- 1Center for Global Infectious Disease Research, Seattle Children's Research Institute, Seattle Children's Hospital, Seattle, WA, United States
- 2Department of Global Health, University of Washington, Seattle, WA, United States
- 3Department of Pediatrics, University of Washington School of Medicine, Seattle, WA, United States
Despite co-evolving with humans for centuries and being intensely studied for decades, the immune correlates of protection against Mycobacterium tuberculosis (Mtb) have yet to be fully defined. This lapse in understanding is a major lag in the pipeline for evaluating and advancing efficacious vaccine candidates. While CD4+ T helper 1 (TH1) pro-inflammatory responses have a significant role in controlling Mtb infection, the historically narrow focus on this cell population may have eclipsed the characterization of other requisite arms of the immune system. Over the last decade, the tuberculosis (TB) research community has intentionally and intensely increased the breadth of investigation of other immune players. Here, we review mechanistic preclinical studies as well as clinical anecdotes that suggest the degree to which different cell types, such as NK cells, CD8+ T cells, γ δ T cells, and B cells, influence infection or disease prevention. Additionally, we categorically outline the observed role each major cell type plays in vaccine-induced immunity, including Mycobacterium bovis bacillus Calmette-Guérin (BCG). Novel vaccine candidates advancing through either the preclinical or clinical pipeline leverage different platforms (e.g., protein + adjuvant, vector-based, nucleic acid-based) to purposefully elicit complex immune responses, and we review those design rationales and results to date. The better we as a community understand the essential composition, magnitude, timing, and trafficking of immune responses against Mtb, the closer we are to reducing the severe disease burden and toll on human health inflicted by TB globally.
Introduction
Pulmonary tuberculosis (TB) caused by Mycobacterium tuberculosis (Mtb) was the leading infectious killer globally for the 4 years (1) predating the severe acute respiratory syndrome corona virus 2 (SARS-CoV-2) pandemic (2). Approximately 1.5 million individuals succumbed to TB in 2020, up from 1.4 million in 2019, marking the first increase in global TB deaths in more than a decade (3, 4). Furthermore, the World Health Organization (WHO) and others estimate that COVID-19-related disruptions in care, including a 21% reduction in people receiving care for TB in 2020, could result in an additional half million deaths (5), likely in low- and middle-income countries (LMICs), which continue to bear a disproportionate burden of TB (6, 7). New therapies or interventions aimed towards prevention of infection (POI), prevention of disease (POD), and subsequent transmission are urgently needed (8). Moreover, Mtb drug resistance (DR) is steadily increasing globally. In each year since 2017, roughly half a million Mtb-infected individuals developed rifampicin resistance and ~80% of those cases had multidrug-resistant (MDR) TB (1, 3, 9). Focused efforts to design and evaluate low-cost and highly effective TB vaccines are urgently needed as current interventions alone are insufficient by many models (10, 11) to achieve the WHO End TB Strategy milestones. Several TB vaccines have been tested in clinical trials; however, only Mycobacterium bovis Bacillus Calmette Guérin (BCG) (0%–80% efficacy) (12) and M72/AS01E (~50% effective against the progression of TB disease) (13, 14) have shown protection in humans. Additionally, no defined correlates of protection (COP) are solidified for Mtb infection or TB disease. Therefore, it is worth taking note of the immune responses elicited by these vaccines (15) and others showing promise in the pipeline. The aim of this review is to focus on immune cells and immunological mediators against Mtb, induced both preclinically and clinically by TB vaccine candidates and BCG, that have been overshadowed by a myopic focus on CD4+ T helper 1 (TH1) cells. We hope that this collection informs future immune efficacy endpoints and helps draw the field closer to predictive COP endpoints.
The TB vaccine landscape is poised to make formidable leaps forward. This is in part due to courageous work by the international research communities and seminal publications demonstrating near sterilizing protection from Mtb challenge in preclinical models (16). Findings of a recent study showing that intravenous (i.v.) BCG prevents or substantially limits Mtb infection in a susceptible rhesus macaque model (16) provides a benchmark against which future vaccines will be evaluated and importantly a new framework to understand the immune correlates and mechanisms of protection against TB. For example, many arms of the immune response are engaged following i.v. BCG delivery compared to intradermal (i.d.) delivery. In the airway (bronchioalveolar lavage fluid), early γδ T-cell, invariant natural killer T cell (iNKT), natural killer (NK) cell, B-cell, neutrophil, myeloid dendritic cell (mDC), and mucosal-associated invariant T (MAIT) cell responses are observed. In the i.v. group, both memory CD4+ and CD8+ T cells producing TH1 and TH17 cytokines were captured, whereas only CD4+ responses are elicited in the i.d. cohort (16). Mucosal airway and peripheral antibody responses (IgG, IgA, and IgM) were also highest in the i.v. group, 4 weeks after vaccination (16, 17). These data help to highlight the diversity of immune responses that may be working in concert to afford protection from Mtb, but to date have not been so well captured across a single study with robust correlating efficacy. Between this seminal investigation and other recent groundbreaking discoveries of immune COP, the research community is highlighting the critical roles of commonly overlooked and bypassed immune responses.
Cell Subsets, Widening the View
While most primary endpoints for vaccine immunogenicity preferentially evaluate anti-Mtb specific TH1-type responses (18), the full mechanism of protection has yet to be determined (19). Despite many candidates inducing robust classical TH1 CD4+ T-cell responses in preclinical and clinical trials, no candidate has met the target product profile for an efficacious TB vaccine, so we need to collectively look beyond this subset. In a phase 2b safety and efficacy trial of candidate MVA85A, researchers observed robust TH1 responses but a dramatic lack of efficacy in previously BCG-vaccinated neonates (20). This is a recent example of how the reliance on this primary endpoint has led to disappointing efficacy results and stalling of funding support for clinical candidates. Indeed, protection from Mtb infection and TB disease is likely a multifaceted process involving many cell types beyond canonical CD4+ T cells and their main proinflammatory cytokine interferon gamma (IFNγ). In a 2017 review of polyfunctional CD4+ T cells induced by BCG and TB vaccine candidates in preclinical and clinical studies, Lewinsohn and colleagues conclude that this subset is likely not sufficient for protective efficacy (18) and suggested that further studies were warranted to specifically address their mechanistic role in protection. Now, several years later, in the collaborative cross mouse model (21), researchers have succinctly uncoupled the magnitude of IFNγ expression and subsequent control of Mtb, where a proportion of genotypes evaluated for Mtb susceptibility had low IFNγ production but still controlled the infection (22). This dissociation found in mice has also been recently observed in human resister (RSTR) cohorts. A prospective household contact study in Uganda found that RSTRs consistently test negative by tuberculin skin testing (TST) despite constant Mtb challenge, suggesting IFNγ-independent immunity in this population (23). Given these findings, it is even more important to diversify our understanding of the cellular contributors to Mtb immunity. Here, we have reviewed the effector functions, role(s) during infection, and what is known about specific cell subsets’ [alveolar macrophages (AM), neutrophils, DCs, NK cells, B cells, CD8+ T cells, and γδ T cells] induction with different vaccine candidates in the preclinical or clinical pipeline (Figure 1 and Table 1). While animal models of TB do not fully represent the spectrum of human infection and disease pathogenesis or align within the preclinical pipeline itself, they are improving and can be informative (95); therefore, the information presented here reflects a review of primary in vitro or ex vivo data from animal models as well as observations from human clinical studies.
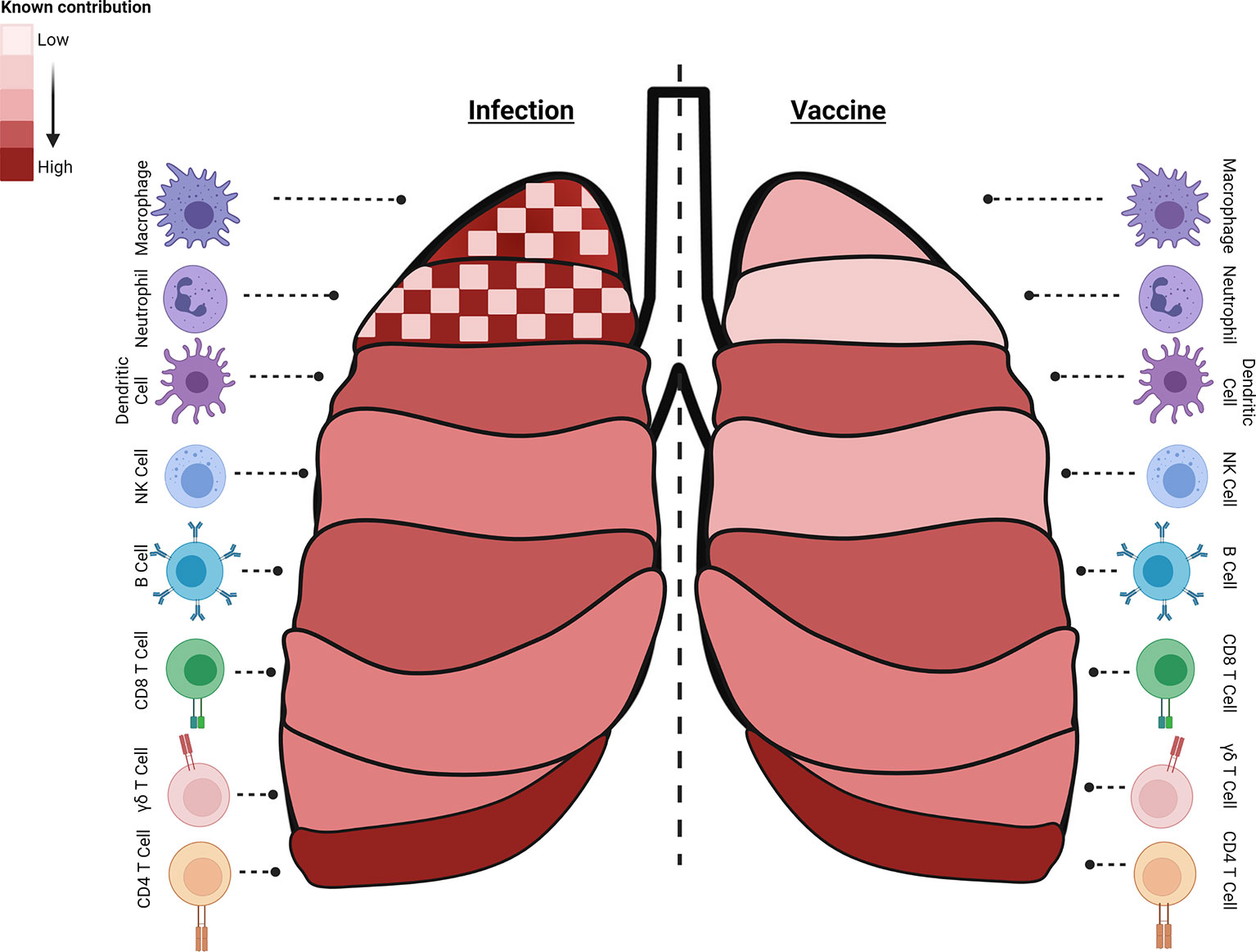
Figure 1 Immune cell subsets with critical roles during different stages of Mtb infection or generated by specific vaccine strategies. Known contributions (heatmap of light: low, dark: high, checked: mixed) of specific subsets are outlined during infection (left) or following vaccine induction (right). Macrophages, neutrophils, and B cells have known and defined contributions to controlling infection and preventing disease. Interestingly, both macrophages and neutrophils can contribute to Mtb control or serve as a niche for bacterial growth and dissemination and their dual role is highlighted (checked). Dendritic cells participate in early stages of infection and post vaccination as an APC. NK cells contribute moderately to infection control and little is known about their induction with different vaccination regimens. Antigen-specific cytolytic CD8+ T target Mtb and Mtb-infected cells, and their part in controlling latent infection, important in POD vaccination strategies, is expanding. While a multi-faceted immune response is induced to control Mtb infection, vaccine-induced immune responses essential for protection by many of these cell subsets are still understudied as endpoints. The summary presented here is a collection of information from many models and clinical data and do not reflect the data known or observed for each model (created with BioRender.com).
Neutrophils/PMNs
Effector functions. Innate immune cells such as neutrophils have been shown to help mediate early inflammatory responses that are critical for controlling infection (96–98). Initial immunology work focused on the role of specific phagocytes in infection, leaving neutrophils understudied and underappreciated. However, neutrophils account for 50%–80% of all circulating white blood cells in humans and contribute to the innate immune response via phagocytosis of invading bacteria, degranulation, and subsequent secretion of cytokines such as tumor-necrosis factor-alpha (TNF-α) and interleukin 1 (IL-1) (96, 99–102). Neutrophils provide a non-specific immediate innate response that helps contain and control invading pathogens, preventing dissemination and recruiting other cell types. Discovery of the various functions of neutrophils such as neutrophil extracellular trap (NET) formation, phagocytosis, heterogeneity, and plasticity has increased and opened new avenues in recent neutrophil research (103). For example, NET structures, made up of sticky extruded DNA, are unique to neutrophils and not only limit microbial spread and dissemination, but also enhance the concentration of microbicidal agents in human ex vivo evaluations (104–107). Neutrophils secrete reactive oxygen species (ROS), elastase, collagenase, and myeloperoxidase, factors that can both combat invading mycobacteria and, when overabundant, damage host cells in a nonselective manner (98, 102, 108).
Neutrophils in circulation can be recruited to lung parenchyma by cytokines, leading to their activation and phagocytosis of pathogen. In preclinical rodent models, CXC chemokines are a potent chemoattractant pulling neutrophils from circulation into an infected or damaged lung space (109). Neutrophils are also an important amplifying cell as they are a significant source of specific cytokines that help promote early recruitment and activation of other innate immune cells, contributing to cellular immunity against mycobacterial infection, as observed in a Balb/c mouse model challenged with H37Rv Mtb (110) (Figure 2). For example, ex vivo human neutrophils have been shown to modulate the effector mechanisms of resident AM (111), another crucial cell for control of Mtb, through the localization of antimicrobials and proteins like heat shock protein 72 (Hsp72), which induce inflammatory responses in AMs. Neutrophils employ an arsenal of bactericidal proteolytic enzymes that are in an arms race with evasive countermeasures deployed by certain bacterial processes (112). Therefore, neutrophil enzyme activation and secretion help shape the early innate immune response during bacterial infection (111).
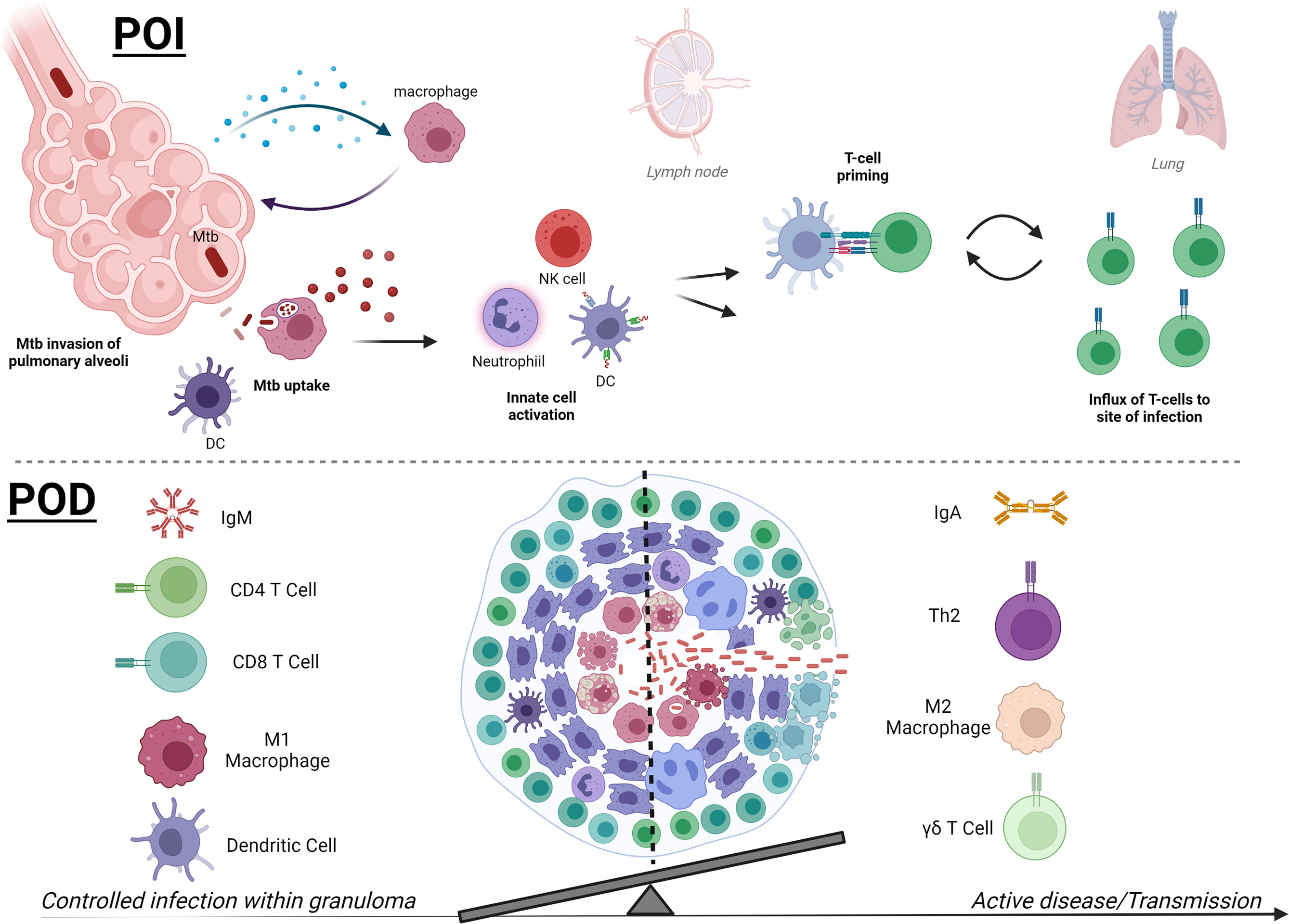
Figure 2 Mtb therapies focus on two main strategies, early prevention of infection or later prevention of disease. In early infection (POI, upper), detection of Mtb bacilli in the pulmonary alveoli by macrophages leads to downstream activation of innate immune cells, which may include neutrophils, NK cells, and DCs. Activated APCs can then prime T cells (CD4+ or CD8+) for further Mtb-specific adaptive responses to target Mtb and Mtb-infected cells and control infection. Novel therapies are working to skew innate immune responses to be protective and less permissive and accelerate T-cell priming and effector responses that traffic to the pulmonary space. Later stages of Mtb infection (POD, lower) and pathology are defined by formation of a granuloma, which contains Mtb by a surrounding composition of immune cells. While a hallmark of disease, there are still questions surrounding the factors that can affect granuloma formation and composition that can help resolve infection and prevent progression to active disease. Containment and POD progression seem to correlate with IgM, robust pulmonary CD4+ and CD8+ T cells, and activated inflammatory M1 macrophages and DCs at the granuloma. In contrast, regulatory TH2 CD4+ T cells, abundance of IgA or IgG4, and M2 macrophages are more associated with loss of control. Higher peripheral γδ T cells are associated with active TB disease in humans, but their direct role in granuloma control or bacterial dissemination requires further study. The balance of both the composition and magnitude of specific cellular and humoral players is becoming clearer and POD vaccine strategies will be able to benchmark these parameters in preclinical and clinical studies. The summary presented here is a collection of information from many models and clinical data and does not reflect the data known or observed for each model (created with BioRender.com).
Role in Mtb infections. While neutrophils provide early protection and participate in the early formation of granuloma structures, they can also be detrimental to the host if overabundant in later TB disease states. Studies of human neutrophils ex vivo observe that this subset can directly kill virulent Mtb lineages and suggest that TNF stimulation can aid this killing and the mechanism may be nonoxidative (113, 114). Indeed, neutrophils from patients with chronic granulomatous disease, which harbor defects in certain oxidase pathways, are equally efficient at Mtb killing compared to normal healthy control cells (113). However, there are many mechanisms by which neutrophils kill Mtb and eliminating one pathway may be compensated for by another. For example, human neutrophils have been shown to release lysosomal enzymes, human neutrophil peptides, and ROS, which can directly lyse Mtb in ex vivo co-culture situations (115, 116). Furthermore, depletion of neutrophils from patient whole blood samples significantly reduces the ability of those samples to restrict mycobacterial growth of both recombinant BCG and Mtb containing luciferase for kinetic evaluations (116). Arguably, neutrophils’ most iconic tool against bacterial pathogens are NETs, which play a significant role in controlling Mtb and subsequent immune activation. Indeed, DNA NET induction has been shown to be induced by Mtb in a guinea pig model (117), be at higher levels in plasma in patients with active TB disease (118), and activate human macrophages ex vivo (105). Mtb-induced NETs can also help sequester toxic contents from dying neutrophils, including lysozyme, ion chelators (calgranulin), and histones (119), to limit damage to surrounding tissue, which have been observed in humans and preclinical models (105, 106). NETs play a vital role in the partnership between neutrophils and macrophages. As mentioned above, Hsp72-containing NETs trigger a pro-inflammatory response in resident AMs, inducing the release of IL-6, TNF-α, and IL-1β (105). Therefore, these important innate immune cells can help contain the infection and contribute to early granuloma formation (Figure 2) (104–107, 113, 114). Despite these many tools for Mtb killing and growth inhibition, there is also evidence of bacterial immune evasion from neutrophils. For instance, neutrophils isolated from healthy human blood have been shown to effectively phagocytose Mtb ex vivo but not kill up to 6 h in co-culture, eluding bacterial escape mechanisms (120). In these studies, neutrophils were activated but also underwent necrosis, which may enable Mtb survival (120).
An effective host innate immune response against Mtb is based not only on successful cell-mediated killing of Mtb, but also on the efficient regulation of innate immune cells, often via cytokines (114, 121). Activated neutrophils express TNF-α, IL-8, and granzyme effectors, which all further influence other cell subsets. TNF-α stimulates dendritic cells and macrophage differentiation and activation, which are vital host responses to Mtb infection (100, 101, 122). Additionally, TNF-α aids in the activation of T cells and helps promote granuloma formation (123, 124). In response to Mtb, neutrophils have also been shown to secrete the chemokine IL-8 (125), which has autocrine properties, enhancing direct neutrophil killing of Mtb, as well as paracrine recruitment of leukocytes to areas of granuloma formation (126–130). Interestingly, IL-8 concentrations are increased following the completion of a 9-month-long antibacterial therapy of Mtb both in vivo and in LPS-stimulated ex vivo plasma. Lastly, neutrophils in macaque and human granulomas have been shown to be significant contributors of granzyme B (GRZB) (131) and that GRZB+ expression in neutrophils positively associated with higher granuloma bacterial loads. GRZB is known to act on intracellular substrates including pro-caspase 3, contributing to pathogen clearance (132), but in the study discussed above, it correlates with a negative outcome (131). These data collectively suggest that based on timing and location, responses from neutrophils can be beneficial or detrimental and that intermediates like cytokines and chemokines play complex roles in these processes (131–134).
The role of neutrophils in TB disease progression is of importance. Whereas early neutrophil responses may be beneficial for reasons outlined above, several inflammatory mediators stemming from neutrophils cause lung damage and contribute to the development of caseous necrotic granulomas leading to active TB disease and transmission of Mtb (Figure 1). An important contribution to understanding the role of neutrophils in the late stage of active TB disease was the human blood IFN signature of active disease derived from neutrophils (135). In addition, a recent review discusses neutrophil-specific mediators that impact destructive inflammation resulting in increased disease and mortality (136). Indeed, disease severity and reduction of long-term pulmonary function seem to correlate with neutrophil mechanisms in patients with TB disease (136). These mediators include matrix metalloproteinases (MMPs), which modify tissue architecture and facilitate transmembrane migration of neutrophils (136). The role of MMPs in TB pathogenesis has been known for some time (137). Several exciting new host-directed therapies against these lung-damaging mediators are being pursued including anti-tuberculosis drugs that also reduce lung damage including the combined use of an eicosanoid inhibitor (used against asthma; zileuton) along with Prostaglandin E2, in addition to other host-directed therapies such as statins, as discussed in the review by Muefong and Sutherland (136).
Vaccine induced. Previous publications have highlighted the importance of the innate immune system during BCG vaccination and have indicated that non-specific effects of BCG vaccination may benefit young children even if protection against Mtb is not attained (138–141). A recent study employing depletion and knockout (KO) mouse studies identified that following subcutaneous BCG inoculation, neutrophils, circulating monocytes, and AMs are sufficient to reduce Mtb burden. This study also provided evidence that neutrophils play a significant role in establishing innate immunity, possibly through an early inflammatory response that initiates the reduction in Mtb burden (142). Specifically, depletion of neutrophils in these mouse models was associated with diminished protection by BCG, which supports findings in humans where intradermal BCG was associated with a neutrophil transcriptional signature and elevated neutrophil counts in BCG-vaccinated infants (143). While BCG is effective at preventing disseminated disease in infants, it confers highly variable efficacy against pulmonary TB in adults, particularly in the developing world (12). A greater understanding of the reasons for this variability, together with a better understanding of the early, innate, and non-antigen-specific mechanisms of protection would facilitate the design and development of more effective vaccines (15). Next-generation live-attenuated vaccines based on BCG that aimed to enhance efficacy and durability are well reviewed here (144).
Emerging evidence about the cross-talk between neutrophils and T cells suggests that a balanced targeting of these cell types may improve vaccine-induced immunity (145). While the role of IL-17 in protection against Mtb infection may be related to its role in the induction of TH1 cells (146), IL-17 is also known to activate and recruit neutrophils (147–150). Furthermore, IL-17 favors the recall of vaccine-specific memory T cells in response to Mtb challenge through an IL-23-dependent mechanism (151), and neutrophils when activated by IL-23 also produce IL-17 and IL-22 (152). The role of neutrophils in the induction of specific TH1 and TH17 cells in response to vaccination against TB and the subsequent protective outcome is being investigated. For example, vaccine candidate M. smegmatis-Ag85C-MPT51-HspX (mc2-CMX) recombinant live vaccine vector has been shown to elicit a humoral and cellular response against Mtb in mice that is superior to BCG (153), largely due to the TH1- and TH17-specific responses promoted by neutrophils (154). Given the tremendous role of neutrophils in eliciting an enhanced immune response in mice vaccinated with mc2-CMX, neutrophils may represent an underappreciated/bystander innate target for TB vaccine candidates, particularly those targeting POI mechanisms.
Monocytes/Macrophages
Effector functions. Lung resident myeloid cells, including specialized AM, have dual and opposing roles in Mtb control: contributing to host resistance, and a niche for infection (Figure 1). Derived from hematopoietic precursors, macrophages are central innate immune cells that function in host defense and maintenance of tissue homeostasis. When tissue homeostasis is perturbed, bone marrow-derived monocytes are recruited from the blood to the affected site where they differentiate into macrophages. Both tissue-resident and monocyte-derived macrophages (MDM) are at the leading edge of an innate immune response through phagocytosis and cytokine release, as well as forming a bridge to adaptive immune responses through antigen presentation (155). Human and animal model macrophages express scavenger receptors and immunoglobulin receptors, which promote phagocytosis (156), antibody-dependent cell phagocytosis (ADCP) (157), and antibody-dependent cell cytotoxicity (ADCC) (158). One of their most robust innate tools are germ-line encoded pattern recognition receptors (PRRs) that sense microbial- or danger-associated molecular patterns (MAMPs/DAMPs). AMs and monocyte-derived macrophages (MDM) are activated by Toll-like receptors (TLRs) TLR2, TLR4, and TLR9 during the early stage of infection with Mtb (159). Furthermore, AM express innate immune receptors, C-type lectin receptors (CLRs), Dectin-1, nucleotide-binding oligomerization domain-like receptors (NLRs), inflammasome-IL-1β activator, and DC-specific intracellular adhesion molecule-3–grabbing nonintegrin (DC-SIGN), for non-specific pathogen recognition and induction of effector functions as observed in ex vivo human macrophage studies and surveys of human genetic variants that influence TB susceptibility and outcomes (160–163). Following PRR stimulation, signal transduction cascades converge on the activation of master transcription factors, proteases, and effectors of phagocytosis, allowing for rapid non-specific innate immune responses (156). PRR engagement also ensures durable responses through trained immunity, which is established by metabolic and epigenetic reprogramming of transcriptional pathways in myeloid progenitors (164).
