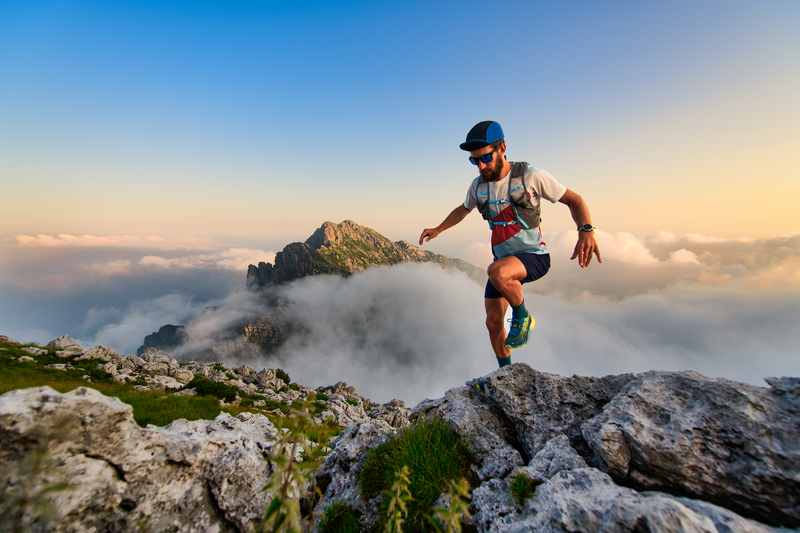
95% of researchers rate our articles as excellent or good
Learn more about the work of our research integrity team to safeguard the quality of each article we publish.
Find out more
REVIEW article
Front. Immunol. , 08 July 2022
Sec. Alloimmunity and Transplantation
Volume 13 - 2022 | https://doi.org/10.3389/fimmu.2022.855263
This article is part of the Research Topic Immunology of machine perfused organs and tissues View all 11 articles
The frequent use of marginal livers forces transplant centres to explore novel technologies to improve organ quality and outcomes after implantation. Organ perfusion techniques are therefore frequently discussed with an ever-increasing number of experimental and clinical studies. Two main approaches, hypothermic and normothermic perfusion, are the leading strategies to be introduced in clinical practice in many western countries today. Despite this success, the number of studies, which provide robust data on the underlying mechanisms of protection conveyed through this technology remains scarce, particularly in context of different stages of ischemia-reperfusion-injury (IRI). Prior to a successful clinical implementation of machine perfusion, the concept of IRI and potential key molecules, which should be addressed to reduce IRI-associated inflammation, requires a better exploration. During ischemia, Krebs cycle metabolites, including succinate play a crucial role with their direct impact on the production of reactive oxygen species (ROS) at mitochondrial complex I upon reperfusion. Such features are even more pronounced under normothermic conditions and lead to even higher levels of downstream inflammation. The direct consequence appears with an activation of the innate immune system. The number of articles, which focus on the impact of machine perfusion with and without the use of specific perfusate additives to modulate the inflammatory cascade after transplantation is very small. This review describes first, the subcellular processes found in mitochondria, which instigate the IRI cascade together with proinflammatory downstream effects and their link to the innate immune system. Next, the impact of currently established machine perfusion strategies is described with a focus on protective mechanisms known for the different perfusion approaches. Finally, the role of such dynamic preservation techniques to deliver specific agents, which appear currently of interest to modulate this posttransplant inflammation, is discussed together with future aspects in this field.
The introduction of an effective immunosuppression was a milestone in the history of organ transplantation. Various modifications of the pharmaceuticals used to modulate the immune system have been reported for most solid organs since that. The general drawback remains however with the putative risk to develop de-novo malignancies, chronic renal failure, and cardiovascular or metabolic diseases (1, 2). In an attempt to reduce such side effects, the weaning of immunosuppression (IS) and the induction of graft tolerance are important targets nowadays (1, 3, 4). Human liver allografts display several unique immunological features, including transplantation across a positive cross match, less vigorous immunosuppressive regimens, the lack of a significant benefit from HLA matching, and the overall low rates of chronic rejection. While some of these characteristics can be explained by the unique regenerative capacity of the liver, only in liver transplantation a significant proportion of patients can be eventually discontinued from maintenance IS without immediate rejection, a phenomenon known as operational tolerance (5). Tailored posttransplant protocols will include biopsies and IS regimens, which are required to individualize such concepts for a successful IS minimization (6). This approach starts however with preventive measures, applied ideally before graft reperfusion to reduce the initial “inflammatory hit” during ischemia-reperfusion. This early activation of the innate immune system is the direct consequence of ischemia-reperfusion-injury (IRI), which is the initial trigger of tissue inflammation in the transplanted organ and also in the entire recipient (7). This complex interplay between graft and recipient triggers various less well explored crosstalks between implanted organ and recipient’s immune system and promotes the establishment of an inflammatory milieu with a chronic component (8, 9). The level of this inflammation strictly depends on the initial donor and organ quality and can be modulated through a different preservation strategy (10). The potential role of machine perfusion technology and the effect on this initial posttransplant inflammation, which is crucial for recipient outcomes, is therefore highly relevant (9). While the first randomized controlled trials (RCT) are now available and demonstrate a reduction of posttransplant complications with the use of machine perfusion, the impact on basic mechanisms of early IRI and subsequent immune response, remains not well explored.
This review focus therefore on the mechanisms of how the innate immune response is triggered after liver transplantation and the role of machine perfusion techniques, individual and as a tool to deliver therapeutic agents to the organ.
Ischemia-reperfusion injury (IRI) is a paradoxical cascade of injury, which occurs during re-oxygenation of an ischemic organ (11, 12). It is now well recognized, that the predominant effector of this injury is an early burst of reactive oxygen species (ROS), occurring across numerous types of tissues, including liver, kidney, lung, heart, muscle, and brain (7, 13–17). While there are several sources of cellular ROS, for example the xanthine oxidase pathway or NADPH oxidase systems, superoxide production during IRI is reported to be the result of a dysregulation of the electron transport chain, with electrons leaking at various sites when oxygen is re -introduced following a period of ischemia (18, 19). The level of previously accumulated succinate, the most important Krebs cycle metabolite in this context, plays a crucial role and associates with the level of released ROS from mitochondrial complex I (20). With prolonged cellular ischemia, succinate accumulates to a higher extent, which also correlates with the energy content of liver cells (11). When mitochondrial succinate levels are high, they trigger an immediate metabolism of this detrimental compound by mitochondrial complex II and the TCA-cycle as soon as oxygen is reintroduced and the respiratory chain reestablishes an electron flow. This is however initially uncoordinated and retrograde and therefore triggers the release of reactive oxygen species (ROS) at complex I (11, 18). Cells, which are severely affected by ROS may die and release further molecules, including Danger-associated molecular pattern (Damps) and cytokines, which trigger the inflammatory response from other surrounding cells, initially less affected (Figure 1) (8). Such Damps are released from all severely injured liver cells in combination with mitochondrial DNA and represent the first level of innate immune system activation (9, 11, 21–23). With the presentation of surface markers on activated macrophages, recipient T cells are attracted and activated, which represent the next level of contribution to the innate immune response (7, 8, 24). The next subchapter provides further details on specific innate immune signaling among involved cells.
Figure 1 Schematic presentation of ischemia-reperfusion-injury with pro-inflammatory signaling. The rapid succinate metabolism at complex II leads to the initial key event of ROS release when oxygen is reintroduced into ischemic tissues. Based on the level of accumulated succinate during ischemia, a number of cells are severely affected and die with subsequent release of mitochondrial DNA and Damps (A). Specific receptors are expressed on liver and immune cells, which trigger transcription and upregulation of pro-cytokines, creating an inflammatory milieu. Various Damps molecules instigate proinflammatory signals and the assembly of the inflammasome (NLRP-3) plus caspase cleavage, which activates available pro-cytokines (B). Such features lead to the injury of previously less activated and affected cells, which releases additional damps and cytokines. ATP, Adenosine-trisphosphate; Damps, danger associated molecular patterns; Hmgb-1, High mobility group box protein-1; IL, Interleukin; ROS, reactive oxygen species; TLR, toll like receptor.
