- 1Research Center in Biodiversity and Genetic Resources (CIBIO-InBIO), University of Porto, Vairão, Portugal
- 2Max von Pettenkofer Institute and Gene Center, Virology, National Reference Center for Retroviruses, Faculty of Medicine, Ludwig Maximilian University of Munich (LMU) München, Munich, Germany
- 3Department of Biology, Faculty of Sciences, University of Porto, Porto, Portugal
- 4BIOPOLIS Program in Genomics, Biodiversity and Land Planning, Research Center in Biodiversity and Genetic Resources (CIBIO), Vairão, Portugal
- 5Center of Investigation in Health Technologies (CITS), CESPU, Gandra, Portugal
Guanylate binding proteins (GBPs) are paramount in the host immunity by providing defense against invading pathogens. Multigene families related to the immune system usually show that the duplicated genes can either undergo deletion, gain new functions, or become non-functional. Here, we show that in muroids, the Gbp genes followed an unusual pattern of gain and loss of genes. Muroids present a high diversity and plasticity regarding Gbp synteny, with most species presenting two Gbp gene clusters. The phylogenetic analyses revealed seven different Gbps groups. Three of them clustered with GBP2, GBP5 and GBP6 of primates. Four new Gbp genes that appear to be exclusive to muroids were identified as Gbpa, b, c and d. A duplication event occurred in the Gbpa group in the common ancestor of Muridae and Cricetidae (~20 Mya), but both copies were deleted from the genome of Mus musculus, M. caroli and Cricetulus griseus. The Gbpb gene emerged in the ancestor of Muridae and Cricetidae and evolved independently originating Gbpb1 in Muridae, Gbpb2 and Gbpb3 in Cricetidae. Since Gbpc appears only in three species, we hypothesize that it was present in the common ancestor and deleted from most muroid genomes. The second Gbp gene cluster, Gbp6, is widespread across all muroids, indicating that this cluster emerged before the Muridae and Cricetidae radiation. An expansion of Gbp6 occurred in M. musculus and M. caroli probably to compensate the loss of Gbpa and b. Gbpd is divided in three groups and is present in most muroids suggesting that a duplication event occurred in the common ancestor of Muridae and Cricetidae. However, in Grammomys surdaster and Mus caroli, Gbpd2 is absent, and in Arvicanthis niloticus, Gbpd1 appears to have been deleted. Our results further demonstrated that primate GBP1, GBP3 and GBP7 are absent from the genome of muroids and showed that the Gbp gene annotations in muroids were incorrect. We propose a new classification based on the phylogenetic analyses and the divergence between the groups. Extrapolations to humans based on functional studies of muroid Gbps should be re-evaluated. The evolutionary analyses of muroid Gbp genes provided new insights about the evolution and function of these genes.
Introduction
The innate ability of cells to resist against invading pathogens is due to the cell-autonomous immunity (1, 2). Upon the recognition of pathogens, production of type I interferon (IFN) and type II IFN increases, which results in the expression of numerous IFN-stimulated genes (3). Several of these genes enhance the efficacy of cell-autonomous immunity, including the guanylate binding proteins (GBPs), which are specialized in the host defense against intracellular pathogens ranging from bacteria to viruses (1, 4). The Gbp gene family belongs to the large dynamin GTPase superfamily that further includes myxoma resistance proteins, immunity-related GTPases proteins and the very large inducible GTPases (4). These proteins present structural and biochemical similarities (5, 6). The mammalian GBP vary from ~65 to 73 kDa in size and are mainly localized in the cytoplasm (4, 7).
Muridae and Cricetidae emerged 20 million years ago (Mya) from a single ancestor and are possibly the most successful mammals as they represent 27% of the total diversity (8). Muridae includes several subfamilies (Murinae, Lophiomyinae, Deomyinae and Gerbillinae) (9). Gerbillinae and Murinae appeared to have split ~17 Mya; however, the precise date still lack consensus (8). Within the Murinae group, rats and mice, diverged ~12.5 Mya (10). In Cricetidae, the first split occurred around 14.6 Mya, originating several subfamilies like Cricetinae, Arvicolinae and Neotominae (8). Muroids are scientifically important as they serve as a model for ecological and biomedical research (8, 11, 12). Further, they are hosts and vectors for many human diseases and evidence for co-speciation between rodents and viruses has been reported (13, 14). Despite its importance, there are some limitations in results’ extrapolation from the mouse model, considering inflammatory diseases, infection, sepsis and acute respiratory distress syndrome in humans (15, 16). Additionally, the phylogeny and the diversification patterns of such a relatively young group are not yet fully resolved, though several studies have been conducted (8, 12, 17, 18).
In mammals, GBP genes are usually organized in tandem on the same chromosome (19, 20). In primates, they are present on a single gene cluster (21), with humans presenting seven GBPs and one pseudogene located on chromosome 1 (1, 4). However, Mus musculus Gbp genes are found on two chromosomes, with Gbp1, Gbp2, Gbp3 Gbp5 and Gbp7 cluster together on chromosome 3, and chromosome 5 encodes Gbp4, Gbp6, Gbp8, Gbp9, Gbp10 and Gbp11 (4, 19).
In mice, GBPs can be induced by IFN, but it has been shown that interleukin (IL)-1α, IL-1β and tumor necrosis factor-α also induce the transcription of Gbps (22). Mouse GBP2, and possibly other GBPs from chromosome 3, has the ability to target vacuoles containing pathogens, like the Salmonella typhimurium, and promote the lysis of such vacuoles liberating the bacteria into the cytoplasm (4). Release of Gram-negative bacteria floods the cytoplasm with lipopolysaccharide (LPS), triggering the activation of NLRP3 inflammasome and leading to the production of pro-inflammatory cytokines, such as IL-1β and IL-18 (5, 7). As such, it was demonstrated that the knockout of Gbp2 reduced IL-18 concentrations, which is crucial for IFN-γ-induced host defense against Francisella novicida, making mice highly susceptible to infections (5, 23, 24). In addition, mouse GBP2 has antiviral activity against vesicular stomatitis virus and encephalomyocarditis virus (25), the same as for human GBP1 (26, 27). Upon infection with pathogenic bacteria, mouse GBP5 regulates the activation of NLRP3 inflammasome (28). Both mouse GBP2 and GBP5 can independently control the pathways that promote AIM2 inflammasome activation during F. novicida infection (24). The deletion of Gbp2, Gbp3, Gbp5 and Gbp7 in mice leads to severe susceptibility for a broad range of pathogens and may also lead to different inflammatory phenotypes, in a similar manner to what occurs in human GBPs-defective cells (5). This highlights the importance of Gbp genes in the immune system of mammals.
