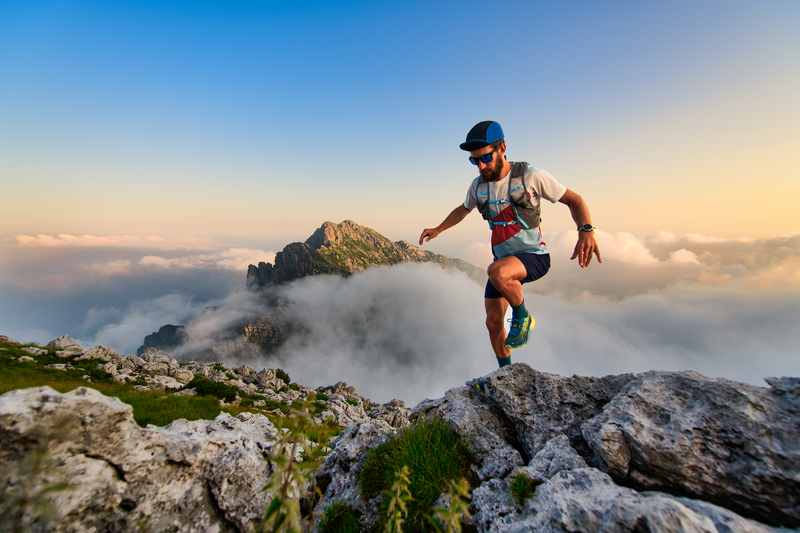
95% of researchers rate our articles as excellent or good
Learn more about the work of our research integrity team to safeguard the quality of each article we publish.
Find out more
REVIEW article
Front. Immunol. , 23 December 2022
Sec. Cancer Immunity and Immunotherapy
Volume 13 - 2022 | https://doi.org/10.3389/fimmu.2022.1075260
This article is part of the Research Topic Extracellular Traps in Cancer Immunity and Immunotherapy View all 7 articles
The tumor immune microenvironment (TIME) controls tumorigenesis. Neutrophils are important components of TIME and control tumor progression and therapy resistance. Neutrophil extracellular traps (NETs) ejected by activated neutrophils are net-like structures composed of decondensed extracellular chromatin filaments decorated with a plethora of granules as well as cytoplasmic proteins. Many of these harbour post translational modifications. Cancer cells reportedly trigger NET formation, and conversely, NETs alter the TIME and promote tumor cell proliferation and migration. The specific interactions between NETs and TIME and the respective effects on tumor progression are still elusive. In certain tumors, a CD4+ T helper (Th) 2 cell-associated TIME induces NETs and exerts immunosuppressive functions via programmed death 1 (PD-1)/PD-L1, both associated with poorer prognosis. In other cases, NETs induce the proliferation of Th1 cells, associated with an improved prognosis in cancer. In addition, NETs can drive macrophage polarization and often rely on macrophages to promote cancer cell invasion and metastasis. In turn, macrophages can swiftly clear NETs in an immunologically silent manner. The aim of this review is to summarize the knowledge about the mutual interaction between NETs and TIME and its impact on tumor growth and therapy.
The tumor immune microenvironment (TIME) is orchestrated by the interaction between immune and tumor cells and is shaped by various cytokines and chemokines (1–3). It plays a pivotal role in the initiation, progression, invasion, and metastasis of cancers. Tumor infiltrating immune cells can promote or inhibit tumorigenesis.
Most studies focused on the role of adaptive immune cells in cancer. Various pre-clinical and clinical models demonstrated that T lymphocytes exert an integral role in tumor immune defence. Cytotoxic CD8+ T cells (CTLs) are prominent components within the TIME and form a homogenous population of cytotoxic cells that secret interferon (IFN)-γ. CTLs drive anti-tumor responses and improve patient prognosis (4). In contrast, regulatory T cells (Tregs) dampen immune responses and, thus, contribute to the immune evasion of tumor cells (5). Th cells come in different flavours and form functionally different populations, such as Th1, Th2 and Th17 cells (6). Th1 cells shape the anti-tumor immunity, induce CTLs and are associated with improved prognosis. On the contrary, Th2 cells promote humoral immunity, restrain Th1 responses and are associated with poorer prognosis (6–8). Th17 cells exhibit heterogeneity in human cancer with the expression of various activated markers, cytokines and transcriptional factors leading to different prognoses of patients. It still remains a challenge to use Th17 cells as a predictor for the prognosis in human cancer (9).
These preclinical studies provided indispensable help for the promising improvement of the prognosis of cancer diseases over the last decades. However, in most solid tumors, particularly when CTL infiltration is low and immunosuppressive immune cell infiltration is high, metastasis is responsible for the majority of cancer-related mortality. Tumor cells may escape current immunotherapies and the resistance to immunotherapy is partly due to the dysregulation of innate immune cells (10), such as dendritic cells (DCs) (11), tumor-associated neutrophils (TANs) (12), tumor-associated macrophages (TAMs) (13), natural killer (NK) cells (14), and myeloid-derived suppressor cells (MDSCs) (15). These innate immune cells participate in the malignant progression from the primary to the metastatic tumor. They display a high plasticity often depending on the type and stage of the different tumors (Figure 1) (16, 17).
Figure 1 The TIME: Immune cells and soluble mediators shape the diversity of the TIME. Innate immunity plays multiple roles in cancer and shows a high plasticity depending on changes of the TIME. In Anti-tumorigenic TIMEs, innate immune cells (1) recognize and present tumor cell-derived antigenic peptides to T cells and activate the adaptive immunity (2); eliminate tumor cells directly by phagocytosis and secrete cytotoxic substances, like perforin and granzyme (3); secrete proinflammatory cytokines and, thus, expand and promote a wide variety of Anti-tumor responses. In a Pro-tumorigenic TIME, innate immune cells (1) differentiate towards an immunosuppressive phenotype with the release of immunosuppressive cytokines (2); induce the infiltration of immunosuppressive adaptive immune cells, such as Tregs and Th2 cells (3); increase the formation of extracellular traps (ETs); and (4) promote angiogenesis (5), epithelial-mesenchymal transition (EMT) and (6) extracellular matrix (ECM) remodelling. Consequently, they enhance tumor invasion and migration. The plasticity of innate immunity allows remodelling of the TIME. CTLA-4, cytotoxic T lymphocyte associated antigen 4; DCs, dendritic cells; ECM, extracellular matrix; EMT, epithelial mesenchymal transition; G-CSF, granulocyte colony stimulating factor; GM-CSF, granulocyte-macrophage colony stimulating factor; IFN, interferon; IL, interleukin; MDSC, myeloid-derived suppressor cells; METs, macrophage extracellular traps; MMPs, matrix metalloproteinases; NETs, neutrophil extracellular traps; NK, natural killing; NO, nitric oxide; PD-L1, programmed death-ligand 1; ROS, reactive oxygen species; TGF, transforming growth factor; TNF, tumor necrosis factor; VEGF, vascular endothelial growth factor.
Neutrophils are the first line of defence against various kinds of pathogens. They primarily act as innate effector cells and account for around 70% of circulating leukocytes in humans (18). In both infections and cancer, their functions are predominantly implemented via degranulation, phagocytosis and the formation of neutrophil extracellular traps (NETs) (19). NETs have originally been described in 2004 as a nucleic acid based structure involved in bacterial defence (20). NETs ejected by activated neutrophils are net-like structures composed of decondensed extracellular chromatin filaments decorated with granular proteins, such as neutrophil elastase (NE), cathepsin G, myeloperoxidase (MPO), matrix metalloproteinases 9 (MMP9) and histones; the latter are often posttranslationally citrullinated (21). NETs were first identified as contributors to the innate immune response, capable of directly immobilizing and killing pathogens or releasing anti-microbial agents (20). Recently, NETs have been reported to play an important role in cancer initiation and progression (22–24). They are essential in the development of pre-metastatic niches, awakening of dormant metastases and may directly promote tumor growth by associated proteases, such as NE and MMP9 through proteolytic remodelling of laminin (12). NETs can also entrap circulating tumor cells and act as adhesion substrate to promote their adhesion, invasion and migration (25, 26). In comparison to the direct impact on cancer cells, only little is known about the mutual interaction of NETs with infiltrating immune cells in the TIME.
Macrophages are large phagocytic cells, not only pivotal for host defence, but also essential for tissue homeostasis (27). The major function of macrophages is to recognize and phagocytose cellular debris and opsonize immune complexes. Macrophages prey proteins, process them and present the respective peptides to T cells and elicit adaptive immune responses. Macrophages are regarded as one of the most important bridges between innate and adaptive immunity. Within innate immune cells, monocyte derived macrophages reflect the Th1/Th2 paradigm through their ability to differentiate into the inflammatory M1 or immune suppressive M2 phenotype in vitro (28). However, several other macrophage subtypes have been described in addition to M1 and M2 representing extremes of a multidimensional/spectral continuum (29). High macrophage infiltration in most solid tumors correlates with poor overall survival. It is associated with changes in cancer-related inflammation, angiogenesis, extracellular matrix (ECM) remodelling, and epithelial mesenchymal transition (EMT) of cancer cells (30–33). Macrophages can also form extracellular traps (ETs), referred to as macrophage extracellular traps (METs) (34). METs can activate the migration and invasion of tumor cells and are an independent risk factor for the prognosis of colorectal cancer (CRC) (35).
Although some studies have shown the biological function of NETs in cancer, the crosstalk between NETs, macrophages, and METs still remains elusive. The contribution of NETs to TIME including innate and adaptive immunity is also underexplored. More effective strategies may be inspired by better understanding of how the TIME and NETs interact. Therefore, this review will integrate the available knowledge in this context aiming to explore the interaction between NETs and TIME on tumor progression.
There are two main models of NET formation: suicidal NETosis and vital NET formation (Figure 2) (36–38).
