front Immunol (2019) 10:1462. doi: 10.3389/fimmu.2019.01462
27. Wu K, Lin K, Li X, Yuan X, Xu P, Ni P, et al. Redefining Tumor-Associated Macrophage Subpopulations and Functions in the Tumor Microenvironment. Front Immunol (2020) 11:1731. doi: 10.3389/fimmu.2020.01731
28. Malyshev I, Malyshev Y. Current Concept and Update of the Macrophage Plasticity Concept: Intracellular Mechanisms of Reprogramming and M3 Macrophage “Switch” Phenotype. BioMed Res Int (2015) 2015:341308. doi: 10.1155/2015/341308
29. Yang F, Zheng Q, Jin L. Dynamic Function and Composition Changes of Immune Cells During Normal and Pathological Pregnancy at the Maternal-Fetal Interface. Front Immunol (2019) 10:2317. doi: 10.3389/fimmu.2019.02317
30. Zulu MZ, Martinez FO, Gordon S, Gray CM. The Elusive Role of Placental Macrophages: The Hofbauer Cell. J Innate Immun (2019) 11(6):447–56. doi: 10.1159/000497416
31. Tauber Z, Foltynkova T, Cizkova K. Morphometric Analysis of Hofbauer Cells in Normal Placenta and Chorioamnionitis in Humans. Anatomia Histol Embryol (2020) 50(2):396–403. doi: 10.1111/ahe.12644
32. Reyes L, Wolfe B, Golos T. Hofbauer Cells: Placental Macrophages of Fetal Origin. Results Probl Cell Diff (2017) 62:45–60. doi: 10.1007/978-3-319-54090-0_3
33. Reyes L, Golos TG. Hofbauer Cells: Their Role in Healthy and Complicated Pregnancy. Front Immunol (2018) 9:2628. doi: 10.3389/fimmu.2018.02628
34. Yang SW, Cho EH, Choi SY, Lee YK, Park JH, Kim MK, et al. DC-SIGN Expression in Hofbauer Cells may Play an Important Role in Immune Tolerance in Fetal Chorionic Villi During the Development of Preeclampsia. J Reprod Immunol (2017) 124:30–7. doi: 10.1016/j.jri.2017.09.012
35. Richardson C, King W, Vashisht K. CD28 Expression in Human CD163(+) Placental Hofbauer Cells. Placenta (2020) 89:8–9. doi: 10.1016/j.placenta.2019.10.005
36. Suryawanshi H, Morozov P, Straus A, Sahasrabudhe N, Max KEA, Garzia A, et al. A Single-Cell Survey of the Human First-Trimester Placenta and Decidua. Sci Adv (2018) 4(10):eaau4788. doi: 10.1126/sciadv.aau4788
37. Liang G, Zhou C, Jiang X, Zhang Y, Huang B, Gao S, et al. De Novo Generation of Macrophage From Placenta-Derived Hemogenic Endothelium. Dev Cell (2021) 56(14):2121–33.e6. doi: 10.1016/j.devcel.2021.06.005
38. Kim SY, Romero R, Tarca AL, Bhatti G, Kim CJ, Lee J, et al. Methylome of Fetal and Maternal Monocytes and Macrophages at the Feto-Maternal Interface. Am J Reprod Immunol (N Y NY: 1989) (2012) 68(1):8–27. doi: 10.1111/j.1600-0897.2012.01108.x
39. Tabas I, Bornfeldt KE. Intracellular and Intercellular Aspects of Macrophage Immunometabolism in Atherosclerosis. Circ Res (2020) 126(9):1209–27. doi: 10.1161/circresaha.119.315939
40. Mathis D, Shoelson SE. Immunometabolism: An Emerging Frontier. Nat Rev Immunol (2011) 11(2):81. doi: 10.1038/nri2922
41. Das Gupta K, Shakespear MR, Curson JEB, Murthy AMV, Iyer A, Hodson MP, et al. Class IIa Histone Deacetylases Drive Toll-Like Receptor-Inducible Glycolysis and Macrophage Inflammatory Responses via Pyruvate Kinase M2. Cell Rep (2020) 30(8):2712–28.e8. doi: 10.1016/j.celrep.2020.02.007
42. Codo AC, Davanzo GG, Monteiro LB, de Souza GF, Muraro SP, Virgilio-da-Silva JV, et al. Elevated Glucose Levels Favor SARS-CoV-2 Infection and Monocyte Response Through a HIF-1α/Glycolysis-Dependent Axis. Cell Metab (2020) 32(3):437–46.e5. doi: 10.1016/j.cmet.2020.07.007
43. Rodríguez-Prados JC, Través PG, Cuenca J, Rico D, Aragonés J, Martín-Sanz P, et al. Substrate Fate in Activated Macrophages: A Comparison Between Innate, Classic, and Alternative Activation. J Immunol (2010) 185(1):605–14. doi: 10.4049/jimmunol.0901698
44. Yu Q, Wang Y, Dong L, He Y, Liu R, Yang Q, et al. Regulations of Glycolytic Activities on Macrophages Functions in Tumor and Infectious Inflammation. Front Cell Infect Microbiol (2020) 10:287. doi: 10.3389/fcimb.2020.00287
45. Deng H, Wu L, Liu M, Zhu L, Chen Y, Zhou H, et al. Bone Marrow Mesenchymal Stem Cell-Derived Exosomes Attenuate LPS-Induced ARDS by Modulating Macrophage Polarization Through Inhibiting Glycolysis in Macrophages. Shock (2020) 54(6):828–43. doi: 10.1097/shk.0000000000001549
