- 1Center for Integrative Biology, Facultad de Ciencias, Universidad Mayor, Santiago, Chile
- 2Escuela de Tecnología Médica, Facultad de Ciencias, Universidad Mayor, Santiago, Chile
- 3Escuela de Biotecnología, Facultad de Ciencias, Universidad Mayor, Santiago, Chile
- 4Department of Medical Genetics, Cumming School of Medicine, University of Calgary, Calgary, AB, Canada
- 5 Department of Biochemistry and Molecular Biology, Cumming School of Medicine, University of Calgary, Calgary, AB, Canada
- 6Alberta Children’s Hospital Research Institute, Calgary, AB, Canada
Infections during pregnancy can seriously damage fetal neurodevelopment by aberrantly activating the maternal immune system, directly impacting fetal neural cells. Increasing evidence suggests that these adverse impacts involve alterations in neural stem cell biology with long-term consequences for offspring, including neurodevelopmental disorders such as autism spectrum disorder, schizophrenia, and cognitive impairment. Here we review how maternal infection with viruses such as Influenza A, Cytomegalovirus, and Zika during pregnancy can affect the brain development of offspring by promoting the release of maternal pro-inflammatory cytokines, triggering neuroinflammation of the fetal brain, and/or directly infecting fetal neural cells. In addition, we review insights into how these infections impact human brain development from studies with animal models and brain organoids. Finally, we discuss how maternal infection with SARS-CoV-2 may have consequences for neurodevelopment of the offspring.
Introduction
The mother’s health and her environment can strongly influence the brain development of her offspring (1–3). For example, viral infection of the mother during pregnancy has been linked to increased risk of neurodevelopmental defects in the offspring, such as microcephaly, autism spectrum disorder (ASD), epilepsy, and schizophrenia (4–33). These effects on the offspring may be a consequence of the mother’s systemic immune response against infection, which includes production of maternal cytokines that can interfere with fetal neural stem/precursor cells (NPCs) and microglia (34–37). Viruses can also infect the placenta and thereby pass vertically from mother to fetus, entering NPCs, microglia, and neural cells in the developing fetal brain (38, 39).
Viruses and pro-inflammatory cytokines can damage the nervous system by inhibiting the genesis of NPCs, which are self-renewing, multipotent stem cells capable of giving rise to neurons, astrocytes, and oligodendrocytes (40). Normally the number and fate of NPCs are determined by a balance between their maintenance as NPCs and their differentiation and commitment to form parts of neural circuits. However, viruses and cytokines can throw off this balance, resulting in neurodevelopmental disorders or cortical defects (41, 42). These factors can also harm microglia, the immune cells of the brain that monitor against inflammation and try to ensure normal functioning of neurons (43). Although not themselves neurons, microglia play important roles in neural circuits, such as by regulating synaptic activity and controlling the pool of NPCs (44–46).
This review focuses on how viral infection of the mother can trigger defects in fetal neurodevelopment. We examine three viruses whose effect on brain development has been well studied in animal and cellular models: Zika virus (ZIKV), Cytomegalovirus (CMV), and Influenza A virus. Next we explore the specific pathways triggered in the fetal brain by pro-inflammatory cytokines produced by the mother in response to viral infection. Finally, we consider the possibility that the emerging severe acute respiratory syndrome coronavirus 2 (SARS-CoV-2) may have long-term consequences on fetal brain development.
Maternal Viral Infections During Fetal Neurodevelopment
Viruses are microorganisms that require the host's cellular machinery to replicate and then infect new cells or integrate their genetic material into the host genome. To enter host cells, viruses must express diverse molecules such as proteins or carbohydrates on their surface that can be recognized by host cell receptors (47). The innate immune system recognizes these same viral surface molecules, which acts as the first line of defense. When so-called “pattern recognition receptors” on the surface of immune cells or inside the cells recognize pathogen-associated molecular patterns on the virus, the immune cells begin to produce pro-inflammatory cytokines and chemokines by recruiting adapter proteins such as myeloid differentiation primary response 88 (MYD88), TIR-domain-containing adapter-inducing interferon-β (TRIF), and translocating chain-associated membrane protein (TRAM) to initiate a signaling cascade that culminates in the activation of nuclear factor kappa B (NF-κB), mitogen-activated protein kinase (MAPK) and interferon regulatory factors (IRFs). These transcription factors induce the expression of interferon (IFN) α and β as well as pro-inflammatory cytokines such as interleukin (IL)-6, tumor necrosis factor (TNF)-α and IL-1β, which recruit more immune cells to destroy infected cells (48, 49) (Figure 1A). The immune cells also induce the adaptive immune system to produce antibodies against the pathogen via signaling involving the major histocompatibility complex II, CD40, CD80 and CD86.
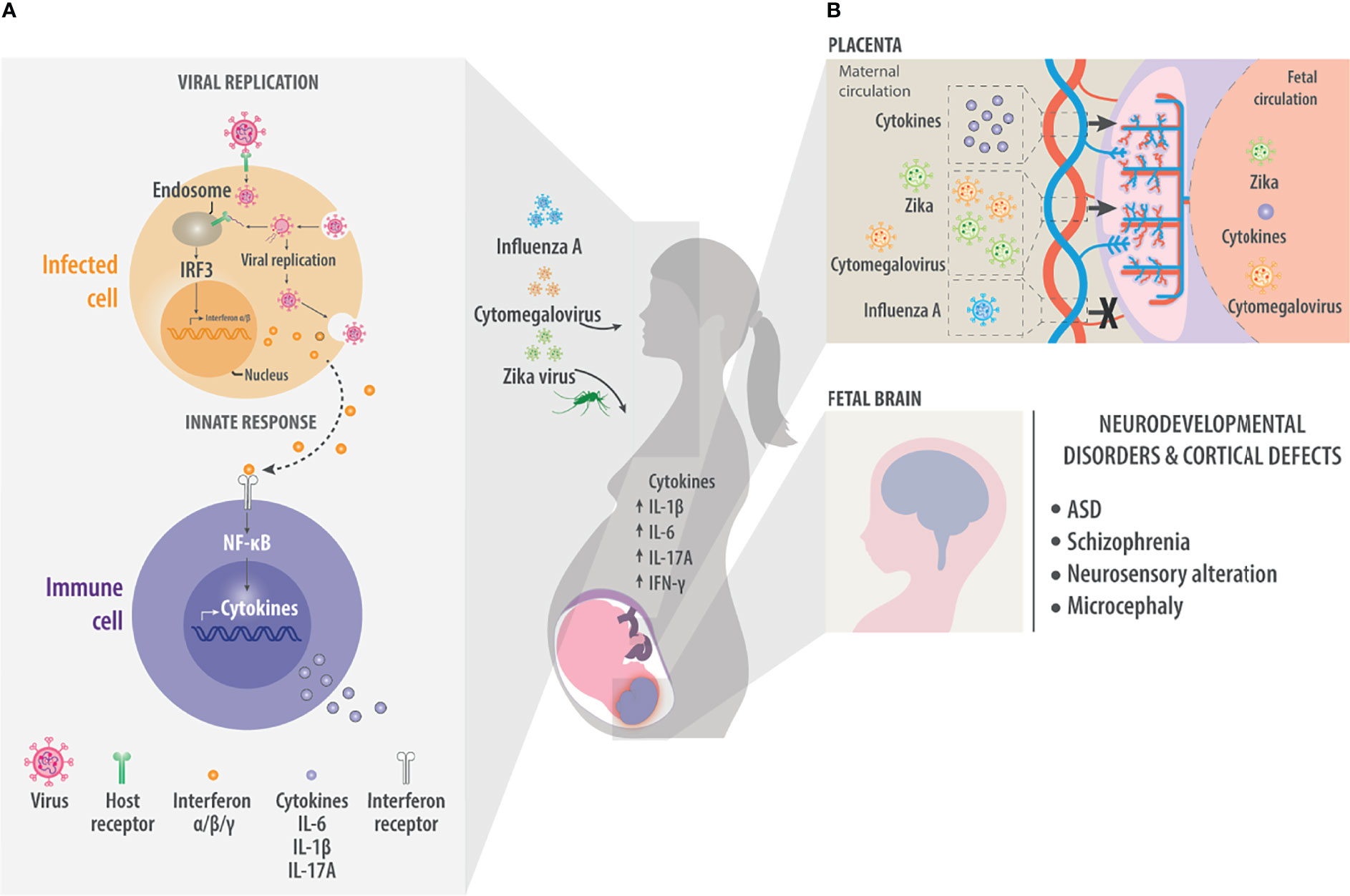
Figure 1 Maternal viral infection. As a result of maternal viral infection, immune responses generate pro-inflammatory cytokines such as IL-1β, IL-6, and IL-17A. These cross the placenta and generate neuroinflammation in the fetal brain. (A) During viral infection, molecules on the viral surface are recognized and trigger a cascade of events in the infected cell. Host receptors recognize viral molecules or nucleic acids and initiate a signaling cascade that ends with the nuclear translocation of transcription factors such as interferon regulatory factor 3 (IRF3), which induce the synthesis of type I interferons α and β, which are ultimately released into the extracellular environment. There the interferons are detected by cells of the innate immune system. Innate immune system cells, such as macrophages or dendritic cells, recognize pathogen-associated molecular patterns (PAMPs) and damage-associated molecular patterns (DAMPs). They also respond to cytokines such as interferons to activate signaling pathways via NF-κB to generate pro-inflammatory cytokines such as IL-6 and IL-1β. These factors trigger inflammation and recruit more cells to inhibit viral replication or kill infected cells. (B) Maternal infection by the Influenza A, Cytomegalovirus or Zika virus generates an increase in maternal pro-inflammatory cytokines that can reach the fetal circulation through the placenta. In the same way, viruses such as Cytomegalovirus or Zika also cross the placenta and directly affect the fetal brain. In the fetal brain, the presence of pro-inflammatory cytokines or viruses can result in neurodevelopmental disorders and defects in the formation of the cerebral cortex, manifesting effects such as ASD, Schizophrenia, neurosensory alteration or microcephaly.
Many viruses can have the potential to extend an effect on the fetal brain and trigger pro-inflammatory responses in this way, with potentially serious consequences for the developing brain. Such pathogens include rubella virus (50, 51), herpes simplex virus (52–54) and parvovirus (55). Three viruses that show strong tropism toward neural cells and linked to neurodevelopmental disorders are ZIKV, Influenza A virus and CMV. All three have been associated with ASD, schizophrenia, neurosensory alterations, hydrocephaly, or microcephaly (Figure 1B) (4–30).
Zika Virus
ZIKV is one of the most well-studied viruses that perturb brain development. It is a single-stranded RNA virus, it belongs to the family Flaviviridae and genus Flavivirus (56). The most evident consequence of ZIKV infection of pregnant mothers is microcephaly in the offspring, and a causal link between the two was implied by the simultaneous presence of the viral genome in cerebrospinal fluid and antibodies in neonates (31–33). Furthermore, animal models in which the virus is injected into the uterus or directly into the fetal brain ventricle have provided important insights into how ZIKV disrupts brain development (Figure 2) (57). These models link virus infection with decreased brain volume, disorganization of neuronal layers in the cortex, and apoptosis in the hippocampus and cortex (58), as well as with disruptions of NPC biology that mimic the etiology of several neurodevelopmental disorders (41, 59). In fact, infection of NPCs induces their death by multiple mechanisms, including apoptosis, pyroptosis, and autophagy, which contribute to microcephaly in the offspring (60–67).
