- 1Laboratory of Cellular and Molecular Immunology, Gavin Herbert Eye Institute, School of Medicine, University of California Irvine, Irvine, CA, United States
- 2Department of Vaccines and Immunotherapies, TechImmune, Limited Liability Company (LLC), University Lab Partners, Irvine, CA, United States
- 3Biomedical Sciences, University of Ottawa, Ottawa, ON, Canada
- 4Department of Molecular Biology & Biochemistry, Institute for Immunology, School of Medicine, University of California Irvine, Irvine, CA, United States
The development of vaccines against herpes simplex virus type 1 and type 2 (HSV1 and HSV-2) is an important goal for global health. In this review we reexamined (i) the status of ocular herpes vaccines in clinical trials; and (ii) discusses the recent scientific advances in the understanding of differential immune response between HSV infected asymptomatic and symptomatic individuals that form the basis for the new combinatorial vaccine strategies targeting HSV; and (iii) shed light on our novel “asymptomatic” herpes approach based on protective immune mechanisms in seropositive asymptomatic individuals who are “naturally” protected from recurrent herpetic diseases. We previously reported that phenotypically and functionally distinct HSV-specific memory CD8+ T cell subsets in asymptomatic and symptomatic HSV-infected individuals. Moreover, a better protection induced following a prime/pull vaccine approach that consists of first priming anti-viral effector memory T cells systemically and then pulling them to the sites of virus reactivation (e.g., sensory ganglia) and replication (e.g., eyes and vaginal mucosa), following mucosal administration of vectors expressing T cell-attracting chemokines. In addition, we reported that a combination of prime/pull vaccine approach with approaches to reverse T cell exhaustion led to even better protection against herpes infection and disease. Blocking PD-1, LAG-3, TIGIT and/or TIM-3 immune checkpoint pathways helped in restoring the function of antiviral HSV-specific CD8+ T cells in latently infected ganglia and increased efficacy and longevity of the prime/pull herpes vaccine. We discussed that a prime/pull vaccine strategy that use of asymptomatic epitopes, combined with immune checkpoint blockade would prove to be a successful herpes vaccine approach.
Introduction
According to the World Health Organization (WHO), over two-thirds of the worldwide population in infected with HSV-1 (commonly known to cause oral herpes or cold sores) and HSV-2 (commonly known to cause genital herpes) (1, 2). The prevalence of HSV-1 and HSV-2 is 47.8% and 11.9%, respectively, for individuals aged 14 to 49 years according to a 2018 February data brief published by the US Centers for Disease Control and Prevention’s National Center for Health Statistics (1, 2). In the United States alone, every year, there are 500,000 HSV-1 oral herpes cases; 300,000 HSV-1 and HSV-2 genital herpes cases; 20,000 HSV-1 ocular herpes cases and 1,500 cases of herpes encephalitis (3, 4). Apart from being the most prevalent sexually transmitted disease, HSV-1 is the leading cause of infectious blindness in Western countries (5). HSV-1 and HSV-2 are neurotropic viruses that infect the anogenital, oral mucosal lining and the skin and the eyes (6) The immune response to HSV typically controls the acute mucosal infection; however, the virus remains latent in the ganglia, and there is a life-long sporadic low-grade shedding of virus from sensory neurons into the mucosa (6). Thus, while HSV hides for a lifetime in the trigeminal, autonomic, or dorsal root ganglia, it reactivates and sheds asymptomatically making the transmission high. In addition to causing painful blisters, HSV-2 can cause encephalitis and death in newborns from vertical transmission and increases the risk for HIV infection two-three-fold times (7). Antiviral drugs are the only current treatment approved by the Food and Drug Administration (FDA) for treatment of herpetic diseases. Due to the cost, virus resistances and limited effectiveness of antiviral drugs, preventive or therapeutic vaccines are highly desirable to control herpes infection and/or diseases (8). The development of a vaccine that proves effective against one type of the HSV would be helpful for the other type due to the genetic similarity between HSV-1 and HSV-2. However, due to virus latency and HSV immune evasion, immunotherapy and vaccine development against the virus have become a real challenge. As of 2018, a number of different HSV vaccine candidates were at different stages of clinical trials (9, 10) (Table 1).
One common denominator in these vaccines is the use of the whole virus or whole virus proteins, which contain both protective “asymptomatic” epitopes and pathogenic “symptomatic” epitopes. Our developed “asymptomatic” herpes vaccine approach which is based on understanding the immune mechanisms by which seropositive asymptomatic individuals are “naturally” protected from recurrent herpes disease throughout their lives. Clinical and pre-clinical studies have proved that the T cell-based immune system in the mucosa lining of the genital tract plays a crucial role in the prevention of HSV acquisition. A better mucosal vaccine approach to boost effector memory T cell responses will serve instrumental in developing an effective HSV vaccine (45). Our latest approach of using adenoviral vectors delivering chemokines and asymptomatic dominant epitopes to induce and pull antiviral CD4+ and CD8+ T cells to the site of reactivation (i.e., ganglia) and replication (i.e., epithelia) would be an effective combinatorial herpes simplex vaccine strategy. Moreover, another combinatorial herpes simplex vaccine strategy that consists of reversing T cell exhaustion by immune checkpoint blockade would be a successful strategy to clear herpes infection (46). In this review, we highlight the current clinical trials in herpes vaccine development and emphasize the significance of using the asymptomatic epitope approach in a combinatorial vaccine strategy.
HSV Vaccines: From Past to Present
The success of vaccines against other alpha herpes, like the chicken-pox and shingles vaccine, has given hope for the development of a vaccine against HSV (47) (Table 1). Four main vaccine approaches have been designed and tested in the past four decades to fight off herpes simplex virus type 1 (HSV-1) and type 2 (HSV-2) infections and diseases (48): (1) Inactivated “killed” HSV vaccines; (2) Live-attenuated HSV vaccines; (3) Replication-defective HSV vaccines; and (4) Subunit HSV vaccines (9, 49–54). Each of these types of vaccine approaches has its pros and cons when it comes to safety, immunogenicity, and protective efficacy.
Inactivated “Killed” HSV Vaccines
HSV is a highly successful neurotropic virus that resides in the nervous system and therefore presents the risk of developing neuro-pathogenesis and life-threatening Herpes Simplex Encephalitis (HSE). Thus, back in the 70s and 80s, the first whole inactivated HSV vaccine approach used “kill” the whole virus after exposure to heat, UV-light (55) or chemicals (56, 57). These whole inactivated HSV vaccines induced antibodies, but not T cells, and as such have not been successful in the protection against recurrent HSV-1 or HSV-2 infections and diseases (58–60). Therefore, the live-attenuated HSV vaccines (61–66) and replication-defective HSV vaccines were introduced (51, 58–60, 67–71).
Live-Attenuated HSV Vaccines
Live-attenuated HSV vaccines contrast inactivated HSV vaccines produced by “killing” the virus and reducing the neurovirulence of HSV-1 or HSV-2, while keeping them viable. In the past 24 years, many live-attenuated HSV vaccines have been introduced and tested in both the mouse and guinea pig models mainly in a prophylactic setting (instead of a therapeutic setting). However, due mostly to safety concerns, only a few of these live vaccines have progressed into clinical trials (63). Live-attenuated HSV vaccines include: (1) The HSV-2 TK(-) mutant reported back in 1995 by Milligan and Bernstein and then by Kiyono in 2014 (72); (2) the RAV 9395 live attenuated recombinant virus; evaluated in guinea pigs and reported by Spaete back in 1998 (70); (3) AD472, a live attenuated recombinant HSV-2 vaccine evaluated in guinea pigs was reported back in 2005 (51); (4) The most studied HSV-1 and HSV-2 ICP0 (-) live-attenuated mutant vaccines, lacking the nuclear localization signal (NLS) on the ICP0 gene (0DeltaNLS), developed in 2010 by Halford and tested in mice and guinea pigs (69, 73–76); (5) The HSV2-gD27 mutant vaccine reported by Cohen in 2012 (77); (6) The HSV-2 gE2-del mutant vaccine reported by Friedman in 2012 (78); (7) The HSV-2 UL24 mutant tested in mice and guinea pigs reported by Visalli in 2014 (67); and (8) The HSV-1 VC2 mutant reported by Kousoulas in 2014 (79).
Replication-Defective HSV Vaccines
Replication-defective virus vaccines, also called DISC (Disabled Infectious Single Cycle) virus vaccines, are defective for one or more genes that are essential for viral genome replication or synthesis and assembly of viral particles. In normal cells, they express viral gene products but do not replicate to form progeny virions. Replication-defective HSV vaccines can stimulate immune responses but produce no progeny viral particles. However, because they do not replicate and spread in the host, replication-defective virus vaccines may be less immunogenic, specifically less T cell stimulators because they have a relatively limited capacity to solicit professional antigen presenting cells (i.e., B, macrophage, and dendritic cells), a prerequisite for the induction of CD4+ and CD8+ T cell responses.
The replication-defective HSV vaccines developed during the last 24 years include: (1) DISC HSV-1 vaccine tested in guinea pigs by McLean, back in 1996 (80); (2) This was followed by another DISC HSV-2 vaccines which consisted of gH-deleted HSV-2 mutant tested in guinea pigs for recurrent genital herpes and reported by McLean in 1997 (81); (3) The HSV-2 mutant engineered by Dr. Knipe back in 1997, by replacing the ICP8 gene of HSV-2 strain 186 with an ICP8-lacZ fusion gene from the HSV-1 HD-2 mutant strain. The resulting HSV-2 5BlacZ mutant was later tested in guinea pigs by the same group as reported in 2001 (61, 62), (4) The most studied replication-defective virus HSV-2 dl5-29 vaccine, was developed by Knipe in 2008 and tested in mice and guinea pigs by Cohen in 2010 (12, 59, 63, 82) and by Londono-Hayes in 2015 (14) and shown to be have a protective effect. Eventually, this vaccine progressed to human trials only to show unsuccessful results in a Phase 1 clinical trial conducted recently by Sanofi Pasteur; (5) The HSV-2 ACAM529 mutant tested in a mouse model of genital herpes challenge and reported by Knipe and others in 2010 and 2012 (12, 83, 84); (6) The HSV-1 Δ gK mutant tested in mouse model of herpes challenge and reported in 2013 by Kousoulas (85); (7) The HSV-1 CJ2-gD2 vaccine, a glycoprotein D-expressing replication-defective and dominant-negative HSV-1 recombinant viral vaccine, tested in mice guinea pigs and reported in 2011 (11) and 2014 by Yao (86); (8) The latest replication defective HSV vaccine is the HSV-2 ΔgD (gD1-/+) reported in 2015 by Herold and Jacobs group as being protective in a mouse model of genital herpes challenge (87). The efficacy of the HSV-2 ΔgD vaccine in prophylactic and therapeutic settings has yet to be evaluated in the guinea pig model of primary and recurrent genital herpes. Compared to clinical trials using adjuvanted subunit vaccines (e.g., the adjuvanted gD/gB vaccine trials), many live attenuated/replication defective vaccines-based Phase 1 trial trials, were either terminated or did not progress to Phase II, because of: (i) A lack of immunogenicity; and/or (ii) Concerns related to safety of using a live virus as vaccine, as detailed above.
