- Department of Thoracic Surgery, Renji Hospital, School of Medicine, Shanghai Jiao Tong University, Shanghai, China
Emerging evidence indicates that the induction of radiotherapy(RT) on the immunogenic cell death (ICD) is not only dependent on its direct cytotoxic effect, changes in the tumor immune microenvironment also play an important role in it. Tumor immune microenvironment (TIME) refers to the immune microenvironment that tumor cells exist, including tumor cells, inflammatory cells, immune cells, various signaling molecules and extracellular matrix. TIME has a barrier effect on the anti-tumor function of immune cells, which can inhibit all stages of anti-tumor immune response. The remodeling of TIME caused by RT may affect the degree of immunogenicity, and make it change from immunosuppressive phenotype to immunostimulatory phenotype. It is of great significance to reveal the causes of immune escape of tumor cells, especially for the treatment of drug-resistant tumor. In this review, we focus on the effect of RT on the TIME, the mechanism of RT in reversing the TIME to suppress intrinsic immunity, and the sensitization effect of the remodeling of TIME caused by RT on the effectiveness of immunotherapy.
Introduction
Characteristics of tumor include sustained proliferation, resistance to cell death, angiogenesis, invasion and metastasis, as well as suppression of inflammation and immunity (1). Among them, immunosuppression, an important feature of the tumor immune microenvironment (TIME), is considered to be an important reason of tumor progression and metastasis and has become a therapeutic target for numerous tumor types. Radiotherapy (RT) with highly effective and non-specific in nature is one of the commonly used therapies in the treatment of malignant tumors. RT is regarded as the most effective cytotoxic therapy for treating patients with solid tumors and is used as first-line treatment in approximately 60% of newly diagnosed patients (2, 3). Firstly, RT directly or indirectly induces DNA damage and endoplasmic reticulum (ER) stress, leading to tumor cell death, which is thought to target cancer cells. In addition, non-targeted and systemic effects of RT have also been identified (4). There is growing body of evidence that RT can remodel TIME to alter the original immunosuppressive state, exert anti-tumor effects, and exhibit enhanced immune responses and therapeutic effects when combined with immunotherapy (5–7). This article partly reviewed the impact of TIME on immunosuppression and the effects of RT on TIME, elaborated the mechanisms of reversal of TIME on the suppression of intrinsic immunity, and the sensitizing effect of the remodeling of TIME on the effectiveness of immunotherapy.
TIME
TIME is the structural and functional niche where tumor cells arise and live, and includes not only tumor cells and extracellular matrix (ECM), but also fibroblasts, epithelial cells (ECs), immune or inflammatory cells, blood and lymphatic vessels, etc (8). TIME, mediated by the secretion of a large variety of factors by a diverse range of cells, forms a local milieu that favors tumor proliferation, infiltration and metastasis. Tumorigenesis is usually accompanied by the activation of innate and adaptive immunity, called functional cancer immunosurveillance, which gradually results in the accumulation of immune or inflammatory cells within the TIME. The immune response plays a dual role in the complex interaction between tumor and host (pro-/anti- tumor) and undergoes cancer immunoediting processes (elimination, equilibrium, and escape), culminating in the formation of an immunosuppressive microenvironment that promotes malignant tumor progression. Natural killer (NK) cells are key cells in innate immunity, relying on granzymes and perforin for direct cell killing without prior sensitization or MHC restriction. In the adaptive immune system, CD4+ T cells and dendritic cells (DCs) are important mediators, while CD8+ cytotoxic T lymphocytes (CTLs) play the ultimate tumor-killing role. CD4+ T cells, mainly T helper cells, broadly play an important adjuvant function in the recognition and clearance of tumor cells, through promoting the proliferation and activation of CTLs, the formation of memory CTLs, and enhancing the antigen presentation of DCs (9, 10). Cytolytic CD4+ T cells recognize antigenic peptides presented by MHC-II molecules mainly on antigen-presenting cells (APCs), and are relevant to antitumor immunity in cancer patients (11, 12). DCs are the most important APCs that initiate adaptive immune responses via activation of naive T cells (13). DCs cross-present MHC-I molecules to CD8+ T cells to induce the production of cytotoxic effector CD8+ T cells, known as CTLs (14). CTLs recognize MHC-I molecules expressed by tumor cells and specifically kill tumor cells through Granule exocytosis and Fas ligand (Fas-L)-mediated apoptosis induction (15). Moreover, CTLs release interferon-γ (IFN-γ) and tumor necrosis factor α (TNF-α) to induce cytotoxicity within tumor cells (16). Tumor-associated macrophages (TAMs) are abundant in TME and are major players in the inflammatory response. In addition to promoting tumor cell proliferation and angiogenesis, TAMs suppress adaptive immune responses (17). Cancer Associated Fibroblasts (CAFs), the plentiful stromal cells in the TME, are a major source of extracellular matrix fibrogenic components such as collagen, hyaluronic acid and fibronectin (18). CAFs actively contribute to cancer invasion by modulating distinct malignant processes (angiogenesis, chronic inflammation and ECM remodeling) and therapeutic resistance (19). CAFs control the functional fate of innate and adaptive immune cells in the TIME by secreting cytokines/chemokines and engaging in direct intercellular interactions (20). Moreover, CAFs play important metabolic effects. The secretion of alanine by CAFs supports malignant cell growth and may also have a positive effect on T cell function (21, 22).
TIME suppresses intrinsic immunity
Although the immune system can clear tumors through the cancer-immune cycle, tumors often evade the body’s immune surveillance by shaping an inhibitory TIME. The complex interactions between the mediators of pro- and anti-tumor in TIME ultimately determine trends of anti-tumor immunity (23, 24). Among these, pro-tumor immune cells include regulatory T cells (Tregs), myeloid-derived suppressor cells (MDSCs), TAMs, CAFs, and tumor-associated neutrophils (TANs). In TIME, CAFs, TAMs and Tregs form an immune barrier to CTLs-mediated anti-tumor immune responses (15). In addition, pro-tumor immune cells and immunosuppressive factors (e.g., transforming growth factor β, interleukin-10) act synergistically to exert important immunosuppressive effects, including inhibition of differentiation and maturation of DCs, inhibition of NK cell toxicity, inhibition of antigen presentation, inactivation of the pro-apoptotic pathway, and disturbance of T cell receptor signaling (25) (Figure 1).
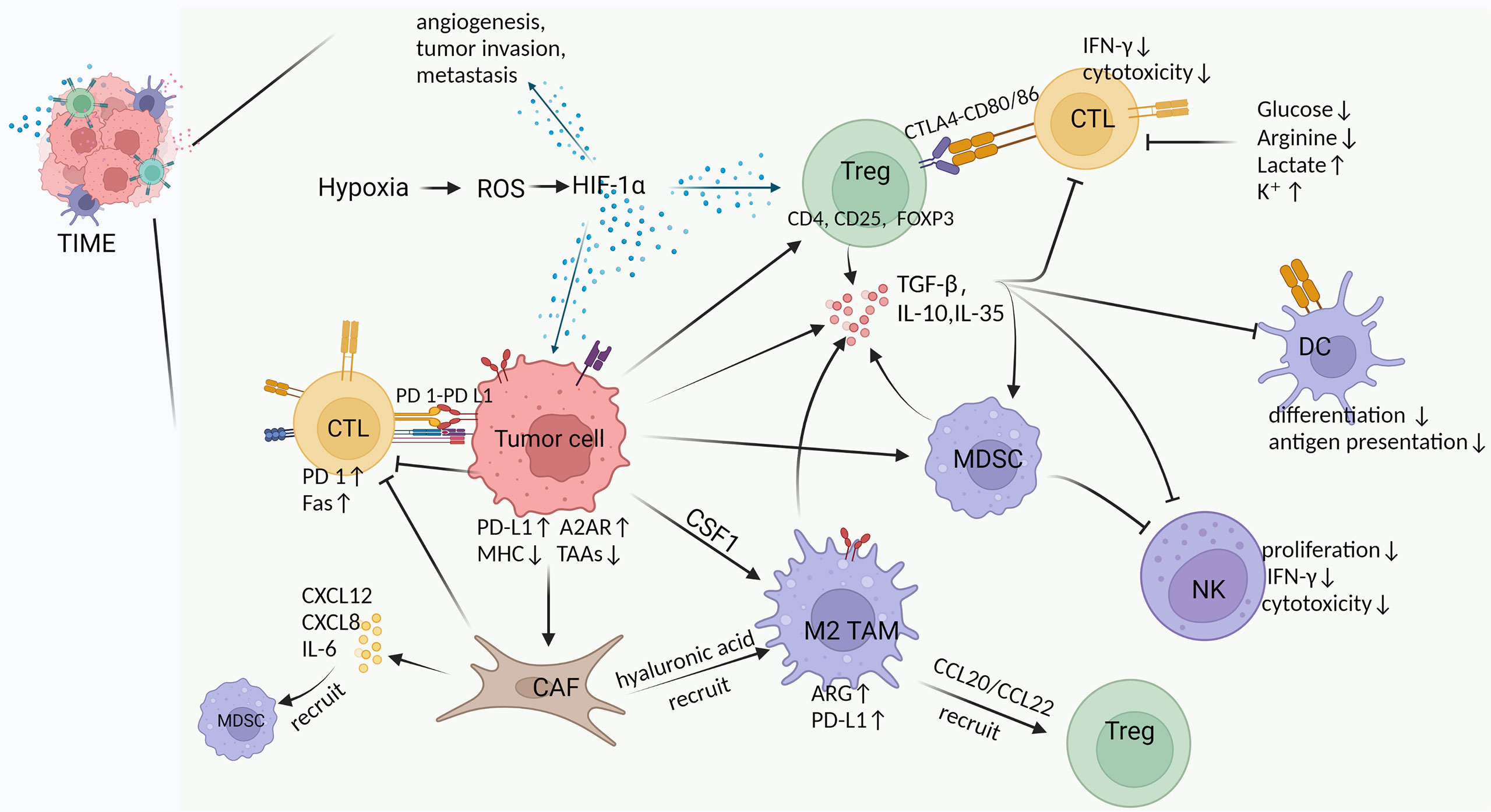
Figure 1 Cross-talk between various components in TIME. Hypoxia is an important feature of TIME in solid tumors, which exerts multiple effects by inducing HIF-1α, such as 1) promoting angiogenesis, tumor invasion and metastasis, 2) increasing Tregs abundance to suppress host immune response, and 3) upregulating PD-L1 and A2AR on tumor cells to evade immunity (26–30). Tumor cells induce and chemotactic immunosuppressive cells (Tregs, M2 TAMs, MDSCs, CAFs) to infiltrate in TIME by secreting TGF-β and CSF (31, 32). Moreover, tumor cells inherently upregulate PD-L1 and A2AR expression to suppress the immune function of CTLs, as well as downregulate MHC and tumor-associated antigens (TAAs) expression to reduce antigen presentation and immune activation (29, 30). Tregs produce multiple immunosuppressive cytokines and express CTLA4 to inhibit CTLs and DCs by binding CD80/86 (33). M2 TAMs in TIME suppress T cell function by upregulating ARG and PD-L1 expression while recruiting Tregs via CCL20/CCL22 (34–36). MDSCs produce inhibitory cytokines and inhibit the formation and cytotoxicity of NK cells by reducing NKG2D expression and IFN-γ secretion (37). CAFs secrete a large number of cytokines, chemokines and ECM, which exhibit immunosuppressive and tumor-supportive effects (31, 38, 39). For example, IL-6 from CAFs recruits MDSCs, CAFs reduce M1 macrophages while recruiting M2 TAMs via hyaluronic acid, upregulated expression of Fas and PD-1 suppresses and depletes T cells (40–42). Suppressive cytokines (e.g., TGF-β, IL-10, IL-35) can be produced by tumor cells and a variety of immunosuppressive cells in TIME to inhibit immune killing of CTLs, suppress the differentiation and antigen presentation of DCs, and restrain the proliferation and cytotoxicity of NK cells (43–46). In addition, glucose and arginine deficiency in TIME, as well as high lactate levels inhibit T cell function (47–51).
Immunosuppression of tumor cells
Cytokines, chemokines and metabolites from tumor cells have a significant impact on TIME, such as transforming growth factor-β (TGF-β) and interleukin (IL)-10. Tumor cells inhibit the function of NK cells, CD8+ T cells and evade recognition and attack by the immune system. Most tumor cells express a large amount of stem cell factor, which induces mast cells to infiltrate the tumor site. Mast cells inactivate T cells and NK cells, as well as inhibit their anti-tumor activity (31). Colony stimulating factor 1 (CSF1) produced by tumor cells promotes differentiation of TAMs and production of granulocyte-specific chemokines in CAFs (31). CSF1 receptor inhibitor and CXCR2 antagonist treatment inhibit the recruitment of MDSCs to TIME, exhibiting significant anti-tumor effects (32). TIME also modify certain inflammatory cell types so that they present a pro-tumor phenotype, in particular many chronic inflammation-associated inflammatory cells promoting tumor progression (52, 53). Hypoxia is a prevalent feature in solid tumor TIME and contributes to the suppression of immune killer cells and protection of tumor cells from immune attack (26, 27). Hypoxia-induced factor 1α (HIF-1α) is a key regulator of adaptive responses to hypoxia, involved in angiogenesis, tumor invasion and metastasis, and increases Tregs abundance by inducing FOXP3 (28). Moreover, HIF-1α also increases PD-L1 expression on tumor cells and suppresses immune cell responses by targeting PD-1 on activated T cells. Tumor cells reduce the expression of MHC or tumor antigens to avoid recognition and clearance by immune cells (29). Adenosine A2a receptor (A2AR) expressed on tumor cells inhibits the activation of immune cells and its expression is associated with cytokines such as HIF-1α. A2AR blockade reduces CD4+ FOXP3+ Tregs infiltration and enhances the anti-tumor response of CD8+ T cells by attenuating hypoxic HIF-1α signaling (30).
Immunosuppression of Regulatory T cells
Tregs are an immunosuppressive subset of CD4+ T cells characteristically expressing CD4, CD25, and FOXP3, and exhibit diversity and functional heterogeneity across tumor types. Hypoxia in TIME increases Tregs abundance by upregulating FOXP3 (28). Tregs, the important Tumor-Promoting Immune Cells, affect a variety of tumor-infiltrating immune cells by producing multiple immunosuppressive cytokines (such as TGF-β, IL-10 and IL-35) and exhibit a significant anti-tumor immune response (43). Tregs inhibit tumor killing by CTLs through TGF-β-dependent cell contact (54), inhibit the production of memory CTLs via cytotoxic-T-lymphocyte-associated protein-4 (CTLA-4) (33), and induction of CTLs death via granzyme B and perforin-dependent manner (55). In addition, Tregs inhibit IFN-γ secretion by CD8+ T Cells and promote the polarization of M2 TAMs (suppressing immunity) (56). Tregs-expressed CTLA-4 binds to CD80/CD86 on DCs to downregulate co-stimulatory signaling and inhibit DCs function (57). Tregs restrain NK cell proliferation, IFN-γ production, degranulation and cytotoxicity (58). Tregs-produced TGF-β and IL-35 enhance the function of MDSCs (59).
Immunosuppression of tumor-associated macrophages
Macrophages account for >50% of tumor-infiltrating immune cells. According to function and cytokine secretion, macrophages are classified as classical activation (M1) with immunostimulatory function and alternative activation (M2) with immunosuppressive and tumor-supportive effects (60). Macrophages are referred to as TAMs in solid tumors, mainly M2, and there is a strong negative correlation between their presence and survival in a variety of solid tumors including breast, colon, bladder and lung cancers (61–64). TAMs are functionally heterogeneous and display remarkable plasticity, which allows macrophages to ‘switching’ into an ‘M2’ phenotype in TIME, associated with immunosuppressive, tumor angiogenic and metastatic consequences (44). In contrast to classical M1 macrophages, these M2 TAMs secrete large amounts of IL-10, and TGF-β, which exert anti-inflammatory effects (44). Hypoxia in TIME also increases arginase 1 (ARG1), VEGF, and macrophage-derived protein kinase signaling by activating mitogen-activated protein kinase signaling in TAMs (34, 65). ARG1 expression is upregulated in TAMs and tumor cells, inhibiting T cell activation by reducing arginine entry into tumor-infiltrating immune cells (34). M2 macrophages-derived CCL20/CCL22 is involved in the recruitment of Tregs (35). M2 TAMs also increases PD-L1 expression to attenuate the effect of CTLs (36).