Mitochondrial ROS are a consequence of mitochondrial disruption in all liver cells, macrophages and T cells. Once oxygen is reintroduced after a period of ischemia, mitochondrial electron transfer complexes I and III serve as the major sites of ROS production (18, 25). Released ROS are directly antibacterial, but also signal the release of inflammatory cytokines. Following their release, ROS molecules first injure subcellular structures within the same cell, which releases further proinflammatory molecules (26). Cells severely affected by the cascade of ischemia-reperfusion-injury (IRI) leak pro-inflammatory molecules, which in turn activate other cells, initially less affected (Figure 1A). Such pro-inflammatory molecules include the release of damage-associated molecular patterns (DAMPs), which activate local antigen presenting cells (21). DAMP receptors are toll-like-receptors (TLR) or receptors for advanced glycation end products (RAGE), which mediate downstream cytokines release with subsequent production of more ROS by non-parenchymal liver cells, mostly Kupffer cells. ROS and DAMP release promotes two main signals, first transcription and upregulation of pro-cytokines in the nucleus (e.g., IL-1β) and second, the assembling of the inflammasome (NLRP-3) and capsase-1 cleavage to finally activate and cleavage such pro-cytokines (Figure 1B) (8, 27, 28). TLR-signaling also involves multiple adaptor proteins MyD88, TIRAP, TRIF, TRAM, and the activation of the transcription factors NFκB, AP-1 and IRF3, which ultimately lead to a maturation of dendritic cells with consecutively presentation of antigens together with the expression of co-stimulatory molecules, which attract immune cells (Figure 2) (21, 29, 30).
Figure 2 Cascade of ischemia-reperfusion-injury with specific downstream activation of the innate immune system; Different stages of ischemia reperfusion injury (IRI) with innate immune activation and resolution with or without pharmacological treatment in liver transplantation are presented. According to the accumulated succinate, ROS are released at reperfusion (1) with subsequent release of mitochondrial DNA and Damps molecules, which link IRI to innate immune response. (2) Subsequently, dendritic cells and macrophages become activated and present their well-known surface receptors to circulating T cells, which become in turn activated (3). Recipient neutrophils and other immune cells are also attracted by activated endothelial cells in the organ. With the involvement of other liver and recipient immune cells, including stellate cells and fibroblasts, T and B cells plus killer cells, the inflammatory milieu achieves a chronic stage (4) and is resolved by pharmacological upstream treatment (5). Chronic processes may also become very advanced and severe with subsequent fibrosis of the implanted organ (6) if resolution mechanisms fail.
T cells appear as main contributors in this context and become activated (T cell receptor signaling), thereby inducing a further increase in mitochondrial ROS production (31, 32). Interestingly, T cell subtypes that lack the Complex-III component RISP are more protected from ROS release. Subsequently, such T cells have a decreased expression of the activation markers CD-25 and CD-69 (31). Pharmacological blocking of this T cell activation process antagonizes their IL-2 production. One subtype of such immune cells appear as regulatory T cells, which are described with a reduced expression of CD-25 and subsequent less IL-2 production. Such T regs are therefore protective from IRI-injury and may help to reduce inflammation (33). The antagonism of CD-25 in Treg deficient mice was shown to protect murine kidneys from IRI-injury (34). Despite such known protective effect, the role of Tregs was described controversial in different stages of brain IRI. While Tregs were found to reduce inflammation and promote tissue repair in the later stage of resolution after IRI, during the acute phase stroke pathogenesis was rather pronounced by Treg (34). Additionally, in other models as for example hindlimb ischemia and reperfusion, Treg depletion through anti-CD25 led to higher levels of inflammation and to a higher number of newly developed vessels.
TCR signaling also leads to the release of calcium, stored in the endoplasmic reticulum, which can be taken up by mitochondria to drive the Krebs cycle enzyme activity, thereby increasing the amounts of NADH and Krebs cycle intermediates, including succinate (35). Mitochondrial calcium influx contributes to mitochondrial ROS generation in T cells, and succinate drives further mitochondrial ROS generation in macrophages (31).
Mitochondrial ROS also function as a signal in B cell activation. B cell activation by antigen and helper T cells induces several processes such as somatic hypermutation, to diversify the B cell receptor for antigen, and class-switch recombination (CSR), which enables B cells to express a particular type of immunoglobulin (36). The ligation of the BCR stimulates calcium release into the cytoplasm, which promotes ROS production. Stimulation with LPS and IL-4 or with anti-CD40 and IL-4 generates a population of B cells with increased mitochondrial mass, membrane potential and ROS production compared with naive B cells (37). Undifferentiated cells within this population undergo CSR after differentiation. Mitochondrial ROS may also act to induce CSR by inhibiting the synthesis of heme, a molecule that decreases CSR by antagonizing BACH2, a key transcription factor for CSR57 (36).
In summary, these observations indicate that T and B cells require ROS production for the generation of an appropriate immune response. Innate immune cells are similarly reliant on ROS production, with Krebs cycle intermediates such as succinate, citrate, fumarate and itaconate emerging as important regulators of the production of this noxious agent, as well as other events (38). Overall, mitochondrial ROS, originating from a variety of mitochondrial sources, are key signaling molecules for immune-cell function.
During ischemia, various Krebs cycle metabolites with important inflammatory signaling function accumulate in all involved cells. These metabolites are present because of the occurrence of break points in the Krebs cycle during ischemia. The interruption at the enzyme succinate dehydrogenase (SDH), which represents mitochondrial complex II, and converts succinate to fumarate, is associated with an accumulation of succinate, a metabolite with proinflammatory activity and roles in immunity (18).
Mitochondrial succinate, once accumulated to a significant extend, triggers two main metabolic features at reoxygenation. First, when high succinate levels are present, mitochondria aim for a rapid metabolism and immediate reestablishment of the electron flow, which is however reverse (RET) with subsequent ROS production from complex I, thereby instigating the inflammatory IRI-cascade (Figure 1, 2) (11, 39, 40). Succinate oxidation by SDH, also leads to HIF-1α activation and HIF-1α-dependent gene expression; a notable example of a gene affected in this way is IL1B, which encodes IL-1β (41). Succinate also limits the production of anti-inflammatory cytokines, particularly of IL-10. Inhibition of SDH with dimethylmalonate (DMM) profoundly inhibits LPS-induced ROS generation and the expression of IL-1β and a range of proinflammatory genes in macrophages, and boosts the expression of IL-10 and anti-inflammatory genes (8, 10, 24, 42). DMM administration in mice treated with LPS results in decreased IL-1β expression and a boost in IL-10 expression systemically (31, 35). LPS treatment boosts the mitochondrial membrane potential in macrophages, which is dependent on increased glycolytic ATP production. Limiting of LPS-induced membrane hyperpolarization, which is required for RET, inhibits IL-1β induction in response to LPS in macrophages (31). The induction of IL-1β by LPS and succinate is also impaired in macrophages, that express the enzyme alternative oxidase (AOX), which limits RET. All of these observations not only confirm a role for mitochondria-derived succinate as a critical proinflammatory mediator, but also shed light on the mechanism by which LPS drives ROS production, and provide further rationales for why macrophages favor aerobic glycolysis and decrease Oxidative Phosphorylation (OXPHOS)
in response to LPS. These metabolic alterations repurpose mitochondria from ATP synthesis to ROS production to promote and maintain a proinflammatory state (18, 21, 23, 25, 43). An antibacterial role of SDH was also suggested by the observation that the inhibition of SDH during bacterial infections rendered mice more susceptible to infection. Taken together, these findings identify complex II (SDH) as one key control point IRI-associated inflammation.
Mitochondrial antiviral signaling protein (MAVS) is another key signaling protein activated by the different RNA sensors, including RIG-I and MDA5 (29). It in turn activates pathways, that regulate the transcription factor NF-κB and IRFs to promote proinflammatory gene expression (8, 29, 44, 45). Interaction with the outer mitochondrial membrane is essential for a fully functional MAVS molecule. Mitochondrial ROS can induce and drive MAVS oligomerization, leading to the production of type I interferon, independent of RNA sensing, which in turn suggests that MAVS might be a key sensor of mitochondrial ROS, that acts to promote the recipient defense and inflammation (25, 29, 31). Furthermore, MAVS associates with Inflammasome (NLRP-3) and promotes its oligomerization, which leads to caspase-1 activation (8, 23, 43, 46–48). Activation of NLRP-3 with the synthetic Toll-like-receptor (TLR) -7 ligand imiquimod has recently been shown to occur as a result of the mitochondria ROS production from complex I and the quinone oxidoreductase NQO2 (31, 48). This effect was independent of potassium efflux, which highlights the importance of mitochondrial ROS for NLRP-3 activation (49, 50). Finally, NLRP-3 is also regulated by cardiolipin, a lipid of the inner mitochondrial membrane, which translocates to the outer membrane, where it recruits NLRP-3 after mitochondrial membrane depolarization (31). This interaction appears crucial for NLRP-3 activation, and suggests that mitochondria function as signaling hubs and activate the innate immunity. NLRP-3 activation in turn leads to mitochondrial damage and subsequent mitochondrial ROS release, which represents a feedback loop between NLRP-3 and mitochondria (23, 48). Mitochondria are therefore critical for signaling by the described three major innate immune signaling pathways.
