- 1Department of Cardiovascular and Metabolic Sciences, Lerner Research Institute, Cleveland Clinic, Cleveland, OH, United States
- 2Department of Microbiology and Immunology, Feinberg School of Medicine, Northwestern University, Chicago, IL, United States
Foxp3+ regulatory T (Treg) cells are a CD4 T cell subset with unique immune regulatory function that are indispensable in immunity and tolerance. Their indisputable importance has been investigated in numerous disease settings and experimental models. Despite the extensive efforts in determining the cellular and molecular mechanisms operating their functions, our understanding their biology especially in vivo remains limited. There is emerging evidence that Treg cells resident in the non-lymphoid tissues play a central role in regulating tissue homeostasis, inflammation, and repair. Furthermore, tissue-specific properties of those Treg cells that allow them to express tissue specific functions have been explored. In this review, we will discuss the potential mechanisms and key cellular/molecular factors responsible for the homeostasis and functions of tissue resident Treg cells under steady-state and inflammatory conditions.
Introduction
The immune system is incessantly confronted by antigens derived not only from invading foreign pathogens but also from self. Highly efficacious processes are in place so that harmful pathogens are cleared by rapid yet indiscriminating innate immunity and by more sophisticated antigen-specific adaptive immunity. For those lymphocytes posing a risk of self-reactivity, they are effectively eliminated during the development within the primary lymphoid tissues. The elimination is incomplete though, thus some lymphocytes with potential self-reactivity still mature and seed the periphery. There comes a secondary screening procedure in the periphery to prevent unwanted activation of self-reactive lymphocytes, predominantly operated through regulatory T (Treg) cells (1, 2).
Treg cells are a subset of CD4 T lymphocytes that plays an indispensable role in maintaining immunity and tolerance. Treg cell development is regulated by the master transcription factor, Foxp3, which forms multiprotein complexes capable of activating or repressing gene transcription responsible for Treg cell differentiation and functions (3). Depending on the site of their generation, Treg cells are primarily divided into two distinct subsets, thymus-derived tTreg and peripherally induced pTreg cells. Foxp3 expression is acquired in developing T cells within the thymus, and the level of self-reactivity is thought to support the differentiation into tTreg lineage cells (4, 5). On the other hand, conventional CD4 T cells activated during immune responses may acquire Foxp3 expression under adequate conditions and become pTreg cells (inducible iTreg cells when generated in vitro) (5, 6). The chief importance of Treg cells in immunity and tolerance is well exemplified from the facts that Foxp3 deficiency or its functional mutation results in systemic lethal autoimmune inflammation (7, 8). Scurfy mice have a missense mutation in the Foxp3 gene due to 2-bp insertion, generating truncated Foxp3 protein and lacking Treg cells, and exhibit a fatal lymphoproliferative disease and premature death (9, 10). Likewise, deletion in Foxp3 gene causes scurfy-like phenotypes (11). Similarly, the immunodysregulation polyendocrinopathy enteropathy x-linked (IPEX) syndrome is a rare recessive disease with life-threatening multi-organ autoimmune inflammation, and mutations in the Foxp3 gene is responsible for the manifestation of the disease (12, 13). More than 70 mutations in the Foxp3 gene have been identified in IPEX syndrome, ranging from a single base substitution through deletion mutations (14, 15). Recapitulation of some of the IPEX mutation in mice results in the development of a fulminant autoimmune syndrome (16). Unlike the scurfy mutation or Foxp3 deletion in mice where Treg cell generation is severely impaired, some IPEX patients still display undisturbed Treg cell generation, suggesting functional defects of Treg cells (17). The contribution of tTreg and pTreg cells to the immunity and tolerance is generally overlapping, although there is evidence that pTreg cells may play an additional role in maintaining inflammation and tolerance especially at the mucosal tissues (18, 19). Indeed, deletion of the conserved noncoding sequence-1 (CNS-1) of the Foxp3 gene causes severe defects in TGFβ-dependent Foxp3 expression in vitro (i.e., iTreg cells) without affecting thymic generation of tTreg cells (20). These animals spontaneously develop Th2 type inflammation at mucosal sites (20, 21), further emphasizing non-overlapping roles of in vivo generated pTreg cells from those of tTreg cells.
Over the last decade, much attention has been paid to the tissue-resident T cell subset, a sessile population of antigen-experienced memory T cells within the non-lymphoid tissues. They play a critical role in protecting the host from pathogens reentering the tissue sites. They are also found to display transcriptionally distinct programs from those of circulating memory counterparts, suggesting a distinct mechanism for their homeostasis and functions (22, 23). It was subsequently reported that certain Treg cells also express tissue-resident properties especially first in the visceral adipose tissue (VAT), and then in numerous non-lymphoid tissues including the skeletal muscles, intestine, skin, and central nervous system. As we begin to understand more about distinct features of Treg cells residing in different tissue sites, this review will discuss various tissue-resident (TR)-Treg cell subsets and the cellular/molecular mechanisms shaping their homeostasis as well as their beneficial or pernicious functions.
Treg Cell-Mediated Immune Suppression
The mechanisms through which Treg cells regulate immunity have extensively been examined over the years. Treg cell-mediated immune regulation is generally achieved via two modalities: contact-dependent and -independent mechanisms. Treg cells secrete copious amounts of anti-inflammatory/regulatory mediators to dampen inflammatory responses. IL-10 produced by Treg cells, although dispensable for tTreg cell development and not required to prevent systemic autoimmune inflammation, play an instrumental role in limiting inflammation especially at the mucosal interface (24). TGFβ1, a pleiotropic cytokine with potent immune suppressive property, is another cytokine produced by Treg cells (25). TGFβ1 produced by Treg cells was shown to be required to inhibit allergic and autoimmune inflammatory responses (26, 27), although the role of Treg cell-derived TGFβ1 has also been challenged (28). IL-35, composed of the IL-12p35 and Ebi3 subunits, is another Treg cell-derived cytokine known to antagonize T cell proliferation (29). Besides producing immune regulatory cytokines, Treg cells are also capable of suppressing effector T cell responses by consuming IL-2 (30). As for contact-dependent mechanisms, Treg cells express numerous inhibitory co-receptors capable of antagonizing immune activation. Lymphocyte activation gene 3 (Lag3) is a CD4-related receptor expressed by activated T cells, and Treg cells are known to express high level of Lag3 (31). Lag3-deficient Treg cells express deficient suppressive activity (31), although the precise underlying mechanism remains unclear. Lag3 may suppress antigen presenting cell maturation by interacting with its ligand, MHCII, thereby diminishing T cell activation (32). Cytotoxic T lymphocyte-associated protein 4 (CTLA4) is a B7 like molecule constitutively expressed in Treg cells and has been implicated in Treg cell suppression (33), although CTLA4-deficient Treg cells were still able to suppress immune responses (34). Treg cells can suppress effector T cell responses though degrading extracellular ATP. Treg cells highly express CD73 and CD39, ATP hydrolyzing ectoenzymes, on their surface. Adenosines and AMPs generated from degrading extracellular ATP have the capacity to inhibit antigen presenting cell maturation and T cell activation (35). In sum, the mode of suppressive mechanisms in Treg cells is likely determined by inflammatory and tissue conditions.
Subsets Of Tissue Resident Treg Cells: Characteristics And Functions In Healthy/Disease Status
Treg Cells in the Visceral Adipose Tissues (VAT)
While there were many reports describing Treg cells detected in tissues from the early era in the Treg cell field, the genesis of TR-Treg cell concept is a seminal study by Feuerer et al. that examined a unique Treg cell population within the VAT (36). First hint came from an unexpected observation that more than 50% of CD4 T cells found in the epididymal fat pads express Foxp3. These Treg cells are equally suppressive, and most importantly display transcriptional profiles that are distinct from Treg cells isolated from the counterparts of lymphoid tissues (36). VAT Treg cells are thought to be originated from the thymus. VAT Treg cells express high levels of Helios and neuropilin-1 (Nrp-1), markers considered for tTreg cells (37). TCR sequencing analysis uncovers little overlap between VAT Treg and conventional CD4 T cells from the lymphoid tissues (36, 37). Likewise, adoptively transferred conventional CD4 T cells do not give rise to VAT Treg cells (38). Notably, Treg cell accumulation in the VAT seems to occur early in life (prior to 3-4 weeks of age), since thymectomy at this age does not affect the numbers of VAT Treg cells (37). The contribution of circulating Treg cells in VAT Treg establishment seems minimal after 3 weeks as replenishing VAT Treg cells by adoptive transfer of Treg cells or using parabiosis model fails to do so (37).
Genes preferentially expressed in VAT Treg cells include transcription factors such as Pparg, Rora, and Gata3, and chemokines/cytokines and their receptors such as Cxcl2, Cxcr6, Ccr1, Ccr2, Il10, Il5, Il1rl1, and Il9r (39, 40). There are co-stimulatory receptors, Pdcd1, and Ctla80, and other proteins related to lipid metabolism including Dgat1, Dgat2, and Cd36 highly expressed in VAT Treg cells (39, 40). Peroxisome proliferator-activated receptor (PPAR) is the master transcriptional regulator that controls adipocyte differentiation (41). It was found that Treg cell expression of the Pparg is essential for VAT Treg cell accumulation, phenotype, and functions (39). Treg cell-specific PPARγ-deficient mice show decreased VAT Treg cell number and loss of their function without affecting Treg cells from other tissues (39). Likewise, mice treated with a PPARγ agonist demonstrate a pronounced increase in the number of VAT Treg cells (39). In addition to the Pparg gene, the Il1rl1 gene encoding ST2 protein, the receptor for IL-33, is also highly expressed in VAT Treg cells (39), suggesting that IL-33 may play a role in VAT Treg cells. Indeed, IL-33 promotes VAT Treg cell accumulation (37, 42). Single-cell RNA sequencing analysis showed trajectory of VAT Treg cells, as the splenic Treg cells expressing low levels of PPARγ contain precursors of TR-Treg cells, which accumulate in non-lymphoid tissue including VAT, further supporting a critical role of PPARγ in tissue adaptation of Treg cells (43).
One notable feature of VAT Treg cells is that they express a clonally expanded TCR repertoire (36, 37), strongly suggesting that there may be antigen(s) expressed within the tissue. By generating TCR Tg mice utilizing VAT Treg clone, Li et al. demonstrated that the Tg mice (named vTreg53) have substantial population of VAT Treg cells expressing the TCR. Consistent with the earlier transcriptional profiles from polyclonal VAT Treg cells, VAT Treg cells from the TCR Tg mice express representative gene signatures that are previously identified (38).
Obesity-associated metabolic diseases are in part driven by chronic inflammation of the VAT, and VAT Treg cells are central regulators of the inflammatory responses (36). In obese patients, the number of Treg cells in the circulation as well as VAT is substantially reduced (44). Eller and colleagues showed that significant decrease in the number of VAT Treg cells is closely associated with an increase in inflammatory mediators and a decrease in insulin sensitivity in VAT (45). In contrast, supplementation with Treg cells reduced VAT inflammation and improved metabolic parameters in obese mice (45). How VAT Treg cells are reduced during obesity has recently been explored. IFNα produced by plasmacytoid dendritic cells could deplete PPARγ+ VAT Treg cells (46). Alternatively, the soluble isoform of IL-33 receptor ST2 (sST2) acts as an obesity induced adipokine capable of antagonizing IL-33 signaling, thereby disrupting VAT Treg cell homeostasis (47).
Cold exposure is another factor that regulates VAT Treg cell accumulation. Short-term cold exposure enhances in vivo Treg cell induction in mice and humans by upregulating C17orf59 that inhibits mTORC1 signaling, thereby enhancing Treg cell generation (48). It has been reported that Treg cells are more enriched in brown adipose tissue (BAT) and subcutaneous adipose tissue (SAT) compared to VAT, implying the impact of cold exposure on generation and accumulation of Treg cells in fat tissues (49). Systemic ablation of Treg cells results in compromised energy expenditure adaptation upon cold exposure, accompanied with increased macrophage infiltration into brown adipose tissue (BAT) (50), indicating a novel role of Treg cells in thermogenesis. Treg cells upregulate thermogenic gene expressions upon cold exposure or β3-adrenergic receptor agonist CL316243 stimulation (50, 51). Interestingly, the role of SAT Treg cells in promoting adipocyte beiging and thermogenesis appears to be more prominent in female mice than male mice (51). This finding suggests a possible sex difference in the role of fat Treg cells. Along the line, the role of sex hormones in regulating VAT Treg cell accumulation has recently been uncovered, as androgen promotes production of IL-33 from stromal cells, which results in local expansion of VAT Treg cells in males (52). The precise contribution of sex hormones during fat Treg cell homeostasis needs to be investigated.
However, there is also emerging evidence that VAT Treg cells may negatively influence insulin resistance. IL-10 secreted from Treg cells, including VAT Treg cells, can drive insulin resistance in obesity by suppressing adipocyte energy expenditure and thermogenesis (53). In support, Treg cell specific IL-10 deficiency leads to increased insulin sensitivity and reduced obesity in male mice fed with high fat diet. Blimp1 is a transcription factor involved in IL-10 expression in Treg cells (54). Blimp1 deficiency in Treg cells reduces IL-10+ Treg cells especially within the adipose tissue, and Blimp1 deficient mice are protected from insulin resistance and obesity (53). Zheng and colleagues reported that VAT Treg cells may play a distinct role during insulin resistance derived from obesity and from aging (55). Indeed, mice deficient in VAT Treg cells, while susceptible to obesity associated insulin resistance, are better protected from age-associated insulin resistance (55), suggesting a complexity of VAT Treg cell-mediated regulation of insulin metabolism. A recent study from Li et al. uncovered using single-cell ATAC-sequencing that insulin signaling triggers the transition of VAT Treg cell subsets (CD73hiST2lo subset into a CD73loST2hi subset) through a HIF-1α-Med23-PPARγ axis (56). In obese and aged mice, CD73hiST2lo VAT Treg cells are markedly decreased (56). In support, deleting Hif1α or Med23 in Treg cells is sufficient to block the transition, resulting in an enrichment of CD73hiST2lo VAT Treg cells, which activate beige fat biogenesis and protect from metabolic disorders (56).
Lastly, the discrepancy on adipose tissue TR-Treg cells among species is a subject of high importance. A study by Laparra et al. directly compared the proportion of Treg cells present in VAT or SAT among mouse models, cynomolgus macaques, and humans (57). Surprisingly, the proportion of fat TR-Treg cells is found low, except C57BL/6 strain which show male-specific and aging-related increase of Treg cells. As C57BL/6 mouse strains are widely used to study VAT Treg cells, this species/strain-related discrepancy needs to be taken into account when translating the findings to humans.
