- 1School of Clinical Medicine, Chengdu University of Traditional Chinese Medicine, Chengdu, China
- 2School of International Education, Chengdu University of Traditional Chinese Medicine, Chengdu, China
- 3School of Basic Medical Sciences, Chengdu University of Traditional Chinese Medicine, Chengdu, China
T Helper 17 (Th17) cells are adaptive immune cells that play myriad roles in the body. Immune–endocrine interactions are vital in endocrine organs during pathological states. Th17 cells are known to take part in multiple autoimmune diseases over the years. Current evidence has moved from minimal to substantial that Th17 cells are closely related to endocrine organs. Diverse tissue Th17 cells have been discovered within endocrine organs, including gut, adipose tissue, liver and bone, and these cells are modulated by various secretions from endocrine organs. Th17 cells in these endocrine organs are key players in the process of an array of metabolic disorders and inflammatory conditions, including obesity, insulin resistance, nonalcoholic fatty liver disease (NAFLD), primary sclerosing cholangitis (PSC), osteoporosis and inflammatory bowel disease (IBD). We reviewed the pathogenetic or protective functions played by Th17 cells in various endocrine tissues and identified potential regulators for plasticity of it. Furthermore, we discussed the roles of Th17 cells in crosstalk of gut-organs axis.
1. Introduction
T lymphocytes can be divided into CD4+ T cells and CD8+T cells. CD4+ T cells are central to the functioning of the entire immune system. CD4+ T cells polarize to different phenotypes in a variety of tissues, including T Helper 17 (Th17) cells, Foxp3+T regulatory cells (Treg), Th1 cells, Th2 cells, T follicular helper (Tfh), Th9 and Th22 cells (1). Th17 cells have unique properties and versatile functions. On the one hand, Th17 cells play vital roles in the etiopathogenesis of various inflammatory diseases. On the other, the adaptive cellular immune responses are reinforced by Th17 cells against extracellular bacteria, fungi, and viruses (2). Increased attention has been attracted by Th17 cells recently in the regulation of chronic inflammation and they further participate in metabolic disorders. The plasticity of Th17 cells is influenced by multiple factors, such as cytokine milieu, microbial products and products of metabolism, and Th17 cells exert pathogenicity via various mechanisms in endocrine organs.
In addition to classic endocrine organs or tissues, plenty of novel endocrine organs were observed in the past few decades. Gut is regarded as a full-blown endocrine organ, as the gut microbiota make a far-reaching influence on the intestinal milieu (3). Gut plays a pivotal role in the endocrine system by interacting with other endocrine organs, including adipose tissue, liver and bone. Adipose tissue, as endocrine organ, is characterized by complicated and dynamic. Adipose tissue consist mainly of adipocytes as well as connective tissue matrix, nerve tissue, stromovascular cells, and immune cells (4). These components work together as a whole unit (4). Indeed, the endocrine system is marked by the production of hormones, and some parts of liver pathology are in accordance with this property (5). The liver, as a key regulator of metabolic homeostasis, perceives and integrates hormone signals and whole body energy status that are triggered by alterations in metabolism (6, 7). In the past, the skeleton that was considered as the regulator of calcium-phosphorus and haematopoiesis homoeostasis, has now been identified as a crucial modulator of metabolism (8). A large number of groundbreaking research confirm that the bone is a real endocrine organ (8).
As such, the plasticity and adaptability of Th17 cells in response to various stimuli make them attractive targets. We reviewed the pathogenetic or protective functions played by Th17 cells in various endocrine tissues and identified potential regulators for plasticity of it (Figure 1). Furthermore, we discussed the roles of Th17 cells in crosstalk of gut-organs axis (Figure 2).
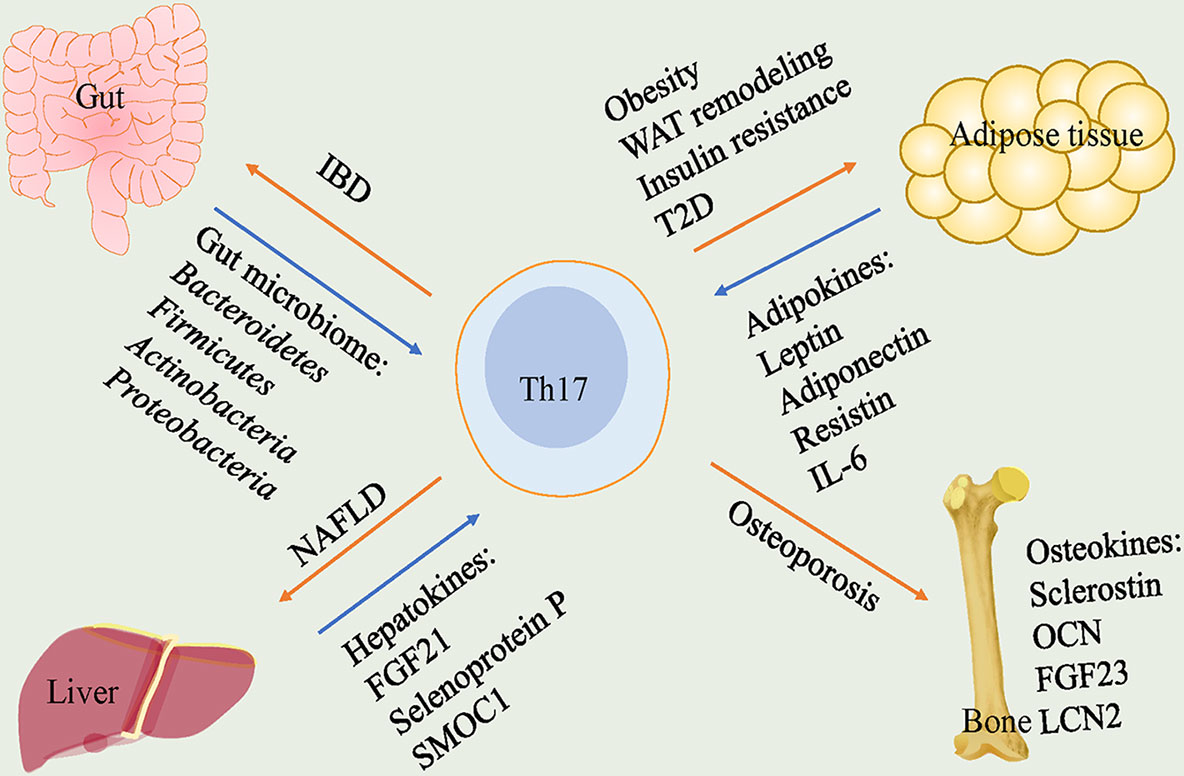
Figure 1 Th17 cells play pathogenetic functions in gut, adipose tissue, liver and bone. In gut, gut microbiome including Bacteroidetes, Firmicutes, Actinobacteria and Proteobacteria lead to a high frequency of Th17 cells that cause IBD. In adipose tissue, adipokines including leptin, adiponectin, resistin and IL-6 participate in the regulation of Th17 cells. Th17 cells trigger obesity, WAT remodeling, insulin resistance and T2D. In liver, hepatokines, FGF21, selenoprotein P and SMOC1, are correlated with levels of Th17 cells and Th17 cells play a crucial role in NAFLD. In bone, Th17 cells are responsible for promoting osteoporosis. Th17, T helper 17; IBD, inflammatory bowel disease; WAT, white adipose tissue; T2D, type 2 diabetes; FGF21, fibroblast growth factor 21; SMOC1, sparc-related modular calcium-binding protein 1; NAFLD, nonalcoholic fatty liver disease; OCN, osteocalcin; FGF23, fibroblast growth factor 23; LCN2, lipocalin-2.
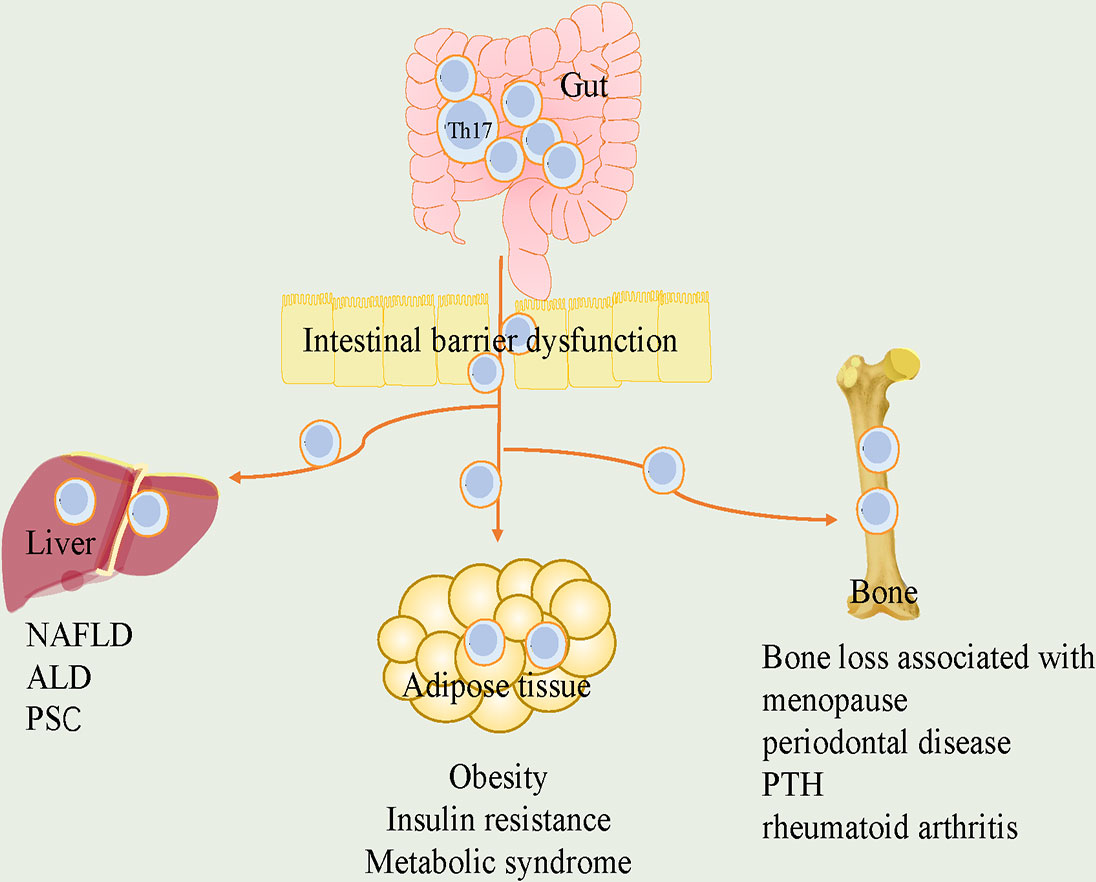
Figure 2 The roles of Th17 cells in the crosstalk of gut and adipose tissue, liver and bone. Loss of gut integrity increases influx of Th17 cells into metabolic tissue. In gut-adipose, due to increased gut permeability, Th17 cells are disseminated from intestine into the adipose. it further stimulates local Th17 cells and contributes to the development of obesity, insulin resistance and metabolic syndrome. In gut-liver, intestinal barrier defects promote liver disease. Th17 cells in intestinal dysbiosis are associated with liver diseases, including NAFLD, ALD and PSC. In gut-bone, intestinal Th17 cells flow into bone, which are critical for bone loss associated with menopause, periodontal disease, PTH and rheumatoid arthritis. Th17, T helper 17; NAFLD, nonalcoholic fatty liver disease; ALD, alcohol-associated liver disease; PSC, primary sclerosing cholangitis; PTH, parathyroid hormone.
2. Endocrine organs
The endocrine system is a complex interconnected system of organs. The primary endocrine organs contain hypothalamus, pituitary, thyroid, pancreas, and ovaries or testes, whose primary function is hormonal production and secretion. Over the past few decades, it is well established that secondary endocrine organs (e.g., gut, adipose tissue, liver and bone) also contribute to the regulation of metabolic homeostasis and inflammatory conditions through secretion of a great deal of hormones, proteins and cytokines.