A relatively novel strategy to decrease the posttransplant inflammation and the need of IS is to approach the crosslink between organ ischemia-reperfusion injury (IRI) and immune response. Instead of focusing on the key instigator of the IRI-cascade in mitochondria, most teams target the suppression of proinflammatory molecules, released in response to ROS later after reperfusion (Table 1). Others focus on the suppression of defense mechanisms. Preventive or therapeutic measures with impact on IRI-associated inflammation were applied for example in the donor as direct treatment or as an additive to the donor flush solution (56). Others have added specific molecules during cold storage or as a treatment in the recipient. Most molecules were however not yet transferred into clinical practice, although the results seen in experimental studies appeared quite promising with impact on IRI-associated inflammation, the effect got either lost or was limited when explored in transplant models (40, 57, 58). The reason behind could be the target of too peripheral individual genes or molecules of the IRI-cascade. Although the underlying mechanisms of IRI are increasingly linked to tissue succinate accumulation during ischemia with subsequent ROS release after reoxygenation, this key metabolite is rarely addressed in studies (40). A significant protection from IRI-associated complications could be achieved through the prevention of succinate accumulation before or during donation (e.g., before ischemia) or also by a slow succinate oxidation before normothermic reperfusion at transplantation (40). Already established succinate levels could be eliminated through a slow activation of complex II, before reintroducing oxygen at normothermic temperatures (Figure 3) (40). Reduced cellular succinate stores prevent the initial burst of ROS release, which therefore eliminates the initial danger event of the IRI- cascade (40). In addition to succinate, other compounds in mitochondria could be the target to reduce IRI. Malonate could for example be used to block complex II (SDH) and limit succinate accumulation and also impact on the rapid succinate oxidation at reperfusion (11, 18, 38). Next, the inhibition of SDH with DMM may also provide a therapeutic benefit by limiting ROS production and proinflammatory responses, and boosting the anti-inflammatory response (19, 31). If the reprogramming would sustain, it could also offer the possibility of inducing remission in chronic inflammatory diseases. In addition, the blockage of the mitochondrial permeability transition pore (MPTP) with Cyclosporin, as tested in mice, could be advanced into human practice. However, despite promising results of a randomized controlled trial (RCT), the effect of this pharmaceutical was limited in a recent phase III study in hearts (59, 60).
Table 1 Clinical studies with the impact of machine perfusion on immune response within the last 3 years.
Figure 3 Timing of treatment modalities to address IRI-associated inflammation. Overview on the current timings when therapeutics are administered from the donor to implantation. Most molecules target genes or downstream receptors beyond the instigating processes, instead of succinate and subsequent ROS release.
Various other molecules and drugs are currently developed to be used to reduce downstream inflammatory processes induced by ROS, Damps and mitochondrial DNA. Most studies appear however older and involve only animal models with only very few that have explored the effect in a transplant model (56).
Next, the natural process of RNA interference (RNAi) with silencing of specific genes was recently explored and introduced into transplantation settings. Various experimental studies were presented with the interference of the specific RNA, targeting genes of IRI-injury and immune activation. Unfortunately, in most studies, RNAi is explored in the donor 1 to 72hrs before ischemia (61). This approach appears however rather unpractical in clinical settings, where donor treatment is prohibited in most countries (62). Only very few preclinical studies involve other treatment routes, including administration through portal vein infusion (61, 63).
In addition to RNAi other molecules, including antioxidants and antibodies are administered to block specific molecular reactions. Li et al. added the CD47m antibody into the liver flush solution during donation. CD47 is a member of the immunoglobulin superfamily and is involved in various pathways related to ROS molecules. Recipients were found with lower rates of acute rejection and a lower severity in this allogenic mouse model (64).
In summary, various treatments are currently explored, frequently targeting rather peripheral processes instead of mitochondrial components. Multiple routes are used, including donor injections, organ flush and recipient treatment. The modulation of the current standard cold storage preservation, with the implementation of machine perfusion technologies, might serve as most attractive tools to supply pre-injured organs with beneficial compounds before implantation in the future. However, prior to successful utilization of machine perfusion as delivery tool, underlying mechanisms of protection and injury should be explored and understood.
Novel organ perfusion strategies appear of interest and various groups have promoted the technological development in the last decade (51, 54, 55, 65–68). Despite this advancement, available data on the solitary impact of machine perfusion (MP) on ROS-mediated allograft oxidative injury and subsequent immune response remain scarce (5, 42).
Novel organ perfusion approaches can be divided into two main strategies, first in-situ perfusions, which include regional perfusion, mainly done at normothermic temperatures and secondly, ex-situ techniques, where organs undergo perfusion on a tailored device either after cold storage (e.g., endischemic) or instead (69). Normothermic regional perfusion (NRP) is applied in the donor immediately after circulatory death (DCD). Leading countries, where this technology is routinely used in DCD liver donors include Spain, France, Italy and a few centers in the United Kingdom (70–73). The early graft evaluation during NRP in the donor helps to select livers with too high injury. This selection is mainly based on the macroscopic liver appearance, the perfusion quality, pH, liver transaminases and lactate measured from the NRP-circuit. DCD liver preserved with NRP were shown to achieve immediate function and recipients experienced reduced levels of biliary complications after transplantation (68, 74). With the overall aim to push regulatory and donor risk boundaries, the limitations of all perfusion techniques are increasingly described. With NRP, DCD livers were well preserved provided that the national regulations of donor and recipient risk were respected. French centres for example described a higher number of graft loss with the use of NRP-treated grafts when the donor warm ischemia time or recipient lab MELD exceeded national criteria (72). With additional cold storage time following NRP and liver procurement, the exact metabolic situation of such organs appears not well known at the time of implantation (69). In this context, prolonged cold ischemia of more than 7hrs was recently described as risk factor for graft loss in Spain, when combined with NRP (75). This risk was even more pronounced when such DCD grafts were implanted into recipients waiting for a retransplantation (75). Comparable to any other technique of normothermic reperfusion, where oxygen is reintroduced into ischemic tissue, during initial NRP, the cells and more specifically mitochondria have the main goal to metabolize high succinate concentrations rapidly with subsequent ROS release from mitochondrial complex-I and downstream inflammation. This has been recently demonstrated in the setting of clinical NRP in Italy with the quantification of cytokine levels in the recirculating NRP-perfusate (76). Based on the known link between IRI and innate immune activation, more data are required to describe the impact of NRP on the innate immune system, and ideally in context of an increased utilization of risky donors.
In contrast, two main ex-situ machine perfusion techniques are currently explored (51, 67, 68). First, the normothermic machine perfusion (NMP) technique using a blood-based perfusate at 37°C, which is ideally applied after liver procurement and instead of cold storage, replacing cold ischemia with a subsequent reduction of liver injury and IRI(62). And secondly, a logistically less challenging perfusion strategy, applied after cold ischemia in the recipient centre using a hypothermic oxygenated perfusion (HOPE) prior to implantation (11, 77). Of note, although various groups have explored the use of such perfusion techniques as delivery tool for molecules or stem cells in ex-vivo models of liver perfusion, the underlying mechanisms of such perfusion techniques are not well enough understood and deserve more recognition (78).
Perfusion techniques done at warm temperatures were found to trigger the same ROS-induced inflammation as seen with the IRI-cascade after liver transplantation. The level of IRI might however be dependent on the perfusate components. The literature describing such features of liver NMP in a transparent way is very scarce (28, 79, 80). Normothermic perfusion strategies induce an inflammatory environment with the release of ROS and DAMPs and consecutive activation of toll-like-receptors (28, 81). Downstream activation of the innate immune system after NMP of livers can be likewise expected, promoting acute organ rejection, particularly when NMP is applied after a relevant period of standard cold storage (82, 83). Recent data from normothermic kidney perfusion support such mechanistical insights (84).
Unfortunately, markers of immune response are frequently not reported in clinical and experimental studies with normothermic perfusion. We were therefore also not able to add clinical studies to Table 1, which assess the impact of NMP on the immune system.
Jassem et al. have recently compared the expression of immune-related pro-inflammatory genes between cold stored livers (n=12) and grafts that underwent NMP instead (n=27) from brain death donors (DBD) (85, 86). Cold stored livers showed a higher expression of genes involved in innate immune activation, neutrophil chemotaxis and platelet activity with higher levels of recipient plasma cytokines (85). Livers, exposed to NMP demonstrated less amounts of specific T cells, known for their production of Interleukins 2, 4 and 17, and Interferon y. The proportion of regulatory T cells was found slightly higher in the NMP group, particularly the tissue-resident subtype CD4posCD25highCD127negFOXP3pos was described with higher concentrations throughout prolonged normothermic perfusion (85). Of note, the replacement of cold storage by NMP led to a lower liver injury in the NMP group, while grafts in the control group were exposed to the entire duration of cold ischemia before implantation and exploring the immune response presented here (67, 85, 87). The work by Scheuermann et al. presents one of the very few studies actually quantifying the inflammatory injury during NMP, compared to other perfusion techniques. Authors have demonstrated that liver perfusion at lower temperatures, e.g., subnormothermic, induces less inflammation compared to NMP (88).
