- 1Guangdong Provincial Key Laboratory of Tumour Interventional Diagnosis and Treatment, Zhuhai Institute of Translational Medicine, Zhuhai People’s Hospital Affiliated with Jinan University, Jinan University, Zhuhai, China
- 2The Biomedical Translational Research Institute, Faculty of Medical Science, Jinan University, Guangzhou, China
- 3Microbiology and Immunology Department, School of Medicine, Faculty of Medical Science, Jinan University, Guangzhou, China
The demise of cells in various ways enables the body to clear unwanted cells. Studies over the years revealed distinctive molecular mechanisms and functional consequences of several key cell death pathways. Currently, the most intensively investigated programmed cell death (PCD) includes apoptosis, necroptosis, pyroptosis, ferroptosis, PANoptosis, and autophagy, which has been discovered to play crucial roles in modulating the immunosuppressive tumor microenvironment (TME) and determining clinical outcomes of the cancer therapeutic approaches. PCD can play dual roles, either pro-tumor or anti-tumor, partly depending on the intracellular contents released during the process. PCD also regulates the enrichment of effector or regulatory immune cells, thus participating in fine-tuning the anti-tumor immunity in the TME. In this review, we focused primarily on apoptosis, necroptosis, pyroptosis, ferroptosis, PANoptosis, and autophagy, discussed the released molecular messengers participating in regulating their intricate crosstalk with the immune response in the TME, and explored the immunological consequence of PCD and its implications in future cancer therapy developments.
Introduction
To maintain the physiological homeostasis in normal or stress-challenged (injury or infection, etc.) states, cells adopt different cell death pathways which generate distinctive morphological and functional outcomes (Table 1). In an adult, approximately 50~70 billion cells die each day to maintain a healthy turnover of cells (48). Programmed Cell Death (PCD) and non-PCD both are demonstrated to participate in this turnover process of the cells. However, PCD is orchestrated by precise molecular circuitry whereas non-PCD such as necrosis is characterized as a premature death caused by injury. We will limit our discussion on PCD and its communication with the immune milieu in the context of the tumor microenvironment (TME) in this review.
According to the ability to initiate further adaptive immune response or not, PCD can be further categorized as immunogenic and non-immunogenic (or tolerogenic) ones (44). Immunogenic PCD alerts the surrounding immune system of potential danger through the release of cellular components, mainly pro-inflammatory cytokines, or other damage-associated molecular patterns (DAMPs). These signals are recognized by the Pattern Recognition Receptors (PRRs) on innate immune cells, thus activating subsequent immune responses. On the other hand, non-immunogenic cell death such as apoptosis maintains the integrity of the cell membrane without leaking cellular contents, therefore leading to a “silent” clearance by phagocytic cells without initiating further inflammation (49).
Besides apoptosis, several other well-recognized PCD pathways, necroptosis (50, 51), pyroptosis (27, 36, 52), and ferroptosis (53–56), etc. have also been found to be tightly regulated and connected with the tumor immunity in TME. Interestingly, one pro-survival strategy to avoid extensive PCD adopted by cells is called autophagy. It’s also worth mentioning that autophagy could convert into yet another type of PCD under certain physiological circumstances (57, 58). Therefore, a game between pro-survival and pro-death pathways shapes the heterogeneity and complexity of the tumor immunity in TMEs. Here, we will constrain our discussion on the following types of PCD, apoptosis, necroptosis, pyroptosis, PANoptosis, ferroptosis, and autophagy, respectively.
Apoptosis
One of the earliest well-recognized non-immunogenic PCD is apoptosis (1, 59–65), which is elegantly orchestrated by the sequential cleavages of the aspartate-specific proteases [caspases (49, 65)]. This leads to cell membrane blebbing and the generation of apoptotic bodies, nucleus condensation, and cellular organelle/DNA fragmentation. These alterations eventually cause cell disintegration followed by the engulfment by phagocytic housekeepers from the innate immunity without releasing proinflammatory cellular contents to the extracellular environment. Although typical apoptosis is non-immunogenic, studies indicated that, under certain conditions such as caspase deficiency, apoptosis could indeed trigger adaptive anti-tumor or anti-viral immune responses by activating NF-κB signaling (66) and cGAS/STING pathway, respectively (67, 68). Moreover, radiotherapy or chemotherapy could induce immunogenic apoptosis as well.
In the TME, drugs or cytotoxic immune cells induced apoptosis has long been considered as the primary way of cancer cell clearance in TME. Unfortunately, drugs showing potent anti-tumor potency in vitro mostly lost their cytotoxicity or quickly develop drug resistance in patients (69). Moreover, the immunosuppressive nature such as low pH, hypoxia, and ROS of the TME also mediates the exhaustion and apoptosis of cytotoxic immune cells at the same time, facilitating the growth of pro-tumoral immune cells such as Treg, M2 macrophage, and myeloid-derived suppressor cells (MDSC) (70–79). As a result, cancer cell apoptosis is commonly attenuated in the TME due to the loss of cytotoxic tumor immunity and/or apoptotic signals of cancer cells (77). Therefore, re-initiation of cancer cell-specific apoptosis in TME is one of the focuses of cancer study (80). For instance, Agonists such as APG350, AMP655 targeting TRAIL (tumor necrosis factor (TNF) related apoptosis-inducing ligand) receptor signaling could selectively induce cancer cell apoptosis in mice models but limited benefits was observed in cancer patients (81–86). It is also worth noticing that few studies evaluated the potential damage of chemotherapy or radiation to the immune cells. In fact, apoptosis of immune cells, such as cytotoxic T cells, can directly undermine the anti-tumor immunity in the TME (87, 88). Thus, careful assessments on different apoptosis-inducing strategies may pave a way for scientists to constrain or clear cancerous cells without compromising the anti-tumor immunity.
Necroptosis
Contrary to necrosis, necroptosis (89–91) belongs to PCD and can trigger inflammation in TME when apoptosis is prohibited (41, 51). Necroptosis differentiates itself from apoptosis in that its progression does not involve caspases activation. It is instead mediated by external signals that trigger activation of Receptor-Interacting Protein 1 (RIP1), RIP3, and Mixed-Lineage Kinase Domain-Like (MLKL) signaling cascade. MLKL pseudokinase is one of the main actors of necroptosis due to its ability to form membrane pores via polymerization and insertion into the plasma membrane. Notably, necroptosis involves the permeabilization of the lysosomal membrane followed by mitochondrial damage and ultimately ends in necrosis-like death both morphologically and biochemically.
Necroptosis is finely tuned and plays various functions. For instance, in physiological states, necroptosis mediates the formation of the mammalian bone plate, generation of megakaryocytes (92), and maintaining epithelial hemostasis (93). Necroptosis has been found to have both pro- and anti-tumor roles in TME (94). On one hand, low expression of necroptosis regulators RIP(K)3 and MLKL correlated with poor prognosis in various types of solid tumors (95–97). Specifically, necroptotic cells have been shown to promote dendric cell maturation (98) and determined cross-priming efficiency thus anti-tumor immunity of CD8+ T cells through RIPK1 and NF-κB signaling (99). In comparison, cells going through passive necrosis cannot effectively activate CD8+ T cells in vivo (99). Notably, RIP(K)3 deletion in mice impaired NKT cells’ cytotoxicity against tumors (100). Thus, triggering well-targeted necroptosis of cancer cells whilst activating cytotoxic T cells becomes one of the novel strategies in cancer therapy. Moreover, vaccination with necroptotic cancer cells could stimulate the maturation of dendritic cells, cross-priming of CD8+ T cells, and IFN-γ production, thereby enhancing anti-tumor immunity (101). On the other hand, inhibiting TCR restimulation-induced necroptosis in T cells could refresh the anti-tumor efficacy of T cells. Moreover, Endothelial cells necroptosis induced by tumor cells could in turn promote tumor metastasis (102). Similarly, necroptosis-induced signaling promotes macrophage-induced T cell suppression in pancreatic ductal adenocarcinoma (PDA) mice models (103). Recently, Jiao et al. demonstrated an elevated level of RIP(K)3-mediated MLKL phosphorylation in breast tumor necrotic area in late stages compared with early stages of breast cancer tumors. Meanwhile, lung metastasis was suppressed in MLKL deficient tumors, further correlating necroptosis with tumor metastasis (104). Thus, the “friend” or “foe” relationship between necroptosis and tumor immunity is highly context-dependent and needs to be carefully differentiated.
Pyroptosis
Pyroptosis (33, 105), similar to necroptosis, is an immunogenic PCD that results in the perforation of plasma membrane followed by the release of pro-inflammatory cellular components. It was first discovered in macrophages upon pathogen infection (106, 107). Since caspase cleavages also orchestrate apoptosis processes, pyroptosis phenotype on macrophage has long been mistaken for apoptosis until the discovery of gasdermin family proteins. Pyroptosis could be initiated through both the pathogen-associated molecular patterns (PAMPs)/danger-associated molecular patterns (DAMPs) activated canonical caspase-1 inflammasome pathway (27, 33, 105) and lipopolysaccharide (LPS) activated non-canonical caspase-4/5/11 inflammasome pathway (108, 109). Activated caspases cleave GSDMD and release its N-terminal fragments, which then oligomerize on the cellular membrane, leading to pore formation. In the meantime, caspase1 cleaves pro-IL-1β/IL-18 and releases the highly immunogenic IL-1β/IL-18 through the GSDMD pore (2, 27, 39, 110). In addition to the above pathways, recent studies indicated that caspase 3, which has long been considered the essential modulator of apoptosis, also regulates pyroptosis induction through GSDME cleavage (3, 111). This discovery further raises the possibility of caspase 3/GSDME signaling might act as a switch between apoptosis and pyroptosis, implying crosstalk of the two (112).
Pyroptosis also has both pro- and anti-tumor functions in regulating anti-tumor immunity in TMEs. Lower levels of caspase-1, IL-1β, and IL-18 were observed in hepatocellular carcinoma (HCC) tissues compared with adjacent normal ones (113), implying the role of pyroptosis in tumorigenicity. Being an immunogenic form of cell death, pyroptosis produces proinflammatory cytokines such as IL-1β, IL18 to facilitate the infiltration of immune cells to the immunosuppressive TME, demonstrating it can be utilized in anti-tumor therapy (114). It has been shown that Nlrp3 and caspase-1 deficient mice, lacking the ability to initiate effective pyroptosis, were more prone to chemical-induced colitis-associated colon cancer (CAC) than the wild type mice (115–117). Applying a bioorthogonal system, which helps investigate the pyroptotic processes in live animals, researchers found that the pyroptosis of less than 15% of cancer cells was enough to strengthen T cell response and eventually achieve the complete remission of solid tumor (118). Nanoparticles can be used as pyroptosis inducers as well, and thus potentiate antitumor immunity by enriching effector-memory T cells and inhibiting tumor growth and metastasis (119, 120). Cytotoxic immune cells such as natural killer cells and CD8+ T cells can also trigger cancer cell pyroptosis through lymphocyte-derived granzyme A (GZMA) or granzyme B (GZMB) but not caspases-mediated cleavage of the GSDM family proteins. The GZMA/GZMB triggered proteolytic cleavages subsequently activate the pyroptosis cascade, thus further recruiting more cytotoxic lymphocytes and amplifying the anti-tumor signals in TME (40, 121). Currently, there are attempts to utilize chemo- or radiotherapy to induce pyroptosis for cancer treatment (122). It should be mentioned here that apoptosis can convert into pyroptosis in the presence of TNF or chemotherapy treatment, in which GSDME cleavage by caspase plays a key role (3).
Alternatively, pyroptosis has also been implicated with cancer immune evasion in TME. Chronic inflammation induced by pro-inflammatory cytokines such as IL-1β, IL6, and IL-18, released via pyroptotic cell death, is considered to drive tumor progression and immune evasion (123, 124). Zhai et al. demonstrated the pro-tumoral aspect of NLRP1 inflammasomes, which promoted tumor growth by suppressing the apoptotic pathway (125). Furthermore, pyroptosis directly mediated immune cell death in cancer and other diseases. Although pyroptosis was initially discovered in macrophages (106) and neutrophils (126) as the host innate immune defense against pathogen invasion, pyroptosis of the adaptive immune cells (CD4+ T cells) was also observed in chronic HIV infected patients (127, 128). CARD8 inflammasome has been linked to T cell pyroptosis via the caspase-1-GSDMD axis (129). Notably, GSDM family gene expressions have been observed in various B, T leukemia cell lines according to Cancer Cell Line Encyclopedia (CCLE) database (Figure 1A). These observations imply that pyroptosis is not limited to innate immunity, adaptive immune cells adopt pyroptosis as well. Thus, careful evaluation of both pros and cons of pyroptosis during the design of cancer treatment strategy will be helpful for better clinical outcomes.
