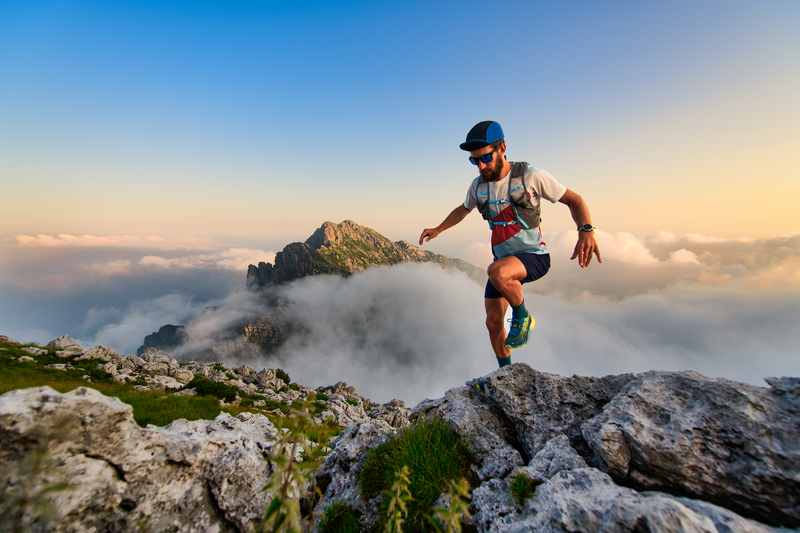
95% of researchers rate our articles as excellent or good
Learn more about the work of our research integrity team to safeguard the quality of each article we publish.
Find out more
REVIEW article
Front. Immunol. , 04 March 2022
Sec. Multiple Sclerosis and Neuroimmunology
Volume 13 - 2022 | https://doi.org/10.3389/fimmu.2022.860070
This article is part of the Research Topic Exploiting New Methods to Study Microglia in Healthy and Diseased Retina View all 6 articles
Traumatic optic neuropathy (TON) refers to a pathological condition caused by a direct or indirect insult to the optic nerves, which often leads to a partial or permanent vision deficit due to the massive loss of retinal ganglion cells (RGCs) and their axonal fibers. Retinal microglia are immune-competent cells residing in the retina. In rodent models of optic nerve crush (ONC) injury, resident retinal microglia gradually become activated, form end-to-end alignments in the vicinity of degenerating RGC axons, and actively internalized them. Some activated microglia adopt an amoeboid morphology that engulf dying RGCs after ONC. In the injured optic nerve, the activated microglia contribute to the myelin debris clearance at the lesion site. However, phagocytic capacity of resident retinal microglia is extremely poor and therefore the clearance of cellular and myelin debris is largely ineffective. The presence of growth-inhibitory myelin debris and glial scar formed by reactive astrocytes inhibit the regeneration of RGC axons, which accounts for the poor visual function recovery in patients with TON. In this Review, we summarize the current understanding of resident retinal microglia in RGC survival and axon regeneration after ONC. Resident retinal microglia play a key role in facilitating Wallerian degeneration and the subsequent axon regeneration after ONC. However, they are also responsible for producing pro-inflammatory cytokines, chemokines, and reactive oxygen species that possess neurotoxic effects on RGCs. Intraocular inflammation triggers a massive influx of blood-borne myeloid cells which produce oncomodulin to promote RGC survival and axon regeneration. However, intraocular inflammation induces chronic neuroinflammation which exacerbates secondary tissue damages and limits visual function recovery after ONC. Activated retinal microglia is required for the proliferation of oligodendrocyte precursor cells (OPCs); however, sustained activation of retinal microglia suppress the differentiation of OPCs into mature oligodendrocytes for remyelination after injury. Collectively, controlled activation of retinal microglia and infiltrating myeloid cells facilitate axon regeneration and nerve repair. Recent advance in single-cell RNA-sequencing and identification of microglia-specific markers could improve our understanding on microglial biology and to facilitate the development of novel therapeutic strategies aiming to switch resident retinal microglia’s phenotype to foster neuroprotection.
The retina is a highly organized tissue that functions to convey visual signals to the brain for both image-forming and non-image-forming visual function. The mammalian retina has more than 60 functionally distinct cell types that mainly reside in three different cellular layers (1, 2). The outer nuclear layer (ONL) consists of two types of photoreceptors: the rods and the cones. Human retina consists of approximately 92 million rod and 4.6 million cone photoreceptors (3), which are responsible for the detection of light stimuli and pass visual information to interneurons. The inner nuclear layer (INL) consists of three major types of interneurons (bipolar cells, horizontal cells and amacrine cells) (4), which process visual information and pass it to retinal ganglion cells (RGCs). The ganglion cell layer (GCL) consists of mainly RGCs which accounts for approximately 1% of the total cells (i.e. ~1.2 million) in the human retina (5). RGCs are primed to deliver visual information to the brain through the optic nerves (6, 7). Recent single-cell RNA-sequencing revealed more than 46 distinct subtypes of RGCs in the adult retina (8, 9). As an extension to the brain and a part of the CNS, mammalian retina also consists of three main types of glial cells: Müller cells, astrocytes and resident microglia; all of these glial cells are essential to maintain retinal homeostasis (10). Müller cells are highly specialized macroglial cells that span across the entire retinae and represent the largest population of glial cells in the mammalian retina (~80-90% of total glial cells) (11). Retinal astrocytes are another macroglial cells that are mainly found in the nerve fiber layer (NFL) in the mammalian retina (12). Retinal microglia were first identified by Lopez Enrique (13) and Marchesani (14) in 1926, and are mainly found in the NFL and IPL of retinae as well as in the optic tract accounting for ~5-20% of total glial cells in the retina (15–17). Retinal microglia possess distinct morphological and immunohistochemical properties, which distinguish them from horizontal cells and macroglial cells in the retina (18). In most cases, microglial cell markers such as CD11b (also known as OX42), F4/80, CD68 (antibody clone ED-1 for detection of rat CD68), and ionized calcium-binding adapter molecule 1 (IBA1) (19, 20) can be used to differentiate microglia from other cells. Retinal microglia are described as active surveillance and immunocompetent cells residing in various layers of healthy retinae including NFL, GCL, inner plexiform layer (IPL), outer plexiform layer (OPL), and INL (10).
The dynamic nature of microglia and their vast phenotypic diversity as reflected by a variety of microglial morphologies in different brain regions, and under diverse pathological conditions. In rodent retinae, microglia started to appear in the GCL and neuroblastic layer of developing retinae at embryonic day 11.5 (E11.5) (21). These resident retinal microglia adopt an amoeboid-like phenotype and actively phagocytized dying RGCs until postnatal day 5 (P5) (21, 22), a time when programed cell death of RGCs has reached its peak during the development of retinae (23). After that, amoeboid microglia transform into a ‘surveying’ phenotype with compact cell bodies and long branching processes, known as ‘ramified microglia’. Ramified microglia mainly reside in the GCL, IPL, INL, and OPL of the healthy adult retina (24) (Figure 1A). Ramified microglia are active and highly motile surveillant cells with their processes undergoing constant extension and retraction to sense the surrounding microenvironment for perturbations (25). Once subtle damage to the tissue is detected, ramified microglia quickly thicken their processes, transform into amoeboid microglia with enlarged cell bodies, and colonize in close proximity to lesions shortly after injuries (26). Amoeboid microglia are regarded as ‘activated microglia’ that are responsible for the production of pro-inflammatory (M1) and anti-inflammatory (M2) cytokines, chemokines, and various neurotrophic factors. They are also involved in the elimination of dysregulated synapses, phagocytosis of dead cells and cellular debris, and antigen presentation (17, 27). Acute and controlled activation of microglia favor neuroprotection, limit secondary tissue damages and facilitate nerve repair. In contrast, persistent microglial activation often leads to excessive production of neurotoxic pro-inflammatory (M1) cytokines and other inflammatory mediators that exacerbate secondary tissue damages. Activation of resident retinal microglia is a common hallmark of many retinal degenerative disorders, including traumatic optic neuropathy, age-related macular degeneration, glaucoma, retinitis pigmentosa, uveoretinitis and diabetic retinopathies (15, 28–31). Targeting microglial activation might therefore represent an attractive therapeutic target for the development of novel therapies in CNS injuries.
Figure 1 Inflammatory responses after optic nerve crush (ONC) injury. (A) In the intact retina, retinal microglia usually resided in NFL, GCL, IPL, INL, and OPL. These resident retinal microglia adopt ramified morphology with which they constantly send and withdraw their processes to sense the surrounding microenvironment. Retinal astrocytes and Müller cells are the two major macroglial cells that resided in retinae for the maintenance of retinal homeostasis. (B) Optic nerve crush (ONC) triggers the activation of resident retinal microglia, as well as retinal astrocytes and Müller cells. Some of the microglia transform into bipolar/rod-shaped microglia and colonize adjacent to the degenerating RGC axons in NFL. These bipolar/rod-shaped microglia actively internalized these degenerating axons after ONC. Some activated microglia adopt amoeboid morphology and actively engulf the dead RGCs in GCL. Also, these activated retinal microglia produce a broad spectrum of pro-inflammatory cytokines (e.g. IL-1β, IL-6 and TNF-α), chemokines (CCL2, CCL3 and CCL5), and reactive oxygen species (ROS), which induced reactive astrocytes to adopt a neurotoxic A1 phenotype and increased production of neurotoxic factors. Activated Müller cells are also responsible for the production of pro-inflammatory cytokines in the injured retinae. Collectively, the number of survived RGCs gradually declined over time, and most of these survived RGCs failed to regenerate across the lesion site, leading to a permanent vision loss after ONC. (C) Intraocular inflammation induced by zymosan or curdlan treatment, or lens injury triggers a massive influx of blood-borne myeloid cells, and robust astrocyte and Müller cell activation. The infiltrating myeloid cells produce a large quantity of growth-promoting factors, such as oncomodulin, which promoted RGC survival after ONC. The reactive astrocytes also elevate the production of CNTF and LIF after intraocular inflammation. More importantly, the macrophage- and astrocyte-derived growth-promoting factors stimulate robust axon regeneration which enabled a partial restoration of some visual-guided behaviors when combined with other manipulations, such as PTEN deletion. NFL, nerve fibre layer; GCL, ganglion cell layer; IPL, inner plexiform layer; INL, inner nuclear layer; OPL, outer plexiform layer; ONL, outer nuclear layer; PRL, photoreceptor layer; RPE, retinal pigment epithelium; RGC, retinal ganglion cell; ROS, reactive oxygen species. CNTF, ciliary neurotrophic factor; LIF, leukemia inhibitory factor.
In this Review, we summarize the current understanding of resident retinal microglia after traumatic optic neuropathy (TON). Compelling evidence suggests that activation of resident retinal microglia and recruitment of infiltrating macrophages are essential for effective clearance of myelin debris and production of growth factors that facilitate nerve repair. In contrast, sustained and unresolved neuroinflammation contributed to production of neurotoxic pro-inflammatory cytokines and persistent secondary tissue damages. To this end, we aim to provide a novel insight into the development of new therapeutic strategies for TON.
TON refers to any direct or indirect insult which causes damage to the optic nerves and its axonal fibres. Permanent visual impairments are seen most commonly in patients resulting from a massive loss in RGCs and axonal connections with their brain after TON (32). In addition, the remaining viable RGCs failed to regenerate axons spontaneously which further limited the restoration of visual function after injuries (33, 34). The availability of well-established rodent models of optic nerve crush (ONC) for TON and glaucomatous injury (35), which enable researchers to study molecular machinery for neuronal survival and axon regeneration for recovery of visual function. In the past decades, a broad spectrum of intrinsic regulators for pro-regenerative growth, and factors that involved in glial inhibition have been identified. Manipulation of these factors enabled sustained and long-distance axon regeneration after ONC (33, 36, 37). However, the roles of resident microglia in RGC survival and axon regeneration after ONC remained largely unexplored.
After ONC, the resident microglia become activated, adopting a reactive phenotype characterized by enlarged cell bodies and shortened processes, and colonized progressively the GCL, IPL and OPL (38, 39) (Figure 1B) as well as the optic nerves (39). Blood-borne macrophages with large and round cell bodies are also recruited to the crushed site of the optic nerve after ONC (40). The resident retinal microglia and infiltrating macrophages are known to be involved in the degradation and removal of myelin debris from the injured optic nerve, a process known as Wallerian degeneration (41). In the peripheral nervous system (PNS), after degradation of axons, myelin sheaths are collapsed, degenerated, and cleared effectively starting within hours and completed in days after peripheral nerve injury (PNI) (42–45). Within hours after PNI, Schwann cells undergo rapid dedifferentiation and proliferation at the distal nerve stump (42). In parallel, a rapid influx of blood-borne macrophages towards the proximal and distal nerve stump occurs in the first 12 hours after PNI (40, 46). Infiltrating macrophages display a tremendous phagocytic capacity to remove myelin debris (43). Successful completion of Wallerian degeneration generates a growth-permissive environment, which enables regenerating motor axons to reinnervate motor endplates at the distal target muscles for motor function recovery after PNI (44, 47–49). In contrast to the PNS, Wallerian degeneration after CNS injuries occurs at an extremely slow rate. Oligodendrocytes are the glial cells responsible for clearance of myelin debris after CNS injuries. However, oligodendrocytes underwent apoptosis soon after ONC due to the loss of retinal inputs from RGCs (50, 51) and the oligodendrocyte precursor cells failed to proliferate as well (52). Injury to the optic nerve had no immediate effect on the immune cell populations, neither colonization of resident microglia, nor influx of infiltrating macrophages were observed in the distal nerve stump at 1 week post-ONC (53). Macrophages became more prominent throughout the optic nerve only at 8 weeks post-ONC (40, 41). In addition, activated retinal microglia displayed diminished phagocytic capacity to remove myelin debris from the degenerating optic nerve (54). As a result, clearance of myelin debris by the resident retinal microglia and infiltrating macrophages become largely ineffective which persists for months after ONC (40, 51).
