- 1Instituto de Investigaciones Biotecnológicas Dr. Rodolfo A. Ugalde, Universidad Nacional de San Martín, Consejo Nacional de Investigaciones Científicas y Técnicas (UNSAM-CONICET), San Martín, Argentina
- 2Instituto de Virología e Innovaciones Tecnológicas (IVIT), Centro de Investigaciones en Ciencias Veterinarias y Agronómicas (CICVyA), Instituto Nacional de Tecnología Agropecuaria (INTA)-Consejo Nacional de Investigaciones Científicas y Técnicas (CONICET), Buenos Aires, Argentina
- 3Department of Entomology, College of Agriculture and Life Sciences, Fralin Life Science Institute, Virginia Polytechnic Institute and State University, Blacksburg, VA, United States
- 4Instituto de Investigaciones Biomédicas en Retrovirus y SIDA (INBIRS, Universidad de Buenos Aires-CONICET), Buenos Aires, Argentina
- 5Center for Emerging, Zoonotic, and Arthropod-borne Pathogens, Virginia Polytechnic Institute and State University, Blacksburg, VA, United States
In this work, we evaluated recombinant receptor binding domain (RBD)-based vaccine formulation prototypes with potential for further clinical development. We assessed different formulations containing RBD plus alum, AddaS03, AddaVax, or the combination of alum and U-Omp19: a novel Brucella spp. protease inhibitor vaccine adjuvant. Results show that the vaccine formulation composed of U-Omp19 and alum as adjuvants has a better performance: it significantly increased mucosal and systemic neutralizing antibodies in comparison to antigen plus alum, AddaVax, or AddaS03. Antibodies induced with the formulation containing U-Omp19 and alum not only increased their neutralization capacity against the ancestral virus but also cross-neutralized alpha, lambda, and gamma variants with similar potency. Furthermore, the addition of U-Omp19 to alum vaccine formulation increased the frequency of RBD-specific geminal center B cells and plasmablasts. Additionally, U-Omp19+alum formulation induced RBD-specific Th1 and CD8+ T-cell responses in spleens and lungs. Finally, this vaccine formulation conferred protection against an intranasal severe acute respiratory syndrome coronavirus 2 (SARS-CoV-2) challenge of K18-hACE2 mice.
Introduction
Severe acute respiratory syndrome coronavirus 2 (SARS-CoV-2) is the causative agent of coronavirus disease 2019 (COVID-19) that developed into a global pandemic causing (as of November 30, 2021) over 260 million cases and over 5.2 million deaths worldwide (Weekly epidemiological update, World Health Organization, WHO). Mass vaccination offers the most efficient public health intervention to control the pandemic. Several vaccines have been shown to be effective and have been either approved or authorized for emergency use in different countries (Status of COVID-19 Vaccines, WHO).
Despite the efforts made to vaccinate people, it is still too early to establish the durability and extent of protection, and recent data on approved vaccines have shown a diminished efficacy 6 months after vaccination (1–3). Most importantly, it is critical to find a way to optimize the existing vaccines to protect against the prevalent SARS-CoV-2 variants of concern (VOC) that are spreading globally (4). Evidence of waning immunity and viral diversification creates a possible need for a booster vaccine dose to protect the population (5), leading advisory health agencies to recommend an additional dose of a COVID-19 vaccine. For all these reasons, there is a need to produce safer, more effective, highly scalable, and more affordable COVID-19 vaccines locally or regionally.
Most of the approved vaccines are mRNA-based, vector-based, or inactivated viruses. Currently, there are a few protein-based subunit vaccine candidates in late phase trials and/or approved (6, 7). Subunit vaccines are a well-known platform, and many subunit vaccines are already in widespread use. Protein subunit vaccines are easy to produce and safe, but in practice, they require a suitable adjuvant to stimulate the host immune response.
Subunit vaccine candidates in development are mainly based on Spike protein or the receptor-binding domain (RBD) from SARS-CoV-2. RBD is located within the S1 subunit of the Spike. Angiotensin-converting enzyme 2 (ACE2) is the functional receptor for SARS-CoV-2 comprising a critical factor for SARS-CoV-2 to enter into target cells, and RBD is a key functional component that is responsible for binding of SARS-CoV-2 to host cells (8, 9). It is therefore not surprising that antibodies directed against the RBD or overlapping with the ACE2 binding region are strongly neutralizing, making the RBD a promising antigen (Ag) for subunit vaccines (10). RBD-based antigens have been described in previous studies for SARS-CoV and MERS-CoV vaccine development (10, 11). RBD from SARS-CoV-2 is an ideal Ag for vaccine formulations because of its high expression levels, ease of manufacturing, stability, and capacity to elicit functional antibodies (12).
Although there is no defined immune correlate of protection from SARS-CoV-2 infection yet, it has been proposed that neutralizing antibody levels are highly predictive of immune protection (13, 14). A strong correlation between vaccine-induced neutralizing antibodies (nAbs) and reduction in viral loads has been found in non-human primates and humans after SARS-CoV-2 infection (15, 16). T-cell responses also play important protective roles in SARS-CoV-2 infection. The depletion of T cells in rhesus macaques has been shown to impair virus clearance (15). In humans, virus-specific CD4+ and CD8+ T-cell responses are associated with milder disease, indicating an involvement in protective immunity against COVID-19. Therefore, an ideal vaccine is expected to induce both the humoral and cellular arms of the immune system.
Vaccine adjuvants can enhance the magnitude, breadth, and durability of the immune response. Following its introduction in the 1920s, alum remained the only adjuvant licensed for human use for the next 70 years; however, five new adjuvants have been included in licensed vaccines until present (17). The design and selection of adjuvants for COVID-19 vaccine formulations are key to induce optimal immune responses with adequate safety profiles. The introduction of novel adjuvants that have been shown to induce both humoral and cellular immune responses could be more favorable.
In previous works, we demonstrated that a bacterial protease inhibitor from Brucella abortus (U-Omp19) can be used as an adjuvant in parenteral and oral vaccine formulations (18–21). U-Omp19 parenteral delivery induces the recruitment of CD11c+ CD8α+ dendritic cells (DCs) and monocytes to lymph nodes where it partially limits in vivo Ag proteolysis inside DCs and increases Ag intracellular half-life. Consequently, U-Omp19 enhances Ag cross-presentation by DCs to CD8+ T cells. Antitumor responses were elicited after U-Omp19 co-administration, increasing survival of mice in a murine melanoma challenge model. Moreover, subcutaneous, or intramuscular co-administration of U-Omp19 with Trypanosoma cruzi Ags conferred protection against virulent parasite challenge, reducing parasitemia and increasing mice survival (20, 22). When U-Omp19 was co-delivered orally, it increased mucosal Th1, Th17, effector CD8+ T cells, and Ab responses and reduced parasite or bacterial loads after oral challenge with virulent Toxoplasma gondii or Salmonella (21). Thus, U-Omp19 is a promising novel adjuvant able to promote specific Th1 and CD8+ T-cell immune responses in addition to Ab responses.
Here, we present preclinical data of a COVID-19 recombinant RBD-based vaccine candidate formulated with alum and U-Omp19 adjuvant with potential for further clinical development. Development of recombinant-protein-based COVID-19 vaccines technology could facilitate its transfer for emerging market vaccine manufacturers in low- and middle-income countries and improve local vaccine availability at affordable costs.
Results
Antigen Expression and Characterization
In this study, RBD was used as the vaccine antigen. A monomeric version of RBD preceded by SARS-CoV-2 spike signal peptide for secretion and a C-terminal hexahistidine (6xHis)-Tag was expressed after plasmid transfection in HEK-293 cells. The RBD segment selected in this study (residues 319-541) contains eight predicted immunodominant CD4+ T-cell epitopes and 17 predicted CD8+ T-cell epitopes in addition to B-cell epitope motifs (23).
Recombinant RBD was purified from cell culture supernatant through a single-step Ni-NTA affinity chromatography. SARS-CoV-2 RBD protein had high expression with remarkable purity (Figure 1A). Noteworthy, purified RBD was recognized by polyclonal antibodies in sera from a convalescent patient infected with SARS-CoV-2 (Figure 1B). Analytical evaluation by size exclusion chromatography revealed that the recombinant protein is monodispersed. The sample eluted as a single peak with an apparent molecular weight of 40.3 kDa, representing >95% of the sample (Figure 1C). Endotoxin levels measured in the purified protein were ≤1.25 EU/mg; this value is significantly lower than the maximum recommended endotoxin level for recombinant subunit human vaccines (24).
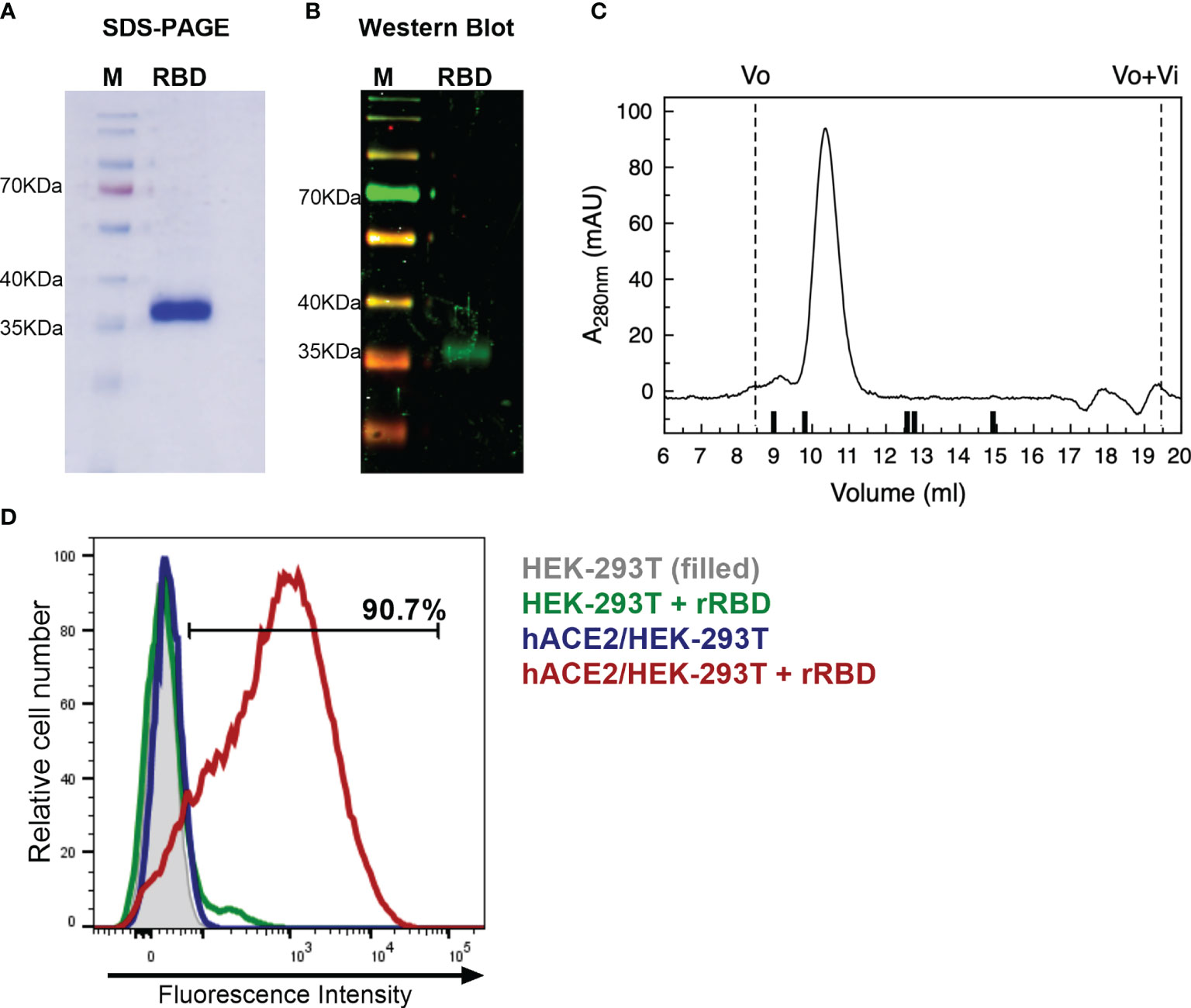
Figure 1 Characterization of recombinant RBD. (A) Coomassie blue-SDS-PAGE stained of reduced RBD. M, page ruler. (B) Western Blot analysis of recombinant RBD produced in HEK-293 cells using a convalescent human serum as primary antibody. M, page ruler. (C) Representative size exclusion chromatography elution profile of recombinant RBD. Black bars represent MWM; from left to right: BSA (66.4 kDa), OVA (44.3 kDa), papain (23 kDa), RNaseA (13.7 kDa), Aprotinin (6.5 kDa), and Vo and Vo+Vi are shown as dashed lines. (D) Binding of recombinant RBD (rRBD) to HEK-293T cells expressing hACE2. HEK-293T were used as control. Histograms and percentage of cells positive for RBD are shown. Results are representative of two independent experiments.
Purified RBD was also assessed for its direct binding to the human ACE2 receptor in ACE2-expressing HEK-293T cells by flow cytometry. Strong binding of recombinant RBD to hACE2-HEK-293T cells was observed (>90%) (Figure 1D). This result confirms that purified RBD binds to cell-associated hACE2 receptor, suggesting that it has a correct folding.
All Vaccine Formulations Induce Serum RBD-Specific IgG Responses, While Only U-Omp19+RBD+Alum Formulation Induces Specific IgA in BAL After Intramuscular Immunization
The immunogenicity of a variety of vaccine formulations comprising recombinant RBD with different adjuvants was evaluated in mice. We assessed formulations containing approved adjuvants for human use, including aluminum hydroxide, AddaS03 (similar to AS03), or AddaVax (similar to MF59), and the combination of alum and U-Omp19, a novel adjuvant developed in our laboratory that demonstrated vaccine adjuvant properties when co-administered with different Ags at pre-clinical stages.
