- 1Department of Clinical Veterinary Medicine, College of Veterinary Medicine, Jilin University, Changchun, China
- 2Portsmouth Abbey School, Portsmouth, RI, United States
- 3Key Laboratory for Zoonosis Research, Ministry of Education, College of Veterinary Medicine, Jilin University, Changchun, China
African swine fever (ASF), a highly contagious, deadly infectious disease, has caused huge economic losses to animal husbandry with a 100% mortality rate of the most acute and acute infection, which is listed as a legally reported animal disease by the World Organization for Animal Health (OIE). African swine fever virus (ASFV) is the causative agent of ASF, which is the only member of the Asfarviridae family. Ornithodoros soft ticks play an important role in ASFV transmission by active biological or mechanical transmission or by passive transport or ingestion, particularly in Africa, Europe, and the United States. First, this review summarized recent reports on (1) tick species capable of transmitting ASFV, (2) the importance of ticks in the transmission and epidemiological cycle of ASFV, and (3) the ASFV strains of tick transmission, to provide a detailed description of tick-borne ASFV. Second, the dynamics of tick infection with ASFV and the tick-induced immune suppression were further elaborated to explain how ticks spread ASFV. Third, the development of the anti-tick vaccine was summarized, and the prospect of the anti-tick vaccine was recapitulated. Then, the marked attenuated vaccine, ASFV-G-ΔI177L, was compared with those of the anti-tick vaccine to represent potential therapeutic or strategies to combat ASF.
Introduction
African swine fever (ASF) is a very serious contagious disease prevalent in pigs. The clinical symptoms of African swine fever virus (ASFV)-infected pigs include high fever, vomiting, diarrhea, skin hemorrhages, cyanosis, and abortion in pregnant sows, resulting in a mortality rate of up to 100% in acute ASF (1). Subacute and chronic forms emerge as respiratory signs, intermittent fever, chronic skin ulcers, and arthritis, which are caused by lower virulent strains and exhibit lower mortality rates. ASF infection in domestic pigs causes significant direct economic losses and associated indirect economic losses, such as trade restrictions. Since it was tested in Liaoning province in 2018, ASFV has spread to all mainland provinces in China, causing local pork prices to rise (2, 3) and global pork supply to be affected (4).
ASFV, the only known DNA arbovirus, belongs to the family Asfarviridae. ASFV is extremely stable and spreads easily through the infected swine, contamination during the trade of pig products, or blood feeding of infected Ornithodoros vector.
In the first report on ASF, Montgomery et al. speculated that the transmission of ASFV might be related to arthropod vectors, since the virus was not transmitted by contacting between warthogs and domestic pigs. Still, many outbreaks on Kenyan farms could only be attributed to warthogs, as the farms are isolated and no pigs or their products were introduced. However, the biological arthropod vectors of ASFV were not published in Spain until 1962, when Sanchez Botija (1963) confirmed that Ornithodoros erraticus could maintain and transmit ASFV (5). Following an investigation by researchers in East Africa, they demonstrated ASFV infection in Ornithodoros moubata complex in warthog-occupied animal burrows in Tanzania (6). Plowright et al. demonstrated ASFV proliferation, transstadial transmission, and transovarial transmission in the ticks and sexual transmission, showing that ticks could be competent vectors of the ASFV (7–10). Based on the common characteristics of Iridoviridae with ASFV, Plowright et al. suggested that ASFV may have originated from arthropod vectors and evolved in them (6). A recent molecular study supported this opinion by demonstrating ASFV genome-like segments existing in archived specimens of the O. moubata complex. Many studies have shown that ticks were important to the transmission of ASFV, and it might contribute to ASFV evolution (11).
Since Ornithodoros play an essential role in ASFV transmission, an anti-tick vaccine could be an alternative to prevent ASF in the absence of commercially available anti-ASFV vaccines. Therefore, a large number of researchers have examined the immune effects of tick components, starting with mixed proteins such as egg vitellin, salivary gland extract, and midgut protein extract, and later with recombinant single proteins. Although there are many kinds of candidate proteins affecting the life cycle of ticks, the ideal anti-tick vaccine antigen has not been screened. It was not until 3 June 2022 that the launch of ASFV-G-ΔI177L became a boon for the pig industry against ASFV. So far, whether anti-tick vaccines prevent ASF needs to be further investigated.
Here, we will summarize the role of ticks in ASFV transmission to evaluate the necessity of anti-tick vaccine development and further compare its advantages and disadvantages with the anti-ASFV vaccine, providing direction and ideas for the prevention of ASF.
Tick species capable of transmitting ASFV
Argasidae
It was demonstrated that Ornithodoros is the only biological ASFV vector (12). Soft ticks repeatedly take blood meals in both the nymph stage and the adult stage, during which both biological and mechanical transmission of pathogens may be involved. Not all Argasidae can transmit ASFV. According to the epidemiological investigation and laboratory infection research results, it has been confirmed that eight taxa had the ability to transmit ASFV, including Ornithodoros marocanus, Ornithodoros moubata porcinus, Ornithodoros puertoricensis, O. erraticus, Ornithodoros moubata complex, Ornithodoros turicata, Ornithodoros savignyi, and Ornithodoros coriaceus (13).
Ornithodoros erraticus was the first tick association with ASFV made by Sanchez-Botija and was later recognized as a key factor in maintaining the enzootic cycle of ASFV in the Iberian Peninsula. Ornithodoros moubata porcinus was identified to transmit ASFV and successful transmit ASFV 469 days post-infection (dpi) through experimental infection (8). Similarly, experimental infections have demonstrated that other Ornithodoros can transmit ASFV, including O. savignyi (14), O. coriaceus (15, 16), O. turicata (16), and O. puertoricensis (16, 17). Ornithodoros marocanus could transmit ASFV out to 588 days post-infection (dpi), although infected ticks had a 73% mortality (18, 19). ASFV was isolated from O. moubata collected in domestic pig sties and houses in certain villages in Mchinji district where there was ASF outbreaks, demonstrating that O. moubata can act as a reservoir and potential vector of ASFV (20). Due to transovarial, transstadial, or sexual transmission of ASFV, the tick served as reservoir of ASFV (21).
Ixodidae
Hard ticks or Ixodidae are one of the most important vectors of veterinary significance in the world. Several species, including Dermacentor reticulatus and Ixodes ricinus, have been assessed for transmitting ASFV by hard ticks. European hard (ixodid) ticks might be a possible vector of ASFV, as ASFV DNA could be measured for 6 weeks or up to 8 weeks in infected I. ricinus or D. reticulatus, respectively (22).
Although DNA segments of ASFV were detected in Dermacentor ticks (23), there is no evidence that it can carry and transmit ASFV (24). The investigation showed that the European hard ticks is not a risk factor of biological transmission of ASFV in the Baltic States (25). Although hard ticks cannot be considered a mechanical vector for ASFV because they only take blood once at each stage, ASFV could be detected within a few days of experimental infection and transmitted orally in some tick species (26). The worry is that infected hard ticks can transmit ASFV over long distances because they attached to a host for a long time.
The role of ticks in the transmission and epidemiological cycle of ASFV
Generally, ASFV transmission occurs through direct contact with infected animals or contaminants or through the bite of a soft tick. Four epidemiological cycles described by Chenais summarize the transmission of ASFV (27). Sylvatic cycle was observed in eastern and southern Africa where the virus was transmitted between warthogs and O. moubata complex (28). Tick–pig cycle mainly occurs in sub-Saharan Africa and Iberian Peninsula (29). Transmission during domestic cycle was only linked with domestic pigs, without the presence of infected free-living pigs or tick vector, such as West Africa and Brazil (30, 31). In wild boar–habitat cycle, ASFV transmission depends on carcasses in the habitat (27). This chapter will detail the role of soft ticks in ASFV epidemiological cycles.
