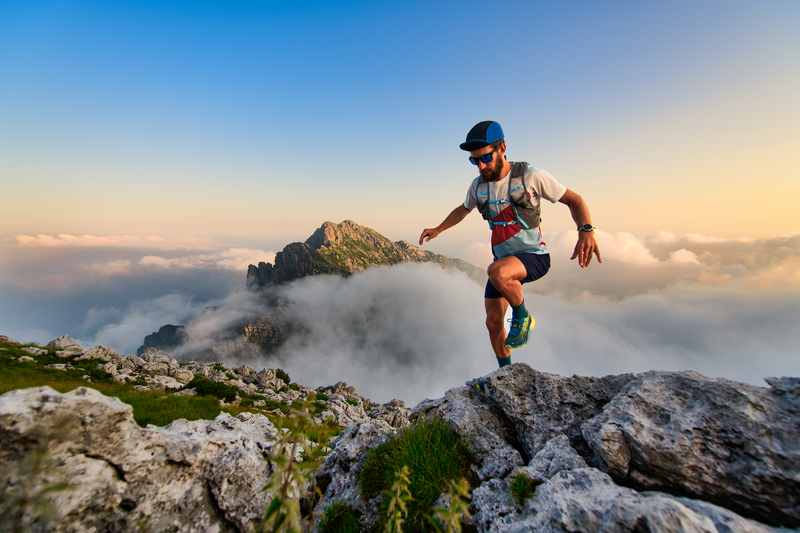
95% of researchers rate our articles as excellent or good
Learn more about the work of our research integrity team to safeguard the quality of each article we publish.
Find out more
REVIEW article
Front. Immunol. , 29 April 2022
Sec. T Cell Biology
Volume 13 - 2022 | https://doi.org/10.3389/fimmu.2022.864628
This article is part of the Research Topic Non-Lymphoid Functions of Regulatory T cells in Health and Disease View all 19 articles
Regulatory T cells (Tregs) restrain excessive immune responses and dampen inflammation. In addition to this classical immune suppressive role, Tregs in non-lymphoid tissues also promote tissue homeostasis, regeneration and repair. In this review, we outline our current understanding of how Tregs migrate to peripheral tissues and the factors required for their maintenance at these sites. We discuss the tissue-specific adaptations of Tregs at barrier and immuno-privileged sites and the mechanisms that regulate their function within these organs. Furthermore, we outline what is known about the interactions of Tregs with non-immune cells in the different peripheral tissues at steady state and upon challenge or tissue damage. A thorough understanding of the tissue-specific adaptations and functions of Tregs will potentially pave the way for therapeutic approaches targeting their regenerative role.
Regulatory T cells (Tregs) are essential for suppressing autoreactive immune responses and controlling the effector immune response to prevent autoimmunity and maintain tissue homeostasis. Tregs are defined by their expression of the transcription factor Foxp3, which confers them with their suppressive function (1). Furthermore, they are marked by constitutive expression of the co-inhibitory receptor CTLA-4 and the high affinity IL-2 receptor α-chain CD25 and strictly depend on extrinsic IL-2 for their survival (2–4). Tregs use a wide array of mechanisms to exert their suppressive activity, including the secretion of inhibitory cytokines, such as IL-10 and TGF-β (5, 6), the modulation of antigen presenting cells (APCs) (7), direct cytolysis (8) as well as metabolic disruption of the target effector cells by cytokine sequestration (8–10). In addition, non-canonical functions, including a direct role in tissue protection and repair, have recently been attributed to Tregs (11, 12). Tregs thus cover a broad functional spectrum and represent a heterogenous population composed of specialized subsets that fulfill these diverse functions.
To exert their tissue protective functions, Tregs home to and localize within the peripheral tissue niche, where they are embedded in a tissue-specific network of immune and non-immune cells. This cellular network constantly interacts with and shapes the Treg population in the respective tissue, while conversely the Tregs shape the tissue niche. In this review, we will outline how Tregs seed the peripheral tissues and highlight the importance of this process for exerting their effector functions. We will further discuss the tissue-specific adaptations of Tregs as well as their functions at barrier and immuno-privileged sites at steady state and upon challenge or tissue damage.
Already 20 years ago it became clear that Tregs show a high degree of heterogeneity regarding their localization and function, when Lehmann and colleagues subdivided the Treg population based on its expression of CD103, the integrin aE (13). Integrin aEb7 binds to E-cadherin, expressed on epithelial cells but not on the endothelium, and was therefore used as a marker for intraepithelial T cells residing in non-lymphoid tissues. Lehmann et al. found that CD103+ Tregs express higher levels of the co-inhibitory receptor CTLA-4, which confers them with a higher suppressive capacity. Subsequent studies additionally used CD25 to further segregate Tregs into phenotypically and functionally distinct subsets. CD103-CD25+ Tregs are enriched for CCR7 and CD62L (L-selectin) and therefore show a naïve phenotype, whereas CD103+ Tregs express CD44, ICOS, Granzyme B (GrzB), Integrin b1 and several chemokine receptors indicating a more active, effector-like phenotype (14) (Figure 1). Other groups used the expression of CD44 and CD62L to divide the Treg population into central Tregs (CD44loCD62Lhi) and effector-like Tregs (CD44hi CD62Llo) (15). These Treg subsets not only differ in their activation status but also in their migratory capacity. Although both the CD103+ CD25+ double-positive and the CD25+ single-positive Tregs migrate towards the CCR7 ligand CCL21, CD103+ Tregs show a higher infiltration to sites of active immune responses by migrating towards CCL17 (CCR4 ligand), CCL20 (CCR6 ligand) and CXCL9 (CXCR3 ligand), which are released upon inflammation (14). In fact, only the CD103+ Tregs can effectively and specifically infiltrate the inflamed skin after hapten 2,4-dinitrofluorobenzene (DNFB) treatment in mice (14), a murine model of contact hypersensitivity. Similarly, only CD103+ Tregs constrain the development of intestinal inflammation after transfer of CD4+ T cells into SCID mice (13). This observation suggested that primarily the CD103+ Tregs react to inflammatory stimuli present at peripheral tissues, while CD25+ Tregs respond to chemokines present in lymphoid organs, allowing for a distinction between naïve CD103-CD25+ and effector/memory-like CD103+CD25- Tregs.
Figure 1 Main markers of naïve, effector and memory Tregs. Surface receptors expressed on Tregs are crucial to determine their migration and residency in peripheral tissues. As depicted in the figure, markers of effector and memory Tregs are to a large degree shared but naïve Tregs show a clearly distinct marker profile. Illustration created with BioRender.com.
Having defined the concept of naïve and effector-like Tregs, Siegmund and colleagues later reported that effector T cell activation and differentiation in the lymph nodes is regulated by CD103- CD25+ Tregs (16). This population is more homogeneous in its expression of chemokine and homing receptors and is found recirculating between blood and lymph nodes, whereas the effector/memory Treg population shows a more heterogeneous pattern reflecting its ability to migrate to different tissues (17). Nevertheless, these are not two different Treg populations originating from a common progenitor, but rather they represent distinct differentiation stages. The T cell maturation process naturally occurring in the thymus gives rise to a homogeneous CD62L+CCR7+CXCR4lo Treg population targeted preferentially to secondary lymphoid organs (SLOs) (18) (Figure 1). Upon antigen-specific priming within the lymphoid organs the expression pattern of homing and chemokine receptors is then altered (18). During the priming phase, on day 3 after immunization, approximately 60% of the Tregs localized in the lymph node lose the expression of CCR7 and CXCR4. In return, they gain CXCR3, CCR4, CCR6 and/or CCR8 expression, allowing them to migrate to peripheral tissues (18). Interestingly, this shift in the chemokine receptors expression pattern in Tregs is more pronounced than the corresponding changes in conventional Foxp3- T cells and also precedes them. This differential expression pattern may represent a mechanism to ensure timely and efficient recruitment of Tregs to the peripheral sites to help maintain tissue integrity. Indeed, Tregs already seed tissues during development and the early post-natal periods. Importantly, their depletion during this time period can cause alterations in tissue structure (19), suggesting that they play an active role in shaping peripheral tissues.
Besides the acquisition of homing receptors in lymph nodes, recent studies showed that also the tissue phenotype of Tregs is primed in SLOs. This phenomenon was studied in the context of visceral adipose tissue (VAT) Tregs, which express the VAT Treg-specific transcription factor PPARγ (20). As they arise in the thymus, Tregs are PPARγ-, but within the SLOs a small Treg population with intermediate PPARγ expression emerges (21, 22). These Tregs already express some aspects of the VAT Treg signature but only become PPARγhi and acquire the full VAT Treg phenotype and epigenetic profile once they enter the VAT (21, 22). This two-step differentiation process was also apparent in a study that analyzed transcriptional trajectories of single Treg cells as they progress from SLOs to non-lymphoid tissues (23). A subset of Tregs in the SLOs already displays tissue-like transcriptional profiles, representing a portion of the tissue-specific transcriptional programs as well as the chemokine receptors that allow for homing to the respective tissues (23). Moreover, these intermediate differentiation stages of tissue Tregs are shared between different Treg subsets, regardless of their terminal tissue (22, 23). Further characterization of these tissue Treg precursors revealed that they depend on the transcription factor Batf for their development and, when present in the SLOs, express Nfil3 (24). Furthermore, acquisition of a tissue Treg phenotype goes along with the loss of the transcription factor ID3 (25). The target tissue of these transitional Treg subsets is determined by their antigen specificity and hence their T cell receptor (TCR) (21). As a consequence, Tregs seeding the VAT, skin or colon represent clonally expanded populations that are also represented in smaller numbers in the lymph nodes, where they are primed before migrating to the peripheral tissue and acquiring their tissue phenotype (21, 24, 26). Single-cell TCR-sequencing confirmed the clonal nature of tissue Tregs as the percentage of polyclonal TCRs decreased with the transition of Nfil3- to Nfil3+ Tregs and was lowest in tissue-skewed Tregs also expressing Klrg1 (24). Thus, tissue Tregs go through a two-step differentiation process, where they acquire a partial tissue Treg signature within the SLOs before seeding their target tissue in a TCR-dependent manner and adopt a terminally differentiated tissue-specific phenotype.
