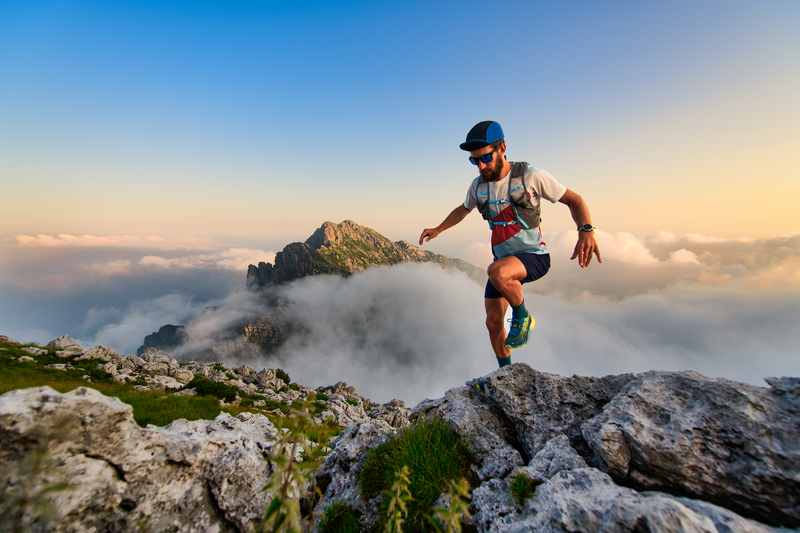
95% of researchers rate our articles as excellent or good
Learn more about the work of our research integrity team to safeguard the quality of each article we publish.
Find out more
REVIEW article
Front. Immunol. , 28 March 2022
Sec. Multiple Sclerosis and Neuroimmunology
Volume 13 - 2022 | https://doi.org/10.3389/fimmu.2022.845243
This article is part of the Research Topic Neuro-Immune Interactions and Neuroinflammation in Neurocritical Care View all 8 articles
An increasing number of studies have focused on the gut microbiota and its relationship with various neurological diseases. The gut microbiota can affect the metabolic status of the body, in addition to having an important impact on blood pressure, blood glucose, and atherosclerosis, all of which are risk factors for ischemic stroke. In this review, we summarized studies that included the physiological function of the gut microbiota and gut microbiota disorders related to the central nervous system, thus providing novel ideas for the prevention and treatment of ischemic stroke.
Ischemic stroke is a common central nervous system (CNS) disease and one of the most serious health problems worldwide. The gut microbiota is an important intestinal microecosystem that plays an important role in the homeostasis of the internal environment. Under normal circumstances, intestinal microorganisms and the host maintain a dynamic ecological balance, and gut microbiota homeostasis is closely associated with the health. In recent years, studies have found that the gut microbiota plays a key role in the occurrence and development of ischemic stroke (1–3). An imbalance within the gut microbiota is not only closely related to gastrointestinal diseases, such as irritable bowel syndrome, ulcerative colitis, colon cancer (4–6), but is also associated with the occurrence and development of hypertension, diabetes, obesity, atherosclerosis and metabolic diseases (7–9), all of which are risk factors for ischemic stroke. In this review, we summarized the influence and mechanisms of gut microbiota disorders on the pathogenesis of chronic metabolic diseases and stroke, and provided new proposals for the prevention or treatment of stroke.
The human gut microbiota is a very complex ecosystem with a large number and wide variety of species involved, which consists of approximately 10 times the number of cells in the human body and > 100 times as many genes as the entire human genome (10). The human gut microbiota is mainly composed of bacteria from the phyla Firmicutes and Bacteroidetes with the rest phyla comprising Actinobacteria, Proteobacteria and Verrucomicrobia (11). The gut microbiota is vital to health and plays a key role in many important physiological functions: (1) physiological nutrition: some probiotics can decompose dietary fiber and starch that cannot be digested in the daily diet and can degrade polysaccharides (12); (2) antagonism: some probiotics reproduce in the intestinal tract and generate various metabolites, such as hydrogen peroxide, carbon dioxide, and acetaldehyde, all of which inhibit or kill intestinal Escherichia coli and Streptococcus spp. (13); (3) immune regulation: probiotics, such as Bifidobacterium spp., can improve the body’s immune response and expression level of multiple cytokines, thus improving the disease-resistance of the host (14); (4) anti-cancer: Lactobacillus and Bifidobacterium spp. and other probiotics can enhance intestinal peristalsis, which may reduce the intestinal retention time of harmful or carcinogenic substances (15); (5) other functions: normal gut microbiota can regulate the development of the brain (15, 16).
Normally, the gut microbiota exists in a dynamically balanced ecological environment. The gut microbiota imbalance may occur for several reasons, such as prolonged drug treatments, stress, or infections by pathogenic bacteria and viruses. Under these conditions, the number, species, proportion, location, and biological characteristics of the gut microbiota will be altered (17).
The colonization of human gut microbiota in the infant is related to many factors including: intrauterine contact, delivery method (vaginal or caesarean delivery), feeding method (breast feeding or formula milk), the mother’s diet, external environment, and the use of antibiotics. These factors have a significant impact on the composition of the neonatal gut microbiota (18–20). The controversy over whether colonization of the human gut microbiota begins at birth or in the womb resulted from the discovery of the presence of bacteria in the placenta and amniotic fluid by Jimenez et al. (19). Prior to this, the fetus was considered to be a completely sterile environment. In a study of pregnant women who had caesarean deliveries, Collado et al. found that the characteristics of the microbiota in the placenta and amniotic fluid were consistent with the meconium of the newborn (21). The delivery mode can also affect the colonization of the neonatal gut. During vaginal delivery, the neonate is exposed to microorganisms that mainly come from the mother’s vagina and feces, while the composition of the baby’s intestinal flora is similar to that of the mother’s skin after caesarean delivery. Lee et al. found that on day 1 and 3 after birth, the richness and diversity of the gut microbiota of newborns delivered vaginally was significantly higher than that of newborns delivered by caesarean section, but the differences gradually decreased over time (22).
The intestinal microbiota undergoes a series of maturation processes in infancy and early childhood; however, by 3 years of age, it has established a structure similar to that of the adult intestinal microbiota (23). During the first 3 years of age, there is substantial synaptogenesis and neural development occurring in the brain (24). In addition, fewer types of gut microbes are present in early childhood and adolescence. The gut microbiota eventually reaches a relative maturity in adulthood and is manifested by an increase in the numbers and types of gut microbiota that display stronger resistance to antibiotics and other disturbances (25). Interactions between the host and gut microbiota have been shown to occur during an important window of brain development, referred to as “early life” (26). Disruption of the gut microbiota during this period may interfere with communication between the intestinal microbiota and brain, which may lead to CNS diseases (27, 28).
The traditional methods used to study the gut microbiota mainly include bacterial cultures and molecular biological techniques that are independent of culturing (29). The former method is mainly used for fecal culture; however, under laboratory conditions, 99% of the microorganisms in feces cannot be isolated and cultured, and these procedures are complicated and have low purification efficiency. These methodologies are laborious, and the bacterial strains obtained are limited. In recent years, real time quantitative PCR, fluorescence in situ hybridization, denatured gradient gel electrophoresis, terminal restriction fragment length polymorphism, second generation of sequencing, metagenomics, metabolomics, 16S rRNA sequencing, and other sequencing technologies have been developed (10, 30, 31). Development of germ-free (GF) animal models is an important method to study gut microbiota. GF mice can be colonized with a specific bacterial strain or transplanted with feces (32, 33). Additionally, antibiotics or probiotics can be used. The former interferes with the composition of the normal gut microbiota in mice, while the latter can be used to treat the mice with brain dysfunction. Using these methods, the differences in various physiological indicators and behaviors of mice after different interventions can be analyzed (34).
The gastrointestinal nervous system is regulated by the CNS, enteric nervous system (ENS), and autonomic nervous system and includes four stages of neural regulation (35). The first stage is the self-regulation of the ENS. The ENS is composed of an intermuscular and submucosal plexus. The sensory and motor neurons of the ENS are connected with each other to process and integrate independent information. The second stage involves the prevertebral ganglion, which accepts information from the ENS and CNS. The third stage involves the CNS, which senses the changes in the environment inside and outside of the intestinal tract. The CNS integrates the information from the brain and spinal cord centers and transmits the signal to the ENS or acts directly on the gastrointestinal effector cells via the autonomic nervous system and neuroendocrine system to regulate the activity of smooth muscle, glands, and blood vessels. The last stage consists of developed brain areas, and information from the cortex and subcortical areas interests at specific brainstem nuclei in the basal ganglia. These neural networks connect the CNS and gastrointestinal tract and represent the structural foundation for interactions between the gut microbiota and CNS (36). A disorder at any level of neural control will affect the transmission of information between the brain and intestinal tract.
The ENS can directly transfer information sensed in the gut to the brain via the enteric and vagal afferent nerves. The vagus nerve pathway is the main pathway by which the gut microbiota affects the CNS. Studies have shown that gut microbes activate the vagus nerve, which plays a key role in brain function, metabolism, and many behavioral changes in animal models (37–39).
Biochemical changes in the brain via the hypothalamic-pituitary-adrenal (HPA) axis can lead to changes in intestinal physiology. The HPA axis activates a stress response that affects intestinal permeability, motility, and mucus production, thereby changing the intestinal environment and affecting the composition and activity of the intestinal microbiota. Sudo et al. showed that the concentrations of corticosteroids and adrenal hormones in GF mice were higher than normal mice under stress conditions, which demonstrated that the gut microbiota was associated with the HPA axis (40). Intestinal colonization by Bifidobacterium spp. only attenuated the HPA axis response early in life, suggesting that exposure to primitive microbes is necessary to inhibit HPA axis regulation (41).