Injury to the CNS is accompanied by reactive gliosis, inflammation and formation of glial scar. Scar tissue and associated deposition of extracellular matrix (ECM) molecules such as chondroitin sulfate proteoglycans (CSPG), and the presence of myelin-associated inhibitors (MAIs), among which Nogo-A, myelin-associated glycoprotein (MAG) and oligodendrocyte-myelin glycoprotein (OMgp) are the major players in CNS myelin that inhibit axon regeneration (36, 55). The reactive astrocytes and the formation of a glial scar (also known as astrocytic scar) facilitate the repair of damaged tissue by limiting the spread of inflammation and separating healthy tissue from pathology following injury. However, the persistence of scarring beyond the acute phase of injury can impede axon regeneration and long-term recovery (55–57). In response to ONC, reactive astrocytes can be found in the retinae (58, 59) and optic nerve (60, 61), producing growth-inhibitory molecules such as CSPG at the crushed site (60, 61). In the presence of glial scar, regenerating axons formed dystrophic and swollen retraction bulbs while trying to penetrate a glial scar, a phenomenon known as growth cone collapse (62). The formation of dystrophic growth cones stalls the axonal growth, and therefore virtually no axons could regenerate beyond the glial scar after ONC. Unfortunately, pharmaceutical blockade or genetic ablation of some of the known MAIs failed to facilitate sustained axon regeneration for function recovery after ONC (63, 64). More importantly, chronic glial scarring has been shown to transform resident microglia and infiltrating macrophages into a neurotoxic M1-like phenotype (65–67), further exacerbating axonal damage secondary to the myelin damage. Therefore, experimental therapies to increase macrophage infiltration by treating the rats with a group B-streptococcus exotoxin, exhibited a rapid infiltration of macrophages into the injured optic nerve and demonstrated a higher phagocytic capacity than the resident retinal microglia (54). The increase in macrophage influx around the crushed site led to the reduction of glial scar and reactive astrocytes, and the effective clearance of myelin debris facilitating axons to regenerate across the glial scar after ONC (68). Furthermore, pre-treating peripheral blood-borne macrophages with sciatic nerve segments activated macrophages and enhanced their phagocytic capacity. Subsequent transplantation of these activated macrophages into injured optic nerve facilitated myelin debris clearance within 7 days after optic nerve transection (69). This raise the possibility that by switching resident retinal microglia and infiltrating macrophage polarization to a neuroprotective M2-like phenotype with higher phagocytic capacity creates a growth permissive microenvironment for axon regeneration after ONC (70). In fact, M2 microglia produce anti-inflammatory cytokines and neurotrophic factors (71, 72) that are neuroprotective in nature promoting neuronal survival and repair after CNS injuries (73, 74). In another study, recombinant interferon-β (IFN-β) augmented the phagocytic capacity of primary microglia and contributed to the active removal of myelin debris by activated microglia in vitro (75). Further investigation is required to examine whether these factors would also prime resident retinal microglia for effective clearance of myelin debris and switch a hostile CNS microenvironment to a growth-permissive microenvironment after ONC.
The resident microglia express a range of surface receptors for recognition and phagocytosis of cell undergoing apoptosis (76). Accumulating evidence suggests that the clearance of myelin debris after CNS injuries involves the activation of complement signaling cascades (77–79). A recent study highlighted that complement activation in resident retinal microglia and infiltrating macrophages are pre-requisite for successful axon regeneration after ONC. Both complement proteins C1q and C3 as well as the complement receptor CR3, were markedly elevated in the optic nerve but not in the mouse retinae shortly after ONC (80). It has been reported that complement protein C1q and C3 promoted axon regeneration and function recovery after SCI (81, 82). Genetic ablation of C1q, C3 and CR3 receptors, completely abolished the growth-promoting effects induced by several manipulations known to promote axon regeneration in adult mice after ONC (80), including intraocular inflammation using zymosan treatment (83–86), zinc chelator TPEN to block the accumulation of mobile zinc ions in injured RGCs (87), and deletion of phosphatase and tensin homolog (PTEN) with oncomodulin treatment (88, 89). Resident microglia are known to express and produce both complement proteins C1q and C3 in the retina (90, 91). To address the roles of resident retinal microglia in the production of complement proteins and activation of complement signaling cascades, the authors attempted to deplete microglia using PLX5622 which allows the studies of RGC axon regeneration in the absence of microglia (80). PLX5622 and its structural homolog PLX3397 could eliminate virtually all microglia from the adult CNS through binding the colony-stimulating factor-1 receptor (CSF1R) (92–94). Both PLX5622 and PLX3397 becomes a valuable tool to study the roles of microglia in the pathogenesis of numerous neurodegenerative diseases such as Alzheimer’s Disease (93), Parkinson’s Disease (95), ischemic stroke (96), amyotrophic lateral sclerosis (97), and Charcot-Marie-Tooth disease (98). A recent study demonstrates that PLX5622 treatment eliminated most of the resident retinal microglia from injured optic nerve and retinae. Subsequently, the expressions of complement proteins C1q and C3, and CR3 receptor, were markedly reduced at the early time points after ONC suggesting that resident retinal microglia are the major source of these complement proteins. More importantly, activated resident retinal microglia with high expression of CR3 receptor, actively removed myelin debris at the lesion site after ONC to create a growth-permissive microenvironment for axon regeneration. In contrast, genetic ablation of CR3 receptor largely impaired the clearance of myelin debris at the lesion site and thus stalled axon regeneration (80). Interestingly, one study suggested that microglial depletion using PLX5622 did not affect lens injury-induced axon growth in adult mice after ONC (94). The reason for this discrepancy is unclear but could possibly be related to differences in the time points being analyzed (14 versus 21 days post-ONC). Nevertheless, another possible explanation is that lens injury induces a group of growth-promoting factors that are unavailable in the zymosan-induced intraocular inflammation, and the lens injury-specific growth-promoting factors support RGC axon regeneration even when the clearance of myelin debris was largely impaired in the absence of retinal microglia. Interestingly, a suboptimal dose of zymosan produced modest level of axon regeneration in C57BL/6 mice; however, administration of the same doses of zymosan in CAST/Ei mice sustained long-distance axons regenerated along the entire length of the optic nerve, reaching the optic chiasm (99). It suggests that genetically diverse inbred mouse strains with varied intrinsic growth capacity response very differently to lens injury and regenerate injured CNS axons at different extent. The reasons for this will require further investigation of the casual link between inflammation and retinal microglia responses across mouse strains.
ONC triggers neuronal apoptosis in the RGCs (100), which activates resident retinal microglia and recruitment of infiltrating macrophages into the retinae and proximal nerve stump (59). In adult female rats, an increased number of microglia was detected in the retinae as early as 2 days after ONC and accompanied by increase production of neurotoxic pro-inflammatory cytokines in the retinae and proximal nerve stump, resulting in widespread neuronal loss and axon degeneration after ONC (38). The number of activated microglia gradually increased over time, with its peak at day 14 after ONC. Sustained microglial activation was observed in the retinae even 2 months after ONC and RGC survival was reduced to 7% (38). At the same time, the expression levels of M2 markers (CD206, Ym1 and Arg1) were substantially declined and expression levels of M1 markers (IL-1β, IL-6 and TNF-α) were elevated after prolonged activation of retinal microglia, which induced neuronal apoptosis and exacerbated secondary tissue damage (101, 102). It is well documented that inducible nitric oxide synthase (iNOS) expression level is upregulated in activated microglia, which can cause RGC death (103, 104). Profound microglial activation was also observed in the proximal nerve stump at day 3 post-ONC, with its peak at day 5 post-ONC, which was accompanied by elevated production of neurotoxic pro-inflammatory cytokine IL-1β and profound axonal damage (105).
ONC triggers not only the activation of resident retinal microglia, but also other macroglia in the retinae, including astrocytes and Müller cells. Growing evidence suggests that resident retinal microglia might interact with these macroglial cells to modulate RGC survival and axon regeneration after ONC (106). After ONC in rats, the intraocular glutamate level became significantly elevated in the first week of injury, which gradually returned to basal level at two weeks post-injury (107). The elevated intraocular glutamate induced activation of Müller cells which led to the release of adenosine triphosphate (ATP) (108), a well-known chemoattractant for microglia and an important mediator for injury-induced microglial responses (26). In primary culture of rat brain microglia, microglia were highly responsive to extracellular ATP with increased expression of neurotoxic pro-inflammatory cytokines IL-1β and TNF-α (109, 110). Co-culture of Müller cells and lipopolysaccharide (LPS)-activated retinal microglia, Müller cells became highly activated with prominent alternation in cell morphology and increased production of a number of neurotrophic factors and pro-inflammatory modulators, including glial cell-derived neurotrophic factor (GDNF), leukemia inhibitory factor (LIF), IL-1β, IL-6 and iNOS (111). Interestingly, conditioned medium derived from activated Müller cells further stimulated retinal microglia to produce more IL-1β, IL-6 and iNOS in cultures (111), which suggests a reciprocal signaling between Müller cells and resident retinal microglia to prolong the local inflammatory responses in the injured retinae. There is increasing evidence that resident retinal microglia might interact with retinal astrocytes directly after ONC. Indeed, a recent study showed that neurotoxic reactive astrocytes were primed by activated microglia in cultures. These effects were accomplished through increased secretion of pro-inflammatory cytokines IL-1α and TNF-α, and classical complement component C1q by activated retinal microglia, which induced reactive astrocytes to adopt a neurotoxic A1 phenotype in the retinae leading to RGC death after ONC in mice (112, 113). Interestingly, resting astrocytes promoted synaptogenesis in axotomized RGC cultures; however, RGCs failed to form synapses when co-cultured with A1 astrocytes. In addition, A1 astrocytes almost completely lose the phagocytic ability to phagocytose synaptosomes and myelin debris in cultures, suggesting potential ineffective synaptic pruning and myelin debris clearance which hampers the axonal remodeling and regrowth (112).
Sustained and unresolved inflammation in the retinae might also affect the permeability of blood-retinal barrier after ONC. The disruption or breakdown of blood-retinal barrier is a clinical feature of several ocular diseases including glaucoma, diabetic retinopathy, age-related macular degeneration, retinal vein occlusion, and uveitis (114, 115), which often lead to macular edema and retinal tissue damage due to increased vascular permeability (115). Accumulating evidence suggests that chronic neuroinflammation and elevated production of pro-inflammatory cytokines in the retinae contributed to the breakdown of blood-retinal barrier in diabetic retinopathy. Elevated levels of pro-inflammatory cytokines such as IL-1β, TNF-α and IL-6 were found in the retinae of patients with diabetic retinopathy (116–118), as well as in mouse or rat models of diabetic retinopathy (119–121). Intravitreal injections of pro-inflammatory cytokines markedly increased the permeability of blood-retinal barrier, and caused a massive influx of leukocytes into the retinae in both rats and rabbits (122–126). Interestingly, the blood-retinal barrier remained relatively intact up to 12 days after ONC (53). Nevertheless, it is yet to determine whether prolonged activation of retinal microglia and elevation of pro-inflammatory cytokines (IL-1β, IL-6 and TNF-α) (101, 102) contributed to the breakdown of blood-retinal barrier after ONC. In a mouse model of TON, expression levels of zonula occludens-1 and occluding (major tight junction proteins present in the blood-retinal barrier) were markedly reduced in the retinae, indicating the dysfunction of blood-retinal barrier. At the same time, elevated levels of pro-inflammatory cytokine TNF-α and IBA-1-positive activated microglia were detected in the retinae at 5 days post-injury (127).
Microglial activation and subsequent inflammation-mediated neurotoxicity are implicated as a target of therapeutic intervention to alleviate neuronal cell death and axonal injury. For instance, arginase 2 was elevated shortly (6 h) after ONC. Genetic ablation of arginase 2 markedly suppressed microglial activation in the injured retina and reduced the mRNA levels of IL-1β as well as its precursor (pro-IL-1β) protein expression. More importantly, arginase 2 ablation and the attenuation of microglial activation protected injured RGCs from apoptosis, and induced a considerable amount of axon regeneration at day 14 post-ONC (128). Oral administration of spermidine, an endogenous free radical scavenger that protected the cells from oxidative stress, markedly inhibited microglial activation and expression of chemokines such as CCL2 and CCL5 after ONC. The expression of iNOS was also suppressed in the activated microglia resulted in greater RGC survival and modest yet significant axon regeneration two weeks after ONC (129). Neutralizing antibodies specific for Sems3A abolished the Sema3A actions and switched the retinal microglial polarization from neurotoxic M1-like phenotype to neuroprotective M2-like phenotype at 3-7 days post-ONC, which promoted RGC survival (102). Interestingly, microglial depletion using PLX5622 exhibited a transient neuroprotective effect against RGC death and axon degeneration at day 5 after ONC (105), possibly due to the reduction of microglia-derived semaphorin 3A (Sema3A) and M1-activated microglia in the retinae (102). However, depletion of retinal microglia using PLX5622 did not further enhanced or reduced RGC survival at later stage (days 7-21) after ONC (94). A recent study showed that by inhibiting the activity of mammalian target of rapamycin (mTOR) using resveratrol (sirtuin-1 activator which inhibits mTOR activity) (130) or by genetic ablation of microglia-specific regulatory-associated protein of mTOR (Raptor) largely suppressed the colonization of activated microglia, which led to a reduced level of IL-1β in proximal nerve stump after ONC. RGC loss and axonal damage was reduced in mice with resveratrol treatment or Raptor deletion in microglia at day 5 post-ONC (105). In another study, intravitreal injection of neutralizing antibodies against microglia-specific secretory factors IL-1α, TNF-α and C1q inhibited the neurotoxic A1 astrocytes formation and the subsequent RGC death after ONC in mice (112, 113). These studies collectively suggest that by counteracting the detrimental role of microglia, instead of complete removal of resident microglia from injured retinae and optic nerve, could elicit a potent neuroprotective and pro-regenerative effect after ONC.
