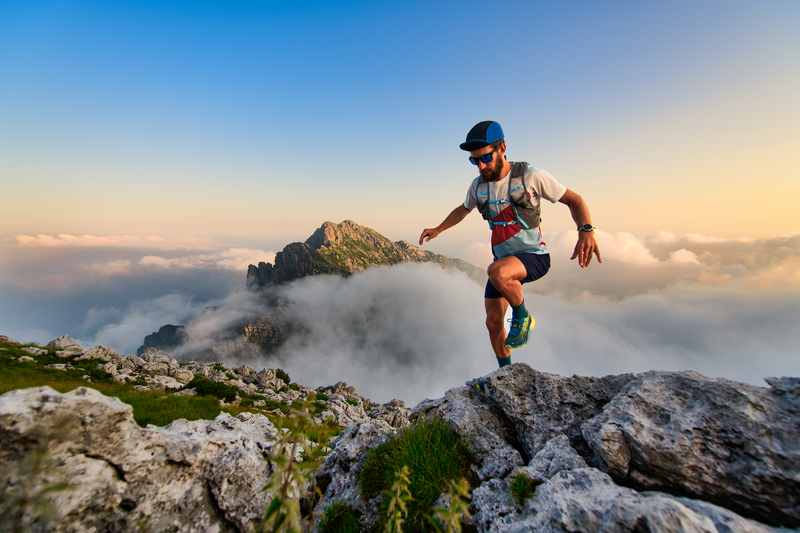
94% of researchers rate our articles as excellent or good
Learn more about the work of our research integrity team to safeguard the quality of each article we publish.
Find out more
REVIEW article
Front. Immunol. , 16 January 2023
Sec. Immunological Tolerance and Regulation
Volume 13 - 2022 | https://doi.org/10.3389/fimmu.2022.1085057
Exosomes, which are nano-sized transport bio-vehicles, play a pivotal role in maintaining homeostasis by exchanging genetic or metabolic information between different cells. Exosomes can also play a vital role in transferring virulent factors between the host and parasite, thereby regulating host gene expression and the immune interphase. The association of inflammation with disease development and the potential of exosomes to enhance or mitigate inflammatory pathways support the notion that exosomes have the potential to alter the course of a disease. Clinical trials exploring the role of exosomes in cancer, osteoporosis, and renal, neurological, and pulmonary disorders are currently underway. Notably, the information available on the signatory efficacy of exosomes in immune-related disorders remains elusive and sporadic. In this review, we discuss immune cell-derived exosomes and their application in immunotherapy, including those against autoimmune connective tissue diseases. Further, we have elucidated our views on the major issues in immune-related pathophysiological processes. Therefore, the information presented in this review highlights the role of exosomes as promising strategies and clinical tools for immune regulation.
Extracellular vesicles encompass a large heterogeneous family of membrane-bound structures, such as microvesicles, exosomes, and apoptotic bodies. The Heine group initially named all vesicles derived from plasma membrane exosomes (1). Earlier studies by the Turbide group reported that reticulocyte culture releases exosomal vesicles that can be harvested by ultracentrifugation. Further analysis of these vesicles revealed the presence of transferrin receptors, nucleoside transporters, glucose transporters, acetylcholinesterase, and Na+-independent amino acid transporter (2). Exosomes are capable of transferring miRNAs and mRNAs between cells (3). Almost all cell types release exosomes with varying molecular compositions and biogenesis pathways. Several bodily fluids, such as saliva, urine, breast milk, amniotic fluid, synovial fluid, serum, and ascites fluid, contain exosomes under physiological and pathological conditions. Exosomes were previously considered cellular waste generated due to cell damage and homeostasis. However, they have been extensively investigated after revealing the antitumor potential of exosomes carrying MHC-I and MHC-II (4).
Exosomes range from 30–150 nm in size (5, 6). Later research ascertained their genesis to be from the inward budding of endosomes, producing multivesicular bodies with intraluminal vesicles. Subsequently, proteins are incorporated into the invaginating membrane, and cytosolic components are engulfed and enclosed within intraluminal vesicles. The contents of exosomes can differ depending on the source and physiological conditions of the cells that release them (7). The fusion of multivesicular bodies with the plasma membrane results in the release of intraluminal vesicles into the extracellular space, giving rise to “exosomes” (2, 8, 9). This is primarily an endosomal sorting complex required for transport (ESCRT)-dependent processes. However, it reportedly functions independently of ESCRT complexes (10, 11). Alternatively, they may also be trafficked to the lysosomes for degradation. These exosomes are mini versions of parent cells and have emerged as critical mediators in inter-cell communication, functioning as vehicles delivering a complex cargo of proteins, lipids, and nucleic acids from the parent cells to other distant cells. Upon reaching recipient cells, they interact either via surface ligands or through the delivery of activated receptors or epigenetic modulation of the recipient cells via the cargo of bioactive cells, consequently modulating the physiology of recipient cells. Thus, exosomes play distinct roles in a multitude of physiological processes, such as immune response, cell differentiation, signal transduction, and antigen presentation. Various cells secrete exosomes; the cargo is unique to its cellular origin and thus can be used as a biomarker. Their ability to package biomolecules within them has facilitated their use as drug delivery systems (12–15).
This review focuses on one of the major physiological roles of exosomes: innate immune regulation. To maintain body function and homeostasis, protection against foreign agents is essential. This function is effectively performed by the immune system, a complex network of cells and organs that protects the organism against infectious agents, such as bacteria, viruses, and other pathogens. This is achieved through a combination of innate and adaptive immunity. Innate immunity is an antigen-non-specific, evolutionarily conserved system in most multicellular organisms, whereas lymphocytes mediate adaptive immunity through antigen specificity and memory. The innate immune system forms the first line of defense and comprises a network of cells, including monocytes/macrophages, dendritic cells, neutrophils, and natural killer cells, facilitating the earliest interactions between the host and pathogens. Upon entry of a foreign object, pattern-recognition receptors on immune cells, such as Toll-like receptors (TLRs), RIG-I-like receptors, and certain DNA sensors, such as cGAS, recognize various molecular signatures of invaders called pathogen-associated molecular patterns (PAMPs), which include numerous molecules, such as lipopolysaccharide (LPS) from gram-negative bacteria, peptidoglycans from gram-positive bacteria, and unmethylated CpG DNA from bacteria and viruses. Upon recognition of the entry of an invader, cell-cell communication is critical for swiftly spreading the message of infection and enabling the innate immune system to mount a broad response against the pathogen. Until recently, cytokines and chemokines have been extensively studied for their role as messengers in innate immunity. However, recent research has revealed that exosomes are also vital in this communication (16). This review mainly focuses on the mechanisms by which exosomes mediate the innate immune response of the host to pathogens (viruses, bacteria, and parasites) as well as act against their cells via autoimmunity. We also focus on using exosomes as diagnostic biomarkers and therapeutic agents. We also focus on using exosomes as diagnostic biomarkers and therapeutic agents (Figure 1).
Figure 1 The role of exosomes in innate immunity, diagnosis and therapy. Created with BioRender.com.
Exosomes are released from pathogens and host cells. They modulate stimulatory or suppressive effects on the innate immune system through exosome-mediated intercellular communications. They are crucial in immune regulation, including antigen presentation, immune activation, immune suppression, and immune tolerance. Their immune activator or suppressor role depends mainly on the source of the exosomes and their biomolecular content. Exosomes derived from healthy human plasma samples contain various RNA species, such as mRNAs and noncoding regulatory RNAs, within these circulating vesicles (17–19). Pathways related to NF-κB activation and TLR cascades differ between exosomal mRNAs from naïve cells compared with those from LPS-stimulated cells, indicating significant changes in adaptive and innate immune processes. Exosomes are also carriers of critical soluble mediators such as cytokines. After LPS stimulation, RAW 264.7 mouse macrophages exhibited increased levels of cytokines, predominantly chemokines. Ten of the 16 cytokines secreted by LPS-stimulated RAW 264.7 cells were from cell-derived exosomes (20).
Mounting evidence from several viral infections suggest that exosomes can transfer viral components, including proteins, genomic molecules, and receptors, from infected to healthy cells, thus promoting infection and inflammation. The delivery of viral receptors to target cells by exosomes renders the cells susceptible to viral entry.
Nef, the HIV protein, is enclosed within exosomes (21, 22); they are activated during the uptake of these exosomes by latent cells, rendering them susceptible to HIV infection (23). Nef also inhibits the generation of CD4+ exosomes from T cells and induces the death of CD4+ T cells. Consequently, immune cells suppress viral recognition (24–26). Mack et al. demonstrated that the transport of viral receptors such as chemokine receptor 5 (CCR5), the main co-receptor for HIV infection, from peripheral blood mononuclear and CCR5+ ovary cells to CCR5-null cells enhances HIV-1 infection (27). Moreover, exosomes produced by megakaryocytes and platelets harbor the HIV co-receptor C-X-C chemokine receptor type 4 (CXCR4) and increase the susceptibility of CXCR4-null cells to X4-HIV infection (28). Exosomes also promote infection by delivering viral nucleic acids to the uninfected cells. Exosomes from HIV-infected cells reportedly transmit transactivation response elements (29, 30). The transactivation response element at the 5′ ends of the HIV transcript copies and interacts with the Tat protein to produce viral RNAs, consequently generating miRNAs. These miRNAs can inhibit a Bcl-2-interacting protein, which ultimately promotes resistance to apoptosis and promotes virus production (29, 30). Conversely, exosomes from infected cells can suppress viral infection. For instance, human cytidine deaminase apolipoprotein B mRNA editing enzyme, catalytic subunit 3G (APOBEC3G) molecules present in exosomes from infected cells cause the deamination of cytosine residues to uracil in the minus strand of viral DNA during reverse transcription. Consequently, the viral infectivity factor of HIV type-1, which is essential for efficient viral replication, is not sorted into exosomes (31). Additionally, exosomes produced by infected cells contain cyclic guanosine monophosphate–adenosine monophosphate (cGAMP), which can trigger an antiviral response via innate immune responses and interferon upregulation (32, 33). HIV-related miRNAs, such as miRNA-88 and miRNA-99, induce endosomal nuclear factor-kappa B (NF-κB) and toll-like receptor 8 (TLR8) signaling, which activate the immune response against HIV via tumor necrosis factor-alpha (TNF-α) production from macrophages (34).
Although many viruses evade the pathogen-sensing pathway, the immune system adopts alternative pathogen-sensing strategies that are not challenged by viral evasion mechanisms. For instance, hepatitis C virus-permissive cells can selectively pack immunostimulatory viral RNA into exosomes and deliver them to neighboring plasmacytoid dendritic cells, triggering an antiviral IFN response in vitro (35).
Although knowledge of host-pathogen interactions in novel coronavirus SARS-CoV-2 infection remains limited, growing evidence suggest the role of exosomes in this process. In an in vitro study wherein, SARS-CoV was cultured in alveolar epithelial type II cells, viral particles were observed within double-membrane vesicles (36, 37). This immune system evasion could be attributed to the reinfection observed in several discharged and fully recovered patients. Exosomes can also transfer angiotensin-converting enzyme 2 (ACE2), the main viral receptor, to recipient cells (38), thereby rendering them susceptible to virus docking. Non-peer-reviewed observations from El-Shennawy et al. reported that exosomal ACE2 competes with cellular ACE2 to neutralize SARS-CoV-2 infection. The ACE2-expressing (ACE2+) exosomes blocked the binding of viral spike (S) protein RBD to ACE2+ cells in a dose-dependent manner. Notably, this was 400–700-fold more effective than that of vesicle-free recombinant human ACE2 extracellular domain protein (rhACE2). They could also prove that ACE2-containing exosomes could prevent SARS-CoV-2 pseudotype virus attachment and could infect human host cells at an efficacy 50–150-fold higher than that of rhACE2. Thus, ACE2+ exosomes serve as competitive inhibitors to block SARS-CoV-2 infection, suggesting a promising therapeutic role (39). The contribution of exosomes in COVID-19 infection has already been proven to be quite significant, and further research is essential to elucidate the exact mechanisms (Figure 2 and Table 1).
Figure 2 Roles of exosomes in virus infection. ACE2, Angiotensin converting enzyme 2. Created with BioRender.com.
Exosomes can promote further infection by delivering bacterial molecules involved in pathogenesis. For instance, Staphylococcus aureus-derived exosomes harbor the bacterial pore-forming molecule α-toxin (40), and exosomes from Bacillus anthracis-infected cells have been observed to transport the lethal toxin virulence factor to sites distal to the infection (41). The work described (41, 42) sheds new light on how exosomes protect host cells by functioning as cellular decoys. The autophagy protein (ATG16L1) is required for protection against Staphylococcus aureus, which expresses α-toxin. This pore-forming toxin binds to the metalloprotease, a disintegrin and metalloproteinase domain-containing protein 10 (ADAM10), on the surface of various target cells and tissues. This study confirmed that ATG16L1 and other ATG proteins mediate protection against α-toxin by releasing ADAM10 on exosomes, which act as scavengers that can bind the toxin and improve host survival (Table 1).
Several protozoan parasites, such as Plasmodium sp., Leishmania sp., and Trypanosoma sp., release exosomes. Malarial disease severity correlates with the production of exosomes from Plasmodium-infected cells (49). Plasmodium falciparum-infected red blood cells communicate with parasites using exosome-like vesicles. This also promotes differentiation into sexual forms (43). In Plasmodium yoelii-infected mice, parasite protein-containing exosomes are released from infected reticulocytes and can induce antigen presentation (44).
Leishmania donovani (promastigote and amastigote forms) and Leishmania primarily release exosomes that can induce interleukin (IL)-8 secretion from macrophages (45). Subsequently, neutrophils are recruited, and Leishmania can invade these cells and gain access to macrophages during the phagocytosis of infected neutrophils (45, 50). Metacyclic trypomastigote and non-infective (epimastigote) forms of Trypanosoma cruzi parasites release exosomes (46) that contain proteins associated with immune modulation and virulence. Leishmania spp. and Trypanosoma cruzi can also induce exosome release from the infected cells. Studies on Leishmania mexicana-treated macrophages in vitro suggested that exosomes released from infected cells can induce phosphorylation of signaling proteins and significantly upregulate immune-related genes, including adenosine receptor 2a (Adora2a) on macrophages (46, 47). Trypanosoma brucei rhodesiense-derived exosomes can transfer serum resistance-associated proteins to Trypanosoma brucei. The parasite requires serum resistance-associated proteins to circumvent the action of host lytic factors, thereby conferring the ability to evade innate immunity (48).
Culturing the intestinal epithelial cells with Cryptosporidium parvum activates TLR4 signaling, leading to an upregulation of exosome secretion from these cells. This process is mediated by synaptosome-associated protein 23 (SNAP23)-associated vesicular exocytosis. These released exosomes contain epithelial antimicrobial peptides that can bind to and decrease the viability and infectivity of C. parvum sporozoites (51), (Table 1).
