- 1Immunology Unit, Institut Pasteur du Cambodge, Pasteur Network, Phnom Penh, Cambodia
- 2Virology Unit, Institut Pasteur du Cambodge, Pasteur Network, Phnom Penh, Cambodia
- 3Epidemiology and Public Health Unit, Institut Pasteur du Cambodge, Pasteur Network, Phnom Penh, Cambodia
- 4 Department of Communicable Disease Control, Ministry of Health (CDC-MoH), Phnom Penh, Cambodia
- 5Institut Pasteur, Université de Paris, CNRS UMR3569, Virus and Immunity Unit, Paris, France
- 6Vaccine Research Institute, Créteil, France
The duration of humoral and cellular immune memory following SARS-CoV-2 infection in populations in least developed countries remains understudied but is key to overcome the current SARS-CoV-2 pandemic. Sixty-four Cambodian individuals with laboratory-confirmed infection with asymptomatic or mild/moderate clinical presentation were evaluated for Spike (S)-binding and neutralizing antibodies and antibody effector functions during acute phase of infection and at 6-9 months follow-up. Antigen-specific B cells, CD4+ and CD8+ T cells were characterized, and T cells were interrogated for functionality at late convalescence. Anti-S antibody titers decreased over time, but effector functions mediated by S-specific antibodies remained stable. S- and nucleocapsid (N)-specific B cells could be detected in late convalescence in the activated memory B cell compartment and are mostly IgG+. CD4+ and CD8+ T cell immune memory was maintained to S and membrane (M) protein. Asymptomatic infection resulted in decreased antibody-dependent cellular cytotoxicity (ADCC) and frequency of SARS-CoV-2-specific CD4+ T cells at late convalescence. Whereas anti-S antibodies correlated with S-specific B cells, there was no correlation between T cell response and humoral immune memory. Hence, all aspects of a protective immune response are maintained up to nine months after SARS-CoV-2 infection and in the absence of re-infection.
Introduction
In December 2019, a cluster of severe pneumonia of unknown cause was reported to the World Health Organization. Investigation into the etiology revealed a novel betacoronavirus, subsequently named Severe Acute Respiratory Syndrome Coronavirus 2 (SARS-CoV-2), the causative agent of Coronavirus Disease 2019 (COVID-19) (1–3).
Upon infection with SARS-CoV-2, humans generate SARS-CoV-2-specific antibodies, memory B cells, and CD4+ and CD8+ T cells, which all have complementary functions in the clearance of SARS-CoV-2 virions and infected cells (4–6). Mainly structural proteins are targeted by the immune response, such as the membrane (M) and spike (S) protein integrated in the virion envelope, and the nucleoprotein (N), which protects the RNA genome (7–9). The S protein consists of two domains. The S1 region contains the receptor binding domain (RBD) which interacts with the host protein Angiotensin-converting enzyme 2 (ACE2) to mediate cell entry, whereas the S2 domain mediates membrane fusion. The S1 domain with the RBD is a major target of neutralizing antibodies (10, 11). Several studies show correlation between antibodies targeting S and functional neutralization (12–14). In animal models, these neutralizing antibodies are protective against secondary infection (15, 16). In humans, anti-S antibodies and neutralizing antibodies can be detected up to one year post infection (17–19).
Besides neutralization, antibodies activate a variety of effector functions mediated by their Fc domain. These include complement activation, killing of infected cells and phagocytosis of viral particles (20). Indeed, it has been shown that symptomatic and asymptomatic SARS-CoV-2 infection elicit polyfunctional antibodies targeting infected cells (21, 22) and Fc mediated effector activity of antibodies correlates with reduced disease severity and mortality after SARS-CoV-2 infection (23). However, the evolution of this response over time requires further investigation (24, 25).
Persistence of serum antibodies may not be the sole determinant of long-lasting immune memory post infection or vaccination. Anamnestic recall of memory T and B cell populations can also reduce infection or disease at re-exposure (4, 26, 27), with increasing importance as antibody titers wane. Virus-specific memory T and B cells can be detected in at least 50% of the individuals more than eight months post infection (4, 27, 28). Several studies suggest that increased severity of COVID-19 induces a stronger SARS-CoV-2-specific CD4+ T cell response (28–30). However, the magnitude, quality, and protective capacity of cellular responses against SARS-CoV-2 requires further definition (31).
Kinetics and duration of the memory immune responses could depend on a number of factors including disease severity, re-infection, cross-reactivity with human seasonal coronaviruses (hCoVs), ethnic background, age and length of antigenic exposure [reviewed in (31)]. Other human betacoronaviruses, such as hCoV OC43 and HKU1, and zoonotic viruses, such as SARS-CoV−1 and Middle East respiratory syndrome-related coronavirus (MERS-CoV), show waning antibody levels as soon as three months post infection. In contrast, T cell responses are detectable up to 17 years later (32, 33).
Most studies analyzing the evolution of the adaptive immune response to SARS-CoV-2 are conducted in Caucasian populations (31). In South-East Asia, very few studies have been performed, which mainly focused on antibody responses (18, 34–36). Paucity of data from at risk areas and populations can hamper global mitigation and vaccination efforts.
We comprehensively characterized long-lived immune memory in 64 Cambodian individuals with laboratory-confirmed infection experiencing mild/moderate or asymptomatic clinical outcome. Cambodia remained almost completely COVID-19-free in 2020 (37), hence additional exposure to SARS-CoV-2 in this cohort during the follow-up period is highly unlikely.
Results
Long-Term Follow-Up of SARS-CoV-2 Imported Cases
Sixty-four individuals with confirmed SARS-CoV-2 were included and re-assessed 6-9 months after infection. SARS-CoV-2 infection was confirmed by positive molecular diagnosis as part of the national surveillance system. Since Cambodia had minimal detection of SARS-CoV-2 during the follow-up period, the probability of re-exposure to SARS-CoV-2 was minimal (37) in 2020. For 33 individuals, we obtained a blood sample 2-9 days after laboratory confirmed infection (Figure S1A). For all 64 study participants, between 1 to 15 follow-up nasopharyngeal/oropharyngeal (NP/OP) swab samplings assessed the duration of SARS-CoV-2 RNA shedding during the acute phase of infection via RT-PCR (38). Based on the duration of SARS-CoV-2 RNA shedding, 53% of individuals were considered “long shedders” with detection of viral RNA in NP/OP swabs for ≥10 days (Figure S1B). Overall, 70% of the patients displayed mild or moderate symptoms, and 30% remained asymptomatic (Table S1). For all assays, samples were selected based on availability and quality.
Asymptomatic and Mild/Moderate Infection Induces a Persisting Anti-Spike Antibody Response
The presence of S-binding antibodies was measured using the S-Flow assay, which sensitively and quantitatively measures anti-S IgG, IgA, and IgM by flow cytometry (21, 39) (Figure 1A). The National Institute for Biological Standards and Control (NIBSC) references were utilized to validate the assays and pre-pandemic samples obtained from nineteen individuals were measured to set the cutoff for each assay (Figure S2). Anti-S IgM, IgG, and IgA titers decreased significantly between acute phase and late convalescence (p=0.02, p<0.0001, p<0.0001, respectively) (Figure 1B). Within the total S-binding antibodies, the percentage of anti-S IgM and IgA decreased whereas anti-S IgG increased over time (p=0.03, Figure 1C). The detection of neutralizing antibodies was achieved by foci reduction neutralization test using a Cambodian SARS-CoV-2 isolate. There was no difference in the titers of SARS-CoV-2 neutralizing antibodies between the acute and convalescent phase, even though titers tended to decrease over time (Figure 1D). Over time, the percentage of individuals positive for anti-S IgM (p<0.0001) and anti-S IgA (p<0.0001) decreased (Figure 1E). In the acute phase, 91% of individuals were positive for anti-S IgG, and only 70% of the individuals were positive for neutralizing antibody titers. Up to nine months post infection, the frequency of individuals positive for anti-S IgG remained stable (88%) whereas the frequency of individuals with neutralizing titers decreased to 56% (p=0.055) (Figure 1E). Analyzing only individuals with paired samples available, revealed similar results as the whole cohort (Figures S3A, B). Taken together, these data show that despite decreases in antibody titers over time, the percentage of individuals positive for anti-S IgG remains stable.
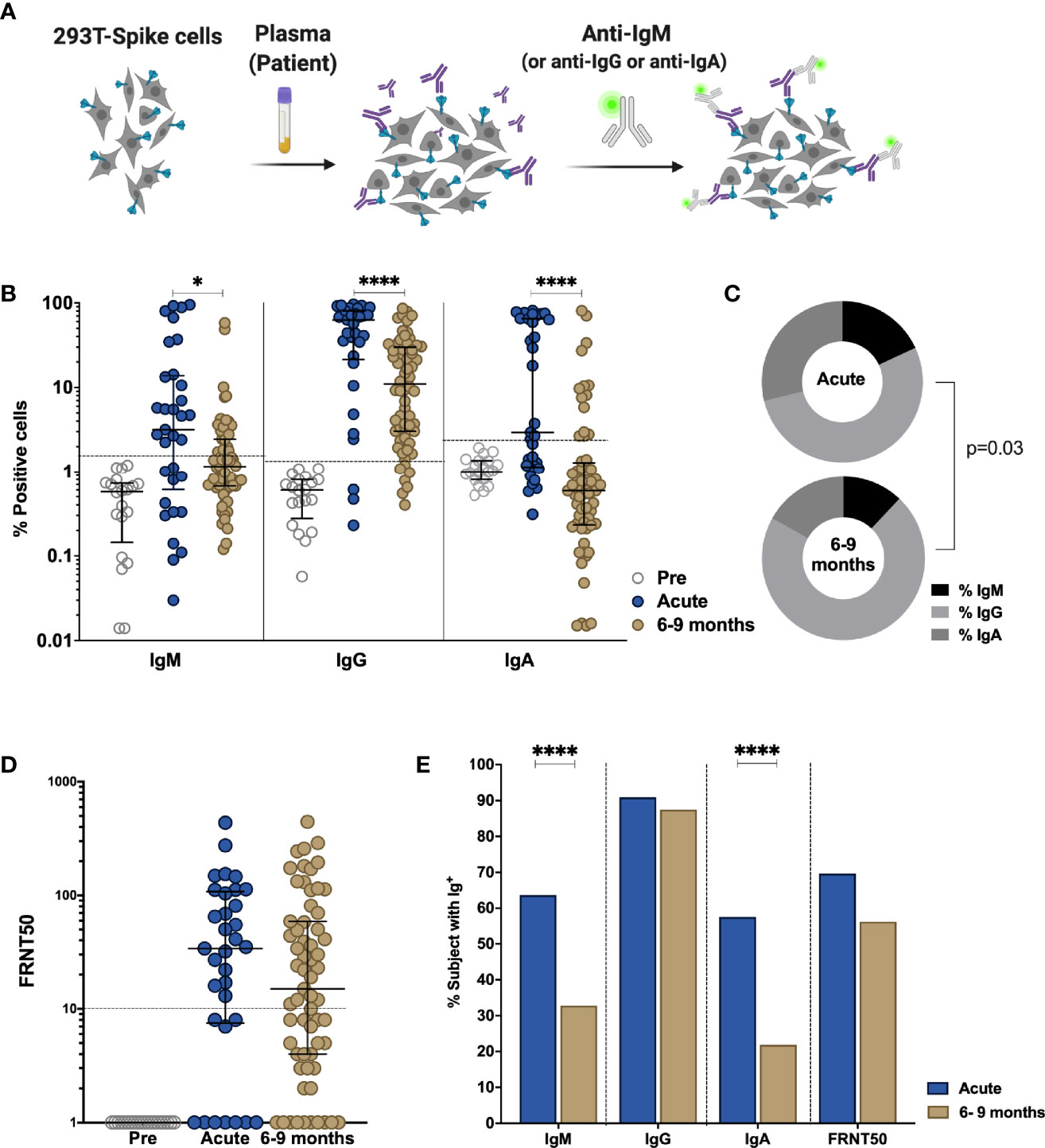
Figure 1 Comparison of antibody response in SARS-CoV-2-infected individuals during the acute phase and 6-9 months post infection. Non-infected samples obtained before the pandemic (pre), and SARS-Cov-2 infected individuals were sampled 2-9 days post laboratory confirmation and 6-9 months later. (A) Schematic model of the S-Flow assay. (B) Amount of antibodies against spike protein were reported as percentage of spike-expressing 293T cells bound by IgM, IgG, IgA in the S-Flow assay. (C) Pie charts show the proportion of anti-S IgM, IgG and IgA antibodies. (D) SARS-CoV-2 neutralizing activity was calculated as FRNT50 titer in foci reduction neutralization test (FRNT). (E) Comparison of the percentage of individuals positive for anti-S IgM, IgG, IgA and FRNT50. Statistical comparisons were performed by Mann Whitney test (B, D) and Chi-square test (C, E). The dashed line indicates the cutoff for positivity based on values calculated following formula: cut-off = % mean positive cells from 19 pre-pandemic samples + 3x standard deviation. Each dot represents the result from a single individual. Lines represent median and IQR. *p < 0.05 and ****p < 0.0001. Pre-pandemic n=19, acute n=33, 6-9 months n=64.
