- 1Genethon, Evry, France
- 2Université Paris-Saclay, Univ Evry, Inserm, Genethon, Integrare research unit UMR_S951, Evry, France
One of the major goals of in vivo gene transfer is to achieve long-term expression of therapeutic transgenes in terminally differentiated cells. The extensive clinical experience and the recent approval of Luxturna® (Spark Therapeutics, now Roche) and Zolgensma® (AveXis, now Novartis) place vectors derived from adeno-associated viruses (AAV) among the best options for gene transfer in multiple tissues. Despite these successes, limitations remain to the application of this therapeutic modality in a wider population. AAV was originally identified as a promising virus to derive gene therapy vectors because, despite infecting humans, it was not associated with any evident disease. Thee large proportion of AAV infections in the human population is now revealing as a limitation because after exposure to wild-type AAV, anti-AAV antibodies develops and may neutralize the vectors derived from the virus. Injection of AAV in humans is generally well-tolerated although the immune system can activate after the recognition of AAV vectors capsid and genome. The formation of high-titer neutralizing antibodies to AAV after the first injection precludes vector re-administration. Thus, both pre-existing and post-treatment humoral responses to AAV vectors greatly limit a wider application of this gene transfer modality. Different methods were suggested to overcome this limitation. The extensive preclinical data available and the large clinical experience in the control of AAV vectors immunogenicity are key to clinical translation and to demonstrate the safety and efficacy of these methods and ultimately bring a curative treatment to patients.
Introduction
Adeno-associated viruses (AAV) are constituted by a 25-nanometer protein icosahedral capsid containing a single-stranded DNA genome flanked by two palindromic inverted terminal repeats (ITR). The 4.7 Kb AAV genome encodes for four different Rep proteins (Rep78, Rep68, Rep52 and Rep40), three Cap proteins (VP1, VP2 and VP3), the assembly activating protein (AAP) and the newly identified membrane-associated accessory protein (MAAP) (1–3). Cap proteins constitute the capsid of the virus and mediate the interaction with the host. The capsid proteins VP1 and VP2 share most of the sequence with VP3 that is the major component of the AAV capsid with 50 out of 60 capsid subunits being VP3 (4). At the time of writing, 13 different AAV serotypes and more than hundred isolates, distinguished by amino acid modifications in the capsid proteins have been identified in different species (1, 5–8).
After its isolation as a contaminant of adenovirus preparations in 1965 (9–11), it took almost 20 years for molecular cloning of the AAV genome thus opening the way to the generation of recombinant AAV (rAAV) vectors from AAV by encapsidating a transgene expression cassette flanked by the ITRs from serotype 2 (12–15). Importantly, through this process, the same transgene expression cassette can be pseudo-typed by virtually any of the natural AAV serotypes. As for the natural virus, capsid composition affects the tissue tropism and the intracellular trafficking of the recombinant virus (1, 16).
The adenovirus-free method of rAAV production is based on transient transfection of mammalian cells with three plasmids (17). Two of the plasmids provide in trans the rep and cap genes and the helper genes, typically from adenovirus (18, 19). A third plasmid contains the transgene expression cassette flanked by the two ITRs. Recombinant AAV vectors can be produced in mammalian cells also through the infection with adenovirus (20) or herpes simplex virus (21). Finally, rAAV can be produced in insect cells infected with baculoviruses carrying all the components necessary for vector production (22). Regardless of the production method, and differently from the wild-type virus (23), rAAV vectors are produced as a mix of full capsids, containing the genomic material, and empty capsids. Several distinct natural serotypes isolated in humans and other mammalian species were produced as well as chimeric AAV obtained through different techniques [recently reviewed in (24)].
As previously mentioned, the transduction properties of rAAV vectors are a direct consequence of the capsid composition. Surface-receptors binding, endocytosis and intracellular trafficking as well as the escape of the vector from the late endosome/lysosomal compartments and the nuclear import contributes to the preference of rAAV vectors for a certain cell type/tissue [reviewed in (25)]. After nuclear translocation, the genome of rAAV do not integrate efficiently and remains in the episomal form (26).
AAVs infect humans and other mammalian species starting from the first years of life (27–33), but are not associated to any known disease (34). Infection with AAVs results in the formation of a humoral response against the virus. Although the frequency of individuals seropositive to AAV may vary, large portions of the human population are infected, with an estimated seroprevalence for neutralizing antibodies (NAb) for the different AAV serotypes in the range of 30-60% (27–29, 35–37). The presence of NAbs due to exposure to the wild-type AAV reduce the number of patients that may benefit from the treatment.
Although multi-year transgene expression was reported in large animals and patients treated with rAAV (38–43), loss of expression at long-term is still possible as a consequence of slow cell replication or mechanisms of inactivation acting on the vector genome (44). The formation of anti-AAV NAbs with long persistence and wide specificity after injection of rAAV (45) represents an important limitation to re-administration of the vectors in patients that have received the rAAV and experienced an expression loss.
This review will then focus on the methods that overcome the limitations imposed by the humoral response to gene transfer with rAAV (Figure 1) perceived as fundamental to expand patients access to therapy and allow for life-long treatment of genetic diseases.
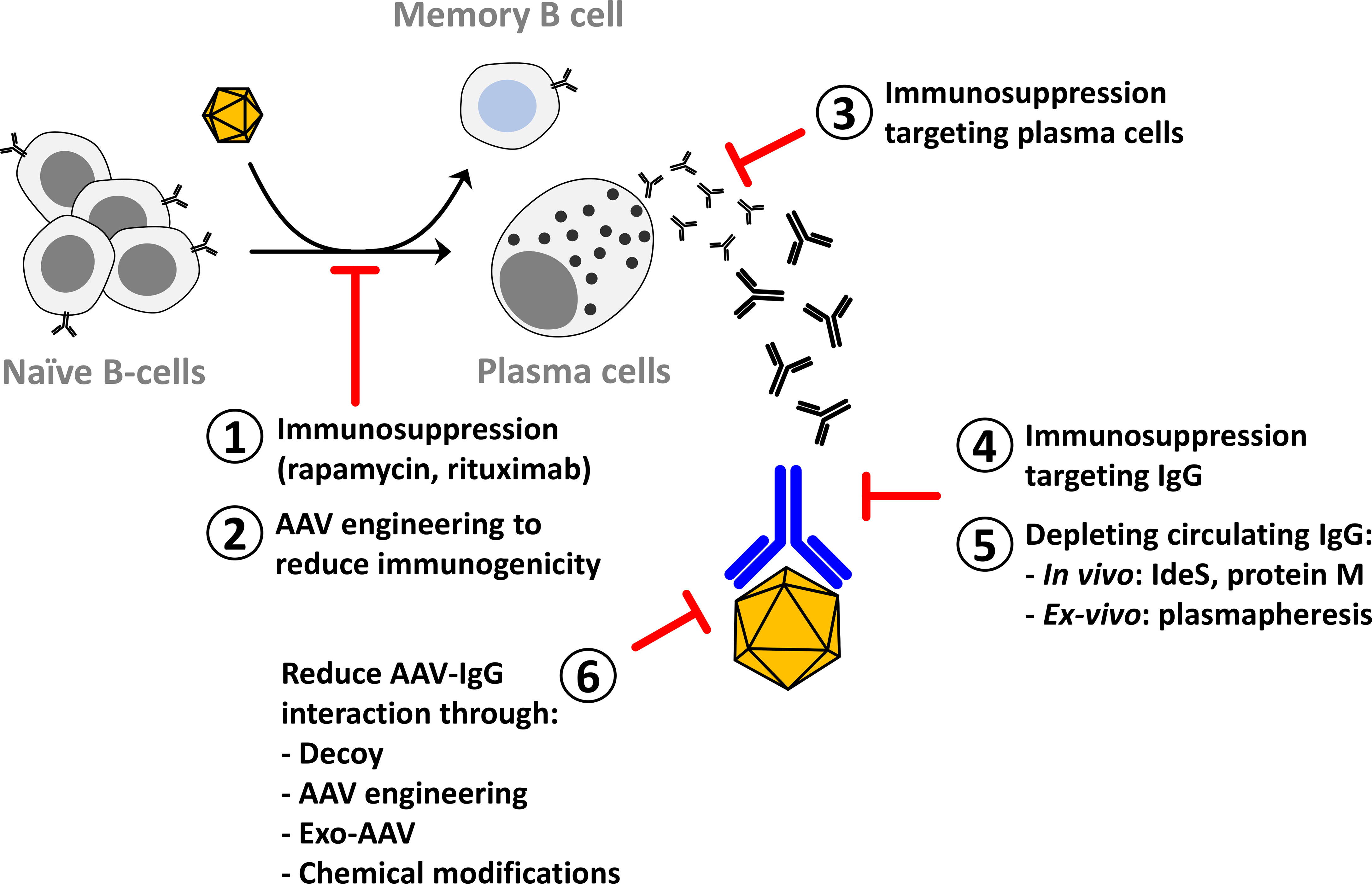
Figure 1 Methods to reduce the impact of anti-AAV neutralizing antibodies on AAV gene transfer. After AAV gene transfer in seronegative individuals naïve B-cells are activated to plasma cells that are specialized in antibody secretion. Part of the activated B-cells become memory B-cells that participate in the long-term stability of the humoral response. Methods to reduce the activation of B-cells include immunosuppression to target the CD4-mediated helper function (1) or to reduce the viability of plasma cells (3) and AAV engineering to reduce the activation of the immune system (2). Immunosuppression to reduce circulating IgG has been proposed in autoimmune diseases and can be applied in AAV gene therapy (4). Circulating IgG can also be reduced through in vivo or in vitro depletion (5). Another method to reduce the impact of anti-AAV IgG on gene transfer efficacy is to modify the vector to evade neutralization (6).
