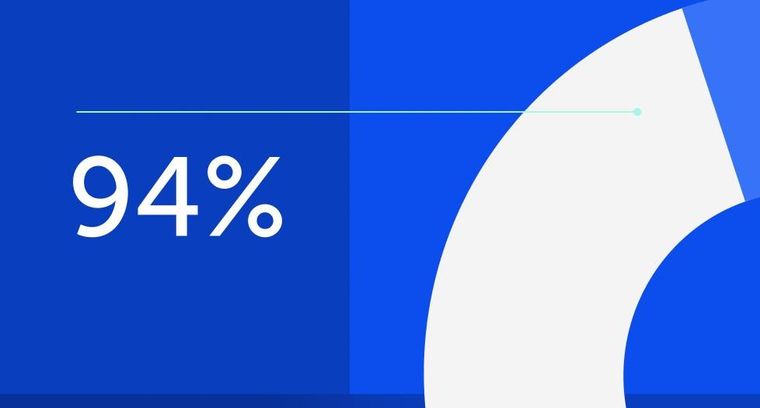
94% of researchers rate our articles as excellent or good
Learn more about the work of our research integrity team to safeguard the quality of each article we publish.
Find out more
REVIEW article
Front. Immunol., 22 November 2022
Sec. Viral Immunology
Volume 13 - 2022 | https://doi.org/10.3389/fimmu.2022.1053437
This article is part of the Research TopicImmune Response to SARS-CoV-2 and Implications for Clinical OutcomeView all 30 articles
Coronavirus disease 2019 (COVID-19) has been a global pandemic, caused by a novel coronavirus strain with strong infectivity, severe acute respiratory syndrome coronavirus 2 (SARS-CoV-2). With the in-depth research, the close relationship between COVID-19 and immune system has been dug out. During the infection, macrophages, dendritic cells, natural killer cells, CD8+ T cells, Th1, Th17, Tfh cells and effector B cells are all involved in the anti-SARS-CoV-2 responses, however, the dysfunctional immune responses will ultimately lead to the excessive inflammation, acute lung injury, even other organ failure. Thus, a detailed understanding of pertinent immune response during COVID-19 will provide insights in predicting disease outcomes and developing appropriate therapeutic approaches. In this review, we mainly clarify the role of immune cells in COVID-19 and the target-vaccine development and treatment.
In December 2019, severe acute respiratory syndrome coronavirus 2 (SARS-CoV-2) emerged in Wuhan, China, a novel coronavirus that has never been reported before.The disease caused by SARS-CoV-2 is known as coronavirus disease 2019 (COVID-19), has spread rapidly in China and all over the world due to the high transmissibility, threatening human health and public safety (1). Clinical symptoms that patients exhibit include pyrexia, fatigue, cough, and even lead to organ function damage (2). Over the past years, the investigation of the interaction between the “attacker” and the “defender” has provided us more supporting evidences on host immune responses and the plan for therapeutic vaccines and drugs in the future. In this review, we mainly discuss the innate and the adaptive immune responses in COVID-19 to provide a deeper insight into the disease pathogenesis, thereby providing more information for COVID-19 treatments exploration.
As a novel coronavirus, 79% and 50% genome sequence identity of SARS-CoV-2 are similar to SARS-CoV and Middle East respiratory syndrome coronavirus (MERS-CoV), respectively (3). There are six functional open reading frames in SARS-CoV-2, including ORF1a/ORF1b, spike (S), envelope (E), membrane (M) and nucleocapsid (N) from 5’ to 3’ in the genome (4). Up to now, real-time reverse transcriptase-polymerase chain reaction (RT-PCR) assays for SARS-CoV-2 nucleic acid mainly targeting ORF1a/1b, N, E and S genes are considered as the gold standard for confirming SARS-CoV-2 infection in patients (5). In addition, the amino acid identity of structural and non-structural proteins encoded by SARS-CoV-2 is similar to SARS-CoV. Apart from spike, another three structural proteins (envelope-membrane-nucleocapsid) have more than 90% amino acid identity with SARS-CoV, and most non-structural proteins are above 85% (3, 6).
The spike (S)-glycoprotein contains two functional domains, the amino (N)-terminal and carboxyl (C)-terminal named S1 subunit and S2 subunit, respectively (7). S1 subunit includes a receptor-binding domain (RBD), which is a key site in mediating the fusion of SARS-CoV-2 and host cell membrane during the infection. Being different from S1 subunit, the S2 subunit is a coiled helix structurethat contains a hydrophobic fusion loop and two heptad repeat regions. Previous reports have revealed these two domains exist as homo-trimer spikes and bind to the human Angiotensin-converting enzyme 2 (ACE2) host cell receptor in an accessible conformation via RBD domain of S1 subunit (8).
ACE2, a well-known receptor of SAR-CoV, has also been revealed to be the receptor of S-glycoprotein in SARS-CoV-2. ACE2 is widely expressed in human, bats, pangolins, pigs, ferrets, rhesus monkeys, civets, cats and dogs, which implies a wide host range of SARS-CoV-2 (9). For humans, the invasion of SARS-CoV-2 was not determined solely by ACE2 expression but also the assist by other factors (such as host proteases). For instance, the expression of ACE2 was limited in the respiratory tract which were favored by SARS-CoV-2 (10).
In the initial stage of infection, S1 protein binds to ACE2 via the RBD and then S1 protein shed from the virus surface, prompting the S2 domain membrane fusion with host cell (Figure 1) (11, 12). This process must be activated by cleavage of the S1 protein by the host protease to activate the S protein, including transmembrane protease serine protease 2 (TMPRSS2), cathepsin L and furin (11, 13). The study suggested that TMPRSS2 could be hired for the priming of SARS-CoV-2 S protein, which contributed to the fusion of the virus with the host cell membrane (13). Also, it could cooperate with cathepsin L to generate the cumulative effects in the fusion process (11). Additionally, other membrane proteins have also been confirmed to involve in this process. Interestingly, researchers from Peking University found that LDLRAD3, TMEM30A and CLEC4G effectively mediated virus invasion into cells in an ACE2-independent manner (14).
Figure 1 The SARS-CoV-2 virion and life cycle. The SARS-CoV-2 virion contains four structural proteins, namely spike (S), envelope (E), membrane (M), and nucleocapsid (N). The positive-sense, single-stranded RNA genome (+ssRNA) is encapsidated by N, whereas M and E ensure its incorporation into the viral particle during the assembly process. The spike (S) protein of SARS-CoV-2 specifically binds to ACE2, together with host factors (such as the cell surface serine protease TMPRSS2), facilitating the uptake and fusion of the virus in the cell or endosomal membrane. Following entry, the release and uncoating of the incoming genomic RNA allow it to the immediate translation of open reading frames. The resulting polyproteins pp1a and pp1ab are co-translated and post-translationally processed into the individual non-structural proteins (NSPs) that form the viral replication and transcription complex. Translated structural proteins translocate into endoplasmic reticulum (ER) membranes and transit through the ER-to-Golgi intermediate compartment (ERGIC), where they interact with N-encapsidated, newly produced genomic RNA and then lead tobudding into the lumen of secretory vesicular compartments. Finally, SARS-CoV-2 are secreted from the infected cell by exocytosis.
Similar to other coronaviruses, variants of SARS-CoV-2 have emerged in the past few years, and the main prevalent variant in the world today is Omicron variant (15–17). Variants might influence the epidemiological characteristics and clinical symptoms of COVID-19, even reducing the protection established by neutralizing antibodies and vaccines. Therefore, comprehending the immune responses to SARS-CoV-2 is of great importance for future vaccination and therapy.
Age is one of the main factors affecting the COVID-19 severity. Previous studies showed that every age group was susceptible to SARS-CoV-2, and in contrast to children (0-14 years old), old adults (above 65 years old), especially those with underlying diseases, are prone to develop severe respiratory diseases or even die (2, 18). Notably, some studies reported that SARS-CoV-2 could be transmitted to the fetus from pregnant women with COVID-19 through the placenta (19–21). Thus, all population are susceptible to SARS-CoV-2 through different ways.
Along with the deepening of research, the role of immune cells in the anti-SARS-CoV-2 response has increasingly being recognized. In the following description, we will discuss both innate and adaptive immune responses in COVID-19 (Figure 2 and Table 1).
Figure 2 Immune response against SARS-CoV-2. The induction of innate immune cells (e.g. neutrophils, monocytes/macrophages and dendritic cells) results in production of various pro-inflammatory cytokines and chemokines. And the adequate production of these factors helps to clear the virus, while over-production can lead to cytokine storm and lung injury. The presentation of antigen by antigen-presenting cells (APCs) can induce humoral and cellular immunity in the human body. Specific CD4+ T cells can be activated and differentiate into Th cells, where Th1 cells promote the activation of macrophage-dependent virus clearance via producing macrophage activating factors (e.g. TNF-α and GM-CSF), and Th2 cells facilitate the humoral immunity. With the help of Th2 cells, B cells produce virus specific antibodies and neutralize viruses. CD8+ T cells differentiate into cytotoxic T lymphocyte (CTL), contributing to virus clearance by lysis of infected cells.
Macrophages are myeloid immune cells that are involve in inflammation and tissue reparation. In lung, macrophages are divided into alveolar macrophages and interstitial macrophages. The former are mainly distributed near type I/II epithelial alveolar cells, while the latter preferentially reside between the microvascular endothelium and alveolar epithelium zone (36, 37). When pathogens and heterologous materials enter into lung, actived macrophages serve as the first line to resist the attackers. It is worth noting that short-lived macrophages can limit the replication of the virus, and once converted into long-lived macrophages, they might become infected resident cells (38).
Upon SASR-CoV-2 infection, the pro-inflammatory activity of macrophages was enhanced, as indicated by the increased expression of inflammatory cytokines (IL-1β, IL-6) and chemokines (CXCL10) by macrophages (39, 40). Macrophages adjacent to areas of endothelial cell damage were the main producers of IFN-I, which might be elicited by the stimulator of interferon genes (STING) induced-IFN-I production and mtDNA release from adjacent endothelial cells (41). Increased pro-inflammatory factors may led to the occurrence of cytokine storm, which was correlated with the poor prognosis of COVID-19 patients (42, 43). Independent infection experiments in human macrophages also corroborated that the induction of IFN-I, TNF, IL-1β and IL-6 generally resulted in IFN-I-mediated cell death (39). Studies have shown that S-protein in SARS-CoV-2 could elicit inflammasome formation and IL-1β production in macrophages from COVID-19 patients, and further exploration revealed that this process needed non-specific activation of monocytes in vivo to trigger NLRP3 inflammatory signaling (40). Macrophages express ACE2, furin and TMPRSS2, whether the three have a synergistic effect in the process of virus infection still need to be explored (44–46). SARS-CoV-2 infection might promote macrophages to adopt a profibrotic phenotype and result in lung fibrosis (22). In vitro, SARS-CoV-2 induced profibrotic programs in classical monocytes, as indicated by a profibrotic proteome profile that was also expressed in idiopathic pulmonary fibrosis (22). There were several articles looking at the future of mesenchymal stem cells (MSC) and MSC-derived extracellular vesicles (MSC-EVs) as living anti-inflammatory therapy for COVID-19, including inhalation of MSC-EVs that promoted the macrophages polarization into a M2 phenotype (47, 48). A novel ACE2-overexpressing microparticles (AO-MPs) could adsorb more virus and then deliver them into alveolar macrophages, consequently quenched in alveolar macrophages and ameliorated macrophage-mediated inflammation (49). Accordingly, macrophages are non-negligible players in SARS-CoV-2-elicited inflammation and lung firbrosis, and macrophages might be a target for COVID-19 treatment.
