- 1Institute of Drug Discovery Technology, Ningbo University, Ningbo, China
- 2Qian Xuesen Collaborative Research Center of Astrochemistry and Space Life Sciences, Ningbo University, Ningbo, China
- 3School of Medicine, Xiamen University, Xiamen, China
Coronavirus Disease 2019 (COVID-19) caused by SARS-CoV-2 has become a global health issue. The clinical presentation of COVID-19 is highly variable, ranging from asymptomatic and mild disease to severe. However, the mechanisms for the high mortality induced by SARS-CoV-2 infection are still not well understood. Recent studies have indicated that the cytokine storm might play an essential role in the disease progression in patients with COVID-19, which is characterized by the uncontrolled release of cytokines and chemokines leading to acute respiratory distress syndrome (ARDS), multi-organ failure, and even death. Cell death, especially, inflammatory cell death, might be the initiation of a cytokine storm caused by SARS-CoV-2 infection. This review summarizes the forms of cell death caused by SARS-CoV-2 in vivo or in vitro and elaborates on the dedication of apoptosis, necroptosis, NETosis, pyroptosis of syncytia, and even SARS-CoV-2 E proteins forming channel induced cell death, providing insights into targets on the cell death pathway for the treatment of COVID-19.
Introduction
Human coronaviruses (HCoVs) are known respiratory pathogens that could cause multiple respiratory diseases, ranging from the common cold and bronchitis to serious pneumonia (1, 2). Three of these viruses have been causing serious symptoms over the last years, including Severe Acute Respiratory Syndrome (SARS), Middle East Respiratory Syndrome Coronavirus (MERS), and now SARS Coronavirus 2 (SARS-CoV-2), especially SARS-CoV-2, which is responsible for the Coronavirus Disease 2019 (COVID-19) and has become a pandemic worldwide, causing millions of deaths and massive property losses (3–7). SARS-CoV-2 is a single-stranded RNA virus; belongs to the β-coronavirus; contains 29,903 nucleotides; encodes 16 non-structural proteins (NSP1–NSP16), 9 putative accessory factors, and 4 structural proteins, i.e., spike (S), envelope (E), membrane (M), and nucleocapsid (N); and spreads via respiratory droplets or close contact, which triggers mild or severe diseases (8–11) (Figure 1A). The main clinical symptoms of infected patients are cough, fever, and tachypnea; a CT scan usually reveals multiple patchy shadows. Severe infection can cause cytokine storms within the body, leading to multi-organ failure and even death (12–14). Cytokine storm is a life-threatening systemic inflammatory response syndrome that can be induced by pathogens, autoimmune disorders, or inflammatory cell death (15–18).
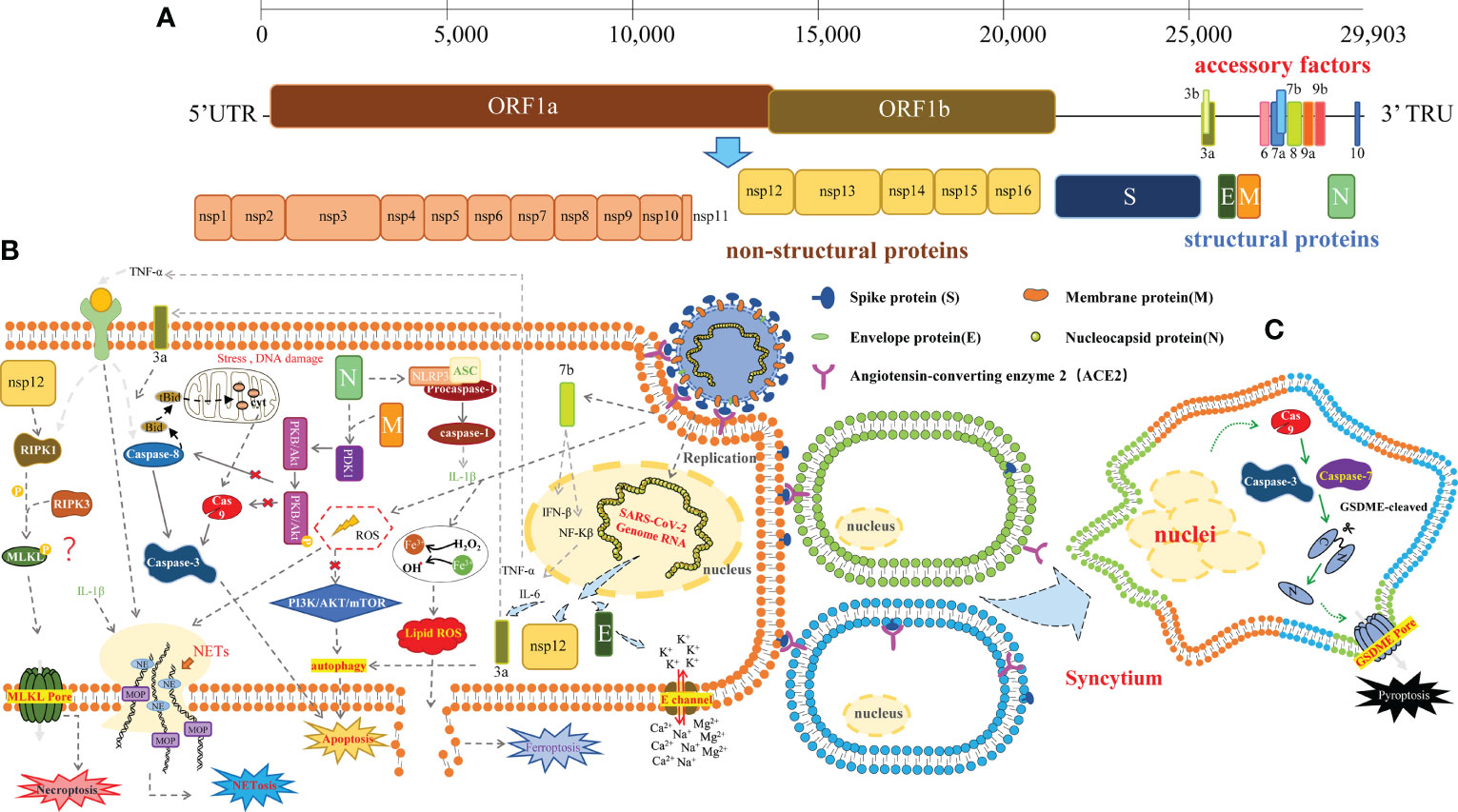
Figure 1 The schematic of SARS-CoV-2-induced cell death. (A) The SARS-CoV-2 virus particle and genome. The genome is a single-stranded RNA genome of which the full length is 29,903 bp. It includes ORF1a and ORF1b, which encode the 16 non-structural proteins, 9 accessory factors, and 4 structural proteins: spike protein (S), envelope protein (E), mbrane protein (M), and nucleocapsid protein (N). (B) SARS-CoV-2 induces various cellular stress: apoptosis, triggered by the extrinsic pathway (death receptor pathway), or the intrinsic pathway (mitochondrial pathway), involving the caspase cleavage. SARS-CoV-2 ORF3a caused apoptosis via the caspase-8/Bid extrinsic pathway; ORF7b can activate TNFα-induced apoptosis. Membrane (M) protein with nucleocapsid (N) protein via interacting with PDK1 and inhibiting the activation of PDK1-PKB/Akt signaling to trigger caspase-dependent apoptosis. Another structural protein spike of SARS-CoV-2 also induced autophagy and apoptosis by ROS-suppressed PI3K/AKT/mTOR signaling; necroptosis, mediated by RIPK1/RIPK3/MLKL. MLKL can be recruited by the autophosphorylated RIPK3 and subsequently phosphorylated by RIPK3 of human MLKL. Phosphorylated MLKL will form an MLKL pore, resulting in necroptosis. Nsp12 interacted with RIPK1 and activated it; NLRP3 inflammasome, consisting of NLRP3, ASC, and caspase-1, activated by N protein of SARS-CoV-2 interacted directly with NLRP3; SARS-CoV-2 E proteins form cation channels to trigger cell death independent of MLKL and gasdermins; NETosis, triggered by neutrophils and formed neutrophil extracellular traps (NETs) to release of chromatin structures containing myeloperoxidase and antimicrobial proteins to neutralize intruders. MPO, myeloperoxidase; NE, Neutrophil Elastase. Ferroptosis, triggered by iron accumulation and overload, or reactive oxygen species (ROS). (C) Pyroptosis, mediated by the gasdermin (GSDM) protein family. The N-terminal fragments of GSDM protein could induce the formation of membrane pores. SARS-CoV-2 S induces cell–cell fusion and syncytia formation driving caspase-9/GSDME-mediated syncytia pyroptosis.
The autopsy of patients infected with SARS-CoV-2 is of great significance to truly understand the pathological changes of COVID-19 (19–27). Gupta et al. have well-reviewed that SARS-CoV-2 infection caused various injuries ranging from substantial respiratory to many extrapulmonary organs failure, including thrombotic complications, myocardial dysfunction and arrhythmia, acute coronary syndromes, acute kidney injury (AKI), gastrointestinal symptoms, hepatocellular injury, hyperglycemia and ketosis, neurologic illnesses, ocular symptoms, and dermatologic complications (28). Here, we mainly summarized how the different types of cell death caused by SARS-CoV-2 infection contribute to the organic failure directly, or indirectly, and discussed the therapy targets on the cell death signaling transduction molecules for treatment for COVID-19.