Recombinant RBD was formulated with aluminum hydroxide (-alum-Alhydrogel 2%) alone, alum plus U-Omp19, AddaS03, or AddaVax. BALB/c mice received two doses at day 0 and 14 via intramuscular (i.m.) route (Figure 2A). After the first dose, animals immunized with RBD+alum or RBD+alum+U-Omp19 produced a specific anti-RBD IgG response in serum (GMT at day 14: 4,222 and 5,572, respectively, Figure 2B). Anti-RBD IgG titers increased after the second dose reaching a plateau (GMT at day 44: 215,269 and 323,050, respectively, Figure 2B). The groups of animals immunized with formulations containing AddaVax or AddaS03 as adjuvants failed to induce specific antibodies after the first dose (Figure 2B) but showed a significant anti-RBD IgG response after two doses (GMT at day 44: 337,794 and 215,269, respectively). RBD-specific IgG subclasses were evaluated 1 month after last immunization, demonstrating that all vaccine formulations induced higher titers of IgG1 than IgG2a in serum of mice (Figure 2C). Ag-specific-IgA at the low respiratory tract has an important role to control virus dissemination (25). Interestingly, levels of RBD-specific IgA in the bronchoalveolar lavage (BAL) of mice were higher in the group that received RBD+alum+U-Omp19 than in groups that received AddaVax or AddaS03 (Figure 2D). Besides, anti-RBD IgA was measured in serum samples revealing that groups containing alum or alum plus U-Omp19 as adjuvants induced significant higher levels compared to phosphate-buffered saline (PBS) or groups containing AddaVax or AddaS03 as adjuvants (Figure 2E).
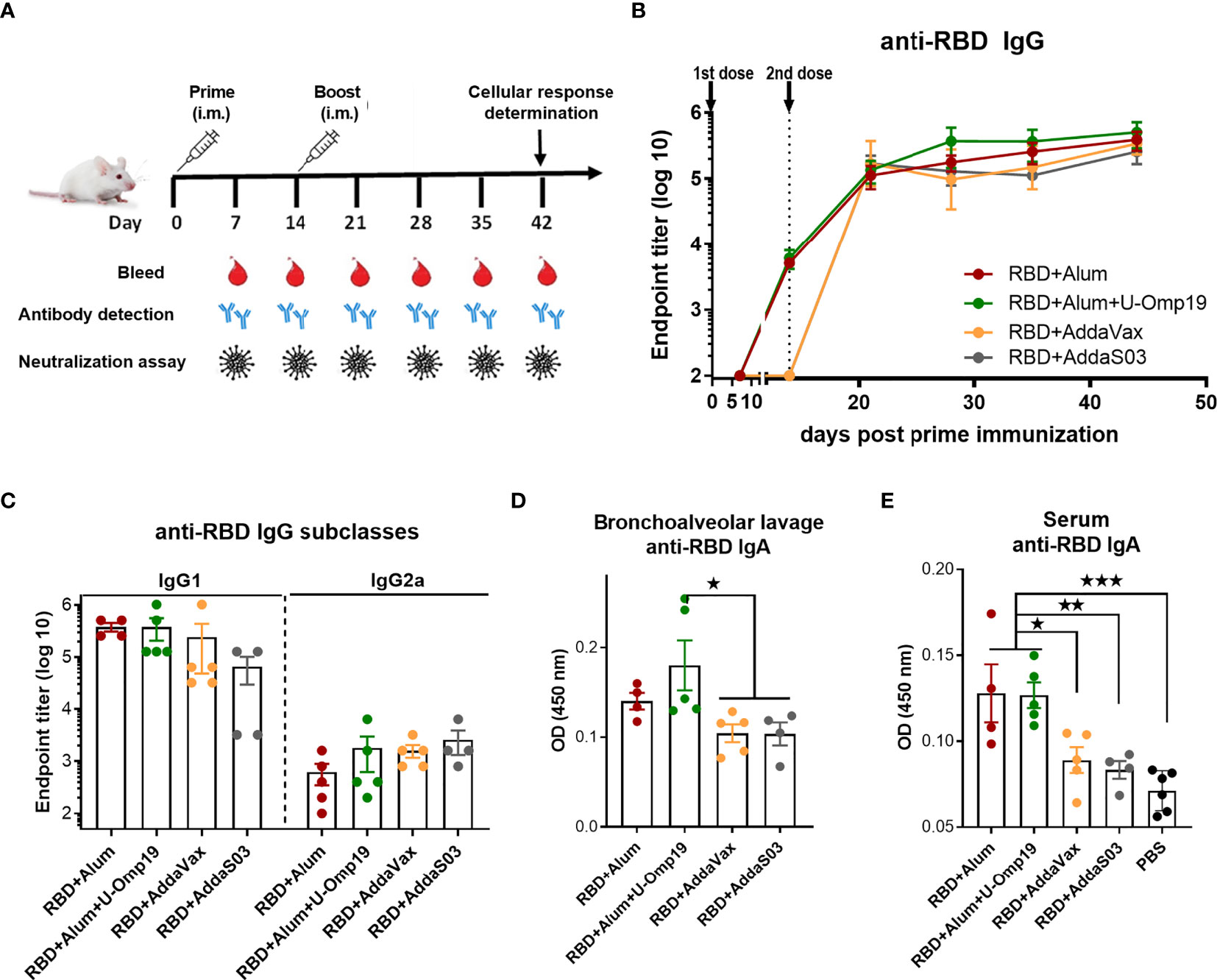
Figure 2 BALB/c mice immunized with RBD plus different adjuvants induced RBD-specific antibodies. (A) Immunization protocol scheme. BALB/c mice were vaccinated at day 0 and day 14 via i.m route with RBD+Alum (n = 5), RBD+Alum+U-Omp19 (n = 5), RBD+AddaVax (n=5), or RBD+AddaS03 (n = 4). Serum samples were obtained at indicated time points for ELISA and neutralization assays. (B) Kinetics of RBD-specific IgG endpoint titers in sera of immunized animals by ELISA. Points are means ± SEM. (C) RBD-specific IgG subclasses (IgG1 and IgG2a) titers in sera of immunized animals at day 44 post prime immunization. Detection of RBD-specific IgA in the bronchoalveolar lavage (D) and serum (E) of immunized mice at day 44 post prime immunization. Samples were diluted at 1/50 for BAL and 1/100 for serum. Data are optical density (OD) at 450 nm. *p < 0.05. **p < 0.01. ***p < 0.001. One-way ANOVA with Bonferroni post-test. Results are representative of two independent experiments.
U-Omp19+Ag+Alum Formulation Significantly Increases Mucosal and Systemic Neutralizing Abs in Comparison to Ag Plus Alum, AddaVax, or AddaS03
Next, the neutralization capacity of the vaccine-induced antibodies was evaluated using an HIV-based pseudovirus neutralization assay (PsVNA). All vaccine formulations induced serum-neutralizing antibodies against the SARS-CoV-2 spike pseudotyped virus (Figure 3A). Remarkably, immunization with two doses of the formulation containing alum plus U-Omp19 as adjuvants induced a 10-fold increase in the neutralization titer (GMT, 325.1; 95%CI, 103.8–1,018) compared to the groups immunized with alum alone as adjuvant, AddaVax, or AddaS03 (GMT alum+RBD group: 34.2; 95%CI, 3.79–308.5, Figure 3A). This increment was statistically significant (p = 0.0257 vs. RBD+alum, p = 0.0259 vs. AddaVax, and p =0.022 vs. AddaS03). AddaVax or AddaS03 as adjuvants induced a titer of neutralizing antibodies similar to alum alone (GMT AddaVax, 69.83; 95%CI, 33.08–147.4; GMT AddaS03, 45; 95%CI, 45–45, Figure 3B).
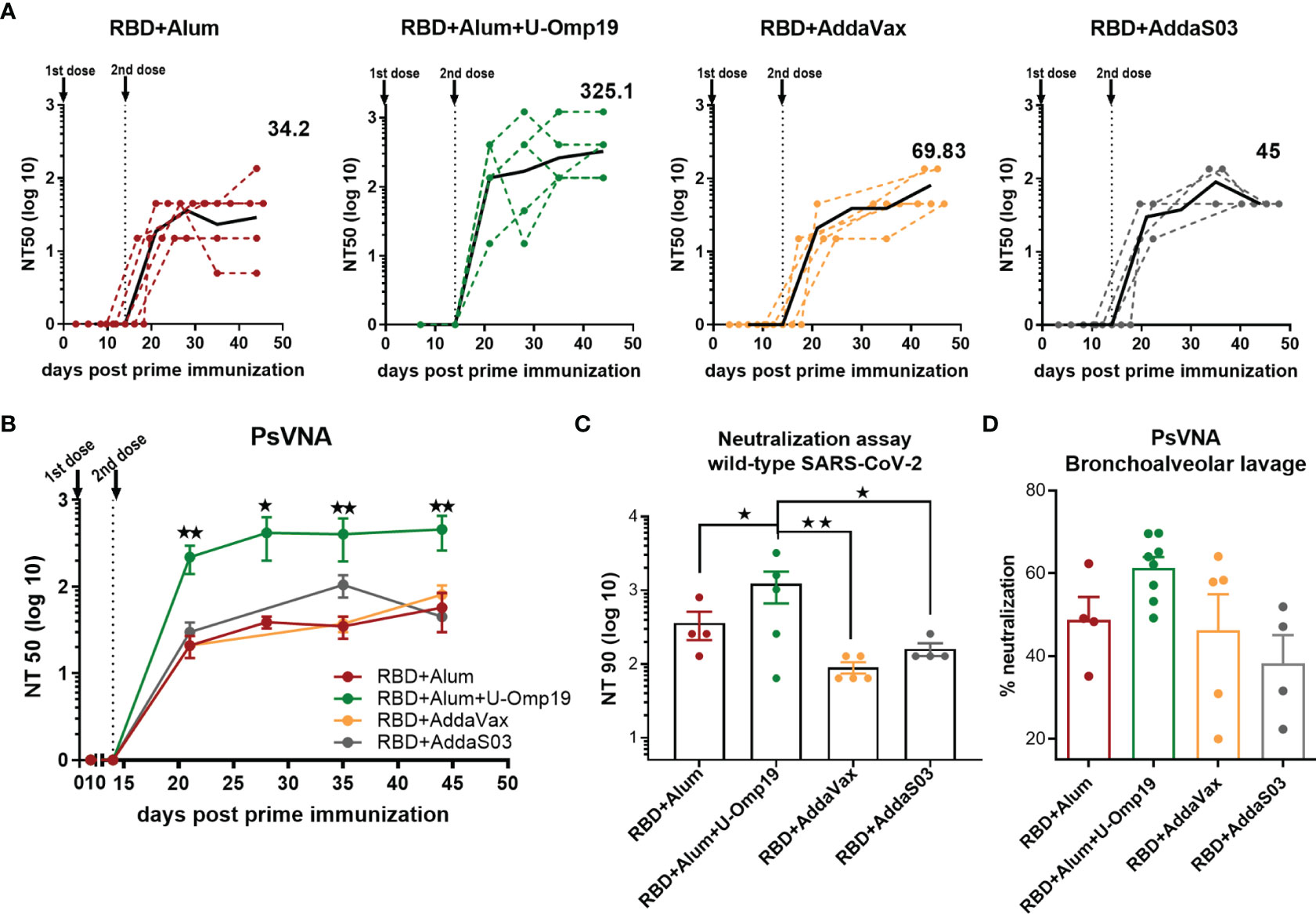
Figure 3 Immunization with RBD+Alum+U-Omp19 increases systemic and mucosal neutralizing antibody titers. (A) BALB/c mice were vaccinated as described in Figure 2. Serum neutralizing-antibody titers determined by pseudo-typed SARS-CoV-2 assay for each group of vaccinated mice at different time points. The black lines represent the geometric mean of all data points, and numbers are GMT on day 44. Dotted lines represent the mean titer of each mouse at indicated time points. Titers correspond to the 50% of virus neutralization (NT50). (B) Kinetics of neutralizing antibody titers of all groups determined by pseudo-typed SARS-CoV-2 assay. Points are means ± SEM. Titers correspond to the 50% of virus neutralization (NT50). *p < 0.05. **p < 0.01. One-way ANOVA with Bonferroni post-test. (C) Neutralizing antibody titers against ancestral SARS-CoV-2 virus at day 44 post prime immunization. Neutralization titer was defined as the highest serum dilution without any cytopathic effect in replicable wells (NT 90). Data are shown as means ± SEM. *p < 0.05. **p < 0.01. One way ANOVA with Bonferroni post-test. (D) BALB/c mice were vaccinated at day 0 and day 14 via i.m. with RBD+Alum (n = 4), RBD+Alum+U-Omp19 (n = 8), RBD+AddaVax (n = 5), or RBD+AddaS03 (n = 4). Determination of neutralizing antibodies in the bronchoalveolar lavage by pseudo-typed SARS-CoV-2 assay at day 44. Data are expressed in percentage of neutralization compared with controls (virus alone). Results are representative of two independent experiments.
To assess the functionality of vaccine-elicited antibodies against the ancestral SARS-CoV-2 (Wuhan reference strain), neutralization assay with sera from immunized animals was performed. Similar to the results obtained using the pseudovirus system, 1 month after the second dose, RBD+alum+U-Omp19 immunized mice had significant higher virus neutralization antibody titers in serum (Figure 3C; GMT, 612.1; 95%CI, 87.80–4267) than mice immunized with RBD+alum (Figure 3C; GMT, 140; 95%CI, 16.34–1,199) or plus commercial adjuvants (AddaVax or AddaS03). These results further confirm the data obtained by PsVNA.
As SARS-CoV-2 initially infects the upper respiratory tract, its first interactions with the immune system must occur predominantly at the respiratory mucosal surfaces. Mucosal responses may be crucial to stop person to person transmission of this virus. Thus, examination of neutralizing activity in BAL was performed using pseudotyped virus system. Adding U-Omp19 as an adjuvant to the formulation induced a slight but not significant increase in ancestral virus neutralization in the BAL of mice compared with the vaccine adjuvanted with alum alone, AddaVax, or AddaS03 (Figure 3D).
