- 1Department of Oncology, Tongji Hospital of Tongji Medical College, Huazhong University of Science and Technology, Wuhan, China
- 2Department of Interventional Radiology, The First Affiliated Hospital of Zhengzhou University, Zhengzhou, China
The immune checkpoint pathway consisting of the cell membrane-bound molecule programmed death protein 1 (PD-1) and its ligand PD-L1 has been found to mediate negative regulatory signals that effectively inhibit T-cell proliferation and function and impair antitumor immune responses. Considerable evidence suggests that the PD-1/PD-L1 pathway is responsible for tumor immune tolerance and immune escape. Blockage of this pathway has been found to reverse T lymphocyte depletion and restore antitumor immunity. Antagonists targeting this pathway have shown significant clinical activity in specific cancer types. Although originally identified as membrane-type molecules, several other forms of PD-1/PD-L1 have been detected in the blood of cancer patients, including soluble PD-1/PD-L1 (sPD-1/sPD-L1) and exosomal PD-L1 (exoPD-L1), increasing the composition and functional complications of the PD-1/PD-L1 signaling pathway. For example, sPD-1 has been shown to block the PD-1/PD-L immunosuppressive pathway by binding to PD-L1 and PD-L2, whereas the role of sPD-L1 and its mechanism of action in cancer remain unclear. In addition, many studies have investigated the roles of exoPD-L1 in immunosuppression, as a biomarker for tumor progression and as a predictive biomarker for response to immunotherapy. This review describes the molecular mechanisms underlying the generation of sPD-1/sPD-L1 and exoPD-L1, along with their biological activities and methods of detection. In addition, this review discusses the clinical importance of sPD-1/sPD-L1 and exoPD-L1 in cancer, including their predictive and prognostic roles and the effects of treatments that target these molecules.
1 Introduction
The immune cycle consists of a series of reduplicative events required by the immune system to generate a robust antitumor immune response (1). Neoantigens on tumors are recognized by antigen-presenting cells (APCs), which activate tumor antigen-specific T lymphocytes to eliminate cancer cells (1). Antitumor immunity is also mediated by several negative factors, such as programmed death protein 1 (PD-1), programmed death ligand-1 (PD-L1), and cytotoxic T-lymphocyte-associated antigen-4 (CTLA-4) (2, 3). The binding of the immunosuppressive molecule PD-1 to its ligand PD-L1 can initiate programmed T cell death, induce the production of Foxp3+CD4+ regulatory T cells (Tregs) and enhance the function of immunosuppressive Tregs (4, 5). The immunosuppressive microenvironment provided by negative regulatory signaling pathways is an important component of tumor escape from the immune system in cancer patients (6).
Although mainly expressed on T cells, PD-1 is also presented on other immune cells including natural killer (NK) cells, monocytes, dendritic cells (DCs), B cells, and Tregs (7). PD-1 expression is induced by multiple cytokines, such as interferon-α (IFN-α), released by DCs and monocytes (8). PD-L1 is mainly expressed on the surface of tumor cells, but is also presented on immune system cells and non-hematopoietic cells, including vascular endothelial and epithelial cells (7). Oncogenic signals, such as PI3K-AKT, MEK-ERK, and EGFR (9–11), as well as several cytokines, such as interleukin-6 (IL-6) and IFN-γ (12, 13), have been found to regulate PD-L1 expression. The binding of PD-L1 to PD-1 triggers PD-1-mediated intracellular signaling and inhibits the PI3K-AKT and MAPK pathways, thereby restricting T-cell proliferation, activation and survival (14, 15). In addition, the complete activation of T cells relies on two signals, with the first signal provided by the specific binding of T-cell receptor (TCR) to major histocompatibility complex (MHC) and the second signal provided by the interaction of CD28 on T cell surfaces with co-stimulatory molecules expressed by APCs (16). The mechanism of PD-1/PD-L1 signaling involves the recruitment of the Src homology 2 domain containing phosphatases 2 (SHP2) to the PD-1 cytoplasmic domain, which dephosphorylates signaling molecules of the proximal TCR and CD28 pathways (17). PD-L1 can also mediate reverse signaling that upregulates the PI3K-AKT signaling cascade and enhances glycolytic metabolism in cancer cells (18).
Many studies have indicated the potential importance of membrane-bound PD-1/PD-L1 (mPD-1/mPD-L1) in antitumor immunotherapy (19, 20). Blocking the interaction of PD-1 with PD-L1 improves T-cell function, restarting and amplifying the cancer-immune cycle (1). Hence, anti-PD-1/PD-L1 antibodies are frequently used to treat various types of solid tumors and have greatly improved the survival of patients with advanced cancers (21, 22). Clinical responses to antitumor treatment strategies, including immunotherapy, have been found to vary widely across different cancer types, and the durability of these responses varies from patient to patient (23–25). This may be due in part to the form of PD-1/PD-L1 presentation (26). In recent years, soluble PD-1/PD-L1 (sPD-1/sPD-L1) and exosomal PD-L1 (exoPD-L1) have been detected in the blood of tumor patients (17, 27), with many studies investigating the exact roles of these soluble molecules in cancer (28, 29). This review discusses the origin, biological mechanisms and methods of detection of sPD-1/sPD-L1 and exoPD-L1 and highlights their predictive and prognostic roles in cancer, as well as the effects of treatments that target these molecules.
2 Generation of sPD-1 and sPD-L1
A variety of immunomodulatory molecules exist as both cell membrane and soluble forms. Many soluble co-stimulatory and co-inhibitory molecules, such as sCTLA-4, sCD80, sCD86, sB7-H3, and sLAG-3, have been detected in the blood of cancer patients (30–35). These soluble molecules are produced either by selective splicing of genes encoding the membrane-bound molecules or by proteolytic cleavage of membrane-bound proteins (36, 37). Soluble forms of PD-1 and PD-L1 (sPD-1/sPD-L1) have also been detected in the blood of cancer patients.
2.1 Generation of sPD-1
sPD-1 is thought to be generated primarily by selective splicing (Figure 1) (38). PD-1 is a type I transmembrane glycoprotein encoded by the Pdcd1 gene on chromosome 2 in humans and chromosome 1 in mice (39). The Pdcd1 gene contains five exons (exons 1-5), which encode a short signal sequence, an immunoglobulin (Ig) domain, the stalk and transmembrane domain, a short 12 amino acid (aa) sequence that marks the beginning of the cytoplasmic domain, the C-terminal intracellular residues and a long 3’UTR, respectively (39). In addition to full length PD-1 (flPD-1), four splice variants of PD-1 mRNA (PD-1Deltaex2, PD-1Deltaex3, PD-1Deltaex2/3, and PD-1Deltaex2/3/4) have been cloned from human peripheral blood mononuclear cells (PBMCs) (38). PD-1Deltaex2, which lacks exon 2, encoding the extracellular domain; PD-1Deltaex2/3, which lacks exons 2 and 3, encoding the extracellular and transmembrane domains; and PD-1Deltaex2/3/4, which lacks exons 2, 3 and exon 4, and contains a premature stop codon in exon 5, are unable to bind to the ligand PD-L1 and transmit inhibitory signals. In contrast, PD-1Deltaex3, which lacks only exon 3, retains the extracellular Ig domain and can be translated to generate sPD-1 (38).
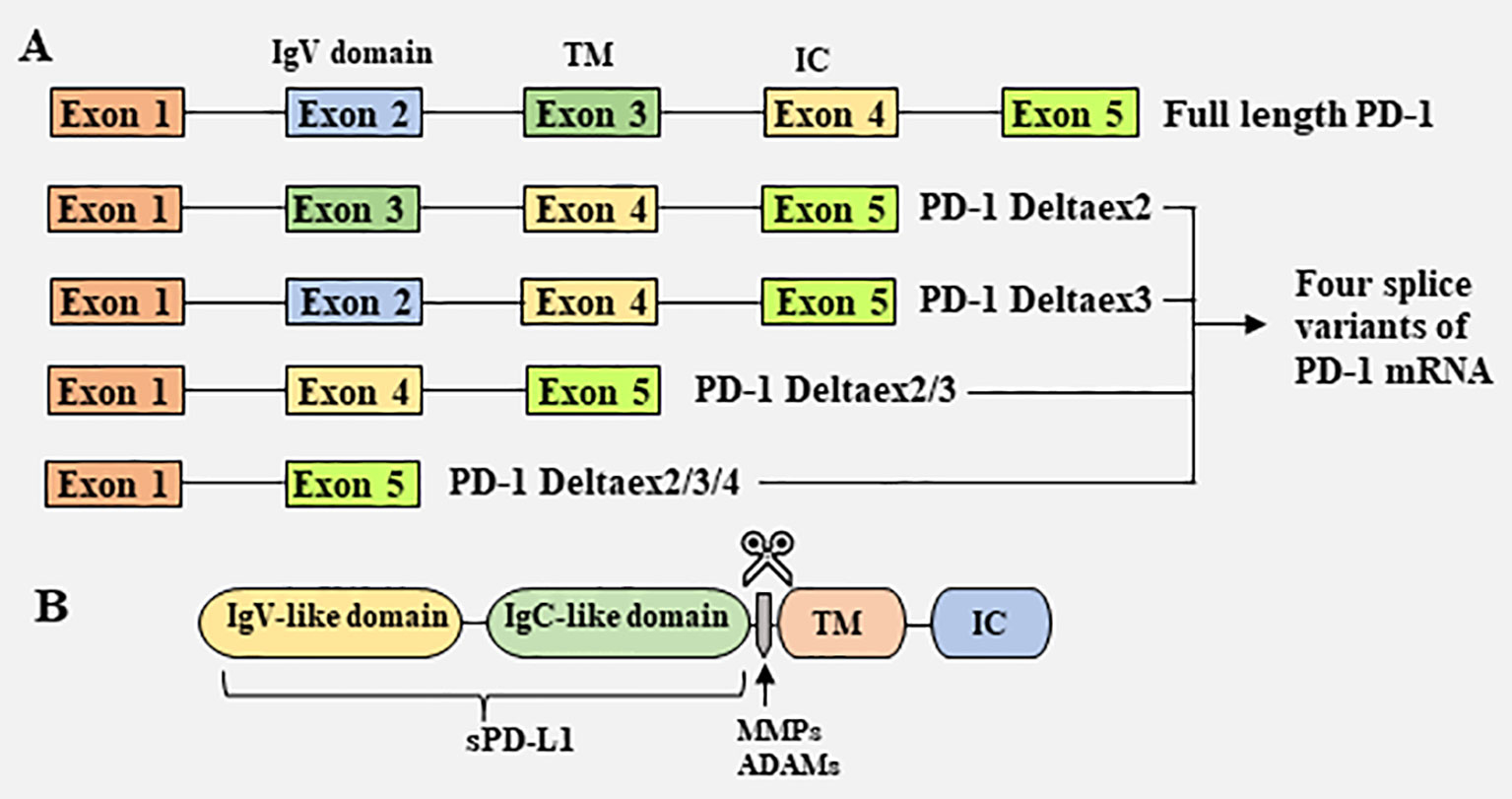
Figure 1 Mechanisms responsible for the generation of soluble PD-1 and PD-L1. (A) Generation of soluble PD-1 from splice variants of PD-1 mRNA. Full length PD-1 (flPD-1) mRNA contains five exons (exons 1–5), which encode a short signal sequence, an immunoglobulin (Ig) domain, the stalk and transmembrane (TM) domain, a 12 amino acid (aa) sequence that marks the beginning of the cytoplasmic domain, the C-terminal intracellular (IC) domain and a long 3’UTR, respectively. Four splice variants of PD-1 mRNA, PD-1Deltaex2, PD-1Deltaex3, PD-1Deltaex2/3, and PD-1Deltaex2/3/4, have been cloned from human peripheral blood mononuclear cells (PBMCs). PD-1Deltaex3 only lacks exon 3, but retains an extracellular Ig domain. Translation of this mRNA results in a soluble form PD-1. (B) Generation of soluble PD-L1 by proteolytic cleavage of membrane-bound PD-L1. Several proteases, including MMPs and ADAMs, are capable of cleaving membrane-bound PD-L1, releasing soluble PD-L1.
