- National Translational Science Center for Molecular Medicine & Department of Cell Biology, Fourth Military Medical University, Xi’an, China
Immuno-checkpoint inhibitors (ICIs) bring a promising prospect for patients with cancers, which restrains the growth of tumor cells by enhancing anti-tumor activity. Nevertheless, not all patients benefit from the administration of ICIs monotherapy. The partial response or resistance to ICIs is mainly due to the complex and heterogenous tumor microenvironment (TME). The combined therapy is necessary for improving the efficacy of tumor treatment. Chemotherapy is reported not only to kill tumor cells directly, but also to stimulate effective anti-tumor immune responses. Several combined therapies of ICIs and chemotherapeutic agents have been approved for the first-line treatment of cancers, including PD-1/PD-L1 inhibitors. This review summarizes the potential mechanisms of the combined therapy of ICIs and chemotherapeutic agents in inducing immunogenic cell death (ICD) and reprogramming TME, and elucidates the possible anti-tumor effects of combined therapy from the perspective of metabolic reprogramming and microbiome reprogramming.
Introduction
Malignant tumors, as a type of incurable diseases, have threatened human health seriously owing to the immunosuppressive tumor microenvironment. The tumor immunotherapies dramatically make a monumental breakthrough for cancer treatment and bring significant improvement for patient survival by boosting effective immune response to eliminate malignant cells (1, 2). Oncolytic virus therapies, cancer vaccines, cytokine therapies, adoptive cell transfer therapies, and ICIs are included, and ICIs, which include inhibitors of the programmed cell death protein 1 (PD-1), programmed cell death-ligand 1 (PD-L1), and the cytotoxic T lymphocyte-associated protein 4 (CTLA-4), have been broadly used in clinical applications and contribute to prolonged survival for lung cancer patients (2–5).
However, not all patients benefit from immune checkpoint blockade therapy (6, 7). Based on response to ICIs, three broad population of patients are identified, which include responders, those that acquire resistance, and those that never respond (8–10). Unfortunately, only 20% of NSCLC patients response to ICIs, which shows a relatively lower clinical effect compared to other cancers (7). The complex and heterogenous TME is reported to be involved in the response to ICIs (11, 12). Based on the status of T cell infiltration, TME is classified as the immune-inflamed phenotype, the immune-excluded phenotype, and the immune-desert phenotype (13, 14). The reactivation and clonal-proliferation of antigen-experienced T cells in the TME are necessary for an effective anti-tumor response after ICI administration. Nevertheless, the tumor-associated macrophages (TAMs), cancer-associated fibroblasts (CAFs), myeloid-derived suppressor cells (MDSCs), and regulated T cells (Tregs) in TME have an inhibitory impact on the infiltration and activation of effector T cells (15). As a result, ICIs combined with other therapies that activate the immune effects of TME seems to be a better choice for tumor treatment. As of December 2021, 4,897 clinical trials are conducted to test the efficacy of PD-1/PD-L1 inhibitors. Among them, 83% are ICIs combined with other therapies, such as chemotherapy, radiotherapy, and other immuno-oncology therapies (16). Several clinical trials show that ICIs combined with chemotherapy have a better clinical effect compared to ICIs monotherapy (17–19). For example, pembrolizumab, a humanized monoclonal antibody against PD-1, plus platinum-based chemotherapy significantly improved overall survival rates of NSCLC patients with a PD-L1 ≥ 50% and negative for genomic alterations in the EGFR and ALK genes, compared with pembrolizumab monotherapy (19). Meanwhile, ICIs combined with chemotherapy also prolong the NSCLC patient survival, compared with chemotherapy (20–23), which have been approved for the first-line treatment in advanced NSCLC patients (24).
In this review, we summarize the synergetic effects of chemotherapy with ICI therapy in tumor treatment from the perspective of inducing ICD, remodeling TME, metabolic reprogramming, and microbiome reprogramming (Figure 1). In addition, further researches need to be conducted to explore the novel mechanisms of above-mentioned therapy in cancers, which may provide a solid foundation for future clinical applications.
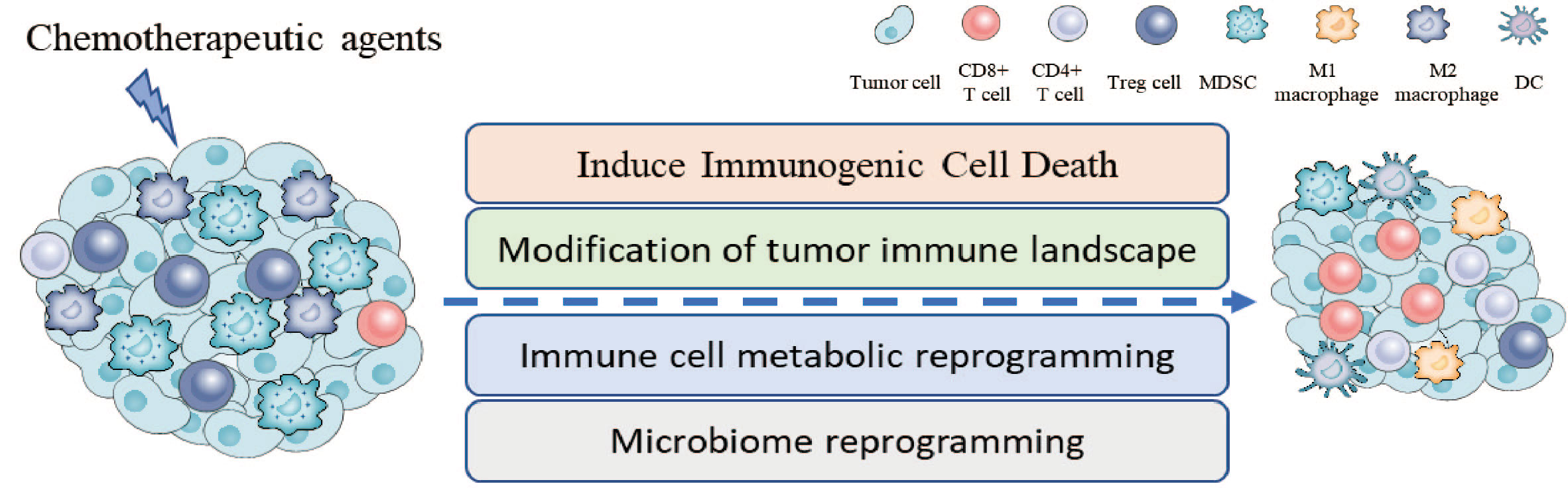
Figure 1 Chemotherapeutic agents enhance the anti-tumor activity of ICIs through several mechanisms, including inducing immunogenic cell death, changing the proportion and activity of immune cells in TME, immune cell metabolic reprogramming, and microbiome reprogramming.