46. Baardman J, Verberk SGS, Prange KHM, van Weeghel M, van der Velden S, Ryan DG, et al. A Defective Pentose Phosphate Pathway Reduces Inflammatory Macrophage Responses During Hypercholesterolemia. Cell Rep (2018) 25(8):2044–52.e5. doi: 10.1016/j.celrep.2018.10.092
47. Nagy C, Haschemi A. Time and Demand are Two Critical Dimensions of Immunometabolism: The Process of Macrophage Activation and the Pentose Phosphate Pathway. Front Immunol (2015) 6:164. doi: 10.3389/fimmu.2015.00164
48. Sun L, Zhou F, Shao Y, Lv Z, Li C. Sedoheptulose Kinase Bridges the Pentose Phosphate Pathway and Immune Responses in Pathogen-Challenged Sea Cucumber Apostichopus Japonicus. Dev Comp Immunol (2020) 109:103694. doi: 10.1016/j.dci.2020.103694
49. Artyomov MN, Sergushichev A, Schilling JD. Integrating Immunometabolism and Macrophage Diversity. Semin Immunol (2016) 28(5):417–24. doi: 10.1016/j.smim.2016.10.004
50. Ryan DG, Murphy MP, Frezza C, Prag HA, Chouchani ET, O’Neill LA, et al. Coupling Krebs Cycle Metabolites to Signalling in Immunity and Cancer. Nat Metab (2019) 1:16–33. doi: 10.1038/s42255-018-0014-7
51. Bailey JD, Diotallevi M, Nicol T, McNeill E, Shaw A, Chuaiphichai S, et al. Nitric Oxide Modulates Metabolic Remodeling in Inflammatory Macrophages Through TCA Cycle Regulation and Itaconate Accumulation. Cell Rep (2019) 28(1):218–30.e7. doi: 10.1016/j.celrep.2019.06.018
52. Michelucci A, Cordes T, Ghelfi J, Pailot A, Reiling N, Goldmann O, et al. Immune-Responsive Gene 1 Protein Links Metabolism to Immunity by Catalyzing Itaconic Acid Production. Proc Natl Acad Sci USA (2013) 110(19):7820–5. doi: 10.1073/pnas.1218599110
53. Hooftman A, Angiari S, Hester S, Corcoran SE, Runtsch MC, Ling C, et al. The Immunomodulatory Metabolite Itaconate Modifies NLRP3 and Inhibits Inflammasome Activation. Cell Metab (2020) 32(3):468–78.e7. doi: 10.1016/j.cmet.2020.07.016
54. Cordes T, Wallace M, Michelucci A, Divakaruni AS, Sapcariu SC, Sousa C, et al. Immunoresponsive Gene 1 and Itaconate Inhibit Succinate Dehydrogenase to Modulate Intracellular Succinate Levels. J Biol Chem (2016) 291(27):14274–84. doi: 10.1074/jbc.M115.685792
55. Mills EL, Kelly B, Logan A, Costa ASH, Varma M, Bryant CE, et al. Succinate Dehydrogenase Supports Metabolic Repurposing of Mitochondria to Drive Inflammatory Macrophages. Cell (2016) 167(2):457–70.e13. doi: 10.1016/j.cell.2016.08.064
56. Divakaruni AS, Hsieh WY, Minarrieta L, Duong TN, Kim KKO, Desousa BR, et al. Etomoxir Inhibits Macrophage Polarization by Disrupting CoA Homeostasis. Cell Metab (2018) 28(3):490–503.e7. doi: 10.1016/j.cmet.2018.06.001
57. Van den Bossche J, O’Neill LA, Menon D. Macrophage Immunometabolism: Where Are We (Going)? Trends Immunol (2017) 38(6):395–406. doi: 10.1016/j.it.2017.03.001
58. Carroll RG, Zasłona Z, Galván-Peña S, Koppe EL, Sévin DC, Angiari S, et al. An Unexpected Link Between Fatty Acid Synthase and Cholesterol Synthesis in Proinflammatory Macrophage Activation. J Biol Chem (2018) 293(15):5509–21. doi: 10.1074/jbc.RA118.001921
59. Zhang Q, Wang H, Mao C, Sun M, Dominah G, Chen L, et al. Fatty Acid Oxidation Contributes to IL-1β Secretion in M2 Macrophages and Promotes Macrophage-Mediated Tumor Cell Migration. Mol Immunol (2018) 94:27–35. doi: 10.1016/j.molimm.2017.12.011
60. Zhang YH, He M, Wang Y, Liao AH. Modulators of the Balance Between M1 and M2 Macrophages During Pregnancy. Front Immunol (2017) 8:120. doi: 10.3389/fimmu.2017.00120
61. Liu PS, Wang H, Li X, Chao T, Teav T, Christen S, et al. α-Ketoglutarate Orchestrates Macrophage Activation Through Metabolic and Epigenetic Reprogramming. Nat Immunol (2017) 18(9):985–94. doi: 10.1038/ni.3796
62. Zangari J, Petrelli F, Maillot B, Martinou JC. The Multifaceted Pyruvate Metabolism: Role of the Mitochondrial Pyruvate Carrier. Biomolecules (2020) 10(7):1068. doi: 10.3390/biom10071068