To ensure close proximity of Tregs and effector cells at the site of their activation, both are directed to peripheral tissues by tissue-specific molecules. These include CLA (cutaneous leucocyte-associated antigen) and the integrin a4b7, directing Tregs to the skin and the intestinal epithelium, respectively. CLA binds E-selectin which is uniquely enriched in the endothelium of the skin and up-regulated upon inflammation, while a4b7 binds to MadCAM-1, and together with the chemokine receptor CCR9, drives migration to the gastrointestinal-associated lymphoid tissue (GALT) (27). Interestingly, a considerable proportion of human circulating Tregs express CLA and CCR4, indicative of their ability to recirculate and migrate between skin and draining lymph nodes at steady-state (28–30). In contrast, very few circulating Tregs express the gut-homing receptors (29), suggesting that they represent a more resident subset. But what is driving the acquisition of the tissue-specific homing receptors? Several groups reported that tissue-specific dendritic cells (DCs) able to migrate to draining lymph nodes were involved in naïve T cell instruction both in vitro and in vivo (27, 31). Gut-tropic DCs are able to convert vitamin A captured from the diet into retinoic acid (RA), which induces the up-regulation of CCR9 and a4b7 in T cells (32). Similarly, IL-12 and TGF-β were suggested as drivers of CLA and other homing receptors in Tregs, such as CCR4, CCR10, P- and E-selectin ligands favoring migration to the skin (33, 34). DCs and soluble factors from distinct tissues can indeed induce expression of homing receptors specific for the same tissue in Tregs in vitro. RA promotes expression of gut-tropic homing receptors, and Tregs treated with RA can migrate to the gut to suppress DSS-induced colitis, an effect that was inhibited when treated with anti-a4b7 antibodies (17). IL-12 instead induces expression of skin-homing receptors enabling IL-12-cultured Tregs to infiltrate the inflamed foodpad (17). However, despite several tissue-specific chemokine receptor patterns on Tregs, some receptors are shared among different peripheral sites, indicating a certain redundancy (see Table 1).
Interestingly, the site of antigen encounter not only has an impact on the homing capacity of Tregs, but also influences their function. In a peanut allergy model, different administration routes for the immunotherapy induced functionally distinct Tregs. These not only expressed different sets of chemokine receptors, but also differed in their expression and dependence on the suppressive mediators IL-10 and CTLA-4 as well as their longevity (43). Whether the site of Treg priming also has an impact on their non-canonical functions is still unclear. However, given that Tregs in peripheral tissues display an enhanced ability to e.g. produce the tissue protective factor amphiregulin (Areg), this will be important to address and may be an interesting therapeutic approach for pathologies marked by severe tissue-damage.
In addition to the site of priming, the chemokine expression pattern and function of Tregs are also affected by the cytokine environment present during an immune response. For instance, during type 1 immune responses, Tregs up-regulate the master transcription factor T-bet, which in turn drives CXCR3 expression, allowing them to migrate to the inflammatory sites (44–46). CXCR3 drives recruitment of T cells to sites of ongoing type 1 immune responses and also acts as a critical lung-homing receptor in respiratory viral infections (47). CXCL10, the ligand of CXCR3, is increased upon infection with different respiratory viruses, including Influenza, SARS-CoV-1 and SARS-CoV-2 (48, 49), allowing for an efficient recruitment of CXCR3+ Tregs to the lung and the site of the effector response. Treg-mediated suppression is strictly dependent on their localization in close proximity to the effector cells at the site of an ongoing immune response. Hence, blockade of any step of the migration process will interfere with Treg function. Nevertheless, as Treg and effector T cell require the same molecular interaction for their migration, any interference with Treg migration would also interfere with migration of effector T cells into the affected tissue.
Several strategies aimed at blocking effector T cell migration have been developed to treat autoimmune disorders. Examples include the monoclonal antibody Natalizumab targeting the α4β1 integrin (50) or the sphingosine-1-phosphate receptor modulator Fingolimod, which sequesters lymphocytes in lymph nodes (51). These therapies are successfully used in the treatment of autoimmune disorders such as Multiple Sclerosis (MS). While Natalizumab blocks infiltration of cells to peripheral sites and does not alter the Treg composition in blood (52), Fingolimod appears to sequester CD4+ Foxp3- T cells in secondary lymphoid tissues more strongly than the Foxp3+ counterparts (53). This is due to the differential expression of S1PRs in the different T cell populations (54). Apart from this, signaling through S1P1R blocks the TGF-β pathway (55), which is crucial for Treg differentiation. Hence, treatment with Fingolimod not only removes potentially pathogenic lymphocytes from the circulation but may also interfere with the generation of Tregs. Another example on how defects in T cell migration could affect the Treg compartment is P-selectin ligand disfunction. PSGL-1-/- mice have an enhanced susceptibility to EAE (experimental autoimmune encephalitis), the animal model for MS, due to the importance of the P-selectin ligand for Treg-mediated control of the autoimmune T cell response (56). In addition to these general migratory mechanisms, there are also some differences in the adhesion molecules required by different T cell subsets to enter peripheral tissues, e.g. the CNS. Th1 cells entail the integrin α4 to infiltrate the CNS whereas Th17 cells and Tregs depend on LFA-1 in the absence of integrin α4 (41, 57). As such, it seems likely that the functional outcome of T cell migration blockade depends on three key points: (i) the relative expression of the targeted molecule in effector T cells and Tregs, (ii) their dependence on that molecule or redundancy in targeting each population to a specific tissue and, iii) the relative importance of Treg recruitment for immune suppression vs. tissue protection and regeneration in the damaged organ (as discussed in detail in the next section). As such, the correct and timely localization of Tregs is a key event in controlling immune activation and maintaining tissue homeostasis. Tissue homing is essential to bring Tregs to the site of action, where they not only perform their immune suppressive functions, but also provide mediators for tissue protection.
Once in the tissue, Tregs can perform a wide range of functions. Their first and main role is to maintain homeostasis, to dampen excessive immune responses, and in this way avoid collateral tissue damage. Recent studies have revealed that in addition to this indirect role in tissue protection, Tregs also actively promote tissue maintenance, healing and regeneration (11, 58) (Figure 2). As such, Tregs e.g. promote hair regeneration by facilitating the function of hair follicle stem cells in the skin (59). Furthermore, Tregs can improve the formation and remodeling of scar tissue and reduce collagen degradation in the heart (60, 61). Similarly, Tregs interact with satellite cells to directly promote repair and regeneration after muscle injury and to counteract fibrosis (11, 58). This active role of Tregs in tissue regeneration has been observed in various tissues and the important pioneering work, describing their function in muscle, adipose tissue and skin, has been summarized in a number of excellent reviews [examples include (62, 63)]. Here, we will summarize our current understanding of this non-canonical function of Tregs in the intestine and the lung as examples of barrier sites as well as the CNS as an immune privileged site.
Figure 2 Treg functions in peripheral tissues. In addition to their main function of suppressing effector immunity, Tregs fulfill additional functions in peripheral organs. These include control of overreactive cells and promotion of tissue repair as illustrated for the brain, lung and intestine. Illustration created with BioRender.com.
Barrier sites are constantly exposed to external antigens and face the challenge of ensuring a potent immune response against pathogens while maintaining tolerance to commensals and harmless antigens. This challenge also extends to Tregs, which at these sites are largely specific for microbial antigens and to a large degree differentiate into Tregs at the barrier site itself (23, 64, 65).
In the gut, the microbiota and the host tissue are separated by a physical barrier of intestinal epithelial cells (IECs). An extensive crosstalk exists between immune cells in the host tissue and IECs that conveys information from the gut lumen to the immune compartment and from immune cells to the IECs and the microbiota in the gut lumen (Figure 3). Foxp3+ Tregs that reside in the intestinal tissue are in their great majority induced from circulating conventional T cells, but there is also a fraction that originates in the thymus and homes to the intestine (66). In the lamina propria of the small intestine, a Treg niche has been characterized and some studies have addressed the possibility that these cells migrate to and are maintained in this organ independently from other immune system cues such as antigen encounter (67). In vivo studies with mice lacking peripheral MHC II expression have shown that, despite a reduction in the total CD4+ T cell count, adult MHC II KO mice have an increased frequency of Foxp3+ Tregs in the lamina propria (67). Despite the absence of MHC II, these Tregs show high CD44 expression, indicative of an activated phenotype. Furthermore, intestinal Tregs have a reduced expression of the IL-2 receptor subunit CD25 and, unlike other Tregs, their proliferation and maintenance is independent of IL-2 (15, 67). Instead, continued ICOS signaling is important for their maintenance (15). In addition, the alarmin IL-33, which is constitutively expressed by epithelial cells at barrier sites (68), acts as an intestinal survival factor for Tregs. The IL-33 receptor ST2 is enriched on colonic Tregs and IL-33 promotes expansion of these ST2+ Tregs (24, 69). Furthermore, IL-33 signaling also enhances TGF-β-mediated differentiation of Tregs and thus promotes Treg accumulation and maintenance in the inflamed gut tissue (69).