Humoral Immunity to AAV
AAVs naturally infect humans and other mammalians starting from infancy. Although the natural history of AAV infection is still poorly characterized, data indicated that, after infection, a large proportion (ranging from 30% to 60%) of the infected population developed cross-reactive anti-AAV NAbs possibly due to successive infections and/or broad cross-reactivity between AAV serotypes (46). The proportion of seropositive individuals increased from early childhood to peak at the age of adolescence (27–29). Infection with wild-type AAV resulted in antibodies from all IgG subclasses with a preference for IgG1. The levels of IgG, in general, correlated with the neutralizing antibody titers measured by an in vitro neutralization test (35, 36) although it was reported the presence of IgG that while binding to AAV vectors did not neutralize their capacity to enter the cells in vitro and in vivo (47). NAbs against AAV2 and AAV1 were reported as the most prevalent in the human population (48) starting from three years of age. Importantly, the occurrence of maternal transfer of Nabs, with neonates being seronegative only between 7 and 11 months, leaves a short time window for AAV gene transfer (27).
At the very beginning of gene therapy with AAV vectors, the role of pre-existing immunity in gene transfer efficacy was uncertain. The presence of neutralizing antibodies to AAV2 was not among the exclusion criteria in the first clinical trial of liver gene transfer after systemic infusion with this vector (49). Although the choice was possibly motivated by the use of a ‘‘local’’ administration of the vector, i.e. portal vein infusion, data showed a robust reduction in transgene expression in one patient having low anti-AAV neutralizing titers (49). Preclinical data obtained in mice and published in the same year supported this finding (50). Later on, experiments in non-human primates (NHP) clearly showed neutralization due to low-titer anti-AAV pre-existing antibodies (51). Based on this experience, exclusion criteria for seropositive patients were included in most of the clinical trials.
In humans, administration of AAV vectors resulted in a fast and robust rise in IgM, followed by IgG with a high neutralizing titer either after intramuscular (52) or portal vein infusion (49). Similarly to the wild-type virus infection, the neutralizing titers in general correlate with the levels of binding antibodies as measured by ELISA (53). Long term follow-up of patients receiving treatment for hemophilia B indicates up to fifteen years of stability of neutralizing anti-AAV antibody titers for the injected AAV (43, 45). Importantly, while higher titers were measured against the injected serotype, the antibodies were neutralizing also against other serotypes although with lower titers (45).
Neutralizing factors, capable of binding to AAV vectors thus reducing their efficacy in vitro were identified as elements of the complement system (54, 55). Important and open questions about neutralizing factors against AAV are their exact nature and role in the immune response and gene transfer efficacy in vivo. Complement activation was reported in ongoing clinical trials for Duchenne muscular dystrophy and spinal muscular atrophy type 1, in which patients administered with high-dose AAV vectors developed serious adverse events including liver dysfunction, acute kidney injury or thrombocytopenia (56–58). More recently, LogicBio reported two cases of thrombotic microangiopathy following the administration of 5 x 1013 vg/kg of AAV-LK03 (59). Both complement proteins and antibodies are likely being investigated for their role in those adverse events. In animal models, neuronal degeneration in the dorsal root ganglia (DRG) were observed few weeks after high-dose intravenous or intrathecal administration in piglets and NHPs (60–62). This neuronal toxicity was not prevented by conventional steroid regimens and was dependent on overabundance of the transgene product and subsequent activation of cellular stress pathway (63). Beside this DRG toxicity, acute liver damage occurred in NHPs 3-4 days after high-dose systemic delivery of AAV9 or AAV-PHP.B, with acute elevations in liver enzymes, thrombocytopenia as well as acute hemorrhage (60, 64). Interestingly, prophylactic steroid treatment mitigates the increase in liver enzymes but not the thrombocytopenia suggesting that these two events may not correlate. Moreover, selection of seronegative NHPs for the studies, timing of this acute toxicity as well as activation of alternative complement pathway support a working hypothesis where classical complement pathway activated by antigen-antibody immune complexes is likely not involved in these deleterious events. Poor availability of predictive animal models to study complement activation and limited clinical experience with AAV vectors administration in seropositive patients may be two of the reasons why, so far, a mechanistic proof of the involvement of these factors in AAV toxicity is still missing.
Regardless of their involvement in unwanted toxicities, the long-term stability and the wide neutralization potential of the humoral immune response to AAV vectors are important limitations of this gene therapy modality as they preclude both vector infusion in seropositive patients and re-administration in patients that received a sub-optimal dose or in whom the efficacy of gene therapy waned overtime.
AAV Capsid Modifications to Reduce Antibody-Mediated Neutralization
Early attempts to prevent capsid neutralization were based on the use of empty capsids as decoys to shield the full particles from neutralization (65). This approach required large amounts of empty AAV capsids thus increasing total vector load. Liver toxicity associated with the death of four patients was recently reported in a clinical trial for X-linked myotubular myopathy (NCT03199469) and likely linked to the high vector dose and pre-existing liver disease. Another potential limitation in the decoy strategy is the lack of efficient methods for the production and purification of empty capsids with limited encapsidation of random cellular components and genomic and plasmid DNA.
The relatively low complexity of the capsid structure and AAV virus genome has allowed for the creation of a multitude of engineered capsids with improved properties. Rational design as well as random mutagenesis and capsid shuffling were used to derive novel serotypes. Peptide insertion in specific VP3 protein position combined with directed evolution, provided a powerful tool to improve AAV capsids biodistribution (66–68). Structure-guided evolution was also used to modify the epitopes recognized by antibodies thus evading neutralization. In particular, the resolution of the structure of AAV2 complexed with a monoclonal antibody (69) enabled rational mutation of the AAV2 sequence to avoid neutralization from this specific clone (70). An evolution of this approach is based on the rational mutation of the regions of AAV capsids involved in the interactions with polyclonal human serums (71). Through this approach, novel AAV capsids were identified with improved evasion of NAbs without compromising vector productivity or biodistribution. Another method to isolate neutralization-resistant AAV vectors is the directed evolution of AAV libraries in an in vitro neutralization setting. Although limited by the poor in vitro transduction of AAV vectors, the use of selective pressure on randomly mutated or shuffled AAV capsid libraries led to the isolation of serotypes that were less neutralized by purified intravenous immunoglobulins (IVIg) (72–74). The use of immune-orthogonal orthologues of AAV has been proposed to overcome the constraints imposed by the humoral response to AAV vectors (75). This approach is limited by the higher complexity of the human immune system that reduces the chance to identify orthologs (76). However, after confirmation in relevant preclinical models, this approach may represent an option to dose seropositive patients.
Another interesting approach to evade antibody-mediated neutralization is the use of AAV vectors co-purified with exosomes derived from the producer cell line. Exosomes are extracellular vesicles naturally produced by various types of cells both in culture and in vivo and used by the cells for communication or exchange of proteins and genetic material (reviewed in (77)). Importantly, some viruses showed the ability to hack this system and acquire a membrane shield to the immune system. Similarly, AAV vectors associated with membranes of the producer cell lines can be purified as exosome-associated AAV vectors or exo-AAV (78). Exo-AAV showed improved cell transduction and reduced sensitivity to neutralization in an in vivo neutralization assay (79–81). One potential limitation associated to the clinical translation of exo-AAV is related to the potential adjuvant effect of the proteins and nucleic acid co-packaged with the exo-AAV and to the challenges regarding the development of a clinical grade manufacturing process and the associated analytical methods.
The high stability of the AAV capsid in extreme conditions led to the development of methods to modify the surface of AAV capsids by cross-linking different chemical molecules. RGD-containing peptides as well as PEG were originally proposed to improve AAV tropism but also to reduce the impact of neutralizing antibodies on transduction (82–86). More recently, amino sugars, known to increase liver targeting were chemically linked to the AAV capsid (87). Although the chemical alteration of AAV vectors may, in principle, extensively modify the capsid surface, only partial evasion of NAbs was reported so far (87). One important limitation of the chemical modification of the AAV capsid and more generally of proteins is that drastic conditions are required to obtain extensive modification of the surface, and this may not be compatible with the stability of the 3D structure of the virus.
Although different methods were proposed to modify the AAV capsid and reduce the impact of neutralizing antibodies, so far, there is no clinical proof of the possibility to administer those modified AAV vectors in seropositive individuals. Perhaps in this context are worth mentioning the recent clinical results suggesting that AAV5 is less sensitive to neutralization in humans (88). Administration of an AAV5 vector expressing human coagulation factor IX in patients who have retrospectively been shown to have significant NAb titer resulted in similar expression levels of the transgene with a lower impact on the transduction compared to what was previously reported for AAV2 (49). Those results were confirmed in a large study in NHPs were different doses of AAV5-hFIX demonstrated efficacy irrespective of the presence of pre-existing anti AAV5 NAb at titers up to 1:1030 (88). The absence of standardized tests to compare neutralizing titers measured in the different clinical trials limits any further conclusion. However, these results suggest inconsistency between the neutralization titers measured with an in vitro assay and the neutralization in vivo after administration of the AAV5 vector in humans. If confirmed in a larger number of patients, the use of AAV5 for liver gene transfer may represent a valid option to treat seropositive patients.
