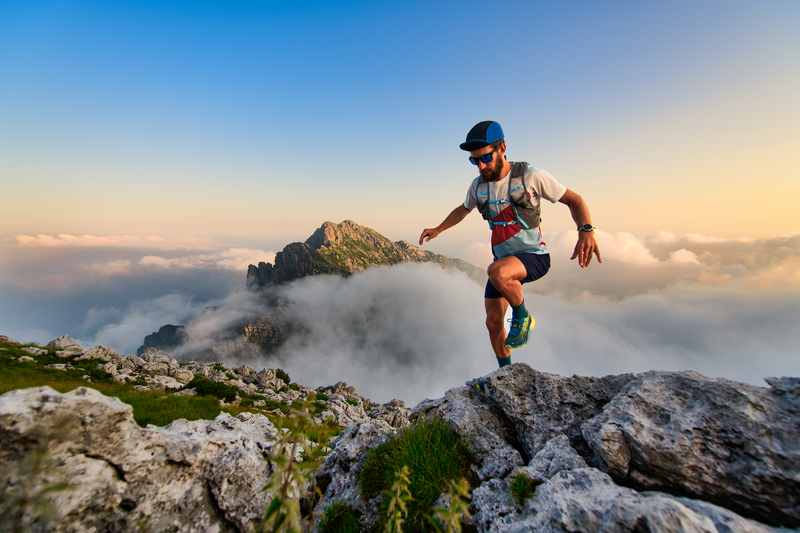
95% of researchers rate our articles as excellent or good
Learn more about the work of our research integrity team to safeguard the quality of each article we publish.
Find out more
PERSPECTIVE article
Front. Immunol. , 03 May 2022
Sec. Viral Immunology
Volume 13 - 2022 | https://doi.org/10.3389/fimmu.2022.842453
Pulmonary surfactant constitutes an important barrier that pathogens must cross to gain access to the rest of the organism via the respiratory surface. The presence of pulmonary surfactant prevents the dissemination of pathogens, modulates immune responses, and optimizes lung biophysical activity. Thus, the application of pulmonary surfactant for the treatment of respiratory diseases provides an effective strategy. Currently, several clinical trials are investigating the use of surfactant preparations to treat patients with coronavirus disease 2019 (COVID-19). Some factors have been considered in the application of pulmonary surfactant for the treatment COVID-19, such as mechanical ventilation strategy, timing of treatment, dose delivered, method of delivery, and preparation utilized. This review supplements this list with two additional factors: accurate measurement of surfactants in patients and proper selection of pulmonary surfactant components. This review provides a reference for ongoing exogenous surfactant trials involving patients with COVID-19 and provides insight for the development of surfactant preparations for the treatment of viral respiratory infections.
The ongoing coronavirus disease 2019 (COVID-19) pandemic, caused by severe acute respiratory syndrome coronavirus 2 (SARS-CoV-2), has affected over 200 million people worldwide (1). SARS-CoV-2 can induce lung injury that involves the airways, alveoli, and pulmonary vessels (2). Autopsies of patients with COVID-19 reveal patchy peripheral hemorrhage of the lung parenchyma, loss of alveolar elasticity (3), and fibrous cords with sticky secretion exuding from cut surfaces of the pulmonary alveoli, bronchi, and tracheae (3). Moreover, pathological examinations demonstrate diffuse alveolar damage, including inflammatory exudate, interstitial inflammation, and infiltrating monocytes, lymphocytes, and macrophages (4). Further, type II alveolar epithelial cell proliferation and focal desquamation of alveolar epithelial cells are observed (3). Severe COVID-19 is associated with multiple changes in immune profiles, affecting the ability of the host to mount a timely and effective immune response against SARS-CoV-2 (5). Eosinopenia and lymphopenia with a severe reduction in the frequency of CD4+ and CD8+ T cells, B cells, and natural killer (NK) cells are common features of patients with severe COVID-19 (5). Additionally, T cell lymphopenia driven by T cell sequestration in tissues or T cell apoptosis as a result of pro-inflammatory cytokines is common in patients with severe COVID-19 (6). Defects in type I IFN response are present in some patients with severe COVID-19 (7). Loss of function variants in loci that control toll-like receptor (TLR)3- and IRF7-dependent type I IFN immunity may lead to defects in type I IFN response in patients with severe COVID-19 (7). In addition, autoantibodies against IFN-α and IFN-ω are present in patients with COVID-19 (8), as well as substantial accumulation of activated immune cells, such as myeloid-derived suppressor cells (MDSCs) (5). Excess circulating immature monocytes, neutrophils, and myeloid progenitors—named emergency myelopoiesis—are almost pathognomonic features of severe disease (9). Circulating myeloid cells produce excessive amounts of inflammatory molecules, often causing a cytokine storm, which promotes multiple organ damage (9). In contrast, lung tissue–resident macrophages, such as alveolar macrophages, which are known to play an important role in tissue homeostasis and repair, are often depleted in patients with severe COVID-19 (10).