Immunosuppression by myeloid-derived suppressor cells
MDSCs represent a heterogeneous population of immature myeloid cells with different transcriptional activity and differentiation states, including granulocytic or polymorphonuclear MDSCs (PMN-MDSCs) and monocytic MDSCs (M-MDSCs) (66). They prevent T cell-mediated adaptive immune responses and killing of tumor cells via the innate immune system mediated by NK cells or TAMs (67). Among them, PMN-MDSCs produce ROS and reduce T-cell responses to antigens (66). M-MDSCs produce nitric oxide or differentiate into immunosuppressive macrophages to suppress immune activation (68, 69). Similar to Tregs, the secretion of IL-10 and TGF-β by MDSCs impairs CTLs function and facilitates the induction of Tregs formation (45, 46). MDSCs also cause arginine deficiency by consuming nutrients in the TIME, which in turn causes Teff cell inactivation (46). In a xenograft mouse model, MDSCs inhibit NK cells formation and cytotoxicity by reducing natural killer group 2 member D (NKG2D) expression and IFN-γ secretion (37).
Immunosuppression of cancer-associated fibroblasts
CAFs generally exhibit immunosuppressive and tumor-supportive functions (70–72). CAFs secrete a large number of immunosuppressive cytokines and chemokines, such as CXCL12, CXCL8, IL-6, TNF, TGF-β, etc. (31). Among these, high levels of IL-6 recruit MDSCs, upregulate PD-L1 expression and induce tumor immunosuppression (40). CAFs inhibit the production of regulatory factors such as IFN-γ and TNF-α by T cells and block the migratory capacity of T cells (73). In addition, factors secreted by CAFs also reduce the migration of M1 macrophages and inhibit the pro-inflammatory function of M1 macrophages (41). Meanwhile, CAFs upregulate Fas and PD-1 expression on T cells and deplete CD8+ T cells by binding PD-L2 and FasL (42). CAFs remodel the ECM and protect tumor cells from CTLs, for example, hyaluronic acid produced by CAFs recruits TAMs to the TIME (38, 74, 75). In short, CAF-derived cytokines/chemokines mediate immune escape, growth and metastasis of tumors (39). The SynCon FAP DNA vaccine reduces the number of FAP+ CAFs by targeting Fibroblast activation protein (FAP), a major marker of CAFs, thereby inducing T cell activation and suppressing tumor metastasis (76, 77).
Immunosuppression of TGF-β
In preinvasive disease, TGF-β mainly acts as a tumor suppressor. Once the tumor has invaded, TGF-β promotes tumor progression through epithelial mesenchymal transition, angiogenesis, tumor metastasis, proliferation and immunosuppression of CAFs in TIME (16, 78–81). RT-mediated reactive oxygen species(ROS) production can activate TGF-β (82). TGF-β promotes immunosuppressive TIME through its effects on all immune subgroups (16). For example, TGF-β promotes stromal fibrosis and immune escape, which exclude T cells from infiltrating into tumor tissue, thereby mediating resistance to T cell-directed immunotherapy (64).
Immunosuppression of nutrient competition, metabolite and ion pooling
The high consumption of glucose and amino acids by tumor cells contributes to the achievement of tumor growth, metastasis and immune tolerance (83). Glucose deficiency leads to a decrease in glycolysis in immune cells, which hinders IFN-γ production and the function of CTLs (47). Arginine is exhausted by MDSCs and macrophages, resulting in arginine deficiency in TIME. The anti-tumor activity of T cells is inhibited due to protein biosynthesis-mediated cellular exhaustion (48, 49). Indoleamine 2,3-dioxygenase (IDO) is an important rate-limiting enzyme expressed in CAFs, macrophages, and tumor cells that catalyzes the production of kynurenine from tryptophan (84, 85). Tryptophan metabolites/enzymes suppress inflammatory responses by recruiting Tregs and inhibiting Teff cells proliferation (86, 87). High levels of extracellular lactate inhibited the proliferation and cytokine production of human CTLs (88). Excess lactate led to an acidic environment that reduced arginine concentrations in TIME by inducing ARG1 expression in macrophages, which in turn inhibited CD8+ T cell proliferation and function (50, 51). Intracellular lactate, a product of glycolysis, inhibits T-cell glycolysis by suppressing the mTORC1-mediated signaling pathway (89). The increase in extracellular fluid potassium ions caused by tumor necrosis leads to severe suppression of T cell effector function (90).
Immunosuppression of blood vessels
The immunosuppressive properties of TIME promote vascular destruction, which limits the infiltration of cytotoxic T lymphocytes into the tumor and exacerbates hypoxia (91). And there is a functional defect in the emerging vascular network in TIME that promotes hypoxia formation (92).
In summary, according to the immune characteristics in TIME, Tumor immunophenotype is usually classified as “cold” or “hot” tumors, which suggests individualized clinical treatment options. In “hot” or “inflamed” tumors, high expression of PD-L1, enrichment of Th1-type chemokines, and a large number of NK cells, CD8+ T cells and APCs are found (93, 94). And it has been established that immune “hot” as a protective factor leads to better clinical outcomes when treated with anti-PD-1/PD-L1 (95). In contrast, “cold” tumors so-called “immune-desert” tumors, are characterized by a high number of Tregs and MDSCs, few NK cells, CD8+ T cells, Th1 cells and DCs, but abundant immunosuppressive cytokines (93, 94).
Effects of RT on the TIME
RT is a form of local ablative physiotherapy, the principle of which was using high-energy radiation to treat localized tumors (96). In addition to damaging tumor cells through different pathways, RT also affects other components of the TIME, including immune cells, CAFs, etc. Besides, RT has both “Non-targeted” and abscopal effects on tumor cells. “Non-targeted” effects, also called bystander effects, are molecular signals from irradiated cells that affect adjacent non-irradiated tissues (97). An abscopal effect, explained by the regression of the tumor occurring at a site far from the radiation, is thought to be the result of a systemic immune response (98). Weichselbaum and colleagues experimentally confirmed that the host immune response was the primary cause of the RT response and not the intrinsic radio-sensitivity of the tumor cells (99). RT is involved in every process of the immune response, the recruitment and accumulation of T cells in tumors, the release and presentation of antigens, the initiation and activation of T lymphocytes, and the recognition and killing of tumor cells by T lymphocytes. The effect of RT on irradiated TIME is immunostimulatory or immunosuppressive, which is primarily influenced by the immune landscape of the tumor as well as the dose and fractionation of RT (100).
RT and tumor cells
RT achieves single- and double-stranded DNA damage, mis-repair and chromosomal aberrations through the induction of ROS and reactive nitrogen species (RNS) (92, 101). When RT-induced damage is limited, cells initiate damage repair mechanisms (including DNA damage response, the unfolded protein response and autophagy) to ensure the survival of irradiated cells and re-entry into the cell cycle (102). However, when damage cannot be resolved by repair mechanisms, the molecular mechanism of adaptation to stress switches from a cytoprotective to a cytostatic or cytotoxic mode, usually in one of these forms, ultimately leading to cellular senescence or regulated cell death (RCD) (103). Moreover, both protective repair mechanisms and senescence or RCD have an impact on the local microenvironment and organismal homeostasis, not only through the production of many different cytokines and chemokines, but also through the involvement of damage-associated molecular patterns (DAMPs), ions, and metabolites (103). RT-driven DNA damage response (DDR) can mediate immunostimulatory effects (104). For example, irradiated cancer cells express NK cell-activating ligands (NKALs) on the cell surface after DDR, which support antigen-independent NK cell activation by binding to specific receptors on NK cells (105). NF-κB is sensitive to intracellular alterations that occur after RT, including DNA damage and oxidative stress (102). RT-induced initiation of NF-κB signaling increased the release of cytokines including TNF and IL-1β (106). Tumor cells and CAFs have a proficient autophagic response and successful autophagic response to RT not only preserves cellular viability but also facilitates the maintenance of immunosuppression by TIME (102). However, apoptotic RCD resulting from failing DDR, UPR and autophagic responses transmits danger signals to TIME via membrane exposure and secreted factors in response to lethally irradiation (102). In addition, radiation also induces a variety of non-apoptotic cell death signals, for example, RT-driven mitotic catastrophe activates cGMP-AMP (cGAMP) synthase (cGAS)-stimulator of interferon genes (STING) signaling via TBK1 and IRF3, thereby facilitating the secretion of large amounts of type I IFN (107, 108). Necroptosis is a major pro-inflammatory RCD modality that may ultimately lead to increased tumor infiltration of myeloid cells and CTLs (103, 109). Genetic data suggested that necroptosis was the predominant RCD mechanism in non-small cell lung cancer (NSCLC) cells expressing high RIPK3 levels after ablative hypo-fractionated RT (110). In contrast, a study by Sandy Adjemian et al. showed that necroptosis was not the predominant form of IR-induced death (111). The intrinsic radio-sensitivity of malignant cells exhibits intra- and inter-cancer variability, which depends not only on intrinsic cell characteristics (including efficient DDR, UPR and autophagic competence) but also on TIME factors (e.g. partial oxygen tension) (112–114). Depending on the radiation dose, high doses tend to trigger powerful cytotoxic effects and a strong immune response, while low doses tend to induce cellular senescence and acquire a senescence-associated secretory phenotype (SASP) mainly promoting immunosuppression (102, 115). Data suggest that when cells are irradiated with doses below 10 Gy, most DNA breaks can be repaired and the cells can resume their cell cycle, divide and remain viable. However, at doses higher than 10 Gy some DNA damage fail to repair, at which point mitotic catastrophe and many different forms of death occur (111).
Abscopal radiation-induced antitumor immune responses are rarely observed in clinical practice (116). Apparently, RT-induced antitumor immunity is dependent on RT-generated immune activation signals and immunosuppressive factors (4). One immunosuppressive component is TGF-β1, which promotes tumor progression, invasion and metastasis. Active TGF-β1 is produced in tumors after RT, particularly in endothelial cells undergoing low-dose ionizing-radiation (82, 117). TGF-β1 induces a phenotype of infiltrating inflammatory cells with immunosuppressive effects, e.g. TANs N2 with a protumor phenotype, TAMs M2 (118, 119). RT induces the expression of immunosuppressive molecules, such as PD-L1, through local cytokine-mediated extrinsic effects or P53-mediated intrinsic mechanisms (120, 121).
RT and lymphocytes
Various subtypes of T cells have different resistance to RT, and unlike Th cells and CTLs, Tregs cells are relatively radioresistant (122). B cells and their precursor cells are highly sensitive to radiation-induced DNA damage (123). However, focal radiation treatment of tumor sites at 12-18 Gy using a mouse model suggests that radiation alters B-cell activation, differentiation and clonogenicity, prompting B cells resistance to tumorigenesis (124). Irradiation induces B cells maturation and activation as well as increases the differentiation of tumor antigen-specific plasma cells (124). RT induces the expression of CD20, a common surface antigen on B cells, which is now used as a target for some therapeutic strategies, such as radio-immunotherapy (125).
RT and macrophages
Monocytes, the source of macrophages and DCs, show a high sensitivity to RT and oxidative damages that can lead to single- and double-strand DNA breaks (126). However, both macrophages and dendritic cells upregulate DNA damage repair mechanisms and display a relatively normal DNA repair damage response, leading to an increase in their radio-resistance (126). In TIME, a low-dose RT (LDRT) of 2 Gy induced the differentiation of iNOS+M1 macrophages promoting a pro-immunogenic environment (127). In contrast, higher RT doses promoted tumor infiltration via pro-tumorigenic M2-TAMs polarization (128, 129). In addition, high-dose RT (>8 Gy) may promote anti-inflammatory activation of macrophages (130) and doses of 20 Gy activate the M2 TAMs with pathogenic properties via induction of the immunosuppressive molecules COX-2/PGE2 and NO (128, 131).
RT and DCs
Higher doses of irradiation (20 Gy) affect the function of DCs, leading to a reduction in the efficiency of antigen presentation and a reduced ability to induce T lymphocyte proliferation (126, 132). According to some reports, irradiated DCs secrete increased amounts of pro-inflammatory cytokines (including IL-1β and IL-12) and decreased amounts of anti-inflammatory cytokines such as IL-10 (133, 134). Fractionated RT along with anti-CTLA4 produced abscopal effects caused in part by an increased number of Batf3 DCs, which were abolished in Batf3-/- mice, confirming the important role of Batf3 DCs in RT-induced anti-tumor immunity (135–137).
RT and Natural Killer Cells
Mature NK cells have been reported to be relatively radioresistant, while their precursors are radiosensitive (138). It is generally accepted that the effect of RT on NK cells is influenced by the radiation dose, with low doses of RT activating NK cells and high doses leading to impaired NK function (139). Low doses of RT (0.075 Gy to 0.15 Gy) triggered increased expression of IFN-γ and TNF-α in vitro, and doses of 0.1 Gy to 0.2 Gy resulted in NK activation in an vivo rat model (139). RT induces an ATM-dependent DNA damage response in NK that promotes immune response and reduces exhaustion (140). Radiation increased the ability of NK cells to kill experimental cells such as MCA105 and K562cells (141, 142), and studies using human primary NK cells or the NK-92 cell line also confirmed increased NK cell-mediated cytotoxicity after radiation (143). In addition, RT also induces the migration of NK cells towards the tumor with the help of the chemokine CXCL16/CXCR6 (144). Clinical data from patients with cervical cancer showed increased cytotoxic activity of circulating NK cells post-RT, suggesting systemic activation of NK cells (145). Some studies have also shown a decrease in circulating NK cells but an increase in robust TIM3+ NK cells after ablative RT (146). Conversely, several clinical studies have shown a decrease in NK cell activity post-RT (147–149).
RT and CAFs
CAFs are extremely radio-resistant, do not trigger apoptosis even at high doses of radiation (e.g. 30 Gy) and maintain a strong immunosuppressive effect on activated T cells (in a single dose of 18 Gy) (73). However, post-RT CAFs become senescent and produce a different combination of immunomodulatory molecules (73). In particular, senescent CAFs secrete high levels of TGF-β1, which mediates T-cell rejection and facilitates the establishment of immunosuppressive TIME (150, 151). RT was associated with increased radio-resistance of tumor cells, including in NSCLC, which may be due to the pro-tumor activity of CAFs (152). The pro-tumorigenic nature of radiated CAFs is achieved by direct tumor cell stimulation and suppression of immune cells, including macrophages, DCs, NK cells and T cells (41, 70, 73). Differently, in vivo models have shown that irradiation of CAF (iCAF) alters pro-cancer characteristics and reduces tumor engraftment and angiogenesis (153). In conclusion, CAFs are the main drivers of becoming established immunosuppressive TIME post-RT.
RT and vasculature, endothelial cells
There is evidence that single radiation doses of 5-10 Gy result in relatively mild vascular changes, while higher doses(>10 Gy) result in significant vascular damage, at which point reduced vascular flow due to endothelial cell death leads to hypoxia, reduced effector T cell recruitment and suppression of local immune responses in TIME (154). In addition, high doses of RT (HDRT) also exhibited pro-tumor effects by inducing HIF-1α/TGF-β signaling, increasing the number of CAFs and promoting fibrosis and remodeling of TIME (94).
RT and chemokines, cytokines, and other soluble factors
Increased expression of type I IFNs post-RT stimulates the expression of chemokines CXCL9 and CXCL10, which recruit CXCR3-expressing T cells to the TIME (94). In addition, type I IFNs promote Battf3-expressing DCs to present antigens to CD8+ T cells and initiate anti-tumor immunity (155). IFN-γ promotes Th1 cells polarization and CTLs activation, but also upregulates PD-L1 expression in TIME (156, 157). HDRT induces the production of tumorigenic cytokines, such as HIF-1α/VEGF-A, which promotes the release of a large number of cytokines including IL-1, IL-6, IL-10, and TGF-β (94, 152). Among these, TGF-β exerts multiple immunosuppressive effects. TGF-β inhibits the expansion and cytotoxicity of CD8+ T cells, suppresses the differentiation of CD4+ T cells and induces Tregs transformation (94). In addition, RT-induced TGF-β signaling increases the number of CAFs whose release of CXCL12 binds to the ligands CXCR4 and CXCR7, exerting pleiotropic pro-tumor activity, including induction of tumor survival, metastasis and affecting immune cell infiltration, and function (158–162). RT-induced hypoxic TIME depletes glucose and essential amino acids, and metabolite accumulation occurs, such as lactate, adenosine, and kynurenine, which can blunt the function of CTLs while promoting the accumulation of Tregs (163).
Furthermore, while radiation initially induces an anti-tumor response, radiation also induces chronic inflammation and rebound immune suppression (64). During this phase, tumor-promoting macrophages are recruited to the tumor in a radiation dose-dependent manner, resulting in an immunosuppressive TIME that supports tumor regeneration or resistance. RT also induces HIF-1α which induces PD-L1 expression in tumor cells and TAMs, leading to resistance to RT and immunosuppression (164, 165). In addition, the inflammatory response induced by RT also induced upregulation of IDO, which increased TAMs and MDSCs in TIME, associated with tumor immunosuppression (166, 167). From this, it appears that radiation may have a temporary effect on the immune response to TIME, where there appears to be a window of anti-tumor response. The clinical data from the PACIFIC trial suggested that patients who started checkpoint suppression within 14 days of completing RT appeared to have better outcomes than those who started later (168).