Immunosuppression to Prevent Anti-AAV Capsid Antibody Formation
The formation of anti-AAV NAbs following AAV gene transfer is dependent on the serotype used and on the route of administration which may influence the presentation of the AAV capsid proteins on antigen-presenting cells (APCs) (89–91). Blocking of classical costimulatory pathways, implicated in B-cell activation, demonstrated efficacy on the inhibition of humoral response and allowed AAV re-administration in mice (92, 93). Early findings supported the involvement of the innate signaling in the formation of the humoral immune response to AAV vectors (94). In particular, loss of MyD88, a central node for the signaling downstream of Toll-like receptor (TLR) and interleukin-1 (IL-1) receptor pathways, significantly reduced anti-AAV NAb titers (95, 96). An isotype switch from IgG2c to IgG1 was also observed in MyD88 deficient mice possibly due to a switch to a Th2-type immune response in the absence of Th1-polarizing stimuli. In human peripheral blood mononuclear cells (PBMCs), stimulation with AAV2 capsid resulted in an interleukin-1b (IL-1b) -dependent B-cell maturation and anti-AAV antibody secretion (97). Importantly, the inhibition of IL-1b through monoclonal antibodies resulted in decreased anti-AAV antibody formation both in vitro, in human PBMCs and in vivo in C57Bl6 mice challenged with an AAV8 vector (97). Based on these data, the inhibition of innate signaling seems to be a promising approach to control adaptive immunity to AAV vectors. Another relevant target for the suppression of the anti-AAV humoral response is the inhibition of the CD4 co-receptor. Both the antioxidant MnTBAP and a non-depleting anti-CD4 antibody were used to reduce the activation of CD4+ T-cells, thus decreasing the anti-AAV humoral response (90, 98). As an alternative, rapamycin (Sirolimus), an immunosuppressive drug largely used in transplantation, was shown to reduce anti-AAV immune response (99). Interestingly, rapamycin formulated in nanoparticles showed greater efficacy in small and large preclinical models and allowed to re-administer an AAV vector in primates while reducing the dose of rapamycin by specific targeting APCs (100–102). B-cell depletion with an anti-CD20 antibody (Rituximab) has also shown efficacy in the reduction of anti-AAV humoral immune response in preclinical models (103, 104). The combination of Sirolimus and Rituximab is being investigated in a clinical protocol for AAV vector re-administration in Pompe disease (NCT02240407). The clinical protocol involves the repeated intramuscular administration of an AAV9 vector expressing GAA in the presence of an immunosuppression regimen combining Rituximab and Sirolimus. Although the results of this trial are not public, a single-case report indicated that simultaneous treatment with these two drugs prevented the formation of anti-AAV1 antibodies when the vector was administered into the diaphragm (105).
One important limitation of the immunosuppression methods described above is that proof of their efficacy was obtained mainly in naïve animals that received the AAV vector for the first time and their efficacy is likely very limited in primed animals were the B-cells are already activated and differentiated in both memory B-cells and plasma cells (PCs). Since PCs are responsible for the maintenance of high levels of circulating antibodies, different strategies, derived from those used in autoimmune diseases and myeloma, were attempted to substantially reduce the number of active PCs. Targeting PCs may result in toxicity and the debate on the risk/benefit ratio of such approaches in AAV gene therapy is still open. Among them, we may cite the use of bortezomib, a proteasome inhibitor approved for the treatment of mantle cell lymphoma and myeloma, that despite its toxicity, has been proposed for the control of anti-AAV immune response (106). Although the combination of bortezomib with AAV vectors was also tested in dogs (107), no significant effect was reported on the pre-existing humoral immunity to AAV vectors and the strategy was never moved used in the clinic. Novel approaches are being developed for the control of PCs proliferation with improved selectivity and reduced toxicity [reviewed in (108)] and based on their improved risk/benefit ratio they may be tested as a pretreatment to reduce the humoral immune response to AAV vectors and allow for vector administration in seropositive patients.
Methods to Reduce the Levels of Circulating Antibodies
As already discussed, plasma cells are a relevant target to reduce the impact of neutralizing antibodies on gene transfer. However, the current approaches to drastically reduce the number of antibody-secreting cells suffer from toxicity and poor selectivity.
A strategy to reduce the impact of NAbs on AAV transduction relies on the isolated perfusion of the liver with a catheter to flush the blood from the liver (109, 110). Although this method allows to reduce the titers of NAbs and increase liver transduction in seropositive monkeys, its clinical translation may prove complex and potential inflammation of the liver related to the procedure may threaten the outcome of gene transfer in humans.
As an alternative, reduction of the circulating levels of IgG is an ideal strategy to reduce the impact of anti-AAV NAbs on AAV gene transfer. Among those methods, the use of blockers of neonatal Fc receptors (FcRn) was proposed for AAV vector administration in seropositive patients (111), although it was never tested in this setting. FcRn receptors have a profound effect on the levels of circulating IgG by increasing their recycling (112). Blocking the action of FcRn led to increased antibody trafficking to lysosomes and degradation and reduced the levels of circulating IgG in both NHPs and humans (113, 114). FcRn-targeting therapeutics demonstrated efficacy in preclinical models of autoimmunity such as antibody-induced arthritis, experimental autoimmune encephalomyelitis or immune thrombocytopenia [reviewed in (115)].
A more invasive alternative to FcRn blockers is the use of plasmapheresis. Plasmapheresis is widely used in autoimmune diseases to reduce the negative effects of auto-antibodies. Early findings indicated that multiple cycles of plasmapheresis were needed in humans to significantly reduce the level of anti-AAV NAbs in humans (116). In NHPs, two cycles of plasmapheresis allowed for muscle targeting after administration of AAVrh74 through isolated limb perfusion (117). More recently, a second study in NHPs demonstrated that three cycles of plasmapheresis reduced the levels of neutralizing antibodies to AAV5 of at least ten-fold on average and allowed for repeated administration of the same vector bearing two distinct transgenes (118). In the same publication, the authors showed that two cycles of plasmapheresis in humans led to a three to five-fold decrease in the anti AAV2 and AAV9 NAb titers (118). Although these results seem to support the use of plasmapheresis for AAV administration in seropositive individuals or for vector re-administration, the procedure is burdensome and results in transient suppression of the immunoglobulin in circulation. For this reason, two specific approaches were recently developed (119, 120). Both of them were based on the immobilization of an AAV capsid (AAV8 and AAV9 respectively) on a chromatography resin then used to specifically sequester anti-AAV antibodies from serum. These proof-of-concept studies clearly demonstrated the advantages of antigen-specific IgG depletion over plasmapheresis. However, the clinical development of this technology may prove complex and the demonstration of efficacy in large animal models is still missing.
An alternative to the use of plasmapheresis could be the reduction of circulating IgG with a bacterial protein, the IgG-degrading enzyme of Streptococcus pyogenes (IdeS) (121, 122). This cysteine endopeptidase is part of the bacterial arsenal to defend themselves from IgG-mediated opsonization (121). IdeS was originally proposed to prevent kidney rejection in HLA-sensitized individuals (123–125), and is now approved for use in this patients’ population (Idefirix®, Hansa Biopharma). The molecular mechanism of action is based on the very fast and efficient cleavage of human IgG into F(ab’)2 fragments and Fc (126, 127). Although the F(ab’)2 fragments still retains some neutralizing activity, in the absence of the Fc portion, they are rapidly eliminated from the circulation. One of the biggest limitations for the identification of the potential of IdeS in gene therapy with AAV is the lack of preclinical models to demonstrate its efficacy. IdeS is highly specific for human IgG, it does not cut murine IgG and it is only partially efficient towards monkey IgG. In vitro digestion of monkey IgG with IdeS resulted in the degradation of the vast majority of the IgG. However, single chain IgGs, intermediates of the digestion reaction with a neutralizing potential and not easily cleared from the circulation, were still present after overnight digestion (128). Recently, we demonstrated the efficacy of IdeS for the degradation of anti-AAV antibodies and the successful administration of AAV vectors in seropositive NHPs (128). In particular, IdeS pre-treatment improved liver transduction efficacy in seropositive NHPs both in the context of first administration in monkeys naturally exposed to AAVs and in a re-administration setting (128). Confirmation of the potential of IdeS in AAV gene therapy was further provided by the use of IdeZ, an IdeS homolog (111). Importantly, although the injection of the bacterial protein induced the formation of anti-IdeS antibodies, they were not neutralizing the protein function, and IdeS was able to degrade IgG in the presence of anti-IdeS antibodies both in vivo and in vitro (128). Another interesting property of IdeS is its capacity to cleave B-cell receptor and temporarily inhibit memory B-cell activation (129) thus providing a further advantage when it comes to the administration of vectors in non-naïve individuals. In conclusion, IdeS has the potential to enable AAV vectors administration in seropositive patients even in the context of repeated administration of the same vector. One limitation toward the clinical use of IdeS in this setting could be that the repeated administration of IdeS may trigger a hypersensitivity reaction. Ongoing work is trying to address this potential issue by developing new IdeS molecules with reduced immunogenicity to unlock the potential of this approach in autoimmune diseases, chronic transplant rejection, oncology and gene therapy.
An orthogonal approach to IdeS, based on a distinct bacterial protein, is the use of protein M, an IgG binding protein isolated in human mycoplasma (130). Very positive preliminary data on the use of this protein to reduce the neutralizing titers against AAV were communicated (131) although no published data are available so far.
Conclusions
The overcoming of humoral immune response to AAVs is key to unlock the full potential of gene transfer with this vector.
The exclusion of patients from clinical trials based on the presence of antibodies against the gene therapy vector raises also important ethical questions. Different methods were developed to evaluate the neutralization activity against AAV vectors in patients (Table 1) , however limitations still exist and validation of those methods across laboratories is required to allow comparison of the data and equal access to the treatment starting from early-stage clinical research.
Different methods were developed in the past to reduce the impact of anti-AAV NAbs (Figure 2). Among them, immune suppression strategies inspired to those developed in transplantation seem to be the one with the highest potential in the clinic. So far, clinical proof of their efficacy in the reduction of anti-AAV antibody titers has been obtained only in individuals that, being seronegative, were likely naïve for AAVs.