2.2 Generation of sPD-L1
sPD-L1 has been reported to be generated in and released from tumor cells and mature DCs, but not from immature DCs, T cells, macrophages, and monocytes (40). Although both myeloid cells and activated T lymphocytes exhibit elevated levels of mPD-L1, only myeloid cells can produce sPD-L1 (40), suggesting differences in the mechanisms that regulate mPD-L1 and sPD-L1 production. High levels of sPD-L1 have been detected in the supernatants of multiple PD-L1+ cell lines (41). Many studies have shown that sPD-L1 is generated by proteolytic cleavage of mPD-L1 by endogenous matrix metalloproteinases (MMPs) (Figure 1) (42, 43). PD-L1/PD-L2 expressed on fibroblasts can be cleaved by MMP-9 and MMP-13, resulting in the lack of a PD-1 binding domain, thereby reversing T-cell depletion and limiting immunosuppressive capacity (42). MMP-7 and MMP-13, which are upregulated in the human head and neck squamous cell carcinoma OSC-20 cell line, can cleave PD-L1, whereas inhibitors that specifically target MMP-13 are able to restore mPD-L1 expression (43). MMP inhibitors have also been found to suppress sPD-L1 secretion into the supernatants of PD-L1 transfected cell lines (41). Members of a disintegrin and metalloproteinase (ADAM) family are also involved in the shedding of extracellular domains of membrane molecules (26). For example, ADAM10 and ADAM17 have been found to cleave mPD-L1 on cancer cells and extracellular vesicles (44–46). Other enzymes may also participate in the hydrolytic shedding of membrane molecules and the release of sPD-L1.
PD-L1 is a type I transmembrane glycoprotein encoded by the CD274 gene on chromosome 9 in humans (47). Transcription of this gene can produce multiple PD-L1 splice variants, including PD-L1 lncRNA splice isoforms and truncated PD-L1 (48, 49). sPD-L1 has been shown to be produced primarily through the exaptation of an intronic LINE-2A (L2A) endogenous retroelement in the CD274 gene, with the resulting CD274-L2A encoded sPD-L1 having a receptor antagonistic effect (50). In addition, exon 4-enriched variants are able to produce a secreted form of PD-L1 in various cancers (49). Secreted PD-L1 binds PD-1 and negatively regulates T-cell function (49). In contrast, one splice variant does not include splicing of the transmembrane domain but secretes PD-L1 with a unique 18 aa tail. This variant is able to homodimerize and more effectively inhibit lymphocyte function (51). A variant lacking exon 2, which encodes the IgV domain, was found in human PBMCs; this was unable to bind PD-1 and to generate sPD-L1 (52).
3 Biological Activity
Immunosuppression induced by mPD-1/mPD-L1 was found to be involved in tumor escape from immune system (6). In addition to being presented at the plasma membrane, PD-1/PD-L1 has been detected in the plasma and serum of patients with several diseases (53–56). The biological activity of sPD-1/sPD-L1 is similar to that of the corresponding membrane-bound molecules. Specifically, sPD-1/sPD-L1 may transfer signals among different types of immune cells, altering their activity and regulating the secretion of cytokines (57, 58).
3.1 Biological Activity of sPD-1
sPD-1 has been found to function as a blocker of PD-1 ligands and to suppress the interactions of PD-1 with PD-L1 and PD-L2 and the interaction of PD-L1 with B7-1 (CD80) (Figure 2) (57). Coadministration of the PD-1/PD-L1 blocker sPD-1 and human papillomavirus-16 E7 DNA vaccine significantly enhanced antitumor activity against E7-expressing tumors (57). Mechanistically, sPD-1 effectively increased the numbers and functional activities of E7-specific CD8+ T cells and the maturation of DCs through the upregulation of MHC class II molecules (57). Similarly, reconstructed adeno-associated virus-mediated delivery of the PD-1 extracellular domain to tumor sites augmented the cytotoxicity of antigen-specific lymphocytes (59). In addition, the recombinant peptide sPD-1-CH50 was able to upregulate the expression of IFN-γ, tumor necrosis factor-α (TNF-α), and inducible nitric oxide synthase (iNOS) and to enhance the lytic activity of macrophages against B7-H1-positive tumor cells (60). The combination of heat shock protein 70 (HSP70) and sPD-1 vaccine increased the expression of the TH1 cytokines IL-2 and IFN-γ and decreased the expression of the negative regulatory molecules Foxp3, IL-10 and TGF-β in tumor-infiltrating lymphocytes of lung metastatic melanoma (61). Residual tumor cells expressing B7-H1 were shown to be responsible for tumor resistance to HSP70 vaccine. Intravenous administration of a plasmid encoding the extracellular domain of sPD-1 was able to block the PD-1 pathway, overcome tumor resistance and reduce lung metastasis of B16F1 melanoma cells (61). A eukaryotic expression plasmid expressing sPD-1 was found to partially enhance the early activation of lymphocytes in vitro, upregulating the levels of TNF-α, IFN-γ, B7.1, and 4-1BB mRNAs and downregulating the levels of OX40 and IL-10 mRNAs (62). Conversely, sPD-1 may also have reverse signaling effects on DCs via the PD-L1/PD-L2 pathway, reducing DC maturation and inhibiting T cell activation and IL-2 production (58, 63).
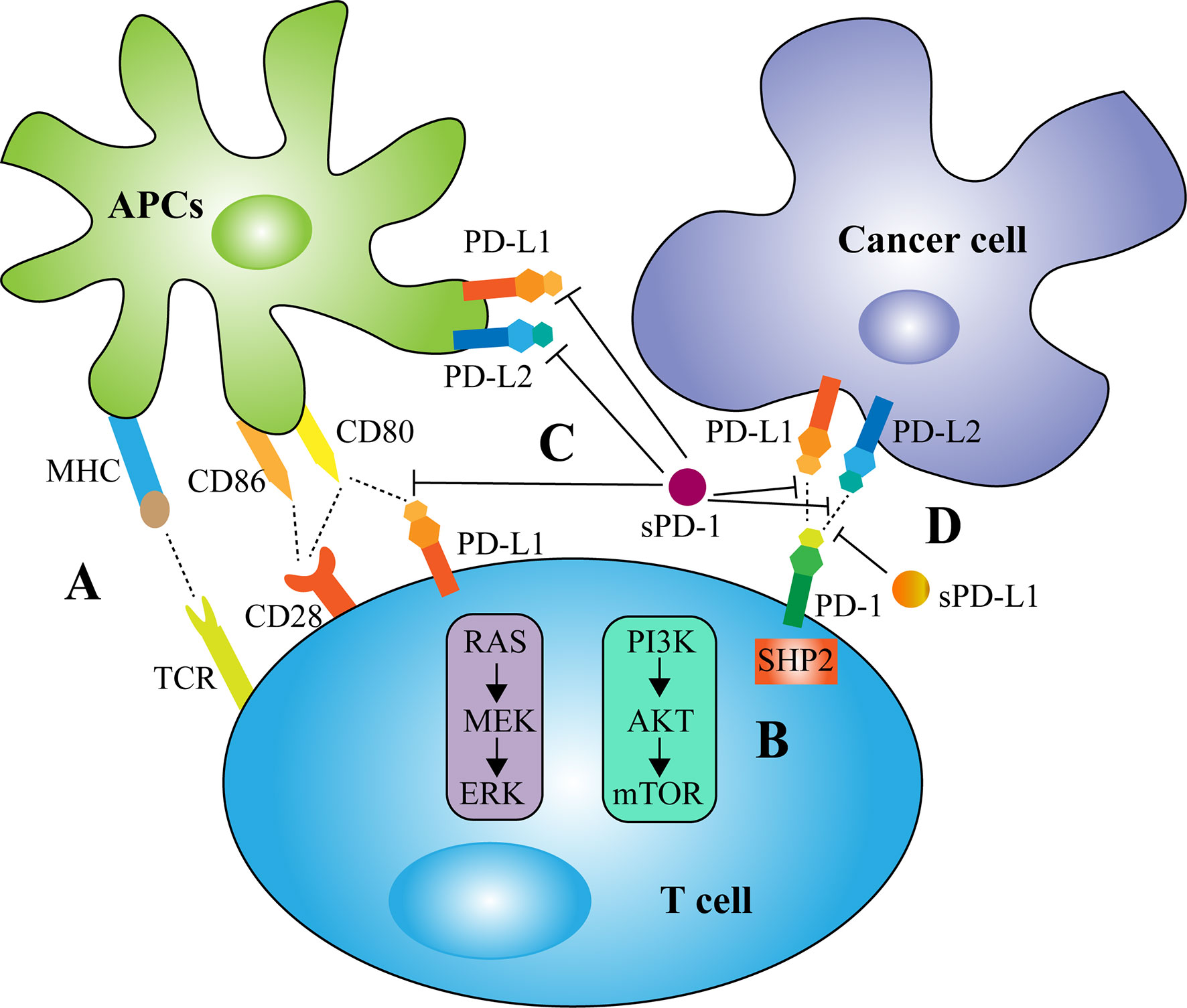
Figure 2 Biological activity of membrane and soluble PD-1/PD-L1 in tumor immunity. (A) The complete activation of T cells relies on two signals, with the first signal provided by the specific binding of T-cell receptor (TCR) to major histocompatibility complex (MHC) and the second signal provided by the interaction of CD28 on T cell surfaces with co-stimulatory molecules CD80/CD86 expressed by antigen-presenting cells (APCs). (B) The mechanism of PD-1/PD-L1 signaling involves the recruitment of the Src homology 2 domain containing phosphatases 2 (SHP2) to the PD-1 cytoplasmic domain, which dephosphorylates signaling molecules of the PI3K-AKT and MAPK pathways, thereby restricting T-cell proliferation, activation and survival. (C) sPD-1 has been found to suppress the interactions of PD-1 with PD-L1 and PD-L2 and the interaction of PD-L1 with CD80. However, sPD-1 may also have reverse signaling effects on APCs via the PD-L1/PD-L2 pathway and inhibits T cell function. (D) sPD-L1, like mPD-L1, binds to PD-1 to transmit negative regulatory signals.
3.2 Biological Activity of sPD-L1
The biological activity of sPD-L1 remains unclear. In most cases, sPD-L1, like mPD-L1, binds to PD-1 to transmit negative regulatory signals (Figure 2) (49). A variety of malignant cells and DC-derived sPD-L1 have been reported to induce apoptosis of T cells and impair their function (40, 49, 64, 65). sPD-L1 in the sera of patients with cystic echinococcosis was found to significantly reduce the expression of TH1 cytokines such as IL-2 and to increase the expression of TH2 cytokines such as IL-4, IL-6, and IL-10 (66). Similarly, sPD-L1 in the supernatants of PD-L1-expressing breast cancer cell lines was found to have a negative regulatory effect on cellular immunity (67). The dimerized form of sPD-L1 was found to possess superior immunosuppressive activity compared with monomeric sPD-L1 (51). However, the impact of sPD-L1 on immune cell function may also depend on the affinity between sPD-L1 and PD-1 (68). Native soluble human PD-L1 (hPD-L1) was found to exert a suppressive effect on activated T cells (68). The soluble hPD-L1 variants L3C7-hPD-L1 and L3B3-hPD-L1, which were generated by directed molecular evolution and had a >20-fold higher affinity to PD-1 than native hPD-L1, were able to attenuate the inhibitory effects of the PD-1 axis on PBMC amplification and IFN-γ release (68). A similar result was observed in patients with diabetic atherosclerotic macrovascular diseases (69). These findings suggest that sPD-L1 may also act as a PD-1 blocker, competitively suppressing the inhibitory effect of mPD-L1. In addition, sPD-L1 levels in maternal blood were found to increase throughout gestation and return to control levels post-partum (70). During placental development, IFN-β secretion by decidual macrophages was shown to enhance the constitutive production of sPD-L1 by trophoblasts, initiating macrophage polarization towards an M2 phenotype and thereby reducing inflammation (71). This may at least partly suppress maternal immunity and play a role in immune tolerance that is critical for the fetus (72).
4 Detection of sPD-1 and sPD-L1
sPD-1 and sPD-L1 circulate in the blood as free proteins and can be measured by enzyme-linked immunosorbent assays (ELISA) (70, 72, 73). ELISA, however, involves the use of monoclonal antibodies, which are costly to produce and time-consuming to isolate and purify (74). In addition, these assays are complicated to perform and have limited reproducibility and sensitivity for clinical applications. Several new, inexpensive, accurate, sensitive and rapid detection methods have been developed to overcome these limitations.
In a fully automated immunoassay system based on chemiluminescent magnetic technology (HISCL system), specific biotinylated antibodies bind to the target proteins, with the resulting complexes mixed homogeneously with alkaline phosphatase (ALP)-coupled antibodies (75). The fluorescent signals are subsequently measured and the chemiluminescent intensity determined (75). This system is rapid, sensitive and reproducible, and can accurately measure sPD-1, sPD-L1 and sCTLA-4 levels in the clinic (75). Another assay uses localized surface plasmon resonance (LSPR) biosensors (74). Gold nanoshells have strong optical properties of absorption and scattering, with changes in the sizes of these gold nanoshells altering the LSPR absorption peaks. These biosensors can be fabricated using excessively tilted fiber gratings (ExTFGs) coupled with large-sized gold nanoshells (∼160 nm) so that it works at the C/L band (1525 nm-1625 nm) (74). Anti-sPD-L1 monoclonal antibodies are bound to the surface of the ExTFG-LSPR sensor using the staphylococcal protein A (SPA) method, resulting in the label-free and specific detection of sPD-L1 (74). The interaction of sPD-L1 with the corresponding monoclonal antibody has also been shown to enhance the LSPR effect and improve specificity (74).