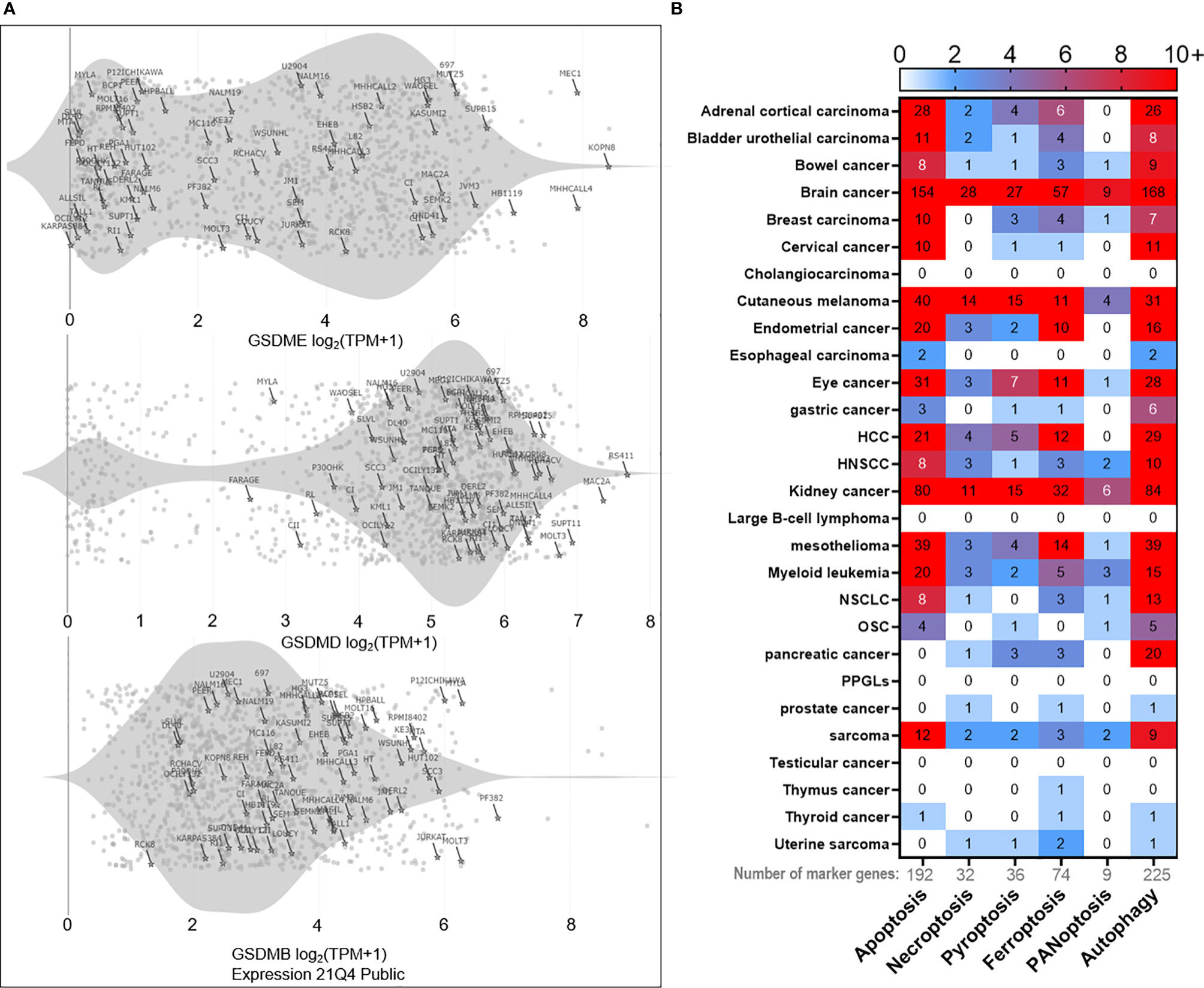
Figure 1 Bioinformatics of PCD landscape. (A) PCD occurs in immune cells as well. For instance, expressions of the pyroptosis marker gene ‘gasdermin’ family (GSDME, GSDMD, GSDMB) can be detected in various T and B leukemia cell lines (marked with the cell line name and highlighted with stars) according to Cancer Cell Line Encyclopedia (CCLE) database. The rest of the points represent other types of cell lines deposited in the database. The gray shade represents the distribution density of T and B cell lines. (B) Based on PCD marker genes (192 for apoptosis, 32 for necroptosis, 36 for pyroptosis, 74 for ferroptosis, 9 for PANoptosis, and 225 for autophagy), we analyzed the correlation between each marker gene and the overall survival in pan-cancer. Only those genes with p < 0.01 significance in correlation are used to plot the heatmap. The numeric mark in the heatmap means the number of marker genes that is significantly (p < 0.01) correlated with the overall survival. The results reveal heterogeneous contributions of each PCD in the overall survival across cancers. In brief, brain cancer ranks first in overall survival correlation with apoptosis, necroptosis, pyroptosis, ferroptosis, and PANoptosis, followed by kidney cancer, cutaneous melanoma, mesothelioma, adrenal cortical cancer, and others. As for autophagy, it positively correlates with the overall survival in most cancers, with brain cancer being the most significantly correlated as well. Clinical data are acquired from the TCGA database. Marker gene lists of respective PCD are provided in the Supplementary Excel File. HNSCC, squamous cell carcinoma of the head and neck; HCC, hepatocellular carcinoma; NSCLC, non-small cell lung cancer; OSC, ovarian serous cystic adenocarcinoma; PPGLs, pheochromocytomas, and paragangliomas.
Ferroptosis
Ferroptosis (130), another emerging immunogenic PCD, is initiated by the excessive accumulation of intracellular reactive oxygen species (ROS) which oxidize polyunsaturated fatty acids (PUFAs) on the plasma membrane in an iron-dependent manner, leading to lipid peroxidation-induced cellular membrane destruction. Glutathione peroxidase 4 (GPX4) is thus far the only enzyme known to prevent membrane lipid peroxidation. Ferroptosis occurs when the balance between the oxidation of the PUFAs and the detoxification of GPX4 is disrupted.
The induction of cancer cell ferroptosis in TME has been explored as a treatment alternative for cancers (131). Interestingly, recent discoveries indicated that cancer stem cells (CSCs) might be sensitive to ferroptosis due to their relatively strong dependency on the lipid intake pathways and higher intracellular iron levels compared with regular cancer cells (132, 133). Therefore, interference with GPX4 pathways seems to sensitize CSCs to ferroptosis (134–136). Furthermore, cytotoxic CD8+ T cells could enhance tumor cell lipid peroxidation caused by ferroptosis, thus achieving higher efficacy of PD1 checkpoint blockade therapy (137). Ferroptosis has also been found to play a crucial role in regulating T cell immunity. Lack of glutathione peroxidase 4 (Gpx4) in CD8+ and CD4+ T cells, the major scavenger of phospholipid hydroperoxide, induces ferroptosis and loss of protection from infection (138, 139) and might facilitate cancer development. Moreover, it’s shown that the overexpression of CD36, which is the fatty acid (AA) transporter on T cells, can lead to tumor-infiltrating CD8+ T cell ferroptosis through excessive lipid peroxidation and eventually impaired anti-tumor immunity (140). As for regulatory T cell (Treg) in TME, it’s well protected from ferroptosis by glutathione peroxidase 4 (Gpx4). Targeted ablation of Gpx4 in Treg inhibited tumor growth and potentiated anti-tumor immunity (141). Thus, the consequences of ferroptosis in the TME need to be carefully evaluated and interpreted (131, 142), which could be highly context-dependent for achieving a sound clinical outcome of anti-tumor therapy.
PANoptosis
From the above discussion, distinct and separate molecular pathways of apoptosis, pyroptosis, and necroptosis are described. Nonetheless, accumulating evidence indicated extensive cross-talk among these PCD pathways (36, 143–147). This led to the hypothesis that master regulators exist to orchestrate the interplay of different PCDs. Recently, the concept of PANoptosis PCD was established and shown to be able to incorporate and co-regulate apoptosis, pyroptosis, and necroptosis through the formation of PANoptosome as part of host innate immune defense (7, 47). PANoptosis could be triggered by the cooperative interactions of AIM2, pyrin, and ZBP1 that drives the formation of AIM2 PANoptosome, Specifically, the PANoptosome protein complex encompasses key signaling molecules of PCDs such as caspase-1, GSDMD, GSDME of pyroptosis, caspase-8, caspase-3, FADD of apoptosis, and RIPK3, MLKL of necroptosis. Therefore, the PANoptosome complex acts as a molecular scaffold to facilitate signal transduction and interplay among these PCDs, providing host protection against virus or bacterial infection (47). Meanwhile, excessive PANoptosis has been found to trigger cytokine release syndrome (CRS) (148) during SARS-CoV-2 infection (149). Lately, emerging studies highlighted the role of PANopotosis in tumorigenesis and anti-tumor therapy. For instance, IFNγ, together with TNFα, could induce PANoptosis in diverse cancer cell lines and reduced tumor size in an immunodeficient mice model (150). Moreover, blocking the interaction of ZBP1, (the key mediator in PANoptosis) with RIPK3 or deletion of key PANoptosis regulatory element IRF1 (Interferon regulatory factor 1) suppressed PANoptosis and promoted tumorigenesis in mice studies (151, 152). Therefore, harnessing the potent immunogenicity of PANoptosis might strengthen anti-tumor immunity in TME. It should be mentioned here that since PANoptosis is a newly established concept of PCD, further mechanistic exploration needs to be done at the single-cell level (single-cell multi-omics techniques etc.) to address the possibility that the observed “PANoptosis phenotype” is due to different cellular subclusters undergoing respective PCDs.
Autophagy
Autophagy (153, 154) is a surviving mechanism adopted by eukaryotic cells under nutrient stress conditions. The autophagic pathway starts with the formation of an autophagosome, a double-membrane structure, which contains autophagic components such as ATG proteins and cellular organelles. Autophagosome then fuses with lysosome for degradation to provide an extra energy source. This pathway could help recycle cellular nutrients and organelles to prevent nutritional stress-induced premature cell death. Although autophagy is normally considered as a pro-survival strategy adopted by cells, it has also been proposed as a “suicide” mechanism committed by cells, including malignant cells, through self-digestion (155). Evidence indicated that excessive autophagy can lead to cell death (autophagy-dependent cell death, ADCD) (156, 157). ADCD should not be mistaken or obscured with the autophagy-associated or autophagy-mediated cell deaths, which coincides with or triggers apoptosis, respectively. ADCD, on the other hand, is defined as ‘a form of regulated cell death that mechanistically depends on the autophagic machinery (or components thereof)’ according to the Nomenclature Committee of Cell Death (45, 57, 58). ADCD has critical physiological role in suppressing the oncogenic transformation by eliminating pre-cancerous cells and is an integral component of the tumor-suppressive machinery (158). However, autophagy is also considered to play crucial role in establishing resistance to cancer therapies (159). Pharmacological inhibition of autophagy slowed pancreatic tumor growths (160, 161). Autophagy can cross-talk with other PCD (e.g. apoptosis), and actively regulate both cancer metastasis (162) and anti-tumor immunity (163, 164). Evidence indicated that autophagy regulated survival, and memory formation of cytotoxic T cells (165–167). Meanwhile, TME has long been known as a nutrient-depleted environment, study indicated that the autophagy of cancer cells rescued itself from T cell-mediated cytotoxicity by blocking cytokine-induced apoptosis (168). Inhibiting cancer cell autophagy could facilitate cancer cell clearance in the TME (169). Interestingly, naïve T cells in ovarian cancer patients could not effectively engage in autophagy under TME challenge, but go through apoptosis instead, leading to poor anti-tumor immunity (170). Therefore, pharmacologic inhibition of overall autophagy in TME, regardless of which type of cells should be precisely targeted in the context of cancer therapy, might be problematic (171). Nevertheless, targeting autophagy might improve and/or synergize the efficacy of current cancer therapies.
Molecular Messengers Released by PCD Tune Tumor Immunity
The occurrence of PCD in the TME is accompanied by the release of intracellular components, including cytokines, small molecules, mtDNA (172), ncRNA (173, 174), and exosomes (175), etc. which are altogether involved in shaping the immune landscape of the TME. Subsequently, we focused on reviewing the effects of a few well-studied “end products” of immunogenic PCD on innate and adaptive immune cells in TME, mainly including cytokines (e.g. IL1) and small molecules (e.g. ATP).
Family Cytokines
As pro-inflammatory cytokines, IL1 family cytokines such as IL1β and IL18 belong to the “end products” of pyroptosis and PANoptosis (27, 47, 52, 176). IL1β is one of the biomarkers for pyroptosis since it is produced from caspase 1 cleavage of pro-IL1β and subsequently secreted from GSDM pores. IL1 signaling cascade activates dendritic cells and macrophages, professional antigen-presenting cells (APCs), as well as regulates Th1/Th17 differentiation of CD4+ T cell and CD8+ T cell effector function (177). Moreover, IL1 signaling disruption in myeloid cells leads to colorectal cancer progression (117, 178). Notably, IL1β also plays beneficial roles in the initiation of anti-tumor immunity in TME (179, 180). Similar to IFNγ, IL1β also plays both anti- and pro-tumoral roles in TME in a highly context-dependent manner. Accumulating evidence suggested the pro-tumoral role of IL1β across a wide range of cancer types (178, 181). This might be due to the increased level of IL1 cytokines, which leads to chronic inflammation and drives tumor development and progression via the stimulation of the epithelial-to-mesenchymal transition (182), the proliferation of cancer cells, and the enrichment of immunosuppressive cell populations in TME. In TME, the IL1 family creates a complex regulating network and orchestrates the local anti-tumor immunity (183). These dichotomous discoveries on the IL1 family emphasized comprehensive evaluations of the pro- and anti-tumor responses of therapies that focus on the induction of pyroptosis and PANoptosis will be necessary to clarify potential benefits and unexpected risks.