The gut microbiota can cause changes in behavior and cognitive function by influencing the release of diversified neurotransmitters, including norepinephrine, dopamine, 5-hydroxytryptophan, and brain-derived neurotrophic factor (42). The gut microbiota secretes various neurotransmitters, such as γ-aminobutyric acid, catecholamine and histamine, and transmits signals to the CNS via intestinal nerves and enterochromaffin cells (43). It has been found that GF mice show less depressive and anxious behaviors, the striatum contains higher 5-hydroxytryptophan content, and the number of enterochromaffin cells is significantly larger compared with that in specific pathogen-free mice (44). Desbonnet et al. (45) demonstrated that, after Bifidobacterium uptake in Sprague-Dawley rats, concentrations of the pro-inflammatory factors like IFN-γ, TNF-α, and IL-6 decreased significantly, but the concentrations of serum tryptophan, 5-hydroxyindoleacetic acid in the frontal cortex, and dopamine in the amygdala increased significantly. These findings suggest that the gut microbiota can influence brain function through complex neurotransmitter pathways, however, confirmation will require additional clinical studies.
Microbes can influence neurophysiological changes in their hosts by metabolizing chemicals that bind to receptors inside and outside of the intestine. Short-chain fatty acids (SCFAs) are produced by microbe-mediated fermentation of dietary fiber and mainly include acetate, propionate, and butyrate (46). SCFAs affect the CNS via the following three pathways: (1) binding to the G-protein coupled receptors GPR41 and GPR43 and acting as signaling molecules to activate special immune cells. The process is involved in response to an acute infection or in an abnormality in the regulating mechanism; therefore, leading to increased intestinal permeability and absorption of active metabolites (47); (2) direct activation of the sympathetic nervous system by the GPR41 located on sympathetic neurons (48); and (3) crossing the blood-brain barrier, which affects both nerve signals and neurotransmitter products, thereby leading to behavioral changes (48, 49). Intraperitoneal injection of sodium butyrate improved the breakdown of the blood-brain barrier (BBB) in a rat ischemic stroke model with transient middle cerebral artery occlusion (50). Studies have shown that intravenous or peritoneal administration of sodium butyrate inhibited histone deacetylation (51), prevented BBB decomposition (52), and promoted angiogenesis and neurogenesis (53, 54).
Intestinal bacteria can metabolize tryptophan into active products. For example, E. coli uses tryptophan to synthesize indoles, which can reduce the virulence and biofilm formation of E. coli and other bacteria in addition to having salutary effects on the intestinal environment (55). Tryptophan metabolites also regulate astrocyte activation and CNS inflammation by activating aromatic hydrocarbon receptors (56).
Dietary phosphatidylcholine, L-carnitine and choline are metabolized by the gut microbiota to produce trimethylamine, which is then oxidized by hepatic flavin monooxygenase to produce trimethylamine N-oxide (TMAO) (57). TMAO can inhibit the reverse transport of cholesterol, affect lipid metabolism in the intestinal tract and liver, activate macrophages, promote the accumulation of foam cells in the blood vessel walls, and increase the risk of atherosclerosis (58). In the past, it was thought that the interactions between gut bacteria and the host mainly took place in the intestine (59); however, recent studies have shown that small amounts of bacteria entering the bloodstream from the gut can cause chronic inflammation throughout the body (60, 61). Systemic chronic inflammation occurs in many chronic metabolic diseases, such as obesity, type 2 diabetes mellitus, and atherosclerosis (62).
The immune system, including both adaptive and innate immunity, plays a crucial role in the gut-brain axis. The gut microbiota can affect the maturation, development, and function of immune cells in the CNS, as well as the activation of peripheral immune cells, involved in both cellular and humoral immunity (63). The gut microbiota affects neurophysiological functions, which includes neural development, CNS immune activation, and BBB integrity, by regulating microglia and astrocyte development and function (64). The gut microbiome also regulates peripheral immune responses and plays a key role in brain inflammation, injury, and behavior (65).
Microglia are the most numerous and abundant immune cells in the brain and account for approximately 5%–20% of all glial cells (66). The immune functions of microglia in the CNS include phagocytosis, antigen presentation, cytokine production, and activation of inflammatory responses (67, 68). The gut microbiota can affect the maturation and function of microglia. Compared with those in normal mice, the numbers of immature microglia in the cortex, hippocampus, olfactory bulb, corpus callosum, and cerebellar gray matter and cerebral white matter in the GF mice were significantly increased (69). Moreover, when compared with normal mice and GF mice, brain microglia showed expanded maturity and increased expression of colony stimulating factor 1 receptor, F4/80, and CD31 in normal mice. The expression of these molecules is present in mature microglia and gradually decrease, and the same phenomenon occurred in normal mice after antibiotic treatment (69). The immune response induced by lipopolysaccharide or choriomeningitis virus included decreased activation of brain microglia, delayed development of immune cells, and reduced production of the inflammatory cytokines IL-6, IL-1β and TNF-α in GF mice compared with normal mice (68).
Astrocytes are the most numerous and largest glial cells in the brain and perform various functions, including regulating BBB integrity, neurotransmitter conversion, ion gradient balance, cerebral blood flow adjustment, and nutrient metabolism (70). Astrocytes integrate information from adjacent glial cells, neurons, blood vessels, and immune cells to regulate neural cells excitability and synaptic formation (71). The intestinal microbiota metabolizes tryptophan, including indole-3-aldehydes and indole-3-propionic acid (72), which in turn, activates the astrocyte aromatic hydrocarbon receptors and regulates astrocyte activity (56).
The intestinal microbiota and mucosal cells regulate the activation of immune molecules that affect the CNS, including the pro-inflammatory factors IL-8 and IL-1 and anti-inflammatory factors IL-10 and TGF-β (73–75). Intestinal mucosal pattern recognition receptors include Toll-like receptors that bind to lipopolysaccharide and other microbial-related molecules and activate immune cells, such as dendritic cells, neutrophils, and macrophages (76). Receptor binding results in the production of pro-inflammatory cytokines that include IL-1α, IL-1β, TNF-α, and IL-6, which in turn can cross the BBB and affect brain function directly (77). One study found that filamentous bacteria promoted the development of T-helper cells that produced IL-17 (Th17) in the small intestine of mice, and further study found that mice lacking normal intestinal flora had very few Th17 cells (78, 79). Lee et al. (80) demonstrated that the lack of normal number of Th17 cells in GF mice resulted in an immune deficiency against pathogenic infections, but the mice had increased resistance to the development of autoimmune diseases, such as autoimmune encephalomyelitis. A study on multiple sclerosis showed that IgA+ B cells from the intestine crossed the BBB, migrated to brain lesions, and then released IgA antibodies (81). These antibodies mainly reacted with diversified bacteria mainly, including MS related bacteria, without attacking self-brain tissue, which may play a protective role in ameliorating neuroinflammation (81). In conclusion, the immune system plays a very important role in the gut-brain axis.
Among intestinal lymphocytes, CD4 + T cells are the main cell population mediating various host protection and homeostasis responses (82). The gut microbiota and its metabolites can directly or indirectly induce the differentiation of CD4+ T cells, including T-bet+ Th1 cells, RORγt+ Th17 cells, Foxp3+ Treg cells and GATA3+ Th2 cells (76). The initial polarization of CD4+ T cells is uncertain and dynamic, mainly characterized by the transformation of key transcription factors and characteristic cytokines. The bacteria-related inflammatory response can reprogram RORγt+ cells to co-express T-bet or Foxp3, promoting the interconversion between Treg cells and Th17 cells (83). The gut microbiota can influence the formation of T cell subsets through specific cytokine combinations, microbial environment, and biogeography. For example, Th13 cells that secrete IFN-γ are formed by expressing T-bet under the influence of IL-12, IL-18, and IL-23, while Th2 cells are formed under the influence of IL-4 or IL-5, express GATA3, and secrete IL-5 and IL-13 (84). Most microorganisms with epithelial cell invasion ability can be phagocytosed by dendritic cells, and can also stimulate dendritic cells to release inflammatory cytokines such as IL-6 and TNF-α, and bind with OX40 and IL-12. These signals can preferentially polarize Th1 cells (85). Th17 cells and Treg cells are two important lymphocyte subsets with opposite functions. Despite having different functional properties, differentiation from naive T cells into Th17 cells and iTreg cells is dependent on TGF-β expression levels. Low expression levels of TGF-β, IL-23, or IL-6 induced the development of naive T cells into Th17 cells, while high expression levels of TGF-β induced iTreg cells (86). The gut microbiota can induce Th17 cell response, thus inhibiting intestinal flora, while regulating Treg cell response to provide tolerance to gut microbiota (87). One study found that 11 strains of bacteria isolated from the feces of healthy people, when colonized in combination in the intestine, effectively induced IFN-γ -producing CD8+ T cells in the intestine and other organs of mice without causing inflammation. The induction is dependent on CD103+ dendritic cells and MHC Ia antigen presenting molecules (88).
Ischemic stroke refers to the obstruction of blood supply to brain tissue caused by various conditions, which induces irreversible damage to brain tissue at the site of insult, and leads to brain tissue necrosis (89). The risk factors for ischemic stroke are complex and diverse. The gut microbiota disorders will change the intestinal environment, affect intestinal metabolism and absorption functions, and cause gut microbiota to affect the risk factors for ischemic stroke by different means.
Hypertension has an obvious induction effect on ischemic stroke, and the incidence and prognosis of ischemic stroke are closely related to the severity degree and duration of hypertension. Therefore, blood pressure is positively associated with the incidence of stroke (90). Many studies have confirmed that hypertension is the most important independent risk factor for ischemic stroke (91, 92).
The gut microbiota can produce substances that affect blood pressure levels, which cannot be produced other organs. Butyric acid and propionic acid can induce vasodilation of the colonic and caudal arteries (93). Acetic acid is widely used in hemodialysis and is related to the development of hypotension and vascular dilatation (7). Yang et al. found that the abundance and diversity of the gut microbiota in hypertensive rats and humans decreased significantly, and the proportion of Firmicutes/Bacteroidetes in the hypertensive rat model increased (93). Another study of mice with spontaneous hypertension demonstrated increased in intestinal wall permeability, decreased intestinal content of tight junction protein, increased numbers of intestinal Streptococcus spp., and a significant decrease in the number of Bifidobacterium spp. (94). SFCAs, metabolites of gut microbiota, can regulate blood pressure by activating cell surface receptors, such as GPR41 (47) and olfactory receptor 78 (95).