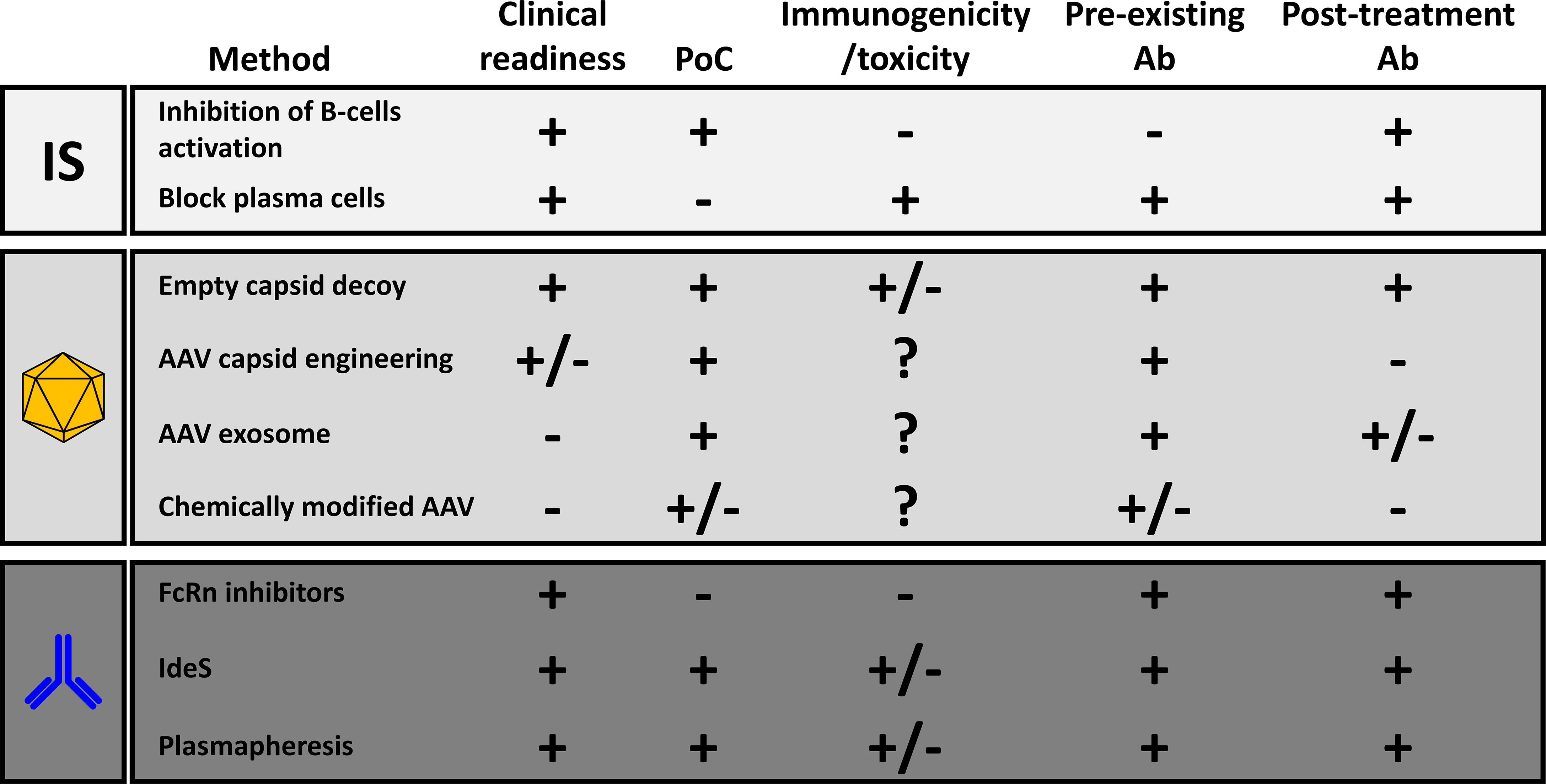
Figure 2 Comparative analysis of the different methods to reduce the anti-AAV humoral response. The last two columns refer respectively to the efficacy of the method in the reduction of the impact of pre-existing neutralizing antibodies or in the inhibition of the anti-AAV humoral immunity after treatment with recombinant vectors. PoC, proof-of-concept with AAV vector gene transfer; Ab, antibodies; FcRn, neonatal Fc-receptors; IdeS, Immunoglobulin G-degrading enzyme of S. Pyogenes.
The modification of the surface of AAV through direct engineering of the capsid sequence, or vector shielding with empty particles, exosomes and through chemical modifications was also proposed to escape neutralization. These techniques can be used to prevent neutralization in seropositive individuals. However, after the first injection the generation of antibodies specific for those modified capsids is likely to prevent vector re-administration. AAV-exosome have the potential to achieve re-administration although data in relevant preclinical models are still missing.
A more specific alternative to immune suppression is to transiently reduce the levels of IgG, known to be the more important class of antibodies neutralizing AAV vectors, to create a window for the delivery of AAV vectors to target organs. The possibility to administer AAV vectors in seropositive monkeys through IdeS pre-treatment supports the hypothesis that by clearing circulating IgG a safe and efficient gene transfer can be achieved in the liver. Similar results were obtained with plasmapheresis in primates further confirming AAVs as vectors with a relatively low immunogenicity also in individuals with pre-existing immunity. Proof-of-concept of the efficacy of these approaches required adaptation due to the low activity of IdeS on monkey IgG and the small size of the animals. A clinical demonstration is likely required to demonstrate the full potential of these approaches.
Intravenous infusion is probably the route of administration where NAbs have the largest negative impact. Other routes of administration of AAV vectors, e.g. intravitreal or intrathecal are being tested in the clinic. For these routes, further studies are needed to better understand the impact of pre-existing NAbs on AAV gene transfer. The spatial constrains, the different composition and density of the fluids in these compartments and potential differences in the antibody composition of vitreous or cerebrospinal fluid may impact the efficacy of the different methods described in this review and they will possibly need to be adapted to the specificities of those compartments.
The administration of AAV vectors in non-naïve individuals is a potential concern when one of these strategies will reach the clinic. Immune responses to AAV vectors are unique in humans and it cannot be excluded that pre-exposed and naïve individuals may react differently to AAV vectors. This is particularly relevant for studies involving large doses of AAV vectors where toxicity was observed, possibly dependent on complement or platelets activation. Importantly, future studies of AAV administration in seropositive individuals will take advantage of the large clinical experience available on the control of AAV vector immunogenicity.
In conclusions, although all the approaches described in this review have the potential to reduce the impact of NAbs on tissue transduction, their efficacy was frequently demonstrated either with low neutralizing titers or through partial reduction of higher titers. Lower neutralizing titers are observed in individuals naturally infected by AAV and this is possibly the first population that will benefit from these approaches. In case of vector re-administration, the titers are in general more elevated and a combination of orthogonal techniques for the reduction of the impact of NAbs is potentially needed.
Despite the challenges imposed by the humoral immune response to AAV gene transfer, the knowledge on the immune response to AAV vectors and the technological advances make possible the clinical validation for some of these approaches. As we learned through the extensive clinical experience with AAV gene transfer, only a confirmation of safety and efficacy in humans could open the way to a generalized use of techniques to decrease the impact of humoral immune response on AAV gene transfer. The stakes are extremely high. On one hand, pre-existing immunity prevents seropositive patients to access a life-changing treatment. On the other hand, setting-up of protocols for safe and efficient AAV vector re-administration will completely change the paradigm of gene therapy, moving from a once-in-a-life treatment to a treatment on-demand. This is a fundamental step to ensure durability of the treatment and provide a better alternative to other treatment like small-molecules or protein replacement therapy.
Author Contributions
D-AG, NT, CL, and GR wrote the manuscript. All authors contributed to the article and approved the submitted version.
Funding
This work was supported by Genethon. GR was supported by a grant from the World anti-doping agency (WADA) and a grant from the IMI2-2019 Call of the Horizon 2020 framework program (ARDAT, ID 945473). D-AG was supported by Agence Nationale de la Recherche (ANR-15-CE15-0005-02), an ATIGE grant from Genopole (Evry, France) and Paris Île-de-France Region (DIM Thérapie Génique).
Conflict of Interest
The authors declare the following competing interest: CL and GR are inventors in patents related to AAV gene therapy and the control of immune responses against AAV vectors.
The remaining authors declare that the research was conducted in the absence of any commercial or financial relationships that could be construed as a potential conflict of interest.
Publisher’s Note
All claims expressed in this article are solely those of the authors and do not necessarily represent those of their affiliated organizations, or those of the publisher, the editors and the reviewers. Any product that may be evaluated in this article, or claim that may be made by its manufacturer, is not guaranteed or endorsed by the publisher.
