- Department of Oncology, The First Affiliated Hospital of Zhengzhou University, Zhengzhou, China
Multiple myeloma (MM) is a malignant plasma cell disorder that remains incurable for most patients, as persistent clonal evolution drives new mutations which confer MM high-risk signatures and resistance to standard care. The past two decades have significantly refashioned the therapeutic options for MM, especially adoptive T cell therapy contributing to impressive response rate and clinical efficacy. Despite great promises achieved from chimeric antigen receptor T-cell (CAR-T) therapy, the poor durability and severe toxicity (cytokine release syndrome and neurotoxicity) are still huge challenges. Therefore, relapsed/refractory multiple myeloma (RRMM), characterized by the nature of clinicopathologic and molecular heterogeneity, is frequently associated with poor prognosis. B Cell Maturation Antigen (BCMA) is the most successful target for CAR-T therapy, and other potential targets either for single-target or dual-target CAR-T are actively being studied in numerous clinical trials. Moreover, mechanisms driving resistance or relapse after CAR-T therapy remain uncharacterized, which might refer to T-cell clearance, antigen escape, and immunosuppressive tumor microenvironment. Engineering CAR T-cell to improve both efficacy and safety continues to be a promising area for investigation. In this review, we aim to describe novel tumor-associated neoantigens for MM, summarize the data from current MM CAR-T clinical trials, introduce the mechanism of disease resistance/relapse after CAR-T infusion, highlight innovations capable of enhanced efficacy and reduced toxicity, and provide potential directions to optimize manufacturing processes.
1. Introduction
Multiple myeloma (MM) is a malignant plasma cell disorder that displays a myriad of manifestations including hypercalcemia, renal insufficiency, anemia, and bone destruction (CRAB) (1, 2). MM is the second most common hematological malignancy with an estimated 32270 new cases and 12830 deaths in the United States in 2020 (3). Genetic abnormities, mostly translocation and hyper-diploidy, result in dysregulated cancer-immunity cycle that allows MM to escape immune surveillance with an uncontrolled cell proliferation (4, 5). The past two decades have significantly refashioned the therapeutic options of MM, such as the availability of proteasome inhibitors (PI), immunomodulatory drugs (IMiDs), histone deacetylase inhibitors (HDACi), anti-CD38 monoclonal antibodies (mABs), antibody-drug conjugates (ADC), and selective inhibitors nuclear export (SINE) (6). However, MM remains incurable for most patients, as persistent clonal evolution drives new mutations which confer MM high-risk signatures and resistance to standard care (7, 8). Therefore, relapsed/refractory multiple myeloma (RRMM), characterized by the nature of clinicopathologic and molecular heterogeneity (9, 10), is frequently associated with poor prognosis (11).
Chimeric antigen receptor T-cell therapy (CAR-T) has shown exceptional success in the treatment of relapsed/refractory B-cell acute lymphoblastic leukaemia (B-ALL), B-cell chronic lymphoblastic leukaemia (B-CLL), and diffuse large B-cell Lymphoma (DLBCL) (12, 13), thereby motivating its application in RRMM (14). T cells are firstly isolated from the patients’ or donors’ blood and genetically modified in the laboratory to encode an artificial receptor, enabling CAR T cells to identify targets better and precisely destroy cancer cells. CAR T-cell functions with two major roles: 1) tumor-associated antigen (TAA) binding; 2) MHC-independent T-cell activation. Emerging as a novel immunotherapy, CAR T-cell therapy consists of an extracellular antigen recognition domain (scFv, Fab, Nb, and NKG2D ligand), a transmembrane domain, and an intracellular domain incorporating co-stimulation (CD28 or a 4-1BB) and signaling components (CD3zeta) (Figure 1) (15, 16). The interplay between tumor cell and CAR gives rise to an immunological synapse. This process could attack target cells through various pathways, such as the release of cytotoxic molecules, and the induction of apoptosis signal pathway, eventually leading to the activation of effector T cells and elimination of tumor cells (17).
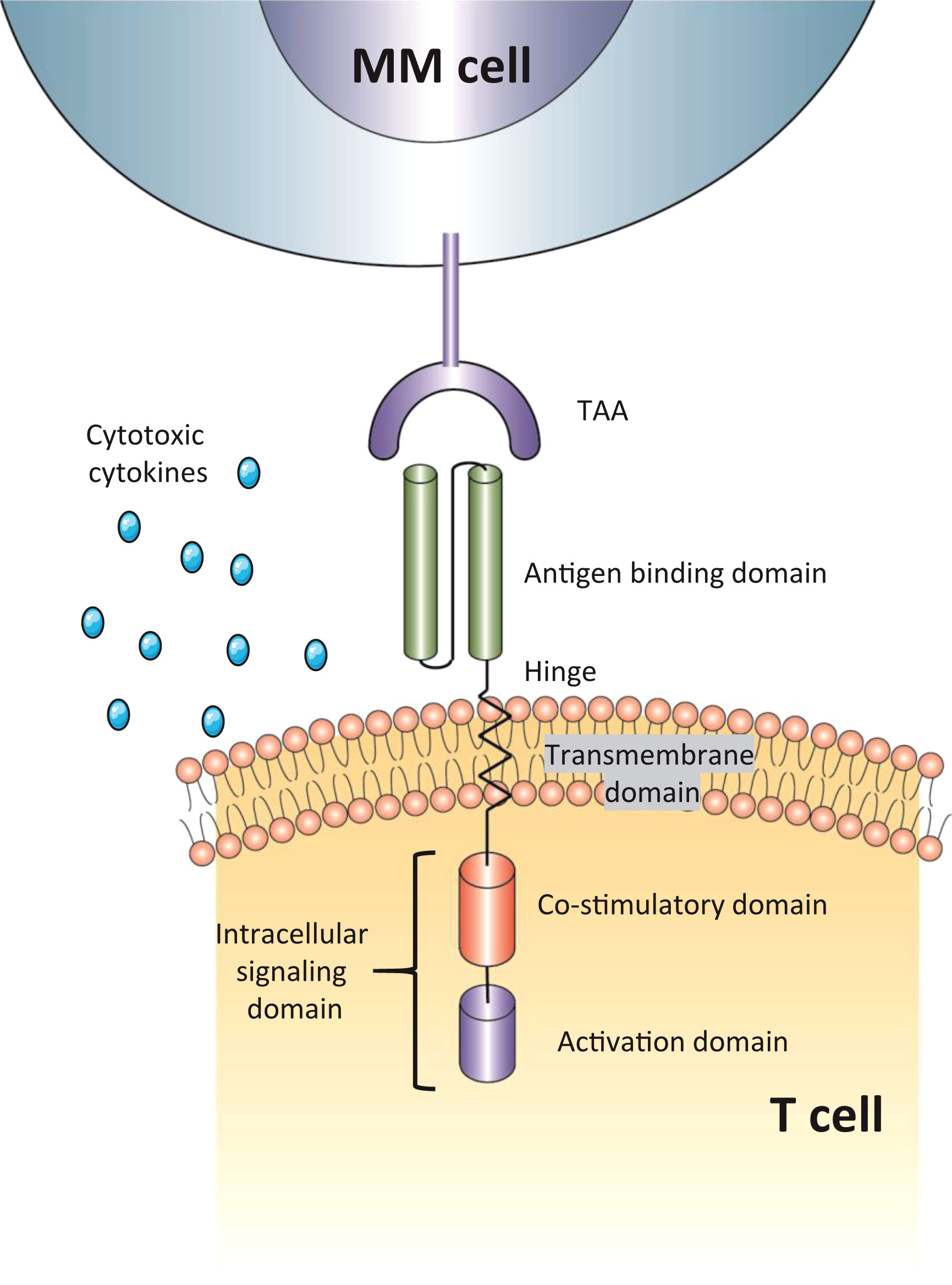
Figure 1 The structure of CAR-T cells. The antigen-binding domain is usually a single-chain variable fragment (scFv) but also has other structures; intracellular signaling domain include both co-stimulatory domain (CD28, 4-1BB) and activation domain (CD3zeta). TAA, tumor-associated antigen; MM cell, multiple myeloma cell.
Despite great promises achieved by CAR-T therapy, the poor durability and severe toxicity are still huge challenges. The mechanisms driving resistance and relapse after MM CAR T-cell therapy remain uncharacterized. Consequently, this review aims to describe candidate tumor-associated neoantigens for MM, provide a summary of efficacy and safety data from clinical trials, introduce mechanisms of disease resistance/relapse to CAR-T, and explore future innovations capable of enhanced efficacy and reduced toxicity, and provide potential directions to optimize manufacturing processes.
2. Candidate targets for multiple myeloma CAR-T
The key to design a successful CAR is to select a surface antigen that presents at high concentration on MM cells, but absent in non-malignant hematopoietic lineages or other tissues (18–21). The most important avenue is to discover novel TAAs to improve CAR-T therapy. Several targetable antigens are currently being evaluated regarding their safety and efficacy in clinical trials (Tables 1, 2). Potential targetable antigens for MM are summarized in Figure 2, including BCMA, CD19, SLAMF7, GPRC5D, CD138, CD38, CD70, NKG2DL, Kappa light chain.
2.1. BCMA
B cell maturation antigen (BCMA), a transmembrane glycoprotein belonging to tumor necrosis factor (TNF) receptor superfamily, is the most commonly used surface antigen target for multiple myeloma CAR-T. BCMA plays a critical role in differentiating B-cell to plasma cell and maintaining the survival of plasma cell (34, 35). BCMA is preferentially expressed on plasma cells, though limited BCMA-positive cells can be identified in normal tissues, such as the spleen, lymph nodes, and the stomach (36, 37). A European study involving 70 MM patients identified that surface BCMA expression on plasma cells (normal or malignant) was significantly higher (P<0.001) than non-plasma cells (38). The high expression of surface BCMA is associated with MM in several preclinical models and humans, making it an attractive target for MM (39–41). However, BCMA could be expressed at high or low concentrations in MM cells (36, 42, 43). In a United Kingdom study, 28 evaluable MM patients all expressed BCMA, and levels differed from low to moderate (42). Similarly, a UK study reported that all 64 patients with MM expressed surface BCMA at varying levels by immunohistochemistry (43). Since there is a considerable variation in BCMA expression on MM cells, patients may respond differently to BCMA-targeted CAR-T therapy. As surface BCMA level may serve as an independent prognostic factor, cytogenetic assessments are of great importance (43). It is anticipated that patients with high levels of BCMA may gain more benefits from BCMA-targeted CAR-T therapy. Thereby, all findings support that BCMA may be a promising target for MM CAR-T therapy.
The first BCMA-targeted MM CAR-T clinical trial was conducted by National Cancer Institute (NCT02215967) (44). A total of 24 patients with RRMM were enrolled. The notable findings of this study were the dose-dependence of efficacy and toxicity. The ORR was 20% among 10 patients receiving the lowest dose of 0.3-3.0 × 106 CAR-T cells/kg. However, of 16 patients treated with high-dose level, the ORR was 81% with 62.5% having very good partial response (VEGF) or better. Notably, the toxicity of low-dose CAR-T was generally modest and no patient with grade 3 or 4 cytokine release syndrome (CRS). By contrast, grade 3-4 CRS and neurotoxicity (NTX) were 25% and 4% among patients treated with highest dose (9 × 106 CAR-T cells/kg). Further, a statistically significant relationship (P = 0.04) between plasma cell burden and severe CRS had been reported from patients with high-dose level of CAR-T cells. Many BCMA-targeted CAR-T clinical trials are ongoing or completed (Table 1). Additionally, combination therapies are evaluated as well, such as associating BCMA CARs with tyrosine kinase inhibitor (NCT04603827), immunomodulators (NCT03070327, NCT04287660), nonspecific immune inhibitors (NCT03943472), and gamma-secrete inhibitor (NCT03502577).
2.2. Non-BCMA targets
Though a majority of MM CAR-T clinical trials target BCMA, but there are several studies focused on non-BCMA MM-associated neoantigens (Table 2).
2.2.1. CD19
Human CD19 antigen belongs to type-I transmembrane glycoprotein of the IgG immunoglobulin superfamily. In normal tissues, CD19 is specifically expressed throughout the development of B-cell lineage except for hematopoietic stem cells and terminal plasma cells, whereas it is absent on other hematopoietic lineages. In B-cell malignancies, its expression is widely distributed in relapsed/refractory B-cell acute lymphoblastic leukemia (R/R B-ALL) and relapsed/refractory B-cell non-Hodgkin lymphoma (R/R B-NHL) (45). Despite low expression of CD19 on MM cells, CD19 is expressed on the minor multiple myeloma stem cell (MMSC) subset that has been reported (46). MMSC is capable of self-renewal and drug-resistance. Thus, CD19 might be a potential target for MM. One clinical trial (NCT02135406) indicated that autologous stem cell transplantation (ASCT) followed by CD19-targeted CAR-T therapy (CTL019) infusion was safe and available in RRMM, leading to a longer PFS compared to patients with ASCT alone (47, 48).
2.2.2. SLAMF7
SLAMF7 belongs to the signaling lymphocyte activation molecule family (SLAMF). SLAMF7 is firstly documented in natural killer cells (49). It is also expressed on T cells, B cells, monocytes, macrophages, and dendritic cells. Over 95% of normal or malignant plasma cells of MM expressed SLAMF7 (50). Since SLAMF7 is also expressed in normal plasma cells, specific attacks on this target inevitably cause normal cell death. Thereby, SLAMF7 is an alternative but suboptimal choice for CAR-T cell therapy.
The function of SLAMF7 is poorly understood, but previous evidence indicates its similar role as growth factor contributing to myeloma cell proliferation (51, 52). It has been reported that SLAMF7-CAR T cells attack myeloma and confer selective fratricide of SLAMF7-positive normal lymphocytes (53). A conceivable side effect is the depletion of SLAMF7+ lymphocytes, including a substantial proportion of T cells, B cells, and NK cells. It would be reasonable to engineer SLAMF7-CAR T cells with a safety switch to terminate fratricide of normal lymphocytes. Inducible caspase 9 or herpes simplex virus thymidine kinase might be preferable choices for safety switch (54, 55).
Several anti-SLAMF7 CAR constricts are evaluated in clinical trials, mostly as monotherapy (NCT03710421, NCT04142619, NCT04541368, NCT03958656, NCT04499339), or as dual CARs targeting both BCMA and SLAMF7 (NCT04795882, NCT04156269).
2.2.3. GPRC5D
The G protein-coupled receptor, class C group 5 member D (GPRC5D), is expressed on 98% of the CD138+ cells by quantitative immunofluorescence (56). Also, this surface receptor is primarily expressed on hair follicles, but also in multiple myeloma cells. Therefore, GPRC5D-targeted CAR-T was constructed by Smith et al., which displayed potent anti-MM effects on MM cell lines and xenografted models (56). Anti-GPRC5D was deemed safe and effective as no alopecia or any skin-related disorders were detected in a preclinical study (57). A series of GPRC5D-CAR T trials are ongoing, such as NCT05219721, NCT04555551, NCT05016778. MCARH109, as the first-in-class GPRC5D-targeted CAR T-cell therapy for MM, has a manageable safety profile and high rates of clinical response (ORR: 83%). More importantly, all 6 patients who relapsed after BCMA-targeted CAR-T responded to MCARH109.
