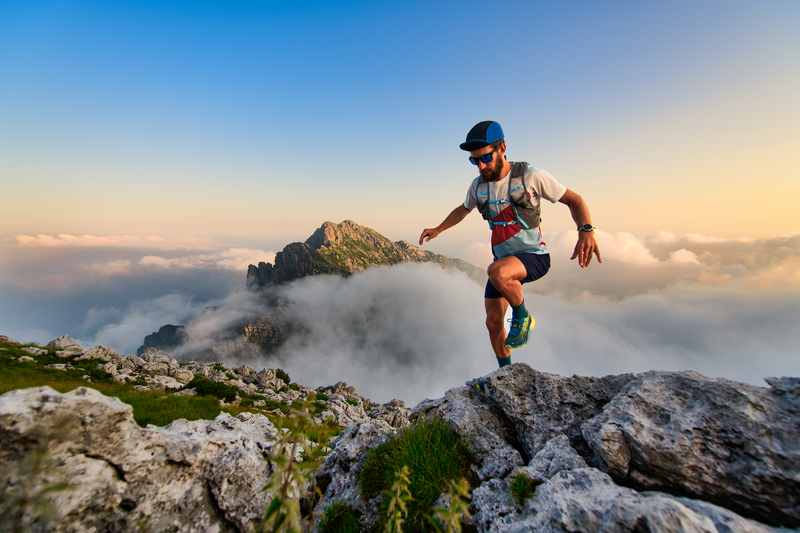
95% of researchers rate our articles as excellent or good
Learn more about the work of our research integrity team to safeguard the quality of each article we publish.
Find out more
REVIEW article
Front. Immunol. , 09 January 2023
Sec. Cancer Immunity and Immunotherapy
Volume 13 - 2022 | https://doi.org/10.3389/fimmu.2022.1070367
This article is part of the Research Topic Tumor Ablation and Immunity View all 36 articles
Despite advancements in chemotherapy, the issue of resistance and non-responsiveness to many chemotherapeutic drugs that are currently in clinical use still remains. Recently, cancer immunotherapy has gathered attention as a novel treatment against select cancers. Immunomodulation is also emerging as an effective strategy to improve efficacy. Natural phytochemicals, with known anticancer properties, been reported to mediate their effects by modulating both traditional cancer pathways and immunity. The mechanism of phytochemical mediated-immunomodulatory activity may be attributed to the remodeling of the tumor immunosuppressive microenvironment and the sensitization of the immune system. This allows for improved recognition and targeting of cancer cells by the immune system and synergy with chemotherapeutics. In this review, we will discuss several well-known plant-derived biomolecules and examine their potential as immunomodulators, and therefore, as novel immunotherapies for cancer treatment.
Cancer remains one of the leading causes of global mortality despite improved diagnostic techniques, identification of novel disease-predictive markers and the approval of a myriad of new therapeutics. Surgical removal, radiotherapy and chemotherapy remain the cornerstones of treatment, each with their own limitations. Chemotherapy has two main limitations, the occurrence of resistance in over 90% of patients and the blunt targeting of pathways also required for normal cellular development and homeostasis (1). Radiotherapy also induces healthy tissue injury, inflammation and oxidative stress with up to 85-95% of patients experiencing skin lesions and 60% experiencing lung injury (2, 3). Both modalities offer a broadly-targeted approach to cancer treatment leaving behind cell death, debris and damage-associated molecular patterns (DAMPs) in both cancer and healthy cells. Alleviating collateral damage, overcoming resistance and finding more targeted approaches are central goals to improving cancer treatment and quality of life. We believe that synergism between natural biomolecules and conventional therapeutics could be a step to achieve these goals for several reasons, which will be discussed later on.
Collateral damage can be lessened by targeting inflammation, a double-edged sword in cancer as chronic inflammation itself promotes tumorigenesis and cancer progression (2). At the same time, wielding the power of the immune system by stimulating cancer cell recognition from the host defense and by inducing immunogenic cell death (4–7) is also an attractive approach for several reasons. As this immune-cancer interplay and particularly evasion of immune recognition is what cancer cells need in order to develop, proliferate and acquire resistance.
The cancer-immunity cycle aims to unmask, locate and kill cancer cells. The first step of the cycle is the release of tumor-derived antigens that are created as a result of genomic instability. Dendritic cells capture tumor-associated antigens and upon full maturation and activation become antigen presenting cells (APCs) to T lymphocytes in the lymph nodes. Dendritic cell maturation includes the increased cell-surface expression of antigen peptides on major histocompatibility complex (MHC) class I components, and of co-stimulatory receptor ligands including CD80 and CD86. Engagement of T cell receptors (TCRs) by MHC class I, as well as of co-stimulatory receptors such as CD28, results in T cell activation, differentiation into tumor-specific cytotoxic T cells and clonal expansion. Proinflammatory mediators including tumor necrosis factor alpha (TNF-α), interferon alpha (IFN-α), IFN-γ, interleukin-2 (IL-2) and IL-12 promote T cell activation. Chemokines such as C-X-C Motif Chemokine Ligand 9 (CXCL9) and CXCL10 stimulate T cell trafficking and infiltration into the tumor, where T-cell-mediated cytotoxicity liberates more tumor-associated antigens to further propagate the cancer-immunity cycle (4, 5, 8).
Antigen-dependent cytotoxicity can be inhibited as effector T cells become exhausted especially when chronic inflammation in the TME leads to continuous activation of TCRs and co-stimulatory receptors (9, 10). This occurs via the upregulation of immune checkpoints including the programmed cell death protein 1 (PD-1) (10, 11) and cytotoxic T-lymphocyte-associated protein 4 (CTLA-4) (12, 13). Like CD28, CTLA-4 binds to dendritic CD80 and CD86, but with markedly higher affinity, and CTLA-4 competitively inhibits CD28-induced effector T cell responses (13). Checkpoint inhibitors, which are aimed at reactivating T cells and re-establishing cytotoxicity, are emerging as the most prominent immunomodulatory agents in cancer chemotherapy (14). They have already shown success in metastatic melanoma, urothelial and non-small cell lung (NSCL) carcinomas (12, 15), but have been associated with the development of immune-related adverse events in 70-90% of patients (16, 17). This necessitates the development of novel agents with improved safety profiles. Natural biomolecules including berberine, triptolide, epigallocatechin gallate (EGCG) and curcumin, reviewed here, have been shown to reduce cancer cell expression of the PD-1 ligand, PD-L1. Could these phytochemicals emerge as novel checkpoint inhibitors with improved safety profiles?
Nonspecific cytotoxicity is primarily executed by natural killer (NK) cells, whose activity is potentiated upon stress-induced stimulation of natural cytotoxicity receptors (NCR). NK cell activity is, however, inhibited upon MHC-class-I-dependent stimulation of killer cell inhibitory immunoglobulin-like receptors (KIR). Cancer cells lacking MHC class I can be recognized as ‘non-self’ and lysed by NK cells. The importance of immune surveillance and cytotoxicity in tumorigenesis and cancer progression is highlighted by findings that increased numbers of antitumor cells such as mature dendritic cells, NK, cytotoxic T, T helper (Th) and B cells in the TME are associated with improved prognosis (18–21) (Figure 1).
Figure 1 Tumorsupressive & supportive cells and cytokines. Immune cells of the tumor microenvironment exert tumorsupportive and tumorsupressive effects. On one side tumorsupportive cells (tumor associated macrophages, myeloid-derived suppressor cells, T regulatory cells) are stimulated to proliferate or switch to immunosuppressive phenotypes by cancer cell secreted cytokines. This interplay promotes immune suppression and ultimately immune escape, proliferation and metastasis. Due to this immune suppression at the tumor microenvironment, the tumor suppressive cells (cytotoxic T cells, NK cells, dendritic cells) are usually desensitized and unable to unmask and kill the cancer. However, again through the secretion of cytokines or through immune checkpoint inhibitors, tumorsupressive cells can be sensitized and potentiated to regain their functionality, exerting antigen presentation and tumor lysis.
Cancer cells can escape immune surveillance and through proliferation of immune-escapee clones give rise to established tumors via immunoediting (7, 22). Malignant cells can lose their antigenicity and evade detection by NK cells or APCs through downregulation of tumor-specific antigens or dysregulated antigen presentation via MHC class I and II. In fact, 20-60% of solid cancers show decreased expression of MHC class I, and reduced MHC I and II expression levels predict poor prognosis in cancers such as breast (23), colorectal (24) and bladder (25). Tumor-specific expression of MHC class II, however, improves immune checkpoint inhibitor efficacy (26, 27). Cancer cells can also take advantage of negative feedback mechanisms that promote immune tolerance including the expression of ligands for immune checkpoint receptors (e.g. PD-L1) that promote T cell exhaustion (28). Cancer cells may also secrete inhibitory, Th2-type cytokines including transforming growth factor β (TGF-β), interleukin 4 (IL-4) and IL-10 that in turn stimulate the recruitment, generation and propagation of tumorsupportive immune cells including Foxp3+ regulatory T (Treg) and B (Breg) cells, M2-polarized tumor associated macrophages (TAMs) and myeloid derived suppressor cells (MDSCs). These tumorsupportive cells promote cancer progression by promoting angiogenesis, migration and metastasis (29–31), and by inhibiting T- and NK-cell-mediated cytotoxicity (32–34) (Figure 2).
Figure 2 T cell exhaustion and PD-L1 checkpoint inhibitors. Expression of PD-L1 or PD-L2 on cancer cells is recognized by PD-1 to promote exhaustion of cytotoxic T cells. Natural biomolecules including berberine, epigallocatechin gallate (EGCG), triptolide and curcumin inhibit expression of PD-L1 and PD-L2 and thereby inhibit T cell exhaustion.
One of the additional targets that have emerged is STAT3 (signal transducer and transcription activator 3). STAT3 is a downstream effector of cytokines including IL-6 and IL-4, growth factors such as EGF, and hypoxia. Phosphorylated STAT3 dimers in cancer cells promote the transcription of genes associated with proliferation, cell cycle regulation, adhesion, metastasis and angiogenesis. Persistent activation of STAT3 is poor prognostic indicator in some cancers. In immune cells, IL-6 induces STAT-3 phosphorylation to promote IL-10 secretion leading to autocrine activation of immune STAT-3 and maintenance a strong immunosuppressive microenvironment. Additionally, IL-6-mediated activation of JAK2/STAT3 within cancer cells promotes the expression of PD-L1 and PD-L2 and checkpoint inhibition. STAT3 has emerged as important target as it promotes tumor cell stemness, malignant transformation, uncontrolled proliferation, evasion of apoptosis, metastasis and most importantly for this scope of this review, immune evasion (35) (Figure 3).
Figure 3 Effects of STAT 3 on cancer and immune cells. STAT3, which is persistently phosphorylated in cancer, leads to transcription of genes associated with proliferation, immunosupression, angiogenesis, migration and evasion of cancer cells. Aditionally STAT3 either directly or indirectly mediates processes that lead to decreased function of immunosuportive cells and increased function of immunosupressive cells.
About half of the clinically-utilized cancer chemotherapeutics ranging from topoisomerase inhibitors, cancer chemotherapeutic antibiotics to microtubule-stabilizing agents, are derived from or inspired from natural sources (36). As new therapeutic targets emerge, interest in the incorporation of novel biomolecules as adjuncts to conventional therapy has increased. While initial investigations focused on dietary compounds which through their antioxidant and anti-inflammatory properties may be cancer preventative, proapoptotic and antiproliferative effects have also been identified, leading to clinical trials. As the spotlight has moved into the development of immunomodulatory cancer chemotherapies, so has the identification of anti-inflammatory and immunomodulatory phytochemicals. In this review, we will highlight phytochemicals with anticancer and immunomodulatory properties (Figures 4, 5). We will focus on evaluating anticancer efficacy demonstrated in clinical trials, immunomodulatory roles, and will only summarize the antineoplastic effects identified in preclinical studies.
Figure 4 Immunoregulatory functions of natural biomolecules. Immune cells of the tumor microenvironment exert tumorsupportive and tumorsupressive effects. Myeloid-derived suppressor cells (MDSC), tumor associated macrophages (TAM) and regulatory T cells (Treg) support tumor growth and metastasis. Natural killer (NK) cells and cytotoxic T cells induce tumor cell cytotoxicity. Dendritic cells recognize tumor-associated antigens and are the principal antigen presenting cells in the tumor microenvironment. The natural biomolecules and inhibit tumorsupportive cells, but induce immune-mediated cytotoxicity are listed.
Artemisinin is a semisynthetic isoprenoid (alternatively sesquiterpene or terpene) from Artemisia annua. It was rediscovered as an antimalarial drug by Chinese chemist and Nobel Laureate Tu Youyou in 1972. Qinghaosu, or artemisinin, was isolated from A. annua by low-temperature, ethanol-based extraction. An inactive, acidic moiety was removed to increase efficacy but reduce toxicity and yield artemisinin. Further structure-activity studies resulted in the discovery of three artemisinin derivatives: artemether, a methyl-ether derivative; artesunate, a hydrophilic derivative; and dihydro-artemisinin, an intermediate and active metabolite of the last two. Artemisinin derivatives, from here on referred to as ACTs, are currently being investigated for cancer therapy. So far, their efficacy has mostly been demonstrated in vitro and in vivo with relatively few human trials, a focus of this review. But, ACTs are ideal drugs for repurposing due to a 50-year safety record as monotherapy or adjunct therapy for malaria. And, the availability of a stable supply chain of semi-synthetic ACTs with a predicable cost, which has been established through the Semi-synthetic Artemisinin Project (37).
To date, human clinical trials for the use of ACTs as cancer chemotherapeutics are limited to small cohorts of breast, colorectal and cervical cancer patients. Nonetheless, results are promising with indications of reduced disease progression in the absence of serious side effects.
Artesunate, a more stable and bioavailable analogue of artemisinin, was utilized as an add-on to guidance-based chemotherapy in patients with metastatic breast cancer. No significant side effects were reported and artesunate exhibited improved safety profile when compared to selective estrogen receptor modifiers (SERMs) (38, 39). Notably, SERMs (e.g. tamoxifen), a staple in breast cancer chemotherapy, are associated with side effects like hepatotoxicity, nephrotoxicity, neurotoxicity, cardiotoxicity, and increased thromboembolic risk (40). Artesunate addition aided in disease stability in some patients. But, the small cohort size and confounders hindered differentiation between drug- and disease-related symptoms. Overall artesunate was found to be safe with unclear benefits (38, 39). Artesunate did reduce expression of Ki67 in colorectal carcinoma, a proliferation marker associated with poor prognosis (41), and resulted in an overall reduction of tumor cell proliferation. No deaths occurred in the artesunate-treated group, but 27.3% of controls had died at the time of analysis (42).
Cervical cancer is one of the most common cancers in women worldwide with 90% of deaths occurring in low-income countries due to lack of screening. Standard-of-care for low-grade lesions and early stage cancer include surgery combined with radio- and chemotherapy (43). Non-surgical treatment could be of significant benefit for all women, but in particular for women with limited access to healthcare. A self-administered and affordable therapeutic option along with the currently-available self-performed screening methods could prove extremely beneficial.
Encouraging results for the use of ACTs in cervical cancer have emerged from clinical trials with intravaginal artesunate (44) or oral artenimol (45). Self-administration of intravaginal artesunate led to regression of cervical intraepithelial neoplasia (CIN) and clearance of human papillomavirus (HPV) (44), the main cause of cervical cancer (43). Oral artenimol induced a 6-month remission in 10 advanced metastatic cervical cancer patients, with sufficient remission to allow hysterectomy in one patient. Importantly, study participates survived for a minimum of 12 months, far longer than the 4-month survival of patients with similar level of care (43, 45). No significant adverse effects were reported. Biopsy analysis revealed reduced vascularization, and reduced expression of p53, epidermal growth factor receptor (EGFR) and Ki-67. But, expression of transferrin receptor 1 (TfR1), a poor prognostic indicator in cervical cancer (46), was increased (45).
ACTs may prove excellent candidates for cervical cancer therapy because; 1) Cervical cancer is a slow growing cancer that is treated by local resection or hysterectomy so pharmacotherapeutic options can be tested without delaying or interfering with standard-of-care treatment. 2) ACTs have demonstrated cytotoxic effects against HPV infected cells with relative selectivity (44, 47). 3) Clinical trials, although with limited number of patients, have shown extremely promising results (44, 45). The results of all clinical trials discussed in this review, including those utilizing ACTs, are presented in (Table 1) below.
ACT-mediated control of the immune response to cancer is yet to be examined in patients, so we summarize the in vitro and in vivo evidence. Artemisinin caused a dose-dependent increase in CD107a, a marker of increased activity and degranulation, in primary human NK cells (70). While, artemisinin pre-treated leukemia and lymphoma cells became more susceptible to cytolysis by human primary NK cells (71). This suggests, that artemisinin employs two independent mechanisms in increasing NK-dependent cytotoxicity; by directly increasing the cytolytic activity of the NK cell predator, while simultaneously increasing the susceptibility of the cancer cell prey to NK-mediated destruction.
ACTs may reprogram immune cells to increase tumorsuppression and tumorlysis. Artesunate inhibited JAK2/STAT3 in human primary monocytes to decrease CD163+/CD206+ M2 macrophage numbers and polarize monocytes to a tumoricidal phenotype, which induced leukemia cell apoptosis (72). ACTs promoted γδ T cell proliferation and cytolytic activity by inducing granzyme B expression (73–75). Immune-derived granzymes enter cancer cells via perforin-formed pores to induce DNA fragmentation and caspase-3-dependent apoptosis. ACT-dependent induction of granzyme B indirectly induced apoptosis of pancreatic (74), liver (75) and breast (73) cancer cells. Concomitantly, artesunate increased hepatocarcinoma susceptibility to γδ T cytolysis by inhibiting STAT3-mediated downregulation of Fas (75). Artesunate suppressed naïve CD4+ T cell proliferation and induced their differentiation into a predominantly Th1 tumoricidal phenotype with increased expression of IFN-γ (76). Artesunate also reduced the expression of IL-2 (76), an anti-inflammatory cytokine produced by Th2 cells that can promote cancer progression by inhibiting IFN-γ release (77). These findings were extended by Chen et al. who, in a murine model of ovarian cancer, showed that artesunate induced Th1 differentiation of CD4+ via miR-142-mediated downregulation of sirtuin 1, resulting in enhanced ovarian cancer apoptosis (78).
In addition to enhancing immune-mediated cytolysis, ACTs can significantly remodel the TME. Artemisinin remodeled breast cancer TME by increasing the numbers of tumorsuppressive CTLs and CD4+/IFN-γ+ Th1 cells, while concomitantly decreasing the numbers of tumorsupportive FOXP3+ Treg and T-bet+ MDSCs (73). Similarly, Yu et al. showed that dihydroartemisinin decreased phosphorylated STAT3, but increased STAT1 phosphorylation in melanoma cells to decrease tumor growth, proliferation and metastasis but induce apoptosis in melanoma-bearing mice. Importantly, dihydroartemisinin-mediated decrease of IL-10 and IL-6 in melanoma inhibited Treg polarization and infiltration in the TME (79). Tregs are an additional source of immunosuppressive cytokines (5, 8), and by decreasing Treg numbers, dihydroartemisinin treatment allowed for increased infiltration of CD8+ CTLs and promoted antitumor immunity (79).