HMGB1
As a type of DAMP molecule released by immunogenic PCD, high-mobility group box 1 (HMGB1) is a chromatin-associated protein first identified in 1973 (184). Since it tightly binds to chromatin, it could only be secreted from cells with destructed membrane structure (185). Once secreted to the extracellular milieu, HMGB1 could interact with various cellular receptors and form complexes with immune activators, regulating both the innate and adaptive immune responses (186). For instance, through binding to the receptor of advanced glycation end products (RAGE) and Toll-like receptors (TLRs), HMGB1 could activate caspase 1 cleavage and induce macrophage pyroptosis (187). HMGB1 is a coactivator for NF-κB as well, regulating inflammatory gene expressions in mice macrophages via epigenetic chromatin remodulation (188). Similar to IL1β, HMGB1 is also involved in dendritic cell (DC) maturation, tumor antigen presentation (189), neutrophil polarization, and cytokine release in TME (190, 191). HMGB1 production positively correlates with tumor antigen-specific T cell response and thus could serve as a biomarker for patient prognosis (192, 193). HMGB1 signaling can directly trigger T (194, 195) and B lymphocytes (196) proliferation, and downregulate immunosuppressive CTLA4 and Foxp3 expression and IL-10 secretion in Tregs via the TLR pathway.
Nonetheless, HMGB1 plays immunosuppressive roles as well. For example, HMBG1 can facilitate the growth and differentiation of MDSCs to promote cancer progression in TME (197, 198). Evidence also indicated HMGB1, together with complement protein, could induce monocyte differentiation into anti-inflammatory macrophage M2, thus regulating immune homeostasis (199). Moreover, HMGB1 and its interaction with the RAGE receptor on tumor cells could also directly regulate tumor cell autophagy and result in HMGB1-mediated tumorigenesis (200). Furthermore, HGMB1 blockade inhibited tumor growth and could work synergistically with checkpoint immunotherapy (201). Interestingly, the expression level of HMGB1 gene is elevated in tumor specimens from TCGA database across almost all cancer types, but it does not significantly correlate with patient prognosis (http://gepia.cancer-pku.cn/). Therefore, the role of HMGB1 in TME needs to be further investigated.
ATP and Its Intermediates
Adenosine triphosphate (ATP) has long been considered as the intracellular currency inside living cells, fueling numerous biological processes. Therefore, the concentration of intracellular ATP (iATP) is very high, ranging from 1-10 mM (202). In stark contrast, the concentration of extracellular ATP (eATP) under normal physiological condition is comparatively low (nM range). The physiological level of eATP does not induce an immune response (203). However, in the context of immunogenic PCD, ATP can leak from the “porous” cells into the extracellular milieu and serve as a type of “alarmins” or “find‐me” and “eat‐me” signals to attract phagocytes. Thus, elevated eATP level is highly pro-inflammatory (204). Notably, eATP can be further converted into the immunosuppressive metabolite adenosine by CD39 and CD73 ectonucleotidases on the cellular membrane. A main function of the extracellular adenosine is to create an immunosuppressive tumor environment by inducing tumor-infiltrating macrophage proliferation (205), regulatory immune cell, and MDSC activation (206–209) while repressing the anti-tumor function of cytotoxic T cells (210, 211). Therefore, PCD-induced release of eATP, together with adenosine, forms an intricate modulatory network of tumor immunity in TME.
Other Immunogenic Molecules
Calreticulin, a calcium-binding chaperone protein, mainly resides in the endoplasmic reticulum (ER) but can translocate to the cellular membrane during immunogenic PCD. It can serve as a DAMP and “eat me” signal for antigen-presenting cells (APCs), thus is a proinflammatory element in TME. Genetic knockdown or antibody ablation of calreticulin attenuated the phagocytosis of cancer cells by APCs, resulting in the elimination of cancer cell immunogenicity (212). Interestingly, chemotherapy drugs can induce calreticulin exposure onto the cancer cell surface, leading to maturation of DC and activation of tumor-specific effector T cells (213).
Another ER chaperone is the heat shock protein (HSP) family, which exposes to the extracellular environment during immunogenic PCD. Like calreticulin, HSP was found to have an immunomodulatory role in the TME. For instance, recombinant rHsp70, combined with radiotherapy, can potentiate DC immunotherapy by inducing tumor-specific T cell response in mice models (214). Furthermore, several HSP protein cancer vaccines have been developed and are under clinical trials (215, 216). However, it’s reported that HSP protein has both pro- and anti-inflammatory functions in TME, implying a sophisticated role of HSP proteins play in regulating tumor immunity.
Together, DAMPs released during immunogenic PCD not only can act as immunogens which lead to pro-inflammatory immune response but also might cause chronic inflammation or immune suppression, thus leading to tumor progression. Thus, it is necessary to comprehensively weigh both the pros and cons of the immune and systemic consequences of immunogenic PCD in tumor therapy designs.
Clinical Benefits and Concerns of PCD
Currently, efforts have been made on designing chemo- or radiotherapies to induce immunogenic PCD in tumor cells. At least 19 clinical trials, mostly chemotherapies, have been completed or are underway in exploring the role of immunogenic PCD in cancer treatments (clinicaltrials.gov). Bleomycin (BLM) (217), Cyclophosphamide (CTX) (218), Shikonin (219), Anthracyclines (213), and Oxaliplatin (220) are examples of immunogenic PCD inducers being studied extensively. These chemo-drugs can stimulate DC maturation, subsequently affecting tumor antigen uptake and presentation of adaptive immune cells. In addition to chemotherapy, radiotherapy (221), phototherapy (222, 223), and targeted nano-drug delivery therapy (224, 225) can induce immunogenic PCD as well. Reports showed that combining a PCD-inducing regimen with immunotherapy could yield promising results (221, 226). However, chimeric antigen receptor T cell (CAR-T) therapy can trigger tumor cell pyroptosis-induced cytokine release syndrome (CRS), leading to mitigated benefits of the cell therapy (227). Moreover, PANoptosis, encompassing features of pyroptosis, has also been found to initiate CRS (148, 149), whether it also contributes to the CRS observed in CAR-T therapy awaits further investigation. Meanwhile, our previous clinical studies demonstrated allogeneic Vδ2+ γδ T cells transfers do not cause CRS, and possess a high safety profile and clinical benefits in terminal cancer patients (228, 229). Therefore, how to utilize PCD to design safe and effective therapy protocol requires further investigation.
Future Prospects
To vividly demonstrate the occurrence probability of respective PCD across cancer types, we profiled maker gene contributions of each PCD and generated a pan-cancer heatmap (Figure 1B). Interestingly, there are significant variations in terms of PCD occurrence in different cancer types, with brain cancer being the most PCD-prone one, prompting one to speculate cancer types might be a crucial factor in determining the response rate and efficacy of PCD-inducing treatments.
In summary, different types of PCD can be triggered by different or similar causes and lead to heterogeneous consequences in the TME, resulting in either immunogenic or non-immunogenic responses and eventually tumor regression or progression (Figure 2A). Importantly, we believe the TME is precisely regulated various types of PCD, including apoptosis, necroptosis, pyroptosis, ferroptosis, PANoptosis, autophagy, and others, as well as PCD-related cytokines, metabolites, and immunogenic molecules, which collaboratively participate in balancing the TME to enrich either anti-tumor effector immune cells (e.g. cytotoxic T cells, NK cells, Vγ9Vδ2 γδ T cells, and M1 macrophages) or regulatory immune cells (e.g. Tregs, MDSCs, Vγ9Vδ1 γδ T cells, and M2 macrophages), eventually lead to tumor regression or progress (Figure 2B). Though most of the current literatures focuses on exploring the role of cancer cell PCD plays in shaping the immune landscape of TME, recently, increasing evidence indicated that both immunogenic and non-immunogenic PCD of immune cells can compromise anti-tumor immunity. Specifically, Zou’s group made an insightful discovery that apoptotic Tregs can exert significantly higher immunosuppressive function than live Tregs (230). Moreover, ferroptosis induced by T cell lipid peroxidation weakened T cell immunity to both virus infection and tumor (138, 231). Similarly, the pyroptosis of CD4+ T cells led to immunodeficiency in HIV (127, 128). Thus, it’s imperative to further explore the immunological consequences of PCD of immune cells in the TME.
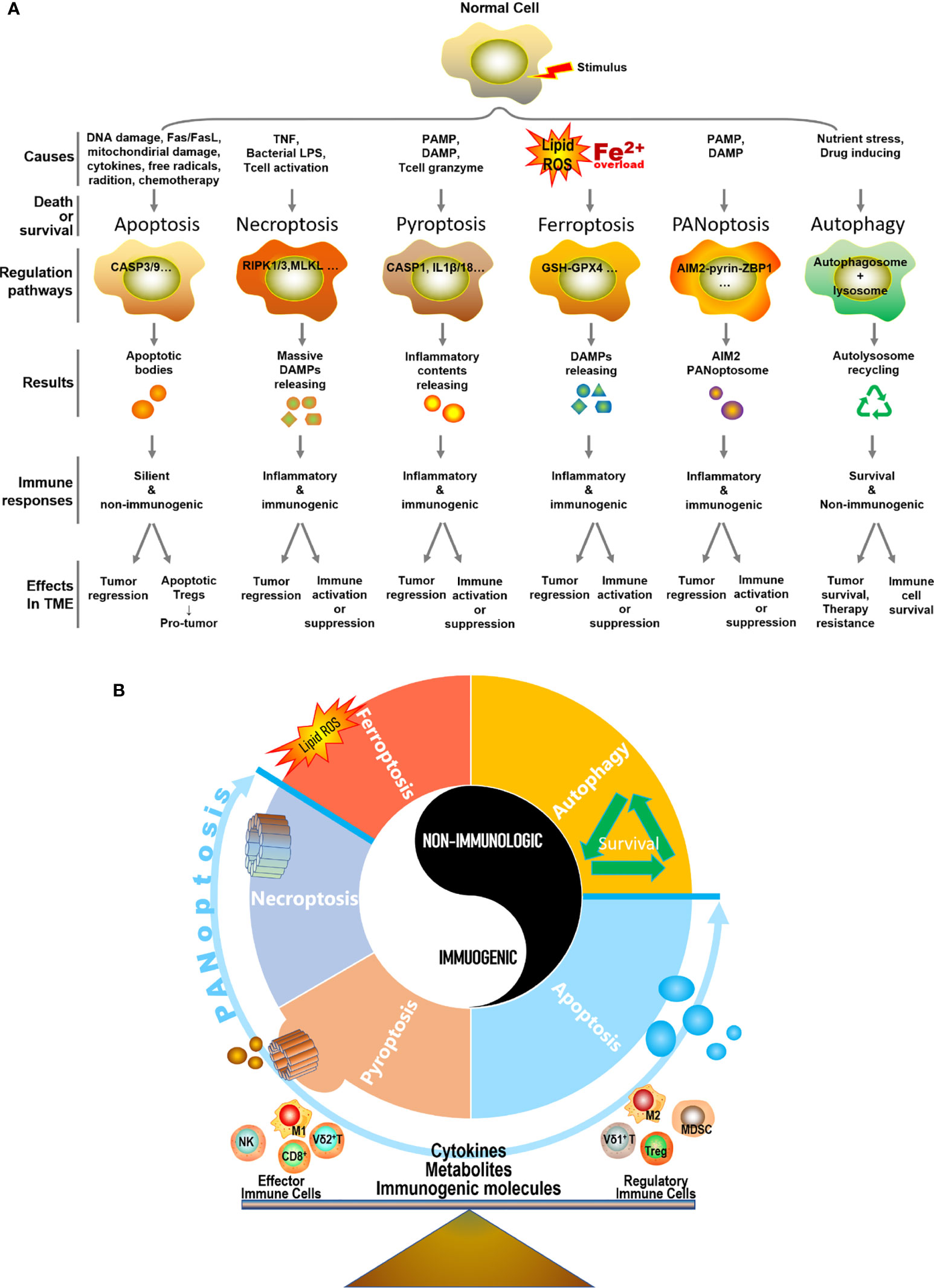
Figure 2 Distinctive hallmarks and mechanisms of five types of PCDs and autophagy. (A) In the context of different types of extracellular stimulus and intracellular signaling, a normal cell may undergo a specific type of cell death or survival. This process is precisely regulated by a set of genes and signaling molecules. Necroptosis, pyroptosis, ferroptosis, and PANoptosis represent four typical ways of immunologic PCD in the TME, which releases various cytokines, metabolites, and immunogenic molecules, thus leading to either tumor regression accompanied by immune activation or tumor progress along with immune suppression. For non-immunologic apoptosis in the TME, it generally connects with tumor regression, however, immune cells in the TME are routinely apoptosis-activated as well and implicate with the depressed immune microenvironment. Particularly, apoptotic regulatory T cell (Treg) can serve as a strong pro-tumor player in the TME. As for autophagy (self-survival dominantly) in the TME, it is closely linked with cancer cell survival, tumor progress, and therapy resistance. Meanwhile, immune cells also adopt autophagy strategy to survive in the stressful condition of the TME, and to eventually perform either pro-tumor or anti-tumor function depending on circumstances. (B) Apoptosis, necroptosis, pyroptosis, ferroptosis, PANoptosis, and autophagy, as well as their respective produced cytokines, metabolites, and immunogenic molecules in the TME, collaboratively participate in balancing the TME to enrich either anti-tumor effector immune cells or regulatory immune cells, eventually lead to tumor regression or progression.
To better understand PCD in the TME, many scientific questions remain to be resolved. A few are listed below.
1. How do the heterogeneous cell populations in TME sense and respond to PCD signals respectively?
2. Do different types of PCD have crosstalk in the TME? What might be the immunological consequences of PCD crosstalk?
3. How do PCDs induce the depletion/deficiency of anti-tumor effector immune cells, while enriching the suppressive immune cells in TME, thus creating a “cold” tumor?