Inducing immunogenic cell death
Several studies have shown that chemotherapeutic agents have the ability of inducing ICD in animal experiments, including anthracyclines, cyclophosphamide, oxaliplatin, pemetrexed, and paclitaxel (25–30). For example, anthracyclines, including doxorubicin, idarubicin and mitoxantrone, are identified as ICD inducers in the mouse models of colorectal cancer (27), and cyclophosphamide can also induce ICD as shown in glioma mouse models (30). In addition, single-agent pemetrexed or docetaxel can induce ICD in 16 NSCLC patients, with increasing plasma concentration of soluble calreticulin (31). ICD is a form of regulated cell death, which activates an adaptive immune response in immunocompetent hosts (32, 33). The hallmarks of ICD include the exposure and release of numerous damage-associated molecular patterns (DAMPs), the phosphorylation of eukaryotic translation initiation factor 2 subunit-α (eIF2α), and the activation of type I IFN signaling and autophagy. Among them, DAMPs play a vital role in stimulating adaptive immune response, which contain increasing extracellular ATP, surface-exposed calreticulin, and released high mobility group box protein 1 (HMGB1) (34, 35). Increasing extracellular ATP, which provides a ‘find me’ signal, attracts antigen-presenting cells through binding with the purinergic receptors (36, 37) and stimulates dendritic cell (DC) maturation (38–41). Moreover, ATP triggers the formation of the NOD-like receptor family, pyrin domain containing-3 protein (NLRP3)-dependent caspase-1 activation complex (42), which promotes the secretion of IL-1β and IL-18 (43), to stimulate adaptive immune response (44). In addition, the P2X7 receptor (P2X7R), is expressed on various immune cells, and its expression sensitizes cells to enhanced ambient ATP concentrations, which modulates energy metabolism and T cell growth and differentiation (45). The exposure of calreticulin from endoplasmic reticulum to cell surface depends on the rapid phosphorylation of eIF2α and ERp57 (46), and provides an ‘eat me’ signal to promote phagocytosis by DCs, provoking the adaptive immune response (27, 47). However, mutant calreticulin with loss of the KDEL sequence is secreted to extracellular space, and may inhibit the phagocytosis of dying cancer cells by DCs through saturating binding sites on DCs (48). Moreover, the interaction between calreticulin and toll-like receptor 4 (TLR4) expressed on tumor cell surface promotes the secretion of TNFα and CCL19, which facilitates the migration and maturation of DCs, to limit the tumor progression in vivo (49). HMGB1, released from dying cancer cells, binds with TLR4 expressed on DCs to strengthen the antigen-presenting activity of DCs through activating the PI3K/AKT/mTOR signaling pathway, and promotes anti-tumor immune response of T cells (50, 51). Different HMGB1 isoforms exert different effects on immune response, and the reduced form is responsible for the activation of DCs (52). In addition, the administration of carboplatin, cisplatin, and gemcitabine increases the PD-L1 expression on tumor cells, and shows a better efficacy when combining with ICIs in NSCLC mouse models (53–55), in which the role of cisplatin has been validated in human NSCLC tumor samples (56). Moreover, cisplatin increases the expression level of MHC class I antigen on tumor cells, and subsequently augment CTL-mediated attack to tumor cells (57, 58). In summary, chemotherapy-induced ICD promotes the cross-presentation of tumor antigen to CD8+ cytotoxic T lymphocytes (CTLs), which limits tumor progression effectively.
Changing the proportion and activity of immune cells in TME
Chemotherapeutic agents have been validated that they can interact directly with immune cells to stimulate anticancer immunity through several mechanisms, which changes the infiltration and activity of immune cells in TME, including the depletion of immunosuppressive cells, the activation of immune effector cells, and promoting the proliferation of immune cells. Tumor-infiltrating Tregs promote tumor progression by inhibiting endogenous cytotoxic T cell responses (59), and it has been reported that some chemotherapy drugs can decrease the amount of Tregs (60–63). For example, the frequencies of intra-tumoral Tregs decreases significantly after the pretreatment of paclitaxel and cisplatin in a murine lung carcinoma model (64). Paclitaxel selectively decreases the size of Treg population in peripheral blood of patients with NSCLC, which may promote the upregulation of CD95 on Tregs, leading to cell apoptosis (65). Moreover, cyclophosphamide also decreases the amount of intra-tumoral Tregs in NSCLC mouse model (66), which is also identified in patients with recurrent prostate cancer (67). In addition, the amount of MDSCs, another immunosuppressive cell that helps tumor cells evade immune destruction (68, 69), is decreased after the administration of docetaxel, gemcitabine and 5-fluorouracil in mouse experiments (70–73). As shown in mouse models of melanoma (B16F10), gemcitabine significantly reduces the immunosuppressive state by decreasing the size of MDSCs and Tregs (70). Moreover, the number of circulating MDSCs in patients with pancreatic cancer decreases after the administration of gemcitabine, which provides precise clinical evidence (74), which is also validated in glioblastoma patients (75). The combination of gemcitabine and cisplatin reduce the amount of Tregs and regulatory B cells in in nasopharyngeal carcinoma patients (76). In addition, cyclophosphamide plus gemcitabine combination chemotherapy reduces the immunosuppressive state through decreasing the number of Tregs and MDSCs, enhancing anti-tumor immune response in colon carcinoma-bearing mice (63). In summary, the use of chemotherapeutic agents impairs the immunosuppressive role of TME by reducing the number of immunosuppressive cells, which enhances the anti-tumor activity.
In addition, several animal studies have validated that some chemotherapeutic agents, including platinum drugs and docetaxel, have the ability to promote the infiltration of CD8+ T cells, enhancing their anti-tumor effects (53, 60, 77–79). It has been reported that the administration of cisplatin and docetaxel increases intra-tumoral CD8+ T cell infiltration in a phase I/II study of neoadjuvant chemotherapy for resectable NSCLC (80). The increasing CD8+ T cell infiltration contributes to enhancing the anti-tumor activity and limits the tumor progression (81). Meanwhile, further studies explaining the mechanisms that platinum drugs increase the infiltration of CD8+ T cells have been conducted. For example, oxaliplatin enhances the secretion of CXCL9, CXCL10, and CXCL11 from tumor cells, which attracts CD8+ effector T cells through interacting with CXCR3 (82, 83), and subsequently promotes T cell infiltration in tumor tissues. In addition, neoadjuvant chemotherapy increases the infiltration of tissue resident memory T cells (TRMs) in resectable NSCLC patients, which will provide long-term anti-tumor immune response (84). TRMs express high levels of inhibitory receptors, such as PD-1 and Tim-3, and it has been validated that TRMs show a significant expansion and enhancing cytotoxic capacity after the administration of PD-1 inhibitors (85–88). Moreover, cisplatin can increase the infiltration of CD8+ T cells via activating cGAS-STING signaling in K-ras-driven tumor cells (77), and enhance the killing effects of CD8+ T cells through Fas/Fas ligand interactions in NSCLC mouse model (89), which may provide an inflammatory environment to enhance the anti-tumor activity of ICIs.
Chemotherapeutic agents also increase the infiltration of APCs in tumor tissues, such as DCs and macrophages, and enhances the anti-tumor immune response. Anthracycline-based chemotherapy increases the intra-tumoral infiltration of DCs in fibrosarcoma and breast cancer mouse models (90, 91). A prospective study suggests that the responsiveness of DCs recovers after anthracycline-based neoadjuvant chemotherapy in breast cancer patients (92). Except for above mentioned ATP signaling, CCL2/CCR2 axis may also be required for the intratumoral recruitment of DCs (90). In addition, platinum (IV) complexes increase the infiltration of M1 macrophages by decreasing the expression of CD47 in lung cancer mouse model, which is overexpressed on tumor cells and limits the antigen-presenting activity of APCs (93, 94). DCs play a vital role in maintaining CD8+ T cell function within tumors, and promote ICIs mediated anti-tumor immunity (95, 96). Furthermore, DCs may license PD-1 blockade via CD28 costimulation (97), and conventional DCs express genes correlated with CXCL9, which is related to PD-1 inhibitor response (98–100). In summary, chemotherapy-induced APCs increase in tumor tissues may contribute to ICI-enhanced anti-tumor activity.
Immune cell metabolic reprogramming
As a crucial hallmark of cancer, metabolism reprogramming provides a favorable immunosuppressive microenvironment for the tumor progression (101–103). Chemotherapy usually influences patients’ nutritional status, and the serum of patients with lung cancer is accompanied by metabolic alterations, including glycolysis and lipid metabolism, phosphatidylcholine biosynthesis as well as amino acid metabolism (104). What’s more, metabolic reprogramming is closely involved in the activation and of T cells. For example, the activation of T cells needs higher levels of glycolysis and mitochondrial respiration (105). Thus, chemotherapy may influence the immune effects of T cells through metabolic processes. Pemetrexed has been validated to increase mitochondrial function of T cells in colon cancer mouse model, which is necessary for the activation of T cells (26, 106). Nevertheless, there is little researches about the influence of other chemotherapeutic agents on metabolic reprogramming of immune cells, and further metabolomics study is necessary to explore this influence in preclinical and clinical studies.
Microbiome reprogramming
Gut microbiome is a complex ecosystem that regulates the interaction of the human and their environment, which has a potential impact on anti-tumor immune responses through various mechanisms (107), and is closely correlated with the efficacy of ICIs in cancer treatment (108–110). Chemotherapy has been validated to change the proportion of gut microbiome. The abundance of the Firmicutes phylum and Enterobacteriaceae increase after the administration of pemetrexed in the patient-lung-derived tumor xenograft mouse models (111). The selected species of Gram-positive bacteria are induced into the secondary lymphoid organs by cyclophosphamide, and stimulates the memory Th1 immune responses, which promotes the anti-tumor immune response (112). Therefore, the microbiome reprogramming induced by chemotherapy may provoke the anti-tumor effects of ICIs. However, the influence of chemotherapy on local microbiome needs to be investigated further, and the impacts of chemotherapy on ICI administration are required to be validated in clinical researches from the perspective of microbiome reprogramming.