Multiple Cell Death Pathways Were Induced in SARS-CoV-2 Infection
The cytopathic effect of cell death caused by the virus invading the host cells is a common result after the infection (29). Cell death in some instances can inhibit viral replication, but in more cases, it can enhance viral dissemination and affect the physiology of cells, leading to tissue and organ damage (30). The replication of coronaviruses in cells is regulated by many host factors, which can induce drastic structural and physiological changes in cells (2). During infection, SARS-CoV-2 could induce diverse cell death pathways (31, 32), such as apoptosis, necroptosis, pyroptosis, and NETosis in the host cells (Figure 1B).
Apoptosis is a major type of programmed cell death, morphologically characterized by cellular shrinkage, nuclear condensation, chromosomal DNA fragmentation, cytosolic membrane blebbing, and apoptotic body formation. It is triggered by the extrinsic (death receptor pathway) pathway, or the intrinsic (mitochondrial pathway) pathway, involving a group of cysteinyl aspartate proteases (caspases) cleavage (activation) (33–37).
SARS-CoV-2 infection can induce apoptosis via a variety of signaling pathways. It has been reported that the accessory protein ORF3a of SARS-CoV-1 caused cell death, vesicle formation, and Golgi fragmentation in VERO cells (38). To survey whether SARS-CoV-2 ORF3a can induce apoptosis, Ren and colleagues (39) overexpressed SARS-CoV-2 ORF3a in cultured HEK293T, HepG2, and VERO E6 cells; then stained the cells by annexin V-fluorescein 5-isothiocyanate (FITC)/propidium iodide (PI); and analyzed the apoptotic cells by flow cytometry. They found that SARS-CoV-2 ORF3a caused apoptosis via the caspase-8/Bid extrinsic pathway, which can be restored by z-VAD-fmk, a pan-caspase inhibitor. Importantly, SARS-CoV-2 ORF3a showed weaker proapoptotic activity than SARS-CoV-1 ORF3a in cultured cells, which might lead the virus to spread more widely. Consistently, two more groups demonstrated that SARS-CoV-2 ORF3a inhibited autophagic flux by blocking the fusion of autophagosomes/amphisomes with lysosomes, causing lysosomal destruction, which allowed the virus to escape the degradation by lysosomal (40, 41). These studies facilitated strategies targeting SARS-CoV-2 ORF3a or autophagic pathway for conferring potential protection against the spread of SARS-CoV-2. In support of this concept, a study by Gassen and colleagues demonstrated that targeting autophagic pathways on the polyamine pathway, and the control of BECN1 abundance through AKT1/SKP2 signaling by exogenous administration of spermidine and spermine, the selective AKT1 inhibitor MK-2206, and the BECN1-stabilizing anthelmintic drug niclosamide inhibited SARS-CoV-2 propagation in vitro and in vivo (42). Thus, both MK-2206 and niclosamide might be promising candidates for clinical trials.
ORF7b is another accessory protein of SARS-CoV-2, which can induce the transcription of IFN-β, TNF-α, and IL-6, activating type-I IFN signaling through IRF3 phosphorylation and activating TNFα-induced apoptosis in HEK293T cells and VERO E6 cells (43).
The membrane glycoprotein M of SARS-CoV-2 could trigger caspase-dependent apoptosis with the assistance of the nucleocapsid (N) protein via interacting with PDK1 and inhibiting the activation of PDK1-PKB/Akt signaling. Disruption of the M–N interaction by certain rationally designed peptides, abolished M-induced apoptosis, shedding light on a new aspect of drug designs on M–N interaction to prevent SARS-CoV-2 infection, which caused apoptosis (44).
Another structural protein spike of SARS-CoV-2 also induced autophagy and apoptosis in human bronchial epithelial and microvascular endothelial cells by reactive oxygen species (ROS)-suppressed PI3K/AKT/mTOR signaling, which then led to inflammatory responses, raising important implications for developing anti-inflammatory therapies, such as ROS and autophagy inhibitors, for COVID-19 patients (45).
In addition, a clinical report showed that a total of 17 of the 18 patients who died of COVID-19 suffered from lymphocytopenia, which is the main feature of severe COVID-19 disease (46). TUNEL staining showed that spleens and hilar lymph nodes (LNs) exhibited many lymphocyte apoptosis processes, which were caused by SARS-CoV-2 promoting Fas-mediated apoptosis of T and B lymphocytes.
Further, elevated serum levels of creatinine, tubular necrosis, and renal inflammation were observed in critically ill COVID-19 patients, consistent with AKI symptoms (47–49). To identify and uncover mechanisms specifically related to a SARS-CoV-2 protein that can induce cell death in AKI after SARS-CoV-2 infection, the SARS-CoV-2 nucleocapsid (N) structural protein-expressing plasmid was delivered into the normal mouse kidneys using a well-established non-invasive ultrasound-microbubble technique, which can induce AKI and exacerbate AKI under ischemic stress conditions. The mechanism lies in SARS-CoV-2 N interacting with Smad3 and enhances TGF-β/Smad3 signaling to arrest the G1 cell cycle leading to renal tubular epithelial cell apoptosis as labeled by TUNEL-positive cells. Moreover, both deletion of Smad3 and treatment with SIS3, the inhibitor of Smad3, can restore the SARS-CoV-2 N-induced AKI, which indicated that targeting Smad3 may represent a novel therapy for COVID-19-associated AKI (50).
Although we have summarized the apoptosis caused via different mechanisms induced by SARS-CoV-2, the underlying mechanisms of the massive inflammatory responses triggered by SARS-CoV-2 are largely limited. In contrast to necrosis, apoptosis is a form of clear cell death because the apoptotic bodies can be cleared through the phagocytic pathway by neighboring cells, without the release of cellular contents (51). We wonder whether inflammatory cell death occurred during SARS-CoV-2 infection. Indeed, analysis of the postmortem lung sections of fatal COVID-19 patients revealed that not only apoptosis but also necroptosis occurred in the lung, and the necrotic cell debris promoted massive inflammatory cell infiltration leading to lung damage in COVID-19 patients (52).
Necroptosis is an inflammatory type of programmed cell death mediated by RIPK1/RIPK3/MLKL. The occurrence of programmed necrosis could induce a series of morphological alterations in cells: with slight changes in the ultrastructure of the nucleus (especially the expansion of the nuclear membrane and the formation of small, irregular, and circumscribed patches by chromatin condensation), with increasing lucent cytoplasm and swelling organelles, the increased permeability of the cell membrane causes the cell to grow in size, resulting in the cell rupturing and the outflow of intracellular contents and provoking the inflammatory response of the surrounding tissues (53, 54). Mixed-lineage kinase domain-like (MLKL) is the main effector protein in necroptosis, which contains an N-terminal coiled-coil domain and a C-terminal kinase-like domain. MLKL can be recruited by the autophosphorylated RIPK3 and subsequently phosphorylated by RIPK3 at the threonine 357 and serine 358 residues of human MLKL (serine at positions 345, 347, and 352 and threonine at position 349 for mouse MLKL) (55–57). Phosphorylated MLKL will oligomerize and traffic to the plasma membrane, forming an MLKL pore, resulting in necroptosis (58).
As an important mediator of inflammation and cell death, RIPK1 can mediate the activation of caspase-8 to promote apoptosis or promote necroptosis by activating RIPK3 and MLKL (59–62). Based on some evidence of RIPK1 activation found in COVID-19 (63–65), Xu et al. used the lung pathological samples of COVID-19 patients and cultured human lung organoids and ACE2 transgenic mice infected by SARS-CoV-2 to explore the role of RIPK1 in SARS-CoV-2 infection. Although autopsy detection revealed that the expression of its downstream signaling molecule RIPK3 was found to be very low, and phosphorylated RIPK3 and MLKL were also undetectable, they found that the RNA-dependent RNA polymerase of SARS-CoV-2, NSP12, directly interacted with RIPK1 to promote its activation, resulting in the transcriptional induction of proinflammatory cytokines and host factors including ACE2 and EGFR, which promote viral entry into cells (66). As multiple RIPK1 inhibitors (Nec-1s, GSK′481/GSK′772, etc.) have been advanced beyond Phase I safety studies in human clinical trials (67, 68), the authors suggested that the RIPK1 kinase inhibitors may provide effective therapy for severe COVID-19.
During SARS-CoV-2 infection, the inhibitor of necroptosis did not completely block IL-1β secretion, suggesting that there may be other pathways involved in the inflammatory responses such as pyroptosis (52).
Pyroptosis is a lytic and inflammatory type of programmed cell death, which is characterized by the swelling of cells, forming a big balloon on the plasma membrane, destructing the cell plasma membrane, releasing the cellular contents, and causing lysis of cells (69, 70). This type of cell death is mediated by the gasdermin (GSDM) protein family (71), which is activated and cleaved by caspase protein or other proteases (72–79). The N-terminal fragments of GSDM protein could induce the formation of membrane pores, disrupting the cell membrane and causing eventual lysis (80, 81).