Sterile mice transplanted with normal intestinal microbiota have an increased risk of obesity and insulin resistance (96). The sensitivity of obese individuals to insulin increased significantly after receiving the gut microbiota from healthy people (97). The ratio of Firmicutes/Bacteroidetes in the intestinal tract of obese people and mice was significantly increased compared with normal people (98, 99). SFCAs are absorbed into the blood by intestinal epithelial cells and then metabolized by the liver, these metabolites can serve as a new energy source and increase energy intake and the risk of obesity (100, 101).
Hyperglycemia is an another independent risk factor for ischemic stroke, and the risk of ischemic stroke in diabetic patients is 1.8-6 times higher than in the general population (102). Changes in gut microbiota composition are related to insulin resistance. An imbalance in the gut microbiota can lead to lower insulin levels, insulin resistance, and a subsequent rise in blood glucose levels (8). Qin et al. found that there were significant differences in gut microbiota enrichment between healthy people and patients with type 2 diabetes mellitus (103). Chronic inflammation is an important pathogenic characteristic of insulin resistance. Gut microbiota imbalance may trigger an inflammatory response, and inflammatory factors may cause insulin resistance by affecting the insulin signaling pathway (104). Lipopolysaccharide can increase the production of pro-inflammatory cytokines after entering the circulation (105). These inflammatory cytokines induce the serine phosphorylation of insulin receptor substrate 1 in muscle and adipose tissue, block the insulin signaling pathway, and cause insulin resistance (106). Some anaerobic bacteria in the intestine convert primary bile acids into secondary bile acids, and a small amount of secondary bile acids can regulate lipid and glucose metabolism in the liver or the whole body by activating G-protein coupled receptors (107).
Intracranial or cervical atherosclerosis is also an important risk factor for ischemic stroke, while hyperlipidemia is a risk factor for atherosclerotic plaques development. High-fat diets, hyperlipidemia, and the gut microbiota are closely related. A long-term high-fat diet may change the types of gut microbes. Relevant studies have found that hyperlipidemia can affect the metabolic status and reproduction of gut microbes, resulting in a decrease in the abundance of beneficial bacteria, such as Bifidobacteria and Lactobacillus spp., while the gut microbiota imbalance can aggravate the lipid metabolic disorders and increase blood lipid levels (108, 109). Compared with a healthy control group matched by age and gender, patients with symptomatic atherosclerosis displayed significantly more Collinsella sp. in the intestinal tract; the intestinal tract was rich in Roseburia and Eubacterium spp. in the healthy control group. Analysis of the functional omics of microorganisms demonstrated that the gut microbiota of patients with symptomatic atherosclerosis were rich in genes encoding the synthesis of peptidoglycan, which is the main component of the bacterial cell wall and may cause atherosclerosis by activating the immune system, particularly neutrophils (110). One study found that the bacterial DNA content in atherosclerotic plaques was related to cardiovascular risk factors, such as low-density lipoprotein cholesterol in serum (111). After transplantation of feces from atherosclerosis-sensitive mice into the intestinal tract of atherosclerosis-resistant mice, atherosclerosis occurred in the atherosclerotic resistant mice, and their serum TMAO levels increased, indicating that atherosclerotic susceptibility can be transferred through fecal microbiota transplantation (112).
Hypertension, obesity, diabetes, and atherosclerosis are independent risk factors for stroke. Gut microbiota disorders and their endotoxins and metabolites play an important role in the pathophysiological processes of obesity, diabetes mellitus, and atherosclerosis (113). In addition, increasing numbers of studies have shown that gut microbiota disorders are directly related to stroke directly.
The gut microbiota can effectively regulate the number of lymphocytes, such as regulatory T (Treg) and γδT cells, and a gut microbiota imbalance will affect Treg cells and IL-17 + γδT cells, both of which are involved in an ischemic brain injury (114). The γδT cells are a group of cells with primary innate immune function, and are situated mainly on the surface of the intestinal epithelium (115). The γδT cells can secrete large amounts of IL-17 after an imbalance in the gut microbiota, which leads to chemokine production from surrounding medullary cells (monocytes and neutrophils) and aggravation of ischemic brain injury (116). Effector T cells may induce focal ischemic brain injury, but Treg cells can play a neuroprotective role by inhibiting post-ischemic inflammation (117). Treg cells inhibit IL-17 + γδT cells by secreting IL-10, thus exerting a neuroprotective effect (118). However, Treg cells have been not shown to enter the brain parenchyma during the acute stage of stroke, indicating that the beneficial role of Treg cells is achieved by regulating the peripheral immune system rather than directly acting on damaged brain tissue (119).
Wang et al. (120) found that oral butyrate reduced brain damage caused by type 2 diabetes. Xu et al. (121) found that cerebral ischemia rapidly caused intestinal ischemia and increased production of nitrate through free radical reactions, which resulted in an intestinal flora disorder accompanied by expansion of bacteria from the family Enterobacteriaceae. Enrichment of Enterobacteriaceae microbes contributes to cerebral infarction injury by enhancing systemic inflammation and represents an independent risk factor for a major adverse outcome in stroke patients. Treatment with superoxide dismutase or aminoguanidine can reduce nitrate production, while treatment with tungstate inhibits nitrate respiration, inhibits overgrowth of Enterobacteriaceae, reduces systemic inflammation, and alleviates cerebral infarction injury.
The beneficial or harmful effect of gut microbiota imbalance on stroke prognosis remains unclear. Pretreatment with antibiotics inhibited the gut microbiota and significantly decreased the α-diversity of the gut microbiota by day 3 after treatment in mice with middle cerebral artery occlusion, resulting in decreased cerebral infarction volume and better prognosis (122). However, another experiment had the opposite result. In the middle cerebral artery occlusion model using C57BL/6 mice, after gut microbiota was inhibited by broad-spectrum antibiotics, the mortality rate of mice with gut microbiota disorders was significantly increased (123). Singh et al. studied the relationship between brain injury, intestinal microbial disorders, and the immune system after ischemic stroke and found that ischemic stroke caused intestinal microbial disorders and impaired gut microbiota function (1). Conversely, changes in the intestinal microbiota affected the prognosis of brain injury caused by inflammation. After ischemic stroke, T cells migrated from the intestinal tract to the damaged brain, suggesting that gut microbiota disorders may be a therapeutic target to alleviate the immune responses after ischemic stroke. Subsequently, Singh et al. used the method of intestinal bacterial transplantation to restore the balance of the gut microbiota, improve the area of cerebral infarction in mice, and reduce the number of Treg cells (1). In a study comparing the effects of oral and intravenous antibiotics on the gut microbiota, both oral and intravenous antibiotics affected the gut microbiota, taking less time to recover in the intravenous antibiotics group than in the oral antibiotics group (124).
The relationship between ischemic stroke and the gut microbiota has been extensively studied in animal models, and progress has also been made in clinical studies. Karlsson et al. studied the genomic composition of the gut microbiota in patients with cerebral ischemic stroke and found that the microbial composition was different from that of normal people. The number of Ruminococcus spp. was significantly increased in patients with cerebral ischemia, while the number of Eubacillus spp. and Bacteroides spp. was significantly decreased (110). The relationship between gut microbiota disorders and ischemic stroke has not been fully clarified. According to current research, gut microbiota disorders may promote the pathological disease processes and the formation of atherosclerotic plaques. A clinical study compared the gut microbiota and plasma TMAO levels in patients with asymptomatic atherosclerosis, transient ischemic stroke, and stroke. The researchers found that plasma TMAO levels and microflora composition were independent of carotid atherosclerotic plaque formation in the asymptomatic atherosclerosis group. However, the differences in microflora composition and plasma TMAO content between the transient ischemic attack and stroke groups were statistically significant (125). A study shows that patients with acute ischemic stroke can experience significant gut microbiota disturbance that lasts for more than 3 weeks, after 4 weeks, the disturbed gut microbiota can gradually recover with a significant decrease in microbiota diversity (3). The alterations in the gut microbiota may be an indication of ischemic stroke incidence, progression, and prognosis (Table 1).
Gut microbiota disorders and intestinal dysfunction can affect the prognosis after a stroke through various mechanisms that include microflora migration, intestinal bacterial metabolites, and immune regulation. After an ischemic stroke, intestinal motility decreases and intestinal permeability increases, and gut microbe translocation into extraintestinal organs leads to local and systemic infection (2). Endotoxins derived from the gut microbiota, such as lipopolysaccharide and peptidoglycan, enter the blood through the highly permeable intestinal wall, activate the innate immune response of the body, and aggravate the inflammatory reaction. Infection and inflammation are detrimental to stroke outcomes. After cerebral apoplexy, the host immune system is severely suppressed (131), and the immune barrier function of the intestinal tract is reduced (132). Intestinal bacteria may be transferred into the blood and external organs, thereby, inducing a systemic inflammatory response and aggravating the adverse outcome of cerebral infarction injury. Crapser et al. found that, in aged mice, ischemic stroke induced gut permeability and enhanced bacterial translocation that resulted in sepsis, while young mice were able to resolve the infection (133).
Fecal microbiota transplantation (FMT) is the transplantation of functional bacteria from healthy donor feces into the gastrointestinal tract of a patient to repair the balance of the gut microbiota, a process that involves a point in time prior to the onset of disease. Filtered feces are collected from healthy donors or recipients themselves (autologous FMT) and transplanted into the intestines of patients with certain diseases (134). Chen et al. showed that transplantation of gut microbiota from normal mice into the intestinal tract of mice with ischemic stroke improved the long-term functional prognosis and survival rate (135). The results of studies on patients with severe stroke showed that the intestinal flora changed significantly after stroke onset leading to homeostasis disorders and immune responses, and FMT significantly improved the prognosis of these stroke patients (1). The mechanisms by which FMT improves the prognosis of ischemic stroke remain unclear, but is thought to be because of the restoration of intestinal homeostasis, which alleviates the inflammatory response and improves the overall condition.