Sylvatic cycle
Warthogs (Phacochoerus africanus), the primary sylvatic host, are asymptomatic but are reservoirs of ASFV. In Africa, the cross-transmission of ASFV between warthogs and soft ticks is a major cause of sylvatic cycle, which is mainly recorded in southern and eastern African countries (Figure 1) (32, 33). ASFV transmission occurs between juvenile warthogs and ticks when the ticks were taking blood meals (6, 34). Experimental infected young warthog with ASFV developed viremias between 102 and 106 HAD50/ml and high virus concentrations in some lymphatic tissues (higher than or equal to 106 HAD50/g) within the first week after infection. The ASFV titer in lymph nodes did not decrease significantly and kept domestic pigs infectious within 33 dpi (35). The sylvatic cycle occurred in eastern and southern Africa where ASFV was transmitted between O. moubata complex and warthogs (28). Because the bush pig’s behavior is less conducive to interaction with soft ticks, it is generally thought to play a smaller role in the sylvatic cycle than the warthog, although it can also be infected and transmit ASFV (32, 33, 36, 37).
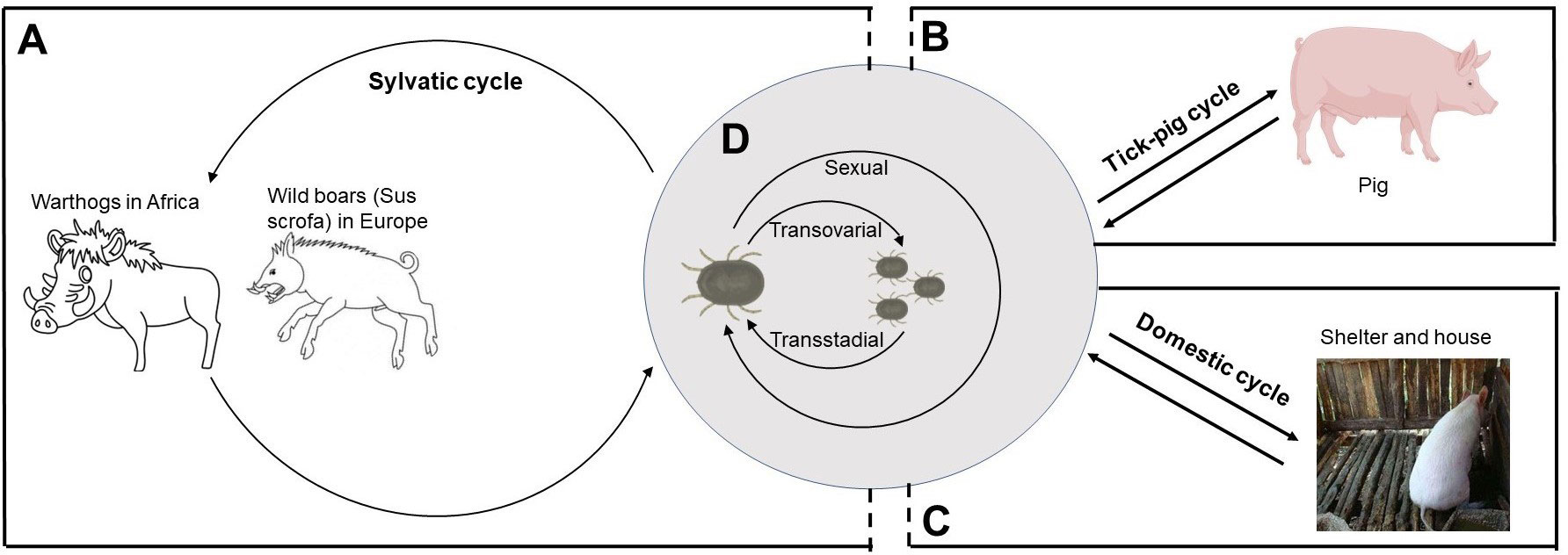
Figure 1 The role of Ornithodoros species in ASFV transmission cycles. (A) The sylvatic cycle occurs in Africa and Europe where ASFV is transmitted between Ornithodoros species and warthogs or wild boars. (B) In sub-Saharan Africa and Iberian Peninsula, tick–pig cycle occurs in areas where there is little or no contact between wild and domestic swine. (C) In domestic cycle, ASFV-infected ticks usually hide in shelters and houses during the days and have a blood meal at night. (D) In Ornithodoros species, ASFV is transmitted by transovarial, transstadial, and sexual routes.
Similarly, the sylvatic cycle was present in Europe, which involved O. erraticus and wild boar (Sus scrofa) (Figure 1) (33). However, unlike the African system, ASFV may persist in wild and domestic pig populations through horizontal transmission without soft ticks (38).
Tick–pig cycle
The tick–pig cycle, described mainly in sub-Saharan Africa, is the best evidence that ticks are important risk factors of ASFV transmission (Figure 1). In Africa and Madagascar, the isolation of infected O. moubata complex in areas with ASF outbreaks suggested that soft ticks were a key risk factor of ASFV maintenance (20, 39, 40). In the Iberian Peninsula, it is also observed that O. erraticus were linked with ASFV maintenance (29, 41–43). Among the risk factors for the 2018–2020 ASF outbreak in China reviewed by Cheng et al., swill feeding, live pig transport, and vehicles were listed as the important risk factors (1). Although there is no evidence to link the outbreak of ASF in China to ticks, soft ticks are still classified as a low risk of ASF outbreak (1). In particular, according to the model prediction of Li et al., the southeast coast or central region of China is suitable for ASF distribution, and its environment is suitable for soft ticks, indicating that the tick–pig cycle may promote the outbreak of ASF in China (44).
Domestic cycle
In the 1980s, there were regular outbreaks of ASF in domestic pigs in Malawi with a low mortality rate, which was called domestic pig–tick cycle since no warthogs or bushpigs in the region were negative for ASFV (20, 45). In shelters of pigs or house of humans, ticks could be found at night but not at day, whose infection rates were comparable with warthog burrows in South Africa, East Africa, and Namibia (Figure 1) (20).
The ASFV strains of tick transmission
ASFV was classified into 8 serogroups and 24 genotypes based on antibody-mediated hemadsorption inhibition and the B646L gene, respectively (46). Here, we summarized the ASFV strains of experimental transmission by the eight ticks mentioned above.
Ornithodoros marocanus larvae became infected with ASFV, isolated from an infected domestic pig in Portugal in 1986, by feeding on a viremia pig. Although virus titers decreased with tick development, adult ticks were still able to transmit ASFV to susceptible pigs 588 days later and could recover ASFV 655 days after the infective blood meal (Table 1) (19). Experimentally infected O. puertoricensis second-instar nymphs with the Dominican Republic isolate (DR-II) of ASFV could transmit virus 239 days after infection (Table 1) (17). Hess et al. demonstrated that it was able to transmit ASFV transstadially and transovarially (Table 1) (16). Ornithodoros coriaceus can be infected with ASFV strain Tengani, Z1, and DR2 together with different mortalities, and infection with the DR2 persisted for 502 days (Table 1) (16). Ornithodoros coriaceus can pass ASFV transstadially but not transovarially (Table 1) (15, 16). Ornithodoros moubata porcinus-transmitted ASFV strains include Z1, Uganda, Tengani, Chiredzi/83/1 (Ch1), Pretoriuskop/96/4/1 (Pr4), Crocodile/96/1 (Cr1), and Nooitverwacht/96/6 (No6), with at least 70% of ticks multiplying and persisting for 13–15 months over “Uganda” strain virus (Table 1) (8, 16, 47). Sexual transmission, stadial transmission, and ovarial transmission of ASFV were involved in O. moubata porcinus (Table 1) (10, 16). ASFV strains Tomar 87, OUR T88/1, and ASFV/P99 isolate were able to be transmitted by O. erraticus, which harbored ASFV and transmitted it to pigs for at least 588 days after infection (Table 1) (43, 48). ASFV strains CHZ T90/1, SIN T90/1, and VIC T90/1, isolated from O. moubata, together with Georgia 2007/1 and Ukr12/Zapo isolated from domestic pigs, were able to be transmitted by O. moubata (Table 1) (37, 49, 50). Ornithodoros moubata could transmit Georgia2007/1 and Liv13/33 strains to naive pigs 8 months post-infection (Table 1) (50). Only O. moubata has been shown to transovarial transmit ASFV (Table 1) (51). Infected O. turicata transmitted ASFV to susceptible pigs 23 days later (Table 1) (16). Infected O. savignyi can transstadially transmit ASFV and maintain it for 106 days or more (Table 1) (14).