The participation of the inflammasome in COVID-19 has been highly speculated as to its main contribution to excessive inflammatory responses upon SARS-CoV-2 infection (82, 83). The NLRP3 inflammasome, consisting of NLRP3, ASC, and caspase-1, is activated in response to SARS-CoV-2 infection and is active in COVID-19 patients, which is associated with the clinical outcome of the disease (84). Furthermore, Pan and colleagues found that the N protein of SARS-CoV-2 interacted directly with NLRP3, promoted the recruitment of ASC, and facilitated NLRP3 inflammasome assembly, which resulted in the maturation of proinflammatory cytokines and triggered proinflammatory responses in cultured HEK293T or A549 cells. Notably, treatment with MCC950 (a specific inhibitor of NLRP3) and Ac-YVAD-cmk (an inhibitor of caspase-1) or genetic deletion of Nlrp3 inhibited N protein-induced lung injury and cytokine production (85). However, in cultured Calu-3 cells, the inhibitors of caspase-1 and NLRP3 had no effects on the production of IL-1β induced by SARS-CoV-2 infection but blocks caspase-8 using the inhibitor, or siRNA knockdown decreased the production and secretion of IL-1β (52). In this scenario, it is important to further determine specific mechanisms by which SARS-CoV-2 triggers the inflammasome activation and investigate which specific inflammasome platforms are activated during the disease for effective therapeutic strategies to target COVID-19.
NETosis, a form of regulated neutrophil death, is characterized by the formation and release of neutrophil extracellular traps (NETs), which are networks of myriad pathogen-associated molecular patterns (PAMPs), consisting of extracellular fibers composed of DNA containing histones and granule-derived enzymes (such as lactoferrin, cathepsins, neutrophil elastase (NE), and myeloperoxidase (MPO)), as well as cytoplasmic and cytoskeletal proteins. In addition to the NADPH oxidase (NOX)/ROS-, peptidylarginine deiminase 4 (PADI4)-, and NE-dependent pathways on the activation of NETosis, RIPK3/MLKL-mediated necroptosis and GSDMD-driven pyroptosis linked the excessive inflammatory response to NETosis (86–89). Emerging evidence from the clinic severe cases of COVID-19 implicated that NETosis and NET formation/release played a central role in the pathophysiology of inflammation, coagulopathy, immunothrombosis, and even organ damage during SARS-CoV-2 infection (90–94). With the growing roles of NETosis and NETs in COVID-19 reported, targeting dysregulated NETosis and NET formation/release is a new aspect of severe COVID-19 treatment. NETosis inhibitors (fostamatinib targeting SYK, etc.), or NET degraders (GSK 484 targeting PAD 4, Dornase alfa degrading cfDNA) were used in preclinical or clinical development as anti-COVID-19 drugs, which was well-summarized by other groups (93, 95–97). Here, we emphasized the Food and Drug Administration (FDA)-approved alcoholism-averting drug, disulfiram, which was identified as an inhibitor of GSDMD pore formation by covalently modifying human/mouse Cys191/Cys192 in GSDMD and preventing IL-1β release and pyroptosis (98). Although the linkage of GSDMD-mediated pyroptosis with NETosis has been reported (99, 100), Egeblad and colleagues recently found that treatment with disulfiram reduced NET formation, as well as lung inflammation and perivascular fibrosis in a golden hamster SARS-CoV-2 infection model via downregulated innate immune and complement/coagulation pathways (101).
SARS-CoV-2 S Induced Cell–Cell Fusion and Syncytia Death
Cell fusion between eukaryotic cells is a common phenomenon, caused by various pathogens, including bacteria, parasites, and viruses, which involves a broad range of physiological and pathological processes (102). The virus-mediated cell–cell fusion will lead to the fusion of cell membrane and cytoplasmic contents between cells, forming the multinucleated giant cells, also known as syncytia. SARS-CoV-2 infection can induce cell–cell fusion and syncytia formation, which has been widely confirmed in the lungs and other tissues of infected patients (103–105), or in vitro cell culture systems (106–108), which was well-summarized by Schwartz and colleagues (109). Syncytia formation was mediated by cell–cell fusion occurring between the surfaces of cell membranes. Within the syncytia, cellular contents from different cells mixed and interacted, triggering various cellular responses. We aimed to discuss the fate determination of syncytia and its role in COVID-19 progress, providing insights into targeting syncytia death on COVID-19 treatment.
Recent works reported that both DNA damage response and cGAS-STING signaling pathway were activated upon cell–cell fusion, which was important for host antiviral responses (110, 111). Furthermore, Zhang and colleagues demonstrated that the multinucleate syncytia formed by SARS-CoV-2 infection could internalize multiple lines of lymphocytes to form typical cell-in-cell structures, remarkably leading to the death of internalized cells (112). Moreover, we found that syncytia formed by HeLa–spike cell fusion with HeLa-ACE2 cells died in parallel with the increased activity of caspase-3/7/9 and the cleavage of GSDME (108). Interestingly, the deletion of caspase-9 not only blocked the cleavage of GSDME and cell death but also abolished the S2′ fragment of SARS-CoV-2-S-Flag induced by cell–cell fusion, indicating a linkage between caspase-9 and SARS-CoV-2 S protein cleavage. Thus, targeting caspase-9 might be a promising strategy to prevent syncytia cell death (Figure 1C). To extend the pathophysiological role of this caspase-9/GSDME-mediated syncytia pyroptosis, single-cell RNA-sequencing (scRNA-Seq) data from eight normal human lung transplant donors with a total of 42,225 cells were analyzed, showing that both ACE2 and GSDME were expressed in AT2 cells in the human lung. Finally, we proposed that this lytic pyroptosis of syncytia may contribute to the excessive inflammatory responses in severe COVID-19 patients. In line with this idea, treatment with caspase-9 selective inhibitor, z-LEHD-fmk, markedly reduced SARS-CoV-2-induced lung damage in K18-hACE2 transgenic mouse model, which was evidenced by the reduced hemorrhage and inflammatory cell infiltration, as well as the alleviated proinflammatory response in the lung (113), while the authors demonstrated that this effect was due to intrinsic apoptosis inhibition by z-LEHD-fmk. Whether apoptosis switched to pyroptosis needs further investigation.
SARS-CoV-2 E Proteins Form Cation Channels to Trigger Cell Death
Interestingly, consistent with the executors of pyroptosis (GSDMs) or necroptosis (p-MLKL) destroying the membrane integrity by forming either pores or channels, the envelope (E) protein, another structural protein of SARS-CoV-2, can form a cation channel to induce rapid cell death in myriad susceptible cell types and robust secretion of cytokines and chemokines in macrophages resulting in acute respiratory distress syndrome (ARDS)-like damages in vitro and in vivo (Figure 1B). Using a planar lipid bilayer recording system, the authors found that BE-12 (berbamine), a type of bisbenzylisoquinoline alkaloid, might be a candidate inhibitor for 2-E channels. Furthermore, to improve the antiviral activity, four more channel inhibitors (BE-30~33) were designed and synthesized based on BE-12. Finally, a new class of 2-E channel inhibitor BE-33 was identified, which exhibited not only high efficiency for antiviral activity both in vitro and in vivo but also negligible cytotoxicity, raising a promising antiviral strategy targeting 2-E channel (114). To discover SARS-CoV-2-E channel inhibitors, Wang and coworkers developed a cell-based high-throughput screening (HTS) assay and screened 4,376 compounds. Proanthocyanidins, a natural product widely used in cosmetics, were identified (115).
Discussion and Perspectives
The abovementioned different types of cell death induced by SARS-CoV-2 infection have been demonstrated in all kinds of cells including epithelial cells, macrophages, and neutrophils. It was reported that ACE2-mediated SARS-CoV-2 spike infection could induce inflammatory responses and apoptosis of human bronchial epithelial and microvascular endothelial cells via enhancing autophagy, which might result in organ dysfunction (45). It was also found that SARS-CoV-2 N protein-mediated AKI may be caused by tubular epithelial cell apoptosis through the TGF/Smad3 signaling-dependent G1 cell cycle arrest (50). Using gene expression profiling, Jha et al. revealed that the apoptosis signaling pathway was activated in SARS-CoV-2-infected human lung epithelial cells, which may lead to cardiovascular complications of COVID-19 (116). A recent study showed that the non-structural protein 6 (NSP6) of SARS-CoV-2 could induce NLRP3-dependent pyroptosis in lung epithelial cells via binding to the vacuolar ATPase proton pump component ATP6AP1, while pharmacological rectification of autophagic flux by 1α,25-dihydroxyvitamin D3, metformin, or polydatin could be a novel therapeutic strategy to reduce pyroptosis in lung epithelial cells and improve clinical outcomes of COVID-19 (117). T- and B-lymphocyte apoptosis was also observed after SARS-CoV-2 infection, which may be the cause of lymphopenia, a common symptom in severe COVID-19 patients (46, 118). Aside from apoptosis, SARS-CoV-2-induced lymphocyte loss may also be due to cell–cell fusion-mediated syncytia death, which could be a potential therapeutic target for antiviral therapy in patients with COVID-19 (112). It was found that apoptotic markers were increased in plasmacytoid dendritic cells (pDCs), a cell type that is specialized in antiviral immunity to produce abundant type I interferons (IFNs). Hence, the diminished pDCs in COVID-19 patients may be associated with increased cell apoptosis (119). Ongoing pyroptosis was also found in circulating monocytes from COVID-19 patients with increased caspase-1 activation and lytic death (120, 121). Abundant cleared caspase-3 positive macrophages have been found in the lungs of patients with COVID-19, indicating that apoptosis may mediate the death of macrophages in COVID-19 lung tissues (66). Furthermore, SARS-CoV-2 spike infection can upregulate caspase-3 and caspase-6 expression to induce apoptosis in THP-1-like macrophages, which is likely mediated by the increase of ROS and intracellular calcium release (122). The pathological investigation of a clinical study demonstrated that SARS-CoV-2 infection caused severe lung injury via cell pyroptosis in pneumocytes and apoptosis in endothelial cells (123). Additionally, SARS-CoV-2 infection-induced apoptosis of endothelial cells may also lead to endotheliitis in various tissues including the lung, heart, kidney, and liver (124). Moreover, SARS-CoV-2 can promote NET formation in neutrophils under a process called NETosis, a form of neutrophil death, leading to multi-organ damage during the pathogenesis of COVID-19. Indeed, increased concentration of NETs has been detected in circulating and lung-infiltrating neutrophils from COVID-19 patients. Mechanistically, SARS-CoV-2-induced release of NETs might be mediated by ACE2, serine protease, virus replication, and PAD-4 (92, 125).