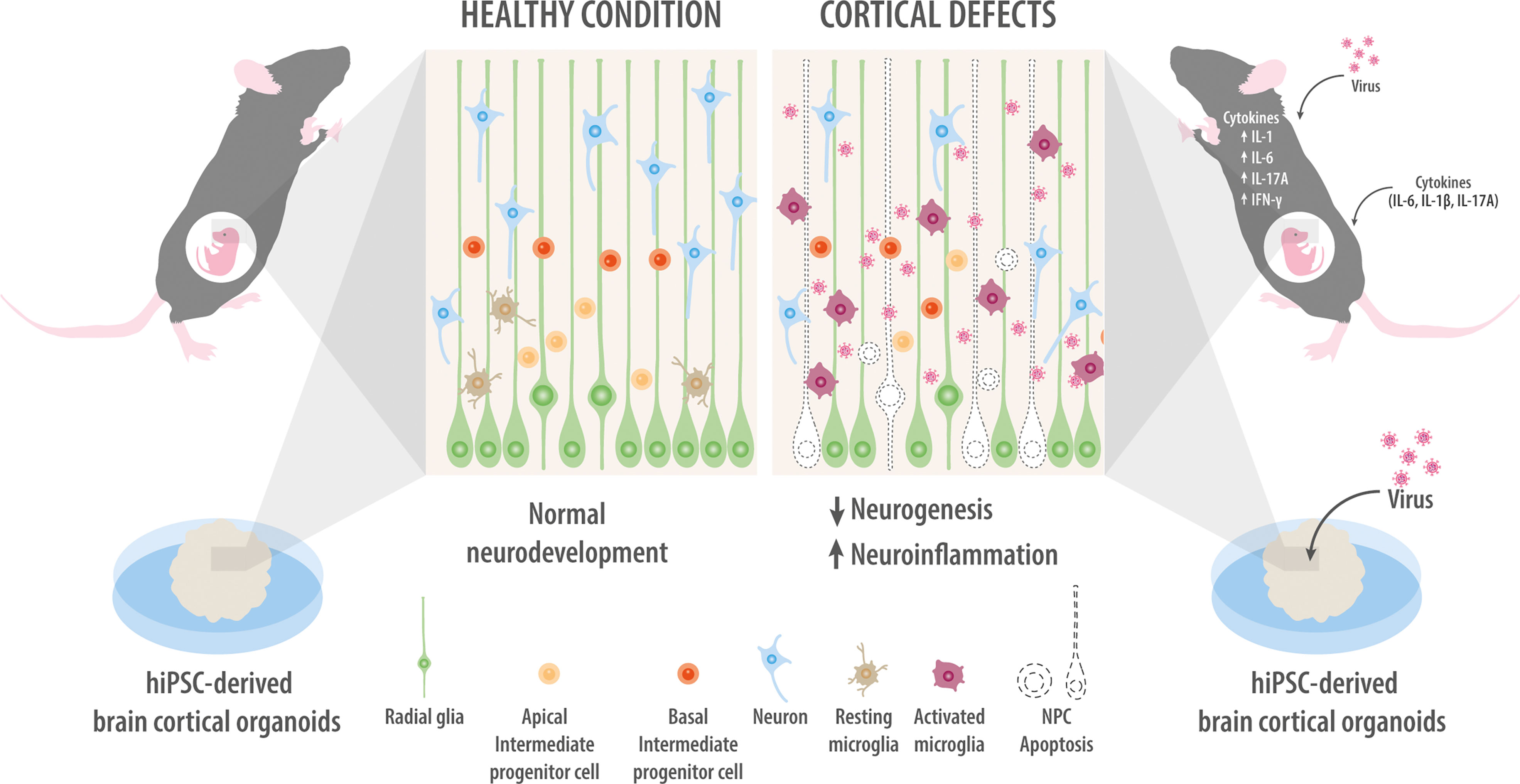
Figure 2 Models of maternal infection. Modeling of maternal infection in mice either by injection of viruses or cytokines (upper right panel). Maternal infection activates microglia and neuroinflammation while inhibiting NPC proliferation and neuronal migration. Infecting brain organoids with Zika virus or Cytomegalovirus inhibits NPC proliferation and promotes their apoptosis, ultimately shrinking the organoid (bottom right panel). For comparison, mock-infected NPCs are intact and show normal organization of the different cortical layers, as well as normal levels of neuronal migration and survival (left panel).
A critical question is how ZIKV comes into contact with NPCs. Presumably the placenta is to blame as the bridge between the mother and fetus. This transient organ begins to form at implantation and supports the passage of nutrition and gases, toxin elimination, and protection against pathogens (68–70). Injecting ZIKV into the uterus of pregnant animals leads to detectable viral antigens in the placenta, indicating that the virus can infect those cells. Indeed, ZIKV infection of the placenta leads to total loss of boundaries between placental layers, and mixing of maternal and fetal blood (71, 72). Once the virus infects the placenta, it can replicate in cytotrophoblasts and infect macrophages (Hofbauer cells), which then produce IFNα, IL-6, and monocyte chemoattractant protein-1 (MCP-1) (38). Ultimately the virus passes through the placenta and enters the brain.
Once inside the fetal central nervous system, ZIKV can infect NPCs, astrocytes, and microglia, but it is toxic only to NPCs. Microglia, in contrast, allow the virus to replicate but do not die, implying that they can serve as a viral reservoir in the fetal brain (73). Given the developmental origin of the microglia from the yolk sac, the virus likely enters the fetus via the maternal vasculature (73, 74). Microglia not only help the virus spread throughout the central nervous system (CNS) (73, 75), but they also induce the secretion of pro-inflammatory cytokines IL-6, IL-1 β, TNF-α, and MCP-1, which contribute to neuroinflammation with long-term consequences (76–78).
Much of what we know about how ZIKV affects the fetal brain comes from studies in cell culture. Given that a Petri dish can be a poor mimic of the diverse, highly structured brain, many researchers have focused on brain organoids (Figure 2). Typically, human inducible pluripotent stem cells (iPSC) are used to derive NPCs in a three-dimensional culture system in which specific brain regions can develop. Brain organoids mimic key characteristics of human neurodevelopment, such as forming a complex progenitor zone with abundant outer radial glial cells (79). Interestingly, infecting brain organoids with ZIKV reduces their volume, analogous to microcephaly (61, 80). Such observations have led to the idea that ZIKV targets NPCs through recognition via Toll-Like receptor 3, but not neurons (81–83), while downregulating genes related to the cell cycle, cell division, neurogenesis, as well as axonal guidance and differentiation, consistent with the characteristics of microcephalic offspring born to ZIKV-infected mothers (84, 85).
Cytomegalovirus
This double-stranded DNA virus belongs to the family Herpesviridae and genus Herpesvirus (86). Congenital CMV infection leads to clinical manifestations in only 10% of newborns, yet 10-15% of apparently asymptomatic newborns have long-term sequelae, such as sensorineural hearing loss, neurodevelopmental disorders, ophthalmic complications, cerebral neoplasms, and ASD (Figure 2) (87–89). The virus moves vertically from mother to fetus by infecting cytotrophoblasts in the placenta, where the infection alters placenta integrity and development (90, 91) and dysregulates gene expression to inhibit placental cell differentiation, self-renewal, and migration (39, 92). Similar to ZIKV, CMV infects and replicates in microglia (93). In animal models, CMV infects fetal macrophages, which infiltrate the fetal brain preferentially in the choroid plexus and in ventricular and subventricular areas, where the macrophages induce inflammation by producing IL-6, IL-1β, and TNF-α (94–97).
Once inside the fetal brain, CMV can also directly infect NPCs, likely through platelet-derived growth factor receptor alpha (PDGFRα) (98). Indeed, expressing this receptor in NPC-derived neurospheres in vitro allows CMV infection (99). Like ZIKV, CMV also inhibits NPC neurogenesis, proliferation, differentiation and migration (100–104), while stimulating their apoptosis (105). This may help explain why maternal CMV infection is linked to hearing loss, intellectual disability, and other cognitive deficits in offspring (106).
Studies with human brain organoids have provided crucial information about how CMV infection disrupts fetal brain development (Figure 2). Exposing brain organoids to CMV alters the structure and organization of the cortex-like component and the distribution of NPCs and neuronal populations in cortical layers (107, 108).
Influenza A Virus
Influenza A virus is a single-stranded RNA virus belonging to the family Orthomyxoviridae and the genus Alphainfluenzavirus (109). A seasonal virus, it causes 3-5 million severe cases globally, with pregnant women at particularly high risk of complications following infection. Occasionally, strains of the Influenza virus have caused pandemics that killed millions of people worldwide, such as in 1918 and 2009 (110). Offspring of mothers infected with Influenza A virus show reduced psychomotor development in the first months of life (111), as well as cognitive problems, schizophrenia, and bipolar disorder (Figure 1B) (9, 11, 13, 15, 18, 112).
Interestingly, the viral genome has yet to be detected in the placenta or fetal brain (113), suggesting that maternal infection with the virus affects the fetus indirectly by triggering immune responses that compromise neurodevelopment. Increases in maternal levels of IL-6, IL-1β, TNF-α, and IFN-β in response to infection can damage the placenta and intrauterine fetal growth restriction caused by hypoxia that can be detected as upregulation of hypoxic-inducible factor-1α (HIF-1α) in the placenta (114). In pregnant women expressing human leukocyte antigen DRB14, this can induce the production of pro-inflammatory cytokines that cause fetal neuroinflammation, which has been linked to increased risk of schizophrenia in offspring (13). Indeed, postmortem histology of schizophrenic offspring shows disturbed neuronal migration, disorganized lamina strata, ectopic pyramidal cells, abnormal expression of neural cell adhesion molecules, no astrogliosis, as well as reduced densities of axons, dendrites, and synapses. Magnetic resonance imaging shows smaller intracranial volume than in offspring born to uninfected mothers as well as less gray and white matter, especially in the cerebral cortex (115). Animal studies confirm that viral infection of the mother induces immune responses in the fetus that alter critical signaling proteins and pathways in neurodevelopment, such as the fragile X mental retardation protein (FMR1), glutamatergic/GABAergic balance, and reelin signaling (116). Those studies further confirm that viral infection of the mother significantly reduces volumes of the prefrontal, frontal, cingulate, insular, parietal and temporal-auditory regions of the cortex (117) and can lead to impairments in exploratory behavior and social interaction in adulthood (118).
SARS-CoV-2
While researchers have explored the effects of maternal infection with ZIKV, CMV and Influenza A virus on fetal neurodevelopment for several years, we are only beginning to examine whether and how the recently emerged SARS-CoV-2 virus, responsible for the current coronavirus disease 2019 (COVID-19) pandemic, may affect newborns in the long term. SARS-CoV-2 infection induces similar cellular responses as the other three viruses, including an increase in pro-inflammatory cytokines associated with disease severity and can be fatal (119). In particular, COVID-19 patients show elevated IL-6 in their serum (120), even prompting the consideration of anti-IL-6 antibodies as a treatment (121, 122). In some patients, peripheral T helper cells also show high levels of interleukin (IL) 17A in the lung (123, 124).
Whether the virus can cross the placenta and infect the fetus is unknown. Several studies have failed to detect viral antigens or RNA in the placenta (125–133), and placental cells do not seem to co- express the two receptors normally required for the virus to enter cells, angiotensin-converting enzyme 2 receptor (ACE2) and transmembrane protease serine 2 (TMPRSS2) (134, 135). On the other hand, others have reported viral infection in the placenta (136–142), but not in the fetal brain, even though ACE2 and TMPRSS2 are expressed in different types of neurons and microglia (143–148) as well as NPCs (145, 149). In fact, NPCs in culture can be artificially infected with SARS-CoV-2 (150, 151). These contradictory findings make it crucial to assess whether SARS-CoV-2 directly infects fetal neural cells. Studies with human iPSCs and brain organoid cultures are essential in this regard, and such work has already described the expression of ACE2 and TMPRSS2 in the brain (152) and choroid plexus (152, 153). Since the choroid plexus is essential for the formation and function of the CNS and cerebrospinal fluid (CSF) (154), SARS-CoV-2 infection of this region leads to the breakdown of the barrier between the blood and CSF, which can allow the passage of virus, immune cells, and cytokines in the brain, where they can promote a pro-inflammatory environment (153) and disrupt the normal choroid plexus function (155). Organoid studies have also shown that SARS-CoV-2 can contribute to neuroinflammation by infecting pericytes and then spreading to neural cells such as astrocytes, in which they induce type I IFN production and ultimately cell death (156).
Substantial circumstantial evidence points to the ability of SARS-CoV-2 to directly affect the developing brain, but much more remains to be clarified, especially with respect to viral effects on NPCs and cortical development. For this work, well-designed studies are needed with cortical organoids and assembloids involving the various actors involved in neurodevelopment, such as immune system cells, choroid plexus and microglia (Figure 3).