Nei and Rooney (29) defined a multigene family as a group of genes that have originated from a common ancestral gene and present similar functions and DNA sequences. For several years, concerted evolution was invoked to explain the evolution of multigene families related to the immune system; however, this did not explain how some immune genes were more closely related between species than within the same species (30). Nei and colleagues proposed the birth-and-death model of evolution for genes of the immune system (30). Duplication of genes can be produced by tandem and gene-block duplication (30). Some duplicated genes may diverge, remaining functional, or even gain new functions, whereas others can suffer deleterious mutations, becoming pseudogenes, or can also be deleted from the genome (30). Considering that Gbps belong to a multigene family of the immune system and the existence of more than 200 orthologs, Gbps most likely follows the birth-and-death model of evolution (7, 21).
Over the past years, it has become clear that GBPs are major players of the host defense and are important against a broad array of pathogens (6, 31) making it relevant to study Gbp genes evolution and function. Despite this, only few studies have focused on the evolution and function of Gbps in muroids. As such, we investigated the Gbp multigene family in muroids to bring new insights about their evolution.
Methods
Phylogenetic Analysis
Complete coding sequences of Gbps were obtained from publicly available databases. We retrieved a total of 182 Gbp nucleotide sequences from 12 different species of Muridae and Cricetidae (124 sequences), Homo sapiens (7 sequences), Tupaia glis (5 sequences) and from 5 different species of primates (34 sequences) to increase the robustness of the analysis. Loxodonta africana Gbps were used as outgroups (12 sequences). Sequences were retrieved from species for which the genomes are available at GenBank and Ensembl (see Supplementary Table 1 for accession numbers). Additionally, BLAST analyses were performed to confirm that all Gbps sequences were retrieved from the species used in this study. An alignment was performed in BioEdit (32) using Clustal W (33), followed by visual inspection. Before the phylogenetic analysis, the dataset was screened for gene conversion using GARD (Genetic Algorithm for Recombination Detection) (34).
Phylogenetic relationships among the GBP amino acid sequences were inferred in MEGA X (35) and RAxML (Randomized Axelerated Maximum Likelihood) v8.2.12 (35–37) using maximum-likelihood (ML) criteria, and with BEAST v1.10.4 for a Bayesian inference (38). The best-fit amino acid substitution model for GBP genes was determined in MEGA X and ProtTest v3.4.2 (39). In MEGA X, bootstrap (1000 replicates) was used to assess reliability and robustness of the phylogenetic tree branches. The best ML phylogeny was further determined using RAxML and branch supports were obtained using 1000 rapid bootstrap replicates as implemented in the method. In addition, two independent replicate runs of 10 million generations were performed in BEAST, using the Yule tree prior and an uncorrelated lognormal relaxed clock (40). The convergence of the BEAST runs was assessed using Tracer v1.7 (41); the resulting tree files were concatenated using LogCombiner v1.10.4, discarding the first 10% as burn-in, and posterior trees were summarized using TreeAnnotator v1.10.4, both included in the BEAST v1.10.4 package.
Sequence alignments can be found in Supplementary Data. Sequences that did not encode a putative functional protein, i.e. pseudogenes, were discarded from the analysis (see Supplementary Table 1 for accession numbers).
Genomic Synteny Analysis
NCBI (https://www.ncbi.nlm.nih.gov/genome/gdv/) and Ensembl (https://www.ensembl.org/index.html) were used to determine the relative syntenic positions and transcription orientation of Gbps across the genomes of Muridae and Cricetidae analyzed. BLAST analysis was performed to ensure that all Gbp genes of muroids were included in the study.
Divergence Analyses
Genetic distances between the groups established based on the ML tree (see Figure 1) were calculated using MEGA X (35). Analyses were conducted using the JTT matrix-based model as determined by the same program (42). All ambiguous positions were removed for each sequence pair (pairwise deletion option). The analysis involved 165 amino acid sequences (Tupaia glis and Loxodonta africana sequences were not included) and there was a total of 676 amino acid positions in the final dataset.
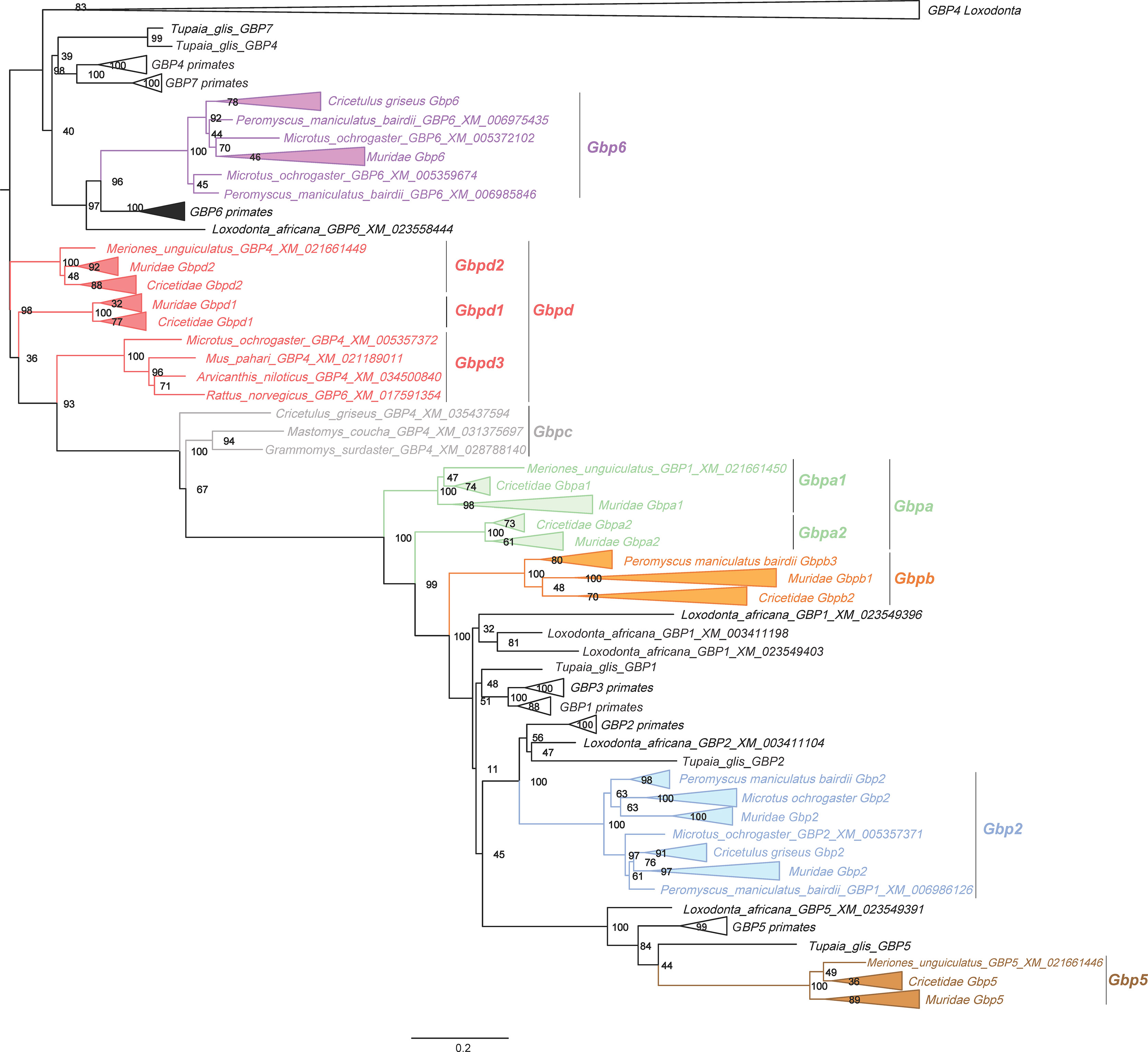
Figure 1 Randomized Axelerated Maximum Likelihood (RAxML) tree of the Gbp genes in Muridae and Cricetidae. The tree was obtained using the RAxML method using 1000 rapid bootstrap and is represented with midpoint root. Numbers on branches are the ML bootstrap values. All new Gbpd groups are also present. In Supplementary Figure 1, a full tree is depicted without collapsed nodes of Muridae and Cricetidae. Scale bar refers to the inferred amount of change per site along branches.