Functional Antibody Response Changes Over Time Post SARS-CoV-2 Infection
Besides neutralization, antibodies can mediate Fc-effector functions, such as complement activation, killing of virus-infected cells and phagocytosis of viral particles (20). To further define the humoral response in these individuals, we assessed antibody effector functions in vitro. The NIBSC references were utilized to validate the assays and nineteen pre-pandemic samples were measured to set the cutoff for each assay. Antibody-dependent cellular phagocytosis (ADCP) assay measures the engulfment of neutravidin beads coated with SARS-CoV-2 derived S1 by THP-1 cells (Figures 2A, S4). A decrease in ADCP can be observed between the acute and late convalescent phase (p=0.005, Figures 2B, C). The percentage of subjects with ADCP activity decreased from 73% to 55% over time. However, when calculating the proportion of ADCP within the total anti-S IgG, we observed a significant increase of the proportion of ADCP over time (p=0.003, Figure 2D).
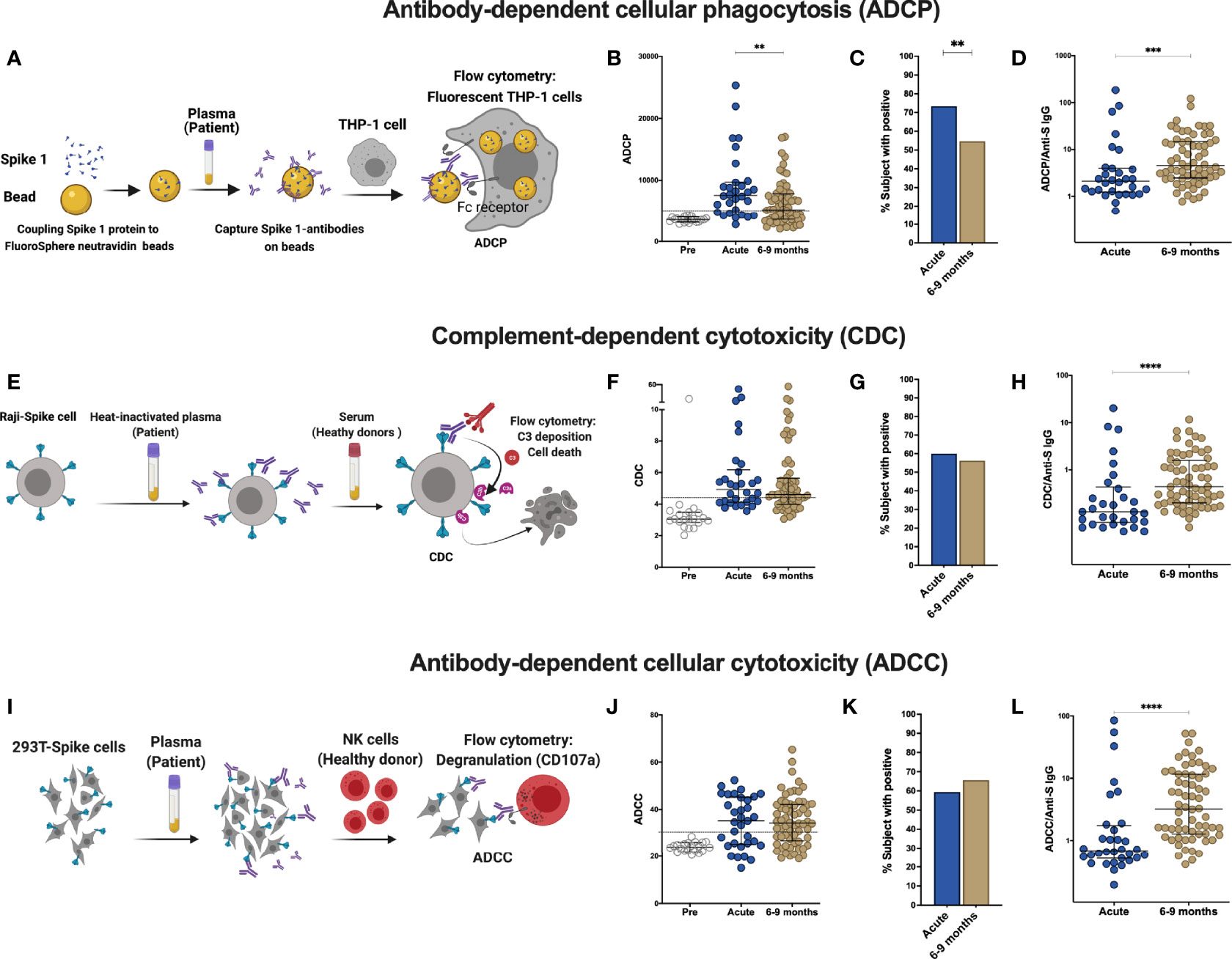
Figure 2 Comparison of effector function profiles of plasma from SARS-CoV-2-infected individuals during the acute phase and 6-9 months post infection. Non-infected samples obtained before the pandemic (pre), and SARS-Cov-2 infected individuals were sampled 2-9 days post laboratory confirmation and 6-9 months later. (A) Schematic representation of the antibody-dependent cellular phagocytosis (ADCP) assay. (B) Comparison of ADCP activity in pre-pandemic samples, SARS-CoV-2-infected individuals in the acute phase of infection and 6-9 months later. (C) Percentage of individuals with ADCP above the cutoff for positivity. (D) Ratio of ADCP to anti-spike IgG measured by S-Flow. (E) Schematic representation of complement-dependent cytotoxicity (CDC) assay. (F) Comparison of CDC activity in pre-pandemic samples, SARS-CoV-2-infected individuals in the acute phase of infection and 6-9 months later. (G) Percentage of individuals with CDC above the cutoff for positivity. (H) Ratio of CDC to anti-spike IgG as measured by S-Flow. (I) Schematic representation of antibody-dependent cellular cytotoxicity (ADCC). SARS-CoV-2 plasma induced NK degranulation as measured by CD107a staining using spike-expressing 293T cells as target cells. NK cells were isolated from healthy donors. (J) Comparison of ADCC activity in pre-pandemic samples, SARS-CoV-2-infected individuals in the acute phase of infection and 6-9 months later. (K) Percentage of individuals with ADCC above the cutoff for positivity. (L) Ratio of ADCC to anti-spike IgG as measured by S-Flow. Statistical comparisons were performed by Mann Whitney test. The dashed line indicates the cutoff for positivity set based on values calculated following formula: cut-off = % mean positive cells from 19 pre-pandemic samples + 3x standard deviation. Each dot represents result from a single individual. Lines represent median and IQR. **p < 0.01, ***p < 0.001, and ****p < 0.0001. Pre-pandemic: n=19, acute ADCP and CDC: n=30, acute ADCC: n=32, 6-9 months ADCP, CDC and ADCC: n=64.
Next, to evaluate the contribution of anti-S antibodies to complement dependent cytotoxicity (CDC), we assessed cell death in Raji cells engineered to express S protein in the presence of normal human serum as source of complement (Figure 2E, S5) (21). No differences in CDC activity was observed between the acute and late convalescent phase, where 60% and 56% of the subjects showed CDC activity, respectively (Figures 2F, G). The proportion of CDC-mediating antibodies within the total anti-S IgG fraction significantly increased between acute and late convalescence (p=0.0002, Figure 2H).
Killing of virus-infected cells can also be mediated by activated NK cells, after binding of immunocomplexes to CD16 (20). Therefore, antibody-dependent cellular cytotoxicity (ADCC) activity was measured using S-expressing 293T cells as target cells with degranulation measured by CD107a staining in primary NK cells as a readout for ADCC (Figures 2I, S6). ADCC activity did not change between the acute and late convalescent phase (Figures 2J, K). At both time points, 59% - 66% of individuals showed anti-S mediated ADCC activity. However, similar to ADCP and CDC, the proportion of ADCC-mediating antibodies within the fraction of anti-S IgG increased significantly over time (p<0.0001, Figure 2L). Analyzing only individuals with paired samples available, revealed similar results as the cohort as a whole (Figures S3C–H). Overall, these data show that antibody effector functions mediated by S-specific antibodies remain stable over time and that the proportion of the functional antibody response within the total anti-S antibodies increases over time.
SARS-CoV-2 Infection Induces a Sustained Memory B Cell Compartment Reacting Against Spike and Nucleocapsid Protein 6–9 Months After Infection
Upon re-infection, memory B cells are rapidly activated to differentiate into antibody-producing plasmablasts and/or re-initiate germinal centers in the case of secondary heterologous infection with antigenically similar pathogens (40). Therefore, they may play an important role in long-term immune memory to SARS-CoV-2 and their evolving variants. We assessed the phenotype and frequency of antigen-specific memory B cells from 40 SARS-CoV-2 infected individuals in the cohort by staining with site-specific biotinylated recombinant S1 and N protein (Figures 3A, S7A, B). At late convalescence, 0.10% of median of the total CD27+ B cells are S1-specific, whereas 0.66% of median are N-specific (p<0.0001, Figure 3B). We observed the highest frequencies of S1-specific and N-specific B cells in the activated memory B cell compartment (CD27+CD38+) compared to plasma blast (CD27+CD38hi) and resting memory compartments (CD27+CD38-) (Figures 3C, D) (41, 42). The proportion of CD27+CD38+ S1-specific B cells (median=75%, IQR=30%) is significantly increased compared to the proportion of CD27+CD38+ N-specific B cells (median=39%, IQR=26%, Mann-Whitney Test, p<0.0001) (Figure 3E). Moreover, the proportion of S1- versus N-specific B cells varies within each CD27+ B cell subset (p<0.0001, Figure 3F). We next analyzed S1- and N-specific B cells within the unswitched (IgD-IgM+) and switched (IgD-IgG+ and IgD-IgA+) B cell compartments (Figure S7A). S1-specific B cells were mainly IgD-IgG+, whereas N-specific B cells were either IgD-IgM+ or IgD-IgG+ (Figures 3G, H). The proportion of IgD-IgG+ S1-specific B cells (median=75%, IQR=24%) was significantly increased compared to the proportion of IgD-IgG+ N-specific B cells (median=37%, IQR=17%) (p<0.0001) (Figure 3I). Therefore, within each switched B cell subset, the proportion of S1- versus N-specific B cells was different (p<0.0001) (Figure 3J). Taken together, SARS-CoV-2 infection induces a robust memory B cell response targeting both S and N.
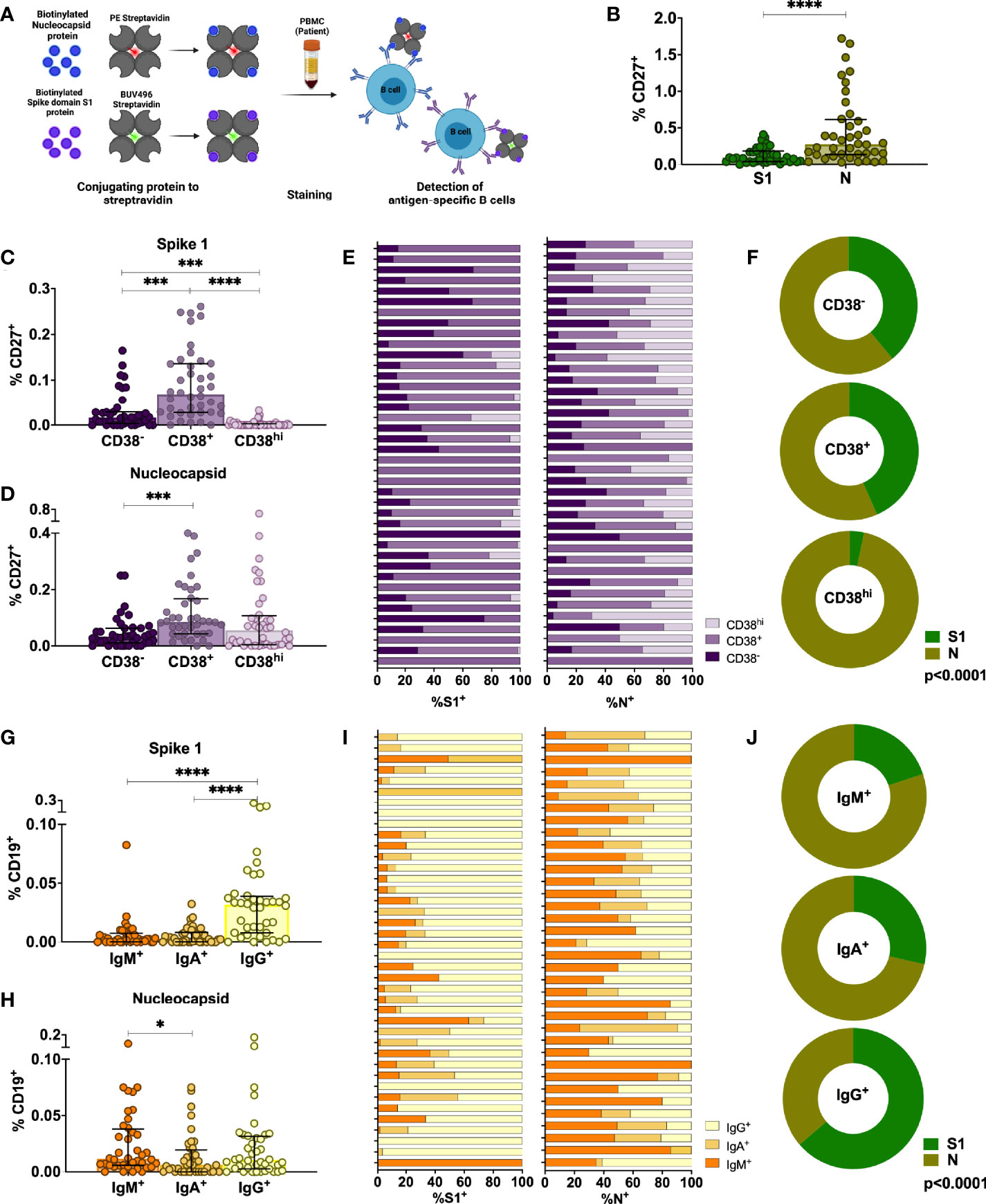
Figure 3 Characterization of antigen-specific memory B cells in the peripheral blood of individuals infected with SARS-CoV-2 6-9 months after infection. (A) Schematic representation of the memory B cell assay. (B) Comparison of percentages of S1-specific or N-specific memory B cells (CD19+CD27+). (C, D) Percentages of S1- and N-specific B cells among resting memory B cells (CD38-), activated memory B cells (CD38+) or plasmablasts within the CD27+ memory B cell population. (E, F) Proportion of S1-specific and N-specific CD27+CD19+ B cell subsets for each individual and the whole cohort (G, H) Percentages of S1- and N-specific cells in non-class-switched B cells (IgD-IgM+) or class-switched B cells (IgD-IgA+ or IgD-IgG+). (I, J) Proportion of S1-specific and N-specific switched and unswitched CD19+ B cells for each individual and for the whole cohort. Statistical comparisons were performed by Mann-Whitney test (B), Wilcoxon Rank Sum test (C, D, G, H) or Chi-square test (F, J). Each dot represents result from a single individual. Lines represent median and IQR (n=40). *p < 0.05, ***p < 0.001, and ****p < 0.0001. n = 40. S1, subunit 1 of spike protein; N, Nucleocapsid protein.