Subunit HSV Vaccines
A variety of subunit HSV vaccine approaches have been developed including proteins, DNA and peptide epitope-based vaccines (88, 89). Traditional protein-based vaccines are safe compared to live-attenuated and replication-defective HSV vaccines. Recombinant soluble HSV-2 glycoprotein D (gD) has been the most promising subunit vaccine that went into extensive clinical evaluation. Over the past 25 years, there has been one Phase II therapeutic genital herpes vaccine and three Phase III clinical trials of prophylactic subunit vaccines, all using the HSV-2 gD (or mixed with gB in one trial) (90–95). Back in 1994, the first therapeutic vaccine trial delivered the gD with aluminum salt (i.e. Alum) adjuvant in 98 symptomatic genital herpes patients who reported 4 to 14 recurrences per year (96). Unfortunately, this vaccine reduced the frequency of recurrences by only 24% despite that the vaccine boosted neutralizing antibodies to HSV-2 four-fold over baseline levels (96). These disappointing results from the first therapeutic gD/Alum vaccine trial suggested that for therapeutic protection; a vaccine must: (1) Induce CD4+ and CD8+ T cell responses, in addition to neutralizing antibodies, (2) Incorporate HSV-2 antigens other than gD; and (3) Must test different adjuvants, other than Alum. Three years later in 1997, the Chiron vaccine trial used a combination of gD and gB delivered together with the MF59 Novartis’ adjuvant, an oil-in-water emulsion of squalene oil, using the same target population of genital herpes patients as in the 1994 trial. This gB/gD/MF59 vaccine did not elicit T cell responses, produced high levels of neutralizing antibody to HSV-2, yet had only a 9% efficacy (94). This trial suggested that: (1) besides neutralizing antibodies, a protective vaccine must induce antiviral CD4+ and CD8+ T cell responses; (2) a therapeutic vaccine must incorporate HSV-2 antigens other than gB and gD; and (3) must test different adjuvants, other than Alum and MF59. Later, two GlaxoSmithKline (GSK) vaccine trials (one reported in 2004 and the other in 2012), used the gD protein delivered together with a different adjuvant, the 3-0-deacylated monophosphoryl lipid A (MPL), a TLR4 agonist (93) together with Alum (gD/MPL/Alum vaccine). The first trial enrolled discordant couples, who have regular partners with genital herpes, while the second trial enrolled HSV seronegative women who have multiple and random partners (93). The first trial, reported in 2004, showed a 74% efficacy against genital herpes disease caused by HSV-2 (93). Unfortunately, later, results using the same gD/MPL/Alum vaccine reported in 2012, showed only 58% efficacy against genital HSV-2 disease (13). The apparent contradictions in efficacy against genital HSV-2 disease, of the two GSK trials that used the same gD/MPL/Alum vaccine, is puzzling. The difference in efficacy in the two clinical trials attributed to different populations enrolled in each trial (i.e. discordant couples vs. random seropositive women with multiple partners) (13). In the first clinical trial, the distinguishing feature of discordant couples was that they were a highly selected group in which the uninfected partner is potentially repeatedly exposed to HSV by the infected partner. This likely increased risk of infection and disease, hence lowering the threshold of seeing a significant effect of the therapeutic vaccine. In other words, the attack rates of HSV-2 genital disease were high among discordant couples making easy to see a significant reduction following therapeutic vaccination. In contrast, the second clinical trial that enrolled random seropositive women, with multiple lifetime sexual partners, in which the attack rate and the risk of infection and disease was much lower and hence likely raised the threshold of seeing a significant effect of the therapeutic vaccine. Regardless of the targeted population, the first GSK vaccine trial that produced 74% protective efficacy also stimulated both T cells and neutralizing antibodies (13). In 2016-2018, a Genocea vaccine trial (designated as Gen-003) used a combination of ICP4 and gD2 truncated proteins with a novel adjuvant, named Matrix M-2 (MM-2) (89). Matrix M is a saponin-based adjuvant that has a balanced B and T cell immuno-stimulatory profile. This trial reported a significant reduction of recurrent herpes lesions and genital viral shedding (90–92). This protection appeared to correlate with blood-derived antiviral CD4+ and CD8+ T cell responses (90–92). Due to ethical and practical limitations, none of the vaccine clinical trials have investigated the local tissue resident CD4+ and CD8+ T cells in dorsal root ganglia (DRG) and vaginal mucosal tissues.
Modified RNA (mRNA) Vaccine Platforms Against HSV-1 and HSV-2
RNA vaccines, during the current pandemic, have emerged as a versatile approach against emerging viral infections to overcome the challenges confronted with the conventional vaccine strategies 1–7. mRNA is the carrier of the genetic information necessary for the endogenous proteins synthesis, it does not integrate into the genome and safely metabolized and eliminated by the cells 8–10. RNA-based vaccines have been shown safe in animal models and in human clinical trials and trigger a strong innate immune response. Many strategies have been used to increase the delivery and immunogenicity of mRNA while diminishing innate immune sensing11. Free and protamine-complexed mRNA were among the first approaches to provide robust antigen expression and immune-stimulation 12–14. This vaccine set-up showed the ability to induce strong immunity and protective efficacy against lethal influenza or rabies viral infections in many animal models 4,15. The first ever prophylactic mRNA-based vaccine (CV7201) in healthy human volunteers was made against rabies. This vaccine was generally safe and led to the induction of neutralizing antibody that waned one year after the first vaccination 8. The success of mRNA vaccines has greatly benefited from the development of lipid- and polymer-based nanoparticles that protect RNA from degradation, enhanced cell uptake and improve delivery to the translational machinery. Currently, lipid nanoparticles (LNPs) are the most frequently used and effective agents for in vivo delivery of mRNA vaccines 9,16,17. Recently, the Food and Drug Administration (FDA) issued an Emergency Use Authorization (EUA) for the Pfizer-BioNTech COVID-19 (BNT162b2) vaccine (Pfizer, Inc; Philadelphia, Pennsylvania), nucleoside-modified mRNA vaccine formulated lipid nanoparticle- encoding the spike glycoprotein of SARS-CoV-2, the virus that causes coronavirus disease 2019 (COVID-19)7. This technology has encouraged other groups working on vaccines against cancer and viral pathogens to use the NLP-formulated mRNA platform. Recently, the Friedman group18 showed that nucleoside-modified mRNA in lipid nanoparticle vaccine encoding for glycoproteins gC, gD, and gE induced strong and protective immunity against acute and latent herpes simplex virus type 2 infection in mice. Indeed, and in a side-by-side experiment they compared two vaccine platforms: (1) Trivalent gC2/gD2/gE purified glycoproteins were given with adjuvants (CpG and Alum) 19and (2) modified mRNA encoding the 3 glycoproteins formulated in lipid nanoparticles (LNP) 20. The RNA was modified to increase the cellular uptake and prevent the innate immunity sensors from inhibiting the translation machinery 21. The mRNA-LPN vaccine demonstrated to induce effective T-follicular helper and germinal center B cell responses translated into high titers and durable antibodies responses 22 that outperform the glycoproteins-based vaccine in preventing HSV-1 and HSV-2 genital infection and in protecting mice and guinea pigs against intravaginal HSV-2 infection 20.
Lessons Learned from Past HSV Vaccine Clinical Trials
The vaccine clinical trials produced valuable lessons that should help improve future herpes subunit vaccines. Specifically, these trials emphasize four major gaps in our current knowledge: (1) The need to incorporate protective herpes protein Ags, other than gB and gD, in the development of a future herpes therapeutic vaccine (3); (2) The need to design a vaccine strategy that induces anti-viral CD4+ and CD8+ T cell-mediated immunity (in addition to HSV-specific neutralizing antibodies) for a better protection against recurrent herpes (3). This includes exploring new adjuvants and antigen delivery systems, and (3) The need to develop a mucosal vaccine strategy that would induce strong tissue resident CD4+ and CD8+ TRM cells (beside mucosal antibodies such as IgA) that would reduce virus reactivation from latently infected dorsal root ganglia (DRG) and subsequent virus shedding in the genital tract and recurrent herpetic disease. This is because of the failure of past parenteral subunit vaccines that elicit systemic immune responses against HSV-2. Although most of these vaccine research trials have not been promising, we have gained a better understanding of the correlates of protective immunity for a therapeutic HSV vaccine, forming the platform for novel combinatorial vaccine strategies against HSV.
Phenotypic and Functionally Differential HSV-Specific Memory CD8+ T Cell Subsets in Asymptomatic and Symptomatic HSV Infected Individuals
Understanding the immune mechanisms by which seropositive asymptomatic individuals are protected from recurrent herpes disease is significantly important as exploiting it can elicit a T cell-based immune response in the mucosa lining the genital tract to prevent HSV acquisition. Recurrent genital herpes disease occurs following periodic reactivation of the virus that travels the axons of DRG neurons to re-infect the genital tract (GT), where lytic replication leads to herpetic lesions and transmission (15). In asymptomatic individuals (ASYMP) HSV reactivation never causes recurrent disease (16–18, 20). In symptomatic individuals (SYMP), HSV reactivation often causes painful recurrent genital disease (17, 19, 21, 22). Reports on HSV therapeutic vaccine trials have shown that both innate and adaptive immunity play an equal role in directing the right immune response to prevent disease by causing a low to no-shedding of the virus. Our research group has explored the differential immune scenarios present in asymptomatic protected individuals that gives them the natural immunity to contain recurrence of herpes. The asymptomatic and symptomatic individuals are strikingly different in their HSV-specific CD8 T memory cell immune-profile. After resolution of primary genital herpes infection, a heterogeneous pool (in terms of anatomic distribution, phenotype and fu) of HSV-specific memory CD8+ T cells develops (23) and can be divided into three major subsets: (1) effector memory CD8+ T cells (TEM) (2) central memory CD8+ T cells (TCM) (24) and (3) tissue-resident memory CD8+ T (TRM) cells. The different CD8 memory T cell subsets in HSV infection is illustrated in Figure 1. Regarding anatomic distribution, effector memory CD8+ TEM cells and central memory CD8+ TCM cells circulate between lymphoid and non-lymphoid tissues, such as the DRG and GT (24). The third subset does not enter circulation, but is instead selectively retained in infected tissues, such as DRG (25–27) and GT (25, 28), as a tissue-resident memory CD8+ TRM cells. These CD8+ TRM cells are poised for immediate response to reactivation from DRG (25, 29) and inhibit virus replication at GT (25). TRM cells have altered T cell trafficking patterns due to the down-regulation of T cell homing molecules CD62L and CCR7 (30–34). The phenotypic profile of TCM cells is CD8CD103lowCD62Lhigh CCR7high. TEM cells are CD8+CD103lowCD62LlowCCR7low. TRM cells are CD8+CD103highCD62LlowCCR7lowCD11ahighCD69high (24, 35, 36). TCM and TEM cells, but not TRM cells, express CD103. TCM cells must proliferate and undergo differentiation for effector function (37–40). In contrast, TEM and TRM cells are already differentiated and poised for immediate effector function (41). We recently discovered that most HSV-specific CD8 T cells from ASYMP individuals expressed low levels of lymphoid homing markers (CD62LlowCCR7low), suggesting that these T cells are predominantly of a CD8+ TEM cell subset. In contrast, most HSV-specific CD8+ T cells from SYMP individuals are predominantly of TCM cell subset (42). Moreover, a decline in the number and function of memory CD8+ T cells positively correlated with severe recurrent genital disease in SYMP individuals.
