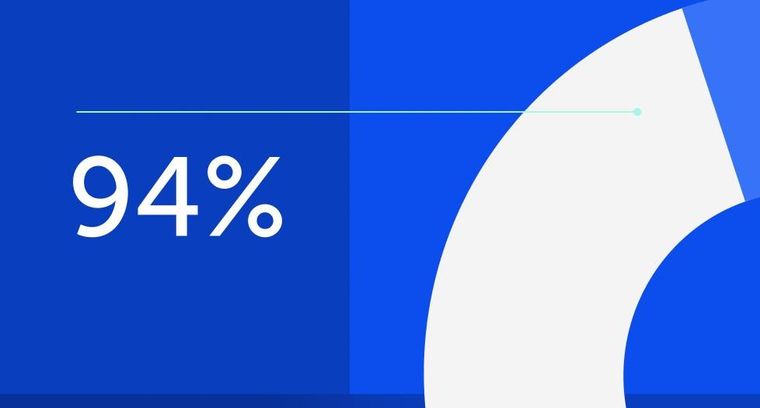
94% of researchers rate our articles as excellent or good
Learn more about the work of our research integrity team to safeguard the quality of each article we publish.
Find out more
ORIGINAL RESEARCH article
Front. Immunol., 24 March 2022
Sec. T Cell Biology
Volume 13 - 2022 | https://doi.org/10.3389/fimmu.2022.839783
This article is part of the Research TopicNew Frontiers in Gene-Modified T Cell TechnologyView all 16 articles
Chimeric antigen receptor (CAR)-engineered T cells can be highly effective in the treatment of hematological malignancies, but mostly fail in the treatment of solid tumors. Thus, approaches using 4th advanced CAR T cells secreting immunomodulatory cytokines upon CAR signaling, known as TRUCKs (“T cells redirected for universal cytokine-mediated killing”), are currently under investigation. Based on our previous development and validation of automated and closed processing for GMP-compliant manufacturing of CAR T cells, we here present the proof of feasibility for translation of this method to TRUCKs. We generated IL-18-secreting TRUCKs targeting the tumor antigen GD2 using the CliniMACS Prodigy® system using a recently described “all-in-one” lentiviral vector combining constitutive anti-GD2 CAR expression and inducible IL-18. Starting with 0.84 x 108 and 0.91 x 108 T cells after enrichment of CD4+ and CD8+ we reached 68.3-fold and 71.4-fold T cell expansion rates, respectively, in two independent runs. Transduction efficiencies of 77.7% and 55.1% was obtained, and yields of 4.5 x 109 and 3.6 x 109 engineered T cells from the two donors, respectively, within 12 days. Preclinical characterization demonstrated antigen-specific GD2-CAR mediated activation after co-cultivation with GD2-expressing target cells. The functional capacities of the clinical-scale manufactured TRUCKs were similar to TRUCKs generated in laboratory-scale and were not impeded by cryopreservation. IL-18 TRUCKs were activated in an antigen-specific manner by co-cultivation with GD2-expressing target cells indicated by an increased expression of activation markers (e.g. CD25, CD69) on both CD4+ and CD8+ T cells and an enhanced release of pro-inflammatory cytokines and cytolytic mediators (e.g. IL-2, granzyme B, IFN-γ, perforin, TNF-α). Manufactured TRUCKs showed a specific cytotoxicity towards GD2-expressing target cells indicated by lactate dehydrogenase (LDH) release, a decrease of target cell numbers, microscopic detection of cytotoxic clusters and detachment of target cells in real-time impedance measurements (xCELLigence). Following antigen-specific CAR activation of TRUCKs, CAR-triggered release IL-18 was induced, and the cytokine was biologically active, as demonstrated in migration assays revealing specific attraction of monocytes and NK cells by supernatants of TRUCKs co-cultured with GD2-expressing target cells. In conclusion, GMP-compliant manufacturing of TRUCKs is feasible and delivers high quality T cell products.
One of the most significant recent developments in cancer therapy is the CAR T cell technology. To enable and improve CAR T cell proliferation, anti-tumor activity, and in vivo persistence, advanced generations of CARs have been developed (1). A promising strategy to target solid tumors with their phenotypic heterogeneity has led to the fourth generation of CARs also known as TRUCKs or armored CARs (2, 3). TRUCKs are CAR T cells that release a transgenic protein upon CAR engagement of cognate antigen and signaling. TRUCKs are thereby used as “living factories” to produce and deposit substances with anti-tumor activity in the targeted tissue. These factors include cytokines, such as IL (interleukin)-12 and IL-18, but also enzymes and costimulatory ligands augmenting T cell activation. Innate immune cells are attracted and activated by IL-12 or IL-18 (4) to eliminate antigen-low expressing or antigen-negative cancer cells within the tumor (2). CAR T cells engineered with inducible IL-18 release improve T cell effector functions towards superior activity against pancreatic and lung tumors in mice that were refractory to CAR T cells without cytokines (5, 6). In this study we focused on the manufacturing of TRUCKs targeting disialoganglioside GD2. Physiological expression of GD2 is restricted to low densities on neurons, skin melanocytes and peripheral pain fibers (7). GD2 is highly and consistently expressed in childhood cancer neuroblastoma and can be found on the cell surface of other solid cancer entities including breast cancer (8), osteosarcoma (9), melanoma (10), glioblastoma (11), small cell lung cancer (12), retinoblastoma (13), soft tissue sarcoma (14) and Ewing sarcoma (15, 16).
GD2 therefore is a promising target for redirected immunotherapy. Initial GD2-CAR T cell clinical studies targeting neuroblastoma by first to third generation CAR T cells showed moderate or transient anti-tumor responses but failed to produce sustained remissions, emphasizing the need to modulate the T cell response (17–19). With the increasing expectation of GD2 as a broad target for CAR T cell therapy and the expected benefit in applying TRUCKs with transgenic IL-18 release, there is a need to manufacture such cellular medicinal products in a safe, validated and reproducible fashion. CAR T cell manufacturing for clinical use is a complex process and places high standard demands on safety, quality and efficacy. Chemistry, Manufacturing and Controls (CMC) even in the preclinical phase of drug development includes significant quality attributes and critical process parameters, including cell composition and transduction efficiency, assessment of potency, product sterility, process validation, stability and production at multiple manufacturing sites (20). The CliniMACS Prodigy® (Miltenyi Biotec B.V. & Co. KG) allows ex vivo magnetic bead-based cell separation followed by activation, transduction, expansion, final formulation and sampling of T cells in one device leading to robust, reproducible and automated, supervised cost-effective manufacturing processes [Process of CAR T cell Therapy in Europe EHA Guidance Document, 2019 (21)]. The feasibility of the T cell transduction (TCT) process for use in automated and closed GMP-compliant manufacturing of CAR T cells on the CliniMACS Prodigy® platform, as shown by us and others (22–25) is here extended to the manufacturing of IL-18 TRUCKs targeting GD2 as an example. Preclinical characterization showed equivalent quality and function of the final clinical-scale products compared to manually produced IL-18 TRUCKs in laboratory-scale.
For the manufacturing of TRUCKs in a clinical-scale process (n=2) on the CliniMACS Prodigy® (Miltenyi Biotec, Bergisch Gladbach, Germany) and for the manufacturing of laboratory-scale TRUCKs (n=3) lymphapheresis products from two healthy donors (D1 and D2) were obtained from the Institute for Transfusion Medicine of Hannover Medical School (MHH) after donors’ written informed consent. According to standard donation requirements, the donors had no signs of acute infection and no previous history of blood transfusion.
The generation of the lentiviral IL-18 TRUCK SIN vector was described previously (26). In brief, the lentiviral 3rd generation SIN vector pCCL.PPT.NFATsyn.hIL18.PGK.GD2CAR.PRE was used. The human codon-usage optimized ORF second-generation CAR containing scFv (14.G2a), human IgG1- hinge, CD28 transmembrane, CD137 (4-1BB), and CD3ζ signaling domains was flanked by restriction enzymes AgeI and SalI and cloned into the lentiviral “all-in-one” SIN vector driven by an hPGK promoter (27). A schematic map of the construct is presented in Supplement Figure S8. The construct was confirmed by sequencing (Microsynth SeqLab, Germany). Lentiviral vector particles were generated as described previously (26, 28). Briefly, 5 × 106 293T cells were used for calcium phosphate transfection in the presence of 25 µM chloroquine. For transfection, the following plasmids were used: lentiviral vector plasmid (10 µg), pcDNA3.HIV-1.GP.4 × CTE (lentiviral gag/pol) (12 µg) (29), pRSV-Rev (5 µg; kindly provided by T. Hope, Northwestern University Chicago, IL), and VSVg-encoding pMD.G (1.5 µg) (30). For better standardization, pcDNA3.HIV-1.GP.4×CTE, pRSV-Rev and pMD.G were produced and purified by PlasmidFactory (Bielefeld, Germany).After 36 h and 48 h of transfection, supernatants were harvested and concentrated via ultracentrifugation at 4°C and 13,238× g or 82,740× g for 16 h or 2 h, respectively. The particles were resuspended in TexMACS™ GMP medium. Lentiviral supernatant was titrated in HT1080 fibroblasts via spinoculation-mediated transduction, i. e. 1 × 105 cells were seeded, the supernatant containing viral particles and 4 µg/ml protamine sulfate (Sigma-Aldrich, St. Louis, USA) were added and cells were centrifuged (1 h, 800× g, 37°C). Three days post transduction, transduction efficiency was determined via flow cytometric staining of GD2-CAR expression and functional viral vector titers were calculated from samples with GD2-CAR expression percentages of ≤ 30% to avoid cells with multiple integrations (26, 31).
GMP-compliant manufacturing of IL-18 TRUCKs was performed using the CliniMACS Prodigy® platform, which allows for automated cell processing in a closed system controlled by operating software version V1.3 and process software for T cell transduction (TCT) version V2.0 (released). For overview of clinical-scale process see Figure 1. Within the scope of the automatically running process, the input of different variable process parameters like time points of transduction, media exchange, culture wash, harvesting and volume of media exchange is possible. Buffer, media, starting cell material and vector were connected directly to TS520 via sterile tubing welder device (TSCDII Terumo BCT).