SARS-CoV-2 Infection Induces Mainly Spike and Membrane Protein-Specific Memory CD4+ and CD8+ T Cells That Are Maintained Up to 6–9 Months After Infection
In addition to humoral immune memory, the generation and maintenance of virus-specific cellular immune responses is critical to help prevent reinfection. Long-term maintenance and phenotypes of SARS-CoV-2-specific memory T cell responses are still under investigation (4, 43, 44). SARS-CoV-2-specific CD4+ and CD8+ T cells were assessed in 33 individuals at late convalescence by incubating PBMCs with peptide pools covering immunodominant sequences of the viral S1, M and N protein (Figure 4A). Post incubation, activation induced marker (AIM) assays identified CD4+ antigen-specific cells using OX40+CD137+ combined with phenotypic markers to measure different memory and T helper (Th) subsets (Figures S8A–D). Percentages of both S1- and M-specific CD4+ T cells were significantly increased compared to the percentage of N-specific cells (p<0.0001, p=0.0002), Figure 4B). Phenotypically, 42% of virus-specific T cells displayed an effector memory phenotype (CD45RA-CCR7+) and 87% of the cells showed a Th1-skewed phenotype (CXCR3+CCR6-) (Figures 4C, D). Comparing the memory phenotype of S1-, M- and N-specific cells, we observed that a lower proportion of S1-specific cells displayed an effector memory phenotype (23%) compared to M-specific cells (41%, p=0.0457) and N-specific cells (58%, p<0.0001) (Figure S9A). Moreover, 97% of M-specific cells showed a Th1-skewed phenotype compared to only 65% (p<0.0001) of the S1-specific cells and 71% (p=0.0130) of the N-specific cells (Figure S9B). In eight individuals, sufficient cell numbers were available to assess functionality by cytokine production after peptide stimulation using a multi-parameter ex vivo intracellular cytokine staining (ICS) assay (Figure S8E). SARS-CoV-2-specific CD4+ T cells produced Interleukin (IL)-2 (36%) or IL-6 (28%) after peptide stimulation, and were polyfunctional (Figures 4E, F). Percentages of IL-2+ and IL-17+ cells were significantly higher after S1 stimulation compared to M stimulation (p=0.046, p=0.017) (Figure S9C).
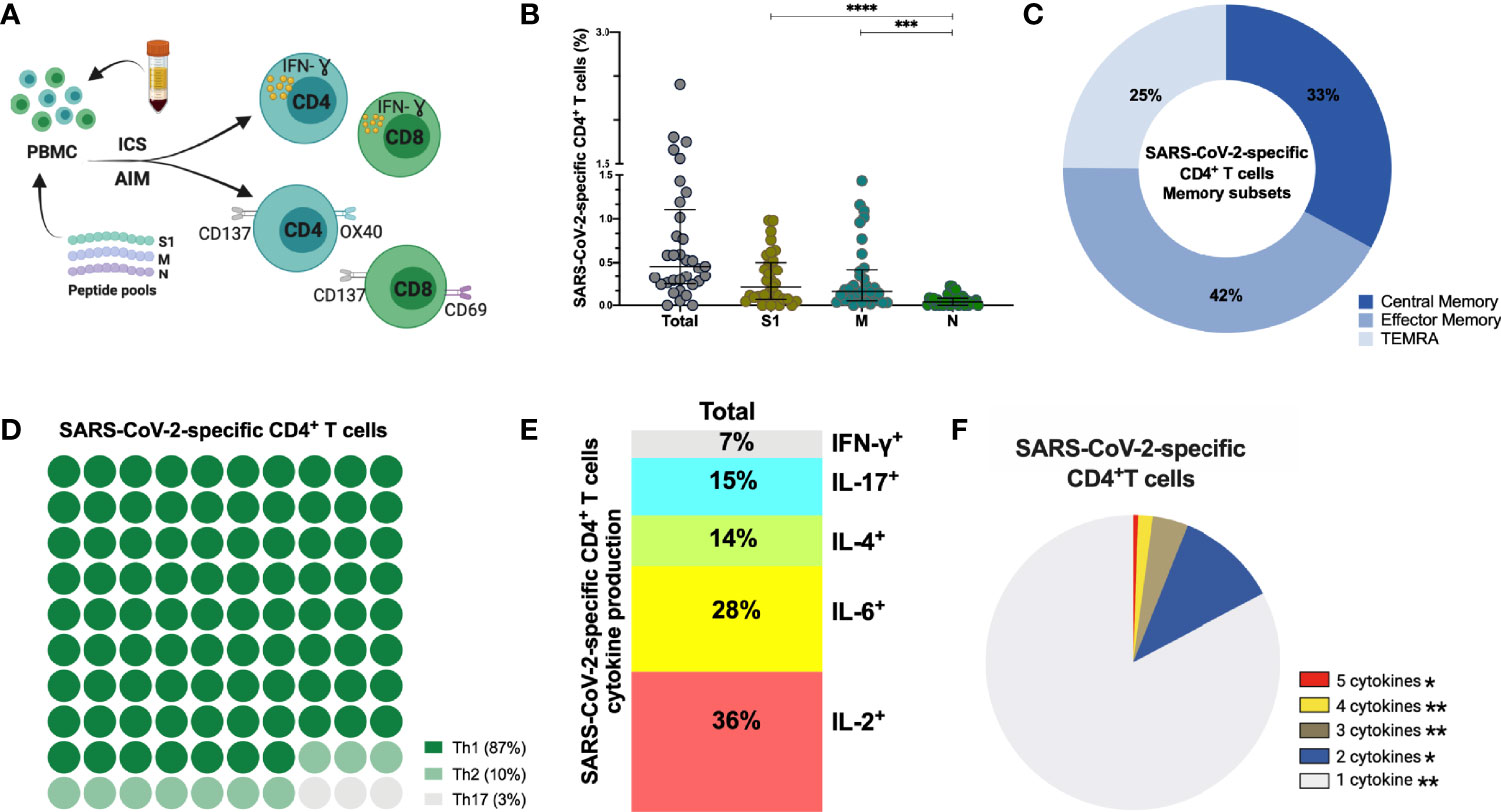
Figure 4 SARS-CoV-2-specific CD4+ T cells 6-9 months post-infection. (A) Schematic representation of the CD4+ T cell assay (B) Frequency (percentage of CD4+ T cells) of total SARS-CoV-2-specific CD4+ T cells after overnight stimulation with S, M and N peptide pools as assessed by induced expression of OX40 and CD137. Each dot represents result from a single individual (n=33). Lines represent median and IQR. (B) Distribution of SARS-CoV-2–specific CD4+ T cells among central memory, effector memory, and terminally differentiated effector memory cells (TEMRA). (C) Frequencies of SARS-CoV-2-specific CD4+ T helper (Th) subset. (D) Cytokine production and (E) pie chart representing the multifunctional SARS-CoV-2-specific CD4+ T cell response assessed by intracellular cytokine staining after incubation with SARS-CoV-2 peptides compared to unstimulated control, n=8. SARS-CoV-2 specific activation and cytokine production were calculated by subtracted the unstimulated control from the SARS-CoV-2 peptide stimulated condition. Statistical comparisons were performed by Kruskal-Wallis test (B) and Wilcoxon Rank Sum test (F). *p < 0.05, **p < 0.01, ***p < 0.001, ****p < 0.0001. S1, subunit 1 of spike protein; M, membrane protein; N, nucleocapsid protein.
Next, we assessed the frequency and phenotype of cytotoxic CD8+ T cells by AIM assay using CD69+CD137+ to identify antigen-specific CD8+ T cells. Frequency of total SARS-CoV-2-specific CD8+ cells is 0.44% (median) (Figure 5A) with 61% of these SARS-CoV-2-specific CD8+ T cells being terminally differentiated effector memory cells (TEMRA, CD45RA+CCR7-) (Figures 5B, S9D). No differences were observed between S1-, M- and N-specific CD8+ T cells. Similar to antigen-specific CD4+ T cells, SARS-CoV-2-specific CD8+ T cells produced either IL-2 (56%) or IL-6 (16%) after peptide stimulation, and were polyfunctional (Figures 5C, D), (Figure S9E). Interestingly, 2/33 (6%) individuals displayed no CD4+ T cell reactivity, and 6/33 (19%) individuals lacked a CD8+ T cell response after stimulation. In summary, sustained and functional CD4+ and CD8+ T cell responses are detected in the study participants, even after experiencing only mild or asymptomatic SARS-CoV-2 infection. These data suggest that SARS-CoV-2 can induce a long-lived cellular immune response, which could confer protection after reinfection or could be reactivated with vaccination.
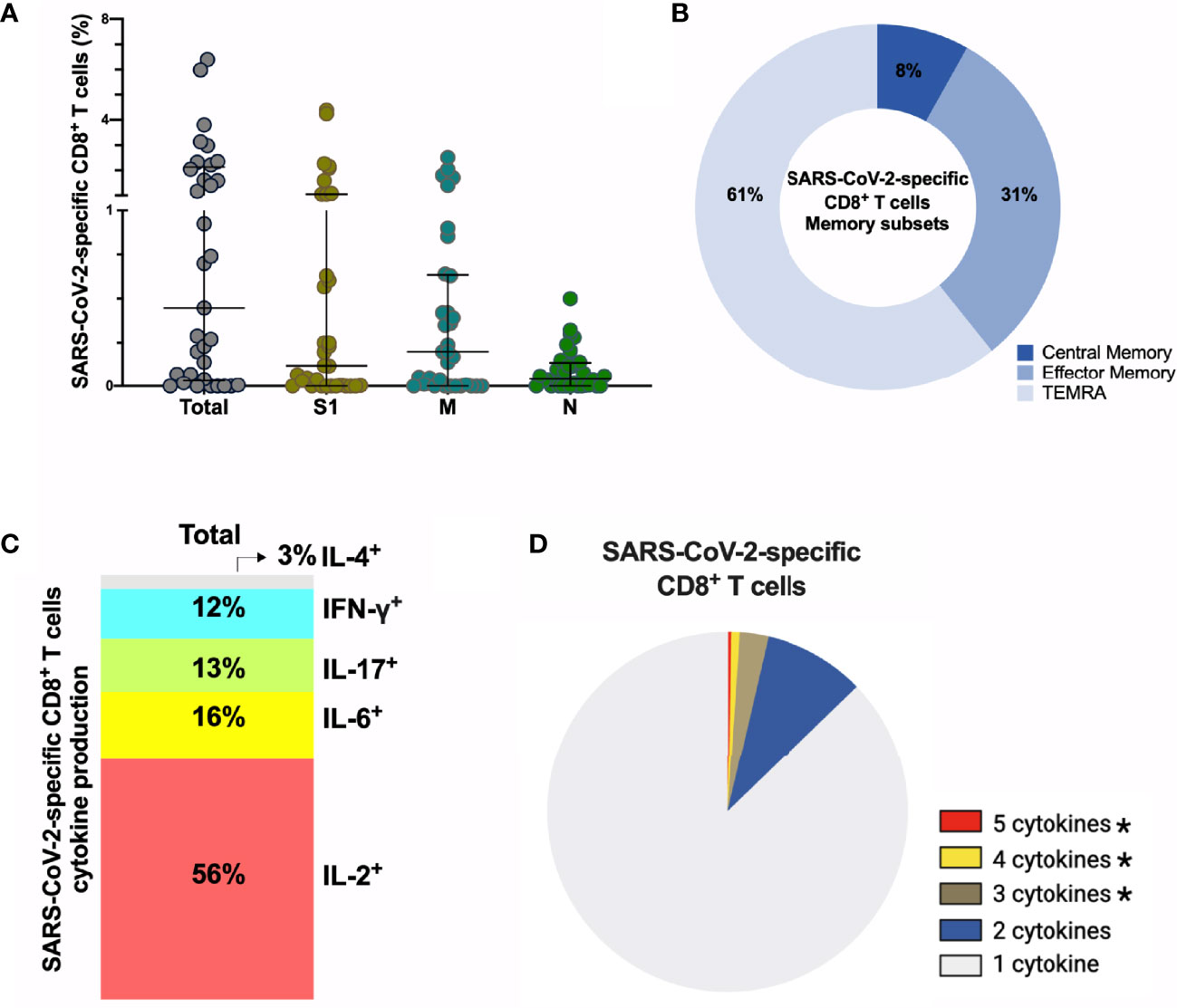
Figure 5 SARS-CoV-2-specific CD8+ T cells 6-9 months post-infection. (A) Frequency (percentage of CD8+ T cells) of total SARS-CoV-2-specific CD8+ T cells after overnight stimulation with S, M and N peptide pools as assessed by induced expression of CD69 and CD137. Each dot represents result of a single individual (n=33). Lines represent median and IQR. (B) Distribution of SARS-CoV-2-specific CD8+ T cells among central memory, effector memory, and terminally differentiated effector memory cells (TEMRA). (C) Cytokine production and (D) pie chart representing the multifunctional CD8+ T of SARS-Cov-2-specific T cells assessed by intracellular cytokine staining after incubation with SARS-CoV-2 peptides compared to unstimulated control, n=8. SARS-CoV-2 specific activation and cytokine production were calculated by subtracted the unstimulated control from the SARS-CoV-2 peptide stimulated condition. (A) Kruskal-Wallis test and (D) Wilcoxon Rank Sum test. *p < 0.05. S1, subunit 1 of spike protein; M, membrane protein; N, nucleocapsid protein.
