- 1Centro de Investigacións Mariñas (CIMA), Consellería do Mar, Xunta de Galicia, Vilanova de Arousa, Spain
- 2MARE - Marine and Environmental Sciences Centre, Laboratório de Ciências do Mar, Universidade de Évora, Sines, Portugal
- 3Departamento de Ciencias de la Vida, Universidad de Alcalá, Alcalá de Henares, Spain
- 4Research Centre for Experimental Marine Biology and Biotechnology, Plentziako Itsas Estazioa (PIE), University of the Basque Country (UPV/EHU), Plentzia, Spain
Bivalve molluscs stand out for their ecological success and their key role in the functioning of aquatic ecosystems, while also constituting a very valuable commercial resource. Both ecological success and production of bivalves depend on their effective immune defence function, in which haemocytes play a central role acting as both the undertaker of the cellular immunity and supplier of the humoral immunity. Bivalves have different types of haemocytes, which perform different functions. Hence, identification of cell subpopulations and their functional characterisation in immune responses is essential to fully understand the immune system in bivalves. Nowadays, there is not a unified nomenclature that applies to all bivalves. Characterisation of bivalve haemocyte subpopulations is often combined with 1) other multiple parameter assays to determine differences between cell types in immune-related physiological activities, such as phagocytosis, oxidative stress and apoptosis; and 2) immune response to different stressors such as pathogens, temperature, acidification and pollution. This review summarises the major and most recent findings in classification and functional characterisation of the main haemocyte types of bivalve molluscs.
1 Introduction
Marine invertebrates constitute the largest group of macroscopic species in the sea (1). The phylum Mollusca is the second most diverse group of animals after Arthropods; among them, Bivalvia class is the second largest group of Mollusca that is worldwide distributed (2). They are often the major macrofauna on rocky substrates of littoral, shallow sub-littoral and deep-sea vents (3, 4). Bivalve molluscs are abundant in marine and freshwater ecosystems and perform important ecological functions. Bivalves have epifaunal or infaunal lifestyles and they are largely filter feeders that couple the water column and benthos. This filter-feeding habit adds greatly to their ecological significance in that bivalves are important calcium and carbon accumulators, they link primary producers (bacteria and phytoplankton) with higher organisms in aquatic food chains and are responsible for filtration of the water body (5, 6). Therefore, bivalve molluscs stand out for their fundamental role in the functioning of the aquatic ecosystems, impact nutrient cycling, create and modify habitat, and affect food webs (6). Moreover, they are used as environmental monitors because of the materials accumulated in their soft tissue and shells (7). Most bivalves, as sessile aquatic organisms, are exposed to an environment in continuous confrontation with pathogenic organisms and stressful conditions, such as dynamic variation in temperature, salinity and prolonged desiccation; so that throughout the evolution, these organisms have developed an array of effective strategies to protect themselves from the attacks of fast-evolving pathogens and environmental stresses, which has allowed them to obtain a high adaptation capacity to the different environments in which they live (8–11). In addition, both climate change and environmental pollution significantly affect the health of molluscs, potentially reducing the capacity of the bivalve immune system and increasing susceptibility to diseases (12–18). Altogether, the evolutionary ecological success of bivalves, showing a considerable resilience and occupying niches in a wide range of aquatic environments, is largely due to a robust, effective and multifaceted immune system which incorporates cellular and humoral components (8–11).
Hence, immune responses in bivalves and the processes that govern them, are important areas of active research. These immunological processes are centrally coordinated by a group of cells known as haemocytes which may act directly or in concert with humoral factors in the haemolymph to defend the animal against infection. Haemocytes constitute the cellular component of the haemolymph; they move through the circulatory system and migrate to other locations, such as the connective tissue and epithelia (19, 20). Among the important functions they perform in bivalves, haemocytes are best known for their primary role in phagocytosis, encapsulation and production of cytotoxic molecules, such as reactive oxygen species, antimicrobial peptides (AMPs) and secretion of inflammatory cytokines involved in pathogen killing and elimination (9, 19, 21–27). In addition to their role in host defence, bivalve haemocytes perform various important physiological functions, including nutrient digestion, transportation and distribution, wound healing, detoxification processes, shell mineralisation and excretion (25, 28, 29). The composition and dynamics of the bivalve haemocyte population, as well as the functional properties of circulating cells, reflect fairly objectively the general physiological and immunological status of bivalve molluscs and have an enormous potential for the study of physiological ecology (29–35). Due to the haemocyte major role in the immune system and homeostasis and the fact that few reports aimed to establish functional relationships between bivalve haemocytes subpopulations and immune response capacity, this review paper aims (i) to summarise current knowledge about bivalve haemocyte subpopulations classification and (ii) to point out functional differences between the main haemocyte types.
2 Bivalve Haemocytes Classification
The classification of haemocyte populations or cell types in bivalve molluscs has been the subject of multiple studies since early 1970s. Research on haemocytes has been hindered by the lack of a consensus on their classification. A plethora of categories have been established on the basis of different parameters and techniques and, as a consequence, it is often difficult to compare results and draw general conclusions from the literature. Various criteria have been considered: cellular morphology (including ultrastructure), enzymatic cytochemistry, physicochemical features and cell population separation, and biological activities and functions. Numerous authors have focused their efforts on developing a classification of the different blood cell types present in bivalves. The researchers Cheng (1981) and Hine (1999) published two of the most important reviews on morphofunctional aspects of haemocytes of the Phylum Mollusca (19, 23). In the early studies, the haemocytes were characterised mainly by morphological and cytochemical criteria, such as the size, nucleus/cytoplasm (N/C) ratio, cytoplasmic complexity and enzyme content (36, 37). More recent trends for identifying haemolymph cell types are focused on flow cytometry, tool that allows determining the size and granularity of haemocytes (38). Most studies have classified bivalve haemocytes into two main groups: granulocytes, cells with granules in the cytoplasm and typically a low N/C ratio, and hyalinocytes (or agranulocytes), cells containing few or no granules within the cytoplasm and a higher N/C ratio (19, 21, 36, 39) (Figure 1). Table 1 brings together the different studies focused on categorising the haemocytes subpopulations in bivalves. Both cell types, granulocytes and hyalinocytes (or agranulocytes), have been found in clams, razor shells, scallops, cockles, mussels, and oysters. In many of these species, granulocytes contain hydrolytic and oxidative enzymes and may be further subclassified into different categories based on granular affinity to specific dyes, such as acidophilic/eosinophilic, basophilic and neutrophilic granulocytes; or different subtypes according to the size and granularity (23, 60, 108, 181). Cheng suggested that the occurrence of various types of granules might be related to differentiation and maturation processes; specifically, basophilic granules were hypothesised to be immature granules which mature and become acidophilic (19). Recently, up to fourteen types of granules were identified in the clam Ruditapes philippinarum granulocytes (75) and up to twelve haemocyte subpopulations were identified in the oyster Crassostrea hongkongensis, the latter based on transcriptomic profile of single cell RNA-seq data (182). One of the most recent morphology classification of bivalve haemocytes was made with a new computational approach that combines fractal formalism with linear methods of image analysis (183, 184); however, results are not easily comparable with classic haemocyte classification.
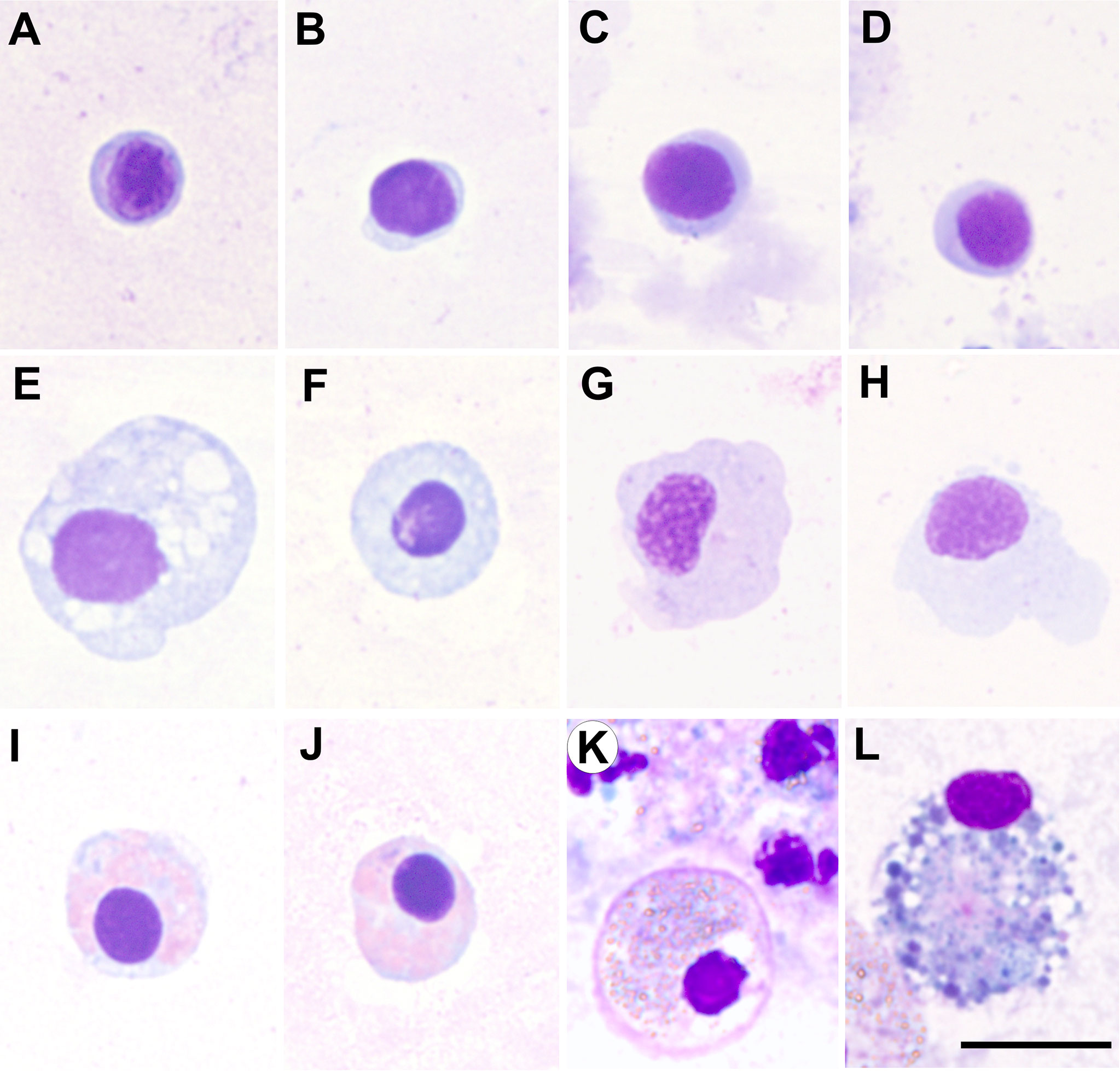
Figure 1 Micrographs of haemocyte types of various bivalve species, all them corresponding to haemolymph samples collected from the adductor muscle, cytocentrifuged onto slides and fixed and stained with the kit Hemacolor® (Merck). (A–D) Blast-like cells of Ruditapes decussatus, Ruditapes philippinarum, Aequipecten opercularis and Mimachlamys varia, respectively. (E–H) Hyalinocytes of R. decussatus, R. philippinarum, A. opercularis and M. varia, respectively. (I–K) Eosinophilic granulocytes of R. decussatus, R. philippinarum and Ostrea edulis, respectively. (L) Basophilic granulocyte of O. edulis. Scale bar: 10 µm.
The relative abundance of each cell type in the haemolymph of bivalve molluscs is variable, being affected by seasonal changes, temperature, size, sex, maturity, food availability and inter-individual variability (43, 70, 185–190). In bivalves, granulocytes are generally considered the most abundant cell type (191). Nevertheless, in some studies a greater number of hyalinocytes (or agranulocytes) have been observed, such as in the marine mussels Perna perna (120), Perna canaliculus (119) and Mytilus chilensis (109); the freshwater mussels Dreissena polymorpha (127) and Diplodon chilensis (128); the clams Chamelea gallina (56), Meretrix meretrix (62) and Ruditapes decussatus (192); the lantern clam Laternula elliptica (46); the boreal tridonta Astarte borealis (40); the geoduck clam Panopea globosa (45); the scallop Argopecten irradians (93); the oysters Crassostrea brasiliana (149), Crassostrea gigas (155), C. hongkongensis (157), Crassostrea rhizophorae (162), Saccostrea glomerata (173), Saccostrea kegaki, Ostrea circumpicta (148), Ostrea edulis (171) and Hyotissa hyotis (148); the wing-shell Pteria hirundo (180); and the pearl oysters Pinctada fucata (176) and Pinctada margaritifera (177).
Although in almost all the studied species it is possible refer to the two cell types as hyalinocytes (agranulocytes) and granulocytes, there are some exceptions and not all haemocyte types occur in each bivalve species. In the clam Macrocallista nimbosa, flow cytometrically characterised haemocytes appeared as an unique population, both in terms of morphology and intracellular parameters (58). Some authors disagree with the existence of granular cells in pectinids (23, 39, 193); however, different studies have found granulocytes in the haemolymph of some scallop species (93–96), thus a sole rule would not apply for all the scallop species. In the scallop Nodipecten subnodosus, classification into main types of haemocytes (hyalinocyte and granulocyte) was not deemed totally correct, considering a haemocyte subpopulation as semi-granular cells (98). Most of ark clams, species of the family Arcidae, also known as “blood clams”, have erythrocytes, haemocytes containing the respiratory pigment haemoglobin, a rare occurrence in invertebrates (194, 195). Although generally shallow-sea veneroid clams have no erythrocytes in the haemolymph, erythrocytes were found as the most abundant cells in the species Phreagena okutanii and Abyssogena phaseoliformis (76). A particular type of haemocyte, without cytoplasm granules, with a large vacuole occupying most of the cytoplasm and peripheral flattened nucleus, has been observed in the common edible cockle Cerastoderma edulis, reported as type III (77, 78), and in the lagoon cockle Cerastoderma glaucum, reported as acidophil granulocyte (79).
Another haemocyte type frequently found in bivalves is the blast-like cell (Figure 1, Table 1), this cell type exhibiting high N/C ratio and small size and apparent low levels in biological activities (hydrolytic enzymes, oxidative activity, phagocytosis, number of lysosomes) (74, 127, 151). Blast-like cells designation remains variable and different authors use different denominations to identify this cell type: lymphoid haemocytes (62, 110, 136), basophils (112), haemoblast-like (104, 123, 162, 173) or small hyalinocytes (170), referring to the same haemocyte type (127). As discussed below, blast-like cells are considered haemocyte precursors according to their morphometric parameters and low organelle content (19, 23, 162).
There is not a unified classification system for haemocytes in bivalve molluscs, thus far. On the contrary, the haemocyte types have been reported differentially between species. Even for the same species, different haemocyte classification has been proposed, as shown in Table 1 (species in bold). This diversity may be in part due to some true differences, but also resulting from the use of different classification criteria or experimental procedures, endogenous and exogenous factors, like age, pollution or the high inter-individual variability (71, 77, 79, 112, 196, 197). Additionally, different nomenclatures adopted by various researchers due to lack of biological markers for specific cell lineages or maturation stages contribute to the problem (198). Moreover, the process of haematopoiesis in bivalves is not completely clear yet, therefore, the lack of evidence on the origin of haemocytes hampers classification because no correspondence between haemocyte subtypes and ontogeny can be made (162).
3 Bivalve Haematopoiesis
Haematopoiesis is a crucial and vital process for homeostasis and immune response against infection in invertebrate animals and therefore for survival (198). Various theories have been proposed to elucidate the lineage of haemocytes in bivalves. Cheng (1981) and Auffret (1988) propose two types of initial cell precursors capable of differentiating into granulocytes and hyalinocytes (19, 39). Alternatively, a model with a single precursor cell type giving rise to hyalinocytes that later mature into granulocytes was suggested first by Mix (199), and then by Hine (23). In the case of the mussel M. galloprovincialis haemolymph, the occurrence of only one haemocyte type represented by two different ageing-related stages has been proposed; specifically, hyalinocytes in a proliferative stage which mature to become granulocytes (200), consistently with the Mix’s one-cell-type model (199). In the clam R. philippinarum, a single population of precursor cells lacking granules in their cytoplasm called blast-like cell has been described; these cells were mitotic haemocytes positive for CD34, a transmembrane glycoprotein characteristic of haematopoietic mammalian cells (74, 201), thus supporting the hypothesis proposed by Hine (23). Unlike granulocytes and hyalinocytes, precursor cells do not contribute to immune response mechanisms such as phagocytosis or encapsulation, and they also lack common intracellular enzyme systems associated with host defence. The presence of few cytoplasmic organelles and low enzyme activity suggests that these precursor cells are immature haemocytes (23, 74) leaving open the possibility that these cells act as stem cells from which derive the two classes of mature haemocytes. In many invertebrates, multiple types of haemocytes appear to derive from the differentiation of stem cells that have a morphology very similar to the cells of S. glomerata that are considered haemocyte precursors (173, 202, 203). Those potential haemocyte precursors, also called blast-like cells, have been widely found in clams, ark clams, scallops, marine mussels, freshwater mussels, oysters, pearl oysters and wing-shells as shown in Table 1 (column B). Rebelo et al. (162) proposed another hypothesis in which the different haemocyte types derive from the same cell type that matures first without granules (hyalinocyte), later produces granules (granulocyte) and finally, eventually, they can lose the granules being an agranular cell again. Thus, different haemocyte subpopulations have been indicated as different stages of one cell type only; theory similar to that proposed by Ottaviani (200). The hypothesis by Rebelo et al. is based on observations made in C. rhizophorae (162) and later in C. virginica (204). A recent study identified different stages of granulocytes in oysters C. hongkongensis, which led authors to propose that several differentiation states may exist within one cell type in the haemocyte formation process (182). Despite various theories, the detailed characterisation of larval and adult haematopoiesis in bivalves will only be possible by sequencing mollusc genomes and identifying the full set of transcription factors and biomarkers that regulate haematopoiesis (198, 205, 206).
Even being an essential process in bivalve immunity, there is no clear haematopoietic organ or cell precursor, although the generally accepted belief is that haemocytes can originate from connective tissue and/or mantle (19, 199, 207). In spite of some haemocytes may mature before entering the circulation (29, 79, 123, 162), evidence is accumulating that mitosis may also occur after haemocyte release into the haemolymph (201). In the oyster C. gigas, important vertebrate embryonic haematopoiesis transcription factors have been found expressed during ontogeny (208, 209), which were observed only in cells attached to the blood vessel endothelium, leading the authors to hypothesise that haematopoietic cells could derive from the vessel and/or artery endothelial cells (209). It has been also proposed that haemocytes can be differentiated from a population of adult somatic cells residing in an irregularly folded structure in the gill of the adult oyster C. gigas (210), suggesting that gills can potentially act as the haematopoietic organ in oysters (211), which bears out the early proposition by Cuénot (212) that bivalve haemocytes originate in the gills. To this extent, a very recent study suggests that stem cells firstly divide and differentiate into pro- haemocytes in gills (213). Also in the oyster C. gigas, some haematopoietic relative genes have been found up-regulated after bacterial infection (214); this evidence also supports the hypothesis that in bivalves the proliferation of circulating haemocytes may occur as a consequence of an immune challenge (215). In addition, in the same species some transcription factors were identified in granulocyte-specific genes with strong potentials in regulation of haematopoiesis (216). In the scallop Azumapecten farreri, several molecules associated with the proliferation and differentiation of pro-haemocytes have been identified and characterised from the circulating haemocytes (217). These molecular studies are providing evidence that precursors of fully differentiated haemocytes can occur in the circulatory system of bivalves, where presumably they continue to mature (218). In oysters C. gigas, a conserved haematopoietic transcription factor has been found expressed in important immune organs, such as gills, mantle and haemocytes (219). Moreover, recently, another transcription factor involved in haematopoiesis has been found highly expressed in gills and haemocytes, with higher abundance in semigranulocytes and agranulocytes (220). This fact, added to that a definitive haematopoietic site common to all bivalves has yet to be identified, lead to the possibility that adult bivalves do not produce haemocyte precursors or mature haemocytes from a centralised organ, as occurs in other molluscan taxa; multiple or ubiquitous sites of haematopoiesis may exist, comprising a system in which stem-like cells receive determining signals from neighbouring specialised cells or tissues (206). Therefore, a bivalve haematopoietic organ is not the norm; haemocytes may instead be formed in various ways (216). Spontaneous mitosis of haemocytes increases during circulation in haemolymph vessels, sinuses, and soft tissues (19, 198), which raises the possibility of observing plasticity during various stages of haemocyte maturation (162, 199, 200).
4 Functional Differences Between Hyalinocytes and Granulocytes
For the purposes of this review, we will refer to the two haemolymph cell types as hyalinocytes (agranulocytes) and granulocytes, while acknowledging that these may be different life stages of the same cell type in some species. In spite that Cheng (36) suggested that different haemocyte types perform distinct functions in the 1980s, the involvement of the different haemocyte subpopulations in immune functions is still far from well understood. Initially, the distinction between hyalinocytes and granulocytes was made based on morphological parameters, years later it has been confirmed that there are also functional differences between both haemolymph cell types after analysis of metabolic and functional parameters (122, 221). Recently, research efforts are focusing on revealing dissimilar immune functions among marine invertebrate haemocyte subpopulations, for example in ascidians (222); crustaceans as shrimps (223–228), lobsters (229) and crabs (230); gastropod molluscs as sea snails (231–234), abalones (235) and sea hares (236); and bivalve molluscs as oysters (182, 216, 237, 238). This review focuses on the major findings in functional differences between the main bivalve haemocyte types.
