- 1Department of Orthodontics II, Affiliated Stomatological Hospital of Zunyi Medical University, Zunyi, China
- 2Oral Disease Research Key Laboratory of Guizhou Tertiary Institution, School of Stomatology, Zunyi Medical University, Zunyi, China
- 3School of Medicine and Technology, Zunyi Medical University, Zunyi, China
Cancer immunotherapy, including the inhibition of immune checkpoints, improves the tumor immune microenvironment and is an effective tool for cancer therapy. More effective and alternative inhibitory targets are critical for successful immune checkpoint blockade therapy. The interaction of the immunomodulatory ligand B7 family with corresponding receptors induces or inhibits T cell responses by sending co-stimulatory and co-inhibitory signals respectively. Blocking the glycosylation of the B7 family members PD-L1, PD-L2, B7-H3, and B7-H4 inhibited the self-stability and receptor binding of these immune checkpoint proteins, leading to immunosuppression and rapid tumor progression. Therefore, regulation of glycosylation may be the “golden key” to relieve tumor immunosuppression. The exploration of a more precise glycosylation regulation mechanism and glycan structure of B7 family proteins is conducive to the discovery and clinical application of antibodies and small molecule inhibitors.
Introduction
Changes in protein glycosylation are a pivotal regulatory part of tumor progression and directly affect cell growth, survival, tumor immune escape and final metastasis (1). Tumor-related crucial glycoproteins (such as EGFR (2, 3), CD44 (4), E-cadherin (5), TGF-β receptor (6, 7), CA199 (8) and MUC-1 (9, 10)), glycan abundance and structural changes profoundly affect tumor cell fate and patient prognosis. Most immune checkpoints are membrane glycoproteins, and increasing attention has been given to how protein glycosylation changes affect the tumor immune microenvironment (11) (12) (13). Programmed cell death ligand 1 (PD-L1) belongs to the B7 protein family and binds to its receptor programmed death receptor 1 (PD-1) on activated T cells to suppress antitumor immunity by counteracting T-cell activation signals (14). The role of glycoprotein PD-L1/PD-1 glycosylation in tumor immune regulation has been extensively studied (15–17). B7 family members also include B7-1, B7-2, PD-L2, B7-H2, B7-H3, B7-H4, B7-H5, BTNL2, B7-H6, B7-H7 and Ig-like domain-containing receptor 2 (ILDR2). These proteins and their receptors play critical roles in cell proliferation, cytokine secretion and tumor immune microenvironment regulation (18–21). B7-1 (22), PD-L2 (23), B7-H3 (24), B7-H4 (25), and B7-H6 (26, 27) were confirmed to be modified by glycosylation. However, current evidence suggests that glycosylation may not be required for the function of B7-1 (22) and B7-H6 (26, 27), and the significance of glycosylation for B7-1 and B7-H6 proteins is largely unknown. Therefore, the significance, mechanism and possibility of targeted therapy of PD-L1, PD-L2, B7-H3, B7-H4 and B7-H6 glycosylation are explored in this review.
Significance, mechanism and possible use of PD-L1 glycosylation as a therapeutic target
The PD-L1 protein consists of an immunoglobulin V-like domain (IgV, F19-T127), an immunoglobulin C-like domain (IgC, P133-V225), a transmembrane domain (TM, T239-F259) and an intracellular short tail domain (R260-T290) (28, 29), and is affected by a variety of posttranslational modifications, including phosphorylation, glycosylation, palmitoylation, acetylation and ubiquitination (30). There are four N-glycan sites in the extracellular domain of PD-L1: N35, N192, N200 and N219 (31), and these N-glycans are critical to the stability of the PD-L1 protein (30, 31). PD-1 and PD-L1 interact through the large hydrophobic surface of their respective Ig-like V-domains, while the Ig-like C2 domain of PD-L1 has no contact with PD-1 (32). The interaction between PD-L1 and PD-1 also requires glycosylation (33). PD-1/PD-L1 binding can induce tumor-specific T-cell apoptosis by inhibiting T-cell activation and is currently one of the most important immunotherapeutic targets (34). The glycosylation site of PD-L1 may not be easily bound by antibodies (35, 36), and understanding the specific mechanism of PD-L1 glycosylation (Figure 1) is of practical significance for targeted therapy.
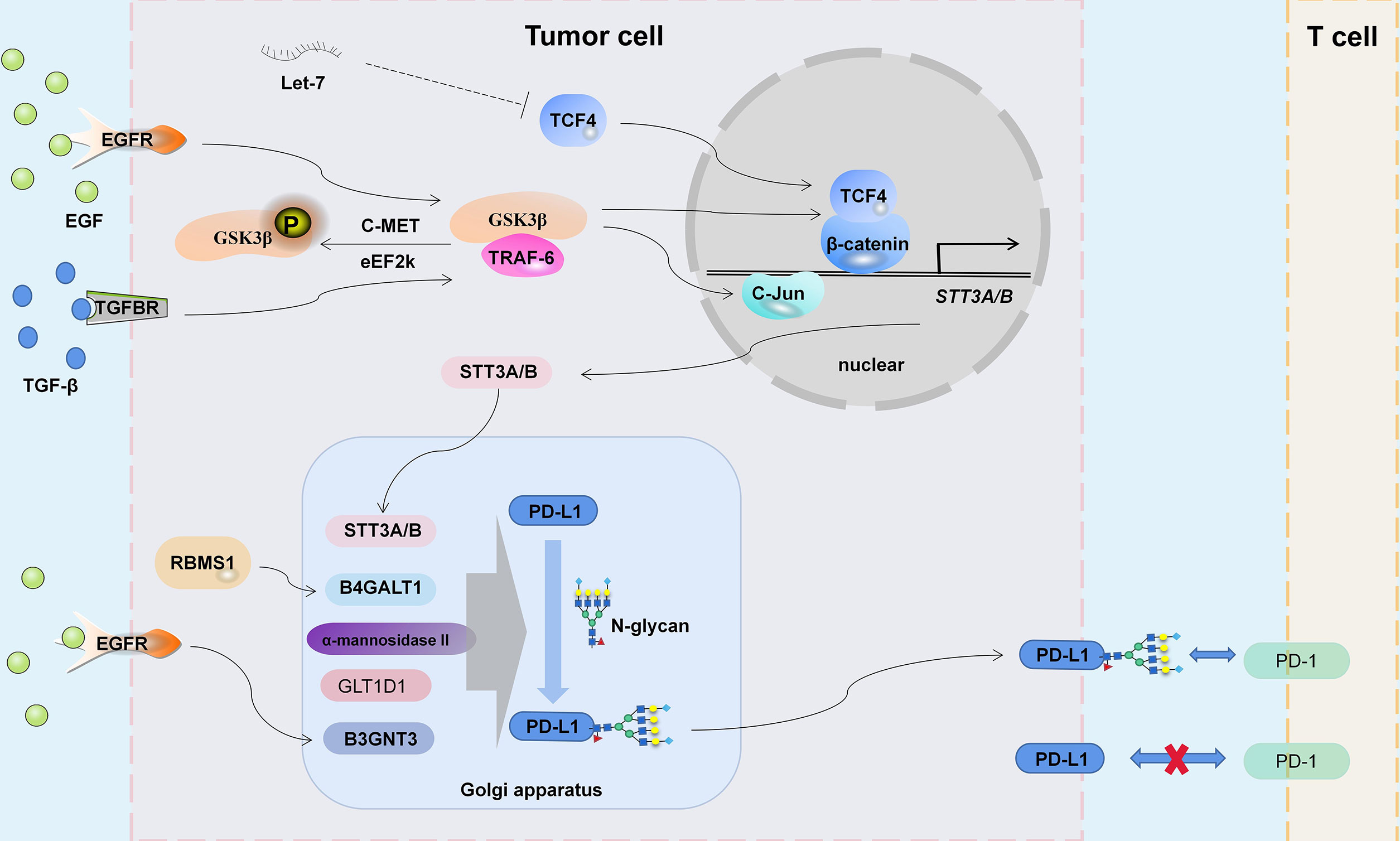
Figure 1 The mechanism of PD-L1 glycosylation. EGFR, TGF-β Signaling and noncoding RNA Let-7 participate in the regulation of STT3A/B expression by C-Jun and TCF4/β-catenin complex. Glycosyltransferases STT3A/B, B4GALT1, α-Mannosidase II, GLT1D1 and B3GNT3 regulate the glycosylation abundance of PD-L1, thus changing the tumor immune microenvironment.
STT3 mediates PD-L1 N-glycosylation
Oligosaccharide transferases (OSTs) generate N-glycosylated proteins by transferring oligosaccharides in lipolinked oligosaccharides (LLOs) to the asparagine residues of the Asn-Xaa-Ser/Thr receptor sequence (37). The mammalian OST subtypes carry STT3A or STT3B catalytic subunits for co-translation or post translation N-glycosylation modification (37). STT3A-OST and STT3B-OST are highly correlated, but there are important differences in their catalytic mechanisms and speed (38–40). The role of STT3A/B in tumor progression has been studied (41–43), and it plays an important role in the N-glycosylation of PD-L1 (44).
At the initial stage of tumor cell metastasis, epithelioid tumor cells transform into mesenchymal-like cells (EMT), resulting in loss of cell-to-cell contact, increased mechanical mobility, and invasion of the surrounding matrix (45). At the same time, the tumor immune microenvironment is also changing (46). The molecular relationship between EMT and tumor immune escape is being explored (47). For example, miR-200/ZEB1-mediated EMT progression can promote PD-L1 expression and subsequent CD8+ T-cell suppression (48). In addition, as an EMT transcription regulator, β-Catenin can also regulate the expression of PD-L1 (49–52). Hsu et al. (53) found that the TGF-β-driven β-catenin/TCF4 complex activates STT3A/B expression on the promoter of the STT3 subtype. In addition, TGF-β1 also promotes c-Jun binding to the promoter of STT3A and regulates STT3A expression at the transcriptional level (54). Early studies have shown that the two STT3 isomers act on peptides sequentially to maximize the efficiency of N-glycosylation (38). In triple-negative breast cancer (TNBC), nasopharyngeal carcinoma and HNSCC, STT3A/B increased the level of PD-L1 N-glycosylation and protein stability (53–55). The β-catenin inhibitor KYA1797K can downregulate the expression of STT3A/B, thereby inhibiting PD-L1 glycosylation and immune escape of colon CSCs (56). However, the difference in STT3A- and STT3B-mediated N-glycan modification and how they are regulated remain to be fully elucidated, and distinguishing the differences in PD-L1 N-glycosylation induced by STT3A and STT3B may provide ideas for more precise targeted therapy.
EGFR/GSK3β mediated PD-L1 glycosylation and ubiquitination
Glycogen synthase kinase 3β (GSK3β) is a serine/threonine protein kinase originally identified as a regulator of glycogen metabolism (57, 58), which is widely believed to be associated with tumors, embryonic development, liver injury and aging (59). GSK3β induces the phosphorylation-dependent proteasome degradation of Snail, Mcl-1, SIRT7, GFI1, CRY1 and EZH2, leading to mesenchymal epithelial transformation, chemotherapy sensitivity, apoptosis and chromosome stability of cancer cells (60–65). Recently, the focus of research in this area has been strictly on decreasing the stability of the PD-L1 protein by inactivating GSK3β (phosphorylation at the T180, S9 and S184 sites), thereby regulating T-cell-mediated tumor immunity (66–70). Inactivation of GSK3β may stabilize the PD-L1 protein by increasing PD-L1 ubiquitination (31). However, the EGF/EGFR pathway not only inactivates GSK3β (31) but also promotes PD-L1 glycosylation (31, 71) and ubiquitination (72). In addition, Li et al. (31) ound that GSK3β binds to phosphorylated, nonglycosylated PD-L1 (N192, N200 and N219 residues are required), which may block PD-L1 glycosylation, and the glycosylation of N192, N200 and N219 antagonizes the interaction between PD-L1 and GSK3β. Furthermore, GSK3 is involved in the regulation of β-catenin (73), and GSK3β/β-catenin/STT3 may be one of the pathways that regulates PD-L1 glycosylation (53). Therefore, the EGFR/GSK3β pathway is required for PD-L1 protein stability and PD-L1/PD-1 interface maintenance.
