- 1Department of Promotion for Blood and Marrow Transplantation, Aichi Medical University School of Medicine, Nagakute, Japan
- 2Department of Pediatric Surgery, Osaka University Graduate School of Medicine, Suita, Japan
- 3International Institute for Bio-Resource Research, Meiji University, Kawasaki, Japan
Xenotransplantation is very attractive strategy for addressing the shortage of donors. While hyper acute rejection (HAR) caused by natural antibodies and complement has been well defined, this is not the case for innate cellular xenogeneic rejection. An increasing body of evidence suggests that innate cellular immune responses contribute to xenogeneic rejection. Various molecular incompatibilities between receptors and their ligands across different species typically have an impact on graft outcome. NK cells are activated by direct interaction as well as by antigen dependent cellular cytotoxicity (ADCC) mechanisms. Macrophages are activated through various mechanisms in xenogeneic conditions. Macrophages recognize CD47 as a “marker of self” through binding to SIRPα. A number of studies have shown that incompatibility of porcine CD47 against human SIRPα contributes to the rejection of xenogeneic target cells by macrophages. Neutrophils are an early responder cell that infiltrates xenogeneic grafts. It has also been reported that neutrophil extracellular traps (NETs) activate macrophages as damage-associated pattern molecules (DAMPs). In this review, we summarize recent insights into innate cellular xenogeneic rejection.
The Pivotal Role of Innate Immunity in Xenogeneic Rejection
While the main cells that infiltrate allograft rejections are cytotoxic T lymphocytes (CTL); xenografts induce the infiltration of NK cells, macrophages and neutrophils (1).
Neutrophils are the most abundant circulating leukocytes and a cell population that responds early to infiltrate cellular and solid organ xenografts following transplantation (2, 3). These cells are subsequently replaced by macrophages and T cells (2, 3).
Neutrophils induce tissue damage under xenogeneic conditions in both antibody-dependent and antibody-independent manners (4–6). In the response to inflammatory stimuli, neutrophils induce a unique type of cell death process that is referred to as “NETosis”. They release network structures that are referred to as neutrophil extracellular traps (NETs), and contains serine proteases and antibacterial peptides. NETs themselves induce tissue damage through the generation of reactive oxygen species (ROS) (7–10) and the release of digestive enzymes (11, 12). Moreover, NETs are recognized by macrophages as damage-associated molecular patterns (DAMPs) which triggers inflammatory signals and IL-1beta production in macrophages (12). It has also been reported that the IL-1beta produced by macrophages enhances NET formation in neutrophils (13). Because macrophages are also activated under xenogeneic conditions and directly reject a xenograft (14, 15), inhibiting this negative chain reaction by inhibiting the action of neutrophils leads to the suppression of innate cellular rejection in a xenograft (Figure 1).
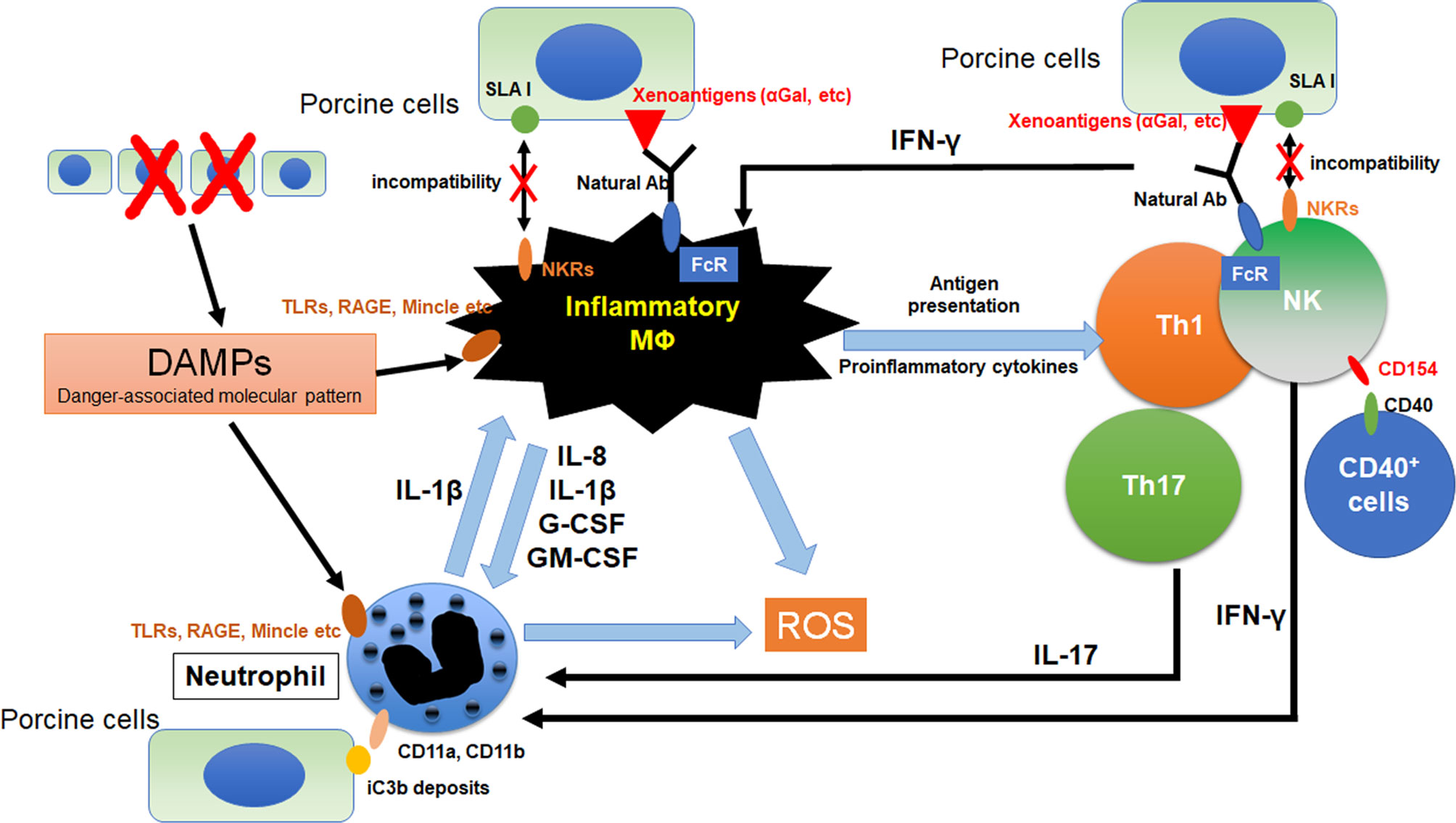
Figure 1 Crosstalk between macrophages and other innate immune cells in xenograft. The immunocomplex of porcine cells and natural antibodies against porcine antigens binds to Fc receptors (FcRs) on macrophages and NK cell. Activated NK cell and macrophage induce antibody-dependent cellular cytotoxicity (ADCC) against porcine cells. Furthermore, incompatibility between SLA1 and NK receptors (NKRs) causes macrophage and NK cell activation because of lack of inhibitory signals. Macrophages were activated by the binding of DAMPs from dead cells to toll-like receptors (TLRs), receptor for advanced glycation end-products (RAGE) and macrophage-inducible C-type lectin (Mincle). Activation signals were induced and various pro-inflammatory cytokines are released from macrophages. These cytokines activate neutrophils and promote NETosis. Especially, IL-8 contributes to the neutrophil recruitment and IL-1β enhances NETosis formation in neutrophils. In addition, NETs from neutrophils also activate macrophages as DAMPs. Macrophages can activate NK cells and IFN-γ from activated NK cells was known to activates both macrophage and neutrophil. CD11a and CD11b on neutrophils bind to iC3b deposits on porcine cells, suggesting that neutrophils can recognize porcine target cells via binding of CD11a,b and iC3b. CD154 on NK cells enhances NK cytotoxicity to CD40 positive target cells.