Figure 1 Schematic diagram of producing IL-18 TRUCKs targeting GD2 utilizing the CliniMACS Prodigy® automated cell processor (clinical-scale process). The entire 12-day process from lymphapheresis material to formulated final products was performed using the CliniMACS Prodigy®. Day -1: Overnight storage of the lymphapheresis at 4°C. Day 0: Immunomagnetic enrichment of CD4+ and CD8+ cells. Start of T cell activation with CD3/CD28 TransAct Beads (day 0-3) and cultivation in TexMACS™ basal medium supplemented with IL-7, IL-15 and human AB serum until day 5. Day 1-3: Lentiviral transduction. Day 3: Culture wash and start of culture agitation. Day 5: Switch to TexMACS™ basal medium supplemented with IL-7, IL-15 without human AB serum for following media exchanges. Day 12: Wash of cells and harvest in 100 ml formulation buffer for following cryopreservation. During T cell expansion several feeding steps by medium exchange were included in the activity matrix.
The applied materials were either medicinal products with a marketing approval (HSA, PEI.H.03272.01.1), GMP-grade reagents and tubing sets from Miltenyi Biotec (designed following the recommendations of USP <1043> on ancillary materials and/or compliant with the requirements laid down in the Ph. Eur. Chapter 5.2.12, where applicable), or approved medical devices (DMSO, Composol, SSP+, transfer bags, connections, syringes). The pool-Human Serum P-HS/Tü was purchased from the Centre for Clinical Transfusion Medicine Tübingen/ZKT and certified as suitable for manufacturing of pharmaceutical products. The single non-regulated reagent was the vector, designed and produced at the Institute of Experimental Hematology, Hannover Medical School, Division of Hematology/Oncology. Detailed information regarding the materials was recorded, including the supplier, lot number, and expiration date. Starting material for manufacturing of IL-18 TRUCKs were CD3+ T cells derived from a non-mobilized lymphapheresis. Cell processing started within 24 h after product collection with immunomagnetic enrichment of 1 x 109 CD4+ and CD8+ T cells using CliniMACS® CD4 Reagent, CliniMACS® CD8 Reagent and CliniMACS® PBS/EDTA buffer supplemented with human serum albumin (HSA, Human albumin 200 g/l Baxalta, Shire Deutschland GmbH, Berlin, Germany) to a final concentration of 0.5%. For cultivation, the basal TexMACS™ GMP medium was supplemented with 12.5 ng/ml MACS GMP Recombinant Human IL-7, 12.5 ng/ml MACS GMP Recombinant Human IL-15 and 3% heat-inactivated human AB serum (pool-human serum P-HS/Tü, Centre for Clinical Transfusion Medicine Tübingen/ZKT, Germany) until day 5. T cells were activated for 72 h (day 0 to day 3) with CD3/CD28 MACS GMP T Cell TransAct Beads. On day 1 of culture transduction took place by adding 10 ml lentiviral vector with a multiplicity of infection (MOI) of 29 D1 and 10 D2 in total volume of 100 ml. At day 3, CD3/CD28 T cell TransAct beads and non-bound vector were washed out (culture wash) and culture volume increased to 200 ml. The culture was fed every 12 – 24 h after day 5 of culture. Hereby the concentration of AB serum in the culture was reduced continuously using supplemented TexMACS™ GMP medium without AB serum for further medium exchange. On day of harvest (day 12), cells were formulated in Composol PS (Fresenius Kabi Deutschland GmbH, Bad Homburg, Germany) with 2.86% (w/v) HSA (D1) or SSP+ (D2) (Maco Pharma International GmbH, Langen, Germany) with 3.33% HSA for later cryopreservation. During cultivation, the temperature and atmosphere was maintained at 37°C with 5% CO2. After 3 days of static culture shaking modus was activated (culture agitation) enabling high cell concentrations in the limited volume of the CentriCult Chamber.
Total cell number and viability was analyzed by flow cytometric analysis as described below. The glucose concentration of the cell-free culture supernatant was determined with the blood glucose meter Accu-Chek® Aviva (Roche, Mannheim, Germany). For analysis of pH during cell cultivation pH-indicator strips MColorpHast™ pH 6.5 - 10.0 (Merck Millipore, Darmstadt, Germany) were used.
Flow cytometric analysis was performed by using anti-human monoclonal antibodies. Cellular composition antibody table is shown in Supplementary Table 1. Transduction efficiency was determined by GD2-CAR detection with the antibody Ganglidiomab, which was conjugated with Phycoerythrin (PE) by Miltenyi Biotec, herein after referred to as Ganglidiomab-PE. The antigen-specific single-chain variable fragment (scFv) of the GD2-CAR is derived from the anti-GD2 antibody 14G2a (32, 33). Ganglidiomab is a monoclonal anti-idiotype antibody to 14G2a and therefore allows for a direct detection of the GD2-CAR on cells. Subsequently antibody staining (10 min at RT) cells were incubated (10 min at RT) with freshly prepared red blood cell lysis solution (Miltenyi Biotec). T cell phenotype antibody panel is shown in Table 1. After antibody staining (10 min at RT in PBS supplemented with 4% FCS) cells were washed and resuspended with PBS supplemented with 4% FCS (both Merck, Darmstadt, Germany). Prior to flow cytometric analysis 7-AAD and Flow-Count Fluorospheres (both Beckman Coulter) were added to the samples for dead cell discrimination and single platform cell quantification, respectively. Flow cytometric analysis was performed with the Navios flow cytometer (Navios 3L 10C, Software 1.3, Beckman Coulter). For gating strategies see Supplement Figures S6, 7.
The cell lines 293T, HT1080, HT1080-GD2 and SH-SY5Y were cultivated as recently described (26). NK-92 cells (human natural killer lymphoma #ACC 488; DSMZ, Braunschweig, Germany) were cultivated in RPMI 1640 medium (Lonza, Basel, Switzerland) supplemented with 10% FBS, 2 mM L-glutamine (c.c.pro, Oberdorla, Germany) and 400 IU/mL human IL-2 (Proleukin S, Novartis Pharma GmbH, Nürnberg, Germany). THP-1 (human acute monocytic leukemia, #ACC 16; DSMZ, Braunschweig, Germany) cells were cultivated in RPMI 1640 medium with 2 mM L-glutamine, 2-mercaptoethanol to a final concentration of 0.05 mM, 10% (v/v) heat-inactivated fetal bovine serum (HI-FBS), and 50 IU/ml penicillin and 50 µg/ml streptomycin. All cells were tested for mycoplasma contamination on a regular basis using the MycoAlert™ Mycoplasma Detection Kit (Lonza, Basel, Switzerland) according to the manufacturer’s protocol.
CD4+ and CD8+ T cells isolated by the CliniMACS Prodigy® (see clinical-scale manufacturing) were transduced and expanded as previously described (34). Briefly, they were activated with anti-CD3/CD28 beads (Thermo Fisher Scientific, Waltham, MA, USA) at a ratio of 1:1 in TexMACS™ (Miltenyi Biotec) with 3% human serum (c.c.pro, Oberdorla, Germany) supplemented with 12.5 ng/ml IL-7 and IL-15 (PeproTech, Rocky Hill, NJ, USA). On the following day, T cells were either left untransduced or transduced with lentiviral particles by spinoculation using an MOI of 7 and addition of 5 µg/ml Polybrene Infection/Transfection Reagent (Merck Millipore, Burlington, MA, USA). The anti-CD3/CD28 beads were removed on the following day and cells were further cultivated in TexMACS™ medium supplemented with 3% human serum, 12.5 ng/ml IL-7 and IL-15 and splitting 1:2 every 2 - 3 days for a total expansion time of 12 days.
Untransduced and transduced T cells were cryopreserved in Composol® PS Fresenius Kabi, Bad Homburg, Germany/2.86% (w/v) HSA (Biotest, Dreieich, Germany) (D1) and SSP+ (Maco Pharma International GmbH, Langen, Germany) supplemented with 3.33% (w/v) HSA (D2), respectively after manufacturing by adjusting the cell counts and addition of DMSO (CryoSure-DMSO, USP grade; WAK Chemie, Steinbach, Germany) to a final concentration of 10% (v/v). After cryopreservation in a < -80°C freezer overnight, the cells were stored in the vapor phase above liquid nitrogen at < -140°C. For T cell phenotype analysis cells were thawed in RPMI 1640 medium with 20% (v/v) FCS (both Merck, Darmstadt, Germany) and rested for 1 h in RPMI 1640 with 10% FCS (37°C, 5% (v/v) CO2) before flow cytometric analysis. For functional analysis of the cryopreserved cells, they were thawed and seeded in TexMACS™ medium in a cell density of 2.5 x 106 cells/ml and rested overnight.
Directly after expansion or after cryopreservation and thawing (cryo), functionality of the laboratory- and clinical-scale IL-18 TRUCKs in comparison to untransduced T cells was assessed by co-culturing them with target cells. For flow cytometry and soluble mediator measurements, 5 x 104 target cells were seeded in 800 µl of their respective culture medium, which was removed after 4 - 24 h followed by addition of effector cells according to the specified effector-to-target (E:T) ratio in 800 µl CTL medium. For cytotoxicity, microscopy and intracellular cytokine assessment, 2 x 104 target cells were seeded in 200µl and co-cultured with effector cells accordingly.
The antibodies used for flow cytometric analysis are listed in Supplement Table S1. The transduction efficiency was analyzed with the Ganglidiomab-PE mAb. Intracellular staining of TNF-α was performed by using the IntraPrep Permeabilization Reagent (Beckman Coulter, Brea, CA, USA) according to the manufacturer’s instructions. Samples were read on a BD FACSCanto™ Flow Cytometer (Becton Dickinson, Franklin Lakes, NJ, USA). To determine cytotoxicity of engineered T cells, target cells were gated as CD3- cells. Percentage of killed cells was normalized to untransduced cell co-cultures using the following formula:
Cytokine concentrations in the supernatant were determined using a customized LEGENDplex™ Multi-Analyte Flow Assay (BioLegend, San Diego, CA, USA), which allowed for the detection of human IL-2, IL-4, IL-10, IL-18, granzyme B, perforin, interferon (IFN)-γ, and tumor necrosis factor (TNF)-α. Samples were analyzed with LEGENDplex v8.0 software (BioLegend, San Diego, CA, USA).