SARS-CoV-2 binds angiotensin-converting enzyme 2 (ACE2), which is expressed by pulmonary epithelial cells, causing acute interstitial pneumonia (11). Pulmonary epithelial cells can produce pulmonary surfactant, which contains a complex mixture of highly reactive compounds (12). Pulmonary surfactant covers the alveolar epithelium, facilitating breathing by reducing the surface tension of the air-water interface within alveoli, thereby preventing alveolar collapse and easing the mechanical work required to breathe (13). Emerging data indicate that pulmonary surfactant plays a pivotal role in the pulmonary host defense against respiratory viral infections, such as influenza and respiratory syncytial virus (RSV) infection (14). Moreover, pulmonary surfactant exerts anti-inflammatory and anti-viral effects against some respiratory viral infections (14–16). Recent studies show that SARS-CoV-2 infection may result in changes in pulmonary surfactant (14, 17). A study analyzing the lung transcriptome of patients with COVID-19 reported that the expression of surfactant proteins was downregulated during SARS-CoV-2 infection (18). Another study reported that surfactant protein production was deregulated in patients with COVID-19, resulting in increased expression of surfactant protein (SP)-A (19). A recent study indicated that levels of pulmonary surfactant lipids were markedly reduced in the bronchoalveolar lavage fluid of patients with COVID-19 compared to that in healthy controls (20). Moreover, SARS-CoV-2 infects alveolar type II cells (AT II cells) by binding to ACE2, thus impacting the production and turnover of pulmonary surfactant in AT II cells (14). Furthermore, SARS-CoV-2 infection may influence the recycling and catabolism of pulmonary surfactant in the alveoli by AT II cells and alveolar macrophages (14). These studies suggest that pulmonary surfactant is altered in patients with COVID-19, which not only influences surface tension-related properties but also impacts the host’s antiviral immunity following viral infection (13–15). Severe respiratory viral infection often causes a disorder of pulmonary surfactant in the lung, which increase the surface tension in the lung, and then induce alveolar collapse at end-expiration (14, 21). Supplemental pulmonary surfactant can reduce surface tension and prevent alveolar collapse, thereby preserving lung function for oxygenation (14). Therefore, pharmacological and therapeutic strategies aimed at readjusting pulmonary surfactant dysfunction during respiratory viral infection not only contribute to preserving lung function, but also inhibiting the pro-inflammatory response and limiting viral infection.
A previous study reported that intratracheal administration of surfactant resulted in improved lung compliance and less oxygen required to maintain acceptable oxygen saturation in RSV pneumonia (22). Moreover, administration of pulmonary surfactant has been used to effectively treat preterm infants with neonatal respiratory distress syndrome (NRDS), which is caused by pulmonary surfactant deficiency (23). Clinical data indicate that severe COVID-19 most commonly manifests as viral pneumonia-induced acute respiratory distress syndrome (ARDS), which is characterized by diffuse inflammatory damage that results in increased vascular permeability and reduced lung compliance (24). Interestingly, a recent study proposed that ARDS in COVID-19 resembled NRDS (17). Thus, some researchers have suggested that exogenous pulmonary surfactants may provide an effective treatment for COVID-19 (17, 25, 26). Accordingly, several studies have investigated the therapeutic value of administering exogenous pulmonary surfactants to patients with COVID-19 (17, 27, 28). Several clinical trials exploring surfactant preparations as a treatment for COVID-19 are ongoing using surfactants often used to treat NRDS (27, 28). To date, seven clinical trials have been conducted using exogenous surfactant preparations to treat patients with COVID-19 (27, 28) (Table 1). Although the outcomes of these trials have not yet been published, some initial results and case reports are available. One pilot study indicated that exogenous surfactant administration via bronchoscopy reduced the duration of mechanical ventilation and 28-day mortality rate of COVID-19, although the differences were not statistically significant (29). Further, a case report indicated that oxygenation was improved in a patient with COVID-19 after exogenous surfactant treatment (30). Despite these promising data, prior trials in which surfactant was administered to adults with ARDS were generally disappointing, with the majority showing no benefit (28). A meta-analysis of randomized controlled trials examining the effect of surfactant administration on adult patients with ARDS revealed no improvement in mortality or oxygenation (31). Therefore, the effectiveness of pulmonary surfactant for COVID-19 treatment remains unclear.
The failure of surfactant preparations in treating adults with ARDS largely curtailed clinical interest in this approach over the last 15 years. However, the emergence of COVID-19-associated ARDS has generated renewed interest in this clinical approach. A recent review indicated that the success of surfactant therapy may be influenced by the dose administered, method of delivery, preparation utilized, mechanical ventilation strategy, and timing of surfactant treatment (28). Additional factors may need to be considered in the effective treatment of patients with COVID-19 using pulmonary surfactant. These factors are reviewed and discussed in this review.
As previously mentioned, SARS-CoV-2 infection may induce changes in pulmonary surfactant. However, due to the complexity of the mixture, the extent of changes in each pulmonary surfactant component caused by SARS-CoV-2 infection remains unknown. Surfactant treatment in children with NRDS enabled infants to start producing endogenous surfactant, in part facilitated by re-utilization of surfactant constituents through recycling mechanisms (28, 32). Current evidence indicates that patients with COVID-19 have dysfunctional, rather than deficient pulmonary surfactant, and the dysfunction is not equivalent to a deficiency.
Pulmonary surfactant levels do not always decrease during viral respiratory infection. A study analyzing pulmonary tissue samples obtained from RSV-infected mice demonstrated changes in 86 surfactant lipids compared to control mice (Fold Change (FC) > 1.5 or FC < 0.67) (33). Among the altered lipids, some lipids displayed decreased abundance, such as diacylglycerols (DGs), triacylglycerols (TGs), and some palmitoylated phosphatidylglycerols (PGs), including PG 16:0_22:5 (FC=0.56) and PG 16:0_22:6 (FC=0.61). However, some lipids were more abundant, such as acylcarnitine (FC=3.77), phosphatidylinositol (PI) 18:0_18:2 (FC=2.53), lysophosphatidylinositol (lysoPI) 16:0 (FC=10.53), and some stearoylated PGs, including PG 18:2_20:4 (FC=10.84), PG 18:2_18:2 (FC=8.23), and PG 18:1_20:4 (FC=6.93) (33). During influenza virus infection, levels of phosphatidylcholine (PC), PG, and phosphatidylethanolamine (PE) in AT II cells were significantly lower in influenza-infected mice compared to those in control animals, while levels of phosphatidylserine (PS), PI, sphingomyelin, cholesterol, and DG were increased (34).
Moreover, levels of pulmonary surfactant proteins do not show a simple decreasing trend during viral respiratory infection. For example, one study reported that SP-A expression was significantly elevated whereas SP-B expression was unchanged in the lungs of patients with COVID-19 compared to those of control patients (19). The authors of this study suggested that the increased expression of SP-A, which was present in condensed masses inside the alveolar spaces, could invalidate the therapeutic efficacy of exogenous surfactant treatment (19).