63. Belizário JE, Faintuch J, Garay-Malpartida M. Gut Microbiome Dysbiosis and Immunometabolism: New Frontiers for Treatment of Metabolic Diseases. Mediators Inflammation (2018) 2018:2037838. doi: 10.1155/2018/2037838
64. Na YR, Je S, Seok SH. Metabolic Features of Macrophages in Inflammatory Diseases and Cancer. Cancer Lett (2018) 413:46–58. doi: 10.1016/j.canlet.2017.10.044
65. Chen D, Xie J, Fiskesund R, Dong W, Liang X, Lv J, et al. Chloroquine Modulates Antitumor Immune Response by Resetting Tumor-Associated Macrophages Toward M1 Phenotype. Nat Commun (2018) 9(1):873. doi: 10.1038/s41467-018-03225-9
66. Boutens L, Hooiveld GJ, Dhingra S, Cramer RA, Netea MG, Stienstra R. Unique Metabolic Activation of Adipose Tissue Macrophages in Obesity Promotes Inflammatory Responses. Diabetologia (2018) 61(4):942–53. doi: 10.1007/s00125-017-4526-6
67. Van den Bossche J, Baardman J, Otto NA, van der Velden S, Neele AE, van den Berg SM, et al. Mitochondrial Dysfunction Prevents Repolarization of Inflammatory Macrophages. Cell Rep (2016) 17(3):684–96. doi: 10.1016/j.celrep.2016.09.008
68. Wang F, Zhang S, Vuckovic I, Jeon R, Lerman A, Folmes CD, et al. Glycolytic Stimulation Is Not a Requirement for M2 Macrophage Differentiation. Cell Metab (2018) 28(3):463–75.e4. doi: 10.1016/j.cmet.2018.08.012
69. Pickles S, Vigié P, Youle RJ. Mitophagy and Quality Control Mechanisms in Mitochondrial Maintenance. Curr Biol: CB (2018) 28(4):R170–r85. doi: 10.1016/j.cub.2018.01.004
70. Esteban-Martínez L, Sierra-Filardi E, McGreal RS, Salazar-Roa M, Mariño G, Seco E, et al. Programmed Mitophagy Is Essential for the Glycolytic Switch During Cell Differentiation. EMBO J (2017) 36(12):1688–706. doi: 10.15252/embj.201695916
71. Zhang J, Hou C, Dou S, Li G, Wang Z, Liu Y, et al. T Cell Immunoglobulin and Mucin Domain Protein 3 Inhibits Glycolysis in RAW 264.7 Macrophages Through Hexokinase 2. Scand J Immunol (2021) 93(2):e12981. doi: 10.1111/sji.12981
72. Hackett EE, Charles-Messance H, O’Leary SM, Gleeson LE, Muñoz-Wolf N, Case S, et al. Mycobacterium Tuberculosis Limits Host Glycolysis and IL-1β by Restriction of PFK-M via MicroRNA-21. Cell Rep (2020) 30(1):124–36.e4. doi: 10.1016/j.celrep.2019.12.015
73. Haas R, Cucchi D, Smith J, Pucino V, Macdougall CE, Mauro C. Intermediates of Metabolism: From Bystanders to Signalling Molecules. Trends Biochem Sci (2016) 41(5):460–71. doi: 10.1016/j.tibs.2016.02.003
74. Chen P, Zuo H, Xiong H, Kolar MJ, Chu Q, Saghatelian A, et al. Gpr132 Sensing of Lactate Mediates Tumor-Macrophage Interplay to Promote Breast Cancer Metastasis. Proc Natl Acad Sci USA (2017) 114(3):580–5. doi: 10.1073/pnas.1614035114
75. Colegio OR, Chu NQ, Szabo AL, Chu T, Rhebergen AM, Jairam V, et al. Functional Polarization of Tumour-Associated Macrophages by Tumour-Derived Lactic Acid. Nature (2014) 513(7519):559–63. doi: 10.1038/nature13490
76. Ohashi T, Aoki M, Tomita H, Akazawa T, Sato K, Kuze B, et al. M2-Like Macrophage Polarization in High Lactic Acid-Producing Head and Neck Cancer. Cancer Sci (2017) 108(6):1128–34. doi: 10.1111/cas.13244
77. Kellum JA, Song M, Li J. Lactic and Hydrochloric Acids Induce Different Patterns of Inflammatory Response in LPS-Stimulated RAW 264.7 Cells. Am J Physiol Regulatory Integr Comp Physiol (2004) 286(4):R686–92. doi: 10.1152/ajpregu.00564.2003
78. Ohashi T, Akazawa T, Aoki M, Kuze B, Mizuta K, Ito Y, et al. Dichloroacetate Improves Immune Dysfunction Caused by Tumor-Secreted Lactic Acid and Increases Antitumor Immunoreactivity. Int J Cancer (2013) 133(5):1107–18. doi: 10.1002/ijc.28114
79. Gao F, Tang Y, Liu WL, Zou MZ, Huang C, Liu CJ, et al. Intra/Extracellular Lactic Acid Exhaustion for Synergistic Metabolic Therapy and Immunotherapy of Tumors. Adv Mater (Deerfield Beach Fla) (2019) 31(51):e1904639. doi: 10.1002/adma.201904639
80. Park YJ, Choe SS, Sohn JH, Kim JB. The Role of Glucose-6-Phosphate Dehydrogenase in Adipose Tissue Inflammation in Obesity. Adipocyte (2017) 6(2):147–53. doi: 10.1080/21623945.2017.1288321