Treg Cells in the Skin
Skin is the largest organ of the body and the importance of cutaneous Treg cells in maintaining tissue homeostasis and immune tolerance especially against skin microbiome has been investigated. In the skin of adult mice, the proportion of Foxp3+ Treg cells is considerably higher than that of circulation or of lymphoid tissues (~40% vs. ~10%) (58). Gratz et al. reported the existence of Treg cells expressing activated effector memory phenotypes that preferentially locate in hair follicles in the skin of mice (59). Memory phenotype Treg cells found in normal human skin are similarly localized in hair follicles (60). Ontogeny of skin resident Treg cells begins early at postnatal period, during which immune tolerance against commensal microbes is thought to be established (58). Preventing Treg cell accumulation in the skin by treating sphingosine-1-phosphate receptor agonist (FTY720) substantially disrupts tolerance against commensal bacteria, upon challenge later (58). A subset of CCR6high Treg cells found within the neonatal thymus are thought to be those seeding the skin during postnatal period (61). Inhibiting the migration during this developmental window results in accumulation of these Treg cells in the thymus, suggesting that they are of thymic origin.
Skin Treg cells are highly heterogeneous. Comparing TCR sequences between conventional memory T helper cells and Treg cells isolated from human skin revealed little homology, indicating that they may recognize different antigens (60). An elegant study from Tomura and colleagues utilized a mouse model expressing a photoconvertible protein to track migratory cells during contact hypersensitivity. From single cell gene and protein expression analyses they identified two populations of skin Treg cells, one subset primarily expressing Nrp1 and another subset that express CD39 together with CD25, granzyme B, or CTLA4 (62). Skin Treg cells are also known to express GATA3 and RORα (63), and they may directly control Treg cell functions because mice deficient in these transcription factors in Treg cells spontaneously develop Th2 type skin inflammation (64).
Skin-specific environmental cues help to promote skin TR-Treg cell development and proper functions. Skin commensal microbes play an important role for the early recruitment of Treg cells to the skin, since germ-free neonates display substantial reduction in skin Treg cells (61). Microbiota are known to stimulate CCL20 production by hair follicles, supporting the migration of Treg cells (61). Phenotypic features of skin resident Treg cells are analogous to that of memory T cells in that they express low levels of CD25 yet higher KLRG1, CTLA4, and CD127 (59). Moreover, various chemokine receptors involved in skin migration are expressed, including CCR2, CCR6, CCR8, CCR10, CXCR4, and CXCR6. IL-15 and dermal fibroblasts, conditions found in chronically inflamed skin, trigger cocultured cutaneous Treg cell proliferation in an antigen-independent manner, indicating that skin Treg cells may directly respond to inflammatory cues to limit inflammatory processes (65). Likewise, IL-33, an alarmin cytokine highly expressed in the skin from patients with atopic dermatitis or psoriasis, is known to drive accumulation and functional maturation of skin Treg cells (66). Interestingly, unlike lymphoid Treg cells, skin Treg cells are found to highly express both IL-2 and IL-7 receptors (59). However, IL-2 is dispensable for their maintenance in the skin. Instead, IL-7 seems crucial for their retention in the skin at steady state (59). Additionally, skin Treg cells express the mitochondrial enzyme, arginase 2 (Arg2) (67). Arg2 augments suppressive functions of Treg cells in part by regulating their metabolic program via mTOR signaling (67). Arg2 deletion in primary human Treg cells results in the loss of Treg transcriptional signature (67), suggesting broad functions of Arg2 in Treg cells.
There is a plethora of evidence emphasizing the importance of skin Treg cells for maintaining tissue homeostasis. Skin Treg cells express Notch ligand family member, Jagged 1, and facilitates hair follicle stem cell function and hair follicle regeneration by supporting stem cell proliferation and differentiation through the CXCL5-IL-17 axis (68). It was recently reported that obesity targets hair follicle stem cells to accelerate hair thinning and loss (69). High-fat diet (HFD) induced IL-1-derived NFkB activation within the stem cells, which inhibits Sonic hedgehog (SHH) signal transduction in the stem cells, thereby inducing depletion of hair follicle and hair loss. Because HFD is known to impair Treg cell function especially in the fat tissues, it is interesting to speculate that HFD inhibits hair follicle stem cells through altering skin Treg cell functions. Skin Treg cells also inhibit myofibroblast activation, which suppress excessive scar formation during wound healing (64). Treg cells can promote the repair of skin trauma by the expression of epidermal growth factor receptor (EGFR) and are involved in stem cell differentiation in the skin (70). In support, the lack of EGFR in Treg cells can lead to delayed wound closure and increase accumulation of pro-inflammatory macrophages (70). Expansion of skin Treg cells with a healing function upon UVB exposure has been reported (71). The expanded Treg cells promote wound healing and suppresses inflammation, via proenkephalin (PENK), an endogenous opioid precursor, and amphiregulin (Areg), the EGFR ligand (71).
Skin Treg cells are fundamentally important in regulating inflammatory responses in the skin. In vitiligo, a T cell mediated autoimmune condition resulting in the loss of melanocytes, cutaneous Treg cells are greatly reduced, suggesting that autoreactive T cell activation is not adequately controlled (72). Diffuse systemic scleroderma/sclerosis (SSc) is an autoimmune condition characterized by excessive fibrosis in barrier tissues like skin (73). Skin Treg cells are similarly reduced in the SSc skin, which seems associated with diminished TGFβ and IL-10 expression (74). However, one study that compared Treg cells from blood and the skin of limited and diffuse SSc patients found that Treg cells in the skin lesions produced pro-fibrotic Th2 cytokines like IL-13 and IL-4 (75). Along the same line, an increase in the frequency of circulating CD25+FoxP3+CD127− Treg cells in SSc patients was observed especially in early phase of the disease. There is also recent evidence indicating that Treg cells could contribute to SSc pathogenesis by conversion into pathogenic effector T cells (76). Fenoglio et al. found a significant correlation between increased circulating Th17 cells and alteration of Treg cell compartment (77). Therefore, these dysfunctional Treg cells may exacerbate the disease. Elevated methylation levels of the FoxP3 promoter, inversely correlated with FoxP3 mRNA expression, are accompanied by reduced proportion of CD4+CD25+FoxP3+ Tregs (78). Indeed, increased methylation levels of the FoxP3 promoter in Ssc patients was found associated with reduced FoxP3 mRNA expression and proportion of CD4+CD25+FoxP3+ Treg cells (79). Treg cells are also able to limit psoriatic inflammation in the skin. Experimental psoriasis induced by topical imiquimod application results in significant accumulation of Treg cells in the skin lesion, as seen in human psoriasis (80). The accumulation is thought to mediate resolution of the inflammation, because Treg cell depletion dramatically exacerbates skin inflammation (80). However, Treg cells may alternatively promote the disease in some cases. The disease-promoting features of Treg cells are attributed to Treg cells’ differentiation into IL-17-producing effector-like cells (81, 82).
Treg Cells in the Lung
As the lung is constantly exposed to microbial and environmental antigens, balancing tolerance and immunity is an important task of lung resident immune cells. The lung is another organ replete with TR-Treg cells. Treg cells resident in the lung tissue express various immune regulatory properties during infection, allergy, and injury. In asthmatic inflammation, Foxp3 expression in TR-Treg cells or the frequency of TR-Treg cells is found diminished (83), although in some cases, Treg cell numbers are instead increased in patients with severe allergic inflammation, suggesting their defective suppressive functions (84). The precise mechanisms underlying the defects is not well understood. However, inflammatory cytokines may destabilize the stability of Treg cells or undermine Treg cell functions. Thymic stromal lymphopoietin (TSLP) highly present in allergic conditions is shown to inhibit IL-10 production by Treg cells, resulting in diminished suppressive functions (85). Similar defective functions and reduced numbers of TR-Treg cells are found in idiopathic pulmonary fibrosis (IPF), a chronic lung disease caused by the accumulation of extracellular matrix in the lung (86). Earlier studies identified that Treg cells in the bronchoalveolar lavage are reduced in patients with IPF (87). Reilkoff et al. reported that Semaphorin (Sema) 7a expression is increased in lymphocytes including Treg cells and that Sema 7a+ Treg cells represent functionally defective Treg cells potentially supporting fibrosis in a TGFβ-driven fibrosis (88), suggesting that targeting Sema 7a+ Treg cells could be a strategy of therapy. In addition, an elegant study from Belperio and colleagues presented the evidence supporting a pathogenic role of Treg cells during fibrosis in the lung. Using bleomycin-induced fibrosis model in mice, treatment of mice with IL-2 complex during intratracheal bleomycin challenge exacerbates lung fibrosis, which was in part due to heightened type 2 immunity triggered by altered Treg cell functions (89). On the other hand, ST2+ Treg cells producing IL-13 in response to IL-33 are shown to be critical in preventing mortality following acute lung injury by limiting inflammatory cytokine production and Ly6C monocyte accumulation (90). Therefore, expanding Treg cells may deviate immune responses to type 2 immunity, yet the impact of type 2 immune responses remains controversial. Unlike bleomycin-induced lung injury model, CD103+ TR-Treg cells in the lung could potentially limit lung fibrosis induced by TR-pathogenic CD4 in Aspergillus infection model (91). Ichikawa et al. demonstrated that in response to chronic exposure to Aspergillus fumigatus CD69+CD103+ TR-Treg cells are generated to limit pathogenic functions of CD69+CD103+ TR-effector memory CD4 T cells to suppress fibrotic inflammatory responses (91). Therefore, the precise roles of TR-Treg cells may be determined by the type of immune responses in the lung.
The contribution of lung TR-Treg cells has also been explored in the model of lung infection. During pulmonary infection with Cryptococcus, antigen specific Treg cells are induced and accumulate in the lung. These Treg cells uniquely express the IRF4 to suppress Th2 type immune responses induced to control fungal replication and pathology. IRF4 expression in Treg cells plays a critical role for their retention in the lung through CCR5 expression (92). In viral infection such as influenza virus, antigen specific Treg cells expressing memory phenotype accumulate in the lung tissue and control anti-viral responses (93). Helios, an Ikaros family member transcription factor and preferentially expressed in Treg cells, was recently reported to be highly expressed in lung Treg cells during influenza virus infection. Importantly, Helios+ Treg cells have the ability to persist in the lung tissues and to more potently suppress virus specific CD8 T cell responses (94). Treg cells are also shown to mediate rapid antigen-specific primary and memory T cells responses (95) and to promote formation of follicular helper T cell and germinal center B cell responses in the lymph nodes by controlling IL-2 availability (96), thereby enhancing anti-viral immune responses. In addition to modulating anti-viral immunity, lung Treg cells promote tissue repair. Arpaia et al. previously reported that inflammatory cytokine IL-18 or alarmin IL-33 is able to induce Areg production from lung Treg cells during lung injury from viral infection, and the production of Areg seems crucial for Treg cell-mediated tissue repair (97). Given that TCR- and IL-2-signaling pathways primarily control Treg cell suppressive function, this study suggests that a distinct pathway triggered by ‘innate’ tissue-derived signals generated during inflammation and tissue injury is critically involved in tissue repair function, independent of the Treg cell suppressive function. Transcriptomic analysis of human TR-Treg cells uncovers that human lung TR-Treg cells differentially express genes associated with the Wnt pathway (specifically Wnt ligands, wnt1, wnt2, wnt7a and Wnt receptor, Fizzled 2), supporting the possibility that lung TR-Treg cell may mediate epithelial cell repair and regeneration (98).
Amid the SARS-CoV2 pandemic, the roles of lung TR-Treg cells during COVID-19 pathogenesis remain to be determined. Circulating Treg cell number is greatly reduced in COVID-19 patients, and importantly, the reduction rate is correlated with disease severity (99–102). A recent study also reported a decrease in airway Treg cells in COVID-19 patients compared to healthy controls (103). The loss of Treg cells in circulation seems related to increased and persistent tissue damage, which often leads to worsen disease outcomes. On the other hand, another study by Galvan-Pena et al., showed increase in proportions and Foxp3 levels in circulating Treg cells, correlating with disease severity (104). These discrepant findings are likely attributed to lung TR-Treg cell heterogeneity, suggesting a need for more precise characterization of lung TR-Treg cells beyond the change in their number or ratio. Evaluating antigen specificity of Treg cells to virus will be necessary based on the protective role of antigen-specific CCR7+ tissue resident memory (Trm) CD8 T cells in the non-hospitalized and convalescent patients, emphasizing the importance of persistent antigen-specific Trm cells (105). Given that lung TR-Treg cells can limit pulmonary immunopathology during respiratory virus infection, understanding the behavior of lung TR-Treg cells from COVID-19 patients may provide better insights into the understanding the pathology of this virus. However, a study from Benoist and colleagues reports that Treg cells from severe COVID-19 patients appear to express distinct phenotypes, unexpectedly characterized by increased Treg cell proportion and Foxp3 expression (104). It was found that these Treg cells overexpress not only suppressive effectors but also pro-inflammatory cytokine such as IL-32. Most strikingly, these Treg cells express similar traits as tumor-infiltrating Treg cells. These results suggest a detrimental role of Treg cells in COVID-19, by interfering with anti-viral immunity (104).
Treg Cells in the Skeletal Muscle
Treg cells present in the skeletal muscle were first reported in 2013 (106). A small proportion of Treg cells (~10% of CD4+ T cells) exist in the muscle of healthy mice (106). In the injured condition, Treg cells rapidly proliferate within 3-4 days and then decrease. However, even after a month after injury, detectable Treg cells remain (106, 107). Accumulation of Treg cells in injured muscle is primarily mediated by IL-33 secreted by injured skeletal muscle (107). IL-33 acts on the cells expressing IL33 receptor, ST2, one of the most strongly up-regulated genes in Treg cells isolated from injured muscle compared to Tregs present in lymphoid tissue (106).