ICIs combined with chemotherapy in various cancers
Considering the limitations of ICIs monotherapy in controlling tumor progression, a large amount of studies are devoted to the safety and effectiveness of ICIs combined with standard-of-care chemotherapies. ICIs combined with chemotherapy has been approved for the treatment of certain cancer types by FDA, and more than 600 ongoing clinical trials are devoted to exploring or optimizing for various oncological indications. In patients with NSCLC, pembrolizumab plus a platinum and pemetrexed provides a better prognosis compared to pembrolizumab monotherapy (113), owing to the immune activation of these chemotherapy drugs. Similar effects are also observed in patients with untreated locally incurable recurrent or metastatic head and neck squamous cell carcinoma treated with pembrolizumab plus a platinum and 5-fluorouracil (114, 115). In addition, atezolizumab plus carboplatin and nab-paclitaxel prolongs the overall survival and progression-free survival in patients with advanced non-squamous NSCLC (116). Neoadjuvant carboplatin and paclitaxel chemotherapy increases the amount of central memory CD8+ T cell in peripheral blood of patients with advanced serous ovarian cancer, enhancing antigen processing and presentation (117). However, the further studies are needed to investigate the corresponding mechanisms of neoadjuvant carboplatin and paclitaxel chemotherapy in inhibiting NSCLC progression. Although ICIs combined with chemotherapy significantly prolongs the survival of patients with tumors, it is necessary for us to evaluate the toxicity of the combination therapies. ICIs combined with chemotherapy may cause hematological, gastrointestinal, and renal toxicity, and contribute to hypothyroidism, hyperthyroidism, pneumonitis, hepatitis, severe skin reactions, colitis, and infusion reactions (118–121). However, the current understanding of adverse reactions of combined therapies is incomplete, and it is necessary to describe the preferable adverse reactions of different combinations, which is beneficial for balancing the safety and efficiency of the corresponding treatment.
Discussion
ICIs have made great contributions to the survival of patients with cancers. However, the low response rate of patients to ICIs prompts us to explore the possibility of ICIs combination with other therapies. As a routine therapy, chemotherapy attracts much attention because of its immune stimulation activity, and ICIs combination with chemotherapy achieves great effects in clinical trials. FDA has approved several combination therapies for the treatment of advanced NSCLC in the first-line setting (122). To clarify the mechanism how chemotherapy promotes curative effect of ICIs is beneficial for the application of the combination therapies. As mentioned above, chemotherapeutic agents-induced ICD and their immune stimulation activity are considered as the main mechanism of combination therapy. However, the impacts of chemotherapy are so complex that chemotherapy may influence ICI-mediated anti-tumor responses through various routes.
With the development of sequencing technology, the landscape of immune cells within tumor tissues has been gradually revealed, and more and more cellular components have been recognized. For example, B cells represent a vital component of infiltrating immune cells in a variety of solid tumors, and play a dual role in modulating anti-tumor immune response (123). Chemotherapy reduces the amount of adenosine-producing B cells, which may reduce potential immunosuppression in TME (124). However, there is little research about the influence of chemotherapy on B cell subpopulations and their activity.
Moreover, cancer-associated fibroblasts (CAFs) account for more than 50% of stroma cells in TME, and various CAF populations have been identified based on the results of single-cell sequencing analyses, including the cancer-associated myofibroblasts (myCAFs), inflammatory-like CAFs (iCAFs), and the antigen-presenting CAFs (apCAFs) (125). CAFs are involved in regulating tumor immune response and the efficacy of immunotherapy through several routes (126). For example, CAFs increase the ratio of FoxP3+ (Tregs) and CD8+ tumor-infiltrating lymphocytes via IL-6 in TME, and IL-6 blockade enhances the immunotherapy efficacy in esophageal cancer models (127). Several studies have reported that CAFs protect tumor cells from apoptosis induced by chemotherapy, while insulin-like growth factors secreted by CAFs enhanced the anti-tumor effects of osimertinib in mice model (125, 128). Moreover, CAFs can activate the NLRP3 inflammasome through sensing DAMPs in breast cancer, which leads to a pro-inflammatory signaling (129). The influence of chemotherapy on diverse CAFs and their association with the immunotherapy efficacy has not been explored comprehensively, and further researches are needed.
Except for the influence of chemotherapeutic agents on novel cellular components in TME, chemotherapy-induced microbiome reprogramming also contributes to the combination of ICIs and chemotherapy. Although the impacts of chemotherapy on gut microbiome have been explained partly in animal experiments, the overall landscape of gut microbiome reprogramming after treating with different chemotherapeutic agents needs to be described in more preclinical and clinical researches. Beyond that, the influence of chemotherapy on local microbiome also needs to be investigated further, and the impacts of chemotherapy on ICI administration are required to be validated in clinical researches from the perspective of microbiome reprogramming.
In addition, the administration approaches of ICIs combination with chemotherapy are necessary to be improved. Firstly, a proper combination therapy can maximize the clinical benefit and minimize the adverse drug reactions. As mentioned above, different chemotherapy drugs stimulate effective anti-tumor immune responses through different mechanisms, and cancer patients may reap more benefits with appropriate combination therapies after evaluating the tumor conditions, including PD-L1 expression level, immune cell infiltration, and tumor mutation burden. However, there is little clinical trials about comparing the effectiveness of combination therapies of different chemotherapeutic drugs and ICIs. Secondly, chemotherapeutic regimens and ICIs are administrated simultaneously in most clinical trials. Nevertheless, it has reported that the sequence of administrating chemotherapeutic agents and ICIs has an impact on the efficiency of the combined therapies in several animal experiments. The administration of anti‐CTLA‐4 antibody after injecting cyclophosphamide significantly inhibits the tumor progression in the CT26 colon carcinoma model, while the reverse administration sequence leads to the apoptosis of anti-tumor CD8+ T cells (130). Apart from this, the time interval from chemotherapy to immunotherapy may also influence the response to ICIs. In the long rest period group, the frequency of the Th1 subset and PD-1 + CD8+ T cells are significantly higher than that in the short rest period group, which provides a novel perspective for the application of combination therapies (131). However, there is no more research about appropriate sequence and proper time interval, and it needs more researches to study the influence of time interval on anti-tumor effects. Lastly, proper dose may be the most important respect to effectively stimulate the maximum anti-tumor immune response and minimize adverse reactions. For example, low dose cisplatin and oxaliplatin increase the number of circulating CD4+ and CD8+ T cells, while high dose regimens decrease the size of lymphocyte in a mouse model of colon cancer (132). Metronomic low dose cyclophosphamide enhance anti-tumor immune response by selectively reducing the amount of Treg cells in tumor patients (133), while high dose cyclophosphamide completely eradicates the hemopoietic cell (134). In addition, a novel administration mode called medium-dose intermittent chemotherapy provokes a striking response depending on the activation of a sustaining anti-tumor immune response (66, 135). Therefore, appropriate dosage for combined therapies is necessary to be investigated in further studies.
In summary, ICIs combination with chemotherapy has shown a better anti-tumor response and provides a more beneficial survival, compared to ICIs monotherapy. This benefit is supported by a strong cancer biology rationale, which induce a better immune response. Hence, the evaluation of the panoramic dynamic immune landscape of TME will be helpful to understand tumor pathogenesis and provide novel approaches for cancer treatment.
Author contributions
All authors have made a direct contribution to the work, and approved it for publication.
Funding
This work was supported by Science and Technology Project of Shaanxi Province (2021PT-047), National Natural Science Foundation of China (82002425, 82273226), and the Young Elite Scientist Sponsorship Program by Cast of China Association for Science and Technology (2020QNRC001).
Conflict of interest
The authors declare that the research was conducted in the absence of any commercial or financial relationships that could be construed as a potential conflict of interest.