B3GNT3 mediates PD-L1 glycosylation
β-1,3-n-acetylglucosamine aminotransferase 3 (B3GNT3) is a type II transmembrane protein in the Golgi apparatus (74) that can form extended core 1 oligosaccharides (75). The relationship between B3GNT3 and tumor immunosuppression is being explored. B3GNT3 overexpression inhibits CD8+ T-cell infiltration in pancreatic cancer and promotes tumor progression (76). A metabolism-related gene pair index (MRGPI) study showed that the high expression of B3GNT3 and low expression of HSD17B6 may have a synergistic reaction in the immune escape of lung adenocarcinoma through the PD-1/PD-L1 pathway (77). The immunohistochemical data of 145 cases of primary lung adenocarcinoma also showed that the expression of B3GNT3 was closely and positively correlated with the expression of PD-L1 and EGFR mutation (78). B3GNT3 also regulates L-selectin ligand function, lymphocyte transport and T-cell homing (75). Li et al. (71) found that B3GNT3 induced by EGF can increase glycosylation at the N192 and N200 sites (poly LacNAc) of PD-L1 and promote PD-L1/PD-1 binding. In addition, B3GNT3 can activate NF-κB signaling (79). Considering the important role of NF-κB in tumor immunity (80, 81), B3GNT3 may regulate tumor immunity through multiple pathways.
B4GALT1 mediates PD-L1 galactosylation
Seven members of the β4-galactosyltransferase (B4GALT) family have different biological functions due to differences in receptor specificity, tissue distribution, and temporal expression (82). B4GALT1 is the main enzyme responsible for the transfer of UDP-galactose residues to terminal N-acetylglucosamine residues in Golgi-processed glycoproteins (83), and its expression is involved in galactosylation of IgG, CDK11p110 and other proteins (84–88). In addition, B4GALT1 can also regulate the expression of glycans on proteins through the JAK signaling pathway (89, 90). B4GALT1 is overexpressed in pathological processes, such as inflammation and proliferation of cancer cells, which makes targetting this enzyme in anticancer therapy possible (91, 92). B4GALT1 expression was positively correlated with PD-L1 and CTLA4 expression in bladder cancer (93). In TNBC, RNA binding motif single strand interacting protein 1 (RBMS1) positively regulates B4GALT1 expression, which is related to the inhibition of inflammation and PD-L1-mediated antitumor immunity (94). Mechanistically, RBMS1 increases the stability of B4GALT1 mRNA and promotes B4GALT1-mediated PD-L1 galactosylation in N-glycans, thereby reducing PD-L1 protein degradation (94).
MAN2A1 mediates PD-L1 glycosylation
The mannosidase α class II member 1 (MAN2A1) gene encodes α-mannosidase II, which can transform the precursor high mannose type N-glycans into mature complex structures and is a key enzyme for N-glycan biosynthesis (95). The MAN2A1 and MAN2A2 genes are widely expressed in the human body at a relatively high level (96). The single deletion of MAN2A1 or MAN2A2 will leads to a relatively mild and organ-specific phenotype, but the simultaneous deletion of both genes will leads to embryonic death and complete lack of complex N-glycans (97). Shi et al. (98) knocked out MAN2A1 in tumor cells, and simple/precursor and heterozygous N-glycans increased, while complex N-glycans decreased. Therefore, the lack of α-mannosidase II weakened PD-L1/PD-1 binding-mediated T-cell immunosuppression (98). The α-mannosidase inhibitor swainsonine makes tumors sensitive to anti-PD-L1 therapy (98). However, α-mannosidase II is highly similar in structure to lysosomal α-mannosidase, and coinhibition of these two proteins was produced when targeting α-mannosidase II and resulted in severe side effects, weakening the potential of α-mannosidase II as a therapeutic target (99).
GLT1D1 is involved in PD-L1 glycosylation
The glycosylation process mainly involves the sequential action of different glycosyltransferase families, and their expression and function are strictly regulated in each cell (100). In addition to STT3, B3GNT3, B4GALT1 and α-mannosidase II, which are involved in PD-L1 glycosylation (54, 71, 94, 98), other glycosyltransferases involved or possibly involved in PD-L1 glycosylation have also been explored. Glycosyltransferase 1 containing domain 1 (GLT1D1) is highly upregulated in incurable B-cell non-Hodgkin’s lymphoma subtypes and early relapsed diffuse large B-cell lymphoma (101) and may be associated with poor prognosis of colon cancer (102) and multiple myeloma (103). GLT1D1 expression is positively correlated with glycosylated PD-L1 levels in B-cell non-Hodgkin’s lymphoma, and high GLT1D1 expression is associated with poor prognosis of patients (101). GLT1D1 plays an important role in the N-glycosylation and stability of the PD-L1 protein. The downregulation of GLT1D1 reduces the glycosylation of the PD-L1 protein, leading to an increase in cytotoxic T-cell infiltration in the tumor microenvironment (101). However, GLT1D1 is an insufficiently studied glycosyltransferase, and its specific modification form is unclear.
Glycosylation is critical for PD-1 stability and its binding to PD-L1
Approximately 20%-90% of protein N-glycans on the cell surface are generated by core fucosylation, which is catalyzed by α-1,6 fucosyltransferase (FUT8) (104, 105). FUT8-mediated core fucosylation modification of the TGF-β receptor and E-cadherin, PD-1 and α3β1 integrin proteins is vital for their function (106). In fact, the four N-glycans of PD-L1 are highly core fucosylated (31), but the significance of FUT8-mediated PD-L1 N-glycan core fucosylation has not been discussed. However the loss of core fucosylation significantly enhanced the ubiquitination of PD-1, which led to the degradation of PD-1 in the proteasome (107). Highly N-glycosylated PD-1 is widely expressed in T cells and is the key to maintaining the stability and cell surface localization of PD-1 protein, especially the glycosylation at the N58 site, which is necessary to mediate its interaction with PD-L1 (16, 108). Monoclonal antibodies STM418 (108), camrelizumab (17), mAb059c (109) and penpulimab (110) specifically target glycosylated PD-1 and have a high binding affinity for PD-1, effectively inhibiting PD-L1/PD-1 binding and enhancing anti-tumor immunity. In addition, adenine base editor (ABE) induces the conversion from a-t to g-c at specific sites, changes the coding sequence of the N74 residue of PDCD1 in CAR-T cells, downregulates the expression and glycosylation of PD-1 in CAR-T cells, and enhances the cytotoxicity in vitro and in vivo (111).
Inhibiting PD-L1 glycosylation to improve tumor immune infiltration
Antibodies targeting the immune checkpoint receptor PD-1 or its ligand PD-L1 are used to treat various types of cancer, and can significantly improve the survival of patients (112). However, drug resistance in tumor immunotherapy forces us to look for more effective inhibitors. Direct/indirect inhibition of PD-L1 glycosylation is a potential strategy to achieve therapeutic effects. The antibodies STM004 and STM108 constructed by Li et al. (71) effectively block the interaction of PD-L1/PD-1. STM108 recognizes the N192 and N200 glycosylation sites, and the amino acid cross-linking is closer to the C-terminal domain of PD-L1 (Y81, K162 and S169); STM004 recognizes the N35 glycosylation site, and amino acid cross-linking is relatively close to the N-terminal domain of PD-L1 (Y56, K62 and K75) (71). STM108 can specifically recognize the B3GNT3 mediated poly LacNAc part on N192 and N200 glycosylation sites of PD-L1, and induce PD-L1 internalization and degradation (71). Metformin can improve the effect of immune checkpoint inhibitor therapy (113), changing the glycan structure of PD-L1 by activating AMPK, thus promoting the degradation of PD-L1 and thereby blocking the immunosuppressive signal (114). 2-deoxyglucose (2-DG) can be used as a glucose analog to reduce PD-L1 glycosylation and reverse the immunosuppression induced by polyadenosine-diphosphate-ribose polymerase (PARP) inhibitor in TNBC (115, 116). In addition, D-mannose can also activate the AMPK pathway to phosphorylate PD-L1 at the S195 site, leading to abnormal glycosylation and degradation of PD-L1 (117).
Resveratrol is a kind of polyphenolic stilbene that is found in grapes, mulberries, peanuts, rhubarb and several other plants and is used to treat diabetes, obesity, cardiovascular disease, neurodegeneration and cancer (118). Resveratrol can affect the expression of PD-L1/PD-1 and the subcellular localization and posttranslational modification of PD-L1 (119). Resveratrol regulates the N-glycosylation modification of PD-L1 by inhibiting α-glucosidase/α-mannosidase, a mannose-rich abnormal glycosylated form of PD-L1 that inhibits binding to PD-1 (120). In addition, resveratrol promotes PD-L1 dimerization by interacting with the inner surface of PD-L1 (111), but dimerized PD-L1 can also bind to PD-1 and regulate T-cell toxicity (121, 122). Poor pharmacokinetics and low potency seem to be the two main bottlenecks of resveratrol (123). However, resveratrol combined with a PD-L1 inhibitor can not only significantly promote the infiltration of CD8+/CD4+ T cells but also significantly inhibit the number of Treg cells and MDSCs at the same glycolysis level (124).
Inhibitors of glycosyltransferase associated with PD-L1 glycosylation have been developed. The small molecule OST inhibitor such as NGI-1 can inhibit the activities of STT3A and STT3B at the same time (125, 126). Puschnik et al. (127) found that 12 inhibitors such as me-3,4-depostatin, hispidin, myricetin and piceatannol can inhibit B4GALT1 activity. And α-Mannosidase II can be inhibited by pyrrolidine compounds and salacinol family compounds (128). These inhibitors are potential drugs to improve tumor immunosuppression mediated by PD-L1 glycosylation. However, the role of these glycosyltransferase inhibitors in the treatment of tumors and PD-L1 deglycosylation still needs to be further explored.
Structure and significance of PD-L2 glycosylation
PD-L1 and PD-L2 (B7-DC) are the two main ligands of PD-1 (129). The binding affinity of PD-L2 to PD-1 is 2-6 times higher than that of PD-L1 to PD-1 (130). In addition, PD-L2 is also the combination partner of repulsive guidance molecule b (RGMb), which improves respiratory tolerance (131). Similar to PD-L1, PD-L2 has an N-terminal IgV domain and a membrane proximate IgC domain. However, the PD-L1 IgV domain contains the C’ and C″β chains, while in the PD-L2 IgV domain the C’ and C″β chains are replaced by a flexible C-D ring (132). The IgV domain of PD-L2 binds to PD-1, and its glycosylation structure regulates its affinity for PD-1 (133). The stability of the PD-L2 protein is related to the N-glycosylation sites N157, N163 and N189 but not N64 (23). The N64 glycan is located in the C-D ring region of PD-L2, and its glycosyl structure and solubility increase the dynamic characteristics of the C-D ring region. Furthermore, the affinity of PD-L2 for PD-1 can be improved by removing the N64 glycan (119). In addition, the “pocket” formed by N64 may be the binding site of PD-L2/PD-1 affinity drugs (134). However, the glycosylation significance of PD-L2 at the N10 and N43 sites has not been clarified (135).