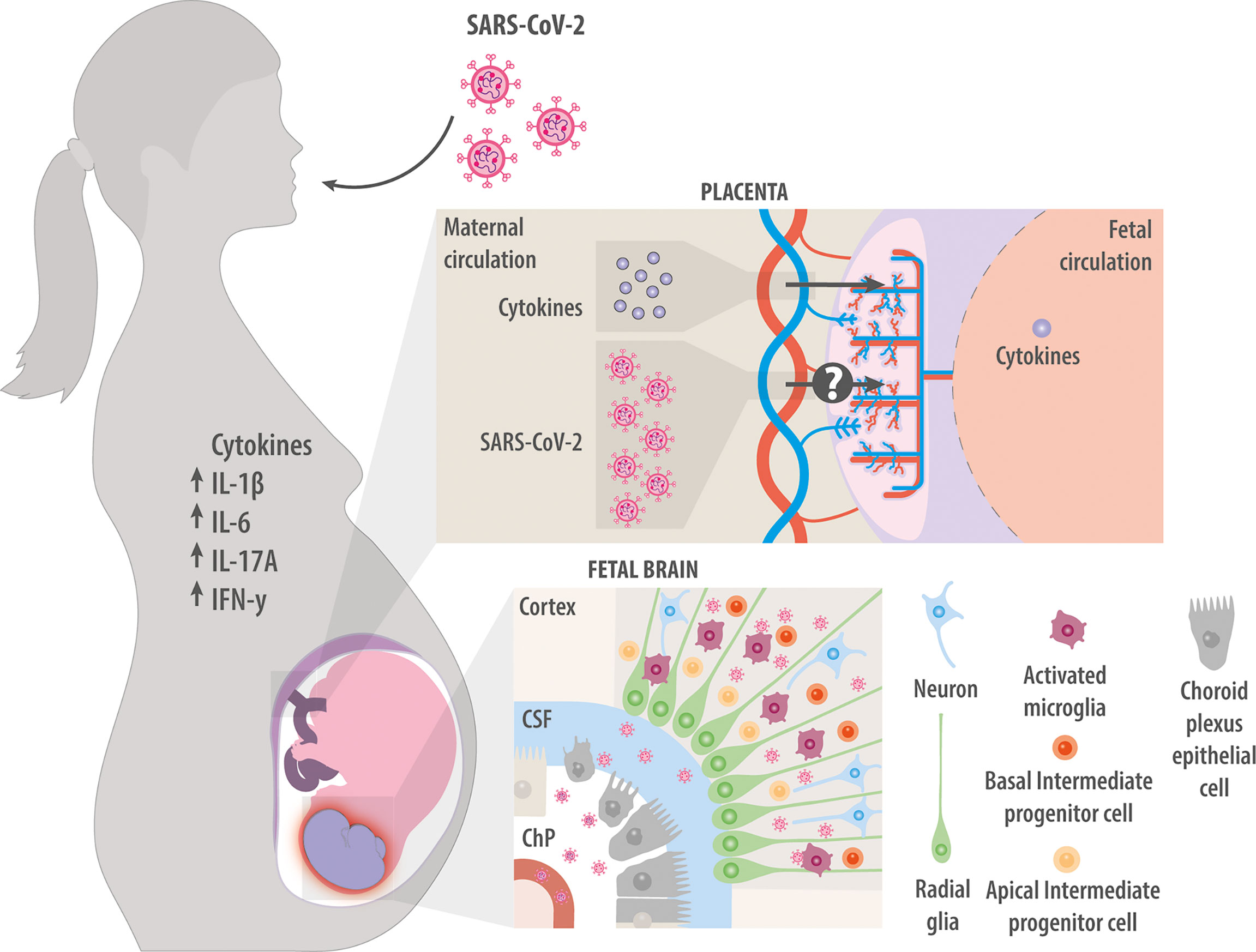
Figure 3 Maternal infection with SARS-CoV-2. Maternal infection with SARS-CoV-2 increases levels of cytokines IL-1β, IL-6, IL-17A, and IFN-γ in the mother. These factors can cross the placenta and induce neuroinflammation in the fetal brain. Whether the virus itself can also cross the placenta and infect the fetus is unclear. Studies in vitro suggest that the virus can infect fetal choroid plexus cells, where they disrupt production of cerebrospinal fluid and compromise the integrity of the blood-brain barrier, allowing immune cells and cytokines to enter the developing brain. Studies in vitro have also suggested that SARS-CoV-2 can directly infect NPCs and microglia.
Ultimately, appropriate animal models are needed, which has been a challenge so far because the viral “spike” protein binds only weakly to the ACE2 receptor in mice (157). Researchers can use the transgenic mouse “B6. Cg-Tg(K18-ACE2)2Prlmn/J”, which was developed during the SARS-CoV epidemic in 2003 and which expresses the human ACE2 receptor (158). The virus can infect epithelial cells in the upper and lower respiratory tract and brain of this mouse. In addition, the “mouse-adapted” recombinant SARS-CoV-2 virus called “SARS-CoV-2 DA” can enter mouse cells (159). These two models will likely provide important insights into how maternal infection with SARS-CoV-2 affects offspring.
Analysis of brain organoids and assembloids may be powerful for elucidating the effects of not only SARS-CoV-2, but also better studied viruses like ZIKV and CMV, on fetal neurodevelopment. For example, combining the use of assembloids and single-cell analysis may clarify how viruses influence the interactions among NPC populations, newborn neurons and microglia in different brain areas. Such work may help identify how viral infection alters gene expression and signaling pathways in NPCs and microglia, and determine whether viral effects on offspring depend on the gestational age at infection. Age-dependent effects may help explain why ZIKV and CMV target the same cells, yet exert different long-term effects on the offspring. Ultimately, such studies may lead to strategies that prevent viral entry and replication in fetal cells, and reduce the production of pro-inflammatory cytokines that can fight the infection and wreak havoc on the developing fetal brain.
Pro-Inflammatory Cytokines And Fetal Neurodevelopment
The release of pro-inflammatory cytokines by maternal immune cells in response to infections can reach the developing fetus through the placenta (160). Furthermore, considering that the primordial fetal blood-brain barrier (BBB) is highly permeable throughout development, the maternal pro-inflammatory cytokines can enter the fetal circulation and profoundly effects the fetal brain (161). This likely explains why elevated levels of pro-inflammatory cytokines in the fetal brain and cerebrospinal fluid have been associated with neuroinflammation and neurodevelopmental diseases (162–166).
IL-6
IL-6 has well-established effects on neurodevelopment. It is produced by various cell types, including fibroblasts, endothelial cells, and immune cells. In the innate immune system, myeloid cells such as neutrophils, monocytes/macrophages, and dendritic cells recognize pathogens through Toll-like receptors, then produce IL-6 (167). Higher levels of maternal IL-6 during pregnancy have been linked to lower cognitive ability in offspring during the first year of life, suggesting an altered front limbic circuit (168). Higher levels of maternal IL-6 during pregnancy have also been linked to an enlarged right amygdala and greater bilateral connectivity of the amygdala to brain regions involved in sensory processing and integration (spindle-shaped somatosensory cortex), salience deletion (insula anterior), and learning and memory (caudate and parahippocampal gyrus) in 2-year-old offspring (169). These findings may reflect the effects on IL-6 on working memory, cognitive function and behavior (169).
Studies in animal models have begun to clarify how IL-6 exerts its effects on fetal neurodevelopment. IL-6 readily crosses the fetal BBB (170, 171). Intraperitoneal administration of IL-6 to pregnant females results in inflammation of the fetal brain and causes social, motor, and learning deficits in the offspring as adults (Figure 2) (172, 173). Intraventricular injection of IL-6 on postnatal day 0.5 increases the formation of excitatory synapses while reducing inhibitory synapses, cognitive and learning functions, and social interactions (174). Transgenic mice that overexpress IL-6 in the brain show reduced neurogenesis in the dentate gyrus of the hippocampus (175). These perturbations are linked to an expansion of NPCs in the adult forebrain and to altered proportions of interneuron subtypes during olfactory neurogenesis in the offspring as adults. Moreover, IL-6 levels in the embryo regulate the size of the NPC pool, and the burst of maternal IL-6 expression upon infection permanently increases this pool, which persists into adulthood (176, 177). Elevated maternal IL-6 may also delay the migration of progenitor cells of GABAergic neurons from the ventral telencephalon to the developing cortical plate (178).
Infection of pregnant mice causes an increase in IL-6 in both maternal serum and fetal brain, and loss of Purkinje cells in lobe VII of the cerebellum. These changes are associated with phosphorylation of signal transducer and activator of transcription (STAT) 3, a regulator of genes related to cell proliferation and apoptosis, in the fetal hindbrain. In support of the role of IL-6 in these brain alterations, ablating the receptor for IL-6 from placental trophoblasts mitigates or even prevents the alterations as well as the accompanying autistic-like behaviors (179). In addition to this receptor in the placenta, microglia help mediate the effects of IL-6: the cytokine increases the branching and vacuole density of microglia, and activated microglia themselves produce IL-6, exacerbating its adverse effects during neurodevelopment (180).
IL-17A
IL-6 and other cytokines help drive the transition from the initial innate immune response to the specific adaptive immune response led by T cells (181). IL-6 in combination with TGF-β promotes the differentiation of naive T cells towards helper T cells with a Th17 phenotype (182). Th17 cells produce IL-17A (183) in response to fungal, bacterial and viral infections (184–186). Infection of pregnant mice has been linked to alterations in IL-17A, which in turn lead to fetal neurodevelopmental problems (187–189). Administering IL-17A to the mother or directly into the embryonic brain activates microglia in the subventricular zone and increases their phagocytic ability (Figure 2) (180), leading to a smaller cortex in the embryo and in the offspring as adults. IL-17A also upregulates gene expression at GABAergic synapses and reduces anxiety-like behavior in offspring (190).
IL-17A binds to NPCs via a specific surface receptor, inhibiting their proliferation as well as their ability to form neurospheres or to differentiate into astrocytes and oligodendrocyte precursor cells (35). In addition, IL-17A acts to disorganize cytoarchitecture in the cortex, reflected in delayed expression of neuronal markers in different cortical layers (191). These cortical alterations are associated with ASD-like behaviors in the offspring, including abnormal ultrasonic vocalization, deficits in social interaction, and repetitive behavior. The tight link between maternal cytokine signaling and fetal neurodevelopment is reflected in the observation that maternal infection upregulates the IL-17 receptor in the fetal cortex, but not if the mother is pretreated with an anti-IL-17A antibody (191).
IL-1β
During an infection, IL-1β is one of the first cytokines released by macrophages, monocytes, and dendritic cells. It is required for efficient innate and adaptive immune responses (192). Intraperitoneal injection of IL-1β into pregnant animals (Figure 2) increases the density of amoeboid microglia in the cortical plate, suggesting inflammation of the fetal brain (193). In addition, IL-1β injection into pregnant female induces CD4+ and CD8+ T cells to infiltrate the placenta and ultimately enter the fetus, where they thin out the cerebral cortex and cause behavioral deficits later in the offspring (194). Decreasing IL-1β expression in the placenta and fetal brain as well as inhibiting the enzyme nitric oxide synthase in the cortex reverse the ability of IL-1β to disorganize the arborization of neuronal dendrites in the offspring (195). The harmful effects of IL-1β and IL-6 on fetal brain can also be reversed by intraperitoneal administration of an interleukin-1 receptor antagonist into pregnant animals (196): the antagonist reduces damage to the placenta, and in the offspring, it reduces the density of microglia expressing ionized calcium-binding adaptor molecule 1 (Iba-1) and improves motor performance.
IFN-γ
IFN-γ is secreted by natural killer cells as part of the innate immune response and by CD4+ Th1 cells and CD8+ T cells as part of the adaptive immune response. In mice, IFN-γ inhibits NPC proliferation by blocking them in G1/S through a pathway that involves STAT1 (197). Injecting IFN-γ into the fetal brain ventricle on embryonic day 9.5 leads to a smaller number of immature neurons and larger number of nestin-positive NPCs on embryonic day 14.5, suggesting that IFN-γ inhibits embryonic neurogenesis (198). These effects can be reversed by inhibiting signaling downstream of the IFN-γ receptor or by knocking down STAT1 results in a reversal of the adverse effects of IFN-γ administration on neurogenesis (198).
In these ways, the maternal inflammatory response to viral infection induces pro-inflammatory cytokines that can reach the developing fetal brain via the placenta and the fetal BBB. These cytokines exert their effects on NPCs and in microglia leading to their activation and long-lasting neuroinflammation. These “indirect” effects of maternal infection add to the direct effects caused by vertical virus transmission, emphasizing the importance of developing vaccines against viruses that affect fetal neurodevelopment and encouraging vaccination among pregnant women or trying to become pregnant. These considerations also highlight the need to develop comprehensive anti-viral strategies that neutralize the virus and dampen the strong maternal immune response that can harm fetal neurodevelopment.