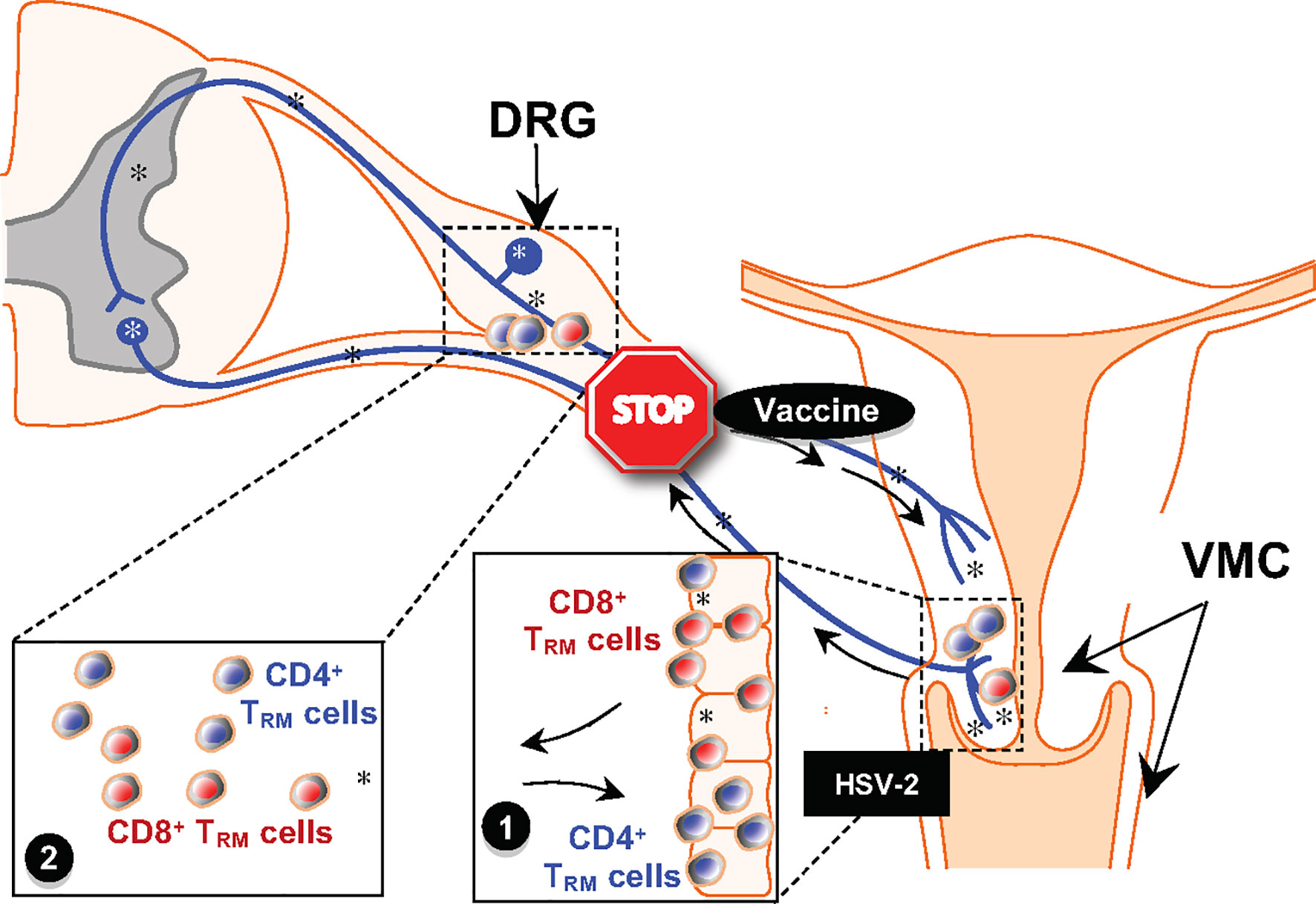
Figure 1 Schematic of Prime-Pull-Keep Therapeutic Vaccine (PPK Vaccine). The PPK vaccine is designed to boost Neutralizing IgG/IgA antibodies (Abs) and boost the number and function of antiviral CD4+ and CD8+ TRM cells within the cervico genital muco-cutaneous [CGMC, (1)] and dorsal root ganglia [DRG (2)] tissues. The PPK vaccine is expected to help STOP the virus reactivation from latently infected DRG, virus shedding and virus replication in CGMC, thus curing or reducing recurrent genital herpes disease. *, represent virus.
The critical role of antigen-specific CD8 T cells has been demonstrated in studies using various animal models (43, 44). We are now beginning to appreciate the differences observed in CD8 T cell memory population in symptomatic and asymptomatic HSV infected individuals, and understand the importance of stimulating tissue-resident memory T cells for prevention of HSV infection in the mouse model (44). T cell-based immunotherapeutic strategies to treat recurrent herpes infection and disease are emerging for HSV, and our laboratory has contributed significantly towards developing human asymptomatic CD8 T cell epitopes for HSV immunotherapy (20, 44, 97, 98). In the last fifteen years of vaccine development, we have succeeded in identifying new HLA-A2*01 restricted “asymptomatic” human CD4+ and CD8+ T cell epitopes from HSV-1 gB and gD glycoproteins and from HSV-1 VP11/12 and VP13/14 tegument proteins. Ocular herpes models using HLA-A2*01 restricted transgenic mouse and rabbits have shown that these asymptomatic human epitopes stimulated protective CD8 T cell responses (21, 99, 100). Presently, we are making significant headway with novel combinatorial approaches to use these epitopes as a SAPN (self-assembling protein nanoparticle) with built-in flagellin domains as a therapeutic HSV vaccine.
Prime and Pull Vaccines Using Adenoviral Vectors Delivering Epitopes Together With T-Cell Chemokines into HSV Infected Tissues
Chemokines are naturally produced by our immune system and could serve as safer and reliable adjuvants (101). Memory CD8+ T cells specific for HSV play an important role in inhibiting HSV-1 reactivation from TG and subsequent viral shedding in tears that trigger the recurrent corneal herpetic disease. The CXC chemokine ligand 10 (CXCL10)/CXC chemokine receptor 3 (CXCR3) pathways are critical in promoting T cell immunity against many viral infections (102). In a “prime and pull” strategy, a topical chemokine was applied to the genital mucosa after subcutaneous vaccination to pull HSV-specific CD8 T cells and was shown to be associated with decreased disease upon challenge with HSV-2 (103). The CXCL10/CXCR3 pathway also affects TG- and cornea-resident CD8+ T cell responses to recurrent ocular herpes virus infection and disease (104). Chemokines can also be co-delivered in a DNA vaccine for immunomodulation. Adenovirus-CCL21 transduced class I peptide-pulsed DC, and autologous DC-adenovirus CCL21 vaccines are currently in Phase I clinical trials for the treatment of malignant melanoma and stage IIIB-IV or recurrent non-small lung cancer respectively while XCL1 along with the IL-2 gene (CHESAT tumor vaccine) is in a clinical trial for neuroblastoma (101). Pre-clinical studies in HSV have shown immuno-potentiation of DNA vaccines by co-delivery of chemokines such as CCR7 ligands and IL-8, RANTES delivered to the mucosa (105, 106). We are in the advent of testing multi-epitope vaccine that co-delivers chemokines using adenovirus vectors. A “Prime-Pull-Keep” Therapeutic Vaccine (PPK Vaccine) is being designed to boost Neutralizing IgG/IgA antibodies and boost the number and function of antiviral CD4+ and CD8+ TRM cells within the cervico genital muco-cutaneous (CGMC) and DRG tissues. The PPK vaccine is expected to help STOP the virus reactivation from latently infected DRG, virus shedding and virus replication in CGMC, thus curing or reducing recurrent genital herpes disease (Figure 1).
Laser Adjuvants
As an alternative to currently used conventional adjuvants, the chemical- and biological-free laser-adjuvant offers a well-tolerated, simple to produce method to enhance mass vaccination for widespread viral infections (107). Studies from our laboratory have reported that skin exposure of B6 mice with the FDA approved non-ablative fractional diode laser (PaloVia Laser), followed by an intradermal delivery of a HSV peptide vaccine, safely induced potent and sustained HSV-specific CD8+ T cells, detected in both the draining lymph nodes (DLN) and in the vaginal mucosa (VM) (108). In the vaginal mucosa of laser-treated and peptide vaccinated mice, we observed more HSV-specific effector memory CD8 T cells. Following an intravaginal HSV-2 challenge, we found decreased genital herpes lesions and increased DC infiltrates around the laser-treated skin area. These findings have important implications for the development of efficient vaccine immunization strategies against HSV-1 and HSV-2.
Immune Checkpoint Blockade Combined With Therapeutic Herpes Vaccine
Total or partial loss of T cell function (dysfunction) occurs following repetitive HSV latent/reactivation cycles (109–111) and exposure to antigens is termed exhaustion (112) and is usually linked with expression of T cell co-inhibitory receptors: PD-1, TIM-3, LAG3 (CD223), TIGIT, PSGL-1, 2B4 (CD244), GITR, CTLA-4 (CD152), CD160, and BTLA (CD272) (113, 114). T cell dysfunction requires two signals: (1) T cell receptor (TCR) engaged by MHC presenting an HSV epitope (113); and a (2) T cell co-inhibitory receptor (e.g., PD-1) engaged by ligand (i.e., PDL-1). In humans, latent HSV in sensory ganglia is accompanied by chronic CD8 T cell infiltrates (115). A portion of viral reactivation in sensory ganglia appears to be controlled by CD8 T cell-mediated mechanisms (111, 116, 117). Recently, we compared the expression levels of eight known T cell co-inhibitory receptors on blood-derived HSV-specific CD8 T cells from symptomatic and asymptomatic HSV infected individuals and discovered that, HSV-specific CD8 T cells from symptomatic individuals expressed significantly higher levels of T cell co-inhibitory receptors like PD-1, LAG-3, TIM-3 and TIGIT (Figure 1). This phenotype correlated with functional exhaustion of HSV-specific CD8 T cells in symptomatic individuals with increased virus titers and severe disease. In mice, like humans, HSV-1 latently infected sensory ganglia have chronic CD8 T cell infiltrates (118). HSV-specific CD8 T cells producing IFN-γ and Granzyme B appear to suppress (or abort) induced viral reactivation in explanted mouse sensory ganglia (118, 119) and may similarly reduce detectable HSV-1 and HSV-2 reactivation in vivo (120–123). During acute (11 days) and latent (30 days) post-infection HSV-1infection of mice, most effector CD8 T cells from sensory ganglia simultaneously express high levels of 2 to 3 immune checkpoint receptors (e.g. PD-1 and LAG-3) (39, 111, 116, 117). This phenotype correlated with functional exhaustion of sensory ganglia-derived CD8 T cells and increased virus reactivation from infected sensory ganglia explants (39, 111, 116, 117).