Lens injury primes adult RGCs into an actively growing state as a result of the activation of resident retinal microglia, astrocytes and Müller cells, and infiltration of peripheral myeloid cells into the retinae, leading to enhanced RGC survival and axon regeneration after ONC in both mice and rats (83, 131, 132). Intravitreal injection of zymosan, a cell wall suspension from yeast, elicits a massive infiltration of myeloid cells into the retina and recapitulates the lens injury-induced growth-promoting effects (83, 84, 99). Meanwhile, the infiltration of myeloid cells into the retinae also activated retinal astrocytes and Müller cells, leading to the production of Müller cell-derived growth factors and robust axon regeneration after intravitreal injections of zymosan and ONC. In stark contrast, intra-optic nerve zymosan injection failed to elicit infiltration of myeloid cells into the retinae and thus the activation of retinal astrocytes and Müller cells became very limited, resulting in poor axon regeneration after ONC (133). These results suggest that there may be crosstalk among resident retinal microglia, astrocytes, Müller cells, and infiltrated myeloid cells in the injured retinae for mediating inflammation-induced growth-promoting effects. However, current knowledge about the major factors contributing to the lens injury-induced and zymosan-induced growth-promoting effects remain limited. For instance, axotomized adult rat RGCs exhibited longer neurites when co-cultured with lens and vitreous body from zymosan-treated mice than vehicle-treated mice, since a large number of infiltrating macrophages were observed in the vitreous body after zymosan treatment. (132). Similarly, conditioned medium derived from zymosan-treated rat macrophage cultures promoted neurite outgrowth from axotomized adult rat RGCs (84). In a follow-up study, oncomodulin was identified as one of the major macrophage-derived growth factors for RGCs, which play a key role in the pro-regenerative processes of injured RGCs (84–86, 134). Intravitreal injections of recombinant oncomodulin with concurrent elevation of cyclic AMP (cAMP) enhanced binding of oncomodulin to RGCs (88) that recapitulated the zymosan-induced growth-promoting effects on axon regeneration (134). Nevertheless, Zymosan treatment alone did not induce sustained axon regeneration to grow over long distances to reinnervate visual target and thereby resulted in poor visual function recovery (89). Combinatorial treatments with zymosan and PTEN deletion that induced robust axon regeneration through activation of mTOR-mediated signaling pathway (61, 135), allowing axon to regenerate down the full length of the optic nerve, and to reinnervate the distal subcortical brain regions including the suprachiasmatic nucleus (SCN), lateral geniculate nucleus (LGN), olivary pretectal nucleus (OPN) and superior colliculus (SC) after ONC in mice (88, 89). The regenerated RGC connections partially rescued visually guided behaviors such as optomotor responses and depth perception (89). Mechanistically, it has been shown that β-glucan/dectin-1 signaling cascade was identified as a critical mediator of zymosan-induced axon regeneration (136). Dectin-1 is a phagocytic receptor expressed on resident microglia and infiltrating macrophages in the retinae (136), which acts as one of the pattern recognition receptors for zymosan (137). Genetic ablation of dectin-1 abolished the zymosan-induced axonal growth after ONC (136). Intravitreal injections of curdlan, a water-insoluble β-glucan which formed complex with dectin-1 (138), recruited retinal microglia and infiltrating myeloid cells that highly expressed dectin-1 to the retinae and induced robust axon regeneration two weeks after ONC. Interestingly, the curdlan-induced axonal growth requires dectin-1 expression in both resident retinal microglia and infiltrating myeloid cells. Genetic ablation of dectin-1 in either resident retinal microglia or infiltrating myeloid cells markedly attenuated the curdlan-induced axonal growth after ONC (136) (Figure 1C). These studies collectively point to the direction that the production of secreted factors might contribute to the lens-injured induced growth-promoting effects by activating resident retinal glial cells such as retinal microglia and inflammatory responses elicited occur in the presence of infiltrating macrophages after ONC (139) that requires further investigation.
Lens injury and intravitreal injections of zymosan trigger intraocular inflammatory responses in the retinae leading to unprecedented levels of axon regeneration following ONC; however, depletion of resident retinal microglia using PLX5622 did not attenuate the degree of axon growth, suggesting the lack of involvement of resident retinal microglia in the pro-regenerative responses associated with lens injury. Also, co-treatment of PLX5622 with liposomal clodronate can be used to deplete both resident retinal microglia and infiltrating macrophages, which only shows modest impairment of intraocular inflammation that are required for axon regeneration (94). One possible explanation is that the presence of other glial cells such as astrocytes produce growth factors contributing to the lens injury- and zymosan-induced pro-regenerative processes. In fact, there was a profound up-regulation of ciliary neurotrophic factor (CNTF) and LIF expression in the reactive astrocytes at the GCL of the injured retinae (58, 140). Deletion of either CNTF or LIF alone, or co-deletion of both, or deletion of STAT3 (the downstream effector of CNTF), abolished the growth-promoting effects of lens injury or zymosan treatment (58, 140, 141). It has been reported that CNTF activated STAT3 in the RGCs via the JAK/STAT signaling pathway, and the activation of JAK/STAT signaling pathway might mediate the neuroprotective effects of CNTF on RGCs directly (142, 143). Intravitreal CNTF administration induced the recruitment of activated retinal microglia and infiltrating macrophages to facilitate its growth-promoting effects on RGC survival and axon regeneration after ONC in adult rats. Depletion of infiltrating macrophages by liposomal clodronate completely abolished the growth-promoting effects of CNTF (144). Yet researchers have long been baffled by CNTF contradictory effects on RGCs. Recombinant CNTF promoted axon outgrowth from neonate RGCs in culture (145), but failed to exert similar effect on cultured mature RGCs (134). Several studies reported that recombinant CNTF promoted optic nerve regeneration (58, 140), however; others found very limited promoting effects unless suppressor of cytokine signaling 3 (SOCS3) was deleted in the RGCs (146, 147). Effective administration of CNTF to RGCs may be hampered by the exceedingly short half-life of CNTF (148). In fact, the use of viral-based vector to transfer CNTF into injured retinae has proven to be a promising method for long-term expression of CNTF results in a robust axon regeneration (149–152), possibly via up-regulation of C-C motif chemokine ligand 5 (CCL5) in macrophage and infiltrating neutrophil. Depletion of neutrophil or pharmaceutical blockade of CCL5 receptor CCR5, completely abolished the growth-promoting effects induced by CNTF overexpression (152). Another plausible explanation for the ineffectiveness of microglia/macrophage depletion to compromise lens injury-induced axon regeneration is that macrophage might not be the only immune cell producing oncomodulin. Previous studies showed that infiltrating macrophages and neutrophils in the aqueous and vitreous humor produced oncomodulin after lens injury or zymosan treatment (85, 86, 134). Blocking the binding of oncomodulin to its receptors or depletion of neutrophils decreased the number of axons regenerating into the distal optic nerve after zymosan treatment, but did not completely abolish the promoting effects (85, 86, 88). High expression of oncomodulin was detected in both GCL and IPL in the retina by immunohistology (134), quantitative RT-PCR, and Western blot analysis (85). Nevertheless, the oncomodulin-positive cells did not overlap with ED-1-positive microglia or infiltrating macrophages (134). It suggests that, other than infiltrating microglia/macrophages, there might be other cells that produce oncomodulin after lens injury or zymosan treatment. Recently, a conditional microglia/macrophage-specific CX3CR1-Cre mouse line, which expresses Cre recombinase under the control of a chemokine receptor CX3CR1 promoter, was established for studying microglia/macrophage (153). By crossing the CX3CR1-Cre transgenic mouse line with a oncomodulin-floxed mouse line (154), oncomodulin could be deleted specifically from most of the resident retinal microglia and infiltrating macrophages. Using the conditional gene knock-out approaches, one might conclude that if infiltrating myeloid cells were the major source of oncomodulin. It might help to address the controversies regarding the importance of oncomodulin and microglia/macrophage activation in promoting axon regeneration (155, 156).
Long-distance axon regeneration induced by combinatorial treatments of zymosan and PTEN deletion partially restored optomotor responses and depth perception; however, most of the mice developed severe cataracts months after ONC possibly due to chronic and unresolved intraocular inflammation (89). Mice with cataracts were unable to regain a proper pupillary light reflex (PLR) and to constrict the pupil in response to light stimulation (89), despite the regenerated axons have already reached the sub-cortical brain target OPN that is responsible for PLR (157, 158). Zymosan and curdlan treatment induced a massive influx of infiltrating macrophages and activation of resident microglia, which caused retinal folding and detachment in the treated retinae (136), a condition similar to experimental autoimmune uveitis (159). Of note, although zymosan treatment triggered intraocular inflammation that promoted RGC survival and robust axon regeneration (83), some secretory factors generated by the infiltrating macrophages exhibited strong growth-inhibitory effects on neurite outgrowth and survival in axotomized adult RGCs in vitro (84). Therefore, a more in-depth study is required to identify myeloid cell subtypes and its associated growth factors, which induce axon regeneration and nerve repair without causing any tissue damages.
Some approaches that promote long-distance axon regeneration also induce a considerable degree of remyelination and support functional restoration of some simple visual tasks after ONC (89, 160). However, remyelination does not necessarily occur immediately after the injured RGC axons regenerated the entire length of the optic nerve. For instance, combinational genetic manipulations by co-deletion of PTEN and SOCS3 and overexpression of CNTF, or overexpression of osteopontin, IGF-1 and CNTF enabled long-distance axon regeneration and formation of functional synapses with their distal brain targets. These regenerating axons provoked significant neural activity at the superior colliculus upon stimulation to the injured axons. However, the regenerated axons remained poorly myelinated leading to poor recovery of visual-associated behaviors such as optomotor acuity after ONC (150), suggesting remyelination is required for visual function restoration.
Optic nerve transection led to a massive loss of oligodendrocytes in adult mice and rats (50). For myelination to occur after CNS injuries, oligodendrocyte precursor cells (OPCs) need to undergo rapid proliferation, differentiate into mature oligodendrocytes and ensheath the regenerating axons for proper nerve conduction (161, 162). Accumulating evidence suggested that resident microglia were involved in the remyelination process after CNS injuries (163). In a mouse model of demyelination, M1 (TNF-α/iNOS-positive) microglia and macrophages were dominant at the early time point when OPCs were recruited and proliferated at the lesion site (164). These M1-polarized microglia and macrophages also expressed high levels of genes related to myelin debris clearance including C1q complement proteins and CX3CR1. Interestingly, these M1-polarized microglia and macrophages underwent necroptosis and most of them were eliminated before the onset of remyelination (165). At the time when OPCs initialized to differentiate into mature oligodendrocytes, these residual microglia and macrophages transformed into M2-like phenotype (165), and expressed distinct M2 markers arginase-1, CD206 and IGF-1 (164). Blocking the CD206 (M2) receptors suppressed M2 polarization in these microglia and macrophages, and inhibited oligodendrocyte differentiation. In contrast, promoting M2 polarization of these microglia and macrophages accelerated the remyelination process (164), suggesting that microglial polarization might be a key determinant for successful oligodendrocyte differentiation and remyelination.
A recent study showed that OPCs were rapidly proliferated at the crushed optic nerve in the first five days, and gradually declined to basal levels at the later time points after ONC. This process largely depends on activation of resident retinal microglia as depleting these activated microglia immediately after ONC largely inhibited the rapid proliferation of OPCs in the first two weeks after ONC. Unfortunately, these proliferating OPCs failed to differentiate into mature oligodendrocytes for remyelination even though robust axon regeneration occurred after growth stimulation by overexpression of osteopontin, IGF-1 and CNTF. One possible reason for this remyelination failure is that the resident retinal microglial remained activated even at later time points (i.e. 21 days) after ONC. These activated microglia adopted a M1-like phenotype and expressed a high level of iNOS. Delayed elimination of activated microglia in the injured optic nerve at 2 weeks post-ONC not only allowed the OPCs to proliferate, but also promote maturation of these OPCs to differentiate into mature oligodendrocytes for remyelination at 6 weeks post-ONC (101). Of note, promoting myelination is crucial to protect the regenerating axons from degeneration and sustain long-distance axon regeneration towards the distal brain targets for reinnervation. Most of the unmyelinated regenerating axons started to degenerate if they failed to innervate their targets in a short period of time (101). Therapeutic interventions that promoted M2 microglial polarization might be beneficial to facilitate robust remyelination after ONC (163).