4.1 Immune Parameters
In the last two decades, the application of flow cytometry analysis and molecular characterisation of different immune-related molecules have greatly improved our knowledge of the functional characterisation of haemocytes, underlying both common and distinct features of the immune system in different bivalve species (9, 148, 165, 221). Although granulocytes were largely suspected to play a prominent role in defence, few reports aimed to establish functional relationships between bivalve haemocyte subpopulations and immune capabilities (114, 170, 239). Therefore, this work attempts to gather information on different immune competencies among types of bivalve haemocytes.
4.1.1 Phagocytosis and Encapsulation
One of the most important mechanisms of pathogen elimination in bivalves is phagocytosis, i.e. the engulfment of those foreign structures by haemocytes and their destruction (240). Phagocytosis of foreign structures by bivalve haemocytes was firstly reported in the oyster C. gigas (241). Studies on phagocytosis activity of bivalve haemocyte subpopulations have found diverse results. In the majority of bivalve species, both granulocytes and hyalinocytes are able to internalise foreign particles and pathogens; however, some studies have found that only granulocytes have phagocytic activity; this is the case of C. edule (77), Tridacna crocea (41), M. galloprovincialis (181), C. virginica (204), P. nobilis (179) and P. fucata (242). Nonetheless, other studies performed in some of the aforementioned species have pointed out that both haemocyte types have phagocytic ability as is indicated below.
Generally, granulocytes show higher phagocytosis capacities than hyalinocytes, which has been documented in oysters C. ariakensis (151), C. gigas (156), C. hongkongensis (157), C. nippona (160), Crassostrea plicatula (163), C. virginica (167), Hyotissa hyotis (148), O. chilensis (169), O. edulis (170), O. circumpicta, S. kegaki (148) and S. glomerata (173); in clams M. lusoria (61), M. mercenaria (66, 67), R. decussatus (72) and R. philippinarum (75); in the boreal tridonta A. borealis (40); in the geoduck clam P. globosa (45); in ark clams A. broughtonii, A. kagoshimensis and T. granosa (82); in the razor clam Sinonovacula constricta (92); in the cockle C. glaucum (79); in scallops A. irradians (93) and A. farreri (96); in marine mussels B. azoricus (101), Mytilisepta virgata (107), M. chilensis (109), M. edulis (110), M. galloprovincialis (113), M. unguiculatus (117), P. canaliculus (119), P. perna (120), P. viridis (122) and X. securis (124); and in freshwater mussels Amblema plicata, Quadrula quadrula (131), D. chilensis (128), L. marginalis (140) and S. cumingii (145) and in the pearl oyster P. imbricata (175). There are also studies where no differences in immune responses between the haemocytes types were found, as it happened in the ark clam A. kagoshimensis (86). In the clam Callista chione no differences in phagocytosis activity between granulocytes and hyalinocytes were observed (55).
Within granulocytes, eosinophilic granular haemocytes exhibited higher phagocytic activity than the basophilic ones in mussels, clams, cockles and oysters (23, 34, 72, 76, 78, 79, 102, 111, 163, 181, 243). Higher activity levels for phenoloxidases, peroxidases and superoxide dismutases, a greater production of superoxide radical (72, 111, 243), and a higher phagosome-lysosome fusion rate in eosinophilic granulocytes than in basophilic ones have been observed (76). Altogether suggest that eosinophilic granulocytes are more immune-reactive than basophilic granulocytes in bivalve immune defence (163).
Encapsulation is another major cellular immune defence process to eliminate foreign particles that are too large to be phagocytosed, by which haemocytes attach to the foreign organism to extracellularly destroy it (244). Different encapsulation activity was witnessed between haemolymph cells. In the oyster C. virginica, several studies reported the role of agranulocytes in encapsulation (245, 246). However, more recent studies propose granulocytes as the predominant cell types involved in such process in the oysters S. glomerata and C. gigas (156, 173).
Altogether it seems clear that in the vast majority of studied bivalves, granulocytes are the most active phagocytic cells. It has been hypothesised that the high phagocytosis activity of granulocytes is associated with the presence of granules with high levels of enzymatic activities which could act to kill and degrade the phagocytosed particles. Moreover, granulocytes had high ability to produce reactive oxygen species (ROS, radicals with microbicidal potential) and to form pseudopods. Finally, granulocytes contained more mitochondria, which could provide energy in the phagocytosis process to result in higher phagocytosis ability (75). However, some studies consider that hyalinocytes, as well as granulocytes, can be regarded as professional phagocytes (247), suggesting that they may target different types of microorganisms. While granulocytes possess a constantly high phagocytic index, the phagocytic index of hyalinocytes seemed related to the nature of the foreign material in the clam R. decussatus (72). The granulocytes of C. gigas were found to exhibit higher levels of phagocytic activity against bacteria and yeast than the agranulocytes, while the agranulocytes exhibited higher levels of phagocytosis against latex beads than granulocytes (248). In the same oyster species, hyalinocyte phagocytosis is regulated by an integrin-dependent mechanism, and it is thought that granulocytes have other receptors, still to be identified, to carry out this function (247). Different degradation pathways could be linked to different cell phagocytic abilities, depending on particle nature, to optimise degradation efficiency (127). Such observations suggest functional differences between haemocyte types and receptor-based initiation of phagocytosis (29).
4.1.2 Enzymatic Lysosomal Content
Quantitative differences in the content of lysosomes between haemocyte types might be related to different cellular functions (148). The intracellular lysosomal enzyme contents of granulocytes have been reported much higher than those of hyalinocytes in several oyster species (148, 159, 169, 173), mussels (117, 122, 123, 181), clams (72, 74), ark clams (82), razor clams (92) and scallops (95). Granulocytes contain higher peroxidase, phenoloxidase and alkaline phosphatase activity than hyalinocytes in the scallop A. farreri (95). Granulocytes of the oyster S. glomerata are the haemocyte subpopulation with greater levels of acid phosphatase and phenoloxidase enzymatic activities (173). Acid phosphatase activity have been found only in granulocytes in the boreal tridonta A. borealis (40). In the pen-shell P. nobilis, granulocytes were more positive to hydrolases than hyalinocytes (179). These results indicate the important role played by granulocytes in immune reactions.
On the contrary, agranulocytes (large and small hyalinocytes) seemed more diverse in protein content than the granulocytes in the oyster O. edulis (249). In the freshwater mussel D. polymorpha, presence of acid phosphatase and non−specific esterase was detected in both hyalinocytes and granulocytes, while ß−glucuronidase was detected only in hyalinocytes (126). The differential distribution of hydrolytic enzymes between granulocytes and hyalinocytes is associated with different physiological and immune responses (74).
4.1.3 Oxidative Activity
The production of radicals with microbicidal activity, such as reactive oxygen species (ROS) and reactive nitrogen species (RNS), is induced after phagocytosis and it may be used to evaluate the immunocompetence in different haemocyte subsets (156). Oxidative activity through ROS production is generally higher in granulocytes than in hyalinocytes, which was observed in oysters C. ariakensis (151), C. gigas (154, 156, 250, 251), C. hongkongensis (157), C. nippona (160), C. virginica (167, 252), O. chilensis (169), Hyotissa hyotis, Ostrea circumpicta, S. kegaki (148), and S. glomerata (173); in clams R. decussatus (73) and R. philippinarum (75); in the geoduck clam P. globosa (45); in ark clams A. broughtonii, A. kagoshimensis and T. granosa (82); in marine mussels M. galloprovincialis (114), M. unguiculatus (117), P. canaliculus (119), P. viridis (122), and X. securis (124); and in freshwater mussels L. marginalis (140). In L. marginalis, granulocytes were identified as the principal phagocytes with prominent activity of superoxide anion (a ROS) and nitric oxide (NO, a RNS) (140). The higher ROS production of granulocytes may be also related to a more active metabolism (253). Moreover, it has been shown that the oxidative process within haemocytes plays a key role in the formation of extracellular DNA traps in oysters (254), clams (255, 256) and mussels (257). These extracellular traps (ETs) carrying AMPs and hydrolases released from granules could surround, entangle and eventually kill the pathogens, operating as antimicrobial effectors during the innate immune response. Thus, in marine bivalves, ETs participate in host defence by capturing large numbers of microbes and preventing their dissemination (254, 258, 259). Differences in ROS production between bivalve haemocyte subpopulations could be related with differences on such immune responses (158); in higher organisms granulocytes seem to be more implicated in ETs formation (260).
Other studies showed that granulocytes and hyalinocytes have the ability to produce different ROS and RNS (148). In the freshwater mussel D. polymorpha, hyalinocytes showed the highest intracellular ROS production (127). Oyster C. gigas hyalinocytes produced more reactive nitrogen species (RNS) than granulocytes (250). These results could indicate that the main haemocyte types have different capabilities for ROS/RNS production response. Both cell types possess NADPH-oxidase and NO-synthase-like pathways to produce ROS/RNS but the NO-synthase pathway seemed more dominant in hyalinocytes, whereas NADPH-oxidase was more active in granulocytes (250). Thus, differences in oxidative activity between the granulocytes and hyalinocytes could be associated with the differential involvement of ROS production pathways of the two haemocyte types. Moreover, regulation of phagocytosis of diverse targets and regulation of ROS and NO production, reveals haemocyte type-specific variations in signalling mechanisms, which could be due to the differential expression of membrane receptors (114). These observations led to hypothesise that mechanisms for killing foreign particles might be different between hyalinocytes and granulocytes (204). It was also suggested that differences in ROS production between haemocyte types may be associated with the functional role and the morphological structure of each cell type (261), being granulocytes more oxygen demanding than agranular cells, because the former type possess more complicated ultrastructure with numerous mitochondria and endoplasmic reticulum (60).
4.1.4 Aggregation and Wound Healing
Even though the hyalinocytes are not as avidly phagocytic as granulocytes, it is believed that they play a central role in haemocyte aggregation processes and wound healing (20, 173, 240, 262, 263). In the giant clam T. crocea, hyalinocytes were located in the core of haemocyte aggregations associated with wound healing (41). In the oyster S. glomerata, hyalinocytes were shown to have a central role in haemocyte aggregation processes (173). Similar results have been observed in the clam R. philippinarum (75). In the mussel P. viridis, hyalinocytes were shown to have a central role in either haemocyte aggregation or coagulation processes (123).
4.1.5 Apoptosis
Granulocytes normally show higher levels of apoptosis than hyalinocytes, possibly due to increased phagocytic activity and respiratory burst (264, 265). Differences in mortality have been found between both cell types, being greater in granulocytes; such difference in mortality may be related to the difference in ROS production (266). In mussels M. galloprovincialis, apoptotic levels seemed to be higher in granulocytes (267). Similarly, apoptosis after pesticides exposure have been found higher in granulocytes than in agranulocytes in the freshwater mussel L. marginalis (268). In the mussel M. galloprovincialis, although both haemocytes subpopulations were susceptible to UV light treatment, the damages induced in hyalinocytes were detected earlier than in granulocytes, which led authors to suggest that the cytoplasmic granules of granulocytes could have some protective effect against apoptosis induced by UV radiation (269). On the contrary, in the oyster O. edulis, granulocytes appeared more affected by apoptosis than hyalinocytes (270); after a proteomic approach more proteins related with apoptosis were identified in granulocytes (237, 238). In the clam R. decussatus, haemocytes stimulation with lipopolysaccharide induced a significant up-regulation of a gene with important roles in the apoptotic process, with a maximum level registered in granulocytes (73). A recent study suggested that apoptosis of C. virginica granulocytes may be Apoptosis protein inhibitor (IAP)-dependent and involve caspase-independent pathways (271).
4.1.6 Immune Molecules and Pathways
Higher content of molecules involved in immune response and higher expression levels of various immune related genes in the granulocytes of the oyster C. gigas compared to semigranulocytes and agranulocytes led to suggest that the granulocytes are the main immunocompetent haemocytes (156). Previous studies have shown that defensins and mytilins are stored mainly in granulocytes (272, 273). Myticin C was found expressed in granulocytes of mussels M. galloprovincialis (274); on the contrary, Myticin C was identified expressed in hyalinocytes and not in granulocytes in the oyster O. edulis (238). Haemolymph cells exhibited distinct inter-specific lectin binding in clams (275), marine mussels (101, 102, 276) and freshwater mussels (132), suggesting that haemocytes subpopulations may express different sugar moieties and perform disparate functions. Oyster C. virginica galectins CvGal1 and CvGal2 and clam R. philippinarum galectin MaGal1 were identified with strong ability to recognise parasites of the genus Perkinsus (277–279); one of them, CvGal1, has been observed to be secreted by granulocytes (277). In oysters O. edulis, the lectins galectin-4 isoform X1 and ß-1,4-N-acetylgalactosaminyltransferase bre-4-like appear to be expressed in hyalinocytes (238). Lectin-like receptors (LLRs) were found to play important roles in the phagocytosis of granulocytes and semigranulocytes in C. gigas (280). In the same way, antimicrobial peptides were found within granular haemocytes in the scallop N. subnodosus (97). ATP-binding cassette (ABC) proteins associated with the multixenobiotic resistance were observed expressed differently between haemocyte types in mussels and oysters (281, 282). Cathepsin L gene expression, involved in the inflammatory response, was strongly associated with the number of circulating granulocytes in C. gigas (283). SPRY (sp1A/ryanodine receptor) domain-containing SOCS box protein (CgSPSB), which play an important role in the regulation of cytokine production in C. gigas, was found mainly distributed in the cytoplasm of granulocytes (284). In the same species, immune related genes, including CgTLR, CgClathrin, CgATPeV, CgLysozyme, CgDefensin and CgIL-17, were mainly expressed in granulocytes (156). CgCaspase-8-2 was found mainly distributed in granulocytes. This protein functioned as important protease to be involved in the anti-bacterial immunity responses through inducing the expressions of cytokines, defensin and autophagy-related genes (285). Aminopeptidase was found expressed in eosinophilic granulocytes from M. edulis (286). Chitinase (Cg-Chit) seems to play redundant functions for the immune responses in C. gigas and it is specifically expressed in granulocytes (287). Alcohol acyltransferase (CgAATase), enzyme involved in immune response, was found to be mainly expressed in granulocytes (288). Glutamic acid decarboxylase (CgGAD), enzyme responsible to catalyse the production of gamma aminobutyric acid (GABA), an important neurotransmitter of the GABAergic system, was dominantly expressed in granulocytes of C. gigas (289). In the same species, dopamine β-hydroxylase (DBH), a norepinephrine synthesising enzyme, was highly expressed in granulocytes and involved in neuroendocrine and immune response (290). Differences in the expression of neuroendocrine immunomodulation (NEI) related proteins between oyster O. edulis haemocyte types have been detected (238). Some antigens reacted differently in granulocytes and agranulocytes in the deep-sea symbiotic mussel B. japonicus (291).
Different proteins with key roles in important immune pathways were identified in the main haemocyte types of the oyster O. edulis, with more proteins involved in the MAPK, Ras and NF-κβ pathways in granulocytes, while in hyalinocytes there were more identified proteins that participate in the Wnt signalling pathway (238). In the oyster C. gigas, the MAPK pathway was found to participate in granulocytes to regulate functional activities prior than in hyalinocytes, also suggesting functional differentiation of haemocyte types (216). A recent study proposed that oyster C. hongkongensis granulocytes mainly participate in immune response through the NF-κB pathway and autophagy process (182).
4.2 Other Physiological Processes
Differences in cellular metabolism suggest, as discussed above, that granulocytes and hyalinocytes may be involved in different physiological functions.
4.2.1 Motility
Motility is crucial for haemocytes to carry out multiple functions, including immune response. Previous reports showed variations related to the locomotion and cytoskeleton properties among haemocyte types of bivalves. In the pearl oyster P. imbricata, granulocytes showed amoeboid locomotion and directional, while hyalinocytes appeared to be less mobile, often adhered to a substrate and spread multidirectionally (175). However, hyalinocytes also showed the ability for amoeboid movement in the oyster C. hongkongensis (157). Motility dynamics (either ameboid or based on pseudopod formation) has been emphasised as an important criterion for morphofunctional classification of mussel M. edulis haemocytes (112).
4.2.2 Spawning
Spawning in marine bivalves is a great energy-demanding process, and it often results in lethal and sublethal stresses during the post-spawning period, including depressed immune capacity (292). Female and male clams R. philippinarum showed different haemocyte populations during the pre-spawning phase, females having a higher fraction of granulocytes and males of hyalinocytes (188). Granulocyte percentage was higher in spring and early summer in the scallop A. farreri, and lower in summer and early autumn, the period corresponding to reproduction completion (293). The phagocytosis capacities of haemocytes were significantly reduced during the post-spawning period in the oyster S. kegaki, being more pronounced in granulocytes (190). In the oyster C. hongkongensis, males exhibited a more powerful cellular immune response than females after spawning, the former showing higher esterase activities, lysosomal masses, nitric oxide levels, and granulocyte numbers (294). Spawning was shown as a stressful activity inducing depressed immunological capacities in the ark clam T. granosa, with dramatical decline of granulocyte phagocytosis capacity in individuals engaged in active spawning while the production of ROS (indicative of stress) of the granulocytes and the erythrocytes type II increased linearly during the post-spawning period (292).
4.2.3 Shell Formation and Repair
Regarding shell regeneration, granulocytes have been shown to participate in the synthesis and transportation of CaCO3 in oysters C. virginica (25, 295) and C. gigas (296, 297), as well as in the deep-sea vent mussel B. azoricus (100, 298) and in pearl oysters P. fucata (242, 299). In the oyster C. gigas, several shell formation-related genes are highly expressed in H2 and H3 haemocytes (similar to granulocytes), thus these haemocyte types are potential players in biomineralisation processes (300). Moreover, the direct involvement of granulocytes in the formation of the prismatic layer has been found in the pearl oyster P. fucata (299). In the same species, Huang et al. (2018) successfully identified numerous calcium-rich vesicles and crystals in granulocytes and assigned to this cell type the strongest ability of migration (242). Altogether, the granulocytes may be a calcium pool and act as a calcium conveyor during shell formation and may participate in the initiating process of bivalve shell mineralisation (301).
5 Differential Response to Stress
Bivalve molluscs are poikilotherm organisms and multiple physiological functions of bivalves show seasonal variation. Consistently, as haemocytes are involved in multiple functions, total haemocyte count and the relative abundance of each haemocyte type in the haemolymph of bivalves also show seasonal variation (161, 302–304), which has to be considered normal. Additionally, the internal responses of bivalves to invasive pathogens, natural environmental impacts, and pollutants are mediated at least in part by haemocytes (23, 39). Furthermore, any effect that environmental stressors exert on the bivalve haemocyte proportions or functioning may ultimately result in a reduction of immune response effectivity, whereas bivalves are able to overcome well exposure to a wide variety of pathogens if the immune system is not over-challenged (147). Changes in total haemocyte count and the relative proportions of granular and agranular cells often are used as indices of bivalve immune status (30, 31, 248). There are many studies focused on the haemocyte immune response to a wide range of stressors in which it is possible to suggest functional differences between haemolymph cell types. Research literature focusing on effects of biotic and abiotic stress on bivalve haemocyte subpopulations is summarised in Tables 2 and 3.
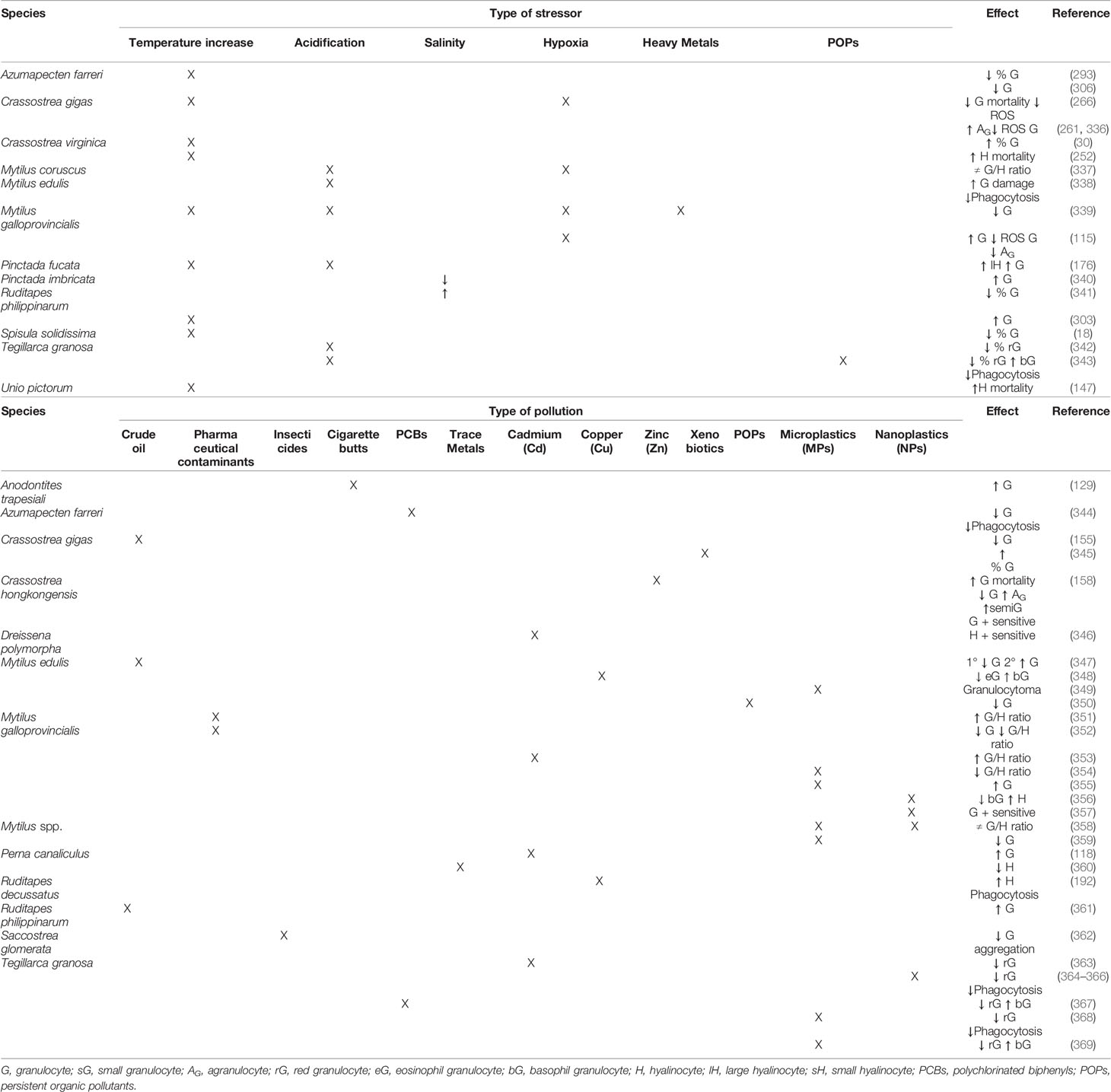
Table 3 Literature survey of reported effects of abiotic stress on bivalve haemocyte subpopulations.