The immune system is vital for the protection of the body and the maintenance of homeostasis. However, this system must be tightly regulated for the normal physiological functioning of the body to avoid response to non-threatening and beneficial antigens (for instance, microbiota), to distinguish self from non-self, and from over-reactivity to antigens (allergy or hyper-immunity). Despite stringent control, the immune system can sometimes react to its cells, tissues, and other components, contributing to autoimmunity. Exosomes also engage in these processes, which are detailed in the subsequent sections. Common auto immune thyroid diseases (AITDs) like Hashimoto’s thyroiditis and Graves’ diseases are class of immune disorders caused by the irregular infiltrations of lymphocytes and over productions of autoantibodies to cause the hypothyroidism or hyperthyroidism (52) and genetic along with environmental factors were acknowledged for contributing to development of AITDs (53, 54). Irregular activation/production of autoantibodies like thyroid peroxidase antibodies (TPOAb), thyroglobulin antibodies (TGAb) and thyrotropin receptor antibodies (TRAb) cause thyroid dysfunction, which greatly affect the quality of life (54–56). So, it is clear that over production of autoantibodies and its governance by regulatory T-cell (Tregs) and T-helper-17 cells (Th17) is prime in controlling immune-induced thyroid pathogenesis. The micro communications between T-cells and AITDs are mainly controlled by plasma microvesicles (57). Intercellular communication mediated by exosomes is helpful in regulating innate and adaptive immunity by delivering the target antigens, inflammatory factors presentation and killer proteins. Recent studies have reported that the crucial role of apoptosis in the maintenance of tissue homeostasis and the pathogenesis of immune diseases and AITDs were known for dysregulation of apoptotic signaling pathways (58). A study reported that excess iodide could induced apoptosis through TNF-related apoptosis-inducing ligand and death receptor expression (59), Iodinization increased TPOAb and TGAb, which lead to increased apoptosis via the Fas-mediated pathway (60). B cell-human lymphoblastoid cell lines derived exosomes enriched with Fas Lignad (FasL), which lead to activation of apoptosis in CD4+ T cells by Fas/FasL interaction, so exosomes can be a could be associated with cell apoptosis in Hashimoto’s thyroiditis (61). Immuno-modulatory actions and immune responses of cells are fine-tuned by exosomal surface protein markers like HSP60 and HSP70, which binds to various receptors like TLR, CD40, and Cd9 of the immune cells (62). However, specific mechanism of trans-communications and molecular switch at receptor level yet to be unraveled.
Graves’ disease is the most common cause of hyperthyroidism with manifestation of thyrotoxicosis and Graves’ ophthalmopathy and highest incidence in 30-50 aged women (63). An interesting studies by Rossi and others found that Dichlorodiphenlytrichloroetahns (DDT) stimulated thyroid follicular cells have active role in exosome mediated transferring the thyrotropin releasing hormones (TSHR) which involved in production of autoantibodies to the TSHR leading to Graves’ diseases (64). As mentioned before epigenetic modification (DNA methylation, histone modifications and noncoding RNAs(ncRNAs) interference) also plays a main role in pathogenesis of Graves’ diseases (65, 66), recent studies revealed that nucleic acids present in exosomes retain their pathological function in a recipient cell after direct transfer exosomal cargoes (67) and different stages of Graves’ diseases patients showed a different noncoding RNAs in exosome compared to healthy (68). Till now, exosomes related AITDs are still in sporadic stage. Till now, understanding of exosomes related AITDs are still in its infancy. Consequently, further research is required to elucidate the snapshot mechanism of exosomes in AITDS thereby delivers the deep understanding of AITDs, diagnosis and prognosis.
Drug induced liver injury (DILI) is common and almost all types of medicines. Recovery from DILI may require discontinuation medicines, hospitalization, or even liver transplantation (69, 70). Exosome are an attractive therapeutics and drug delivery vehicle, recently the hepatocyte and MSCs derived exosomes/EVs have revealed that they are effective in hepatic regeneration in liver injury models (71–73). Hepatocyte exosomes shown to have ceramidase and sphingosine kinase 2 (SK2) in their compartment, increased sphingosine-1-phosphate (S1P) in hepatocyte, which promotes proliferation hepatocytes (71). The MSC-EVs enriched in glutathione peroxidase 1 (GPX1) and knockdown of GPX1 reduced the protective effects of MSC-EVs in DILI model (74, 75). However, more preclinical experiments are still needed to understand roles of exosomes in DILI which can accelerate successful clinical translation of exosome therapy in DILI.
Gut cells are regularly exposed to foreign antigens, such as food molecules and bacteria. Partially digested and undigested food materials putrefy in the intestine through bacterial action, releasing several secondary metabolites of bacterial origin and further challenging the intestinal immune system. Inducing immune tolerance towards harmless food and bacterial antigens is essential to sustain progressive health devoid of routine inflammatory reactions. Defective immune tolerance can lead to inflammatory and autoimmune diseases.
During infection, foreign antigens are presented to T cells by antigen-presenting cells. The dendritic cells play a pivotal role in controlling the effector and regulatory mechanisms of the immune response. A subset of dendritic cells called tolerogenic dendritic cells binds to T cells and suppresses the immune response against harmless food antigens or self-antigens, thus inducing immune tolerance (76). The presentation of antigens by tolerogenic dendritic cells results in the activation and proliferation of regulatory T cells (Treg), consequently leading to immune tolerance against a specific antigen (77). Furthermore, these semi-mature dendritic cells also induce clonal deletion of T cells and cause T cell anergy, resulting in an immunosuppression-dependent peripheral tolerance towards harmless antigens of the gut. Tregs execute their immunosuppressive functions by secreting cytokines (IL-10 and TGF-β) (Figure 3). Besides tolerogenic dendritic cells and Tregs, exosomes also play a vital role in immune response regulation. Exosomes secreted from intestinal epithelial cells (IECs) play a critical role in intestinal immune homeostasis regulation. IECs divert dendritic cells towards the immune tolerance pathway through TGF-β, retinoic acid, and thymic stromal lymphopoietin (IL-7 family). Exosomes secreted by IECs contain immunoregulatory molecules. During an infection, exosomes loaded with peptide-MHC II are secreted by IECs, taken up by antigen-presenting cells, and induce efficient T-cell activation. This would eventually lead to Th1- and Th17-mediated clearing of the foreign antigen/pathogen. IECs exposed to harmless antigens have an entirely different activation pathway that ensures the suppression of inflammation against harmless antigens. In vitro cell culture experiments simulating digestion using cells exposed to ovalbumin and digestive enzymes indicated that IECs have an increase in integrin αvβ6 expression with a corresponding increase in integrin αvβ6 in exosomes when exposed to harmless antigens. The uptake of integrin-loaded exosomes by dendritic cells increases their TGF-β expression and transformation into tolerogenic dendritic cells. This, along with T regulatory cell activation, results in the suppression of the immune response against harmless antigens (78). Additionally, Tregs secrete exosomes that elicit immunosuppressive activity (Figure 3) through the transfer of miRNA Let-7d to Th1 cells (79).
Figure 3 Roles of exosomes in inducing immune tolerance. TGF-b, Transforming growth factor beta; DC, Dendritic cell; IL-10, Interleukin 10; Treg, Regulatory T cells; Th1, T helper type 1; and miRNA, micro Ribonucleic Acid. Created with BioRender.com.
IECs secrete immunomodulatory exosomes, which have increased expression of MHC class II and Fas ligands, into the mesenteric lymph after trauma/hemorrhagic shock. This study also demonstrated that exosomes released after trauma/hemorrhagic shock significantly suppressed lipopolysaccharide-mediated CD80 and CD86 expression on dendritic cells and decreased their antigen-presenting capacity to induce lymphocyte proliferation (80). Exosomes secreted by the intestine after intestinal ischemia/reperfusion activate microglia, increasing the neuronal apoptotic rate and decreasing synaptic stability, thus leading to memory impairment (81). Exosomes produced by gut tropic T-cells regulate T-cell homing to the gut. These exosomes upregulate integrin α4β7 binding to mucosal address in cell adhesion molecule 1 (MAdCAM-1) expressed on endothelial venules in the gut, suppressing MAdCAM-1 expression in the small intestine, thereby inhibiting T cell homing to the gut (82). Human breast milk produces exosomes that decrease inflammation caused by necrotizing enterocolitis (83). Exosomes engage in activating the neuronal cells when intestinal cells are treated and activated with GABA (Gamma-aminobutyric acid). MicroRNAs in exosomes are responsible for this activation (84). These data collectively indicate a crucial role played by exosomes in the gut and gut-brain axis.
Exosomes produced by various cells are also involved in healing various gut-related diseases, such as inflammatory bowel disease (IBD), colorectal cancer, and intestinal barrier dysfunction. Proteasome subunit alpha type 7 was abnormally expressed in salivary exosomes of IBD patients (85). Moreover, exosomes extracted from the saliva of IBD patients contained approximately 2000 proteins, which were significantly altered compared with that in healthy individuals. Further research will reveal the importance of this variation in IBD pathology. Exosomes produced from Curcuma longa have the potential to inactivate the nuclear NF-κB pathway to ameliorate colitis and promote intestinal wound repair, which in turn alleviates IBD (86). Exosome-like nanoparticles obtained from grapes help induce intestinal stem cells and protect against dextran sulfate sodium (DSS)-induced colitis (87). Another study reported that oxaliplatin resistance in colorectal cancer was reduced with exosomes delivering miR-128-3p, consequently increasing the chemosensitivity of these cells (88). Mesenchymal stem cell (MSC)-derived exosomal miR-34a/c-5p and miR-29b-3p reportedly improve intestinal damage by targeting the Snail/Claudin signaling pathway. These exosomes increase the expression of Claudin-3, Claudin-2, and ZO-1 (89) (Table 2).
Asthma is a non-contagious disease that can develop owing to allergies. It is characterized by bronchial hyperresponsiveness, mucosal edema, and airflow restriction (94). Asthma progression requires interaction between resident and inflammatory cells (95). Exosomes released from eosinophils and innate immune cells are critical in regulating and enhancing asthma pathophysiology. Pathological changes in asthma are caused by the activation of structural lung cells and airway remodeling, which contain enzymes such as eosinophil cationic protein, eosinophil peroxidase, or major basic proteins (96) that cause epithelial damage (a hallmark of asthma). Apart from this, the exosomes also contain molecules such as nitric oxide (NO), lipid mediators, and ROS, which are responsible for inflammation (97). Previous reports confirm (97, 98) the abundance of exosomes containing these enzymes in individuals with asthma than in healthy individuals.
T lymphocytes, key players in the inflammatory response in asthma, are also known to produce exosomes. The exosomes produced by T cells activate and degranulate mast cells and release cytokines, leading to tissue remodeling and airway hyper-responsiveness (99). Similarly, exosomes released by B lymphocytes transport specific molecules of antigen-presenting cells, enabling these exosomes to present the antigen to induce T-cell responses and release IL-5 and IL-13 cytokines (100).
The expression of several proteins involved in allergic responses increases owing to the transfer of exosomes from airway epithelial cells to human tracheobronchial cells, which causes aggravation of asthmatic symptoms. Extracellular vesicles aid in disease progression by promoting dendritic cell maturation and increasing T helper cell 2 (Th2) proliferation (101). MSC-derived exosomes have disease-alleviating effects, such as decreased production of Type-2 innate lymphoid cells, Th2 cytokines, and mucus. In contrast, exosomes derived from bronchoalveolar lavage fluid inhibit specific immunoglobulins such as IgE and IgG1 (102).
Another study (103) suggested that asthma induction enhanced the levels of CD63 and acetylcholine esterase activity (exosome-associated enzyme), indicating an increase in exosome biogenesis and its secretory pathways. In asthmatic tissue samples, IL-4 levels were increased, triggering the central pro-inflammatory responses that regulate eosinophil transendothelial migration and IgE production. This can further increase mucus secretion and also accelerate Th2 differentiation. In contrast, IL-10 levels were decreased, which inhibited Treg activity (104). TNF-α, a pro-inflammatory cytokine, was also increased in the tissue samples. This correlates with an increase in exosome secretion and its participation in inflammatory pathways that function in asthma (100).
Arthritis is the inflammation of the joints caused mainly by immune system dysfunction. It is an umbrella term for several diseases, such as rheumatoid arthritis and osteoarthritis. Osteoarthritis is a degenerative disease that damages the synovial joints of the hands, feet, knees, and hips, which in many instances, are disabled (105–108). The different tissues around the joints have various effects on the pathology of osteoarthritis, especially inflammatory cytokines from the synovial fibroblasts of inflammatory cells. IL-1β, a pro-inflammatory mediator, and bone-regulatory factors, including BMP-2 (bone morphogenetic protein-2) (67), promote articular cartilage damage and hasten osteoarthritis development by facilitating the release of various proteolytic enzymes (109).
Bone homeostasis is balanced by resorption and formation, and exosomes produced by osteoblasts regulate this process. These exosomes contain osteogenic signals, such as molecules of the eukaryotic initiation factor-2 pathway, which help bone formation (110). Exosomes produced from specialized cells, such as mineralized osteoblasts, promote the differentiation of bone marrow stromal cells into osteoblasts (111). MSC-derived exosomes also help maintain bone homeostasis by preventing cells from undergoing apoptosis during stress conditions, such as hypoxia and serum starvation (112). They also assist in healing fractures via the sprout-related EVH1 domain containing 1 (SPRED1)/Ras/Erk signaling pathway (113, 114).
IL-1β treatment reduced the expression of the anti-inflammatory gene TGF-β. Notably, exposure to exosomes derived from bone MSCs recovered TGFβ expression. This phenomenon was again confirmed by the observation that abnormally high expression of several pro-inflammatory genes, such as NF-κB and TNF-α, can be reduced by co-culture with exosomes (115). By exploiting this function of exosomes, their clinical applications for the treatment of bone disorders are being explored. As the cargo in the exosome is highly dependent on the release of host cells, changes, if any, in the tissue microenvironment are reflected in the exosomal cargo.
Psoriasis is a non-contagious, immune-mediated disease that causes scaly, raised patches on the skin owing to systemic inflammation. The immunopathology of psoriasis is characterized by an increase in the CD4+ and CD8+ T cells, neutrophils, natural killer cells, mast cells, and macrophages (116–118). Discoveries in this field have shed light on the increased numbers of IL-17-secreting T cells and elevated levels of the Th17-polarizing cytokine IL-23 in psoriatic lesions (119). Furthermore, small extracellular vesicles released from psoriatic keratinocytes transfer miR-381-3p to CD4+ T cells, which induces the polarization of Th1 and Th17 cells and eventually contributes to psoriasis development (120). Patients with generalized pustular psoriasis have a higher neutrophil-to-lymphocyte ratio than in healthy individuals. These neutrophils consequently induce a higher expression of inflammatory genes, including IL-1β, IL-18, and TNF-α. This occurs via the increased secretion of exosomes from neutrophils, which are then rapidly internalized by keratinocytes, thus increasing the expression of these inflammatory molecules via activating the NF-κB and MAPK signaling pathways (121).
Langerhans cells, prominently present in the epidermal layer of the skin, play a pivotal role in the pathogenesis of psoriasis. Unlike MHC receptors that present only peptides, the CD1a receptor of Langerhans cells presents a broad spectrum of lipid antigens to T-cells, which amplify inflammation in psoriasis mouse models (122). The T-cell-mediated inflammatory response in the skin is mediated by increased phospholipase A2 activity, and phospholipase A2 levels were reportedly high in patients with psoriasis. IFN-α increases the release of exosomes from mast cells. T cells associated with psoriasis identify exosomes carrying lipid antigens/phospholipases originating from mast cells. This can lead to increased IL-22 and IL-17A production by CD1a-autoreactive T cells (123). Neutrophils (NETs) released during NETosis are a major source of increased IL-17 levels in psoriasis (124, 125). TNF-α, IFN-γ, IL-2, IL-6, IL-8, IL-18, and IL-22 levels were higher in patients with psoriasis than in healthy individuals. However, this was not the case for IL-1β, IL-4, IL-10, IL-12, IL-17A, IL-21, and IL-23 (126). Another prominent feature of patients with psoriasis is that the levels of iron, hepcidin, and total iron-binding capacity of the exosomes were significantly lower. In contrast, the soluble transferrin receptor and heme oxygenase-1 levels were significantly overexpressed (127).