Another assay system involves the use of an integrated surface enhanced Raman scattering (SERS) microfluidics device with high specificity for the detection of multiple immune checkpoints (76, 77). These biosensors offer several advantages. First, the replacement of monoclonal antibodies with engineered high-affinity nano yeast single chain variable fragments (scFv) results in high efficiency and stability, along with lower cost. In addition, the graphene oxide functionalized surface reverses the general biotin-streptavidin chemistry paradigm; the microfluidics platform induces nanofluidic mixing by ac-electrohydrodynamics (ac-EHD) and integrates microfluidic sandwich immunoassays, which enhance scFv-target specific binding and minimize non-specific binding (76, 77). This biosensor is capable of analyzing up to 28 samples simultaneously in <2 hours and requires only 20 µl of sample per target immune checkpoint (77).
5 Clinical Importance of sPD-1 and sPD-L1 in Cancer
5.1 Predictive and Prognostic Significance
The levels of sPD-1 and sPD-L1 in cancer patients have been reported to correlate with disease severity, clinicopathological characteristics, survival and response to treatment. Because of their clinical importance, sPD-1 and sPD-L1 have been widely utilized as biomarkers to predict treatment efficacy and patient prognosis (Tables 1, 2).
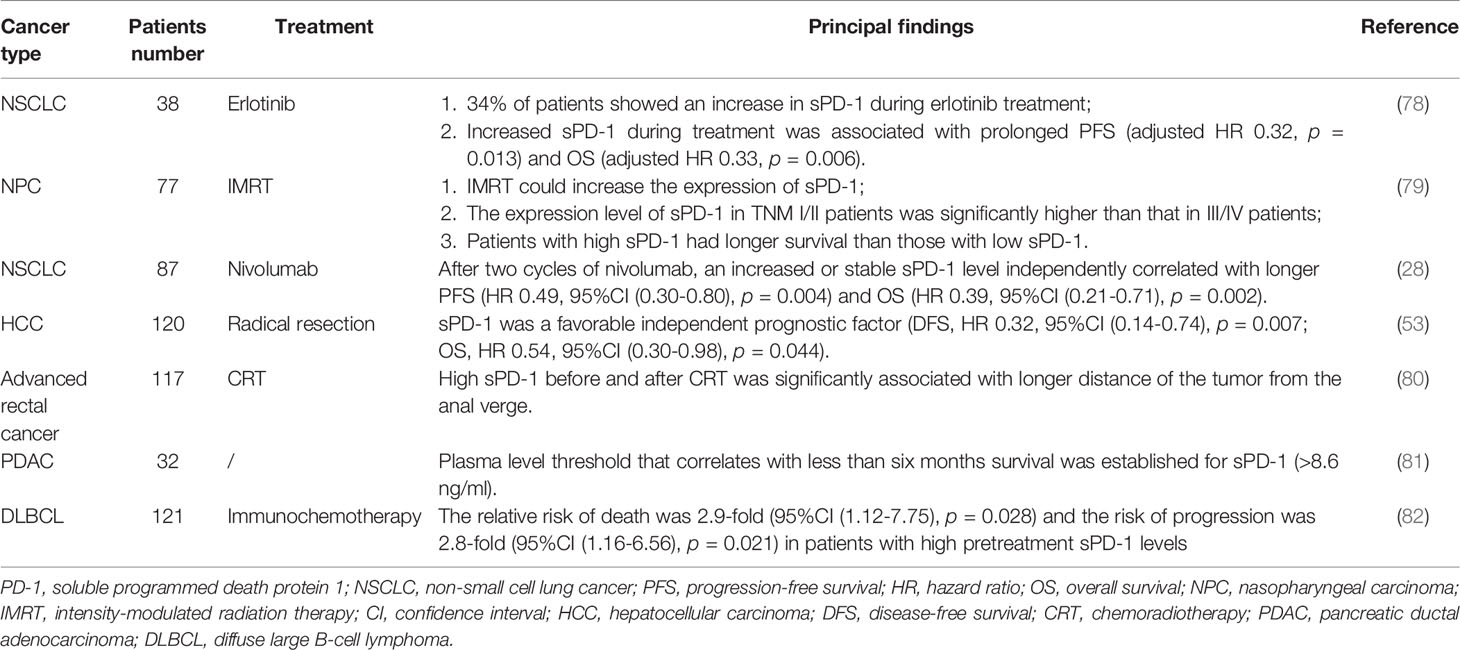
Table 1 Soluble PD-1 expression levels in different cancers and their correlation with disease prognosis and efficacy prediction.
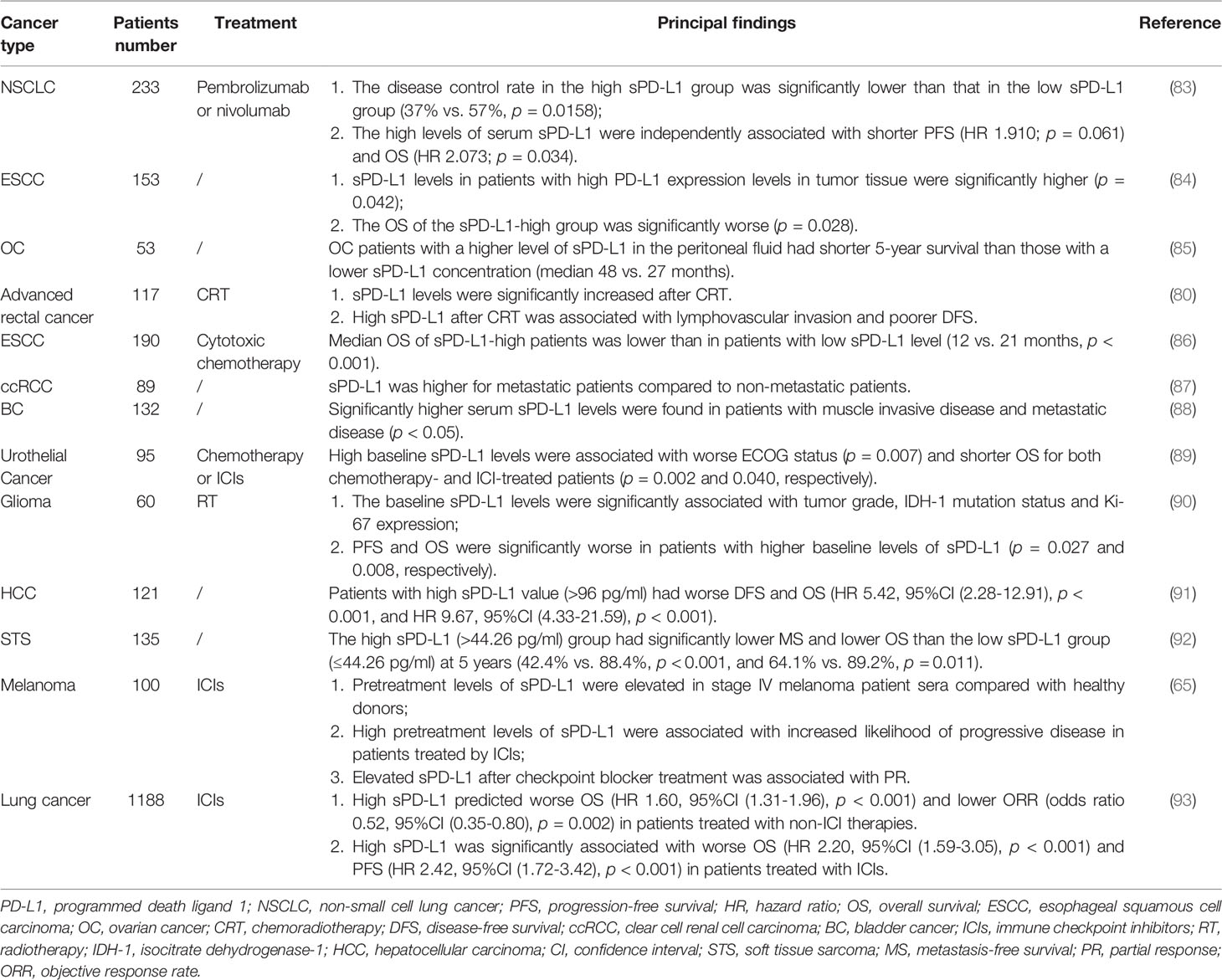
Table 2 Soluble PD-L1 expression levels in different cancers and their correlation with disease prognosis and efficacy prediction.
5.1.1 Predictive and Prognostic Significance of sPD-1
Erlotinib is a tyrosine kinase inhibitor that inhibits the activity of the epidermal growth factor receptor (EGFR) (94). In a study of patients with non-small cell lung cancer (NSCLC), 34% of erlotinib-treated patients showed elevated sPD-1 during treatment, with these patients experiencing prolonged progression-free survival (PFS) and overall survival (OS) compared with patients without elevated sPD-1 (78). Similarly, sPD-1 was significantly elevated in patients with nasopharyngeal carcinoma after intensity-modulated radiation therapy (IMRT), with survival being longer in patients with high than with low sPD-1 (79). In addition, sPD-1 expression was significantly higher in TNM I/II patients than in TNM III/IV patients (79). Radiotherapy may enhance the antigen-presentation process, which increases the number of tumor-specific T lymphocytes and sPD-1 production (95, 96). NSCLC patients with increased or stable sPD-1 after anti-PD-1 therapy also tended to have favorable outcomes (28). In addition, a retrospective study of patients with hepatocellular carcinoma (HCC) undergoing radical resection reported that enhanced sPD-1 was associated with longer survival (53). The mechanisms by which sPD-1 is increased and patient survival improved after specific treatments, such as targeted therapy, radiotherapy, and immunotherapy, are not fully understood. These treatments may affect the cancer-immune cycle, such as by increasing antigen presentation, restoring tumor-specific cytotoxic T-cell activity and enhancing anti-cancer immunity (95–98). In contrast, elevated sPD-1 in untreated cancer patients was found to predict unfavorable survival outcomes. High sPD-1 in patients with advanced rectal cancer correlated with a longer distance of the tumor from the anal verge (80). In addition, plasma sPD-1 concentration >8.6 ng/ml was found to be associated with OS < 6 months in 27 patients newly diagnosed with pancreatic ductal adenocarcinoma (81). Pretreatment sPD-1 levels are a predictor of adverse outcomes after dose-dense immunochemotherapy and can predict the risk of disease progression in patients with diffuse large B-cell lymphoma (DLBCL) (82). Additional studies are required determine the precise mechanism underlying the relationship between malignancy and sPD-1.
5.1.2 Predictive and Prognostic Significance of sPD-L1
In general, elevated sPD-L1 in cancer patients is indicative of poor prognosis or resistance to treatment (83–85). High sPD-L1 levels before neoadjuvant chemoradiotherapy (CRT) were found to be associated with younger age, and significantly increased sPD-L1 levels after CRT were associated with lymphovascular infiltration and shorter disease-free survival (DFS) (80). High pretreatment sPD-L1 concentration may be a marker of poor prognosis in patients with locally advanced or metastatic esophageal squamous cell carcinoma treated with cytotoxic chemotherapy (86). Higher sPD-L1 levels were observed in patients with metastatic than non-metastatic clear cell renal cell carcinoma (87), and sPD-L1 levels were significantly elevated in patients with muscle-invasive and metastatic urinary bladder cancer (88). Similarly, high baseline sPD-L1 levels were associated with poorer ECOG status and shorter OS in patients treated with chemotherapy and immune checkpoint inhibitors (ICIs) (89). Higher baseline sPD-L1 levels in patients with glioma treated with radiotherapy were associated with poorer PFS and OS (90), and high sPD-L1 was considered a biomarker of poor prognosis in HCC patients undergoing curative treatment (91). High sPD-L1 concentrations were also found to predict future metastases in patients with soft tissue sarcoma (92), and high pretreatment sPD-L1 was associated with an increased likelihood of disease progression in patients with malignant melanoma (65). In addition, high sPD-L1 was significantly associated with poorer OS and PFS in lung cancer patients treated with ICIs (93).
5.2 Therapeutic Value
In recent years, immune checkpoint blockade has attracted considerable attention in cancer immunotherapy, with anti-PD-1/PD-L1 treatments associated with high response rates in multiple advanced-stage cancers (99–103). sPD-1 and sPD-L1 were found to have immunomodulatory activities, suggesting that agents that modify their activities may have application in cancer treatment. These agents have been tested in animal models, with the results of these studies possibly providing new insights for future anti-cancer therapy in humans.