Where these Treg cells are originated from remains unclear. Treatment of FTY720 before inducing muscle injury reduced the absolute number of accumulated Treg cells, but not their proportion among CD4+ T cells in the muscle (107), suggesting accumulation of muscle Treg cells in response to injury may be dependent on the recruitment from the circulating T cell pool. However, this does not rule out the possibility that local expansion of Treg cells that are already found in the muscle. The TCR sequences of muscle Treg cells were distinct from muscle conventional T cells and a substantial proportion (20-40%) of muscle Treg cells is clonally expanded (106). Using a transgenic mice carrying rearranged transgenes encoding the TCRα and TCRβ chains from a muscle TR-Treg clone, Cho et al. showed a muscle specific accumulation and proliferation of Treg cells, indicating TCR-dependent development of muscle Treg cells (108). Therefore, muscle Treg cells may arise from the mixed origins, and this needs to be further examined.
Skeletal muscle regeneration requires the activation and differentiation of myogenic stem cells, called muscle satellite cells (109). Upon muscle injury, proinflammatory (M1 type) macrophages immediately infiltrate the tissue, followed by anti-inflammatory (M2 type) macrophages (110). M1 macrophages stimulate satellite cell proliferation and exacerbate inflammation, while M2 macrophages induce differentiation of satellite cells and promote tissue regeneration (110). Muscle Treg cells play a crucial role in M1-to-M2 switch in macrophages as this transition is absent when Treg cells are depleted (106). Treg cells also limit IFNγ production, reducing inflammation and fibrosis (111). Furthermore, Treg cells may directly regulate activity of muscle satellite cells. In vitro coculture with Treg cells induces enhanced proliferation and reduced myogenic differentiation of muscle satellite cells (106). Regulatory role of Treg cells on tissue regeneration seems to be dependent on a growth factor, Areg. Areg is overexpressed by muscle Treg cells and administration of Areg to Treg-depleted mice at the time of muscle injury induces expression of genes involved in muscle repair (106). Addition of Areg in the muscle satellite cell culture also enhances differentiation of myogenic differentiation (106), suggesting a critical role of Areg, particularly derived from Treg cells, on muscle repair.
Little is known about Treg cells in muscle disease. Treg cells were highly accumulated in skeletal muscles of mdx dystrophin-deficient mice, a model of human Duchenne muscular dystrophy (DMD), in which muscle injury and inflammation is mitigated by expansion of the Treg population but exacerbated by Treg-cell depletion (112). The P2X7 purinergic receptor (P2RX7) appears to be especially important in this respect. Genetic ablation of the P2X7 gene in mdx mice or P2RX7 antagonist administration leads to improved muscle structure and function, with reduction of inflammation and fibrosis, and increased muscle strength and endurance (113, 114). Muscle Treg cells also support muscle repair after myocardial infarction directly via promoting cardiomyocyte proliferation (115) and indirectly via modulating M1/M2 differentiation (116). More work is needed to understand TR-Treg cell biology in the muscle.
Treg Cells in the Intestine
The gut tissues are separated from microbial and food antigens by single layer of intestinal epithelial cells (117). Therefore, the epithelial layers as well as underlying tissues, lamina propria, are heavily populated with various immune cells particularly with regulatory properties. In support, the intestines are most frequently affected by the loss-of-function mutations of the FOXP3 gene in IPEX patients, highlighting the key role of Treg cells in the intestinal tolerance (118). Intestinal TR-Treg cells are heterogeneous and can be divided into the three subsets based on transcription factor expression: GATA3+Helios+, RORγt+, and RORγt-Helios- Treg cells (119). Wang et al. reported that Treg cell-specific deletion of GATA3 results in a spontaneous inflammatory disorder (120). These Treg cells display defective suppressive function with diminished Foxp3 expression, instead acquire Th17 phenotypes (120). In contrast, Wohlfert et al. reported that Treg cell-specific Gata3-/- mice do not exhibit any inflammatory conditions at steady state. However, the frequency of Treg cells in the intestinal tract is found markedly diminished in these mice (121). Consistent with this observation, Gata3-/- Treg cells fail to protect animal from colitis (121). Particularly, GATA3 expression in Treg cells plays an important role in limiting expression of effector T cell markers such as RORγt and IL-17A especially under inflammatory settings (121). GATA3 also induces expression of ST2 and loss of GATA3 markedly reduces ST2 expression in Treg cells (122). RORγt, a transcription factor involved in Th17 cell differentiation, is also found highly expressed in colonic Treg cells compared to splenic Treg cells at steady state and its expression is microbiome-dependent (123, 124). RORγt supports Foxp3 expression in colonic Treg cells in part by suppressing the effector programs (125). RORγt-deficient Treg cells transferred into Rag-/- mice induced for colitis fail to protect mice from the disease, as these Treg cells express Th1-like effector phenotypes and subsequently lose suppressive activity (125).
Intestine TR-Treg cells likely have mixed origin. Approximately 10% of CD4+ T cells express Foxp3 in germ-free mice, while ~35% of colonic CD4+ T cells are Treg cells in specific pathogen-free (SPF) mice (126), suggesting that the majority is likely of locally generated pTreg cells. Indeed, analysis on colonic Treg cells in SPF mice showed low expression of Helios and Nrp-1, markers of tTreg cells (107, 126, 127). Adoptive transfer of naïve conventional T cells from lymph node (LN) to Treg-depleted mice successfully restored RORγt+ or Helios+ colonic Treg cells upon interaction with microbiota (128), indicating microbiome-derived signals are required for inducing certain subset of Treg cells in the gut. However, some Treg cells are likely of thymic origin. In support, TCR repertoire of intestine TR-Treg cells is similar to LN Treg cells but distinct from intestinal conventional T cells (129).
Inflammatory bowel disease (IBD) is a group of chronic inflammatory diseases of the intestine, including ulcerative colitis and Crohn’s disease. IBD patients have increased Treg cells in the mucosa of gut; however, the suppressive function of Treg cells from IBD patients seems impaired (130). Increased expressions of Tbet and RORγt are associated with elevated levels of proinflammatory cytokines including IL-17, IL-6, and IL-1β, resulting in a decreased suppressive Treg cell function and severe inflammation in IBD (131–133). Areg is also highly expressed by intestinal Treg cells; however, Treg-specific ablation of Areg does not alter the phenotypes nor functions of Treg cells, suggesting dispensable role of Treg cell-derived Areg in the intestine (97). Dysregulated Treg cell functions appear to contribute to the pathogenesis, although the precise role of dysregulated Treg cells in IBD etiology remains to be determined.
Treg cells play an opposite role in the context of colorectal cancer (CRC), where an increased accumulation of Treg cells is associated with worse prognosis and Treg cells suppress anti-tumor effector T cell immunity (134). However, it is also possible that Treg cells can be protective in CRC as Treg cells are the major source of IL-10, which reduce polyposis (135). Oral administration of IL-10 to a mouse model of spontaneous gastrointestinal polyposis ameliorates disease and enhances survival, via regulating the ratio between RORγt+IL-17+ and RORgt-IL-17- Treg cell subsets (136). This opposite role of Treg cells might be due to their extremely high heterogeneity in intestines. Single-cell RNA sequencing analysis on intratumoral Tregs from CRC patients revealed Treg cells with low activity of the MondoA-thioredoxin-interacting protein (TXNIP) axis and increased glucose uptake (137). Treg cells with low MondoA-TXNIP axis are Th17-like and hyperglycolytic, which promote tumor growth. This seems to be a result of adaptation to glucose-depleting tumor microenvironment. It was recently reported that TCF-1 plays an essential role in regulating Treg cell development and functions (138). Interestingly, TCF-1-deficient Treg cells, while fully competent to suppress T cell proliferation and cytotoxicity, were unable to control CD4 T cell polarization and inflammation (138). In mice with polyposis, the lack of TCF-1 expression in Treg cells promotes tumor growth. Likewise, in CRC patients TCF-1low Treg cells and elevated Th17 immunity were found (138). In a mouse model of environmental enteric dysfunction (EED), a gastrointestinal inflammatory disease caused by malnutrition and chronic infection, Hand and colleagues recently reported that mice with EED harbor increased RORγt+ Treg cells in the small intestine, contributing to poor efficacy of oral vaccines (139). Deletion of RORγt expression in Treg cells was sufficient to restore small intestinal vaccine specific CD4 T cell responses and vaccine induced protection, suggesting a detrimental role of Treg cells. However, the lack of these Treg cells was associated with enhanced susceptibility to EED (139). These findings highlight the complex and diverse mechanisms mediated by intestine TR-Treg cells in different disease settings.
Treg Cells in Tumors
?A3B2 twb .24w?>Treg cells are abundant in tumor tissues. It is generally known that intratumoral Treg cells hinder effective anti-tumor immunity, representing a critical barrier to successful anti-tumor immunity and immunotherapy. De Simone et al. compared Treg cells infiltrating colorectal or non-small cell lung carcinomas and found that intratumoral Treg cells are highly suppressive compared to Treg cells isolated from normal tissues or the peripheral blood of the same patients (140). Phenotypically, these Treg cells express immune checkpoint surface receptors (Lag3, CTLA4, PD1, TIM3). Expression of tumor Treg cell signature genes, such as Layn, Mageh1, and Ccr8, is found negatively correlated with poor patient survival (140). Similar features of intratumoral Treg cells are also found in human breast cancer, namely, high suppressive ability and their gene expression pattern, notably CCR8 (141). In prostate cancer patients, CCR4+ Treg cells are found abundant in the tumor tissue and associate with poor prognosis (142). Tumor microenvironment secretes chemokines selectively recruiting Treg cells to tumor sites. Hypoxia induces CCL28 in ovarian cancer and liver cancer, recruiting CCR10+ Treg cells (143). Pancreatic ductal adenocarcinoma secretes CCL5 to recruit CCR5+ Treg cells and blocking CCL5 expression could improve anti-tumor immunity (144). Anti-CCR4 antibody treatment enhances antitumor immune responses mainly by altering tumor infiltrating Treg cells in an ovarian cancer model (145). Similarly, anti-CCR4 antibody selectively depletes intratumoral Treg cells, promoting anti-tumor T cell responses (146).
TCR repertoire analyses uncover little overlap between effector T cells and intratumoral Treg cells in human breast cancer and melanoma patients (141, 147). Instead, tumor Treg cells display substantial overlap with circulating Treg cells, suggesting that they are likely of tTreg cell origin (147). Alternatively, tumor microenvironment can promote the differentiation of pTreg cells from naïve CD4+ T cells due to enriched signals including TGF-β, IL-10, and indoleamine 2,3-dioxygenase (IDO), all of which are known Treg cell inducers. For example, acute myeloid leukemia cells express IDO, supporting the intratumoral Treg cell conversion (148). The precise origin of intratumoral Treg cells may thus differ depending on the tumor cell types.
Treg cells accelerate immune evasion by tumor cells, leading to tumor development and progression in various types of cancer. Treg cells known to restrict the anti-tumor immune response through multiple mechanisms such as CTLA4 mediated suppression of antigen presenting cell function, consumption of IL-2, production of immunosuppressive cytokines, and expression of immune checkpoint inhibitory receptors. Lag3 is highly expressed in intratumoral Treg cells. Lag3 expressed on Treg cells was initially reported to play an important role in mediating Treg cell functions (31). Paradoxically, Lag3 expression in Treg cells was shown to limit Treg cell function and survival in a mouse model of autoimmune diabetes (149). Whether Lag3 expression in intratumoral Treg cells plays positive or negative role in Treg cell functions need to be examined. If Lag3 is an inhibitory receptor interfering with Treg cell function as observed in diabetes, targeting Lag3 in intratumoral Treg cells will enforce the function, further limiting anti-tumor immunity.
Treg Cells in Transplantation
Treg cells derived from recipients are generally considered critical in preventing allograft rejection, as a selective depletion of recipient Treg cells but not donor Treg cells abrogates graft acceptance (150, 151). Using a murine cardiac allograft model, Hancock and colleagues found that ~100-fold higher intragraft Foxp3 levels in recipients tolerized with anti-CD154 antibody and donor-specific transfusion, compared to those rejecting allograft (152). Treg cells are further found indispensable for tolerance induction, because anti-CD25 antibody injection or peritransplant CD25+ Treg cell depletion prevents long-term graft survival (152). Similar protective roles of Treg cells were also reported in liver and lung transplantation (153, 154). In case of kidney transplantation, allografts are spontaneously accepted, which is associated with increased Foxp3 expression (155). Interestingly, donor-derived TR-Treg cells were more potent than the recipient-derived Treg cells (156). The precise mechanisms supporting the formation of TR-Treg cells and the cellular and molecular signatures of TR-Treg cells within different transplant organs remain to be determined.
Crosstalk Between Treg Cells And Tissues
Once activated Treg cells enter the inflamed or infected target tissues, they receive additional tissue-derived signals that allow them to further differentiate into TR-Treg cells. These signals are tissue-specific, and likely turn on the ‘tissue-specific’ signatures on Treg cells (Figure 1). For example, IL-33, a member of the IL-1 family and an alarmin, is mainly produced by epithelial and endothelial cells following damage or cell stress and function as a ‘pan-TR-Treg cell factor’ as they are expressed in multiple tissue sites (157). A study by Shiering et al. has found that IL-33 produced by inflamed intestines acts on colonic Treg cells that highly express the ST2 and supports Treg cell function and, most importantly, provides a signal for Treg cell accumulation and maintenance in the sites (122). Similar mechanism was also observed in intestinal cancer. Pastille et al. demonstrated that tumor infiltrating Treg cells preferentially express ST2 and that IL-33 stimulated intratumoral Treg cells display more activated phenotypes and better accumulate in the tumor (158). IL-33 also shapes Treg cell stability by curtailing IL-17 production by Treg cells. IL-33’s ability to modulate Treg cell homeostasis is also found in VAT. Vasanthakumar et al. reported that IL-33 expressed in the VAT is able to induce VAT TR-Treg cell proliferation and maintain Treg cell identity, because both proportions and Foxp3 expression of VAT TR-Treg cells are greatly diminished in Myd88-/- mice (159). Expression of the Pparg, a master transcription factor essential for VAT TR-Treg cells, is maintained through IL-33 stimulation, further supporting the IL-33’s support for VAT Treg cells (159). Last example of IL-33 function as a regulator of TR-Treg cells is from skeletal muscle. Kuswanto et al. demonstrated that IL-33 is rapidly produced after acute muscle injury and that IL-33 is primarily produced by CD31+ endothelial cells (107). IL-33 signaling is particularly important for muscle TR-Treg cell accumulation, as Treg cell-specific ST2-deficient mice exhibit delayed muscle repair (107). Most notably, tissue regenerative activities of Treg cells, for instance to induce tissue repair factors such as Myog (muscle transcription factor) and Adam8 (constructing extracellular matrix) are significantly decreased in the absence of ST2 on Treg cells (107). In addition to soluble mediators like cytokine IL-33, there are tissue-specific mechanisms involved in TR-Treg cell trafficking, accumulation, and retention. FucT-VII (alpha-1,3-fucosyltransferase VII) is an enzyme required for PSGL-1 glycosylation to facilitate TR-Treg cell accumulation in tissues like skin and lamina propria (160, 161). ATP released by necrotic muscle cells during muscle injury inhibits TR-Treg cell generation and functions through purinergic P2X receptors, delaying myofiber regeneration (113, 114). In the CNS, serotonin (5-HT) produced by brain cells activates 5-HT7 receptor expressing Treg cells, supporting Treg cell expansion (162). Meanwhile, Treg cells infiltrating the brain during ischemic injury release osteopontin that enhances the repair activity of microglia (162). A protein known as cellular communication network factor 3 (CCN3) is another cellular factor secreted from CNS TR-Treg cells, promoting oligodendrocyte differentiation and remyelination (163).