In summary, in vitro and in vivo studies have identified a number of immunomodulatory roles for ACTs that lead to an overall increase of the antitumor response. ACTs directly polarize immune cells towards tumoricidal, and away from tumorsupportive populations. Concomitantly, ACTs act on cancer cells to increase their susceptibility to immune-mediated cytolysis. It remains to be seen whether these promising results translate to the clinic (Table 2).
In addition to altering immunosurveillance, ACTs target cancer cells directly. They induce ROS generation, autophagy and cell death, but inhibit proliferation, growth and migration in vitro and in vivo (83). Here, we will only summarize the major mechanistic insights into the direct antineoplastic effects of ACTs.
ACTs have recently emerged as potential inhibitors of STAT3 in cancer cells in vitro. Ilamathi et al. demonstrated that artesunate exhibited similar potency to the established STAT3 blocker S31-201 in inhibiting both constitutive and IL-6-dependent dimerization of STAT3 to induce apoptosis of hepatocellular carcinoma (84). Dihydroartemisinin inhibited both IL-6-mediated and EGFR-mediated activation of JAK2/STAT3 in head and neck squamous cell carcinoma (HNCC) in vivo and in vitro. Dihydroartemisinin-dependent inhibition of STAT3 decreased the levels of antiapoptotic (Bcl-xL, Mcl-1), proproliferative (cyclin D1), prometastatic (MMP-2/9) and proangiogenic (VEGF) proteins resulting in inhibited xenograft tumor growth (85). Similar results were observed with artesunate-dependent inhibition of STAT3 in AML (81), diffuse large B cell lymphoma (82) and melanoma (86) cell lines. HNCC resistance to cisplatin is associated with STAT3 phosphorylation (87). Importantly, dihydroartemisinin sensitized HNCC cells to cisplatin and acted synergistically with cisplatin to arrest HNCC in G0/G1 (85). Two independent studies have demonstrated that by inhibiting STAT3, dihydroartemisinin acted synergistically with the Bcl-2 inhibitor ABT-263 (88) and with the tyrosine kinase inhibitor gefitinib (89) to induce apoptosis in EGFR and RAS mutant NSCLC cells. In addition to inhibiting STAT3 activity, dihydroartemisinin has emerged as an inhibitor of STAT3 translation. Dihydroartemisinin increased miR-130b-3p to reduce STAT3 protein expression and block IL-6-dependent EMT of laryngeal squamous cell carcinoma (90). These findings identify ACTs as novel regulators of STAT3 expression and activity that can target a variety of cancers both alone and in combination with established chemotherapy.
Cancer cells evade apoptosis and many traditional chemotherapeutics are aimed at inducing this form of cell death. Efferth et al. were the first to report that ACTs promote leukemia apoptosis in vitro (91). Since, ACTs have been shown to induce apoptosis in numerous cancer cell lines via activation of both intrinsic and extrinsic factors (83). Importantly, immune cells are spared from ACT-induced apoptosis (73, 74, 92), suggesting that side effects such as lymphopenia, which has been associated with poor responses to chemotherapy and prognosis (93, 94), may be avoided. However, apoptosis was unaltered in artesunate-treated colorectal cancer patients (42). A reason for the discrepancy could be that in vitro ACTs are used in concentrations far beyond those achievable in human plasma. Alternatively, ACTs do cause cell death, but via alternative pathways.
Ferroptosis is a caspase-independent programmed cell death that can be triggered through intracellular Fe2+ accumulation and dysregulation of iron homeostasis (95). Cancer cells are enriched in iron. Additionally, increased abundance of transferrin receptors indicates poor prognosis and correlates with immune infiltration (96) suggesting that ferroptosis may selectively kill cancer cells while sparing healthy tissue. ACTs induced intracellular iron accumulation and triggered ferroptosis in NSCL, colon, renal, ovarian, CNS, leukemia, melanoma, prostate, and breast cancer cell lines by altering the mRNA expression of over 20 genes regulating iron homeostasis (80). Canonical ferroptosis is triggered by lipid ROS generated through lipid peroxidation and opposed by of glutathione peroxidase 4 (GPX4), a promising chemotherapeutic target that reduces toxic lipid peroxides by oxidizing glutathione (96). Dihydroartemisinin inhibited GPX4 to trigger ferroptosis in glioblastoma in vitro (97). Ferroptosis is an attractive alternative in inducing cell death especially in apoptosis-resistant, and conventional chemotherapy resistant cancers. ACTs have been shown to induce cell death through ferroptosis in cisplatin-resistant bladder and head and neck cancer cells (98) in addition to various other cancer subtypes. The emergence of ACTs as inducers of ferroptosis may therefore confer selectivity of these natural biomolecules against cancer with decreased side effect profile compared to conventional chemotherapy. To date, the effect of ACTs on ferroptosis has never been examined in humans, but we propose that it should not be ignored in future ACT therapy clinical trials.
A number of preclinical studies have identified that ACTs inhibit tumor cell growth and viability via autophagy. Autophagy occurs when microtubule-associated protein 1A/1B-light chain 3 (LC3) is incorporated in pre-autophagosomal membranes, where it is acted upon by autophagy related proteins (ATG) to generate LC3-I, LC3-II and mature autophagosomes (99). ACTs increased LC3, LC3-I, LC3-II and autophagosome maturation in cancer cell lines including, but not limited to, leukemia, esophageal, ovarian, pancreatic, and colon. Target recognition in autophagy is accomplished through autophagy receptors including P62/SQSTM1 (sequestosome 1), whose sustained upregulation or decreased degradation have been linked to tumorigenesis (99). Dihydroartemisinin decreased P62/SQSTM1 to induce autophagy-mediated death of tongue squamous cell carcinoma (100). P62/SQSTM1 is a target gene of NF-E2-related factor 2 (Nrf2). The dihydroartemisinin derivative DC32 (101) and a (NHC)gold(I)–artemisinin hybrid complex (102) inhibited Nrf2-mediated P62/SQSTM1 transcription. Artesunate activated AMPK (AMP-activated protein kinase) to induce autophagy-dependent apoptosis in bladder cancer cell lines (103), and suppressed Akt-mTOR signaling to induce in hepatoblastoma senescence (104). In summary, numerous in vitro studies have established a role for ACTs in increasing autophagy. But, given that autophagy can be either tumorsupportive or tumorsuppressive (99), caution should be taken when considering ACTs as adjuncts to chemotherapy.
Since resistance to conventional chemotherapeutics is a major contributing factor to patient mortality, agents that sensitize resistant cells to chemotherapy or circumvent resistance altogether are of therapeutic value. Artemisinin acted synergistically with oxaliplatin to decrease tumor growth, migration and invasion of esophageal carcinoma (105). It also induced DNA damage, autophagy, cell cycle arrest and apoptosis in cisplatin-resistant breast cancer cells (106). Interestingly, artemisinin-free A. annua extract exhibited potency against triple-negative human breast cancer cells (83) suggesting that not just artemisinin but A. annua as a whole may be of chemotherapeutic interest. Drug resistance can be circumvented by the covalent linking of two bioactive molecules via hybridization. In two studies, Fröhlich et al. reported that tamoxifen-artemisinin or estrogen-artemisinin hybrids were more potent than the parent drugs or cisplatin against human prostate, breast and cervical cancer cell lines (107). These results highlight the utility of ACTs not only as adjunct therapy, but as a natural biomolecule that can be utilized in drug hybridization to improve the potency and potentially reduce the resistance of standard chemotherapeutic agents. Furthermore, artemisinin and oxaliplatin synergistically decreased invasion, migration, and tumor growth of esophageal cancer cells (105).
Berberine is an alkaloid found in Berberidaceae and thus the barberry fruit (Berberis vulgaris). Berberine use as an antioxidant, chemoprotective and anthelminthic agent in the treatment of infections and skin conditions dates back to 650BC. In modern medicine, berberine is primarily investigated for its potential in controlling metabolic syndrome and associated conditions like obesity, diabetes, hyperglycemia, hyperlipidemia, and cancer (108, 109). But low oral bioavailability and complicated pharmacokinetics, which are significantly influenced intestinal microflora, need to be considered. Importantly, the bioactivity of berberine does not stem from the parent molecule, but from its primary metabolites which bioaccumulate (110, 111). Thus, despite its low bioavailability, oral berberine could be of therapeutic value through its bioaccumulating metabolites, but perhaps with a loading dose.
To date, several clinical trials have examined the effects of berberine, however only a few have done so in the context of cancer and none have tested its cancer chemotherapeutic efficacy. Chen et al. used berberine or placebo in patients with confirmed colorectal adenomas (not carcinomas) who had undergone a complete polypectomy. Berberine reduced risk of recurrent adenoma in the absence of side effects other than constipation (48). Given that colorectal adenomas are benign, but demonstrate potential for malignant transformation into carcinomas especially in patients with metabolic syndrome and inflammatory conditions (41), berberine could be effective in preventing the development of these tumors. Notably, none of the adenoma study participants developed carcinoma during the follow-up period (48) (Table 1).
Potential clinical benefits of phytochemicals are not limited to antineoplastic effects, but include chemo- and radioprotective potential. Berberine exerted protective effects against radiation-induced injury without significant side effects (112, 113). Berberine or placebo were administered to patients treated from seminoma, lymphoma and cervical cancer via abdominal radiotherapy. Although the study was small, it did demonstrate that berberine significantly delayed onset and reduced the incidence and severity of radiation-induced acute abdominal syndrome (112). Berberine also reduced the incidence of radiation-induced lung injury, the abundance of TGF-β and intercellular adhesion molecule-1 (ICAM-1), and thus inflammation in radiotherapy-treated NSCL patients (113). Neither clinical trial included mechanistic insight, but they did provide a proof-of-principle for the protective role of berberine as an adjunct to radiation. Clinical evidence of the protective role of berberine and other biomolecules discussed here is presented in (Table 3).
Radiation therapy leads to inflammasome activation in macrophages, dendritic, NK, B and T cells. Moreover, NLRP3 inflammasome activation accelerates inflammation and fibrosis, induces pyroptosis and is necessary for radiation-induced injury (122). Berberine inhibited NLRP3 inflammasome activation and pyroptosis in nonalcoholic steatohepatitis, a condition associated with liver cancer development (123). It inhibited influenza-induced NLRP3 inflammasome activation in macrophages by inhibiting mitophagy and mitochondrial ROS production (124). Berberine also inhibited AMPK-dependent autophagy in adipose tissue macrophages, which reduced palmitate-induced inflammasome activation, IL-1β release, insulin resistance (125). Beneficial effects of berberine-mediated inflammasome inhibition have been reported in Parkinson’s disease, endovascular injury, drug-induced hepatotoxicity and diabetic nephropathy (by suppressing epithelial to mesenchymal transition (EMT) (126). Given the inhibitory effects of berberine on inflammasome activation and the importance of this pathway in radiation-induced injury, we propose that berberine may protect patients from radiation-induced lung and abdominal injury (112, 113) by inflammasome inhibition. Studies on the effects of berberine on cancer through inflammasome modulation are still limited. However, Yao et al. reported the berberine inhibited the NLRP3 inflammasome; reduced secretion of proinflammatory interleukins and TNF-α; and inhibited tumor colony formation and migration in triple-negative breast cancer cells in vitro (127).
Molecular docking analysis revealed that berberine binds STAT3 directly to inhibit STAT3 phosphorylation and dimerization. Berberine-mediated STAT3 inhibition reduced IL-10 expression in melanoma cells; reprogrammed M2 tumor-associated macrophages to a tumorsuppressive M1 phenotype; increased tumor cell recognition by T cells; and ultimately reduced tumor burden in melanoma-bearing mice (128). Berberine also inhibits the expression of protein arginine deaminase 4 (PADI4) in macrophages. Overexpression of PADI4 induces macrophage activation and promotes EMT. PADI4 expression is directly correlated to the number of lung nodules in a murine model of lung cancer. By reducing PADI4 expression, berberine dose-dependently reduced lung nodules and improved lung function suggesting a putative tumor-preventative role of berberine (129). Inflammation, oxidative stress and intestinal flora imbalance are associated with colitis and colorectal cancer development and progression. Given that fecal excretion is the predominant route of berberine excretion, berberine can reach the colon in sufficient concentrations to achieve efficacy against inflammation and malignancy (130). Berberine remodeled intestinal microflora to favor beneficial over pathogenic bacteria and reduce proinflammatory cytokine secretion. Berberine-induced inhibition of the TLR4/NF-κB/IL-6/STAT3 pathway reduced malignant transformation and cancer recurrence in colitis-associated colorectal carcinoma models (131, 132).
Tumor progression is associated with immunoediting, which is promoted by increased PD-L1 expression within tumors. Liu et al. recently reported a novel function for berberine as a PD-1/PD-L1 checkpoint inhibitor. Berberine directly bound to the fifth subunit of the COP9 signalosome complex (CSN5) and inhibited CSN5-mediated PD-L1 deubiquitylation and stabilization within NSCL cells. Berberine-induced PD-L1 degradation increased cancer cell susceptibility to tumor-infiltrating T cells in mouse xenografts. Additionally, berberine limited the tumorsupportive functions of Treg and MDSCs further solidifying its potential as a chemotherapeutic and immunomodulating agent (133) (Table 4).
Berberine has been shown to promote tumor cell apoptosis, cell cycle arrest, mitochondrial dysfunction, ROS generation, apoptosis and autophagy in a variety of in vitro and in vivo cancer models (109, 134). A detailed examination of these studies has been performed recently (109), and is beyond the scope of this review.
Berberine inhibited colorectal adenoma transformation to colorectal cancer by binding to pyruvate kinase isozyme type M2 (PKM2) and inhibiting the Warburg effect (137). Berberine-mediated inhibition of STAT3 decreased MMP2/9 expression to inhibit metastasis of established colorectal carcinomas (138). Protective effects of berberine have also been reported for other gastrointestinal cancers including gastric (139) and cholangiocarcinoma (140, 141). Notably, molecular docking experiments identified STAT3, Akt, Erk and EGFR as putative berberine binding partners (141), suggesting that berberine may act as a multi-kinase inhibitor.
Berberine targets nine different oncomiRs including miR-17-5p and miR-21 which upregulate oncogenic pathways including STAT3, NF-κB, PI3K/Akt, PTEN and downregulate tumorsuppressive programmed cell death 4 (PDCD4) among others (136, 142, 143). Berberine downregulated miR-21 to induce expression of PDCD4, which in turn promoted multiple myeloma apoptosis by suppressing p53 (136). Similarly, berberine induced cytotoxicity in bladder cancer cells via miR-17-5p upregulation. Notably, p53 expression determined the type of berberine-induced cell death, such that p53-expressing cells underwent senescence, while p53-deficient cells – apoptosis (143), suggesting that berberine exhibits context-dependent pleiotropy. miR-17-5p is downregulated in cervical cancer and malignant pleural mesothelioma. But increased miR-17-5p expression is a poor prognostic indicator for colon, colorectal, gastric, pancreatic, nasopharyngeal, and lung cancers (142). Thus miR-17-5p acts as a double-edge sword in cancer progression and the cell-specific effects of berberine on this miRNA may determine the efficacy of berberine against different malignancies.
Berberine protected against intestinal injury induced by the conventional chemotherapeutic methotrexate. Mechanistically, berberine protected against methotrexate-induced upregulation of JAK1, STAT3 and Gsk-3β, downregulation of Akt/mTOR, oxidative stress and inflammation (144). Similarly, berberine ameliorated doxorubicin toxicity, and a berberine/doxorubicin nanoformulation synergistically decreased tumor growth and metastasis in breast cancer xenografts (145). But berberine also demonstrated synergistic effects with conventional chemotherapeutics. Berberine and 5-fluoruracil synergistically inhibited STAT3 and surviving to induce gastric cancer cell death (146). Berberine induced autophagy in NSCL cells with resistance to EGFR inhibitors like icotinib. Berberine synergized with icotinib to decrease EGFR expression and activity, induce autophagy and apoptosis, and reduce metastasis in xenograft models (147). Similarly, berberine inhibited EGFR-induced STAT3 activity to increase gastric cancer sensitivity to the EGFR inhibitors cetuximab and erlotinib (148). Moreover, berberine reversed resistance to cisplatin (149) of gastric cancer (149); to doxorubicin of lung (150) and breast cancers (145, 151, 152); and to temozolomide of glioblastoma (153). The rapidly mounting evidence of the chemosensitizing potential of berberine warrants the inclusion of this phytochemical as an adjunct to conventional chemotherapy.
Bioavailability and efficacy of berberine have been enhanced through novel formulations. 9-O-substitutted berberine derivatives decreased leukemia cell proliferation 30 fold in comparison to non-substituted berberine and enhanced apoptosis 6 fold (135). 9-/13-dodecyl berberine derivatives displayed selective photocytotoxicity, destabilized the mitochondrial membrane potential and increased ROS production, cell cycle arrest and apoptosis of liver, colon and bladder cancer cell lines. Notably efficacy was proportional to alkyl-chain length and lipophilicity. Importantly, substituted derivatives exhibited cytotoxicity against colon cancer, but not healthy cells (154).
Clinical safety and efficacy for berberine have been established in diabetes and metabolic syndrome (108, 155), but as of today its antineoplastic efficacy has not be tested in patients. The promising effects of berberine in metabolic syndrome together with the correlation between obesity and metabolic syndrome with carcinogenesis (108, 155) establish a role for berberine in preventative medicine (123). Berberine could be of great promise in several stages of tumorigenesis. It is a novel PD-L1 checkpoint inhibitor with direct immunomodulatory actions that can promote T-cell-mediated cytolysis in resistant cancers (133). It has demonstrated synergistic and sensitizing effects with traditional chemotherapeutics. But, berberine is also protective and has already proven efficacious in preventing radiation-induced injury in patients (112, 113). We conclude that berberine is of great promise as a chemotherapeutic or radiotherapy add on.
Triptolide is a diterpene triepoxide derived from Tripterygium wilfordii with antimicrobial, anti-inflammatory, immunomodulatory and antitumor effects. However, its therapeutic potential is hindered by water insolubility and a narrow therapeutic index due to substantial hepato-, cardio- and nephrotoxicity. An array of triptolide derivatives aimed at improving bioavailability, limiting toxicity, but retaining efficacy has been synthesized. Modifications at C14 of triptolide have yielded the most promise and notable examples are (5R)-5-hydroxytriptolide (LLDT-8) and the prodrug minnelide (156).
LLDT-8 has not yet been tested on cancer patients, but has yielded promising phase II results in rheumatoid arthritis patients with poor responses to the standard-of-care methotrexate (156). Minnelide is currently undergoing a phase I in patients with advanced GI tumors (NCT01927965) and a multicenter phase II trial in patients with refractory pancreatic cancer (NCT03117920). Recruiting is ongoing for a phase II trial for advanced refractory pancreatic adenosquamous carcinoma (NCT04896073), as well as for phase I trials for EGFR-mutant NSCL (NCT05166616), for relapsed or refractory leukemia (NCT03760523) and in conjunction with paclitaxel for solid tumors (NCT03129139). There is limited trial reporting, but so far sufficient bioavailability of minnelide and its metabolite triptolide were achieved, with complete elimination of triptolide within 6 hours. A common side effect was reversible, rapid onset neutropenia, while reversible acute cerebellar toxicity was reported in two patients (157). Not all triptolide derivatives are suitable for clinical use as exemplified by F60008, whose high pharmacokinetic variability between patients with advanced solid tumors led to two fatalities, resulting in the termination of a phase I clinical trial (Table 1).