The dynamics of ASFV-infected tick
ASFV can continuously infect natural hosts and domestic pigs, which is of great significance in epidemiological studies. As an important transmission medium of ASF, the study of ASFV infection dynamics in ticks has attracted many researchers’ interests. Here, O. porcinus was taken as an example to describe the dynamics after ASFV infection.
ASFV infection happened when O. porcinus porcinus feed on blood of viremic pigs. After infection, the midgut was the first site of ASFV replication as determined by Greig, although there were immunofluorescence and virus titration detection of dissected tissues (52). Although the mechanism has not been reported, it could be speculated that the entry of ASFV into midgut epithelial cells may be related to erythrocyte phagocytosis because intact erythrocytes infected with ASFV were detected in the midgut epithelial cells phagolysosomes. It is important to note that most viremic pigs are related to erythrocytic fractions, and almost all field isolates of ASFV are hemadsorbing (53–57). An entry mechanism unrelated to erythrocyte phagocytosis might exist because non-hemadsorbing ASFV was isolated from O. porcinus porcinus and experimental ASFV infection could also occur in feeding on an artificial membrane with cell-free virus preparations (47, 47). Greig demonstrated that after being ingested by ticks, ASFV rapidly crosses the gut wall and infect the hemocoel, coxal sac, rectal ampulla, and salivary gland, together with ASFV recovered from the hemocoel and other tissues within 24–48 h of infection, suggesting that there was no true gut barrier to ASFV (52). However, Kleiboeker’s study showed a delay of 15–21 days for ticks to reach generalization of ASFV infection, suggesting the presence of midgut barrier (47).
After transmission and infection in multiple different tissues, the ASFV titer remained at 6 log10 HAD50/mg of body weight for 290 days of sampling period, which is closely related to that in naturally infected ticks (34, 40, 47). ASFV titers of the reproductive tissue and salivary glands rose to 5–6 log10 HAD50/mg after 91 dpi, which was the highest of any tissues (47).
Successful ASFV transmission from infected O. porcinus porcinus to pigs was associated with high titers in salivary and coxal glands and excretion virus into secretions (8). Surprisingly, coxal secretions were more ASFV positive and had higher ASFV titer than salivary secretions, showing that they were also an important source of ASFV transmission (47). Regurgitation was unlikely to be an effective transmission mechanism for ASFV because the esophageal terminus of argasid species had a proventricular valve, although the tick midgut had high titers of ASFV (58).
Tick-induced immune suppression
The skin represents the interface where ticks bite and be a site of tick-borne pathogen transmission. Saliva or salivary glands (SG) play a key role in the transmission of most tick-borne pathogens. Tick saliva is a critical biological cocktail that inhibits host defenses and promotes blood flow, containing a large number of molecules involved in cytolytic, anti-coagulant, anti-inflammatory, anti-chemokine, anti-pain, and vasodilating activities (59).
Tick saliva has the ability to suppress innate immune response, complement system, and adaptive immunity of host. In terms of innate immunity, tick saliva strongly suppresses the recruitment of blood-borne innate immune cells. Evasin-1, evasin-2, and evasin-3 are chemokine-binding proteins from Rhipicephalus sanguineus SG, which present selectively for different chemokines (60, 61). Ir-LBP, a lipocalin from I. ricinus, interfered with neutrophil chemotaxi and activation (62). The saliva of several tick species also contains macrophage migration inhibitory factor (MIF), which inhibit migration of macrophages (63, 64). Tick saliva also inhibits inflammation by reducing or enhancing pro- or anti-inflammatory cytokines secretion, respectively. Hyalomin-A and hyalomin-B exerted significant anti-inflammatory functions by inhibiting the secretion of C–C motif chemokine ligand 2 (CCL2), tumor necrosis factor alpha (TNF-α), and interferon gamma (IFN-γ) and increasing the secretion of interleukin (IL)-10 (65). PGE2 and purine nucleoside adenosine (Ado) also impaired the production of IL-12p40 and TNF-α and increased IL-10 level by murine DC (66).
The complement system links host innate and adaptive immune responses. IRAC I and II from I. ricinus inhibited C3 convertase formation of the alternative pathway through blocking complement factor B binding to complement C3b (67, 68). Ornithodoros moubata OmCI, TSGP2, and TSGP3 specifically target C5 activation (69, 70). Salp14 and Salp9Pac from I. scapularis inhibited mannan-binding lectin binding to the polysaccharide mannan, preventing complement pathway activation (71).
Tick saliva also suppressed the adaptive immunity, including cellular immune response and humoral immune response (72). Iripin-3 from I. ricinus reduced IL-6 production by MPs and reduced T-helper type 1 immune response (73). Sialostatin L (SialoL) from I. scapularis reduced IFN-γ and IL-17 production and impaired specific T-cell proliferation (74). Salp14 also inhibits pro-inflammatory cytokine production and impaired T-cell proliferation (75). Tick salivary components inhibited humoral immunity by interfering with B-cell-derived immune responses; for example, several ticks could secrete a series of IgG-binding proteins (IGBPs) to suppress specific antibodies (76).
In addition to suppressing immune responses, tick saliva has the ability to block hemostasis and mitigate itching and pain.
The development of anti-tick vaccine
ASF causes major economic losses with mortality rates approaching 100% and limiting pork production. In addition to anti-viral vaccines, anti-tick vaccine is theoretically an alternative to prevent ASF. In the last decade of the twentieth century, two commercial anti-tick vaccines (TickGARD© and GAVAC©) against Rhipicephalus microplus were developed based on the glycoprotein antigen Bm86. Regarding soft ticks, antigens that can be used as candidate vaccines are being developed to prevent tick-borne diseases (77).
To date, vaccine development for soft ticks has been significantly less studied than that for hard ticks, and only a few Ornithodoros and Argas genera species have been involved. Here, the protective effects of candidate antigen of eight soft ticks associated with ASFV transmission are summarized, which include salivary and concealed proteins.
Concealed antigens
Chinzei and Minoura verified that the egg vitellin of soft ticks was the first recorded study toward concealed antigen, which provided a protective response with 50% reduced fecundity of female tick in immunized rabbits (Table 2) (78). Manzano-Román confirmed that the membrane protein extracts of O. erraticus midgut epithelial cells had a protective efficacy in mice, pigs, and rabbits (Table 2) (79). Oe45, a 45-kDa protein, provided a protective effect on vaccinated host; the mechanism is that anti-Oe45 antibodies immobilize the host complement system and activate it on the intestinal membrane (Table 2) (79–81).
RNA interference with the subolesin gene orthologues had no effect on feeding and survival of O. moubata and O. erraticus but strongly inhibited tick oviposition, and its recombinant vaccine induced a strong but low protective humoral response (reduced oviposition by 5%–24.5%) in the host (Table 2) (82). Then, coupled keyhole limpet hemocyanin (KLH) with four synthetic peptides (OM1, OM2, OE1, and OE2), based on subolesin sequence unrecognized/disordered regions, induced specific antibodies and provided up to 83.1% or 70.1% protective effect in O. moubata or O. erraticus, respectively (Table 2) (83).