Figure 2 NET formation: suicidal NETosis and vital NET formation establish two main types of chromatin release by neutrophils. Suicidal NETosis is characterized by ROS generation and rupture of neutrophils. Neutrophils are activated by stimuli, such as PMA, cholesterol crystals, certain autoantibodies or immune complexes. These extracellular signals induce the phosphorylation of the NOX complex and the release of ROS. This process depends on a high Ca2+ concentration. Subsequently, PAD4 is activated and causes the translocation of NE and MPO from azurophilic granules to the nucleus. NE and MPO combined with PAD4 result in the citrullination of histones and chromatin decondensation. After rupture of the nuclear membrane, the decondensed chromatin enters the cytoplasm mixed with granular proteins. Finally, the cytoplasma membrane gets leaky, the modified chromatin is released from neutrophils and forms NETs. In contrast, Vital NET formation is executed in a shorter time after activation of neutrophils and can also occur in the absence of the NOX complex and ROS. Vital NET formation is initiated by stimuli, such as S. aureus through TLR2 and complement receptors, or LPS from gram negative bacteria through TLR4 or indirectly through TLR4-activated platelets. PAD4 is activated and NE and MPO translocate to the nucleus to promote chromatin decondensation. The decondensed chromatin decorated with granular proteins and histones is packed in vesicles that bud from nuclei. Subsequently, these vesicles are expelled from intact neutrophils and form NETs in the vicinity of the neutrophils. In consequence, neutrophils stay intact and can exert further functions, such as phagocytosis. Figure adopted with modifications from [36], with permission from Springer Nature, Nature medicine © [2017]. Abs, antibodies; FcR, Fc receptor; GP, glycoprotein; MPO, myeloperoxidase; NE, neutrophil elastase; NOX, NADPH oxidase; P, phosphorylation; PAD4, protein-arginine deiminase 4; PMA, phorbol-12-myristate-13-acetate; ROS, reactive oxygen species; S.aureus, staphylococcus aureus; TLR, toll like receptor.
Suicidal NETosis begins with the activation of neutrophils by stimuli, such as immune complexes, certain autoantibodies, calcium-salt or cholesterol crystals, or phorbol-12-myristate-13-acetate (PMA) (39). These stimuli activate the NADPH oxidase (NOX) complex and lead to subsequent formation of reactive oxygen species (ROS) through Raf/MEK/ERK signalling, along with an increase in cytosolic Ca2+ concentrations. Then, NOX and ROS complexes induce the translocation of NE and MPO from neutrophil granules into the nucleus together with the activation of protein-arginine deiminase 4 (PAD4), which reduces positive charges from histones. This causes chromatin decondensation in the nuclei of neutrophils (39, 40). Decondensed chromatin enters the cytoplasm, mixes with granular and cytosolic proteins and is finally expelled outside neutrophils accompanied by cellular lysis. Here, it forms NETs. Suicidal NETosis causes the death of the respective cell due to membrane disintegration and this process can take several hours to complete (41).
Vital NET formation occurs independently of cell death in the absence of membrane disruption within minutes after stimulation of neutrophils (42). The process is initiated by stimuli such as S. aureus or lipopolysaccharide (LPS) from gram negative bacteria through toll-like receptors (TLRs) and complement receptors (CR) (36). Vital NET formation does not rely on the NOX complex or ROS. The release of nuclear DNA in vital NET formation is associated with characteristic morphological changes (1): nuclear envelope growth and the release of vesicles (2); nuclear decondensation, and (3) nuclear envelope disruption (43–45). Vital NET formation is observed more often in infectious than in non-infectious diseases. This is supported by the observation that neutrophils stay alive and are still able to perform anti-microbial functions such as chemotaxis, phagocytosis, and killing of bacteria (46). In a specific type of vital NET formation, mitochondrial DNA may also be released, and this is dependent on ROS. This process results in NET formation from 80% of the neutrophils within 15 min following stimulation with C5a or LPS (47, 48).
Regardless of the type of NET formation certain factors, such as PAD4, NE and MPO are commonly involved in NET formation (49). However, not all of them are strictly required. PAD4 is a calcium-dependent enzyme dispersed in the nucleus, cytoplasm, and secretory granules of neutrophils. Nuclear PAD4 converts arginine in proteins (e.g. in histones H3, H2A, and H4) to citrulline. Every citrullination neutralizes one positive charge of histones and decreases their affinity for nucleic acids and concomitantly supports chromatin decondensation (50). In naive neutrophils, NE and MPO are stored in azurophilic granules (51, 52). In activated neutrophils, NE enters the nucleus, where it clips the tails of certain histones further supporting chromatin decondensation (20). Although MPO has only a minor effect on chromatin decondensation on its own, it binds to DNA and catalyzes oxidative reactions that promote NE relocation (40). Thus, MPO synergizes with NE in chromatin decondensation. Furthermore, both NE and MPO reportedly decorate the DNA backbone of NET fibers (20).
Histone citrullination is a characteristic feature of NET formation and the detection of citrullinated histones on extracellular chromatin is often used to identify NETs in tissues (53, 54). As an example, citrullinated NETs were significantly associated with high histopathological tumor grades and lymph node metastasis in human CRC (Figure 3) (55).
Figure 3 NETs in colorectal cancer identified by interstitial H3cit are associated with high histopathological tumor grades and lymph node metastasis in human CRC. (A, B) NE, H2B, and H3cit were used to detect NET formation on consecutive sections of CRC by immunofluorescence. Draq5 served as counterstain. Notably, regions with extranuclear H3cit colocalize with NE H2B in consecutive sections. Extracellular DNA detected by anti-DNA antibody is restricted to H3cit positive tissues. Scale bar: 75µm. (C) In human CRC tissues, NETs identified by H3cit positively correlated with high histopathological grading and lymph node metastasis. Results taken from [55], © [2022] Pathological Society of Great Britain and Ireland, first published by John Wiley & Sons Ltd. H2B, histone H2B; H3cit, citrullinated histone 3; NE, neutrophil elastase; NETs, neutrophil extracellular traps. ****p < 0.0001, *p < 0.05.
There are several reports documenting the presence of NETs in tumor tissues (56–58). In agreement with the co-cultivation of cancer cells with neutrophils resulting in NET formation within 3 hours, electron microscopy showed that neutrophils were destructed and did not provide evidence for DNA-containing vesicles budding from intact neutrophils (58). Moreover, the cancer cell-induced NET formation depended on NOX activity (58). Altogether these findings indicated that cancer cells induced suicidal rather than vital NET formation (58).
The TME is rich in factors that can promote NET formation from both TAN and granulocytic myeloid-derived suppressor cells (GR-MDSCs), such as granulocyte-colony-stimulating factor (G-CSF) and interleukin-8 (IL-8) (59–62). G-CSF is a cytokine produced by leukocytes, macrophages, endothelium, fibroblasts and cancer cells. The expression of G-CSF is highly increased in both murine and human tumor cells (63–65). G-CSF overexpression predisposes neutrophil recruitment into metastatic lesions and enhances migration and invasion of tumor cells via generation of ROS, NET formation and production of other pro-tumor proteins (59, 66–68). IL8 is a chemokine of the CXC glutamic acid-leucine-arginine motif bearing (ELR+) family and was initially identified as a powerful chemotactic factor for neutrophils (69, 70). IL8 is produced in large amounts by several human tumors and as a main agonist of CXCR1 and CXCR2 was mostly implicated in the recruitment of neutrophils and MDSCs (61, 71). In many types of human cancer, such as bladder cancer, non-small cell lung cancer (NSCLC) and metastatic melanoma NETs show a positive association with IL-8 in tumor tissues and serum (72). In patients with diffuse large B-cell lymphoma (DLBCL), DLBCL-derived IL8 interacting with CXCR2 on neutrophils resulted in NET formation via Src, p38 and ERK signalling. Blocking of the IL8–CXCR2 axis inhibited the formation of NETs (73). Conditioned media (CM) harvested from different cancer cell lines also induced NET formation, both from neutrophils and granulocytic myeloid-derived suppressor cells (GR-MDSCs). Blocking of CXCR1 and CXCR2 with Reparixin or a CXCR1 blocking monoclonal antibody (mAb) inhibited NET formation induced by the respective CM (61, 74). Besides G-CSF and IL-8, many additional pro-inflammatory cytokines and damage-associated molecular pattern (DAMP) present in the TIME are well established to promote NET formation. In consequence, it is generally accepted that the TIME plays a critical role in the development of malignant tumors and that NETs exhibit a significant impact on tumorigenesis (75). However, how the initiation and progression of tumors is regulated by NETs and how these functions are affected by different TIMEs remains elusive.
In the following we will discuss the impact of different cell populations of the adaptive immune system on the formation and tumorigenic activities of NETs.
T cells are among the most plastic cells in response to different TIMEs as they can be rendered dysfunctional and exhausted by chronic persistent antigen stimulation allowing immune escape and augmenting tumor development (76). CD8+ T cells of the adaptive immune system are the most potent effectors in the anti-cancer immune response and serve as executors of cancer immunotherapies with a large impact on the outcome of many different tumors (77). An interplay between NETs and CD8+ T cells in the TIME was suggested by de Andrea and colleagues who reported that NET density in human tumor tissues and NET concentrations in the serum of cancer patients negatively correlated with CD8+ T cell counts in tumor tissues (72). In analogy, Kaltenmeier and colleagues reported that NET-rich TIMEs, both in a murine liver ischemia/reperfusion (IR) metastasis model and in a murine subcutaneous tumor model induced exhaustion and dysfunction of CD8+ T cells (78). This was accompanied by an increase of the exhaustion markers PD-1, Tim3, and Lag3 together with a diminished production of the effector cytokines IL-2, IFN-γ, and TNF-α, and altered metabolic profiles including decreased mitochondrial function, glucose uptake, and upregulated fatty acid intake (78). Continuous exposure of murine T cells to NETs in vitro induced an exhaustive phenotype as well, supporting direct effects of NETs on CD8+ T cells (78). The assessment of mechanisms how NETs regulate T cell function in the TIME identified that PD-L1 was embedded in the NET chromatin of wild type (WT) bone marrow (BM) derived-neutrophils treated with PMA (79). Immune inhibitory receptor PD-1 and its ligand PD-L1 were previously recognized as an immune inhibitory axis on the surface of T cells promoting the depletion of functional T cells and tumor immune escape (79). Accordingly, NETs may directly provoke the exhaustion of T cells via the PD-1/PD-L1 axis. Consequently, targeting of PD-L1 in NETs restored functional T cells and ameliorated the tumor burden (78).
Apart from direct effects of NETs on T cell exhaustion, NETs are also able to impair the cytotoxic function of T cells by physical shielding. Teijeira et al. identified in a co-culture system and a mouse model that NETs alone cannot impact tumor cell spheroid survival or proliferation (61). However, coating of tumor cells with NETs impaired cytotoxicity of CD8+ T cells and protected the tumor cells from direct contact with CTLs (61). Several other studies confirmed that NETs protect tumor cells by forming a physical barrier at the tumor/stroma interface. This abrogates the infiltration of CD8+ T cells into the tumor cell areas (58, 80, 81). Apparently, this barrier function of NETs can protect tumors from cytotoxic immune attacks.