Discussion
Maternal infections during pregnancy can have severe effects on fetal brain development, causing long-term consequences manifesting as ASD, schizophrenia, social disturbances, cognitive impairment, and other disorders. This review has discussed two of the major mechanisms by which a maternal infection can alter neurodevelopment. First, vertical transmission of virus between mother and fetus via the placenta allows the virus to enter the fetal circulation, from where it infects cells important for neurogenesis, such as NPCs and microglia. Second, viral infection in the mother triggers an increase in the levels of pro-inflammatory cytokines in her systemic circulation, which then enter the fetal circulation and ultimately reach the developing brain. These cytokines alter NPC biology and activate microglia, causing them to produce even more pro-inflammatory cytokines that maintain neuroinflammation. Whether fetal brain cells are directly infected by virus or affected by cytokines coming from other infected cells, the fetal cells undergo various changes in response to maternal infection, leading to altered cortical structure, brain size, or connectivity between brain areas. These perturbations can ultimately affect the cognitive ability and behavior of the offspring into adulthood.
The severe SARS-CoV-2 pandemic has brought new urgency to research into how maternal infection with virus can affect fetal neurodevelopment. It is imperative that we understand whether SARS-CoV-2 can pass from mother to fetus, and whether it can directly infect fetal neural cells and microglia. However, as this review illustrates, the virus may affect fetal neurodevelopment even without directly infecting it, so studies should examine whether maternal infection with SARS-CoV2 is associated with neurodevelopmental disorders. Such studies should examine COVID-19 patients but also exploit the experimental tools of human iPSC-derived brain organoids and assembloids, which have proven so powerful in research on ZIKV and CMV. Such studies should also make use of appropriate mouse models. The overarching goal is to prevent or safely manage viral infections during pregnancy and minimize their long-term consequences on the offspring.
Author Contributions
DE and GC drafted the manuscript, which all authors revised. All authors contributed to the article and approved the submitted version.
Funding
DE is supported by a Postdoctoral Grant from ANID FONDECYT (3200846), and GC by a Returnee Fellowship from IBRO. This work was funded by Regular Grants from ANID FONDECYT (1161374, 1210507), a REDES Grant from ANID PCI (180113), and a Start-Up Grant from Universidad Mayor. PM is supported by ANID FONDECYT Iniciación (11190258) ER is supported by an ANID FONDECYT Regular 1191526 grant.
Conflict of Interest
The authors declare that the research was conducted in the absence of any commercial or financial relationships that could be construed as a potential conflict of interest.
Publisher’s Note
All claims expressed in this article are solely those of the authors and do not necessarily represent those of their affiliated organizations, or those of the publisher, the editors and the reviewers. Any product that may be evaluated in this article, or claim that may be made by its manufacturer, is not guaranteed or endorsed by the publisher.
Acknowledgments
We thank David R. Kaplan for his thoughtful comments.
Abbreviations
ASD, autism spectrum disorder; BBB, blood-brain-barrier; CDn, cluster of differentiation CMV, Cytomegalovirus; CNS, central nervous system; COVID-19, coronavirus disease 2019; CSF, cerebral spinal fluid; IFNn, interferon; IL-1β, interleukin 1 beta; IL-6, interleukin 6; IL-17A, interleukin 17A; iPSC, inducible pluripotent stem cells, NPCs, neural stem/precursor cells; SARS-CoV-2, severe acute respiratory syndrome coronavirus 2; THn, T helper; TMPRSS2, transmembrane protease serine 2; TNF-α, tumor necrosis factor alpha; ZIKV, Zika virus.
References
1. Bolton JL, Marinero S, Hassanzadeh T, Natesan D, Le D, Belliveau C, et al. Gestational Exposure to Air Pollution Alters Cortical Volume, Microglial Morphology, and Microglia-Neuron Interactions in a Sex-Specific Manner. Front Synaptic Neurosci (2017) 9:10. doi: 10.3389/fnsyn.2017.00010
2. Edlow AG. Maternal Obesity and Neurodevelopmental and Psychiatric Disorders in Offspring. Prenatal Diagn (2017) 37(1):95–110. doi: 10.1002/pd.4932
3. Andersen SL, Laurberg P, Wu CS, Olsen J. Attention Deficit Hyperactivity Disorder and Autism Spectrum Disorder in Children Born to Mothers With Thyroid Dysfunction: A Danish Nationwide Cohort Study. Aarhus, Denmark: Epidemiology (2014). pp. 1365–74. doi: 10.1111/1471-0528.12681.
4. Khandaker GM, Zimbron J, Lewis G, Jones PB. Prenatal Maternal Infection, Neurodevelopment and Adult Schizophrenia: A Systematic Review of Population-Based Studies. Psychol Med (2013) 43(2):239–57. doi: 10.1017/S0033291712000736
5. Fatemi SH, Reutiman TJ, Folsom TD, Huang H, Oishi K, Mori S, et al. Maternal Infection Leads to Abnormal Gene Regulation and Brain Atrophy in Mouse Offspring: Implications for Genesis of Neurodevelopmental Disorders. Schizophr Res (2008) 99(1–3):56–70. doi: 10.1016/j.schres.2007.11.018
6. Nielsen-Saines K, Brasil P, Kerin T, Vasconcelos Z, Gabaglia CR, Damasceno L, et al. Delayed Childhood Neurodevelopment and Neurosensory Alterations in the Second Year of Life in a Prospective Cohort of ZIKV-Exposed Children. Nat Med (2019) 25(8):1213–7. doi: 10.1038/s41591-019-0496-1
7. Miller BJ, Culpepper N, Rapaport MH, Buckley P. Prenatal Inflammation and Neurodevelopment in Schizophrenia: A Review of Human Studies. Prog Neuropsychopharmacol Biol Psychiatry (2013) 42:92–100. doi: 10.1016/j.pnpbp.2012.03.010
8. Atladóttir HjördisÓ, Thorsen P, Østergaard L, Schendel DE, Lemcke S, Abdallah M, et al. Maternal Infection Requiring Hospitalization During Pregnancy and Autism Spectrum Disorders. J Autism Dev Disord (2010) 40(12):1423–30. doi: 10.1007/s10803-010-1006-y
9. Atladóttir HjördisÓsk, Henriksen TB, Schendel DE, Parner ET. Autism After Infection, Febrile Episodes, and Antibiotic Use During Pregnancy: An Exploratory Study. Pediatrics (2012) 130(6):1447–54. doi: 10.1542/peds.2012-1107
10. Garofoli F, Lombardi G, Orcesi S, Pisoni C, Mazzucchelli I, Angelini M, et al. An Italian Prospective Experience on the Association Between Congenital Cytomegalovirus Infection and Autistic Spectrum Disorder. J Autism Dev Disord (2017) 47(5):1490–5. doi: 10.1007/s10803-017-3050-3
11. Mahic M, Che X, Susser E, Levin B, Reichborn-Kjennerud T, Magnus P, et al. Epidemiological and Serological Investigation Into the Role of Gestational Maternal Influenza Virus. MSphere (2017) 2(3):e00159–17. doi: 10.1128/mSphere.00159-17
12. Slawinski BL, Talge N, Ingersoll B, Smith A, Glazier A, Kerver J, et al. Maternal Cytomegalovirus Sero-Positivity and Autism Symptoms in Children. Am J Reprod Immunol (2018) 79(5):1–7. doi: 10.1111/aji.12840
13. Brown AS, Begg MD, Gravenstein S, Schaefer CA, Wyatt RJ, Bresnahan M, et al. Serologic Evidence of Prenatal Influenza in the Etiology of Schizophrenia. Arch Gen Psychiatry (2004) 61(8):774–80. doi: 10.1001/archpsyc.61.8.774
14. Brown AS, Schaefer CA, Quesenberry CP, Liu L, Babulas VP, Susser ES. Maternal Exposure to Toxoplasmosis and Risk of Schizophrenia in Adult Offspring. Am J Psychiatry (2005) 162(4):767–73. doi: 10.1176/appi.ajp.162.4.767
15. Kępińska AP, Iyegbe CO, Vernon AC, Yolken R, Murray RM, Pollak TA. Schizophrenia and Influenza at the Centenary of the 1918-1919 Spanish Influenza Pandemic: Mechanisms of Psychosis Risk. Front Psychiatry (2020) 11:72. doi: 10.3389/fpsyt.2020.00072
16. Mortensen PB, Nørgaard-Pedersen B, Waltoft BL, Sørensen TL, Hougaard D, Torrey EF, et al. Toxoplasma Gondii as a Risk Factor for Early-Onset Schizophrenia: Analysis of Filter Paper Blood Samples Obtained at Birth. Biol Psychiatry (2007) 61(5):688–93. doi: 10.1016/j.biopsych.2006.05.024
17. Buka SL, Tsuang MT, Torrey EF, Klebanoff MA, Bernstein D, Yolken RH. Maternal Infections and Subsequent Psychosis Among Offspring. Arch Gen Psychiatry (2001) 58(11):1032–7. doi: 10.1001/archpsyc.58.11.1032
18. Canetta SE, Bao Y, Co MDT, Ennis FA, Cruz J, Terajima M, et al. Serological Documentation of Maternal Influenza Exposure and Bipolar Disorder in Adult Offspring. Am J Psychiatry (2014) 171(5):557–63. doi: 10.1176/appi.ajp.2013.13070943
19. De Oliveira Melo AS, Aguiar RS, Amorim MMR, Arruda MB, De Oliveira Melo F, Ribeiro STC, et al. Congenital Zika Virus Infection: Beyond Neonatal Microcephaly. JAMA Neurol (2016) 73(12):1407–16. doi: 10.1001/jamaneurol.2016.3720
20. Mlakar J, Korva M, Tul N, Popović M, Poljšak-Prijatelj M, Mraz J, et al. Zika Virus Associated With Microcephaly. N Engl J Med (2016) 374(10):951–8. doi: 10.1056/nejmoa1600651
21. Sharma V, Sharma M, Dhull D, Sharma Y, Kaushik S, Kaushik S. Zika Virus: An Emerging Challenge to Public Health Worldwide. Can J Microbiol (2020) 66(2):87–98. doi: 10.1139/cjm-2019-0331
22. Antoniou E, Orovou E, Sarella A, Iliadou M, Rigas N, Palaska E, et al. Zika Virus and the Risk of Developing Microcephaly in Infants: A Systematic Review. Int J Environ Res Public Health (2020) 17(11):1–12. doi: 10.3390/ijerph17113806