Despite the sparse clinical trial data, triptolide and its analogues are included in this review for their immunomodulatory potential. Triptolide is an immune checkpoint inhibitor. It inhibits IFN-γ-induced expression of PD-L1 on the surface of glioma (158), breast (159) and oral cancer cells (160). Triptolide-mediated repression of PD-L1 reduced tumor growth of patient-derived tumor xenografts and was mediated via inhibition of JAK2/STAT (160). Triptolide-induced downregulation of glioma PD-L1 increased IFN-γ and IL-2 and reversed glioma-induced inhibition of CD4+ helper T cells (158). Taken together these findings suggest a therapeutic potential of triptolide in reversing T cell exhaustion, and thus, immune evasion of tumor cells. Further studies are needed to elucidate the effect of triptolide, if any, on other immune checkpoints such as CTLA-4.
Triptolide can also remodel the TME in vivo. Triptolide inhibited melanoma growth by reducing Foxp3 mRNA levels in the spleen and auxiliary lymph nodes, as well as TGF-β, IL-10, VEGF and Tregs within the TME (161). Triptolide significantly increased the populations of monocytes, macrophages, B and T cells in a murine leukemia model (162). Recently, triptolide downregulated CXCL12 in subcutaneous colon cancer tumors, which in turn reduced TAM infiltration, reduced tumorsupportive M2 polarization, and decreased the expression of CD206 and IL-10 (163).
By reversing immune checkpoint inhibition and tumorsupportive immunity in the TME, triptolide analogues are attractive adjuncts to cancer chemotherapeutics especially for advanced-stage, chemotherapy-resistant cancers. Despite this, traditionally triptolide is used as an immunosuppressor with therapeutic potential in autoimmune diseases, which are linked to predisposition to leukemia and lymphoma (164). Triptolide inhibited IL-6/STAT3 to reduce ascites formation and organ infiltration of primary effusion lymphoma (165). Mutations in FIP1L1-PDGFRα (FIP1-Like-1 (FIP1L1)-platelet-derived growth factor receptor α (PDGFRα) confers resistance to imatinib in hypereosinophilic syndrome. Triptolide suppressed FIP1L1-PDGFRα expression, as well as downstream activation of STAT3, Erk and Akt to induce apoptosis of imatinib-resistant hypereosinophilic syndrome cells (166). Additionally, triptolide was cytotoxic to acute myeloid leukemia cells carrying the oncogenic fusion gene UBA2-WTIP (167). Since fusion gene expression is inherently limited to cancer cells, by targeting fusion gene function triptolide may exhibit selective toxicity against chemotherapy-resistant malignancies.
Promising radioprotective effects of triptolide have emerged from preclinical studies. Triptolide inhibited of IKKβ/NF-κB-mediated lysyl oxidase (LOX) production to reduce profibrotic IL-1β, IL-13 and TGF-β to diminish radiation-induced pulmonary fibrosis, and to improve mouse survival following radiation (168, 169). Triptolide additionally reduced pulmonary fibrosis by reducing alveolar macrophage infiltration, phagocytosis and proinflammatory cytokine and chemokine production (170). Thoracic radiation shows therapeutic benefit in the treatment of breast, lung and esophageal cancers, but is accompanied by pneumonitis in early stages and pulmonary fibrosis in late stages, which reduce quality of life and patient survival. To date, effective therapy is lacking highlighting the urgent need for novel therapeutic modalities (3). Triptolide itself is too toxic to be a viable therapeutic option, but if less toxic triptolide analogues show similar radioprotective roles, they may prove viable candidates for translational medicine (Table 5).
In vitro an in vivo studies have established that triptolide is capable of eliciting direct antitumor effects by inhibiting proliferation, dysregulating metabolism, promoting ROS generation and autophagy, apoptosis and pyroptosis (156, 173–176). Although preclinical effects are clear and have led to clinical trials, the limiting parameter of human triptolide use is its toxicity, which should not be ignored. For chemotherapeutic success, studies on triptolide should focus on its synergistic and chemosensitizing actions, as well as, on reformulation aimed at minimizing systemic toxicity and targeted-tumor release. We focus on such preclinical studies in our discussion below.
Minnelide and triptolide demonstrated synergistic effects with gemcitabine and Nab-paclitaxel pancreatic cancer animal models and cell lines. Specifically for pancreatic cancer, both have been described as inhibitors of super-enhancers, which are tumorigenic regulatory DNA sequences that function as binding platforms for transcription factors and coactivators (177, 178). Triptolide/paclitaxel and minnelide/paclitaxel/gemcitabine combinations decreased cell viability, increased apoptosis, and cell cycle arrest in vitro and in vivo respectively (179, 180). Notably, synergistic effects were absent in cells with silenced NF-κB suggesting that triptolide-mediated inhibition of NF-κB is necessary for synergism (179). Triptolide-induced sensitization of pancreatic cancer cell lines to gemcitabine was similarly dependent on NF-κB inhibition by triptolide (181). Addition of minnelide to gemcitabine-paclitaxel therapy allowed for the administration of lower conventional-chemotherapeutic doses, while decreasing cancer progression and metastasis, but increasing survival of tumor-bearing mice (180). Synergistic effects of triptolide have also been observed with idarubicin in acute myeloid leukemia (171, 172); with cisplatin in gastric (182); with 5-fluorouracil (183) and with oxaliplatin (184) in colon; with paclitaxel in lung (185); and with gemcitabine in bladder cancers (186).
Triptolide potentially sensitizes resistant cancers to conventional chemotherapeutics in vivo. Minnelide inhibited DNA repair and reversed resistance to oxaliplatin of pancreatic adenocarcinoma (187) and to cisplatin in triple-negative breast cancer cells (188). Triptolide inhibited Toll-like receptor 4 (TLR4)/NF-κB signaling to enhance pancreatic-cancer-cell-line sensitivity to gemcitabine, which resulted in decreased tumor cell proliferation, viability and xenograft tumor volume (181). Similarly, triptolide-induced inhibition of NF-κB reversed resistance to taxol in lung adenocarcinoma (189). Taken together these findings identify NF-κB pathway inhibition as a key mediator of triptolide-induced sensitization of malignant cells to conventional cancer chemotherapy. Similar effects of chemotherapy sensitization were observed when using triptolide on doxorubicin-resistant breast (190), cisplatin-resistant ovarian (175, 191) and bladder (192), and cisplatin- and 5-fluoruracil-resistant liver (193) cancers.
Triptolide derivatives with improved toxicity and bioavailability like minnelide are currently in clinical trials. However, minnelide is a prodrug of triptolide, and although its bioavailability has convincingly been improved, serious side effects have been reported in humans. Targeted drug delivery strategies take advantage of features that are unique to cancer cells. Recently, Kong et al. took advantage of the increased micropinocytosis and lysosomal activity of KRAS-mutant pancreatic cancer cells to directly deliver triptolide encapsulated in ultra-pH-sensitive micelles (T-UPSM). T-UPSM demonstrated rapid intratumor drug release with superior safety and antitumor efficacy in KRAS mutant mice (194). Triptolide conjugated to a generation-4 hydroxyl-terminated PolyAMidoAMine dendrimer was targeted to TAMs in an orthotopic glioblastoma model. Dendrimer-conjugated triptolide repolarized TAMs to a tumorsuppressive phenotype, decreased glioblastoma burden, while simultaneously limiting triptolide-associated cardiac and hepatic toxicity (195). Safety and efficacy of various dendrimers is currently being evaluated for a number of diseases with optimistic results (196) suggesting that dendrimer-triptolide conjugates may enter clinical testing soon. A nanocarrier system carrying triptolide and the active metabolite of irinotecan, SN38 inhibited NF-κB in both gastric cancer cells and cancer-associated fibroblasts (CAF) to inhibit CAF-induced cancer cell proliferation, migration and SN38-resistance, resulting in significant reduction in tumor burden in vivo (197). A biomimetic triptolide-paclitaxel nanodelivery system also caused significant antineoplastic effects against gastric cancer (198). Biomimetic nanotriptolide was also used with sorafenib in the treatment of hepatocellular carcinoma in vivo resulting in potent proapoptotic synergy resulting in reduced tumor burden (199).
Graviola (Annona muricata) is a fruit tree of the tropical and subtropical regions of the Americas. It is used in indigenous medicine against cancer, parasites, malaria, and depression. A. muricata extracts can be derived from the fruit, the seeds, the roots or the leaves of the plant and consist of over 200 phytochemicals including but not limited acetogenins, flavonols, phenolic compounds and glycopeptides (200). We will first review studies utilizing graviola extracts and then we will focus on the anticancer properties of select graviola phytochemicals.
As of today, graviola has been utilized in only one clinical trial, while two more – are currently recruiting. Graviola leaf extract or placebo was ingested by colorectal carcinoma patients who had already undergone surgical treatment. Safety was established as bone marrow, weight, nutritional status, liver and kidney function were not affected (49). Serum from study participants decreased colorectal carcinoma cell populations in vitro, demonstrating antineoplastic effects of bioavailable graviola phytochemicals and metabolites (50). However, no pharmacokinetic data, mechanistic insight, nor long-term effects of graviola on disease outcome or patient quality of life were reported (49, 50). Anecdotal evidence of the antitumor effects of graviola comes from a case report of a patient with refractory metastatic breast cancer, who achieved disease stability for 5 years after self-medicating with a daily ingestion of graviola leaf extract (201). As of right now, the clinical evidence of the anticancer efficacy of graviola is inconclusive. But, a combination of graviola and ellagic acid may prevent preneoplastic cervical lesion development as lower incidence of abnormal Pap smears was reported HPV-infected women treated for 1 year (202) (Table 1).
Graviola extracts have demonstrated immunomodulatory potential in vitro and in vivo. Graviola extracts increased TNF-α and IL-1β expression in cultured macrophages via MAPK activation suggesting that graviola may boost macrophage immune activity (203). Najmuddin et al. showed that graviola extracts increased CD8+, CD4+ and Nk1.1 cells in a breast cancer mouse model suggesting that graviola may potentiate anticancer immunity. In breast cancer cells, graviola reduced Fas ligand, proinflammatory IL-1β, TNF-α, nitric oxide and malondialdehyde, a biproduct of lipid peroxidation associated with DNA damage. Graviola-extract treatment increased tumor cell apoptosis, reduced tumor cell proliferation and metastasis, reduced angiogenesis and tumor burden in vivo (204). Taken together these results demonstrate that graviola exerts direct effects on breast cancer cells, as well as increases antitumor immunity (Table 6).
The antineoplastic effects of graviola have been elucidated in a plethora of in vitro and in vivo studies and have been extensively reviewed elsewhere (200). Graviola has demonstrated significant potency against breast cancer in vitro and in vivo. In vitro, it induced apoptosis in breast cancer, but not normal kidney cells (205), suggesting that graviola exhibits selective anticancer cytotoxicity. Similar findings were observed with ionic liquid, leaf-methanol, as well as seed-methanol extracts of graviola, which additionally induced G0/G1 cell cycle arrest (206, 207). Furthermore, Daddaiouaissa et al. showed that although less potent, ionic liquid graviola extract was more efficacious than taxol in inducing growth arrest (206). Graviola decreased estrogen receptor (EGFR) expression, tumor marker (CA15-3) antigenicity, ROS production and lipid peroxidation, but promoted apoptosis in 7,12-dimethylbenz[a]anthracene (DMBA)-induced breast cancer in vivo (208). Graviola acted alone, but also synergistically, with doxorubicin to induce cell cycle arrest and decrease viability of triple-negative breast cancer in vitro (209). Graviola-mediated effects in triple-negative breast cancer cells may be through inhibition of EGFR signaling (210).
Antiproliferative and proapoptotic activities of various graviola extracts have been observed against prostate cancer cells. Graviola pulp fruit extract decreased HIF-1α expression and NADPH oxidase activity to inhibit proliferation and colony formation of prostate cancer cell lines (211). Graviola leaf extract gavage of male rats increased apoptosis in the testes and glandular epithelium, decreased seminal index and reduced prostate size (212). Graviola synergized with doxorubicin to induce ROS production, apoptosis and potentially inhibit metastasis by downregulating VEGF (213). Furthermore, synergistic effects of graviola and low-dose radiation resulted in reduced TGF-β and CD44 expression, increased apoptosis, and overall reduced solid tumor burden in mouse xenografts (214). Graviola suppressed mucin 4 (MUC4) and HIF-1α-mediated expression of glycolytic genes and VEGF to promote tumor cell necrosis, but decrease metastasis, of orthotopically-implanted pancreatic tumors (215). MUC4 is selective tumor antigen of pancreatic, ovarian and head and neck carcinoma MUC4 vaccines are investigated as novel anticancer therapies (216). MUC4 has also been implicated in antitumor immunity, however, its exact function in this context is still unclear (216).
Over 200 phytochemicals have been identified in graviola extracts with most of the antitumor effects being attributed to flavonoids and acetogenins. Acetogenins are cytotoxic against carcinomas of the breast, pancreas, prostate, colon, liver and kidney in vitro and in vivo (200). Annonacin, a potent complex 1 inhibitor, inhibited EGFR activity when used alone, and sensitized multidrug-resistance breast cancer cells to tamoxifen to reduce xenograft tumor burden (217). Annosquacin and bullatacin targeted multidrug-resistance breast cancer by modulating MAPK and depleting ATP respectively (218, 219). But, acetogenins exhibit significant neurotoxicity induce atypical Parkinsonism in humans (220). Thus, although acetogenins are largely responsible for the antitumor efficacy of graviola, utilization of these in the clinic may lead to significant toxicity. Acetogenin-enriched graviola extracts exhibited superior potency and efficacy to flavonoid-enriched extracts, but with significant lethality. Importantly, co-administration of the two extracts did result in antitumor cytotoxicity, but with reduced side effects and lethality. The authors attributed the protective role of flavonoid-enriched extracts to rutin-mediated induction of P glycoprotein, which promoted acetogenin efflux from the brain (221). This seminal study demonstrates that the temptation to utilize the most active ingredients of graviola in developing novel chemotherapeutics should be avoided, and that whole extracts should be favored instead. Perhaps nature knows best.
Graviola extract has been recently formulated with silver nanoparticles aiming at targeted drug delivery with limited toxicity. Graviola-silver nanoparticles (GRANAg) promoted apoptosis, decreased proliferation and increased autophagy via IL-1β reduction and NLRP3 inflammasome activation (222). GRANAg peel extract demonstrated superior potency than graviola leaf extract on colon, breast and melanoma cell lines (223). Furthermore, GRANAg induced cell cycle arrest in NSCLC (224) and promoted apoptosis in cervical and prostate cancer cell line (225). In vivo studies are still needed to establish GRANAg potency, efficacy and safety before ascertaining its suitability for clinical translation.
Despite the limited clinical trial data, graviola has demonstrated safety and bioavailability in cancer patients. Graviola has consistently demonstrated proapoptotic, antiproliferative and immunomodulatory antitumor activity, but large-scale clinical trials are urgently needed in order to fully elucidate its chemotherapeutic potential.
A number of epidemiological studies and meta-analyses have linked green tea consumption to reduced prostate, breast, thyroid, colorectal and stomach cancer risk (226). Although green tea is a complex mixture of phytochemicals, EGCG has been documented as the most abundant and most bioactive (227), and thus has been studied extensively for its chemopreventative and chemotherapeutic effects (226).
A number of clinical trials have identified green tea extract, and EGCG in particular as a potential cancer chemopreventative phytochemical.
Green-tea-extract supplementation for 1 year in over 1000 healthy postmenopausal women with increased risk of breast cancer significantly reduced percent mammographic density (PMD) (i.e. proportion of fibroglandular tissue within the breast) in younger women (51). Importantly, women with more than 75% PMD are 4-6fold more likely to develop breast cancer (228), and the age-dependent EGCG effect was similar to what had been previously reported for tamoxifen (40, 51). EGCG demonstrated antiproliferative effects in patients with early breast cancer, where decreased Ki-67 correlated with high plasma EGCG levels (229). EGCG is metabolized by catechol-O-methyl transferase (COMT) and UDP-glucuronosyltransferase (UGT). Epidemiological studies revealed that green tea drinkers, especially those with low COMT-mediated catechin metabolism, exhibit lower PMD and lower breast cancer risk (52). Notably, COMT-mediated metabolism also reduced anticarcinogenic efficacy against prostate cancer (230). This findings are contradicted by a small phase II trial of EGCG-containing polyphenol formulation of green tea (Polyphenon E) in bladder cancer patients, where the pharmacogenomic relationship between EGCG pharmacokinetics and COMT or UGT1A1 polymorphisms was absent (53). Polyphenon E reduced PCNA (53) warranting further investigation of EGCG efficacy against bladder cancer. However, lack of EGCG efficacy has been reported in patients with high-grade prostatic intraepithelial neoplasia and/or atypical small acinar proliferation (54), in patients of high risk of recurrent colonic neoplasia (55), and in patients with colorectal adenomas (56). EGCG-enriched double-brewed green tea also did not protect against recurrence women with advance-stage ovarian cancer who had previously achieved complete response with surgical and chemotherapeutic interventions (57). EGCG did show promising results in chronic lymphocytic leukemia (CLL). EGCG supplementation resulted in a sustained decline in lymphocyte numbers and/or lymphadenopathy in asymptomatic Rai stage 0-II CLL patients (58). Furthermore, in Rai stage 0 CLL patients, EGCG reduced circulating Tregs, suggesting that EGCG may control lymphocytosis and delay disease progression at early disease stages (59). In summary, clinical trial data demonstrate EGCG-mediated chemoprotection against CLL, breast and bladder cancers, but not against prostate, colorectal or ovarian cancers (Table 1).
EGCG has also demonstrated radioprotective potential in the clinic. It alleviated acute radiation-induced esophagitis in advanced, unresectable lung (114) and in esophageal cancer patients (115). Similar results were observed for post-resection radiotherapy-induced dermatitis in breast cancer patients (116). EGCG mouthwash reduced radiation-induced oral mucosal injury in radiotherapy-treated head and neck cancer patients in phase I (117). Overall, EGCG has demonstrated significant efficacy in reducing the incidence and severity of radiation-induced injury without significant adverse effects suggesting that it should be considered as an adjuvant to radiotherapy (Table 3).
EGCG-mediated radioprotection likely stems from its anti-inflammatory effects, which have ameliorated inflammatory conditions including multiple sclerosis, lupus nephritis, rheumatoid arthritis and colitis in vivo (227). EGCG binds to the extracellular domain of the 67-kDa laminin receptor (67LR) (231), a non-integrin cell surface receptor, to inhibit TLR4 signaling via EGCG/67-kDa-induced expression of Tollip, a negative regulator of TLR4. This inhibited MAPK and NF-κB-mediated expression of proinflammatory cytokines and dendritic cell maturation (232). EGCG can also inhibit NF-κB activity directly, through a covalent interaction with NF-κB-p65 sulfhydryls (233). Some additional anti-inflammatory functions of EGCG include inhibition of LPS-induced M1-to-M2 macrophage polarization (234), and inhibition of activator-protein-1-mediated proinflammatory cytokine production in lymphocytes (235). Molecular docking studies have identified that EGCG, and also curcumin, directly bind NRLP3 components to inhibit inflammasome assembly (236), which can be triggered by radiation. EGCG exhibited radioprotective effects in both rat hippocampi and intestinal epithelia. These effects were mediated by ROS scavenging, activation of the Nrf2- antioxidant pathway and Bcl-2 (237, 238).