A recent study revealed that patients with COVID-19 exhibited a defective DC response (24). In severe COVID-19 cases, plasmacytoid DCs (pDCs, critical effectors of antiviral immunity) exhibited increased pro-apoptotic pathways than those in moderate cases, accompanied by the downregulation of antiviral interferon-stimulated genes (ISGs) and innate sensors, TLR9, IFNAR and DHX36 (23, 24). pDCs are one of the main producers of IFN-I, which might produce more IFN-I than other immune cells or infected cells (50). Infiltration of pDCs (sensing SARS-CoV-2) were positively correlated with IFN-I production, contributing to the macrophage-mediated cytokine storm in the lungs of COVID-19 patients and might increase the lung inflammation (51). In detail, IFN-α production following TLR7 sensing of SARS-CoV-2 in pDCs mediated the transcriptional and epigenetic changes in macrophages, which facilitated their hyperactivation upon environmental stimuli (51). Comparing with moderate cases, conventional DCs (cDCs) from patients with severe COVID-19 exhibited decreased IFN signature and MHC II expression, contrary to pro-inflammatory pathways (24). Further exploration suggested that this reduction was correlated with low expression of MHC-II regulators (RFX5, RFXANK and CIITA) in severe cases. This viral inhibition of antigen presentation may explain the disease aggravation in severe cases and provide novel targets to restore the defective antiviral defense (24). Above all, DCs may have the potential to be a target to enhance the protective effects triggered by vaccines.
NK cells from healthy individuals showed anti-viral activity, as indicated by the reduced SARS-CoV-2 protein levels in cocultured infected cells (52). In addition to the anti-viral activity, NK cells also exhibited antifibrotic activity by the decreased expression of profibrotic genes COL1A1 and ACTA2 in lung fibroblasts (25). In contrast to the healthy individuals, NK cells from patients with COVID-19 showed limited anti-viral activity with an exhausted phenotype (53, 54). Previous studies have reported that the quantity of NK cells was significantly reduced in COVID-19 patients, displaying an exhausted phenotype by increased expression of NK inhibitory marker NKG2A (53, 55). Nevertheless, increased expression of NKG2A led to the reduced levels of CD107a (degranulation marker), IFN-γ, IL-2, granzyme B, and TNF-α in NK cells from COVID-19 patients (53). COVID-19-associated NK cells display a genetic profile similar to that of pulmonary NK cells from patients with pulmonary fibrosis, accompanied by the decreased ability to suppress the profibrotic gene expression (mentioned above) in lung fibroblasts (25). As an important anti-viral effector molecule, IFN-α suppressed the IFN-γ production by NK cells, but neutralization of IFN-α was unable to rescue the function of NK cells.
Based on the reduced anti-viral activity and antifibrotic activity of NK cells in patients with COVID-19, recovering their activities might be promising therapies against SAR-CoV-2. A recent study revealed that BNT162b1 mRNA vaccine significantly increased the quantity of CD56bright, CD56dim, and CD56dim/CD16dim NK cells, and with an increase in IFN-γ, perforin and granzyme content (56). This result offered further support to end the SARS-CoV-2 pandemic with worldwide vaccination efforts (56). Therefore, the benefits of NK cell-based immunotherapy in patients with COVID-19 require further study.
Immunity against viral infection mainly begins with the recognition by pattern recognition receptor (PRRs) that are widely distributed on a variety of immune and non-immune cells. Signaling via PRRs induce the production of type I/III IFNs and pro-inflammatory cytokines, which trigger the transcription of ISGs to restrict viral infection. Valuable descriptive and correlative information about COVID-19 and innate immune responses (monocytes/macrophages, dendritic cells and NK cells) have been gathered during the pandemic. In addition to the above discussion, other innate immune cells (including neutrophils, eosinophils and epithelial cells) also have an important role in COVID-19 prediction and development. For example, neutrophils responses and neutrophil extracellular traps (NETs, contain nuclear component, microbicidal proteins and oxidative enzymes derived from neutrophils to limit infection) are correlated with the hyperinflammation in COVID-19 patients (57). Thus, targeting innate immunity may become a new strategy for the treatment of COVID-19.
Virus-specific cellular and humoral responses are the main weapons in protecting patients after SARS-CoV-2 infection. Next, we will comprehensively discuss the role of adaptive immunity in the fight against SARS-CoV-2.
Different CD4+ T cell subsets can play beneficial or detrimental roles in the progression of COVID-19. Highly activated, functional CD4+ T cells and functionally exhausted CD4+ T cells have been detected simultaneously in individuals with severe COVID-19 (58). Previous studies have demonstrated that 83% of CD4+ T cells in patients with COVID-19 were S reactive, which could respond to both N and C terminal of S protein (59). Thus, the interest in studying S protein-specific antiviral immune responses (including T-cell responsive COVID-19 vaccine) has been stimulated (60, 61). SARS-CoV-2-specific CD4+ T cells were associated with ameliorated COVID-19 disease severity, and the induction of SARS-CoV-2-specific CD4+ T cells was correlated with rapid decrease of viral load (62, 63). Another study also confirmed that early viral clearance and less critical illness were correlated with the higher counts of CD4+ T cells, particularly naïve CD4+ T cells (64).
The disproportionality of T helper type 1 (Th1) or Th2 cells has been well elucidated in patients suffered from COVID-19 pandemic and non-infected people who are at maximum risk of infection (26). In an observational study,COVID-19 patients have been reported to have lower proportion of Th1 and Th17 cells and a higher proportion of activated Th2 cells compared with control population (27). In addition, higher proportion of senescent (PD1+/ICOS-) Th2 cells in dead patients than in survivors (27). By evaluating the relationship between Th subsets and mortality, they found that the total lymphocytes and the higher percentages of senescent Th2 cells seem to be independent and significant risk factors for death (27). In the stage of recovery, the proportion of both Th1 and Th2 cells in patients were decreased than in healthy controls (27, 65). Thus, the dynamic change of Th cells might be a tool to monitor the development of COVID-19.
Th17 cells play pivotal roles in tissue damage and excessive inflammation via IL-17 and IL-23 (66). Researchers have found that high levels of Th17 cells in the periphery of patients infected with SARS-CoV-2 (30, 67, 68). A new study revealed that IL-17A expression was significantly associated with the severity of the disease (69). The results of studies also suggested that a systemic neutrophil environment may preferentially skew CD4+ T cells toward Th17 and IL-17A promotion, thereby exacerbating inflammation (69, 70). Another study’s results were consistent with this phenomenon, further experiment provided the evidence that this Th17 promotion could be reversed by inhibitors of arginase-1 (Arg-1), NO synthase (NOS), and reactive oxygen species (ROS), all produced by myeloid cells under oxidative stress (71). Thus, for clinical benefit, targeting Th17 promotion and blocking IL-17 signaling to manage COVID-19 patients, particularly those presenting with cytokine storm syndrome is promising.
Among CD4+ Th cell subsets, follicular helper T (Tfh) cells participate in humoral immunity via interacting with B cells in the germinal center (GC), facilitating the differentiation of B cells into plasma cells to product high-affinity neutralizing antibodies (28). Researchers revealed the absence of GC in thoracic lymph nodes and spleens from COVID-19 patients, accompanied by the reduction of Bcl-6+ GC B cells in both organs (72). The reduction of CD4+CXCR5+Bcl-6+ germinal center Tfh cells might explain the loss of GCs and the accumulation of non-GC-derived activated B cells (72). However, SARS-CoV-2 infection increased the number of peripheral CD4+ Tfh and GC responses in rhesus macaques (73). In clinical studies, convalescent individuals who experienced severe COVID-19 showed a higher induction of CXCR3+ Tfh cells when compared with those non-severe COVID-19-convalescent individuals. And the frequencies of them were positively associated with neutralizing antibody titers in convalescent individuals (74). In addition, they intriguingly found that circulating Tfh cells were S-protein specific and functional in spleen (73, 74). Moreover, researchers found that circulating S-specific Tfh cells could persist in constant numbers in the blood and lymph nodes for more than six months (75). In this study, they highlighted the importance of virus-specific Tfh cell responses in mRNA vaccine-induced robust and durable immune protection against SARS-CoV-2 (75). Furthermore, in Tfh-deleted mice immunized with S protein vaccination, Tfh cells also played an indispensable role for the formation of optimal SARS-CoV-2 Spike-specific B cell in GCs and for somatic hypermutation (SHM) (76).
In addition to the above protective effects, Tfh cells could also accelerate the disease progression in certain cases. Compared to non-hospitalized COVID-19 patients, severe cases showed increased cytotoxic Tfh cells which manifested high levels of IFN-γ, IL-2, and TNF-α (77). In an observational study, the results showed that CCR4+ and CCR6+ Tfh cells could be pathogenic in COVID-19 patients (78). In addition, attention needs to be focused on COVID-19 patients those who have Tfh-associated diseases, such as HIV, autoimmune diseases, who are more likely to develop severe disease (79, 80).
Regulatory T cells (Tregs) keep the immune homeostasis via multiple mechanisms thus the role of Tregs has not allow to ignore in these processes (81). Flow cytometric analysis showed an increased proportion of Tregs in peripheral blood mononuclear cells (PBMCs) with enhanced expression of FoxP3, which correlated with poor prognosis (82). In mechanically ventilated patients with COVID-19 acute respiratory distress syndrome (ARDS, hypoxemic respiratory failure caused by inflammation within the lung), in sharp contrast to the lymphopenia in both subsets of CD4+ and CD8+ T cells, the proportions of Tregs were increased in the lungs and PBMCs (83). The recruitment of Tregs into the lungs of COVID-19 patients may determine the severity of the disease since patients with more Treg cells experienced the milder disease (84). However, recent clinical studies showed that CD3+CD4+CD25hiCD127loFoxP3+ Tregs in PBMCs markedly reduced in severe COVID-19 patients (77, 85, 86). In ICU patients with COVID-19, the quantity of Tregs in PBMCs and their functional molecules (TGF-β and IL-10) were significantly reduced, accompanied by an increased ratio of Th17/Treg cells, RORγt/FoxP3, and IL‐17/IL‐10 in patients compared with the controls (30). In adults and children with severe COVID-19, the proportion of Tregs in CD4+ T was also decreased (87). When recovered from mild COVID-19, patients seem to exhibit decreased levels of Tregs compared to themselves during hospitalization, and severe convalescents resulted in perturbances in CD4+ Tregs (88, 89). Moreover, Treg-targeted therapies have been proposed and might be promising, including the adoptive transfer of Tregs and rIL-2 administration (NCT04468971 and NCT04357444, ClinicalTrials.gov) (90). Above all, a comprehensive understanding of the roles of Treg in COVID-19, and identification of the mechanisms regulating the balance between Tregs and other Th subsets may offer therapeutic novelties for COVID-19 infection.
It was reported that SARS-CoV-2-specific CD8+ T-cell responses might be associated with disease severity during the acute phase, and decreased number has been observed in severe cases (31, 62, 91). For example, a study suggested that weak CD8+ T cell responses, despite high antibody titers, might contribute to the pathogenesis of acute COVID-19 (54). Depletion of CD8+ T cells in vaccinated macaques prior to SARS-CoV-2 infection led to the higher levels of peak viral load and day 4 in both the upper and lower respiratory tracts (92). CD8+ T cells from COVID-19 patients exerted enhanced production of IL-2, IL-17, and the expression of degranulation marker CD107a upon anti-CD3/CD28 stimulation when compared to cells from healthy controls (70). In contrast to the previous conclusion, several studies reported that CD8+ T cells from COVID-19 patients exhibited a decreased cytokine-producing capacity (53, 91). However, all this information was not based on SARS-CoV-2 antigen stimulation. There was increasing evidence that circulating CD8+ T cells from severe COVID-19 patients with exhibited an exhausted phenotype characterized by increased expression of PD-1, TIM-3, LAG-3, CTLA-4, NKG2A, and CD39, and there was an inverse relationship between the expression of PD-1 and CD38 (an activation marker) in CD8+ T cells (53, 93–96). Accumulated evidence revealed that the expression of PD-1 in CD8+ T cells from COVID-19 patients was increased, especially ICU patients (95). Moreover, a recent study revealed that SARS-Cov-2-specific CD8+PD-1+ T cells produced more IFN-γ than PD-1-negative populations regardless of disease severity, indicating a functional state of SARS-CoV-2-specific CD8+PD-1+ T cells (96).