The roles of ramified and amoeboid microglia have been extensively studied under normal and pathogenic conditions. However, little is known about the functional role of bipolar/rod-shaped microglia largely due to the lack of in vitro system to enrich bipolar/rod-shaped microglia (166, 167) and a highly reproducible in vivo model (168–170). Bipolar/rod-shaped microglia were first classified as an activated form of microglia by Nissl in 1899 (171) with slim cell bodies and indefinitely long processes. Bipolar/rod-shaped microglia were identified in postmortem cerebral cortices from patients with paralytic dementia, syphilis, typhus infections, and sleeping disorders (171, 172). The occurrence of these infectious diseases was dramatically reduced due to the rapid development of penicillin and other antibiotics, and thus reports on these bipolar/rod-shaped microglia became scarce until they were “rediscovered” in an experimental model of traumatic brain injury in adult rats (173). After a midline fluid percussion injury (mFPI), the resting ramified microglia quickly adopted a ‘rod-like’ morphology and colonized in primary somatosensory barrel field (S1BF) which formed trains of end-to-end alignment spanning perpendicular to the dura surface across the cortical layers at day 1 post-injury (173, 174). The formation of the trains of bipolar/rod-shaped microglia became more pronounced and with its peak at day 7 post-injury (173). Bipolar/rod-shaped microglia are morphologically distinct from other microglia subtypes, with an increase in cell length to width ratio and significantly fewer side processes (174). These bipolar/rod-shaped microglia formed alignment in close proximity to injured axons and expressed a high level of CD68 (a phagocytic marker) (173). The formation of the end-to-end alignment of bipolar/rod-shaped microglia was also found in other neuropathological disorders such as Alzheimer’s Disease, Parkinson’s Disease, Huntington’s Disease, viral encephalitis, lead encephalopathy, subacute sclerosing panencephalitis, and ischemic stroke. The possible functional roles of the bipolar/rod-shaped microglia in these disorders have been extensively discussed in recent reviews by us and others (168–170). The current Review only focuses on the role of bipolar/rod-shaped microglia in retinal disorders, especially on the pathological conditions in TOP.
Compelling evidence suggested that traumatic injuries to the optic nerve and retinal degeneration induced the formation of end-to-end alignments of bipolar/rod-shaped microglia in the retinae. In a rodent ocular hypertension (OHT) model of glaucoma, microglia quickly adopted bipolar/rod-shaped morphology with their processes aligned end-to-end in the NFL of OHT retinae, but not the contralateral eye (175). The bipolar/rod-shaped microglia in the OHT retinae exhibited high immunoreactivity of CD68 (phagocytic marker) and MHC-II (marker for antigen-presenting cells), but relatively low immunoreactivity of CD86 (M1 marker) and Ym1 (M2 marker) (175, 176). In contrast, amoeboid microglia were found in the OHT retinae with high immunoreactivity of both CD86 and Ym1 (176), whereas ramified microglia were restricted in the contralateral eye with low immunoreactivity of CD68 and MHC-II (175). The results suggest that bipolar/rod-shaped microglia are immunocompetent cells with distinct immunophenotypic profiles when compared with ramified (resting) and amoeboid (activated) microglia. After optic nerve transection in rats, microglia became activated and gradually adopted an amoeboid morphology as soon as at day 1 post-injury (38, 177). Amoeboid microglia actively engulf dying RGCs which have gradually lost the expression of Brn3a (RGC marker) (38). Interestingly, some of the activated microglia begin to transform into bipolar/rod-shaped microglia with elongated cell bodies and form end-to-end alignments in the GCL and NFL of retinae after ONC (Figure 1B). Trains of bipolar/rod-shaped microglia started to appear at day 3 post-injury, became more prominent at days 7 to 21 post-injury, and gradually disappeared at 6 weeks post-injury (38, 177). These bipolar/rod-shaped microglia were highly proliferative and aligned in close proximity to the βIII-tubulin-positive RGC axon fibres (177), in which they preferentially internalized the degenerating RGC axons, but not the RGC cell bodies (38, 177). Collectively, these studies suggest that bipolar/rod-shaped microglia are phagocytic cells that contribute to the clearance of degenerating RGC axons after optic nerve injuries.
Enriched ramified and amoeboid microglia are prepared by culturing primary microglia in a fibronectin-coated and laminin-coated surface, respectively (178). It allows researchers to examine the molecular and cellular responses of microglia with different morphologies to various cytokines, chemokines, chemoattractant, endotoxin, and so forth (168). However, the lack of a highly reproducible in vitro model system to enrich bipolar/rod-shaped microglia largely limited the studies on the functional characterization of this form of microglia in an isolated system. Recently, we have developed a cost-effective method to enrich bipolar/rod-shaped microglia in vitro with high reproducibility (166, 167). Primary microglia purified from postnatal mice were seeded onto a poly-D-lysine and laminin-coated surface with multiple scratches. Physical scratches removed laminin coating from the surface (167) and microglia colonized in the laminin-free scratched surface to form bipolar processes and end-to-end alignments in a direction parallel to the physical scratches (166, 167). Primary microglia colonized in the laminin-rich non-scratched surface displayed an amoeboid morphology that actively digested the surrounding laminin by up-regulation of laminin-cleaving proteins ADAM9 and CTSS (167). Compared with amoeboid-enriched primary microglia, bipolar/rod-shaped microglia were highly proliferative and exhibited decreased levels of both M1 (TNF-α, IL-1β, CD32 and CD86) and M2 (IL-10 and TGF-β) markers (166). Upon LPS challenge, bipolar/rod-shaped microglia could quickly transform into amoeboid morphology and elevate the expression of M1 markers (TNF-α and IL-1β) (166) and laminin-cleaving proteins ADAM9 and CTSS (167). Taken together, our in vitro model system successfully produces highly-enriched bipolar/rod-shaped microglia which are highly resemble the in vivo end-to-end alignment of bipolar/rod-shaped microglia in the brain after CNS injuries. Cultured bipolar/rod-shaped microglia exhibit key morphological and phenotypic characteristics (i.e. highly proliferative with distinct M1/M2 polarization) reminiscent of bipolar/rod-shaped microglia in S1BF after mFPI (173, 174) and injured retinae after axotomy (38, 177). In recent years, primary retinal microglia have been successfully isolated and purified from the postnatal (179, 180) and adult (181) mice. We believe that our in vitro system could be used to enrich bipolar/rod-shaped microglia from primary retinal microglia for gene expression profiling and cellular property studies.
Traumatic injuries trigger activation of resident microglia, as well as a massive influx of blood-borne macrophages (38, 39). It remains a technical challenge to distinguish between resident microglia and infiltrating myeloid cells from the pool of activated microglia/macrophage residing in the injured retinae and optic nerves. Both resident retinal microglia and infiltrating myeloid cells expressed high levels of CD11b, CD45, F4/80, and IBA-1 (20, 182). One might manage to separate CD11b-positive resident microglia expressing a relatively low level of CD45 (i.e. CD11b+/CD45low) from those CD11b-positive infiltrating macrophages expressing a relatively high level of CD45 (i.e. CD11b+/CD45high) by fluorescence-activated cell sorting analysis (183). However, the expression of CD45 gradually increased in the resident microglia over the course of neuroinflammation, making it difficult to separate the resident microglia from infiltrating macrophages at the advanced stages of neuroinflammation (184). Despite the fact that both resident microglia and blood-borne macrophages share similar biological functions such as antigen presentation, phagocytosis of cellular debris, and production of inflammatory cytokines and chemokines (185). A recent study highlighted that there was a substantial difference between resident microglia and blood-borne macrophages in gene expression profiles. It implies that these two populations of cells have clear functional differences under normal and pathogenic conditions (186). Therefore, a microglia-specific marker is required to distinguish resident microglia from infiltrating myeloid cells and thus researchers can study the unique functions and cellular responses of resident microglia after CNS injuries.
A recent study identified TMEM119 as a microglia-specific marker that specifically labeled resident retinal microglia with no immunoreactivity in infiltrating myeloid cells. TMEM119 was exclusively expressed in the resident microglia in the brain parenchyma, but not expressed in blood-borne macrophages that abundantly residing in the liver and spleen (187). In a subsequent experiment, ONC was performed in CCR2-RFP mice (187) with which the red fluorescent protein (RFP) was expressed in infiltrating myeloid cells only (188). After ONC, two distinct populations of IBA-1-positive cells migrated to colonize the crushed site. A population of IBA-1-positive cells displayed distinctive TMEM119 immunoreactivity, but no RFP expression, were characterized as resident retinal microglia. Those IBA-1- and RFP-positive cells representing the population of infiltrating myeloid cells, which showed no TMEM119 immunoreactivity. It therefore suggests that TMEM119 is a microglia-specific marker labeling both resting and activated microglia (187).
In another study, P2RY12 was characterized as a resident microglia marker (186). The expression of P2RY12 was dramatically reduced during the progression of neurodegenerative diseases such as Alzheimer’s disease and multiple sclerosis (189–192), suggesting that the expression level of P2RY12 might be used as an indicator of resident microglia and activated microglia (i.e. TMEM119-positive and P2RY12-negative) after CNS injuries. A recently developed TMEM119-tdTomato and TMEM119-eGFP reporter mice enabled the isolation and purification of resident retinal microglia for transcriptomic studies (193, 194), which might facilitate the identification of potential growth mediators associated with activated microglia after intraocular inflammation. In addition, TMEM119-CreERT2 transgenic mice expressed Cre recombinase only in the resident microglia (193). By crossing the TMEM119-CreERT2 transgenic mice with transgenic mice expressing Cre-inducible diphtheria toxin receptor (DTR) (195), microglial depletion could be achieved after intraperitoneal injections of tamoxifen (to induce the expression of Cre recombinase in resident microglia and the expression of DTR) followed by a low dose of diphtheria toxin to deplete resident microglia. This transgenic mouse model allows the study of RGC survival and axon regeneration after ONC in the absence of resident retinal microglia without the need for a continued supply of PLX3397- or PLX5622-containing chow diet.
Current available strategies for analyzing gene expression perturbation from bulk cell population (e.g., CD11b+/CD45low resident microglia and CD11b+/CD45high infiltrating macrophages) are not sufficient to resolve cell-to-cell heterogeneity in complex immune responses (e.g., ONC and intraocular inflammation). Intraocular inflammation triggers the production of growth-promoting factors that accelerated axon regeneration after ONC (84, 85, 134), and growth-inhibitory factors that induce axon degeneration, secondary tissue damages and apoptotic cell death (84, 136). In the injured retinae, it is believed that a specialized subset of activated microglia and macrophages contribute to the production of neuroprotective agents, while the other subsets contribute to the production of neurotoxic factors. Transcriptome deconvolution allows the evaluation of relative changes in cell-type proportions after ONC based on transcriptomic data generated from the bulk microglial population, subject to the availability of readily available and well-characterized marker genes from each microglial subset (196, 197). In fact, the availability of single-cell genomic technologies such as single-cell RNA-sequencing enables a more comprehensive and unbiased molecular characterization of diverse microglial subsets under normal and pathogenic conditions. It also allows the identification of novel markers representing microglial subpopulations with different activation statuses (198). Using single-cell RNA-sequencing, a recent study successfully identified a novel microglial subtype, known as disease-associated microglia (DAM), which contributed to the progression of Alzheimer’s Disease. Upon the disease progression, resident microglia gradually lost their homeostatic identity with down-regulation of P2ry12, P2ry13 and Cx3cr1, and increased expression of DAM marker genes (Apoe, Lpl, Cd9, Cst7 and Trem2) (189). With the availability of several microglia-specific reporter mouse lines (193, 194), one can purify the resident retinal microglia from injured retinae and optic nerves, and to further identify microglial subtypes that contribute to the neuroprotective and growth-promoting effects after ONC. This strategy provides an important foundation for future development of novel therapeutic interventions by switching the resident retinal microglia’s phenotype to foster neuroprotection.
NPBA and CHEM discussed and developed the idea of the review. NA performed the literature review and wrote the first draft of the manuscript under the supervision of CHEM. CHEM revised the manuscript for final submission. All the authors have read the final version of the manuscript and approved for submission.
This work was supported in part by a General Research Fund (GRF) from The Research Grant Council of the Government of the Hong Kong Special Administrative Region (CityU 11100519, and CityU 11100318); the Health and Medical Research Fund (HMRF), Food and Health Bureau, Hong Kong Special Administrative Region Government (07181356); and the National Natural Science Foundation of China (NSFC) (81971149) awarded to CHEM.
The authors declare that the research was conducted in the absence of any commercial or financial relationships that could be construed as a potential conflict of interest.
All claims expressed in this article are solely those of the authors and do not necessarily represent those of their affiliated organizations, or those of the publisher, the editors and the reviewers. Any product that may be evaluated in this article, or claim that may be made by its manufacturer, is not guaranteed or endorsed by the publisher.
Schematic illustrations are created with BioRender.com.