In contrast, hypothermic oxygenated perfusion (HOPE), is performed at 6-10°C using an artificial perfusate, which is highly oxygenated (60-80kPa) (89, 90). HOPE therefore was shown to reprogram mitochondrial during the introduction of oxygen at such low temperatures. During HOPE the electron flow is reestablished with subsequent steady and slow metabolism of accumulated succinate and ATP reloading at the same time. While the respiratory chain is functioning the high load of NADH at complex I is also reduced by metabolism to NAD, which is a surrogate marker of a functioning complex I and energy recharge (91). Mitochondria, which underwent reoxygenation at hypothermic temperatures are switched and can handle the reperfusion with blood at normothermic temperatures without thew known detrimental rapid succinate metabolism and with much lower ROS production (11, 81, 92, 93). HOPE-treatment achieves this by increasing the activity of mitochondrial complex proteins (11). Based on these significant changes during HOPE, mitochondria are prepared and experience less oxidative stress and subsequently trigger less Damps release with less TLR activation and a reduced innate immune response (28, 79). Ex-vivo graft treatment by HOPE was consecutively also found to prevent downstream T-cell activation in allogenic liver and kidney transplant models, without any additional immune suppressive treatment (17, 42). Combined experimental protocols allowed to reduce the immunosuppression to one third of the normal dosage when IS was combined with HOPE. An excerpt of the results found after transplantation with various IS and HOPE combinations in this allogeneic liver model is shown in Figure 4 (42). Normal livers from Lewis rats were procured and with minimal cold ischemia transplanted into Brown Norway recipient rats, which is well-described in the literature as a known model of allogeneic liver transplantation. Of note, recipients without any immunosuppression achieved poor survival, expectedly shorter than two weeks in most. In contrast, HOPE treatment in these allogeneic rodent livers led to similar survival rates as seen in recipient, which received the normal immunosuppressive treatment with Tacrolimus (42). In combination with a reduced Tacrolimus dosage, allogeneic liver recipients achieved prolonged survival when combined with HOPE treatment. Of note, similar findings are described in the literature for allogeneic models of kidney transplantation using endischemic HOPE (17). These findings from preclinical studies are now also paralleled by an increasing body of clinical studies with and without a randomized design. Table 1 summarizes clinical studies reporting an impact of HOPE on the immune activation after human liver transplantation. The recently presented RCT from the Groningen group found as a secondary endpoint a 9% reduction of acute rejections after DCD liver transplantation with dual HOPE treatment compared to cold storage controls (51). A similar tendency was described by another RCT from Germany, where the team described a reduction of acute rejections from 26% in the cold storage study arm to 17% after HOPE (52). Earlier retrospective studies have already demonstrated similar results. The Zurich group compared the effect of HOPE in extended DCD liver grafts with unperfused, cold stored controls from the United Kingdom. Of note, livers with HOPE presented an acute rejection in 4%, which is a significant reduction from 28% seen in unperfused controls (p=0.0019)(Table 1) (90). Although the impact of machine perfusion on costs is not well enough explored yet, a more than 10-20% reduction of acute rejections will most likely reduce transplant related costs with less requirements of liver graft biopsies and readmissions. In addition to the impact on biliary and overall complications, further results are awaited with regard to costs. Another important factor is the perfusion duration of HOPE. A recent multi-centre study has collected outcomes of 93 human liver transplants (50 DCD, 43 DBD) with a prolonged HOPE treatment of >4hrs and a median overall preservation of 10hrs. Outcomes were excellent and comparable to other series reported with shorter endischemic HOPE (94). Of note, prolonged HOPE of >4hrs was also shown after more than 10hrs of cold storage in extended criteria donor livers in Germany and in combination with high MELD recipients in Brasil (95). A prospective study on prolonged HOPE is currently ongoing in the Netherlands to confirm the safe prolongation to compensate logistical issues (96).
Figure 4 Impact of HOPE on innate immune response after allogeneic liver transplantation; To address the accumulated succinate with a slow oxidation at complex II is a key mechanism to avoid the massive ROS release and subsequent IRI cascade with complications after transplantation. Machine perfusion is therefore a well explored new method to improve and assess metabolic processes. Hypothermic oxygenated perfusion (HOPE) before implantation was shown to reduce the accumulated succinate and to improve complex I and II function. Using a model of allogeneic liver transplantation, the protective effect of HOPE on the innate immune system was demonstrated with a lower number of activated of Kupffer cells (through less Damps release) and subsequently a lower number of infiltrating T cells in transplanted livers, compared to untreated controls (without HOPE and without immunosuppression). Of note, HOPE treatment achieved the best protection from Kupffer cell and dendritic cell activation early after implantation, e.g., at 24hrs, also compared to the group with full dosage of immunosuppression, which requires time until the most effective blood levels are seen. The protective effect of HOPE was still present 4 weeks after implantation, although the delayed immune response became visible. HOPE treatment was therefore combined with a low dose of immunosuppression, which led to acute rejection when applied alone. HOPE with reduced immunosuppression protected recipients from innate immune activation and acute rejection, similarly to recipients which received the full dose of tacrolimus (immunosuppression). These images were obtained from samples from the study presented in reference (42) (samples and histological images were not published before in this reference). CD, cluster of differentiation.
Recirculating perfusates may provide two main benefits during machine perfusion. First, injured and dying cells release specific molecules, which might signal functional deficits and could be used to assess organ viability (97). And secondly, perfusate could be used to carry specific molecules into the cells and subcellular compounds to prevent production and release of IRI-associated compounds. Various therapies, such as pharmacological agents, genes, stem cells and nanoparticles were administered into organs through this route in preclinical studies (56, 57). The technique of normothermic perfusion is more frequently applied because of the assumed better transport and uptake of such molecules at 37°C, when compared to hypothermic perfusion settings. This last subchapter describes studies within the last 3 years, where machine perfusion was used to deliver compounds with an impact on the innate immune response (Table 2). Only very few studies explore the impact in a transplant model. Cao et al. exposed DCD livers with 30 minutes of donor warm ischemia time to 4hrs of NMP with the addition of bone marrow-derived mesenchymal stem cells, which were found to inhibit the release of Hmgb-1 with subsequent reduction of TLR-4 activation. Authors demonstrated a clear effect on the early innate immune response through NMP with such stem cells compared to unperfused cold stored controls (98). This work was paralleled by Yu et al, who blocked NLRP-3 in a pig DCD liver transplant model. All experimental groups underwent hypothermic perfusion and the NLRP-3 blocker was administered either during perfusion or after implantation in the recipient. Groups with additional NLRP-3 blockage were found with lower innate immune response independent from the administration route compared to hypothermic perfusion alone. Of note the group where the recipient received the NRLP-3 blocker was superior to all other groups. This work demonstrated first, that the addition of molecules with a blocking effect on specific receptors is also effective during HMP or HOPE and secondly, that the already protective HOPE-effect on mitochondria can be even more enhanced with additional reduction of downstream inflammatory pathways (99). Other groups have for example demonstrated, that a selective cytokine blockage, e.g., IL-10 and TGF-β also reduces immune responses, however mainly in models with liver machine perfusion but without subsequent transplantation (100). Another approach is the addition of stem cells to perfusates. Laing et al. have added mesenchymal stem cells to NMP perfusates and show feasibility and comparable cell settling in perfused livers, when administered through different inflow vessels (78). Other agents, including defatting cocktails, were found to reduce the overall inflammation induced by NMP. Boteon et al. demonstrated an effect on the early innate immune response during NMP. Both, CD14, as found in macrophages and neutrophils, and pro-inflammatory cytokines were reduced by defatting cocktails in NMP perfusates (101). Another attractive approach is increasingly discussed with RNA interference. The group of Paulo Martins has recently demonstrated the first successful administration of siRNA during both, hypothermic and normothermic perfusion. The hepatocyte transfection was achieved through siRNA coating with lipid nanoparticles (102, 103). Of note, this is another evidence that such treatments are feasible in both perfusion approaches, warm and cold (53, 55, 103). The impact of such selective gene down regulation might however trigger responsive upregulation of other genes and subsequent cytokine and mediator release, where more studies also to identify the best pathways in humans are needed (Figure 5).
Table 2 Experimental studies exploring the impact of machine perfusion with or without specific perfusate additives on the immune system in liver transplantation.
Figure 5 Therapeutic strategies to target ischemia-reperfusion injury and improve outcomes after liver transplantation; This figure provides an overview of currently applied pharmacological and non-pharmacological modalities with impact on IRI associated features. From the donor, procurement to preservation and reperfusion (transplantation) modalities, their targets and results as well as challenges are presented. The majority of strategies affects individual genes or receptors, which might lead to an even higher proinflammatory response by other genes not affected.