4. How can we strengthen the anti-tumor immunity of immunologic PCDs at the same time avoiding chronic inflammation?
5. How would PCD of tumor-infiltrating immune cells affect the therapeutic efficacy and patient prognosis in different cancers?
Currently, although certain “biomarkers” or “morphological characteristics” were identified to differentiate individual PCD, it is hard to exquisitely extinguish them apart from one another. Therefore, it’s still difficult to develop highly targeted pharmacological inhibitors for each PCD without causing unwanted “off-target” effects. However, multi-omics technologies at the single-cell level allow us to clarify the characteristics of individual cells in the TME. This might greatly benefit researchers to gain thorough understanding of the above questions, which then facilitate the design of optimal cancer treatment strategies.
Author Contributions
Work supervision and project design, YH and YW. Bioinformatics, YH and YW. Literature compiling and summary, JL. Manuscript drafting, proof-reading, and revision: YH and YW. Discussion, MH, YL, and DC. All authors contributed to the article and approved the submitted version.
Funding
YH is supported by the National Natural Science Foundation of China (82002787). YW is supported by the Startup Foundation of the Zhuhai People’s Hospital (YNXM20210305), the Natural Science Foundation of Guangdong Province (2020A1515010132), and partially by the Key Program of the National Natural Science Foundation of China (32030036).
Conflict of Interest
The authors declare that the research was conducted in the absence of any commercial or financial relationships that could be construed as a potential conflict of interest.
Publisher’s Note
All claims expressed in this article are solely those of the authors and do not necessarily represent those of their affiliated organizations, or those of the publisher, the editors and the reviewers. Any product that may be evaluated in this article, or claim that may be made by its manufacturer, is not guaranteed or endorsed by the publisher.
Supplementary Material
The Supplementary Material for this article can be found online at: https://www.frontiersin.org/articles/10.3389/fimmu.2022.847345/full#supplementary-material
References
1. Kerr JF, Wyllie AH, Currie AR. Apoptosis: A Basic Biological Phenomenon With Wide-Ranging Implications in Tissue Kinetics. Br J Cancer (1972) 26(4):239–57. doi: 10.1038/bjc.1972.33
2. Orning P, Weng D, Starheim K, Ratner D, Best Z, Lee B, et al. Pathogen Blockade of TAK1 Triggers Caspase-8-Dependent Cleavage of Gasdermin D and Cell Death. Science (2018) 362(6418):1064–9. doi: 10.1126/science.aau2818
3. Wang Y, Gao W, Shi X, Ding J, Liu W, He H, et al. Chemotherapy Drugs Induce Pyroptosis Through Caspase-3 Cleavage of a Gasdermin. Nature (2017) 547(7661):99–103. doi: 10.1038/nature22393
4. Jorgensen I, Zhang Y, Krantz BA, Miao EA. Pyroptosis Triggers Pore-Induced Intracellular Traps (PITs) That Capture Bacteria and Lead to Their Clearance by Efferocytosis. J Exp Med (2016) 213(10):2113–28. doi: 10.1084/jem.20151613
5. Riegman M, Sagie L, Galed C, Levin T, Steinberg N, Dixon SJ, et al. Ferroptosis Occurs Through an Osmotic Mechanism and Propagates Independently of Cell Rupture. Nat Cell Biol (2020) 22(9):1042–8. doi: 10.1038/s41556-020-0565-1
6. Magtanong L, Ko PJ, Dixon SJ. Emerging Roles for Lipids in non-Apoptotic Cell Death. Cell Death Differ (2016) 23(7):1099–109. doi: 10.1038/cdd.2016.25
7. Malireddi RKS, Kesavardhana S, Kanneganti TD. ZBP1 and TAK1: Master Regulators of NLRP3 Inflammasome/Pyroptosis, Apoptosis, and Necroptosis (PAN-Optosis). Front Cell Infect Microbiol (2019) 9:406. doi: 10.3389/fcimb.2019.00406
8. Mizushima N, Komatsu M. Autophagy: Renovation of Cells and Tissues. Cell (2011) 147(4):728–41. doi: 10.1016/j.cell.2011.10.026
9. Xia H, Green DR, Zou W. Autophagy in Tumour Immunity and Therapy. Nat Rev Cancer (2021) 21(5):281–97. doi: 10.1038/s41568-021-00344-2
10. Zhang Y, Chen X, Gueydan C, Han J. Plasma Membrane Changes During Programmed Cell Deaths. Cell Res (2018) 28(1):9–21. doi: 10.1038/cr.2017.133
11. Chen X, He WT, Hu L, Li J, Fang Y, Wang X, et al. Pyroptosis is Driven by non-Selective Gasdermin-D Pore and its Morphology is Different From MLKL Channel-Mediated Necroptosis. Cell Res (2016) 26(9):1007–20. doi: 10.1038/cr.2016.100
12. Xie Y, Hou W, Song X, Yu Y, Huang J, Sun X, et al. Ferroptosis: Process and Function. Cell Death Differ (2016) 23(3):369–79. doi: 10.1038/cdd.2015.158
13. Latunde-Dada GO. Ferroptosis: Role of Lipid Peroxidation, Iron and Ferritinophagy. Biochim Biophys Acta Gen Subj (2017) 1861(8):1893–900. doi: 10.1016/j.bbagen.2017.05.019
14. Debnath J, Baehrecke EH, Kroemer G. Does Autophagy Contribute to Cell Death? Autophagy (2005) 1(2):66–74. doi: 10.4161/auto.1.2.1738
15. Wang C, Youle RJ. The Role of Mitochondria in Apoptosis*. Annu Rev Genet (2009) 43:95–118. doi: 10.1146/annurev-genet-102108-134850
16. Li Q, Shi N, Cai C, Zhang M, He J, Tan Y, et al. The Role of Mitochondria in Pyroptosis. Front Cell Dev Biol (2020) 8:630771. doi: 10.3389/fcell.2020.630771
17. Zhang YF, Zhou L, Mao HQ, Yang FH, Chen Z, Zhang L. Mitochondrial DNA Leakage Exacerbates Odontoblast Inflammation Through Gasdermin D-Mediated Pyroptosis. Cell Death Discovery (2021) 7(1):381. doi: 10.1038/s41420-021-00770-z
18. Wang H, Liu C, Zhao Y, Gao G. Mitochondria Regulation in Ferroptosis. Eur J Cell Biol (2020) 99(1):151058. doi: 10.1016/j.ejcb.2019.151058
19. Gao M, Yi J, Zhu J, Minikes AM, Monian P, Thompson CB, et al. Role of Mitochondria in Ferroptosis. Mol Cell (2019) 73(2):354–63.e3. doi: 10.1016/j.molcel.2018.10.042
20. Gan B. Mitochondrial Regulation of Ferroptosis. J Cell Biol (2021) 220(9):e202105043. doi: 10.1083/jcb.202105043
21. Wu C, Zhao W, Yu J, Li S, Lin L, Chen X. Induction of Ferroptosis and Mitochondrial Dysfunction by Oxidative Stress in PC12 Cells. Sci Rep (2018) 8(1):574. doi: 10.1038/s41598-017-18935-1
22. Battaglia AM, Chirillo R, Aversa I, Sacco A, Costanzo F, Biamonte F. Ferroptosis and Cancer: Mitochondria Meet the “Iron Maiden” Cell Death. Cells (2020) 9(6):1505. doi: 10.3390/cells9061505
23. Karch J, Kanisicak O, Brody MJ, Sargent MA, Michael DM, Molkentin JD. Necroptosis Interfaces With MOMP and the MPTP in Mediating Cell Death. PloS One (2015) 10(6):e0130520. doi: 10.1371/journal.pone.0130520
24. Rambold AS, Lippincott-Schwartz J. Mechanisms of Mitochondria and Autophagy Crosstalk. Cell Cycle (2011) 10(23):4032–8. doi: 10.4161/cc.10.23.18384
25. Lee J, Giordano S, Zhang J. Autophagy, Mitochondria and Oxidative Stress: Cross-Talk and Redox Signalling. Biochem J (2012) 441(2):523–40. doi: 10.1042/BJ20111451
26. Matassov D, Kagan T, Leblanc J, Sikorska M, Zakeri Z. Measurement of Apoptosis by DNA Fragmentation. Methods Mol Biol (2004) 282:1–17. doi: 10.1385/1-59259-812-9:001
27. Yu P, Zhang X, Liu N, Tang L, Peng C, Chen X. Pyroptosis: Mechanisms and Diseases. Signal Transduct Target Ther (2021) 6(1):128. doi: 10.1038/s41392-021-00507-5
28. Vande Walle L, Lamkanfi M. Pyroptosis. Curr Biol (2016) 26(13):R568–72. doi: 10.1016/j.cub.2016.02.019
29. Tang D, Chen X, Kang R, Kroemer G. Ferroptosis: Molecular Mechanisms and Health Implications. Cell Res (2021) 31(2):107–25. doi: 10.1038/s41422-020-00441-1
30. Shostak K, Jiang Z, Charloteaux B, Mayer A, Habraken Y, Tharun L, et al. The X-Linked Trichothiodystrophy-Causing Gene RNF113A Links the Spliceosome to Cell Survival Upon DNA Damage. Nat Commun (2020) 11(1):1270. doi: 10.1038/s41467-020-15003-7
31. Challa S, Chan FK. Going Up in Flames: Necrotic Cell Injury and Inflammatory Diseases. Cell Mol Life Sci (2010) 67(19):3241–53. doi: 10.1007/s00018-010-0413-8
32. Hewitt G, Korolchuk VI. Repair, Reuse, Recycle: The Expanding Role of Autophagy in Genome Maintenance. Trends Cell Biol (2017) 27(5):340–51. doi: 10.1016/j.tcb.2016.11.011
33. Fink SL, Cookson BT. Apoptosis, Pyroptosis, and Necrosis: Mechanistic Description of Dead and Dying Eukaryotic Cells. Infect Immun (2005) 73(4):1907–16. doi: 10.1128/IAI.73.4.1907-1916.2005
34. Dhuriya YK, Sharma D. Necroptosis: A Regulated Inflammatory Mode of Cell Death. J Neuroinflamm (2018) 15(1):199. doi: 10.1186/s12974-018-1235-0
35. Jung S, Jeong H, Yu SW. Autophagy as a Decisive Process for Cell Death. Exp Mol Med (2020) 52(6):921–30. doi: 10.1038/s12276-020-0455-4
36. Bertheloot D, Latz E, Franklin BS. Necroptosis, Pyroptosis and Apoptosis: An Intricate Game of Cell Death. Cell Mol Immunol (2021) 18(5):1106–21. doi: 10.1038/s41423-020-00630-3
37. Legrand AJ, Konstantinou M, Goode EF, Meier P. The Diversification of Cell Death and Immunity: Memento Mori. Mol Cell (2019) 76(2):232–42. doi: 10.1016/j.molcel.2019.09.006
38. Wang Y, Kanneganti TD. From Pyroptosis, Apoptosis and Necroptosis to PANoptosis: A Mechanistic Compendium of Programmed Cell Death Pathways. Comput Struct Biotechnol J (2021) 19:4641–57. doi: 10.1016/j.csbj.2021.07.038
39. Shi J, Zhao Y, Wang K, Shi X, Wang Y, Huang H, et al. Cleavage of GSDMD by Inflammatory Caspases Determines Pyroptotic Cell Death. Nature (2015) 526(7575):660–5. doi: 10.1038/nature15514
40. Zhou Z, He H, Wang K, Shi X, Wang Y, Su Y, et al. Granzyme A From Cytotoxic Lymphocytes Cleaves GSDMB to Trigger Pyroptosis in Target Cells. Science (2020) 368(6494):eaaz7548. doi: 10.1126/science.aaz7548
41. Feoktistova M, Leverkus M. Programmed Necrosis and Necroptosis Signalling. FEBS J (2015) 282(1):19–31. doi: 10.1111/febs.13120
42. Giampietri C, Starace D, Petrungaro S, Filippini A, Ziparo E. Necroptosis: Molecular Signalling and Translational Implications. Int J Cell Biol 2014 (2014) p:490275. doi: 10.1155/2014/490275
43. Zhou J, Tan SH, Nicolas V, Bauvy C, Yang ND, Zhang J, et al. Activation of Lysosomal Function in the Course of Autophagy via Mtorc1 Suppression and Autophagosome-Lysosome Fusion. Cell Res (2013) 23(4):508–23. doi: 10.1038/cr.2013.11
44. Green DR, Ferguson T, Zitvogel L, Kroemer G. Immunogenic and Tolerogenic Cell Death. Nat Rev Immunol (2009) 9(5):353–63. doi: 10.1038/nri2545