1. Masland RH. The Fundamental Plan of the Retina. Nat Neurosci (2001) 4:877–86. doi: 10.1038/nn0901-877
2. Goldman D. Muller Glial Cell Reprogramming and Retina Regeneration. Nat Rev Neurosci (2014) 15:431–42. doi: 10.1038/nrn3723
3. Curcio CA, Sloan KR, Kalina RE, Hendrickson AE. Human Photoreceptor Topography. J Comp Neurol (1990) 292:497–523. doi: 10.1002/cne.902920402
4. Masri RA, Weltzien F, Purushothuman S, Lee SCS, Martin PR, Grunert U. Composition of the Inner Nuclear Layer in Human Retina. Invest Ophthalmol Vis Sci (2021) 62:22. doi: 10.1167/iovs.62.9.22
5. Watson AB. A Formula for Human Retinal Ganglion Cell Receptive Field Density as a Function of Visual Field Location. J Vis (2014) 14:15. doi: 10.1167/14.7.15
6. Jeon CJ, Strettoi E, Masland RH. The Major Cell Populations of the Mouse Retina. J Neurosci (1998) 18:8936–46. doi: 10.1523/JNEUROSCI.18-21-08936.1998
7. Masland RH. The Neuronal Organization of the Retina. Neuron (2012) 76:266–80. doi: 10.1016/j.neuron.2012.10.002
8. Rheaume BA, Jereen A, Bolisetty M, Sajid MS, Yang Y, Renna K, et al. Single Cell Transcriptome Profiling of Retinal Ganglion Cells Identifies Cellular Subtypes. Nat Commun (2018) 9:2759. doi: 10.1038/s41467-018-05134-3
9. Tran NM, Shekhar K, Whitney IE, Jacobi A, Benhar I, Hong G, et al. Single-Cell Profiles of Retinal Ganglion Cells Differing in Resilience to Injury Reveal Neuroprotective Genes. Neuron (2019) 104:1039–55.e1012. doi: 10.1016/j.neuron.2019.11.006
10. Vecino E, Rodriguez FD, Ruzafa N, Pereiro X, Sharma SC. Glia-Neuron Interactions in the Mammalian Retina. Prog Retin Eye Res (2016) 51:1–40. doi: 10.1016/j.preteyeres.2015.06.003
11. Bringmann A, Pannicke T, Grosche J, Francke M, Wiedemann P, Skatchkov SN, et al. Muller Cells in the Healthy and Diseased Retina. Prog Retin Eye Res (2006) 25:397–424. doi: 10.1016/j.preteyeres.2006.05.003
12. Watanabe T, Raff MC. Retinal Astrocytes are Immigrants From the Optic Nerve. Nature (1988) 332:834–7. doi: 10.1038/332834a0
13. Lopez Enrique M. Existencia De Celulas De Hortega" Microglia" En La Retina Y Vias Opticas. Bol Real Soc Esp Hist Nat (1926) 26:294–301.
14. Marchesani O. Die Morphologie Der Glia Im Nervus Opticus Und in Der Retina, Dargestellt Nach Den Neuesten Untersuchungsmethoden Und Untersuchungsergebnissen I. Mitteilung. Albrecht von Graefes Archiv für Ophthalmologie (1926) 117:575–605. doi: 10.1007/BF01863969
15. Langmann T. Microglia Activation in Retinal Degeneration. J Leukoc Biol (2007) 81:1345–51. doi: 10.1189/jlb.0207114
16. Karlstetter M, Scholz R, Rutar M, Wong WT, Provis JM, Langmann T. Retinal Microglia: Just Bystander or Target for Therapy? Prog Retin Eye Res (2015) 45:30–57. doi: 10.1016/j.preteyeres.2014.11.004
17. Rathnasamy G, Foulds WS, Ling EA, Kaur C. Retinal Microglia - A Key Player in Healthy and Diseased Retina. Prog Neurobiol (2019) 173:18–40. doi: 10.1016/j.pneurobio.2018.05.006
18. Boycott BB, Hopkins JM. Microglia in the Retina of Monkey and Other Mammals: Its Distinction From Other Types of Glia and Horizontal Cells. Neuroscience (1981) 6:679–88. doi: 10.1016/0306-4522(81)90151-2
19. Hopperton KE, Mohammad D, Trepanier MO, Giuliano V, Bazinet RP. Markers of Microglia in Post-Mortem Brain Samples From Patients With Alzheimer's Disease: A Systematic Review. Mol Psychiatry (2018) 23:177–98. doi: 10.1038/mp.2017.246
20. Jurga AM, Paleczna M, Kuter KZ. Overview of General and Discriminating Markers of Differential Microglia Phenotypes. Front Cell Neurosci (2020) 14:198. doi: 10.3389/fncel.2020.00198
21. Santos AM, Calvente R, Tassi M, Carrasco MC, Martin-Oliva D, Marin-Teva JL, et al. Embryonic and Postnatal Development of Microglial Cells in the Mouse Retina. J Comp Neurol (2008) 506:224–39. doi: 10.1002/cne.21538
22. Hume DA, Perry VH, Gordon S. Immunohistochemical Localization of a Macrophage-Specific Antigen in Developing Mouse Retina: Phagocytosis of Dying Neurons and Differentiation of Microglial Cells to Form a Regular Array in the Plexiform Layers. J Cell Biol (1983) 97:253–7. doi: 10.1083/jcb.97.1.253
23. Thanos S. The Relationship of Microglial Cells to Dying Neurons During Natural Neuronal Cell Death and Axotomy-Induced Degeneration of the Rat Retina. Eur J Neurosci (1991) 3:1189–207. doi: 10.1111/j.1460-9568.1991.tb00054.x
24. Zeng XX, Ng YK, Ling EA. Neuronal and Microglial Response in the Retina of Streptozotocin-Induced Diabetic Rats. Vis Neurosci (2000) 17:463–71. doi: 10.1017/S0952523800173122
25. Nimmerjahn A, Kirchhoff F, Helmchen F. Resting Microglial Cells are Highly Dynamic Surveillants of Brain Parenchyma In Vivo. Science (2005) 308:1314–8. doi: 10.1126/science.1110647
26. Davalos D, Grutzendler J, Yang G, Kim JV, Zuo Y, Jung S, et al. ATP Mediates Rapid Microglial Response to Local Brain Injury In Vivo. Nat Neurosci (2005) 8:752–8. doi: 10.1038/nn1472
27. Rashid K, Akhtar-Schaefer I, Langmann T. Microglia in Retinal Degeneration. Front Immunol (2019) 10:1975. doi: 10.3389/fimmu.2019.01975
28. Gupta N, Brown KE, Milam AH. Activated Microglia in Human Retinitis Pigmentosa, Late-Onset Retinal Degeneration, and Age-Related Macular Degeneration. Exp eye Res (2003) 76:463–71. doi: 10.1016/S0014-4835(02)00332-9
29. Zhou T, Huang Z, Sun X, Zhu X, Zhou L, Li M, et al. Microglia Polarization With M1/M2 Phenotype Changes in Rd1 Mouse Model of Retinal Degeneration. Front Neuroanat (2017) 11:77. doi: 10.3389/fnana.2017.00077
30. Chen H, Cho KS, Vu THK, Shen CH, Kaur M, Chen G, et al. Commensal Microflora-Induced T Cell Responses Mediate Progressive Neurodegeneration in Glaucoma. Nat Commun (2018) 9:3209. doi: 10.1038/s41467-018-05681-9
31. Yu C, Roubeix C, Sennlaub F, Saban DR. Microglia Versus Monocytes: Distinct Roles in Degenerative Diseases of the Retina. Trends Neurosci (2020) 43:433–49. doi: 10.1016/j.tins.2020.03.012
32. Levin LA, Beck RW, Joseph MP, Seiff S, Kraker R. The Treatment of Traumatic Optic Neuropathy: The International Optic Nerve Trauma Study. Ophthalmology (1999) 106:1268–77. doi: 10.1016/S0161-6420(99)00707-1
33. He Z, Jin Y. Intrinsic Control of Axon Regeneration. Neuron (2016) 90:437–51. doi: 10.1016/j.neuron.2016.04.022
34. Mahar M, Cavalli V. Intrinsic Mechanisms of Neuronal Axon Regeneration. Nat Rev Neurosci (2018) 19:323–37. doi: 10.1038/s41583-018-0001-8
35. Cameron EG, Xia X, Galvao J, Ashouri M, Kapiloff MS, Goldberg JL. Optic Nerve Crush in Mice to Study Retinal Ganglion Cell Survival and Regeneration. Bio Protoc (2020) 10. doi: 10.21769/BioProtoc.3559
36. Lang BT, Wang J, Filous AR, Au NP, Ma CH, Shen Y. Pleiotropic Molecules in Axon Regeneration and Neuroinflammation. Exp Neurol (2014) 258:17–23. doi: 10.1016/j.expneurol.2014.04.031
37. Curcio M, Bradke F. Axon Regeneration in the Central Nervous System: Facing the Challenges From the Inside. Annu Rev Cell Dev Biol (2018) 34:495–521. doi: 10.1146/annurev-cellbio-100617-062508
38. Nadal-Nicolas FM, Jimenez-Lopez M, Salinas-Navarro M, Sobrado-Calvo P, Vidal-Sanz M, Agudo-Barriuso M. Microglial Dynamics After Axotomy-Induced Retinal Ganglion Cell Death. J Neuroinflamm (2017) 14:218. doi: 10.1186/s12974-017-0982-7
39. Heuss ND, Pierson MJ, Roehrich H, Mcpherson SW, Gram AL, Li L, et al. Optic Nerve as a Source of Activated Retinal Microglia Post-Injury. Acta neuropathologica Commun (2018) 6:1–19. doi: 10.1186/s40478-018-0571-8
40. Perry VH, Brown MC, Gordon S. The Macrophage Response to Central and Peripheral Nerve Injury. A Possible Role for Macrophages in Regeneration. J Exp Med (1987) 165:1218–23. doi: 10.1084/jem.165.4.1218
41. Stoll G, Trapp BD, Griffin JW. Macrophage Function During Wallerian Degeneration of Rat Optic Nerve: Clearance of Degenerating Myelin and Ia Expression. J Neurosci (1989) 9:2327–35. doi: 10.1523/JNEUROSCI.09-07-02327.1989
42. Coleman MP, Freeman MR. Wallerian Degeneration, Wld(s), and Nmnat. Annu Rev Neurosci (2010) 33:245–67. doi: 10.1146/annurev-neuro-060909-153248
43. Gaudet AD, Popovich PG, Ramer MS. Wallerian Degeneration: Gaining Perspective on Inflammatory Events After Peripheral Nerve Injury. J Neuroinflamm (2011) 8:110. doi: 10.1186/1742-2094-8-110
44. Painter MW, Brosius Lutz A, Cheng YC, Latremoliere A, Duong K, Miller CM, et al. Diminished Schwann Cell Repair Responses Underlie Age-Associated Impaired Axonal Regeneration. Neuron (2014) 83:331–43. doi: 10.1016/j.neuron.2014.06.016
45. Asthana P, Vong JS, Kumar G, Chang RC, Zhang G, Sheikh KA, et al. Dissecting the Role of Anti-Ganglioside Antibodies in Guillain-Barre Syndrome: An Animal Model Approach. Mol Neurobiol (2016) 53:4981–91. doi: 10.1007/s12035-015-9430-9
46. Seitz RJ, Reiners K, Himmelmann F, Heininger K, Hartung HP, Toyka KV. The Blood-Nerve Barrier in Wallerian Degeneration: A Sequential Long-Term Study. Muscle Nerve (1989) 12:627–35. doi: 10.1002/mus.880120803
47. Ma CH, Omura T, Cobos EJ, Latremoliere A, Ghasemlou N, Brenner GJ, et al. Accelerating Axonal Growth Promotes Motor Recovery After Peripheral Nerve Injury in Mice. J Clin Invest (2011) 121:4332–47. doi: 10.1172/JCI58675
48. Sakuma M, Gorski G, Sheu SH, Lee S, Barrett LB, Singh B, et al. Lack of Motor Recovery After Prolonged Denervation of the Neuromuscular Junction is Not Due to Regenerative Failure. Eur J Neurosci (2016) 43:451–62. doi: 10.1111/ejn.13059
49. Asthana P, Zhang G, Sheikh KA, Him Eddie Ma C. Heat Shock Protein is a Key Therapeutic Target for Nerve Repair in Autoimmune Peripheral Neuropathy and Severe Peripheral Nerve Injury. Brain Behav Immun (2021) 91:48–64. doi: 10.1016/j.bbi.2020.08.020
50. Barres BA, Jacobson MD, Schmid R, Sendtner M, Raff MC. Does Oligodendrocyte Survival Depend on Axons? Curr Biol (1993) 3:489–97. doi: 10.1016/0960-9822(93)90039-Q
51. Vargas ME, Barres BA. Why is Wallerian Degeneration in the CNS So Slow? Annu Rev Neurosci (2007) 30:153–79. doi: 10.1146/annurev.neuro.30.051606.094354
52. Barres BA, Raff MC. Proliferation of Oligodendrocyte Precursor Cells Depends on Electrical Activity in Axons. Nature (1993) 361:258–60. doi: 10.1038/361258a0
53. Garcia-Valenzuela E, Sharma SC. Laminar Restriction of Retinal Macrophagic Response to Optic Nerve Axotomy in the Rat. J Neurobiol (1999) 40:55–66. doi: 10.1002/(SICI)1097-4695(199907)40:1<55::AID-NEU5>3.0.CO;2-E
54. Kuhlmann T, Wendling U, Nolte C, Zipp F, Maruschak B, Stadelmann C, et al. Differential Regulation of Myelin Phagocytosis by Macrophages/Microglia, Involvement of Target Myelin, Fc Receptors and Activation by Intravenous Immunoglobulins. J Neurosci Res (2002) 67:185–90. doi: 10.1002/jnr.10104
55. Silver J, Miller JH. Regeneration Beyond the Glial Scar. Nat Rev Neurosci (2004) 5:146–56. doi: 10.1038/nrn1326
56. Fawcett JW, Asher RA. The Glial Scar and Central Nervous System Repair. Brain Res Bull (1999) 49:377–91. doi: 10.1016/S0361-9230(99)00072-6
57. Cregg JM, Depaul MA, Filous AR, Lang BT, Tran A, Silver J. Functional Regeneration Beyond the Glial Scar. Exp Neurol (2014) 253:197–207. doi: 10.1016/j.expneurol.2013.12.024
58. Muller A, Hauk TG, Fischer D. Astrocyte-Derived CNTF Switches Mature RGCs to a Regenerative State Following Inflammatory Stimulation. Brain (2007) 130:3308–20. doi: 10.1093/brain/awm257
59. Mac Nair CE, Schlamp CL, Montgomery AD, Shestopalov VI, Nickells RW. Retinal Glial Responses to Optic Nerve Crush are Attenuated in Bax-Deficient Mice and Modulated by Purinergic Signaling Pathways. J Neuroinflamm (2016) 13:93. doi: 10.1186/s12974-016-0558-y
60. Bahr M, Przyrembel C, Bastmeyer M. Astrocytes From Adult Rat Optic Nerves are Nonpermissive for Regenerating Retinal Ganglion Cell Axons. Exp Neurol (1995) 131:211–20. doi: 10.1016/0014-4886(95)90043-8
61. Park KK, Liu K, Hu Y, Smith PD, Wang C, Cai B, et al. Promoting Axon Regeneration in the Adult CNS by Modulation of the PTEN/mTOR Pathway. Science (2008) 322:963–6. doi: 10.1126/science.1161566
62. Erturk A, Hellal F, Enes J, Bradke F. Disorganized Microtubules Underlie the Formation of Retraction Bulbs and the Failure of Axonal Regeneration. J Neurosci (2007) 27:9169–80. doi: 10.1523/JNEUROSCI.0612-07.2007
63. Bartsch U, Bandtlow CE, Schnell L, Bartsch S, Spillmann AA, Rubin BP, et al. Lack of Evidence That Myelin-Associated Glycoprotein is a Major Inhibitor of Axonal Regeneration in the CNS. Neuron (1995) 15:1375–81. doi: 10.1016/0896-6273(95)90015-2
64. Fischer D, He Z, Benowitz LI. Counteracting the Nogo Receptor Enhances Optic Nerve Regeneration If Retinal Ganglion Cells are in an Active Growth State. J Neurosci (2004) 24:1646–51. doi: 10.1523/JNEUROSCI.5119-03.2004
65. Kigerl KA, Gensel JC, Ankeny DP, Alexander JK, Donnelly DJ, Popovich PG. Identification of Two Distinct Macrophage Subsets With Divergent Effects Causing Either Neurotoxicity or Regeneration in the Injured Mouse Spinal Cord. J Neurosci (2009) 29:13435–44. doi: 10.1523/JNEUROSCI.3257-09.2009
66. Pruss H, Kopp MA, Brommer B, Gatzemeier N, Laginha I, Dirnagl U, et al. Non-Resolving Aspects of Acute Inflammation After Spinal Cord Injury (SCI): Indices and Resolution Plateau. Brain Pathol (2011) 21:652–60. doi: 10.1111/j.1750-3639.2011.00488.x
67. Bradbury EJ, Burnside ER. Moving Beyond the Glial Scar for Spinal Cord Repair. Nat Commun (2019) 10:3879. doi: 10.1038/s41467-019-11707-7
68. Ohlsson M, Mattsson P, Wamil BD, Hellerqvist CG, Svensson M. Macrophage Stimulation Using a Group B-Streptococcus Exotoxin (CM101) Leads to Axonal Regrowth in the Injured Optic Nerve. Restor Neurol Neurosci (2004) 22:33–41.