Machine perfusion is an attractive tool to directly treat human livers prior to implantation and also to deliver specific molecules with impact on outcomes. The underlying mechanisms will however require more studies in the future to understand the individual impact of different perfusion strategies on the immune system. This review has mainly focused on the innate immune response, where an effect of HOPE perfusion is described in the current literature. The potential effect on the B cell response remains however entirely unknown. Most administered compounds during MP remain currently experimental and studies to identify the best route and dosage for administration are still lacking, particularly in humans. This is also valid for the spectrum of nanoparticles with various available types and formulations to be explored in the future.
RP, MC and AS designed the review and the figures. RP, MC, DD and AS: wrote the first draft. All coauthors discussed the content, revised the manuscript and approved the final version.
The authors declare that the research was conducted in the absence of any commercial or financial relationships that could be construed as a potential conflict of interest.
All claims expressed in this article are solely those of the authors and do not necessarily represent those of their affiliated organizations, or those of the publisher, the editors and the reviewers. Any product that may be evaluated in this article, or claim that may be made by its manufacturer, is not guaranteed or endorsed by the publisher.
ALT, Alanine Aminotransferase; AP-1, Activator Proteine-1; AS, Anastomotic strictures; AST, Aspartate-Aminotransferase; ATP, Adenosine-trisphosphate; BACH2, transcription regulator protein; CD, Cluster of differentiation; CIT, Cold ischemia time; CS, cold storage; CSR, Class switch recombination; Damp`s, Danger associated molecular pattern`s; DBD, donation after brain death; DCD, Donation after circulatory death; DMM, dimethylmalonate; DWIT, donor warm ischemia time; EAD, Early allograft dysfunction; ECD, Extended Criteria Donor; FMN, Flavin-mononucleotide; HA, Hepatic artery; GSH, Glutathione; HAR, hexa-ammineruthenium; HAT, Hepatic artery thrombosis; HMGB-1, High mobility group box-1 protein; HMP, Hypothermic machine perfusion; HOPE, Hypothermic oxygenated perfusion; H&E, Hematoxylin and Eosin; IC, Ischemic cholangiopathy, ICAM-1, Intercellular adhesion molecule-1; IL, Interleukin; IRF3, Interferon regulatory factor 3; IRI, Ischemia-reperfusion injury; IS, Immunosuppression; ITBL, Ischemic type biliary lesions; KC`s, Kupffer cells; LPS, Lipopolysaccharide; LT, Liver Transplantation; MAVS, Mitochondrial antiviral signaling (protein); MELD, Model of end stage liver disease; MPS, Machine perfusion solution; MPT pore, Mitochondria permeability transition pore; mtROS, mitochondrial reactive oxygen species; MyD88, Myeloid differentiation primary response 88; NAD/NADH, nicotine adenine dinucleotide (oxidized/reduced); NFkB, nuclear factor kappa-light-chain-enhancer; NOX4, NMP, Normothermic machine perfusion; NRP, normothermic regional perfusion; PNF, Primary non function; PV, portal vein; RCT, Randomized controlled trial; RET, reverse electron flow; ROS, reactive oxygen species; SCS, standard cold storage; SDH, Succinate dehydrogenase; SEC, sinusoidal endothelial cells; TIRAP, Toll-interleukin 1 receptor (TIR) domain containing adaptor protein; TLR-4 (9), Toll-like-receptor-4; 8-OHdG, 8-hydroxy-2-deoxy Guanosine
1. Srinivas TR, Meier-Kriesche HU. Minimizing Immunosuppression, an Alternative Approach to Reducing Side Effects: Objectives and Interim Result. Clin J Am Soc Nephrol (2008) 3(Supplement 2):S101–16. doi: 10.2215/CJN.03510807
2. Colaneri J. An Overview of Transplant Immunosuppression–History, Principles, and Current Practices in Kidney Transplantation. Nephrol Nurs J (2014) 41:549–60; quiz 561.
3. Londoño MC, Rimola A, O’Grady J, Sanchez-Fueyo A. Immunosuppression Minimization vs. Complete Drug Withdrawal in Liver Transplantation. J Hepatol (2013) 59:872–9. doi: 10.1016/j.jhep.2013.04.003
4. Doherty DG. Immunity, Tolerance and Autoimmunity in the Liver: A Comprehensive Review. J Autoimmun (2016) 66:60–75. doi: 10.1016/j.jaut.2015.08.020
5. Clavien PA, Muller X, de Oliveira ML, Dutkowski P, Sanchez-Fueyo A. Can Immunosuppression be Stopped After Liver Transplantation? Lancet Gastroenterol Hepatol (2017) 2:531–7. doi: 10.1016/S2468-1253(16)30208-4
6. Saunders EA, Engel B, Höfer A, Hartleben B, Vondran FWR, Richter N, et al. Outcome and Safety of a Surveillance Biopsy Guided Personalized Immunosuppression Program After Liver Transplantation. Am J Transplant (2022) 22:519–31. doi: 10.1111/AJT.16817
7. Land WG. Emerging Role of Innate Immunity in Organ Transplantation. Part I: Evolution of Innate Immunity and Oxidative Allograft Injury. Transplant Rev (2012) 26:60–72. doi: 10.1016/j.trre.2011.05.001
8. Kubes P, Mehal WZ. Sterile Inflammation in the Liver. Gastroenterology (2012) 143:1158–72. doi: 10.1053/J.GASTRO.2012.09.008
9. van Golen RF, Reiniers MJ, Olthof PB, van Gulik TM HM. Sterile Inflammation in Hepatic Ischemia/Reperfusion Injury: Present Concepts and Potential Therapeutics. J Gastroenterol Hepatol (2013) 3:394–400. doi: 10.1111/jgh.12072
10. Land WG. Innate Immunity-Mediated Allograft Rejection and Strategies to Prevent it. Transpl Proc (2007) 39:667–72. doi: 10.1016/j.transproceed.2007.01.052
11. Schlegel A, Muller X, Mueller M, Stepanova A, Kron P, de Rougemont O, et al. Hypothermic Oxygenated Perfusion Protects From Mitochondrial Injury Before Liver Transplantation. EBioMedicine (2020) 60:103014. doi: 10.1016/J.EBIOM.2020.103014
12. Boteon YL, Afford SC. Machine Perfusion of the Liver: Which is the Best Technique to Mitigate Ischaemia-Reperfusion Injury? World J Transplant (2019) 9:14–20. doi: 10.5500/WJT.V9.I1.14
13. Kaushik D, Roychoudhury A. Reactive Oxygen Species (ROS) and Response of Antioxidants as ROS-Scavengers During Environmental Stress in Plants. Front Environ Sci (2014) 2:53. doi: 10.3389/fenvs.2014.00053
14. Nakajima D, Chen F, Yamada T, Sakamoto J, Osumi A, Fujinaga T, et al. Hypothermic Machine Perfusion Ameliorates Ischemia-Reperfusion Injury in Rat Lungs From non-Heart-Beating Donors. Transplantation (2011) 92:858–63. doi: 10.1097/TP.0b013e31822d8778
15. Wyss R, Méndez Carmona N, Arnold M, Segiser A, Mueller M, Dutkowski P, et al. Hypothermic, Oxygenated Perfusion (HOPE) Provides Cardioprotection via Succinate Oxidation Prior to Normothermic Perfusion in a Rat Model of Donation After Circulatory Death (DCD). Am J Transplant (2021) 21:1003–11. doi: 10.1111/ajt.16258