Symptomatic Infection Is Associated With Increased ADCC Activity and Increased Frequency of SARS-CoV-2-Specific CD4+ T Cells Observed 6–9 Months After Infection
In order to assess if symptomatic disease is associated to altered immune memory formation, we compared the functional immune response between asymptomatic and symptomatic patients with mild/moderate clinical presentation. Overall, no differences occurred in the titers of anti-S IgM, IgA, IgG, neutralization or antibody-effector functions assessed in the acute phase of infection (Figures 6A, S10). At late convalescence, symptomatic disease resulted in increased ADCC activity compared to asymptomatic individuals (p=0.0034) (Figure 6A). Other Fc-mediated effector functions, such as ADCP and CDC and neutralizing titers were not different between the patients (Figure S10). Percentages of N-specific CD27+ B cells, but not S1-specific, were increased in asymptomatic individuals versus patients who were symptomatic (p=0.051) (Figure 6B). Symptomatic disease resulted in increased percentage of SARS-CoV-2-specific CD4+ T cells (p=0.0018) with a central memory phenotype (p=0.0498) (Figures 6C, D), but no differences were observed in the CD8+ T cell compartment (Figure S10).
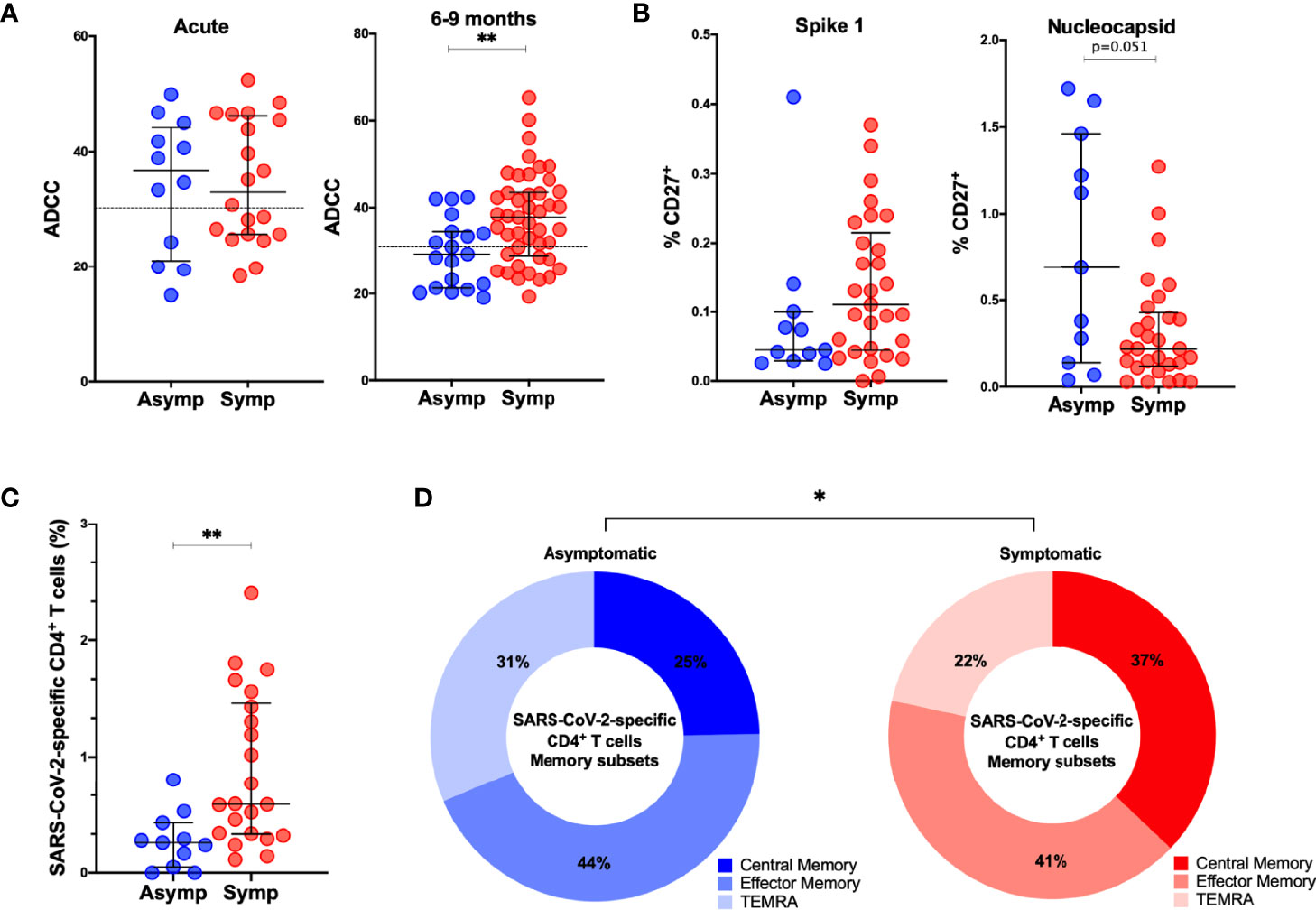
Figure 6 Comparison of adaptive immune memory in asymptomatic and symptomatic individuals. (A). Comparisons of ADCC activity in asymptomatic (asymp; n=12) versus symptomatic (symp; n=20) individuals in the acute phase and 6-9 months after confirmed infection using 293T-spike cells as target cell. Percentage of CD107a positive cells is measured as readout for ADCC. (B) Comparison of percentages of S1-specific or N-specific memory B cells (CD19+CD27+) between 11 asymptomatic individuals and 29 symptomatic individuals. (C) Frequency (percentage of CD4+ T cells) of total SARS-CoV-2-specific CD4+ T cells after overnight stimulation with peptide pools comparing asymptomatic individuals (asymp; n=11) with symptomatic patients (symp; n=22). (D) Comparison of CD4+ T cell memory phenotype between asymptomatic individuals (asymp; n=11) and symptomatic patients (symp; n=22). Statistical comparisons were performed by (A–C) Mann Whitney tests and (D) Chi-square test for trend *p < 0.05, **p < 0.01.
These data suggest that the outcome of acute infection has an imprint on the memory immune response with implications for response to subsequent infection or vaccination.
Correlations Between Various Aspects of the Functional Anti-Viral Memory Response
In order to assess the relation between antibody titers, functional humoral immune memory, and the cellular T and B cell compartment we performed extensive correlation analysis (Figure 7A). Age correlated to anti-S antibody titers and S1-specific CD19+IgD-IgG+ and CD19+CD27+B cells. In the acute phase of infection, anti-S IgG, IgM and IgA titers, functionality, measured by seroneutralization, and effector functions correlated. Seroneutralization, anti-S IgA, and ADCC correlated over time, albeit not very strong. Of note, viral shedding did not correlate with anti-SARS-CoV-2 immunity (Figure 7A), and subdividing individuals into short or long viral RNA shedding using an arbitrary cut-off of 10 days did not yield any differences in SARS-CoV-2 specific immune responses (Figure S11).
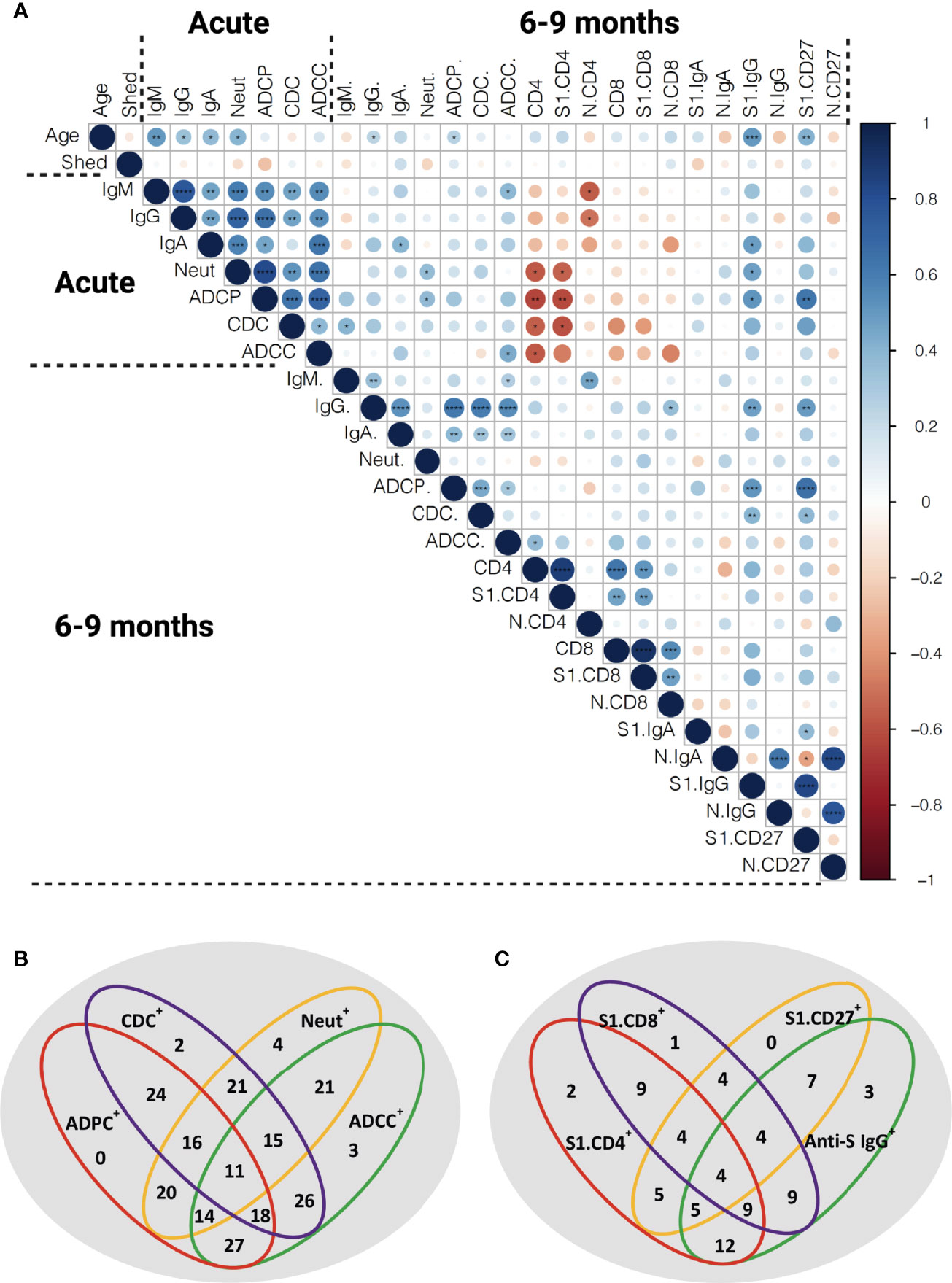
Figure 7 Correlation of the functional anti-SARS-CoV-2 immune responses. (A) Spearman correlation matrix showing humoral immune memory and effector functions measured in the acute phase and 6-9 months post infection were correlated to each other and to frequencies of antigen-specific B and T cells measured 6-9 months after infection. Red represents a negative correlation between two variables and blue indicates a positive correlation. The size of the dot represents the magnitude of the correlation coefficient. Statistical analysis was performed with spearman correlation test. *p <0.05, **p < 0.01, ***p < 0.001, ****p < 0.001. IgM: anti-S IgM titers, IgG: anti-S IgG titers, IgA: anti-S IgA titers, Neut: FRNT50 titers, CD4: total SARS-CoV-2 specific CD4+ T cells, S1.CD4: S-specific CD4+ T cells, N.CD4: N-specific CD4+ T cells, CD8: total SARS-CoV-2 specific CD8+ T cells, S1.CD8: S-specific CD8+ T cells, N.CD8: N-specific CD8+ T cells, S1.IgA: S1-specific IgD-IgA+ B cells, N.IgA: N-specific IgD-IgA+ B cells, S1. IgG: S1-specific IgD-IgG+ B cells, N.IgG: N-specific IgD-IgG+ B cells, S1.CD27: S1-specific CD27+ B cells, N.CD27: N-specific CD27+ B cells. (B) Venn diagram showing the relation of anti-S humoral immune responses at late convalescence. All individuals with detectable anti-S IgG titers are included (n=56). (C) Venn diagram showing the relation of anti-S IgG, S1-specific CD27+ B cells and S1-specific T cells at late convalescence. The cutoffs for S1-specific CD27+ B cell response and S-specific CD4+ and CD8+ T cell responses were arbitrarily set above 0.1% (n =20).