CD4+ T cells are essential regulators in cancer immunosurveillance. They modulate the tumor microenvironment and eradicate tumor cells. NETs can exert an immunosuppressive function by inhibition of CD4+ T cells (78). CD4+ T cells are highly heterogeneous and exert different immune responses to various pathogens (82). Three major subsets of CD4+ T cells have been identified so far: Th cells, Tregs and follicular helper T cells (TFH) (83). Th cells have drawn a lot of attention since their integral roles in the TIME was demonstrated by several pre-clinical and clinical models (84, 85). Th1 and Th2 are the two predominant categories of CD4+ Th cells. Th1 and Th2 are characterized by high levels of IFN-γ and IL-4 expression, respectively (86). For Th1 polarization, the signal transducer and activator of transcription-1 (STAT1) and STAT4 activated by IL-12 and IFN-γ induce the T-box transcription factor (T-bet) that drives Th1 differentiation and suppresses Th2 differentiation. IFN-γ in turn secreted from Th1 cells stabilizes Th1 differentiation forming a positive feedback loop (86, 87). Th2 differentiation is dependent on the expression of IL-4, which results in STAT6-mediated activation of the GATA3 transcription factor that stimulates Th2 polarization and suppresses Th1 differentiation. Similar to Th1 differentiation, an autocrine positive feedback loop of IL-4 stabilizes Th2 differentiation (87, 88). The balance between Th1 and Th2 CD4+ T cells is critically regulating tumorigenesis. A dominance of a Th1 and Th2 TIME is associated with improved or poor prognosis, respectively (88).
It has been shown that in some non-cancer diseases, such as type 1 diabetes (T1D), rheumatoid arthritis (RA), and chronic pneumonia NETs exacerbated Th1 responses by activation of dendritic cells (89–91). Moreover, NETs may also promote a Th1-like TIME through activation of peripheral blood mononuclear cells (PBMCs), which contributed to the recruitment of T cells and monocytes-macrophages and prevented tumor growth (92). In a murine model of bladder cancer Bacillus Calmette-Guerin (BCG) caused NET-induced apoptosis, cell-cycle arrest, and inhibited migration of tumor cells (92). In contrast, Zheng and colleagues found that in a murine breast cancer model with lung metastasis Th2 cytokines stimulated NET formation by maintaining complement 3 (C3) highly expressed in lung mesenchymal stromal cells (LMSCs) through the STAT6 signalling pathway (93). The Th2-STAT6-C3-NET cascade promoted lung metastasis and NET formation was essentially required for lung metastasis in the Th2 prominent TIME (93). Th2 cells are regarded as contributors to the immunosuppression and tumor escape, corresponding to NETs, which lead to the exhaustion and dysfunction of CLTs. The partially conflicting results indicate that different TIMEs and prognoses can be associated with NETs. However, it is clear that NETs can regulate the differentiation of TIME.
Th1 cells are the main cytotoxic subtype of CD4+ T cells. Tregs are critical for immune tolerance and homeostasis limiting potential collateral tissue damage after the initial CTL response. The central differentiation factor of Tregs is the transcription factor forkhead box protein P3 (FOXP3) (94). Wang and colleagues observed that in the non-alcoholic steatohepatitis-hepatocellular carcinoma model (NASH-HCC model, STAM) the overall number of CD4+ T cells dropped while the number of Tregs sharply increased coincident with more severe tumor burden. After depletion of Tregs by diphtheria toxin (DT) Th1 cells counts increased and tumor burden decreased (95). Meanwhile, it has been repeatedly confirmed that Tregs impair cancer immunosurveillance by suppressing immunity and hampering immune responses; that fosters tumor development and progression (96–99). Altogether, Tregs establish an immunosuppressive subset of CD4+ T cells and play an important role in the TIME. Interestingly, in murine models of liver cancer [non-alcoholic fatty liver disease (NAFLD), non-alcoholic steatohepatitis (NASH)] NETs co-localized with Tregs, occurred in early stages, and persisted during cancer development (95). Also in humans a positive correlation between the presence of the NET marker H3cit and FoxP3 was observed in livers of patients with NASH or NASH-HCC (95). From this it has been suggested that Tregs may induce NET formation (95). Vice versa, NETs can also induce Treg differentiation from naïve CD4+ T cells in the NASH liver microenvironment through the reprogramming of metabolic pathways involved in the oxidative phosphorylation (OXPHOS) of mitochondrial respiration (95). NETs activated TLR4 on the surface of naïve CD4+ T cells and subsequently modulated OXPHOS by enhancing oxidation of NADH to NAD+, which was required for Treg differentiation (95, 100). This was further confirmed by NET ablation, which was associated with a decrease of Treg-specific FoxP3 protein levels in the liver of STAM mice followed by alleviated HCC development and progression (95).
In conclusion, most studies summarized in the chapter above indicate that NETs exert immunosuppressive functions on CD8+ T cells and CD4+ T cells augmenting the progression of tumors, further supporting an important role of NETs in the TIME. Enigmatically, in certain non-cancer diseases NETs appear pro-inflammatory through eliciting immune functions.
In the following we will discuss the impact of cell subtypes of the innate immune system on the formation and tumorigenic activities of NETs.
The innate immunity establishes the first line of anti-microbial defence with a major impact on the TIME. Various kinds of macrophages together with dendritic cells, histiocytes, Kupffer cells, and mast cells are the gate keepers of innate immunity. Besides long-lived sessile tissue macrophages, monocytes from peripheral blood differentiate into macrophages after recruitment to tissues. Macrophages make up the majority of myeloid immune cells in the TIME (101, 102). They possess phagocytic functions and serve as antigen presenters in the TIME. Dependent on their activation, macrophages can be polarized into many distinct subsets, with M1 and M2 macrophages being the extremes of a multidimensional continuum (29).
M1 macrophages are induced in a Th1 TIME by pro-inflammatory cytokines, such as IFN-γ. They mediate the defence of the host from a variety of antigens and have roles in the anti-tumor immunity (103). M2 macrophages are induced in a Th2 TIME and exert an anti-inflammatory function and immunosuppressive activity (103).
Beyond their antigen-presenting capabilities and phagocytic functions, macrophages regulate NET formation and clearance. Haider and colleagues analysed abdominal aortic aneurysm (AAA) and found macrophage counts to be inversely correlated with NETs in both the intraluminal thrombi and the vessel wall (104). Macrophages, when seeded on blood-clots coated by NETs in vitro showed the ability to digest NETs by secreted deoxyribonucleases (DNases) followed by phagocytosis of the NET remnants (104). Specially, M1 macrophages were most active in NET clearance by macropinocytosis (104). Expanding on macrophage-dependent NET degradation, Fadeel and colleagues reported that physiological concentrations of extracellular DNase I were not sufficient to completely clear NETs and the cytosolic exonuclease TREX 1 (DNase III) in macrophages was required for the digestion of NETs after phagocytic uptake (105, 106).
In contrast, other reports indicated that macrophages can also induce the formation of NETs. It was shown that exosomal miR-146a from oxidized low-density lipoprotein (oxLDL)-stimulated macrophages promoted the excessive release of NETs, concomitant with the overexpression of ROS and high levels of pro-inflammatory cytokines such as IL-8, TNF-α, IL-6, and IL-1β (107). This indicated that according to their stimulation macrophages may decrease or increase the concentrations of NETs in tissues. In a third scenario macrophages may also mediate the activity of NETs. For example in an in vitro co-culture system of macrophages and A549 lung cancer cells, Zhang and colleagues reported that addition of purified NETs promoted migration and invasion of A549 lung cancer cells (108). They suggested that this partly depended on the cytokines IL-1β, IL-6, IL-18 and TNF-α, which were released from the macrophages (108). Interestingly, a subsequent study showed that the treatment of macrophages with NETs also increased extracellular M1 macrophage-derived DNA, likely representing METs (109). Accordingly, METs may have contributed to the activation of migration and invasion of tumor cells in the co-culture experiment.
In conclusion, it is best established that macrophages exert disintegrating functions on NETs. However, sporadic reports on different effects of macrophages on the formation and the activity of NETs indicate that the specific outcome of the interaction of NETs with macrophages may be TIME related, warranting further investigations.
Not only neutrophils but also macrophages can form net-like structures, referred to as METs. Similar to NETs, METs are composed of DNA and extracellular proteins and are produced in response to various pathogens and chemical stimuli. METs are generated in the course of a unique cell death program of macrophages (METosis) and until now have only been observed in the inflammatory M1 subset after treatment with IFN-γ and LPS (109, 110).
Only few differences have been reported between NETs and METs. King and colleagues noted at the morphological level that chromatin fragments are longer in NETs as compared to METs (111). In addition, METs are associated with the macrophage marker protein CD68 and the matrix metalloproteinase (MMP) 12 (111–113). Moreover, differences have been reported at the signalling processes responsible for extracellular trap formation. For example, Aulik and colleagues reported that after stimulation of cells MET formation occurs faster than NET formation (114). Finally, PAD4 and PAD2 are considered to be the main promoters of NET and MET formation, respectively (115, 116). Notably, elastase and MPO, which are often regarded as neutrophil-specific markers, have also been identified in METs (117, 118). Accordingly, co-staining of H3cit together with CD68 seems to be at present the most specific approach to distinguish METs and NETs (111–113). The differences between NETs and METs may cause their different roles in host defence.
NETs and METs generally protect against microorganisms by immobilizing them (119). However, at present it is not clear whether this immobilization is specifically microbicidal, because MET formation has also been found to promote pathogen growth by providing a scaffold for aggregation rather than killing pathogens (114, 119, 120).
In tumor tissues, macrophages are often present in higher numbers as compared to neutrophils (121). However, the presence of METs and NETs was not found to be significantly related with the infiltration of the respective cell types (122). Accordingly, the presence of METs and NETs may be more strongly related to the responsiveness of the respective cell types to TIME-derived stimuli and to different stability of NETs and METs. The first hypothesis was supported by the fact that higher concentrations of PMA are required to induce the formation of METs compared with NETs and that this process is accompanied by a higher ROS production in macrophages (111, 114).
To specifically address the relevance of METs in cancers, a retrospective study of 135 patients after radical resection of non-functioning pancreatic neuroendocrine tumors (pNETs) was analysed and showed that both NETs and METs were associated with shorter recurrence-free survival (RFS) (122). In univariate and multivariate Cox regression analyses NETs and METs were independent prognostic factors for shorter RFS and better indicators than WHO grade and TNM stage to predict the prognosis for patients (122). This finding was confirmed in another study with 116 CRC patients. In a training and a validation cohort (n=94), METs were identified as an independent prognostic factor to predict the 5-year overall survival rate in CRC and significantly associated with distant metastasis but not with local tumor invasion and lymph node metastasis (35).