81. Yuan Y, Chen Y, Peng T, Li L, Zhu W, Liu F, et al. Mitochondrial ROS-Induced Lysosomal Dysfunction Impairs Autophagic Flux and Contributes to M1 Macrophage Polarization in a Diabetic Condition. Clin Sci (London England: 1979) (2019) 133(15):1759–77. doi: 10.1042/cs20190672
82. Liang Y, Dong B, Pang N, Hu J. ROS Generation and DNA Damage Contribute to Abamectin-Induced Cytotoxicity in Mouse Macrophage Cells. Chemosphere (2019) 234:328–37. doi: 10.1016/j.chemosphere.2019.06.031
83. West AP, Brodsky IE, Rahner C, Woo DK, Erdjument-Bromage H, Tempst P, et al. TLR Signalling Augments Macrophage Bactericidal Activity Through Mitochondrial ROS. Nature (2011) 472(7344):476–80. doi: 10.1038/nature09973
84. Rendra E, Riabov V, Mossel DM, Sevastyanova T, Harmsen MC, Kzhyshkowska J. Reactive Oxygen Species (ROS) in Macrophage Activation and Function in Diabetes. Immunobiology (2019) 224(2):242–53. doi: 10.1016/j.imbio.2018.11.010
85. Ham M, Choe SS, Shin KC, Choi G, Kim JW, Noh JR, et al. Glucose-6-Phosphate Dehydrogenase Deficiency Improves Insulin Resistance With Reduced Adipose Tissue Inflammation in Obesity. Diabetes (2016) 65(9):2624–38. doi: 10.2337/db16-0060
86. Ham M, Lee JW, Choi AH, Jang H, Choi G, Park J, et al. Macrophage Glucose-6-Phosphate Dehydrogenase Stimulates Proinflammatory Responses With Oxidative Stress. Mol Cell Biol (2013) 33(12):2425–35. doi: 10.1128/mcb.01260-12
87. Bermúdez-Muñoz JM, Celaya AM, Hijazo-Pechero S, Wang J, Serrano M, Varela-Nieto I. G6PD Overexpression Protects From Oxidative Stress and Age-Related Hearing Loss. Aging Cell (2020) 19(12):e13275. doi: 10.1111/acel.13275
88. Choi I, Son H, Baek JH. Tricarboxylic Acid (TCA) Cycle Intermediates: Regulators of Immune Responses. Life (Basel Switzerland) (2021) 11(1):69. doi: 10.3390/life11010069
89. Noe JT, Mitchell RA. Tricarboxylic Acid Cycle Metabolites in the Control of Macrophage Activation and Effector Phenotypes. J Leukoc Biol (2019) 106(2):359–67. doi: 10.1002/jlb.3ru1218-496r
90. Zanluqui NG, Lovo-Martins MI, Malvezi AD, Panis C, da Silva RV, Tatakihara VLH, et al. Concanavalin-A Stimulates IL-17 and Nitric Oxide Production and Induces Macrophage Polarization and Resistance to Trypanosoma Cruzi Infection. Life Sci (2020) 258:118137. doi: 10.1016/j.lfs.2020.118137
91. Palmieri EM, Gonzalez-Cotto M, Baseler WA, Davies LC, Ghesquière B, Maio N, et al. Nitric Oxide Orchestrates Metabolic Rewiring in M1 Macrophages by Targeting Aconitase 2 and Pyruvate Dehydrogenase. Nat Commun (2020) 11(1):698. doi: 10.1038/s41467-020-14433-7
92. De Souza DP, Achuthan A, Lee MK, Binger KJ, Lee MC, Davidson S, et al. Autocrine IFN-I Inhibits Isocitrate Dehydrogenase in the TCA Cycle of LPS-Stimulated Macrophages. J Clin Invest (2019) 129(10):4239–44. doi: 10.1172/jci127597
93. Li Y, Chen X, Zhang H, Xiao J, Yang C, Chen W, et al. 4-Octyl Itaconate Alleviates Lipopolysaccharide-Induced Acute Lung Injury in Mice by Inhibiting Oxidative Stress and Inflammation. Drug Design Dev Ther (2020) 14:5547–58. doi: 10.2147/dddt.S280922
94. Sun KA, Li Y, Meliton AY, Woods PS, Kimmig LM, Cetin-Atalay R, et al. Endogenous Itaconate Is Not Required for Particulate Matter-Induced NRF2 Expression or Inflammatory Response. eLife (2020) 9:e54877. doi: 10.7554/eLife.54877
95. Yi Z, Deng M, Scott MJ, Fu G, Loughran PA, Lei Z, et al. Immune-Responsive Gene 1/Itaconate Activates Nuclear Factor Erythroid 2-Related Factor 2 in Hepatocytes to Protect Against Liver Ischemia-Reperfusion Injury. Hepatology (2020) 72(4):1394–411. doi: 10.1002/hep.31147
96. Zhang S, Jiao Y, Li C, Liang X, Jia H, Nie Z, et al. Dimethyl Itaconate Alleviates the Inflammatory Responses of Macrophages in Sepsis. Inflammation (2020) 44(2):549–57. doi: 10.1007/s10753-020-01352-4
97. ElAzzouny M, Tom CT, Evans CR, Olson LL, Tanga MJ, Gallagher KA, et al. Dimethyl Itaconate Is Not Metabolized Into Itaconate Intracellularly. J Biol Chem (2017) 292(12):4766–9. doi: 10.1074/jbc.C117.775270