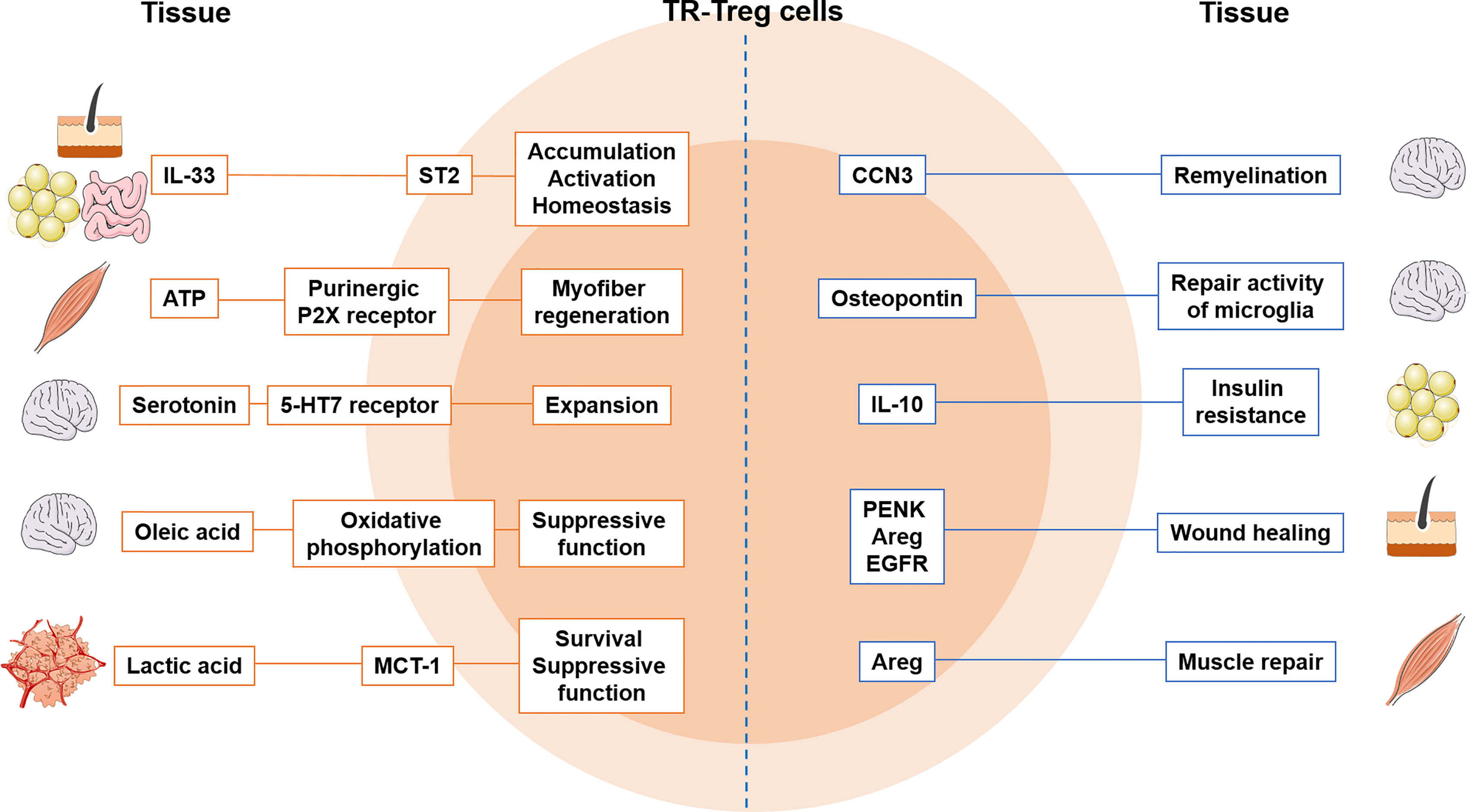
Figure 1 Tissue-specific adaptation of TR-Treg cells and crosstalk between tissue and Treg cells. The factors produced from tissues shape Treg cells’ ability to control in the tissue. Treg cells express markers to become more tissue specific and produce factors that regulate tissue function. MCT-1, lactate transporter; Areg, amphiregulin; 5-HT7, serotonin receptor; CCN3, cellular communication network factor 3; PENK, proenkephalin; EGFR, epidermal growth factor receptor.
The evidence that local metabolites critically modulate Treg cell functions continues to rise (164, 165). Treg cells heavily rely on oxidative phosphorylation for their homeostasis and functions (165, 166). Hafler and colleagues recently reported that tissue-derived lipid molecule plays a key role in maintaining Treg cell homeostasis (167). Oleic acid, the most prevalent free fatty acid, amplifies Treg cell-oxidative phosphorylation by increasing Foxp3 expression as well as Stat5 phosphorylation, enhancing Treg cell suppressive functions. Indeed, Treg cells isolated from multiple sclerosis (MS) patients express different transcriptomic signatures that resemble those exposed to proinflammatory arachidonic acid (167). In support, the concentration of oleic acid was significantly reduced, and exposing MS patient Treg cells to oleic acid restores defects in suppressive functions. Tumors provide hostile environments for recruited immune cells (metabolite-depleted, hypoxic, and acidic conditions). An elegant study by Delgoffe and colleagues recently reported that intratumoral Treg cells unexpectedly utilize glycolysis by-product, lactic acid as an alternative energy source (168). Treg cell expression of a lactate transporter MCT-1 is thus essential for Treg cell survival and function within tumors, as Treg cell specific MCT1 deletion results in slow tumor growth, and more importantly, in an increased response to immunotherapy. How metabolic rewiring of intratumoral Treg cells favors Treg cell proliferation, survival, and suppressive functions will require further investigation. Therefore, tissue-specific metabolites may play a fundamental role in modulating Treg cell homeostasis and functions by reprogramming gene expression profiles of TR-Treg cells to equip them with cellular machineries ideal for their adequate maintenance.
Therapeutic Interventions
Therapeutic approaches targeting Treg cells have gained much attention and multiple clinical trials are underway to apply preclinical findings in clinical settings (Table 1). Strategies leveraging Treg cells vary depending on the disease type, as augmentation of Treg cell number and function is critical for autoimmune diseases whereas depleting or suppressing Treg cells is required for successful cancer treatment. Depletion of Treg cells has been traditional attempts predominantly by targeting CD25. The approach has expanded to other Treg cell markers such as CD122, CD44, 4-1BB, and CCR8 in order to improve the specificity of treatments (192). Recent studies suggest a new approach rather to weaken Treg cell function via stimulating costimulatory molecules such as OX40 and GITR (193, 194). Now that TR-Treg cells may acquire tissue-specific signatures in phenotypes and gene profiles, developing more specialized tools targeting tissue-specific TR-Treg cells, particularly in tumors of different tissue sites, will be needed. Combining Treg cell target approaches with other immunotherapies such as anti-PD1 and anti-PDL1 therapies has also been attempted in cancer patients. Of note, depleting Treg cells may trigger compensation program such as an increased infiltration of macrophage in tumor or a reduction in conventional T cells (195, 196), reducing the efficacy of immunotherapy. Therefore, modulating Treg cells has to be performed in consideration of multiple Treg cell-extrinsic factors.
Likewise, CD25 can also be a target to promote Treg cell expansion as treatment with low dose IL-2 or IL-2:anti-IL-2 complexes that selectively expand Treg cells including TR-Treg cells (197). However, IL-2 can still be used by CD25+ effector T cells, or TR-Treg cells in different tissues may utilize other factors (e.g. IL-7 or TGFβ) to maintain in certain tissues. Therefore, more specialized and tailored approaches to different tissues are necessary to support Treg cell homeostasis.
Conclusion and Outstanding Questions
It is obvious that TR-Treg cells are critical regulators of tissue functions including homeostasis, inflammation, and repair, or a significant barrier antagonizing effective anti-tumor immunity. Over the years, the field has significantly grown and identified TR-Treg cells within different tissue sites, primarily skin, muscle, and VAT. We now begin to understand the origin of TR-Treg cells and key tissue-derived factors that promote the generation and maintenance of TR-Treg cells. There are several important questions that warrant future investigations. First, we need to better understand the mechanisms involved in TR-Treg cell retention within the tissues. Tissue retention is an important feature of tissue resident lymphocytes and appears to be mediated by multiple mechanisms depending on the tissue types and the nature of immune responses. Treg cell retention at the site of Leishmania infection is in part mediated by CD103, the αE chain of the αEβ7 integrin that is highly expressed on Treg cells (198). Sphingosine 1-phosphate receptor 5 (S1PR5) has recently been identified as a key regulator of the peripheral retention of TR-memory CD8 T cells (199). TR-Treg cells may share similar mechanisms for efficient tissue retention. Second, there is increasing evidence that local tissue antigen stimulation is critical for TR-Treg cell maintenance. A TCR transgenic mice specific for VAT-specific antigens has opened new opportunity to understand VAT TR-Treg cell biology (38). More rigorous TCR repertoire analyses and identification of key tissue specific antigens supporting TR-Treg cell differentiation will obviously be the next step.
Author Contributions
JL, DK, and BM wrote the manuscript. All authors contributed to the article and approved the submitted version.
Funding
This work was supported by NIH grant AI125247 and NMSS grant RG1806-31374 (BM).
Conflict of Interest
The authors declare that the research was conducted in the absence of any commercial or financial relationships that could be construed as a potential conflict of interest.
Publisher’s Note
All claims expressed in this article are solely those of the authors and do not necessarily represent those of their affiliated organizations, or those of the publisher, the editors and the reviewers. Any product that may be evaluated in this article, or claim that may be made by its manufacturer, is not guaranteed or endorsed by the publisher.
References
1. Sakaguchi S, Mikami N, Wing JB, Tanaka A, Ichiyama K, Ohkura N. Regulatory T Cells and Human Disease. Annu Rev Immunol (2020) 38:541–66. doi: 10.1146/annurev-immunol-042718-041717
2. Akkaya B, Shevach EM. Regulatory T Cells: Master Thieves of the Immune System. Cell Immunol (2020) 355:104160. doi: 10.1016/j.cellimm.2020.104160
3. Rudensky AY. Regulatory T Cells and Foxp3. Immunol Rev (2011) 241(1):260–8. doi: 10.1111/j.1600-065X.2011.01018.x
4. Chen W, Konkel JE. Development of Thymic Foxp3(+) Regulatory T Cells: TGF-β Matters. Eur J Immunol (2015) 45(4):958–65. doi: 10.1002/eji.201444999
5. Shevach EM, Thornton AM. Ttregs, Ptregs, and Itregs: Similarities and Differences. Immunol Rev (2014) 259(1):88–102. doi: 10.1111/imr.12160
6. Kanamori M, Nakatsukasa H, Okada M, Lu Q, Yoshimura A. Induced Regulatory T Cells: Their Development, Stability, and Applications. Trends Immunol (2016) 37(11):803–11. doi: 10.1016/j.it.2016.08.012
7. Hori S, Nomura T, Sakaguchi S. Control of Regulatory T Cell Development by the Transcription Factor Foxp3. Sci (New York NY) (2003) 299(5609):1057–61. doi: 10.1126/science.1079490
8. Fontenot JD, Rasmussen JP, Williams LM, Dooley JL, Farr AG, Rudensky AY. Regulatory T Cell Lineage Specification by the Forkhead Transcription Factor Foxp3. Immunity (2005) 22(3):329–41. doi: 10.1016/j.immuni.2005.01.016
9. Godfrey VL, Wilkinson JE, Rinchik EM, Russell LB. Fatal Lymphoreticular Disease in the Scurfy (Sf) Mouse Requires T Cells That Mature in a Sf Thymic Environment: Potential Model for Thymic Education. Proc Natl Acad Sci United States America (1991) 88(13):5528–32. doi: 10.1073/pnas.88.13.5528
10. Godfrey VL, Wilkinson JE, Russell LB. X-Linked Lymphoreticular Disease in the Scurfy (Sf) Mutant Mouse. Am J Pathol (1991) 138(6):1379–87.