For their role in host defense and inflammatory resolution, activated macrophages from various mouse backgrounds have been divided into two major subgroups from in vitro phenotypes. First are classically activated macrophages (M1) associated with inflammatory responses, which can be induced by stimulating resting macrophages with lipopolysaccharide (LPS) and IFNγ. Second, alternatively activated macrophages (M2) are associated with tissue remodeling, resolution of inflammation, and anti-inflammatory responses, and are generated in vitro using IL-4 and/or IL-13 (165). While an M1/M2 classification is useful for mapping metabolic pathways of differentially activated macrophages, this does not necessarily reflect the heterogeneity of macrophages present in vivo. Macrophages in vivo responding to specific external stimuli result in unique subsets that fall between the two extremes of M1 and M2 on a continuum of phenotypes observed in humans (166–168). Metabolic intermediates may have some role as effector molecules like nitric oxide (NO), reactive oxygen species (ROS), and tricarboxylic acid (TCA) derivatives have been shown to regulate macrophage phenotypes and functions by modulating signaling pathways, leading to the production of cytokines, anti-microbial peptides, or tissue repair factors (169).
Role in Mtb infections. Macrophages and specifically AM serve as both the first line of defense against Mtb as well as a major intracellular niche for long-term survival in the host (Figures 1, 2). This dual role of macrophages in vivo appears to be driven by the cellular activation state. Although a nonselective depletion of macrophages after Mtb challenge in mice improved the outcome of disease, a specific depletion of activated macrophages led to impaired resistance, as reflected by enhanced mycobacterial outgrowth (170). Interestingly, alveolar epithelial type II cells (AECII) have a similar dichotomy in Mtb clearance or protection depending on the progression of infection. While the prevailing dogma is that Mtb is phagocytosed by AMs and dendritic cells once entering the alveolar sac, AECs have also been found to uptake Mtb despite not being professional antigen-presenting cells (APCs). Infected AECs may provide some early innate signaling and cross-talk with innate immune cells, including neutrophils and AMs, and those hypotheses are well discussed here (171). While we focus on the role of AMs in the sections below, it is of note that there is an active and well-warranted study of AECs, and their roles during Mtb infection or control may well be intertwined.
Macrophages are stimulated by PRRs, NLRs, and TLRs to endocytose/phagocytose Mtb and sequester the pathogen in intracellular phagosomes (160, 161, 163, 172). A proinflammatory status activates the intrinsic capacity of macrophages to generate reactive oxygen intermediates (ROI), phagosome maturation, and consequent microbicidal activity (173, 174). Once activated, macrophages in the airway and lamina propria produce a range of pro-inflammatory cytokines and chemokines in response to and in defense against Mtb (160, 161, 175). Within this defense, there is a constant battle of metals going on inside the phagosome of the macrophage between the host cell and Mtb (176). The macrophage delivers an overload of copper and zinc, which are toxic to Mtb deploys a series of protective mechanisms to capture metals, including oxidation and by promoting an increase in metal efflux (176). The upregulation of ctpC gene encoding for the P-type ATPase that regulates the intra-bacterial levels of zinc is another example of how Mtb manages to prevent heavy metal poisoning, observed in an ex vivo human macrophage model of challenge (177). As a countermeasure, the macrophage then attempts to block the arrival of nutrients to Mtb, including iron and manganese (176).
A central role of macrophages is the production of TNF and its importance to immunity against Mtb. One of the key pieces of evidence that TNF plays a role in this function comes from the neutralization of TNF in cynomolgus macaques, where TNF neutralization leads to disseminated disease within 8 weeks of infection following adalimumab, also known as Humera® (178). Furthermore, TNF neutralization during latency using a soluble TNF inhibitor, p55-TNFR1, in latently infected nonhuman primates (NHPs) leads to increased lung pathology, bacterial burden, and reactivation (178). Whereas in mice the neutralization of TNF appears to affect the organization of the granuloma leading to lack of control of Mtb (179), the mechanism appears different in NHP, where the granuloma structure does not appear to be impacted (178); however, more neutrophils appear to be present in the granulomas of these NHPs. Immune suppression via TNF inhibitors seems to universally increase susceptibility to TB disease across humans and preclinical models. Indeed, in humans, it is well known that the use of TNFa inhibitors are associated with an increased risk of TB disease; therefore, careful screening is applied to individuals requiring treatment for immune-mediated inflammatory diseases needing this treatment course (180).
While IFNγ has been observed to increase survival of mouse bone marrow-derived macrophages by inhibiting bacterial replication (181), it has also been shown to struggle against the ESX system, a sophisticated Mtb secretion system also involved in reducing phagosome maturation (182). Interestingly, host Vitamin D allows the macrophage to increase phagosome maturation (183). In human macrophages, activated Vitamin D3 induces autophagy, as well as direct Mtb killing via cathelicidin activation, an antimicrobial peptide that activates the transcription of autophagy-related genes (184). Indeed, previous clinical studies have indicated that Vitamin D deficiency or receptor polymorphisms are associated with an increased risk of Mtb (185–188), and a meta-analysis in 2008 found that low serum Vitamin D levels are associated with a higher risk of active TB (189). A more recent study showed that a serum Vitamin D concentration ≤ 25 nmol/L was significantly associated with an increased risk of active TB, while the range 51–75 nmol/L was not associated with an increased risk of TB (190), suggesting that a threshold of Vitamin D can help promote host anti-Mtb activity.
A strong innate host defense tool against Mtb is bystander macrophages that use efferocytosis for infected cells, which is an efficient means of killing the intracellular bacilli and disposal of debris (191, 192). Apoptosis of Mtb-containing macrophages may result from endoplasmic reticulum (ER) stress and subsequent accumulation of misfolded proteins. ER stress may also induce apoptosis via macrophage signaling factors such as inositol-requiring-1α (IRE-1α), double-stranded RNA-dependent protein kinase (PKR)-like ER kinase (PERK), and activating transcription factor-6 (ATF-6) (192). A clinical trial with 185 TB patients showed that clinical Mtb isolates induce autophagosome formation in ex vivo macrophages to a variable degree, as measured by LC3II marker protein. Severity of active TB disease in this cohort was inversely related to levels of LC3II production. Collectively, these data indicate a significant protection–mitigation from TB in humans, owing to a macrophage autophagy response (193).
Alternative receptor engagement and immune evasion can lead to different outcomes post macrophage challenge with Mtb. For example, mannose receptor (MR) recognizes mannose present on Mtb, which does not induce bactericidal ROS or phagosome maturation but instead produces anti-inflammatory cytokine signals that help set up a more permissive cell state for Mtb to survive in this intracellular immune niche (172, 174). Mtb can also subvert bactericidal activity in macrophages by escaping the phagosome and persisting in the cytosol of the cell, and specific Mtb antigens can limit or induce macrophage apoptosis and autophagy (174, 182, 194–196). Furthermore, the relatively low dose of challenge with Mtb in normal transmission settings means AM are the first cells infected by Mtb for several days and, in the absence of other inflammatory signals, can be preferentially skewed to promote survival and dissemination, as observed in a mouse aerosol challenge model (197). The dissemination of infected AM from the airway into lung tissues is driven largely by the ESX-1 secretion system in Mtb (197). Work done by Rothchild and colleagues helped demonstrate that AM have an inherent delay in proinflammatory gene transcription post in vivo challenge in mice and they instead induce an antioxidant transcriptional program more conducive to Mtb survival (198). Similarly tissue resident macrophages M1 and M2 differentially allow mycobacterial growth, where M2 cells allow more robust Mtb survival than M1 cells, and this seems linked to metabolic profiles in the host (199). Interestingly, Mtb uses cholesterol and fatty acids from the host; therefore, host cells in divergent metabolic states are differentially able to control or support Mtb fitness (199). So, while events following the initial infection of specialized macrophages with Mtb are worth noting, in the absence of activation, metabolic reprogramming, or other proinflammatory signals, these cells become the main permissive hosts for Mtb.
Vaccine induced. BCG vaccination has been shown to result in an increase in inflammatory mediators produced by monocytes from healthy volunteers, correlating with histone modification and specific gene activation (138). Indeed, healthy volunteers vaccinated with BCG were observed to have hyper Mtb responsive circulating monocytes 2 and 3 weeks post immunization. This immune potentiation was monitored by secretion of pro-inflammatory cytokines, upregulation of PRRs, and distinct myeloid markers such as CD14, CD11b, and TLR4. Immunized participant PBMCs, when stimulated ex vivo with sonicated Mtb lysate, induced a sevenfold increase in IFNγ secretion when compared with basal levels from donors before their BCG vaccination. Furthermore, monocyte secretion of TNF-α and IL-1β was augmented 2-fold. Such trained immune effects associated with histone epigenetic reprogramming depended on the activation of NOD2 receptor, increasing methylation of histone 2 at lysine 4 (H3K4m3) through methyltransferase (138). A follow-up study determined that BCG-trained immunity can be long-lived and effective over 1 year in healthy volunteers’ post-vaccination. Trained monocytes upregulated their PRRs and other innate activation markers, such as myeloid CD14+ TH cells, complement receptor 3 integrin-CD11b+, TLR4, and C-type-1 lectin mannose receptor. These data indicate that non-specific TH cells as well as BCG primed monocytes may collaborate in host protection against Mtb, which help inform and influence vaccine candidate design (200).
To enhance the efficacy, recombinant BCG (rBCG) vaccines (201, 202) are being thoroughly explored and developed. VPM1002, for example, is a rBCG expressing listeriolysin (LLO, encoded by the hly gene used by Listeria monocytogenes as a virulence factor for cell to cell spread), which is a clinical stage candidate (28, 203) (Table 1). LLO combined with a urease c gene (urec) allows for escape of BCG antigens into the cytosol through perforation of the phagosomal membrane in an optimal pH of 5.5 (urease), promoting MHCI binding of antigens as well as apoptosis induction (203). Another rBCG evaluated in a phase 1 clinical trial was rBCG30 (204). In this case, the strategy was to overexpress Ag85b to increase host immunity to an immunodominant TB antigen. In the Phase 1 clinical trial, both Ag85b-specific CD4+ and CD8+ Th1 immunity in addition to memory T cells were induced and the vaccine was determined to be safe in humans (204). Since this clinical trial, however, rBCG30 has not progressed further.
While BCG is incredibly safe, safer vaccines are needed for those with underlying diseases such as human immunodeficiency virus (HIV), and any acquired or inherited immunodeficiencies where attenuated vaccines can cause unchecked disseminated infection (205, 206). Subunit vaccines, for example, are safe, induce strong antigen-specific immune responses, and have shown promise against TB disease in humans. Protein antigens, which are, on average, weak immune stimulants, are partnered with immune driving adjuvants that classically target PRR signaling pathways that are abundant on macrophages (207, 208). Several subunit vaccines are currently in the clinical pipeline (19, 209), and the recent success of candidate M72+AS01E has provided confidence that an adjuvanted subunit vaccine is a feasible approach for prevention of TB disease (13, 14). The AS01E adjuvant includes bacterially derived monophosphoryl lipid A (MPL) from Salmonella minnesota, in addition to saponin QS-21 (210). Vaccinating mice with AS01 directs monocytes to help dendritic cells as well as activation of adaptive immune players like CD8+ T cells or NK cells (57). Another promising adjuvant contains a synthetic version of MPL, glucopyranosyl lipid adjuvant (GLA), that, when combined with a stable emulsion (SE) and ID93 antigen, constitutes a candidate with documented efficacy in several animal models including mice, guinea pigs, and NHPs, and is in late Phase 2 clinical trials (40–49, 211, 212). The mechanism of action of GLA-SE has been previously described, where its drive of innate immune responses via TLR4 promotes the generation of antigen-specific TH1 CD4+ T cells in mice (50, 51, 213, 214). Another candidate subunit vaccine in the TB vaccine clinical pipeline is H56:IC31 (215). IC31 is a two-component adjuvant that contains a KLK antimicrobial synthetic cationic peptide vehicle along with TLR9 agonist phosphodiester-backboned oligodeoxynucleotide (ODN1a), which induces type 1 IFN (69). Unlike the MPL-based adjuvants discussed above, in mouse models of vaccination, IC31 has been shown to induce both TH1 and TH17 polarizing T-cell response (216–218). While these subunit vaccine adjuvants (AS01E, GLA-SE, and IC31) drive some innate responses in macrophages, their main influence is on dendritic cells (51, 69, 218), which will be discussed at length below (Table 1). In addition to adjuvant strategies designed to drive innate immune responses, Mtb antigen selection in vaccine candidates can also be used to combat Mtb immune evasion tools specifically at the macrophage. For example, ESAT-6 is a well-studied candidate antigen included in subunit vaccines, and is encoded by esxA in genetic locus region of difference 1 (RD1) (219). ESAT-6 has been shown to inhibit autophagic flux by impeding autophagosome–lysosome fusion in ex vivo cell culture, which assists in Mtb immune escape from macrophages (220–222). Preclinical studies in Balb/c mice show that vaccination with ESAT-6 and c-di-AMP regulate macrophage autophagy, resulting in the inhibition of Mtb growth in macrophages during early infection (223).
Beyond vaccination regimens, therapeutic-based strategies are being developed to target macrophages and overcome metabolic barriers to Mtb killing in these cells (224, 225). There is growing interest in the induction of autophagy in Mtb-infected host cells using autophagy-inducing compounds (AICs). Nanoparticles (NPs) can be utilized as a delivery system to improve the activity of AICs against intracellular Mtb by transporting the encapsulated AICs to their target sites while protecting them from biodegradation and enhancing their absorption across biological barriers (226, 227), which has been demonstrated nicely for delivery of rifampicin to macrophages in vitro (227). NPs sustain drug release in the target tissues, allowing for the reduction of dosing frequency and lessening drug-associated side effects in the process (228). In addition, the materials used to synthesize the NPs can possess autophagy-inducing activity or the surface of the NPs can be integrated with an AIC (229). Upadhyay et al. reported the capacity of yeast-derived glucan particles (GP) loaded with high payload of rifabutin (RB) NPs [(RB-NPs)-GP] to induce anti-mycobacterial and cellular activation responses in Mtb-infected J774 macrophage cells. The exposure to (RB-NPs)-GP triggered strong innate immune responses in Mtb-infected macrophages including the induction of apoptosis, autophagy, as well as ROS and NOS. Macrophage targeting particles containing rapamycin are also in development to induce Mtb killing in infected cells via mTOR inhibition to drive autophagy, and have shown promise as spray dried formulations delivered to mice (225, 230). Careful examination of bacterial evasion and host regulation can lead to more promising candidates for targeted therapies. For example, the absence of Alox5 leads to increased CD8+ and CD4+ T-cell responses in mice by cross-presentation and MHCII antigen presentation pathways (231), which may be carefully exploited. However, additional investigations in vivo as well as studies to target and deliver known AICs are required to advance these therapies (232).
Dendritic Cells
Effector functions. Dendritic cells (DCs) are considered APCs and immature or less differentiated DCs are present in many tissues. Like macrophages, DCs use PRRs to survey for potential antigenic material and leverage phagocytosis as well as macropinocytosis, receptor-mediated endocytosis, or direct contact with damaged or infected cells (233–235). In contrast to other phagocytes, once DCs receive activation signals resulting from pathogenic interaction or inflammatory stimuli, they mature and migrate to secondary lymphoid organs for antigen presentation (236) (Figure 2). Antigens can be prepared by lysosomal proteases within the endocytic pathway or proteosomes for MCH I or II presentation, allowing for both naïve CD4+ and CD8+ T-cell priming (236). Additionally, endocytosed proteins can be cross-presented via MHCI (235, 237). Activated DCs serve as a bridge between innate immune surveillance and adaptive immune activation.
In both humans and preclinical mouse models, DCs are observed to be a heterogeneous population containing subsets with unique surface markers and specialized functions (238). There are two overarching subsets to which human and mouse DCs are traditionally characterized: conventional DCs (cDCs) and non-conventional DCs (239). While certain markers and subsets of human and mouse DCs may differ, their general functions are similar. Conventional DCs, derived from conventional DC progenitors, are characterized by their APC functionality and can be found within lymphoid tissues and barrier tissues (lung, skin, intestinal tract, kidney, and liver) (240, 241). Further development of cDCs is directed by transcription factors differentiating the cDC1 subset, which cross present to naïve CD8+ T cells, or cDC2 subset, with focused presentation to naïve CD4+ T cells (240–245). Plasmacytoid cells (pDCs), derived from common DC progenitors, largely encompass the non-conventional DC subset. pDCs primarily circulate in the blood and secondary lymphoid organs, but also localize to skin, intestine, and epithelial tissue during inflammation (246). pDCs are characterized by large production of type I IFNs during viral infection, and when activated, pDCs secrete TNF-α, IL-1, and IL-6 (246).
Role in Mtb infections. DCs are critical for initiating anti-Mtb responses as primary APCs that present antigens and induce adaptive immunity. From the bone marrow, DC progenitors enter the bloodstream and home to different epithelial tissues, including airway epithelium and lung parenchyma, the primary site of Mtb challenge. Like macrophages, human DCs are observed to use a series of PRRs to bind and phagocytose Mtb in ex vivo co-culture conditions; this includes complement receptors, MR, TLRs, and DC-SIGN (247–249). Mtb uptake by DCs leads to DC maturation and upregulation of MHCI, MHCII, CD40, CD54, CD58, and CD80 (248). After reaching draining-secondary lymphoid organs, DCs present antigens to prime naïve T cells activating Mtb-specific responses and effector differentiation (Figure 2). While this is the common response to infection, studies have shown that DC activity may vary depending on Mtb strain. Transcriptional analysis of human monocyte-derived DCs (moDCs) infected with different Mtb strains found that infection with Mtb H37Rv led to significant upregulation of EBI3 and, compared to other mycobacteria, induced the least level of IL-10 expression (250). In this same ex vivo moDC challenge model, BCG-Japan induced CCR7 and TNF-α expression and showed considerably lower intracellular growth, suggesting that DC responses reflect challenge strain virulence. Corroborating these findings, a study by Ramos-Martinez et al. reported that human moDCs infected with a hypervirulent lineage 3 Mtb strain neutralized intracellular growth of the bacilli, underwent decreased apoptosis, and led to poor T-cell expansion compared to an Mtb H37Rv reference strain (250). In contrast, human moDCs infected with hypovirulent lineage 4 strain displayed high apoptosis and consequently this precluded their capacity to expand T cells. The relationship between host cell responses and immune evasion strategies by virulent mycobacteria is still an active area of research. However, as DCs help drive adaptive immunity, they are a logical target for specific vaccine strategies, which are discussed below.
Vaccine induced. Robust T-cell responses following vaccination is vital for successful durable immunity against Mtb. Delayed or low T-cell activity in the mouse model has been observed and suggested as a bottleneck for protection following vaccination (251). In order to overcome this delay, researchers evaluated the adoptive transfer of stimulated DCs intratracheally into BCG vaccinated mice and observed that this group had a ~1.5 CFU log reduction compared to BCG vaccinated only controls (252). Transfer of activated DCs into non-vaccinated mice saw a reduction in lung CFU comparable to the vaccinated controls, demonstrating the profound influence of DCs in the correct location on maturing a protective immune response. While mechanistically informative, adoptive transfer of activated DCs is not a robust strategy for global TB protection, but certain vaccine approaches instead preferentially target DC activation. For example, one strategy is the selection of DC-activating proteins into a recombinant vaccine (253). The mycobacterial heat-shock protein Rv2299c (heat-shock protein 90 family) in mice leads to DC maturation via the TLR4 pathway, with TH1 leaning towards cytokine production and enhanced expression of MHCI and MHCII. Administration of Rv2299c fused with the T-cell-stimulating antigen ESAT-6 following BCG immunization in mice showed significant reduction in lung bacterial burden and inflammation compared to BCG control 16 weeks post challenge with Mtb HN878. This study helps to underscore the importance and promise of DC activity particularly in multiantigen vaccine designs. Additional studies have investigated other novel antigens that target DC maturation and enhance TH1 responses, including a DosR regulon-encoded protein (Rv2005c) fused with macrophage-activating protein, Rv2882c, and Rv1876 (254, 255). Common to these mouse immunological studies is enhanced DC maturation, increased expression of IFNγ, IL-2, and nitric oxide, and decreased bacterial burden. Given the importance of DCs for priming T cells and in turn robust T-cell activity for protection, new vaccine approaches have wisely targeted DCs via antigen selection and other vaccine components to boost downstream responses.
DCs are also targeted through use of immunogenic adjuvants. For example, clinical stage saponin-based adjuvant, AS01, composed of QS21 and TLR4 agonist MPL, leads to release of alarmins and subsequent DC activation in mice (57). This study of acute responses in mice after immunizations found that the addition of AS01 to antigen led to an 8.6-fold increase of MHCII+ DCs within the draining lymph node compared to antigen alone, as well as enhanced T-cell priming, making DCs a crucial target of the AS01-induced immune response (57). AS01E is being utilized in human vaccines including the shingles recombinant zoster vaccine (Shingrix), the RTS,S malaria vaccine, as well as the M72 Mtb vaccine candidate. In a study that analyzed the RNA expression in PBMCs and whole blood from participants who had received the M72/AS01E vaccine, researchers found that these samples were enriched in activated dendritic cells compared to baseline measurements up to 31 days post-injection (256). Despite the multiple safety and immunogenicity studies completed for the M72/ASO1E candidate, there are little data phenotyping the vaccine-induced DC response in humans. Following the M72/AS01E vaccine candidate was the release of a similar protein-adjuvant strategy leveraging vaccine candidate ID93+GLA-SE. Early studies on GLA showed its ability to stimulate both mouse and human DCs leading to high production of TNF, IL-6, and IL-12p40 (51). Administration of GLA-SE through intradermal injection of human skin in an explant model showed that DC enhanced CD4+ T-cell proliferation (257). Indeed, three major subunit vaccine adjuvants (AS01E, GLA-SE, and IC31) rely on DC activation for optimal protective responses in candidate vaccines as they are the most efficient APCs for adaptive immune responses (51, 69, 218).
As for DC responses to BCG, which is currently the only licensed TB vaccine, an in vitro study found that BCG-infected human DCs produced TNF-α, IL-1β, IL-6, and IL-10 (258). However, when BCG-DCs were co-cultured with human cord-blood mononuclear cells, they produced anti-inflammatory cytokine IL-4, which may partially contribute to low protection garnered by BCG. In the elderly, a study surveying whole blood and plasma samples 1 month post BCG immunization observed enhanced plasmacytoid and myeloid DCs (259). Despite this, plasma levels of type I IFNs (IFNα/β) were significantly decreased, while type III IFNs (l), which comprise anti-viral cytokines (also named IL-28A, IL28B, and IL-29), were significantly enhanced, potentially showcasing the off-target effects of BCG (259). Next-generation BCG vaccine candidates may also work to improve and direct BCG-induced inflammatory DC activation as a proposal for enhanced protection.
Natural Killer/NKT Cells
Effector functions. Natural killer (NK) cells are lymphocytes within the innate immune system harboring potent cytotoxic effector functions. NK cells help control diseased states by identifying and attacking infected or malignant cells directly through cellular toxicity and indirectly via cytokine signaling (260). NK cell activation is regulated by activating inhibitory cell surface receptors (261, 262). MHCI expressed by healthy nucleated cells bind NK inhibitory receptors [killer cell Ig-like receptors (KIRs), leukocyte Ig-like receptor (LIR), and CD94/NKG2 receptors] and prevent cytotoxic activity (262). Conversely, infected cells downregulate surface MHCI and upregulate co-stimulatory ligands to engage NK cell-activating receptors. The cytokine milieu induced downstream by other activated innate cell subsets can also direct and help influence NK cell activity. In vitro studies with mouse and human-derived cells have shown enhanced NK cell activation from DC-produced IL-12, IL-18, IL-15, and IFN-α/β (263, 264).
Once activated, NK cells have multiple mechanisms of cytotoxicity that are largely shared with CD8+ T cells, including release of granzymes, perforin, and utilization of death receptors (265, 266). Perforin, a glycoprotein, binds the cell membrane of the target cell and forms pores (267). The pores can disrupt the cell membrane and subsequently destabilize mineral homeostasis, which can indirectly induce apoptosis. Additionally, perforin can aid in other targeted cytotoxic effects, including the entry of granzyme through perforin-formed pores (268, 269). Granzymes, a family of serine proteases, cleave proteins such as procaspases, which initiate intrinsic apoptotic signaling cascades and induce target cell death (270). NK cells can also induce extrinsic apoptotic signaling via two primary TNF receptors, Fas (CD95) and TNF-related apoptosis-inducing ligand (TRAIL) (271). Binding of Fas, widely expressed on tissues, and Fas ligand, expressed by activated NK cells and cytotoxic T lymphocytes, results in nuclear condensation, membrane blebbing, caspase activation, and eventual cell death (272). Similarly, cross-linking of TRAIL, and TRAIL-R1 and -R2, leads to caspase activation and apoptosis (273–275).
In addition to cytotoxic effects on infected cell targets, NK cells can regulate immune responses via T-cell activity, indirectly via IFNγ signaling or directly via cytotoxic-mediated killing (276). In vitro and in vivo studies have found that IFNγ produced by NK cells promote DC production of IL-12, leading to downstream enhanced CD8+ T-cell activity (277, 278). In addition, NK cells can regulate T-cell activity indirectly via IFNγ signaling or directly via cytotoxic-mediated killing (276). In vitro studies of human cells and in vivo mouse models have found that IFNγ produced by NK cells promote DC production of IL-12, leading to downstream enhanced CD8+ T-cell activity (277, 278). IFNγ signaling also is known to promote CD4+T cell TH1 differentiation (276, 279–281). Beyond downstream cytokine activity, both in vivo and in vitro studies have shown that NK cells can target T cells via secretion of cytolytic granules or death receptors (282–284).
Role in Mtb infection. As discussed above, NK cells have multiple roles in driving immune responses, illustrating their importance in combatting infection. While NK cells have been known to play a large part in immune responses to viral infections, there has been growing interest in the role NK cells play during Mtb infection (Figure 1). In vitro studies have found that upregulation of stress-induced UL-16 binding protein by Mtb-infected human monocytes leads to recognition by NKG2D-activating receptor followed by NK cell-mediated lysis (285, 286). Interestingly, while granzyme A-producing NK cells are observed after challenge with Mtb in an in vivo mouse challenge model, it did not correlate with protection from bacterial burden (287). These data together may highlight the differences between models, or suggest many compensating pathways derived from NK cells that contribute to Mtb control. For example, NK cells have been observed to restrict intracellular Mtb growth in infected human monocytes through direct contact and partially mediated through secretion of soluble factors such as IL-22 and IFNγ (288–290). In vivo studies in C57BL/6 mice infected with Mtb showed that NK cell depletion had no significant effect on bacterial burden (291). However, T-cell-deficient Rag mice with further NK cell depletion demonstrated enhanced bacterial burden post challenge, which may highlight the importance of NK cells in immunocompromised environments and possible overlap of NK and cytotoxic T-cell functions that make parsing out direct contributions difficult. NK cells are also found in later stages of Mtb infection and have been identified to infiltrate granuloma lesions in Mtb-infected patients (292). The direct influence of NK cells, like neutrophils, may depend on their location and timing with respect to challenge with Mtb.
Adjacent to NK cells are NK T cells, which co-express the T-cell receptor (TCR) and NK cell surface receptors (293, 294). This co-expression confers both adaptive and innate immune activity (294). Invariant NKT cells (iNKT) are the primary subset of NK T cells identified. iNKT cells recognize self and foreign lipids presented by the glycoprotein CD1d (295). As summarized by Ruibal et al., there has been growing interest in iNKT cells’ role in controlling Mtb infection (296), largely due to the observation that iNKT cells have increased activated phenotypes in TB patients compared to LTBI and healthy controls (297). Additionally, the number of NKT cells measured in patients during TB diagnosis correlated with faster responses to antibiotic treatment (298). Given these dichotomies of NK/NKT cell activation and numbers in different patient populations and outcomes, they may serve as an important predictive correlate for TB protection and should be examined closely in vaccine candidate evaluations.