23. Martins MM, da Cunha AJLA, Robaina JR, Raymundo CE, Barbosa AP, de Andrade Medronho R. Fetal, Neonatal, and Infant Outcomes Associated With Maternal Zika Virus Infection During Pregnancy: A Systematic Review and Meta-Analysis. PloS One (2021) 16:1–26. doi: 10.1371/journal.pone.0246643
24. Robbiani DF, Olsen PC, Costa F, Wang Q, Oliveira TY, Nery N, et al. Risk of Zika Microcephaly Correlates With Features of Maternal Antibodies. J Exp Med (2019) 216(10):2302–15. doi: 10.1084/jem.20191061
25. Brady OJ, Osgood-Zimmerman A, Kassebaum NJ, Ray SE, De Araùjo VEM, Da Nóbrega AA, et al. The Association Between Zika Virus Infectionand Microcephaly in Brazil 2015–2017: Anobservational Analysis of Over 4 Million Births. PloS Med (2019) 16(3):1–21. doi: 10.1371/journal.pmed.1002755
26. Kagan KO, Hamprecht K. Cytomegalovirus Infection in Pregnancy. Arch Gynecol Obstetrics (2017) 296(1):15–26. doi: 10.1007/s00404-017-4380-2
27. Cheeran MCJ, Lokensgard JR, Schleiss MR. Neuropathogenesis of Congenital Cytomegalovirus Infection: Disease Mechanisms and Prospects for Intervention. Clin Microbiol Rev (2009) 22(1):99–126. doi: 10.1128/CMR.00023-08
28. Nicloux M, Peterman L, Parodi M, Magny JF. Outcome and Management of Newborns With Congenital Cytomegalovirus Infection. Arch Pediatrie (2020) 27(3):160–5. doi: 10.1016/j.arcped.2020.01.006
29. Somerville L, Basile K, Dwter D, Kok J. The Impact of Influenza Virus Infection in Pregnancy. Future Microbiol (2018) 13(2):263–74. doi: 10.2217/fmb-2017-0096
30. Spini VBMG, Ferreira FR, Gomes AO, Duarte RMF, Oliveira VHS, Costa NB, et al. Maternal Immune Activation With H1N1 or Toxoplasma Gondii Antigens Induces Behavioral Impairments Associated With Mood Disorders in Rodents. Neuropsychobiology (2021) 80(3):234–41. doi: 10.1159/000510791
31. de Araújo TVB, Ximenes RA, de A, Miranda-Filho DdeB, Souza WV, Montarroyos UR, et al. Association Between Microcephaly, Zika Virus Infection, and Other Risk Factors in Brazil: Final Report of a Case-Control Study. Lancet Infect Dis (2018) 3099(17):1–9. doi: 10.1016/S1473-3099(17)30727-2
32. Krow-Lucal ER, de Andrade MR, Cananéa JNA, Moore CA, Leite PL, Biggerstaff BJ, et al. Association and Birth Prevalence of Microcephaly Attributable to Zika Virus Infection Among Infants in Paraíba, Brazil, in 2015–16: A Case-Control Study. Lancet Child Adolesc Health (2018) 2(3):205–13. doi: 10.1016/S2352-4642(18)30020-8
33. Rocha SGMO, Correia LL, Cunha AJLA, Rocha HAL, Leite Á.JM, Campos JS, et al. Zika Virus Infection and Microcephaly: A Case-Control Study in Brazil. Ann Global Health (2019) 85(1):1–11. doi: 10.5334/aogh.2394
34. Nandi S, Gokhan S, Dai XM, Wei S, Enikolopov G, Lin H, et al. The CSF-1 Receptor Ligands IL-34 and CSF-1 Exhibit Distinct Developmental Brain Expression Patterns and Regulate Neural Progenitor Cell Maintenance and Maturation. Dev Biol (2012) 367:100–13. doi: 10.1016/j.ydbio.2012.03.026
35. Li Z, Li K, Zhu L, Kan Q, Yan Y, Kumar P, et al. Inhibitory Effect of IL-17 on Neural Stem Cell Proliferation and Neural Cell Differentiation. BMC Immunol (2013) 14:1–10. doi: 10.1186/1471-2172-14-20
36. Ozaki K, Kato D, Ikegami A, Hashimoto A, Sugio S, Guo Z, et al. Maternal Immune Activation Induces Sustained Changes in Fetal Microglia Motility. Sci Rep (2020) 10(1):1–19. doi: 10.1038/s41598-020-78294-2
37. Prins JR, Eskandar S, Eggen BJL, Scherjon SA. Microglia, the Missing Link in Maternal Immune Activation and Fetal Neurodevelopment; and a Possible Link in Preeclampsia and Disturbed Neurodevelopment? J Reprod Immunol (2018) 126(December 2017):18–22. doi: 10.1016/j.jri.2018.01.004
38. Quicke KM, Bowen JR, Johnson EL, McDonald CE, Ma H, O’Neal JT, et al. Zika Virus Infects Human Placental Macrophages. Cell Host Microbe (2016) 20(1):83–90. doi: 10.1016/j.chom.2016.05.015
39. Tabata T, Petitt M, Zydek M, Fang-Hoover J, Larocque N, Tsuge M, et al. Human Cytomegalovirus Infection Interferes With the Maintenance and Differentiation of Trophoblast Progenitor Cells of the Human Placenta. J Virol (2015) 89(9):5134–47. doi: 10.1128/jvi.03674-14
40. Aarum J, Sandberg K, Haeberlein SLB, Persson MAA. Migration and Differentiation of Neural Precursor Cells can be Directed by Microglia. Proc Natl Acad Sci USA (2003) 100(26):15983–8. doi: 10.1073/pnas.2237050100
41. Ernst C. Proliferation and Differentiation Deficits are a Major Convergence Point for Neurodevelopmental Disorders. Trends Neurosci (2016) 39(5):290–9. doi: 10.1016/j.tins.2016.03.001
42. Juric-Sekhar G, Hevner RF. Malformations of Cerebral Cortex Development: Molecules and Mechanisms. Annu Rev Pathol: Mech Dis (2019) 14:293–318. doi: 10.1146/annurev-pathmechdis-012418-012927
43. Salter MW, Stevens B. Microglia Emerge as Central Players in Brain Disease. Nat Med (2017) 23(9):1018–27. doi: 10.1038/nm.4397
44. Yeo W, Gautier J. Early Neural Cell Death: Dying to Become Neurons. Dev Biol (2004) 274(2):233–44. doi: 10.1016/j.ydbio.2004.07.026
45. Cunningham CL, Martínez-Cerdeño V, Noctor SC. Microglia Regulate the Number of Neural Precursor Cells in the Developing Cerebral Cortex. J Neurosci (2013) 33(10):4216–33. doi: 10.1523/JNEUROSCI.3441-12.2013
46. Schafer DP, Lehrman EK, Kautzman AG, Koyama R, Mardinly AR, Yamasaki R, et al. Microglia Sculpt Postnatal Neural Circuits in an Activity and Complement-Dependent Manner. Neuron (2012) 74(4):691–705. doi: 10.1016/j.neuron.2012.03.026
47. Maginnis MS. Virus–Receptor Interactions: The Key to Cellular Invasion Melissa. J Mol Biol (2018) 430:2590–611. doi: 10.1016/j.jmb.2018.06.024
48. Thompson MR, Kaminski JJ, Kurt-Jones EA, Fitzgerald KA. Pattern Recognition Receptors and the Innate Immune Response to Viral Infection. Viruses (2011) 3(6):920–40. doi: 10.3390/v3060920
49. Aoshi T, Koyama S, Kobiyama K, Akira S, Ishii KJ. Innate and Adaptive Immune Responses to Viral Infection and Vaccination. Curr Opin Virol (2011) 1(4):226–32. doi: 10.1016/j.coviro.2011.07.002
50. Shuid AN, Jayusman PA, Shuid N, Ismail J, Nor NK, Mohamed IN. Association Between Viral Infections and Risk of Autistic Disorder: An Overview. Int J Environ Res Public Health (2021) 18(6):1–17. doi: 10.3390/ijerph18062817
51. Mawson AR, Croft AM. Rubella Virus Infection, the Congenital Rubella Syndrome, and the Link to Autism. Int J Environ Res Public Health (2019) 16(19):1–28. doi: 10.3390/ijerph16193543
52. Enright AM, Prober CG. Neonatal Herpes Infection: Diagnosis, Treatment and Prevention. Semin Neonatol (2002) 7(4):283–91. doi: 10.1053/siny.2002.0115
53. Silasi M, Cardenas I, Kwon JY, Racicot K, Aldo P, Mor G. Viral Infections During Pregnancy. Am J Reprod Immunol (2015) 73(3):199–213. doi: 10.1111/aji.12355
54. Fa F, Laup L, Mandelbrot L, Sibiude J, Picone O. Fetal and Neonatal Abnormalities Due to Congenital Herpes Simplex Virus Infection: A Literature Review. Prenatal Diagn (2020) 40(4):408–14. doi: 10.1002/pd.5587
55. Ornoy A, Ergaz Z. Parvovirus B19 Infection During Pregnancy and Risks to the Fetus. Birth Defects Res (2017) 109(5):311–23. doi: 10.1002/bdra.23588
56. Baud D, Gubler DJ, Schaub B, Lanteri MC, Musso D. An Update on Zika Virus Infection. Lancet (2017) 390(10107):2099–109. doi: 10.1016/S0140-6736(17)31450-2
57. Shi Y, Li S, Wu Q, Sun L, Zhang J, Pan N, et al. Vertical Transmission of the Zika Virus Causes Neurological Disorders in Mouse Offspring. Sci Rep (2018) 8(1):1–14. doi: 10.1038/s41598-018-21894-w
58. Sherer ML, Khanal P, Talham G, Brannick EM, Parcells MS, Schwarz JM. Zika Virus Infection of Pregnant Rats and Associated Neurological Consequences in the Offspring. PloS One (2019) 14(6):1–16. doi: 10.1371/journal.pone.0218539
59. Starr JM. Ageing and Epigenetics: Linking Neurodevelopmental and Neurodegenerative Disorders. Dev Med Child Neurol (2019) 61(10):1134–8. doi: 10.1111/dmcn.14210
60. Li C, Xu D, Hong S, Jiang Y, Liu X, Zhang N, et al. Zika Virus Disrupts Neural Progenitor Development and Leads to Microcephaly in Mice. Cell Stem Cell (2016) 19(1):120–6. doi: 10.1016/j.stem.2016.04.017
61. Cugola FR, Fernandes IR, Russo FB, Freitas BC, Dias JLM, Guimarães KP, et al. The Brazilian Zika Virus Strain Causes Birth Defects in Experimental Models. Nature (2016) 534(7606):267–71. doi: 10.1038/nature18296
62. Shao Q, Herrlinger S, Yang SL, Lai F, Moore JM, Brindley MA, et al. Zika Virus Infection Disrupts Neurovascular Development and Results in Postnatal Microcephaly With Brain Damage. Dev (Cambridge) (2016) 143(22):4127–36. doi: 10.1242/dev.143768
63. Souza BSF, Sampaio GLA, Pereira CS, Campos GS, Sardi SI, Freitas LAR, et al. Zika Virus Infection Induces Mitosis Abnormalities and Apoptotic Cell Death of Human Neural Progenitor Cells. Sci Rep (2016) 6:1–13. doi: 10.1038/srep39775
64. Yang S, Gorshkov K, Lee EM, Xu M, Cheng YS, Sun N, et al. Zika Virus-Induced Neuronal Apoptosis via Increased Mitochondrial Fragmentation. Front Microbiol (2020) 11:598203. doi: 10.3389/fmicb.2020.598203
65. Zhang F, Hammack C, Ogden SC, Cheng Y, Lee EM, Wen Z, et al. Molecular Signatures Associated With ZIKV Exposure in Human Cortical Neural Progenitors. Nucleic Acids Res (2016) 44(18):8610–20. doi: 10.1093/nar/gkw765
66. He Z, An S, Chen J, Zhang S, Tan C, Yu J, et al. Neural Progenitor Cell Pyroptosis Contributes to Zika Virus-Induced Brain Atrophy and Represents a Therapeutic Target. Proc Natl Acad Sci USA (2020) 117(38):23869–78. doi: 10.1073/pnas.2007773117
67. Tiwari SK, Dang JW, Lin N, Qin Y, Wang S, Rana TM. Zika Virus Depletes Neural Stem Cells and Evades Selective Autophagy by Suppressing the Fanconi Anemia Protein FANCC. EMBO Rep (2020) 21(12):1–17. doi: 10.15252/embr.201949183