11. Ramsdell F, Ziegler SF. FOXP3 and Scurfy: How It All Began. Nat Rev Immunol (2014) 14(5):343–9. doi: 10.1038/nri3650
12. Wildin RS, Freitas A. IPEX and FOXP3: Clinical and Research Perspectives. J Autoimmun (2005) 25 Suppl:56–62. doi: 10.1016/j.jaut.2005.04.008
13. Ochs HD, Gambineri E, Torgerson TR. IPEX, FOXP3 and Regulatory T-Cells: A Model for Autoimmunity. Immunologic Res (2007) 38(1-3):112–21. doi: 10.1007/s12026-007-0022-2
14. van der Vliet HJ, Nieuwenhuis EE. IPEX as a Result of Mutations in FOXP3. Clin Dev Immunol (2007) 2007:89017. doi: 10.1155/2007/89017
15. Bacchetta R, Barzaghi F, Roncarolo MG. From IPEX Syndrome to FOXP3 Mutation: A Lesson on Immune Dysregulation. Ann New York Acad Sci (2018) 1417(1):5–22. doi: 10.1111/nyas.13011
16. Van Gool F, Nguyen MLT, Mumbach MR, Satpathy AT, Rosenthal WL, Giacometti S, et al. A Mutation in the Transcription Factor Foxp3 Drives T Helper 2 Effector Function in Regulatory T Cells. Immunity (2019) 50(2):362–77.e6. doi: 10.1016/j.immuni.2018.12.016
17. Bacchetta R, Passerini L, Gambineri E, Dai M, Allan SE, Perroni L, et al. Defective Regulatory and Effector T Cell Functions in Patients With FOXP3 Mutations. J Clin Invest (2006) 116(6):1713–22. doi: 10.1172/JCI25112
18. Traxinger BR, Richert-Spuhler LE, Lund JM. Mucosal Tissue Regulatory T Cells Are Integral in Balancing Immunity and Tolerance at Portals of Antigen Entry. Mucosal Immunol (2021), 1–10. doi: 10.1038/s41385-021-00471-x
19. Schmitt E, Williams C. Generation and Function of Induced Regulatory T Cells. Front Immunol (2013) 4. doi: 10.3389/fimmu.2013.00152
20. Josefowicz SZ, Niec RE, Kim HY, Treuting P, Chinen T, Zheng Y, et al. Extrathymically Generated Regulatory T Cells Control Mucosal TH2 Inflammation. Nature (2012) 482(7385):395–9. doi: 10.1038/nature10772
21. Zheng Y, Josefowicz S, Chaudhry A, Peng XP, Forbush K, Rudensky AY. Role of Conserved Non-Coding DNA Elements in the Foxp3 Gene in Regulatory T-Cell Fate. Nature (2010) 463(7282):808–12. doi: 10.1038/nature08750
22. Mackay LK, Rahimpour A, Ma JZ, Collins N, Stock AT, Hafon ML, et al. The Developmental Pathway for CD103(+)CD8+ Tissue-Resident Memory T Cells of Skin. Nat Immunol (2013) 14(12):1294–301. doi: 10.1038/ni.2744
23. Schenkel JM, Masopust D. Tissue-Resident Memory T Cells. Immunity (2014) 41(6):886–97. doi: 10.1016/j.immuni.2014.12.007
24. Cosovanu C, Neumann C. The Many Functions of Foxp3(+) Regulatory T Cells in the Intestine. Front Immunol (2020) 11:600973. doi: 10.3389/fimmu.2020.600973
25. Wan YY, Flavell RA. 'Yin-Yang' Functions of Transforming Growth Factor-Beta and T Regulatory Cells in Immune Regulation. Immunol Rev (2007) 220:199–213. doi: 10.1111/j.1600-065X.2007.00565.x
26. Li MO, Wan YY, Flavell RA. T Cell-Produced Transforming Growth Factor-Beta1 Controls T Cell Tolerance and Regulates Th1- and Th17-Cell Differentiation. Immunity (2007) 26(5):579–91. doi: 10.1016/j.immuni.2007.03.014
27. Wang J, Li T, Wang JL, Xu Z, Meng W, Wu QF. Talpid3-Mediated Centrosome Integrity Restrains Neural Progenitor Delamination to Sustain Neurogenesis by Stabilizing Adherens Junctions. Cell Rep (2020) 33(11):108495. doi: 10.1016/j.celrep.2020.108495
28. Turner JA, Stephen-Victor E, Wang S, Rivas MN, Abdel-Gadir A, Harb H, et al. Regulatory T Cell-Derived TGF-β1 Controls Multiple Checkpoints Governing Allergy and Autoimmunity. Immunity (2020) 53(6):1202–14.e6. doi: 10.1016/j.immuni.2020.10.002
29. Collison LW, Workman CJ, Kuo TT, Boyd K, Wang Y, Vignali KM, et al. The Inhibitory Cytokine IL-35 Contributes to Regulatory T-Cell Function. Nature (2007) 450(7169):566–9. doi: 10.1038/nature06306
30. Eggenhuizen PJ, Ng BH, Ooi JD. Treg Enhancing Therapies to Treat Autoimmune Diseases. Int J Mol Sci (2020) 21(19):7015. doi: 10.3390/ijms21197015
31. Huang CT, Workman CJ, Flies D, Pan X, Marson AL, Zhou G, et al. Role of LAG-3 in Regulatory T Cells. Immunity (2004) 21(4):503–13. doi: 10.1016/j.immuni.2004.08.010
32. Buisson S, Triebel F. LAG-3 (CD223) Reduces Macrophage and Dendritic Cell Differentiation From Monocyte Precursors. Immunology (2005) 114(3):369–74. doi: 10.1111/j.1365-2567.2004.02087.x
33. Read S, Greenwald R, Izcue A, Robinson N, Mandelbrot D, Francisco L, et al. Blockade of CTLA-4 on CD4+CD25+ Regulatory T Cells Abrogates Their Function In Vivo. J Immunol (2006) 177(7):4376–83. doi: 10.4049/jimmunol.177.7.4376
34. Schmidt A, Oberle N, Krammer PH. Molecular Mechanisms of Treg-Mediated T Cell Suppression. Front Immunol (2012) 3:51. doi: 10.3389/fimmu.2012.00051
35. Ohta A, Sitkovsky M. Extracellular Adenosine-Mediated Modulation of Regulatory T Cells. Front Immunol (2014) 5:304. doi: 10.3389/fimmu.2014.00304
36. Feuerer M, Herrero L, Cipolletta D, Naaz A, Wong J, Nayer A, et al. Lean, But Not Obese, Fat is Enriched for a Unique Population of Regulatory T Cells That Affect Metabolic Parameters. Nat Med (2009) 15(8):930–9. doi: 10.1038/nm.2002
37. Kolodin D, van Panhuys N, Li C, Magnuson AM, Cipolletta D, Miller CM, et al. Antigen- and Cytokine-Driven Accumulation of Regulatory T Cells in Visceral Adipose Tissue of Lean Mice. Cell Metab (2015) 21(4):543–57. doi: 10.1016/j.cmet.2015.03.005
38. Li C, DiSpirito JR, Zemmour D, Spallanzani RG, Kuswanto W, Benoist C, et al. TCR Transgenic Mice Reveal Stepwise, Multi-Site Acquisition of the Distinctive Fat-Treg Phenotype. Cell (2018) 174(2):285–99.e12. doi: 10.1016/j.cell.2018.05.004
39. Cipolletta D, Feuerer M, Li A, Kamei N, Lee J, Shoelson SE, et al. PPAR-γ is a Major Driver of the Accumulation and Phenotype of Adipose Tissue Treg Cells. Nature (2012) 486(7404):549–53. doi: 10.1038/nature11132
40. DiSpirito JR, Zemmour D, Ramanan D, Cho J, Zilionis R, Klein AM, et al. Molecular Diversification of Regulatory T Cells in Nonlymphoid Tissues. Sci Immunol (2018) 3(27):eaat5861. doi: 10.1126/sciimmunol.aat5861
41. Tontonoz P, Spiegelman BM. Fat and Beyond: The Diverse Biology of PPARgamma. Annu Rev Biochem (2008) 77:289–312. doi: 10.1146/annurev.biochem.77.061307.091829
42. Han JM, Wu D, Denroche HC, Yao Y, Verchere CB, Levings MK. IL-33 Reverses an Obesity-Induced Deficit in Visceral Adipose Tissue ST2+ T Regulatory Cells and Ameliorates Adipose Tissue Inflammation and Insulin Resistance. J Immunol (2015) 194(10):4777–83. doi: 10.4049/jimmunol.1500020
43. Li C, Muñoz-Rojas AR, Wang G, Mann AO, Benoist C, Mathis D. Pparγ Marks Splenic Precursors of Multiple Nonlymphoid-Tissue Treg Compartments. Proc Natl Acad Sci (2021) 118(13):e2025197118. doi: 10.1073/pnas.2025197118
44. Wagner NM, Brandhorst G, Czepluch F, Lankeit M, Eberle C, Herzberg S, et al. Circulating Regulatory T Cells Are Reduced in Obesity and may Identify Subjects at Increased Metabolic and Cardiovascular Risk. Obes (Silver Spring Md) (2013) 21(3):461–8. doi: 10.1002/oby.20087
45. Eller K, Kirsch A, Wolf AM, Sopper S, Tagwerker A, Stanzl U, et al. Potential Role of Regulatory T Cells in Reversing Obesity-Linked Insulin Resistance and Diabetic Nephropathy. Diabetes (2011) 60(11):2954–62. doi: 10.2337/db11-0358
46. Li C, Wang G, Sivasami P, Ramirez RN, Zhang Y, Benoist C, et al. Interferon-α-Producing Plasmacytoid Dendritic Cells Drive the Loss of Adipose Tissue Regulatory T Cells During Obesity. Cell Metab (2021) 33(8):1610–23.e5. doi: 10.1016/j.cmet.2021.06.007
47. Zhao XY, Zhou L, Chen Z, Ji Y, Peng X, Qi L, et al. The Obesity-Induced Adipokine Sst2 Exacerbates Adipose T(reg) and ILC2 Depletion and Promotes Insulin Resistance. Sci advances (2020) 6(20):eaay6191. doi: 10.1126/sciadv.aay6191
48. Becker M, Serr I, Salb VK, Ott VB, Mengel L, Blüher M, et al. Short-Term Cold Exposure Supports Human Treg Induction In Vivo. Mol Metab (2019) 28:73–82. doi: 10.1016/j.molmet.2019.08.002
49. Kälin S, Becker M, Ott VB, Serr I, Hosp F, Mollah MMH, et al. A Stat6/Pten Axis Links Regulatory T Cells With Adipose Tissue Function. Cell Metab (2017) 26(3):475–92.e7. doi: 10.1016/j.cmet.2017.08.008
50. Medrikova D, Sijmonsma TP, Sowodniok K, Richards DM, Delacher M, Sticht C, et al. Brown Adipose Tissue Harbors a Distinct Sub-Population of Regulatory T Cells. PloS One (2015) 10(2):e0118534. doi: 10.1371/journal.pone.0118534
51. Fang W, Deng Z, Benadjaoud F, Yang D, Yang C, Shi GP. Regulatory T Cells Promote Adipocyte Beiging in Subcutaneous Adipose Tissue. FASEB J (2020) 34(7):9755–70. doi: 10.1096/fj.201902518R
52. Vasanthakumar A, Chisanga D, Blume J, Gloury R, Britt K, Henstridge DC, et al. Sex-Specific Adipose Tissue Imprinting of Regulatory T Cells. Nature (2020) 579(7800):581–5. doi: 10.1038/s41586-020-2040-3
53. Beppu LY, Mooli RGR, Qu X, Marrero GJ, Finley CA, Fooks AN, et al. Tregs Facilitate Obesity and Insulin Resistance via a Blimp-1/IL-10 Axis. JCI Insight (2021) 6(3):e140644. doi: 10.1172/jci.insight.140644
54. Cretney E, Xin A, Shi W, Minnich M, Masson F, Miasari M, et al. The Transcription Factors Blimp-1 and IRF4 Jointly Control the Differentiation and Function of Effector Regulatory T Cells. Nat Immunol (2011) 12(4):304–11. doi: 10.1038/ni.2006
55. Bapat SP, Myoung Suh J, Fang S, Liu S, Zhang Y, Cheng A, et al. Depletion of Fat-Resident Treg Cells Prevents Age-Associated Insulin Resistance. Nature (2015) 528(7580):137–41. doi: 10.1038/nature16151
56. Li Y, Lu Y, Lin S-H, Li N, Han Y, Huang Q, et al. Insulin Signaling Establishes a Developmental Trajectory of Adipose Regulatory T Cells. Nat Immunol (2021) 22(9):1175–85. doi: 10.1038/s41590-021-01010-3
57. Laparra A, Tricot S, Le Van M, Damouche A, Gorwood J, Vaslin B, et al. The Frequencies of Immunosuppressive Cells in Adipose Tissue Differ in Human, Non-Human Primate, and Mouse Models. Front Immunol (2019) 10. doi: 10.3389/fimmu.2019.00117
58. Scharschmidt TC, Vasquez KS, Truong HA, Gearty SV, Pauli ML, Nosbaum A, et al. A Wave of Regulatory T Cells Into Neonatal Skin Mediates Tolerance to Commensal Microbes. Immunity (2015) 43(5):1011–21. doi: 10.1016/j.immuni.2015.10.016
59. Gratz IK, Truong HA, Yang SH, Maurano MM, Lee K, Abbas AK, et al. Cutting Edge: Memory Regulatory T Cells Require IL-7 and Not IL-2 for Their Maintenance in Peripheral Tissues. J Immunol (2013) 190(9):4483–7. doi: 10.4049/jimmunol.1300212