In the acute phase of COVID-19, SARS-CoV-2-specific CD8+ T cells expressed activation markers (CD38 and HLA-DR) and secreted cytotoxic molecules (perforins and granzyme B), indicating these cells were activated with cytotoxic functions (31). Nasal-resident SARS-CoV-2-specific CD8+ cells could be detected almost exclusively in vaccinees who experienced SARS-CoV-2 breakthrough infection (persisted for ≥140d) (12). And these CD8+ T cells were predominantly tissue-resident memory T cells which had an elevated proportion of activated cells (97, 98). Moreover, researchers have found that memory CD8+ T cells were required for the clearance of SARS-CoV-2, which was reflected by a delayed decreased of viral loads in the respiratory tract in CD8+ T cells-depleted and SARS-CoV-2 rechallenged convalescent rhesus macaques (99). It’s worth noting that memory CD8+ T cells found in a study of UK convalescent individuals following COVID-19 would support an understanding of protective immunity and may contribute to put forward potential immune-based therapeutics (100). Vaccine-induced CD8+ T cells also help to fight against SARS-CoV-2 re-infection (101–103). One week after prime vaccination with BNT162b2, a stable and fully functional CD8+ T cell response was mobilized, as indicated by the increased production of IFN-γ and TNF-α (104). In addition, boost vaccination-induced highly differentiated effector CD8+ T cells and vaccination-induced early memory T cells exhibited similar capacities (104).
The alterations of B cell subpopulations in COVID-19 have been reported, either immature or terminally differentiated. Compared to healthy subjects, the frequencies of CD19+ B cells in PBMCs seem to be slightly increased in severe and critical cases (105). The double negative (DN) fraction (CD27-IgD-) is one of B cell compartments that showed significant alterations in COVID-19 (105). Depending on the disease severity, mean frequencies of DN1 (CD21+CD11c-), DN2 (CD21-CD11c+), and DN3 (CD21-CD11c-) subsets exhibited shifted distributions. DN1 B cells showed a significant reduction in severe and critical cases. DN2 B cells displayed a significant increase in severe cases vs mild/moderate and critical cases while DN3 B cells were significantly increased as disease severity increased. In addition, immature, activated naïve B cells and the DN3 fraction showed a strong correlation with ventilatory parameters, such as respiratory rate, SpO2, and PaO2/FiO2 (105). Another study also revealed similar phenomenon about DN2 B cells in acute patients with COVID-19 (106). This study also revealed that severe patients displayed enhanced extrafollicular B cell activation with accelerated inflammation, while mild patients counteracted the disease through the timely induction of mitochondrial dysfunction in B cells, which suppressed the extrafollicular responses, resulting in increased neutralizing potency index and reduced inflammation (106).
Germinal centers (GCs) are transient microstructures formed within the follicles of secondary lymphoid tissues. Within GCs, activated B cells undergo clonal expansion and affinity maturation and get further help from Tfh cells to differentiate into memory B cells or long-lived plasma cells, which is of vital importance for the generation of humoral immunity (107). In severe SARS-CoV-2 infection, GC responses were suppressed, which inhibited the production of neutralizing antibody (nAb). Multicolor histological assessments revealed that GCs were also largely absent in thoracic lymph nodes and spleens from patients succumbing to COVID-19. There were also the absence of BCL6+ B cells or Tfh cells, both were the contributors to GCs (72). Furthermore, they also speculated that excess TNF-α inhibited the formation of GC responses in COVID-19 via blocking Tfh cell differentiation (72, 108, 109).
Following infection with SARS-CoV-2, naïve B cells and existed memory B cells (upon early exposure of other coronaviruses) will be activated and differentiate into antibody-secreting cells (ASCs). Discrete increments of ASCs were seen in patients with mild/moderate COVID-19 and were significantly amplified with disease severity (105). In contrast to the majority infection, the serum IgG appeared at approximately the same time as serum IgM and IgA responses to SARS-CoV-2 S and N, usually within the first 2 weeks after symptom onset (32–34). Importantly, the memory B cell is the only B cell subset that correlated with a clinical outcome in hospitalization period (105). A recent study revealed that individuals recovered from COVID-19 developed SARS-CoV-2-specific immunoglobulin (IgG) antibodies, neutralizing plasma, and memory B and memory T cells which could persist for at least 3 months. Moreover, SARS-CoV-2-specific IgG memory B cells (MBCs) increased over time (110). S-RBD-specific class-switched MBCs were detected in 13 of 14 participants, failing only in the individual with the lowest plasma levels of anti-S-RBD IgG and neutralizing antibodies. Resting MBCs (rMBCs) made up the largest proportion of S-RBD-specific MBCs patients with mild disease and hospitalized patients with moderate to severe disease (111). Another study found that in mild and severe COVID-19 patients, serum neutralizing antibody (nAb) responses waned rapidly but spike (S)-specific IgG+ memory B cells (MBCs) remained stable or increased. Analysis of 1,213 monoclonal antibodies (mAbs) isolated from S-specific MBCs displayed increased somatic hypermutation, binding affinity, and neutralization potency over time, indicating prolonged protection via humoral immunity (111). A recent study introduced that the effect of MBCs elicited by mRNA vaccines in preventing SARS-CoV-2 variants (including Omicron variants), highlighting the protective roles of MBCs in disease (112).
The adaptive immune system is important for control of most viral infections. We have discussed the roles of Th, Tfh, CD8+ T and B cells in the pathogenesis of COVID-19, and other adaptive immune cells, such as Tfr cells, also contribute to optimize humoral immunity, meaning their importance to avoid the overactivation of immune responses. Whether viral-inhibiting or viral-promoting effects of immune cells in COVID-19, these researches state the importance of them in anti-SARS-CoV-2 and define emergence of new paradigms in anti-viral activity.
Vaccination is considered as one of the greatest successes in the history of medicine, it could decrease the disease incidence, or at least suppress its detrimental or even fatal clinical manifestations. At present, there are mainly five kinds of SARS-CoV-2 vaccines, including inactivated vaccines, recombinant protein vaccines, viral vector-based vaccines, live attenuated vaccines and nucleic acid vaccines (113). As the first inactivated SARS-CoV-2 vaccine, PiCoVacc induced SARS-CoV-2-specific neutralizing antibodies in mice, rats and non-human primates, and these antibodies showed a broader neutralizing ability against other 10 representative SARS-CoV-2 strains (114). In vivo, vaccinated macaques related symptom ameliorated, as indicated by the mild and focal histopathological changes and viral load compared with the controls (114). In phase 1/2 clinical trials, CoronaVac has been confirmed to be safe and well-tolerated, inducing humoral responses in children and adolescents aged 3-17 years and healthy adults (≥18 years old) (115–117). The phase 3 trial in Turkey also revealed a vaccine efficacy of 83.5% in the vaccine group with good safety and tolerability profile (118). In phase 4 studies, scientists found that low antibody concentrations 6 months after previous immunisation with two doses of CoronaVac. However, a third dose induced a significant increase of neutralizing antibodies, which could improve protection against infection. Importantly, heterologous boosting produce a more stronger immune response than homologous boosting (119). The BNT162b2 mRNA and mRNA-1273 COVID-19 vaccine are the most widely used mRNA vaccines, and both vaccines showed more than 90% protective efficiency (120, 121). Viral vector-based adenoviral vaccines have also been explored, including adenovirus-vectored vaccines. Exploration conducted by Chen’s team showed that the recombinant adenovirus type 5 transmitted COVID-19 vaccine was safe in a single dose, and induced robust immune responses in healthy adults aged 18 years or older and children or adolescents (122–124). Humoral responses peaked at day 28 post-vaccination in healthy adults, and rapid specific T-cell responses were noted from day 14 post-vaccination (122). Another adenovirus-vectored vaccine, ChAdOx1 nCoV-19 vaccine also exerted protective effective against SARS-CoV-2 (125, 126). Recently, recombinant tandem-repeat dimeric RBD-based protein subunit vaccine (such as ZF2001) also have been investigated and showed a good protective effect (127, 128). Scientists evaluated the SARS-CoV-2-spike-specific immune responses to Moderna mRNA-1273, Pfizer/BioNTech BNT162b2, Janssen Ad26.COV2.S, and Novavax NVX-CoV2373 (129). 100% of individuals made memory CD4+ T cells, and cTfh and CD4-CTL highly represented only after mRNA or NVX-CoV2373 vaccination, and a differentiating feature of Ad26.COV2.S immunization was a high frequency of CXCR3+ memory B cells (129). mRNA vaccinees had substantial declines in antibodies, while memory T and B cells were comparatively stable (129). A recent study demonstrated that Omicron infection enhances pre-existing immunity elicited by vaccines and, higher neutralization titres against all variants of concern (VOC) (130). Thus, Omicron infection may confer broad protection against non-Omicron variants in vaccinated individuals (130). Although there are different variants (Beta, Delta, Omicron and others), these vaccines are still effective especially in preventing the occurrence of severe cases (131–134).
Although vaccines can prevent the occurrence of severe diseases, accumulated evidence still showed that additional protective measures should be maintained to fight against variants with increased infectivity (135–137). In particular, as time goes on, the vaccine induced immune response will naturally decline.
Currently, COVID-19 pandemic is one of the greatest threats to human health with more than 611 million confirmed cases and over 6.51 million deaths reported worldwide (as of September 23, 2022, sources from WHO). Various drugs were proclaimed to be effective against SARS-CoV-2, such as remdesivir, paxlovid, lopinavir/ritonavir, molnupiravir, and some monoclonal antibodies (138).
Monoclonal antibodies are used as a potential therapeutic and preventive drug for viral infection due to its high specificity and the ability to enhance immune response. Bamlanivimab (LY-CoV555, IgG1), a potent anti-spike neutralizing antibody, block the binding and entry to human cells (139). For primary outcomes, no differences were observed in bamlanivimab vs placebo, but bamlanivimab treatment was associated with lower day 3 nasopharyngeal viral levels and faster reductions in inflammatory markers and viral decay (140). One of three doses of bamlanivimab appeared to promote the natural decline in viral load over time (141). Moreover, compared to the placebo group, patients within LY-CoV555 group had a lower ratio of COVID-19-related hospitalization or visit to an emergency department (141). When combined with another monoclonal antibody, Etesevimab, the incidence of COVID-19-related hospitalization and death was decreased than the placebo group, accompanied by the decline of viral load (142). Sotrovimab (IgG1), casirivimab (IgG1-κ) and imdevimab (IgG1-λ), as S protein RBD specific antibodies which could prevent the binding of SARS-CoV-2 to the human ACE-2 receptor, may contribute to COVID-19 patients recover (143–145). Tocilizumab, a monoclonal antibody binds specifically to both soluble and membrane-bound receptors for IL-6and has been explored in the treatment of COVID-19. The results showed that the mortality in the tocilizumab group was lower than not treated with tocilizumab (146).
Remdesivir, an adenosine analogue, can be metabolized to its active metabolite remdesivir triphosphatea (an analogue of ATP), and integrate into the nascent viral RNA via RNA polymerase, resulting in delayed chain termination during replication, thereby inhibiting viral replication (147). In 2020, a study reported that patients with severe COVID-19 receiving remdesivir had a numerically faster time to clinical improvement than those patients receiving placebo when the duration of symptoms was 10 days or less (148). The ratio of adverse events was similar in both groups, but the proportion of patients who stopped clinical trial was higher in remdesivir group (12%) than placebo group (5%) (148). A 5 day remdesivir therapy of COVID-19 patients showed greater clinical improvement than patients in placebo group, but not different from a 10 day therapy (149). For cases treated in the ICU, remdesivir use within 9 days from symptom onset reduced mortality risk compared with control group (150).