2.1. Gut
The gut microbiome contains a large amount of information. Secretin, gastrin and cholecystokinin were identified as gut hormones for the first time (9). Nowadays, with the discovery of multiple gut hormone genes and bioactive peptides, gut is recognized as the largest endocrine organ in the body (9). It is well known that humans have trillions of microorganisms. A union made up of bacteria, fungi, protozoa, and viruses live in several areas of the body, including the lungs, urinary tract vagina, and skin, however, the largest microbial flora exists in the intestine (10). Increasing evidence has suggested that these microbes almost play the role of an extra organ by actively participating in shaping and sustaining our physiological functions (3, 11). Nowadays, it is generally believed that the metabolic ability of the gut microbiota is far superior to that of the human host (12). Interactive diversity between the gut microbiota and the pathophysiology of the host makes it clear that the gut microbiota not only affects the intestinal milieu but also has ability to influence distant tissues and pathways (13).
2.2. Adipose Tissue
The discovery of leptin established the status of adipose tissue as an indispensable endocrine organ in 1994 (14). Since then, researches have discovered numerous peptide and non-peptide hormones derived from adipose tissue with autocrine, paracrine or endocrine (15), which make the adipose tissue a complex and dynamic endocrine organ. Adipose tissue not just reacts to afferent signals from classical hormone systems and the central nervous system. It also secretes factors that exert essential endocrine functions (16). Adipose tissue displays a wide range of biological functions, including the regulation of energy consumption, appetite control, glucose homeostasis, insulin sensitivity, inflammation and tissue repair by releasing endocrine factors (17). In addition to the traditional polypeptide adipokines and cytokines, extracellular vesicles (EVs) that are released from a variety of cell types in adipose tissue also mediate the crosstalk between cells and organs (18).
2.3. Liver
The liver is a highly active organ that plays key roles in numerous pathological processes, the secretions from liver are well known to have an influence on glucose and lipid metabolism. The role of liver as a crucial secretory organ has long been appreciated (19), which has multiple endocrine functions, including production of hormone and hepatokine, hormone metabolism, synthesis of binding proteins (20). Proteins that are secreted from hepatocytes can affect the process of metabolism through autocrine, paracrine and endocrine signals (21). These proteins include sex hormone-binding globulin (SHBG), fibroblast growth factor 21 (FGF21) and adropin (21). Similar to the functional proteins that are derived from adipose tissue and skeletal muscle, these liver-derived proteins are called hepatokines (22). On account of its anatomy, the liver might play a crucial role in interorgan crosstalk. The liver participates in reapportionment of hormones, lipoproteins, carbohydrates as well as fatty acids to other tissues, as it receives a dual blood supply from the hepatic portal vein and the hepatic arteries (23). Furthermore, the location of “open-pore” sinusoids is between hepatocyte planes, which let exchange of hepatokines (23).
2.4. Bone
Bone has classically been known as a “dull” organ over the years. Most recent, from evidence that the skeleton behaves as an endocrine organ capable of influencing bone metabolism and physical stability through the secretion of cytokines (24, 25). Studies have revealed the novel endocrine functions of bone, and bone secretes cytokines that can regulate glucose, energy and phosphate metabolism (26). It has been discovered that osteocytes have a variety of functions, such as remodeling the bone by modulating both osteoclast and osteoblast activity, and also acting as an endocrine cell, which releases factors to sustain skeletal homeostasis through endocrine and paracrine functions (27). Bone-derived cytokines or hormones, such as sclerostin, osteocalcin (OCN), fibroblast growth factor 23 (FGF23) and lipocalin-2 (LCN2), target cells on the bone surface and distant tissues, such as kidney, muscle, and other tissues (28, 29).
3. Th17 in endocrine organs
3.1. Th17 and Gut
There are two different subsets of Th17 cells in the intestine, and their metabolic mechanisms and inflammatory outputs are different. Homeostatic Th17 cells have a mild inflammatory property, which is a defender of intestinal barrier, while pathogenic Th17 cells secrete plenty of inflammatory cytokines that trigger severe inflammatory response (30). Th17 cells exist in the whole intestinal lamina propria and maintain intestinal homeostasis in a microbiome-dependent manner. By stimulating antigen-activated T cells with TGF-β and IL-6 or IL-21, which can induce STAT3 phosphorylation, the homeostatic Th17 cells can be differentiated (31). Countless factors including microbiome, proteins, and transcription factors influence Th17 cells development and plasticity in the gut and render it pathogenic (32).
Gut is a complicated ecosystem that is composed of intestinal epithelium, immune cells, mucus layer, and resident microbiota. The gut microbiome is the microbial community colonizing the gut, including dominant bacteria, archaea, protists, fungi, and viruses (33). In the adult gut, over 90% of the bacteria are the phyla of Firmicutes and Bacteroidetes (34). Increasing evidences have shown that T cell subsets in the intestine dynamically present the signals of microbiota (35). Particular components of the gut microbiome take part in the production of Th17 cells and subsequent production of inflammatory response (33).
Intestinal draining lymph nodes are located in the mesentery of the small intestine and colon, where adaptive immune responses are further formed by the intestinal symbionts, and naïve T cells are differentiated within the mesenteric lymph nodes (MLNs) (36). As for differentiation of CD4+ T cells, especially Th17 cells that have a preference for the gut, firstly, dendritic cells reach MLNs, then they interact with naïve T cells and stimulate them (36). Dysbiosis can result in damage of immune responses with destruction of mucosal barriers (37). Migration of gut microbiome to the MLNs and change of the cytokine milieu in intestinal mucosa make MLNs tend to an inflammatory phenotype with activation of Th17 cells, which lead to the inflow of neutrophils and give rise to a severe inflammatory state (37).
Inflammatory bowel disease (IBD), including Crohn’s disease and ulcerative colitis, is a chronic inflammatory disease. A number of metabolic disorders have been proposed to be related to IBD. Studies have revealed analogies of pathophysiological characteristics between metabolic disorders and IBD, including adipose tissue disorder, immune response dysfunction, chronic inflammation and liver metabolic disorder (38, 39).
Researches have showed that IBD is closely connected with the immune response and may alter intestine barrier function that is resulted from gut microbiome disorder (40). Although the mechanism of bacterial reactive pathogenic T cells in IBD is still not completely clear, bacteria-reactive CD4+ T cells gather in the intestinal mucosa of patients with IBD and are considered to play a key role in the pathogenesis of diseases (41). Among these CD4+ T cells, Th17 cells have been found to accumulate in the intestinal mucosa and are reactive to resident bacteria (42). The level of Th17 cells induced by gut microbiota was a prediction of IBD and stated the severity of disease in the Rag1-/- colitis model (43). Bacteria including those that adhere to epithelial cells and several clostridium species can alter differentiation of Th17 cells and segmented filamentous bacteria (SFB) are the main driving force of Th17 responses (44, 45).
In terms of bacterial composition at the phylum level, Bacteroidetes, Firmicutes, Actinobacteria and Proteobacteria are closely related to pro-inflammatory Th17 cells in IBD. Studies have exhibited a relatively low abundance of Firmicutes phylum (46, 47) and Bacteroidetes (46, 48, 49), which showed a high frequency of Th17 cells in IBD. However, a relatively increased abundance of the Firmicutes phylum (48) and Bacteroidetes (47) was observed in IBD, which also enhanced Th17 cells. Luo et al. (47) and Tong et al. (49) reported that Proteobacteria displayed a relatively high abundance in ulcerative colitis with increase of Th17 cells. In addition, totally opposite roles of Actinobacteria (47, 48) that increased the level of Th17 cells, was found in ulcerative colitis.
At genus level, a relatively high abundance of Bacteroides (40, 46), Actinobacterium Eggerthella lenta (50) and Bacteroides ovatus (51) was found in IBD, which induced high levels of intestinal pro-inflammatory Th17 cells. Lachnospiraceae (40), Alistipes (40), Lactobacillus (40), Bilophila (40), Desulfovibrio (40), Alloprevotella (46), Butyricicoccus pullicaecorum (47), Enterorhabdus (49) and unclassified_Bacteroidia (48) displayed a relatively low abundance, which resulted in a higher frequency of pathogenic Th17 cells in a gut microbiota-dependent manner in IBD. In addition, a relatively high abundance of Bifidobacterium (48) but a relatively low abundance of Lactobacillus (52), Oscillibacter (52), Pseudoflavonifractor (52), Clostridium XIVa (52), Johnsonella (52) and Rothia (52) were found to promote Th17 cells on different diet. Treatment of microbiota-reactive CD4+ T cells with IL-1β, IL-6, or IL-23 during stimulation with E coli, S typhimurium, L acidophilus, or B animalis caused an increase in IL-17A production, which suggested that these cytokines might promote Th17 polarization in bacteria-reactive manner (53). Research findings on the gut microbiota and pathogenic Th17 cells are summarized in Table 1.
Moreover, the hormones secreted from intestinal epithelium also have significant impact on Th17 function. The colonic epithelium plays a role in innate and adaptive mucosal immunity and form a mucus barrier (54). Serum amyloid A (SAA) proteins that promote adaptive immunity, are family of retinol-binding proteins expressed in the intestinal epithelium and liver (55, 56). In the differentiation of Th17 cells, IL-6 can combine with the SAAs instead of TGF-β, which result in a pathogenic pro-inflammatory Th17 cell differentiation program (57). In addition to promote local Th17 cell proliferation and/or retention, SAA may promote Th17 cytokine production, such as IL-17 (58).
Glucagon-like peptide-1 (GLP-1) is an incretin hormone secreted from enteroendocrine cells that reside within intestine epithelium (59). Targeting GLP-1 may be an effective therapeutic strategy for Th17/Treg-mediated inflammatory diseases (60). A clinical research showed that GLP-1 appeared to decrease production of Th17-related cytokines in people with obesity and asthma (61).
3.2. Th17 and Adipose Tissue
Adipose tissue (AT), as endocrine organ, is a crucial regulator of excessive fat storage, energy intake and consumption through releasing multiple factors, including adipokines, chemokines and cytokines (62). From the cell perspective, AT contains only 10-30% adipocytes, while the rest of the tissue is made up of various cells, which is called the stromal vascular fraction (SVF) (63), a producer of a series of metabolic diseases. Adipose tissue divides into three types, including white adipose tissue (WAT), beige adipose tissue and brown adipose tissue (BAT). White adipose tissue which mainly lies in subcutaneous or intravisceral sites, is the major energy store. Brown adipose tissue, that locates in the interscapular region, is responsible for energy consumption. Beige adipose tissue are scattered in WAT and can present a brown-like phenotype (64). These three types of adipose tissues also have endocrine functions and play major roles in whole body metabolism especially in obesity.
In 1993, the concept of AT inflammation was first proposed in Science (65). AT inflammation is largely caused or exacerbated by the following factors, including immune cell recruitment rapidly, remodeling of the AT stromal immune components (e.g., immune cells, endothelial cells, fibroblasts and adipocyte progenitors), and AT immune cell dysfunction (66). Plentiful experiments have demonstrated that adipose tissue expansion brings about a complicated and extensive immune response, including innate and adaptive immune system, which play essential roles in the modulation of inflammation (67). The infiltration of immune cells into the AT, including macrophages, dendritic cells, neutrophils, T and B cells, give rise to AT inflammation in obesity (68). Studies have shown that the T cells that are skewed towards a Th17 phenotype, generally accumulate in obese adipose tissue in humans and mice (69–72). Moreover, most recent finding has suggested that obesity convert the classical Th2-predominant disease to a more severe disease with prominent Th17 inflammation (73).
Adipocytes are related to production of Th17 cells, including antigen presentation, costimulatory molecule and further proliferation and differentiation. Major histocompatibilty complex (MHC) class II molecules and costimulators, which are regarded as antigen-presenting cells (APCs) to promote the activation of CD4+ T cells, are overexpressed in obese adipocytes (74). In mice, adipocyte MHCII increased within 2 weeks on high-fat diet (HFD) and adipocytes can activate T cells directly via the MHCII pathway (75). Large adipocytes, which highly expressed MHCII and stimulated CD4+ T cells as APC, were the major contributors to adipose tissue inflammation (76). CD40 (74), CD80 (74), CD86 (74) and OX40 (77) were induced in adipocytes of obese human or mice, and may co-stimulate adipose resident T cells in obesity. Additionally, adipose tissue, as a key endocrine organ, is a modulator of Th17 cells differentiation and pathogenicity by secreting plentiful bioactive substances, termed adipose-derived secreted factors or adipokines.