Publisher’s note
All claims expressed in this article are solely those of the authors and do not necessarily represent those of their affiliated organizations, or those of the publisher, the editors and the reviewers. Any product that may be evaluated in this article, or claim that may be made by its manufacturer, is not guaranteed or endorsed by the publisher.
References
1. Esfahani K, Roudaia L, Buhlaiga N, Del Rincon SV, Papneja N, Miller W H Jr. A review of cancer immunotherapy: From the past, to the present, to the future Curr Oncol (2020) 27:S87–97 doi: 10.3747/co.27.5223
2. Zhang Y, Zhang Z. The history and advances in cancer immunotherapy: Understanding the characteristics of tumor-infiltrating immune cells and their therapeutic implications. Cell Mol Immunol (2020) 17:807–21. doi: 10.1038/s41423-020-0488-6
3. Gettinger S, Horn L, Jackman D, Spigel D, Antonia S, Hellmann M, et al. Five-year follow-up of nivolumab in previously treated advanced non-Small-Cell lung cancer: Results from the CA209-003 study. J Clin Oncol (2018) 36:1675–84. doi: 10.1200/JCO.2017.77.0412
4. Garon EB, Hellmann MD, Rizvi NA, Carcereny E, Leighl NB, Ahn MJ, et al. Five-year overall survival for patients with advanced NonSmall-cell lung cancer treated with pembrolizumab: Results from the phase I KEYNOTE-001 study. J Clin Oncol (2019) 37:2518–27. doi: 10.1200/JCO.19.00934
5. Horn L, Mansfield AS, Szczesna A, Havel L, Krzakowski M, Hochmair MJ, et al. First-line atezolizumab plus chemotherapy in extensive-stage small-cell lung cancer. N Engl J Med (2018) 379:2220–9. doi: 10.1056/NEJMoa1809064
6. Haslam A, Prasad V. Estimation of the percentage of US patients with cancer who are eligible for and respond to checkpoint inhibitor immunotherapy drugs. JAMA Netw Open (2019) 2:e192535. doi: 10.1001/jamanetworkopen.2019.2535
7. Ribas A, Wolchok JD. Cancer immunotherapy using checkpoint blockade. Science (2018) 359:1350–5. doi: 10.1126/science.aar4060
8. Jenkins RW, Barbie DA, Flaherty KT. Mechanisms of resistance to immune checkpoint inhibitors. Br J Cancer (2018) 118:9–16. doi: 10.1038/bjc.2017.434
9. Gide TN, Wilmott JS, Scolyer RA, Long GV. Primary and acquired resistance to immune checkpoint inhibitors in metastatic melanoma. Clin Cancer Res (2018) 24:1260–70. doi: 10.1158/1078-0432.CCR-17-2267
10. Sharma P, Hu-Lieskovan S, Wargo JA, Ribas A. Primary, adaptive, and acquired resistance to cancer immunotherapy. Cell (2017) 168:707–23. doi: 10.1016/j.cell.2017.01.017
11. Petitprez F, Meylan M, de Reynies A, Sautes-Fridman C, Fridman WH. The tumor microenvironment in the response to immune checkpoint blockade therapies. Front Immunol (2020) 11:784. doi: 10.3389/fimmu.2020.00784
12. Wu T, Dai Y. Tumor microenvironment and therapeutic response. Cancer Lett (2017) 387:61–8. doi: 10.1016/j.canlet.2016.01.043
13. Chen DS, Mellman I. Elements of cancer immunity and the cancer-immune set point. Nature (2017) 541:321–30. doi: 10.1038/nature21349
14. Herbst RS, Soria JC, Kowanetz M, Fine GD, Hamid O, Gordon MS, et al. Predictive correlates of response to the anti-PD-L1 antibody MPDL3280A in cancer patients. Nature (2014) 515:563–7. doi: 10.1038/nature14011
15. Horvath L, Thienpont B, Zhao L, Wolf D, Pircher A. Overcoming immunotherapy resistance in non-small cell lung cancer (NSCLC) - novel approaches and future outlook. Mol Cancer (2020) 19:141. doi: 10.1186/s12943-020-01260-z
16. Upadhaya S, Neftelinov ST, Hodge J, Campbell J. Challenges and opportunities in the PD1/PDL1 inhibitor clinical trial landscape. Nat Rev Drug Discovery (2022) 21:482–3. doi: 10.1038/d41573-022-00030-4
17. Chen Y, Yang Z, Wang Y, Hu M, Zhang B, Zhang Y, et al. Pembrolizumab plus chemotherapy or anlotinib vs. pembrolizumab alone in patients with previously treated EGFR-mutant NSCLC. Front Oncol (2021) 11:671228. doi: 10.1016/S0140-6736(20)32531-9
18. Kim R, Keam B, Hahn S, Ock CY, Kim M, Kim TM, et al. First-line pembrolizumab versus pembrolizumab plus chemotherapy versus chemotherapy alone in non-small-cell lung cancer: A systematic review and network meta-analysis. Clin Lung Cancer (2019) 20:331–338 e4. doi: 10.1016/j.cllc.2019.05.009
19. Chen Y, Wang Y, Yang Z, Hu M, Zhang Y, Qian F, et al. Pembrolizumab alone or combined with chemotherapy in advanced NSCLC with PD-L1 >/=50%: Results of a retrospective study. Front Oncol (2021) 11:691519. doi: 10.1016/S0140-6736(19)32591-7
20. Rodriguez-Abreu D, Powell SF, Hochmair MJ, Gadgeel S, Esteban E, Felip E, et al. Pemetrexed plus platinum with or without pembrolizumab in patients with previously untreated metastatic nonsquamous NSCLC: Protocol-specified final analysis from KEYNOTE-189. Ann Oncol (2021) 32:881–95. doi: 10.1016/j.annonc.2021.04.008
21. Reck M, Mok TSK, Nishio M, Jotte RM, Cappuzzo F, Orlandi F, et al. Atezolizumab plus bevacizumab and chemotherapy in non-small-cell lung cancer (IMpower150): key subgroup analyses of patients with EGFR mutations or baseline liver metastases in a randomised, open-label phase 3 trial. Lancet Respir Med (2019) 7:387–401. doi: 10.1016/S2213-2600(19)30084-0
22. Paz-Ares L, Vicente D, Tafreshi A, Robinson A, Soto Parra H, Mazieres J, et al. Placebo-controlled trial of pembrolizumab plus chemotherapy in patients with metastatic squamous NSCLC: Protocol-specified final analysis of KEYNOTE-407. J Thorac Oncol (2020) 15:1657–69. doi: 10.1016/j.jtho.2020.06.015
23. West H, McCleod M, Hussein M, Morabito A, Rittmeyer A, Conter HJ, et al. Atezolizumab in combination with carboplatin plus nab-paclitaxel chemotherapy compared with chemotherapy alone as first-line treatment for metastatic non-squamous non-small-cell lung cancer (IMpower130): a multicentre, randomised, open-label, phase 3 trial. Lancet Oncol (2019) 20:924–37. doi: 10.1016/S1470-2045(19)30167-6
24. Kim SY, Halmos B. Choosing the best first-line therapy: NSCLC with no actionable oncogenic driver. Lung Cancer Manag (2020) 9:LMT36. doi: 10.2217/lmt-2020-0003
25. Tesniere A, Schlemmer F, Boige V, Kepp O, Martins I, Ghiringhelli F, et al. Immunogenic death of colon cancer cells treated with oxaliplatin. Oncogene (2009) 29:482–91. doi: 10.1038/onc.2009.356
26. Schaer DA, Geeganage S, Amaladas N, Lu ZH, Rasmussen ER, Sonyi A, et al. The folate pathway inhibitor pemetrexed pleiotropically enhances effects of cancer immunotherapy. Clin Cancer Res (2019) 25:7175–88. doi: 10.1158/1078-0432.CCR-19-0433
27. Obeid M, Tesniere A, Ghiringhelli F, Fimia GM, Apetoh L, Perfettini JL, et al. Calreticulin exposure dictates the immunogenicity of cancer cell death. Nat Med (2007) 13:54–61. doi: 10.1038/nm1523