Reversal of RT: From immunosuppression to immunostimulation
A growing number of studies have confirmed that radiation increases the amount of MHC on the cell surface, leading to the expression and release of immunostimulatory cytokines and danger signals, which in turn leads to the activation of innate and adaptive immune responses (92, 169). (Variations of multiple factors in TIME post-RT are summarized in Table 1.) RT acts in several aspects of the immune response, transforming the immunosuppressed state into an immune activated state, e.g. increased infiltration of immune cells in TIME, activation of innate and adaptive immunity, enhanced existing T cell responses, neoantigen-induced immune responses and diminished immunosuppression.
Increased immune cell infiltration in TIME
A single irradiation with 2 Gy increased the ability of tumor-specific CD4+ and CD8+ T cells to migrate into the tumor (127). LDRT-induced expression of inflammatory cytokines (IL-1β, TNF-α and type I and II IFN) and endothelial cell-activated adhesion molecules (ICAM1 and VCAM1) facilitates extravasation and activation of immune cells (92, 193). RT induced the expression of E- and P-selectin on the surface of vascular endothelial cells, facilitating lymphocyte homing (195). RT induced polarization of M1 macrophages and secretion of NO via iNOS activation, promoting normalization of blood vessels and facilitating adhesion and infiltration into the TIME (181). Cytoplasmic DNA produced by irradiated tumor cells is sensed by cGAS, which enhances the expression of type I IFNs through cGAS-STING signaling in host immune cells and tumor cells (188, 189). Increased expression of type I IFNs after RT stimulates the expression of chemokines CXCL9 and CXCL10, which recruit CXCR3-expressing T cells to the TIME (94). Similarly, RT induced CXCL16 to interact with CD8+ T cells to promote their recruitment activity (191). Tumor cells with senescent characteristics induce targeted migration of CD56dim NK cells by secreting CXCL8, which in turn initiates an innate anti-tumor immune response (192). In addition, radiation generates a pro-inflammatory microenvironment with remodeling of the vasculature, allowing T cells extravasation and tumor destruction (212). RT makes refractory “cold” tumors sensitive to immune checkpoint inhibitors by promoting the recruitment of anti-tumor T cells (213). One study showed that HDRT reshaped the immunosuppressive tumor microenvironment, leading to a significant increase in CD8+ T cell tumor infiltration, while suppressing MDSCs, however, the number of CD8+ T cells decreased when extended fractionated radiation was given (170). In studies on oral squamous cell carcinomas, metastatic renal cell carcinomas, and soft tissue sarcomas, neo-adjuvant RT increased the number of locally infiltrating immune cells in a variety of tumors, including CD4+, CD8+, and CD20+ TILs (64, 171, 172). Recent experiments in both mouse models and patient tumors have found that LDRT induces predominantly infiltration of CD4+ T cells with Th1 signatures in TIME (179). (Figure 2)
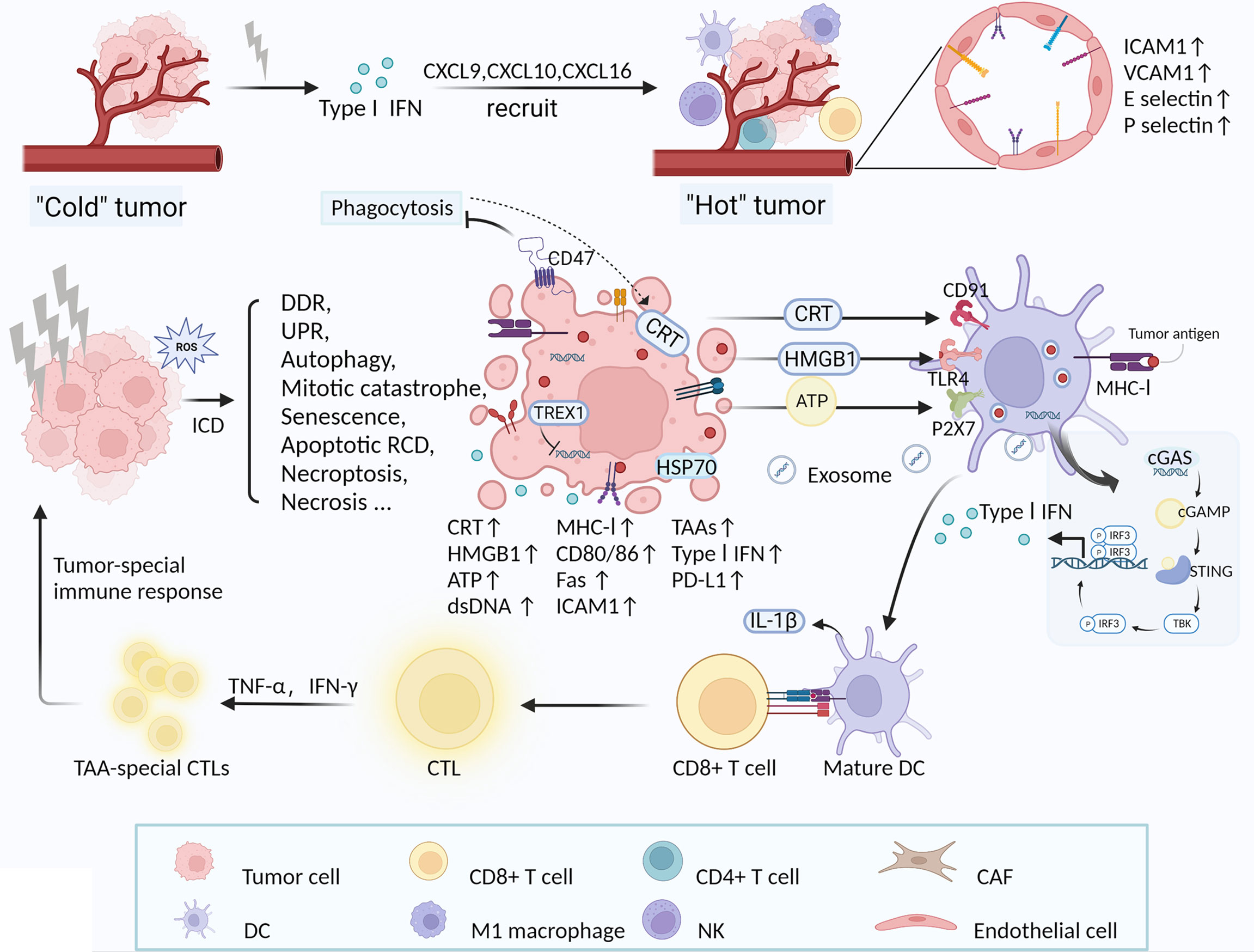
Figure 2 RT-induced increase in immune cells in TIME and tumor-special immune response activated by ICD. LDRT induces increased expression of a variety of molecules, including inflammatory cytokines (type I IFN), chemokines (CXCL9, CXCL10, CXCL16), adhesion molecules (ICAM1 and VCAM1) and E-/P-selectin, which facilitate the recruitment of multiple immune cells (DCs, NK cells, M1 macrophages, CD8+ and CD4+ T cells) (92, 94, 191, 193, 195). Concurrently, RT-induced vascular normalization also promotes immune cell infiltration in the TIME (181). Ultimately, RT transforms “cold” tumors (lymphocyte deficiency) into “hot” tumors sensitive to immunotherapy. RT directly or indirectly causes DNA damage in tumor cells and induces various forms of cellular responses and death, such as DDR, UPR, autophagy, mitotic catastrophe, senescence, apoptotic RCD, necroptosis, necrosis, etc (102, 103, 107, 108). Exposure and release of multiple DAMPs are critical for RT-induced ICD, including CRT (binding to CD91), HMGB1 (binding to TLR4), ATP (binding to P2Y2), and dsDNA (entering DCs) (25, 196–198, 214). DAMPs or danger signals recruit DCs and other APCs into irradiated TIME and to promote the maturation and activation of DCs. Mature DCs enhance uptake of tumor-associated antigens (TAAs) and subsequent cross-presentation with CD8+ CTLs, thereby initiating a tumor-specific adaptive immune response (181–183). During the process, dsDNA from irradiated cells activates the cGAS-STING pathway signaling via TBK1 and IRF3 in host DCs and tumor cells, culminating in the production of type I IFN (188, 189, 199, 200). Type I IFN in TIME is thought to be crucial for the induction of anti-tumor immune responses by RT (165, 190). In addition, RT enhances the immunogenicity of tumor cells by modulating the expression of cell surface molecules and receptors, and enhances the existing immune response, such as MHC-I, stimulatory molecules (CD80, CD86), adhesion molecules (ICAM1), death receptors (Fas) (204, 207, 215–218). PD-L1 expression is increased on tumor cells post-RT, which is one of the important targets for ICB therapy (120, 121, 164, 165, 176).
Innate and adaptive immune response activation
A series of studies have demonstrated that radiation induces innate and adaptive immune response activation, of which RT-induced ICD is a very important mechanism that alters intracellular immunogenicity through external stimulation (182, 214, 219). ICD is characterized by the translocation of the calreticulin (CRT), the release of high-mobility group box 1 (HMGB1) protein and the release of ATP following apoptosis (214). Among these, the ER-derived proteins CRT translocated from the ER to the cell surface are key to the ICD, which binds to CD91 receptors as prophagocytic signals for macrophages and DCs (196, 197). HMGB1 stimulates the TLR4/MyD88/TRIIF pathway and activates T cells (25). In TIME, these factors act synergistically as DAMPs or danger signals to recruit DCs and other APCs into irradiated TIME and to promote the maturation and activation of DCs. Mature DCs enhance uptake of tumor-associated antigens (TAAs) and subsequent cross-presentation with CD8+ CTLs, thereby initiating a tumor-specific adaptive immune response, activating T cells and forming memory phenotypes (181–183). The current study demonstrates that during RT-mediated ICD, tumor-derived dsDNA enters the cytoplasm of DCs and activates the cGAS-STING DNA-sensing pathway signaling a type-I IFN response in which TREX1 exerts an inhibitory effect by degrading DNA (199, 200). STING induces IFN-β transcription and type I IFN expression, which are required for DC activation, ultimately leading to cross-presentation of TAAs and initiation of tumor-specific CTLs (155, 190). In addition to host immune cells, DNA-sensing pathways in tumor cells were also activated, which increase type I IFN production (188, 189). Inflammatory pathways activated by STING ligands have adjuvant activity enhancing tumor-specific adaptive immune responses post-RT (220). ATP is involved in the recruitment of monocytes into tumors (via P2Y2 receptor) and in the production of IL-1β (via P2RX7 receptor and inflammasome NLRP3), which is required for the activation of T cells (198). Unlike Apoptotic cells, which are normally cleared via the anti-inflammatory pathway, necrotic cells are immunogenic due to loss of membrane integrity and sustained release of DAMPs, inducing strong immune and inflammatory responses (207, 221). For example, the apoptosis inhibitor zVAD-fmk effectively blocked programmed cell death and induced necrosis as a form of ICD, and its combination with radiation altered the infiltration of immune cells in TIME, i.e. increased DCs and CD8+ T cells and decreased Tregs and MDSCs (173).(Figure 2)
Enhancement of existing T-cell responses
Preclinical studies have shown that SBRT can also enhance immunogenicity by modulating the expression of cell surface molecules and receptors to reinforce existing immune responses, such as MHC-I, stimulatory molecules (e.g. CD80, CD86), adhesion molecules (e.g. ICAM1), death receptors (e.g. Fas), NKG2D ligands, heat-shock proteins (e.g. HSP70), endoplasmic reticulum (ER)-derived calreticulin, etc. (204, 207, 215–218). Garnett et al. investigated the increase in five cell surface antigen proteins (including Fas, MHC-I, ICAM-1, CEA, or mucin-1) post-RT in 23 human carcinoma cell lines and the results suggested that 91% of human carcinoma cell lines showed dose-dependent increases in at least one antigen (201). Among other things, radiation increased Fas gene expression in tumor cells of CEA-expressing mice, thereby enhancing their sensitivity to CEA-specific CTL-mediated killing (201).
Several studies have confirmed that radiation increases the expression of tumor cell surface antigens, particularly MHC class I/II antigens, and that its expression upregulation is dose-dependent (222, 223). RT upregulates MHC-I molecules and generates unique MHC-I antigenic peptides that promote antigen-specific CTLs responses, one of the important mechanisms of RT induced immune sensitization (201, 204). RT has also been shown to enhance the susceptibility of tumor cells to immune-mediated cytotoxicity via the Fas/FasL pathway, a key mechanism for cell death mediated by NK cells and CTLs (202). Chakraborty et al. demonstrated that RT can upregulate Fas and ICAM-1 expression on MC38 mouse colon cancer cell lines in a dose-dependent manner (194). Hsp70 translocates from the cytoplasm to the extracellular matrix by binding to CD14, CD40, CD91, Lox1 and Toll-like receptors to activate monocytes, macrophages and DCs (205).
NKG2D is an essential costimulatory receptor expressed mainly on CD8+ T cells and NK cells, contributing to enhanced cytotoxicity of T cells and prevention of Fas-mediated autophagy (224–226). In addition, NKG2D+ CD4+ T cells were found in cervical carcinoma while missing on CD4+ T cells in the physiological state (179, 206). Tumors evade NKG2D through multiple mechanisms and soluble NKG2D ligands improve ICB effects, suggesting an significant anti-tumor function of the NKG2D pathway (227–230). RT was also found to upregulate NGK2D ligand expression on tumor cells, making them more sensitive to NK cell-mediated cytotoxicity (207, 208). Recently Fernanda G Herrera and colleagues found an increase of NKG2D+ CD4+ T cells in TIME post-LDRT and exhibited proliferative capacity (179). Furthermore, an elevated expression of NKG2D ligand RAE1 was observed in DCs, supporting a functional cross-talk between DCs and CD4+ T cells via NGK2D pathway (179).
Neoantigen-induced immune responses
Silvia C Formenti et al. reported that RT in combination with CTLA-4 blockade induced anti-tumor responses in chemotherapy-refractory metastatic non-small cell lung cancer (NSCLC), where TCR-Seq analysis of responding patients suggested that CD8+ T cells expanded rapidly in vivo due to the recognition of a new antigen encoded by a gene that was upregulated by radiation (209). RT upregulated the expression of genes containing immunogenic mutations in a mouse model of triple-negative breast cancer with poor immunogenicity and increased tumor cell surface death receptors Fas and DR5, with the result that neoantigen-specific CD8+ T cells and CD4+ T cells preferentially killed irradiated tumor cells as well as promoted epitope spreading (203). This suggests that exposure to RT-induced immunogenic mutations stimulates a systemic anti-tumor response. Factors released from dead cells may be the source of radiation-associated antigenic proteins (RAAPs) (231). RT is able to modulate the peptide repertoire of irradiated cells, and in particular, radiation induces the expression of novel proteins that result in unique MHC-I antigenic peptides that enhance polyclonal antigen-specific CTLs responses (204).Whole-exome sequencing of NSCLC treated with PD-1 blockade confirmed treatment response was better when there was increased mutational burden, higher neo-antigenic burden and mutations in the DNA repair pathway (232). This suggests that the response to immunotherapy after RT is associated with irradiation-induced neoantigens.
Decreased immunosuppression
Radiation also reduces the immunosuppressive properties to achieve remodeling of the TIME. TNF production by radiation-activated T cells leads to direct elimination of MDSCs locally and in the system (187). In contrast, found in experiments of RT combined with DNA vaccines, RT induced a decrease in Tregs, but not MDSCs (174). The transmembrane protein CD47 is overexpressed in most cancer cells and acts as an anti-phagocytic signal to promote immune evasion, with downregulation of expression in the presence of radiation exposure (210).