60. Sanchez Rodriguez R, Pauli ML, Neuhaus IM, Yu SS, Arron ST, Harris HW, et al. Memory Regulatory T Cells Reside in Human Skin. J Clin Invest (2014) 124(3):1027–36. doi: 10.1172/JCI72932
61. Scharschmidt TC, Vasquez KS, Pauli ML, Leitner EG, Chu K, Truong H-A, et al. Commensal Microbes and Hair Follicle Morphogenesis Coordinately Drive Treg Migration Into Neonatal Skin. Cell Host Microbe (2017) 21(4):467–77.e5. doi: 10.1016/j.chom.2017.03.001
62. Ikebuchi R, Fujimoto M, Nakanishi Y, Okuyama H, Moriya T, Kusumoto Y, et al. Functional Phenotypic Diversity of Regulatory T Cells Remaining in Inflamed Skin. Front Immunol (2019) 10:1098. doi: 10.3389/fimmu.2019.01098
63. Ikebuchi R, Teraguchi S, Vandenbon A, Honda T, Shand FHW, Nakanishi Y, et al. A Rare Subset of Skin-Tropic Regulatory T Cells Expressing Il10/Gzmb Inhibits the Cutaneous Immune Response. Sci Rep (2016) 6(1):35002. doi: 10.1038/srep35002
64. Kalekar LA, Cohen JN, Prevel N, Sandoval PM, Mathur AN, Moreau JM, et al. Regulatory T Cells in Skin Are Uniquely Poised to Suppress Profibrotic Immune Responses. Sci Immunol (2019) 4(39):eaaw2910. doi: 10.1126/sciimmunol.aaw2910
65. Clark RA, Kupper TS. IL-15 and Dermal Fibroblasts Induce Proliferation of Natural Regulatory T Cells Isolated From Human Skin. Blood (2007) 109(1):194–202. doi: 10.1182/blood-2006-02-002873
66. Kawai K, Uchiyama M, Hester J, Issa F. IL-33 Drives the Production of Mouse Regulatory T Cells With Enhanced In Vivo Suppressive Activity in Skin Transplantation. Am J Transplant (2021) 21(3):978–92. doi: 10.1111/ajt.16266
67. Lowe MM, Boothby I, Clancy S, Ahn RS, Liao W, Nguyen DN, et al. Regulatory T Cells Use Arginase 2 to Enhance Their Metabolic Fitness in Tissues. JCI Insight (2019) 4(24):e129756. doi: 10.1172/jci.insight.129756
68. Ali N, Zirak B, Rodriguez RS, Pauli ML, Truong HA, Lai K, et al. Regulatory T Cells in Skin Facilitate Epithelial Stem Cell Differentiation. Cell (2017) 169(6):1119–29.e11. doi: 10.1016/j.cell.2017.05.002
69. Morinaga H, Mohri Y, Grachtchouk M, Asakawa K, Matsumura H, Oshima M, et al. Obesity Accelerates Hair Thinning by Stem Cell-Centric Converging Mechanisms. Nature (2021) 595(7866):266–71. doi: 10.1038/s41586-021-03624-x
70. Nosbaum A, Prevel N, Truong HA, Mehta P, Ettinger M, Scharschmidt TC, et al. Cutting Edge: Regulatory T Cells Facilitate Cutaneous Wound Healing. J Immunol (Baltimore Md 1950) (2016) 196(5):2010–4. doi: 10.4049/jimmunol.1502139
71. Shime H, Odanaka M, Tsuiji M, Matoba T, Imai M, Yasumizu Y, et al. Proenkephalin+ Regulatory T Cells Expanded by Ultraviolet B Exposure Maintain Skin Homeostasis With a Healing Function. Proc Natl Acad Sci (2020) 117(34):20696–705. doi: 10.1073/pnas.2000372117
72. Klarquist J, Denman CJ, Hernandez C, Wainwright DA, Strickland FM, Overbeck A, et al. Reduced Skin Homing by Functional Treg in Vitiligo. Pigment Cell melanoma Res (2010) 23(2):276–86. doi: 10.1111/j.1755-148X.2010.00688.x
73. Benfaremo D, Svegliati S, Paolini C, Agarbati S, Moroncini G. Systemic Sclerosis: From Pathophysiology to Novel Therapeutic Approaches. Biomedicines (2022) 10(1):163. doi: 10.3390/biomedicines10010163
74. Antiga E, Quaglino P, Bellandi S, Volpi W, Del Bianco E, Comessatti A, et al. Regulatory T Cells in the Skin Lesions and Blood of Patients With Systemic Sclerosis and Morphoea. Br J Dermatol (2010) 162(5):1056–63. doi: 10.1111/j.1365-2133.2010.09633.x
75. MacDonald KG, Dawson NAJ, Huang Q, Dunne JV, Levings MK, Broady R. Regulatory T Cells Produce Profibrotic Cytokines in the Skin of Patients With Systemic Sclerosis. J Allergy Clin Immunol (2015) 135(4):946–55.e9. doi: 10.1016/j.jaci.2014.12.1932
76. Liu X, Gao N, Li M, Xu D, Hou Y, Wang Q, et al. Elevated Levels of CD4(+)CD25(+)FoxP3(+) T Cells in Systemic Sclerosis Patients Contribute to the Secretion of IL-17 and Immunosuppression Dysfunction. PloS One (2013) 8(6):e64531. doi: 10.1371/journal.pone.0064531
77. Fenoglio D, Battaglia F, Parodi A, Stringara S, Negrini S, Panico N, et al. Alteration of Th17 and Treg Cell Subpopulations Co-Exist in Patients Affected With Systemic Sclerosis. Clin Immunol (2011) 139(3):249–57. doi: 10.1016/j.clim.2011.01.013
78. Polansky JK, Kretschmer K, Freyer J, Floess S, Garbe A, Baron U, et al. DNA Methylation Controls Foxp3 Gene Expression. Eur J Immunol (2008) 38(6):1654–63. doi: 10.1002/eji.200838105
79. Wang YY, Wang Q, Sun XH, Liu RZ, Shu Y, Kanekura T, et al. DNA Hypermethylation of the Forkhead Box Protein 3 (FOXP3) Promoter in CD4+ T Cells of Patients With Systemic Sclerosis. Br J Dermatol (2014) 171(1):39–47. doi: 10.1111/bjd.12913
80. Hartwig T, Zwicky P, Schreiner B, Yawalkar N, Cheng P, Navarini A, et al. Regulatory T Cells Restrain Pathogenic T Helper Cells During Skin Inflammation. Cell Rep (2018) 25(13):3564–72.e4. doi: 10.1016/j.celrep.2018.12.012
81. Bovenschen HJ, van de Kerkhof PC, van Erp PE, Woestenenk R, Joosten I, Koenen HJ. Foxp3+ Regulatory T Cells of Psoriasis Patients Easily Differentiate Into IL-17A-Producing Cells and Are Found in Lesional Skin. J Invest Dermatol (2011) 131(9):1853–60. doi: 10.1038/jid.2011.139
82. Koenen HJ, Smeets RL, Vink PM, van Rijssen E, Boots AM, Joosten I. Human CD25highFoxp3pos Regulatory T Cells Differentiate Into IL-17-Producing Cells. Blood (2008) 112(6):2340–52. doi: 10.1182/blood-2008-01-133967
83. Hartl D, Koller B, Mehlhorn AT, Reinhardt D, Nicolai T, Schendel DJ, et al. Quantitative and Functional Impairment of Pulmonary CD4+CD25hi Regulatory T Cells in Pediatric Asthma. J Allergy Clin Immunol (2007) 119(5):1258–66. doi: 10.1016/j.jaci.2007.02.023
84. Faustino L, Mucida D, Keller AC, Demengeot J, Bortoluci K, Sardinha LR, et al. Regulatory T Cells Accumulate in the Lung Allergic Inflammation and Efficiently Suppress T-Cell Proliferation But Not Th2 Cytokine Production. Clin Dev Immunol (2012) 2012:721817. doi: 10.1155/2012/721817
85. Nguyen KD, Vanichsarn C, Nadeau KC. TSLP Directly Impairs Pulmonary Treg Function: Association With Aberrant Tolerogenic Immunity in Asthmatic Airway. Allergy Asthma Clin Immunol (2010) 6(1):4. doi: 10.1186/1710-1492-6-4
86. Moore MW, Herzog EL. Regulatory T Cells in Idiopathic Pulmonary Fibrosis: Too Much of a Good Thing? Am J Pathol (2016) 186(8):1978–81. doi: 10.1016/j.ajpath.2016.06.002
87. Kotsianidis I, Nakou E, Bouchliou I, Tzouvelekis A, Spanoudakis E, Steiropoulos P, et al. Global Impairment of CD4+CD25+FOXP3+ Regulatory T Cells in Idiopathic Pulmonary Fibrosis. Am J Respir Crit Care Med (2009) 179(12):1121–30. doi: 10.1164/rccm.200812-1936OC
88. Reilkoff RA, Peng H, Murray LA, Peng X, Russell T, Montgomery R, et al. Semaphorin 7a+ Regulatory T Cells Are Associated With Progressive Idiopathic Pulmonary Fibrosis and Are Implicated in Transforming Growth Factor-β1-Induced Pulmonary Fibrosis. Am J Respir Crit Care Med (2013) 187(2):180–8. doi: 10.1164/rccm.201206-1109OC
89. Birjandi SZ, Palchevskiy V, Xue YY, Nunez S, Kern R, Weigt SS, et al. CD4(+)CD25(hi)Foxp3(+) Cells Exacerbate Bleomycin-Induced Pulmonary Fibrosis. Am J Pathol (2016) 186(8):2008–20. doi: 10.1016/j.ajpath.2016.03.020
90. Liu Q, Dwyer GK, Zhao Y, Li H, Mathews LR, Chakka AB, et al. IL-33–Mediated IL-13 Secretion by ST2+ Tregs Controls Inflammation After Lung Injury. JCI Insight (2019) 4(6):e123919. doi: 10.1172/jci.insight.123919
91. Ichikawa T, Hirahara K, Kokubo K, Kiuchi M, Aoki A, Morimoto Y, et al. CD103(hi) T(reg) Cells Constrain Lung Fibrosis Induced by CD103(lo) Tissue-Resident Pathogenic CD4 T Cells. Nat Immunol (2019) 20(11):1469–80. doi: 10.1038/s41590-019-0494-y
92. Wiesner DL, Smith KD, Kotov DI, Nielsen JN, Bohjanen PR, Nielsen K. Regulatory T Cell Induction and Retention in the Lungs Drives Suppression of Detrimental Type 2 Th Cells During Pulmonary Cryptococcal Infection. J Immunol (2016) 196(1):365–74. doi: 10.4049/jimmunol.1501871
93. Brincks EL, Roberts AD, Cookenham T, Sell S, Kohlmeier JE, Blackman MA, et al. Antigen-Specific Memory Regulatory CD4+Foxp3+ T Cells Control Memory Responses to Influenza Virus Infection. J Immunol (Baltimore Md 1950) (2013) 190(7):3438–46. doi: 10.4049/jimmunol.1203140
94. Lu C, Chen W. Influenza Virus Infection Selectively Triggers the Accumulation and Persistence of More Potent Helios-Expressing Foxp3(+) Regulatory T Cells in the Lungs. Immunol Cell Biol (2021) 99(10):1011–25. doi: 10.1111/imcb.12492
95. Betts RJ, Prabhu N, Ho AW, Lew FC, Hutchinson PE, Rotzschke O, et al. Influenza A Virus Infection Results in a Robust, Antigen-Responsive, and Widely Disseminated Foxp3+ Regulatory T Cell Response. J Virol (2012) 86(5):2817–25. doi: 10.1128/JVI.05685-11
96. León B, Bradley JE, Lund FE, Randall TD, Ballesteros-Tato A. FoxP3+ Regulatory T Cells Promote Influenza-Specific Tfh Responses by Controlling IL-2 Availability. Nat Commun (2014) 5:3495. doi: 10.1038/ncomms4495
97. Arpaia N, Green JA, Moltedo B, Arvey A, Hemmers S, Yuan S, et al. A Distinct Function of Regulatory T Cells in Tissue Protection. Cell (2015) 162(5):1078–89. doi: 10.1016/j.cell.2015.08.021
98. Niedzielska M, Israelsson E, Angermann B, Sidders BS, Clausen M, Catley M, et al. Differential Gene Expression in Human Tissue Resident Regulatory T Cells From Lung, Colon, and Blood. Oncotarget (2018) 9(90):36166–84. doi: 10.18632/oncotarget.26322
99. Chen G, Wu D, Guo W, Cao Y, Huang D, Wang H, et al. Clinical and Immunological Features of Severe and Moderate Coronavirus Disease 2019. J Clin Invest (2020) 130(5):2620–9. doi: 10.1172/JCI137244
100. Wang F, Hou H, Luo Y, Tang G, Wu S, Huang M, et al. The Laboratory Tests and Host Immunity of COVID-19 Patients With Different Severity of Illness. JCI Insight (2020) 5(10):e137799. doi: 10.1172/jci.insight.137799
101. Qin C, Zhou L, Hu Z, Zhang S, Yang S, Tao Y, et al. Dysregulation of Immune Response in Patients With Coronavirus 2019 (COVID-19) in Wuhan, China. Clin Infect Dis (2020) 71(15):762–8. doi: 10.1093/cid/ciaa248
102. Sadeghi A, Tahmasebi S, Mahmood A, Kuznetsova M, Valizadeh H, Taghizadieh A, et al. Th17 and Treg Cells Function in SARS-CoV2 Patients Compared With Healthy Controls. J Cell Physiol (2021) 236(4):2829–39. doi: 10.1002/jcp.30047