Pembrolizumab and nivolumab are the first of the anti-PD-1 pathway family of checkpoint inhibitors to obtain FDA approval for the treatment of melanoma. The FDA has also granted approval of nivolumab for squamous cell lung cancer and Hodgkin lymphoma (HL), and MPDL-3280A, for bladder cancer and non–small cell lung cancer (124). From 2014-2017, the FDA approved several different anti-PD-1 mAbs opening the field of next vogues of so-called “immune checkpoint therapy mAbs” (125–127). Blocking the PD-1/PD-L1 (128–135) pathway in animal models demonstrated an improvement in CD8+ T cell effector function against persistent viral infections (136). Recent reports show that the natural constitutive PD-L1 expression on corneal cells impacts the HSV-1 infection of corneas. Genetic deficiency in PD-L1 using B7-H12/2 mice and the use of anti–PD-L1 blocking Ab significantly enhanced HSV-1 clearance from corneas of C57BL/6 mice mediated mainly by monocytes/macrophages (137). Based on our preliminary data of PD-L1 and GAL-9 blockade, we hypothesized that blocking PD-1, LAG-3, TIGIT and/or TIM-3 immune checkpoint pathways will help in restoring the function of HSV-specific CD8+ T cells in latently infected DRG and increasing efficacy and longevity of a therapeutic herpes vaccine.
Herpes Vaccine- Safety Evaluation
Safety concerns for vaccines include: (i) the potential inherent toxicities of the antigen and the adjuvants, as well as potential toxicities due to interactions of the components present in the final formulation; and (ii) the possibility that the vaccine induces inflammatory responses that may lead to undesired toxic side effects. Some adjuvants may elicit elevated levels of proinflammatory cytokines and other mediators of toxicity, irrespective of the immune response against the antigen. Preclinical standard repeated-dose toxicology studies performed in animals will identify whether intrinsic toxicity and immunotoxicity are: (i) confined primarily to the sites of injection; (ii) caused by the delivery method (i.e., the side effects are seen in both control and vaccinated animals) or (iii) caused by the intended immune responses to the vaccine (i.e., side effects occur with greater frequency and severity in vaccinated animals compared to controls). (1) Parameters for monitoring of systemic toxicity: Toxicity studies, repeated-dose toxicity studies, address the potential for systemic toxicity including, but not limited to, the systemic effects on the immune system. A broad spectrum of information should be obtained from the toxicity study, and both in-life and postmortem data should be collected. This routinely includes careful monitoring of body weight and food consumption, body temperature, histopathology, clinical chemistry, hematology, coagulation parameters and acute phase reactants. (2) Parameters for monitoring of local reactogenicity: Local toxicity studies of intramuscularly administered vaccines should preferably be conducted in animals with sufficient muscle mass, (such as rabbits) to test the full human dose of the final vaccine formulation.
Conclusions
Since most of the current HSV vaccine candidates were not promising individually in clinical trials, combinatorial vaccine approach seems to be the most appropriate in the present scenario to further advance HSV vaccine trials. Combinatorial application practically poses many problems and hence requires optimization in animal models. For example, one such approach optimized in the guinea pig model in our laboratory, is illustrated in Figure 1.
Results from clinical trials of the HSV vaccine indicate that it is essential to explore combinatorial approaches in the discovery of an effective therapeutic vaccine. Our long-term goal is to develop a long-lasting immunotherapeutic vaccine against genital herpes. HSV-specific CD8+ T cells are critical in preventing HSV reactivations from neurons of DRG and in limiting the severity of GT inflammatory lesions by reducing HSV replication (138–142). By harnessing the immune mechanisms active in seropositive asymptomatic individuals that make them “naturally” protected from recurrent herpes disease, we came up with a multiple-asymptomatic/protective epitope-based vaccine strategy, a promising HSV vaccine candidate when combined with other T cell-based immunotherapies like immune-checkpoint blockade or immunomodulation using various chemokines.
Expert Review
▪ The latest failures of most of the clinical herpes vaccines indicate that immunotherapeutic vaccine against HSV should be efficient in eliciting antigen-specific immune responses that contain reactivation of the virus, to control both recurrent lesions and viral shedding. Our vaccine research approach is based on the understanding and harnessing of immune strategies that make the seropositive asymptomatic individuals “naturally” protected from recurrent herpes disease throughout their life. We realized that the best strategy for an effective HSV vaccine would be to elicit a T cell-based immune response that boosts HSV specific effector memory T cell functionalities in the mucosal lining to prevent HSV-1/HSV-2 acquisition/reactivation.
▪ Much remains unknown about the protective immune effector of herpes, however, improved knowledge of HSV immuno-epidemiology, and immunopathology should help guide new vaccine strategies for HSV. In the last fifteen years of vaccine development, we have succeeded in identifying many protective “asymptomatic” human CD4+ and CD8+ T cell epitopes from HSV-1 gB and gD glycoproteins and from HSV-1 VP11/12 and VP13/14 tegument proteins. We are currently progressing with novel combinatorial approaches to use these epitopes as a SAPN with built-in flagellin domains as therapeutic HSV vaccine. A Prime-Pull-Keep Therapeutic Vaccine (PPK Vaccine) is designed to boost Neutralizing IgG/IgA antibodies (Abs) and boost the number and function of antiviral CD4+ and CD8+ TRM cells within the cervico genital muco-cutaneous (CGMC) and dorsal root ganglia (DRG) tissues. PPK vaccine is expected to help STOP the virus reactivation from latently infected DRG, virus shedding and virus replication in CGMC, thus curing or reducing recurrent genital herpes disease.
▪ Since most of the current HSV vaccine candidates were not promising individually in clinical trials, a combinatorial vaccine approach seems to be the most appropriate in the present scenario to further advance HSV vaccine trials. Combinatorial application practically poses many problems and hence requires optimization. We are currently optimizing these combinatorial approaches in animal models. We came up with multiple-asymptomatic/protective epitope-based vaccine strategy which will be a promising HSV vaccine candidate when combined with other T cell-based immunotherapy-like immune-checkpoint blockade or immunomodulation using various chemokines.
Author Contributions
AC, ND, RS, SP, P-GC, and LB: conceived and designed the experiments, performed the experiments, contributed reagents, materials, and analysis tools. AC, ND, RS, SP, P-GC, LZ, HV, HC, KH-C, and LB wrote the paper. All authors contributed to the article and approved the submitted version.
Funding
This work is supported by Public Health Service Research R01 Grants EY026103, EY019896 and EY024618 from National Eye Institute (NEI) and R21 Grant AI158060, AI150091, AI143348, AI147499, AI143326, AI138764, AI124911 and AI110902 from National Institutes of allergy and Infectious Diseases (NIAID) (to LB), and in part by The Discovery Center for Eye Research (DCER) and the Research to Prevent Blindness (RPB) grant.
Disclaimer
The authors alone are responsible for the views expressed in this review article, and they do not necessarily represent the decisions, policy, or views of the institutions, with which they are affiliated.
Conflict of Interest
Author HV was employed by TechImmune, LLC.
The remaining authors declare that the research was conducted in the absence of any commercial or financial relationships that could be construed as a potential conflict of interest.
Publisher’s Note
All claims expressed in this article are solely those of the authors and do not necessarily represent those of their affiliated organizations, or those of the publisher, the editors and the reviewers. Any product that may be evaluated in this article, or claim that may be made by its manufacturer, is not guaranteed or endorsed by the publisher.
Acknowledgments
This work is dedicated to the memory of late Professor Steven L. Wechsler “Steve” (1948-2016), whose numerous pioneering works on herpes infection and immunity laid the foundation to this line of research.
References
1. Looker KJ, Magaret AS, May MT, Turner KM, Vickerman P, Gottlieb SL, et al. Global and Regional Estimates of Prevalent and Incident Herpes Simplex Virus Type 1 Infections in 2012. PloS One (2015) 10:e0140765. doi: 10.1371/journal.pone.0140765
2. McQuillan G, Kruszon-Moran D, Flagg EW, Paulose-Ram R. Prevalence of Herpes Simplex Virus Type 1 and Type 2 in Persons Aged 14-49: United States, 2015-2016. NCHS Data Brief (2018) (304):1–8.