The release of lactate dehydrogenase (LDH) into the cell culture supernatant was assessed by using the Cytotoxicity Detection Kit (Roche, Basel, Switzerland). Cells lysed by adding Triton X-100 (Merck, Darmstadt, Germany) to a final concentration of 1% to all control wells served as maximum controls. Absorbance was assessed at a wavelength of 490 nm with a reference wavelength of 690 nm on a Synergy 2 Multi-Mode Microplate Reader (Biotek, Winooski, VT, USA). LDH release (%) was calculated according to the manufacturer’s protocol.
Transmitted-light microscope images of co-cultures of target and effector cells were taken with an Olympus IX81 microscope combined with a digital B/W camera using 10x objective lenses and analyzed with Xcellence Pro image software (all from Olympus, Hamburg, Germany). Representative pictures are shown.
Target cell killing by cryopreserved and thawed TRUCKs was furthermore determined with the XCelligence RTCA S16 Real Time Cell Analyzer and using E-Plates 16 PET (both ACEA Biosciences, San Diego, CA, USA). Background impedance of all wells was assessed with cell culture medium measurements. Afterwards, target cells were seeded in an amount of 1 x 105 cells (SH-SY5Y) or 2 x 104 cells (HT1080, HT1080-GD2) in 200 µl of their respective culture medium and adhesion was checked by measurements every 30 min. T cells were added after target cell adhesion shown by a constant impedance after 19 – 25 h. For this, 150 µl medium were carefully removed and replaced by T cells in the specified E:T-ratios in 150 µl CTL medium. Impedance was measured every 30 min. Cell indices were normalized to the respective indices after T cell addition.
To test the chemo-attractive potential of supernatants that were derived from co-culture experiments of primary human T cells transduced with the IL-18 TRUCK vector and target cells, migration assays with THP-1 or NK-92 cells were performed using a Boyden chamber (NeuroProbe, Gaithersburg, MD, USA) as recently described (26). The cell number was calculated using an Olympus IX71 microscope and imageJ 1.53k software. To normalize results from different plates, cell numbers of migrated cells towards untransduced T cells as background migration were subtracted from all values for each plate.
Genomic DNA was isolated from 1 x 106 transduced or untransduced cells (-20°C frozen cell pellets) with the QIAamp® DNA blood mini kit (Qiagen, Hilden, Germany) according to the manufacturer’s protocol. The determination of the VCN by qPCR was performed as recently described (35).
Statistical analysis was performed with GraphPad Prism V.9.1.2 using the Kruskal-Wallis and uncorrected Dunn’s test. Only mean ranks of preselected data sets were compared: large-scale TRUCKs cultured alone or co-cultured with different target cells, large-scale TRUCKs co-cultured with different target cells immediately after generation (indicated by black asterisks) or cryopreservation (indicated by grey asterisks), large-scale TRUCKs co-cultured with different target cells in comparison to the same co-cultures with all laboratory-scale manufactured cells. Significant differences are shown (*p ≤ 0.05, **p ≤ 0.01).
To assess feasibility, we performed two complete GMP-compliant processes (D1 and D2 with two individual donor lymphocyte products) to manufacture IL-18 TRUCKs targeting GD2 using an automated TCT protocol for CliniMACS Prodigy® as shown in Figure 1.
Starting with the lymphapheresis, 1.14 x 109 D1 and 1.17 x 109 D2, respectively, CD4+ and CD8+ cells (20.6% D1 and 46.1% D2 of unstimulated short time lymphapheresis) were applied for enrichment. An amount of 0.67 x 109 D1 and 0.88 x 109 D2 CD4+ and CD8+ cells were obtained representing a recovery of 58.8% D1 and 75.2% D2. Thereby, the isolation of CD4+ cells with a recovery of 63.9% D1 and 84.04% D2 was more effective than enrichment of CD8+ cells with 46.8% D1 and 61.7% D2 recovery, respectively (Figure 2A). The achieved T cell purities and cell compositions are shown in Figure 2B. Due to the CD8 based isolation procedure, NK as well as NKT cells were not completely depleted releasing 7.6% D1 and 4.4% D2 contaminating NKT cells in the final cell product (Figure 2B). The CD4/CD8 ratio pre-enrichment was 2.1 D1 and 1.6 D2 and post-enrichment 2.9 D1 and 2.2 D2 reflecting a superior recovery of CD4+ cells (Figure 2D).
Figure 2 Automated GMP-compliant manufacturing of IL-18 TRUCKs targeting GD2 using the CliniMACS Prodigy® (clinical-scale process). (A) Recovery (cell population in percent of cell population pre-enrichment) of CD4+ and CD8+ cells after enrichment. (B) Cell composition after enrichment of CD4+ and CD8+ cells and in the final products. The achieved T cell purity in the positive fraction (PF) was 79.1% D1 and 87.7% D2 with contaminating CD56+CD3+ NKT cells (12.0% D1 and 3.8% D2), CD56+CD3- NK cells (6.4% D1 and 6.8% D2), CD14+ monocytes (1.9% D1 and 0.6% D2) and CD20+ B cells (0.2% D1 and 0.5% D2). Cell composition of the final products (FP) with a purity of 92.1% D1 and 95.0% D2 T cells (CD3+/CD56-) and impurities with NKT cells (7.6% D1 and 4.4% D2) as well as residual 0.1% D1 and 0.2% D2 NK cells. Residual B cells (0.05% D2) were detected but no monocytes in the final products. (C) Expansion and viability of T cells (CD3+ CD56-) during 12-day manufacturing. (D) CD4/CD8 ratio during cultivation. The CD4/CD8 ratio changed during the manufacturing process (post-enrichment) from 2.9 to 3.3 D1 and 2.2 to 2.0 D2 in the final products. (E) Transduction rate of CD3+ cells and CD4+ CD8+ subtypes in the final products. (F) T cell phenotypic analysis of the starting material on day 0 (lymphapheresis) and in the final products on day 12. The analyses were performed by flow cytometry based on the expression of CD45RO, CCR7 and CD95 among viable CD3+ cells to define naïve (TN: CD45RO-, CCR7+, CD95-), stem-cell memory (TSCM: CD45RO-, CCR7+, CD95+), central memory (TCM: CD45RO+, CCR7+ CD95+), effector memory (TEM: CD45RO+, CCR7- CD95+) and effector (TEF: CD45RO-, CCR7- CD95+) T cell subsets. TN (33.1% D1 and 30.0% D2), TCM (25.1% and 11.8% D2) and TEM (37.6% D1 and 53.0% D2) cells were present in the initial product with differences in the T cell subsets (TSCM: 2.2% D1 and 0.62% D2, TEF: 2.1% D1 and 4.62% D2). In contrast, the final TRUCK products harbored cells with less differentiated memory phenotypes with a TCM (56.9% D1 and 8.17% D2) and TSCM (18.3% D1 and 8.62% D2) cells, TEM (23.6% D1 and 72.74% D2) and TEF (1.2% D1 and 9.9% D2) cells and the decrease of TN (<0.1% D1 and 0.54% D2) cells For cell composition, cells (B, D) were gated as viable CD45+ cells using lineage-specific markers: T cells (CD3+ CD56-), monocytes (CD14+), NK cells (CD56+ CD16+), NKT cells (CD56+ CD3+), B cells (CD20+). n = 2.
In accordance with the TCT protocol, the cell expansion started with 0.84 x 108 D1 and 0.9 x 108 D2 viable T cells (corresponding 1.18 x 108 D1 and 1.0 x 108 D2 viable WBC) after enrichment of CD4+ and CD8+ cells. The expansion rate during the 12-day manufacturing process was 68.3-fold D1 and 71.4-fold D2 for T cells. Viability of the cells was >96% during the whole process until final harvest (Figure 2C). Cell concentration increased in a constant culture volume from 3.8 x 106/ml D1 on day 5 and 5.5 x 106/ml D2 to 31.2 x 106/ml D1 and 34.2 x 106/ml D2 in the final products (Figure S1A). Monitoring of cell culture condition revealed a pH of 7.0 - 7.1 and a glucose level above the critical level of 100 mg/dl. (Figure S1B). T cell purity and cell composition of the final products is shown in Figure 2B. During expansion, the CD4/CD8 ratio increased in the first run from 2.9 to 3.3 D1 but decreased slightly in the second run from 2.2 to 2.0 D2 (Figure 2D). The proportion of T cell phenotypes during ex vivo cell expansion was analyzed by comparing T cell phenotypes in the initial product post CD4+ and CD8+ enrichment at process day 0 and in the final product at process day 12 as shown in Figure 2F.
The recently described “all-in-one” lentiviral vector (26) encoding constitutive GD2 CAR and inducible IL-18 expression was used for cell transduction on day 1 of the manufacturing process with a multiplicity of infection (MOI) of 29 for D1 and 10 for D2 derived T cells. The percentage of transduced CD3+ cells in the final product, was 74.9% D1 and 52.2% D2, while CD4+ cells exhibited a higher transduction efficiency (80.6% D1 and 65.8% D2) compared to CD8+ cells (57.2% D1 and 26.5% D2) (Figure 2E). The VCN determined by qPCR of genomic DNA in the final products were 2.6 D1 and 2.4 D2 copies/cell, respectively.