SARS-CoV-2 infection can cause severe respiratory tract disease and lung injury and threaten human life, while there is still no special prevention or treatment at present. When the virus infects cells, many factors are involved in the pathogenesis of the host disease, leading to human death. In this review, we focused on the multiple types of cell death such as apoptosis, necroptosis, pyroptosis, NETosis, and other undefined death triggered by SARS-CoV-2 infection in vivo or in vitro; then we discussed the relationship between inflammatory cell death and cytokine storm, raising the possibility for targeting cell death pathway for the treatment of COVID-19 (Figure 2). Among these, we highlighted some potential compounds or drugs targeting the molecules of cell death pathway, such as RIPK1 (66), caspase-9 (108), Smad (50), SARS-CoV-2 Orf3a (39–41), and E protein (114). What is more, iron metabolism dysfunction has also been found in COVID-19 patients; for example, the serum ferritin levels were higher in severe COVID-19 patients than in mild cases, which may cause iron accumulation and overload, which trigger ferroptosis (126). Yang and Lai hypothesized that ferroptosis might serve as a new treatment target, and the improved ferrostatin-1 and liproxstatin-1 analogs might be potential drug candidates for COVID-19 (127). Interestingly, disulfiram, an alcoholism-averting drug approved by the FDA, was recommended to be a potential therapeutic target for SARS-CoV-2 infection in Phase 2 clinical trials by targeting SARS-CoV-2 main protease, 3CLpro (128–130). However, it has been identified that disulfiram is covalently targeted on human/mouse Cys191/Cys192 of GSDMD protein leading to blocking the GSDMD pore formation, IL-1β release, and pyroptosis (98). Furthermore, another study showed that disulfiram can inhibit the NET formation and protect rodents from SARS-CoV-2 infection (101). All these raise a common point that one drug/compound might target various proteins even on multiple signaling pathways to either synergically exert effects or trigger off-target toxicities. Therefore, we need to deeply explore the cell types of death caused by SARS-CoV-2 infection, reveal the molecular mechanism of cell death, and accurately regulate cell death by using specific pharmacological therapies to reduce the occurrence and prognosis of COVID-19.
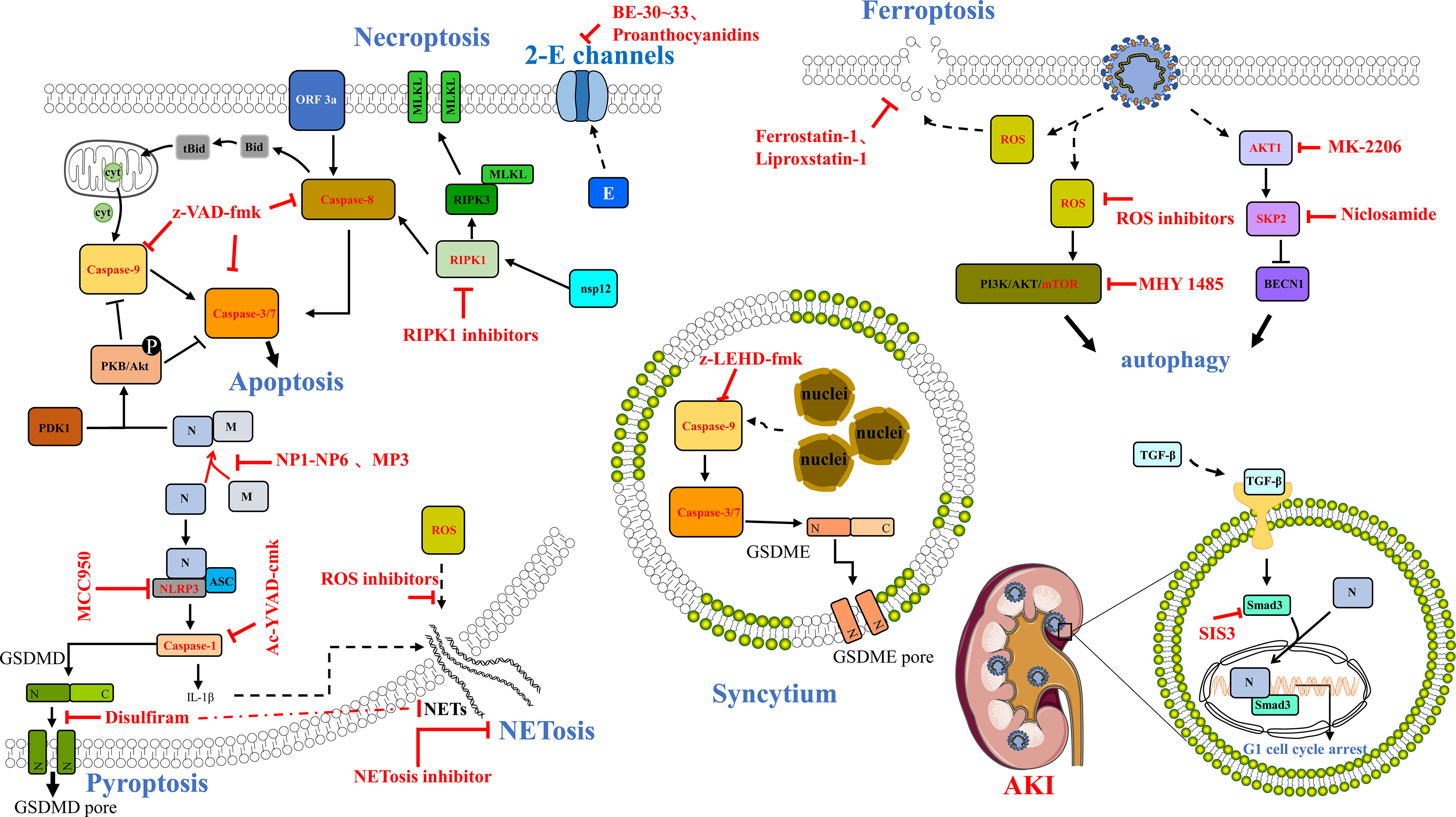
Figure 2 Potential compounds or drugs that targeted different cell death pathways. The potential therapeutics of drugs or compounds to inhibit cell death, including the use of z-VAD-fmk, a pan-caspase inhibitor, to reduce the caspase activity and block the apoptosis induced by SARS-CoV-2 ORF3a, and the use of RIPK1 inhibitors (Nec-1s, GSK′481/GSK′772, etc.), as well as using peptides such as NP1-NP6 and MP3 to disrupt the M–N interaction, and abolish the activity of N on the M-triggered apoptosis. In addition, a specific inhibitor of NLRP3 called MCC950 and an inhibitor of caspase-1 named Ac-YVAD-cmk can block NLRP3 inflammasome activation induced by SARS-CoV-2 N protein. Interestingly, disulfiram was revealed as an inhibitor of GSDMD that can effectively block pyroptosis and NET formation. BE-30~33 and proanthocyanidins could inhibit 2-E channel activity as channel inhibitors. Autophagy could be regulated using various treatments, such as the ROS inhibitor MHY1485, the AKT1 inhibitor MK-2206, and the BECN1-stabilizing anthelmintic drug/SKP2 inhibitor, niclosamide. Ferroptosis inhibitors including ferrostatin-1 and liproxstatin-1 might also be potential drug candidates for COVID-19. Further, z-LEHD-fmk, the caspase-9 selective inhibitor could suppress syncytium formation. SIS3, Smad3 pharmacological inhibitor, can inhibit SARS-CoV-2 N-induced AKI. The compounds or drugs are marked in bold and red.
Nevertheless, the impact of the damaged or dead cells on the injured tissues and organs is still not well understood. Furthermore, whether cytokines released by cell death participate in cytokine storms has not been well described yet. However, research on the mechanism of SARS-CoV-2 infection-induced cell death may provide additional perspectives for antiviral therapies and the development of anti-SARS-CoV-2 drugs.
Author Contributions
LL, JW, YZ, and HM: conceptualization. ZZ and JS: investigation and writing—original draft preparation. LL, JW, and HM: writing—review and editing. All authors have read and agreed to the published version of the manuscript.
Funding
This work was supported by the Natural Science Foundation of Zhejiang Province (No. LQ21H030002), the Scientific Research Grant of Ningbo University (215–432000282), and the Ningbo Top Talent Project (215–432094250).
Conflict of Interest
The authors declare that the research was conducted in the absence of any commercial or financial relationships that could be construed as a potential conflict of interest.
Publisher’s Note
All claims expressed in this article are solely those of the authors and do not necessarily represent those of their affiliated organizations, or those of the publisher, the editors and the reviewers. Any product that may be evaluated in this article, or claim that may be made by its manufacturer, is not guaranteed or endorsed by the publisher.