2.2.4. CD138
As a major extracellular matrix (ECM) receptor, CD138 (syndecan-1) plays an important role in cell-cell and cell-matrix adhesion, and cell proliferation (58, 59). CD138 is widely expressed on normal and malignant plasma cells (60), but also expressed on the surface of mature epithelial cells that might cause skin toxicity. A prior study found that a high concentration of CD138 might be poor prognostic factor for MM (61). A CD138-directed CAR-T (CART-138) has been built incorporating with a 4-1BB domain (62). Relevant CD138-targeted CAR trials include single-target (NCT01886976, NCT03672318, NCT03196414, NCT03778346) and multi-target CAR-T products (NCT03271632). Based on current data (NCT01886976), the ORR achieved 80% and no toxicity has been reported, manifesting a good efficacy and tolerability. However, CD138 shedding and skin toxicity are major barriers for wide application of CD138-targeted CAR-T.
2.2.5. CD38
CD38, a transmembrane glycoprotein, is known to mediate cell adhesion, signal transduction, and Ca2+ regulation (63). CD38 is highly expressed on the surface of MM cells, though its expression in normal hematopoietic cells also have been detected, such as T cells, precursors of B cells, NK cells, and myeloid precursors (63). Some monoclonal antibodies against CD38 have been approved by FDA to treat multiple myeloma, such as Daratumumab. The success of mAb targeting CD38 in the treatment of MM has encouraged the development of CD38-targeted CAR T cells. Light-chain exchange technology brings potential to avoid accident damage to CD38+ normal cells (64). A clinical trial (NCT0346491) investigated CD38-targeted CAR-T as a monotherapy for RRMM. In addition, dual CAR products are also tested in clinical trials, combing CD38 and BCMA (NCT03767751), CD38 and CD19 (NCT03125577).
2.2.6. CD70
Aberrant expression of CD70 has been found in hematological malignancies and solid tumors (65). Because of its limited expression on normal cells, CD70 holds great promises for monoclonal antibody-based therapy. A preclinical study supported that CD70-targeted CAR T-cell therapy was safe and effective (66). Further, related publications manifested that CD70 targeting CAR-T cells caused robust anti-tumor activity in both human cancer cells and animal models (67, 68). It is worth noting that a clinical trial (NCT04662294) on CD70 is recruiting RRMM patients, although no data has been reported yet. Importantly, an obvious advantage is a low risk of fratricidal killing caused by CD70 antibody, mainly because of the transient expression of CD70 on immune cells (8).
2.2.7. NKG2DL
NKG2D, a cell surface receptor binding to several ligands, is predominantly expressed on immune cytotoxic cells, such as NK cell and CD8+ cytotoxic T cells. NKG2D ligands, such as MIC-A, MIC-B, and UL-16, are upregulated in many solid tumors or hematologic malignancies but absent on healthy tissue. NKG2D binds to corresponding ligands to prompt the secretion of proinflammatory cytokines and the activation of cytotoxic cells, leading to immune elimination of MM cells (69). Due to the presence of a natural costimulatory domain, DAP10, there is no need to add this specific domain to NKG2D CARs. But a potential challenge is the poor persistency of T cells. To resolve this problem, patients should be treated with high doses or multiple infusions without compromising the toxicity (70). Satisfactorily, higher doses have the same safety profile with low doses, with no reports of CRS or NTX so far. We have identified one NKG2D CAR study (NCT03018405) in MM with an enrollment of 12 patients, but efficacy profile has not been published.
2.2.8. Kappa light chain
Although cell surface immunoglobulins are not expressed on all plasma cells, it is recognized that MM stem cells express surface immunoglobulins (71). Thereby, kappa light chain might be an ideal target for MM (71). Several monoclonal antibodies targeting kappa light chain have been developed and tested in clinical trials, such as MDX-1097 (72). But CAR-targeting kapa light chain is still a less explored field. In one trial conducted by Ramos et al., 4 of 7 RRMM patients responded to kappa-targeted CAR-T cell therapy, keeping disease stable for 2-17 months. In a phase-I trial of κ-CAR-T cells (NCT00881920), 16 patients with non-Hodgkin lymphoma/chronic lymphocytic leukemia or MM were enrolled. Notably, 4 of 7 patients with relapsed or refractory MM kept disease stable for 2-17 months (71).
3. Mechanisms of disease resistance/relapse after MM CAR-T
Despite the impressive ORR, over 50% of patients after BCMA-directed CAR-T would relapse or progress within 1-year (73). Another study showed a consistent preliminary trend that most MM patients who achieved MRD-negative to bb2121 have progressed in follow-up period (74). Thus, though CAR-T cells have the robust cytoreductive capacity to treat multiple myeloma, they cannot produce lasting immune surveillance. Currently, exact mechanism of disease resistance/relapse after MM CAR-T remains elusive, but there are several deductive mechanisms stated as following: 1) T cell-dependent resistance; 2) antigen-driven resistance (antigen escape, antigen shedding); 3) TME-related resistance. Some mechanisms are presented in Figure 3.
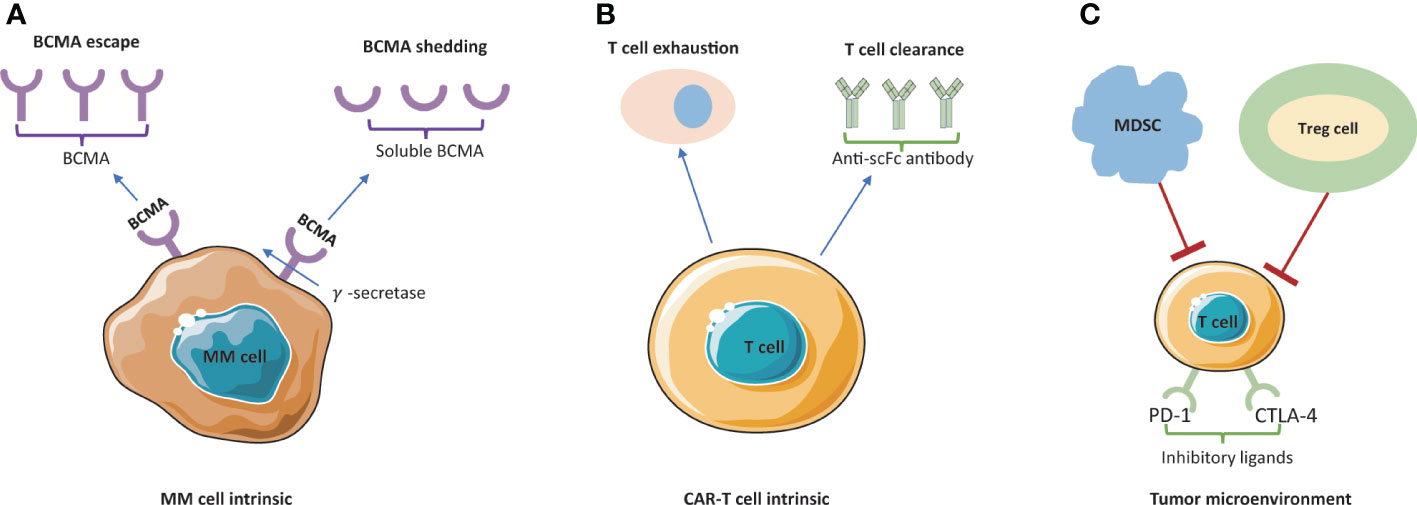
Figure 3 Mechanism of resistance/relapse to BCMA-targeted CAR-T cell therapy. (A) BCMA escape and BCMA shedding are blocking the antigen recognition by CAR-T cells. Membrane BCMA can be cleaved by γ–secretase and released to the plasma as soluble BCMA (sBCMA). (B) Poor persistence of CAR-T cells is mainly caused by T cell exhaustion and T cell clearance. (C) The tumor immunosuppressive microenvironment is mainly led by inhibitory ligands (PD-1 and CTLA-4) and suppressive immune cells (MDSC and Treg cells). MM, multiple myeloma; MDCS, myeloid-derived suppressor cells; Treg cell, T regulatory cells.
3.1. Poor persistence of CAR T cells
One study suggested that CAR-T cells were detectable up to 3 months after CAR-T injection and were gradually eliminated (73). At 12 months after infusion, only approximately 20% of patients had detectable engineered T cells (73). A lot of efforts have been made to figure out potential mechanisms leading to short persistence of CAR-T cells (73).
3.1.1. T cell clearance
CAR-T cells are immunogenicity, thereby they might be eliminated by adaptive immune response over time. Single-chain fragment variable (ScFv) is the most common antigen-binding counterpart in CAR-T constructs. Most of ScFvs in BCMA-directed CAR-T are derived from non-human species (73), which induce immunogenicity and thereby potentially limit the T-cell persistence. In legend-2 study (75), anti-ScFv antibodies were detected in 7 of 17 MM patients after receiving bi-epitope BCMA-targeting CAR-T (LCAR-B38M), and 6 of them had decreased CAR-T cells and experienced tumor recurrence. More specifically, camelid-derived ScFvs were used to assemble LCAR-B38M, specifically targeting two different epitopes of BCMA on MM cell surface. There are agreements that non-human ScFv can induce immunological reaction to produce anti-CAR antibodies, which eventually lead to T-cell clearance and constitute a higher risk of relapse after CAR-T. This observation also highlights the importance of manufacturing humanized ScFV.
3.1.2. Lack of memory characteristics
The differentiation stage of CAR-T cells affects their proliferation and survival, strongly correlating with their anti-tumor activity (76–78). The immunophenotype of T-cell used to manufacture CAR-T is considerably pivotal for T-cell persistence. Each subset of T cells possessed heterogeneity of proliferation and longevity (79). For example, naïve T-cells, stem memory T-cells, and central memory T-cells present the best proliferation capacity and delayed exhaustion or senescence (80). The enrichment of CD27+/CD45RO-/CD8+ T cells with memory-like features is correlated with long-term remission (81, 82). Also, a high percentage of cytotoxic CD8+ T cells with a naïve or stem memory characteristic are found to persist much longer and expand better in vivo, achieving superior outcomes after BCMA-targeted CAR-T treatment (23).
This view keeps in line with a previous finding that longer persistence of CAR-T cells in vivo expansion has been associated with better clinical remission and survival for recipient patients (83–86). One study also indicated that persistent CAR-T cells detected in peripheral blood tend to generate superior clinical response even among patients with high-grade diseases (87). Therefore, naïve cells and memory cells are important for CAR-T cell manufacture, mainly because they display sustained proliferation and longer persistence in vivo.
3.1.3. Impaired T cell fitness
The quality of T cells also profoundly affects their life span in vivo. Notably, malignancy itself and chemotherapy-related myelosuppression could hamper T-cell fitness (88). When patients receive many lines of myeloma treatments, the composition of T cells would change over time. Furthermore, patients who underwent more lines of chemotherapies tended to have less early memory T cells in vivo (89).
3.1.4. T cell exhaustion
T-cell exhaustion is another potential culprit, mainly because of constitutive antigen-independent tonic signaling by CAR-T. A variety of factors are able to induce tonic signaling to form activating clusters, leading to off-target activation and T-cell exhaustion (90, 91). Optimizing the CAR to limit antigen-independent tonic signaling and increase antigen-dependent recognition could be beneficial for T-cell persistence. In an anti-GPRC5D model of CAR-T, an IgG4/IgG2-derived spacer with modifications has been raised by Smith and colleagues, which might delay T-cell exhaustion (57).
3.2. Antigen escape and shedding
Antigen escape and shedding are the most common causes of the failure of CAR-T cell strategy. First, downregulation of tumor antigen reduces the CAR-T cell targeting ability, weakening the tumor-killing effects. Second, increased antigen shedding into a soluble form could negatively affect the efficacy of CAR-T therapy.
BCMA represents an important target. Theoretically, nearly all MM patients express BCMA irrespective of newly diagnosed or relapsed (38). It remains controversial about whether BCMA expression level is associated with the response rate to BCMA-directed CAR-T cells. However, loss of BCMA expression was suspected in post-treatment residual MM cells. Based on existing findings, there is a transient phenomenon that BCMA disappeared after initial response and subsequently remerged over MM progression (92). Though MM relapse is mainly caused by BCMA-positive clones, cases of recurrence led by BCMA-negative target cells have been noticed (22, 93). For example, a recent study pointed out that BCMA-negative was suspected in 3 of 71 patients at disease progression (94).
BCMA shedding from plasma cells is mediated by γ-secretase, producing the soluble-BCMA (sBCMA) that serves as a circulatory biomarker. Previous literatures have demonstrated that sBCMA is associated with the tumor burden and the prognosis (41, 95). High levels of soluble-BCMA might competitively bind to ScFv and consequently interfere the precise recognition of MM cells by CAR-T cells (96). Inhibitors of γ-secretase avoid BCMA shedding from MM cells and reduce the interference of soluble BCMA. Intriguingly, based on preclinical data, soluble BCMA does not affect the function of novel BCMA-CAR T in vitro and in vivo (37). Up to date, there is no clear clinical evidence that the level of sBCMA could negatively affect the efficacy of BCMA-targeted CAR-T therapy.
Likewise, high levels of soluble SLAMF7 are associated with a worse response to elotuzumab, along with a shorter survival (97). In addition, soluble CD38 could reduce the anti-MM response of daratumumab (98). However, as a seven-transmembrane protein, the likelihood of GPRC5D shedding into serum is low (57, 99). It is interesting to find that GPRC5D expression is independent from BCMA, therefore it might be an alternative target for relapsed MM patients after BCMA-directed therapy due to BCMA loss or shedding (57).
3.3. TME suppression
The tumor microenvironment (TME) plays a critical role in drug-resistance mechanism. CAR T cells need to overcome inhibitory signals and immunosuppressive cells existing in the TME. Immunosuppressive cells consist of T regulatory cells (Treg), B regulatory cells, myeloid-derived suppressor cells (MDSC), and plasmacytoid dendritic cells. These subsets may negatively affect the function of CAR-T cells (100–102). Besides, inaccessibility of MM cells by CAR-T cells forms another barrier. It is true MM cells generally reside in bone marrow microenvironment involving various cell types and extracellular matrix (ECM), which make CAR-T cells difficult to access MM (103). A recent study (104) about B-cell lymphoma reported a similar observation that many CD19-targted CAR-T cells did not successfully reach their target destination. Although mature CAR-T tracing method are still unmet needs, it is widely accepted that MM exploits immunosuppressive TME to block the efficacy of CAR-T cells and consequently lead to high risks of recurrence. PD1-PDL1 axis is another major cause of CAR T-cell dysfunction (105, 106). PD-1 expressed on activated T cells, is capable of binding with PD-L1 expressed by MM cells, eventually leading to exhausted state of T cells (107).