IL-18 TRUCKs targeting GD2 manufactured using the CliniMACS Prodigy® (referred to as clinical-scale TRUCKs) were compared to the respective TRUCKs generated under laboratory conditions (referred to as laboratory-scale TRUCKs). These cells were manufactured manually as previously described (34) using the same isolated CD4+ and CD8+ T cell starting population, lentiviral vector, expansion media, cytokines, and experimental timing. Untransduced T cells as well as GD2 TRUCKs with inducible EGFP expression (referred to as EGFP-TRUCKs) were generated in laboratory scale and served as controls. From a starting fraction of 0.6 x 106 cells, laboratory-scale TRUCKs expanded to a cell number of 48 x 106 cells, which was slightly lower compared to untransduced T cells and EGFP-TRUCKs with final cell numbers of 53 x 106 and 54 x 106 cells, respectively (Figure S2A). Both, laboratory- and clinical-scale IL-18 TRUCKs, showed an enhanced frequency of CD4+ T cells after expansion with CD4/CD8 ratios of 2.2 for clinical- and 2.1 for laboratory-scale TRUCKs, whereas it was 0.79 for untransduced T cells (Figures 3A, B). Utilizing the recently described “all-in-one” vector” (26), we obtained a high percentage of transduced cells with 65% and 85% under clinical scale as well as under laboratory conditions, respectively, with a higher frequency of transduced CD4+ T cells than CD8+ T cells (Figures 3C, D). Activation marker expression on clinical-scale TRUCKs, assessed after expansion of T cells with anti-CD3/CD28 stimulation and IL-7 and IL-15 supplementation, revealed CD25 and CD69 expression on CD3+ T cells (45% and 24%, respectively), moderate CD154 expression on CD4+ cells (13%) and absent CD137 expression on CD8+ cells (Figures 3E–H); similar data were obtained for laboratory-scale cells. Clinical use of modified cells often requires cryopreservation to allow for centralized manufacturing and administration flexibility (36). To assess the feasibility of cryopreservation of the obtained T cell product, the manufactured T cells were also characterized after freezing and thawing. Cryopreservation of clinical-scale IL-18 TRUCKs did not significantly change their activation state, CD4/CD8 ratios and frequency of transduced cells (Figures 3A, B, D–H).
Figure 3 Clinical-scale-manufactured IL-18 TRUCKs targeting GD2 are transduced and pre-activated at similar efficacies compared to laboratory-scale-manufactured TRUCKs. IL-18 TRUCKs targeting GD2 were generated using the CliniMACS Prodigy® (clinical-scale TRUCK; n=2) or under laboratory conditions (lab.-scale TRUCK; n=3). Untransduced T cells (lab.-scale untransduced; n=3) as well as GD2 TRUCKs with inducible EGFP expression (EGFP-TRUCK; n=3) served as control. The manufactured cells were either characterized directly after the generation process (d12) or after cryopreservation and thawing (cryo). (A, B) Frequency of (A) CD4+ and (B) CD8+ T cells in the final cell product. (C, D) Percentage of CAR+ cells of (C) CD3+ cells shown as representative plots and (D) CD3+, CD4+ and CD8+ cells as assessed by staining of the scFv-domain of TRUCKs with a Ganglidiomab antibody after expansion. (E–H) Expression of the activation markers (E) CD25 on CD3+, (F) CD69 on CD3+, (G) CD137 on CD8+ and (H) CD154 on CD4+ T cells. (A, B, D–H) Data are shown as mean ± SD. Statistical differences of large-scale TRUCKs directly after generation or cryopreservation as well as in comparison to laboratory-scale manufactured cells were assessed by Kruskal-Wallis and Dunn’s test, significant differences are shown (*p ≤ 0.05).
We tested the ability of the generated TRUCKs to recognize and react towards target cells. Manufactured cells were co-cultivated either with GD2- HT1080, HT1080 cells expressing GD2 or with the GD2+ neuroblastoma cell line SH-SY5Y as target cells and examined for activation marker expression. Clinical-scale IL-18 TRUCKs targeting GD2 responded with increased expression of CD25 and CD69 by 50 – 64% and 35 – 45%, respectively, on all CD3+ T cells to both GD2-expressing target cells, whereas no specific response towards unmodified HT1080 cells was detected (Figures 4B, C and S3A–D). The slightly lower activation response towards SH-SY5Y in comparison to HT1080-GD2 coincides with the lower GD2 expression levels (26). Clinical-scale compared to laboratory-scale TRUCKs exhibited a similar activation marker increase for all markers and target cells, and also cryopreserved TRUCKs did not show significantly different responses regarding expression of CD137 on CD8+ and CD154 on CD4+ T cells, the same effects were observed, indicating cells of the CD4+ and CD8+ populations in manufactured IL-18 TRUCKs were specifically and effectively activated in response to target cells (Figures 4A, D, E and S3E–H). Assessment of respective activation marker expression levels as mean fluorescence intensity (MFI) or in further effector-to-target (E:T) ratios confirmed the observed results (Figures S3A–H).
Figure 4 Clinical-scale-manufactured IL-18 TRUCKs targeting GD2 specifically respond to target cells with an increase of activation marker expression. IL-18 TRUCKs targeting GD2 were generated using the CliniMACS Prodigy® (clinical-scale TRUCK; n=2) or under laboratory conditions (lab.-scale TRUCK; n=3). Untransduced T cells (lab.-scale untransduced; n=3) as well as GD2 TRUCKs with inducible EGFP expression (EGFP-TRUCK; n=3) served as control. The manufactured cells were tested for GD2-CAR-mediated activation either directly after the generation process (d12) or after cryopreservation and thawing (cryo) by co-cultivation with the indicated target cells for 48h in an effector-to-target (E:T) ratio of 4:1 or cultivation alone (T cells only). (A–E) Frequency of (A) CD137+ of CD8+ as representative plots, (B) CD25+ of CD3+, (C) CD69+ of CD3+, (D) CD137+ of CD8+ and (E) CD154+ of CD4+ T cells as determined by flow cytometry. (B–E) A dashed line indicates background levels of the respective expression by untransduced T cells (grey), EGFP-TRUCKs (blue), as well as clinical-scale (red) and laboratory-scale (green) TRUCKs cultured alone. Data is shown as mean ± SD. Statistical differences of clinical-scale TRUCKs co-cultured with different target cells after generation or cryopreservation as well as in comparison to laboratory-scale manufactured cells were assessed by Kruskal-Wallis and Dunn’s test, significant differences are shown (*p ≤ 0.05, **p ≤ 0.01).
The release of cytokines by manufactured cells into the cell co-culture supernatants upon target contact was assessed. In co-cultures with HT1080-GD2 cells and, to a lower extent also with SH-SY5Y cells, the release of several cytokines and cytolytic factors was upregulated in clinical-scale IL-18 TRUCKs compared to background secretion: IL-2 (108 and 25-fold), IL-4 (39 and 23-fold), IL-10 (5.8 and 2.2-fold), interferon (IFN)-γ (70 and 49-fold), granzyme B (290 and 150-fold), perforin (3.7 and 2.7-fold) and tumor necrosis factor (TNF)-α (430 and 380-fold; all in an E:T ratio of 8:1) (Figures 5 and S4). Thereby, antigen-induced cytokine release by clinical-scale TRUCKs was similar compared to laboratory-scale TRUCKs and, furthermore, cryopreservation of cells did not significantly change the secretion pattern. A higher expression of TNF-α, by IL-18 TRUCKs upon specific target contact was confirmed by intracellular staining (Figure S3I).
Figure 5 Clinical-scale-manufactured IL-18 TRUCKs targeting GD2 specifically react to target recognition with increased release of soluble mediators. IL-18 TRUCKs targeting GD2 were generated using the CliniMACS Prodigy® (clinical-scale TRUCK; n=2) or under laboratory conditions (lab.-scale TRUCK; n=3). Untransduced T cells (lab.-scale untransduced; n=3) as well as GD2 TRUCKs with inducible EGFP expression (EGFP-TRUCK; n=3) served as control. The manufactured cells were tested for functionality either directly after the generation process (d12) or after cryopreservation and thawing (cryo) by co-cultivation with the indicated target cells in an effector-to-target (E:T) ratio of 8:1 or cultivation of T cells only. The concentration of released cytokines (A) IL-2, (B) IL-4, (C) IL-10, (D) granzyme B, (E) IFN-γ, (F) perforin, and (G) TNF-α in the cell culture supernatants after 48 h was assessed by LEGENDPlex™. (F) A dashed line indicates background levels of the respective cytokine release by untransduced T cells (grey), EGFP-TRUCKs (blue), as well as clinical-scale (red) and laboratory-scale (green) TRUCKs cultured alone. (A–G) Data are shown as mean ± SD. Statistical differences of clinical-scale TRUCKs co-cultured with different target cells after generation or cryopreservation as well as in comparison to all laboratory-scale manufactured cells were assessed by Kruskal-Wallis and Dunn’s test, significant differences are shown (*p ≤ 0.05, **p ≤ 0.01).
Finally, the killing capacity of manufactured IL-18 TRUCKs targeting GD2 was determined by flow cytometric analysis of target cells in the co-cultures. Relative to co-cultures of untransduced T cells with HT1080-GD2 and SH-SY5Y, clinical-scale TRUCKs eliminated 69 - 88% of HT1080-GD2 and 65 - 86% of SH-SY5Y cells at different E:T ratios, whereas the frequency of HT1080 in co-cultures with the clinical-scale TRUCKs was on the level of untransduced T cells or laboratory-scale manufactured cells, likely representing expected allo-reactivity (Figure 6A). Compared to TRUCKs generated in the laboratory-scale, clinical-scale-manufactured TRUCKs exhibited a similar cytotoxic ability to eliminate target cells, which was moreover not impeded by cryopreservation. The release of lactate dehydrogenase (LDH) into the supernatant as parameter for cytolysis confirmed the result; cytotoxicity was enhanced in co-cultures of both IL-18 TRUCKs with HT1080-GD2 cells (18-23% and 0-17% for large- and laboratory-scale TRUCKs, respectively) compared to the respective co-cultures with unmodified HT1080 cells, in which LDH was not released above background of cells cultured alone (Figure S5A). Target cell death by LDH release in co-cultures of clinical-scale TRUCKs with SH-SY5Y was also enhanced (6-16%) and similar to the cytotoxicity by laboratory-scale TRUCKs (2-8%). LDH measurements in the co-cultures with cryopreserved T cells revealed a similar cytotoxic capability towards target cells. Real-time measurement of target cell viability confirmed these results. After addition of both TRUCKs to adherent SH-SY5Y cells, the cell index was rapidly reduced, resulting in almost complete absence of adherent target cells after co-cultivation with laboratory- or clinical-scale TRUCKs for 60 h in different ratios (Figures 6B, C). Transmitted-light microscopy visualized the process of target cell elimination. In co-cultures of HT1080 with all T cell products, cells are distributed equally, and the target cells stayed viable in all E:T ratios (Figure 6D). In co-cultures of both TRUCKs with HT1080-GD2 or SH-SY5Y, the target cells were diminished or even absent after 72 h and T cells formed large clusters around the target cells. For thawed TRUCKs, the clusters tended to be even larger and already appeared at low E:T ratios in co-cultures with SH-SY5Y cells (Figure S5B).