PD-L2 is expressed on immune cells, dendritic cells and other types of hematopoietic and non hematopoietic cells (136) (137) (138). PD-L2 and PD-1 inhibit T-cell proliferation mediated by the T-cell receptor (TCR) and cytokine production (139). Although the frequency or intensity of PD-L2 expression may not be as high as that of PD-L1 in most tumors (140), PD-L2 can be expressed without PL-L1 expression in some specific tumors. PD-L2 is expressed or strongly expressed in 51.7% of esophageal adenocarcinomas, while PD-L1 is expressed in 2% of esophageal adenocarcinomas (141). PD-L2 was expressed in 62.7% of HNSCCs (more than twice as many as for PD-L1), and 61.4% of HNSCCs were PD-L1-negative (142). In addition, Xu et al. (23) found that PD-L2 was N-glycosylated and upregulated in tumor tissues of HNSCC patients resistant to cetuximab. Deglycosylation inhibited the expression of PD-L2 in colorectal cancer cells (143). In HNSCC, the STAT3 pathway activates FUT8-mediated PD-L2 glycosylation, which stabilizes the PD-L2 protein by blocking ubiquitin-dependent lysosome degradation, thereby promoting its combination with PD-1 and immune escape (23). Moreover, glycosylated PD-L2 forms a complex with EGFR, which leads to the activation of EGFR/STAT3 signaling and reduces the binding affinity of cetuximab for EGFR (23).
Significance of B7-H3 glycosylation
As a type I transmembrane protein, B7-H3 consists of an extracellular domain, a transmembrane domain and a short intracellular domain. The human B7-H3 protein has two isomers determined by the extracellular domain: 4IgB7-H3 and 2IgB7-H3. 4IgB7-H3 consists of two pairs of identical IgV-like domains and IgC-like domains, 2IgB7-H3 consists of one pair of identical IgV-like domains and IgC-like domains, and 4IgB7-H3 is more common in human cells (144). B7-H3 is abnormally highly expressed in lung (145), ovarian (146), glioblastoma (147), colorectal (148), gastric (149), prostate (150), urothelial (151), brain (152), pancreatic (153), breast (154), cholangiocarcinoma (155), hepatocellular (156), oral (157) and renal (158) cancer cells and can be induced to be expressed on antigen presenting cells (APCs), including dendritic cells (DC) and macrophages (159). In the tumor microenvironment, B7-H3 inhibits CD4+ and CD8+ T cell responses by inhibiting IFN-γ, IL2, IL-10 and IL-13 (160) and promotes the immune escape of tumor cells (161, 162). In addition, B7-H3 also has nonimmunogenic effects, promoting tumor cell migration, invasion, angiogenesis, drug resistance, and EMT and regulating cell metabolism (144). These results make B7-H3 a potential target for tumor therapy.
B7-H3 is a highly glycosylated protein. Human B7-H3 protein can have eight N-glycan sites, N91, N104, N189, N215, N309, N322, N407 and N433 (Figure 2) (24, 163). Chen et al. (157) found that the glycan of B7-H3 in an OSCC cell line contains a more diversified N-glycan structure and a terminal α-galactose, and the glycan rich structure of B7-H3 may allow it to play an important role in the progression of oral cancer. In esophageal squamous cell carcinoma (ESCC), the increased level of B7-H3 protein N-glycan fucosylation promotes the occurrence and development of tumors (164). Huang et al. (24) found that the N-glycosylation of B7-H3 at the NXT motif site is related to protein stability and triple-negative breast cancer (TNBC) immunosuppression. In breast cancer, there are more than 140 N-glycan core fucosylated glycoproteins mediated by FUT8 (165, 166). Knockout of FUT8 inhibits the immunosuppressive function mediated by N-glycosylated B7-H3 in TNBC cells (24). Moreover, the combination of the core fucosylation inhibitor 2F-Fuc and anti-PD-L1 can enhance the therapeutic effect of B7-H3-positive TNBC (24).
Significance of B7-H4 glycosylation
The B7-H4 (also known as B7x, B7S1 or VTCN1) protein consists of 282 amino acids, including an extracellular domain, a large hydrophobic transmembrane domain and an intracellular domain composed of only two amino acid residues (132). The B7-H4 protein has the overall structure of a type I transmembrane protein. Similar to other B7 family members, its extracellular domain has a pair of Ig-like domains. However, the homology of this protein with other B7 family members is only approximately 25% (132). Different from other B7 family members with restricted mRNA expression, B7-H4 mRNA is widely expressed in the brain, heart, kidney, liver, lung, ovary, pancreas, placenta, prostate, skeletal muscle, skin, thymus and uterus (167). Although B7-H4 mRNA is widely expressed in normal human cells, the distribution of B7-H4 protein on the surface of normal cells is rare (168). However, the B7-H4 protein is highly expressed in human tumors and is associated with the clinicopathological features of patients (157). The expression of B7-H4 in gastric (169, 170), breast (171, 172), lung (145), prostate (173), pancreatic (174), bladder (175), colorectal (176), ovarian (177), renal (178), urothelial (179), esophageal (180), and gallbladder (181) cancers is associated with tumor size, primary tumor grade, TNM stage, low survival rate, drug resistance and a decreased number of tumor-infiltrating T cells. B7-H4 inhibits the proliferation, cell cycle progression and cytokine production of CD4+/CD8+ T cells (168, 182), attenuates the inflammatory response, and enables tumor cells to evade the immune system (132, 183).
Salceda et al. (184) found that the highly glycosylated B7-H4 protein was overexpressed in most serous ovarian cancers and breast cancers but was hardly expressed in normal tissues, mucous or low-grade malignant ovarian cancers. The accumulation of glycosylated B7-H4 expression in immunocompetent breast cancer was negatively correlated with the expression of PD-L1 (25), and similar results were also found in glioma (185), lung (186) and pancreatic (187) cancer. Therefore, B7-H4 may be the key to treating PD-L1-negative cold tumors, and B7-H4 glycosylation sites are potential therapeutic targets. In 293T cells, B7-H4 has five N-glycans (N112, N140, N156, N160 and N255) and two ubiquitination sites (K146 and K138) (25). Glycosylation can stabilize the structure of the B7-H4 protein, blocking the phosphorylation of eIF2α, reducing the exposure of calreticulin, and thus inhibiting the immunogenicity of cancer cells (Figure 3) (25). Moreover, the B7-H4 ubiquitination site can only be detected in the presence of PNGas F, and glucosyltransferase STT3A/UGGG1-mediated N-glycosylation at the asparagine site interferes with the ubiquitination of lysine residues (Figure 3) (25).
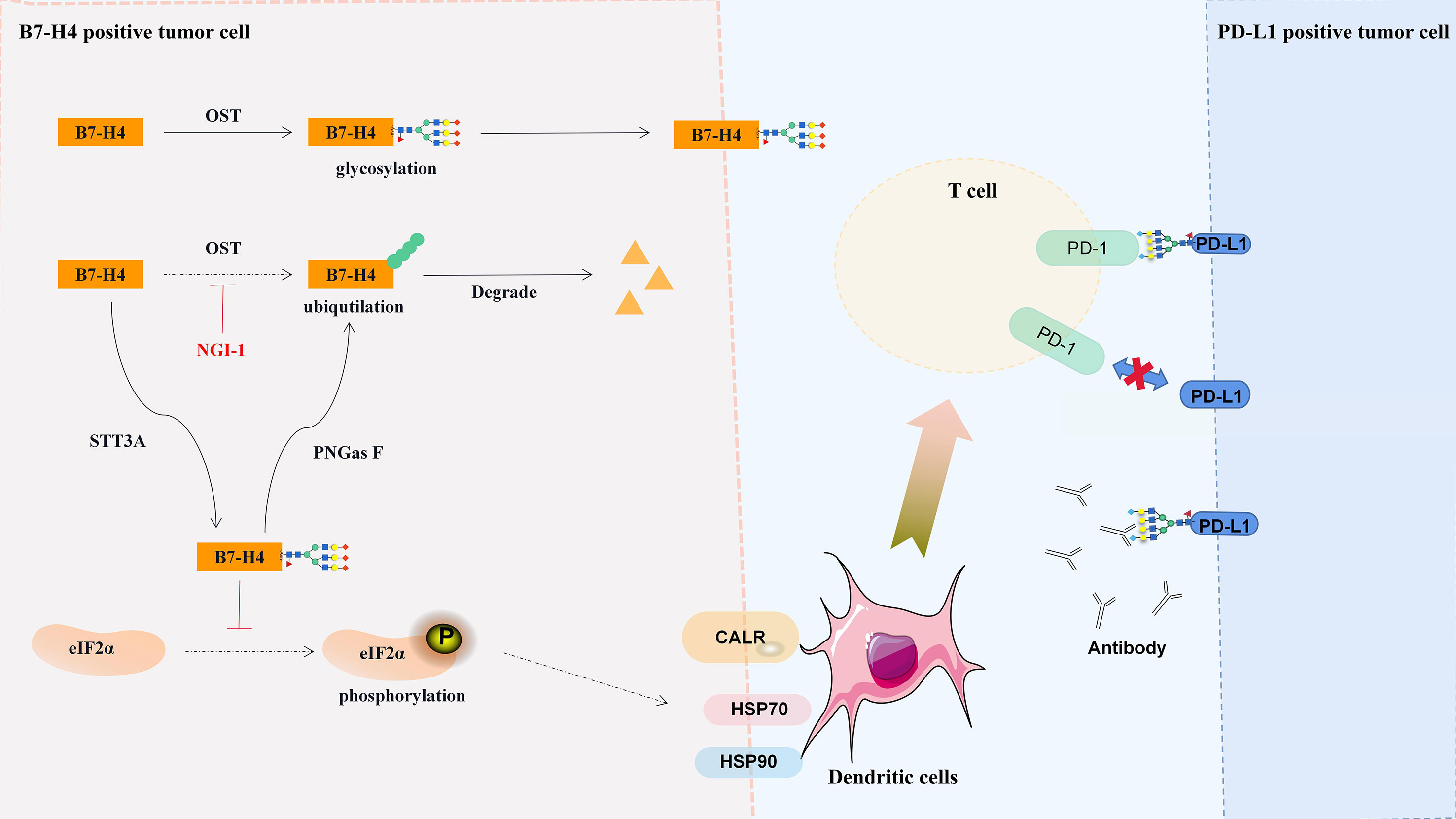
Figure 3 Regulation and significance of B7-H4 glycosylation. Glycosylated B7-H4 inhibits the phosphorylation of eIF2α and dendritic cell recruit, and deglycosylation lead to the ubiquitination and degradation of B7-H4. CALR, calreticulin; HSK70, heat shock protein 70; HSK90, heat shock protein 90.
Glycosylation mediates the interaction between B7-H6 and NKp30
B7-H6 is a type I transmembrane protein consisting of two extracellular IgG-like domains (IgV and IgC), an α-helical transmembrane domain, and homologous C-terminal sequences of population specific antigen (GAG) proteins (26). The B7-H6 C-terminal sequence has a variety of signal motifs, including ITIM, SH-2 and SH-3 binding motifs, which can trigger signal transduction after binding with natural killer protein 30 (NKp30) (26, 27) and activate NK cells and cytokine secretion (188). The closest structural homolog of B7-H6 is PD-L1, and both are highly glycosylated glycoproteins (26, 27). However, although the B7-H6 protein has five predicted N-glycosylation sites, Skořepa et al. (189) found that the removal of the B7-H6 N-glycan did not affect its crosslinking with NKp30. In contrast, NKp30 glycosylation modification is essential for the interaction between NKp30 and B7-H6 (190). The removal of the N68 glycosylation site of NKp30 reduced its affinity for the B7-H6 ligand, while the removal of the N42 glycosylation site of NKp30 almost completely eliminated its binding to the B7-H6 ligand (179). Targeting NKp30 to treat tumors has proven to be effective, and CAR-T cells expressing chimeric NKp30 receptors can destroy B7-H6+ cells (191, 192).