Results
Gbp Phylogeny
A first screening of the dataset was performed using GARD to detect gene conversion/recombination. Overall, gene conversion was not detected (data not shown) and all sequences were included in the phylogenetic analyses. The obtained ML phylogenetic tree from RAxML (Figure 1, some Muridae and Cricetidae branches are collapsed, see Supplementary Figure 1 for full tree) shows that primate and Tupaia GBP1, GBP3 and GBP7 are absent from the genomes of muroids as none of the retrieved Muridae and Cricetidae sequences clustered together with the corresponding GBPs from primates (Figure 1; Supplementary Figures 1–3). This suggests that the Gbp genes of muroids previously classified as Gbp1, Gbp3 and Gbp7 had been misclassified and that their reclassification is necessary. Furthermore, some Gbps within the major clusters also seem to have been misclassified. As such, we propose a new classification system for Gbps in Muridae and Cricetidae (see Supplementary Table 2). Following the obtained results, we propose a total of 87 changes; Gbp5 classification remained unchanged.
From the analysis, muroids seem to encode a total of seven different Gbps. Muroid Gbp2 (Figure 1, in blue) is present in all analyzed species. Duplication events seem to have occurred in Gbp2 in most species with Mus musculus, Mastomys coucha, Microtus ochrogaster and Cricetulus griseus having two copies, Mus caroli, Peromyscus maniculatus bairdii and Mus pahari with three copies and Arvicanthis niloticus with four copies (Figure 1 and Supplementary Figure 1). Rattus norvegicus and Rattus rattus have only one copy. Gbp5 is present in all twelve species analyzed as a single copy gene. For M. musculus, two Gbp5 sequences are available (Gbp5 and Gbp5a; see Supplementary Figure 1). However, only one Gbp5 gene was detected in the genome. These two sequences are most likely allelic variations of Gbp5 since the M. musculus genome has one of the highest genome sequencing coverages. Our ML tree results further indicate that rodent Gbp2 (Figure 1, in blue) and Gbp5 (Figure 1, in brown) are most likely orthologs of GBP2 and GBP5 from primates, with bootstrap values of 100 (Figure 1). In addition, some muroid Gbps within the Gbp2 group seem to have also been misclassified (see Supplementary Table 2).
The Gbp6 (Figure 1, in purple) is present in all families analyzed, suggesting it emerged in the common ancestor of Muridae and Cricetidae. It is only absent in M. pahari and R. rattus, while an expansion seems to have occurred in M. caroli and M. musculus, which have four and six copies, respectively (see Supplementary Figure 1). In the case of M. musculus, the Gbp6 group includes the previously classified Gbp4, 8, 9, 10 and 11 genes that are located on chromosome 5 (Supplementary Table 2). Indeed, all genes clustered in the Gbp6 group are located on the second Gbp gene cluster (see Figure 2). Similarly, to Gbp2 and 5, Gbp6 appears to be ortholog of primates GBP6 clustering together with bootstrap value of 96 (Figure 1 and Supplementary Figures 1, 3).