Co-cultivation of the CRC tumor cell lines HCT-116 and SW480 with macrophages and a neutrophil-deficient mouse model were used to investigate the crosstalk between METs and CRC cell lines. These studies revealed that METs in the absence of NETs can activate the migration and invasion of CRC tumor cells and promote liver metastases (35). For example, the inhibition of PAD2 impeded MET formation from macrophages, blocked the crosstalk between CRC cells and METs and reduced liver metastasis (35). From this it can be concluded that targeting of both METs and NETs expands the palette of new therapeutic targets for cancer patients.
NK cells are innate cytotoxic lymphoid cells acting against tumor cells and pathogens. Activated NK cells exert their cytotoxic functions by releasing perforin and granzymes. They secrete cytokines, such as IFN-γ that participate in the orchestration of the adaptive immune response (123, 124). NK cells, unlike CTLs, are not restricted by the major histocompatibility complex (MHC). They can identify and destroy tumor cells without exposing tumor-specific antigens. This considerably accelerates the anti-cancer immune response (125, 126). The interaction between neutrophils and NK cells has emerged as an important mechanism for the modulation of immunological responses (127). NK cell-derived IFN-γ, GM-CSF and TNF-α not only prolong neutrophil survival and induce neutrophil activation, but are also essential for NET formation (128, 129). Of note, surgery promotes the production of fibrin and platelet clots coating tumor cell emboli. This limits NK cell-mediated tumor clearance (130, 131). In addition, surgery was found to stimulate NET formation and exacerbated distal organ injury by the activation of a systemic procoagulant state and diffuse microvascular immune thrombi (132–134). Clinical trials showed convincing therapeutic effects of NK cell infusion in various hematological malignancies (135). However, NET-coated tumor spheroids protected tumor cells from cytotoxicity of NK cells (61). In vitro, NETs inhibited migration and motility of NK cells indicating a direct effect of NETs (61). In a NET-rich TIME the therapeutic efficiency of NK cells was clearly impaired (135). Moreover, the inhibition of NETs in a murine model of HCC enhanced the anti-tumor immunity mediated by NK cells (135). Besides physical shielding tumor cells from attacks of NK cells, further mechanisms may exist that impair the anti-tumor function of NK cells. For example, NETs may activate platelets to impair the NK cell mediated tumorilytic function by increasing the secretion of transforming growth factor (TGF)-β, which can inhibit the mobilization of NK cells (136, 137). MMP-9 in NETs may also be implicated in NK cell dysfunction resulting in tumor immune evasion (138). All of these results indicate a mutual interaction of NETs and NK cells, which in an inflammatory TIME fosters the tumorigenic functions of NETs by counteracting NK cell activity.
Although DCs constitute a rare immune cell population in tumors and lymphoid organs, these cells are crucial for specialized antigen-presentation, the regulation of innate immunity, and the initiation of the adaptive immune response (129, 139). DCs as antigen-presenting cells are required for T cell responses and are widely used in vaccination. DCs include several subsets, such as plasmacytoid DCs (pDCs), conventional DCs (cDCs) [also referred to as myeloid DCs (mDCs)], and monocyte-derived DCs (moDCs). cDCs are the major DC population and they play a critical role in anti-tumor responses. They endocytose apoptotic and necrotic tumor cells and present tumor-related antigens to T cells (140). This may happen either in an activating or a tolerogenic manner. While pDCs mainly produce large amounts of IFN-α and IFN-β and can also be stimulated to directly activate T cells (141, 142). In patients with psoriasis and systemic lupus erythematosus NETs modulate the crosstalk between innate and adaptive immune responses and activate pDCs via TLR9 (143, 144). Sangaletti and colleagues described co-cultures of mDCs with inflammatory polymorphonuclear leukocytes (PMNs) were prone for NET formation, which induced a stable interaction of NETs and mDCs, subsequently resulting in mDCs loaded with NET components, including extracellular proteins and DNA (145). Thus, mDCs were capable to take up antigens of NETs for potential antigen processing and presentation (145). In vivo, immunization with mDCs co-cultured with NETs or apoptotic/necrotic PMNs induced anti-neutrophil cytoplasmic antibodies (ANCAs) and anti-dsDNA autoantibodies, while mDCs or NETs alone had no effect (145). Grippingly, ANCA-related autoimmune vasculitis was exclusively found in the renal and pulmonary parenchyma of mice inoculated by NET-loaded mDCs, although measurable amounts of ANCA were also detected in mice inoculated by mDCs co-cultured with apoptotic/secondarily necrotic PMNs (145). This indicated that the structural integrity of NETs was required for the transfer of cytoplasmic neutrophil antigens to mDCs (145). The autoimmune features observed in immunized mice were shared by human autoimmune systemic vasculitis (145). The interaction between NETs and mDCs might lead to autoimmunity and underlies the dynamics of ANCA induction in humans.
In cancer DCs acquire, process and present tumor-associated antigens (TAAs) on MHC molecules and shape adaptive immune responses (141). However, the investigation of the interaction of NETs and DCs in tumors is still at the beginning. Tripodo and colleagues described that NETs can boost DC vaccination against acute myeloid leukemia (AML) (146). They established a h-MRP8-NPM1+ (NPMc+) transgenic mouse model, which developed myeloproliferation without inducing overt AML (146). DCs co-cultured with NETs from NPMc+ mice were used to treat NPMc+ mice, thereby reducing NPMc+ myeloproliferation with a deceleration of myeloid expansion and a reduction of myeloid blasts by triggering immune activation (146). To further evaluate the impact of DCs uploaded with NPMc+ NETs on AML, NPMc+ mice were implanted subcutaneously with the leukemia cell line C1498 with mutant NPM1 (C1498-NPMc+) followed by a treatment with NPMc+ NETs/DC vaccine. A reduced tumor growth and stronger CTL cytotoxicity against NPMc was observed (146). NPMc+ NETs/DC vaccination enhanced anti-tumor immunity and prevented growth of leukemia transplants (146). These data indicated that NETs improved anti-tumor vaccination and tumor antigens trapped by NETs could be used to boost immune responses to cancer vaccines.
The studies above provided new insights into the role of NETs in the TIME. Currently, it is established that under certain conditions NETs foster immunosuppression and abrogate the efficacy of immunotherapy. Zhang and colleagues reported that in pancreatic ductal adenocarcinomas (PDAC), neutrophils were recruited by IL-17, subsequently released NETs, and thereby induced immunosuppression (147). This was attributed to the activation of immune checkpoints, depletion of CTLs, and direct protective functions of NETs that protected tumor cells from cytotoxic attack (147). The deletion of NETs together with the application of immune checkpoint inhibition fostered anti-tumorigenic responses in the PDAC mouse model dramatically as compared to the treatment with immune checkpoint inhibition alone (147). This indicated that the removal of NETs might overcome the resistance of immune checkpoint inhibitors and restore adaptive immune responses in certain conditions. Similarly, Zhang and colleagues reported that the degradation of NETs with DNase I highly increased therapeutic benefits of anti-PD-1 immune treatment in a MC38-bearing mouse model of CRC (148). Mechanistically, the combination of DNase I and PD-1 inhibition resulted in the increased CD8+ T cell infiltration and cytotoxicity, eventually overcoming the resistance to anti-PD-1 monotherapy (148). However, considering its short biologic half-life, repeated daily injections of DNase I that are required to ensure an adequate drug level limits its therapeutic application. To overcome this limitation Xia et al. created an adeno-associated virus (AAV) gene therapy vector that expressed DNase I selectively in the liver as potential anti-tumor therapy (149). In this study, a single intravenous injection of AAV maintained sufficient long-term hepatic expression of DNase I (149). In a corresponding murine model CD8+ T cell counts and local immune responses were restored in the TIME, and the development of liver metastases was suppressed (149).
In addition to adaptive immune therapy, innate immune therapy also has been advocated as an appealing option for cancer treatment. NK cell infusion is considered as a potential option in cancer therapy. However, the acidic TIME and NETs severely counteract its efficacy (135). Cheng at el. designed an in situ injectable dual pH-responsive adhesive hemostatic hydrogel that neutralized tumor acidity and digested NETs to improve the therapeutic efficiency of adoptive NK cells for the prevention of HCC recurrence after surgery (135). In more detail, they applied biocompatible mesoporous bioactive glass nanoparticles (MBGNs) loaded with DNase I. Subsequently, the nanoparticles were incorporated into a hydrogel (GODM-gel). Injection of this GODM-gel into liver resection margins in the orthotopic HCC murine model neutralized tumor acidity, destructed NETs and significantly augmented NK cell infiltration and prevented HCC recurrence after surgery (135). These findings indicate that NETs in the TIME are a promising target to improve immune checkpoint therapy and to avoid disease recurrence after tumor surgery.
According to the available data, the tight relationship between NETs, tumor cells, and TIME sheds light on the pivotal function of NETs in cancer progression and metastasis. In most cancer related diseases, NETs emerge as villains that drive metastasis by suppressing innate and adaptive immune responses. Immune therapies combined with the targeting of NETs was proven to enhance anti-tumor efficacy and to reduce drug resistance, providing new therapeutic strategies for patients with cancer. It is necessary to further elucidate the crosstalk between NETs, other extracellular traps and TIME. Table 1 summarizes some key questions in this effort. Based on these open questions more studies are warranted to implement novel pharmacological interventions that target NETs.
Author contributions: QF, MH and MS elaborated the subject of the review. QF wrote the manuscript. AS, EN, JK, MH and MS helped writing, provided helpful ideas and corrected the manuscript. All authors contributed to the article and approved the submitted version.
The work of the authors is supported by grants from the German Research Foundation (DFG) FOR 2438 (subproject 2 to EN and MS), FOR 2886 PANDORA (subproject B3 to MH), SFB/TRR 241 (subproject A06 to MS and subproject B04 to MH, project-ID 375876048), CRC1181- 261193037 (subproject C03 to MH), STU 238/10-1 (to MS), TRR 305 (subproject B08 to EN), the W. Lutz Stiftung (to MS), the Forschungsstiftung Medizin am Universitätsklinikum Erlangen (to MS), the European Council H2020-FETOPEN-2018-2019-2020-01, 861878 “NeutroCure” to MH, the Volkswagen Foundation (grant 97744 to MH), and the Bayerisches Wissenschaftsministerium Corona-Forschung to MH. QF is sponsored by a MD doctoral scholarship from the China Scholarship Council. The present work was performed in (partial) fulfilment of the requirements for obtaining the degree Dr. med. for first-author QF.
The authors declare that the research was conducted in the absence of any commercial or financial relationships that could be construed as a potential conflict of interest.