The family of adipokines is very broad, including leptin, adiponectin, resistin, IL-6 and hundreds more (78, 79). What’s more, some novel adipokines were proposed in recent two years, including Isthmin 1 (80) and leucine-rich alpha-2-glycoprotein 1 (LRG1) (81), which played an active role in the regulation of hepatosteatosis, insulin resistance, glucose uptake and glucose tolerance. However, interaction between these novel adipokines and Th17 cells requires further exploration.
3.2.1. Leptin
Leptin is a pro-inflammatory factor that is involved in both adaptive and innate immunity (82). Increasing evidences have suggested that leptin promotes Th17 cells differentiation (83–85) and this process may be achieved by NF-κB, MAPK, JNK pathway and β3 integrin receptor (86). Furthermore, leptin polarized Th17 cells indirectly through the secretion of pro-inflammatory cytokines, such as IL-6, IL-12, TNF-α (85) and ILC2, which promoted retinoic acid-related orphan receptor γt (RORγt) expression (a transcription factor for Th17 cell differentiation) (87).
3.2.2. Adiponectin
Adiponectin is a negative regulator of T cells activity. Adiponectin can inhibit production of T cells through decreasing the expression of MHCII, CD80, CD86 (88) and proinflammatory cytokines, including IL-6 and TNF-α (89). Data have shown that adiponectin can inhibit Th17 cell differentiation and limit inflammation through upregulation of sirtuin 1 (SIRT1) and peroxisome proliferator-activated receptor γ (PPARγ) and suppression of RORγt (90).
3.2.3. Resistin
Resistin is mainly derived from the adipose tissue. Resistin exerts proinflammatory role mostly through infiltrating immune cells in adipose tissue (91). The positive role of resistin in CD4+ T cells was detected in resistin-induced cell migration (92). Furthermore, proinflammatory Th17 and Th1 can be activated by resistin (91).
3.2.4. IL-6
It has been reported that AT provides about 30% of circulating IL-6 (93). The level of IL-6 produced by visceral AT is higher than that of subcutaneous AT (93). It is all accepted that Th17 cells express RORγ and RORα, which are induced by TGFβ and IL-6 in a STAT3-dependent manner (94).
Obesity is a state of pathological accumulation of adipose tissue. The underlying cause of obesity and overweight is an energy imbalance between the calorie intake and the calorie consumption (95). Globally, there has been an increased intake of high-fat and high-sugar foods and an decrease in physical activity (95). Obesity in many senses is regarded as an inflammatory predisposition (96). Under white adipocyte stress, local infiltration of immune cells and enhanced production of pro-inflammatory cytokines together reduce metabolic flexibility and lead to insulin resistance in obesity (97). It actually is demonstrated in research that Th17 cells polarization is essential to maintain AT inflammation and link obesity to the development of metabolic diseases through AT inflammation (98, 99). An increase in the number of Th17 cells derived from obesity was observed in adipose tissue (83, 100, 101) or peripheral blood mononuclear cells (PBMCs) (102), which supported insulin resistance, blood glucose control and type 2 diabetes (T2D). Evidence also showed that combination of fatty acid metabolites and reduced β oxidation could stimulate Th17 inflammation in patients with T2D, which may predict the metabolic status of people with obesity (103). Additionally, increased expansion of Th17 cells significantly has been displayed to delay diabetic wound healing (104). Dendritic cells have functionality and could modulate AT inflammation via regulating the transformation of Th17 cell responses in obesity-associated insulin resistance (105). Obesity-induced loss of Rab4b in adipose T cells may be involved in maladaptive white adipose tissue reshaping and insulin resistance by increasing adipose Th17 (106). Furthermore, Th17 cells are characterized by production of IL-17. Study has demonstrated that IL-17 receptor knockout markedly limits the body and visceral fat pad weights of mice on HFD and ameliorates AT inflammation and insulin sensitivity (107).
When the positive energy balance is sustained and obesity progresses, a chronic, low-grade inflammatory status is established in WAT and leads to insulin resistance (97). Compared to WAT, brown adipose tissue is less susceptible to developing local inflammation in response to obesity (97). BAT-mediated thermogenesis can protect against obesity via promoting energy expenditure (108). However, pro-inflammatory cytokines may alter the specific thermogenic activity of BAT. Inflammation that infiltrates into BAT may both cause insulin resistance and reduce thermogenesis through weakening its energy consumption and glucose uptake capacity (109, 110).
Although mainly composed of brown adipocytes and pre-adipocytes, BAT also consists of a variety of immune cells (110). Kälin et al. (111) showed that Treg cells are critical for BAT thermogenic capacity and lipolytic function. It was believed that GRIM19 inhibited the progression of obesity by regulating BAT differentiation and the Th17/Treg balance (112). However, the relationship of BAT and Th17 cell are not mentioned. In addition, normal brown adipose tissue has the ability to regulate immunity. Moon J et al. (113) demonstrated that brown adipose tissue ameliorated autoimmune arthritis through inhibition of Th17 cells.
IFN-γ are believed to promote beige fat development. given that Th1 cells are major producers of IFN-γ, it is possible that Th1 cells may also be involved in the regulation of energy expenditure (114).
3.3. Th17 and Liver
The liver acts as an endocrine organ through secreting factors, which is known as hepatokines. The identification of hepatokines has greatly broadened the field of metabolic physiology. Hepatokines are liver-derived proteins who are a modulator of liver and systemic metabolism by autocrine, paracrine and endocrine signaling to affect lipid metabolism, cell function, peripheral insulin action and glucose control (19). Aside from classic hepatokines, such as adropin, angiopoietin-related proteins (ANGPTLs), fetuins and FGF21, novel hepatokines, including sex hormone-binding globulin(SHBG), apolipoprotein J (ApoJ), mesencephalic astrocyte-derived neurotrophic factor (Manf), fibronectin type III domain containing 4 (FNDC4), pregnancy zone protein (PZP) and sparc-related modular calcium-binding protein 1(SMOC1) play a key role in maintaining nutrient homeostasis by directly affecting glucose, lipid metabolism and insulin action.
Careful deduction of the Mendelian randomization results suggested that SHBG, as a hepatokine, plays a direct, causal role in the pathogenesis of female type 2 diabetes (115). Liver is the main source of circulating ApoJ. As a novel hepatokine, ApoJ aims at muscle glucose metabolism and insulin sensitivity through a low-density lipoprotein receptor-related protein-2 (LRP2)-dependent mechanism and the insulin receptor signaling cascade (116). Manf is a feeding-induced hepatokine. Manf specific overexpression in liver improved high-fat diet-induced obesity, promoted browning of subcutaneous white AT via the p38 MAPK pathway and also have relationship with the improvement of insulin sensitivity and hepatic steatosis (117). Liver primarily controls the circulating levels of soluble FNDC4 (sFNDC4). Data provided evidence that sFNDC4 acted as a hepatokine (118). The reduction of liver FNDC4 mRNA corresponded with sFNDC4-circulating levels, which subsequently resulted in prediabetes in mice (118). A novel hepatokine, PZP, was identified to promote diet-induced thermogenesis through activating brown adipose tissue (119).
Among those molecules, FGF21 and SMOC1, are involved in the regulation of Th17 cells.
3.3.1. FGF21
FGF21 is a stress-inducible hormone that plays a crucial role in the modulation of energy balance, glucose and lipid metabolism. The liver is the main organ that controls the secretion and actions of FGF21 (120). Studies revealed that the liver regulated carbohydrate intake through production of the hepatokine FGF21 (7). It was observed that there were significant improvements of dyslipidaemia, hepatic fat fractions and serum markers of liver fibrosis in patients with non-alcoholic steatohepatitis (NASH) with administration of FGF21 (121). Hepatic inflammation was greatly reduced in NASH mice with the treatment of FGF21, which was related to suppression of IL-17A expression in Th17 cells (122). In addition, FGF21 depended on adiponectin or STAT3/RORγt pathway (123) to exert its inhibition of Th17 cell differentiation and IL-17A expression (122).
3.3.2. SMOC1
SMOC1 was identified as a glucose-responsive hepatokine and a regulator of glucose metabolism, which was dysregulated in the setting of NAFLD in mice (124). However, Ghodsian et al. (125) proposed that the hepatokine SMOC1 may not regulated by NAFLD and may not modulate glucose-insulin homeostasis in humans. The different results suggest that SMOC1 in the regulation of metabolism in rodents may not always translate to human biology. Proof showed that the expression of SMOC1 was negatively correlated with levels of CD4+ T cells (126). Additionally, SMOC1 co-expressed genes were suppressed in processes such as adaptive immune response, T cell activation and pathways including Th17 cell differentiation (126).
NAFLD is regarded as an ectopic accumulation of fat in the liver. It is confirmed by imaging or histology that there is no known cause of secondary hepatic fat accumulation such as drinking, steatogenic drugs or genetic diseases (127). NAFLD consists of a great variety of histological spectrum ranging from benign simple steatosis to NASH. Persistent hepatic inflammation plays an essential role in this process (128). It is generally believed that changes of T cell responses and related cytokines may cause the progress of NASH. Notably, a higher frequency of Th17 cells was found in liver, which was the sign of progression from nonalcoholic fatty liver (NAFL) to NASH (129). The steatotic liver microenvironment promotes the production and metabolism of Th17 cells. Th17 cells are capable of transforming into an inflammatory hepatic CXCR3+Th17 (ihTh17) cell, which is adequate to aggravate NAFLD pathogenesis (130). The increased expression of hepatic Th17 cells and IL-17 were detected in NASH mice and patients, separately (131). Obesity promotes peripheral Th17 cell expansion and infiltration into liver (130). DNA damage in hepatocytes was found to trigger inflammation through Th17 cells and IL-17A, which induced the release of fatty acid who stored in liver as triglycerides, causing NASH (132). RNA clustering analysis of liver demonstrated that high-fat high-fructose diet-induced elevated Th17 cells in liver, especially through up-regulation of Rorc (coding for the canonical transcription factor RORγt) in murine nonalcoholic steatohepatitis. Moreover, pathway analysis showed enrichment of the Th17 activation pathway in liver, thus causing inflammation and NASH (72). Converted double negative T cells could limit inflammation in the liver and NASH development by lowering the ratio and survival of CD4+ T cell, and Th17 cell differentiation in the liver (133).
3.4. Th17 and Bone
The skeleton, as an endocrine organ, modulates various energy metabolism. The three main cell types in the bone, osteoblasts, osteocytes, and osteoclasts, regulate metabolism by production of molecules. Recently, multiple factors secreted by bone, known as osteokines, have been considered as regulators systemically. OCN is particularly secreted by osteoblasts, and is the most plentiful non-collagenous protein in bone (134). As a hormone, OCN was identified to inhibit bone formation and function in the regulation of glycometabolism in the pancreas, testosterone synthesis in the testis and muscle mass (134). Indeed, FGF23 play a crucial role in the modulation of other organs through being released into the circulation by osteoblasts/osteocytes, and it also create sophisticated endocrine feedback loops that regulate mineral and energy metabolism (135). Recent study demonstrated that osteoblast-derived LCN2 sustains glucose homeostasis through the regulation of glucose tolerance, insulin sensitivity, and insulin secretion (136). Sclerostin is a secreted glycoprotein primarily expressed by mature osteocytes and is widely regarded as a negative regulator of osteogenesis (137). Evidence showed that sclerostin can exert endocrine effects on stimulating adipogenesis (137). However, association between theses osteokines and Th17 cells remains to be seen.