Luteolin could be used in treating psoriasis as it heals skin lesions and alleviates psoriatic symptoms by reducing the effects of IFN-γ, inhibits the expression and exosome secretion of HSP90, and modulates the proportion of T-cells in the plasma (128). Furthermore, topical application of MSC exosomes, known for their immunomodulatory properties, reduced IL-17 and terminal complement activation complex C5b-9 (129). Another study used MSC-derived exosomes to alleviate psoriasis-like skin inflammation via the IL-23/IL-17 axis. Exposure to exosomes reduces the levels of STAT3/p-STAT3, IL-17, IL-23, and CCL20, suggesting that exosomes are a potential therapeutic candidate (130).
Type-1 diabetes mellitus is an autoimmune disease characterized by the selective killing of pancreatic beta cells by the selective infiltration of T lymphocytes. Lymphocytes release exosomes containing miRNAs, which are involved in the differentiation and maturation of beta cells and regulate insulin release and survival. Exosomes released from pancreatic cells could be associated with cytokine stimulation and recreation, as exosomes contain diabetic autoantigens (92, 131). Exosomes released by adipose tissue can regulate gene expression, thus modulating interorgan communication (132). The various miRNAs in exosomes associated with diabetes mellitus include miR-142-3p, miR-142-5p, and miR-155 from lymphocytes that can cause selective death of insulin-secreting beta cells (133–135). Microarray evaluation revealed 25 miRNAs dysregulated in patients with diabetes, including miR-326, miR-186, miR-21, miR-126, miR-16, let-7a, let-7f, and let-7g, compared with that in the control. A correlation was seen connecting HbA1c, miR-326, and let-7a between plasma glucose levels and let-7f (132, 136). Individuals affected by type2 diabetes exhibit altered miR-20b-5p expression, which eventually leads to alterations in protein kinase-B (AKT) and glycogen synthase kinase (GSK), the main components of the insulin signaling pathway (137). Individuals with type 1 diabetes contained decreased miR-16-5p, miRNA-302d-3p, and miR-574-5p expressions, and increased miR-25-3p expression (138).
However, studies have reported that it does not affect the function of pancreatic glucagon-producing α-cells. The intracellular β-cell autoantigens in type 1 diabetes mellitus, namely, GAD65, IA-2, and proinsulin present in exosomes, are taken up by dendritic cells and, consequently, activated. Thus, the exosomal release of intracellular autoantigens and immunostimulatory chaperones induced by stress may initiate autoimmune responses in type1 diabetes mellitus (139). Pancreatic β-cells transfer secretory vesicles to phagocytic cells for presentation to T cells. The criterion for transfer requires the positioning of beta cells in close interaction with phagocytes under low-glucose conditions. This transfer is promoted by increased glucose concentration, as it increases cytosolic Ca2+ levels. Secretory vesicles from the pancreas transfer two sets of vesicles, one with insulin and another containing its catabolites (140), eventually facilitating their access to the immune system. Exosomes and exosome-like vesicles are used to diagnose and treat insulin resistance in type2 diabetes mellitus (141). Exosome-loaded immunomodulatory biomaterials have been used to alleviate the immune response in immunocompetent diabetic mice after islet xenotransplantation (141, 142). However, the comprehensive characterization of exosome-like vesicles is challenging, and their effects are not specific, which can lead to undesired consequences (141).
For end-stage organ diseases, transplantation becomes the only therapeutic option left with patients (143). Regardless of advancement in the field of medicine and surgery, substantial portion of patients continue to suffer various post-transplant complications and rejection of transplants. In recent years, EVs (including exosomes) have fascinated boundless attention of the scientist and surgeons as exosomes becoming a potential tool for understanding the transplanted organ physiology and diseases. Numerous studies have shown that exosomes released by various antigen presenting cells, which involves in humoral and cellular immune system (144).
The transplanted organ releases exosomes (allograft-exo) with donor major histocompatibility complex (MHC) molecules and they go out from the graft to the recipient’s lymphoid system (145, 146). These allograft-exo are internalized by recipient antigen-presenting cells (APCs), which leads to presence of donor MHC molecules on recipient APCs’ surface. This is called as semi-direct pathways in T cell-mediated rejection without direct contact between donor cells with recipient APCs (146–148). In a skin graft mice model, the donor MHC molecules are transported by EVs to the host’s lymphoid organs, then dendritic cells/B cells take up this donor MHC molecules (MHC-I and MHC-II) and presented to T cells, which leads to a direct inflammatory alloresponse in mice (146, 149). In case of solid organ transplantation, for example the heart transplant, recipient’s blood vessels are connected to the transplant during surgery. This leading to donor passenger leukocytes from graft may move to recipient, reported that donor dendritic cells was present with in the spleen of allogeneic heart transplanted mice (150), this was further reported in other studies that allo-MHC cross-dressed cells in lymphoid organs in vivo after cardiac transplantation (151, 152), allograft-exo may have been involved as well. Some studies show that allogeneic exosomes under certain circumstances involved in tolerance rather than rejection of allografts (153, 154). However, exosome involvement in organ transplantation still has not been elucidated and extensively studies are needed.
Inborn or innate immunity is inherited immunity consists of cellular and hormonal arms. The intercellular communication during the human genesis is first drafted by cell “language” communication system which is mediated by exosomes to maintain the rigidity of the cell. The effective immune response to foreign materials, like pathogen associated molecular patterns (PAMPS), danger associate molecular pattern (DAMPS), or life style associated molecular pattern (LAMPS), is controlled by exosome communication language. PAMPS and DAMPS are structurally diverse external molecules from pathogenic bacteria which includes, lipids, proteins, carbohydrates and material by products. Mega receptors in defense cell like leukocytes, first will have defense mechanism during the bacteria, virus, parasites and fungi invasion. Cellular recognition patterns such as Toll like receptors (TLRs) are expressed in various immune cells like leukocytes, macrophages, dendritic cells, B cells, T cells, and even non-immune cells like fibroblasts and epithelial cells (155). TLR first binds to PAMPS, and DAMPS thereby ignites the cell signaling cascades as combat mechanism. Under sterile inflammation condition, parenchymal and stromal cells communicate via extracellular vesicles through innate system. For example, during noxious gas inhalation, in lung, epithelial cell produces more exosomes with excess loading of microRNA17/221 (miRNA17/221) thereby promoting the local inflammation through the recruitment of M0 macrophage (156). Type 1 transmembrane protein, TLR has two domains like leucine-rich repeats (LRR) motif and Toll/LI-1R (TRR) as cytoplasmic domain in all immune cells and having different sub types like TLR-1, 2, 4, 5, 6 as cell surface members and TLR-3, 7, 8 and 9 as intracellular components (157). Among all these TLR-2 recognizes pathogens metabolites like lipoproteins/lipopeptides, peptidoglycan, glycosyphosphatidylinositol, phenol soluble modulin, zymosan and glycolipids (158). Double stranded RNA (dsRNA) of viruses will be recognized by TLR-3, while TLR-7 perceive GU rich single stranded RNA (ssRNA) but TLR-5 spots the bacterial flagellin (159–161). In tumor development and subsequent metastatic microenvironment, series of coordinated events are taking place between exosomes and TLR, thereby it plays an important role in the cancer development process. In cancer microenvironment scenario, TLR-8 plays important role by transmitting ECmiRNAs like miRNA 21 and miRNA 29a because of highest binding capacity with TLR-8 receptors thereby activates the receptors in immune cells and subsequently leads to the premetastatic inflammatory cytokines (162).
Despite the evidence that exosomes do play important role in the intercellular communications, the production and transport rely on the donor cells physiological conditions and receptor activations of receiver cells. TLR is known to be an importance factor in acute kidney diseases like urinary tract infections, ischemia-reperfusion (IR) injury, lupus nephritis and diabetic nephropathy (163). Micromanagement of exosomes in renal physiology have been studied both in vitro and in vivo condition. Miyazawa et al. first reported the role of exosome mediated aquaporin 1 and 2 (AQP-1, AQP-2) transport in collecting duct cells (164). Lipopolysaccharide (LPA) induced renal tubular epithelial cells showed the elevated level of exosome miRNA 19b-3p thereby induce the macrophage dependent tubulointerstitial inflammation (165). Extensive investigation on exosome mediated urolithiasis (kidney stone disease) remains under investigated, indeed, decreased the level of miRNA-21and Let-7 was noticed in lupus nephritis patients (166). However, genetic manipulations of specific transcriptomes aided with exosome engineering would shed better understanding of kidney stone disease (167).
Potential role of exosomes in immunomodulation disorder in blood cancer or the induction of hematological diseases in polycythemia vera (PV), essential thrombocythemia (ET) myelofibrosis (MF) (168). Chronic Myeloid leukemia (CML) cells (K562) derived exosomes promoted the angiogenesis, which was inhibited by dasatinib through inhibition of exosome release from K562 CML cells and their microenvironments (169). This in vitro study strongly supports hypothesis that microenvironment driven by K562 CML cells derived exosomes governs the clinical manifestation.
These exosomes also contains angiogeneic miRNAs such as mir-92 which helps in tumor cell migration during hypoxic condition (170). Disturbed HIF-1 pathways with upstream and downstream associated gene regulations by miR-1555, mir210, and mir135b also plays important role in migration of the CML cells by suppress or activation of factor inhibiting hypoxia inducible factor 1 (FIH-1). Taken altogether, cumulative factors such as EVs, growth factors cytokines and miRNAs play a fascinating role in the leukemogenesis, and cross-talks between various cell populations via each of the indicated components promote the formation of different types of hematological malignancies, including acute myeloid leukemia (AML), acute lymphoblastic leukemia (ALL), chronic lymphocytic leukemia (CLL), chronic myeloid leukemia (CML), lymphoma, and multiple myeloma (MM) (171).
The body uses autophagy to eliminate unhealthy cells so that it can replace them with new, healthier ones. In normal conditions, long-lived proteins and old organelles were degraded by autophagy for restoration of cellular contents. But under stressful conditions, for example hypoxic (lower oxygen) conditions, autophagy is activated to recycle molecules for providing energy and nutrients (172–174). Studies on exosomes have enlightened our understanding of their biological functions and recently several studies have shown that cancer exosomes play a great role in tumor progression and metastasis (175, 176). The studies have reported that knockdown of both autophagy related 16 Like 1 and autophagy related 5 in breast cancer cells shown to produce and release lesser exosome, this leads to decrease tumor metastasis (177, 178). Studies on G alpha interacting protein (GAIP) and GAIP interacting protein C-terminus have been shown involves in stimulation of biogenesis of exosome and autophagy flux in pancreatic tumor cells (179, 180). Inhibition of autophagy related 12–autophagy related 3, a complex plays important role in late step of autophagosome formation, reduce the exosome biogenesis by disrupting late endosome trafficking in multivesicular body (MVB) through interacting with Alix (172, 181). Several studies have well documented that autophagy, exosome plays important role in tumor progression (172, 182, 183). Autophagy and exosome release are robustly activated in tumors, which strongly showing that both these pathways are play a interplay in between and they are as part of hallmark of cancer cells.
Recently the scientists are focusing on inhibition of tumors progression and metastasis by targeting the autophagy and exosome biogenesis. A study reported that sulfisoxazole targeting the endothelin receptor A, which lead to inhibition of exosome biogenesis through increased degradation of MVB via the autophagy–lysosome pathway (184). A Study suggested that an inhibitor of ULKs (a serine/threonine protein kinase) leads to accumulation of immature early autophagosomal structures (185); ULK1 are involved in the trafficking of autophagy related 9, which is main player in intraluminal vesicle formation within amphisomes and autolysosomes (186, 187). Likewise other several inhibitors have been studied to inhibit biogenesis of exosomes (185, 188). Still several preclinical and clinical studies are required to understand the role of cancer exosome and autophagy and their targeting in cancer treatments.
Exosomes are released by cells, and their components provide a brief story about the microenvironment in and around the cell. These could be signals, exosomes presenting antigens, or even cancer-promoting factors. This property has previously been exploited for diagnostic purposes. The increased and highly stable exosome expression in patients with cancer renders it an up-and-coming player for cancer diagnosis. Various groups have used exosomes present in the plasma for diagnostic purposes. Different immunological markers of exosomes have been characterized in patients with chronic lung allograft dysfunction. Lung transplant recipients who displayed both the phenotypes of obstructive bronchiolitis obliterans syndrome and restrictive allograft syndrome had exosomes with distinct molecular and immunological profiles. Upon further testing, restrictive allograft syndrome samples were observed to have a higher concentration of pro-inflammatory factors, indicating severe allograft injury (189). Exosomes have emerged as prominent biomarkers for cancer diagnoses. Another group investigated the prominence of plasma-derived exosomal miR-19b in the diagnosis of pancreatic cancer. The results suggested that the levels of Exo-miR-19b normalized using miR-1228 were comparatively lower in patients with pancreatic cancer than in healthy individuals. This indicates that exosomes are promising candidates as biomarkers (190). This property of exosomes is utilized to diagnose various types of cancers, such as non-small cell lung cancer (NSCLC), prostate cancer, osteosarcoma, and cervical cancer. Lower expression of circulating miR-651 was observed in patients with cancer than in healthy individuals. Moreover, exosomes collected from HeLa cells were rich in CD63, CD9, and CD81 proteins, which are cancer cell hallmarks (191).
Long non-coding RNA (lncRNA) expression in urinary exosomes of NSCLC patients and healthy individuals as a potential lung cancer diagnosis has been explored. The results indicated that differential lncRNAs in urinary exosomes are NSCLC biomarkers because lncRNAs are enriched in specific pathways that might be involved in tumor cell proliferation and other processes associated with NSCLC pathogenesis (192). Liquid biopsy of exosomes isolated from patients with prostate cancer revealed that exosomes are enriched for genes that are hallmarks of prostate cancer, such as androgen receptor, kallikreins (KLK2), cyclin-dependent kinase inhibitor 1A (CDKN1A), KLK10, JUN, and B2M (β2 microglobulin). These observations indicate that exosomes transport critical disease RNA transcripts and can be used as non-invasive diagnostic biomarkers (193). Exosomes of patients with prostate cancer had higher amounts of survivin, an apoptosis inhibitor, which is another biomarker for early detection of prostate cancer (194). These examples suggest that this is an untapped niche for early cancer diagnosis and has potential.
One of the first examples of exosome biodistribution in the body by in vivo positron emission tomography (PET) for non-invasive monitoring of copper-64 (64Cu)-radiolabeled polyethylene glycol (PEG)-modified exosomes was demonstrated with high imaging quality and quantitative measurements of blood residence and tumor retention. A fluorescent dye, amine-reactive Alexa Fluor 488 (NHS-Alexa Fluor 488), was conjugated to exosomes to form Alexa Fluor 488−exosome and Alexa Fluor 488−exosome−PEG. PEGylation confers an enhanced pharmacokinetic profile and higher tumor accumulation in exosomes compared with that of native exosomes. However, it also reduces premature hepatic sequestration and clearance of exosomes, thereby highlighting their improved therapeutic delivery efficacy and safety (195). Notably, this study provides crucial guidelines for obtaining precise and quantitative information on the biodistribution of exosomes via surface engineering, radiochemistry, and molecular imaging, which may aid future exosome research.