All claims expressed in this article are solely those of the authors and do not necessarily represent those of their affiliated organizations, or those of the publisher, the editors and the reviewers. Any product that may be evaluated in this article, or claim that may be made by its manufacturer, is not guaranteed or endorsed by the publisher.
1. Galon J, Lanzi A. Immunoscore and its introduction in clinical practice. Q J Nucl Med Mol Imaging. (2020) 64(2):152–61. doi: 10.23736/s1824-4785.20.03249-5
2. Naschberger E, Liebl A, Schellerer VS, Schütz M, Britzen-Laurent N, Kölbel P, et al. Matricellular protein SPARCL1 regulates tumor microenvironment–dependent endothelial cell heterogeneity in colorectal carcinoma. J Clin Invest (2016) 126(11):4187–204. doi: 10.1172/jci78260
3. Naschberger E, Croner RS, Merkel S, Dimmler A, Tripal P, Amann KU, et al. Angiostatic immune reaction in colorectal carcinoma: Impact on survival and perspectives for antiangiogenic therapy. Int J Cancer. (2008) 123(9):2120–9. doi: 10.1002/ijc.23764
4. St. Paul M, Ohashi PS. The roles of CD8+ T cell subsets in antitumor immunity. Trends Cell Biol (2020) 30(9):695–704. doi: 10.1016/j.tcb.2020.06.003
5. Li C, Jiang P, Wei S, Xu X, Wang J. Regulatory T cells in tumor microenvironment: new mechanisms, potential therapeutic strategies and future prospects. Mol Cancer. (2020) 19(1):116. doi: 10.1186/s12943-020-01234-1
6. Zhu X, Zhu J. CD4 T helper cell subsets and related human immunological disorders. Int J Mol Sci (2020) 21(21):8011. doi: 10.3390/ijms21218011
7. Matsuo K, Yoshie O, Nakayama T. Multifaceted roles of chemokines and chemokine receptors in tumor immunity. Cancers (2021) 13(23):6132. doi: 10.3390/cancers13236132
8. Chatzileontiadou DSM, Sloane H, Nguyen AT, Gras S, Grant EJ. The many faces of CD4+ T cells: Immunological and structural characteristics. Int J Mol Sci (2020) 22(1):73. doi: 10.3390/ijms22010073
9. Chang SH. T Helper 17 (Th17) cells and interleukin-17 (IL-17) in cancer. Arch Pharm Res (2019) 42(7):549–59. doi: 10.1007/s12272-019-01146-9
10. Güç E, Pollard JW. Redefining macrophage and neutrophil biology in the metastatic cascade. Immunity (2021) 54(5):885–902. doi: 10.1016/j.immuni.2021.03.022
11. Tran Janco JM, Lamichhane P, Karyampudi L, Knutson KL. Tumor-infiltrating dendritic cells in cancer pathogenesis. J Immunol (2015) 194(7):2985–91. doi: 10.4049/jimmunol.1403134
12. Albrengues J, Shields MA, Ng D, Park CG, Ambrico A, Poindexter ME, et al. Neutrophil extracellular traps produced during inflammation awaken dormant cancer cells in mice. Science (2018) 361(6409):eaao4227. doi: 10.1126/science.aao4227
13. Lin Y, Xu J, Lan H. Tumor-associated macrophages in tumor metastasis: biological roles and clinical therapeutic applications. J Hematol Oncol (2019) 12(1):76. doi: 10.1186/s13045-019-0760-3
14. Woan KV, Miller JS. Harnessing natural killer cell antitumor immunity: From the bench to bedside. Cancer Immunol Res (2019) 7(11):1742–7. doi: 10.1158/2326-6066.Cir-19-0404
15. Kumar V, Patel S, Tcyganov E, Gabrilovich DI. The nature of myeloid-derived suppressor cells in the tumor microenvironment. Trends Immunol (2016) 37(3):208–20. doi: 10.1016/j.it.2016.01.004
16. Sharma P, Hu-Lieskovan S, Wargo JA, Ribas A. Primary, adaptive, and acquired resistance to cancer immunotherapy. Cell (2017) 168(4):707–23. doi: 10.1016/j.cell.2017.01.017
17. Maiorino L, Daßler-Plenker J, Sun L, Egeblad M. Innate immunity and cancer pathophysiology. Annu Rev Pathol: Mech Disease. (2022) 17(1):425–57. doi: 10.1146/annurev-pathmechdis-032221-115501
18. Wang J, Jia Y, Wang N, Zhang X, Tan B, Zhang G, et al. The clinical significance of tumor-infiltrating neutrophils and neutrophil-to-CD8+ lymphocyte ratio in patients with resectable esophageal squamous cell carcinoma. J Transl Med (2014) 12:7. doi: 10.1186/1479-5876-12-7
19. Burn GL, Foti A, Marsman G, Patel DF, Zychlinsky A. The neutrophil. Immunity (2021) 54(7):1377–91. doi: 10.1016/j.immuni.2021.06.006
20. Brinkmann V, Reichard U, Goosmann C, Fauler B, Uhlemann Y, Weiss DS, et al. Neutrophil extracellular traps kill bacteria. Science (2004) 303(5663):1532–5. doi: 10.1126/science.1092385
21. Leshner M, Wang S, Lewis C, Zheng H, Chen XA, Santy L, et al. PAD4 mediated histone hypercitrullination induces heterochromatin decondensation and chromatin unfolding to form neutrophil extracellular trap-like structures. Front Immunol (2012) 3:307. doi: 10.3389/fimmu.2012.00307
22. Arpinati L, Shaul ME, Kaisar-Iluz N, Mali S, Mahroum S, Fridlender ZG. NETosis in cancer: a critical analysis of the impact of cancer on neutrophil extracellular trap (NET) release in lung cancer patients vs. mice. Cancer Immunol Immunother (2020) 69(2):199–213. doi: 10.1007/s00262-019-02474-x
23. Masucci MT, Minopoli M, Del Vecchio S, Carriero MV. The emerging role of neutrophil extracellular traps (NETs) in tumor progression and metastasis. Front Immunol (2020) 11:1749. doi: 10.3389/fimmu.2020.01749
24. Homa-Mlak I, Majdan A, Mlak R, Malecka-Massalska T. Metastatic potential of NET in neoplastic disease. Postepy Hig Med Dosw (Online). (2016) 70(0):887–95. doi: 10.5604/17322693.1216275
25. Monti M, De Rosa V, Iommelli F, Carriero MV, Terlizzi C, Camerlingo R, et al. Neutrophil extracellular traps as an adhesion substrate for different tumor cells expressing RGD-binding integrins. Int J Mol Sci (2018) 19(8):2350. doi: 10.3390/ijms19082350
26. Kanamaru R, Ohzawa H, Miyato H, Yamaguchi H, Hosoya Y, Lefor AK, et al. Neutrophil extracellular traps generated by low density neutrophils obtained from peritoneal lavage fluid mediate tumor cell growth and attachment. J Visualized Experiments (2018) 138:e58201. doi: 10.3791/58201
27. Wynn TA, Chawla A, Pollard JW. Macrophage biology in development, homeostasis and disease. Nature (2013) 496(7446):445–55. doi: 10.1038/nature12034
28. Kim J, Bae J-S. Tumor-associated macrophages and neutrophils in tumor microenvironment. Mediators Inflammation. (2016) 2016:1–11. doi: 10.1155/2016/6058147
29. Murray PJ. Macrophage polarization. Annu Rev Physiol (2017) 79(1):541–66. doi: 10.1146/annurev-physiol-022516-034339
30. De Palma M, Venneri MA, Galli R, Sergi LS, Politi LS, Sampaolesi M, et al. Tie2 identifies a hematopoietic lineage of proangiogenic monocytes required for tumor vessel formation and a mesenchymal population of pericyte progenitors. Cancer Cell (2005) 8(3):211–26. doi: 10.1016/j.ccr.2005.08.002
31. Gentles AJ, Newman AM, Liu CL, Bratman SV, Feng W, Kim D, et al. The prognostic landscape of genes and infiltrating immune cells across human cancers. Nat Med (2015) 21(8):938–45. doi: 10.1038/nm.3909
32. Hoque MO, Zhang Q-, Liu L, Gong C-Y, Shi H-S, Zeng Y-H, et al. Prognostic significance of tumor-associated macrophages in solid tumor: A meta-analysis of the literature. PloS One (2012) 7(12):e50946. doi: 10.1371/journal.pone.0050946
33. Su S, Liu Q, Chen J, Chen J, Chen F, He C, et al. A positive feedback loop between mesenchymal-like cancer cells and macrophages is essential to breast cancer metastasis. Cancer Cell (2014) 25(5):605–20. doi: 10.1016/j.ccr.2014.03.021
34. Solinas G, Germano G, Mantovani A, Allavena P. Tumor-associated macrophages (TAM) as major players of the cancer-related inflammation. J Leukocyte Biol (2009) 86(5):1065–73. doi: 10.1189/jlb.0609385
35. Chen T, Wang Y, Nan Z, Wu J, Li A, Zhang T, et al. Interaction between macrophage extracellular traps and colon cancer cells promotes colon cancer invasion and correlates with unfavorable prognosis. Front Immunol (2021) 12:779325. doi: 10.3389/fimmu.2021.779325
36. Jorch SK, Kubes P. An emerging role for neutrophil extracellular traps in noninfectious disease. Nat Med (2017) 23(3):279–87. doi: 10.1038/nm.4294
37. Delgado-Rizo V, Martínez-Guzmán MA, Iñiguez-Gutierrez L, García-Orozco A, Alvarado-Navarro A, Fafutis-Morris M. Neutrophil extracellular traps and its implications in inflammation: An overview. Front Immunol (2017) 8:81. doi: 10.3389/fimmu.2017.00081
38. Ronchetti L, Boubaker NS, Barba M, Vici P, Gurtner A, Piaggio G. Neutrophil extracellular traps in cancer: not only catching microbes. J Exp Clin Cancer Res (2021) 40(1):231. doi: 10.1186/s13046-021-02036-z
39. Lewis HD, Liddle J, Coote JE, Atkinson SJ, Barker MD, Bax BD, et al. Inhibition of PAD4 activity is sufficient to disrupt mouse and human NET formation. Nat Chem Biol (2015) 11(3):189–91. doi: 10.1038/nchembio.1735