4. Strategies to improve the efficacy
For RRMM patients, poor persistence of T cells, antigen escape, and TME suppression restrict the durability of immune response and consequently limit the efficacy of CAR-T therapy in clinical settings. However, recently initiated studies have incorporated innovations to address above barriers (Figure 4, Table 3).
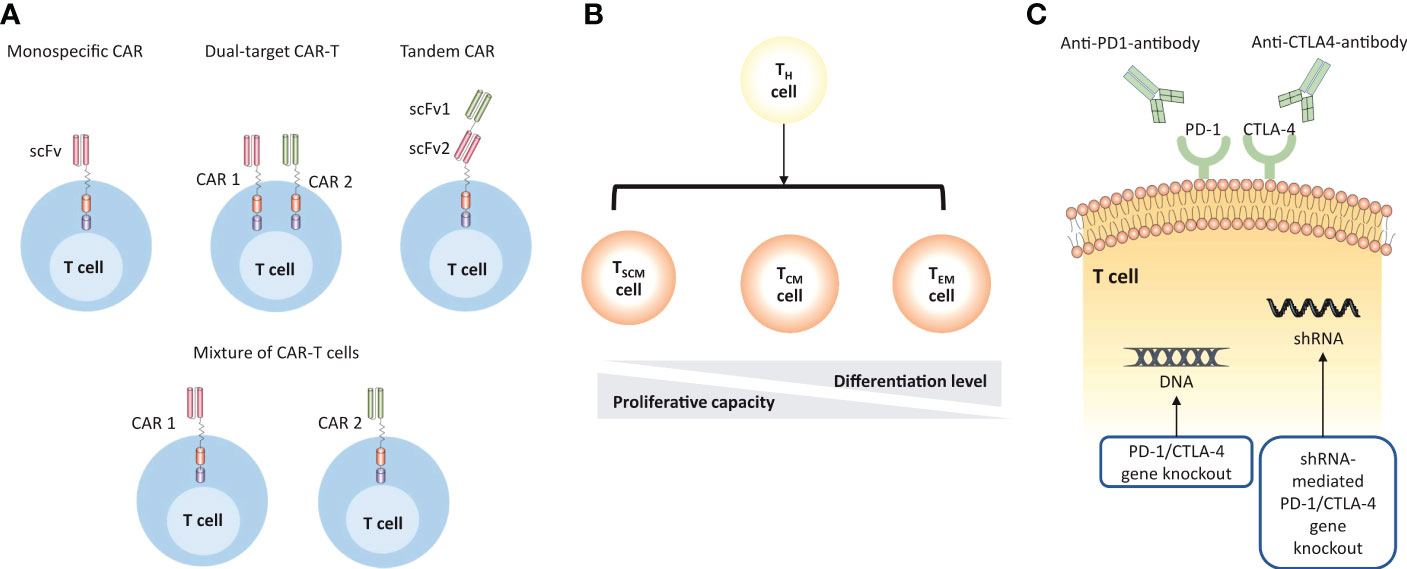
Figure 4 Strategies to improve the efficacy of MM CAR-T cell therapy. (A) CAR-T cell products are designed to target multiple TAAs to overcome antigen escape. The monospecific CAR has a single scFv; the dual-target CAR construct includes two separate monospecific CARs on the surface of T cells; the tandem CAR has two antigen-binding domains that are linked tandemly on one CAR protein; the mixture of CAR-T cells describes the simultaneous transduction of different types of CAR-T cells in vivo. (B) The persistence of CAR-T cells can be enhanced by using less-differentiated T cell subsets. (C) CAR-T cells can be engineered to overcome the immunosuppressive microenvironment by using immune checkpoint inhibitors or direct gene knockout.
4.1. Enhancing CAR-T cell persistence
Optimizing CAR-T design is a potential strategy to enhance CAR-T cell persistence. The utility of fully human recognition domains, rather than those derived from mouse antibodies, is an attempt to reduce immunogenicity which usually leads to clearance of CAR-T cells by patients’ immune system (22, 44, 75, 108, 109). Importantly, this strategy not only improves CAR-T cell persistence, but also simultaneously reduces cytokine storm. Besides, several studies demonstrated that the transmembrane region and co-stimulatory domain confer different properties of CAR-T cells that may influence efficacy and toxicity as well (110–125).
Another promising approach is to use less-differentiated T cells subsets that have a good proliferative capacity, such as naïve T cells, stem cell memory T cells (TSCM), central memory T cells (TCM). According to preclinical studies, CAR-T cells with memory phenotype presented superior engraftment, proliferation, and longevity compared to general CAR-T components (126, 127). Further, those who are treated with a defined ratio (1:1) of CD4+/CD8+ CAR-T cells, were monitored with more potent T cell expansion and fewer toxicities in vivo (128, 129).
In addition, lymphodepleting regimen may enhance the expansion of adoptively transferred T cells leading to superior persistence (130). First, lymphocytes depletion therapy before CAR-T could greatly reduce the risk of anti-CAR immune response. Second, a lymphodepleting environment is suitable for CAR-T cell expansion and persistence (80). It is known that IL-7 could assist CD8+ cytotoxic T-cell to preserve a stem memory phenotype in vivo (131), which is critical for T-cell expansion. All these data support the conclusion that more intense lymphodepletion may induce better CAR-T persistence and expansion.
4.2. Countering antigen escape
Increasing the density of BCMA expression is a critical area to counter antigen escape. The use of γ-secretase inhibitor (GSI) is able to increase BCMA expression on MM cells and reduce sBCMA levels by inhibiting the cleavage of surface BCMA (132). Preclinical models (133) have demonstrated that the presence of GSI could lead to a threefold to fivefold increase of BCMA expression level in MM cell lines. Particularly, when the density of BCMA is relatively low on the target cells, the administration of GSI may enhance the capacity of identifying MM cells. Great advancements in the efficacy of BCMA-targeted CAR T cells in combination with GSI have been observed in mouse models (133). Currently, several GSIs are being tested in clinical trials, even including patients with solid tumors (134). Future studies might discover other approaches to upregulate BCMA expression.
To address BCMA-negative clones, targeting two or more distinct antigens is underway. Due to the heterogeneity nature, targeting only one antigen at a time may not produce a long-lasting immunosurveillance in a large number of MM patients (135–137). More specifically, single target CAR-T only displays one single-chain variable fragment (ScFv) for antigen recognition, whereas dual-target CAR-T simultaneously contains co-stimulatory domain or tandem CAR molecules to overcome antigen escape and guarantee better identification. There are several strategies to achieve dual-target CAR-T products: 1) sequentially infusion of two CAR-T cells that respectively target different MM-associated antigen; 2) the same T cell displays two different CAR products; 3) One tandem CAR construct containing two antigen recognition moieties incorporated with one activation region (138). Available dual CAR products involved a combination of BCMA and CD19 (NCT04236011, NCT04162353), BCMA and SLAMF7 (NCT04662099, NCT04156269), BCMA and CD38 (NCT03767751). More details could be seen in Table 4.
4.3. Overcoming immunosuppression in the TME
CAR-T cells should preliminarily overcome direct T cell inhibitory signals presented in the TME. PD1-PDL1 is the best characterized pathway. Inhibition of the PD-1 signals could produce dramatic clinical benefits in a variety types of tumors (139). Recent studies have demonstrated that coadministration of immune checkpoint inhibitors (ICI) with CAR-T therapy brought increased efficacy in preclinical models (105). In addition to ICI, knockout of the PD-1 coding gene could be engineered by gene silencing techniques, such as short hairpin RNAs (140) and CRISPR-Cas9 (141). Also, armoured CAR T cells secreting cytokines or chemokines are able to alter the inflammatory microenvironment and support the functionality of CAR T cells (142). Further, the metabolic competition between tumor and immune cells in the TME may restrict nutrient availability and cause microenvironment acidosis, which could trigger T cell inhibitory pathways or otherwise hinder immune cell function (143). Intriguingly, the expression of the antioxidant enzyme catalase in CAR-T cells may overcome granulocyte-mediated oxidative stress in vitro (144). Modifying T cell metabolism is a promising area to boost efficacy, but further validation is needed in clinical application.
5. Strategies to reduce the toxicity
Overall, treatment-related toxicity of MM CAR-T therapy involves two major categories: 1) general toxicity caused by T cell activation and following systemic cytokine storm; 2) specific toxicity caused by the interaction between CARs and TAAs expressed on non-tumor cells, which is also termed as ‘on-target, off-tumor’ toxicity.
5.1. Systemic cytokine storm
The rapid immune activation responsible for the success of CAR-T strategy also stimulates treatment-related toxicity. The clinical complications caused by different CAR-T in MM are similar to those led by CD19-targeted CAR-T in ALL and DLBCL (84, 145, 146), including cytokine release syndrome (CRS) and neurotoxicity (NTX), and hematologic cytopenia, which might limit the wide application of CAR-T cell therapy in MM.
The most frequent toxicity is cytokine release syndrome (CRS), a constellation of symptoms involving fever, myalgia, hypoxia and hypertension, resulting from increased inflammatory cytokines like IL-6. IL-6 receptor antagonism via Tocilizumab and short-course steroids could be used for CRS management (147). Besides, CAR-T cell-associated HLH/MAS is a more severe systemic hyperinflammatory syndrome. CAR-T cell-induced HLH/MAS may be resistant to IL-6 receptor inhibitors, of which condition chemotherapy would be required (145).
Neurotoxicity (NTX), is the second major adverse effect, mainly because of the disruption of the blood-brain barrier and increased cerebrospinal fluid cytokine levels (148). NTX frequently occurs with or following CRS, presenting encephalopathy, delirium, aphasia, seizures, and life-threatening cerebral oedema (149). The consensus grading scheme proposed by ASBMT was applied extensively (149). Notably, the grade 3-4 CRS and NTX could be effectively managed by tocilizumab and supportive care. Also, management of NTX comprises of corticosteroids and IL-6 pathway antagonisms (145). A special form of NTX is referred to immune effector cell-associated neurotoxicity syndrome (ICANS), as transient encephalopathy, which is attributed to off-target cytokine production, as well as immune response of central nervous system (CNS). A mounting evidence suggests that ICANS could be characterized by atypical features and prolonged timeframes (150). And its management coincides with CRS interventions, such as cytokine inhibitors and corticosteroids. However, current understanding of ICANS is still limited. The mechanisms for ICANS after BCMA-targeted therapy need further elucidation (151).
Hematologic cytopenia is commonly reported following BCMA CAR-T cell therapy, manifesting as leukopenia, lymphopenia, anemia, neutropenia, and thrombocytopenia, which could increase the risks of infection, bleeding, fever, and bruising (146, 152–154). After infusion, CAR-T cells not only activate tumor-specific T-cell, but also induce non-specific T or B clones that target hematopoietic stem cell (HSC), neutrophils, platelets, and erythroid cells (155). Besides, the release of cytokines could drive differentiation but arrest maturation of HSC (156). Therefore, the IL-6 blockade may control hematologic cytopenia as well. The management of cytopenia also includes transfusion of blood cells and growth factors of hematopoietic stem cell transplantation (HSCT) (157, 158).
To counter systemic cytokine toxicity, CAR-T cells must reach a threshold level for activation but not exceed the level that would result in a series of cytokine secretion. Thus, therapeutic window for each CAR should be carefully considered. Researchers are currently engineering several innovations to control CAR expression or activity (Figure 5).
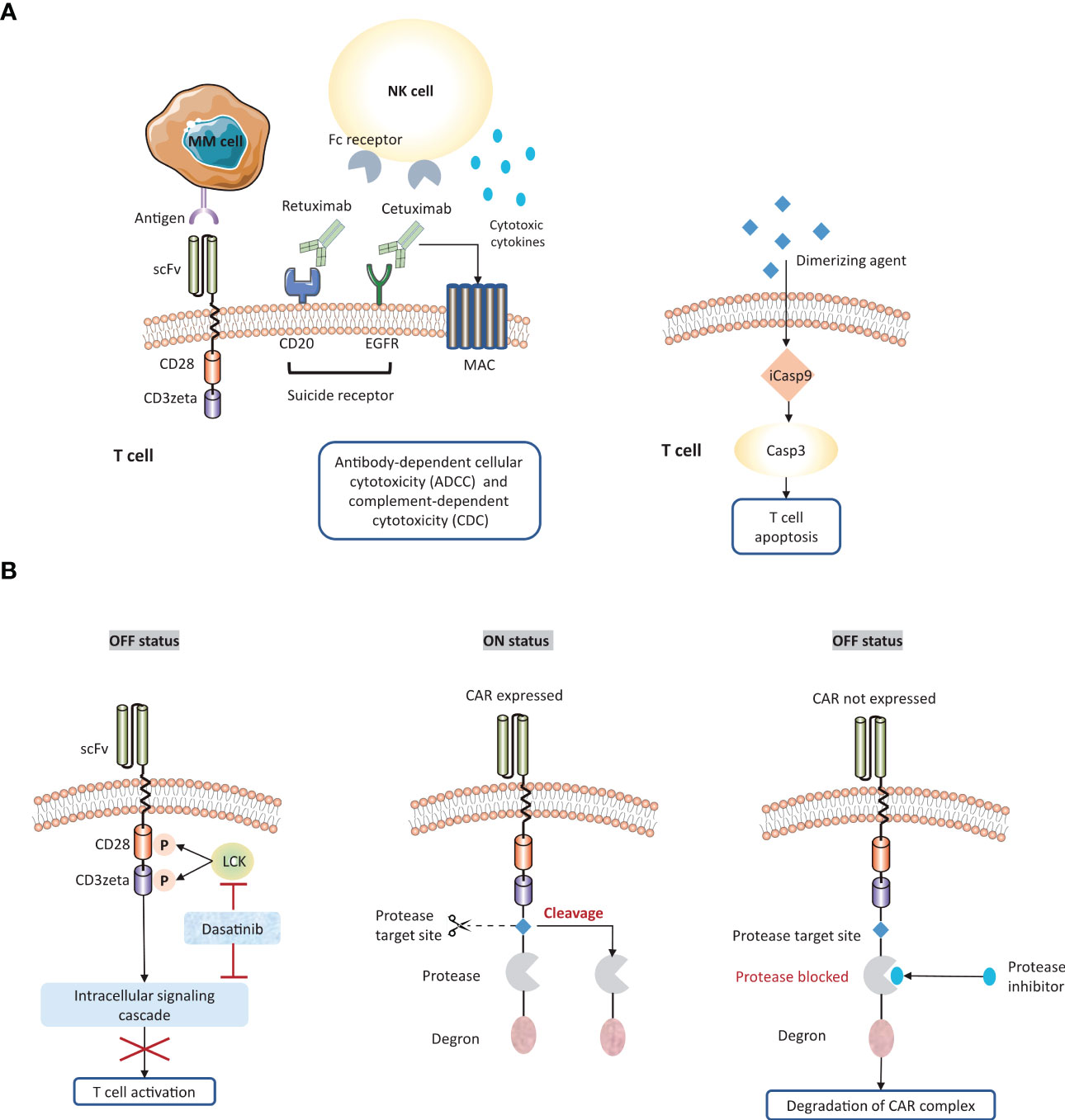
Figure 5 Strategies to overcome systemic cytokine toxicity. To counter systemic cytokine storm, several approaches are engineered to adjust CAR expression or activity. (A) Suicide gene system enables the elimination of CAR-T cells by following strategies: a) the activation of antibody-dependent cellular cytotoxicity (ADCC) or complement-dependent cytotoxicity (CDC); b) the induction of apoptosis pathway. (B) The ‘ON/OFF switches’ of CAR-T cells could be regulated by small molecular agents. scFv, single chain fragment variable; MAC, membrane attack complex; iCasp9, inducible caspase 9.