At late convalescence, anti-S IgG correlated with all three effector functions, but not with neutralizing capacity. Within the B cell compartment, N-specific IgD-IgG+, IgD-IgA+ and CD27+ B cells correlated to one another, as did S1-specific IgD-IgG+, IgD-IgA+ and CD27+ B cells. No correlation was identified between S1- and N-specific B cells. Anti-S IgG titers, ADCP, and CDC correlated with S1-specific IgD-IgG+ and CD27+ B cells. The S-specific CD4+ T cell responses correlated with S-specific CD8+ T cell responses, but did not correlate to antibody titers nor to effector functions or to S1-specific B cells.
Next, we assessed in more detail the relationship between the different effector functions at late convalescence. We included 56 individuals with measurable anti-S IgG above the cutoff based on the pre-pandemic samples (Figures 1, 7B). We found that plasma from each individual could induce neutralization or at least one antibody-effector function (ADCP, CDC and ADCC). In 11 out of the 56 individuals (19.6%), both neutralization titers and all antibody-effector functions could be measured. Eighteen out of the 56 individuals (32,1%) had measurable antibody-effector functions, but no neutralizing titers, while in 4 out of the 56 (7.1%) individuals only neutralizing titers were detected (Figure 7B).
We further detailed if individuals showed measurable immune responses in one or multiple immune compartments at 6-9 months post infection. In twenty individuals we assessed in parallell anti-S IgG, S1-specific B cells and S-specific T cells. We set an arbitrary cutoff for positive B and T cell responses at 0.1% of S-specific CD27+ B, CD4+ T and CD8+ T cells. Four out of the 20 individuals (20.0%) had responses in all compartments. Three individuals (15.0%) showed only measurable anti-S IgG titers. Moreover, 7 individuals (35.0%) had anti-S IgG titers and detectable S1-specific CD27+ B cells but no S-specific T cell responses. In contrast, 9 (45.0%) individuals had measurable S-specific T cell responses, but no S1-specific B cell responses or anti-S antibody titers (Figure 7C).
Overall, different aspects of a functional immune memory response do not fully correlate with one another and require separate evaluation when considering long-term immune memory to SARS-CoV-2.
Discussion
In this study, we investigated a partially asymptomatic cohort of Cambodian individuals in the acute and late convalescent phase for anti-S antibody titers, neutralization and effector functions, as well as SARS-CoV-2-specific B and T cell responses. As Cambodia was relatively COVID-19-free throughout 2020 (45), it is highly unlikely this cohort had additional exposure events after inclusion in this study, that could have boosted their immune memory to SARS-CoV-2. One limitation is the uncertainty of the exact timing of exposure/infection, as infections were identified by screening at entry into Cambodia rather than in a direct surveillance or community cohort.
Studies assessing long-term immune memory to SARS-CoV-2 in Asian populations are scarce (18, 34–36, 46). Historically, the population in East Asia seems to be more exposed to coronavirus-like viruses as only East Asian population show genetic adaptation to coronaviruses (47). The main natural reservoir of SARS-related coronaviruses is believed to be Horseshoe bats (genus Rhinolophus), which are endemic to Southeast Asia and China (48–51). Whether possible cross-reactivity to other coronavirus-like viruses or hCoVs may have influenced the adaptive immune response to SARS-CoV-2 in Southeast Asian populations remained to be investigated.
As shown in previous studies anti-S IgM, IgG and IgA titers declined over time and anti-S IgG becomes the major isotype at late convalescence (4, 52, 53). In this study, IgA titers were the most affected over time. The formation of anti-S IgA is shown to be dependent on local lung inflammation (6, 54, 55) hence titers decline the strongest in asymptomatic/mild patients. Titers of neutralizing antibodies are reported to reach their maximum within the first month after infection and then decay, but mostly remain detectable six months and even up to one year after infection (4, 12). A relatively low rate of individuals retained neutralizing antibodies at late convalescence in this cohort (56%) as most longitudinal studies found 76-98% of individuals remaining positive (4, 21). This might be attributed to the absence of possible re-exposure and/or the consequence of asymptomatic/mild infection (12, 27, 43, 53). One caveat is that it is possible that the humoral responses for some donors were still on the rise and had not peaked by the detection at the acute timepoint.
In contrast with other papers, neutralizing titers did not correlate to anti-S IgG antibodies at late convalescence. This might be due to the genetic background or previous immunity to other hCoVs of the participants inducing antibodies binding to different viral epitopes, or could be due to the different technique to measure anti-S binding and neutralizing antibodies (56, 57).
Fc-mediated effector functions contribute to clearance of virus-infected cells but are often critically overlooked. SARS-CoV-2 infection induces Fc-mediated effector functions irrespective of disease outcome (21–23). Antibody effector functions develop rapidly after infection and correlate with anti-S IgG and neutralizing titers in the acute phase and at late convalescence (21, 22). In this current study, we assessed three antibody effector functions using cell-based assays. Between 55-66% of individuals showed antibody effector function activity up to nine months after infection. Also, ADCC persisted in a higher percentage of individuals compared to neutralization or other effector functions (24, 25). We report here the maintenance of CDC over time suggesting that both ADCC and CDC can contribute to protection from re-infection. ADCP levels decreased over time which could have consequences for antigen presentation and macrophage activation upon re-infection (58). Interestingly, the ratio of S-mediated effector functions over total anti-S IgG increases over time. Together with reports showing the evolution of the BCR repertoire over time (27, 59, 60), these data indicate ongoing affinity maturation and evolution of the antibody response to a more functional response. Therefore, measurement of only S-binding antibodies at late convalescence does not reflect their function.
S-, RBD- and N-specific memory B cells are maintained more than six months post symptom onset and their frequency increased over time (4, 61, 62). In this cohort, S1-and N-specific memory B cells persisted up to 6-9 months post infection with some variability between individuals. One of the caveats of this study is that the cut-off for positive staining in antigen-specific B/T cells is arbitrary, which may lead to over-estimate the true number. The percentage of S1-specific IgG B cells correlated with S-specific IgG antibodies, and S1-specific B cells displayed an activated phenotype. This suggests that these B cells could be recruited after secondary exposure with SARS-CoV-2 and might confer some level of protection against infection with new variants or could be re-activated after vaccination via additional diversification trough germinal center responses (40, 63).
Anti-SARS-CoV-2 T cell immune memory was assessed by AIM, which is a sensitive assay that provides a broader picture of the overall antigen-specific T cell response, compared to cytokine-detection based assays (8, 64). Persistence of functional memory T cells after SARS-CoV-2 infection has been reported, also after asymptomatic infection (4, 65). Similar to other reports, virus-specific memory CD4+ T cells were skewed to a Th1 or Th1/Th17 profile and displayed mainly an effector memory (CD45RA-CCR7-) phenotype (28, 65, 66). Virus-specific CD8+ T cells consisted mostly of cells with a TEMRA phenotype, a compartment of cytotoxic CD8+ T with limited proliferative potential (66). Polyfunctional virus-specific CD4+ and CD8+ T cells could be detected, mainly secreting IL-2 (18, 43), albeit we could only include few individuals in this analysis. Similar to other long-term cohorts, virus-specific CD4+ and CD8+ cells can be detected in up to 90% - 70% of the individuals, respectively (31). Hence, cellular immune responses might confer protection after reinfection with variants of concern (67).
Differences in frequency and phenotype of N- and S-specific B and T cells has been reported before (4, 5, 28, 43). This might be due to the difference in antigen availability, persistence, and immunological context. Together with other envelope proteins, S proteins cover the surface of the virus and bind to the host cell, while the N protein underlies viral packaging and hence is less accessible (68). The N protein is more conserved among coronaviruses (68), whereas S protein and especially the RBD-bearing S1 subunit are more prone for acquiring mutations (69, 70). Consequently, anti-N IgG rather than anti-S1 IgG can be found in individuals not exposed to SARS-CoV-2 (68, 71, 72). This might explain the observed higher frequency of N-specific B cells in our study.
Correlations between CD4+ T cells and humoral responses can be observed in some long-term cohorts (28, 30, 73, 74) but not all (4). In this study, there was no correlation between the S-specific cellular and humoral immune compartment at late convalescence. Importantly, in 45% of the individuals anti-S CD4+ and CD8+ T cell responses could be detected but their anti-S antibody titers were below the pre-pandemic detection threshold. Therefore, neither anti-S IgG nor neutralizing antibodies are a good proxy to determine the cellular response to SARS-CoV-2. Interestingly, we showed that 32% of the individuals had measurable antibody-effector functions, but no neutralizing titers, while in 7% of the individuals only neutralizing titers were detected. Hence, subtle differences in anti-S antibody titers, neutralization and Fc-related functions might lead to a different disease outcome upon re-exposure. Therefore, serological testing alone might not be sufficient to understand the full spectrum of long-term immune memory generated after SARS-CoV-2 infection.
In this cohort, the duration of SARS-CoV-2 RNA shedding did not correlate with the magnitude of anti-SARS-CoV-2 immunity, either measured in the acute phase or at late convalescence, which is in contrast to previous studies (12, 34, 75) and could be explained by the inclusion of mainly asymptomatic and mild cases.
The development, characteristics and functionality of the totality of long-term immune memory in asymptomatic infected individuals remains to be further characterized. We observed an increase of ADCC at late convalescence in patients who had mild/moderate disease compared to asymptomatic individuals. This observation is in line with studies showing increased anti-S IgG afucosylation in severe patients compared to mild and asymptomatic cases (76, 77). Indeed, afucosylated monoclonal antibodies can cause elevated ADCC though increased IgG-FcγRIIIa affinity (78, 79). More severe COVID-19 induced a stronger SARS-CoV-2-specific CD4+ T cell response (28, 29). We confirm and extend these data as we observed lower levels of virus-specific CD4+ T cells in asymptomatic individuals compared to mild/moderate cases. These data suggest that different disease outcome after infection results in altered long-term immune memory, which could shape the response to subsequent infection or vaccination.
Taken together, our work shows additional evidence of long-term and persistent immune memory after asymptomatic and mild SARS-CoV-2 infection. Furthermore, this cohort describes the immune response in individuals of Asian origin and in the absence of re-exposure to SARS-CoV-2. We show the persistence of humoral immune memory, antibody effector functions, and virus-specific memory T and B cells 6-9 months after infection, which do not correlate to each other. These data enhance our understanding of long-term functional immune memory.
Materials and Methods
Human Subjects
Ethical approval for the study was obtained from the National Ethics Committee of Health Research of Cambodia. Written informed consent was obtained from all participants prior to inclusion in the study. Pre-pandemic blood samples were obtained from clinically healthy individuals included in the dengue vaccine initiative study in 2015-2016. Clinically healthy adult volunteers who presented at the International Vaccination Centre, Institut Pasteur du Cambodge before the onset of the pandemic were included to validate the antigen-specific B and T cell staining. Acute SARS-CoV-2 infected patients were identified via screening of imported cases in Cambodia between 6th March to 12th August 2020. All laboratory confirmed cases are quarantined and monitored for symptoms. Moreover, 1-15 follow-up nasopharyngeal/oropharyngeal swab samplings for SARS-CoV-2 detection were conducted to assess SARS-CoV-2 RNA shedding. Patients were only discharged after two consecutive negative RT-PCR tests within 48h. Symptomatic patients displayed mild/moderate symptoms such as running nose, cough, fever and difficult to breath. In total, we included 64 individuals for follow up. In 33 individuals, 2-9 days after laboratory confirmation, a blood sample was obtained. A second blood sample was obtained 6-9 months later from all 64 study participants. Participant characteristics and clinical signs are summarized in Table S1. Plasma was collected and stored at -80°C, The PBMCs were isolated via Ficoll-Paque separation, cryopreserved in 10% DMSO/FBS and stored in liquid nitrogen until analysis. The National Institute for Biological Standards and Control (NIBSC) 20/130 (research reagent) and 20/118 (reference panel) have been obtained from WHO Solidarity II, the global serologic study for COVID-19.
SARS-CoV-2 Detection
Molecular detection of SARS-CoV-2 in combined nasopharyngeal/oropharyngeal swabs was performed as previously described (38). Briefly, RNA was extracted with the QIAamp Viral RNA Mini Kit (Qiagen) and real-time RT-PCR assays for SARS-CoV-2 RNA detection were performed in using primers/probes from Charité Virologie [Berlin, Germany (80)] to detect both E and RdRp genes.