28. Lau TS, Chan LKY, Man GCW, Wong CH, Lee JHS, Yim SF, et al. Paclitaxel induces immunogenic cell death in ovarian cancer via TLR4/IKK2/SNARE-dependent exocytosis. Cancer Immunol Res (2020) 8:1099–111. doi: 10.1158/2326-6066.CIR-19-0616
29. Galluzzi L, Humeau J, Buque A, Zitvogel L, Kroemer G. Immunostimulation with chemotherapy in the era of immune checkpoint inhibitors. Nat Rev Clin Oncol (2020) 17:725–41. doi: 10.1038/s41571-020-0413-z
30. Du B, Waxman DJ. Medium dose intermittent cyclophosphamide induces immunogenic cell death and cancer cell autonomous type I interferon production in glioma models. Cancer Lett (2020) 470:170–80. doi: 10.1016/j.canlet.2019.11.025
31. Furukawa R, Inoue H, Yoneshima Y, Tsutsumi H, Iwama E, Ikematsu Y, et al. Cytotoxic chemotherapeutic agents and the EGFR-TKI osimertinib induce calreticulin exposure in non-small cell lung cancer. Lung Cancer (2021) 155:144–50. doi: 10.1016/j.lungcan.2021.03.018
32. Galluzzi L, Vitale I, Aaronson SA, Abrams JM, Adam D, Agostinis P, et al. Molecular mechanisms of cell death: Recommendations of the nomenclature committee on cell death 2018. Cell Death Differ (2018) 25:486–541. doi: 10.1038/s41418-017-0012-4
33. Galluzzi L, Vitale I, Warren S, Adjemian S, Agostinis P, Martinez AB, et al. Consensus guidelines for the definition, detection and interpretation of immunogenic cell death. J Immunother Cancer (2020) 8(1):e000337. doi: 10.1136/jitc-2019-000337corr1
34. Galluzzi L, Buque A, Kepp O, Zitvogel L, Kroemer G. Immunogenic cell death in cancer and infectious disease. Nat Rev Immunol (2017) 17:97–111. doi: 10.1038/nri.2016.107
35. Kroemer G, Galassi C, Zitvogel L, Galluzzi L. Immunogenic cell stress and death. Nat Immunol (2022) 23:487–500. doi: 10.1038/s41590-022-01132-2
36. Elliott MR, Chekeni FB, Trampont PC, Lazarowski ER, Kadl A, Walk SF, et al. Nucleotides released by apoptotic cells act as a find-me signal to promote phagocytic clearance. Nature (2009) 461:282–6. doi: 10.1038/nature08296
37. Saez PJ, Vargas P, Shoji KF, Harcha PA, Lennon-Dumenil AM, Saez JC. ATP promotes the fast migration of dendritic cells through the activity of pannexin 1 channels and P2X7 receptors. Sci Signal 10 (2017) 10:eaah7107. doi: 10.1126/scisignal.aah7107
38. Idzko M, Hammad H, van Nimwegen M, Kool M, Willart MA, Muskens F, et al. Extracellular ATP triggers and maintains asthmatic airway inflammation by activating dendritic cells. Nat Med (2007) 13:913–9. doi: 10.1038/nm1617
39. Kroemer G, Galluzzi L, Kepp O, Zitvogel L. Immunogenic cell death in cancer therapy. Annu Rev Immunol (2013) 31:51–72. doi: 10.1146/annurev-immunol-032712-100008
40. Yu Y, Feng S, Wei S, Zhong Y, Yi G, Chen H, et al. Extracellular ATP activates P2X7R-NF-kappaB (p65) pathway to promote the maturation of bone marrow-derived dendritic cells of mice. Cytokine (2019) 119:175–81. doi: 10.1016/j.cyto.2019.03.019
41. Wilkin F, Duhant X, Bruyns C, Suarez-Huerta N, Boeynaems JM, Robaye B. The P2Y11 receptor mediates the ATP-induced maturation of human monocyte-derived dendritic cells. J Immunol (2001) 166:7172–7. doi: 10.4049/jimmunol.166.12.7172
42. Ghiringhelli F, Apetoh L, Tesniere A, Aymeric L, Ma Y, Ortiz C, et al. Activation of the NLRP3 inflammasome in dendritic cells induces IL-1beta-dependent adaptive immunity against tumors. Nat Med (2009) 15:1170–8. doi: 10.1038/nm.2028
43. Zhang Y, Yang W, Li W, Zhao Y. NLRP3 inflammasome: Checkpoint connecting innate and adaptive immunity in autoimmune diseases. Front Immunol (2021) 12:732933. doi: 10.3389/fimmu.2021.732933
44. Garlanda C, Dinarello CA, Mantovani A. The interleukin-1 family: Back to the future. Immunity (2013) 39:1003–18. doi: 10.1016/j.immuni.2013.11.010
45. Di Virgilio F, Dal Ben D, Sarti AC, Giuliani AL, Falzoni S. The P2X7 receptor in infection and inflammation. Immunity (2017) 47:15–31. doi: 10.1016/j.immuni.2017.06.020
46. Panaretakis T, Joza N, Modjtahedi N, Tesniere A, Vitale I, Durchschlag M, et al. The co-translocation of ERp57 and calreticulin determines the immunogenicity of cell death. Cell Death Different (2008) 15:1499–509. doi: 10.1038/cdd.2008.67
47. Obeid M, Panaretakis T, Tesniere A, Joza N, Tufi R, Apetoh L, et al. Leveraging the immune system during chemotherapy: Moving calreticulin to the cell surface converts apoptotic death from “silent” to immunogenic. Cancer Res (2007) 67:7941–4. doi: 10.1158/0008-5472.CAN-07-1622
48. Liu P, Zhao L, Loos F, Marty C, Xie W, Martins I, et al. Immunosuppression by mutated calreticulin released from malignant cells. Mol Cell (2020) 77:748–760.e9. doi: 10.1016/j.molcel.2019.11.004
49. Chen R, Huang M, Yang X, Chen XH, Shi MY, Li ZF, et al. CALR-TLR4 complex inhibits non-small cell lung cancer progression by regulating the migration and maturation of dendritic cells. Front Oncol (2021) 11:743050. doi: 10.3389/fonc.2021.743050
50. Li R, Zou X, Huang H, Yu Y, Zhang H, Liu P, et al. HMGB1/PI3K/Akt/mTOR signaling participates in the pathological process of acute lung injury by regulating the maturation and function of dendritic cells. Front Immunol (2020) 11. doi: 10.3389/fimmu.2020.01104
51. Apetoh L, Ghiringhelli F, Tesniere A, Obeid M, Ortiz C, Criollo A, et al. Toll-like receptor 4-dependent contribution of the immune system to anticancer chemotherapy and radiotherapy. Nat Med (2007) 13:1050–9. doi: 10.1038/nm1622
52. Hubert P, Roncarati P, Demoulin S, Pilard C, Ancion M, Reynders C, et al. Extracellular HMGB1 blockade inhibits tumor growth through profoundly remodeling immune microenvironment and enhances checkpoint inhibitorbased immunotherapy. J Immunother Cancer (2021) 9(3):e001966. doi: 10.1136/jitc-2020-001966
53. Zhou L, Xu Q, Huang L, Jin J, Zuo X, Zhang Q, et al. Low-dose carboplatin reprograms tumor immune microenvironment through STING signaling pathway and synergizes with PD-1 inhibitors in lung cancer. Cancer Lett (2021) 500:163–71. doi: 10.1016/j.canlet.2020.11.049
54. Du B, Wen X, Wang Y, Lin M, Lai J. Gemcitabine and checkpoint blockade exhibit synergistic anti-tumor effects in a model of murine lung carcinoma. Int Immunophar (2020) 86:106694. doi: 10.1016/j.intimp.2020.106694
55. Lu CS, Lin CW, Chang YH, Chen HY, Chung WC, Lai WY, et al. Antimetabolite pemetrexed primes a favorable tumor microenvironment for immune checkpoint blockade therapy. J Immunother Cancer (2020) 8(2):e001392. doi: 10.1136/jitc-2020-001392
56. Fournel L, Wu Z, Stadler N, Damotte D, Lococo F, Boulle G, et al. Cisplatin increases PD-L1 expression and optimizes immune check-point blockade in non-small cell lung cancer. Cancer Lett (2019) 464:5–14. doi: 10.1016/j.canlet.2019.08.005