Figure 6 Clinical-scale-manufactured IL-18 TRUCKs targeting GD2 specifically eliminate target cells. IL-18 TRUCKs targeting GD2 were generated using the CliniMACS Prodigy® (clinical-scale TRUCK; n=2) or under laboratory conditions (lab.-scale TRUCK; n=3). Untransduced T cells (lab.-scale untransduced; n=3) as well as GD2 TRUCKs with inducible EGFP expression (EGFP-TRUCK; n=3) served as control. The manufactured cells were tested for cytotoxicity either directly after the generation process (d12) or after cryopreservation and thawing (cryo) by co-cultivation with the indicated target cells and in the indicated effector-to-target (E:T) ratios. (A) Target cell killing after 48h was measured by flow cytometry as percentage of killed CD3- cells relative to those eliminated in co-cultures with untransduced T cells. Data is shown as mean ± SD. Statistical differences of large-scale TRUCKs co-cultured with different target cells directly after generation or cryopreservation as well as in comparison to all laboratory-scale manufactured cells were assessed by Kruskal-Wallis and Dunn’s test, whereby only significant differences are shown (*p ≤ 0.05, **p ≤ 0.01). (B, C) Killing of SH-SY5Y cells by the generated cells (B) directly after their generation (here: lab.-scale untransduced n=2, clinical-scale TRUCK n=1) or (C) after cryopreservation and thawing was determined with the XCelligence Real-Time Cell Analyzer. Cell indices were normalized to the respective indices after T cell addition. Data is shown as mean. (D) Representative transmitted-light microscope images of co-cultures of fresh effector cells with target cells taken after 48 h by an Olympus IX81 microscope combined with a digital B/W camera using 10x objective lenses.
To address the ability of the generated TRUCKs to selectively release IL-18 following CAR engagement of GD2 target antigen, the cytokine was measured in the co-culture supernatants. Importantly, the release of IL-18 into the cell culture supernatant by freshly-generated or cryopreserved clinical-scale TRUCKs was induced in a target-specific manner up to 41 pg/ml upon HT1080-GD2 and 18 pg/ml upon SH-SY5Y encounter (Figure 7A). To assess the effect of anti-GD2 IL-18 TRUCK-induced cytokines with respect to the recruitment of innate immune cells, we used a modified Boyden chamber assay to compare the migration potential of cell supernatants collected from co-culture experiments of IL-18 TRUCKs vs. untransduced T cells and target cells (HT1080-GD2 and SH-SY5Y) to promote the recruitment of monocytes (THP-1 cells) and NK cells (NK-92 cells). The capacity of IL-18 containing supernatants to recruit innate immune cells was shown by positive Giemsa staining of the transwell membrane through which migrating cells were recruited (Figure 7B). Giemsa staining of migrated cells revealed that increased numbers of the monocytic cells THP-1 as well as NK-92 cells were recruited by supernatants collected from IL-18 TRUCKs co-cultured with HT1080-GD2 or SH-SY5Y target cells compared to untransduced T cells, EGFP-TRUCKs or upon co-culture with target cells, likely as a result of induced IL-18 cytokine secretion (Figures 7C, D). Taken together, supernatants from TRUCKs releasing IL-18 in an inducible manner by CAR activation upon antigen recognition exhibit an innate immune cell recruitment potential in vitro indicating IL-18 biological activity.
Figure 7 Clinical-scale-manufactured IL-18 TRUCKs targeting GD2 released IL-18 in a target-specific manner leading to innate immune cell attraction. IL-18 TRUCKs targeting GD2 were generated using the CliniMACS Prodigy® (clinical-scale TRUCK; n=2) or under laboratory conditions (lab.-scale TRUCK; n=3). Untransduced T cells (lab-scale untransduced; n=3) as well as GD2 TRUCKs with inducible EGFP expression (EGFP-TRUCK; n=3 [except for D, in which n=1]) served as control. The manufactured cells were tested for functionality either directly after the generation process (d12) or after cryopreservation and thawing (cryo) by co-cultivation with the indicated target cells in the indicated effector-to-target (E:T) ratios or cultivation alone (T cells only). (A) The concentration of released cytokines in the cell culture supernatants after 48h was assessed by LEGENDPlex™. The chemoattractive potential of IL-18 released by TRUCKs upon target recognition in terms of the migration of (B, C) THP-1 and (D) NK-92 cells was assessed. Supernatant of engineered T cells cultured alone (T cells only) or together with the indicated target cells for 48 h was placed in a Boyden chamber, covered with an 8 µm polycarbonate membrane and incubated for another 4h. Medium served as the control supernatant. Cells migrated through the membranes were Giemsa stained. (B) Representative pictures of Giemsa stained THP-1 cells (violet) on the bottom of the membrane. (C, D) The number of cells that migrated through the membrane was determined. To normalize results from different plates, cell numbers of migrated cells towards untransduced T cells only were subtracted from all values. (A, C, D) Data is shown as mean ± SD. A dashed line indicates background levels of the respective cytokine release by untransduced T cells (grey), EGFP-TRUCKs (blue), as well as clinical-scale (red) and laboratory-scale (green) TRUCKs cultured alone. Statistical differences of large-scale TRUCKs co-cultured with different target cells after generation or cryopreservation as well as in comparison to laboratory-scale manufactured cells were assessed by Kruskal-Wallis and Dunn’s test, significant differences are shown (*p ≤ 0.05).
In general, CAR and TRUCK vector design is a critical aspect for the generation of engineered T cells. Especially, the costimulatory domains within the CAR might be of high interest to improve CAR T-cell activity and long-lasting persistence with reduced T cell exhaustion, also with respect to the targeted tumor antigen (37). TRUCKs additionally combine the redirected CAR T cell attack with the on-site release of a biologically active protein while avoiding its systemic toxicity, thereby holding promise to modulate the environment of the targeted solid tumor. The cytokine of choice within the TRUCK concept should be chosen with respect to the desired immune response within the tumor microenvironment (TME). The IL-18 cytokine creates a proinflammatory environment, recruits bystander effector cells to the tumor site and enhances cytolytic activity (4–6). Therefore, IL-18 was an attractive cytokine for the development of the “all-in-one” lentiviral vector combining constitutive anti-GD2 CAR expression and inducible IL-18 (26) as well as for the automated and closed processing for GMP-compliant manufacturing process for CAR T cells (24, 25).
TRUCKs combine the redirected CAR T cell attack with the on-site release of a biologically active protein while avoiding its systemic toxicity, thereby holding promise to modulate the environment of the targeted solid tumor. Further development of the strategy is based on design and broad in vitro characterization of the “all-in-one” lentiviral vector combining constitutive anti-GD2 CAR expression and inducible IL-18 (26) as well as on an automated and closed processing for GMP-compliant manufacturing process for CAR T cells (24, 25). We present the proof of feasibility for translation of the method to activate and expand IL-18 TRUCKs targeting GD2 for clinical application.
Our protocol is optimized to produce CAR-engineered T cells in clinically sufficient numbers under GMP-compliance using the CliniMACS Prodigy® platform that integrates different steps of manufacturing including cell isolation, activation, transduction, cell washing, cultivation and formulation of the final product in a single process and thus minimizes variability emanating from various manual work steps. The fully integrated modular system allows for flexibility and standardized procedure at the same time, which is the key for the production of personalized cell products of various kinds. Multiple steps are required to produce gene modified effector cells starting with enrichment of CD4+ and CD8+ T cell subset followed by activation, transduction and expansion of effector cells. CD4+ and CD8+ enrichment is regarded as a safety procedure to decrease blast counts in the culture (38). CD4+ and CD8+ enrichment also decreases contaminating cells such as monocytes, which inhibit CAR T cell expansion (39). The primary objective was the feasibility of cell production for 3 dose levels (e. g. 5 × 105, 1 ×106, and 3 × 106 anti-GD2 IL-18 TRUCKs/kg). For enrichment of CD4+ and CD8+ cells the TCT process is limited to a maximum of 3 x 109 target cells. This means for our two processes that only a part (20.6% D1 and 46.1% D2) of the lymphapheresis from a healthy donor was used for enrichment. Likewise, only part of enriched cells (12.5% D1 and 10.0% D2) could be applied for activation and expansion. Due to the limited culture volume and growth area, it is recommended to start with 1 x 108 cells. Any remainder may be frozen as backup for the patient.
After CD4+ and CD8+ enrichment we found high T cell purities with low contaminating cell populations of CD8+ NK and NKT cells which are not removed during the CD4+ and CD8+ enrichment step also described by other groups (23, 38). With a T cell expansion rate of 68.3-fold D1 and 71.4-fold D2 and a transduction rate of 77.7% D1 and 55.1% D2 we reached sufficient cell doses of 4.8 x 109 D1 and 3.7 x 109 D2 IL-18 TRUCKs. This would allow for the application of 60.0 x 106 cells/kg respectively 46.0 x 106 cells/kg IL-18 TRUCKs targeting GD2 to a recipient weighing 80 kg even in a multi-dose base. We and others show the eligibility of the T cell transduction (TCT) process developed by Miltenyi Biotec with fixed process parts (enrichment, activation and transduction) as well as the possibility of variable culture set up by modular programming of the activity matrix (22–25, 38, 40–43).