The effect of RT-induced reconfiguration of the TIME on the effectiveness of immunotherapy sensitization
Immunotherapies designed to activate the patient’s immune system to kill cancer cells include chimeric antigen receptor T-cell therapy (CAR-T), immune-checkpoint blockade (ICB), and tumor vaccines. ICB is the most commonly used immunotherapy option. Immune checkpoints are a series of inhibitory pathways present in the immune system that are essential for the maintenance of self-tolerance and facilitate the regulation of duration and amplitude of physiological immune responses in order to mitigate additional tissue damage. Unfortunately, tumors use certain immune checkpoint pathways as the primary mechanism of immune resistance (211). CTLA-4 binds to its ligands B7-1 (CD80) and B7-2 (CD86) to generate inhibitory signals that suppress T cell activation and cytokine production and protect tumor cells from T cell attack (233). PD-1, mainly expressed in activated T cells, B cells and macrophages, binds to ligands (PD-L1 and PD-L2) to inhibit T cell activity, induce apoptosis of tumor-specific T cells and suppress Tregs apoptosis (234). PD-L1 is expressed on tumor cells, immune cells and epithelial cells, whereas PD-L2 is only induced on antigen-presenting cells (235). PD-L1 is overexpressed on tumor cells and is thought to be associated with immune escape. ICB alleviate the functional suppression of T cells and have been used to shift the balance of TIME from an immunosuppressed to an immune activated state, resulting in a sustained and durable anti-tumor response at multiple lesion sites (236). Anti-PD-1, anti-PD-L1 and anti-CTLA-4 are currently FDA-approved treatment options for a variety of cancer types (237). In addition, some emerging immune target studies have recently emerged, for example, lymphocyte activation gene 3 (LAG-3) overexpression in Tregs produces the immunosuppressive cytokines IL-10 and TGF-β, which inhibit the activity of effector T cells, and whose expression levels correlate with tumor progression and poor prognosis (238). Dual blockade of LAG-3 and PD-1 also increased the number of tumor-infiltrating CD8+ T cells and reduced Tregs, thereby synergistically enhancing anti-tumor immunity (239). Dual blockade of PI3k-γ and CSF-1R promotes a shift in polarization state from M2 TAMs to M1 macrophages, reduces infiltration of MDSCs and enhances CD8+ T cell activation in TIME (240). DC-based vaccines can activate T-cell responses by removing inhibition of antigen presentation (241).
As radiotherapy can produce anti-tumor immune response and a control mechanism of suppressive tumor immune response, thus the combination of RT and drugs targeting tumor immunosuppression enhances the anti-tumor immune response and improves the efficacy of single modality therapy (64). Currently, numerous preclinical and clinical studies reveal the synergistic effect of RT with ICB (155, 165, 179, 180, 209, 242–245), and part of the relevant clinical trials are summarized in Table 2. Jing Zeng and colleagues showed that the combination of PD-1 blockade and local RT led to long-term survival in mice with in situ brain tumors compared to single radiation or immunotherapy, and that immunological data showed increased infiltration of CTLs(CD8+/IFN-γ+/TNF-α+) and reduced infiltration of Tregs (CD4+/FOXP3) in the combined treatment group (175). Single-cell RNA-sequencing revealed a significant increase in B cells germinal center formation after PD-1 blockade and radiotherapy (124). To take advantage of the enhanced radiation-induced endogenous anti-tumor immune response, increased PD-L1 expression on tumor cells or infiltrating immune cells must be counteracted by blocking the PD-1/PD-L1 pathway (211). In studies on NSCLC, PD-L1 expression was increased both in vitro and in vivo after conventionally fractionated radiation. Further studies showed that RT combined with anti-PD-L1 antibody enhanced anti-tumor immune responses by promoting CD8+ T cell infiltration and reducing MDSCs and Tregs cell aggregation (176). Xiaoqiang Qi et al.’s study of the therapeutic effect of Minimally invasive radiofrequency ablation (RFA) combined with sunitinib in an HCC model showed that the combined treatment increased the frequency of CD8+ T cells and DCs, reduced Tregs infiltration, and activated tumor-specific antigen (TSA) immune response, ultimately favoring inhibition of HCC growth (177). In addition, RFA caused the upregulation of PD-1 in tumor-infiltrating T cells by promoting hepatocyte growth factor (HGF) expression, which was inhibited by sunitinib treatment (177). The combination of anti-CTLA-4 antibody and fractionated RT regimens showed an enhanced antitumor response at the primary site in situ and an abscopal effect was observed (135). The frequency of CD8+ T cells producing tumor-specific IFN-γ correlates with secondary tumor suppression (135). Ming-Cheng Chang et al. demonstrated that local RT stimulated DCs by inducing apoptosis and HMGB-1 release. RT combined with DNA vaccine increased the number of antigen-specific CD8+ CTLs and enhanced antitumor efficacy and suggested that biweekly moderate radiation dose was a more optimal choice (174). Chemoradiotherapy-exposed TIMEs were highly enriched with newly infiltrated tumor-specific CD8+ T cells and tissue-resident memory T cells, moreover, the authors found that chemoradiotherapy combined with dual CTLA-4 and PD-1 blockade achieved optimal anti-tumor effects (254). As recent studies have shown, LDRT combined with ICB improved the anti-tumor outcome of ICB by supporting M1 macrophages polarization, enhancing NK cells infiltration and reducing TGF-β levels. Moreover, Depletion of CD4+ T cells and NK cells attenuated this anti-tumor effect, suggesting a key role of both cells in the anti-tumor immunity (180). Similarly, Preclinical and clinical studies supported LDRT induces predominantly infiltration of CD4+ T cells with Th1 signatures in TIME (179).
It is currently believed that HDRT (>5 Gy per fraction) is of limited value in tumor immunomodulation due to the presence of inherent toxicity and immunosuppression, whereas more recent studies have elaborated that LDRT (<3Gy per fraction) stimulates innate and adaptive immune responses, as well as improves the sensitivity of primary and metastatic lesions to ICBs, which is expected to improve cancer treatment outcomes by combining ICB (7). Three recent preclinical studies (1 Gy in lung adenocarcinoma model, 2.5 Gy in melanoma tumors model, 0.5-2 Gy in ovarian cancer model) all elucidated that LDRT acts as a modifier of immune response, remodeling TIME, significantly increasing infiltration of effector immune cells including tumor-infiltrating myeloid cells, DCs, NK cells, CD4+ and CD8+ effector T cells, etc., and was superior to either treatment alone in combination with ICB (178–180). Currently, for “cold” tumors ICB is not effective (255, 256). The corresponding Phase I clinical studies(https://clinicaltrials.gov/ct2/show/NCT02710253, https://clinicaltrials.gov/ct2/show/NCT03728179) were conducted in patients with a variety of “immune desert” tumors, including but not limited to advanced melanoma, anaplastic thyroid carcinoma, and metastatic ovarian cancer, demonstrating the safety, feasibility, and significant therapeutic efficacy of RT in combination with ICB.
It is generally accepted that TGF-β signaling is a strong regulator of radiation response in normal and tumor tissues (257). A preclinical study showed that concurrent administration of TGF-β blockade and RT followed by a PD 1 inhibitor improved tumor control and prolonged survival in a mouse model of metastatic cancer (258). The combination of RT and TGF-β blockade thus offers a new direction for personalized cancer therapy. In recent years, multiple studies using radiosensitizer have revealed potent RT-induced antitumor immunity, while also providing new options for radio-immunotherapy. Experiments by Kaiyuan Ni et al. observed that intra-tumor injection of radiosensitizer repolarized M2 TAMs to M1 macrophages, reduced intra-tumor TGF-β and collagen density, as well as inactivated CAFs. When intravenous radiosensitizer was combined with ICB, the mouse model exhibited enhanced T cells infiltration and a robust abscopal effect (186). Radiosensitizer acting on the STING pathway significantly promoted the activation of DCs and enhanced systemic immune responses against primary and metastatic tumors (184). Recently developed biogenetic gold nanoparticles (Au@MC38), a radiosensitizer, intensified radiation-induced DNA damage and ROS production, exacerbated apoptosis and necrosis, enhanced ICD-mediated immune responses, and achieved a satisfactory survival benefit in combination with ICB (259). Recently, additional pathways have been identified that may be involved in the radio-immunotherapy process. For example, tumor-induced CD45-Ter119+CD71+ erythroid progenitor cells (Ter cells) promote tumor progression by secreting artemin (ARTN), a neurotrophic peptide. Both topical RT and anti-PD-L1 treatment reduced Ter cell abundance and ARTN secretion in mice by an IFN- and CD8+ T cell-dependent manner (260).
Regarding the fractionation and dose of RT, M Zahidunnabi Dewan et al. showed that fractionated but not single-dose RT induced local and systemic anti-tumor immune responses when in combination with anti-CTLA-4 antibody (135). Single radiation doses (>12 Gy) may attenuate immunogenicity through TREX1 induction, while hypo-fractionated regimens (i.e., 8 Gy × 3) may be more effective when used in combination with immunotherapy (188). Fractionated doses of 2.5 Gy×4 and 15 Gy×2 produced higher NK cytotoxicity than single doses (e.g. 30 Gy or 10 Gy) (185). Differently, a study by Byron C Burnette and colleagues suggested that local high single dose RT promotes type I IFN production, initiating a cascade of innate and adaptive immune attacks against tumors by enhancing the ability to prime trans-tumor infiltrating dendritic cells (TIDC) (189). Latest animal and clinical studies indicated that when tumor burden was high, it was necessary to combine high-dose RT, low-dose RT and ICB therapy to achieve optimal therapeutic effects, specifically, HDRT (12 Gy×3) to target primary tumors that had activated T cells, while LDRT (1 Gy×2) targeted metastatic lesions to modulate immunosuppressive stroma and sensitize ICB (180).Thus, fractions and doses can significantly alter the immune response to TIME radiation. Primarily, immune cells must be recruited into the tumor by RT and immune activation achieved, followed by additional immunotherapy in order to exert a stronger anti-tumor immune effect. However, more data are urgently needed to draw more consistent conclusions about RT activation of the immune response and the optimal dose and fractionation in combination therapies with immunotherapy. And there may not be a so-called optimal RT fraction and dose, but different fractions and doses may be the most effective way to utilize the immunogenic properties of radiation in multimodal tumor therapies (4). Regarding the timing of immunotherapy after RT, studies have shown that immune cells migrate into the TIME within two days after the last radiation and remain there for several days, suggesting that immunotherapy is best applied in the middle to end of the treatment cycle (96). Additional studies have also shown that the combination of anti-PD-1 Ab one week after the last irradiation did not improve the tumor effects of RT (165). Thus, hypo-fractionated RT may predominate and longer radiation pauses allow time for the immune system to activate and function (96).
Conclusion
RT remodels the suppressive TIME and mobilizes immune response, which creates the conditions for immunotherapy to work better and thus act locally and systematically against tumors. RT in combination with additional immunotherapy is a promising approach to induce specific anti-tumor immune responses. Accumulating clinical and preclinical data suggest that the immunogenic effects of radiotherapy may convert “cold” tumor into “hot” lesion with massive immune cell infiltration, thereby sensitizing unresponsive tumors to immunotherapy (213). There is a very delicate balance between activation of the immune system and RT-induced immunosuppression, depending on the specific radiation timing, fractionation, and dosing regimen. There is a need to initiate clinical trials and preclinical studies aimed at systematically evaluating the effects of different grading and treatment regimens to gain more insight into the optimal dose and schedule that may be able to induce synergy between immunotherapy and RT. As different immune cell types, with different states of differentiation, exhibit different radio-sensitivities, the selection of the most suitable radiotherapy regimen for combination with immunotherapy must carefully consider the radio-sensitivity of TIME and circulating lymphocytes. In addition to this, which LDRT technology is preferable and which drug combinations benefit the most in radio-immunotherapy are critical issues to be explored more thoroughly in the future. Overall, although there is strong evidence from preclinical work that radiotherapy and immunotherapy are synergistic, clinical reports detailing the interaction of radiotherapy and immunotherapy are limited, and are currently under development.
Author contributions
SZ, YW, and JT wrote and edited the manuscript. MC designed the study and modified grammatical errors. All authors contributed to the article and approved the submission.
Conflict of interest
The authors declare that the research was conducted in the absence of any commercial or financial relationships that could be construed as a potential conflict of interest.
Publisher’s note
All claims expressed in this article are solely those of the authors and do not necessarily represent those of their affiliated organizations, or those of the publisher, the editors and the reviewers. Any product that may be evaluated in this article, or claim that may be made by its manufacturer, is not guaranteed or endorsed by the publisher.
References
1. Hanahan D, Weinberg RA. Hallmarks of cancer: The next generation. Cell (2011) 144(5):646–74. doi: 10.1016/j.cell.2011.02.013
2. Herrera FG, Bourhis J, Coukos G. Radiotherapy combination opportunities leveraging immunity for the next oncology practice. CA Cancer J Clin (2017) 67(1):65–85. doi: 10.3322/caac.21358
3. Schaue D, McBride WH. Opportunities and challenges of radiotherapy for treating cancer. Nat Rev Clin Oncol (2015) 12(9):527–40. doi: 10.1038/nrclinonc.2015.120
4. Frey B, Ruckert M, Deloch L, Ruhle PF, Derer A, Fietkau R, et al. Immunomodulation by ionizing radiation-impact for design of radio-immunotherapies and for treatment of inflammatory diseases. Immunol Rev (2017) 280(1):231–48. doi: 10.1111/imr.12572