Figure 3 Pathways and molecules involved in Treg function in non-lymphoid organs. Tregs which home to the brain, lung and intestine acquire phenotypic characteristics that potentiate their function in these organs. Among these, the production of Areg and anti-inflammatory cytokines such as IL-10 and TGF-β, mediate Treg protective and reparative action. Illustration created with BioRender.com.
Interestingly, IL-33 not only plays an important role as a local survival factor for Tregs, but can also modulates their function by inducing Areg production (12). Areg promotes tissue regeneration by signaling through the epidermal growth factor receptor and multiple studies have demonstrated the ability of Tregs to produce Areg and promote tissue regeneration (11, 12, 70, 71). However, whether ST2+ Tregs in the gut also produce Areg and exert similar tissue protective and regenerative functions still remains to be determined. Besides Areg, which would directly act on barrier tissues, Tregs also produce immunomodulatory cytokines to promote homeostasis. One such anti-inflammatory cytokine is IL-10, which plays a crucial role in maintaining tolerance to commensal microbiota in the gut (5). IL-10 promotes tissue integrity by dampening effector immune responses against bacteria, viruses and parasites and hence dampening immuno-pathology. At the same time, it can also enable pathogen persistence, which may result in pathogen-induced pathology (72). Selective deficiency of IL-10 in Foxp3+ Tregs results in spontaneous colitis (73), suggesting that IL-10 is a key effector molecule for Treg-mediated tissue regeneration and protection. This likely not only holds true for the regulation of immune responses against microbiota, but also for those elicited upon infection (Figure 3). Overall, intestinal Tregs represent a highly specialized Treg subset that is essential for limiting inflammation through its secretion of anti-inflammatory cytokines. Furthermore, additional mediators of tissue protection, such as Areg, may enable them to actively promote tissue regeneration and thereby maintain tissue integrity.
Non-canonical Treg functions are strictly associated with their localization and tissue adaptation as well as a fine-tuned crosstalk with the surrounding tissue. A good example of this is the gut, where continuous exposure to microbe- and food-derived antigens shapes the Treg niche to undergo a specialization driven by the tissue and food antigens present at this site. As such, intestinal Tregs can lose expression of Foxp3 and convert into non-inflammatory CD4+ intestinal epithelium lymphocytes (IELs) once they have migrated to the damaged epithelium (Figure 2). In this environment, they can co-operate with the remaining Foxp3+ Tregs, performing complementary roles in balancing intestinal inflammation and promoting an anti-inflammatory state (74). Notably, these local Tregs strictly depend on the intestinal microenvironment, i.e. microorganism-derived signals and defined components of the microbiota, for proper function and development (75). In vitro studies using a reporter cell line for TCR engagement showed that TCR activation in Tregs did not occur in the absence of microorganism-derived antigens (64). Nevertheless, germ-free mice have similar Treg frequencies and numbers as specific pathogen-free mice (76). In contrast, antigen-free mice harbor a dramatically reduced Treg population. Despite this dramatic reduction, antigen-free mice still maintained a small intestinal Treg population suggesting that not the entire Treg population in the gut is induced by local antigen (76). Besides providing antigen, commensal microorganisms in the gut also produce metabolites, such as the short-chain fatty acid (SCFA) butyrate, that promote Treg differentiation in vivo (77). A similar function has been assigned to food-derived fatty acids (78, 79). Dietary-derived SCFAs expand gut Tregs by suppressing the JNK and p38 signaling pathways (80). Conversely, dietary long-chain fatty acids (LCFAs) can induce Th1/Th17 differentiation, while decreasing SCFAs in the gut (80). LCFA treatment resulted in exacerbated autoimmune disease in the EAE model, which could be rescued by treatment with SCFAs via differentiation of lamina propria-derived Tregs (80). In addition to directly enhancing Treg differentiation, SCFAs produced by the microbiota enhance TGF-β1 production by IECs (81) (Figure 3). TGF-β1 then directly drives the conversion of conventional T cells into Foxp3+ Tregs in peripheral organs (82). Thus, SCFA-induced TGF-β1 production generates an environment that also indirectly promotes Treg induction in vivo. Specific symbionts in the mouse gut microbiome have been found to induce a subset of Tregs that co-expresses the transcription factor Rorγ together with Foxp3 (65, 83). This Treg subset plays an important role in controlling colonic inflammation. Intestinal Rorγ+ Tregs are mostly Helios- and express high levels of IL-10, ICOS and CTLA-4 (65, 83), which mediate the strong suppressive capacity of these specialized Tregs. Nevertheless, whether these microbiota-induced Tregs only contribute to tissue homeostasis by limiting effector T cell activation or whether they also directly promote tissue protection and regeneration through release of tissue regenerative factors such as Areg still needs to be investigated. However, the studies we summarized here highlight the heterogeneity and plasticity of T cells and Tregs in the intestine as well as the fact that these Tregs are shaped by microbiota- and food-derived antigens and specialize to limit inflammation of the intestinal tissue and potentially participate in its renewal when damaged.
The lung represents another non-lymphoid organ where Tregs have shown a strong involvement in tissue maintenance beyond the canonical immune homeostasis (Figure 2). Like the gut, the lung is highly exposed to external pathogens and microorganisms, which can potentially cause harm. Adaptive immune cells including Tregs colonize the respiratory tract and like in the gut, the Tregs have to maintain the delicate balance between allowing efficient immunity against pathogenic threats while maintaining tissue homeostasis and integrity.
Non-classical roles of Tregs in the lung tissue are still under investigation, but several independent studies have demonstrated their active involvement in lung epithelium regeneration (84, 85). Indeed, multiple studies have shown the ability of lung Tregs to produce Areg in response to IL-33 (11, 12, 70, 71). During lung inflammation, ST2+ Tregs exert tissue protective and regenerative functions through Areg production. Furthermore, Foxp3+ Tregs in the lung produce keratinocyte growth factor upon LPS stimulation. This factor, together with Areg, induces epithelial cell proliferation and subsequent tissue regeneration upon injury (85) (Figure 3).
Tregs have also been studied in acute lung injury (ALI), which leads to a progressive influx of CD3+ T cells including Tregs into the respiratory tract. Importantly, Treg influx into the alveolar compartment correlates with the transition from injury to resolution and Treg infusion promotes resolution of LPS-induced lung injury in mice, resulting in increased survival and reduced BAL protein and cell count (84). Interestingly, Tregs directly act on alveolar epithelial cells during the recovery phase of ALI by enhancing their proliferation and thereby accelerating resolution and lung tissue regeneration (86) (Figure 3). Furthermore, IL-10-producing Tregs play an important role in limiting type 2 inflammation in the lung. In an OVA-induced lung inflammation model, lack of Treg-derived IL-10 results in exacerbated immune pathology characterized by increased mucus production, Goblet cell expansion, edema, as well as lymphocyte and eosinophil infiltration (73). Nevertheless, Tregs can also have a negative impact on the lung tissue by promoting fibrosis through secretion of IL-10 and TGF-β (87). This once again highlights the need for a fine-tuned balance to allow prevention of immune-pathology while promoting tissue regeneration.
In addition to limiting lung pathology in settings of sterile inflammation, Tregs also play an important role in mitigating lung damage upon infection. Like upon injury, lung Tregs release Areg in response to Influenza Virus infection, thereby counteracting pathogen-induced as well as immune-mediated pathology (12). Importantly, this tissue protective effect is independent of the immune suppressive function of Tregs and their classical regulatory role (12). In addition, Tregs have been addressed in the context of SARS-CoV-2 infection and several studies revealed that in patients with severe COVID-19, marked by potent cytokine storm, Tregs might be beneficial for the lung environment, dampening the pro-inflammatory cues and restoring tissue integrity (88) (Figure 3). On the other hand, excessive immune regulation by Tregs may hamper an effective immune response against SARS-CoV-2 and thereby exacerbate disease severity (89). Of note, Tregs in COVID patients are phenotypically distinct from Tregs observed upon Influenza Virus and Respiratory Syncytial Virus infection (89, 90). As mentioned earlier, Tregs up-regulate CXCR3 in response to the infection, which directs them to the lung as the site of an ongoing immune response (44). While the recruitment of T-bet-expressing CXCR3+ Tregs to the site of type 1 immune responses is essential for the efficient control of these responses (44, 45), it is still unknown whether the same Treg subset also exerts the tissue protective functions observed upon infection (12). Understanding the exact functions of each Treg population will be particularly important in infectious settings in which immune-mediated damage correlates with disease severity, such as Influenza or COVID-19 (91, 92). In conclusion, Treg function may contribute to pathology if they excessively limit immunity to infection. Nevertheless, Tregs also play an important role in limiting sterile, immune-mediated, or pathogen-induced tissue damage by shaping the immune environment but also by acting directly on epithelial cells.