3. Knipe DM, Corey L, Cohen JI, Deal CD. Summary and Recommendations From a National Institute of Allergy and Infectious Diseases (NIAID) Workshop on "Next Generation Herpes Simplex Virus Vaccines". Vaccine (2014) 32:1561–2. doi: 10.1016/j.vaccine.2014.01.052
4. Awasthi S, Friedman HM. Status of Prophylactic and Therapeutic Genital Herpes Vaccines. Curr Opin Virol (2014) 6C:6–12. doi: 10.1016/j.coviro.2014.02.006
5. Wald A, Link K. Risk of Human Immunodeficiency Virus Infection in Herpes Simplex Virus Type 2-Seropositive Persons: A Meta-Analysis. J Infect Dis (2002) 185:45–52. doi: 10.1086/338231
6. Cunningham AL, Diefenbach RJ, Miranda-Saksena M, Bosnjak L, Kim M, Jones C, et al. The Cycle of Human Herpes Simplex Virus Infection: Virus Transport and Immune Control. J Infect Dis (2006) 194 Suppl 1:S11–8. doi: 10.1086/505359
7. Samandary S, Kridane-Miledi H, Sandoval JS, Choudhury Z, Langa-Vives F, Chentoufi AA, et al. Associations of HLA-A, HLA-B and HLA-C Alleles Frequency With Prevalence of Herpes Simplex Virus Infections and Diseases Across Global Populations: Implication for the Development of an Universal CD8+ T-Cell Epitope-Based Vaccine. Hum Immunol (2014) 75(8):7157–29. doi: 10.1016/j.humimm.2014.04.016
8. Schiffer JT, Swan DA, Corey L, Wald A. Rapid Viral Expansion and Short Drug Half-Life Explain the Incomplete Effectiveness of Current Herpes Simplex Virus 2-Directed Antiviral Agents. Antimicrob Agents Chemother (2013) 57:5820–9. doi: 10.1128/AAC.01114-13
9. Sandgren KJ, Bertram K, Cunningham AL. Understanding Natural Herpes Simplex Virus Immunity to Inform Next-Generation Vaccine Design. Clin Transl Immunol (2016) 5:e94. doi: 10.1038/cti.2016.44
10. Johnston C, Gottlieb SL, Wald A. Status of Vaccine Research and Development of Vaccines for Herpes Simplex Virus. Vaccine (2016) 34:2948–52. doi: 10.1016/j.vaccine.2015.12.076
11. Akhrameyeva NV, Zhang P, Sugiyama N, Behar SM, Yao F. Development of a Glycoprotein D-Expressing Dominant-Negative and Replication-Defective Herpes Simplex Virus 2 (HSV-2) Recombinant Viral Vaccine Against HSV-2 Infection in Mice. J Virol (2011) 85:5036–47. doi: 10.1128/JVI.02548-10
12. Reszka NJ, Dudek T, Knipe DM. Construction and Properties of a Herpes Simplex Virus 2 Dl5-29 Vaccine Candidate Strain Encoding an HSV-1 Virion Host Shutoff Protein. Vaccine (2010) 28:2754–62. doi: 10.1016/j.vaccine.2010.01.030
13. Belshe PB, Leone PA, Bernstein DI, Wald A, Levin MJ, Stapleton JT, et al. Efficacy Results of a Trial of a Herpes Simplex Vaccine. N Engl J Med (2012) 366:34–43. doi: 10.1056/NEJMoa1103151
14. Bernard MC, Barban V, Pradezynski F, de Montfort A, Ryall R, Caillet C, et al. Immunogenicity, Protective Efficacy, and Non-Replicative Status of the HSV-2 Vaccine Candidate HSV529 in Mice and Guinea Pigs. PloS One (2015) 10:e0121518. doi: 10.1371/journal.pone.0121518
15. Ohashi M, Bertke AS, Patel A, Krause PR. Spread of Herpes Simplex Virus to the Spinal Cord is Independent of Spread to Dorsal Root Ganglia. J Virol (2011) 85:3030–2. doi: 10.1128/JVI.02426-10
16. Dasgupta G, Chentoufi AA, Kalantari M, Falatoonzadeh P, Chun S, Lim CH, et al. Immunodominant "Asymptomatic" Herpes Simplex Virus 1 and 2 Protein Antigens Identified by Probing Whole-ORFome Microarrays With Serum Antibodies From Seropositive Asymptomatic Versus Symptomatic Individuals. J Virol (2012) 86:4358–69. doi: 10.1128/JVI.07107-11
17. Dasgupta G, Nesburn AB, Wechsler SL, BenMohamed L. Developing an Asymptomatic Mucosal Herpes Vaccine: The Present and the Future. Future Microbiol (2010) 5:1–4. doi: 10.2217/fmb.09.101
18. Chentoufi AA, BenMohamed L. Future Viral Vectors for the Delivery of Asymptomatic Herpes Epitope-Based Immunotherapeutic Vaccines. Future Virol (2010) 5:525–8. doi: 10.2217/fvl.10.44
19. Schiffer JT, Abu-Raddad L, Mark KE, Zhu J, Selke S, Koelle DM, et al. Mucosal Host Immune Response Predicts the Severity and Duration of Herpes Simplex Virus-2 Genital Tract Shedding Episodes. Proc Natl Acad Sci U S A (2010) 107:18973–8. doi: 10.1073/pnas.1006614107
20. Chentoufi AA, Binder NR, Berka N, Durand G, Nguyen A, Bettahi I, et al. Asymptomatic Human CD4+ Cytotoxic T-Cell Epitopes Identified From Herpes Simplex Virus Glycoprotein B. J Virol (2008) 82:11792–802. doi: 10.1128/JVI.00692-08
21. Dervillez X, Qureshi H, Chentoufi AA, Khan AA, Kritzer K, Yu DC, et al. “Asymptomatic” HLA-A*02:01-Restricted Epitopes From Herpes Simplex Virus Glycoprotein B Preferentially Recall Polyfunctional CD8+ T Cells From Seropositive Asymptomatic Individuals and Protect HLA Transgenic Mice Against Ocular Herpes. J Immunol (2013) 191:5124–38. doi: 10.4049/jimmunol.1301415
22. Dervillez X, Gottimukkala C, Kabbara KW, Nguyen C, Badakhshan T, Kim SM, et al. Future of an "Asymptomatic" T-Cell Epitope-Based Therapeutic Herpes Simplex Vaccine. Future Virol (2012) 7:371–8. doi: 10.2217/fvl.12.22
23. Pope C, Kim SK, Marzo A, Masopust D, Williams K, Jiang J, et al. Organ-Specific Regulation of the CD8 T Cell Response to Listeria Monocytogenes Infection. J Immunol (2001) 166:3402–9. doi: 10.4049/jimmunol.166.5.3402
24. Gebhardt T, Whitney PG, Zaid A, Mackay LK, Brooks AG, Heath WR, et al. Different Patterns of Peripheral Migration by Memory CD4+ and CD8+ T Cells. Nature (2011) 477:216–9. doi: 10.1038/nature10339
25. Nelson MH, Bird MD, Chu CF, Johnson AJ, Friedrich BM, Allman WR, et al. Rapid Clearance of Herpes Simplex Virus Type 2 by CD8+ T Cells Requires High Level Expression of Effector T Cell Functions. J Reprod Immunol (2011) 89:10–7. doi: 10.1016/j.jri.2011.01.013
26. Bertke AS, Patel A, Imai Y, Apakupakul K, Margolis TP, Krause PR. Latency-Associated Transcript (LAT) Exon 1 Controls Herpes Simplex Virus Species-Specific Phenotypes: Reactivation in the Guinea Pig Genital Model and Neuron Subtype-Specific Latent Expression of LAT. J Virol (2009) 83:10007–15. doi: 10.1128/JVI.00559-09
27. Schiffer JT, Corey L. Rapid Host Immune Response and Viral Dynamics in Herpes Simplex Virus-2 Infection. Nat Med (2013) 19:280–90. doi: 10.1038/nm.3103
28. Tang VA, Rosenthal KL. Intravaginal Infection With Herpes Simplex Virus Type-2 (HSV-2) Generates a Functional Effector Memory T Cell Population That Persists in the Murine Genital Tract. J Reprod Immunol (2010) 87:39–44. doi: 10.1016/j.jri.2010.06.155
29. van Lint A, Ayers M, Brooks AG, Coles RM, Heath WR, Carbone FR. Herpes Simplex Virus-Specific CD8+ T Cells can Clear Established Lytic Infections From Skin and Nerves and can Partially Limit the Early Spread of Virus After Cutaneous Inoculation. J Immunol (2004) 172:392–7. doi: 10.4049/jimmunol.172.1.392
30. Rott LS, Briskin MJ, Andrew DP, Berg EL, Butcher EC. A Fundamental Subdivision of Circulating Lymphocytes Defined by Adhesion to Mucosal Addressin Cell Adhesion Molecule-1. Comparison With Vascular Cell Adhesion Molecule-1 and Correlation With Beta 7 Integrins and Memory Differentiation. J Immunol (1996) 156:3727–36.
31. Mebius RE, Streeter PR, Michie S, Butcher EC, Weissman IL. A Developmental Switch in Lymphocyte Homing Receptor and Endothelial Vascular Addressin Expression Regulates Lymphocyte Homing and Permits CD4+ CD3- Cells to Colonize Lymph Nodes. Proc Natl Acad Sci USA (1996) 93:11019–24. doi: 10.1073/pnas.93.20.11019
32. Mackay CR, Andrew DP, Briskin M, Ringler DJ, Butcher EC. Phenotype, and Migration Properties of Three Major Subsets of Tissue Homing T Cells in Sheep. Eur J Immunol (1996) 26:2433–9. doi: 10.1002/eji.1830261025
33. Abitorabi MA, Mackay CR, Jerome EH, Osorio O, Butcher EC, Erle DJ. Differential Expression of Homing Molecules on Recirculating Lymphocytes From Sheep Gut, Peripheral, and Lung Lymph. J Immunol (1996) 156:3111–7.
34. von Andrian UH, Mackay CR. T-Cell Function and Migration. Two Sides of the Same Coin. N Engl J Med (2000) 343:1020–34. doi: 10.1056/NEJM200010053431407
35. Mackay LK, Wakim L, van Vliet CJ, Jones CM, Mueller SN, Bannard O, et al. Maintenance of T Cell Function in the Face of Chronic Antigen Stimulation and Repeated Reactivation for a Latent Virus Infection. J Immunol (2012) 188:2173–8. doi: 10.4049/jimmunol.1102719
36. Mackay LK, Stock AT, Ma JZ, Jones CM, Kent SJ, Mueller SN, et al. Long-Lived Epithelial Immunity by Tissue-Resident Memory T (TRM) Cells in the Absence of Persisting Local Antigen Presentation. Proc Natl Acad Sci U S A (2012) 109:7037–42. doi: 10.1073/pnas.1202288109
37. Masopust D, Picker LJ. Hidden Memories: Frontline Memory T Cells and Early Pathogen Interception. J Immunol (2012) 188:5811–7. doi: 10.4049/jimmunol.1102695
38. Suni MA, Ghanekar SA, Houck DW, Maecker HT, Wormsley SB, Picker LJ, et al. CD4(+)CD8(dim) T Lymphocytes Exhibit Enhanced Cytokine Expression, Proliferation and Cytotoxic Activity in Response to HCMV and HIV-1 Antigens. Eur J Immunol (2001) 31:2512–20. doi: 10.1002/1521-4141(200108)31:8<2512::AID-IMMU2512>3.0.CO;2-M
39. Jiang X, Chentoufi AA, Hsiang C, Carpenter D, Osorio N, Benmohamed L, et al. The Herpes Simplex Virus Type 1 Latency Associated Transcript (LAT) can Protect Neuronal Derived C1300 and Neuro2A Cells From Granzyme B Induced Apoptosis and CD8 T-Cell Killing. J Virol (2010) 91(Pt 4):858-66. doi: 10.1128/JVI.01791-10
40. Harari A, Enders FB, Cellerai C, Bart PA, Pantaleo G. Distinct Profiles of Cytotoxic Granules in Memory CD8 T Cells Correlate With Function, Differentiation Stage, and Antigen Exposure. J Virol (2009) 83:2862–71. doi: 10.1128/JVI.02528-08
41. Jameson SC, Masopust D. Diversity in T Cell Memory: An Embarrassment of Riches. Immunity (2009) 31:859–71. doi: 10.1016/j.immuni.2009.11.007
42. Khan AA, Srivastava R, Spencer D, Garg S, Fremgen D, Vahed H, et al. Phenotypic and Functional Characterization of Herpes Simplex Virus Glycoprotein B Epitope-Specific Effector and Memory CD8+ T Cells From Ocular Herpes Symptomatic and Asymptomatic Individuals. J Virol (2015)89(7):3776–92. doi: 10.1128/JVI.03419-14
43. Shin H, Iwasaki A. A Vaccine Strategy That Protects Against Genital Herpes by Establishing Local Memory T Cells. Nat (2012) 491:463–7. doi: 10.1038/nature11522
44. Khan AA, Srivastava R, Chentoufi AA, Kritzer E, Chilukuri S, Garg S, et al. Bolstering the Number and Function of HSV-1-Specific CD8(+) Effector Memory T Cells and Tissue-Resident Memory T Cells in Latently Infected Trigeminal Ganglia Reduces Recurrent Ocular Herpes Infection and Disease. J Immunol (2017) 199:186–203. doi: 10.4049/jimmunol.1700145
45. Khan AA, Srivastava R, Lopes PP, Wang C, Pham TT, Cochrane J, et al. Asymptomatic Memory CD8 T Cells: From Development and Regulation to Consideration for Human Vaccines and Immunotherapeutics. Hum Vaccin Immunother (2014) 10(4):845–63. 10. doi: 10.4161/hv.27762
46. Hashimoto M, Kamphorst AO, Im SJ, Kissick HT, Pillai RN, Ramalingam SS, et al. CD8 T Cell Exhaustion in Chronic Infection and Cancer: Opportunities for Interventions. Annu Rev Med (2018) 69:301–18. doi: 10.1146/annurev-med-012017-043208
47. Lal H, Cunningham AL, Godeaux O, Chlibek R, Diez-Domingo J, Hwang SJ, et al. Efficacy of an Adjuvanted Herpes Zoster Subunit Vaccine in Older Adults. N Engl J Med (2015) 372:2087–96. doi: 10.1056/NEJMoa1501184