FMT is still in its infancy, and it remains unknown whether FMT may result in the transfer of harmful microbes to potential subjects. There is a great deal of uncertainty regarding intestinal microbiota composition, which is complex combined with probiotics. As with any treatment, there are potential adverse effects or serious risks associated with FMT. Generally speaking, FMT is relatively safe even for individuals with low immune function or at high risk for inflammatory bowel disease. Common adverse events in the digestive tract, such as abdominal discomfort, flatulence, short-term low fever, change in defecation habits, abdominal bowel sounds, nausea, and vomiting, are mostly mild and self-limited (136). Serious adverse events, including death, are rare and are mainly caused by comorbidities or FMT manipulation problems (137). More research is needed to understand the potential side effects of FMT, such as the initiation of chronic disease or transmission of pathogens because of changes in the gut microbiota (138). FMT therapy for ischemic strokes provides a new approach, but further research will be required for extensive clinical applications.
Probiotics refer to edible microorganisms that are generally believed to have positive benefits to the host after ingestion, mainly including Lactobacillus spp. and Bifidobacterium spp (139). Probiotics entering the intestine from food sources will lead to the increase of SCFAs producing bacteria, resulting in a decrease in the number of protein-producing bacteria, thus promoting the increase of SCFAs and butyrate synthesis, reducing the amount of protein synthesis, resulting in changes in carbohydrate metabolism, and ultimately affecting blood glucose and blood lipids (140, 141). Probiotics such as Lactobacillus spp. and Bifidobacterium spp. can regulate renin-angiotensin system by producing angiotensin converting enzyme inhibitory peptide, SCFAs, conjugated linoleic acid and γ -aminobutyric acid, and have a certain antihypertensive effect (142). Another study showed that probiotics can treat gastrointestinal symptoms such as diarrhea and constipation after stroke (143).
Dietary phosphatidylcholine is closely related to TMAO production, and TMAO production depends on intestinal microbiota metabolism (144), while TMAO plays an important role in atherosclerosis (145). By ingesting probiotics and adjusting our daily diet, we can prevent and treat high-risk factors for ischemic stroke.
The gut microbiota can interact with the brain through various mechanisms, and gut microbiota imbalance can promote the occurrence of strokes, which in turn may aggravate the gut microbiota dysbiosis. The prognosis after a stroke can be improved through multiple interventions (Figure 1). The large number of gut microbes and variety of metabolites may change the impact of the gut microbiota on stroke outcomes. At present, the treatment of stroke by altering gut microbiota has substantial limitations, and more in-depth research will still be required.
Figure 1 General concept of interactions between the gut microbiota and ischemic stroke. The brain and gut microbiota interact with each other through neural, endocrine, metabolic and immune pathways. A stroke can cause a series of reactions, such as gut microbiota disorder, microbial composition changes, and immune responses, while gut dysbiosis can lead to metabolic and immune response changes, systemic inflammation, and other reactions that result in increased neuroinflammation and poor stroke outcomes. Furthermore, hypertension, obesity, diabetes, and atherosclerosis are independent risk factors for stroke, and the gut microbiota may indirectly influence stroke by affecting these risk factors. Fecal microbiota transplantation, probiotics, butyrate, and tryptophane can improve the prognosis of stroke by improving the composition of the gut microbiota.
There are many symbiotic microorganisms in the gut whose composition and abundance may affect both the autonomic nervous system and CNS. In recent years, many studies have confirmed that intestinal microbiome disorders are associated with certain central nervous system diseases, such as stroke, Alzheimer’s disease, Parkinson’s disease, and multiple sclerosis (146, 147). A stroke is the consequence of many complex factors, including obesity, hypertension, hyperlipidemia, insulin resistance, and atherosclerosis. Gut microbiota disorders are not only involved in the pathological processes of these risk factors, but are also directly related to strokes. A diet high in fat and sugar leads to changes in the proportion of symbiotic bacteria and imbalance of the gut microbiota (148). Therefore, maintaining a reasonable diet may reduce the incidence of ischemic stroke. After the occurrence of an ischemic stroke, the application of antibiotics for gut microbiota treatment and the restoration of intestinal homeostasis may control the development of the disease and improve its prognosis.
Although many studies have been conducted to evaluate the relationship between the gut microbiota and the occurrence and development of strokes, the following limitations remain: (1) Many studies have shown that different bacterial populations are associated with particular clinical symptoms; however, in most cases, it is not clear whether these differences cause disease. (2) Few breakthroughs have been made. Most studies have focused only on the correlations between the gut microbiota and diseases. Further studies are still needed to determine the mechanisms by which intestinal microbiota dysfunction affects the brain. (3) There is no research on chemical drugs that can effectively regulate the gut microbiota. Furthermore, the causal relationships between specific intestinal flora and specific diseases as well as detailed mechanisms should be further clarified to provide theoretical support for clinical disease prevention or treatments related to gut microbiota regulation.
The drug research on microbial flora has been performed for a considerable length of time and the present study mainly includes the following four types: (1) the FMT, which involves extracting gut microbes from healthy donor stools through different preparation processes, such as adding a protective agent to intestinal soluble capsules; (2) bacteria formula, which involves isolating strains one by one through artificial intelligence calculations and functional screenings and creating combinations of strains to treat diseases; (3) bacterial metabolites, small molecule regulators, and drugs similar to small molecules and peptides; and (4) artificial modification of bacteria through synthetic biology and genetic engineering methods to enhance the pharmaceutical function. These disease-specific microbiological drug studies require a deep understanding of the gut microbiota and the pathogenesis of various diseases, including stroke.
Microbe-targeted interventions, such as antibiotics, probiotics, and FMT, have been shown to have a beneficial effect on host health. External interventions with intestinal flora may change the outcome of some refractory diseases, including stroke, indicating that the gut microbiota has substantial therapeutic potential in clinical practice. Restoring gut microbiota homeostasis to treat ischemic stroke may represent a major breakthrough.
Future research will include defining mechanisms of microbe-microbe and microbe-host interactions in complex microbial-human gut ecosystems, using high-throughput sequencing of gut microbial genomes, and developing drugs based on these data. All of these studies will have a significant impact on treatment potential for various diseases, including stroke. Finally, it will be necessary to perform tightly designed, prospective, and longitudinal studies to transform these studies to clinical applications.
JW, HZ, and XX edited the manuscript. JH directed the project, and revised the manuscript. All authors read and approved the final manuscript.
This work was supported by the National Natural Science Foundation of China (no. 82171336, 81870939 to XX) and Health Commission of Jiangxi Provincial (no.202130032 to JH).
The authors declare that the research was conducted in the absence of any commercial or financial relationships that could be construed as a potential conflict of interest.