Macrophages are activated via various mechanisms under xenogeneic conditions through various mechanisms. As an antibody-dependent mechanism, immunocomplexes of porcine cells with xenogeneic antigen-specific antibodies such as anti-α1,3Gal natural antibodies bind to FcγR and induce the production of an activation signal (16, 17). As an antibody-independent mechanism, the binding of galectin-3 to α1,3Gal on porcine cells has been reported to function as an activation signal in macrophages (18–20). Macrophages are also activated by interactions with neutrophils, NK cells and Th1 cells (21, 22). DAMPs arising from damaged porcine cells also contribute to macrophage-mediated rejection (23). Activated macrophages induce tissue damage in grafts via the secretion of various proinflammatory cytokines, reactive oxygen and nitrogen species, and complement factors (24).
NK cells rapidly infiltrate porcine xenografts that have been perfused ex vivo with human blood and reject xenogeneic cells by direct interaction or by antibody dependent cellular cytotoxicity (ADCC) mechanisms. NK cells which are activated in an antibody-independent manner, induce cytotoxicity mainly by the release of perforin and granzymes (25–27). The direct cytotoxicity of NK cells is regulated by the balance between the activation and the inhibition of signal pathways that are mediated by various NK receptors (28). The activating NK receptors NKG2D (29) and pULBP-1 (30) bind to the porcine ligand NKp44 and an unidentified molecule, respectively, resulting in the release of lytic granules. However, the inhibitory receptors on human NK cells, KIR, ILT2, and CD94/NKG2A, do not efficiently identify swine leukocyte antigen I (SLAI, swine MHC class I), leading to invalidating inhibitory signals for NK cell activation (31, 32). The deposits of natural and elicited antibodies on the graft endothelial cells are recognized by the Fc-receptors (FcRs) on NK cells. Interaction between FcRs and immunocomplex of porcine cell and antibody causes the release of perforin and granzymes from NK cells and, in turn, triggers target cell apoptosis (33). In addition to xenoantibodies that are bound on the endothelial cells, the anti-swine leukocyte antigen class I (anti-SLA classI) antibodies also induce antibody-dependent cellular cytotoxicity (ADCC) (28, 34).
Inhibition of NK Cell-Mediated Xenograft Rejection
NK cells are activated in antibody-dependent and -independent manners under xenogeneic conditions. The knock out of alpha1,3-galactosyltoransferase (αGalT) is known to suppress NK-mediated ADCC but not antibody independent cytotoxicity (35, 36). NK cells are known to be regulated by the balance between activation signals and inhibitory signals and receive inhibitory signals from MHC class I molecules. In the case of xenografts between humans and pigs, the inhibitory receptors on NK cells fail to deliver inhibitory signals due to the lack of human leukocyte antigens (HLA) on target cells. To protect porcine target cells from being killed by human NK cells, classical and non-classical human MHC class I molecules were forcibly expressed in porcine cells and, in a number of studies, have been reported to suppress NK xenocytotoxicity (37–40). HLA-G1 is recognized by CD158d and immunoglobulin-like transcripts (ILT) -2,4. It is known that HLA-E functions as a ligand for the CD94/NKG2A inhibitory receptor and the CD94/NKG2C activation receptor. These receptors have inhibitory immunoreceptor tyrosine-based inhibitory motifs (ITIMs) in their cytoplasmic tail and induce inhibitory signal in NK cells. Therefore, the modification of HLA-G1 and HLA-E cDNA for transfection for it to only bind to inhibitory receptors would be expected to improve the efficacy of non-classical HLA strategies.
An anti-CD154 monoclonal antibody, a co-stimulation blockade agent, has recently been reported to be effective in xenogeneic rejections (41, 42). IL-2-activated human NK cells express CD154 (the CD40 ligand, CD40L) and the crosslinking of CD154 on NK cells enhances the NK cytotoxicity to CD40 positive target cells (43). NK cells also contribute to antibody-mediated rejection by interaction with marginal zone B cells in the spleen via the CD40-CD154 pathway and the production of T cell-mediated αGal-independent antibodies (44). These findings indicate that anti-CD154 mAb may also suppress NK-mediated xenogeneic rejection. We recently demonstrated that prenylated quinolinecarboxylic acid (PQA)-18, a p21-activated kinase 2 (PAK2) inhibitor, suppresses the expression of CD40 on macrophages (45). A combination therapy of anti-CD154mAb and PQA-18 would therefore be expected to induce a greater suppression in xenogeneic rejection.
Inhibition of Macrophage-Mediated Xenograft Rejection
CD47-SIRPα has been reported to play a critical role in signaling by virtue of its ability to prevent phagocytosis (46–48). CD47 binds to SIRPα and prevents the clearance of cells by the immune system. SIRP-α is expressed by macrophages and neutrophils and recognizes CD47 as a marker of self. The cytoplasmic domain of SIRP-α contains ITIMs that function to regulate their immunological function by recruiting the tyrosine phosphatase Src homology 2 domain-containing protein tyrosine phosphatase-1 (SHP-1). CD47-SIRPα signaling acts as a “do not eat me” signal and reduces the macrophage-mediated phagocytosis of opsonized cells. In addition, a large number of studies have shown that pig to human molecular incompatibility of SIRPα-CD47 interactions contribute to the rejection of xenogeneic cells by macrophages (49–53). The ectopic expression of human CD47 on swine endothelial cells has been reported to lead to significant reduction of the human macrophage-mediated phagocytosis of xenogeneic cells. However, a recent study by Chen et al. reported that thrombospondin (TSP)-1-CD47 signaling may stimulate vascularized allograft rejection (54). TSP-1 also binds to CD47 and inhibits the binding of SIRP-α to CD47 in macrophages. TSP-1 blocks hCD47- SIRP-α signaling and increases phagocytosis in macrophages. Moreover, TSP-1 contributes to platelet aggregation (55) and up-regulates the expression of TSP-1 in ischemic tissues and damaged allografts, resulting in vasculopathy and rejection (56–58). The strong and week points of CD47 in xenografts will likely be revealed in future studies.
Human inflammatory monocyte-derived macrophages that are generated from peripheral blood monocytes express CD94/NKG2A and ILT-2,4, which are inhibitory NK receptors for HLA-E and G1 (59, 60). The expression of both transgenic HLA-E and HLA-G1 on porcine cells significantly suppresses macrophage-mediated xenogeneic cytotoxicity. Furthermore, both HLA-E and G1 were reported to suppress the production of proinflammatory cytokines by macrophages. Because non-classical MHC class I molecules suppress both macrophage and NK rejection, generating HLA-E and G1 transgenic pigs would be an excellent strategy for inhibiting innate cellular rejection.