References
1. Balakrishnan B, Jayandharan GR. Basic Biology of Adeno-Associated Virus (AAV) Vectors Used in Gene Therapy. Curr Gene Ther (2014) 14(2):86–100. doi: 10.2174/1566523214666140302193709
2. Ogden PJ, Kelsic ED, Sinai S, Church GM. Comprehensive AAV Capsid Fitness Landscape Reveals a Viral Gene and Enables Machine-Guided Design. Science (2019) 366(6469):1139–43. doi: 10.1126/science.aaw2900
3. Elmore ZC, Patrick Havlik L, Oh DK, Anderson L, Daaboul G, Devlin GW, et al. The Membrane Associated Accessory Protein is an Adeno-Associated Viral Egress Factor. Nat Commun (2021) 12(1):6239. doi: 10.1038/s41467-021-26485-4
4. Snijder J, van de Waterbeemd M, Damoc E, Denisov E, Grinfeld D, Bennett A, et al. Defining the Stoichiometry and Cargo Load of Viral and Bacterial Nanoparticles by Orbitrap Mass Spectrometry. J Am Chem Soc (2014) 136(20):7295–9. doi: 10.1021/ja502616y
5. Gao G, Vandenberghe LH, Wilson JM. New Recombinant Serotypes of AAV Vectors. Curr Gene Ther (2005) 5(3):285–97. doi: 10.2174/1566523054065057
6. Gao G, Vandenberghe LH, Alvira MR, Lu Y, Calcedo R, Zhou X, et al. Clades of Adeno-Associated Viruses are Widely Disseminated in Human Tissues. J Virol (2004) 78(12):6381–8. doi: 10.1128/JVI.78.12.6381-6388.2004
7. Gao G, Alvira MR, Somanathan S, Lu Y, Vandenberghe LH, Rux JJ, et al. Adeno-Associated Viruses Undergo Substantial Evolution in Primates During Natural Infections. Proc Natl Acad Sci USA (2003) 100(10):6081–6. doi: 10.1073/pnas.0937739100
8. Gao GP, Alvira MR, Wang L, Calcedo R, Johnston J, Wilson JM. Novel Adeno-Associated Viruses From Rhesus Monkeys as Vectors for Human Gene Therapy. Proc Natl Acad Sci USA (2002) 99(18):11854–9. doi: 10.1073/pnas.182412299
9. Atchison RW, Casto BC, Hammon WM. Adenovirus-Associated Defective Virus Particles. Science (1965) 149(3685):754–6. doi: 10.1126/science.149.3685.754
10. Rose JA, Berns KI, Hoggan MD, Koczot FJ. Evidence for a Single-Stranded Adenovirus-Associated Virus Genome: Formation of a DNA Density Hybrid on Release of Viral DNA. Proc Natl Acad Sci USA (1969) 64(3):863–9. doi: 10.1073/pnas.64.3.863
11. Blacklow NR, Hoggan MD, Rowe WP. Isolation of Adenovirus-Associated Viruses From Man. Proc Natl Acad Sci USA (1967) 58(4):1410–5. doi: 10.1073/pnas.58.4.1410
12. Rabinowitz JE, Rolling F, Li C, Conrath H, Xiao W, Xiao X, et al. Cross-Packaging of a Single Adeno-Associated Virus (AAV) Type 2 Vector Genome Into Multiple AAV Serotypes Enables Transduction With Broad Specificity. J Virol (2002) 76(2):791–801. doi: 10.1128/JVI.76.2.791-801.2002
13. Senapathy P, Carter BJ. Molecular Cloning of Adeno-Associated Virus Variant Genomes and Generation of Infectious Virus by Recombination in Mammalian Cells. J Biol Chem (1984) 259(7):4661–6. doi: 10.1016/S0021-9258(17)43097-3
14. Dixit M, Tillery MK, Plonk SG, Ohi S. The Recombinant Human Parvoviruses for Gene Therapy of Hemoglobinopathies. SAAS Bull Biochem Biotechnol (1990) 3:63–8.
15. Tratschin JD, Miller IL, Smith MG, Carter BJ. Adeno-Associated Virus Vector for High-Frequency Integration, Expression, and Rescue of Genes in Mammalian Cells. Mol Cell Biol (1985) 5(11):3251–60. doi: 10.1128/mcb.5.11.3251-3260.1985
16. Shen X, Storm T, Kay MA. Characterization of the Relationship of AAV Capsid Domain Swapping to Liver Transduction Efficiency. Mol Ther (2007) 15(11):1955–62. doi: 10.1038/sj.mt.6300293
17. Matsushita T, Elliger S, Elliger C, Podsakoff G, Villarreal L, Kurtzman GJ, et al. Adeno-Associated Virus Vectors can be Efficiently Produced Without Helper Virus. Gene Ther (1998) 5(7):938–45. doi: 10.1038/sj.gt.3300680
18. Wright JF. Manufacturing and Characterizing AAV-Based Vectors for Use in Clinical Studies. Gene Ther (2008) 15(11):840–8. doi: 10.1038/gt.2008.65
19. Grieger JC, Samulski RJ. Adeno-Associated Virus Vectorology, Manufacturing, and Clinical Applications. Methods Enzymol (2012) 507:229–54. doi: 10.1016/B978-0-12-386509-0.00012-0
20. Gao GP, Qu G, Faust LZ, Engdahl RK, Xiao W, Hughes JV, et al. High-Titer Adeno-Associated Viral Vectors From a Rep/Cap Cell Line and Hybrid Shuttle Virus. Hum Gene Ther (1998) 9(16):2353–62. doi: 10.1089/hum.1998.9.16-2353
21. Conway JE, Zolotukhin S, Muzyczka N, Hayward GS, Byrne BJ. Recombinant Adeno-Associated Virus Type 2 Replication and Packaging is Entirely Supported by a Herpes Simplex Virus Type 1 Amplicon Expressing Rep and Cap. J Virol (1997) 71(11):8780–9. doi: 10.1128/jvi.71.11.8780-8789.1997
22. Marek M, van Oers MM, Devaraj FF, Vlak JM, Merten OW. Engineering of Baculovirus Vectors for the Manufacture of Virion-Free Biopharmaceuticals. Biotechnol Bioeng (2011) 108(5):1056–67. doi: 10.1002/bit.23028
23. Zeltner N, Kohlbrenner E, Clement N, Weber T, Linden RM. Near-Perfect Infectivity of Wild-Type AAV as Benchmark for Infectivity of Recombinant AAV Vectors. Gene Ther (2010) 17(7):872–9. doi: 10.1038/gt.2010.27
24. Wang D, Tai PWL, Gao G. Adeno-Associated Virus Vector as a Platform for Gene Therapy Delivery. Nat Rev Drug Discovery (2019) 18(5):358–78. doi: 10.1038/s41573-019-0012-9
25. Riyad JM, Weber T. Intracellular Trafficking of Adeno-Associated Virus (AAV) Vectors: Challenges and Future Directions. Gene Ther (2021) 28(12):683–96. doi: 10.1038/s41434-021-00243-z
26. Giraud C, Winocour E, Berns KI. Recombinant Junctions Formed by Site-Specific Integration of Adeno-Associated Virus Into an Episome. J Virol (1995) 69(11):6917–24. doi: 10.1128/jvi.69.11.6917-6924.1995
27. Calcedo R, Morizono H, Wang L, McCarter R, He J, Jones D, et al. Adeno-Associated Virus Antibody Profiles in Newborns, Children, and Adolescents. Clin Vaccine Immunol (2011) 18(9):1586–8. doi: 10.1128/CVI.05107-11
28. Erles K, Sebokova P, Schlehofer JR. Update on the Prevalence of Serum Antibodies (IgG and IgM) to Adeno-Associated Virus (AAV). J Med Virol (1999) 59(3):406–11. doi: 10.1002/(SICI)1096-9071(199911)59:3<406::AID-JMV22>3.0.CO;2-N
29. Li C, Narkbunnam N, Samulski RJ, Asokan A, Hu G, Jacobson LJ, et al. Neutralizing Antibodies Against Adeno-Associated Virus Examined Prospectively in Pediatric Patients With Hemophilia. Gene Ther (2011) 19(3):288–94. doi: 10.1038/gt.2011.90
30. Fu H, Meadows AS, Pineda RJ, Kunkler KL, Truxal KV, McBride KL, et al. Differential Prevalence of Antibodies Against Adeno-Associated Virus in Healthy Children and Patients With Mucopolysaccharidosis III: Perspective for AAV-Mediated Gene Therapy. Hum Gene Ther Clin Dev (2017) 28(4):187–96. doi: 10.1089/humc.2017.109
31. Calcedo R, Wilson JM. AAV Natural Infection Induces Broad Cross-Neutralizing Antibody Responses to Multiple AAV Serotypes in Chimpanzees. Hum Gene Ther Clin Dev (2016) 27(2):79–82. doi: 10.1089/humc.2016.048
32. Dai Y, Kavita U, Lampen MH, Gielen S, Banks G, Levesque PC, et al. Prevalence of Pre-Existing Neutralizing Antibodies Against AAV Serotypes 1, 2, 5, 6, 8, and 9 in Sera of Different Pig Strains. Hum Gene Ther (2021). doi: 10.1089/hum.2021.213
33. Li P, Boenzli E, Hofmann-Lehmann R, Helfer-Hungerbuehler AK. Pre-Existing Antibodies to Candidate Gene Therapy Vectors (Adeno-Associated Vector Serotypes) in Domestic Cats. PloS One (2019) 14(3):e0212811. doi: 10.1371/journal.pone.0212811
34. Smith RH. Adeno-Associated Virus Integration: Virus Versus Vector. Gene Ther (2008) 15(11):817–22. doi: 10.1038/gt.2008.55
35. Kruzik A, Fetahagic D, Hartlieb B, Dorn S, Koppensteiner H, Horling FM, et al. Prevalence of Anti-Adeno-Associated Virus Immune Responses in International Cohorts of Healthy Donors. Mol Ther Methods Clin Dev (2019) 14:126–33. doi: 10.1016/j.omtm.2019.05.014
36. Leborgne C, Latournerie V, Boutin S, Desgue D, Quere A, Pignot E, et al. Prevalence and Long-Term Monitoring of Humoral Immunity Against Adeno-Associated Virus in Duchenne Muscular Dystrophy Patients. Cell Immunol (2019) 342:103780. doi: 10.1016/j.cellimm.2018.03.004