48. Us D. [Herpes Simplex Virus Vaccine Studies: From Past to Present]. Mikrobiyol Bul (2006) 40:413–33.
49. Corey L, Langenberg AG, Ashley R, Sekulovich RE, Izu AE, Douglas JM Jr, et al. Recombinant Glycoprotein Vaccine for the Prevention of Genital HSV-2 Infection: Two Randomized Controlled Trials. Chiron HSV Vaccine Study Group. Jama (1999) 282:331–40. doi: 10.1001/jama.282.4.331
50. Bourne N, Bravo FJ, Francotte M, Bernstein DI, Myers MG, Slaoui M, et al. Herpes Simplex Virus (HSV) Type 2 Glycoprotein D Subunit Vaccines and Protection Against Genital HSV-1 or HSV-2 Disease in Guinea Pigs. J Infect Dis (2003) 187:542–9. doi: 10.1086/374002
51. Prichard MN, Kaiwar R, Jackman WT, Quenelle DC, Collins DJ, Kern ER, et al. Evaluation of AD472, a Live Attenuated Recombinant Herpes Simplex Virus Type 2 Vaccine in Guinea Pigs. Vaccine (2005) 23:5424–31. doi: 10.1016/j.vaccine.2005.02.028
52. Dutton JL, Li B, Woo WP, Marshak JO, Xu Y, Huang ML, et al. A Novel DNA Vaccine Technology Conveying Protection Against a Lethal Herpes Simplex Viral Challenge in Mice. PloS One (2013) 8:e76407. doi: 10.1371/journal.pone.0076407
53. Wang K, Goodman KN, Li DY, Raffeld M, Chavez M, Cohen JI. A Herpes Simplex Virus 2 (HSV-2) gD Mutant Impaired for Neural Tropism Is Superior to an HSV-2 gD Subunit Vaccine To Protect Animals From Challenge With HSV-2. J Virol (2016) 90:562–74. doi: 10.1128/JVI.01845-15
54. Gilbert PB, Excler JL, Tomaras GD, Carpp LN, Haynes BF, Liao HX, et al. Antibody to HSV gD Peptide Induced by Vaccination Does Not Protect Against HSV-2 Infection in HSV-2 Seronegative Women. PloS One (2017) 12:e0176428. doi: 10.1371/journal.pone.0176428
55. Cappel R. Comparison of the Humoral and Cellular Immune Response After Immunization With Live, UV Inactivated Herpes Simplex Virus and a Subunit Vaccine and Efficacy of These Immunizations. Arch Virol (1976) 52:29–35. doi: 10.1007/BF01317862
56. Metcalf JF. Protection From Experimental Ocular Herpetic Keratitis by a Heat-Killed Virus Vaccine. Arch Ophthalmol (1980) 98:893–6. doi: 10.1001/archopht.1980.01020030887017
57. Rajcáni J, Kutinová L, Vonka V. Restriction of Latent Herpes Virus Infection in Rabbits Immunized With Subviral Herpes Simplex Virus Vaccine. Acta Virol (1980) 24:183–93.
58. Dudek T, Knipe DM. Replication-Defective Viruses as Vaccines and Vaccine Vectors. Virol (2006) 344:230–9. doi: 10.1016/j.virol.2005.09.020
59. Hoshino Y, Pesnicak L, Dowdell KC, Lacayo J, Dudek T, Knipe DM, et al. Comparison of Immunogenicity and Protective Efficacy of Genital Herpes Vaccine Candidates Herpes Simplex Virus 2 Dl5-29 and Dl5-29-41L in Mice and Guinea Pigs. Vaccine (2008) 26:4034–40. doi: 10.1016/j.vaccine.2008.05.022
60. Liu X, Broberg E, Watanabe D, Dudek T, Deluca N, Knipe DM. Genetic Engineering of a Modified Herpes Simplex Virus 1 Vaccine Vector. Vaccine (2009) 27:2760–7. doi: 10.1016/j.vaccine.2009.03.003
61. Da Costa XJ, Bourne N, Stanberry LR, Knipe DM. Construction and Characterization of a Replication-Defective Herpes Simplex Virus 2 ICP8 Mutant Strain and its Use in Immunization Studies in a Guinea Pig Model of Genital Disease. Virol (1997) 232:1–12. doi: 10.1006/viro.1997.8564
62. Da Costa XJ, Morrison LA, Knipe DM. Comparison of Different Forms of Herpes Simplex Replication-Defective Mutant Viruses as Vaccines in a Mouse Model of HSV-2 Genital Infection. Virol (2001) 288:256–63. doi: 10.1006/viro.2001.1094
63. Diaz F, Gregory S, Nakashima H, Viapiano MS, Knipe DM. Intramuscular Delivery of Replication-Defective Herpes Simplex Virus Gives Antigen Expression in Muscle Syncytia and Improved Protection Against Pathogenic HSV-2 Strains. Virol (2018) 513:129–35. doi: 10.1016/j.virol.2017.10.011
64. Hoshino Y, Pesnicak L, Dowdell KC, Burbelo PD, Knipe DM, Straus SE, et al. Protection From Herpes Simplex Virus (HSV)-2 Infection With Replication-Defective HSV-2 or Glycoprotein D2 Vaccines in HSV-1-Seropositive and HSV-1-Seronegative Guinea Pigs. J Infect Dis (2009) 200:1088–95. doi: 10.1086/605645
65. Morrison LA. Replication-Defective Virus Vaccine-Induced Protection of Mice From Genital Herpes Simplex Virus 2 Requires CD4 T Cells. Virol (2008) 376:205–10. doi: 10.1016/j.virol.2008.03.010
66. Vagvala SP, Thebeau LG, Wilson SR, Morrison LA. Virus-Encoded B7-2 Costimulation Molecules Enhance the Protective Capacity of a Replication-Defective Herpes Simplex Virus Type 2 Vaccine in Immunocompetent Mice. J Virol (2009) 83:953–60. doi: 10.1128/JVI.02022-08
67. Awasthi S, Zumbrun EE, Si H, Wang F, Shaw CE, Cai M, et al. Live Attenuated Herpes Simplex Virus 2 Glycoprotein E Deletion Mutant as a Vaccine Candidate Defective in Neuronal Spread. J Virol (2012) 86:4586–98. doi: 10.1128/JVI.07203-11
68. Brittle EE, Wang F, Lubinski JM, Bunte RM, Friedman HM. A Replication-Competent, Neuronal Spread-Defective, Live Attenuated Herpes Simplex Virus Type 1 Vaccine. J Virol (2008) 82:8431–41. doi: 10.1128/JVI.00551-08
69. Royer DJ, Carr MM, Chucair-Elliott AJ, Halford WP, Carr DJ. Impact of Type I Interferon on the Safety and Immunogenicity of an Experimental Live-Attenuated Herpes Simplex Virus 1 Vaccine in Mice. J Virol (2017) 91(7). doi: 10.1128/JVI.02342-16
70. Spector FC, Kern ER, Palmer J, Kaiwar R, Cha TA, Brown P, et al. Evaluation of a Live Attenuated Recombinant Virus RAV 9395 as a Herpes Simplex Virus Type 2 Vaccine in Guinea Pigs. J Infect Dis (1998) 177:1143–54. doi: 10.1086/515278
71. Stanfield BA, Rider PJF, Caskey J, Del Piero F, Kousoulas KG. Intramuscular Vaccination of Guinea Pigs With the Live-Attenuated Human Herpes Simplex Vaccine VC2 Stimulates a Transcriptional Profile of Vaginal Th17 and Regulatory Tr1 Responses. Vaccine (2018) 36:2842–9. doi: 10.1016/j.vaccine.2018.03.075
72. Sato A, Suwanto A, Okabe M, Sato S, Nochi T, Imai T, et al. Vaginal Memory T Cells Induced by Intranasal Vaccination are Critical for Protective T Cell Recruitment and Prevention of Genital HSV-2 Disease. J Virol (2014) 88:13699–708. doi: 10.1128/JVI.02279-14
73. Halford WP, Puschel R, Gershburg E, Wilber A, Gershburg S, Rakowski B. A Live-Attenuated HSV-2 ICP0 Virus Elicits 10 to 100 Times Greater Protection Against Genital Herpes Than A Glycoprotein D Subunit Vaccine. PloS One (2011) 6:e17748. doi: 10.1371/journal.pone.0017748
74. Halford WP, Puschel R, Rakowski B. Herpes Simplex Virus 2 ICP0 Mutant Viruses are Avirulent and Immunogenic: Implications for a Genital Herpes Vaccine. PloS One (2010) 5:e12251. doi: 10.1371/journal.pone.0012251
75. Geltz JJ, Gershburg E, Halford WP. Herpes Simplex Virus 2 (HSV-2) Infected Cell Proteins are Among the Most Dominant Antigens of a Live-Attenuated HSV-2 Vaccine. PloS One (2015) 10:e0116091. doi: 10.1371/journal.pone.0116091
76. Halford WP, Geltz J, Gershburg E. Pan-HSV-2 IgG Antibody in Vaccinated Mice and Guinea Pigs Correlates With Protection Against Herpes Simplex Virus 2. PloS One (2013) 8:e65523. doi: 10.1371/journal.pone.0065523
77. Wang K, Kappel JD, Canders C, Davila WF, Sayre D, Chavez M, et al. A Herpes Simplex Virus 2 Glycoprotein D Mutant Generated by Bacterial Artificial Chromosome Mutagenesis is Severely Impaired for Infecting Neuronal Cells and Infects Only Vero Cells Expressing Exogenous HVEM. J Virol (2012) 86:12891–902. doi: 10.1128/JVI.01055-12
78. Visalli RJ, Natuk RJ, Kowalski J, Guo M, Blakeney S, Gangolli S, et al. Vaccination With a HSV-2 UL24 Mutant Induces a Protective Immune Response in Murine and Guinea Pig Vaginal Infection Models. Vaccine (2014) 32:1398–406. doi: 10.1016/j.vaccine.2013.10.079
79. Stanfield BA, Stahl J, Chouljenko VN, Subramanian R, Charles AS, Saied AA, et al. A Single Intramuscular Vaccination of Mice With the HSV-1 VC2 Virus With Mutations in the Glycoprotein K and the Membrane Protein UL20 Confers Full Protection Against Lethal Intravaginal Challenge With Virulent HSV-1 and HSV-2 Strains. PloS One (2014) 9:e109890. doi: 10.1371/journal.pone.0109890
80. McLean CS, Ni Challanain D, Duncan I, Boursnell ME, Jennings R, Inglis SC. Induction of a Protective Immune Response by Mucosal Vaccination With a DISC HSV-1 Vaccine. Vaccine (1996) 14:987–92. doi: 10.1016/0264-410X(95)00259-4