103. Szabo PA, Dogra P, Gray JI, Wells SB, Connors TJ, Weisberg SP, et al. Longitudinal Profiling of Respiratory and Systemic Immune Responses Reveals Myeloid Cell-Driven Lung Inflammation in Severe COVID-19. Immunity (2021) 54(4):797–814.e6. doi: 10.1016/j.immuni.2021.03.005
104. Galván-Peña S, Leon J, Chowdhary K, Michelson DA, Vijaykumar B, Yang L, et al. Profound Treg Perturbations Correlate With COVID-19 Severity. Proc Natl Acad Sci (2021) 118(37):e2111315118. doi: 10.1073/pnas.2111315118
105. Grau-Expósito J, Sánchez-Gaona N, Massana N, Suppi M, Astorga-Gamaza A, Perea D, et al. Peripheral and Lung Resident Memory T Cell Responses Against SARS-CoV-2. Nat Commun (2021) 12(1):3010. doi: 10.1038/s41467-021-23333-3
106. Burzyn D, Kuswanto W, Kolodin D, Shadrach JL, Cerletti M, Jang Y, et al. A Special Population of Regulatory T Cells Potentiates Muscle Repair. Cell (2013) 155(6):1282–95. doi: 10.1016/j.cell.2013.10.054
107. Kuswanto W, Burzyn D, Panduro M, Wang KK, Jang YC, Wagers AJ, et al. Poor Repair of Skeletal Muscle in Aging Mice Reflects a Defect in Local, Interleukin-33-Dependent Accumulation of Regulatory T Cells. Immunity (2016) 44(2):355–67. doi: 10.1016/j.immuni.2016.01.009
108. Cho J, Kuswanto W, Benoist C, Mathis D. T Cell Receptor Specificity Drives Accumulation of a Reparative Population of Regulatory T Cells Within Acutely Injured Skeletal Muscle. Proc Natl Acad Sci USA (2019) 116(52):26727–33. doi: 10.1073/pnas.1914848116
109. Brack AS, Rando TA. Tissue-Specific Stem Cells: Lessons From the Skeletal Muscle Satellite Cell. Cell Stem Cell (2012) 10(5):504–14. doi: 10.1016/j.stem.2012.04.001
110. Arnold L, Henry A, Poron F, Baba-Amer Y, van Rooijen N, Plonquet A, et al. Inflammatory Monocytes Recruited After Skeletal Muscle Injury Switch Into Antiinflammatory Macrophages to Support Myogenesis. J Exp Med (2007) 204(5):1057–69. doi: 10.1084/jem.20070075
111. Panduro M, Benoist C, Mathis D. T(reg) Cells Limit IFN-γ Production to Control Macrophage Accrual and Phenotype During Skeletal Muscle Regeneration. Proc Natl Acad Sci USA (2018) 115(11):E2585–e93. doi: 10.1073/pnas.1800618115
112. Villalta SA, Rosenthal W, Martinez L, Kaur A, Sparwasser T, Tidball JG, et al. Regulatory T Cells Suppress Muscle Inflammation and Injury in Muscular Dystrophy. Sci Transl Med (2014) 6(258):258ra142. doi: 10.1126/scitranslmed.3009925
113. Raffaghello L, Principi E, Baratto S, Panicucci C, Pintus S, Antonini F, et al. P2X7 Receptor Antagonist Reduces Fibrosis and Inflammation in a Mouse Model of Alpha-Sarcoglycan Muscular Dystrophy. Pharmaceuticals (Basel) (2022) 15(1):89. doi: 10.3390/ph15010089
114. Gazzerro E, Baldassari S, Assereto S, Fruscione F, Pistorio A, Panicucci C, et al. Enhancement of Muscle T Regulatory Cells and Improvement of Muscular Dystrophic Process in Mdx Mice by Blockade of Extracellular ATP/P2X Axis. Am J Pathol (2015) 185(12):3349–60. doi: 10.1016/j.ajpath.2015.08.010
115. Zacchigna S, Martinelli V, Moimas S, Colliva A, Anzini M, Nordio A, et al. Paracrine Effect of Regulatory T Cells Promotes Cardiomyocyte Proliferation During Pregnancy and After Myocardial Infarction. Nat Commun (2018) 9(1):2432. doi: 10.1038/s41467-018-04908-z
116. Weirather J, Hofmann UD, Beyersdorf N, Ramos GC, Vogel B, Frey A, et al. Foxp3+ CD4+ T Cells Improve Healing After Myocardial Infarction by Modulating Monocyte/Macrophage Differentiation. Circ Res (2014) 115(1):55–67. doi: 10.1161/CIRCRESAHA.115.303895
117. Okumura R, Takeda K. Roles of Intestinal Epithelial Cells in the Maintenance of Gut Homeostasis. Exp Mol Med (2017) 49(5):e338–e. doi: 10.1038/emm.2017.20
118. Gambineri E, Torgerson TR, Ochs HD. Immune Dysregulation, Polyendocrinopathy, Enteropathy, and X-Linked Inheritance (IPEX), a Syndrome of Systemic Autoimmunity Caused by Mutations of FOXP3, a Critical Regulator of T-Cell Homeostasis. Curr Opin Rheumatol (2003) 15(4):430–5. doi: 10.1097/00002281-200307000-00010
119. Cho I, Lui PP, Ali N. Treg Regulation of the Epithelial Stem Cell Lineage. J Immunol Regener Med (2020) 8:100028. doi: 10.1016/j.regen.2020.100028
120. Wang Y, Su MA, Wan YY. An Essential Role of the Transcription Factor GATA-3 for the Function of Regulatory T Cells. Immunity (2011) 35(3):337–48. doi: 10.1016/j.immuni.2011.08.012
121. Wohlfert EA, Grainger JR, Bouladoux N, Konkel JE, Oldenhove G, Ribeiro CH, et al. GATA3 Controls Foxp3⁺ Regulatory T Cell Fate During Inflammation in Mice. J Clin Invest (2011) 121(11):4503–15. doi: 10.1172/JCI57456
122. Schiering C, Krausgruber T, Chomka A, Fröhlich A, Adelmann K, Wohlfert EA, et al. The Alarmin IL-33 Promotes Regulatory T-Cell Function in the Intestine. Nature (2014) 513(7519):564–8. doi: 10.1038/nature13577
123. Sefik E, Geva-Zatorsky N, Oh S, Konnikova L, Zemmour D, McGuire AM, et al. Individual Intestinal Symbionts Induce a Distinct Population of RORg⁺ Regulatory T Cells. Science (2015) 349(6251):993–7. doi: 10.1126/science.aaa9420
124. Yang BH, Hagemann S, Mamareli P, Lauer U, Hoffmann U, Beckstette M, et al. Foxp3(+) T Cells Expressing Rorγt Represent a Stable Regulatory T-Cell Effector Lineage With Enhanced Suppressive Capacity During Intestinal Inflammation. Mucosal Immunol (2016) 9(2):444–57. doi: 10.1038/mi.2015.74
125. Bhaumik S, Mickael ME, Moran M, Spell M, Basu R. Rorγt Promotes Foxp3 Expression by Antagonizing the Effector Program in Colonic Regulatory T Cells. J Immunol (2021) 207(8):2027–38. doi: 10.4049/jimmunol.2100175
126. Atarashi K, Tanoue T, Shima T, Imaoka A, Kuwahara T, Momose Y, et al. Induction of Colonic Regulatory T Cells by Indigenous Clostridium Species. Sci (New York NY) (2011) 331(6015):337–41. doi: 10.1126/science.1198469
127. Weiss JM, Bilate AM, Gobert M, Ding Y, Curotto de Lafaille MA, Parkhurst CN, et al. Neuropilin 1 Is Expressed on Thymus-Derived Natural Regulatory T Cells, But Not Mucosa-Generated Induced Foxp3+ T Reg Cells. J Exp Med (2012) 209(10):1723–42, s1. doi: 10.1084/jem.20120914
128. Pratama A, Schnell A, Mathis D, Benoist C. Developmental and Cellular Age Direct Conversion of CD4+ T Cells Into Rorγ+ or Helios+ Colon Treg Cells. J Exp Med (2020) 217(1):e20190428. doi: 10.1084/jem.20190428
129. Cebula A, Seweryn M, Rempala GA, Pabla SS, McIndoe RA, Denning TL, et al. Thymus-Derived Regulatory T Cells Contribute to Tolerance to Commensal Microbiota. Nature (2013) 497(7448):258–62. doi: 10.1038/nature12079
130. Lord JD, Shows DM, Chen J, Thirlby RC. Human Blood and Mucosal Regulatory T Cells Express Activation Markers and Inhibitory Receptors in Inflammatory Bowel Disease. PloS One (2015) 10(8):e0136485. doi: 10.1371/journal.pone.0136485
131. Ueno A, Jijon H, Chan R, Ford K, Hirota C, Kaplan GG, et al. Increased Prevalence of Circulating Novel IL-17 Secreting Foxp3 Expressing CD4+ T Cells and Defective Suppressive Function of Circulating Foxp3+ Regulatory Cells Support Plasticity Between Th17 and Regulatory T Cells in Inflammatory Bowel Disease Patients. Inflammation Bowel Dis (2013) 19(12):2522–34. doi: 10.1097/MIB.0b013e3182a85709
132. Cao AT, Yao S, Stefka AT, Liu Z, Qin H, Liu H, et al. TLR4 Regulates IFN-γ and IL-17 Production by Both Thymic and Induced Foxp3+ Tregs During Intestinal Inflammation. J Leukoc Biol (2014) 96(5):895–905. doi: 10.1189/jlb.3A0114-056RR
133. Eastaff-Leung N, Mabarrack N, Barbour A, Cummins A, Barry S. Foxp3+ Regulatory T Cells, Th17 Effector Cells, and Cytokine Environment in Inflammatory Bowel Disease. J Clin Immunol (2010) 30(1):80–9. doi: 10.1007/s10875-009-9345-1
134. Zhang X, Kelaria S, Kerstetter J, Wang J. The Functional and Prognostic Implications of Regulatory T Cells in Colorectal Carcinoma. J gastrointestinal Oncol (2015) 6(3):307–13. doi: 10.3978/j.issn.2078-6891.2015.017
135. Dennis KL, Wang Y, Blatner NR, Wang S, Saadalla A, Trudeau E, et al. Adenomatous Polyps Are Driven by Microbe-Instigated Focal Inflammation and Are Controlled by IL-10-Producing T Cells. Cancer Res (2013) 73(19):5905–13. doi: 10.1158/0008-5472.CAN-13-1511
136. Chung AY, Li Q, Blair SJ, De Jesus M, Dennis KL, LeVea C, et al. Oral Interleukin-10 Alleviates Polyposis via Neutralization of Pathogenic T-Regulatory Cells. Cancer Res (2014) 74(19):5377–85. doi: 10.1158/0008-5472.CAN-14-0918
137. Lu Y, Li Y, Liu Q, Tian N, Du P, Zhu F, et al. MondoA-Thioredoxin-Interacting Protein Axis Maintains Regulatory T-Cell Identity and Function in Colorectal Cancer Microenvironment. Gastroenterology (2021) 161(2):575–91.e16. doi: 10.1053/j.gastro.2021.04.041
138. Osman A, Yan B, Li Y, Pavelko KD, Quandt J, Saadalla A, et al. TCF-1 Controls T(reg) Cell Functions That Regulate Inflammation, CD8(+) T Cell Cytotoxicity and Severity of Colon Cancer. Nat Immunol (2021) 22(9):1152–62. doi: 10.1038/s41590-021-00987-1
139. Bhattacharjee A, Burr AHP, Overacre-Delgoffe AE, Tometich JT, Yang D, Huckestein BR, et al. Environmental Enteric Dysfunction Induces Regulatory T Cells That Inhibit Local CD4+ T Cell Responses and Impair Oral Vaccine Efficacy. Immunity (2021) 54(8):1745–57.e7. doi: 10.1016/j.immuni.2021.07.005
140. De Simone M, Arrigoni A, Rossetti G, Gruarin P, Ranzani V, Politano C, et al. Transcriptional Landscape of Human Tissue Lymphocytes Unveils Uniqueness of Tumor-Infiltrating T Regulatory Cells. Immunity (2016) 45(5):1135–47. doi: 10.1016/j.immuni.2016.10.021
141. Plitas G, Konopacki C, Wu K, Bos PD, Morrow M, Putintseva EV, et al. Regulatory T Cells Exhibit Distinct Features in Human Breast Cancer. Immunity (2016) 45(5):1122–34. doi: 10.1016/j.immuni.2016.10.032
142. Watanabe M, Kanao K, Suzuki S, Muramatsu H, Morinaga S, Kajikawa K, et al. Increased Infiltration of CCR4-Positive Regulatory T Cells in Prostate Cancer Tissue is Associated With a Poor Prognosis. Prostate (2019) 79(14):1658–65. doi: 10.1002/pros.23890
143. Facciabene A, Peng X, Hagemann IS, Balint K, Barchetti A, Wang LP, et al. Tumour Hypoxia Promotes Tolerance and Angiogenesis via CCL28 and T(reg) Cells. Nature (2011) 475(7355):226–30. doi: 10.1038/nature10169