Recently, Parasitología et al. sequenced the midgut transcriptomes and proteomes of female O. moubata at two physiological conditions, namely, before feeding and 48 h post-feeding, providing a valuable research basis for screening candidate vaccine molecules (84, 85). Next, Prosper screened and recombined five of these candidate proteins (namely, Om17, Om86, OM99, OM85, and OM03), formulated with Freund S adjuvant, and evaluated their immunogenicity and protective effect by vaccinated rabbits. Although these candidate genes had low protective effect against O. moubata infection (<39%), they were more effective against O. erraticus infection (ranging from 20% to 66%). Two of the five antigens (OM03 and OM85) were considered as an effective anti-tick vaccine and worthy of further study (Table 2) (86). Ricardo selected and designed synthetic immunogenic peptides based on the four theoretical candidates, including one selenoprotein T (OeSEL), one ABC transporter (OeABC), and two aquaporins (OeAQP and OeAQP1), which induced humoral responses in vaccinated rabbit, leading to decreased feeding and fertility of tick (Table 2) (87). Each of these recombinant proteins for five theoretical candidate antigens, including one chitinase (CHI), one secreted protein PK-4 (PK4), the ribosomal protein P0 (RPP0), and two tetraspanins (TSPs), induced strong humoral responses in vaccinated rabbit, providing a protective effect to O. erraticus infestations about 30.2% (CHI), 57.8% (PK4), 57.5% (RPP0), and 56% (TSPs) and cross-protection to O. moubata infestations about 19.6% (CHI), 8.1% (PK4), 0% (RPP0), and 11.1% (TSPs). The joint vaccine of these candidates showed a stronger protective effect, with 66.3% protection or 25.6% cross-protection to O. erraticus or to O. moubata, respectively (Table 2) (88).
Salivary antigens
Astigarraga et al. vaccinated pigs with O. erraticus salivary gland extract (SGE), the best effect of which was reduced female feeding and fecundity by 50%. This protective effect is related to three mainly silencing antigens, including proteins of 70, 50, and 20 kDa (89). Subsequently, these proteins were purified to test its protective potential. The pigs produced specific antibodies toward the induced antigens after being vaccinated with either 70- or 50-kDa proteins, thus reducing female feeding and fertility. In contrast, the 20-kDa protein has a poor ability to induce specific antibody responses in pigs (Table 3) (79).
Regarding the vaccines against O. moubata, Salat et al. showed 39% reduction in feeding and 15% increase in mortality after nymphs-1 took blood meal of recombinant OmC2 cystatin vaccinated C3H/HeN mice (Table 3) (90). Similarly, SGE induced various homogeneous protective responses in vaccinated pigs, of which a protein of 44 kDa (named Om44) was the key antigen (89). Purified Om44 vaccinated pigs and rabbits inhibited the feeding of O. moubata by up to 54% (Table 3) (91). Díaz found that recombinant enolase (rOMENO) vaccinated rabbits caused 18.1% reduction in female oviposition and a rising mortality rates for nymphs-4 and nymphs-3 (Table 3) (92), and recombinant proteins of a secreted phospholipaseA2 (PLA2), an apyrase (APY), a mougrin (MOU), a riboprotein 60S L10 (RP-60S), and a 7DB-like protein (7DB-like) induced strong humoral responses, providing protective efficacy of 44.2%, 43.2%, 27.2%, 19.9%, and 17.3%, respectively (Table 3) (93). Manzano et al. showed that the recombinant protein TSGP4 (present in the saliva of O. moubata) in Freund’s adjuvants provided a 14.1% protective efficacy by activating humoral immunity in a vaccinated host (Table 3) (94).
Discussion
Current strategies to control ASF depend on rapid testing for the virus and the policies of quarantine and slaughter, which result in large numbers of animals being culled and are ineffective. Therefore, prevention is the best way to protect pigs against ASF. Strengthening feeding management, avoiding contact with ASFV-infected pigs, and strictly controlling the quality of pig feed are effective ways to control ASF (95).
In areas where ticks are involved, either in a domestic or sylvatic cycle, tick prevention is also an important part of ASF prevention. Using chemical acaricides is the most basic method of tick control, which has serious shortcomings, including pollution of the environment, contamination of animal products, and the emergence of drug-resistant tick strains (96–98). In addition, there is no guarantee that acaricides will completely kill hidden ticks because they have a nidicolous/endophilic lifestyle, making the use of this strategy inefficient (89). The high cost of developing and commercializing acaricides has prompted the search for alternative methods to control tick.
Ticks are vectors of many zoonotic pathogens, including viruses, parasites, and bacteria, making them a major threat to human and animal health. Alarmingly, more tick-borne diseases are being discovered, such as Alongshan virus and Songling virus (99, 100). Therefore, immunization control with anti-tick vaccines is a promising strategy to prevent tick-borne diseases. Bm86 antigen was identified in Boophilus microplus and used as a commercial vaccine against the same tick species. Studies showed that BM86 can effectively inhibit the weight and egg-laying capacity of female adult ticks and total weight of nymphs. Calves immunized with the BM86 vaccine have an ability to resist B. microplus, Boophilus decoloratus, and Hyalomma dromedarii (101). In addition to damaging the life cycle of ticks, it needs to be evaluated whether anti-tick vaccines block pathogens transmission. Malan showed that Rhipicephalus appendiculatus cement protein (64TRP) protected mice against the lethal challenge by infected ticks, which had a protective effect comparable to commercial TBEV vaccine and a better effect in transmission blocking (102). Andaleeb and his colleagues demonstrated that I. scapularis salivate gland protein (19ISP) mRNA vaccine inhibited the blood feeding of I. scapularis and impeded Borrelia burgdorferi transmission from I. scapularis to immunized guinea pigs (103). These two cases fully demonstrate the advantages of anti-tick vaccines. The hope for a vaccine against ticks is that it will both reduce tick bites, affect the life stages of ticks after they feed on blood, and reduce pathogens transmission.
The current challenge of an anti-tick vaccine against ASFV transmission is that no candidate anti-tick vaccine has been reported to inhibit transmission of ASFV from ticks to pigs. All the successful anti-tick vaccines described above are used for hard ticks, which differ greatly from soft ticks in their blood feeding habits. Hard ticks attach to hosts for blood meals and last up to 10 days for adulthood (24). Unlike hard ticks, soft ticks feed for a short time; for example, O. porcinus porcinus ticks feed in 1 h or less (47). Therefore, it is uncertain whether anti-tick vaccines can prevent ASFV transmission from ticks to pigs. It takes the nymphs 36 h to transmit B. burgdorferi to the naive host after feeding on the host, whereas guinea pigs immunized with the 19ISP mRNA vaccine reported by Andaleeb elicited an immune response as early as 18 h after tick challenge, thus blocking B. burgdorferi transmission (103, 104). The transmission of the virus is usually within a few hours, so it is necessary to confirm whether the immunized animal can induce an effective immune response to prevent the blood feeding of soft ticks. The effectiveness of the candidate vaccine reported by Ricardo et al. in inhibiting tick blood feeding is limited, so the ability of the vaccine against ASFV transmission is also questionable, which is a problem with all anti-soft tick candidate vaccines reported so far. On the other hand, vaccines against soft ticks have been reported to be effective in the reduction in survival and female fecundity, which is beneficial in reducing tick populations and the risk of ASF and other tick-borne diseases. Interestingly, Jennifer et al. demonstrated that pigs co-inoculated with ASFV and SGE of O. porcinus presented increased fever, and SGE had corresponding regulatory effects on skin tissue lesions, Langerhans cells disappearance in epidermis, macrophages recruitment in dermis, and virus dissemination in ASFV infection, suggesting the important role of SGE in the transmission of ASFV (105). Therefore, we are very optimistic about the screening of soft tick antigen and the development of anti-tick vaccine.
Anti-ASFV vaccine is also one of the effective means of prevention and control of ASF. ASFV-G-ΔI177L, marked at 3 June 2022, has become the first commercially available anti-ASFV vaccine due to its protective effect and good safety against Georgia strain. Manuel et al. discovered that ASFV-G-ΔI177L was clinically asymptomatic during the 28-day observational period and exhibited an efficacious protection against the epidemiologically relevant ASFV Georgia isolate (106). Interestingly, Oronasal administration of ASFV-G-ΔI177L provides a protective effect similar to intramuscular administration through mediating ASFV-specific antibody response, such as IgG1, IgG2, and IgM (107). Surprisingly, ASFV-G-ΔI177L also protected pigs from the isolated virulent ASFV circulated and produced in Vietnam (108). Fortunately, virulence regression studies of domestic pigs and large-scale tests of virus shedding and transmission confirmed that ASFV-G-ΔI177L is a safe vaccine (109).