98. Swain A, Bambouskova M, Kim H, Andhey PS, Duncan D, Auclair K, et al. Comparative Evaluation of Itaconate and its Derivatives Reveals Divergent Inflammasome and Type I Interferon Regulation in Macrophages. Nat Metab (2020) 2(7):594–602. doi: 10.1038/s42255-020-0210-0
99. Keiran N, Ceperuelo-Mallafré V, Calvo E, Hernández-Alvarez MI, Ejarque M, Núñez-Roa C, et al. SUCNR1 Controls an Anti-Inflammatory Program in Macrophages to Regulate the Metabolic Response to Obesity. Nat Immunol (2019) 20(5):581–92. doi: 10.1038/s41590-019-0372-7
100. Wu JY, Huang TW, Hsieh YT, Wang YF, Yen CC, Lee GL, et al. Cancer-Derived Succinate Promotes Macrophage Polarization and Cancer Metastasis via Succinate Receptor. Mol Cell (2020) 77(2):213–27.e5. doi: 10.1016/j.molcel.2019.10.023
101. Guo Y, Xie C, Li X, Yang J, Yu T, Zhang R, et al. Succinate and its G-Protein-Coupled Receptor Stimulates Osteoclastogenesis. Nat Commun (2017) 8:15621. doi: 10.1038/ncomms15621
102. Mills E, O’Neill LA. Succinate: A Metabolic Signal in Inflammation. Trends Cell Biol (2014) 24(5):313–20. doi: 10.1016/j.tcb.2013.11.008
103. Chen F, Chen J, Yang L, Liu J, Zhang X, Zhang Y, et al. Extracellular Vesicle-Packaged HIF-1α-Stabilizing lncRNA From Tumour-Associated Macrophages Regulates Aerobic Glycolysis of Breast Cancer Cells. Nat Cell Biol (2019) 21(4):498–510. doi: 10.1038/s41556-019-0299-0
104. Zeng H, Qi X, Xu X, Wu Y. TAB1 Regulates Glycolysis and Activation of Macrophages in Diabetic Nephropathy. Inflammation Res: Off J Eur Histamine Res Soc (2020) 69(12):1215–34. doi: 10.1007/s00011-020-01411-4
105. Song W, Li D, Tao L, Luo Q, Chen L. Solute Carrier Transporters: The Metabolic Gatekeepers of Immune Cells. Acta Pharm Sin B (2020) 10(1):61–78. doi: 10.1016/j.apsb.2019.12.006
106. Yu Y, Cai W, Zhou J, Lu H, Wang Y, Song Y, et al. Anti-Arthritis Effect of Berberine Associated With Regulating Energy Metabolism of Macrophages Through AMPK/HIF-1α Pathway. Int Immunopharmacol (2020) 87:106830. doi: 10.1016/j.intimp.2020.106830
107. Wei Y, Chen J, Cai GE, Lu W, Xu W, Wang R, et al. Rosmarinic Acid Regulates Microglial M1/M2 Polarization via the PDPK1/Akt/HIF Pathway Under Conditions of Neuroinflammation. Inflammation (2020) 44(1):129–47. doi: 10.1007/s10753-020-01314-w
108. Marín-Hernández A, Gallardo-Pérez JC, Ralph SJ, Rodríguez-Enríquez S, Moreno-Sánchez R. HIF-1alpha Modulates Energy Metabolism in Cancer Cells by Inducing Over-Expression of Specific Glycolytic Isoforms. Mini Rev Med Chem (2009) 9(9):1084–101. doi: 10.2174/138955709788922610
109. Ménégaut L, Jalil A, Thomas C, Masson D. Macrophage Fatty Acid Metabolism and Atherosclerosis: The Rise of PUFAs. Atherosclerosis (2019) 291:52–61. doi: 10.1016/j.atherosclerosis.2019.10.002
110. Qian X, Yang Z, Mao E, Chen E. Regulation of Fatty Acid Synthesis in Immune Cells. Scand J Immunol (2018) 88(5):e12713. doi: 10.1111/sji.12713
111. Nakayama Y, Fujiu K, Yuki R, Oishi Y, Morioka MS, Isagawa T, et al. A Long Noncoding RNA Regulates Inflammation Resolution by Mouse Macrophages Through Fatty Acid Oxidation Activation. Proc Natl Acad Sci USA (2020) 117(25):14365–75. doi: 10.1073/pnas.2005924117
112. Batista-Gonzalez A, Vidal R, Criollo A, Carreño LJ. New Insights on the Role of Lipid Metabolism in the Metabolic Reprogramming of Macrophages. Front Immunol (2019) 10:2993. doi: 10.3389/fimmu.2019.02993
113. Malandrino MI, Fucho R, Weber M, Calderon-Dominguez M, Mir JF, Valcarcel L, et al. Enhanced Fatty Acid Oxidation in Adipocytes and Macrophages Reduces Lipid-Induced Triglyceride Accumulation and Inflammation. Am J Physiol Endocrinol Metab (2015) 308(9):E756–69. doi: 10.1152/ajpendo.00362.2014
114. Su P, Wang Q, Bi E, Ma X, Liu L, Yang M, et al. Enhanced Lipid Accumulation and Metabolism Are Required for the Differentiation and Activation of Tumor-Associated Macrophages. Cancer Res (2020) 80(7):1438–50. doi: 10.1158/0008-5472.Can-19-2994
115. Ménégaut L, Thomas C, Lagrost L, Masson D. Fatty Acid Metabolism in Macrophages: A Target in Cardio-Metabolic Diseases. Curr Opin Lipidol (2017) 28(1):19–26. doi: 10.1097/mol.0000000000000370