Vaccine induced. NK cell’s ability to target infected cells and regulate T-cell activity has enhanced interest in vaccine-induced NK cell responses. An in vivo study found that C57BL/6 mice immunized with BCG and subsequently challenged with Mtb H37Rv showed increased IFNγ- and IL-22-producing NK cells compared to controls (299). To further determine if these cells played a role in protection afforded by BCG, researchers treated mice with 0.3 mg of anti-NK1.1 for NK cell depletion during BCG vaccination. This NK depletion led to an increase of immunosuppressive T-regulatory cells, increase in bacterial burden, and reduced T-cell activity after Mtb H37Rv challenge. However, supplementing with IL-22 restored some BCG-induced protection, exhibiting the importance of the IL-22–NK cell axis in protection (299). Unfortunately, NK cells have been an underappreciated subset of cells that should be evaluated in POI and POD vaccine candidate screens.
B Cells
Effector functions. B cells are an immune target of many vaccines, and their main effectors, antibodies, are crucial particularly for viral and extracellular bacterial infections. B cells are, however, also important for protection against intracellular bacteria including Mtb as reviewed in depth by Chan et al. (300). T-cell regulation, for example, can be geared towards regulation or different helper T-cell phenotypes through cytokines produced by B cells. Like T cells, B cells are also classified into different subsets: B effector 1 (Be1) producing IFNγ, IL-12, TNF, IL-10, and IL6; B effector 2 (Be2) producing IL-2, lymphotoxin, IL-4, IL-13, IL-10, and IL-6, as reviewed previously (300).
The effector functions of B cells during tuberculosis infection are several-fold as discussed in a recent review by Rijnink et al. (301). These properties include the production of antibodies, antigen presentation, as well as cytokine production. Antibodies, or immunoglobulins (Ig), can be cell bound or secreted, make up five classes or isotypes (IgG, IgA, IgE, IgM, and IgD), and have numerous functional capabilities due to the interaction with other immune cells via constant fragment receptors (FcR) and components that facilitate the host response to infection. Since these B-cell and antibody functions have been extensively reviewed in the context of tuberculosis by others in the field (301–303), this section is only meant to serve as a broad overview and highlight specific findings.
B cells, which constitutively express major histocompatibility complex class II (MHCII), are in the “professional” APC category along with DCs, monocytes, and macrophages (304). As eloquently described in the review by Ghosh et al., once a foreign antigen is recognized by the B-cell receptor (BCR), this leads to internalization and ubiquitination steps that transport the Ag-BCR clusters to MHCII loading compartments within the cell in either endosomes or lysosomes. The MHCII molecule assembles in the endoplasmic reticulum along with trimers of invariant chain (Ii; CD74), which, when Ii is processed a place-holder peptide (class II invariant chain peptide, or CLIP), binds into the MHCII groove. Following a number of processes outside the scope of this review, the antigen undergoes proteolytic processing followed by a loading of higher-affinity peptide from the pathogen (or other immunogen) into peptide/MHCII complexes, displacing CLIP. These complexes are exported to the surface of the cells and presented to CD4+ T cells (304). B cells present antigen to T follicular helper cells (Tfh), and subsequent differentiation of Tfh is dependent on B-cell cytokine production following stimulation from pathogens. Antigen presentation on B cells can also impact T-cell memory responses. Our group has shown that B-cell-deficient mice fail to generate vaccine (ID93+GLA-SE)-induced MPEC (memory “precursor” effector CD4+ T cells) in mice, and this deficiency reduces memory T-cell recall responses (305). This is in alignment with other studies that have shown evidence for decreased T-cell responses or protective immunity against intracellular pathogens in animal models with B-cell deficiencies (306–310).
Role in Mtb infections. There are several animal models, particularly mouse models, and experimental studies that have helped elucidate the effects of immune cells on the outcome of Mtb infection. The use of B-cell-deficient mice for understanding the role of B cells and antibodies has been reported by several investigators, utilizing several different Mtb strains, doses, and routes of infection, all leading to different conclusions regarding the importance of B cells and/or antibody responses on early, chronic, or disseminated TB [reviewed here (301)]. As increased age has been associated with recrudescence of TB, Turner et al. investigated the progression of TB disease in the aerosol model using Mtb Erdman challenge in B-cell-disrupted C57BL/6j-IgH-6 mice, IL-4 gene-disrupted mice, or in wild-type mice (311). An aging immune response results in increasing numbers of B cells and Th2-biased responses. In this study, bacterial burden was similar in the lungs of the mice, regardless of the defect. In an acute Mtb mouse study done in B-cell-deficient mice, infection with Mtb CDC1551 resulted in a delay in bacterial dissemination to the spleen and liver, and decreased lung pathology (312). In contrast, in another study performed in B-cell-deficient mMT mice infected with Mtb Erdman, researchers observed increased recruitment of neutrophils and worsened immunopathology, and increased IL-10 within the lung, suggesting that B cells contribute to the modulation of inflammation and enhancement of immunity against infection (313). With an increased infectious dose of Mtb Erdman (300 CFU), B-cell-deficient mice were slightly more susceptible to infection (40% mortality compared to 0% mortality in the wild-type mice), demonstrated increased pathology within the lung, had increased neutrophil numbers in the lung, and enhanced IL-10 levels as well in the lungs. Vordermeier et al. also demonstrated that, in µMT B-cell-deficient mice given high-dose (106/mouse in 0.1 ml) i.v. injection of Mtb H37Rv, B cells play a role in containing infection in the lung, spleen, and liver (313), although higher mortality was not shown in the B-cell-deficient mice over 18 weeks following infection (data not shown, but communicated in the manuscript). In addition, vaccination of the B-cell-deficient mice with BCG 4 weeks prior to challenge with Mtb provided protection, suggesting that T-cell responses were not impaired over that short period of time.
Vaccine induced. The design of preclinical vaccines against µtb has typically relied on ways to generate a potent TH1 cellular response. The correlates of vaccine efficacy against tuberculosis are unclear, however, and attempts to determine these correlates could aid in developing a vaccine that provides long-lived protection on its own or as a vaccine that suitably boosts BCG. While few dispute the benefits of generating a cellular response as a requirement for immunity against TB, B cells and antibodies have convincingly shown beneficial protective effects against TB. Recent research has shed additional light on the role of B cells against TB and may in fact warrant further investigation particularly during vaccine development (Figures 1, 2). A lot of attention has recently focused on the results of superior (nearly sterilizing) protection following i.v. BCG vaccination in rhesus macaques (16), where the model has suggested prioritizing interesting correlates of protection. The antibody responses induced from i.v. BCG immunized rhesus macaques were thoroughly investigated (17). In this study, significantly enhanced IgG1, IgA, and IgM titers were observed against lipoarabinomannan (LAM) in the lungs (bronchoalveolar lavage) 4 and 16 weeks after i.v. immunization, and increased anti-LAM IgG1 and IgA responses at 8 and 24 weeks, and higher anti-LAM responses 8 weeks following i.v. immunization in the plasma, all compared to i.d. administration. In addition, various antibody responses to HspX and the glycoproteins PstS1 and Apa were also elevated in the i.v. BCG-immunized NHPs compared to the standard i.d. route. Enhanced functional antibody responses in the BAL 4 weeks after immunization was also importantly demonstrated, including an increase in FcγR2A binding, NK cell degranulation, and intracellular antibody-dependent Mtb killing within macrophages with i.v. immunization in these studies (17). Lastly, plasma LAM-specific IgG1 (week 8) and LAM-specific IgM (week 24), in addition to HspX-specific IgM (week 4), were predictive of vaccine-induced protection (<1,000 CFU) using partial least-squares discriminant analysis. A comprehensive analysis of the role of anti-LAM antibodies is well reviewed here (314). While i.v. BCG is not being considered as a viable route of immunization, these findings are valuable indicators of potential humoral correlates of protection that can be tested for other preclinical candidates and clinically in historical or planned trials.
The route of immunization can also play a role in vaccine-specific antibody responses. Heat-killed MTBVAC (MTBVAC HK) delivered mucosally in mice (via the intranasal route) and in NHP (intrabronchial route) induces mucosal IgA, IgM, and IgG responses (315). When BCG-primed C57BL/6 mice were given intranasal immunization with MTBVAC HK, enhanced survival following high-dose Mtb H37Rv was observed compared to BCG (given s.c.) alone. One month following MTBVAC HK immunization, antigen-specific IL-17 in addition to IFNγ was induced in the lung and spleen. Our group has also shown that mucosal delivery with ID93+GLA-SE leads to antigen-specific IL-17 production and increased protection 4 weeks after the last immunization; however, both intramuscular and intranasal delivery led to increased protection in the lungs of immunized mice infected with low-dose Mtb H37Rv (52). Although antibody responses were not measured following mucosal delivery, i.m. immunization with ID93+GLA-SE routinely induces antigen-specific IgG2 and IgG1 responses, and induces TH1 CD4+ T cell cytokine responses (IFNγ, TNF, and IL-2) (40, 42).
A correlate of vaccine-induced protection against Mtb would enable down-selection of vaccines that enter into the Mtb vaccine clinical pipeline and would accelerate lengthy clinical trials, and antibody responses should not be overlooked in defining these correlates. A United Kingdom (U.K.) study conducted over 10 years showed that people with hypogammaglobulinemia have an increased risk of contracting Mtb (316). Several different functional roles of antigen-specific antibodies against Mtb have recently been described by the Alter lab in Lu et al (303). Fc-mediated effector responses are dictated by the interactions of the Fc domain of the antibody with the activating or inhibitory Fc receptors on innate cells such as NKs, monocytes, and neutrophils (303). PPD-specific responses from people latently infected with Mtb had higher PPD-specific NK cell enhancement, ADCC, and NK cell degranulation in comparison to those with active TB. Different antibody Fc effector profiles were also shown to correlate with different states of TB disease utilizing a systems serology approach. Antibodies may hold the key for biomarker indicators that correlate with TB disease, providing an efficacy screen for treatments, which, in turn, could help reduce the spread of Mtb.
Different TB-specific antibody subclasses and glycosylation patterns have been characterized, offering interesting perspectives on how a reduction in a humoral marker of TB disease (IgG4) can potentially track the success of drug treatment (317). Grace et al. have recently reported striking differences in the TB-specific antibodies in four human cohorts in different stages of TB: healthy, latent TB infection (LTBI), active TB (ATB), and treated active TB (txATB) (317). ATB subjects were enrolled within 1 week of treatment with isoniazid, rifampicin, ethambutol, and pyrazinamide for 2 months followed by 4 months of isoniazid and rifampicin. The treated cohort were patients that completed 6-month antibiotic treatment, and were culture negative at 2 and 6 months of therapy. LTBI patients were contacts of patients with ATB, with positive QuantiFERON-TB Gold results but no symptoms of TB. Antibody profiling included responses to several TB antigens including PPD, recombinant Ag85A/B (1:1 ratio), ESAT6/CFP10 (1:1 ratio), GroES, glcB, and HspX (317). Of note, enrichment of PPD-specific IgG4 was shown in the ATB cohort, and depleting this specific subclass led to increases in neutrophil and NK effector functions. In addition, this marker of TB disease (PPD-IgG4) was decreased in the txATB cohort. Besides the enrichment of PPD-specific IgG4 in ATB, higher levels of Ag85-specific IgG were observed in the ATB cohort. In the treated group, PPD-specific phagocytosis, and increased HspX-IgG and Ag85-IgM were seen in the txATB subjects. In the ATB group, expanded IgA titers to Ag85A/B whereas higher PPD-IgM and HspX-IgG1 were significantly higher in the txATB cohort, which may be indicative of effector functions used to control infection (317).
One vaccine candidate success story warranting further humoral endpoint evaluations is the M72+AS01E clinical trial, which has shown ~50% efficacy against the development of pulmonary TB disease (13, 14). For humoral immunity, only the geometric mean anti-M72 IgG antibody responses were assessed, where the participants were seropositive at 2 months (highest responses) and had detectable anti-M72 titers through 36 months (13). Given the IgM correlation described above in NHPs, it will be of interest to measure anti-M72 IgM responses in these cohorts.
Another success story is that of ID93+GLA-SE. This vaccine is currently in Phase 2 and has shown promise both for safety and immunogenicity (45). In the phase 1 trial, all ID93+GLA-SE recipients showed significantly greater ID93-specific IgG titers after one injection compared to the protein (ID93) alone, and after three vaccinations, these responses persisted until Day 238. There was a predominance of anti-ID93 IgG1 and IgG3 subtypes. Anti-ID93 IgM responses were also measured following immunization. Interestingly, stronger IgM responses were observed against ID93, with some anti-IgM response against Rv2608 and Rv1813, and low IgM responses to Rv3620 and Rv3619 components of ID93 in the ID93+GLA-SE-immunized cohorts. Antibody effector functions were also measured in ID93+GLA-SE-immunized cohorts, including antibody-dependent cellular cytotoxicity (ADCC), and ID93-specific antibody-mediated NK cell degranulation and activation determined by the enhanced IFNγ, MIP1β, and CD107a upregulation. In addition, antibody-mediated cellular phagocytosis (ADCP) of ID93-coated beads was increased from the sera of those vaccinated with ID93-GLA-SE compared to ID93 alone (sera was analyzed 28 days after the third immunization). Antibody functions in this study were correlated with multiple subclasses and isotypes rather than one. These responses also suggest that the adjuvant, GLA-SE, is able to augment antibody effector functions as these responses were not elicited in the ID93 protein alone group. To our knowledge, this was the first candidate TB vaccine that was analyzed for antibody effector functions. Specific antibody-mediated immunity is well reviewed here (301, 318) and provides ample rationale of why these endpoints should be expanded for clinical TB vaccine candidates.
CD8+ T Cells
Effector functions. T cells expressing CD8 are largely known as killer T cells with effector functions related to T-cell receptor (TCR) engagement by antigen presented on MHC class I molecules, helping to fight intracellular pathogens. CD8+ T cells have an arsenal of cytotoxic molecules, including Fas ligand (binding Fas [CD95] on target cells and inducing apoptosis), perforin (driving membrane holes in target cells), and granzymes (protease enzymes that induce target cell apoptosis and usually enter perforin-induced holes) (319). Perforin and granzyme are stored in lytic granules at the ready, while other membrane-associated receptors and cytokines are produced de novo upon receptor binding. Activated CD8+ T cells express proinflammatory cytokines, notably IFNγ and TNF family members (319), as well as paracrine and autocrine proliferation inducing IL-2, which combine to help activate local and distal responses.
After activation, human CD8+ T-cell expansion and contraction are regulated by distinct changes in metabolic pathways and cell death induction (320), namely, restimulation-induced cell death (RICD) (321) and cytokine withdrawal-induced cell death (CWID) (322). Those cells that survive these expansion and contraction phases make up the memory compartment, which is leveraged for faster subsequent pathogen encounters, the hallmark of adaptive immunity. Memory CD8+ T cells comprise a spectrum of subset lineages with variable longevity, localization, and reactivity, which are well reviewed here (323). Given their role in combating intracellular pathogens and ability to form T-cell memory, CD8+ T cells and their effector functions have been well studied in Mtb infection and vaccine-induced immunity (321).
Role in Mtb infections. Mtb antigen-specific CD8+ T cells have been isolated post challenge in preclinical models and can migrate to the lung post infection in both preclinical and clinical evaluations (324–326), induce IFNγ and lyse Mtb-infected macrophages in vitro (327–331). Depletion or disruption of either MHC class I or CD8+ T cells significantly enhances Mtb susceptibility in mouse models, and IFNγ derived from CD8+ T cells contributes to controlling bacteria in vivo (331–335). In mice, granzyme A (GZMA)-producing CD8+ T cells are observed after challenge with Mtb in vivo (287); however, GZMA deficient (GZMA-/-) mice are not more susceptible to Mtb infection or TB morbidity than wild-type mice. These data suggest that while GZMA may play a role in infection and immunity, there may be other pathways that compensate in its absence, and indeed candidate vaccine MTBVAC-induced protection was not reduced in GZMA-/- mice compared to wild-type mice (287). Importantly, CD8+ T cells generate pulmonary immune memory and can be activated post challenge, as demonstrated by a C57BL/6 mouse model of i.v. Mtb infection, drug treatment, and Mtb aerosol rechallenge (329). It may be that the contribution of CD8+ T cells in controlling Mtb has been underestimated because they are more involved in the latent phases than acute phases of infection and depletion studies may have lacked the dynamic resolution to study these kinetics (Figures 1, 2). In an exceptional murine study design from Pinxteren and colleagues, depletion of CD8+ T cells during the acute stages (days 1–21 post challenge) of infection did not result in higher bacterial burden, whereas depletion during a latent phase (11 weeks post drug treatment) increased pulmonary bacterial burden 10-fold (336).
In a screen of human cohorts with positive tuberculin skin tests (TST), T cells were stimulated with synthetic peptide pools (337) and 74 different Mtb proteins, 58 novel, were determined by IFNγ ELISPOT to have CD8+ immunodominant antigens (338). In addition to antigen recognition, ex vivo human Mtb-antigen specific CD8+ T-cell lines lyse Mtb-infected macrophages and inhibit intracellular persistence (327). Antigen specificity and localization may indeed be critical components of CD8+ T-cell vaccine designs as a cohort of TB-infected individuals from the Gambia showed reduced CD8+ T-cell activation and cytotoxicity by flow cytometry after stimulation with Mtb H37Rv compared to healthy BCG-vaccinated controls (326). While the overall percent lysis of target cells infected with Mtb H37Rv ex vivo was equal between cohorts, the TB-infected group did have a significantly higher specific cell lysis against target cells with recombinant vaccinia virus (rVV)-ESAT6 compared to healthy controls (326). Furthermore, the specific phenotype of CD8+ T cells has been observed to track with progressive disease where ex vivo stimulated CD8+ T cells from participants with active TB disease have increased TGFβ and IL-10 and decreased granzyme B expression that correlates with bacterial load in induced sputum samples (339).
CD4+ T cells are decidedly not the focus of this review; however it is critical to note their role in maintenance of other cells, including CD8+ T cells across a variety of infectious disease models (340). Interestingly, Lu and colleagues recently demonstrated that in a mouse model, CD4+ T cells provide help to CD8+ T cells, reducing exhaustion and improving control of Mtb in vivo (341). These studies suggest that the relative contribution of CD8+ T cells in CD4+ preclinical KO experiments may have underestimated their role. Furthermore, with the advancement of in situ analyses, single-cell evaluations, and TCR sequencing, we will further unravel the complexities of T-cell subsets and their role(s) in protection and disease. For example, recent single-cell analysis of TB pleural effusion in humans demonstrated that CD8+ T cells expressing GRZM K are enriched in pleural fluid and may contribute to this disease state (342). Balance of CD8+ T-cell phenotypes, localization, and abundance are likely important for driving immunity versus pathogenesis.
Vaccine induced. While RNA vaccine platforms (343) are in development for TB vaccine candidates, many vaccine strategies designed to induce robust anti-Mtb CD8+ T cells have been evaluated and are well reviewed here (344–346). These platforms include recombinant BCG encoding listeriolysin O (VPM1002), recombinant adenoviruses containing Ag85A (Ad5Ag85A) and Ad35 containing Ag85A, Ag85B, and TB10.4 (Aeras-402), chimpanzee adenoviral vectored vaccine (ChAdOx185A), recombinant vaccinia virus (rVV), modified vaccinia virus Ankara (MVA85A), vaccination with HSP65 DNA (DNAhsp65), and Cytomegalovirus vector approaches such as RhCMV/TB (CMV-6Ag) and MTBVAC (344, 345). Conversely, for protein-adjuvant strategies like vaccine candidate ID93+GLA-SE, which contains Mtb antigens confirmed to contain immunodominant CD8+ T-cell epitopes (338), preclinical and clinical trial data to date suggest that a paucity of this subset is driven by this platform delivered intramuscularly (45, 46). Some strategies, like that for VMP1002, a recombinant BCG vaccine, are designed to drive CD8+ T-cell responses by enhancing vector apoptosis and autophagy, resulting in greater cytosolic antigen availability for MHCI presentation (29), which are only weakly induced in human infants by parental BCG vaccines (347, 348).
Along with platform of vaccination, route of delivery (also discussed further below) may play a critical role in eliciting pulmonary CD8+ T cells. In a mouse model, intranasal (i.n.) vaccination with an adenovirus vaccine drives mucosal CD8+ Mtb-antigen specific T cells that have distinct effector memory phenotypes and are maintained separate from circulating populations (325), suggesting that CD8+ T cells can be elicited at mucosal sites via vaccination and may persist. In the remarkable paper showing that 6 of 10 NHPs were protected from infection with Mtb in the cohort receiving i.v. BCG, CD8+ T cells were enriched in the airways compared to i.d. vaccinated groups (16). Importantly, vaccine-induced CD8+ T cells contribute to reducing bacterial burden in preclinical models of TB (325, 331, 345, 349). Our group has shown that a protein prime/Ad5 vector boost induces both CD4+ and CD8+ T cells and induces long-lived immunity in a preclinical mouse model of TB (350). Other groups have similarly demonstrated that this heterologous “prime-boost” strategy helps drive CD8+ T-cell responses in vivo (350–355). With the advancement of novel and existing viral vectored and nucleic-acid-based vaccines, the focused targeting of Mtb-antigen-specific CD8+ T-cell responses is set to expand (344). We expect that the identification of high-priority immunodominant Mtb antigens containing CD8+ T-cell epitopes (337, 338, 356, 357) will further help drive candidate design for the heterologous immunity contributing to Mtb control.
γδ T Cells
Effector functions. CD3+ T cells that express a unique combination of Vγ and Vδ in their TCR are known as γδ T cells. They depend heavily on junctional diversity, and in humans, there is an interesting preference for Vγ9Vδ2 pairs (358). They are less abundant than other T-cell types, making up 2%–10% of total circulating T cells in healthy individuals (359), and seem to fill a niche in peripheral tissues including mucosal airways, gastrointestinal tract, and epithelium (360). This mucosal preference makes them an enticing cell type to study for TB vaccine strategies. γδ T cells interact with many other cell subsets including DCs, NKs, and CD8+ T cells (359). Their location along with the ability to produce proinflammatory cytokines, including IL-17 and IFNγ, make them well suited to help in tissue repair and early recognition and combat of pathogens (360).
Unlike conventional αβ CD3+ T cells that recognize peptide antigens via MHC presentation, γδ T-cell subsets recognize non-peptide-based phosphorylated antigens and metabolic intermediate molecules with co-stimulation but without antigen processing or MHC presentation (361, 362). This categorical difference may help explain why γδ T cells are able to quickly respond to stimuli. However, in human age stratification studies, γδ T cells are able to form resident memory and persist in tissues after pathogen clearance (363), making them an interesting adaptive subset with early innate characteristics (361).
Role in Mtb infections. In preclinical models, γδ T cells have been shown to traffic to the airways and express IFNγ and IL-17 early after Mtb challenge (364). Indeed, early induction of IL-17, in particular, seems to be dominantly produced by γδ T cells and not CD4+ T cells, promoting early control of Mtb and subsequent granuloma formation (360, 361, 365). IL-17 is known to be protective against Mtb, as mice with depleted IL-17 are more susceptible to Mtb (366) and humans with specific IL-17 allelic genotypes are at a decreased risk of TB disease (367). There is an interesting dichotomy between the reduction in IL-17 production and an observed increase in T-cell exhaustion, including PD-1 expression, in individuals with TB disease, suggesting that beyond its pro-inflammatory role, IL-17 may also help regulate T-cell exhaustion profiles during disease states (368). In several clinical studies, adult and adolescent patients with active TB have been shown to harbor robustly higher cytolytic and IL-17-producing γδ T cells compared with healthy controls (369–371) (Figure 2), and this expansion decreases with TB drug treatment (362). Interestingly, some studies have reported a progression of TB disease severity to correspond with a decrease in circulating γδ T cells (372). Connecting these trends is difficult as TCR sequencing has revealed that distinct clones populate the periphery and lung (371), suggesting that there may be dedicated roles for local and disseminated control. Importantly, human Vγ9Vδ2 T cells are able to kill intracellular and extracellular Mtb in vitro via granulysin and perforin (373) and therefore may play both a direct and an indirect role in pathogen control.
Vaccine induced. Readouts for functional γδ T cells have largely been left out of many TB vaccine candidate assessments. Interestingly, while γδ T cells can respond to BCG immunization, depletion of this subset did not reduce protection from BCG challenge in a mouse model (371, 374). However, like CD8+ T cells, this may be an underappreciation of their full contribution to TB control. For instance, Vγ9Vδ2 T cells have been shown to provide help to CD8+ and CD4+ T cells (375), and this help may be largely unrecognized in preclinical models at singular time points. Indeed, rhesus macaques that received a mucosal immunization of vaccine candidate L. monocytogenes (Lm ΔactA prfA*) expressing Vγ2Vδ2 T-cell antigen (E)-4-hydroxy-3-methyl-but-2-enyl pyrophosphate (HMBPP) had a more rapid TH1 CD4+ and CD8+ T-cell response (376). These nonhuman primates also demonstrated reduced dissemination of Mtb post challenge compared to cohorts not immunized with the HMBPP vaccine candidate (376).
Early in vitro studies suggested that human monocytes infected with live Mtb were superior at activating and expanding Vγ2Vδ2 T cells compared to heat-killed Mtb preparations or protein antigens (377). These data may help explain why the majority of vaccine studies that note γδ T-cell contributions are replicating platforms. For example, a non-statistically significant increase in IL-17-producing γδ T cells was observed in cohorts of rhesus macaques immunized intradermally with MTBVAC and BCG compared to saline controls (24). In mouse models, it has been shown that γδ T cells are critical for vaccinia virus-induced CD8+ T-cell responses (378), which may have further implications for nucleic acid- or viral-based TB vaccine platforms (Figure 1). As tools for studying γδ T cells expand, the research community will better understand and dissect their possible role(s) in protective immunity.
Review of Clinical Stage Vaccine Candidates and Strategies
Efficacious, durable, and globally available TB vaccines are on the horizon. The diverse representation of candidate platforms and antigen usage in the pipeline demonstrate how the research community is advancing despite the absence of robust continual funding needed. An update of completed and ongoing TB vaccine clinical trials is presented here (379). This is not a trivial endeavor as the Mtb genome encodes thousands of proteins, so protective antigen selection alone is a hurdle. For example, antigen selection and dose have been shown to directly influence T-cell differentiation and subsequent protection in therapeutic mouse models where too high antigen burden in vivo reduces vaccine efficacy and drives more terminal differentiation and less memory formation of T cells (380–382). When we examine clinical stage TB vaccine candidates (based on the Tuberculosis Vaccine Initiative Pipeline Tracker 2021) for preclinical or clinical evidence of the cell subsets reviewed above, it is evident that there is a paucity of surveillance for most innate subsets and a lack of uniformity for exploring adaptive responses (Table 1). Not surprisingly, most vaccine candidates that have evaluated a reported innate subset induction were subunit strategies that are affirming adjuvant properties. In Table 1, we included data generated from in vivo or ex vivo animal models or human clinical trial data that specifically identify subset-specific results. Some data that were excluded were T-cell ELISPOTs or proliferative assays, which did not specify a subset or phenotype assayed. Many of these subsets may fall into secondary endpoints for clinical studies but are not well followed up or reported if the findings are negative. Diversifying secondary immunogenicity endpoints and including early innate markers of vaccine-induced responses would only help the field continue to down-select those cell subsets or combinations that most significantly correlate with specific types of protection.
While Table 1 captures an overview of cell subsets identified to be vaccine-induced in preclinical or clinical studies, there are particularly important nuances not detailed including the following: response kinetics, magnitude, lung homing, or memory and effector phenotypes, which are sure to influence protection. For example, route of vaccine delivery, may specifically skew mucosal immunity. The compartmentalization of immune responses between peripheral and local mucosa is well reviewed here (383). Recent studies have shown that aerosol and mucosal delivery of TB vaccine candidates help drive some IL-17-dependent cellular immunity (384) and increase vaccine-induced protective IgM antibody responses (385). Aerosol delivery of an adenovirus TB vaccine candidate has been described as safe and induces enhanced polyfunctional airway responses, including CD8+ T cells, outperforming intramuscular delivery of the same vaccine in mouse models of Mtb challenge (349, 386) and a phase 1 human clinical trial (387). A similar observation was made for an influenza vectored TB vaccine candidate in an i.n. administration to mice (388). Our own group has demonstrated that mucosal delivery of ID93+GLA-SE in mice converts the response from a TH1 CD4+ T-cell response typically seen following parenteral immunization, to a TH17 CD4+ T-cell response (52). The field has also demonstrated that i.n. BCG administration provides enhanced protection in mice and guinea pigs compared to subcutaneous delivery (389–391). Both the adaptive (392) and, most recently, trained innate immune responses (393) have been independently characterized for pulmonary BCG delivery-induced protection. Heat-killed MTBVAC delivered intranasally induces mucosal IgA, IgM, and IgG responses in both mouse and NHP models (315). In summary, mucosal delivery fundamentally changes the composition, localization, and functionality of vaccine-induced responses. In addition to inducing robust airway responses, mucosal delivery of TB vaccine candidates allow for dose sparing (394), which is an essential consideration for products intended for LMICs.