As discussed, the main function of Tregs at barrier sites is to maintain tolerance where continuous environmental cues are faced. In addition, accumulating evidence for a memory phenotype of Tregs at these sites has emerged. A clear phenotypical characterization of a Treg memory subset is still lacking but, in the past years, evidence of the persistence of memory-like Tregs in peripheral lymphoid and non-lymphoid tissues has arisen, especially at barrier sites (93). Functional Treg memory was assessed in the lung, where adoptive transfer of memory but not naïve Tregs from SLOs attenuated lung pathology following Influenza infection (94). Similarly, memory Tregs capable of suppressing a secondary inflammatory response upon antigen re-encounter have been observed in pregnancy. In this context, fetal antigen-specific Tregs persist in SLOs even beyond the pregnancy. These Tregs re-expand following re-encounter with the same antigen in a subsequent pregnancy and maintain fetal tolerance (95). However, whether fetal-antigen-specific Tregs are also maintained as a tissue-resident memory population or are only present in SLOs still needs to be determined. Guo and colleagues defined a subset of CD4+CD25+Foxp3+ T cells in the lamina propria which has effector/memory-like properties and is characterized by low expression of CD62L and CD45RB while expressing CD44 and the chemokine receptors CCR4 and CCR9, which are not exclusively expressed on memory cells but mark circulating and tissue-infiltrating lymphocytes (96) (Figure 1). However, whether these memory Tregs are maintained in the tissue and represent a truly tissue-resident population is unclear. Moreover, a mouse model allowing for the inducible expression of a self-antigen in the skin clearly showed that a memory Treg population is maintained following antigen clearance and contraction of the effector cytokine response (97). A follow-up study then also identified the corresponding memory Treg subset in humans (98). Moreover, IL-2 has been shown not to be required for the generation of memory Tregs in the skin, while IL-7 is essential for maintaining them at steady state in this organ (99). While these studies showed that memory Tregs persist in the skin, it is still unclear whether they are replenished from SLOs or whether they represent a truly tissue-resident memory population that is maintained locally.
The existence of memory Tregs has been explored for decades now, but a distinct characterization of their phenotype, maintenance, and mechanism of action in the peripheral tissue is still missing and will require further investigation. Nevertheless, as highlighted above, accumulating data suggest that Treg memory may play a key role in tissue protection in peripheral organs. However, as memory Tregs share phenotypical characteristics and functional features with effector Tregs, further studies are needed to clearly define their markers to allow for a clear identification of these cells. Furthermore, it will be important to determine whether memory Tregs residing in peripheral tissues can recirculate or whether they are tightly restricted to the tissues and represent a tissue-resident population.
In contrast to barrier tissues, the central nervous system (CNS) is shielded from external antigens and holds immune privilege, conferring protection from potential damage of the peripheral immune system to this site. As such, Treg numbers in brain tissue at steady state are very low, under 100 cells in most of the cases. Despite this low count, Tregs are present and show a similar phenotype as those observed at barrier sites in association with tissue repair (Figure 2). Like barrier Tregs, CNS Tregs express the IL-33 receptor ST2. In addition, they also express the serotonin receptor as a tissue specific receptor and can survive and proliferate in absence of IL-2 upon IL-33 and serotonin sensing (40).
While the CNS is well guarded from immune-mediated insults, some pathogens may exploit the immune privilege and target the CNS to expand. When that happens, Tregs (and other T cells) can infiltrate the brain upon inflammation, following the CCL1 and CCL20 gradient produced by astrocytes (40). Effector Th1 cells and memory cells may enter the CNS through the blood-brain barrier, while Tregs and Th17 can reach the CNS through the choroid plexus (100), emphasizing a difference between these subsets of CD4+ T cells. This variation in the entry site could influence their final location, and may therefore influence their main function and purpose as well (101, 102). After infiltration, the main role of Tregs is to suppress ongoing immune responses and dampen inflammation to protect neural integrity. Nevertheless, other functions have been attributed to them, making Tregs a key protective cell population of the nervous system (Figure 2). To start with, Tregs can control overreactive microglia, either by reducing their activation and cytokine secretion, by decreasing their production of neurotoxic factors (e.g. nitric oxide, NO, and inducible nitric oxide synthase, iNOS) through driving a shift to the anti-inflammatory M2 phenotype or by inducing their apoptosis (103–105). As NO is one of the major causes of neuronal apoptosis, this role of Tregs is crucial. On the other hand, astrogliosis (or abnormal astrocyte growth) can also be dampened by Tregs through the release of Areg (40) (Figure 3). Moreover, Areg can potentiate neural stem cell proliferation (106). Again, the fact that Areg also acts in immune privileged tissues highlights the importance of Treg-derived Areg in tissue protection. During sterile CNS damage as it may occur during a stroke, Tregs can contribute to the resolution of the acute phase either directly or by promoting macrophages to adopt a M2 phenotype (107, 108). Moreover, Treg depletion during EAE impairs remyelination, a property that can be regained upon Treg transfer (109), and explains why a diet restricted in SCFAs reduces axonal damage through Treg induction, as discussed in detail in an earlier section (80). In addition, the fact that Treg numbers do not decrease during the remission phase, as it occurs for CD4+ effector T cells, highlights that the role of Tregs in tissue protection continues beyond the inflammatory phase (110).
Despite their important role in maintaining CNS homeostasis, Tregs are a double-edged sword when it comes to CNS infection: their proliferation and activation can dampen effector immune responses, allowing e.g. viruses to spread in the CNS (111). On the other hand, Treg depletion can allow excessive infiltration and activation of effector T cells in the brain, causing strong immune pathology and damage (112). Furthermore, Tregs have been observed to directly act on infected innate immune cells and drive their apoptosis via the caspase-3 and the perforin/granzyme B pathway (61). Hence, Tregs can be involved in viral clearance from the brain, as observed in Human Immunodeficiency Virus (HIV) infection (113). Moreover, during HIV infection, brain-infiltrating Tregs can potentially promote the conversion of M1 to M2 macrophages, which adopt an anti-inflammatory phenotype, and thereby protect the morphology of the dendrites (114). IL-10 secretion by Tregs has also been associated with neuroprotection upon viral infection, independent from immune suppression (115). In the case of Herpesvirus, Tregs from acute murine cytomegalovirus (MCMV)-infected animals prevent neural damage by restraining microgliosis and astrogliosis and can avoid hippocampal injury and cognitive impairment (116). Interestingly, Tregs also promote memory T cell formation in the CNS after MCMV-infection (117). Therefore, besides acting on effector T cells to suppress collateral T cell damage, Tregs can actively support formation of immunological memory. Treg depletion prior infection with the model coronavirus MHV (mouse hepatitis virus) did not interfere with T cell infiltration but led to increased apoptosis of neurons and myelin loss (118), suggesting that rather than being mainly involved in T cell suppression, CNS Tregs have a central role in tissue protection after infection. In line with this, it is tempting to hypothesize that brain Tregs could ameliorate the neurological symptoms derived from COVID-19 infection (119).
All in all, the potential beneficial roles of Tregs in CNS will depend on the circumstances. Most studies focus on the role of Tregs in limiting effector T cell activation and immunopathology, while little is known about their direct impact on neural cells. Regarding viral infections, we understand that Tregs hamper viral clearance in the periphery and may thereby facilitate virus infiltration in the CNS. Nevertheless, depending on the type of virus, Tregs can also promote anti-viral effects and may play a favorable role, particularly during the late stage of encephalic disease, when their tissue protective functions take the lead.
Tregs are critical for maintaining tissue homeostasis both by inhibiting excessive immune activation and by directly interacting with the cellular network in the tissue niche to promote tissue renewal and repair. While a core set of features is shared between Tregs in lymphoid organs and peripheral tissues, many non-lymphoid tissues harbor phenotypically distinct Treg subsets with unique functions that we are only beginning to understand. Nevertheless, some general concepts have started emerging.
First, Treg activity is strictly dependent on their localization at a given tissue site, which is controlled by their specificity and site of priming, their migration to a specific tissue, and the availability of growth and survival factors within that tissue. As a part of their adaptation to non-lymphoid tissues, Tregs become less dependent on IL-2 and are instead maintained by other cytokines such as IL-7 and IL-33 or by tissue specific factors like serotonin (40, 69, 99). Furthermore, the milieu and cellular network present in different tissues, such as microbiota derived factors and abundance of TGF-β at barrier sites, promote Treg differentiation and thereby control the amount and functional impact of Tregs at that site (65, 75, 77).
Second, classical immune regulation by Tregs at peripheral sites and their direct tissue protective effects rely on distinct functional programs. While immune suppression involving co-inhibitory receptors and the modulation of APCs come into play during immune suppression in lymphoid tissues, suppressive cytokines like IL-10 and TGF-β are important functional mediators in both lymphoid and non-lymphoid organs (5). In addition, Tregs in peripheral tissues produce Areg - and likely also other factors still to be identified - that directly act on tissue cells to regulate their regeneration and function (11). Whether the classical immune suppressive and the regenerative function of Tregs are carried out by the same Treg population still needs to be determined. Similarly, the conditions that allow for Tregs to acquire this regenerative capacity, which may include the site and conditions present during their initial priming or simply the presence of the right stimuli at the tissue site, remain unclear. A better understanding of these processes will potentially allow for the manipulation of Tregs in therapeutic approaches targeting their regenerative role.
All authors wrote and revised the manuscript. All authors contributed to the article and approved the submitted version.
This work was supported by the European Research Council (grant No. 677200 to NJ).
The authors declare that the research was conducted in the absence of any commercial or financial relationships that could be construed as a potential conflict of interest.
All claims expressed in this article are solely those of the authors and do not necessarily represent those of their affiliated organizations, or those of the publisher, the editors and the reviewers. Any product that may be evaluated in this article, or claim that may be made by its manufacturer, is not guaranteed or endorsed by the publisher.