57. Zhou Y, Bastian IN, Long MD, Dow M, Li W, Liu T, et al. Activation of NF-kappaB and p300/CBP potentiates cancer chemoimmunotherapy through induction of MHC-I antigen presentation. Proc Natl Acad Sci U.S.A. (2021) 118(8):e2025840118. doi: 10.1073/pnas.2025840118
58. Okita R, Yukawa T, Nojima Y, Maeda A, Saisho S, Shimizu K, et al. MHC class I chain-related molecule a and b expression is upregulated by cisplatin and associated with good prognosis in patients with non-small cell lung cancer. Cancer Immunol Immunother (2016) 65:499–509. doi: 10.1007/s00262-016-1814-9
59. Ganesan AP, Johansson M, Ruffell B, Yagui-Beltran A, Lau J, Jablons DM, et al. Tumor-infiltrating regulatory T cells inhibit endogenous cytotoxic T cell responses to lung adenocarcinoma. J Immunol (2013) 191:2009–17. doi: 10.4049/jimmunol.1301317
60. Ye J, Zou MM, Li P, Lin XJ, Jiang QW, Yang Y, et al. Oxymatrine and cisplatin synergistically enhance anti-tumor immunity of CD8(+) T cells in non-small cell lung cancer. Front Oncol (2018) 8:631. doi: 10.3389/fonc.2018.00631
61. Leonetti A, Wever B, Mazzaschi G, Assaraf YG, Rolfo C, Quaini F, et al. Molecular basis and rationale for combining immune checkpoint inhibitors with chemotherapy in non-small cell lung cancer. Drug Resist Update (2019) 46:100644. doi: 10.1016/j.drup.2019.100644
62. Ghiringhelli F, Larmonier N, Schmitt E, Parcellier A, Cathelin D, Garrido C, et al. CD4+CD25+ regulatory T cells suppress tumor immunity but are sensitive to cyclophosphamide which allows immunotherapy of established tumors to be curative. Eur J Immunol (2004) 34:336–44. doi: 10.1002/eji.200324181
63. Tongu M, Harashima N, Monma H, Inao T, Yamada T, Kawauchi H, et al. Metronomic chemotherapy with low-dose cyclophosphamide plus gemcitabine can induce anti-tumor T cell immunity in vivo. Cancer Immunol Immunother (2013) 62:383–91. doi: 10.1007/s00262-012-1343-0
64. Huang X, Huang G, Song H, Chen L. Preconditioning chemotherapy with paclitaxel and cisplatin enhances the antitumor activity of cytokine induced-killer cells in a murine lung carcinoma model. Int J Cancer (2011) 129:648–58. doi: 10.1002/ijc.25702
65. Zhang L, Dermawan K, Jin M, Liu R, Zheng H, Xu L, et al. Differential impairment of regulatory T cells rather than effector T cells by paclitaxel-based chemotherapy. Clin Immunol (2008) 129:219–29. doi: 10.1016/j.clim.2008.07.013
66. Zhong H, Lai Y, Zhang R, Daoud A, Feng Q, Zhou J, et al. Low dose cyclophosphamide modulates tumor microenvironment by TGF-beta signaling pathway. Int J Mol Sci (2020) 21(3):957. doi: 10.3390/ijms21030957
67. Laheurte C, Thiery-Vuillemin A, Calcagno F, Legros A, Simonin H, Boullerot L, et al. Metronomic cyclophosphamide induces regulatory T cells depletion and PSA-specific T cells reactivation in patients with biochemical recurrent prostate cancer. Int J Cancer (2020) 147:1199–205. doi: 10.1002/ijc.32803
68. Gabrilovich DI. Myeloid-derived suppressor cells. Cancer Immunol Res (2017) 5:3–8. doi: 10.1158/2326-6066.CIR-16-0297
69. Kumar V, Patel S, Tcyganov E, Gabrilovich DI. The nature of myeloid-derived suppressor cells in the tumor microenvironment. Trends Immunol (2016) 37:208–20. doi: 10.1016/j.it.2016.01.004
70. Zhang Y, Bush X, Yan B, Chen JA. Gemcitabine nanoparticles promote antitumor immunity against melanoma. Biomaterials (2019) 189:48–59. doi: 10.1016/j.biomaterials.2018.10.022
71. Kodumudi KN, Woan K, Gilvary DL, Sahakian E, Wei S, Djeu JY. A novel chemoimmunomodulating property of docetaxel: Suppression of myeloid-derived suppressor cells in tumor bearers. Clin Cancer Res (2010) 16:4583–94. doi: 10.1158/1078-0432.CCR-10-0733
72. Suzuki E, Kapoor V, Jassar AS, Kaiser LR, Albelda SM. Gemcitabine selectively eliminates splenic gr-1+/CD11b+ myeloid suppressor cells in tumor-bearing animals and enhances antitumor immune activity. Clin Cancer Res (2005) 11:6713–21. doi: 10.1158/1078-0432.CCR-05-0883
73. Vincent J, Mignot G, Chalmin F, Ladoire S, Bruchard M, Chevriaux A, et al. 5-fluorouracil selectively kills tumor-associated myeloid-derived suppressor cells resulting in enhanced T cell-dependent antitumor immunity. Cancer Res (2010) 70:3052–61. doi: 10.1158/0008-5472.CAN-09-3690
74. Eriksson E, Wenthe J, Irenaeus S, Loskog A, Ullenhag G. Gemcitabine reduces MDSCs, tregs and TGFbeta-1 while restoring the teff/treg ratio in patients with pancreatic cancer. J Transl Med (2016) 14:282. doi: 10.1186/s12967-016-1037-z
75. Peereboom DM, Alban TJ, Grabowski MM, Alvarado AG, Otvos B, Bayik D, et al. Metronomic capecitabine as an immune modulator in glioblastoma patients reduces myeloid-derived suppressor cells. JCI Insight (2019) 4(22):e130748. doi: 10.1172/jci.insight.130748
76. Li XM, Zhang XM, Li JY, Jiang N, Chen L, Tang LL, et al. The immune modulation effects of gemcitabine plus cisplatin induction chemotherapy in nasopharyngeal carcinoma. Cancer Med (2022) 11:3437–44. doi: 10.1002/cam4.4705
77. Glorieux C, Xia X, You X, Wang Z, Han Y, Yang J, et al. Cisplatin and gemcitabine exert opposite effects on immunotherapy with PD-1 antibody in K-ras-driven cancer. J Adv Res (2022) 40:109–24. doi: 10.1016/j.jare.2021.12.005
78. Gao Q, Wang S, Chen X, Cheng S, Zhang Z, Li F, et al. Cancer-cell-secreted CXCL11 promoted CD8(+) T cells infiltration through docetaxel-induced-release of HMGB1 in NSCLC. J Immunother Cancer (2019) 7:42. doi: 10.1186/s40425-019-0511-6
79. Fang Y, Sun H, Xiao X, Tang M, Tian Z, Wei H, et al. Low-dose immunogenic chemotherapeutics promotes immune checkpoint blockade in microsatellite stability colon cancer. Front Immunol (2022) 13:1040256. doi: 10.3389/fimmu.2022.1040256
80. Cascone T, Sepesi B, Lin HY, Kalhor N, Parra ER, Jiang M, et al. A phase I/II study of neoadjuvant cisplatin, docetaxel, and nintedanib for resectable non-small cell lung cancer. Clin Cancer Res (2020) 26:3525–36. doi: 10.1158/1078-0432.CCR-19-4180
81. Xin M, Lin D, Yan N, Li H, Li J, Huang Z. Oxaliplatin facilitates tumor-infiltration of T cells and natural-killer cells for enhanced tumor immunotherapy in lung cancer model. Anticancer Drugs (2022) 33:117–23. doi: 10.1097/CAD.0000000000001248
82. Karin N. CXCR3 ligands in cancer and autoimmunity, chemoattraction of effector T cells, and beyond. Front Immunol (2020) 11:976. doi: 10.3389/fimmu.2020.00976
83. Agostini C, Calabrese F, Rea F, Facco M, Tosoni A, Loy M, et al. CXCR3 and its ligand CXCL10 are expressed by inflammatory cells infiltrating lung allografts and mediate chemotaxis of T cells at sites of rejection. Am J Pathol (2001) 158:1703–11. doi: 10.1016/S0002-9440(10)64126-0