37. Boutin S, Monteilhet V, Veron P, Leborgne C, Benveniste O, Montus MF, et al. Prevalence of Serum IgG and Neutralizing Factors Against Adeno-Associated Virus (AAV) Types 1, 2, 5, 6, 8, and 9 in the Healthy Population: Implications for Gene Therapy Using AAV Vectors. Hum Gene Ther (2010) 21(6):704–12. doi: 10.1089/hum.2009.182
38. Nguyen GN, Everett JK, Kafle S, Roche AM, Raymond HE, Leiby J, et al. A Long-Term Study of AAV Gene Therapy in Dogs With Hemophilia A Identifies Clonal Expansions of Transduced Liver Cells. Nat Biotechnol (2021) 39(1):47–55. doi: 10.1038/s41587-020-0741-7
39. George LA, Monahan PE, Eyster ME, Sullivan SK, Ragni MV, Croteau SE, et al. Multiyear Factor VIII Expression After AAV Gene Transfer for Hemophilia A. N Engl J Med (2021) 385(21):1961–73. doi: 10.1056/NEJMoa2104205
40. Gernoux G, Guilbaud M, Devaux M, Journou M, Pichard V, Jaulin N, et al. AAV8 Locoregional Delivery Induces Long-Term Expression of an Immunogenic Transgene in Macaques Despite Persisting Local Inflammation. Mol Ther Methods Clin Dev (2021) 20:660–74. doi: 10.1016/j.omtm.2021.02.003
41. Jiang H, Pierce GF, Ozelo MC, de Paula EV, Vargas JA, Smith P, et al. Evidence of Multiyear Factor IX Expression by AAV-Mediated Gene Transfer to Skeletal Muscle in an Individual With Severe Hemophilia B. Mol Ther (2006) 14(3):452–5. doi: 10.1016/j.ymthe.2006.05.004
42. Marco S, Haurigot V, Jaen ML, Ribera A, Sanchez V, Molas M, et al. Seven-Year Follow-Up of Durability and Safety of AAV CNS Gene Therapy for a Lysosomal Storage Disorder in a Large Animal. Mol Ther Methods Clin Dev (2021) 23:370–89. doi: 10.1016/j.omtm.2021.09.017
43. Nathwani AC, Reiss UM, Tuddenham EG, Rosales C, Chowdary P, McIntosh J, et al. Long-Term Safety and Efficacy of Factor IX Gene Therapy in Hemophilia B. N Engl J Med (2014) 371(21):1994–2004. doi: 10.1056/NEJMoa1407309
44. Das A, Vijayan M, Walton EM, Stafford VG, Fiflis DN, Asokan A. Epigenetic Silencing of Recombinant AAV Genomes by NP220 and the HUSH Complex. J Virol (2022) 96(4):e02039–21. doi: 10.1128/jvi.02039-21
45. George LA, Ragni MV, Rasko JEJ, Raffini LJ, Samelson-Jones BJ, Ozelo M, et al. Long-Term Follow-Up of the First in Human Intravascular Delivery of AAV for Gene Transfer: AAV2-Hfix16 for Severe Hemophilia B. Mol Ther (2020) 28(9):2073–82. doi: 10.1016/j.ymthe.2020.06.001
46. Wang L, Calcedo R, Wang H, Bell P, Grant R, Vandenberghe LH, et al. The Pleiotropic Effects of Natural AAV Infections on Liver-Directed Gene Transfer in Macaques. Mol Ther (2010) 18(1):126–34. doi: 10.1038/mt.2009.245
47. Fitzpatrick Z, Leborgne C, Barbon E, Masat E, Ronzitti G, van Wittenberghe L, et al. Influence of Pre-Existing Anti-Capsid Neutralizing and Binding Antibodies on AAV Vector Transduction. Mol Ther Methods Clin Dev (2018) 9:119–29. doi: 10.1016/j.omtm.2018.02.003
48. Calcedo R, Vandenberghe LH, Gao G, Lin J, Wilson JM. Worldwide Epidemiology of Neutralizing Antibodies to Adeno-Associated Viruses. J Infect Dis (2009) 199(3):381–90. doi: 10.1086/595830
49. Manno CS, Pierce GF, Arruda VR, Glader B, Ragni M, Rasko JJ, et al. Successful Transduction of Liver in Hemophilia by AAV-Factor IX and Limitations Imposed by the Host Immune Response. Nat Med (2006) 12(3):342–7. doi: 10.1038/nm1358
50. Scallan CD, Jiang H, Liu T, Patarroyo-White S, Sommer JM, Zhou S, et al. Human Immunoglobulin Inhibits Liver Transduction by AAV Vectors at Low AAV2 Neutralizing Titers in SCID Mice. Blood (2006) 107(5):1810–7. doi: 10.1182/blood-2005-08-3229
51. Jiang H, Couto LB, Patarroyo-White S, Liu T, Nagy D, Vargas JA, et al. Effects of Transient Immunosuppression on Adenoassociated, Virus-Mediated, Liver-Directed Gene Transfer in Rhesus Macaques and Implications for Human Gene Therapy. Blood (2006) 108(10):3321–8. doi: 10.1182/blood-2006-04-017913
52. Kay MA, Manno CS, Ragni MV, Larson PJ, Couto LB, McClelland A, et al. Evidence for Gene Transfer and Expression of Factor IX in Haemophilia B Patients Treated With an AAV Vector. Nat Genet (2000) 24(3):257–61. doi: 10.1038/73464
53. Murphy SL, Li H, Mingozzi F, Sabatino DE, Hui DJ, Edmonson SA, et al. Diverse IgG Subclass Responses to Adeno-Associated Virus Infection and Vector Administration. J Med Virol (2009) 81(1):65–74. doi: 10.1002/jmv.21360
54. Zaiss AK, Cotter MJ, White LR, Clark SA, Wong NC, Holers VM, et al. Complement is an Essential Component of the Immune Response to Adeno-Associated Virus Vectors. J Virol (2008) 82(6):2727–40. doi: 10.1128/JVI.01990-07
55. Denard J, Marolleau B, Jenny C, Rao TN, Fehling HJ, Voit T, et al. C-Reactive Protein (CRP) is Essential for Efficient Systemic Transduction of Recombinant Adeno-Associated Virus Vector 1 (rAAV-1) and rAAV-6 in Mice. J Virol (2013) 87(19):10784–91. doi: 10.1128/JVI.01813-13
56. SolidBio. Available at: https://investors.solidbio.com/news-releases/news-releasedetails/solid-biosciences-provides-data-update-sgt-001-development.
57. FDA. Available at: https://www.fda.gov/media/126109/download.
58. Pfizer. Available at: https://pfizer2016ir.q4web.com/investor-news/press-release-details/2019/Pfizer-Presents-Initial-Clinical-Data-on-Phase-1b-Gene-Therapy-Study-for-Duchenne-Muscular-Dystrophy-DMD/default.aspx.
59. LogicBio. Available at: https://investor.logicbio.com/news-releases/news-release-details/logicbio-therapeutics-provides-update-lb-001-clinical.
60. Hinderer C, Katz N, Buza EL, Dyer C, Goode T, Bell P, et al. Severe Toxicity in Nonhuman Primates and Piglets Following High-Dose Intravenous Administration of an Adeno-Associated Virus Vector Expressing Human SMN. Hum Gene Ther (2018) 29(3):285–98. doi: 10.1089/hum.2018.015
61. Hordeaux J, Hinderer C, Goode T, Buza EL, Bell P, Calcedo R, et al. Toxicology Study of Intra-Cisterna Magna Adeno-Associated Virus 9 Expressing Iduronate-2-Sulfatase in Rhesus Macaques. Mol Ther Methods Clin Dev (2018) 10:68–78. doi: 10.1016/j.omtm.2018.06.004
62. Hordeaux J, Hinderer C, Goode T, Katz N, Buza EL, Bell P, et al. Toxicology Study of Intra-Cisterna Magna Adeno-Associated Virus 9 Expressing Human Alpha-L-Iduronidase in Rhesus Macaques. Mol Ther Methods Clin Dev (2018) 10:79–88. doi: 10.1016/j.omtm.2018.06.003
63. Hordeaux J, Buza EL, Jeffrey B, Song C, Jahan T, Yuan Y, et al. MicroRNA-Mediated Inhibition of Transgene Expression Reduces Dorsal Root Ganglion Toxicity by AAV Vectors in Primates. Sci Transl Med (2020) 12(569). doi: 10.1126/scitranslmed.aba9188
64. Hordeaux J, Wang Q, Katz N, Buza EL, Bell P, Wilson JM, et al. The Neurotropic Properties of AAV-PHP.B Are Limited to C57BL/6J Mice. Mol Ther (2018) 26(3):664–8. doi: 10.1016/j.ymthe.2018.01.018
65. Mingozzi F, Anguela XM, Pavani G, Chen Y, Davidson RJ, Hui DJ, et al. Overcoming Preexisting Humoral Immunity to AAV Using Capsid Decoys. Sci Transl Med (2013) 5(194):194ra92. doi: 10.1126/scitranslmed.3005795
66. Girod A, Ried M, Wobus C, Lahm H, Leike K, Kleinschmidt J, et al. Genetic Capsid Modifications Allow Efficient Re-Targeting of Adeno-Associated Virus Type 2. Nat Med (1999) 5(9):1052–6. doi: 10.1038/12491
67. Grifman M, Trepel M, Speece P, Gilbert LB, Arap W, Pasqualini R, et al. Incorporation of Tumor-Targeting Peptides Into Recombinant Adeno-Associated Virus Capsids. Mol Ther (2001) 3(6):964–75. doi: 10.1006/mthe.2001.0345
68. Michelfelder S, Lee MK, deLima-Hahn E, Wilmes T, Kaul F, Muller O, et al. Vectors Selected From Adeno-Associated Viral Display Peptide Libraries for Leukemia Cell-Targeted Cytotoxic Gene Therapy. Exp Hematol (2007) 35(12):1766–76. doi: 10.1016/j.exphem.2007.07.018
69. Tseng YS, Gurda BL, Chipman P, McKenna R, Afione S, Chiorini JA, et al. Adeno-Associated Virus Serotype 1 (AAV1)- and AAV5-Antibody Complex Structures Reveal Evolutionary Commonalities in Parvovirus Antigenic Reactivity. J Virol (2015) 89(3):1794–808. doi: 10.1128/JVI.02710-14
70. Li C, Diprimio N, Bowles DE, Hirsch ML, Monahan PE, Asokan A, et al. Single Amino Acid Modification of Adeno-Associated Virus Capsid Changes Transduction and Humoral Immune Profiles. J Virol (2012) 86(15):7752–9. doi: 10.1128/JVI.00675-12