Firstly, 4-1BB co-stimulatory domain is associated with a much slower onset of T cell activation, increased T cell durability, and a lower risk of cytokine-related toxicity compared to CD28 domain. Therefore, inclusion of 4-1BB co-stimulatory domains might be less toxic in patients with heavy tumor burden. But CD28 is necessary to achieve the required threshold for T cell activation, especially for MM with a relatively low density of antigen or a low-affinity antigen-binding domain. Overall, the choice of co-stimulatory domain is critical to balance the efficacy and safety in CAR-T cell therapy.
Secondly, engineering ‘suicide genes’ into the CAR construct could induce apoptosis to eliminate CAR-T cells when treatment-related toxicity occurs. Co-expression of suicide receptors on MM CAR-T cells, such as CD20 and EGFR, could be attacked by rituximab (159–161) and cetuximab (162), respectively. Therefore, these FDA-approved antibodies provide a mean to deactivate CAR-T cells. Another strategy is to incorporate apoptosis-triggering fusion protein into CAR-T cells. iCasp9 is a well-characterized example, which can be triggered by dimerizing agents and subsequently drive rapid T cell depletion (163).
Thirdly, administration of small-molecular agents could control ‘on or off switch’ on CAR-T cells. Dasatinib, a tyrosine kinase inhibitor for CML and ALL. This agent enables the inhibition of LCK or intracellular signaling cascade, followed by destroying the downstream signal of activated CD3zeta. It has been demonstrated that dasatinib rapidly and reversibly hinder CAR-T cell activation, which provides a well-tolerated pharmacological toxicity switch without eradication of T cells (164). Alternatively, switch-off CARs (SMASh-CARs) provide another strategy to dynamically regulate T cell functionality via embedding a protease target, a protease, and a degron moiety (165). In the ‘OFF’ state, the degron moiety promotes the degradation of CAR-protease-degron complex. Protease inhibitors may function as the similar role to retain the degron structure. In the ‘On’ state, the protease target site is cleavage by protease leading to the removal of the degron from CAR protein, and consequently the CAR is expressed on the surface of T cells.
In addition, a more direct antagonism way is knockout of cytokine genes or expression of cytokine antagonists, both of which might provide opportunities to avert systemic toxicities. For example, the macrophage-activating and monocyte-activating cytokine GM-CSF can be antagonized by mutational inactivation and antibody lenzilumab, both of which can increase CAR T cell persistence while decreasing the risk of CRS.
5.2. On-target, off-tumor toxicity
Typically, CAR T cells are designed to target tumor-associated antigens (TAA). However, some TAAs are also expressed on the normal cells, leading to mistaken recognition and attack by CAR T cells. BCMA is a prominent TAA for CAR-T cell therapy in MM. However, the public transcriptomic datasets confirmed BCMA RNA expression in the caudate of normal human brains (166), indicating an on-target effect of anti-BCMA CAR-T therapy. Given the reports of phase-II cilta-cel study, 12 of 97 patients were reported with non-ICANS neurotoxicity. 5 of 97 (5.2%) patients suffered from a cluster of movement and neurocognitive symptoms (3 with ≥ Grade 3 parkinsonism) (167). Among them, one patient developed a progressive movement disorder with symptoms of parkinsonism around three months after BCMA-targeted CAR-T cell infusion. By analyzing this case, one study demonstrated that BCMA expression on neurons and astrocytes in the basal ganglia (166). Therefore, BCMA-targeted CAR-T cells may hold the potential to cross the blood-brain barrier and induce a progressive neurocognitive or movement disorder by targeting the basal ganglia. Close monitoring of neurotoxicity is necessary in patients with BCMA-targeted CAR-T cell therapies.
Engineering strategies aims to overcome on-target, off-tumor toxicity mediated by CAR-T cell therapy (Figure 6). The first strategy is to enhance the specificity of antigen recognition. Targeting multiple TAA is a promising approach. Specifically, CAR protein could be disassembled into two separate receptors, one with CD3zeta domain and another with a co-stimulatory domain. Both receptors need to recognize different TAAs for CAR T cells activation. Preclinical models have observed the promises in such a strategy (168–170). Alternatively, the inhibitory CAR (iCAR) contains a special inhibitory region that is generally derived from immune checkpoint proteins, such as PD-1 and CTLA-4. The inhibitory signal could recognize an antigen expressed on healthy tissues but absent on tumor cells (171). Moreover, engineering chimeric co-stimulatory receptor enables T cells to recognize antigens that are enriched on tumor cells. The second strategy is to utilize logic gating or conditional system to control CAR-T cell activation, such as the phospho-antigens that could be identified by T cell receptor. For example, HIF-1α degradation pathway is exploited to restrict CAR expression to CAR-T cells located in hypoxia TME, thereby avoiding adverse effects on healthy tissues which are normally non-hypoxic (172).
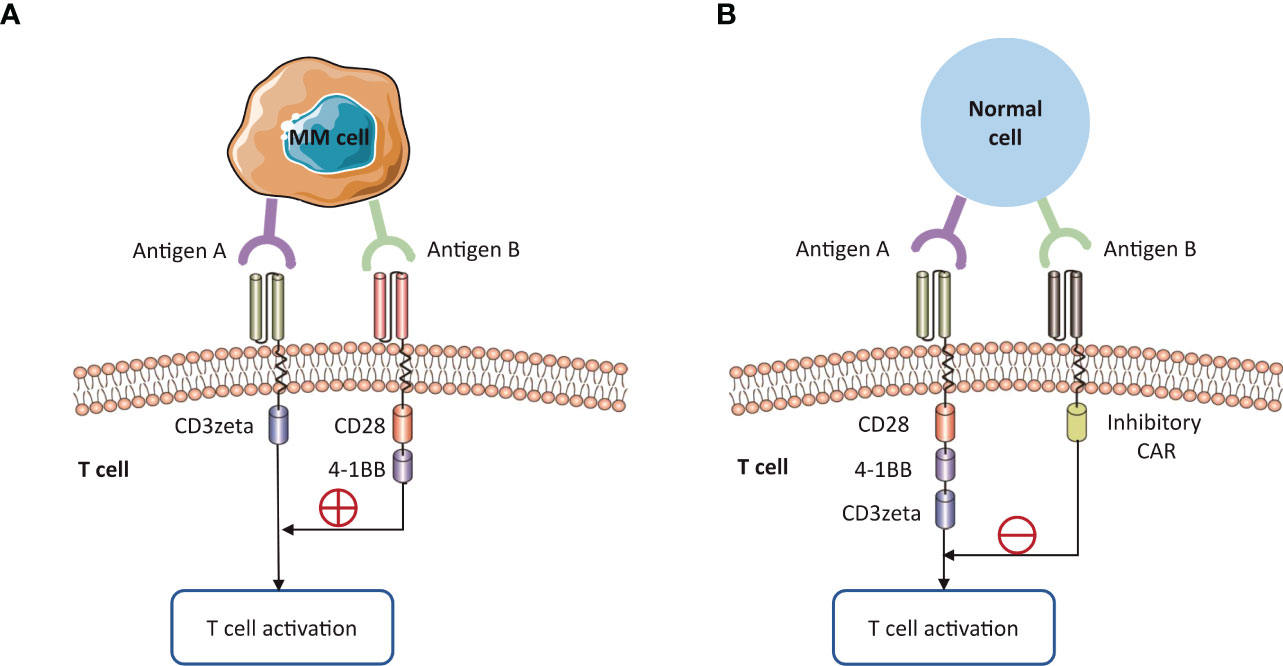
Figure 6 Strategies to overcome on-target, off-tumor toxicity. The expression of tumor-associated antigens on healthy tissues can lead to ‘on-target, off-tumor’ toxicity. (A) The specificity of CAR T cells is enhanced by targeting multiple TAAs. The activation domain and co-stimulatory domain should respectively bind to different antigens on MM cells for CAR T cell activation. (B) Alternative strategy is to use the inhibitory CAR against a specific non-tumor antigen, requiring the absence of this antigen on MM cells.
6. Innovations of MM CAR-T manufacture
Novel agents and CAR-T manufacture platforms are especially noteworthy. Table 5 specifically focused on data of novel therapeutic agents for RRMM presented at major oncology meeting between 2020 and 2022, including Annual Society of Hematology (ASH) and American Society of Clinical Oncology (ASCO).
6.1. Role of allogeneic CAR-T
Currently, all FDA-approved CAR-T constructs are manufactured within autologous T cells isolated from the patients’ blood. However, this individualized production process is somewhat costly and time-consuming, limiting the number of MM patients who can benefit from CAR-T therapy. First, the manufacture time of autological CAR-T cells is lengthy. Many patients with advanced stage of MM may be unable to benefit from this therapy (84, 186). Second, the production failure may be attributable to the insufficient T cells obtained from MM patients, as patients who previously received chemotherapy tend to undergo bone marrow suppression and lymphodepletion (88, 187). Third, the heterogeneity of apheresis CAR product is another underlying cause of preparation failure. There is a phenomenon that dysfunctional T subsets could result in inferior CAR-T products, consequently leading to poor efficacy and response rates (81, 188–191).
Allogeneic donor T cells provides an alternative to autological CAR-T cell therapy, which might potentially solve the manufacturing issues of inadequate T-cell number and suboptimal T-cell fitness for CAR-T production. CAR-T cells could be derived from HLA-matched allogeneic hemopoietic stem cell donors. Nevertheless, allogeneic CAR-T cell therapy has been associated with graft-versus-host (GVHD) and graft rejection. The engrafted allogeneic donor cells could launch an attack on recipient cells (192), whereas the host immune cells are able to eliminate allogeneic CAR-T cells. Recently, genetic modifications are explored to cope with T cell alloreactivity, such as TCR disruption and safety switch insertions (176). Genome-editing technologies include ZFN, TALEN, and CRISPR-Cas 9, all of which are used to generate universal CAR-T cells (193). Ongoing clinical trials provide novel armamentarium for MM immunotherapy.
At ASH 2021, updated results of an open-label, phase-I clinical study (UNIVERSAL, NCT04093596) were reported to validate the feasibility of allogeneic anti-BCMA ALLO-715 for RRMM (176). ALLO-715 is a genetically modified anti-BCMA CAR-T product which employed TALEN technology to disrupt the TCR constant gene and CD52 gene to prevent GVHD and allow the use of anti-CD52 based lymphodepletion (194). At the time of data cut-off, 47 patients were enrolled; 42 patients received ALLO-715 infusion. Efficacy outcomes presented 61.5% ORR among patients with high doses. Safety prolife showed CRS occurred in 52.4% and there was no grade 4-5 CRS. Overall, the UNIVERSAL trial demonstrates the proof for allogeneic CAR-T therapy for MM, which might bring meaningful efficacy and tolerable toxicity. But this trial continues to enroll more patients and follow-up data will be updated in the future (176).
6.2. Rapid CAR-T manufacture platform
In the process of commercial manufacture, patients need to wait for around 3-4 weeks until CAR-T infusion, in whom disease might progress while waiting for CAR production. The first-in-human dual BCMA and CD19 targeted CAR was manufactured by a novel platform (FAST CAR platform) that significantly reduced the production time to only 24-36 hours (195). Latest results of this trial (NCT04236011) showed a high response with 100% (DL-1: 1×105/kg), 80% (DL-1: 2×105/kg), and 93.8% (DL-1: 3×105/kg) ORR, respectively. Also, 23 out of 28 patients (82.1%) suffered from grade 1-2 CRS and 2 patients (7.1%) with grade 3. The data presented promising efficacy and favorable safety of the BCMA-CD19 dual fast CAR-T for RRMM patients (182). This clinical trial is still ongoing and recruiting more patients.
At ASH 2021, a rapid manufacturing process that could both preserve the stemness of T cells to ensure longer durability and provide timely access for patients with aggressive disease, has been presented (28). Researchers developed a superior anti-BCMA CAR-T construct (PHE885) carrying a fully human anti-BCMA ScFv fused to 4-1BB/CD3zeta signaling domains and an innovative T-Charge manufacturing platform, which enables rapid and reliable patient access. More specifically, this novel manufacturing platform allows PHE885 to preserve a higher percentage of naïve/TSCM cells, leading to effectively engraft, expand, and reject tumors. Based on this principle, a phase-I trial (NCT04318327) has been initiated and early data of this study will be presented in the future.
6.3. Modified manufacturing process to harvest early memory T-cell
CAR-T cells start to disappear at first 3-6 months after infusion, subsequently leading to the loss of disease control. An innovation is to enrich early memory T cells by modification of manufacturing process. JCARH125 is a well elaborated example. Its production is optimized to harvest early memory T-cell and increase T-cell fitness. Relevant clinical trial (EVOLVE) data have been previously presented in ASH 2018. According to the latest reporting at ASCO 2020 (174), a total of 44 patients who received higher doses (300 × 106, 450 × 106, 600 × 106) respectively achieved the ORR of 95%, 94%, and 71%. A promising finding is that functional CAR-T cells could be detected in 69% of cases at 6 months. P-BCMA-101 is an autologous BCMA-targeted CAR construct that consisted of a large number of stem cell memory cells. P-BCMA-101 was manufactured by a novel virus-free transposon “piggy-Bac” technology that preferentially transfect early memory T cells (196), thereby increasing efficacy while minimizing toxicity (173). A phase I-II study of P-BCMA-101 (NCT03288493) is being tested in RRMM patients and early data were reported in ASH 2018 (197). Current clinical data keep consistent with preclinical findings that the modifications of CAR production appear to have notably improved efficacy.
7. Conclusion
In this review, we summarized the current status and future innovations in CAR-T therapy for multiple myeloma. Clinical benefits of using CAR-T therapy to treat MM has been confirmed, but it does not lead to favorable durability and safety with current technologies. Numerous promising engineering approaches are underway to improve the efficacy and safety of CAR-T cell therapy, expanding this technology for a wider range of application and bring more benefits for MM patients.
Author contributions
YQ and YJ designed the study and reviewed the manuscript. ZW and CC participated in study design and wrote the original draft of the manuscript. ZW and CC was mainly responsible for the design of tables and figures. LW contributed to the conception of the paper. All authors contributed to the article and approved the submitted version.