It was recently reported that the overexpression of human CD200 on porcine cells suppresses xenogeneic macrophage-mediated cytotoxicity and phagocytosis (61, 62). CD200 and its receptor, CD200R, are both members of the immunoglobulin superfamily. CD200 induces immunosuppression via binding to CD200R, which contains inhibitory NPXY signaling motifs in the cytoplasmic region. The suppression of allogeneic graft rejection by CD200-CD200R signaling has been reported in animal models (63, 64). CD200 on porcine cells not only suppresses macrophage-mediated phagocytosis and cytotoxicity, but also suppresses the release of proinflammatory cytokines from macrophages under xenogeneic conditions. It has been reported that the recombinant fusion protein CD200-Ig (OX2) functions to prolong allograft survival (65). The function of OX2 is expected to be confirmed in a xenogeneic model.
The surfactant proteins (SP)-A and SP-D are members of a family of molecules that induce innate immune responce as pathogen-associated molecular patterns (PAMPs) (66, 67). On the contrary, it has also been reported that SP-A and -D have anti-inflammatory properties (68, 69). While the ligation of the N-terminal collagen domains of SP-A and -D with the calreticulin/CD91 receptor complex induce inflammation, the binding of the C-terminal heads to SIRPα induces an inhibitory signal in innate immune responses (70, 71). We recently demonstrated that the forcible expression of carbohydrate recognition (CRD) of SP-D on porcine endothelial cells significantly suppresses macrophage-mediated cytotoxicity and the production of proinflammatory cytokine from macrophages (72). Because SP-A and D also bind to SIRPα on neutrophils, SP-A and D may also suppress neutrophil-mediated xenogeneic rejection.
Cellular xenografts are rejected predominantly by cellular immune responses. This response is initiated by CD4+ T cells (73–76) and macrophages have been reported to be the most important effector cells that are involved in this response (77). The JAKs inhibitor, Tofacitinib, has been reported to inhibit the production of macrophage-mediated inflammatory cytokines (78, 79). In addition, Kim et al. reported that Tofacitinib suppress allogenic rejection in islet transplantation (80). We recently reported that both PQA-18, a PAK2 inhibitor, and Tofacitinib suppress both the differentiation of macrophages and xenogeneic macrophage-mediated cytotoxicity (45). Furthermore, both PQA-18 and Tofacitinib suppress the induction of xenogeneic cytotoxic T lymphocytes (CTLs) to a considerable extent in mixed lymphocyte reactions (MLR). These findings suggest that PQA-18 and Tofacitinib suppress not only innate immunity but also acquired immunity under xenogeneic conditions and may be effective in both cellular and organ xenograft rejection.
Inhibition of Neutrophil-Mediated Xenogeneic Rejection
A number of studies dealing with human neutrophil migration and adherence to pig endothelial cells have been reported (81–83). al-Mohanna et al. reported that the binding of neutrophils to xenogeneic cells is dependent on the interaction between CD11a and Mac-1 (CD11b/CD18, an iC3b receptor) and their ligands for CD11a and CD11b/CD18 suppress neutrophil adhesion in vitro (84). MacNally et al. It is known that activated neutrophils are attached to foreign surfaces through the interaction of CD11b/CD18 with surface-bound iC3b (85), suggesting that neutrophils are able to recognize xenogeneic cells via the binding iC3b to CD11b/CD18 on neutrophils. A recent study by Bastian et al. supported this finding. They found that, when decellularized porcine heart valveswere incubated in plasma, iC3b was deposited and a significant adhesion of neutrophils was observed (86). CD82, one of the tetraspanins, was also reported to contribute to the adhesion of human neutrophils to porcine cells (87). Saleh et al. reported that CD82 contributes to the Galα1,3-Gal-independent adhesion of neutrophils to porcine endothelial cells. Indeed, the compstatin analogue Cp40, a potent complement C3 inhibitor, was found to suppress CD11b expression on neutrophils and neutrophil adhesion to xenogeneic target cells (88).
Wang et al. recently reported that the ectopic expression of human CD31 on porcine cells suppress xenogeneic neutrophil NETosis and cytotoxicity (89). CD31 (PECAM-1) is well known as an adhesion molecule and is ubiquitously expressed by various inflammatory cells (90). CD31 homophilic ligation induces signal transduction via its immunoreceptor tyrosine-based inhibition motif (ITIMs) to attenuate tyrosine kinase-mediated signaling pathways (91–93). Because CD31 has been reported to be incompatible between human and pig (94, 95), human CD31 may be a good candidate for suppressing neutrophil-mediated xenogeneic rejection.
Conclusion
The infiltration of various inflammatory leukocytes into α1,3-galactosyltransferase knockout (αGalTKO) neonatal porcine islets (96), which suggests that suppressing antibody dependent rejection is not sufficient to prevent xenogeneic cellular innate immunity. Therefore, a strategy that involves the suppression of direct recognition by innate immune cells is essential for clinical applications of xenografts. A variety of strategies for suppressing NK and macrophage-mediated rejection have been proposed, but there appears to be quite a bit of room for improvement. Neutrophils contribute, not only to the induction of inflammation but also to the resolution of inflammation. The phagocytosis of apoptotic cells by macrophages plays a pivotal role in the resolution of an inflammatory response and apoptotic neutrophils themselves exert anti-inflammatory effects (97, 98). The Bcl-2 homologue, Mcl-1, which functions to prevent intrinsic apoptosis, is central to the ability of neutrophils to undergo rapid apoptosis (99). Hence the induction of a Bcl-2 proapoptotic homologue such as Bax in important in terms of inducing apoptosis of neutrophils. The death receptor ligand, TRAIL, has been reported to induce apoptosis in neutrophils (100). A leucine zipper-tagged form of TRAIL may therefore be a good strategy for induce the resolution of inflammation.
In conclusion, significant advances have been made in the field of xenogeneic cellular immune responses in the past 20-30 years (101). Various transgenic and knockout pigs were generated to suppress the xenogeneic rejection. The strategies against innate cellular xenogeneic rejection were summarized in Figure 2. Further improvements will be needed if better effects are to be achieved in the future.