EGCG also promotes anticancer immunity by inhibiting STAT3 activity in immune cells. EGCG reduced STAT3 and CXCL8 expression in colon-cancer-patient-derived neutrophils to inhibit the formation of neutrophil extracellular traps and suppress colon cancer migration in vitro (239). EGCG remodeled breast cancer TME in vivo by increasing the numbers of tumorsuppressive lymphocytes, but inducing MDSC apoptosis and alleviating MDSC-mediated tumorsuppression (240). Another mode by which ECGG promotes anticancer activity and immunotherapy is through the inhibition of PD-L1 as shown in NSCL mouse xenografts (241) (Table 7).
A critical target of EGCG is 67LR, which is overexpressed in cancers including of the blood, prostate, breast, bile duct, pancreas, and colon, and has been associated with tumor cell migration, invasion, and metastasis (244). EGCG inhibited EMT-like transition of glioblastoma (245) It inhibited melanoma growth and proliferation via 67LR-mediated activation of the tumor suppressor merlin (246). EGCG/67LR-mediated dephosphorylation and activation of myosin phosphatase target subunit (MYPT1) results in myosin II regulatory light chain (MRLC) inactivation and overall inhibition of cancer cell cytokinesis (247). EGCG suppressed STAT3 transcription in colorectal cancer cell in vitro to inhibit migration, but promote apoptosis (248) EGCG/67LR promoted apoptosis of multiple myeloma, acute and chronic myeloid leukemias via multiple downstream signaling cascades including p38-MAPK/JNK and JAK2/STAT3 (242–244). EGCG/67LR inhibited phosphodiesterase 5 (PDE5) to increase cGMP levels and induce multiple myeloma cell death via PKCδ and acid sphingomyelinase (243). Notably, PDE-overexpressing breast, pancreatic, and gastric cancer cells are resistant to EGCG-induced apoptosis. Co-administration of PDE5 inhibitors, including sildenafil and vardenafil, sensitized cells to EGCG and inhibited xenograft tumor growth. EGCG/PDE5-inhibitor combination therapy also reduced EGCG dosage requirements and decreased EGCG-induced hepatotoxicity (243, 249). Finally, EGCG reduced metastasis of nasopharyngeal carcinoma cells through Src-mediated downregulation of MMPs (250). EGCG also remodels the TME to inhibit cancer development and progression. Notably, it inhibited adipogenesis and homing of triple-negative breast cancer cells (251). EGCG also synergized with curcumin to reduce tumor-supportive endothelial transition. EGCG/curcumin also inhibited JAK/STAT3 in tumor endothelial cells to inhibit angiogenesis and colon cancer metastasis (252).
EGCG targeted STAT3 to downregulate multidrug resistance 1 (MDR1) or c-Myb and increase sensitivity to cisplatin of cisplatin-resistant oral and ovarian cancers (253, 254). EGCG reduced cisplatin-induced ototoxicity (255). Taken together, these data demonstrate that EGCG could act as an adjunct to cisplatin to reduce chemotherapeutic resistance and cisplatin-induced hearing loss.
Curcumin is a polyphenol derived from turmeric, a spice made from the roots of Curcuma longa. Turmeric has a long history of use as a remedy in Ayurveda and traditional Chinese medicine. Perhaps it is the historical significance of turmeric which sparked the very large volume of research aimed at evaluating the potential of curcuminoids as antioxidant, anti-inflammatory, immunomodulatory and anticancer agents (256–258). Given the overwhelming number of studies and significant anticancer activity against different cancers types in vitro and in vivo (257), curcumin cannot be ignored in this review. Due to its preclinical success and safety even in relatively high doses (259), curcumin has been clinically tested as an adjuvant for chemotherapy (61, 63, 64). Despite extensive research and a total of 75 human clinical trials, the clinical efficacy of curcumin against cancer is still inconclusive. We will briefly discuss these findings here, and attempt to identify reasons for the difficulty in translating curcumin into clinical use.
Clinical trials have examined the effects of curcumin as an adjuvant to radiotherapy. Curcumin was an effective alternative to radiosensitizing agents in cervical cancer (60). Curcumin was also examined for its radioprotective potential. In head and neck cancer patients, nanomicellar curcumin significantly reduced pain, severity and occurrence of radiotherapy-induced oral mucositis (120), while curcumin mouthwash was as effective as benzydamine and delayed oral mucositis occurrence (121). Results from trials the effects of curcumin in ameliorating radiotherapy-induced dermatitis in breast cancer patients have been lacklustre at best. Topical curcumin administration reduced radiotherapy-induced dermatitis only in a limited number of large breast separation patients (118), while a much larger trial found no difference between oral curcumin and placebo (119).
Curcumin has also been used as an adjuvant. Curcumin was found safe in a small cohort of metastatic colorectal cancer patients receiving folinic acid/5-fluorouracil/oxaliplatin (FOLFOX) ± bevacizumab, with half additionally receiving curcumin. It did not significantly affect quality of life and neurotoxicity scores, but did significantly improve overall patient survival. However, caution is warranted as significantly fewer patients in the curcumin arm had more than two metastatic loci, which have been shown to increase the likelihood of death (61). Again in colorectal cancer, supplementation with curcuminoids improved quality of life and inflammatory markers ESR and CRP (62), with the latter also noted in breast cancer patients (260). In metastatic castration-resistant prostate cancer (CRPC), curcumin/docetaxel combination demonstrated safety along with a response in PSA (66), a prostate cancer marker which may also predict recurrence of it post treatment (261). Synergy of curcumin and docetaxel in promoting autophagy and apoptosis via PI3K/Akt/mTOR downregulation has been demonstrated in oesophageal carcinoma in vitro (262). Yet, another human trial showed no significant benefit and was discontinued due to futility (67). No significant differences were found in off treatment duration, levels of PSA or testosterone, quality of life scores and adverse drug reactions when curcumin was added to intermittent androgen deprivation. However, adverse events and notably PSA progression were reduced in the curcumin treatment group (68). Coadministration of curcumin and gemcitabine in advanced pancreatic cancer patients yielded inconclusive results, significant gastrointestinal symptoms and forced several patients to decrease or discontinue curcumin (63). Dhillon et al. also obtained lacklustre results with only 2 of 21 patients demonstrating activity and variable plasma curcumin concentrations highlighting the problem of low oral bioavailability (65). Interestingly a similar trial (with no controls) using patented curcumin along with gemcitabine found it to be safe with a good response rate in pancreatic cancer (64) (Table 1).
It can be argued that inconclusive results are due to poor pharmacokinetics and that the safety along with the volume of in vitro evidence, prompt the development of different formulations and not the abandonment of curcumin. Indeed, these problems were circumvented by a clinical trial assessing the tolerability, safety and efficacy of patented IV liposomal curcumin on 32 patients with metastatic cancer. No adverse effects were reported for 26 patients at doses of 100-300mg. However even at that dose, which is significantly lower than in vitro dosing, hemolysis and a decrease in hemoglobin occurred in one and three patients respectively. Furthermore, curcumin plasma concentrations became undetectable rapidly after the infusion. There was no tumor reduction but there was a response in tumor markers (69).
Perhaps clinical trial focus should be shifted to more potent and bioavailable analogues which can be standardized and have shown greater potency in vitro and in vivo (263–269). Promising analogues include GO-Y30 and WZ30. GO-Y30 modulated TGF-β and Treg function, supressed STAT3 in breast and pancreatic cancer cells, and inhibited the PD-1 immune checkpoint to increase the efficacy of cancer immunotherapy (263–265, 267). WZ30 demonstrated superior activity to curcumin and synergy with cisplatin (266). Nanomicellar curcumin/docetaxel achieved targeted and sustained delivery and synergy to reduce cancer cell viability, proliferation and angiogenesis in ovarian cancer xenografts (268). A similar combination of curcumin and docetaxel synergistically reduced tumor volume in chemoresistant breast and ovarian cancer cells (269).
Through analogues like GO-Y30, perhaps the immunomodulatory aspect of curcumin can be clarified. Overall curcumin (non-analogue) has been shown to reduce STAT3 activation in both immune and cancer cells and potentiate anticancer immunity. It also exhibited synergy with chemotherapy and decreased resistance. Curcumin inhibited tumor-derived IL-6 to inhibit dendritic cell STAT3 and restore dendritic cell-mediated stimulation of T cell immunity. Coadministration of PD-1/PD-L1 antibodies with curcumin achieved further therapeutic benefit in colon cancer xenografts (270). Curcumin decreased 5-fluorouracil resistance of gastric carcinoma by suppressing STAT3 in cancer associated fibroblasts (271). Again, in gastric cancer cells curcumin (and also berberine) given alone or with fluorouracil downregulated pSTAT3, survivin and synergistically decreases cancer cell viability of cancer (146). Curcumin/IFN-β/retinoic acid combination also showed synergistic antiproliferative effects by suppressing STAT3, Bcl-2, COX-2, MMP-9 and survivin, but upregulating GADD153 and GRIM-19 (272). Similarly in haematological malignancies curcumin has decreased STAT3 activation in multiple myeloma cells, acute myeloid leukaemia and chronic myelogenous leukemia (273–275). Curcumin also establishes a tumorsuppressive TME by TAM and CTL repolarization. Curcumin-induced TAM1 repolarization to tumoricidal M1 phenotype increase MCP-1 secretion and NK cell recruitment. NK cells in turn secrete IFN-γ and further support M2-to-M1 TAM repolarization. In addition, curcumin-mediated decrease in TGF-β and IL-10 reduced Treg, but increased CTL numbers and antitumor immunity (258) (Table 8).
In conclusion, curcumin has successfully demonstrated antitumor activity in vivo and in vitro, but clinical trial efficacy, if any, was limited to reduced inflammatory markers, which are not always corelated to improved outcomes. The chasm between preclinical potential and the clinical failure could be attributed to a troubled compound or study design. Variability in curcumin extraction, curcuminoid mixture, poor and unpredictable pharmacokinetics hinder data reproducibility (257).
Curcumin has been classified as Pan Assay Interference (PAINS) compound and an Invalid Metabolic Panacea (IMPS). PAINS and IMPs are compounds that give false leads through various types of assay interference due to their high reactivity, indiscriminate-binding to substrates, inherent fluorescence or denaturation properties (276). These issues along with questionable efficacy, bring the utility of curcumin for cancer treatment and its use in further trials into question. Despite this, repeated successful preclinical studies (257) have led researches to invest in improving the delivery and pharmacokinetics of the molecule, rather than abandoning it. Novel curcumin formulations and analogues are still being made perhaps in hopes of salvaging such an expensive and potentially false lead. While both these avenues appear very promising the pitfalls of curcumin research (PAINS, IMPS, non-standardization) may be lurking yet again and caution is advised for the yellow powder that may have cried wolf.
Natural phytochemicals are known to mediate multiple cancer pathways and reduce tumor growth. This is done through well-known processes such as the induction of ROS and apoptosis, but also through novel targets such as immune modulation, including PD-L1 and STAT3 inhibition. Apart from immunomodulatory agents, natural biomolecules are also able to act synergistically with or offer protection from chemo- and radiotherapy. They are able to augment the tumoricidal capacity of immune cells while also decreasing proinflammatory responses that promote tumorigenesis or injury (Figures 2, 4). Therefore phytochemicals possess a lot of qualities rendering them attractive as adjuvants to established therapeutic modalities. They also possess some advantages over monoclonal therapies, such as wide sources, less toxic side effects, and diverse immunomodulatory activities. One important area that needs to be resolved, is that phytochemicals have a wide range of pharmacological effects, but their targets and molecular mechanisms relevant to tumor immunity have not been fully researched. Further research for screening natural plant-derived biomolecules for targeted antitumor effects having immunomodulatory properties could be performed with the aid of advanced novel drug delivery technologies, and computer-aided design techniques. Both the guidance of computer assisted drug design and the trojan-horse of drug encapsulation are ideal tools for furthering this field of research and the efficacy of natural biomolecules. We believe that the role of natural biomolecules in tumor immunotherapy and as therapeutic adjuvants is emerging, has great potential and will hopefully lead to clinically applied pharmaceuticals.
All authors listed have made a substantial, direct, and intellectual contribution to the work and approved it for publication.
The authors declare that the research was conducted in the absence of any commercial or financial relationships that could be construed as a potential conflict of interest.
All claims expressed in this article are solely those of the authors and do not necessarily represent those of their affiliated organizations, or those of the publisher, the editors and the reviewers. Any product that may be evaluated in this article, or claim that may be made by its manufacturer, is not guaranteed or endorsed by the publisher.
1. Emran TB, Shahriar A, Mahmud AR, Rahman T, Abir MH, Siddiquee Mohd FR, et al. Multidrug resistance in cancer: Understanding molecular mechanisms, immunoprevention and therapeutic approaches. Front Oncol (2022) 12:891652. doi: 10.3389/fonc.2022.891652
2. Nepon H, Safran T, Reece EM, Murphy AM, Vorstenbosch J, Davison PG. Radiation-induced tissue damage: Clinical consequences and current treatment options. Semin Plast Surg (2021) 35(3):181–8. doi: 10.1055/s-0041-1731464
3. Arroyo-Hernández M, Maldonado F, Lozano-Ruiz F, Muñoz-Montaño W, Nuñez-Baez M, Arrieta O. Radiation-induced lung injury: Current evidence. BMC Pulmon Med (2021) 21(1):9. doi: 10.1186/s12890-020-01376-4
4. Ahmed A, Tait SWG. Targeting immunogenic cell death in cancer. Mol Oncol (2020) 14(12):2994–3006. doi: 10.1002/1878-0261.12851
5. Greten FR, Grivennikov SI. Inflammation and cancer: Triggers, mechanisms and consequences. Immunity (2019) 51(1):27–41. doi: 10.1016/j.immuni.2019.06.025
6. Valle ASd, Naval J, Anel A, Marzo I. Novel forms of immunomodulation for cancer therapy. Trends Cancer (2020) 6(6):518–32. doi: 10.1016/j.trecan.2020.02.015
7. Schreiber RD, Old LJ, Smyth MJ. Cancer immunoediting: Integrating immunity’s roles in cancer suppression and promotion. Science (2011) 331(6024):1565–70. doi: 10.1126/science.1203486
8. Chen DS, Mellman I. Oncology meets immunology: The cancer-immunity cycle. Immunity (2013) 39(1):1–10. doi: 10.1016/j.immuni.2013.07.012
9. Day CL, Kaufmann DE, Kiepiela P, Brown JA, Moodley ES, Reddy S, et al. PD-1 expression on HIV-specific T cells is associated with T-cell exhaustion and disease progression. Nature (2006) 443(7109):350–4. doi: 10.1038/nature05115
10. Baitsch L, Baumgaertner P, Devêvre E, Raghav SK, Legat A, Barba L, et al. Exhaustion of tumor-specific CD8+ T cells in metastases from melanoma patients. J Clin Invest (2011) 121(6):2350–60. doi: 10.1172/JCI46102
11. Ishida Y, Agata Y, Shibahara K, Honjo T. Induced expression of PD-1, a novel member of the immunoglobulin gene superfamily, upon programmed cell death. EMBO J (1992) 11(11):3887–95. doi: 10.1002/j.1460-2075.1992.tb05481.x
12. Curran MA, Montalvo W, Yagita H, Allison JP. PD-1 and CTLA-4 combination blockade expands infiltrating T cells and reduces regulatory T and myeloid cells within B16 melanoma tumors. Proc Natl Acad Sci U S A (2010) 107(9):4275–80. doi: 10.1073/pnas.0915174107
13. Krummel MF, Allison JP. CD28 and CTLA-4 have opposing effects on the response of T cells to stimulation. J Exp Med (1995) 182(2):459–65. doi: 10.1084/jem.182.2.459
14. Kraehenbuehl L, Weng CH, Eghbali S, Wolchok JD, Merghoub T. Enhancing immunotherapy in cancer by targeting emerging immunomodulatory pathways. Nat Rev Clin Oncol (2022) 19(1):37–50. doi: 10.1038/s41571-021-00552-7
15. Necchi A, Joseph RW, Loriot Y, Hoffman-Censits J, Perez-Gracia JL, Petrylak DP, et al. Atezolizumab in platinum-treated locally advanced or metastatic urothelial carcinoma: Post-progression outcomes from the phase II IMvigor210 study. Ann Oncol: Off J Eur Soc Med Oncol (2017) 28(12):3044–50. doi: 10.1093/annonc/mdx518
16. Trinh S, Le A, Gowani S, La-Beck NM. Management of immune-related adverse events associated with immune checkpoint inhibitor therapy: A minireview of current clinical guidelines. Asia Pac J Oncol Nurs (2019) 6(2):154–60. doi: 10.4103/apjon.apjon_3_19
17. Pillai RN, Behera M, Owonikoko TK, Kamphorst AO, Pakkala S, Belani CP, et al. Comparison of the toxicity profile of PD-1 versus PD-L1 inhibitors in non-small cell lung cancer: A systematic analysis of the literature. Cancer (2018) 124(2):271–7. doi: 10.1002/cncr.31043
18. Imai K, Matsuyama S, Miyake S, Suga K, Nakachi K. Natural cytotoxic activity of peripheral-blood lymphocytes and cancer incidence: An 11-year follow-up study of a general population. Lancet ‘ (2000) 356(9244):1795–9. doi: 10.1016/S0140-6736(00)03231-1
19. Truxova I, Kasikova L, Hensler M, Skapa P, Laco J, Pecen L, et al. Mature dendritic cells correlate with favorable immune infiltrate and improved prognosis in ovarian carcinoma patients. J Immunother Cancer (2018) 6(1):139. doi: 10.1186/s40425-018-0446-3
20. Hwang WT, Adams SF, Tahirovic E, Hagemann IS, Coukos G. Prognostic significance of tumor-infiltrating T cells in ovarian cancer: A meta-analysis. Gynecol Oncol (2012) 124(2):192–8. doi: 10.1016/j.ygyno.2011.09.039
21. Kroeger DR, Milne K, Nelson BH. Tumor-infiltrating plasma cells are associated with tertiary lymphoid structures, cytolytic T-cell responses, and superior prognosis in ovarian cancer. Clin Cancer Res (2016) 22(12):3005–15. doi: 10.1158/1078-0432.CCR-15-2762
22. Teng MWL, Galon J, Fridman WH, Smyth MJ. From mice to humans: Developments in cancer immunoediting. J Clin Invest (2015) 125(9):3338–46. doi: 10.1172/JCI80004
23. Forero A, Li Y, Chen D, Grizzle WE, Updike KL, Merz ND, et al. Expression of the MHC class II pathway in triple-negative breast cancer tumor cells is associated with a good prognosis and infiltrating lymphocytes. Cancer Immunol Res (2016) 4(5):390–9. doi: 10.1158/2326-6066.CIR-15-0243
24. Sconocchia G, Eppenberger-Castori S, Zlobec I, Karamitopoulou E, Arriga R, Coppola A, et al. HLA class II antigen expression in colorectal carcinoma tumors as a favorable prognostic marker. Neoplasia (2014) 16(1):31–42. doi: 10.1593/neo.131568
25. Yi R, Hong S, Zhang Y, Lin A, Ying H, Zou W, et al. MHC-II signature correlates with anti-tumor immunity and predicts anti-PD-L1 response of bladder cancer. Front Cell Dev Biol (2022) 10:757137. doi: 10.3389/fcell.2022.757137
26. Roemer MGM, Redd RA, Cader FZ, Pak CJ, Abdelrahman S, Ouyang J, et al. Major histocompatibility complex class II and programmed death ligand 1 expression predict outcome after programmed death 1 blockade in classic Hodgkin lymphoma. J Clin Oncol (2018) 36(10):942–50. doi: 10.1200/JCO.2017.77.3994
27. Rodig SJ, Gusenleitner D, Jackson DG, Gjini E, Giobbie-Hurder A, Jin C, et al. MHC proteins confer differential sensitivity to CTLA-4 and PD-1 blockade in untreated metastatic melanoma. Sci Trans Med (20180) 10(450):eaar3342. doi: 10.1126/scitranslmed.aar3342
28. Buchbinder EI, Desai A. CTLA-4 and PD-1 pathways: Similarities, differences, and implications of their inhibition. Am J Clin Oncol (2016) 39(1):98–106. doi: 10.1097/COC.0000000000000239
29. Chen Y, Song Y, Du W, Gong L, Chang H, Zou Z. Tumor-associated macrophages: An accomplice in solid tumor progression. J Biomed Sci (2019) 26(1):78. doi: 10.1186/s12929-019-0568-z
30. Verma A, Mathur R, Farooque A, Kaul V, Gupta S, Dwarakanath BS. T-Regulatory cells in tumor progression and therapy. Cancer Manag Res (2019) 11:10731–47. doi: 10.2147/CMAR.S228887
31. Shang J, Zha H, Sun Y. Phenotypes, functions, and clinical relevance of regulatory b cells in cancer. Front Immunol (2020) 11:582657. doi: 10.3389/fimmu.2020.582657
32. Ruffell B, Coussens LM. Macrophages and therapeutic resistance in cancer. Cancer Cell (2015) 27(4):462–72. doi: 10.1016/j.ccell.2015.02.015
33. Ostrand-Rosenberg S, Fenselau C. Myeloid-derived suppressor cells: Immune-suppressive cells that impair antitumor immunity and are sculpted by their environment. J Immunol (2018) 200(2):422–31. doi: 10.4049/jimmunol.1701019
34. Togashi Y, Shitara K, Nishikawa H. Regulatory T cells in cancer immunosuppression — implications for anticancer therapy. Nat Rev Clin Oncol (2019) 16(6):356–71. doi: 10.1038/s41571-019-0175-7
35. Zou S, Tong Q, Liu B, Huang W, Tian Y, Fu X. Targeting STAT3 in cancer immunotherapy. Mol Cancer (2020) 19:145. doi: 10.1186/s12943-020-01258-7
36. Newman DJ, Cragg GM. Natural products as sources of new drugs from 1981 to 2014. J Nat Prod (2016) 79(3):629–61. doi: 10.1021/acs.jnatprod.5b01055
37. Lu F, He XL, Richard C, Cao J. A brief history of artemisinin: Modes of action and mechanisms of resistance. Chin J Nat Med (2019) 17(5):331–6. doi: 10.1016/S1875-5364(19)30038-X
38. von Hagens C, Walter-Sack I, Goeckenjan M, Osburg J, Storch-Hagenlocher B, Sertel S, et al. Prospective open uncontrolled phase I study to define a well-tolerated dose of oral artesunate as add-on therapy in patients with metastatic breast cancer (ARTIC M33/2). Breast Cancer Res Treat (2017) 164(2):359–69. doi: 10.1007/s10549-017-4261-1
39. von Hagens C, Walter-Sack I, Goeckenjan M, Storch-Hagenlocher B, Sertel S, Elsässer M, et al. Long-term add-on therapy (compassionate use) with oral artesunate in patients with metastatic breast cancer after participating in a phase I study (ARTIC M33/2). Phytomedicine (2019) 54:140–8. doi: 10.1016/j.phymed.2018.09.178
40. Cuzick J, Warwick J, Pinney E, Warren RML, Duffy SW. Tamoxifen and breast density in women at increased risk of breast cancer. J Natl Cancer Inst (2004) 96(8):621–8. doi: 10.1093/jnci/djh106
41. Kuipers EJ, Grady WM, Lieberman D, Seufferlein T, Sung JJ, Boelens PG, et al. Colorectal cancer. Nat Rev Dis Primers (2015) 1(1):1–25. doi: 10.1038/nrdp.2015.65
42. Krishna S, Ganapathi S, Ster IC, Saeed MEM, Cowan M, Finlayson C, et al. A randomised, double blind, placebo-controlled pilot study of oral artesunate therapy for colorectal cancer. EBioMedicine (2015) 2(1):82–90. doi: 10.1016/j.ebiom.2014.11.010
43. Ferrall L, Lin KY, Roden RBS, Hung CF, Wu TC. Cervical cancer immunotherapy: Facts and hopes. Clin Cancer Res (2021) 27(18):4953–73. doi: 10.1158/1078-0432.CCR-20-2833
44. Trimble CL, Levinson K, Maldonado L, Donovan MJ, Clark KT, Fu J, et al. A first-in-human proof-of-concept trial of intravaginal artesunate to treat cervical intraepithelial neoplasia 2/3 (CIN2/3). Gynecol Oncol (2020) 157(1):188–94. doi: 10.1016/j.ygyno.2019.12.035
45. Jansen FH, Adoubi I, Kouassi Commoe JC, De Cnodder T, Jansen N, Tschulakow A, et al. First study of oral artenimol-r in advanced cervical cancer: Clinical benefit, tolerability and tumor markers. Anticancer Res (2011) 31(12):4417–22.