Funding
This study was supported by the National Natural Science Foundation of China (grant no. 81872264).
Conflict of interest
The authors declare that the research was conducted in the absence of any commercial or financial relationships that could be construed as a potential conflict of interest.
Publisher’s note
All claims expressed in this article are solely those of the authors and do not necessarily represent those of their affiliated organizations, or those of the publisher, the editors and the reviewers. Any product that may be evaluated in this article, or claim that may be made by its manufacturer, is not guaranteed or endorsed by the publisher.
References
1. Kehrer M, Koob S, Strauss A, Wirtz DC, Schmolders J. [Multiple myeloma - current status in diagnostic testing and therapy]. Z fur Orthopadie und Unfallchirurgie (2017) 155(5):575–86. doi: 10.1055/s-0043-110224
2. Michels TC, Petersen KE. Multiple myeloma: Diagnosis and treatment. Am Family Physician (2017) 95(6):373–83.
3. Padala SA, Barsouk A, Barsouk A, Rawla P, Vakiti A, Kolhe R, et al. Epidemiology, staging, and management of multiple myeloma. Med Sci (Basel Switzerland) (2021) 9(1):3. doi: 10.3390/medsci9010003
4. Kumar SK, Rajkumar V, Kyle RA, van Duin M, Sonneveld P, Mateos MV, et al. Multiple myeloma. Nat Rev Dis Primers (2017) 3:17046. doi: 10.1038/nrdp.2017.46
5. Casey M, Nakamura K. The cancer-immunity cycle in multiple myeloma. ImmunoTargets Ther (2021) 10:247–60. doi: 10.2147/itt.S305432
6. Kegyes D, Constantinescu C, Vrancken L, Rasche L, Gregoire C, Tigu B, et al. Patient selection for car T or bite therapy in multiple myeloma: Which treatment for each patient? J Hematol Oncol (2022) 15(1):78. doi: 10.1186/s13045-022-01296-2
7. Abramson HN. The multiple myeloma drug pipeline-2018: A review of small molecules and their therapeutic targets. Clin lymphoma Myeloma Leukemia (2018) 18(9):611–27. doi: 10.1016/j.clml.2018.06.015
8. Wu C, Zhang L, Brockman QR, Zhan F, Chen L. Chimeric antigen receptor T cell therapies for multiple myeloma. J Hematol Oncol (2019) 12(1):120. doi: 10.1186/s13045-019-0823-5
9. Ye CJ, Chen J, Liu G, Heng HH. Somatic genomic mosaicism in multiple myeloma. Front Genet (2020) 11:388. doi: 10.3389/fgene.2020.00388
10. Heng J, Heng HH. Genome chaos: Creating new genomic information essential for cancer macroevolution. Semin Cancer Biol (2022) 81:160–75. doi: 10.1016/j.semcancer.2020.11.003
11. Kumar SK, Dimopoulos MA, Kastritis E, Terpos E, Nahi H, Goldschmidt H, et al. Natural history of relapsed myeloma, refractory to immunomodulatory drugs and proteasome inhibitors: A multicenter imwg study. Leukemia (2017) 31(11):2443–8. doi: 10.1038/leu.2017.138
12. Kochenderfer JN, Somerville RPT, Lu T, Yang JC, Sherry RM, Feldman SA, et al. Long-duration complete remissions of diffuse Large b cell lymphoma after anti-Cd19 chimeric antigen receptor T cell therapy. Mol Therapy: J Am Soc Gene Ther (2017) 25(10):2245–53. doi: 10.1016/j.ymthe.2017.07.004
13. Park JH, Rivière I, Gonen M, Wang X, Sénéchal B, Curran KJ, et al. Long-term follow-up of Cd19 car therapy in acute lymphoblastic leukemia. New Engl J Med (2018) 378(5):449–59. doi: 10.1056/NEJMoa1709919
14. Choi T, Kang Y. Chimeric antigen receptor (Car) T-cell therapy for multiple myeloma. Pharmacol Ther (2022) 232:108007. doi: 10.1016/j.pharmthera.2021.108007
15. van der Stegen SJ, Hamieh M, Sadelain M. The pharmacology of second-generation chimeric antigen receptors. Nat Rev Drug Discovery (2015) 14(7):499–509. doi: 10.1038/nrd4597
16. Jensen MC, Riddell SR. Designing chimeric antigen receptors to effectively and safely target tumors. Curr Opin Immunol (2015) 33:9–15. doi: 10.1016/j.coi.2015.01.002
17. Benmebarek MR, Karches CH, Cadilha BL, Lesch S, Endres S, Kobold S. Killing mechanisms of chimeric antigen receptor (Car) T cells. Int J Mol Sci (2019) 20(6):1283. doi: 10.3390/ijms20061283
18. Sadelain M, Rivière I, Riddell S. Therapeutic T cell engineering. Nature (2017) 545(7655):423–31. doi: 10.1038/nature22395
19. Srivastava S, Riddell SR. Engineering car-T cells: Design concepts. Trends Immunol (2015) 36(8):494–502. doi: 10.1016/j.it.2015.06.004
20. Lim WA, June CH. The principles of engineering immune cells to treat cancer. Cell (2017) 168(4):724–40. doi: 10.1016/j.cell.2017.01.016
21. Brudno JN, Kochenderfer JN. Recent advances in car T-cell toxicity: Mechanisms, manifestations and management. Blood Rev (2019) 34:45–55. doi: 10.1016/j.blre.2018.11.002
22. Brudno JN, Maric I, Hartman SD, Rose JJ, Wang M, Lam N, et al. T Cells genetically modified to express an anti-B-Cell maturation antigen chimeric antigen receptor cause remissions of poor-prognosis relapsed multiple myeloma. J Clin Oncology: Off J Am Soc Clin Oncol (2018) 36(22):2267–80. doi: 10.1200/jco.2018.77.8084
23. Cohen AD, Garfall AL, Stadtmauer EA, Melenhorst JJ, Lacey SF, Lancaster E, et al. B cell maturation antigen-specific car T cells are clinically active in multiple myeloma. J Clin Invest (2019) 129(6):2210–21. doi: 10.1172/jci126397
24. Usmani SZ, Berdeja JG, Truppel-Hartmann A, Fei Y, Wortman-Vayn H, Shelat S, et al. Karmma-4: Idecabtagene vicleucel (Ide-cel, Bb2121), a bcma-directed car T-cell therapy in high-risk newly diagnosed multiple myeloma. J Clin Oncol (2021) 39(15_suppl):TPS8053–TPS. doi: 10.1200/JCO.2021.39.15_suppl.TPS8053
25. Kumar SK, Baz RC, Orlowski RZ, Anderson LD Jr., Ma H, Shrewsbury A, et al. Results from lummicar-2: A phase 1b/2 study of fully human b-cell maturation antigen-specific car T cells (Ct053) in patients with relapsed and/or refractory multiple myeloma. Blood (2020) 136(Supplement 1):28–9. doi: 10.1182/blood-2020-139802
26. Jie J, Hao S, Jiang S, Li Z, Yang M, Zhang W, et al. Phase 1 trial of the safety and efficacy of fully human anti-bcma car T cells in Relapsed/Refractory multiple myeloma. Blood (2019) 134(Supplement_1):4435. doi: 10.1182/blood-2019-126104
27. An G, Sui W, Wang T, Qu X, Zhang X, Yang J, et al. An anti-bcma car T-cell therapy (C-Car088) shows promising safety and efficacy profile in relapsed or refractory multiple myeloma. Blood (2020) 136(Supplement 1):29–30. doi: 10.1182/blood-2020-138734
28. Sperling AS, Nikiforow S, Nadeem O, Mo CC, Laubach JP, Anderson KC, et al. Phase I study of Phe885, a fully human bcma-directed car-T cell therapy for Relapsed/Refractory multiple myeloma manufactured in ≪2 days using the T-charge Tm platform. Blood (2021) 138(Supplement 1):3864. doi: 10.1182/blood-2021-146646
29. Han L, Gao Q, Zhou K, Zhou J, Yin Q-S, Fang B, et al. The clinical study of anti-bcma car-T with single-domain antibody as antigen binding domain. J Clin Oncol (2021) 39(15_suppl):8025. doi: 10.1200/JCO.2021.39.15_suppl.8025
30. Liu Y, Chen Z, Wei R, Shi L, He F, Shi Z, et al. Remission observed from a phase 1 clinical study of car-T therapy with safety switch targeting bcma for patients with Relapsed/Refractory multiple myeloma. J Clin Oncol (2018) 36(15_suppl):8020. doi: 10.1200/JCO.2018.36.15_suppl.8020
31. Zhao WH, Wang BY, Chen LJ, Fu WJ, Xu J, Liu J, et al. Four-year follow-up of lcar-B38m in relapsed or refractory multiple myeloma: A phase 1, single-arm, open-label, multicenter study in China (Legend-2). J Hematol Oncol (2022) 15(1):86. doi: 10.1186/s13045-022-01301-8
32. Madduri D, Berdeja JG, Usmani SZ, Jakubowiak A, Agha M, Cohen AD, et al. Cartitude-1: Phase 1b/2 study of ciltacabtagene autoleucel, a b-cell maturation antigen-directed chimeric antigen receptor T cell therapy, in Relapsed/Refractory multiple myeloma. Blood (2020) 136(Supplement 1):22–5. doi: 10.1182/blood-2020-136307
33. Hao S, Jin J, Jiang S, Li Z, Zhang W, Yang M, et al. Two-year follow-up of investigator-initiated phase 1 trials of the safety and efficacy of fully human anti-bcma car T cells (Ct053) in Relapsed/Refractory multiple myeloma. Blood (2020) 136(Supplement 1):27–8. doi: 10.1182/blood-2020-140156
34. Novak AJ, Darce JR, Arendt BK, Harder B, Henderson K, Kindsvogel W, et al. Expression of bcma, taci, and baff-r in multiple myeloma: A mechanism for growth and survival. Blood (2004) 103(2):689–94. doi: 10.1182/blood-2003-06-2043
35. Roex G, Timmers M, Wouters K, Campillo-Davo D, Flumens D, Schroyens W, et al. Safety and clinical efficacy of bcma car-T-Cell therapy in multiple myeloma. J Hematol Oncol (2020) 13(1):164. doi: 10.1186/s13045-020-01001-1
36. Bu DX, Singh R, Choi EE, Ruella M, Nunez-Cruz S, Mansfield KG, et al. Pre-clinical validation of b cell maturation antigen (Bcma) as a target for T cell immunotherapy of multiple myeloma. Oncotarget (2018) 9(40):25764–80. doi: 10.18632/oncotarget.25359
37. Carpenter RO, Evbuomwan MO, Pittaluga S, Rose JJ, Raffeld M, Yang S, et al. B-cell maturation antigen is a promising target for adoptive T-cell therapy of multiple myeloma. Clin Cancer Res: an Off J Am Assoc Cancer Res (2013) 19(8):2048–60. doi: 10.1158/1078-0432.Ccr-12-2422
38. Seckinger A, Delgado JA, Moser S, Moreno L, Neuber B, Grab A, et al. Target expression, generation, preclinical activity, and pharmacokinetics of the bcma-T cell bispecific antibody Em801 for multiple myeloma treatment. Cancer Cell (2017) 31(3):396–410. doi: 10.1016/j.ccell.2017.02.002
39. Tai YT, Acharya C, An G, Moschetta M, Zhong MY, Feng X, et al. April And bcma promote human multiple myeloma growth and immunosuppression in the bone marrow microenvironment. Blood (2016) 127(25):3225–36. doi: 10.1182/blood-2016-01-691162
40. Sanchez E, Gillespie A, Tang G, Ferros M, Harutyunyan NM, Vardanyan S, et al. Soluble b-cell maturation antigen mediates tumor-induced immune deficiency in multiple myeloma. Clin Cancer Res: an Off J Am Assoc Cancer Res (2016) 22(13):3383–97. doi: 10.1158/1078-0432.Ccr-15-2224
41. Sanchez E, Li M, Kitto A, Li J, Wang CS, Kirk DT, et al. Serum b-cell maturation antigen is elevated in multiple myeloma and correlates with disease status and survival. Br J Haematol (2012) 158(6):727–38. doi: 10.1111/j.1365-2141.2012.09241.x
42. Quinn J, Glassford J, Percy L, Munson P, Marafioti T, Rodriguez-Justo M, et al. April Promotes cell-cycle progression in primary multiple myeloma cells: Influence of d-type cyclin group and translocation status. Blood (2011) 117(3):890–901. doi: 10.1182/blood-2010-01-264424
43. Lee L, Bounds D, Paterson J, Herledan G, Sully K, Seestaller-Wehr LM, et al. Evaluation of b cell maturation antigen as a target for antibody drug conjugate mediated cytotoxicity in multiple myeloma. Br J Haematol (2016) 174(6):911–22. doi: 10.1111/bjh.14145
44. Ali SA, Shi V, Maric I, Wang M, Stroncek DF, Rose JJ, et al. T Cells expressing an anti-B-Cell maturation antigen chimeric antigen receptor cause remissions of multiple myeloma. Blood (2016) 128(13):1688–700. doi: 10.1182/blood-2016-04-711903
45. Wang K, Wei G, Liu D. Cd19: A biomarker for b cell development, lymphoma diagnosis and therapy. Exp Hematol Oncol (2012) 1(1):36. doi: 10.1186/2162-3619-1-36
46. Johnsen HE, Bøgsted M, Schmitz A, Bødker JS, El-Galaly TC, Johansen P, et al. The myeloma stem cell concept, revisited: From phenomenology to operational terms. Haematologica (2016) 101(12):1451–9. doi: 10.3324/haematol.2015.138826
47. Garfall AL, Stadtmauer EA, Hwang WT, Lacey SF, Melenhorst JJ, Krevvata M, et al. Anti-Cd19 car T cells with high-dose melphalan and autologous stem cell transplantation for refractory multiple myeloma. JCI Insight (2018) 3(8):e120505. doi: 10.1172/jci.insight.120505
48. Garfall AL, Maus MV, Hwang WT, Lacey SF, Mahnke YD, Melenhorst JJ, et al. Chimeric antigen receptor T cells against Cd19 for multiple myeloma. New Engl J Med (2015) 373(11):1040–7. doi: 10.1056/NEJMoa1504542
49. Boles KS, Mathew PA. Molecular cloning of Cs1, a novel human natural killer cell receptor belonging to the Cd2 subset of the immunoglobulin superfamily. Immunogenetics (2001) 52(3-4):302–7. doi: 10.1007/s002510000274
50. Hsi ED, Steinle R, Balasa B, Szmania S, Draksharapu A, Shum BP, et al. Cs1, a potential new therapeutic antibody target for the treatment of multiple myeloma. Clin Cancer Res: an Off J Am Assoc Cancer Res (2008) 14(9):2775–84. doi: 10.1158/1078-0432.Ccr-07-4246
51. Tai YT, Dillon M, Song W, Leiba M, Li XF, Burger P, et al. Anti-Cs1 humanized monoclonal antibody Huluc63 inhibits myeloma cell adhesion and induces antibody-dependent cellular cytotoxicity in the bone marrow milieu. Blood (2008) 112(4):1329–37. doi: 10.1182/blood-2007-08-107292
52. Kikuchi J, Hori M, Iha H, Toyama-Sorimachi N, Hagiwara S, Kuroda Y, et al. Soluble Slamf7 promotes the growth of myeloma cells Via homophilic interaction with surface Slamf7. Leukemia (2020) 34(1):180–95. doi: 10.1038/s41375-019-0525-6
53. Gogishvili T, Danhof S, Prommersberger S, Rydzek J, Schreder M, Brede C, et al. Slamf7-car T cells eliminate myeloma and confer selective fratricide of Slamf7(+) normal lymphocytes. Blood (2017) 130(26):2838–47. doi: 10.1182/blood-2017-04-778423
54. Bonini C, Ferrari G, Verzeletti S, Servida P, Zappone E, Ruggieri L, et al. Hsv-tk gene transfer into donor lymphocytes for control of allogeneic graft-Versus-Leukemia. Sci (New York NY) (1997) 276(5319):1719–24. doi: 10.1126/science.276.5319.1719
55. Hoyos V, Savoldo B, Quintarelli C, Mahendravada A, Zhang M, Vera J, et al. Engineering Cd19-specific T lymphocytes with interleukin-15 and a suicide gene to enhance their anti-Lymphoma/Leukemia effects and safety. Leukemia (2010) 24(6):1160–70. doi: 10.1038/leu.2010.75
56. Smith EL, Harrington K, Staehr M, Masakayan R, Jones J, Long T, et al. T Cell therapy targeting G protein-coupled receptor class c group 5 member d (Gprc5d), a novel target for the immunotherapy of multiple myeloma. Blood (2018) 132(Supplement 1):589. doi: 10.1182/blood-2018-99-110471
57. Smith EL, Harrington K, Staehr M, Masakayan R, Jones J, Long TJ, et al. Gprc5d is a target for the immunotherapy of multiple myeloma with rationally designed car T cells. Sci Trans Med (2019) 11(485):eaau7746. doi: 10.1126/scitranslmed.aau7746
58. Sanderson RD, Turnbull JE, Gallagher JT, Lander AD. Fine structure of heparan sulfate regulates syndecan-1 function and cell behavior. J Biol Chem (1994) 269(18):13100–6. doi: 10.1016/S0021-9258(17)36804-7
59. Wijdenes J, Vooijs WC, Clément C, Post J, Morard F, Vita N, et al. A plasmocyte selective monoclonal antibody (B-B4) recognizes syndecan-1. Br J Haematol (1996) 94(2):318–23. doi: 10.1046/j.1365-2141.1996.d01-1811.x
60. Kambham N, Kong C, Longacre TA, Natkunam Y. Utility of syndecan-1 (Cd138) expression in the diagnosis of undifferentiated malignant neoplasms: A tissue microarray study of 1,754 cases. Appl Immunohistochem Mol Morphol: AIMM (2005) 13(4):304–10. doi: 10.1097/01.pai.0000159773.50905.7b
61. O’Connell FP, Pinkus JL, Pinkus GS. Cd138 (Syndecan-1), a plasma cell marker immunohistochemical profile in hematopoietic and nonhematopoietic neoplasms. Am J Clin Pathol (2004) 121(2):254–63. doi: 10.1309/617d-wb5g-nfwx-hw4l
62. Guo B, Chen M, Han Q, Hui F, Dai H, Zhang W, et al. Cd138-directed adoptive immunotherapy of chimeric antigen receptor (Car)-modified T cells for multiple myeloma. J Cell Immunother (2016) 2(1):28–35. doi: 10.1016/j.jocit.2014.11.001
63. Fernàndez JE, Deaglio S, Donati D, Beusan IS, Corno F, Aranega A, et al. Analysis of the distribution of human Cd38 and of its ligand Cd31 in normal tissues. J Biol Regul Homeostatic Agents (1998) 12(3):81–91.