Osteoporosis is a metabolic bone disease, which can increase the risk of fragility fractures. Osteoporosis is characterized by low bone mass and deterioration of bone microarchitecture (138). Aging and estrogen deficiency may be two of the most key risk factors in the development of osteoporosis and both of them have impact on immune function (139). Recently, the immune system and immune factors play vital roles in the occurrence and development of osteoporosis, especially in the differentiation of osteoclast (140). Large quantities of studies have demonstrated that differentiation and activation of osteoclasts that are out of control lead to bone erosion (141). Bone destruction is directly or indirectly modulated by CD4+ T cells that infiltrate into the lesion (142). There is a possibility that the Th lineage that is in charge of pathogenic mechanism of oestrogen-deficient osteoporosis contribute to the major sources of inflammatory cytokines to promote bone loss (143). However, as a candidate Th lineage, the Th1/Th2 pattern fails to meet those requirements (143). Studies indicated that Th17 cells play essential roles in the regulation of the process of bone reconstruction and are responsible for promoting osteoclastogenesis (140, 144). Evidence showed that elevated Th17 cell frequency and IL-17 level were related to low bone mineral density (145). Interaction between Myeloid-derived suppressor cells (MDSC) and Th17 cells potentiated concurrently abnormal inflammation and osteoclastogenesis (141). Increased proportion of anti-osteoclastogenic T lymphocytes (Treg cells) and decreased osteoclastogenic T lymphocytes (Th17 cells) were found to enhance bone health in ovariectomy mice (146). In terms of mechanism, receptor activator of nuclear factor kappa B ligand (RANKL) is a vital factor that connects the bone and immune systems (147). The stimulation of RANKL activated a signal pathway downstream of RANK, which can evaluate the degree of bone resorption via inducing osteoclast maturation (147). Activated T cells are primary sources of RANKL (148). Th17 cells are one of the main causes of bone loss through expressing high RANKL level (149). Besides, Th17 cells enhanced the expression of RANKL on osteoblasts and fibroblasts by the production of inflammatory cytokines, such as IL-6, IL-17 and TNF-α, and subsequently promoted osteoclast-mediated bone resorption (150). Additionally, RANKL/RANK/osteoprotegerin (OPG) system has been proposed to be of great importance to periodontitis bone metabolism, and its relationship with the Th17/Treg cell imbalance made periodontal bone metabolism and the immune system closely connected (151).
4. Th17 in gut-organs axis
Recently, it has been well documented that the gut may influence other organs through the production of microbiota capable of regulating immune cells. Gut microbiota may also trigger systemic immune responses through immune cell (36). Gut interacts with peripheral organs such as the adipose tissue, liver and bone to control diverse processes, including lipid metabolism, insulin resistance, bone loss, and inflammation conditions. Among immune cells, Th17 cells in draining MLNs of the gut, get into systemic circulation to promote immune responses of other organs in resistance to the same organism or other antigens in cross-reaction to similar epitopes (152). Migratory Th17 cells have remarkable plasticity in function on the basis of the existing local state (153).
4.1. Th17 in gut-adipose
Gut microbiota influence host metabolism via crosstalk with the adipose tissue, which contribute to an alteration in metabolism related to obesity. Gut could regulate several events of adipose tissue function, including lipid metabolism, endocrine function (154) and inflammation (155).
Growing evidences revealed immunological crosstalk between the gut and AT. It is found in obesity that the immunomodulatory characteristics of the gut microbiota make it attractive in the context of metainflammation (156). Obesity is related to crucial alterations in the adaptive immune compartment in the gut, which leads to abnormal inflammatory skewing (42, 157). During obesity, bowel inflammation may directly contribute to visceral AT inflammation and insulin resistance (155). Pro-inflammatory changes in immune cells, including Th17, Th1 and IL-17-producing γδ T cells, contribute to increased gut permeability (155). Lack of gut integrity allows bacterial products to flow into metabolic tissue, such as the visceral AT, where it further stimulates local immune cells and contributes to the chronic inflammation of adipose tissue that is associated with the development of insulin resistance, and other conditions related to the metabolic syndrome (158).
There are homeostatic tissue-resident and inflammatory Th17 cells with different functions in the intestine (30). In contrast to homeostatic Th17 cells, infection-induced Th17 cells in intestine displayed widespread plasticity towards pro-inflammatory cytokines, and distributed widely from intestine to the periphery (30). Diet regulates gut microbiota and Th17 cells, and plays a crucial role in the regulation of adipose tissue. A significant drop was detected in Th17 cells that accumulate in visceral adipose tissue on ketogenic diet, which may be associated with gut microbial-induced alterations in the intestinal immune environment (47). A HFD-derived ileum microbiota was found to trigger a drop in Th17 cells of the lamina propria, which contributed to metabolic inflammation by the means of transferring from the gut across the damaged intestinal barrier to diverse metabolic organs, such as the liver and adipose tissue (159, 160). Secretion of IL‐17 is mediated largely by IL‐17‐producing Th17 cells of intestinal lamina propria. IL‐17 receptor (IL‐17R) deficiency on the HFD contributed to inflammation and damage in the gut, which prevented the absorption and subsequent deposition of lipids in adipose tissue (161).
Besides, Th17 cells also play a protective role in gut and AT, and most of them are homeostatic gut-resident Th17 cells. Carlos et al. (162) proved that intestinal Th17 response limited intestinal microbiome disorder and lipopolysaccharide (LPS) translocation to visceral AT, which protected against metabolic syndrome. Gut-homing property of the transferred TH17 cells reversed the weight gain and decreased the fat mass (163).
4.2. Th17 in gut-liver
As 70% of its blood supply comes from the portal vein, the liver is physiologically exposed to intestinal microorganisms and metabolites (164). Th17 cells in intestinal dysbiosis are associated with liver diseases, including alcohol-associated liver disease(ALD), primary sclerosing cholangitis (PSC) and NAFLD. One hypothesis is that intestinal inflammation and barrier dysfunction are responsible for liver disease as the bacteria and inflammatory cells flow into the liver (165). Alcohol intake induced a pro-inflammatory shift in Th17 cells in the gut, which resulted in ALD via Sphingosine-1-Phosphate (S1P)/S1P receptor 1 (S1PR1) signaling, moreover, the decreased level of intestinal Th17 cells limited liver damage (166). PSC coexists frequently with IBD, which identifies the gut-liver axis as the core of pathogenesis (167). It also exemplifies the ‘leaky gut’ hypothesis. Gut microbiota contributes to the impairment of the first intestinal barrier, and a second trigger, such as colitis or hepatobiliary injury, further promotes the TH17-mediated disease development in PSC (168).
In parallel with the ‘leaky gut’ hypothesis, a ‘gut lymphocyte homing’ hypothesis has displayed the pathogenesis of gut–liver axis (169). Mucosal T cells produced by intestine were abnormally activated by the commensal microbiome, which further translocated to the liver and cross-reacted with antigens in the liver (169, 170). In accordance with this hypothesis, plenty of studies have been performed. Research about PSC patients with concurrent inflammatory bowel disease (PSC-IBD) clearly showed that T cells infiltration in the gut and liver of PSC-IBD patients were clonally correlative and may be able to recognize the same antigens (171). Gut-derived memory T cells that infiltrated in liver were related to enhanced Th17 cytokines (172). Co-culture assays showed MLNs CD4+ T cells from HFD-fed mice skewed toward migrating to the liver and contributed to hepatic inflammation (173). Adoptive transfer of MLNs CD4+ T cells from NAFLD mice to HFD-fed mice led to elevated transaminase, more severe hepatic inflammation and lipid accumulation (173).
4.3. Th17 in gut-bone
During the past 20 years, inflammatory diseases associated with gut have been proposed to be related to a drop in bone mass, which suggested that the gut may be interlinked with the bone. A growing body of evidences support an essential role of Th17 cells in gut-bone axis, especially in bone loss associated with menopause, periodontal disease, parathyroid hormone (PTH) and rheumatoid arthritis. It is obvious that the crosstalk between the immune system and the gut microbiota have extensive impact on bone health (174). Gut microbiome is responsible for pathological process of osteoporosis. The molecular mechanisms mainly include: 1) Intestinal barrier and nutrient absorption (involving short-chain-fatty acids), 2) Immunoregulation (Th17 and Treg cells balance), 3) Modulation of intestinal-brain axis (involving 5-HT) (175).
Estrogen deficiency is the etiology of postmenopausal osteoporosis (PMO). It has been widely accepted that estrogen plays an key role in the regulation of the immune system and that immune cells and associated cytokines have major influences on bone cells (176). More recent work has implicated that T cells in the gut are a proximal target of sex steroid deficiency relevant to bone loss (177). The possible mechanism is that ovariectomy increased intestinal Th17 cells and TNF+ T cells, enhanced their S1PR1-mediated excretion from the intestine, and further promoted their influx into the bone marrow (BM) through CXCR3- and CCL20-mediated mechanisms (177). These data emphasize the role that played by the gut microbiota in triggering intestinal Th17 cells migration that are critical for bone loss.
Bone density in vertebrae and long bone is reduced by oestrogen deficiency, which also exacerbates alveolar bone loss related to inflammatory (178). Recent evidence has suggested that gut microbiome disorder associated with oestrogen deficiency enhanced gut permeability with increased serum LPS (167). Subsequent inflammatory responses induced an imbalance of Th17/Treg cells in the bone marrow and aggravated alveolar bone loss (178).
Bone loss is a common complication of hyperparathyroidism. PTH is one of the osteoregulatory hormones that depends on gut microbiome to exert its bone catabolic and bone anabolic effects (179). It has been proved that PTH contributes to Th17 cell proliferation in the small intestine and results in bone loss (180). A specific bacterial strain, SFB, that causes Th17 cell expansion in the gut, negatively influences skeletal maturation (181). PTH only led to bone loss in mice whose gut microbiota was enriched by the Th17 cell-induced SFB (180).
Recent data indicate that osteoporosis and joint disease associated with inflammation share a joint immune component (175). Th17 cells differentiation have been shown to play a key role in rheumatoid arthritis and IBD induced bone loss (182).
5. Conclusion
In summary, Th17 cells are vital regulators in various endocrine organs, and contribute to crosstalk between gut and other organs, including adipose tissue, liver and bone, and eventually trigger multiple metabolic disorders and inflammatory conditions. Re-thinking about metabolic diseases relevant to endocrine organs from Th17 cells point of view may produce additional understanding of the disease mechanisms. Th17 cells have its dual roles, which are not only pathogenic in many inflammatory diseases, but also protect against extracellular bacteria, fungi, and viruses. Targeting pathological Th17 cells may be a promising treatment for systematic diseases, including IBD, obesity, insulin resistance, NAFLD, PSC and osteoporosis. Although therapies on the basis of Th17 cells are still at the initial stage of research, a great deal of studies have broadened our comprehension of Th17 cells in pathophysiology.
However, current understanding of the crosstalk between Th17 cells and endocrine system is far from enough. For example, few researches focus on the effects of osteokines on Th17 cells. Besides, plenty of factors, including diet, temperature and heredity, participate in the modulation of gut on adipose tissue. The role of Th17 cells that are influenced by temperature and heredity in gut-adipose axis is not fully expounded.
Author contributions
CZ wrote the manuscript. DW arranged literature. LH and YG reviewed the manuscript. All authors contributed to the article and approved the submitted version.
Funding
This study was supported by Young Scientists Fund of National Natural Science Foundation of China (NO.82004242).
Conflict of interest
The authors declare that the research was conducted in the absence of any commercial or financial relationships that could be construed as a potential conflict of interest.
Publisher’s note
All claims expressed in this article are solely those of the authors and do not necessarily represent those of their affiliated organizations, or those of the publisher, the editors and the reviewers. Any product that may be evaluated in this article, or claim that may be made by its manufacturer, is not guaranteed or endorsed by the publisher.