All claims expressed in this article are solely those of the authors and do not necessarily represent those of their affiliated organizations, or those of the publisher, the editors and the reviewers. Any product that may be evaluated in this article, or claim that may be made by its manufacturer, is not guaranteed or endorsed by the publisher.
1. Singh V, Roth S, Llovera G, Sadler R, Garzetti D, Stecher B, et al. Microbiota Dysbiosis Controls the Neuroinflammatory Response After Stroke. J Neurosci (2016) 36(28):7428–40. doi: 10.1523/JNEUROSCI.1114-16.2016
2. Stanley D, Mason LJ, Mackin KE, Srikhanta YN, Lyras D, Prakash MD, et al. Translocation and Dissemination of Commensal Bacteria in Post-Stroke Infection. Nat Med (2016) 22(11):1277–84. doi: 10.1038/nm.4194
3. Xia GH, You C, Gao XX, Zeng XL, Zhu JJ, Xu KY, et al. Stroke Dysbiosis Index (SDI) in Gut Microbiome Are Associated With Brain Injury and Prognosis of Stroke. Front Neurol (2019) 10:397. doi: 10.3389/fneur.2019.00397
4. Collins SM. The Intestinal Microbiota in the Irritable Bowel Syndrome. Int Rev Neurobiol (2016) 131:247–61. doi: 10.1016/bs.irn.2016.08.003
5. Butto LF, Haller D. Dysbiosis in Intestinal Inflammation: Cause or Consequence. Int J Med Microbiol (2016) 306(5):302–9. doi: 10.1016/j.ijmm.2016.02.010
6. Irrazábal T, Belcheva A, Girardin SE, Martin A, J. %J D. Molecular Cell Philpott: The Multifaceted Role of the Intestinal Microbiota in Colon Cancer. Mol Cell (2014) 54(2):309–20. doi: 10.1016/j.molcel.2014.03.039
7. Pevsner-Fischer M, Blacher E, Tatirovsky E, Ben-Dov IZ, Elinav E. The Gut Microbiome and Hypertension. Curr Opin Nephrol Hypertens (2017) 26(1):1–8. doi: 10.1097/MNH.0000000000000293
8. Baothman OA, Zamzami MA, Taher I, Abubaker J, Abu-Farha M. The Role of Gut Microbiota in the Development of Obesity and Diabetes. Lipids Health Dis (2016) 15:108. doi: 10.1186/s12944-016-0278-4
9. Grill JP, Cayuela C, Antoine JM, Schneider F. Effects of Lactobacillus Amylovorus and Bifidobacterium Breve on Cholesterol. Lett Appl Microbiol (2010) 31(2):154–6. doi: 10.1046/j.1365-2672.2000.00792.x
10. Qin J, Li R, Raes J, Arumugam M, Burgdorf KS, Manichanh C, et al. A Human Gut Microbial Gene Catalogue Established by Metagenomic Sequencing. Nature (2010) 464(7285):59–65. doi: 10.1038/nature08821
11. Eckburg PB, Bik EM, Bernstein CN, Purdom E, Dethlefsen L, Sargent M, et al. Diversity of the Human Intestinal Microbial Flora. Science (2005) 308(5728):1635. doi: 10.1126/science.1110591
12. Hooper LV, Midtvedt T, Gordon JI. How Host-Microbial Interactions Shape the Nutrient Environment of the Mammalian Intestine. Annu Rev Nutr (2002) 22:283–307. doi: 10.1146/annurev.nutr.22.011602.092259
13. Shokryazdan P, Faseleh Jahromi M, Liang JB, Ho YW. Probiotics: From Isolation to Application. J Am Coll Nutr (2017) 36(8):666–76. doi: 10.1080/07315724.2017.1337529
14. Cerf-Bensussan N, Gaboriau-Routhiau V. The Immune System and the Gut Microbiota: Friends or Foes? Nat Rev Immunol (2010) 10(10):735–44. doi: 10.1038/nri2850
15. Curtis H, Dirk G, Rob K, Sahar A, Jonathan HB, Asif TC, et al. Human Microbiome Project Consortium: Structure, Function and Diversity of the Healthy Human Microbiome. Nature (2012) 486(7402):207–14. doi: 10.1038/nature11234
16. Sjogren K, Engdahl C, Henning P, Lerner UH, Tremaroli V, Lagerquist MK, et al. The Gut Microbiota Regulates Bone Mass in Mice. J Bone Miner Res (2012) 50(6):S91–2. doi: 10.1016/j.bone.2012.02.272
17. Yang Y, Jobin C. Microbial Imbalance and Intestinal Pathologies: Connections and Contributions. Dis Model Mech (2014) 7(10):1131–42. doi: 10.1242/dmm.016428
18. Matamoros S, Gras-Leguen C, Le Vacon F, G. Potel and MF, Cochetiere de la. Development of Intestinal Microbiota in Infants and its Impact on Health. Trends Microbiol (2013) 21(4):167–73. doi: 10.1016/j.tim.2012.12.001
19. Jimenez E, Marin ML, Martin R, Odriozola JM, Olivares M, Xaus J, et al. Is Meconium From Healthy Newborns Actually Sterile? Res Microbiol (2008) 159(3):187–93. doi: 10.1016/j.resmic.2007.12.007
20. Aagaard K, Ma J, Antony KM, Ganu R, J. Petrosino and J. Versalovic: The Placenta Harbors a Unique Microbiome. Sci Transl Med (2014) 6(237):237ra65. doi: 10.1126/scitranslmed.3008599
21. Collado MC, Rautava S, Aakko J, Isolauri E, Salminen S. Human Gut Colonisation may be Initiated In Utero by Distinct Microbial Communities in the Placenta and Amniotic Fluid. Sci Rep (2016) 6:23129. doi: 10.1038/srep23129
22. Lee E, Kim BJ, Kang MJ, Choi KY, Cho HJ, Kim Y, et al. Dynamics of Gut Microbiota According to the Delivery Mode in Healthy Korean Infants. Allergy Asthma Immunol Res (2016) 8(5):471–7. doi: 10.4168/aair.2016.8.5.471
23. Yatsunenko T, Rey FE, Manary MJ, Trehan I, Dominguez-Bello MG, Contreras M, et al. Human Gut Microbiome Viewed Across Age and Geography. Nature (2012) 486(7402):222–7. doi: 10.1038/nature11053
24. Tau GZ, Peterson BS. Normal Development of Brain Circuits. Neuropsychopharmacology (2010) 35(1):147–68. doi: 10.1038/npp.2009.115
25. Agans R, Rigsbee L, Kenche H, Michail S, Khamis HJ, Paliy O. Distal Gut Microbiota of Adolescent Children Is Different From That of Adults. FEMS Microbiol Ecol (2011) 77(2):404–12. doi: 10.1111/j.1574-6941.2011.01120.x
26. Jasarevic E, Howerton CL, Howard CD, Bale TL. Alterations in the Vaginal Microbiome by Maternal Stress Are Associated With Metabolic Reprogramming of the Offspring Gut and Brain. Endocrinology (2015) 156(9):3265–76. doi: 10.1210/en.2015-1177
27. Rapoport JL, Giedd JN, Gogtay N. Neurodevelopmental Model of Schizophrenia: Update 2012. Mol Psychiatry (2012) 17(12):1228–38. doi: 10.1038/mp.2012.23
28. Ben-Ari Y. Neuropaediatric and Neuroarchaeology: Understanding Development to Correct Brain Disorders. Acta Paediatr (2013) 102(4):331–4. doi: 10.1111/apa.12161
29. Austin B. The Value of Cultures to Modern Microbiology. Antonie Van Leeuwenhoek (2017) 110(10):1247–56. doi: 10.1007/s10482-017-0840-8
30. Tuohy KM, Gougoulias C, Shen Q, Walton G, Fava F, Ramnani P. Studying the Human Gut Microbiota in the Trans-Omics Era–Focus on Metagenomics and Metabonomics. Curr Pharm Des (2009) 15(13):1415–27. doi: 10.2174/138161209788168182
31. Salonen A, Nikkila J, Jalanka-Tuovinen J, Immonen O, Rajilic-Stojanovic M, Kekkonen RA, et al. Comparative Analysis of Fecal DNA Extraction Methods With Phylogenetic Microarray: Effective Recovery of Bacterial and Archaeal DNA Using Mechanical Cell Lysis. J Microbiol Methods (2010) 81(2):127–34. doi: 10.1016/j.mimet.2010.02.007
32. Luczynski P, McVey Neufeld KA, Oriach CS, Clarke G, Dinan TG, Cryan JF. Growing Up in a Bubble: Using Germ-Free Animals to Assess the Influence of the Gut Microbiota on Brain and Behavior. Int J Neuropsychopharmacol (2016) 19(8):pyw020. doi: 10.1093/ijnp/pyw020
33. Clarke G, Grenham S, Scully P, Fitzgerald P, Moloney RD, Shanahan F, et al. The Microbiome-Gut-Brain Axis During Early Life Regulates the Hippocampal Serotonergic System in a Sex-Dependent Manner. Mol Psychiatry (2013) 18(6):666–73. doi: 10.1038/mp.2012.77
34. Liang S, Wang T, Hu X, Luo J, Li W, Wu X, et al. Administration of Lactobacillus Helveticus NS8 Improves Behavioral, Cognitive, and Biochemical Aberrations Caused by Chronic Restraint Stress. Neuroscience (2015) 310:561–77. doi: 10.1016/j.neuroscience.2015.09.033
35. Mulak A, Bonaz B. Irritable Bowel Syndrome: A Model of the Brain-Gut Interactions. Med Sci Monit (2004) 10(4):RA55–62. doi: 10.1016/j.gcb.2009.07.006
36. Forsythe P, Bienenstock J, Kunze WA. Vagal Pathways for Microbiome-Brain-Gut Axis Communication. Adv Exp Med Biol (2014) 817:115–33. doi: 10.1007/978-1-4939-0897-4_5
37. Bonaz B, Bazin T, Pellissier S. The Vagus Nerve at the Interface of the Microbiota-Gut-Brain Axis. Front Neurosci (2018) 12:49. doi: 10.3389/fnins.2018.00049
38. Bravo JA, Forsythe P, Chew MV, Escaravage E, Savignac HM, Dinan TG, et al. Ingestion of Lactobacillus Strain Regulates Emotional Behavior and Central GABA Receptor Expression in a Mouse via the Vagus Nerve. Proc Natl Acad Sci USA (2011) 108(38):16050–5. doi: 10.1073/pnas.1102999108
39. Sherwin E, Bordenstein SR, Quinn JL, Dinan TG, Cryan JF. Microbiota and the Social Brain. Science (2019) 366(6465):eaar2016. doi: 10.1126/science.aar2016