64. Drent E, Themeli M, Poels R, de Jong-Korlaar R, Yuan H, de Bruijn J, et al. A rational strategy for reducing on-target off-tumor effects of Cd38-chimeric antigen receptors by affinity optimization. Mol Therapy: J Am Soc Gene Ther (2017) 25(8):1946–58. doi: 10.1016/j.ymthe.2017.04.024
65. McEarchern JA, Smith LM, McDonagh CF, Klussman K, Gordon KA, Morris-Tilden CA, et al. Preclinical characterization of sgn-70, a humanized antibody directed against Cd70. Clin Cancer Res: an Off J Am Assoc Cancer Res (2008) 14(23):7763–72. doi: 10.1158/1078-0432.Ccr-08-0493
66. Wang QJ, Yu Z, Hanada KI, Patel K, Kleiner D, Restifo NP, et al. Preclinical evaluation of chimeric antigen receptors targeting Cd70-expressing cancers. Clin Cancer Res: an Off J Am Assoc Cancer Res (2017) 23(9):2267–76. doi: 10.1158/1078-0432.Ccr-16-1421
67. Ge H, Mu L, Jin L, Yang C, Chang YE, Long Y, et al. Tumor associated Cd70 expression is involved in promoting tumor migration and macrophage infiltration in gbm. Int J Cancer (2017) 141(7):1434–44. doi: 10.1002/ijc.30830
68. Jin L, Ge H, Long Y, Yang C, Chang YE, Mu L, et al. Cd70, a novel target of car T-cell therapy for gliomas. Neuro-oncology (2018) 20(1):55–65. doi: 10.1093/neuonc/nox116
69. Wensveen FM, Jelenčić V, Polić B. Nkg2d: A master regulator of immune cell responsiveness. Front Immunol (2018) 9:441. doi: 10.3389/fimmu.2018.00441
70. Nikiforow S, Werner L, Murad J, Jacobs M, Johnston L, Patches S, et al. Safety data from a first-in-Human phase 1 trial of Nkg2d chimeric antigen receptor-T cells in Aml/Mds and multiple myeloma. Blood (2016) 128(22):4052–. doi: 10.1182/blood.V128.22.4052.4052
71. Ramos CA, Savoldo B, Torrano V, Ballard B, Zhang H, Dakhova O, et al. Clinical responses with T lymphocytes targeting malignancy-associated K light chains. J Clin Invest (2016) 126(7):2588–96. doi: 10.1172/jci86000
72. Hutchinson AT, Jones DR, Raison RL. Preclinical and clinical development of an anti-kappa free light chain mab for multiple myeloma. Mol Immunol (2015) 67(2 Pt A):89–94. doi: 10.1016/j.molimm.2015.04.013
73. Raje N, Berdeja J, Lin Y, Siegel D, Jagannath S, Madduri D, et al. Anti-bcma car T-cell therapy Bb2121 in relapsed or refractory multiple myeloma. New Engl J Med (2019) 380(18):1726–37. doi: 10.1056/NEJMoa1817226
74. Raje NS, Berdeja JG, Lin Y, Munshi NC, Siegel DSD, Liedtke M, et al. Bb2121 anti-bcma car T-cell therapy in patients with Relapsed/Refractory multiple myeloma: Updated results from a multicenter phase I study. J Clin Oncol (2018) 36(15_suppl):8007. doi: 10.1200/JCO.2018.36.15_suppl.8007
75. Xu J, Chen LJ, Yang SS, Sun Y, Wu W, Liu YF, et al. Exploratory trial of a biepitopic car T-targeting b cell maturation antigen in Relapsed/Refractory multiple myeloma. Proc Natl Acad Sci United States America (2019) 116(19):9543–51. doi: 10.1073/pnas.1819745116
76. Gattinoni L, Lugli E, Ji Y, Pos Z, Paulos CM, Quigley MF, et al. A human memory T cell subset with stem cell-like properties. Nat Med (2011) 17(10):1290–7. doi: 10.1038/nm.2446
77. Hinrichs CS, Borman ZA, Cassard L, Gattinoni L, Spolski R, Yu Z, et al. Adoptively transferred effector cells derived from naive rather than central memory Cd8+ T cells mediate superior antitumor immunity. Proc Natl Acad Sci United States America (2009) 106(41):17469–74. doi: 10.1073/pnas.0907448106
78. Gattinoni L, Klebanoff CA, Palmer DC, Wrzesinski C, Kerstann K, Yu Z, et al. Acquisition of full effector function in vitro paradoxically impairs the in vivo antitumor efficacy of adoptively transferred Cd8+ T cells. J Clin Invest (2005) 115(6):1616–26. doi: 10.1172/jci24480
79. Gattinoni L, Speiser DE, Lichterfeld M, Bonini C. T Memory stem cells in health and disease. Nat Med (2017) 23(1):18–27. doi: 10.1038/nm.4241
80. McLellan AD, Ali Hosseini Rad SM. Chimeric antigen receptor T cell persistence and memory cell formation. Immunol Cell Biol (2019) 97(7):664–74. doi: 10.1111/imcb.12254
81. Fraietta JA, Lacey SF, Orlando EJ, Pruteanu-Malinici I, Gohil M, Lundh S, et al. Determinants of response and resistance to Cd19 chimeric antigen receptor (Car) T cell therapy of chronic lymphocytic leukemia. Nat Med (2018) 24(5):563–71. doi: 10.1038/s41591-018-0010-1
82. Gattinoni L, Klebanoff CA, Restifo NP. Paths to stemness: Building the ultimate antitumour T cell. Nat Rev Cancer (2012) 12(10):671–84. doi: 10.1038/nrc3322
83. Hsieh EM, Scherer LD, Rouce RH. Replacing car-T cell resistance with persistence by changing a single residue. J Clin Invest (2020) 130(6):2806–8. doi: 10.1172/jci136872
84. Maude SL, Laetsch TW, Buechner J, Rives S, Boyer M, Bittencourt H, et al. Tisagenlecleucel in children and young adults with b-cell lymphoblastic leukemia. New Engl J Med (2018) 378(5):439–48. doi: 10.1056/NEJMoa1709866
85. Porter DL, Hwang WT, Frey NV, Lacey SF, Shaw PA, Loren AW, et al. Chimeric antigen receptor T cells persist and induce sustained remissions in relapsed refractory chronic lymphocytic leukemia. Sci Trans Med (2015) 7(303):303ra139. doi: 10.1126/scitranslmed.aac5415
86. Kershaw MH, Westwood JA, Parker LL, Wang G, Eshhar Z, Mavroukakis SA, et al. A phase I study on adoptive immunotherapy using gene-modified T cells for ovarian cancer. Clin Cancer Res: an Off J Am Assoc Cancer Res (2006) 12(20 Pt 1):6106–15. doi: 10.1158/1078-0432.Ccr-06-1183
87. Louis CU, Savoldo B, Dotti G, Pule M, Yvon E, Myers GD, et al. Antitumor activity and long-term fate of chimeric antigen receptor–positive T cells in patients with neuroblastoma. Blood (2011) 118(23):6050–6. doi: 10.1182/blood-2011-05-354449
88. Das RK, Vernau L, Grupp SA, Barrett DM. Naïve T-cell deficits at diagnosis and after chemotherapy impair cell therapy potential in pediatric cancers. Cancer Discovery (2019) 9(4):492–9. doi: 10.1158/2159-8290.Cd-18-1314
89. Dancy E, Garfall AL, Cohen AD, Fraietta JA, Davis M, Levine BL, et al. Clinical predictors of T cell fitness for car T cell manufacturing and efficacy in multiple myeloma. Blood (2018) 132(Supplement 1):1886. doi: 10.1182/blood-2018-99-115319
90. Ajina A, Maher J. Strategies to address chimeric antigen receptor tonic signaling. Mol Cancer Ther (2018) 17(9):1795–815. doi: 10.1158/1535-7163.Mct-17-1097
91. Hudecek M, Sommermeyer D, Kosasih PL, Silva-Benedict A, Liu L, Rader C, et al. The nonsignaling extracellular spacer domain of chimeric antigen receptors is decisive for in vivo antitumor activity. Cancer Immunol Res (2015) 3(2):125–35. doi: 10.1158/2326-6066.Cir-14-0127
92. Susanibar Adaniya SP, Cohen AD, Garfall AL. Chimeric antigen receptor T cell immunotherapy for multiple myeloma: A review of current data and potential clinical applications. Am J Hematol (2019) 94(S1):S28–33. doi: 10.1002/ajh.25428
93. Green DJ, Pont M, Sather BD, Cowan AJ, Turtle CJ, Till BG, et al. Fully human bcma targeted chimeric antigen receptor T cells administered in a defined composition demonstrate potency at low doses in advanced stage high risk multiple myeloma. Blood (2018) 132(Supplement 1):1011. doi: 10.1182/blood-2018-99-117729
94. Munshi NC, Anderson LD Jr., Shah N, Madduri D, Berdeja J, Lonial S, et al. Idecabtagene vicleucel in relapsed and refractory multiple myeloma. New Engl J Med (2021) 384(8):705–16. doi: 10.1056/NEJMoa2024850
95. Ghermezi M, Li M, Vardanyan S, Harutyunyan NM, Gottlieb J, Berenson A, et al. Serum b-cell maturation antigen: A novel biomarker to predict outcomes for multiple myeloma patients. Haematologica (2017) 102(4):785–95. doi: 10.3324/haematol.2016.150896
96. Chen CI, Bahlis N, Gasparetto C, Tuchman SA, Lipe BC, Baljevic M, et al. Selinexor, pomalidomide, and dexamethasone (Spd) in patients with relapsed or refractory multiple myeloma. Blood (2019) 134(Supplement_1):141. doi: 10.1182/blood-2019-122907
97. Ishibashi M, Soeda S, Sasaki M, Handa H, Imai Y, Tanaka N, et al. Clinical impact of serum soluble Slamf7 in multiple myeloma. Oncotarget (2018) 9(78):34784–93. doi: 10.18632/oncotarget.26196
98. Nooka AK, Kaufman JL, Hofmeister CC, Joseph NS, Heffner TL, Gupta VA, et al. Daratumumab in multiple myeloma. Cancer (2019) 125(14):2364–82. doi: 10.1002/cncr.32065
99. Kodama T, Kochi Y, Nakai W, Mizuno H, Baba T, Habu K, et al. Anti-Gprc5d/Cd3 bispecific T-Cell-Redirecting antibody for the treatment of multiple myeloma. Mol Cancer Ther (2019) 18(9):1555–64. doi: 10.1158/1535-7163.Mct-18-1216
100. Oliva S, Troia R, D’Agostino M, Boccadoro M, Gay F. Promises and pitfalls in the use of pd-1/Pd-L1 inhibitors in multiple myeloma. Front Immunol (2018) 9:2749. doi: 10.3389/fimmu.2018.02749
101. Bonello F, D’Agostino M, Moscvin M, Cerrato C, Boccadoro M, Gay F. Cd38 as an immunotherapeutic target in multiple myeloma. Expert Opin Biol Ther (2018) 18(12):1209–21. doi: 10.1080/14712598.2018.1544240
102. Chauhan D, Singh AV, Brahmandam M, Carrasco R, Bandi M, Hideshima T, et al. Functional interaction of plasmacytoid dendritic cells with multiple myeloma cells: A therapeutic target. Cancer Cell (2009) 16(4):309–23. doi: 10.1016/j.ccr.2009.08.019
103. Hideshima T, Mitsiades C, Tonon G, Richardson PG, Anderson KC. Understanding multiple myeloma pathogenesis in the bone marrow to identify new therapeutic targets. Nat Rev Cancer (2007) 7(8):585–98. doi: 10.1038/nrc2189
104. Cazaux M, Grandjean CL, Lemaître F, Garcia Z, Beck RJ, Milo I, et al. Single-cell imaging of car T cell activity in vivo reveals extensive functional and anatomical heterogeneity. J Exp Med (2019) 216(5):1038–49. doi: 10.1084/jem.20182375
105. Yoon DH, Osborn MJ, Tolar J, Kim CJ. Incorporation of immune checkpoint blockade into chimeric antigen receptor T cells (Car-ts): Combination or built-in car-T. Int J Mol Sci (2018) 19(2):340. doi: 10.3390/ijms19020340
106. Moon EK, Wang LC, Dolfi DV, Wilson CB, Ranganathan R, Sun J, et al. Multifactorial T-cell hypofunction that is reversible can limit the efficacy of chimeric antigen receptor-transduced human T cells in solid tumors. Clin Cancer Res: an Off J Am Assoc Cancer Res (2014) 20(16):4262–73. doi: 10.1158/1078-0432.Ccr-13-2627
107. Gay F, D’Agostino M, Giaccone L, Genuardi M, Festuccia M, Boccadoro M, et al. Immuno-oncologic approaches: Car-T cells and checkpoint inhibitors. Clin lymphoma Myeloma Leukemia (2017) 17(8):471–8. doi: 10.1016/j.clml.2017.06.014
108. Lam N, Trinklein ND, Buelow B, Patterson GH, Ojha N, Kochenderfer JN. Anti-bcma chimeric antigen receptors with fully human heavy-Chain-Only antigen recognition domains. Nat Commun (2020) 11(1):283. doi: 10.1038/s41467-019-14119-9
109. Smith EL, Staehr M, Masakayan R, Tatake IJ, Purdon TJ, Wang X, et al. Development and evaluation of an optimal human single-chain variable fragment-derived bcma-targeted car T cell vector. Mol Therapy: J Am Soc Gene Ther (2018) 26(6):1447–56. doi: 10.1016/j.ymthe.2018.03.016
110. Shimasaki N, Jain A, Campana D. Nk cells for cancer immunotherapy. Nat Rev Drug Discovery (2020) 19(3):200–18. doi: 10.1038/s41573-019-0052-1
111. Liu E, Marin D, Banerjee P, Macapinlac HA, Thompson P, Basar R, et al. Use of car-transduced natural killer cells in Cd19-positive lymphoid tumors. New Engl J Med (2020) 382(6):545–53. doi: 10.1056/NEJMoa1910607