Selection of secondary immunogenicity endpoints and alignment across vaccine studies will likely vary slightly between POI and POD strategies. POD endpoints will likely more heavily rely on memory adaptive immunity whereas POI will be more innate and mucosal based. However, many candidates are being evaluated for both POI and POD indications (Table 1) and so comprehensive testing across the spectrum of cells discussed is likely warranted. Many other target population characteristics are also important to consider and may dramatically change the vaccine-induced immune response. TB vaccine candidates can be designed to be broadly effective across population heterogeneity or specific for different groups of people including naïve individuals or those who are BCG vaccinated, persons living with HIV, and individuals with latent TB, recovered from TB, or with TB disease recurrence (395). For example, more balanced CD4+ and CD8+ T-cell responses were observed in BCG primed individuals receiving Ad5Ag85A than BCG naïve individuals (32). Furthermore, the Ad5Ag85A candidate is likely best as a targeted childhood boost of BCG, as anti-Ad5 vector immunity increases with age and may reduce efficacy. “Prevention”, therefore, will have different definitions depending on the strategy as described above. Phase 3 clinical trials evaluating prevention are difficult as they require large numbers of participants followed for extended (2–5 years for POD) lengths of time. POI and POD vaccine strategies, target populations, efficacy endpoints, and novel strategies are outlined below.
POI target populations are largely pre-infected or naïve/unchallenged and a primary efficacy readout for these clinical studies would be remaining IGRA negative for a specific follow-up period. Prevention for POI in this case is absence of infection and likely a lack of measurable infection-mediated adaptive immune responses (e.g., IGRA-negative RSTRs). Populations with regular high exposures to Mtb, such as healthcare workers and household contacts of persons with active disease, are ideal for POI studies since they have a higher chance of becoming challenged than the general population, allowing for smaller trial numbers. For example, the recent phase 2b trial of ID93+GLA-SE completed in BCG-vaccinated healthcare workers in South Korea required just 107 participants to study two doses of vaccine against placebo (ClinicalTrials.gov Identifier: NCT03806686). These community participant trials are needed because there is currently no uniform human challenge model of Mtb, although robust advances in the last few years have made this more feasible. Experimental medicine studies in healthy volunteers leveraging BCG for pulmonary mycobacterial challenge may help serve as a bridge in the field and advances in this model are being made in Cape Town, South Africa (396, 397) as well as Oxford, UK (ClinicalTrials.gov Identifier: NCT02709278 and NCT03912207).
Conversely, POD strategies have a target population, which is post-Mtb challenge, and a primary efficacy readout is an absence of clinically active TB symptoms whereby the individual clears the infection (e.g., IGRA reversion) or remains latently infected for a period of follow-up. The complexities of the spectrum of latency and the research communities’ dated definitions of latency (harboring live bacteria that may reactivate versus a memory immune response to infection) and cure (clearance of bacteria) are recently discussed here (398). These definitions are not trivial as they are composed of information collected from clinical, pathological, microbial, and immunological sources but importantly inform vaccine trial endpoints (398). Better agreement in defining and testing for POD in latent individuals in particular would be beneficial for the field. Advances in host correlate of risk (COR) for progression to active disease signatures (399–402) are being evaluated as more short-term endpoints that can inform efficacy outcomes for drug and vaccine candidate regimens.
Exceptional efforts to mathematically model which vaccine strategies will most disrupt the global burden of TB disease have largely been led by the TB Modeling Group from the London School of Hygiene and Tropical Medicine. Most recently, the population-level impact of candidate POD or POI TB vaccines was modeled for China, South Africa, and India as representative high-burden regions with different demographics, healthcare infrastructures, and epidemiological trends. Interestingly, these models suggest that a candidate POD vaccine with ~70% efficacy for adolescents/adults already infected with Mtb would have the largest effect of incidence rate reduction (IRR) in all populations tested over a targeted 10-year mass vaccination campaign period (403), whereas the IRR was more variable by population for POI vaccine candidates depending on amount of transmission and less effective in regions modeled with reactivation as a primary driver (403). These findings do not seem unique to drug-sensitive Mtb, as this same Modeling Group next examined the influence of POI or POD vaccine candidates on emergence of DR in China and India as two representative regions that account for nearly 40% of the total global distribution of Mtb drug resistance (9). Interestingly, a vaccine with POI and POD efficacy had the largest influence of reducing DR incidence rates in both India and China (>70% in both models) with a predicted 2 million cases prevented in both regions (404), whereas a targeted vaccine with POI efficacy alone was nearly cut in half comparatively (404). Cost-effectiveness of these vaccine strategies against DR Mtb was dependent on regional wealth scenarios, efficacy, and POI or POD strategies, suggesting that a low-cost and age-targeted vaccine should still be a main priority for the pipeline (404, 405). Indeed, modeling deployment of M72/AS01E for populations in South Africa and India suggests that this vaccine candidate would be cost-effective in all scenarios for South Africa based on thresholds for disability-adjusted life-years (DALY) avoided, but that adolescent vaccine campaigns in India would be most cost-effective (406).
Animal Modeling for Vaccine Efficacy
The integration of animal models across the pipeline will only help to facilitate translational advancement of vaccine candidates. While each main model used (mouse, guinea pig, and NHP) has their own caveats for lack of fidelity to human infection, disease progression, and pathology, they do still make up a functional pipeline with tools for Mtb challenge, immunological study, and pathology interrogations. A summary of strengths and weaknesses of each model is presented here (407). Homogeneity of in-bred models is a common complaint or worry for preclinical modeling. However, the Collaborative Cross (CC) and Diversity Outbred (DO) mouse models are elegant systems to examine host–pathogen interactions and uncover more detailed mechanisms of vaccine-mediated protection (408), including those in TB (409, 410), and we expect these genotypes to be further leveraged to examine the contribution of cellular subsets to vaccine-mediated immunity in the near future. These detailed studies should carefully profile and capture the kinetics of cell activation, metabolic states, trafficking, and contribution to containment in early, middle, and late time points post challenge. The ultra-low dose challenge mouse infection (1–3 CFU per mouse), well defined and leveraged by the Urdahl research team, is another recent advancement bringing greater harmony across models that are more physiologically relevant and representative of human infections with resulting heterogeneous acute bacterial loads (411). Historically, the mouse model represents acute and chronic TB disease, but offered little symmetry with human latent TB immunology and protection against reinfection. Recently, researchers have pioneered a small animal model of “contained Mtb infection” (CMTB). In this model, live Mtb is administered into a mouse ear where it persists, which will be exceedingly useful for differentiating immune profiles of active and latent disease and for evaluating protective responses (412, 413). The next model in the three-tiered pipeline is commonly rabbits or guinea pigs, which are readily infected with aerosolized Mtb and demonstrate more robust pathology, including human-like necrotic granulomas, than mice. A lack of immunological resources makes these models less than ideal for evaluating immune mechanism(s) of protection; however, they are a readily available model for vaccine and drug safety (PPD and DTH responses) and efficacy evaluations (414). With the more widespread adoption of in situ transcriptional evaluations and pioneering researchers generating more immunological tools, the guinea pig will likely become more beneficial for COP interrogations in the coming decade. NHPs are the final model in the preclinical pipeline, which demonstrate the closest alignment with human TB disease and immunology. These surrogates are used for a wide array of human diseases and interventional studies, which can significantly hamper their availability, as experienced during the SARS-CoV-2 pandemic (415). NHPs serve as not only an ideal model for late-stage vaccine candidates but also a valuable tool for dissecting specific immune COP, especially in light of the best protection data in NHPs observed to date with the administration of i.v. BCG (16). AS surrogate endpoints become more predictive of efficacy for human protection, with or without reliance on mechanism, we expect them to be evaluated and even back translated through the pipeline. An example are host risk signatures predicting progression to active disease, which were identified in human clinical cohorts (416–421) and evaluated in preclinical models (422).
Discussion
This review is not exhaustive of all cellular compartments or agents and many subsets have been well reviewed elsewhere. Indeed, many of these cells or antimicrobial mediators may play significant roles in the prevention of Mtb infection, as signs of measurable memory immune responses in some cases are not evident, and could explain why some individuals are resistant to infection. For example, while trained innate immunity has been widely studied in the HIV research community, it is more recently being comprehensively followed by the TB research community (423). Trained innate immune cells, including myeloid lineages and NK cells, are those that display a “memory-like” phenotype with metabolic and epigenetic reprogramming (164, 423, 424). This strategy is of particular interest for live-attenuated vaccine strategies like BCG and BCG-based candidates and strategies with adjuvants that differentiate innate cells through PRR pathways and induce specific metabolic phenotypes (425). Donor-unrestricted T cells (DURTs), including γδ T-cell subsets discussed above, are exceedingly unique in that they are not restricted by MHC presentation of antigen but rather recognize non-peptide antigens on unique non-polymorphic molecules including Cluster of Differentiation 1 (CD1), MHC-related 1 (MR1 molecules), HLAE, and butyrophilin 3A1 (296, 426). These non-MHC molecules are genetically shared across heterogenous populations and so the responding cells are not donor-restricted in the way classical TCRs are, making them attractive vaccine targets as they may be more widely immunogenic across diverse regions. Generating tools to better study DURTs are a significant research priority for the field, including generation of DURT antigens and amenable vaccine formulations (426). Indeed, the non-peptide nature of DURT antigens makes them candidates for incorporation into formulations such as emulsions and nanoparticles, which could further be complexed with classical vaccine T-cell antigens as a novel vaccine strategy. MAIT cells are T cells that are similarly non-classically restricted but with a propensity for mucosal homing, recognizing antigens presented on MR1 with rapid response capabilities and the functional capacity to kill infected cells (296, 427, 428). However, their active role is somewhat confusing as some clinical data demonstrate that individuals with TB have decreased numbers of circulating MAIT cells with reduced activation (429), whereas others are detected with tetramers at similar frequencies in peripheral blood from TB-infected and uninfected individuals (427). In mouse models of TB challenge, MAIT cells have been shown to delay T-cell priming early during challenge but are efficient targets of host-directed therapies (428), making them an interesting target in vaccine and drug therapy strategies. Beyond cell subsets, antimicrobial peptides (AMP) likely play a significant role in POI mechanisms. AMP are a wide array of multi-functional peptides secreted by innate immune cells. They are capable of targeting bacteria, viruses, and fungi. AMPs are broadly classified by their structural characteristics and amino acid composition, but all share similar mechanisms for targeting pathogens. AMPs are known to primarily target the pathogen membrane through permeation, although there has been evidence of AMPs also disrupting DNA/RNA synthesis and inducing ATP loss (430). AMPs identified in the human defense against mycobacteria include cathelicidin, defensins, hepcidin, lactoferrin, azurocidin, elastases, antimicrobial RNases, eosinophil peroxidase, cathepsins, granulysin, and lipocalin2 (430). While these AMPs are derived from many different types of cells, the overwhelming majority are innate and likely play early roles in protection from mycobacteria and may contribute to RSTR phenotypes. More work needs to be done to determine if genetics plays a role in abundance or efficacy of AMPs against Mtb.
While many vaccine strategies are benchmarked against induction of robust Mtb-antigen-specific CD4+ TH1 responses, the data reviewed here and elsewhere make a strong case for inclusion of many other subsets depending on POI or POD strategy. While heterologous vaccine strategies such as subunit + adjuvant prime with vector-based candidate boost may better drive multifaceted adaptive immunity, their clinical deployment may be further delayed than present candidates. However, developing and screening vaccine platforms that induce specific combinations of immunity against high-priority Mtb protective antigens should be a major strategy for the pipeline (Table 2). While it is true that candidates that show efficacy in the preclinical pipeline may not ultimately be successful in clinical trials (20), if we begin to look across candidates and compile more data, trends that better predict success may emerge. When we evaluate the many cell subsets that contribute to Mtb control, it is essential to consider (1) the time in which they contribute, (2) the way they interact with other cell subsets, and (3) how they may be preferentially induced by a vaccine regimen platform, route of delivery, and dosing interval (Table 1). As a research community, we need better immune correlates that are mechanistic or that better predict clinical efficacy. The simultaneous scrutiny of vaccines and their potential for public health impact on a global scale have never been higher. As national and international consortiums of TB vaccine developers and researchers align across the pipeline, we may soon have reliable models and vaccine endpoints that more readily steer candidates to approval and clinical deployment, relegating TB disease to a thing of the past.
Author Contributions
All authors (SL, DW, MR, RC, and SB) helped conceptualize the manuscript. SL, DW, MR, and SB drafted the manuscript. SL, DW, and MR drafted figures and tables. All authors (SL, DW, MR, RC, and SB) reviewed the manuscript and contributed to edits. All authors contributed to the article and approved the submitted version.
Funding
This review was supported by the National Institute of Allergy and Infectious Diseases (NIAID) of the National Institutes of Health (NIH) under award number R01AI125160. BDW was supported through the University of Washington, Diseases of Public Health Importance training grant number T32AI007509.. The content is solely the responsibility of the authors and does not necessarily represent the official views of the National Institutes of Health.
Conflict of Interest
The authors declare that the research was conducted in the absence of any commercial or financial relationships that could be construed as a potential conflict of interest.
Publisher’s Note
All claims expressed in this article are solely those of the authors and do not necessarily represent those of their affiliated organizations, or those of the publisher, the editors and the reviewers. Any product that may be evaluated in this article, or claim that may be made by its manufacturer, is not guaranteed or endorsed by the publisher.
Acknowledgments
The authors would like to express their gratitude to Seattle Children’s Research Institute Leadership for their guidance, compassion, and mentorship.
References
1. World Health Organization. Global Tuberculosis Report 2018, Geneva: World Health Organization (2018).
2. Cucinotta D, Vanelli M. WHO Declares COVID-19 a Pandemic. Acta BioMed (2020) 91:157–60. doi: 10.23750/abm.v91i1.9397
3. World Health Organization. Global Tuberculosis Report 2020: Executive Summary. Geneva: World Health Organization (2020).
4. World Health Organization. Global Tuberculosis Report 2021, Geneva: World Health Organization (2021).
5. World Health Organization Global TB Programme. Impact of the Covid-19 Pandemic on Tb Detection and Mortality in 2020, Geneva: World Health Organization (2021).
6. Zaman K. Tuberculosis: A Global Health Problem. J Health Popul Nutr (2010) 28:111–3. doi: 10.3329/jhpn.v28i2.4879
7. Pai M, Kasaeva T, Swaminathan S. Covid-19’s Devastating Effect on Tuberculosis Care — A Path to Recovery. N Engl J Med (2022). doi: 10.1056/NEJMp2118145
8. Arregui S, Iglesias MJ, Samper S, Marinova D, Martin C, Sanz J, et al. Data-Driven Model for the Assessment of Mycobacterium Tuberculosis Transmission in Evolving Demographic Structures. Proc Natl Acad Sci USA (2018) 115:E3238–e3245. doi: 10.1073/pnas.1720606115
10. Houben R, Menzies NA, Sumner T, Huynh GH, Arinaminpathy N, Goldhaber-Fiebert JD, et al. Feasibility of Achieving the 2025 WHO Global Tuberculosis Targets in South Africa, China, and India: A Combined Analysis of 11 Mathematical Models. Lancet Glob Health (2016) 4:e806–15. doi: 10.1016/S2214-109X(16)30199-1
11. Menzies NA, Gomez GB, Bozzani F, Chatterjee S, Foster N, Baena IG, et al. Cost-Effectiveness and Resource Implications of Aggressive Action on Tuberculosis in China, India, and South Africa: A Combined Analysis of Nine Models. Lancet Glob Health (2016) 4:e816–26. doi: 10.1016/S2214-109X(16)30265-0
12. Fine PEM. Variation in Protection by BCG: Implications of and for Heterologous Immunity. Lancet (1995) 346:1339–45. doi: 10.1016/S0140-6736(95)92348-9
13. Tait DR, Hatherill M, van der Meeren O, Ginsberg AM, Van Brakel E, Salaun B, et al. Final Analysis of a Trial of M72/AS01E Vaccine to Prevent Tuberculosis. N Engl J Med (2019) 381:2429–39. doi: 10.1056/NEJMoa1909953
14. Van Der Meeren O, Hatherill M, Nduba V, Wilkinson RJ, Muyoyeta M, Van Brakel E, et al. Phase 2b Controlled Trial of M72/AS01(E) Vaccine to Prevent Tuberculosis. N Engl J Med (2018) 379:1621–34. doi: 10.1056/NEJMoa1803484
15. Dockrell HM, Butkeviciute E. Can What Have We Learnt About BCG Vaccination in the Last 20 Years Help Us to Design a Better Tuberculosis Vaccine? Vaccine (2021). doi: 10.1016/j.vaccine.2021.01.068
16. Darrah PA, Zeppa JJ, Maiello P, Hackney JA, Wadsworth MH, et al. Prevention of Tuberculosis in Macaques After Intravenous BCG Immunization. Nature (2020) 577:95–102. doi: 10.1038/s41586-019-1817-8
17. Irvine EB, O'Neil A, Darrah PA, Shin S, Choudhary A, Li W, et al. Robust IgM Responses Following Intravenous Vaccination With Bacille Calmette-Guérin Associate With Prevention of Mycobacterium Tuberculosis Infection in Macaques. Nat Immunol (2021) 22:1515–23. doi: 10.1038/s41590-021-01066-1
18. Lewinsohn DA, Lewinsohn DM, Scriba TJ. Polyfunctional CD4(+) T Cells As Targets for Tuberculosis Vaccination. Front Immunol (2017) 8:1262. doi: 10.3389/fimmu.2017.01262
19. Martin C, Aguilo N, Marinova D, Gonzalo-Asensio J. Update on TB Vaccine Pipeline. Appl Sci (2020) 10:2632. doi: 10.3390/app10072632
20. Tameris MD, Hatherill M, Landry BS, Scriba TJ, Snowden MA, Lockhart S, et al. Safety and Efficacy of MVA85A, a New Tuberculosis Vaccine, in Infants Previously Vaccinated With BCG: A Randomised, Placebo-Controlled Phase 2b Trial. Lancet (2013) 381:1021–8. doi: 10.1016/S0140-6736(13)60177-4
21. Saul MC, Philip VM, Reinholdt LG, Chesler EJ. High-Diversity Mouse Populations for Complex Traits. Trends Genet (2019) 35:501–14. doi: 10.1016/j.tig.2019.04.003
22. Smith CM, Baker RE, Proulx MK, Mishra BB, Long JE, Park SW, et al. Host-Pathogen Genetic Interactions Underlie Tuberculosis Susceptibility. bioRxiv (2021) 2020.2012.2001.405514. doi: 10.1101/2020.12.01.405514
23. Lu LL, Smith MT, Yu KKQ, Luedemann C, Suscovich TJ, Grace PS, et al. IFN-γ-Independent Immune Markers of Mycobacterium Tuberculosis Exposure. Nat Med (2019) 25:977–87. doi: 10.1038/s41591-019-0441-3
24. White AD, White AD, Sibley L, Sarfas C, Morrison A, Gullick J, Clark S, et al. MTBVAC Vaccination Protects Rhesus Macaques Against Aerosol Challenge With M. Tuberculosis and Induces Immune Signatures Analogous to Those Observed in Clinical Studies. NPJ Vaccines (2021) 6:4–4. doi: 10.1038/s41541-020-00262-8
25. Spertini F, Audran R, Chakour R, Karoui O, Steiner-Monard V, Thierry AC, et al. Safety of Human Immunisation With a Live-Attenuated Mycobacterium Tuberculosis Vaccine: A Randomised, Double-Blind, Controlled Phase I Trial. Lancet Respir Med (2015) 3:953–62. doi: 10.1016/s2213-2600(15)00435-x
26. Tameris M, Mearns H, Penn-Nicholson A, Gregg Y, Bilek N, Mabwe S, et al. Live-Attenuated Mycobacterium Tuberculosis Vaccine MTBVAC Versus BCG in Adults and Neonates: A Randomised Controlled, Double-Blind Dose-Escalation Trial. Lancet Respir Med (2019) 7:757–70. doi: 10.1016/s2213-2600(19)30251-6
27. Dijkman K, Aguilo N, Boot C, Hofman SO, Sombroek CC, Vervenne RAW, et al. Pulmonary MTBVAC Vaccination Induces Immune Signatures Previously Correlated With Prevention of Tuberculosis Infection. Cell Rep Med (2021) 2:100187. doi: 10.1016/j.xcrm.2020.100187
28. Grode L, Ganoza CA, Brohm C, Weiner J, Eisele B, Kaufmann SHE. Safety and Immunogenicity of the Recombinant BCG Vaccine VPM1002 in a Phase 1 Open-Label Randomized Clinical Trial. Vaccine (2013) 31:1340–8. doi: 10.1016/j.vaccine.2012.12.053
29. Nieuwenhuizen NE, Kulkarni PS, Shaligram U, Cotton MF, Rentsch CA, Eisele B, et al. The Recombinant Bacille Calmette–Guérin Vaccine VPM1002: Ready for Clinical Efficacy Testing. Front Immunol (2017) 8:1147. doi: 10.3389/fimmu.2017.01147
30. Loxton AG, Knaul JK, Grode L, Gutschmidt A, Meller C, Eisele B, et al. Safety and Immunogenicity of the Recombinant Mycobacterium Bovis BCG Vaccine VPM1002 in HIV-Unexposed Newborn Infants in South Africa. Clin Vaccine Immunol (2017) 24. doi: 10.1128/cvi.00439-16
31. Desel C, Dorhoi A, Bandermann S, Grode L, Eisele B, Kaufmann SH. Recombinant BCG Δurec Hly+ Induces Superior Protection Over Parental BCG by Stimulating a Balanced Combination of Type 1 and Type 17 Cytokine Responses. J Infect Dis (2011) 204:1573–84. doi: 10.1093/infdis/jir592
32. Smaill F, Jeyanathan M, Smieja M, Medina MF, Thanthrige-Don N, Zganiacz A, et al. A Human Type 5 Adenovirus Based Tuberculosis Vaccine Induces Robust T Cell Responses in Humans Despite Preexisting Anti-Adenovirus Immunity. Sci Trans Med (2013) 5:205ra134. doi: 10.1126/scitranslmed.3006843
33. Metcalfe HJ, Steinbach S, Jones GJ, Connelley T, Morrison WI, Vordermeier M, et al. Protection Associated With a TB Vaccine Is Linked to Increased Frequency of Ag85A-Specific CD4(+) T Cells But No Increase in Avidity for Ag85A. Vaccine (2016) 34:4520–5. doi: 10.1016/j.vaccine.2016.07.055
34. Smaill F, Xing Z. Human Type 5 Adenovirus-Based Tuberculosis Vaccine: Is the Respiratory Route of Delivery the Future? Expert Rev Vaccines (2014) 13:927–30. doi: 10.1586/14760584.2014.929947
35. Wilkie M, Satti I, Minhinnick A, Harris S, Riste M, Ramon RL, et al. A Phase I Trial Evaluating the Safety and Immunogenicity of a Candidate Tuberculosis Vaccination Regimen, ChAdOx1 85A Prime - MVA85A Boost in Healthy UK Adults. Vaccine (2020) 38:779–89. doi: 10.1016/j.vaccine.2019.10.102
36. Pinpathomrat N, Bull N, Pasricha J, Harrington-Kandt R, McShane H, Stylianou E. Using an Effective TB Vaccination Regimen to Identify Immune Responses Associated With Protection in the Murine Model. Vaccine (2021) 39:1452–62. doi: 10.1016/j.vaccine.2021.01.034