45. Galluzzi L, Vitale I, Aaronson SA, Abrams JM, Adam D, Agostinis P, et al. Molecular Mechanisms of Cell Death: Recommendations of the Nomenclature Committee on Cell Death 2018. Cell Death Differ (2018) 25(3):486–541. doi: 10.1038/s41418-018-0102-y
46. Tang D, Kang R, Berghe TV, Vandenabeele P, Kroemer G. The Molecular Machinery of Regulated Cell Death. Cell Res (2019) 29(5):347–64. doi: 10.1038/s41422-019-0164-5
47. Lee S, Karki R, Wang Y, Nguyen LN, Kalathur RC, Kanneganti TD. AIM2 Forms a Complex With Pyrin and ZBP1 to Drive PANoptosis and Host Defence. Nature (2021) 597(7876):415–9. doi: 10.1038/s41586-021-03875-8
48. Reed JC. Drug Insight: Cancer Therapy Strategies Based on Restoration of Endogenous Cell Death Mechanisms. Nat Clin Pract Oncol (2006) 3(7):388–98. doi: 10.1038/ncponc0538
49. Taylor RC, Cullen SP, Martin SJ. Apoptosis: Controlled Demolition at the Cellular Level. Nat Rev Mol Cell Biol (2008) 9(3):231–41. doi: 10.1038/nrm2312
50. Galluzzi L, Kepp O, Chan FK, Kroemer G. Necroptosis: Mechanisms and Relevance to Disease. Annu Rev Pathol (2017) 12:103–30. doi: 10.1146/annurev-pathol-052016-100247
51. Pasparakis M, Vandenabeele P. Necroptosis and its Role in Inflammation. Nature (2015) 517(7534):311–20. doi: 10.1038/nature14191
52. Bergsbaken T, Fink SL, Cookson BT. Pyroptosis: Host Cell Death and Inflammation. Nat Rev Microbiol (2009) 7(2):99–109. doi: 10.1038/nrmicro2070
53. Zheng J, Conrad M. The Metabolic Underpinnings of Ferroptosis. Cell Metab (2020) 32(6):920–37. doi: 10.1016/j.cmet.2020.10.011
54. Stockwell BR, Friedmann Angeli JP, Bayir H, Bush AI, Conrad M, Dixon SJ, et al. Ferroptosis: A Regulated Cell Death Nexus Linking Metabolism, Redox Biology, and Disease. Cell (2017) 171(2):273–85. doi: 10.1016/j.cell.2017.09.021
55. Chen X, Li J, Kang R, Klionsky DJ, Tang D. Ferroptosis: Machinery and Regulation. Autophagy (2021) 17(9):2054–81. doi: 10.1080/15548627.2020.1810918
56. Xu S, Min J, Wang F. Ferroptosis: An Emerging Player in Immune Cells. Sci Bull (2021) 66:2257–60. doi: 10.1016/j.scib.2021.02.026
57. Klionsky DJ, Abdelmohsen K, Abe A, Abedin MJ, Abeliovich H, Acevedo Arozena A, et al. Guidelines for the Use and Interpretation of Assays for Monitoring Autophagy (3rd Edition). Autophagy (2016) 12(1):1–222. doi: 10.1080/15548627.2015.1100356
58. Galluzzi L, Baehrecke EH, Ballabio A, Boya P, Bravo-San Pedro JM, Cecconi F, et al. Molecular Definitions of Autophagy and Related Processes. EMBO J (2017) 36(13):1811–36. doi: 10.15252/embj.201796697
59. Ellis HM, Horvitz HR. Genetic Control of Programmed Cell Death in the Nematode C. Elegans. Cell (1986) 44(6):817–29. doi: 10.1016/0092-8674(86)90004-8
60. Boldin MP, Varfolomeev EE, Pancer Z, Mett IL, Camonis JH, Wallach D. A Novel Protein That Interacts With the Death Domain of Fas/APO1 Contains a Sequence Motif Related to the Death Domain. J Biol Chem (1995) 270(14):7795–8. doi: 10.1074/jbc.270.14.7795
61. Chinnaiyan AM, O'Rourke K, Tewari M, Dixit VM. FADD, a Novel Death Domain-Containing Protein, Interacts With the Death Domain of Fas and Initiates Apoptosis. Cell (1995) 81(4):505–12. doi: 10.1016/0092-8674(95)90071-3
62. Kischkel FC, Hellbardt S, Behrmann I, Germer M, Pawlita M, Krammer PH, et al. Cytotoxicity-Dependent APO-1 (Fas/CD95)-Associated Proteins Form a Death-Inducing Signaling Complex (DISC) With the Receptor. EMBO J (1995) 14(22):5579–88. doi: 10.1002/j.1460-2075.1995.tb00245.x
63. Boldin MP, Goncharov TM, Goltsev YV, Wallach D. Involvement of MACH, a Novel MORT1/FADD-Interacting Protease, in Fas/APO-1- and TNF Receptor-Induced Cell Death. Cell (1996) 85(6):803–15. doi: 10.1016/S0092-8674(00)81265-9
64. Muzio M, Chinnaiyan AM, Kischkel FC, O'Rourke K, Shevchenko A, Ni J, et al. FLICE, a Novel FADD-Homologous ICE/CED-3-Like Protease, is Recruited to the CD95 (Fas/APO-1) Death–Inducing Signaling Complex. Cell (1996) 85(6):817–27. doi: 10.1016/S0092-8674(00)81266-0
65. Zou H, Henzel WJ, Liu X, Lutschg A, Wang X. Apaf-1, a Human Protein Homologous to C. Elegans CED-4, Participates in Cytochrome C-Dependent Activation of Caspase-3. Cell (1997) 90(3):405–13. doi: 10.1016/S0092-8674(00)80501-2
66. Giampazolias E, Zunino B, Dhayade S, Bock F, Cloix C, Cao K, et al. Mitochondrial Permeabilization Engages NF-kappaB-Dependent Anti-Tumour Activity Under Caspase Deficiency. Nat Cell Biol (2017) 19(9):1116–29. doi: 10.1038/ncb3596
67. Rongvaux A, Jackson R, Harman CC, Li T, West AP, de Zoete MR, et al. Apoptotic Caspases Prevent the Induction of Type I Interferons by Mitochondrial DNA. Cell (2014) 159(7):1563–77. doi: 10.1016/j.cell.2014.11.037
68. White MJ, McArthur K, Metcalf D, Lane RM, Cambier JC, Herold MJ, et al. Apoptotic Caspases Suppress mtDNA-Induced STING-Mediated Type I IFN Production. Cell (2014) 159(7):1549–62. doi: 10.1016/j.cell.2014.11.036
69. Yaacoub K, Pedeux R, Tarte K, Guillaudeux T. Role of the Tumor Microenvironment in Regulating Apoptosis and Cancer Progression. Cancer Lett (2016) 378(2):150–9. doi: 10.1016/j.canlet.2016.05.012
70. O’Donnell JS, Teng MWL, Smyth MJ. Cancer Immunoediting and Resistance to T Cell-Based Immunotherapy. Nat Rev Clin Oncol (2019) 16(3):151–67. doi: 10.1038/s41571-018-0142-8
71. Leone RD, Powell JD. Metabolism of Immune Cells in Cancer. Nat Rev Cancer (2020) 20(9):516–31. doi: 10.1038/s41568-020-0273-y
72. Bunse L, Pusch S, Bunse T, Sahm F, Sanghvi K, Friedrich M, et al. Suppression of Antitumor T Cell Immunity by the Oncometabolite (R)-2-Hydroxyglutarate. Nat Med (2018) 24(8):1192–203. doi: 10.3410/f.733617626.793550723
73. Zhao E, Maj T, Kryczek I, Li W, Wu K, Zhao L, et al. Cancer Mediates Effector T Cell Dysfunction by Targeting microRNAs and EZH2 via Glycolysis Restriction. Nat Immunol (2016) 17(1):95–103. doi: 10.1038/ni.3313
74. Xiao Z, Dai Z, Locasale JW. Metabolic Landscape of the Tumor Microenvironment at Single Cell Resolution. Nat Commun (2019) 10(1):3763. doi: 10.1038/s41467-019-11738-0
75. Li C, Jiang P, Wei S, Xu X, Wang J. Regulatory T Cells in Tumor Microenvironment: New Mechanisms, Potential Therapeutic Strategies and Future Prospects. Mol Cancer (2020) 19(1):116. doi: 10.1186/s12943-020-01234-1
76. Jiang Y, Li Y, Zhu B. T-Cell Exhaustion in the Tumor Microenvironment. Cell Death Dis (2015) 6:e1792. doi: 10.1038/cddis.2015.162
77. Hanahan D, Weinberg RA. Hallmarks of Cancer: The Next Generation. Cell (2011) 144(5):646–74. doi: 10.1016/j.cell.2011.02.013
78. Hanahan D. Hallmarks of Cancer: New Dimensions. Cancer Discovery (2022) 12(1):31–46. doi: 10.1158/2159-8290.CD-21-1059
79. Horton BL, Gajewski TF. Back From the Dead: TIL Apoptosis in Cancer Immune Evasion. Br J Cancer (2018) 118(3):309–11. doi: 10.1038/bjc.2017.483
80. Carneiro BA, El-Deiry WS. Targeting Apoptosis in Cancer Therapy. Nat Rev Clin Oncol (2020) 17(7):395–417. doi: 10.1038/s41571-020-0341-y
81. de Looff M, de Jong S, Kruyt FAE. Multiple Interactions Between Cancer Cells and the Tumor Microenvironment Modulate TRAIL Signaling: Implications for TRAIL Receptor Targeted Therapy. Front Immunol (2019) 10:1530. doi: 10.3389/fimmu.2019.01530
82. de Miguel D, Lemke J, Anel A, Walczak H, Martinez-Lostao L. Onto Better TRAILs for Cancer Treatment. Cell Death Differ (2016) 23(5):733–47. doi: 10.1038/cdd.2015.174
83. Legler K, Hauser C, Egberts JH, Willms A, Heneweer C, Boretius S, et al. The Novel TRAIL-Receptor Agonist APG350 Exerts Superior Therapeutic Activity in Pancreatic Cancer Cells. Cell Death Dis (2018) 9(5):445. doi: 10.1038/s41419-018-0478-0
84. Fuchs CS, Fakih M, Schwartzberg L, Cohn AL, Yee L, Dreisbach L, et al. TRAIL Receptor Agonist Conatumumab With Modified FOLFOX6 Plus Bevacizumab for First-Line Treatment of Metastatic Colorectal Cancer: A Randomized Phase 1b/2 Trial. Cancer (2013) 119(24):4290–8. doi: 10.1002/cncr.28353
85. Paz-Ares L, Balint B, de Boer RH, van Meerbeeck JP, Wierzbicki R, De Souza P, et al. A Randomized Phase 2 Study of Paclitaxel and Carboplatin With or Without Conatumumab for First-Line Treatment of Advanced non-Small-Cell Lung Cancer. J Thorac Oncol (2013) 8(3):329–37. doi: 10.1097/JTO.0b013e31827ce554
86. Kindler HL, Richards DA, Garbo LE, Garon EB, Stephenson JJ Jr, Rocha-Lima CM, et al. A Randomized, Placebo-Controlled Phase 2 Study of Ganitumab (AMG 479) or Conatumumab (AMG 655) in Combination With Gemcitabine in Patients With Metastatic Pancreatic Cancer. Ann Oncol (2012) 23(11):2834–42. doi: 10.1093/annonc/mds142
87. Horton BL, Williams JB, Cabanov A, Spranger S, Gajewski TF. Intratumoral CD8(+) T-Cell Apoptosis Is a Major Component of T-Cell Dysfunction and Impedes Antitumor Immunity. Cancer Immunol Res (2018) 6(1):14–24. doi: 10.1158/2326-6066.CIR-17-0249
88. Jarosz-Biej M, Smolarczyk R, Cichon T, Kulach N. Tumor Microenvironment as A “Game Changer” in Cancer Radiotherapy. Int J Mol Sci (2019) 20(13):3212. doi: 10.3390/ijms20133212
89. Degterev A, Huang Z, Boyce M, Li Y, Jagtap P, Mizushima N, et al. Chemical Inhibitor of Nonapoptotic Cell Death With Therapeutic Potential for Ischemic Brain Injury. Nat Chem Biol (2005) 1(2):112–9. doi: 10.1038/nchembio711
90. Degterev A, Hitomi J, Germscheid M, Ch'en IL, Korkina O, Teng X, et al. Identification of RIP1 Kinase as a Specific Cellular Target of Necrostatins. Nat Chem Biol (2008) 4(5):313–21. doi: 10.1038/nchembio.83
91. Galluzzi L, Kroemer G. Necroptosis: A Specialized Pathway of Programmed Necrosis. Cell (2008) 135(7):1161–3. doi: 10.1016/j.cell.2008.12.004
92. Moujalled D, Gangatirkar P, Kauppi M, Corbin J, Lebois M, Murphy JM, et al. The Necroptotic Cell Death Pathway Operates in Megakaryocytes, But Not in Platelet Synthesis. Cell Death Dis (2021) 12(1):133. doi: 10.1038/s41419-021-03418-z
93. Dannappel M, Vlantis K, Kumari S, Polykratis A, Kim C, Wachsmuth L, et al. RIPK1 Maintains Epithelial Homeostasis by Inhibiting Apoptosis and Necroptosis. Nature (2014) 513(7516):90–4. doi: 10.1038/nature13608
94. Qin X, Ma D, Tan YX, Wang HY, Cai Z. The Role of Necroptosis in Cancer: A Double-Edged Sword? Biochim Biophys Acta Rev Cancer (2019) 1871(2):259–66. doi: 10.1016/j.bbcan.2019.01.006
95. Park JE, Lee JH, Lee SY, Hong MJ, Choi JE, Park S, et al. Expression of Key Regulatory Genes in Necroptosis and its Effect on the Prognosis in non-Small Cell Lung Cancer. J Cancer (2020) 11(18):5503–10. doi: 10.7150/jca.46172