Virus Neutralization Assay
The detection of neutralizing antibodies was achieved by foci reduction neutralization test (FRNT) similar as described before (81) and adapted to SARS-CoV-2. Briefly, serial diluted, heat-treated plasma samples were incubated with a Cambodian SARS-CoV-2 isolate (ancestral strain; GISAID: EPI_ISL_956384 (38);) for 30min at 37°C and 5% CO2. The mixtures were distributed on African green monkey kidney cells (VeroE6; ATCC CRL-1586) and incubated again for 30min 37°C and 5% CO2. Afterwards, the mixtures were replaced by an overlay medium containing 2% carboxymethyl cellulose (Sigma-Aldrich) in Dulbecco’s modified Eagle medium (DMEM; Sigma-Aldrich) supplemented with 3% FBS (Gibco) and 100 U/mL penicillin-streptomycin (Gibco). Infection was visualized 16-18h after inoculation by staining of infected cells with a SARS-CoV-2-specific antibody (rabbit, antibodies-online GmbH), targeting the S2 subunit of the viral spike protein, and afterwards with antibody anti-rabbit IgG HRP conjugate (goat; antibodies-online GmbH). Finally, cells were incubated with TrueBlue TMB substrate (KPL), and infection events appear as stained foci and were counted with an ELISPOT reader (AID Autoimmune Diagnostika GmbH, Strassberg, Germany). The amount of neutralizing antibodies is expressed as the reciprocal serum dilution that induces 50% reduction of infection (FRNT50) compared to the positive control (virus only) and is calculated by log probit regression analysis (SPSS for Windows, Version 16.0, SPSS Inc., Chicago, IL, USA). FRNT50 titers below 10 are considered negative.
S-Expressing Cell Lines
Transfected cell lines, Raji (ATCC® CCL‐86™) and 293T (ATCC® CRL‐3216™), with SARS-Cov-2 spike plasmid or a control plasmid using Lipofectamine 2000 (Life technologies) are kind gifts from Olivier Schwartz, Institut Pasteur, Paris, France (21). Spike-expressing Raji cells and Raji control cells were cultured at 37°C, 5% CO2 in RPMI medium while 293T-spike cells and 293T control cells were cultured in DMEM medium. All media were completed with 10% FBS (Gibco, MT, USA), 1% L glutamine (Gibco), 1% penicillin/streptomycin and puromycin (1 μg/mL, Gibo™) for cell selection during the culture.
S-Flow Assay
The S-Flow assay was performed as previously described (39). Briefly, plasma samples were diluted (1:200) in 1xPBS with 2mM EDTA and 0.5% BSA (PBS/BSA/EDTA) and incubated with 293T-spike cells (80000 cells/100µl) for 30 minutes on ice. The cells were washed with PBS/BSA/EDTA and stained either with anti-IgM PE (dilution 1:100, Biolegend) and anti-IgG Alexa Fluor™ 647 (dilution 1:600, Thermo Fisher) or anti-IgA Alexa Fluor 647 (dilution 1:800, Jakson ImmunoResearch) for 30 minutes on ice. The cells were washed with 1xPBS and fixed using buffer of the True-Nuclear Transcription Factor Staining kit (Biolegend). After fixing, the cells were washed and resuspended in 1xPBS. The results were acquired using FACS Canto II, BD Biosciences. The gating strategy for anti-IgM, anti-IgG or anti-IgA positive cells was based on the 293T control cells incubated with negative SARS-CoV-2 reference plasma. The data were reported as percentage of positive cells for anti-IgM, anti-IgG or anti-IgA. The NIBSC Research Reagent (20/130) and panel (20/118) (WHO Solidarity II) was utilized to set the cutoff for positivity based on the background staining of the negative SARS-CoV-2 plasma and calculated following formula: cut-off= % positive cells + 2x standard deviation.
Antibody Dependent Cellular Phagocytosis (ADCP) Assay
THP-1 cells (ATCC® TIB-202™) were used as phagocytic cells. For this, 1 µg of biotinylated S1 protein (Genscripts) was used to saturate the binding sites on 1 µl of FluoroSphere neutravidin beads (Thermo Fisher) overnight at 4°C. Excess protein was removed by washing the pelleted beads. The protein-coated beads were incubated with 40 µl heated-inactivated plasma diluted in complete RPMI (1:40) for 15 minutes at room temperature. Then, 5x104 THP-1 cells suspended in 50 µl complete RPMI were added to the complex and incubated for 16 hours at 37°C, 5% CO2. After incubation, the cells were washed with 1xPBS and fixed using buffer in True-Nuclear Transcription Factor Staining kit (Biolegend). After fixing, the cells were washed and resuspended in 1xPBS. The samples were analyzed using FACS Canto II, BD Biosciences. Phagocytosis activity was scored by the integrate mean fluorescence intensity (iMFI) value (% positive fluorescence THP-1 cells x MFI of the positive fluorescence THP-1 cells).
Complement Dependent Cytotoxicity (CDC) Assay
The assay used spike-expressing Raji cells as target cells, pooled serum (4 healthy donors) as complement source and heated-inactivated patient plasma as antibody source. In short, 50 µl of heated-inactivated plasma (1:50) were incubated with Raji-spike cells for 30 minutes at 37°C, 5% CO2. Afterward, 50 µl of complete RPMI containing 15% of pooled serum was added into the cells and incubated at 37°C, 5% CO2 for 14 hours. The cells were washed with PBS and stained with Zombie Aqua viability dye (BioLegend) for 20 minutes on ice and then stained anti-APC C3/C3b/iC3b antibody (Cedarlane) for 30 minutes on ice. The cells were fixed with fixation buffer in True-Nuclear Transcription Factor Staining kit (Biolegend) for 20 minutes on ice. After fixing, the cells were washed and resuspended in 1xPBS. The samples were acquired using FACS Canto II, BD Biosciences. The results were reported as percentage of cell death and MFI of C3 deposition on the cells.
Antibody-Dependent Cellular Cytotoxicity (ADCC) Assay
The assay used 293T-spike cells as a target cell and purified NK cells from healthy donor PMBCs as effector cells. First, 293T-spike cells were incubated with heated-inactivated patient plasma diluted in complete DMEM medium (1:50) at 37°C, 5% CO2 for 30 minutes. The NK cells were enriched by magnetic negative selection (Miltenyi) according to manufactor’s instruction. The 293T-spike cells were washed five times with complete RPMI medium. The NK cells were mixed with 293T-spike cells at a ratio 1:1 at final volume of 100 µl complete RPMI. Anti-CD107a and Monensin (Biolegend) 1:1000 dilution were added to the suspension and incubated at 37°C, 5% CO2 for 6 hours. The cells were washed with 1xPBS and stained with Zombie Aqua viability dye (BioLegend) for 20 minutes on ice. Then the cells were stained with anti-CD3 and anti-CD56 for 30 minutes on ice. The cells were washed and fixed/permeabilized using True-Nuclear Transcription Factor Staining kit (Biolegend) for 20 minutes on ice. After staining, the cells were washed and resuspended in 1xPBS. The samples were acquired using FACS Canto II, BD Biosciences.
Detection of Antigen-Specific Memory B Cells
Biotinylated SARS-CoV-2 S1 protein and biotinylated SARS-CoV-2 N protein were purchased from GenScript. The biotinylated proteins were combined with different streptavidin (SA) fluorophore conjugates, BUV496 (BD Biosciences) and PE (Biolegend), respectively, at 1:1 molar ratio. Briefly, each SA was added gradually (3 times, every 20 minutes) to 20 µl of each biotinylated protein (1 µM) on ice. The reaction was quenched with D-biotin (GeneCopeia) at 50:1 molar ratio to SA for a total probe volume of 30 µl for 30 minutes on ice. Probes were then used immediately for staining. Each staining used 5 µl of probe. Shortly, patient PBMCs was washed with 1xPBS and stained with Zombie Aqua viability dye (BioLegend) for 10 minutes on ice. The cells were stained with the probes. Then the cells were washed and stained with anti-IgG antibody, for 30 minutes on ice. After that, the cells were washed and stained with master mix containing of anti-CD3, anti-CD19, anti-CD27, anti-CD38, anti-IgD, anti-IgM and anti-IgA antibodies for 30 minutes on ice Antibodies are listed in Table S2. After staining, the cells were washed and resuspended in 1xPBS with 2% FBS. The samples were analyzed using FACS Aria, BD Biosciences. The flow cytometry gating strategy to classify memory B cell subsets and switched B cells is shown in Figure S7. Overall, 40 samples were of sufficient quality and were included in the analysis.
Activation-Induced Markers (AIM) T Cell Assay
Antigen-specific CD4+ and CD8+ T cells, as well as memory T cells and T helper subsets were assessed by Activation-Induced Marker (AIM) assay (4, 8). Cells were cultured at 37°C, 5% CO2, in the presence of SARS-CoV-2-specific S1, M and N protein pools [1 µg/mL] (PepTivator® SARS-CoV-2 regents; Miltenyi Biotec) in 96-well U-bottom plates at 0,5-1x106 PBMCs per well. After 24 hours, cells were washed in 1xPBS supplemented with 0.5% bovine serum albumin (BSA) and 2 mM EDTA (FACS buffer) and stained with Zombie Aqua Fixable Viability kit (Biolegend) and incubated for 20 min at 4°C followed by surface staining for 30 min at 4°C. Stained cells were washed and resuspended in FACS buffer and analyzed using a FACSAria Fusion (BD Biosciences). Antibodies are listed in Table S2. Negative controls without peptide stimulation were included for each donor. Antigen-specific CD4+ and CD8+ T cells were measured subtracting the background (unstimulated control) from the peptide-stimulated sample. Negative results were set to zero. Data were analyzed with FlowJo software version 10.7.1 (FlowJo LLC). Overall, 33 samples were of sufficient quality and were included in the analysis.
Intracellular Staining (ICS) Assay
Functional SARS-CoV-2-specific CD4+ and CD8+ T cells were assessed by surface and intracellular staining in a subset of individuals if sufficient amount of PBMCs were obtained (n=8). Cells were cultured at 37°C, 5% CO2, in the presence of SARS-CoV-2-specific S1, M and N protein pools separately [1 µg/mL each] (PepTivator® SARS-CoV-2 reagents; Miltenyi Biotec), Monensin (Biolegend) 1:1000 dilution and anti-Human CD28/CD49d purified [100 µg/mL] (BD Bioscience) in 96-well U-bottom plates at 0,5-1x106 PBMCs per well. After 6 hours, cells were washed in FACS buffer and stained using a Zombie Aqua Fixable Viability kit (Biolegend) and incubated for 20 minutes at 4°C. Cells were then washed in PBS and fixed/permeabilized with True-Nuclear™ Transcription Factor Buffer Set (Biolegend). Surface (CD3, CD4 and CD8) and intracellular markers (IFN-γ, IL-2, IL-4, IL-6 and IL-17) were detected via the subsequent addition of directly conjugated antibodies incubating for 30 minutes at 4°C. Antibodies are listed in Table S2. Stained cells were finally washed and resuspended in FACS buffer and analyzed using a FACSAria Fusion (BD Biosciences). Antigen-specific CD4+ and CD8+ T cells were measured subtracting the background (unstimulated control) from the peptide-stimulated sample. Negative results were set to zero. Data were analyzed with FlowJo software version 10.7.1 (FlowJo LLC).
Statistical Analysis
Calculations, figures and statistics were made using Prism 9 (GraphPad Software) or RStudio (Version 1.2.1335). The data were tested for statistical normality before applying the appropriate statistical tests. All information about sample sizes and statistical tests performed were shown in the figure legends. Spearman correlation plot was calculated and visualized with the following packages: FactoMineR, factoextra (https://cran.r-project.org/web/packages/factoextra/index.html) and corrplot (https://github.com/taiyun/corrplot) in R (Version 3.6.1) and RStudio (Version 1.2.1335).
Data Availability Statement
The original contributions presented in the study are included in the article/Supplementary Material. Further inquiries can be directed to the corresponding author.
Ethics Statement
The studies involving human participants were reviewed and approved by National Ethics Committee of Health Research Cambodia (approval number 2020-316). The patients/participants provided their written informed consent to participate in this study.
Author Contributions
Conceptualization: TC, PD, and EK. Methodology: HV, AM, HA, SovL, SSa, NY, and PP. Investigation: HV, AM, HA, TB, VD, TC, and EK. Visualization: HV, AM, HA, and SokL. Funding acquisition: TC, HA, and PD. Patient inclusion: HS and SSo. Cohort management and patient selection: SovL and SowL. Project administration: TC, EK, PP, and PD. Supervision: HV, AM, BT, OS, VD, EK, and TC. Writing: original draft: HV, AM, HA, TB, EK, and TC. Writing: review and editing: HV, AM, HA, TB, VD, OS, EK, and TC. All authors contributed to the article and approved the submitted version.
Funding
The Howard Hughes Medical Institute (HHMI)–Wellcome Trust (208710/Z/17/Z to TC), « URGENCE COVID-19 » fundraising campaign of Institut Pasteur (TC, HA, and PD). HA is supported by the German Centre for International Migration and Development (CIM).
Conflict of Interest
The authors declare that the research was conducted in the absence of any commercial or financial relationships that could be construed as a potential conflict of interest.