5.2.1 Therapeutic Value of sPD-1
sPD-1 has been found to enhance the effector functions of T lymphocytes and other immune cells (57). Constitutive sPD-1-secreting sPD-1 chimeric antigen receptor (CAR) T cells were found to mitigate tumor burden and prolong OS in NOD-SCID-IL2rg mice bearing NALM-6-PD-L1 (104). Intramuscular injection of a eukaryotic expression plasmid expressing sPD-1 (pPD-1A) was shown to significantly inhibit the growth of H22 HCC cells in mice (62). Moreover, a test of local gene therapy showed that inoculation of a recombinant adeno-associated viral vector (rAAV)/sPD-1 construct at the site of H22 hepatoma cell inoculation inhibited tumor growth (59).
The combination of sPD-1 with other therapies demonstrated more robust antitumor effects than the other therapies alone. For example, local treatment of murine H22 HCC ectopic tumors by injection of naked plasmids expressing the co-stimulatory molecules 4-1BBL and sPD-1 eradicated a small number of pre-existing tumor cells in murine tumors and larger amounts of pre-existing tumor cells in approximately 60% of mice (105). An adenovirus expressing sPD-1-Ig markedly enhanced CD8+ T cell-mediated tumor rejection compared with adenovirus expressing herpes simplex virus thymidine kinase (HSVtk) alone (106). The fibronectin CH50-sPD-1 recombinant peptide was found to increase the cytolytic activity of cytotoxic T-lymphocytes and macrophages against tumors (60). Coadministration of sPD-1 with human papillomavirus-16 E7 DNA vaccine markedly improved E7-specific CD8+ T-cell responses and enhanced immunological cytotoxic killing effect on E7-expressing tumors (57). These findings demonstrate that sPD-1 plays a vaccine type-independent adjuvant role in suppressing tumor grown (57). In addition, sPD-1 enhanced the antitumor activity induced by other treatments, such as secondary lymphoid chemokines (SLC, CCL21) (107). Local gene transfer of IL-21 in combination with sPD-1 into murine H22 HCC tumors greatly facilitated the antitumor immune responses and inhibited tumor growth in mice (108). Similarly, co-delivery of miR-34a and sPD-1 by ultrasound complexed with microbubbles enhanced antitumor immunity and inhibited tumor tissue growth in mice bearing U14 cervical carcinomas (109). Pre-injection of a senescent tumor cell vaccine expressing sPD-1 (STCV/sPD-1) was found to protect mice from challenge with 4T1 murine breast cancer cells, delaying tumorigenesis and inhibiting early-stage tumor progression (110). Both in vitro and in vivo analyses demonstrated that high-affinity sPD-1 molecule blocked PD-L1- and PD-L2-mediated immune evasion and reduced tumor growth in immune-competent mouse models of ovarian cancer (111). Nanobubbles (NBs) containing sPD-1/dihydroporphyrin e6 (Ce6) were found to enhance tumor suppression by increasing tumor-targeted accumulation of Ce6 and sPD-1 and inducing ultrasound-targeted NB destruction (112). Transfection of tumor cells with sPD-1 delivered by NBs was found to downregulate PD-L1 expression, improving PD-1/PD-L1 signaling pathway-mediated T cell function (112). In addition, ICIs bound to Ce6 were shown to induce immunogenic cell death by translocating calreticulin to the cell surface, followed by synergistic enhancement of the antitumor immune response (112). A case-control analysis of patients with HBV-related HCC showed that median sPD-1 levels were significantly higher in men than in women patients (443.3 vs 307.3 pg/mL) (113). Moreover, high sPD-1 levels were associated elevated viral load, further increasing the risk of HCC in men (113). Taken together, these studies indicate that sPD-1 has potential clinical application in cancer treatment.
5.2.2 Therapeutic Value of sPD-L1
The active sPD-L1 fragment generated by ADAM10 and ADAM17 cleavage of mPD-L1 was found to attenuate the killing of tumor cells by CD8+ T cells, revealing a novel mechanism of resistance to anti-PD-(L)1 antibodies (114). Two secreted PD-L1 splicing variants (PD-L1v242 and PD-L1v229) lacking the transmembrane domain were found to act as “decoys” of anti-PD-L1 antibodies, resulting in resistance to PD-L1 inhibitors (115). Anti-PD-1 antibodies, however, reversed the resistance induced by PD-L1 splicing variants (115). Small molecule inhibitors, such as BMS-1001 and BMS-1166, were found to impair the ability of sPD-L1 to inhibit T-lymphocyte activation (116). Because few studies have evaluated the biological activity of sPD-L1, no anticancer therapy directly targeting sPD-L1 has been tested in humans. Tumor-specific antigenic peptides presented by MHC molecules trigger TCR signaling and mediate T-cell activation. In addition, a deficiency in MHC molecules can result in sPD-L1 having subtle immunosuppressive effects (16). Targeting sPD-L1 alone may not effectively hamper tumor immune evasion (117). In-depth investigations of the functions of sPD-L1 and its use as a target for clinical anticancer therapy are urgently needed.
6 Exosomal PD-L1
In addition to PD-L1, which is free in solution, extracellular PD-L1 exists in another important form, exoPD-L1 (17, 118, 119). Exosomes are produced by double invagination of the plasma membrane and the formation of intracellular multivesicular bodies (MVBs) (120, 121). Following fusion of MVBs with the plasma membrane, these exosomes are secreted into the extracellular space and microenvironment by exocytosis (120, 121). Secreted exosomes transport their contents, including nucleic acids, lipids, and proteins, to target cells, thereby mediating intercellular signaling, drug resistance and immune regulation (122–126). Tumor cells can produce and secreted large amounts of exosomes with cancer-promoting contents (127, 128). Moreover, tumor-derived exosomes may act as regulatory elements that can reprogram the immune microenvironment (129, 130). Cancer-derived exosomes have been shown to present antigens to APCs to activate T-cell function and improve anti-tumor responses (130). ELISA and immunoelectron microscopy demonstrated that exoPD-L1 has the same membrane topology as mPD-L1, with many studies showing that tumor cell-derived exosomes carry bioactive PD-L1 and are able to bind to T cell surface PD-1 to deliver inhibitory signals (119). Exosomes also express other proteins that are crucial for T-cell signaling, such as MHC proteins, which interact with TCRs to deliver activation signals (17). Thus, exosomes co-expressing PD-L1 and MHC molecules may mediate more potent immunosuppressive effects than free sPD-L1 (131).
6.1 Immunosuppressive and Anti-Tumor Roles of ExoPD-L1
ExoPD-L1 inhibits immune cell-associated immune responses and promotes the growth of various tumor types. Replacing exogenously expressed PD-L1 on Raji B cells with exosomal PD-L1 from PC3 cells was shown to inhibit the activation of Jurkat T cells and reduce IL-2 secretion (118). Similarly, tumor-derived exoPD-L1 was found to inhibit T-cell activation in draining lymph nodes in vivo and the introduction of exogenous exoPD-L1 promoted tumor growth (118). In vitro co-culture of PD-L1high exosomes with T cells effectively inhibited T cell activation and reduced CD69 expression on CD8+ T cells (132). ExoPD-L1 in the supernatant of murine or human HNSCC cell lines was found to reduce the infiltration of CD4+ and CD8+ T cells into tumor sites (133). In addition, exoPD-L1 levels correlated with disease progression in HNSCC patients (133). ExoPD-L1 derived from LLC Lewis lung or 4T1 breast cancer cells inhibited the differentiation of bone marrow precursor cells to DCs and their maturation, decreasing CD4+ IFN-γ+ Th1 differentiation while increasing the percentage of Tregs (134). Breast cancer-derived exosomes were able to translocate functional PD-L1, not only from PD-L1-positive to PD-L1-negative breast cancer cells, but also to other cell types, including macrophages and DCs, to regulate immune surveillance (135). ExoPD-L1 was found to significantly inhibit CD3/CD28-induced ERK phosphorylation and NF-κB activation in T cells in a dose-dependent manner and to reduce the secretion of IL-2 and of granzyme B, a marker of killing activity (135). PD-L1-expressing extracellular vehicles (EVs) of glioblastoma (GBM) cells have been reported to show dose-dependent effects, with low doses of EVs resulting in an immunostimulatory phenotype and higher doses of EVs having immunosuppressive effects (136). Similarly, exoPD-L1 in NSCLC patients inhibited CD8+ T cell activity in a dose-dependent manner, inducing CD8+ T cell apoptosis and reducing IL-2 and IFN-γ production (137).
6.2 Predictive and Prognostic Significance of ExoPD-L1
ExoPD-L1 has been identified as a biomarker of disease status and the clinical effects of immunotherapy. High levels of exoPD-L1 have been reported to correlate with advanced tumor stage, larger tumor size (> 2.5 cm), lymph node metastasis and distant metastasis in patients with NSCLC (138). In addition, exoPD-L1 has been associated with poor prognosis in patients with pancreatic ductal adenocarcinoma (139). Metastatic melanoma patients with a > 2.43-fold change in exoPD-L1 levels during immunotherapy were found to be more likely to respond successfully to treatment (119). The increase in exoPD-L1 during anti-PD-1 treatment is thought to result from IFN-γ stimulation produced by reinvigorated CD8+ T cells, indicating that the change in tumor-derived exoPD-L1 is an adaptive response of tumor cells to T cell regeneration (140). A >100 pg/mL change in exoPD-L1 concentration in melanoma patients was found to have a 91% positive predictive value for disease progression (141). Detection of exoPD-L1 and N-cadherin in the sera of patients with osteosarcoma may predict the progression of pulmonary metastases (142). Significantly elevated plasma levels of PD-L1-positive EVs may be a biomarker predictive of survival outcomes in patients with DLBCL (143).
6.3 Therapeutic Value of ExoPD-L1
Evidence in tumor-bearing mice suggests that exosome elimination mitigates tumor burden and enhances the potency of anti-PD-1/PD-L1 antibodies, with exoPD-L1 elimination restoreing T-cell activation (144). In an established xenograft mouse model of PD-L1 knockdown in the TRAMP-C2 cell line, the percentage of CD8+ T cells in draining lymph nodes was upregulated and the proportions of cells expressing granzyme B and Ki67 were increased, while the percentage of cells expressing the exhaustion marker Tim3 was downregulated (118). ExoPD-L1 deficiency not only inhibited local tumor growth but also blocked the ability of wild-type tumor cells to attack the other flank, indicating a potent anti-tumor memory response (118). Treatment of 4T1 cells with the exosomal secretion inhibitor GW4869 or tetracycline-induced Rab27 knockdown significantly inhibited tumor growth and enhanced anti-PD-L1 efficacy (135). These findings suggest that binding of exoPD-L1 to anti-PD-L1 antibodies prevents these antibodies from sequestering PD-L1 on the surface of tumor cells (131, 144). In addition, exosome removal from the circulation by hemofiltration may have anti-tumor effects (131). IFN-γ increases the amount of PD-L1 on exosomes released from metastatic melanoma, thereby inhibiting the function of CD8+ T cells, whereas anti-PD-1 antibodies reverse these effects and halt tumor progression (119). Similarly, anti-PD-1 antibodies were shown to significantly reverse the exosome-mediated blockade of T-cell activation in glioblastoma (145), suggesting that these anti-PD-1 antibodies compete with exoPD-L1 to bind to PD-1 on T cells (131). These findings suggest that exosome elimination may be a viable and necessary concomitant therapy for anti-PD-1/PD-L1 antibody potentiation.
7 Conclusion and Perspective
The PD-1/PD-L1 pathway is critical for inducing tumor escape from the immune system. Immunotherapy targeting PD-1/PD-L1 has revolutionized the paradigm of cancer therapy. Biomarkers predicting the efficacy of immunotherapy have also been extensively investigated (146–148). sPD-1/PD-L1 and exoPD-L1 molecules have been detected in the blood of cancer patients. sPD-1/PD-L1 are generated by proteolytic cleavage of membrane-bound molecules or by selective splicing during gene transcription. These molecules may be important components of immune regulation, although their exact biochemical properties and functions have not yet been determined. Several methods have been developed to assess these molecules. Current investigations have focused mainly on the use of these biomarkers to assess patient prognosis and to predict the efficacy of treatment (149, 150). Accumulated data indicate that these molecules may correlate with disease progression and patient survival. Changes in their levels after certain treatments, including surgery, radiotherapy, targeted therapy, and immunotherapy, suggest their potential as biomarkers for predicting treatment efficacy. In addition, survival may correlate with resistance to PD-(L)1 inhibitors. The characteristics of sPD-1 suggest that it may attenuate immunosuppression by blocking ligands. Lower sPD-1 levels have fewer side effects than monoclonal antibodies while exerting strong therapeutic effects. In vivo and in vitro studies have indicated that delivery of sPD-1 into the tumor microenvironment induces antitumor immunity. In addition, combination therapeutic strategies have shown synergistic effects. Similar to mPD-L1, sPD-L1 and exoPD-L1 bind to mPD-1 to exert immunosuppressive effects, suggesting that these reactions may be targets of anticancer treatment. Few studies to date have evaluated the potential of targeting sPD-1/PD-L1 and exoPD-L1 in cancer therapy. Results to date seem promising, suggesting new clinical antitumor strategies.