Neutralizing Antibodies Last Over 5–6 Months After Immunization With U-Omp19+Ag+Alum
Duration of vaccine immunity is key to estimate how long protection lasts. To this effect, we evaluated the level of total antibodies over 175 days after prime immunization with the vaccine formulation containing U-Omp19 and alum as adjuvants. Interestingly, titers of anti-RBD IgG antibodies remained stable at least 5–6 months after i.m. immunization of mice with this formulation (Figure 4A). Remarkably, neutralizing capacity of the antibodies remained stable till day 175 post prime immunization (Figure 4B).
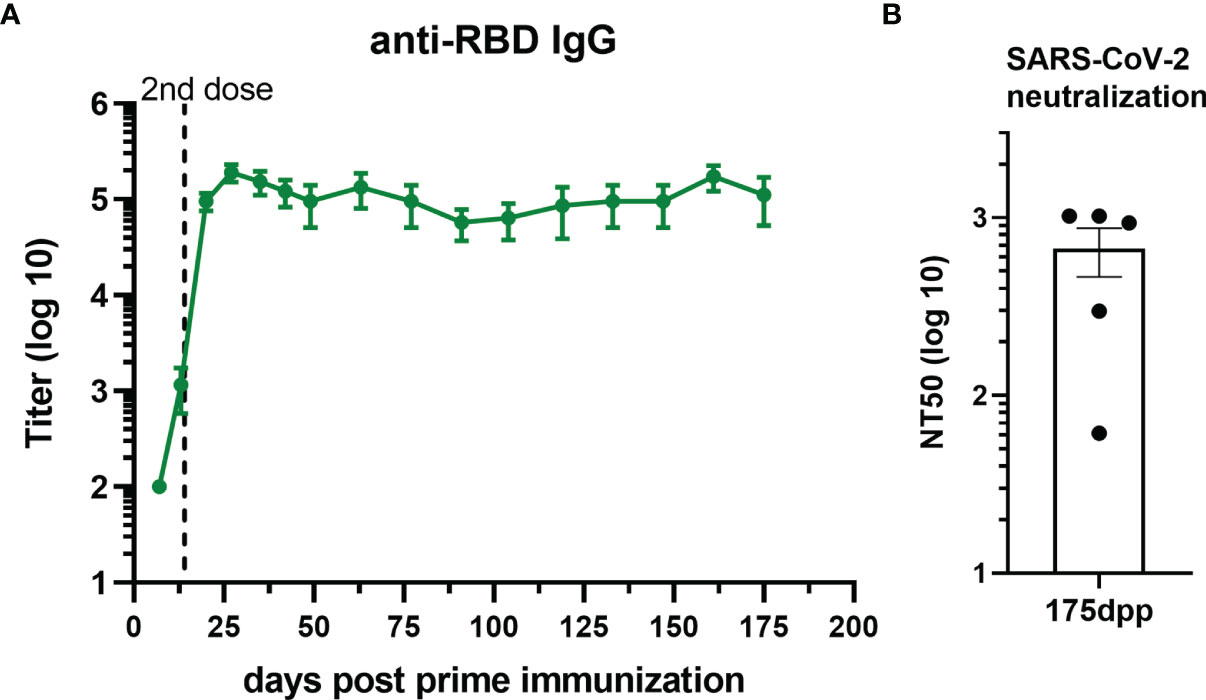
Figure 4 RBD+Alum+U-Omp19 immunization induces a long-term antibody response. (A) Kinetics of RBD-specific IgG endpoint titers in sera of RBD+Alum+U-Omp19 BALB/c immunized animals (n = 5) by ELISA. Points are means ± SEM. (B) Bar plot of neutralizing-antibody titer against ancestral SARS-CoV-2 at day 175 post prime immunization (dpp). Neutralization titer was defined as the serum dilution that reduces 50% the cytopathic effect (NT50). Bar is the mean ± SEM.
U-Omp19+Ag+Alum Formulation Induces Neutralizing Abs Against Multiple SARS-CoV-2 Variants
To adequately address the public health impact that newly emerging COVID-19 variants present, there is a need for vaccine-elicited antibodies that can cross-neutralize different SARS-CoV-2 variants. Thus, neutralization activity of sera against prevalent circulating variants of SARS-CoV-2 in our region, namely, alpha (B.1.1.7, first identified in UK), gamma (P.1, first identified in Manaos, Brazil), and lambda (C.37, first identified in Peru), was evaluated and compared to neutralizing activity against ancestral reference strain. In particular, gamma and lambda variants have been shown to partially escape neutralization by antibodies triggered by previously circulating variants or vaccine induced antibodies (26, 27). Noteworthy, antibodies induced after vaccination with the formulation containing U-Omp19 not only neutralize the ancestral virus but also cross-neutralized alpha, lambda, and gamma variants (Figure 5). In contrast, antibodies produced by mice immunized with RBD+alum could neutralize the ancestral SARS-CoV-2 and alpha variant but showed significantly lower neutralizing activity against gamma and lambda variants (Figure 5). AddaVax and AddaS03 adjuvanted formulations induced similar neutralizing antibody titers against the ancestral, gamma, and lambda variants (Figure 5).
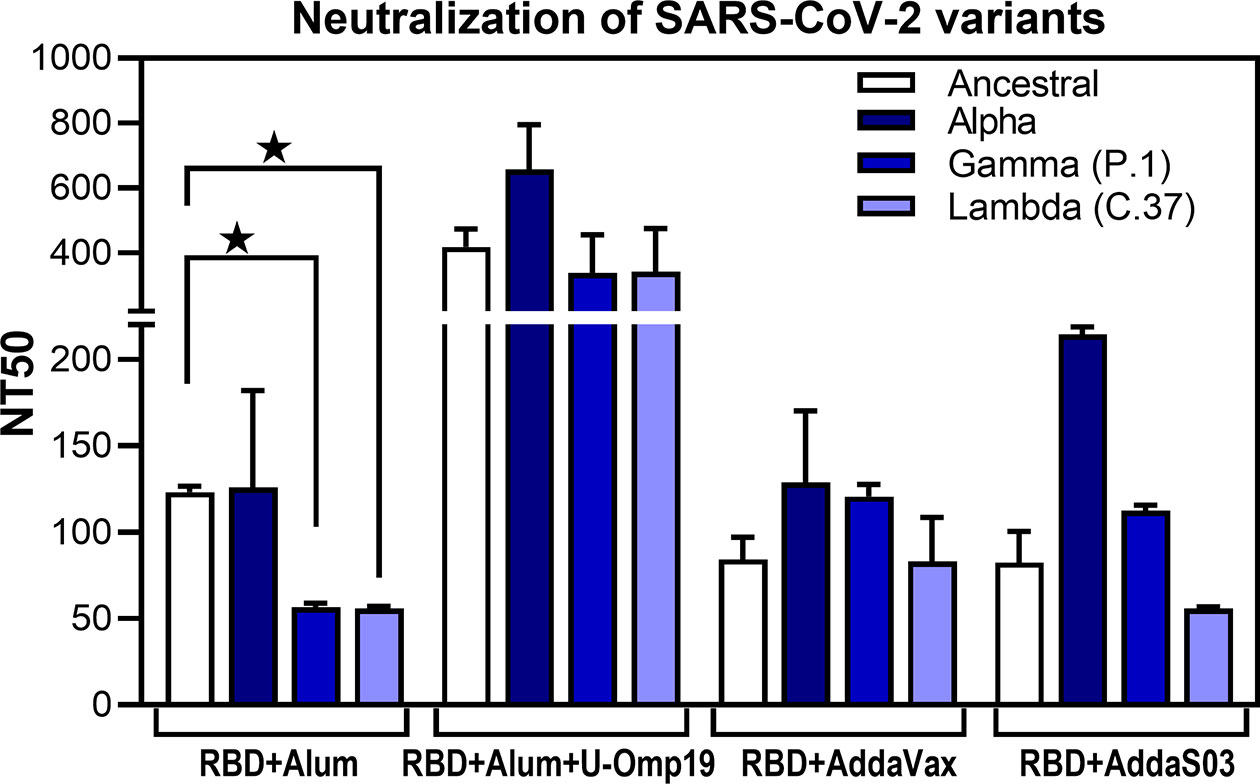
Figure 5 U-Omp19+Ag+Alum formulation induces neutralizing Abs against multiple SARS-CoV-2 variants. BALB/c mice were vaccinated as described in Figure 2. Neutralizing-antibody titers against ancestral (Wuhan reference strain) SARS-CoV-2 and alpha, gamma (P.1), and lambda (C.37) variants were assessed 30 days post second dose. Neutralization titer was defined as the serum dilution that reduces 50% the cytopathic effect (NT50). Bars represent means ± SEM. *p < 0.05. One-way ANOVA with Bonferroni post-test. Results are representative of two independent experiments.
Altogether these results demonstrate that addition of U-Omp19 to the alum plus RBD vaccine formulation increases virus neutralizing antibodies, specific IgA in BAL, and neutralizing antibodies of the virus in BAL. Neutralizing antibodies are proposed as the best correlate of protection; thus, we focused the next studies on the vaccine formulation containing U-Omp19 as adjuvant.
U-Omp19+RBD+Alum Formulation Induces Ag-Specific Th1 and CD8+ T Cells in Spleen and Lung
In addition to memory B cells and neutralizing antibodies, induction of specific T-cell immune responses could have a role in protection against SARS-CoV-2 infection (28).
To determine T-cell-mediated immune responses, splenocytes and lung cells from RBD+alum or RBD+alum+U-Omp19 immunized mice were stimulated with RBD or medium alone, and then, cytokines levels in the supernatants were measured. Both formulations were able to induce Ag-specific cytokine secretion at spleen (Figure 6A). Importantly, the levels of interferon (IFN)-γ were higher than that of interleukin (IL)-5 at the spleens of both vaccine formulations. In the lungs, immunization with RBD+alum+U-Omp19 promoted a significant increment in IFN-γ secretion compared with the formulation containing RBD+alum (Figure 6B). However, the alum-adjuvanted vaccine elicited a higher amount of IL-5 in the lung (Figure 6B). These results suggest that U-Omp19 as adjuvant promotes a specific T-cell response biased to a Th1 profile in the lung.
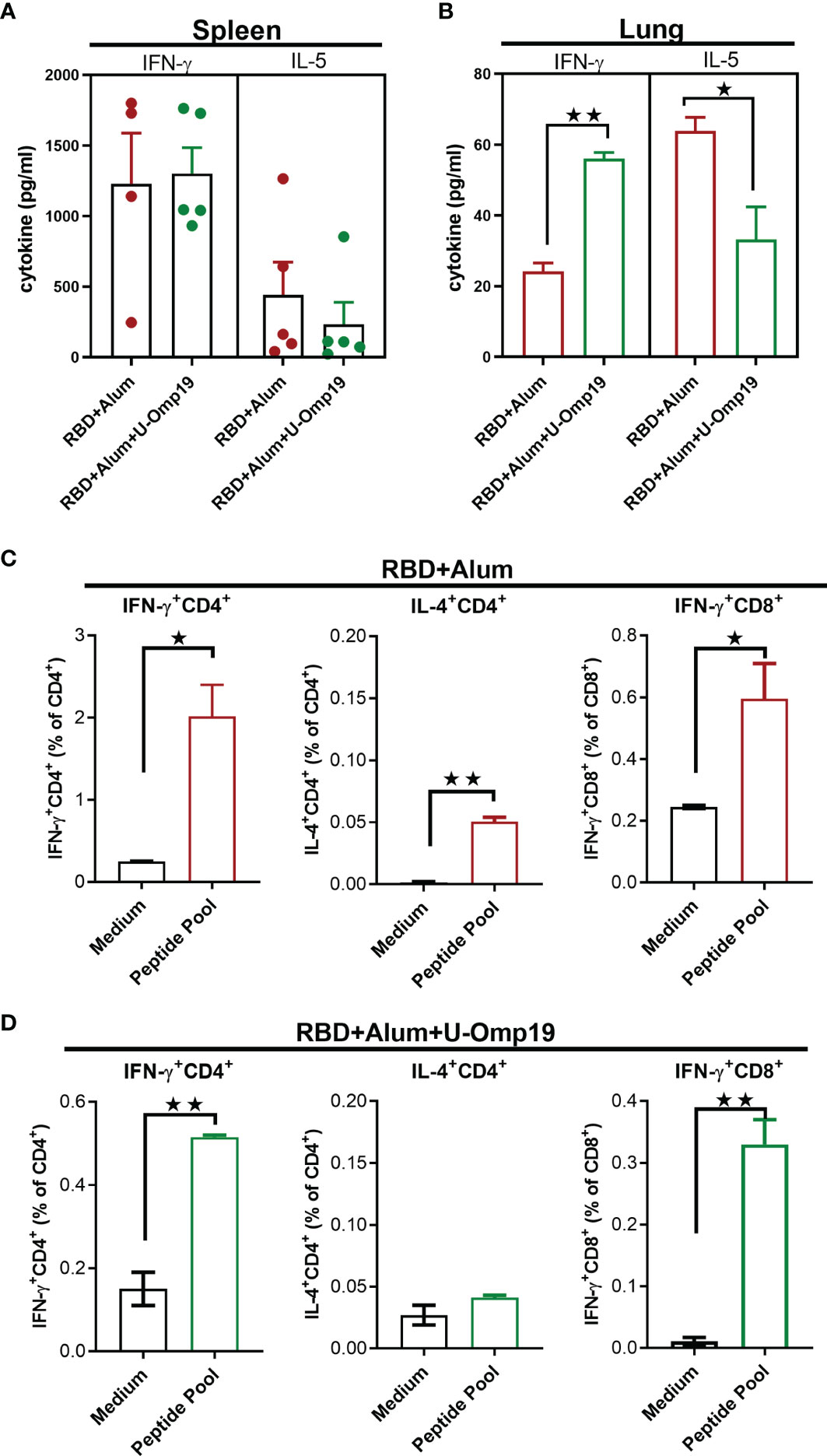
Figure 6 U-Omp19+RBD+Alum formulation induces Ag-specific Th1 and CD8+ T cells in the spleen and lung. BALB/c mice were vaccinated as described in Figure 2. Mice were sacrificed 44 days after the first immunization to obtain spleens and lungs, and T-cell response was evaluated. Levels of secreted IFN-γ and IL-5 following splenocytes (A) or lung cells (B) stimulation with medium or recombinant RBD were determined by ELISA. Bars are means ± SEM of pg/ml of IFN-γ and IL-5 after subtracting the amount in medium stimulated cells. *p < 0.05, **p < 0.01. t-test. (C, D) Intracellular flow cytometry analysis of cytokine secreting T cells. Splenocytes from groups RBD+Alum (C) or RBD+Alum+U-Omp19 (D) were stimulated with complete medium or RBD-peptides pool for 18 h, and then, brefeldin A was added for 5 h. Afterward, cells were harvested and stained with specific Abs anti-CD8, and anti-CD4, fixed, permeabilized, and stained intracellularly with anti-IFN-γ and anti-IL-4. Results are presented as percentage of IFN-γ or IL-4-producing T lymphocytes. Bars are means ± SEM. *p < 0.05, **p < 0.01 vs. medium. t-test. Results are representative of two independent experiments.