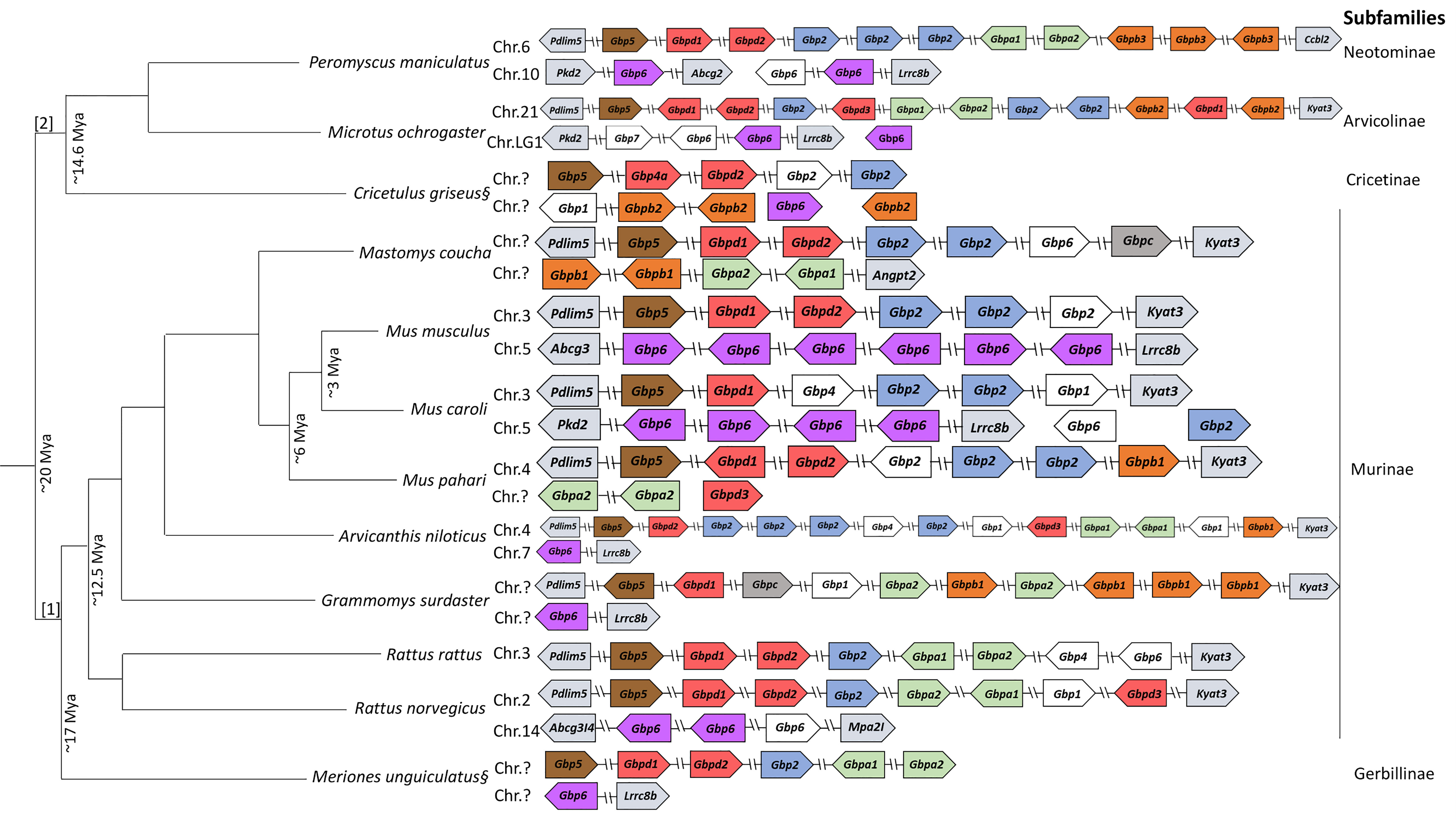
Figure 2 Gbp gene family synteny in Muridae and Cricetidae. Organization of the Gbp gene family in the species studied according to genomes available in NCBI (www.ncbi.nlm.nih.org) and Ensembl (wwww.ensembl.org). Arrows represent transcription orientation. White boxes indicate pseudogenes. The diagram is not to drawn to scale. Chromosomes are indicated when information is available. § Putative representation of the Gbp gene family in Cricetulus griseus and Meriones unguiculatus. [1]: Muridae; [2]: Cricetidae. Color scheme: - Gbp5;
- Gbp2;
- Gbpa;
- Gbpb;
- Gbpc;
- Gbp6;
- Gbpd.
We identified four new groups of Gbps that appear to be only present in Muridae and Cricetidae and classified them as Gbpa (Figure 1, in green), Gbpb (Figure 1, in orange), Gbpc (Figure 1, in grey) and Gbpd (Figure 1, in red). These new groups are very-well supported with bootstrap values of ≥94 (Figure 1). Gbpa (Figure 1, in green) is divided into two highly supported subgroups, Gbpa1 and Gbpa2 (Figure 1; bootstrap value of 100). It seems that Gbpa duplicated in the common ancestor of Muridae and Cricetidae and evolved differently, originating the two Gbpa groups. The establishment of these two groups is also supported by the MEGAX ML tree and the BEAST analysis (see Supplementary Figures 2.1, 3.2, respectively). Indeed, several amino acid differences are found between them (see Table 1). For M. pahari and Grammomys surdaster, the two sequences are grouped in the Gbpa2 group, which can be explained by an old duplication event followed by concerted evolution, however, we cannot exclude a recent duplication event in this species. Additionally, in three species (M. musculus, M. caroli and C. griseus), this gene was not detected in the genome, which suggests that it could have been independently deleted from the genome.
Gbpb is divided into three clusters, Gbpb1, Gbpb2 and Gbpb3 (bootstrap values of 100, 70 and 80 respectively, Figure 1, in orange). This gene seems to have been present before the split of Murinae and Cricetidae, but appears to be absent in Meriones unguiculatus, suggesting that it was deleted from the genome. Gbpb1 includes only sequences from Murinae and is absent in the genus Rattus, in M. musculus and M. caroli. For Grammomys surdaster, four copies have been identified, which suggests that for this species several duplication events may have occurred. Gbpb2 encompasses only sequences from Cricetidae (Cricetulus griseus, and Microtus ochrogaster; see Supplementary Figure 1, in orange). For Cricetulus griseus, five copies have been identified, also suggesting species-specific duplication events. Interestingly, Gbpb3 is composed by three copies of Peromyscus maniculatus bairdii, this suggests that Gbpb has evolved independently in this species originating Gbpb3 (Supplementary Figure 1).
The Gbpc group showed an unusual composition. In fact, by using RaxML analysis only two sequences appear clustered from Murinae species Grammomys surdaster and Mastomys coucha. However, using BEAST and ML all three sequences clustered together and are well supported (bootstrap 99; in grey; Supplementary Figure 2.2 in grey) which indicates that Gbpc is composed of three sequences. The most likely explanation is the emergence of this gene in the ancestor of Muridae and Cricetidae family followed by a loss of this gene in most rodents species.
We consider that Gbpd from Muridae and Cricetidae is exclusive to these two families (Figure 1, in red). The Gbpd is divided into three groups that are well supported with bootstrap values of 100 (Figure 1, in red). It is composed of muroid sequences previously classified as Gbp3, 4, 6 and 7 (see Supplementary Figure 1). These three groups include sequences from all muroids, suggesting that these three groups originated from an old duplication event that occurred before the radiation of Muridae and Cricetidae. The only exceptions are the absence of sequences from Grammomys surdaster and M. caroli in the Gbpd2 group and the absence of Arvicanthis niloticus in Gbpd1. Gbpd3 is composed of four sequences (R. norvegicus, Microtus ochrogaster, Mus pahari and Arvicanthis niloticus). We propose that this gene emerged in the ancestor of Muridae and Cricetidae family and was then lost in most rodents species.
Synteny Analysis
Despite presenting similarities, muroids have a high plasticity regarding their Gbps synteny (Figure 2). Indeed, several rodent Gbp genes are clustered in more than one chromosome (e.g., Mus musculus, Rattus norvegicus, Microtus ochrogaster and Mus caroli). Rattus rattus seems to be an exception as it has, similar to primates, all Gbp copies in a single gene cluster on chromosome 3 (Figure 2).