116. Namgaladze D, Brüne B. Fatty Acid Oxidation is Dispensable for Human Macrophage IL-4-Induced Polarization. Biochim Biophys Acta (2014) 1841(9):1329–35. doi: 10.1016/j.bbalip.2014.06.007
117. Nomura M, Liu J, Rovira II, Gonzalez-Hurtado E, Lee J, Wolfgang MJ, et al. Fatty Acid Oxidation in Macrophage Polarization. Nat Immunol (2016) 17(3):216–7. doi: 10.1038/ni.3366
118. Gonzalez-Hurtado E, Lee J, Choi J, Selen Alpergin ES, Collins SL, Horton MR, et al. Loss of Macrophage Fatty Acid Oxidation Does Not Potentiate Systemic Metabolic Dysfunction. Am J Physiol Endocrinol Metab (2017) 312(5):E381–e93. doi: 10.1152/ajpendo.00408.2016
119. Mills CD, Kincaid K, Alt JM, Heilman MJ, Hill AM. M-1/M-2 Macrophages and the Th1/Th2 Paradigm. J Immunol (2000) 164(12):6166–73. doi: 10.4049/jimmunol.164.12.6166
120. Pekarova M, Lojek A. The Crucial Role of L-Arginine in Macrophage Activation: What You Need to Know About It. Life Sci (2015) 137:44–8. doi: 10.1016/j.lfs.2015.07.012
121. Brunner JS, Vulliard L, Hofmann M, Kieler M, Lercher A, Vogel A, et al. Environmental Arginine Controls Multinuclear Giant Cell Metabolism and Formation. Nat Commun (2020) 11(1):431. doi: 10.1038/s41467-020-14285-1
122. Fall F, Lamy E, Brollo M, Naline E, Lenuzza N, Thévenot E, et al. Metabolic Reprograming of LPS-Stimulated Human Lung Macrophages Involves Tryptophan Metabolism and the Aspartate-Arginosuccinate Shunt. PLoS One (2020) 15(4):e0230813. doi: 10.1371/journal.pone.0230813
123. Murray PJ. Amino Acid Auxotrophy as a System of Immunological Control Nodes. Nat Immunol (2016) 17(2):132–9. doi: 10.1038/ni.3323
124. Lanaspa MA, Ishimoto T, Cicerchi C, Tamura Y, Roncal-Jimenez CA, Chen W, et al. Endogenous Fructose Production and Fructokinase Activation Mediate Renal Injury in Diabetic Nephropathy. J Am Soc Nephrol: JASN (2014) 25(11):2526–38. doi: 10.1681/asn.2013080901
125. Gleissner CA, Sanders JM, Nadler J, Ley K. Upregulation of Aldose Reductase During Foam Cell Formation as Possible Link Among Diabetes, Hyperlipidemia, and Atherosclerosis. Arteriosclerosis Thrombosis Vasc Biol (2008) 28(6):1137–43. doi: 10.1161/atvbaha.107.158295
126. Zhang K, Lu WC, Zhang M, Zhang Q, Xian PP, Liu FF, et al. Reducing Host Aldose Reductase Activity Promotes Neuronal Differentiation of Transplanted Neural Stem Cells at Spinal Cord Injury Sites and Facilitates Locomotion Recovery. Neural Regeneration Res (2022) 17(8):1814–20. doi: 10.4103/1673-5374.330624
127. Liang W, Ouyang S, Shaw N, Joachimiak A, Zhang R, Liu ZJ. Conversion of D-Ribulose 5-Phosphate to D-Xylulose 5-Phosphate: New Insights From Structural and Biochemical Studies on Human RPE. FASEB J: Off Publ Fed Am Societies Exp Biol (2011) 25(2):497–504. doi: 10.1096/fj.10-171207
128. Rmaileh AA, Solaimuthu B, Tanna M, Khatib A, Yosef MB, Hayashi A, et al. Large-Scale Differential Gene Expression Transcriptomic Analysis Identifies a Metabolic Signature Shared by All Cancer Cells. Biomolecules (2020) 10(5):701. doi: 10.3390/biom10050701
129. Zhang D, Tang Z, Huang H, Zhou G, Cui C, Weng Y, et al. Metabolic Regulation of Gene Expression by Histone Lactylation. Nature (2019) 574(7779):575–80. doi: 10.1038/s41586-019-1678-1
130. Huang C, Wang J, Liu H, Huang R, Yan X, Song M, et al. Ketone Body β-Hydroxybutyrate Ameliorates Colitis by Promoting M2 Macrophage Polarization Through the STAT6-Dependent Signaling Pathway. BMC Med (2022) 20(1):148. doi: 10.1186/s12916-022-02352-x
131. Yang K, Fan M, Wang X, Xu J, Wang Y, Tu F, et al. Lactate Promotes Macrophage HMGB1 Lactylation, Acetylation, and Exosomal Release in Polymicrobial Sepsis. Cell Death Differ (2022) 29(1):133–46. doi: 10.1038/s41418-021-00841-9
132. Sun S, Xu X, Liang L, Wang X, Bai X, Zhu L, et al. Lactic Acid-Producing Probiotic Saccharomyces Cerevisiae Attenuates Ulcerative Colitis via Suppressing Macrophage Pyroptosis and Modulating Gut Microbiota. Front Immunol (2021) 12:777665. doi: 10.3389/fimmu.2021.777665