5. De Martino M, Daviaud C, Vanpouille-Box C. Radiotherapy: An immune response modifier for immuno-oncology. Semin Immunol (2021) 52:101474. doi: 10.1016/j.smim.2021.101474
6. Cytlak UM, Dyer DP, Honeychurch J, Williams KJ, Travis MA, Illidge TM. Immunomodulation by radiotherapy in tumour control and normal tissue toxicity. Nat Rev Immunol (2022) 22(2):124–38. doi: 10.1038/s41577-021-00568-1
7. Herrera FG, Romero P, Coukos G. Lighting up the tumor fire with low-dose irradiation. Trends Immunol (2022) 43(3):173–9. doi: 10.1016/j.it.2022.01.006
8. Wu T, Dai Y. Tumor microenvironment and therapeutic response. Cancer Lett (2017) 387:61–8. doi: 10.1016/j.canlet.2016.01.043
9. Bennett SR, Carbone FR, Karamalis F, Miller JF, Heath WR. Induction of a CD8+ cytotoxic T lymphocyte response by cross-priming requires cognate CD4+ T cell help. J Exp Med (1997) 186(1):65–70. doi: 10.1084/jem.186.1.65
10. Bourgeois C, Rocha B, Tanchot C. A role for CD40 expression on CD8+ T cells in the generation of CD8+ T cell memory. Science (2002) 297(5589):2060–3. doi: 10.1126/science.1072615
11. Kitano S, Tsuji T, Liu C, Hirschhorn-Cymerman D, Kyi C, Mu Z, et al. Enhancement of tumor-reactive cytotoxic CD4+ T cell responses after ipilimumab treatment in four advanced melanoma patients. Cancer Immunol Res (2013) 1(4):235–44. doi: 10.1158/2326-6066.CIR-13-0068
12. Oh DY, Kwek SS, Raju SS, Li T, McCarthy E, Chow E, et al. Intratumoral CD4(+) T cells mediate anti-tumor cytotoxicity in human bladder cancer. Cell (2020) 181(7):1612–25 e13. doi: 10.1016/j.cell.2020.05.017
13. Fu C, Jiang A. Dendritic cells and CD8 T cell immunity in tumor microenvironment. Front Immunol (2018) 9:3059. doi: 10.3389/fimmu.2018.03059
14. Spranger S, Gajewski TF. Impact of oncogenic pathways on evasion of antitumour immune responses. Nat Rev Cancer (2018) 18(3):139–47. doi: 10.1038/nrc.2017.117
15. Farhood B, Najafi M, Mortezaee K. CD8(+) cytotoxic T lymphocytes in cancer immunotherapy: A review. J Cell Physiol (2019) 234(6):8509–21. doi: 10.1002/jcp.27782
16. Thomas DA, Massague J. TGF-beta directly targets cytotoxic T cell functions during tumor evasion of immune surveillance. Cancer Cell (2005) 8(5):369–80. doi: 10.1016/j.ccr.2005.10.012
17. Solinas G, Germano G, Mantovani A, Allavena P. Tumor-associated macrophages (TAM) as major players of the cancer-related inflammation. J Leukoc Biol (2009) 86(5):1065–73. doi: 10.1189/jlb.0609385
18. Pereira BA, Vennin C, Papanicolaou M, Chambers CR, Herrmann D, Morton JP, et al. CAF subpopulations: A new reservoir of stromal targets in pancreatic cancer. Trends Cancer (2019) 5(11):724–41. doi: 10.1016/j.trecan.2019.09.010
19. Augsten M. Cancer-associated fibroblasts as another polarized cell type of the tumor microenvironment. Front Oncol (2014) 4:62. doi: 10.3389/fonc.2014.00062
20. Monteran L, Erez N. The dark side of fibroblasts: Cancer-associated fibroblasts as mediators of immunosuppression in the tumor microenvironment. Front Immunol (2019) 10:1835. doi: 10.3389/fimmu.2019.01835
21. Sousa CM, Biancur DE, Wang X, Halbrook CJ, Sherman MH, Zhang L, et al. Pancreatic stellate cells support tumour metabolism through autophagic alanine secretion. Nature (2016) 536(7617):479–83. doi: 10.1038/nature19084
22. Ron-Harel N, Ghergurovich JM, Notarangelo G, LaFleur MW, Tsubosaka Y, Sharpe AH, et al. T Cell activation depends on extracellular alanine. Cell Rep (2019) 28(12):3011–21.e4. doi: 10.1016/j.celrep.2019.08.034
23. Chen DS, Mellman I. Oncology meets immunology: the cancer-immunity cycle. Immunity (2013) 39(1):1–10. doi: 10.1016/j.immuni.2013.07.012
24. Locy H, de Mey S, de Mey W, De Ridder M, Thielemans K, Maenhout SK. Immunomodulation of the tumor microenvironment: Turn foe into friend. Front Immunol (2018) 9:2909. doi: 10.3389/fimmu.2018.02909
25. Shevtsov M, Sato H, Multhoff G, Shibata A. Novel approaches to improve the efficacy of immuno-radiotherapy. Front Oncol (2019) 9:156. doi: 10.3389/fonc.2019.00156
26. Joyce JA, Pollard JW. Microenvironmental regulation of metastasis. Nat Rev Cancer (2009) 9(4):239–52. doi: 10.1038/nrc2618
27. Palazon A, Martinez-Forero I, Teijeira A, Morales-Kastresana A, Alfaro C, Sanmamed MF, et al. The HIF-1alpha hypoxia response in tumor-infiltrating T lymphocytes induces functional CD137 (4-1BB) for immunotherapy. Cancer Discov (2012) 2(7):608–23. doi: 10.1158/2159-8290.CD-11-0314
28. Clambey ET, McNamee EN, Westrich JA, Glover LE, Campbell EL, Jedlicka P, et al. Hypoxia-inducible factor-1 alpha-dependent induction of FoxP3 drives regulatory T-cell abundance and function during inflammatory hypoxia of the mucosa. Proc Natl Acad Sci USA (2012) 109(41):E2784–93. doi: 10.1073/pnas.1202366109
29. Garcia-Lora A, Algarra I, Garrido F. MHC class I antigens, immune surveillance, and tumor immune escape. J Cell Physiol (2003) 195(3):346–55. doi: 10.1002/jcp.10290
30. Ma SR, Deng WW, Liu JF, Mao L, Yu GT, Bu LL, et al. Blockade of adenosine A2A receptor enhances CD8(+) T cells response and decreases regulatory T cells in head and neck squamous cell carcinoma. Mol Cancer (2017) 16(1):99. doi: 10.1186/s12943-017-0665-0
31. Zhang J, Shi Z, Xu X, Yu Z, Mi J. The influence of microenvironment on tumor immunotherapy. FEBS J (2019) 286(21):4160–75. doi: 10.1111/febs.15028
32. Kumar V, Donthireddy L, Marvel D, Condamine T, Wang F, Lavilla-Alonso S, et al. Cancer-associated fibroblasts neutralize the anti-tumor effect of CSF1 receptor blockade by inducing PMN-MDSC infiltration of tumors. Cancer Cell (2017) 32(5):654–68.e5. doi: 10.1016/j.ccell.2017.10.005
33. Kalia V, Penny LA, Yuzefpolskiy Y, Baumann FM, Sarkar S. Quiescence of memory CD8(+) T cells is mediated by regulatory T cells through inhibitory receptor CTLA-4. Immunity (2015) 42(6):1116–29. doi: 10.1016/j.immuni.2015.05.023
34. Carmona-Fontaine C, Deforet M, Akkari L, Thompson CB, Joyce JA, Xavier JB. Metabolic origins of spatial organization in the tumor microenvironment. Proc Natl Acad Sci USA (2017) 114(11):2934–9. doi: 10.1073/pnas.1700600114
35. Najafi M, Hashemi Goradel N, Farhood B, Salehi E, Nashtaei MS, Khanlarkhani N, et al. Macrophage polarity in cancer: A review. J Cell Biochem (2019) 120(3):2756–65. doi: 10.1002/jcb.27646
36. Bloch O, Crane CA, Kaur R, Safaee M, Rutkowski MJ, Parsa AT. Gliomas promote immunosuppression through induction of B7-H1 expression in tumor-associated macrophages. Clin Cancer Res (2013) 19(12):3165–75. doi: 10.1158/1078-0432.CCR-12-3314
37. Sadegh L, Chen PW, Brown JR, Han Z, Niederkorn JY. NKT cells act through third party bone marrow-derived cells to suppress NK cell activity in the liver and exacerbate hepatic melanoma metastases. Int J Cancer (2015) 137(5):1085–94. doi: 10.1002/ijc.29480
38. Joyce JA, Fearon DT. T Cell exclusion, immune privilege, and the tumor microenvironment. Science (2015) 348(6230):74–80. doi: 10.1126/science.aaa6204
39. Cazet AS, Hui MN, Elsworth BL, Wu SZ, Roden D, Chan CL, et al. Targeting stromal remodeling and cancer stem cell plasticity overcomes chemoresistance in triple negative breast cancer. Nat Commun (2018) 9(1):2897. doi: 10.1038/s41467-018-05220-6
40. Liu H, Shen J, Lu K. IL-6 and PD-L1 blockade combination inhibits hepatocellular carcinoma cancer development in mouse model. Biochem Biophys Res Commun (2017) 486(2):239–44. doi: 10.1016/j.bbrc.2017.02.128
41. Berzaghi R, Ahktar MA, Islam A, Pedersen BD, Hellevik T, Martinez-Zubiaurre I. Fibroblast-mediated immunoregulation of macrophage function is maintained after irradiation. Cancers (Basel) (2019) 11(5):689. doi: 10.3390/cancers11050689
42. Lakins MA, Ghorani E, Munir H, Martins CP, Shields JD. Cancer-associated fibroblasts induce antigen-specific deletion of CD8 (+) T cells to protect tumour cells. Nat Commun (2018) 9(1):948. doi: 10.1038/s41467-018-03347-0
43. Wei X, Zhang J, Gu Q, Huang M, Zhang W, Guo J, et al. Reciprocal expression of IL-35 and IL-10 defines two distinct effector treg subsets that are required for maintenance of immune tolerance. Cell Rep (2017) 21(7):1853–69. doi: 10.1016/j.celrep.2017.10.090
44. Goswami KK, Bose A, Baral R. Macrophages in tumor: An inflammatory perspective. Clin Immunol (2021) 232:108875. doi: 10.1016/j.clim.2021.108875
45. Krishnamoorthy M, Gerhardt L, Maleki Vareki S. Immunosuppressive effects of myeloid-derived suppressor cells in cancer and immunotherapy. Cells (2021) 10(5):1170. doi: 10.3390/cells10051170
46. Novitskiy SV, Pickup MW, Gorska AE, Owens P, Chytil A, Aakre M, et al. TGF-beta receptor II loss promotes mammary carcinoma progression by Th17 dependent mechanisms. Cancer Discov (2011) 1(5):430–41. doi: 10.1158/2159-8290.CD-11-0100
47. Cham CM, Driessens G, O'Keefe JP, Gajewski TF. Glucose deprivation inhibits multiple key gene expression events and effector functions in CD8+ T cells. Eur J Immunol (2008) 38(9):2438–50. doi: 10.1002/eji.200838289
48. Fletcher M, Ramirez ME, Sierra RA, Raber P, Thevenot P, Al-Khami AA, et al. L-arginine depletion blunts antitumor T-cell responses by inducing myeloid-derived suppressor cells. Cancer Res (2015) 75(2):275–83. doi: 10.1158/0008-5472.CAN-14-1491
49. Rodriguez PC, Quiceno DG, Ochoa AC. L-arginine availability regulates T-lymphocyte cell-cycle progression. Blood (2007) 109(4):1568–73. doi: 10.1182/blood-2006-06-031856
50. Fischer K, Hoffmann P, Voelkl S, Meidenbauer N, Ammer J, Edinger M, et al. Inhibitory effect of tumor cell-derived lactic acid on human T cells. Blood (2007) 109(9):3812–9. doi: 10.1182/blood-2006-07-035972
51. Ohashi T, Akazawa T, Aoki M, Kuze B, Mizuta K, Ito Y, et al. Dichloroacetate improves immune dysfunction caused by tumor-secreted lactic acid and increases antitumor immunoreactivity. Int J Cancer (2013) 133(5):1107–18. doi: 10.1002/ijc.28114
52. Mantovani A, Allavena P, Sica A, Balkwill F. Cancer-related inflammation. Nature (2008) 454(7203):436–44. doi: 10.1038/nature07205
53. Medzhitov R. Origin and physiological roles of inflammation. Nature (2008) 454(7203):428–35. doi: 10.1038/nature07201
54. Budhu S, Schaer DA, Li Y, Toledo-Crow R, Panageas K, Yang X, et al. Blockade of surface-bound TGF-beta on regulatory T cells abrogates suppression of effector T cell function in the tumor microenvironment. Sci Signal (2017) 10(494):eaak9702. doi: 10.1126/scisignal.aak9702
55. Cao X, Cai SF, Fehniger TA, Song J, Collins LI, Piwnica-Worms DR, et al. Granzyme b and perforin are important for regulatory T cell-mediated suppression of tumor clearance. Immunity (2007) 27(4):635–46. doi: 10.1016/j.immuni.2007.08.014
56. Liu C, Chikina M, Deshpande R, Menk AV, Wang T, Tabib T, et al. Treg cells promote the SREBP1-dependent metabolic fitness of tumor-promoting macrophages via repression of CD8(+) T cell-derived interferon-gamma. Immunity (2019) 51(2):381–97.e6. doi: 10.1016/j.immuni.2019.06.017
57. Walker LS, Sansom DM. The emerging role of CTLA4 as a cell-extrinsic regulator of T cell responses. Nat Rev Immunol (2011) 11(12):852–63. doi: 10.1038/nri3108
58. Sarhan D, Hippen KL, Lemire A, Hying S, Luo X, Lenvik T, et al. Adaptive NK cells resist regulatory T-cell suppression driven by IL37. Cancer Immunol Res (2018) 6(7):766–75. doi: 10.1158/2326-6066.CIR-17-0498
59. Li C, Jiang P, Wei S, Xu X, Wang J. Regulatory T cells in tumor microenvironment: new mechanisms, potential therapeutic strategies and future prospects. Mol Cancer (2020) 19(1):116. doi: 10.1158/1557-3125.HIPPO19-B11
60. Cassetta L, Pollard JW. Targeting macrophages: therapeutic approaches in cancer. Nat Rev Drug Discov (2018) 17(12):887–904. doi: 10.1038/nrd.2018.169
61. Shankaran V, Ikeda H, Bruce AT, White JM, Swanson PE, Old LJ, et al. IFNgamma and lymphocytes prevent primary tumour development and shape tumour immunogenicity. Nature (2001) 410(6832):1107–11. doi: 10.1038/35074122
62. Steidl C, Lee T, Shah SP, Farinha P, Han G, Nayar T, et al. Tumor-associated macrophages and survival in classic hodgkin's lymphoma. N Engl J Med (2010) 362(10):875–85. doi: 10.1056/NEJMoa0905680
63. Hanada T, Nakagawa M, Emoto A, Nomura T, Nasu N, Nomura Y. Prognostic value of tumor-associated macrophage count in human bladder cancer. Int J Urol (2000) 7(7):263–9. doi: 10.1046/j.1442-2042.2000.00190.x
64. Monjazeb AM, Schalper KA, Villarroel-Espindola F, Nguyen A, Shiao SL, Young K. Effects of radiation on the tumor microenvironment. Semin Radiat Oncol (2020) 30(2):145–57. doi: 10.1016/j.semradonc.2019.12.004
65. Kang FB, Wang L, Li D, Zhang YG, Sun DX. Hepatocellular carcinomas promote tumor-associated macrophage M2-polarization via increased B7-H3 expression. Oncol Rep (2015) 33(1):274–82. doi: 10.3892/or.2014.3587
66. Gabrilovich DI. Myeloid-derived suppressor cells. Cancer Immunol Res (2017) 5(1):3–8. doi: 10.1158/2326-6066.CIR-16-0297
67. Gabrilovich DI, Nagaraj S. Myeloid-derived suppressor cells as regulators of the immune system. Nat Rev Immunol (2009) 9(3):162–74. doi: 10.1038/nri2506
68. Veglia F, Perego M, Gabrilovich D. Myeloid-derived suppressor cells coming of age. Nat Immunol (2018) 19(2):108–19. doi: 10.1038/s41590-017-0022-x
69. Kwak T, Wang F, Deng H, Condamine T, Kumar V, Perego M, et al. Distinct populations of immune-suppressive macrophages differentiate from monocytic myeloid-derived suppressor cells in cancer. Cell Rep (2020) 33(13):108571. doi: 10.1016/j.celrep.2020.108571
70. Martinez-Zubiaurre I, Chalmers AJ, Hellevik T. Radiation-induced transformation of immunoregulatory networks in the tumor stroma. Front Immunol (2018) 9:1679. doi: 10.3389/fimmu.2018.01679
71. Kraman M, Bambrough PJ, Arnold JN, Roberts EW, Magiera L, Jones JO, et al. Suppression of antitumor immunity by stromal cells expressing fibroblast activation protein-alpha. Science (2010) 330(6005):827–30. doi: 10.1126/science.1195300
72. Ragunathan K, Upfold NLE, Oksenych V. Interaction between fibroblasts and immune cells following DNA damage induced by ionizing radiation. Int J Mol Sci (2020) 21(22):8635. doi: 10.3390/ijms21228635
73. Gorchs L, Hellevik T, Bruun JA, Camilio KA, Al-Saad S, Stuge TB, et al. Cancer-associated fibroblasts from lung tumors maintain their immunosuppressive abilities after high-dose irradiation. Front Oncol (2015) 5:87. doi: 10.3389/fonc.2015.00087
74. Pearce OMT, Delaine-Smith RM, Maniati E, Nichols S, Wang J, Bohm S, et al. Deconstruction of a metastatic tumor microenvironment reveals a common matrix response in human cancers. Cancer Discov (2018) 8(3):304–19. doi: 10.1158/2159-8290.CD-17-0284
75. Kobayashi N, Miyoshi S, Mikami T, Koyama H, Kitazawa M, Takeoka M, et al. Hyaluronan deficiency in tumor stroma impairs macrophage trafficking and tumor neovascularization. Cancer Res (2010) 70(18):7073–83. doi: 10.1158/0008-5472.CAN-09-4687
76. Duperret EK, Trautz A, Ammons D, Perales-Puchalt A, Wise MC, Yan J, et al. Alteration of the tumor stroma using a consensus DNA vaccine targeting fibroblast activation protein (FAP) synergizes with antitumor vaccine therapy in mice. Clin Cancer Res (2018) 24(5):1190–201. doi: 10.1158/1078-0432.CCR-17-2033