68. Burton GJ, Jauniaux E. What is the Placenta? Am J Obstetrics Gynecol (2015) 213(4):S6.e1–4. doi: 10.1016/j.ajog.2015.07.050
69. Heerema-McKenney A. Defense and Infection of the Human Placenta. Apmis (2018) 126(7):570–88. doi: 10.1111/apm.12847
70. Costa ML, de Moraes Nobrega G, Antolini-Tavares A. Key Infections in the Placenta. Obstetrics Gynecol Clinics North America (2020) 47(1):133–46. doi: 10.1016/j.ogc.2019.10.003
71. Tabata T, Pettit M, Puerta-Guardo H, Michlmayr D, Wang C, Fang-Hoover J, et al. Zika Virus Targests Differents Primary Human Placental Cells, Suggesting Two Routes for Vertical Transmission. Cell Hosts Microbe (2016) 20(2):155–66. doi: 10.1016/j.chom.2016.07.002
72. Vermillion MS, Lei J, Shabi Y, Baxter VK, Crilly NP, McLane M, et al. Intrauterine Zika Virus Infection of Pregnant Immunocompetent Mice Models Transplacental Transmission and Adverse Perinatal Outcomes. Nat Commun (2017) 8:1–14. doi: 10.1038/ncomms14575
73. Muffat J, Li Y, Omer A, Durbin A, Bosch I, Bakiasi G, et al. Human Induced Pluripotent Stem Cell-Derived Glial Cells and Neural Progenitors Display Divergent Responses to Zika and Dengue Infections. Proc Natl Acad Sci USA (2018) 115(27):7117–22. doi: 10.1073/pnas.1719266115
74. Xu P, Shan C, Dunn TJ, Xie X, Xia H, Gao J, et al. Role of Microglia in the Dissemination of Zika Virus From Mother to Fetal Brain. PloS Neglected Trop Dis (2020) 14(7):1–21. doi: 10.1371/journal.pntd.0008413
75. Mesci P, Macia A, LaRock CN, Tejwani L, Fernandes IR, Suarez NA, et al. Modeling Neuro-Immune Interactions During Zika Virus Infection. Hum Mol Genet (2018) 27(1):41–52. doi: 10.1093/hmg/ddx382
76. Lum FM, Low DKS, Fan Y, Tan JJL, Lee B, Chan JKY, et al. Zika Virus Infects Human Fetal Brain Microglia and Induces Inflammation. Clin Infect Dis (2017) 64(7):914–20. doi: 10.1093/cid/ciw878
77. Diop F, Vial T, Ferraris P, Wichit S, Bengue M, Hamel R, et al. Zika Virus Infection Modulates the Metabolomic Profile of Microglial Cells. PloS One (2018) 13(10):1–16. doi: 10.1371/journal.pone.0206093
78. Wang J, Liu J, Zhou R, Ding X, Zhang Q, Zhang C, et al. Zika Virus Infected Primary Microglia Impairs NPCs Proliferation and Differentiation. Biochem Biophys Res Commun (2018) 497(2):619–25. doi: 10.1016/j.bbrc.2018.02.118
79. Lancaster MA, Renner M, Martin C, Wenzel D, Bicknell LS, Hurles ME, et al. Cerebral Organoids Model Human Brain Development and Microcephaly. Nature (2013) 501(7467):373–9. doi: 10.1038/nature12517
80. Gabriel E, Ramani A, Karow U, Gottardo M, Natarajan K, Gooi LM, et al. Recent Zika Virus Isolates Induce Premature Differentiation of Neural Progenitors in Human Brain Organoids. Cell Stem Cell (2017) 20(3):397–406.e5. doi: 10.1016/j.stem.2016.12.005
81. Qian X, Nguyen HN, Song MM, Hadiono C, Ogden SC, Hammack C, et al. Brain-Region-Specific Organoids Using Mini-Bioreactors for Modeling ZIKV Exposure. Cell (2016) 165(5):1238–54. doi: 10.1016/j.cell.2016.04.032
82. Dang J, Tiwari SK, Lichinchi G, Qin Y, Patil VS, Eroshkin AM, et al. Zika Virus Depletes Neural Progenitors in Human Cerebral Organoids Through Activation of the Innate Immune Receptor Tlr3. Cell Stem Cell (2016) 19(2):258–65. doi: 10.1016/j.stem.2016.04.014
83. Matsumoto M, Funami K, Oshiumi H, Seya T. Toll-Like Receptor 3: A Link Between Toll-Like Receptor, Interferon and Viruses. Microbiol Immunol (2004) 48(3):147–54. doi: 10.1111/j.1348-0421.2004.tb03500.x
84. Garcez PP, Loiola EC, Da Costa RM, Higa LM, Trindade P, Delvecchio R, et al. Zika Virus: Zika Virus Impairs Growth in Human Neurospheres and Brain Organoids. Science (2016) 352(6287):816–8. doi: 10.1126/science.aaf6116
85. Yoon KJ, Song G, Qian X, Pan J, Xu D, Rho HS, et al. Zika-Virus-Encoded NS2A Disrupts Mammalian Cortical Neurogenesis by Degrading Adherens Junction Proteins. Cell Stem Cell (2017) 21(3):349–358.e6. doi: 10.1016/j.stem.2017.07.014
86. Sinnot J, Cancio M. Topics in Clinical Microbiology: Pseudomonas Cepacia. Infect Control (1986) 7(5):281–4. doi: 10.1017/s0195941700064213
87. Zhang XY, Fang F. Congenital Human Cytomegalovirus Infection and Neurologic Diseases in Newborns. Chin Med J (2019) 132(17):2109–18. doi: 10.1097/CM9.0000000000000404
88. Tsutsui Y. Effects of Cytomegalovirus Infection on Embryogenesis and Brain Development. Congenital Anomalies (2009) 49(2):47–55. doi: 10.1111/j.1741-4520.2009.00222.x
89. Lazarini F, Katsimpardi L, Levivien S, Wagner S, Gressens P, Teissier N, et al. Congenital Cytomegalovirus Infection Alters Olfaction Before Hearing Deterioration in Mice. J Neurosci (2018) 38(49):10424–37. doi: 10.1523/JNEUROSCI.0740-18.2018
90. Fisher S, Genbacev O, Maidji E, Pereira L. Human Cytomegalovirus Infection of Placental Cytotrophoblasts In Vitro and In Utero: Implications for Transmission and Pathogenesis. J Virol (2000) 74(15):6808–20. doi: 10.1128/jvi.74.15.6808-6820.2000
91. Maidji E, Percivalle E, Gerna G, Fisher S, Pereira L. Transmission of Human Cytomegalovirus From Infected Uterine Microvascular Endothelial Cells to Differentiating/Invasive Placental Cytotrophoblasts. Virology (2002) 304(1):53–69. doi: 10.1006/viro.2002.1661
92. Pereira L, Tabata T, Petitt M, Fang-Hoover J. Congenital Cytomegalovirus Infection Undermines Early Development and Functions of the Human Placenta. Placenta (2017) 59:S8–S16. doi: 10.1016/j.placenta.2017.04.020
93. Schut RL, Gekker G, Hu S, Chao CC, Pomeroy C, Jordan MC, et al. Cytomegalovirus Replication in Murine Microglial Cell Cultures: Suppression of Permissive Infection by Interferon-γ. J Infect Dis (1994) 169(5):1092–6. doi: 10.1093/infdis/169.5.1092
94. Sakao-Suzuki M, Kawasaki H, Akamatsu T, Meguro S, Miyajima H, Iwashita T, et al. Aberrant Fetal Macrophage/Microglial Reactions to Cytomegalovirus Infection. Ann Clin Trans Neurol (2014) 1(8):570–88. doi: 10.1002/acn3.88
95. Seleme MC, Kosmac K, Jonjic S, Britt WJ. Tumor Necrosis Factor Alpha-Induced Recruitment of Inflammatory Mononuclear Cells Leads to Inflammation and Altered Brain Development in Murine Cytomegalovirus-Infected Newborn Mice. J Virol (2017) 91(8):1–22. doi: 10.1128/jvi.01983-16
96. Cloarec R, Bauer S, Luche H, Buhler E, Pallesi-Pocachard E, Salmi M, et al. Cytomegalovirus Infection of the Rat Developing Brain In Utero Prominently Targets Immune Cells and Promotes Early Microglial Activation. PloS One (2016) 11(7):1–20. doi: 10.1371/journal.pone.0160176
97. Cloarec R, Bauer S, Teissier N, Schaller F, Luche H, Courtens S, et al. In Utero Administration of Drugs Targeting Microglia Improves the Neurodevelopmental Outcome Following Cytomegalovirus Infection of the Rat Fetal Brain. Front Cell Neurosci (2018) 12:55. doi: 10.3389/fncel.2018.00055
98. Soroceanu L, Akhavan A, Cobbs CS. Platelet-Derived Growth Factor-α Receptor Activation is Required for Human Cytomegalovirus Infection. Nature (2008) 455(7211):391–5. doi: 10.1038/nature07209
99. Berger AA, Gil Y, Panet A, Weisblum Y, Oiknine-Djian E, Gropp M, et al. Transition Toward Human Cytomegalovirus Susceptibility in Early Human Embryonic Stem Cell-Derived Neural Precursors. J Virol (2015) 89(21):11159–64. doi: 10.1128/jvi.01742-15
100. Li X-J, Liu X-J, Yang B, Fu Y-R, Zhao F, Shen Z-Z, et al. Human Cytomegalovirus Infection Dysregulates the Localization and Stability of NICD1 and Jag1 in Neural Progenitor Cells. J Virol (2015) 89(13):6792–804. doi: 10.1128/jvi.00351-15
101. Mutnal MB, Cheeran MCJ, Hu S, Lokensgard JR. Murine Cytomegalovirus Infection of Neural Stem Cells Alters Neurogenesis in the Developing Brain. PloS One (2011) 6(1):1–12. doi: 10.1371/journal.pone.0016211
102. Han D, Byun S, Kim J, M K, Pleasure SJ, Ahn J, et al. Human Cytimegalovirus IE2 Protein Disturbs Brain Development by the Dysregulation of Neural Stem Cell Maintenance and the Polarization of Migrating Neurons. J Virol (2017) 91(17):1–11. doi: 10.1128/JVI.00799-17
103. Rolland M, Li X, Sellier Y, Martin H, Perez-Berezo T, Rauwel B, et al. Pparγ Is Activated During Congenital Cytomegalovirus Infection Ans Inhibits Neuronogenesis From Human Neural Stem Cells. PloS Pathologens (2016) 12(4):1–30. doi: 10.1371/journal.ppat.1005547
104. Rolland M, Martin H, Bergamelli M, Sellier Y, Bessieres B, Aziza J, et al. Human Cytomegalovirus Infection is Associated With Increased in Neural Stem Cells Ans Congenitally Infected Brains. J Pathol (2021) 254(1):92–102. doi: 10.1002/path.5640
105. Nakamura H, Liao H, Minami K, Toyoda M, Akutsu H, Miyagawa Y, et al. Human Cytomegalovirus Induces Apoptosis in Neural Stem/Progenitor Cells Derived From Induced Pluripotent Stem Cells by Generating Mitochondrial Dysfunction and Endoplasmic Reticulum Stress. Herpesviridae (2013) 4(1):2. doi: 10.1186/2042-4280-4-2
106. Odeberg J, Wolmer N, Falci S, Westgren M, Seiger Å., Söderberg-Nauclér C. Human Cytomegalovirus Inhibits Neuronal Differentiation and Induces Apoptosis in Human Neural Precursor Cells. J Virol (2006) 80(18):8929–39. doi: 10.1128/jvi.00676-06
107. Brown RM, Rana PSJB, Jaeger HK, O’Dowd JM, Balemba OB, Fortunato EA. Human Cytomegalovirus Compromises Development of Cerebral Organoids. J Virol (2019) 93(17):1–21. doi: 10.1128/jvi.00957-19
108. Sison SL, O’Brien BS, Johnson AJ, Seminary ER, Terhune SS, Ebert AD. Human Cytomegalovirus Disruption of Calcium Signaling in Neural Progenitor Cells and Organoids. J Virol (2019) 93(17):1–23. doi: 10.1128/jvi.00954-19