1. Fontenot JD, Gavin MA, Rudensky AY. Foxp3 Programs the Development and Function of CD4+CD25+ Regulatory T Cells. Nat Immunol (2003) 4(4):330–36. doi: 10.1038/ni904
2. Furtado GC, De Curotto Lafaille MA, Kutchukhidze N, Lafaille JJ. Interleukin 2 Signaling is Required for CD4+ Regulatory T Cell Function. J Exp Med (2002) 196(6):851–7. doi: 10.1084/jem.20020190
3. Read S, Malmström V, Powrie F. Cytotoxic T Lymphocyte–Associated Antigen 4 Plays an Essential Role in the Function of Cd25+Cd4+ Regulatory Cells That Control Intestinal Inflammation. J Exp Med (2000) 192(2):295–302. doi: 10.1084/jem.192.2.295
4. Takahashi T, Tagami T, Yamazaki S, Uede T, Shimizu J, Sakaguchi N, et al. Immunologic Self-Tolerance Maintained by CD25(+)CD4(+) Regulatory T Cells Constitutively Expressing Cytotoxic T Lymphocyte-Associated Antigen 4. J Exp Med (2000) 192(2):303–10. doi: 10.1084/jem.192.2.303
5. Asseman C, Mauze S, Leach MW, Coffman RL, Powrie F. An Essential Role for Interleukin 10 in the Function of Regulatory T Cells That Inhibit Intestinal Inflammation. J Exp Med (1999) 190(7):995–1004. doi: 10.1084/jem.190.7.995
6. Fahlén L, Read S, Gorelik L, Hurst SD, Coffman RL, Flavell RA, et al. T Cells That Cannot Respond to TGF-Beta Escape Control by CD4(+)CD25(+) Regulatory T Cells. J Exp Med (2005) 201(5):737–46. doi: 10.1084/jem.20040685
7. Qureshi OS, Zheng Y, Nakamura K, Attridge K, Manzotti C, Schmidt EM, et al. Trans-Endocytosis of CD80 and CD86: A Molecular Basis for the Cell-Extrinsic Function of CTLA-4. Science (2011) 332(6029):600–3. doi: 10.1126/science.1202947
8. Gondek DC, Lu L-F, Quezada SA, Sakaguchi S, Noelle RJ. Cutting Edge: Contact-Mediated Suppression by CD4 + CD25 + Regulatory Cells Involves a Granzyme B-Dependent, Perforin-Independent Mechanism. J Immunol (2005) 174(4):1783–6. doi: 10.4049/jimmunol.174.4.1783
9. Duthoit CT, Mekala DJ, Alli RS, Geiger TL. Uncoupling of IL-2 Signaling From Cell Cycle Progression in Naive CD4 + T Cells by Regulatory CD4 + CD25 + T Lymphocytes. J Immunol (2005) 174(1):155–63. doi: 10.4049/jimmunol.174.1.155
10. Pandiyan P, Zheng L, Ishihara S, Reed J, Lenardo MJ. CD4+CD25+Foxp3+ Regulatory T Cells Induce Cytokine Deprivation-Mediated Apoptosis of Effector CD4+ T Cells. Nat Immunol (2007) 8(12):1353–62. doi: 10.1038/ni1536
11. Burzyn D, Kuswanto W, Kolodin D, Shadrach JL, Cerletti M, Jang Y, et al. A Special Population of Regulatory T Cells Potentiates Muscle Repair. Cell (2013) 155(6):1282–95. doi: 10.1016/j.cell.2013.10.054
12. Arpaia N, Green JA, Moltedo B, et al. A Distinct Function of Regulatory T Cells in Tissue Protection. Cell (2015) 162(5):1078–89. doi: 10.1016/j.cell.2015.08.021
13. Lehmann J, Huehn J, de la Rosa M, Maszyna F, Kretschmer U, Krenn V, et al. Expression of the Integrin αeβ7 Identifies Unique Subsets of CD25+ as Well as CD25- Regulatory T Cells. Proc Natl Acad Sci USA (2002) 99(20):13031–6. doi: 10.1073/pnas.192162899
14. Huehn J, Siegmund K, Lehmann JCU, Siewert C, Haubold U, Feuerer M, et al. Developmental Stage, Phenotype, and Migration Distinguish Naive- and Effector/Memory-Like CD4+ Regulatory T Cells. J Exp Med (2004) 199(3):303–13. doi: 10.1084/jem.20031562
15. Smigiel KS, Richards E, Srivastava S, Thomas KR, Dudda JC, Klonowski KD, et al. CCR7 Provides Localized Access to IL-2 and Defines Homeostatically Distinct Regulatory T Cell Subsets. J Exp Med (2014) 211(1):121–36. doi: 10.1084/jem.20131142
16. Siegmund K, Feuerer M, Siewert C, Ghani S, Haubold U, Dankof A, et al. Migration Matters: Regulatory T-Cell Compartmentalization Determines Suppressive Activity In Vivo. Blood (2005) 106(9):3097–104. doi: 10.1182/blood-2005-05-1864
17. Siewert C, Menning A, Dudda J, Siegmund K, Lauer U, Floess S, et al. Induction of Organ-Selective CD4+ Regulatory T Cell Homing. Eur J Immunol (2007) 37(4):978–89. doi: 10.1002/eji.200636575
18. Lee JH, Kang SG, Kim CH. FoxP3 + T Cells Undergo Conventional First Switch to Lymphoid Tissue Homing Receptors in Thymus But Accelerated Second Switch to Nonlymphoid Tissue Homing Receptors in Secondary Lymphoid Tissues. J Immunol (2007) 178(1):301–11. doi: 10.4049/jimmunol.178.1.301
19. Boothby IC, Kinet MJ, Boda DP, Kwan EY, Clancy S, Cohen JN, et al. Early-Life Inflammation Primes a T Helper 2 Cell–Fibroblast Niche in Skin. Nature (2021) 599(7886):667–72. doi: 10.1038/s41586-021-04044-7
20. Cipolletta D, Feuerer M, Li A, Kamei N, Lee J, Shoelson SE, et al. PPAR-γ is a Major Driver of the Accumulation and Phenotype of Adipose Tissue T Reg Cells. Nature (2012) 486(7404):549–53. doi: 10.1038/nature11132
21. Li C, DiSpirito JR, Zemmour D, Spallanzani RG, Kuswanto W, Benoist C, et al. TCR Transgenic Mice Reveal Stepwise, Multi-Site Acquisition of the Distinctive Fat-Treg Phenotype. Cell (2018) 174(2):285–99.e12. doi: 10.1016/j.cell.2018.05.004
22. Li C, Muñoz-Rojas AR, Wang G, Mann AO, Benoist C, Mathis D. Pparγ Marks Splenic Precursors of Multiple Nonlymphoid-Tissue Treg Compartments. Proc Natl Acad Sci USA (2021) 118(13):e2025197118. doi: 10.1073/pnas.2025197118
23. Miragaia RJ, Gomes T, Chomka A, Jardine L, Riedel A, Hegazy AN, et al. Single-Cell Transcriptomics of Regulatory T Cells Reveals Trajectories of Tissue Adaptation. Immunity (2019) 50(2):493–504.e7. doi: 10.1016/j.immuni.2019.01.001
24. Delacher M, Imbusch CD, Hotz-Wagenblatt A, Mallm JP, Bauer K, Simon M, et al. Precursors for Nonlymphoid-Tissue Treg Cells Reside in Secondary Lymphoid Organs and Are Programmed by the Transcription Factor BATF. Immunity (2020) 52(2):295–312.e11. doi: 10.1016/j.immuni.2019.12.002
25. Sullivan JM, Höllbacher B, Campbell DJ. Cutting Edge: Dynamic Expression of Id3 Defines the Stepwise Differentiation of Tissue-Resident Regulatory T Cells. J Immunol (2019) 202(1):31–6. doi: 10.4049/jimmunol.1800917
26. Sivasami P, Li C. Derivation and Differentiation of Adipose-Tissue Regulatory T Cells: A Stepwise, Multi-Site Process. Front Immunol (2020) 11:1–6. doi: 10.3389/fimmu.2020.599277
27. Mora JR, von Andrian UH. T-Cell Homing Specificity and Plasticity: New Concepts and Future Challenges. Trends Immunol (2006) 27(5):235–43. doi: 10.1016/j.it.2006.03.007
28. Tomura M, Honda T, Tanizaki H, Otsuka A, Egawa G, Tokura Y, et al. Activated Regulatory T Cells are the Major T Cell Type Emigrating From the Skin During a Cutaneous Immune Response in Mice. J Clin Invest (2010) 120(3):883–93. doi: 10.1172/JCI40926
29. Iellem A, Colantonio L, D’Ambrosio D. Skin-Versus Gut-Skewed Homing Receptor Expression and Intrinsic CCR4 Expression on Human Peripheral Blood CD4+CD25+ Suppressor T Cells. Eur J Immunol (2003) 33(6):1488–96. doi: 10.1002/eji.200323658
30. Hirahara K, Liu L, Clark RA, Yamanaka K, Fuhlbrigge RC, Kupper TS. The Majority of Human Peripheral Blood CD4+CD25highFoxp3+ Regulatory T Cells Bear Functional Skin-Homing Receptors. J Immunol (2006) 177(7):4488–94. doi: 10.4049/jimmunol.177.7.4488
31. Stagg AJ, Kamm MA, Knight SC. Intestinal Dendritic Cells Increase T Cell Expression of Alpha4beta7 Integrin. Eur J Immunol (2002) 32(5):1445–54. doi: 10.1002/1521-4141(200205)32:5<1445::AID-IMMU1445>3.0.CO;2-E
32. Iwata M, Hirakiyama A, Eshima Y, Kagechika H, Kato C, Song S-Y. Retinoic Acid Imprints Gut-Homing Specificity on T Cells. Immunity (2004) 21(4):527–38. doi: 10.1016/j.immuni.2004.08.011
33. Lim YC, Henault L, Wagers AJ, Kansas GS, Luscinskas FW, Lichtman AH. Expression of Functional Selectin Ligands on Th Cells is Differentially Regulated by IL-12 and IL-4. J Immunol (1999) 162(6):3193–201.