Nevertheless, because it remains unclear whether pulmonary surfactant is deficient in patients with COVID-19, using pulmonary surfactant to treat COVID-19 may be unreasonable. Such attempts may put patients at risk, since the lungs of patients with severe COVID-19 are considerably damaged and highly susceptible to further injury (21). Additionally, the use of pulmonary surfactant to treat COVID-19 may further disturb the pulmonary microenvironment and aggravate lung burden. For example, due to pulmonary surfactant is associated with the sputum formation (35), administration of exogenous pulmonary surfactant may lead to the formation of sputum thrombi. In fact, previous trials with exogenous surfactant in patients with non-SARS-CoV-2-induced ARDS were unsuccessful (36), often because intervention took place when the lungs had already suffered irreparable damage (25). Thus, studies suggest that early use of exogenous surfactants is necessary for COVID-19 treatment (28).
Clarifying changes in pulmonary surfactant components during SARS-CoV-2 infection is needed before conducting related trials. Some researchers have suggested that pulmonary surfactants in patients should be assessed prior to initiating treatment (17). A point-of-care, rapid test that measures surfactant levels at birth has been developed for premature babies (37). This method may also be suitable for measuring surfactant levels in tracheal fluid obtained from patients with COVID-19. Other detection technologies, such as mass spectrometry, can also be used to measure surfactant components. In summary, accurate measurement and understanding of surfactant trends in COVID-19 may help determine the therapeutic application of pulmonary surfactants.
Pulmonary surfactant is a lipoprotein complex composed by weight of 80% phospholipids (PLs), 10% neutral lipids (mainly cholesterol), and 10% surfactant-associated proteins, named SP-A, SP-B, SP-C, and SP-D (14). The major PL components include PC (approximately 80%), PG (approximately 7–15%), and small quantities of PI, PE, and PS (approximately 5%) (14). The hydrophobic surfactant proteins SP-B and SP-C along with dipalmitoyl PC (DPPC) mainly confer surface tension–lowering properties to pulmonary surfactant (14). Meanwhile, the hydrophilic surfactant proteins SP-A and SP-D participate in pulmonary host defense and modify immune responses during respiratory viral infection (14). The host defensive functions of pulmonary surfactant components, including proteins and lipids, are summarized in Table 2.
SP-A and SP-D are known to protect against viral and other pathogenic infections by blocking the entry of numerous viruses, such as influenza, RSV, and human immunodeficiency virus (HIV), into host cells (16). SP-A and SP-D play roles in modulating coronavirus infection by binding to human coronavirus 229E (HCoV-229E) virions and preventing HCoV-229E from infecting host cells (40, 45). SP-A and SP-D can also bind to and neutralize SARS-CoV by interacting with the spike protein (45). Recombinant fragments of human SP-D (rfhSP-D) can compete with ACE-2 for binding to the S1 spike protein subunit of SARS-CoV-2, thereby reducing SARS-CoV-2 infection (66, 67). These results suggest that SP-A and SP-D may have therapeutic potential for the treatment of COVID-19.
Therapeutic pulmonary surfactants can be natural or synthetic (27). Natural pulmonary surfactants have been isolated from bovine, porcine, and human amniotic fluids (27). A previous study reported that natural (animal-derived) surfactants were more effective than synthetic surfactants (68, 69) because natural preparations contained all the surfactant phospholipids and hydrophobic proteins (SP-B and SP-C) needed to facilitate rapid formation of a functional surface film (28). However, the use of natural surfactants is accompanied by inherent risks, such as the transmission of infectious agents, immunogenicity, and impurities (70). Removal of highly immunogenic proteins such as SP-A and SP-D, terminal sterilization, and screening of animal sources have been used to minimize the potential risks (70). Interestingly, a previous study suggested that surfactant preparations containing SP-A and SP-D might have better efficiency (28). In contrast, completely synthetic surfactants possess a greater degree of chemical purity, thus avoiding some potential risks (70). Additionally, synthetic surfactants relieve the potential resource limitations of animal-derived surfactants, avoid religious factors, and have lower manufacturing costs (28). Theoretically, a synthetic surfactant could be formulated to contain SP-A and SP-D (28). Moreover, SP-B and SP-C are difficult to synthesize and synthetic surfactant preparations without these components display limited functionality (28). Studies suggest that synthesized forms and recombinant fragments of SP-A and SP-D may be feasible for therapeutic use (28, 67). Recombinant SP-D fragments have the advantage of smaller size, thus increasing the probability of reaching distal lung locations, and show higher resistance to proteases and collagenases than full-length SP-D (67). Therefore, synthesized forms and recombinant fragments of SP-A and SP-D may be considered in addition to natural pulmonary surfactants.
Pulmonary surfactant lipids also play a pivotal role in pulmonary host defense responses to respiratory viral infection (14). Recent studies reported that intranasal administration of some pulmonary surfactant lipids, such as PG and PI, prevented influenza and RSV infections (50, 51, 53, 54). PG and PI can markedly suppress RSV replication by binding to the virus with high affinity (50, 51, 53, 54). PG can block the replication of H1N1-PR8 and H3N2 influenza by binding to influenza virus with high affinity (52, 55). PI can prevent H1N1 spread from infected to non-infected cells in tissue culture by binding to H1N1 influenza with high affinity (52, 55). Further, plasmalogens can potentially be used as antiviral therapeutic and prophylactic agents against human cytomegalovirus (HCMV), influenza, WNV, and SARS-CoV-2 infections (71). Pulmonary surfactant lipids also exert anti-inflammatory effects against viral infection (72). For example, PC can inhibit multiple pro-inflammatory mediators to alleviate tissue damage (72), and DPPC inhibits LPS-induced pro-inflammatory cytokine secretion in airway epithelial cells and monocytes (72). Furthermore, PI and PG can inhibit pro-inflammatory cytokine responses in macrophages by blocking the TLR2 and TLR7 pathways. These studies suggest that pulmonary surfactant lipids may possess potential antiviral and anti-inflammatory properties against SARS-CoV-2 infection.