16. Leemkuil M, Lier G, Engelse MA, Ploeg RJ, EJP de Koning EJP, ‘t Hart NA, et al. Hypothermic Oxygenated Machine Perfusion of the Human Donor Pancreas. Transplant Direct (2018) 4(10):e388. doi: 10.1097/TXD.0000000000000829
17. Kron P, Schlegel A, Muller X, Gaspert A, Clavien PA, Dutkowski P. Hypothermic Oxygenated Perfusion: A Simple and Effective Method to Modulate the Immune Response in Kidney Transplantation. Transplantation (2019) 103:e128–36. doi: 10.1097/TP.0000000000002634
18. Chouchani ET, Pell VR, Gaude E, Aksentijević D, Sundier SY, Robb EL, et al. Ischaemic Accumulation of Succinate Controls Reperfusion Injury Through Mitochondrial ROS. Nature (2014) 515:431–5. doi: 10.1038/nature13909
19. Dröse S. Differential Effects of Complex II on Mitochondrial ROS Production and Their Relation to Cardioprotective Pre- and Postconditioning. Biochim Biophys Acta - Bioenergetics (2013) 1827(5):578–87. doi: 10.1016/j.bbabio.2013.01.004
20. Murphy MP. How Mitochondria Produce Reactive Oxygen Species. Biochem J (2009) 417:1–13. doi: 10.1042/BJ20081386
21. Zhang Q, Raoof M, Chen Y, Sumi Y, Sursal T, Junger W, et al. Circulating Mitochondrial DAMPs Cause Inflammatory Responses to Injury. Nature (2010) 464:104–7. doi: 10.1038/nature08780
22. van Golen RF, van Gulik TM, Heger M. Mechanistic Overview of Reactive Species-Induced Degradation of the Endothelial Glycocalyx During Hepatic Ischemia/Reperfusion Injury. Free Radical Biol Med (2012) 52:1382–402. doi: 10.1016/j.freeradbiomed.2012.01.013
23. Shimada K, Crother T, Karlin J, Dagvadorj J, Chiba N, Chen S, et al. Oxidized Mitochondrial DNA Activates the NLRP3 Inflammasome During Apoptosis. Immunity (2012) 36:401–14. doi: 10.1016/J.IMMUNI.2012.01.009
24. Próchnicki T, Latz E. Inflammasomes on the Crossroads of Innate Immune Recognition and Metabolic Control. Cell Metab (2017) 26(1):71–93. doi: 10.1016/j.cmet.2017.06.018
25. Sorbara M, Girardin S. Mitochondrial ROS Fuel the Inflammasome. Cell Res (2011) 21:558–60. doi: 10.1038/CR.2011.20
26. Zhang J, Wang X, Vikash V, Ye Q, Wu D, Liu Y, et al. ROS and ROS-Mediated Cellular Signaling. Oxid Med Cell Longevity (2016) 2016:4350965. doi: 10.1155/2016/4350965
27. Jha AK, Huang SCC, Sergushichev A, Lampropoulou V, Ivanova Y, Loginicheva E, et al. Network Integration of Parallel Metabolic and Transcriptional Data Reveals Metabolic Modules That Regulate Macrophage Polarization. Immunity (2015) 42(3):419–30. doi: 10.1016/j.immuni.2015.02.005
28. Schlegel A, Kron P, Graf R, Dutkowski P, Clavien PA. Warm vs. Cold Perfusion Techniques to Rescue Rodent Liver Grafts. J Hepatol (2014) 61:1267–75. doi: 10.1016/j.jhep.2014.07.023
29. Seth RB, Sun L, Ea CK, Chen ZJ. Identification and Characterization of MAVS, a Mitochondrial Antiviral Signaling Protein That Activates NF-κb and IRF3. Cell (2005) 122(5):669–82. doi: 10.1016/j.cell.2005.08.012
30. Eltzschig HK, Eckle T. Ischemia and Reperfusion–From Mechanism to Translation. Nat Med (2011) 17:1391–401. doi: 10.1038/NM.2507
31. Mills EL, Kelly B, O’Neill LAJ. Mitochondria are the Powerhouses of Immunity. Nat Immunol (2017) 18:488–98. doi: 10.1038/NI.3704
32. Chao T, Wang H, Ho PC. Mitochondrial Control and Guidance of Cellular Activities of T Cells. Front Immunol (2017) 8:473. doi: 10.3389/FIMMU.2017.00473
33. Jun C, Ke W, Qingshu L, Ping L, Jun D, Jie L, et al. Protective Effect of CD4+CD25highCD127low Regulatory T Cells in Renal Ischemia–Reperfusion Injury. Cell Immunol (2014) 289:106–11. doi: 10.1016/J.CELLIMM.2014.04.002
34. Rao J, Lu L, Zhai Y. T Cells in Organ Ischemia Reperfusion Injury. Cur Opin in Org Transplant (2014) 19(2):115–20 doi: 10.1097/MOT.0000000000000064
35. Mills EL, Kelly B, Logan A, Costa ASH, Varma M, Bryant CE, et al. Succinate Dehydrogenase Supports Metabolic Repurposing of Mitochondria to Drive Inflammatory Macrophages. Cell (2016) 167:457–70.e13. doi: 10.1016/j.cell.2016.08.064
36. Watanabe-Matsui M, Muto A, Matsui T, Itoh-Nakadai A, Nakajima O, Murayama K, et al. Heme Regulates B-Cell Differentiation, Antibody Class Switch, and Heme Oxygenase-1 Expression in B Cells as a Ligand of Bach2. Blood (2011) 117 (20):5438–48. doi: 10.1182/blood-2010-07-296483
37. Jang KJ, Mano H, Aoki K, Hayashi T, Muto A, Nambu Y, et al. Mitochondrial Function Provides Instructive Signals for Activation-Induced B-Cell Fates. Nat Commun (2015) 6:6750. doi: 10.1038/ncomms7750
38. Martin J, Costa A, Gruszczyk A, Beach T, Allen F, Prag H, et al. Succinate Accumulation Drives Ischaemia-Reperfusion Injury During Organ Transplantation. Nat Metab (2019) 1:966–74. doi: 10.1038/s42255-019-0115-y
39. Stepanova A, Kahl A, Konrad C, Ten V, Starkov AS, Galkin A. Reverse Electron Transfer Results in a Loss of Flavin From Mitochondrial Complex I: Potential Mechanism for Brain Ischemia Reperfusion Injury. J Cereb Blood Flow Metab (2017) 37(12):3649–58. doi: 10.1177/0271678X17730242
40. Saeb-Parsy K, Martin JL, Summers DM, Watson CJE, Krieg T, Murphy MP. Mitochondria as Therapeutic Targets in Transplantation. Trends Mol Med (2021) 27:185–98. doi: 10.1016/j.molmed.2020.08.001
41. Sukumar M, Liu J, Mehta GU, Patel SJ, Roychoudhuri R, Crompton JG, et al. Mitochondrial Membrane Potential Identifies Cells With Enhanced Stemness for Cellular Therapy. Cell Metab (2016) 23:63–76. doi: 10.1016/j.cmet.2015.11.002
42. Schlegel A, Kron P, Graf R, Clavien P-A, Dutkowski P. Hypothermic Oxygenated Perfusion (HOPE) Downregulates the Immune Response in a Rat Model of Liver Transplantation. Ann Surg (2014) 260:931–7. doi: 10.1097/SLA.0000000000000941
43. Gong Z, Pan J, Shen Q, Li M, Peng Y. Mitochondrial Dysfunction Induces NLRP3 Inflammasome Activation During Cerebral Ischemia/Reperfusion Injury. J Neuroinflamm (2018) 15:242. doi: 10.1186/S12974-018-1282-6
44. Davis BK, Wen H, Ting JP-Y. The Inflammasome NLRs in Immunity, Inflammation, and Associated Diseases. Annurev-Immunol (2011) 29:707–35. doi: 10.1146/ANNUREV-IMMUNOL-031210-101405
45. He G, Karin M. NF-κb and STAT3- Key Players in Liver Inflammation and Cancer. Cell Res (2011) 21:159–68. doi: 10.1038/cr.2010.183
46. Ricci J-E, Muñ Oz-Pinedo C, Fitzgerald P, Atrice Bailly-Maitre B, Perkins GA, Yadava N, et al. Disruption of Mitochondrial Function During Apoptosis Is Mediated by Caspase Cleavage of the P75 Subunit of Complex I of the Electron Transport Chain. Cell (2004) 117:773–86. doi: 10.1016/j.cell.2004.05.008
47. Martinvalet D, Dykxhoorn DM, Ferrini R, Lieberman J. Granzyme A Cleaves a Mitochondrial Complex I Protein to Initiate Caspase-Independent Cell Death. Cell (2008) 133:681–92. doi: 10.1016/j.cell.2008.03.032