112. Depil S, Duchateau P, Grupp SA, Mufti G, Poirot L. ‘Off-the-Shelf’ allogeneic car T cells: Development and challenges. Nat Rev Drug Discovery (2020) 19(3):185–99. doi: 10.1038/s41573-019-0051-2
113. Ying Z, Huang XF, Xiang X, Liu Y, Kang X, Song Y, et al. A safe and potent anti-Cd19 car T cell therapy. Nat Med (2019) 25(6):947–53. doi: 10.1038/s41591-019-0421-7
114. Weinkove R, George P, Dasyam N, McLellan AD. Selecting costimulatory domains for chimeric antigen receptors: Functional and clinical considerations. Clin Trans Immunol (2019) 8(5):e1049. doi: 10.1002/cti2.1049
115. Rezvani K. Adoptive cell therapy using engineered natural killer cells. Bone Marrow Transplant (2019) 54(Suppl 2):785–8. doi: 10.1038/s41409-019-0601-6
116. Salter AI, Ivey RG, Kennedy JJ, Voillet V, Rajan A, Alderman EJ, et al. Phosphoproteomic analysis of chimeric antigen receptor signaling reveals kinetic and quantitative differences that affect cell function. Sci Signaling (2018) 11(544):eaat6753. doi: 10.1126/scisignal.aat6753
117. Brudno JN, Kochenderfer JN. Chimeric antigen receptor T-cell therapies for lymphoma. Nat Rev Clin Oncol (2018) 15(1):31–46. doi: 10.1038/nrclinonc.2017.128
118. Alabanza L, Pegues M, Geldres C, Shi V, Wiltzius JJW, Sievers SA, et al. Function of novel anti-Cd19 chimeric antigen receptors with human variable regions is affected by hinge and transmembrane domains. Mol Therapy: J Am Soc Gene Ther (2017) 25(11):2452–65. doi: 10.1016/j.ymthe.2017.07.013
119. Sabatino M, Hu J, Sommariva M, Gautam S, Fellowes V, Hocker JD, et al. Generation of clinical-grade Cd19-specific car-modified Cd8+ memory stem cells for the treatment of human b-cell malignancies. Blood (2016) 128(4):519–28. doi: 10.1182/blood-2015-11-683847
120. Geldres C, Savoldo B, Dotti G. Chimeric antigen receptor-redirected T cells return to the bench. Semin Immunol (2016) 28(1):3–9. doi: 10.1016/j.smim.2015.12.001
121. Zhao Z, Condomines M, van der Stegen SJC, Perna F, Kloss CC, Gunset G, et al. Structural design of engineered costimulation determines tumor rejection kinetics and persistence of car T cells. Cancer Cell (2015) 28(4):415–28. doi: 10.1016/j.ccell.2015.09.004
122. Teh BW, Harrison SJ, Worth LJ, Spelman T, Thursky KA, Slavin MA. Risks, severity and timing of infections in patients with multiple myeloma: A longitudinal cohort study in the era of immunomodulatory drug therapy. Br J Haematol (2015) 171(1):100–8. doi: 10.1111/bjh.13532
123. Blimark C, Holmberg E, Mellqvist UH, Landgren O, Björkholm M, Hultcrantz M, et al. Multiple myeloma and infections: A population-based study on 9253 multiple myeloma patients. Haematologica (2015) 100(1):107–13. doi: 10.3324/haematol.2014.107714
124. Xu Y, Zhang M, Ramos CA, Durett A, Liu E, Dakhova O, et al. Closely related T-memory stem cells correlate with in vivo expansion of Car.Cd19-T cells and are preserved by il-7 and il-15. Blood (2014) 123(24):3750–9. doi: 10.1182/blood-2014-01-552174
125. Zhang X, Sun S, Hwang I, Tough DF, Sprent J. Potent and selective stimulation of memory-phenotype Cd8+ T cells in vivo by il-15. Immunity (1998) 8(5):591–9. doi: 10.1016/s1074-7613(00)80564-6
126. Petersen CT, Hassan M, Morris AB, Jeffery J, Lee K, Jagirdar N, et al. Improving T-cell expansion and function for adoptive T-cell therapy using ex vivo treatment with Pi3kδ inhibitors and vip antagonists. Blood Adv (2018) 2(3):210–23. doi: 10.1182/bloodadvances.2017011254
127. Morgan MA, Schambach A. Engineering car-T cells for improved function against solid tumors. Front Immunol (2018) 9:2493. doi: 10.3389/fimmu.2018.02493
128. Turtle CJ, Hanafi LA, Berger C, Hudecek M, Pender B, Robinson E, et al. Immunotherapy of non-hodgkin’s lymphoma with a defined ratio of Cd8+ and Cd4+ Cd19-specific chimeric antigen receptor-modified T cells. Sci Trans Med (2016) 8(355):355ra116. doi: 10.1126/scitranslmed.aaf8621
129. Turtle CJ, Hanafi LA, Berger C, Gooley TA, Cherian S, Hudecek M, et al. Cd19 car-T cells of defined Cd4+:Cd8+ composition in adult b cell all patients. J Clin Invest (2016) 126(6):2123–38. doi: 10.1172/jci85309
130. Susanibar Adaniya S, Stadtmauer EA, Cohen AD. Car T cell therapy for multiple myeloma: What have we learned? Leukemia (2022) 36(6):1481–4. doi: 10.1038/s41375-022-01539-8
131. Cieri N, Camisa B, Cocchiarella F, Forcato M, Oliveira G, Provasi E, et al. Il-7 and il-15 instruct the generation of human memory stem T cells from naive precursors. Blood (2013) 121(4):573–84. doi: 10.1182/blood-2012-05-431718
132. Laurent SA, Hoffmann FS, Kuhn PH, Cheng Q, Chu Y, Schmidt-Supprian M, et al. Γ-secretase directly sheds the survival receptor bcma from plasma cells. Nat Commun (2015) 6:7333. doi: 10.1038/ncomms8333
133. Pont MJ, Hill T, Cole GO, Abbott JJ, Kelliher J, Salter AI, et al. Γ-secretase inhibition increases efficacy of bcma-specific chimeric antigen receptor T cells in multiple myeloma. Blood (2019) 134(19):1585–97. doi: 10.1182/blood.2019000050
134. Ran Y, Hossain F, Pannuti A, Lessard CB, Ladd GZ, Jung JI, et al. Γ-secretase inhibitors in cancer clinical trials are pharmacologically and functionally distinct. EMBO Mol Med (2017) 9(7):950–66. doi: 10.15252/emmm.201607265
135. Furukawa Y, Kikuchi J. Molecular basis of clonal evolution in multiple myeloma. Int J Hematol (2020) 111(4):496–511. doi: 10.1007/s12185-020-02829-6
136. Kumar SK, Rajkumar SV. The multiple myelomas - current concepts in cytogenetic classification and therapy. Nat Rev Clin Oncol (2018) 15(7):409–21. doi: 10.1038/s41571-018-0018-y
137. Bolli N, Avet-Loiseau H, Wedge DC, Van Loo P, Alexandrov LB, Martincorena I, et al. Heterogeneity of genomic evolution and mutational profiles in multiple myeloma. Nat Commun (2014) 5:2997. doi: 10.1038/ncomms3997
138. Yu S, Yi M, Qin S, Wu K. Next generation chimeric antigen receptor T cells: Safety strategies to overcome toxicity. Mol Cancer (2019) 18(1):125. doi: 10.1186/s12943-019-1057-4
139. Postow MA, Callahan MK, Wolchok JD. Immune checkpoint blockade in cancer therapy. J Clin Oncology: Off J Am Soc Clin Oncol (2015) 33(17):1974–82. doi: 10.1200/jco.2014.59.4358
140. Cherkassky L, Morello A, Villena-Vargas J, Feng Y, Dimitrov DS, Jones DR, et al. Human car T cells with cell-intrinsic pd-1 checkpoint blockade resist tumor-mediated inhibition. J Clin Invest (2016) 126(8):3130–44. doi: 10.1172/jci83092
141. Rupp LJ, Schumann K, Roybal KT, Gate RE, Ye CJ, Lim WA, et al. Crispr/Cas9-mediated pd-1 disruption enhances anti-tumor efficacy of human chimeric antigen receptor T cells. Sci Rep (2017) 7(1):737. doi: 10.1038/s41598-017-00462-8
142. Chmielewski M, Hombach AA, Abken H. Of cars and trucks: Chimeric antigen receptor (Car) T cells engineered with an inducible cytokine to modulate the tumor stroma. Immunol Rev (2014) 257(1):83–90. doi: 10.1111/imr.12125
143. Xu X, Gnanaprakasam JNR, Sherman J, Wang R. A metabolism toolbox for car T therapy. Front Oncol (2019) 9:322. doi: 10.3389/fonc.2019.00322
144. Ando T, Mimura K, Johansson CC, Hanson MG, Mougiakakos D, Larsson C, et al. Transduction with the antioxidant enzyme catalase protects human T cells against oxidative stress. J Immunol (Baltimore Md: 1950) (2008) 181(12):8382–90. doi: 10.4049/jimmunol.181.12.8382
145. Neelapu SS, Tummala S, Kebriaei P, Wierda W, Gutierrez C, Locke FL, et al. Chimeric antigen receptor T-cell therapy - assessment and management of toxicities. Nat Rev Clin Oncol (2018) 15(1):47–62. doi: 10.1038/nrclinonc.2017.148
146. Brudno JN, Kochenderfer JN. Toxicities of chimeric antigen receptor T cells: Recognition and management. Blood (2016) 127(26):3321–30. doi: 10.1182/blood-2016-04-703751
147. Grupp SA, Kalos M, Barrett D, Aplenc R, Porter DL, Rheingold SR, et al. Chimeric antigen receptor-modified T cells for acute lymphoid leukemia. New Engl J Med (2013) 368(16):1509–18. doi: 10.1056/NEJMoa1215134
148. Santomasso BD, Park JH, Salloum D, Riviere I, Flynn J, Mead E, et al. Clinical and biological correlates of neurotoxicity associated with car T-cell therapy in patients with b-cell acute lymphoblastic leukemia. Cancer Discovery (2018) 8(8):958–71. doi: 10.1158/2159-8290.Cd-17-1319
149. Lee DW, Santomasso BD, Locke FL, Ghobadi A, Turtle CJ, Brudno JN, et al. Astct consensus grading for cytokine release syndrome and neurologic toxicity associated with immune effector cells. Biol Blood Marrow Transpl: J Am Soc Blood Marrow Transplant (2019) 25(4):625–38. doi: 10.1016/j.bbmt.2018.12.758
150. Mohyuddin GR, Banerjee R, Alam Z, Berger KE, Chakraborty R. Rethinking mechanisms of neurotoxicity with bcma directed therapy. Crit Rev Oncol/Hematol (2021) 166:103453. doi: 10.1016/j.critrevonc.2021.103453
151. Garcia Borrega J, Gödel P, Rüger MA, Onur ÖA, Shimabukuro-Vornhagen A, Kochanek M, et al. In the eye of the storm: Immune-mediated toxicities associated with car-T cell therapy. HemaSphere (2019) 3(2):e191. doi: 10.1097/hs9.0000000000000191
152. Strati P, Varma A, Adkins S, Nastoupil LJ, Westin J, Hagemeister FB, et al. Hematopoietic recovery and immune reconstitution after axicabtagene ciloleucel in patients with Large b-cell lymphoma. Haematologica (2021) 106(10):2667–72. doi: 10.3324/haematol.2020.254045
153. Nahas GR, Komanduri KV, Pereira D, Goodman M, Jimenez AM, Beitinjaneh A, et al. Incidence and risk factors associated with a syndrome of persistent cytopenias after car-T cell therapy (Pctt). Leuk lymphoma (2020) 61(4):940–3. doi: 10.1080/10428194.2019.1697814
154. Fried S, Avigdor A, Bielorai B, Meir A, Besser MJ, Schachter J, et al. Early and late hematologic toxicity following Cd19 car-T cells. Bone Marrow Transplant (2019) 54(10):1643–50. doi: 10.1038/s41409-019-0487-3
155. Hegde M, Joseph SK, Pashankar F, DeRenzo C, Sanber K, Navai S, et al. Tumor response and endogenous immune reactivity after administration of Her2 car T cells in a child with metastatic rhabdomyosarcoma. Nat Commun (2020) 11(1):3549. doi: 10.1038/s41467-020-17175-8
156. Juluri KR, Wu QV, Voutsinas J, Hou J, Hirayama AV, Mullane E, et al. Severe cytokine release syndrome is associated with hematologic toxicity following Cd19 car T-cell therapy. Blood Adv (2022) 6(7):2055–68. doi: 10.1182/bloodadvances.2020004142
157. Zhang X, Zhu L, Zhang H, Chen S, Xiao Y. Car-T cell therapy in hematological malignancies: Current opportunities and challenges. Front Immunol (2022) 13:927153. doi: 10.3389/fimmu.2022.927153
158. Huang R, Wang X, Zhang X. Unity brings strength: Combination of car-T cell therapy and hsct. Cancer Lett (2022) 549:215721. doi: 10.1016/j.canlet.2022.215721
159. Philip B, Kokalaki E, Mekkaoui L, Thomas S, Straathof K, Flutter B, et al. A highly compact epitope-based Marker/Suicide gene for easier and safer T-cell therapy. Blood (2014) 124(8):1277–87. doi: 10.1182/blood-2014-01-545020
160. Griffioen M, van Egmond EH, Kester MG, Willemze R, Falkenburg JH, Heemskerk MH. Retroviral transfer of human Cd20 as a suicide gene for adoptive T-cell therapy. Haematologica (2009) 94(9):1316–20. doi: 10.3324/haematol.2008.001677