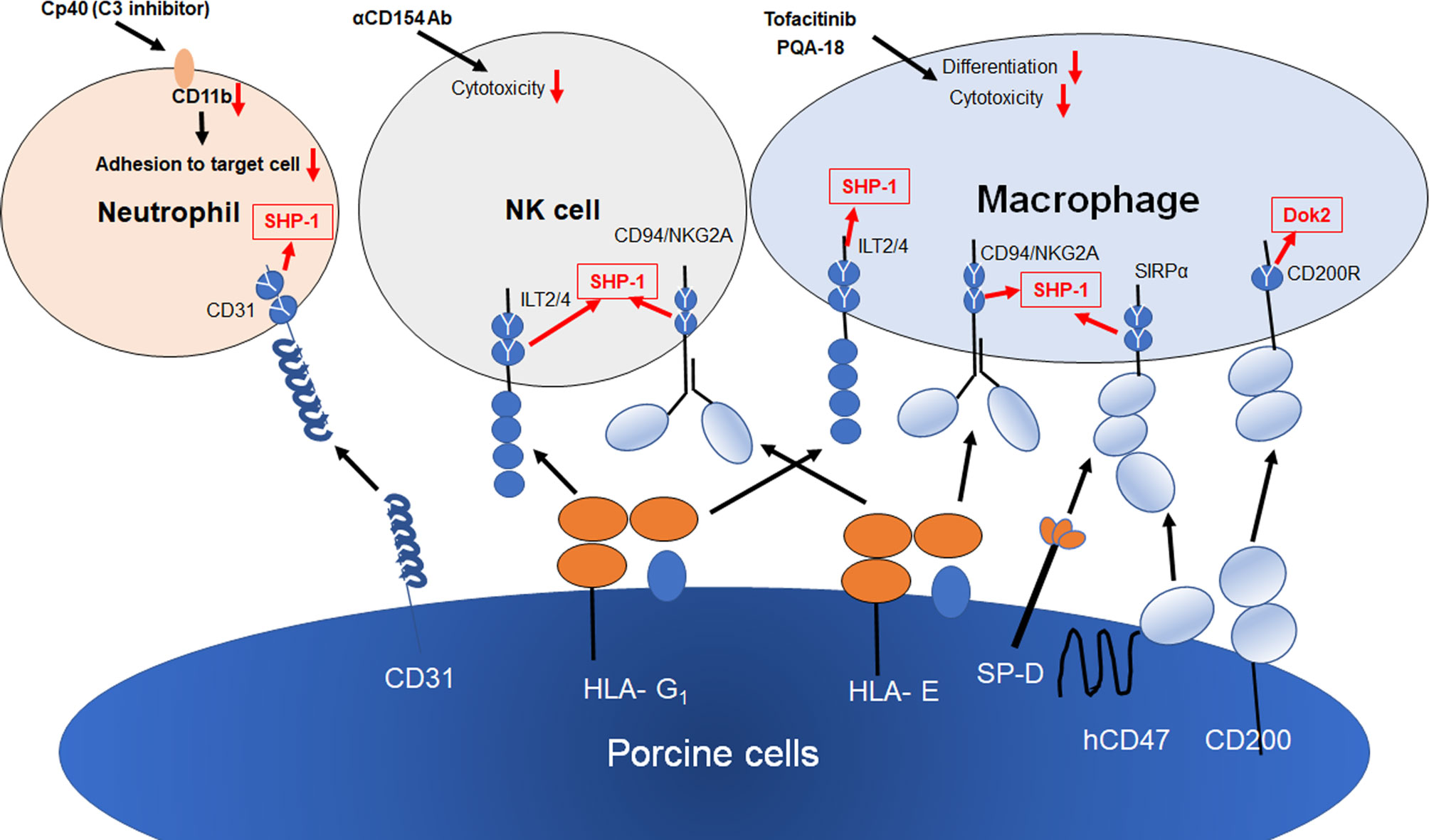
Figure 2 Strategies for innate cellular xenogeneic rejection. Both HLA-E and G1 on porcine endothelial cells suppress NK and macrophage-mediated xenogeneic rejection. The transgenic expression of human CD47, SP-D and CD200 on porcine cells results in the suppression of macrophage-mediated cytotoxicity and inflammation. Human CD31 inhibits NETosis in neutrophils via the homophilic binding to CD31 on neutrophils. Both Tofacitinib (JAK inhibitor) and PQA-18 (PAK2 inhibitor) have been reported to suppress macrophage-mediated cytotoxicity and differentiation of macrophages. Anti-CD154 Ab suppress NK cytotoxicity against CD40+ target cells. Cp40 (C3 inhibitor) suppress CD11b expression, resulting in suppression of neutrophil adhesion to porcine cell. Y shows an inhibitory intracellular signaling motif of inhibitory receptors.
Author Contributions
Collection of manuscript was done by AM while SK, CT, P-CL, CO, RY, KM, MK, HE, and MW collected relevant literature. HN, HO, and SM edited and approved of the manuscript. All authors contributed to the article and approved the submitted version.
Funding
This work was supported by Grants-in Aid for Scientific Research, and Health and Labor Sciences Research Grants, Japan (grant number is 19K9092).
Conflict of Interest
The authors declare that the research was conducted in the absence of any commercial or financial relationships that could be construed as a potential conflict of interest.
Publisher’s Note
All claims expressed in this article are solely those of the authors and do not necessarily represent those of their affiliated organizations, or those of the publisher, the editors and the reviewers. Any product that may be evaluated in this article, or claim that may be made by its manufacturer, is not guaranteed or endorsed by the publisher.
Acknowledgments
The authors wish to thank Dr. Milton. S. Feather for his editing of the manuscript. This work was supported by Grants-in Aid for Scientific Research, and Health and Labor Sciences Research Grants, Japan.
References
1. Lin Y, Vandeputte M, Waer M. Natural Killer Cell- and Macrophage-Mediated Rejection of Concordant Xenografts in the Absence of T and B Cell Responses. J Immunol (1997) 158:5658–67.
2. Shimizu A, Yamada K, Robson SC, Sachs DH, Colvin RB. Pathologic Characteristics of Transplanted Kidney Xenografts. J Am Soc Nephrol (2012) 23:225–35. doi: 10.1681/ASN.2011040429
3. Ezzelarab M, Garcia B, Azimzadeh A, Sun H, Lin CC, Hara H, et al. The Innate Immune Response and Activation of Coagulation in Alpha1,3-Galactosyltransferase Gene-Knockout Xenograft Recipients. Transplantation (2009) 87:805–12. doi: 10.1097/TP.0b013e318199c34f
4. Al-Mohanna FA, Collison KS, Allen SP, Stern D, Yacoub MH. Naive Neutrophils and Xenotransplantation. Lancet (1996) 348:1246. doi: 10.1016/S0140-6736(05)65524-9
5. Al-Mohanna F, Collison K, Parhar R, Kwaasi A, Meyer B, Saleh S, et al. Activation of Naïve Xenogeneic But Not Allogeneic Endothelial Cells by Human Naïve Neutrophils: A Potential Occult Barrier to Xenotransplantation. Am J Pathol (1997) 151:111–20.