Janus activated kinase (JAK) inhibitors also have been applied to the treatment of COVID-19 (151, 152). Baricitinib is a selective inhibitor of JAK1 and JAK2, both of which mediate signaling for inflammatory cytokines involved in inflammation and the immune response of COVID-19 (151). The results indicated a lower case fatality rate within 2 weeks in the baricitinib group (0%) than the control (6.4%) (152). In addition, the ICU admission rate was lower in patients receiving baricitinib treatment (0.88%) than controls (17.9%) (152). Tofacitinib is another inhibitor of JAK, which has been shown to inhibit the activity of JAK1, JAK2, JAK3, and to a lesser extent of Tyk2 (153). The results of a clinical trial have demonstrated that tofacitinib led to a lower risk of death or respiratory failure through day 28 than placebo (18.1% vs 29.0%), implying the therapeutic effects of tofacitinib in COVID-19 (154). By day 28, death occurred in 2.8% of patients in the tofacitinib group and in 5.5% of patients in the placebo group (154). Thus, the use of JAK inhibitors in hospitalized patients with COVID-19 should be considered to improve the clinical outcome and prevent death.
Paxlovid is a therapeutic combination consisting of two compounds: nirmatrelvir and ritonavir (155). Ritonavir could inhibit the metabolism of nirmatrelvir and allow the administration of lower doses of this substance (156). A recent study revealed that among patients who received paxlovid treatment within three days of symptom onset, only 0.8% patients were admitted to hospital by day 28 after randomization and no deaths. In comparison, these two values in the control group were 7% and 7 (157). In participants treated within five days of symptom onset, the effect of paxlovid was still significant. In addition, no deaths were reported in the paxlovid group as compared to 10 deaths (1.6%) in the placebo group (157). During the pandemic of COVID-19 in Shanghai (2022), scientists found that unvaccinated, delayed use of paxlovid (started 5 days after diagnosis) and immunosuppressive status were independent risk factors for delayed virus clearance (158). Compared with patients who delayed paxlovid treatment, immunosuppressive patients who began to use paxlovid within 5 days after diagnosis had an earlier virus clearance time of about 6 days. Moreover, correlation analysis showed that the earlier paxlovid was used, the more rapidly the virus was cleared (158). Although the benefit of paxlovid has been confirmed and has been approved in some contries, the furture of paxlovid still need further exploration (159).
Molnupiravir is a new oral antiviral drug that has recently been tested in COVID-19 (160). The results of phase clinical trial (NCT04575597) of molnupiravir showed that it can significantly reduce the risk of hospitalization and mortality in mild to moderate COVID-19 patients. Compared with placebo, patients taking molnupiravir had a 50% lower risk of hospitalization or death. More impressively, no deaths were reported in the group of patients taking molnupiravir, while there were 8 deaths in the placebo group. In terms of side effects, there was no difference between the two groups (161–163). Also, molnupiravir accelerated SARS-CoV-2 RNA clearance and elimination of infectious virus in patients with COVID-19 (median 14 days vs 15 days, log rank p= 0.013) (164). In this study, molnupiravir was well tolerated across all doses (200/400/800 mg), and the adverse events were mainly low-drade that were similar to those reported by participants in placebo group (164). For SARS-CoV-2 variants, a recent study found that SARS-CoV-2 Omicron variant was highly sensitive to molnupiravir, nirmatrelvir, and the combination (165). These evidence suggest molnupiravir is a promising drug in treating COVID-19.
Azvudine, a nucleoside reverse transcriptase inhibitor that previously been used in HIV-1 infection (166). In recent years, the effects of azvudine in COVID-19 have been revealed (167, 168). A randomized, open-label, controlled clinical trial (ChiCTR2000029853, http://www.chictr.org.cn) contained 20 mild and common COVID-19 patients showed that the mean times of the first nucleic acid negative conversion (NANC) of azvudine group and the control group were 2.6 days and 5.6 days, respectively (p = 0.008) (167). Also, the mean time of the first NANC of newly diagnosed subjects in the azvudine group was shorter (167). Thus, azvudine is an effective anti-SARS-CoV-2 drug in treating COVID-19 patients, and it has been approved to treat COVID-19 in China (169).
Diamidobenzimidazole 4 (diABZI-4), an STING agonist, has been confirmed to induce a rapid short-lived activation of STING, then the transient expression of proinflammatory cytokines and lymphocyte activation (170). In vitro, diABZI-4 significantly inhibited SARS-CoV-2 replication in infected cells (170, 171). In mouse model, the results showed that the viral load in both nasal cavity and lung was lower than the control, consequently prevented the disease progression (171). For variants (B.1.351), diABZI-4 showed good antiviral effect as well (171). In this process, attention needs to be taken to avoid excessive production of pro-inflammatory cytokines and cytokine storm.
As an important part of world medicine, Chinese traditional medicine is safe and effective in the treatment of COVID-19. The results showed that after 14 days of treatment with Lianhua Qingwen capsule, the cure rate of main clinical symptoms (fever, fatigue and cough) in the treatment group was significantly higher than the control group, reaching 57.7% on the 7th day, 80.3% on the 10th day and 91.5% on the 14th day. The duration of single symptoms of fever, fatigue and cough was also shortened. In terms of reducing the proportion of patients with severe disease, the treatment group of Lianhua Qingwen capsule was significantly lower than the control group (Lianhua Qingwen treatment group: 2.1%, control group: 4.2%) (172). Another study using network pharmacological strategy revealed that AKT1 is a promising drug target to reduce tissue damage and help eliminate virus infection (173). Practically, Chinese traditional medicine have been written up in COVID-19 diagnosis and treatment plan in China (173).
The current knowledge about SASR-CoV-2 indicates that the immune system plays a crucial role in setting the severity of COVID-19. Although an extraordinary amount has been accomplished during the last three years, the relationship between immune responses and the severity of diseases still remains to be explored. Appropriate responses against SARS-CoV-2 could ameliorate the symptoms of COVID-19 and prevent the occurrence of severe diseases, while excessive responses elicited cytokine storm and pathogenic B cell activation, increasing the risk of death. Thus, in immune-based therapeutics, avoiding the excessive activation of immune cells will also be the proposition of our future research. However, understanding heterogeneous disease manifestations of COVID-19 remains a major knowledge gap, and exploring the relationships between COVID-19 and immunity is a priority. The emergence of new mutant strains has also brought unprecedented pressure to vaccine development. With the advent of new technologies such as next-generation sequencing (NGS), CRISPR-based assays and nanotechnology, enhanced the the accuracy and sensitivity of COVID-19 diagnosis and treatments. Furthermore, duration of immune memory and protective immunity to SARS-CoV-2 after infection or vaccination will be a high priority for years to come.
QZ drafted the manuscript. YX and TW discussed and revised the manuscript. FX conceived the topic and revised the manuscript. All authors contributed to the article and approved the submitted version.
The authors declare that the research was conducted in the absence of any commercial or financial relationships that could be construed as a potential conflict of interest.
All claims expressed in this article are solely those of the authors and do not necessarily represent those of their affiliated organizations, or those of the publisher, the editors and the reviewers. Any product that may be evaluated in this article, or claim that may be made by its manufacturer, is not guaranteed or endorsed by the publisher.
1. Yang J, Chen X, Deng X, Chen Z, Gong H, Yan H, et al. Disease burden and clinical severity of the first pandemic wave of covid-19 in wuhan, China. Nat Commun (2020) 11(1):5411. doi: 10.1038/s41467-020-19238-2
2. Guan WJ, Ni ZY, Hu Y, Liang WH, Ou CQ, He JX, et al. Clinical characteristics of coronavirus disease 2019 in China. New Engl J Med (2020) 382(18):1708–20. doi: 10.1056/NEJMoa2002032
3. Lu R, Zhao X, Li J, Niu P, Yang B, Wu H, et al. Genomic characterisation and epidemiology of 2019 novel coronavirus: Implications for virus origins and receptor binding. Lancet (2020) 395(10224):565–74. doi: 10.1016/S0140-6736(20)30251-8
4. Tsai PH, Wang ML, Yang DM, Liang KH, Chou SJ, Chiou SH, et al. Genomic variance of open reading frames (Orfs) and spike protein in severe acute respiratory syndrome coronavirus 2 (Sars-Cov-2). J Chin Med Association: JCMA (2020) 83(8):725–32. doi: 10.1097/jcma.0000000000000387