A technique was devised for in vivo neuroimaging and exosome tracking using gold nanoparticle labeling (196). Exosomes may be directly labeled with glucose-coated nanoparticles (GNP) without the need to label parent cells, and this labeling occurs through an active mechanism linked to the GLUT-1 (glucose transporter-1). Intranasal delivery is more effective than intravenous injection for brain accumulation. The noninvasive intranasal delivery route has multiple benefits and is a potential and effective treatment option for various CNS disorders. In contrast to the non-lesioned brain, the detection and accumulation of MSC-derived, GNP-labeled exosome labeling in the stroke region of the brain up to 24 h after administration was observed. Multifunctional exosomes for cancer theranostics were developed by electroporating urinary exosomes and ultra-small gold nanoparticles with Ce6 (chlorin e6) to obtain real-time fluorescence imaging and improve photodynamic therapy (197). The nanocomposites cloaked with exosomes exhibited enhanced long-term retention, biocompatibility, and penetrating behavior than that of free Ce6. A comprehensive study of the theranostic application of exosomes in cancer has been described by Ailuno et al. (198).
Circulating tumor exosomes with specific biomolecules have been recently used for detecting cancers and assessing therapy response and they are becoming more common as diagnostic targets (199–201). In recent times an ExoChip (with an anti-CD63 antibody), microfluidics device constructed to capture and collect specific exosomes, it showed that exosomes were significantly higher from individuals with cancerous diseases than healthy controls (202). Liquid biopsy with exosomal IGF-1R expression is used in individuals with lung cancers instead of invasive traditional tissue biopsies, which was achieved by microfluidics exosome analysis platform (203). A microfluidics chip is used for evaluation of circulating EpCAM-positive exosomes with plasma exosomes. EpCAM-positive exosomes was higher in individuals with breast cancer than healthy individuals (204). These studies and several other studies suggest that microfluidics-based exosome isolation and detection methods are more reliable (205) but still further discoveries are needed for its routine clinical translation.
The role of exosomes for therapeutic purposes requires further elucidation; nonetheless, their potential has been well-established. Exosomes did not exhibit toxicity upon injection, and nano-sized membrane-bound vesicles were well tolerated by the body. These properties make them excellent candidates for contact delivery because they do not undergo degradation. Both targeted and non-targeted exosome deliveries successfully alter protein expression in cancer cells (206).
There are several methods for isolating and purifying exosomes. Based on yield and purity, acoustic nanofilter (207), ExoSearch chip (208), immunoprecipitation (209), density gradient (210), precipitation kits, and ultracentrifugation methods are in use. A recent study demonstrated a separation method based on acoustofluidics by integrating acoustics and microfluidics approaches to isolate exosomes from blood directly [140]. This approach combines cell removal with exosome isolation. By integrating these modules into a single chip, exosomes were isolated with a blood cell removal rate of over 99.999% (211). Another study described a novel approach using microfluidic devices to isolate exosomes from whole blood, directly facilitating translation to the clinic (212). The choice of the device has a significant impact on the yield and purity of exosomes in the case of cell lines and complex biological fluids. The isolation of exosomes can be validated using nanosight tracking analysis, transmission electron microscopy, or western blotting. The revolutionized development of different approaches for exosome isolation and purification has made procedures more feasible for early diagnosis and therapeutics.
The off-target binding of drugs leads to several adverse effects (213). Several research groups are investigating targeted drug delivery to obtain high efficacy and low toxicity. Exosomes play a significant role in the targeted delivery of drugs and drug-like molecules. Similarly, paclitaxel (PTX)-loaded AS1411-chol exosomes (AS1411: a nucleolin-targeting aptamer) were delivered to target cancer cells with high efficiency in a recent study (214). Surface engineering, genetic engineering, chemical modification, and membrane fusion are some approaches adopted for the targeted delivery of exosomes (12). By using targeted surface engineering delivery and increasing exosome concentration at disease sites, this approach can reduce toxicity and increase therapeutic efficacy (215). In genetic engineering, ligands or homing peptides can be fused with TMP (5,10,15,20-tetrakis (1-methyl-4-pyridinio)), which is expressed on the surface of exosomes (216). In chemical modification, any functional group can be modified by another; for example, alkynes can be modified by an amine group. The exosomal lipid bilayer membrane can spontaneously fuse with other membranes using a membrane fusion approach.
Targeting exosomes to deliver chemotherapeutics, such as doxorubicin, to breast cancer tumor tissues in mice has been evaluated. The results were substantial as the treatment caused enhanced and rapid tumor regression without toxicity than that with standard therapy (217). Another study successfully used exosomes as a delivery vector to transport PLK-1 siRNA to bladder cancer cells in vitro, resulting in selective gene silencing of PLK-1 (218). ACTN4 is highly expressed in exosomes from patients with castration-resistant prostate cancer (CRPC). RNA interference-mediated ACTN4 downregulation significantly attenuates cell proliferation and tumor invasion, thereby confirming its role in prostate cancer development (219). Exosomes produced by adipose-derived stromal cells (ASCs) can also be used in prostate cancer therapy. ASC-derived exosomal miR-145 could reduce Bcl-xL activity and promote prostate cancer cell apoptosis via the caspase-3/7 pathway (220).
Phosphatidylserine (PS) expressed on the surface of exosomes is linked to T-cell immune suppression. Recently, a novel PS-binding molecule was developed that successfully blocked the immunosuppressive activity of human ovarian tumors and melanoma-associated exosomes. Upon treatment with ExoBlock, T-cell-mediated tumor suppression was significantly enhanced along with an increase in the number and function of CD4 and CD8 T-cells, which are involved in reducing tumor metastasis, thus providing promising antitumor therapy (221). Exosomes isolated from human umbilical cord MSCs were transfected with miR-3182. Exosomal miR-3182 significantly reduced cell migration and proliferation in triple-negative breast cancer (TNBC) cells. We also observed that miR-3182 loaded exosomes induced apoptosis in TNBC cells by downregulating mTOR and S6KB1 expression. Thus, this treatment can reduce the invasiveness of TNBC cells (222). Exosome therapy has also been used to treat other diseases.
In psoriasis, phospholipases on exosomes are associated with disease progression. Inhibition of phospholipases suppresses psoriasis progression (223). Inflammatory cytokines in the IL-23/Th17 axis and TNF-α signaling can be targeted. However, these can cause toxic side effects over an extended period (224). Alternatively, exosomes derived from MSCs have been used as potential treatments. This was tested in a mouse skin psoriasis model. The levels of pathology-associated IL-17, IL-23, terminal complement complex, and C5b-9 complex were reduced in the skin of mice treated with exosomes relative to that in the control (129).
Exosomes derived from adipose-derived MSCs (ADSCs) have therapeutic applications in hepatic I/R injury. ADSC-derived exosomes improved liver function by maintaining mitochondrial homeostasis through mitochondrial fission inhibition and promoting mitochondrial fusion and biogenesis. This may be attributed to the exosome-induced increase in the expression of mitochondrial fusion proteins such as OPA-1, MFN-1, and MFN-2. In contrast, they decreased DRP-1 and Fis-1 mRNA and protein expression related to mitochondrial fission. Additionally, exosomes significantly increase the expression of PGC-1α, NRF-1, and TFAM genes and proteins related to mitochondrial biogenesis (225). Another study in hindlimb ischemic animal models reported that MSC-derived extracellular vesicles activated VEGF receptors in endothelial cells, increased neovascularization at the site of ischemia, and accelerated recovery (226). In an associated study, extracellular vesicles derived from RAW 264.7, macrophages could also induce angiogenesis in vitro and in vivo (227). Exosomes derived from MSCs can target endogenous MSCs to enhance osteogenic differentiation and reduce adipogenic differentiation, which is a promising therapy for osteoporosis (228). Other possible treatment methods could be effective in initiating bone repair, regulating the immune response, and preventing bone resorption. Similarly, MSC-derived exosomes have also been used to treat inflammatory disorders, such as neurological disorders. This is accomplished using mRNAs, miRNAs, and immunosuppressive factors sourced from MSCs (229). Exosomes produced by MSCs stimulated by IFNγ reduced demyelination, decreased neuroinflammation, and upregulated the number of CD4, CD25, FOXP3, and regulatory T cells within the spinal cord. Moreover, exosomes reduce the levels of pro-inflammatory Th1 and Th17 cytokines; hence, exosomes can also serve as therapeutic targets for neurodegenerative disorders (230). Another promising study in this direction is from the Shetty group, which demonstrated that extracellular vesicles isolated from induced pluripotent stem cells effectively induce neurogenesis and treat neurodegenerative disorders in animal models (231). The hallmark of patients with pulmonary embolism is that pulmonary epithelial cells (PECs) are resistant to apoptosis. Mao et al. used exosomal miR-28-3p, which upregulated miR-28-3p expression and increased apoptosis by targeting apoptosis inhibitor 5 (API5) in PECs (229).
Milk-derived exosomes (mExosomes) are one of the most economical and promising drug delivery systems. Milk, an easily accessible raw material, can be prepared in substantially large amounts. Uniform particle size of ~100 nm, unique phospholipid layer (232), low immunogenicity, and inflammatory response (233, 234) make mExosomes an attractive research topic and an ideal vehicle for drug delivery in the future. The anticancer properties of drug-encapsulated mExosomes demonstrated in vitro growth inhibitory action in breast (T47D and MDA-MB-231) and human lung (A549 and H 1299) cancer cell lines (235). Animal studies on anti-tumor activity in mice bearing xenografts of A549 lung tumors exhibited low cytotoxicity and high bioavailability of mExosomes in the organs of the experimental model. A similar study reported that curcumin-loaded mExosomes exhibit enhanced anti-inflammatory and antitumor activities. This study also revealed that curcumin-loaded mExosomes have higher bioavailability than free curcumin (236).
RNA-based therapy has recently gained attention, but the challenge involves the delivery of highly unstable RNA molecules to biological systems. Gene expression studies have demonstrated the compatibility of mExosomes in delivering miR-148a-3p to hepatic (HepG2) and intestinal (Caco-2) cell lines (236), suggesting that mExosomes can be utilized as carriers of functional microRNAs. In a recent study (237), the mucus penetrability of siRNA-loaded exosomes was enhanced by adding a hydrophilic surface coating of PEG. PEGylated mExosomes exhibit improved penetrability and stability in an acidic gut environment. Bovine mExosomes are reportedly considered therapeutic agents against arthritis. Studies have demonstrated that spontaneous polyarthritis in IL-1Ra-deficient mice and collagen-induced arthritis is diminished by oral administration of mExosomes (238). However, the mechanism underlying the delayed disease onset remains unknown. Bovine mExosomes containing immunoregulatory miRNAs and anti-inflammatory proteins might have targeted inflammatory pathways. These are just a few examples of using exosomes for therapeutic purposes; further discoveries and breakthroughs have yet to be made.
Research on exosomes suggest that they are representative of the cellular condition and play a crucial role in upregulating, stabilizing, or down-regulating that condition. Exosomes secreted from a diseased cell would support disease progression, such as promoting angiogenesis for cancer or enhancing receptor expression to support viral infection or enhance inflammation. Exosomes secreted from stem cells reflect their purpose of supporting regeneration, healing, and growth. This information indicates that exosomes could be the next-generation technology that could receive large-scale public acceptance owing to their success in non-invasive diagnostics and non-toxic, target-specific drug delivery techniques used in clinical trials.
PG, HM and B-CA contributed to the conception, writing, and discussion of this manuscript. All authors wrote the initial draft of the manuscript. All authors Contributed to the critical conclusion of the manuscript. All authors contributed to the article and approved the submitted version.
This research was supported by the Basic Science Research Program of the National Research Foundation of Korea (NRF), funded by the Ministry of Education (NRF-2021R1I1A1A01040732, NRF-2022R1I1A3068477, and NRF-2022R1I1A1A01068652).
The authors declare that the research was conducted in the absence of any commercial or financial relationships that could be construed as a potential conflict of interest.
All claims expressed in this article are solely those of the authors and do not necessarily represent those of their affiliated organizations, or those of the publisher, the editors and the reviewers. Any product that may be evaluated in this article, or claim that may be made by its manufacturer, is not guaranteed or endorsed by the publisher.