144. Deng G. Tumor-Infiltrating Regulatory T Cells: Origins and Features. Am J Clin Exp Immunol (2018) 7(5):81–7.
145. Chang DK, Peterson E, Sun J, Goudie C, Drapkin RI, Liu JF, et al. Anti-CCR4 Monoclonal Antibody Enhances Antitumor Immunity by Modulating Tumor-Infiltrating Tregs in an Ovarian Cancer Xenograft Humanized Mouse Model. Oncoimmunology (2016) 5(3):e1090075. doi: 10.1080/2162402X.2015.1090075
146. Sugiyama D, Nishikawa H, Maeda Y, Nishioka M, Tanemura A, Katayama I, et al. Anti-CCR4 mAb Selectively Depletes Effector-Type FoxP3+CD4+ Regulatory T Cells, Evoking Antitumor Immune Responses in Humans. Proc Natl Acad Sci USA (2013) 110(44):17945–50. doi: 10.1073/pnas.1316796110
147. Ahmadzadeh M, Pasetto A, Jia L, Deniger DC, Stevanović S, Robbins PF, et al. Tumor-Infiltrating Human CD4(+) Regulatory T Cells Display a Distinct TCR Repertoire and Exhibit Tumor and Neoantigen Reactivity. Sci Immunol (2019) 4(31):eaao4310. doi: 10.1126/sciimmunol.aao4310
148. Curti A, Pandolfi S, Valzasina B, Aluigi M, Isidori A, Ferri E, et al. Modulation of Tryptophan Catabolism by Human Leukemic Cells Results in the Conversion of CD25- Into CD25+ T Regulatory Cells. Blood (2007) 109(7):2871–7. doi: 10.1182/blood-2006-07-036863
149. Zhang Q, Chikina M, Szymczak-Workman AL, Horne W, Kolls JK, Vignali KM, et al. LAG3 Limits Regulatory T Cell Proliferation and Function in Autoimmune Diabetes. Sci Immunol (2017) 2(9):eaah4569. doi: 10.1126/sciimmunol.aah4569
150. Jiang X, Morita M, Sugioka A, Harada M, Kojo S, Wakao H, et al. The Importance of CD25+ CD4+ Regulatory T Cells in Mouse Hepatic Allograft Tolerance. Liver Transpl (2006) 12(7):1112–8. doi: 10.1002/lt.20787
151. Li W, Kuhr CS, Zheng XX, Carper K, Thomson AW, Reyes JD, et al. New Insights Into Mechanisms of Spontaneous Liver Transplant Tolerance: The Role of Foxp3-Expressing CD25+CD4+ Regulatory T Cells. Am J Transplant (2008) 8(8):1639–51. doi: 10.1111/j.1600-6143.2008.02300.x
152. Lee I, Wang L, Wells AD, Dorf ME, Ozkaynak E, Hancock WW. Recruitment of Foxp3+ T Regulatory Cells Mediating Allograft Tolerance Depends on the CCR4 Chemokine Receptor. J Exp Med (2005) 201(7):1037–44. doi: 10.1084/jem.20041709
153. Li Y, Zhao X, Cheng D, Haga H, Tsuruyama T, Wood K, et al. The Presence of Foxp3 Expressing T Cells Within Grafts of Tolerant Human Liver Transplant Recipients. Transplantation (2008) 86(12):1837–43. doi: 10.1097/TP.0b013e31818febc4
154. Taubert R, Danger R, Londoño MC, Christakoudi S, Martinez-Picola M, Rimola A, et al. Hepatic Infiltrates in Operational Tolerant Patients After Liver Transplantation Show Enrichment of Regulatory T Cells Before Proinflammatory Genes Are Downregulated. Am J Transplant (2016) 16(4):1285–93. doi: 10.1111/ajt.13617
155. Wang C, Cordoba S, Hu M, Bertolino P, Bowen DG, Sharland AF, et al. Spontaneous Acceptance of Mouse Kidney Allografts is Associated With Increased Foxp3 Expression and Differences in the B and T Cell Compartments. Transpl Immunol (2011) 24(3):149–56. doi: 10.1016/j.trim.2010.12.004
156. Prosser AC, Kallies A, Lucas M. Tissue-Resident Lymphocytes in Solid Organ Transplantation: Innocent Passengers or the Key to Organ Transplant Survival? Transplantation (2018) 102(3):378–86. doi: 10.1097/TP.0000000000002001
157. Lui PP, Cho I, Ali N. Tissue Regulatory T Cells. Immunology (2020) 161(1):4–17. doi: 10.1111/imm.13208
158. Pastille E, Wasmer MH, Adamczyk A, Vu VP, Mager LF, Phuong NNT, et al. The IL-33/ST2 Pathway Shapes the Regulatory T Cell Phenotype to Promote Intestinal Cancer. Mucosal Immunol (2019) 12(4):990–1003. doi: 10.1038/s41385-019-0176-y
159. Vasanthakumar A, Moro K, Xin A, Liao Y, Gloury R, Kawamoto S, et al. The Transcriptional Regulators IRF4, BATF and IL-33 Orchestrate Development and Maintenance of Adipose Tissue-Resident Regulatory T Cells. Nat Immunol (2015) 16(3):276–85. doi: 10.1038/ni.3085
160. Dudda JC, Perdue N, Bachtanian E, Campbell DJ. Foxp3+ Regulatory T Cells Maintain Immune Homeostasis in the Skin. J Exp Med (2008) 205(7):1559–65. doi: 10.1084/jem.20072594
161. Abadier M, Pramod AB, McArdle S, Marki A, Fan Z, Gutierrez E, et al. Effector and Regulatory T Cells Roll at High Shear Stress by Inducible Tether and Sling Formation. Cell Rep (2017) 21(13):3885–99. doi: 10.1016/j.celrep.2017.11.099
162. Ito M, Komai K, Mise-Omata S, Iizuka-Koga M, Noguchi Y, Kondo T, et al. Brain Regulatory T Cells Suppress Astrogliosis and Potentiate Neurological Recovery. Nature (2019) 565(7738):246–50. doi: 10.1038/s41586-018-0824-5
163. Dombrowski Y, O'Hagan T, Dittmer M, Penalva R, Mayoral SR, Bankhead P, et al. Regulatory T Cells Promote Myelin Regeneration in the Central Nervous System. Nat Neurosci (2017) 20(5):674–80. doi: 10.1038/nn.4528
164. Shi H, Chi H. Metabolic Control of Treg Cell Stability, Plasticity, and Tissue-Specific Heterogeneity. Front Immunol (2019) 10:2716. doi: 10.3389/fimmu.2019.02716
165. Kempkes RWM, Joosten I, Koenen H, He X. Metabolic Pathways Involved in Regulatory T Cell Functionality. Front Immunol (2019) 10:2839. doi: 10.3389/fimmu.2019.02839
166. Howie D, Cobbold SP, Adams E, Ten Bokum A, Necula AS, Zhang W, et al. Foxp3 Drives Oxidative Phosphorylation and Protection From Lipotoxicity. JCI Insight (2017) 2(3):e89160. doi: 10.1172/jci.insight.89160
167. Pompura SL, Wagner A, Kitz A, LaPerche J, Yosef N, Dominguez-Villar M, et al. Oleic Acid Restores Suppressive Defects in Tissue-Resident FOXP3 Tregs From Patients With Multiple Sclerosis. J Clin Invest (2021) 131(2):e138519. doi: 10.1172/JCI138519
168. Watson MJ, Vignali PDA, Mullett SJ, Overacre-Delgoffe AE, Peralta RM, Grebinoski S, et al. Metabolic Support of Tumour-Infiltrating Regulatory T Cells by Lactic Acid. Nature (2021) 591(7851):645–51. doi: 10.1038/s41586-020-03045-2
169. Foss F. Clinical Experience With Denileukin Diftitox (ONTAK). Semin Oncol (2006) 33(1 Suppl 3):S11–6. doi: 10.1053/j.seminoncol.2005.12.017
170. Cohan SL, Lucassen EB, Romba MC, Linch SN. Daclizumab: Mechanisms of Action, Therapeutic Efficacy, Adverse Events and Its Uncovering the Potential Role of Innate Immune System Recruitment as a Treatment Strategy for Relapsing Multiple Sclerosis. Biomedicines (2019) 7(1):18. doi: 10.3390/biomedicines7010018
171. Kreitman RJ, Stetler-Stevenson M, Jaffe ES, Conlon KC, Steinberg SM, Wilson W, et al. Complete Remissions of Adult T-Cell Leukemia With Anti-CD25 Recombinant Immunotoxin LMB-2 and Chemotherapy to Block Immunogenicity. Clin Cancer Res (2016) 22(2):310–8. doi: 10.1158/1078-0432.CCR-15-1412
172. Sharma M, Khong H, Fa'ak F, Bentebibel SE, Janssen LME, Chesson BC, et al. Bempegaldesleukin Selectively Depletes Intratumoral Tregs and Potentiates T Cell-Mediated Cancer Therapy. Nat Commun (2020) 11(1):661. doi: 10.1038/s41467-020-14471-1
173. Till BG, Press OW. Depletion of Tregs for Adoptive T-Cell Therapy Using CD44 and CD137 as Selection Markers. Immunotherapy (2012) 4(5):483–5. doi: 10.2217/imt.12.33
174. Bulliard Y, Jolicoeur R, Zhang J, Dranoff G, Wilson NS, Brogdon JL. OX40 Engagement Depletes Intratumoral Tregs via Activating Fcγrs, Leading to Antitumor Efficacy. Immunol Cell Biol (2014) 92(6):475–80. doi: 10.1038/icb.2014.26
175. Duhen R, Ballesteros-Merino C, Frye AK, Tran E, Rajamanickam V, Chang SC, et al. Neoadjuvant Anti-OX40 (MEDI6469) Therapy in Patients With Head and Neck Squamous Cell Carcinoma Activates and Expands Antigen-Specific Tumor-Infiltrating T Cells. Nat Commun (2021) 12(1):1047. doi: 10.1038/s41467-021-21383-1
176. Gutierrez M, Moreno V, Heinhuis KM, Olszanski AJ, Spreafico A, Ong M, et al. OX40 Agonist BMS-986178 Alone or in Combination With Nivolumab and/or Ipilimumab in Patients With Advanced Solid Tumors. Clin Cancer Res (2021) 27(2):460–72. doi: 10.1158/1078-0432.CCR-20-1830
177. Balmanoukian AS, Infante JR, Aljumaily R, Naing A, Chintakuntlawar AV, Rizvi NA, et al. Safety and Clinical Activity of MEDI1873, a Novel GITR Agonist, in Advanced Solid Tumors. Clin Cancer Res (2020) 26(23):6196–203. doi: 10.1158/1078-0432.CCR-20-0452
178. Heinhuis KM, Carlino M, Joerger M, Di Nicola M, Meniawy T, Rottey S, et al. Safety, Tolerability, and Potential Clinical Activity of a Glucocorticoid-Induced TNF Receptor-Related Protein Agonist Alone or in Combination With Nivolumab for Patients With Advanced Solid Tumors: A Phase 1/2a Dose-Escalation and Cohort-Expansion Clinical Trial. JAMA Oncol (2020) 6(1):100–7. doi: 10.1001/jamaoncol.2019.3848
179. Kurose K, Ohue Y, Wada H, Iida S, Ishida T, Kojima T, et al. Phase Ia Study of FoxP3+ CD4 Treg Depletion by Infusion of a Humanized Anti-CCR4 Antibody, KW-0761, in Cancer Patients. Clin Cancer Res (2015) 21(19):4327–36. doi: 10.1158/1078-0432.CCR-15-0357
180. Wang Z, Wei M, Zhang H, Chen H, Germana S, Huang CA, et al. Diphtheria-Toxin Based Anti-Human CCR4 Immunotoxin for Targeting Human CCR4(+) Cells In Vivo. Mol Oncol (2015) 9(7):1458–70. doi: 10.1016/j.molonc.2015.04.004
181. Van Damme H, Dombrecht B, Kiss M, Roose H, Allen E, Van Overmeire E, et al. Therapeutic Depletion of CCR8(+) Tumor-Infiltrating Regulatory T Cells Elicits Antitumor Immunity and Synergizes With Anti-PD-1 Therapy. J Immunother Cancer (2021) 9(2):e001749. doi: 10.1136/jitc-2020-001749
182. Campbell JR, McDonald BR, Mesko PB, Siemers NO, Singh PB, Selby M, et al. Fc-Optimized Anti-CCR8 Antibody Depletes Regulatory T Cells in Human Tumor Models. Cancer Res (2021) 81(11):2983–94. doi: 10.1158/0008-5472.CAN-20-3585
183. Rosenzwajg M, Salet R, Lorenzon R, Tchitchek N, Roux A, Bernard C, et al. Low-Dose IL-2 in Children With Recently Diagnosed Type 1 Diabetes: A Phase I/II Randomised, Double-Blind, Placebo-Controlled, Dose-Finding Study. Diabetologia (2020) 63(9):1808–21. doi: 10.1007/s00125-020-05200-w
184. He J, Zhang R, Shao M, Zhao X, Miao M, Chen J, et al. Efficacy and Safety of Low-Dose IL-2 in the Treatment of Systemic Lupus Erythematosus: A Randomised, Double-Blind, Placebo-Controlled Trial. Ann Rheum Dis (2020) 79(1):141–9. doi: 10.1136/annrheumdis-2019-215396
185. Rosenzwajg M, Lorenzon R, Cacoub P, Pham HP, Pitoiset F, El Soufi K, et al. Immunological and Clinical Effects of Low-Dose Interleukin-2 Across 11 Autoimmune Diseases in a Single, Open Clinical Trial. Ann Rheum Dis (2019) 78(2):209–17. doi: 10.1136/annrheumdis-2018-214229
186. Long SA, Rieck M, Sanda S, Bollyky JB, Samuels PL, Goland R, et al. Rapamycin/IL-2 Combination Therapy in Patients With Type 1 Diabetes Augments Tregs Yet Transiently Impairs β-Cell Function. Diabetes (2012) 61(9):2340–8. doi: 10.2337/db12-0049
187. Revilla-López E, Berastegui C, Méndez A, Sáez-Giménez B, Ruiz de Miguel V, López-Meseguer M, et al. Long-Term Results of Sirolimus Treatment in Lymphangioleiomyomatosis: A Single Referral Centre Experience. Sci Rep (2021) 11(1):10171. doi: 10.1038/s41598-021-89562-0
188. Bluestone JA, Buckner JH, Fitch M, Gitelman SE, Gupta S, Hellerstein MK, et al. Type 1 Diabetes Immunotherapy Using Polyclonal Regulatory T Cells. Sci Transl Med (2015) 7(315):315ra189. doi: 10.1126/scitranslmed.aad4134
189. Dong S, Hiam-Galvez KJ, Mowery CT, Herold KC, Gitelman SE, Esensten JH, et al. The Effect of Low-Dose IL-2 and Treg Adoptive Cell Therapy in Patients With Type 1 Diabetes. JCI Insight (2021) 6(18):e147474. doi: 10.1172/jci.insight.147474
190. Sicard A, Lamarche C, Speck M, Wong M, Rosado-Sánchez I, Blois M, et al. Donor-Specific Chimeric Antigen Receptor Tregs Limit Rejection in Naive But Not Sensitized Allograft Recipients. Am J Transplant (2020) 20(6):1562–73. doi: 10.1111/ajt.15787
191. Rosado-Sánchez I, Levings MK. Building a CAR-Treg: Going From the Basic to the Luxury Model. Cell Immunol (2020) 358:104220. doi: 10.1016/j.cellimm.2020.104220
192. Togashi Y, Shitara K, Nishikawa H. Regulatory T Cells in Cancer Immunosuppression - Implications for Anticancer Therapy. Nat Rev Clin Oncol (2019) 16(6):356–71. doi: 10.1038/s41571-019-0175-7
193. Kitamura N, Murata S, Ueki T, Mekata E, Reilly RT, Jaffee EM, et al. OX40 Costimulation can Abrogate Foxp3+ Regulatory T Cell-Mediated Suppression of Antitumor Immunity. Int J Cancer (2009) 125(3):630–8. doi: 10.1002/ijc.24435
194. Coe D, Begom S, Addey C, White M, Dyson J, Chai JG. Depletion of Regulatory T Cells by Anti-GITR mAb as a Novel Mechanism for Cancer Immunotherapy. Cancer immunology immunotherapy CII (2010) 59(9):1367–77. doi: 10.1007/s00262-010-0866-5
195. Gyori D, Lim EL, Grant FM, Spensberger D, Roychoudhuri R, Shuttleworth SJ, et al. Compensation Between CSF1R+ Macrophages and Foxp3+ Treg Cells Drives Resistance to Tumor Immunotherapy. JCI Insight (2018) 3(11):e120631. doi: 10.1172/jci.insight.120631
196. Curtin JF, Candolfi M, Fakhouri TM, Liu C, Alden A, Edwards M, et al. Treg Depletion Inhibits Efficacy of Cancer Immunotherapy: Implications for Clinical Trials. PloS One (2008) 3(4):e1983. doi: 10.1371/journal.pone.0001983
197. Rosenzwajg M, Churlaud G, Mallone R, Six A, Dérian N, Chaara W, et al. Low-Dose Interleukin-2 Fosters a Dose-Dependent Regulatory T Cell Tuned Milieu in T1D Patients. J Autoimmun (2015) 58:48–58. doi: 10.1016/j.jaut.2015.01.001
198. Suffia I, Reckling SK, Salay G, Belkaid Y. A Role for CD103 in the Retention of CD4+CD25+ Treg and Control of Leishmania Major Infection. J Immunol (2005) 174(9):5444–55. doi: 10.4049/jimmunol.174.9.5444
Keywords: regulatory T cell, tissue residency, inflammation, homeostasis, Foxp3
Citation: Lee J, Kim D and Min B (2022) Tissue Resident Foxp3+ Regulatory T Cells: Sentinels and Saboteurs in Health and Disease. Front. Immunol. 13:865593. doi: 10.3389/fimmu.2022.865593
Received: 30 January 2022; Accepted: 22 February 2022;
Published: 11 March 2022.
Edited by:
Dipayan Rudra, ShanghaiTech University, ChinaReviewed by:
Louise D’Cruz, University of Pittsburgh, United StatesYangyang Li, ShanghaiTech University, China
Copyright © 2022 Lee, Kim and Min. This is an open-access article distributed under the terms of the Creative Commons Attribution License (CC BY). The use, distribution or reproduction in other forums is permitted, provided the original author(s) and the copyright owner(s) are credited and that the original publication in this journal is cited, in accordance with accepted academic practice. No use, distribution or reproduction is permitted which does not comply with these terms.
*Correspondence: Booki Min, booki.min@northwestern.edu