To further evaluate the Th1/2 balance, IFN-γ- and IL-4-producing cells were measured by intracellular cytokine staining. Spleen cells from immunized mice were stimulated with a pool of SARS-CoV-2 RBD peptides to detect antigen-specific T-cell responses. Percentages of IFN-γ-producing CD4+ and CD8+ T cells were increased in both groups of mice while IL-4-producing CD4+ T cells were only increased after RBD+alum administration (Figures 6C, D). These results support the induction of Th1 and CD8+ T-cell immune responses after immunization with RBD adjuvanted with U-Omp19 in combination with alum.
U-Omp19 as Adjuvant Increases Neutralizing Antibodies in C57BL/6 Mice
Immunogenicity of vaccine formulations using alum alone or combining both adjuvants (alum and U-Omp19) with RBD as Ag was also evaluated in the C57BL/6 mouse strain. Vaccine formulations were administered following the same schedule used for BALB/c mice, two doses every 14 days.
Both vaccine formulations induced high anti-RBD IgG titers in sera (Figure 7A). Remarkably, anti-RBD IgA levels in the BAL of RBD+alum+U-Omp19 immunized mice were higher than in the RBD+alum immunized mice (Figure 7B, p<0.05). There were no differences in specific IgG levels in the BAL between both groups (Figure 7B).
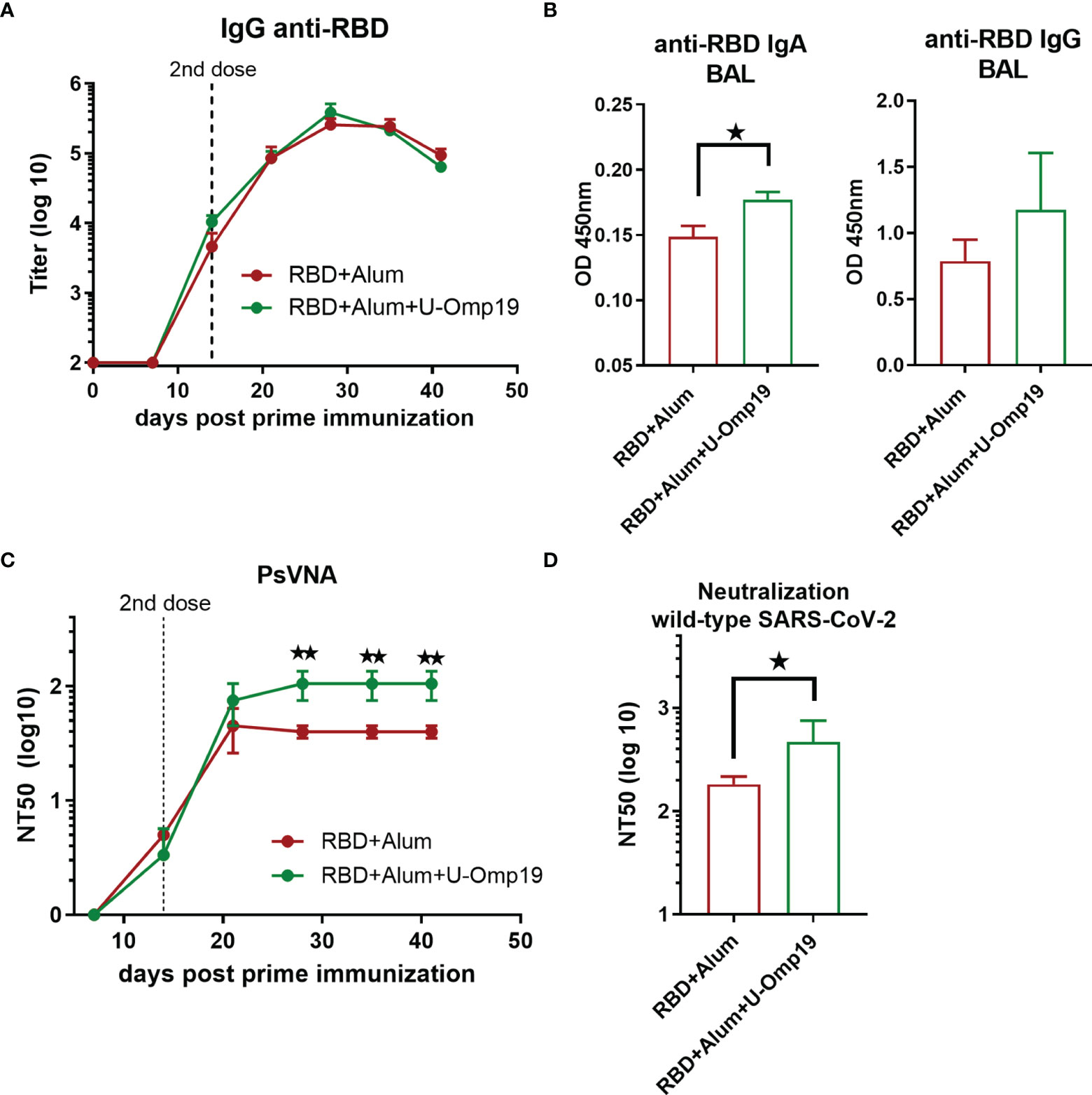
Figure 7 U-Omp19 as adjuvant increases neutralizing antibodies in C57BL/6. Mice (n = 3–5/group) were vaccinated at days 0 and 14 via i.m route. with RBD+Alum or RBD+Alum+U-Omp19. (A) Kinetics of RBD-specific IgG endpoint titer in sera of immunized animals by ELISA. Points are means ± SEM. (B) Detection of RBD-specific IgA and IgG at the bronchoalveolar lavage of immunized mice at day 42 post prime immunization. Data are optical density (OD) at 450 nm. *p < 0.05. Mann–Whitney test. (C) Kinetics of neutralizing-antibody titers determined by pseudo-typed SARS-CoV-2 assay. Neutralization titer was defined as the reciprocal serum dilution that causes a 50% reduction of transduction efficiency (NT50). **p < 0.01. t-test. (D) Neutralization titers against ancestral SARS-CoV-2 virus at day 42. Neutralization titer was defined as the serum dilution that reduces 50% the cytopathic effect (NT50). Data are shown as means ± SEM. *p < 0.05. t-test.
Formulation containing RBD+U-Omp19+alum induced higher neutralizing antibody titers than RBD+alum formulation (Figure 7C; GMT at 44 days post prime dose, 93.60; 95%CI, 19.36–452.5 and 37.47; 95%CI, 23.40–59.99, respectively).
The addition of U-Omp19 to RBD plus alum vaccine increased the neutralizing antibody titers against authentic ancestral virus. Four weeks after second dose, sera of mice immunized with RBD+alum+U-Omp19 produced a threefold increase in the viral neutralizing antibodies titer (GMT, 93.60; 95%CI, 19.36–452.5, Figure 7D) compared with mice receiving RBD + alum (Figure 7D; GMT, 37.47; 95%CI, 23.40–59.99). These results validated the data obtained in BALB/c mice and further indicate that U-Omp19 can be used under different genetic backgrounds.
U-Omp19 Adjuvanted Vaccine Increases RBD-Specific Germinal Center B Cells and Plasmablasts in the Spleen
A persistent germinal center (GC) B cell response enables the generation of robust humoral immunity (29). Therefore, specific GC B cells were evaluated in spleens from vaccinated mice 1 month after second dose; Figure 8A shows the gating strategy used. There were no differences between groups in the total CG cells (B220+ CD19+ IgD− CD95+ GL7+ cells) among spleen samples (Figure 8B). Of note, there were differences in the frequency of RBD+-specific GC cells, since mice immunized with RBD+alum+U-Omp19 increased the percentage of RBD+-specific GC cells in comparison with RBD+alum (Figure 8C). Besides, the percentages of RBD+-specific plasma blasts (B220+ CD19+ IgD− CD138+ cells) were also higher in animals from RBD+alum+U-Omp19 than from RBD+alum (Figure 8D). These results indicate a better performance of the vaccine formulation containing U-Omp19 to induce specific GC and secretory B cells 1 month after immunization.
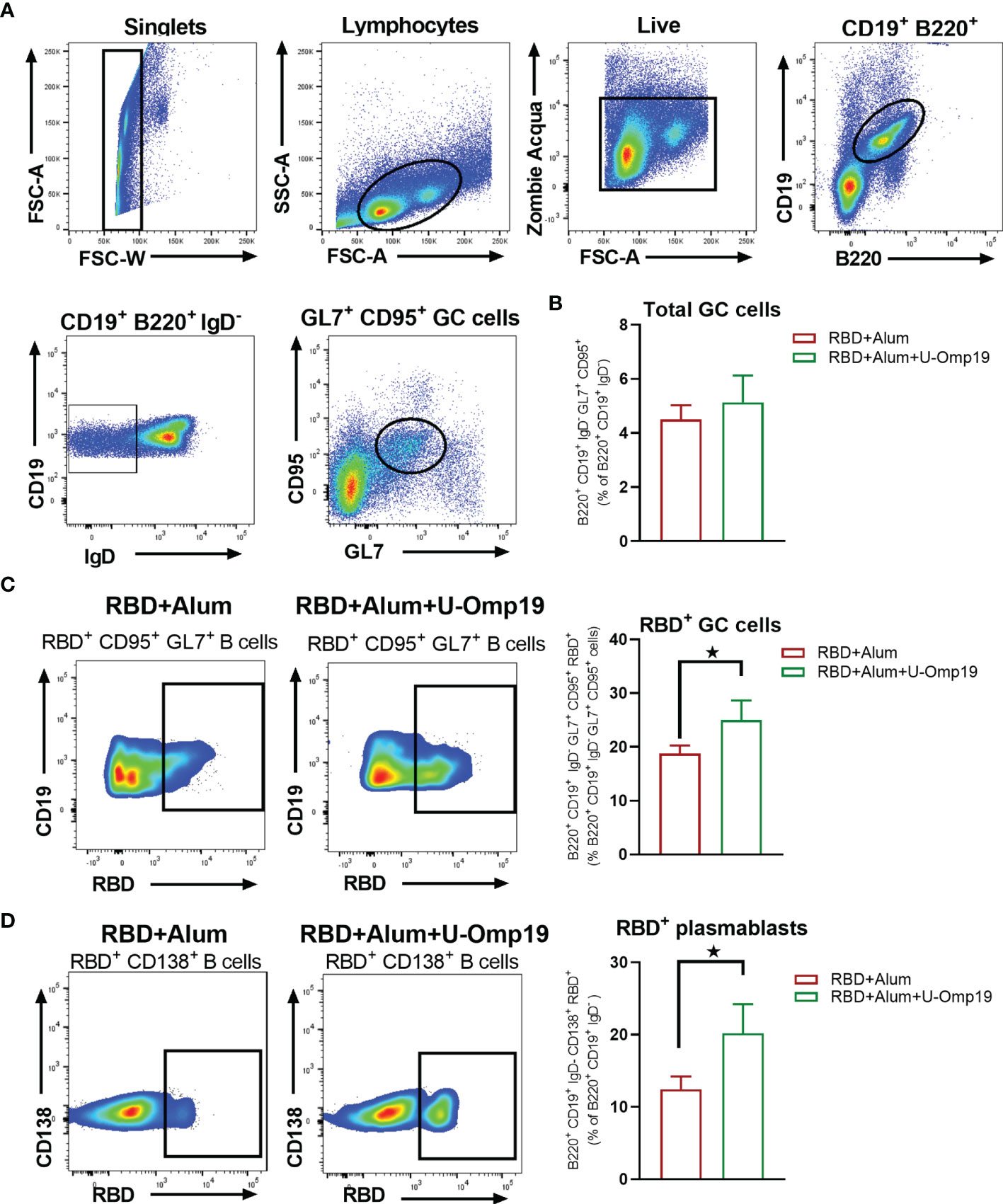
Figure 8 U-Omp19 as adjuvant increases RBD specific germinal center B cells and plasmablasts. Mice were vaccinated as described in Figure 7. Flow cytometry analysis of different B-cell populations at spleen of vaccinated mice were performed using anti-CD19, anti-B220, anti-IgD, anti-CD138, anti-GL7, and anti-CD95 antibodies. Specific cells were determined by binding to fluorescent RBD. Gating strategy is shown in Panel (A). (B) Results are presented as percentage of total GC cells (B220+ CD19+ IgD− GL7+ CD95+). Bars are means ± SEM. (C) Dot plots for each group are shown (right), and results are presented as percentage of RBD-specific GC cells (B220+ CD19+ IgD− GL7+ CD95+ RBD+). Bars are means ± SEM. *p < 0.05. t-test. (D) Dot plots for each group are shown (right), and results are presented as percentage of RBD-specific plasmablasts (B220+ CD19+ IgD− CD138+ RBD+). Bars are means ± SEM. *p < 0.05. t-test. Results are representative of two independent experiments.