1. Trams EG, Lauter CJ, Norman Salem, Heine U. Exfoliation of membrane ecto-enzymes in the form of micro-vesicles. Biochim Biophys Acta (BBA) - Biomembr (1981) 645:63–70. doi: 10.1016/0005-2736(81)90512-5
2. Johnstone RM, Adam M, Hammond JR, Orr L, Turbide C. Vesicle formation during reticulocyte maturation. association of plasma membrane activities with released vesicles (exosomes). J Biol Chem (1987) 262:9412–20. doi: 10.1016/S0021-9258(18)48095-7
3. Valadi H, Ekström K, Bossios A, Sjöstrand M, Lee JJ, Lötvall JO. Exosome-mediated transfer of mRNAs and microRNAs is a novel mechanism of genetic exchange between cells. Nat Cell Biol (2007) 9:654–9. doi: 10.1038/ncb1596
4. Hsu D-H, Paz P, Villaflor G, Rivas A, Mehta-Damani A, Angevin E, et al. Exosomes as a tumor vaccine: Enhancing potency through direct loading of antigenic peptides. J Immunother (2003) 26:440–50. doi: 10.1097/00002371-200309000-00007
5. Mathivanan S, Ji H, Simpson RJ. Exosomes: extracellular organelles important in intercellular communication. J Proteomics (2010) 73:1907–20. doi: 10.1016/j.jprot.2010.06.006
6. Théry C, Ostrowski M, Segura E. Membrane vesicles as conveyors of immune responses. Nat Rev Immunol (2009) 9:581–93. doi: 10.1038/nri2567
7. Théry C, Zitvogel L, Amigorena S. Exosomes: composition, biogenesis and function. Nat Rev Immunol (2002) 2:569–79. doi: 10.1038/nri855
8. Pan BT, Teng K, Wu C, Adam M, Johnstone RM. Electron microscopic evidence for externalization of the transferrin receptor in vesicular form in sheep reticulocytes. J Cell Biol (1985) 101:942–8. doi: 10.1083/jcb.101.3.942
9. Trowbridge IS, Collawn JF, Hopkins CR. Signal-dependent membrane protein trafficking in the endocytic pathway. Annu Rev Cell Biol (1993) 9:129–61. doi: 10.1146/annurev.cb.09.110193.001021
10. van Niel G, D’Angelo G, Raposo G. Shedding light on the cell biology of extracellular vesicles. Nat Rev Mol Cell Biol (2018) 19:213–28. doi: 10.1038/nrm.2017.125
11. Kenific CM, Zhang H, Lyden D. An exosome pathway without an ESCRT. Cell Res (2021) 31:105–6. doi: 10.1038/s41422-020-00418-0
12. Gangadaran P, Gunassekaran GR, Rajendran RL, Oh JM, Vadevoo SMP, Lee HW, et al. Interleukin-4 receptor targeting peptide decorated extracellular vesicles as a platform for In vivo drug delivery to thyroid cancer. Biomedicines (2022) 10:1978. doi: 10.3390/biomedicines10081978
13. Gangadaran P, Rajendran RL, Kwack MH, Jeyaraman M, Hong CM, Sung YK, et al. Application of cell-derived extracellular vesicles and engineered nanovesicles for hair growth: From mechanisms to therapeutics. Front Cell Dev Biol (2022) 10:963278. doi: 10.3389/fcell.2022.963278
14. Krishnan A, Gangadaran P, Chavda VP, Jogalekar MP, Muthusamy R, Valu D, et al. Convalescent serum-derived exosomes: Attractive niche as COVID-19 diagnostic tool and vehicle for mRNA delivery. Exp Biol Med (Maywood) (2022) 247:1244–52. doi: 10.1177/15353702221092984
15. Rajendran RL, Paudel S, Gangadaran P, Oh JM, Oh EJ, Hong CM, et al. Extracellular vesicles act as nano-transporters of tyrosine kinase inhibitors to revert iodine avidity in thyroid cancer. Pharmaceutics (2021) 13:248. doi: 10.3390/pharmaceutics13020248
16. Gangadaran P, Ahn B-C. Extracellular vesicle- and extracellular vesicle mimetics-based drug delivery systems: New perspectives, challenges, and clinical developments. Pharmaceutics (2020) 12:E442. doi: 10.3390/pharmaceutics12050442
17. Huang X, Yuan T, Tschannen M, Sun Z, Jacob H, Du M, et al. Characterization of human plasma-derived exosomal RNAs by deep sequencing. BMC Genomics (2013) 14:319. doi: 10.1186/1471-2164-14-319
18. Mittelbrunn M, Gutiérrez-Vázquez C, Villarroya-Beltri C, González S, Sánchez-Cabo F, González MÁ, et al. Unidirectional transfer of microRNA-loaded exosomes from T cells to antigen-presenting cells. Nat Commun (2011) 2:282. doi: 10.1038/ncomms1285
19. Nolte-’t Hoen ENM, Buermans HPJ, Waasdorp M, Stoorvogel W, Wauben MHM, ‘t Hoen PAC. Deep sequencing of RNA from immune cell-derived vesicles uncovers the selective incorporation of small non-coding RNA biotypes with potential regulatory functions. Nucleic Acids Res (2012) 40:9272–85. doi: 10.1093/nar/gks658
20. McDonald MK, Tian Y, Qureshi RA, Gormley M, Ertel A, Gao R, et al. Functional significance of macrophage-derived exosomes in inflammation and pain. Pain (2014) 155:1527–39. doi: 10.1016/j.pain.2014.04.029
21. Ali SA, Huang M-B, Campbell PE, Roth WW, Campbell T, Khan M, et al. Genetic characterization of HIV type 1 nef-induced vesicle secretion. AIDS Res Hum Retroviruses (2010) 26:173–92. doi: 10.1089/aid.2009.0068
22. Raymond AD, Campbell-Sims TC, Khan M, Lang M, Huang MB, Bond VC, et al. HIV Type 1 nef is released from infected cells in CD45(+) microvesicles and is present in the plasma of HIV-infected individuals. AIDS Res Hum Retroviruses (2011) 27:167–78. doi: 10.1089/aid.2009.0170
23. Arenaccio C, Anticoli S, Manfredi F, Chiozzini C, Olivetta E, Federico M. Latent HIV-1 is activated by exosomes from cells infected with either replication-competent or defective HIV-1. Retrovirology (2015) 12:87. doi: 10.1186/s12977-015-0216-y
24. de Carvalho JV, de Castro RO, da Silva EZM, Silveira PP, da Silva-Januário ME, Arruda E, et al. Nef neutralizes the ability of exosomes from CD4+ T cells to act as decoys during HIV-1 infection. PloS One (2014) 9:e113691. doi: 10.1371/journal.pone.0113691
25. Lenassi M, Cagney G, Liao M, Vaupotic T, Bartholomeeusen K, Cheng Y, et al. HIV Nef is secreted in exosomes and triggers apoptosis in bystander CD4+ T cells. Traffic (2010) 11:110–22. doi: 10.1111/j.1600-0854.2009.01006.x
26. Arenaccio C, Chiozzini C, Columba-Cabezas S, Manfredi F, Affabris E, Baur A, et al. Exosomes from human immunodeficiency virus type 1 (HIV-1)-Infected cells license quiescent CD4+ T lymphocytes to replicate HIV-1 through a nef- and ADAM17-dependent mechanism. J Virol (2014) 88:11529–39. doi: 10.1128/JVI.01712-14
27. Mack M, Kleinschmidt A, Brühl H, Klier C, Nelson PJ, Cihak J, et al. Transfer of the chemokine receptor CCR5 between cells by membrane-derived microparticles: A mechanism for cellular human immunodeficiency virus 1 infection. Nat Med (2000) 6:769–75. doi: 10.1038/77498
28. Rozmyslowicz T, Majka M, Kijowski J, Murphy SL, Conover DO, Poncz M, et al. Platelet- and megakaryocyte-derived microparticles transfer CXCR4 receptor to CXCR4-null cells and make them susceptible to infection by X4-HIV. AIDS (2003) 17:33–42. doi: 10.1097/00002030-200301030-00006
29. Narayanan A, Iordanskiy S, Das R, Van Duyne R, Santos S, Jaworski E, et al. Exosomes derived from HIV-1-infected cells contain trans-activation response element RNA. J Biol Chem (2013) 288:20014–33. doi: 10.1074/jbc.M112.438895
30. He N, Liu M, Hsu J, Xue Y, Chou S, Burlingame A, et al. HIV-1 tat and host AFF4 recruit two transcription elongation factors into a bifunctional complex for coordinated activation of HIV-1 transcription. Mol Cell (2010) 38:428–38. doi: 10.1016/j.molcel.2010.04.013
31. Khatua AK, Taylor HE, Hildreth JEK, Popik W. Exosomes packaging APOBEC3G confer human immunodeficiency virus resistance to recipient cells. J Virol (2009) 83:512–21. doi: 10.1128/JVI.01658-08
32. Gentili M, Kowal J, Tkach M, Satoh T, Lahaye X, Conrad C, et al. Transmission of innate immune signaling by packaging of cGAMP in viral particles. Science (2015) 349:1232–6. doi: 10.1126/science.aab3628
33. Bridgeman A, Maelfait J, Davenne T, Partridge T, Peng Y, Mayer A, et al. Viruses transfer the antiviral second messenger cGAMP between cells. Science (2015) 349:1228–32. doi: 10.1126/science.aab3632
34. Bernard MA, Zhao H, Yue SC, Anandaiah A, Koziel H, Tachado SD. Novel HIV-1 MiRNAs stimulate TNFα release in human macrophages via TLR8 signaling pathway. PloS One (2014) 9:e106006. doi: 10.1371/journal.pone.0106006
35. Dreux M, Garaigorta U, Boyd B, Décembre E, Chung J, Whitten-Bauer C, et al. Short-range exosomal transfer of viral RNA from infected cells to plasmacytoid dendritic cells triggers innate immunity. Cell Host Microbe (2012) 12:558–70. doi: 10.1016/j.chom.2012.08.010
36. Mason RJ. Pathogenesis of COVID-19 from a cell biology perspective. Eur Respir J (2020) 55:2000607. doi: 10.1183/13993003.00607-2020
37. Qian Z, Travanty EA, Oko L, Edeen K, Berglund A, Wang J, et al. Innate immune response of human alveolar type II cells infected with severe acute respiratory syndrome-coronavirus. Am J Respir Cell Mol Biol (2013) 48:742–8. doi: 10.1165/rcmb.2012-0339OC
38. Wang J, Chen S, Bihl J. Exosome-mediated transfer of ACE2 (Angiotensin-converting enzyme 2) from endothelial progenitor cells promotes survival and function of endothelial cell. Oxid Med Cell Longev (2020) 2020:4213541. doi: 10.1155/2020/4213541
39. El-Shennawy L, Hoffmann AD, Dashzeveg NK, McAndrews KM, Mehl PJ, Cornish D, et al. Circulating ACE2-expressing extracellular vesicles block broad strains of SARS-CoV-2. Nat Commun (2022) 13:405. doi: 10.1038/s41467-021-27893-2
40. Husmann M, Beckmann E, Boller K, Kloft N, Tenzer S, Bobkiewicz W, et al. Elimination of a bacterial pore-forming toxin by sequential endocytosis and exocytosis. FEBS Lett (2009) 583:337–44. doi: 10.1016/j.febslet.2008.12.028
41. Abrami L, Brandi L, Moayeri M, Brown MJ, Krantz BA, Leppla SH, et al. Hijacking multivesicular bodies enables long-term and exosome-mediated long-distance action of anthrax toxin. Cell Rep (2013) 5:986–96. doi: 10.1016/j.celrep.2013.10.019
42. Keller MD, Ching KL, Liang F-X, Dhabaria A, Tam K, Ueberheide BM, et al. Decoy exosomes provide protection against bacterial toxins. Nature (2020) 579:260–4. doi: 10.1038/s41586-020-2066-6
43. Regev-Rudzki N, Wilson DW, Carvalho TG, Sisquella X, Coleman BM, Rug M, et al. Cell-cell communication between malaria-infected red blood cells via exosome-like vesicles. Cell (2013) 153:1120–33. doi: 10.1016/j.cell.2013.04.029
44. Martin-Jaular L, Nakayasu ES, Ferrer M, Almeida IC, Del Portillo HA. Exosomes from plasmodium yoelii-infected reticulocytes protect mice from lethal infections. PloS One (2011) 6:e26588. doi: 10.1371/journal.pone.0026588
45. Silverman JM, Clos J, de’Oliveira CC, Shirvani O, Fang Y, Wang C, et al. An exosome-based secretion pathway is responsible for protein export from leishmania and communication with macrophages. J Cell Sci (2010) 123:842–52. doi: 10.1242/jcs.056465
46. Bayer-Santos E, Aguilar-Bonavides C, Rodrigues SP, Cordero EM, Marques AF, Varela-Ramirez A, et al. Proteomic analysis of trypanosoma cruzi secretome: Characterization of two populations of extracellular vesicles and soluble proteins. J Proteome Res (2013) 12:883–97. doi: 10.1021/pr300947g
47. Hassani K, Olivier M. Immunomodulatory impact of leishmania-induced macrophage exosomes: A comparative proteomic and functional analysis. PloS Negl Trop Dis (2013) 7:e2185. doi: 10.1371/journal.pntd.0002185
48. Szempruch AJ, Sykes SE, Kieft R, Dennison L, Becker AC, Gartrell A, et al. Extracellular vesicles from trypanosoma brucei mediate virulence factor transfer and cause host anemia. Cell (2016) 164:246–57. doi: 10.1016/j.cell.2015.11.051
49. Combes V, Taylor TE, Juhan-Vague I, Mège J-L, Mwenechanya J, Tembo M, et al. Circulating endothelial microparticles in malawian children with severe falciparum malaria complicated with coma. JAMA (2004) 291:2542–4. doi: 10.1001/jama.291.21.2542-b
50. van Zandbergen G, Klinger M, Mueller A, Dannenberg S, Gebert A, Solbach W, et al. Cutting edge: neutrophil granulocyte serves as a vector for leishmania entry into macrophages. J Immunol (2004) 173:6521–5. doi: 10.4049/jimmunol.173.11.6521
51. Hu G, Gong A-Y, Roth AL, Huang BQ, Ward HD, Zhu G, et al. Release of luminal exosomes contributes to TLR4-mediated epithelial antimicrobial defense. PloS Pathog (2013) 9:e1003261. doi: 10.1371/journal.ppat.1003261
52. Tomer Y. Mechanisms of autoimmune thyroid diseases: from genetics to epigenetics. Annu Rev Pathol (2014) 9:147–56. doi: 10.1146/annurev-pathol-012513-104713
53. Bogusławska J, Godlewska M, Gajda E, Piekiełko-Witkowska A. Cellular and molecular basis of thyroid autoimmunity. Eur Thyroid J (2022) 11. doi: 10.1530/ETJ-21-0024
54. Schultheiss UT, Teumer A, Medici M, Li Y, Daya N, Chaker L, et al. A genetic risk score for thyroid peroxidase antibodies associates with clinical thyroid disease in community-based populations. J Clin Endocrinol Metab (2015) 100:E799–807. doi: 10.1210/jc.2014-4352
55. Brčić L, Barić A, Gračan S, Brdar D, Torlak Lovrić V, Vidan N, et al. Association of established thyroid peroxidase autoantibody (TPOAb) genetic variants with hashimoto’s thyroiditis. Autoimmunity (2016) 49:480–5. doi: 10.1080/08916934.2016.1191475
56. Franco J-S, Amaya-Amaya J, Anaya J-M. Thyroid disease and autoimmune diseases (2013). El Rosario University Press. Available at: https://www.ncbi.nlm.nih.gov/books/NBK459466/ (Accessed December 2, 2022).
57. Zou J, Peng H, Liu Y. The roles of exosomes in immunoregulation and autoimmune thyroid diseases. Frontiers in immunology(2021) (Accessed November 28, 2022).
58. Andrikoula M, Tsatsoulis A. The role of fas-mediated apoptosis in thyroid disease. Eur J Endocrinol (2001) 144:561–8. doi: 10.1530/eje.0.1440561
59. Zaletel K, Gaberšček S. Hashimoto’s thyroiditis: From genes to the disease. Curr Genomics (2011) 12:576–88. doi: 10.2174/138920211798120763
60. Fröhlich E, Wahl R. Thyroid autoimmunity: Role of anti-thyroid antibodies in thyroid and extra-thyroidal diseases. Front Immunol (2017) 8:521. doi: 10.3389/fimmu.2017.00521
61. Klinker MW, Lizzio V, Reed TJ, Fox DA, Lundy SK. Human b cell-derived lymphoblastoid cell lines constitutively produce fas ligand and secrete MHCII(+)FasL(+) killer exosomes. Front Immunol (2014) 5:144. doi: 10.3389/fimmu.2014.00144
62. Caruso Bavisotto C, Cappello F, Macario AJL, Conway de Macario E, Logozzi M, Fais S, et al. Exosomal HSP60: A potentially useful biomarker for diagnosis, assessing prognosis, and monitoring response to treatment. Expert Rev Mol Diagn (2017) 17:815–22. doi: 10.1080/14737159.2017.1356230
63. Smith TJ, Hegedüs L. Graves’ disease. N Engl J Med (2016) 375:1552–65. doi: 10.1056/NEJMra1510030
64. Rossi M, Taddei AR, Fasciani I, Maggio R, Giorgi F. The cell biology of the thyroid-disrupting mechanism of dichlorodiphenyltrichloroethane (DDT). J Endocrinol Invest (2018) 41:67–73. doi: 10.1007/s40618-017-0716-9
65. Cañas CA, Cañas F, Bonilla-Abadía F, Ospina FE, Tobón GJ. Epigenetics changes associated to environmental triggers in autoimmunity. Autoimmunity (2016) 49:1–11. doi: 10.3109/08916934.2015.1086996
66. Wang B, Shao X, Song R, Xu D, Zhang J-A. The emerging role of epigenetics in autoimmune thyroid diseases. Front Immunol (2017) 8:396. doi: 10.3389/fimmu.2017.00396
67. Mathiessen A, Conaghan PG. Synovitis in osteoarthritis: current understanding with therapeutic implications. Arthritis Res Ther (2017) 19:18. doi: 10.1186/s13075-017-1229-9
68. Hiratsuka I, Yamada H, Munetsuna E, Hashimoto S, Itoh M. Circulating MicroRNAs in graves’ disease in relation to clinical activity. Thyroid (2016) 26:1431–40. doi: 10.1089/thy.2016.0062