77. Xia Q, Zhang FF, Geng F, Liu CL, Xu P, Lu ZZ, et al. Anti-tumor effects of DNA vaccine targeting human fibroblast activation protein alpha by producing specific immune responses and altering tumor microenvironment in the 4T1 murine breast cancer model. Cancer Immunol Immunother (2016) 65(5):613–24. doi: 10.1007/s00262-016-1827-4
78. David CJ, Huang YH, Chen M, Su J, Zou Y, Bardeesy N, et al. TGF-beta tumor suppression through a lethal EMT. Cell (2016) 164(5):1015–30. doi: 10.1016/j.cell.2016.01.009
79. Sounni NE, Dehne K, van Kempen L, Egeblad M, Affara NI, Cuevas I, et al. Stromal regulation of vessel stability by MMP14 and TGFbeta. Dis Model Mech (2010) 3(5-6):317–32. doi: 10.1242/dmm.003863
80. Friedl P, Alexander S. Cancer invasion and the microenvironment: plasticity and reciprocity. Cell (2011) 147(5):992–1009. doi: 10.1016/j.cell.2011.11.016
81. Calon A, Espinet E, Palomo-Ponce S, Tauriello DV, Iglesias M, Cespedes MV, et al. Dependency of colorectal cancer on a TGF-beta-driven program in stromal cells for metastasis initiation. Cancer Cell (2012) 22(5):571–84. doi: 10.1016/j.ccr.2012.08.013
82. Barcellos-Hoff MH, Derynck R, Tsang ML, Weatherbee JA. Transforming growth factor-beta activation in irradiated murine mammary gland. J Clin Invest (1994) 93(2):892–9. doi: 10.1172/JCI117045
83. Altman BJ, Stine ZE, Dang CV. From Krebs to clinic: Glutamine metabolism to cancer therapy. Nat Rev Cancer (2016) 16(11):749. doi: 10.1038/nrc.2016.71
84. Cheong JE, Sun L. Targeting the IDO1/TDO2-KYN-AhR pathway for cancer immunotherapy - challenges and opportunities. Trends Pharmacol Sci (2018) 39(3):307–25. doi: 10.1016/j.tips.2017.11.007
85. Moffett JR, Namboodiri MA. Tryptophan and the immune response. Immunol Cell Biol (2003) 81(4):247–65. doi: 10.1046/j.1440-1711.2003.t01-1-01177.x
86. Prendergast GC, Metz R, Muller AJ. IDO recruits tregs in melanoma. Cell Cycle (2009) 8(12):1818–9. doi: 10.4161/cc.8.12.8887
87. Romani L, Bistoni F, Perruccio K, Montagnoli C, Gaziano R, Bozza S, et al. Thymosin alpha1 activates dendritic cell tryptophan catabolism and establishes a regulatory environment for balance of inflammation and tolerance. Blood (2006) 108(7):2265–74. doi: 10.1182/blood-2006-02-004762
88. Mendler AN, Hu B, Prinz PU, Kreutz M, Gottfried E, Noessner E. Tumor lactic acidosis suppresses CTL function by inhibition of p38 and JNK/c-jun activation. Int J Cancer (2012) 131(3):633–40. doi: 10.1002/ijc.26410
89. Duvel K, Yecies JL, Menon S, Raman P, Lipovsky AI, Souza AL, et al. Activation of a metabolic gene regulatory network downstream of mTOR complex 1. Mol Cell (2010) 39(2):171–83. doi: 10.1016/j.molcel.2010.06.022
90. Eil R, Vodnala SK, Clever D, Klebanoff CA, Sukumar M, Pan JH, et al. Ionic immune suppression within the tumour microenvironment limits T cell effector function. Nature (2016) 537(7621):539–43. doi: 10.1038/nature19364
91. Wennerberg E, Vanpouille-Box C, Bornstein S, Yamazaki T, Demaria S, Galluzzi L. Immune recognition of irradiated cancer cells. Immunol Rev (2017) 280(1):220–30. doi: 10.1111/imr.12568
92. Jarosz-Biej M, Smolarczyk R, Cichon T, Kulach N. Tumor microenvironment as a "Game changer" in cancer radiotherapy. Int J Mol Sci (2019) 20(13):3212. doi: 10.3390/ijms20133212
93. Junttila MR, de Sauvage FJ. Influence of tumour micro-environment heterogeneity on therapeutic response. Nature (2013) 501(7467):346–54. doi: 10.1038/nature12626
94. Portella L, Scala S. Ionizing radiation effects on the tumor microenvironment. Semin Oncol (2019) 46(3):254–60. doi: 10.1053/j.seminoncol.2019.07.003
95. Pan X, Zhang C, Wang J, Wang P, Gao Y, Shang S, et al. Epigenome signature as an immunophenotype indicator prompts durable clinical immunotherapy benefits in lung adenocarcinoma. Brief Bioinform (2022) 23(1):bbab481. doi: 10.1093/bib/bbab481
96. Derer A, Frey B, Fietkau R, Gaipl US. Immune-modulating properties of ionizing radiation: Rationale for the treatment of cancer by combination radiotherapy and immune checkpoint inhibitors. Cancer Immunol Immunother (2016) 65(7):779–86. doi: 10.1007/s00262-015-1771-8
97. Mukherjee S, Chakraborty A. Radiation-induced bystander phenomenon: insight and implications in radiotherapy. Int J Radiat Biol (2019) 95(3):243–63. doi: 10.1080/09553002.2019.1547440
98. Demaria S, Ng B, Devitt ML, Babb JS, Kawashima N, Liebes L, et al. Ionizing radiation inhibition of distant untreated tumors (abscopal effect) is immune mediated. Int J Radiat Oncol Biol Phys (2004) 58(3):862–70. doi: 10.1016/j.ijrobp.2003.09.012
99. Weichselbaum RR, Liang H, Deng L, Fu YX. Radiotherapy and immunotherapy: a beneficial liaison? Nat Rev Clin Oncol (2017) 14(6):365–79. doi: 10.1038/nrclinonc.2016.211
100. Romano E, Honeychurch J, Illidge TM. Radiotherapy-immunotherapy combination: How will we bridge the gap between pre-clinical promise and effective clinical delivery? Cancers (Basel) (2021) 13(3):457. doi: 10.3390/cancers13030457
101. Baumann M, Krause M, Overgaard J, Debus J, Bentzen SM, Daartz J, et al. Radiation oncology in the era of precision medicine. Nat Rev Cancer (2016) 16(4):234–49. doi: 10.1038/nrc.2016.18
102. Rodriguez-Ruiz ME, Vitale I, Harrington KJ, Melero I, Galluzzi L. Immunological impact of cell death signaling driven by radiation on the tumor microenvironment. Nat Immunol (2020) 21(2):120–34. doi: 10.1038/s41590-019-0561-4
103. Galluzzi L, Vitale I, Aaronson SA, Abrams JM, Adam D, Agostinis P, et al. Molecular mechanisms of cell death: Recommendations of the nomenclature committee on cell death 2018. Cell Death Differ (2018) 25(3):486–541. doi: 10.1038/s41418-017-0012-4
104. Wilkins AC, Patin EC, Harrington KJ, Melcher AA. The immunological consequences of radiation-induced DNA damage. J Pathol (2019) 247(5):606–14. doi: 10.1002/path.5232
105. Lopez-Soto A, Gonzalez S, Smyth MJ, Galluzzi L. Control of metastasis by NK cells. Cancer Cell (2017) 32(2):135–54. doi: 10.1016/j.ccell.2017.06.009
106. Janus P, Szoltysek K, Zajac G, Stokowy T, Walaszczyk A, Widlak W, et al. Pro-inflammatory cytokine and high doses of ionizing radiation have similar effects on the expression of NF-kappaB-dependent genes. Cell Signal (2018) 46:23–31. doi: 10.1016/j.cellsig.2018.02.011
107. Mackenzie KJ, Carroll P, Martin CA, Murina O, Fluteau A, Simpson DJ, et al. cGAS surveillance of micronuclei links genome instability to innate immunity. Nature (2017) 548(7668):461–5. doi: 10.1038/nature23449
108. Harding SM, Benci JL, Irianto J, Discher DE, Minn AJ, Greenberg RA. Mitotic progression following DNA damage enables pattern recognition within micronuclei. Nature (2017) 548(7668):466–70. doi: 10.1038/nature23470
109. Snyder AG, Hubbard NW, Messmer MN, Kofman SB, Hagan CE, Orozco SL, et al. Intratumoral activation of the necroptotic pathway components RIPK1 and RIPK3 potentiates antitumor immunity. Sci Immunol (2019) 4(36):eaaw2004. doi: 10.1126/sciimmunol.aaw2004
110. Wang HH, Wu ZQ, Qian D, Zaorsky NG, Qiu MH, Cheng JJ, et al. Ablative hypofractionated radiation therapy enhances non-small cell lung cancer cell killing via preferential stimulation of necroptosis In vitro and in vivo. Int J Radiat Oncol Biol Phys (2018) 101(1):49–62. doi: 10.1016/j.ijrobp.2018.01.036
111. Adjemian S, Oltean T, Martens S, Wiernicki B, Goossens V, Vanden Berghe T, et al. Ionizing radiation results in a mixture of cellular outcomes including mitotic catastrophe, senescence, methuosis, and iron-dependent cell death. Cell Death Dis (2020) 11(11):1003. doi: 10.1038/s41419-020-03209-y
112. Yard BD, Adams DJ, Chie EK, Tamayo P, Battaglia JS, Gopal P, et al. A genetic basis for the variation in the vulnerability of cancer to DNA damage. Nat Commun (2016) 7:11428. doi: 10.1038/ncomms11428
113. Good JS, Harrington KJ. The hallmarks of cancer and the radiation oncologist: updating the 5Rs of radiobiology. Clin Oncol (R Coll Radiol) (2013) 25(10):569–77. doi: 10.1016/j.clon.2013.06.009
114. Hill RP. The changing paradigm of tumour response to irradiation. Br J Radiol (2017) 90(1069):20160474. doi: 10.1259/bjr.20160474
115. Faget DV, Ren Q, Stewart SA. Unmasking senescence: context-dependent effects of SASP in cancer. Nat Rev Cancer (2019) 19(8):439–53. doi: 10.1038/s41568-019-0156-2
116. McBride S, Sherman E, Tsai CJ, Baxi S, Aghalar J, Eng J, et al. Randomized phase II trial of nivolumab with stereotactic body radiotherapy versus nivolumab alone in metastatic head and neck squamous cell carcinoma. J Clin Oncol (2021) 39(1):30–7. doi: 10.1200/JCO.20.00290
117. Rodel F, Frey B, Capalbo G, Gaipl U, Keilholz L, Voll R, et al. Discontinuous induction of X-linked inhibitor of apoptosis in EA.hy.926 endothelial cells is linked to NF-kappaB activation and mediates the anti-inflammatory properties of low-dose ionising-radiation. Radiother Oncol (2010) 97(2):346–51. doi: 10.1016/j.radonc.2010.01.013
118. Fridlender ZG, Sun J, Kim S, Kapoor V, Cheng G, Ling L, et al. Polarization of tumor-associated neutrophil phenotype by TGF-beta: "N1" versus "N2" TAN. Cancer Cell (2009) 16(3):183–94. doi: 10.1016/j.ccr.2009.06.017
119. Zhang F, Wang H, Wang X, Jiang G, Liu H, Zhang G, et al. TGF-beta induces M2-like macrophage polarization via SNAIL-mediated suppression of a pro-inflammatory phenotype. Oncotarget (2016) 7(32):52294–306. doi: 10.18632/oncotarget.10561
120. Deng L, Liang H, Burnette B, Weicheslbaum RR, Fu YX. Radiation and anti-PD-L1 antibody combinatorial therapy induces T cell-mediated depletion of myeloid-derived suppressor cells and tumor regression. Oncoimmunology (2014) 3:e28499. doi: 10.4161/onci.28499
121. Cortez MA, Ivan C, Valdecanas D, Wang X, Peltier HJ, Ye Y, et al. PDL1 regulation by p53 via miR-34. J Natl Cancer Inst (2016) 108(1):djv303. doi: 10.1093/jnci/djv303
122. Liu S, Sun X, Luo J, Zhu H, Yang X, Guo Q, et al. Effects of radiation on T regulatory cells in normal states and cancer: Mechanisms and clinical implications. Am J Cancer Res (2015) 5(11):3276–85.
123. Nishii K, Gibbons DL, Titley I, Papworth D, Goodhead DT, Greaves M. Regulation of the apoptotic response to radiation damage in b cell development. Cell Death Differ (1998) 5(1):77–86. doi: 10.1038/sj.cdd.4400317
124. Kim SS, Shen S, Miyauchi S, Sanders PD, Franiak-Pietryga I, Mell L, et al. B cells improve overall survival in HPV-associated squamous cell carcinomas and are activated by radiation and PD-1 blockade. Clin Cancer Res (2020) 26(13):3345–59. doi: 10.1158/1078-0432.CCR-19-3211
125. Kunala S, Macklis RM. Ionizing radiation induces CD20 surface expression on human b cells. Int J Cancer (2001) 96(3):178–81. doi: 10.1002/ijc.1018
126. Bauer M, Goldstein M, Christmann M, Becker H, Heylmann D, Kaina B. Human monocytes are severely impaired in base and DNA double-strand break repair that renders them vulnerable to oxidative stress. Proc Natl Acad Sci USA (2011) 108(52):21105–10. doi: 10.1073/pnas.1111919109
127. Klug F, Prakash H, Huber PE, Seibel T, Bender N, Halama N, et al. Low-dose irradiation programs macrophage differentiation to an iNOS(+)/M1 phenotype that orchestrates effective T cell immunotherapy. Cancer Cell (2013) 24(5):589–602. doi: 10.1016/j.ccr.2013.09.014
128. Tsai CS, Chen FH, Wang CC, Huang HL, Jung SM, Wu CJ, et al. Macrophages from irradiated tumors express higher levels of iNOS, arginase-I and COX-2, and promote tumor growth. Int J Radiat Oncol Biol Phys (2007) 68(2):499–507. doi: 10.1016/j.ijrobp.2007.01.041
129. Vitale I, Manic G, Coussens LM, Kroemer G, Galluzzi L. Macrophages and metabolism in the tumor microenvironment. Cell Metab (2019) 30(1):36–50. doi: 10.1016/j.cmet.2019.06.001
130. Meziani L, Deutsch E, Mondini M. Macrophages in radiation injury: a new therapeutic target. Oncoimmunology (2018) 7(10):e1494488. doi: 10.1080/2162402X.2018.1494488
131. Hellevik T, Martinez-Zubiaurre I. Radiotherapy and the tumor stroma: the importance of dose and fractionation. Front Oncol (2014) 4:1. doi: 10.3389/fonc.2014.00001
132. Cao MD, Chen ZD, Xing Y. Gamma irradiation of human dendritic cells influences proliferation and cytokine profile of T cells in autologous mixed lymphocyte reaction. Cell Biol Int (2004) 28(3):223–8. doi: 10.1016/j.cellbi.2003.12.006
133. Persa E, Szatmari T, Safrany G, Lumniczky K. In vivo irradiation of mice induces activation of dendritic cells. Int J Mol Sci (2018) 19(8):2391. doi: 10.3390/ijms19082391
134. Chun SH, Park GY, Han YK, Kim SD, Kim JS, Lee CG, et al. Effect of low dose radiation on differentiation of bone marrow cells into dendritic cells. Dose Response (2012) 11(3):374–84. doi: 10.2203/dose-response.12-041.Lee
135. Dewan MZ, Galloway AE, Kawashima N, Dewyngaert JK, Babb JS, Formenti SC, et al. Fractionated but not single-dose radiotherapy induces an immune-mediated abscopal effect when combined with anti-CTLA-4 antibody. Clin Cancer Res (2009) 15(17):5379–88. doi: 10.1158/1078-0432.CCR-09-0265
136. Desai P, Tahiliani V, Abboud G, Stanfield J, Salek-Ardakani S. Batf3-dependent dendritic cells promote optimal CD8 T cell responses against respiratory poxvirus infection. J Virol (2018) 92(16):e00495-18. doi: 10.1128/JVI.00495-18
137. Hildner K, Edelson BT, Purtha WE, Diamond M, Matsushita H, Kohyama M, et al. Batf3 deficiency reveals a critical role for CD8alpha+ dendritic cells in cytotoxic T cell immunity. Science (2008) 322(5904):1097–100. doi: 10.1126/science.1164206
138. Hochman PS, Cudkowicz G, Dausset J. Decline of natural killer cell activity in sublethally irradiated mice. J Natl Cancer Inst (1978) 61(1):265–8. doi: 10.1093/jnci/61.1.265
139. Chen J, Liu X, Zeng Z, Li J, Luo Y, Sun W, et al. Immunomodulation of NK cells by ionizing radiation. Front Oncol (2020) 10:874. doi: 10.3389/fonc.2020.00874
140. Alvarez M, Simonetta F, Baker J, Pierini A, Wenokur AS, Morrison AR, et al. Regulation of murine NK cell exhaustion through the activation of the DNA damage repair pathway. JCI Insight (2019) 5(14):e127729. doi: 10.1172/jci.insight.127729
141. Begovic M, Herberman R, Gorelik E. Increase in immunogenicity and sensitivity to natural cell-mediated cytotoxicity following in vitro exposure of MCA105 tumor cells to ultraviolet radiation. Cancer Res (1991) 51(19):5153–9.
142. Uchida A, Mizutani Y, Nagamuta M, Ikenaga M. Effects of X-ray irradiation on natural killer (NK) cell system. i. elevation of sensitivity of tumor cells and lytic function of NK cells. Immunopharmacol Immunotoxicol (1989) 11(2-3):507–19. doi: 10.3109/08923978909005381
143. Yang KL, Wang YS, Chang CC, Huang SC, Huang YC, Chi MS, et al. Reciprocal complementation of the tumoricidal effects of radiation and natural killer cells. PloS One (2013) 8(4):e61797. doi: 10.1371/journal.pone.0061797
144. Yoon MS, Pham CT, Phan MT, Shin DJ, Jang YY, Park MH, et al. Irradiation of breast cancer cells enhances CXCL16 ligand expression and induces the migration of natural killer cells expressing the CXCR6 receptor. Cytotherapy (2016) 18(12):1532–42. doi: 10.1016/j.jcyt.2016.08.006
145. Pinel MI, Esteves EB, Rumjanek VM. Increased natural killer cell activity in uterine cervix cancer patients undergoing radiation therapy. Nat Immun (1995) 14(4):216–24.