RBD+Alum+U-Omp19 Induces Protection Against Intranasal SARS-CoV-2 Challenge
To determine vaccine efficacy, we used a severe disease model using K-18-hACE2 transgenic mice. Infection of transgenic mice with SARS-CoV-2 results in lung disease with signs of diffuse alveolar damage and variable spread to the central nervous system (30). The lethal dose 50% (LD50) is estimated to be 10 (4) plaque-forming units (PFU) (31). Vaccine formulation efficacy was evaluated in K18-hACE2 mice vaccinated with RBD+alum+U-Omp19 or PBS (control) and challenged intranasally with 2 × 105 PFU of SARS-CoV-2. At day 5 post-infection, some animals were euthanized to assess the viral load in lungs and brains. The presence of the SARS-CoV-2 virus was not detected in the lungs, while very low virus titers were detected in the brains of animals vaccinated with RBD+alum+U-Omp19 (Figure 9A). In contrast, a high viral load was detected in the lungs and brains of animals immunized with PBS (Figure 9A). It is noteworthy that most of mice vaccinated with RBD+alum+U-Omp19 did not lose weight after challenge (Figure 9B). Thus, the RBD+alum+U-Omp19 vaccine induced protection against the experimental challenge with SARS-CoV-2.
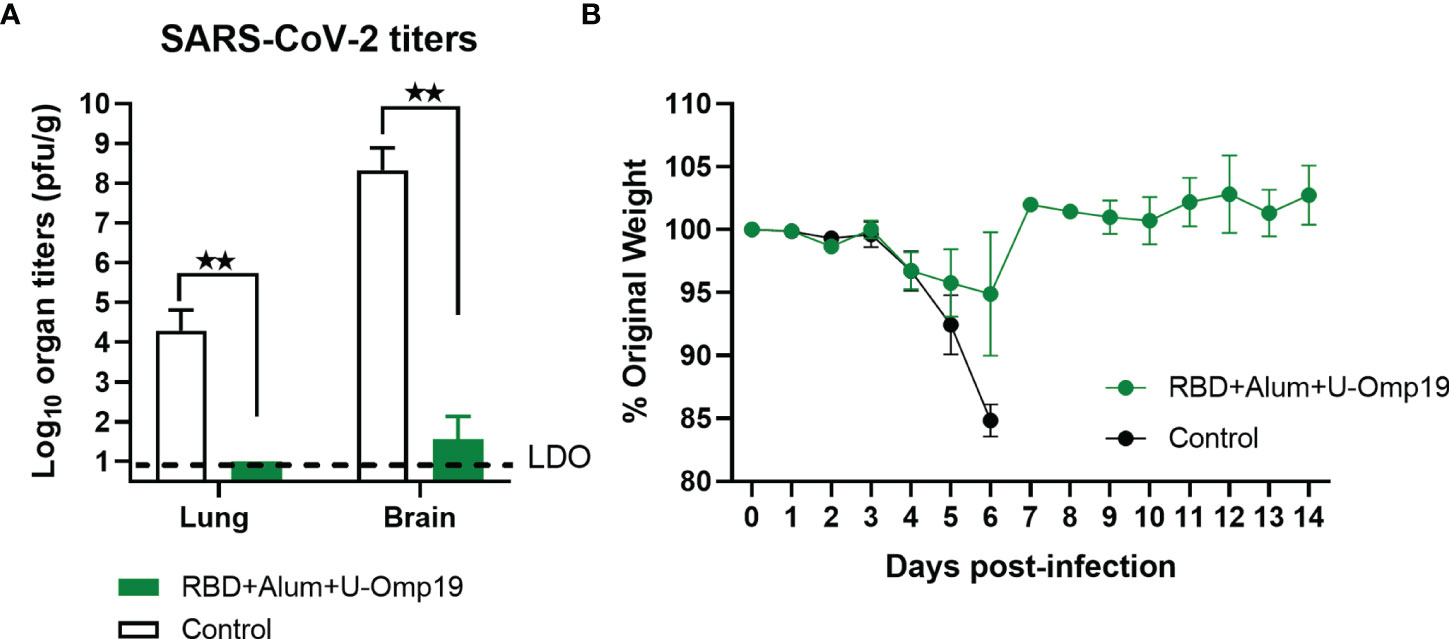
Figure 9 Vaccination with RBD+Alum+U-Omp19 protects K18-hACE2 transgenic mice against SARS-CoV-2 infection. Mice received PBS (Control) (n = 7) or RBD+Alum+U-Omp19 (n = 8) administered via i.m. route at days 0 and 14. Mice in each group included similar number of males and females. Four weeks following immunization, K18-hACE2 mice were intranasally infected with 2 × 105 PFU of SARS-CoV-2. (A) Five days after infection, lungs and brains (n=3) were obtained from groups of mice, and SARS-CoV-2 virus was titrated. Bars represent the mean ± SEM. Dotted line: limit of detection (LOD). **p < 0.01. t-test. (B) Weight loss outcomes in K18-hACE2 transgenic mice vaccinated and challenge with SARS-CoV-2. Weight changes in mice were monitored daily until day 14 after infection. Points are means ± SEM of percentage of original weight.
Discussion
There is an urgent need to develop safe, effective, and affordable COVID-19 vaccines for low- and middle-income countries. Such vaccines should rely on proven technologies such as recombinant-protein-based vaccines to facilitate their transfer to emerging market vaccine manufacturers. Protein-based vaccines are classic vaccine platforms and are considered a very safe vaccine strategy. There are licensed anti-viral vaccines that are protein-based vaccines, such as hepatitis B and human papillomavirus subunit vaccines, which have been widely administered and present an exceptional safety profile (32).
Our group has been working on the development of a recombinant-protein-based vaccine to prevent COVID-19. We selected the SARS-CoV-2 spike protein RBD as an immunogen, since it offers advantages for rational vaccine design both immunologically and from a manufacturability point of view (33). RBD-targeted binding antibodies correlate very strongly with virus-neutralizing activity in natural infections and vaccinations (34). Therefore, selection of RBD as antigen may induce a higher proportion of neutralizing antibodies, compared to full-length Spike protein immunization. Indeed, B-cell repertoire analysis after RBD immunization has been shown to induce a higher proportion of neutralizing antibodies than full-length Spike protein immunization (35). Moreover, RBD binding antibodies account for more than 90% of the neutralizing activity in COVID-19 convalescent and vaccinated individuals (34, 36). Previous studies have found that both SARS-CoV and MERS-CoV display antibody-dependent enhancement (ADE) (37), where non-neutralizing antibodies produced in response to a vaccine mediate virus infection via the fragment crystallizable (Fc) receptor and thus increase the risk of vaccinations enhancing viral infection (38). Although ADE has not been reported for the existing COVID-19 vaccines, a recent study has shown that antibodies against the S protein N-terminal domain enhanced the binding capacity of S protein to ACE2 and infectivity of SARS-CoV-2 (39). To mitigate the ADE effect, minimizing non-neutralizing epitopes, we decided to work with the SARS-CoV-2 spike protein RBD. Furthermore, in our hands (data not shown) and as described by others (40), yields of recombinant RBD were much higher than those of full-length Spike, an important factor in delivering the vaccine to global population.
Different cell types have been used to produce RBD antigens, such as yeast, plant, and insect cells (33, 41–44). However, production in mammalian cells (12, 45–49) may produce an RBD antigenic domain that more closely resembles that generated during virus infection in human cells (including post-translational modifications such as glycosylation and correct folding) (50). Our results showing binding to hACE2-expressing cells and the induction of neutralizing antibody titers confirm the preservation of the RBD structure and the suitable exposition of the receptor binding motif (RBM).
In this work, we have assessed the immunogenicity in mice of four different formulations containing the SARS-CoV-2 spike protein RBD with: (i) human-approved vaccine adjuvants, Alhydrogel (alum), an α-tocopherol and squalene-based containing oil-in-water emulsion (AddaS03) or a squalene-based oil-in-water nano-emulsion (AddaVax) or (ii) a combination of alum and a novel adjuvant called U-Omp19, a bacterial protease inhibitor with adjuvant properties.
All adjuvanted formulations induced robust anti-SARS-CoV-2 antibody responses. However, only alum containing formulations resulted in detectable antibody titers after the first immunization. This result is in line with literature reporting low immunogenicity in mice after a single dose when RBD is formulated with AddaVax (51–54), but significant seroconversion titers after single dose when formulated with alum (41, 54) or after two doses when formulated with AddaVax or AddaS03 (40, 47, 51, 52, 54). The overall response was dominated by the IgG1 subclass in all immunized groups.
RBD-immunization with alum, AddaS03, AddaVax, or U-Omp19 + alum induced substantial neutralizing antibody titers against Spike‐pseudotyped virions and ancestral SARS-CoV-2. Notably, the formulation adjuvanted with alum + U-Omp19 induced stronger neutralizing antibody titers when compared to formulations adjuvanted with alum, AddaVax, or AddaS03. In our experience, U-Omp19 as adjuvant alone in combination with a model Ag like OVA or with T. cruzi Ags administered parenterally did not increase serum Ab titers against the co-administered Ag. However, U-Omp19 was capable of changing the T-helper profile to Th1 (20). Although an effect of U-Omp19 in antibody functionality was seen with recombinant subunit vaccine against T. cruzi, a formulation adjuvanted with U-Omp19, despite inducing lower antibody titers, showed strong antibody-mediated lytic activity, which, together with the induction of a Th1-biased immune response, may account for the better elicited protection of this formulation (22). Given the importance of COVID-19 vaccine to induce a high titer of antibodies, in this work, we decided to combine U-Omp19 with alum and optimize current alum-adjuvanted subunit vaccines in development.
The improvement in antibody function by U-Omp19 addition to formulations could also be due to its protease inhibitor activity. U-Omp19 can inhibit neutrophil elastase (19), and Kim et al. have recently shown that coadministration of a neutrophil elastase inhibitor enhances the affinity and function of antibodies induced by alum as adjuvant (55). In the same work, Kim et al. showed that neutrophil elastase inhibitor supplementation can improve the efficacy of alum-adsorbed anti-SARS-CoV-2 vaccines by promoting the induction of IgA in the serum and mucosal secretions (55). Of note, U-Omp19 adjuvanted group presented the highest levels of RBD-specific IgA in serum and BAL and the highest neutralizing activity against SARS‐CoV‐2 Spike‐pseudotyped virions in BAL. This local immune response in the lungs may be of vital importance in neutralizing the virus before infection establishment, since it has been suggested that IgA-mediated mucosal immunity may be a critical defense mechanism against SARS-CoV-2 that may reduce infectivity of human secretions and consequently viral transmission as well (25). Moreover, secretory dimeric IgA found in mucosa has been shown to be a more potent SARS-CoV-2 neutralizer than serum IgA (56).
Recently, new variants of concern (VOC) or interest (VOI) of SARS-CoV-2 have been identified worldwide, and many of them have been shown to partially escape neutralization by antibodies induced by infection with previous circulating variants or vaccines (26, 27). These variants harbor mutations in RBD and N-terminal domain (NTD) of the spike protein that could impair the neutralizing activity of vaccine-induced antibodies (4). Therefore, we also evaluated the neutralizing activity induced by the vaccine formulations against highly circulating SARS-CoV-2 variants in our region: alpha (B.1.1.7), gamma (P1), and lambda (C.37). The neutralizing activity of antibodies elicited by the vaccine formulation with alum alone was twofold lower for gamma and lambda SARS-CoV-2 variants compared to D614G and alpha SARS-CoV-2 variants; similar or higher variant escape to vaccine-induced neutralizing antibodies was described for several vaccines (40, 57, 58). Interestingly, the AddaVax and AddaS03 adjuvanted formulations induced similar neutralizing antibody titers against the ancestral, gamma, and lambda variants. These results are in line with published data for CHO-cell-expressed RBD formulated with AddaS03 (40). More importantly, RBD adjuvanted with U-Omp19 + alum induced significantly higher and broader neutralizing activity. These data agree with other works showing that adjuvants not only enhance immunogenicity but also may have different potential to elicit neutralizing antibodies that provide a greater breadth of neutralization (59).
Most effective vaccines generate prolonged immunity by eliciting long-lived plasma cells (LLPCs) and memory B cells (MBCs) (60). LLPCs and MBCs with high affinity for the antigen are formed during germinal center reactions. In this study, we have shown that RBD immunization with alum+U-Omp19 induced higher levels of RBD-specific GC B cells than RBD formulated with alum alone. It has been reported that RBD-specific GC responses strongly correlate with neutralizing antibody production (61). It has also been suggested that prolonged antigen availability along with continuous presentation of antigens via major histocompatibility complex class II can improve GC reactions (61). We have previously demonstrated that U-Omp19 increases antigen half-life in antigen presenting cells (20, 62) and thus may increase GC reactions and CD4+ T-cell activation. In addition, it has been shown that neutrophil elastase inhibitor supplementation to alum formulations increases the frequency of GC B cells and the size of GC (55), suggesting that enrichment of RBD-specific GC B cells induced by U-Omp19 could be related to its ability to inhibit neutrophil elastase. U-Omp19 ability to increase GC B cell reactions was also demonstrated in the context of a rabies vaccine, in which the addition of U-Omp19 resulted in enhanced immunogenicity through increasing dendritic cells activation and germinal center formation (63).
Although antibodies have been shown to play a critical role in protection against coronavirus infections, the T-cell response is still indispensable for virus clearance, decreasing severe illness, and prognostic recovery (64). A study by McMahan et al. in rhesus macaques suggested that vaccine-induced memory T-cell responses contribute to protection against SARS-CoV-2, especially when antibodies work sub-optimally (15). In this study, we have investigated the intensity and diversity of T cells elicited in the lungs and the spleens in response to vaccination with RBD formulated with alum or with alum + U-Omp19. Interestingly, immunization with RBD adjuvanted with alum alone induced a Th2-biased response in the lungs and a Th1/Th2 profile in the spleens; the addition of U-Omp19 biased the response to a Th1 profile, with predominance of IFN-γ production in the lung. Both vaccine formulations induced both IFN-γ+ CD4+ and IFN-γ+ CD8+ T-cell responses, while IL-4+ CD4+ were induced only after immunization with RBD + alum. Similar results have been reported for a formulation containing RBD and alum that induced a mixed Th1/Th2 immune response (41). Interestingly, an RBD dimer vaccine formulated with AddaVax was unable to elicit a T-cell response in the mouse model (65). Our results highlight that Th1 or Th2 responses are mainly dependent on the type of adjuvant. Notably, SARS-CoV-2-enhanced immunopathology was associated with Th2-biased responses (66). Therefore, the addition of U-Omp19 may be a way to prevent immunopathology during SARS-CoV-2 infection in vaccinated individuals.