Not all pulmonary surfactant lipids protect against viral infection; some lipids facilitate viral infection (14). One study reported that PE was required for the replication of (+)RNA viruses, such as hepatitis C virus, dengue virus, and WNV (56). Further, the replication of some RNA viruses, such as tomato bushy stunt virus, depends on PE enrichment at replication sites in subcellular membranes (57). Cholesterol promotes the entry of several coronaviruses into host cells (58), such as SARS-CoV (59), murine coronavirus (60), porcine deltacoronavirus (61), and infectious bronchitis virus (62). Thus, cholesterol may contribute to coronavirus replication by acting as a key component in viral entry (73). Moreover, cholesterol may participate in the entry of other viruses into host cells. For example, Ebola virus glycoprotein interacts with cholesterol to enhance membrane fusion and cell entry (64), while hepatitis C virus replication depends on endosomal cholesterol homeostasis (63). Therefore, some cholesterol-lowering drugs, such as statins, can reduce viral infectivity (58). Statins may also serve as potential main protease inhibitors of SARS-CoV-2, thereby contributing to the control of viral infection (58). PS can promote poxvirus infectivity (48) through apoptotic cell mimicry (49). Some pulmonary surfactant preparations used for the clinical treatment of COVID-19 contain cholesterol, PE, or PS. Although there is no evidence that cholesterol, PE, or PS influence SARS-CoV-2 infection, this possibility should be considered before administering surfactants that contain these lipids.
The biological functions of some pulmonary surfactant components have been clarified. Therefore, components selected for surfactant preparation should refer to their biological functions. Additionally, further studies are warranted to explore the potential functions of other pulmonary surfactant components in order to guide appropriate selection for surfactant preparations.
The lung epithelium is constantly exposed to the environment and protected by pulmonary surfactant, which provides an important barrier against pathogen infection. Pulmonary surfactant prevents the dissemination of pathogens, modulates immune responses, and optimizes lung biophysical activity. Additionally, pulmonary surfactant may mitigate and reverse ARDS by reducing alveolar surface tension and improving pulmonary mechanical properties, while also exerting anti-inflammatory and antiviral effects (Figure 1). Thus, the application of pulmonary surfactant may provide an effective strategy for the treatment of respiratory diseases. This review highlights two new factors for consideration when selecting pulmonary surfactant therapy for COVID-19, namely accurate assessment of pulmonary surfactants in patients and appropriate selection of pulmonary surfactant components. This review provides a reference for ongoing trials investigating the use of exogenous surfactant in patients with COVID-19.
Figure 1 Therapeutic mechanisms of exogenous pulmonary surfactant for SARS-CoV-2 infection. Pulmonary surfactant can (1) mitigate acute respiratory distress syndrome by reducing alveolar surface tension and improving pulmonary mechanical properties; (2) suppress pro-inflammatory cytokine secretion; and (3) potentially inhibit SARS-CoV-2 replication and restrict SARS-CoV-2 infection.
Pharmacological and therapeutic strategies to improve pulmonary surfactant dysfunction can prevent alveolar collapse at end-expiration, inhibit the pro-inflammatory response, and limit viral infection. Several clinical trials are currently exploring the use of surfactant preparations to treat COVID-19. In our opinion, accurate measurement of surfactants in patients and proper selection of pulmonary surfactant components should be considered prior to the clinical use of pulmonary surfactants. The rapid development of surfactant lipidomics has facilitated accurate measurement of pulmonary surfactants (33). Identifying pulmonary surfactant changes in patients with COVID-19 and modifying surfactant preparations accordingly can mitigate potential risks. Some components of pulmonary surfactant possess anti-inflammatory or antiviral properties and help prevent alveolar collapse, such as PG and SP-D (14, 16, 74). Several studies have reported that these components exert therapeutic effects against viral respiratory infection (14, 74). Clinical trial has been conducted to evaluate the safety and tolerated of AT-100 (rhSP-D) in patients with COVID-19 (Table 1). These studies suggest that a single lung surfactant component may effectively treat COVID-19. Moreover, using a single surfactant component may help avoid some potential risks. Taken together, this review provides important insight for the development of pulmonary surfactant preparations for the treatment of respiratory viral infections, including SARS-CoV-2.
The original contributions presented in the study are included in the article/supplementary material. Further inquiries can be directed to the corresponding authors.
JJ wrote the manuscript. XW and DL assisted with the manuscript preparation. SW, JS, and YL revised and polished the manuscript. All authors contributed to the article and approved the submitted version.
This work was sponsored by the National Natural Science Foundation of China (82004204, 81774156), Qinglan Project of Jiangsu Province of China, Natural Science Foundation of Jiangsu Province of China (BK20210681), and Colleges and universities in Jiangsu Province Natural Science Research (21KJB310007).
The authors declare that the research was conducted in the absence of any commercial or financial relationships that could be construed as a potential conflict of interest.
All claims expressed in this article are solely those of the authors and do not necessarily represent those of their affiliated organizations, or those of the publisher, the editors and the reviewers. Any product that may be evaluated in this article, or claim that may be made by its manufacturer, is not guaranteed or endorsed by the publisher.