Publisher’s Note
All claims expressed in this article are solely those of the authors and do not necessarily represent those of their affiliated organizations, or those of the publisher, the editors and the reviewers. Any product that may be evaluated in this article, or claim that may be made by its manufacturer, is not guaranteed or endorsed by the publisher.
Acknowledgments
This publication has been supported by WHO Solidarity II, global serologic study for COVID-19, with funding from the German Federal Ministry of Health (BMG) COVID-19 Research and development to WHO. We would like to acknowledge all patients that participated to the study. We would like to thank Borita Heng for her technical assistance.
Supplementary Material
The Supplementary Material for this article can be found online at: https://www.frontiersin.org/articles/10.3389/fimmu.2022.817905/full#supplementary-material
References
1. Chen N, Zhou M, Dong X, Qu J, Gong F, Han Y, et al. Epidemiological and Clinical Characteristics of 99 Cases of 2019 Novel Coronavirus Pneumonia in Wuhan, China: A Descriptive Study. Lancet (2020) 395:507–13. doi: 10.1016/S0140-6736(20)30211-7
2. Guan WJ, Ni ZY, Hu Y, Liang WH, Ou CQ, He JX, et al. Clinical Characteristics of Coronavirus Disease 2019 in China. N Engl J Med (2020) 382:1708–20. doi: 10.1056/NEJMoa2002032
3. Zhu N, Zhang D, Wang W, Li X, Yang B, Song J, et al. A Novel Coronavirus From Patients With Pneumonia in China, 2019. N Engl J Med (2020) 382:727–33. doi: 10.1056/NEJMoa2001017
4. Dan JM, Mateus J, Kato Y, Hastie KM, Yu ED, Faliti CE, et al. Immunological Memory to SARS-CoV-2 Assessed for Up to 8 Months After Infection. Science (2021) 371:eabf4063. doi: 10.1126/science.abf4063
5. Hartley GE, Edwards ESJ, Aui PM, Varese N, Stojanovic S, McMahon J, et al. Rapid Generation of Durable B Cell Memory to SARS-CoV-2 Spike and Nucleocapsid Proteins in COVID-19 and Convalescence. Sci Immunol (2020) 5:e01991-20. doi: 10.1126/sciimmunol.abf8891
6. Sherina N, Piralla A, Du L, Wan H, Kumagai-Braesch M, Andréll J, et al. Persistence of SARS-CoV-2-Specific B and T Cell Responses in Convalescent COVID-19 Patients 6-8 Months After the Infection. Med (N Y) (2021) 2:281–95.e284. doi: 10.1016/j.medj.2021.02.001
7. Wang M-Y, Zhao R, Gao LJ, Gao XF, Wang DP, Cao JM. SARS-CoV-2: Structure, Biology, and Structure-Based Therapeutics Development. Front Cell Infect Microbiol (2020) 10:724. doi: 10.3389/fcimb.2020.587269
8. Grifoni A, Weiskopf D, Ramirez SI, Mateus J, Dan JM, Moderbacher CR, et al. Targets of T Cell Responses to SARS-CoV-2 Coronavirus in Humans With COVID-19 Disease and Unexposed Individuals. Cell (2020) 181:1489–501.e1415. doi: 10.1016/j.cell.2020.05.015
9. Yoshida S, Ono C, Hayashi H, Fukumoto S, Shiraishi S, Tomono K, et al. SARS-CoV-2-Induced Humoral Immunity Through B Cell Epitope Analysis in COVID-19 Infected Individuals. Sci Rep (2021) 11:5934. doi: 10.1038/s41598-021-85202-9
10. Wang Q, Zhang Y, Wu L, Niu S, Song C, Zhang Z, et al. Structural and Functional Basis of SARS-CoV-2 Entry by Using Human Ace2. Cell (2020) 181:894–904.e899. doi: 10.1016/j.cell.2020.03.045
11. Yan R, Zhang Y, Li Y, Xia L, Guo Y, Zhou Q. Structural Basis for the Recognition of SARS-CoV-2 by Full-Length Human ACE2. Science (2020) 367:1444–8. doi: 10.1126/science.abb2762
12. Dispinseri S, Secchi M, Pirillo MF, Tolazzi M, Borghi M, Brigatti C, et al. Neutralizing Antibody Responses to SARS-CoV-2 in Symptomatic COVID-19 is Persistent and Critical for Survival. Nat Commun (2021) 12:2670. doi: 10.1038/s41467-021-22958-8
13. Wajnberg A, Amanat F, Firpo A, Altman DR, Bailey MJ, Mansour M, et al. Robust Neutralizing Antibodies to SARS-CoV-2 Infection Persist for Months. Science (2020) 370:1227. doi: 10.1126/science.abd7728
14. Legros V, Denolly S, Vogrig M, Boson B, Siret E, Rigaill J, et al. A Longitudinal Study of SARS-CoV-2-Infected Patients Reveals a High Correlation Between Neutralizing Antibodies and COVID-19 Severity. Cell Mol Immunol (2021) 18:318–27. doi: 10.1038/s41423-020-00588-2
15. Yu J, Tostanoski LH, Peter L, Mercado NB, McMahan K, Mahrokhian SH, et al. DNA Vaccine Protection Against SARS-CoV-2 in Rhesus Macaques. Science (2020) 369:806–11. doi: 10.1126/science.abc6284
16. Schäfer A, Muecksch F, Lorenzi JCC, Leist SR, Cipolla M, Bournazos S, et al. Antibody Potency, Effector Function, and Combinations in Protection and Therapy for SARS-CoV-2 Infection In Vivo. J Exp Med (2021) 218:e20201993. doi: 10.1084/jem.20201993
17. Capetti AF, Borgonovo F, Mileto D, Gagliardi G, Mariani C, Lupo A, et al. One-Year Durability of Anti-Spike IgG to SARS-CoV-2: Preliminary Data From the AntiCROWN Prospective Observational Study One Year Durability of COVID-19 Anti-Spike IgG. J Infect (2021) 83:237–79. doi: 10.1016/j.jinf.2021.05.023
18. Kang CK, Kim M, Lee S, Kim G, Choe PG, Park WB, et al. Longitudinal Analysis of Human Memory T-Cell Response According to the Severity of Illness Up to 8 Months After SARS-CoV-2 Infection. J Infect Dis (2021) 224:39–48. doi: 10.1093/infdis/jiab159
19. Wang Z, Muecksch F, Schaefer-Babajew D, Finkin S, Viant C, Gaebler C, et al. Naturally Enhanced Neutralizing Breadth Against SARS-CoV-2 One Year After Infection. Nature (2021) 595:426–31. doi: 10.1038/s41586-021-03696-9
20. Bournazos S, Ravetch JV. Fcγ Receptor Function and the Design of Vaccination Strategies. Immunity (2017) 47:224–33. doi: 10.1016/j.immuni.2017.07.009
21. Dufloo J, Grzelak L, Staropoli I, Madec Y, Tondeur L, Anna F, et al. Asymptomatic and Symptomatic SARS-CoV-2 Infections Elicit Polyfunctional Antibodies. Cell Rep Med (2021) 2:100275. doi: 10.1016/j.xcrm.2021.100275
22. Natarajan H, Crowley AR, Butler SE, Xu S, Weiner JA, Bloch EM, et al. Markers of Polyfunctional SARS-CoV-2 Antibodies in Convalescent Plasma. mBio (2021) 12:e00765-00721. doi: 10.1128/mBio.00765-21
23. Zohar T, Loos C, Fischinger S, Atyeo C, Wang C, Slein MD, et al. Compromised Humoral Functional Evolution Tracks With SARS-CoV-2 Mortality. Cell (2020) 183:1508–19.e1512. doi: 10.1016/j.cell.2020.10.052
24. Anand SP, Prévost J, Nayrac M, Beaudoin-Bussières G, Benlarbi M, Gasser R, et al. Longitudinal Analysis of Humoral Immunity Against SARS-CoV-2 Spike in Convalescent Individuals Up to Eight Months Post-Symptom Onset. Cell Rep Med (2021) 2:100290. doi: 10.1016/j.xcrm.2021.100290
25. Lee WS, Selva KJ, Davis SK, Wines BD, Reynaldi A, Esterbauer R, et al. Decay of Fc-Dependent Antibody Functions After Mild to Moderate COVID-19. Cell Rep Med (2021) 2(6):100296. doi: 10.1016/j.xcrm.2021.100296
26. Bonifacius A, Tischer-Zimmermann S, Dragon AC, Gussarow D, Vogel A, Krettek U, et al. COVID-19 Immune Signatures Reveal Stable Antiviral T Cell Function Despite Declining Humoral Responses. Immunity (2021) 54:340–54.e346. doi: 10.1016/j.immuni.2021.01.008
27. Gaebler C, Wang Z, Lorenzi JCC, Muecksch F, Finkin S, Tokuyama M, et al. Evolution of Antibody Immunity to SARS-CoV-2. Nature (2021) 591:639–44. doi: 10.1038/s41586-021-03207-w
28. Zuo J, Dowell AC, Pearce H, Verma K, Long HM, Begum J, et al. Robust SARS-CoV-2-Specific T Cell Immunity is Maintained at 6 Months Following Primary Infection. Nat Immunol (2021) 22:620–6. doi: 10.1038/s41590-021-00902-8
29. Wheatley AK, Juno JA, Wang JJ, Selva KJ, Reynaldi A, Tan HX, et al. Evolution of Immune Responses to SARS-CoV-2 in Mild-Moderate COVID-19. Nat Commun (2021) 12:1162. doi: 10.1038/s41467-021-21444-5
30. Peluso MJ, Deitchman AN, Torres L, Iyer NS, Munter SE, Nixon CC, et al. Long-Term SARS-CoV-2-Specific Immune and Inflammatory Responses in Individuals Recovering From COVID-19 With and Without Post-Acute Symptoms. Cell Rep (2021) 36:109518. doi: 10.1016/j.celrep.2021.109518
31. Sette A, Crotty S. Adaptive Immunity to SARS-CoV-2 and COVID-19. Cell (2021) 184:861–80. doi: 10.1016/j.cell.2021.01.007
32. Le Bert N, Tan AT, Kunasegaran K, Tham CYL, Hafezi M, Chia A, et al. SARS-CoV-2-Specific T Cell Immunity in Cases of COVID-19 and SARS, and Uninfected Controls. Nature (2020) 584:457–62. doi: 10.1038/s41586-020-2550-z
33. Sariol A, Perlman S. Lessons for COVID-19 Immunity From Other Coronavirus Infections. Immunity (2020) 53:248–63. doi: 10.1016/j.immuni.2020.07.005
34. Noh JY, Kwak JE, Yang JS, Hwang SY, Yoon JG, Seong H, et al. Longitudinal Assessment of Anti-SARS-CoV-2 Immune Responses for Six Months Based on the Clinical Severity of COVID-19. J Infect Dis (2021) 224:754–63. doi: 10.2139/ssrn.3719075
35. Ravichandran S, Lee Y, Grubbs G, Coyle EM, Klenow L, Akasaka O, et al. Longitudinal Antibody Repertoire in "Mild" Versus "Severe" COVID-19 Patients Reveals Immune Markers Associated With Disease Severity and Resolution. Sci Adv (2021) 7:eabf2467. doi: 10.1126/sciadv.abf2467
36. Long Q-X, Jia YJ, Wang X, Deng HJ, Cao XX, Yuan J, et al. Immune Memory in Convalescent Patients With Asymptomatic or Mild COVID-19. Cell Discov (2021) 7:18. doi: 10.1038/s41421-021-00250-9
38. Auerswald H, Yann S, Dul S, In S, Dussart P, Martin NJ, et al. Assessment of Inactivation Procedures for SARS-CoV-2. J Gen Virol (2021) 102:001539. doi: 10.1099/jgv.0.001539
39. Grzelak L, Temmam S, Planchais C, Demeret C, Tondeur L, Huon C, et al. A Comparison of Four Serological Assays for Detecting Anti-SARS-CoV-2 Antibodies in Human Serum Samples From Different Populations. Sci Transl Med (2020) 12:eabc3103. doi: 10.1126/scitranslmed.abc3103
40. Mesin L, Schiepers A, Ersching J, Barbulescu A, Cavazzoni CB, Angelini A, et al. Restricted Clonality and Limited Germinal Center Reentry Characterize Memory B Cell Reactivation by Boosting. Cell (2020) 180:92–106.e111. doi: 10.1016/j.cell.2019.11.032
41. Bohnhorst J, Bjørgan MB, Thoen JE, Natvig JB, Thompson KM. Bm1-Bm5 Classification of Peripheral Blood B Cells Reveals Circulating Germinal Center Founder Cells in Healthy Individuals and Disturbance in the B Cell Subpopulations in Patients With Primary Sjögren’s Syndrome. J Immunol (2001) 167:3610–8. doi: 10.4049/jimmunol.167.7.3610
42. Wei C, Anolik J, Cappione A, Zheng B, Pugh-Bernard A, Brooks J, et al. A New Population of Cells Lacking Expression of CD27 Represents a Notable Component of the B Cell Memory Compartment in Systemic Lupus Erythematosus. J Immunol (2007) 178:6624–33. doi: 10.4049/jimmunol.178.10.6624
43. Rodda LB, Netland J, Shehata L, Pruner KB, Morawski PA, Thouvenel CD, et al. Functional SARS-CoV-2-Specific Immune Memory Persists After Mild COVID-19. Cell (2021) 184:169–83.e117. doi: 10.1016/j.cell.2020.11.029
44. Peng Y, Mentzer AJ, Liu G, Yao X, Yin Z, Dong D, et al. Broad and Strong Memory CD4+ and CD8+ T Cells Induced by SARS-CoV-2 in UK Convalescent Individuals Following COVID-19. Nat Immunol (2020) 21:1336–45. doi: 10.1038/s41590-020-0782-6
45. WHO. COVID-19 Situation Report. Geneva: WHO Coronavirus (COVID-19). (2021). Available at: https://www.who.int/emergencies/diseases/novel-coronavirus-2019/situation-reports/ Assessed in 21st.June.2021.