37. Stylianou E, Griffiths KL, Poyntz HC, Harrington-Kandt R, Dicks MD, Stockdale L, et al. Improvement of BCG Protective Efficacy With a Novel Chimpanzee Adenovirus and a Modified Vaccinia Ankara Virus Both Expressing Ag85A. Vaccine (2015) 33:6800–8. doi: 10.1016/j.vaccine.2015.10.017
38. Lu JB, Chen BW, Wang GZ, Fu LL, Shen XB, Su C, et al. Recombinant Tuberculosis Vaccine AEC/BC02 Induces Antigen-Specific Cellular Responses in Mice and Protects Guinea Pigs in a Model of Latent Infection. J Microbiol Immunol Infect (2015) 48:597–603. doi: 10.1016/j.jmii.2014.03.005
39. Sereinig S, Stukova M, Zabolotnyh N, Ferko B, Kittel C, Romanova J, et al. Influenza Virus NS Vectors Expressing the Mycobacterium Tuberculosis ESAT-6 Protein Induce CD4+ Th1 Immune Response and Protect Animals Against Tuberculosis Challenge. Clin Vaccine Immunol (2006) 13:898–904. doi: 10.1128/cvi.00056-06
40. Baldwin SL, Bertholet S, Reese VA, Ching LK, Reed SG, Coler RN. The Importance of Adjuvant Formulation in the Development of a Tuberculosis Vaccine. J Immunol (2012) 188:2189–97. doi: 10.4049/jimmunol.1102696
41. Baldwin SL, Reese VA, Huang PW, Beebe EA, Podell BK, Reed SG, et al. Protection and Long-Lived Immunity Induced by the ID93/GLA-SE Vaccine Candidate Against a Clinical Mycobacterium Tuberculosis Isolate. Clin Vaccine Immunol (2016) 23:137–47. doi: 10.1128/CVI.00458-15
42. Bertholet S, Ireton GC, Ordway DJ, Windish HP, Pine SO, Kahn M, et al. A Defined Tuberculosis Vaccine Candidate Boosts BCG and Protects Against Multidrug-Resistant Mycobacterium Tuberculosis. Sci Transl Med (2010) 2:53ra74. doi: 10.1126/scitranslmed.3001094
43. Cha SB, Kim WS, Kim JS, Kim H, Kwon KW, Han SJ, et al. Pulmonary Immunity and Durable Protection Induced by the ID93/GLA-SE Vaccine Candidate Against the Hyper-Virulent Korean Beijing Mycobacterium Tuberculosis Strain K. Vaccine (2016) 34:2179–87. doi: 10.1016/j.vaccine.2016.03.029
44. Coler RN, Bertholet S, Pine SO, Orr MT, Reese V, Windish HP, et al. Therapeutic Immunization Against Mycobacterium Tuberculosis Is an Effective Adjunct to Antibiotic Treatment. J Infect Dis (2013) 207:1242–52. doi: 10.1093/infdis/jis425
45. Coler RN, Day TA, Ellis R, Piazza FM, Beckmann AM, Vergara J, et al. The TLR-4 Agonist Adjuvant, GLA-SE, Improves Magnitude and Quality of Immune Responses Elicited by the ID93 Tuberculosis Vaccine: First-in-Human Trial. NPJ Vaccines (2018) 3:34–4. doi: 10.1038/s41541-018-0057-5
46. Day TA, Penn-Nicholson A, Luabeya AKK, Fiore-Gartland A, Du Plessis N, Loxton AG, et al. Safety and Immunogenicity of the Adjunct Therapeutic Vaccine ID93 + GLA-SE in Adults Who Have Completed Treatment for Tuberculosis: A Randomised, Double-Blind, Placebo-Controlled, Phase 2a Trial. Lancet Respir Med (2020). doi: 10.1016/s2213-2600(20)30319-2
47. Kwon KW, Lee A, Larsen SE, Baldwin SL, Coler RN, Reed SG, et al. Long-Term Protective Efficacy With a BCG-Prime ID93/GLA-SE Boost Regimen Against the Hyper-Virulent Mycobacterium Tuberculosis Strain K in a Mouse Model. Sci Rep (2019) 9:15560. doi: 10.1038/s41598-019-52146-0
48. Larsen SE, Baldwin SL, Orr MT, Reese VA, Pecor T, Granger B, et al. Enhanced Anti-Mycobacterium Tuberculosis Immunity Over Time With Combined Drug and Immunotherapy Treatment. Vaccines (Basel) (2018) 6. doi: 10.3390/vaccines6020030
49. Penn-Nicholson A, Tameris M, Smit E, Day TA, Musvosvi M, Jayashankar L, et al. Safety and Immunogenicity of the Novel Tuberculosis Vaccine ID93 + GLA-SE in BCG-Vaccinated Healthy Adults in South Africa: A Randomised, Double-Blind, Placebo-Controlled Phase 1 Trial. Lancet Respir Med (2018) 6:287–98. doi: 10.1016/S2213-2600(18)30077-8
50. Desbien AL, Reed SJ, Bailor HR, Cauwelaert ND, Laurance JD, Orr MT, et al. Squalene Emulsion Potentiates the Adjuvant Activity of the TLR4 Agonist, GLA, via Inflammatory Caspases, IL-18, and IFN-γ. Eur J Immunol (2015) 45:407–17. doi: 10.1002/eji.201444543
51. Coler RN, Bertholet S, Moutaftsi M, Guderian JA, Windish HP, Baldwin SL, et al. Development and Characterization of Synthetic Glucopyranosyl Lipid Adjuvant System as a Vaccine Adjuvant. PloS One (2011) 6:e16333. doi: 10.1371/journal.pone.0016333
52. Orr MT, Beebe EA, Hudson TE, Argilla D, Huang PW, Reese VA, et al. Mucosal Delivery Switches the Response to an Adjuvanted Tuberculosis Vaccine From Systemic TH1 to Tissue-Resident TH17 Responses Without Impacting the Protective Efficacy. Vaccine (2015) 33:6570–8. doi: 10.1016/j.vaccine.2015.10.115
53. Rodo MJ, Rozot V, Nemes E, Dintwe O, Hatherill M, Little F, et al. A Comparison of Antigen-Specific T Cell Responses Induced by Six Novel Tuberculosis Vaccine Candidates. PloS Pathog (2019) 15:e1007643. doi: 10.1371/journal.ppat.1007643
54. Tkachuk AP, Gushchin VA, Potapov VD, Demidenko AV, Lunin VG, Gintsburg AL. Multi-Subunit BCG Booster Vaccine GamTBvac: Assessment of Immunogenicity and Protective Efficacy in Murine and Guinea Pig TB Models. PloS One (2017) 12:e0176784. doi: 10.1371/journal.pone.0176784
55. Vasina DV, Kleymenov DA, Manuylov VA, Mazunina EP, Koptev EY, Tukhovskaya EA, et al. First-In-Human Trials of GamTBvac, a Recombinant Subunit Tuberculosis Vaccine Candidate: Safety and Immunogenicity Assessment. Vaccines (Basel) (2019) 7. doi: 10.3390/vaccines7040166
56. Tkachuk AP, Bykonia EN, Popova LI, Kleymenov DA, Semashko MA, Chulanov VP, et al. Safety and Immunogenicity of the GamTBvac, the Recombinant Subunit Tuberculosis Vaccine Candidate: A Phase II, Multi-Center, Double-Blind, Randomized, Placebo-Controlled Study. Vaccines (Basel) (2020) 8. doi: 10.3390/vaccines8040652
57. Didierlaurent AM, Collignon C, Bourguignon P, Wouters S, Fierens K, Fochesato M, et al. Enhancement of Adaptive Immunity by the Human Vaccine Adjuvant AS01 Depends on Activated Dendritic Cells. J Immunol (2014) 193:1920–30. doi: 10.4049/jimmunol.1400948
58. Penn-Nicholson A, Geldenhuys H, Burny W, van der Most R, Day CL, Jongert E, et al. Safety and Immunogenicity of Candidate Vaccine M72/AS01E in Adolescents in a TB Endemic Setting. Vaccine (2015) 33:4025–34. doi: 10.1016/j.vaccine.2015.05.088
59. Montoya J, Solon JA, Cunanan SR, Acosta L, Bollaerts A, Moris P, et al. A Randomized, Controlled Dose-Finding Phase II Study of the M72/AS01 Candidate Tuberculosis Vaccine in Healthy PPD-Positive Adults. J Clin Immunol (2013) 33:1360–75. doi: 10.1007/s10875-013-9949-3
60. Day CL, Tameris M, Mansoor N, van Rooyen M, de Kock M, Geldenhuys H, et al. Induction and Regulation of T-Cell Immunity by the Novel Tuberculosis Vaccine M72/AS01 in South African Adults. Am J Respir Crit Care Med (2013) 188:492–502. doi: 10.1164/rccm.201208-1385OC
61. Kumarasamy N, Poongulali S, Beulah FE, Akite EJ, Ayuk LN, Bollaerts A, et al. Long-Term Safety and Immunogenicity of the M72/AS01E Candidate Tuberculosis Vaccine in HIV-Positive and -Negative Indian Adults: Results From a Phase II Randomized Controlled Trial. Med (Baltimore) (2018) 97:e13120. doi: 10.1097/md.0000000000013120
62. Idoko OT, Owolabi OA, Owiafe PK, Moris P, Odutola A, Bollaerts A, et al. Safety and Immunogenicity of the M72/AS01 Candidate Tuberculosis Vaccine When Given as a Booster to BCG in Gambian Infants: An Open-Label Randomized Controlled Trial. Tuberculosis (Edinb) (2014) 94:564–78. doi: 10.1016/j.tube.2014.07.001
63. Leroux-Roels I, Forgus S, De Boever F, Clement F, Demoitié MA, Mettens P, et al. Improved CD4⁺ T Cell Responses to Mycobacterium Tuberculosis in PPD-Negative Adults by M72/AS01 as Compared to the M72/AS02 and Mtb72F/AS02 Tuberculosis Candidate Vaccine Formulations: A Randomized Trial. Vaccine (2013) 31:2196–206. doi: 10.1016/j.vaccine.2012.05.035
64. Bosteels C, Fierens K, De Prijck S, Van Moorleghem J, Vanheerswynghels M, De Wolf C, et al. CCR2- and Flt3-Dependent Inflammatory Conventional Type 2 Dendritic Cells Are Necessary for the Induction of Adaptive Immunity by the Human Vaccine Adjuvant System As01. Front Immunol (2020) 11:606805. doi: 10.3389/fimmu.2020.606805
65. Detienne S, Welsby I, Collignon C, Wouters S, Coccia M, Delhaye S, et al. Central Role of CD169(+) Lymph Node Resident Macrophages in the Adjuvanticity of the QS-21 Component of AS01. Sci Rep (2016) 6:39475. doi: 10.1038/srep39475
66. Lahey T, Laddy D, Hill K, Schaeffer J, Hogg A, Keeble J, et al. Immunogenicity and Protective Efficacy of the DAR-901 Booster Vaccine in a Murine Model of Tuberculosis. PloS One (2016) 11:e0168521. doi: 10.1371/journal.pone.0168521
67. Masonou T, Hokey DA, Lahey T, Halliday A, Berrocal-Almanza LC, Wieland-Alter WF, et al. CD4+ T Cell Cytokine Responses to the DAR-901 Booster Vaccine in BCG-Primed Adults: A Randomized, Placebo-Controlled Trial. PloS One (2019) 14:e0217091. doi: 10.1371/journal.pone.0217091
68. von Reyn CF, Lahey T, Arbeit RD, Landry B, Kailani L, Adams LV, et al. Safety and Immunogenicity of an Inactivated Whole Cell Tuberculosis Vaccine Booster in Adults Primed With BCG: A Randomized, Controlled Trial of DAR-901. PloS One (2017) 12:e0175215. doi: 10.1371/journal.pone.0175215
69. Szabo A, Gogolak P, Pazmandi K, Kis-Toth K, Riedl K, Wizel B, et al. The Two-Component Adjuvant IC31® Boosts Type I Interferon Production of Human Monocyte-Derived Dendritic Cells via Ligation of Endosomal TLRs. PloS One (2013) 8:e55264. doi: 10.1371/journal.pone.0055264
70. Luabeya AK, Kagina BM, Tameris MD, Geldenhuys H, Hoff ST, Shi Z, et al. First-In-Human Trial of the Post-Exposure Tuberculosis Vaccine H56:IC31 in Mycobacterium Tuberculosis Infected and Non-Infected Healthy Adults. Vaccine (2015) 33:4130–40. doi: 10.1016/j.vaccine.2015.06.051
71. Lin PL, Dietrich J, Tan E, Abalos RM, Burgos J, Bigbee C, et al. The Multistage Vaccine H56 Boosts the Effects of BCG to Protect Cynomolgus Macaques Against Active Tuberculosis and Reactivation of Latent Mycobacterium Tuberculosis Infection. J Clin Invest (2012) 122:303–14. doi: 10.1172/JCI46252
72. Suliman S, Luabeya AKK, Geldenhuys H, Tameris M, Hoff ST, Shi Z, et al. Dose Optimization of H56:IC31 Vaccine for Tuberculosis-Endemic Populations. A Double-Blind, Placebo-Controlled, Dose-Selection Trial. Am J Respir Crit Care Med (2019) 199:220–31. doi: 10.1164/rccm.201802-0366OC
73. Jenum S, Tonby K, Rueegg CS, Rühwald M, Kristiansen MP, Bang P, et al. A Phase I/II Randomized Trial of H56:IC31 Vaccination and Adjunctive Cyclooxygenase-2-Inhibitor Treatment in Tuberculosis Patients. Nat Commun (2021) 12:6774. doi: 10.1038/s41467-021-27029-6
74. Bekker LG, Dintwe O, Fiore-Gartland A, Middelkoop K, Hutter J, Williams A, et al. A Phase 1b Randomized Study of the Safety and Immunological Responses to Vaccination With H4:IC31, H56:IC31, and BCG Revaccination in Mycobacterium Tuberculosis-Uninfected Adolescents in Cape Town, South Africa. EClinicalMedicine (2020) 21:100313. doi: 10.1016/j.eclinm.2020.100313
75. Knudsen NP, Olsen A, Buonsanti C, Follmann F, Zhang Y, Coler RN, et al. Different Human Vaccine Adjuvants Promote Distinct Antigen-Independent Immunological Signatures Tailored to Different Pathogens. Sci Rep (2016) 6:19570. doi: 10.1038/srep19570
76. Olafsdottir TA, Lindqvist M, Nookaew I, Andersen P, Maertzdorf J, Persson J, et al. Comparative Systems Analyses Reveal Molecular Signatures of Clinically Tested Vaccine Adjuvants. Sci Rep (2016) 6:39097. doi: 10.1038/srep39097
77. Aagaard C, Hoang T, Dietrich J, Cardona PJ, Izzo A, Dolganov G, et al. A Multistage Tuberculosis Vaccine That Confers Efficient Protection Before and After Exposure. Nat Med (2011) 17:189–94. doi: 10.1038/nm.2285
78. Thakur A, Pinto FE, Hansen HS, Andersen P, Christensen D, Janfelt C, et al. Intrapulmonary (I.Pulmon.) Pull Immunization With the Tuberculosis Subunit Vaccine Candidate H56/CAF01 After Intramuscular (I.M.) Priming Elicits a Distinct Innate Myeloid Response and Activation of Antigen-Presenting Cells Than I.M. Or I.Pulmon. Prime Immunization Alone. Front Immunol (2020) 11:803. doi: 10.3389/fimmu.2020.00803
79. Schellack C, Prinz K, Egyed A, Fritz JH, Wittmann B, Ginzler M, et al. IC31, a Novel Adjuvant Signaling via TLR9, Induces Potent Cellular and Humoral Immune Responses. Vaccine (2006) 24:5461–72. doi: 10.1016/j.vaccine.2006.03.071
80. Rakshit S, Ahmed A, Adiga V, Sundararaj BK, Sahoo PN, Kenneth J, et al. BCG Revaccination Boosts Adaptive Polyfunctional Th1/Th17 and Innate Effectors in IGRA+ and IGRA- Indian Adults. JCI Insight (2019) 4. doi: 10.1172/jci.insight.130540
81. Nemes E, Geldenhuys H, Rozot V, Rutkowski KT, Ratangee F, Bilek N, et al. Prevention of M. Tuberculosis Infection With H4:IC31 Vaccine or BCG Revaccination. N Engl J Med (2018) 379:138–49. doi: 10.1056/NEJMoa1714021
82. Suliman S, Geldenhuys H, Johnson JL, Hughes JE, Smit E, Murphy M, et al. Bacillus Calmette-Guérin (BCG) Revaccination of Adults With Latent Mycobacterium Tuberculosis Infection Induces Long-Lived BCG-Reactive NK Cell Responses. J Immunol (2016) 197:1100–10. doi: 10.4049/jimmunol.1501996
83. Husain AA, Warke SR, Kalorey DR, Daginawala HF, Taori GM, Kashyap RS, et al. Comparative Evaluation of Booster Efficacies of BCG, Ag85B, and Ag85B Peptides Based Vaccines to Boost BCG Induced Immunity in BALB/c Mice: A Pilot Study. Clin Exp Vaccine Res (2015) 4:83–7. doi: 10.7774/cevr.2015.4.1.83
84. Chen T, Blanc C, Eder AZ, Prados-Rosales R, Souza AC, Kim RS, et al. Association of Human Antibodies to Arabinomannan With Enhanced Mycobacterial Opsonophagocytosis and Intracellular Growth Reduction. J Infect Dis (2016) 214:300–10. doi: 10.1093/infdis/jiw141
85. Gupta A, Ahmad FJ, Ahmad F, Gupta UD, Natarajan M, Katoch V, et al. Efficacy of Mycobacterium Indicus Pranii Immunotherapy as an Adjunct to Chemotherapy for Tuberculosis and Underlying Immune Responses in the Lung. PloS One (2012) 7:e39215. doi: 10.1371/journal.pone.0039215
86. Gupta A, Geetha N, Mani J, Upadhyay P, Katoch VM, Natrajan M, et al. Immunogenicity and Protective Efficacy of “Mycobacterium W” Against Mycobacterium Tuberculosis in Mice Immunized With Live Versus Heat-Killed M. W by the Aerosol or Parenteral Route. Infect Immun (2009) 77:223–31. doi: 10.1128/iai.00526-08
87. Gupta A, Saqib M, Singh B, Pal L, Nishikanta A, Bhaskar S. Mycobacterium Indicus Pranii Induced Memory T-Cells in Lung Airways Are Sentinels for Improved Protection Against M.tb Infection. Front Immunol (2019) 10:2359. doi: 10.3389/fimmu.2019.02359
88. Gupta A, Ahmad FJ, Ahmad F, Gupta UD, Natarajan M, Katoch VM, et al. Protective Efficacy of Mycobacterium Indicus Pranii Against Tuberculosis and Underlying Local Lung Immune Responses in Guinea Pig Model. Vaccine (2012) 30:6198–209. doi: 10.1016/j.vaccine.2012.07.061
89. Guleria I, Mukherjee R, Kaufmann SH. In Vivo Depletion of CD4 and CD8 T Lymphocytes Impairs Mycobacterium W Vaccine-Induced Protection Against M. Tuberculosis in Mice. Med Microbiol Immunol (1993) 182:129–35. doi: 10.1007/bf00190265
90. Sharma SK, Katoch K, Sarin R, Balambal R, Kumar Jain N, Patel N, et al. Efficacy and Safety of Mycobacterium Indicus Pranii as an Adjunct Therapy in Category II Pulmonary Tuberculosis in a Randomized Trial. Sci Rep (2017) 7:3354. doi: 10.1038/s41598-017-03514-1
91. Das S, Chowdhury BP, Goswami A, Parveen S, Jawed J, Pal N, et al. Mycobacterium Indicus Pranii (MIP) Mediated Host Protective Intracellular Mechanisms Against Tuberculosis Infection: Involvement of TLR-4 Mediated Signaling. Tuberculosis (Edinb) (2016) 101:201–9. doi: 10.1016/j.tube.2016.09.027
92. Vilaplana C, Montané E, Pinto S, Barriocanal AM, Domenech G, Torres F, et al. Double-Blind, Randomized, Placebo-Controlled Phase I Clinical Trial of the Therapeutical Antituberculous Vaccine RUTI. Vaccine (2010) 28:1106–16. doi: 10.1016/j.vaccine.2009.09.134
93. Guirado E, Gil O, Cáceres N, Singh M, Vilaplana C, Cardona PJ, et al. Induction of a Specific Strong Polyantigenic Cellular Immune Response After Short-Term Chemotherapy Controls Bacillary Reactivation in Murine and Guinea Pig Experimental Models of Tuberculosis. Clin Vaccine Immunol (2008) 15:1229–37. doi: 10.1128/cvi.00094-08
94. Prabowo SA, Painter H, Zelmer A, Smith SG, Seifert K, Amat M, et al. RUTI Vaccination Enhances Inhibition of Mycobacterial Growth Ex Vivo and Induces a Shift of Monocyte Phenotype in Mice. Front Immunol (2019) 10:894. doi: 10.3389/fimmu.2019.00894
95. Karp CL, Wilson CB, Stuart LM. Tuberculosis Vaccines: Barriers and Prospects on the Quest for a Transformative Tool. Immunol Rev (2015) 264:363–81. doi: 10.1111/imr.12270
96. Morris D, Nguyen T, Kim J, Kassissa C, Khurasany M, Luong J, et al. An Elucidation of Neutrophil Functions Against Mycobacterium Tuberculosis Infection. Clin Dev Immunol (2013) 2013:959650. doi: 10.1155/2013/959650
97. Hilda JN, Narasimhan M, Das SD. Neutrophils From Pulmonary Tuberculosis Patients Show Augmented Levels of Chemokines MIP-1α, IL-8 and MCP-1 Which Further Increase Upon In Vitro Infection With Mycobacterial Strains. Hum Immunol (2014) 75:914–22. doi: 10.1016/j.humimm.2014.06.020
98. Jiang LN, Yao CY, Jin QL, He WX, Li BQ. The Enhanceing Effect of IL-12 on Phagocytosis and Killing of Mycobacterium Tuberculosis by Neutrophils in Tuberculosis Patients. Xi Bao Yu Fen Zi Mian Yi Xue Za Zhi (2011) 27:1191–4.
99. Bainton DF, Ullyot JL, Farquhar MG. The Development of Neutrophilic Polymorphonuclear Leukocytes in Human Bone Marrow. J Exp Med (1971) 134:907–34. doi: 10.1084/jem.134.4.907
100. Bennouna S, Bliss SK, Curiel TJ, Denkers EY. Cross-Talk in the Innate Immune System: Neutrophils Instruct Recruitment and Activation of Dendritic Cells During Microbial Infection. J Immunol (2003) 171:6052–8. doi: 10.4049/jimmunol.171.11.6052
101. van Gisbergen KP, Sanchez-Hernandez M, Geijtenbeek TB, van Kooyk Y. Neutrophils Mediate Immune Modulation of Dendritic Cells Through Glycosylation-Dependent Interactions Between Mac-1 and DC-SIGN. J Exp Med (2005) 201:1281–92. doi: 10.1084/jem.20041276
102. DeLeo FR. Modulation of Phagocyte Apoptosis by Bacterial Pathogens. Apoptosis (2004) 9:399–413. doi: 10.1023/B:APPT.0000031448.64969.fa
103. Kolaczkowska E, Kubes P. Neutrophil Recruitment and Function in Health and Inflammation. Nat Rev Immunol (2013) 13:159–75. doi: 10.1038/nri3399
104. Francis RJ, Butler RE, Stewart GR. Mycobacterium Tuberculosis ESAT-6 is a Leukocidin Causing Ca2+ Influx, Necrosis and Neutrophil Extracellular Trap Formation. Cell Death Dis (2014) 5:e1474. doi: 10.1038/cddis.2014.394
105. Braian C, Hogea V, Stendahl O. Mycobacterium Tuberculosis- Induced Neutrophil Extracellular Traps Activate Human Macrophages. J Innate Immun (2013) 5:591–602. doi: 10.1159/000348676
106. Brinkmann V, Reichard U, Goosmann C, Fauler B, Uhlemann Y, Weiss DS, et al. Neutrophil Extracellular Traps Kill Bacteria. Science (2004) 303:1532–5. doi: 10.1126/science.1092385
107. Papayannopoulos V, Zychlinsky A. NETs: A New Strategy for Using Old Weapons. Trends Immunol (2009) 30:513–21. doi: 10.1016/j.it.2009.07.011
108. Klebanoff SJ. Myeloperoxidase: Friend and Foe. J Leukoc Biol (2005) 77:598–625. doi: 10.1189/jlb.1204697
109. Quinton LJ, Nelson S, Zhang P, Boé DM, Happel KI, Pan W, et al. Selective Transport of Cytokine-Induced Neutrophil Chemoattractant From the Lung to the Blood Facilitates Pulmonary Neutrophil Recruitment. Am J Physiol Lung Cell Mol Physiol (2004) 286:L465–72. doi: 10.1152/ajplung.00153.2003
110. Barrios-Payán J, Aguilar-León D, Lascurain-Ledezma R, Hernández-Pando R. Neutrophil Participation in Early Control and Immune Activation During Experimental Pulmonary Tuberculosis. Gac Med Mex (2006) 142:273–81.
111. Warren E, Teskey G, Venketaraman V. Effector Mechanisms of Neutrophils Within the Innate Immune System in Response to Mycobacterium Tuberculosis Infection. J Clin Med (2017) 6. doi: 10.3390/jcm6020015
112. Kobayashi SD, Malachowa N, DeLeo FR. Neutrophils and Bacterial Immune Evasion. J Innate Immun (2018) 10:432–41. doi: 10.1159/000487756
113. Jones GS, Amirault HJ, Andersen BR. Killing of Mycobacterium Tuberculosis by Neutrophils: A Nonoxidative Process. J Infect Dis (1990) 162:700–4. doi: 10.1093/infdis/162.3.700
114. Kisich KO, Higgins M, Diamond G, Heifets L. Tumor Necrosis Factor Alpha Stimulates Killing of Mycobacterium Tuberculosis by Human Neutrophils. Infect Immun (2002) 70:4591–9. doi: 10.1128/iai.70.8.4591-4599.2002
115. May ME, Spagnuolo PJ. Evidence for Activation of a Respiratory Burst in the Interaction of Human Neutrophils With Mycobacterium Tuberculosis. Infect Immun (1987) 55:2304–7. doi: 10.1128/iai.55.9.2304-2307.1987
116. Martineau AR, Newton SM, Wilkinson KA, Kampmann B, Hall BM, Nawroly N, et al. Neutrophil-Mediated Innate Immune Resistance to Mycobacteria. J Clin Invest (2007) 117:1988–94. doi: 10.1172/jci31097
117. Filio-Rodríguez G, Estrada-García I, Arce-Paredes P, Moreno-Altamirano MM, Islas-Trujillo S, Ponce-Regalado MD, et al. In Vivo Induction of Neutrophil Extracellular Traps by Mycobacterium Tuberculosis in a Guinea Pig Model. Innate Immun (2017) 23:625–37. doi: 10.1177/1753425917732406
118. Schechter MC, Buac K, Adekambi T, Cagle S, Celli J, Ray SM, et al. Neutrophil Extracellular Trap (NET) Levels in Human Plasma Are Associated With Active TB. PloS One (2017) 12:e0182587. doi: 10.1371/journal.pone.0182587
119. Xu J, Zhang X, Pelayo R, Monestier M, Ammollo CT, Semeraro F, et al. Extracellular Histones Are Major Mediators of Death in Sepsis. Nat Med (2009) 15:1318–21. doi: 10.1038/nm.2053
120. Corleis B, Korbel D, Wilson R, Bylund J, Chee R, Schaible UE. Escape of Mycobacterium Tuberculosis From Oxidative Killing by Neutrophils. Cell Microbiol (2012) 14:1109–21. doi: 10.1111/j.1462-5822.2012.01783.x
121. Poveda F, Camacho J, Arnalich F, Codoceo R, del Arco A, Martínez-Hernández P. Circulating Cytokine Concentrations in Tuberculosis and Other Chronic Bacterial Infections. Infection (1999) 27:272–4. doi: 10.1007/s150100050028
122. Tsuda Y, Takahashi H, Kobayashi M, Hanafusa T, Herndon DN, Suzuki F. Three Different Neutrophil Subsets Exhibited in Mice With Different Susceptibilities to Infection by Methicillin-Resistant Staphylococcus Aureus. Immunity (2004) 21:215–26. doi: 10.1016/j.immuni.2004.07.006
123. Appelberg R, Castro AG, Pedrosa J, Minóprio P. Role of Interleukin-6 in the Induction of Protective T Cells During Mycobacterial Infections in Mice. Immunology (1994) 82:361–4.
124. Falcone V, Bassey EB, Toniolo A, Conaldi PG, Collins FM. Differential Release of Tumor Necrosis Factor-Alpha From Murine Peritoneal Macrophages Stimulated With Virulent and Avirulent Species of Mycobacteria. FEMS Immunol Med Microbiol (1994) 8:225–32. doi: 10.1111/j.1574-695X.1994.tb00447.x
125. Kasahara K, Sato I, Ogura K, Takeuchi H, Kobayashi K, Adachi M, et al. Expression of Chemokines and Induction of Rapid Cell Death in Human Blood Neutrophils by Mycobacterium Tuberculosis. J Infect Dis (1998) 178:127–37. doi: 10.1086/515585
126. Riedel DD, Kaufmann SH. Chemokine Secretion by Human Polymorphonuclear Granulocytes After Stimulation With Mycobacterium Tuberculosis and Lipoarabinomannan. Infect Immun (1997) 65:4620–3. doi: 10.1128/iai.65.11.4620-4623.1997
127. Strieter RM, Kasahara K, Allen RM, Standiford TJ, Rolfe MW, Becker FS, et al. Cytokine-Induced Neutrophil-Derived Interleukin-8. Am J Pathol (1992) 141:397–407.
128. Bazzoni F, Cassatella MA, Rossi F, Ceska M, Dewald B, Baggiolini M. Phagocytosing Neutrophils Produce and Release High Amounts of the Neutrophil-Activating Peptide 1/Interleukin 8. J Exp Med (1991) 173:771–4. doi: 10.1084/jem.173.3.771
129. Friedland JS, Hartley JC, Hartley CG, Shattock RJ, Griffin GE. Cytokine Secretion In Vivo and Ex Vivo Following Chemotherapy of Mycobacterium Tuberculosis Infection. Trans R Soc Trop Med Hyg (1996) 90:199–203. doi: 10.1016/s0035-9203(96)90141-8
130. Nibbering PH, Pos O, Stevenhagen A, Van Furth R. Interleukin-8 Enhances Nonoxidative Intracellular Killing of Mycobacterium Fortuitum by Human Granulocytes. Infect Immun (1993) 61:3111–6. doi: 10.1128/iai.61.8.3111-3116.1993
131. Mattila JT, Maiello P, Sun T, Via LE, Flynn JL. Granzyme B-Expressing Neutrophils Correlate With Bacterial Load in Granulomas From Mycobacterium Tuberculosis-Infected Cynomolgus Macaques. Cell Microbiol (2015) 17:1085–97. doi: 10.1111/cmi.12428
132. Trapani JA, Smyth MJ. Functional Significance of the Perforin/Granzyme Cell Death Pathway. Nat Rev Immunol (2002) 2:735–47. doi: 10.1038/nri911
133. Ma Y, Chen HD, Wang Y, Wang Q, Li Y, Zhao Y, et al. Interleukin 24 as a Novel Potential Cytokine Immunotherapy for the Treatment of Mycobacterium Tuberculosis Infection. Microbes Infect (2011) 13:1099–110. doi: 10.1016/j.micinf.2011.06.012
134. Pokkali S, Rajavelu P, Sudhakar R, Das SD. Phenotypic Modulation in Mycobacterium Tuberculosis Infected Neutrophil During Tuberculosis. Indian J Med Res (2009) 130:185–92.