COVID-19, coronavirus disease 2019; SARS-CoV-2, severe acute respiratory syndrome coronavirus 2; ACE2, angiotensin converting enzyme 2; RSV, respiratory syncytial virus; AT II cells, alveolar type II cells; SP, surfactant protein; NRDS, neonatal respiratory distress syndrome; ARDS, acute respiratory distress syndrome; PI, phosphatidylinositol; PE, phosphatidylethanolamine; PG, phosphatidylglycerol; PC, phosphatidylcholine; PS, phosphatidylserine; PL, phospholipid; HIV, human immunodeficiency virus; HCoV-229E, human coronavirus 229E; rfh SP-D, Recombinant fragments of human SP-D; WNV, West Nile virus; HCMV, human cytomegalovirus; TLR, toll-like receptor; POPG, 1-Palmitoyl-2-oleoyl-sn-glycero-3-phosphatidylglycerol; DPPC, dipalmitoyl PC; lysoPI, lysophosphatidylinositol; DG, diacylglycerol; TG, triacylglycerol; MDSCs, myeloid-derived suppressor cells; NK, natural killer; TLR, toll-like receptor; FC, Fold Change; BLES, Bovine Lipid Extract Surfactant.
1. The Lancet Infectious D. Challenges of Coronavirus Disease 2019. Lancet Infect Dis (2020) 20(3):261. doi: 10.1016/S1473-3099(20)30072-4
2. Scendoni R, Gattari D, Cingolani M. COVID-19 Pulmonary Pathology, Ventilator-Induced Lung Injury (VILI), or Sepsis-Induced Acute Respiratory Distress Syndrome (ARDS)? Healthcare Considerations Arising From an Autopsy Case and Miny-Review. Clin Pathol (2022) 15:2632010X221083223. doi: 10.1177/2632010X221083223
3. Calabrese F, Pezzuto F, Fortarezza F, Hofman P, Kern I, Panizo A, et al. Pulmonary Pathology and COVID-19: Lessons From Autopsy. The Experience of European Pulmonary Pathologists. Virchows Arch (2020) 477(3):359–72. doi: 10.1007/s00428-020-02886-6
4. Rendeiro AF, Ravichandran H, Bram Y, Chandar V, Kim J, Meydan C, et al. The Spatial Landscape of Lung Pathology During COVID-19 Progression. Nature (2021) 593(7860):564–9. doi: 10.1038/s41586-021-03475-6
5. Merad M, Blish CA, Sallusto F, Iwasaki A. The Immunology and Immunopathology of COVID-19. Science (2022) 375(6585):1122–7. doi: 10.1126/science.abm8108
6. Zhou R, To KK, Wong YC, Liu L, Zhou B, Li X, et al. Acute SARS-CoV-2 Infection Impairs Dendritic Cell and T Cell Responses. Immunity (2020) 53(4):864–77.e5. doi: 10.1016/j.immuni.2020.07.026
7. Zhang Q, Bastard P, Liu Z, Le Pen J, Moncada-Velez M, Chen J, et al. Inborn Errors of Type I IFN Immunity in Patients With Life-Threatening COVID-19. Science (2020) 370(6515):eabd4570-85. doi: 10.1126/science.abd4570
8. Wang EY, Mao T, Klein J, Dai Y, Huck JD, Jaycox JR, et al. Diverse Functional Autoantibodies in Patients With COVID-19. Nature (2021) 595(7866):283–8. doi: 10.1038/s41586-021-03631-y
9. Schulte-Schrepping J, Reusch N, Paclik D, Bassler K, Schlickeiser S, Zhang B, et al. Severe COVID-19 Is Marked by a Dysregulated Myeloid Cell Compartment. Cell (2020) 182(6):1419–40.e23. doi: 10.1016/j.cell.2020.08.001
10. Liao M, Liu Y, Yuan J, Wen Y, Xu G, Zhao J, et al. Single-Cell Landscape of Bronchoalveolar Immune Cells in Patients With COVID-19. Nat Med (2020) 26(6):842–4. doi: 10.1038/s41591-020-0901-9
11. Zhang H, Rostami MR, Leopold PL, Mezey JG, O’Beirne SL, Strulovici-Barel Y, et al. Expression of the SARS-CoV-2 ACE2 Receptor in the Human Airway Epithelium. Am J Respir Crit Care Med (2020) 202(2):219–29. doi: 10.1164/rccm.202003-0541OC
12. Rose F, Zwick K, Ghofrani HA, Sibelius U, Seeger W, Walmrath D, et al. Prostacyclin Enhances Stretch-Induced Surfactant Secretion in Alveolar Epithelial Type II Cells. Am J Respir Crit Care Med (1999) 160(3):846–51. doi: 10.1164/ajrccm.160.3.9812155
13. Autilio C, Perez-Gil J. Understanding the Principle Biophysics Concepts of Pulmonary Surfactant in Health and Disease. Arch Dis Child Fetal Neonatal Ed (2019) 104(4):F443–51. doi: 10.1136/archdischild-2018-315413
14. Ji J, Sun L, Luo Z, Zhang Y, Xianzheng W, Liao Y, et al. Potential Therapeutic Applications of Pulmonary Surfactant Lipids in the Host Defence Against Respiratory Viral Infections. Front Immunol (2021) 12:730022. doi: 10.3389/fimmu.2021.730022
15. Han S, Mallampalli RK. The Role of Surfactant in Lung Disease and Host Defense Against Pulmonary Infections. Ann Am Thorac Soc (2015) 12(5):765–74. doi: 10.1513/AnnalsATS.201411-507FR
16. Watson A, Madsen J, Clark HW. SP-A and SP-D: Dual Functioning Immune Molecules With Antiviral and Immunomodulatory Properties. Front Immunol (2020) 11:622598. doi: 10.3389/fimmu.2020.622598
17. Schousboe P, Wiese L, Heiring C, Verder H, Poorisrisak P, Verder P, et al. Assessment of Pulmonary Surfactant in COVID-19 Patients. Crit Care (2020) 24(1):552. doi: 10.1186/s13054-020-03268-9