34. Austrup F, Vestweber D, Borges E, Löhning M, Bräuer R, Herz U, et al. P- and E-Selectin Mediate Recruitment of T-Helper-1 But Not T-Helper-2 Cells Into Inflammed Tissues. Nature (1997) 385(6611):81–3. doi: 10.1038/385081a0
35. Yurchenko E, Tritt M, Hay V, Shevach EM, Belkaid Y, Piccirillo CA. CCR5-Dependent Homing of Naturally Occurring CD4+ Regulatory T Cells to Sites of Leishmania Major Infection Favors Pathogen Persistence. J Exp Med (2006) 203(11):2451–60. doi: 10.1084/jem.20060956
36. Scharschmidt TC, Leitner KS, Pauli ML, Leitner EG, Chu K, Truong H, et al. Commensal Microbes and Hair Follicle Morphogenesis Coordinately Drive Treg Migration into Neonatal Skin. Cell Host Microbe (2017) 21(4):467–77.e5. doi: 10.1016/j.chom.2017.03.001
37. Zou L, Barnett B, Safah H, Larussa VF, Evdemon-Hogan S, Mottram P, et al. Bone Marrow is a Reservoir for CD4+CD25+ Regulatory T Cells That Traffic Through CXCL12/CXCR4 Signals. Cancer Res (2004) 64(22):8451–5. doi: 10.1158/0008-5472.CAN-04-1987
38. Shetty S, Weston CJ, Oo YH, Westerlund N, Stamataki Z, Youster J, et al. Common Lymphatic Endothelial and Vascular Endothelial Receptor-1 Mediates the Transmigration of Regulatory T Cells Across Human Hepatic Sinusoidal Endothelium. J Immunol (2011) 186(7):4147–55. doi: 10.4049/jimmunol.1002961
39. Lee I, Wang L, Wells AD, Dorf ME, Ozkaynak E, Hancock WW. Recruitment of Foxp3+ T Regulatory Cells Mediating Allograft Tolerance Depends on the CCR4 Chemokine Receptor. J Exp Med (2005) 201(7):1037–44. doi: 10.1084/jem.20041709
40. Ito M, Komai K, Mise-Omata S, Iizuka-Koga M, Noguchi Y, Kondo T, et al. Brain Regulatory T Cells Suppress Astrogliosis and Potentiate Neurological Recovery. Nature (2019) 565(7738):246–50. doi: 10.1038/s41586-018-0824-5
41. Glatigny S, Duhen R, Arbelaez C, Kumari S, Bettelli E. Integrin Alpha L Controls the Homing of Regulatory T Cells During CNS Autoimmunity in the Absence of Integrin Alpha 4. Sci Rep (2015) 5:7834. doi: 10.1038/srep07834
42. Kluger MA, Nosko A, Ramcke T, Nosko A, Ramcke T, Goerke B, et al. RORγt Expression in T(regs) Promotes Systemic Lupus Erythematosus via IL-17 Secretion, Alteration of T(reg) Phenotype and Suppression of Th2 Responses. Clin Exp Immunol (2017) 188(1):63–78. doi: 10.1111/cei.12905
43. Dioszeghy V, Mondoulet L, Puteaux E, Dhelft V, Ligouis M, Plaquet C, et al. Differences in Phenotype, Homing Properties and Suppressive Activities of Regulatory T Cells Induced by Epicutaneous, Oral or Sublingual Immunotherapy in Mice Sensitized to Peanut. Cell Mol Immunol (2017) 14(9):770–82. doi: 10.1038/cmi.2016.14
44. Koch MA, Tucker-Heard G, Perdue NR, Killebrew JR, Urdahl KB, Campbell DJ. The Transcription Factor T-Bet Controls Regulatory T Cell Homeostasis and Function During Type 1 Inflammation. Nat Immunol (2009) 10(6):595–602. doi: 10.1038/ni.1731
45. Levine AG, Medoza A, Hemmers S, Moltedo B, Niec RE, Schizas M, et al. Stability and Function of Regulatory T Cells Expressing the Transcription Factor T-Bet. Nature (2017) 546(7658):421–5. doi: 10.1038/nature22360
46. Littringer K, Moresi C, Rakebrandt N, Zhou X, Schorer M, Dolowschiak T, et al. Common Features of Regulatory T Cell Specialization During Th1 Responses. Front Immunol (2018) 9:1344(JUN). doi: 10.3389/fimmu.2018.01344
47. Brownlie D, Rødahl I, Varnaite R, Asgeirsson H, Glans H, Falck-Jones S, et al. Distinct Lung-Homing Receptor Expression and Activation Profiles on NK Cell and T Cell Subsets in COVID-19 and Influenza. bioRxiv (2021) 2021.01.13.426553. doi: 10.1101/2021.01.13.426553
48. Chu H, Chan JF-W, Wang Y, Yuen TT-T, Chai Y, Hou Y, et al. Comparative Replication and Immune Activation Profiles of SARS-CoV-2 and SARS-CoV in Human Lungs: An Ex Vivo Study With Implications for the Pathogenesis of COVID-19. Clin Infect Dis an Off Publ Infect Dis Soc Am (2020) 71(6):1400–9. doi: 10.1093/cid/ciaa410
49. Peiris JSM, Yu WC, Leung CW, Cheung CY, Ng WF, Nicholls JM, et al. Re-Emergence of Fatal Human Influenza A Subtype H5N1 Disease. Lancet (London England) (2004) 363(9409):617–9. doi: 10.1016/S0140-6736(04)15595-5
50. Khoy K, Mariotte D, Defer G, Petit G, Toutirais O, Le Mauff B. Natalizumab in Multiple Sclerosis Treatment: From Biological Effects to Immune Monitoring. Front Immunol (2020) 11:549842. doi: 10.3389/fimmu.2020.549842
51. Chun J, Hartung H-P. Mechanism of Action of Oral Fingolimod (FTY720) in Multiple Sclerosis. Clin Neuropharmacol (2010) 33(2):91–101. doi: 10.1097/WNF.0b013e3181cbf825
52. Stenner M-P, Waschbisch A, Buck D, Doerck S, Einsele H, Toyka KV, et al. Effects of Natalizumab Treatment on Foxp3+ T Regulatory Cells. PloS One (2008) 3(10):e3319. doi: 10.1371/journal.pone.0003319
53. Muls N, Dang HA, Sindic CJM, van Pesch V. Fingolimod Increases CD39-Expressing Regulatory T Cells in Multiple Sclerosis Patients. PloS One (2014) 9(11):e113025. doi: 10.1371/journal.pone.0113025
54. Sawicka E, Dubois G, Jarai G, Edwards M, Thomas M, Nicholls A, et al. The Sphingosine 1-Phosphate Receptor Agonist FTY720 Differentially Affects the Sequestration of CD4+/CD25+ T-Regulatory Cells and Enhances Their Functional Activity. J Immunol (2005) 175(12):7973–80. doi: 10.4049/jimmunol.175.12.7973
55. Liu G, Burns S, Huang G, Boyd K, Proia RL, Flavell RA, et al. The Receptor S1P1 Overrides Regulatory T Cell-Mediated Immune Suppression Through Akt-mTOR. Nat Immunol (2009) 10(7):769–77. doi: 10.1038/ni.1743
56. Angiari S, Rossi B, Piccio L, Zinselmeyer BH, Budui S, Zenaro E, et al. Regulatory T Cells Suppress the Late Phase of the Immune Response in Lymph Nodes Through P-Selectin Glycoprotein Ligand-1. J Immunol (2013) 191(11):5489–500. doi: 10.4049/jimmunol.1301235
57. Glatigny S, Duhen R, Oukka M, Bettelli E. Cutting Edge: Loss of α4 Integrin Expression Differentially Affects the Homing of Th1 and Th17 Cells. J Immunol (2011) 187(12):6176–9. doi: 10.4049/jimmunol.1102515
58. Kuswanto W, Burzyn D, Panduro M, Wang KK, Jang YC, Wagers AJ, et al. Poor Repair of Skeletal Muscle in Aging Mice Reflects a Defect in Local, Interleukin-33-Dependent Accumulation of Regulatory T Cells. Immunity (2016) 44(2):355–67. doi: 10.1016/j.immuni.2016.01.009
59. Ali N, Zirak B, Rodriguez RS, Pauli ML, Truong HA, Lai K, et al. Regulatory T Cells in Skin Facilitate Epithelial Stem Cell Differentiation. Cell (2017) 169(6):1119–29.e11. doi: 10.1016/j.cell.2017.05.002
60. Weirather J, Hofmann UDW, Beyersdorf N, Ramos GC, Vogel B, Frey A, et al. Foxp3+ CD4+ T Cells Improve Healing After Myocardial Infarction by Modulating Monocyte/Macrophage Differentiation. Circ Res (2014) 115(1):55–67. doi: 10.1161/CIRCRESAHA.115.303895
61. Tang T-T, Yuan J, Zhu Z-F, Zhang W-C, Xiao H, Xia N, et al. Regulatory T Cells Ameliorate Cardiac Remodeling After Myocardial Infarction. Basic Res Cardiol (2012) 107(1):232. doi: 10.1007/s00395-011-0232-6
62. Muñoz-Rojas AR, Mathis D. Tissue Regulatory T Cells: Regulatory Chameleons. Nat Rev Immunol (2021) 21(9):597–611. doi: 10.1038/s41577-021-00519-w
63. Boothby IC, Cohen JN, Rosenblum MD. Regulatory T Cells in Skin Injury: At the Crossroads of Tolerance and Tissue Repair. Sci Immunol (2020) 5(47):e2025197118. doi: 10.1126/sciimmunol.aaz9631