5. Rahbari R, Moradi N, Abdi M. Rrt-pcr for sars-Cov-2: Analytical considerations. Clin Chim Acta (2021) 516:1–7. doi: 10.1016/j.cca.2021.01.011
6. Chan JF, Kok KH, Zhu Z, Chu H, To KK, Yuan S, et al. Genomic characterization of the 2019 novel human-pathogenic coronavirus isolated from a patient with atypical pneumonia after visiting wuhan. Emerg Microbes Infect (2020) 9(1):221–36. doi: 10.1080/22221751.2020.1719902
7. Gui M, Song W, Zhou H, Xu J, Chen S, Xiang Y, et al. Cryo-electron microscopy structures of the sars-cov spike glycoprotein reveal a prerequisite conformational state for receptor binding. Cell Res (2017) 27(1):119–29. doi: 10.1038/cr.2016.152
8. Wrapp D, Wang N, Corbett Kizzmekia S, Goldsmith Jory A, Hsieh C-L, Abiona O, et al. Cryo-em structure of the 2019-ncov spike in the prefusion conformation. Science (2020) 367(6483):1260–3. doi: 10.1126/science.abb2507
9. Saponaro F, Rutigliano G, Sestito S, Bandini L, Storti B, Bizzarri R, et al. Ace2 in the era of sars-Cov-2: Controversies and novel perspectives. Front Mol Biosci (2020) 7:588618. doi: 10.3389/fmolb.2020.588618
10. Hikmet F, Mear L, Edvinsson A, Micke P, Uhlen M, Lindskog C. The protein expression profile of Ace2 in human tissues. Mol Syst Biol (2020) 16(7):e9610. doi: 10.15252/msb.20209610
11. Shang J, Wan Y, Luo C, Ye G, Geng Q, Auerbach A, et al. Cell entry mechanisms of sars-Cov-2. Proc Natl Acad Sci USA (2020) 117(21):11727–34. doi: 10.1073/pnas.2003138117
12. Lim JME, Tan AT, Le Bert N, Hang SK, Low JGH, Bertoletti A. Sars-Cov-2 breakthrough infection in vaccinees induces virus-specific nasal-resident Cd8+ and Cd4+ T cells of broad specificity. J Exp Med (2022) 219(10):e20220780. doi: 10.1084/jem.20220780
13. Hoffmann M, Kleine-Weber H, Schroeder S, Krüger N, Herrler T, Erichsen S, et al. Sars-Cov-2 cell entry depends on Ace2 and Tmprss2 and is blocked by a clinically proven protease inhibitor. Cell (2020) 181(2):271–80.e8. doi: 10.1016/j.cell.2020.02.052
14. Zhu S, Liu Y, Zhou Z, Zhang Z, Xiao X, Liu Z, et al. Genome-wide crispr activation screen identifies candidate receptors for sars-Cov-2 entry. Sci China Life Sci (2022) 65(4):701–17. doi: 10.1007/s11427-021-1990-5
15. Tao K, Tzou PL, Nouhin J, Gupta RK, de Oliveira T, Kosakovsky Pond SL, et al. The biological and clinical significance of emerging sars-Cov-2 variants. Nat Rev Genet (2021) 22(12):757–73. doi: 10.1038/s41576-021-00408-x
16. Callaway E. Omicron likely to weaken covid vaccine protection. Nature (2021) 600(7889):367–8. doi: 10.1038/d41586-021-03672-3
17. Uraki R, Kiso M, Iida S, Imai M, Takashita E, Kuroda M, et al. Characterization and antiviral susceptibility of sars-Cov-2 omicron ba. 2 Nat (2022) 607(7917):119–27. doi: 10.1038/s41586-022-04856-1
18. Huang C, Wang Y, Li X, Ren L, Zhao J, Hu Y, et al. Clinical features of patients infected with 2019 novel coronavirus in wuhan, China. Lancet (2020) 395(10223):497–506. doi: 10.1016/S0140-6736(20)30183-5
19. Vivanti AJ, Vauloup-Fellous C, Prevot S, Zupan V, Suffee C, Do Cao J, et al. Transplacental transmission of sars-Cov-2 infection. Nat Commun (2020) 11(1):3572. doi: 10.1038/s41467-020-17436-6
20. Chen H, Guo J, Wang C, Luo F, Yu X, Zhang W, et al. Clinical characteristics and intrauterine vertical transmission potential of covid-19 infection in nine pregnant women: A retrospective review of medical records. Lancet (2020) 395(10226):809–15. doi: 10.1016/S0140-6736(20)30360-3
21. Bandyopadhyay T, Sharma A, Kumari P, Maria A, Choudhary R. Possible early vertical transmission of covid-19 from an infected pregnant female to her neonate: A case report. J Trop Pediatr (2021) 67(1):fmaa094. doi: 10.1093/tropej/fmaa094
22. Wendisch D, Dietrich O, Mari T, von Stillfried S, Ibarra IL, Mittermaier M, et al. Sars-Cov-2 infection triggers profibrotic macrophage responses and lung fibrosis. Cell (2021) 184(26):6243–61.e27. doi: 10.1016/j.cell.2021.11.033
23. Hadjadj J, Yatim N, Barnabei L, Corneau A, Boussier J, Smith N, et al. Impaired type I interferon activity and inflammatory responses in severe covid-19 patients. Sci (New York NY) (2020) 369(6504):718–24. doi: 10.1126/science.abc6027
24. Saichi M, Ladjemi MZ, Korniotis S, Rousseau C, Ait Hamou Z, Massenet-Regad L, et al. Single-cell rna sequencing of blood antigen-presenting cells in severe covid-19 reveals multi-process defects in antiviral immunity. Nat Cell Biol (2021) 23(5):538–51. doi: 10.1038/s41556-021-00681-2
25. Kramer B, Knoll R, Bonaguro L, ToVinh M, Raabe J, Astaburuaga-Garcia R, et al. Early ifn-alpha signatures and persistent dysfunction are distinguishing features of nk cells in severe covid-19. Immunity (2021) 54(11):2650–69.e14. doi: 10.1016/j.immuni.2021.09.002
26. Supriya R, Gao Y, Gu Y, Baker JS. Role of exercise intensity on Th1/Th2 immune modulations during the covid-19 pandemic. Front Immunol (2021) 12:761382. doi: 10.3389/fimmu.2021.761382
27. Gil-Etayo FJ, Suarez-Fernandez P, Cabrera-Marante O, Arroyo D, Garcinuno S, Naranjo L, et al. T-Helper cell subset response is a determining factor in covid-19 progression. Front Cell Infect Microbiol (2021) 11:624483. doi: 10.3389/fcimb.2021.624483
28. Crotty S. Follicular helper Cd4 T cells (Tfh). Annu Rev Immunol (2011) 29:621–63. doi: 10.1146/annurev-immunol-031210-101400
29. Zahran AM, Abdel-Rahim MH, Nasif KA, Hussein S, Hafez R, Ahmad AB, et al. Association of follicular helper T and follicular regulatory T cells with severity and hyperglycemia in hospitalized covid-19 patients. Virulence (2022) 13(1):569–77. doi: 10.1080/21505594.2022.2047506
30. Sadeghi A, Tahmasebi S, Mahmood A, Kuznetsova M, Valizadeh H, Taghizadieh A, et al. Th17 and treg cells function in sars-Cov2 patients compared with healthy controls. J Cell Physiol (2021) 236(4):2829–39. doi: 10.1002/jcp.30047
31. Sekine T, Perez-Potti A, Rivera-Ballesteros O, Stralin K, Gorin JB, Olsson A, et al. Robust T cell immunity in convalescent individuals with asymptomatic or mild covid-19. Cell (2020) 183(1):158–68.e14. doi: 10.1016/j.cell.2020.08.017
32. Röltgen K, Nielsen SCA, Arunachalam PS, Yang F, Hoh RA, Wirz OF, et al. Mrna vaccination compared to infection elicits an igg-predominant response with greater sars-Cov-2 specificity and similar decrease in variant spike recognition. medRxiv preprint server Health Sci [Preprint] (2021). doi: 10.1101/2021.04.05.21254952
33. Long QX, Liu BZ, Deng HJ, Wu GC, Deng K, Chen YK, et al. Antibody responses to sars-Cov-2 in patients with covid-19. Nat Med (2020) 26(6):845–8. doi: 10.1038/s41591-020-0897-1
34. Iyer AS, Jones FK, Nodoushani A, Kelly M, Becker M, Slater D, et al. Persistence and decay of human antibody responses to the receptor binding domain of sars-Cov-2 spike protein in covid-19 patients. Sci Immunol (2020) 5(52):eabe0367. doi: 10.1126/sciimmunol.abe0367
35. Cervantes-Díaz R, Sosa-Hernández VA, Romero-Ramírez S, Torres-Ruiz J, Pérez-Fragoso A, Meza-Sánchez DE, et al. Circulating B10 regulatory cells are decreased in severe and critical covid-19. J leukocyte Biol (2022) 112(2):333–7. doi: 10.1002/jlb.5covcra0721-387rr
36. Schneberger D, Aharonson-Raz K, Singh B. Monocyte and macrophage heterogeneity and toll-like receptors in the lung. Cell Tissue Res (2011) 343(1):97–106. doi: 10.1007/s00441-010-1032-2
37. Wang C, Xie J, Zhao L, Fei X, Zhang H, Tan Y, et al. Alveolar macrophage dysfunction and cytokine storm in the pathogenesis of two severe covid-19 patients. EBioMedicine (2020) 57:102833. doi: 10.1016/j.ebiom.2020.102833
38. Nikitina E, Larionova I, Choinzonov E, Kzhyshkowska J. Monocytes and macrophages as viral targets and reservoirs. Int J Mol Sci (2018) 19(9):2821. doi: 10.3390/ijms19092821
39. Zheng J, Wang Y, Li K, Meyerholz DK, Allamargot C, Perlman S. Severe acute respiratory syndrome coronavirus 2-induced immune activation and death of monocyte-derived human macrophages and dendritic cells. J Infect Dis (2021) 223(5):785–95. doi: 10.1093/infdis/jiaa753
40. Theobald SJ, Simonis A, Georgomanolis T, Kreer C, Zehner M, Eisfeld HS, et al. Long-lived macrophage reprogramming drives spike protein-mediated inflammasome activation in covid-19. EMBO Mol Med (2021) 13(8):e14150. doi: 10.15252/emmm.202114150
41. Domizio JD, Gulen MF, Saidoune F, Thacker VV, Yatim A, Sharma K, et al. The cgas-sting pathway drives type I ifn immunopathology in covid-19. Nature (2022) 603(7899):145–51. doi: 10.1038/s41586-022-04421-w
42. Vaninov N. In the eye of the covid-19 cytokine storm. Nat Rev Immunol (2020) 20(5):277. doi: 10.1038/s41577-020-0305-6
43. Fajgenbaum DC, June CH. Cytokine storm. New Engl J Med (2020) 383(23):2255–73. doi: 10.1056/NEJMra2026131
44. Keewan E, Beg S, Naser SA. Anti-Tnf-A agents modulate sars-Cov-2 receptors and increase the risk of infection through notch-1 signaling. Front Immunol (2021) 12:641295. doi: 10.3389/fimmu.2021.641295
45. Verdecchia P, Cavallini C, Spanevello A, Angeli F. The pivotal link between Ace2 deficiency and sars-Cov-2 infection. Eur J Internal Med (2020) 76:14–20. doi: 10.1016/j.ejim.2020.04.037