71. Tse LV, Klinc KA, Madigan VJ, Castellanos Rivera RM, Wells LF, Havlik LP, et al. Structure-Guided Evolution of Antigenically Distinct Adeno-Associated Virus Variants for Immune Evasion. Proc Natl Acad Sci USA (2017) 114(24):E4812–21. doi: 10.1073/pnas.1704766114
72. Grimm D, Lee JS, Wang L, Desai T, Akache B, Storm TA, et al. In Vitro and In Vivo Gene Therapy Vector Evolution via Multispecies Interbreeding and Retargeting of Adeno-Associated Viruses. J Virol (2008) 82(12):5887–911. doi: 10.1128/JVI.00254-08
73. Li C, Wu S, Albright B, Hirsch M, Li W, Tseng YS, et al. Development of Patient-Specific AAV Vectors After Neutralizing Antibody Selection for Enhanced Muscle Gene Transfer. Mol Ther (2016) 24(1):53–65. doi: 10.1038/mt.2015.134
74. Paulk NK, Pekrun K, Zhu E, Nygaard S, Li B, Xu J, et al. Bioengineered AAV Capsids With Combined High Human Liver Transduction In Vivo and Unique Humoral Seroreactivity. Mol Ther (2018) 26(1):289–303. doi: 10.1016/j.ymthe.2017.09.021
75. Moreno AM, Palmer N, Aleman F, Chen G, Pla A, Jiang N, et al. Immune-Orthogonal Orthologues of AAV Capsids and of Cas9 Circumvent the Immune Response to the Administration of Gene Therapy. Nat BioMed Eng (2019) 3(10):806–16. doi: 10.1038/s41551-019-0431-2
77. Fevrier B, Raposo G. Exosomes: Endosomal-Derived Vesicles Shipping Extracellular Messages. Curr Opin Cell Biol (2004) 16(4):415–21. doi: 10.1016/j.ceb.2004.06.003
78. Maguire CA, Balaj L, Sivaraman S, Crommentuijn MH, Ericsson M, Mincheva-Nilsson L, et al. Microvesicle-Associated AAV Vector as a Novel Gene Delivery System. Mol Ther (2012) 20(5):960–71. doi: 10.1038/mt.2011.303
79. Gyorgy B, Fitzpatrick Z, Crommentuijn MH, Mu D, Maguire CA. Naturally Enveloped AAV Vectors for Shielding Neutralizing Antibodies and Robust Gene Delivery In Vivo. Biomaterials (2014) 35(26):7598–609. doi: 10.1016/j.biomaterials.2014.05.032
80. Meliani A, Boisgerault F, Fitzpatrick Z, Marmier S, Leborgne C, Collaud F, et al. Enhanced Liver Gene Transfer and Evasion of Preexisting Humoral Immunity With Exosome-Enveloped AAV Vectors. Blood Adv (2017) 1(23):2019–31. doi: 10.1182/bloodadvances.2017010181
81. Wassmer SJ, Carvalho LS, Gyorgy B, Vandenberghe LH, Maguire CA. Exosome-Associated AAV2 Vector Mediates Robust Gene Delivery Into the Murine Retina Upon Intravitreal Injection. Sci Rep (2017) 7:45329. doi: 10.1038/srep45329
82. Carlisle RC, Benjamin R, Briggs SS, Sumner-Jones S, McIntosh J, Gill D, et al. Coating of Adeno-Associated Virus With Reactive Polymers can Ablate Virus Tropism, Enable Retargeting and Provide Resistance to Neutralising Antisera. J Gene Med (2008) 10(4):400–11. doi: 10.1002/jgm.1161
83. Kelemen RE, Mukherjee R, Cao X, Erickson SB, Zheng Y, Chatterjee A. A Precise Chemical Strategy To Alter the Receptor Specificity of the Adeno-Associated Virus. Angew Chem Int Ed Engl (2016) 55(36):10645–9. doi: 10.1002/anie.201604067
84. Le HT, Yu QC, Wilson JM, Croyle MA. Utility of PEGylated Recombinant Adeno-Associated Viruses for Gene Transfer. J Control Release (2005) 108(1):161–77. doi: 10.1016/j.jconrel.2005.07.019
85. Lee GK, Maheshri N, Kaspar B, Schaffer DV. PEG Conjugation Moderately Protects Adeno-Associated Viral Vectors Against Antibody Neutralization. Biotechnol Bioeng (2005) 92(1):24–34. doi: 10.1002/bit.20562
86. Zhang C, Yao T, Zheng Y, Li Z, Zhang Q, Zhang L, et al. Development of Next Generation Adeno-Associated Viral Vectors Capable of Selective Tropism and Efficient Gene Delivery. Biomaterials (2016) 80:134–45. doi: 10.1016/j.biomaterials.2015.11.066
87. Mevel M, Bouzelha M, Leray A, Pacouret S, Guilbaud M, Penaud-Budloo M, et al. Chemical Modification of the Adeno-Associated Virus Capsid to Improve Gene Delivery. Chem Sci (2019) 11(4):1122–31. doi: 10.1039/C9SC04189C
88. Majowicz A, Nijmeijer B, Lampen MH, Spronck L, de Haan M, Petry H, et al. Therapeutic hFIX Activity Achieved After Single AAV5-hFIX Treatment in Hemophilia B Patients and NHPs With Pre-Existing Anti-AAV5 NABs. Mol Ther Methods Clin Dev (2019) 14:27–36. doi: 10.1016/j.omtm.2019.05.009
89. Chirmule N, Xiao W, Truneh A, Schnell MA, Hughes JV, Zoltick P, et al. Humoral Immunity to Adeno-Associated Virus Type 2 Vectors Following Administration to Murine and Nonhuman Primate Muscle. J Virol (2000) 74(5):2420–5. doi: 10.1128/JVI.74.5.2420-2425.2000
90. McIntosh JH, Cochrane M, Cobbold S, Waldmann H, Nathwani SA, Davidoff AM, et al. Successful Attenuation of Humoral Immunity to Viral Capsid and Transgenic Protein Following AAV-Mediated Gene Transfer With a non-Depleting CD4 Antibody and Cyclosporine. Gene Ther (2012) 19(1):78–85. doi: 10.1038/gt.2011.64
91. Xiao W, Chirmule N, Schnell MA, Tazelaar J, Hughes JV, Wilson JM. Route of Administration Determines Induction of T-Cell-Independent Humoral Responses to Adeno-Associated Virus Vectors. Mol Ther (2000) 1(4):323–9. doi: 10.1006/mthe.2000.0045
92. Lorain S, Gross DA, Goyenvalle A, Danos O, Davoust J, Garcia L. Transient Immunomodulation Allows Repeated Injections of AAV1 and Correction of Muscular Dystrophy in Multiple Muscles. Mol Ther (2008) 16(3):541–7. doi: 10.1038/sj.mt.6300377
93. Zhong C, Jiang W, Wang Y, Sun J, Wu X, Zhuang Y, et al. Repeated Systemic Dosing of Adeno-Associated Virus Vectors in Immunocompetent Mice After Blockade of T Cell Costimulatory Pathways. Hum Gene Ther (2021) 33(5-6):290–300. doi: 10.1089/hum.2021.129
94. Zhu J, Huang X, Yang Y. The TLR9-MyD88 Pathway is Critical for Adaptive Immune Responses to Adeno-Associated Virus Gene Therapy Vectors in Mice. J Clin Invest (2009) 119(8):2388–98. doi: 10.1172/JCI37607
95. Rogers GL, Suzuki M, Zolotukhin I, Markusic DM, Morel LM, Lee B, et al. Unique Roles of TLR9- and MyD88-Dependent and -Independent Pathways in Adaptive Immune Responses to AAV-Mediated Gene Transfer. J Innate Immun (2015) 7(3):302–14. doi: 10.1159/000369273
96. Sudres M, Cire S, Vasseur V, Brault L, Da Rocha S, Boisgerault F, et al. MyD88 Signaling in B Cells Regulates the Production of Th1-Dependent Antibodies to AAV. Mol Ther (2012) 20(8):1571–81. doi: 10.1038/mt.2012.101
97. Kuranda K, Jean-Alphonse P, Leborgne C, Hardet R, Collaud F, Marmier S, et al. Exposure to Wild-Type AAV Drives Distinct Capsid Immunity Profiles in Humans. J Clin Invest (2018) 128(12):5267–79. doi: 10.1172/JCI122372
98. Da Rocha S, Bigot J, Onodi F, Cosette J, Corre G, Poupiot J, et al. Temporary Reduction of Membrane CD4 With the Antioxidant MnTBAP Is Sufficient to Prevent Immune Responses Induced by Gene Transfer. Mol Ther Methods Clin Dev (2019) 14:285–99. doi: 10.1016/j.omtm.2019.06.011
99. Shi X, Aronson SJ, Ten Bloemendaal L, Duijst S, Bakker RS, de Waart DR, et al. Efficacy of AAV8-Hugt1a1 With Rapamycin in Neonatal, Suckling, and Juvenile Rats to Model Treatment in Pediatric CNs Patients. Mol Ther Methods Clin Dev (2021) 20:287–97. doi: 10.1016/j.omtm.2020.11.016
100. Haddadi A, Elamanchili P, Lavasanifar A, Das S, Shapiro J, Samuel J. Delivery of Rapamycin by PLGA Nanoparticles Enhances its Suppressive Activity on Dendritic Cells. J BioMed Mater Res A (2008) 84(4):885–98. doi: 10.1002/jbm.a.31373
101. Maldonado RA, LaMothe RA, Ferrari JD, Zhang AH, Rossi RJ, Kolte PN, et al. Polymeric Synthetic Nanoparticles for the Induction of Antigen-Specific Immunological Tolerance. Proc Natl Acad Sci USA (2015) 112(2):E156–65. doi: 10.1073/pnas.1408686111
102. Meliani A, Boisgerault F, Hardet R, Marmier S, Collaud F, Ronzitti G, et al. Antigen-Selective Modulation of AAV Immunogenicity With Tolerogenic Rapamycin Nanoparticles Enables Successful Vector Re-Administration. Nat Commun (2018) 9(1):4098. doi: 10.1038/s41467-018-06621-3
103. Biswas M, Palaschak B, Kumar SRP, Rana J, Markusic DM. B Cell Depletion Eliminates FVIII Memory B Cells and Enhances AAV8-Cof8 Immune Tolerance Induction When Combined With Rapamycin. Front Immunol (2020) 11:1293. doi: 10.3389/fimmu.2020.01293