146. McGee HM, Daly ME, Azghadi S, Stewart SL, Oesterich L, Schlom J, et al. Stereotactic ablative radiation therapy induces systemic differences in peripheral blood immunophenotype dependent on irradiated site. Int J Radiat Oncol Biol Phys (2018) 101(5):1259–70. doi: 10.1016/j.ijrobp.2018.04.038
147. Yamazaki H, Yoshioka Y, Inoue T, Tanaka E, Nishikubo M, Sato T, et al. Changes in natural killer cell activity by external radiotherapy and/or brachytherapy. Oncol Rep (2002) 9(2):359–63. doi: 10.3892/or.9.2.359
148. Mozaffari F, Lindemalm C, Choudhury A, Granstam-Bjorneklett H, Helander I, Lekander M, et al. NK-cell and T-cell functions in patients with breast cancer: Effects of surgery and adjuvant chemo- and radiotherapy. Br J Cancer (2007) 97(1):105–11. doi: 10.1038/sj.bjc.6603840
149. McGinnes K, Florence J, Penny R. The effect of radiotherapy on the natural killer (NK)-cell activity of cancer patients. J Clin Immunol (1987) 7(3):210–7. doi: 10.1007/BF00915726
150. Mariathasan S, Turley SJ, Nickles D, Castiglioni A, Yuen K, Wang Y, et al. TGFbeta attenuates tumour response to PD-L1 blockade by contributing to exclusion of T cells. Nature (2018) 554(7693):544–8. doi: 10.1038/nature25501
151. Tauriello DVF, Palomo-Ponce S, Stork D, Berenguer-Llergo A, Badia-Ramentol J, Iglesias M, et al. TGFbeta drives immune evasion in genetically reconstituted colon cancer metastasis. Nature (2018) 554(7693):538–43. doi: 10.1038/nature25492
152. Wennerberg E, Lhuillier C, Vanpouille-Box C, Pilones KA, Garcia-Martinez E, Rudqvist NP, et al. Barriers to radiation-induced In situ tumor vaccination. Front Immunol (2017) 8:229. doi: 10.3389/fimmu.2017.00229
153. Grinde MT, Vik J, Camilio KA, Martinez-Zubiaurre I, Hellevik T. Ionizing radiation abrogates the pro-tumorigenic capacity of cancer-associated fibroblasts co-implanted in xenografts. Sci Rep (2017) 7:46714. doi: 10.1038/srep46714
154. Park HJ, Griffin RJ, Hui S, Levitt SH, Song CW. Radiation-induced vascular damage in tumors: implications of vascular damage in ablative hypofractionated radiotherapy (SBRT and SRS). Radiat Res (2012) 177(3):311–27. doi: 10.1667/RR2773.1
155. Deng L, Liang H, Xu M, Yang X, Burnette B, Arina A, et al. STING-dependent cytosolic DNA sensing promotes radiation-induced type I interferon-dependent antitumor immunity in immunogenic tumors. Immunity (2014) 41(5):843–52. doi: 10.1016/j.immuni.2014.10.019
156. Mandai M, Hamanishi J, Abiko K, Matsumura N, Baba T, Konishi I. Dual faces of IFNgamma in cancer progression: A role of PD-L1 induction in the determination of pro- and antitumor immunity. Clin Cancer Res (2016) 22(10):2329–34. doi: 10.1158/1078-0432.CCR-16-0224
157. Spranger S, Spaapen RM, Zha Y, Williams J, Meng Y, Ha TT, et al. Up-regulation of PD-L1, IDO, and t(regs) in the melanoma tumor microenvironment is driven by CD8(+) T cells. Sci Transl Med (2013) 5(200):200ra116. doi: 10.1126/scitranslmed.3006504
158. Wang Z, Tang Y, Tan Y, Wei Q, Yu W. Cancer-associated fibroblasts in radiotherapy: challenges and new opportunities. Cell Commun Signal (2019) 17(1):47. doi: 10.1186/s12964-019-0362-2
159. Ziani L, Chouaib S, Thiery J. Alteration of the antitumor immune response by cancer-associated fibroblasts. Front Immunol (2018) 9:414. doi: 10.3389/fimmu.2018.00414
160. Scala S. Molecular pathways: Targeting the CXCR4-CXCL12 axis–untapped potential in the tumor microenvironment. Clin Cancer Res (2015) 21(19):4278–85. doi: 10.1158/1078-0432.CCR-14-0914
161. Guo F, Wang Y, Liu J, Mok SC, Xue F, Zhang W. CXCL12/CXCR4: A symbiotic bridge linking cancer cells and their stromal neighbors in oncogenic communication networks. Oncogene (2016) 35(7):816–26. doi: 10.1038/onc.2015.139
162. Orimo A, Gupta PB, Sgroi DC, Arenzana-Seisdedos F, Delaunay T, Naeem R, et al. Stromal fibroblasts present in invasive human breast carcinomas promote tumor growth and angiogenesis through elevated SDF-1/CXCL12 secretion. Cell (2005) 121(3):335–48. doi: 10.1016/j.cell.2005.02.034
163. Renner K, Singer K, Koehl GE, Geissler EK, Peter K, Siska PJ, et al. Metabolic hallmarks of tumor and immune cells in the tumor microenvironment. Front Immunol (2017) 8:248. doi: 10.3389/fimmu.2017.00248
164. Noman MZ, Desantis G, Janji B, Hasmim M, Karray S, Dessen P, et al. PD-L1 is a novel direct target of HIF-1alpha, and its blockade under hypoxia enhanced MDSC-mediated T cell activation. J Exp Med (2014) 211(5):781–90. doi: 10.1084/jem.20131916
165. Dovedi SJ, Adlard AL, Lipowska-Bhalla G, McKenna C, Jones S, Cheadle EJ, et al. Acquired resistance to fractionated radiotherapy can be overcome by concurrent PD-L1 blockade. Cancer Res (2014) 74(19):5458–68. doi: 10.1158/0008-5472.CAN-14-1258
166. Monjazeb AM, Kent MS, Grossenbacher SK, Mall C, Zamora AE, Mirsoian A, et al. Blocking indolamine-2,3-Dioxygenase rebound immune suppression boosts antitumor effects of radio-immunotherapy in murine models and spontaneous canine malignancies. Clin Cancer Res (2016) 22(17):4328–40. doi: 10.1158/1078-0432.CCR-15-3026
167. Li A, Barsoumian HB, Schoenhals JE, Caetano MS, Wang X, Menon H, et al. IDO1 inhibition overcomes radiation-induced "Rebound immune suppression" by reducing numbers of IDO1-expressing myeloid-derived suppressor cells in the tumor microenvironment. Int J Radiat Oncol Biol Phys (2019) 104(4):903–12. doi: 10.1016/j.ijrobp.2019.03.022
168. Antonia SJ, Villegas A, Daniel D, Vicente D, Murakami S, Hui R, et al. Durvalumab after chemoradiotherapy in stage III non-Small-Cell lung cancer. N Engl J Med (2017) 377(20):1919–29. doi: 10.1056/NEJMoa1709937
169. Ngwa W, Irabor OC, Schoenfeld JD, Hesser J, Demaria S, Formenti SC. Using immunotherapy to boost the abscopal effect. Nat Rev Cancer (2018) 18(5):313–22. doi: 10.1038/nrc.2018.6
170. Filatenkov A, Baker J, Mueller AM, Kenkel J, Ahn GO, Dutt S, et al. Ablative tumor radiation can change the tumor immune cell microenvironment to induce durable complete remissions. Clin Cancer Res (2015) 21(16):3727–39. doi: 10.1158/1078-0432.CCR-14-2824
171. Suwa T, Saio M, Umemura N, Yamashita T, Toida M, Shibata T, et al. Preoperative radiotherapy contributes to induction of proliferative activity of CD8+ tumor-infiltrating T-cells in oral squamous cell carcinoma. Oncol Rep (2006) 15(4):757–63. doi: 10.3892/or.15.4.757
172. Singh AK, Winslow TB, Kermany MH, Goritz V, Heit L, Miller A, et al. A pilot study of stereotactic body radiation therapy combined with cytoreductive nephrectomy for metastatic renal cell carcinoma. Clin Cancer Res (2017) 23(17):5055–65. doi: 10.1158/1078-0432.CCR-16-2946
173. Werthmoller N, Frey B, Wunderlich R, Fietkau R, Gaipl US. Modulation of radiochemoimmunotherapy-induced B16 melanoma cell death by the pan-caspase inhibitor zVAD-fmk induces anti-tumor immunity in a HMGB1-, nucleotide- and T-cell-dependent manner. Cell Death Dis (2015) 6:e1761. doi: 10.1038/cddis.2015.129
174. Chang MC, Chen YL, Lin HW, Chiang YC, Chang CF, Hsieh SF, et al. Irradiation enhances abscopal anti-tumor effects of antigen-specific immunotherapy through regulating tumor microenvironment. Mol Ther (2018) 26(2):404–19. doi: 10.1016/j.ymthe.2017.11.011
175. Zeng J, See AP, Phallen J, Jackson CM, Belcaid Z, Ruzevick J, et al. Anti-PD-1 blockade and stereotactic radiation produce long-term survival in mice with intracranial gliomas. Int J Radiat Oncol Biol Phys (2013) 86(2):343–9. doi: 10.1016/j.ijrobp.2012.12.025
176. Gong X, Li X, Jiang T, Xie H, Zhu Z, Zhou F, et al. Combined radiotherapy and anti-PD-L1 antibody synergistically enhances antitumor effect in non-small cell lung cancer. J Thorac Oncol (2017) 12(7):1085–97. doi: 10.1016/j.jtho.2017.04.014
177. Qi X, Yang M, Ma L, Sauer M, Avella D, Kaifi JT, et al. Synergizing sunitinib and radiofrequency ablation to treat hepatocellular cancer by triggering the antitumor immune response. J Immunother Cancer (2020) 8(2):e001038. doi: 10.1136/jitc-2020-001038
178. Patel RB, Hernandez R, Carlson P, Grudzinski J, Bates AM, Jagodinsky JC, et al. Low-dose targeted radionuclide therapy renders immunologically cold tumors responsive to immune checkpoint blockade. Sci Transl Med (2021) 13(602):eabb3631. doi: 10.1126/scitranslmed.abb3631
179. Herrera FG, Ronet C, Ochoa de Olza M, Barras D, Crespo I, Andreatta M, et al. Low-dose radiotherapy reverses tumor immune desertification and resistance to immunotherapy. Cancer Discov (2022) 12(1):108–33. doi: 10.1158/2159-8290.CD-21-0003
180. Barsoumian HB, Ramapriyan R, Younes AI, Caetano MS, Menon H, Comeaux NI, et al. Low-dose radiation treatment enhances systemic antitumor immune responses by overcoming the inhibitory stroma. J Immunother Cancer (2020) 8(2):e000537. doi: 10.1136/jitc-2020-000537
181. Marciscano AE, Haimovitz-Friedman A, Lee P, Tran PT, Tome WA, Guha C, et al. Immunomodulatory effects of stereotactic body radiation therapy: Preclinical insights and clinical opportunities. Int J Radiat Oncol Biol Phys (2021) 110(1):35–52. doi: 10.1016/j.ijrobp.2019.02.046
182. Golden EB, Pellicciotta I, Demaria S, Barcellos-Hoff MH, Formenti SC. The convergence of radiation and immunogenic cell death signaling pathways. Front Oncol (2012) 2:88. doi: 10.3389/fonc.2012.00088
183. Lee Y, Auh SL, Wang Y, Burnette B, Wang Y, Meng Y, et al. Therapeutic effects of ablative radiation on local tumor require CD8+ T cells: changing strategies for cancer treatment. Blood (2009) 114(3):589–95. doi: 10.1182/blood-2009-02-206870
184. Yan J, Wang G, Xie L, Tian H, Li J, Li B, et al. Engineering radiosensitizer-based metal-phenolic networks potentiate STING pathway activation for advanced radiotherapy. Adv Mater (2022) 34(10):e2105783. doi: 10.1002/adma.202105783
185. Hietanen T, Pitkanen M, Kapanen M, Kellokumpu-Lehtinen PL. Effects of single and fractionated irradiation on natural killer cell populations: Radiobiological characteristics of viability and cytotoxicity in vitro. Anticancer Res (2015) 35(10):5193–200.
186. Ni K, Xu Z, Culbert A, Luo T, Guo N, Yang K, et al. Synergistic checkpoint-blockade and radiotherapy-radiodynamic therapy via an immunomodulatory nanoscale metal-organic framework. Nat BioMed Eng (2022) 6(2):144–56. doi: 10.1038/s41551-022-00846-w
187. Kaminski JM, Shinohara E, Summers JB, Niermann KJ, Morimoto A, Brousal J. The controversial abscopal effect. Cancer Treat Rev (2005) 31(3):159–72. doi: 10.1016/j.ctrv.2005.03.004
188. Vanpouille-Box C, Alard A, Aryankalayil MJ, Sarfraz Y, Diamond JM, Schneider RJ, et al. DNA Exonuclease Trex1 regulates radiotherapy-induced tumour immunogenicity. Nat Commun (2017) 8:15618. doi: 10.1038/ncomms15618
189. Burnette BC, Liang H, Lee Y, Chlewicki L, Khodarev NN, Weichselbaum RR, et al. The efficacy of radiotherapy relies upon induction of type i interferon-dependent innate and adaptive immunity. Cancer Res (2011) 71(7):2488–96. doi: 10.1158/0008-5472.CAN-10-2820
190. Deng L, Liang H, Fu S, Weichselbaum RR, Fu YX. From DNA damage to nucleic acid sensing: A strategy to enhance radiation therapy. Clin Cancer Res (2016) 22(1):20–5. doi: 10.1158/1078-0432.CCR-14-3110
191. Matsumura S, Wang B, Kawashima N, Braunstein S, Badura M, Cameron TO, et al. Radiation-induced CXCL16 release by breast cancer cells attracts effector T cells. J Immunol (2008) 181(5):3099–107. doi: 10.4049/jimmunol.181.5.3099
192. Walle T, Kraske JA, Liao B, Lenoir B, Timke C, von Bohlen Und Halbach E, et al. Radiotherapy orchestrates natural killer cell dependent antitumor immune responses through CXCL8. Sci Adv (2022) 8(12):eabh4050. doi: 10.1126/sciadv.abh4050
193. Liao JK. Linking endothelial dysfunction with endothelial cell activation. J Clin Invest (2013) 123(2):540–1. doi: 10.1172/JCI66843
194. Chakraborty M, Abrams SI, Camphausen K, Liu K, Scott T, Coleman CN, et al. Irradiation of tumor cells up-regulates fas and enhances CTL lytic activity and CTL adoptive immunotherapy. J Immunol (2003) 170(12):6338–47. doi: 10.4049/jimmunol.170.12.6338
195. Gaugler MH, Squiban C, van der Meeren A, Bertho JM, Vandamme M, Mouthon MA. Late and persistent up-regulation of intercellular adhesion molecule-1 (ICAM-1) expression by ionizing radiation in human endothelial cells in vitro. Int J Radiat Biol (1997) 72(2):201–9. doi: 10.1080/095530097143428
196. Frey B, Rubner Y, Wunderlich R, Weiss EM, Pockley AG, Fietkau R, et al. Induction of abscopal anti-tumor immunity and immunogenic tumor cell death by ionizing irradiation - implications for cancer therapies. Curr Med Chem (2012) 19(12):1751–64. doi: 10.2174/092986712800099811
197. Obeid M, Tesniere A, Ghiringhelli F, Fimia GM, Apetoh L, Perfettini JL, et al. Calreticulin exposure dictates the immunogenicity of cancer cell death. Nat Med (2007) 13(1):54–61. doi: 10.1038/nm1523
198. Ma Y, Pitt JM, Li Q, Yang H. The renaissance of anti-neoplastic immunity from tumor cell demise. Immunol Rev (2017) 280(1):194–206. doi: 10.1111/imr.12586
199. Diamond JM, Vanpouille-Box C, Spada S, Rudqvist NP, Chapman JR, Ueberheide BM, et al. Exosomes shuttle TREX1-sensitive IFN-stimulatory dsDNA from irradiated cancer cells to DCs. Cancer Immunol Res (2018) 6(8):910–20. doi: 10.1158/2326-6066.CIR-17-0581
200. Hemphill WO, Simpson SR, Liu M, Salsbury FR Jr., Hollis T, Grayson JM, et al. TREX1 as a novel immunotherapeutic target. Front Immunol (2021) 12:660184. doi: 10.3389/fimmu.2021.660184
201. Garnett CT, Palena C, Chakraborty M, Tsang KY, Schlom J, Hodge JW. Sublethal irradiation of human tumor cells modulates phenotype resulting in enhanced killing by cytotoxic T lymphocytes. Cancer Res (2004) 64(21):7985–94. doi: 10.1158/0008-5472.CAN-04-1525
202. Nagata S. Fas ligand-induced apoptosis. Annu Rev Genet (1999) 33:29–55. doi: 10.1146/annurev.genet.33.1.29
203. Lhuillier C, Rudqvist NP, Yamazaki T, Zhang T, Charpentier M, Galluzzi L, et al. Radiotherapy-exposed CD8+ and CD4+ neoantigens enhance tumor control. J Clin Invest (2021) 131(5):e138740. doi: 10.1172/JCI138740
204. Reits EA, Hodge JW, Herberts CA, Groothuis TA, Chakraborty M, Wansley EK, et al. Radiation modulates the peptide repertoire, enhances MHC class I expression, and induces successful antitumor immunotherapy. J Exp Med (2006) 203(5):1259–71. doi: 10.1084/jem.20052494
205. Hekim N, Cetin Z, Nikitaki Z, Cort A, Saygili EI. Radiation triggering immune response and inflammation. Cancer Lett (2015) 368(2):156–63. doi: 10.1016/j.canlet.2015.04.016
206. Garcia-Chagollan M, Jave-Suarez LF, Haramati J, Bueno-Topete MR, Aguilar-Lemarroy A, Estrada-Chavez C, et al. An approach to the immunophenotypic features of circulating CD4(+)NKG2D(+) T cells in invasive cervical carcinoma. J BioMed Sci (2015) 22:91. doi: 10.1186/s12929-015-0190-7
207. Tesniere A, Panaretakis T, Kepp O, Apetoh L, Ghiringhelli F, Zitvogel L, et al. Molecular characteristics of immunogenic cancer cell death. Cell Death Differ (2008) 15(1):3–12. doi: 10.1038/sj.cdd.4402269
208. Kim JY, Son YO, Park SW, Bae JH, Chung JS, Kim HH, et al. Increase of NKG2D ligands and sensitivity to NK cell-mediated cytotoxicity of tumor cells by heat shock and ionizing radiation. Exp Mol Med (2006) 38(5):474–84. doi: 10.1038/emm.2006.56
209. Formenti SC, Rudqvist NP, Golden E, Cooper B, Wennerberg E, Lhuillier C, et al. Radiotherapy induces responses of lung cancer to CTLA-4 blockade. Nat Med (2018) 24(12):1845–51. doi: 10.1038/s41591-018-0232-2
210. Vermeer DW, Spanos WC, Vermeer PD, Bruns AM, Lee KM, Lee JH. Radiation-induced loss of cell surface CD47 enhances immune-mediated clearance of human papillomavirus-positive cancer. Int J Cancer (2013) 133(1):120–9. doi: 10.1002/ijc.28015
211. Pardoll DM. The blockade of immune checkpoints in cancer immunotherapy. Nat Rev Cancer (2012) 12(4):252–64. doi: 10.1038/nrc3239
212. Ganss R, Ryschich E, Klar E, Arnold B, Hammerling GJ. Combination of T-cell therapy and trigger of inflammation induces remodeling of the vasculature and tumor eradication. Cancer Res (2002) 62(5):1462–70.