46. Zhao G, Yang W, Wu J, Chen B, Yang X, Chen J, et al. Influence of a coronary artery disease-associated genetic variant on furin expression and effect of furin on macrophage behavior. Arteriosclerosis Thrombosis Vasc Biol (2018) 38(8):1837–44. doi: 10.1161/atvbaha.118.311030
47. Cao Y, Wu H, Zhai W, Wang Y, Li M, Li M, et al. A safety consideration of mesenchymal stem cell therapy on covid-19. Stem Cell Res (2020) 49:102066. doi: 10.1016/j.scr.2020.102066
48. Zhao R, Wang L, Wang T, Xian P, Wang H, Long Q. Inhalation of msc-evs is a noninvasive strategy for ameliorating acute lung injury. J Controlled Release (2022) 345:214–30. doi: 10.1016/j.jconrel.2022.03.025
49. Wang Z, Lv J, Yu P, Qu Y, Zhou Y, Zhou L, et al. Sars-Cov-2 treatment effects induced by Ace2-expressing microparticles are explained by the oxidized cholesterol-increased endosomal ph of alveolar macrophages. Cell Mol Immunol (2022) 19(2):210–21. doi: 10.1038/s41423-021-00813-6
50. Fitzgerald-Bocarsly P, Dai J, Singh S. Plasmacytoid dendritic cells and type I ifn: 50 years of convergent history. Cytokine Growth Factor Rev (2008) 19(1):3–19. doi: 10.1016/j.cytogfr.2007.10.006
51. Laurent P, Yang C, Rendeiro AF, Nilsson-Payant BE, Carrau L, Chandar V, et al. Sensing of sars-Cov-2 by pdcs and their subsequent production of ifn-I contribute to macrophage-induced cytokine storm during covid-19. Sci Immunol (2022) 7(75):eadd4906. doi: 10.1126/sciimmunol.add4906
52. Krämer B, Knoll R, Bonaguro L, ToVinh M, Raabe J, Astaburuaga-García R, et al. Early ifn-A signatures and persistent dysfunction are distinguishing features of nk cells in severe covid-19. Immunity (2021) 54(11):2650–69.e14. doi: 10.1016/j.immuni.2021.09.002
53. Zheng M, Gao Y, Wang G, Song G, Liu S, Sun D, et al. Functional exhaustion of antiviral lymphocytes in covid-19 patients. Cell Mol Immunol (2020) 17(5):533–5. doi: 10.1038/s41423-020-0402-2
54. Zhou R, To KK, Wong YC, Liu L, Zhou B, Li X, et al. Acute sars-Cov-2 infection impairs dendritic cell and T cell responses. Immunity (2020) 53(4):864–77.e5. doi: 10.1016/j.immuni.2020.07.026
55. Wang F, Nie J, Wang H, Zhao Q, Xiong Y, Deng L, et al. Characteristics of peripheral lymphocyte subset alteration in covid-19 pneumonia. J Infect Dis (2020) 221(11):1762–9. doi: 10.1093/infdis/jiaa150
56. Saresella M, Piancone F, Marventano I, Hernis A, Trabattoni D, Invernizzi M, et al. Innate immune responses to three doses of the Bnt162b2 mrna sars-Cov-2 vaccine. Front Immunol (2022) 13:947320. doi: 10.3389/fimmu.2022.947320
57. Zuo Y, Yalavarthi S, Shi H, Gockman K, Zuo M, Madison JA, et al. Neutrophil extracellular traps in covid-19. JCI Insight (2020) 5(11):e138999. doi: 10.1172/jci.insight.138999
58. Adamo S, Chevrier S, Cervia C, Zurbuchen Y, Raeber ME, Yang L, et al. Profound dysregulation of T cell homeostasis and function in patients with severe covid-19. Allergy (2021) 76(9):2866–81. doi: 10.1111/all.14866
59. Braun J, Loyal L, Frentsch M, Wendisch D, Georg P, Kurth F, et al. Sars-Cov-2-Reactive T cells in healthy donors and patients with covid-19. Nature (2020) 587(7833):270–4. doi: 10.1038/s41586-020-2598-9
60. Krammer F. Sars-Cov-2 vaccines in development. Nature (2020) 586(7830):516–27. doi: 10.1038/s41586-020-2798-3
61. Zhang C, Zhou C, Shi L, Liu G. Perspectives on development of vaccines against severe acute respiratory syndrome coronavirus 2 (Sars-Cov-2). Hum Vaccin Immunother (2020) 16(10):2366–9. doi: 10.1080/21645515.2020.1787064
62. Rydyznski Moderbacher C, Ramirez SI, Dan JM, Grifoni A, Hastie KM, Weiskopf D, et al. Antigen-specific adaptive immunity to sars-Cov-2 in acute covid-19 and associations with age and disease severity. Cell (2020) 183(4):996–1012 e19. doi: 10.1016/j.cell.2020.09.038
63. Tan AT, Linster M, Tan CW, Le Bert N, Chia WN, Kunasegaran K, et al. Early induction of functional sars-Cov-2-Specific T cells associates with rapid viral clearance and mild disease in covid-19 patients. Cell Rep (2021) 34(6):108728. doi: 10.1016/j.celrep.2021.108728
64. Zlei M, Sidorov IA, Joosten SA, Heemskerk MHM, Myeni SK, Pothast CR, et al. Immune determinants of viral clearance in hospitalised covid-19 patients: Reduced circulating naïve Cd4+ T cell counts correspond with delayed viral clearance. Cells (2022) 11(17):2743. doi: 10.3390/cells11172743
65. Fathi F, Sami R, Mozafarpoor S, Hafezi H, Motedayyen H, Arefnezhad R, et al. Immune system changes during covid-19 recovery play key role in determining disease severity. Int J Immunopathol Pharmacol (2020) 34:2058738420966497. doi: 10.1177/2058738420966497
66. Martonik D, Parfieniuk-Kowerda A, Rogalska M, Flisiak R. The role of Th17 response in covid-19. Cells (2021) 10(6):1550. doi: 10.3390/cells10061550
67. Wu D, Yang XO. Th17 responses in cytokine storm of covid-19: An emerging target of Jak2 inhibitor fedratinib. J microbiol immunol infection = Wei mian yu gan ran za zhi (2020) 53(3):368–70. doi: 10.1016/j.jmii.2020.03.005
68. Xu Z, Shi L, Wang Y, Zhang J, Huang L, Zhang C, et al. Pathological findings of covid-19 associated with acute respiratory distress syndrome. Lancet Respir Med (2020) 8(4):420–2. doi: 10.1016/s2213-2600(20)30076-x
69. Choto TA, Makupe I, Cakana AZ, Sibanda EN, Mduluza T. Excessive neutrophil recruitment promotes typical T-helper 17 responses in coronavirus disease 2019 patients. PloS One (2022) 17(8):e0273186. doi: 10.1371/journal.pone.0273186
70. De Biasi S, Meschiari M, Gibellini L, Bellinazzi C, Borella R, Fidanza L, et al. Marked T cell activation, senescence, exhaustion and skewing towards Th17 in patients with covid-19 pneumonia. Nat Commun (2020) 11(1):3434. doi: 10.1038/s41467-020-17292-4
71. Parackova Z, Bloomfield M, Klocperk A, Sediva A. Neutrophils mediate Th17 promotion in covid-19 patients. J Leukocyte Biol (2021) 109(1):73–6. doi: 10.1002/jlb.4covcra0820-481rrr
72. Kaneko N, Kuo HH, Boucau J, Farmer JR, Allard-Chamard H, Mahajan VS, et al. Loss of bcl-6-Expressing T follicular helper cells and germinal centers in covid-19. Cell (2020) 183(1):143–57.e13. doi: 10.1016/j.cell.2020.08.025
73. Shaan Lakshmanappa Y, Elizaldi SR, Roh JW, Schmidt BA, Carroll TD, Weaver KD, et al. Sars-Cov-2 induces robust germinal center Cd4 T follicular helper cell responses in rhesus macaques. Nat Commun (2021) 12(1):541. doi: 10.1038/s41467-020-20642-x
74. Zhang J, Wu Q, Liu Z, Wang Q, Wu J, Hu Y, et al. Spike-specific circulating T follicular helper cell and cross-neutralizing antibody responses in covid-19-Convalescent individuals. Nat Microbiol (2021) 6(1):51–8. doi: 10.1038/s41564-020-00824-5
75. Mudd PA, Minervina AA, Pogorelyy MV, Turner JS, Kim W, Kalaidina E, et al. Sars-Cov-2 mrna vaccination elicits a robust and persistent T follicular helper cell response in humans. Cell (2022) 185(4):603–13.e15. doi: 10.1016/j.cell.2021.12.026
76. Cavazzoni CB, Hanson BL, Podestà MA, Bechu ED, Clement RL, Zhang H, et al. Follicular T cells optimize the germinal center response to sars-Cov-2 protein vaccination in mice. Cell Rep (2022) 38(8):110399. doi: 10.1016/j.celrep.2022.110399
77. Meckiff BJ, Ramírez-Suástegui C, Fajardo V, Chee SJ, Kusnadi A, Simon H, et al. Imbalance of regulatory and cytotoxic sars-Cov-2-Reactive Cd4(+) T cells in covid-19. Cell (2020) 183(5):1340–53.e16. doi: 10.1016/j.cell.2020.10.001
78. Fenoglio D, Dentone C, Parodi A, Di Biagio A, Bozzano F, Vena A, et al. Characterization of T lymphocytes in severe covid-19 patients. J Med Virol (2021) 93(9):5608–13. doi: 10.1002/jmv.27037
79. Picchianti-Diamanti A, Aiello A, Laganà B, Agrati C, Castilletti C, Meschi S, et al. Immunosuppressivetherapies differently modulate humoral- and T-Cell-Specific responses to covid-19 mrna vaccine in rheumatoid arthritis patients. Front Immunol (2021) 12:740249. doi: 10.3389/fimmu.2021.740249
80. Riou C, du Bruyn E, Stek C, Daroowala R, Goliath RT, Abrahams F, et al. Relationship of sars-Cov-2-Specific Cd4 response to covid-19 severity and impact of hiv-1 and tuberculosis coinfection. J Clin Invest (2021) 131(12):e149125. doi: 10.1172/jci149125
81. Corthay A. How do regulatory T cells work? Scandinavian J Immunol (2009) 70(4):326–36. doi: 10.1111/j.1365-3083.2009.02308.x
82. Galvan-Pena S, Leon J, Chowdhary K, Michelson DA, Vijaykumar B, Yang L, et al. Profound treg perturbations correlate with covid-19 severity. Proc Natl Acad Sci U.S.A. (2021) 118(37):e2111315118. doi: 10.1073/pnas.2111315118
83. Ronit A, Berg RMG, Bay JT, Haugaard AK, Ahlstrom MG, Burgdorf KS, et al. Compartmental immunophenotyping in covid-19 Ards: A case series. J Allergy Clin Immunol (2021) 147(1):81–91. doi: 10.1016/j.jaci.2020.09.009
84. Taefehshokr N, Taefehshokr S, Heit B. Mechanisms of dysregulated humoral and cellular immunity by sars-Cov-2. Pathog (Basel Switzerland) (2020) 9(12):1027. doi: 10.3390/pathogens9121027
85. Kratzer B, Trapin D, Ettel P, Kormoczi U, Rottal A, Tuppy F, et al. Immunological imprint of covid-19 on human peripheral blood leukocyte populations. Allergy (2021) 76(3):751–65. doi: 10.1111/all.14647
86. Mohebbi SR, Baghaei K, Rostami-Nejad M, Nazemalhosseini Mojarad E, Mirjalali H, Yadegar A, et al. Significant changes of Cd4, Foxp3, Cd25, and Il6 expression level in Iranian covid-19 patients. Gastroenterol Hepatol bed to bench (2020) 13(4):388–92.