40. Sudo N, Chida Y, Aiba Y, Sonoda J, Oyama N, Yu XN, et al. Postnatal Microbial Colonization Programs the Hypothalamic-Pituitary-Adrenal System for Stress Response in Mice. J Physiol (2004) 558(Pt 1):263–75. doi: 10.1113/jphysiol.2004.063388
41. Cryan JF, Dinan TG. Mind-Altering Microorganisms: The Impact of the Gut Microbiota on Brain and Behaviour. Nat Rev Neurosci (2012) 13(10):701–12. doi: 10.1038/nrn3346
42. Freestone PP, Sandrini SM, Haigh RD, Lyte M. Microbial Endocrinology: How Stress Influences Susceptibility to Infection. Trends Microbiol (2008) 16(2):55–64. doi: 10.1016/j.tim.2007.11.005
43. Frye RE, Rose S, Slattery J, MacFabe DF. Gastrointestinal Dysfunction in Autism Spectrum Disorder: The Role of the Mitochondria and the Enteric Microbiome. Microb Ecol Health Dis (2015) 26:27458. doi: 10.3402/mehd.v26.27458
44. Kaneko Y, Onda N, Watanabe Y, . Shibutani M. Identification of 5-Hydroxytryptamine-Producing Cells by Detection of Fluorescence in Paraffin-Embedded Tissue Sections. Eur J Histochem (2016) 60(3):2684. doi: 10.4081/ejh.2016.2684
45. Desbonnet L, Garrett L, Clarke G, Bienenstock J, . Dinan TG. The Probiotic Bifidobacteria Infantis: An Assessment of Potential Antidepressant Properties in the Rat. J Psychiatr Res (2008) 43(2):164–74. doi: 10.1016/j.jpsychires.2008.03.009
46. Martin-Gallausiaux C, Marinelli L, Blottiere HM, P. Larraufie and N. Lapaque: SCFA: Mechanisms and Functional Importance in the Gut. Proc Nutr Soc (2021) 80(1):37–49. doi: 10.1017/S0029665120006916
47. Kim MH, Kang SG, Park JH, Yanagisawa M, Kim CH. Short-Chain Fatty Acids Activate GPR41 and GPR43 on Intestinal Epithelial Cells to Promote Inflammatory Responses in Mice. Gastroenterology (2013) 145(2):396–406 e1-10. doi: 10.1053/j.gastro.2013.04.056
48. Frost G, Sleeth ML, Sahuri-Arisoylu M, Lizarbe B, Cerdan S, Brody L, et al. The Short-Chain Fatty Acid Acetate Reduces Appetite via a Central Homeostatic Mechanism. Nat Commun (2014) 5:3611. doi: 10.1038/ncomms4611
49. Kimura I, Inoue D, Maeda T, Hara T, Ichimura A, Miyauchi S, et al. Short-Chain Fatty Acids and Ketones Directly Regulate Sympathetic Nervous System via G Protein-Coupled Receptor 41 (GPR41). Proc Natl Acad Sci USA (2011) 108(19):8030–5. doi: 10.1073/pnas.1016088108
50. Patnala R, Arumugam TV, Gupta N, . Dheen ST. HDAC Inhibitor Sodium Butyrate-Mediated Epigenetic Regulation Enhances Neuroprotective Function of Microglia During Ischemic Stroke. Mol Neurobiol (2017) 54(8):6391–411. doi: 10.1007/s12035-016-0149-z
51. Levenson JM, O'Riordan KJ, Brown KD, Trinh MA, Molfese DL, Sweatt JD. Regulation of Histone Acetylation During Memory Formation in the Hippocampus. J Biol Chem (2004) 279(39):40545–59. doi: 10.1074/jbc.M402229200
52. Fessler EB, Chibane FL, Wang Z, Chuang DM. Potential Roles of HDAC Inhibitors in Mitigating Ischemia-Induced Brain Damage and Facilitating Endogenous Regeneration and Recovery. Curr Pharm Des (2013) 19(28):5105–20. doi: 10.2174/1381612811319280009
53. Kim HJ, Leeds P, Chuang DM. The HDAC Inhibitor, Sodium Butyrate, Stimulates Neurogenesis in the Ischemic Brain. J Neurochem (2009) 110(4):1226–40. doi: 10.1111/j.1471-4159.2009.06212.x
54. Yoo DY, Kim W, Nam SM, Kim DW, Chung JY, Choi SY, et al. Synergistic Effects of Sodium Butyrate, a Histone Deacetylase Inhibitor, on Increase of Neurogenesis Induced by Pyridoxine and Increase of Neural Proliferation in the Mouse Dentate Gyrus. Neurochem Res (2011) 36(10):1850–7. doi: 10.1007/s11064-011-0503-5
55. Li X, Yang Q, Dierckens K, Milton DL, Defoirdt T. RpoS and Indole Signaling Control the Virulence of Vibrio Anguillarum Towards Gnotobiotic Sea Bass (Dicentrarchus Labrax) Larvae. PloS One (2014) 9(10):e111801. doi: 10.1371/journal.pone.0111801
56. Rothhammer V, Mascanfroni ID, Bunse L, Takenaka MC, Kenison JE, Mayo L, et al. Type I Interferons and Microbial Metabolites of Tryptophan Modulate Astrocyte Activity and Central Nervous System Inflammation via the Aryl Hydrocarbon Receptor. Nat Med (2016) 22(6):586–97. doi: 10.1038/nm.4106
57. Wu WK, Chen CC, Liu PY, Panyod S, Liao BY, Chen PC, et al. Identification of TMAO-Producer Phenotype and Host-Diet-Gut Dysbiosis by Carnitine Challenge Test in Human and Germ-Free Mice. Gut (2019) 68(8):1439–49. doi: 10.1136/gutjnl-2018-317155
58. Koeth RA, Wang Z, Levison BS, Buffa JA, Org E, Sheehy BT, et al. Intestinal Microbiota Metabolism of L-Carnitine, a Nutrient in Red Meat, Promotes. Nat Med (2013) 1546-170X(Electronic):576–85. doi: 10.1038/nm.3145
59. Baumler AJ, Sperandio V. Interactions Between the Microbiota and Pathogenic Bacteria in the Gut. Nature (2016) 535(7610):85–93. doi: 10.1038/nature18849
60. Dickson RP, Singer BH, Newstead MW, Falkowski NR, Erb-Downward JR, Standiford TJ, et al. Enrichment of the Lung Microbiome With Gut Bacteria in Sepsis and the Acute Respiratory Distress Syndrome. Nat Microbiol (2016) 1(10):16113. doi: 10.1038/nmicrobiol.2016.113
61. Spadoni I, Zagato E, Bertocchi A, Paolinelli R, Hot E, Di Sabatino A, et al. A Gut-Vascular Barrier Controls the Systemic Dissemination of Bacteria. Science (2015) 350(6262):830–4. doi: 10.1126/science.aad0135
62. Neves AL, Coelho J, Couto L, Leite-Moreira A, Jr. Roncon-Albuquerque R. Metabolic Endotoxemia: A Molecular Link Between Obesity and Cardiovascular Risk. J Mol Endocrinol (2013) 1479-6813(Electronic):R51–64. doi: 10.1530/JME-13-0079
63. Wastyk HC, Fragiadakis GK, Perelman D, Dahan D, Merrill BD, Yu FB, et al. Gut-Microbiota-Targeted Diets Modulate Human Immune Status. Cell (2021) 184(16):4137–4153 e14. doi: 10.1016/j.cell.2021.06.019
64. Milani C, Duranti S, Bottacini F, Casey E, Turroni F, Mahony J, et al. The First Microbial Colonizers of the Human Gut: Composition, Activities, and Health Implications of the Infant Gut Microbiota. Microbiol Mol Biol Rev (2017) 81(4):e00036–17. doi: 10.1128/MMBR.00036-17
65. Cryan JF, O'Riordan KJ, Cowan CSM, Sandhu KV, Bastiaanssen TFS, Boehme M, et al. The Microbiota-Gut-Brain Axis. Physiol Rev (2019) 99(4):1877–2013. doi: 10.1152/physrev.00018.2018
66. Borst K, Dumas AA, Prinz M. Microglia: Immune and non-Immune Functions. Immunity (2021) 54(10):2194–208. doi: 10.1016/j.immuni.2021.09.014
67. Nayak D, Roth TL, McGavern DB. Microglia Development and Function. Annu Rev Immunol (2014) 32:367–402. doi: 10.1146/annurev-immunol-032713-120240
68. Matcovitch-Natan O, Winter DR, Giladi A, Vargas Aguilar S, Spinrad A, Sarrazin S, et al. Microglia Development Follows a Stepwise Program to Regulate Brain Homeostasis. Science (2016) 353(6301):aad8670. doi: 10.1126/science.aad8670
69. Erny D, Hrabe de Angelis AL, Jaitin D, Wieghofer P, Staszewski O, David E, et al. Host Microbiota Constantly Control Maturation and Function of Microglia in the CNS. Nat Neurosci (2015) 18(7):965–77. doi: 10.1038/nn.4030
70. Stogsdill JA, Ramirez J, Liu D, Kim YH, Baldwin KT, Enustun E, et al. Astrocytic Neuroligins Control Astrocyte Morphogenesis and Synaptogenesis. Nature (2017) 551(7679):192–7. doi: 10.1038/nature24638
71. Sofroniew MV. Astrocyte Reactivity: Subtypes, States, and Functions in CNS Innate Immunity. Trends Immunol (2020) 41(9):758–70. doi: 10.1016/j.it.2020.07.004
72. Rothhammer V, Borucki DM, Tjon EC, Takenaka MC, Chao CC, Ardura-Fabregat A, et al. Microglial Control of Astrocytes in Response to Microbial Metabolites. Nature (2018) 557(7707):724–8. doi: 10.1038/s41586-018-0119-x
73. Shi N, Li N, Duan X, Niu H. Interaction Between the Gut Microbiome and Mucosal Immune System. Mil Med Res (2017) 4:14. doi: 10.1186/s40779-017-0122-9
74. Kayama H, Okumura R, Takeda K. Interaction Between the Microbiota, Epithelia, and Immune Cells in the Intestine. Annu Rev Immunol (2020) 38:23–48. doi: 10.1146/annurev-immunol-070119-115104
75. Wang L, Zhu L, Qin S. Gut Microbiota Modulation on Intestinal Mucosal Adaptive Immunity. J Immunol Res (2019) 2019:4735040. doi: 10.1155/2019/4735040
76. Honda K, Littman DR. The Microbiota in Adaptive Immune Homeostasis and Disease. Nature (2016) 535(7610):75–84. doi: 10.1038/nature18848
77. Camara-Lemarroy CR, Silva C, Greenfield J, Liu WQ, Metz LM, Yong VW. Biomarkers of Intestinal Barrier Function in Multiple Sclerosis are Associated With Disease Activity. Mult Scler (2020) 26(11):1340–50. doi: 10.1177/1352458519863133
78. Ivanov II, Atarashi K, Manel N, Brodie EL, Shima T, Karaoz U, et al. Induction of Intestinal Th17 Cells by Segmented Filamentous Bacteria. Cell (2009) 139(3):485–98. doi: 10.1016/j.cell.2009.09.033