Conclusion
The binding of antibodies to PD-L1 was affected by the degree of protein glycosylation. Deglycosylation of PD-L1 enhances binding to 28-8, CAL10, CAL10, SP142, atezolizumab and SP142 MAbs (193, 194) but is not conducive for binding to avelumab (195). Targeting PD-L1 glycosylation promotes its degradation and inhibits the binding of some antibodies to PD-1 but also increases the therapeutic effect of some antibodies. The complex network composed of STT3A/B, EGFR/GSK3β, B3GNT3, B4GALT1, α-mannosidase and GLT1D1 regulates PD-L1 glycosylation and participates in PD-L1 stabilization and binding to PD-1 (Figure 1). Glycosylation is also important for the protein function and stability of PD-L2, B7-H3 and B7-H4. Blocking or targeting PD-L1/2 and B7-H3/4 protein glycosylation may be an important supplement for tumor immunotherapy. A more comprehensive study of the glycosylation modification of the B7 protein family will reveal a new direction for the translational application of glycobiology in tumor immunotherapy.
Author contributions
CL, LX, XG and JL contributed to the conception and design of the review. CL and LX wrote the manuscript. YG, JL and QW validate the manuscript. MX, QL, CY and LC contributed to draw the figures. All authors contributed to the article and approved the submitted version.
Funding
This study was supported by the National Key R&D Program (2016YFC1102800); Guizhou Province Medical Biomaterials R&D Talent Base (QianRenLingFa (2018) No. 3); the Sixth Talent Foundation in Guizhou province (rcjd2019-9); Zunyi Medical Biomaterials R&D and Innovative Talent Base (ZunWei [2019] No. 69); the Youth Science and Technology Talents Growth Project of Guizhou Education Department (Qian-Jiao-He KY ZI [2018] 236); Zunyi City and Zunyi Medical University Unite Fund [QianShiKeHe HZ Zi (2022) 390 and 393]; Outstanding Young Talent Project of Zunyi Medical University (17zy-002); Master Fund of Zunyi Medical University (S-81) and Technological Talents in Colleges and Universities of Guizhou Province (Qian Jiao He KY ZI [2021] 215).
Conflict of interest
The authors declare that the research was conducted in the absence of any commercial or financial relationships that could be construed as a potential conflict of interest.
Publisher’s note
All claims expressed in this article are solely those of the authors and do not necessarily represent those of their affiliated organizations, or those of the publisher, the editors and the reviewers. Any product that may be evaluated in this article, or claim that may be made by its manufacturer, is not guaranteed or endorsed by the publisher.
References
1. Stowell SR, Ju T, Cummings RD. Protein glycosylation in cancer. Annu Rev Pathol (2015) 10:473–510. doi: 10.1146/annurev-pathol-012414-040438
2. Duarte HO, Reis CA, Gomes J. Insights on ErbB glycosylation - contributions to precision oncology. Trends Cancer (2022) 8(6):448–55. doi: 10.1016/j.trecan.2022.02.003
3. Wang Q, Liao C, Tan Z, Li X, Guan X, Li H, et al. FUT6 inhibits the proliferation, migration, invasion, and EGF-induced EMT of head and neck squamous cell carcinoma (HNSCC) by regulating EGFR/ERK/STAT signaling pathway. Cancer Gene Ther (2022). [Epub ahead of print]. doi: 10.1038/s41417-022-00530-w
4. Liao C, Wang Q, An J, Chen J, Li X, Long Q, et al. CD44 glycosylation as a therapeutic target in oncology. Front Oncol (2022) 12:883831. doi: 10.3389/fonc.2022.883831
5. Pinho SS, Seruca R, Gärtner F, Yamaguchi Y, Gu J, Taniguchi N, et al. Modulation of e-cadherin function and dysfunction by n-glycosylation. Cell Mol Life Sci (2011) 68(6):1011–20. doi: 10.1007/s00018-010-0595-0
6. Sun X, He Z, Guo L, Wang C, Lin C, Ye L, et al. ALG3 contributes to stemness and radioresistance through regulating glycosylation of TGF-β receptor II in breast cancer. J Exp Clin Cancer Res (2021) 40(1):149. doi: 10.1186/s13046-021-01932-8
7. Zhang J, Ten Dijke P, Wuhrer M, Zhang T. Role of glycosylation in TGF-β signaling and epithelial-to-mesenchymal transition in cancer. Protein Cell (2021) 12(2):89–106. doi: 10.1007/s13238-020-00741-7
8. Luo G, Jin K, Deng S, Cheng H, Fan Z, Gong Y, et al. Roles of CA19-9 in pancreatic cancer: Biomarker, predictor and promoter. Biochim Biophys Acta Rev Cancer (2021) 1875(2):188409. doi: 10.1016/j.bbcan.2020.188409
9. Nath S, Mukherjee P. MUC1: a multifaceted oncoprotein with a key role in cancer progression. Trends Mol Med (2014) 20(6):332–42. doi: 10.1016/j.molmed.2014.02.007
10. Posey AD Jr, Schwab RD, Boesteanu AC, Steentoft C, Mandel U, Engels B, et al. Engineered CAR T cells targeting the cancer-associated tn-glycoform of the membrane mucin MUC1 control adenocarcinoma. Immunity (2016) 44(6):1444–54. doi: 10.1016/j.immuni.2016.05.014
11. Mereiter S, Balmaña M, Campos D, Gomes J, Reis CA. Glycosylation in the era of cancer-targeted therapy: Where are we heading? Cancer Cell (2019) 36(1):6–16. doi: 10.1016/j.ccell.2019.06.006
12. Sun R, Kim AMJ, Lim SO. Glycosylation of immune receptors in cancer. Cells (2021) 10(5):1100. doi: 10.3390/cells10051100
13. De Bousser E, Meuris L, Callewaert N, Festjens N. Human T cell glycosylation and implications on immune therapy for cancer. Hum Vaccin Immunother (2020) 16(10):2374–88. doi: 10.1080/21645515.2020.1730658
14. Sun C, Mezzadra R, Schumacher TN. Regulation and function of the PD-L1 checkpoint. Immunity (2018) 48(3):434–52. doi: 10.1016/j.immuni.2018.03.014
15. Wang YN, Lee HH, Hsu JL, Yu D, Hung MC. The impact of PD-L1 N-linked glycosylation on cancer therapy and clinical diagnosis. J BioMed Sci (2020) 27(1):77. doi: 10.1186/s12929-020-00670-x
16. Sun L, Li CW, Chung EM, Yang R, Kim YS, Park AH, et al. Targeting glycosylated PD-1 induces potent antitumor immunity. Cancer Res (2020) 80(11):2298–310. doi: 10.1158/0008-5472.CAN-19-3133
17. Liu K, Tan S, Jin W, Guan J, Wang Q, Sun H, et al. N-glycosylation of PD-1 promotes binding of camrelizumab. EMBO Rep (2020) 21(12):e51444. doi: 10.15252/embr.202051444
18. Zhao Y, Zheng Q, Jin L. The role of B7 family molecules in maternal-fetal immunity. Front Immunol (2020) 11:458. doi: 10.3389/fimmu.2020.00458
19. Greenwald RJ, Freeman GJ, Sharpe AH. The B7 family revisited. Annu Rev Immunol (2005) 23:515–48. doi: 10.1146/annurev.immunol.23.021704.115611
20. Ni L, Dong C. New B7 family checkpoints in human cancers. Mol Cancer Ther (2017) 16(7):1203–11. doi: 10.1158/1535-7163.MCT-16-0761
21. Zhang W, Qiu Y, Xie X, Fu Y, Wang L, Cai Z. B7 family members in lymphoma: Promising novel targets for tumor immunotherapy? Front Oncol (2021) 11:647526. doi: 10.3389/fonc.2021.647526
22. Chen A, Meyerson HJ, Salvekar A, Tykocinski ML. Non-glycosylated human B7-1(CD80) retains the capacity to bind its counter-receptors. FEBS Lett (1998) 428(3):127–34. doi: 10.1016/s0014-5793(98)00460-8
23. Xu Y, Gao Z, Hu R, Wang Y, Wang Y, Su Z, et al. PD-L2 glycosylation promotes immune evasion and predicts anti-EGFR efficacy. J Immunother Cancer (2021) 9(10):e002699. doi: 10.1136/jitc-2021-002699
24. Huang Y, Zhang HL, Li ZL, Du T, Chen YH, Wang Y, et al. FUT8-mediated aberrant n-glycosylation of B7H3 suppresses the immune response in triple-negative breast cancer. Nat Commun (2021) 12(1):2672. doi: 10.1038/s41467-021-22618-x
25. Song X, Zhou Z, Li H, Xue Y, Lu X, Bahar I, et al. Pharmacologic suppression of B7-H4 glycosylation restores antitumor immunity in immune-cold breast cancers. Cancer Discovery (2020) 10(12):1872–93. doi: 10.1158/2159-8290.CD-20-0402
26. Brandt CS, Baratin M, Yi EC, Kennedy J, Gao Z, Fox B, et al. The B7 family member B7-H6 is a tumor cell ligand for the activating natural killer cell receptor NKp30 in humans. J Exp Med (2009) 206(7):1495–503. doi: 10.1084/jem.20090681
27. Kaifu T, Escalière B, Gastinel LN, Vivier E, Baratin M. B7-H6/NKp30 interaction: a mechanism of alerting NK cells against tumors. Cell Mol Life Sci (2011) 68(21):3531–9. doi: 10.1007/s00018-011-0802-7
28. Wang H, Yao H, Li C, Shi H, Lan J, Li Z, et al. HIP1R targets PD-L1 to lysosomal degradation to alter T cell-mediated cytotoxicity. Nat Chem Biol (2019) 15(1):42–50. doi: 10.1038/s41589-018-0161-x
29. Dong H, Zhu G, Tamada K, Chen L. B7-H1, a third member of the B7 family, co-stimulates T-cell proliferation and interleukin-10 secretion. Nat Med (1999) 5(12):1365–9. doi: 10.1038/70932
30. Zhou S, Zhu J, Xu J, Gu B, Zhao Q, Luo C, et al. Anti-tumour potential of PD-L1/PD-1 post-translational modifications. Immunology (2022) 167(4):471–81. doi: 10.1111/imm.13573
31. Li CW, Lim SO, Xia W, Lee HH, Chan LC, Kuo CW, et al. Glycosylation and stabilization of programmed death ligand-1 suppresses T-cell activity. Nat Commun (2016) 7:12632. doi: 10.1038/ncomms12632
32. Lin DY, Tanaka Y, Iwasaki M, Gittis AG, Su HP, Mikami B, et al. The PD-1/PD-L1 complex resembles the antigen-binding fv domains of antibodies and T cell receptors. Proc Natl Acad Sci U.S.A. (2008) 105(8):3011–6. doi: 10.1073/pnas.0712278105
33. Lee HH, Wang YN, Xia W, Chen CH, Rau KM, Ye L, et al. Removal of n-linked glycosylation enhances PD-L1 detection and predicts anti-PD-1/PD-L1 therapeutic efficacy. Cancer Cell (2019) 36(2):168–78. doi: 10.1016/j.ccell.2019.06.008
34. Yi M, Zheng X, Niu M, Zhu S, Ge H, Wu K. Combination strategies with PD-1/PD-L1 blockade: current advances and future directions. Mol Cancer (2022) 21(1):28. doi: 10.1186/s12943-021-01489-2