Safety aspects in the clinical use of Advanced Therapy Medicinal Products (ATMPs) have high priority. Lentiviral vectors are known to have a lower risk for mutational oncogenesis than γ-retroviral vectors (38). The European Medicines Agency (28) reflection paper (EMA/CAT/190186/2012) on the management of clinical risks deriving from insertional mutagenesis highlighted the VCN as a risk factor for oncogenesis and recommended risk assessment and management of the integration copy numbers, integration profile and sites in cellular products. The IL-18 TRUCK final products contained 2.6 D1 and 2.4 D2 copies/cell, respectively, which is below 5 copies/cell that is considered to be safe (44). The transduction rate of 74.9% D1 and 52.2% D2 in the final products was higher than reported by other groups using CliniMACS Prodigy® for production of CAR T cells (22, 23, 25, 38, 40–43, 45). Transduction efficiency was higher for CD4+ compared to CD8+ T cells as also shown by previous reports (25, 43).
To prolong the in vivo persistence of CAR T cells in patients, enrichment of cell products with less differentiated T cell subsets such as central memory (TCM) or stem cell memory T (TSCM) T cells is thought to be crucial. These subpopulations have gained substantial attention, as the adoptive transfer of even low numbers of T cells from these subsets can reconstitute robust and long-term maintained immune responses (46, 47). IL-18 TRUCK final product T cells from Donor 1 showed a Tcm/Tscm phenotype while Donor 2 showed predominantly a TEM phenotype. CD3/CD28 activation and culture of naïve T (48) cells in presence of IL-7 and IL-15 promotes the acquisition of TCM or TSCM phenotypes (22, 23, 38, 49). This is in line with previous reports (23, 43). In addition, whereas T cells expressing CARs with CD28 domains predominantly differentiate into effector memory T (TEM) cells, in vitro expansion of 4-1BB-containing CAR T cells as the TRUCKs used here produces a higher proportion of TCM cells (50). We also demonstrate, that the TRUCK manufacturing process did not lead to unspecific secretion of IL-18 even after T cell activation with TransAct (CD3/CD28) and cytokines (IL-7 and IL-15).
In vitro characterization of the obtained engineered T cell product revealed an upregulation of activation markers (CD25, CD69, CD137 and CD154) on both CD4+ and CD8+ T cells and a variety of pro-inflammatory cytokines and cytotoxic mediators (IL-2, IL-4, granzyme B, perforin, IFN-γ, TNF-α) upon specific recognition of GD2-expressing target cells, but not after co-cultivation with a control cell line lacking the target. In addition, TRUCKs induced release of the engineered cytokine IL-18 in an antigen-dependent manner and mediated a very low background secretion upon co-cultivation with target-negative cells or spontaneous release without target cells. The risk of toxicity for IL-18 TRUCKs is different to IL-12 TRUCKs that revealed severe toxicity of T cells engineered with inducible IL-12 in a melanoma mouse model due to off-target cytokine secretion (4). However, other studies with IL-12 TRUCKs reported a safe administration into mice and such toxicities were not observed for IL-18 TRUCKs (2, 3, 5, 51). GMP-compliant manufactured IL-18 TRUCKs targeting GD2 showed a high cytotoxic capability and were able to eliminate tumor cells while forming clusters around target cells. IL-18 TRUCKs show higher toxicities against high GD2-expressing target cells confirming the in vitro studies by Wiebel et al. (52).
To accurately quantify and confirm cytotoxicity towards target cells, we combined different methodologies including the label-free, real-time monitoring by impedance measurements, flow cytometric analysis allowing for concurrent phenotypic evaluation of TRUCKs, and detection of pro-inflammatory cytokines as indirect and cytotoxic mediators as direct parameter of cell lysis in the co-culture. Kiesgen (53) et al. comprehensively compare the power and limitation of different cytotoxicity assays and emphasize that especially impedance-based assays display a superior sensitivity and signal-to-background ratio over the “gold standard” 51chromium-release assay making it possible to evaluate even low E:T ratios as most appropriate to resemble physiological conditions. Such low E:T ratios would be interesting to evaluate in further experiments, since the manufactured TRUCKs exhibited rapid elimination of SH-SY5Y cells in the lowest E:T ratio of 1:1. Moreover, in vitro tests using repeated antigen stimulation or inclusion of immunosuppressive factors present in the TME are increasingly being used and could give an insight about persistence and exhaustion level of the generated TRUCKs upon high antigen stress.
We show similar manufacturing of clinical-scale and laboratory-scaled IL-18 TRUCKs concerning transduction and amplification efficiency and cellular functionality. After cryopreservation of the T cell products, the specificity and cytotoxicity of TRUCKs was maintained. Attempts to treat solid tumors with redirected T cells have largely failed so far, with very few patients responding and with only transient and partial tumor regression (17, 18, 48, 54–57). The poor clinical outcome is thought to be due at least in part to an unfavorable environment in the tumor tissue that suppresses CAR T cell responses. TRUCK-secreted cytokine IL-18 led to increased recruitment of monocytes and NK cells in an in vitro cell migration assay. This may contribute to reprogramming the tumor stroma towards a more favorable environment for CAR T cell function, thereby enhancing their efficacy in the treatment of solid tumors. Furthermore, IL-18 was shown to polarize TRUCKs towards more potent pro-inflammatory effector cells that do not drive into functional exhaustion in the long term (5).
In conclusion, GMP-compliant manufacturing of IL-18 TRUCKs targeting GD2 using the automated closed CliniMACS Prodigy® system is feasible and enables the manufacturing of a sufficient number of cells for clinical application. The automatic mode of operation improves standardization and robustness of the manufacturing process. This benefits the manufacturing at different sites for an academia-initiated multicenter trial. The smooth adaption of the process established and validated for the manufacturing of CAR T cells to generate IL-18 TRUCKs encourages the translation of the procedure to other cells and targets.
The original contributions presented in the study are included in the article/Supplementary Material. Further inquiries can be directed to the corresponding authors.
For this study the donors gave their written informed consent and approval was granted by the corresponding ethics committee (No. 2829-2015). The study was approved by the ethics committee of Hannover Medical School (2519-2014, 2830-2015, 3639-2017).
Conceptualization: RE, WG, BE-V, AD, UK, KZ, and ASc. Methodology: AD, WG, RE, MM, AM-E, CK, ASt, KZ, and ASc. Formal analysis: WG, AD, RE, AM-E, MM, CK, ASt, KZ, and KA. Resources: HL, NS, and CR. Writing original draft application: WG, AD, RE, and AM-E. Writing review and editing: all authors. Supervision: HA, LA, TM, RB, LG, ASc, BE-V, and UK. Funding acquisition: BE-V, HA, TM, RB, ASc, UK, and CR. All authors have read and agreed to the published version of the manuscript.
This work was supported by the following grants: “From CARs to TRUCKs: Induction of a concerted antitumor immune response by engineered T cells” (Deutsche Krebshilfe/German Cancer Aid-Priority Program in Translational Oncology #111975) and DFG-funded SFB738 (projects C4 & C9) and Cluster of Excellence REBIRTH (EXC62/2).
HA, ASc, and KZ have submitted a patent application describing the “all-in-one” TRUCK vector technology. The University Medicine of Greifswald licensed Ganglidiomab to AnYxis Immuno-oncology for commercialization.
The remaining authors declare that the research was conducted in the absence of any commercial or financial relationships that could be construed as a potential conflict of interest.
All claims expressed in this article are solely those of the authors and do not necessarily represent those of their affiliated organizations, or those of the publisher, the editors and the reviewers. Any product that may be evaluated in this article, or claim that may be made by its manufacturer, is not guaranteed or endorsed by the publisher.
The pQPCR-Stdx4”-plasmid was kindly provided by Michael Rothe. We thank Anna-Magdalena Bartels, Andrea Quaiser, Sarina Lukis and Elvira Schulde for excellent technical assistance. Furthermore, we would like to thank Dr. Bastian Schirmer and Prof. Dr. Detlef Neumann (Institute of Pharmacology, Hannover Medical School) for providing the XCelligence RTCA S16 Real Time Cell Analyzer. We thank Thea Boehm and Caren Clark (Miltenyi Biotec) for helpful discussions and valuable technical advice for the development of process protocol for automated cell-manufacturing. We also thank Carole Batkai for editing the manuscript.