5.1 Biotic Stress: Pathogens and Harmful Algal Blooms
The relative abundance of each cell type in the haemolymph of bivalve molluscs is variable and is influenced by the presence of certain pathogens (321). Regarding the reaction against bacteria, juvenile oyster disease was correlated with altered cell ratios resulting in an increase in the percentage of granulocytes in oysters C. virginica (31). Clams R. philippinarum challenged with various Vibrio species showed significant decrease of hyalinocytes and increase of granulocytes, suggesting different involvement of each haemocyte type in antibacterial defence (315). On the contrary, the granulocyte concentration decreased in the same clam species after infection with Vibrio tapetis (316). In the oyster C. gigas, strong enhancement of haemocyte ROS production following bacterial infection was observed, which was higher in granulocytes than hyalinocytes (307). In the same species, modifications of the proportion of the haemolymph cells have been observed after bacterial challenge (308). Granulocytes were reported as the main haemocyte type involved in antibacterial response in the freshwater mussel A. cygnea (370). In the marine mussel M. galloprovincialis, the haemocyte types exhibited distinct responses to infection by various bacterial species of the genera Vibrio and Micrococcus (221, 371). The infection with bacteria Listonella anguilarum also induced changes in the relative abundance of haemocyte types of the oyster O. edulis haemolymph, favouring granulocytes (312). In the clam Mya arenaria, independent modifications after bacterial infection were observed in the proportions of haemocyte subpopulations established by their lysosomal content, suggesting specific modulation of bivalve responses against pathogenic bacteria that would include degranulation (310). Bacterial challenge produced an increase in the percentage of granulocytes and a decrease in that of hyalinocytes in the oyster C. madrasensis, suggesting the main involvement of granulocytes in immune response (309). Similar results were found in the freshwater mussel S. cumingii, with total haemocyte count increase, especially the proportion of granulocytes, after bacterial infection (146). On the contrary, granulocyte count decreased in the scallop A. farreri when challenged with bacteria; such decline increased at high temperature (306). Regarding metazoan pathogens, it has been suggested that the bivalve immune system responds to trematode invasion by recruiting granulocytes (305). Thus, in the mussel P. perna a decrease in the percentage of granulocytes in the circulating haemolymph was observed associated with trematode infection (314). Trematodes also caused change in the proportions of the haemocyte types in the ark clam A. trapezia (87). A decrease in the percentage of granulocytes upon infestation by copepods was found in the mussel M. galloprovincialis, which was interpreted as a tendency for invertebrate haemocytes to degranulate in response to parasitism (185). Regarding responses to protistan pathogens, Perkinsus spp. appear to induce changes in the proportions of haemocyte types in the oyster C. brasiliana (149), suppression of granulocyte apoptosis in C. virginica (271) and a significant increase in granulocyte concentration in clams R. philippinarum (303). In the oyster O. edulis, increase of the proportion of small hyalinocytes and decrease of that of granulocytes associated with Bonamia ostreae infection was observed (171, 313). Hyalinocytes were abundant in tissues heavily infected with Haplosporidium sp. in the oyster S. cucullata (172). In the mussel M. galloprovincialis, lower percentage of granulocytes was observed in the presence of Marteilia spp. parasites (311).
A more precise perspective on the relevance of the haemocyte types in the response against pathogens can be attained from comparison between resistant (or tolerant) and susceptible hosts (372). Oysters C. virginica resistant to the protistan Haplosporidium nelsoni showed significantly higher percentage of granulocytes (317). In the same species, higher percentage of granulocytes was found in the haemolymph of oysters with higher tolerance to infection with the protistan Perkinsus marinus (318). Similarly, clams R. philippinarum resistant to Vibrio tapetis had relatively more granular haemocytes, resulting in increased phagocytic capacity (191). Oysters O. edulis with increased resistance to B. ostreae showed different haemocyte counts than susceptible ones (320), displaying higher percentage of granulocytes (313). Remarkably, the intracellular parasite B. ostreae multiplies more successfully within hyalinocytes than granulocytes (321); similarly, hyalinocytes of the oyster O. chilensis are known to be preferentially infected by Bonamia exitiosa (319). In oysters S. glomerata, resistance to the protistan Marteilia sydneyi is linked to increased frequencies of granulocytes, likely due to higher phagocytic activity and higher levels of phenoloxidase in this haemocyte type (322) and higher ROS production (174). A positive health effect of treatment of C. gigas with brown and red seaweeds has been suggested; treated oysters showed significant increase in granulocyte count and a low pathogen prevalence (373).
Several studies have reported modulation of bivalve haemocyte variables in response to harmful algal bloom (HAB) exposure (374). Exposure to toxin-producing dinoflagellates increased oyster C. virginica haemocyte phagocytosis ability and granulocyte subpopulation (328). In the case of the scallop A. irradians, a biphasic effect was detected, with initial increase of hyalinocyte count and decrease of that of granulocytes and posterior hyalinocyte decrease and increase of granulocyte count (323). Variation of the differential haemocyte count was also detected in the mussel M. edulis, in which eosinophilic granulocytes decreased at the beginning of exposure but increased after a few days (330). In the same mussel species, degranulation of eosinophilic granulocytes associated with exposure to toxin-producing dinoflagellates was reported (331). Other reported effects of exposure to toxin-producing dinoflagellates were drastic increase of granulocytes (324), increase of granulocyte size (325), and changes in the haemocyte type relative abundance in the oyster C. gigas (326); doubling number of granulocytes in clams R. philippinarum (335); decrease in the percentage of granulocytes in the mussel P. perna (334); and anomalous decrement of granulocytes (332) and variations of the granulocytes/hyalinocytes (G/H) ratio in the mussel M. galloprovincialis (333). In vitro exposure to saxitoxin, a neurotoxin produced by dinoflagellates, affected more granulocytes than hyalinocytes of the mussel M. chilensis (109), while oyster C. gigas hyalinocytes were found to be highly responsive (327). Exposure to cyanotoxin producing bacteria increased the relative proportion of granulocytes in the freshwater mussel D. polymorpha (329).
5.2 Abiotic Stress: Temperature, Salinity, Acidification, Hypoxia and Pollution
High water temperature can influence haemocyte parameters in bivalves, including haemocyte number, motility, viability, adhesive capacity, phagocytic ability, membrane permeability, and intracellular enzyme activities, which may result in weakened ability to mount an immune defence (375, 376). Temperature increase was associated with higher percentage of granulocytes in the oyster C. virginica (30) and higher mortality of hyalinocytes (252). A positive correlation between temperature and granulocyte counts was observed in the clam R. philippinarum (303). High values of granulocyte percentage were observed in the scallop A. farreri during the period of favourable water temperature, whereas low values were found during the period of high water temperature (293). Increase of the percentage of large hyalinocytes and granulocytes in the pearl oyster P. fucata was proposed to be associated with warming and ocean acidification (176). On the contrary, increasing temperature was found associated with decrease of the percentage of granulocytes in the clam Spisula solidissima (18). Hyalinocyte mortality was significantly increased at high temperature while no effect of temperature was evident in the granulocyte mortality of the freshwater mussel Unio pictorum (147). The phagocytic capacity of granulocytes from the mussels M. virgata under the heatwave condition decreased to one-third of the values in control mussels (107). Regarding salinity effects, decrease of the number of granulocytes in the clam R. philippinarum was reported as consequence of salinity increment (341). Similarly, the frequency of granulocytes increased significantly when pearl oysters P. imbricata were stressed by hypo-saline conditions (340). In the oyster Crassostrea corteziensis, hyalinocyte and granulocyte counts have higher values in hyposaline stress conditions and lower values in hypersaline stress conditions; however, these haemocyte type counts change at a different rate (377). Realistic pH reduction, as expected with ocean acidification, induced a decrease of the percentage of red granulocytes in the ark clam T. granosa (342) and an increment of the ratio of damaged granulocytes in mussels M. edulis (338). Mussels M. galloprovincialis co-exposed to high temperature, acidification and cadmium experienced significantly reduction of the granulocyte proportion (339). Changes of the three subpopulations of the mussel M. coruscus under a short-term exposure to acidification and hypoxia have been observed (337). Hypoxia also induced substantial increase of granulocytes and a decrease of agranulocytes and intracellular ROS production in granulocytes in the mussel M. galloprovincialis (115). However, in the oyster C. gigas, hypoxia influenced agranular and granular cells differently, with a higher decrease of ROS production in granulocytes and an increase of agranulocytes number (261, 336). In the same species, O2 deprivation resulted in a strong decrease of granulocyte mortality potentially linked with a decrease of ROS production (266).
Bivalves have been widely used as sentinel organisms in the biomonitoring of aquatic pollution (7). Pollution may result in the death of haemocytes owing to lysis and in changes in the proportions of their main cell types; this fact had been observed in mussels, oysters and clams (155, 348, 378). Moreover, the formation of granulocytomas is an inflammatory cellular response associated with environmental pollution. Granulocytomas are a bioindicator of the haemocytic response to pollutants as well as a general loss of health in bivalves (379, 380). Thus, morphometric alterations of granulocytes may be used in a biomarker battery in aquatic environmental monitoring (381). The clam M. arenaria collected from polluted sediments had a higher proportion of granulocytes compared to those from a relatively clean area, indicating possible haemocyte involvement in sequestration of chemical pollutants (382). Also in the mussel M. galloprovincialis, the numbers of eosinophilic and basophilic granulocytes were higher in polluted than in clean areas (383). In the mussel M. kurilensis, pollution resulted in significantly decrease of the percentage of agranulocytes and phagocytic activity and the formation of granulocytomas (35). In mussels M. edulis exposed to crude oil, initial reduction of granulocytes followed by granulocyte increase was observed, which was considered an adaptive response to stress (347). One year after a disastrous oil spill in Korea, decreased granulocyte count was reported in the oyster C. gigas (155), while higher proportion of granulocytes in the clam R. philippinarum was reported two years after the spill (361). Different types of pharmaceutical contaminants produce variation in the mussel M. galloprovincialis physiological response, particularly inducing changes of the G/H ratio, some compounds increasing (351), while decreasing it others (352). Insecticides also impair bivalve physiology and, in the oyster S. glomerata, granulocytes and hyalinocytes respond differently to different concentrations of pesticides (362). Pollution by leachates from cigarette butts induced increase of granulocyte proportion in the freshwater mussel A. trapesiali (129). A variety of responses in differential haemocyte count have been previously reported in bivalves upon exposure to metal toxicants. The proportion of red granulocytes were significantly reduced after 10 days exposure of the ark clam T. granosa to Cd2+ spiked seawater, which suggested significant immunotoxicity of Cd2+ to this species (363). In mussels M. edulis, decrease of the proportion of circulating eosinophilic granulocytes and increase of basophils after copper exposure was observed (348). In mussels M. galloprovincialis, granular haemocytes were found less sensitive to genotoxic damage compared with agranular haemocytes (384). Exposure to Cd increased the proportion of granulocytes in the mussel P. canaliculus (118) and the G/H ratio in M. galloprovincialis (353). Also in the mussel P. canaliculus, metal pollution induced hyalinocyte decrease (360). In freshwater mussels D. polymorpha, the different response of haemocyte types to Cd led to consider granulocytes with higher capacity to regulate oxidative stress and greater involvement in essential metal transport or sequestration of heavy metals (346). In the clam R. decussatus, higher phagocytic activity in hyalinocytes than in granulocytes was reported when the cells were in vitro exposed to CuSO4 (192). Zinc-contaminated oysters C. hongkongensis showed increase of granulocyte mortality, which suggested that granulocytes were the most sensitive cell type in responding to Zn; moreover, the granulocyte number decreased whereas those of semigranulocytes and agranulocytes increased (158). In the same species, specific responses of granulocyte, semigranulocyte and hyalinocyte have been detected to copper; granulocyte was the most important responsive cell type and displayed heterogeneity responses of its two distinguished subtypes (385). In the oyster C. gigas, granulocyte percentage increased in the presence of hydrocarbons, which led authors to hypothesise that granulocytes may be more resistant than hyalinocytes (345). The proportion of granulocytes and phagocytosis ability decreased while the proportion of hyalinocytes increased in the scallop A. farreri after exposure to polychlorinated biphenyls (PCBs) (344). In the ark clam T. granosa, exposure to high doses of PCB led to red granulocyte percentage decrease and basophil granulocyte increase (367). Nanoplastics (NPs) and microplastics (MPs) had a significant effect on G/H ratios of Mytilus spp. (356, 358, 359), with a decrease of granulocyte concentrations by MPs (359), a decrease in basophil granulocytes and an increase in hyalinocytes in M. galloprovincialis by NPs (356). Exposure of mussels M. galloprovincialis to benzo[a]pyrene (B[a]P) and MPs increased granulocytes proportion (355), while, in another study, MPs induced decrease of the G/H ratio (354). In mussels M. edulis, MPs caused the formation of granulocytomas, an inflammatory response mainly due to eosinophilic granulocytes (349). Exposure to NPs causes different immune responses between haemocyte subpopulations. In fact, granulocytes of the mussel M. galloprovincialis appeared to be more sensitive than hyalinocytes (357). Ark clams T. granosa showed phagocytic activity and red granulocytes ratio significantly reduced after exposure to NPs (364–366) and B[a]P (368). Similarly, exposure to MPs and polycyclic aromatic hydrocarbons (PAHs) led to a significant decrease of the proportion of red granulocytes and increase of basophil granulocytes in T. granosa. Moreover, the ark clams coexposed to MPs and PAHs showed significantly lower proportion of red granulocytes and higher of basophil granulocytes than clams exposed to MPs or PAHs alone (369). In the same species, a significant decrease detected in phagocytosis when exposed to B[a]P under low pH simulating future ocean acidification scenarios may be attributed to significant reduction of red granulocyte count (343). Long-time-exposure to persistent organic pollutants (POPs) in mussels M. edulis may decrease the proportion of granulocytes, suggesting that such haemocyte type may be more sensitive to these pollutants (350).
Considering the important effects of biotic and abiotic stressors on the bivalve haemolymph cells, extensive monitoring studies of the morphofunctional properties of the haemocytes of bivalves in the natural environment would be useful to state reliable criteria for diagnosing the physiological status of bivalves (34).
6 Conclusion
Due to bivalve mollusc diversity and functional heterogeneity, haemocyte types vary from species to species and, nowadays, there is not a unified nomenclature that applies to all bivalves. Moreover, functions of each haemocyte type cannot be reliably extrapolated among all species. Haemocyte subpopulations own distinct properties that should be considered when characterising the overall immune related functions of bivalves. Granulocytes and hyalinocytes display differences in their metabolism and immune abilities, which implies that they play different physiological and immunological roles that should be deeply explored. Infections induce changes in the proportions of haemocyte types, which points out differential involvement of the haemocyte types in immune response. Abiotic stressors also alter the relative abundance of haemocyte types, which highlights functional differences. The ratio of cell types in the haemocyte community could be noted as indicator of immune function, being an important immune parameter to assess the bivalve health-status.
Author Contributions
NB: conceptualisation, methodology, literature review, and writing – original draft. FM: conceptualisation, literature review, writing – review and editing. AC: writing - review and editing. AV: conceptualisation, literature review, writing – review and editing, and supervision. All authors contributed to the article and approved the submitted version.
Conflict of Interest
The authors declare that the research was conducted in the absence of any commercial or financial relationships that could be construed as a potential conflict of interest.
Publisher’s Note
All claims expressed in this article are solely those of the authors and do not necessarily represent those of their affiliated organizations, or those of the publisher, the editors and the reviewers. Any product that may be evaluated in this article, or claim that may be made by its manufacturer, is not guaranteed or endorsed by the publisher.
References
1. Ruppert EE, Fox RS, Barnes RD. Invertebrate Zoology: A Functional Evolutionary Approach. Syst Biol (2004) 53:662–4. doi: 10.1080/10635150490472977
2. Zhang Z-Q. Animal Biodiversity: An Update of Classification and Diversity in 2013. Zootaxa (2013) 3703:5. doi: 10.11646/zootaxa.3703.1.3
3. Lutz RA, Kennish MJ. Ecology of Deep-Sea Hydrothermal Vent Communities: A Review. Rev Geophys (1993) 31:211. doi: 10.1029/93RG01280
4. Gosling E. Bivalve Molluscs. Biology, Ecology and Culture. Oxford, UK: Publishing Fishing News Books (2003). p. 443.
5. Newell RIE. Ecosystem Influences of Natural and Cultivated Populations of Suspension-Feeding Bivalve Molluscs: A Review. J Shellfish Res (2004) 23:51–61.
6. Vaughn CC, Hoellein TJ. Bivalve Impacts in Freshwater and Marine Ecosystems. Annu Rev Ecol Evol Syst (2018) 49:183–208. doi: 10.1146/annurev-ecolsys-110617-062703
7. Sheehan D, McDonagh B. Oxidative Stress and Bivalves: A Proteomic Approach. Invertebr Surviv J (2008) 5:110–23.
8. Loker ES, Adema CM, Zhang SM, Kepler TB. Invertebrate Immune Systems - Not Homogeneous, Not Simple, Not Well Understood. Immunol Rev (2004) 198:10–24. doi: 10.1111/j.0105-2896.2004.0117.x
9. Song L, Wang L, Qiu L, Zhang H. Bivalve Immunity. Adv Exp Med Biol (2010) 708:44–65. doi: 10.1007/978-1-4419-8059-5_3
10. Venier P, Varotto L, Rosani U, Millino C, Celegato B, Bernante F, et al. Insights Into the Innate Immunity of the Mediterranean Mussel Mytilus galloprovincialis. BMC Genomics (2011) 12:69. doi: 10.1186/1471-2164-12-69
11. Wang L, Song X, Song L. The Oyster Immunity. Dev Comp Immunol (2018) 80:99–118. doi: 10.1016/j.dci.2017.05.025
13. Dyrynda EA, Pipe RK, Burt GR, Ratcliffe NA. Modulations in the Immune Defences of Mussels (Mytilus edulis) From Contaminated Sites in the UK. Aquat Toxicol (1998) 42:169–85. doi: 10.1016/S0166-445X(97)00095-7
14. Dyrynda EA, Law RJ, Dyrynda PEJ, Kelly CA, Pipe RK, Ratcliffe NA. Changes in Immune Parameters of Natural Mussel Mytilus edulis Populations Following a Major Oil Spill (“Sea Empress”, Wales, Uk). Mar Ecol Prog Ser (2000) 206:155–70. doi: 10.3354/meps206155
15. Cherkasov AS, Grewal S, Sokolova IM. Combined Effects of Temperature and Cadmium Exposure on Haemocyte Apoptosis and Cadmium Accumulation in the Eastern Oyster Crassostrea virginica (Gmelin). J Therm Biol (2007) 32:162–70. doi: 10.1016/j.jtherbio.2007.01.005
16. Anestis A, Pörtner HO, Lazou A, Michaelidis B. Metabolic and Molecular Stress Responses of Sublittoral Bearded Horse Mussel Modiolus barbatus to Warming Sea Water: Implications for Vertical Zonation. J Exp Biol (2008) 211:2889–98. doi: 10.1242/jeb.016782
17. Sokolova I, Lannig G. Interactive Effects of Metal Pollution and Temperature on Metabolism in Aquatic Ectotherms: Implications of Global Climate Change. Clim Res (2008) 37:181–201. doi: 10.3354/cr00764
18. Hornstein J, Pales Espinosa E, Cerrato RM, Lwiza KMM, Allam B. The Influence of Temperature Stress on the Physiology of the Atlantic Surfclam, Spisula solidissima. Comp Biochem Physiol A (2018) 222:66–73. doi: 10.1016/j.cbpa.2018.04.011
19. Cheng TC. Bivalves. In: Ratcliffe NA, Rowley AF, editors. Invertebrate Blood Cells. London, UK: Academic Press (1981). p. 233–300.
20. Lau YT, Sussman L, Pales Espinosa E, Katalay S, Allam B. Characterization of Hemocytes From Different Body Fluids of the Eastern Oyster Crassostrea virginica. Fish Shellfish Immunol (2017) 71:372–9. doi: 10.1016/j.fsi.2017.10.025
21. Fisher WS. Structure and Functions of Oyster Hemocytes. In: Brehélin M, editor. Immunity in Invertebrates. Berlin, Germany: Springer (1986). p. 25–35. doi: 10.1007/978-3-642-70768-1
22. Cheng TC. Hemocytes: Forms and Functions. In: Kennedy VS, Newell RIE, Eble AF, editors. The Eastern Oyster: Crassostrea virginica. College Park, MD: Maryland Sea Grant Book (1996). p. 772.