84. Gaudreau PO, Negrao MV, Mitchell KG, Reuben A, Corsini EM, Li J, et al. Neoadjuvant chemotherapy increases cytotoxic T cell, tissue resident memory T cell, and b cell infiltration in resectable NSCLC. J Thorac Oncol (2021) 16:127–39. doi: 10.1016/j.jtho.2020.09.027
85. Edwards J, Wilmott JS, Madore J, Gide TN, Quek C, Tasker A, et al. CD103(+) tumor-resident CD8(+) T cells are associated with improved survival in immunotherapy-naive melanoma patients and expand significantly during anti-PD-1 treatment. Clin Cancer Res (2018) 24:3036–45. doi: 10.1158/1078-0432.CCR-17-2257
86. Djenidi F, Adam J, Goubar A, Durgeau A, Meurice G, de Montpreville V, et al. CD8+CD103+ tumor-infiltrating lymphocytes are tumor-specific tissue-resident memory T cells and a prognostic factor for survival in lung cancer patients. J Immunol (2015) 194:3475–86. doi: 10.4049/jimmunol.1402711
87. Ganesan AP, Clarke J, Wood O, Garrido-Martin EM, Chee SJ, Mellows T, et al. Tissue-resident memory features are linked to the magnitude of cytotoxic T cell responses in human lung cancer. Nat Immunol (2017) 18:940–50. doi: 10.1038/ni.3775
88. Mami-Chouaib F, Tartour E. Editorial: Tissue resident memory T cells. Front Immunol (2019) 10:1018. doi: 10.3389/fimmu.2019.01018
89. Merritt RE, Mahtabifard A, Yamada RE, Crystal RG, Korst RJ. Cisplatin augments cytotoxic t-lymphocyte–mediated antitumor immunity in poorly immunogenic murine lung cancer. J Thorac Cardiovasc Surg (2003) 126:1609–17. doi: 10.1016/S0022-5223(03)00707-4
90. Ma Y, Mattarollo SR, Adjemian S, Yang H, Aymeric L, Hannani D, et al. CCL2/CCR2-dependent recruitment of functional antigen-presenting cells into tumors upon chemotherapy. Cancer Res (2014) 74:436–45. doi: 10.1158/0008-5472.CAN-13-1265
91. Ma Y, Adjemian S, Mattarollo SR, Yamazaki T, Aymeric L, Yang H, et al. Anticancer chemotherapy-induced intratumoral recruitment and differentiation of antigen-presenting cells. Immunity (2013) 38:729–41. doi: 10.1016/j.immuni.2013.03.003
92. Bernal-Estevez DA, Garcia O, Sanchez R, Parra-Lopez CA. Monitoring the responsiveness of T and antigen presenting cell compartments in breast cancer patients is useful to predict clinical tumor response to neoadjuvant chemotherapy. BMC Cancer (2018) 18:77. doi: 10.1186/s12885-017-3982-1
93. Tan Y, Chen H, Zhang J, Cai L, Jin S, Song D, et al. Platinum(IV) complexes as inhibitors of CD47-SIRPalpha axis for chemoimmunotherapy of cancer. Eur J Med Chem (2022) 229:114047. doi: 10.1016/j.ejmech.2021.114047
94. Lian S, Xie R, Ye Y, Xie X, Li S, Lu Y, et al. Simultaneous blocking of CD47 and PD-L1 increases innate and adaptive cancer immune responses and cytokine release. EBioMedicine (2019) 42:281–95. doi: 10.1016/j.ebiom.2019.03.018
95. Salmon H, Idoyaga J, Rahman A, Leboeuf M, Remark R, Jordan S, et al. Expansion and activation of CD103(+) dendritic cell progenitors at the tumor site enhances tumor responses to therapeutic PD-L1 and BRAF inhibition. Immunity (2016) 44:924–38. doi: 10.1016/j.immuni.2016.03.012
96. Spranger S, Bao R, Gajewski TF. Melanoma-intrinsic beta-catenin signalling prevents anti-tumour immunity. Nature (2015) 523:231–5. doi: 10.1038/nature14404
97. Duraiswamy J, Turrini R, Minasyan A, Barras D, Crespo I, Grimm AJ, et al. Myeloid antigen-presenting cell niches sustain antitumor T cells and license PD-1 blockade via CD28 costimulation. Cancer Cell (2021) 39:1623–1642.e20. doi: 10.1016/j.ccell.2021.10.008
98. Gardner A, de Mingo Pulido A, Ruffell B. Dendritic cells and their role in immunotherapy. Front Immunol (2020) 11:924. doi: 10.3389/fimmu.2020.00924
99. de Mingo Pulido A, Gardner A, Hiebler S, Soliman H, Rugo HS, Krummel MF, et al. TIM-3 regulates CD103(+) dendritic cell function and response to chemotherapy in breast cancer. Cancer Cell (2018) 33:60–74.e6. doi: 10.1016/j.ccell.2017.11.019
100. Chow MT, Ozga AJ, Servis RL, Frederick DT, Lo JA, Fisher DE, et al. Intratumoral activity of the CXCR3 chemokine system is required for the efficacy of anti-PD-1 therapy. Immunity (2019) 50:1498–1512.e5. doi: 10.1016/j.immuni.2019.04.010
101. Hanahan D, Weinberg RA. Hallmarks of cancer: the next generation. Cell (2011) 144:646–74. doi: 10.1016/j.cell.2011.02.013
102. Cascone T, McKenzie JA, Mbofung RM, Punt S, Wang Z, Xu C, et al. Increased tumor glycolysis characterizes immune resistance to adoptive T cell therapy. Cell Metab (2018) 27:977–987 e4. doi: 10.1016/j.cmet.2018.02.024
103. Renner K, Singer K, Koehl GE, Geissler EK, Peter K, Siska PJ, et al. Metabolic hallmarks of tumor and immune cells in the tumor microenvironment. Front Immunol (2017) 8. doi: 10.3389/fimmu.2017.00248
104. Ma Y, Yang H, Pitt JM, Kroemer G, Zitvogel L. Therapy-induced microenvironmental changes in cancer. J Mol Med (Berl) (2016) 94:497–508. doi: 10.1007/s00109-016-1401-8
105. Buck MD, O’Sullivan D, Pearce EL. T Cell metabolism drives immunity. J Exp Med (2015) 212:1345–60. doi: 10.1084/jem.20151159
106. Tan H, Yang K, Li Y, Shaw TI, Wang Y, Blanco DB, et al. Integrative proteomics and phosphoproteomics profiling reveals dynamic signaling networks and bioenergetics pathways underlying T cell activation. Immunity (2017) 46:488–503. doi: 10.1016/j.immuni.2017.02.010
107. McCoy KD, Mager LF. Impact of the microbiome on tumor immunity. Curr Opin Immunol (2021) 69:39–46. doi: 10.1016/j.coi.2021.01.002
108. Jin Y, Dong H, Xia L, Yang Y, Zhu Y, Shen Y, et al. The diversity of gut microbiome is associated with favorable responses to anti-programmed death 1 immunotherapy in Chinese patients with NSCLC. J Thorac Oncol (2019) 14:1378–89. doi: 10.1016/j.jtho.2019.04.007
109. Gopalakrishnan V, Spencer CN, Nezi L, Reuben A, Andrews MC, Karpinets TV, et al. Gut microbiome modulates response to anti-PD-1 immunotherapy in melanoma patients. Science (2018) 359:97–103. doi: 10.1126/science.aan4236
110. Peng Z, Cheng S, Kou Y, Wang Z, Jin R, Hu H, et al. The gut microbiome is associated with clinical response to anti-PD-1/PD-L1 immunotherapy in gastrointestinal cancer. Cancer Immunol Res (2020) 8:1251–61. doi: 10.1158/2326-6066.CIR-19-1014
111. Pensec C, Gillaizeau F, Guenot D, Bessard A, Carton T, Leuillet S, et al. Impact of pemetrexed chemotherapy on the gut microbiota and intestinal inflammation of patient-lung-derived tumor xenograft (PDX) mouse models. Sci Rep (2020) 10:9094. doi: 10.1038/s41598-020-65792-6
112. Viaud S, Saccheri F, Mignot G, Yamazaki T, Daillere R, Hannani D, et al. The intestinal microbiota modulates the anticancer immune effects of cyclophosphamide. Science (2013) 342:971–6. doi: 10.1126/science.1240537