46. Chia WN, Zhu F, Ong SWX, Young BE, Fong SW, Le Bert N, et al. Dynamics of SARS-CoV-2 Neutralising Antibody Responses and Duration of Immunity: A Longitudinal Study. Lancet Microbe (2021) 2:e240–9. doi: 10.1016/S2666-5247(21)00025-2
47. Zeberg H, Pääbo S. The Major Genetic Risk Factor for Severe COVID-19 is Inherited From Neanderthals. Nature (2020) 587:610–2. doi: 10.1038/s41586-020-2818-3
48. Zhou P, Yang XL, Wang XG, Hu B, Zhang L, Zhang W, et al. A Pneumonia Outbreak Associated With a New Coronavirus of Probable Bat Origin. Nature (2020) 579:270–3. doi: 10.1038/s41586-020-2012-7
49. Wacharapluesadee S, Tan CW, Maneeorn P, Duengkae P, Zhu F, Joyjinda Y, et al. Evidence for SARS-CoV-2 Related Coronaviruses Circulating in Bats and Pangolins in Southeast Asia. Nat Commun (2021) 12:972. doi: 10.1038/s41467-021-21240-1
50. Delaune D, Hul V, Karlsson EA, Hassanin A, Ou TP, Baidaliuk A, et al. A Novel SARS-CoV-2 Related Coronavirus in Bats From Cambodia. Nat Commun (2021) 12:6563. doi: 10.1038/s41467-021-26809-4
51. Souilmi Y, Lauterbur ME, Tobler R, Huber CD, Johar AS, Moradi SV, et al. An Ancient Viral Epidemic Involving Host Coronavirus Interacting Genes More Than 20,000 Years Ago in East Asia. Curr Biol (2021) 31:3504–14.e3509. doi: 10.1016/j.cub.2021.05.067
52. Van Elslande J, Gruwier L, Godderis L, Vermeersch P. Estimated Half-Life of SARS-CoV-2 Anti-Spike Antibodies More Than Double the Half-Life of Anti-Nucleocapsid Antibodies in Healthcare Workers. Clin Infect Dis (2021) 73:2366–8. doi: 10.1093/cid/ciab219
53. Harrington WE, Trakhimets O, Andrade DV, Dambrauskas N, Raappana A, Jiang Y, et al. Rapid Decline of Neutralizing Antibodies is Associated With Decay of IgM in Adults Recovered From Mild COVID-19. Cell Rep Med (2021) 2:100253. doi: 10.1016/j.xcrm.2021.100253
54. Guo L, Ren L, Yang S, Xiao M, Chang D, Yang F, et al. Profiling Early Humoral Response to Diagnose Novel Coronavirus Disease (COVID-19). Clin Infect Dis (2020) 71:778–85. doi: 10.1093/cid/ciaa310
55. Cervia C, Nilsson J, Zurbuchen Y, Valaperti A, Schreiner J, Wolfensberger A, et al. Systemic and Mucosal Antibody Responses Specific to SARS-CoV-2 During Mild Versus Severe COVID-19. J Allergy Clin Immunol (2021) 147:545–57.e549. doi: 10.1016/j.jaci.2020.10.040
56. Sterlin D, Mathian A, Miyara M, Mohr A, Anna F, Claër L, et al. IgA Dominates the Early Neutralizing Antibody Response to SARS-CoV-2. Sci Transl Med (2021) 13:eabd2223. doi: 10.1126/scitranslmed.abd2223
57. Gasser R, Cloutier M, Prévost J, Fink C, Ducas É, Ding S, et al. Major Role of IgM in the Neutralizing Activity of Convalescent Plasma Against SARS-CoV-2. Cell Rep (2021) 34:108790. doi: 10.1016/j.celrep.2021.108790
58. Tay MZ, Wiehe K, Pollara J. Antibody-Dependent Cellular Phagocytosis in Antiviral Immune Responses. Front Immunol (2019) 10:332. doi: 10.3389/fimmu.2019.00332
59. Sakharkar M, Rappazzo CG, Wieland-Alter WF, Hsieh CL, Wrapp D, Esterman ES, et al. Prolonged Evolution of the Human B Cell Response to SARS-CoV-2 Infection. Sci Immunol (2021) 6:eabg6916. doi: 10.1126/sciimmunol.abg6916
60. Dugan HL, Stamper CT, Li L, Changrob S, Asby NW, Halfmann PJ, et al. Profiling B Cell Immunodominance After SARS-CoV-2 Infection Reveals Antibody Evolution to Non-Neutralizing Viral Targets. Immunity (2021) 54:1290–303.e1297. doi: 10.1016/j.immuni.2021.05.001
61. Robbiani DF, Gaebler C, Muecksch F, Lorenzi JCC, Wang Z, Cho A, et al. Convergent Antibody Responses to SARS-CoV-2 in Convalescent Individuals. Nature (2020) 584:437–42. doi: 10.1038/s41586-020-2456-9
62. Kreer C, Zehner M, Weber T, Ercanoglu MS, Gieselmann L, Rohde C, et al. Longitudinal Isolation of Potent Near-Germline SARS-CoV-2-Neutralizing Antibodies From COVID-19 Patients. Cell (2020) 182:843–54.e812. doi: 10.1016/j.cell.2020.06.044
63. Perugino CA, Liu H, Feldman J, Hauser BM, Jacob-Dolan C, Nathan A, et al. Preferential Expansion of Cross-Reactive Pre-Existing Switched Memory B Cells That Recognize the SARS-CoV-2 Omicron Variant Spike Protein. medRxiv (2022) 2021:2012.2030.21268554. doi: 10.1101/2021.12.30.21268554
64. Reiss S, Baxter AE, Cirelli KM, Dan JM, Morou A, Daigneault A, et al. Comparative Analysis of Activation Induced Marker (AIM) Assays for Sensitive Identification of Antigen-Specific CD4 T Cells. PloS One (2017) 12:e0186998. doi: 10.1371/journal.pone.0186998
65. Sekine T, Perez-Potti A, Rivera-Ballesteros O, Strålin K, Gorin JB, Olsson A, et al. Robust T Cell Immunity in Convalescent Individuals With Asymptomatic or Mild COVID-19. Cell (2020) 183:158–68.e114. doi: 10.1016/j.cell.2020.08.017
66. Cohen KW, Linderman SL, Moodie Z, Czartoski J, Lai L, Mantus G, et al. Longitudinal Analysis Shows Durable and Broad Immune Memory After SARS-CoV-2 Infection With Persisting Antibody Responses and Memory B and T Cells. Cell Rep Med (2021) 2:100354. doi: 10.1016/j.xcrm.2021.100354
67. Keeton R, Tincho MB, Ngomti A, Baguma R, Benede N, Suzuki A, et al. SARS-CoV-2 Spike T Cell Responses Induced Upon Vaccination or Infection Remain Robust Against Omicron. medRxiv (2021) 2021:2012.2026.21268380. doi: 10.1101/2021.12.26.21268380
68. Cubuk J, Alston JJ, Incicco JJ, Singh S, Stuchell-Brereton MD, Ward MD, et al. The SARS-CoV-2 Nucleocapsid Protein is Dynamic, Disordered, and Phase Separates With RNA. Nat Commun (2021) 12:1936. doi: 10.1038/s41467-021-21953-3
69. Laha S, Chakraborty J, Das S, Manna SK, Biswas S, Chatterjee R. Characterizations of SARS-CoV-2 Mutational Profile, Spike Protein Stability and Viral Transmission. Infect Genet Evol (2020) 85:104445. doi: 10.1016/j.meegid.2020.104445
70. Korber B, Fischer WM, Gnanakaran S, Yoon H, Theiler J, Abfalterer W, et al. Tracking Changes in SARS-CoV-2 Spike: Evidence That D614G Increases Infectivity of the COVID-19 Virus. Cell (2020) 182:812–27.e819. doi: 10.1016/j.cell.2020.06.043
71. Ng KW, Faulkner N, Cornish GH, Rosa A, Harvey R, Hussain S, et al. Preexisting and De Novo Humoral Immunity to SARS-CoV-2 in Humans. Science (2020) 370:1339. doi: 10.1126/science.abe1107
72. Song G, He WT, Callaghan S, Anzanello F, Huang D, Ricketts J, et al. Cross-Reactive Serum and Memory B-Cell Responses to Spike Protein in SARS-CoV-2 and Endemic Coronavirus Infection. Nat Commun (2021) 12:2938. doi: 10.1038/s41467-021-23074-3
73. Bartsch YC, Fischinger S, Siddiqui SM, Chen Z, Yu J, Gebre M, et al. Discrete SARS-CoV-2 Antibody Titers Track With Functional Humoral Stability. Nat Commun (2021) 12:1018. doi: 10.1038/s41467-021-21336-8
74. Pušnik J, Richter E, Schulte B, Dolscheid-Pommerich R, Bode C, Putensen C, et al. Memory B Cells Targeting SARS-CoV-2 Spike Protein and Their Dependence on CD4(+) T Cell Help. Cell Rep (2021) 34(13):109320. doi: 10.1016/j.celrep.2021.109320
75. Vibholm LK, Nielsen SSF, Pahus MH, Frattari GS, Olesen R, Andersen R, et al. SARS-CoV-2 Persistence is Associated With Antigen-Specific CD8 T-Cell Responses. EBioMedicine (2021) 64:103230. doi: 10.1016/j.ebiom.2021.103230
76. Chakraborty S, Gonzalez J, Edwards K, Mallajosyula V, Buzzanco AS, Sherwood R, et al. Proinflammatory IgG Fc Structures in Patients With Severe COVID-19. Nat Immunol (2021) 22:67–73. doi: 10.1038/s41590-020-00828-7
77. Larsen MD, de Graaf EL, Sonneveld ME, Plomp HR, Nouta J, Hoepel W, et al. Afucosylated IgG Characterizes Enveloped Viral Responses and Correlates With COVID-19 Severity. Science (2021) 371:eabc8378. doi: 10.1126/science.abc8378
78. Shields RL, Lai J, Keck R, O'Connell LY, Hong K, Meng YG, et al. Lack of Fucose on Human IgG1 N-Linked Oligosaccharide Improves Binding to Human Fcgamma RIII and Antibody-Dependent Cellular Toxicity. J Biol Chem (2002) 277:26733–40. doi: 10.1074/jbc.M202069200
79. Pereira NA, Chan KF, Lin PC, Song Z. The "Less-is-More" in Therapeutic Antibodies: Afucosylated Anti-Cancer Antibodies With Enhanced Antibody-Dependent Cellular Cytotoxicity. MAbs (2018) 10:693–711. doi: 10.1080/19420862.2018.1466767
80. Corman VM, Landt O, Kaiser M, Molenkamp R, Meijer A, Chu DK, et al. Detection of 2019 Novel Coronavirus (2019-Ncov) by Real-Time RT-PCR. Euro Surveill (2020) 25:2000045. doi: 10.2807/1560-7917.ES.2020.25.3.2000045
Keywords: SARS-CoV-2, B cell immunity, T cell immunity, antibody effector function, long term immune response
Citation: Vo HTM, Maestri A, Auerswald H, Sorn S, Lay S, Seng H, Sann S, Ya N, Pean P, Dussart P, Schwartz O, Ly S, Bruel T, Ly S, Duong V, Karlsson EA and Cantaert T (2022) Robust and Functional Immune Memory Up to 9 Months After SARS-CoV-2 Infection: A Southeast Asian Longitudinal Cohort. Front. Immunol. 13:817905. doi: 10.3389/fimmu.2022.817905
Received: 18 November 2021; Accepted: 10 January 2022;
Published: 03 February 2022.
Edited by:
Kihyuck Kwak, Yonsei University, South KoreaReviewed by:
Christopher Sundling, Karolinska Institutet (KI), SwedenConstantin Joachim Thieme, Charité Medical University of Berlin, Germany
Copyright © 2022 Vo, Maestri, Auerswald, Sorn, Lay, Seng, Sann, Ya, Pean, Dussart, Schwartz, Ly, Bruel, Ly, Duong, Karlsson and Cantaert. This is an open-access article distributed under the terms of the Creative Commons Attribution License (CC BY). The use, distribution or reproduction in other forums is permitted, provided the original author(s) and the copyright owner(s) are credited and that the original publication in this journal is cited, in accordance with accepted academic practice. No use, distribution or reproduction is permitted which does not comply with these terms.
*Correspondence: Tineke Cantaert, dGluZWtlLmNhbnRhZXJ0QHBhc3RldXIuZnI=
†These authors have contributed equally to this work
‡These authors have contributed equally to this work