References
1. Zhu J. T Helper cell differentiation, heterogeneity, and plasticity. Cold Spring Harb Perspect Biol (2018) 10(10):a030338. doi: 10.1101/cshperspect.a030338
2. Van Herck MA, Weyler J, Kwanten WJ, Dirinck EL, De Winter BY, Francque SM, et al. The differential roles of T cells in non-alcoholic fatty liver disease and obesity. Front Immunol (2019) 10:82. doi: 10.3389/fimmu.2019.00082
3. Qi X, Yun C, Pang Y, Qiao J. The impact of the gut microbiota on the reproductive and metabolic endocrine system. Gut Microbes (2021) 13(1):1–21. doi: 10.1080/19490976.2021.1894070
4. Kershaw EE, Flier JS. Adipose tissue as an endocrine organ. J Clin Endocrinol Metab (2004) 89(6):2548–56. doi: 10.1210/jc.2004-0395
5. Asa SL, Mete O. Endocrine pathology: Past, present and future. Pathology (2018) 50(1):111–8. doi: 10.1016/j.pathol.2017.09.003
6. Toews JNC, Hammond GL, Viau V. Liver at the nexus of rat postnatal hpa axis maturation and sexual dimorphism. J Endocrinol (2021) 248(1):R1–r17. doi: 10.1530/joe-20-0286
7. von Holstein-Rathlou S, BonDurant LD, Peltekian L, Naber MC, Yin TC, Claflin KE, et al. Fgf21 mediates endocrine control of simple sugar intake and sweet taste preference by the liver. Cell Metab (2016) 23(2):335–43. doi: 10.1016/j.cmet.2015.12.003
8. Oldknow KJ, MacRae VE, Farquharson C. Endocrine role of bone: Recent and emerging perspectives beyond osteocalcin. J Endocrinol (2015) 225(1):R1–19. doi: 10.1530/JOE-14-0584
9. Ahlman H, Nilsson. The gut as the largest endocrine organ in the body. Ann Oncol Off J Eur Soc Med Oncol (2001) 12 Suppl 2:S63–8. doi: 10.1093/annonc/12.suppl_2.s63
10. Busnelli M, Manzini S, Chiesa G. The gut microbiota affects host pathophysiology as an endocrine organ: A focus on cardiovascular disease. Nutrients (2019) 12(1):79. doi: 10.3390/nu12010079
11. Pernigoni N, Zagato E, Calcinotto A, Troiani M, Mestre RP, Calì B, et al. Commensal bacteria promote endocrine resistance in prostate cancer through androgen biosynthesis. Science (2021) 374(6564):216–24. doi: 10.1126/science.abf8403
12. Li J, Jia H, Cai X, Zhong H, Feng Q, Sunagawa S, et al. An integrated catalog of reference genes in the human gut microbiome. Nat Biotechnol (2014) 32(8):834–41. doi: 10.1038/nbt.2942
13. Neuman H, Debelius JW, Knight R, Koren O. Microbial endocrinology: The interplay between the microbiota and the endocrine system. FEMS Microbiol Rev (2015) 39(4):509–21. doi: 10.1093/femsre/fuu010
14. Zhang Y, Proenca R, Maffei M, Barone M, Leopold L, Friedman JM. Positional cloning of the mouse obese gene and its human homologue. Nature (1994) 372(6505):425–32. doi: 10.1038/372425a0
15. Kahn CR, Wang G, Lee KY. Altered adipose tissue and adipocyte function in the pathogenesis of metabolic syndrome. J Clin Invest (2019) 129(10):3990–4000. doi: 10.1172/JCI129187
16. Funcke JB, Scherer PE. Beyond adiponectin and leptin: Adipose tissue-derived mediators of inter-organ communication. J Lipid Res (2019) 60(10):1648–84. doi: 10.1194/jlr.R094060
17. Scheja L, Heeren J. The endocrine function of adipose tissues in health and cardiometabolic disease. Nat Rev Endocrinol (2019) 15(9):507–24. doi: 10.1038/s41574-019-0230-6
18. Huang Z, Xu A. Adipose extracellular vesicles in intercellular and inter-organ crosstalk in metabolic health and diseases. Front Immunol (2021) 12:608680. doi: 10.3389/fimmu.2021.608680
19. Watt MJ, Miotto PM, De Nardo W, Montgomery MK. The liver as an endocrine organ-linking nafld and insulin resistance. Endocrine Rev (2019) 40(5):1367–93. doi: 10.1210/er.2019-00034
20. Jensen-Cody SO, Potthoff MJ. Hepatokines and metabolism: Deciphering communication from the liver. Mol Metab (2021) 44:101138. doi: 10.1016/j.molmet.2020.101138
21. Meex RCR, Watt MJ. Hepatokines: Linking nonalcoholic fatty liver disease and insulin resistance. Nat Rev Endocrinol (2017) 13(9):509–20. doi: 10.1038/nrendo.2017.56
22. Stefan N, Häring HU. The role of hepatokines in metabolism. Nat Rev Endocrinol (2013) 9(3):144–52. doi: 10.1038/nrendo.2012.258
23. Sabaratnam R, Wojtaszewski JFP, Hojlund K. Factors mediating exercise-induced organ crosstalk. Acta Physiol (Oxf) (2022) 234(2):e13766. doi: 10.1111/apha.13766
24. Fukumoto S, Martin TJ. Bone as an endocrine organ. Trends Endocrinol metabolism: TEM (2009) 20(5):230–6. doi: 10.1016/j.tem.2009.02.001
25. Mizokami A, Kawakubo-Yasukochi T, Hirata M. Osteocalcin and its endocrine functions. Biochem Pharmacol (2017) 132:1–8. doi: 10.1016/j.bcp.2017.02.001
26. Wang H, Zheng X, Zhang Y, Huang J, Zhou W, Li X, et al. The endocrine role of bone: Novel functions of bone-derived cytokines. Biochem Pharmacol (2021) 183:114308. doi: 10.1016/j.bcp.2020.114308
27. Chande S, Bergwitz C. Role of phosphate sensing in bone and mineral metabolism. Nat Rev Endocrinol (2018) 14(11):637–55. doi: 10.1038/s41574-018-0076-3
29. Lin X, Onda DA, Yang CH, Lewis JR, Levinger I, Loh K. Roles of bone-derived hormones in type 2 diabetes and cardiovascular pathophysiology. Mol Metab (2020) 40:101040. doi: 10.1016/j.molmet.2020.101040
30. Omenetti S, Bussi C, Metidji A, Iseppon A, Lee S, Tolaini M, et al. The intestine harbors functionally distinct homeostatic tissue-resident and inflammatory Th17 cells. Immunity (2019) 51(1):77–89.e6. doi: 10.1016/j.immuni.2019.05.004
31. Ivanov II, McKenzie BS, Zhou L, Tadokoro CE, Lepelley A, Lafaille JJ, et al. The orphan nuclear receptor rorgammat directs the differentiation program of proinflammatory il-17+ T helper cells. Cell (2006) 126(6):1121–33. doi: 10.1016/j.cell.2006.07.035
32. Ueno A, Jeffery L, Kobayashi T, Hibi T, Ghosh S, Jijon H. Th17 plasticity and its relevance to inflammatory bowel disease. J Autoimmun (2018) 87:38–49. doi: 10.1016/j.jaut.2017.12.004
33. Kiner E, Willie E, Vijaykumar B, Chowdhary K, Schmutz H, Chandler J, et al. Gut Cd4(+) T cell phenotypes are a continuum molded by microbes, not by T(H) archetypes. Nat Immunol (2021) 22(2):216–28. doi: 10.1038/s41590-020-00836-7
34. Chen P, Tang X. Gut microbiota as regulators of Th17/Treg balance in patients with myasthenia gravis. Front Immunol (2021) 12:803101. doi: 10.3389/fimmu.2021.803101
35. Human Microbiome Project Consortium. Structure, function and diversity of the healthy human microbiome. Nature (2012) 486(7402):207–14. doi: 10.1038/nature11234
36. Gopalakrishnan V, Helmink BA, Spencer CN, Reuben A, Wargo JA. The influence of the gut microbiome on cancer, immunity, and cancer immunotherapy. Cancer Cell (2018) 33(4):570–80. doi: 10.1016/j.ccell.2018.03.015
37. Levy M, Kolodziejczyk AA, Thaiss CA, Elinav E. Dysbiosis and the immune system. Nat Rev Immunol (2017) 17(4):219–32. doi: 10.1038/nri.2017.7
38. Lv WJ, Liu C, Yu LZ, Zhou JH, Li Y, Xiong Y, et al. Melatonin alleviates neuroinflammation and metabolic disorder in dss-induced depression rats. Oxid Med Cell Longev (2020) 2020:1241894. doi: 10.1155/2020/1241894
39. Argollo M, Gilardi D, Peyrin-Biroulet C, Chabot JF, Peyrin-Biroulet L, Danese S. Comorbidities in inflammatory bowel disease: A call for action. Lancet Gastroenterol Hepatol (2019) 4(8):643–54. doi: 10.1016/S2468-1253(19)30173-6
40. Zhang W, Cheng C, Han Q, Chen Y, Guo J, Wu Q, et al. Flos abelmoschus manihot extract attenuates dss-induced colitis by regulating gut microbiota and Th17/Treg balance. BioMed Pharmacother (2019) 117:109162. doi: 10.1016/j.biopha.2019.109162
41. Kitamoto S, Nagao-Kitamoto H, Jiao Y, Gillilland MG 3rd, Hayashi A, Imai J, et al. The intermucosal connection between the mouth and gut in commensal pathobiont-driven colitis. Cell (2020) 182(2):447–62.e14. doi: 10.1016/j.cell.2020.05.048
42. Atarashi K, Tanoue T, Ando M, Kamada N, Nagano Y, Narushima S, et al. Th17 cell induction by adhesion of microbes to intestinal epithelial cells. Cell (2015) 163(2):367–80. doi: 10.1016/j.cell.2015.08.058
43. Britton GJ, Contijoch EJ, Mogno I, Vennaro OH, Llewellyn SR, Ng R, et al. Microbiotas from humans with inflammatory bowel disease alter the balance of gut Th17 and rorgammat(+) regulatory T cells and exacerbate colitis in mice. Immunity (2019) 50(1):212–24 e4. doi: 10.1016/j.immuni.2018.12.015
44. Schirmer M, Garner A, Vlamakis H, Xavier RJ. Microbial genes and pathways in inflammatory bowel disease. Nat Rev Microbiol (2019) 17(8):497–511. doi: 10.1038/s41579-019-0213-6
45. Hang S, Paik D, Yao L, Kim E, Trinath J, Lu J, et al. Bile acid metabolites control Th17 and treg cell differentiation. Nature (2019) 576(7785):143–8. doi: 10.1038/s41586-019-1785-z
46. Liu YJ, Tang B, Wang FC, Tang L, Lei YY, Luo Y, et al. Parthenolide ameliorates colon inflammation through regulating Treg/Th17 balance in a gut microbiota-dependent manner. Theranostics (2020) 10(12):5225–41. doi: 10.7150/thno.43716
47. Luo S, Wen R, Wang Q, Zhao Z, Nong F, Fu Y, et al. Rhubarb peony decoction ameliorates ulcerative colitis in mice by regulating gut microbiota to restoring Th17/Treg balance. J Ethnopharmacol (2019) 231:39–49. doi: 10.1016/j.jep.2018.08.033
48. Ang QY, Alexander M, Newman JC, Tian Y, Cai J, Upadhyay V, et al. Ketogenic diets alter the gut microbiome resulting in decreased intestinal Th17 cells. Cell (2020) 181(6):1263–75.e16. doi: 10.1016/j.cell.2020.04.027
49. Tong L, Hao H, Zhang Z, Lv Y, Liang X, Liu Q, et al. Milk-derived extracellular vesicles alleviate ulcerative colitis by regulating the gut immunity and reshaping the gut microbiota. Theranostics (2021) 11(17):8570–86. doi: 10.7150/thno.62046
50. Alexander M, Ang QY, Nayak RR, Bustion AE, Sandy M, Zhang B, et al. Human gut bacterial metabolism drives Th17 activation and colitis. Cell Host Microbe (2022) 30(1):17–30 e9. doi: 10.1016/j.chom.2021.11.001
51. Lavoie S, Conway KL, Lassen KG, Jijon HB, Pan H, Chun E, et al. The crohn's disease polymorphism, Atg16l1 T300a, alters the gut microbiota and enhances the local Th1/Th17 response. Elife (2019) 8:e39982. doi: 10.7554/eLife.39982