6. Sachs UJ, Andrei-Selmer CL, Maniar A, Weiss T, Paddock C, Orlova VV, et al. The Neutrophil-Specific Antigen CD177 Is a Counter-Receptor for Platelet Endothelial Cell Adhesion Molecule-1 (Cd31). J Biol Chem (2007) 10:23603–12. doi: 10.1074/jbc.M701120200
7. Schofield ZV, Woodruff TM, Halai M, Wu MC, Cooper MA. Neutrophils—A Key Component of Ischemia-Reperfusion Injury. Shock (2013) 40:463–70. doi: 10.1097/SHK.0000000000000044
8. Ferrari RS, Andrade CF. Oxidative Stress and Lung Ischemia-Reperfusion Injury. Oxid Med Cell Longev (2015) 2015:590987. doi: 10.1155/2015/590987
9. Loukogeorgakis SP, van den Berg MJ, Sofat R, Nitsch D, Charakida M, Haiyee B, et al. Role of NADPH Oxidase in Endothelial Ischemia/Reperfusion Injury in Humans. Circulation (2010) 121:2310–6. doi: 10.1161/CIRCULATIONAHA.108.814731
10. Duilio C, Ambrosio G, Kuppusamy P, DiPaula A, Becker LC, Zweier JL, et al. Neutrophils are Primary Source of O2 Radicals During Reperfusion After Prolonged Myocardial Ischemia. Am J Physiol Heart Circ Physiol (2001) 280:2649–57. doi: 10.1152/ajpheart.2001.280.6.H2649
11. Kimura K, Shirabe K, Yoshizumi T, Takeishi K, Itoh S, Harimoto N, et al. Ischemia-Reperfusion Injury in Fatty Liver is Mediated by Activated NADPH Oxidase 2 in Rats. Transplantation (2016) 100:791–800. doi: 10.1097/TP.0000000000001130
12. Uchida Y, Freitas MCS, Zhao D, Busuttil RW, Kupiec-Weglinski JW. The Protective Function of Neutrophil Elastase Inhibitor in Liver Ischemia and Reperfusion Injury. Transplantation (2010) 89:1050–6. doi: 10.1097/TP.0b013e3181d45a98
13. Hardison MT, Galin FS, Calderon CE, Djekic UV, Parker SB, Wille KM, et al. The Presence of a Matrix-Derived Neutrophil Chemoattractant in Bronchiolitis Obliterans Syndrome After Lung Transplantation. J Immunol (2009) 182:4423–31. doi: 10.4049/jimmunol.0802457
14. Sil P, Wicklum H, Surell C, Rada B. Macrophage-Derived IL-1β Enhances Monosodium Urate Crystal-Triggered NET Formation. Inflamm Res (2017) 66:227–37. doi: 10.1007/s00011-016-1008-0
15. Hu Z, Murakami T, Tamura H, Reich J, Kuwahara-Arai K, Iba T, et al. Neutrophil Extracellular Traps Induce IL-1β Production by Macrophages in Combination With Lipopolysaccharide. Int J Mol Med (2017) 39:549–58. doi: 10.3892/ijmm.2017.2870
16. Candinas D, Belliveau S, Koyamada N, Miyatake T, Hechenleitner P, Mark W, et al. T Cell Independence of Macrophage and Natural Killer Cell Infiltration, Cytokine Production, and Endotherial Activation During Delayed Xenograft Rejection. Transplantation (1996) 62:1920–7. doi: 10.1097/00007890-199612270-00042
17. Fox A, Mountford J, Braakhuis A and Harrison LC. Innate and Adaptive Immune Responses to Nonvascular Xenografts: Evidence That Macrophages are Direct Effectors of Xenograft Rejection. J Immunol (2001) 166:2133–40. doi: 10.4049/jimmunol.166.3.2133
18. Basker M, Alwayn IP, Buhler L, Harper D, Abraham S, Kruger Gray H, et al. Clearance of Mobilized Porcine Peripheral Blood Progenitor Cells is Delayed by Depletion of the Phagocytic Reticuloendothelial System in Baboons. Transplantation (2001) 72:1278–85. doi: 10.1097/00007890-200110150-00017
19. Hu Z, Van Rooijen N, Yang YG. Macrophages Prevent Human Red Blood Cell Reconstitution in Immunodeficient Mice. Blood (2011) 118:5938–46. doi: 10.1182/blood-2010-11-321414
20. Peterson MD, Jin R, Hyduk S, Duchesneau P, Cybulsky MI, Waddell TK. Monocyte Adhesion to Xenogeneic Endothelium During Laminar Flow is Dependent on Alpha-Gal-Mediated Monocyte Activation. J Immunol (2005) 174:8072–81. doi: 10.4049/jimmunol.174.12.8072
21. Jin R, Greenwald A, Peterson MD, Waddell TK. Human Monocytes Recognize Porcine Endothelium via the Interaction of Galectin 3 and Alpha-GAL. J Immunol (2006) 177:1289–95. doi: 10.4049/jimmunol.177.2.1289
22. Greenwald AG, Jin R, Waddell TK. Galectin-3-Mediated Xenoactivation of Human Monocytes. Transplantation (2009) 87:44–51. doi: 10.1097/TP.0b013e318191e6b4
23. Xu XC, Goodman J, Sasaki H, Lowell J, Mohanakumar T. Activation of Natural Killer Cells and Macrophages by Porcine Endothelial Cells Augments Specific T-Cell Xenoresponse. Am J Transplant (2002) 2:314–22. doi: 10.1034/j.1600-6143.2002.20405.x
24. Adams S, van der Laan LJ, Vernon-Wilson E, Renardel de Lavalette C, Doep EA, Dijkstra CD, et al. Signal-Regulatory Protein Is Selectively Expressed by Myeloid and Neuronal Cells. J Immunol (1998) 161:1853–9.
25. Itoh T, Hata Y, Nishinakamura H, Kumano K, Takahashi H, Kodama S. Islet-Derived Damage-Associated Molecular Pattern Molecule Contributes to Immune Responses Following Microencapsulated Neonatal Porcine Islet Xenotransplantation in Mice. Xenotransplantation (2016) 23:393–404. doi: 10.1111/xen.12253
26. Schneider MK, Forte P, Seebach JD. Adhesive Interactions Between Human NK Cells and Porcine Endothelial Cells. Scand J Immunol (2001) 54:70–5. doi: 10.1046/j.1365-3083.2001.00966.x
27. Matter-Reissmann UB, Forte P, Schneider MK, Filgueira L, Groscurth P, Seebach JD. Xenogeneic Human NK Cytotoxicity Against Porcine Endothelial Cells Is Perforin/Granzyme B Dependent and Not Inhibited by Bcl-2 Overexpression. Xenotransplantation (2002) 9:325–37. doi: 10.1034/j.1399-3089.2002.01074.x
28. Resch T, Fabiritius C, Ebner S, Ritschl P, Kotsch K. The Role of Natural Killer Cells in Humoral Rejection. Transplantation (2015) 99:1335–40.
29. Watzl C. How to Trigger a Killer: Modulation of Natural Killer Cell Reactivity on Many Levels. Adv Immunol (2014) 124:137–70. doi: 10.1016/B978-0-12-800147-9.00005-4
30. Lilienfeld BG, Garcia-Borges C, Crew MD, Seebach JD. Porcine UL16-Binding Protein 1 Expressed on the Surface of Endothelial Cells Triggers Human NK Cytotoxicity Through NKG2D. J Immunol (2006) 177:2146–52. doi: 10.4049/jimmunol.177.4.2146
31. Forte P, Lilienfeld BG, Baumann BC, Seebach JD. Human NK Cytotoxicity Against Porcine Cells is Triggered by NKp44 and NKG2D. J Immunol (2005) 175:5463–70. doi: 10.4049/jimmunol.175.8.5463
32. Sullivan JA, Oettinger HF, Sachs DH, Edge AS. Analysis of Polymorphism in Porcine MHC Class I Genes: Alterations in Signals Recognized by Human Cytotoxic Lymphocytes. J Immunol (1997) 159:2318–26.