135. Berry MP, Graham CM, McNab FW, Xu Z, Bloch SA, Oni T, et al. An Interferon-Inducible Neutrophil-Driven Blood Transcriptional Signature in Human Tuberculosis. Nature (2010) 466:973–7. doi: 10.1038/nature09247
136. Muefong CN, Sutherland JS. Neutrophils in Tuberculosis-Associated Inflammation and Lung Pathology. Front Immunol (2020) 11:962. doi: 10.3389/fimmu.2020.00962
137. Elkington PT, Ugarte-Gil CA, Friedland JS. Matrix Metalloproteinases in Tuberculosis. Eur Respir J (2011) 38:456–64. doi: 10.1183/09031936.00015411
138. Kleinnijenhuis J, Quintin J, Preijers F, Joosten LA, Ifrim DC, Saeed S, et al. Bacille Calmette-Guerin Induces NOD2-Dependent Nonspecific Protection From Reinfection via Epigenetic Reprogramming of Monocytes. Proc Natl Acad Sci USA (2012) 109:17537–42. doi: 10.1073/pnas.1202870109
139. Aaby P, Benn CS. Saving Lives by Training Innate Immunity With Bacille Calmette-Guerin Vaccine. Proc Natl Acad Sci USA (2012) 109:17317–8. doi: 10.1073/pnas.1215761109
140. Dye C. Making Wider Use of the World’s Most Widely Used Vaccine: Bacille Calmette-Guerin Revaccination Reconsidered. J R Soc Interface (2013) 10:20130365. doi: 10.1098/rsif.2013.0365
141. Iglesias MJ, Martin C. Editorial Commentary: Nonspecific Beneficial Effects of BCG Vaccination in High-Income Countries, Should We Extend Recommendation of BCG Vaccination? Clin Infect Dis (2015) 60:1620–1. doi: 10.1093/cid/civ148
142. Bickett TE, McLean J, Creissen E, Izzo L, Hagan C, Izzo AJ, et al. Characterizing the BCG Induced Macrophage and Neutrophil Mechanisms for Defense Against Mycobacterium Tuberculosis. Front Immunol (2020) 11:1202. doi: 10.3389/fimmu.2020.01202
143. Cirovic B, de Bree LCJ, Groh L, Blok BA, Chan J, van der Velden W, et al. BCG Vaccination in Humans Elicits Trained Immunity via the Hematopoietic Progenitor Compartment. Cell Host Microbe (2020) 28:322–34.e325. doi: 10.1016/j.chom.2020.05.014
144. Nieuwenhuizen NE, Kaufmann SHE. Next-Generation Vaccines Based on Bacille Calmette-Guérin. Front Immunol (2018) 9:121. doi: 10.3389/fimmu.2018.00121
145. Lyadova IV. Neutrophils in Tuberculosis: Heterogeneity Shapes the Way? Mediators Inflamm (2017) 2017:8619307. doi: 10.1155/2017/8619307
146. Kolls JK, Khader SA. The Role of Th17 Cytokines in Primary Mucosal Immunity. Cytokine Growth Factor Rev (2010) 21:443–8. doi: 10.1016/j.cytogfr.2010.11.002
147. Ye P, Rodriguez FH, Kanaly S, Stocking KL, Schurr J, Schwarzenberger P, et al. Requirement of Interleukin 17 Receptor Signaling for Lung CXC Chemokine and Granulocyte Colony-Stimulating Factor Expression, Neutrophil Recruitment, and Host Defense. J Exp Med (2001) 194:519–27. doi: 10.1084/jem.194.4.519
148. Hu S, He W, Du X, Yang J, Wen Q, Zhong XP, et al. IL-17 Production of Neutrophils Enhances Antibacteria Ability But Promotes Arthritis Development During Mycobacterium Tuberculosis Infection. EBioMedicine (2017) 23:88–99. doi: 10.1016/j.ebiom.2017.08.001
149. Griffin GK, Newton G, Tarrio ML, Bu DX, Maganto-Garcia E, Azcutia V, et al. IL-17 and TNF-α Sustain Neutrophil Recruitment During Inflammation Through Synergistic Effects on Endothelial Activation. J Immunol (2012) 188:6287–99. doi: 10.4049/jimmunol.1200385
150. Lombard R, Doz E, Carreras F, Epardaud M, Le Vern Y, Buzoni-Gatel D, et al. IL-17RA in Non-Hematopoietic Cells Controls CXCL-1 and 5 Critical to Recruit Neutrophils to the Lung of Mycobacteria-Infected Mice During the Adaptive Immune Response. PloS One (2016) 11:e0149455. doi: 10.1371/journal.pone.0149455
151. Monin L, Griffiths KL, Slight S, Lin Y, Rangel-Moreno J, Khader SA. Immune Requirements for Protective Th17 Recall Responses to Mycobacterium Tuberculosis Challenge. Mucosal Immunol (2015) 8:1099–109. doi: 10.1038/mi.2014.136
152. Chen F, Cao A, Yao S, Evans-Marin HL, Liu H, Wu W, et al. mTOR Mediates IL-23 Induction of Neutrophil IL-17 and IL-22 Production. J Immunol (2016) 196:4390–9. doi: 10.4049/jimmunol.1501541
153. Oliveira FM, Trentini MM, Junqueira-Kipnis AP, Kipnis A. The Mc2-CMX Vaccine Induces an Enhanced Immune Response Against Mycobacterium Tuberculosis Compared to Bacillus Calmette-Guérin But With Similar Lung Inflammatory Effects. Mem Inst Oswaldo Cruz (2016) 111:223–31. doi: 10.1590/0074-02760150411
154. Trentini MM, de Oliveira FM, Kipnis A, Junqueira-Kipnis AP. The Role of Neutrophils in the Induction of Specific Th1 and Th17 During Vaccination Against Tuberculosis. Front Microbiol (2016) 7:898. doi: 10.3389/fmicb.2016.00898
155. Lavin Y, Mortha A, Rahman A, Merad M. Regulation of Macrophage Development and Function in Peripheral Tissues. Nat Rev Immunol (2015) 15:731–44. doi: 10.1038/nri3920
156. Kumar V. Phagocytosis: Phenotypically Simple Yet a Mechanistically Complex Process. Int Rev Immunol (2020) 39:118–50. doi: 10.1080/08830185.2020.1732958
157. Gül N, van Egmond M. Antibody-Dependent Phagocytosis of Tumor Cells by Macrophages: A Potent Effector Mechanism of Monoclonal Antibody Therapy of Cancer. Cancer Res (2015) 75:5008–13. doi: 10.1158/0008-5472.Can-15-1330
158. Yeap WH, Wong KL, Shimasaki N, Teo EC, Quek JK, Yong HX, et al. CD16 Is Indispensable for Antibody-Dependent Cellular Cytotoxicity by Human Monocytes. Sci Rep (2016) 6:34310. doi: 10.1038/srep34310
159. Rivas-Santiago B, Wong KL, Shimasaki N, Teo EC, Quek JK, Yong HX, et al. Expression of Cathelicidin LL-37 During Mycobacterium Tuberculosis Infection in Human Alveolar Macrophages, Monocytes, Neutrophils, and Epithelial Cells. Infect Immun (2008) 76:935–41. doi: 10.1128/iai.01218-07
160. Li Y, Wang Y, Liu X. The Role of Airway Epithelial Cells in Response to Mycobacteria Infection. Clin Dev Immunol (2012) 2012:791392. doi: 10.1155/2012/791392
161. Lerner TR, Borel S, Gutierrez MG. The Innate Immune Response in Human Tuberculosis. Cell Microbiol (2015) 17:1277–85. doi: 10.1111/cmi.12480
162. Kleinnijenhuis J, Oosting M, Joosten LAB, Netea MG, Van Crevel R. Innate Immune Recognition of Mycobacterium Tuberculosis. Clin Dev Immunol (2011) 2011:405310. doi: 10.1155/2011/405310
163. Brooks MN, Rajaram MV, Azad AK, Amer AO, Valdivia-Arenas MA, Park JH, et al. NOD2 Controls the Nature of the Inflammatory Response and Subsequent Fate of Mycobacterium Tuberculosis and M. Bovis BCG in Human Macrophages. Cell Microbiol (2011) 13:402–18. doi: 10.1111/j.1462-5822.2010.01544.x
164. Netea MG, Domínguez-Andrés J, Barreiro LB, Chavakis T, Divangahi M, Fuchs E, et al. Defining Trained Immunity and its Role in Health and Disease. Nat Rev Immunol (2020) 20:375–88. doi: 10.1038/s41577-020-0285-6
165. Mills CD, Kincaid K, Alt JM, Heilman MJ, Hill AM. M-1/M-2 Macrophages and the Th1/Th2 Paradigm. J Immunol (2000) 164:6166–73. doi: 10.4049/jimmunol.164.12.6166
166. Xue J, Schmidt SV, Sander J, Draffehn A, Krebs W, Quester I, et al. Transcriptome-Based Network Analysis Reveals a Spectrum Model of Human Macrophage Activation. Immunity (2014) 40:274–88. doi: 10.1016/j.immuni.2014.01.006
167. Guilliams M, Mildner A, Yona S. Developmental and Functional Heterogeneity of Monocytes. Immunity (2018) 49:595–613. doi: 10.1016/j.immuni.2018.10.005
168. Bonnardel J, Guilliams M. Developmental Control of Macrophage Function. Curr Opin Immunol (2018) 50:64–74. doi: 10.1016/j.coi.2017.12.001
169. O’Neill LA, Kishton RJ, Rathmell J. A Guide to Immunometabolism for Immunologists. Nat Rev Immunol (2016) 16:553–65. doi: 10.1038/nri.2016.70
170. Leemans JC, Thepen T, Weijer S, Florquin S, van Rooijen N, van de Winkel JG, et al. Macrophages Play a Dual Role During Pulmonary Tuberculosis in Mice. J Infect Dis (2005) 191:65–74. doi: 10.1086/426395
171. Scordo JM, Knoell DL, Torrelles JB. Alveolar Epithelial Cells in Mycobacterium Tuberculosis Infection: Active Players or Innocent Bystanders? J Innate Immun (2016) 8:3–14. doi: 10.1159/000439275
172. Queval CJ, Brosch R, Simeone R. The Macrophage: A Disputed Fortress in the Battle Against Mycobacterium Tuberculosis. Front Microbiol (2017) 8:2284. doi: 10.3389/fmicb.2017.02284
173. Zuñiga J, Torres-García D, Santos-Mendoza T, Rodriguez-Reyna TS, Granados J, Yunis EJ. Cellular and Humoral Mechanisms Involved in the Control of Tuberculosis. Clin Dev Immunol (2012) 2012:193923. doi: 10.1155/2012/193923
174. Upadhyay S, Mittal E, Philips JA. Tuberculosis and the Art of Macrophage Manipulation. Pathog Dis (2018) 76. doi: 10.1093/femspd/fty037
175. Verrall AJ, Netea MG, Alisjahbana B, Hill PC, van Crevel R. Early Clearance of Mycobacterium Tuberculosis: A New Frontier in Prevention. Immunology (2014) 141:506–13. doi: 10.1111/imm.12223
176. Neyrolles O, Wolschendorf F, Mitra A, Niederweis M. Mycobacteria, Metals, and the Macrophage. Immunol Rev (2015) 264:249–63. doi: 10.1111/imr.12265
177. Botella H, Peyron P, Levillain F, Poincloux R, Poquet Y, Brandli I, et al. Mycobacterial P(1)-Type ATPases Mediate Resistance to Zinc Poisoning in Human Macrophages. Cell Host Microbe (2011) 10:248–59. doi: 10.1016/j.chom.2011.08.006
178. Lin PL, Myers A, Smith L, Bigbee C, Bigbee M, Fuhrman C, et al. Tumor Necrosis Factor Neutralization Results in Disseminated Disease in Acute and Latent Mycobacterium Tuberculosis Infection With Normal Granuloma Structure in a Cynomolgus Macaque Model. Arthritis Rheum (2010) 62:340–50. doi: 10.1002/art.27271
179. Lin PL, Plessner HL, Voitenok NN, Flynn JL. Tumor Necrosis Factor and Tuberculosis. J Invest Dermatol Symposium Proc (2007) 12:22–5. doi: 10.1038/sj.jidsymp.5650027
180. Dobler CC, Schlossberg D. Biologic Agents and Tuberculosis. Microbiol Spectr (2016) 4:4.6.49. doi: 10.1128/microbiolspec.TNMI7-0026-2016
181. Lee J, Kornfeld H. Interferon-γ Regulates the Death of M. Tuberculosis-Infected Macrophages. J Cell Death (2010) 3:1–11. doi: 10.4137/jcd.s2822
182. Gröschel MI, Sayes F, Simeone R, Majlessi L, Brosch R. ESX Secretion Systems: Mycobacterial Evolution to Counter Host Immunity. Nat Rev Microbiol (2016) 14:677–91. doi: 10.1038/nrmicro.2016.131
183. Gou X, Pan L, Tang F, Gao H, Xiao D. The Association Between Vitamin D Status and Tuberculosis in Children: A Meta-Analysis. Med (Baltimore) (2018) 97:e12179. doi: 10.1097/md.0000000000012179
184. Yuk JM, Shin DM, Lee HM, Yang CS, Jin HS, Kim KK, et al. Vitamin D3 Induces Autophagy in Human Monocytes/Macrophages via Cathelicidin. Cell Host Microbe (2009) 6:231–43. doi: 10.1016/j.chom.2009.08.004
185. Kim JH, Park JS, Cho YJ, Yoon HI, Song JH, Lee CT, et al. Low Serum 25-Hydroxyvitamin D Level: An Independent Risk Factor for Tubercxulosis? Clin Nutr (2014) 33:1081–6. doi: 10.1016/j.clnu.2013.11.014
186. Wilkinson RJ, Llewelyn M, Toossi Z, Patel P, Pasvol G, Lalvani A, et al. Influence of Vitamin D Deficiency and Vitamin D Receptor Polymorphisms on Tuberculosis Among Gujarati Asians in West London: A Case-Control Study. Lancet (2000) 355:618–21. doi: 10.1016/s0140-6736(99)02301-6
187. Ho-Pham LT, Nguyen ND, Nguyen TT, Nguyen DH, Bui PK, Nguyen VN, et al. Association Between Vitamin D Insufficiency and Tuberculosis in a Vietnamese Population. BMC Infect Dis (2010) 10:306. doi: 10.1186/1471-2334-10-306
188. Hong JY, Kim SY, Chung KS, Kim EY, Jung JY, Park MS, et al. Association Between Vitamin D Deficiency and Tuberculosis in a Korean Population. Int J Tuberc Lung Dis (2014) 18:73–8. doi: 10.5588/ijtld.13.0536
189. Nnoaham KE, Clarke A. Low Serum Vitamin D Levels and Tuberculosis: A Systematic Review and Meta-Analysis. Int J Epidemiol (2008) 37:113–9. doi: 10.1093/ije/dym247
190. Zeng J, Wu G, Yang W, Gu X, Liang W, Yao Y, et al. A Serum Vitamin D Level <25nmol/L Pose High Tuberculosis Risk: A Meta-Analysis. PloS One (2015) 10:e0126014. doi: 10.1371/journal.pone.0126014
191. Srinivasan L, Ahlbrand S, Briken V. Interaction of Mycobacterium Tuberculosis With Host Cell Death Pathways. Cold Spring Harb Perspect Med (2014) 4. doi: 10.1101/cshperspect.a022459
192. Lam A, Prabhu R, Gross CM, Riesenberg LA, Singh V, Aggarwal S. Role of Apoptosis and Autophagy in Tuberculosis. Am J Physiol Lung Cell Mol Physiol (2017) 313:L218–l229. doi: 10.1152/ajplung.00162.2017
193. Li F, Gao B, Xu W, Chen L, Xiong S. The Defect in Autophagy Induction by Clinical Isolates of Mycobacterium Tuberculosis Is Correlated With Poor Tuberculosis Outcomes. PloS One (2016) 11:e0147810. doi: 10.1371/journal.pone.0147810
194. Velmurugan K, Chen B, Miller JL, Azogue S, Gurses S, Hsu T, et al. Mycobacterium Tuberculosis nuoG Is a Virulence Gene That Inhibits Apoptosis of Infected Host Cells. PloS Pathog (2007) 3:e110. doi: 10.1371/journal.ppat.0030110
195. Gröschel MI, Sayes F, Shin SJ, Frigui W, Pawlik A, Orgeur M, et al. Recombinant BCG Expressing ESX-1 of Mycobacterium Marinum Combines Low Virulence With Cytosolic Immune Signaling and Improved TB Protection. Cell Rep (2017) 18:2752–65. doi: 10.1016/j.celrep.2017.02.057
196. Aguilo JI, Alonso H, Uranga S, Marinova D, Arbués A, de Martino A, et al. ESX-1-Induced Apoptosis Is Involved in Cell-to-Cell Spread of Mycobacterium Tuberculosis. Cell Microbiol (2013) 15:1994–2005. doi: 10.1111/cmi.12169
197. Cohen SB, Gern BH, Delahaye JL, Adams KN, Plumlee CR, Winkler JK, et al. Alveolar Macrophages Provide an Early Mycobacterium Tuberculosis Niche and Initiate Dissemination. Cell Host Microbe (2018) 24:439–46.e434. doi: 10.1016/j.chom.2018.08.001
198. Rothchild AC, Olson GS, Nemeth J, Amon LM, Mai D, Gold ES, et al. Alveolar Macrophages Generate a Noncanonical NRF2-Driven Transcriptional Response to Mycobacterium Tuberculosis In Vivo. Sci Immunol (2019) 4:eaaw6693. doi: 10.1126/sciimmunol.aaw6693
199. Huang L, Nazarova EV, Russell DG. Mycobacterium Tuberculosis: Bacterial Fitness Within the Host Macrophage. Microbiol Spectr (2019) 7. doi: 10.1128/microbiolspec.BAI-0001-2019
200. Kleinnijenhuis J, Quintin J, Preijers F, Benn CS, Joosten LA, Jacobs C, et al. Long-Lasting Effects of BCG Vaccination on Both Heterologous Th1/Th17 Responses and Innate Trained Immunity. J Innate Immun (2014) 6:152–8. doi: 10.1159/000355628
201. da Costa A, Nogueira S, Kipnis A, Junqueira-Kipnis AP. Recombinant BCG: Innovations on an Old Vaccine. Scope of BCG Strains and Strategies to Improve Long-Lasting Memory. Front Immunol (2014) 5:152. doi: 10.3389/fimmu.2014.00152
202. Whitlow E, Mustafa AS, Hanif SNM. An Overview of the Development of New Vaccines for Tuberculosis. Vaccines (2020) 8:586. doi: 10.3390/vaccines8040586
203. Osborne SE, Brumell JH. Listeriolysin O: From Bazooka to Swiss Army Knife. Philos Trans R Soc Lond B Biol Sci (2017) 372. doi: 10.1098/rstb.2016.0222
204. Hoft DF, Quintin J, Preijers F, Benn CS, Joosten LA, Jacobs C, et al. A New Recombinant Bacille Calmette-Guérin Vaccine Safely Induces Significantly Enhanced Tuberculosis-Specific Immunity in Human Volunteers. J Infect Dis (2008) 198:1491–501. doi: 10.1086/592450
205. Khalili N, Mohammadzadeh I, Khalili N, Heredia RJ, Zoghi S, Boztug K, et al. BCGitis as the Primary Manifestation of Chronic Granulomatous Disease. IDCases (2021) 23:e01038. doi: 10.1016/j.idcr.2020.e01038
206. Fekrvand S, Yazdani R, Olbrich P, Gennery A, Rosenzweig SD, Condino-Neto A, et al. Primary Immunodeficiency Diseases and Bacillus Calmette-Guérin (BCG)-Vaccine-Derived Complications: A Systematic Review. J Allergy Clin Immunol Pract (2020) 8:1371–86. doi: 10.1016/j.jaip.2020.01.038
207. Coffman RL, Sher A, Seder RA. Vaccine Adjuvants: Putting Innate Immunity to Work. Immunity (2010) 33:492–503. doi: 10.1016/j.immuni.2010.10.002
208. Duthie MS, Windish HP, Fox CB, Reed SG. Use of Defined TLR Ligands as Adjuvants Within Human Vaccines. Immunol Rev (2011) 239:178–96. doi: 10.1111/j.1600-065X.2010.00978.x
209. Roordink D, Williams A, Fritzell B, Laddy DJ, Gerdil E, Graffin AM, et al. The TB Vaccine Development Pathway – An Innovative Approach to Accelerating Global TB Vaccine Development. Tuberculosis (2021) 126:102040. doi: 10.1016/j.tube.2020.102040
210. Garçon N, Van Mechelen M. Recent Clinical Experience With Vaccines Using MPL- and QS-21-Containing Adjuvant Systems. Expert Rev Vaccines (2011) 10:471–86. doi: 10.1586/erv.11.29
211. Baldwin SL, Reese V, Granger B, Orr MT, Ireton GC, Coler RN, et al. The ID93 Tuberculosis Vaccine Candidate Does Not Induce Sensitivity to Purified Protein Derivative. Clin Vaccine Immunol (2014) 21:1309–13. doi: 10.1128/CVI.00372-14
212. Orr MT, Beebe EA, Hudson TE, Moon JJ, Fox CB, Reed SG, et al. A Dual TLR Agonist Adjuvant Enhances the Immunogenicity and Protective Efficacy of the Tuberculosis Vaccine Antigen ID93. PloS One (2014) 9:e83884. doi: 10.1371/journal.pone.0083884
213. Dubois Cauwelaert N, Desbien AL, Hudson TE, Pine SO, Reed SG, Coler RN, et al. The TLR4 Agonist Vaccine Adjuvant, GLA-SE, Requires Canonical and Atypical Mechanisms of Action for TH1 Induction. PloS One (2016) 11:e0146372. doi: 10.1371/journal.pone.0146372
214. Orr MT, Duthie MS, Windish HP, Lucas EA, Guderian JA, Hudson TE, et al. MyD88 and TRIF Synergistic Interaction Is Required for TH1-Cell Polarization With a Synthetic TLR4 Agonist Adjuvant. Eur J Immunol (2013) 43:2398–408. doi: 10.1002/eji.201243124
215. Perez-Martinez AP, Ong E, Zhang L, Marrs CF, He Y, Yang Z. Conservation in Gene Encoding Mycobacterium Tuberculosis Antigen Rv2660 and a High Predicted Population Coverage of H56 Multistage Vaccine in South Africa. Infect Genet Evol (2017) 55:244–50. doi: 10.1016/j.meegid.2017.09.023
216. Agger EM, Rosenkrands I, Olsen AW, Hatch G, Williams A, Kritsch C, et al. Protective Immunity to Tuberculosis With Ag85B-ESAT-6 in a Synthetic Cationic Adjuvant System IC31. Vaccine (2006) 24:5452–60. doi: 10.1016/j.vaccine.2006.03.072
217. Kamath AT, Rochat AF, Valenti MP, Agger EM, Lingnau K, Andersen P, et al. Adult-Like Anti-Mycobacterial T Cell and In Vivo Dendritic Cell Responses Following Neonatal Immunization With Ag85B-ESAT-6 in the IC31 Adjuvant. PloS One (2008) 3:e3683. doi: 10.1371/journal.pone.0003683
218. Kamath AT, Valenti MP, Rochat AF, Agger EM, Lingnau K, von Gabain A, et al. Protective Anti-Mycobacterial T Cell Responses Through Exquisite In Vivo Activation of Vaccine-Targeted Dendritic Cells. Eur J Immunol (2008) 38:1247–56. doi: 10.1002/eji.200737889
219. Abdallah AM, Gey van Pittius NC, Champion PA, Cox J, Luirink J, Vandenbroucke-Grauls CM, et al. Type VII Secretion–Mycobacteria Show the Way. Nat Rev Microbiol (2007) 5:883–91. doi: 10.1038/nrmicro1773
220. Dong H, Jing W, Runpeng Z, Xuewei X, Min M, Ru C, et al. ESAT6 Inhibits Autophagy Flux and Promotes BCG Proliferation Through MTOR. Biochem Biophys Res Commun (2016) 477:195–201. doi: 10.1016/j.bbrc.2016.06.042
221. Peng X, Sun J. Mechanism of ESAT-6 Membrane Interaction and Its Roles in Pathogenesis of Mycobacterium Tuberculosis. Toxicon (2016) 116:29–34. doi: 10.1016/j.toxicon.2015.10.003
222. Wong KW. The Role of ESX-1 in Mycobacterium Tuberculosis Pathogenesis. Microbiol Spectr (2017) 5. doi: 10.1128/microbiolspec.TBTB2-0001-2015
223. Ning H, Zhang W, Kang J, Ding T, Liang X, Lu Y, et al. Subunit Vaccine ESAT-6:C-Di-AMP Delivered by Intranasal Route Elicits Immune Responses and Protects Against Mycobacterium Tuberculosis Infection. Front Cell Infect Microbiol (2021) 11:647220. doi: 10.3389/fcimb.2021.647220
224. Sheedy FJ, Divangahi M. Targeting Immunometabolism in Host Defence Against Mycobacterium Tuberculosis. Immunology (2021) 162:145–59. doi: 10.1111/imm.13276
225. Dara Y, Volcani D, Shah K, Shin K, Venketaraman V. Potentials of Host-Directed Therapies in Tuberculosis Management. J Clin Med (2019) 8:1166. doi: 10.3390/jcm8081166
226. Dube A, Lemmer Y, Hayeshi R, Balogun M, Labuschagne P, Swai H, et al. State of the Art and Future Directions in Nanomedicine for Tuberculosis. Expert Opin Drug Deliv (2013) 10:1725–34. doi: 10.1517/17425247.2014.846905
227. Tukulula M, Gouveia L, Paixao P, Hayeshi R, Naicker B, Dube A. Functionalization of PLGA Nanoparticles With 1,3-β-Glucan Enhances the Intracellular Pharmacokinetics of Rifampicin in Macrophages. Pharm Res (2018) 35:111. doi: 10.1007/s11095-018-2391-8
228. Grabowski N, Hillaireau H, Vergnaud J, Santiago LA, Kerdine-Romer S, Pallardy M, et al. Toxicity of Surface-Modified PLGA Nanoparticles Toward Lung Alveolar Epithelial Cells. Int J Pharm (2013) 454:686–94. doi: 10.1016/j.ijpharm.2013.05.025
229. Bekale RB, Du Plessis SM, Hsu NJ, Sharma JR, Sampson SL, Jacobs M, et al. Mycobacterium Tuberculosis and Interactions With the Host Immune System: Opportunities for Nanoparticle Based Immunotherapeutics and Vaccines. Pharm Res (2018) 36:8. doi: 10.1007/s11095-018-2528-9
230. Gupta A, Pant G, Mitra K, Madan J, Chourasia MK, Misra A. Inhalable Particles Containing Rapamycin for Induction of Autophagy in Macrophages Infected With Mycobacterium Tuberculosis. Mol Pharm (2014) 11:1201–7. doi: 10.1021/mp4006563
231. Divangahi M, Desjardins D, Nunes-Alves C, Remold HG, Behar SM. Eicosanoid Pathways Regulate Adaptive Immunity to Mycobacterium Tuberculosis. Nat Immunol (2010) 11:751–8. doi: 10.1038/ni.1904
232. Upadhyay TK, Fatima N, Sharma A, Sharma D, Sharma R. Nano-Rifabutin Entrapment Within Glucan Microparticles Enhances Protection Against Intracellular Mycobacterium Tuberculosis. Artif Cells Nanomed Biotechnol (2019) 47:427–35. doi: 10.1080/21691401.2018.1559180
233. Banchereau J, Steinman RM. Dendritic Cells and the Control of Immunity. Nature (1998) 392:245–52. doi: 10.1038/32588
234. Steinman RM. The Dendritic Cell System and Its Role in Immunogenicity. Annu Rev Immunol (1991) 9:271–96. doi: 10.1146/annurev.iy.09.040191.001415
235. ten Broeke T, Wubbolts R, Stoorvogel W. MHC Class II Antigen Presentation by Dendritic Cells Regulated Through Endosomal Sorting. Cold Spring Harb Perspect Biol (2013) 5:a016873. doi: 10.1101/cshperspect.a016873
236. Guermonprez P, Valladeau J, Zitvogel L, Théry C, Amigorena S. Antigen Presentation and T Cell Stimulation by Dendritic Cells. Annu Rev Immunol (2002) 20:621–67. doi: 10.1146/annurev.immunol.20.100301.064828
237. Cresswell P, Ackerman AL, Giodini A, Peaper DR, Wearsch PA. Mechanisms of MHC Class I-Restricted Antigen Processing and Cross-Presentation. Immunol Rev (2005) 207:145–57. doi: 10.1111/j.0105-2896.2005.00316.x
238. Shortman K, Liu YJ. Mouse and Human Dendritic Cell Subtypes. Nat Rev Immunol (2002) 2:151–61. doi: 10.1038/nri746
239. Shortman K, Naik SH. Steady-State and Inflammatory Dendritic-Cell Development. Nat Rev Immunol (2007) 7:19–30. doi: 10.1038/nri1996
240. Kushwah R, Hu J. Complexity of Dendritic Cell Subsets and Their Function in the Host Immune System. Immunology (2011) 133:409–19. doi: 10.1111/j.1365-2567.2011.03457.x
241. Sichien D, Lambrecht BN, Guilliams M, Scott CL. Development of Conventional Dendritic Cells: From Common Bone Marrow Progenitors to Multiple Subsets in Peripheral Tissues. Mucosal Immunol (2017) 10:831–44. doi: 10.1038/mi.2017.8
242. Guilliams M, Ginhoux F, Jakubzick C, Naik SH, Onai N, Schraml BU, et al. Dendritic Cells, Monocytes and Macrophages: A Unified Nomenclature Based on Ontogeny. Nat Rev Immunol (2014) 14:571–8. doi: 10.1038/nri3712
243. Murphy KM. Transcriptional Control of Dendritic Cell Development. Adv Immunol (2013) 120:239–67. doi: 10.1016/b978-0-12-417028-5.00009-0
244. Sichien D, Scott CL, Martens L, Vanderkerken M, Van Gassen S, Plantinga M, et al. IRF8 Transcription Factor Controls Survival and Function of Terminally Differentiated Conventional and Plasmacytoid Dendritic Cells, Respectively. Immunity (2016) 45:626–40. doi: 10.1016/j.immuni.2016.08.013