81. Boursnell ME, Entwisle C, Blakeley D, Roberts C, Duncan IA, Chisholm SE, et al. A Genetically Inactivated Herpes Simplex Virus Type 2 (HSV-2) Vaccine Provides Effective Protection Against Primary and Recurrent HSV-2 Disease. J Infect Dis (1997) 175:16–25. doi: 10.1093/infdis/175.1.16
82. Dudek T, Mathews LC, Knipe DM. Disruption of the U(L)41 Gene in the Herpes Simplex Virus 2 Dl5-29 Mutant Increases its Immunogenicity and Protective Capacity in a Murine Model of Genital Herpes. Virol (2008) 372:165–75. doi: 10.1016/j.virol.2007.10.014
83. Delagrave S, Hernandez H, Zhou C, Hamberger JF, Mundle ST, Catalan J, et al. Immunogenicity and Efficacy of Intramuscular Replication-Defective and Subunit Vaccines Against Herpes Simplex Virus Type 2 in the Mouse Genital Model. PloS One (2012) 7:e46714. doi: 10.1371/journal.pone.0046714
84. Mundle ST, Hernandez H, Hamberger J, Catalan J, Zhou C, Stegalkina S, et al. High-Purity Preparation of HSV-2 Vaccine Candidate ACAM529 is Immunogenic and Efficacious In Vivo. PloS One (2013) 8:e57224. doi: 10.1371/journal.pone.0057224
85. Iyer AV, Pahar B, Chouljenko VN, Walker JD, Stanfield B, Kousoulas KG. Single Dose of Glycoprotein K (Gk)-Deleted HSV-1 Live-Attenuated Virus Protects Mice Against Lethal Vaginal Challenge With HSV-1 and HSV-2 and Induces Lasting T Cell Memory Immune Responses. Virol J (2013) 10:317. doi: 10.1186/1743-422X-10-317
86. Zhang P, Xie L, Balliet JW, Casimiro DR, Yao F. A Herpes Simplex Virus 2 (HSV-2) Glycoprotein D-Expressing Nonreplicating Dominant-Negative HSV-2 Virus Vaccine is Superior to a Gd2 Subunit Vaccine Against HSV-2 Genital Infection in Guinea Pigs. PloS One (2014) 9:e101373. doi: 10.1371/journal.pone.0101373
87. Petro C, González PA, Cheshenko N, Jandl T, Khajoueinejad N, Bénard A, et al. Herpes Simplex Type 2 Virus Deleted in Glycoprotein D Protects Against Vaginal, Skin and Neural Disease. Elife (2015) 4:e06054. doi: 10.7554/eLife.06054
88. Veselenak RL, Shlapobersky M, Pyles RB, Wei Q, Sullivan SM, Bourne N. A Vaxfectin((R))-Adjuvanted HSV-2 Plasmid DNA Vaccine is Effective for Prophylactic and Therapeutic Use in the Guinea Pig Model of Genital Herpes. Vaccine (2012) 30:7046–51. doi: 10.1016/j.vaccine.2012.09.057
89. Skoberne M, Cardin R, Lee A, Kazimirova A, Zielinski V, Garvie D, et al. An Adjuvanted Herpes Simplex Virus 2 Subunit Vaccine Elicits a T Cell Response in Mice and is an Effective Therapeutic Vaccine in Guinea Pigs. J Virol (2013) 87:3930–42. doi: 10.1128/JVI.02745-12
90. Van Wagoner N, Fife K, Leone PA, Bernstein DI, Warren T, Panther L, et al. Effects of Different Doses of GEN-003, A Therapeutic Vaccine for Genital Herpes Simplex Virus-2, on Viral Shedding and Lesions: Results of a Randomized Placebo-Controlled Trial. J Infect Dis (2018) 218:1890–9. doi: 10.1093/infdis/jiy415
91. Flechtner JB, Long D, Larson S, Clemens V, Baccari A, Kien L, et al. Immune Responses Elicited by the GEN-003 Candidate HSV-2 Therapeutic Vaccine in a Randomized Controlled Dose-Ranging Phase 1/2a Trial. Vaccine (2016) 34:5314–20. doi: 10.1016/j.vaccine.2016.09.001
92. Bernstein DI, Wald A, Warren T, Fife K, Tyring S, Lee P, et al. Therapeutic Vaccine for Genital Herpes Simplex Virus-2 Infection: Findings From a Randomized Trial. J Infect Dis (2017) 215:856–64. doi: 10.1093/infdis/jix004
93. Stanberry LR. Clinical Trials of Prophylactic and Therapeutic Herpes Simplex Virus Vaccines. Herpes (2004) 11 Suppl 3:161A–9A.
94. Straus SE, Wald A, Kost RG, McKenzie R, Langenberg AG, Hohman P, et al. Immunotherapy of Recurrent Genital Herpes With Recombinant Herpes Simplex Virus Type 2 Glycoproteins D and B: Results of a Placebo-Controlled Vaccine Trial. J Infect Dis (1997) 176:1129–34. doi: 10.1086/514103
95. Langenberg AG, Burke RL, Adair SF, Sekulovich R, Tigges M, Dekker CL, et al. A Recombinant Glycoprotein Vaccine for Herpes Simplex Virus Type 2: Safety and Immunogenicity [Corrected]. Ann Intern Med (1995) 122:889–98. doi: 10.7326/0003-4819-122-12-199506150-00001
96. Straus SE, Corey L, Burke RL, Savarese B, Barnum G, Krause PR, et al. Placebo-Controlled Trial of Vaccination With Recombinant Glycoprotein D of Herpes Simplex Virus Type 2 for Immunotherapy of Genital Herpes. Lancet (1994) 343:1460–3. doi: 10.1016/S0140-6736(94)92581-X
97. Chentoufi AA, Zhang X, Lamberth K, Dasgupta G, Bettahi I, Nguyen A, et al. HLA-A*0201-Restricted CD8+ Cytotoxic T Lymphocyte Epitopes Identified From Herpes Simplex Virus Glycoprotein D. J Immunol (2008) 180:426–37. doi: 10.4049/jimmunol.180.1.426
98. Kuo T, Wang C, Badakhshan T, Chilukuri S, BenMohamed L. The Challenges and Opportunities for the Development of a T-Cell Epitope-Based Herpes Simplex Vaccine. Vaccine (2014) 32:6733–45. doi: 10.1016/j.vaccine.2014.10.002
99. Srivastava R, Khan AA, Garg S, Syed SA, Furness JN, Vahed H, et al. Human Asymptomatic Epitopes Identified From the Herpes Simplex Virus Tegument Protein VP13/14 (UL47) Preferentially Recall Polyfunctional Effector Memory CD44high CD62Llow CD8+ TEM Cells and Protect Humanized HLA-A*02:01 Transgenic Mice Against Ocular Herpesvirus Infection. J Virol (2017) 91:e01796-16. doi: 10.1128/JVI.01793-16
100. Khan AA, Srivastava R, Chentoufi AA, Geertsema R, Thai NT, Dasgupta G, et al. Therapeutic Immunization With a Mixture of Herpes Simplex Virus 1 Glycoprotein D-Derived "Asymptomatic" Human CD8+ T-Cell Epitopes Decreases Spontaneous Ocular Shedding in Latently Infected HLA Transgenic Rabbits: Association With Low Frequency of Local PD-1+ TIM-3+ CD8+ Exhausted T Cells. J Virol (2015) 89:6619–32. doi: 10.1128/JVI.00788-15
101. Mohan T, Zhu W, Wang Y, Wang BZ. Applications of Chemokines as Adjuvants for Vaccine Immunotherapy. Immunobiology (2018) 223:477–85. doi: 10.1016/j.imbio.2017.12.001
102. Liu C, Luo D, Reynolds BA, Meher G, Katritzky AR, Lu B, et al. Chemokine Receptor CXCR3 Promotes Growth of Glioma. Carcinogenesis (2011) 32:129–37. doi: 10.1093/carcin/bgq224
103. Khan AA, Srivastava R, Vahed H, Roy S, Walia SS, Kim GJ, et al. Human Asymptomatic Epitope Peptide/CXCL10-Based Prime/Pull Vaccine Induces Herpes Simplex Virus-Specific Gamma Interferon-Positive CD107(+) CD8(+) T Cells That Infiltrate the Corneas and Trigeminal Ganglia of Humanized HLA Transgenic Rabbits and Protect Against Ocular Herpes Challenge. J Virol (2018) 92. doi: 10.1128/JVI.00535-18
104. Srivastava R, Khan AA, Chilukuri S, Syed SA, Tran TT, Furness J, et al. CXCL10/CXCR3-Dependent Mobilization of Herpes Simplex Virus-Specific CD8(+) TEM and CD8(+) TRM Cells Within Infected Tissues Allows Efficient Protection Against Recurrent Herpesvirus Infection and Disease. J Virol (2017) 91:e00278-17. doi: 10.1128/JVI.00278-17
105. Sin J, Kim JJ, Pachuk C, Satishchandran C, Weiner DB. DNA Vaccines Encoding Interleukin-8 and RANTES Enhance Antigen-Specific Th1-Type CD4(+) T-Cell-Mediated Protective Immunity Against Herpes Simplex Virus Type 2 In Vivo. J Virol (2000) 74:11173–80. doi: 10.1128/JVI.74.23.11173-11180.2000
106. Eo SK, Kumaraguru U, Rouse BT. Plasmid DNA Encoding CCR7 Ligands Compensate for Dysfunctional CD8+ T Cell Responses by Effects on Dendritic Cells. J Immunol (2001) 167:3592–9. doi: 10.4049/jimmunol.167.7.3592
107. Kashiwagi S, Brauns T, Gelfand J, Poznansky MC. Laser Vaccine Adjuvants. History, Progress, and Potential. Hum Vaccin Immunother (2014) 10:1892–907. doi: 10.4161/hv.28840
108. Lopes PP, Todorov G, Pham TT, Nesburn AB, Bahraoui E, BenMohamed L. Laser Adjuvant-Assisted Peptide Vaccine Promotes Skin Mobilization of Dendritic Cells and Enhances Protective CD8(+) TEM and TRM Cell Responses Against Herpesvirus Infection and Disease. J Virol (2018) 92. doi: 10.1128/JVI.02156-17
109. Frank GM, Lepisto AJ, Freeman ML, Sheridan BS, Cherpes TL, Hendricks RL. Early CD4(+) T Cell Help Prevents Partial CD8(+) T Cell Exhaustion and Promotes Maintenance of Herpes Simplex Virus 1 Latency. J Immunol (2010) 184:277–86. doi: 10.4049/jimmunol.0902373
110. Allen SJ, Mott KR, Zandian M, Ghiasi H. Immunization With Different Viral Antigens Alters the Pattern of T Cell Exhaustion and Latency in Herpes Simplex Virus Type 1-Infected Mice. J Virol (2010) 84:12315–24. doi: 10.1128/JVI.01600-10
111. Chentoufi AA, Dervillez X, Dasgupta G, Nguyen C, Kabbara KW, Jiang X, et al. The Herpes Simplex Virus Type 1 Latency-Associated Transcript Inhibits Phenotypic and Functional Maturation of Dendritic Cells. Viral Immunol (2012) 25(3):204–15. in press. doi: 10.1089/vim.2011.0091