33. Long EO, Kim HS, Liu D, Peterson ME, Rajagopalan S. Controlling Natural Killer Cell Responses: Integration of Signals for Activation and Inhibition. Annu Rev Immunol (2013) 31:227–58. doi: 10.1146/annurev-immunol-020711-075005
34. Martens GR, Reyes LM, Li P, Butler JR, Ladowski JM, Estrada JL, et al. Humoral Reactivity of Renal Transplant-Waitlisted Patients to Cells From GGTA1/CMAH/B4GalNT2, and SLA Class I Knockout Pigs. Transplantation (2017) 101:e86–92. doi: 10.1097/TP.0000000000001646
35. Bauman BC, Forte P, Hawley RJ, Rieben R, Schneider MHJ, Seebach JD. Lack of Galactose-α-1,3-Galactose Expression on Porcine Endothelial Cells Prevents Conplement-Induced Lysis But Not Direct Xenogeneic NK Cytotoxicity. J Immunol (2004) 172:6460–7. doi: 10.4049/jimmunol.172.10.6460
36. Bauman BC, Stussi G, Huggel K, Rieben R and Seebach JD. Reactivity of Human Natural Antibodies to Endothelial Cells From Gal(1,3)gal-Deficient Pigs. Transplantation (2007) 83:193–201. doi: 10.1097/01.tp.0000250478.00567.e5
37. Seebach JD, Comrack C, Germana S, LeGuern C, Sachs DH, DerSimonian H. HLA-Cw3 Expression on Porcine Endothelial Cells Protects Against Xenogeneic Cytotoxicity Mediated by a Subset of Human NK Cells. J Immunol (1997) 159:3655–61.
38. Forte P, Baumann BC, Schneider MKJ, Seebach JD. HLA-Cw4 Expression on Porcine Endothelial Cells Reduces Cytotoxicity and Adhesion Mediated by CD158a+ Human NK Cells. Xenotransplantation (2009) 16:19–26. doi: 10.1111/j.1399-3089.2009.00510.x
39. Matsunami K, Miyagawa S, Nakai R, Murase A, Shirakura R. The Possible Use of HLAG1 and G3 in the Inhibition of NK Cell-Mediated Swine Endothelial Cell Lysis. Clin Exp Immunol (2001) 126:165–72. doi: 10.1046/j.1365-2249.2001.01622.x
40. Matsunami K, Miyagawa S, Nakai R, Yamada M, Shirakura R. Modulation of the Leader Peptide Sequence of the HLA-E Gene Up-Regulates its Expression and Down-Regulates Natural Killer Cell-Mediated Swine Endothelial Cell Lysis. Transplantation (2002) 73:1582–9. doi: 10.1097/00007890-200205270-00010
41. Bottino R, Knoll MF, Graeme-Wilson J, Klein EC, Ayares D, Trucco M, et al. Safe Use of Anti-CD154 Monoclonal Antibody in Pig Islet Xenotransplantation in Monkeys. Xenotransplantation (2017) 24:10. doi: 10.1111/xen.12283
42. Cooper DKC, Foote JB, Javed M, Nguyen HQ, Bikhet MH, Hansen-Estruch C, et al. Initial Evidence That Blockade of the CD40/CD154 Costimulation Pathway Alone is Sufficient as Maintenance Therapy in Xenotransplantation. Xenotransplantation (2021) 8:e12721. doi: 10.1111/xen.12721
43. Carbone E, Ruggiero G, Terrazzano G, Palomba C, Manzo C, Fontana F, et al. A New Mechanism of NK Cell Cytotoxicity Activation: The CD40-CD40 Ligand Interaction. J Exp Med (1997) 185:2053–60. doi: 10.1084/jem.185.12.2053
44. Li S, Yan Y, Lin Y, Bullens DM, Rutgeerts O, Goebels J, et al. Rapidly Induced, T-Cell Independent Xenoantibody Production is Mediated by Marginal Zone B Cells and Requires Help From NK Cells. Blood (2007) 110:3926–35. doi: 10.1182/blood-2007-01-065482
45. Lo PC, Maeda A, Kodama T, Takakura C, Yoneyama T, Sakai R, et al. The Novel Immunosuppressant Prenylated Quinolinecarboxylic Acid-18 (PQA-18) Suppresses Macrophage Differentiation and Cytotoxicity in Xenotransplantation. Immunobiology (2019) 224:575–84. doi: 10.1016/j.imbio.2019.04.003
46. Oldenborg PA, Zheleznyak A, Fang YF, Lagenaur CF, Gresham HD, Lindberg FP, et al. Role of CD47 as a Marker of Self on Red Blood Cells. Science (2000) 288:2051–4. doi: 10.1126/science.288.5473.2051
47. Oldenborg PA, Gresham HD, Lindberg FP. CD47-Signal Regulatory Protein Alpha (SIRPalpha) Regulates Fcgamma and Complement Receptor-Mediated Phagocytosis. J Exp Med (2001) 193:855–62. doi: 10.1084/jem.193.7.855
48. Blazar BR, Lindberg FP, Ingulli E, Panoskaltsis-Mortari A, Oldenborg PA, Iizuka K, et al. CD47 (Integrin-Associated Protein) Engagement of Dendritic Cell and Macrophage Counterreceptors is Required to Prevent the Clearance of Donor Lymphohematopoietic Cells. J Exp Med (2001) 194:541–9. doi: 10.1084/jem.194.4.541
49. Ide K, Wang H, Tahara H, Liu J, Wang X, Asahara T, et al. Role for CD47-SIRPalpha Signaling in Xenograft Rejection by Macrophages. Proc Natl Acad Sci USA (2007) 104:5062–6. doi: 10.1073/pnas.0609661104
50. Wang H, VerHalen J, Madariaga ML, Xiang S, Wang S, Lan P, et al. Attenuation of Phagocytosis of Xenogeneic Cells by Manipulating CD47. Blood (2007) 109:836–42. doi: 10.1182/blood-2006-04-019794
51. Cooper DKC, Hara H, Iwase H, Yamamoto T, Li Q, Ezzelarab M, et al. Justification of Specific Genetic Modifications in Pigs for Clinical Organ Enotransplantation. Xenotransplantation (2019) 26:e12516. doi: 10.1111/xen.12516
52. Navarro-Alvarez N, Yang YG. Lack of CD47 on Donor Hepatocytes Promotes Innate Immune Cell Activation and Graft Loss: A Potential Barrier to Hepatocyte Xenotransplantation. Cell Transplant (2014) 23:345–54. doi: 10.3727/096368913X663604
53. Teraoka Y, Ide K, Morimoto H, Tahara H, Ohdan H. Expression of Recipient CD47 on Rat Insulinoma Cell Xenografts Prevents Macrophage-Mediated Rejection Through Sirpα Inhibitory Signaling in Mice. PloS One (2013) 8:e58359. doi: 10.1371/journal.pone.0058359
54. Chen M, Wang Y, Wang H, Sun L, Fu Y, Yang YG. Elimination of Donor CD47 Protects Against Vascularized Allograft Rejection in Mice. Xenotransplantation (2019) 26:e12459. doi: 10.1111/xen.12459
55. Carpizo D, Iruela-Arispe ML. Endogenous Regulators of Angiogenesis–Emphasis on Proteins With Thrombospondin–Type I Motifs. Cancer Metastasis Rev (2000) 19:159–65. doi: 10.1023/A:1026570331022
56. Thakar CV, Zahedi K, Revelo MP, Wang Z, Burnham CE, Barone S, et al. Identification of Thrombospondin 1 (TSP-1) as a Novel Mediator of Cell Injury in Kidney Ischemia. J Clin Invest (2005) 115:3451–9. doi: 10.1172/JCI25461
57. Lario S, Bescós M, Campos B, Mur C, Luque P, Alvarez R, et al. Thrombospondin-1 mRNA Expression in Experimental Kidney Transplantation With Heart-Beating and Non-Heart-Beating Donors. J Nephrol (2007) 20:588–95.