245. Carotta S, Dakic A, D'Amico A, Pang SH, Greig KT, Nutt SL, et al. The Transcription Factor PU.1 Controls Dendritic Cell Development and Flt3 Cytokine Receptor Expression in a Dose-Dependent Manner. Immunity (2010) 32:628–41. doi: 10.1016/j.immuni.2010.05.005
246. McKenna K, Beignon AS, Bhardwaj N. Plasmacytoid Dendritic Cells: Linking Innate and Adaptive Immunity. J Virol (2005) 79:17–27. doi: 10.1128/jvi.79.1.17-27.2005
247. Mihret A. The Role of Dendritic Cells in Mycobacterium Tuberculosis Infection. Virulence (2012) 3:654–9. doi: 10.4161/viru.22586
248. Mihret A, Mamo G, Tafesse M, Hailu A, Parida S. Dendritic Cells Activate and Mature After Infection With Mycobacterium Tuberculosis. BMC Res Notes (2011) 4:247. doi: 10.1186/1756-0500-4-247
249. Tailleux L, Schwartz O, Herrmann J-L, Pivert E, Jackson M, Amara A, et al. DC-SIGN Is the Major Mycobacterium Tuberculosis Receptor on Human Dendritic Cells. J Exp Med (2003) 197:121–7. doi: 10.1084/jem.20021468
250. Ramos-Martinez AG, Valtierra-Alvarado MA, Garcia-Hernandez MH, Hernandez-Pando R, Castañeda-Delgado JE, Cougoule C, et al. Variability in the Virulence of Specific Mycobacterium Tuberculosis Clinical Isolates Alters the Capacity of Human Dendritic Cells to Signal for T Cells. Mem Inst Oswaldo Cruz (2019) 114:e190102. doi: 10.1590/0074-02760190102
251. Khader SA, Bell GK, Pearl JE, Fountain JJ, Rangel-Moreno J, Cilley GE, et al. IL-23 and IL-17 in the Establishment of Protective Pulmonary CD4+ T Cell Responses After Vaccination and During Mycobacterium Tuberculosis Challenge. Nat Immunol (2007) 8:369–77. doi: 10.1038/ni1449
252. Griffiths KL, Ahmed M, Das S, Gopal R, Horne W, Connell TD, et al. Targeting Dendritic Cells to Accelerate T-Cell Activation Overcomes a Bottleneck in Tuberculosis Vaccine Efficacy. Nat Commun (2016) 7:13894. doi: 10.1038/ncomms13894
253. Choi HG, Choi S, Back YW, Paik S, Park HS, Kim WS, et al. Rv2299c, a Novel Dendritic Cell-Activating Antigen of Mycobacterium Tuberculosis, Fused-ESAT-6 Subunit Vaccine Confers Improved and Durable Protection Against the Hypervirulent Strain HN878 in Mice. Oncotarget (2017) 8:19947–67. doi: 10.18632/oncotarget.15256
254. Choi S, Choi S, Back YW, Paik S, Park HS, Kim WS, et al. A Dendritic Cell-Activating Rv1876 Protein Elicits Mycobacterium Bovis BCG-Prime Effect via Th1-Immune Response. Biomolecules (2021) 11. doi: 10.3390/biom11091306
255. Back YW, Shin KW, Choi S, Park HS, Lee KI, Choi HG, et al. Mycobacterium Tuberculosis Rv2005c Induces Dendritic Cell Maturation and Th1 Responses and Exhibits Immunotherapeutic Activity by Fusion With the Rv2882c Protein. Vaccines (Basel) (2020) 8. doi: 10.3390/vaccines8030370
256. van den Berg RA, De Mot L, Leroux-Roels G, Bechtold V, Clement F, Coccia M, et al. Adjuvant-Associated Peripheral Blood mRNA Profiles and Kinetics Induced by the Adjuvanted Recombinant Protein Candidate Tuberculosis Vaccine M72/AS01 in Bacillus Calmette-Guérin-Vaccinated Adults. Front Immunol (2018) 9:564. doi: 10.3389/fimmu.2018.00564
257. Schneider LP, Schoonderwoerd AJ, Moutaftsi M, Howard RF, Reed SG, de Jong EC, et al. Intradermally Administered TLR4 Agonist GLA-SE Enhances the Capacity of Human Skin DCs to Activate T Cells and Promotes Emigration of Langerhans Cells. Vaccine (2012) 30:4216–24. doi: 10.1016/j.vaccine.2012.04.051
258. Martino A, Sacchi A, Sanarico N, Spadaro F, Ramoni C, Ciaramella A, et al. Dendritic Cells Derived From BCG-Infected Precursors Induce Th2-Like Immune Response. J Leukoc Biol (2004) 76:827–34. doi: 10.1189/jlb.0703313
259. Kumar NP, Padmapriyadarsini C, Rajamanickam A, Bhavani PK, Nancy A, Jeyadeepa B, et al. BCG Vaccination Induces Enhanced Frequencies of Dendritic Cells and Altered Plasma Levels of Type I and Type III Interferons in Elderly Individuals. Int J Infect Dis (2021) 110:98–104. doi: 10.1016/j.ijid.2021.07.041
260. Zhang Y, Huang B. The Development and Diversity of ILCs, NK Cells and Their Relevance in Health and Diseases. Adv Exp Med Biol (2017) 1024:225–44. doi: 10.1007/978-981-10-5987-2_11
261. Biassoni R, Cantoni C, Marras D, Giron-Michel J, Falco M, Moretta L, et al. Human Natural Killer Cell Receptors: Insights Into Their Molecular Function and Structure. J Cell Mol Med (2003) 7:376–87. doi: 10.1111/j.1582-4934.2003.tb00240.x
262. Moretta L, Biassoni R, Bottino C, Mingari MC, Moretta A. Human NK-Cell Receptors. Immunol Today (2000) 21:420–2. doi: 10.1016/s0167-5699(00)01673-x
263. Walzer T, Dalod M, Robbins SH, Zitvogel L, Vivier E. Natural-Killer Cells and Dendritic Cells: “L’union Fait La Force”. Blood (2005) 106:2252–8. doi: 10.1182/blood-2005-03-1154
264. Lucas M, Schachterle W, Oberle K, Aichele P, Diefenbach A. Dendritic Cells Prime Natural Killer Cells by Trans-Presenting Interleukin 15. Immunity (2007) 26:503–17. doi: 10.1016/j.immuni.2007.03.006
265. Prager I, Watzl C. Mechanisms of Natural Killer Cell-Mediated Cellular Cytotoxicity. J Leukoc Biol (2019) 105:1319–29. doi: 10.1002/jlb.Mr0718-269r
266. Degli-Esposti M. To Die or Not to Die–the Quest of the TRAIL Receptors. J Leukoc Biol (1999) 65:535–42. doi: 10.1002/jlb.65.5.535
267. Lichtenheld MG, Olsen KJ, Lu P, Lowrey DM, Hameed A, Hengartner H, et al. Structure and Function of Human Perforin. Nature (1988) 335:448–51. doi: 10.1038/335448a0
268. Lopez JA, Susanto O, Jenkins MR, Lukoyanova N, Sutton VR, Law RH, et al. Perforin Forms Transient Pores on the Target Cell Plasma Membrane to Facilitate Rapid Access of Granzymes During Killer Cell Attack. Blood (2013) 121:2659–68. doi: 10.1182/blood-2012-07-446146
269. Kurschus FC, Fellows E, Stegmann E, Jenne DE. Granzyme B Delivery via Perforin Is Restricted by Size, But Not by Heparan Sulfate-Dependent Endocytosis. Proc Natl Acad Sci USA (2008) 105:13799–804. doi: 10.1073/pnas.0801724105
270. Trapani JA. Granzymes: A Family of Lymphocyte Granule Serine Proteases. Genome Biol (2001) 2:Reviews3014. doi: 10.1186/gb-2001-2-12-reviews3014
271. Zamai L, Ahmad M, Bennett IM, Azzoni L, Alnemri ES, Perussia B. Natural Killer (NK) Cell-Mediated Cytotoxicity: Differential Use of TRAIL and Fas Ligand by Immature and Mature Primary Human NK Cells. J Exp Med (1998) 188:2375–80. doi: 10.1084/jem.188.12.2375
272. Smyth MJ, Cretney E, Kelly JM, Westwood JA, Street SE, Yagita H, et al. Activation of NK Cell Cytotoxicity. Mol Immunol (2005) 42:501–10. doi: 10.1016/j.molimm.2004.07.034
273. Wiley SR, Schooley K, Smolak PJ, Din WS, Huang CP, Nicholl JK, et al. Identification and Characterization of a New Member of the TNF Family That Induces Apoptosis. Immunity (1995) 3:673–82. doi: 10.1016/1074-7613(95)90057-8
274. Sedger LM, Shows DM, Blanton RA, Peschon JJ, Goodwin RG, Cosman D, et al. IFN-Gamma Mediates a Novel Antiviral Activity Through Dynamic Modulation of TRAIL and TRAIL Receptor Expression. J Immunol (1999) 163:920–6.
275. Chester C, Fritsch K, Kohrt HE. Natural Killer Cell Immunomodulation: Targeting Activating, Inhibitory, and Co-Stimulatory Receptor Signaling for Cancer Immunotherapy. Front Immunol (2015) 6:601. doi: 10.3389/fimmu.2015.00601
276. Crouse J, Xu HC, Lang PA, Oxenius A. NK Cells Regulating T Cell Responses: Mechanisms and Outcome. Trends Immunol (2015) 36:49–58. doi: 10.1016/j.it.2014.11.001
277. Mocikat R, Braumüller H, Gumy A, Egeter O, Ziegler H, Reusch U, et al. Natural Killer Cells Activated by MHC Class I(low) Targets Prime Dendritic Cells to Induce Protective CD8 T Cell Responses. Immunity (2003) 19:561–9. doi: 10.1016/s1074-7613(03)00264-4
278. Gerosa F, Baldani-Guerra B, Nisii C, Marchesini V, Carra G, Trinchieri G. Reciprocal Activating Interaction Between Natural Killer Cells and Dendritic Cells. J Exp Med (2002) 195:327–33. doi: 10.1084/jem.20010938
279. Martín-Fontecha A, Thomsen LL, Brett S, Gerard C, Lipp M, Lanzavecchia A, et al. Induced Recruitment of NK Cells to Lymph Nodes Provides IFN-Gamma for T(H)1 Priming. Nat Immunol (2004) 5:1260–5. doi: 10.1038/ni1138
280. Morandi B, Bougras G, Muller WA, Ferlazzo G, Münz C. NK Cells of Human Secondary Lymphoid Tissues Enhance T Cell Polarization via IFN-Gamma Secretion. Eur J Immunol (2006) 36:2394–400. doi: 10.1002/eji.200636290
281. Laouar Y, Sutterwala FS, Gorelik L, Flavell RA. Transforming Growth Factor-Beta Controls T Helper Type 1 Cell Development Through Regulation of Natural Killer Cell Interferon-Gamma. Nat Immunol (2005) 6:600–7. doi: 10.1038/ni1197
282. Waggoner SN, Taniguchi RT, Mathew PA, Kumar V, Welsh RM. Absence of Mouse 2B4 Promotes NK Cell-Mediated Killing of Activated CD8+ T Cells, Leading to Prolonged Viral Persistence and Altered Pathogenesis. J Clin Invest (2010) 120:1925–38. doi: 10.1172/jci41264
283. Lang PA, Sutterwala FS, Gorelik L, Flavell RA. Natural Killer Cell Activation Enhances Immune Pathology and Promotes Chronic Infection by Limiting CD8+ T-Cell Immunity. Proc Natl Acad Sci USA (2012) 109:1210–5. doi: 10.1073/pnas.1118834109
284. Crouse J, Bedenikovic G, Wiesel M, Ibberson M, Xenarios I, Von Laer D, et al. Type I Interferons Protect T Cells Against NK Cell Attack Mediated by the Activating Receptor NCR1. Immunity (2014) 40:961–73. doi: 10.1016/j.immuni.2014.05.003
285. Vankayalapati R, Garg A, Porgador A, Griffith DE, Klucar P, Safi H, et al. Role of NK Cell-Activating Receptors and Their Ligands in the Lysis of Mononuclear Phagocytes Infected With an Intracellular Bacterium. J Immunol (2005) 175:4611–7. doi: 10.4049/jimmunol.175.7.4611
286. Esin S, Batoni G. Natural Killer Cells: A Coherent Model for Their Functional Role in Mycobacterium Tuberculosis Infection. J Innate Immun (2015) 7:11–24. doi: 10.1159/000363321
287. Uranga S, Marinova D, Martin C, Pardo J, Aguilo N. Granzyme A Is Expressed in Mouse Lungs During Mycobacterium Tuberculosis Infection But Does Not Contribute to Protection In Vivo. PloS One (2016) 11:e0153028. doi: 10.1371/journal.pone.0153028
288. Dhiman R, Indramohan M, Barnes PF, Nayak RC, Paidipally P, Rao LV, et al. IL-22 Produced by Human NK Cells Inhibits Growth of Mycobacterium Tuberculosis by Enhancing Phagolysosomal Fusion. J Immunol (2009) 183:6639–45. doi: 10.4049/jimmunol.0902587
289. Brill KJ, Li Q, Larkin R, Canaday DH, Kaplan DR, Boom WH, et al. Human Natural Killer Cells Mediate Killing of Intracellular Mycobacterium Tuberculosis H37Rv via Granule-Independent Mechanisms. Infect Immun (2001) 69:1755–65. doi: 10.1128/iai.69.3.1755-1765.2001
290. Yoneda T, Ellner JJ. CD4(+) T Cell and Natural Killer Cell-Dependent Killing of Mycobacterium Tuberculosis by Human Monocytes. Am J Respir Crit Care Med (1998) 158:395–403. doi: 10.1164/ajrccm.158.2.9707102
291. Feng CG, Kaviratne M, Rothfuchs AG, Cheever A, Hieny S, Young HA, et al. NK Cell-Derived IFN-Gamma Differentially Regulates Innate Resistance and Neutrophil Response in T Cell-Deficient Hosts Infected With Mycobacterium Tuberculosis. J Immunol (2006) 177:7086–93. doi: 10.4049/jimmunol.177.10.7086
292. Portevin D, Via LE, Eum S, Young D. Natural Killer Cells are Recruited During Pulmonary Tuberculosis and Their Ex Vivo Responses to Mycobacteria Vary Between Healthy Human Donors in Association With KIR Haplotype. Cell Microbiol (2012) 14:1734–44. doi: 10.1111/j.1462-5822.2012.01834.x
293. Makino Y, Kanno R, Ito T, Higashino K, Taniguchi M. Predominant Expression of Invariant V Alpha 14+ TCR Alpha Chain in NK1.1+ T Cell Populations. Int Immunol (1995) 7:1157–61. doi: 10.1093/intimm/7.7.1157
294. Tupin E, Kinjo Y, Kronenberg M. The Unique Role of Natural Killer T Cells in the Response to Microorganisms. Nat Rev Microbiol (2007) 5:405–17. doi: 10.1038/nrmicro1657
295. Brigl M, Brenner MB. CD1: Antigen Presentation and T Cell Function. Annu Rev Immunol (2004) 22:817–90. doi: 10.1146/annurev.immunol.22.012703.104608
296. Ruibal P, Voogd L, Joosten SA, Ottenhoff THM. The Role of Donor-Unrestricted T-Cells, Innate Lymphoid Cells, and NK Cells in Anti-Mycobacterial Immunity. Immunol Rev (2021) 301:30–47. doi: 10.1111/imr.12948
297. Montoya CJ, Cataño JC, Ramirez Z, Rugeles MT, Wilson SB, Landay AL. Invariant NKT Cells From HIV-1 or Mycobacterium Tuberculosis-Infected Patients Express an Activated Phenotype. Clin Immunol (2008) 127:1–6. doi: 10.1016/j.clim.2007.12.006
298. Veenstra H, Baumann R, Carroll NM, Lukey PT, Kidd M, Beyers N, et al. Changes in Leucocyte and Lymphocyte Subsets During Tuberculosis Treatment; Prominence of CD3dimCD56+ Natural Killer T Cells in Fast Treatment Responders. Clin Exp Immunol (2006) 145:252–60. doi: 10.1111/j.1365-2249.2006.03144.x
299. Dhiman R, Periasamy S, Barnes PF, Jaiswal AG, Paidipally P, Barnes AB, et al. NK1.1+ Cells and IL-22 Regulate Vaccine-Induced Protective Immunity Against Challenge With Mycobacterium Tuberculosis. J Immunol (2012) 189:897–905. doi: 10.4049/jimmunol.1102833
300. Chan J, Mehta S, Bharrhan S, Chen Y, Achkar JM, Casadevall A, et al. The Role of B Cells and Humoral Immunity in Mycobacterium Tuberculosis Infection. Semin Immunol (2014) 26:588–600. doi: 10.1016/j.smim.2014.10.005
301. Rijnink WF, Ottenhoff THM, Joosten SA. B-Cells and Antibodies as Contributors to Effector Immune Responses in Tuberculosis. Front Immunol (2021) 12:640168. doi: 10.3389/fimmu.2021.640168
302. Kozakiewicz L, Phuah J, Flynn J, Chan J. The Role of B Cells and Humoral Immunity in Mycobacterium Tuberculosis Infection. Adv Exp Med Biol (2013) 783:225–50. doi: 10.1007/978-1-4614-6111-1_12
303. Lu LL, Chung AW, Rosebrock TR, Ghebremichael M, Yu WH, Grace PS, et al. A Functional Role for Antibodies in Tuberculosis. Cell (2016) 167:433–43.e414. doi: 10.1016/j.cell.2016.08.072
304. Ghosh D, Jiang W, Mukhopadhyay D, Mellins ED. New Insights Into B Cells as Antigen Presenting Cells. Curr Opin Immunol (2021) 70:129–37. doi: 10.1016/j.coi.2021.06.003
305. Dubois Cauwelaert N, Baldwin SL, Orr MT, Desbien AL, Gage E, Hofmeyer KA, et al. Antigen Presentation by B Cells Guides Programing of Memory CD4(+) T-Cell Responses to a TLR4-Agonist Containing Vaccine in Mice. Eur J Immunol (2016) 46:2719–29. doi: 10.1002/eji.201646399
306. Misumi I, Whitmire JK. B Cell Depletion Curtails CD4+ T Cell Memory and Reduces Protection Against Disseminating Virus Infection. J Immunol (2014) 192:1597–608. doi: 10.4049/jimmunol.1302661
307. Whitmire JK, Asano MS, Kaech SM, Sarkar S, Hannum LG, Shlomchik MJ, et al. Requirement of B Cells for Generating CD4+ T Cell Memory. J Immunol (2009) 182:1868–76. doi: 10.4049/jimmunol.0802501
308. Matsuzaki G, Vordermeier HM, Hashimoto A, Nomoto K, Ivanyi J. The Role of B Cells in the Establishment of T Cell Response in Mice Infected With an Intracellular Bacteria, Listeria Monocytogenes. Cell Immunol (1999) 194:178–85. doi: 10.1006/cimm.1999.1503
309. Elkins KL, Bosio CM, Rhinehart-Jones TR. Importance of B Cells, But Not Specific Antibodies, in Primary and Secondary Protective Immunity to the Intracellular Bacterium Francisella Tularensis Live Vaccine Strain. Infect Immun (1999) 67:6002–7. doi: 10.1128/iai.67.11.6002-6007.1999
310. Vordermeier HM, Venkataprasad N, Harris DP, Ivanyi J. Increase of Tuberculous Infection in the Organs of B Cell-Deficient Mice. Clin Exp Immunol (1996) 106:312–6. doi: 10.1046/j.1365-2249.1996.d01-845.x
311. Turner J, Frank AA, Brooks JV, Gonzalez-Juarrero M, Orme IM. The Progression of Chronic Tuberculosis in the Mouse Does Not Require the Participation of B Lymphocytes or Interleukin-4. Exp Gerontol (2001) 36:537–45. doi: 10.1016/s0531-5565(00)00257-6
312. Bosio CM, Gardner D, Elkins KL. Infection of B Cell-Deficient Mice With CDC 1551, a Clinical Isolate of Mycobacterium Tuberculosis: Delay in Dissemination and Development of Lung Pathology. J Immunol (2000) 164:6417–25. doi: 10.4049/jimmunol.164.12.6417
313. Maglione PJ, Xu J, Chan J. B Cells Moderate Inflammatory Progression and Enhance Bacterial Containment Upon Pulmonary Challenge With Mycobacterium Tuberculosis. J Immunol (2007) 178:7222–34. doi: 10.4049/jimmunol.178.11.7222
314. Correia-Neves M, Sundling C, Cooper A, Källenius G. Lipoarabinomannan in Active and Passive Protection Against Tuberculosis. Front Immunol (2019) 10:1968. doi: 10.3389/fimmu.2019.01968
315. Aguilo N, Uranga S, Mata E, Tarancon R, Gómez AB, Marinova D, et al. Respiratory Immunization With a Whole Cell Inactivated Vaccine Induces Functional Mucosal Immunoglobulins Against Tuberculosis in Mice and Non-Human Primates. Front Microbiol (2020) 11:1339. doi: 10.3389/fmicb.2020.01339
316. Hypogammaglobulinaemia in the United Kingdom. Summary Report of a Medical Research Council Working-Party. Lancet (1969) 1:163–8.
317. Grace PS, Dolatshahi S, Lu LL, Cain A, Palmieri F, Petrone L, et al. Antibody Subclass and Glycosylation Shift Following Effective TB Treatment. Front Immunol (2021) 12:679973. doi: 10.3389/fimmu.2021.679973
318. Li H, Javid B. Antibodies and Tuberculosis: Finally Coming of Age? Nat Rev Immunol (2018) 18:591–6. doi: 10.1038/s41577-018-0028-0
319. Janeway CA Jr, Travers P, Walport M, et al. Immunobiology: The Immune System in Health and Disease. 5th Eddition. New York: Garland Science (2001).
320. Voss K, Larsen SE, Snow AL. Metabolic Reprogramming and Apoptosis Sensitivity: Defining the Contours of a T Cell Response. Cancer Lett (2017) 408:190–6. doi: 10.1016/j.canlet.2017.08.033
321. Larsen SE, Bilenkin A, Tarasenko TN, Arjunaraja S, Stinson JR, McGuire PJ, et al. Sensitivity to Restimulation-Induced Cell Death Is Linked to Glycolytic Metabolism in Human T Cells. J Immunol (2017) 198:147–55. doi: 10.4049/jimmunol.1601218
322. Larsen SE, Voss K, Laing ED, Snow AL. Differential Cytokine Withdrawal-Induced Death Sensitivity of Effector T Cells Derived From Distinct Human CD8(+) Memory Subsets. Cell Death Discov (2017) 3:17031. doi: 10.1038/cddiscovery.2017.31
323. Muroyama Y, Wherry EJ. Memory T-Cell Heterogeneity and Terminology. Cold Spring Harb Perspect Biol (2021) 13. doi: 10.1101/cshperspect.a037929
324. Kamath AB, Woodworth J, Xiong X, Taylor C, Weng Y, Behar SM. Cytolytic CD8+ T Cells Recognizing CFP10 Are Recruited to the Lung After Mycobacterium Tuberculosis Infection. J Exp Med (2004) 200:1479–89. doi: 10.1084/jem.20041690
325. Jeyanathan M, Mu J, McCormick S, Damjanovic D, Small CL, Shaler CR, et al. Murine Airway Luminal Antituberculosis Memory CD8 T Cells by Mucosal Immunization Are Maintained via Antigen-Driven In Situ Proliferation, Independent of Peripheral T Cell Recruitment. Am J Respir Crit Care Med (2010) 181:862–72. doi: 10.1164/rccm.200910-1583OC
326. Smith SM, Klein MR, Malin AS, Sillah J, Huygen K, Andersen P, et al. Human CD8(+) T Cells Specific for Mycobacterium Tuberculosis Secreted Antigens in Tuberculosis Patients and Healthy BCG-Vaccinated Controls in The Gambia. Infect Immun (2000) 68:7144–8. doi: 10.1128/iai.68.12.7144-7148.2000
327. Cho S, Mehra V, Thoma-Uszynski S, Stenger S, Serbina N, Mazzaccaro RJ, et al. Antimicrobial Activity of MHC Class I-Restricted CD8+ T Cells in Human Tuberculosis. Proc Natl Acad Sci (2000) 97:12210–5. doi: 10.1073/pnas.210391497
328. Serbina NV, Liu CC, Scanga CA, Flynn JL. CD8+ CTL From Lungs of Mycobacterium Tuberculosis-Infected Mice Express Perforin In Vivo and Lyse Infected Macrophages. J Immunol (2000) 165:353–63. doi: 10.4049/jimmunol.165.1.353
329. Serbina NV, Flynn JL. CD8(+) T Cells Participate in the Memory Immune Response to Mycobacterium Tuberculosis. Infect Immun (2001) 69:4320–8. doi: 10.1128/iai.69.7.4320-4328.2001
330. Brookes RH, Pathan AA, McShane H, Hensmann M, Price DA, Hill AV. CD8+ T Cell-Mediated Suppression of Intracellular Mycobacterium Tuberculosis Growth in Activated Human Macrophages. Eur J Immunol (2003) 33:3293–302. doi: 10.1002/eji.200324109
331. Lazarevic V, Flynn J. CD8+ T Cells in Tuberculosis. Am J Respir Crit Care Med (2002) 166:1116–21. doi: 10.1164/rccm.2204027
332. Tascon RE, Stavropoulos E, Lukacs KV, Colston MJ. Protection Against Mycobacterium Tuberculosis Infection by CD8+ T Cells Requires the Production of Gamma Interferon. Infect Immun (1998) 66:830–4. doi: 10.1128/iai.66.2.830-834.1998
333. Müller I, Cobbold SP, Waldmann H, Kaufmann SH. Impaired Resistance to Mycobacterium Tuberculosis Infection After Selective In Vivo Depletion of L3T4+ and Lyt-2+ T Cells. Infect Immun (1987) 55:2037–41. doi: 10.1128/iai.55.9.2037-2041.1987
334. Orme IM, Collins FM. Adoptive Protection of the Mycobacterium Tuberculosis-Infected Lung. Dissociation Between Cells That Passively Transfer Protective Immunity and Those That Transfer Delayed-Type Hypersensitivity to Tuberculin. Cell Immunol (1984) 84:113–20. doi: 10.1016/0008-8749(84)90082-0
335. Flynn JL, Chan J. Immunology of Tuberculosis. Annu Rev Immunol (2001) 19:93–129. doi: 10.1146/annurev.immunol.19.1.93
336. van Pinxteren LA, Cassidy JP, Smedegaard BH, Agger EM, Andersen P. Control of Latent Mycobacterium Tuberculosis Infection Is Dependent on CD8 T Cells. Eur J Immunol (2000) 30:3689–98. doi: 10.1002/1521-4141(200012)30:12<3689::Aid-immu3689>3.0.Co;2-4
337. Lewinsohn DM, Swarbrick GM, Cansler ME, Null MD, Rajaraman V, Frieder MM, et al. Human Mycobacterium Tuberculosis CD8 T Cell Antigens/Epitopes Identified by a Proteomic Peptide Library. PloS One (2013) 8:e67016. doi: 10.1371/journal.pone.0067016
338. Lewinsohn DA, Swarbrick GM, Park B, Cansler ME, Null MD, Toren KG, et al. Comprehensive Definition of Human Immunodominant CD8 Antigens in Tuberculosis. NPJ Vaccines (2017) 2:8. doi: 10.1038/s41541-017-0008-6
339. Silva BDS, Trentini MM, da Costa AC, Kipnis A, Junqueira-Kipnis AP. Different Phenotypes of CD8+ T Cells Associated With Bacterial Load in Active Tuberculosis. Immunol Lett (2014) 160:23–32. doi: 10.1016/j.imlet.2014.03.009
340. Smith CM, Wilson NS, Waithman J, Villadangos JA, Carbone FR, Heath WR, et al. Cognate CD4(+) T Cell Licensing of Dendritic Cells in CD8(+) T Cell Immunity. Nat Immunol (2004) 5:1143–8. doi: 10.1038/ni1129
341. Lu Y-J, Barreira-Silva P, Boyce S, Powers J, Cavallo K, Behar SM. CD4 T Cell Help Prevents CD8 T Cell Exhaustion and Promotes Control of Mycobacterium Tuberculosis Infection. Cell Rep (2021) 36:109696. doi: 10.1016/j.celrep.2021.109696
342. Cai Y, Wang Y, Shi C, Dai Y, Li F, Xu Y, et al. Single-Cell Immune Profiling Reveals Functional Diversity of T Cells in Tuberculous Pleural Effusion. J Exp Med (2022) 219. doi: 10.1084/jem.20211777
343. Verbeke R, Lentacker I, De Smedt SC, Dewitte H. The Dawn of mRNA Vaccines: The COVID-19 Case. J Control Release (2021) 333:511–20. doi: 10.1016/j.jconrel.2021.03.043
344. Behar SM, Woodworth JS, Wu Y. Next Generation: Tuberculosis Vaccines That Elicit Protective CD8+ T Cells. Expert Rev Vaccines (2007) 6:441–56. doi: 10.1586/14760584.6.3.441
345. Behar SM. Antigen-Specific CD8(+) T Cells and Protective Immunity to Tuberculosis. Adv Exp Med Biol (2013) 783:141–63. doi: 10.1007/978-1-4614-6111-1_8
346. Lin PL, Flynn JL. CD8 T Cells and Mycobacterium Tuberculosis Infection. Semin Immunopathol (2015) 37:239–49. doi: 10.1007/s00281-015-0490-8
347. Zodpey SP, Shrikhande SN, Maldhure BR, Vasudeo ND, Kulkarni SW. Effectiveness of Bacillus Calmette Guerin (BCG) Vaccination in the Prevention of Childhood Pulmonary Tuberculosis: A Case Control Study in Nagpur, India. Southeast Asian J Trop Med Public Health (1998) 29:285–8.