Several duplication events are observed across all families, e.g., Gbp6 in M. musculus and M. caroli, Gbp2 in M. musculus, M. caroli, M. pahari, Arvicanthis niloticus and Mastomys coucha. Some genes seem to have lost their function and became pseudogenes, e.g., Gbp2 in Cricetulus griseus and M. pahari and M. musculus. Gbp5 and Gbpd appear to be the only genes from this multigene family that are present on the same location across each gene cluster from all species analyzed, adjacent to the Pdlim5 gene (Figure 2). For the rest of this gene cluster, no specific patterns could be detected.
Considering the second cluster of Gbp genes, which is located on chromosome 5 in M. musculus, our results suggest that, at least for well-characterized species, the newly found genes belong to the Gbp6 group. Since this occurred in all rodent species analyzed, this second gene cluster was most likely already existing in the common ancestor of these species. In M. musculus and M. caroli, a duplication event occurred in Gbp6. Surprisingly, this gene was not detected in several other species like Mastomys coucha, M. pahari and R. rattus. This might be explained by a genome deletion or a bad quality of the sequenced genomes (Figure 2).
Divergence Analysis
Despite being phylogenetically close to their primate counterparts (Figure 1) and having a common ancestor, muroid and primates GBP2, GBP5 and GBP6 show high divergence values (~22%, ~30% and ~19%, respectively; Table 2). For instance, primates GBP1 and pGBP3 are considered as different groups with a divergence as low as 7% (Table 2). For muroids, a divergence of at least 21% exists between the different Gbp groups (Table 2). Considering that Gbp groups are well-supported in the phylogenetic trees (Figure 1 and Supplementary Figures 1–3), several amino acid differences can be found between the muroid groups and several common amino acids can be identified within each established group in this work (see Table 1), our data support the newly proposed classification for muroid Gbps.
Discussion
The GBP multigene family has an important role in the innate immune response against invading pathogens such as bacteria and viruses (1, 4). Rounds of duplication and the birth-and-death process shape the evolution of GBPs. Indeed, more than 200 GBPs orthologs have been described, but variable numbers of GBP genes exist in different species distributed in one or two chromosomal regions (7, 19). For example, in humans, seven GBP genes exist in a single cluster on chromosome 5, while in mice, 11 Gbps have been described scattered on two chromosomes, five on chromosome 3 and six on the second chromosome (19). Recently, we showed that GBP7 genes are unique to primates and emerged following a duplication of GBP4, while GBP3 is restricted to simians and originated from GBP1 and GBP6 duplicated in Tarsiiformes, with both copies remaining functional in Cebidae and Cercopithecidae (21).
The present study demonstrated that Gbp3 and Gbp7 are not present in rodents, consistent with our previous findings in primates (21). Additionally, Gbp1 also appears to be absent from the muroid genomes, suggesting that other muroid Gbps might present similar biological activities. In contrast, Gbp2, Gbp5 and Gbp6 orthologs are present in Muridae and Cricetidae as confirmed by their clustering in the phylogenetic trees with primates GBP2, GBP5 and GBP6, respectively (Figure 1 and Supplementary Figures 1–3). This indicates that Gbp2, Gbp5 and Gbp6 were already present in the ancestor of rodents and primates at least ~96 Mya (43). Maintenance of these genes for such a long period of time might be explained by their importance in regulating the immune system against a broad range of pathogens. Indeed, GBP2 and GBP5 appear to have important roles against viral and bacterial infections and to induce immune responses in mouse and humans (24, 25, 28, 44). To our knowledge no studies have been performed about the function of mouse Gbp6.
Our ML phylogenetic tree strongly supports the existence of four new muroid Gbp groups and for which we suggest a new nomenclature as Gbpa, Gbpb, Gbpc and Gbpd (see Supplementary Table 2). All these groups are phylogenetically well-supported with high bootstrap values and show high levels of genetic divergence. Additionally, these groups present characteristic amino acids (Table 1). Interestingly, Gbpa, Gbpb and Gbpc are not present in Mus musculus and M. caroli genomes (Figure 1, highlighted in green, orange and grey, respectively). To our knowledge, these four new groups had not been described. This indicates that, within these groups, Gbps are poorly studied and incorrectly annotated. Indeed, several sequences previously classified as Gbp1-7 were clustered in these new groups.
Gbpa appears to be present since the emergence of Muridae and Cricetidae (~20 Mya; 8); yet, M. musculus, M. caroli and C. griseus do not encode Gbpa. Thus, we hypothesize that evolutionary pressures led to its disappearance in these species. Gbpb emerged before the separation of Muridae with Cricetidae (~20 Mya) [Figures 1 and 2; (8)]. After their separation, Gbpb independently evolved in each family originating Gbpb1 in Muridae and Gbpb2 and Gbpb3 in Cricetidae, the latest is only present in Peromyscus maniculatus bairdii. The Gbpc cluster is strongly supported (bootstrap value of 94) and has an amino acidic genetic distance of at least 23% from all the other groups (see Table 2). Interestingly, the Gbpc is present in only three species, two belonging to the Murinae (Grammomys surdaster and Mastomys coucha) and one to the Cricetinae (Cricetulus griseus). This evolutionary pattern is quite puzzling and two different hypotheses might explain it: i) an event of convergent evolution where the gene emerged independently in three different lineages, or, most likely, ii) it emerged in Muridae and Cricetidae at least ~20 Mya but was deleted from the genome in most of the rodent species. The Gbp6 gene is widespread across all rodents in the second Gbp gene cluster (Figure 2), indicating that the second cluster emerged before the ancestor of Muridae and Cricetidae, as it clustered with primates Gbp6. Despite this, the gene is not present in the genome of M. pahari, Mastomys coucha and R. rattus. The second gene cluster could have been lost in these three species; however, further genome analyses are required since the genome might be poorly assembled. The Gbp evolution in the genus Mus shows some interesting features. Indeed, both Gbpa and Gbpb are not present in M. musculus and M. caroli, but exist in M. pahari, indicating that both genes were deleted from the genome after the divergence of M. pahari from M. musculus and M. caroli (~6 Mya) and the split between M. musculus and M. caroli around 3 Mya (10; Figure 2). The expansion of Gbp6 in M. musculus (six copies) and M. caroli (four copies) might have been a strategy to compensate for the loss of Gbpa and Gbpb.