40. Papayannopoulos V, Metzler KD, Hakkim A, Zychlinsky A. Neutrophil elastase and myeloperoxidase regulate the formation of neutrophil extracellular traps. J Cell Biol (2010) 191(3):677–91. doi: 10.1083/jcb.201006052
41. Fuchs TA, Abed U, Goosmann C, Hurwitz R, Schulze I, Wahn V, et al. Novel cell death program leads to neutrophil extracellular traps. J Cell Biol (2007) 176(2):231–41. doi: 10.1083/jcb.200606027
42. Clark SR, Ma AC, Tavener SA, McDonald B, Goodarzi Z, Kelly MM, et al. Platelet TLR4 activates neutrophil extracellular traps to ensnare bacteria in septic blood. Nat Med (2007) 13(4):463–9. doi: 10.1038/nm1565
43. Yipp BG, Kubes P. NETosis: how vital is it? Blood (2013) 122(16):2784–94. doi: 10.1182/blood-2013-04-457671
44. Pilsczek FH, Salina D, Poon KKH, Fahey C, Yipp BG, Sibley CD, et al. A novel mechanism of rapid nuclear neutrophil extracellular trap formation in response toStaphylococcus aureus. J Immunol (2010) 185(12):7413–25. doi: 10.4049/jimmunol.1000675
45. Rochael NC, Guimarães-Costa AB, Nascimento MTC, DeSouza-Vieira TS, Oliveira MP, Garcia e Souza LF, et al. Classical ROS-dependent and early/rapid ROS-independent release of neutrophil extracellular traps triggered by leishmania parasites. Sci Rep (2015) 5(1):18302. doi: 10.1038/srep18302
46. Yipp BG, Petri B, Salina D, Jenne CN, Scott BNV, Zbytnuik LD, et al. Infection-induced NETosis is a dynamic process involving neutrophil multitasking in vivo. Nat Med (2012) 18(9):1386–93. doi: 10.1038/nm.2847
47. Lood C, Blanco LP, Purmalek MM, Carmona-Rivera C, De Ravin SS, Smith CK, et al. Neutrophil extracellular traps enriched in oxidized mitochondrial DNA are interferogenic and contribute to lupus-like disease. Nat Med (2016) 22(2):146–53. doi: 10.1038/nm.4027
48. Yousefi S, Mihalache C, Kozlowski E, Schmid I, Simon HU. Viable neutrophils release mitochondrial DNA to form neutrophil extracellular traps. Cell Death Differentiation. (2009) 16(11):1438. doi: 10.1038/cdd.2009.96
49. Snoderly HT, Boone BA, Bennewitz MF. Neutrophil extracellular traps in breast cancer and beyond: current perspectives on NET stimuli, thrombosis and metastasis, and clinical utility for diagnosis and treatment. Breast Cancer Res (2019) 21(1):145. doi: 10.1186/s13058-019-1237-6
50. Wang Y, Li M, Stadler S, Correll S, Li P, Wang D, et al. Histone hypercitrullination mediates chromatin decondensation and neutrophil extracellular trap formation. J Cell Biol (2009) 184(2):205–13. doi: 10.1083/jcb.200806072
51. Lominadze G, Powell DW, Luerman GC, Link AJ, Ward RA, McLeish KR. Proteomic analysis of human neutrophil granules. Mol Cell Proteomics. (2005) 4(10):1503–21. doi: 10.1074/mcp.M500143-MCP200
52. Borregaard N, Cowland JB. Granules of the human neutrophilic polymorphonuclear leukocyte. Blood (1997) 89(10):3503–21. doi: 10.1182/blood.V89.10.3503
53. Li P, Li M, Lindberg MR, Kennett MJ, Xiong N, Wang Y. PAD4 is essential for antibacterial innate immunity mediated by neutrophil extracellular traps. J Exp Med (2010) 207(9):1853–62. doi: 10.1084/jem.20100239
54. Chang X, Han J, Pang L, Zhao Y, Yang Y, Shen Z. Increased PADI4 expression in blood and tissues of patients with malignant tumors. BMC Cancer. (2009) 9(1). doi: 10.1186/1471-2407-9-40
55. Stehr AM, Wang G, Demmler R, Stemmler MP, Krug J, Tripal P, et al. Neutrophil extracellular traps drive epithelial-mesenchymal transition of human colon cancer. J Pathol (2022) 256(4):455–67. doi: 10.1002/path.5860
56. Palaniyar N, Arelaki S, Arampatzioglou A, Kambas K, Papagoras C, Miltiades P, et al. Gradient infiltration of neutrophil extracellular traps in colon cancer and evidence for their involvement in tumour growth. PloS One (2016) 11(5):e0154484. doi: 10.1371/journal.pone.0154484
57. Cools-Lartigue J, Spicer J, McDonald B, Gowing S, Chow S, Giannias B, et al. Neutrophil extracellular traps sequester circulating tumor cells and promote metastasis. J Clin Invest (2013) 123(8):3446–58. doi: 10.1172/jci67484
58. Park J, Wysocki RW, Amoozgar Z, Maiorino L, Fein MR, Jorns J, et al. Cancer cells induce metastasis-supporting neutrophil extracellular DNA traps. Sci Trans Med (2016) 8(361):361ra138. doi: 10.1126/scitranslmed.aag1711
59. Demers M, Krause DS, Schatzberg D, Martinod K, Voorhees JR, Fuchs TA, et al. Cancers predispose neutrophils to release extracellular DNA traps that contribute to cancer-associated thrombosis. Proc Natl Acad Sci (2012) 109(32):13076–81. doi: 10.1073/pnas.1200419109
60. Gonzalez-Aparicio M, Alfaro C. Influence of interleukin-8 and neutrophil extracellular trap (NET) formation in the tumor microenvironment: Is there a pathogenic role? J Immunol Res (2019) 2019:1–7. doi: 10.1155/2019/6252138
61. Teijeira Á, Garasa S, Gato M, Alfaro C, Migueliz I, Cirella A, et al. CXCR1 and CXCR2 chemokine receptor agonists produced by tumors induce neutrophil extracellular traps that interfere with immune cytotoxicity. Immunity (2020) 52(5):856–871.e8. doi: 10.1016/j.immuni.2020.03.001
62. Cedervall J, Zhang Y, Huang H, Zhang L, Femel J, Dimberg A, et al. Neutrophil extracellular traps accumulate in peripheral blood vessels and compromise organ function in tumor-bearing animals. Cancer Res (2015) 75(13):2653–62. doi: 10.1158/0008-5472.CAN-14-3299
63. Yang X. Expression of granulocyte colony stimulating factor receptor in human colorectal cancer. Postgraduate Med J (2005) 81(955):333–7. doi: 10.1136/pgmj.2004.024646
64. Fukui Y, Kawashima M, Kawaguchi K, Takeuchi M, Hirata M, Kataoka TR, et al. Granulocyte-colony-stimulating factor-producing metaplastic carcinoma of the breast with significant elevation of serum interleukin-17 and vascular endothelial growth factor levels. Int Cancer Conf J (2018) 7(3):107–13. doi: 10.1007/s13691-018-0330-5
65. Ruffolo LI, Jackson KM, Kuhlers PC, Dale BS, Figueroa Guilliani NM, Ullman NA, et al. GM-CSF drives myelopoiesis, recruitment and polarisation of tumour-associated macrophages in cholangiocarcinoma and systemic blockade facilitates antitumour immunity. Gut (2022) 71(7):1386–98. doi: 10.1136/gutjnl-2021-324109
66. Powell DR, Huttenlocher A. Neutrophils in the tumor microenvironment. Trends Immunol (2016) 37(1):41–52. doi: 10.1016/j.it.2015.11.008
67. Kowanetz M, Wu X, Lee J, Tan M, Hagenbeek T, Qu X, et al. Granulocyte-colony stimulating factor promotes lung metastasis through mobilization of Ly6G+Ly6C+ granulocytes. Proc Natl Acad Sci (2010) 107(50):21248–55. doi: 10.1073/pnas.1015855107
68. Avalos BR, Gasson JC, Hedvat C, Quan SG, Baldwin GC, Weisbart RH, et al. Human granulocyte colony-stimulating factor: biologic activities and receptor characterization on hematopoietic cells and small cell lung cancer cell lines. Blood (1990) 75(4):851–7. doi: 10.1182/blood.V75.4.851.851
69. Dunican A, Grutkoski P, Leuenroth S, Ayala A, Simms HH. Neutrophils regulate their own apoptosis via preservation of CXC receptors. J Surg Res (2000) 90(1):32–8. doi: 10.1006/jsre.2000.5829
70. Van Damme J, Van Beeumen J, Opdenakker G, Billiau A. A novel, NH2-terminal sequence-characterized human monokine possessing neutrophil chemotactic, skin-reactive, and granulocytosis-promoting activity. J Exp Med (1988) 167(4):1364–76. doi: 10.1084/jem.167.4.1364
71. Teijeira A, Garasa S, Ochoa MC, Villalba M, Olivera I, Cirella A, et al. IL8, neutrophils, and NETs in a collusion against cancer immunity and immunotherapy. Clin Cancer Res (2021) 27(9):2383–93. doi: 10.1158/1078-0432.Ccr-20-1319
72. de Andrea CE, Ochoa MC, Villalba-Esparza M, Teijeira A, Schalper KA, Abengozar-Muela M, et al. Heterogenous presence of neutrophil extracellular traps in human solid tumours is partially dependent on IL-8. J Pathol (2021) 255(2):190–201. doi: 10.1002/path.5753
73. Nie M, Yang L, Bi X, Wang Y, Sun P, Yang H, et al. Neutrophil extracellular traps induced by IL8 promote diffuse Large b-cell lymphoma progression via the TLR9 signaling. Clin Cancer Res (2019) 25(6):1867–79. doi: 10.1158/1078-0432.Ccr-18-1226
74. Alfaro C, Teijeira A, Oñate C, Pérez G, Sanmamed MF, Andueza MP, et al. Tumor-produced interleukin-8 attracts human myeloid-derived suppressor cells and elicits extrusion of neutrophil extracellular traps (NETs). Clin Cancer Res (2016) 22(15):3924–36. doi: 10.1158/1078-0432.Ccr-15-2463
75. Rayes RF, Mouhanna JG, Nicolau I, Bourdeau F, Giannias B, Rousseau S, et al. Primary tumors induce neutrophil extracellular traps with targetable metastasis promoting effects. JCI Insight (2019) 5(16):e128008. doi: 10.1172/jci.insight.128008