113. Gandhi L, Rodriguez-Abreu D, Gadgeel S, Esteban E, Felip E, De Angelis F, et al. Pembrolizumab plus chemotherapy in metastatic non-Small-Cell lung cancer. N Engl J Med (2018) 378:2078–92. doi: 10.1056/NEJMoa1801005
114. Burtness B, Harrington KJ, Greil R, Soulières D, Tahara M, de Castro G, et al. Pembrolizumab alone or with chemotherapy versus cetuximab with chemotherapy for recurrent or metastatic squamous cell carcinoma of the head and neck (KEYNOTE-048): a randomised, open-label, phase 3 study. Lancet (2019) 394:1915–28. doi: 10.1016/S0140-6736(19)32591-7
115. Leduc C, Adam J, Louvet E, Sourisseau T, Dorvault N, Bernard M, et al. TPF induction chemotherapy increases PD-L1 expression in tumour cells and immune cells in head and neck squamous cell carcinoma. ESMO Open (2018) 3:e000257. doi: 10.1136/esmoopen-2017-000257
116. Jotte R, Cappuzzo F, Vynnychenko I, Stroyakovskiy D, Rodriguez-Abreu D, Hussein M, et al. Atezolizumab in combination with carboplatin and nab-paclitaxel in advanced squamous NSCLC (IMpower131): Results from a randomized phase III trial. J Thorac Oncol (2020) 15:1351–60. doi: 10.1016/j.jtho.2020.03.028
117. Liu M, Tayob N, Penter L, Sellars M, Tarren A, Chea V, et al. Improved T-cell immunity following neoadjuvant chemotherapy in ovarian cancer. Clin Cancer Res (2022) 28:3356–66. doi: 10.1158/1078-0432.CCR-21-2834
118. Hoffner B, Leighl NB, Davies M. Toxicity management with combination chemotherapy and programmed death 1/programmed death ligand 1 inhibitor therapy in advanced lung cancer. Cancer Treat Rev (2020) 85:101979. doi: 10.1016/j.ctrv.2020.101979
119. Schmid P, Salgado R, Park YH, Munoz-Couselo E, Kim SB, Sohn J, et al. Pembrolizumab plus chemotherapy as neoadjuvant treatment of high-risk, early-stage triple-negative breast cancer: Results from the phase 1b open-label, multicohort KEYNOTE-173 study. Ann Oncol (2020) 31:569–81. doi: 10.1016/j.annonc.2020.01.072
120. Hu F, Zhai Y, Yuan L, Liang J, Xu J, Guo X, et al. Renal toxicities in immune checkpoint inhibitors with or without chemotherapy: An observational, retrospective, pharmacovigilance study leveraging US FARES database. Cancer Med (2021) 10:8754–62. doi: 10.1002/cam4.4343
121. Cui W, Luo Q, Sun SL, Xu JL, Gu H. Efficacy and safety of PD-1/PD-L1 inhibitors plus chemotherapy for triple-negative breast cancer: A systematic review and meta-analysis. Recenti Prog Med (2022) 113:722–32. doi: 10.1701/3914.38974
122. Segal EM. Immunotherapy in the frontline management of advanced and metastatic NSCLC. Am J Manag Care (2021) 27:S323–32. doi: 10.37765/ajmc.2021.88769
123. Leong TL, Bryant VL. B cells in lung cancer-not just a bystander cell: A literature review. Transl Lung Cancer Res (2021) 10:2830–41. doi: 10.21037/tlcr-20-788
124. Ziebart A, Huber U, Jeske S, Laban S, Doescher J, Hoffmann TK, et al. The influence of chemotherapy on adenosine-producing b cells in patients with head and neck squamous cell carcinoma. Oncotarget (2018) 9:5834–47. doi: 10.18632/oncotarget.23533
125. Barrett RL, Pure E. Cancer-associated fibroblasts and their influence on tumor immunity and immunotherapy. Elife (2020) 9:e57243. doi: 10.7554/eLife.57243
126. Barrett R, Pure E. Cancer-associated fibroblasts: Key determinants of tumor immunity and immunotherapy. Curr Opin Immunol (2020) 64:80–7. doi: 10.1016/j.coi.2020.03.004
127. Kato T, Noma K, Ohara T, Kashima H, Katsura Y, Sato H, et al. Cancer-associated fibroblasts affect intratumoral CD8(+) and FoxP3(+) T cells Via IL6 in the tumor microenvironment. Clin Cancer Res (2018) 24:4820–33. doi: 10.1158/1078-0432.CCR-18-0205
128. Remsing Rix LL, Sumi NJ, Hu Q, Desai B, Bryant AT, Li X, et al. IGF-binding proteins secreted by cancer-associated fibroblasts induce context-dependent drug sensitization of lung cancer cells. Sci Signal (2022) 15:eabj5879. doi: 10.1126/scisignal.abj5879
129. Ershaid N, Sharon Y, Doron H, Raz Y, Shani O, Cohen N, et al. NLRP3 inflammasome in fibroblasts links tissue damage with inflammation in breast cancer progression and metastasis. Nat Commun (2019) 10:4375. doi: 10.1038/s41467-019-12370-8
130. Iida Y, Harashima N, Motoshima T, Komohara Y, Eto M, Harada M. Contrasting effects of cyclophosphamide on anti-CTL-associated protein 4 blockade therapy in two mouse tumor models. Cancer Sci (2017) 108:1974–84. doi: 10.1111/cas.13337
131. Kang DH, Choi SW, Sun P, Chung C, Park D, Lee SI, et al. The rest period between chemotherapy and immunotherapy influences the efficacy of immune checkpoint inhibitors in lung cancer. Thorac Cancer (2022) 13:2346–54. doi: 10.1111/1759-7714.14568
132. Fu D, Wu J, Lai J, Liu Y, Zhou L, Chen L, et al. T Cell recruitment triggered by optimal dose platinum compounds contributes to the therapeutic efficacy of sequential PD-1 blockade in a mouse model of colon cancer. Am J Cancer Res (2020) 10:473–90.
133. Ghiringhelli F, Menard C, Puig PE, Ladoire S, Roux S, Martin F, et al. Metronomic cyclophosphamide regimen selectively depletes CD4+CD25+ regulatory T cells and restores T and NK effector functions in end stage cancer patients. Cancer Immunol Immunother (2007) 56:641–8. doi: 10.1007/s00262-006-0225-8
134. Ahlmann M, Hempel G. The effect of cyclophosphamide on the immune system: implications for clinical cancer therapy. Cancer Chemother Pharmacol (2016) 78:661–71. doi: 10.1007/s00280-016-3152-1
Keywords: immuno-checkpoint inhibitors, chemotherapy, tumor microenvironment, immunogenic cell death, anti-tumor activity
Citation: Shi M-Y, Liu H-G, Chen X-H, Tian Y, Chen Z-N and Wang K (2023) The application basis of immuno-checkpoint inhibitors combined with chemotherapy in cancer treatment. Front. Immunol. 13:1088886. doi: 10.3389/fimmu.2022.1088886
Received: 03 November 2022; Accepted: 20 December 2022;
Published: 10 January 2023.
Edited by:
Jian Zhang, Southern Medical University, ChinaReviewed by:
Jake ODonnell, The University of Queensland, AustraliaDalil Hannani, Grenoble (TIMC-IMAG), France
Jian Qiao, University of Texas Southwestern Medical Center, United States
Copyright © 2023 Shi, Liu, Chen, Tian, Chen and Wang. This is an open-access article distributed under the terms of the Creative Commons Attribution License (CC BY). The use, distribution or reproduction in other forums is permitted, provided the original author(s) and the copyright owner(s) are credited and that the original publication in this journal is cited, in accordance with accepted academic practice. No use, distribution or reproduction is permitted which does not comply with these terms.
*Correspondence: Ke Wang, wangke@fmmu.edu.cn; Zhi-Nan Chen, znchen@fmmu.edu.cn
†These authors have contributed equally to this work