The Supplementary Material for this article can be found online at: https://www.frontiersin.org/articles/10.3389/fimmu.2022.839783/full#supplementary-material
1. Kagoya Y, Tanaka S, Guo T, Anczurowski M, Wang CH, Saso K, et al. A Novel Chimeric Antigen Receptor Containing a JAK-STAT Signaling Domain Mediates Superior Antitumor Effects. Nat Med (2018) 24(3):352–9. doi: 10.1038/nm.4478
2. Chmielewski M, Kopecky C, Hombach AA, Abken H. IL-12 Release by Engineered T Cells Expressing Chimeric Antigen Receptors can Effectively Muster an Antigen-Independent Macrophage Response on Tumor Cells That Have Shut Down Tumor Antigen Expression. Cancer Res (2011) 71(17):5697–706. doi: 10.1158/0008-5472.CAN-11-0103
3. Chinnasamy D, Yu Z, Kerkar SP, Zhang L, Morgan RA, Restifo NP, et al. Local Delivery of Interleukin-12 Using T Cells Targeting VEGF Receptor-2 Eradicates Multiple Vascularized Tumors in Mice. Clin Cancer Res (2012) 18(6):1672–83. doi: 10.1158/1078-0432.CCR-11-3050
4. Kunert A, Chmielewski M, Wijers R, Berrevoets C, Abken H, Debets R. Intra-Tumoral Production of IL18, But Not IL12, by TCR-Engineered T Cells is non-Toxic and Counteracts Immune Evasion of Solid Tumors. Oncoimmunology (2017) 7(1):e1378842. doi: 10.1080/2162402X.2017.1378842
5. Chmielewski M, Abken H. CAR T Cells Releasing IL-18 Convert to T-Bet(high) FoxO1(low) Effectors That Exhibit Augmented Activity Against Advanced Solid Tumors. Cell Rep (2017) 21(11):3205–19. doi: 10.1016/j.celrep.2017.11.063
6. Tokarew N, Ogonek J, Endres S, von Bergwelt-Baildon M, Kobold S. Teaching an Old Dog New Tricks: Next-Generation CAR T Cells. Br J Cancer (2019) 120(1):26–37. doi: 10.1038/s41416-018-0325-1
7. Svennerholm L, Bostrom K, Fredman P, Jungbjer B, Lekman A, Mansson JE, et al. Gangliosides and Allied Glycosphingolipids in Human Peripheral Nerve and Spinal Cord. Biochim Biophys Acta (1994) 1214(2):115–23. doi: 10.1016/0005-2760(94)90034-5
8. Seitz CM, Schroeder S, Knopf P, Krahl AC, Hau J, Schleicher S, et al. GD2-Targeted Chimeric Antigen Receptor T Cells Prevent Metastasis Formation by Elimination of Breast Cancer Stem-Like Cells. Oncoimmunology (2020) 9(1):1683345. doi: 10.1080/2162402X.2019.1683345
9. Chulanetra M, Morchang A, Sayour E, Eldjerou L, Milner R, Lagmay J, et al. GD2 Chimeric Antigen Receptor Modified T Cells in Synergy With Sub-Toxic Level of Doxorubicin Targeting Osteosarcomas. Am J Cancer Res (2020) 10(2):674–87.
10. Navid F, Santana VM, Barfield RC. Anti-GD2 Antibody Therapy for GD2-Expressing Tumors. Curr Cancer Drug Targets (2010) 10(2):200–9. doi: 10.2174/156800910791054167
11. Golinelli G, Grisendi G, Prapa M, Bestagno M, Spano C, Rossignoli F, et al. Targeting GD2-Positive Glioblastoma by Chimeric Antigen Receptor Empowered Mesenchymal Progenitors. Cancer Gene Ther (2020) 27(7-8):558–70. doi: 10.1038/s41417-018-0062-x
12. Grant SC, Kostakoglu L, Kris MG, Yeh SD, Larson SM, Finn RD, et al. Targeting of Small-Cell Lung Cancer Using the Anti-GD2 Ganglioside Monoclonal Antibody 3F8: A Pilot Trial. Eur J Nucl Med (1996) 23(2):145–9. doi: 10.1007/BF01731837
13. Andersch L, Radke J, Klaus A, Schwiebert S, Winkler A, Schumann E, et al. CD171- and GD2-Specific CAR-T Cells Potently Target Retinoblastoma Cells in Preclinical In Vitro Testing. BMC Cancer (2019) 19(1):895. doi: 10.1186/s12885-019-6131-1
14. Chang HR, Cordon-Cardo C, Houghton AN, Cheung NK, Brennan MF. Expression of Disialogangliosides GD2 and GD3 on Human Soft Tissue Sarcomas. Cancer (1992) 70(3):633–8. doi: 10.1002/1097-0142(19920801)70:3<633::AID-CNCR2820700315>3.0.CO;2-F
15. Kailayangiri S, Altvater B, Spurny C, Jamitzky S, Schelhaas S, Jacobs AH, et al. Targeting Ewing Sarcoma With Activated and GD2-Specific Chimeric Antigen Receptor-Engineered Human NK Cells Induces Upregulation of Immune-Inhibitory HLA-G. Oncoimmunology (2017) 6(1):e1250050. doi: 10.1080/2162402X.2016.1250050
16. Casey DL, Lin TY, Cheung NV. Exploiting Signaling Pathways and Immune Targets Beyond the Standard of Care for Ewing Sarcoma. Front Oncol (2019) 9:537. doi: 10.3389/fonc.2019.00537
17. Heczey A, Louis CU, Savoldo B, Dakhova O, Durett A, Grilley B, et al. CAR T Cells Administered in Combination With Lymphodepletion and PD-1 Inhibition to Patients With Neuroblastoma. Mol Ther (2017) 25(9):2214–24. doi: 10.1016/j.ymthe.2017.05.012
18. Louis CU, Savoldo B, Dotti G, Pule M, Yvon E, Myers GD, et al. Antitumor Activity and Long-Term Fate of Chimeric Antigen Receptor-Positive T Cells in Patients With Neuroblastoma. Blood (2011) 118(23):6050–6. doi: 10.1182/blood-2011-05-354449
19. Straathof K, Flutter B, Wallace R, Jain N, Loka T, Depani S, et al. Antitumor Activity Without on-Target Off-Tumor Toxicity of GD2-Chimeric Antigen Receptor T Cells in Patients With Neuroblastoma. Sci Transl Med (2020) 12(571). doi: 10.1126/scitranslmed.abd6169
20. Cauchon NS, Oghamian S, Hassanpour S, Abernathy M. Innovation in Chemistry, Manufacturing, and Controls-A Regulatory Perspective From Industry. J Pharm Sci (2019) 108(7):2207–37. doi: 10.1016/j.xphs.2019.02.007
21. Chomienne C, Sierra J, Einsele H, Jäger U. EHA Guidance Document: The Process of CAR-T Cell Therapy in Europe. HemaSphere (2019).
22. Mock U, Nickolay L, Philip B, Cheung GW, Zhan H, Johnston ICD, et al. Automated Manufacturing of Chimeric Antigen Receptor T Cells for Adoptive Immunotherapy Using CliniMACS Prodigy. Cytotherapy (2016) 18(8):1002–11. doi: 10.1016/j.jcyt.2016.05.009
23. Lock D, Mockel-Tenbrinck N, Drechsel K, Barth C, Mauer D, Schaser T, et al. Automated Manufacturing of Potent CD20-Directed Chimeric Antigen Receptor T Cells for Clinical Use. Hum Gene Ther (2017) 28(10):914–25. doi: 10.1089/hum.2017.111
24. Priesner C, Aleksandrova K, Esser R, Mockel-Tenbrinck N, Leise J, Drechsel K, et al. Automated Enrichment, Transduction, and Expansion of Clinical-Scale CD62L(+) T Cells for Manufacturing of Gene Therapy Medicinal Products. Hum Gene Ther (2016) 27(10):860–9. doi: 10.1089/hum.2016.091
25. Aleksandrova K, Leise J, Priesner C, Melk A, Kubaink F, Abken H, et al. Functionality and Cell Senescence of CD4/ CD8-Selected CD20 CAR T Cells Manufactured Using the Automated CliniMACS Prodigy(R) Platform. Transfus Med Hemother (2019) 46(1):47–54. doi: 10.1159/000495772
26. Zimmermann K, Kuehle J, Dragon AC, Galla M, Kloth C, Rudek LS, et al. Design and Characterization of an "All-In-One" Lentiviral Vector System Combining Constitutive Anti-GD2 CAR Expression and Inducible Cytokines. Cancers (Basel) (2020) 12(2):375–96. doi: 10.3390/cancers12020375
27. Kailayangiri S, Altvater B, Lesch S, Balbach S, Gottlich C, Kuhnemundt J, et al. EZH2 Inhibition in Ewing Sarcoma Upregulates GD2 Expression for Targeting With Gene-Modified T Cells. Mol Ther (2019) 27(5):933–46. doi: 10.1016/j.ymthe.2019.02.014
28. Klapdor R, Wang S, Hacker U, Buning H, Morgan M, Dork T, et al. Improved Killing of Ovarian Cancer Stem Cells by Combining a Novel Chimeric Antigen Receptor-Based Immunotherapy and Chemotherapy. Hum Gene Ther (2017) 28(10):886–96. doi: 10.1089/hum.2017.168
29. Schambach A, Bohne J, Chandra S, Will E, Margison GP, Williams DA, et al. Equal Potency of Gammaretroviral and Lentiviral SIN Vectors for Expression of O6-Methylguanine-DNA Methyltransferase in Hematopoietic Cells. Mol Ther (2006) 13(2):391–400. doi: 10.1016/j.ymthe.2005.08.012
30. Yang Y, Vanin EF, Whitt MA, Fornerod M, Zwart R, Schneiderman RD, et al. Inducible, High-Level Production of Infectious Murine Leukemia Retroviral Vector Particles Pseudotyped With Vesicular Stomatitis Virus G Envelope Protein. Hum Gene Ther (1995) 6(9):1203–13. doi: 10.1089/hum.1995.6.9-1203
31. Fehse B, Kustikova OS, Bubenheim M, Baum C. Pois(s)on–it's a Question of Dose. Gene Ther (2004) 11(11):879–81. doi: 10.1038/sj.gt.3302270
32. Lode HN, Schmidt M, Seidel D, Huebener N, Brackrock D, Bleeke M, et al. Vaccination With Anti-Idiotype Antibody Ganglidiomab Mediates a GD(2)-Specific Anti-Neuroblastoma Immune Response. Cancer Immunol Immunother (2013) 62(6):999–1010. doi: 10.1007/s00262-013-1413-y
33. Siebert N, Seidel D, Eger C, Brackrock D, Reker D, Schmidt M, et al. Validated Detection of Anti-GD2 Antibody Ch14.18/CHO in Serum of Neuroblastoma Patients Using Anti-Idiotype Antibody Ganglidiomab. J Immunol Methods (2013) 398-399:51–9. doi: 10.1016/j.jim.2014.04.001