23. Hine PM. The Inter-Relationships of Bivalve Haemocytes. Fish Shellfish Immunol (1999) 9:367–85. doi: 10.1006/fsim.1998.0205
24. Canesi L, Gallo G, Gavioli M, Pruzzo C. Bacteria-Hemocyte Interactions and Phagocytosis in Marine Bivalves. Microsc Res Tech (2002) 57:469–76. doi: 10.1002/jemt.10100
25. Mount AS, Wheeler AP, Paradkar RP, Snider D. Hemocyte-Mediated Shell Mineralization in the Eastern Oyster. Science (80-) (2004) 304:297–300. doi: 10.1126/science.1090506
26. Soudant P, Chu FE, Volety A. Host – Parasite Interactions : Marine Bivalve Molluscs and Protozoan Parasites, Perkinsus Species. J Invertebr Pathol (2013) 114:196–216. doi: 10.1016/j.jip.2013.06.001
27. Oyanedel D, Gonzalez R, Brokordt K, Schmitt P, Mercado L. Insight Into the Messenger Role of Reactive Oxygen Intermediates in Immunostimulated Hemocytes From the Scallop Argopecten purpuratus. Dev Comp Immunol (2016) 65:226–30. doi: 10.1016/j.dci.2016.07.015
28. Matozzo V, Ballarin L, Pampanin DM, Marin MG. Effects of Copper and Cadmium Exposure on Functional Responses of Hemocytes in the Clam, Tapes philippinarum. Arch Environ Contam Toxicol (2001) 41:163–70. doi: 10.1007/s002440010234
29. Donaghy L, Lambert C, Choi K-S, Soudant P. Hemocytes of the Carpet Shell Clam (Ruditapes decussatus) and the Manila Clam (Ruditapes philippinarum): Current Knowledge and Future Prospects. Aquaculture (2009) 297:10–24. doi: 10.1016/j.aquaculture.2009.09.003
30. Chu F, La Peyre J. Perkinsus marinus Susceptibility and Defense-Related Activities in Eastern Oysters Crassostrea virginica Temperature Effects. Dis Aquat Organ (1993) 16:223–34. doi: 10.3354/dao016223
31. Paillard C, Ashton-Alcox KA, Ford SE. Changes in Bacterial Densities and Hemocyte Parameters in Eastern Oysters, Crassostrea virginica, Affected by Juvenile Oyster Disease. Aquat Living Resour (1996) 9:145–58. doi: 10.1051/alr:1996018
32. Chu FLE. Defense Mechanisms of Marine Bivalves. In: Fingerman M, Nagabhushanam R, editors. Recent Advances in Marine Biotechnology: Immunobiology and Pathology. Enfield, NH: Science Publishers Inc (2000). p. 1–42.
33. Auffret M. Bivalves as Models for Marine Immunotoxicology. In: Tryphonas H, Fournier M, Blakley BR, Smits JEG, Brousseau P, editors. Investigative Immunotoxicology. New York, NY: Taylor and Francis (2005). p. 29–48.
34. Anisimova AA. Morphofunctional Parameters of Hemocytes in the Assessment of the Physiological Status of Bivalves. Russ J Mar Biol (2013) 39:381–91. doi: 10.1134/S1063074013060023
35. Kumeiko VV, Sokolnikova YN, Grinchenko AV, Mokrina MS, Kniazkina MI. Immune State Correlates With Histopathological Level and Reveals Molluscan Health in Populations of Modiolus kurilensis by Integral Health Index (IHI). J Invertebr Pathol (2018) 154:42–57. doi: 10.1016/j.jip.2018.03.014
36. Cheng TC. A Classification of Molluscan Hemocytes Based on Functional Evidences. In: Cheng TC, editor. Invertebrate Blood. Comparative Pathobiology, vol. 6 . Boston, MA: Springer (1984). p. 111–46. doi: 10.1007/978-1-4684-4766-8_5
37. Bachère E, Chagot D, Grizel H. Separation of Crassostrea gigas Hemocytes by Density Gradient Centrifugation and Counterflow Centrifugal Elutriation. Dev Comp Immunol (1988) 12:549–59. doi: 10.1016/0145-305X(88)90071-7
38. Soudant P, Leite R, Chu F, Villalba A, Cancela L. Bivalves – Perkinsus Spp. Interactions. In: Villalba A, editor. Workshop for the Analysis of the Impact of Perkinsosis to the European Shellfish Industry. Vilanova de Arousa, Spain: Centro de Investigacións Mariñas, Consellería de Pesca e Asuntos Marítimos da Xunta de Galicia and Centro Tecnológico del Mar – Fundación CETMAR (2008). p. 79–109.
40. Yu J-H, Park K-I, Park S-W. Characterization of Haemocytes in the Surf Clam Mactra veneriformis. J Fish Pathol (2009) 22:305–16.
41. Nakayama K, Nomoto AM, Nishijima M, Maruyama T. Morphological and Functional Characterization of Hemocytes in the Giant Clam, Tridacna crocea. Dev Comp Immunol (1997) 21:67. doi: 10.1016/s0145-305x(97)87961-x
42. Reade P, Reade E. Phagocytosis in Invertebrates: Studies on the Hemocytes of the Clam Tridacna maxima. J Invertebr Pathol (1976) 28:281–90. doi: 10.1016/0022-2011(76)90001-X
43. Anisimova AA, Ponomareva AL, Grinchenko AV, Kirsanova IA, Kravchenko DN. The Composition and Seasonal Dynamics of the Hemocyte Cell Population in the Clams Corbicula japonica Prime (1864) of the Kievka River (the Basin of the Sea of Japan). Russ J Mar Biol (2017) 43:156–63. doi: 10.1134/S106307401702002X
44. Suresh K, Mohandas A. Number and Types of Hemocytes in Sunetta scripta and Villorita cyprinoides Var. cochinensis (Bivalvia), and Leukocytosis Subsequent to Bacterial Challenge. J Invertebr Pathol (1990) 55:312–8. doi: 10.1016/0022-2011(90)90072-E
45. Hernández-Méndez LS, Castro-Longoria E, Araujo-Palomares CL, García-Esquivel Z, Castellanos-Martínez S. Hemocyte Cell Types of the Cortes Geoduck, Panopea globosa (Dall 1898), From the Gulf of California, Mexico. Fish Shellfish Immunol (2020) 100:230–7. doi: 10.1016/j.fsi.2020.03.013
46. Husmann G, Philipp EER, Rosenstiel P, Vazquez S, Abele D. Immune Response of the Antarctic Bivalve Laternula elliptica to Physical Stress and Microbial Exposure. J Exp Mar Bio Ecol (2011) 398:83–90. doi: 10.1016/j.jembe.2010.12.013
47. Pan H, Gao R, Wu L, Li W. Flow Cytometry Studies on Haemocyte Classification in Three Species of Bivalve Mollusks. J Fujian Norm Univ (2011) 4:133–36.
48. Santos JJS, Carvalho YB, Lopes DL de A, Romano LA. Immunological Profile of the Yellow Clam Mesodesma mactroides (Mesodesmatidae) From the Southern Coast of Rio Grande do Sul, Brazil. J Aquat Anim Health (2016) 28:11–20. doi: 10.1080/08997659.2015.1116471
49. Ross PM, Pande A, Jones JB, Cope J, Flowers G. First Detection of Gas Bubble Disease and Rickettsia -Like Organisms in Paphies ventricosa, a New Zealand Surf Clam. J Fish Dis (2018) 41:187–90. doi: 10.1111/jfd.12684
50. Huffman JE, Tripp MR. Cell Types and Hydrolytic Enzymes of Soft Shell Clam (Mya arenaria) Hemocytes. J Invertebr Pathol (1982) 40:68–74. doi: 10.1016/0022-2011(82)90038-6
51. Brousseau P, Pellerin J, Morin Y, Cyr D, Blakley B, Boermans H, et al. Flow Cytometry as a Tool to Monitor the Disturbance of Phagocytosis in the Clam Mya arenaria Hemocytes Following In Vitro Exposure to Heavy Metals. Toxicology (1999) 142:145–56. doi: 10.1016/S0300-483X(99)00165-1
52. Wootton EC, Pipe RK. Structural and Functional Characterisation of the Blood Cells of the Bivalve Mollusc, Scrobicularia plana. Fish Shellfish Immunol (2003) 15:249–62. doi: 10.1016/S1050-4648(02)00164-X
53. da Silva PM, Cremonte F, Sabry RC, Rosa RD, Cantelli L, Barracco MA. Presence and Histopathological Effects of the Parvatrema Sp. (Digenea, Gymnophallidae) in the Stout Razor Clam Tagelus plebeius (Bivalvia, Psammobiidae). J Invertebr Pathol (2009) 102:14–20. doi: 10.1016/j.jip.2009.05.014
54. Shoukry NM. Hemocytes of the Marine Bivalve Macoma edentula as Biomarkers of Environmental Quality in Lake Timsah, Ismailia, Egypt. Egypt J Zool (2013) 60:267–94. doi: 10.12816/0003294
55. Matozzo V, Bailo L. A First Insight Into Haemocytes of the Smooth Venus Clam Callista chione. Fish Shellfish Immunol (2015) 42:494–502. doi: 10.1016/j.fsi.2014.11.034
56. Pampanin DM, Marin MG, Ballarin L. Morphological and Cytoenzymatic Characterization of Haemocytes of the Venus Clam Chamelea gallina. Dis Aquat Organ (2002) 49:227–34. doi: 10.3354/dao049227
57. Montenegro D, Valdés J, González MT. Histopathological Lesions, Pathogens and Parasites as Health Indicators of an Edible Clam (Protothaca thaca) Inhabiting a Bay Exposed to Anthropogenic Activities in Northern Chile. Environ Monit Assess (2019) 191:536–50. doi: 10.1007/s10661-019-7678-7
58. Jauzein C, Donaghy L, Volety AK. Flow Cytometric Characterization of Hemocytes of the Sunray Venus Clam Macrocallista nimbosa and Influence of Salinity Variation. Fish Shellfish Immunol (2013) 35:716–24. doi: 10.1016/j.fsi.2013.06.003
59. Chiou-Ming W, Guang-Hsiung K, Shiu-Nan C. Light and Electron Microscopy of Hemocyte of the Hard Clam, Meretrix lusoria (Röding). Comp Biochem Physiol A (1994) 108:279–86. doi: 10.1016/0300-9629(94)90096-5
60. Chang S, Tseng S, Chou H. Morphological Characterization via Light and Electron Microscopy of the Hemocytes of Two Bivalves: A Comparison Study Between the Hard Clam Meterix lusoria and the Pacific Oyster Crassostrea gigas. Zool Stud (2005) 44:144–52.
61. Tu CY, Hung SW, Tsou LT, Chang YC, Wang WS. Simultaneous Flow Cytometric Assessment for Cellular Types and Phagocytic Abilities of the Haemocytes of the Hard Clam, Meretrix lusoria. Fish Shellfish Immunol (2007) 23:16–23. doi: 10.1016/j.fsi.2006.08.021
62. Zhang Y, Ren S, Wang D, Song W. Structure and Classification of Haemocytes in the Bivalve Mollusc Meretrix meretrix. J Ocean Univ China (2006) 5:132–6. doi: 10.1007/BF02919211
63. Kim WS, Hwang IJ, Yoon JH, Kim BJ, Kwon JY, Lim HJ, et al. Proper Infiltrate the Substrate Composition and the Stocking Density of the Endlily, Meretrix petechialis (Lamarck) Form at the Immunological Response of a Blood Cell. Korean J Malacol (2017) 23:223–31. doi: 10.9710/kjm.2017.33.4.223
64. Rodrick GE, Ulrich SA. Microscopical Studies on the Hemocytes of Bivalves and Their Phagocytic Interaction With Selected Bacteria. Helgoländer Meeresun (1984) 176:167–76. doi: 10.1007/BF01989301
65. Cheng TC, Foley DA. Hemolymph Cells of the Bivalve Mollusc Mercenaria mercenaria: An Electron Microscopical Study. J Invertebr Pathol (1975) 26:341–51. doi: 10.1016/0022-2011(75)90232-3
66. Moore CA, Eble AF. Cytochemical Aspects of Mercenaria mercenaria Hemocytes. Biol Bull (1977) 152:105–19. doi: 10.2307/1540730
67. Tripp MR. Phagocytosis by Hemocytes of the Hard Clam, Mercenaria mercenaria. J Invertebr Pathol (1992) 59:222–7. doi: 10.1016/0022-2011(92)90125-N
68. Zeng Y, Huo Y, Yang H. Immunological Assays of Hemocytes in the Northern Quahog Mercenaria mercenaria. Fish Shellfish Immunol (2021) 118:261–9. doi: 10.1016/j.fsi.2021.09.006
69. Lin T, Yao Z, Hao Z, Lai Q, Zhou K. Classification of Granulocytes in Three Bivalve Species Using Neutral Red Staining. J Shellfish Res (2013) 32:861–5. doi: 10.2983/035.032.0330
70. Gajbhiye DS, Khandeparker L. Immunoecology of the Short Neck Clam Paphia malabarica (Chemnitz, 1782) in a Tropical Monsoon-Influenced Estuary. Mar Environ Res (2019) 143:60–70. doi: 10.1016/j.marenvres.2018.11.004
71. López C, Carballal MJ, Azevedo C, Villalba A. Morphological Characterization of the Hemocytes of the Clam, Ruditapes decussatus (Mollusca: Bivalvia). J Invertebr Pathol (1997) 69:51–7. doi: 10.1006/jipa.1996.4639
72. López C, Carballal M, Azevedo C, Villalba A. Differential Phagocytic Ability of the Circulating Haemocyte Types of the Carpet Shell Clam Ruditapes decussatus (Mollusca : Bivalvia). Dis Aquat Organ (1997) 30:209–15. doi: 10.3354/dao030209
73. Prado-Alvarez M, Romero A, Balseiro P, Dios S, Novoa B, Figueras A. Morphological Characterization and Functional Immune Response of the Carpet Shell Clam (Ruditapes decussatus) Haemocytes After Bacterial Stimulation. Fish Shellfish Immunol (2012) 32:69–78. doi: 10.1016/j.fsi.2011.10.019
74. Cima F, Matozzo V, Marin MG, Ballarin L. Haemocytes of the Clam Tapes philippinarum (Adams & Reeve, 1850): Morphofunctional Characterisation. Fish Shellfish Immunol (2000) 10:677–93. doi: 10.1006/fsim.2000.0282
75. Liu J, Zhao Y. Morphological and Functional Characterization of Clam Ruditapes philippinarum Haemocytes. Fish Shellfish Immunol (2018) 82:136–46. doi: 10.1016/j.fsi.2018.08.019
76. Tame A, Ozawa G, Maruyama T, Yoshida T. Morphological and Functional Characterization of Hemocytes From Two Deep-Sea Vesicomyid Clams Phreagena okutanii and Abyssogena phaseoliformis. Fish Shellfish Immunol (2018) 74:281–94. doi: 10.1016/j.fsi.2017.12.058
77. Russell-Pinto F, Reimão R, de Sousa M. Haemocytes in Cerastoderma edule (Mollusca, Bivalvia): Distinct Cell Types Engage in Different Responses to Sheep Erythrocytes. Fish Shellfish Immunol (1994) 4:383–97. doi: 10.1006/fsim.1994.1033
78. Wootton E, Dyrynda E, Ratcliffe N. Bivalve Immunity: Comparisons Between the Marine Mussel (Mytilus edulis), the Edible Cockle (Cerastoderma edule) and the Razor-Shell (Ensis siliqua). Fish Shellfish Immunol (2003) 15:195–210. doi: 10.1016/S1050-4648(02)00161-4
79. Matozzo V, Rova G, Marin MG. Haemocytes of the Cockle Cerastoderma glaucum: Morphological Characterisation and Involvement in Immune Responses. Fish Shellfish Immunol (2007) 23:732–46. doi: 10.1016/j.fsi.2007.01.020
80. Hameed A, Muhammad F, Muhammad AA, Shafi M, Sultana R. Morphological and Structural Characterization of Blood Cells of Anadara antiquata. Iran J Fish Sci (2018) 17:613–9. doi: 10.22092/IJFS.2018.119523
81. Zhou L, Yang A, Wang Q, Liu Z, Wu B, Tian J, et al. Studies on the Hemocytes Types and Their Immunological Functions in Bloody Clam (Scapharca broughtonii). J Fish China (2013) 37:599–606. doi: 10.3724/SP.J.1231.2013.38321
82. Kim J-H, Lee H-M, Cho Y-G, Shin J-S, You J-W, Choi K-S, et al. Flow Cytometric Characterization of the Hemocytes of Blood Cockles Anadara broughtonii (Schrenck, 1867), Anadara kagoshimensis (Lischke, 1869), and Tegillarca granosa (Linnaeus, 1758) as a Biomarker for Coastal Environmental Monitoring. Mar Pollut Bull (2020) 160:111654. doi: 10.1016/j.marpolbul.2020.111654
83. Holden JA, Pipe RK, Quaglia A, Ciani G. Blood Cells of the Arcid Clam, Scapharca inaequivalvis. J Mar Biol Assoc United Kingdom (1994) 74:287–99. doi: 10.1017/S0025315400039333
84. Suganthi K, Bragadeeswaran S, Prabhu K, Rani SS. In Vitro Assessment of Haemocyte and Thrombocyte Count From the Blood Clam of Anadara inequivalvis. Middle East J Sci Res (2009) 4:163–7.
85. Zhi-hong LIU, Shicui Z, Aiguo Y. Immunological Study of Phagocytosis and Serum Lectin of Scapharca subcrenata. In: Walker P, Lester R, Bondad-Reantaso MG, editors. Diseases in Asian Aquaculture V. Manila, Philippines. Fish Health Section, Asian Fisheries Society (2005) p. 495–502.
86. Kladchenko ES, Andreyeva AY, Kukhareva TA, Soldatov AA. Morphologic, Cytometric and Functional Characterisation of Anadara kagoshimensis Hemocytes. Fish Shellfish Immunol (2020) 98:1030–2. doi: 10.1016/j.fsi.2019.11.061
87. Dang C, Cribb TH, Osborne G, Kawasaki M, Bedin AS, Barnes AC. Effect of a Hemiuroid Trematode on the Hemocyte Immune Parameters of the Cockle Anadara trapezia. Fish Shellfish Immunol (2013) 35:951–6. doi: 10.1016/j.fsi.2013.07.010
88. Gabriel U, Akinrotimi O, Orlu E. Haematological Characteristics of the Bloody Cockle Anadara senilis From Andoni Flats, Niger Delta, Nigeria. Sci World J (2011) 6:1–4. doi: 10.4314/swj.v6i1.70305
89. Zhu Z, Xu L, Wu X, Zhang Z, Wu L, Lou H. Morphological, Structural Characteristics and Phagocytic and Enzymatic Activities of Haemocytes in Blood Clam Tegillarca granosa. J Fish China (2011) 35:1494–504.
90. Mohite S, Mesram AM. On Haematological Characteristics of Blood Clam, Tegillarca rhombea (Born, 1778). J Aquac Mar Biol (2015) 3:00065. doi: 10.15406/jamb.2015.03.00065
91. Preziosi BM, Bowden TJ. Morphological Characterization via Light and Electron Microscopy of Atlantic Jackknife Clam (E directus) Hemocytes. Micron (2016) 84:96–106. doi: 10.1016/j.micron.2016.03.003
92. Nguyen DH, Niu D, Chen Z, Peng M, Tran NT, Li J. Classification and Morphology of Circulating Haemocytes in the Razor Clam Sinonovacula constricta. Aquac Fish (2022) 7:52–8. doi: 10.1016/j.aaf.2020.06.007
93. Zhang W, Wu X, Wang M. Morphological, Structural, and Functional Characterization of the Haemocytes of the Scallop, Argopecten irradians. Aquaculture (2006) 251:19–32. doi: 10.1016/j.aquaculture.2005.05.020
94. González M, Arenas G. Caracterización De La Respuesta Inmune En El Ostión Del Norte Argopecten purpuratus (Lamarck, 1819) (Mollusca: Bivalvia). Cienc Mar (2002) 28:247–55. doi: 10.7773/cm.v28i3.227
95. Xing J, Bin ZW, Zhou L. Endoenzymes Associated With Haemocyte Types in the Scallop (Chlamys farreri). Fish Shellfish Immunol (2002) 13:271–8. doi: 10.1006/fsim.2001.0402
96. Zhang W, Wu X, Sun J, Li D, Zhang W, Wu X, et al. Morphological and Functional Characterization of the Hemocytes of the Scallop, Chlamys farreri. J Shellfish Res (2005) 24:931–6. doi: 10.2983/0730-8000(2005)24[931:mafcot]2.0.co;2
97. Schleder DD, Kayser M, Sühnel S, Ferreira JF, Rupp GS, Barracco MA. Evaluation of Hemato-Immunological Parameters During the Reproductive Cycle of the Scallop Nodipecten nodosus in Association With a Carotenoid-Enriched Diet. Aquaculture (2008) 280:256–63. doi: 10.1016/j.aquaculture.2008.05.001
98. Estrada N, Velázquez E, Rodríguez-Jaramillo C, Ascencio F. Morphofunctional Study of Hemocytes From Lions-Paw Scallop Nodipecten subnodosus. Immunobiology (2013) 218:1093–103. doi: 10.1016/j.imbio.2013.03.004
99. Caza F, Betoulle S, Auffret M, Brousseau P, Fournier M, St-Pierre Y. Comparative Analysis of Hemocyte Properties From Mytilus edulis desolationis and Aulacomya ater in the Kerguelen Islands. Mar Environ Res (2015) 110:174–82. doi: 10.1016/j.marenvres.2015.09.003
100. Kadar E. Haemocyte Response Associated With Induction of Shell Regeneration in the Deep-Sea Vent Mussel Bathymodiolus azoricus (Bivalvia: Mytilidae). J Exp Mar Bio Ecol (2008) 362:71–8. doi: 10.1016/j.jembe.2008.05.014
101. Bettencourt R, Dando P, Collins P, Costa V, Allam B, Serrão Santos R. Innate Immunity in the Deep Sea Hydrothermal Vent Mussel Bathymodiolus azoricus. Comp Biochem Physiol A (2009) 152:278–89. doi: 10.1016/j.cbpa.2008.10.022
102. Tame A, Yoshida T, Ohishi K, Maruyama T. Phagocytic Activities of Hemocytes From the Deep-Sea Symbiotic Mussels Bathymodiolus japonicus, B. Platifrons, and B. Septemdierum. Fish Shellfish Immunol (2015) 45:146–56. doi: 10.1016/j.fsi.2015.03.020
103. Parisi M, Vizzini A, Toubiana M, Sarà G, Cammarata M. Identification, Cloning and Environmental Factors Modulation of a αβ Defensin From the Lessepsian Invasive Mussel Brachidontes pharaonis (Bivalvia: Mytilidae). Invertebr Surviv J (2015) 12:264–73.