PD-1/PD-L1 blockade alone, however, is likely insufficient to obtain optimal results in a large proportion of patients. In addition to PD-1/PD-L1, other stimulatory and inhibitory molecules are involved in immune regulation. The various forms of these molecules, including soluble, exosome-associated, and membrane-associated molecules, play roles in the tumor microenvironment (27). For example, sCTLA-4 binds to the co-stimulatory ligand B7 on APCs and prevents B7 from binding to CD28 on T cells, thereby inhibiting T cell function (151). sCD86 is thought to bind to CTLA-4 and deliver negative signals to T lymphocytes (152). sB7-H3 not only significantly inhibits T cell proliferation but also activates NF-κB signaling by upregulating TLR4 expression, which induces IL-8 and VEGF expression, thereby promoting neovascularization and cancer cell invasion and metastasis (153). In addition, tumor-secreted sCD137 inhibits T-lymphocyte activation by blocking the interaction of CD137L with CD137 on T-lymphocytes (154). Additional studies of inhibitory and stimulatory molecules in cancer are necessary, including studies on the various forms of these molecules, their complex interactions, and their changes in concentration during tumor progression.
Author Contributions
MN and YL drafted the manuscript and prepared the figure and tables. MY helped in revising it critically for important intellectual content. DJ and KW designed this review and revised the manuscript. All authors contributed to the article and approved the submitted version.
Funding
This work was supported by the National Natural Science Foundation of China (No.81874120, 82073370).
Conflict of Interest
The authors declare that the research was conducted in the absence of any commercial or financial relationships that could be construed as a potential conflict of interest.
Publisher’s Note
All claims expressed in this article are solely those of the authors and do not necessarily represent those of their affiliated organizations, or those of the publisher, the editors and the reviewers. Any product that may be evaluated in this article, or claim that may be made by its manufacturer, is not guaranteed or endorsed by the publisher.
References
1. Chen DS, Mellman I. Oncology Meets Immunology: The Cancer-Immunity Cycle. Immunity (2013) 39:1–10. doi: 10.1016/j.immuni.2013.07.012
2. Freeman GJ, Long AJ, Iwai Y, Bourque K, Chernova T, Nishimura H, et al. Engagement of the PD-1 Immunoinhibitory Receptor by a Novel B7 Family Member Leads to Negative Regulation of Lymphocyte Activation. J Exp Med (2000) 192:1027–34. doi: 10.1084/jem.192.7.1027
3. Teft WA, Kirchhof MG, Madrenas J. A Molecular Perspective of CTLA-4 Function. Annu Rev Immunol (2006) 24:65–97. doi: 10.1146/annurev.immunol.24.021605.090535
4. Dong H, Strome SE, Salomao DR, Tamura H, Hirano F, Flies DB, et al. Tumor-Associated B7-H1 Promotes T-Cell Apoptosis: A Potential Mechanism of Immune Evasion. Nat Med (2002) 8:793–800. doi: 10.1038/nm730
5. Wang L, Pino-Lagos K, de Vries VC, Guleria I, Sayegh MH, Noelle RJ. Programmed Death 1 Ligand Signaling Regulates the Generation of Adaptive Foxp3+CD4+ Regulatory T Cells. Proc Natl Acad Sci USA (2008) 105:9331–6. doi: 10.1073/pnas.0710441105
6. Jiang X, Wang J, Deng X, Xiong F, Ge J, Xiang B, et al. Role of the Tumor Microenvironment in PD-L1/PD-1-Mediated Tumor Immune Escape. Mol Cancer (2019) 18:10. doi: 10.1186/s12943-018-0928-4
7. Sharpe AH, Pauken KE. The Diverse Functions of the PD-1 Inhibitory Pathway. Nat Rev Immunol (2018) 18:153–67. doi: 10.1038/nri.2017.108
8. Terawaki S, Chikuma S, Shibayama S, Hayashi T, Yoshida T, Okazaki T, et al. IFN-α Directly Promotes Programmed Cell Death-1 Transcription and Limits the Duration of T Cell-Mediated Immunity. J Immunol (2011) 186:2772–9. doi: 10.4049/jimmunol.1003208
9. Akbay EA, Koyama S, Carretero J, Altabef A, Tchaicha JH, Christensen CL, et al. Activation of the PD-1 Pathway Contributes to Immune Escape in EGFR-Driven Lung Tumors. Cancer Discov (2013) 3:1355–63. doi: 10.1158/2159-8290.Cd-13-0310
10. Ota K, Azuma K, Kawahara A, Hattori S, Iwama E, Tanizaki J, et al. Induction of PD-L1 Expression by the EML4-ALK Oncoprotein and Downstream Signaling Pathways in non-Small Cell Lung Cancer. Clin Cancer Res (2015) 21:4014–21. doi: 10.1158/1078-0432.Ccr-15-0016
11. Yi M, Niu M, Xu L, Luo S, Wu K. Regulation of PD-L1 Expression in the Tumor Microenvironment. J Hematol Oncol (2021) 14:10. doi: 10.1186/s13045-020-01027-5
12. Fang W, Zhang J, Hong S, Zhan J, Chen N, Qin T, et al. EBV-Driven LMP1 and IFN-γ Up-Regulate PD-L1 in Nasopharyngeal Carcinoma: Implications for Oncotargeted Therapy. Oncotarget (2014) 5:12189–202. doi: 10.18632/oncotarget.2608
13. Xu L, Chen X, Shen M, Yang DR, Fang L, Weng G, et al. Inhibition of IL-6-JAK/Stat3 Signaling in Castration-Resistant Prostate Cancer Cells Enhances the NK Cell-Mediated Cytotoxicity via Alteration of PD-L1/NKG2D Ligand Levels. Mol Oncol (2018) 12:269–86. doi: 10.1002/1878-0261.12135
14. Zhao R, Song Y, Wang Y, Huang Y, Li Z, Cui Y, et al. PD-1/PD-L1 Blockade Rescue Exhausted CD8+ T Cells in Gastrointestinal Stromal Tumours via the PI3K/Akt/mTOR Signalling Pathway. Cell Prolif (2019) 52:e12571. doi: 10.1111/cpr.12571
15. Patsoukis N, Brown J, Petkova V, Liu F, Li L, Boussiotis VA. Selective Effects of PD-1 on Akt and Ras Pathways Regulate Molecular Components of the Cell Cycle and Inhibit T Cell Proliferation. Sci Signal (2012) 5:ra46. doi: 10.1126/scisignal.2002796
16. Chen L, Flies DB. Molecular Mechanisms of T Cell Co-Stimulation and Co-Inhibition. Nat Rev Immunol (2013) 13:227–42. doi: 10.1038/nri3405
17. Daassi D, Mahoney KM, Freeman GJ. The Importance of Exosomal PD-L1 in Tumour Immune Evasion. Nat Rev Immunol (2020) 20:209–15. doi: 10.1038/s41577-019-0264-y
18. Chang CH, Qiu J, O’Sullivan D, Buck MD, Noguchi T, Curtis JD, et al. Metabolic Competition in the Tumor Microenvironment Is a Driver of Cancer Progression. Cell (2015) 162:1229–41. doi: 10.1016/j.cell.2015.08.016
19. Topalian SL, Drake CG, Pardoll DM. Targeting the PD-1/B7-H1(PD-L1) Pathway to Activate Anti-Tumor Immunity. Curr Opin Immunol (2012) 24:207–12. doi: 10.1016/j.coi.2011.12.009
20. Yi M, Zhang J, Li A, Niu M, Yan Y, Jiao Y, et al. The Construction, Expression, and Enhanced Anti-Tumor Activity of YM101: A Bispecific Antibody Simultaneously Targeting TGF-β and PD-L1. J Hematol Oncol (2021) 14:27. doi: 10.1186/s13045-021-01045-x
21. Nghiem PT, Bhatia S, Lipson EJ, Kudchadkar RR, Miller NJ, Annamalai L, et al. PD-1 Blockade With Pembrolizumab in Advanced Merkel-Cell Carcinoma. N Engl J Med (2016) 374:2542–52. doi: 10.1056/NEJMoa1603702
22. Middleton G, Brock K, Savage J, Mant R, Summers Y, Connibear J, et al. Pembrolizumab in Patients With non-Small-Cell Lung Cancer of Performance Status 2 (PePS2): A Single Arm, Phase 2 Trial. Lancet Respir Med (2020) 8:895–904. doi: 10.1016/s2213-2600(20)30033-3
23. Robert C, Long GV, Brady B, Dutriaux C, Maio M, Mortier L, et al. Nivolumab in Previously Untreated Melanoma Without BRAF Mutation. N Engl J Med (2015) 372:320–30. doi: 10.1056/NEJMoa1412082
24. Powles T, Eder JP, Fine GD, Braiteh FS, Loriot Y, Cruz C, et al. MPDL3280A (Anti-PD-L1) Treatment Leads to Clinical Activity in Metastatic Bladder Cancer. Nature (2014) 515:558–62. doi: 10.1038/nature13904
25. Persico P, Lorenzi E, Dipasquale A, Pessina F, Navarria P, Politi LS, et al. Checkpoint Inhibitors as High-Grade Gliomas Treatment: State of the Art and Future Perspectives. J Clin Med (2021) 10:1367. doi: 10.3390/jcm10071367
26. Bailly C, Thuru X, Quesnel B. Soluble Programmed Death Ligand-1 (sPD-L1): A Pool of Circulating Proteins Implicated in Health and Diseases. Cancers (Basel) (2021) 13:3034. doi: 10.3390/cancers13123034
27. Gu D, Ao X, Yang Y, Chen Z, Xu X. Soluble Immune Checkpoints in Cancer: Production, Function and Biological Significance. J Immunother Cancer (2018) 6:132. doi: 10.1186/s40425-018-0449-0
28. Tiako Meyo M, Jouinot A, Giroux-Leprieur E, Fabre E, Wislez M, Alifano M, et al. Predictive Value of Soluble PD-1, PD-L1, VEGFA, CD40 Ligand and CD44 for Nivolumab Therapy in Advanced non-Small Cell Lung Cancer: A Case-Control Study. Cancers (Basel) (2020) 12:473. doi: 10.3390/cancers12020473
29. Feng Y, Jing C, Yu X, Cao X, Xu C. Predicting Treatment Response of Patients With Extranodal Natural Killer/T-Cell Lymphoma Based on Levels of PD-L1 mRNA and Soluble PD-L1. Hematol Oncol (2020) 38:467–77. doi: 10.1002/hon.2758
30. Ward FJ, Dahal LN, Wijesekera SK, Abdul-Jawad SK, Kaewarpai T, Xu H, et al. The Soluble Isoform of CTLA-4 as a Regulator of T-Cell Responses. Eur J Immunol (2013) 43:1274–85. doi: 10.1002/eji.201242529
31. Hock BD, Starling GC, Patton WN, Salm N, Bond K, McArthur LT, et al. Identification of a Circulating Soluble Form of CD80: Levels in Patients With Hematological Malignancies. Leuk Lymphoma (2004) 45:2111–8. doi: 10.1080/10428190410001712199
32. Hock BD, Patton WN, Budhia S, Mannari D, Roberts P, McKenzie JL. Human Plasma Contains a Soluble Form of CD86 Which is Present at Elevated Levels in Some Leukaemia Patients. Leukemia (2002) 16:865–73. doi: 10.1038/sj.leu.2402466
33. Chen L, Zhang G, Sheng S, Zhou Q, Pan Y, Guan S. Upregulation of Soluble B7-H3 in NSCLC-Derived Malignant Pleural Effusion: A Potential Diagnostic Biomarker Correlated With NSCLC Staging. Clin Chim Acta (2016) 457:81–5. doi: 10.1016/j.cca.2016.04.009
34. Triebel F, Hacene K, Pichon MF. A Soluble Lymphocyte Activation Gene-3 (sLAG-3) Protein as a Prognostic Factor in Human Breast Cancer Expressing Estrogen or Progesterone Receptors. Cancer Lett (2006) 235:147–53. doi: 10.1016/j.canlet.2005.04.015