69. Lazarov-Spiegler O, Solomon AS, Schwartz M. Peripheral Nerve-Stimulated Macrophages Simulate a Peripheral Nerve-Like Regenerative Response in Rat Transected Optic Nerve. Glia (1998) 24:329–37. doi: 10.1002/(SICI)1098-1136(199811)24:3<329::AID-GLIA7>3.0.CO;2-X
70. Raposo C, Schwartz M. Glial Scar and Immune Cell Involvement in Tissue Remodeling and Repair Following Acute CNS Injuries. Glia (2014) 62:1895–904. doi: 10.1002/glia.22676
71. Wang N, Liang H, Zen K. Molecular Mechanisms That Influence the Macrophage M1-M2 Polarization Balance. Front Immunol (2014) 5:614. doi: 10.3389/fimmu.2014.00614
72. Tang Y, Le W. Differential Roles of M1 and M2 Microglia in Neurodegenerative Diseases. Mol Neurobiol (2016) 53:1181–94. doi: 10.1007/s12035-014-9070-5
73. Lipsky RH, Marini AM. Brain-Derived Neurotrophic Factor in Neuronal Survival and Behavior-Related Plasticity. Ann N Y Acad Sci (2007) 1122:130–43. doi: 10.1196/annals.1403.009
74. Zhou Z, Peng X, Insolera R, Fink DJ, Mata M. IL-10 Promotes Neuronal Survival Following Spinal Cord Injury. Exp Neurol (2009) 220:183–90. doi: 10.1016/j.expneurol.2009.08.018
75. Kocur M, Schneider R, Pulm AK, Bauer J, Kropp S, Gliem M, et al. IFNbeta Secreted by Microglia Mediates Clearance of Myelin Debris in CNS Autoimmunity. Acta Neuropathol Commun (2015) 3:20. doi: 10.1186/s40478-015-0192-4
76. Neumann H, Kotter MR, Franklin RJ. Debris Clearance by Microglia: An Essential Link Between Degeneration and Regeneration. Brain (2009) 132:288–95. doi: 10.1093/brain/awn109
77. Bruck W, Bruck Y, Friede RL. TNF-Alpha Suppresses CR3-Mediated Myelin Removal by Macrophages. J Neuroimmunol (1992) 38:9–17. doi: 10.1016/0165-5728(92)90085-Y
78. Anderson AJ, Robert S, Huang W, Young W, Cotman CW. Activation of Complement Pathways After Contusion-Induced Spinal Cord Injury. J Neurotrauma (2004) 21:1831–46. doi: 10.1089/neu.2004.21.1831
79. Kopper TJ, Gensel JC. Myelin as an Inflammatory Mediator: Myelin Interactions With Complement, Macrophages, and Microglia in Spinal Cord Injury. J Neurosci Res (2018) 96:969–77. doi: 10.1002/jnr.24114
80. Peterson SL, Li Y, Sun CJ, Wong KA, Leung KS, De Lima S, et al. Retinal Ganglion Cell Axon Regeneration Requires Complement and Myeloid Cell Activity Within the Optic Nerve. J Neurosci (2021) 41:8508–31. doi: 10.1523/JNEUROSCI.0555-21.2021
81. Peterson SL, Nguyen HX, Mendez OA, Anderson AJ. Complement Protein C1q Modulates Neurite Outgrowth In Vitro and Spinal Cord Axon Regeneration In Vivo. J Neurosci (2015) 35:4332–49. doi: 10.1523/JNEUROSCI.4473-12.2015
82. Brennan FH, Jogia T, Gillespie ER, Blomster LV, Li XX, Nowlan B, et al. Complement Receptor C3aR1 Controls Neutrophil Mobilization Following Spinal Cord Injury Through Physiological Antagonism of CXCR2. JCI Insight (2019) 4. doi: 10.1172/jci.insight.98254
83. Leon S, Yin Y, Nguyen J, Irwin N, Benowitz LI. Lens Injury Stimulates Axon Regeneration in the Mature Rat Optic Nerve. J Neurosci (2000) 20:4615–26. doi: 10.1523/JNEUROSCI.20-12-04615.2000
84. Yin Y, Cui Q, Li Y, Irwin N, Fischer D, Harvey AR, et al. Macrophage-Derived Factors Stimulate Optic Nerve Regeneration. J Neurosci (2003) 23:2284–93. doi: 10.1523/JNEUROSCI.23-06-02284.2003
85. Yin Y, Cui Q, Gilbert HY, Yang Y, Yang Z, Berlinicke C, et al. Oncomodulin Links Inflammation to Optic Nerve Regeneration. Proc Natl Acad Sci USA (2009) 106:19587–92. doi: 10.1073/pnas.0907085106
86. Kurimoto T, Yin Y, Habboub G, Gilbert HY, Li Y, Nakao S, et al. Neutrophils Express Oncomodulin and Promote Optic Nerve Regeneration. J Neurosci (2013) 33:14816–24. doi: 10.1523/JNEUROSCI.5511-12.2013
87. Li Y, Andereggen L, Yuki K, Omura K, Yin Y, Gilbert HY, et al. Mobile Zinc Increases Rapidly in the Retina After Optic Nerve Injury and Regulates Ganglion Cell Survival and Optic Nerve Regeneration. Proc Natl Acad Sci USA (2017) 114:E209–18. doi: 10.1073/pnas.1616811114
88. Kurimoto T, Yin Y, Omura K, Gilbert HY, Kim D, Cen LP, et al. Long-Distance Axon Regeneration in the Mature Optic Nerve: Contributions of Oncomodulin, cAMP, and Pten Gene Deletion. J Neurosci (2010) 30:15654–63. doi: 10.1523/JNEUROSCI.4340-10.2010
89. de Lima S, Koriyama Y, Kurimoto T, Oliveira JT, Yin Y, Li Y, et al. Full-Length Axon Regeneration in the Adult Mouse Optic Nerve and Partial Recovery of Simple Visual Behaviors. Proc Natl Acad Sci USA (2012) 109:9149–54. doi: 10.1073/pnas.1119449109
90. Stevens B, Allen NJ, Vazquez LE, Howell GR, Christopherson KS, Nouri N, et al. The Classical Complement Cascade Mediates CNS Synapse Elimination. Cell (2007) 131:1164–78. doi: 10.1016/j.cell.2007.10.036
91. Schafer DP, Lehrman EK, Kautzman AG, Koyama R, Mardinly AR, Yamasaki R, et al. Microglia Sculpt Postnatal Neural Circuits in an Activity and Complement-Dependent Manner. Neuron (2012) 74:691–705. doi: 10.1016/j.neuron.2012.03.026
92. Elmore MR, Najafi AR, Koike MA, Dagher NN, Spangenberg EE, Rice RA, et al. Colony-Stimulating Factor 1 Receptor Signaling is Necessary for Microglia Viability, Unmasking a Microglia Progenitor Cell in the Adult Brain. Neuron (2014) 82:380–97. doi: 10.1016/j.neuron.2014.02.040
93. Dagher NN, Najafi AR, Kayala KM, Elmore MR, White TE, Medeiros R, et al. Colony-Stimulating Factor 1 Receptor Inhibition Prevents Microglial Plaque Association and Improves Cognition in 3xtg-AD Mice. J Neuroinflamm (2015) 12:139. doi: 10.1186/s12974-015-0366-9
94. Hilla AM, Diekmann H, Fischer D. Microglia Are Irrelevant for Neuronal Degeneration and Axon Regeneration After Acute Injury. J Neurosci (2017) 37:6113–24. doi: 10.1523/JNEUROSCI.0584-17.2017
95. George S, Rey NL, Tyson T, Esquibel C, Meyerdirk L, Schulz E, et al. Microglia Affect Alpha-Synuclein Cell-to-Cell Transfer in a Mouse Model of Parkinson's Disease. Mol Neurodegener (2019) 14:34. doi: 10.1186/s13024-019-0335-3
96. Jin WN, Shi SX, Li Z, Li M, Wood K, Gonzales RJ, et al. Depletion of Microglia Exacerbates Postischemic Inflammation and Brain Injury. J Cereb Blood Flow Metab (2017) 37:2224–36. doi: 10.1177/0271678X17694185
97. Spiller KJ, Restrepo CR, Khan T, Dominique MA, Fang TC, Canter RG, et al. Microglia-Mediated Recovery From ALS-Relevant Motor Neuron Degeneration in a Mouse Model of TDP-43 Proteinopathy. Nat Neurosci (2018) 21:329–40. doi: 10.1038/s41593-018-0083-7
98. Klein D, Patzko A, Schreiber D, Van Hauwermeiren A, Baier M, Groh J, et al. Targeting the Colony Stimulating Factor 1 Receptor Alleviates Two Forms of Charcot-Marie-Tooth Disease in Mice. Brain (2015) 138:3193–205. doi: 10.1093/brain/awv240
99. Omura T, Omura K, Tedeschi A, Riva P, Painter MW, Rojas L, et al. Robust Axonal Regeneration Occurs in the Injured CAST/Ei Mouse CNS. Neuron (2015) 86:1215–27. doi: 10.1016/j.neuron.2015.05.005
100. Mansour-Robaey S, Clarke DB, Wang YC, Bray GM, Aguayo AJ. Effects of Ocular Injury and Administration of Brain-Derived Neurotrophic Factor on Survival and Regrowth of Axotomized Retinal Ganglion Cells. Proc Natl Acad Sci USA (1994) 91:1632–6. doi: 10.1073/pnas.91.5.1632
101. Wang J, He X, Meng H, Li Y, Dmitriev P, Tian F, et al. Robust Myelination of Regenerated Axons Induced by Combined Manipulations of GPR17 and Microglia. Neuron (2020) 108:876–886 e874. doi: 10.1016/j.neuron.2020.09.016
102. Yun-Jia L, Xi C, Jie-Qiong Z, Jing-Yi Z, Sen L, Jian Y. Semaphorin3A Increases M1-Like Microglia and Retinal Ganglion Cell Apoptosis After Optic Nerve Injury. Cell Biosci (2021) 11:97. doi: 10.1186/s13578-021-00603-7
103. Block ML, Zecca L, Hong JS. Microglia-Mediated Neurotoxicity: Uncovering the Molecular Mechanisms. Nat Rev Neurosci (2007) 8:57–69. doi: 10.1038/nrn2038
104. Brown GC, Neher JJ. Inflammatory Neurodegeneration and Mechanisms of Microglial Killing of Neurons. Mol Neurobiol (2010) 41:242–7. doi: 10.1007/s12035-010-8105-9
105. Mou Q, Yao K, Ye M, Zhao B, Hu Y, Lou X, et al. Modulation of Sirt1-Mtorc1 Pathway in Microglia Attenuates Retinal Ganglion Cell Loss After Optic Nerve Injury. J Inflammation Res (2021) 14:6857. doi: 10.2147/JIR.S338815