113. Day CL, Kaufmann DE, Kiepiela P, Brown JA, Moodley ES, Reddy S, et al. PD-1 Expression on HIV-Specific T Cells is Associated With T-Cell Exhaustion and Disease Progression. Nat (2006) 443:350–4. doi: 10.1038/nature05115
114. Anderson AC, Joller N, Kuchroo VK. Lag-3, Tim-3, and TIGIT: Co-Inhibitory Receptors With Specialized Functions in Immune Regulation. Immunity (2016) 44:989–1004. doi: 10.1016/j.immuni.2016.05.001
115. Held K, Eiglmeier I, Himmelein S, Sinicina I, Brandt T, Theil D, et al. Clonal Expansions of CD8(+) T Cells in Latently HSV-1-Infected Human Trigeminal Ganglia. J Neurovirol (2012) 18:62–8. doi: 10.1007/s13365-011-0067-9
116. Chentoufi AA, Kritzer E, Tran MV, Dasgupta G, Lim CH, Yu DC, et al. The Herpes Simplex Virus 1 Latency-Associated Transcript Promotes Functional Exhaustion of Virus-Specific CD8+ T Cells in Latently Infected Trigeminal Ganglia: A Novel Immune Evasion Mechanism. J Virol (2011) 85:9127–38. doi: 10.1128/JVI.00587-11
117. Allen SJ, Hamrah P, Gate D, Mott KR, Mantopoulos D, Zheng L, et al. The Role of LAT in Increased CD8+ T Cell Exhaustion in Trigeminal Ganglia of Mice Latently Infected With Herpes Simplex Virus 1. J Virol (2011) 85:4184–97. doi: 10.1128/JVI.02290-10
118. Sheridan BS, Cherpes TL, Urban J, Kalinski P, Hendricks RL. Reevaluating the CD8 T-Cell Response to Herpes Simplex Virus Type 1: Involvement of CD8 T Cells Reactive to Subdominant Epitopes. J Virol (2009) 83:2237–45. doi: 10.1128/JVI.01699-08
119. Liu T, Khanna KM, Chen X, Fink DJ, Hendricks RL. CD8(+) T Cells can Block Herpes Simplex Virus Type 1 (HSV-1) Reactivation From Latency in Sensory Neurons. J Exp Med (2000) 191:1459–66. doi: 10.1084/jem.191.9.1459
120. Liu T, Khanna KM, Carriere BN, Hendricks RL. Gamma Interferon can Prevent Herpes Simplex Virus Type 1 Reactivation From Latency in Sensory Neurons. J Virol (2001) 75:11178–84. doi: 10.1128/JVI.75.22.11178-11184.2001
121. Divito S, Cherpes TL, Hendricks RL. A Triple Entente: Virus, Neurons, and CD8+ T Cells Maintain HSV-1 Latency. Immunol Res (2006) 36:119–26. doi: 10.1385/IR:36:1:119
122. Khanna KM, Lepisto AJ, Hendricks RL. Immunity to Latent Viral Infection: Many Skirmishes But Few Fatalities. Trends Immunol (2004) 25:230–4. doi: 10.1016/j.it.2004.02.010
123. Knickelbein JE, Khanna KM, Yee MB, Baty CJ, Kinchington PR, Hendricks RL. Noncytotoxic Lytic Granule-Mediated CD8+ T Cell Inhibition of HSV-1 Reactivation From Neuronal Latency. Science (2008) 322:268–71. doi: 10.1126/science.1164164
124. Mahoney KM, Rennert PD, Freeman GJ. Combination Cancer Immunotherapy and New Immunomodulatory Targets. Nat Rev Drug Discov (2015) 14:561–84. doi: 10.1038/nrd4591
125. Garon EB, Rizvi NA, Hui R, Leighl N, Balmanoukian AS, Eder JP, et al. Pembrolizumab for the Treatment of Non-Small-Cell Lung Cancer. N Engl J Med (2015) 372:2018–28. doi: 10.1056/NEJMoa1501824
126. Rizvi NA, Hellmann MD, Snyder A, Kvistborg P, Makarov V, Havel JJ, et al. Cancer Immunology. Mutational Landscape Determines Sensitivity to PD-1 Blockade in Non-Small Cell Lung Cancer. Science (2015) 348:124–8. doi: 10.1126/science.aaa1348
127. Rizvi NA, Mazieres J, Planchard D, Stinchcombe TE, Dy GK, Antonia SJ, et al. Activity and Safety of Nivolumab, an Anti-PD-1 Immune Checkpoint Inhibitor, for Patients With Advanced, Refractory Squamous Non-Small-Cell Lung Cancer (CheckMate 063): A Phase 2, Single-Arm Trial. Lancet Oncol (2015) 16:257–65. doi: 10.1016/S1470-2045(15)70054-9
128. Ishida M, Iwai Y, Tanaka Y, Okazaki T, Freeman GJ, Minato N, et al. Differential Expression of PD-L1 and PD-L2, Ligands for an Inhibitory Receptor PD-1, in the Cells of Lymphohematopoietic Tissues. Immunol Lett (2002) 84:57–62. doi: 10.1016/S0165-2478(02)00142-6
129. Iwai Y, Ishida M, Tanaka Y, Okazaki T, Honjo T, Minato N. Involvement of PD-L1 on Tumor Cells in the Escape From Host Immune System and Tumor Immunotherapy by PD-L1 Blockade. Proc Natl Acad Sci U.S.A (2002) 99:12293–7. doi: 10.1073/pnas.192461099
130. Agata Y, Kawasaki A, Nishimura H, Ishida Y, Tsubata T, Yagita H, et al. Expression of the PD-1 Antigen on the Surface of Stimulated Mouse T and B Lymphocytes. Int Immunol (1996) 8:765–72. doi: 10.1093/intimm/8.5.765
131. Kasagi S, Kawano S, Okazaki T, Honjo T, Morinobu A, Hatachi S, et al. Anti-Programmed Cell Death 1 Antibody Reduces CD4+PD-1+ T Cells and Relieves the Lupus-Like Nephritis of NZB/W F1 Mice. J Immunol (2010) 184:2337–47. doi: 10.4049/jimmunol.0901652
132. Boenisch O, D'Addio F, Watanabe T, Elyaman W, Magee CN, Yeung MY, et al. TIM-3: A Novel Regulatory Molecule of Alloimmune Activation. J Immunol (2010) 185:5806–19. doi: 10.4049/jimmunol.0903435
133. Sehrawat S, Reddy PB, Rajasagi N, Suryawanshi A, Hirashima M, Rouse BT. Galectin-9/TIM-3 Interaction Regulates Virus-Specific Primary and Memory CD8 T Cell Response. PloS Pathog (2010) 6:e1000882. doi: 10.1371/journal.ppat.1000882
134. Sehrawat S, Suryawanshi A, Hirashima M, Rouse BT. Role of Tim-3/Galectin-9 Inhibitory Interaction in Viral-Induced Immunopathology: Shifting the Balance Toward Regulators. J Immunol (2009) 182:3191–201. doi: 10.4049/jimmunol.0803673
135. Dai SY, Nakagawa R, Itoh A, Murakami H, Kashio Y, Abe H, et al. Galectin-9 Induces Maturation of Human Monocyte-Derived Dendritic Cells. J Immunol (2005) 175:2974–81. doi: 10.4049/jimmunol.175.5.2974
136. Sakuishi K, Apetoh L, Sullivan JM, Blazar BR, Kuchroo VK, Anderson AC. Targeting Tim-3 and PD-1 Pathways to Reverse T Cell Exhaustion and Restore Anti-Tumor Immunity. J Exp Med (2010) 207:2187–94. doi: 10.1084/jem.20100643
137. Jeon S, Rowe AM, Carroll KL, Harvey SAK, Hendricks RL. PD-L1/B7-H1 Inhibits Viral Clearance by Macrophages in HSV-1-Infected Corneas. J Immunol (2018) 200:3711–9. doi: 10.4049/jimmunol.1700417
138. Banerjee K, Biswas PS, Rouse BT. Elucidating the Protective and Pathologic T Cell Species in the Virus-Induced Corneal Immunoinflammatory Condition Herpetic Stromal Keratitis. J Leukoc Biol (2005) 77:24–32. doi: 10.1189/jlb.0904486
139. Zhang X, Dervillez X, Chentoufi AA, Badakhshan T, Bettahi I, Benmohamed L. Targeting the Genital Tract Mucosa With a Lipopeptide/Recombinant Adenovirus Prime/Boost Vaccine Induces Potent and Long-Lasting CD8+ T Cell Immunity Against Herpes: Importance of Myd88. J Immunol (2012) 189:4496–509. doi: 10.4049/jimmunol.1201121
140. Laing KJ, Magaret AS, Mueller DE, Zhao L, Johnston C, De Rosa SC, et al. Diversity in CD8(+) T Cell Function and Epitope Breadth Among Persons With Genital Herpes. J Clin Immunol (2010) 30:703–22. doi: 10.1007/s10875-010-9441-2
141. Zhang X, Chentoufi AA, Dasgupta G, Nesburn AB, Wu M, Zhu X, et al. A Genital Tract Peptide Epitope Vaccine Targeting TLR-2 Efficiently Induces Local and Systemic CD8+ T Cells and Protects Against Herpes Simplex Virus Type 2 Challenge. Mucosal Immunol (2009) 2:129–43. doi: 10.1038/mi.2008.81
Keywords: herpes simplex virus, clinical trials, vaccines, asymptomatic, immune checkpoint blockade
Citation: Chentoufi AA, Dhanushkodi NR, Srivastava R, Prakash S, Coulon P-GA, Zayou L, Vahed H, Chentoufi HA, Hormi-Carver KK and BenMohamed L (2022) Combinatorial Herpes Simplex Vaccine Strategies: From Bedside to Bench and Back. Front. Immunol. 13:849515. doi: 10.3389/fimmu.2022.849515
Received: 06 January 2022; Accepted: 18 March 2022;
Published: 25 April 2022.
Edited by:
Susmit Suvas, Wayne State University, United StatesReviewed by:
Elmostafa Bahraoui, U1043 Centre de Physiopathologie de Toulouse Purpan (INSERM), FrancePamela Rosato, Dartmouth College, United States
Copyright © 2022 Chentoufi, Dhanushkodi, Srivastava, Prakash, Coulon, Zayou, Vahed, Chentoufi, Hormi-Carver and BenMohamed. This is an open-access article distributed under the terms of the Creative Commons Attribution License (CC BY). The use, distribution or reproduction in other forums is permitted, provided the original author(s) and the copyright owner(s) are credited and that the original publication in this journal is cited, in accordance with accepted academic practice. No use, distribution or reproduction is permitted which does not comply with these terms.
*Correspondence: Lbachir BenMohamed, TGJlbm1vaGFAdWNpLmVkdQ==