References
1. Lim YX, Ng YL, Tam JP, Liu DX. Human Coronaviruses: A Review of Virus-Host Interactions. Diseases (2016) 4(3):26. doi: 10.3390/diseases4030026
2. Fung TS, Liu DX. Human Coronavirus: Host-Pathogen Interaction. Annu Rev Microbiol (2019) 73:529–57. doi: 10.1146/annurev-micro-020518-115759
3. Harrison AG, Lin T, Wang P. Mechanisms of SARS-CoV-2 Transmission and Pathogenesis. Trends Immunol (2020) 41(12):1100–15. doi: 10.1016/j.it.2020.10.004
4. Tang D, Comish P, Kang R. The Hallmarks of Covid-19 Disease. PLoS Pathog (2020) 16(5):e1008536. doi: 10.1371/journal.ppat.1008536
5. Hu B, Guo H, Zhou P, Shi ZL. Characteristics of SARS-CoV-2 and Covid-19. Nat Rev Microbiol (2020) 19:1–14. doi: 10.1038/s41579-020-00459-7
6. Zhou P, Yang XL, Wang XG, Hu B, Zhang L, Zhang W, et al. A Pneumonia Outbreak Associated With a New Coronavirus of Probable Bat Origin. Nature (2020) 579(7798):270–3. doi: 10.1038/s41586-020-2012-7
7. Gorbalenya AE, Baker SC, Baric RS, de Groot RJ, Drosten C, Gulyaeva AA, et al. The Species Severe Acute Respiratory Syndrome-Related Coronavirus: Classifying 2019-Ncov and Naming it SARS-CoV-2. Nat Microbiol (2020) 5(4):536–44. doi: 10.1038/s41564-020-0695-z
8. Ashour HM, Elkhatib WF, Rahman MM, Elshabrawy HA. Insights Into the Recent 2019 Novel Coronavirus (SARS-CoV-2) in Light of Past Human Coronavirus Outbreaks. Pathogens (2020) 9(3):186. doi: 10.3390/pathogens9030186
9. Wu F, Zhao S, Yu B, Chen YM, Wang W, Song ZG, et al. A New Coronavirus Associated With Human Respiratory Disease in China. Nature (2020) 579(7798):265–9. doi: 10.1038/s41586-020-2008-3
10. Kim D, Lee JY, Yang JS, Kim JW, Kim VN, Chang H. The Architecture of SARS-CoV-2 Transcriptome. Cell (2020) 181(4):914–21.e10. doi: 10.1016/j.cell.2020.04.011
11. Gordon DE, Jang GM, Bouhaddou M, Xu J, Obernier K, White KM, et al. A Sars-Cov-2 Protein Interaction Map Reveals Targets for Drug Repurposing. Nature (2020) 583(7816):459–68. doi: 10.1038/s41586-020-2286-9
12. Guan WJ, Ni ZY, Hu Y, Liang WH, Ou CQ, He JX, et al. Clinical Characteristics of Coronavirus Disease 2019 in China. N Engl J Med (2020) 382(18):1708–20. doi: 10.1056/NEJMoa2002032
13. Zhou F, Yu T, Du R, Fan G, Liu Y, Liu Z, et al. Clinical Course and Risk Factors for Mortality of Adult Inpatients With Covid-19 in Wuhan, China: A Retrospective Cohort Study. Lancet (2020) 395(10229):1054–62. doi: 10.1016/s0140-6736(20)30566-3
14. Merad M, Martin JC. Pathological Inflammation in Patients With Covid-19: A Key Role for Monocytes and Macrophages. Nat Rev Immunol (2020) 20(6):355–62. doi: 10.1038/s41577-020-0331-4
15. Fajgenbaum DC, June CH. Cytokine Storm. N Engl J Med (2020) 383(23):2255–73. doi: 10.1056/NEJMra2026131
16. Long Q-X, Tang X-J, Shi Q-L, Li Q, Deng H-J, Yuan J, et al. Clinical and Immunological Assessment of Asymptomatic SARS-CoV-2 Infections. Nat Med (2020) 26(8):1200–+. doi: 10.1038/s41591-020-0965-6
17. Wilk AJ, Rustagi A, Zhao NQ, Roque J, Martinez-Colon GJ, McKechnie JL, et al. A Single-Cell Atlas of the Peripheral Immune Response in Patients With Severe Covid-19. Nat Med (2020) 26(7):1070–+. doi: 10.1038/s41591-020-0944-y
18. Jose RJ, Manuel A. Covid-19 Cytokine Storm: The Interplay Between Inflammation and Coagulation. Lancet Respir Med (2020) 8(6):e46–7. doi: 10.1016/s2213-2600(20)30216-2
19. Bian XW, Team C-P. Autopsy of Covid-19 Patients in China. Natl Sci Rev (2020) 7(9):1414–8. doi: 10.1093/nsr/nwaa123
20. Liu Q, Shi Y, Cai J, Duan Y, Wang R, Zhang H, et al. Pathological Changes in the Lungs and Lymphatic Organs of 12 Covid-19 Autopsy Cases. Natl Sci Rev (2020) 7(12):1868–78. doi: 10.1093/nsr/nwaa247
21. Wichmann D, Sperhake J-P, Luegehetmann M, Steurer S, Edler C, Heinemann A, et al. Autopsy Findings and Venous Thromboembolism in Patients With Covid-19. Ann Internal Med (2020) 173(4):268–+. doi: 10.7326/m20-2003
22. Franks TJ, Chong PY, Chui P, Galvin JR, Lourens RM, Reid AH, et al. Lung Pathology of Severe Acute Respiratory Syndrome (Sars): A Study of 8 Autopsy Cases From Singapore. Hum Pathol (2003) 34(8):743–8. doi: 10.1016/s0046-8177(03)00367-8
23. Edler C, Schroeder AS, Aepfelbacher M, Fitzek A, Heinemann A, Heinrich F, et al. Dying With Sars-Cov-2 Infection-An Autopsy Study of the First Consecutive 80 Cases in Hamburg, Germany. Int J Legal Med (2020) 134(4):1275–84. doi: 10.1007/s00414-020-02317-w
24. Hanley B, Lucas SB, Youd E, Swift B, Osborn M. Autopsy in Suspected Covid-19 Cases. J Clin Pathol (2020) 73(5):239–42. doi: 10.1136/jclinpath-2020-206522
25. Fox SE, Akmatbekov A, Harbert JL, Li G, Brown JQ, Heide RSV. Pulmonary and Cardiac Pathology in African American Patients With Covid-19: An Autopsy Series From New Orleans. Lancet Respir Med (2020) 8(7):681–6. doi: 10.1016/s2213-2600(20)30243-5
26. Bussani R, Schneider E, Zentilin L, Collesi C, Ali H, Braga L, et al. Persistence of Viral Rna, Pneumocyte Syncytia and Thrombosis are Hallmarks of Advanced Covid-19 Pathology. EBioMedicine (2020) 61:103104. doi: 10.1016/j.ebiom.2020.103104
27. Tian S, Hu W, Niu L, Liu H, Xu H, Xiao S-Y. Pulmonary Pathology of Early-Phase 2019 Novel Coronavirus (Covid-19) Pneumonia in Two Patients With Lung Cancer. J Thorac Oncol (2020) 15(5):700–4. doi: 10.1016/j.jtho.2020.02.010
28. Gupta A, Madhavan MV, Sehgal K, Nair N, Mahajan S, Sehrawat TS, et al. Extrapulmonary Manifestations of Covid-19. Nat Med (2020) 26(7):1017–32. doi: 10.1038/s41591-020-0968-3
29. Fung SY, Yuen KS, Ye ZW, Chan CP, Jin DY. A Tug-Of-War Between Severe Acute Respiratory Syndrome Coronavirus 2 and Host Antiviral Defence: Lessons From Other Pathogenic Viruses. Emerg Microbes Infect (2020) 9(1):558–70. doi: 10.1080/22221751.2020.1736644
30. Danthi P. Viruses and the Diversity of Cell Death. Annu Rev Virol (2016) 3(1):533–53. doi: 10.1146/annurev-virology-110615-042435
31. Bader SM, Cooney JP, Pellegrini M, Doerflinger M. Programmed Cell Death: The Pathways to Severe Covid-19? Biochem J (2022) 479(5):609–28. doi: 10.1042/bcj20210602
32. Morais da Silva M, Lira de Lucena AS, Paiva Júnior SSL, Florêncio De Carvalho VM, Santana de Oliveira PS, da Rosa MM, et al. Cell Death Mechanisms Involved in Cell Injury Caused by Sars-Cov-2. Rev Med Virol (2021):e2292. doi: 10.1002/rmv.2292
33. Denecker G, Vercammen D, Declercq W, Vandenabeele P. Apoptotic and Necrotic Cell Death Induced by Death Domain Receptors. Cell Mol Life Sci (2001) 58(3):356–70. doi: 10.1007/pl00000863
34. Jiang X, Wang X. Cytochrome C-Mediated Apoptosis. Annu Rev Biochem (2004) 73:87–106. doi: 10.1146/annurev.biochem.73.011303.073706
35. Green DR, Llambi F. Cell Death Signaling. Cold Spring Harbor Perspect Biol (2015) 7(12):a006080. doi: 10.1101/cshperspect.a006080
36. Galluzzi L, Vitale I, Aaronson SA, Abrams JM, Adam D, Agostinis P, et al. Molecular Mechanisms of Cell Death: Recommendations of the Nomenclature Committee on Cell Death 2018. Cell Death Differ (2018) 25(3):486–541. doi: 10.1038/s41418-017-0012-4
37. Xu X, Lai Y, Hua ZC. Apoptosis and Apoptotic Body: Disease Message and Therapeutic Target Potentials. Biosci Rep (2019) 39(1):BSR20180992. doi: 10.1042/bsr20180992
38. Freundt EC, Yu L, Goldsmith CS, Welsh S, Cheng A, Yount B, et al. The Open Reading Frame 3a Protein of Severe Acute Respiratory Syndrome-Associated Coronavirus Promotes Membrane Rearrangement and Cell Death. J Virol (2010) 84(2):1097–109. doi: 10.1128/jvi.01662-09
39. Ren Y, Shu T, Wu D, Mu J, Wang C, Huang M, et al. The Orf3a Protein of Sars-Cov-2 Induces Apoptosis in Cells. Cell Mol Immunol (2020) 17(8):881–3. doi: 10.1038/s41423-020-0485-9
40. Miao G, Zhao H, Li Y, Ji M, Chen Y, Shi Y, et al. Orf3a of the Covid-19 Virus Sars-Cov-2 Blocks Hops Complex-Mediated Assembly of the Snare Complex Required for Autolysosome Formation. Dev Cell (2021) 56(4):427–42.e5. doi: 10.1016/j.devcel.2020.12.010