Importantly, the in vivo functionality of RBD+alum+U-Omp19 vaccine elicited immune responses was evaluated in a severe disease COVID-19 murine model showing that this vaccine was able to confer protection in lungs and brains from i.n. SARS-CoV-2 challenged K18-hACE2 mice.
Vaccine formulation presented in this study can be further updated against new SARS-CoV-2 variants and be used as primary immunization and also as heterologous booster for other vaccines. Interestingly, priming with full-length Spike and then boosting with SARS-CoV-2 RBD “immuno-focuses” neutralizing antibody responses to the RBD protein in mice and macaques (51) and might represent an approach to redirect immunity against SARS-CoV-2 variants.
While RBD+alum induces significant immune responses, it has been suggested that its immunogenicity should be increased. Different approaches have been proposed to increase its immunogenicity, such as (i) expression as dimer (43, 49, 65, 67) or trimers (45), (ii) fusion to carrier proteins like human IgG Fc moiety (44, 67), tetanus toxoid (12), and interferon-α (67), (iii) addition of pan HLA-DR-binding epitope to enhance helper T cell responses (67), (iv) using nanoparticles as delivery system (42, 46, 48, 53, 59), or (v) addition of immunopotentiators as CpG (42, 43, 47, 59), MPLA (44), 3M-052, or a TLR-7/8 agonist (45, 59). Here, we demonstrated that the addition of U-Omp19 to RBD + alum formulation was able to increase the induction and breadth of SARS-CoV-2 neutralizing antibody responses, increase the frequency of RBD-specific germinal center B cells, and induce antigen-specific Th1 and CD8+ T cells. Safety and stability studies of this formulation will be needed prior to evaluate its use in humans. Together, our results highlight that the addition of U-Omp19 could be another approach to improve vaccine formulations comprising an antigen and alum.
Materials and Methods
Antigen Expression and Purification
Codon-optimized RBD containing spike signal peptide (residues 1-14) fused to the RBD domain (residues 319–541), and a C-terminal 6xHis tag was obtained. The protein was expressed from a pcDNA 3.1 plasmid in HEK 293 cells. Cells grown in monolayer were transfected with polyethylenimine (PEI), and 3 days after transfection, the supernatant was harvested and clarified by centrifugation at 1,500 × g for 15 min. The recombinant proteins were purified from supernatants by affinity chromatography with a Ni-agarose column (HisTrapTM HP, GE Healthcare, Chicago, IL), dialyzed against PBS, quantified, and stored at −80°C. LPS contamination from RBD was adsorbed with Sepharose–polymyxin B (Sigma Aldrich, St Louis, MO). Endotoxin determination was performed with a Limulus amebocyte chromogenic assay (Lonza, Basel, Switzerland).
SDS-PAGE and Western Blot Analysis
RBD samples were run under reducing conditions by sodium dodecyl sulfate–polyacrylamide gel electrophoresis (SDS-PAGE). Samples were mixed with Laemmli sample buffer with β-mercaptoethanol. The samples were incubated at 95°C for 5 min. Protein bands were visualized by staining with Coomassie blue R250. Bands were then transferred to a nitrocellulose membrane (GE, Healthcare, Chicago, IL), blocked with Tris-buffered saline (TBS)–Tween 0.05%, and incubated with human convalescent serum (1/100 dilution). An anti-human IRDye 800 (1/2,000 dilution) was used as a secondary antibody for infrared fluorescence detection on the Odyssey Imaging System.
Size Exclusion Chromatography Analysis of the Spike RBD Domain
SEC runs were performed on a Superdex 75 column by injecting 150 µg of RBD protein in 20 mM sodium phosphate buffer, pH 7.0 and 0.2M NaCl in the absence of DTT. Runs were performed at a flowrate of 0.4 ml/min. Apparent molecular weight was calculated by calibrating the column with molecular weight markers: bovine serum albumin (66.4 kDa), ovalbumin (44.3 kDa), papain (23 kDa), Ribonuclease A (13.7 kDa), and Aprotinin (6.5 kDa) with Vo = 8.1 ml and Vo+Vi = 19.5 ml.
Adjuvants and Vaccine Formulations
Recombinant U-Omp19 was expressed in Escherichia coli cells and purified as previously described in Ref. 18. LPS contamination from RBD was adsorbed with sepharose–polymyxin B (Sigma Aldrich, St Louis, MO). Endotoxin determination was performed with a Limulus amebocyte chromogenic assay (Lonza, Basel, Switzerland). U-Omp19 preparations used contained <0.1 endotoxin units per milligram protein.
AddaVax or AddaS03 was purchased from InvivoGen (San Diego, CA, USA), and Alhydrogel 2% was kindly provided by CRODA (Newcastle, DE, USA). In vaccine formulations, Ag and U-Omp19 were absorbed to Alhydrogel (CRODA, Inc., Newcastle, DE, USA). Protein adsorption was analyzed by sodium dodecyl sulfate–polyacrylamide gel electrophoresis, followed by Coomassie staining. Protein concentration was determined by the bicinchoninic acid method.
Ethics Statement
All experimental protocols with animals were conducted in strict accordance with international ethical standards for animal experimentation (Helsinki Declaration and its amendments, Amsterdam Protocol of welfare and animal protection and National Institutes of Health, USA NIH, guidelines). The protocols performed were also approved by the Institutional Committee for the use and care of experimental animals (CICUAE) from National University of San Martin (UNSAM) (01/2020).
Animals
Eight-week-old female BALB/c or C57BL/6 mice were obtained from IIB UNSAM animal facility. Four-week-old K18-hACE2 male and female mice were obtained from Jackson Laboratory.
Immunizations and Sample Collection
Mice were intramuscularly (i.m) inoculated at day 0 and 14 with (i) RBD + Alhydrogel, (ii) RBD + Alhydrogel + U-Omp19, (iii) RBD + AddaVax, and (iv) RBD + AddaS03. The dose of RBD was 20 µg in the first inoculation and 10 µg in the second inoculation. Dose of adjuvants in the formulations were Alhydrogel, 500 µg; U-Omp19, 100 µg; AddaVax, 50 µl (InvivoGen); and AddaS03, 50 µl (InvivoGen). Blood samples were collected weekly to measure total and neutralizing antibody titers. Four weeks post prime immunization, animals were sacrificed, and the spleens, lungs, and bronchoalveolar lavages (BAL) were obtained.
Determination of Antibody Levels in Serum and BAL
RBD-specific antibody responses (IgA, IgG, IgG1, and IgG2a) were evaluated by indirect ELISA. Ninety-six-well plates were coated with 0.1 µg/well of RBD in phosphate-buffered saline (PBS) overnight at 4°C. Plates were washed with PBS–Tween 0.05% and blocked with PBS–Tween 0.01% 1% non-fat milk for 1 h. Plates were then incubated with sera or BAL (diluted in PBS–Tween 0.01% containing 1% non-fat milk) for 1 h, and then plates, were washed and incubated with horseradish peroxidase (HRP)-conjugated anti-mouse IgA, IgG (Sigma, St. Louis, MO, USA), IgG1, or IgG2a (Thermo Fisher Scientific, Waltham, MA) for 1 h at 37°C. Then, TMB (3,3í,5,5í-tetramethylbenzidine) was added, and reaction was stopped with 4 N H2SO4 and immediately read at 450 nm to collect end-point ELISA data. End-point cutoff values for serum titer determination were calculated as the mean specific optical density (OD) plus three standard deviations (SD) from sera of saline-immunized mice, and titers were established as the reciprocal of the last dilution yielding an OD higher than the cutoff.
Plasmids
Plasmid pCMV14-3X-Flag-SARS-CoV-2 S was a gift from Zhaohui Qian (Addgene plasmid # 145780) (68), psPAX2 was a gift from Didier Trono (Addgene plasmid # 12260), and pLB-GFP was a gift from Stephan Kissler (Addgene plasmid # 11619).
Cell Lines
Human embryonic kidney cell line 293T expressing the SV40 T-antigen (HEK-293T, ATCC #CRL-11268) was kindly provided by Cecilia Frecha (Instituto de Medicina Traslacional e Ingeniería Biomédica, Hospital Italiano de Buenos Aires) and maintained in complete Dulbecco’s modified Eagle’s medium (DMEM, Gibco, Thermofisher Scientific. Waltham, MA USA) containing 10% (vol/vol) fetal bovine serum (FBS, Internegocios, Buenos Aires, Argentina), 100 IU/ml penicillin, and 100 μg/ml streptomycin (Gibco). For lentivirus production, HEK-293T cells were maintained in DMEM10 containing 100 µg/ml G418 (Sigma Aldrich, St Louis, MO). African green monkey kidney cell line Vero E6 ATCC #CRL-1586 were cultured at 37°C in 5% CO2 in Dulbecco’s modified Eagle’s high glucose medium (Sigma Aldrich) supplemented with 5% fetal bovine serum (FBS) (Sigma Aldrich).
HEK-293T cells expressing the SARS-CoV-2 receptor protein ACE2 (HEK-hACE2) were established in our laboratory by lentivirus transduction. Clonal selection of HEK-hACE2 was achieved by limit dilution and selection with 200 µg/ml of hygromycin.
SARS-CoV-2 Pseudovirus Production
For SARS-CoV-2 pseudovirus production, 5 × 106 HEK-293T cells were seeded in complete DMEM in 10-cm dishes and incubated 24 h at 37°C and 5% CO2. Pseudoviruses were obtained by co-transfection with psPAX2, pCMV14-3xFlag SARS-CoV-2 S, and pLB GFP by using polyetherimide (PEI) (1:2, DNA/PEI). The supernatants were harvested at 72 h post-transfection and centrifuged at 3,000×g for 15 min at 4°C. Then, HEPES was added to a final concentration of 20 mM to the supernatant, which was then passed through a 0.45-μm filter. When necessary, pseudovirus suspensions were concentrated by an overnight centrifugation at 3,000×g at 4°C. Pseudovirus (PsV) was titrated in HEK-hACE2 cells and stored at −70°C until use.
Pseudovirus Neutralization Assay
HEK-hACE2 cells were seeded in 96-well plates in DMEM10 and incubated 24 h at 37°C and 5% CO2. SARS-CoV-2 PsV (500–800 focus-forming units, FFU) were preincubated with serially diluted sera for 1 h at 37°C. Then, PsV-sera mixture was added to HEK-hACE2 and centrifuged at 2,500 rpm for 1 h at 26°C. After a 72-h incubation at 37°C and 5% CO2, cultures were fixed with 4% paraformaldehyde for 20 min. Transduced cells express GFP, and neutralization titer 50 (NT50) was defined as the reciprocal serum dilution that causes a 50% reduction of transduction efficiency.
Viruses
SARS-CoV-2 reference strain -ancestral- (hCoV-19/Argentina/PAIS-G0001/2020 GISAID Accession ID: EPI_ISL_499083) was obtained from Dr. Sandra Gallegos (InViV working group). SARS-CoV-2 Gamma P.1 (GISAID Accession ID: EPI_ISL_2756556) and alpha (GISAID Accession ID: EPI_ISL_2756558) were isolated in Instituto de Investigaciones Biomédicas en Retrovirus y SIDA (INBIRS, UBA-CONICET) from nasopharyngeal swabs of patients. SARS-CoV-2 Lambda C.37 (hCoV-19/Argentina/PAIS-A0612/2021 GISAID Accession ID: EPI_ISL_3320903) was isolated at INBIRS from a sample of nasopharyngeal swabs kindly transferred by Dr. Viegas and Proyecto PAIS. Virus was amplified in Vero E6 cells, and each stock was fully sequenced. Studies using SARS-CoV-2 were done in a Biosafety Level 3 laboratory, and the protocol was approved by the INBIRS Institutional Biosafety Committee.
SARS-CoV-2 Neutralization Assay
Serum samples were heat inactivated at 56°C for 30 min. Serial dilutions were performed and then incubated for 1 h at 37°C in the presence of SARS-CoV-2 in DMEM 2% FBS. Fifty microliters of the mixtures were then added to Vero cells monolayers for an hour at 37°C (MOI = 0.004). Infectious media were removed and replaced for DMEM 2% FBS. After 72 h, cells were fixed with paraformaldehyde (PFA) 4% (4°C, 20 min) and stained with crystal violet solution in methanol. The cytopathic effect (CPE) of the virus on the cell monolayer was assessed visually, if even a minor damage to the monolayer (1–2 «plaques») was observed in the well; this well was considered as a well with a manifestation of CPE. Neutralization titer was defined as the highest serum dilution without any CPE in two of three replicable wells. Otherwise, plates were scanned for determination of media absorbance at 585 nm, and non-linear curves were fitted to obtain the titer corresponding to the 50% of neutralization (NT50). Neutralization assays to compare neutralization among different SARS-CoV-2 variants (alpha, gamma, and lambda) were performed in the same plate for each sample.
Determination of T-Cell Immune Responses
Four weeks after the second dose, mice were sacrificed to study cellular responses. Intracellular cytokine determination: splenocytes were cultured (4×106 cells/well) in the presence of stimulus medium (complete medium supplemented with anti-CD28 and anti-CD49d) or Ag stimuli (stimulus medium + RBD-peptides + RBD protein) for 18 h. Next, brefeldin A was added for 5 h to the samples. After that, cells were washed, fixed, permeabilized, stained, and analyzed by flow cytometry. The cells were stained with Viability dye (Zombie Acqua), anti-mouse-CD8a Alexa Fluor 488, anti-mouse-CD4 Alexa Fluor 647, anti-IL-4 Brilliant Violet 421, and anti-IFN-γ PE (Biolegend, San Diego, CA).