46. Xu X, Liu T, Wu J, Wang Y, Hong Y, Zhou H. Transferrin receptor-involved HIF-1 signaling pathway in cervical cancer. Cancer Gene Ther (2019) 26(11–12):356–65. doi: 10.1038/s41417-019-0078-x
47. Disbrow GL, Baege AC, Kierpiec KA, Yuan H, Centeno JA, Thibodeaux CA, et al. Dihydroartemisinin is cytotoxic to papillomavirus-expressing epithelial cells in vitro and in vivo. Cancer Res (2005) 1;65(23):10854–61. doi: 10.1158/0008-5472.CAN-05-1216
48. Chen YX, Gao QY, Zou TH, Wang BM, Liu SD, Sheng JQ, et al. Berberine versus placebo for the prevention of recurrence of colorectal adenoma: A multicentre, double-blinded, randomised controlled study. Lancet Gastroenterol Hepatol (2020) 5(3):267–75. doi: 10.1016/S2468-1253(19)30409-1
49. Indrawati L, Purwantyastuti P, Abdullah M, Surono IS, Basir I. Safety of annona muricata extract supplementation for colorectal cancer patients. InaJGHE (2017) 17(3):170–5. doi: 10.24871/1732016170-175
50. Indrawati L, Ascobat P, Bela B, Abdullah M, Surono IS. The effect of an annona muricata leaf extract on nutritional status and cytotoxicity in colorectal cancer: a randomized controlled trial. Asia Pac J Clin Nutr (2017) 26(4):606–12. doi: 10.6133/apjcn.062016.02
51. Samavat H, Ursin G, Emory TH, Lee E, Wang R, Torkelson CJ, et al. A randomized controlled trial of green tea extract supplementation and mammographic density in postmenopausal women at increased risk of breast cancer. Cancer Prev Res (Phila) (2017) 10(12):710–8. doi: 10.1158/1940-6207.CAPR-17-0187
52. Wu AH, Tseng CC, Van Den Berg D, Yu MC. Tea intake, COMT genotype, and breast cancer in Asian-American women. Cancer Res (2003) 63(21):7526–9.
53. Gee JR, Saltzstein DR, Kim K, Kolesar J, Huang W, Havighurst TC, et al. A phase II randomized, double-blind, presurgical trial of polyphenon e in bladder cancer patients to evaluate pharmacodynamics and bladder tissue biomarkers. Cancer Prev Res (Phila) (2017) 10(5):298–307. doi: 10.1158/1940-6207.CAPR-16-0167
54. Kumar NB, Pow-Sang J, Egan KM, Spiess PE, Dickinson S, Salup R, et al. Randomized, placebo-controlled trial of green tea catechins for prostate cancer prevention. Cancer Prev Res (Phila) (2015) 8(10):879–87. doi: 10.1158/1940-6207.CAPR-14-0324
55. Sinicrope FA, Viggiano TR, Buttar NS, Song LMWK, Schroeder KW, Kraichely RE, et al. Randomized phase II trial of polyphenon e versus placebo in patients at high risk of recurrent colonic neoplasia. Cancer Prev Res (Phila) (2021) 14(5):573–80. doi: 10.1158/1940-6207.CAPR-20-0598
56. Seufferlein T, Ettrich TJ, Menzler S, Messmann H, Kleber G, Zipprich A, et al. Green tea extract to prevent colorectal adenomas, results of a randomized, placebo-controlled clinical trial. Am J Gastroenterol (2022) 117(6):884–94. doi: 10.14309/ajg.0000000000001706
57. Trudel D, Labbé DP, Araya-Farias M, Doyen A, Bazinet L, Duchesne T, et al. A two-stage, single-arm, phase II study of EGCG-enriched green tea drink as a maintenance therapy in women with advanced stage ovarian cancer. Gynecol Oncol (2013) 131(2):357–61. doi: 10.1016/j.ygyno.2013.08.019
58. Shanafelt TD, Call TG, Zent CS, Leis JF, LaPlant B, Bowen DA, et al. Phase 2 trial of daily, oral polyphenon e in patients with asymptomatic, rai stage 0-II chronic lymphocytic Leukemia(CLL). Cancer (2013) 119(2):363–70. doi: 10.1002/cncr.27719
59. D’Arena G, Simeon V, De Martino L, Statuto T, D’Auria F, Volpe S, et al. Regulatory T-cell modulation by green tea in chronic lymphocytic leukemia. Int J Immunopathol Pharmacol (2013) 26(1):117–25. doi: 10.1177/039463201302600111
60. Hidayat YM, Wagey F, Suardi D, Susanto H, Laihad BJ, Tobing MDL. Analysis of curcumin as a radiosensitizer in cancer therapy with serum survivin examination: Randomised control trial. Asian Pac J Cancer Prev (2021) 22(1):139–43. doi: 10.31557/APJCP.2021.22.1.139
61. Howells LM, Iwuji COO, Irving GRB, Barber S, Walter H, Sidat Z, et al. Curcumin combined with FOLFOX chemotherapy is safe and tolerable in patients with metastatic colorectal cancer in a randomized phase IIa trial. J Nutr (2019) 149(7):1133–9. doi: 10.1093/jn/nxz029
62. Panahi Y, Saberi-Karimian M, Valizadeh O, Behnam B, Saadat A, Jamialahmadi T, et al. Effects of curcuminoids on systemic inflammation and quality of life in patients with colorectal cancer undergoing chemotherapy: A randomized controlled trial. Adv Exp Med Biol (2021) 1328:1–9. doi: 10.1007/978-3-030-73234-9_1
63. Epelbaum R, Schaffer M, Vizel B, Badmaev V, Bar-Sela G. Curcumin and gemcitabine in patients with advanced pancreatic cancer. Nutr Cancer (2010) 62(8):1137–41. doi: 10.1080/01635581.2010.513802
64. Pastorelli D, Fabricio ASC, Giovanis P, D’Ippolito S, Fiduccia P, Soldà C, et al. Phytosome complex of curcumin as complementary therapy of advanced pancreatic cancer improves safety and efficacy of gemcitabine: Results of a prospective phase II trial. Pharmacol Res (2018) 132:72–9. doi: 10.1016/j.phrs.2018.03.013
65. Dhillon N, Aggarwal BB, Newman RA, Wolff RA, Kunnumakkara AB, Abbruzzese JL, et al. Phase II trial of curcumin in patients with advanced pancreatic cancer. Clin Cancer Res (2008) 14(14):4491–9. doi: 10.1158/1078-0432.CCR-08-0024
66. Mahammedi H, Planchat E, Pouget M, Durando X, Curé H, Guy L, et al. The new combination docetaxel, prednisone and curcumin in patients with castration-resistant prostate cancer: A pilot phase II study. Oncology (2016) 90(2):69–78. doi: 10.1159/000441148
67. Passildas-Jahanmohan J, Eymard JC, Pouget M, Kwiatkowski F, Van Praagh I, Savareux L, et al. Multicenter randomized phase II study comparing docetaxel plus curcumin versus docetaxel plus placebo in first-line treatment of metastatic castration-resistant prostate cancer. Cancer Med (2021) 10(7):2332–40. doi: 10.1002/cam4.3806
68. Choi YH, Han DH, Kim SW, Kim MJ, Sung HH, Jeon HG, et al. A randomized, double-blind, placebo-controlled trial to evaluate the role of curcumin in prostate cancer patients with intermittent androgen deprivation. Prostate (2019) 79(6):614–21. doi: 10.1002/pros.23766
69. Greil R, Greil-Ressler S, Weiss L, Schönlieb C, Magnes T, Radl B, et al. A phase 1 dose-escalation study on the safety, tolerability and activity of liposomal curcumin (LipocurcTM) in patients with locally advanced or metastatic cancer. Cancer Chemother Pharmacol (2018) 82(4):695–706. doi: 10.1007/s00280-018-3654-0
70. Houh YK, Kim KE, Park S, Hur DY, Kim S, Kim D, et al. The effects of artemisinin on the cytolytic activity of natural killer (NK) cells. Int J Mol Sci (2017) 18(7):E1600. doi: 10.3390/ijms18071600
71. Lu Z, Bi J, Wan X. Artemisinin sensitizes tumor cells to NK cell-mediated cytolysis. Biochem Biophys Res Commun (2020) 524(2):418–23. doi: 10.1016/j.bbrc.2020.01.094
72. Mancuso RI, Olalla Saad ST, Azambuja JH. Artesunate switches monocytes to an inflammatory phenotype with the ability to kill leukemic cells. Int J Mol Sci (2021) 22(2):E608. doi: 10.3390/ijms22020608
73. Cao Y, Feng YH, Gao LW, Li XY, Jin QX, Wang YY, et al. Artemisinin enhances the anti-tumor immune response in 4T1 breast cancer cells in vitro and in vivo. Int Immunopharmacol (2019) 70:110–6. doi: 10.1016/j.intimp.2019.01.041
74. Zhou ZH, Chen FX, Xu WR, Qian H, Sun LQ, Lü XT, et al. Enhancement effect of dihydroartemisinin on human γδ T cell proliferation and killing pancreatic cancer cells. Int Immunopharmacol (2013) 17(3):850–7. doi: 10.1016/j.intimp.2013.09.015
75. Qian P, Zhang YW, Zhou ZH, Liu JQ, Yue SY, Guo XL, et al. Artesunate enhances γδ T-cell-mediated antitumor activity through augmenting γδ T-cell function and reversing immune escape of HepG2 cells. Immunopharmacol Immunotoxicol (2018) 40(2):107–16. doi: 10.1080/08923973.2017.1386212
76. Lee SH, Cho YC, Kim KH, Lee IS, Choi HJ, Kang BY. Artesunate inhibits proliferation of naïve CD4(+) T cells but enhances function of effector T cells. Arch Pharm Res (2015) 38(6):1195–203. doi: 10.1007/s12272-014-0491-5
77. Narsale A, Moya R, Davies JD. Human CD4+ CD25+ CD127hi cells and the Th1/Th2 phenotype. Clin Immunol (2018) 188:103–12. doi: 10.1016/j.clim.2018.01.003
78. Chen X, Zhang XL, Zhang GH, Gao YF. Artesunate promotes Th1 differentiation from CD4+ T cells to enhance cell apoptosis in ovarian cancer via miR-142. Braz J Med Biol Res (2019) 52(5):e7992. doi: 10.1590/1414-431x20197992
79. Yu R, Jin L, Li F, Fujimoto M, Wei Q, Lin Z, et al. Dihydroartemisinin inhibits melanoma by regulating CTL/Treg anti-tumor immunity and STAT3-mediated apoptosis via IL-10 dependent manner. J Dermatol Sci (2020) 99(3):193–202. doi: 10.1016/j.jdermsci.2020.08.001
80. Ooko E, Saeed MEM, Kadioglu O, Sarvi S, Colak M, Elmasaoudi K, et al. Artemisinin derivatives induce iron-dependent cell death (ferroptosis) in tumor cells. Phytomedicine (2015) 22(11):1045–54. doi: 10.1016/j.phymed.2015.08.002
81. Tan M, Rong Y, Su Q, Chen Y. Artesunate induces apoptosis via inhibition of STAT3 in THP-1 cells. Leuk Res (2017) 62:98–103. doi: 10.1016/j.leukres.2017.09.022
82. Chen Y, Wang F, Wu P, Gong S, Gao J, Tao H, et al. Artesunate induces apoptosis, autophagy and ferroptosis in diffuse large b cell lymphoma cells by impairing STAT3 signaling. Cell Signal (2021) 88:110167. doi: 10.1016/j.cellsig.2021.110167
83. Lang SJ, Schmiech M, Hafner S, Paetz C, Steinborn C, Huber R, et al. Antitumor activity of an artemisia annua herbal preparation and identification of active ingredients. Phytomedicine (2019) 62::152962. doi: 10.1016/j.phymed.2019.152962
84. Ilamathi M, Santhosh S, Sivaramakrishnan V. Artesunate as an anti-cancer agent targets stat-3 and favorably suppresses hepatocellular carcinoma. Curr Top Med Chem (2016) 16(22):2453–63. doi: 10.2174/1568026616666160212122820
85. Jia L, Song Q, Zhou C, Li X, Pi L, Ma X, et al. Dihydroartemisinin as a putative STAT3 inhibitor, suppresses the growth of head and neck squamous cell carcinoma by targeting Jak2/STAT3 signaling. PloS One (2016) 11(1):e0147157. doi: 10.1371/journal.pone.0147157
86. Berköz M, Özkan-Yılmaz F, Özlüer-Hunt A, Krośniak M, Türkmen Ö, Korkmaz D, et al. Artesunate inhibits melanoma progression in vitro via suppressing STAT3 signaling pathway. Pharmacol Rep (2021) 73(2):650–63. doi: 10.1007/s43440-021-00230-6
87. Gu F, Ma Y, Zhang Z, Zhao J, Kobayashi H, Zhang L, et al. Expression of Stat3 and Notch1 is associated with cisplatin resistance in head and neck squamous cell carcinoma. Oncol Rep (2010) 23(3):671–6. doi: 10.3892/or_00000683
88. Yan X, Li P, Zhan Y, Qi M, Liu J, An Z, et al. Dihydroartemisinin suppresses STAT3 signaling and mcl-1 and survivin expression to potentiate ABT-263-induced apoptosis in non-small cell lung cancer cells harboring EGFR or RAS mutation. Biochem Pharmacol (2018) 150:72–85. doi: 10.1016/j.bcp.2018.01.031
89. Jin H, Jiang AY, Wang H, Cao Y, Wu Y, Jiang XF. Dihydroartemisinin and gefitinib synergistically inhibit NSCLC cell growth and promote apoptosis via the Akt/mTOR/STAT3 pathway. Mol Med Rep (2017) 16(3):3475–81. doi: 10.3892/mmr.2017.6989
90. Sun Y, Lu X, Li H, Li X. Dihydroartemisinin inhibits IL-6-induced epithelial–mesenchymal transition in laryngeal squamous cell carcinoma via the miR-130b-3p/STAT3/β-catenin signaling pathway. J Int Med Res (2021) 49(11):03000605211009494. doi: 10.1177/03000605211009494
91. Efferth T, Rücker G, Falkenberg M, Manns D, Olbrich A, Fabry U, et al. Detection of apoptosis in KG-1a leukemic cells treated with investigational drugs. Arzneimittelforschung (1996) 46(2):196–200.