348. Murray RA, Mansoor N, Harbacheuski R, Soler J, Davids V, Soares A, et al. Bacillus Calmette Guerin Vaccination of Human Newborns Induces a Specific, Functional CD8+ T Cell Response. J Immunol (2006) 177:5647–51. doi: 10.4049/jimmunol.177.8.5647
349. Wang J, Thorson L, Stokes RW, Santosuosso M, Huygen K, Zganiacz A, et al. Single Mucosal, But Not Parenteral, Immunization With Recombinant Adenoviral-Based Vaccine Provides Potent Protection From Pulmonary Tuberculosis. J Immunol (2004) 173:6357–65. doi: 10.4049/jimmunol.173.10.6357
350. Baldwin SL, Ching LK, Pine SO, Moutaftsi M, Lucas E, Vallur A, et al. Protection Against Tuberculosis With Homologous or Heterologous Protein/Vector Vaccine Approaches Is Not Dependent on CD8+ T Cells. J Immunol (2013) 191:2514–25. doi: 10.4049/jimmunol.1301161
351. Duthie MS, Van Hoeven N, MacMillen Z, Picone A, Mohamath R, Erasmus J, et al. Heterologous Immunization With Defined RNA and Subunit Vaccines Enhances T Cell Responses That Protect Against Leishmania Donovani. Front Immunol (2018) 9:2420. doi: 10.3389/fimmu.2018.02420
352. Schell JB, Rose NF, Bahl K, Diller K, Buonocore L, Hunter M, et al. Significant Protection Against High-Dose Simian Immunodeficiency Virus Challenge Conferred by a New Prime-Boost Vaccine Regimen. J Virol (2011) 85:5764–72. doi: 10.1128/JVI.00342-11
353. McShane H, Pathan AA, Sander CR, Keating SM, Gilbert SC, Huygen K, et al. Recombinant Modified Vaccinia Virus Ankara Expressing Antigen 85A Boosts BCG-Primed and Naturally Acquired Antimycobacterial Immunity in Humans. Nat Med (2004) 10:1240–4. doi: 10.1038/nm1128
354. Xu R, Nasar F, Megati S, Luckay A, Lee M, Udem SA, et al. Prime-Boost Vaccination With Recombinant Mumps Virus and Recombinant Vesicular Stomatitis Virus Vectors Elicits an Enhanced Human Immunodeficiency Virus Type 1 Gag-Specific Cellular Immune Response in Rhesus Macaques. J Virol (2009) 83:9813–23. doi: 10.1128/JVI.00550-09
355. Walczak M, de Mare A, Riezebos-Brilman A, Regts J, Hoogeboom B-N, Visser JT, et al. Heterologous Prime-Boost Immunizations With a Virosomal and an Alphavirus Replicon Vaccine. Mol Pharmaceutics (2011) 8:65–77. doi: 10.1021/mp1002043
356. Nair SK, Tomaras GD, Sales AP, Boczkowski D, Chan C, Plonk K, et al. High-Throughput Identification and Dendritic Cell-Based Functional Validation of MHC Class I-Restricted Mycobacterium Tuberculosis Epitopes. Sci Rep (2014) 4:4632–2. doi: 10.1038/srep04632
357. Cayabyab MJ, Qin L, Kashino SS, Izzo A, Campos-Neto A. An Unbiased Peptide-Wide Discovery Approach to Select Mycobacterium Tuberculosis Antigens That Target CD8+ T Cell Response During Infection. Vaccine (2013) 31:4834–40. doi: 10.1016/j.vaccine.2013.07.077
358. Pauza CD, Cairo C. Evolution and Function of the TCR Vgamma9 Chain Repertoire: It’s Good to be Public. Cell Immunol (2015) 296:22–30. doi: 10.1016/j.cellimm.2015.02.010
359. Xi X, Han X, Li L, Zhao Z. γδ T Cells Response to Mycobacterium Tuberculosis in Pulmonary Tuberculosis Patients Using Preponderant Complementary Determinant Region 3 Sequence. Indian J Med Res (2011) 134:356–61.
360. Ribot JC, Lopes N, Silva-Santos B. γδ T Cells in Tissue Physiology and Surveillance. Nat Rev Immunol (2021) 21:221–32. doi: 10.1038/s41577-020-00452-4
361. Meraviglia S, El Daker S, Dieli F, Martini F, Martino A. γδ T Cells Cross-Link Innate and Adaptive Immunity in Mycobacterium Tuberculosis Infection. Clin Dev Immunol (2011) 2011:587315–5. doi: 10.1155/2011/587315
362. Poccia F, Malkovsky M, Pollak A, Colizzi V, Sireci G, Salerno A, et al. In Vivo Gammadelta T Cell Priming to Mycobacterial Antigens by Primary Mycobacterium Tuberculosis Infection and Exposure to Nonpeptidic Ligands. Mol Med (1999) 5:471–6. doi: 10.1007/BF03403540
363. Khairallah C, Chu TH, Sheridan BS. Tissue Adaptations of Memory and Tissue-Resident Gamma Delta T Cells. Front Immunol (2018) 9:2636. doi: 10.3389/fimmu.2018.02636
364. Bhatt K, Verma S, Ellner JJ, Salgame P. Quest for Correlates of Protection Against Tuberculosis. Clin Vaccine Immunol (2015) 22:258–66. doi: 10.1128/cvi.00721-14
365. Lockhart E, Green AM, Flynn JL. IL-17 Production Is Dominated by Gammadelta T Cells Rather Than CD4 T Cells During Mycobacterium Tuberculosis Infection. J Immunol (2006) 177:4662–9. doi: 10.4049/jimmunol.177.7.4662
366. Segueni N, Tritto E, Bourigault ML, Rose S, Erard F, Le Bert M, et al. Controlled Mycobacterium Tuberculosis Infection in Mice Under Treatment With Anti-IL-17A or IL-17F Antibodies, in Contrast to Tnfα Neutralization. Sci Rep (2016) 6:36923. doi: 10.1038/srep36923
367. Milano M, Moraes MO, Rodenbusch R, Carvalho CX, Delcroix M, Mousquer G, et al. Single Nucleotide Polymorphisms in IL17A and IL6 Are Associated With Decreased Risk for Pulmonary Tuberculosis in Southern Brazilian Population. PloS One (2016) 11:e0147814. doi: 10.1371/journal.pone.0147814
368. Shen H, Chen ZW. The Crucial Roles of Th17-Related Cytokines/Signal Pathways in M. Tuberculosis Infection. Cell Mol Immunol (2018) 15:216–25. doi: 10.1038/cmi.2017.128
369. Peng MY, Wang ZH, Yao CY, Jiang LN, Jin QL, Wang J, et al. Interleukin 17-Producing Gamma Delta T Cells Increased in Patients With Active Pulmonary Tuberculosis. Cell Mol Immunol (2008) 5:203–8. doi: 10.1038/cmi.2008.25
370. Ordway DJ, Pinto L, Costa L, Martins M, Leandro C, Viveiros M, et al. Gamma Delta T Cell Responses Associated With the Development of Tuberculosis in Health Care Workers. FEMS Immunol Med Microbiol (2005) 43:339–50. doi: 10.1016/j.femsim.2004.09.005
371. Ogongo P, Steyn AJC, Karim F, Dullabh KJ, Awala I, Madansein R, et al. Differential Skewing of Donor-Unrestricted and γδ T Cell Repertoires in Tuberculosis-Infected Human Lungs. J Clin Invest (2020) 130:214–30. doi: 10.1172/JCI130711
372. Yan L, Cui H, Xiao H, Zhang Q. Anergic Pulmonary Tuberculosis Is Associated With Contraction of the Vd2+T Cell Population, Apoptosis and Enhanced Inhibitory Cytokine Production. PloS One (2013) 8:e71245. doi: 10.1371/journal.pone.0071245
373. Dieli F, Troye-Blomberg M, Ivanyi J, Fournié JJ, Krensky AM, Bonneville M, et al. Granulysin-Dependent Killing of Intracellular and Extracellular Mycobacterium Tuberculosis by Vgamma9/Vdelta2 T Lymphocytes. J Infect Dis (2001) 184:1082–5. doi: 10.1086/323600
374. Nabeshima S, Hiromatsu K, Matsuzaki G, Mukasa A, Takada H, Yoshida S, et al. Infection of Mycobacterium Bovis Bacillus Calmette-Guérin in Antibody-Mediated Gamma Delta T-Cell-Depleted Mice. Immunology (1995) 84:317–21.
375. Hoft DF, Brown RM, Roodman ST. Bacille Calmette-Guérin Vaccination Enhances Human Gamma Delta T Cell Responsiveness to Mycobacteria Suggestive of a Memory-Like Phenotype. J Immunol (1998) 161:1045–54.
376. Shen L, Frencher J, Huang D, Wang W, Yang E, Chen CY, et al. Immunization of Vγ2vδ2 T Cells Programs Sustained Effector Memory Responses That Control Tuberculosis in Nonhuman Primates. Proc Natl Acad Sci USA (2019) 116:6371–8. doi: 10.1073/pnas.1811380116
377. Havlir DV, Ellner JJ, Chervenak KA, Boom WH. Selective Expansion of Human Gamma Delta T Cells by Monocytes Infected With Live Mycobacterium Tuberculosis. J Clin Invest (1991) 87:729–33. doi: 10.1172/jci115053
378. Dai R, Huang X, Yang Y. γδt Cells Are Required for CD8+ T Cell Response to Vaccinia Viral Infection. Front Immunol (2021) 12:727046. doi: 10.3389/fimmu.2021.727046
379. Saramago S, Magalhães J, Pinheiro M. Tuberculosis Vaccines: An Update of Recent and Ongoing Clinical Trials. Appl Sci (2021) 11:9250. doi: 10.3390/app11199250
380. Billeskov R, Lindenstrøm T, Woodworth J, Vilaplana C, Cardona PJ, Cassidy JP, et al. High Antigen Dose Is Detrimental to Post-Exposure Vaccine Protection Against Tuberculosis. Front Immunol (2017) 8:1973. doi: 10.3389/fimmu.2017.01973
381. Clemmensen HS, Dube JY, McIntosh F, Rosenkrands I, Jungersen G, Aagaard C, et al. In Vivo Antigen Expression Regulates CD4 T Cell Differentiation and Vaccine Efficacy Against Mycobacterium Tuberculosis Infection. bioRxiv (2021). doi: 10.1101/2021.02.02.429488
382. Moguche AO, Musvosvi M, Penn-Nicholson A, Plumlee CR, Mearns H, Geldenhuys H, et al. Antigen Availability Shapes T Cell Differentiation and Function During Tuberculosis. Cell Host Microbe (2017) 21:695–706.e695. doi: 10.1016/j.chom.2017.05.012
383. Morrison H, McShane H. Local Pulmonary Immunological Biomarkers in Tuberculosis. Front Immunol (2021) 12:640916. doi: 10.3389/fimmu.2021.640916
384. Counoupas C, Ferrell KC, Ashhurst A, Bhattacharyya ND, Nagalingam G, Stewart EL, et al. Mucosal Delivery of a Multistage Subunit Vaccine Promotes Development of Lung-Resident Memory T Cells and Affords Interleukin-17-Dependent Protection Against Pulmonary Tuberculosis. NPJ Vaccines (2020) 5:105. doi: 10.1038/s41541-020-00255-7
385. Irvine EB, O’Neil A, Darrah PA, Shin S, Choudhary A, Li W, et al. Robust IgM Responses Following Vaccination Are Associated With Prevention of Mycobacterium Tuberculosis Infection in Macaques. bioRxiv (2021) 2021.2005.2006.442979. doi: 10.1101/2021.05.06.442979
386. Santosuosso M, Zhang X, McCormick S, Wang J, Hitt M, Xing Z. Mechanisms of Mucosal and Parenteral Tuberculosis Vaccinations: Adenoviral-Based Mucosal Immunization Preferentially Elicits Sustained Accumulation of Immune Protective CD4 and CD8 T Cells Within the Airway Lumen. J Immunol (2005) 174:7986–94. doi: 10.4049/jimmunol.174.12.7986
387. Jeyanathan M, Fritz DK, Afkhami S, Aguirre E, Howie KJ, Zganiacz A, et al. Aerosol Delivery, But Not Intramuscular Injection, of Adenovirus-Vectored Tuberculosis Vaccine Induces Respiratory-Mucosal Immunity in Humans. JCI Insight (2022). doi: 10.1172/jci.insight.155655
388. Vasilyev K, Shurygina AP, Zabolotnykh N, Sergeeva M, Romanovskaya-Romanko E, Pulkina A, et al. Enhancement of the Local CD8(+) T-Cellular Immune Response to Mycobacterium Tuberculosis in BCG-Primed Mice After Intranasal Administration of Influenza Vector Vaccine Carrying TB10.4 and HspX Antigens. Vaccines (Basel) (2021) 9. doi: 10.3390/vaccines9111273
389. Lagranderie M, Ravisse P, Marchal G, Gheorghiu M, Balasubramanian V, Weigeshaus EH, et al. BCG-Induced Protection in Guinea Pigs Vaccinated and Challenged Via the Respiratory Route. Tuber Lung Dis (1993) 74:38–46. doi: 10.1016/0962-8479(93)90067-8
390. Aguilo N, Alvarez-Arguedas S, Uranga S, Marinova D, Monzón M, Badiola J, et al. Pulmonary But Not Subcutaneous Delivery of BCG Vaccine Confers Protection to Tuberculosis-Susceptible Mice by an Interleukin 17-Dependent Mechanism. J Infect Dis (2016) 213:831–9. doi: 10.1093/infdis/jiv503
391. Perdomo C, Zedler U, Kühl AA, Lozza L, Saikali P, Sander LE, et al. Mucosal BCG Vaccination Induces Protective Lung-Resident Memory T Cell Populations Against Tuberculosis. mBio (2016) 7. doi: 10.1128/mBio.01686-16
392. Dijkman K, Sombroek CC, Vervenne RAW, Hofman SO, Boot C, Remarque EJ, et al. Prevention of Tuberculosis Infection and Disease by Local BCG in Repeatedly Exposed Rhesus Macaques. Nat Med (2019) 25:255–62. doi: 10.1038/s41591-018-0319-9
393. Mata E, Tarancon R, Guerrero C, Moreo E, Moreau F, Uranga S, et al. Pulmonary BCG Induces Lung-Resident Macrophage Activation and Confers Long-Term Protection Against Tuberculosis. Sci Immunol (2021) 6:eabc2934. doi: 10.1126/sciimmunol.abc2934
394. Ferrell KC, Stewart EL, Counoupas C, Ashhurst TM, Britton WJ, Petrovsky N, et al. Intrapulmonary Vaccination With Delta-Inulin Adjuvant Stimulates non-Polarised Chemotactic Signalling and Diverse Cellular Interaction. Mucosal Immunol (2021) 14:762–73. doi: 10.1038/s41385-021-00379-6
395. Schrager LK, Harris RC, Vekemans J. Research and Development of New Tuberculosis Vaccines: A Review. F1000Res (2018) 7:1732. doi: 10.12688/f1000research.16521.2
396. Davids M, Pooran A, Hermann C, Mottay L, Thompson F, Cardenas J, et al. A Human Lung Challenge Model to Evaluate the Safety and Immunogenicity of PPD and Live Bacillus Calmette-Guérin. Am J Respir Crit Care Med (2020) 201:1277–91. doi: 10.1164/rccm.201908-1580OC
397. McShane H. Controlled Human Infection Models: Is It Really Feasible to Give People Tuberculosis? Am J Respir Crit Care Med (2020) 201:1180–1. doi: 10.1164/rccm.201912-2408ED
398. Behr MA, Kaufmann E, Duffin J, Edelstein PH, Ramakrishnan L. Latent Tuberculosis: Two Centuries of Confusion. Am J Respir Crit Care Med (2021) 204:142–8. doi: 10.1164/rccm.202011-4239PP
399. Tabone O, Verma R, Singhania A, Chakravarty P, Branchett WJ, Graham CM, et al. Blood Transcriptomics Reveal the Evolution and Resolution of the Immune Response in Tuberculosis. J Exp Med (2021) 218. doi: 10.1084/jem.20210915
400. Sumner T, Fiore-Gartland A, Hatherill M, Houben R, Scriba TJ, White RG. The Effect of New Mycobacterium Tuberculosis Infection on the Sensitivity of Prognostic TB Signatures. Int J Tuberc Lung Dis (2021) 25:1001–5. doi: 10.5588/ijtld.21.0323
401. Burel JG, Babor M, Pomaznoy M, Lindestam Arlehamn CS, Khan N, Sette A, et al. Host Transcriptomics as a Tool to Identify Diagnostic and Mechanistic Immune Signatures of Tuberculosis. Front Immunol (2019) 10:221. doi: 10.3389/fimmu.2019.00221
402. Burel JG, Singhania A, Dubelko P, Muller J, Tanner R, Parizotto E, et al. Distinct Blood Transcriptomic Signature of Treatment in Latent Tuberculosis Infected Individuals at Risk of Developing Active Disease. Tuberculosis (Edinb) (2021) 131:102127. doi: 10.1016/j.tube.2021.102127
403. Harris RC, Sumner T, Knight GM, Zhang H, White RG. Potential Impact of Tuberculosis Vaccines in China, South Africa, and India. Sci Trans Med (2020) 12:eaax4607. doi: 10.1126/scitranslmed.aax4607
404. Weerasuriya CK, Harris RC, McQuaid CF, Bozzani F, Ruan Y, Li R, et al. The Epidemiologic Impact and Cost-Effectiveness of New Tuberculosis Vaccines on Multidrug-Resistant Tuberculosis in India and China. BMC Med (2021) 19:60. doi: 10.1186/s12916-021-01932-7
405. Weerasuriya CK, Harris RC, Quaife M, McQuaid CF, White RG, Gomez GB. Affordability of Adult Tuberculosis Vaccination in India and China: A Dynamic Transmission Model-Based Analysis. Vaccines (2021) 9:245.
406. Harris RC, Quaife M, Weerasuriya C, Gomez GB, Sumner T, Bozzani F, et al. Cost-Effectiveness of Routine Adolescent Vaccination With an M72/AS01E-Like Tuberculosis Vaccine in South Africa and India. Nat Commun (2022) 13:602. doi: 10.1038/s41467-022-28234-7
407. Li J, Zhao A, Tang J, Wang G, Shi Y, Zhan L, et al. Tuberculosis Vaccine Development: From Classic to Clinical Candidates. Eur J Clin Microbiol Infect Dis (2020) 39:1405–25. doi: 10.1007/s10096-020-03843-6
408. Noll KE, Ferris MT, Heise MT. The Collaborative Cross: A Systems Genetics Resource for Studying Host-Pathogen Interactions. Cell Host Microbe (2019) 25:484–98. doi: 10.1016/j.chom.2019.03.009
409. Smith CM, Proulx MK, Lai R, Kiritsy MC, Bell TA, Hock P, et al. Functionally Overlapping Variants Control Tuberculosis Susceptibility in Collaborative Cross Mice. mBio (2019) 10:e02791–02719. doi: 10.1128/mBio.02791-19
410. Smith CM, Proulx MK, Olive AJ, Laddy D, Mishra BB, Moss C, et al. Tuberculosis Susceptibility and Vaccine Protection Are Independently Controlled by Host Genotype. mBio (2016) 7:e01516–01516. doi: 10.1128/mBio.01516-16
411. Plumlee CR, Duffy FJ, Gern BH, Delahaye JL, Cohen SB, Stoltzfus CR, et al. Ultra-Low Dose Aerosol Infection of Mice With Mycobacterium Tuberculosis More Closely Models Human Tuberculosis. Cell Host Microbe (2021) 29:68–82.e65. doi: 10.1016/j.chom.2020.10.003
412. Nemeth J, Olson GS, Rothchild AC, Jahn AN, Mai D, Duffy FJ, et al. Contained Mycobacterium Tuberculosis Infection Induces Concomitant and Heterologous Protection. PloS Pathog (2020) 16:e1008655. doi: 10.1371/journal.ppat.1008655
413. Duffy FJ, Olson GS, Gold ES, Jahn A, Aderem A, Aitchison JD, et al. Use of a Contained Mycobacterium Tuberculosis Mouse Infection Model to Predict Active Disease and Containment in Humans. J Infect Dis (2021). doi: 10.1093/infdis/jiab130
414. Clark S, Hall Y, Williams A. Animal Models of Tuberculosis: Guinea Pigs. Cold Spring Harbor Perspect Med (2014) 5:a018572–a018572. doi: 10.1101/cshperspect.a018572
415. Hild SA, Chang MC, Murphy SJ, Grieder FB. Nonhuman Primate Models for SARS-CoV-2 Research: Infrastructure Needs for Pandemic Preparedness. Lab Anim (2021) 50:140–1. doi: 10.1038/s41684-021-00760-9
416. Zak DE, Penn-Nicholson A, Scriba TJ, Thompson E, Suliman S, Amon LM, et al. A Blood RNA Signature for Tuberculosis Disease Risk: A Prospective Cohort Study. Lancet (2016) 387:2312–22. doi: 10.1016/S0140-6736(15)01316-1
417. Duffy FJ, Weiner J 3rd, Hansen S, Tabb DL, Suliman S, Thompson E, et al. Immunometabolic Signatures Predict Risk of Progression to Active Tuberculosis and Disease Outcome. Front Immunol (2019) 10:527. doi: 10.3389/fimmu.2019.00527
418. Fiore-Gartland A, Carpp LN, Naidoo K, Thompson E, Zak DE, Self S, et al. Considerations for Biomarker-Targeted Intervention Strategies for Tuberculosis Disease Prevention. Tuberculosis (Edinburgh Scotland) (2018) 109:61–8. doi: 10.1016/j.tube.2017.11.009
419. Penn-Nicholson A, Mbandi SK, Thompson E, Mendelsohn SC, Suliman S, Chegou NN, et al. RISK6, a 6-Gene Transcriptomic Signature of TB Disease Risk, Diagnosis and Treatment Response. Sci Rep (2020) 10:8629. doi: 10.1038/s41598-020-65043-8
420. Suliman S, Thompson E, Sutherland J, Weiner Rd J, Ota MOC, Shankar S, et al. Four-Gene Pan-African Blood Signature Predicts Progression to Tuberculosis. Am J Respir Crit Care Med (2018) 197:1198–208. doi: 10.1164/rccm.201711-2340OC
421. Thompson EG, Du Y, Malherbe ST, Shankar S, Braun J, Valvo J, et al. Host Blood RNA Signatures Predict the Outcome of Tuberculosis Treatment. Tuberculosis (Edinb) (2017) 107:48–58. doi: 10.1016/j.tube.2017.08.004
422. Ahmed M, Thirunavukkarasu S, Rosa BA, Thomas KA, Das S, Rangel-Moreno J, et al. Immune Correlates of Tuberculosis Disease and Risk Translate Across Species. Sci Trans Med (2020) 12:eaay0233. doi: 10.1126/scitranslmed.aay0233
423. Khader SA, Divangahi M, Hanekom W, Hill PC, Maeurer M, Makar KW, et al. Targeting Innate Immunity for Tuberculosis Vaccination. J Clin Invest (2019) 129:3482–91. doi: 10.1172/jci128877
424. Divangahi M, Aaby P, Khader SA, Barreiro LB, Bekkering S, Chavakis T, et al. Trained Immunity, Tolerance, Priming and Differentiation: Distinct Immunological Processes. Nat Immunol (2021) 22:2–6. doi: 10.1038/s41590-020-00845-6
425. Ferluga J, Yasmin H, Al-Ahdal MN, Bhakta S, Kishore U. Natural and Trained Innate Immunity Against Mycobacterium Tuberculosis. Immunobiology (2020) 225:151951. doi: 10.1016/j.imbio.2020.151951
426. Joosten SA, Ottenhoff THM, Lewinsohn DM, Hoft DF, Moody DB, Seshadri C, et al. Harnessing Donor Unrestricted T-Cells for New Vaccines Against Tuberculosis. Vaccine (2019) 37:3022–30. doi: 10.1016/j.vaccine.2019.04.050
427. Suliman S, Gela A, Mendelsohn SC, Iwany SK, Tamara KL, Mabwe S, et al. Peripheral Blood Mucosal-Associated Invariant T Cells in Tuberculosis Patients and Healthy Mycobacterium Tuberculosis-Exposed Controls. J Infect Dis (2020) 222:995–1007. doi: 10.1093/infdis/jiaa173
428. Sakai S, Kauffman KD, Oh S, Nelson CE, Barry CE, Barber DL. MAIT Cell-Directed Therapy of Mycobacterium Tuberculosis Infection. Mucosal Immunol (2021) 14:199–208. doi: 10.1038/s41385-020-0332-4
429. Balfour A, Schutz C, Goliath R, Wilkinson KA, Sayed S, Sossen B, et al. Functional and Activation Profiles of Mucosal-Associated Invariant T Cells in Patients With Tuberculosis and HIV in a High Endemic Setting. Front Immunol (2021) 12:648216. doi: 10.3389/fimmu.2021.648216
Keywords: infection, immunity, vaccines, BCG, Mycobacterium tuberculosis, prevention of infection (POI), prevention of disease (POD)
Citation: Larsen SE, Williams BD, Rais M, Coler RN and Baldwin SL (2022) It Takes a Village: The Multifaceted Immune Response to Mycobacterium tuberculosis Infection and Vaccine-Induced Immunity. Front. Immunol. 13:840225. doi: 10.3389/fimmu.2022.840225
Received: 20 December 2021; Accepted: 08 February 2022;
Published: 10 March 2022.
Edited by:
Deepak Kaushal, Southwest National Primate Research Center (SNPRC), United StatesReviewed by:
Carmen Fernández, Stockholm University, SwedenArshad Khan, Houston Methodist Research Institute, United States
Copyright © 2022 Larsen, Williams, Rais, Coler and Baldwin. This is an open-access article distributed under the terms of the Creative Commons Attribution License (CC BY). The use, distribution or reproduction in other forums is permitted, provided the original author(s) and the copyright owner(s) are credited and that the original publication in this journal is cited, in accordance with accepted academic practice. No use, distribution or reproduction is permitted which does not comply with these terms.
*Correspondence: Susan L. Baldwin, U3VzYW4uQmFsZHdpbkBTZWF0dGxlY2hpbGRyZW5zLm9yZw==