104. Mingozzi F, Chen Y, Murphy SL, Edmonson SC, Tai A, Price SD, et al. Pharmacological Modulation of Humoral Immunity in a Nonhuman Primate Model of AAV Gene Transfer for Hemophilia B. Mol Ther (2012) 20(7):1410–6. doi: 10.1038/mt.2012.84
105. Corti M, Elder M, Falk D, Lawson L, Smith B, Nayak S, et al. B-Cell Depletion is Protective Against Anti-AAV Capsid Immune Response: A Human Subject Case Study. Mol Ther Methods Clin Dev (2014) 1. doi: 10.1038/mtm.2014.33
106. Finn JD, Hui D, Downey HD, Dunn D, Pien GC, Mingozzi F, et al. Proteasome Inhibitors Decrease AAV2 Capsid Derived Peptide Epitope Presentation on MHC Class I Following Transduction. Mol Ther (2010) 18(1):135–42. doi: 10.1038/mt.2009.257
107. Sun J, Shao W, Chen X, Merricks EP, Wimsey L, Abajas YL, et al. An Observational Study From Long-Term AAV Re-Administration in Two Hemophilia Dogs. Mol Ther Methods Clin Dev (2018) 10:257–67. doi: 10.1016/j.omtm.2018.07.011
108. Schrezenmeier E, Jayne D, Dorner T. Targeting B Cells and Plasma Cells in Glomerular Diseases: Translational Perspectives. J Am Soc Nephrol (2018) 29(3):741–58. doi: 10.1681/ASN.2017040367
109. Mimuro J, Mizukami H, Hishikawa S, Ikemoto T, Ishiwata A, Sakata A, et al. Minimizing the Inhibitory Effect of Neutralizing Antibody for Efficient Gene Expression in the Liver With Adeno-Associated Virus 8 Vectors. Mol Ther (2013) 21(2):318–23. doi: 10.1038/mt.2012.258
110. Zabaleta N, Salas D, Paramo M, Hommel M, Sier-Ferreira V, Hernandez-Alcoceba R, et al. Improvement of Adeno-Associated Virus-Mediated Liver Transduction Efficacy by Regional Administration in Macaca Fascicularis. Hum Gene Ther Clin Dev (2017) 28(2):68–73. doi: 10.1089/humc.2016.183
111. Elmore ZC, Oh DK, Simon KE, Fanous MM, Asokan A. Rescuing AAV Gene Transfer From Neutralizing Antibodies With an IgG-Degrading Enzyme. JCI Insight (2020) 5(19):e139881. doi: 10.1172/jci.insight.139881
112. Roopenian DC, Akilesh S. FcRn: The Neonatal Fc Receptor Comes of Age. Nat Rev Immunol (2007) 7(9):715–25. doi: 10.1038/nri2155
113. Blumberg LJ, Humphries JE, Jones SD, Pearce LB, Holgate R, Hearn A, et al. Blocking FcRn in Humans Reduces Circulating IgG Levels and Inhibits IgG Immune Complex-Mediated Immune Responses. Sci Adv (2019) 5(12):eaax9586. doi: 10.1126/sciadv.aax9586
114. Kiessling P, Lledo-Garcia R, Watanabe S, Langdon G, Tran D, Bari M, et al. The FcRn Inhibitor Rozanolixizumab Reduces Human Serum IgG Concentration: A Randomized Phase 1 Study. Sci Transl Med (2017) 9(414). doi: 10.1126/scitranslmed.aan1208
115. Zuercher AW, Spirig R, Baz Morelli A, Rowe T, Kasermann F. Next-Generation Fc Receptor-Targeting Biologics for Autoimmune Diseases. Autoimmun Rev (2019) 18(10):102366. doi: 10.1016/j.autrev.2019.102366
116. Monteilhet V, Saheb S, Boutin S, Leborgne C, Veron P, Montus MF, et al. A 10 Patient Case Report on the Impact of Plasmapheresis Upon Neutralizing Factors Against Adeno-Associated Virus (AAV) Types 1, 2, 6, and 8. Mol Ther (2011) 19(11):2084–91. doi: 10.1038/mt.2011.108
117. Chicoine LG, Montgomery CL, Bremer WG, Shontz KM, Griffin DA, Heller KN, et al. Plasmapheresis Eliminates the Negative Impact of AAV Antibodies on Microdystrophin Gene Expression Following Vascular Delivery. Mol Ther (2014) 22(2):338–47. doi: 10.1038/mt.2013.244
118. Salas D, Kwikkers KL, Zabaleta N, Bazo A, Petry H, van Deventer SJ, et al. Immunoadsorption Enables Successful Raav5-Mediated Repeated Hepatic Gene Delivery in Nonhuman Primates. Blood Adv (2019) 3(17):2632–41. doi: 10.1182/bloodadvances.2019000380
119. Bertin B, Veron P, Leborgne C, Deschamps JY, Moullec S, Fromes Y, et al. Capsid-Specific Removal of Circulating Antibodies to Adeno-Associated Virus Vectors. Sci Rep (2020) 10(1):864. doi: 10.1038/s41598-020-57893-z
120. Orlowski A, Katz MG, Gubara SM, Fargnoli AS, Fish KM, Weber T. Successful Transduction With AAV Vectors After Selective Depletion of Anti-AAV Antibodies by Immunoadsorption. Mol Ther Methods Clin Dev (2020) 16:192–203. doi: 10.1016/j.omtm.2020.01.004
121. von Pawel-Rammingen U, Johansson BP, Bjorck L. IdeS, a Novel Streptococcal Cysteine Proteinase With Unique Specificity for Immunoglobulin G. EMBO J (2002) 21(7):1607–15. doi: 10.1093/emboj/21.7.1607
122. Wenig K, Chatwell L, von Pawel-Rammingen U, Bjorck L, Huber R, Sondermann P. Structure of the Streptococcal Endopeptidase IdeS, a Cysteine Proteinase With Strict Specificity for IgG. Proc Natl Acad Sci U.S.A. (2004) 101(50):17371–6. doi: 10.1073/pnas.0407965101
123. Jordan SC, Lorant T, Choi J. IgG Endopeptidase in Highly Sensitized Patients Undergoing Transplantation. N Engl J Med (2017) 377(17):1693–4. doi: 10.1056/NEJMc1711335
124. Lorant T, Bengtsson M, Eich T, Eriksson BM, Winstedt L, Jarnum S, et al. Safety, Immunogenicity, Pharmacokinetics, and Efficacy of Degradation of Anti-HLA Antibodies by IdeS (Imlifidase) in Chronic Kidney Disease Patients. Am J Transplant (2018) 18(11):2752–62. doi: 10.1111/ajt.14733
125. Winstedt L, Jarnum S, Nordahl EA, Olsson A, Runstrom A, Bockermann R, et al. Complete Removal of Extracellular IgG Antibodies in a Randomized Dose-Escalation Phase I Study With the Bacterial Enzyme IdeS–A Novel Therapeutic Opportunity. PloS One (2015) 10(7):e0132011. doi: 10.1371/journal.pone.0132011
126. Ryan MH, Petrone D, Nemeth JF, Barnathan E, Bjorck L, Jordan RE. Proteolysis of Purified IgGs by Human and Bacterial Enzymes In Vitro and the Detection of Specific Proteolytic Fragments of Endogenous IgG in Rheumatoid Synovial Fluid. Mol Immunol (2008) 45(7):1837–46. doi: 10.1016/j.molimm.2007.10.043
127. Vindebro R, Spoerry C, von Pawel-Rammingen U. Rapid IgG Heavy Chain Cleavage by the Streptococcal IgG Endopeptidase IdeS is Mediated by IdeS Monomers and is Not Due to Enzyme Dimerization. FEBS Lett (2013) 587(12):1818–22. doi: 10.1016/j.febslet.2013.04.039
128. Leborgne C, Barbon E, Alexander JM, Hanby H, Delignat S, Cohen DM, et al. IgG-Cleaving Endopeptidase Enables In Vivo Gene Therapy in the Presence of Anti-AAV Neutralizing Antibodies. Nat Med (2020) 26(7):1096–101. doi: 10.1038/s41591-020-0911-7
129. Jarnum S, Bockermann R, Runstrom A, Winstedt L, Kjellman C. The Bacterial Enzyme IdeS Cleaves the IgG-Type of B Cell Receptor (BCR), Abolishes BCR-Mediated Cell Signaling, and Inhibits Memory B Cell Activation. J Immunol (2015) 195(12):5592–601. doi: 10.4049/jimmunol.1501929
130. Grover RK, Zhu X, Nieusma T, Jones T, Boreo I, MacLeod AS, et al. A Structurally Distinct Human Mycoplasma Protein That Generically Blocks Antigen-Antibody Union. Science (2014) 343(6171):656–61. doi: 10.1126/science.1246135
Keywords: AAV vectors, gene therapy, immunogenicity, humoral response, B-cells, neutralizing antibodies
Citation: Gross D-A, Tedesco N, Leborgne C and Ronzitti G (2022) Overcoming the Challenges Imposed by Humoral Immunity to AAV Vectors to Achieve Safe and Efficient Gene Transfer in Seropositive Patients. Front. Immunol. 13:857276. doi: 10.3389/fimmu.2022.857276
Received: 18 January 2022; Accepted: 16 March 2022;
Published: 07 April 2022.
Edited by:
Sophie Tourdot, Pfizer, United StatesReviewed by:
Moanaro Biswas, Indiana University, United StatesZuben E. Sauna, United States Food and Drug Administration, United States
Copyright © 2022 Gross, Tedesco, Leborgne and Ronzitti. This is an open-access article distributed under the terms of the Creative Commons Attribution License (CC BY). The use, distribution or reproduction in other forums is permitted, provided the original author(s) and the copyright owner(s) are credited and that the original publication in this journal is cited, in accordance with accepted academic practice. No use, distribution or reproduction is permitted which does not comply with these terms.
*Correspondence: Giuseppe Ronzitti, Z3JvbnppdHRpQGdlbmV0aG9uLmZy