52. Wilck N, Matus MG, Kearney SM, Olesen SW, Forslund K, Bartolomaeus H, et al. Salt-responsive gut commensal modulates Th17 axis and disease. Nature (2017) 551(7682):585–9. doi: 10.1038/nature24628
53. Hegazy AN, West NR, Stubbington MJT, Wendt E, Suijker KIM, Datsi A, et al. Circulating and tissue-resident Cd4(+) T cells with reactivity to intestinal microbiota are abundant in healthy individuals and function is altered during inflammation. Gastroenterology (2017) 153(5):1320–37.e16. doi: 10.1053/j.gastro.2017.07.047
54. Parikh K, Antanaviciute A, Fawkner-Corbett D, Jagielowicz M, Aulicino A, Lagerholm C, et al. Colonic epithelial cell diversity in health and inflammatory bowel disease. Nature (2019) 567(7746):49–55. doi: 10.1038/s41586-019-0992-y
55. Derebe MG, Zlatkov CM, Gattu S, Ruhn KA, Vaishnava S, Diehl GE, et al. Serum amyloid a is a retinol binding protein that transports retinol during bacterial infection. Elife (2014) 3:e03206. doi: 10.7554/eLife.03206
56. Bang YJ, Hu Z, Li Y, Gattu S, Ruhn KA, Raj P, et al. Serum amyloid a delivers retinol to intestinal myeloid cells to promote adaptive immunity. Science (2021) 373(6561):eabf9232. doi: 10.1126/science.abf9232
57. Lee JY, Hall JA, Kroehling L, Wu L, Najar T, Nguyen HH, et al. Serum amyloid a proteins induce pathogenic Th17 cells and promote inflammatory disease. Cell (2020) 180(1):79–91.e16. doi: 10.1016/j.cell.2019.11.026
58. Sano T, Huang W, Hall JA, Yang Y, Chen A, Gavzy SJ, et al. An il-23r/Il-22 circuit regulates epithelial serum amyloid a to promote local effector Th17 responses. Cell (2016) 164(1-2):324. doi: 10.1016/j.cell.2015.12.047
59. Worthington JJ, Reimann F, Gribble FM. Enteroendocrine cells-sensory sentinels of the intestinal environment and orchestrators of mucosal immunity. Mucosal Immunol (2018) 11(1):3–20. doi: 10.1038/mi.2017.73
60. da Silva EM, Yariwake VY, Alves RW, de Araujo DR, Andrade-Oliveira V. Crosstalk between incretin hormones, Th17 and treg cells in inflammatory diseases. Peptides (2022) 155:170834. doi: 10.1016/j.peptides.2022.170834
61. Liao SY, Linderholm A, Showalter MR, Chen CH, Fiehn O, Kenyon NJ. L-arginine as a potential glp-1-Mediated immunomodulator of Th17-related cytokines in people with obesity and asthma. Obes Sci Pract (2021) 7(3):339–45. doi: 10.1002/osp4.500
62. Wang Q, Wang Y, Xu D. The roles of T cells in obese adipose tissue inflammation. Adipocyte (2021) 10(1):435–45. doi: 10.1080/21623945.2021.1965314
63. Sun W, Modica S, Dong H, Wolfrum C. Plasticity and heterogeneity of thermogenic adipose tissue. Nat Metab (2021) 3(6):751–61. doi: 10.1038/s42255-021-00417-4
64. Hildebrand S, Stümer J, Pfeifer A. Pvat and its relation to brown, beige, and white adipose tissue in development and function. Front Physiol (2018) 9:70. doi: 10.3389/fphys.2018.00070
65. Hotamisligil GS, Shargill NS, Spiegelman BM. Adipose expression of tumor necrosis factor-alpha: Direct role in obesity-linked insulin resistance. Science (1993) 259(5091):87–91. doi: 10.1126/science.7678183
66. Winn NC, Cottam MA, Wasserman DH, Hasty AH. Exercise and adipose tissue immunity: Outrunning inflammation. Obes (Silver Spring) (2021) 29(5):790–801. doi: 10.1002/oby.23147
67. Unamuno X, Gomez-Ambrosi J, Rodriguez A, Becerril S, Fruhbeck G, Catalan V. Adipokine dysregulation and adipose tissue inflammation in human obesity. Eur J Clin Invest (2018) 48(9):e12997. doi: 10.1111/eci.12997
68. Gong Z, Zhang X, Su K, Jiang R, Sun Z, Chen W, et al. Deficiency in Aim2 induces inflammation and adipogenesis in white adipose tissue leading to obesity and insulin resistance. Diabetologia (2019) 62(12):2325–39. doi: 10.1007/s00125-019-04983-x
69. Lu J, Zhao J, Meng H, Zhang X. Adipose tissue-resident immune cells in obesity and type 2 diabetes. Front Immunol (2019) 10:1173. doi: 10.3389/fimmu.2019.01173
70. Han SJ, Glatman Zaretsky A, Andrade-Oliveira V, Collins N, Dzutsev A, Shaik J, et al. White adipose tissue is a reservoir for memory T cells and promotes protective memory responses to infection. Immunity (2017) 47(6):1154–68.e6. doi: 10.1016/j.immuni.2017.11.009
71. Sidles SJ, Xiong Y, Young MRI, LaRue AC. High-fat diet alters immunogenic properties of circulating and adipose tissue-associated myeloid-derived Cd45(+)Ddr2(+) cells. Mediators Inflammation (2019) 2019:1648614. doi: 10.1155/2019/1648614
72. Van Herck MA, Vonghia L, Kwanten WJ, Julé Y, Vanwolleghem T, Ebo DG, et al. Diet reversal and immune modulation show key role for liver and adipose tissue T cells in murine nonalcoholic steatohepatitis. Cell Mol Gastroenterol Hepatol (2020) 10(3):467–90. doi: 10.1016/j.jcmgh.2020.04.010
73. Bapat SP, Whitty C, Mowery CT, Liang Y, Yoo A, Jiang Z, et al. Obesity alters pathology and treatment response in inflammatory disease. Nature (2022) 604(7905):337–42. doi: 10.1038/s41586-022-04536-0
74. Song J, Deng T. The adipocyte and adaptive immunity. Front Immunol (2020) 11:593058. doi: 10.3389/fimmu.2020.593058
75. Deng T, Lyon CJ, Minze LJ, Lin J, Zou J, Liu JZ, et al. Class ii major histocompatibility complex plays an essential role in obesity-induced adipose inflammation. Cell Metab (2013) 17(3):411–22. doi: 10.1016/j.cmet.2013.02.009
76. Xiao L, Yang X, Lin Y, Li S, Jiang J, Qian S, et al. Large Adipocytes function as antigen-presenting cells to activate Cd4(+) T cells Via upregulating mhcii in obesity. Int J Obes (2005) (2016) 40(1):112–20. doi: 10.1038/ijo.2015.145
77. Liu B, Yu H, Sun G, Sun X, Jin H, Zhang C, et al. Ox40 promotes obesity-induced adipose inflammation and insulin resistance. Cell Mol Life Sci (2017) 74(20):3827–40. doi: 10.1007/s00018-017-2552-7
78. Ouchi N, Parker JL, Lugus JJ, Walsh K. Adipokines in inflammation and metabolic disease. Nat Rev Immunol (2011) 11(2):85–97. doi: 10.1038/nri2921
79. Fasshauer M, Blüher M. Adipokines in health and disease. Trends Pharmacol Sci (2015) 36(7):461–70. doi: 10.1016/j.tips.2015.04.014
80. Jiang Z, Zhao M, Voilquin L, Jung Y, Aikio MA, Sahai T, et al. Isthmin-1 is an adipokine that promotes glucose uptake and improves glucose tolerance and hepatic steatosis. Cell Metab (2021) 33(9):1836–52.e11. doi: 10.1016/j.cmet.2021.07.010
81. He S, Ryu J, Liu J, Luo H, Lv Y, Langlais PR, et al. Lrg1 is an adipokine that mediates obesity-induced hepatosteatosis and insulin resistance. J Clin Invest (2021) 131(24):e148545. doi: 10.1172/jci148545
82. Naylor C, Petri WA Jr. Leptin regulation of immune responses. Trends Mol Med (2016) 22(2):88–98. doi: 10.1016/j.molmed.2015.12.001
83. Chuang HC, Sheu WH, Lin YT, Tsai CY, Yang CY, Cheng YJ, et al. Hgk/Map4k4 deficiency induces Traf2 stabilization and Th17 differentiation leading to insulin resistance. Nat Commun (2014) 5:4602. doi: 10.1038/ncomms5602
84. Villarreal-Calderón JR, Castillo EC, Cuellar-Tamez RX, García-Garza M, Elizondo-Montemayor L, García-Rivas G. Reduced Th1 response is associated with lower glycolytic activity in activated peripheral blood mononuclear cells after metabolic and bariatric surgery. J endocrinological Invest (2021) 44(12):2819–30. doi: 10.1007/s40618-021-01587-4
85. Tsigalou C, Vallianou N, Dalamaga M. Autoantibody production in obesity: Is there evidence for a link between obesity and autoimmunity? Curr Obes Rep (2020) 9(3):245–54. doi: 10.1007/s13679-020-00397-8
86. Liu W, Zeng Q, Zhou L, Li Y, Chen Y, Luo R. Leptin/Osteopontin axis contributes to enhanced T helper 17 type responses in allergic rhinitis. Pediatr Allergy Immunol Off Publ Eur Soc Pediatr Allergy Immunol (2018) 29(6):622–9. doi: 10.1111/pai.12926
87. Wen Y, Zeng Q, Luo X, Ma R, Tang Y, Liu W. Leptin promoted il-17 production from Ilc2s in allergic rhinitis. Mediators Inflammation (2020) 2020:9248479. doi: 10.1155/2020/9248479
88. Tsang JY, Li D, Ho D, Peng J, Xu A, Lamb J, et al. Novel immunomodulatory effects of adiponectin on dendritic cell functions. Int Immunopharmacol (2011) 11(5):604–9. doi: 10.1016/j.intimp.2010.11.009
89. Esser N, Legrand-Poels S, Piette J, Scheen AJ, Paquot N. Inflammation as a link between obesity, metabolic syndrome and type 2 diabetes. Diabetes Res Clin Pract (2014) 105(2):141–50. doi: 10.1016/j.diabres.2014.04.006
90. Zhang K, Guo Y, Ge Z, Zhang Z, Da Y, Li W, et al. Adiponectin suppresses T helper 17 cell differentiation and limits autoimmune cns inflammation Via the Sirt1/Pparγ/Rorγt pathway. Mol Neurobiol (2017) 54(7):4908–20. doi: 10.1007/s12035-016-0036-7
91. Guzik TJ, Skiba DS, Touyz RM, Harrison DG. The role of infiltrating immune cells in dysfunctional adipose tissue. Cardiovasc Res (2017) 113(9):1009–23. doi: 10.1093/cvr/cvx108
92. Walcher D, Hess K, Berger R, Aleksic M, Heinz P, Bach H, et al. Resistin: A newly identified chemokine for human Cd4-positive lymphocytes. Cardiovasc Res (2010) 85(1):167–74. doi: 10.1093/cvr/cvp278
93. Fantuzzi G. Adipose tissue, adipokines, and inflammation. J Allergy Clin Immunol (2005) 115(5):911–9. doi: 10.1016/j.jaci.2005.02.023
94. Yang XO, Pappu BP, Nurieva R, Akimzhanov A, Kang HS, Chung Y, et al. T Helper 17 lineage differentiation is programmed by orphan nuclear receptors ror alpha and ror gamma. Immunity (2008) 28(1):29–39. doi: 10.1016/j.immuni.2007.11.016
95. Blüher M. Obesity: Global epidemiology and pathogenesis. Nat Rev Endocrinol (2019) 15(5):288–98. doi: 10.1038/s41574-019-0176-8
96. Ahmed M, Gaffen SL. Il-17 in obesity and adipogenesis. Cytokine Growth factor Rev (2010) 21(6):449–53. doi: 10.1016/j.cytogfr.2010.10.005
97. Villarroya F, Cereijo R, Gavaldà-Navarro A, Villarroya J, Giralt M. Inflammation of Brown/Beige adipose tissues in obesity and metabolic disease. J Internal Med (2018) 284(5):492–504. doi: 10.1111/joim.12803