133. Zhu Y, Zhang S, Sun J, Wang T, Liu Q, Wu G, et al. Cigarette Smoke Promotes Oral Leukoplakia via Regulating Glutamine Metabolism and M2 Polarization of Macrophage. Int J Oral Sci (2021) 13(1):25. doi: 10.1038/s41368-021-00128-2
134. Merlin J, Ivanov S, Dumont A, Sergushichev A, Gall J, Stunault M, et al. Non-Canonical Glutamine Transamination Sustains Efferocytosis by Coupling Redox Buffering to Oxidative Phosphorylation. Nat Metab (2021) 3(10):1313–26. doi: 10.1038/s42255-021-00471-y
135. Menga A, Favia M, Spera I, Vegliante MC, Gissi R, De Grassi A, et al. N-Acetylaspartate Release by Glutaminolytic Ovarian Cancer Cells Sustains Protumoral Macrophages. EMBO Rep (2021) 22(9):e51981. doi: 10.15252/embr.202051981
136. Li Z, Zhang S, Zhang Y, Chen J, Wu F, Liu G, et al. Applications and Mechanism of 3-Hydroxybutyrate (3HB) for Prevention of Colonic Inflammation and Carcinogenesis as a Food Supplement. Mol Nutr Food Res (2021) 65(24):e2100533. doi: 10.1002/mnfr.202100533
137. Houser BL, Tilburgs T, Hill J, Nicotra ML, Strominger JL. Two Unique Human Decidual Macrophage Populations. J Immunol (2011) 186(4):2633–42. doi: 10.4049/jimmunol.1003153
138. Guerin L, Wu V, Houser B, Tilburgs T, de Jong A, Moody DB, et al. CD1 Antigen Presentation and Autoreactivity in the Pregnant Human Uteru. Am J Reprod Immunol (2015) 74(2):126–35. doi: 10.1111/aji.12375
139. Jiang X, Du MR, Li M, Wang H. Three Macrophage Subsets Are Identified in the Uterus During Early Human Pregnancy. Cell Mol Immunol (2018) 15(12):1027–37. doi: 10.1038/s41423-018-0008-0
140. Gill N, Leng Y, Romero R, Xu Y, Panaitescu B, Miller D, et al. The Immunophenotype of Decidual Macrophages in Acute Atherosis. Am J Reprod Immunol (N Y NY: 1989) (2019) 81(4):e13098. doi: 10.1111/aji.13098
141. Mantovani A, Sozzani S, Locati M, Allavena P, Sica A. Macrophage Polarization: Tumor-Associated Macrophages as a Paradigm for Polarized M2 Mononuclear Phagocytes. Trends Immunol (2002) 23(11):549–55. doi: 10.1016/s1471-4906(02)02302-5
142. Chen X, Zhou J, Li X, Wang X, Lin Y, Wang X. Exosomes Derived From Hypoxic Epithelial Ovarian Cancer Cells Deliver microRNAs to Macrophages and Elicit a Tumor-Promoted Phenotype. Cancer Lett (2018) 435:80–91. doi: 10.1016/j.canlet.2018.08.001
143. Wang L, Li YS, Yu LG, Zhang XK, Zhao L, Gong FL, et al. Galectin-3 Expression and Secretion by Tumor-Associated Macrophages in Hypoxia Promotes Breast Cancer Progression. Biochem Pharmacol (2020) 178:114113. doi: 10.1016/j.bcp.2020.114113
144. Yu X, Li Z, Zhang Y, Xu M, Che Y, Tian X, et al. β-Elemene Inhibits Radiation and Hypoxia-Induced Macrophages Infiltration via Prx-1/NF-κb/HIF-1α Signaling Pathway. Onco Targets Ther (2019) 12:4203–11. doi: 10.2147/ott.S196910
145. Ahmed M, de Winther MPJ, Van den Bossche J. Epigenetic Mechanisms of Macrophage Activation in Type 2 Diabetes. Immunobiology (2017) 222(10):937–43. doi: 10.1016/j.imbio.2016.08.011
146. Xie Z, Wang X, Liu X, Du H, Sun C, Shao X, et al. Adipose-Derived Exosomes Exert Proatherogenic Effects by Regulating Macrophage Foam Cell Formation and Polarization. J Am Heart Assoc (2018) 7(5):e007442. doi: 10.1161/jaha.117.007442
147. Zhu X, Owen JS, Wilson MD, Li H, Griffiths GL, Thomas MJ, et al. Macrophage ABCA1 Reduces MyD88-Dependent Toll-Like Receptor Trafficking to Lipid Rafts by Reduction of Lipid Raft Cholesterol. J Lipid Res (2010) 51(11):3196–206. doi: 10.1194/jlr.M006486
148. Feduska JM, Tse HM. The Proinflammatory Effects of Macrophage-Derived NADPH Oxidase Function in Autoimmune Diabetes. Free Radical Biol Med (2018) 125:81–9. doi: 10.1016/j.freeradbiomed.2018.04.581
149. Bories GFP, Leitinger N. Macrophage Metabolism in Atherosclerosis. FEBS Lett (2017) 591(19):3042–60. doi: 10.1002/1873-3468.12786
150. Ali L, Schnitzler JG, Kroon J. Metabolism: The Road to Inflammation and Atherosclerosis. Curr Opin Lipidol (2018) 29(6):474–80. doi: 10.1097/mol.0000000000000550
151. Chistiakov DA, Bobryshev YV, Orekhov AN. Changes in Transcriptome of Macrophages in Atherosclerosis. J Cell Mol Med (2015) 19(6):1163–73. doi: 10.1111/jcmm.12591