18. Islam A, Khan MA. Lung Transcriptome of a COVID-19 Patient and Systems Biology Predictions Suggest Impaired Surfactant Production Which may be Druggable by Surfactant Therapy. Sci Rep (2020) 10(1):19395. doi: 10.1038/s41598-020-76404-8
19. Gerosa C, Fanni D, Cau F, Ravarino A, Senes G, Demontis R, et al. Immunohistochemical Findings in the Lungs of COVID-19 Subjects: Evidence of Surfactant Dysregulation. Eur Rev Med Pharmacol Sci (2021) 25(13):4639–43. doi: 10.26355/eurrev_202107_26257
20. Schousboe P, Ronit A, Nielsen HB, Benfield T, Wiese L, Scoutaris N, et al. Reduced Levels of Pulmonary Surfactant in COVID-19 ARDS. Sci Rep (2022) 12(1):4040. doi: 10.1038/s41598-022-07944-4
21. Leist SR, Dinnon KH 3rd, Schafer A, Tse LV, Okuda K, Hou YJ, et al. A Mouse-Adapted SARS-CoV-2 Induces Acute Lung Injury and Mortality in Standard Laboratory Mice. Cell (2020) 183(4):1070–85.e12. doi: 10.1016/j.cell.2020.09.050
22. Vos GD, Rijtema MN, Blanco CE. Treatment of Respiratory Failure Due to Respiratory Syncytial Virus Pneumonia With Natural Surfactant. Pediatr Pulmonol (1996) 22(6):412–5. doi: 10.1002/(SICI)1099-0496(199612)22:6<412::AID-PPUL11>3.0.CO;2-C
23. Vento G, Ventura ML, Pastorino R, van Kaam AH, Carnielli V, Cools F, et al. Lung Recruitment Before Surfactant Administration in Extremely Preterm Neonates With Respiratory Distress Syndrome (IN-REC-SUR-E): A Randomised, Unblinded, Controlled Trial. Lancet Respir Med (2021) 9(2):159–66. doi: 10.1016/S2213-2600(20)30179-X
24. Bos LDJ, Sjoding M, Sinha P, Bhavani SV, Lyons PG, Bewley AF, et al. Longitudinal Respiratory Subphenotypes in Patients With COVID-19-Related Acute Respiratory Distress Syndrome: Results From Three Observational Cohorts. Lancet Respir Med (2021) 9(12):1377–86. doi: 10.1016/S2213-2600(21)00365-9
25. Koumbourlis AC, Motoyama EK. Lung Mechanics in COVID-19 Resemble Respiratory Distress Syndrome, Not Acute Respiratory Distress Syndrome: Could Surfactant Be a Treatment? Am J Respir Crit Care Med (2020) 202(4):624–6. doi: 10.1164/rccm.202004-1471LE
26. Takano H. Pulmonary Surfactant Itself Must be a Strong Defender Against SARS-CoV-2. Med Hypotheses (2020) 144:110020. doi: 10.1016/j.mehy.2020.110020
27. Wang S, Li Z, Wang X, Zhang S, Gao P, Shi Z. The Role of Pulmonary Surfactants in the Treatment of Acute Respiratory Distress Syndrome in COVID-19. Front Pharmacol (2021) 12:698905. doi: 10.3389/fphar.2021.698905
28. Veldhuizen RAW, Zuo YY, Petersen NO, Lewis JF, Possmayer F. The COVID-19 Pandemic: A Target for Surfactant Therapy? Expert Rev Respir Med (2021) 15(5):597–608. doi: 10.1080/17476348.2021.1865809
29. Piva S, DiBlasi RM, Slee AE, Jobe AH, Roccaro AM, Filippini M, et al. Surfactant Therapy for COVID-19 Related ARDS: A Retrospective Case-Control Pilot Study. Respir Res (2021) 22(1):20. doi: 10.1186/s12931-020-01603-w
30. Heching M, Lev S, Shitenberg D, Dicker D, Kramer MR. Surfactant for the Treatment of ARDS in a Patient With COVID-19. Chest (2021) 160(1):e9–e12. doi: 10.1016/j.chest.2021.01.028
31. Ballard PL, Keller RL, Truog WE, Chapin C, Horneman H, Segal MR, et al. Surfactant Status and Respiratory Outcome in Premature Infants Receiving Late Surfactant Treatment. Pediatr Res (2019) 85(3):305–11. doi: 10.1038/s41390-018-0144-3
32. Lewis JF, Veldhuizen R. The Role of Exogenous Surfactant in the Treatment of Acute Lung Injury. Annu Rev Physiol (2003) 65:613–42. doi: 10.1146/annurev.physiol.65.092101.142434
33. Shan J, Qian W, Shen C, Lin L, Xie T, Peng L, et al. High-Resolution Lipidomics Reveals Dysregulation of Lipid Metabolism in Respiratory Syncytial Virus Pneumonia Mice. RSC Adv (2018) 8(51):29368–77. doi: 10.1039/C8RA05640D
34. Woods PS, Doolittle LM, Rosas LE, Joseph LM, Calomeni EP, Davis IC. Lethal H1N1 Influenza A Virus Infection Alters the Murine Alveolar Type II Cell Surfactant Lipidome. Am J Physiol Lung Cell Mol Physiol (2016) 311(6):L1160–L9. doi: 10.1152/ajplung.00339.2016
35. Mazur W, Toljamo T, Ohlmeier S, Vuopala K, Nieminen P, Kobayashi H, et al. Elevation of Surfactant Protein A in Plasma and Sputum in Cigarette Smokers. Eur Respir J (2011) 38(2):277–84. doi: 10.1183/09031936.00110510
36. Dushianthan A, Cusack R, Goss V, Postle AD, Grocott MP. Clinical Review: Exogenous Surfactant Therapy for Acute Lung Injury/Acute Respiratory Distress Syndrome–Where do We Go From Here? Crit Care (2012) 16(6):238. doi: 10.1186/cc11512
37. Heiring C, Verder H, Schousboe P, Jessen TE, Bender L, Ebbesen F, et al. Predicting Respiratory Distress Syndrome at Birth Using a Fast Test Based on Spectroscopy of Gastric Aspirates: 2. Clin part Acta Paediatr (2020) 109(2):285–90. doi: 10.1111/apa.14831