64. Lathrop SK, Bloom SM, Rao SM, Nutsch K, Lio C-W, Santacruz N, et al. Peripheral Education of the Immune System by Colonic Commensal Microbiota. Nature (2011) 478(7368):250–4. doi: 10.1038/nature10434
65. Sefik E, Geva-Zatorsky N, Oh S, Konnikova L, Zemmour D, McGuire AM, et al. Individual Intestinal Symbionts Induce a Distinct Population of Rorγ+ Regulatory T Cells. Science (2015) 349(6251):993–7. doi: 10.1126/science.aaa9420
66. Cebula A, Seweryn M, Rempala GA, Pabla SS, McIndoe RA, Denning TL, et al. Thymus-Derived Regulatory T Cells Contribute to Tolerance to Commensal Microbiota. Nature (2013) 497(7448):258–62. doi: 10.1038/nature12079
67. Korn LL, Hubbeling HG, Porrett PM, Yang Q, Barnett LG, Laufer TM. Regulatory T Cells Occupy an Isolated Niche in the Intestine That is Antigen Independent. Cell Rep (2014) 9(5):1567–73. doi: 10.1016/j.celrep.2014.11.006
68. Pichery M, Mirey E, Mercier P, Lefrancais E, Dujardin A, Ortega N, et al. Endogenous IL-33 Is Highly Expressed in Mouse Epithelial Barrier Tissues, Lymphoid Organs, Brain, Embryos, and Inflamed Tissues: In Situ Analysis Using a Novel Il-33-LacZ Gene Trap Reporter Strain. J Immunol (2012) 188(7):3488–95. doi: 10.4049/jimmunol.1101977
69. Schiering C, Krausgruber T, Chomka A, et al. The Alarmin IL-33 Promotes Regulatory T-Cell Function in the Intestine. Nature (2014) 513(7519):564–568. doi: 10.1038/nature13577
70. Zaiss DMW, van Loosdregt J, Gorlani A, Bekker CPJ, Gröne A, Sibilia M, et al. Amphiregulin Enhances Regulatory T Cell-Suppressive Function via the Epidermal Growth Factor Receptor. Immunity (2013) 38(2):275–84. doi: 10.1016/j.immuni.2012.09.023
71. Harb H, Benamar M, Lai PS, Contini P, Griffith JW, Crestani E, et al. Notch4 Signaling Limits Regulatory T-Cell-Mediated Tissue Repair and Promotes Severe Lung Inflammation in Viral Infections. Immunity (2021) 54(6):1186–99.e7. doi: 10.1016/j.immuni.2021.04.002
72. Couper KN, Blount DG, Riley EM. IL-10: The Master Regulator of Immunity to Infection. J Immunol (2008) 180(9):5771–7. doi: 10.4049/jimmunol.180.9.5771
73. Rubtsov YP, Rasmussen JP, Chi EY, Fontenot J, Castelli L, Ye X, et al. Regulatory T Cell-Derived Interleukin-10 Limits Inflammation at Environmental Interfaces. Immunity (2008) 28(4):546–58. doi: 10.1016/j.immuni.2008.02.017
74. Bilate AM, Bousbaine D, Mesin L, Agudelo M, Leube J, Kratzert A, et al. Tissue-Specific Emergence of Regulatory and Intraepithelial T Cells From a Clonal T Cell Precursor. Sci Immunol (2016) 1(2):eaaf7471. doi: 10.1126/sciimmunol.aaf7471
75. Atarashi K, Tanoue T, Shima T, Imaoka A, Kuwahara T, Momose Y, et al. Induction of Colonic Regulatory T Cells by Indigenous Clostridium Species. Science (2011) 331(6015):337–41. doi: 10.1126/science.1198469
76. Kim KS, Hong S-W, Han D, Yi J, Jung J, Yang B-G, et al. Dietary Antigens Limit Mucosal Immunity by Inducing Regulatory T Cells in the Small Intestine. Science (2016) 351(6275):858–63. doi: 10.1126/science.aac5560
77. Arpaia N, Campbell C, Fan X, Dikiy S, Van Der Veeken J, Deroos P, et al. Metabolites Produced by Commensal Bacteria Promote Peripheral Regulatory T-Cell Generation. Nature (2013) 504(7480):451–5. doi: 10.1038/nature12726
78. Pompura SL, Wagner A, Kitz A, LaPerche J, Yosef N, Dominguez-Villar M, et al. Oleic Acid Restores Suppressive Defects in Tissue-Resident FOXP3 Tregs From Patients With Multiple Sclerosis. J Clin Invest (2021) 131(2):e138519. doi: 10.1172/JCI138519
79. Hao F, Tian M, Zhang X, Jin X, Jiang Y, Sun X, et al. Butyrate Enhances CPT1A Activity to Promote Fatty Acid Oxidation and Itreg Differentiation. Proc Natl Acad Sci (2021) 118(22):e2014681118. doi: 10.1073/pnas.2014681118
80. Haghikia A, Jörg S, Duscha A, Berg J, Manzel A, Waschbisch A, et al. Dietary Fatty Acids Directly Impact Central Nervous System Autoimmunity via the Small Intestine. Immunity (2015) 43(4):817–29. doi: 10.1016/j.immuni.2015.09.007
81. Atarashi K, Tanoue T, Oshima K, Suda W, Nagano Y, Nishikawa H, et al. Treg Induction by a Rationally Selected Mixture of Clostridia Strains From the Human Microbiota. Nature (2013) 500(7461):232–6. doi: 10.1038/nature12331
82. Chen W, Jin W, Hardegen N, Lei K-J, Li L, Marinos N, et al. Conversion of Peripheral CD4+CD25- Naive T Cells to CD4+CD25+ Regulatory T Cells by TGF-Beta Induction of Transcription Factor Foxp3. J Exp Med (2003) 198(12):1875–86. doi: 10.1084/jem.20030152
83. Ohnmacht C, Park J-H, Cording S, Wing JB, Atarashi K, Obata Y, et al. MUCOSAL IMMUNOLOGY. The Microbiota Regulates Type 2 Immunity Through Rorγt+ T Cells. Science (2015) 349(6251):989–93. doi: 10.1126/science.aac4263
84. D’Alessio FR, Tsushima K, Aggarwal NR, West EE, Willett MH, Britos MF, et al. CD4+CD25+Foxp3+ Tregs Resolve Experimental Lung Injury in Mice and are Present in Humans With Acute Lung Injury. J Clin Invest (2009) 119(10):2898–913. doi: 10.1172/JCI36498
85. Dial CF, Tune MK, Doerschuk CM, Mock JR. Foxp3(+) Regulatory T Cell Expression of Keratinocyte Growth Factor Enhances Lung Epithelial Proliferation. Am J Respir Cell Mol Biol (2017) 57(2):162–73. doi: 10.1165/rcmb.2017-0019OC
86. Mock JR, Dial CF, Tune MK, Gilmore RC, O'Neal WK, Dang H, et al. Impact of Regulatory T Cells on Type 2 Alveolar Epithelial Cell Transcriptomes During Resolution of Acute Lung Injury and Contributions of IFN-γ. Am J Respir Cell Mol Biol (2020) 63(4):464–77. doi: 10.1165/rcmb.2019-0399OC
87. Liu F, Liu J, Weng D, Chen Y, Song L, He Q, et al. CD4+CD25+Foxp3+ Regulatory T Cells Depletion May Attenuate the Development of Silica-Induced Lung Fibrosis in Mice. PloS One (2010) 5(11):e15404. doi: 10.1371/journal.pone.0015404
88. Stephen-Victor E, Das M, Karnam A, Pitard B, Gautier JF, Bayry J. Potential of Regulatory T-Cell-Based Therapies in the Management of Severe COVID-19. Eur Respir J (2020) 56(3):3–6. doi: 10.1183/13993003.02182-2020
89. Galván-Peña S, Leon J, Chowdhary K, Michelson DA, Vijaykumar B, Yang L, et al. Profound Treg Perturbations Correlate With COVID-19 Severity. Proc Natl Acad Sci USA (2021) 118(37):1–9. doi: 10.1073/pnas.2111315118
90. Vick SC, Frutoso M, Mair F, Konecny AJ, Greene E, Wolf CR, et al. A Differential Regulatory T Cell Signature Distinguishes the Immune Landscape of COVID-19 Hospitalized Patients From Those Hospitalized With Other Respiratory Viral Infections. medRxiv (2021) 2021:03.25.21254376
91. Paules C, Subbarao K. Influenza. Lancet (2017) 390(10095):697–708. doi: 10.1016/S0140-6736(17)30129-0
92. Zhou F, Yu T, Du R, Fan G, Liu Y, Liu Z, et al. Clinical Course and Risk Factors for Mortality of Adult Inpatients With COVID-19 in Wuhan, China: A Retrospective Cohort Study. Lancet (2020) 395(10229):1054–62. doi: 10.1016/S0140-6736(20)30566-3
93. Gratz IK, Campbell DJ. Organ-Specific and Memory Treg Cells: Specificity, Development, Function, and Maintenance. Front Immunol (2014) 5:1–17. doi: 10.3389/fimmu.2014.00333
94. Lu C, Zanker D, Lock P, Jiang X, Deng J, Duan M, et al. Memory Regulatory T Cells Home to the Lung and Control Influenza A Virus Infection. Immunol Cell Biol (2019) 97(9):774–86. doi: 10.1111/imcb.12271