104. Anisimova AA. Flow Cytometric and Light Microscopic Identification of Hemocyte Subpopulations in Modiolus kurilensis (Bernard, 1983) (Bivalvia: Mytilidae). Russ J Mar Biol (2012) 38:406–15. doi: 10.1134/S1063074012050021
105. Anisimova AA, Chaika VV, Kuznetsov VL, Golokhvast KS. Study of the Influence of Multiwalled Carbon Nanotubes (12–14 Nm) on the Main Target Tissues of the Bivalve Modiolus modiolus. Nanotechnol Russ (2015) 10:278–87. doi: 10.1134/S1995078015020020
106. de Oliveira David JA, Salaroli RB, Fontanetti CS. Fine Structure of Mytella falcata (Bivalvia) Gill Filaments. Micron (2008) 39:329–36. doi: 10.1016/j.micron.2007.06.002
107. Hong H-K, Kim CW, Kim J-H, Kajino N, Choi K-S. Effect of Extreme Heatwaves on the Mortality and Cellular Immune Responses of Purplish Bifurcate Mussel Mytilisepta virgata (Wiegmann, 1837) (=Septifer virgatus) in Indoor Mesocosm Experiments. Front Mar Sci (2021) 8:794168. doi: 10.3389/fmars.2021.794168
108. Bayne CJ, Moore MN, Carefoot TH, Thompson RJ. Hemolymph Functions in Mytilus californianus: The Cytochemistry of Hemocytes and Their Responses to Foreign Implants and Hemolymph Factors in Phagocytosis. J Invertebr Pathol (1979) 34:1–20. doi: 10.1016/0022-2011(79)90048-X
109. Astuya A, Carrera C, Ulloa V, Aballay A, Núñez-Acuña G, Hégaret H, et al. Saxitoxin Modulates Immunological Parameters and Gene Transcription in Mytilus chilensis Hemocytes. Int J Mol Sci (2015) 16:15235–50. doi: 10.3390/ijms160715235
110. Moore MN, Lowe DM. The Cytology and Cytochemistry of the Hemocytes of Mytilus edulis and Their Responses to Experimentally Injected Carbon Particles. J Invertebr Pathol (1977) 29:18–30. doi: 10.1016/0022-2011(77)90167-7
111. Pipe RK, Farley SR, Coles JA. The Separation and Characterisation of Haemocytes From the Mussel Mytilus edulis. Cell Tissue Res (1997) 289:537–45. doi: 10.1007/s004410050899
112. Le Foll F, Rioult D, Boussa S, Pasquier J, Dagher Z, Leboulenger F. Characterisation of Mytilus edulis Hemocyte Subpopulations by Single Cell Time-Lapse Motility Imaging. Fish Shellfish Immunol (2010) 28:372–86. doi: 10.1016/j.fsi.2009.11.011
113. Carballal M, Lopez M, Azevedo C, Villalba A. Hemolymph Cell Types of the Mussel Mytilus galloprovincialis. Dis Aquat Organ (1997) 29:127–35. doi: 10.3354/dao029127
114. García-García E, Prado-Álvarez M, Novoa B, Figueras A, Rosales C. Immune Responses of Mussel Hemocyte Subpopulations Are Differentially Regulated by Enzymes of the PI 3-K, PKC, and ERK Kinase Families. Dev Comp Immunol (2008) 32:637–53. doi: 10.1016/j.dci.2007.10.004
115. Andreyeva AY, Efremova ES, Kukhareva TA. Morphological and Functional Characterization of Hemocytes in Cultivated Mussel (Mytilus galloprovincialis) and Effect of Hypoxia on Hemocyte Parameters. Fish Shellfish Immunol (2019) 89:361–7. doi: 10.1016/j.fsi.2019.04.017
116. Vassilenko E, Baldwin SA. Using Flow Cytometry to Detect Haemic Neoplasia in Mussels (Mytilus trossulus) From the Pacific Coast of Southern British Columbia, Canada. J Invertebr Pathol (2014) 117:68–72. doi: 10.1016/j.jip.2014.02.002
117. Yang HS, Hong HK, Donaghy L, Noh CH, Park HS, Kim DS, et al. Morphology and Immune-Related Activities of Hemocytes of the Mussel Mytilus coruscus (Gould, 1861) From East Sea of Korea. Ocean Sci J (2015) 50:77–85. doi: 10.1007/s12601-015-0006-4
118. Chandurvelan R, Marsden ID, Gaw S, Glover CN. Waterborne Cadmium Impacts Immunocytotoxic and Cytogenotoxic Endpoints in Green-Lipped Mussel, Perna canaliculus. Aquat Toxicol (2013) 142–143:283–93. doi: 10.1016/j.aquatox.2013.09.002
119. Rolton A, Ragg NLC. Green-Lipped Mussel (Perna canaliculus) Hemocytes: A Flow Cytometric Study of Sampling Effects, Sub-Populations and Immune-Related Functions. Fish Shellfish Immunol (2020) 103:181–9. doi: 10.1016/j.fsi.2020.05.019
120. Barracco MA, Medeiros ID, Moreira FM. Some Haemato-Immunological Parameters in the Mussel Perna perna. Fish Shellfish Immunol (1999) 9:387–404.
121. Fonseca VB, Cruz BP, Silveira da Silva S, Soares MP, Cañedo AD, Vargas MA, et al. Morphological Characterization of Hemocytes of the Brown Mussel Perna perna: An Update. Fish Shellfish Immunol (2022) 120:139–41. doi: 10.1016/j.fsi.2021.11.030
122. Donaghy L, Volety AK. Functional and Metabolic Characterization of Hemocytes of the Green Mussel, Perna viridis: In Vitro Impacts of Temperature. Fish Shellfish Immunol (2011) 31:808–14. doi: 10.1016/j.fsi.2011.07.018
123. Wang Y, Hu M, Chiang MWL, Shin PKS, Cheung SG. Characterization of Subpopulations and Immune-Related Parameters of Hemocytes in the Green-Lipped Mussel Perna viridis. Fish Shellfish Immunol (2012) 32:381–90. doi: 10.1016/j.fsi.2011.08.024
124. Romero A, Aranguren R, Moreira R, Novoa B, Figueras A. Integrated Transcriptomic and Functional Immunological Approach for Assessing the Invasiveness of Bivalve Alien Species. Sci Rep (2019) 9:1–13. doi: 10.1038/s41598-019-56421-y
125. Evariste L, David E, Cloutier PL, Brousseau P, Auffret M, Desrosiers M, et al. Field Biomonitoring Using the Zebra Mussel Dreissena polymorpha and the Quagga Mussel Dreissena bugensis Following Immunotoxic Reponses. Is There a Need to Separate the Two Species? Environ Pollut (2018) 238:706–16. doi: 10.1016/j.envpol.2018.03.098
126. Giamberini L, Auffret M, Pihan JC. Haemocytes of the Freshwater Mussel, Dreissena polymorpha Pallas: Cytology, Cytochemistry and X-Ray Microanalysis. J Molluscan Stud (1996) 62:367–79. doi: 10.1093/mollus/62.3.367
127. Evariste L, Auffret M, Audonnet S, Geffard A, David E, Brousseau P, et al. Functional Features of Hemocyte Subpopulations of the Invasive Mollusk Species Dreissena polymorpha. Fish Shellfish Immunol (2016) 56:144–54. doi: 10.1016/j.fsi.2016.06.054
128. Castro JM, Bianchi VA, Pascual MM, Almeida C, Venturino A, Luquet CM. Immune and Biochemical Responses in Hemolymph and Gills of the Patagonian Freshwater Mussel Diplodon chilensis, Against Two Microbiological Challenges: Saccharomyces cerevisiae and Escherichia coli. J Invertebr Pathol (2018) 157:36–44. doi: 10.1016/j.jip.2018.08.005
129. Montalvão MF, Chagas TQ, da Silva Alvarez TG, Mesak C, da Costa Araújo AP, Gomes AR, et al. How Leachates From Wasted Cigarette Butts Influence Aquatic Life? A Case Study on Freshwater Mussel Anodontites trapesiali. Sci Total Environ (2019) 689:381–9. doi: 10.1016/j.scitotenv.2019.06.385
130. Valentine KH. Methods in Health Assessment of Freshwater Mussels, Amblema plicata and Quadrula Spp. (2011). Available at: http://rave.ohiolink.edu/etdc/view?acc_num=osu1293654676 (Accessed May 28, 2020).
131. Steinagel AC, Burkhard MJ, Kuehnl KF, Thomas Watters G, Rajala-Schultz PJ, Valentine KH, et al. Hematological and Biochemical Assessment of Two Species of Freshwater Mussels, Quadrula quadrula and Amblema plicata, Following Translocation. J Aquat Anim Health (2018) 30:119–29. doi: 10.1002/aah.10016
132. Hinzmann M, Lopes-Lima M, Cerca F, Correia A, Machado J, Vilanova M. Identification of Distinct Haemocyte Populations From the Freshwater Bivalves Swan Mussel (Anodonta cygnea) and Duck Mussel (Anodonta anatina) Using Wheat-Germ Agglutinin. Can J Zool (2017) 95:937–47. doi: 10.1139/cjz-2017-0006
133. Soares-Da-Silva IM, Ribeiro J, Valongo C, Pinto R, Vilanova M, Bleher R, et al. Cytometric, Morphologic and Enzymatic Characterisation of Haemocytes in Anodonta cygnea. Comp Biochem Physiol A (2002) 132:541–53. doi: 10.1016/S1095-6433(02)00039-9
134. Salimi L, Jamili S, Motalebi A, Eghtesadi-Araghi P, Rabbani M, Rostami-Beshman M. Morphological Characterization and Size of Hemocytes in Anodonta cygnea. J Invertebr Pathol (2009) 101:81–5. doi: 10.1016/j.jip.2009.03.003
135. Shi A, Qiu A, Tang M, Yu Y, Zhang H, Machii A. Hemoculture of Anodonta woodiana pacifica. Acta Hydrobiol Sin (2001) 25:116–22.
136. Xie Y, Hu B, Wen C, Mu S. Morphology and Phagocytic Ability of Hemocytes From Cristaria plicata. Aquaculture (2011) 310:245–51. doi: 10.1016/j.aquaculture.2010.09.034
137. François G, Mélanie D, Marlène F, Michel F. Effects of a Municipal Effluent on the Freshwater Mussel Elliptio complanata Following Challenge With Vibrio anguillarum. J Environ Sci (China) (2015) 37:91–9. doi: 10.1016/j.jes.2015.03.029
138. Soimalaitong S, Srakaew N, Kovitvadhi U, Kovitvadhi S. Morphology of the Hemocytes in the Freshwater Pearl Mussel Hyriopsis bialata Simpson, 1900. J Kasetsart Vet (2018) 28:29–38.
139. Das K, Ray M, Ray S. Cypermethrin Induced Dynamics of Hemocyte Density of Indian Mollusc Lamellidens marginalis. Anim Biol J (2012) 3:39–49. doi: 10.1002/9783527678679.dg02126
140. Ray M, Bhunia NS, Bhunia AS, Ray S. A Comparative Analyses of Morphological Variations, Phagocytosis and Generation of Cytotoxic Agents in Flow Cytometrically Isolated Hemocytes of Indian Molluscs. Fish Shellfish Immunol (2013) 34:244–53. doi: 10.1016/j.fsi.2012.11.006
141. Shiver MA. Reproduction and Propagation of the Neosho Mucket, Lampsilis rafinesqueana. Cape Girardeau (MO: Southwest Missouri State University (2002). [Dissertation/Naster’s Thesis].
142. Burkhard MJ, Leavell S, Weiss RB, Kuehnl K, Valentine H, Watters GT, et al. Analysis and Cytologic Characterization of Hemocytes From Freshwater Mussels (Quadrula Sp.). Vet Clin Pathol (2009) 38:426–36. doi: 10.1111/j.1939-165X.2009.00148.x
143. Li Q, Zhang G, Wei K, Wang Y, Guo X, Chen Z, et al. A Preliminary Study on Morphology and Phagocytic Ability of Hemocytes From Solenaia oleivora. J Hidroecol (2012) 33:116–21.
144. Guo L, Sheng J-Q, Hong Y-J, Wang Y-F, Guo H-J, Wang J-H. Microscopic Observing on Hemocytes of Hyriopsis schlegeli. Acta Hydrobiol Sin (2008) 32:840–44. doi: 10.3724/SP.J.1035.2008.00839
145. Li W, Shi Z, He X. Study on Immune Regulation in Hyriopsis cumingii Lea: Effect of Pearl-Nucleus Insertion in the Visceral Mass on Immune Factors Present in the Hemolymph. Fish Shellfish Immunol (2010) 28:789–94. doi: 10.1016/j.fsi.2010.01.019
146. Yang Q, Yu X, Du C, Ni X, Li W, Yao W, et al. Bacterial Challenge Undermines the Innate Immune Response in Hyriopsis cumingii. Aquaculture (2021) 530:735783. doi: 10.1016/j.aquaculture.2020.735783
147. Beggel S, Hinzmann M, Machado J, Geist J. Combined Impact of Acute Exposure to Ammonia and Temperature Stress on the Freshwater Mussel Unio pictorum. Water (Switzerland) (2017) 9:3–5. doi: 10.3390/w9070455
148. Hong H-K, Kang H-S, Le TC, Choi K-S. Comparative Study on the Hemocytes of Subtropical Oysters Saccostrea kegaki (Torigoe & Inaba, 1981), Ostrea circumpicta (Pilsbry, 1904), and Hyotissa hyotis (Linnaeus, 1758) in Jeju Island, Korea: Morphology and Functional Aspects. Fish Shellfish Immunol (2013) 35:2020–5. doi: 10.1016/j.fsi.2013.09.022
149. Queiroga FR, Marques-Santos LF, Hégaret H, Soudant P, Farias ND, Schlindwein AD, et al. Immunological Responses of the Mangrove Oysters Crassostrea gasar Naturally Infected by Perkinsus Sp. In the Mamanguape Estuary, Paraíba State (Northeastern, Brazil). Fish Shellfish Immunol (2013) 35:319–27. doi: 10.1016/j.fsi.2013.04.034
150. Sun J, Wu X, Zhang W. Morphological, Structural and Functional Characteristics of the Hemocytes of the Oyster, Crassostrea ariakensis. J Shellfish Res (2006) 25:55–64. doi: 10.2983/0730-8000(2006)25[55:MSAFCO]2.0.CO;2
151. Donaghy L, Kim BK, Hong HK, Park HS, Choi KS. Flow Cytometry Studies on the Populations and Immune Parameters of the Hemocytes of the Suminoe Oyster, Crassostrea ariakensis. Fish Shellfish Immunol (2009) 27:296–301. doi: 10.1016/j.fsi.2009.05.010
152. Hurtado MA, Ramírez JL, Rodríguez-Jaramillo C, Tovar D, Ibarra AM, Soudant P, et al. Comparison of Continuous and Batch Feeding Systems on Maturation, Biochemical Composition and Immune Variables of the Oyster Crassostrea corteziensis (Hertlein 1951). Aquac Res (2009) 40:464–72. doi: 10.1111/j.1365-2109.2008.02117.x
153. Auffret M. Comparative Study of the Hemocytes of Two Oyster Species: The European Flat Oyster, Ostrea edulis, Linnaeus, 1750 and the Pacific Oyster, Crassostrea gigas (Thunberg, 1793). J Shellfish Res (1989) 8:367–73.
154. Lambert C, Soudant P, Choquet G, Paillard C. Measurement of Crassostrea gigas Hemocyte Oxidative Metabolism by Flow Cytometry and the Inhibiting Capacity of Pathogenic Vibrios. Fish Shellfish Immunol (2003) 15:225–40. doi: 10.1016/S1050-4648(02)00160-2
155. Donaghy L, Hong H-K, Lee H-J, Jun J-C, Park Y-J, Choi K-S. Hemocyte Parameters of the Pacific Oyster Crassostrea gigas a Year After the Hebei Spirit Oil Spill Off the West Coast of Korea. Helgol Mar Res (2010) 64:349–55. doi: 10.1007/s10152-010-0190-7
156. Wang W, Li M, Wang L, Chen H, Liu Z, Jia Z, et al. The Granulocytes Are the Main Immunocompetent Hemocytes in Crassostrea gigas. Dev Comp Immunol (2017) 67:221–8. doi: 10.1016/j.dci.2016.09.017
157. Li J, Zhang Y, Mao F, Lin Y, Xiao S, Xiang Z, et al. The First Morphologic and Functional Characterization of Hemocytes in Hong Kong Oyster, Crassostrea hongkongensis. Fish Shellfish Immunol (2018) 81:423–9. doi: 10.1016/j.fsi.2018.05.062
158. Luo Y, Wang W-X. Immune Responses of Oyster Hemocyte Subpopulations to In Vitro and In Vivo Zinc Exposure. Aquat Toxicol (2021) 242:106022. doi: 10.1016/j.aquatox.2021.106022
159. Ittoop G, George KC, Sanil NK, George RM, Sobhana KS, Nisha PC. Characterization of Haemocytes of the Indian Edible Oyster, Crassostrea madrasensis (Preston). Aquac Res (2006) 37:1636–43. doi: 10.1111/j.1365-2109.2006.01606.x
160. Hong H-K, Donaghy L, Choi K-S. Flow Cytometric Studies on the Morphology and Immunological Functions of Hemocytes in the Iwagaki Oyster Crassostrea nippona. Fish Sci (2014) 80:969–76. doi: 10.1007/s12562-014-0777-z
161. Barth T, Moraes N, Barracco MA. Evaluation of Some Hemato-Immunological Parameters in the Mangrove Oyster Crassostrea rhizophorae of Different Habitats of Santa Catarina Island, Brazil. Aquat Living Resour (2005) 18:179–86. doi: 10.1051/alr:2005019
162. Rebelo M de F, Figueiredo E de S, Mariante RM, Nóbrega A, de Barros CM, Allodi S. New Insights From the Oyster Crassostrea rhizophorae on Bivalve Circulating Hemocytes. PloS One (2013) 8:e57384. doi: 10.1371/journal.pone.0057384
163. Lin T, Zhang D, Lai Q, Sun M, Quan W, Zhou K. A Modified Method to Detect the Phagocytic Ability of Eosinophilic and Basophilic Haemocytes in the Oyster Crassostrea plicatula. Fish Shellfish Immunol (2014) 40:337–43. doi: 10.1016/j.fsi.2014.07.016
164. Foley DA, Cheng TC. Interaction of Molluscs and Foreign Substances: The Morphology and Behavior of Hemolymph Cells of the American Oyster, Crassostrea virginica, In Vitro. J Invertebr Pathol (1972) 19:383–94. doi: 10.1016/0022-2011(72)90238-8
165. Allam B, Ashton-Alcox KA, Ford SE. Flow Cytometric Comparison of Haemocytes From Three Species of Bivalve Molluscs. Fish Shellfish Immunol (2002) 13:141–58. doi: 10.1006/fsim.2001.0389
166. Hégaret H, Wikfors GH, Soudant P. Flow-Cytometric Analysis of Haemocytes From Eastern Oysters, Crassostrea virginica, Subjected to a Sudden Temperature Elevation I. Haemocyte Types and Morphology. J Exp Mar Bio Ecol (2003) 293:237–48. doi: 10.1016/S0022-0981(03)00236-3
167. Goedken M, De Guise S. Flow Cytometry as a Tool to Quantify Oyster Defence Mechanisms. Fish Shellfish Immunol (2004) 16:539–52. doi: 10.1016/j.fsi.2003.09.009
168. Hine PM, Wesney B. Interaction of Phagocytosed Bonamia Sp. (Haplosporidia) With Haemocytes of Oysters Tiostrea chilensis. Dis Aquat Organ (1994) 20:219–29. doi: 10.3354/dao020219
169. Rolton A, Delisle L, Berry J, Venter L, Webb SC, Adams S, et al. Flow Cytometric Characterization of Hemocytes of the Flat Oyster, Ostrea chilensis. Fish Shellfish Immunol (2020) 97:411–20. doi: 10.1016/j.fsi.2019.12.071
170. Xue QG, Renault T, Chilmonczyk S. Flow Cytometric Assessment of Haemocyte Sub-Populations in the European Flat Oyster, Ostrea edulis, Haemolymph. Fish Shellfish Immunol (2001) 11:557–67. doi: 10.1006/fsim.2001.0335
171. Comesaña P, Casas SM, Cao A, Abollo E, Arzul I, Morga B, et al. Comparison of Haemocytic Parameters Among Flat Oyster Ostrea edulis Stocks With Different Susceptibility to Bonamiosis and the Pacific Oyster Crassostrea gigas. J Invertebr Pathol (2012) 109:274–86. doi: 10.1016/j.jip.2011.12.007
172. Hine PM, Thorne T. Haplosporidium Sp. (Alveolata: Haplosporidia) Associated With Mortalities Among Rock Oysters Saccostrea cuccullata in North Western Australia. Dis Aquat Organ (2002) 51:123–33. doi: 10.3354/dao051123
173. Aladaileh S, Nair SV, Birch D, Raftos DA. Sydney Rock Oyster (Saccostrea glomerata) Hemocytes: Morphology and Function. J Invertebr Pathol (2007) 96:48–63. doi: 10.1016/j.jip.2007.02.011
174. Dang C, Lambert C, Soudant P, Delamare-Deboutteville J, Chan J, Zhang MM, et al. Immune Parameters of QX-Resistant and Wild Caught Saccostrea glomerata Hemocytes in Relation to Marteilia sydneyi Infection. Fish Shellfish Immunol (2011) 31:1034–40. doi: 10.1016/j.fsi.2011.09.003
175. Kuchel RP, Raftos DA, Birch D, Vella N. Haemocyte Morphology and Function in the Akoya Pearl Oyster, Pinctada imbricata. J Invertebr Pathol (2010) 105:36–48. doi: 10.1016/j.jip.2010.04.011
176. Li S, Liu Y, Liu C, Huang J, Zheng G, Xie L, et al. Morphology and Classification of Hemocytes in Pinctada fucata and Their Responses to Ocean Acidification and Warming. Fish Shellfish Immunol (2015) 45:194–202. doi: 10.1016/j.fsi.2015.04.006
177. Tan TLS, Paul-Pont I, Evans OM, Watterson D, Young P, Whittington R, et al. Resistance of Black-Lip Learl Oyster, Pinctada margaritifera, to Infection by Ostreid Herpes Virus 1μvar Under Experimental Challenge may be Mediated by Humoral Antiviral Activity. Fish Shellfish Immunol (2015) 44:232–40. doi: 10.1016/j.fsi.2015.02.026
178. Henry M, Vicente N, Houache N. Caracterisation Des Hemocytes D’’un Mollusque Bivalve Marin, La Nacre, Pinna nobilis L. 1758. In: Aspects Récents De La Biologie Des Mollusques. Société Française De Malacologie. Actes De Colloques 13. Brest, France: Ifremer (1992). p. 97–106.