35. Lawson NL, Dix CI, Scorer PW, Stubbs CJ, Wong E, Hutchinson L, et al. Mapping the binding sites of antibodies utilized in programmed cell death ligand-1 predictive immunohistochemical assays for use with immuno-oncology therapies. Mod Pathol (2020) 33(4):518–30. doi: 10.1038/s41379-019-0372-z
36. Xu J, Yang X, Mao Y, Mei J, Wang H, Ding J, et al. Removal of n-linked glycosylation enhances PD-L1 detection in colon cancer: Validation research based on immunohistochemistry analysis. Technol Cancer Res Treat (2021) 20:15330338211019442. doi: 10.1177/15330338211019442
37. Lu H, Fermaintt CS, Cherepanova NA, Gilmore R, Yan N, Lehrman MA. Mammalian STT3A/B oligosaccharyltransferases segregate n-glycosylation at the translocon from lipid-linked oligosaccharide hydrolysis. Proc Natl Acad Sci U.S.A. (2018) 115(38):9557–62. doi: 10.1073/pnas.1806034115
38. Ruiz-Canada C, Kelleher DJ, Gilmore R. Cotranslational and posttranslational n-glycosylation of polypeptides by distinct mammalian OST isoforms. Cell (2009) 136(2):272–83. doi: 10.1016/j.cell.2008.11.047
39. Shrimal S, Ng BG, Losfeld ME, Gilmore R, Freeze HH. Mutations in STT3A and STT3B cause two congenital disorders of glycosylation. Hum Mol Genet (2013) 22(22):4638–45. doi: 10.1093/hmg/ddt312
40. Kelleher DJ, Karaoglu D, Mandon EC, Gilmore R. Oligosaccharyltransferase isoforms that contain different catalytic STT3 subunits have distinct enzymatic properties. Mol Cell (2003) 12(1):101–11. doi: 10.1016/s1097-2765(03)00243-0
41. Sigstad E, Paus E, Bjøro T, Berner A, Grøholt KK, Jørgensen LH, et al. The new molecular markers DDIT3, STT3A, ARG2 and FAM129A are not useful in diagnosing thyroid follicular tumors. Mod Pathol (2012) 25(4):537–47. doi: 10.1038/modpathol.2011.188
42. Ding J, Xu J, Deng Q, Ma W, Zhang R, He X, et al. Knockdown of oligosaccharyltransferase subunit ribophorin 1 induces endoplasmic-Reticulum-Stress-Dependent cell apoptosis in breast cancer. Front Oncol (2021) 11:722624. doi: 10.3389/fonc.2021.722624
43. Liu J, Ma T, Gao M, Liu Y, Liu J, Wang S, et al. Proteomic characterization of proliferation inhibition of well-differentiated laryngeal squamous cell carcinoma cells under below-background radiation in a deep underground environment. Front Public Health (2020) 8:584964. doi: 10.3389/fpubh.2020.584964
44. Liao C, An J, Tan Z, Xu F, Liu J, Wang Q. Changes in protein glycosylation in head and neck squamous cell carcinoma. J Cancer (2021) 12(5):1455–66. doi: 10.7150/jca.51604
45. Liao C, Wang Q, An J, Long Q, Wang H, Xiang M, et al. Partial EMT in squamous cell carcinoma: A snapshot. Int J Biol Sci (2021) 17(12):3036–47. doi: 10.7150/ijbs.61566
46. Hanahan D, Coussens LM. Accessories to the crime: functions of cells recruited to the tumor microenvironment. Cancer Cell (2012) 21(3):309–22. doi: 10.1016/j.ccr.2012.02.022
47. Jiang Y, Zhan H. Communication between EMT and PD-L1 signaling: New insights into tumor immune evasion. Cancer Lett (2020) 468:72–81. doi: 10.1016/j.canlet.2019.10.013
48. Chen L, Gibbons DL, Goswami S, Cortez MA, Ahn YH, Byers LA, et al. Metastasis is regulated via microRNA-200/ZEB1 axis control of tumour cell PD-L1 expression and intratumoral immunosuppression. Nat Commun (2014) 5:5241. doi: 10.1038/ncomms6241
49. Du L, Lee JH, Jiang H, Wang C, Wang S, Zheng Z, et al. β-catenin induces transcriptional expression of PD-L1 to promote glioblastoma immune evasion. J Exp Med (2020) 217(11):e20191115. doi: 10.1084/jem.20191115
50. Gan S, Ye J, Li J, Hu C, Wang J, Xu D, et al. LRP11 activates β-catenin to induce PD-L1 expression in prostate cancer. J Drug Target (2020) 28(5):508–15. doi: 10.1080/1061186X.2019.1687710
51. Lim WJ, Lee M, Oh Y, Fang XQ, Lee S, Lim CH, et al. Statins decrease programmed death-ligand 1 (PD-L1) by inhibiting AKT and β-catenin signaling. Cells (2021) 10(9):2488. doi: 10.3390/cells10092488
52. Yu W, Hua Y, Qiu H, Hao J, Zou K, Li Z, et al. PD-L1 promotes tumor growth and progression by activating WIP and β-catenin signaling pathways and predicts poor prognosis in lung cancer. Cell Death Dis (2020) 11(7):506. doi: 10.1038/s41419-020-2701-z
53. Hsu JM, Xia W, Hsu YH, Chan LC, Yu WH, Cha JH, et al. STT3-dependent PD-L1 accumulation on cancer stem cells promotes immune evasion. Nat Commun (2018) 9(1):1908. doi: 10.1038/s41467-018-04313-6
54. Ma XM, Luo YF, Zeng FF, Su C, Liu X, Li XP, et al. TGF-β1-Mediated PD-L1 glycosylation contributes to immune escape via c-Jun/STT3A pathway in nasopharyngeal carcinoma. Front Oncol (2022) 12:815437. doi: 10.3389/fonc.2022.815437
55. Yu D, Liu X, Han G, Liu Y, Zhao X, Wang D, et al. The let-7 family of microRNAs suppresses immune evasion in head and neck squamous cell carcinoma by promoting PD-L1 degradation. Cell Commun Signal (2019) 17(1):173. doi: 10.1186/s12964-019-0490-8
56. Ruan Z, Liang M, Lai M, Shang L, Deng X, Su X. KYA1797K down-regulates PD-L1 in colon cancer stem cells to block immune evasion by suppressing the β-catenin/STT3 signaling pathway. Int Immunopharmacol (2020) 78:106003. doi: 10.1016/j.intimp.2019.106003
57. Doble BW, Woodgett JR. GSK-3: tricks of the trade for a multi-tasking kinase. J Cell Sci (2003) 116(Pt 7):1175–86. doi: 10.1242/jcs.00384
58. Frame S, Cohen P. GSK3 takes centre stage more than 20 years after its discovery. Biochem J (2001) 359(Pt 1):1–16. doi: 10.1042/0264-6021:3590001
59. Mancinelli R, Carpino G, Petrungaro S, Mammola CL, Tomaipitinca L, Filippini A, et al. Multifaceted roles of GSK-3 in cancer and autophagy-related diseases. Oxid Med Cell Longev (2017) 2017:4629495. doi: 10.1155/2017/4629495
60. Zhou BP, Deng J, Xia W, Xu J, Li YM, Gunduz M, et al. Dual regulation of snail by GSK-3beta-mediated phosphorylation in control of epithelial-mesenchymal transition. Nat Cell Biol (2004) 6(10):931–40. doi: 10.1038/ncb1173
61. Ding Q, He X, Hsu JM, Xia W, Chen CT, Li LY, et al. Degradation of mcl-1 by beta-TrCP mediates glycogen synthase kinase 3-induced tumor suppression and chemosensitization. Mol Cell Biol (2007) 27(11):4006–17. doi: 10.1128/MCB.00620-06
62. Ko HW, Lee HH, Huo L, Xia W, Yang CC, Hsu JL, et al. GSK3β inactivation promotes the oncogenic functions of EZH2 and enhances methylation of H3K27 in human breast cancers. Oncotarget (2016) 7(35):57131–44. doi: 10.18632/oncotarget.11008
63. Tang X, Li G, Shi L, Su F, Qian M, Liu Z, et al. Combined intermittent fasting and ERK inhibition enhance the anti-tumor effects of chemotherapy via the GSK3β-SIRT7 axis. Nat Commun (2021) 12(1):5058. doi: 10.1038/s41467-021-25274-3
64. Kuai X, Li L, Chen R, Wang K, Chen M, Cui B, et al. SCFFBXW7/GSK3β-mediated GFI1 degradation suppresses proliferation of gastric cancer cells. Cancer Res (2019) 79(17):4387–98. doi: 10.1158/0008-5472.CAN-18-4032
65. Kim YY, Jang H, Lee G, Jeon YG, Sohn JH, Han JS, et al. Hepatic GSK3β-dependent CRY1 degradation contributes to diabetic hyperglycemia. Diabetes (2022) 71(7):1373–87. doi: 10.2337/db21-0649
66. Chen X, Wang K, Jiang S, Sun H, Che X, Zhang M, et al. eEF2K promotes PD-L1 stabilization through inactivating GSK3β in melanoma. J Immunother Cancer (2022) 10(3):e004026. doi: 10.1136/jitc-2021-004026
67. Wu M, Xia X, Hu J, Fowlkes NW, Li S. WSX1 act as a tumor suppressor in hepatocellular carcinoma by downregulating neoplastic PD-L1 expression. Nat Commun (2021) 12(1):3500. doi: 10.1038/s41467-021-23864-9
68. Li H, Li CW, Li X, Ding Q, Guo L, Liu S, et al. MET inhibitors promote liver tumor evasion of the immune response by stabilizing PDL1. Gastroenterology (2019) 156(6):1849–61. doi: 10.1053/j.gastro.2019.01.252
69. Jiao S, Xia W, Yamaguchi H, Wei Y, Chen MK, Hsu JM, et al. PARP inhibitor upregulates PD-L1 expression and enhances cancer-associated immunosuppression. Clin Cancer Res (2017) 23(14):3711–20. doi: 10.1158/1078-0432.CCR-16-3215
70. Schulz D, Stancev I, Sorrentino A, Menevse AN, Beckhove P, Brockhoff G, et al. Increased PD-L1 expression in radioresistant HNSCC cell lines after irradiation affects cell proliferation due to inactivation of GSK-3beta. Oncotarget (2019) 10(5):573–83. doi: 10.18632/oncotarget.26542
71. Li CW, Lim SO, Chung EM, Kim YS, Park AH, Yao J, et al. Eradication of triple-negative breast cancer cells by targeting glycosylated PD-L1. Cancer Cell (2018) 33(2):187–201. doi: 10.1016/j.ccell.2018.01.009
72. Horita H, Law A, Hong S, Middleton K. Identifying regulatory posttranslational modifications of PD-L1: A focus on monoubiquitinaton. Neoplasia (2017) 19(4):346–53. doi: 10.1016/j.neo.2017.02.006
73. Clevers H. Wnt/beta-catenin signaling in development and disease. Cell (2006) 127(3):469–80. doi: 10.1016/j.cell.2006.10.018
74. Hennet T, Dinter A, Kuhnert P, Mattu TS, Rudd PM, Berger EG. Genomic cloning and expression of three murine UDP-galactose: beta-n-acetylglucosamine beta1,3-galactosyltransferase genes. J Biol Chem (1998) 273(1):58–65. doi: 10.1074/jbc.273.1.58
75. Yeh JC, Hiraoka N, Petryniak B, Nakayama J, Ellies LG, Rabuka D, et al. Novel sulfated lymphocyte homing receptors and their control by a Core1 extension beta 1,3-n-acetylglucosaminyltransferase. Cell (2001) 105(7):957–69. doi: 10.1016/s0092-8674(01)00394-4
76. Zhuang H, Zhou Z, Zhang Z, Chen X, Ma Z, Huang S, et al. B3GNT3 overexpression promotes tumor progression and inhibits infiltration of CD8+ T cells in pancreatic cancer. Aging (Albany NY) (2020) 13(2):2310–29. doi: 10.18632/aging.202255