As for the anti-ASFV vaccine, it is undoubtedly the best way to prevent ASF. However, marketed vaccines must be safe without the risk of regaining virulence, which is unpredictable with attenuated vaccines. Since ASFV-G-ΔI177L is a proven safe and effective vaccine, its marketing is good news for pig producers around the world. As there are many serotypes of ASF, the broad spectrum of ASFV-G-ΔI177L needs to be verified. In addition, the duration after vaccination also needs to be verified. Thus, although there is one vaccine available, the development of vaccines for different serotypes in different regions needs to be carried out.
In conclusion, given the critical role of soft ticks in ASFV transmission, the development of both anti-tick and anti-ASFV vaccines is an important strategy for preventing ASF.
Author contributions
YC, WZ and TL conceived and designed the study. TL, XX, NS, SZ, YD, KL, LD, XC and SJ wrote the manuscript. All authors contributed to the article and approved the submitted version.
Funding
This work was supported by grants from Jilin University (419021422B16) and National Natural Science Foundation of China (32172872).
Conflict of interest
The authors declare that the research was conducted in the absence of any commercial or financial relationships that could be construed as a potential conflict of interest.
Publisher’s note
All claims expressed in this article are solely those of the authors and do not necessarily represent those of their affiliated organizations, or those of the publisher, the editors and the reviewers. Any product that may be evaluated in this article, or claim that may be made by its manufacturer, is not guaranteed or endorsed by the publisher.
References
1. Cheng J, Ward MP. Risk factors for the spread of African swine fever in China: A systematic review of Chinese-language literature. Transbound Emerg Dis (2022) 69:e1289–98. doi: 10.1111/tbed.14573
2. Wang T, Sun Y, Qiu HJ. African Swine fever: an unprecedented disaster and challenge to China. Infect Dis Poverty (2018) 7:111. doi: 10.1186/s40249-018-0495-3
3. Lu G, Pan J, Zhang G. African Swine fever virus in Asia: Its rapid spread and potential threat to unaffected countries. J Infect (2020) 80:350–71. doi: 10.1016/j.jinf.2019.11.011
4. Brookes VJ, Barrett TE, Ward MP, Roby JA, Hernandez-Jover M, Cross EM, et al. A scoping review of African swine fever virus spread between domestic and free-living pigs. Transboundary Emerging Dis (2021) 68:2643–56. doi: 10.1111/tbed.13993
5. Penrith ML, Vosloo W. Review of African swine fever: transmission, spread and control. J S Afr Vet Assoc (2009) 80:58–62. doi: 10.4102/jsava.v80i2.172
6. Plowright W, Parker J, Peirce MA. African Swine fever virus in ticks (Ornithodoros moubata, murray) collected from animal burrows in Tanzania. Nature (1969) 221:1071–3. doi: 10.1038/2211071a0
7. Parker J, Plowright W, Pierce MA. The epizootiology of African swine fever in Africa. Vet Rec (1969) 85:668–74.
8. Plowright W, Perry CT, Peirce MA, Parker J. Experimental infection of the argasid tick, ornithodoros moubata porcinus, with African swine fever virus. Arch Gesamte Virusforsch (1970) 31:33–50. doi: 10.1007/BF01241664
9. Plowright W, Perry CT, Peirce MA. Transovarial infection with African swine fever virus in the argasid tick, ornithodoros moubata porcinus, Walton. Res Vet Sci (1970) 11:582–4. doi: 10.1016/S0034-5288(18)34259-0
10. Plowright W, Perry CT, Greig A. Sexual transmission of African swine fever virus in the tick, ornithodoros moubata porcinus, Walton. Res Vet Sci (1974) 17:106–13. doi: 10.1016/S0034-5288(18)33716-0
11. Forth JH, Forth LF, Lycett S, Bell-Sakyi L, Keil GM, Blome S, et al. Identification of African swine fever virus-like elements in the soft tick genome provides insights into the virus' evolution. BMC Biol (2020) 18:136. doi: 10.1186/s12915-020-00865-6
12. Burrage TG. African Swine fever virus infection in ornithodoros ticks. Virus Res (2013) 173:131–9. doi: 10.1016/j.virusres.2012.10.010
13. Golnar AJ, Martin E, Wormington JD, Kading RC, Teel PD, Hamer SA, et al. Reviewing the potential vectors and hosts of African swine fever virus transmission in the united states. Vector-Borne Zoonot (2019) 19:512–24. doi: 10.1089/vbz.2018.2387
14. Mellor PS, Wilkinson PJ. Experimental transmission of African swine fever virus by ornithodoros savignyi (Audouin). Res Vet Sci (1985) 39:353–6. doi: 10.1016/S0034-5288(18)31726-0
15. Groocock CM, Hess WR, Gladney WJ. Experimental transmission of African swine fever virus by ornithodoros coriaceus, an argasid tick indigenous to the united states. Am J Vet Res (1980) 41:591–4.
16. Hess WR, Endris RG, Haslett TM, Monahan MJ, McCoy JP. Potential arthropod vectors of African swine fever virus in north America and the Caribbean basin. Vet Parasitol (1987) 26:145–55. doi: 10.1016/0304-4017(87)90084-7
17. Endris RG, Haslett TM, Hess WR. Experimental transmission of African swine fever virus by the tick ornithodoros (Alectorobius) puertoricensis (Acari: Argasidae). J Med Entomol (1991) 28:854–8. doi: 10.1093/jmedent/28.6.854
18. Endris RG, Hess WR, Caiado JM. African Swine fever virus infection in the Iberian soft tick, ornithodoros (Pavlovskyella) marocanus (Acari: Argasidae). J Med Entomol (1992) 29:874–8. doi: 10.1093/jmedent/29.5.874
19. Endris RG, Hess WR. Experimental transmission of African swine fever virus by the soft tick ornithodoros (Pavlovskyella) marocanus (Acari: Ixodoidea: Argasidae). J Med Entomol (1992) 29:652–6. doi: 10.1093/jmedent/29.4.652
20. Haresnape JM, Wilkinson PJ, Mellor PS. Isolation of African swine fever virus from ticks of the ornithodoros-moubata complex (Ixodoidea, argasidae) collected within the African swine fever enzootic area of Malawi. Epidemiol Infect (1988) 101:173–85. doi: 10.1017/S0950268800029332
21. Kleiboeker SB, Scoles GA, Burrage TG, Sur JH. African Swine fever virus replication in the midgut epithelium is required for infection of ornithodoros ticks. J Virol (1999) 73:8587–98. doi: 10.1128/JVI.73.10.8587-8598.1999
22. Ferreira HCD, Zuquetec ST, Wijnveld M, Weesendorp E, Jongejan F, Stegeman A, et al. No evidence of African swine fever virus replication in hard ticks. Ticks Tick-Borne Dis (2014) 5:582–9. doi: 10.1016/j.ttbdis.2013.12.012
23. Chen Z, Xu XF, Wang YF, Bei JL, Jin XF, Dou WH, et al. DNA Segments of African swine fever virus detected for the first time in hard ticks from sheep and bovines. Syst Appl Acarol-Uk (2019) 24:180–4. doi: 10.11158/saa.24.1.13
24. Bonnet SI, Bouhsira E, De Regge N, Fite J, Etore F, Garigliany MM, et al. Putative role of arthropod vectors in African swine fever virus transmission in relation to their bio-ecological properties. Viruses (2020) 12:778. doi: 10.3390/v12070778
25. Frant M, Wozniakowski G, Pejsak Z. African Swine fever (ASF) and ticks. no risk of tick-mediated ASF spread in Poland and Baltic states. J Vet Res (2017) 61:375–80. doi: 10.1515/jvetres-2017-0055
26. Pereira De Oliveira R, Hutet E, Duhayon M, Guionnet JM, Paboeuf F, Vial L, et al. Successful infection of domestic pigs by ingestion of the European soft tick o. erraticus that fed on African swine fever virus infected pig. Viruses (2020) 12:300. doi: 10.3390/v12030300
27. Chenais E, Stahl K, Guberti V, Depner K. Identification of wild boar-habitat epidemiologic cycle in African swine fever epizootic. Emerg Infect Dis (2018) 24:810–2. doi: 10.3201/eid2404.172127
29. Boinas FS, Wilson AJ, Hutchings GH, Martins C, Dixon LJ. The persistence of African swine fever virus in field-infected ornithodoros erraticus during the ASF endemic period in Portugal. PloS One (2011) 6:e20283. doi: 10.1371/journal.pone.0020383
30. Brown AA, Penrith ML, Fasina FO, Beltran-Alcrudo D. The African swine fever epidemic in West Africa, 1996-2002. Transboundary Emerging Dis (2018) 65:64–76. doi: 10.1111/tbed.12673
31. Moura JA, McManus CM, Bernal FEM, de Melo CB. An analysis of the 1978 African swine fever outbreak in Brazil and its eradication. Rev Sci Tech Oie (2010) 29:549–63. doi: 10.20506/rst.29.3.1992
32. Penrith ML, Bastos AD, Etter EMC, Beltran-Alcrudo D. Epidemiology of African swine fever in Africa today: Sylvatic cycle versus socio-economic imperatives. Transbound Emerg Dis (2019) 66:672–86. doi: 10.1111/tbed.13117
33. Jori F, Bastos AD. Role of wild suids in the epidemiology of African swine fever. Ecohealth (2009) 6:296–310. doi: 10.1007/s10393-009-0248-7
34. Thomson GR, Gainaru MD, Vandellen AF. Experimental-infection of warthog (Phacochoerus-aethiopicus) with African swine fever virus. Onderstepoort J Vet (1980) 47:19–22.