58. Zhao XM, Hu Y, Miller GG, Mitchell RN, Libby P. Association of Thrombospondin-1 and Cardiac Allograft Vasculopathy in Human Cardiac Allografts. Circulation (2001) 103:525–31. doi: 10.1161/01.CIR.103.4.525
59. Maeda A, Kawamura T, Ueno T, Usui N, Eguchi H, Miyagawa S. The Suppression of Inflammatory Macrophage-Mediated Cytotoxicity and Proinflammatory Cytokine Production by Transgenic Expression of HLA-E. Transpl Immunol (2013) 29:76–81. doi: 10.1016/j.trim.2013.08.001
60. Esquivel EL, Maeda A, Eguchi H, Asada M, Sugiyama M, Manabe C, et al. Suppression of Human Macrophage-Mediated Cytotoxicity by Transgenic Swine Endothelial Cell Expression of HLA-G. Transpl Immunol (2015) 32:109–15. doi: 10.1016/j.trim.2014.12.004
61. Sakai R, Maeda A, Choi TV, Lo PC, Jiaravuthisan P, Shabri AM, et al. Human CD200 Suppresses Macrophage-Mediated Xenogeneic Cytotoxicity and Phagocytosis. Surg Today (2018) 48:119–26. doi: 10.1007/s00595-017-1546-2
62. Yan JJ, Koo TY, Lee HS, Lee WB, Kang B, Lee JG, et al. Role of Human CD200 Overexpression in Pig-to-Human Xenogeneic Immune Response Compared With Human CD47 Overexpression. Transplantation (2018) 102:406–16. doi: 10.1097/TP.0000000000001966
63. Gorczynski RM. Transplant Tolerance Modifying Antibody to CD200 Receptor, But Not CD200, Alters Cytokine Production Profile From Stimulated Macrophages. Eur J Immunol (2001) 31:2331–7. doi: 10.1002/1521-4141(200108)31:8<2331::AID-IMMU2331>3.0.CO;2-#
64. Gorczynski RM. CD200 and its Receptors as Targets for Immunoregulation. Curr Opin Investig Drugs (2005) 6:483–8.
65. Gorczynski RM, Cattral MS, Chen Z, Hu J, Lei J, Min WP, et al. An Immunoadhesin Incorporating the Molecule OX-2 Is a Potent Immunosuppressant That Prolongs Allo- and Xenograft Survival. J Immunol (1999) 163:1654–60.
66. LeVine AM, Whitsett JA. Pulmonary Collectins and Innate Host Defense of the Lung. Microbes Infect (2001) 3:161–6. doi: 10.1016/S1286-4579(00)01363-0
67. Kremlev SG, Phelps DS. Surfactant Protein A Stimulation of Inflammatory Cytokine and Immunoglobulin Production. Am J Physiol (1994) 267:L712–9. doi: 10.1152/ajplung.1994.267.6.L712
68. Bridges JP, Davis HW, Damodarasamy M, Kuroki Y, Howles G, Hui DY, et al. Pulmonary Surfactant Proteins A and D Are Potent Endogenous Inhibitors of Lipid Peroxidation and Oxidative Cellular Injury. J Biol Chem (2000) 275:38848–55. doi: 10.1074/jbc.M005322200
69. Borron P, McIntosh JC, Korfhagen TR, Whitsett JA, Taylor J, Wright JP. Surfactant-Associated Protein A Inhibits LPS-Induced Cytokine and Nitric Oxide Production In Vivo. Am J Physiol Lung Cell Mol Physiol (2000) 278:L840–7. doi: 10.1152/ajplung.2000.278.4.L840
70. Gardai SJ, Xiao YQ, Dickinson M, Nick JA, Voelker DR, Greene KE, et al. By Binding SIRPalpha or Calreticulin/CD91, Lung Collectins Act as Dual Function Surveillance Molecules to Suppress or Enhance Inflammation. Cell (2003) 115:13–23. doi: 10.1016/S0092-8674(03)00758-X
71. Janssen WJ, McPhillips KA, Dickinson MG, Linderman DJ, Morimoto K, Xiao YQ, et al. Surfactant Proteins A and D Suppress Alveolar Macrophage Phagocytosis via Interaction With SIRP Alpha. Am J Respir Crit Care Med (2008) 178:158–67. doi: 10.1164/rccm.200711-1661OC
72. Jiaravuthisan P, Maeda A, Takakura C, Wang HT, Sakai R, Shabri AM, et al. A Membrane-Type Surfactant Protein D (SP-D) Suppresses Macrophage-Mediated Cytotoxicity in Swine Endothelial Cells. Transpl Immunol (2018) 47:44–8. doi: 10.1016/j.trim.2018.02.003
73. Pierson RN III, Winn HJ, Russell PS, Auchincloss H Jr. Xenogeneic Skin Graft Rejection is Especially Dependent on CD4+ T Cells. J Exp Med (1989) 170:991–6. doi: 10.1084/jem.170.3.991
74. Morris CF, Simeonovic CJ, Fung MC, Wilson JD, Hapel AJ. Intragraft Expression of Cytokine Transcripts During Pig Proislet Xenograft Rejection and Tolerance in Mice. J Immunol (1995) 154:2470–82.
75. Loudovaris T, Mandel TE, Charlton B. CD4+ T-Cell Mediated Destruction of Xenografts Within Cell-Impermeable Membranes in the Absence of CD8+ T Cells and B Cells. Transplantation (1996) 61:1678–84. doi: 10.1097/00007890-199606270-00003
76. Yi S, Horthone WJ, Lehnert AM, Ha H, Wong JKW, Rooijen NV, et al. T Cell-Activated Macrophages Are Capable of Both Recognition and Rejection of Pancreatic Islet Xenografts. J Immunol (2003) 170:2750–8. doi: 10.4049/jimmunol.170.5.2750
77. Fox A, Koulmanda M, Mandel TE, van Rooijen N, Harrison LC. Evidence That Macrophages are Required for T-Cell Infiltration and Rejection of Fetal Pig Pancreas Xenografts in Nonobese Diabetic Mice. Transplantation (1998 1998) 66:1407. doi: 10.1097/00007890-199812150-00002
78. Ghoreschi K, Jesson MI, Li X, Lee JL, Ghosh S, Alsup JW, et al. Modulation of Innate and Adaptive Immune Responses by Tofacitinib (CP-690,550). J Immunol (2011) 186:4234–43. doi: 10.4049/jimmunol.1003668
79. Nishimura K, Saegusa J, Matsuki F, Akashi K, Kageyama G, Morinobu A. Tofacitinib Facilitates the Expansion of Myeloid-Derived Suppressor Cells and Ameliorates Arthritis in SKG Mice. Arthritis Rheumatol (2015) 67:893–902. doi: 10.1002/art.39007
80. Kim JM, Shin JS, Min BH, Kang SJ, Yoon IH, Chung H, et al. JAK3 Inhibitor-Based Immunosuppression in Allogeneic Islet Transplantation in Cynomolgus Monkeys. Islets (2019) 11:119–28. doi: 10.1080/19382014.2019.1650580
81. Gilli UO, Schneider MK, Loetscher P, Seebach JD. Human Polymorphonuclear Neutrophils are Recruited by Porcine Chemokines Acting on CXC Chemokine Receptor 2, and Platelet-Activating Factor. Transplantation (2005) 79:1324–31. doi: 10.1097/01.TP.0000155429.44902.44
82. Warrens AN, Simon AR, Theodore PR, Sykes M. Human-Porcine Receptor-Ligand Compatibility Within the Immune System: Relevance for Xenotransplantation. Xenotransplantation (1999) 6:75–8. doi: 10.1034/j.1399-3089.1999.00020.x
83. Cruzado JM, Torras J, Riera M, Lloberas N, Herrero I, Condom E, et al. Effect of a Platelet-Activating Factor (PAF) Receptor Antagonist on Hyperacute Xenograft Rejection; Evaluation in a Pig Kidney-Human Blood Xenoperfusion Model. Clin Exp Immunol (1998) 113:136–44. doi: 10.1046/j.1365-2249.1998.00634.x
84. al-Mohanna F, Collison K, Parhar R, Kwaasi A, Meyer B, Saleh S, et al. Activation of Naive Xenogeneic But Not Allogeneic Endothelial Cells by Human Naive Neutrophils: A Potential Occult Barrier to Xenotransplantation. Am J Pathol (1997) 151:111–20.