92. Cabello CM, Lamore SD, Bair WB, Qiao S, Azimian S, Lesson JL, et al. The redox antimalarial dihydroartemisinin targets human metastatic melanoma cells but not primary melanocytes with induction of NOXA-dependent apoptosis. Invest New Drugs (2012) 30(4):1289–301. doi: 10.1007/s10637-011-9676-7
93. Verma R, Foster RE, Horgan K, Mounsey K, Nixon H, Smalle N, et al. Lymphocyte depletion and repopulation after chemotherapy for primary breast cancer. Breast Cancer Res (2016) 18:10. doi: 10.1186/s13058-015-0669-x
94. Ménétrier-Caux C, Ray-Coquard I, Blay JY, Caux C. Lymphopenia in cancer patients and its effects on response to immunotherapy: An opportunity for combination with cytokines? J ImmunoTher Cancer (2019) 7(1):85. doi: 10.1186/s40425-019-0549-5
95. Dixon SJ, Lemberg KM, Lamprecht MR, Skouta R, Zaitsev EM, Gleason CE, et al. Ferroptosis: An iron-dependent form of nonapoptotic cell death. Cell (2012) 149(5):1060–72. doi: 10.1016/j.cell.2012.03.042
96. Wei J, Nai GY, Dai Y, Huang XJ, Xiong MY, Yao XY, et al. Dipetidyl peptidase-4 and transferrin receptor serve as prognostic biomarkers for acute myeloid leukemia. Ann Trans Med (2021) 9(17):1381–1. doi: 10.21037/atm-21-3368
97. Yi R, Wang H, Deng C, Wang X, Yao L, Niu W, et al. Dihydroartemisinin initiates ferroptosis in glioblastoma through GPX4 inhibition. Biosci Rep (2020) 40(6):BSR20193314. doi: 10.1042/BSR20193314
98. Roh JL, Kim EH, Jang H, Shin D. Nrf2 inhibition reverses the resistance of cisplatin-resistant head and neck cancer cells to artesunate-induced ferroptosis. Redox Biol (2017) 11:254–62. doi: 10.1016/j.redox.2016.12.010
99. Yun CW, Lee SH. The roles of autophagy in cancer. Int J Mol Sci (2018) 19(11):3466. doi: 10.3390/ijms19113466
100. Shi X, Wang L, Ren L, Li J, Li S, Cui Q, et al. Dihydroartemisinin, an antimalarial drug, induces absent in melanoma 2 inflammasome activation and autophagy in human hepatocellular carcinoma HepG2215 cells. Phytother Res (2019) 33(5):1413–25. doi: 10.1002/ptr.6332
101. Fan M, Li Y, Yao C, Liu X, Liu J, Yu B. DC32, a dihydroartemisinin derivative, ameliorates collagen-induced arthritis through an Nrf2-p62-Keap1 feedback loop. Front Immunol (2018) 9:2762. doi: 10.3389/fimmu.2018.02762
102. Zhang C, Fortin PY, Barnoin G, Qin X, Wang X, Fernandez Alvarez A, et al. An Artemisinin-Derivative–(NHC)Gold(I) hybrid with enhanced cytotoxicity through inhibition of NRF2 transcriptional activity. Angewandte Chemie Int Edition (2020) 59(29):12062–8. doi: 10.1002/anie.202002992
103. Zhou X, Chen Y, Wang F, Wu H, Zhang Y, Liu J, et al. Artesunate induces autophagy dependent apoptosis through upregulating ROS and activating AMPK-mTOR-ULK1 axis in human bladder cancer cells. Chem Biol Interact (2020) 331:109273. doi: 10.1016/j.cbi.2020.109273
104. Zou J, Ma Q, Sun R, Cai J, Liao H, Xu L, et al. Dihydroartemisinin inhibits HepG2.2.15 proliferation by inducing cellular senescence and autophagy. BMB Rep (2019) 52(8):520–5. doi: 10.5483/BMBRep.2019.52.8.058
105. Wang T, Wang J, Ren W, Liu ZL, Cheng YF, Zhang XM. Combination treatment with artemisinin and oxaliplatin inhibits tumorigenesis in esophageal cancer EC109 cell through wnt/β-catenin signaling pathway. Thorac Cancer (2020) 11(8):2316–24. doi: 10.1111/1759-7714.13570
106. Guan X, Guan Y. Artemisinin induces selective and potent anticancer effects in drug resistant breast cancer cells by inducing cellular apoptosis and autophagy and G2/M cell cycle arrest. J BUON (2020) 25(3):1330–6.
107. Fröhlich T, Mai C, Bogautdinov RP, Morozkina SN, Shavva AG, Friedrich O, et al. Synthesis of tamoxifen-artemisinin and estrogen-artemisinin hybrids highly potent against breast and prostate cancer. Chem Med Chem (2020) 15(15):1473–9. doi: 10.1002/cmdc.202000174
108. Neag MA, Mocan A, Echeverría J, Pop RM, Bocsan CI, Crişan G, et al. Berberine: Botanical occurrence, traditional uses, extraction methods, and relevance in cardiovascular, metabolic, hepatic, and renal disorders. Front Pharmacol (2018) 9:557. doi: 10.3389/fphar.2018.00557
109. Rauf A, Abu-Izneid T, Khalil AA, Imran M, Shah ZA, Emran TB, et al. Berberine as a potential anticancer agent: A comprehensive review. Molecules (2021) 26(23):7368. doi: 10.3390/molecules26237368
110. Zuo F, Nakamura N, Akao T, Hattori M. Pharmacokinetics of berberine and its main metabolites in conventional and pseudo germ-free rats determined by liquid chromatography/ion trap mass spectrometry. Drug Metab Dispos (2006) 34(12):2064–72. doi: 10.1124/dmd.106.011361
111. Cao S, Zhou Y, Xu P, Wang Y, Yan J, Bin W, et al. Berberine metabolites exhibit triglyceride-lowering effects via activation of AMP-activated protein kinase in hep G2 cells. J Ethnopharmacol (2013) 149(2):576–82. doi: 10.1016/j.jep.2013.07.025
112. Li Gh, Wang Dl, Hu Y, Pu P, Li Dz, Wang Wd, et al. Berberine inhibits acute radiation intestinal syndrome in human with abdomen radiotherapy. Med Oncol (2010) 27(3):919–25. doi: 10.1007/s12032-009-9307-8
113. Liu Y, Yu H, Zhang C, Cheng Y, Hu L, Meng X, et al. Protective effects of berberine on radiation-induced lung injury via intercellular adhesion molecular-1 and transforming growth factor-beta-1 in patients with lung cancer. Eur J Cancer (2008) 44(16):2425–32. doi: 10.1016/j.ejca.2008.07.040
114. Zhao H, Jia L, Chen G, Li X, Meng X, Zhao X, et al. A prospective, three-arm, randomized trial of EGCG for preventing radiation-induced esophagitis in lung cancer patients receiving radiotherapy. Radiother Oncol (2019) 137:186–91. doi: 10.1016/j.radonc.2019.02.022
115. Li X, Xing L, Zhang Y, Xie P, Zhu W, Meng X, et al. Phase II trial of epigallocatechin-3-Gallate in acute radiation-induced esophagitis for esophagus cancer. J Med Food (2020) 23(1):43–9. doi: 10.1089/jmf.2019.4445
116. Zhao H, Zhu W, Zhao X, Li X, Zhou Z, Zheng M, et al. Efficacy of epigallocatechin-3-Gallate in preventing dermatitis in patients with breast cancer receiving postoperative radiotherapy: A double-blind, placebo-controlled, phase 2 randomized clinical trial. JAMA Dermatol (2022) 158(7):779–86. doi: 10.1001/jamadermatol.2022.1736
117. Zhu W, Mei H, Jia L, Zhao H, Li X, Meng X, et al. Epigallocatechin-3-gallate mouthwash protects mucosa from radiation-induced mucositis in head and neck cancer patients: A prospective, non-randomised, phase 1 trial. Invest New Drugs (2020) 38(4):1129–36. doi: 10.1007/s10637-019-00871-8
118. Ryan Wolf J, Gewandter JS, Bautista J, Heckler CE, Strasser J, Dyk P, et al. Utility of topical agents for radiation dermatitis and pain: a randomized clinical trial. Support Care Cancer (2020) 28(7):3303–11. doi: 10.1007/s00520-019-05166-5
119. Ryan Wolf J, Heckler CE, Guido JJ, Peoples AR, Gewandter JS, Ling M, et al. Oral curcumin for radiation dermatitis: A URCC NCORP study of 686 breast cancer patients. Support Care Cancer (2018) 26(5):1543–52. doi: 10.1007/s00520-017-3957-4
120. Delavarian Z, Pakfetrat A, Ghazi A, Jaafari MR, Homaei Shandiz F, Dalirsani Z, et al. Oral administration of nanomicelle curcumin in the prevention of radiotherapy-induced mucositis in head and neck cancers. Spec Care Dentist (2019) 39(2):166–72. doi: 10.1111/scd.12358
121. Shah S, Rath H, Sharma G, Senapati SN, Mishra E. Effectiveness of curcumin mouthwash on radiation-induced oral mucositis among head and neck cancer patients: A triple-blind, pilot randomised controlled trial. Indian J Dent Res (2020) 31(5):718–27. doi: 10.4103/ijdr.IJDR_822_18
122. Wei J, Wang H, Wang H, Wang B, Meng L, Xin Y, et al. The role of NLRP3 inflammasome activation in radiation damage. BioMed Pharmacother (2019) 118:109217. doi: 10.1016/j.biopha.2019.109217
123. Mai W, Xu Y, Xu J, Zhao D, Ye L, Yu G, et al. Berberine inhibits nod-like receptor family pyrin domain containing 3 inflammasome activation and pyroptosis in nonalcoholic steatohepatitis via the ROS/TXNIP axis. Front Pharmacol (2020) 11:185. doi: 10.3389/fphar.2020.00185
124. Liu H, You L, Wu J, Zhao M, Guo R, Zhang H, et al. Berberine suppresses influenza virus-triggered NLRP3 inflammasome activation in macrophages by inducing mitophagy and decreasing mitochondrial ROS. J Leukoc Biol (2020) 108(1):253–66. doi: 10.1002/JLB.3MA0320-358RR
125. Zhou H, Feng L, Xu F, Sun Y, Ma Y, Zhang X, et al. Berberine inhibits palmitate-induced NLRP3 inflammasome activation by triggering autophagy in macrophages: A new mechanism linking berberine to insulin resistance improvement. BioMed Pharmacother (2017) 89:864–74. doi: 10.1016/j.biopha.2017.03.003
126. Sarbadhikary P, George BP, Abrahamse H. Inhibitory role of berberine, an isoquinoline alkaloid, on NLRP3 inflammasome activation for the treatment of inflammatory diseases. Molecules (2021) 26(20):6238. doi: 10.3390/molecules26206238
127. Yao M, Fan X, Yuan B, Takagi N, Liu S, Han X, et al. Berberine inhibits NLRP3 inflammasome pathway in human triple-negative breast cancer MDA-MB-231 cell. BMC Complement Altern Med (2019) 19(1):216. doi: 10.1186/s12906-019-2615-4
128. Shah D, Challagundla N, Dave V, Patidar A, Saha B, Nivsarkar M, et al. Berberine mediates tumor cell death by skewing tumor-associated immunosuppressive macrophages to inflammatory macrophages. Phytomedicine (2022) 99:153904. doi: 10.1016/j.phymed.2021.153904
129. Gu W, Zhang M, Gao F, Niu Y, Sun L, Xia H, et al. Berberine regulates PADI4-related macrophage function to prevent lung cancer. Int Immunopharmacol (2022), 110:108965. doi: 10.1016/j.intimp.2022.108965
130. Ashrafizadeh M, Najafi M, Mohammadinejad R, Farkhondeh T, Samarghandian S. Berberine administration in treatment of colitis: A review. Curr Drug Targets (2020) 21(13):1385–93. doi: 10.2174/1389450121666200621193758
131. Yan S, Chang J, Hao X, Liu J, Tan X, Geng Z, et al. Berberine regulates short-chain fatty acid metabolism and alleviates the colitis-associated colorectal tumorigenesis through remodeling intestinal flora. Phytomedicine (2022) 102:154217. doi: 10.1016/j.phymed.2022.154217
132. Deng J, Zhao L, Yuan X, Li Y, Shi J, Zhang H, et al. Pre-administration of berberine exerts chemopreventive effects in AOM/DSS-induced colitis-associated carcinogenesis mice via modulating inflammation and intestinal microbiota. Nutrients (2022) 14(4):726. doi: 10.3390/nu14040726
133. Liu Y, Liu X, Zhang N, Yin M, Dong J, Zeng Q, et al. Berberine diminishes cancer cell PD-L1 expression and facilitates antitumor immunity via inhibiting the deubiquitination activity of CSN5. Acta Pharm Sin B (2020) 10(12):2299–312. doi: 10.1016/j.apsb.2020.06.014
134. Liu J, Liu P, Xu T, Chen Z, Kong H, Chu W, et al. Berberine induces autophagic cell death in acute lymphoblastic leukemia by inactivating AKT/mTORC1 signaling. Drug Des Devel Ther (2020) 14:1813–23. doi: 10.2147/DDDT.S239247
135. Milata V, Svedova A, Barbierikova Z, Holubkova E, Cipakova I, Cholujova D, et al. Synthesis and anticancer activity of novel 9-O-Substituted berberine derivatives. Int J Mol Sci (2019) 20(9):2169. doi: 10.3390/ijms20092169
136. Luo X, Gu J, Zhu R, Feng M, Zhu X, Li Y, et al. Integrative analysis of differential miRNA and functional study of miR-21 by seed-targeting inhibition in multiple myeloma cells in response to berberine. BMC Syst Biol (2014) 8:82. doi: 10.1186/1752-0509-8-82
137. Yan SH, Hu LM, Hao XH, Liu J, Tan XY, Geng ZR, et al. Chemoproteomics reveals berberine directly binds to PKM2 to inhibit the progression of colorectal cancer. iScience (2022) 25(8):104773. doi: 10.1016/j.isci.2022.104773
138. Liu X, Ji Q, Ye N, Sui H, Zhou L, Zhu H, et al. Berberine inhibits invasion and metastasis of colorectal cancer cells via COX-2/PGE2 mediated JAK2/STAT3 signaling pathway. PloS One (2015) 10(5):e0123478. doi: 10.1371/journal.pone.0123478
139. Xu M, Ren L, Fan J, Huang L, Zhou L, Li X, et al. Berberine inhibits gastric cancer development and progression by regulating the JAK2/STAT3 pathway and downregulating IL-6. Life Sci (2022) 290:120266. doi: 10.1016/j.lfs.2021.120266
140. Puthdee N, Seubwai W, Vaeteewoottacharn K, Boonmars T, Cha’on U, Phoomak C, et al. Berberine induces cell cycle arrest in cholangiocarcinoma cell lines via inhibition of NF-κB and STAT3 pathways. Biol Pharm Bull (2017) 40(6):751–7. doi: 10.1248/bpb.b16-00428
141. Obchoei S, Detarya M, Boonnate P, Saranaruk P, Vaeteewoottacharn K, Mahalapbutr P, et al. Low dose berberine suppresses cholangiocarcinoma cell progression as a multi-kinase inhibitor. Asian Pac J Cancer Prev (2022) 23(10):3379–86. doi: 10.31557/APJCP.2022.23.10.3379
142. Bobbili MR, Mader RM, Grillari J, Dellago H. OncomiR-17-5p: alarm signal in cancer? Oncotarget (2017) 8(41):71206–22. doi: 10.18632/oncotarget.19331
143. Xia Y, Chen S, Cui J, Wang Y, Liu X, Shen Y, et al. Berberine suppresses bladder cancer cell proliferation by inhibiting JAK1-STAT3 signaling via upregulation of miR-17-5p. Biochem Pharmacol (2021) 188:114575. doi: 10.1016/j.bcp.2021.114575
144. Hassanein EHM, Kamel EO, Ali FEM, Ahmed MAR. Berberine and/or zinc protect against methotrexate-induced intestinal damage: Role of GSK-3β/NRF2 and JAK1/STAT-3 signaling pathways. Life Sci (2021) 281:119754. doi: 10.1016/j.lfs.2021.119754
145. Zheng X, Zhao Y, Jia Y, Shao D, Zhang F, Sun M, et al. Biomimetic co-assembled nanodrug of doxorubicin and berberine suppresses chemotherapy-exacerbated breast cancer metastasis. Biomaterials (2021) 271:120716. doi: 10.1016/j.biomaterials.2021.120716
146. Pandey A, Vishnoi K, Mahata S, Tripathi SC, Misra SP, Misra V, et al. Berberine and curcumin target survivin and STAT3 in gastric cancer cells and synergize actions of standard chemotherapeutic 5-fluorouracil. Nutr Cancer (2015) 67(8):1293–304. doi: 10.1080/01635581.2015.1085581
147. Chen P, Dai CH, Shi ZH, Wang Y, Wu JN, Chen K, et al. Synergistic inhibitory effect of berberine and icotinib on non-small cell lung cancer cells via inducing autophagic cell death and apoptosis. Apoptosis (2021) 26(11–12):639–56. doi: 10.1007/s10495-021-01694-w
148. Wang J, Yang S, Cai X, Dong J, Chen Z, Wang R, et al. Berberine inhibits EGFR signaling and enhances the antitumor effects of EGFR inhibitors in gastric cancer. Oncotarget (2016) 7(46):76076–86. doi: 10.18632/oncotarget.12589
149. Kou Y, Tong B, Wu W, Liao X, Zhao M. Berberine improves chemo-sensitivity to cisplatin by enhancing cell apoptosis and repressing PI3K/AKT/mTOR signaling pathway in gastric cancer. Front Pharmacol (2020) 11:616251. doi: 10.3389/fphar.2020.616251
150. Zhu T, Li LL, Xiao GF, Luo QZ, Liu QZ, Yao KT, et al. Berberine increases doxorubicin sensitivity by suppressing STAT3 in lung cancer. Am J Chin Med (2015) 43(07):1487–502. doi: 10.1142/S0192415X15500846
151. Qian K, Tang CY, Chen LY, Zheng S, Zhao Y, Ma LS, et al. Berberine reverses breast cancer multidrug resistance based on fluorescence pharmacokinetics in vitro and In vivo. ACS Omega (2021) 6(16):10645–54. doi: 10.1021/acsomega.0c06288
152. Pan Y, Zhang F, Zhao Y, Shao D, Zheng X, Chen Y, et al. Berberine enhances chemosensitivity and induces apoptosis through dose-orchestrated AMPK signaling in breast cancer. J Cancer (2017) 8(9):1679–89. doi: 10.7150/jca.19106
153. Qu H, Song X, Song Z, Jiang X, Gao X, Bai L, et al. Berberine reduces temozolomide resistance by inducing autophagy via the ERK1/2 signaling pathway in glioblastoma. Cancer Cell Int (2020) 20(1):592. doi: 10.1186/s12935-020-01693-y
154. Lin HJ, Ho JH, Tsai LC, Yang FY, Yang LL, Kuo CD, et al. Synthesis and In vitro photocytotoxicity of 9-/13-Lipophilic substituted berberine derivatives as potential anticancer agents. Molecules (2020) 25(3):677. doi: 10.3390/molecules25030677
155. Zhang Y, Gu Y, Ren H, Wang S, Zhong H, Zhao X, et al. Gut microbiome-related effects of berberine and probiotics on type 2 diabetes (the PREMOTE study). Nat Commun (2020) 11(1):5015. doi: 10.1038/s41467-020-18414-8