76. Han Y, Liu D, Li L. PD-1/PD-L1 pathway: current researches in cancer. Am J Cancer Res (2020) 10(3):727–42.
77. Raskov H, Orhan A, Christensen JP, Gögenur I. Cytotoxic CD8+ T cells in cancer and cancer immunotherapy. Br J Cancer. (2020) 124(2):359–67. doi: 10.1038/s41416-020-01048-4
78. Kaltenmeier C, Yazdani HO, Morder K, Geller DA, Simmons RL, Tohme S. Neutrophil extracellular traps promote T cell exhaustion in the tumor microenvironment. Front Immunol (2021) 12:785222. doi: 10.3389/fimmu.2021.785222
79. Vesely MD, Zhang T, Chen L. Resistance mechanisms to anti-PD cancer immunotherapy. Annu Rev Immunol (2022) 40(1):45–74. doi: 10.1146/annurev-immunol-070621-030155
80. Shinde-Jadhav S, Mansure JJ, Rayes RF, Marcq G, Ayoub M, Skowronski R, et al. Role of neutrophil extracellular traps in radiation resistance of invasive bladder cancer. Nat Commun (2021) 12(1):2776. doi: 10.1038/s41467-021-23086-z
81. Schauer C, Janko C, Munoz LE, Zhao Y, Kienhofer D, Frey B, et al. Aggregated neutrophil extracellular traps limit inflammation by degrading cytokines and chemokines. Nat Med (2014) 20(5):511–7. doi: 10.1038/nm.3547
82. Geginat J, Paroni M, Facciotti F, Gruarin P, Kastirr I, Caprioli F, et al. The CD4-centered universe of human T cell subsets. Semin Immunol (2013) 25(4):252–62. doi: 10.1016/j.smim.2013.10.012
83. Geginat J, Paroni M, Maglie S, Alfen JS, Kastirr I, Gruarin P, et al. Plasticity of human CD4 T cell subsets. Front Immunol (2014) 5:630. doi: 10.3389/fimmu.2014.00630
84. Tay RE, Richardson EK, Toh HC. Revisiting the role of CD4+ T cells in cancer immunotherapy–new insights into old paradigms. Cancer Gene Ther (2020) 28(1-2):5–17. doi: 10.1038/s41417-020-0183-x
85. Borst J, Ahrends T, Bąbała N, Melief CJM, Kastenmüller W. CD4+ T cell help in cancer immunology and immunotherapy. Nat Rev Immunol (2018) 18(10):635–47. doi: 10.1038/s41577-018-0044-0
86. Zhou L, Chong MMW, Littman DR. Plasticity of CD4+ T cell lineage differentiation. Immunity (2009) 30(5):646–55. doi: 10.1016/j.immuni.2009.05.001
87. Saravia J, Chapman NM, Chi H. Helper T cell differentiation. Cell Mol Immunol (2019) 16(7):634–43. doi: 10.1038/s41423-019-0220-6
88. Basu A, Ramamoorthi G, Albert G, Gallen C, Beyer A, Snyder C, et al. Differentiation and regulation of TH cells: A balancing act for cancer immunotherapy. Front Immunol (2021) 12:669474. doi: 10.3389/fimmu.2021.669474
89. Parackova Z, Zentsova I, Vrabcova P, Klocperk A, Sumnik Z, Pruhova S, et al. Neutrophil extracellular trap induced dendritic cell activation leads to Th1 polarization in type 1 diabetes. Front Immunol (2020) 11:661. doi: 10.3389/fimmu.2020.00661
90. Qiu S-L, Zhang H, Q-y T, Bai J, He Z-Y, Zhang J-Q, et al. Neutrophil extracellular traps induced by cigarette smoke activate plasmacytoid dendritic cells. Thorax (2017) 72(12):1084–93. doi: 10.1136/thoraxjnl-2016-209887
91. Papadaki G, Kambas K, Choulaki C, Vlachou K, Drakos E, Bertsias G, et al. Neutrophil extracellular traps exacerbate Th1-mediated autoimmune responses in rheumatoid arthritis by promoting DC maturation. Eur J Immunol (2016) 46(11):2542–54. doi: 10.1002/eji.201646542
92. Liu K, Sun E, Lei M, Li L, Gao J, Nian X, et al. BCG-Induced formation of neutrophil extracellular traps play an important role in bladder cancer treatment. Clin Immunol (2019) 201:4–14. doi: 10.1016/j.clim.2019.02.005
93. Zheng Z, Li Y-n, Jia S, Zhu M, Cao L, Tao M, et al. Lung mesenchymal stromal cells influenced by Th2 cytokines mobilize neutrophils and facilitate metastasis by producing complement C3. Nat Commun (2021) 12(1):6202. doi: 10.1038/s41467-021-26460-z
94. Sakaguchi S, Mikami N, Wing JB, Tanaka A, Ichiyama K, Ohkura N. Regulatory T cells and human disease. Annu Rev Immunol (2020) 38(1):541–66. doi: 10.1146/annurev-immunol-042718-041717
95. Wang H, Zhang H, Wang Y, Brown ZJ, Xia Y, Huang Z, et al. Regulatory T-cell and neutrophil extracellular trap interaction contributes to carcinogenesis in non-alcoholic steatohepatitis. J Hepatol (2021) 75(6):1271–83. doi: 10.1016/j.jhep.2021.07.032
96. Kang T-W, Yevsa T, Woller N, Hoenicke L, Wuestefeld T, Dauch D, et al. Senescence surveillance of pre-malignant hepatocytes limits liver cancer development. Nature (2011) 479(7374):547–51. doi: 10.1038/nature10599
97. Roychoudhuri R, Eil RL, Restifo NP. The interplay of effector and regulatory T cells in cancer. Curr Opin Immunol (2015) 33:101–11. doi: 10.1016/j.coi.2015.02.003
98. Vesely MD, Kershaw MH, Schreiber RD, Smyth MJ. Natural innate and adaptive immunity to cancer. Annu Rev Immunol (2011) 29(1):235–71. doi: 10.1146/annurev-immunol-031210-101324
99. Togashi Y, Shitara K, Nishikawa H. Regulatory T cells in cancer immunosuppression — implications for anticancer therapy. Nat Rev Clin Oncol (2019) 16(6):356–71. doi: 10.1038/s41571-019-0175-7
100. González-Navajas JM, Fine S, Law J, Datta SK, Nguyen KP, Yu M, et al. TLR4 signaling in effector CD4+ T cells regulates TCR activation and experimental colitis in mice. J Clin Invest (2010) 120(2):570–81. doi: 10.1172/jci40055
101. Muraoka D, Seo N, Hayashi T, Tahara Y, Fujii K, Tawara I, et al. Antigen delivery targeted to tumor-associated macrophages overcomes tumor immune resistance. J Clin Invest (2019) 129(3):1278–94. doi: 10.1172/jci97642
102. Broz Miranda L, Binnewies M, Boldajipour B, Nelson Amanda E, Pollack Joshua L, Erle David J, et al. Dissecting the tumor myeloid compartment reveals rare activating antigen-presenting cells critical for T cell immunity. Cancer Cell (2014) 26(5):638–52. doi: 10.1016/j.ccell.2014.09.007
103. Murray PJ, Wynn TA. Protective and pathogenic functions of macrophage subsets. Nat Rev Immunol (2011) 11(11):723–37. doi: 10.1038/nri3073
104. Haider P, Kral-Pointner JB, Mayer J, Richter M, Kaun C, Brostjan C, et al. Neutrophil extracellular trap degradation by differently polarized macrophage subsets. Arteriosclerosis Thrombosis Vasc Biol (2020) 40(9):2265–78. doi: 10.1161/atvbaha.120.314883
105. Farrera C, Fadeel B. Macrophage clearance of neutrophil extracellular traps is a silent process. J Immunol (2013) 191(5):2647–56. doi: 10.4049/jimmunol.1300436
106. Lazzaretto B, Fadeel B. Intra- and extracellular degradation of neutrophil extracellular traps by macrophages and dendritic cells. J Immunol (2019) 203(8):2276–90. doi: 10.4049/jimmunol.1800159
107. Zhang Y-G, Song Y, Guo X-L, Miao R-Y, Fu Y-Q, Miao C-F, et al. Exosomes derived from oxLDL-stimulated macrophages induce neutrophil extracellular traps to drive atherosclerosis. Cell Cycle (2019) 18(20):2672–82. doi: 10.1080/15384101.2019.1654797
108. Zhang L, Yi H, Chen J, Li H, Luo Y, Cheng T, et al. Neutrophil extracellular traps facilitate A549 cell invasion and migration in a macrophage-maintained inflammatory microenvironment. BioMed Res Int (2022) 2022:1–11. doi: 10.1155/2022/8316525
109. Nakazawa D, Shida H, Kusunoki Y, Miyoshi A, Nishio S, Tomaru U, et al. The responses of macrophages in interaction with neutrophils that undergo NETosis. J Autoimmun (2016) 67:19–28. doi: 10.1016/j.jaut.2015.08.018
110. Doster RS, Rogers LM, Gaddy JA, Aronoff DM. Macrophage extracellular traps: A scoping review. J Innate Immunity. (2018) 10(1):3–13. doi: 10.1159/000480373
111. King PT, Sharma R, O’Sullivan KM, Callaghan J, Dousha L, Thomas B, et al. Deoxyribonuclease 1 reduces pathogenic effects of cigarette smoke exposure in the lung. Sci Rep (2017) 7(1):12128. doi: 10.1038/s41598-017-12474-5
112. King PT, Sharma R, O’Sullivan K, Selemidis S, Lim S, Radhakrishna N, et al. Nontypeable haemophilus influenzae induces sustained lung oxidative stress and protease expression. PloS One (2015) 10(3):e0120371. doi: 10.1371/journal.pone.0120371
113. Pertiwi KR, de Boer OJ, Mackaaij C, Pabittei DR, de Winter RJ, Li X, et al. Extracellular traps derived from macrophages, mast cells, eosinophils and neutrophils are generated in a time-dependent manner during atherothrombosis. J Pathol (2019) 247(4):505–12. doi: 10.1002/path.5212
114. Aulik NA, Hellenbrand KM, Czuprynski CJ, Urban JF. Mannheimia haemolytica and its leukotoxin cause macrophage extracellular trap formation by bovine macrophages. Infection Immunity. (2012) 80(5):1923–33. doi: 10.1128/iai.06120-11
115. Zhou Y, Chen B, Mittereder N, Chaerkady R, Strain M, An LL, et al. Spontaneous secretion of the citrullination enzyme PAD2 and cell surface exposure of PAD4 by neutrophils. Front Immunol (2017) 8:1200. doi: 10.3389/fimmu.2017.01200
116. Mohanan S, Horibata S, McElwee JL, Dannenberg AJ, Coonrod SA. Identification of macrophage extracellular trap-like structures in mammary gland adipose tissue: a preliminary study. Front Immunol (2013) 4:67. doi: 10.3389/fimmu.2013.00067
117. Halder LD, Abdelfatah MA, Jo EA, Jacobsen ID, Westermann M, Beyersdorf N, et al. Factor h binds to extracellular DNA traps released from human blood monocytes in response to candida albicans. Front Immunol (2016) 7:671. doi: 10.3389/fimmu.2016.00671