95. Rowe JH, Ertelt JM, Xin L, Way SS. Pregnancy Imprints Regulatory Memory That Sustains Anergy to Fetal Antigen. Nature (2012) 490(7418):102–6. doi: 10.1038/nature11462
96. Guo Z, Jang MH, Otani K, Bai Z, Umemoto E, Matsumoto M, et al. CD4+CD25+ Regulatory T Cells in the Small Intestinal Lamina Propria Show an Effector/Memory Phenotype. Int Immunol (2008) 20(3):307–15. doi: 10.1093/intimm/dxm143
97. Rosenblum MD, Gratz IK, Paw JS, Lee K, Marshak-Rothstein A, Abbas AK. Response to Self Antigen Imprints Regulatory Memory in Tissues. Nature (2011) 480(7378):538–42. doi: 10.1038/nature10664
98. Sanchez Rodriguez R, Pauli ML, Neuhaus IM, Yu SS, Arron ST, Harris HW, et al. Memory Regulatory T Cells Reside in Human Skin. J Clin Invest (2014) 124(3):1027–1036. doi: 10.1172/JCI72932
99. Gratz IK, Truong H-A, Yang SH-Y, Maurano MM, Lee K, Abbas AK, et al. Cutting Edge: Memory Regulatory T Cells Require IL-7 and Not IL-2 for Their Maintenance in Peripheral Tissues. J Immunol (2013) 190(9):4483–87. doi: 10.4049/jimmunol.1300212
100. Sallusto F, Impellizzieri D, Basso C, Laroni A, Uccelli A, Lanzavecchia A, et al. T-Cell Trafficking in the Central Nervous System. Immunol Rev (2012) 248(1):216–27. doi: 10.1111/j.1600-065X.2012.01140.x
101. O’Brien CA, Overall C, Konradt C, O'Hara Hall AC, Hayes NW, Wagage S, et al. CD11c-Expressing Cells Affect Regulatory T Cell Behavior in the Meninges During Central Nervous System Infection. J Immunol (2017) 198(10):4054–61. doi: 10.4049/jimmunol.1601581
102. Müller M, Carter SL, Hofer MJ, Manders P, Getts DR, Getts MT, et al. CXCR3 Signaling Reduces the Severity of Experimental Autoimmune Encephalomyelitis by Controlling the Parenchymal Distribution of Effector and Regulatory T Cells in the Central Nervous System. J Immunol (2007) 179(5):2774–86. doi: 10.4049/jimmunol.179.5.2774
103. Zhao J, Zhao J, Fett C, Trandem K, Fleming E, Perlman S. IFN-γ- and IL-10-Expressing Virus Epitope-Specific Foxp3(+) T Reg Cells in the Central Nervous System During Encephalomyelitis. J Exp Med (2011) 208(8):1571–7. doi: 10.1084/jem.20110236
104. Tiemessen MM, Jagger AL, Evans HG, van Herwijnen MJC, John S, Taams LS. CD4+CD25+Foxp3+ Regulatory T Cells Induce Alternative Activation of Human Monocytes/Macrophages. Proc Natl Acad Sci USA (2007) 104(49):19446–51. doi: 10.1073/pnas.0706832104
105. Beers DR, Henkel JS, Zhao W, Wang J, Huang A, Wen S, et al. Endogenous Regulatory T Lymphocytes Ameliorate Amyotrophic Lateral Sclerosis in Mice and Correlate With Disease Progression in Patients With Amyotrophic Lateral Sclerosis. Brain (2011) 134(Pt 5):1293–314. doi: 10.1093/brain/awr074
106. Kimura H, Schubert D. Schwannoma-Derived Growth Factor Promotes the Neuronal Differentiation and Survival Of PC12 Cells. J Cell Biol (1992) 116(3):777–83. doi: 10.1083/jcb.116.3.777
107. Liesz A, Suri-Payer E, Veltkamp C, Doerr H, Sommer C, Rivest S, et al. Regulatory T Cells are Key Cerebroprotective Immunomodulators in Acute Experimental Stroke. Nat Med (2009) 15(2):192–9. doi: 10.1038/nm.1927
108. Ito M, Komai K, Nakamura T, Srirat T, Yoshimura A. Tissue Regulatory T Cells and Neural Repair. Int Immunol (2019) 31(6):361–9. doi: 10.1093/intimm/dxz031
109. Dombrowski Y, O’Hagan T, Dittmer M, Penalva R, Mayoral SR, Bankhead P, et al. Regulatory T Cells Promote Myelin Regeneration in the Central Nervous System. Nat Neurosci (2017) 20(5):674–80. doi: 10.1038/nn.4528
110. Korn T, Reddy J, Gao W, Bettelli E, Awasthi A, Petersen TR, et al. Myelin-Specific Regulatory T Cells Accumulate in the CNS But Fail to Control Autoimmune Inflammation. Nat Med (2007) 13(4):423–31. doi: 10.1038/nm1564
111. Reuter D, Sparwasser T, Hünig T, Schneider-Schaulies J. Foxp3+ Regulatory T Cells Control Persistence of Viral CNS Infection. PloS One (2012) 7(3):e33989. doi: 10.1371/journal.pone.0033989
112. Anghelina D, Zhao J, Trandem K, Perlman S. Role of Regulatory T Cells in Coronavirus-Induced Acute Encephalitis. Virology (2009) 385(2):358–67. doi: 10.1016/j.virol.2008.12.014
113. Liu J, Gong N, Huang X, Reynolds AD, Mosley RL, Gendelman HE. Neuromodulatory Activities of CD4+CD25+ Regulatory T Cells in a Murine Model of HIV-1-Associated Neurodegeneration. J Immunol (2009) 182(6):3855–65. doi: 10.4049/jimmunol.0803330
114. Huang X, Stone DK, Yu F, Zeng Y, Gendelman HE. Functional Proteomic Analysis for Regulatory T Cell Surveillance of the HIV-1-Infected Macrophage. J Proteome Res (2010) 9(12):6759–73. doi: 10.1021/pr1009178
115. Uhde A-K, Ciurkiewicz M, Herder V, Khan MA, Hensel N, Claus P, et al. Intact Interleukin-10 Receptor Signaling Protects From Hippocampal Damage Elicited by Experimental Neurotropic Virus Infection of SJL Mice. Sci Rep (2018) 8(1):6106. doi: 10.1038/s41598-018-24378-z
116. Mutnal MB, Hu S, Schachtele SJ, Lokensgard JR. Infiltrating Regulatory B Cells Control Neuroinflammation Following Viral Brain Infection. J Immunol (2014) 193(12):6070–80. doi: 10.4049/jimmunol.1400654
117. Prasad S, Hu S, Sheng WS, Singh A, Lokensgard JR. Tregs Modulate Lymphocyte Proliferation, Activation, and Resident-Memory T-Cell Accumulation Within the Brain During MCMV Infection. PloS One (2015) 10(12):e0145457. doi: 10.1371/journal.pone.0145457
118. Cervantes-Barragán L, Firner S, Bechmann I, Waisman A, Lahl K, Sparwasser T, et al. Regulatory T Cells Selectively Preserve Immune Privilege of Self-Antigens During Viral Central Nervous System Infection. J Immunol (2012) 188(8):3678–85. doi: 10.4049/jimmunol.1102422
Keywords: regulatory T cells, homing, tissue homeostasis, tissue repair, immune regulation
Citation: Estrada Brull A, Panetti C and Joller N (2022) Moving to the Outskirts: Interplay Between Regulatory T Cells and Peripheral Tissues. Front. Immunol. 13:864628. doi: 10.3389/fimmu.2022.864628
Received: 28 January 2022; Accepted: 25 March 2022;
Published: 29 April 2022.
Edited by:
Dipayan Rudra, ShanghaiTech University, ChinaReviewed by:
Chaoran Li, Emory University, United StatesCopyright © 2022 Estrada Brull, Panetti and Joller. This is an open-access article distributed under the terms of the Creative Commons Attribution License (CC BY). The use, distribution or reproduction in other forums is permitted, provided the original author(s) and the copyright owner(s) are credited and that the original publication in this journal is cited, in accordance with accepted academic practice. No use, distribution or reproduction is permitted which does not comply with these terms.
*Correspondence: Nicole Joller, bmljb2xlLmpvbGxlckB1emguY2g=
†These authors have contributed equally to this work
Disclaimer: All claims expressed in this article are solely those of the authors and do not necessarily represent those of their affiliated organizations, or those of the publisher, the editors and the reviewers. Any product that may be evaluated in this article or claim that may be made by its manufacturer is not guaranteed or endorsed by the publisher.
Research integrity at Frontiers
Learn more about the work of our research integrity team to safeguard the quality of each article we publish.