Determination of Ag-Specific B Cells
Ag-specific B cells (plasmablasts and germinal center B cells) present in the spleens were determined by flow cytometry. Splenocytes were plated (2×106 cells/well) and stained with Viability dye (Zombie Acqua), anti-B220 Alexa Fluor 594, anti-CD19 APC/Cy7, anti-CD138 Brilliant Violet 785, anti-IgD Brilliant Violet 605, anti-GL7 Alexa Fluor 488, and anti-CD95 PE (Biolegend, San Diego, CA). For Ag-specific detection, cells were also stained with fluorescent RBD (RBD conjugated to Alexa Fluor 647 succinimidil ester). Next, cells were washed, fixed, and analyzed by flow cytometry.
Vaccine Efficacy in K18-hACE2 Mice
Four-week-old K18-hACE2 mice from Jackson Laboratory were used for evaluating vaccine efficacy. Mice were separated into two groups: (i) control, inoculated with PBS (n = 7), and (ii) vaccine, inoculated with RBD+alum+U-Omp19 (n = 8). Mice in each group included males and females. They were i.m. immunized at day 0 and 14 as described for immune assays. Four weeks post second vaccination, mice were challenged intranasally (i.n.) with 105 PFU of SARS-CoV-2 strain WA1/2020 in each nare. Then, they were monitored daily for weight loss and signs of disease for 2 weeks post-challenge. Three mice per group were euthanized at day 5 post-challenge to evaluate organ viral loads, by plaque assay on Vero E6 cells.
Statistical Analysis
Statistical analysis and plotting were performed using GraphPad Prism 8 software (GraphPad Software, San Diego, CA). In experiments with more than two groups, data were analyzed using one-way ANOVA with a Bonferroni post-test. When necessary, a logarithmic transformation was applied prior to the analysis to obtain data with a normal distribution. In experiments with two groups, an unpaired t-test or Mann–Whitney U-test were used. A p-value <0.05 was considered significant. When bars were plotted, results were expressed as means ± SEM for each group.
Data Availability Statement
The raw data supporting the conclusions of this article will be made available by the authors, without undue reservation.
Ethics Statement
The animal study was reviewed and approved by Institutional Committee for the use and care of experimental animals (CICUAE) from National University of San Martin (UNSAM) (01/2020).
Author Contributions
JC and KAP were responsible for overall experimental design and supervision of studies. DEA constructed and expressed the RBD protein, designed, and supervised neutralization studies. LMC designed and conducted experiments, collected data, and performed data analysis. LMS, LAB, and MLD purified RBD, formulated vaccine, and conducted experiments. EFC performed pseudovirus neutralization studies and data analysis. CPC conducted humoral and cellular studies and data analysis. AJA designed, supervised, and conducted animal challenge studies and data analysis. WS performed animal challenge studies and data analysis. JA conducted long-term humoral response studies. PSP and IM performed neutralization studies with ancestral and SARS-CoV-2 variants. AV and MS isolated SARS-CoV-2 variants. LBC performed RBD characterization by size exclusion chromatography. LMC, KAP, and JC wrote the manuscript. All authors contributed to the article and approved the submitted version.
Funding
This work was supported by grants from Agencia Nacional de Promoción de la Investigación, el Desarrollo Tecnológico y la Innovación (AGENCIA I+D+i) and Ministerio de Ciencia, Tecnología e Innovación (IP COVID-260 and FONARSEC 0001); the Bill and Melinda Gates Foundation through the Grand Challenges Explorations Initiative (OPP1119024) to JC and from National Institute of Allergy and Infectious Diseases of the National Institutes of Health under Award Number R01AI153433 to AA.
Conflict of Interest
LC, KP and JC are inventors of a patent related to U-Omp19 “Adjuvant for vaccines, vaccines that comprise it and uses thereof” PCT/ES2010/070667. The owner of this patent is the National Research Council CONICET. The existence of the patent did not have any role in experimental design, data collection and analysis, decision to publish, or preparation of this manuscript.
The remaining authors declare that the research was conducted in the absence of any commercial or financial relationships that could be construed as a potential conflict of interest.
The handling Editor declared a shared affiliation, with no collaboration, with several of the authors, namely, PP, IM, AV, and MS, at the time of review.
Publisher’s Note
All claims expressed in this article are solely those of the authors and do not necessarily represent those of their affiliated organizations, or those of the publisher, the editors and the reviewers. Any product that may be evaluated in this article, or claim that may be made by its manufacturer, is not guaranteed or endorsed by the publisher.
Acknowledgments
The authors thank to the staff at the Instituto de Investigaciones Biotecnológicas and the Animal Facility of Universidad de San Martin who facilitate animal studies in this work. The authors would also like to thank Danielle Porier, Krisangel López, and Manette Tanelus for technical assistance with the SARS-CoV-2 challenge studies.
References
1. Liu Y, Liu J, Xia H, Zhang X, Fontes-Garfias CR, Swanson KA, et al. Neutralizing Activity of BNT162b2-Elicited Serum. N Engl J Med (2021) 384:1466–8. doi: 10.1056/nejmc2102017
2. Thomas SJ, Moreira ED, Kitchin N, Absalon J, Gurtman A, Lockhart S, et al. Safety and Efficacy of the BNT162b2 mRNA Covid-19 Vaccine Through 6 Months. N Engl J Med (2021)385:1761–73. doi: 10.1056/nejmoa2110345
3. Levin EG, Lustig Y, Cohen C, Fluss R, Indenbaum V, Amit S, et al. Waning Immune Humoral Response to BNT162b2 Covid-19 Vaccine Over 6 Months. N Engl J Med (2021)) 385:e84. doi: 10.1056/nejmoa2114583
4. Harvey WT, Carabelli AM, Jackson B, Gupta RK, Thomson EC, Harrison EM, et al. SARS-CoV-2 Variants, Spike Mutations and Immune Escape. Nat Rev Microbiol (2021) 19:409–24. doi: 10.1038/s41579-021-00573-0
5. Falsey AR, Frenck RW, Walsh EE, Kitchin N, Absalon J, Gurtman A, et al. SARS-CoV-2 Neutralization With BNT162b2 Vaccine Dose 3. N Engl J Med (2021) 385:1627–9. doi: 10.1056/nejmc2113468
6. Mahase E. Covid-19: Novavax Vaccine Efficacy is 86% Against UK Variant and 60% Against South African Variant. BMJ (2021) 372:n296. doi: 10.1136/bmj.n296
7. Pollet J, et al. SARS−CoV-2 RBD219-N1C1: A Yeast-Expressed SARS-CoV-2 Recombinant Receptor-Binding Domain Candidate Vaccine Stimulates Virus Neutralizing Antibodies and T-Cell Immunity in Mice. Hum Vaccines Immunother (2021) 17:2356–66. doi: 10.1080/21645515.2021.1901545
8. Letko M, Marzi A, Munster V. Functional Assessment of Cell Entry and Receptor Usage for SARS-CoV-2 and Other Lineage B Betacoronaviruses. Nat Microbiol (2020) 5:562–9. doi: 10.1038/s41564-020-0688-y
9. Hoffmann M, Kleine-Weber H, Schroeder S, Krüger N, Herrler T, Erichsen S, et al. SARS-CoV-2 Cell Entry Depends on ACE2 and TMPRSS2 and Is Blocked by a Clinically Proven Protease Inhibitor. Cell (2020) 181:271–80.e278. doi: 10.1016/j.cell.2020.02.052
10. Dai L, Gao GF. Viral Targets for Vaccines Against COVID-19. Nat Rev Immunol (2021) 21:73–82. doi: 10.1038/s41577-020-00480-0
11. Jiang S, Bottazzi ME, Du L, Lustigman S, Tseng C-TK, Curti E, et al. Roadmap to Developing a Recombinant Coronavirus S Protein Receptor-Binding Domain Vaccine for Severe Acute Respiratory Syndrome. Expert Rev Vaccines (2012) 11:1405–13. doi: 10.1586/erv.12.126
12. Valdes-Balbin Y, Santana-Mederos D, Quintero L, Fernandez S, Rodriguez L, Sanchez Ramirez B, et al. SARS-CoV-2 RBD-Tetanus Toxoid Conjugate Vaccine Induces a Strong Neutralizing Immunity in Preclinical Studies. ACS Chem Biol (2021) 16:1223–33. doi: 10.1021/acschembio.1c00272
13. Khoury DS, Cromer D, Reynaldi A, Schlub TE, Wheatley AK, Juno JA, et al. Neutralizing Antibody Levels are Highly Predictive of Immune Protection From Symptomatic SARS-CoV-2 Infection. Nat Med (2021) 27:1205–11. doi: 10.1038/s41591-021-01377-8
14. Earle KA, Ambrosino DM, Fiore-Gartland A, Goldblatt D, Gilbert PB, Siber GR, et al. Evidence for Antibody as a Protective Correlate for COVID-19 Vaccines. Vaccine (2021) 39:4423–8. doi: 10.1016/j.vaccine.2021.05.063
15. McMahan K, Yu J, Mercado NB, Loos C, Tostanoski LH, Chandrashekar A, et al. Correlates of Protection Against SARS-CoV-2 in Rhesus Macaques. Nature (2021) 590:630–4. doi: 10.1038/s41586-020-03041-6
16. Wang H, Zhang Y, Huang B, Deng W, Quan Y, Wang W, et al. Development of an Inactivated Vaccine Candidate, BBIBP-CorV, With Potent Protection Against SARS-CoV-2. Cell (2020) 182:713–21.e719. doi: 10.1016/j.cell.2020.06.008
17. Pulendran B, Arunachalam S, O’Hagan DT. Emerging Concepts in the Science of Vaccine Adjuvants. Nat Rev Drug Discov (2021) 20:454–75. doi: 10.1038/s41573-021-00163-y
18. Pasquevich KA, Ibanez AE, Coria LM, Garcia Samartino C, Estein SM, Zwerdling A, et al. An Oral Vaccine Based on U-Omp19 Induces Protection Against B. Abortus Mucosal Challenge by Inducing an Adaptive IL-17 Immune Response in Mice. PloS One (2011) 6:e16203. doi: 10.1371/journal.pone.0016203
19. Ibanez AE, Coria LM, Carabajal MV, Delpino MV, Risso GS, Cobiello PG, et al. A Bacterial Protease Inhibitor Protects Antigens Delivered in Oral Vaccines From Digestion While Triggering Specific Mucosal Immune Responses. J Control Release (2015) 220:18–28. doi: 10.1016/j.jconrel.2015.10.011
20. Coria LM, Ibanez AE, Pasquevich KA, Cobiello PLG, Frank FM, Giambartolomei GH, et al. Brucella Abortus Omp19 Recombinant Protein Subcutaneously Co-Delivered With an Antigen Enhances Antigen-Specific T Helper 1 Memory Responses and Induces Protection Against Parasite Challenge. Vaccine (2016) 34:430–7. doi: 10.1016/j.vaccine.2015.12.012
21. Risso GS, Carabajal MV, Bruno LA, Ibanez AE, Coria LM, Pasquevich KA, et al. U-Omp19 From Brucella Abortus Is a Useful Adjuvant for Vaccine Formulations Against Salmonella Infection in Mice. Front Immunol (2017) 8:171. doi: 10.3389/fimmu.2017.00171
22. Caeiro LD, Masip YE, Rizzi M, Rodriguez ME, Pueblas Castro C, Sanchez DO, et al. The Trypanosoma Cruzi TcTASV-C Protein Subfamily Administrated With U-Omp19 Promotes a Protective Response Against a Lethal Challenge in Mice. Vaccine (2020) 38:7645–53. doi: 10.1016/j.vaccine.2020.10.006
23. Grifoni A, Sidney J, Vita R, Peters B, Crotty S, Weiskopf D, et al. SARS-CoV-2 Human T Cell Epitopes: Adaptive Immune Response Against COVID-19. Cell Host Microbe (2021) 29:1076–92. doi: 10.1016/j.chom.2021.05.010
24. Brito LA, Singh M. Acceptable Levels of Endotoxin in Vaccine Formulations During Preclinical Research. J Pharm Sci (2011) 100:34–7. doi: 10.1002/jps.22267
25. Sterlin D, Mathian A, Miyara M, Mohr A, Anna F, Claer L, et al. IgA Dominates the Early Neutralizing Antibody Response to SARS-CoV-2. Sci Transl Med (2021) 13(577):eabd2223. doi: 10.1126/scitranslmed.abd2223
26. Acevedo ML, Alonso-Palomares L, Bustamante A, Gaggero A, Paredes F, Cortés CP, et al. Infectivity and Immune Escape of the New Sars-Cov-2 Variant of Interest Lambda. medRxiv (2021) 2021.06.28.21259673. doi: 10.1101/2021.06.28.21259673
27. Sabino EC, Buss LF, Carvalho MPS, Prete CA, Crispim MAE, Fraiji NA, et al. Resurgence of COVID-19 in Manaus, Brazil, Despite High Seroprevalence. Lancet (2021) 397:452–5. doi: 10.1016/s0140-6736(21)00183-5