213. Demaria S, Coleman CN, Formenti SC. Radiotherapy: Changing the game in immunotherapy. Trends Cancer (2016) 2(6):286–94. doi: 10.1016/j.trecan.2016.05.002
214. Kroemer G, Galluzzi L, Kepp O, Zitvogel L. Immunogenic cell death in cancer therapy. Annu Rev Immunol (2013) 31:51–72. doi: 10.1146/annurev-immunol-032712-100008
215. Gameiro SR, Jammeh ML, Wattenberg MM, Tsang KY, Ferrone S, Hodge JW. Radiation-induced immunogenic modulation of tumor enhances antigen processing and calreticulin exposure, resulting in enhanced T-cell killing. Oncotarget (2014) 5(2):403–16. doi: 10.18632/oncotarget.1719
216. Gehrmann M, Radons J, Molls M, Multhoff G. The therapeutic implications of clinically applied modifiers of heat shock protein 70 (Hsp70) expression by tumor cells. Cell Stress Chaperones (2008) 13(1):1–10. doi: 10.1007/s12192-007-0006-0
217. Bottcher JP, Bonavita E, Chakravarty P, Blees H, Cabeza-Cabrerizo M, Sammicheli S, et al. NK cells stimulate recruitment of cDC1 into the tumor microenvironment promoting cancer immune control. Cell (2018) 172(5):1022–37 e14. doi: 10.1016/j.cell.2018.01.004
218. Molla M, Gironella M, Miquel R, Tovar V, Engel P, Biete A, et al. Relative roles of ICAM-1 and VCAM-1 in the pathogenesis of experimental radiation-induced intestinal inflammation. Int J Radiat Oncol Biol Phys (2003) 57(1):264–73. doi: 10.1016/S0360-3016(03)00523-6
219. Ahmed A, Tait SWG. Targeting immunogenic cell death in cancer. Mol Oncol (2020) 14(12):2994–3006. doi: 10.1002/1878-0261.12851
220. Baird JR, Friedman D, Cottam B, Dubensky TW Jr., Kanne DB, Bambina S, et al. Radiotherapy combined with novel STING-targeting oligonucleotides results in regression of established tumors. Cancer Res (2016) 76(1):50–61. doi: 10.1158/0008-5472.CAN-14-3619
221. Munoz LE, Frey B, Pausch F, Baum W, Mueller RB, Brachvogel B, et al. The role of annexin A5 in the modulation of the immune response against dying and dead cells. Curr Med Chem (2007) 14(3):271–7. doi: 10.2174/092986707779941131
222. Chiriva-Internati M, Grizzi F, Pinkston J, Morrow KJ, D'Cunha N, Frezza EE, et al. Gamma-radiation upregulates MHC class I/II and ICAM-I molecules in multiple myeloma cell lines and primary tumors. In Vitro Cell Dev Biol Anim (2006) 42(3-4):89–95. doi: 10.1290/0508054.1
223. Ma ZF, Huang JR, Cui NP, Wen M, Chen BP, Li Z, et al. [Expressions of immunogenic molecules in low-dose radiotherapy-treated human renal clear cell carcinoma 786-0 cells]. Zhonghua Yi Xue Za Zhi (2013) 93(30):2385–7.
224. Maasho K, Opoku-Anane J, Marusina AI, Coligan JE, Borrego F. NKG2D is a costimulatory receptor for human naive CD8+ T cells. J Immunol (2005) 174(8):4480–4. doi: 10.4049/jimmunol.174.8.4480
225. Markiewicz MA, Carayannopoulos LN, Naidenko OV, Matsui K, Burack WR, Wise EL, et al. Costimulation through NKG2D enhances murine CD8+ CTL function: Similarities and differences between NKG2D and CD28 costimulation. J Immunol (2005) 175(5):2825–33. doi: 10.4049/jimmunol.175.5.2825
226. Groh V, Smythe K, Dai Z, Spies T. Fas-ligand-mediated paracrine T cell regulation by the receptor NKG2D in tumor immunity. Nat Immunol (2006) 7(7):755–62. doi: 10.1038/ni1350
227. Lazarova M, Steinle A. Impairment of NKG2D-mediated tumor immunity by TGF-beta. Front Immunol (2019) 10:2689. doi: 10.3389/fimmu.2019.02689
228. Groh V, Wu J, Yee C, Spies T. Tumour-derived soluble MIC ligands impair expression of NKG2D and T-cell activation. Nature (2002) 419(6908):734–8. doi: 10.1038/nature01112
229. Paczulla AM, Rothfelder K, Raffel S, Konantz M, Steinbacher J, Wang H, et al. Absence of NKG2D ligands defines leukaemia stem cells and mediates their immune evasion. Nature (2019) 572(7768):254–9. doi: 10.1038/s41586-019-1410-1
230. Zhang J, Larrocha PS, Zhang B, Wainwright D, Dhar P, Wu JD. Antibody targeting tumor-derived soluble NKG2D ligand sMIC provides dual co-stimulation of CD8 T cells and enables sMIC(+) tumors respond to PD1/PD-L1 blockade therapy. J Immunother Cancer (2019) 7(1):223. doi: 10.1186/s40425-019-0693-y
231. Ozpiskin OM, Zhang L, Li JJ. Immune targets in the tumor microenvironment treated by radiotherapy. Theranostics (2019) 9(5):1215–31. doi: 10.7150/thno.32648
232. Rizvi NA, Hellmann MD, Snyder A, Kvistborg P, Makarov V, Havel JJ, et al. Cancer immunology. mutational landscape determines sensitivity to PD-1 blockade in non-small cell lung cancer. Science (2015) 348(6230):124–8. doi: 10.1126/science.aaa1348
233. Sobhani N, Tardiel-Cyril DR, Davtyan A, Generali D, Roudi R, Li Y. CTLA-4 in regulatory T cells for cancer immunotherapy. Cancers (Basel) (2021) 13(6):1440. doi: 10.3390/cancers13061440
234. Han Y, Liu D, Li L. PD-1/PD-L1 pathway: Current researches in cancer. Am J Cancer Res (2020) 10(3):727–42.
235. Kim JM, Chen DS. Immune escape to PD-L1/PD-1 blockade: Seven steps to success (or failure). Ann Oncol (2016) 27(8):1492–504. doi: 10.1093/annonc/mdw217
236. Topalian SL, Drake CG, Pardoll DM. Immune checkpoint blockade: A common denominator approach to cancer therapy. Cancer Cell (2015) 27(4):450–61. doi: 10.1016/j.ccell.2015.03.001
237. Mehdizadeh S, Bayatipoor H, Pashangzadeh S, Jafarpour R, Shojaei Z, Motallebnezhad M. Immune checkpoints and cancer development: Therapeutic implications and future directions. Pathol Res Pract (2021) 223:153485. doi: 10.1016/j.prp.2021.153485
238. Chocarro L, Blanco E, Zuazo M, Arasanz H, Bocanegra A, Fernandez-Rubio L, et al. Understanding LAG-3 signaling. Int J Mol Sci (2021) 22(10):5282. doi: 10.3390/ijms22105282
239. Qin S, Xu L, Yi M, Yu S, Wu K, Luo S. Novel immune checkpoint targets: moving beyond PD-1 and CTLA-4. Mol Cancer (2019) 18(1):155. doi: 10.1186/s12943-019-1091-2
240. Li M, Li M, Yang Y, Liu Y, Xie H, Yu Q, et al. Remodeling tumor immune microenvironment via targeted blockade of PI3K-gamma and CSF-1/CSF-1R pathways in tumor associated macrophages for pancreatic cancer therapy. J Control Release (2020) 321:23–35. doi: 10.1016/j.jconrel.2020.02.011
241. Mastelic-Gavillet B, Balint K, Boudousquie C, Gannon PO, Kandalaft LE. Personalized dendritic cell vaccines-recent breakthroughs and encouraging clinical results. Front Immunol (2019) 10:766. doi: 10.3389/fimmu.2019.00766
242. Demaria S, Kawashima N, Yang AM, Devitt ML, Babb JS, Allison JP, et al. Immune-mediated inhibition of metastases after treatment with local radiation and CTLA-4 blockade in a mouse model of breast cancer. Clin Cancer Res (2005) 11(2 Pt 1):728–34. doi: 10.1158/1078-0432.728.11.2
243. Deng L, Liang H, Burnette B, Beckett M, Darga T, Weichselbaum RR, et al. Irradiation and anti-PD-L1 treatment synergistically promote antitumor immunity in mice. J Clin Invest (2014) 124(2):687–95. doi: 10.1172/JCI67313
244. Twyman-Saint Victor C, Rech AJ, Maity A, Rengan R, Pauken KE, Stelekati E, et al. Radiation and dual checkpoint blockade activate non-redundant immune mechanisms in cancer. Nature (2015) 520(7547):373–7. doi: 10.1038/nature14292
245. Postow MA, Callahan MK, Barker CA, Yamada Y, Yuan J, Kitano S, et al. Immunologic correlates of the abscopal effect in a patient with melanoma. N Engl J Med (2012) 366(10):925–31. doi: 10.1056/NEJMoa1112824
246. Theelen W, Peulen HMU, Lalezari F, van der Noort V, de Vries JF, Aerts J, et al. Effect of pembrolizumab after stereotactic body radiotherapy vs pembrolizumab alone on tumor response in patients with advanced non-small cell lung cancer: Results of the PEMBRO-RT phase 2 randomized clinical trial. JAMA Oncol (2019) 5(9):1276–82. doi: 10.1001/jamaoncol.2019.1478
247. Theelen WSME, Chen D, Verma V, Hobbs BP, Peulen HMU, Aerts JGJV, et al. Pembrolizumab with or without radiotherapy for metastatic non-small-cell lung cancer: A pooled analysis of two randomised trials [published correction appears in Lancet Respir Med. Lancet Respir Med (2021) 9(5):467–465. doi: 10.1016/S2213-2600(20)30391-X
248. Shaverdian N, Lisberg AE, Bornazyan K, Veruttipong D, Goldman JW, Formenti SC, et al. Previous radiotherapy and the clinical activity and toxicity of pembrolizumab in the treatment of non-small-cell lung cancer: A secondary analysis of the KEYNOTE-001 phase 1 trial. Lancet Oncol (2017) 18(7):895–903. doi: 10.1016/S1470-2045(17)30380-7
249. Hua Y, You R, Wang Z, Huang P, Lin M, Ouyang Y, et al. Toripalimab plus intensity-modulated radiotherapy for recurrent nasopharyngeal carcinoma: an open-label single-arm, phase II trial. J Immunother Cancer (2021) 9(11):e003290. doi: 10.1136/jitc-2021-003290
250. Siva S, Bressel M, Wood ST, Shaw MG, Loi S, Sandhu SK, et al. Stereotactic radiotherapy and short-course pembrolizumab for oligometastatic renal cell carcinoma-the RAPPORT trial. Eur Urol (2022) 81(4):364–72. doi: 10.1016/j.eururo.2021.12.006
251. Ho AY, Barker CA, Arnold BB, Powell SN, Hu ZI, Gucalp A, et al. A phase 2 clinical trialassessing theefficacy and safety of pembrolizumab and radiotherapy in patients with metastatic triple-negative breast cancer. Cancer (2020) 126(4):850–60. doi: 10.1002/cncr.32599
252. Tang C, Welsh JW, de Groot P, Massarelli E, Chang JY, Hess KR, et al. Ipilimumab with stereotactic ablative radiation therapy: Phase I results and immunologic correlates from peripheral T cells. Clin Cancer Res (2017) 23(6):1388–96. doi: 10.1158/1078-0432.CCR-16-1432
253. Menon H, Chen D, Ramapriyan R, Verma V, Barsoumian HB, Cushman TR, et al. Influence of low-dose radiation on abscopal responses in patients receiving high-dose radiation and immunotherapy. J Immunother Cancer (2019) 7(1):237. doi: 10.1186/s40425-019-0718-6
254. Lauret Marie Joseph E, Kirilovsky A, Lecoester B, El Sissy C, Boullerot L, Rangan L, et al. Chemoradiation triggers antitumor Th1 and tissue resident memory-polarized immune responses to improve immune checkpoint inhibitors therapy. J Immunother Cancer (2021) 9(7):e002256. doi: 10.1136/jitc-2020-002256
255. Tumeh PC, Harview CL, Yearley JH, Shintaku IP, Taylor EJ, Robert L, et al. PD-1 blockade induces responses by inhibiting adaptive immune resistance. Nature (2014) 515(7528):568–71. doi: 10.1038/nature13954
256. Herbst RS, Soria JC, Kowanetz M, Fine GD, Hamid O, Gordon MS, et al. Predictive correlates of response to the anti-PD-L1 antibody MPDL3280A in cancer patients. Nature (2014) 515(7528):563–7. doi: 10.1038/nature14011
257. Wang J, Xu Z, Wang Z, Du G, Lun L. TGF-beta signaling in cancer radiotherapy. Cytokine (2021) 148:155709. doi: 10.1016/j.cyto.2021.155709
258. Vanpouille-Box C, Diamond JM, Pilones KA, Zavadil J, Babb JS, Formenti SC, et al. TGFbeta is a master regulator of radiation therapy-induced antitumor immunity. Cancer Res (2015) 75(11):2232–42. doi: 10.1158/0008-5472.CAN-14-3511
259. Qin X, Yang C, Xu H, Zhang R, Zhang D, Tu J, et al. Cell-derived biogenetic gold nanoparticles for sensitizing radiotherapy and boosting immune response against cancer. Small (2021) 17(50):e2103984. doi: 10.1002/smll.202103984
Keywords: radiotherapy (RT), tumor immune microenvironment (TIME), immunogenic cell death (ICD), immune-checkpoint blockade (ICB), tumor
Citation: Zhu S, Wang Y, Tang J and Cao M (2022) Radiotherapy induced immunogenic cell death by remodeling tumor immune microenvironment. Front. Immunol. 13:1074477. doi: 10.3389/fimmu.2022.1074477
Received: 19 October 2022; Accepted: 15 November 2022;
Published: 01 December 2022.
Edited by:
Haojun Chen, First Affiliated Hospital of Xiamen University, ChinaReviewed by:
Chentian Shen, Shanghai Sixth People’s Hospital, ChinaZhongmin Tang, University of Wisconsin-Madison, United States
Weiyu Chen, Zhejiang University, China
Copyright © 2022 Zhu, Wang, Tang and Cao. This is an open-access article distributed under the terms of the Creative Commons Attribution License (CC BY). The use, distribution or reproduction in other forums is permitted, provided the original author(s) and the copyright owner(s) are credited and that the original publication in this journal is cited, in accordance with accepted academic practice. No use, distribution or reproduction is permitted which does not comply with these terms.
*Correspondence: Min Cao, ZHJjYW9taW5AMTI2LmNvbQ==
†These authors have contributed equally to this work