156. Hou W, Liu B, Xu H. Triptolide: Medicinal chemistry, chemical biology and clinical progress. Eur J Med Chem (2019) 176:378–92. doi: 10.1016/j.ejmech.2019.05.032
157. Khanipour Roshan S, Spano AD, McKinney AM, Nascene DR. Potentially reversible acute cerebellar toxicity associated with minnelide. Neuroradiology (2017) 59(4):419–21. doi: 10.1007/s00234-017-1809-z
158. Zhang L, Yu JS. Triptolide reverses helper T cell inhibition and down-regulates IFN-γ induced PD-L1 expression in glioma cell lines. J Neurooncol (2019) 143(3):429–36. doi: 10.1007/s11060-019-03193-0
159. Liang M, Fu J. Triptolide inhibits interferon-gamma-induced programmed death-1-ligand 1 surface expression in breast cancer cells. Cancer Lett (2008) 270(2):337–41. doi: 10.1016/j.canlet.2008.05.025
160. Kuo CS, Yang CY, Lin CK, Lin GJ, Sytwu HK, Chen YW. Triptolide suppresses oral cancer cell PD-L1 expression in the interferon-γ-modulated microenvironment in vitro, in vivo, and in clinical patients. Biomed Pharmacother (2021) 133:111057. doi: 10.1016/j.biopha.2020.111057
161. Liu B, Zhang H, Li J, Lu C, Chen G, Zhang G, et al. Triptolide downregulates treg cells and the level of IL-10, TGF-β, and VEGF in melanoma-bearing mice. Planta Med (2013) 79(15):1401–7. doi: 10.1055/s-0033-1350708
162. Chan SF, Chen YY, Lin JJ, Liao CL, Ko YC, Tang NY, et al. Triptolide induced cell death through apoptosis and autophagy in murine leukemia WEHI-3 cells in vitro and promoting immune responses in WEHI-3 generated leukemia mice in vivo. Environ Toxicol (2017) 32(2):550–68. doi: 10.1002/tox.22259
163. Jiang X, Cao G, Gao G, Wang W, Zhao J, Gao C. Triptolide decreases tumor-associated macrophages infiltration and M2 polarization to remodel colon cancer immune microenvironment via inhibiting tumor-derived CXCL12. J Cell Physiol (2021) 236(1):193–204. doi: 10.1002/jcp.29833
164. Yuan K, Li X, Lu Q, Zhu Q, Jiang H, Wang T, et al. Application and mechanisms of triptolide in the treatment of inflammatory diseases–a review. Front Pharmacol (2019) 10:1469. doi: 10.3389/fphar.2019.01469
165. Long C, Guo W, Zhou H, Wang J, Wang H, Sun X. Triptolide decreases expression of latency-associated nuclear antigen 1 and reduces viral titers in kaposi’s sarcoma-associated and herpesvirus-related primary effusion lymphoma cells. Int J Oncol (2016) 48(4):1519–30. doi: 10.3892/ijo.2016.3353
166. Jin Y, Chen Q, Lu Z, Chen B, Pan J. Triptolide abrogates oncogene FIP1L1-PDGFRalpha addiction and induces apoptosis in hypereosinophilic syndrome. Cancer Sci (2009) 100(11):2210–7. doi: 10.1111/j.1349-7006.2009.01283.x
167. Lu X, Zhuang H, Yu Q, Zhang X, Wu Z, Zhang L, et al. Identification of the UBA2-WTIP fusion gene in acute myeloid leukemia. Exp Cell Res (2018) 371(2):409–16. doi: 10.1016/j.yexcr.2018.08.035
168. Guo K, Chen J, Chen Z, Luo G, Yang S, Zhang M, et al. Triptolide alleviates radiation-induced pulmonary fibrosis via inhibiting IKKβ stimulated LOX production. Biochem Biophys Res Commun (2020) 527(1):283–8. doi: 10.1016/j.bbrc.2020.04.023
169. Yang S, Zhang M, Chen C, Cao Y, Tian Y, Guo Y, et al. Triptolide mitigates radiation-induced pulmonary fibrosis. Radiat Res (2015) 184(5):509–17. doi: 10.1667/RR13831.1
170. Chen C, Yang S, Zhang M, Zhang Z, Zhang SB, Wu B, et al. Triptolide mitigates radiation-induced pneumonitis via inhibition of alveolar macrophages and related inflammatory molecules. Oncotarget (2017) 8(28):45133–42. doi: 10.18632/oncotarget.16456
171. Liu Y, Chen F, Wang S, Guo X, Shi P, Wang W, et al. Low-dose triptolide in combination with idarubicin induces apoptosis in AML leukemic stem-like KG1a cell line by modulation of the intrinsic and extrinsic factors. Cell Death Dis (2013) 4(12):e948. doi: 10.1038/cddis.2013.467
172. Shi P, Zha J, Feng J, Jiang Z, Zhao H, Deng M, et al. Low-dose triptolide enhanced activity of idarubicin against acute myeloid leukemia stem-like cells via inhibiting DNA damage repair response. Stem Cell Rev Rep (2021) 17(2):616–27. doi: 10.1007/s12015-020-10054-1
173. Cai J, Yi M, Tan Y, Li X, Li G, Zeng Z, et al. Natural product triptolide induces GSDME-mediated pyroptosis in head and neck cancer through suppressing mitochondrial hexokinase-II. J Exp Clin Cancer Res (2021) 40(1):190. doi: 10.1186/s13046-021-02100-8
174. Dai H, Jiang Y, Luo Y, Bie P, Chen Z. Triptolide enhances TRAIL sensitivity of pancreatic cancer cells by activating autophagy via downregulation of PUM1. Phytomedicine (2019), 62:152953. doi: 10.1016/j.phymed.2019.152953
175. Zhong Y, Le F, Cheng J, Luo C, Zhang X, Wu X, et al. Triptolide inhibits JAK2/STAT3 signaling and induces lethal autophagy through ROS generation in cisplatin−resistant SKOV3/DDP ovarian cancer cells. Oncol Rep (2021) 45(5):69. doi: 10.3892/or.2021.8020
176. Yu D, Liu Y, Zhou Y, Ruiz-Rodado V, Larion M, Xu G, et al. Triptolide suppresses IDH1-mutated malignancy via Nrf2-driven glutathione metabolism. Proc Natl Acad Sci U S A (2020) 117(18):9964–72. doi: 10.1073/pnas.1913633117
177. Noel P, Hussein S, Ng S, Antal CE, Lin W, Rodela E, et al. Triptolide targets super-enhancer networks in pancreatic cancer cells and cancer-associated fibroblasts. Oncogenesis (2020) 9(11):1–12. doi: 10.1038/s41389-020-00285-9
178. Lovén J, Hoke HA, Lin CY, Lau A, Orlando DA, Vakoc CR, et al. Selective inhibition of tumor oncogenes by disruption of super-enhancers. Cell (2013) 153(2):320–34. doi: 10.1016/j.cell.2013.03.036
179. Wang W, Lin W, Wang Q, Zhuang X, Luo J. The enhanced antitumor effect of combined triptolide and paclitaxel on pancreatic cancer cell lines. JCO (2014) 32(3_suppl):335–5. doi: 10.1200/jco.2014.32.3_suppl.335
180. Modi S, Giri B, Gupta VK, Lavania S, Sethi V, Sharma NS, et al. Minnelide synergizes with conventional chemotherapy by targeting both cancer and associated stroma components in pancreatic cancer. Cancer Lett (2022) 537:215591. doi: 10.1016/j.canlet.2022.215591
181. Ma JX, Sun YL, Yu Y, Zhang J, Wu HY, Yu XF. Triptolide enhances the sensitivity of pancreatic cancer PANC-1 cells to gemcitabine by inhibiting TLR4/NF-κB signaling. Am J Transl Res (2019) 11(6):3750–60.
182. Li CJ, Chu CY, Huang LH, Wang MH, Sheu LF, Yeh JI, et al. Synergistic anticancer activity of triptolide combined with cisplatin enhances apoptosis in gastric cancer in vitro and in vivo. Cancer Lett (2012) 319(2):203–13. doi: 10.1016/j.canlet.2012.01.006
183. Tang X, Zhu Y, Tao W, Wei B, Lin X. Synergistic effect of triptolide combined with 5-fluorouracil on colon carcinoma. Postgrad Med J (2007) 83(979):338–43. doi: 10.1136/pgmj.2006.055426
184. Liu Y, Xiao E, Yuan L, Li G. Triptolide synergistically enhances antitumor activity of oxaliplatin in colon carcinoma in vitro and in vivo. DNA Cell Biol (2014) 33(7):418–25. doi: 10.1089/dna.2014.2356
185. Liu J, Cheng H, Han L, Qiang Z, Zhang X, Gao W, et al. Synergistic combination therapy of lung cancer using paclitaxel- and triptolide-coloaded lipid–polymer hybrid nanoparticles. DDDT (2018), 12:3199–209. doi: 10.2147/DDDT.S172199
186. Yang Y, Zhang LJ, Bai XG, Xu HJ, Jin ZL, Ding M. Synergistic antitumour effects of triptolide plus gemcitabine in bladder cancer. BioMed Pharmacother (2018) 106:1307–16. doi: 10.1016/j.biopha.2018.07.083
187. Modi S, Kir D, Giri B, Majumder K, Arora N, Dudeja V, et al. Minnelide overcomes oxaliplatin resistance by downregulating DNA repair pathway in pancreatic cancer. J Gastrointest Surg (2016) 20(1):13–24. doi: 10.1007/s11605-015-3000-3
188. Zhang Z, Sun C, Zhang L, Chi X, Ji J, Gao X, et al. Triptolide interferes with XRCC1/PARP1-mediated DNA repair and confers sensitization of triple-negative breast cancer cells to cisplatin. BioMed Pharmacother (2019) 109:1541–6. doi: 10.1016/j.biopha.2018.11.008
189. Jiang N, Dong XP, Zhang SL, You QY, Jiang XT, Zhao XG. Triptolide reverses the taxol resistance of lung adenocarcinoma by inhibiting the NF-κB signaling pathway and the expression of NF-κB-regulated drug-resistant genes. Mol Med Rep (2016) 13(1):153–9. doi: 10.3892/mmr.2015.4493
190. Deng Y, Li F, He P, Yang Y, Yang J, Zhang Y, et al. Triptolide sensitizes breast cancer cells to doxorubicin through the DNA damage response inhibition. Mol Carcinog (2018) 57(6):807–14. doi: 10.1002/mc.22795
191. Huang G, Hu H, Zhang Y, Zhu Y, Liu J, Tan B, et al. Triptolide sensitizes cisplatin-resistant human epithelial ovarian cancer by inhibiting the phosphorylation of AKT. J Cancer (2019) 10(13):3012–20. doi: 10.7150/jca.30669
192. Ho JN, Byun SS, Lee S, Oh JJ, Hong SK, Lee SE, et al. Synergistic antitumor effect of triptolide and cisplatin in cisplatin resistant human bladder cancer cells. J Urol (2015) 193(3):1016–22. doi: 10.1016/j.juro.2014.09.007
193. Li Y, Hu S. Triptolide sensitizes liver cancer cell lines to chemotherapy in vitro and in vivo. Panminerva Med (2014) 56(3):211–20.
194. Kong C, Li Y, Liu Z, Ye J, Wang Z, Zhang L, et al. Targeting the oncogene KRAS mutant pancreatic cancer by synergistic blocking of lysosomal acidification and rapid drug release. ACS Nano (2019) 13(4):4049–63. doi: 10.1021/acsnano.8b08246
195. Liaw K, Sharma R, Sharma A, Salazar S, Appiani La Rosa S, Kannan RM. Systemic dendrimer delivery of triptolide to tumor-associated macrophages improves anti-tumor efficacy and reduces systemic toxicity in glioblastoma. J Control Release (2021) 329:434–44. doi: 10.1016/j.jconrel.2020.12.003
196. Caminade AM. Dendrimers, an emerging opportunity in personalized medicine? J Pers Med (2022) 12(8):1334. doi: 10.3390/jpm12081334
197. Zheng S, Wang J, Ding N, Chen W, Chen H, Xue M, et al. Prodrug polymeric micelles integrating cancer-associated fibroblasts deactivation and synergistic chemotherapy for gastric cancer. J Nanobiotechnol (2021) 19(1):381. doi: 10.1186/s12951-021-01127-5
198. Wang S, Jiang H, Wang J, Wu H, Wu T, Ni M, et al. Superior in vitro anticancer effect of biomimetic paclitaxel and triptolide co-delivery system in gastric cancer. J BioMed Res (2021) 35(4):327–38. doi: 10.7555/JBR.35.20210102
199. Li Z, Yang G, Han L, Wang R, Gong C, Yuan Y. Sorafenib and triptolide loaded cancer cell-platelet hybrid membrane-camouflaged liquid crystalline lipid nanoparticles for the treatment of hepatocellular carcinoma. J Nanobiotechnol (2021) 19(1):360. doi: 10.1186/s12951-021-01095-w
200. Ilango S, Sahoo DK, Paital B, Kathirvel K, Gabriel JI, Subramaniam K, et al. A review on annona muricata and its anticancer activity. Cancers (2022) 14(18):4539. doi: 10.3390/cancers14184539
201. Hansra DM, Silva O, Mehta A, Ahn E. Patient with metastatic breast cancer achieves stable disease for 5 years on graviola and xeloda after progressing on multiple lines of therapy. Adv Breast Cancer Res (2014) 3(3):84–7. doi: 10.4236/abcr.2014.33012
202. Morosetti G, Criscuolo AA, Santi F, Perno CF, Piccione E, Ciotti M. Ellagic acid and annona muricata in the chemoprevention of HPV-related pre-neoplastic lesions of the cervix. Oncol Lett (2017) 13(3):1880–4. doi: 10.3892/ol.2017.5634
203. Kim GT, Tran NKS, Choi EH, Song YJ, Song JH, Shim SM, et al. Immunomodulatory efficacy of standardized annona muricata (Graviola) leaf extract via activation of mitogen-activated protein kinase pathways in RAW 264.7 macrophages. Evidence-Based Complement Altern Med (2016) 2016:e2905127. doi: 10.1155/2016/2905127
204. Syed Najmuddin SUF, Romli MF, Hamid M, Alitheen NB, Nik Abd Rahman NMA. Anti-cancer effect of annona muricata Linn leaves crude extract (AMCE) on breast cancer cell line. BMC Complement Altern Med (2016) 16(1):311. doi: 10.1186/s12906-016-1290-y
205. Hadisaputri YE, Habibah U, Abdullah FF, Halimah E, Mutakin M, Megantara S, et al. Antiproliferation activity and apoptotic mechanism of soursop (Annona muricata l.) leaves extract and fractions on MCF7 breast cancer cells. Breast Cancer (Dove Med Press) (2021) 13:447–57. doi: 10.2147/BCTT.S317682
206. Daddiouaissa D, Amid A, Kabbashi NA, Fuad FAA, Elnour AM, Epandy MAKMS. Antiproliferative activity of ionic liquid-graviola fruit extract against human breast cancer (MCF-7) cell lines using flow cytometry techniques. J Ethnopharmacol (2019) 236:466–73. doi: 10.1016/j.jep.2019.03.003
207. Naik AV, Sellappan K. In vitro evaluation of annona muricata l. (Soursop) leaf methanol extracts on inhibition of tumorigenicity and metastasis of breast cancer cells. Biomarkers (2020) 25(8):701–10. doi: 10.1080/1354750X.2020.1836025
208. Zeweil MM, Sadek KM, Taha NM, El-Sayed Y, Menshawy S. Graviola attenuates DMBA-induced breast cancer possibly through augmenting apoptosis and antioxidant pathway and downregulating estrogen receptors. Environ Sci pollut Res Int (2019) 26(15):15209–17. doi: 10.1007/s11356-019-04920-w
209. Salsabila IA, Nugraheni N, Ahlina FN, Haryanti S, Meiyanto E. Synergistic cotreatment potential of soursop (Annona muricata l.) leaves extract with doxorubicin on 4T1 cells with antisenescence and anti-reactive-oxygen-species properties. Iran J Pharm Res (2021) 20(2):57–67. doi: 10.22037/ijpr.2020.112485.13788
210. Alshaeri HK, Alasmari MM, Natto ZS, Pino-Figueroa A. Effects of annona muricata extract on triple-negative breast cancer cells mediated through EGFR signaling. Cancer Manag Res (2020) 12:12519–26. doi: 10.2147/CMAR.S278647
211. Deep G, Kumar R, Jain AK, Dhar D, Panigrahi GK, Hussain A, et al. Graviola inhibits hypoxia-induced NADPH oxidase activity in prostate cancer cells reducing their proliferation and clonogenicity. Sci Rep (2016) 6:23135. doi: 10.1038/srep23135
212. Asare GA, Afriyie D, Ngala RA, Abutiate H, Doku D, Mahmood SA, et al. Antiproliferative activity of aqueous leaf extract of annona muricata l. on the prostate, BPH-1 cells, and some target genes. Integr Cancer Ther (2015) 14(1):65–74. doi: 10.1177/1534735414550198
213. Foster K, Oyenihi O, Rademan S, Erhabor J, Matsabisa M, Barker J, et al. Selective cytotoxic and anti-metastatic activity in DU-145 prostate cancer cells induced by annona muricata l. bark extract and phytochemical, annonacin. BMC Complement Med Ther (2020) 20(1):375. doi: 10.1186/s12906-020-03130-z
214. El Tawiil GA, Noaman EA, Askar MA, El Fatih NM, Mohamed HE. Anticancer and apoptogenic effect of graviola and low-dose radiation in tumor xenograft in mice. Integr Cancer Ther (2020) 19:1534735419900930. doi: 10.1177/1534735419900930
215. Torres MP, Rachagani S, Purohit V, Pandey P, Joshi S, Moore ED, et al. Graviola: A novel promising natural-derived drug that inhibits tumorigenicity and metastasis of pancreatic cancer cells in vitro and in vivo through altering cell metabolism. Cancer Lett (2012) 323(1):29–40. doi: 10.1016/j.canlet.2012.03.031
216. Gautam SK, Kumar S, Dam V, Ghersi D, Jain M, Batra SK. MUCIN-4 (MUC4) is a novel tumor antigen for pancreatic cancer immunotherapy. Semin Immunol (2020) 47:101391. doi: 10.1016/j.smim.2020.101391
217. Ko YM, Wu TY, Wu YC, Chang FR, Guh JY, Chuang LY. Annonacin induces cell cycle-dependent growth arrest and apoptosis in estrogen receptor-α-related pathways in MCF-7 cells. J Ethnopharmacol (2011) 137(3):1283–90. doi: 10.1016/j.jep.2011.07.056
218. Oberlies NH, Croy VL, Harrison ML, McLaughlin JL. The annonaceous acetogenin bullatacin is cytotoxic against multidrug-resistant human mammary adenocarcinoma cells. Cancer Lett (1997) 115(1):73–9. doi: 10.1016/S0304-3835(97)04716-2
219. Yuan F, Bai G, Miao Y, Chen Y, Li X, Chen J. Annosquacin b induces mitochondrial apoptosis in multidrug resistant human breast cancer cell line MCF-7/ADR through selectively modulating MAPKs pathways. Pharm Biol (2016) 54(12):3040–5. doi: 10.1080/13880209.2016.1200634