79. Gaboriau-Routhiau V, Rakotobe S, Lecuyer E, Mulder I, Lan A, Bridonneau C, et al. The Key Role of Segmented Filamentous Bacteria in the Coordinated Maturation of Gut Helper T Cell Responses. Immunity (2009) 31(4):677–89. doi: 10.1016/j.immuni.2009.08.020
80. Lee YK, Menezes JS, Umesaki Y, Mazmanian SK. Proinflammatory T-Cell Responses to Gut Microbiota Promote Experimental Autoimmune Encephalomyelitis. Proc Natl Acad Sci USA (2011) 108(Suppl 1):4615–22. doi: 10.1073/pnas.1000082107
81. Probstel AK, Zhou X, Baumann R, Wischnewski S, Kutza M, Rojas OL, et al. Gut Microbiota-Specific IgA(+) B Cells Traffic to the CNS in Active Multiple Sclerosis. Sci Immunol (2020) 5(53):eabc7191. doi: 10.1126/sciimmunol.abc7191
82. Shale M, Schiering C, Powrie F. CD4(+) T-Cell Subsets in Intestinal Inflammation. Immunol Rev (2013) 252(1):164–82. doi: 10.1111/imr.12039
83. Sprouse ML, Bates NA, Felix KM, Wu HJ. Impact of Gut Microbiota on Gut-Distal Autoimmunity: A Focus on T Cells. Immunology (2019) 156(4):305–18. doi: 10.1111/imm.13037
84. Geva-Zatorsky N, Sefik E, Kua L, Pasman L, Tan TG, Ortiz-Lopez A, et al. Mining the Human Gut Microbiota for Immunomodulatory Organisms. Cell (2017) 168(5):928–943 e11. doi: 10.1016/j.cell.2017.01.022
85. Brown EM, Kenny DJ, Xavier RJ. Gut Microbiota Regulation of T Cells During Inflammation and Autoimmunity. Annu Rev Immunol (2019) 37:599–624. doi: 10.1146/annurev-immunol-042718-041841
86. Gagliani N, Amezcua Vesely MC, Iseppon A, Brockmann L, Xu H, Palm NW, et al. Th17 Cells Transdifferentiate Into Regulatory T Cells During Resolution of Inflammation. Nature (2015) 523(7559):221–5. doi: 10.1038/nature14452
87. Gefen T, Geva-Zatorsky N. What Came First: The Microbiota or the Tr(egg) Cells? Immunity (2018) 48(6):1072–4. doi: 10.1016/j.immuni.2018.06.005
88. Tanoue T, Morita S, Plichta DR, Skelly AN, Suda W, Sugiura Y, et al. A Defined Commensal Consortium Elicits CD8 T Cells and Anti-Cancer Immunity. Nature (2019) 565(7741):600–5. doi: 10.1038/s41586-019-0878-z
89. Liu M, Wu B, Wang WZ, Lee LM, Zhang SH, %J LZ. Lancet Neurology Kong: Stroke in China: Epidemiology, Prevention, and Management Strategies. Lancet Neurol (2007) 6(5):456–64. doi: 10.1016/S1474-4422(07)70004-2
90. Powers WJ, Clarke WR, Grubb RL, Videen TO, Adams HP, Derdeyn CP. %J Neurology: Lower Stroke Risk With Lower Blood Pressure in Hemodynamic Cerebral Ischemia. Neurology (2014) 82(12):1027–32. doi: 10.1212/WNL.0000000000000238
91. Li J, Zhao F, Wang Y, Chen J, Tao J, Tian G, et al. Gut Microbiota Dysbiosis Contributes to the Development of Hypertension. Microbiome (2017) 5(1):14. doi: 10.1186/s40168-016-0222-x
92. Cipolla MJ, Liebeskind DS, Chan SL. The Importance of Comorbidities in Ischemic Stroke: Impact of Hypertension on the Cerebral Circulation. J Cereb Blood Flow Metab (2018) 38(12):2129–49. doi: 10.1177/0271678X18800589
93. Yang T, Santisteban MM, Rodriguez V, Li E, Ahmari N, Carvajal JM, et al. Gut Dysbiosis is Linked to Hypertension. Hypertension (2015) 65(6):1331–40. doi: 10.1161/HYPERTENSIONAHA.115.05315
94. Santisteban MM, Qi Y, Zubcevic J, Kim S, Yang T, Shenoy V, et al. Hypertension-Linked Pathophysiological Alterations in the Gut. Circ Res (2017) 120(2):312–23. doi: 10.1161/CIRCRESAHA.116.309006
95. Pluznick J. %J Gut Microbes: A Novel SCFA Receptor, the Microbiota, and Blood Pressure Regulation. Gut Microbes (2014) 5(2):202–7. doi: 10.4161/gmic.27492
96. Chenhong Z, Zhang M, Pang X, Zhao Y, Wang L, Liping %J. Isme Journal Zhao: Structural Resilience of the Gut Microbiota in Adult Mice Under High-Fat Dietary Perturbations. ISME J (2012) 6(10):1848–57. doi: 10.1038/ismej.2012.27
97. Vrieze A, Van Nood E, Holleman F, Salojärvi J, Kootte RS, Bartelsman JFWM, et al. Transfer of Intestinal Microbiota From Lean Donors Increases Insulin Sensitivity in Individuals With Metabolic Syndrome. Gastroenterology (2012) 143(4):913–6. doi: 10.1053/j.gastro.2012.06.031
98. Martinez Kristina B, Leone V, Eugene B. %J Gut Microbes Chang: Western Diets, Gut Dysbiosis, and Metabolic Diseases: Are They Linked? Gut Microbes (2017) 8(2):130–42. doi: 10.1080/19490976.2016.1270811
99. Ley RE, Turnbaugh PJ, Klein S, Gordon JI. Microbial Ecology: Human Gut Microbes Associated With Obesity. Nature (2006) 444(7122):1022–3. doi: 10.1038/4441022a
100. Turnbaugh Peter J, Ley RE, Mahowald MA, Magrini V, Mardis ER, Gordon JI. An Obesity-Associated Gut Microbiome With Increased Capacity for Energy Harvest. Nature (2006) 444(7122):1027–31. doi: 10.1038/nature05414
101. Backhed F, Ding H, Wang T, Hooper LV, Koh GY, Nagy A, et al. The Gut Microbiota as an Environmental Factor That Regulates Fat Storage. Proc Natl Acad Sci USA (2004) - 0027-8424(Print):15718–23. doi: 10.1073/pnas.0407076101
102. Backhed F, Ley Re JL, Sonnenburg DA, Peterson JI, Gordon JI. Host-Bacterial Mutualism in the Human Intestine. Science (2005) 307(5717):1915–20. doi: 10.1126/science.1104816
103. Junjie Q, Li Y, Cai Z, Li S, Zhu J, Zhang F, et al. A Metagenome-Wide Association Study of Gut Microbiota in Type 2 Diabetes. Nature (2012) 490(7418):55–60. doi: 10.1038/nature11450
104. Saad MJ, Santos A, Prada PO. Linking Gut Microbiota and Inflammation to Obesity and Insulin Resistance. Physiol (Bethesda) (2016) 31(4):283–93. doi: 10.1152/physiol.00041.2015
105. Siebler J, Galle PR, Weber MM. The Gut-Liver-Axis: Endotoxemia, Inflammation, Insulin Resistance and NASH. J Hepatol (2008) 48(6):1032–4. doi: 10.1016/j.jhep.2008.03.007
106. Hotamisligil GS, Peraldi P, Budavari A, Ellis R, White MF, Spiegelman BM. IRS-1-Mediated Inhibition of Insulin Receptor Tyrosine Kinase Activity in TNF-Alpha- and Obesity-Induced Insulin Resistance. Science (1996) 271(5249):665–8. doi: 10.1126/science.271.5249.665
107. Thomas C, Gioiello A, Noriega L, Strehle A, Oury J, Rizzo G, et al. TGR5-Mediated Bile Acid Sensing Controls Glucose Homeostasis. Cell Metab (2009) 1932-7420(Electronic):167–77. doi: 10.1016/j.cmet.2009.08.001
108. Hidenori H, Takahashi R, Nishi T, Sakamoto M, Benno Y. molecular analysis of jejunal, ileal, caecal and Recto-Sigmoidal Human Colonic Microbiota Using 16S rRNA Gene Libraries and Terminal Restriction Fragment Length Polymorphism. J Med Microbiol (2005) 54(11):1093–101. doi: 10.1099/jmm.0.45935-0
109. Martínez Inés, Grant W, Chaomei Z, Ryan L, Benson Andrew K, Carr Timothy P, et al. Diet-Induced Metabolic Improvements in a Hamster Model of Hypercholesterolemia Are Strongly Linked to Alterations of the Gut Microbiota. Appl Environ Microbiol (2009) 75(12):4175. doi: 10.1128/AEM.00380-09
110. Karlsson FH, Fåk F, Nookaew I, Tremaroli V, Fagerberg B, Petranovic D, et al. Symptomatic Atherosclerosis Is Associated With an Altered Gut Metagenome. Nat Commun (2012) 3(4):1245. doi: 10.1038/ncomms2266
111. Spinler SA, Cziraky MJ, Willey VJ, Tang F, Maddox TM, Thomas T, et al. Frequency of Attainment of Low-Density Lipoprotein Cholesterol and Non-High-Density Lipoprotein Cholesterol Goals in Cardiovascular Clinical Practice (From the National Cardiovascular Data Registry PINNACLE Registry). Am J Cardiol (2015) 116(4):547–53. doi: 10.1016/j.amjcard.2015.05.011
112. Gregory JC, Buffa JA, Elin O, Zeneng W, Levison BS, Weifei Z, et al. Transmission of Atherosclerosis Susceptibility With Gut Microbial Transplantation. J Biol Chem (2015) 290(9):5647–60. doi: 10.1074/jbc.M114.618249
113. Prame KK, Wong CH. Imbalance in the Force: The Dark Side of the Microbiota on Stroke Risk and Progression. Curr Opin Neurobiol (2020) 62:10–6. doi: 10.1016/j.conb.2019.10.002
114. Costantino I, Anrather J. The Immunology of Stroke: From Mechanisms to Translation. Nat Med (2012) 17(7):796–808. doi: 10.1038/nm.2399
115. Immo P, Silva Santos B, Pennington DJ. Functional Development of γδ T Cells. Eur J Immunol (2013) 43(8):1988–94. doi: 10.1002/eji.201343759
116. Shichita T, Sugiyama Y, Ooboshi H. Pivotal Role of Cerebral Interleukin-17-Producing gammadeltaT Cells in the Delayed Phase of Ischemic Brain Injury. Nat Med (2009) 15(8):946–50. doi: 10.1038/nm.1999
117. Liesz A, Hu X, Kleinschnitz C, Offner H. Functional Role of Regulatory Lymphocytes in Stroke: Facts and Controversies. Stroke (2015) 46(5):1422–30. doi: 10.1161/STROKEAHA.114.008608