109. Shim JM, Kim J, Tenson T, Min JY, Kainov DE. Influenza Virus Infection, Interferon Response, Viral Counter-Response, and Apoptosis. Viruses (2017) 9(8):1–12. doi: 10.3390/v9080223
110. Labella AM, Merel SE. Influenza. Med Clinics North Am (2013) 97(4):621–45. doi: 10.1016/j.mcna.2013.03.001
111. Borren I, Tambs K, Gustavson K, Schjølberg S, Eriksen W, Håberg SE, et al. Early Prenatal Exposure to Pandemic Influenza A (H1N1) Infection and Child Psychomotor Development at 6 Months – A Population-Based Cohort Study. Early Hum Dev (2018) 122:1–7. doi: 10.1016/j.earlhumdev.2018.05.005
112. Cordeiro CN, Simis MT, Burd I. Infections and Brain Development. Pediatr Emergency Care (2015) 33(12):792–3. doi: 10.1097/01.pec.0000526609.89886.37
113. Shi L, Tu N, Patterson PH. Maternal Influenza Infection is Likely to Alter Fetal Brain Development Indirectly: The Virus is Not Detected in the Fetus. Int J Dev Neurosci (2005) 23(2-3 SPEC. ISS.):299–305. doi: 10.1016/j.ijdevneu.2004.05.005
114. Liong S, Oseghale O, To EE, Brassington K, Erlich JR, Luong R, et al. Influenza A Virus Causes Maternal and Fetal Pathology via Innate and Adaptive Vascular Inflammation in Mice. Proc Natl Acad Sci USA (2020) 117(40):24964–73. doi: 10.1073/pnas.2006905117
115. Fruntes V, Limosin F. Schizophrenia and Viral Infection During Neurodevelopment: A Pathogenesis Model? Med Sci Monitor (2008) 14(6):71–7. doi: 10.1038/sj.mp.4000956
116. Fatemi SH, Folsom TD, Liesch SB, Kneeland RE, Karkhane Yousefi M, Thuras PD. The Effects of Prenatal H1N1 Infection at E16 on FMRP, Glutamate, GABA, and Reelin Signaling Systems in Developing Murine Cerebellum. J Neurosci Res (2017) 95(5):1110–22. doi: 10.1002/jnr.23949
117. Short SJ, Lubach GR, Karasin AI, Olsen CW, Styner M, Knickmeyer RC, et al. Maternal Influenza Infection During Pregnancy Impacts Postnatal Brain Development in the Rhesus Monkey. Biol Psychiatry (2010) 67(10):965–73. doi: 10.1016/j.biopsych.2009.11.026
118. Shi L, Fatemi H, Sidwell RW, Patterson PH. Maternal Influenza Infection Causes Marked Behavioral and Pharmacological Changes in the Offspring. J Neurosci (2003) 23(1):297–302. doi: 10.1523/jneurosci.23-01-00297.2003
119. Hu B, Huang S, Yin L. The Cytokine Storm and COVID-19. J Med Virol (2020) 0:0. doi: 10.1002/jmv.26232
120. Mcgonagle D, Sharif K, Regan AO, Bridgewood C. The Role of Cytokines Including Interleukin-6 in COVID-19 Induced Pneumonia and Macrophage Activation Syndrome-Like Disease. Autoimmun Rev (2020) 19(March):1–7. doi: 10.1016/j.autrev.2020.102537
121. Zhang C, Wu Z, Li J, Zhao H, Wang G. Cytokine Release Syndrome in Severe COVID-19 : Interleukin-6 Receptor Antagonist Tocilizumab may be the Key to Reduce Mortality. Int J Antimucrob Angents (2020) 55:1–6. doi: 10.1016/j.ijantimicag.2020.105954
122. Giamarellos-Bourboulis EJ, Netea MG, Mikoletta R, Nikolaos K, Gogos C, Koutsoukou A. Complex Immune Dysregulation in COVID-19 Patients With Severe Respiratory Failure. Cell Host Microbe (2020) 27:992–1000. doi: 10.1016/j.chom.2020.04.009
123. Xu Z, Shi L, Wang Y, Zhang J, Huang L, Zhang C, et al. Pathological Findings of COVID-19 Associated With Acute Respiratory Distress Syndrome. Case Rep (2020) 8:420–2. doi: 10.1016/S2213-2600(20)30076-X
124. Biasi S, Meschiari M, Gibellini L, Bellinazzi C, Borella R, Fidanza L, et al. Marked T Cell Activation, Senescence, Exhaustion and Skewing Towards TH17 in Patients With COVID-19 Pneumonia. Nat Communications1 (2020) 11:1–17. doi: 10.1038/s41467-020-17292-4
125. Cimolai N. A Comprehensive Analysis of Maternal and Newborn Disease and Related Control for COVID-19. SN Compr Clin Med (2021) 3:1272–94. doi: 10.1007/s42399-021-00836-0
126. Edlow AG, Li JZ, Collier ARY, Atyeo C, James KE, Boatin AA, et al. Assessment of Maternal and Neonatal SARS-CoV-2 Viral Load, Transplacental Antibody Transfer, and Placental Pathology in Pregnancies During the COVID-19 Pandemic. JAMA Network Open (2020) 3(12):e2030455. doi: 10.1001/jamanetworkopen.2020.30455
127. Chen H, Guo J, Wang C, Luo F, Yu X, Zhang W, et al. Clinical Characteristics and Intrauterine Vertical Transmission Potential of COVID-19 Infection in Nine Pregnant Women: A Retrospective Review of Medical Records. Lancet (2020) 395(10226):809–15. doi: 10.1016/S0140-6736(20)30360-3
128. Lamouroux A, Attie-Bitach T, Martinovic J, Leruez-Ville M, Ville Y. Evidence for and Against Vertical Transmission for Severe Acute Respiratory Syndrome Coronavirus 2. Am J Obstetrics Gynecol (2020) 223(1):91.e1–4. doi: 10.1016/j.ajog.2020.04.039
129. Liu W, Wang J, Li W, Zhou Z, Liu S, Rong Z. Clinical Characteristics of 19 Neonates Born to Mothers With COVID-19. Front Med (2020) 14(2):193–8. doi: 10.1007/s11684-020-0772-y
130. Schwartz DA. An Analysis of 38 Pregnant Women With COVID-19, Their Newborn Infants, and Maternal-Fetal Transmission of SARS-CoV-2: Maternal Coronavirus Infections and Pregnancy Outcomes. Arch Pathol Lab Med (2020) 144(7):799–805. doi: 10.5858/arpa.2020-0901-SA
131. Shanes ED, Mithal LB, Otero S, Azad HA, Miller ES, Goldstein JA. Placental Pathology in COVID-19. Am J Clin Pathol (2020) 154(1):23–32. doi: 10.1093/ajcp/aqaa089
132. Yan J, Guo J, Fan C, Juan J, Yu X, Li J, et al. Coronavirus Disease 2019 in Pregnant Women: A Report Based on 116 Cases. Am J Obstetrics Gynecol (2020) 223(1):111.e1–111.e14. doi: 10.1016/j.ajog.2020.04.014
133. Zhu H, Wang L, Fang C, Peng S, Zhang L, Chang G, et al. Clinical Analysis of 10 Neonates Born to Mothers With 2019-Ncov Pneumonia. Trans Pediatr (2020) 9(1):51–60. doi: 10.21037/tp.2020.02.06
134. Hoffmann M, Kleine-Weber H, Schroeder S, Krüger N, Herrler T, Erichsen S, et al. SARS-CoV-2 Cell Entry Depends on ACE2 and TMPRSS2 and Is Blocked by a Clinically Proven Protease Inhibitor. Cell (2020) 181(2):271–80.e8. doi: 10.1016/j.cell.2020.02.052
135. Pique-Regi R, Romero R, Tarca AL, Luca F, Xu Y, Alazizi A, et al. Does the Human Placenta Express the Canonical Cell Entry Mediators for Sars-Cov-2? ELife (2020) 9:1–15. doi: 10.7554/ELIFE.58716
136. Vivanti AJ, Vauloup-fellous C, Prevot S, Zupan V, Suffee C, Cao J, et al. Transplacental Transmission of SARS-CoV-2 Infection. Nat Commun (2020) 11(2020):1–7. doi: 10.1038/s41467-020-17436-6
137. Hosier H, Farhadian SF, Morotti RA, Deshmukh U, Lu-Culligan A, Campbell KH, et al. SARS-CoV-2 Infection of the Placenta. J Clin Invest (2020) 130(9):4947–53. doi: 10.1172/JCI139569
138. Penfield CA, Brubaker SG, Limaye MA, Lighter J, Ratner AJ, Thomas KM, et al. Detection of Severe Acute Respiratory Syndrome Coronavirus 2 in Placental and Fetal Membrane Samples. Am J Obstetrics Gynecol MFM (2020) 2(3):100133. doi: 10.1016/j.ajogmf.2020.100133
139. Hsu AL, Guan M, Johannesen E, Stephens AJ, Khaleel N, Kagan N, et al. Placental SARS-CoV-2 in a Pregnant Woman With Mild COVID-19 Disease. J Med Virol (2021) 93(2):1038–44. doi: 10.1002/jmv.26386
140. Debelenko L, Katsyv I, Chong AM, L P, Szabolcs M, Uhlemann A. Trophoblast Damage With Acutw and Chronic Intervillositis: Disruption of the Placental Barrier by Severe Acure Respiratory Syndrome Coronavirus 2. Ann Oncol (2020) 109:69–79. doi: 10.1016/j.humpath.2020.12.004
141. Algarroba GN, Rekawek P, Vahanian SA, Khullar P, Palaia T, Peltier MR, et al. Visualization of Severe Acute Respiratory Syndrome Coronavirus 2 Invading the Human Placenta Using Electron Microscopy. Am J Obstetrics Gynecol (2020) 223(2):275–8. doi: 10.1016/j.ajog.2020.05.023
142. Fenizia C, Biasin M, Cetin I, Vergani P, Mileto D, Spinillo A, et al. Analysis of SARS-CoV-2 Vertical Transmission During Pregnancy. Nat Commun (2020) 11(1):1–10. doi: 10.1038/s41467-020-18933-4
143. Baig AM, Khaleeq A, Ali U, Syeda H. Evidence of the COVID-19 Virus Targeting the CNS: Tissue Distribution, Host-Virus Interaction, and Proposed Neurotropic Mechanisms. ACS Chem Neurosci (2020) 11(7):995–8. doi: 10.1021/acschemneuro.0c00122
144. Jakhmola S, Indari O, Chatterjee S, Jha HC. SARS-CoV-2, an Underestimated Pathogen of the Nervous System. SN Compr Clin Med (2020) 2(11):2137–46. doi: 10.1007/s42399-020-00522-7
145. Tiwari SK, Wang S, Smith D, Carlin AF, Rana TM. Revealing Tissue-Specific SARS-CoV-2 Infection and Host Responses Using Human Stem Cell-Derived Lung and Cerebral Organoids. Stem Cell Rep (2021) 16(3):437–45. doi: 10.1016/j.stemcr.2021.02.005
146. Bao L, Deng W, Huang B, Gao H, Liu J, Ren L, et al. The Pathogenicity of SARS-CoV-2 in hACE2 Transgenic Mice. Nature (2020) 583:830–3. doi: 10.1038/s41586-020-2312-y
147. Yang L, Han Y, Nilsson-payant BE, Evans T, Schwartz RE. A Human Pluripotent Stem Cell-Based Platform to Study SARS-CoV-2 Tropism and Model Virus Infection in Human Cells and Organoids. Cell Stem Cell (2020) 27:125–36. doi: 10.1016/j.stem.2020.06.015
148. Cui C, Xu P, Li G, Qiao Y, Han W, Geng C, et al. Vitamin D Receptor Activation Regulates Microglia Polarization and Oxidative Stress in Spontaneously Hypertensive Rats and Angiotensin II-Exposed Microglial Cells: Role of Renin-Angiotensin System. Redox Biol (2019) 26(August):101295. doi: 10.1016/j.redox.2019.101295
149. Bullen CK, Hogberg HT, Bahadirli-talbott A, Bishai WR, Keuthan C, Looney MM, et al. Infectability of Human BrainSphere Neurons Suggests Neurotropism of SARS-CoV-2 *. Baltimore, USA: ALTEX (Alternatives to animal experimentation) (2020). pp. 1–8. doi: 10.14573/altex.2006111.