28. Bange EM, Han NA, Wileyto P, Kim JY, Gouma S, Robinson J, et al. CD8+ T Cells Contribute to Survival in Patients With COVID-19 and Hematologic Cancer. Nat Med (2021) 27:1280–9. doi: 10.1038/s41591-021-01386-7
29. Turner JS, O’Halloran JA, Kalaidina E, Kim W, Schmitz AJ, Zhou JQ, et al. SARS-CoV-2 mRNA Vaccines Induce Persistent Human Germinal Centre Responses. Nature (2021) 596:109–13. doi: 10.1038/s41586-021-03738-2
30. Winkler ES, Bailey AL, Kafai NM, Nair S, McCune BT, Yu J, et al. SARS-CoV-2 Infection of Human ACE2-Transgenic Mice Causes Severe Lung Inflammation and Impaired Function. Nat Immunol (2020) 21:1327–35. doi: 10.1038/s41590-020-0778-2
31. Jiang R-D, Liu M-Q, Chen Y, Shan C, Zhou Y-W, Shen X-R, et al. Pathogenesis of SARS-CoV-2 in Transgenic Mice Expressing Human Angiotensin-Converting Enzyme 2. Cell (2020) 182:50–58.e58. doi: 10.1016/j.cell.2020.05.027
32. Kyriakidis NC, Lopez-Cortes A, Gonzalez EV, Grimaldos AB, Prado EO. SARS-CoV-2 Vaccines Strategies: A Comprehensive Review of Phase 3 Candidates. NPJ Vaccines (2021) 6:28. doi: 10.1038/s41541-021-00292-w
33. Hotez PJ, Bottazzi ME. Developing a Low-Cost and Accessible COVID-19 Vaccine for Global Health. PloS Negl Trop Dis (2020) 14:e0008548. doi: 10.1371/journal.pntd.0008548
34. Piccoli L, Park YJ, Tortorici MA, Czudnochowski N, Walls AC, Beltramello M, et al. Mapping Neutralizing and Immunodominant Sites on the SARS-CoV-2 Spike Receptor-Binding Domain by Structure-Guided High-Resolution Serology. Cell (2020) 183:1024–42.e1021. doi: 10.1016/j.cell.2020.09.037
35. Tian S, Ji K, Wang M, Wang F, Wang H, Huang W, et al. Distinct BCR Repertoires Elicited by SARS-CoV-2 RBD and S Vaccinations in Mice. Cell Discov (2021) 7:91. doi: 10.1038/s41421-021-00331-9
36. Greaney AJ, Loes AN, Gentles LE, Crawford KHD, Starr TN, Malone KD, et al. Antibodies Elicited by mRNA-1273 Vaccination Bind More Broadly to the Receptor Binding Domain Than Do Those From SARS-CoV-2 Infection. Sci Transl Med (2021) 13. doi: 10.1126/scitranslmed.abi9915
37. Smatti MK, Al Thani AA, Yassine HM. Viral-Induced Enhanced Disease Illness. Front Microbiol (2018) 9:2991:2991. doi: 10.3389/fmicb.2018.02991
38. Wan Y, Shang J, Sun S, Tai W, Chen J, Geng Q, et al. Molecular Mechanism for Antibody-Dependent Enhancement of Coronavirus Entry. J Virol (2020) 94(5):e02015–19. doi: 10.1128/JVI.02015-19
39. Liu Y, Soh WT, Kishikawa JI, Hirose M, Nakayama EE, Li S, et al. An Infectivity-Enhancing Site on the SARS-CoV-2 Spike Protein Targeted by Antibodies. Cell (2021) 184:3452–66.e3418. doi: 10.1016/j.cell.2021.05.032
40. Law JLM, Logan M, Joyce MA, Landi A, Hockman D, Crawford K, et al. SARS-COV-2 Recombinant Receptor-Binding-Domain (RBD) Induces Neutralizing Antibodies Against Variant Strains of SARS-CoV-2 and SARS-CoV-1. Vaccine (2021) 39:5769–79. doi: 10.1016/j.vaccine.2021.08.081
41. Yang J, Wang W, Chen Z, Lu S, Yang F, Bi Z, et al. A Vaccine Targeting the RBD of the S Protein of SARS-CoV-2 Induces Protective Immunity. Nature (2020) 586:572–7. doi: 10.1038/s41586-020-2599-8
42. Dalvie NC, Rodriguez-Aponte SA, Hartwell BL, Tostanoski LH, Biedermann AM, Crowell LE, et al. Engineered SARS-CoV-2 Receptor Binding Domain Improves Manufacturability in Yeast and Immunogenicity in Mice. Proc Natl Acad Sci USA (2021) 118(38):e2106845118. doi: 10.1073/pnas.2106845118
43. Zang J, Zhu Y, Zhou Y, Gu C, Yi Y, Wang S, et al. Yeast-Produced RBD-Based Recombinant Protein Vaccines Elicit Broadly Neutralizing Antibodies and Durable Protective Immunity Against SARS-CoV-2 Infection. Cell Discov (2021) 7:71. doi: 10.1038/s41421-021-00315-9
44. Siriwattananon K, Manopwisedjaroen S, Shanmugaraj B, Prompetchara E, Ketloy C, Buranapraditkun S, et al. Immunogenicity Studies of Plant-Produced SARS-CoV-2 Receptor Binding Domain-Based Subunit Vaccine Candidate With Different Adjuvant Formulations. Vaccines (Basel) (2021) 9(7):744. doi: 10.3390/vaccines9070744
45. Routhu NK, Cheedarla N, Bollimpelli VS, Gangadhara S, Edara VV, Lai L, et al. SARS-CoV-2 RBD Trimer Protein Adjuvanted With Alum-3M-052 Protects From SARS-CoV-2 Infection and Immune Pathology in the Lung. Nat Commun (2021) 12:3587. doi: 10.1038/s41467-021-23942-y
46. Salzer R, Clark JJ, Vaysburd M, Chang VT, Albecka A, Kiss L, et al. Single-Dose Immunisation With a Multimerised SARS-CoV-2 Receptor Binding Domain (RBD) Induces an Enhanced and Protective Response in Mice. FEBS Lett (2021) 595:2323–40. doi: 10.1002/1873-3468.14171
47. Nanishi E, Borriello F, O'Meara TR, McGrath ME, Saito Y, Haupt RE, et al. Alum:CpG Adjuvant Enables SARS-CoV-2 RBD-Induced Protection in Aged Mice and Synergistic Activation of Human Elder Type 1 Immunity. bioRxiv (2021). doi: 10.1101/2021.05.20.444848
48. Halfmann PJ, Castro A, Loeffler K, Frey SJ, Chiba S, Kawaoka Y, et al. Potent Neutralization of SARS-CoV-2 Including Variants of Concern by Vaccines Presenting the Receptor-Binding Domain Multivalently From Nanoscaffolds. Bioeng Transl Med (2021) 6:e10253. doi: 10.1002/btm2.10253
49. Pan X, Shi J, Hu X, Wu Y, Zeng L, Yao Y, et al. RBD-Homodimer, a COVID-19 Subunit Vaccine Candidate, Elicits Immunogenicity and Protection in Rodents and Nonhuman Primates. Cell Discov (2021) 7:82. doi: 10.1038/s41421-021-00320-y
50. Starr TN, Greaney AJ, Hilton SK, Ellis D, Crawford KHD, Dingens AS, et al. Deep Mutational Scanning of SARS-CoV-2 Receptor Binding Domain Reveals Constraints on Folding and ACE2 Binding. Cell (2020) 182:1295–310.e1220. doi: 10.1016/j.cell.2020.08.012
51. Tan HX, Juno JA, Lee WS, Barber-Axthelm I, Kelly HG, Wragg KM, et al. Immunogenicity of Prime-Boost Protein Subunit Vaccine Strategies Against SARS-CoV-2 in Mice and Macaques. Nat Commun (2021) 12:1403. doi: 10.1038/s41467-021-21665-8
52. Mandolesi M, Sheward DJ, Hanke L, Ma J, Pushparaj P, Perez Vidakovics L, et al. SARS-CoV-2 Protein Subunit Vaccination of Mice and Rhesus Macaques Elicits Potent and Durable Neutralizing Antibody Responses. Cell Rep Med (2021) 2:100252. doi: 10.1016/j.xcrm.2021.100252
53. Walls AC, Fiala B, Schafer A, Wrenn S, Pham MN, Murphy M, et al. Elicitation of Potent Neutralizing Antibody Responses by Designed Protein Nanoparticle Vaccines for SARS-CoV-2. Cell (2020) 183:1367–82.e1317. doi: 10.1016/j.cell.2020.10.043
54. Shrivastava T, Singh B, Rizvi ZA, Verma R, Goswami S, Vishwakarma P, et al. Comparative Immunomodulatory Evaluation of the Receptor Binding Domain of the SARS-CoV-2 Spike Protein; a Potential Vaccine Candidate Which Imparts Potent Humoral and Th1 Type Immune Response in a Mouse Model. Front Immunol (2021) 12:641447. doi: 10.3389/fimmu.2021.641447
55. Kim E, Attia Z, Woodfint RM, Zeng C, Kim SH, Steiner HE, et al. Inhibition of Elastase Enhances the Adjuvanticity of Alum and Promotes Anti-SARS-CoV-2 Systemic and Mucosal Immunity. Proc Natl Acad Sci USA (2021) 118(34):118. doi: 10.1073/pnas.2102435118
56. Wang Z, Lorenzi JCC, Muecksch F, Finkin S, Viant C, Gaebler C, et al. Enhanced SARS-CoV-2 Neutralization by Dimeric IgA. Sci Transl Med (2021) 13():eabf1555. doi: 10.1126/scitranslmed.abf1555
57. Hoffmann M, Arora P, Gross R, Seidel A, Hornich BF, Hahn AS, et al. SARS-CoV-2 Variants B.1.351 and P.1 Escape From Neutralizing Antibodies. Cell (2021) 184:2384–2393 e2312. doi: 10.1016/j.cell.2021.03.036
58. Wang GL, Wang ZY, Duan LJ, Meng QC, Jiang MD, Cao J, et al. Susceptibility of Circulating SARS-CoV-2 Variants to Neutralization. N Engl J Med (2021) 384:2354–6. doi: 10.1056/NEJMc2103022
59. Arunachalam PS, Walls AC, Golden N, Atyeo C, Fischinger S, Li C, et al. Adjuvanting a Subunit COVID-19 Vaccine to Induce Protective Immunity. Nature (2021) 594:253–8. doi: 10.1038/s41586-021-03530-2
60. Sallusto F, Lanzavecchia A, Araki K, Ahmed R. From Vaccines to Memory and Back. Immunity (2010) 33:451–63. doi: 10.1016/j.immuni.2010.10.008
61. Lederer K, Castano D, Gomez Atria D, Oguin TH, et al. SARS-CoV-2 mRNA Vaccines Foster Potent Antigen-Specific Germinal Center Responses Associated With Neutralizing Antibody Generation. Immunity (2020) 53:1281–95.e1285. doi: 10.1016/j.immuni.2020.11.009
62. Coria LM, Ibanez AE, Tkach M, Sabbione F, Bruno L, Carabajal MV, et al. A Brucella Spp. Protease Inhibitor Limits Antigen Lysosomal Proteolysis, Increases Cross-Presentation, and Enhances CD8+ T Cell Responses. J Immunol (2016) 196:4014–29. doi: 10.4049/jimmunol.1501188
63. Zhao J, Zhang Y, Chen Y, Zhang J, Pei J, Cui M, et al. A Novel Oral Rabies Vaccine Enhances the Immunogenicity Through Increasing Dendritic Cells Activation and Germinal Center Formation by Expressing U-OMP19 in a Mouse Model. Emerg Microbes Infect (2021) 10:913–28. doi: 10.1080/22221751.2021.1923341
64. Jung MK, Shin EC. Phenotypes and Functions of SARS-CoV-2-Reactive T Cells. Mol Cells (2021) 44:401–7. doi: 10.14348/molcells.2021.0079
65. Dai L, Zheng T, Xu K, Han Y, Xu L, Huang E, et al. A Universal Design of Betacoronavirus Vaccines Against COVID-19, MERS, and SARS. Cell (2020) 182:722–733 e711. doi: 10.1016/j.cell.2020.06.035
66. Simon HU, Karaulov AV, Bachmann MF. Strategies to Prevent SARS-CoV-2-Mediated Eosinophilic Disease in Association With COVID-19 Vaccination and Infection. Int Arch Allergy Immunol (2020) 181:624–8. doi: 10.1159/000509368
67. Sun S, Cai Y, Song TZ, Pu Y, Cheng L, Xu H, et al. Interferon-Armed RBD Dimer Enhances the Immunogenicity of RBD for Sterilizing Immunity Against SARS-CoV-2. Cell Res (2021) 31:1011–23. doi: 10.1038/s41422-021-00531-8
Keywords: adjuvant, protease inhibitor, SARS- CoV-2, U-Omp19, germinal center cells
Citation: Coria LM, Saposnik LM, Pueblas Castro C, Castro EF, Bruno LA, Stone WB, Pérez PS, Darriba ML, Chemes LB, Alcain J, Mazzitelli I, Varese A, Salvatori M, Auguste AJ, Álvarez DE, Pasquevich KA and Cassataro J (2022) A Novel Bacterial Protease Inhibitor Adjuvant in RBD-Based COVID-19 Vaccine Formulations Containing Alum Increases Neutralizing Antibodies, Specific Germinal Center B Cells and Confers Protection Against SARS-CoV-2 Infection in Mice. Front. Immunol. 13:844837. doi: 10.3389/fimmu.2022.844837
Received: 28 December 2021; Accepted: 01 February 2022;
Published: 28 February 2022.
Edited by:
Pablo C. Baldi, University of Buenos Aires, ArgentinaReviewed by:
Bernard Vanhove, Centre National de la Recherche Scientifique (CNRS), FranceMaripat Corr, University of California, San Diego, United States
Copyright © 2022 Coria, Saposnik, Pueblas Castro, Castro, Bruno, Stone, Pérez, Darriba, Chemes, Alcain, Mazzitelli, Varese, Salvatori, Auguste, Álvarez, Pasquevich and Cassataro. This is an open-access article distributed under the terms of the Creative Commons Attribution License (CC BY). The use, distribution or reproduction in other forums is permitted, provided the original author(s) and the copyright owner(s) are credited and that the original publication in this journal is cited, in accordance with accepted academic practice. No use, distribution or reproduction is permitted which does not comply with these terms.
*Correspondence: Karina A. Pasquevich, a3Bhc3F1ZXZpY2hAaWliLnVuc2FtLmVkdS5hcg==; Juliana Cassataro, anVjYXNzYXRhcm9AaWliLnVuc2FtLmVkdS5hcg==
†These authors have contributed equally to this work
‡These authors have contributed equally to this work