161. Serafini M, Manganini M, Borleri G, Bonamino M, Imberti L, Biondi A, et al. Characterization of Cd20-transduced T lymphocytes as an alternative suicide gene therapy approach for the treatment of graft-Versus-Host disease. Hum Gene Ther (2004) 15(1):63–76. doi: 10.1089/10430340460732463
162. Wang X, Chang WC, Wong CW, Colcher D, Sherman M, Ostberg JR, et al. A transgene-encoded cell surface polypeptide for selection, in vivo tracking, and ablation of engineered cells. Blood (2011) 118(5):1255–63. doi: 10.1182/blood-2011-02-337360
163. Di Stasi A, Tey SK, Dotti G, Fujita Y, Kennedy-Nasser A, Martinez C, et al. Inducible apoptosis as a safety switch for adoptive cell therapy. New Engl J Med (2011) 365(18):1673–83. doi: 10.1056/NEJMoa1106152
164. Mestermann K, Giavridis T, Weber J, Rydzek J, Frenz S, Nerreter T, et al. The tyrosine kinase inhibitor dasatinib acts as a pharmacologic on/Off switch for car T cells. Sci Trans Med (2019) 11(499):eaau5907. doi: 10.1126/scitranslmed.aau5907
165. Juillerat A, Tkach D, Busser BW, Temburni S, Valton J, Duclert A, et al. Modulation of chimeric antigen receptor surface expression by a small molecule switch. BMC Biotechnol (2019) 19(1):44. doi: 10.1186/s12896-019-0537-3
166. Van Oekelen O, Aleman A, Upadhyaya B, Schnakenberg S, Madduri D, Gavane S, et al. Neurocognitive and hypokinetic movement disorder with features of parkinsonism after bcma-targeting car-T cell therapy. Nat Med (2021) 27(12):2099–103. doi: 10.1038/s41591-021-01564-7
167. Berdeja JG, Madduri D, Usmani SZ, Jakubowiak A, Agha M, Cohen AD, et al. Ciltacabtagene autoleucel, a b-cell maturation antigen-directed chimeric antigen receptor T-cell therapy in patients with relapsed or refractory multiple myeloma (Cartitude-1): A phase 1b/2 open-label study. Lancet (London England) (2021) 398(10297):314–24. doi: 10.1016/s0140-6736(21)00933-8
168. Lanitis E, Poussin M, Klattenhoff AW, Song D, Sandaltzopoulos R, June CH, et al. Chimeric antigen receptor T cells with dissociated signaling domains exhibit focused antitumor activity with reduced potential for toxicity in vivo. Cancer Immunol Res (2013) 1(1):43–53. doi: 10.1158/2326-6066.Cir-13-0008
169. Kloss CC, Condomines M, Cartellieri M, Bachmann M, Sadelain M. Combinatorial antigen recognition with balanced signaling promotes selective tumor eradication by engineered T cells. Nat Biotechnol (2013) 31(1):71–5. doi: 10.1038/nbt.2459
170. Wilkie S, van Schalkwyk MC, Hobbs S, Davies DM, van der Stegen SJ, Pereira AC, et al. Dual targeting of Erbb2 and Muc1 in breast cancer using chimeric antigen receptors engineered to provide complementary signaling. J Clin Immunol (2012) 32(5):1059–70. doi: 10.1007/s10875-012-9689-9
171. Fedorov VD, Themeli M, Sadelain M. Pd-1- and ctla-4-Based inhibitory chimeric antigen receptors (Icars) divert off-target immunotherapy responses. Sci Trans Med (2013) 5(215):215ra172. doi: 10.1126/scitranslmed.3006597
172. Juillerat A, Marechal A, Filhol JM, Valogne Y, Valton J, Duclert A, et al. An oxygen sensitive self-decision making engineered car T-cell. Sci Rep (2017) 7:39833. doi: 10.1038/srep39833
173. Costello CL, Cohen AD, Patel KK, Ali SS, Berdeja JG, Shah N, et al. Phase 1/2 study of the safety and response of p-Bcma-101 car-T cells in patients with Relapsed/Refractory (R/R) multiple myeloma (Mm) (Prime) with novel therapeutic strategies. Blood (2020) 136(Supplement 1):29–30. doi: 10.1182/blood-2020-142695
174. Mailankody S, Jakubowiak AJ, Htut M, Costa LJ, Lee K, Ganguly S, et al. Orvacabtagene autoleucel (Orva-cel), a b-cell maturation antigen (Bcma)-directed car T cell therapy for patients (Pts) with Relapsed/Refractory multiple myeloma (Rrmm): Update of the phase 1/2 evolve study (Nct03430011). J Clin Oncol (2020) 38(15_suppl):8504. doi: 10.1200/JCO.2020.38.15_suppl.8504
175. Berdeja JG, Madduri D, Usmani SZ, Singh I, Zudaire E, Yeh T-M, et al. Update of cartitude-1: A phase Ib/Ii study of jnj-4528, a b-cell maturation antigen (Bcma)-directed car-T-Cell therapy, in Relapsed/Refractory multiple myeloma. J Clin Oncol (2020) 38(15_suppl):8505. doi: 10.1200/JCO.2020.38.15_suppl.8505
176. Mailankody S, Liedtke M, Sidana S, Matous JV, Chhabra S, Oluwole OO, et al. Universal updated phase 1 data validates the feasibility of allogeneic anti-bcma allo-715 therapy for Relapsed/Refractory multiple myeloma. Blood (2021) 138(Supplement 1):651. doi: 10.1182/blood-2021-145572
177. Chen W, Fu C, Cai Z, Li Z, Wang H, Yan L, et al. Sustainable efficacy and safety results from lummicar study 1: A phase 1/2 study of fully human b-cell maturation antigen-specific car T cells (Ct053) in Chinese subjects with relapsed and/or refractory multiple myeloma. Blood (2021) 138(Supplement 1):2821. doi: 10.1182/blood-2021-150124
178. Fernandez de Larrea C, Gonzalez-Calle V, Cabañas V, Oliver-Caldes A, Español-Rego M, Rodriguez-Otero P, et al. Results from a pilot study of Ari0002h, an academic bcma-directed car-T cell therapy with fractionated initial infusion and booster dose in patients with relapsed and/or refractory multiple myeloma. Blood (2021) 138(Supplement 1):2837. doi: 10.1182/blood-2021-147188
179. Bu D, Bennett P, Barton N, Bradshaw L, Pinon-Ortiz M, Li X, et al. Identification and development of Phe885: A novel and highly potent fully human anti-bcma car-T manufactured with a novel T-charge Tm platform for the treatment of multiple myeloma. Blood (2021) 138(Supplement 1):2770. doi: 10.1182/blood-2021-148390
180. Li C, Wang D, Song Y, Li J, Huang H, Chen B, et al. A phase 1/2 study of a novel fully human b-cell maturation antigen-specific car T cells (Ct103a) in patients with relapsed and/or refractory multiple myeloma. Blood (2021) 138(Supplement 1):547. doi: 10.1182/blood-2021-152576
181. Larry D, Anderson J, Munshi NC, Shah N, Jagannath S, Berdeja JG, et al. Idecabtagene vicleucel (Ide-cel, Bb2121), a bcma-directed car T cell therapy, in relapsed and refractory multiple myeloma: Updated karmma results. J Clin Oncol (2021) 39(15_suppl):8016. doi: 10.1200/JCO.2021.39.15_suppl.8016
182. Du J, Jiang H, Dong B, Gao L, Liu L, Ge J, et al. Updated results of a multicenter first-in-Human study of Bcma/Cd19 dual-targeting fast car-T Gc012f for patients with Relapsed/Refractory multiple myeloma (Rrmm). J Clin Oncol (2022) 40(16_suppl):8005. doi: 10.1200/JCO.2022.40.16_suppl.8005
183. Frigault MJ, Rosenblatt J, Cook D, Cho HN, Depinho GD, Logan E, et al. Phase 1 study of cart-ddbcma in relapsed or refractory multiple myeloma. J Clin Oncol (2022) 40(16_suppl):8003. doi: 10.1200/JCO.2022.40.16_suppl.8003
184. Huang H, Hu Y, Zhang M, Ding X, Tang Y, He X, et al. Phase I open-label single arm study of Gprc5d car T-cells (Oricar-017) in patients with Relapsed/Refractory multiple myeloma (Polaris). J Clin Oncol (2022) 40(16_suppl):8004. doi: 10.1200/JCO.2022.40.16_suppl.8004
185. Donk NWCJVD, Agha ME, Cohen AD, Cohen YC, Anguille S, Kerre T, et al. Biological correlative analyses and updated clinical data of ciltacabtagene autoleucel (Cilta-cel), a bcma-directed car-T cell therapy, in patients with multiple myeloma (Mm) and early relapse after initial therapy: Cartitude-2, cohort b. J Clin Oncol (2022) 40(16_suppl):8029. doi: 10.1200/JCO.2022.40.16_suppl.8029
186. Schuster SJ, Svoboda J, Chong EA, Nasta SD, Mato AR, Anak Ö, et al. Chimeric antigen receptor T cells in refractory b-cell lymphomas. New Engl J Med (2017) 377(26):2545–54. doi: 10.1056/NEJMoa1708566
187. Singh N, Perazzelli J, Grupp SA, Barrett DM. Early memory phenotypes drive T cell proliferation in patients with pediatric malignancies. Sci Trans Med (2016) 8(320):320ra3. doi: 10.1126/scitranslmed.aad5222
188. Elavia N, Panch SR, McManus A, Bikkani T, Szymanski J, Highfill SL, et al. Effects of starting cellular material composition on chimeric antigen receptor T-cell expansion and characteristics. Transfusion (2019) 59(5):1755–64. doi: 10.1111/trf.15287
189. Philip M, Fairchild L, Sun L, Horste EL, Camara S, Shakiba M, et al. Chromatin states define tumour-specific T cell dysfunction and reprogramming. Nature (2017) 545(7655):452–6. doi: 10.1038/nature22367
190. Schietinger A, Philip M, Krisnawan VE, Chiu EY, Delrow JJ, Basom RS, et al. Tumor-specific T cell dysfunction is a dynamic antigen-driven differentiation program initiated early during tumorigenesis. Immunity (2016) 45(2):389–401. doi: 10.1016/j.immuni.2016.07.011
191. Tötterman TH, Carlsson M, Simonsson B, Bengtsson M, Nilsson K. T-Cell activation and subset patterns are altered in b-cll and correlate with the stage of the disease. Blood (1989) 74(2):786–92. doi: 10.1182/blood.V74.2.786.786
192. Kebriaei P, Singh H, Huls MH, Figliola MJ, Bassett R, Olivares S, et al. Phase I trials using sleeping beauty to generate Cd19-specific car T cells. J Clin Invest (2016) 126(9):3363–76. doi: 10.1172/jci86721
193. Zhao J, Lin Q, Song Y, Liu D. Universal cars, universal T cells, and universal car T cells. J Hematol Oncol (2018) 11(1):132. doi: 10.1186/s13045-018-0677-2
194. Mailankody S, Matous JV, Liedtke M, Sidana S, Malik S, Nath R, et al. Universal: An allogeneic first-in-Human study of the anti-bcma allo-715 and the anti-Cd52 allo-647 in Relapsed/Refractory multiple myeloma. Blood (2020) 136(Supplement 1):24–5. doi: 10.1182/blood-2020-140641
195. Jiang H, Dong B, Gao L, Liu L, Ge J, He A, et al. Clinical results of a multicenter study of the first-in-Human dual bcma and Cd19 targeted novel platform fast car-T cell therapy for patients with Relapsed/Refractory multiple myeloma. Blood (2020) 136(Supplement 1):25–6. doi: 10.1182/blood-2020-138614
196. Hermanson DL, Barnett BE, Rengarajan S, Codde R, Wang X, Tan Y, et al. A novel bcma-specific, centyrin-based car-T product for the treatment of multiple myeloma. Blood (2016) 128(22):2127–. doi: 10.1182/blood.V128.22.2127.2127
Keywords: multiple myeloma, immunotherapy, chimeric antigen receptor cell therapy, resistance mechanism, efficacy, toxicity
Citation: Wang Z, Chen C, Wang L, Jia Y and Qin Y (2022) Chimeric antigen receptor T-cell therapy for multiple myeloma. Front. Immunol. 13:1050522. doi: 10.3389/fimmu.2022.1050522
Received: 21 September 2022; Accepted: 08 December 2022;
Published: 22 December 2022.
Edited by:
Shao-An Xue, Xi’an University, ChinaReviewed by:
Nausheen Ahmed, University of Kansas, United StatesEstefanía García-Guerrero, Institute of Biomedicine of Seville (CSIC), Spain
Copyright © 2022 Wang, Chen, Wang, Jia and Qin. This is an open-access article distributed under the terms of the Creative Commons Attribution License (CC BY). The use, distribution or reproduction in other forums is permitted, provided the original author(s) and the copyright owner(s) are credited and that the original publication in this journal is cited, in accordance with accepted academic practice. No use, distribution or reproduction is permitted which does not comply with these terms.
*Correspondence: Yongxu Jia, jiayongxu111@126.com; Yanru Qin, yanruqin@163.com
†These authors have contributed equally to this work and share first authorship