70. Smith DA, Schmid EF. Drug withdrawals and the lessons within. Curr Opin Drug Discovery Devel (2006) 9:38–46.
71. Nojima H, Freeman CM, Schuster RM, Japtok L, Kleuser B, Edwards MJ, et al. Hepatocyte exosomes mediate liver repair and regeneration via sphingosine-1-phosphate. J Hepatol (2016) 64:60–8. doi: 10.1016/j.jhep.2015.07.030
72. Tan CY, Lai RC, Wong W, Dan YY, Lim S-K, Ho HK. Mesenchymal stem cell-derived exosomes promote hepatic regeneration in drug-induced liver injury models. Stem Cell Res Ther (2014) 5:76. doi: 10.1186/scrt465
73. Damania A, Jaiman D, Teotia AK, Kumar A. Mesenchymal stromal cell-derived exosome-rich fractionated secretome confers a hepatoprotective effect in liver injury. Stem Cell Res Ther (2018) 9:31. doi: 10.1186/s13287-017-0752-6
74. Yan Y, Jiang W, Tan Y, Zou S, Zhang H, Mao F, et al. hucMSC exosome-derived GPX1 is required for the recovery of hepatic oxidant injury. Mol Ther (2017) 25:465–79. doi: 10.1016/j.ymthe.2016.11.019
75. Zhao L, Wang Y, Zhang Y. The potential diagnostic and therapeutic applications of exosomes in drug-induced liver injury. Toxicol Lett (2021) 337:68–77. doi: 10.1016/j.toxlet.2020.11.021
76. Takenaka MC, Quintana FJ. Tolerogenic dendritic cells. Semin Immunopathol (2017) 39:113–20. doi: 10.1007/s00281-016-0587-8
77. Hasegawa H, Matsumoto T. Mechanisms of tolerance induction by dendritic cells. In Vivo Front Immunol (2018) 9:350. doi: 10.3389/fimmu.2018.00350
78. Chen X, Song C-H, Feng B-S, Li T-L, Li P, Zheng P-Y, et al. Intestinal epithelial cell-derived integrin αβ6 plays an important role in the induction of regulatory T cells and inhibits an antigen-specific Th2 response. J Leukoc Biol (2011) 90:751–9. doi: 10.1189/jlb.1210696
79. Okoye IS, Coomes SM, Pelly VS, Czieso S, Papayannopoulos V, Tolmachova T, et al. MicroRNA-containing T-regulatory-cell-derived exosomes suppress pathogenic T helper 1 cells. Immunity (2014) 41:89–103. doi: 10.1016/j.immuni.2014.05.019
80. Kojima M, Costantini TW, Eliceiri BP, Chan TW, Baird A, Coimbra R. Gut epithelial cell-derived exosomes trigger posttrauma immune dysfunction. J Trauma Acute Care Surg (2018) 84:257–64. doi: 10.1097/TA.0000000000001748
81. Chen X-D, Zhao J, Yang X, Zhou B-W, Yan Z, Liu W-F, et al. Gut-derived exosomes mediate memory impairment after intestinal Ischemia/Reperfusion via activating microglia. Mol Neurobiol (2021) 58:4828–41. doi: 10.1007/s12035-021-02444-4
82. Park EJ, Prajuabjinda O, Soe ZY, Darkwah S, Appiah MG, Kawamoto E, et al. Exosomal regulation of lymphocyte homing to the gut. Blood Adv (2019) 3:1–11. doi: 10.1182/bloodadvances.2018024877
83. Miyake H, Lee C, Chusilp S, Bhalla M, Li B, Pitino M, et al. Human breast milk exosomes attenuate intestinal damage. Pediatr Surg Int (2020) 36:155–63. doi: 10.1007/s00383-019-04599-7
84. Inotsuka R, Uchimura K, Yamatsu A, Kim M, Katakura Y. γ-aminobutyric acid (GABA) activates neuronal cells by inducing the secretion of exosomes from intestinal cells. Food Funct (2020) 11:9285–90. doi: 10.1039/D0FO01184C
85. Zheng X, Chen F, Zhang Q, Liu Y, You P, Sun S, et al. Salivary exosomal PSMA7: a promising biomarker of inflammatory bowel disease. Protein Cell (2017) 8:686–95. doi: 10.1007/s13238-017-0413-7
86. Zhang M, Merlin D. Su1836 - curcuma longa-derived nanoparticles reduce colitis and promote intestinal wound repair by inactivating the NF-ΚB pathway. Gastroenterology (2017) 152:S567. doi: 10.1016/S0016-5085(17)32052-8
87. Ju S, Mu J, Dokland T, Zhuang X, Wang Q, Jiang H, et al. Grape exosome-like nanoparticles induce intestinal stem cells and protect mice from DSS-induced colitis. Mol Ther (2013) 21:1345–57. doi: 10.1038/mt.2013.64
88. Liu T, Zhang X, Du L, Wang Y, Liu X, Tian H, et al. Exosome-transmitted miR-128-3p increase chemosensitivity of oxaliplatin-resistant colorectal cancer. Mol Cancer (2019) 18:43. doi: 10.1186/s12943-019-0981-7
89. Li Y-Y, Xu Q-W, Xu P-Y, Li W-M. MSC-derived exosomal miR-34a/c-5p and miR-29b-3p improve intestinal barrier function by targeting the Snail/Claudins signaling pathway. Elsevier Inc (2020). doi: 10.1016/j.lfs.2020.118017
90. Kurakevich E, Hennet T, Hausmann M, Rogler G, Borsig L. Milk oligosaccharide sialyl(α2,3)lactose activates intestinal CD11c+ cells through TLR4. Proc Natl Acad Sci U.S.A. (2013) 110:17444–9. doi: 10.1073/pnas.1306322110
91. Zhao Y, Tao M, Wei M, Du S, Wang H, Wang X. Mesenchymal stem cells derived exosomal miR-323-3p promotes proliferation and inhibits apoptosis of cumulus cells in polycystic ovary syndrome (PCOS). Artif Cells Nanomed Biotechnol (2019) 47:3804–13. doi: 10.1080/21691401.2019.1669619
92. Palmisano G, Jensen SS, Le Bihan M-C, Lainé J, McGuire JN, Pociot F, et al. Characterization of membrane-shed microvesicles from cytokine-stimulated β-cells using proteomics strategies. Mol Cell Proteomics (2012) 11:230–43. doi: 10.1074/mcp.M111.012732
93. Bozdag G, Mumusoglu S, Zengin D, Karabulut E, Yildiz BO. The prevalence and phenotypic features of polycystic ovary syndrome: a systematic review and meta-analysis. Hum Reprod (2016) 31:2841–55. doi: 10.1093/humrep/dew218
94. Mims JW. Asthma: definitions and pathophysiology. Int Forum Allergy Rhinol (2015) 5 Suppl 1:S2–6. doi: 10.1002/alr.21609
95. Lambrecht BN, Hammad H. The immunology of asthma. Nat Immunol (2015) 16:45–56. doi: 10.1038/ni.3049
96. Melo RCN, Spencer LA, Dvorak AM, Weller PF. Mechanisms of eosinophil secretion: large vesiculotubular carriers mediate transport and release of granule-derived cytokines and other proteins. J Leukoc Biol (2008) 83:229–36. doi: 10.1189/jlb.0707503
97. Luna-Gomes T, Bozza P, Bandeira-Melo C. Eosinophil recruitment and activation: the role of lipid mediators. frontiers in pharmacology (2013) (Accessed September 29, 2022).
98. Mazzeo C, Cañas JA, Zafra MP, Rojas Marco A, Fernández-Nieto M, Sanz V, et al. Exosome secretion by eosinophils: A possible role in asthma pathogenesis. J Allergy Clin Immunol (2015) 135:1603–13. doi: 10.1016/j.jaci.2014.11.026
99. Ventimiglia LN, Alonso MA. Biogenesis and function of T cell-derived exosomes. Front Cell Dev Biol (2016) 4:84. doi: 10.3389/fcell.2016.00084
100. Cañas JA, Rodrigo-Muñoz JM, Gil-Martínez M, Sastre B, del Pozo V. Exosomes: A key piece in asthmatic inflammation. Int J Mol Sci (2021) 22:963. doi: 10.3390/ijms22020963
101. Bartel S, La Grutta S, Cilluffo G, Perconti G, Bongiovanni A, Giallongo A, et al. Human airway epithelial extracellular vesicle miRNA signature is altered upon asthma development. Allergy (2020) 75:346–56. doi: 10.1111/all.14008
102. Prado N, Marazuela EG, Segura E, Fernández-García H, Villalba M, Théry C, et al. Exosomes from bronchoalveolar fluid of tolerized mice prevent allergic reaction. J Immunol (2008) 181:1519–25. doi: 10.4049/jimmunol.181.2.1519
103. Almohammai A, Rahbarghazi R, Keyhanmanesh R, Rezaie J, Ahmadi M. Asthmatic condition induced the activity of exosome secretory pathway in rat pulmonary tissues. J Inflammation (Lond) (2021) 18:14. doi: 10.1186/s12950-021-00275-7
104. Ogawa Y, Duru EA, Ameredes BT. Role of IL-10 in the resolution of airway inflammation. Curr Mol Med (2008) 8:437–45. doi: 10.2174/156652408785160907
105. Myszka A, Krenz-Niedbała M, Tomczyk J, Zalewska M. Osteoarthritis: A problematic disease in past human populations. A dependence between entheseal changes, body size, age, sex, and osteoarthritic changes development. Anat Rec (Hoboken) (2020) 303:2357–71. doi: 10.1002/ar.24316
106. Jeyaraman M, Muthu S, Shehabaz S, Jeyaraman N, Rajendran RL, Hong CM, et al. Current understanding of MSC-derived exosomes in the management of knee osteoarthritis. Exp Cell Res (2022) 418:113274. doi: 10.1016/j.yexcr.2022.113274
107. Prajwal GS, Jeyaraman N, Kanth VK, Jeyaraman M, Muthu S, Rajendran SNS, et al. Lineage differentiation potential of different sources of mesenchymal stem cells for osteoarthritis knee. Pharm (Basel) (2022) 15:386. doi: 10.3390/ph15040386
108. Muthu S, Kartheek RR, Jeyaraman N, Rajendran RL, Khanna M, Jeyaraman M, et al. Is culture expansion necessary in autologous mesenchymal stromal cell therapy to obtain superior results in the management of knee osteoarthritis?-Meta-Analysis of randomized controlled trials. Bioeng (Basel) (2021) 8:220. doi: 10.3390/bioengineering8120220
109. Feng Z, Li X, Lin J, Zheng W, Hu Z, Xuan J, et al. Oleuropein inhibits the IL-1β-induced expression of inflammatory mediators by suppressing the activation of NF-κB and MAPKs in human osteoarthritis chondrocytes. Food Funct (2017) 8:3737–44. doi: 10.1039/C7FO00823F
110. Ge M, Ke R, Cai T, Yang J, Mu X. Identification and proteomic analysis of osteoblast-derived exosomes. Biochem Biophys Res Commun (2015) 467:27–32. doi: 10.1016/j.bbrc.2015.09.135
111. Cui Y, Luan J, Li H, Zhou X, Han J. Exosomes derived from mineralizing osteoblasts promote ST2 cell osteogenic differentiation by alteration of microRNA expression. FEBS Lett (2016) 590:185–92. doi: 10.1002/1873-3468.12024
112. Ren L, Song Z-J, Cai Q-W, Chen R-X, Zou Y, Fu Q, et al. Adipose mesenchymal stem cell-derived exosomes ameliorate hypoxia/serum deprivation-induced osteocyte apoptosis and osteocyte-mediated osteoclastogenesis in vitro. Biochem Biophys Res Commun (2019) 508:138–44. doi: 10.1016/j.bbrc.2018.11.109
113. Liu W, Li L, Rong Y, Qian D, Chen J, Zhou Z, et al. Hypoxic mesenchymal stem cell-derived exosomes promote bone fracture healing by the transfer of miR-126. Acta Biomater (2020) 103:196–212. doi: 10.1016/j.actbio.2019.12.020
114. Ni Z, Zhou S, Li S, Kuang L, Chen H, Luo X, et al. Exosomes: roles and therapeutic potential in osteoarthritis. Bone Res (2020) 8:25. doi: 10.1038/s41413-020-0100-9
115. Zhou L, Ye H, Liu L, Chen Y. Human bone mesenchymal stem cell-derived exosomes inhibit IL-1β-Induced inflammation in osteoarthritis chondrocytes. Cell J (2021) 23:485–94. doi: 10.22074/cellj.2021.7127
116. Griffiths CEM, Voorhees JJ. Immunological mechanisms involved in psoriasis. Springer Seminars in Immunopathology (1992) 13, 441–54. doi: 10.1007/BF00200540
117. Cameron AL, Kirby B, Fei W, Griffiths CEM. Natural killer and natural killer-T cells in psoriasis. Arch Dermatol Res (2002) 294:363–9. doi: 10.1007/s00403-002-0349-4
118. Grän F, Kerstan A, Serfling E, Goebeler M, Muhammad K. Current developments in the immunology of psoriasis. Yale J Biol Med (2020) 93:97–110.