96. Bozec D, Iuga AC, Roda G, Dahan S, Yeretssian G. Critical Function of the Necroptosis Adaptor RIPK3 in Protecting From Intestinal Tumorigenesis. Oncotarget (2016) 7(29):46384–400. doi: 10.18632/oncotarget.10135
97. He L, Peng K, Liu Y, Xiong J, Zhu FF. Low Expression of Mixed Lineage Kinase Domain-Like Protein is Associated With Poor Prognosis in Ovarian Cancer Patients. Onco Targets Ther (2013) 6:1539–43. doi: 10.2147/OTT.S52805
98. Schmidt SV, Seibert S, Walch-Ruckheim B, Vicinus B, Kamionka EM, Pahne-Zeppenfeld J, et al. RIPK3 Expression in Cervical Cancer Cells is Required for PolyIC-Induced Necroptosis, IL-1alpha Release, and Efficient Paracrine Dendritic Cell Activation. Oncotarget (2015) 6(11):8635–47. doi: 10.18632/oncotarget.3249
99. Yatim N, Jusforgues-Saklani H, Orozco S, Schulz O, Barreira da Silva R, Reis e Sousa C, et al. RIPK1 and NF-kappaB Signaling in Dying Cells Determines Cross-Priming of CD8(+) T Cells. Science (2015) 350(6258):328–34. doi: 10.1126/science.aad0395
100. Kang YJ, Bang BR, Han KH, Hong L, Shim EJ, Ma J, et al. Regulation of NKT Cell-Mediated Immune Responses to Tumours and Liver Inflammation by Mitochondrial PGAM5-Drp1 Signalling. Nat Commun (2015) 6:8371. doi: 10.1038/ncomms9371
101. Aaes TL, Kaczmarek A, Delvaeye T, De Craene B, De Koker S, Heyndrickx L, et al. Vaccination With Necroptotic Cancer Cells Induces Efficient Anti-Tumor Immunity. Cell Rep (2016) 15(2):274–87. doi: 10.1016/j.celrep.2016.03.037
102. Strilic B, Yang L, Albarran-Juarez J, Wachsmuth L, Han K, Muller UC, et al. Tumour-Cell-Induced Endothelial Cell Necroptosis via Death Receptor 6 Promotes Metastasis. Nature (2016) 536(7615):215–8. doi: 10.1038/nature19076
103. Seifert L, Werba G, Tiwari S, Giao Ly NN, Alothman S, Alqunaibit D, et al. The Necrosome Promotes Pancreatic Oncogenesis via CXCL1 and Mincle-Induced Immune Suppression. Nature (2016) 532(7598):245–9. doi: 10.1038/nature17403
104. Jiao D, Cai Z, Choksi S, Ma D, Choe M, Kwon HJ, et al. Necroptosis of Tumor Cells Leads to Tumor Necrosis and Promotes Tumor Metastasis. Cell Res (2018) 28(8):868–70. doi: 10.1038/s41422-018-0058-y
105. Cookson BT, Brennan MA. Pro-Inflammatory Programmed Cell Death. Trends Microbiol (2001) 9(3):113–4. doi: 10.1016/S0966-842X(00)01936-3
106. Zychlinsky A, Prevost MC, Sansonetti PJ. Shigella Flexneri Induces Apoptosis in Infected Macrophages. Nature (1992) 358(6382):167–9. doi: 10.1038/358167a0
107. Shi J, Gao W, Shao F. Pyroptosis: Gasdermin-Mediated Programmed Necrotic Cell Death. Trends Biochem Sci (2017) 42(4):245–54. doi: 10.1016/j.tibs.2016.10.004
108. Kayagaki N, Wong MT, Stowe IB, Ramani SR, Gonzalez LC, Akashi-Takamura S, et al. Noncanonical Inflammasome Activation by Intracellular LPS Independent of TLR4. Science (2013) 341(6151):1246–9. doi: 10.1126/science.1240248
109. Downs KP, Nguyen H, Dorfleutner A, Stehlik C. An Overview of the non-Canonical Inflammasome. Mol Aspects Med (2020) 76:100924. doi: 10.1016/j.mam.2020.100924
110. Kayagaki N, Stowe IB, Lee BL, O'Rourke K, Anderson K, Warming S, et al. Caspase-11 Cleaves Gasdermin D for non-Canonical Inflammasome Signalling. Nature (2015) 526(7575):666–71. doi: 10.1038/nature15541
111. Yu J, Li S, Qi J, Chen Z, Wu Y, Guo J, et al. Cleavage of GSDME by Caspase-3 Determines Lobaplatin-Induced Pyroptosis in Colon Cancer Cells. Cell Death Dis (2019) 10(3):193. doi: 10.1038/s41419-019-1441-4
112. Jiang M, Qi L, Li L, Li Y. The Caspase-3/GSDME Signal Pathway as a Switch Between Apoptosis and Pyroptosis in Cancer. Cell Death Discovery (2020) 6:112. doi: 10.1038/s41420-020-00349-0
113. Chu Q, Jiang Y, Zhang W, Xu C, Du W, Tuguzbaeva G, et al. Pyroptosis is Involved in the Pathogenesis of Human Hepatocellular Carcinoma. Oncotarget (2016) 7(51):84658–65. doi: 10.18632/oncotarget.12384
114. Tan Y, Chen Q, Li X, Zeng Z, Xiong W, Li G, et al. Pyroptosis: A New Paradigm of Cell Death for Fighting Against Cancer. J Exp Clin Cancer Res (2021) 40(1):153. doi: 10.1186/s13046-021-01959-x
115. Dupaul-Chicoine J, Yeretssian G, Doiron K, Bergstrom KS, McIntire CR, LeBlanc PM, et al. Control of Intestinal Homeostasis, Colitis, and Colitis-Associated Colorectal Cancer by the Inflammatory Caspases. Immunity (2010) 32(3):367–78. doi: 10.1016/j.immuni.2010.02.012
116. Hu B, Elinav E, Huber S, Booth CJ, Strowig T, Jin C, et al. Inflammation-Induced Tumorigenesis in the Colon is Regulated by Caspase-1 and NLRC4. Proc Natl Acad Sci USA (2010) 107(50):21635–40. doi: 10.1073/pnas.1016814108
117. Allen IC, TeKippe EM, Woodford RM, Uronis JM, Holl EK, Rogers AB, et al. The NLRP3 Inflammasome Functions as a Negative Regulator of Tumorigenesis During Colitis-Associated Cancer. J Exp Med (2010) 207(5):1045–56. doi: 10.1084/jem.20100050
118. Wang Q, Wang Y, Ding J, Wang C, Zhou X, Gao W, et al. A Bioorthogonal System Reveals Antitumour Immune Function of Pyroptosis. Nature (2020) 579(7799):421–6. doi: 10.1038/s41586-020-2079-1
119. Ding B, Sheng J, Zheng P, Li C, Li D, Cheng Z, et al. Biodegradable Upconversion Nanoparticles Induce Pyroptosis for Cancer Immunotherapy. Nano Lett (2021) 21(19):8281–9. doi: 10.1021/acs.nanolett.1c02790
120. Zhao P, Wang M, Chen M, Chen Z, Peng X, Zhou F, et al. Programming Cell Pyroptosis With Biomimetic Nanoparticles for Solid Tumor Immunotherapy. Biomaterials (2020) 254:120142. doi: 10.1016/j.biomaterials.2020.120142
121. Zhang Z, Zhang Y, Xia S, Kong Q, Li S, Liu X, et al. Gasdermin E Suppresses Tumour Growth by Activating Anti-Tumour Immunity. Nature (2020) 579(7799):415–20. doi: 10.1038/s41586-020-2071-9
122. Johnson DC, Taabazuing CY, Okondo MC, Chui AJ, Rao SD, Brown FC, et al. DPP8/DPP9 Inhibitor-Induced Pyroptosis for Treatment of Acute Myeloid Leukemia. Nat Med (2018) 24(8):1151–6. doi: 10.1038/s41591-018-0082-y
123. Coussens LM, Werb Z. Inflammation and Cancer. Nature (2002) 420(6917):860–7. doi: 10.1038/nature01322
124. Greten FR, Grivennikov SI. Inflammation and Cancer: Triggers, Mechanisms, and Consequences. Immunity (2019) 51(1):27–41. doi: 10.1016/j.immuni.2019.06.025
125. Zhai Z, Liu W, Kaur M, Luo Y, Domenico J, Samson JM, et al. NLRP1 Promotes Tumor Growth by Enhancing Inflammasome Activation and Suppressing Apoptosis in Metastatic Melanoma. Oncogene (2017) 36(27):3820–30. doi: 10.1038/onc.2017.26
126. Liu L, Sun B. Neutrophil Pyroptosis: New Perspectives on Sepsis. Cell Mol Life Sci (2019) 76(11):2031–42. doi: 10.1007/s00018-019-03060-1
127. Doitsh G, Galloway NL, Geng X, Yang Z, Monroe KM, Zepeda O, et al. Cell Death by Pyroptosis Drives CD4 T-Cell Depletion in HIV-1 Infection. Nature (2014) 505(7484):509–14. doi: 10.1038/nature12940
128. Zhang C, Song JW, Huang HH, Fan X, Huang L, Deng JN, et al. NLRP3 Inflammasome Induces CD4+ T Cell Loss in Chronically HIV-1-Infected Patients. J Clin Invest (2021) 131(6):e138861. doi: 10.1172/JCI138861
129. Linder A, Bauernfried S, Cheng Y, Albanese M, Jung C, Keppler OT, et al. CARD8 Inflammasome Activation Triggers Pyroptosis in Human T Cells. EMBO J (2020) 39(19):e105071. doi: 10.15252/embj.2020105071
130. Dixon SJ, Lemberg KM, Lamprecht MR, Skouta R, Zaitsev EM, Gleason CE, et al. Ferroptosis: An Iron-Dependent Form of Nonapoptotic Cell Death. Cell (2012) 149(5):1060–72. doi: 10.1016/j.cell.2012.03.042
131. Friedmann Angeli JP, Krysko DV, Conrad M. Ferroptosis at the Crossroads of Cancer-Acquired Drug Resistance and Immune Evasion. Nat Rev Cancer (2019) 19(7):405–14. doi: 10.1038/s41568-019-0149-1
132. Bystrom LM, Guzman ML, Rivella S. Iron and Reactive Oxygen Species: Friends or Foes of Cancer Cells? Antioxid Redox Signal (2014) 20(12):1917–24. doi: 10.1089/ars.2012.5014
133. Wu S, Li T, Liu W, Huang Y. Ferroptosis and Cancer: Complex Relationship and Potential Application of Exosomes. Front Cell Dev Biol (2021) 9:733751. doi: 10.3389/fcell.2021.733751
134. Recalcati S, Gammella E, Cairo G. Dysregulation of Iron Metabolism in Cancer Stem Cells. Free Radic Biol Med (2019) 133:216–20. doi: 10.1016/j.freeradbiomed.2018.07.015
135. Visweswaran M, Arfuso F, Warrier S, Dharmarajan A. Aberrant Lipid Metabolism as an Emerging Therapeutic Strategy to Target Cancer Stem Cells. Stem Cells (2020) 38(1):6–14. doi: 10.1002/stem.3101
136. Cosialls E, El Hage R, Dos Santos L, Gong C, Mehrpour M, Hamai A. Ferroptosis: Cancer Stem Cells Rely on Iron Until “To Die for” it. Cells (2021) 10(11):2981. doi: 10.3390/cells10112981
137. Wang W, Green M, Choi JE, Gijon M, Kennedy PD, Johnson JK, et al. CD8(+) T Cells Regulate Tumour Ferroptosis During Cancer Immunotherapy. Nature (2019) 569(7755):270–4. doi: 10.1038/s41586-019-1170-y
138. Matsushita M, Freigang S, Schneider C, Conrad M, Bornkamm GW, Kopf M. T Cell Lipid Peroxidation Induces Ferroptosis and Prevents Immunity to Infection. J Exp Med (2015) 212(4):555–68. doi: 10.1084/jem.20140857
139. Yao Y, Chen Z, Zhang H, Chen C, Zeng M, Yunis J, et al. Selenium-GPX4 Axis Protects Follicular Helper T Cells From Ferroptosis. Nat Immunol (2021) 22(9):1127–39. doi: 10.1038/s41590-021-00996-0
140. Xu S, Chaudhary O, Rodriguez-Morales P, Sun X, Chen D, Zappasodi R, et al. Uptake of Oxidized Lipids by the Scavenger Receptor CD36 Promotes Lipid Peroxidation and Dysfunction in CD8(+) T Cells in Tumors. Immunity (2021) 54(7):1561–1577 e7. doi: 10.1016/j.immuni.2021.05.003
141. Xu C, Sun S, Johnson T, Qi R, Zhang S, Zhang J, et al. The Glutathione Peroxidase Gpx4 Prevents Lipid Peroxidation and Ferroptosis to Sustain Treg Cell Activation and Suppression of Antitumor Immunity. Cell Rep (2021) 35(11):109235. doi: 10.1016/j.celrep.2021.109235
142. Chen X, Kang R, Kroemer G, Tang D. Broadening Horizons: The Role of Ferroptosis in Cancer. Nat Rev Clin Oncol (2021) 18(5):280–96. doi: 10.1038/s41571-020-00462-0
143. Hou J, Zhao R, Xia W, Chang CW, You Y, Hsu JM, et al. PD-L1-Mediated Gasdermin C Expression Switches Apoptosis to Pyroptosis in Cancer Cells and Facilitates Tumour Necrosis. Nat Cell Biol (2020) 22(10):1264–75. doi: 10.1038/s41556-020-0575-z
144. Snyder AG, Oberst A. The Antisocial Network: Cross Talk Between Cell Death Programs in Host Defense. Annu Rev Immunol (2021) 39:77–101. doi: 10.1146/annurev-immunol-112019-072301