35. Qin S, Xu L, Yi M, Yu S, Wu K, Luo S. Novel Immune Checkpoint Targets: Moving Beyond PD-1 and CTLA-4. Mol Cancer (2019) 18:155. doi: 10.1186/s12943-019-1091-2
36. Zhang G, Hou J, Shi J, Yu G, Lu B, Zhang X. Soluble CD276 (B7-H3) is Released From Monocytes, Dendritic Cells and Activated T Cells and is Detectable in Normal Human Serum. Immunology (2008) 123:538–46. doi: 10.1111/j.1365-2567.2007.02723.x
37. Jeannin P, Magistrelli G, Aubry JP, Caron G, Gauchat JF, Renno T, et al. Soluble CD86 is a Costimulatory Molecule for Human T Lymphocytes. Immunity (2000) 13:303–12. doi: 10.1016/s1074-7613(00)00030-3
38. Nielsen C, Ohm-Laursen L, Barington T, Husby S, Lillevang ST. Alternative Splice Variants of the Human PD-1 Gene. Cell Immunol (2005) 235:109–16. doi: 10.1016/j.cellimm.2005.07.007
39. Keir ME, Butte MJ, Freeman GJ, Sharpe AH. PD-1 and its Ligands in Tolerance and Immunity. Annu Rev Immunol (2008) 26:677–704. doi: 10.1146/annurev.immunol.26.021607.090331
40. Frigola X, Inman BA, Krco CJ, Liu X, Harrington SM, Bulur PA, et al. Soluble B7-H1: Differences in Production Between Dendritic Cells and T Cells. Immunol Lett (2012) 142:78–82. doi: 10.1016/j.imlet.2011.11.001
41. Chen Y, Wang Q, Shi B, Xu P, Hu Z, Bai L, et al. Development of a Sandwich ELISA for Evaluating Soluble PD-L1 (CD274) in Human Sera of Different Ages as Well as Supernatants of PD-L1+ Cell Lines. Cytokine (2011) 56:231–8. doi: 10.1016/j.cyto.2011.06.004
42. Dezutter-Dambuyant C, Durand I, Alberti L, Bendriss-Vermare N, Valladeau-Guilemond J, Duc A, et al. A Novel Regulation of PD-1 Ligands on Mesenchymal Stromal Cells Through MMP-Mediated Proteolytic Cleavage. Oncoimmunology (2016) 5:e1091146. doi: 10.1080/2162402x.2015.1091146
43. Hira-Miyazawa M, Nakamura H, Hirai M, Kobayashi Y, Kitahara H, Bou-Gharios G, et al. Regulation of Programmed-Death Ligand in the Human Head and Neck Squamous Cell Carcinoma Microenvironment is Mediated Through Matrix Metalloproteinase-Mediated Proteolytic Cleavage. Int J Oncol (2018) 52:379–88. doi: 10.3892/ijo.2017.4221
44. Romero Y, Wise R, Zolkiewska A. Proteolytic Processing of PD-L1 by ADAM Proteases in Breast Cancer Cells. Cancer Immunol Immunother (2020) 69:43–55. doi: 10.1007/s00262-019-02437-2
45. Pichler R, Lindner AK, Schäfer G, Tulchiner G, Staudacher N, Mayr M, et al. Expression of ADAM Proteases in Bladder Cancer Patients With BCG Failure: A Pilot Study. J Clin Med (2021) 10:764. doi: 10.3390/jcm10040764
46. Yunusova NV, Patysheva MR, Molchanov SV, Zambalova EA, Grigor’eva AE, Kolomiets LA, et al. Metalloproteinases at the Surface of Small Extrcellular Vesicles in Advanced Ovarian Cancer: Relationships With Ascites Volume and Peritoneal Canceromatosis Index. Clin Chim Acta (2019) 494:116–22. doi: 10.1016/j.cca.2019.03.1621
47. Roemer MGM, Redd RA, Cader FZ, Pak CJ, Abdelrahman S, Ouyang J, et al. Major Histocompatibility Complex Class II and Programmed Death Ligand 1 Expression Predict Outcome After Programmed Death 1 Blockade in Classic Hodgkin Lymphoma. J Clin Oncol (2018) 36:942–50. doi: 10.1200/jco.2017.77.3994
48. Qu S, Jiao Z, Lu G, Yao B, Wang T, Rong W, et al. PD-L1 lncRNA Splice Isoform Promotes Lung Adenocarcinoma Progression. Via Enhancing c-Myc Activity Genome Biol (2021) 22:104. doi: 10.1186/s13059-021-02331-0
49. Hassounah NB, Malladi VS, Huang Y, Freeman SS, Beauchamp EM, Koyama S, et al. Identification and Characterization of an Alternative Cancer-Derived PD-L1 Splice Variant. Cancer Immunol Immunother (2019) 68:407–20. doi: 10.1007/s00262-018-2284-z
50. Ng KW, Attig J, Young GR, Ottina E, Papamichos SI, Kotsianidis I, et al. Soluble PD-L1 Generated by Endogenous Retroelement Exaptation is a Receptor Antagonist. eLife (2019) 8:e50256. doi: 10.7554/eLife.50256
51. Mahoney KM, Shukla SA, Patsoukis N, Chaudhri A, Browne EP, Arazi A, et al. A Secreted PD-L1 Splice Variant That Covalently Dimerizes and Mediates Immunosuppression. Cancer Immunol Immunother (2019) 68:421–32. doi: 10.1007/s00262-018-2282-1
52. He XH, Xu LH, Liu Y. Identification of a Novel Splice Variant of Human PD-L1 mRNA Encoding an Isoform-Lacking Igv-Like Domain. Acta Pharmacol Sin (2005) 26:462–8. doi: 10.1111/j.1745-7254.2005.00086.x
53. Chang B, Huang T, Wei H, Shen L, Zhu D, He W, et al. The Correlation and Prognostic Value of Serum Levels of Soluble Programmed Death Protein 1 (sPD-1) and Soluble Programmed Death-Ligand 1 (sPD-L1) in Patients With Hepatocellular Carcinoma. Cancer Immunol Immunother (2019) 68:353–63. doi: 10.1007/s00262-018-2271-4
54. Kruger S, Legenstein ML, Rösgen V, Haas M, Modest DP, Westphalen CB, et al. Serum Levels of Soluble Programmed Death Protein 1 (sPD-1) and Soluble Programmed Death Ligand 1 (sPD-L1) in Advanced Pancreatic Cancer. Oncoimmunology (2017) 6:e1310358. doi: 10.1080/2162402x.2017.1310358
55. Zhou L, Li X, Huang X, Chen L, Gu L, Huang Y. Soluble Programmed Death-1 is a Useful Indicator for Inflammatory and Fibrosis Severity in Chronic Hepatitis B. J Viral Hepat (2019) 26:795–802. doi: 10.1111/jvh.13055
56. Liu C, Jiang J, Gao L, Wang X, Hu X, Wu M, et al. Soluble PD-1 Aggravates Progression of Collagen-Induced Arthritis Through Th1 and Th17 Pathways. Arthritis Res Ther (2015) 17:340. doi: 10.1186/s13075-015-0859-z
57. Song MY, Park SH, Nam HJ, Choi DH, Sung YC. Enhancement of Vaccine-Induced Primary and Memory CD8+ T-Cell Responses by Soluble PD-1. J Immunother (2011) 34:297–306. doi: 10.1097/CJI.0b013e318210ed0e
58. Kuipers H, Muskens F, Willart M, Hijdra D, van Assema FB, Coyle AJ, et al. Contribution of the PD-1 Ligands/PD-1 Signaling Pathway to Dendritic Cell-Mediated CD4+ T Cell Activation. Eur J Immunol (2006) 36:2472–82. doi: 10.1002/eji.200635978
59. Elhag OA, Hu XJ, Wen-Ying Z, Li X, Yuan YZ, Deng LF, et al. Reconstructed Adeno-Associated Virus With the Extracellular Domain of Murine PD-1 Induces Antitumor Immunity. Asian Pac J Cancer Prev (2012) 13:4031–6. doi: 10.7314/apjcp.2012.13.8.4031
60. Qiu H, Liu S, Xie C, Long J, Feng Z. Regulating Immunity and Inhibiting Tumor Growth by the Recombinant Peptide sPD-1-Ch50. Anticancer Res (2009) 29:5089–94.
61. Geng H, Zhang GM, Xiao H, Yuan Y, Li D, Zhang H, et al. HSP70 Vaccine in Combination With Gene Therapy With Plasmid DNA Encoding sPD-1 Overcomes Immune Resistance and Suppresses the Progression of Pulmonary Metastatic Melanoma. Int J Cancer (2006) 118:2657–64. doi: 10.1002/ijc.21795
62. He L, Zhang G, He Y, Zhu H, Zhang H, Feng Z. Blockade of B7-H1 With sPD-1 Improves Immunity Against Murine Hepatocarcinoma. Anticancer Res (2005) 25:3309–13. doi: 10.4103/0377-4929.116164
63. Wang X, Zhang Z, Zhang S, Fu J, Yao J, Jiao Y, et al. B7-H1 Up-Regulation Impairs Myeloid DC and Correlates With Disease Progression in Chronic HIV-1 Infection. Eur J Immunol (2008) 38:3226–36. doi: 10.1002/eji.200838285
64. Frigola X, Inman BA, Lohse CM, Krco CJ, Cheville JC, Thompson RH, et al. Identification of a Soluble Form of B7-H1 That Retains Immunosuppressive Activity and is Associated With Aggressive Renal Cell Carcinoma. Clin Cancer Res (2011) 17:1915–23. doi: 10.1158/1078-0432.Ccr-10-0250
65. Zhou J, Mahoney KM, Giobbie-Hurder A, Zhao F, Lee S, Liao X, et al. Soluble PD-L1 as a Biomarker in Malignant Melanoma Treated With Checkpoint Blockade. Cancer Immunol Res (2017) 5:480–92. doi: 10.1158/2326-6066.Cir-16-0329
66. Li Y, Xiao Y, Su M, Zhang R, Ding J, Hao X, et al. Role of Soluble Programmed Death-1 (sPD-1) and sPD-Ligand 1 in Patients With Cystic Echinococcosis. Exp Ther Med (2016) 11:251–6. doi: 10.3892/etm.2015.2876
67. Han B, Dong L, Zhou J, Yang Y, Guo J, Xuan Q, et al. The Clinical Implication of Soluble PD-L1 (sPD-L1) in Patients With Breast Cancer and its Biological Function in Regulating the Function of T Lymphocyte. Cancer Immunol Immunother (2021) 70:2893–909. doi: 10.1007/s00262-021-02898-4
68. Liang Z, Tian Y, Cai W, Weng Z, Li Y, Zhang H, et al. High-Affinity Human PD-L1 Variants Attenuate the Suppression of T Cell Activation. Oncotarget (2017) 8:88360–75. doi: 10.18632/oncotarget.21729
69. Shi B, Du X, Wang Q, Chen Y, Zhang X. Increased PD-1 on CD4+CD28- T Cell and Soluble PD-1 Ligand-1 in Patients With T2DM: Association With Atherosclerotic Macrovascular Diseases. Metabolism (2013) 62:778–85. doi: 10.1016/j.metabol.2012.12.005
70. Enninga EAL, Harrington SM, Creedon DJ, Ruano R, Markovic SN, Dong H, et al. Immune Checkpoint Molecules Soluble Program Death Ligand 1 and Galectin-9 are Increased in Pregnancy. Am J Reprod Immunol (2018) 79:e12795. doi: 10.1111/aji.12795
71. Zhang YH, Aldo P, You Y, Ding J, Kaislasuo J, Petersen JF, et al. Trophoblast-Secreted Soluble-PD-L1 Modulates Macrophage Polarization and Function. J Leukoc Biol (2020) 108:983–98. doi: 10.1002/jlb.1a0420-012rr
72. Okuyama M, Mezawa H, Kawai T, Urashima M. Elevated Soluble PD-L1 in Pregnant Women’s Serum Suppresses the Immune Reaction. Front Immunol (2019) 10:86. doi: 10.3389/fimmu.2019.00086
73. Huang X, Liu X, Ye Y, Zhang T, Mei S, Zhu T, et al. Polymorphisms and Circulating Plasma Protein Levels of Immune Checkpoints (CTLA-4 and PD-1) are Associated With Posner-Schlossman Syndrome in Southern Chinese. Front Immunol (2021) 12:607966. doi: 10.3389/fimmu.2021.607966
74. Luo B, Wang Y, Lu H, Wu S, Lu Y, Shi S, et al. Label-Free and Specific Detection of Soluble Programmed Death Ligand-1 Using a Localized Surface Plasmon Resonance Biosensor Based on Excessively Tilted Fiber Gratings. Biomed Opt Express (2019) 10:5136–48. doi: 10.1364/boe.10.005136
75. Goto M, Chamoto K, Higuchi K, Yamashita S, Noda K, Iino T, et al. Analytical Performance of a New Automated Chemiluminescent Magnetic Immunoassays for Soluble PD-1, PD-L1, and CTLA-4 in Human Plasma. Sci Rep (2019) 9:10144. doi: 10.1038/s41598-019-46548-3