179. Matozzo V, Pagano M, Spinelli A, Caicci F, Faggio C. Pinna nobilis: A Big Bivalve With Big Haemocytes? Fish Shellfish Immunol (2016) 55:529–34. doi: 10.1016/j.fsi.2016.06.039
180. Vieira GC, da Silva PM, Barracco MA, Hering AF, de Albuquerque MCP, Coelho JR, et al. Morphological and Functional Characterization of the Hemocytes From the Pearl Oyster Pteria hirundo and Their Immune Responses Against Vibrio Infections. Fish Shellfish Immunol (2017) 70:750–8. doi: 10.1016/j.fsi.2017.09.040
181. Carballal M, Lopez M, Azevedo C, Villalba A. In Vitro Study of Phagocytic Ability of Mytilus galloprovincialis Lmk. Haemocytes. Fish Shellfish Immunol (1997) 7:403–16. doi: 10.1006/fsim.1997.0094
182. Meng J, Zhang G, Wang W. Functional Heterogeneity of Immune Defenses in Molluscan Oysters Crassostrea hongkongensis Revealed by High-Throughput Single-Cell Transcriptome. Fish Shellfish Immunol (2022) 120:202–13. doi: 10.1016/j.fsi.2021.11.027
183. Karetin YA, Kalitnik AA, Safonova AE, Cicinskas E. Description and Classification of Bivalve Mollusks Hemocytes: A Computational Approach. PeerJ (2019) 2019:1–15. doi: 10.7717/peerj.7056
184. Karetin YA, Pimenova EA, Kalitnik AA. A Comparative Morphological Classification of Marine Invertebrate Immune Cells. Russ J Mar Biol (2020) 46:1–11. doi: 10.1134/S1063074020010034
185. Santarem M, Robledo J, Figueras A. Seasonal Changes in Hemocytes and Serum Defense Factors in the Blue Mussel Mytilus galloprovincialis. Dis Aquat Organ (1994) 18:217–22. doi: 10.3354/dao018217
186. Ashton-Alcox KA, Ford SE. Variability in Molluscan Hemocytes: A Flow Cytometric Study. Tissue Cell (1998) 30:195–204. doi: 10.1016/S0040-8166(98)80068-2
187. Soudant P, Paillard C, Choquet G, Lambert C, Reid HI, Marhic A, et al. Impact of Season and Rearing Site on the Physiological and Immunological Parameters of the Manila Clam Venerupis (=Tapes, =Ruditapes) philippinarum. Aquaculture (2004) 229:401–18. doi: 10.1016/S0044-8486(03)00352-1
188. Matozzo V, Marin MG. First Evidence of Gender-Related Differences in Immune Parameters of the Clam Ruditapes philippinarum (Mollusca, Bivalvia). Mar Biol (2010) 157:1181–9. doi: 10.1007/s00227-010-1398-4
189. Mosca F, Narcisi V, Cargini D, Calzetta A, Tiscar PG. Age Related Properties of the Adriatic Clam Chamelea gallina (L. 1758) Hemocytes. Fish Shellfish Immunol (2011) 31:1106–12. doi: 10.1016/j.fsi.2011.09.017
190. Hong H-K, Choi K-S. Temporal Changes in Hemocyte Functions of the Oyster Saccostrea kegaki (Torigoe & Inaba, 1981) on Jeju Island Off the South Coast of Korea Are Closely Associated With Annual Gametogenesis. Mar Pollut Bull (2020) 152:110780. doi: 10.1016/j.marpolbul.2019.110780
191. Allam B, Ashton-Alcox KA, Ford SE. Haemocyte Parameters Associated With Resistance to Brown Ring Disease in Ruditapes Spp. Clams. Dev Comp Immunol (2001) 25:365–75. doi: 10.1016/S0145-305X(00)00072-0
192. Ladhar-Chaabouni R, Ayadi W, Sahli E, Mokdad-Gargouri R. Establishment of Primary Cell Culture of Ruditapes decussatus Haemocytes for Metal Toxicity Assessment. Vitr Cell Dev Biol - Anim (2021) 57:477–84. doi: 10.1007/s11626-021-00561-x
193. Mortensen S, Glette J. Phagocytic Activity of Scallop (Pecten maximus) Haemocytes Maintained In vitro. Fish Shellfish Immunol (1996) 6:111–21. doi: 10.1006/fsim.1996.0012
194. Sasakawa S, Walter H. Blood Clam (Anadara inflata) Red Cells. Partition in Aqueoues Two-Polymer Phase Systems. Biochim Biophys Acta - Gen Subj (1971) 244:452–60. doi: 10.1016/0304-4165(71)90249-2
195. Dam T, Sarkar M, Ghosal J, Choudhury A. A Novel Galactosyl-Binding Lectin From the Plasma of the Blood Clam, Anadara granosa (L) and a Study of Its Combining Site. Mol Cell Biochem (1992) 117:1–9. doi: 10.1007/BF00230405
196. Foley DA, Cheng TC. Morphology, Hematologic Parameters, and Behavior of Hemolymph Cells of the Quahaug Clam, Mercenaria mercenaria. Biol Bull (1974) 146:343–56. doi: 10.2307/1540410
197. McCormick-Ray MG, Howard T. Morphology and Mobility of Oyster Hemocytes: Evidence for Seasonal Variations. J Invertebr Pathol (1991) 58:219–30. doi: 10.1016/0022-2011(91)90066-Y
198. Pila EA, Sullivan JT, Wu XZ, Fang J, Rudko SP, Gordy MA, et al. Haematopoiesis in Molluscs: A Review of Haemocyte Development and Function in Gastropods, Cephalopods and Bivalves. Dev Comp Immunol (2016) 58:119–28. doi: 10.1016/j.dci.2015.11.010
199. Mix M. A General Model for Leukocyte Cell Renewal in Bivalve Mollusks. Mar Fish Rev (1976) 38:37–41.
200. Ottaviani E, Franchini A, Barbieri D, Kletsas D. Comparative and Morphofunctional Studies on Mytilus galloprovincialis Hemocytes: Presence of Two Aging-Related Hemocyte Stages. Ital J Zool (1998) 65:349–54. doi: 10.1080/11250009809386772
201. Matozzo V, Marin M, Cima F, Ballarin L. First Evidence of Cell Division in Circulating Haemocytes From the Manila Clam Tapes philippinarum. Cell Biol Int (2008) 32:865–8. doi: 10.1016/j.cellbi.2008.03.008
202. Johansson MW, Keyser P, Sritunyalucksana K, Söderhäll K. Crustacean Haemocytes and Haematopoiesis. Aquaculture (2000) 191:45–52. doi: 10.1016/S0044-8486(00)00418-X
203. Meister M. Blood Cells of Drosophila: Cell Lineages and Role in Host Defence. Curr Opin Immunol (2004) 16:10–5. doi: 10.1016/j.coi.2003.11.002
204. Wikfors GH, Alix JH. Granular Hemocytes Are Phagocytic, But Agranular Hemocytes Are Not, in the Eastern Oyster Crassostrea virginica. Invertebr Immun (2014) 1:15–21. doi: 10.2478/invim-2014-0001
205. Bassim S, Genard B, Gauthier-Clerc S, Moraga D, Tremblay R. Ontogeny of Bivalve Immunity: Assessing the Potential of Next-Generation Sequencing Techniques. Rev Aquac (2014) 7:197–217. doi: 10.1111/raq.12064
206. Dyachuk VA. Hematopoiesis in Bivalvia Larvae: Cellular Origin, Differentiation of Hemocytes, and Neoplasia. Dev Comp Immunol (2016) 65:253–7. doi: 10.1016/j.dci.2016.07.019
207. Elston R. Functional Morphology of the Coelomocytes of the Larval Oysters (Crassostrea virginica and Crassostrea gigas). J Mar Biol Assoc UK (1980) 60:947–57. doi: 10.1017/S0025315400042004
208. Barreau-Roumiguière C, Montagnani C, Escoubas JM. Characterization of a Tal/SCL-Like Transcription Factor in the Pacific Oyster Crassostrea gigas. Dev Comp Immunol (2003) 27:793–800. doi: 10.1016/S0145-305X(03)00075-2
209. Tirapé A, Bacque C, Brizard R, Vandenbulcke F, Boulo V. Expression of Immune-Related Genes in the Oyster Crassostrea gigas During Ontogenesis. Dev Comp Immunol (2007) 31:859–73. doi: 10.1016/j.dci.2007.01.005
210. Jemaa M, Morin N, Cavelier P, Cau J, Strub J-MM, Delsert C, et al. Adult Somatic Progenitor Cells and Hematopoiesis in Oysters. J Exp Biol (2014) 217:3067–77. doi: 10.1242/jeb.106575
211. Li Y, Song X, Wang W, Wang L, Yi Q, Jiang S, et al. The Hematopoiesis in Gill and Its Role in the Immune Response of Pacific Oyster Crassostrea gigas Against Secondary Challenge With Vibrio splendidus. Dev Comp Immunol (2017) 71:59–69. doi: 10.1016/j.dci.2017.01.024
212. Cuénot L. Le Sang Et Les Glandes Lymphatiques. In: Lacaze-Duthiers H, editor. Archives De Zoologie Expérimentale Et Générale. Paris, France: Académie des Sciences (1981). p. 13–90.
213. Jia Z, Jiang S, Wang M, Wang X, Liu Y, Lv Z, et al. Identification of a Novel Pattern Recognition Receptor DM9 Domain Containing Protein 4 as a Marker for Pro-Hemocyte of Pacific Oyster Crassostrea gigas. Front Immunol (2021) 11:603270. doi: 10.3389/fimmu.2020.603270
214. Zhang T, Qiu L, Sun Z, Wang L, Zhou Z, Liu R, et al. The Specifically Enhanced Cellular Immune Responses in Pacific Oyster (Crassostrea gigas) Against Secondary Challenge With Vibrio Splendidus. Dev Comp Immunol (2014) 45:141–50. doi: 10.1016/j.dci.2014.02.015
215. Bachère E, Gueguen Y, Gonzalez M, de Lorgeril J, Garnier J, Romestand B. Insights Into the Anti-Microbial Defense of Marine Invertebrates: The Penaeid Shrimps and the Oyster Crassostrea gigas. Immunol Rev (2004) 198:149–68. doi: 10.1111/j.0105-2896.2004.00115.x
216. Mao F, Wong N, Lin Y, Zhang X, Liu K. Transcriptomic Evidence Reveals the Molecular Basis for Functional Differentiation of Hemocytes in a Marine Invertebrate, Crassostrea gigas. Front Immunol (2020) 11:911. doi: 10.3389/fimmu.2020.00911
217. Yue F, Zhou Z, Wang L, Sun R, Jiang Q, Yi Q, et al. The Essential Roles of Core Binding Factors CfRunt and Cfcbfβ in Hemocyte Production of Scallop Chlamys farreri. Dev Comp Immunol (2014) 44:291–302. doi: 10.1016/j.dci.2014.01.008
218. Smith VJ, Accorsi A, Malagoli D. Hematopoiesis and Hemocytes in Pancrustacean and Molluscan Models. In: Malagoli D, editor. The Evolution of the Immune System: Conservation and Diversification. Amsterdam, The Netherlands: Academic Press/Elsevier (2016). p. 1–28. doi: 10.1016/B978-0-12-801975-7.00001-3
219. Song X, Wang H, Chen H, Sun M, Liang Z, Wang L, et al. Conserved Hemopoietic Transcription Factor Cg-SCL Delineates Hematopoiesis of Pacific Oyster Crassostrea gigas. Fish Shellfish Immunol (2016) 51:180–8. doi: 10.1016/j.fsi.2016.02.023
220. Sun W, Song X, Dong M, Liu Z, Song Y, Wang L, et al. DNA Binding Protein CgIkaros-Like Regulates the Proliferation of Agranulocytes and Granulocytes in Oyster (Crassostrea gigas). Dev Comp Immunol (2021) 124:104201. doi: 10.1016/j.dci.2021.104201
221. Parisi MG, Li H, Jouvet LBPP, Dyrynda EA, Parrinello N, Cammarata M, et al. Differential Involvement of Mussel Hemocyte Sub-Populations in the Clearance of Bacteria. Fish Shellfish Immunol (2008) 25:834–40. doi: 10.1016/j.fsi.2008.09.005
222. Donaghy L, Hong H, Park K, Nobuhisa K, Youn S, Kang C, et al. Flow Cytometric Characterization of Hemocytes of the Solitary Ascidian, Halocynthia roretzi. Fish Shellfish Immunol (2017) 66:289–99. doi: 10.1016/j.fsi.2017.05.009
223. Zhu L, Chang Y, Xing J, Tang X, Sheng X, Zhan W. Comparative Proteomic Analysis Between Two Haemocyte Subpopulations in Shrimp Fenneropenaeus chinensis. Fish Shellfish Immunol (2018) 72:325–33. doi: 10.1016/j.fsi.2017.09.074
224. Zhu L, Tang X, Xing J, Sheng X, Zhan W. Differential Proteome of Haemocyte Subpopulations Responded to White Spot Syndrome Virus Infection in Chinese Shrimp Fenneropenaeus chinensis. Dev Comp Immunol (2018) 84:82–93. doi: 10.1016/j.dci.2018.02.003
225. Sun M, Li S, Zhang X, Xiang J, Li F. Isolation and Transcriptome Analysis of Three Subpopulations of Shrimp Hemocytes Reveals the Underlying Mechanism of Their Immune Functions. Dev Comp Immunol (2020) 108:103689. doi: 10.1016/j.dci.2020.103689
226. Cui C, Liang Q, Tang X, Xing J, Sheng X, Zhan W. Differential Apoptotic Responses of Hemocyte Subpopulations to White Spot Syndrome Virus Infection in Fenneropenaeus chinensis. Front Immunol (2020) 11:594390. doi: 10.3389/fimmu.2020.594390
227. Cui C, Zhu L, Tang X, Xing J, Sheng X, Zhan W. Molecular Characterization of Prohibitins and Their Differential Responses to WSSV Infection in Hemocyte Subpopulations of Fenneropenaeus chinensis. Fish Shellfish Immunol (2020) 106:296–306. doi: 10.1016/j.fsi.2020.07.048
228. Koiwai K, Koyama T, Suzuki H, Kawano R, Tsuda S, Toyoda A, et al. Single-Cell RNA-Seq Analysis Reveals Penaeid Shrimp Hemocyte Subpopulations and Cell Differentiation Process. eLife (2021) 10:1–27. doi: 10.7554/eLife.66954
229. Day RD, Fitzgibbon QP, Gardner C. The Impact of Holding Stressors on the Immune Function and Haemolymph Biochemistry of Southern Rock Lobsters (Jasus edwardsii). Fish Shellfish Immunol (2019) 89:660–71. doi: 10.1016/j.fsi.2019.03.043
230. Qyli M, Aliko V, Faggio C. Physiological and Biochemical Responses of Mediterranean Green Crab, Carcinus aestuarii, to Different Environmental Stressors: Evaluation of Hemocyte Toxicity and Its Possible Effects on Immune Response. Comp Biochem Physiol C (2020) 231:108739. doi: 10.1016/j.cbpc.2020.108739
231. Di G, Li Y, Zhao X, Wang N, Fu J, Li M, et al. Differential Proteomic Profiles and Characterizations Between Hyalinocytes and Granulocytes in Ivory Shell Babylonia areolata. Fish Shellfish Immunol (2019) 92:405–20. doi: 10.1016/j.fsi.2019.06.036
232. Di G, Zhu G, Chen X, Miao X, Li M, Fu J, et al. Quantitative Proteomic Analyses Provide Insights Into the Hyalinocytes and Granulocytes Phagocytic Killing of Ivory Shell Babylonia areolata In Vitro. Aquaculture (2021) 542:736898. doi: 10.1016/j.aquaculture.2021.736898
233. Zhang Y, Xu S, Jiang N, Tang H, Dong H, Zhao Q-P. Morphology and Activities of Cell Populations of Haemocytes in Oncomelania hupensis Following Schistosoma japonicum Infection. J Invertebr Pathol (2021) 181:107590. doi: 10.1016/j.jip.2021.107590
234. Bank B, Tawangrejo D, Binangun K. Hemocyte Profile of Susuh Kura (Sulcospira testudinaria) in Order to Evaluate the Water Quality of Badher Bank Conservation Area, Tawangrejo Village, Binangun District, Blitar. JFMR-J Fish Mar Res (2021) 5:106–18. doi: 10.21776/ub.jfmr.2021.005.01.16
235. Hong H, Donaghy L, Choi K. Flow Cytometric Characterization of Hemocytes of the Abalone Haliotis diversicolor (Reeve, 1846) and E Ff Ects of Air Exposure Stresses on Hemocyte Parameters. Aquaculture (2019) 506:401–9. doi: 10.1016/j.aquaculture.2019.04.001
236. Kajino N, Choi K-S, Hong H-K. Flow Cytometric Characterization of the Hemocytes of Sea Hares from Tidal Pools in Jeju Island off the South Coast of Korea. Fish Shellfish Immunol (2022) 122:409–18. doi: 10.1016/j.fsi.2022.02.026.