38. Benne CA, Kraaijeveld CA, van Strijp JA, Brouwer E, Harmsen M, Verhoef J, et al. Interactions of Surfactant Protein A With Influenza A Viruses: Binding and Neutralization. J Infect Dis (1995) 171(2):335–41. doi: 10.1093/infdis/171.2.335
39. LeVine AM, Gwozdz J, Stark J, Bruno M, Whitsett J, Korfhagen T. Surfactant Protein-A Enhances Respiratory Syncytial Virus Clearance In Vivo. J Clin Invest (1999) 103(7):1015–21. doi: 10.1172/JCI5849
41. Walls AC, Park YJ, Tortorici MA, Wall A, McGuire AT, Veesler D. Structure, Function, and Antigenicity of the SARS-CoV-2 Spike Glycoprotein. Cell (2020) 181(2):281–92.e6. doi: 10.1016/j.cell.2020.02.058
42. Ujma S, Carse S, Chetty A, Horsnell W, Clark H, Madsen J, et al. Surfactant Protein A Impairs Genital HPV16 Pseudovirus Infection by Innate Immune Cell Activation in A Murine Model. Pathogens (2019) 8(4):288. doi: 10.3390/pathogens8040288
43. Hartshorn KL, Webby R, White MR, Tecle T, Pan C, Boucher S, et al. Role of Viral Hemagglutinin Glycosylation in Anti-Influenza Activities of Recombinant Surfactant Protein D. Respir Res (2008) 9:65. doi: 10.1186/1465-9921-9-65
44. LeVine AM, Elliott J, Whitsett JA, Srikiatkhachorn A, Crouch E, DeSilva N, et al. Surfactant Protein-D Enhances Phagocytosis and Pulmonary Clearance of Respiratory Syncytial Virus. Am J Respir Cell Mol Biol (2004) 31(2):193–9. doi: 10.1165/rcmb.2003-0107OC
45. Leth-Larsen R, Zhong F, Chow VT, Holmskov U, Lu J. The SARS Coronavirus Spike Glycoprotein is Selectively Recognized by Lung Surfactant Protein D and Activates Macrophages. Immunobiology (2007) 212(3):201–11. doi: 10.1016/j.imbio.2006.12.001
46. Madsen J, Gaiha GD, Palaniyar N, Dong T, Mitchell DA, Clark HW. Surfactant Protein D Modulates HIV Infection of Both T-Cells and Dendritic Cells. PloS One (2013) 8(3):e59047. doi: 10.1371/journal.pone.0059047
47. Sanaki T, Wakabayashi M, Yoshioka T, Yoshida R, Shishido T, Hall WW, et al. Inhibition of Dengue Virus Infection by 1-Stearoyl-2-Arachidonoyl-Phosphatidylinositol In Vitro. FASEB J (2019) 33(12):13866–81. doi: 10.1096/fj.201901095RR
48. Coil DA, Miller AD. Enhancement of Enveloped Virus Entry by Phosphatidylserine. J Virol (2005) 79(17):11496–500. doi: 10.1128/JVI.79.17.11496-11500.2005
49. Mercer J, Helenius A. Vaccinia Virus Uses Macropinocytosis and Apoptotic Mimicry to Enter Host Cells. Science (2008) 320(5875):531–5. doi: 10.1126/science.1155164
50. Numata M, Chu HW, Dakhama A, Voelker DR. Pulmonary Surfactant Phosphatidylglycerol Inhibits Respiratory Syncytial Virus-Induced Inflammation and Infection. Proc Natl Acad Sci USA (2010) 107(1):320–5. doi: 10.1073/pnas.0909361107
51. Numata M, Nagashima Y, Moore ML, Berry KZ, Chan M, Kandasamy P, et al. Phosphatidylglycerol Provides Short-Term Prophylaxis Against Respiratory Syncytial Virus Infection. J Lipid Res (2013) 54(8):2133–43. doi: 10.1194/jlr.M037077
52. Numata M, Kandasamy P, Nagashima Y, Posey J, Hartshorn K, Woodland D, et al. Phosphatidylglycerol Suppresses Influenza A Virus Infection. Am J Respir Cell Mol Biol (2012) 46(4):479–87. doi: 10.1165/rcmb.2011-0194OC
53. Numata M, Kandasamy P, Nagashima Y, Fickes R, Murphy RC, Voelker DR. Phosphatidylinositol Inhibits Respiratory Syncytial Virus Infection. J Lipid Res (2015) 56(3):578–87. doi: 10.1194/jlr.M055723
54. Voelker DR, Numata M. Phospholipid Regulation of Innate Immunity and Respiratory Viral Infection. J Biol Chem (2019) 294(12):4282–9. doi: 10.1074/jbc.AW118.003229
55. Numata M, Mitchell JR, Tipper JL, Brand JD, Trombley JE, Nagashima Y, et al. Pulmonary Surfactant Lipids Inhibit Infections With the Pandemic H1N1 Influenza Virus in Several Animal Models. J Biol Chem (2020) 295(6):1704–15. doi: 10.1074/jbc.RA119.012053
56. Belov GA. Less Grease, Please. Phosphatidylethanolamine Is the Only Lipid Required for Replication of a (+)RNA Virus. Viruses (2015) 7(7):3500–5. doi: 10.3390/v7072784
57. Xu K, Nagy PD. RNA Virus Replication Depends on Enrichment of Phosphatidylethanolamine at Replication Sites in Subcellular Membranes. Proc Natl Acad Sci USA (2015) 112(14):E1782–91. doi: 10.1073/pnas.1418971112
58. Radenkovic D, Chawla S, Pirro M, Sahebkar A, Banach M. Cholesterol in Relation to COVID-19: Should We Care About it? J Clin Med (2020) 9(6):1909. doi: 10.3390/jcm9061909
59. Lu Y, Liu DX, Tam JP. Lipid Rafts are Involved in SARS-CoV Entry Into Vero E6 Cells. Biochem Biophys Res Commun (2008) 369(2):344–9. doi: 10.1016/j.bbrc.2008.02.023
60. Choi KS, Aizaki H, Lai MM. Murine Coronavirus Requires Lipid Rafts for Virus Entry and Cell-Cell Fusion But Not for Virus Release. J Virol (2005) 79(15):9862–71. doi: 10.1128/JVI.79.15.9862-9871.2005