98. Endo Y, Yokote K, Nakayama T. The obesity-related pathology and Th17 cells. Cell Mol Life Sci (2017) 74(7):1231–45. doi: 10.1007/s00018-016-2399-3
99. Pandolfi JB, Ferraro AA, Sananez I, Gancedo MC, Baz P, Billordo LA, et al. Atp-induced inflammation drives tissue-resident Th17 cells in metabolically unhealthy obesity. J Immunol (Baltimore Md 1950) (2016) 196(8):3287–96. doi: 10.4049/jimmunol.1502506
100. Fabbrini E, Cella M, McCartney SA, Fuchs A, Abumrad NA, Pietka TA, et al. Association between specific adipose tissue Cd4+ T-cell populations and insulin resistance in obese individuals. Gastroenterology (2013) 145(2):366–74.e1-3. doi: 10.1053/j.gastro.2013.04.010
101. Touch S, Clément K, André S. T Cell populations and functions are altered in human obesity and type 2 diabetes. Curr Diabetes Rep (2017) 17(9):81. doi: 10.1007/s11892-017-0900-5
102. Zhang S, Gang X, Yang S, Cui M, Sun L, Li Z, et al. The alterations in and the role of the Th17/Treg balance in metabolic diseases. Front Immunol (2021) 12:678355. doi: 10.3389/fimmu.2021.678355
103. Nicholas DA, Proctor EA, Agrawal M, Belkina AC, Van Nostrand SC, Panneerseelan-Bharath L, et al. Fatty acid metabolites combine with reduced Β oxidation to activate Th17 inflammation in human type 2 diabetes. Cell Metab (2019) 30(3):447–61.e5. doi: 10.1016/j.cmet.2019.07.004
104. Yang X, Mathis BJ, Huang Y, Li W, Shi Y. Klf4 promotes diabetic chronic wound healing by suppressing Th17 cell differentiation in an mdsc-dependent manner. J Diabetes Res (2021) 2021:7945117. doi: 10.1155/2021/7945117
105. Bertola A, Ciucci T, Rousseau D, Bourlier V, Duffaut C, Bonnafous S, et al. Identification of adipose tissue dendritic cells correlated with obesity-associated insulin-resistance and inducing Th17 responses in mice and patients. Diabetes (2012) 61(9):2238–47. doi: 10.2337/db11-1274
106. Gilleron J, Bouget G, Ivanov S, Meziat C, Ceppo F, Vergoni B, et al. Rab4b deficiency in T cells promotes adipose Treg/Th17 imbalance, adipose tissue dysfunction, and insulin resistance. Cell Rep (2018) 25(12):3329–41.e5. doi: 10.1016/j.celrep.2018.11.083
107. Lee M, Song SJ, Choi MS, Yu R, Park T. Il-7 receptor deletion ameliorates diet-induced obesity and insulin resistance in mice. Diabetologia (2015) 58(10):2361–70. doi: 10.1007/s00125-015-3684-7
108. Feldmann HM, Golozoubova V, Cannon B, Nedergaard J. Ucp1 ablation induces obesity and abolishes diet-induced thermogenesis in mice exempt from thermal stress by living at thermoneutrality. Cell Metab (2009) 9(2):203–9. doi: 10.1016/j.cmet.2008.12.014
109. Sakamoto T, Nitta T, Maruno K, Yeh YS, Kuwata H, Tomita K, et al. Macrophage infiltration into obese adipose tissues suppresses the induction of Ucp1 level in mice. Am J Physiol Endocrinol Metab (2016) 310(8):E676–e87. doi: 10.1152/ajpendo.00028.2015
110. Omran F, Christian M. Inflammatory signaling and brown fat activity. Front Endocrinol (2020) 11:156. doi: 10.3389/fendo.2020.00156
111. Kälin S, Becker M, Ott VB, Serr I, Hosp F, Mollah MMH, et al. A Stat6/Pten axis links regulatory T cells with adipose tissue function. Cell Metab (2017) 26(3):475–92.e7. doi: 10.1016/j.cmet.2017.08.008
112. Jhun J, Woo JS, Lee SH, Jeong JH, Jung K, Hur W, et al. Grim19 impedes obesity by regulating inflammatory white fat browning and promoting Th17/Treg balance. Cells (2021) 10(1):162. doi: 10.3390/cells10010162
113. Moon J, Kim D, Kim EK, Lee SY, Na HS, Kim GN, et al. Brown adipose tissue ameliorates autoimmune arthritis Via inhibition of Th17 cells. Sci Rep (2020) 10(1):12374. doi: 10.1038/s41598-020-68749-x
114. Zhou H, Liu F. Regulation, communication, and functional roles of adipose tissue-resident Cd4(+) T cells in the control of metabolic homeostasis. Front Immunol (2018) 9:1961. doi: 10.3389/fimmu.2018.01961
115. Simons P, Valkenburg O, Stehouwer CDA, Brouwers M. Sex hormone-binding globulin: Biomarker and hepatokine? Trends Endocrinol metabolism: TEM (2021) 32(8):544–53. doi: 10.1016/j.tem.2021.05.002
116. Seo JA, Kang MC, Yang WM, Hwang WM, Kim SS, Hong SH, et al. Apolipoprotein j is a hepatokine regulating muscle glucose metabolism and insulin sensitivity. Nat Commun (2020) 11(1):2024. doi: 10.1038/s41467-020-15963-w
117. Wu T, Liu Q, Li Y, Li H, Chen L, Yang X, et al. Feeding-induced hepatokine, manf, ameliorates diet-induced obesity by promoting adipose browning Via P38 mapk pathway. J Exp Med (2021) 218(6):e20201203. doi: 10.1084/jem.20201203
118. Georgiadi A, Lopez-Salazar V, Merahbi RE, Karikari RA, Ma X, Mourão A, et al. Orphan Gpr116 mediates the insulin sensitizing effects of the hepatokine Fndc4 in adipose tissue. Nat Commun (2021) 12(1):2999. doi: 10.1038/s41467-021-22579-1
119. Lin J, Jiang X, Dong M, Liu X, Shen Q, Huang Y, et al. Hepatokine pregnancy zone protein governs the diet-induced thermogenesis through activating brown adipose tissue. Advanced Sci (Weinheim Baden-Wurttemberg Germany) (2021) 8(21):e2101991. doi: 10.1002/advs.202101991
120. Markan KR, Naber MC, Ameka MK, Anderegg MD, Mangelsdorf DJ, Kliewer SA, et al. Circulating Fgf21 is liver derived and enhances glucose uptake during refeeding and overfeeding. Diabetes (2014) 63(12):4057–63. doi: 10.2337/db14-0595
121. Geng L, Lam KSL, Xu A. The therapeutic potential of Fgf21 in metabolic diseases: From bench to clinic. Nat Rev Endocrinol (2020) 16(11):654–67. doi: 10.1038/s41574-020-0386-0
122. Bao L, Yin J, Gao W, Wang Q, Yao W, Gao X. A long-acting Fgf21 alleviates hepatic steatosis and inflammation in a mouse model of non-alcoholic steatohepatitis partly through an Fgf21-Adiponectin-Il17a pathway. Br J Pharmacol (2018) 175(16):3379–93. doi: 10.1111/bph.14383
123. Li SM, Yu YH, Li L, Wang WF, Li DS. Treatment of cia mice with Fgf21 down-regulates Th17-Il-17 axis. Inflammation (2016) 39(1):309–19. doi: 10.1007/s10753-015-0251-9
124. Montgomery MK, Bayliss J, Devereux C, Bezawork-Geleta A, Roberts D, Huang C, et al. Smoc1 is a glucose-responsive hepatokine and therapeutic target for glycemic control. Sci Trans Med (2020) 12(559):eaaz8048. doi: 10.1126/scitranslmed.aaz8048
125. Ghodsian N, Gagnon E, Bourgault J, Gobeil É, Manikpurage HD, Perrot N, et al. Blood levels of the Smoc1 hepatokine are not causally linked with type 2 diabetes: A bidirectional mendelian randomization study. Nutrients (2021) 13(12):4208. doi: 10.3390/nu13124208
126. Wang J, Xia S, Zhao J, Gong C, Xi Q, Sun W. Prognostic potential of secreted modular calcium-binding protein 1 in low-grade glioma. Front Mol Biosci (2021) 8:666623. doi: 10.3389/fmolb.2021.666623
127. Chalasani N, Younossi Z, Lavine JE, Diehl AM, Brunt EM, Cusi K, et al. The diagnosis and management of non-alcoholic fatty liver disease: Practice guideline by the American gastroenterological association, American association for the study of liver diseases, and American college of gastroenterology. Gastroenterology (2012) 142(7):1592–609. doi: 10.1053/j.gastro.2012.04.001
128. Chackelevicius CM, Gambaro SE, Tiribelli C, Rosso N. Th17 involvement in nonalcoholic fatty liver disease progression to non-alcoholic steatohepatitis. World J Gastroenterol (2016) 22(41):9096–103. doi: 10.3748/wjg.v22.i41.9096
129. Taniki N, Nakamoto N, Chu PS, Ichikawa M, Teratani T, Kanai T. Th17 cells in the liver: Balancing autoimmunity and pathogen defense. Semin immunopathology (2022) 44(4):509–26. doi: 10.1007/s00281-022-00917-9
130. Moreno-Fernandez ME, Giles DA, Oates JR, Chan CC, Damen M, Doll JR, et al. Pkm2-dependent metabolic skewing of hepatic Th17 cells regulates pathogenesis of non-alcoholic fatty liver disease. Cell Metab (2021) 33(6):1187–204.e9. doi: 10.1016/j.cmet.2021.04.018
131. Rau M, Schilling AK, Meertens J, Hering I, Weiss J, Jurowich C, et al. Progression from nonalcoholic fatty liver to nonalcoholic steatohepatitis is marked by a higher frequency of Th17 cells in the liver and an increased Th17/Resting regulatory T cell ratio in peripheral blood and in the liver. J Immunol (Baltimore Md 1950) (2016) 196(1):97–105. doi: 10.4049/jimmunol.1501175
132. Gomes AL, Teijeiro A, Burén S, Tummala KS, Yilmaz M, Waisman A, et al. Metabolic inflammation-associated il-17a causes non-alcoholic steatohepatitis and hepatocellular carcinoma. Cancer Cell (2016) 30(1):161–75. doi: 10.1016/j.ccell.2016.05.020
133. Sun G, Zhao X, Li M, Zhang C, Jin H, Li C, et al. Cd4 derived double negative T cells prevent the development and progression of nonalcoholic steatohepatitis. Nat Commun (2021) 12(1):650. doi: 10.1038/s41467-021-20941-x
134. Komori T. Functions of osteocalcin in bone, pancreas, testis, and muscle. Int J Mol Sci (2020) 21(20):7513. doi: 10.3390/ijms21207513
135. Pi M, Quarles LD. Novel bone endocrine networks integrating mineral and energy metabolism. Curr osteoporosis Rep (2013) 11(4):391–9. doi: 10.1007/s11914-013-0178-8
136. Mosialou I, Shikhel S, Liu JM, Maurizi A, Luo N, He Z, et al. Mc4r-dependent suppression of appetite by bone-derived lipocalin 2. Nature (2017) 543(7645):385–90. doi: 10.1038/nature21697
137. Wang JS, Mazur CM, Wein MN. Sclerostin and osteocalcin: Candidate bone-produced hormones. Front Endocrinol (2021) 12:584147. doi: 10.3389/fendo.2021.584147
138. Anam AK, Insogna K. Update on osteoporosis screening and management. Med Clinics North America (2021) 105(6):1117–34. doi: 10.1016/j.mcna.2021.05.016
139. Clowes JA, Riggs BL, Khosla S. The role of the immune system in the pathophysiology of osteoporosis. Immunol Rev (2005) 208:207–27. doi: 10.1111/j.0105-2896.2005.00334.x
140. Zhu L, Hua F, Ding W, Ding K, Zhang Y, Xu C. The correlation between the Th17/Treg cell balance and bone health. Immun Ageing I A (2020) 17:30. doi: 10.1186/s12979-020-00202-z
141. Chen S, Guo C, Wang R, Feng Z, Liu Z, Wu L, et al. Monocytic mdscs skew Th17 cells toward a pro-osteoclastogenic phenotype and potentiate bone erosion in rheumatoid arthritis. Rheumatol (Oxford England) (2021) 60(5):2409–20. doi: 10.1093/rheumatology/keaa625