118. Liesz A, Suri-Payer E, Veltkamp C, Doerr H, Sommer C, Rivest S, et al. Regulatory T Cells Are Key Cerebroprotective Immunomodulators in Acute. Nat Med (2009) - 1546-170X(Electronic):192–9. doi: 10.1038/nm.1927
119. Li P, Gan Y, Sun BL, Zhang F, Lu B, Gao Y, et al. Adoptive Regulatory T-Cell Therapy Protects Against Cerebral Ischemia. Ann Neurol (2013) - 1531-8249(Electronic):458–71. doi: 10.1002/ana.23815
120. Wang H, Song W, Wu Q, Gao X, Li J, Tan C, et al. Fecal Transplantation From Db/Db Mice Treated With Sodium Butyrate Attenuates Ischemic Stroke Injury. Microbiol Spectr (2021) 9(2):e0004221. doi: 10.1128/Spectrum.00042-21
121. Xu K, Gao X, Xia G, Chen M, Zeng N, Wang S, et al. Rapid Gut Dysbiosis Induced by Stroke Exacerbates Brain Infarction in Turn. Gut (2021), gutjnl-2020-323263. doi: 10.1136/gutjnl-2020-323263
122. Benakis C, Brea D, Caballero S, Faraco G, Moore J, Murphy M, et al. Commensal Microbiota Affects Ischemic Stroke Outcome by Regulating Intestinal γδt Cells. Nat Med (2016) 22(5):516–23. doi: 10.1038/nm.4068
123. Winek K, Odilo E, Priscilla K, Heimesaat MM, Fischer A, Bereswill S, et al. Depletion of Cultivatable Gut Microbiota by Broad-Spectrum Antibiotic Pretreatment Worsens Outcome After Murine Stroke. Stroke (2016) 47(5):1354–63. doi: 10.1161/STROKEAHA.115.011800
124. Kelly SA, Nzakizwanayo J, Rodgers AM, Zhao L, Weiser R, Tekko IA, et al. Antibiotic Therapy and the Gut Microbiome: Investigating the Effect of Delivery Route on Gut Pathogens. ACS Infect Dis (2021) 7(5):1283–96. doi: 10.1021/acsinfecdis.1c00081
125. Yin J, Liao SX, He Y, Wang S, Xia GH, Liu FT, et al. Dysbiosis of Gut Microbiota With Reduced Trimethylamine-N-Oxide Level in Patients With Large-Artery Atherosclerotic Stroke or Transient Ischemic Attack. J Am Heart Assoc (2015) 4(11):e002699. doi: 10.1161/JAHA.115.002699
126. Tan C, Wu Q, Wang H, Gao X, Xu R, Cui Z, et al. Dysbiosis of Gut Microbiota and Short-Chain Fatty Acids in Acute Ischemic Stroke and the Subsequent Risk for Poor Functional Outcomes. JPEN J Parenter Enteral Nutr (2021) 45(3):518–29. doi: 10.1002/jpen.1861
127. Li N, Wang X, Sun C, Wu X, Lu M, Si Y, et al. Change of Intestinal Microbiota in Cerebral Ischemic Stroke Patients. BMC Microbiol (2019) 19(1):191. doi: 10.1186/s12866-019-1552-1
128. Stanley D, Moore RJ, Wong CHY. An Insight Into Intestinal Mucosal Microbiota Disruption After Stroke. Sci Rep (2018) 8(1):568. doi: 10.1038/s41598-017-18904-8
129. Yamashiro K, Tanaka R, Urabe T, Ueno Y, Yamashiro Y, Nomoto K, et al. Correction: Gut Dysbiosis Is Associated With Metabolism and Systemic Inflammation in Patients With Ischemic Stroke. PloS One (2017) 12(4):e0176062. doi: 10.1371/journal.pone.0176062
130. Houlden A, Goldrick M, Brough D, Vizi ES, Lenart N, Martinecz B, et al. Brain Injury Induces Specific Changes in the Caecal Microbiota of Mice via Altered Autonomic Activity and Mucoprotein Production. Brain Behav Immun (2016) 57:10–20. doi: 10.1016/j.bbi.2016.04.003
131. Meisel C, Schwab JM, Prass K, A. Meisel and U. Dirnagl: Central Nervous System Injury-Induced Immune Deficiency Syndrome. Nat Rev Neurosci (2005) 6(10):775–86. doi: 10.1038/nrn1765
132. Brea D, Poon C, Benakis C, Lubitz G, Murphy M, Iadecola C, et al. Stroke Affects Intestinal Immune Cell Trafficking to the Central Nervous System. Brain Behav Immun (2021) 96:295–302. doi: 10.1016/j.bbi.2021.05.008
133. Crapser J, Ritzel R, Verma R, Venna VR, Liu F, Chauhan A, et al. Ischemic Stroke Induces Gut Permeability and Enhances Bacterial Translocation Leading to Sepsis in Aged Mice. Aging (Albany NY) (2016) 8(5):1049–63. doi: 10.18632/aging.100952
134. Zeng W, Shen J, Bo T, Peng L, Xu H, Nasser MI, et al. Cutting Edge: Probiotics and Fecal Microbiota Transplantation in Immunomodulation. J Immunol Res (2019) 2019:1603758. doi: 10.1155/2019/1603758
135. Chen R, Xu Y, Wu P, Zhou H, Lasanajak Y, Fang Y, et al. Transplantation of Fecal Microbiota Rich in Short Chain Fatty Acids and Butyric Acid Treat Cerebral Ischemic Stroke by Regulating Gut Microbiota. Pharmacol Res (2019) 148:104403. doi: 10.1016/j.phrs.2019.104403
136. Gupta A, Khanna S. Fecal Microbiota Transplantation. JAMA (2017) 318(1):102. doi: 10.1001/jama.2017.6466
137. Ooijevaar RE, Terveer EM, Verspaget HW, Kuijper EJ, Keller JJ. Clinical Application and Potential of Fecal Microbiota Transplantation. Annu Rev Med (2019) 70:335–51. doi: 10.1146/annurev-med-111717-122956
138. Dailey FE, Turse EP, Daglilar E, Tahan V. The Dirty Aspects of Fecal Microbiota Transplantation: A Review of Its Adverse Effects and Complications. Curr Opin Pharmacol (2019) 49:29–33. doi: 10.1016/j.coph.2019.04.008
139. Hill C, Guarner F, Reid G, Gibson GR, Merenstein DJ, Pot B, et al. Expert Consensus Document. The International Scientific Association for Probiotics and Prebiotics Consensus Statement on the Scope and Appropriate Use of the Term Probiotic. Nat Rev Gastroenterol Hepatol (2014) 11(8):506–14. doi: 10.1038/nrgastro.2014.66
140. Derrien M, van Hylckama Vlieg JE. Fate, Activity, and Impact of Ingested Bacteria Within the Human Gut Microbiota. Trends Microbiol (2015) 23(6):354–66. doi: 10.1016/j.tim.2015.03.002
141. Ebel B, Lemetais G, Beney L, Cachon R, Sokol H, Langella P, et al. Impact of Probiotics on Risk Factors for Cardiovascular Diseases. A review. Crit Rev Food Sci Nutr (2014) 54(2):175–89. doi: 10.1080/10408398.2011.579361
142. Muralitharan RR, Jama HA, Xie L, Peh A, Snelson M, Marques FZ. Microbial Peer Pressure: The Role of the Gut Microbiota in Hypertension and Its Complications. Hypertension (2020) 76(6):1674–87. doi: 10.1161/HYPERTENSIONAHA.120.14473
143. Liu Y, Kong C, Gong L, Zhang X, Zhu Y, Wang H, et al. The Association of Post-Stroke Cognitive Impairment and Gut Microbiota and its Corresponding Metabolites. J Alzheimers Dis (2020) 73(4):1455–66. doi: 10.3233/JAD-191066
144. Tang WH, Wang Z, Levison BS, Koeth RA, Britt EB, Fu X, et al. Intestinal Microbial Metabolism of Phosphatidylcholine and Cardiovascular Risk. N Engl J Med (2013) 368(17):1575–84. doi: 10.1056/NEJMoa1109400
145. Koeth RA, Wang Z, Levison BS, Buffa JA, Org E, Sheehy BT, et al. Intestinal Microbiota Metabolism of L-Carnitine, a Nutrient in Red Meat, Promotes Atherosclerosis. Nat Med (2013) 19(5):576–85. doi: 10.1038/nm.3145
146. Morais LH, th Schreiber HL, Mazmanian SK. The Gut Microbiota-Brain Axis in Behaviour and Brain Disorders. Nat Rev Microbiol (2021) 19(4):241–55. doi: 10.1038/s41579-020-00460-0
147. Berer K, Gerdes LA, Cekanaviciute E, Jia X, Xiao L, Xia Z, et al. Gut Microbiota From Multiple Sclerosis Patients Enables Spontaneous Autoimmune Encephalomyelitis in Mice. Proc Natl Acad Sci USA (2017) 114(40):10719–24. doi: 10.1073/pnas.1711233114
Keywords: ischemic stroke, gut microbiota, gut-brain axis communication, neuro inflammation, fecal microbiota transplantation
Citation: Wang J, Zhang H, He J and Xiong X (2022) The Role of the Gut Microbiota in the Development of Ischemic Stroke. Front. Immunol. 13:845243. doi: 10.3389/fimmu.2022.845243
Received: 29 December 2021; Accepted: 02 March 2022;
Published: 28 March 2022.
Edited by:
Junlei Chang, Shenzhen Institutes of Advanced Technology (CAS), ChinaReviewed by:
Jing Zhao, Chongqing Medical University, ChinaCopyright © 2022 Wang, Zhang, He and Xiong. This is an open-access article distributed under the terms of the Creative Commons Attribution License (CC BY). The use, distribution or reproduction in other forums is permitted, provided the original author(s) and the copyright owner(s) are credited and that the original publication in this journal is cited, in accordance with accepted academic practice. No use, distribution or reproduction is permitted which does not comply with these terms.
*Correspondence: Jianying He, aGVqaWFueWluZ2xhb3NoaUAxNjMuY29t
†These authors share first authorship
Disclaimer: All claims expressed in this article are solely those of the authors and do not necessarily represent those of their affiliated organizations, or those of the publisher, the editors and the reviewers. Any product that may be evaluated in this article or claim that may be made by its manufacturer is not guaranteed or endorsed by the publisher.
Research integrity at Frontiers
Learn more about the work of our research integrity team to safeguard the quality of each article we publish.