61. Jeon JH, Lee C. Cholesterol is Important for the Entry Process of Porcine Deltacoronavirus. Arch Virol (2018) 163(11):3119–24. doi: 10.1007/s00705-018-3967-7
62. Guo H, Huang M, Yuan Q, Wei Y, Gao Y, Mao L, et al. The Important Role of Lipid Raft-Mediated Attachment in the Infection of Cultured Cells by Coronavirus Infectious Bronchitis Virus Beaudette Strain. PloS One (2017) 12(1):e0170123. doi: 10.1371/journal.pone.0170123
63. Stoeck IK, Lee JY, Tabata K, Romero-Brey I, Paul D, Schult P, et al. Hepatitis C Virus Replication Depends on Endosomal Cholesterol Homeostasis. J Virol (2018) 92(1):e01196–17. doi: 10.1128/JVI.01196-17
64. Lee J, Kreutzberger AJB, Odongo L, Nelson EA, Nyenhuis DA, Kiessling V, et al. Ebola Virus Glycoprotein Interacts With Cholesterol to Enhance Membrane Fusion and Cell Entry. Nat Struct Mol Biol (2021) 28(2):181–9. doi: 10.1038/s41594-020-00548-4
65. Sun X, Whittaker GR. Role for Influenza Virus Envelope Cholesterol in Virus Entry and Infection. J Virol (2003) 77(23):12543–51. doi: 10.1128/jvi.77.23.12543-12551.2003
66. Hsieh MH, Beirag N, Murugaiah V, Chou YC, Kuo WS, Kao HF, et al. Human Surfactant Protein D Binds Spike Protein and Acts as an Entry Inhibitor of SARS-CoV-2 Pseudotyped Viral Particles. Front Immunol (2021) 12:641360. doi: 10.3389/fimmu.2021.641360
67. Madan T, Biswas B, Varghese PM, Subedi R, Pandit H, Idicula-Thomas S, et al. A Recombinant Fragment of Human Surfactant Protein D Binds Spike Protein and Inhibits Infectivity and Replication of SARS-CoV-2 in Clinical Samples. Am J Respir Cell Mol Biol (2021) 65(1):41–53. doi: 10.1165/rcmb.2021-0005OC
68. Zuo YY, Veldhuizen RA, Neumann AW, Petersen NO, Possmayer F. Current Perspectives in Pulmonary Surfactant–Inhibition, Enhancement and Evaluation. Biochim Biophys Acta (2008) 1778(10):1947–77. doi: 10.1016/j.bbamem.2008.03.021
69. Curstedt T, Johansson J. New Synthetic Surfactants–Basic Science. Biol Neonate (2005) 87(4):332–7. doi: 10.1159/000084881
70. Suresh GK, Soll RF. Lung Surfactants for Neonatal Respiratory Distress Syndrome: Animal-Derived or Synthetic Agents? Paediatr Drugs (2002) 4(8):485–92. doi: 10.2165/00128072-200204080-00001
71. Deng Y, Angelova A. Coronavirus-Induced Host Cubic Membranes and Lipid-Related Antiviral Therapies: A Focus on Bioactive Plasmalogens. Front Cell Dev Biol (2021) 9:630242. doi: 10.3389/fcell.2021.630242
72. Fessler MB, Summer RS. Surfactant Lipids at the Host-Environment Interface. Metabolic Sensors, Suppressors, and Effectors of Inflammatory Lung Disease. Am J Respir Cell Mol Biol (2016) 54(5):624–35. doi: 10.1165/rcmb.2016-0011PS
73. Katsiki N, Banach M, Mikhailidis DP. Lipid-Lowering Therapy and Renin-Angiotensin-Aldosterone System Inhibitors in the Era of the COVID-19 Pandemic. Arch Med Sci (2020) 16(3):485–9. doi: 10.5114/aoms.2020.94503
Keywords: pulmonary surfactant, COVID-19, ARDS, therapeutic applications, respiratory viral infections
Citation: Li D, Wang X, Liao Y, Wang S, Shan J and Ji J (2022) Insights Gained Into the Treatment of COVID19 by Pulmonary Surfactant and Its Components. Front. Immunol. 13:842453. doi: 10.3389/fimmu.2022.842453
Received: 23 December 2021; Accepted: 11 April 2022;
Published: 03 May 2022.
Edited by:
Taruna Madan, National Institute for Research in Reproductive Health (ICMR), IndiaReviewed by:
Shantibhusan Senapati, Institute of Life Sciences (ILS), IndiaCopyright © 2022 Li, Wang, Liao, Wang, Shan and Ji. This is an open-access article distributed under the terms of the Creative Commons Attribution License (CC BY). The use, distribution or reproduction in other forums is permitted, provided the original author(s) and the copyright owner(s) are credited and that the original publication in this journal is cited, in accordance with accepted academic practice. No use, distribution or reproduction is permitted which does not comply with these terms.
*Correspondence: Shouchuan Wang, d3NjbmpAbmp1Y20uZWR1LmNu; Jianjian Ji, amlqakBuanVjbS5lZHUuY24=; Jinjun Shan, anNoYW5Abmp1Y20uZWR1LmNu
†These authors have contributed equally to this work
Disclaimer: All claims expressed in this article are solely those of the authors and do not necessarily represent those of their affiliated organizations, or those of the publisher, the editors and the reviewers. Any product that may be evaluated in this article or claim that may be made by its manufacturer is not guaranteed or endorsed by the publisher.
Research integrity at Frontiers
Learn more about the work of our research integrity team to safeguard the quality of each article we publish.