119. Kagami S, Rizzo HL, Lee JJ, Koguchi Y, Blauvelt A. Circulating Th17, Th22, and Th1 cells are increased in psoriasis. J Invest Dermatol (2010) 130:1373–83. doi: 10.1038/jid.2009.399
120. Jiang M, Fang H, Dang E, Zhang J, Qiao P, Yu C, et al. Small extracellular vesicles containing miR-381-3p from keratinocytes promote T helper type 1 and T helper type 17 polarization in psoriasis. J Invest Dermatol (2021) 141:563–74. doi: 10.1016/j.jid.2020.07.009
121. Shao S, Fang H, Zhang J, Jiang M, Xue K, Ma J, et al. Neutrophil exosomes enhance the skin autoinflammation in generalized pustular psoriasis via activating keratinocytes. FASEB J (2019) 33:6813–28. doi: 10.1096/fj.201802090RR
122. Cumberbatch M, Singh M, Dearman RJ, Young HS, Kimber I, Griffiths CEM. Impaired langerhans cell migration in psoriasis. J Exp Med (2006) 203:953–60. doi: 10.1084/jem.20052367
123. Cheung KL, Jarrett R, Subramaniam S, Salimi M, Gutowska-Owsiak D, Chen Y-L, et al. Psoriatic T cells recognize neolipid antigens generated by mast cell phospholipase delivered by exosomes and presented by CD1a. J Exp Med (2016) 213:2399–412. doi: 10.1084/jem.20160258
124. Hu SC-S, Yu H-S, Yen F-L, Lin C-L, Chen G-S, Lan C-CE. Neutrophil extracellular trap formation is increased in psoriasis and induces human β-defensin-2 production in epidermal keratinocytes. Sci Rep (2016) 6:31119. doi: 10.1038/srep31119
125. Blauvelt A, Chiricozzi A. The immunologic role of IL-17 in psoriasis and psoriatic arthritis pathogenesis. Clin Rev Allergy Immunol (2018) 55:379–90. doi: 10.1007/s12016-018-8702-3
126. Bai F, Zheng W, Dong Y, Wang J, Garstka MA, Li R, et al. Serum levels of adipokines and cytokines in psoriasis patients: A systematic review and meta-analysis. Oncotarget (2017) 9:1266–78. doi: 10.18632/oncotarget.22260
127. El-Rifaie A-AA, Sabry D, Doss RW, Kamal MA, Abd El Hassib DM. Heme oxygenase and iron status in exosomes of psoriasis patients. Arch Dermatol Res (2018) 310:651–6. doi: 10.1007/s00403-018-1852-6
128. Lv J, Zhou D, Wang Y, Sun W, Zhang C, Xu J, et al. Effects of luteolin on treatment of psoriasis by repressing HSP90. Int Immunopharmacol (2020) 79:106070. doi: 10.1016/j.intimp.2019.106070
129. Zhang B, Lai RC, Sim WK, Choo ABH, Lane EB, Lim SK. Topical application of mesenchymal stem cell exosomes alleviates the imiquimod induced psoriasis-like inflammation. Int J Mol Sci (2021) 22:E720. doi: 10.3390/ijms22020720
130. Zhang Y, Yan J, Li Z, Zheng J, Sun Q. Exosomes derived from human umbilical cord mesenchymal stem cells alleviate psoriasis-like skin inflammation. J Interferon Cytokine Res (2022) 42:8–18. doi: 10.1089/jir.2021.0146
131. Sheng H, Hassanali S, Nugent C, Wen L, Hamilton-Williams E, Dias P, et al. Insulinoma-released exosomes or microparticles are immunostimulatory and can activate autoreactive T cells spontaneously developed in non-obese diabetes mice. J Immunol (2011) 187:1591–600. doi: 10.4049/jimmunol.1100231
132. Castaño C, Novials A, Párrizas M. Exosomes and diabetes. Diabetes Metab Res Rev (2019) 35:e3107. doi: 10.1002/dmrr.3107
133. Dumortier O, Hinault C, Van Obberghen E. MicroRNAs and metabolism crosstalk in energy homeostasis. Cell Metab (2013) 18:312–24. doi: 10.1016/j.cmet.2013.06.004
134. Guay C, Kruit JK, Rome S, Menoud V, Mulder NL, Jurdzinski A, et al. Lymphocyte-derived exosomal MicroRNAs promote pancreatic β cell death and may contribute to type 1 diabetes development. Cell Metab (2019) 29:348–361.e6. doi: 10.1016/j.cmet.2018.09.011
135. Guay C, Roggli E, Nesca V, Jacovetti C, Regazzi R. Diabetes mellitus, a microRNA-related disease? Transl Res (2011) 157:253–64. doi: 10.1016/j.trsl.2011.01.009
136. Santovito D, De Nardis V, Marcantonio P, Mandolini C, Paganelli C, Vitale E, et al. Plasma exosome microRNA profiling unravels a new potential modulator of adiponectin pathway in diabetes: Effect of glycemic control. J Clin Endocrinol Metab (2014) 99:E1681–1685. doi: 10.1210/jc.2013-3843
137. Katayama M, Wiklander OPB, Fritz T, Caidahl K, El-Andaloussi S, Zierath JR, et al. Circulating exosomal miR-20b-5p is elevated in type 2 diabetes and could impair insulin action in human skeletal muscle. Diabetes (2019) 68:515–26. doi: 10.2337/db18-0470
138. Garcia-Contreras M, Shah SH, Tamayo A, Robbins PD, Golberg RB, Mendez AJ, et al. Plasma-derived exosome characterization reveals a distinct microRNA signature in long duration type 1 diabetes. Sci Rep (2017) 7:5998. doi: 10.1038/s41598-017-05787-y
139. Cianciaruso C, Phelps EA, Pasquier M, Hamelin R, Demurtas D, Alibashe Ahmed M, et al. Primary human and rat β-cells release the intracellular autoantigens GAD65, IA-2, and proinsulin in exosomes together with cytokine-induced enhancers of immunity. Diabetes (2017) 66:460–73. doi: 10.2337/db16-0671
140. Vomund AN, Zinselmeyer BH, Hughes J, Calderon B, Valderrama C, Ferris ST, et al. Beta cells transfer vesicles containing insulin to phagocytes for presentation to T cells. Proc Natl Acad Sci U.S.A. (2015) 112:E5496–5502. doi: 10.1073/pnas.1515954112
141. Ge Q, Xie XX, Xiao X, Li X. Exosome-like vesicles as new mediators and therapeutic targets for treating insulin resistance and β-cell mass failure in type 2 diabetes mellitus. J Diabetes Res (2019) 2019:3256060. doi: 10.1155/2019/3256060
142. Mohammadi MR, Rodriguez SM, Luong JC, Li S, Cao R, Alshetaiwi H, et al. Exosome loaded immunomodulatory biomaterials alleviate local immune response in immunocompetent diabetic mice post islet xenotransplantation. Commun Biol (2021) 4:1–17. doi: 10.1038/s42003-021-02229-4
143. Black CK, Termanini KM, Aguirre O, Hawksworth JS, Sosin M. Solid organ transplantation in the 21st century. Ann Transl Med (2018) 6:409. doi: 10.21037/atm.2018.09.68
144. Hwang B, Bryers J, Mulligan MS. Potential role of exosome-based allorecognition pathways involved in lung transplant rejection. J Thorac Cardiovasc Surg (2021) 161:e129–34. doi: 10.1016/j.jtcvs.2020.04.183
145. Liu Q, Rojas-Canales DM, Divito SJ, Shufesky WJ, Stolz DB, Erdos G, et al. Donor dendritic cell-derived exosomes promote allograft-targeting immune response. J Clin Invest (2016) 126:2805–20. doi: 10.1172/JCI84577
146. Marino J, Babiker-Mohamed MH, Crosby-Bertorini P, Paster JT, LeGuern C, Germana S, et al. Donor exosomes rather than passenger leukocytes initiate alloreactive T cell responses after transplantation. Sci Immunol (2016) 1:aaf8759. doi: 10.1126/sciimmunol.aaf8759
147. Benichou G, Valujskikh A, Heeger PS. Contributions of direct and indirect T cell alloreactivity during allograft rejection in mice. J Immunol (1999) 162:352–8.
148. Mirzakhani M, Shahbazi M, Oliaei F, Mohammadnia-Afrouzi M. Immunological biomarkers of tolerance in human kidney transplantation: An updated literature review. J Cell Physiol (2019) 234:5762–74. doi: 10.1002/jcp.27480
149. Marino J, Paster J, Benichou G. Allorecognition by T lymphocytes and allograft rejection. frontiers in immunology (2016) (Accessed December 8, 2022).
150. Larsen CP, Morris PJ, Austyn JM. Migration of dendritic leukocytes from cardiac allografts into host spleens. A novel pathway for initiation of rejection. J Exp Med (1990) 171:307–14. doi: 10.1084/jem.171.1.307
151. Brown K, Sacks SH, Wong W. Coexpression of donor peptide/recipient MHC complex and intact donor MHC: evidence for a link between the direct and indirect pathways. Am J Transplant (2011) 11:826–31. doi: 10.1111/j.1600-6143.2011.03437.x
152. Brown K, Sacks SH, Wong W. Extensive and bidirectional transfer of major histocompatibility complex class II molecules between donor and recipient cells in vivo following solid organ transplantation. FASEB J (2008) 22:3776–84. doi: 10.1096/fj.08-107441
153. Bracamonte-Baran W, Florentin J, Zhou Y, Jankowska-Gan E, Haynes WJ, Zhong W, et al. Modification of host dendritic cells by microchimerism-derived extracellular vesicles generates split tolerance. Proc Natl Acad Sci U.S.A. (2017) 114:1099–104. doi: 10.1073/pnas.1618364114
154. Yu X, Huang C, Song B, Xiao Y, Fang M, Feng J, et al. CD4+CD25+ regulatory T cells-derived exosomes prolonged kidney allograft survival in a rat model. Cell Immunol (2013) 285:62–8. doi: 10.1016/j.cellimm.2013.06.010
155. Akira S, Uematsu S, Takeuchi O. Pathogen recognition and innate immunity. Cell (2006) 124:783–801. doi: 10.1016/j.cell.2006.02.015
156. Lee H, Zhang D, Wu J, Otterbein LE, Jin Y. Lung epithelial cell–derived microvesicles regulate macrophage migration via MicroRNA-17/221–induced integrin β1 recycling. J Immunol (2017) 199:1453–64. doi: 10.4049/jimmunol.1700165
157. Bowie A, O’Neill LA. The interleukin-1 receptor/Toll-like receptor superfamily: signal generators for pro-inflammatory interleukins and microbial products. J Leukoc Biol (2000) 67:508–14. doi: 10.1002/jlb.67.4.508
158. Takeda K, Akira S. Toll-like receptors in innate immunity. Int Immunol (2005) 17:1–14. doi: 10.1093/intimm/dxh186
159. Matsumoto M, Funami K, Oshiumi H, Seya T. Toll-like receptor 3: A link between toll-like receptor, interferon and viruses. Microbiol Immunol (2004) 48:147–54. doi: 10.1111/j.1348-0421.2004.tb03500.x
160. Heil F, Hemmi H, Hochrein H, Ampenberger F, Kirschning C, Akira S, et al. Species-specific recognition of single-stranded RNA via toll-like receptor 7 and 8. Science (2004) 303:1526–9. doi: 10.1126/science.1093620
161. Miao EA, Andersen-Nissen E, Warren SE, Aderem A. TLR5 and ipaf: dual sensors of bacterial flagellin in the innate immune system. Semin Immunopathol (2007) 29:275–88. doi: 10.1007/s00281-007-0078-z
162. Liang H, Kidder K, Liu Y. Extracellular microRNAs initiate immunostimulation via activating toll-like receptor signaling pathways. ExRNA (2019) 1:9. doi: 10.1186/s41544-019-0009-x
163. Smith KD. Toll-like receptors in kidney disease. Curr Opin Nephrol Hypertens (2009) 18:189–96. doi: 10.1097/MNH.0b013e32832a1d5f
164. Miyazawa Y, Mikami S, Yamamoto K, Sakai M, Saito T, Yamamoto T, et al. AQP2 in human urine is predominantly localized to exosomes with preserved water channel activities. Clin Exp Nephrol (2018) 22:782–8. doi: 10.1007/s10157-018-1538-6
165. Lv L-L, Feng Y, Wu M, Wang B, Li Z-L, Zhong X, et al. Exosomal miRNA-19b-3p of tubular epithelial cells promotes M1 macrophage activation in kidney injury. Cell Death Differ (2020) 27:210–26. doi: 10.1038/s41418-019-0349-y
166. Tangtanatakul P, Klinchanhom S, Sodsai P, Sutichet T, Promjeen C, Avihingsanon Y, et al. Down-regulation of let-7a and miR-21 in urine exosomes from lupus nephritis patients during disease flare. Asian Pac J Allergy Immunol (2019) 37:189–97. doi: 10.12932/AP-130318-0280
167. Harishkumar M, Radha M, Yuichi N, Muthukalianan GK, Kaoru O, Shiomori K, et al. Designer exosomes: Smart nano-communication tools for translational medicine. Bioeng (Basel) (2021) 8:158. doi: 10.3390/bioengineering8110158
168. Bernardi S, Farina M. Exosomes and extracellular vesicles in myeloid neoplasia: The multiple and complex roles played by these “Magic bullets.” Biol (Basel) (2021) 10:105. doi: 10.3390/biology10020105
169. Mineo M, Garfield SH, Taverna S, Flugy A, De Leo G, Alessandro R, et al. Exosomes released by K562 chronic myeloid leukemia cells promote angiogenesis in a src-dependent fashion. Angiogenesis (2012) 15:33–45. doi: 10.1007/s10456-011-9241-1
170. Kore RA, Edmondson JL, Jenkins SV, Jamshidi-Parsian A, Dings RPM, Reyna NS, et al. Hypoxia-derived exosomes induce putative altered pathways in biosynthesis and ion regulatory channels in glioblastoma cells. Biochem Biophys Rep (2018) 14:104–13. doi: 10.1016/j.bbrep.2018.03.008
171. Abbaszade Dibavar M, Pourbagheri-Sigaroodi A, Asemani Y, Salari S, Bashash D. Extracellular vesicles (EVs): What we know of the mesmerizing roles of these tiny vesicles in hematological malignancies? Life Sci (2021) 271:119177. doi: 10.1016/j.lfs.2021.119177
172. Colletti M, Ceglie D, Di Giannatale A, Nazio F. Autophagy and exosomes relationship in cancer: Friends or foes? frontiers in cell and developmental biology (2021) (Accessed November 28, 2022).
173. Salimi L, Akbari A, Jabbari N, Mojarad B, Vahhabi A, Szafert S, et al. Synergies in exosomes and autophagy pathways for cellular homeostasis and metastasis of tumor cells. Cell Biosci (2020) 10:64. doi: 10.1186/s13578-020-00426-y
174. Wu Q, Li J, Li Z, Sun S, Zhu S, Wang L, et al. Exosomes from the tumour-adipocyte interplay stimulate beige/brown differentiation and reprogram metabolism in stromal adipocytes to promote tumour progression. J Exp Clin Cancer Res (2019) 38:223. doi: 10.1186/s13046-019-1210-3
175. Liu Y, Shi K, Chen Y, Wu X, Chen Z, Cao K, et al. Exosomes and their role in cancer progression. frontiers in oncology (2021) (Accessed December 12, 2022).