118. Munoz-Caro T, Silva LM, Ritter C, Taubert A, Hermosilla C. Besnoitia besnoiti tachyzoites induce monocyte extracellular trap formation. Parasitol Res (2014) 113(11):4189–97. doi: 10.1007/s00436-014-4094-3
119. Palaniyar N, Liu P, Wu X, Liao C, Liu X, Du J, et al. Escherichia coli and candida albicans induced macrophage extracellular trap-like structures with limited microbicidal activity. PloS One (2014) 9(2):e90042. doi: 10.1371/journal.pone.0090042
120. Herrmann JL, Je S, Quan H, Yoon Y, Na Y, Kim B-J, et al. Mycobacterium massiliense induces macrophage extracellular traps with facilitating bacterial growth. PloS One (2016) 11(5):e0155685. doi: 10.1371/journal.pone.0155685
121. Rahat MA, Shakya J. Parallel aspects of the microenvironment in cancer and autoimmune disease. Mediators Inflamm (2016) 2016:4375120. doi: 10.1155/2016/4375120
122. Xu S-S, Li H, Li T-J, Li S, Xia H-Y, Long J, et al. Neutrophil extracellular traps and macrophage extracellular traps predict postoperative recurrence in resectable nonfunctional pancreatic neuroendocrine tumors. Front Immunol (2021) 12:577517. doi: 10.3389/fimmu.2021.577517
123. Molgora M, Supino D, Mavilio D, Santoni A, Moretta L, Mantovani A, et al. The yin-yang of the interaction between myelomonocytic cells and NK cells. Scandinavian J Immunol (2018) 88(3):e12705. doi: 10.1111/sji.12705
124. Vivier E, Tomasello E, Baratin M, Walzer T, Ugolini S. Functions of natural killer cells. Nat Immunol (2008) 9(5):503–10. doi: 10.1038/ni1582
125. Bald T, Krummel MF, Smyth MJ, Barry KC. The NK cell–cancer cycle: advances and new challenges in NK cell–based immunotherapies. Nat Immunol (2020) 21(8):835–47. doi: 10.1038/s41590-020-0728-z
126. Ahn YH, Ren L, Kim SM, Seo S-H, Jung C-R, Kim DS, et al. A three-dimensional hyaluronic acid-based niche enhances the therapeutic efficacy of human natural killer cell-based cancer immunotherapy. Biomaterials (2020) 247:119960. doi: 10.1016/j.biomaterials.2020.119960
127. Costantini C, Cassatella MA. The defensive alliance between neutrophils and NK cells as a novel arm of innate immunity. J Leukocyte Biol (2011) 89(2):221–33. doi: 10.1189/jlb.0510250
128. Bhatnagar N, Hong HS, Krishnaswamy JK, Haghikia A, Behrens GM, Schmidt RE, et al. Cytokine-activated NK cells inhibit PMN apoptosis and preserve their functional capacity. Blood (2010) 116(8):1308–16. doi: 10.1182/blood-2010-01-264903
129. Riise RE, Bernson E, Aurelius J, Martner A, Pesce S, Della Chiesa M, et al. TLR-stimulated neutrophils instruct NK cells to trigger dendritic cell maturation and promote adaptive T cell responses. J Immunol (2015) 195(3):1121–8. doi: 10.4049/jimmunol.1500709
130. Palumbo JS, Talmage KE, Massari JV, La Jeunesse CM, Flick MJ, Kombrinck KW, et al. Platelets and fibrin(ogen) increase metastatic potential by impeding natural killer cell–mediated elimination of tumor cells. Blood (2005) 105(1):178–85. doi: 10.1182/blood-2004-06-2272
131. Seth R, Tai L-H, Falls T, de Souza CT, Bell JC, Carrier M, et al. Surgical stress promotes the development of cancer metastases by a coagulation-dependent mechanism involving natural killer cells in a murine model. Ann Surgery. (2013) 258(1):158–68. doi: 10.1097/SLA.0b013e31826fcbdb
132. Zhang H, Goswami J, Varley P, van der Windt DJ, Ren J, Loughran P, et al. Hepatic surgical stress promotes systemic immunothrombosis that results in distant organ injury. Front Immunol (2020) 11:987. doi: 10.3389/fimmu.2020.00987
133. Gould TJ, Vu TT, Swystun LL, Dwivedi DJ, Mai SHC, Weitz JI, et al. Neutrophil extracellular traps promote thrombin generation through platelet-dependent and platelet-independent mechanisms. Arteriosclerosis Thrombosis Vasc Biol (2014) 34(9):1977–84. doi: 10.1161/atvbaha.114.304114
134. Boettcher M, Schönfeld L, Heuer A, Elrod J, Stiel C, Raluy LP, et al. (2021). doi: 10.21203/rs.3.rs-1077792/v1
135. Cheng Y, Gong Y, Chen X, Zhang Q, Zhang X, He Y, et al. Injectable adhesive hemostatic gel with tumor acidity neutralizer and neutrophil extracellular traps lyase for enhancing adoptive NK cell therapy prevents post-resection recurrence of hepatocellular carcinoma. Biomaterials (2022) 284:121506. doi: 10.1016/j.biomaterials.2022.121506
136. Nieswandt B, Hafner M, Echtenacher B, Mannel DN. Lysis of tumor cells by natural killer cells in mice is impeded by platelets. Cancer Res (1999) 59(6):1295–300.
137. Egan K, Cooke N, Kenny D. Living in shear: platelets protect cancer cells from shear induced damage. Clin Exp Metastasis. (2014) 31(6):697–704. doi: 10.1007/s10585-014-9660-7
138. Peng YP, Zhang JJ, Liang WB, Tu M, Lu ZP, Wei JS, et al. Elevation of MMP-9 and IDO induced by pancreatic cancer cells mediates natural killer cell dysfunction. BMC Cancer. (2014) 14:738. doi: 10.1186/1471-2407-14-738
139. Collin M, Bigley V. Human dendritic cell subsets: an update. Immunology (2018) 154(1):3–20. doi: 10.1111/imm.12888
140. Mildner A, Jung S. Development and function of dendritic cell subsets. Immunity (2014) 40(5):642–56. doi: 10.1016/j.immuni.2014.04.016
141. Wculek SK, Cueto FJ, Mujal AM, Melero I, Krummel MF, Sancho D. Dendritic cells in cancer immunology and immunotherapy. Nat Rev Immunol (2019) 20(1):7–24. doi: 10.1038/s41577-019-0210-z
142. Gardner A, Ruffell B. Dendritic cells and cancer immunity. Trends Immunol (2016) 37(12):855–65. doi: 10.1016/j.it.2016.09.006
143. Skrzeczynska-Moncznik J, Wlodarczyk A, Zabieglo K, Kapinska-Mrowiecka M, Marewicz E, Dubin A, et al. Secretory leukocyte proteinase inhibitor-competent DNA deposits are potent stimulators of plasmacytoid dendritic cells: Implication for psoriasis. J Immunol (2012) 189(4):1611–7. doi: 10.4049/jimmunol.1103293
144. Lande R, Ganguly D, Facchinetti V, Frasca L, Conrad C, Gregorio J, et al. Neutrophils activate plasmacytoid dendritic cells by releasing self-DNA-peptide complexes in systemic lupus erythematosus. Sci Transl Med (2011) 3(73):73ra19. doi: 10.1126/scitranslmed.3001180
145. Sangaletti S, Tripodo C, Chiodoni C, Guarnotta C, Cappetti B, Casalini P, et al. Neutrophil extracellular traps mediate transfer of cytoplasmic neutrophil antigens to myeloid dendritic cells toward ANCA induction and associated autoimmunity. Blood (2012) 120(15):3007–18. doi: 10.1182/blood-2012-03-416156
146. Bassani B, Tripodo C, Jachetti E, Cancila V, Chiodoni C, Portararo P, et al. Neutrophil extracellular traps arm DC vaccination against NPM-mutant myeloproliferation. eLife (2022) 11:e69257. doi: 10.7554/eLife.69257
147. Zhang Y, Chandra V, Riquelme Sanchez E, Dutta P, Quesada PR, Rakoski A, et al. Interleukin-17–induced neutrophil extracellular traps mediate resistance to checkpoint blockade in pancreatic cancer. Journal of experimental medicine. (2020) 217(12):e20190354. doi: 10.1084/jem.20190354
148. Zhang H, Wang Y, Onuma A, He J, Wang H, Xia Y, et al. Neutrophils extracellular traps inhibition improves PD-1 blockade immunotherapy in colorectal cancer. Cancers (2021) 13(21):5333. doi: 10.3390/cancers13215333
Keywords: tumor microenvironment, cancer, neutrophil extracellular traps, neutrophils, macrophages, adaptive immunity, innate immunity, immunotherapy
Citation: Fang Q, Stehr AM, Naschberger E, Knopf J, Herrmann M and Stürzl M (2022) No NETs no TIME: Crosstalk between neutrophil extracellular traps and the tumor immune microenvironment. Front. Immunol. 13:1075260. doi: 10.3389/fimmu.2022.1075260
Received: 20 October 2022; Accepted: 05 December 2022;
Published: 23 December 2022.
Edited by:
Michal Amit Rahat, Technion-Israel Institute of Technology, IsraelReviewed by:
Anna-Karin Olsson, Uppsala University, SwedenCopyright © 2022 Fang, Stehr, Naschberger, Knopf, Herrmann and Stürzl. This is an open-access article distributed under the terms of the Creative Commons Attribution License (CC BY). The use, distribution or reproduction in other forums is permitted, provided the original author(s) and the copyright owner(s) are credited and that the original publication in this journal is cited, in accordance with accepted academic practice. No use, distribution or reproduction is permitted which does not comply with these terms.
*Correspondence: Michael Stürzl, bWljaGFlbC5zdHVlcnpsQHVrLWVybGFuZ2VuLmRl
†These authors have contributed equally to this work and share last authorship
Disclaimer: All claims expressed in this article are solely those of the authors and do not necessarily represent those of their affiliated organizations, or those of the publisher, the editors and the reviewers. Any product that may be evaluated in this article or claim that may be made by its manufacturer is not guaranteed or endorsed by the publisher.
Research integrity at Frontiers
Learn more about the work of our research integrity team to safeguard the quality of each article we publish.