77. Hu J, Yu H, Sun L, Yan Y, Zhang L, Jiang G, et al. Identification of an individualized metabolism prognostic signature and related therapy regimens in early stage lung adenocarcinoma. Front Oncol (2021) 11:650853. doi: 10.3389/fonc.2021.650853
78. Leng X, Wei S, Mei J, Deng S, Yang Z, Liu Z, et al. Identifying the prognostic significance of B3GNT3 with PD-L1 expression in lung adenocarcinoma. Transl Lung Cancer Res (2021) 10(2):965–80. doi: 10.21037/tlcr-21-146
79. Xu J, Guo Z, Yuan S, Li H, Luo S. Upregulation of B3GNT3 is associated with immune infiltration and activation of NF-κB pathway in gynecologic cancers. J Reprod Immunol (2022) 152:103658. doi: 10.1016/j.jri.2022.103658
80. Sun SC. The non-canonical NF-κB pathway in immunity and inflammation. Nat Rev Immunol (2017) 17(9):545–58. doi: 10.1038/nri.2017.52
81. Hayden MS, Ghosh S. NF-κB in immunobiology. Cell Res (2011) 21(2):223–44. doi: 10.1038/cr.2011.13
82. Zhou H, Ma H, Wei W, Ji D, Song X, Sun J, et al. B4GALT family mediates the multidrug resistance of human leukemia cells by regulating the hedgehog pathway and the expression of p-glycoprotein and multidrug resistance-associated protein 1. Cell Death Dis (2013) 4(6):e654. doi: 10.1038/cddis.2013.186
83. Hansske B, Thiel C, Lübke T, Hasilik M, Höning S, Peters V, et al. Deficiency of UDP-galactose:N-acetylglucosamine beta-1,4-galactosyltransferase I causes the congenital disorder of glycosylation type IId. J Clin Invest (2002) 109(6):725–33. doi: 10.1172/JCI14010
84. Shinzaki S, Iijima H, Fujii H, Kuroki E, Tatsunaka N, Inoue T, et al. Altered oligosaccharide structures reduce colitis induction in mice defective in β-1,4-galactosyltransferase. Gastroenterology (2012) 142(5):1172–82. doi: 10.1053/j.gastro.2012.02.008
85. Chen Y, Su L, Huang C, Wu S, Qiu X, Zhao X, et al. Galactosyltransferase B4GALT1 confers chemoresistance in pancreatic ductal adenocarcinomas by upregulating n-linked glycosylation of CDK11p110. Cancer Lett (2021) 500:228–43. doi: 10.1016/j.canlet.2020.12.006
86. Nilius V, Killer MC, Timmesfeld N, Schmitt M, Moll R, Lorch A, et al. High β-1,4-Galactosyltransferase-I expression in peripheral T-lymphocytes is associated with a low risk of relapse in germ-cell cancer patients receiving high-dose chemotherapy with autologous stem cell reinfusion. Oncoimmunology (2018) 7(5):e1423169. doi: 10.1080/2162402X.2017.1423169
87. Sha J, Zhang R, Fan J, Gu Y, Pan Y, Han J, et al. The b-Cell-Specific ablation of B4GALT1 reduces cancer formation and reverses the changes in serum IgG glycans during the induction of mouse hepatocellular carcinoma. Cancers (Basel) (2022) 14(5):1333. doi: 10.3390/cancers14051333
88. Lauc G, Huffman JE, Pučić M, Zgaga L, Adamczyk B, Mužinić A, et al. Loci associated with n-glycosylation of human immunoglobulin G show pleiotropy with autoimmune diseases and haematological cancers. PloS Genet (2013) 9(1):e1003225. doi: 10.1371/journal.pgen.1003225
89. Giannini S, Lee-Sundlov MM, Rivadeneyra L, Di Buduo CA, Burns R, Lau JT, et al. β4GALT1 controls β1 integrin function to govern thrombopoiesis and hematopoietic stem cell homeostasis. Nat Commun (2020) 11(1):356. doi: 10.1038/s41467-019-14178-y
90. Qasba PK, Ramakrishnan B, Boeggeman E. Structure and function of beta -1,4-galactosyltransferase. Curr Drug Targets (2008) 9(4):292–309. doi: 10.2174/138945008783954943
91. Al-Obaide MA, Alobydi H, Abdelsalam AG, Zhang R, Srivenugopal KS. Multifaceted roles of 5’-regulatory region of the cancer associated gene B4GALT1 and its comparison with the gene family. Int J Oncol (2015) 47(4):1393–404. doi: 10.3892/ijo.2015.3136
92. Yamashita H, Kubushiro K, Ma J, Fujii T, Tsukazaki K, Iwamori M, et al. Alteration in the metastatic potential of ovarian cancer cells by transfection of the antisense gene of beta-1,4-galactosyltransferase. Oncol Rep (2003) 10(6):1857–62. doi: 10.3892/or.10.6.1857
93. Xie H, Zhu Y, Zhang J, Liu Z, Fu H, Cao Y, et al. B4GALT1 expression predicts prognosis and adjuvant chemotherapy benefits in muscle-invasive bladder cancer patients. BMC Cancer (2018) 18(1):590. doi: 10.1186/s12885-018-4497-0
94. Zhang J, Zhang G, Zhang W, Bai L, Wang L, Li T, et al. Loss of RBMS1 promotes anti-tumor immunity through enabling PD-L1 checkpoint blockade in triple-negative breast cancer. Cell Death Differ (2022) 29(11):2247–61. doi: 10.1038/s41418-022-01012-0
95. Chui D, Oh-Eda M, Liao YF, Panneerselvam K, Lal A, Marek KW, et al. Alpha-mannosidase-II deficiency results in dyserythropoiesis and unveils an alternate pathway in oligosaccharide biosynthesis. Cell (1997) 90(1):157–67. doi: 10.1016/s0092-8674(00)80322-0
96. Fagerberg L, Hallström BM, Oksvold P, Kampf C, Djureinovic D, Odeberg J, et al. Analysis of the human tissue-specific expression by genome-wide integration of transcriptomics and antibody-based proteomics. Mol Cell Proteomics (2014) 13(2):397–406. doi: 10.1074/mcp.M113.035600
97. Akama TO, Nakagawa H, Wong NK, Sutton-Smith M, Dell A, Morris HR, et al. Essential and mutually compensatory roles of {alpha}-mannosidase II and {alpha}-mannosidase IIx in n-glycan processing in vivo in mice. Proc Natl Acad Sci U.S.A. (2006) 103(24):8983–8. doi: 10.1073/pnas.0603248103
98. Shi S, Gu S, Han T, Zhang W, Huang L, Li Z, et al. Inhibition of MAN2A1 enhances the immune response to anti-PD-L1 in human tumors. Clin Cancer Res (2020) 26(22):5990–6002. doi: 10.1158/1078-0432.CCR-20-0778
99. Lee ZY, Loo JSE, Wibowo A, Mohammat MF, Foo JB. Targeting cancer via golgi α-mannosidase II inhibition: How far have we come in developing effective inhibitors? Carbohydr Res (2021) 508:108395. doi: 10.1016/j.carres.2021.108395
100. Mohamed Abd-El-Halim Y, El Kaoutari A, Silvy F, Rubis M, Bigonnet M, Roques J, et al. A glycosyltransferase gene signature to detect pancreatic ductal adenocarcinoma patients with poor prognosis. EBioMedicine (2021) 71:103541. doi: 10.1016/j.ebiom.2021.103541
101. Liu X, Zhang Y, Han Y, Lu W, Yang J, Tian J, et al. Overexpression of GLT1D1 induces immunosuppression through glycosylation of PD-L1 and predicts poor prognosis in b-cell lymphoma. Mol Oncol (2020) 14(5):1028–44. doi: 10.1002/1878-0261.12664
102. Gylfe AE, Kondelin J, Turunen M, Ristolainen H, Katainen R, Pitkänen E, et al. Identification of candidate oncogenes in human colorectal cancers with microsatellite instability. Gastroenterology (2013) 145(3):540–3. doi: 10.1053/j.gastro.2013.05.015
103. Krzeminski P, Corchete LA, García JL, López-Corral L, Fermiñán E, García EM, et al. Integrative analysis of DNA copy number, DNA methylation and gene expression in multiple myeloma reveals alterations related to relapse. Oncotarget (2016) 7(49):80664–79. doi: 10.18632/oncotarget.13025
104. Agrawal P, Fontanals-Cirera B, Sokolova E, Jacob S, Vaiana CA, Argibay D, et al. A systems biology approach identifies FUT8 as a driver of melanoma metastasis. Cancer Cell (2017) 31(6):804–819.e7. doi: 10.1016/j.ccell.2017.05.007
105. Fujii H, Shinzaki S, Iijima H, Wakamatsu K, Iwamoto C, Sobajima T, et al. Core fucosylation on T cells, required for activation of T-cell receptor signaling and induction of colitis in mice, is increased in patients with inflammatory bowel disease. Gastroenterology (2016) 150(7):1620–32. doi: 10.1053/j.gastro.2016.03.002
106. Liao C, An J, Yi S, Tan Z, Wang H, Li H, et al. FUT8 and protein core fucosylation in tumours: From diagnosis to treatment. J Cancer (2021) 12(13):4109–20. doi: 10.7150/jca.58268
107. Zhang N, Li M, Xu X, Zhang Y, Liu Y, Zhao M, et al. Loss of core fucosylation enhances the anticancer activity of cytotoxic T lymphocytes by increasing PD-1 degradation. Eur J Immunol (2020) 50(11):1820–33. doi: 10.1002/eji.202048543
108. Okada M, Chikuma S, Kondo T, Hibino S, Machiyama H, Yokosuka T, et al. Blockage of core fucosylation reduces cell-surface expression of PD-1 and promotes anti-tumor immune responses of T cells. Cell Rep (2017) 20(5):1017–28. doi: 10.1016/j.celrep.2017.07.027
109. Liu J, Wang G, Liu L, Wu R, Wu Y, Fang C, et al. Study of the interactions of a novel monoclonal antibody, mAb059c, with the hPD-1 receptor. Sci Rep (2019) 9(1):17830. doi: 10.1038/s41598-019-54231-w
110. Huang Z, Pang X, Zhong T, Qu T, Chen N, Ma S, et al. Penpulimab, an fc-engineered IgG1 anti-PD-1 antibody, with improved efficacy and low incidence of immune-related adverse events. Front Immunol (2022) 13:924542. doi: 10.3389/fimmu.2022.924542
111. Shi X, Zhang D, Li F, Zhang Z, Wang S, Xuan Y, et al. Targeting glycosylation of PD-1 to enhance CAR-T cell cytotoxicity. J Hematol Oncol (2019) 12(1):127. doi: 10.1186/s13045-019-0831-5
112. Yamaguchi H, Hsu JM, Yang WH, Hung MC. Mechanisms regulating PD-L1 expression in cancers and associated opportunities for novel small-molecule therapeutics. Nat Rev Clin Oncol (2022) 19(5):287–305. doi: 10.1038/s41571-022-00601-9
113. Liu W, Wang Y, Luo J, Liu M, Luo Z. Pleiotropic effects of metformin on the antitumor efficiency of immune checkpoint inhibitors. Front Immunol (2021) 11:586760. doi: 10.3389/fimmu.2020.586760
114. Cha JH, Yang WH, Xia W, Wei Y, Chan LC, Lim SO, et al. Metformin promotes antitumor immunity via endoplasmic-Reticulum-Associated degradation of PD-L1. Mol Cell (2018) 71(4):606–20.e7. doi: 10.1016/j.molcel.2018.07.030
115. Shao B, Li CW, Lim SO, Sun L, Lai YJ, Hou J, et al. Deglycosylation of PD-L1 by 2-deoxyglucose reverses PARP inhibitor-induced immunosuppression in triple-negative breast cancer. Am J Cancer Res (2018) 8(9):1837–46.