48. Tschopp J, Schroder K. NLRP3 Inflammasome Activation: The Convergence of Multiple Signalling Pathways on ROS Production? Nat Rev Immunol (2010) 10:3 2010. doi: 10.1038/nri2725
49. Groß CJ, Mishra R, Schneider KS, Médard G, Wettmarshausen J, Dittlein DC, et al. K + Efflux-Independent NLRP3 Inflammasome Activation by Small Molecules Targeting Mitochondria. Immunity (2016) 45:761–73. doi: 10.1016/J.IMMUNI.2016.08.010
50. Schön MP, Schön M. Immune Modulation and Apoptosis Induction: Two Sides of the Antitumoral Activity of Imiquimod. Apoptosis (2004) 9:291–8. doi: 10.1023/B:APPT.0000025805.55340.C3
51. van Rijn R, Schurink I, de Vries Y, van den Berg A, Cortes Cerisuelo M, Darwish M, et al. Hypothermic Machine Perfusion in Liver Transplantation — A Randomized Trial. New Engl J Med (2021) 384:1391–401. doi: 10.1056/NEJMoa2031532
52. Czigany Z, Pratschke J, Froněk J, Guba M, Schöning W, Raptis D, et al. Hypothermic Oxygenated Machine Perfusion (HOPE) Reduces Early Allograft Injury and Improves Post-Transplant Outcomes in Extended Criteria Donation (ECD) Liver Transplantation From Donation After Brain Death (DBD): Results From a Multicenter Randomized Con. Ann Surg (2021) 274(5):705–12. doi: 10.1097/SLA.0000000000005110
53. Ravaioli M, de Pace V, Angeletti A, Comai G, Vasuri F, Baldassarre M, et al. Hypothermic Oxygenated New Machine Perfusion System in Liver and Kidney Transplantation of Extended Criteria Donors:First Italian Clinical Trial. Sci Rep (2020) 10:6063. doi: 10.1038/s41598-020-62979-9
54. Schlegel A, Muller X, Kalisvaart M, Muellhaupt B, Perera MTPR, Isaac JR, et al. Outcomes of DCD Liver Transplantation Using Organs Treated by Hypothermic Oxygenated Perfusion Before Implantation. J Hepatol (2019) 70(1):50–7. doi: 10.1016/j.jhep.2018.10.005
55. Patrono D, Surra A, Catalano G, Rizza G, Berchialla P, Martini S, et al. Hypothermic Oxygenated Machine Perfusion of Liver Grafts From Brain-Dead Donors. Sci Rep (2019) 9:9337. doi: 10.1038/s41598-019-45843-3
56. Zhou J, Chen J, Wei Q, Saeb-Parsy K, Xu X. The Role of Ischemia/Reperfusion Injury in Early Hepatic Allograft Dysfunction. Liver Transpl (2020) 26:1034–48. doi: 10.1002/LT.25779
57. Zulpaite R, Miknevicius P, Leber B, Strupas K, Stiegler P, Schemmer P. Ex-Vivo Kidney Machine Perfusion: Therapeutic Potential. Front Med (Lausanne) (2021) 8:808719. doi: 10.3389/FMED.2021.808719
58. de Rougemont O, Dutkowski P, Clavien P-A. Biological Modulation of Liver Ischemia-Reperfusion Injury. Curr Opin Organ Transplant (2010) 15:183–9. doi: 10.1097/MOT.0b013e3283373ced
59. Cung T-T, Morel O, Cayla G, Rioufol G, Garcia-Dorado D, Angoulvant D, et al. Cyclosporine Before PCI in Patients With Acute Myocardial Infarction. New Engl J Med (2015) 373:1021–31. doi: 10.1056/nejmoa1505489
60. Ottani F, Latini R, Staszewsky L, la Vecchia L, Locuratolo N, Sicuro M, et al. Cyclosporine A in Reperfused Myocardial Infarction: The Multicenter, Controlled, Open-Label CYCLE Trial. J Am Coll Cardiol (2016) 67:365–74. doi: 10.1016/J.JACC.2015.10.081
61. Brüggenwirth IMA, Martins PN. RNA Interference Therapeutics in Organ Transplantation: The Dawn of a New Era. Am J Transplant (2020) 20:931–41. doi: 10.1111/AJT.15689
62. Schlegel A, Foley D, Savier E, Flores Carvalho M, de Carlis M, Heaton N, et al. Recommendations for Donor and Recipient Selection and Risk Prediction: Working Group Report From the ILTS Consensus Conference in DCD Liver Transplantation. Transplantation (2021) 105:1892–903. doi: 10.1097/TP.0000000000003825
63. Fire A, Xu S, Montgomery MK, Kostas SA, Driver SE, Mello CC. Potent and Specific Genetic Interference by Double-Stranded RNA in Caenorhabditis Elegans. Nature (1998) 391:806–11. doi: 10.1038/35888
64. Li DY, Xie SL, Wang GY, Dang XW. CD47 Blockade Alleviates Acute Rejection of Allogeneic Mouse Liver Transplantation by Reducing Ischemia/Reperfusion Injury. Biomedicine Pharmacother = Biomedecine Pharmacotherapie (2020) 123:109793. doi: 10.1016/J.BIOPHA.2019.109793
65. Guarrera JV, Henry SD, Samstein B, Odeh-Ramadan R, Kinkhabwala M, Goldstein MJ, et al. Hypothermic Machine Preservation in Human Liver Transplantation: The First Clinical Series. Am J Transplant (2010) 10:372–81. doi: 10.1111/j.1600-6143.2009.02932.x
66. Watson CJE, Jochmans I. From “Gut Feeling” to Objectivity: Machine Preservation of the Liver as a Tool to Assess Organ Viability. Curr Transplant Rep (2018) 5:72–81. doi: 10.1007/s40472-018-0178-9
67. Nasralla D, Coussios CC, Mergental H, Akhtar MZ, Butler AJ, Ceresa CDL, et al. A Randomized Trial of Normothermic Preservation in Liver Transplantation. Nature (2018) 557:50–6 . doi: 10.1038/s41586-018-0047-9
68. Hessheimer AJ, Coll E, Torres F, Ruíz P, Gastaca M, Rivas JI, et al. Normothermic Regional Perfusion vs. Super-Rapid Recovery in Controlled Donation After Circulatory Death Liver Transplantation. J Hepatol (2019) 70:658–65. doi: 10.1016/j.jhep.2018.12.013
69. Schlegel AA, Kalisvaart M, Muiesan P. Machine Perfusion in Liver Transplantation: An Essential Treatment or Just an Expensive Toy? Minerva Anestesiologica (2018) 84:236–45. doi: 10.23736/S0375-9393.17.12016-X
70. de Beule J, Vandendriessche K, Pengel L, Bellini M, Dark J, Hessheimer A, et al. A Systematic Review and Meta-Analyses of Regional Perfusion in Donation After Circulatory Death Solid Organ Transplantation. Transpl Int (2021) 34(11):2046–60. doi: 10.1111/TRI.14121
71. de Carlis R, Schlegel A, Frassoni S, Olivieri T, Ravaioli M, Camagni S, et al. How to Preserve Liver Grafts From Circulatory Death With Long Warm Ischemia? A Retrospective Italian Cohort Study With Normothermic Regional Perfusion and Hypothermic Oxygenated Perfusion. Transplantation (2021) 105:2385–96. doi: 10.1097/TP.0000000000003595
72. Antoine C, Jasseron C, Dondero F, Savier E. Liver Transplantation From Controlled Donors After Circulatory Death Using Normothermic Regional Perfusion: An Initial French Experience. Liver Transpl (2020) 26:1516–21. doi: 10.1002/LT.25818
73. Schlegel A, van Reeven M, Croome K, Parente A, Dolcet A, Widmer J, et al. A Multicentre Outcome Analysis to Define Global Benchmarks for Donation After Circulatory Death Liver Transplantation. J Hepatol (2021) 76(2):371–82. doi: 10.1016/J.JHEP.2021.10.004
74. Watson C, Hunt F, Butler A, Sutherland A, Upponi S, Currie I, et al. Normothermic Regional Perfusion (NRP) for DCD Liver Transplantation in the UK: Better Graft Survival With No Cholangiopathy. (ILTS Conference:Lisbon Portugal) (2018).