35. Thomson GR, Gainaru MD, Van Dellen AF. Experimental infection of warthos (Phacochoerus aethiopicus) with African swine fever virus. Onderstepoort J Vet Res (1980) 47:19–22.
36. Costard S, Mur L, Lubroth J, Sanchez-Vizcaino JM, Pfeiffer DU. Epidemiology of African swine fever virus. Virus Res (2013) 173:191–7. doi: 10.1016/j.virusres.2012.10.030
37. Anderson EC, Hutchings GH, Mukarati N, Wilkinson PJ. African Swine fever virus infection of the bushpig (Potamochoerus porcus) and its significance in the epidemiology of the disease. Vet Microbiol (1998) 62:1–15. doi: 10.1016/S0378-1135(98)00187-4
38. Pietschmann J, Mur L, Blome S, Beer M, Perez-Sanchez R, Oleaga A, et al. African Swine fever virus transmission cycles in central Europe: Evaluation of wild boar-soft tick contacts through detection of antibodies against ornithodoros erraticus saliva antigen. BMC Vet Res (2016) 12:1. doi: 10.1186/s12917-015-0629-9
39. Ravaomanana J, Michaud V, Jori F, Andriatsimahavandy A, Roger F, Albina E, et al. First detection of African swine fever virus in ornithodoros porcinus in Madagascar and new insights into tick distribution and taxonomy. Parasite Vector (2010) 3:115. doi: 10.1186/1756-3305-3-115
40. Wilkinson PJ, Pegram RG, Perry BD, Lemche J, Schels HF. The distribution of African swine fever virus isolated from ornithodoros-moubata in Zambia. Epidemiol Infect (1988) 101:547–64. doi: 10.1017/S0950268800029423
41. Perezsanchez R, Astigarraga A, Oleagaperez A, Encinasgrandes A. Relationship between the persistence of African swine fever and the distribution of ornithodoros-erraticus in the province of salamanca, Spain. Vet Rec (1994) 135:207–9. doi: 10.1136/vr.135.9.207
42. Boinas F, Ribeiro R, Madeira S, Palma M, de Carvalho IL, Nuncio S, et al. The medical and veterinary role of ornithodoros erraticus complex ticks (Acari: Ixodida) on the Iberian peninsula. J Vector Ecol (2014) 39:238–48. doi: 10.1111/jvec.12098
43. Basto AP, Nix RJ, Boinas F, Mencles S, Silva MJ, Cartaxeiro C, et al. Kinetics of African swine fever virus infection in ornithodoros erraticus ticks. J Gen Virol (2006) 87:1863–71. doi: 10.1099/vir.0.81765-0
44. Li YP, Gao X, An Q, Sun Z, Wang HB. Ecological niche modeling based on ensemble algorithms to predicting current and future potential distribution of African swine fever virus in China. Sci Rep-Uk 12 (2022) 12:15614. doi: 10.1038/s41598-022-20008-x
45. Haresnape JM, Lungu SA, Mamu FD. A four-year survey of African swine fever in Malawi. J Hyg (Lond) (1985) 95:309–23. doi: 10.1017/S0022172400062732
46. Qu H, Ge S, Zhang Y, Wu X, Wang Z. A systematic review of genotypes and serogroups of African swine fever virus. Virus Genes (2022) 58:77–87. doi: 10.1007/s11262-021-01879-0
47. Kleiboeker SB, Burrage TG, Scoles GA, Fish D, Rock DL. African Swine fever virus infection in the argasid host, ornithodoros porcinus porcinus. J Virol (1998) 72:1711–24. doi: 10.1128/JVI.72.3.1711-1724.1998
48. Ribeiro R, Otte J, Madeira S, Hutchings GH, Boinas F. Experimental infection of ornithodoros erraticus sensu stricto with two Portuguese African swine fever virus strains. study of factors involved in the dynamics of infection in ticks. PloS One (2015) 10:e0137718. doi: 10.1371/journal.pone.0137718
49. Rennie L, Wilkinson PJ, Mellor PS. Effects of infection of the tick ornithodoros moubata with African swine fever virus. Med Vet Entomol (2000) 14:355–60. doi: 10.1046/j.1365-2915.2000.00251.x
50. Pereira de Oliveira R, Hutet E, Paboeuf F, Duhayon M, Boinas F, Perez de Leon A, et al. Comparative vector competence of the Afrotropical soft tick ornithodoros moubata and palearctic species, o. erraticus and o. verrucosus, for African swine fever virus strains circulating in Eurasia. PloS One (2019) 14:e0225657. doi: 10.1371/journal.pone.0225657
51. Rennie L, Wilkinson PJ, Mellor PS. Transovarial transmission of African swine fever virus in the argasid tick ornithodoros moubata. Med Vet Entomol (2001) 15:140–6. doi: 10.1046/j.1365-2915.2001.00282.x
52. Greig A. The localization of African swine fever virus in the tick ornithodoros moubata porcinus. Arch Gesamte Virusforsch (1972) 39:240–7. doi: 10.1007/BF01241546
53. Malmquist WA, Hay D. Hemadsorption and cytopathic effect produced by African swine fever virus in swine bone marrow and buffy coat cultures. Am J Vet Res (1960) 21:104–8.
54. Borca MV, Carrillo C, Zsak L, Laegreid WW, Kutish GF, Neilan JG, et al. Deletion of a CD2-like gene, 8-DR, from African swine fever virus affects viral infection in domestic swine. J Virol (1998) 72:2881–9. doi: 10.1128/JVI.72.4.2881-2889.1998
55. Genovesi EV, Knudsen RC, Whyard TC, Mebus CA. Moderately virulent African swine fever virus infection: blood cell changes and infective virus distribution among blood components. Am J Vet Res (1988) 49:338–44.