34. Dragon AC, Zimmermann K, Nerreter T, Sandfort D, Lahrberg J, Kloth C, et al. CAR-T Cells and TRUCKs That Recognize an EBNA-3C-Derived Epitope Presented on HLA-B*35 Control Epstein-Barr Virus-Associated Lymphoproliferation. J Immunother Cancer (2020) 8(2). doi: 10.1136/jitc-2020-000736
35. Bialek-Waldmann JK, Domning S, Esser R, Glienke W, Mertens M, Aleksandrova K, et al. Induced Dendritic Cells Co-Expressing GM-CSF/IFN-Alpha/Twt1 Priming T and B Cells and Automated Manufacturing to Boost GvL. Mol Ther Methods Clin Dev (2021) 21:621–41. doi: 10.1016/j.omtm.2021.04.004
36. Panch SR, Srivastava SK, Elavia N, McManus A, Liu S, Jin P, et al. Effect of Cryopreservation on Autologous Chimeric Antigen Receptor T Cell Characteristics. Mol Ther (2019) 27(7):1275–85. doi: 10.1016/j.ymthe.2019.05.015
37. Quintarelli C, Orlando D, Boffa I, Guercio M, Polito VA, Petretto A, et al. Choice of Costimulatory Domains and of Cytokines Determines CAR T-Cell Activity in Neuroblastoma. Oncoimmunology (2018) 7(6):e1433518. doi: 10.1080/2162402X.2018.1433518
38. Blaeschke F, Stenger D, Kaeuferle T, Willier S, Lotfi R, Kaiser AD, et al. Induction of a Central Memory and Stem Cell Memory Phenotype in Functionally Active CD4(+) and CD8(+) CAR T Cells Produced in an Automated Good Manufacturing Practice System for the Treatment of CD19(+) Acute Lymphoblastic Leukemia. Cancer Immunol Immunother (2018) 67(7):1053–66. doi: 10.1007/s00262-018-2155-7
39. Stroncek DF, Lee DW, Ren J, Sabatino M, Highfill S, Khuu H, et al. Elutriated Lymphocytes for Manufacturing Chimeric Antigen Receptor T Cells. J Transl Med (2017) 15(1):59. doi: 10.1186/s12967-017-1160-5
40. Zhu F, Shah N, Xu H, Schneider D, Orentas R, Dropulic B, et al. Closed-System Manufacturing of CD19 and Dual-Targeted CD20/19 Chimeric Antigen Receptor T Cells Using the CliniMACS Prodigy Device at an Academic Medical Center. Cytotherapy (2018) 20(3):394–406. doi: 10.1016/j.jcyt.2017.09.005
41. Zhang W, Jordan KR, Schulte B, Purev E. Characterization of Clinical Grade CD19 Chimeric Antigen Receptor T Cells Produced Using Automated CliniMACS Prodigy System. Drug Des Devel Ther (2018) 12:3343–56. doi: 10.2147/DDDT.S175113
42. Castella M, Boronat A, Martin-Ibanez R, Rodriguez V, Sune G, Caballero M, et al. Development of a Novel Anti-CD19 Chimeric Antigen Receptor: A Paradigm for an Affordable CAR T Cell Production at Academic Institutions. Mol Ther Methods Clin Dev (2019) 12:134–44. doi: 10.1016/j.omtm.2018.11.010
43. Castella M, Caballero-Banos M, Ortiz-Maldonado V, Gonzalez-Navarro EA, Sune G, Antonana-Vidosola A, et al. Point-Of-Care CAR T-Cell Production (ARI-0001) Using a Closed Semi-Automatic Bioreactor: Experience From an Academic Phase I Clinical Trial. Front Immunol (2020) 11:482. doi: 10.3389/fimmu.2020.00482
44. Zhao Y, Stepto H, Schneider CK. Development of the First World Health Organization Lentiviral Vector Standard: Toward the Production Control and Standardization of Lentivirus-Based Gene Therapy Products. Hum Gene Ther Methods (2017) 28(4):205–14. doi: 10.1089/hgtb.2017.078
45. Vedvyas Y, McCloskey JE, Yang Y, Min IM, Fahey TJ, Zarnegar R, et al. Manufacturing and Preclinical Validation of CAR T Cells Targeting ICAM-1 for Advanced Thyroid Cancer Therapy. Sci Rep (2019) 9(1):10634. doi: 10.1038/s41598-019-46938-7
46. Busch DH, Frassle SP, Sommermeyer D, Buchholz VR, Riddell SR. Role of Memory T Cell Subsets for Adoptive Immunotherapy. Semin Immunol (2016) 28(1):28–34. doi: 10.1016/j.smim.2016.02.001
47. Rafiq S, Hackett CS, Brentjens RJ. Engineering Strategies to Overcome the Current Roadblocks in CAR T Cell Therapy. Nat Rev Clin Oncol (2020) 17(3):147–67. doi: 10.1038/s41571-019-0297-y
48. Keu KV, Witney TH, Yaghoubi S, Rosenberg J, Kurien A, Magnusson R, et al. Reporter Gene Imaging of Targeted T Cell Immunotherapy in Recurrent Glioma. Sci Transl Med (2017) 9(373). doi: 10.1126/scitranslmed.aag2196
49. Martinez M, Moon EK. CAR T Cells for Solid Tumors: New Strategies for Finding, Infiltrating, and Surviving in the Tumor Microenvironment. Front Immunol (2019) 10:128. doi: 10.3389/fimmu.2019.00128
50. Kawalekar OU, O'Connor RS, Fraietta JA, Guo L, McGettigan SE, Posey AD Jr, et al. Distinct Signaling of Coreceptors Regulates Specific Metabolism Pathways and Impacts Memory Development in CAR T Cells. Immunity (2016) 44(3):712. doi: 10.1016/j.immuni.2016.02.023
51. Zhang L, Kerkar SP, Yu Z, Zheng Z, Yang S, Restifo NP, et al. Improving Adoptive T Cell Therapy by Targeting and Controlling IL-12 Expression to the Tumor Environment. Mol Ther (2011) 19(4):751–9. doi: 10.1038/mt.2010.313
52. Wiebel M, Kailayangiri S, Altvater B, Meltzer J, Grobe K, Kupich S, et al. Surface Expression of the Immunotherapeutic Target GD2 in Osteosarcoma Depends on Cell Confluency. Cancer Rep (Hoboken) (2021) e1394. doi: 10.1002/cnr2.1394
53. Kiesgen S, Messinger JC, Chintala NK, Tano Z, Adusumilli PS. Comparative Analysis of Assays to Measure CAR T-Cell-Mediated Cytotoxicity. Nat Protoc (2021) 16(3):1331–42. doi: 10.1038/s41596-020-00467-0
54. Ahmed N, Brawley VS, Hegde M, Robertson C, Ghazi A, Gerken C, et al. Human Epidermal Growth Factor Receptor 2 (HER2) -Specific Chimeric Antigen Receptor-Modified T Cells for the Immunotherapy of HER2-Positive Sarcoma. J Clin Oncol (2015) 33(15):1688–96. doi: 10.1200/JCO.2014.58.0225
55. Feng K, Guo Y, Dai H, Wang Y, Li X, Jia H, et al. Chimeric Antigen Receptor-Modified T Cells for the Immunotherapy of Patients With EGFR-Expressing Advanced Relapsed/Refractory Non-Small Cell Lung Cancer. Sci China Life Sci (2016) 59(5):468–79. doi: 10.1007/s11427-016-5023-8
56. Beatty GL, Haas AR, Maus MV, Torigian DA, Soulen MC, Plesa G, et al. Mesothelin-Specific Chimeric Antigen Receptor mRNA-Engineered T Cells Induce Anti-Tumor Activity in Solid Malignancies. Cancer Immunol Res (2014) 2(2):112–20. doi: 10.1158/2326-6066.CIR-13-0170
57. O'Rourke DM, Nasrallah MP, Desai A, Melenhorst JJ, Mansfield K, Morrissette JJD, et al. A Single Dose of Peripherally Infused EGFRvIII-Directed CAR T Cells Mediates Antigen Loss and Induces Adaptive Resistance in Patients With Recurrent Glioblastoma. Sci Transl Med (2017) 9(399). doi: 10.1126/scitranslmed.aaa0984
Keywords: TRUCK, IL-18, GD2-CAR, Prodigy, GMP, 4th generation CAR
Citation: Glienke W, Dragon AC, Zimmermann K, Martyniszyn-Eiben A, Mertens M, Abken H, Rossig C, Altvater B, Aleksandrova K, Arseniev L, Kloth C, Stamopoulou A, Moritz T, Lode HN, Siebert N, Blasczyk R, Goudeva L, Schambach A, Köhl U, Eiz-Vesper B and Esser R (2022) GMP-Compliant Manufacturing of TRUCKs: CAR T Cells targeting GD2 and Releasing Inducible IL-18. Front. Immunol. 13:839783. doi: 10.3389/fimmu.2022.839783
Received: 20 December 2021; Accepted: 25 February 2022;
Published: 24 March 2022.
Edited by:
Francesca Del Bufalo, Bambino Gesù Children’s Hospital (IRCCS), ItalyReviewed by:
Paul G. Schlegel, University Children’s Hospital Würzburg, GermanyCopyright © 2022 Glienke, Dragon, Zimmermann, Martyniszyn-Eiben, Mertens, Abken, Rossig, Altvater, Aleksandrova, Arseniev, Kloth, Stamopoulou, Moritz, Lode, Siebert, Blasczyk, Goudeva, Schambach, Köhl, Eiz-Vesper and Esser. This is an open-access article distributed under the terms of the Creative Commons Attribution License (CC BY). The use, distribution or reproduction in other forums is permitted, provided the original author(s) and the copyright owner(s) are credited and that the original publication in this journal is cited, in accordance with accepted academic practice. No use, distribution or reproduction is permitted which does not comply with these terms.
*Correspondence: Wolfgang Glienke, d2dsaWVua2VAeWFob28uZGU=; Axel Schambach, U2NoYW1iYWNoLkF4ZWxAbWgtaGFubm92ZXIuZGU=
†These authors have contributed equally to this work and share first authorship
‡These authors share senior authorship
Disclaimer: All claims expressed in this article are solely those of the authors and do not necessarily represent those of their affiliated organizations, or those of the publisher, the editors and the reviewers. Any product that may be evaluated in this article or claim that may be made by its manufacturer is not guaranteed or endorsed by the publisher.
Research integrity at Frontiers
Learn more about the work of our research integrity team to safeguard the quality of each article we publish.