106. Wang M, Wong WT. Microglia-Muller Cell Interactions in the Retina. Adv Exp Med Biol (2014) 801:333–8. doi: 10.1007/978-1-4614-3209-8_42
107. Yoles E, Schwartz M. Elevation of Intraocular Glutamate Levels in Rats With Partial Lesion of the Optic Nerve. Arch Ophthalmol (1998) 116:906–10. doi: 10.1001/archopht.116.7.906
108. Uckermann O, Wolf A, Kutzera F, Kalisch F, Beck-Sickinger AG, Wiedemann P, et al. Glutamate Release by Neurons Evokes a Purinergic Inhibitory Mechanism of Osmotic Glial Cell Swelling in the Rat Retina: Activation by Neuropeptide Y. J Neurosci Res (2006) 83:538–50. doi: 10.1002/jnr.20760
109. Ferrari D, Chiozzi P, Falzoni S, Hanau S, Di Virgilio F. Purinergic Modulation of Interleukin-1 Beta Release From Microglial Cells Stimulated With Bacterial Endotoxin. J Exp Med (1997) 185:579–82. doi: 10.1084/jem.185.3.579
110. Hide I, Tanaka M, Inoue A, Nakajima K, Kohsaka S, Inoue K, et al. Extracellular ATP Triggers Tumor Necrosis Factor-Alpha Release From Rat Microglia. J Neurochem (2000) 75:965–72. doi: 10.1046/j.1471-4159.2000.0750965.x
111. Wang M, Ma W, Zhao L, Fariss RN, Wong WT. Adaptive Muller Cell Responses to Microglial Activation Mediate Neuroprotection and Coordinate Inflammation in the Retina. J Neuroinflamm (2011) 8:173. doi: 10.1186/1742-2094-8-173
112. Liddelow SA, Guttenplan KA, Clarke LE, Bennett FC, Bohlen CJ, Schirmer L, et al. Neurotoxic Reactive Astrocytes are Induced by Activated Microglia. Nature (2017) 541:481–7. doi: 10.1038/nature21029
113. Guttenplan KA, Stafford BK, El-Danaf RN, Adler DI, Munch AE, Weigel MK, et al. Neurotoxic Reactive Astrocytes Drive Neuronal Death After Retinal Injury. Cell Rep (2020) 31:107776. doi: 10.1016/j.celrep.2020.107776
114. Kaur C, Foulds WS, Ling EA. Blood-Retinal Barrier in Hypoxic Ischaemic Conditions: Basic Concepts, Clinical Features and Management. Prog Retin Eye Res (2008) 27:622–47. doi: 10.1016/j.preteyeres.2008.09.003
115. Klaassen I, Van Noorden CJ, Schlingemann RO. Molecular Basis of the Inner Blood-Retinal Barrier and its Breakdown in Diabetic Macular Edema and Other Pathological Conditions. Prog Retin Eye Res (2013) 34:19–48. doi: 10.1016/j.preteyeres.2013.02.001
116. Limb GA, Chignell AH, Green W, Leroy F, Dumonde DC. Distribution of TNF Alpha and its Reactive Vascular Adhesion Molecules in Fibrovascular Membranes of Proliferative Diabetic Retinopathy. Br J Ophthalmol (1996) 80:168–73. doi: 10.1136/bjo.80.2.168
117. Demircan N, Safran BG, Soylu M, Ozcan AA, Sizmaz S. Determination of Vitreous Interleukin-1 (IL-1) and Tumour Necrosis Factor (TNF) Levels in Proliferative Diabetic Retinopathy. Eye (Lond) (2006) 20:1366–9. doi: 10.1038/sj.eye.6702138
118. Mocan MC, Kadayifcilar S, Eldem B. Elevated Intravitreal Interleukin-6 Levels in Patients With Proliferative Diabetic Retinopathy. Can J Ophthalmol (2006) 41:747–52. doi: 10.3129/i06-070
119. Joussen AM, Poulaki V, Mitsiades N, Kirchhof B, Koizumi K, Dohmen S, et al. Nonsteroidal Anti-Inflammatory Drugs Prevent Early Diabetic Retinopathy via TNF-Alpha Suppression. FASEB J (2002) 16:438–40. doi: 10.1096/fj.01-0707fje
120. Vincent JA, Mohr S. Inhibition of Caspase-1/Interleukin-1beta Signaling Prevents Degeneration of Retinal Capillaries in Diabetes and Galactosemia. Diabetes (2007) 56:224–30. doi: 10.2337/db06-0427
121. Behl Y, Krothapalli P, Desta T, Dipiazza A, Roy S, Graves DT. Diabetes-Enhanced Tumor Necrosis Factor-Alpha Production Promotes Apoptosis and the Loss of Retinal Microvascular Cells in Type 1 and Type 2 Models of Diabetic Retinopathy. Am J Pathol (2008) 172:1411–8. doi: 10.2353/ajpath.2008.071070
122. Brosnan CF, Litwak MS, Schroeder CE, Selmaj K, Raine CS, Arezzo JC. Preliminary Studies of Cytokine-Induced Functional Effects on the Visual Pathways in the Rabbit. J Neuroimmunol (1989) 25:227–39. doi: 10.1016/0165-5728(89)90141-0
123. Martiney JA, Litwak M, Berman JW, Arezzo JC, Brosnan CF. Pathophysiologic Effect of Interleukin-1b in the Rabbit Retina. Am J Pathol (1990) 137:1411–23.
124. Martiney JA, Berman JW, Brosnan CF. Chronic Inflammatory Effects of Interleukin-1 on the Blood-Retina Barrier. J Neuroimmunol (1992) 41:167–76. doi: 10.1016/0165-5728(92)90067-U
125. Claudio L, Martiney JA, Brosnan CF. Ultrastructural Studies of the Blood-Retina Barrier After Exposure to Interleukin-1 Beta or Tumor Necrosis Factor-Alpha. Lab Invest (1994) 70:850–61.
126. Bamforth SD, Lightman S, Greenwood J. The Effect of TNF-Alpha and IL-6 on the Permeability of the Rat Blood-Retinal Barrier. vivo Acta Neuropathol (1996) 91:624–32. doi: 10.1007/s004010050476
127. Nadeem M, Kindelin A, Mahady L, Bhatia K, Hoda MN, Ducruet AF, et al. Remote Ischemic Post-Conditioning Therapy is Protective in Mouse Model of Traumatic Optic Neuropathy. Neuromolecular Med (2021) 23:371–82. doi: 10.1007/s12017-020-08631-1
128. Xu Z, Fouda AY, Lemtalsi T, Shosha E, Rojas M, Liu F, et al. Retinal Neuroprotection From Optic Nerve Trauma by Deletion of Arginase 2. Front Neurosci (2018) 12:970. doi: 10.3389/fnins.2018.00970
129. Noro T, Namekata K, Kimura A, Guo X, Azuchi Y, Harada C, et al. Spermidine Promotes Retinal Ganglion Cell Survival and Optic Nerve Regeneration in Adult Mice Following Optic Nerve Injury. Cell Death Dis (2015) 6:e1720–0. doi: 10.1038/cddis.2015.93
130. Zhu X, Liu Q, Wang M, Liang M, Yang X, Xu X, et al. Activation of Sirt1 by Resveratrol Inhibits TNF-Alpha Induced Inflammation in Fibroblasts. PloS One (2011) 6:e27081. doi: 10.1371/journal.pone.0027081
131. Fischer D, Heiduschka P, Thanos S. Lens-Injury-Stimulated Axonal Regeneration Throughout the Optic Pathway of Adult Rats. Exp Neurol (2001) 172:257–72. doi: 10.1006/exnr.2001.7822
132. Lorber B, Berry M, Logan A. Lens Injury Stimulates Adult Mouse Retinal Ganglion Cell Axon Regeneration via Both Macrophage- and Lens-Derived Factors. Eur J Neurosci (2005) 21:2029–34. doi: 10.1111/j.1460-9568.2005.04034.x
133. Ahmed Z, Aslam M, Lorber B, Suggate EL, Berry M, Logan A. Optic Nerve and Vitreal Inflammation are Both RGC Neuroprotective But Only the Latter is RGC Axogenic. Neurobiol Dis (2010) 37:441–54. doi: 10.1016/j.nbd.2009.10.024
134. Yin Y, Henzl MT, Lorber B, Nakazawa T, Thomas TT, Jiang F, et al. Oncomodulin is a Macrophage-Derived Signal for Axon Regeneration in Retinal Ganglion Cells. Nat Neurosci (2006) 9:843–52. doi: 10.1038/nn1701
135. Sun F, Park KK, Belin S, Wang D, Lu T, Chen G, et al. Sustained Axon Regeneration Induced by Co-Deletion of PTEN and SOCS3. Nature (2011) 480:372–5. doi: 10.1038/nature10594
136. Baldwin KT, Carbajal KS, Segal BM, Giger RJ. Neuroinflammation Triggered by Beta-Glucan/Dectin-1 Signaling Enables CNS Axon Regeneration. Proc Natl Acad Sci USA (2015) 112:2581–6. doi: 10.1073/pnas.1423221112
137. Tsoni SV, Brown GD. Beta-Glucans and Dectin-1. Ann N Y Acad Sci (2008) 1143:45–60. doi: 10.1196/annals.1443.019
138. Li X, Utomo A, Cullere X, Choi MM, Milner DA Jr, Venkatesh D, et al. The Beta-Glucan Receptor Dectin-1 Activates the Integrin Mac-1 in Neutrophils via Vav Protein Signaling to Promote Candida Albicans Clearance. Cell Host Microbe (2011) 10:603–15. doi: 10.1016/j.chom.2011.10.009
139. Cui Q, Yin Y, Benowitz LI. The Role of Macrophages in Optic Nerve Regeneration. Neuroscience (2009) 158:1039–48. doi: 10.1016/j.neuroscience.2008.07.036
140. Leibinger M, Muller A, Andreadaki A, Hauk TG, Kirsch M, Fischer D. Neuroprotective and Axon Growth-Promoting Effects Following Inflammatory Stimulation on Mature Retinal Ganglion Cells in Mice Depend on Ciliary Neurotrophic Factor and Leukemia Inhibitory Factor. J Neurosci (2009) 29:14334–41. doi: 10.1523/JNEUROSCI.2770-09.2009
141. Leibinger M, Andreadaki A, Diekmann H, Fischer D. Neuronal STAT3 Activation is Essential for CNTF- and Inflammatory Stimulation-Induced CNS Axon Regeneration. Cell Death Dis (2013) 4:e805. doi: 10.1038/cddis.2013.310
142. Cui Q, Lu Q, So KF, Yip HK. CNTF, Not Other Trophic Factors, Promotes Axonal Regeneration of Axotomized Retinal Ganglion Cells in Adult Hamsters. Invest Ophthalmol Vis Sci (1999) 40:760–6.