116. Repas J, Zupin M, Vodlan M, Veranič P, Gole B, Potočnik U, et al. Dual effect of combined metformin and 2-Deoxy-D-Glucose treatment on mitochondrial biogenesis and PD-L1 expression in triple-negative breast cancer cells. Cancers (Basel) (2022) 14(5):1343. doi: 10.3390/cancers14051343
117. Zhang R, Yang Y, Dong W, Lin M, He J, Zhang X, et al. D-mannose facilitates immunotherapy and radiotherapy of triple-negative breast cancer via degradation of PD-L1. Proc Natl Acad Sci U.S.A. (2022) 119(8):e2114851119. doi: 10.1073/pnas.2114851119
118. Malaguarnera L. Influence of resveratrol on the immune response. Nutrients (2019) 11(5):946. doi: 10.3390/nu11050946
119. Delmas D, Hermetet F, Aires V. PD-1/PD-L1 checkpoints and resveratrol: A controversial new way for a therapeutic strategy. Cancers (Basel) (2021) 13(18):4509. doi: 10.3390/cancers13184509
120. Verdura S, Cuyàs E, Cortada E, Brunet J, Lopez-Bonet E, Martin-Castillo B, et al. Resveratrol targets PD-L1 glycosylation and dimerization to enhance antitumor T-cell immunity. Aging (Albany NY) (2020) 12(1):8–34. doi: 10.18632/aging.102646
121. Zhang X, Schwartz JC, Almo SC, Nathenson SG. Crystal structure of the receptor-binding domain of human B7-2: insights into organization and signaling. Proc Natl Acad Sci U.S.A. (2003) 100(5):2586–91. doi: 10.1073/pnas.252771499
122. Zhou L, Chai F, He Y, Zhou Z, Guo S, Li P, et al. Homodimerized cytoplasmic domain of PD-L1 regulates its complex glycosylation in living cells. Commun Biol (2022) 5(1):887. doi: 10.1038/s42003-022-03845-4
123. Ren B, Kwah MX, Liu C, Ma Z, Shanmugam MK, Ding L, et al. Resveratrol for cancer therapy: Challenges and future perspectives. Cancer Lett (2021) 515:63–72. doi: 10.1016/j.canlet.2021.05.001
124. Jia L, Gao Y, Zhou T, Zhao XL, Hu HY, Chen DW, et al. Enhanced response to PD-L1 silencing by modulation of TME via balancing glucose metabolism and robust co-delivery of siRNA/Resveratrol with dual-responsive polyplexes. Biomaterials (2021) 271:120711. doi: 10.1016/j.biomaterials.2021.120711
125. Rinis N, Golden JE, Marceau CD, Carette JE, Van Zandt MC, Gilmore R, et al. Editing n-glycan site occupancy with small-molecule oligosaccharyltransferase inhibitors. Cell Chem Biol (2018) 25(10):1231–41.e4. doi: 10.1016/j.chembiol.2018.07.005
126. Lu H, Cherepanova NA, Gilmore R, Contessa JN, Lehrman MA. Targeting STT3A-oligosaccharyltransferase with NGI-1 causes herpes simplex virus 1 dysfunction. FASEB J (2019) 33(6):6801–12. doi: 10.1096/fj.201802044RR
127. Kumagai K, Kojima H, Okabe T, Nagano T. Development of a highly sensitive, high-throughput assay for glycosyltransferases using enzyme-coupled fluorescence detection. Anal Biochem (2014) 447:146–55. doi: 10.1016/j.ab.2013.11.025
128. Rose DR. Structure, mechanism and inhibition of golgi α-mannosidase II. Curr Opin Struct Biol (2012) 22(5):558–62. doi: 10.1016/j.sbi.2012.06.005
129. Tan S, Zhang CW, Gao GF. Seeing is believing: anti-PD-1/PD-L1 monoclonal antibodies in action for checkpoint blockade tumor immunotherapy. Signal Transduct Target Ther (2016) 1:16029. doi: 10.1038/sigtrans.2016.29
130. Keir ME, Butte MJ, Freeman GJ, Sharpe AH. PD-1 and its ligands in tolerance and immunity. Annu Rev Immunol (2008) 26:677–704. doi: 10.1146/annurev.immunol.26.021607.090331
131. Xiao Y, Yu S, Zhu B, Bedoret D, Bu X, Francisco LM, et al. RGMb is a novel binding partner for PD-L2 and its engagement with PD-L2 promotes respiratory tolerance. J Exp Med (2014) 211(5):943–59. doi: 10.1084/jem.20130790
132. Zang X, Loke P, Kim J, Murphy K, Waitz R, Allison JP. B7x: a widely expressed B7 family member that inhibits T cell activation. Proc Natl Acad Sci U.S.A. (2003) 100(18):10388–92. doi: 10.1073/pnas.1434299100
133. Philips EA, Garcia-España A, Tocheva AS, Ahearn IM, Adam KR, Pan R, et al. The structural features that distinguish PD-L2 from PD-L1 emerged in placental mammals. J Biol Chem (2020) 295(14):4372–80. doi: 10.1074/jbc.AC119.011747
134. Tang S, Kim PS. A high-affinity human PD-1/PD-L2 complex informs avenues for small-molecule immune checkpoint drug discovery. Proc Natl Acad Sci U.S.A. (2019) 116(49):24500–6. doi: 10.1073/pnas.1916916116
135. Di Maro A, Chambery A, Carafa V, Costantini S, Colonna G, Parente A. Structural characterization and comparative modeling of PD-ls 1-3, type 1 ribosome-inactivating proteins from summer leaves of phytolacca dioica l. Biochimie (2009) 91(3):352–63. doi: 10.1016/j.biochi.2008.10.008
136. Solinas C, Garaud S, De Silva P, Boisson A, Van den Eynden G, de Wind A, et al. Immune checkpoint molecules on tumor-infiltrating lymphocytes and their association with tertiary lymphoid structures in human breast cancer. Front Immunol (2017) 8:1412. doi: 10.3389/fimmu.2017.01412
137. Good-Jacobson KL, Szumilas CG, Chen L, Sharpe AH, Tomayko MM, Shlomchik MJ. PD-1 regulates germinal center b cell survival and the formation and affinity of long-lived plasma cells. Nat Immunol (2010) 11(6):535–42. doi: 10.1038/ni.1877
138. Francisco LM, Sage PT, Sharpe AH. The PD-1 pathway in tolerance and autoimmunity. Immunol Rev (2010) 236:219–42. doi: 10.1111/j.1600-065X.2010.00923.x
139. Latchman Y, Wood CR, Chernova T, Chaudhary D, Borde M, Chernova I, et al. PD-L2 is a second ligand for PD-1 and inhibits T cell activation. Nat Immunol (2001) 2(3):261–8. doi: 10.1038/85330
140. Solinas C, Aiello M, Rozali E, Lambertini M, Willard-Gallo K, Migliori E. Programmed cell death-ligand 2: A neglected but important target in the immune response to cancer? Transl Oncol (2020) 13(10):100811. doi: 10.1016/j.tranon.2020.100811
141. Derks S, Nason KS, Liao X, Stachler MD, Liu KX, Liu JB, et al. Epithelial PD-L2 expression marks barrett’s esophagus and esophageal adenocarcinoma. Cancer Immunol Res (2015) 3:1123–9. doi: 10.1158/2326-6066.CIR-15-0046
142. Qiao Y, Liu C, Zhang X, Zhou Q, Li Y, Xu Y, et al. PD-L2 based immune signature confers poor prognosis in HNSCC. Oncoimmunology (2021) 10(1):1947569. doi: 10.1080/2162402X.2021.1947569
143. Wang H, Yao H, Li C, Liang L, Zhang Y, Shi H, et al. PD-L2 expression in colorectal cancer: Independent prognostic effect and targetability by deglycosylation. Oncoimmunology (2017) 6(7):e1327494. doi: 10.1080/2162402X.2017.1327494
144. Kontos F, Michelakos T, Kurokawa T, Sadagopan A, Schwab JH, Ferrone CR, et al. B7-H3: An attractive target for antibody-based immunotherapy. Clin Cancer Res (2021) 27(5):1227–35. doi: 10.1158/1078-0432.CCR-20-2584
145. Carvajal-Hausdorf D, Altan M, Velcheti V, Gettinger SN, Herbst RS, Rimm DL, et al. Expression and clinical significance of PD-L1, B7-H3, B7-H4 and TILs in human small cell lung cancer (SCLC). J Immunother Cancer (2019) 7(1):65. doi: 10.1186/s40425-019-0540-1
146. Kovaleva OV, Belova TP, Korotkova EA, Kushlinskii DN, Gratchev AN, Petrikova NA, et al. Soluble B7-H3 in ovarian cancer and its predictive value. Bull Exp Biol Med (2021) 171(4):472–4. doi: 10.1007/s10517-021-05253-w
147. Digregorio M, Coppieters N, Lombard A, Lumapat PN, Scholtes F, Rogister B. The expression of B7-H3 isoforms in newly diagnosed glioblastoma and recurrence and their functional role. Acta Neuropathol Commun (2021) 9(1):59. doi: 10.1186/s40478-021-01167-w
148. Fan H, Zhu JH, Yao XQ. Prognostic significance of B7-H3 expression in patients with colorectal cancer: A meta-analysis. Pak J Med Sci (2016) 32(6):1568–73. doi: 10.12669/pjms.326.11511
149. Li ZY, Wang JT, Chen G, Shan ZG, Wang TT, Shen Y, et al. Expression, regulation and clinical significance of B7-H3 on neutrophils in human gastric cancer. Clin Immunol (2021) 227:108753. doi: 10.1016/j.clim.2021.108753
150. Nunes-Xavier CE, Kildal W, Kleppe A, Danielsen HE, Waehre H, Llarena R, et al. Immune checkpoint B7-H3 protein expression is associated with poor outcome and androgen receptor status in prostate cancer. Prostate (2021) 81(12):838–48. doi: 10.1002/pros.24180
151. Koyama Y, Morikawa T, Miyama Y, Miyakawa J, Kawai T, Kume H, et al. B7-H3 expression in upper tract urothelial carcinoma associates with adverse clinicopathological features and poor survival. Pathol Res Pract (2020) 216(12):153219. doi: 10.1016/j.prp.2020.153219
152. Haydar D, Houke H, Chiang J, Yi Z, Odé Z, Caldwell K, et al. Cell-surface antigen profiling of pediatric brain tumors: B7-H3 is consistently expressed and can be targeted via local or systemic CAR T-cell delivery. Neuro Oncol (2021) 23(6):999–1011. doi: 10.1093/neuonc/noaa278
153. Inamura K, Takazawa Y, Inoue Y, Yokouchi Y, Kobayashi M, Saiura A, et al. Tumor B7-H3 (CD276) Expression and Survival in Pancreatic Cancer. J Clin Med (2018) 7(7):172. doi: 10.3390/jcm7070172
154. Fang J, Chen F, Liu D, Gu F, Chen Z, Wang Y. Prognostic value of immune checkpoint molecules in breast cancer. Biosci Rep (2020) 40(7):BSR20201054. doi: 10.1042/BSR20201054
155. Cheng R, Chen Y, Zhou H, Wang B, Du Q, Chen Y. B7-H3 expression and its correlation with clinicopathologic features, angiogenesis, and prognosis in intrahepatic cholangiocarcinoma. APMIS (2018) 126(5):396–402. doi: 10.1111/apm.12837
156. Zheng Y, Liao N, Wu Y, Gao J, Li Z, Liu W, et al. High expression of B7-H2 or B7-H3 is associated with poor prognosis in hepatocellular carcinoma. Mol Med Rep (2019) 19(5):4315–25. doi: 10.3892/mmr.2019.10080
157. Chen JT, Chen CH, Ku KL, Hsiao M, Chiang CP, Hsu TL, et al. Glycoprotein B7-H3 overexpression and aberrant glycosylation in oral cancer and immune response. Proc Natl Acad Sci U.S.A. (2015) 112(42):13057–62. doi: 10.1073/pnas.1516991112