A fourth Gbp gene named in this work, Gbpd, it is divided into three well-supported groups designated as Gbpd1, Gbpd2 and Gbpd3. All groups contain sequences from all species analyzed, which suggests that these three groups originated from an old duplication event that happened before the Muridae and Cricetidae radiation at least ~20 Mya. The only exceptions are the absence of Gbpd2 in Grammomys surdaster and M. caroli and the absence of Arvicanthis niloticus in Gbpd1. Gbpd3 sequences were only detected in R. norvegicus, Arvicanthis niloticus and Mus pahari (Muridae) and Microtus ochrogaster (Cricetidae), which suggests that this gene emerged in the ancestor of Muridae and Cricetidae family and was then lost in most muroid species.
The observed heterogeneity in the number of Gbps and gene copy numbers in Muridae and Cricetidae might be explained by a combination of: i) selective pressures in genes belonging to the immune system due to invading pathogens, that, as a consequence, drive host-specific adaptations and promote expansion and complexity of the immunological repertoire (5); ii) rodents have accelerated diversification rates which lead to the morphological, taxonomical, ecological and physiological diversity found within this group and allow them to explore and adapt to an array of different ecosystems being exposed to different environmental constraints (8, 11). In fact, the high divergence observed between the Gbp groups in Muridae and Cricetidae ranging from 21% to 75% and the emergence of five new Gbp unique to Muridae and Cricetidae support the high selective pressures imposed on this multigene family in muroids.
The number of genes in the major histocompatibility complex (MHC) and immunoglobulins (Ig) varies extensively across species, demonstrating that duplications and deletions are common in multigene families (29). This diversity is crucial in their function to defend the host against a broad range of invading pathogens that will act as the evolutionary pressure to promote the diversification of genes (29). Hence, gene duplication, mutation and diversifying selection are key mechanisms in the evolution of genes of the immune system (29). Our results suggest that the diversity found within Gbps is consistent with their role in triggering the host defense against various pathogens. Although some Gbps have become pseudogenes (Figure 2, in white) or have been lost (e.g. no Gbp6 in M. pahari and R. rattus and no Gbpa and Gbpb in M. caroli and M. musculus), it is reasonable to consider that Gbp genes in muroids follow the birth-and-death model of evolution proposed by Nei and colleagues (30).
Finally, the presence of characteristic amino acids in each of the new proposed groups (Table 2) further supports their classification. Interestingly, most of these characteristic motifs were found downstream the amino acid position 307, which marks the beginning of the C-terminal domain or helical domain (45) where a post-translational modification (isoprenylation) can occur in human GBP 1, 2 and 5 and in mouse GBP2 and 5 (22, 46). This suggests that most GBPs are more conserved in their N-terminal, including their GTPase activity (45, 46). However, future studies should be conducted to assess the structure of these proteins and provide insights about their potential function.
In summary, and based on our results, the nomenclature of the Gbp multigene family in muroids requires an update. Indeed, and as noted by Vestal and Jeyaratnam, similar GBPs are not always the most closely related ones between species (22). The incorrect annotation of Gbps can be problematic, particularly since studies have evaluated the function of mouse Gbps by considering them as orthologs of the human genes. However, our phylogenetic results and estimated amino acid divergences suggest that many are not homologs to the human genes. As such, their biological functions might greatly differ from those of humans and translational studies using muroid Gbps might not have a correct biological meaning. Therefore, a new nomenclature, as the one proposed in this study, will lead to a proper Gbp gene annotation, specifically in muroids, and contribute to a better understanding of their evolution and function. Besides the different evolutionary patterns observed in this mammalian group, it is highly likely that most of them have an important function in the immune system. As such, new studies revealing the structural organization and new functional assays would bring new knowledge about the role of GBPs.
Conclusion
Overall, rodents express seven different Gbp genes. Gbp2, Gbp5 and Gbp6 appear to be phylogenetically similar to their human counterparts. The primate Gbp1, Gbp3 and GBbp7 genes are not present in muroids. Four new Gbp genes exclusive to Muridae and Cricetidae were identified: Gbpa, Gbpb, Gbpc and Gbpd.
The distribution and number of Gbp genes across the different Muridae and Cricetidae genomes differs widely, with duplicated, deleted and pseudogenized genes. This indicates that the Gbp multigene family in muroids evolved under a very strong selective pressure with different evolutionary histories within and between the two muroid taxa.
Some muroid Gbps are phylogenetically different to those of humans and most likely have different functions. This means that translational studies from muroids to human should be re-evaluated. Additionally, this study provides new insight into the evolution of Gbps in muroids and demonstrates that Gbp genes in Muridae and Cricetidae have been poorly annotated. The new proposed classification better matches the evolution of Gbps in muroids and opens new research opportunities to study the evolution and function of the Gbp multigene family in rodents.
Data Availability Statement
The original contributions presented in the study are included in the article/Supplementary Material. Further inquiries can be directed to the corresponding author.
Author Contributions
JVC-R analyzed the data and wrote the manuscript. JM-F analyzed and discussed the data. H-MB and JA discussed the data. PE conceived the study, analyzed, and discussed the data. All authors edited the manuscript and approved the final draft.
Funding
This work was funded by Fundação para a Ciência e Tecnologia (FCT) which supported JVC-R PhD fellowship (DFA/BD/4965/2020), the Assistant Researcher grant of JA (CEECIND/00078/2017), JM-F with the CEEC contract (CEECIND/00372/2018), the Principal Researcher grant of PE (CEECIND/01495/2020) and the project UIDB/50027/2020 (Base). H-MB acknowledges funding from the DFG (BA-6820/1-1).
Conflict of Interest
The authors declare that the research was conducted in the absence of any commercial or financial relationships that could be construed as a potential conflict of interest.
Publisher’s Note
All claims expressed in this article are solely those of the authors and do not necessarily represent those of their affiliated organizations, or those of the publisher, the editors and the reviewers. Any product that may be evaluated in this article, or claim that may be made by its manufacturer, is not guaranteed or endorsed by the publisher.