76. Reza KK, Sina AA, Wuethrich A, Grewal YS, Howard CB, Korbie D, et al. A SERS Microfluidic Platform for Targeting Multiple Soluble Immune Checkpoints. Biosens Bioelectron (2019) 126:178–86. doi: 10.1016/j.bios.2018.10.044
77. Wuethrich A, Rajkumar AR, Shanmugasundaram KB, Reza KK, Dey S, Howard CB, et al. Single Droplet Detection of Immune Checkpoints on a Multiplexed Electrohydrodynamic Biosensor. Analyst (2019) 144:6914–21. doi: 10.1039/c9an01450k
78. Sorensen SF, Demuth C, Weber B, Sorensen BS, Meldgaard P. Increase in Soluble PD-1 is Associated With Prolonged Survival in Patients With Advanced EGFR-Mutated Non-Small Cell Lung Cancer Treated With Erlotinib. Lung Cancer (2016) 100:77–84. doi: 10.1016/j.lungcan.2016.08.001
79. Ruan Y, Hu W, Li W, Lu H, Gu H, Zhang Y, et al. Analysis of Plasma EBV-DNA and Soluble Checkpoint Proteins in Nasopharyngeal Carcinoma Patients After Definitive Intensity-Modulated Radiotherapy. BioMed Res Int (2019) 2019:3939720. doi: 10.1155/2019/3939720
80. Tominaga T, Akiyoshi T, Yamamoto N, Taguchi S, Mori S, Nagasaki T, et al. Clinical Significance of Soluble Programmed Cell Death-1 and Soluble Programmed Cell Death-Ligand 1 in Patients With Locally Advanced Rectal Cancer Treated With Neoadjuvant Chemoradiotherapy. PloS One (2019) 14:e0212978. doi: 10.1371/journal.pone.0212978
81. Bian B, Fanale D, Dusetti N, Roque J, Pastor S, Chretien AS, et al. Prognostic Significance of Circulating PD-1, PD-L1, Pan-BTN3As, BTN3A1 and BTLA in Patients With Pancreatic Adenocarcinoma. Oncoimmunology (2019) 8:e1561120. doi: 10.1080/2162402x.2018.1561120
82. Vajavaara H, Mortensen JB, Leivonen SK, Hansen IM, Ludvigsen M, Holte H, et al. Soluble PD-1 Butnot PD-L1 Levels Predict Poor Outcome in Patients With High-Risk Diffuse Large B-Cell Lymphoma. Cancers (Basel) (2021) 13:398. doi: 10.3390/cancers13030398
83. Murakami S, Shibaki R, Matsumoto Y, Yoshida T, Goto Y, Kanda S, et al. Association Between Serum Level Soluble Programmed Cell Death Ligand 1 and Prognosis in Patients With Non-Small Cell Lung Cancer Treated With Anti-PD-1 Antibody. Thorac Cancer (2020) 11:3585–95. doi: 10.1111/1759-7714.13721
84. Shiraishi T, Toyozumi T, Sakata H, Murakami K, Kano M, Matsumoto Y, et al. Soluble PD-L1 Concentration Is Proportional to the Expression of PD-L1 in Tissue and is Associated With a Poor Prognosis in Esophageal Squamous Cell Carcinoma. Oncology (2022) 100:39–47. doi: 10.1159/000518740
85. Pawłowska A, Kwiatkowska A, Suszczyk D, Chudzik A, Tarkowski R, Barczyński B, et al. Clinical and Prognostic Value of Antigen-Presenting Cells With PD-L1/PD-L2 Expression in Ovarian Cancer Patients. Int J Mol Sci (2021) 22:11563. doi: 10.3390/ijms222111563
86. Fu R, Jing CQ, Li XR, Tan ZF, Li HJ. Prognostic Significance of Serum PD-L1 Level in Patients With Locally Advanced or Metastatic Esophageal Squamous Cell Carcinoma Treated With Combination Cytotoxic Chemotherapy. Cancer Manag Res (2021) 13:4935–46. doi: 10.2147/cmar.S312690
87. Larrinaga G, Solano-Iturri JD, Errarte P, Unda M, Loizaga-Iriarte A, Pérez-Fernández A, et al. Soluble PD-L1 is an Independent Prognostic Factor in Clear Cell Renal Cell Carcinoma. Cancers (Basel) (2021) 13:667. doi: 10.3390/cancers13040667
88. Vikerfors A, Davidsson S, Frey J, Jerlström T, Carlsson J. Soluble PD-L1 in Serum and Urine in Urinary Bladder Cancer Patients. Cancers (Basel) (2021) 13:5841. doi: 10.3390/cancers13225841
89. Krafft U, Olah C, Reis H, Kesch C, Darr C, Grünwald V, et al. High Serum PD-L1 Levels are Associated With Poor Survival in Urothelial Cancer Patients Treated With Chemotherapy and Immune Checkpoint Inhibitor Therapy. Cancers (Basel) (2021) 13:2548. doi: 10.3390/cancers13112548
90. Ding XC, Wang LL, Zhu YF, Li YD, Nie SL, Yang J, et al. The Change of Soluble Programmed Cell Death-Ligand 1 in Glioma Patients Receiving Radiotherapy and its Impact on Clinical Outcomes. Front Immunol (2020) 11:580335. doi: 10.3389/fimmu.2020.580335
91. Mocan T, Ilies M, Nenu I, Craciun R, Horhat A, Susa R, et al. Serum Levels of Soluble Programmed Death-Ligand 1 (sPD-L1): A Possible Biomarker in Predicting Post-Treatment Outcomes in Patients With Early Hepatocellular Carcinoma. Int Immunopharmacol (2021) 94:107467. doi: 10.1016/j.intimp.2021.107467
92. Asanuma K, Nakamura T, Hayashi A, Okamoto T, Iino T, Asanuma Y, et al. Soluble Programmed Death-Ligand 1 Rather Than PD-L1 on Tumor Cells Effectively Predicts Metastasis and Prognosis in Soft Tissue Sarcomas. Sci Rep (2020) 10:9077. doi: 10.1038/s41598-020-65895-0
93. Cheng Y, Wang C, Wang Y, Dai L. Soluble PD-L1 as a Predictive Biomarker in Lung Cancer: A Systematic Review and Meta-Analysis. Future Oncol (2022) 18:261–73. doi: 10.2217/fon-2021-0641
94. Dowell J, Minna JD, Kirkpatrick P. Erlotinib Hydrochloride. Nat Rev Drug Discov (2005) 4:13–4. doi: 10.1038/nrd1612
95. Spiotto M, Fu YX, Weichselbaum RR. The Intersection of Radiotherapy and Immunotherapy: Mechanisms and Clinical Implications. Sci Immunol (2016) 1:EAAG1266. doi: 10.1126/sciimmunol.aag1266
96. Reits EA, Hodge JW, Herberts CA, Groothuis TA, Chakraborty M, Wansley EK, et al. Radiation Modulates the Peptide Repertoire, Enhances MHC Class I Expression, and Induces Successful Antitumor Immunotherapy. J Exp Med (2006) 203:1259–71. doi: 10.1084/jem.20052494
97. Srivastava RM, Trivedi S, Concha-Benavente F, Hyun-Bae J, Wang L, Seethala RR, et al. STAT1-Induced HLA Class I Upregulation Enhances Immunogenicity and Clinical Response to Anti-EGFR mAb Cetuximab Therapy in HNC Patients. Cancer Immunol Res (2015) 3:936–45. doi: 10.1158/2326-6066.Cir-15-0053
98. Garrido G, Rabasa A, Garrido C, Chao L, Garrido F, García-Lora ÁM, et al. Upregulation of HLA Class I Expression on Tumor Cells by the Anti-EGFR Antibody Nimotuzumab. Front Pharmacol (2017) 8:595. doi: 10.3389/fphar.2017.00595
99. Ribas A, Puzanov I, Dummer R, Schadendorf D, Hamid O, Robert C, et al. Pembrolizumab Versus Investigator-Choice Chemotherapy for Ipilimumab-Refractory Melanoma (KEYNOTE-002): A Randomised, Controlled, Phase 2 Trial. Lancet Oncol (2015) 16:908–18. doi: 10.1016/s1470-2045(15)00083-2
100. Herbst RS, Baas P, Kim DW, Felip E, Pérez-Gracia JL, Han JY, et al. Pembrolizumab Versus Docetaxel for Previously Treated, PD-L1-Positive, Advanced Non-Small-Cell Lung Cancer (KEYNOTE-010): A Randomised Controlled Trial. Lancet (2016) 387:1540–50. doi: 10.1016/s0140-6736(15)01281-7
101. Galsky MD, Arija JÁA, Bamias A, Davis ID, De Santis M, Kikuchi E, et al. Atezolizumab With or Without Chemotherapy in Metastatic Urothelial Cancer (IMvigor130): A Multicentre, Randomised, Placebo-Controlled Phase 3 Trial. Lancet (2020) 395:1547–57. doi: 10.1016/s0140-6736(20)30230-0
102. Burtness B, Harrington KJ, Greil R, Soulières D, Tahara M, de Castro G Jr., et al. Pembrolizumab Alone or With Chemotherapy Versus Cetuximab With Chemotherapy for Recurrent or Metastatic Squamous Cell Carcinoma of the Head and Neck (KEYNOTE-048): A Randomised, Open-Label, Phase 3 Study. Lancet (2019) 394:1915–28. doi: 10.1016/s0140-6736(19)32591-7
103. Chen R, Zinzani PL, Lee HJ, Armand P, Johnson NA, Brice P, et al. Pembrolizumab in Relapsed or Refractory Hodgkin Lymphoma: 2-Year Follow-Up of KEYNOTE-087. Blood (2019) 134:1144–53. doi: 10.1182/blood.2019000324
104. Zhang A, Sun Y, Wang S, Du J, Gao X, Yuan Y, et al. Secretion of Human Soluble Programmed Cell Death Protein 1 by Chimeric Antigen Receptor-Modified T Cells Enhances Anti-Tumor Efficacy. Cytotherapy (2020) 22:734–43. doi: 10.1016/j.jcyt.2020.05.007
105. Xiao H, Huang B, Yuan Y, Li D, Han LF, Liu Y, et al. Soluble PD-1 Facilitates 4-1BBL-Triggered Antitumor Immunity Against Murine H22 Hepatocarcinoma. Vivo Clin Cancer Res (2007) 13:1823–30. doi: 10.1158/1078-0432.Ccr-06-2154
106. Shin SP, Seo HH, Shin JH, Park HB, Lim DP, Eom HS, et al. Adenovirus Expressing Both Thymidine Kinase and Soluble PD-1 Enhances Antitumor Immunity by Strengthening CD8 T-Cell Response. Mol Ther (2013) 21:688–95. doi: 10.1038/mt.2012.252
107. He YF, Zhang GM, Wang XH, Zhang H, Yuan Y, Li D, et al. Blocking Programmed Death-1 Ligand-PD-1 Interactions by Local Gene Therapy Results in Enhancement of Antitumor Effect of Secondary Lymphoid Tissue Chemokine. J Immunol (2004) 173:4919–28. doi: 10.4049/jimmunol.173.8.4919
108. Pan XC, Li L, Mao JJ, Yao W, Zheng JN, Liu M, et al. Synergistic Effects of Soluble PD-1 and IL-21 on Antitumor Immunity Against H22 Murine Hepatocellular Carcinoma. Oncol Lett (2013) 5:90–6. doi: 10.3892/ol.2012.966
109. Qin YE, Tang WF, Xu Y, Wan FR, Chen AH. Ultrasound-Mediated Co-Delivery of miR-34a and sPD-1 Complexed With Microbubbles for Synergistic Cancer Therapy. Cancer Manag Res (2020) 12:2459–69. doi: 10.2147/cmar.S238643
110. Chen Z, Hu K, Feng L, Su R, Lai N, Yang Z, et al. Senescent Cells Re-Engineered to Express Soluble Programmed Death Receptor-1 for Inhibiting Programmed Death Receptor-1/Programmed Death Ligand-1 as a Vaccination Approach Against Breast Cancer. Cancer Sci (2018) 109:1753–63. doi: 10.1111/cas.13618
111. Miao YR, Thakkar KN, Qian J, Kariolis MS, Huang W, Nandagopal S, et al. Neutralization of PD-L2 is Essential for Overcoming Immune Checkpoint Blockade Resistance in Ovarian Cancer. Clin Cancer Res (2021) 27:4435–48. doi: 10.1158/1078-0432.Ccr-20-0482
112. Tan Y, Yang S, Ma Y, Li J, Xie Q, Liu C, et al. Nanobubbles Containing sPD-1 and Ce6 Mediate Combination Immunotherapy and Suppress Hepatocellular Carcinoma in Mice. Int J Nanomed (2021) 16:3241–54. doi: 10.2147/ijn.S305857