152. Luo Y, Duan H, Qian Y, Feng L, Wu Z, Wang F, et al. Macrophagic CD146 Promotes Foam Cell Formation and Retention During Atherosclerosis. Cell Res (2017) 27(3):352–72. doi: 10.1038/cr.2017.8
153. Smigiel KS, Parks WC. Macrophages, Wound Healing, and Fibrosis: Recent Insights. Curr Rheumatol Rep (2018) 20(4):17. doi: 10.1007/s11926-018-0725-5
154. Eming SA, Wynn TA, Martin P. Inflammation and Metabolism in Tissue Repair and Regeneration. Science (2017) 356(6342):1026–30. doi: 10.1126/science.aam7928
155. Care AS, Diener KR, Jasper MJ, Brown HM, Ingman WV, Robertson SA. Macrophages Regulate Corpus Luteum Development During Embryo Implantation in Mice. J Clin Invest (2013) 123(8):3472–87. doi: 10.1172/jci60561
156. Ning F, Liu H, Lash GE. The Role of Decidual Macrophages During Normal and Pathological Pregnancy. Am J Reprod Immunol (N Y NY: 1989) (2016) 75(3):298–309. doi: 10.1111/aji.12477
157. Movahedi K, Van Ginderachter JA. The Ontogeny and Microenvironmental Regulation of Tumor-Associated Macrophages. Antioxid Redox Signal (2016) 25(14):775–91. doi: 10.1089/ars.2016.6704
158. Genin M, Clement F, Fattaccioli A, Raes M, Michiels C. M1 and M2 Macrophages Derived From THP-1 Cells Differentially Modulate the Response of Cancer Cells to Etoposide. BMC Cancer (2015) 15:577. doi: 10.1186/s12885-015-1546-9
159. Weiss JM. The Promise and Peril of Targeting Cell Metabolism for Cancer Therapy. Cancer Immunol Immunother: CII (2020) 69(2):255–61. doi: 10.1007/s00262-019-02432-7
160. Dang CP, Leelahavanichkul A. Over-Expression of miR-223 Induces M2 Macrophage Through Glycolysis Alteration and Attenuates LPS-Induced Sepsis Mouse Model, the Cell-Based Therapy in Sepsis. PLoS One (2020) 15(7):e0236038. doi: 10.1371/journal.pone.0236038
161. Chiang CF, Chao TT, Su YF, Hsu CC, Chien CY, Chiu KC, et al. Metformin-Treated Cancer Cells Modulate Macrophage Polarization Through AMPK-NF-κb Signaling. Oncotarget (2017) 8(13):20706–18. doi: 10.18632/oncotarget.14982
162. McLaren JE, Michael DR, Ashlin TG, Ramji DP. Cytokines, Macrophage Lipid Metabolism and Foam Cells: Implications for Cardiovascular Disease Therapy. Prog Lipid Res (2011) 50(4):331–47. doi: 10.1016/j.plipres.2011.04.002
163. Liu Q, Li Y, Niu Z, Zong Y, Wang M, Yao L, et al. Atorvastatin (Lipitor) Attenuates the Effects of Aspirin on Pancreatic Cancerogenesis and the Chemotherapeutic Efficacy of Gemcitabine on Pancreatic Cancer by Promoting M2 Polarized Tumor Associated Macrophages. J Exp Clin Cancer Res: CR (2016) 35:33. doi: 10.1186/s13046-016-0304-4
164. Calle RA, Amin NB, Carvajal-Gonzalez S, Ross TT, Bergman A, Aggarwal S, et al. ACC Inhibitor Alone or Co-Administered With a DGAT2 Inhibitor in Patients With non-Alcoholic Fatty Liver Disease: Two Parallel, Placebo-Controlled, Randomized Phase 2a Trials. Nat Med (2021) 27(10):1836–48. doi: 10.1038/s41591-021-01489-1
Keywords: macrophage, polarization, metabolism, maternal-fetal interface, pregnancy
Citation: Sun J-X, Xu X-H and Jin L (2022) Effects of Metabolism on Macrophage Polarization Under Different Disease Backgrounds. Front. Immunol. 13:880286. doi: 10.3389/fimmu.2022.880286
Received: 21 February 2022; Accepted: 21 June 2022;
Published: 14 July 2022.
Edited by:
Alexei Gratchev, Russian Cancer Research Center NN Blokhin, RussiaReviewed by:
Tatyana Veremeyko, The Chinese University of Hong Kong, ChinaWanfu Xu, Guangzhou Medical University, China
Copyright © 2022 Sun, Xu and Jin. This is an open-access article distributed under the terms of the Creative Commons Attribution License (CC BY). The use, distribution or reproduction in other forums is permitted, provided the original author(s) and the copyright owner(s) are credited and that the original publication in this journal is cited, in accordance with accepted academic practice. No use, distribution or reproduction is permitted which does not comply with these terms.
*Correspondence: Liping Jin, amlubHAwMUAxNjMuY29t; Xiang-Hong Xu, eGlhbmdob25neHUyMDE0QDE2My5jb20=