85. McNally AK, Anderson JM. Complement C3 Participation in Monocyte Adhesion to Different Surfaces. Proc Natl Acad Sci USA (1994) 91:10119–23. doi: 10.1073/pnas.91.21.10119
86. Bastian F, Stelzmuller ME, Kratochwill K, Kasimir MT, Simon P, Weigel G. IgG Deposition and Activation of the Classical Complement Pathway Involvement in the Activation of Human Granulocytes by Decellularized Porcine Heart Valve Tissue. Biomaterials (2008) 29:1824–32. doi: 10.1016/j.biomaterials.2008.01.005
87. Saleh SM, Parhar RS, Al-Hejailan RS, Backheet RH, Khaleel HS, Khalak HG, et al. Identification of the Tetraspanin CD82 as a New Barrier to Xenotransplantation. J Immunol (2013) 191:2796–805. doi: 10.4049/jimmunol.1300601
88. Kourtzelis I, Ferreira A, Mitroulis I, Ricklin D, Bornstein SR, Waskow C, et al. Complement Inhibition in a Xenogeneic Model of Interactions Between Human Whole Blood and Porcine Endothelium. Horm Metab Res (2015) 47:36–42. doi: 10.1055/s-0034-1390452
89. Wang HT, Maeda A, Sakai R, Lo PC, Takakura C, Jiaravuthisan P, et al. Human CD31 on Porcine Cells Suppress Xenogeneic Neutrophil-Mediated Cytotoxicity via the Inhibition of NETosis. Xenotransplantation (2018) 25:e12396. doi: 10.1111/xen.12396
91. Jackson DE, Ward CM, Wang R, Newman PJ. The Protein-Tyrosine Phosphatase SHP-2 Binds Platelet/Endothelial Cell Adhesion Molecule-1 (PECAM-1) and Forms a Distinct Signaling Complex During Platelet Aggregation: Evidence for a Mechanistic Link Between PECAM-1 and Integrin-Mediated Cellular Signaling. J Biol Chem (1997) 272:6986–93. doi: 10.1074/jbc.272.11.6986
92. Hua CT, Gamble JR, Vadas MA, Jackson DE. Recruitment and Activation of SHP-1 Protein-Tyrosine Phosphatase by Human Platelet Endothelial Cell Adhesion Molecule-1 (PECAM-1): Identification of Immunoreceptor Tyrosine-Based Inhibitory Motif-Like Binding Motifs and Substrates. J Biol Chem (1998) 273:28332–40. doi: 10.1074/jbc.273.43.28332
93. Jackson DE, Kupcho KR, Newman PJ. Characterization of Phosphotyrosine Binding Motifs in the Cytoplasmic Domain of Platelet/Endothelial Cell Adhesion Molecule-1 (PECAM-1) That Are Required for the Cellular Association and Activation of the Protein-Tyrosine Phosphatase, SHP-2. J Biol Chem (1997) 272:24868–75. doi: 10.1074/jbc.272.40.24868
94. Henshall TL, Jones KL, Wilkinson R, Jackson DE. SHP-1 and SHP-2 Protein-Tyrosine Phosphatases Are Required for Platelet Endothelial Cell Adhesion Molecule-1 (PECAM-1)-Mediated Inhibitory Signaling. J Immunol (2001) 166:3098–106. doi: 10.4049/jimmunol.166.5.3098
95. Nasu K, Whyte A, Green SJ, Evans PC, Kilshaw PJ. Alpha-Galactosyl-Mediated Activation of Porcine Endothelial Cells: Studies on CD31 and VE-Cadherin in Adhesion and Signaling. Transplantation (1999) 68:861–7. doi: 10.1097/00007890-199909270-00020
96. Martin BM, Samy KP, Lowe MC, Thompson PW, Cano J, Farris AB, et al. Dual Islet Transplantation Modeling of the Islet Blood-Mediated Inflammatory Reaction. Am J Transplant (2015) 15:1241–52. doi: 10.1111/ajt.13098
97. Kennedy AD, DeLeo FR. Neutrophil Apoptosis and the Resolution of Infection. Immunol Res (2009) 43:25–61. doi: 10.1007/s12026-008-8049-6
98. Fox S, Leitch AE, Duffin R, Haslett C, Rossi AG. Neutrophil Apoptosis: Relevance to the Innate Immune Response and Inflammatory Disease. J Innate Immun (2010) 2:216–27. doi: 10.1159/000284367
99. Edwards SW, Derouet M, Howse M, Moots RJ. Regulation of Neutrophil Apoptosis by Mcl-1. Biochem Soc Trans (2004) 32:489–92. doi: 10.1042/bst0320489
100. Renshaw SA, Parmar JS, Singleton V, Rowe SJ, Dockrell DH, Dowker SK, et al. Acceleration of Human Neutrophil Apoptosis by TRAIL. J Immunol (2003) 170:1027–33. doi: 10.4049/jimmunol.170.2.1027
Keywords: xenotransplantation, macrophage-mediated xenogeneic rejection, innate cellular response, NETosis, ITIMs
Citation: Maeda A, Kogata S, Toyama C, Lo P-C, Okamatsu C, Yamamoto R, Masahata K, Kamiyama M, Eguchi H, Watanabe M, Nagashima H, Okuyama H and Miyagawa S (2022) The Innate Cellular Immune Response in Xenotransplantation. Front. Immunol. 13:858604. doi: 10.3389/fimmu.2022.858604
Received: 20 January 2022; Accepted: 23 February 2022;
Published: 28 March 2022.
Edited by:
Geraldo Aleixo Passos, University of São Paulo, BrazilReviewed by:
Mee Kum Kim, Seoul National University, South KoreaCopyright © 2022 Maeda, Kogata, Toyama, Lo, Okamatsu, Yamamoto, Masahata, Kamiyama, Eguchi, Watanabe, Nagashima, Okuyama and Miyagawa. This is an open-access article distributed under the terms of the Creative Commons Attribution License (CC BY). The use, distribution or reproduction in other forums is permitted, provided the original author(s) and the copyright owner(s) are credited and that the original publication in this journal is cited, in accordance with accepted academic practice. No use, distribution or reproduction is permitted which does not comply with these terms.
*Correspondence: Akira Maeda, WlVMMDY3MjBAbmlmdHkuY29t