Supplementary Material
The Supplementary Material for this article can be found online at: https://www.frontiersin.org/articles/10.3389/fimmu.2022.752186/full#supplementary-material
References
1. Man SM, Place DE, Kuriakose T, Kanneganti TD. Interferon-Inducible Guanylate-Binding Proteins at the Interface of Cell-Autonomous Immunity and Inflammasome Activation. J Leukoc Biol (2017) 101(1):143–50. doi: 10.1189/jlb.4MR0516-223R
2. Kohler KM, Kutsch M, Piro AS, Wallace GD, Coers J, Barber MF. A Rapidly Evolving Polybasic Motif Modulates Bacterial Detection by Guanylate Binding Proteins. mBio (2020) 11(3):e00340–00320. doi: 10.1128/mBio.00340-20
3. Santos JC, Broz P. Sensing of Invading Pathogens by GBPs: At the Crossroads Between Cell-Autonomous and Innate Immunity. J Leukoc Biol (2018) 104(4):729–35. doi: 10.1002/jlb.4mr0118-038r
4. Ngo CC, Man SM. Mechanisms and Functions of Guanylate-Binding Proteins and Related Interferon-Inducible GTPases: Roles in Intracellular Lysis of Pathogens. Cell Microbiol (2017) 19(12):e12791. doi: 10.1111/cmi.12791
5. Kim B-H, Chee JD, Bradfield CJ, Park E-S, Kumar P, MacMicking JD. Interferon-Induced Guanylate-Binding Proteins in Inflammasome Activation and Host Defense. Nat Immunol (2016) 17(5):481–9. doi: 10.1038/ni.3440
6. Praefcke GJ. Regulation of Innate Immune Functions by Guanylate-Binding Proteins. Int J Med Microbiol (2018) 308(1):237–45. doi: 10.1016/j.ijmm.2017.10.013
7. Huang S, Meng Q, Maminska A, MacMicking JD. Cell-Autonomous Immunity by IFN-Induced GBPs in Animals and Plants. Curr Opin Immunol (2019) 60:71–80. doi: 10.1016/j.coi.2019.04.017
8. Steppan SJ, Schenk JJ. Muroid Rodent Phylogenetics: 900-Species Tree Reveals Increasing Diversification Rates. PloS One (2017) 12(8):e0183070. doi: 10.1371/journal.pone.0183070
9. Alhajeri BH, Hunt OJ, Steppan SJ. Molecular Systematics of Gerbils and Deomyines (Rodentia: Gerbillinae, Deomyinae) and a Test of Desert Adaptation in the Tympanic Bulla. J Zool Systematics Evolutionary Res (2015) 53(4):312–30. doi: 10.1111/jzs.12102
10. Thybert D, Roller M, Navarro FCP, Fiddes I, Streeter I, Feig C, et al. Repeat Associated Mechanisms of Genome Evolution and Function Revealed by the Mus Caroli and Mus Pahari Genomes. Genome Res (2018) 28(4):448–59. doi: 10.1101/gr.234096.117
11. Steppan SJ, Adkins RM, Anderson J. Phylogeny and Divergence-Date Estimates of Rapid Radiations in Muroid Rodents Based on Multiple Nuclear Genes. Systematic Biol (2004) 53(4):533–53. doi: 10.1080/10635150490468701
12. Steppan SJ, Adkins RM, Spinks PQ, Hale C. Multigene Phylogeny of the Old World Mice, Murinae, Reveals Distinct Geographic Lineages and the Declining Utility of Mitochondrial Genes Compared to Nuclear Genes. Mol Phylogenet Evol (2005) 37(2):370–88. doi: 10.1016/j.ympev.2005.04.016
13. Miles J, Bowen M, Nichol S. African Arenaviruses-Coevolution Between Virus and Murid Host. Belgian J Zool (1997) 127:19–28.
14. Hughes AL, Friedman R. Evolutionary Diversification of Protein-Coding Genes of Hantaviruses. Mol Biol Evol (2000) 17(10):1558–68. doi: 10.1093/oxfordjournals.molbev.a026254
15. Burkhardt AM, Zlotnik A. Translating Translational Research: Mouse Models of Human Disease. Cell Mol Immunol (2013) 10(5):373–4. doi: 10.1038/cmi.2013.19
16. Seok J, Warren HS, Cuenca AG, Mindrinos MN, Baker HV, Xu W, et al. Genomic Responses in Mouse Models Poorly Mimic Human Inflammatory Diseases. Proc Natl Acad Sci USA (2013) 110(9):3507–12. doi: 10.1073/pnas.1222878110
17. Fabre P-H, Hautier L, Dimitrov D, P Douzery EJ. A Glimpse on the Pattern of Rodent Diversification: A Phylogenetic Approach. BMC Evolutionary Biol (2012) 12(1):88. doi: 10.1186/1471-2148-12-88
18. Schenk JJ, Rowe KC, Steppan SJ. Ecological Opportunity and Incumbency in the Diversification of Repeated Continental Colonizations by Muroid Rodents. Systematic Biol (2013) 62(6):837–64. doi: 10.1093/sysbio/syt050
19. Olszewski MA, Gray J, Vestal DJ. In Silico Genomic Analysis of the Human and Murine Guanylate-Binding Protein (GBP) Gene Clusters. J Interferon Cytokine Res (2006) 26(5):328–52. doi: 10.1089/jir.2006.26.328
20. Li G, Zhang J, Sun Y, Wang H, Wang Y. The Evolutionarily Dynamic IFN-Inducible GTPase Proteins Play Conserved Immune Functions in Vertebrates and Cephalochordates. Mol Biol Evol (2009) 26(7):1619–30. doi: 10.1093/molbev/msp074
21. Côrte-Real JV, Baldauf HM, Abrantes J, Esteves PJ. Evolution of the Guanylate Binding Protein (GBP) Genes: Emergence of GBP7 Genes in Primates and Further Acquisition of a Unique GBP3 Gene in Simians. Mol Immunol (2021) 132:79–81. doi: 10.1016/j.molimm.2021.01.025
22. Vestal DJ, Jeyaratnam JA. The Guanylate-Binding Proteins: Emerging Insights Into the Biochemical Properties and Functions of This Family of Large Interferon-Induced Guanosine Triphosphatase. J Interferon Cytokine Res (2011) 31(1):89–97. doi: 10.1089/jir.2010.0102
23. Pierini R, Perret M, Djebali S, Juruj C, Michallet MC, Förster I, et al. ASC Controls IFN-γ Levels in an IL-18-Dependent Manner in Caspase-1-Deficient Mice Infected With Francisella Novicida. J Immunol (2013) 191(7):3847–57. doi: 10.4049/jimmunol.1203326
24. Meunier E, Wallet P, Dreier RF, Costanzo S, Anton L, Rühl S, et al. Guanylate-Binding Proteins Promote Activation of the AIM2 Inflammasome During Infection With Francisella Novicida. Nat Immunol (2015) 16(5):476–84. doi: 10.1038/ni.3119
25. Carter CC, Gorbacheva VY, Vestal DJ. Inhibition of VSV and EMCV Replication by the Interferon-Induced GTPase, mGBP-2: Differential Requirement for Wild-Type GTP Binding Domain. Arch Virol (2005) 150(6):1213–20. doi: 10.1007/s00705-004-0489-2
26. Anderson SL, Carton JM, Lou J, Xing L, Rubin BY. Interferon-Induced Guanylate Binding Protein-1 (GBP-1) Mediates an Antiviral Effect Against Vesicular Stomatitis Virus and Encephalomyocarditis Virus. Virology (1999) 256(1):8–14. doi: 10.1006/viro.1999.9614
27. Pan W, Zuo X, Feng T, Shi X, Dai J. Guanylate-Binding Protein 1 Participates in Cellular Antiviral Response to Dengue Virus. Virol J (2012) 9:292. doi: 10.1186/1743-422x-9-292
28. Shenoy AR, Wellington DA, Kumar P, Kassa H, Booth CJ, Cresswell P, et al. GBP5 Promotes NLRP3 Inflammasome Assembly and Immunity in Mammals. Science (2012) 336(6080):481–5. doi: 10.1126/science.1217141
29. Nei M, Rooney AP. Concerted and Birth-and-Death Evolution of Multigene Families. Annu Rev Genet (2005) 39:121–52. doi: 10.1146/annurev.genet.39.073003.112240
30. Nei M, Gu X, Sitnikova T. Evolution by the Birth-and-Death Process in Multigene Families of the Vertebrate Immune System. Proc Natl Acad Sci (1997) 94(15):7799–806. doi: 10.1073/pnas.94.15.7799
31. Pilla-Moffett D, Barber MF, Taylor GA, Coers J. Interferon-Inducible GTPases in Host Resistance, Inflammation and Disease. J Mol Biol (2016) 428(17):3495–513. doi: 10.1016/j.jmb.2016.04.032
32. Hall TA. BioEdit: A User-Friendly Biological Sequence Alignment Editor and Analysis Program for Windows 95/98/NT. Nucleic Acids symposium Ser (1999) 41(41):95–8.