87. Jia R, Wang X, Liu P, Liang X, Ge Y, Tian H, et al. Mild cytokine elevation, moderate Cd4(+) T cell response and abundant antibody production in children with covid-19. Virologica Sin (2020) 35(6):734–43. doi: 10.1007/s12250-020-00265-8
88. Seepathomnarong P, Ongarj J, Sophonmanee R, Seeyankem B, Chusri S, Surasombatpattana S, et al. Regulatory T cells decreased during recovery from mild covid-19. Viruses (2022) 14(8):1688. doi: 10.3390/v14081688
89. Wiech M, Chroscicki P, Swatler J, Stepnik D, De Biasi S, Hampel M, et al. Remodeling of T cell dynamics during long covid is dependent on severity of sars-Cov-2 infection. Front Immunol (2022) 13:886431. doi: 10.3389/fimmu.2022.886431
90. Stephen-Victor E, Das M, Karnam A, Pitard B, Gautier JF, Bayry J. Potential of regulatory T-Cell-Based therapies in the management of severe covid-19. Eur Respir J (2020) 56(3):2002182. doi: 10.1183/13993003.02182-2020
91. Mazzoni A, Salvati L, Maggi L, Capone M, Vanni A, Spinicci M, et al. Impaired immune cell cytotoxicity in severe covid-19 is il-6 dependent. J Clin Invest (2020) 130(9):4694–703. doi: 10.1172/jci138554
92. Liu J, Yu J, McMahan K, Jacob-Dolan C, He X, Giffin V, et al. Cd8 T cells contribute to vaccine protection against sars-Cov-2 in macaques. Sci Immunol (2022) 7(77):eabq7647. doi: 10.1126/sciimmunol.abq7647
93. Song JW, Zhang C, Fan X, Meng FP, Xu Z, Xia P, et al. Immunological and inflammatory profiles in mild and severe cases of covid-19. Nat Commun (2020) 11(1):3410. doi: 10.1038/s41467-020-17240-2
94. Zheng HY, Zhang M, Yang CX, Zhang N, Wang XC, Yang XP, et al. Elevated exhaustion levels and reduced functional diversity of T cells in peripheral blood may predict severe progression in covid-19 patients. Cell Mol Immunol (2020) 17(5):541–3. doi: 10.1038/s41423-020-0401-3
95. Diao B, Wang C, Tan Y, Chen X, Liu Y, Ning L, et al. Reduction and functional exhaustion of T cells in patients with coronavirus disease 2019 (Covid-19). Front Immunol (2020) 11:827. doi: 10.3389/fimmu.2020.00827
96. Rha MS, Jeong HW, Ko JH, Choi SJ, Seo IH, Lee JS, et al. Pd-1-Expressing sars-Cov-2-Specific Cd8(+) T cells are not exhausted, but functional in patients with covid-19. Immunity (2021) 54(1):44–52.e3. doi: 10.1016/j.immuni.2020.12.002
97. Szabo PA, Dogra P, Gray JI, Wells SB, Connors TJ, Weisberg SP, et al. Longitudinal profiling of respiratory and systemic immune responses reveals myeloid cell-driven lung inflammation in severe covid-19. Immunity (2021) 54(4):797–814.e6. doi: 10.1016/j.immuni.2021.03.005
98. Kuse N, Zhang Y, Chikata T, Nguyen HT, Oka S, Gatanaga H, et al. Long-term memory Cd8(+) T cells specific for sars-Cov-2 in individuals who received the Bnt162b2 mrna vaccine. Nat Commun (2022) 13(1):5251. doi: 10.1038/s41467-022-32989-4
99. McMahan K, Yu J, Mercado NB, Loos C, Tostanoski LH, Chandrashekar A, et al. Correlates of protection against sars-Cov-2 in rhesus macaques. Nature (2021) 590(7847):630–4. doi: 10.1038/s41586-020-03041-6
100. Peng Y, Mentzer AJ, Liu G, Yao X, Yin Z, Dong D, et al. Broad and strong memory Cd4(+) and Cd8(+) T cells induced by sars-Cov-2 in uk convalescent individuals following covid-19. Nat Immunol (2020) 21(11):1336–45. doi: 10.1038/s41590-020-0782-6
101. Kalimuddin S, Tham CYL, Qui M, de Alwis R, Sim JXY, Lim JME, et al. Early T cell and binding antibody responses are associated with covid-19 rna vaccine efficacy onset. Med (N Y) (2021) 2(6):682–8.e4. doi: 10.1016/j.medj.2021.04.003
102. Rydyznski Moderbacher C, Kim CJ, Mateus J, Plested JS, Zhu M, Cloney-Clark S, et al. Nvx-Cov2373 vaccination induces functional sars-Cov-2-Specific Cd4+ and Cd8+ T cell responses. J Clin Invest (2022) 132(19):e160898. doi: 10.1172/jci160898
103. Reinscheid M, Luxenburger H, Karl V, Graeser A, Giese S, Ciminski K, et al. Covid-19 mrna booster vaccine induces transient Cd8+ T effector cell responses while conserving the memory pool for subsequent reactivation. Nat Commun (2022) 13(1):4631. doi: 10.1038/s41467-022-32324-x
104. Oberhardt V, Luxenburger H, Kemming J, Schulien I, Ciminski K, Giese S, et al. Rapid and stable mobilization of Cd8(+) T cells by sars-Cov-2 mrna vaccine. Nature (2021) 597(7875):268–73. doi: 10.1038/s41586-021-03841-4
105. Sosa-Hernández VA, Torres-Ruíz J, Cervantes-Díaz R, Romero-Ramírez S, Páez-Franco JC, Meza-Sánchez DE, et al. B cell subsets as severity-associated signatures in covid-19 patients. Front Immunol (2020) 11:611004. doi: 10.3389/fimmu.2020.611004
106. Cao T, Liu L, To KK, Lim CY, Zhou R, Ming Y, et al. Mitochondrial regulation of acute extrafollicular b-cell responses to covid-19 severity. Clin Trans Med (2022) 12(9):e1025. doi: 10.1002/ctm2.1025
107. Huang C. Germinal center reaction. Adv Exp Med Biol (2020) 1254:47–53. doi: 10.1007/978-981-15-3532-1_4
108. Ryg-Cornejo V, Ioannidis LJ, Ly A, Chiu CY, Tellier J, Hill DL, et al. Severe malaria infections impair germinal center responses by inhibiting T follicular helper cell differentiation. Cell Rep (2016) 14(1):68–81. doi: 10.1016/j.celrep.2015.12.006
109. Popescu M, Cabrera-Martinez B, Winslow GM. Tnf-A contributes to lymphoid tissue disorganization and germinal center b cell suppression during intracellular bacterial infection. J Immunol (Baltimore Md 1950) (2019) 203(9):2415–24. doi: 10.4049/jimmunol.1900484
110. Rodda LB, Netland J, Shehata L, Pruner KB, Morawski PA, Thouvenel CD, et al. Functional sars-Cov-2-Specific immune memory persists after mild covid-19. Cell (2021) 184(1):169–83.e17. doi: 10.1016/j.cell.2020.11.029
111. Ogega CO, Skinner NE, Blair PW, Park HS, Littlefield K, Ganesan A, et al. Durable sars-Cov-2 b cell immunity after mild or severe disease. J Clin Invest (2021) 131(7):e145516. doi: 10.1172/jci145516
112. Sokal A, Broketa M, Barba-Spaeth G, Meola A, Fernández I, Fourati S, et al. Analysis of mrna vaccination-elicited rbd-specific memory b cells reveals strong but incomplete immune escape of the sars-Cov-2 omicron variant. Immunity (2022) 55(6):1096–104.e4. doi: 10.1016/j.immuni.2022.04.002
113. Awadasseid A, Wu Y, Tanaka Y, Zhang W. Current advances in the development of sars-Cov-2 vaccines. Int J Biol Sci (2021) 17(1):8–19. doi: 10.7150/ijbs.52569
114. Gao Q, Bao L, Mao H, Wang L, Xu K, Yang M, et al. Development of an inactivated vaccine candidate for sars-Cov-2. Science (2020) 369(6499):77–81. doi: 10.1126/science.abc1932
115. Zhang Y, Zeng G, Pan H, Li C, Hu Y, Chu K, et al. Safety, tolerability, and immunogenicity of an inactivated sars-Cov-2 vaccine in healthy adults aged 18-59 years: A randomised, double-blind, placebo-controlled, phase 1/2 clinical trial. Lancet Infect Dis (2021) 21(2):181–92. doi: 10.1016/s1473-3099(20)30843-4
116. Wu Z, Hu Y, Xu M, Chen Z, Yang W, Jiang Z, et al. Safety, tolerability, and immunogenicity of an inactivated sars-Cov-2 vaccine (Coronavac) in healthy adults aged 60 years and older: A randomised, double-blind, placebo-controlled, phase 1/2 clinical trial. Lancet Infect Dis (2021) 21(6):803–12. doi: 10.1016/s1473-3099(20)30987-7
117. Han B, Song Y, Li C, Yang W, Ma Q, Jiang Z, et al. Safety, tolerability, and immunogenicity of an inactivated sars-Cov-2 vaccine (Coronavac) in healthy children and adolescents: A double-blind, randomised, controlled, phase 1/2 clinical trial. Lancet Infect Dis (2021) 21(12):1645–53. doi: 10.1016/s1473-3099(21)00319-4
118. Tanriover MD, Doganay HL, Akova M, Guner HR, Azap A, Akhan S, et al. Efficacy and safety of an inactivated whole-virion sars-Cov-2 vaccine (Coronavac): Interim results of a double-blind, randomised, placebo-controlled, phase 3 trial in Turkey. Lancet (2021) 398(10296):213–22. doi: 10.1016/S0140-6736(21)01429-X
119. Costa Clemens SA, Weckx L, Clemens R, Almeida Mendes AV, Ramos Souza A, Silveira MBV, et al. Heterologous versus homologous covid-19 booster vaccination in previous recipients of two doses of coronavac covid-19 vaccine in Brazil (Rhh-001): A phase 4, non-inferiority, single blind, randomised study. Lancet (2022) 399(10324):521–9. doi: 10.1016/S0140-6736(22)00094-0
120. Polack FP, Thomas SJ, Kitchin N, Absalon J, Gurtman A, Lockhart S, et al. Safety and efficacy of the Bnt162b2 mrna covid-19 vaccine. New Engl J Med (2020) 383(27):2603–15. doi: 10.1056/NEJMoa2034577
121. Baden LR, El Sahly HM, Essink B, Kotloff K, Frey S, Novak R, et al. Efficacy and safety of the mrna-1273 sars-Cov-2 vaccine. New Engl J Med (2021) 384(5):403–16. doi: 10.1056/NEJMoa2035389
122. Zhu FC, Li YH, Guan XH, Hou LH, Wang WJ, Li JX, et al. Safety, tolerability, and immunogenicity of a recombinant adenovirus type-5 vectored covid-19 vaccine: A dose-escalation, open-label, non-randomised, first-in-Human trial. Lancet (2020) 395(10240):1845–54. doi: 10.1016/S0140-6736(20)31208-3
123. Zhu FC, Guan XH, Li YH, Huang JY, Jiang T, Hou LH, et al. Immunogenicity and safety of a recombinant adenovirus type-5-Vectored covid-19 vaccine in healthy adults aged 18 years or older: A randomised, double-blind, placebo-controlled, phase 2 trial. Lancet (2020) 396(10249):479–88. doi: 10.1016/S0140-6736(20)31605-6
124. Zhu F, Jin P, Zhu T, Wang W, Ye H, Pan H, et al. Safety and immunogenicity of a recombinant adenovirus type-5-Vectored covid-19 vaccine with a homologous prime-boost regimen in healthy participants aged 6 years and above: A randomised, double-blind, placebo-controlled, phase 2b trial. Clin Infect Dis (2021) 75(1):e783–91. doi: 10.1093/cid/ciab845
125. Hung IFN, Poland GA. Single-dose Oxford-astrazeneca covid-19 vaccine followed by a 12-week booster. Lancet (2021) 397(10277):854–5. doi: 10.1016/S0140-6736(21)00528-6
126. Folegatti PM, Ewer KJ, Aley PK, Angus B, Becker S, Belij-Rammerstorfer S, et al. Safety and immunogenicity of the Chadox1 ncov-19 vaccine against sars-Cov-2: A preliminary report of a phase 1/2, single-blind, randomised controlled trial. Lancet (2020) 396(10249):467–78. doi: 10.1016/S0140-6736(20)31604-4
127. Yang S, Li Y, Dai L, Wang J, He P, Li C, et al. Safety and immunogenicity of a recombinant tandem-repeat dimeric rbd-based protein subunit vaccine (Zf2001) against covid-19 in adults: Two randomised, double-blind, placebo-controlled, phase 1 and 2 trials. Lancet Infect Dis (2021) 21(8):1107–19. doi: 10.1016/S1473-3099(21)00127-4
128. Cao Y, Yisimayi A, Bai Y, Huang W, Li X, Zhang Z, et al. Humoral immune response to circulating sars-Cov-2 variants elicited by inactivated and rbd-subunit vaccines. Cell Res (2021) 31(7):732–41. doi: 10.1038/s41422-021-00514-9
129. Zhang Z, Mateus J, Coelho CH, Dan JM, Moderbacher CR, Gálvez RI, et al. Humoral and cellular immune memory to four covid-19 vaccines. Cell (2022) 185(14):2434–51.e17. doi: 10.1016/j.cell.2022.05.022
130. Suryawanshi RK, Chen IP, Ma T, Syed AM, Brazer N, Saldhi P, et al. Limited cross-variant immunity from sars-Cov-2 omicron without vaccination. Nature (2022) 607(7918):351–5. doi: 10.1038/s41586-022-04865-0