113. Cheng HY, Kang PJ, Chuang YH, Wang YH, Jan MC, Wu CF, et al. Circulating Programmed Death-1 as a Marker for Sustained High Hepatitis B Viral Load and Risk of Hepatocellular Carcinoma. PloS One (2014) 9:e95870. doi: 10.1371/journal.pone.0095870
114. Orme JJ, Jazieh KA, Xie T, Harrington S, Liu X, Ball M, et al. ADAM10 and ADAM17 Cleave PD-L1 to Mediate PD-(L)1 Inhibitor Resistance. Oncoimmunology (2020) 9:1744980. doi: 10.1080/2162402x.2020.1744980
115. Gong B, Kiyotani K, Sakata S, Nagano S, Kumehara S, Baba S, et al. Secreted PD-L1 Variants Mediate Resistance to PD-L1 Blockade Therapy in Non-Small Cell Lung Cancer. J Exp Med (2019) 216:982–1000. doi: 10.1084/jem.20180870
116. Skalniak L, Zak KM, Guzik K, Magiera K, Musielak B, Pachota M, et al. Small-Molecule Inhibitors of PD-1/PD-L1 Immune Checkpoint Alleviate the PD-L1-Induced Exhaustion of T-Cells. Oncotarget (2017) 8:72167–81. doi: 10.18632/oncotarget.20050
117. Ying H, Zhang X, Duan Y, Lao M, Xu J, Yang H, et al. Non-Cytomembrane PD-L1: An Atypical Target for Cancer. Pharmacol Res (2021) 170:105741. doi: 10.1016/j.phrs.2021.105741
118. Poggio M, Hu T, Pai CC, Chu B, Belair CD, Chang A, et al. Suppression of Exosomal PD-L1 Induces Systemic Anti-Tumor Immunity and Memory. Cell (2019) 177:414–27.e413. doi: 10.1016/j.cell.2019.02.016
119. Chen G, Huang AC, Zhang W, Zhang G, Wu M, Xu W, et al. Exosomal PD-L1 Contributes to Immunosuppression and is Associated With Anti-PD-1 Response. Nature (2018) 560:382–6. doi: 10.1038/s41586-018-0392-8
120. Kalluri R, LeBleu VS. The Biology, Function, and Biomedical Applications of Exosomes. Science (2020) 367:eaau6977. doi: 10.1126/science.aau6977
121. Li S, Yi M, Dong B, Tan X, Luo S, Wu K. The Role of Exosomes in Liquid Biopsy for Cancer Diagnosis and Prognosis Prediction. Int J Cancer (2021) 148:2640–51. doi: 10.1002/ijc.33386
122. Pegtel DM, Gould SJ. Exosomes. Annu Rev Biochem (2019) 88:487–514. doi: 10.1146/annurev-biochem-013118-111902
123. Meldolesi J. Exosomes and Ectosomes in Intercellular Communication. Curr Biol (2018) 28:R435-r444. doi: 10.1016/j.cub.2018.01.059
124. Zhang L, Yu D. Exosomes in Cancer Development, Metastasis, and Immunity. Biochim Biophys Acta Rev Cancer (2019) 1871:455–68. doi: 10.1016/j.bbcan.2019.04.004
125. Li S, Yi M, Dong B, Jiao Y, Luo S, Wu K. The Roles of Exosomes in Cancer Drug Resistance and Its Therapeutic Application. Clin Transl Med (2020) 10:e257. doi: 10.1002/ctm2.257
126. Lyu T, Wang Y, Li D, Yang H, Qin B, Zhang W, et al. Exosomes From BM-MSCs Promote Acute Myeloid Leukemia Cell Proliferation, Invasion and Chemoresistance via Upregulation of S100A4. Exp Hematol Oncol (2021) 10:24. doi: 10.1186/s40164-021-00220-7
127. Thakur A, Parra DC, Motallebnejad P, Brocchi M, Chen HJ. Exosomes: Small Vesicles With Big Roles in Cancer, Vaccine Development, and Therapeutics. Bioact Mater (2022) 10:281–94. doi: 10.1016/j.bioactmat.2021.08.029
128. Dai J, Su Y, Zhong S, Cong L, Liu B, Yang J, et al. Exosomes: Key Players in Cancer and Potential Therapeutic Strategy. Signal Transduct Target Ther (2020) 5:145. doi: 10.1038/s41392-020-00261-0
129. Qadir F, Aziz MA, Sari CP, Ma H, Dai H, Wang X, et al. Transcriptome Reprogramming by Cancer Exosomes: Identification of Novel Molecular Targets in Matrix and Immune Modulation. Mol Cancer (2018) 17:97. doi: 10.1186/s12943-018-0846-5
130. Yan W, Jiang S. Immune Cell-Derived Exosomes in the Cancer-Immunity Cycle. Trends Cancer (2020) 6:506–17. doi: 10.1016/j.trecan.2020.02.013
131. Yin Z, Yu M, Ma T, Zhang C, Huang S, Karimzadeh MR, et al. Mechanisms Underlying Low-Clinical Responses to PD-1/PD-L1 Blocking Antibodies in Immunotherapy of Cancer: A Key Role of Exosomal PD-L1. J Immunother Cancer (2021) 9:e001698. doi: 10.1136/jitc-2020-001698
132. Theodoraki MN, Yerneni SS, Hoffmann TK, Gooding WE, Whiteside TL. Clinical Significance of PD-L1+ Exosomes in Plasma of Head and Neck Cancer Patients. Clin Cancer Res (2018) 24:896–905. doi: 10.1158/1078-0432.Ccr-17-2664
133. Razzo BM, Ludwig N, Hong CS, Sharma P, Fabian KP, Fecek RJ, et al. Tumor-Derived Exosomes Promote Carcinogenesis of Murine Oral Squamous Cell Carcinoma. Carcinogenesis (2020) 41:625–33. doi: 10.1093/carcin/bgz124
134. Ning Y, Shen K, Wu Q, Sun X, Bai Y, Xie Y, et al. Tumor Exosomes Block Dendritic Cells Maturation to Decrease the T Cell Immune Response. Immunol Lett (2018) 199:36–43. doi: 10.1016/j.imlet.2018.05.002
135. Yang Y, Li CW, Chan LC, Wei Y, Hsu JM, Xia W, et al. Exosomal PD-L1 Harbors Active Defense Function to Suppress T Cell Killing of Breast Cancer Cells and Promote Tumor Growth. Cell Res (2018) 28:862–4. doi: 10.1038/s41422-018-0060-4
136. Hellwinkel JE, Redzic JS, Harland TA, Gunaydin D, Anchordoquy TJ, Graner MW. Glioma-Derived Extracellular Vesicles Selectively Suppress Immune Responses. Neuro Oncol (2016) 18:497–506. doi: 10.1093/neuonc/nov170
137. Kim DH, Kim H, Choi YJ, Kim SY, Lee JE, Sung KJ, et al. Exosomal PD-L1 Promotes Tumor Growth Through Immune Escape in non-Small Cell Lung Cancer. Exp Mol Med (2019) 51:1–13. doi: 10.1038/s12276-019-0295-2
138. Li C, Li C, Zhi C, Liang W, Wang X, Chen X, et al. Clinical Significance of PD-L1 Expression in Serum-Derived Exosomes in NSCLC Patients. J Transl Med (2019) 17:355. doi: 10.1186/s12967-019-2101-2
139. Lux A, Kahlert C, Grützmann R, Pilarsky C. C-Met and PD-L1 on Circulating Exosomes as Diagnostic and Prognostic Markers for Pancreatic Cancer. Int J Mol Sci (2019) 20:3305. doi: 10.3390/ijms20133305
140. Morrissey SM, Yan J. Exosomal PD-L1: Roles in Tumor Progression and Immunotherapy. Trends Cancer (2020) 6:550–8. doi: 10.1016/j.trecan.2020.03.002
141. Cordonnier M, Nardin C, Chanteloup G, Derangere V, Algros MP, Arnould L, et al. Tracking the Evolution of Circulating Exosomal-PD-L1 to Monitor Melanoma Patients. J Extracell Vesicles (2020) 9:1710899. doi: 10.1080/20013078.2019.1710899
142. Wang J, Zhang H, Sun X, Wang X, Ren T, Huang Y, et al. Exosomal PD-L1 and N-Cadherin Predict Pulmonary Metastasis Progression for Osteosarcoma Patients. J Nanobiotechnol (2020) 18:151. doi: 10.1186/s12951-020-00710-6
143. Li JW, Shi D, Wan XC, Hu J, Su YF, Zeng YP, et al. Universal Extracellular Vesicles and PD-L1+ Extracellular Vesicles Detected by Single Molecule Array Technology as Circulating Biomarkers for Diffuse Large B Cell Lymphoma. Oncoimmunology (2021) 10:1995166. doi: 10.1080/2162402x.2021.1995166
144. Xie F, Xu M, Lu J, Mao L, Wang S. The Role of Exosomal PD-L1 in Tumor Progression and Immunotherapy. Mol Cancer (2019) 18:146. doi: 10.1186/s12943-019-1074-3
145. Ricklefs FL, Alayo Q, Krenzlin H, Mahmoud AB, Speranza MC, Nakashima H, et al. Immune Evasion Mediated by PD-L1 on Glioblastoma-Derived Extracellular Vesicles. Sci Adv (2018) 4:eaar2766. doi: 10.1126/sciadv.aar2766
146. Yi M, Jiao D, Xu H, Liu Q, Zhao W, Han X, et al. Biomarkers for Predicting Efficacy of PD-1/PD-L1 Inhibitors. Mol Cancer (2018) 17:129. doi: 10.1186/s12943-018-0864-3
147. Niu M, Yi M, Li N, Luo S, Wu K. Predictive Biomarkers of Anti-PD-1/PD-L1 Therapy in NSCLC. Exp Hematol Oncol (2021) 10:18. doi: 10.1186/s40164-021-00211-8
148. Yi M, Li A, Zhou L, Chu Q, Luo S, Wu K. Immune Signature-Based Risk Stratification and Prediction of Immune Checkpoint Inhibitor’s Efficacy for Lung Adenocarcinoma. Cancer Immunol Immunother (2021) 70:1705–19. doi: 10.1007/s00262-020-02817-z
149. Khan M, Zhao Z, Arooj S, Fu Y, Liao G. Soluble PD-1: Predictive, Prognostic, and Therapeutic Value for Cancer Immunotherapy. Front Immunol (2020) 11:587460. doi: 10.3389/fimmu.2020.587460
150. Huang P, Hu W, Zhu Y, Wu Y, Lin H. The Prognostic Value of Circulating Soluble Programmed Death Ligand-1 in Cancers: A Meta-Analysis. Front Oncol (2020) 10:626932. doi: 10.3389/fonc.2020.626932
151. Ward FJ, Dahal LN, Khanolkar RC, Shankar SP, Barker RN. Targeting the Alternatively Spliced Soluble Isoform of CTLA-4: Prospects for Immunotherapy? Immunotherapy (2014) 6:1073–84. doi: 10.2217/imt.14.73
152. Fló J, Tisminetzky S, Baralle F. Codelivery of DNA Coding for the Soluble Form of CD86 Results in the Down-Regulation of the Immune Response to DNA Vaccines. Cell Immunol (2001) 209:120–31. doi: 10.1006/cimm.2001.1784
153. Xie C, Liu D, Chen Q, Yang C, Wang B, Wu H. Soluble B7-H3 Promotes the Invasion and Metastasis of Pancreatic Carcinoma Cells Through the TLR4/NF-κb Pathway. Sci Rep (2016) 6:27528. doi: 10.1038/srep27528
Keywords: soluble PD-1, soluble PD-L1, cancer, biological activity, efficacy prediction, prognosis, immunotherapy, exosomal PD-L1
Citation: Niu M, Liu Y, Yi M, Jiao D and Wu K (2022) Biological Characteristics and Clinical Significance of Soluble PD-1/PD-L1 and Exosomal PD-L1 in Cancer. Front. Immunol. 13:827921. doi: 10.3389/fimmu.2022.827921
Received: 02 December 2021; Accepted: 21 February 2022;
Published: 21 March 2022.
Edited by:
Antonio Riva, Institute of Hepatology, United KingdomReviewed by:
Alessandro Poggi, San Martino Hospital (IRCCS), ItalyElizabeth Ann Lieser Enninga, Mayo Clinic, United States
Copyright © 2022 Niu, Liu, Yi, Jiao and Wu. This is an open-access article distributed under the terms of the Creative Commons Attribution License (CC BY). The use, distribution or reproduction in other forums is permitted, provided the original author(s) and the copyright owner(s) are credited and that the original publication in this journal is cited, in accordance with accepted academic practice. No use, distribution or reproduction is permitted which does not comply with these terms.
*Correspondence: Kongming Wu, a213dUB0amgudGptdS5lZHUuY24=; Dechao Jiao, amlhb2RlY2hhbzAwN0AxMjYuY29t
†These authors have contributed equally to this work