158. Saeednejad Zanjani L, Madjd Z, Axcrona U, Abolhasani M, Rasti A, Asgari M, et al. Cytoplasmic expression of B7-H3 and membranous EpCAM expression are associated with higher grade and survival outcomes in patients with clear cell renal cell carcinoma. Ann Diagn Pathol (2020) 46:151483. doi: 10.1016/j.anndiagpath.2020.151483
159. Feng R, Chen Y, Liu Y, Zhou Q, Zhang W. The role of B7-H3 in tumors and its potential in clinical application. Int Immunopharmacol (2021) 101(Pt B):108153. doi: 10.1016/j.intimp.2021.108153
160. Yamato I, Sho M, Nomi T, Akahori T, Shimada K, Hotta K, et al. Clinical importance of B7-H3 expression in human pancreatic cancer. Br J Cancer (2009) 101(10):1709–16. doi: 10.1038/sj.bjc.6605375
161. Kanchan RK, Doss D, Khan P, Nasser MW, Mahapatra S. To kill a cancer: Targeting the immune inhibitory checkpoint molecule, B7-H3. Biochim Biophys Acta Rev Cancer (2022) 1877(5):188783. doi: 10.1016/j.bbcan.2022.188783
162. Zhou WT, Jin WL. B7-H3/CD276: An emerging cancer immunotherapy. Front Immunol (2021) 12:701006. doi: 10.3389/fimmu.2021.701006
163. Chen R, Jiang X, Sun D, Han G, Wang F, Ye M, et al. Glycoproteomics analysis of human liver tissue by combination of multiple enzyme digestion and hydrazide chemistry. J Proteome Res (2009) 8(2):651–61. doi: 10.1021/pr8008012
164. Gao Y, Shen L, Dong T, Yang X, Cui H, Guo Y, et al. An n-glycoproteomic site-mapping analysis reveals glycoprotein alterations in esophageal squamous cell carcinoma. J Transl Med (2022) 20(1):285. doi: 10.1186/s12967-022-03489-2
165. Tu CF, Li FA, Li LH, Yang RB. Quantitative glycoproteomics analysis identifies novel FUT8 targets and signaling networks critical for breast cancer cell invasiveness. Breast Cancer Res (2022) 24(1):21. doi: 10.1186/s13058-022-01513-3
166. Tu CF, Wu MY, Lin YC, Kannagi R, Yang RB. FUT8 promotes breast cancer cell invasiveness by remodeling TGF-β receptor core fucosylation. Breast Cancer Res (2017) 19(1):111. doi: 10.1186/s13058-017-0904-8
167. Podojil JR, Miller SD. Potential targeting of B7-H4 for the treatment of cancer. Immunol Rev (2017) 276(1):40–51. doi: 10.1111/imr.12530
168. Sica GL, Choi IH, Zhu G, Tamada K, Wang SD, Tamura H, et al. B7-H4, a molecule of the B7 family, negatively regulates T cell immunity. Immunity (2003) 18(6):849–61. doi: 10.1016/s1074-7613(03)00152-3
169. Bolandi N, Derakhshani A, Hemmat N, Baghbanzadeh A, Asadzadeh Z, Afrashteh Nour M, et al. The positive and negative immunoregulatory role of B7 family: Promising novel targets in gastric cancer treatment. Int J Mol Sci (2021) 22(19):10719. doi: 10.3390/ijms221910719
170. Mansorunov D, Apanovich N, Apanovich P, Kipkeeva F, Muzaffarova T, Kuzevanova A, et al. Expression of immune checkpoints in malignant tumors: Therapy targets and biomarkers for the gastric cancer prognosis. Diagn (Basel) (2021) 11(12):2370. doi: 10.3390/diagnostics11122370
171. Altan M, Kidwell KM, Pelekanou V, Carvajal-Hausdorf DE, Schalper KA, Toki MI, et al. Association of B7-H4, PD-L1, and tumor infiltrating lymphocytes with outcomes in breast cancer. NPJ Breast Cancer (2018) 4:40. doi: 10.1038/s41523-018-0095-1
172. Wang L, Yang C, Liu XB, Wang L, Kang FB. B7-H4 overexpression contributes to poor prognosis and drug-resistance in triple-negative breast cancer. Cancer Cell Int (2018) 18:100. doi: 10.1186/s12935-018-0597-9
173. Li H, Piao L, Liu S, Cui Y, Xuan Y. B7-H4 is a potential prognostic biomarker of prostate cancer. Exp Mol Pathol (2020) 114:104406. doi: 10.1016/j.yexmp.2020.104406
174. Chen X, Tao L, Yuan C, Xiu D. The prognostic value of B7-H4 in pancreatic cancer: Systematic review and meta-analysis. Med (Baltimore) (2018) 97(12):e0088. doi: 10.1097/MD.0000000000010088
175. Liu Z, Jin K, Zeng H, Shao F, Chang Y, Wang Y, et al. B7-H4 correlates with clinical outcome and immunotherapeutic benefit in muscle-invasive bladder cancer. Eur J Cancer (2022) 171:133–42. doi: 10.1016/j.ejca.2022.05.022
176. Ding S, Lv X, Liu Z, Zhan S, Xu Y, Zhang X, et al. Overexpression of B7-H4 is associated with infiltrating immune cells and poor prognosis in metastatic colorectal cancer. Int Immunopharmacol (2021) 90:107144. doi: 10.1016/j.intimp.2020.107144
177. Fauci JM, Straughn JM Jr, Ferrone S, Buchsbaum DJ. A review of B7-H3 and B7-H4 immune molecules and their role in ovarian cancer. Gynecol Oncol (2012) 127(2):420–5. doi: 10.1016/j.ygyno.2012.08.017
178. Fukuda T, Kamai T, Masuda A, Nukui A, Abe H, Arai K, et al. Higher preoperative serum levels of PD-L1 and B7-H4 are associated with invasive and metastatic potential and predictable for poor response to VEGF-targeted therapy and unfavorable prognosis of renal cell carcinoma. Cancer Med (2016) 5(8):1810–20. doi: 10.1002/cam4.754
179. Podojil JR, Glaser AP, Baker D, Courtois ET, Fantini D, Yu Y, et al. Antibody targeting of B7-H4 enhances the immune response in urothelial carcinoma. Oncoimmunology (2020) 9(1):1744897. doi: 10.1080/2162402X.2020.1744897
180. Chen LJ, Sun J, Wu HY, Zhou SM, Tan Y, Tan M, et al. B7-H4 expression associates with cancer progression and predicts patient’s survival in human esophageal squamous cell carcinoma. Cancer Immunol Immunother (2011) 60(7):1047–55. doi: 10.1007/s00262-011-1017-3
181. Liu CL, Zang XX, Huang H, Zhang H, Wang C, Kong YL, et al. The expression of B7-H3 and B7-H4 in human gallbladder carcinoma and their clinical implications. Eur Rev Med Pharmacol Sci (2016) 20(21):4466–73.
182. Choi IH, Zhu G, Sica GL, Strome SE, Cheville JC, Lau JS, et al. Genomic organization and expression analysis of B7-H4, an immune inhibitory molecule of the B7 family. J Immunol (2003) 171(9):4650–4. doi: 10.4049/jimmunol.171.9.4650
183. Prasad DV, Richards S, Mai XM, Dong C. B7S1, a novel B7 family member that negatively regulates T cell activation. Immunity (2003) 18(6):863–73. doi: 10.1016/s1074-7613(03)00147-x
184. Salceda S, Tang T, Kmet M, Munteanu A, Ghosh M, Macina R, et al. The immunomodulatory protein B7-H4 is overexpressed in breast and ovarian cancers and promotes epithelial cell transformation. Exp Cell Res (2005) 306(1):128–41. doi: 10.1016/j.yexcr.2005.01.018
185. Chen D, Li G, Ji C, Lu Q, Qi Y, Tang C, et al. Enhanced B7-H4 expression in gliomas with low PD-L1 expression identifies super-cold tumors. J Immunother Cancer (2020) 8(1):e000154. doi: 10.1136/jitc-2019-000154
186. Schalper KA, Carvajal-Hausdorf D, McLaughlin J, Altan M, Velcheti V, Gaule P, et al. Differential expression and significance of PD-L1, IDO-1, and B7-H4 in human lung cancer. Clin Cancer Res (2017) 23(2):370–8. doi: 10.1158/1078-0432.CCR-16-0150
187. Yang J, Tian Z, Gao H, Xiong F, Cao C, Yu J, et al. Clinical significance and correlation of PD-L1, B7-H3, B7-H4, and TILs in pancreatic cancer. BMC Cancer (2022) 22(1):584. doi: 10.1186/s12885-022-09639-5
188. Ponath V, Hoffmann N, Bergmann L, Mäder C, Alashkar Alhamwe B, Preußer C, et al. Secreted ligands of the NK cell receptor NKp30: B7-H6 is in contrast to BAG6 only marginally released via extracellular vesicles. Int J Mol Sci (2021) 22(4):2189. doi: 10.3390/ijms22042189
189. Skořepa O, Pazicky S, Kalousková B, Bláha J, Abreu C, Ječmen T, et al. Natural killer cell activation receptor NKp30 oligomerization depends on its n-glycosylation. Cancers (Basel) (2020) 12(7):1998. doi: 10.3390/cancers12071998
190. Hartmann J, Tran TV, Kaudeer J, Oberle K, Herrmann J, Quagliano I, et al. The stalk domain and the glycosylation status of the activating natural killer cell receptor NKp30 are important for ligand binding. J Biol Chem (2012) 287(37):31527–39. doi: 10.1074/jbc.M111.304238
191. Zhang T, Wu MR, Sentman CL. An NKp30-based chimeric antigen receptor promotes T cell effector functions and antitumor efficacy in vivo. J Immunol (2012) 189(5):2290–9. doi: 10.4049/jimmunol.1103495
192. Pinheiro PF, Justino GC, Marques MM. NKp30-a prospective target for new cancer immunotherapy strategies. Br J Pharmacol (2020) 177(20):4563–80. doi: 10.1111/bph.15222
193. Mei J, Xu J, Yang X, Gu D, Zhou W, Wang H, et al. A comparability study of natural and deglycosylated PD-L1 levels in lung cancer: evidence from immunohistochemical analysis. Mol Cancer (2021) 20(1):11. doi: 10.1186/s12943-020-01304-4
194. Ou-Yang F, Li CL, Chen CC, Shen YC, Moi SH, Luo CW, et al. De-glycosylated membrane PD-L1 in tumor tissues as a biomarker for responsiveness to atezolizumab (Tecentriq) in advanced breast cancer patients. Am J Cancer Res (2022) 12(1):123–37.
Keywords: glycosylation, PD-L1, PD-L2, B7-H3, B7-H4, B7-H6, PD-1
Citation: Xiao L, Guan X, Xiang M, Wang Q, Long Q, Yue C, Chen L, Liu J and Liao C (2022) B7 family protein glycosylation: Promising novel targets in tumor treatment. Front. Immunol. 13:1088560. doi: 10.3389/fimmu.2022.1088560
Received: 03 November 2022; Accepted: 22 November 2022;
Published: 06 December 2022.
Edited by:
Olga O. Zaytseva, Genos Glycoscience Research Laboratory, CroatiaReviewed by:
Mou Peng, Department of Urology, Central South University, ChinaNaoe Taira Nihira, St. Marianna University School of Medicine, Japan
Copyright © 2022 Xiao, Guan, Xiang, Wang, Long, Yue, Chen, Liu and Liao. This is an open-access article distributed under the terms of the Creative Commons Attribution License (CC BY). The use, distribution or reproduction in other forums is permitted, provided the original author(s) and the copyright owner(s) are credited and that the original publication in this journal is cited, in accordance with accepted academic practice. No use, distribution or reproduction is permitted which does not comply with these terms.
*Correspondence: Chengcheng Liao, bGNjXzk1MDMzMEAxNjMuY29t; Jianguo Liu, MTMwODc4OTEwMDFAMTYzLmNvbQ==
†These authors have contributed equally to this work and share first authorship