41. Zhang Y, Sun H, Pei R, Mao B, Zhao Z, Li H, et al. The SARS-CoV-2 Protein Orf3a Inhibits Fusion of Autophagosomes With Lysosomes. Cell Discov (2021) 7(1):31. doi: 10.1038/s41421-021-00268-z
42. Gassen NC, Papies J, Bajaj T, Emanuel J, Dethloff F, Chua RL, et al. Sars-Cov-2-Mediated Dysregulation of Metabolism and Autophagy Uncovers Host-Targeting Antivirals. Nat Commun (2021) 12(1):3818. doi: 10.1038/s41467-021-24007-w
43. Yang R, Zhao Q, Rao J, Zeng F, Yuan S, Ji M, et al. SARS-CoV-2 Accessory Protein Orf7b Mediates Tumor Necrosis Factor-Α-Induced Apoptosis in Cells. Front Microbiol (2021) 12:654709. doi: 10.3389/fmicb.2021.654709
44. Ren Y, Wang A, Fang Y, Shu T, Wu D, Wang C, et al. SARS-CoV-2 Membrane Glycoprotein M Triggers Apoptosis With the Assistance of Nucleocapsid Protein N in Cells. Front Cell Infect Microbiol (2021) 11:706252. doi: 10.3389/fcimb.2021.706252
45. Li F, Li J, Wang PH, Yang N, Huang J, Ou J, et al. SARS-CoV-2 Spike Promotes Inflammation and Apoptosis Through Autophagy by Ros-Suppressed Pi3k/Akt/Mtor Signaling. Biochim Biophys Acta Mol Basis Dis (2021) 1867(12):166260. doi: 10.1016/j.bbadis.2021.166260
46. Xiang Q, Feng Z, Diao B, Tu C, Qiao Q, Yang H, et al. SARS-CoV-2 Induces Lymphocytopenia by Promoting Inflammation and Decimates Secondary Lymphoid Organs. Front Immunol (2021) 12:661052. doi: 10.3389/fimmu.2021.661052
47. Diao B, Wang C, Wang R, Feng Z, Zhang J, Yang H, et al. Human Kidney Is a Target for Novel Severe Acute Respiratory Syndrome Coronavirus 2 Infection. Nat Commun (2021) 12(1):2506. doi: 10.1038/s41467-021-22781-1
48. Pei G, Zhang Z, Peng J, Liu L, Zhang C, Yu C, et al. Renal Involvement and Early Prognosis in Patients With Covid-19 Pneumonia. J Am Soc Nephrol JASN (2020) 31(6):1157–65. doi: 10.1681/asn.2020030276
49. Cheng Y, Luo R, Wang K, Zhang M, Wang Z, Dong L, et al. Kidney Disease Is Associated With in-Hospital Death of Patients With Covid-19. Kidney Int (2020) 97(5):829–38. doi: 10.1016/j.kint.2020.03.005
50. Wang W, Chen J, Hu D, Pan P, Liang L, Wu W, et al. SARS-CoV-2 N Protein Induces Acute Kidney Injury Via Smad3-Dependent G1 Cell Cycle Arrest Mechanism. Adv Sci (Weinh) (2021) 9(3):e2103248. doi: 10.1002/advs.202103248
51. Boada-Romero E, Martinez J, Heckmann BL, Green DR. The Clearance of Dead Cells by Efferocytosis. Nat Rev Mol Cell Biol (2020) 21(7):398–414. doi: 10.1038/s41580-020-0232-1
52. Li S, Zhang Y, Guan Z, Li H, Ye M, Chen X, et al. Sars-Cov-2 Triggers Inflammatory Responses and Cell Death Through Caspase-8 Activation. Signal Transduct Tar Ther (2020) 5(1):235. doi: 10.1038/s41392-020-00334-0
53. Xia X, Lei L, Wang S, Hu J, Zhang G. Necroptosis and Its Role in Infectious Diseases. Apoptosis (2020) 25(3-4):169–78. doi: 10.1007/s10495-019-01589-x
54. Gong Y, Fan Z, Luo G, Yang C, Huang Q, Fan K, et al. The Role of Necroptosis in Cancer Biology and Therapy. Mol Cancer (2019) 18(1):100. doi: 10.1186/s12943-019-1029-8
55. Zhang J, Yang Y, He W, Sun L. Necrosome Core Machinery: Mlkl. Cell Mol Life Sci (2016) 73(11-12):2153–63. doi: 10.1007/s00018-016-2190-5
56. Xu Y, Zhang J, Ma L, Zhao S, Li S, Huang T, et al. The Pathogenesis of Necroptosis-Dependent Signaling Pathway in Cerebral Ischemic Disease. Behav Neurol (2018) 2018:6814393. doi: 10.1155/2018/6814393
57. Moriwaki K, Chan FK. Rip3: A Molecular Switch for Necrosis and Inflammation. Genes Dev (2013) 27(15):1640–9. doi: 10.1101/gad.223321.113
58. Petrie EJ, Czabotar PE, Murphy JM. The Structural Basis of Necroptotic Cell Death Signaling. Trends Biochem Sci (2019) 44(1):53–63. doi: 10.1016/j.tibs.2018.11.002
59. Chan FK, Luz NF, Moriwaki K. Programmed Necrosis in the Cross Talk of Cell Death and Inflammation. Annu Rev Immunol (2015) 33:79–106. doi: 10.1146/annurev-immunol-032414-112248
60. Lin J, Kumari S, Kim C, Van TM, Wachsmuth L, Polykratis A, et al. Ripk1 Counteracts Zbp1-Mediated Necroptosis to Inhibit Inflammation. Nature (2016) 540(7631):124–8. doi: 10.1038/nature20558
61. Newton K, Wickliffe KE, Maltzman A, Dugger DL, Strasser A, Pham VC, et al. Ripk1 Inhibits Zbp1-Driven Necroptosis During Development. Nature (2016) 540(7631):129–33. doi: 10.1038/nature20559
62. Dannappel M, Vlantis K, Kumari S, Polykratis A, Kim C, Wachsmuth L, et al. Ripk1 Maintains Epithelial Homeostasis by Inhibiting Apoptosis and Necroptosis. Nature (2014) 513(7516):90–4. doi: 10.1038/nature13608
63. Riebeling T, Jamal K, Wilson R, Kolbrink B, von Samson-Himmelstjerna FA, Moerke C, et al. Primidone Blocks Ripk1-Driven Cell Death and Inflammation. Cell Death Differ (2021) 28(5):1610–26. doi: 10.1038/s41418-020-00690-y
64. Feng L, Yin YY, Liu CH, Xu KR, Li QR, Wu JR, et al. Proteome-Wide Data Analysis Reveals Tissue-Specific Network Associated With SARS-CoV-2 Infection. J Mol Cell Biol (2020) 12(12):946–57. doi: 10.1093/jmcb/mjaa033
65. Belyaeva A, Cammarata L, Radhakrishnan A, Squires C, Yang KD, Shivashankar GV, et al. Causal Network Models of Sars-Cov-2 Expression and Aging to Identify Candidates for Drug Repurposing. Nat Commun (2021) 12(1):1024. doi: 10.1038/s41467-021-21056-z
66. Xu G, Li Y, Zhang S, Peng H, Wang Y, Li D, et al. Sars-Cov-2 Promotes Ripk1 Activation to Facilitate Viral Propagation. Cell Res (2021) 31:1230–43. doi: 10.1038/s41422-021-00578-7
67. Mifflin L, Ofengeim D, Yuan J. Receptor-Interacting Protein Kinase 1 (Ripk1) as a Therapeutic Target. Nat Rev Drug Discov (2020) 19(8):553–71. doi: 10.1038/s41573-020-0071-y
68. Liu L, Lalaoui N. 25 Years of Research Put Ripk1 in the Clinic. Semin Cell Dev Biol (2021) 109:86–95. doi: 10.1016/j.semcdb.2020.08.007
69. Fink SL, Cookson BT. Apoptosis, Pyroptosis, and Necrosis: Mechanistic Description of Dead and Dying Eukaryotic Cells. Infect Immun (2005) 73(4):1907–16. doi: 10.1128/IAI.73.4.1907-1916.2005
70. Jorgensen I, Miao EA. Pyroptotic Cell Death Defends Against Intracellular Pathogens. Immunol Rev (2015) 265(1):130–42. doi: 10.1111/imr.12287
71. Shi J, Gao W, Shao F. Pyroptosis: Gasdermin-Mediated Programmed Necrotic Cell Death. Trends Biochem Sci (2017) 42(4):245–54. doi: 10.1016/j.tibs.2016.10.004
72. Shi J, Zhao Y, Wang K, Shi X, Wang Y, Huang H, et al. Cleavage of Gsdmd by Inflammatory Caspases Determines Pyroptotic Cell Death. Nature (2015) 526(7575):660–5. doi: 10.1038/nature15514
73. He WT, Wan H, Hu L, Chen P, Wang X, Huang Z, et al. Gasdermin D is an Executor of Pyroptosis and Required for Interleukin-1β Secretion. Cell Res (2015) 25(12):1285–98. doi: 10.1038/cr.2015.139
74. Rogers C, Fernandes-Alnemri T, Mayes L, Alnemri D, Cingolani G, Alnemri ES. Cleavage of Dfna5 by Caspase-3 During Apoptosis Mediates Progression to Secondary Necrotic/Pyroptotic Cell Death. Nat Commun (2017) 8:14128. doi: 10.1038/ncomms14128
75. Wang Y, Gao W, Shi X, Ding J, Liu W, He H, et al. Chemotherapy Drugs Induce Pyroptosis Through Caspase-3 Cleavage of a Gasdermin. Nature (2017) 547(7661):99–103. doi: 10.1038/nature22393
76. Fritsch M, Gunther SD, Schwarzer R, Albert MC, Schorn F, Werthenbach JP, et al. Caspase-8 Is the Molecular Switch for Apoptosis, Necroptosis and Pyroptosis. Nature (2019) 575(7784):683–7. doi: 10.1038/s41586-019-1770-6
77. Sarhan J, Liu BC, Muendlein HI, Li P, Nilson R, Tang AY, et al. Caspase-8 Induces Cleavage of Gasdermin D to Elicit Pyroptosis During Yersinia Infection. Proc Natl Acad Sci USA (2018) 115(46):E10888–97. doi: 10.1073/pnas.1809548115
78. Zhou Z, He H, Wang K, Shi X, Wang Y, Su Y, et al. Granzyme a From Cytotoxic Lymphocytes Cleaves Gsdmb to Trigger Pyroptosis in Target Cells. Science (2020) 368(6494):eaaz7548. doi: 10.1126/science.aaz7548. New York, NY.