142. Talaat RM, Sidek A, Mosalem A, Kholief A. Effect of bisphosphonates treatment on cytokine imbalance between Th17 and treg in osteoporosis. Inflammopharmacology (2015) 23(2-3):119–25. doi: 10.1007/s10787-015-0233-4
143. Zhao R. Immune regulation of bone loss by Th17 cells in oestrogen-deficient osteoporosis. Eur J Clin Invest (2013) 43(11):1195–202. doi: 10.1111/eci.12158
144. Redlich K, Smolen JS. Inflammatory bone loss: Pathogenesis and therapeutic intervention. Nat Rev Drug Discovery (2012) 11(3):234–50. doi: 10.1038/nrd3669
145. Bhadricha H, Patel V, Singh AK, Savardekar L, Patil A, Surve S, et al. Increased frequency of Th17 cells and il-17 levels are associated with low bone mineral density in postmenopausal women. Sci Rep (2021) 11(1):16155. doi: 10.1038/s41598-021-95640-0
146. Sapra L, Dar HY, Bhardwaj A, Pandey A, Kumari S, Azam Z, et al. Lactobacillus rhamnosus attenuates bone loss and maintains bone health by skewing treg-Th17 cell balance in ovx mice. Sci Rep (2021) 11(1):1807. doi: 10.1038/s41598-020-80536-2
147. Honma M, Ikebuchi Y, Suzuki H. Rankl as a key figure in bridging between the bone and immune system: Its physiological functions and potential as a pharmacological target. Pharmacol Ther (2021) 218:107682. doi: 10.1016/j.pharmthera.2020.107682
148. Kong YY, Feige U, Sarosi I, Bolon B, Tafuri A, Morony S, et al. Activated T cells regulate bone loss and joint destruction in adjuvant arthritis through osteoprotegerin ligand. Nature (1999) 402(6759):304–9. doi: 10.1038/46303
149. Srivastava RK, Dar HY, Mishra PK. Immunoporosis: Immunology of osteoporosis-role of T cells. Front Immunol (2018) 9:657. doi: 10.3389/fimmu.2018.00657
150. Okamoto K, Takayanagi H. Regulation of bone by the adaptive immune system in arthritis. Arthritis Res Ther (2011) 13(3):219. doi: 10.1186/ar3323
151. Deng J, Lu C, Zhao Q, Chen K, Ma S, Li Z. The Th17/Treg cell balance: Crosstalk among the immune system, bone and microbes in periodontitis. J periodontal Res (2022) 57(2):246–55. doi: 10.1111/jre.12958
152. Stary G, Olive A, Radovic-Moreno AF, Gondek D, Alvarez D, Basto PA, et al. Vaccines. A mucosal vaccine against chlamydia trachomatis generates two waves of protective memory T cells. Science (2015) 348(6241):aaa8205. doi: 10.1126/science.aaa8205
153. Hirota K, Duarte JH, Veldhoen M, Hornsby E, Li Y, Cua DJ, et al. Fate mapping of il-17-Producing T cells in inflammatory responses. Nat Immunol (2011) 12(3):255–63. doi: 10.1038/ni.1993
154. Rosendo-Silva D, Matafome P. Gut-adipose tissue crosstalk: A bridge to novel therapeutic targets in metabolic syndrome? Obes Rev an Off J Int Assoc Study Obes (2021) 22(2):e13130. doi: 10.1111/obr.13130
155. Luck H, Tsai S, Chung J, Clemente-Casares X, Ghazarian M, Revelo XS, et al. Regulation of obesity-related insulin resistance with gut anti-inflammatory agents. Cell Metab (2015) 21(4):527–42. doi: 10.1016/j.cmet.2015.03.001
156. Scheithauer TPM, Rampanelli E, Nieuwdorp M, Vallance BA, Verchere CB, van Raalte DH, et al. Gut microbiota as a trigger for metabolic inflammation in obesity and type 2 diabetes. Front Immunol (2020) 11:571731. doi: 10.3389/fimmu.2020.571731
157. Gomes AC, Hoffmann C, Mota JF. The human gut microbiota: Metabolism and perspective in obesity. Gut Microbes (2018) 9(4):308–25. doi: 10.1080/19490976.2018.1465157
158. Dabke K, Hendrick G, Devkota S. The gut microbiome and metabolic syndrome. J Clin Invest (2019) 129(10):4050–7. doi: 10.1172/jci129194
159. Garidou L, Pomié C, Klopp P, Waget A, Charpentier J, Aloulou M, et al. The gut microbiota regulates intestinal Cd4 t cells expressing rorγt and controls metabolic disease. Cell Metab (2015) 22(1):100–12. doi: 10.1016/j.cmet.2015.06.001
160. Tilg H, Zmora N, Adolph TE, Elinav E. The intestinal microbiota fuelling metabolic inflammation. Nat Rev Immunol (2020) 20(1):40–54. doi: 10.1038/s41577-019-0198-4
161. Pérez MM, Martins LMS, Dias MS, Pereira CA, Leite JA, Gonçalves ECS, et al. Interleukin-17/Interleukin-17 receptor axis elicits intestinal neutrophil migration, restrains gut dysbiosis and lipopolysaccharide translocation in high-fat diet-induced metabolic syndrome model. Immunology (2019) 156(4):339–55. doi: 10.1111/imm.13028
162. Carlos D, Pérez MM, Leite JA, Rocha FA, Martins LMS, Pereira CA, et al. Nod2 deficiency promotes intestinal Cd4+ T lymphocyte imbalance, metainflammation, and aggravates type 2 diabetes in murine model. Front Immunol (2020) 11:1265. doi: 10.3389/fimmu.2020.01265
163. Hong CP, Park A, Yang BG, Yun CH, Kwak MJ, Lee GW, et al. Gut-specific delivery of T-helper 17 cells reduces obesity and insulin resistance in mice. Gastroenterology (2017) 152(8):1998–2010. doi: 10.1053/j.gastro.2017.02.016
164. Wiest R, Albillos A, Trauner M, Bajaj JS, Jalan R. Targeting the gut-liver axis in liver disease. J Hepatol (2017) 67(5):1084–103. doi: 10.1016/j.jhep.2017.05.007
165. Balmer ML, Slack E, de Gottardi A, Lawson MA, Hapfelmeier S, Miele L, et al. The liver may act as a firewall mediating mutualism between the host and its gut commensal microbiota. Sci Trans Med (2014) 6(237):237ra66. doi: 10.1126/scitranslmed.3008618
166. Chu S, Sun R, Gu X, Chen L, Liu M, Guo H, et al. Inhibition of sphingosine-1-Phosphate-Induced Th17 cells ameliorates alcohol-associated steatohepatitis in mice. Hepatol (Baltimore Md) (2021) 73(3):952–67. doi: 10.1002/hep.31321
167. Little R, Wine E, Kamath BM, Griffiths AM, Ricciuto A. Gut microbiome in primary sclerosing cholangitis: A review. World J Gastroenterol (2020) 26(21):2768–80. doi: 10.3748/wjg.v26.i21.2768
168. Nakamoto N, Sasaki N, Aoki R, Miyamoto K, Suda W, Teratani T, et al. Gut pathobionts underlie intestinal barrier dysfunction and liver T helper 17 cell immune response in primary sclerosing cholangitis. Nat Microbiol (2019) 4(3):492–503. doi: 10.1038/s41564-018-0333-1
169. Li B, Selmi C, Tang R, Gershwin ME, Ma X. The microbiome and autoimmunity: A paradigm from the gut-liver axis. Cell Mol Immunol (2018) 15(6):595–609. doi: 10.1038/cmi.2018.7
170. Grant AJ, Lalor PF, Salmi M, Jalkanen S, Adams DH. Homing of mucosal lymphocytes to the liver in the pathogenesis of hepatic complications of inflammatory bowel disease. Lancet (London England) (2002) 359(9301):150–7. doi: 10.1016/s0140-6736(02)07374-9
171. Henriksen EK, Jørgensen KK, Kaveh F, Holm K, Hamm D, Olweus J, et al. Gut and liver T-cells of common clonal origin in primary sclerosing cholangitis-inflammatory bowel disease. J Hepatol (2017) 66(1):116–22. doi: 10.1016/j.jhep.2016.09.002
172. Graham JJ, Mukherjee S, Yuksel M, Sanabria Mateos R, Si T, Huang Z, et al. Aberrant hepatic trafficking of gut-derived T cells is not specific to primary sclerosing cholangitis. Hepatol (Baltimore Md) (2022) 75(3):518–30. doi: 10.1002/hep.32193
173. Su L, Wu Z, Chi Y, Song Y, Xu J, Tan J, et al. Mesenteric lymph node Cd4(+) T lymphocytes migrate to liver and contribute to non-alcoholic fatty liver disease. Cell Immunol (2019) 337:33–41. doi: 10.1016/j.cellimm.2019.01.005
174. Yan J, Charles JF. Gut microbiome and bone: To build, destroy, or both? Curr osteoporosis Rep (2017) 15(4):376–84. doi: 10.1007/s11914-017-0382-z
175. Ding K, Hua F, Ding W. Gut microbiome and osteoporosis. Aging Dis (2020) 11(2):438–47. doi: 10.14336/ad.2019.0523
176. Lorenzo J. From the gut to bone: Connecting the gut microbiota with Th17 T lymphocytes and postmenopausal osteoporosis. J Clin Invest (2021) 131(5):e146619. doi: 10.1172/jci146619
177. Yu M, Pal S, Paterson CW, Li JY, Tyagi AM, Adams J, et al. Ovariectomy induces bone loss Via microbial-dependent trafficking of intestinal tnf+ T cells and Th17 cells. J Clin Invest (2021) 131(4):e143137. doi: 10.1172/jci143137
178. Jia L, Tu Y, Jia X, Du Q, Zheng X, Yuan Q, et al. Probiotics ameliorate alveolar bone loss by regulating gut microbiota. Cell proliferation (2021) 54(7):e13075. doi: 10.1111/cpr.13075
179. Pacifici R. Role of gut microbiota in the skeletal response to pth. J Clin Endocrinol Metab (2021) 106(3):636–45. doi: 10.1210/clinem/dgaa895
180. Yu M, Malik Tyagi A, Li JY, Adams J, Denning TL, Weitzmann MN, et al. Pth induces bone loss Via microbial-dependent expansion of intestinal tnf(+) T cells and Th17 cells. Nat Commun (2020) 11(1):468. doi: 10.1038/s41467-019-14148-4
181. Tyagi AM, Darby TM, Hsu E, Yu M, Pal S, Dar H, et al. The gut microbiota is a transmissible determinant of skeletal maturation. Elife (2021) 10:e64237. doi: 10.7554/eLife.64237
Keywords: Th17 cells, gut, adipose tissue, liver, bone, endocrine organs.
Citation: Zi C, Wang D, Gao Y and He L (2023) The role of Th17 cells in endocrine organs: Involvement of the gut, adipose tissue, liver and bone. Front. Immunol. 13:1104943. doi: 10.3389/fimmu.2022.1104943
Received: 22 November 2022; Accepted: 28 December 2022;
Published: 16 January 2023.
Edited by:
Lifei Hou, Harvard Medical School, United StatesReviewed by:
Haopeng Yang, University of Texas MD Anderson Cancer Center, United StatesJingjing Chen, Dana–Farber Cancer Institute, United States
Copyright © 2023 Zi, Wang, Gao and He. This is an open-access article distributed under the terms of the Creative Commons Attribution License (CC BY). The use, distribution or reproduction in other forums is permitted, provided the original author(s) and the copyright owner(s) are credited and that the original publication in this journal is cited, in accordance with accepted academic practice. No use, distribution or reproduction is permitted which does not comply with these terms.
*Correspondence: Yongxiang Gao, ZHJnYW95eEBjZHV0Y20uZWR1LmNu; Lisha He, anFoZWxpc2hhQDE2My5jb20=
†These authors have contributed equally to this work