237. de la Ballina NR, Villalba A, Cao A. Differences in Proteomic Profile Between Two Haemocyte Types, Granulocytes and Hyalinocytes, of the Flat Oyster Ostrea edulis. Fish Shellfish Immunol (2020) 100:456–66. doi: 10.1016/j.fsi.2020.03.033
238. de la Ballina NR, Villalba A, Cao A. Shotgun Analysis to Identify Differences in Protein Expression Between Granulocytes and Hyalinocytes of the European Flat Oyster Ostrea edulis. Fish Shellfish Immunol (2021) 119:678–91. doi: 10.1016/j.fsi.2021.10.045
239. Friedl FE, Alvarez MR, Johnson JS, Gratzner HG. Cytometric Investigations on Hemocytes of the American Oyster, Crassostrea virginica. Tissue Cell (1988) 20:933–9. doi: 10.1016/0040-8166(88)90034-1
241. Feng SY. Pinocytosis of Proteins by Oyster Leucocytes. Biol Bull (1965) 129:95–105. doi: 10.2307/1539770
242. Huang J, Li S, Liu Y, Liu C, Xie L, Zhang R. Hemocytes in the Extrapallial Space of Pinctada fucata Are Involved in Immunity and Biomineralization. Sci Rep (2018) 8:4657. doi: 10.1038/s41598-018-22961-y
243. Friebel B, Renwrantz L. Application of Density Gradient Centrifugation for Separation of Eosinophilic and Basophilic Hemocytes From Mytilus edulis and Characterization of Both Cell Groups. Comp Biochem Physiol A (1995) 112:81–90. doi: 10.1016/0300-9629(95)00086-M
244. Nguyen TV, Alfaro AC. Applications of Omics to Investigate Responses of Bivalve Haemocytes to Pathogen Infections and Environmental Stress. Aquaculture (2020) 518:734488. doi: 10.1016/j.aquaculture.2019.734488
245. Rifkin E, Cheng TC, Hohl HR. An Electron-Microscope Study of the Constituents Encapsulating Cysts in the American Oyster, Crassostrea virginica, Formed in Response to Tylocephalum Metacestodes. J Invertebr Pathol (1969) 14:211–26. doi: 10.1016/0022-2011(69)90108-6
246. Feng SY, Feng JS, Burke CN, Khairallah LH. Light and Electron Microscopy of the Leucocytes of Crassostrea virginica (Mollusca: Pelecypoda). Z. Zellforsch (1971) 245:222–45. doi: 10.1007/BF00335537
247. Terahara K, Takahashi KG, Nakamura A, Osada M, Yoda M, Hiroi T, et al. Differences in Integrin-Dependent Phagocytosis Among Three Hemocyte Subpopulations of the Pacific Oyster “Crassostrea gigas”. Dev Comp Immunol (2006) 30:667–83. doi: 10.1016/j.dci.2005.09.009
248. Takahashi KG, Mori K. Functional Profiles of Hemocytes in Bio-Defense Process of the Pacific Oyster, Crassostrea gigas. Tohoku J Agric Res (2000) 51:15–27. doi: 10.3147/jsfp.35.15
249. Xue Q, Renault T, Cochennec N, Gerard A. Separation of European Flat Oyster, Ostrea edulis, Haemocytes by Density Gradient Centrifugation and SDS-PAGE Characterisation of Separated Haemocyte Sub-Populations. Fish Shellfish Immunol (2000) 10:155–65. doi: 10.1006/fsim.1999.0234
250. Lambert C, Soudant P, Jegaden M, Delaporte M, Labreuche Y, Moal J, et al. In Vitro Modulation of Reactive Oxygen and Nitrogen Intermediate (ROI/RNI) Production in Crassostrea gigas Hemocytes. Aquaculture (2007) 270:413–21. doi: 10.1016/j.aquaculture.2007.04.074
251. Donaghy L, Kraffe E, Le Goïc N, Lambert C, Volety AK, Soudant P. Reactive Oxygen Species in Unstimulated Hemocytes of the Pacific Oyster Crassostrea gigas: A Mitochondrial Involvement. PloS One (2012) 7:e46594. doi: 10.1371/journal.pone.0046594
252. Hégaret H, Wikfors GH, Soudant P. Flow Cytometric Analysis of Haemocytes From Eastern Oysters, Crassostrea virginica, Subjected to a Sudden Temperature Elevation II. Haemocyte Functions: Aggregation, Viability, Phagocytosis, and Respiratory Burst. J Exp Mar Bio Ecol (2003) 293:249–65. doi: 10.1016/S0022-0981(03)00235-1
253. Sussarellu R, Fabioux C, Camacho Sanchez M, Le Goïc N, Lambert C, Soudant P, et al. Molecular and Cellular Response to Short-Term Oxygen Variations in the Pacific Oyster Crassostrea gigas. J Exp Mar Bio Ecol (2012) 412:87–95. doi: 10.1016/j.jembe.2011.11.007
254. Poirier AC, Schmitt P, Rosa RD, Vanhove AS, Kieffer-Jaquinod S, Rubio TP, et al. Antimicrobial Histones and DNA Traps in Invertebrate Immunity: Evidences in Crassostrea gigas. J Biol Chem (2014) 289:24821–31. doi: 10.1074/jbc.M114.576546
255. Han Y, Chen L, Zhang Q, Yu D, Yang D, Zhao J. Hemocyte Extracellular Traps of Manila Clam Ruditapes philippinarum: Production Characteristics and Antibacterial Effects. Dev Comp Immunol (2021) 116:103953. doi: 10.1016/j.dci.2020.103953
256. Han Y, Zhang Q, Chen L, Yang D, Zhao J. Mitochondria Are Essential for Antibacterial Extracellular Trap Formation Mediated by Zymosan in Hemocytes of Ruditapes philippinarum. Dev Comp Immunol (2021) 121:104094. doi: 10.1016/j.dci.2021.104094
257. Romero A, Novoa B, Figueras A. Extracellular Traps (ETosis) can be Activated Through NADPH-Dependent and -Independent Mechanisms in Bivalve Mollusks. Dev Comp Immunol (2020) 106:103585. doi: 10.1016/j.dci.2019.103585
258. Auguste M, Balbi T, Ciacci C, Canesi L. Conservation of Cell Communication Systems in Invertebrate Host–Defence Mechanisms: Possible Role in Immunity and Disease. Biol (Basel) (2020) 9:1–14. doi: 10.3390/biology9080234
259. Han Y, Hu G, Chen Y, Chen L, Yu D, Zhang Q, et al. Antimicrobial Defensin and DNA Traps in Manila Clam Ruditapes philippinarum: Implications for Their Roles in Immune Responses. Front Mar Sci (2021) 8:690879. doi: 10.3389/fmars.2021.690879
260. Pijanowski L, Golbach L, Kolaczkowska E, Scheer M, Verburg-van Kemenade BML, Chadzinska M. Carp Neutrophilic Granulocytes Form Extracellular Traps via ROS-Dependent and Independent Pathways. Fish Shellfish Immunol (2013) 34:1244–52. doi: 10.1016/j.fsi.2013.02.010
261. Andreyeva AY, Kladchenko ES, Vyalova OY, Kukhareva TA. Functional Characterization of the Pacific Oyster, Crassostrea gigas (Bivalvia: Ostreidae), Hemocytes Under Normoxia and Short-Term Hypoxia. Turkish J Fish Aquat Sci (2020) 21:125–33. doi: 10.4194/1303-2712-v21_3_03
262. Ruddell CL. Elucidation of the Nature and Function of the Granular Oyster Amebocytes Through Histochemical Studies of Normal and Traumatized Oyster Tissues. Histochem Cell Biol (1971) 26:98–112. doi: 10.1007/BF00293500
263. Suzuki T, Yoshinaka R, Mizuta S, Funakoshi S, Wada K. Extracellular Matrix Formation by Amebocytes During Epithelial Regeneration in the Pearl Oyster Pinctada fucata. Cell Tissue Res (1991) 266:75–82. doi: 10.1007/BF00678713
264. Sunila I, LaBanca J. Apoptosis in the Pathogenesis of Infectious Diseases of the Eastern Oyster Crassostrea virginica. Dis Aquat Organ (2003) 56:163–70. doi: 10.3354/dao056163
265. Goedken M, Morsey B, Sunila I, Dungan C, De Guise S. The Effects of Temperature and Salinity on Apoptosis of Crassostrea virginica Hemocytes and Perkinsus marinus. J Shellfish Res (2005) 24:177–83. doi: 10.2983/0730-8000(2005)24[177:TEOTAS]2.0.CO;2
266. Donaghy L, Artigaud S, Sussarellu R, Lambert C, Le Goïc N, Hégaret H, et al. Tolerance of Bivalve Mollusc Hemocytes to Variable Oxygen Availability: A Mitochondrial Origin? Aquat Living Resour (2013) 26:257–61. doi: 10.1051/alr/2013054
267. Estévez-Calvar N, Romero A, Figueras A, Novoa B. Genes of the Mitochondrial Apoptotic Pathway in Mytilus galloprovincialis. PloS One (2013) 8:e61502. doi: 10.1371/journal.pone.0061502
268. Ray M, Bhunia AS, Bhunia NS, Ray S. Density Shift, Morphological Damage, Lysosomal Fragility and Apoptosis of Hemocytes of Indian Molluscs Exposed to Pyrethroid Pesticides. Fish Shellfish Immunol (2013) 35:499–512. doi: 10.1016/j.fsi.2013.05.008
269. Romero A, Estévez-Calvar N, Dios S, Figueras A, Novoa B. New Insights Into the Apoptotic Process in Mollusks: Characterization of Caspase Genes in Mytilus galloprovincialis. PloS One (2011) 6:e17003. doi: 10.1371/journal.pone.0017003
270. Gervais O, Renault T, Arzul I. Induction of Apoptosis by UV in the Flat Oyster, Ostrea edulis. Fish Shellfish Immunol (2015) 46:232–42. doi: 10.1016/j.fsi.2015.05.046
271. Witkop EM, Wikfors GH, Proestou DA, Lundgren KM, Sullivan M, Gomez-Chiarri M. Perkinsus marinus Suppresses In Vitro Eastern Oyster Apoptosis via IAP-Dependent and Caspase-Independent Pathways Involving TNFR, NF-Kb, and Oxidative Pathway Crosstalk. Dev Comp Immunol (2022) 129:104339. doi: 10.1016/j.dci.2022.104339
272. Mitta G, Vandenbulcke F, Hubert F, Roch P. Mussel Defensins Are Synthesised and Processed in Granulocytes Then Released Into the Plasma After Bacterial Challenge. J Cell Sci (1999) 112:4233–42. doi: 10.1242/jcs.112.23.4233
273. Mitta G, Vandenbulcke F, Noël T, Romestand B, Beauvillain JC, Salzet M, et al. Differential Distribution and Defence Involvement of Antimicrobial Peptides in Mussel. J Cell Sci (2000) 113:2759–69. doi: 10.1242/jcs.113.15.2759
274. Rey-Campos M, Moreira R, Valenzuela-Muñoz V, Gallardo-Escárate C, Novoa B, Figueras A. High Individual Variability in the Transcriptomic Response of Mediterranean Mussels to Vibrio Reveals the Involvement of Myticins in Tissue Injury. Sci Rep (2019) 9:1–15. doi: 10.1038/s41598-019-39870-3
275. Cima F, Matozzo V. Proliferation and Differentiation of Circulating Haemocytes of Ruditapes philippinarum as a Response to Bacterial Challenge. Fish Shellfish Immunol (2018) 81:73–82. doi: 10.1016/j.fsi.2018.07.010
276. Pipe RK. Differential Binding of Lectins to Haemocytes of the Mussel Mytilus edulis. Cell Tissue Res (1990) 261:261–8. doi: 10.1007/BF00318667
277. Tasumi S, Vasta GR. A Galectin of Unique Domain Organization From Hemocytes of the Eastern Oyster (Crassostrea virginica) Is a Receptor for the Protistan Parasite Perkinsus marinus. J Immunol (2007) 179:3086–98. doi: 10.4049/jimmunol.179.5.3086
278. Feng C, Ghosh A, Amin MN, Giomarelli B, Shridhar S, Banerjee A, et al. The Galectin CvGal1 From the Eastern Oyster (Crassostrea virginica) Binds to Blood Group a Oligosaccharides on the Hemocyte Surface. J Biol Chem (2013) 288:24394–409. doi: 10.1074/jbc.M113.476531
279. Vasta GR, Feng C, Tasumi S, Abernathy K, Bianchet MA, Wilson IBH, et al. Biochemical Characterization of Oyster and Clam Galectins: Selective Recognition of Carbohydrate Ligands on Host Hemocytes and Perkinsus Parasites. Front Chem (2020) 8:98. doi: 10.3389/fchem.2020.00098
280. Jiang S, Jia Z, Zhang T, Wang L, Qiu L, Sun J, et al. Functional Characterisation of Phagocytes in the Pacific Oyster Crassostrea gigas. PeerJ (2016) 4:e2590. doi: 10.7717/peerj.2590
281. Rioult D, Pasquier J, Boulangé-lecomte C, Poret A. The Multi-Xenobiotic Resistance (MXR) Efflux Activity in Hemocytes of Mytilus edulis is Mediated by an ATP Binding Cassette Transporter of Class C (ABCC) Principally Inducible in Eosinophilic Granulocytes. Aquat Toxicol (2013) 153:98–109. doi: 10.1016/j.aquatox.2013.11.012
282. Marques-santos LF, Hégaret H, Lima-Santos L, Queiroga FR, da Silva PM. ABCB1 and ABCC1-Like Transporters in Immune System Cells From Sea Urchins Echinometra Lucunter and Echinus Esculentus and Oysters Crassostrea gasar and Crassostrea gigas. Fish Shellfish Immunol (2017) 70:195–203. doi: 10.1016/j.fsi.2017.09.014
283. Roberts SB, Sunila I, Wikfors GH. Immune Response and Mechanical Stress Susceptibility in Diseased Oysters, Crassostrea virginica. J Comp Physiol B (2012) 182:41–8. doi: 10.1007/s00360-011-0605-z
284. Wang M, Liu C, Wang W, Dong M, Zhang P, Liu Y, et al. A SPRY Domain-Containing SOCS Box Protein 3 (SPSB3) Involved in the Regulation of Cytokine Production in Granulocytes of Crassostrea gigas. Dev Comp Immunol (2019) 95:28–37. doi: 10.1016/j.dci.2019.01.013
285. Leng J, Li Y, Yang W, Sun J, Huang S, Yang C, et al. The Involvement of CgCaspase-8-2 in Regulating the Expressions of Cytokines, Antibacterial Peptide and Autophagy-Related Genes in Oysters. Fish Shellfish Immunol (2021) 119:145–53. doi: 10.1016/j.fsi.2021.09.037
286. Renwrantz L, Lam A. Soluble and Surface-Bound Aminopeptidase in Eosinophilic Blood Cells From Mytilus edulis. J Invertebr Pathol (2010) 103:68–70. doi: 10.1016/j.jip.2009.10.001
287. Okada Y, Yamaura K, Suzuki T, Itoh N, Osada M, Takahashi KG. Molecular Characterization and Expression Analysis of Chitinase From the Pacific Oyster Crassostrea gigas. Comp Biochem Physiol B (2013) 165:83–9. doi: 10.1016/j.cbpb.2013.03.008
288. Dong M, Song X, Wang M, Wang W, Zhang P, Liu Y, et al. CgAATase With Specific Expression Pattern can be Used as a Potential Surface Marker for Oyster Granulocytes. Fish Shellfish Immunol (2019) 87:96–104. doi: 10.1016/j.fsi.2019.01.003
289. Li M, Wang L, Qiu L, Wang W, Xin L, Xu J, et al. A Glutamic Acid Decarboxylase (CgGAD) Highly Expressed in Hemocytes of Pacific Oyster Crassostrea gigas. Dev Comp Immunol (2016) 63:56–65. doi: 10.1016/j.dci.2016.05.010
290. Li M, Dong M, Wang W, Li H, Liu Z, Wang L, et al. A Membrane-Bound Dopamine β-Hydroxylase Highly Expressed in Granulocyte of Pacific Oyster Crassostrea gigas. Dev Comp Immunol (2020) 104:103563. doi: 10.1016/j.dci.2019.103563
291. Sekine D, Ohishi K, Nakamura Y, Kusaka C, Tame A, Inoue K, et al. Monoclonal Antibodies to Hemocytes of the Deep-Sea Symbiotic Mussel, Bathymodiolus japonicus. JAMSTEC Rep Res Dev (2016) 23:27–33. doi: 10.5918/jamstecr.23.27
292. Kim J-H, Lee H-M, Cho Y-G, Shin J-S, Yoo J-W, Hong H-K, et al. Effects of Spawning Stress on the Immune Capacity of Blood Cockle Tegillarca granosa Occurring on the South Coast of Korea. Fish Shellfish Immunol (2022) 120:15–22. doi: 10.1016/j.fsi.2021.11.013
293. Lin T, Zhou K, Lai Q, Yao Z, Li Z, Xing J. Seasonal Variations of Water Temperature, Food Availability, Size, and Reproduction on the Hemocyte Parameters in the Scallop Chlamys farreri. J Shellfish Res (2012) 31:663–70. doi: 10.2983/035.031.0309
294. Lu J, Shi Y, Yao T, Bai C, Jiang J, Ye L. Gender Differences in Hemocyte Immune Parameters of Hong Kong Oyster Crassostrea hongkongensis During Immune Stress. Front Immunol (2021) 12:659469. doi: 10.3389/fimmu.2021.659469
295. Johnstone MB, Gohad NV, Falwell EP, Hansen DC, Hansen KM, Mount AS. Cellular Orchestrated Biomineralization of Crystalline Composites on Implant Surfaces by the Eastern Oyster, Crassostrea virginica (Gmelin, 1791). J Exp Mar Bio Ecol (2015) 463:8–16. doi: 10.1016/j.jembe.2014.10.014
296. Cho S-M, Lee Y-M, Jeong W-G. Effect of Polycyclic Aromatic Hydrocarbon (PAH) on Shell Repair in the Pacific Oyster, Crassostrea gigas. Korean J Malacol (2011) 27:35–42. doi: 10.9710/kjm.2011.27.1.035
297. Sillanpää JK, Ramesh K, Melzner F, Sundh H, Sundell K. Calcium Mobilisation Following Shell Damage in the Pacific Oyster, Crassostrea gigas. Mar Genomics (2016) 27:75–83. doi: 10.1016/j.margen.2016.03.001
298. Kadar E, Lobo-da-Cunha A, Azevedo C. Mantle-To-Shell CaCO3 Transfer During Shell Repair at Different Hydrostatic Pressures in the Deep-Sea Vent Mussel Bathymodiolus azoricus (Bivalvia: Mytilidae). Mar Biol (2009) 156:959–67. doi: 10.1007/s00227-009-1140-2
299. Li S, Liu Y, Liu C, Huang J, Zheng G, Xie L, et al. Hemocytes Participate in Calcium Carbonate Crystal Formation, Transportation and Shell Regeneration in the Pearl Oyster Pinctada fucata. Fish Shellfish Immunol (2016) 51:263–70. doi: 10.1016/j.fsi.2016.02.027
300. Ivanina AV, Falfushynska HI, Beniash E, Piontkivska H, Sokolova IM. Biomineralization-Related Specialization of Hemocytes and Mantle Tissues of the Pacific Oyster Crassostrea gigas. J Exp Biol (2017) 220:3209–21. doi: 10.1242/jeb.160861
301. Song X, Liu Z, Wang L, Song L. Recent Advances of Shell Matrix Proteins and Cellular Orchestration in Marine Molluscan Shell Biomineralization. Front Mar Sci (2019) 6:41. doi: 10.3389/fmars.2019.00041
302. Carballal MJ, Villalba A, López C. Seasonal Variation and Effects of Age, Food Availability, Size, Gonadal Development, and Parasitism on the Hemogram of Mytilus galloprovincialis. J Invertebr Pathol (1998) 72:304–12. doi: 10.1006/jipa.1998.4779
303. Flye-Sainte-Marie J, Soudant P, Lambert C, Le Goïc N, Goncalvez M, Travers M-A, et al. Variability of the Hemocyte Parameters of Ruditapes philippinarum in the Field During an Annual Cycle. J Exp Mar Bio Ecol (2009) 377:1–11. doi: 10.1016/j.jembe.2009.06.003
304. Hong HK, Do JH, HS K, Choi KS. Seasonal Variations in the Hemocyte Parameters, Gonad Development, Energy Storage and Utilization of the Giant Honeycomb Oyster Hyotissa hyotis (Linnaeus 1758) in Jeju Island Off the South Coast of Korea. Aquac Rep (2020) 17:100299. doi: 10.1016/j.aqrep.2020.100299
305. Magalhães L, de Montaudouin X, Figueira E, Freitas R. Interactive Effects of Contamination and Trematode Infection in Cockles Biochemical Performance. Environ Pollut (2018) 243:1469–78. doi: 10.1016/j.envpol.2018.09.102
306. Chen Z, Zhang D, Xing J, Zhan W. Effects of Temperature on Haemocyte and Granulocyte Counts and Expressions of Immunity-Related Genes in Haemocytes of Scallop Chlamys farreri After Vibrio anguillarum Infection. J Ocean Univ China (2019) 18:1163–73. doi: 10.1007/s11802-019-4180-3
307. Labreuche Y, Lambert C, Soudant P, Boulo V, Huvet A, Nicolas JL. Cellular and Molecular Hemocyte Responses of the Pacific Oyster, Crassostrea gigas, Following Bacterial Infection With Vibrio Aestuarianus Strain 01/32. Microbes Infect (2006) 8:2715–24. doi: 10.1016/j.micinf.2006.07.020
308. Haddaji N, Chakroun I, Fdhila K, Smati H, Bakhrouf A, Mzoughi R. Pathogenic Impacts of Bacillus Cereus Strains on Crassostrea gigas. Foodborne Pathog Dis (2022) 19:1–8. doi: 10.1089/fpd.2021.0050
309. Ittoop G, George KC, George RM, Sobhana KS, Sanil NK, Nisha PC. Modulation of Selected Hemolymph Factors in the Indian Edible Oyster Crassostrea madrasensis (Preston) Upon Challenge by Vibrio alginolyticus. Indian J Fish (2010) 57:55–60.
310. Mateo DR, Spurmanis A, Siah A, Araya MT, Kulka M, Berthe FCJ, et al. Changes Induced by Two Strains of Vibrio splendidus in Haemocyte Subpopulations of Mya arenaria, Detected by Flow Cytometry With LysoTracker. Dis Aquat Organ (2009) 86:253–62. doi: 10.3354/dao02121
311. Canonico C, Barchiesi F. Depuration Capacity of Mussels (Mytilus galloprovincialis) in Presence of Marteilia Spp. Parasites. J Mar Sci Res Dev (2016) 6:1000187. doi: 10.4172/2155-9910.1000187
312. Hauton C, Hawkins LE, Hutchinson S. The Effects of Salinity on the Interaction Between a Pathogen (Listonella anguillarum) and Components of a Host (Ostrea edulis) Immune System. Comp Biochem Physiol B (2000) 127:203–12. doi: 10.1016/S0305-0491(00)00251-0
313. da Silva PM, Comesaña P, Fuentes J, Villalba A. Variability of Haemocyte and Haemolymph Parameters in European Flat Oyster Ostrea edulis Families Obtained From Brood Stocks of Different Geographical Origins and Relation With Infection by the Protozoan Bonamia ostreae. Fish Shellfish Immunol (2008) 24:551–63. doi: 10.1016/j.fsi.2007.11.003
314. da Silva PM, Magalhaes ARM, Barracco MA. Effects of Bucephalus Sp. (Trematoda: Bucephalidae) on Perna perna Mussels From a Culture Station in Ratones Grande Island, Brazil. J Invertebr Pathol (2002) 79:154–62. doi: 10.1016/S0022-2011(02)00026-5
315. Oubella R, Maes P, Allam B, Paillard C, Auffret M. Selective Induction of Hemocytic Response in Ruditapes philippinarum (Bivalvia) by Different Species of Vibrio (Bacteria). Aquat Living Resour (1996) 9:137–43. doi: 10.1051/alr:1996017
316. Paul-Pont I, De Montaudouin X, Gonzalez P, Jude F, Raymond N, Paillard C, et al. Interactive Effects of Metal Contamination and Pathogenic Organisms on the Introduced Marine Bivalve Ruditapes philippinarum in European Populations. Environ Pollut (2010) 158:3401–10. doi: 10.1016/j.envpol.2010.07.028
317. Ford SE, Kanaley S, Littlewood D. Cellular Responses of Oysters Infected With Haplosporidium nelsoni: Changes in Circulating and Tissue-Infiltrating Hemocytes. J Invertebr Pathol (1993) 61:49–57. doi: 10.1006/jipa.1993.1009
318. Chu F-L, La Peyre J. Development of Disease Caused by the Parasite, Perkinsus marinus and Defense-Related Hemolymph Factors in 3 Populations of Oysters From the Chesapeake Bay, USA. J Shellfish Res (1993) 12:21.