79. Kayagaki N, Stowe IB, Lee BL, O’Rourke K, Anderson K, Warming S, et al. Caspase-11 Cleaves Gasdermin D for Non-Canonical Inflammasome Signalling. Nature (2015) 526(7575):666–71. doi: 10.1038/nature15541
80. Ding J, Wang K, Liu W, She Y, Sun Q, Shi J, et al. Pore-Forming Activity and Structural Autoinhibition of the Gasdermin Family. Nature (2016) 535(7610):111–6. doi: 10.1038/nature18590
81. Broz P, Pelegrín P, Shao F. The Gasdermins, A Protein Family Executing Cell Death and Inflammation. Nat Rev Immunol (2020) 20(3):143–57. doi: 10.1038/s41577-019-0228-2
82. Freeman TL, Swartz TH. Targeting the Nlrp3 Inflammasome in Severe Covid-19. Front Immunol (2020) 11:1518. doi: 10.3389/fimmu.2020.01518
83. van den Berg DF, Te Velde AA. Severe Covid-19: Nlrp3 Inflammasome Dysregulated. Front Immunol (2020) 11:1580. doi: 10.3389/fimmu.2020.01580
84. Rodrigues TS, de Sá KSG, Ishimoto AY, Becerra A, Oliveira S, Almeida L, et al. Inflammasomes Are Activated in Response to SARS-CoV-2 Infection and Are Associated With Covid-19 Severity in Patients. J Exp Med (2021) 218(3):e20201707. doi: 10.1084/jem.20201707
85. Pan P, Shen M, Yu Z, Ge W, Chen K, Tian M, et al. SARS-CoV-2 N Protein Promotes Nlrp3 Inflammasome Activation to Induce Hyperinflammation. Nat Commun (2021) 12(1):4664. doi: 10.1038/s41467-021-25015-6
86. Brinkmann V, Reichard U, Goosmann C, Fauler B, Uhlemann Y, Weiss DS, et al. Neutrophil Extracellular Traps Kill Bacteria. Science (2004) 303(5663):1532–5. doi: 10.1126/science.1092385
87. Fuchs TA, Abed U, Goosmann C, Hurwitz R, Schulze I, Wahn V, et al. Novel Cell Death Program Leads to Neutrophil Extracellular Traps. J Cell Biol (2007) 176(2):231–41. doi: 10.1083/jcb.200606027
88. Gupta S, Kaplan MJ. The Role of Neutrophils and Netosis in Autoimmune and Renal Diseases. Nat Rev Nephrol (2016) 12(7):402–13. doi: 10.1038/nrneph.2016.71
89. Papayannopoulos V. Neutrophil Extracellular Traps in Immunity and Disease. Nat Rev Immunol (2018) 18(2):134–47. doi: 10.1038/nri.2017.105
90. Arcanjo A, Logullo J, Menezes CCB, de Souza Carvalho Giangiarulo TC, Dos Reis MC, de Castro GMM, et al. The Emerging Role of Neutrophil Extracellular Traps in Severe Acute Respiratory Syndrome Coronavirus 2 (Covid-19). Sci Rep (2020) 10(1):19630. doi: 10.1038/s41598-020-76781-0
91. Middleton EA, He XY, Denorme F, Campbell RA, Ng D, Salvatore SP, et al. Neutrophil Extracellular Traps Contribute to Immunothrombosis in Covid-19 Acute Respiratory Distress Syndrome. Blood (2020) 136(10):1169–79. doi: 10.1182/blood.2020007008
92. Veras FP, Pontelli MC, Silva CM, Toller-Kawahisa JE, de Lima M, Nascimento DC, et al. Sars-Cov-2-Triggered Neutrophil Extracellular Traps Mediate Covid-19 Pathology. J Exp Med (2020) 217(12):e20201129. doi: 10.1084/jem.20201129
93. Ackermann M, Anders H-J, Bilyy R, Bowlin GL, Daniel C, De Lorenzo R, et al. Patients With Covid-19: In the Dark-Nets of Neutrophils. Cell Death Differ (2021) 28(11):3125–39. doi: 10.1038/s41418-021-00805-z
94. Hong W, Yang J, Zou J, Bi Z, He C, Lei H, et al. Histones Released by Netosis Enhance the Infectivity of Sars-Cov-2 by Bridging the Spike Protein Subunit 2 and Sialic Acid on Host Cells. Cell Mol Immunol (2022) 19:577–87. doi: 10.1038/s41423-022-00845-6
95. Gillot C, Favresse J, Mullier F, Lecompte T, Dogné J-M, Douxfils J. Netosis and the Immune System in Covid-19: Mechanisms and Potential Treatments. Front Pharmacol (2021) 12:708302. doi: 10.3389/fphar.2021.708302
96. Zhu Y, Chen X, Liu X. Netosis and Neutrophil Extracellular Traps in Covid-19: Immunothrombosis and Beyond. Front Immunol (2022) 13:838011. doi: 10.3389/fimmu.2022.838011
97. Pastorek M, Dúbrava M, Celec P. On the Origin of Neutrophil Extracellular Traps in Covid-19. Front Immunol (2022) 13:821007. doi: 10.3389/fimmu.2022.821007
98. Hu JJ, Liu X, Xia S, Zhang Z, Zhang Y, Zhao J, et al. Fda-Approved Disulfiram Inhibits Pyroptosis by Blocking Gasdermin D Pore Formation. Nat Immunol (2020) 21(7):736–45. doi: 10.1038/s41590-020-0669-6
99. Kambara H, Liu F, Zhang X, Liu P, Bajrami B, Teng Y, et al. Gasdermin D Exerts Anti-Inflammatory Effects by Promoting Neutrophil Death. Cell Rep (2018) 22(11):2924–36. doi: 10.1016/j.celrep.2018.02.067
100. Chen KW, Monteleone M, Boucher D, Sollberger G, Ramnath D, Condon ND, et al. Noncanonical Inflammasome Signaling Elicits Gasdermin D-Dependent Neutrophil Extracellular Traps. Sci Immunol (2018) 3(26):eaar6676. doi: 10.1126/sciimmunol.aar6676
101. Adrover JM, Carrau L, Daßler-Plenker J, Bram Y, Chandar V, Houghton S, et al. Disulfiram Inhibits Neutrophil Extracellular Trap Formation and Protects Rodents From Acute Lung Injury and Sars-Cov-2 Infection. JCI Insight (2022) 7(5):e157342. doi: 10.1172/jci.insight.157342
102. Leroy H, Han M, Woottum M, Bracq L, Bouchet J, Xie M, et al. Virus-Mediated Cell-Cell Fusion. Int J Mol Sci (2020) 21(24):9644. doi: 10.3390/ijms21249644
103. Sanders DW, Jumper CC, Ackerman PJ, Bracha D, Donlic A, Kim H, et al. Sars-Cov-2 Requires Cholesterol for Viral Entry and Pathological Syncytia Formation. Elife (2021) 10:e65962. doi: 10.7554/eLife.65962
104. Asarnow D, Wang B, Lee WH, Hu Y, Huang CW, Faust B, et al. Structural Insight Into Sars-Cov-2 Neutralizing Antibodies and Modulation of Syncytia. Cell (2021) 184(12):3192–204.e16. doi: 10.1016/j.cell.2021.04.033
105. Stadlmann S, Hein-Kuhnt R, Singer G. Viropathic Multinuclear Syncytial Giant Cells in Bronchial Fluid From a Patient With Covid-19. J Clin Pathol (2020) 73(9):607–8. doi: 10.1136/jclinpath-2020-206657
106. Buchrieser J, Dufloo J, Hubert M, Monel B, Planas D, Rajah MM, et al. Syncytia Formation by Sars-Cov-2-Infected Cells. EMBO J (2020) 39(23):e106267. doi: 10.15252/embj.2020106267
107. Braga L, Ali H, Secco I, Chiavacci E, Neves G, Goldhill D, et al. Drugs That Inhibit Tmem16 Proteins Block Sars-Cov-2 Spike-Induced Syncytia. Nature (2021) 594:88–93. doi: 10.1038/s41586-021-03491-6
108. Ma H, Zhu Z, Lin H, Wang S, Zhang P, Li Y, et al. Pyroptosis of Syncytia Formed by Fusion of Sars-Cov-2 Spike and Ace2-Expressing Cells. Cell Discov (2021) 7(1):73. doi: 10.1038/s41421-021-00310-0