145. Doerflinger M, Deng Y, Whitney P, Salvamoser R, Engel S, Kueh AJ, et al. Flexible Usage and Interconnectivity of Diverse Cell Death Pathways Protect Against Intracellular Infection. Immunity (2020) 53(3):533–547 e7. doi: 10.1016/j.immuni.2020.07.004
146. Schwarzer R, Jiao H, Wachsmuth L, Tresch A, Pasparakis M. FADD and Caspase-8 Regulate Gut Homeostasis and Inflammation by Controlling MLKL- and GSDMD-Mediated Death of Intestinal Epithelial Cells. Immunity (2020) 52(6):978–993 e6. doi: 10.1016/j.immuni.2020.04.002
147. Taabazuing CY, Okondo MC, Bachovchin DA. Pyroptosis and Apoptosis Pathways Engage in Bidirectional Crosstalk in Monocytes and Macrophages. Cell Chem Biol (2017) 24(4):507–514 e4. doi: 10.1016/j.chembiol.2017.03.009
148. Karki R, Kanneganti TD. The ‘Cytokine Storm’: Molecular Mechanisms and Therapeutic Prospects. Trends Immunol (2021) 42(8):681–705. doi: 10.1016/j.it.2021.06.001
149. Karki R, Sharma BR, Tuladhar S, Williams EP, Zalduondo L, Samir P, et al. Synergism of TNF-Alpha and IFN-Gamma Triggers Inflammatory Cell Death, Tissue Damage, and Mortality in SARS-CoV-2 Infection and Cytokine Shock Syndromes. Cell (2021) 184(1):149–168 e17. doi: 10.1016/j.cell.2020.11.025
150. Malireddi RKS, Karki R, Sundaram B, Kancharana B, Lee S, Samir P, et al. Inflammatory Cell Death, PANoptosis, Mediated by Cytokines in Diverse Cancer Lineages Inhibits Tumor Growth. Immunohorizons (2021) 5(7):568–80. doi: 10.4049/immunohorizons.2100059
151. Karki R, Sundaram B, Sharma BR, Lee S, Malireddi RKS, Nguyen LN, et al. ADAR1 Restricts ZBP1-Mediated Immune Response and PANoptosis to Promote Tumorigenesis. Cell Rep (2021) 37(3):109858. doi: 10.1016/j.celrep.2021.109858
152. Karki R, Sharma BR, Lee E, Banoth B, Malireddi RKS, Samir P, et al. Interferon Regulatory Factor 1 Regulates PANoptosis to Prevent Colorectal Cancer. JCI Insight (2020) 5(12):e136720. doi: 10.1172/jci.insight.136720
153. Noda T, Suzuki K, Ohsumi Y. Yeast Autophagosomes: De Novo Formation of a Membrane Structure. Trends Cell Biol (2002) 12(5):231–5. doi: 10.1016/S0962-8924(02)02278-X
154. Harnett MM, Pineda MA, Latre de Late P, Eason RJ, Besteiro S, Harnett W, et al. From Christian De Duve to Yoshinori Ohsumi: More to Autophagy Than Just Dining at Home. BioMed J (2017) 40(1):9–22. doi: 10.1016/j.bj.2016.12.004
155. Strasser A, Vaux DL. Cell Death in the Origin and Treatment of Cancer. Mol Cell (2020) 78(6):1045–54. doi: 10.1016/j.molcel.2020.05.014
156. Tsujimoto Y, Shimizu S. Another Way to Die: Autophagic Programmed Cell Death. Cell Death Differ (2005) 12 Suppl 2:1528–34. doi: 10.1038/sj.cdd.4401777
157. Denton D, Kumar S. Autophagy-Dependent Cell Death. Cell Death Differ (2019) 26(4):605–16. doi: 10.1038/s41418-018-0252-y
158. Nassour J, Radford R, Correia A, Fuste JM, Schoell B, Jauch A, et al. Autophagic Cell Death Restricts Chromosomal Instability During Replicative Crisis. Nature (2019) 565(7741):659–63. doi: 10.1038/s41586-019-0885-0
159. Amaravadi RK, Kimmelman AC, Debnath J. Targeting Autophagy in Cancer: Recent Advances and Future Directions. Cancer Discovery (2019) 9(9):1167–81. doi: 10.1158/2159-8290.CD-19-0292
160. Yang A, Kimmelman AC. Inhibition of Autophagy Attenuates Pancreatic Cancer Growth Independent of TP53/TRP53 Status. Autophagy (2014) 10(9):1683–4. doi: 10.4161/auto.29961
161. Yang S, Wang X, Contino G, Liesa M, Sahin E, Ying H, et al. Pancreatic Cancers Require Autophagy for Tumor Growth. Genes Dev (2011) 25(7):717–29. doi: 10.1101/gad.2016111
162. Su Z, Yang Z, Xu Y, Chen Y, Yu Q. Apoptosis, Autophagy, Necroptosis, and Cancer Metastasis. Mol Cancer (2015) 14:48. doi: 10.1186/s12943-015-0321-5
163. Maiuri MC, Zalckvar E, Kimchi A, Kroemer G. Self-Eating and Self-Killing: Crosstalk Between Autophagy and Apoptosis. Nat Rev Mol Cell Biol (2007) 8(9):741–52. doi: 10.1038/nrm2239
164. Mukhopadhyay S, Panda PK, Sinha N, Das DN, Bhutia SK. Autophagy and Apoptosis: Where do They Meet? Apoptosis (2014) 19(4):555–66. doi: 10.1007/s10495-014-0967-2
165. Dowling SD, Macian F. Autophagy and T Cell Metabolism. Cancer Lett (2018) 419:20–6. doi: 10.1016/j.canlet.2018.01.033
166. Puleston DJ, Zhang H, Powell TJ, Lipina E, Sims S, Panse I, et al. Autophagy is a Critical Regulator of Memory CD8(+) T Cell Formation. Elife (2014) 3:e03706. doi: 10.7554/eLife.03706
167. Xu X, Araki K, Li S, Han JH, Ye L, Tan WG, et al. Autophagy is Essential for Effector CD8(+) T Cell Survival and Memory Formation. Nat Immunol (2014) 15(12):1152–61. doi: 10.1038/ni.3025
168. Young TM, Reyes C, Pasnikowski E, Castanaro C, Wong C, Decker CE, et al. Autophagy Protects Tumors From T Cell-Mediated Cytotoxicity via Inhibition of TNFalpha-Induced Apoptosis. Sci Immunol (2020) 5(54):eabb9561. doi: 10.1126/sciimmunol.abb9561
169. Pellegrini P, Strambi A, Zipoli C, Hagg-Olofsson M, Buoncervello M, Linder S, et al. Acidic Extracellular pH Neutralizes the Autophagy-Inhibiting Activity of Chloroquine: Implications for Cancer Therapies. Autophagy (2014) 10(4):562–71. doi: 10.4161/auto.27901
170. Xia H, Wang W, Crespo J, Kryczek I, Li W, Wei S, et al. Suppression of FIP200 and Autophagy by Tumor-Derived Lactate Promotes Naive T Cell Apoptosis and Affects Tumor Immunity. Sci Immunol (2017) 2(17):eaan4631. doi: 10.1126/sciimmunol.aan4631
171. Bryant KL, Stalnecker CA, Zeitouni D, Klomp JE, Peng S, Tikunov AP, et al. Combination of ERK and Autophagy Inhibition as a Treatment Approach for Pancreatic Cancer. Nat Med (2019) 25(4):628–40. doi: 10.1038/s41591-019-0368-8
172. Oresta B, Pozzi C, Braga D, Hurle R, Lazzeri M, Colombo P, et al. Mitochondrial Metabolic Reprogramming Controls the Induction of Immunogenic Cell Death and Efficacy of Chemotherapy in Bladder Cancer. Sci Transl Med (2021) 13(575):eaba6110. doi: 10.1126/scitranslmed.aba6110
173. He X, Qi Y, Zhang X, Liu X, Li X, Li S, et al. Current Landscape of Tumor-Derived Exosomal ncRNAs in Glioma Progression, Detection, and Drug Resistance. Cell Death Dis (2021) 12(12):1145. doi: 10.1038/s41419-021-04430-z
174. Villegas-Pineda JC, Lizarazo-Taborda MDR, Ramirez-de-Arellano A, Pereira-Suarez AL. Exosomal miRNAs and lncRNAs: The Modulator Keys of Cancer-Associated Fibroblasts in the Genesis and Progression of Malignant Neoplasms. Front Cell Dev Biol (2021) 9:717478. doi: 10.3389/fcell.2021.717478
175. Abu N, Rus Bakarurraini NAA. The Interweaving Relationship Between Extracellular Vesicles and T Cells in Cancer. Cancer Lett (2021) 12. doi: 10.3389/fimmu.2021.740548
176. Man SM, Karki R, Kanneganti TD. Molecular Mechanisms and Functions of Pyroptosis, Inflammatory Caspases and Inflammasomes in Infectious Diseases. Immunol Rev (2017) 277(1):61–75. doi: 10.1111/imr.12534
177. Ben-Sasson SZ, Hogg A, Hu-Li J, Wingfield P, Chen X, Crank M, et al. IL-1 Enhances Expansion, Effector Function, Tissue Localization, and Memory Response of Antigen-Specific CD8 T Cells. J Exp Med (2013) 210(3):491–502. doi: 10.1084/jem.20122006
178. Baker KJ, Houston A, Brint E. IL-1 Family Members in Cancer; Two Sides to Every Story. Front Immunol (2019) 10:1197. doi: 10.3389/fimmu.2019.01197
179. Haabeth OA, Lorvik KB, Hammarstrom C, Donaldson IM, Haraldsen G, Bogen B, et al. Inflammation Driven by Tumour-Specific Th1 Cells Protects Against B-Cell Cancer. Nat Commun (2011) 2:240. doi: 10.1038/ncomms1239
180. Haabeth OA, Lorvik KB, Yagita H, Bogen B, Corthay A. Interleukin-1 is Required for Cancer Eradication Mediated by Tumor-Specific Th1 Cells. Oncoimmunology (2016) 5(1):e1039763. doi: 10.1080/2162402X.2015.1039763
181. Apte RN, Krelin Y, Song X, Dotan S, Recih E, Elkabets M, et al. Effects of Micro-Environment- and Malignant Cell-Derived Interleukin-1 in Carcinogenesis, Tumour Invasiveness and Tumour-Host Interactions. Eur J Cancer (2006) 42(6):751–9. doi: 10.1016/j.ejca.2006.01.010
182. Li R, Ong SL, Tran LM, Jing Z, Liu B, Park SJ, et al. Chronic IL-1beta-Induced Inflammation Regulates Epithelial-to-Mesenchymal Transition Memory Phenotypes via Epigenetic Modifications in non-Small Cell Lung Cancer. Sci Rep (2020) 10(1):377. doi: 10.1038/s41598-019-57285-y
183. Mantovani A, Dinarello CA, Molgora M, Garlanda C. Interleukin-1 and Related Cytokines in the Regulation of Inflammation and Immunity. Immunity (2019) 50(4):778–95. doi: 10.1016/j.immuni.2019.03.012
184. Goodwin GH, Sanders C, Johns EW. A New Group of Chromatin-Associated Proteins With a High Content of Acidic and Basic Amino Acids. Eur J Biochem (1973) 38(1):14–9. doi: 10.1111/j.1432-1033.1973.tb03026.x
185. Scaffidi P, Misteli T, Bianchi ME. Release of Chromatin Protein HMGB1 by Necrotic Cells Triggers Inflammation. Nature (2002) 418(6894):191–5. doi: 10.1038/nature00858
186. Kang R, Zhang Q, Zeh HJ, MT L, Tang D. HMGB1 in Cancer: Good, Bad, or Both? Clin Cancer Res (2013) 19(15):4046–57. doi: 10.1158/1078-0432.CCR-13-0495
187. Xu J, Jiang Y, Wang J, Shi X, Liu Q, Liu Z, et al. Macrophage Endocytosis of High-Mobility Group Box 1 Triggers Pyroptosis. Cell Death Differ (2014) 21(8):1229–39. doi: 10.1038/cdd.2014.40
188. Ma S, Ming Z, Gong AY, Wang Y, Chen X, Hu G, et al. A Long Noncoding RNA, lincRNA-Tnfaip3, Acts as a Coregulator of NF-kappaB to Modulate Inflammatory Gene Transcription in Mouse Macrophages. FASEB J (2017) 31(3):1215–25. doi: 10.1096/fj.201601056R
189. Gao Q, Li F, Wang S, Shen Z, Cheng S, Ping Y, et al. A Cycle Involving HMGB1, IFN-Gamma and Dendritic Cells Plays a Putative Role in Anti-Tumor Immunity. Cell Immunol (2019) 343:103850. doi: 10.1016/j.cellimm.2018.08.011
190. Wang Z, Yang C, Li L, Jin X, Zhang Z, Zheng H, et al. Tumor-Derived HMGB1 Induces CD62L(dim) Neutrophil Polarization and Promotes Lung Metastasis in Triple-Negative Breast Cancer. Oncogenesis (2020) 9(9):82. doi: 10.1038/s41389-020-00267-x
191. Zha C, Meng X, Li L, Mi S, Qian D, Li Z, et al. Neutrophil Extracellular Traps Mediate the Crosstalk Between Glioma Progression and the Tumor Microenvironment via the HMGB1/RAGE/IL-8 Axis. Cancer Biol Med (2020) 17(1):154–68. doi: 10.20892/j.issn.2095-3941.2019.0353
192. Suzuki Y, Mimura K, Yoshimoto Y, Watanabe M, Ohkubo Y, Izawa S, et al. Immunogenic Tumor Cell Death Induced by Chemoradiotherapy in Patients With Esophageal Squamous Cell Carcinoma. Cancer Res (2012) 72(16):3967–76. doi: 10.1158/0008-5472.CAN-12-0851