143. Peterson WM, Wang Q, Tzekova R, Wiegand SJ. Ciliary Neurotrophic Factor and Stress Stimuli Activate the Jak-STAT Pathway in Retinal Neurons and Glia. J Neurosci (2000) 20:4081–90. doi: 10.1523/JNEUROSCI.20-11-04081.2000
144. Cen LP, Luo JM, Zhang CW, Fan YM, Song Y, So KF, et al. Chemotactic Effect of Ciliary Neurotrophic Factor on Macrophages in Retinal Ganglion Cell Survival and Axonal Regeneration. Invest Ophthalmol Vis Sci (2007) 48:4257–66. doi: 10.1167/iovs.06-0791
145. Jo SA, Wang E, Benowitz LI. Ciliary Neurotrophic Factor is an Axogenesis Factor for Retinal Ganglion Cells. Neuroscience (1999) 89:579–91. doi: 10.1016/S0306-4522(98)00546-6
146. Pernet V, Di Polo A. Synergistic Action of Brain-Derived Neurotrophic Factor and Lens Injury Promotes Retinal Ganglion Cell Survival, But Leads to Optic Nerve Dystrophy In Vivo. Brain (2006) 129:1014–26. doi: 10.1093/brain/awl015
147. Smith PD, Sun F, Park KK, Cai B, Wang C, Kuwako K, et al. SOCS3 Deletion Promotes Optic Nerve Regeneration In Vivo. Neuron (2009) 64:617–23. doi: 10.1016/j.neuron.2009.11.021
148. Ghasemi M, Alizadeh E, Saei Arezoumand K, Fallahi Motlagh B, Zarghami N. Ciliary Neurotrophic Factor (CNTF) Delivery to Retina: An Overview of Current Research Advancements. Artif Cells Nanomed Biotechnol (2018) 46:1694–707. doi: 10.1080/21691401.2017.1391820
149. Leaver SG, Cui Q, Plant GW, Arulpragasam A, Hisheh S, Verhaagen J, et al. AAV-Mediated Expression of CNTF Promotes Long-Term Survival and Regeneration of Adult Rat Retinal Ganglion Cells. Gene Ther (2006) 13:1328–41. doi: 10.1038/sj.gt.3302791
150. Bei F, Lee HHC, Liu X, Gunner G, Jin H, Ma L, et al. Restoration of Visual Function by Enhancing Conduction in Regenerated Axons. Cell (2016) 164:219–32. doi: 10.1016/j.cell.2015.11.036
151. Bray ER, Yungher BJ, Levay K, Ribeiro M, Dvoryanchikov G, Ayupe AC, et al. Thrombospondin-1 Mediates Axon Regeneration in Retinal Ganglion Cells. Neuron (2019) 103:642–657 e647. doi: 10.1016/j.neuron.2019.05.044
152. Xie L, Yin Y, Benowitz L. Chemokine CCL5 Promotes Robust Optic Nerve Regeneration and Mediates Many of the Effects of CNTF Gene Therapy. Proc Natl Acad Sci USA (2021) 118. doi: 10.1073/pnas.2017282118
153. Yona S, Kim KW, Wolf Y, Mildner A, Varol D, Breker M, et al. Fate Mapping Reveals Origins and Dynamics of Monocytes and Tissue Macrophages Under Homeostasis. Immunity (2013) 38:79–91. doi: 10.1016/j.immuni.2012.12.001
154. Tong B, Hornak AJ, Maison SF, Ohlemiller KK, Liberman MC, Simmons DD. Oncomodulin, an EF-Hand Ca2+ Buffer, Is Critical for Maintaining Cochlear Function in Mice. J Neurosci (2016) 36:1631–5. doi: 10.1523/JNEUROSCI.3311-15.2016
155. Cui Q, Benowitz L, Yin Y. Does CNTF Mediate the Effect of Intraocular Inflammation on Optic Nerve Regeneration? Brain (2008) 131:e96–6. doi: 10.1093/brain/awn027
156. Fischer D. What are the Principal Mediators of Optic Nerve Regeneration After Inflammatory Stimulation in the Eye? Proc Natl Acad Sci USA (2010) 107:E8; author reply E9. doi: 10.1073/pnas.0912942107
157. Guler AD, Ecker JL, Lall GS, Haq S, Altimus CM, Liao HW, et al. Melanopsin Cells are the Principal Conduits for Rod-Cone Input to Non-Image-Forming Vision. Nature (2008) 453:102–5. doi: 10.1038/nature06829
158. Chen SK, Badea TC, Hattar S. Photoentrainment and Pupillary Light Reflex are Mediated by Distinct Populations of ipRGCs. Nature (2011) 476:92–5. doi: 10.1038/nature10206
159. Horai R, Caspi RR. Microbiome and Autoimmune Uveitis. Front Immunol (2019) 10:232. doi: 10.3389/fimmu.2019.00232
160. Marin MA, De Lima S, Gilbert HY, Giger RJ, Benowitz L, Rasband MN. Reassembly of Excitable Domains After CNS Axon Regeneration. J Neurosci (2016) 36:9148–60. doi: 10.1523/JNEUROSCI.1747-16.2016
161. Chang KJ, Redmond SA, Chan JR. Remodeling Myelination: Implications for Mechanisms of Neural Plasticity. Nat Neurosci (2016) 19:190–7. doi: 10.1038/nn.4200
162. Monje M. Myelin Plasticity and Nervous System Function. Annu Rev Neurosci (2018) 41:61–76. doi: 10.1146/annurev-neuro-080317-061853
163. Traiffort E, Kassoussi A, Zahaf A, Laouarem Y. Astrocytes and Microglia as Major Players of Myelin Production in Normal and Pathological Conditions. Front Cell Neurosci (2020) 14:79. doi: 10.3389/fncel.2020.00079
164. Miron VE, Boyd A, Zhao J-W, Yuen TJ, Ruckh JM, Shadrach JL, et al. M2 Microglia and Macrophages Drive Oligodendrocyte Differentiation During CNS Remyelination. Nat Neurosci (2013) 16:1211–8. doi: 10.1038/nn.3469
165. Lloyd AF, Davies CL, Holloway RK, Labrak Y, Ireland G, Carradori D, et al. Central Nervous System Regeneration is Driven by Microglia Necroptosis and Repopulation. Nat Neurosci (2019) 22:1046–52. doi: 10.1038/s41593-019-0418-z
166. Tam WY, Ma CH. Bipolar/rod-Shaped Microglia are Proliferating Microglia With Distinct M1/M2 Phenotypes. Sci Rep (2014) 4:7279. doi: 10.1038/srep07279
167. Tam WY, Au NP, Ma CH. The Association Between Laminin and Microglial Morphology In Vitro. Sci Rep (2016) 6:28580. doi: 10.1038/srep28580
168. Au NPB, Ma CHE. Recent Advances in the Study of Bipolar/Rod-Shaped Microglia and Their Roles in Neurodegeneration. Front Aging Neurosci (2017) 9:128. doi: 10.3389/fnagi.2017.00128
169. Holloway OG, Canty AJ, King AE, Ziebell JM. Rod Microglia and Their Role in Neurological Diseases. Semin Cell Dev Biol (2019) 94:96–103. doi: 10.1016/j.semcdb.2019.02.005
170. Giordano KR, Denman CR, Dubisch PS, Akhter M, Lifshitz J. An Update on the Rod Microglia Variant in Experimental and Clinical Brain Injury and Disease. Brain Commun (2021) 3:fcaa227. doi: 10.1093/braincomms/fcaa227
171. Nissl F. Über Einige Beziehungen Zwischen Nervenzellerkrankungen Und Gliösen Erscheinungen Bei Verschiedenen Psychosen. Arch f Psychiatr (1899) 32:656–76.
172. Spielmeyer W. Histopathologie Des Nervensystems. J Springer (1922). doi: 10.1007/978-3-642-50703-8
173. Ziebell JM, Taylor SE, Cao T, Harrison JL, Lifshitz J. Rod Microglia: Elongation, Alignment, and Coupling to Form Trains Across the Somatosensory Cortex After Experimental Diffuse Brain Injury. J Neuroinflamm (2012) 9:247. doi: 10.1186/1742-2094-9-247
174. Taylor SE, Morganti-Kossmann C, Lifshitz J, Ziebell JM. Rod Microglia: A Morphological Definition. PloS One (2014) 9:e97096. doi: 10.1371/journal.pone.0097096
175. De Hoz R, Gallego BI, Ramirez AI, Rojas B, Salazar JJ, Valiente-Soriano FJ, et al. Rod-Like Microglia are Restricted to Eyes With Laser-Induced Ocular Hypertension But Absent From the Microglial Changes in the Contralateral Untreated Eye. PloS One (2013) 8:e83733. doi: 10.1371/journal.pone.0083733
176. Rojas B, Gallego BI, Ramirez AI, Salazar JJ, De Hoz R, Valiente-Soriano FJ, et al. Microglia in Mouse Retina Contralateral to Experimental Glaucoma Exhibit Multiple Signs of Activation in All Retinal Layers. J Neuroinflamm (2014) 11:133. doi: 10.1186/1742-2094-11-133
177. Yuan TF, Liang YX, Peng B, Lin B, So KF. Local Proliferation is the Main Source of Rod Microglia After Optic Nerve Transection. Sci Rep (2015) 5:10788. doi: 10.1038/srep10788
178. Chamak B, Mallat M. Fibronectin and Laminin Regulate the In Vitro Differentiation of Microglial Cells. Neuroscience (1991) 45:513–27. doi: 10.1016/0306-4522(91)90267-R
179. Ma W, Zhao L, Fontainhas AM, Fariss RN, Wong WT. Microglia in the Mouse Retina Alter the Structure and Function of Retinal Pigmented Epithelial Cells: A Potential Cellular Interaction Relevant to AMD. PloS One (2009) 4:e7945. doi: 10.1371/journal.pone.0007945
180. Ma W, Coon S, Zhao L, Fariss RN, Wong WT. A2E Accumulation Influences Retinal Microglial Activation and Complement Regulation. Neurobiol Aging (2013) 34:943–60. doi: 10.1016/j.neurobiolaging.2012.06.010
181. Devarajan G, Chen M, Muckersie E, Xu H. Culture and Characterization of Microglia From the Adult Murine Retina. ScientificWorldJournal (2014):894368. doi: 10.1155/2014/894368
182. Amici SA, Dong J, Guerau-De-Arellano M. Molecular Mechanisms Modulating the Phenotype of Macrophages and Microglia. Front Immunol (2017) 8:1520. doi: 10.3389/fimmu.2017.01520
183. Grabert K, Michoel T, Karavolos MH, Clohisey S, Baillie JK, Stevens MP, et al. Microglial Brain Region-Dependent Diversity and Selective Regional Sensitivities to Aging. Nat Neurosci (2016) 19:504–16. doi: 10.1038/nn.4222
184. Benmamar-Badel A, Owens T, Wlodarczyk A. Protective Microglial Subset in Development, Aging, and Disease: Lessons From Transcriptomic Studies. Front Immunol (2020) 11:430. doi: 10.3389/fimmu.2020.00430
185. Prinz M, Jung S, Priller J. Microglia Biology: One Century of Evolving Concepts. Cell (2019) 179:292–311. doi: 10.1016/j.cell.2019.08.053
186. Hickman SE, Kingery ND, Ohsumi TK, Borowsky ML, Wang LC, Means TK, et al. The Microglial Sensome Revealed by Direct RNA Sequencing. Nat Neurosci (2013) 16:1896–905. doi: 10.1038/nn.3554
187. Bennett ML, Bennett FC, Liddelow SA, Ajami B, Zamanian JL, Fernhoff NB, et al. New Tools for Studying Microglia in the Mouse and Human CNS. Proc Natl Acad Sci USA (2016) 113:E1738–1746. doi: 10.1073/pnas.1525528113
188. Saederup N, Cardona AE, Croft K, Mizutani M, Cotleur AC, Tsou CL, et al. Selective Chemokine Receptor Usage by Central Nervous System Myeloid Cells in CCR2-Red Fluorescent Protein Knock-in Mice. PloS One (2010) 5:e13693. doi: 10.1371/journal.pone.0013693
189. Keren-Shaul H, Spinrad A, Weiner A, Matcovitch-Natan O, Dvir-Szternfeld R, Ulland TK, et al. A Unique Microglia Type Associated With Restricting Development of Alzheimer's Disease. Cell (2017) 169:1276–1290 e1217. doi: 10.1016/j.cell.2017.05.018
190. Mildner A, Huang H, Radke J, Stenzel W, Priller J. P2Y12 Receptor is Expressed on Human Microglia Under Physiological Conditions Throughout Development and is Sensitive to Neuroinflammatory Diseases. Glia (2017) 65:375–87. doi: 10.1002/glia.23097
191. Zrzavy T, Hametner S, Wimmer I, Butovsky O, Weiner HL, Lassmann H. Loss of 'Homeostatic' Microglia and Patterns of Their Activation in Active Multiple Sclerosis. Brain (2017) 140:1900–13. doi: 10.1093/brain/awx113
192. Walker DG, Tang TM, Mendsaikhan A, Tooyama I, Serrano GE, Sue LI, et al. Patterns of Expression of Purinergic Receptor P2RY12, a Putative Marker for Non-Activated Microglia, in Aged and Alzheimer's Disease Brains. Int J Mol Sci (2020) 21:678. doi: 10.3390/ijms21020678
193. Kaiser T, Feng G. Tmem119-EGFP and Tmem119-CreERT2 Transgenic Mice for Labeling and Manipulating Microglia. eNeuro (2019) 6. doi: 10.1101/624825
194. Ruan C, Sun L, Kroshilina A, Beckers L, De Jager P, Bradshaw EM, et al. A Novel Tmem119-Tdtomato Reporter Mouse Model for Studying Microglia in the Central Nervous System. Brain Behav Immun (2020) 83:180–91. doi: 10.1016/j.bbi.2019.10.009
195. Buch T, Heppner FL, Tertilt C, Heinen TJ, Kremer M, Wunderlich FT, et al. A Cre-Inducible Diphtheria Toxin Receptor Mediates Cell Lineage Ablation After Toxin Administration. Nat Methods (2005) 2:419–26. doi: 10.1038/nmeth762
196. Androvic P, Kirdajova D, Tureckova J, Zucha D, Rohlova E, Abaffy P, et al. Decoding the Transcriptional Response to Ischemic Stroke in Young and Aged Mouse Brain. Cell Rep (2020) 31:107777. doi: 10.1016/j.celrep.2020.107777
197. Avila Cobos F, Alquicira-Hernandez J, Powell JE, Mestdagh P, De Preter K. Benchmarking of Cell Type Deconvolution Pipelines for Transcriptomics Data. Nat Commun (2020) 11:5650. doi: 10.1038/s41467-020-19015-1
Keywords: optic neuropathy, microglia, retinal degeneration, astrocyte, Müller cell, neuroinflammation
Citation: Au NPB and Ma CHE (2022) Neuroinflammation, Microglia and Implications for Retinal Ganglion Cell Survival and Axon Regeneration in Traumatic Optic Neuropathy. Front. Immunol. 13:860070. doi: 10.3389/fimmu.2022.860070
Received: 22 January 2022; Accepted: 14 February 2022;
Published: 04 March 2022.
Edited by:
Kin-Sang CHO, Harvard Medical School, United StatesReviewed by:
Jacky Kwong, UCLA Stein Eye Institute, United StatesCopyright © 2022 Au and Ma. This is an open-access article distributed under the terms of the Creative Commons Attribution License (CC BY). The use, distribution or reproduction in other forums is permitted, provided the original author(s) and the copyright owner(s) are credited and that the original publication in this journal is cited, in accordance with accepted academic practice. No use, distribution or reproduction is permitted which does not comply with these terms.
*Correspondence: Chi Him Eddie Ma, ZWRkaWVtYUBjaXR5dS5lZHUuaGs=
Disclaimer: All claims expressed in this article are solely those of the authors and do not necessarily represent those of their affiliated organizations, or those of the publisher, the editors and the reviewers. Any product that may be evaluated in this article or claim that may be made by its manufacturer is not guaranteed or endorsed by the publisher.
Research integrity at Frontiers
Learn more about the work of our research integrity team to safeguard the quality of each article we publish.