150. Yi SA, Nam KH, Yun J, Gim D, Joe D, Kim YH, et al. Infection of Brain Organoids and 2D Cortical Neurons With SARS-CoV-2 Pseudovirus. Viruses (2020) 12(9):1–11. doi: 10.3390/v12091004
151. Zhang BZ, Chu H, Han S, Shuai H, Deng J, Hu Y, et al. SARS-CoV-2 Infects Human Neural Progenitor Cells and Brain Organoids. Cell Res (2020) 30(10):928–31. doi: 10.1038/s41422-020-0390-x
152. Chen R, Wang K, Yu J, Howard D, French L, Chen Z, et al. The Spatial and Cell-Type Distribution of SARS-CoV-2 Receptor ACE2 in the Human and Mouse Brains. Front Neurol (2021) 11:573095. doi: 10.3389/fneur.2020.573095
153. Pellegrini L, Albecka A, Mallery DL, Kellner MJ, Paul D, Carter AP, et al. SARS-CoV-2 Infects the Brain Choroid Plexus and Disrupts the Blood-CSF-Barrier in Human Brain Organoids. Cell Stem Cell (2020) 27:1–11. doi: 10.1016/j.stem.2020.10.001
154. Lun MP, Monuki ES, Lehtinen MK. Development and Functions of the Choroid Plexus-Cerebrospinal Fluid System. Nat Rev Neurosci (2015) 16(8):445–57. doi: 10.1038/nrn3921
155. Jacob F, Pather SR, Huang WK, Zhang F, Wong SZH, Zhou H, et al. Human Pluripotent Stem Cell-Derived Neural Cells and Brain Organoids Reveal SARS-CoV-2 Neurotropism Predominates in Choroid Plexus Epithelium. Cell Stem Cell (2020) 27:1–14. doi: 10.1016/j.stem.2020.09.016
156. Wang L, Sievert D, Clark AE, Lee S, Federman H, Gastfriend BD, et al. A Human Three-Dimensional Neural-Perivascular Assembloid Promotes Astrocytic Development and Enables Modeling of SARS-CoV-2 Neuropathology. Nat Med (2021) 27:1600–06. doi: 10.1038/s41591-021-01443-1
157. Wan Y, Shang J, Graham R, Baric RS, Li F. Receptor Recognition by the Novel Coronavirus From Wuhan: An Analysis Based on Decade-Long Structural Studies of SARS Coronavirus. J Virol (2020) 94(7):1–9. doi: 10.1128/jvi.00127-20
158. McCray PB, Pewe L, Wohlford-Lenane C, Hickey M, Manzel L, Shi L, et al. Lethal Infection of K18- Hace2 Mice Infected With Severe Acute Respiratory Syndrome Coronavirus. J Virol (2007) 81(2):813–21. doi: 10.1128/jvi.02012-06
159. Dinnon KH, Leist SR, Schäfer A, Edwards CE, Martinez DR, Montgomery SA, et al. A Mouse-Adapted Model of SARS-CoV-2 to Test COVID-19 Countermeasures. Nature (2020) 586(7830):560–6. doi: 10.1038/s41586-020-2708-8
160. Maltepe E, Fisher SJ. PLACENTA : The Forgotten Organ. Annu Rev Cell Dev Biol (2015) 31:1–30. doi: 10.1146/annurev-cellbio-100814-125620
161. Urs HL, Ayloo S, Chenghua Gu. Development and Cell Biology of the Blood-Brain Barrier. Annu Rev Cell Dev Biol (2019) 35:1–23. doi: 10.1146/annurev-cellbio-100617-062608
162. Morgan JT, Chana G, Pardo CA, Achim C, Semendeferi K, Buckwalter J, et al. Microglial Activation and Increased Microglial Density Observed in the Dorsolateral Prefrontal Cortex in Autism. Biol Psychiatry (2010) 68(4):368–76. doi: 10.1016/j.biopsych.2010.05.024
163. Suzuki K, Sugihara G, Ouchi Y, Nakamura K, Futatsubashi M, Takebayashi K, et al. Microglial Activation in Young Adults With Autism Spectrum Disorder. Arch Gen Psychiatry (2013) 70(1):49–58. doi: 10.1001/jamapsychiatry.2013.272
164. Vargas DL, Nascimbene C, Krishnan C, Zimmerman AW, Pardo CA. Neuroglial Activation and Neuroinflammation in the Brain of Patients With Autism. Ann Neurol (2005) 57(1):67–81. doi: 10.1002/ana.20315
165. Chez MG, Dowling T, Patel PB, Khanna P, Kominsky M. Elevation of Tumor Necrosis Factor-Alpha in Cerebrospinal Fluid of Autistic Children. Pediatr Neurol (2007) 36(6):361–5. doi: 10.1016/j.pediatrneurol.2007.01.012
166. Li X, Chauhan A, Sheikh AM, Patil S, Chauhan V, Li XM, et al. Elevated Immune Response in the Brain of Autistic Patients. J Neuroimmunol (2009) 207(1–2):111–6. doi: 10.1016/j.jneuroim.2008.12.002
167. Kang S, Tanaka T, Narazaki M, Kishimoto T. Targeting Interleukin-6 Signaling in Clinic. Immunity (2019) 50(4):1007–23. doi: 10.1016/j.immuni.2019.03.026
168. Rasmussen JM, Graham AM, Entringer S, Gilmore JH, Fair DA, Wadhwa PD, et al. Maternal Interleukin-6 Concentration During Pregnancy is Associated With Variation in Frontolimbic White Matter and Cognitive Development in Early Life. Neuroimage (2020) 49(0):825–35. doi: 10.1016/j.neuroimage.2018.04.020.Maternal
169. Rudolph MD, Graham AM, Feczko E, Miranda-Dominguez O, Rasmussen JM, Nardos R, et al. Maternal IL-6 During Pregnancy can be Estimated From Newborn Brain Connectivity and Predicts Future Working Memory in Offspring. Nat Neurosci (2018) 21(5):765–72. doi: 10.1038/s41593-018-0128-y
170. Banks WA, Kastin AJ, Gutierrez EG. Penetration of Interleukin-6 Across the Murine Blood-Brain Barrier. Neurosci Lett (1994) 179(1–2):53–6. doi: 10.1016/0304-3940(94)90933-4
171. Dahlgren J, Samuelsson AM, Jansson T, Holmäng A. Interleukin-6 in the Maternal Circulation Reaches the Rat Fetus in Mid-Gestation. Pediatr Res (2006) 60(2):147–51. doi: 10.1203/01.pdr.0000230026.74139.18
172. Smith SEP, Li J, Garbett K, Mirnics K, Patterson PH. Maternal Immune Activation Alters Fetal Brain Development Through Interleukin-6. J Neurosci (2007) 27(40):10695–702. doi: 10.1523/JNEUROSCI.2178-07.2007
173. Zhao Q, Wang Q, Wang J, Tang M, Huang S, Peng K, et al. Maternal Immune Activation-Induced PPARγ-Dependent Dysfunction of Microglia Associated With Neurogenic Impairment and Aberrant Postnatal Behaviors in Offspring. Neurobiol Dis (2019) 125:1–13. doi: 10.1016/j.nbd.2019.01.005
174. Wei H, Chadman KK, McCloskey DP, Sheikh AM, Malik M, Brown WT, et al. Brain IL-6 Elevation Causes Neuronal Circuitry Imbalances and Mediates Autism-Like Behaviors. Biochim Biophys Acta - Mol Basis Dis (2012) 1822(6):831–42. doi: 10.1016/j.bbadis.2012.01.011
175. Valliéres L, Campbell IL, Gage FH, Sawchenko PE. Reduced Hippocampal Neurogenesis in Adult Transgenic Mice With Chronic Astrocytic Production of Interleukin-6. J Neurosci (2002) 22(2):486–92. doi: 10.1523/jneurosci.22-02-00486.2002
176. Storer MA, Gallagher D, Fatt MP, Simonetta JV, Kaplan DR, Miller FD. Interleukin-6 Regulates Adult Neural Stem Cell Numbers During Normal and Abnormal Post-Natal Development. Stem Cell Rep (2018) 10(5):1464–80. doi: 10.1016/j.stemcr.2018.03.008
177. Gallagher D, Norman AA, Woodard CL, Yang G, Gauthier-Fisher A, Fujitani M, et al. Transient Maternal IL-6 Mediates Long-Lasting Changes in Neural Stem Cell Pools by Deregulating an Endogenous Self-Renewal Pathway. Cell Stem Cell (2013) 13(5):564–76. doi: 10.1016/j.stem.2013.10.002
178. Gumusoglu SB, Fine RS, Murray SJ, Bittle JL, Stevens HE. The Role of IL-6 in Neurodevelopment After Prenatal Stress. Brain Behav Immun (2017) 65:274–83. doi: 10.1016/j.bbi.2017.05.015
179. Wu W, Hsiao EY, Yan Z, Mazmanian SK, Patterson PH, Engineering B, et al. The Placental Interleukin-6 Signaling Controls Fetal Brain Development and Behavior. Brain Behav Immun (2018) 62:11–23. doi: 10.1016/j.bbi.2016.11.007.The
180. Czeh M, Gressens P, Kaindl AM. The Yin and Yang of Microglia. Dev Neurosci (2011) 33(3–4):199–209. doi: 10.1159/000328989
181. Hoebe K, Janssen E, Beutler B. The Interface Between Innate and Adaptative Immunity. Nat Immunol (2004) 5(10):971–4. doi: 10.1038/ni1004-971
182. Bettelli E, Carrier Y, Gao W, Korn T, Strom TB, Oukka M, et al. Reciprocal Developmental Pathways for the Generation of Pathogenic Effector TH17 and Regulatory T Cells. Nature (2006) 441(7090):235–8. doi: 10.1038/nature04753
183. Camporeale A, Poli V. IL-6, IL-17 and STAT3: A Holy Trinity in Auto-Immunity? Front Biosci (2012) 17(4):2306–26. doi: 10.2741/4054
184. Bystrom J, Al-Adhoubi N, Al-Bogami M, Jawad AS, Mageed RA. Th17 Lymphocytes in Respiratory Syncytial Virus Infection. Viruses (2013) 5(3):777–91. doi: 10.3390/v5030777
185. Wang J, Li F, Wei H, Lian Z, Sun R. Respiratory Influenza Virus Infection Induces Intestinal Immune Injury via Microbiota- Mediated Th17 Cell – Dependent Inflammation. J Exp Med (2014) 211:1–14. doi: 10.1084/jem.20140625
186. Paiva IA, Badolato-Corrêa J, Familiar-Macedo D, de-Oliveira-Pinto LM. Th17 Cells in Viral Infections-Friend or Foe? Cells (2021) 10(5):1–24. doi: 10.3390/cells10051159
187. Estes ML, Mcallister AK. Maternal Immune Activation: Implications for Neuropsychiatric Disorders. Science (2016) 353(6301):772–7. doi: 10.1126/science.aag3194
188. Connor CM, Dincer A, Straubhaar J, Galler JR, Houston IB, Akbarian S. Maternal Immune Activation Alters Behavior in Adult Offspring, With Subtle Changes in the Cortical Transcriptome and Epigenome. Schizophr Res (2012) 140(1–3):175–84. doi: 10.1016/j.schres.2012.06.037
189. Bergdolt L, Dunaevsky A. Brain Changes in a Maternal Immune Activation Model of Neurodevelopmental Brain Disorders. Prog Neurobiol (2019) 175(January 2018):1–19. doi: 10.1016/j.pneurobio.2018.12.002
190. Gumusoglu SB, Wen B, Hing Q, Sri A, Chilukuri S, Dewitt JJ, et al. Chronic Maternal Interleukin-17 and Autism-Related Cortical Gene Expression, Neurobiology, and Behavior. Neuropsychopharmacology (2020) 45(February):1008–17. doi: 10.1038/s41386-020-0640-0
191. Choi GB, Yim YS, Wong H, Kim S, Kim H, Kim SV, et al. The Maternal Interleukin-17a Pathway in Mice Promotes Autism-Like Phenotypes in Offspring. Science (2016) 351(6276):933–9. doi: 10.1126/science.aad0314
192. Bent R, Moll L, Grabbe S, Bros M. Interleukin-1 Beta—A Friend or Foe in Malignancies? Int J Mol Sci (2018) 19(8):1–34. doi: 10.3390/ijms19082155
193. Bittle J, Stevens HE. The Role of Glucocorticoid, Interleukin-1β, and Antioxidants in Prenatal Stress Effects on Embryonic Microglia. J Neuroinflamm (2018) 15(1):1–6. doi: 10.1186/s12974-018-1079-7
194. Novak CM, Lee JY, Ozen M, Tsimis ME, Kucirka LM, McLane MW, et al. Increased Placental T Cell Trafficking Results in Adverse Neurobehavioral Outcomes in Offspring Exposed to Sub-Chronic Maternal Inflammation. Brain Behav Immun (2019) 75:129–36. doi: 10.1016/j.bbi.2018.09.025
195. Leitner K, Al Shammary M, Mclane M, Johnston MV, Elovitz MA, Burd I. IL-1 Receptor Blockade Prevents Fetal Cortical Brain Injury But Not Preterm Birth in a Mouse Model of Inflammation-Induced Preterm Birth and Perinatal Brain Injury. Am J Reprod Immunol (2014) 71(5):418–26. doi: 10.1111/aji.12216
196. Girard S, Tremblay L, Lepage M, Sébire G. IL-1 Receptor Antagonist Protects Against Placental and Neurodevelopmental Defects Induced by Maternal Inflammation. J Immunol (2010) 184(7):3997–4005. doi: 10.4049/jimmunol.0903349
197. Kulkarni A, Scully TJ, O’Donnell LA. The Antiviral Cytokine Interferon-Gamma Restricts Neural Stem/Progenitor Cell Proliferation Through Activation of STAT1 and Modulation of Retinoblastoma Protein Phosphorylation. J Neurosci Res (2017) 95(8):1582–601. doi: 10.1002/jnr.23987
Keywords: maternal infections, virus, cytokines, neuroinflammation, neurodevelopmental disorders, cortical development, SARS – CoV – 2, zika virus
Citation: Elgueta D, Murgas P, Riquelme E, Yang G and Cancino GI (2022) Consequences of Viral Infection and Cytokine Production During Pregnancy on Brain Development in Offspring. Front. Immunol. 13:816619. doi: 10.3389/fimmu.2022.816619
Received: 22 November 2021; Accepted: 02 March 2022;
Published: 07 April 2022.
Edited by:
Amy Lovett-Racke, The Ohio State University, United StatesReviewed by:
Cécile E. Malnou, Université Toulouse III Paul Sabatier, FranceJohn R. Lukens, University of Virginia, United States
Copyright © 2022 Elgueta, Murgas, Riquelme, Yang and Cancino. This is an open-access article distributed under the terms of the Creative Commons Attribution License (CC BY). The use, distribution or reproduction in other forums is permitted, provided the original author(s) and the copyright owner(s) are credited and that the original publication in this journal is cited, in accordance with accepted academic practice. No use, distribution or reproduction is permitted which does not comply with these terms.
*Correspondence: Gonzalo I. Cancino, Z29uemFsby5jYW5jaW5vQHVtYXlvci5jbA==