193. Huang CY, Chiang SF, Ke TW, Chen TW, Lan YC, You YS, et al. Cytosolic High-Mobility Group Box Protein 1 (HMGB1) and/or PD-1+ TILs in the Tumor Microenvironment may be Contributing Prognostic Biomarkers for Patients With Locally Advanced Rectal Cancer Who Have Undergone Neoadjuvant Chemoradiotherapy. Cancer Immunol Immunother (2018) 67(4):551–62. doi: 10.1007/s00262-017-2109-5
194. Sundberg E, Fasth AE, Palmblad K, Harris HE, Andersson U. High Mobility Group Box Chromosomal Protein 1 Acts as a Proliferation Signal for Activated T Lymphocytes. Immunobiology (2009) 214(4):303–9. doi: 10.1016/j.imbio.2008.09.006
195. Li G, Liang X, Lotze MT. HMGB1: The Central Cytokine for All Lymphoid Cells. Front Immunol (2013) 4:68. doi: 10.3389/fimmu.2013.00068
196. Avalos AM, Kiefer K, Tian J, Christensen S, Shlomchik M, Coyle AJ, et al. RAGE-Independent Autoreactive B Cell Activation in Response to Chromatin and HMGB1/DNA Immune Complexes. Autoimmunity (2010) 43(1):103–10. doi: 10.3109/08916930903384591
197. Parker KH, Sinha P, Horn LA, Clements VK, Yang H, Li J, et al. HMGB1 Enhances Immune Suppression by Facilitating the Differentiation and Suppressive Activity of Myeloid-Derived Suppressor Cells. Cancer Res (2014) 74(20):5723–33. doi: 10.1158/0008-5472.CAN-13-2347
198. Parker KH, Horn LA, Ostrand-Rosenberg S. High-Mobility Group Box Protein 1 Promotes the Survival of Myeloid-Derived Suppressor Cells by Inducing Autophagy. J Leukoc Biol (2016) 100(3):463–70. doi: 10.1189/jlb.3HI0715-305R
199. Son M, Porat A, He M, Suurmond J, Santiago-Schwarz F, Andersson U, et al. C1q and HMGB1 Reciprocally Regulate Human Macrophage Polarization. Blood (2016) 128(18):2218–28. doi: 10.1182/blood-2016-05-719757
200. Khambu B, Hong H, Liu S, Liu G, Chen X, Dong Z, et al. The HMGB1-RAGE Axis Modulates the Growth of Autophagy-Deficient Hepatic Tumors. Cell Death Dis (2020) 11(5):333. doi: 10.1038/s41419-020-2536-7
201. Hubert P, Roncarati P, Demoulin S, Pilard C, Ancion M, Reynders C, et al. Extracellular HMGB1 Blockade Inhibits Tumor Growth Through Profoundly Remodeling Immune Microenvironment and Enhances Checkpoint Inhibitor-Based Immunotherapy. J Immunother Cancer (2021) 9(3):e001966. doi: 10.1136/jitc-2020-001966
202. Huang H, Zhang X, Li S, Liu N, Lian W, McDowell E, et al. Physiological Levels of ATP Negatively Regulate Proteasome Function. Cell Res (2010) 20(12):1372–85. doi: 10.1038/cr.2010.123
203. Trabanelli S, Ocadlikova D, Gulinelli S, Curti A, Salvestrini V, Vieira RP, et al. Extracellular ATP Exerts Opposite Effects on Activated and Regulatory CD4+ T Cells via Purinergic P2 Receptor Activation. J Immunol (2012) 189(3):1303–10. doi: 10.4049/jimmunol.1103800
204. Cauwels A, Rogge E, Vandendriessche B, Shiva S, Brouckaert P. Extracellular ATP Drives Systemic Inflammation, Tissue Damage and Mortality. Cell Death Dis (2014) 5:e1102. doi: 10.1038/cddis.2014.70
205. Wang J, Wang Y, Chu Y, Li Z, Yu X, Huang Z, et al. Tumor-Derived Adenosine Promotes Macrophage Proliferation in Human Hepatocellular Carcinoma. J Hepatol (2021) 74(3):627–37. doi: 10.1016/j.jhep.2020.10.021
206. Ni C, Fang QQ, Chen WZ, Jiang JX, Jiang Z, Ye J, et al. Breast Cancer-Derived Exosomes Transmit lncRNA SNHG16 to Induce CD73+gammadelta1 Treg Cells. Signal Transduct Target Ther (2020) 5(1):41. doi: 10.1038/s41392-020-0129-7
207. Ernst PB, Garrison JC, Thompson LF. Much Ado About Adenosine: Adenosine Synthesis and Function in Regulatory T Cell Biology. J Immunol (2010) 185(4):1993–8. doi: 10.4049/jimmunol.1000108
208. Neo SY, Yang Y, Record J, Ma R, Chen X, Chen Z, et al. CD73 Immune Checkpoint Defines Regulatory NK Cells Within the Tumor Microenvironment. J Clin Invest (2020) 130(3):1185–98. doi: 10.1172/JCI128895
209. Groth C, Hu X, Weber R, Fleming V, Altevogt P, Utikal J, et al. Immunosuppression Mediated by Myeloid-Derived Suppressor Cells (MDSCs) During Tumour Progression. Br J Cancer (2019) 120(1):16–25. doi: 10.1038/s41416-018-0333-1
210. Cekic C, Linden J. Adenosine A2A Receptors Intrinsically Regulate CD8+ T Cells in the Tumor Microenvironment. Cancer Res (2014) 74(24):7239–49. doi: 10.1158/0008-5472.CAN-13-3581
211. Young A, Ngiow SF, Barkauskas DS, Sult E, Hay C, Blake SJ, et al. Co-Inhibition of CD73 and A2AR Adenosine Signaling Improves Anti-Tumor Immune Responses. Cancer Cell (2016) 30(3):391–403. doi: 10.1016/j.ccell.2016.06.025
212. Obeid M, Tesniere A, Ghiringhelli F, Fimia GM, Apetoh L, Perfettini JL, et al. Calreticulin Exposure Dictates the Immunogenicity of Cancer Cell Death. Nat Med (2007) 13(1):54–61. doi: 10.1038/nm1523
213. Fucikova J, Kralikova P, Fialova A, Brtnicky T, Rob L, Bartunkova J, et al. Human Tumor Cells Killed by Anthracyclines Induce a Tumor-Specific Immune Response. Cancer Res (2011) 71(14):4821–33. doi: 10.1158/0008-5472.CAN-11-0950
214. Wang YS, Liu SJ, Huang SC, Chang CC, Huang YC, Fong WL, et al. Recombinant Heat Shock Protein 70 in Combination With Radiotherapy as a Source of Tumor Antigens to Improve Dendritic Cell Immunotherapy. Front Oncol (2012) 2:149. doi: 10.3389/fonc.2012.00149
215. Shimizu Y, Yoshikawa T, Kojima T, Shoda K, Nosaka K, Mizuno S, et al. Heat Shock Protein 105 Peptide Vaccine Could Induce Antitumor Immune Reactions in a Phase I Clinical Trial. Cancer Sci (2019) 110(10):3049–60. doi: 10.1111/cas.14165
216. Kelly M, McNeel D, Fisch P, Malkovsky M. Immunological Considerations Underlying Heat Shock Protein-Mediated Cancer Vaccine Strategies. Immunol Lett (2018) 193:1–10. doi: 10.1016/j.imlet.2017.11.001
217. Bugaut H, Bruchard M, Berger H, Derangere V, Odoul L, Euvrard R, et al. Bleomycin Exerts Ambivalent Antitumor Immune Effect by Triggering Both Immunogenic Cell Death and Proliferation of Regulatory T Cells. PloS One (2013) 8(6):e65181. doi: 10.1371/journal.pone.0065181
218. Schiavoni G, Sistigu A, Valentini M, Mattei F, Sestili P, Spadaro F, et al. Cyclophosphamide Synergizes With Type I Interferons Through Systemic Dendritic Cell Reactivation and Induction of Immunogenic Tumor Apoptosis. Cancer Res (2011) 71(3):768–78. doi: 10.1158/0008-5472.CAN-10-2788
219. Chen HM, Wang PH, Chen SS, Wen CC, Chen YH, Yang WC, et al. Shikonin Induces Immunogenic Cell Death in Tumor Cells and Enhances Dendritic Cell-Based Cancer Vaccine. Cancer Immunol Immunother (2012) 61(11):1989–2002. doi: 10.1007/s00262-012-1258-9
220. Tesniere A, Schlemmer F, Boige V, Kepp O, Martins I, Ghiringhelli F, et al. Immunogenic Death of Colon Cancer Cells Treated With Oxaliplatin. Oncogene (2010) 29(4):482–91. doi: 10.1038/onc.2009.356
221. Hwang WL, Pike LRG, Royce TJ, Mahal BA, Loeffler JS. Safety of Combining Radiotherapy With Immune-Checkpoint Inhibition. Nat Rev Clin Oncol (2018) 15(8):477–94. doi: 10.1038/s41571-018-0046-7
222. Garg AD, Krysko DV, Verfaillie T, Kaczmarek A, Ferreira GB, Marysael T, et al. A Novel Pathway Combining Calreticulin Exposure and ATP Secretion in Immunogenic Cancer Cell Death. EMBO J (2012) 31(5):1062–79. doi: 10.1038/emboj.2011.497
223. Galluzzi L, Kepp O, Kroemer G. Enlightening the Impact of Immunogenic Cell Death in Photodynamic Cancer Therapy. EMBO J (2012) 31(5):1055–7. doi: 10.1038/emboj.2012.2
224. Huang J, Xiao Z, An Y, Han S, Wu W, Wang Y, et al. Nanodrug With Dual-Sensitivity to Tumor Microenvironment for Immuno-Sonodynamic Anti-Cancer Therapy. Biomaterials (2021) 269:120636. doi: 10.1016/j.biomaterials.2020.120636
225. Ding D, Zhong H, Liang R, Lan T, Zhu X, Huang S, et al. Multifunctional Nanodrug Mediates Synergistic Photodynamic Therapy and MDSCs-Targeting Immunotherapy of Colon Cancer. Adv Sci (Weinh) (2021) 8(14):e2100712. doi: 10.1002/advs.202100712
226. Srivastava S, Furlan SN, Jaeger-Ruckstuhl CA, Sarvothama M, Berger C, Smythe KS, et al. Immunogenic Chemotherapy Enhances Recruitment of CAR-T Cells to Lung Tumors and Improves Antitumor Efficacy When Combined With Checkpoint Blockade. Cancer Cell (2021) 39(2):193–208.e10. doi: 10.1016/j.ccell.2020.11.005
227. Liu Y, Fang Y, Chen X, Wang Z, Liang X, Zhang T, et al. Gasdermin E-Mediated Target Cell Pyroptosis by CAR T Cells Triggers Cytokine Release Syndrome. Sci Immunol (2020) 5(43):eaax7969. doi: 10.1126/sciimmunol.aax7969
228. Alnaggar M, Xu Y, Li J, He J, Chen J, Li M, et al. Allogenic Vgamma9Vdelta2 T Cell as New Potential Immunotherapy Drug for Solid Tumor: A Case Study for Cholangiocarcinoma. J Immunother Cancer (2019) 7(1):36. doi: 10.1186/s40425-019-0501-8
229. Xu Y, Xiang Z, Alnaggar M, Kouakanou L, Li J, He J, et al. Allogeneic Vgamma9Vdelta2 T-Cell Immunotherapy Exhibits Promising Clinical Safety and Prolongs the Survival of Patients With Late-Stage Lung or Liver Cancer. Cell Mol Immunol (2021) 18(2):427–39. doi: 10.1038/s41423-020-0515-7
230. Maj T, Wang W, Crespo J, Zhang H, Wang W, Wei S, et al. Oxidative Stress Controls Regulatory T Cell Apoptosis and Suppressor Activity and PD-L1-Blockade Resistance in Tumor. Nat Immunol (2017) 18(12):1332–41. doi: 10.1038/ni.3868
Keywords: apoptosis, necroptosis, pyroptosis, ferroptosis, PANoptosis, autophagy, tumor microenvironment, tumor immunotherapy
Citation: Liu J, Hong M, Li Y, Chen D, Wu Y and Hu Y (2022) Programmed Cell Death Tunes Tumor Immunity. Front. Immunol. 13:847345. doi: 10.3389/fimmu.2022.847345
Received: 02 January 2022; Accepted: 28 February 2022;
Published: 30 March 2022.
Edited by:
Ana Paula Lepique, University of São Paulo, BrazilReviewed by:
Thirumala-Devi Kanneganti, St. Jude Children’s Research Hospital, United StatesDuanwu Zhang, Fudan University, China
Copyright © 2022 Liu, Hong, Li, Chen, Wu and Hu. This is an open-access article distributed under the terms of the Creative Commons Attribution License (CC BY). The use, distribution or reproduction in other forums is permitted, provided the original author(s) and the copyright owner(s) are credited and that the original publication in this journal is cited, in accordance with accepted academic practice. No use, distribution or reproduction is permitted which does not comply with these terms.
*Correspondence: Yi Hu, eWlodTIwMjBAam51LmVkdS5jbg==; Yangzhe Wu, dHl6d3VAam51LmVkdS5jbg==