109. Rajah MM, Bernier A, Buchrieser J, Schwartz O. The Mechanism and Consequences of SARS-CoV-2 Spike-Mediated Fusion and Syncytia Formation. J Mol Biol (2021) 434(6):167280. doi: 10.1016/j.jmb.2021.167280
110. Zhou Z, Zhang X, Lei X, Xiao X, Jiao T, Ma R, et al. Sensing of Cytoplasmic Chromatin by Cgas Activates Innate Immune Response in Sars-Cov-2 Infection. Signal Transduct Target Ther (2021) 6(1):382. doi: 10.1038/s41392-021-00800-3
111. Ren H, Ma C, Peng H, Zhang B, Zhou L, Su Y, et al. Micronucleus Production, Activation of DNA Damage Response and Cgas-Sting Signaling in Syncytia Induced by Sars-Cov-2 Infection. Biol direct (2021) 16(1):20. doi: 10.1186/s13062-021-00305-7
112. Zhang Z, Zheng Y, Niu Z, Zhang B, Wang C, Yao X, et al. SARS-CoV-2 Spike Protein Dictates Syncytium-Mediated Lymphocyte Elimination. Cell Death Differ (2021) 28:2765–77. doi: 10.1038/s41418-021-00782-3
113. Chu H, Shuai H, Hou Y, Zhang X, Wen L, Huang X, et al. Targeting Highly Pathogenic Coronavirus-Induced Apoptosis Reduces Viral Pathogenesis and Disease Severity. Sci Adv (2021) 7(25):eabf8577. doi: 10.1126/sciadv.abf8577
114. Xia B, Shen X, He Y, Pan X, Liu FL, Wang Y, et al. SARS-CoV-2 Envelope Protein Causes Acute Respiratory Distress Syndrome (Ards)-Like Pathological Damages and Constitutes an Antiviral Target. Cell Res (2021) 31(8):847–60. doi: 10.1038/s41422-021-00519-4
115. Wang Y, Fang S, Wu Y, Cheng X, Zhang LK, Shen XR. Discovery of Sars-Cov-2-E Channel Inhibitors as Antiviral Candidates. Acta Pharmacol Sin (2021) 43:781–87. doi: 10.1038/s41401-021-00732-2
116. Jha PK, Vijay A, Halu A, Uchida S, Aikawa M. Gene Expression Profiling Reveals the Shared and Distinct Transcriptional Signatures in Human Lung Epithelial Cells Infected With Sars-Cov-2, Mers-Cov, or Sars-Cov: Potential Implications in Cardiovascular Complications of Covid-19. Front Cardiovasc Med (2020) 7:623012. doi: 10.3389/fcvm.2020.623012
117. Sun X, Liu Y, Huang Z, Xu W, Hu W, Yi L, et al. Sars-Cov-2 Non-Structural Protein 6 Triggers Nlrp3-Dependent Pyroptosis by Targeting Atp6ap1. Cell Death Differ (2022). doi: 10.1038/s41418-021-00916-7
118. Xiong Y, Liu Y, Cao L, Wang D, Guo M, Jiang A, et al. Transcriptomic Characteristics of Bronchoalveolar Lavage Fluid and Peripheral Blood Mononuclear Cells in Covid-19 Patients. Emerg Microbes Infect (2020) 9(1):761–70. doi: 10.1080/22221751.2020.1747363
119. Saichi M, Ladjemi MZ, Korniotis S, Rousseau C, Ait Hamou Z, Massenet-Regad L, et al. Single-Cell Rna Sequencing of Blood Antigen-Presenting Cells in Severe Covid-19 Reveals Multi-Process Defects in Antiviral Immunity. Nat Cell Biol (2021) 23(5):538–51. doi: 10.1038/s41556-021-00681-2
120. Junqueira C, Crespo A, Ranjbar S, Lewandrowski M, Ingber J, de Lacerda LB, et al. SARS-CoV-2 Infects Blood Monocytes to Activate Nlrp3 and Aim2 Inflammasomes, Pyroptosis and Cytokine Release. Res Sq (2021). doi: 10.21203/rs.3.rs-153628/v1
121. Ferreira AC, Soares VC, de Azevedo-Quintanilha IG, Dias S, Fintelman-Rodrigues N, Sacramento CQ, et al. Sars-Cov-2 Engages Inflammasome and Pyroptosis in Human Primary Monocytes. Cell Death Discov (2021) 7(1):43. doi: 10.1038/s41420-021-00428-w
122. Barhoumi T, Alghanem B, Shaibah H, Mansour FA, Alamri HS, Akiel MA, et al. Sars-Cov-2 Coronavirus Spike Protein-Induced Apoptosis, Inflammatory, and Oxidative Stress Responses in Thp-1-Like-Macrophages: Potential Role of Angiotensin-Converting Enzyme Inhibitor (Perindopril). Front Immunol (2021) 12:728896. doi: 10.3389/fimmu.2021.728896
123. Li S, Jiang L, Li X, Lin F, Wang Y, Li B, et al. Clinical and Pathological Investigation of Patients With Severe Covid-19. JCI Insight (2020) 5(12):e138070. doi: 10.1172/jci.insight.138070
124. Varga Z, Flammer AJ, Steiger P, Haberecker M, Andermatt R, Zinkernagel AS, et al. Endothelial Cell Infection and Endotheliitis in Covid-19. Lancet (2020) 395(10234):1417–8. doi: 10.1016/S0140-6736(20)30937-5
125. Gillot C, Favresse J, Mullier F, Lecompte T, Dogne JM, Douxfils J. Netosis and the Immune System in Covid-19: Mechanisms and Potential Treatments. Front Pharmacol (2021) 12:708302. doi: 10.3389/fphar.2021.708302
126. Phua J, Weng L, Ling L, Egi M, Lim CM, Divatia JV, et al. Intensive Care Management of Coronavirus Disease 2019 (Covid-19): Challenges and Recommendations. Lancet Respir Med (2020) 8(5):506–17. doi: 10.1016/s2213-2600(20)30161-2
127. Yang M, Lai CL. Sars-Cov-2 Infection: Can Ferroptosis be a Potential Treatment Target for Multiple Organ Involvement? Cell Death Discov (2020) 6:130. doi: 10.1038/s41420-020-00369-w
128. Fillmore N, Bell S, Shen C, Nguyen V, La J, Dubreuil M, et al. Disulfiram Use Is Associated With Lower Risk of Covid-19: A Retrospective Cohort Study. PloS One (2021) 16(10):e0259061. doi: 10.1371/journal.pone.0259061
129. Lobo-Galo N, Terrazas-López M, Martínez-Martínez A, Díaz-Sánchez ÁG. Fda-Approved Thiol-Reacting Drugs That Potentially Bind Into the Sars-Cov-2 Main Protease, Essential for Viral Replication. J Biomol Struct Dyn (2021) 39(9):3419–27. doi: 10.1080/07391102.2020.1764393
Keywords: SARS-CoV-2, cell death, apoptosis, necroptosis, syncytia pyroptosis
Citation: Zhu Z, Shi J, Li L, Wang J, Zhao Y and Ma H (2022) Therapy Targets SARS-CoV-2 Infection-Induced Cell Death. Front. Immunol. 13:870216. doi: 10.3389/fimmu.2022.870216
Received: 06 February 2022; Accepted: 19 April 2022;
Published: 17 May 2022.
Edited by:
Tengchuan Jin, University of Science and Technology of China, ChinaReviewed by:
Zhenlong Liu, McGill University, CanadaAhmed Yaqinuddin, Alfaisal University, Saudi Arabia
Copyright © 2022 Zhu, Shi, Li, Wang, Zhao and Ma. This is an open-access article distributed under the terms of the Creative Commons Attribution License (CC BY). The use, distribution or reproduction in other forums is permitted, provided the original author(s) and the copyright owner(s) are credited and that the original publication in this journal is cited, in accordance with accepted academic practice. No use, distribution or reproduction is permitted which does not comply with these terms.
*Correspondence: Long Li, bGlsb25nQG5idS5lZHUuY24=; Jinling Wang, d2psMDkyMEBxcS5jb20=; Huabin Ma, bWFodWFiaW5AbmJ1LmVkdS5jbg==