56. Heuschele WP. Studies on the pathogenesis of African swine fever. i. quantitative studies on the sequential development of virus in pig tissues. Arch Gesamte Virusforsch (1967) 21:349–56. doi: 10.1007/BF01241735
57. Wardley RC, Wilkinson PJ. The association of African swine fever virus with blood components of infected pigs. Arch Virol (1977) 55:327–34. doi: 10.1007/BF01315054
58. Cupp EW. Biology of ticks. Vet Clin North Am Small Anim Pract (1991) 21:1–26. doi: 10.1016/S0195-5616(91)50001-2
59. Simo L, Kazimirova M, Richardson J, Bonnet SI. The essential role of tick salivary glands and saliva in tick feeding and pathogen transmission. Front Cell Infect Microbiol (2017) 7:281. doi: 10.3389/fcimb.2017.00281
60. Frauenschuh A, Power CA, Deruaz M, Ferreira BR, Silva JS, Teixeira MM, et al. Molecular cloning and characterization of a highly selective chemokine-binding protein from the tick rhipicephalus sanguineus. J Biol Chem (2007) 282:27250–8. doi: 10.1074/jbc.M704706200
61. Deruaz M, Frauenschuh A, Alessandri AL, Dias JM, Coelho FM, Russo RC, et al. Ticks produce highly selective chemokine binding proteins with antiinflammatory activity. J Exp Med (2008) 205:2019–31. doi: 10.1084/jem.20072689
62. Beaufays J, Adam B, Menten-Dedoyart C, Fievez L, Grosjean A, Decrem Y, et al. Ir-LBP, an ixodes ricinus tick salivary LTB4-binding lipocalin, interferes with host neutrophil function. PloS One (2008) 3:e3987 doi: 10.1371/journal.pone.0003987
63. Wasala NB, Jaworski DC. Dermacentor variabilis: Characterization and modeling of macrophage migration inhibitory factor with phylogenetic comparisons to other ticks, insects and parasitic nematodes. Exp Parasitol (2012) 130:232–8. doi: 10.1016/j.exppara.2011.12.010
64. Umemiya R, Hatta T, Liao M, Tanaka M, Zhou JL, Inoue N, et al. Haemaphysalis longicornis: Molecular characterization of a homologue of the macrophage migration inhibitory factor from the partially fed ticks. Exp Parasitol (2007) 115:135–42. doi: 10.1016/j.exppara.2006.07.006
65. Wu J, Wang YP, Liu H, Yang HL, Ma DY, Li JX, et al. Two immunoregulatory peptides with antioxidant activity from tick salivary glands. J Biol Chem (2010) 285:16606–13. doi: 10.1074/jbc.M109.094615
66. Oliveira CJF, Sa-Nunes A, Francischetti IMB, Carregaro V, Anatriello E, Silva JS, et al. Deconstructing tick saliva NON-PROTEIN MOLECULES WITH POTENT IMMUNOMODULATORY PROPERTIES. J Biol Chem (2011) 286:10960–9. doi: 10.1074/jbc.M110.205047
67. Daix V, Schroeder H, Praet N, Georgin JP, Chiappino I, Gillet L, et al. Ixodes ticks belonging to the ixodes ricinus complex encode a family of anticomplement proteins. Insect Mol Biol (2007) 16:155–66. doi: 10.1111/j.1365-2583.2006.00710.x
68. Couvreur B, Beaufays J, Charon C, Lahaye K, Gensale F, Denis V, et al. Variability and action mechanism of a family of anticomplement proteins in ixodes ricinus. PloS One (2008) 3:e1400. doi: 10.1371/journal.pone.0001400
69. Nunn MA, Sharma A, Paesen GC, Adamson S, Lissina O, Willis AC, et al. Complement inhibitor of C5 activation from the soft tick ornithodoros moubata. J Immunol (2005) 174:2084–91. doi: 10.4049/jimmunol.174.4.2084
70. Mans BJ, Ribeiro JMC. Function, mechanism and evolution of the moubatin-clade of soft tick lipocalins. Insect Biochem Molec (2008) 38:841–52. doi: 10.1016/j.ibmb.2008.06.007
71. Denisov SS, Ippel JH, Castoldi E, Mans BJ, Hackeng TM, Dijkgraaf I. Molecular basis of anticoagulant and anticomplement activity of the tick salivary protein Salp14 and its homologs. J Biol Chem (2021) 297:100865. doi: 10.1016/j.jbc.2021.100865
72. Ali A, Zeb I, Alouffi A, Zahid H, Almutairi MM, Ayed Alshammari F, et al. Host immune responses to salivary components - a critical facet of tick-host interactions. Front Cell Infect Microbiol (2022) 12:809052. doi: 10.3389/fcimb.2022.809052
73. Chlastakova A, Kotal J, Berankova Z, Kascakova B, Martins LA, Langhansova H, et al. Iripin-3, a new salivary protein isolated from ixodes ricinus ticks, displays immunomodulatory and anti-hemostatic properties in vitro. Front Immunol (2021) 12:626200. doi: 10.3389/fimmu.2021.626200
74. Sa-Nunes A, Bafica A, Antonelli LR, Choi EY, Francischetti IM, Andersen JF, et al. The immunomodulatory action of sialostatin l on dendritic cells reveals its potential to interfere with autoimmunity. J Immunol (2009) 182:7422–9. doi: 10.4049/jimmunol.0900075
75. Pham M, Underwood J, Oliva Chavez AS. Changing the recipe: Pathogen directed changes in tick saliva components. Int J Environ Res Public Health (2021) 18:1806. doi: 10.3390/ijerph18041806
76. Andrade BB, Teixeira CR, Barral A, Barral-Netto M. Haematophagous arthropod saliva and host defense system: a tale of tear and blood. Acad Bras Cienc (2005) 77:665–93. doi: 10.1590/S0001-37652005000400008
77. Willadsen P. Anti-tick vaccines. Parasitology (2004) 129:S367–S387. doi: 10.1017/S0031182003004657
78. Chinzei Y, Minoura H. Reduced oviposition in ornithodoros moubata (Acari: Argasidae) fed on tick-sensitized and vitellin-immunized rabbits. J Med Entomol (1988) 25:26–31. doi: 10.1093/jmedent/25.1.26
79. Román RM. Vacuna anti-ornithodoros erraticus. Spain: Universidad de Salamanca (2002). Available at: http://hdl.handle.net/10261/10366.