75. Hessheimer AJ, de la Rosa G, Gastaca M, Ruíz P, Otero A, Gómez M, et al. Abdominal Normothermic Regional Perfusion in Controlled Donation After Circulatory Determination of Death Liver Transplantation: Outcomes and Risk Factors for Graft Loss. Am J Transplant (2022) 22:1169–81. doi: 10.1111/AJT.16899
76. Baroni S, Marudi A, Rinaldi S, Ghedini S, Magistri P, Piero Guerrini G, et al. Cytokine Mass Balance Levels in Donation After Circulatory Death Donors Using Hemoadsorption: Case Series Report. Int J Artif Organs (2022) 45(7):642–46. doi: 10.1177/03913988221091288
77. Dutkowski P, Schlegel A, de Oliveira M, Müllhaupt B, Neff F, Clavien PA. HOPE for Human Liver Grafts Obtained From Donors After Cardiac Death. J Hepatol (2014) 60:765–72. doi: 10.1016/j.jhep.2013.11.023
78. Laing RW, Stubblefield S, Wallace L, Roobrouck VD, Bhogal RH, Schlegel A, et al. The Delivery of Multipotent Adult Progenitor Cells to Extended Criteria Human Donor Livers Using Normothermic Machine Perfusion. Front Immunol (2020) 11:1226. doi: 10.3389/FIMMU.2020.01226
79. Boteon YL, Laing RW, Schlegel A, Wallace L, Smith A, Attard J, et al. Combined Hypothermic and Normothermic Machine Perfusion Improves Functional Recovery of Extended Criteria Donor Livers. Liver Transplant (2018) 24(12):1699–715. doi: 10.1002/lt.25315
80. Linares-Cervantes I, Echeverri J, Cleland S, Kaths JM, Rosales R, Goto T, et al. Predictor Parameters of Liver Viability During Porcine Normothermic Ex Situ Liver Perfusion in a Model of Liver Transplantation With Marginal Grafts. Am J Transplant (2019) 19:2991–3005. doi: 10.1111/ajt.15395
81. Kron P, Schlegel A, Mancina L, Clavien PA, Dutkowski P. Hypothermic Oxygenated Perfusion (HOPE) for Fatty Liver Grafts in Rats and Humans. J Hepatol (2018) 68:82–91. doi: 10.1016/j.jhep.2017.08.028
82. Watson C, Kosmoliaptsis V, Pley C, Randle L, Fear C, Crick K, et al. Observations on the Ex Situ Perfusion of Livers for Transplantation. Am J Transplant (2018) 18:2005–20. doi: 10.1111/ajt.14687
83. Mergental H, Laing RW, Kirkham AJ, Perera MTPR, Boteon YL, Attard J, et al. Transplantation of Discarded Livers Following Viability Testing With Normothermic Machine Perfusion. Nat Commun (2020) 11:2. doi: 10.1038/s41467-020-16251-3
84. Hosgood SA, Brown RJ, Nicholson ML. Advances in Kidney Preservation Techniques and Their Application in Clinical Practice. Transplantation (2021) 105:E202–14. doi: 10.1097/TP.0000000000003679
85. Jassem W, Xystrakis E, Ghnewa Y, Yuksel M, Pop O, Martinez-Llordella M, et al. Normothermic Machine Perfusion (NMP) Inhibits Proinflammatory Responses in the Liver and Promotes Regeneration. Hepatology (2018) 70(2):682–95. doi: 10.1002/hep.30475
86. Parente A, Osei-Bordom DC, Ronca V, Perera MTPR, Mirza D. Organ Restoration With Normothermic Machine Perfusion and Immune Reaction. Front Immunol (2020) 11:565616. doi: 10.3389/FIMMU.2020.565616
87. Schlegel A, Dutkowski P. Letter to Editor: Repair or Prevent: What Is the Real Impact of Normothermic Machine Perfusion in Liver Transplantation? Hepatology (2019) 70:2231–2. doi: 10.1002/HEP.30567
88. Scheuermann U, Zhu M, Song M, Yerxa J, Gao Q, Davis RP, et al. Damage-Associated Molecular Patterns Induce Inflammatory Injury During Machine Preservation of the Liver: Potential Targets to Enhance a Promising Technology. Liver Transpl (2019) 25:610–26. doi: 10.1002/LT.25429
89. Schlegel A, Kron P, Dutkowski P. Hypothermic Oxygenated Liver Perfusion: Basic Mechanisms and Clinical Application. Curr Transplant Rep (2015) 2:52–62. doi: 10.1007/s40472-014-0046-1
90. Schlegel AA, Muller X, Kalisvaart M, Muellhaupt B, Perera M, Isaac J, et al. Outcomes of Liver Transplantations From Donation After Circulatory Death (DCD) Treated by Hypothermic Oxygenated Perfusion (HOPE) Before Implantation. J Hepatol (2019), 50–7. doi: 10.1016/j.jhep.2018.10.005
91. Schlegel A, de Rougemont O, Graf R, Clavien PA, Dutkowski P. Protective Mechanisms of End-Ischemic Cold Machine Perfusion in DCD Liver Grafts. J Hepatol (2013) 58:278–86. doi: 10.1016/j.jhep.2012.10.004
92. van Rijn R, Karimian N, Matton A, Burlage L, Wetserkamp A, van den Berg A, et al. Dual Hypothermic Oxygenated Machine Perfusion in Liver Transplants Donated After Circulatory Death. Br J Surg (2017) 104(7):907–17. doi: 10.1002/bjs.10515
93. Lee CY, Jain S, Duncan HM, Zhang JX, Jones JW, Southard JH, et al. Survival Transplantation of Preserved non-Heart-Beating Donor Rat Livers: Preservation by Hypothermic Machine Perfusion. Transplantation (2003) 76:1432–6. doi: 10.1097/01.TP.0000088674.23805.0F
94. Brüggenwirth IMA, Mueller M, Lantinga VA, Camagni S, de Carlis R, de Carlis L, et al. Prolonged Preservation by Hypothermic Machine Perfusion Facilitates Logistics in Liver Transplantation: A European Observational Cohort Study. Am J Transplant (2022) 00:1–10. doi: 10.1111/AJT.17037
95. Pavicevic S, Uluk D, Reichelt S, Fikatas P, Globke B, Raschzok N, et al. Hypothermic Oxygenated Machine Perfusion for Extended Criteria Donor Allografts: Preliminary Experience With Extended Organ Preservation Times in the Setting of Organ Reallocation. Artif Organs (2022) 46:306–11. doi: 10.1111/AOR.14103
96. Brüggenwirth IMA, Lantinga VA, Rayar M, van den Berg AP, Blokzijl H, Reyntjens KMEM, et al. Prolonged Dual Hypothermic Oxygenated Machine Preservation (DHOPE-PRO) in Liver Transplantation: Study Protocol for a Stage 2, Prospective, Dual-Arm, Safety and Feasibility Clinical Trial. BMJ Open Gastroenterol (2022) 9:e000842. doi: 10.1136/BMJGAST-2021-000842
97. Panconesi R, Flores Carvalho M, Mueller M, Meierhofer D, Dutkowski P, Muiesan P, et al. Viability Assessment in Liver Transplantation—What Is the Impact of Dynamic Organ Preservation? Biomedicines (2021) 9(2):161. doi: 10.3390/biomedicines9020161
98. Cao H, Yang L, Yang L, Hou B, Hou B, Sun D, et al. Heme Oxygenase-1-Modified Bone Marrow Mesenchymal Stem Cells Combined With Normothermic Machine Perfusion to Protect Donation After Circulatory Death Liver Grafts. Stem Cell Res Ther (2020) 11:218. doi: 10.1186/S13287-020-01736-1
99. Yu Y, Cheng Y, Pan Q, Zhang YJ, Jia DG, Liu YF. Effect of the Selective NLRP3 Inflammasome Inhibitor Mcc950 on Transplantation Outcome in a Pig Liver Transplantation Model With Organs From Donors After Circulatory Death Preserved by Hypothermic Machine Perfusion. Transplantation (2019) 103:353–62. doi: 10.1097/TP.0000000000002461
100. Carlson KN, Pavan-Guimaraes J, Verhagen JC, Chlebeck P, Verhoven B, Jennings H, et al. Interleukin-10 and Transforming Growth Factor-β Cytokines Decrease Immune Activation During Normothermic Ex Vivo Machine Perfusion of the Rat Liver. Liver Transpl (2021) 27:1577–91. doi: 10.1002/LT.26206
101. Boteon YL, Attard J, Boteon APCS, Wallace L, Reynolds G, Hubscher S, et al. Manipulation of Lipid Metabolism During Normothermic Machine Perfusion: Effect of Defatting Therapies on Donor Liver Functional Recovery. Liver Transpl (2019) 5:1007–22. doi: 10.1002/lt.25439
102. Thijssen MF, Brüggenwirth IMA, Gillooly A, Khvorova A, Kowalik TF, Martins PN. Gene Silencing With Small Interfering RNA: A New Therapeutic Option During Ex Vivo Machine Liver Perfusion Preservation. Liver Transpl (2019) 25:140–51. doi: 10.1002/lt.25383
Keywords: ischemia reperfusion injury, innate immune activation, machine perfusion, mitochondrial injury, hypothermic oxygenated perfusion, marginal livers
Citation: Panconesi R, Flores Carvalho M, Dondossola D, Muiesan P, Dutkowski P and Schlegel A (2022) Impact of Machine Perfusion on the Immune Response After Liver Transplantation – A Primary Treatment or Just a Delivery Tool. Front. Immunol. 13:855263. doi: 10.3389/fimmu.2022.855263
Received: 15 January 2022; Accepted: 31 May 2022;
Published: 08 July 2022.
Edited by:
Stefan Schneeberger, Innsbruck Medical University, AustriaReviewed by:
Jeanette Villanueva, Victor Chang Cardiac Research Institute, AustraliaCopyright © 2022 Panconesi, Flores Carvalho, Dondossola, Muiesan, Dutkowski and Schlegel. This is an open-access article distributed under the terms of the Creative Commons Attribution License (CC BY). The use, distribution or reproduction in other forums is permitted, provided the original author(s) and the copyright owner(s) are credited and that the original publication in this journal is cited, in accordance with accepted academic practice. No use, distribution or reproduction is permitted which does not comply with these terms.
*Correspondence: Andrea Schlegel, U2NobGVnZWwuYW5kcmVhQG91dGxvb2suZGU=
Disclaimer: All claims expressed in this article are solely those of the authors and do not necessarily represent those of their affiliated organizations, or those of the publisher, the editors and the reviewers. Any product that may be evaluated in this article or claim that may be made by its manufacturer is not guaranteed or endorsed by the publisher.
Research integrity at Frontiers
Learn more about the work of our research integrity team to safeguard the quality of each article we publish.