319. Hine PM, Wesney B. The Functional Cytology of Bonamia Sp. (Haplosporidia) Infecting Oysters Tiostrea chilensis: An Ultracytochemical Study. Dis Aquat Organ (1994) 20:207–17. doi: 10.3354/dao020207
320. Naciri-Graven Y, Martin AG, Baud JP, Renault T, Gérard A. Selecting the Flat Oyster Ostrea edulis (L.) for Survival When Infected With the Parasite Bonamia ostreae. J Exp Mar Bio Ecol (1998) 224:91–107. doi: 10.1016/S0022-0981(97)00171-8
321. Cochennec-Laureau N, Auffret M, Renault T, Langlade A. Changes in Circulating and Tissue-Infiltrating Hemocyte Parameters of European Flat Oysters, Ostrea edulis, Naturally Infected With Bonamia ostreae. J Invertebr Pathol (2003) 83:23–30. doi: 10.1016/s0022-2011(03)00015-6
322. Butt D, Raftos D. Phenoloxidase-Associated Cellular Defence in the Sydney Rock Oyster, Saccostrea glomerata, Provides Resistance Against QX Disease Infections. Dev Comp Immunol (2008) 32:299–306. doi: 10.1016/j.dci.2007.06.006
323. Hégaret H, Wikfors GH. Time-Dependent Changes in Hemocytes of Eastern Oysters, Crassostrea virginica, and Northern Bay Scallops, Argopecten irradians irradians, Exposed to a Cultured Strain of Prorocentrum minimum. Harmful Algae (2005) 4:187–99. doi: 10.1016/j.hal.2003.12.004
324. Haberkorn H, Lambert C, Le Goïc N, Guéguen M, Moal J, Palacios E, et al. Effects of Alexandrium minutum Exposure Upon Physiological and Hematological Variables of Diploid and Triploid Oysters, Crassostrea gigas. Aquat Toxicol (2010) 97:96–108. doi: 10.1016/j.aquatox.2009.12.006
325. Mello DF, da SPM, MA B, Soudant P, Hégaret H. Effects of the Dinoflagellate Alexandrium minutum and Its Toxin (Saxitoxin) on the Functional Activity and Gene Expression of Crassostrea gigas Hemocytes. Harmful Algae (2013) 26:45–51. doi: 10.1016/j.hal.2013.03.003
326. Castrec J, Soudant P, Payton L, Tran D, Miner P, Lambert C, et al. Bioactive Extracellular Compounds Produced by the Dinoflagellate Alexandrium minutum Are Highly Detrimental for Oysters. Aquat Toxicol (2018) 199:188–98. doi: 10.1016/j.aquatox.2018.03.034
327. Abi-Khalil C, Finkelstein DS, Conejero G, Du Bois J, Destoumieux-Garzon D, Rolland JL. The Paralytic Shellfish Toxin, Saxitoxin, Enters the Cytoplasm and Induces Apoptosis of Oyster Immune Cells Through a Caspase-Dependent Pathway. Aquat Toxicol (2017) 190:133–41. doi: 10.1016/j.aquatox.2017.07.001
328. Hégaret H, Wikfors GH. Effects of Natural and Field-Simulated Blooms of the Dinoflagellate Prorocentrum minimum Upon Hemocytes of Eastern Oysters, Crassostrea virginica, From Two Different Populations. Harmful Algae (2005) 4:201–9. doi: 10.1016/j.hal.2003.12.005
329. Juhel G, Ramsay RM, Davenport J, O’Halloran J, Culloty SC. Effect of the Microcystin-Producing Cyanobacterium, Microcystis aeruginosa, on Immune Functions of the Zebra Mussel Dreissena polymorpha. J Shellfish Res (2015) 34:433–42. doi: 10.2983/035.034.0227
330. Galimany E, Place AR, Ramón M, Jutson M, Pipe RK. The Effects of Feeding Karlodinium veneficum (PLY # 103; Gymnodinium veneficum Ballantine) to the Blue Mussel Mytilus edulis. Harmful Algae (2008) 7:91–8. doi: 10.1016/j.hal.2007.05.004
331. Galimany E, Sunila I, Hégaret H, Ramón M, Wikfors GH. Experimental Exposure of the Blue Mussel (Mytilus edulis, L.) to the Toxic Dinoflagellate Alexandrium fundyense: Histopathology, Immune Responses, and Recovery. Harmful Algae (2008) 7:702–11. doi: 10.1016/j.hal.2008.02.006
332. Gorbi S, Avio GC, Benedetti M, Totti C, Accoroni S, Pichierri S, et al. Effects of Harmful Dinoflagellate Ostreopsis Cf. Ovata Exposure on Immunological, Histological and Oxidative Responses of Mussels Mytilus galloprovincialis. Fish Shellfish Immunol (2013) 35:941–50. doi: 10.1016/j.fsi.2013.07.003
333. Giuliani ME, Accoroni S, Mezzelani M, Lugarini F, Bacchiocchi S, Siracusa MT, et al. Biological Effects of the Azaspiracid-Producing Dinoflagellate Azadinium dexteroporum in Mytilus galloprovincialis From the Mediterranean Sea. Mar Drugs (2019) 17:595. doi: 10.3390/md17100595
334. Mello DF, Proença LA de O, Barracco MA. Comparative Study of Various Immune Parameters in Three Bivalve Species During a Natural Bloom of Dinophysis acuminata in Santa Catarina Island, Brazil. Toxins (Basel) (2010) 2:1166–78. doi: 10.3390/toxins2051166
335. Bricelj VM, Ford SE, Lambert C, Barbou A, Paillard C. Effects of Toxic Alexandrium tamarense on Behavior, Hemocyte Responses and Development of Brown Ring Disease in Manila Clams. Mar Ecol Prog Ser (2011) 430:35–48. doi: 10.3354/meps09111
336. Andreyeva AY, Kladchenko ES, Kukhareva TA. Shift in Functional and Morphological Parameters of the Pacific Oyster Hemocytes After Exposure to Hypoxia. Reg Stud Mar Sci (2021) 48:102062. doi: 10.1016/j.rsma.2021.102062
337. Sui Y, Kong H, Shang Y, Huang X, Wu FL, Hu M, et al. Effects of Short-Term Hypoxia and Seawater Acidification on Hemocyte Responses of the Mussel Mytilus coruscus. Mar Pollut Bull (2016) 108:46–52. doi: 10.1016/j.marpolbul.2016.05.001
338. Sun T, Tang X, Jiang Y, Wang Y. Seawater Acidification Induced Immune Function Changes of Haemocytes in Mytilus edulis: A Comparative Study of CO 2 and HCl Enrichment. Sci Rep (2017) 7:1–10. doi: 10.1038/srep41488
339. Nardi A, Benedetti M, D’Errico G, Fattorini D, Regoli F. Effects of Ocean Warming and Acidification on Accumulation and Cellular Responsiveness to Cadmium in Mussels Mytilus galloprovincialis: Importance of the Seasonal Status. Aquat Toxicol (2018) 204:171–9. doi: 10.1016/j.aquatox.2018.09.009
340. Kuchel RP, Raftos DA, Nair S. Immunosuppressive Effects of Environmental Stressors on Immunological Function in Pinctada imbricata. Fish Shellfish Immunol (2010) 29:930–6. doi: 10.1016/j.fsi.2010.07.033
341. Reid HI, Soudant P, Lambert C, Paillard C, Birkbeck TH. Salinity Effects on Immune Parameters of Ruditapes philippinarum Challenged With Vibrio tapetis. Dis Aquat Organ (2003) 56:249–58. doi: 10.3354/dao056249
342. Liu S, Shi W, Guo C, Zhao X, Han Y, Peng C, et al. Ocean Acidification Weakens the Immune Response of Blood Clam Through Hampering the NF-Kappa β and Toll-Like Receptor Pathways. Fish Shellfish Immunol (2016) 54:322–7. doi: 10.1016/j.fsi.2016.04.030
343. Su W, Zha S, Wang Y, Shi W, Xiao G, Chai X, et al. Benzo[a]pyrene Exposure Under Future Ocean Acidification Scenarios Weakens the Immune Responses of Blood Clam, Tegillarca granosa. Fish Shellfish Immunol (2017) 63:465–70. doi: 10.1016/j.fsi.2017.02.046
344. Liu J, Pan L-Q, Zhang L, Miao J, Wang J. Immune Responses, ROS Generation and the Haemocyte Damage of Scallop Chlamys farreri Exposed to Aroclor 1254. Fish Shellfish Immunol (2009) 26:422–8. doi: 10.1016/j.fsi.2009.01.002
345. Gagnaire B, Thomas-Guyon H, Burgeot T, Renault T. Pollutant Effects on Pacific Oyster, Crassostrea gigas (Thunberg), Hemocytes: Screening of 23 Molecules Using Flow Cytometry. Cell Biol Toxicol (2006) 22:1–14. doi: 10.1007/s10565-006-0011-6
346. Evariste L, Rioult D, Brousseau P, Geffard A, David E, Auffret M, et al. Differential Sensitivity to Cadmium of Immunomarkers Measured in Hemocyte Subpopulations of Zebra Mussel Dreissena polymorpha. Ecotoxicol Environ Saf (2017) 137:78–85. doi: 10.1016/j.ecoenv.2016.11.027
347. McCormick-Ray MG. Hemocytes of Mytilus edulis Affected by Prudhoe Bay Crude Oil Emulsion. Mar Environ Res (1987) 22:107–22. doi: 10.1016/0141-1136(87)90031-6
348. Pipe RK, Coles JA, Carissan FMM, Ramanathan K. Copper Induced Immunomodulation in the Marine Mussel, Mytilus edulis. Aquat Toxicol (1999) 46:43–54. doi: 10.1016/S0166-445X(98)00114-3
349. Von Moos N, Burkhardt-Holm P, Köhler A. Uptake and Effects of Microplastics on Cells and Tissue of the Blue Mussel Mytilus edulis L. After an Experimental Exposure. Environ Sci Technol (2012) 46:11327–35. doi: 10.1021/es302332w
350. Jiang Y, Tang X, Sun T, Wang Y. BDE-47 Exposure Changed the Immune Function of Haemocytes in Mytilus edulis: An Explanation Based on ROS-Mediated Pathway. Aquat Toxicol (2017) 182:58–66. doi: 10.1016/j.aquatox.2016.11.010
351. Mezzelani M, Gorbi S, Fattorini D, Errico G, Benedetti M, Milan M, et al. Transcriptional and Cellular Effects of non-Steroidal Anti-Inflammatory Drugs (NSAIDs) in Experimentally Exposed Mussels, Mytilus galloprovincialis. Aquat Toxicol (2016) 180:306–19. doi: 10.1016/j.aquatox.2016.10.006
352. Mezzelani M, Nardi A, Bernardini I, Milan M, Peruzza L, d’Errico G, et al. Environmental Pharmaceuticals and Climate Change: The Case Study of Carbamazepine in M. galloprovincialis Under Ocean Acidification Scenario. Environ Int (2021) 146:106269. doi: 10.1016/j.envint.2020.106269
353. Nardi A, Benedetti M, Gorbi S, Regoli F. Interactive Immunomodulation in the Mediterranean Mussel Mytilus galloprovincialis Under Thermal Stress and Cadmium Exposure. Front Mar Sci (2021) 8:751983. doi: 10.3389/fmars.2021.751983
354. Avio CG, Gorbi S, Milan M, Benedetti M, Fattorini D, Pauletto M, et al. Pollutants Bioavailability and Toxicological Risk From Microplastics to Marine Mussels. Environ Pollut (2015) 198:211–22. doi: 10.1016/j.envpol.2014.12.021
355. Pittura L, Avio CG, Giuliani ME, d’Errico G, Keiter SH, Cormier B, et al. Microplastics as Vehicles of Environmental PAHs to Marine Organisms: Combined Chemical and Physical Hazards to the Mediterranean Mussels, Mytilus galloprovincialis. Front Mar Sci (2018) 5:103. doi: 10.3389/fmars.2018.00103
356. Bouallegui Y, Ben YR, Turki F, Oueslati R. Impact of Exposure Time, Particle Size and Uptake Pathway on Silver Nanoparticle Effects on Circulating Immune Cells in Mytilus galloprovincialis. J Immunotoxicol (2017) 14:116–24. doi: 10.1080/1547691X.2017.1335810
357. Sendra M, Carrasco-Braganza MI, Yeste PM, Vila M, Blasco J. Immunotoxicity of Polystyrene Nanoplastics in Different Hemocyte Subpopulations of Mytilus galloprovincialis. Sci Rep (2020) 10:8637. doi: 10.1038/s41598-020-65596-8
358. Cole M, Liddle C, Consolandi G, Drago C, Hird C, Lindeque PK, et al. Microplastics, Microfibres and Nanoplastics Cause Variable Sub-Lethal Responses in Mussels (Mytilus Spp.). Mar Pollut Bull (2020) 160:111552. doi: 10.1016/j.marpolbul.2020.111552
359. Paul-Pont I, Lacroix C, González Fernández C, Hégaret H, Lambert C, Le Goïc N, et al. Exposure of Marine Mussels Mytilus Spp. To Polystyrene Microplastics: Toxicity and Influence on Fluoranthene Bioaccumulation. Environ Pollut (2016) 216:724–37. doi: 10.1016/j.envpol.2016.06.039
360. Chandurvelan R, Marsden ID, Glover CN, Gaw S. Assessment of a Mussel as a Metal Bioindicator of Coastal Contamination: Relationships Between Metal Bioaccumulation and Multiple Biomarker Responses. Sci Total Environ (2015) 511:663–75. doi: 10.1016/j.scitotenv.2014.12.064
361. Hong HK, Donaghy L, Kang CK, Kang HS, Lee HJ, Park HS, et al. Substantial Changes in Hemocyte Parameters of Manila Clam Ruditapes philippinarum Two Years After the Hebei Spirit Oil Spill Off the West Coast of Korea. Mar Pollut Bull (2016) 108:171–9. doi: 10.1016/j.marpolbul.2016.04.033
362. Ewere EE, Reichelt-Brushett A, Benkendorff K. The Neonicotinoid Insecticide Imidacloprid, But Not Salinity, Impacts the Immune System of Sydney Rock Oyster, Saccostrea glomerata. Sci Total Environ (2020) 742:140538. doi: 10.1016/j.scitotenv.2020.140538
363. Shi W, Guan X, Han Y, Guo C, Rong J, Su W, et al. Waterborne Cd2+ Weakens the Immune Responses of Blood Clam Through Impacting Ca2+ Signaling and Ca2+ Related Apoptosis Pathways. Fish Shellfish Immunol (2018) 77:208–13. doi: 10.1016/j.fsi.2018.03.055
364. Shi W, Han Y, Guo C, Zhao X, Liu S, Su W, et al. Immunotoxicity of Nanoparticle Ntio2 to a Commercial Marine Bivalve Species, Tegillarca granosa. Fish Shellfish Immunol (2017) 66:300–6. doi: 10.1016/j.fsi.2017.05.036
365. Shi W, Guan X, Han Y, Zha S, Fang J, Xiao G, et al. The Synergic Impacts of TiO2 Nanoparticles and 17β-Estradiol (E2) on the Immune Responses, E2 Accumulation, and Expression of Immune-Related Genes of the Blood Clam, Tegillarca granosa. Fish Shellfish Immunol (2018) 81:29–36. doi: 10.1016/j.fsi.2018.07.009
366. Zha S, Rong J, Guan X, Tang Y, Han Y, Liu G. Immunotoxicity of Four Nanoparticles to a Marine Bivalve Species, Tegillarca granosa. J Hazard Mater (2019) 377:237–48. doi: 10.1016/j.jhazmat.2019.05.071
367. Zha S, Shi W, Su W, Guan X, Liu G. Exposure to 2,3,7,8-Tetrachlorodibenzo-Paradioxin (TCDD) Hampers the Host Defense Capability of a Bivalve Species, Tegillarca granosa. Fish Shellfish Immunol (2019) 86:368–73. doi: 10.1016/j.fsi.2018.11.058
368. Tang Y, Zhou W, Sun S, Du X, Han Y, Shi W, et al. Immunotoxicity and Neurotoxicity of Bisphenol A and Microplastics Alone or in Combination to a Bivalve Species, Tegillarca granosa. Environ Pollut (2020) 265:115115. doi: 10.1016/j.envpol.2020.115115
369. Sun S, Shi W, Tang Y, Han Y, Du X, Zhou W, et al. The Toxic Impacts of Microplastics (MPs) and Polycyclic Aromatic Hydrocarbons (PAHs) on Haematic Parameters in a Marine Bivalve Species and Their Potential Mechanisms of Action. Sci Total Environ (2021) 783:147003. doi: 10.1016/j.scitotenv.2021.147003
370. Antunes F, Hinzmann M, Lopes-Lima M, Vaz-Pires P, Ferreira S, Domingues B, et al. Antibacterial Effects of Anodonta cygnea Fluids on Escherichia coli and Enterococci Multi-Drug-Resistant Strains: Environmental Implications. Toxicol Environ Chem (2014) 96:880–9. doi: 10.1080/02772248.2014.989853
371. Li H, Parisi MG, Toubiana M, Cammarata M, Roch P. Lysozyme Gene Expression and Hemocyte Behaviour in the Mediterranean Mussel, Mytilus galloprovincialis, After Injection of Various Bacteria or Temperature Stresses. Fish Shellfish Immunol (2008) 25:143–52. doi: 10.1016/j.fsi.2008.04.001
372. Peters R, Raftos DA. The Role of Phenoloxidase Suppression in QX Disease Outbreaks Among Sydney Rock Oysters (Saccostrea glomerata). Aquaculture (2003) 223:29–39. doi: 10.1016/S0044-8486(03)00169-8
373. Lynch SA, Breslin R, Bookelaar B, Rudtanatip T, Wongprasert K, Culloty SC. Immunomodulatory and Antiviral Effects of Macroalgae Sulphated Polysaccharides: Case Studies Extend Knowledge on Their Importance in Enhancing Shellfish Health, and the Control of a Global Viral Pathogen Ostreid Herpesvirus-1 Microvar. Polysaccharides (2021) 2:202–17. doi: 10.3390/polysaccharides2020014
374. Lassudrie M, Hégaret H, Wikfors GH, da Silva PM. Effects of Marine Harmful Algal Blooms on Bivalve Cellular Immunity and Infectious Diseases: A Review. Dev Comp Immunol (2020) 108:103660. doi: 10.1016/j.dci.2020.103660
375. Fisher WS. Environmental Influence on Bivalve Hemocyte Function. Am Fish Soc Spec Publ (1988) 18:225–37.
376. Monari M, Matozzo V, Foschi J, Cattani O, Serrazanetti GP, Marin MG. Effects of High Temperatures on Functional Responses of Haemocytes in the Clam Chamelea gallina. Fish Shellfish Immunol (2007) 22:98–114. doi: 10.1016/j.fsi.2006.03.016
377. Pérez–Velasco R, Manzano–Sarabia M, Hurtado–Oliva MÁ. Effect of Hypo– and Hypersaline Stress Conditions on Physiological, Metabolic, and Immune Responses in the Oyster Crassostrea corteziensis (Bivalvia: Ostreidae). Fish Shellfish Immunol (2022) 120:252–60. doi: 10.1016/j.fsi.2021.11.033
378. Kolyuchkina GA, Ismailov AD. Morpho-Functional Characteristics of Bivalve Mollusks Under the Experimental Environmental Pollution by Heavy Metals. Oceanology (2011) 51:804–13. doi: 10.1134/S0001437011050092
379. De Vico G, Carella F. Morphological Features of the Inflammatory Response in Molluscs. Res Vet Sci (2012) 93:1109–15. doi: 10.1016/j.rvsc.2012.03.014
380. Sendra M, Sparaventi E, Novoa B, Figueras A. An Overview of the Internalization and Effects of Microplastics and Nanoplastics as Pollutants of Emerging Concern in Bivalves. Sci Total Environ (2021) 753:142024. doi: 10.1016/j.scitotenv.2020.142024
381. Calisi A, Lionetto MG, Caricato R, Giordano ME, Schettino T. Morphometric Alterations in Mytilus galloprovincialis Granulocytes: A New Biomarker. Environ Toxicol Chem (2007) 27:1435–41. doi: 10.1897/07-396
382. Seiler GR, Morse MP. Kidney and Hemocytes of Mya arenaria (Bivalvia): Normal and Pollution-Related Ultrastructural Morphologies. J Invertebr Pathol (1988) 52:201–14. doi: 10.1016/0022-2011(88)90127-9
383. Ayhan MM, Katalay S, Günal AÇ. How Pollution Effects the Immune Systems of Invertebrate Organisms (Mytilus galloprovincialis Lamark, 1819). Mar Pollut Bull (2021) 172:25–30. doi: 10.1016/j.marpolbul.2021.112750
384. Dolcetti L, Venier P. Susceptibility to Genetic Damage and Cell Types in Mediterranean Mussels. Mar Environ Res (2002) 54:487–91. doi: 10.1016/S0141-1136(02)00142-3
Keywords: granulocyte, hyalinocyte, immune response, haematopoiesis, phagocytosis
Citation: de la Ballina NR, Maresca F, Cao A and Villalba A (2022) Bivalve Haemocyte Subpopulations: A Review. Front. Immunol. 13:826255. doi: 10.3389/fimmu.2022.826255
Received: 30 November 2021; Accepted: 23 February 2022;
Published: 08 April 2022.
Edited by:
Mathilde Knight, George Washington University, United StatesReviewed by:
Timothy James Green, Vancouver Island University, CanadaJose A Fernandez Robledo, Bigelow Laboratory For Ocean Sciences, United States
Copyright © 2022 de la Ballina, Maresca, Cao and Villalba. This is an open-access article distributed under the terms of the Creative Commons Attribution License (CC BY). The use, distribution or reproduction in other forums is permitted, provided the original author(s) and the copyright owner(s) are credited and that the original publication in this journal is cited, in accordance with accepted academic practice. No use, distribution or reproduction is permitted which does not comply with these terms.
*Correspondence: Antonio Villalba, YW50b25pby52aWxsYWxiYS5nYXJjaWFAeHVudGEuZ2Fs
†Present address: Nuria R. de la Ballina, Centre Oceanogràfic de les Balears, Instituto Español de Oceanografía, Palma, Spain