33. Thompson JD, Higgins DG, Gibson TJ. CLUSTAL W: Improving the Sensitivity of Progressive Multiple Sequence Alignment Through Sequence Weighting, Position-Specific Gap Penalties and Weight Matrix Choice. Nucleic Acids Res (1994) 22(22):4673–80. doi: 10.1093/nar/22.22.4673
34. Kosakovsky Pond SL, Posada D, Gravenor MB, Woelk CH, Frost SD. Automated Phylogenetic Detection of Recombination Using a Genetic Algorithm. Mol Biol Evol (2006) 23(10):1891–901. doi: 10.1093/molbev/msl051
35. Kumar S, Stecher G, Li M, Knyaz C, Tamura K. MEGA X: Molecular Evolutionary Genetics Analysis Across Computing Platforms. Mol Biol Evol (2018) 35(6):1547–9. doi: 10.1093/molbev/msy096
36. Stamatakis A. RAxML Version 8: A Tool for Phylogenetic Analysis and Post-Analysis of Large Phylogenies. Bioinformatics (2014) 30(9):1312–3. doi: 10.1093/bioinformatics/btu033
37. Stamatakis A. Using RAxML to Infer Phylogenies. Curr Protoc Bioinf (2015) 51(1):6.14.11–16.14.14. doi: 10.1002/0471250953.bi0614s51
38. Drummond AJ, Rambaut A. BEAST: Bayesian Evolutionary Analysis by Sampling Trees. BMC Evolutionary Biol (2007) 7(1):214. doi: 10.1186/1471-2148-7-214
39. Darriba D, Taboada GL, Doallo R, Posada D. ProtTest 3: Fast Selection of Best-Fit Models of Protein Evolution. Bioinformatics (2011) 27(8):1164–5. doi: 10.1093/bioinformatics/btr088
40. Drummond AJ, Ho SYW, Phillips MJ, Rambaut A. Relaxed Phylogenetics and Dating With Confidence. PloS Biol (2006) 4(5):e88. doi: 10.1371/journal.pbio.0040088
41. Rambaut A, Drummond AJ, Xie D, Baele G, Suchard MA. Posterior Summarization in Bayesian Phylogenetics Using Tracer 1.7. Syst Biol (2018) 67(5):901–4. doi: 10.1093/sysbio/syy032
42. Jones DT, Taylor WR, Thornton JM. The Rapid Generation of Mutation Data Matrices From Protein Sequences. Comput Appl Biosci (1992) 8(3):275–82. doi: 10.1093/bioinformatics/8.3.275
43. Nei M, Xu P, Glazko G. Estimation of Divergence Times From Multiprotein Sequences for a Few Mammalian Species and Several Distantly Related Organisms. Proc Natl Acad Sci USA (2001) 98(5):2497–502. doi: 10.1073/pnas.051611498
44. Degrandi D, Kravets E, Konermann C, Beuter-Gunia C, Klümpers V, Lahme S, et al. Murine Guanylate Binding Protein 2 (Mgbp2) Controls Toxoplasma Gondii Replication. Proc Natl Acad Sci USA (2013) 110(1):294–9. doi: 10.1073/pnas.1205635110
45. Prakash B, Praefcke GJK, Renault L, Wittinghofer A, Herrmann C. Structure of Human Guanylate-Binding Protein 1 Representing a Unique Class of GTP-Binding Proteins. Nature (2000) 403(6769):567–71. doi: 10.1038/35000617
Keywords: evolution, multigene family, GBP, innate immunity, muroids
Citation: Côrte-Real JV, Baldauf H-M, Melo-Ferreira J, Abrantes J and Esteves PJ (2022) Evolution of Guanylate Binding Protein (GBP) Genes in Muroid Rodents (Muridae and Cricetidae) Reveals an Outstanding Pattern of Gain and Loss. Front. Immunol. 13:752186. doi: 10.3389/fimmu.2022.752186
Received: 02 August 2021; Accepted: 20 January 2022;
Published: 09 February 2022.
Edited by:
Ram Savan, University of Washington, United StatesReviewed by:
Jaime Eugenio Figueroa, Austral University of Chile, ChileClaudio Casola, Texas A&M University, United States
Copyright © 2022 Côrte-Real, Baldauf, Melo-Ferreira, Abrantes and Esteves. This is an open-access article distributed under the terms of the Creative Commons Attribution License (CC BY). The use, distribution or reproduction in other forums is permitted, provided the original author(s) and the copyright owner(s) are credited and that the original publication in this journal is cited, in accordance with accepted academic practice. No use, distribution or reproduction is permitted which does not comply with these terms.
*Correspondence: Pedro José Esteves, cGplc3RldmVzQGNpYmlvLnVwLnB0