131. Ranzani OT, Hitchings MDT, Dorion M, D'Agostini TL, de Paula RC, de Paula OFP, et al. Effectiveness of the coronavac vaccine in older adults during a gamma variant associated epidemic of covid-19 in Brazil: Test negative case-control study. BMJ (Clinical Res ed) (2021) 374:n2015. doi: 10.1136/bmj.n2015
132. Madhi SA, Baillie V, Cutland CL, Voysey M, Koen AL, Fairlie L, et al. Efficacy of the Chadox1 ncov-19 covid-19 vaccine against the b. 1.351 variant. New Engl J Med (2021) 384(20):1885–98. doi: 10.1056/NEJMoa2102214
133. Lopez Bernal J, Andrews N, Gower C, Gallagher E, Simmons R, Thelwall S, et al. Effectiveness of covid-19 vaccines against the B.1.617.2 (Delta) variant. New Engl J Med (2021) 385(7):585–94. doi: 10.1056/NEJMoa2108891
134. Cheng SMS, Mok CKP, Leung YWY, Ng SS, Chan KCK, Ko FW, et al. Neutralizing antibodies against the sars-Cov-2 omicron variant Ba.1 following homologous and heterologous coronavac or Bnt162b2 vaccination. Nat Med (2022) 28(3):486–9. doi: 10.1038/s41591-022-01704-7
135. Lapointe HR, Mwimanzi F, Cheung PK, Sang Y, Yaseen F, Kalikawe R, et al. Serial infection with sars-Cov-2 omicron Ba.1 and Ba.2 following three-dose covid-19 vaccination. Front Immunol (2022) 13:947021. doi: 10.3389/fimmu.2022.947021
136. Hajnik RL, Plante JA, Liang Y, Alameh MG, Tang J, Bonam SR, et al. Dual spike and nucleocapsid mrna vaccination confer protection against sars-Cov-2 omicron and delta variants in preclinical models. Sci Trans Med (2022) 14(662):eabq1945. doi: 10.1126/scitranslmed.abq1945
137. Barchuk A, Cherkashin M, Bulina A, Berezina N, Rakova T, Kuplevatskaya D, et al. Vaccine effectiveness against referral to hospital after sars-Cov-2 infection in st. Petersburg, Russia, during the delta variant surge: A test-negative case-control study. BMC Med (2022) 20(1):312. doi: 10.1186/s12916-022-02509-8
138. Drożdżal S, Rosik J, Lechowicz K, Machaj F, Szostak B, Przybyciński J, et al. An update on drugs with therapeutic potential for sars-Cov-2 (Covid-19) treatment. Drug resistance updates Rev commentaries antimicrobial Anticancer chemother (2021) 59:100794. doi: 10.1016/j.drup.2021.100794
139. Kuritzkes DR. Bamlanivimab for prevention of covid-19. Jama (2021) 326(1):31–2. doi: 10.1001/jama.2021.7515
140. Chew KW, Moser C, Daar ES, Wohl DA, Li JZ, Coombs RW, et al. Antiviral and clinical activity of bamlanivimab in a randomized trial of non-hospitalized adults with covid-19. Nat Commun (2022) 13(1):4931. doi: 10.1038/s41467-022-32551-2
141. Chen P, Nirula A, Heller B, Gottlieb RL, Boscia J, Morris J, et al. Sars-Cov-2 neutralizing antibody ly-Cov555 in outpatients with covid-19. New Engl J Med (2021) 384(3):229–37. doi: 10.1056/NEJMoa2029849
142. Dougan M, Nirula A, Azizad M, Mocherla B, Gottlieb RL, Chen P, et al. Bamlanivimab plus etesevimab in mild or moderate covid-19. New Engl J Med (2021) 385(15):1382–92. doi: 10.1056/NEJMoa2102685
143. Gupta A, Gonzalez-Rojas Y, Juarez E, Crespo Casal M, Moya J, Falci DR, et al. Early treatment for covid-19 with sars-Cov-2 neutralizing antibody sotrovimab. New Engl J Med (2021) 385(21):1941–50. doi: 10.1056/NEJMoa2107934
145. RECOVERY Collaborative Group. Casirivimab and imdevimab in patients admitted to hospital with covid-19 (Recovery): A randomised, controlled, open-label, platform trial. Lancet (2022) 399(10325):665–76. doi: 10.1016/s0140-6736(22)00163-5
146. Malgie J, Schoones JW, Pijls BG. Decreased mortality in coronavirus disease 2019 patients treated with tocilizumab: A rapid systematic review and meta-analysis of observational studies. Clin Infect Dis (2021) 72(11):e742–e9. doi: 10.1093/cid/ciaa1445
147. Lamb YN. Remdesivir: First approval. Drugs (2020) 80(13):1355–63. doi: 10.1007/s40265-020-01378-w
148. Wang Y, Zhang D, Du G, Du R, Zhao J, Jin Y, et al. Remdesivir in adults with severe covid-19: A randomised, double-blind, placebo-controlled, multicentre trial. Lancet (2020) 395(10236):1569–78. doi: 10.1016/s0140-6736(20)31022-9
149. Lai CC, Chen CH, Wang CY, Chen KH, Wang YH, Hsueh PR. Clinical efficacy and safety of remdesivir in patients with covid-19: A systematic review and network meta-analysis of randomized controlled trials. J Antimicrobial Chemother (2021) 76(8):1962–8. doi: 10.1093/jac/dkab093
150. Hanafusa M, Nawa N, Goto Y, Kawahara T, Miyamae S, Ueki Y, et al. Effectiveness of remdesivir with corticosteroids for covid-19 patients in intensive care unit: A hospital-based observational study. J Med Virol (2022). doi: 10.1002/jmv.28168
151. Stebbing J, Krishnan V, de Bono S, Ottaviani S, Casalini G, Richardson PJ, et al. Mechanism of baricitinib supports artificial intelligence-predicted testing in covid-19 patients. EMBO Mol Med (2020) 12(8):e12697. doi: 10.15252/emmm.202012697
152. Cantini F, Niccoli L, Nannini C, Matarrese D, Natale MED, Lotti P, et al. Beneficial impact of baricitinib in covid-19 moderate pneumonia; multicentre study. J infection (2020) 81(4):647–79. doi: 10.1016/j.jinf.2020.06.052
153. Dowty ME, Lin J, Ryder TF, Wang W, Walker GS, Vaz A, et al. The pharmacokinetics, metabolism, and clearance mechanisms of tofacitinib, a janus kinase inhibitor, in humans. Drug Metab disposition: Biol fate chemicals (2014) 42(4):759–73. doi: 10.1124/dmd.113.054940
154. Guimarães PO, Quirk D, Furtado RH, Maia LN, Saraiva JF, Antunes MO, et al. Tofacitinib in patients hospitalized with covid-19 pneumonia. New Engl J Med (2021) 385(5):406–15. doi: 10.1056/NEJMoa2101643
155. Extance A. Covid-19: What is the evidence for the antiviral paxlovid? BMJ (Clinical Res ed) (2022) 377:o1037. doi: 10.1136/bmj.o1037
156. Halford B. The path to paxlovid. ACS Cent Sci (2022) 8(4):405–7. doi: 10.1021/acscentsci.2c00369
157. Mahase E. Covid-19: Pfizer's paxlovid is 89% effective in patients at risk of serious illness, company reports. BMJ (Clinical Res ed) (2021) 375:n2713. doi: 10.1136/bmj.n2713
158. Sun F, Lin Y, Wang X, Gao Y, Ye S. Paxlovid in patients who are immunocompromised and hospitalised with sars-Cov-2 infection. Lancet Infect Dis (2022) 22(9):1279. doi: 10.1016/s1473-3099(22)00430-3
159. Burki T. The future of paxlovid for covid-19. Lancet Respir Med (2022) 10(7):e68. doi: 10.1016/s2213-2600(22)00192-8
160. Graham F. Daily briefing: Inside merck's covid drug, molnupiravir. Nature (2021). doi: 10.1038/d41586-021-02792-0
161. Johnson MG, Puenpatom A, Moncada PA, Burgess L, Duke ER, Ohmagari N, et al. Effect of molnupiravir on biomarkers, respiratory interventions, and medical services in covid-19 : A randomized, placebo-controlled trial. Ann Internal Med (2022) 175(8):1126–34. doi: 10.7326/m22-0729
162. Jayk Bernal A, Gomes da Silva MM, Musungaie DB, Kovalchuk E, Gonzalez A, Delos Reyes V, et al. Molnupiravir for oral treatment of covid-19 in nonhospitalized patients. New Engl J Med (2022) 386(6):509–20. doi: 10.1056/NEJMoa2116044
163. Mahase E. Covid-19: Molnupiravir reduces risk of hospital admission or death by 50% in patients at risk, msd reports. BMJ (Clinical Res ed) (2021) 375:n2422. doi: 10.1136/bmj.n2422
164. Fischer WA 2nd, Eron JJ Jr., Holman W, MS C, Fang L, LJ S, et al. A phase 2a clinical trial of molnupiravir in patients with covid-19 shows accelerated sars-Cov-2 rna clearance and elimination of infectious virus. Sci Trans Med (2022) 14(628):eabl7430. doi: 10.1126/scitranslmed.abl7430
165. Li P, Wang Y, Lavrijsen M, Lamers MM, de Vries AC, Rottier RJ, et al. Sars-Cov-2 omicron variant is highly sensitive to molnupiravir, nirmatrelvir, and the combination. Cell Res (2022) 32(3):322–4. doi: 10.1038/s41422-022-00618-w
166. Sun L, Peng Y, Yu W, Zhang Y, Liang L, Song C, et al. Mechanistic insight into antiretroviral potency of 2'-Deoxy-2'-B-Fluoro-4'-Azidocytidine (Fnc) with a long-lasting effect on hiv-1 prevention. J medicinal Chem (2020) 63(15):8554–66. doi: 10.1021/acs.jmedchem.0c00940
167. Ren Z, Luo H, Yu Z, Song J, Liang L, Wang L, et al. A randomized, open-label, controlled clinical trial of azvudine tablets in the treatment of mild and common covid-19, a pilot study. Advanced Sci (Weinheim Baden-Wurttemberg Germany) (2020) 7(19):e2001435. doi: 10.1002/advs.202001435
168. Yu B, Chang J. Azvudine (Fnc): A promising clinical candidate for covid-19 treatment. Signal Transduct Target Ther (2020) 5(1):236. doi: 10.1038/s41392-020-00351-z
169. Zhang JL, Li YH, Wang LL, Liu HQ, Lu SY, Liu Y, et al. Azvudine is a thymus-homing anti-Sars-Cov-2 drug effective in treating covid-19 patients. Signal Transduct Target Ther (2021) 6(1):414. doi: 10.1038/s41392-021-00835-6
170. Humphries F, Shmuel-Galia L, Jiang Z, Wilson R, Landis P, Ng SL, et al. A diamidobenzimidazole sting agonist protects against sars-Cov-2 infection. Sci Immunol (2021) 6(59):eabi9002. doi: 10.1126/sciimmunol.abi9002
171. Li M, Ferretti M, Ying B, Descamps H, Lee E, Dittmar M, et al. Pharmacological activation of sting blocks sars-Cov-2 infection. Sci Immunol (2021) 6(59):eabi9007. doi: 10.1126/sciimmunol.abi9007
172. Hu K, Guan WJ, Bi Y, Zhang W, Li L, Zhang B, et al. Efficacy and safety of lianhua qingwen capsules, a repurposed Chinese herb, in patients with coronavirus disease 2019: A multicenter, prospective, randomized controlled trial [Phytomedicine 85 (2021) 153242]. Phytomedicine (2022) 94:153800. doi: 10.1016/j.phymed.2021.153800
Keywords: COVID-19, SARS-CoV-2, immune responses, vaccination, treatment
Citation: Zhu Q, Xu Y, Wang T and Xie F (2022) Innate and adaptive immune response in SARS-CoV-2 infection-Current perspectives. Front. Immunol. 13:1053437. doi: 10.3389/fimmu.2022.1053437
Received: 25 September 2022; Accepted: 09 November 2022;
Published: 22 November 2022.
Edited by:
Ralf Braun, Danube Private University, AustriaReviewed by:
Stephen Crooke, Centers for Disease Control and Prevention (CDC), United StatesCopyright © 2022 Zhu, Xu, Wang and Xie. This is an open-access article distributed under the terms of the Creative Commons Attribution License (CC BY). The use, distribution or reproduction in other forums is permitted, provided the original author(s) and the copyright owner(s) are credited and that the original publication in this journal is cited, in accordance with accepted academic practice. No use, distribution or reproduction is permitted which does not comply with these terms.
*Correspondence: Feiting Xie, eGllZmVpdGluZ0B6anUuZWR1LmNu
Disclaimer: All claims expressed in this article are solely those of the authors and do not necessarily represent those of their affiliated organizations, or those of the publisher, the editors and the reviewers. Any product that may be evaluated in this article or claim that may be made by its manufacturer is not guaranteed or endorsed by the publisher.
Research integrity at Frontiers
Learn more about the work of our research integrity team to safeguard the quality of each article we publish.