220. Höllerhage M, Rösler TW, Berjas M, Luo R, Tran K, Richards KM, et al. Neurotoxicity of dietary supplements from annonaceae species. Int J Toxicol (2015) 34(6):543–50. doi: 10.1177/1091581815602252
221. Yang C, Gundala SR, Mukkavilli R, Vangala S, Reid MD, Aneja R. Synergistic interactions among flavonoids and acetogenins in graviola (Annona muricata) leaves confer protection against prostate cancer. Carcinogenesis (2015) 36(6):656. doi: 10.1093/carcin/bgv046
222. Jabir MS, Saleh YM, Sulaiman GM, Yaseen NY, Sahib UI, Dewir YH, et al. Green synthesis of silver nanoparticles using annona muricata extract as an inducer of apoptosis in cancer cells and inhibitor for NLRP3 inflammasome via enhanced autophagy. Nanomaterials (Basel) (2021) 11(2):384. doi: 10.3390/nano11020384
223. González-Pedroza MG, Argueta-Figueroa L, García-Contreras R, Jiménez-Martínez Y, Martínez-Martínez E, Navarro-Marchal SA, et al. Silver nanoparticles from annona muricata peel and leaf extracts as a potential potent, biocompatible and low cost antitumor tool. Nanomaterials (Basel) (2021) 11(5):1273. doi: 10.3390/nano11051273
224. Meenakshisundaram S, Krishnamoorthy V, Jagadeesan Y, Vilwanathan R, Balaiah A. Annona muricata assisted biogenic synthesis of silver nanoparticles regulates cell cycle arrest in NSCLC cell lines. Bioorg Chem (2020) 95:103451. doi: 10.1016/j.bioorg.2019.103451
225. Gavamukulya Y, Maina EN, El-Shemy HA, Meroka AM, Kangogo GK, Magoma G, et al. Annona muricata silver nanoparticles exhibit strong anticancer activities against cervical and prostate adenocarcinomas through regulation of CASP9 and the CXCL1/CXCR2 genes axis. Tumour Biol (2021) 43(1):37–55. doi: 10.3233/TUB-200058
226. Aggarwal V, Tuli HS, Tania M, Srivastava S, Ritzer EE, Pandey A, et al. Molecular mechanisms of action of epigallocatechin gallate in cancer: Recent trends and advancement. Semin Cancer Biol (2022) 80:256–75. doi: 10.1016/j.semcancer.2020.05.011
227. Kochman J, Jakubczyk K, Antoniewicz J, Mruk H, Janda K. Health benefits and chemical composition of matcha green tea: A review. Molecules (2020) 26(1):85. doi: 10.3390/molecules26010085
228. McCormack VA, dos Santos Silva I. Breast density and parenchymal patterns as markers of breast cancer risk: A meta-analysis. Cancer Epidemiol Biomarkers Prev (2006) 15(6):1159–69. doi: 10.1158/1055-9965.EPI-06-0034
229. Lazzeroni M, Guerrieri-Gonzaga A, Gandini S, Johansson H, Serrano D, Cazzaniga M, et al. A presurgical study of lecithin formulation of green tea extract in women with early breast cancer. Cancer Prev Res (Phila) (2017) 10(6):363–70. doi: 10.1158/1940-6207.CAPR-16-0298
230. Wang P, Aronson WJ, Huang M, Zhang Y, Lee RP, Heber D, et al. Green tea polyphenols and metabolites in prostatectomy tissue: Implications for cancer prevention. Cancer Prev Res (Phila) (2010) 3(8):985–93. doi: 10.1158/1940-6207.CAPR-09-0210
231. Tachibana H, Koga K, Fujimura Y, Yamada K. A receptor for green tea polyphenol EGCG. Nat Struct Mol Biol (2004) 11(4):380–1. doi: 10.1038/nsmb743
232. Byun EB, Choi HG, Sung NY, Byun EH. Green tea polyphenol epigallocatechin-3-gallate inhibits TLR4 signaling through the 67-kDa laminin receptor on lipopolysaccharide-stimulated dendritic cells. Biochem Biophys Res Commun (2012) 426(4):480–5. doi: 10.1016/j.bbrc.2012.08.096
233. Lakshmi SP, Reddy AT, Kodidhela LD, Varadacharyulu NC. The tea catechin epigallocatechin gallate inhibits NF-κB-mediated transcriptional activation by covalent modification. Arch Biochem Biophys (2020) 695:108620. doi: 10.1016/j.abb.2020.108620
234. Hu Y, Gu J, Lin J, Wang Y, Yang F, Yin J, et al. (-)-Epigallocatechin-3-gallate (EGCG) modulates polarized macrophages to suppress M1 phenotype and promote M2 polarization in vitro and in vivo. J Funct Foods (2021) 87:104743. doi: 10.1016/j.jff.2021.104743
235. Huang SC, Kao YH, Shih SF, Tsai MC, Lin CS, Chen LW, et al. Epigallocatechin-3-gallate exhibits immunomodulatory effects in human primary T cells. Biochem Biophys Res Commun (2021) 550:70–6. doi: 10.1016/j.bbrc.2021.02.132
236. Jena AB, Dash UC, Duttaroy AK. An in silico investigation on the interactions of curcumin and epigallocatechin-3-gallate with NLRP3 inflammasome complex. BioMed Pharmacother (2022) 156:113890. doi: 10.1016/j.biopha.2022.113890
237. El-Missiry MA, Othman AI, El-Sawy MR, Lebede MF. Neuroprotective effect of epigallocatechin-3-gallate (EGCG) on radiation-induced damage and apoptosis in the rat hippocampus. Int J Radiat Biol (2018) 94(9):798–808. doi: 10.1080/09553002.2018.1492755
238. Xie LW, Cai S, Zhao TS, Li M, Tian Y. Green tea derivative (-)-epigallocatechin-3-gallate (EGCG) confers protection against ionizing radiation-induced intestinal epithelial cell death both in vitro and in vivo. Free Radic Biol Med (2020) 161:175–86. doi: 10.1016/j.freeradbiomed.2020.10.012
239. Zhang Z, Zhu Q, Wang S, Shi C. Epigallocatechin-3-gallate inhibits the formation of neutrophil extracellular traps and suppresses the migration and invasion of colon cancer cells by regulating STAT3/CXCL8 pathway. Mol Cell Biochem (2022). doi: 10.1007/s11010-022-04550-w
240. Xu P, Yan F, Zhao Y, Chen X, Sun S, Wang Y, et al. Green tea polyphenol EGCG attenuates MDSCs-mediated immunosuppression through canonical and non-canonical pathways in a 4T1 murine breast cancer model. Nutrients (2020) 12(4):1042. doi: 10.3390/nu12041042
241. Rawangkan A, Wongsirisin P, Namiki K, Iida K, Kobayashi Y, Shimizu Y, et al. Green tea catechin is an alternative immune checkpoint inhibitor that inhibits PD-L1 expression and lung tumor growth. Molecules (2018) 23(8):2071. doi: 10.3390/molecules23082071
242. Xiao X, Jiang K, Xu Y, Peng H, Wang Z, Liu S, et al. (-)-Epigallocatechin-3-gallate induces cell apoptosis in chronic myeloid leukaemia by regulating Bcr/Abl-mediated p38-MAPK/JNK and JAK2/STAT3/AKT signalling pathways. Clin Exp Pharmacol Physiol (2019) 46(2):126–36. doi: 10.1111/1440-1681.13037
243. Kumazoe M, Sugihara K, Tsukamoto S, Huang Y, Tsurudome Y, Suzuki T, et al. 67-kDa laminin receptor increases cGMP to induce cancer-selective apoptosis. J Clin Invest (2013) 123(2):787–99. doi: 10.1172/JCI64768
244. Negri A, Naponelli V, Rizzi F, Bettuzzi S. Molecular targets of epigallocatechin–gallate (EGCG): A special focus on signal transduction and cancer. Nutrients (2018) 10(12):1936. doi: 10.3390/nu10121936
245. Djediai S, Gonzalez Suarez N, El Cheikh-Hussein L, Rodriguez Torres S, Gresseau L, Dhayne S, et al. MT1-MMP cooperates with TGF-β receptor-mediated signaling to trigger SNAIL and induce epithelial-to-Mesenchymal-like transition in U87 glioblastoma cells. Int J Mol Sci (2021) 22(23):13006. doi: 10.3390/ijms222313006
246. Tsukamoto S, Huang Y, Umeda D, Yamada S, Yamashita S, Kumazoe M, et al. 67-kDa laminin receptor-dependent protein phosphatase 2A (PP2A) activation elicits melanoma-specific antitumor activity overcoming drug resistance. J Biol Chem (2014) 289(47):32671–81. doi: 10.1074/jbc.M114.604983
247. Umeda D, Yano S, Yamada K, Tachibana H. Green tea polyphenol epigallocatechin-3-gallate signaling pathway through 67-kDa laminin receptor. J Biol Chem (2008) 283(6):3050–8. doi: 10.1074/jbc.M707892200
248. Luo KW, Xia J, Cheng BH, Gao HC, Fu LW, Luo XL. Tea polyphenol EGCG inhibited colorectal-cancer-cell proliferation and migration via downregulation of STAT3. Gastroenterol Rep (Oxf) (2021) 9(1):59–70. doi: 10.1093/gastro/goaa072
249. Fujimura Y, Kumazoe M, Tachibana H. 67-kDa laminin receptor-mediated cellular sensing system of green tea polyphenol EGCG and functional food pairing. Molecules (2022) 27(16):5130. doi: 10.3390/molecules27165130
250. Ho HC, Huang CC, Lu YT, Yeh CM, Ho YT, Yang SF, et al. Epigallocatechin-3-gallate inhibits migration of human nasopharyngeal carcinoma cells by repressing MMP-2 expression. J Cell Physiol (2019) 234(11):20915–24. doi: 10.1002/jcp.28696
251. Gonzalez Suarez N, Rodriguez Torres S, Ouanouki A, El Cheikh-Hussein L, Annabi B, Inhibits Adipose-Derived Mesenchymal Stem Cells Differentiation into Adipocytes EGCG. And prevents a STAT3-mediated paracrine oncogenic control of triple-negative breast cancer cell invasive phenotype. Molecules (2021) 26(6):1506. doi: 10.3390/molecules26061506
252. Jin G, Yang Y, Liu K, Zhao J, Chen X, Liu H, et al. Combination curcumin and (-)-epigallocatechin-3-gallate inhibits colorectal carcinoma microenvironment-induced angiogenesis by JAK/STAT3/IL-8 pathway. Oncogenesis (2017) 6(10):e384. doi: 10.1038/oncsis.2017.84
253. Yuan CH, Horng CT, Lee CF, Chiang NN, Tsai FJ, Lu CC, et al. Epigallocatechin gallate sensitizes cisplatin-resistant oral cancer CAR cell apoptosis and autophagy through stimulating AKT/STAT3 pathway and suppressing multidrug resistance 1 signaling. Environ Toxicol (2017) 32(3):845–55. doi: 10.1002/tox.22284
254. Tian M, Tian D, Qiao X, Li J, Zhang L. Modulation of myb-induced NF-kB -STAT3 signaling and resulting cisplatin resistance in ovarian cancer by dietary factors. J Cell Physiol (2019) 234(11):21126–34. doi: 10.1002/jcp.28715
255. Borse V, Al Aameri RFH, Sheehan K, Sheth S, Kaur T, Mukherjea D, et al. Epigallocatechin-3-gallate, a prototypic chemopreventative agent for protection against cisplatin-based ototoxicity. Cell Death Dis (2017) 8(7):e2921. doi: 10.1038/cddis.2017.314
256. Nelson KM, Dahlin JL, Bisson J, Graham J, Pauli GF, Walters MA. The essential medicinal chemistry of curcumin. J Med Chem (20170) 60(5):1620–37. doi: 10.1021/acs.jmedchem.6b00975
257. Amalraj A, Pius A, Gopi S, Gopi S. Biological activities of curcuminoids, other biomolecules from turmeric and their derivatives - a review. J Tradit Complement Med (2017) 7(2):205–33. doi: 10.1016/j.jtcme.2016.05.005
258. Mukherjee S, Baidoo JNE, Fried A, Banerjee P. Using curcumin to turn the innate immune system against cancer. Biochem Pharmacol (2020) 176:113824. doi: 10.1016/j.bcp.2020.113824
259. Lao CD, Ruffin MT, Normolle D, Heath DD, Murray SI, Bailey JM, et al. Dose escalation of a curcuminoid formulation. BMC Complement Altern Med (2006) 6:10. doi: 10.1186/1472-6882-6-10
260. Martínez N, Herrera M, Frías L, Provencio M, Pérez-Carrión R, Díaz V, et al. A combination of hydroxytyrosol, omega-3 fatty acids and curcumin improves pain and inflammation among early stage breast cancer patients receiving adjuvant hormonal therapy: Results of a pilot study. Clin Transl Oncol (2019) 21(4):489–98. doi: 10.1007/s12094-018-1950-0
261. Kohaar I, Petrovics G, Srivastava S. A rich array of prostate cancer molecular biomarkers: Opportunities and challenges. Int J Mol Sci (2019) 20(8):E1813. doi: 10.3390/ijms20081813
262. Deng L, Wu X, Zhu X, Yu Z, Liu Z, Wang J, et al. Combination effect of curcumin with docetaxel on the PI3K/AKT/mTOR pathway to induce autophagy and apoptosis in esophageal squamous cell carcinoma. Am J Transl Res (2021) 13(1):57–72.
263. Hutzen B, Friedman L, Sobo M, Lin L, Cen L, De Angelis S, et al. Curcumin analogue GO-Y030 inhibits STAT3 activity and cell growth in breast and pancreatic carcinomas. Int J Oncol (2009) 35(4):867–72. doi: 10.3892/ijo_00000401
264. MaruYama T, Kobayashi S, Nakatsukasa H, Moritoki Y, Taguchi D, Sunagawa Y, et al. The curcumin analog GO-Y030 controls the generation and stability of regulatory T cells. Front Immunol (2021) 12:687669. doi: 10.3389/fimmu.2021.687669
265. Sato A, Kudo C, Yamakoshi H, Uehara Y, Ohori H, Ishioka C, et al. Curcumin analog GO-Y030 is a novel inhibitor of IKKβ that suppresses NF-κB signaling and induces apoptosis. Cancer Sci (2011) 102(5):1045–51. doi: 10.1111/j.1349-7006.2011.01886.x
266. He W, Xia Y, Cao P, Hong L, Zhang T, Shen X, et al. Curcuminoid WZ35 synergize with cisplatin by inducing ROS production and inhibiting TrxR1 activity in gastric cancer cells. J Exp Clin Cancer Res (2019) 38(1):207. doi: 10.1186/s13046-019-1215-y
267. MaruYama T, Kobayashi S, Shibata H, Chen W, Owada Y. Curcumin analog GO-Y030 boosts the efficacy of anti-PD-1 cancer immunotherapy. Cancer Sci (2021) 112(12):4844–52. doi: 10.1111/cas.15136
268. Hu Y, Ran M, Wang B, Lin Y, Cheng Y, Zheng S. Co-Delivery of docetaxel and curcumin via nanomicelles for enhancing anti-ovarian cancer treatment. IJN (2020) 15:9703–15. doi: 10.2147/IJN.S274083
269. Kim CH, Kim BD, Lee TH, Kim HK, Lyu MJ, Yoon YI, et al. Synergistic co-administration of docetaxel and curcumin to chemoresistant cancer cells using PEGylated and RIPL peptide-conjugated nanostructured lipid carriers. Cancer Nanotechnol (2022) 13(1):17. doi: 10.1186/s12645-022-00119-w
270. Hayakawa T, Yaguchi T, Kawakami Y. Enhanced anti-tumor effects of the PD-1 blockade combined with a highly absorptive form of curcumin targeting STAT3. Cancer Sci (2020) 111(12):4326–35. doi: 10.1111/cas.14675
271. Ham IH, Wang L, Lee D, Woo J, Kim TH, Jeong HY, et al. Curcumin inhibits the cancer−associated fibroblast−derived chemoresistance of gastric cancer through the suppression of the JAK/STAT3 signaling pathway. Int J Oncol (2022) 61(1):85. doi: 10.3892/ijo.2022.5375
272. Ren M, Wang Y, Wu X, Ge S, Wang B. Curcumin synergistically increases effects of β-interferon and retinoic acid on breast cancer cells in vitro and in vivo by up-regulation of GRIM-19 through STAT3-dependent and STAT3-independent pathways. J Drug Targeting (2017) 25(3):247–54. doi: 10.1080/1061186X.2016.1242132
273. Bharti AC, Donato N, Aggarwal BB. Curcumin (diferuloylmethane) inhibits constitutive and IL-6-inducible STAT3 phosphorylation in human multiple myeloma cells. J Immunol (2003) 171(7):3863–71. doi: 10.4049/jimmunol.171.7.3863
274. Mohammadi Kian M, Salemi M, Bahadoran M, Haghi A, Dashti N, Mohammadi S, et al. Curcumin combined with thalidomide reduces expression of STAT3 and bcl-xL, leading to apoptosis in acute myeloid leukemia cell lines. Drug Des Devel Ther (2020) 14:185–94. doi: 10.2147/DDDT.S228610
275. Blasius R, Reuter S, Henry E, Dicato M, Diederich M. Curcumin regulates signal transducer and activator of transcription (STAT) expression in K562 cells. Biochem Pharmacol (2006) 72(11):1547–54. doi: 10.1016/j.bcp.2006.07.029
Keywords: anticancer phytochemicals, artemisinin derivatives, berberine, triptolide derivatives, graviola, EGCG, curcumin, STAT3
Citation: Bernitsa S, Dayan R, Stephanou A, Tzvetanova ID and Patrikios IS (2023) Natural biomolecules and derivatives as anticancer immunomodulatory agents. Front. Immunol. 13:1070367. doi: 10.3389/fimmu.2022.1070367
Received: 14 October 2022; Accepted: 05 December 2022;
Published: 09 January 2023.
Edited by:
Nikolai Korpan, International Institute of Cryosurgery, AustriaReviewed by:
Sankar Bhattacharyya, Sidho Kanho Birsha University, IndiaCopyright © 2023 Bernitsa, Dayan, Stephanou, Tzvetanova and Patrikios. This is an open-access article distributed under the terms of the Creative Commons Attribution License (CC BY). The use, distribution or reproduction in other forums is permitted, provided the original author(s) and the copyright owner(s) are credited and that the original publication in this journal is cited, in accordance with accepted academic practice. No use, distribution or reproduction is permitted which does not comply with these terms.
*Correspondence: Iva D. Tzvetanova, aS50enZldGFub3ZhQGV1Yy5hYy5jeQ==; Ioannis S. Patrikios, aS5wYXRyaWtpb3NAZXVjLmFjLmN5
Disclaimer: All claims expressed in this article are solely those of the authors and do not necessarily represent those of their affiliated organizations, or those of the publisher, the editors and the reviewers. Any product that may be evaluated in this article or claim that may be made by its manufacturer is not guaranteed or endorsed by the publisher.
Research integrity at Frontiers
Learn more about the work of our research integrity team to safeguard the quality of each article we publish.