176. Jiang C, Zhang N, Hu X, Wang H. Tumor-associated exosomes promote lung cancer metastasis through multiple mechanisms. Mol Cancer (2021) 20:117. doi: 10.1186/s12943-021-01411-w
177. Guo H, Chitiprolu M, Roncevic L, Javalet C, Hemming FJ, Trung MT, et al. Atg5 disassociates the V1V0-ATPase to promote exosome production and tumor metastasis independent of canonical macroautophagy. Dev Cell (2017) 43:716–730.e7. doi: 10.1016/j.devcel.2017.11.018
178. Nishimura T, Tooze SA. Emerging roles of ATG proteins and membrane lipids in autophagosome formation. Cell Discovery (2020) 6:32. doi: 10.1038/s41421-020-0161-3
179. De Vries L, Lou X, Zhao G, Zheng B, Farquhar MG. GIPC, a PDZ domain containing protein, interacts specifically with the c terminus of RGS-GAIP. Proc Natl Acad Sci U.S.A. (1998) 95:12340–5. doi: 10.1073/pnas.95.21.12340
180. Bhattacharya S, Pal K, Sharma AK, Dutta SK, Lau JS, Yan IK, et al. GAIP interacting protein c-terminus regulates autophagy and exosome biogenesis of pancreatic cancer through metabolic pathways. PloS One (2014) 9:e114409. doi: 10.1371/journal.pone.0114409
181. Murrow L, Malhotra R, Debnath J. ATG12-ATG3 interacts with alix to promote basal autophagic flux and late endosome function. Nat Cell Biol (2015) 17:300–10. doi: 10.1038/ncb3112
182. Xu J, Camfield R, Gorski SM. The interplay between exosomes and autophagy – partners in crime. J Cell Sci (2018) 131:jcs215210. doi: 10.1242/jcs.215210
183. V.a. S, Bairwa RK, Sharma PK, Bissa B. Cancer cell’s internal and external warriors: Autophagosomes and exosomes. Life Sci (2022) 300:120552. doi: 10.1016/j.lfs.2022.120552
184. Im E-J, Lee C-H, Moon P-G, Rangaswamy GG, Lee B, Lee JM, et al. Sulfisoxazole inhibits the secretion of small extracellular vesicles by targeting the endothelin receptor a. Nat Commun (2019) 10:1387. doi: 10.1038/s41467-019-09387-4
185. Petherick KJ, Conway OJL, Mpamhanga C, Osborne SA, Kamal A, Saxty B, et al. Pharmacological inhibition of ULK1 kinase blocks mammalian target of rapamycin (mTOR)-dependent autophagy. J Biol Chem (2015) 290:11376–83. doi: 10.1074/jbc.C114.627778
186. Young ARJ, Chan EYW, Hu XW, Köchl R, Crawshaw SG, High S, et al. Starvation and ULK1-dependent cycling of mammalian Atg9 between the TGN and endosomes. J Cell Sci (2006) 119:3888–900. doi: 10.1242/jcs.03172
187. Bader CA, Shandala T, Ng YS, Johnson IRD, Brooks DA. Atg9 is required for intraluminal vesicles in amphisomes and autolysosomes. Biol Open (2015) 4:1345–55. doi: 10.1242/bio.013979
188. Raudenska M, Balvan J, Masarik M. Crosstalk between autophagy inhibitors and endosome-related secretory pathways: a challenge for autophagy-based treatment of solid cancers. Mol Cancer (2021) 20:140. doi: 10.1186/s12943-021-01423-6
189. Bansal S, Arjuna A, Perincheri S, Poulson C, Bremner RM, Smith MA, et al. Restrictive allograft syndrome vs bronchiolitis obliterans syndrome: Immunological and molecular characterization of circulating exosomes. J Heart Lung Transplant (2022) 41:24–33. doi: 10.1016/j.healun.2021.09.001
190. Wang L, Wu J, Ye N, Li F, Zhan H, Chen S, et al. Plasma-derived exosome MiR-19b acts as a diagnostic marker for pancreatic cancer. Front Oncol (2021) 11:739111. doi: 10.3389/fonc.2021.739111
191. Zhu X, Long L, Xiao H, He X. Cancer-derived exosomal miR-651 as a diagnostic marker restrains cisplatin resistance and directly targets ATG3 for cervical cancer. Dis Markers (2021) 2021:1544784. doi: 10.1155/2021/1544784
192. Lin Q, Xie D, Pan L, Lou Y, Shi M. Urinary exosomal long noncoding RNAs serve as biomarkers for early detection of non-small cell lung cancer. Biosci Rep (2021) 41:BSR20210908. doi: 10.1042/BSR20210908
193. Gaglani S, Gonzalez-Kozlova E, Lundon DJ, Tewari AK, Dogra N, Kyprianou N. Exosomes as a next-generation diagnostic and therapeutic tool in prostate cancer. Int J Mol Sci (2021) 22:10131. doi: 10.3390/ijms221810131
194. Khan S, Jutzy JMS, Valenzuela MMA, Turay D, Aspe JR, Ashok A, et al. Plasma-derived exosomal survivin, a plausible biomarker for early detection of prostate cancer. PloS One (2012) 7:e46737. doi: 10.1371/journal.pone.0046737
195. Shi S, Li T, Wen X, Wu SY, Xiong C, Zhao J, et al. Copper-64 labeled PEGylated exosomes for In vivo positron emission tomography and enhanced tumor retention. Bioconjug Chem (2019) 30:2675–83. doi: 10.1021/acs.bioconjchem.9b00587
196. Betzer O, Perets N, Angel A, Motiei M, Sadan T, Yadid G, et al. In vivo neuroimaging of exosomes using gold nanoparticles. ACS Nano (2017) 11:10883–93. doi: 10.1021/acsnano.7b04495
197. Pan S, Pei L, Zhang A, Zhang Y, Zhang C, Huang M, et al. Passion fruit-like exosome-PMA/Au-BSA@Ce6 nanovehicles for real-time fluorescence imaging and enhanced targeted photodynamic therapy with deep penetration and superior retention behavior in tumor. Biomaterials (2020) 230:119606. doi: 10.1016/j.biomaterials.2019.119606
198. Ailuno G, Baldassari S, Lai F, Florio T, Caviglioli G. Exosomes and extracellular vesicles as emerging theranostic platforms in cancer research. Cells (2020) 9:2569. doi: 10.3390/cells9122569
199. Rountree RB, Mandl SJ, Nachtwey JM, Dalpozzo K, Do L, Lombardo JR, et al. Exosome targeting of tumor antigens expressed by cancer vaccines can improve antigen immunogenicity and therapeutic efficacy. Cancer Res (2011) 71:5235–44. doi: 10.1158/0008-5472.CAN-10-4076
200. Raposo G, Stoorvogel W. Extracellular vesicles: Exosomes, microvesicles, and friends. J Cell Biol (2013) 200:373–83. doi: 10.1083/jcb.201211138
201. Keller S, Ridinger J, Rupp A-K, Janssen JW, Altevogt P. Body fluid derived exosomes as a novel template for clinical diagnostics. J Trans Med (2011) 9:86. doi: 10.1186/1479-5876-9-86
202. Kanwar SS, Dunlay CJ, Simeone DM, Nagrath S. Microfluidic device (ExoChip) for on-chip isolation, quantification and characterization of circulating exosomes. Lab Chip (2014) 14:1891–900. doi: 10.1039/C4LC00136B
203. He M, Crow J, Roth M, Zeng Y, Godwin AK. Integrated immunoisolation and protein analysis of circulating exosomes using microfluidic technology. Lab Chip (2014) 14:3773–80. doi: 10.1039/C4LC00662C
204. Galindo-Hernandez O, Villegas-Comonfort S, Candanedo F, González-Vázquez M-C, Chavez-Ocaña S, Jimenez-Villanueva X, et al. Elevated concentration of microvesicles isolated from peripheral blood in breast cancer patients. Arch Med Res (2013) 44:208–14. doi: 10.1016/j.arcmed.2013.03.002
205. Mousavi SM, Mahdian SMA, Ebrahimi MS, Taghizadieh M, Vosough M, Nahand JS, et al. Microfluidics for detection of exosomes and microRNAs in cancer: State of the art. Mol Ther - Nucleic Acids (2022) 28:758–91. doi: 10.1016/j.omtn.2022.04.011
206. Son SH, Gangadaran P, Ahn B-C. A novel strategy of transferring NIS protein to cells using extracellular vesicles leads to increase in iodine uptake and cytotoxicity. Int J Nanomed (2019) 14:1779–87. doi: 10.2147/IJN.S189738
207. Lee K, Shao H, Weissleder R, Lee H. Acoustic purification of extracellular microvesicles. ACS Nano (2015) 9:2321–7. doi: 10.1021/nn506538f
208. Zhao Z, Yang Y, Zeng Y, He M. A microfluidic ExoSearch chip for multiplexed exosome detection towards blood-based ovarian cancer diagnosis. Lab Chip (2016) 16:489–96. doi: 10.1039/C5LC01117E
209. Tauro BJ, Greening DW, Mathias RA, Ji H, Mathivanan S, Scott AM, et al. Comparison of ultracentrifugation, density gradient separation, and immunoaffinity capture methods for isolating human colon cancer cell line LIM1863-derived exosomes. Methods (2012) 56:293–304. doi: 10.1016/j.ymeth.2012.01.002
210. Zhang Z, Wang C, Li T, Liu Z, Li L. Comparison of ultracentrifugation and density gradient separation methods for isolating Tca8113 human tongue cancer cell line-derived exosomes. Oncol Lett (2014) 8:1701–6. doi: 10.3892/ol.2014.2373
211. Wu M, Ouyang Y, Wang Z, Zhang R, Huang P-H, Chen C, et al. Isolation of exosomes from whole blood by integrating acoustics and microfluidics. Proc Natl Acad Sci (2017) 114:10584–9. doi: 10.1073/pnas.1709210114
212. Sancho-Albero M, Sebastián V, Sesé J, Pazo-Cid R, Mendoza G, Arruebo M, et al. Isolation of exosomes from whole blood by a new microfluidic device: proof of concept application in the diagnosis and monitoring of pancreatic cancer. J Nanobiotechnol (2020) 18:150. doi: 10.1186/s12951-020-00701-7
213. Giri AK, Ianevski A, Aittokallio T. Genome-wide off-targets of drugs: risks and opportunities. Cell Biol Toxicol (2019) 35:485–7. doi: 10.1007/s10565-019-09491-7
214. Liang X, Wang Y, Shi H, Dong M, Han H, Li Q. Nucleolin-targeting AS1411 aptamer-modified micelle for the Co-delivery of doxorubicin and miR-519c to improve the therapeutic efficacy in hepatocellular carcinoma treatment. Int J Nanomed (2021) 16:2569–84. doi: 10.2147/IJN.S304526
215. Luan X, Sansanaphongpricha K, Myers I, Chen H, Yuan H, Sun D. Engineering exosomes as refined biological nanoplatforms for drug delivery. Acta Pharmacol Sin (2017) 38:754–63. doi: 10.1038/aps.2017.12
216. Chen H, Wang L, Zeng X, Schwarz H, Nanda HS, Peng X, et al. Exosomes, a new star for targeted delivery. frontiers in cell and developmental biology (2021) (Accessed September 30, 2022).
217. Tian Y, Li S, Song J, Ji T, Zhu M, Anderson GJ, et al. A doxorubicin delivery platform using engineered natural membrane vesicle exosomes for targeted tumor therapy. Biomaterials (2014) 35:2383–90. doi: 10.1016/j.biomaterials.2013.11.083
218. Greco KA, Franzen CA, Foreman KE, Flanigan RC, Kuo PC, Gupta GN. PLK-1 silencing in bladder cancer by siRNA delivered with exosomes. Urology (2016) 91:241.e1–7. doi: 10.1016/j.urology.2016.01.028
219. Ishizuya Y, Uemura M, Narumi R, Tomiyama E, Koh Y, Matsushita M, et al. The role of actinin-4 (ACTN4) in exosomes as a potential novel therapeutic target in castration-resistant prostate cancer. Biochem Biophys Res Commun (2020) 523:588–94. doi: 10.1016/j.bbrc.2019.12.084
220. Takahara K, Ii M, Inamoto T, Nakagawa T, Ibuki N, Yoshikawa Y, et al. microRNA-145 mediates the inhibitory effect of adipose tissue-derived stromal cells on prostate cancer. Stem Cells Dev (2016) 25:1290–8. doi: 10.1089/scd.2016.0093
221. Bhatta M, Shenoy GN, Loyall JL, Gray BD, Bapardekar M, Conway A, et al. Novel phosphatidylserine-binding molecule enhances antitumor T-cell responses by targeting immunosuppressive exosomes in human tumor microenvironments. J Immunother Cancer (2021) 9:e003148. doi: 10.1136/jitc-2021-003148
222. Khazaei-Poul Y, Shojaei S, Koochaki A, Ghanbarian H, Mohammadi-Yeganeh S. Evaluating the influence of human umbilical cord mesenchymal stem cells-derived exosomes loaded with miR-3182 on metastatic performance of triple negative breast cancer cells. Life Sci (2021) 286:120015. doi: 10.1016/j.lfs.2021.120015
223. Gao Y, Lu J, Bao X, Yi X, Peng C, Chen W, et al. Inhibition of phospholipases suppresses progression of psoriasis through modulation of inflammation. Exp Biol Med (Maywood) (2021) 246:1253–62. doi: 10.1177/1535370221993424
224. Rendon A, Schäkel K. Psoriasis pathogenesis and treatment. Int J Mol Sci (2019) 20:E1475. doi: 10.3390/ijms20061475
225. Zhang Q, Piao C, Ma H, Xu J, Wang Y, Liu T, et al. Exosomes from adipose-derived mesenchymal stem cells alleviate liver ischaemia reperfusion injury subsequent to hepatectomy in rats by regulating mitochondrial dynamics and biogenesis. J Cell Mol Med (2021) 25:10152–63. doi: 10.1111/jcmm.16952
226. Gangadaran P, Rajendran RL, Lee HW, Kalimuthu S, Hong CM, Jeong SY, et al. Extracellular vesicles from mesenchymal stem cells activates VEGF receptors and accelerates recovery of hindlimb ischemia. J Control Release (2017) 264:112–26. doi: 10.1016/j.jconrel.2017.08.022
227. Gangadaran P, Rajendran RL, Oh JM, Hong CM, Jeong SY, Lee S-W, et al. Extracellular vesicles derived from macrophage promote angiogenesis In vitro and accelerate new vasculature formation In vivo. Exp Cell Res (2020) 394:112146. doi: 10.1016/j.yexcr.2020.112146
228. Chen C, Wang D, Moshaverinia A, Liu D, Kou X, Yu W, et al. Mesenchymal stem cell transplantation in tight-skin mice identifies miR-151-5p as a therapeutic target for systemic sclerosis. Cell Res (2017) 27:559–77. doi: 10.1038/cr.2017.11
229. Harrell CR, Jovicic N, Djonov V, Arsenijevic N, Volarevic V. Mesenchymal stem cell-derived exosomes and other extracellular vesicles as new remedies in the therapy of inflammatory diseases. Cells (2019) 8:E1605. doi: 10.3390/cells8121605
230. Riazifar M, Mohammadi MR, Pone EJ, Yeri A, Lässer C, Segaliny AI, et al. Stem cell-derived exosomes as nanotherapeutics for autoimmune and neurodegenerative disorders. ACS Nano (2019) 13:6670–88. doi: 10.1021/acsnano.9b01004
231. Upadhya R, Madhu LN, Attaluri S, Gitaí DLG, Pinson MR, Kodali M, et al. Extracellular vesicles from human iPSC-derived neural stem cells: miRNA and protein signatures, and anti-inflammatory and neurogenic properties. J Extracell Vesicles (2020) 9:1809064. doi: 10.1080/20013078.2020.1809064
232. Li S, Tang Y, Dou Y. The potential of milk-derived exosomes for drug delivery. Curr Drug Delivery (2021) 18:688–99. doi: 10.2174/1567201817666200817112503
233. Munagala R, Aqil F, Jeyabalan J, Gupta RC. Bovine milk-derived exosomes for drug delivery. Cancer Lett (2016) 371:48–61. doi: 10.1016/j.canlet.2015.10.020
234. Somiya M, Yoshioka Y, Ochiya T. Biocompatibility of highly purified bovine milk-derived extracellular vesicles. J Extracell Vesicles (2018) 7:1440132. doi: 10.1080/20013078.2018.1440132
235. Aqil F, Munagala R, Jeyabalan J, Agrawal AK, Gupta R. Exosomes for the enhanced tissue bioavailability and efficacy of curcumin. AAPS J (2017) 19:1691–702. doi: 10.1208/s12248-017-0154-9
236. Del Pozo-Acebo L, Hazas M-CL de L, Tomé-Carneiro J, Gil-Cabrerizo P, San-Cristobal R, Busto R, et al. Bovine milk-derived exosomes as a drug delivery vehicle for miRNA-based therapy. Int J Mol Sci (2021) 22:1105. doi: 10.3390/ijms22031105
237. Warren MR, Zhang C, Vedadghavami A, Bokvist K, Dhal PK, Bajpayee AG. Milk exosomes with enhanced mucus penetrability for oral delivery of siRNA. Biomater Sci (2021) 9:4260–77. doi: 10.1039/D0BM01497D
Keywords: exosomes, secretory cells, tissue inflammation, circulation, therapy
Citation: Gangadaran P, Madhyastha H, Madhyastha R, Rajendran RL, Nakajima Y, Watanabe N, Velikkakath AKG, Hong CM, Gopi RV, Muthukalianan GK, Valsala Gopalakrishnan A, Jeyaraman M and Ahn B-C (2023) The emerging role of exosomes in innate immunity, diagnosis and therapy. Front. Immunol. 13:1085057. doi: 10.3389/fimmu.2022.1085057
Received: 31 October 2022; Accepted: 16 December 2022;
Published: 16 January 2023.
Edited by:
Lei Huang, Newcastle University, United KingdomReviewed by:
Yukihiro Yamaguchi, University of North Carolina at Chapel Hill, United StatesCopyright © 2023 Gangadaran, Madhyastha, Madhyastha, Rajendran, Nakajima, Watanabe, Velikkakath, Hong, Gopi, Muthukalianan, Valsala Gopalakrishnan, Jeyaraman and Ahn. This is an open-access article distributed under the terms of the Creative Commons Attribution License (CC BY). The use, distribution or reproduction in other forums is permitted, provided the original author(s) and the copyright owner(s) are credited and that the original publication in this journal is cited, in accordance with accepted academic practice. No use, distribution or reproduction is permitted which does not comply with these terms.
*Correspondence: Harishkumar Madhyastha, aGt1bWFyQG1lZC5taXlhemFraS11LmFjLmpw; Byeong-Cheol Ahn, YWJjMjAwMEBrbnUuYWMua3I=
†Present address: Madhan Jeyaraman, Department of Orthopaedics, ACS Hospital, Dr. MGR Educational and Research Institute University, Chennai, Tamil Nadu, India
Disclaimer: All claims expressed in this article are solely those of the authors and do not necessarily represent those of their affiliated organizations, or those of the publisher, the editors and the reviewers. Any product that may be evaluated in this article or claim that may be made by its manufacturer is not guaranteed or endorsed by the publisher.
Research integrity at Frontiers
Learn more about the work of our research integrity team to safeguard the quality of each article we publish.