80. Manzano-Roman R, Encinas-Grandes A, Perez-Sanchez R. Antigens from the midgut membranes of ornithodoros erraticus induce lethal anti-tick immune responses in pigs and mice. Vet Parasitol (2006) 135:65–79. doi: 10.1016/j.vetpar.2005.08.004
81. Manzano-Roman R, Garcia-Varas S, Encinas-Grandes A, Perez-Sanchez R. Purification and characterization of a 45-kDa concealed antigen from the midgut membranes of ornithodoros erraticus that induces lethal anti-tick immune responses in pigs. Vet Parasitol (2007) 145:314–25. doi: 10.1016/j.vetpar.2007.01.011
82. Manzano-Roman R, Diaz-Martin V, Oleaga A, Siles-Lucas M, Perez-Sanchez R. Subolesin/akirin orthologs from ornithodoros spp. soft ticks: cloning, RNAi gene silencing and protective effect of the recombinant proteins. Vet Parasitol (2012) 185:248–59. doi: 10.1016/j.vetpar.2011.10.032
83. Manzano-Roman R, Diaz-Martin V, Oleaga A, Perez-Sanchez R. Identification of protective linear b-cell epitopes on the subolesin/akirin orthologues of ornithodoros spp. soft ticks. Vaccine (2015) 33:1046–55. doi: 10.1016/j.vaccine.2015.01.015
84. Oleaga A, Obolo-Mvoulouga P, Manzano-Roman R, Perez-Sanchez R. Functional annotation and analysis of the ornithodoros moubata midgut genes differentially expressed after blood feeding. Ticks Tick-Borne Dis (2017) 8:693–708. doi: 10.1016/j.ttbdis.2017.05.002
85. Oleaga A, Obolo-Mvoulouga P, Manzano-Roman R, Perez-Sanchez R. A proteomic insight into the midgut proteome of ornithodoros moubata females reveals novel information on blood digestion in argasid ticks. Parasite Vector (2017) 10:366. doi: 10.1186/s13071-017-2300-8
86. Obolo-Mvoulouga P, Oleaga A, Manzano-Roman R, Perez-Sanchez R. Evaluation of the protective efficacy of ornithodoros moubata midgut membrane antigens selected using omics and in silico prediction algorithms. Ticks Tick-Borne Dis (2018) 9:1158–72. doi: 10.1016/j.ttbdis.2018.04.015
87. Perez-Sanchez R, Manzano-Roman R, Obolo-Mvoulouga P, Oleaga A. Function-guided selection of midgut antigens from ornithodoros erraticus ticks and an evaluation of their protective efficacy in rabbits. Vet Parasitol (2019) 272:1–12. doi: 10.1016/j.vetpar.2019.06.016
88. Perez-Sanchez R, Manzano-Roman R, Obolo-Mvoulouga P, Oleaga A. In silico selection of functionally important proteins from the mialome of ornithodoros erraticus ticks and assessment of their protective efficacy as vaccine targets. Parasite Vector (2019) 12:508. doi: 10.1186/s13071-019-3768-1
89. Astigarraga A, Oleagaperez A, Perezsanchez R, Encinasgrandes A. A study of the vaccinal value of various extracts of concealed antigens and salivary-gland extracts against ornithodoros-erraticus and ornithodoros-moubata. Vet Parasitol (1995) 60:133–47. doi: 10.1016/0304-4017(94)00772-5
90. Salat J, Paesen GC, Rezacova P, Kotsyfakis M, Kovarova Z, Sanda M, et al. Crystal structure and functional characterization of an immunomodulatory salivary cystatin from the soft tick ornithodoros moubata. Biochem J (2010) 429:103–12. doi: 10.1042/BJ20100280
91. Garcia-Varas S, Manzano-Roman R, Fernandez-Soto P, Encinas-Grandes A, Oleaga A, Perez-Sanchez R. Purification and characterisation of a p-selectin-binding molecule from the salivary glands of ornithodoros moubata that induces protective anti-tick immune responses in pigs. Int J Parasitol (2010) 40:313–26. doi: 10.1016/j.ijpara.2009.08.011
92. Diaz-Martin V, Manzano-Roman R, Oleaga A, Encinas-Grandes A, Perez-Sanchez R. Cloning and characterization of a plasminogen-binding enolase from the saliva of the argasid tick ornithodoros moubata. Vet Parasitol (2013) 191:301–14. doi: 10.1016/j.vetpar.2012.09.019
93. Diaz-Martin V, Manzano-Roman R, Oleaga A, Perez-Sanchez R. New salivary anti-haemostatics containing protective epitopes from ornithodoros moubata ticks: Assessment of their individual and combined vaccine efficacy. Vet Parasitol (2015) 212:336–49. doi: 10.1016/j.vetpar.2015.08.005
94. Manzano-Roman R, Diaz-Martin V, Oleaga A, Obolo-Mvoulouga P, Perez-Sanchez R. TSGP4 from ornithodoros moubata: molecular cloning, phylogenetic analysis and vaccine efficacy of a new member of the lipocalin clade of cysteinyl leukotriene scavengers. Vet Parasitol (2016) 227:130–7. doi: 10.1016/j.vetpar.2016.08.005
95. Sanchez-Vizcaino JM, Mur L, Bastes ADS, Penrith ML. New insights into the role of ticks in African swine fever epidemiology. Rev Sci Tech Oie (2015) 34:503–11. doi: 10.20506/rst.34.2.2375
96. George JE, Pound JM, Davey RB. Chemical control of ticks on cattle and the resistance of these parasites to acaricides. Parasitology (2004) 129:S353–66. doi: 10.1017/S0031182003004682
97. Ghosh S, Azhahianambi P, Yadav MP. Upcoming and future strategies of tick control: a review. J Vector Borne Dis (2007) 44:79–89.
98. Graf JF, Gogolewski R, Leach-Bing N, Sabatini GA, Molento MB, Bordin EL, et al. Tick control: an industry point of view. Parasitology (2004) 129(Suppl):S427–42. doi: 10.1017/S0031182004006079
99. Wang ZD, Wang B, Wei F, Han SZ, Zhang L, Yang ZT, et al. A new segmented virus associated with human febrile illness in China. New Engl J Med (2019) 380:2116–25. doi: 10.1056/NEJMoa1805068
100. Ma J, Lv XL, Zhang X, Han SZ, Wang ZD, Li L, et al. Identification of a new orthonairovirus associated with human febrile illness in China (vol 27, pg 434, 2021). Nat Med (2021) 27:926–6. doi: 10.1038/s41591-021-01364-z
101. De Vos S, Zeinstra L, Taoufik A, Willadsen P, Jongejan F. Evidence for the utility of the Bm86 antigen from boophilus microplus in vaccination against other tick species. Exp Appl Acarol (2001) 25:245–61. doi: 10.1023/A:1010609007009
102. Labuda M, Trimnell AR, Lickova M, Kazimirova M, Davies GM, Lissina O, et al. An antivector vaccine protects against a lethal vector-borne pathogen. PloS Pathog (2006) 2:251–9. doi: 10.1371/journal.ppat.0020027
103. Sajid A, Matias J, Arora G, Kurokawa C, DePonte K, Tang X, et al. mRNA vaccination induces tick resistance and prevents transmission of the Lyme disease agent. Sci Transl Med (2021) 13:eabj9827. doi: 10.1126/scitranslmed.abj9827
104. Stanek G, Wormser GP, Gray J, Strle F. Lyme Borreliosis. Lancet (2012) 379:461–73. doi: 10.1016/S0140-6736(11)60103-7
105. Bernard J, Hutet E, Paboeuf F, Randriamparany T, Holzmuller P, Lancelot R, et al. Effect of o. porcinus tick salivary gland extract on the African swine fever virus infection in domestic pig. PloS One (2016) 11:e0147869. doi: 10.1371/journal.pone.0147869
106. Borca MV, Ramirez-Medina E, Silva E, Vuono E, Rai A, Pruitt S, et al. Development of a highly effective African swine fever virus vaccine by deletion of the I177L gene results in sterile immunity against the current epidemic Eurasia strain. J Virol (2020) 94:e02017–19. doi: 10.1128/JVI.02017-19
107. Borca MV, Ramirez-Medina E, Silva E, Vuono E, Rai A, Pruitt S, et al. ASFV-g- increment I177L as an effective oral nasal vaccine against the Eurasia strain of Africa swine fever. Viruses-Basel (2021) 13:765. doi: 10.3390/v13050765
108. Tran XH, Le TTP, Nguyen QH, Do TT, Nguyen V, Gay CG, et al. African Swine fever virus vaccine candidate ASFV-G-Delta I177L efficiently protects European and native pig breeds against circulating Vietnamese field strain. Transboundary Emerging Dis (2022) 69:E497–504. doi: 10.1111/tbed.14329
Keywords: African swine fever, Ornithodoros soft ticks, transmission, anti-tick vaccine, ASFV-G-ΔI177L, prevention
Citation: Lv T, Xie X, Song N, Zhang S, Ding Y, Liu K, Diao L, Chen X, Jiang S, Li T, Zhang W and Cao Y (2022) Expounding the role of tick in Africa swine fever virus transmission and seeking effective prevention measures: A review. Front. Immunol. 13:1093599. doi: 10.3389/fimmu.2022.1093599
Received: 09 November 2022; Accepted: 29 November 2022;
Published: 16 December 2022.
Edited by:
Tao Lin, New Hope Group, United StatesCopyright © 2022 Lv, Xie, Song, Zhang, Ding, Liu, Diao, Chen, Jiang, Li, Zhang and Cao. This is an open-access article distributed under the terms of the Creative Commons Attribution License (CC BY). The use, distribution or reproduction in other forums is permitted, provided the original author(s) and the copyright owner(s) are credited and that the original publication in this journal is cited, in accordance with accepted academic practice. No use, distribution or reproduction is permitted which does not comply with these terms.
*Correspondence: Yongguo Cao, eWdjYW84MkBqbHUuZWR1LmNu; Wenlong Zhang, endlbmxvbmcxMjNAMTI2LmNvbQ==
†These authors have contributed equally to this work